- 1Faculty of Science, School of Life Sciences, University of Technology Sydney, Sydney, NSW, Australia
- 2Discipline of Pharmacy, Graduate School of Health, University of Technology Sydney, Sydney, NSW, Australia
Intercellular communication is a normal feature of most physiological interactions between cells in healthy organisms. While cells communicate directly through intimate physiology contact, other mechanisms of communication exist, such as through the influence of soluble mediators such as growth factors, cytokines and chemokines. There is, however, yet another mechanism of intercellular communication that permits the exchange of information between cells through extracellular vesicles (EVs). EVs are microscopic (50 nm−10 μM) phospholipid bilayer enclosed entities produced by virtually all eukaryotic cells. EVs are abundant in the intracellular space and are present at a cells' normal microenvironment. Irrespective of the EV “donor” cell type, or the mechanism of EV biogenesis and production, or the size and EV composition, cancer cells have the potential to utilize EVs in a manner that enhances their survival. For example, cancer cell EV overproduction confers benefits to tumor growth, and tumor metastasis, compared with neighboring healthy cells. Herein, we summarize the current status of knowledge on different populations of EVs. We review the situations that regulate EV release, and the factors that instruct differential packaging or sorting of EV content. We then highlight the functions of cancer-cell derived EVs as they impact on cancer outcomes, promoting tumor progression, metastases, and the mechanisms by which they facilitate the creation of a pre-metastatic niche. The review finishes by focusing on the beneficial (and challenging) features of tumor-derived EVs that can be adapted and utilized for cancer treatments, including those already being investigated in human clinical trials.
Introduction
Several mechanisms of cell-to-cell and cell-to-microenvironment communication are used to maintain physiological processes in healthy organisms. These processes are numerous and often involve the production of soluble molecules such as cytokines and growth factors (1, 2). There is also an intercellular communication mechanism involving extracellular vesicles (EVs) (3). EVs are ubiquitous, but unlike soluble cytokines and growth factor molecules, EVs function as a vehicular-mediated exchange of surface and/or intracellular contents, delivering proteins, lipids, nucleic-acid based molecules and metabolites, between adjacent or distant cells, including within a tumor microenvironment (4, 5). In this review we discuss EV biology, the mechanisms of intercellular horizontal vesicular transfer and the impacts of EVs in cancer biology and cancer treatments.
EVs are microscopic phospholipid bilayer enclosed spherical bodies of approximately 50 nm – 10 μm in size. They are abundant particles that are often present in culture supernatants (in vitro) or present within tissue extracellular space (in vivo) between cells (6). The term EVs generally represents all kinds of vesicles released from any cell type, i.e. irrespective of the “donor” producer cell type, the biogenesis mechanism, the particle size, its composition or cargo. EVs are produced in normal cell physiology as well as in many pathological conditions. With respect to cancer, however, EVs play a role in tumor pathogenesis, starting from cancer initiation, propagation, formation of a pre-metastatic niche, and tumor migration, invasion and in cancer metastasis (7–9). As recent research has enabled a more in-depth understanding of the biology of EVs in cancer, it has become evident that EVs offer significant diagnostic and therapeutic potential. For example, cancer cell derived EVs can be used as a cancer biomarker(s) or to monitor the efficacy of cancer treatments (10). EVs are even being harnessed for cell-targeted drug delivery. Indeed, EVs are already being utilized in innovative biomedical and biotechnological applications including regenerative medicine, and tissue engineering, where they are being exploited for targeted drug delivery (11–13). So too, many other novel uses of EVs in cancer are being developed where they are being used for cancer drug monitoring or used as a cancer vaccine (14). Here we review the biology of EVs with respect to cancer and cancer treatments.
Extracellular Vesicles: Exosomes, Microvesicles, Oncosomes and More
There is no unanimous consensus on the nomenclature of EVs largely because they are heterogeneous in nature. Generic terms such as “exosomes” and “microvesicles” have been broadly used, with different definitions depending on the context of the study. For example, descriptors such as tolerosomes (15), prostasomes (16), epididymosomes (17), etc., have been used to reflect tissue origin or a specific EV function (6). Here we will adhere, as much as possible, to the traditional nomenclatures of extracellular vesicles (EVs): microparticles (MPs) (or microvesicles, MVs) and exosomes.
EVs are best defined based on their physical nature, size and biogenesis origin (Figure 1). Nevertheless, due to the biogenesis mechanisms, EVs are classified as either endosomes or ectosomes. The endosome as an organelle comprises internal membranes within the mammalian cell that ultimately fuses with the cells' plasma membrane, forming multi-vesicular bodies (MVB). These are categorized as intraluminal vesicles (ILVs) when present in the cytoplasm, or as exosomes when released into the extracellular milieu. Endosomal vesicles typically range between 40 and 100 nm in diameter (18), whereas ectosomes are shed directly by blebbing and budding mechanisms from the plasma membrane, and are considerably smaller, ranging from 100 nm to 10 μm (Figure 1). Ectosomes have also been referred to as microvesicles (MVs), microparticles (MPs), oncosomes, shedding vesicles, exosome-like vesicles or nanoparticles. Ectosomes include apoptotic bodies that are released from dying cells by blebbing and fragmentation of cell membranes; apoptotic bodies are typically 50–5000 nm in size.
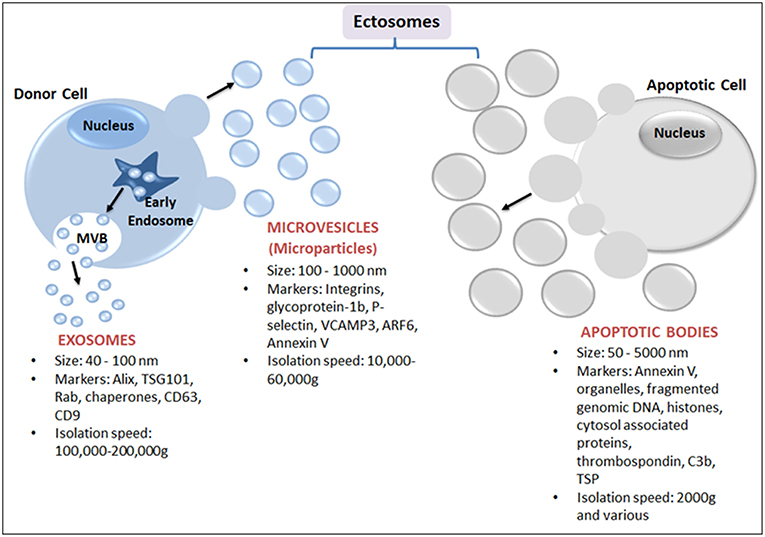
Figure 1. Schematic representation of major subtypes of EVs: exosomes, microparticles and apoptotic bodies. Exosomes, the smallest EVs, originate from within a cell by fusion followed by exocytosis of multivesicular bodies (MVB) from the cell membrane into the extracellular space. MVB are formed by the accumulation of luminal vesicles within endosomes. Ectosomes are assembled at, and pinched off from, the plasma membrane by a process of budding. Ectosomes include microvesicles (or MPs) released from activated cells and/or apoptotic bodies (produced from dying cells).
EV terminology is sometimes reflective of EV cargoes. “Oncosomes” are 100–400 nm vesicles carrying abnormal and transforming macromolecules such as oncogenic proteins (19, 20). In other cases, EVs are known as large oncosomes (LO) since they are distinct from other EVs and typically 1–10 μm in size (21). LOs can be produced from tumor tissues including human prostate cancer (22) and breast cancer (23). However, naming EVs according to their tissue of origin raises confusion because both malignant and non-malignant cells can produce EVs. For example, prostate epithelial cells release EVs that are present in semen (16, 24) and although these EVs are sometimes referred to as “prostasomes,” they are either exosomes or microvesicles—depending on their endocytic or plasma membrane origin (16). Moreover, the term prostasome broadly refers to all EV-like particles that are present in semen plasma i.e., EVs produced from any male urogenital cell type (16, 24). Indeed, the tendency of naming EVs based simply on the biological fluid from which they were isolated has resulted in a somewhat confusing set descriptive terms such as epididymosomes, migrasomes, promininosomes, vexosomes, dexosomes, cardiosomes, texosomes etc. (17, 25, 26). It is important to realize that these terms show no relationship to EV biogenesis or EV functions.
EV-like particles can also be produced from virus-infected cells, such as Herpes virus and retrovirus infected cells. These EVs are typically produced from the host cell plasma membrane and they contain viral-gene encoded molecules (27, 28) but generally lack viral genomes, making them non-infective (29) - for review see (30). Additionally, Golgi organelle membrane-derived EVs known as “gesicles” are released from vesicular stomatitis virus (VSV) DNA transfected cells. These EVs contain the VSV glycoprotein that confers fusogenicity (31, 32) and have a lower density relative to conventional exosomes (33). Nevertheless, non-infected cells can also produce Golgi vesicle derived EVs that are present in body fluids, contain Golgi and endoplasmic reticulum (ER) proteins, and are packaged and secreted as transport vesicles (34). The extent to which virus-induced oncogenesis influences EV production, for example, in HPV-induced head and neck cancer, or HPV-induced cervical cancer, is still unknown and this requires significant further investigation.
Sources of Extracellular Vesicles
EVs are secreted constitutively or following cellular activation and are identifiable in in vitro cell culture supernatants and in in vivo biofluids. EVs can be produced by virtually any mammalian cell type - irrespective of the health status of the cell. EVs are present within blood (35) [plasma (36)], semen (37), urine (38) saliva (39), sputum (40), breast milk (41), amniotic fluid (42), ascites fluid (43), cerebrospinal fluid (44), bile (45), bronchoalveolar fluid (46), malignant ascites (47), lymphatic fluid (48), nasal secretions (49), in tears (50), and are even abundant in feces (51). EVs in body fluids reflect the normal biochemical and metabolic processes of their origin cells. However, EVs may or may not primarily be representative of the most predominant cell type within a specific tissue. For example, EVs in blood have properties of blood vessel endothelial cells, or of the cellular components of the blood itself such as leukocytes, erythrocytes or platelets and the relative abundance of each of these EVs can change depending on the physiological situation (52). In humans EVs are often most abundant in biological fluids that are released externally, such as breast milk, saliva and urine, and they are less abundant in non-secretory type fluids i.e. physically enclosed or contained fluids such as blood and cerebrospinal fluid (53). The fact that EVs are molecularly reflective of their tissue of origin is particularly significant in the context of cancer because tumor cell derived EVs contain molecules that are often specific to their neoplastic origin. For example, exosomes in the blood of brain tumor patients contain more neural cell adhesion molecules and brain tumor antigen L1NCAM (CD171) relative to EVs in blood of healthy individuals (54). In other examples, exosomes from melanoma patients contain Melan-A/Mart1 (55), and EVs in urine from urogenital cancer patients can contain elevated CD36, CD44, 5T4, basigin, CD73, which are all markers of specific malignancies (56–59).
Modulation of EV Production
EV production and release can be altered and regulated; EV production can be triggered by internal cellular process or external stimuli. On the other hand, normal EV production can also be suppressed. Interestingly, there is evidence that cancer cells produce greater numbers of EVs compared to non-transformed healthy cells (60–62) and the likely stimuli for this phenomenon are many. For example, EV production can be enhanced by chemotherapy or photo-dynamic treatments, and sometimes this contributes to the disease burden of the patient (63). Interestingly, a single cell type can produce several types of EVs, as shown for platelets (64), endothelial cells (65) and breast cancer cells (66) that produce both exosomes and MVs. Furthermore, different stimuli can change the production of EV types and vary cargo levels, or even post-transcriptional cargo modifications (67, 68). Thus, factors such as the stimuli that triggers EV release, the donor cell type, and its normal physiological or disease condition, or the biogenesis pathway(s), influence the characteristics and abundance of the EVs present in human biological fluids, especially in situations of pathology including cancer.
Factors Stimulating EV Production
Multiple factors can influence EV shedding (see Table 1). The involvement of fusion machinery such as the SNARE (soluble NSF [N-ethylmaleimide-sensitive factor] attachment protein) receptor SNAP, and tethering factors, are indicated in stimulating EV biogenesis and release [reviewed in (98)]. However, factors such as temperature, cell membrane receptor activation status, infection, or stress, also contribute to the process. For example, lipopolysaccharide-stimulated dendritic cells, and antigen or mitogen activated B and T lymphocytes increase EV production in vitro (75, 99). This has implications in the setting of cancer immunotherapy where immune-modulating biologics are showing impressive efficacy in previously difficult-to-treat cancers, and where EVs are likely to be useful for cancer treatment monitoring. Cell stress is a particularly important factor in cancer because stress-induces intracellular calcium triggers and increased EV production from cancer cells (79). Interestingly, certain gene polymorphisms can correlate with increased EV production capability. Mechanisms vary but in the case of the A348T P2X7R polymorphism, this amino acid substitution results in ATP increased IL-1β secretion by monocytes, and IL-1α IL-1β and IL-18 which stimulates increased secretion of EVs (100, 101).
Factors Attenuating EV Production
EV release may be negatively modulated. This can be important in the context of EV-mediated disease pathogenesis since decreasing production may alleviate the extent of disease burden, e.g., in cancer patients. Drugs that block EV biogenesis, inhibit EV release, EV uptake (by recipient cells), or that interfering with EV-specific recipient cell signaling, are already known. Although the potential to regulate EV production for therapeutic benefit is still very much in its infancy, this approach appears to have particular relevance to cancer pathology, as well as to cancer detection, and cancer treatment outcomes—as is evident in the latter sections of this review.
Blocking EV Biogenesis
Effector molecules that are associated with the membrane vesicle formation or biogenesis processes may be targeted to inhibit the microvesiculation process. As expected intracellular vesicular transport is integral to EV biogenesis, as demonstrated in cells treated with dimethyl amiloride (a drug for clinically managing hypertension) that lowers the yield of tumor-derived EVs by interfering with the recycling of endocytic vesicles (102). This drug functionally abrogates EV-mediated immunosuppressive effects in vivo in a mouse tumor model (102). The lipid translocase enzyme systems are equally important. These include the lipid membrane flippases, scramblases and floppases that are involved in the process of plasma membrane processing to maintain phospholipid symmetry, cytoskeletal modeling and vesicle budding (5). The critical importance of scramblases and sheddases to EV production has been demonstrated via the reduction of MV shedding in human erythrocytes in the presence of the R5421 scramblase inhibitor (103), or the ADAM17 disintegrin metalloproteinase i.e., typical sheddase enzymes (104). Sphingomyelinase-2 plays an important role, too, as it controls the accumulation of ceramide—a physiological trigger for apoptotic cell membrane blebbing and budding of exosomes from multivesicular endosome membranes (105). Indeed, sphingomyelinase-2 inhibitor GW4869 influences exosome-mediated tumor growth, lowering the number of lung metastases in tumor bearing mice (106, 107). Similarly, neutral sphingomyelinase (N-SMase) contributes to exosome biogenesis as demonstrated in oligodendrocytes whereas acid sphingomyelinase (A-SMase) activity is required for MP release from microglial cells (95, 105). Consistent with these findings, an A-SMase inhibitor, imipramine, blocks microvesiculation processes in prostate cancer cells by preventing the activation and movement of A-SMase to the plasma membrane (108). Together these studies demonstrate that different SMase-family enzymes are specific for modulating the production and release of discrete populations of EVs.
Endocytosis inhibitors can influence EV production. Chlorpromazine and methyl-β-cyclodextrin impede the release of exosomes in human prostate cancer cells in vitro (108). The suppression of the RhoA/ROCK-dependent signaling pathway by a ROCK inhibitor Y-27632 reduces the secretion of MVs from human breast cancer cells, human primary glioblastoma cells and EGF-stimulated HeLa cells in vitro (88). RhoA and the highly-related GTPases Rac and Cdc42 are implicated in regulating MV shedding from transformed cell lines and controlling the packaging of specific cargo into MVs (109). Interestingly, the calcium-dependent activation of peptidylarginine deiminase enzymes are elevated in cancer (48) but this can be pharmacologically inhibited by Cl-amidine, which is a peptidylarginine deiminase inhibitor that reduces microvesiculation (108).
Blocking EV Release
Inhibition of vesicular release can be achieved in vitro by targeting many steps of vesicular body processing at the plasma membrane. For example, MV shedding is inhibited in various tumor cell lines (including human melanoma, colon cancer, prostate adenocarcinoma and breast tumor cell lines) where the GTP-binding protein ARF6 activation is inhibited; ARF6 regulates plasma membrane endosomal trafficking (66). In vitro experimental knock-down of Rab27a or Rab27b impairs exosomal secretion in Hela cells without effecting normal physiological protein secretion (110), and Rab11 and Rab35 GTPases prevent exosome release by compromising the integration of multi-vessicular bodies with the cells plasma membrane (111). Consistent with this, decreased endothelial cell MP release can be achieved by treatment with the Y27632 Rho-kinase inhibitor (112). Indeed, drugs such as bisindolylmaleimide-I (a protein kinase C inhibitor) prevent the release of EVs, by inhibiting the externalization of phosphatidylserine (113); the subsequent non-externalization results in the suppression of exosome and MV release from prostate cancer cells (108).
Coincident with the many successful examples of in vitro drug-induced suppression of EV production and/or release there are now numerous exciting clinical examples where this has also been achieved in vivo. The significance is both with respect to EVs in disease pathogenesis, and as biomarkers of cancer cure and/or relapse. For example, a decrease in platelet MV production has been reported with calpain inhibitors calpeptin (85), calpastatin, MDL 28, 170, E64d (114) or thiosulfinates (115). Pre-treatment of endothelial cells with anti-oxidants pyrrolidine dithiocarbamate and N-acetylcysteine reduces the release of thrombogenic tissue factor-bearing MPs, and decreases apoptosis and reactive oxygen species production (94). Other therapeutic targets include intracellular Ca2+ channels because channel inhibitors such as nifedipine and benidipine are effective in decreasing EV release (116), and, moreover, proton-pump inhibitors result in an acidic environment and thus inhibition of melanoma cell exosome release (117). Furthermore, vitamin C therapy inhibits MP plasma levels (118), and since a number of cytokines including interleukin-1β and tumor necrosis factor can induce EV release from specific cells, then, so too, suppression of cytokine synthesis or blocking cytokine receptor function can ultimately reduce EV production (95, 119).
Taken together there is a large body of data documenting a diverse spectrum of EV inhibitory agents, which indicates that certain drugs may tend to act on all types or categories of EVs. There are, however, some pharmacological molecules have been shown to impact the release of specific types of EVs. For example, cytochalasin D appears to decrease in exosome size whilst increasing the production in MV sized vesicles (108). The same study also demonstrated the inhibition of exosome release (but not MVs) by methyl-β-cyclodextrin, whereas Y27632 decreased MV release alone (not influencing exosome production/release). Because many proteins associated with the biogenesis and trafficking of EVs are important in normal functions, therapeutic inhibition of EV production may have unfavorable consequences or off-target effects in vitro and in vivo. Achieving a therapeutic inhibition of EV production is the focus of much research and several clinical trials (see Table 2).
Biochemical Features of Extracellular Vesicles
EVs package many bioactive materials such as nucleic acids comprising of DNA and RNA molecules—including coding RNAs (e.g., messenger RNAs), as well as non-coding RNAs e.g., long non-coding RNAs (lncRNAs), microRNAs (miRNAs) and circular RNAs (120). Transmembrane surface proteins e.g., signal-transducing receptors, or intracellular proteins including a range of cytoplasmic transcriptional factors have all been demonstrated to be present in EVs. Consistent with this, even carbohydrates and glycans, and lipid-based molecules, are packaged in EVs, along with biochemical metabolites (121).
The diverse range of EV cargo is well documented in online listings: ExoCarta (www.exocarta.org) (122) and Vesiclepedia (www.microvesicles.org) (123). Vesiclepedia currently lists 349,988 proteins, 27,646 mRNA, 10,520 miRNAs and 639 lipids, spanning 41 species (both animals and plants) from approximately 1254 independent studies (database accessed on January 2019). A review of this register reveals that certain proteins appear to more frequently packaged and transported by certain types of EVs. Furthermore, the EV registers enable the identification of specific molecules as markers of the different types of EV. For instance, exosome biomarker proteins comprise ESCRT proteins Alix, TSG101, CD9, CD63, CD81, chaperones HSC70 and HSP90, ceramide, flotillin, Rab, and tetraspanin family members (67, 105, 124, 125), and MVs, owing to their plasma membrane origin, appear to predominantly contain integrins, glycoprotein-Ib, P-selectin, VCAMP3, and ARF6 (27, 64, 126). MVs are typically characterized by the presence of externalized phosphatidyl serine—like apoptotic bodies (127). (Of note: EVs are distinguishable from MPS because, apoptotic bodies contain fragmented genomic DNA and even histones,—unlike the exosomes or MPs—and additional phenotypic markers of apoptotic bodies are thrombospondin, and complement component C3b (27, 128–130).
In the same manner certain proteins and miRNAs can identify LO's, including cytokeratin 18 (in prostate cancer derived LOs) or the miR-1227 (in prostrate epithelial cell LOs), compared to smaller sized EVs (21, 131). Of note: LO cargoes are functional and fully capable of inducing an EV-recipient cell effect, just like other EVs (131). However, various “EV type” markers are not exclusive and considerable overlap exists. This is attributable to the heterogeneity in the EV populations, and/or to imperfect EV isolation procedures.
Packaging and Sorting of EV Content
The compartment of origin of EVs, and the EV cargo, are both influential to the types of intercellular interaction and information that is delivered to a recipient cell. For example, MVB-derived exosomes (compared to plasma membrane derived MVs) differ in their cargo content (66, 132, 133). This sorting or selective content packaging is regulated at multiple levels. The endosomal sorting complex required for transport (ESCRT)-dependent pathway is involved in the selection and distribution of proteins within exosomes (134). CD63-dependent process may also be involved in sorting EV cargo (135) and ARF6-regulated recycling pathways affect the packaging of major histocompatibility class I (MHC-I) molecules, integrin receptors, vesicle associated protein-3 and membrane matrix metalloproteinases, whereas ARF6 directs cargo selection in MVs (66). The sorting of nucleic acids in EVs is less well understood but ribonucleoproteins are involved in RNA molecule sorting (136–138). So, too, the RNA-induced silencing complex (or RISC) (136) and heterogeneous nuclear ribonucleoprotein hnRNPA2B1, as these load miRNA into EVs (137), while the Y-box protein-1 aides encasing of miRNA into exosomes (138). The presence of the RNA biogenesis machinery in EVs, suggests that miRNA biogenesis can occur within the EV, which provides additional significance as this offers the capacity for altering recipient cell gene expression (i.e., by newly produced miRNA within the recipient cell) (139).
Since the protein and RNA content of EVs closely matches the donor cell, thus the presence and absence of such molecules offer a snapshot of the molecular circumstances of the donor cell—precisely at the time when the EV is produced. However, as noted earlier, certain conditions or factors, such as hypoxia, heat stress, oxidative stress (140, 141), infection (142) or cell activation (143) [including the activation of specific signaling pathways (144)] result in alterations in EV cargos. Indeed, hypoxia induces alterations of both protein and RNA from endothelial (145) and tumor cell-derived exosomes (72). Also, exosomes from human glioblastoma multiforme patients (serum and tumor samples), or primary glioblastoma cell lines, are elevated in VEGF2 and EGRFA2, in response to hypoxia, and this induces endothelial cell sprouting (in vitro)—an indication of in vivo angiogenesis potential (146). Furthermore, stress and apoptosis induce modulation of mRNA content molecules in exosomes (141), particularly heat-shock proteins (HSPs) (140). In other cancer-related examples, the expression of oncogenes such as mutant KRAS (147) or HRAS (148) modulates the composition of exosomes, and cytotoxic chemotherapy induces expression of phosphatidyl serine and tissue factor in MPs (149), and ERBB2/Her2 oncogene overexpression in EVs mediates transformation toward a malignant phenotype (150). Of note, on a gram per gram basis many comparisons of donor cells and their EVs show differential expression of certain molecules, suggestive of molecule enrichment via a selective packaging mechanism. Knowledge of why the EVs might contain more or less of a specific molecule (relative to its donor cells) is still incomplete. There may, however, be benefits or disadvantages to the host organism, or these differences may merely be reflective of the membrane: cytoplasm ratio and the inherent properties of each molecule.
EV Target Cell Interactions
EVs have the capacity to interact with a wide variety of recipient cells i.e., any cell that engages with EVs, be it within tissue extracellular space, during or after blood or lymphatic distribution. This means that either the original EV donor tissue cells, or blood or lymphatic endothelium, or a distant tissue cell can be influenced by EVs. EVs therefore serve as an important vector of intercellular molecular exchange in diverse but both physiologically linked and unrelated tissues.
EVs as Carriers of Nucleic Acids or Proteomic Molecules
It has long been known that EVs are carriers of genetic and proteomic information. It has not, however, always been appreciated that EV trafficked molecules can be present in a relatively concentrated form. In this regard EVs are frequently said to be “rich” in expression of certain molecules, for example, we and others have shown, EVs can contain high concentrations of certain molecules, such as p-glycoprotein - relative to the EV donor cells (151) Examples of this enrichment phenomenon include proteins, miRNAs and even metabolites (58, 121, 152, 153) and this engenders EVs with genuine capacity to confer functional effects on recipient cells.
The EV cargos influence the recipient cells in multiple positive ways; in normal circumstances EVs are important in tissue homeostasis and organogenesis (52). For example, platelet derived microvesicles induce angiogenesis in vivo (in mice) by facilitating the formation of endothelial capillaries (154) (by virtue of their cytokines-VEGF, bFGF, and PDGF cargo). In pathological situations, however, certain EV functions are implicated in contributing to acute and chronic diseases. In malignancies, the released EVs propagate cancer-signaling molecules such as oncoproteins including epidermal growth factor receptor-III (EGFRvIII), mutant Ras family members, or c-Met etc. (19, 148, 155). Oncogenic transcripts potentially contribute to the horizontal transformation mechanisms and phenotypic reprogramming of the recipient cells (156). Clinically they can serve as cancer biomarker(s) i.e., tumor presence, or as an indication of cure or remission (21, 157). They can also be indicative of cancer staging at the time of diagnosis (158).
EV-Recipient Cell Uptake
The function(s) of EVs depends on their ability to interact with a recipient cell in a manner that inherently involves direct contact of membranes. When encountering a recipient cell, EVs may simply adhere and stably associate with the recipient cell membrane surface, then either dissociate, or become incorporated within the recipient cell membrane (159). Recipient cell EV internalization occurs via multiple mechanisms. Both passive processes of membrane fusion (160), and active processes involving clathrin- and caveolin-dependent internalization, are used in EV-recipient cell interactions and the process typically involves membrane lipid rafts and endocytosis (161–163). EV internalization can also occur by phagocytosis (164), micropinocytosis (165), or macropinocytosis (166). The EV:recipient cell interaction can additionally involve specific ligand-receptor type interactions, such as between membrane-bound cytokines and their cytokine-specific receptors, including TNF-family molecules TNF, TRAIL or FasL and their receptors (167). The EV:recipient cell interaction is therefore both generic and specific. Furthermore, the involvement of transmembrane proteins explains the capacity of EVs to act as activators of specific intracellular signaling pathways (168) that can can be highly specific, even for receptor isoforms and downstream signaling events, as has been shown for exosome VEGF in activating VEGFR (159).
It is unclear whether EV internalization is selective or a completely random process. Current evidence suggests a degree of cellular control of EV internalization, dependent on factors such as the target cell type, the location, or physiological and environmental conditions, or even dependent even on the molecule being received/internalized e.g., nucleic acid, protein, carbohydrate, lipid. In most cases, however, the recipient cell specific interactions with EVs are regulated by adhesion molecules (161). For example, EV phosphatidyl serine, tetraspanin (169), ICAM-1, galactin-5 and galectin-9 (170), TIM4 phosphatidyl serine-specific receptors (164, 171) and heparan sulfate proteoglycans, on the recipient cell surface are involved in the internalization of EVs (172). Additionally, EVs expressing syncytin 2 (also present on a number of tumor cells) (173) bind to a recipient cell specific receptor MFSD2a (Major Facilitator Superfamily Domain 2a), permitting fusion of the EV:recipient cell membranes (168). Fusion permits the exchange of transmembrane proteins such as transfer major-histocompatibility class-II molecules, which, with respect to cancer, will transiently change the antigenicity (antigen presentation capacity) of the recipient cell (170) (also see section Current Challenges in the Use of EVs in Oncology). It has become clear that the number of EV transmembrane molecules engage with, and are exchanged with, recipient cells, and the temperature at which these interactions occurs contributes to the efficiency of EV uptake and the types of molecular membrane exchanges (165). The significance of these interactions is that the release of EV cargo into the recipient cells' cytoplasm can confer a new functional capacity in the recipient cell. This does not necessarily complete the intercellular communication process as the transferred cargo may accumulate into recipient cell vesicles that are subsequently released i.e. liberated to fuse with yet another target cell. Again, in the context of cancer, the EV-mediated intercellular dissemination of bioactive oncogene proteins from one cell type to other cell may in turn contribute to the development of secondary tumors at distant sites (155, 174). This can occur even though the germline DNA mutation of the oncoprotein is not germline encoded within the recipient cell per se.
Biological Effects of Tumor-Derived EVs in Cancer Progression, Migration and Oncogenic Survival
Tumorigenesis was historically attributed to genetic and epigenetic alterations in the genome of an organism but there is compelling evidence that EVs are integral to cancer cell communication and cancer progression. This is essentially due to EV contents because when produced from tumor cells EVs are a reservoir of cancer-associated molecules. Not only can EVs aide tumor cell survival (8, 175, 176) but certain cargoes educate or condition the recipient cell toward a tumor-promoting phenotype to facilitate the establishment of a pre-metastatic niche, thus promoting metastasis (155, 174). In fact, EVs can act as abettors of transformed cells, promoting their proliferation, propagation, even their chemotherapeutic drug resistance phenotype, their capacity for increased angiogenesis and stromal remodeling, and even in the evasion of immune detection (Figure 2).
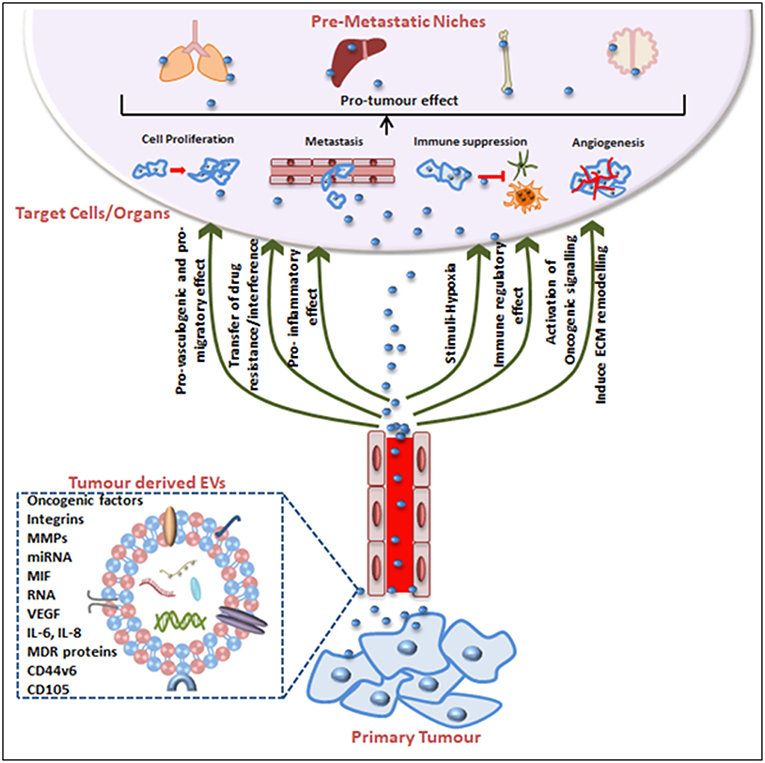
Figure 2. Tumor-derived EVs in pre-metastatic niche (PMN) formation and tumorigenesis. Tumor-derived EVs express surface and cytosolic molecules originating from the primary tumor and are carried to recipient cell/organs via the circulation. The EV surface molecules and cargo confer pro-angiogenic, pro-migratory, pro-inflammatory effects, and chemotherapeutic drug interfering or immune-regulatory effects. EV movement to target organs is generally organotropic and determined by the inherent tumor cell and EV cargos. Finally, EVs contribute to the pre-conditioning of the target site via inducing extracellular matrix remodeling, changes in blood and lymphatic vessels barrier integrity, transfer of immune inhibitory or activating factors and transfer of oncogenic factors. Together these mechanisms explain EV contributions to cancer progression and the impact on cancer treatment efficacy including treatment failures.
Direct Oncogenic Phenotypes
EVs can exert a cargo-dependent oncogenic response in recipient cells. For example, glioma cells display EGF-RvIII, the oncogenic form of the EGF receptor, which induces mitogen-activated protein kinase (MAPK) and Akt pathways promoting anchorage independent growth and survival (19). When compared with EV-donor cells deficient in EGF-RvIII expression, EGF-RvIII-expressing EVs display EGF-R-dependent responses, i.e., AKT and ERK signaling and oncogenic phenotype in EGF-RvIII-null recipient cells (19). Moreover, EGF-RvIII was demonstrated to subsequently present in the EV-interacting recipient cells (19). In another example, apoptotic body type EVs derived from H-rasV12 and c-myc oncogene transfected cells conferred tumorigenicity in vivo (in mice) via a mechanism involving oncogene transfer into recipient cells (177). The EV-donated DNA oncogene cargo is likely present episomally (rather than inserted in cis), but nevertheless transferred DNA can have oncogenic potential via DNA-encoding oncogenes or the oncoproteins themselves, in some respects resembling certain instances of non-integrating viral transformation (178).
Other pro-tumor EV effects include the role of the pro-coagulant transmembrane tissue factor molecule that modulates angiogenesis as well as metastasis in cancer [reviewed in (179)] and tissue factor-bearing MVs derived from colorectal carcinoma cells can contribute to a K-ras dependent cancer progression (180). These tumor EVs can be transferred between dissimilar populations of cancer cells, meaning EVs can potentially enable the propagation of aggressive/oncogenic phenotype between various sub-populations of cancer cells present within heterogeneous tumors (181). Tissue factor-bearing EVs also contribute to cancer-induced thrombosis (182). Thus, tissue factor-expressing MPs are mediators of tumor aggressiveness, a trigger of thrombogenesis and abnormal coagulation in cancer. These types of EV interactions are now being targeted in cancer-associated venous thromboembolism therapies (183, 184).
As alluded to above, tumor-derived EVs can cause the activation of specific pathways that support tumor growth and survival. Tumor proliferation can be promoted by gastric cancer-derived exosomes through the activation of the phosphoinositide 3-kinase (PI3K), Akt, and mitogen-activated protein kinase/extracellular-regulated protein kinase (MAPK/ERK) pathways (185). Cancer cell line-derived exosomes can activate the mitogen-activated protein kinase Ras-Raf-MEK-ERK pathway in monocytes, through the transport of receptor tyrosine kinases EGFR and Her2, and promote the survival of tumor-associated monocytes (186). This mechanism of cancer progression alters monocyte survival prior to the formation of tumor-associated macrophages and occurs directly within the tumor microenvironment (186). Theoretically, this mechanism would also have the capacity to action distally e.g., via circulating monocytes.
Pro- and Anti-inflammatory Effects
Malignant cells modulate the tumor microenvironment via both their pro- or anti-inflammatory effects. The immunomodulatory effects of EVs are particularly well studied in macrophages due to their high propensity for endocytosis and the abundance and accessibility of their precursors - blood monocytes. Melanoma-derived exosomes have been shown to be capable of inducing a pro-inflammatory recipient cell effect by altering the cytokine and chemokine profiles of the target macrophage cells (187). Similarly, breast cancer-derived exosomes can induce increases in mRNAs of pro-inflammatory cytokines such as interleukin (IL)-6, tumor necrosis factor (TNF), granulocyte colony stimulating factor (G-CSF) and chemokine CCL2, through a Toll-like receptor (TLR)-2 stimulation and NF-κB in macrophages (188). In a separate study, NF-κB-dependent expression of IL-6 and TNF was present in gastric cancer-derived exosome cultured macrophages (189). We have also recently demonstrated that MPs secreted from both drug-resistant and drug-sensitive breast cancer cells polarize macrophages toward a pro-inflammatory state, producing increased IL-6 and TNF, and we hypothesize that this contributes to the establishment of a pre-metastatic niche (PMN) especially at secondary tumor sites (176). Furthermore, lung tumor-derived exosomes express surface HSP70 and activates a pro-inflammatory phenotype defined as elevated IL-6, IL-8, and monocyte chemoattractant MCP-1 (190), and TLR-dependent NF-κB activation (190).
There are also reports of an anti-inflammatory role of tumor cell-derived EVs. Melanoma and colorectal carcinoma cell line derived EVs can stimulate the release of transforming growth factor-β (TGFβ) from tolerogenic T-cells that promotes the generation of myeloid-derived suppressor cells (191). This suggests a mechanism that establishes an “immunosuppressive circuit” within the cancer, but with this knowledge comes the potential als for therapeutic modulation, e.g., by interfering with or blocking the interaction, i.e., to re-arm T-cell control of tumors (191). The challenge, however, resides in how to specifically inhibit only EV:TGFβ-producing tolerogenic T cells and not tumor-specific cytotoxic T lymphocytes, and or anti-tumor Natural Killer (NK) cells. Such interventions would need to be cytokine-specific. Other anti-inflammatory mechanisms of EVs includes gastric carcinoma cell-derived MPs interacting with monocytes resulting the production of the immunosuppressive cytokine IL-10. This is directly immune suppressive and additionally acts indirectly to decrease pro-inflammatory cytokines GM-CSF and TNF (via feed-back loops). Hence, EVs skew the tumor cytokine milieu to either an anti- or pro-inflammatory signature (192). Interestingly, EVs have been artificially packaged to deliver anti-inflammatory agent curcumin, i.e. to be delivered to distant site for the treatment of inflammatory conditions (193); curcumin has potent anti-inflammatory properties such as being capable of inhibiting the production of TNF (194). In fact the idea and potential potency of curcumin as an anti-cancer agent has led to the generation of synthetic curcumin analogs and these are being investigated in cancer (195, 196). Of note, however, curcumin itself appears to be capable of altering the EV cargo e.g., for enrichment of miRNAs with anti-cancer properties (197).
Drug Interference and Resistance
Tumor-derived EVs can act as direct mediators of cancer resistance to chemotherapy, via a multimodal process. Horizontal transmission of drug resistance occurs through transfer of drug-resistance conferring molecules: the ATP-binding cassette (ABC) transporter p-glycoprotein (MDR1/ABCB1) (58, 139, 151), multi-drug resistance associated protein-1 (MRP1/ABCC1) (198), the multidrug resistance efflux transporter ABCG2 (199), the ABC transporter-3 (ABCA3) (200), the P-gp inducer- of Ca2+-permeable channel transient receptor potential channel-5 (TrpC5) (201), and the Her2 receptor protein ERBB2/EGFR2 (77). The significance is that EVs containing these cargoes confer a cancer drug resistance phenotype to recipient cells i.e., resistance to multiple tumoricidal drugs (58, 151). Moreover, we and others have demonstrated that modulation and transfer of certain miRNA, lncRNA and mRNA nucleic acids in EVs further regulate drug-resistance traits of tumor cells (139, 202, 203). EVs also function to attenuate the effectiveness of chemotherapeutics by sequestering the administered drug before it reaches the intended tumor. This occurs through the presence of the drug receptor or a drug-interacting protein within the EV, that essentially creates a sublethal concentration of the drug in the circulation of the patient (204). Others have shown that in the case of cisplatin-containing exosomes produced from melanoma cells this helps to generate a cisplatin-resistant melanoma phenotype (117). It is thus appears that cancer cells can directly accumulate drugs within the vesicular compartment and then eliminate the drug by EV shedding, and furthermore, that enhanced shedding correlates with increasing EV drug concentrations (205). In the case of Her2-expressing breast cancer, exosomes bind to trastuzumab antibody, resulting in drug resistance (77). Indeed, in vitro activation of Her2 (by heterodimerisation with EGFR or Her3) with ligands epidermal growth factor (EGF) and heregulin results in the increased production of exosomes (77). This suggests that cancer cells specially upregulate their EVs secretion in the presence of the drug. In the case of Her2-positive tumors, EV sequestration of trastuzumab also inhibits leucocyte activation and cytotoxic effector mechanisms for Her2-expressing tumors (206). Similar effects have been shown for B-cell lymphoma exosomes displaying CD20 with subsequent resistance to the anti-CD20 chimeric antibody rituximab (200).
Effects on Immune Regulation Facilitating Tumor Progression
The production of EVs constitutes a mechanism of tumor-specific immune suppression. This is a complex situation involving multiple mechanisms. Firstly, the packaging of immunosuppressive soluble mediators such as TGFβ in EVs, can directly incapacitate cytotoxic anti-tumor T lymphocytes and NK cells (207, 208), or EV-derived IL-10 can activate myeloid-derived suppressor cells (MDSCs) (191, 209). So too, EVs carrying death-inducing cytotoxic cytokines TNF, TNF-related apoptosis-inducing ligand, TRAIL, and Fas ligand (FasL) induces apoptosis of tumor-specific T cells (210), and exosomal EVs block NKG2D to inhibit NK cell activation and NK tumoricidal activity (211–213). In other examples, MV surface TGF-β1 suppresses NK cell and T cell proliferation through adenosine production, and miRNAs such as miR-23a (214) and miR-4498 regulates CD83 expression (215)(CD83 is a dendritic cell activation/maturation molecule). Furthermore, tumor EVs are similar to the tumor cells themselves, in that they can contain tumor specific antigens such as melan-A and carcinoembryonic antigen (CEA) that are capable of suppressing tumor-specific responses (216). This has been dramatically demonstrated in vivo (in mice) using a model tumor antigen of ovalbumin (OVA)-in melanoma cell derived exosomes (217). Likewise, circulating, tumor-derived MHC Class-II bearing exosomes suppressed tumor-antigen specific responses in tumor-bearing mice (218).
EV-derived immunosuppression also occurs via tumor cell EVs promoting FoxP3+ T regulatory (Treg) cell proliferation or enhancing their suppressive capacities (219). Moreover, nasopharyngeal carcinoma-derived exosomes cause the conversion of naïve T-cells into the immunosuppressive Treg cells and promoted Treg recruitment via chemokine CCL20 (220). This is well demonstrated in an in vivo mouse study where the presence of metastatic breast cancer-derived exosomes leads to the establishment of a microenvironment augmenting cancer metastasis to the lungs and liver, in mice (221). Here, the continuous uptake of breast cancer exosomes in these organs led to the recruitment of immature myeloid cells, decreased numbers of T-cells and NK-cells, correlating with increased cancer progression and mortality (221). Similarly, glioma stem cell-derived exosomes suppresses T cells through monocytes (or myeloid-derived suppressor cells) leading to glioma immune-evasion (222). These are not considered uncommon findings or physiologically unlikely effects in humans, since the tumor-specific EV immunosuppressive effects can be demonstrated for EVs present in body fluids such as malignant effusions or the sera of cancer patients (191).
Finally, cancer-derived EVs can influence macrophage phenotype, converting M1 pro-inflammatory anti-cancer macrophages into M2 phenotype macrophages that better support tumor survival. For example, colorectal cancer-derived MVs regulated the differentiation of monocytes into regulatory M2 macrophages after prolonged contact (223), and glioblastoma-derived EVs induced a modified phenotype of monocytic cells to resemble myeloid-derived suppressor cells (MDCS) in the brain cancer patients (224). Finally, miRNA are potent regulators of immunity, and epithelial ovarian cell-derived exosomal miR-222, and pancreatic cancer-derived exosomes, have both been demonstrated to promote M2 macrophage phenotypes (225, 226). Conversely, over-expression of miR-155 and mI-125b-2 promotes an M1 macrophage phenotype (225). Thus, immunosuppressive tumor-derived EVs and their miRNA cargos modulate tumor immune responses and potently direct tumor immune evasion. A deeper investigation of EV-directed immune evasion mechanisms will be needed in order to harness EVs as effective adjunct cancer therapeutics.
Pro-tumor Effect on Microenvironment
The interplay between the malignant cells and their neighboring healthy cells, stromal cells, endothelial cells, and the immune cells that girt and infiltrate a tumor, comprise the tumor microenvironment. Outcomes of such interactions are fundamental to the growth and spread of the tumor (227), and in this context EVs and EV-derived molecules critically modulate this microenvironment in a number of ways, including via an angiogenic effect, an invasive effect, and a metastatic effect.
Angiogenic Effects
Tumors release angiogenic factors that support the development of new blood vessels to provide an adequate nutrient supply that facilitates tumor growth and metastasis. Tumor angiogenesis is stimulated by the proliferation of endothelial cells within the tumor (228) and also by the recruitment of precursor endothelial cells (from bone marrow) (229), where vessel endothelial cell pericytes aide blood vessel function and the capacity to generate new capillary structures. A role for EVs in microvasculature biology is evident within the tumor microenvironment where tumor-derived EV cargo molecules impact on angiogenic-modulating processes. For example, tumor-derived EVs carry pro-angiogenic factors such as IL-6 and VEGF stimulate endothelial cell proliferation and neo-vascularization within the growing tumor (71, 230), and EV nSMase2 stimulates endothelial cells and angiogenesis (231). Endothelial cell tube formation is stimulated by exosomes when the EVs are released from leukemic cells under hypoxic conditions, and develop within the central regions of growing necrotic tumors (146, 232). A deeper and more precise understanding of the molecular interactions between EV from cancer cells are currently being revealed: a recent mass spectrometry examination of EVs from Glioblastoma cell lines identified over 1000 proteins, including EGFRvIII, to exert angiogenic and tumor-invasive characteristics (233). Other EV angiogenesis promoting molecules include sphingomyelin (234), tetraspanin (235), and miRNAs miR-210, miR-9, miR-92a (232), viral oncoproteins (236), clotting factors and tissue factor (237), cytokines (238), and the oncogene Wnt5A (76) that promote tube formation by human vascular endothelial HUVEC cells (239). The list of clinically used and newly trialed chemotherapeutic agents for cancer, must therefore now consider not only the effect(s) of the drug on the tumor cells, but the effects of EVs on blood vessel endothelium physiology. Blood vessel permeability is particularly important in the setting of brain cancer, where increased intracranial blood pressures is an important clinical presentation, requiring clinical management through the co-delivery of anti-hypertensive agents. It is not surprising therefore that exosomes are also of interest in a number of related settings in neurology including cerebral ischemia where vascular function is integral to the pathology (240).
Invasive Effects
The process of tumor cell invasion is assisted by matrix-degrading proteases and because EVs act as a constant inter-cellular communication system between the tumor and the stroma, EVs play an important role facilitating tumor invasiveness. Tumor-derived EVs promote extracellular matrix degradation, thereby assisting in tumor tissue infiltration. Such molecules include matrix metalloproteinases (MMP)-2, MMP-9, and MT1-MMP (241), their zymogens urokinase-type plasminogen activator (uPA) (242) and extracellular matrix metalloproteinase inducer EMMPRIN (89). Interestingly, malignant ovarian ascites samples from patients with stage-I to -IV ovarian cancer contain proteases MMP-2-, MMP-9-, and uPA- loaded EVs with highly invasive properties (61). In fact, there is a correlation between proteolytic and in vitro invasive capacity of EVs from breast cancer cell lines (242). Moreover, we have shown that breast cancer-derived MP cargo modulates miRNA-503 and proline-rich tyrosine kinase-2, leading to an ennhanced tumor migratory and invasive capacity (243), and it is well known that lung tumor derived-MVs modulate stromal fibroblasts and endothelial cells to promote tumor growth in vitro (86, 244). Indeed, tumor exosomes exert both stromal inducing pro-angiogenic effects as well as pro-invasive effects (245, 246) that aides tumor growth in vivo.
Signaling Tumor Growth and Metastatic Effects
Metastasis involves the dissemination of cancer cells to adjacent or distant tissue sites. Tumor-derived EVs can deliver autocrine, paracrine, endocrine signals facilitating tumor growth and metastasis. Gastric cancer-derived exosomes stimulate the proliferation of other recipient gastric cancer cells, at least in part, through the activation of the PI3K/Akt and MAPK/ERK kinase pathways (185), and exosomes mediate the disposal of a tumor suppressor miRNA miR-23b that aids in the dissemination of cancer cell metastasis (247). Hypoxia-inducible factors and small GTPase Rab22A that mediate microvesicle biogenesis also facilitate breast cancer invasion and metastasis (248). So, too, overexpression of the ERBB2/Her2 oncogene in breast cancer-derived EV alters the vesicular contents toward a malignant phenotype (150). There are several examples of this phenomenon from in vivo models of cancer. Pancreatic carcinoma cell derived exosomes carrying CD44v6 promote tumor growth and metastasis in lymph nodes and lungs of rats (249). One of the main mechanisms for metastasis promotion appears to involve EV signaling in the tumor-surrounding stroma i.e., in the tumor microenvironment, with or without a role for transforming viruses. For example, gamma herpes virus Epstein-Barr virus infected cells secretion EVs containing integrins, actin, interferons and NF-κB that directly modulate the tumor microenvironment (236). In other in vivo examples, miRNAs such as miR-181c and miR-105 EV cargos of breast cancer cell-derived exosomes induce, or facilitate, metastasis to the brain by modulation blood-brain-barrier function (250, 251). Furthermore cancer-derived exosomes that promote lung metastasis use mechanism(s) involving the activation of TLR-signaling by the MyD88 adaptor protein, producing IL-6 and TNF-mediated activation of MDSCs (252). In yet a final example, lung cancer MVs promote metastasis via their expression of proteolytic matrix metalloprotease-9 (MMP-9) from fibroblasts (86) and through a fibronectin-integrin based pathway (253). Hence, it is generally accepted that EVs can enhance the creation circumstances that pre-conditions or educates the secondary metastatic site [for another recent review see (254)].
Tumor-Secreted Effectors of Cross-Boundary Communication Leading to Organotropism and Pre-metastatic Niche Formation
EVs can influence remote organs and distal sites that are otherwise protected by physiological barriers. The creation of a pre-metastatic niche, i.e., a tumor supportive pre-conditioned environment, generally involves bone marrow-derived immunosuppressive cells - and a complex interplay between tumor cell determinants such as tumor-derived factors, and colonization pathways, and changes to metastatic site cells. In certain circumstances tumor-derived EVs can dictate the organ-specific migration of tumors (255–257), or, pre-metastatic niche creating EVs may originate from non-transformed healthy cells (258). It is generally thought that organ- or tissue- specific metastatic preference is due to endogenous niche components within the target organ (259–261). The role of EVs in organ-specific metastasis has been demonstrated by i.v. (tail vein) melanoma-derived exosomes that preferentially migrate to common melanoma metastatic sites of lung, spleen, bone marrow, and liver (155). Fusion of integrin-expressing exosomes (derived from lung-, liver-, and brain-tropic cancer cells) with organ-specific inhabitant cells results in Src-phosphorylation and pro-inflammatory S100 gene expression (174). In this example, the specificity follows the general rules of integrin-homing, where the exosomal integrins α6β1 and α6β4 determines metastasis to the lung, while integrin αvβ6 promotes liver metastasis (174).
Metalloproteases are important in in cancer metastasis and tumor derived-EVs modulate the expressions of MMP-2, MMP-9, and VEGF-A directly at the metastatic site (241, 262, 263). MMPS can also act on blood (or lymphatic) vessels that aiding tumor cell recruitment and tissue matrix remodeling (264). There are also reports of pancreatic cancer exosome-mediated transfer of macrophage migration inhibitory factor (MIF) to liver Kupffer cells, subsequent to increasing TGF-β and fibronectin production, thus aiding tissue remodeling and future tumor metastasis (265). In breast cancer, MV-mediated transfer of miR-122 and inhibition of pyruvate kinase prevents glucose uptake and favors brain metastasis (266). Other examples of EV cargos that influence metastasis are miR-200 (267), tyrosine-kinase receptor expression and silenced Rab27A (155), and mesenchymal stem cell marker CD105- containing MVs (262); these factors all function to prime distant organs for tumor migration.
Essentially the ex vivo isolated tumor EVs have a pro-tumor effect by inducing modifications in remote organs, followed by the relocation of metastatic tumor cells. Thus there is a need to design strategies that manipulate pre-metastatic niche and thereby to prevent seeding and metastasis in vivo [for review see (268)]. Recent exciting advancements in this area highlight the capacity for oncomaterials that modulate tumor cell behavior at the metastatic engraftment site. Several oncomaterials, both natural products such as bone fragments, silk, collagen, lung/liver matrix, as well as synthetic derivatives including poly-L-lactic acid, hydroxyapatite, polyacrylamide, are already approved by the US Federal Drug Administration for their application to humans for tissue bio-engineering applications (268). This is a particularly exciting area of EV research and translational medicine for cancer patients, as it offers the future potential to prevent tumor metastasis.
Tumor-Derived Extracellular Vesicles: Cancer's Friends or Foe?
One application of tumor-derived EVs is that they can constitute novel tumor-specific diagnostic targets, where their contents are diagnostic targets that indicate the presence (or absence) of a tumor. The EVs themselves, or the EV cargo molecules, can be used to track cancer risk, cancer presence, and as marker of treatment outcomes—cancer cure or relapse. In certain circumstances it would be highly beneficial to selectively diminish or deplete tumor-specific EVs in circulation, without affecting non-tumor EVs that have other physiologically important functions. A technology currently in development is capable of removing soluble factors and exosomes from the peripheral blood circulation (269) and is marketed under the term ADAPT™ (adaptive dialysis-like affinity platform technology). It is capable of removing soluble factors and exosomes from the peripheral blood circulation (269). For example, Her2osome™ therapy diminishes tumor-derived Her2-containing exosomes that functional inhibit Her2-binding drugs. This is an impressive example of the potential of this approach, however, there are many other exciting opportunities to selectively target EVs, or their contents, in acute or chronic disease—not just in cancer patients.
On the other hand, the production and presence of tumor-derived EVs can have undesirable effects for the host organism, although, the deleterious effects can also be modulated for better treatment outcomes for cancer patients. Thus, there can be benefits to developing and using compounds that inhibit or interfere with tumor cell EV biogenesis, release and recipient cell uptake. These include reagents that block phosphatidyl serine, surface heparan sulfate, proteoglycans, ICAM1 interactions with their receptors i.e., molecules that are critical for EV engulfment (172). For example, the blocking EV phosphatidyl serine by diannexin suppresses the growth of human glioma in murine xenografts (270, 271). In another example, targeting of exosomal FasL (via FasL-specific monoclonal antibodies) can reduce melanoma tumor growth (272). There are actually many such reagents that are being investigated by biotechnology and pharmaceutical companies worldwide; many are currently being assessed in human clinical trials (Table 2).
EVs as Disease Biomarkers
EV cargoes are stable and have a long half-life to their encapsulation within the vesicular membrane. Even the EV themselves are relatively stable in bodily fluids, and they withstand long-term in vitro storage conditions including several freeze-thaw cycles, making them excellent biomarkers of disease (273). Indeed, the EV stable biomarker cargo, be it protein, lipid, nucleic acid, can include molecular entities that relatively short-lived or highly labile in the cytoplasm of donor cells. In cancer these molecules can be reflective of both the tumors presence and also of cancer staging. For example, EVs in blood and urine of prostate cancer patients contain unique prostate-cancer specific contents that are biomarkers of prostate cancer (274, 275) but even where the diagnosis per se does not necessarily equate to a need for treatment (as in prostate cancer), markers of cancer metastasis are particularly valuable to cancer clinicians. Periostin, is an example of an EV metastatic cancer bio-marker. Periostin is concentrated at high levels in EVs derived from metastatic cells in vitro and abundantly present in plasma exosomes in breast cancer patients with lymph node metastasis (276). Periostin is also present in muscle-invasive bladder cancers, and urinary EV from patients is a prognostic biomarker of muscle invasiveness (277). Moreover, in vitro periostin knock-down experiments demonstrate reduced tumor migration and invasion, in model systems (277), thus EV periostin maybe also functionally important in tumor progression. EVs are proving to be valuable diagnostic biomarker in pancreatic cancer; flow cytometry coupled with mass spectrometry analysis of exosome glypican-1 can distinguish benign disease from early and late stage cancer (278). The challenge now is how to implement these technologies into routine laboratory testing.
Where the important EV cargo is nucleic acids quantitative PCR and/or next-generation sequencing is easily possible. To date, ssDNA, dsDNA and retrotransposon content of EVs have been profiled by PCR (279). However, the presence of 8 miRNAs of diagnostic importance in ovarian cancer, can be specifically amplified and detected by reverse-transcription PCR, even in as yet asymptomatic patients (56). Other similar examples continue to be reported: human lncRNAs profiling of prostate cancer cell lines and their exosomes has identified several cancer-specific RNAs in exosomes (280). Independent validation of these markers is required to progress the development of diagnostic assays for future routine use in pathology laboratories.
EV DNA nucleic acid is not transcriptionally competent since whilst in the EV as there is no RNA-polymerase-II transcriptional complex present there. The EV DNA is simply present and potentially being transferred to a recipient cell. Once transferred, this DNA may have potential for nuclear import and at some point, could theoretically regain transcriptional potential, even if only present episomally. Similarly, EV mRNAs are not translated whilst within EV, as cellular ribosomes are not present within EVs. There is evidence, however, that once transferred, mRNA can potentially be expressed in the recipient cell (281). In contrast, however, we have shown that EV miRNA has the potential to be carried and produced within the EV, because the miRNA biogenesis molecules are also present within the EV (139). The significance of EV RNA transfer, including non-coding RNAs, is clearly that it may bring a regulatory RNA into the recipient cell i.e. that is otherwise not expressed endogenously within the recipient cell. EV transferred nucleic acids can therefore change the protein expression profiles of recipient cells.
EVs as Therapeutics for Targeted Cancer Drug Delivery and More
That EVs strongly preserve diverse bioactive cargos immediately places them as high-utility delivery vehicles, targeted to particularly recipient cells. Arguably one of the most easily achievable applications of therapeutic EVs is that they can be manufactured to deliver cancer chemotherapeutic drugs, such as doxorubicin, paclitaxel, methotrexate or cisplatin, etc., singly or in combination. This can be achieved by an approach as simple as preparing the EVs from cells that are cultured in the desired chemotherapy drug, or combination of such drugs (282) and early indications are that this targeted delivery may reduce toxicity-related adverse effects (283–285). At this stage there would seem to be a broad range of molecules that can be manufactured for delivery via therapy EV. Examples to date include anti-cancer agents such as the angiogenesis and cancer growth inhibitor withaferin A (286), or the plant-based triterpenoid-celastrol (287). In both of these cases, delivery of the therapy EV resulted in a stronger anti-tumor effect in a human lung tumor mouse xenograft model compared to the free drug alone, presumably due to better tumor-specific drug targeting (286, 287). In other examples, curcumin, has been loaded into EL-4 lymphoma cell-derived exosomes and delivered to activated myeloid cells, for protection against LPS-induced inflammation in mice (288), and intra-nasal exosomal delivery of curcumin, or a signal transducer and activator of transcription-3 (STAT3) inhibitor JSI124, has been shown to cross the blood-brain-barrier and suppress GL26 brain tumors in a syngeneic C57BL mouse model of brain cancer (193). Others have prepared EVs directly from plants, and a clinical trial of plant-derived EVs is already underway (see Table 2). While these results are exciting developments, especially for patients with poor-prognosis tumors like glioblastoma, much more information is required to better understand the immunological ramifications of EV therapy, especially in the central nervous system. Although it might be necessary to employ one or more monocyte/macrophage phagocytosis blocking strategies, the small size of EVs has been demonstrated to bypass the blood-brain-barrier endothelium for CNS tissue delivery (289) (for review see (240).
EVs are being pursued as intercellular vectors for RNA-based therapy (both miRNAs and siRNAs), with efficacy documented in animal models of disease. For example, in vivo suppression of breast cancer and prostate cancer has been achieved through exosome delivery of the tumor suppressor miRNAs miR-let-7a and miR-143 in mice (290, 291). Other examples include exosome transfer of miR-146b to glioma cells to inhibit glioma cell growth (292), or exosomes released synthetic miR-143 to osteosarcoma cells to limit migration (293). In these circumstances the RNA cargo need not be a naturally occurring miRNA within the recipient cell. Moreover, siRNA-loaded EVs can specifically deliver anti-tumor effects (294). siRNAs targeting RAD51 (295), c-Myc (296), and PLK-1 (297) inhibit the proliferation of breast cancer, lymphoma, and bladder cancer cells, and “suicide gene” mRNA MVs have efficacy for treatment of pre-established nerve sheath tumors (schwannoma) in an orthotopic mouse model (298).
Although there is an expanding list of these proof-of-principle type experiments, there are currently also a list of challenges that remain, especially in the era of individualized or person-specific targeting of cancer. There is also the issue of what happens in situations of cancer recurrence, and whether chemotherapy-loaded EVs can be re-administered or have immunogenicity in their own right. This is especially of concern, if or when EVs are phagocytosed by macrophages and tissue-resident dendritic cells as this could work to raise antigenicity of the tumor-specific EV-delivered molecule, or the (modified) EVs themselves. If the therapy EVs were autologous (and patient specific) this would help address the potential for immunogenicity. Interestingly, the present thinking appears not to consider EV HLA-expression as being a significant factor, but we have evidence of some degree of immune cell activation by non-HLA-matched EVs (Jaiswal and Sedger, unpublished). A deeper understanding of the benefits and/or limitations of EV immunogenicity may prove to be beneficial to the success of therapy EVs, and it may prove to be beneficial to examine this more deeply in current cancer clinical trials and more human data is desperately needed.
The issue of immunogenicity is a double-edged sword. The potential for therapy EVs to enhance immunogenicity is particularly useful given that EVs are well known to carry tumor-associated antigens (TAAs). Therapy EVs have a documented capacity to elicit TAA-specific anti-tumor immune responses, which improves cancer survival in primary and metastatic mouse tumor models (299). Furthermore, the tumor antigen human mucin-1 (hMUC1), delivered via therapy EVs, has been utilized in dendritic cell-based immunotherapy (300). Hence, TAAs can elicit a strong anti-tumor immune response when fused with the C1C2 domain of lactadherin that binds to the phosphatidyl serine on the EV surface (301, 302). Significantly, the TAA laden EVs can result in “cross-presentation” of tumor-specific antigens in recipient cells (301, 302) in a manner that closely resembles that of apoptotic bodies. This means that an endogenous pathway of antigen processing and presentation can be affected by EVs for tumor cytotoxic T cell recognition. The enhanced immunogenic potential of this approach has been demonstrated for the TAAs carcinoembryonic antigen (CEA) and Her2 (303, 304). Also, tumor-derived MPs inducing the type-I interferons (through the cGAS/STING pathway) resulted in increased dendritic cell maturation, and in enhanced tumor specific T-cell tumor lysis (305). Hence there are many ways in which tumor MPs exert adjuvant effects. Indeed, there is already exciting evidence that exosomes isolated from the ascites fluid of cancer patients can function as a personalized vaccine for cancer (43) and phase-I clinical trials have been performed to evaluate autologous dendritic cell-derived cancer exosome therapy for lung and melanoma cancers (306). This is an active area in clinical cancer medicine; a list of current human clinical trials utilizing EVs in cancer is provided in Table 2.
Current Challenges in the Use of EVs in Oncology
As with any new or emerging biotechnology there are technical issues surrounding its use in the clinic. For EVs these include difficulties in standardization of EV isolation techniques and EV detection methodologies, as well as issues related to the GMP production of therapy EVs.
Challenges in Assessing Biomarker EVs of Clinical Relevance
The potential uses of EVs as biomarkers is exciting but caution is needed as there are many different methods of isolating, detecting and using EVs in cancer detection and treatment (307, 308). This immediately highlights the need for methodological standardization for EV assessment and its introduction into routine pathology laboratories, in order to achieve reproducibility and clinician confidence in the data. Secondly, the assessment methodologies require determination of the level of sensitivity, and the false discovery rate, as these are important for accurate clinical use (309, 310). Detection of proteins can be problematic unless there are high quality monoclonal antibodies for affinity methods, whereas nucleic acid is easily quantified with high degree of accuracy. Furthermore, EV isolation methods and detection efficiencies are integrally linked, because the EV isolation method can impact on EV phenotype and cargo detection capabilities (311). This is partly due to inherent heterogeneity of the EVs in the starting biological sample, and the remaining sample heterogeneity after EV purification [see Table 3 and 4 in (310)]. Traditionally, centrifugation-based methods have been used for EV isolation in research settings. However, the type of centrifuge (with fixed or swinging bucket rotors), the centrifugation matrix/medium, centrifugation speed and time, influences the quality of the EV isolation [thoroughly reviewed in (312)]. The viscosity of the starting sample (and hence both the sample and centrifugation temperature) also impacts on centrifugal methods (313). Step-wise density centrifugation and ultrafiltration offers some improvements (314), including the capacity to add artificial standards, but density gradient centrifugation is time-consuming and has limitations in laboratory scale up (312). Other methods for EV isolation include size-exclusion chromatography, or polymer-based precipitation especially by substances such as polyethylene glycol and sodium chloride or commercial products. The number of methods highlight the difficulties in standardization for EV preparation, and several groups are now reporting optimized isolation methodologies based on the biological sample type and the intended EV use (315), including EV isolation for miRNA or protein profiling (316, 317). Furthermore, given the known presence of specific molecules in certain EVs, immuno-affinity-based methods are also now common, with both “positive” or “negative” affinity selection methods available, offering high selectiivity and high purity (318, 319). The advantage of the immune-affinity approach is that EV particle size heterogeneity is no longer an issue; any particle with the antibody-targeted molecule can be purified. One disadvantage, however, is that not all EVs produced from a cancer may express the designated isolation marker(s), such that certain useful EVs will be lost by antigen-specific immune-affinity based isolation procedures [reviewed in (312)].
Additional innovative methods of EV detection and characterization include various microfluidic (e.g., “lab-on-chip”) approaches that allow for efficient molecular capture and quantification from relatively small volumes of circulating exosomes (320). Indeed, EVs can now be detected even as little as one microliter of patient plasma, which is achieved by coupling antibody-conjugated gold nanospheres and chip-based microfluidic technology (321). In this regard innovative nano-plasmonic sensors (322), or tunable-resistive pulse sensing have been developed (323) and a nanoplasmon-enhanced scattering assay has been successfully applied to staging tumor progression and therapy responsiveness (324). However, despite these successes, there is still the significant problem that few detection assays incorporate biological reference materials - required for inter-laboratory standardization. It is currently possible to produce an EV reference material from synthetic sources, such as liposomes; these can be relatively easily prepared to a desired size by either ultrasonication or polycarbonate filtration (310). EV reference material can also be produced from naturally occurring biological sources such as mammalian erythrocytes (310). Various biological reference materials are currently being investigated for diagnostic assay purposes [for a recent review on this topic see (310)]. Hence, despite the available technologies to detect EVs, there is a clear need to establish assay performance quality control and “best-practice” for clinical laboratory EV detection and quantitation.
Challenges in Using Therapeutic EVs
One of the earliest applications of EVs in cancer was the use of dendritic cell secreted exosomes. The idea is that cancer-peptide-loaded major histocompatibility together with co-stimulatory molecules born by exosomes confer EVs with the properties required for use as a cancer vaccine (299). Their size, structure, cargo, and mode of recipient cell uptake, make EVs prime vehicles to induce antigen cross-presentation to enhance tumor antigenicity (55). Pre-clinical mouse tumor studies showed efficacy of dendritic cell EVs, and now a number of human clinical trials are underway [(308, 325, 326) see Table 2]. Nevertheless, one of the limitations of using therapy EVs is obtaining sufficient quantities i.e. of tissue- or disease- specific, and functionally intact, preparations of EVs from body fluids. Even the relative abundance of urine as an EV bio-fluid source has a limit, and both patient and cell culture-derived EVs need to be “defined,” measured, and determined with high assurance for safe use in patients. Also, the use of EVs as cancer vaccines, requires a priori the existence of true immunogenic “cancer antigens” but these are usually not well defined, if defined at all. The “antigen” might constitute a re-activated endogenous retroviral antigen or cancer-specific neo-antigen. Whether cancer-EVs are pan-specific (i.e., for a selected cancer type) or whether they must be individually identified and isolated and used autologously, i.e., each individual patient, currently remains unclear. Furthermore, the optimal mode of EV delivery is also unclear in terms of achieving optimal clinical outcomes; delivery might be via i.v. infusion (single or repeated dose), or via an implanted medical device—for sustained release. Indeed, optimal delivery will possibly depend on the setting in which the EV vaccine is to be applied—an infectious disease, a certain cancer type, a primary cancer diagnosis or a cancer re-occurrence. On the other hand, the potential clinical use of tumor EVs carries with it potential dangers because of certain cargoes. For example, cancer cell derived EV can contain p-glycoprotein that confers resistance to chemotherapy drugs (5, 151), and certain EV cargo molecules enhance a tumor's metastatic characteristics (327, 328) (as discussed earlier). Clearly, therapy EVs require careful evaluation prior to their use in cancer clinical trials. Finally, in order to address the limited supply, and the various safety issues, plants and various food substances, including milk, have been investigated as a source of therapy EVs (286, 329, 330). An advantage of this source of EVs is the ease of scalability for clinical production. However, there is also the capacity to produce engineered EVs for specific purposes [recently reviewed in (331)], e.g., cancer chemotherapeutic agents such as paclitaxel and taxol can be easily incorporated into EVs (332). Finally, EV engineering has demonstrated the possibility to load the EV with a biosensor agent for sensitive in vivo monitoring to track EV bio-distribution (333).
Conclusions
A vast repertoire of proteins and nucleic acid molecules are present within EVs and this reflects the broad potential of EV as therapeutic and diagnostic (and/or prognostic) agents. With the value of more precise analysis technologies, our understanding of the biological interactions of EVs for intercellular communication is rapidly expanding. This new knowledge has high relevance and high value for the use of EVs in cancer, including for innovative, even patient-specific “designer” cancer treatments. These developments offer the promise of more specific, less toxic, high-efficacy cancer treatments, especially in this era of personalized medicine.
Author Contributions
RJ wrote the first draft of the manuscript. RJ and LS both contributed to manuscript revisions, read and approved the final version.
Conflict of Interest Statement
The authors declare that the research was conducted in the absence of any commercial or financial relationships that could be construed as a potential conflict of interest.
Acknowledgments
RJ is the recipient of a UTS Chancellor's Postdoctoral Research Fellowship award.
Abbreviations
EV, extracellular vesicles; LO, large oncosome; MP, microparticle; MV, microvesicle.
References
1. Barrow AD, Edeling MA, Trifonov V, Luo J, Goyal P, Bohl B, et al. (2018). Natural killer cells control tumor growth by sensing a growth factor. Cell. 172, 534–48 e519. doi: 10.1016/j.cell.2017.11.037
2. Terra M, Oberkampf M, Fayolle C, Rosenbaum P, Guillerey C, Dadaglio G, et al. Tumor-derived TGFbeta alters the ability of plasmacytoid dendritic cells to respond to innate immune signaling. Cancer Res. (2018) 78:3014–26. doi: 10.1158/0008-5472.can-17-2719
3. Alberts B, Johnson A, Lewis JJ. General Principles of Cell Communication. New York, NY: Garland Science (2002).
4. Fruhbeis C, Frohlich D, Kuo WP, Kramer-Albers EM. Extracellular vesicles as mediators of neuron-glia communication. Front Cell Neurosci. (2013) 7:182. doi: 10.3389/fncel.2013.00182
5. Jaiswal R, Raymond Grau GE, Bebawy M. Cellular communication via microparticles: role in transfer of multidrug resistance in cancer. Future Oncol. (2014) 10:655–69. doi: 10.2217/fon.13.230
6. Gould SJ, Raposo G. As we wait: coping with an imperfect nomenclature for extracellular vesicles. J Extracell Vesicles. (2013) 2:20389. doi: 10.3402/jev.v2i0.20389
7. Muralidharan-Chari V, Clancy JW, Sedgwick A, D'souza-Schorey C. (2010). Microvesicles: mediators of extracellular communication during cancer progression. J Cell Sci. 123, 1603–1611. doi: 10.1242/jcs.064386
8. Gong J, Jaiswal R, Dalla P, Luk F, Bebawy M. Microparticles in cancer: A review of recent developments and the potential for clinical application. Semin Cell Dev Biol. (2015) 40:35–40. doi: 10.1016/j.semcdb.2015.03.009
9. Meehan K, Vella LJ. The contribution of tumour-derived exosomes to the hallmarks of cancer. Crit Rev Clin Lab Sci. (2016) 53:121–31. doi: 10.3109/10408363.2015.1092496
10. Armstrong D, Wildman DE. Extracellular vesicles and the promise of continuous liquid biopsies. J Pathol Transl Med. (2018) 52:1–8. doi: 10.4132/jptm.2017.05.21
11. Stremersch S, De Smedt SC, Raemdonck K. Therapeutic and diagnostic applications of extracellular vesicles. J Control Release. (2016) 244:167–83. doi: 10.1016/j.jconrel.2016.07.054
12. Bjorge IM, Kim SY, Mano JF, Kalionis B, Chrzanowski W. Extracellular vesicles, exosomes and shedding vesicles in regenerative medicine - a new paradigm for tissue repair. Biomater Sci. (2017) 6:60–78. doi: 10.1039/c7bm00479f
13. Syn NL, Wang L, Chow EK, Lim CT, Goh BC. Exosomes in cancer nanomedicine and immunotherapy: Prospects and challenges. Trends Biotechnol. (2017) 35:665–76. doi: 10.1016/j.tibtech.2017.03.004
14. Yang Y, Hong Y, Cho E, Kim GB, Kim IS. Extracellular vesicles as a platform for membrane-associated therapeutic protein delivery. J Extracell Vesicles. (2018) 7:1440131. doi: 10.1080/20013078.2018.1440131
15. Ostman S, Taube M, Telemo E. Tolerosome-induced oral tolerance is MHC dependent. Immunology. (2005) 116:464–76. doi: 10.1111/j.1365-2567.2005.02245.x
16. Aalberts M, Stout TA, Stoorvogel W. Prostasomes: extracellular vesicles from the prostate. Reproduction. (2014) 147:R1–14. doi: 10.1530/rep-13-0358
17. Sullivan R, Frenette G, Girouard J. Epididymosomes are involved in the acquisition of new sperm proteins during epididymal transit. Asian J Androl. (2007) 9:483–91. doi: 10.1111/j.1745-7262.2007.00281.x
18. Cocucci E, Meldolesi J. Ectosomes and exosomes: shedding the confusion between extracellular vesicles. Trends Cell Biol. (2015) 25:364–72. doi: 10.1016/j.tcb.2015.01.004
19. Al-Nedawi K, Meehan B, Micallef J, Lhotak V, May L, Guha A, et al. Intercellular transfer of the oncogenic receptor EGFRvIII by microvesicles derived from tumour cells. Nat Cell Biol. (2008) 10:619–24. doi: 10.1038/ncb1725
20. Di Vizio D, Kim J, Hager MH, Morello M, Yang W, Lafargue CJ, et al. Oncosome formation in prostate cancer: association with a region of frequent chromosomal deletion in metastatic disease. Cancer Res. (2009) 69:5601–9. doi: 10.1158/0008-5472.CAN-08-3860
21. Minciacchi VR, You S, Spinelli C, Morley S, Zandian M, Aspuria PJ, et al. Large oncosomes contain distinct protein cargo and represent a separate functional class of tumor-derived extracellular vesicles. Oncotarget. (2015b) 6:11327–41. doi: 10.18632/oncotarget.3598
22. Di Vizio D, Morello M, Dudley AC, Schow PW, Adam RM, Morley S, et al. Large oncosomes in human prostate cancer tissues and in the circulation of mice with metastatic disease. Am J Pathol. (2012) 181:1573–84. doi: 10.1016/j.ajpath.2012.07.030
23. Wright PK, Jones SB, Ardern N, Ward R, Clarke RB, Sotgia F, et al. 17beta-estradiol regulates giant vesicle formation via estrogen receptor-alpha in human breast cancer cells. Oncotarget. (2014) 5:3055–65. doi: 10.18632/oncotarget.1824
24. Ronquist G. Prostasomes are mediators of intercellular communication: from basic research to clinical implications. J Intern Med. (2012) 271:400–13. doi: 10.1111/j.1365-2796.2011.02487.x
25. Al-Nedawi K, Meehan B, Rak J. Microvesicles: messengers and mediators of tumor progression. Cell Cycle. (2009b) 8:2014–8. doi: 10.4161/cc.8.13.8988
26. Da Rocha-Azevedo B, Schmid SL. Migrasomes: a new organelle of migrating cells. Cell Res. (2015) 25:1–2. doi: 10.1038/cr.2014.146
27. Akers JC, Gonda D, Kim R, Carter BS, Chen CC. Biogenesis of extracellular vesicles (EV): exosomes, microvesicles, retrovirus-like vesicles, and apoptotic bodies. J Neurooncol. (2013) 113:1–11. doi: 10.1007/s11060-013-1084-8
28. Roberts JA, Thorley BR, Bruggink LD, Marshall JA. Electron microscope detection of an endogenous infection of retrovirus-like particles in L20B cells. Microscopy (Oxf). (2013) 62:485–6. doi: 10.1093/jmicro/dfs133
29. Pincetic A, Leis J. The mechanism of budding of retroviruses from cell membranes. Adv Virol. (2009) 2009:6239691–9. doi: 10.1155/2009/623969
30. Wurdinger T, Gatson NN, Balaj L, Kaur B, Breakefield XO, Pegtel DM. Extracellular vesicles and their convergence with viral pathways. Adv Virol. (2012) 2012:767694. doi: 10.1155/2012/767694
31. Carneiro FA, Stauffer F, Lima CS, Juliano MA, Juliano L, Da Poian AT. Membrane fusion induced by vesicular stomatitis virus depends on histidine protonation. J Biol Chem. (2003) 278:13789–94. doi: 10.1074/jbc.M210615200
32. Mangeot PE, Dollet S, Girard M, Ciancia C, Joly S, Peschanski M, et al. Protein transfer into human cells by VSV-G-induced nanovesicles. Mol Ther. (2011) 19:1656–66. doi: 10.1038/mt.2011.138
33. Breakefield XO, Frederickson RM, Simpson RJ. Gesicles: Microvesicle “cookies” for transient information transfer between cells. Mol Ther. (2011) 19:1574–6. doi: 10.1038/mt.2011.169
34. Nawaz M, Camussi G, Valadi H, Nazarenko I, Ekstrom K, Wang X, et al. The emerging role of extracellular vesicles as biomarkers for urogenital cancers. Nat Rev Urol. (2014) 11:688–701. doi: 10.1038/nrurol.2014.301
35. Caby MP, Lankar D, Vincendeau-Scherrer C, Raposo G, Bonnerot C. Exosomal-like vesicles are present in human blood plasma. Int Immunol. (2005) 17:879–87. doi: 10.1093/intimm/dxh267
36. Arraud N, Linares R, Tan S, Gounou C, Pasquet JM, Mornet S, et al. Extracellular vesicles from blood plasma: determination of their morphology, size, phenotype and concentration. J Thromb Haemost. (2014) 12:614–27. doi: 10.1111/jth.12554
37. Aalberts M, Van Dissel-Emiliani FM, Van Adrichem NP, Van Wijnen M, Wauben MH, Stout TA, et al. Identification of distinct populations of prostasomes that differentially express prostate stem cell antigen, annexin A1, and GLIPR2 in humans. Biol Reprod. (2012) 86:82. doi: 10.1095/biolreprod.111.095760
38. Pisitkun T, Shen RF, Knepper MA. Identification and proteomic profiling of exosomes in human urine. Proc Natl Acad Sci USA. (2004) 101:13368–73. doi: 10.1073/pnas.0403453101
39. Ogawa Y, Miura Y, Harazono A, Kanai-Azuma M, Akimoto Y, Kawakami H, et al. Proteomic analysis of two types of exosomes in human whole saliva. Biol Pharm Bull. (2011) 34:13–23. doi: 10.1248/bpb.34.13
40. Lacedonia D, Carpagnano GE, Trotta T, Palladino GP, Panaro MA, Zoppo LD, et al. Microparticles in sputum of COPD patients: a potential biomarker of the disease? Int J Chron Obstruct Pulmon Dis. (2016) 11:527–33. doi: 10.2147/copd.s99547
41. Admyre C, Johansson SM, Qazi KR, Filen JJ, Lahesmaa R, Norman M, et al. Exosomes with immune modulatory features are present in human breast milk. J Immunol. (2007) 179:1969–78. doi: 10.4049/jimmunol.179.3.1969
42. Asea A, Jean-Pierre C, Kaur P, Rao P, Linhares IM, Skupski D, et al. Heat shock protein-containing exosomes in mid-trimester amniotic fluids. J Reprod Immunol. (2008) 79:12–7. doi: 10.1016/j.jri.2008.06.001
43. Andre F, Schartz NE, Movassagh M, Flament C, Pautier P, Morice P, et al. Malignant effusions and immunogenic tumour-derived exosomes. Lancet. (2002) 360:295–305. doi: 10.1016/s0140-6736(02)09552-1
44. Chiasserini D, Van Weering JR, Piersma SR, Pham TV, Malekzadeh A, Teunissen CE, et al. Proteomic analysis of cerebrospinal fluid extracellular vesicles: a comprehensive dataset. J Proteomics. (2014) 106:191–204. doi: 10.1016/j.jprot.2014.04.028
45. Li L, Masica D, Ishida M, Tomuleasa C, Umegaki S, Kalloo AN, et al. Human bile contains microRNA-laden extracellular vesicles that can be used for cholangiocarcinoma diagnosis. Hepatology. (2014) 60:896–907. doi: 10.1002/hep.27050
46. Shin TS, Kim JH, Kim YS, Jeon SG, Zhu Z, Gho YS, et al. Extracellular vesicles are key intercellular mediators in the development of immune dysfunction to allergens in the airways. Allergy. (2010) 65:1256–65. doi: 10.1111/j.1398-9995.2010.02359.x
47. Runz S, Keller S, Rupp C, Stoeck A, Issa Y, Koensgen D, et al. Malignant ascites-derived exosomes of ovarian carcinoma patients contain CD24 and EpCAM. Gynecol Oncol. (2007) 107:563–71. doi: 10.1016/j.ygyno.2007.08.064
48. Milasan A, Tessandier N, Tan S, Brisson A, Boilard E, Martel C. Extracellular vesicles are present in mouse lymph and their level differs in atherosclerosis. J Extracell Vesicles. (2016) 5:10.3402/jev.v3405.31427. doi: 10.3402/jev.v5.31427
49. Lasser C, O'neil SE, Ekerljung L, Ekstrom K, Sjostrand M, Lotvall J. RNA-containing exosomes in human nasal secretions. Am J Rhinol All. (2011) 25:89–93. doi: 10.2500/ajra.2011.25.3573
50. Grigor'eva AE, Tamkovich SN, Eremina AV, Tupikin AE, Kabilov MR, Chernykh VV, et al. Exosomes in tears of healthy individuals: isolation, identification, and characterization. Biochem Suppl B Biomed Chem. (2016) 10:165–72. doi: 10.1134/s1990750816020049
51. Koga Y, Yasunaga M, Moriya Y, Akasu T, Fujita S, Yamamoto S, et al. Exosome can prevent RNase from degrading microRNA in feces. J Gastrointest Oncol. (2011) 2:215–22. doi: 10.3978/j.issn.2078-6891.2011.015
52. Yuana Y, Sturk A, Nieuwland R. Extracellular vesicles in physiological and pathological conditions. Blood Rev. (2013) 27:31–9. doi: 10.1016/j.blre.2012.12.002
53. Kalra H, Drummen GP, Mathivanan S. Focus on extracellular vesicles: introducing the next small big thing. Int J Mol Sci. (2016) 17:170. doi: 10.3390/ijms17020170
54. Graner MW, Alzate O, Dechkovskaia AM, Keene JD, Sampson JH, Mitchell DA, et al. Proteomic and immunologic analyses of brain tumor exosomes. FASEB J. (2009) 23:1541–57. doi: 10.1096/fj.08-122184
55. Wolfers J, Lozier A, Raposo G, Regnault A, Thery C, Masurier C, et al. Tumor-derived exosomes are a source of shared tumor rejection antigens for CTL cross-priming. Nat Med. (2001) 7:297–303. doi: 10.1038/85438
56. Taylor DD, Gercel-Taylor C. MicroRNA signatures of tumor-derived exosomes as diagnostic biomarkers of ovarian cancer. Gynecol Oncol. (2008) 110:13–21. doi: 10.1016/j.ygyno.2008.04.033
57. Welton JL, Khanna S, Giles PJ, Brennan P, Brewis IA, Staffurth J, et al. Proteomics analysis of bladder cancer exosomes. Mol Cell Proteomics. (2010) 9:1324–38. doi: 10.1074/mcp.M000063-MCP201
58. Jaiswal R, Gong J, Sambasivam S, Combes V, Mathys JM, Davey R, et al. Microparticle-associated nucleic acids mediate trait dominance in cancer. FASEB J. (2012a) 26:420–9. doi: 10.1096/fj.11-186817
59. Kobayashi M, Salomon C, Tapia J, Illanes SE, Mitchell MD, Rice GE. Ovarian cancer cell invasiveness is associated with discordant exosomal sequestration of Let-7 miRNA and miR-200. J Transl Med. (2014) 12:4. doi: 10.1186/1479-5876-12-4
60. Kim HK, Song KS, Park YS, Kang YH, Lee YJ, Lee KR, et al. Elevated levels of circulating platelet microparticles, VEGF, IL-6 and RANTES in patients with gastric cancer: possible role of a metastasis predictor. Eur J Cancer. (2003) 39:184–91. doi: 10.1016/S0959-8049(02)00596-8
61. Graves LE, Ariztia EV, Navari JR, Matzel HJ, Stack MS, Fishman DA. Proinvasive properties of ovarian cancer ascites-derived membrane vesicles. Cancer Res. (2004) 64:7045–9. doi: 10.1158/0008-5472.CAN-04-1800
62. Van Doormaal FF, Kleinjan A, Di Nisio M, Buller HR, Nieuwland R. Cell-derived microvesicles and cancer. Neth J Med. (2009) 67:266–73.
63. Aubertin K, Silva AK, Luciani N, Espinosa A, Djemat A, Charue D, et al. Massive release of extracellular vesicles from cancer cells after photodynamic treatment or chemotherapy. Sci Rep. (2016) 6:35376. doi: 10.1038/srep35376
64. Heijnen HF, Schiel AE, Fijnheer R, Geuze HJ, Sixma JJ. Activated platelets release two types of membrane vesicles: microvesicles by surface shedding and exosomes derived from exocytosis of multivesicular bodies and alpha-granules. Blood. (1999) 94:3791–9.
65. Deregibus MC, Cantaluppi V, Calogero R, Lo Iacono M, Tetta C, Biancone L, et al. Endothelial progenitor cell derived microvesicles activate an angiogenic program in endothelial cells by a horizontal transfer of mRNA. Blood. (2007) 110:2440–8. doi: 10.1182/blood-2007-03-078709
66. Muralidharan-Chari V, Clancy J, Plou C, Romao M, Chavrier P, Raposo G, et al. ARF6-regulated shedding of tumor cell-derived plasma membrane microvesicles. Curr Biol. (2009) 19:1875–85. doi: 10.1016/j.cub.2009.09.059
67. Thery C, Boussac M, Veron P, Ricciardi-Castagnoli P, Raposo G, Garin J, et al. Proteomic analysis of dendritic cell-derived exosomes: a secreted subcellular compartment distinct from apoptotic vesicles. J Immunol. (2001) 166:7309–18. doi: 10.4049/jimmunol.166.12.7309
68. Claridge B, Kastaniegaard K, Stensballe A, Greening DW. Post-translational and transcriptional dynamics - regulating extracellular vesicle biology. Expert Rev Proteomics. (2018) 20:1–15. doi: 10.1080/14789450.2019.1551135
69. Sohda M, Misumi Y, Oda K. TNFα triggers release of extracellular vesicles containing TNFR1 and TRADD, which can modulate TNFα responses of the parental cells. Arch Biochem Biophys. (2015) 587:31–7. doi: 10.1016/j.abb.2015.10.009
70. Montermini L, Meehan B, Garnier D, Lee WJ, Lee TH, Guha A, et al. Inhibition of oncogenic epidermal growth factor receptor kinase triggers release of exosome-like extracellular vesicles and impacts their phosphoprotein and DNA content. J Biol Chem. (2015) 290:24534–46. doi: 10.1074/jbc.M115.679217
71. Thompson CA, Purushothaman A, Ramani VC, Vlodavsky I, Sanderson RD. Heparanase regulates secretion, composition, and function of tumor cell-derived exosomes. J Biol Chem. (2013) 288:10093–9. doi: 10.1074/jbc.C112.444562
72. King HW, Michael MZ, Gleadle JM. Hypoxic enhancement of exosome release by breast cancer cells. BMC Cancer. (2012) 12:421. doi: 10.1186/1471-2407-12-421
73. Faure J, Lachenal G, Court M, Hirrlinger J, Chatellard-Causse C, Blot B, et al. Exosomes are released by cultured cortical neurones. Mol Cell Neurosci. (2006) 31:642–8. doi: 10.1016/j.mcn.2005.12.003
74. Lachenal G, Pernet-Gallay K, Chivet M, Hemming FJ, Belly A, Bodon G, et al. Release of exosomes from differentiated neurons and its regulation by synaptic glutamatergic activity. Mol Cell Neurosci. (2011) 46:409–18. doi: 10.1016/j.mcn.2010.11.004
75. Blanchard N, Lankar D, Faure F, Regnault A, Dumont C, Raposo G, et al. TCR activation of human T cells induces the production of exosomes bearing the TCR/CD3/zeta complex. J Immunol. (2002) 168:3235–41. doi: 10.4049/jimmunol.168.7.3235
76. Ekstrom EJ, Bergenfelz C, Von Bulow V, Serifler F, Carlemalm E, Jonsson G, et al. WNT5A induces release of exosomes containing pro-angiogenic and immunosuppressive factors from malignant melanoma cells. Mol Cancer. (2014) 13:88. doi: 10.1186/1476-4598-13-88
77. Ciravolo V, Huber V, Ghedini GC, Venturelli E, Bianchi F, Campiglio M, et al. Potential role of HER2-overexpressing exosomes in countering trastuzumab-based therapy. J Cell Physiol. (2012) 227:658–67. doi: 10.1002/jcp.22773
78. Bhattacharya S, Pal K, Sharma AK, Dutta SK, Lau JS, Yan IK, et al. GAIP interacting protein C-terminus regulates autophagy and exosome biogenesis of pancreatic cancer through metabolic pathways. PLoS ONE. (2014) 9:e114409. doi: 10.1371/journal.pone.0114409
79. Savina A, Furlan M, Vidal M, Colombo MI. Exosome release is regulated by a calcium-dependent mechanism in K562 cells. J Biol Chem. (2003) 278:20083–90. doi: 10.1074/jbc.M301642200
80. Savina A, Fader CM, Damiani MT, Colombo MI. Rab11 promotes docking and fusion of multivesicular bodies in a calcium-dependent manner. Traffic. (2005) 6:131–43. doi: 10.1111/j.1600-0854.2004.00257.x
81. Raposo G, Tenza D, Mecheri S, Peronet R, Bonnerot C, Desaymard C. Accumulation of major histocompatibility complex class II molecules in mast cell secretory granules and their release upon degranulation. Mol Biol Cell. (1997) 8:2631–45.
82. Bianco F, Pravettoni E, Colombo A, Schenk U, Moller T, Matteoli M, et al. Astrocyte-derived ATP induces vesicle shedding and IL-1 beta release from microglia. J Immunol. (2005) 174:7268–77. doi: 10.4049/jimmunol.174.11.7268
83. Mackenzie A, Wilson HL, Kiss-Toth E, Dower SK, North RA, Surprenant A. Rapid secretion of interleukin-1beta by microvesicle shedding. Immunity. (2001) 15:825–35. doi: 10.1016/S1074-7613(01)00229-1
84. Wilson HL, Francis SE, Dower SK, Crossman DC. Secretion of intracellular IL-1 receptor antagonist (type 1) is dependent on P2X7 receptor activation. J Immunol. (2004) 173:1202–8. doi: 10.4049/jimmunol.173.2.1202
85. Crespin M, Vidal C, Picard F, Lacombe C, Fontenay M. Activation of PAK1/2 during the shedding of platelet microvesicles. Blood Coagul Fibrinolysis. (2009) 20:63–70. doi: 10.1097/MBC.0b013e32831bc310
86. Wysoczynski M, Ratajczak MZ. Lung cancer secreted microvesicles: under-appreciated modulators of microenvironment in expanding tumors. Int J Cancer. (2009) 125:1595–603. doi: 10.1002/ijc.24479
87. Kholia S, Jorfi S, Thompson PR, Causey CP, Nicholas AP, Inal JM, et al. (2015). A novel role for peptidylarginine deiminases in microvesicle release reveals therapeutic potential of PAD inhibition in sensitizing prostate cancer cells to chemotherapy. J Extracell Vesicles 4:10.3402/jev.v3404.26192. doi: 10.3402/jev.v4.26192
88. Li B, Antonyak MA, Zhang J, Cerione RA. RhoA triggers a specific signaling pathway that generates transforming microvesicles in cancer cells. Oncogene. (2012) 31:4740–9. doi: 10.1038/onc.2011.636
89. Baj-Krzyworzeka M, Szatanek R, Weglarczyk K, Baran J, Urbanowicz B, Branski P, et al. Tumour-derived microvesicles carry several surface determinants and mRNA of tumour cells and transfer some of these determinants to monocytes. Cancer Immunol Immunother. (2006) 55:808–18. doi: 10.1007/s00262-005-0075-9
90. Thomas LM, Salter RD. Activation of macrophages by P2X(7)-induced microvesicles from myeloid cells is mediated by phospholipids and is partially dependent on TLR4. J Immunol. (2010) 185:3740–9. doi: 10.4049/jimmunol.1001231
91. Eltom S, Dale N, Raemdonck KR, Stevenson CS, Snelgrove RJ, Sacitharan PK, et al. Respiratory infections cause the release of extracellular vesicles: implications in exacerbation of asthma/COPD. PLoS ONE. (2014) 9:e101087. doi: 10.1371/journal.pone.0101087
92. Liao CF, Lin SH, Chen HC, Tai CJ, Chang CC, Li LT, et al. CSE1L, a novel microvesicle membrane protein, mediates Ras-triggered microvesicle generation and metastasis of tumor cells. Mol Med. (2012) 18:1269–80. doi: 10.2119/molmed.2012.00205
93. Sapet C, Simoncini S, Loriod B, Puthier D, Sampol J, Nguyen C, et al. Thrombin-induced endothelial microparticle generation: identification of a novel pathway involving ROCK-II activation by caspase-2. Blood. (2006) 108:1868–76. doi: 10.1182/blood-2006-04-014175
94. Szotowski B, Antoniak S, Goldin-Lang P, Tran QV, Pels K, Rosenthal P, et al. Antioxidative treatment inhibits the release of thrombogenic tissue factor from irradiation- and cytokine-induced endothelial cells. Cardiovasc Res. (2007) 73:806–12. doi: 10.1016/j.cardiores.2006.12.018
95. Bianco F, Perrotta C, Novellino L, Francolini M, Riganti L, Menna E, et al. Acid sphingomyelinase activity triggers microparticle release from glial cells. Embo J. (2009) 28:1043–54. doi: 10.1038/emboj.2009.45
96. Obregon C, Rothen-Rutishauser B, Gitahi SK, Gehr P, Nicod LP. Exovesicles from human activated dendritic cells fuse with resting dendritic cells, allowing them to present alloantigens. Am J Pathol. (2006) 169:2127–36. doi: 10.2353/ajpath.2006.060453
97. Kim J, Morley S, Le M, Bedoret D, Umetsu DT, Di Vizio D, et al. Enhanced shedding of extracellular vesicles from amoeboid prostate cancer cells: potential effects on the tumor microenvironment. Cancer Biol Ther. (2014) 15:409–18. doi: 10.4161/cbt.27627
98. Colombo M, Raposo G, Thery C. Biogenesis, secretion, and intercellular interactions of exosomes and other extracellular vesicles. Annu Rev Cell Dev Biol. (2014) 30:255–89. doi: 10.1146/annurev-cellbio-101512-122326
99. Thery C, Ostrowski M, Segura E. Membrane vesicles as conveyors of immune responses. Nat Rev Immunol. (2009) 9:581–93. doi: 10.1038/nri2567
100. Stokes L, Fuller SJ, Sluyter R, Skarratt KK, Gu BJ, Wiley JS. Two haplotypes of the P2X(7) receptor containing the Ala-348 to Thr polymorphism exhibit a gain-of-function effect and enhanced interleukin-1β secretion. FASEB J. (2010) 24:2916–27. doi: 10.1096/fj.09-150862
101. Gulinelli S, Salaro E, Vuerich M, Bozzato D, Pizzirani C, Bolognesi G, et al. IL-18 associates to microvesicles shed from human macrophages by a LPS/TLR-4 independent mechanism in response to P2X receptor stimulation. Eur J Immunol. (2012) 42:3334–45. doi: 10.1002/eji.201142268
102. Chalmin F, Ladoire S, Mignot G, Vincent J, Bruchard M, Remy-Martin JP, et al. Membrane-associated Hsp72 from tumor-derived exosomes mediates STAT3-dependent immunosuppressive function of mouse and human myeloid-derived suppressor cells. J Clin Invest. (2010) 120:457–71. doi: 10.1172/jci40483
103. Gonzalez LJ, Gibbons E, Bailey RW, Fairbourn J, Nguyen T, Smith SK, et al. The influence of membrane physical properties on microvesicle release in human erythrocytes. PMC Biophys. (2009) 2:7. doi: 10.1186/1757-5036-2-7
104. Fong KP, Barry C, Tran AN, Traxler EA, Wannemacher KM, Tang HY, et al. Deciphering the human platelet sheddome. Blood. (2011) 117:e15–26. doi: 10.1182/blood-2010-05-283838
105. Trajkovic K, Hsu C, Chiantia S, Rajendran L, Wenzel D, Wieland F, et al. Ceramide triggers budding of exosome vesicles into multivesicular endosomes. Science. (2008) 319:1244–7. doi: 10.1126/science.1153124
106. Kosaka N, Iguchi H, Yoshioka Y, Takeshita F, Matsuki Y, Ochiya T. Secretory mechanisms and intercellular transfer of microRNAs in living cells. J Biol Chem. (2010) 285:17442–52. doi: 10.1074/jbc.M110.107821
107. Fabbri M, Paone A, Calore F, Galli R, Gaudio E, Santhanam R, et al. MicroRNAs bind to Toll-like receptors to induce prometastatic inflammatory response. Proc Natl Acad Sci USA. (2012) 109:E2110–2116. doi: 10.1073/pnas.1209414109
108. Kosgodage US, Trindade RP, Thompson PR, Inal JM, Lange S. Chloramidine/bisindolylmaleimide-I-mediated inhibition of exosome and microvesicle release and enhanced efficacy of cancer chemotherapy. Int J Mol Sci. (2017) 18:E1007. doi: 10.3390/ijms18051007
109. Antonyak MA, Wilson KF, Cerione RA. R(h)oads to microvesicles. Small GTPases. (2012) 3:219–24. doi: 10.4161/sgtp.20755
110. Ostrowski M, Carmo NB, Krumeich S, Fanget I, Raposo G, Savina A, et al. (2010). Rab27a and Rab27b control different steps of the exosome secretion pathway. Nat Cell Biol. 12, 19–30. doi: 10.1038/ncb2000
111. Hsu C, Morohashi Y, Yoshimura S, Manrique-Hoyos N, Jung S, Lauterbach MA, et al. Regulation of exosome secretion by Rab35 and its GTPase-activating proteins TBC1D10A-C. J Cell Biol. (2010) 189:223–32. doi: 10.1083/jcb.200911018
112. Latham SL, Chaponnier C, Dugina V, Couraud PO, Grau GE, Combes V. Cooperation between beta- and gamma-cytoplasmic actins in the mechanical regulation of endothelial microparticle formation. FASEB J. (2013) 27:672–83. doi: 10.1096/fj.12-216531
113. Smith IM, Hoshi N. ATP competitive protein kinase C inhibitors demonstrate distinct state-dependent inhibition. PLoS ONE. (2011) 6:e26338. doi: 10.1371/journal.pone.0026338
114. Croce K, Flaumenhaft R, Rivers M, Furie B, Furie BC, Herman IM, et al. Inhibition of calpain blocks platelet secretion, aggregation, and spreading. J Biol Chem. (1999) 274:36321–7.
115. Rendu F, Brohard-Bohn B, Pain S, Bachelot-Loza C, Auger J. Thiosulfinates inhibit platelet aggregation and microparticle shedding at a calpain-dependent step. Thromb Haemost. (2001) 86:1284–91. doi: 10.1055/s-0037-1616063
116. Nomura S, Shouzu A, Omoto S, Nishikawa M, Iwasaka T. Benidipine improves oxidized LDL-dependent monocyte and endothelial dysfunction in hypertensive patients with type 2 diabetes mellitus. J Hum Hypertens. (2005) 19:551–7. doi: 10.1038/sj.jhh.1001863
117. Federici C, Petrucci F, Caimi S, Cesolini A, Logozzi M, Borghi M, et al. Exosome release and low pH belong to a framework of resistance of human melanoma cells to cisplatin. PLoS ONE. (2014) 9:e88193. doi: 10.1371/journal.pone.0088193
118. Rossig L, Hoffmann J, Hugel B, Mallat Z, Haase A, Freyssinet JM, et al. Vitamin C inhibits endothelial cell apoptosis in congestive heart failure. Circulation. (2001) 104:2182–7. doi: 10.1161/hc4301.098284
119. Curtis AM, Wilkinson PF, Gui M, Gales TL, Hu E, Edelberg JM. p38 mitogen-activated protein kinase targets the production of proinflammatory endothelial microparticles. J Thromb Haemost. (2009) 7:701–9. doi: 10.1111/j.1538-7836.2009.03304.x
120. Kim KM, Abdelmohsen K, Mustapic M, Kapogiannis D, Gorospe M. RNA in extracellular vesicles. Wiley Interdiscip Rev RNA. (2017) 8:e1413. doi: 10.1002/wrna.1413
121. Puhka M, Takatalo M, Nordberg ME, Valkonen S, Nandania J, Aatonen M, et al. Metabolomic profiling of extracellular vesicles and alternative normalization methods reveal enriched metabolites and strategies to study prostate cancer-related changes. Theranostics. (2017) 7:3824–41. doi: 10.7150/thno.19890
122. Keerthikumar S, Chisanga D, Ariyaratne D, Al Saffar H, Anand S, Zhao K, et al. ExoCarta: a web-based compendium of exosomal cargo. J Mol Biol. (2016) 428:688–92. doi: 10.1016/j.jmb.2015.09.019
123. Kalra H, Simpson RJ, Ji H, Aikawa E, Altevogt P, Askenase P, et al. Vesiclepedia: a compendium for extracellular vesicles with continuous community annotation. PLoS Biol. (2012) 10:e1001450. doi: 10.1371/journal.pbio.1001450
124. Savina A, Vidal M, Colombo MI. The exosome pathway in K562 cells is regulated by Rab11. J Cell Sci. (2002) 115:2505–15.
125. Schorey JS, Bhatnagar S. Exosome function: from tumor immunology to pathogen biology. Traffic. (2008) 9:871–81. doi: 10.1111/j.1600-0854.2008.00734.x
126. Ghossoub R, Lembo F, Rubio A, Gaillard CB, Bouchet J, Vitale N, et al. Syntenin-ALIX exosome biogenesis and budding into multivesicular bodies are controlled by ARF6 and PLD2. Nat Commun. (2014) 5:3477. doi: 10.1038/ncomms4477
127. Liu R, Klich I, Ratajczak J, Ratajczak MZ, Zuba-Surma EK. Erythrocyte-derived microvesicles may transfer phosphatidylserine to the surface of nucleated cells and falsely 'mark' them as apoptotic. Eur J Haematol. (2009) 83:220–9. doi: 10.1111/j.1600-0609.2009.01271.x
128. Flugel-Koch C, Ohlmann A, Piatigorsky J, Tamm ER. Disruption of anterior segment development by TGF-beta1 overexpression in the eyes of transgenic mice. Dev Dyn. (2002) 225:111–25. doi: 10.1002/dvdy.10144
129. Cheung AK, Yang AK, Ngai BH, Yu SS, Gao M, Lau PM, et al. Quantitative detection of eryptosis in human erythrocytes using tunable resistive pulse sensing and annexin-V-beads. Analyst. (2015) 140:1337–48. doi: 10.1039/c4an02079k
130. Roy P, Periasamy AP, Lin CY, Her GM, Chiu WJ, Li CL, et al. Photoluminescent graphene quantum dots for in vivo imaging of apoptotic cells. Nanoscale. (2015) 7:2504–10. doi: 10.1039/c4nr07005d
131. Morello M, Minciacchi VR, De Candia P, Yang J, Posadas E, Kim H, et al. Large oncosomes mediate intercellular transfer of functional microRNA. Cell Cycle. (2013) 12:3526–36. doi: 10.4161/cc.26539
132. Corrado C, Raimondo S, Chiesi A, Ciccia F, De Leo G, Alessandro R. Exosomes as intercellular signaling organelles involved in health and disease: basic science and clinical applications. Int J Mol Sci. (2013) 14:5338–66. doi: 10.3390/ijms14035338
133. Tauro BJ, Greening DW, Mathias RA, Mathivanan S, Ji H, Simpson RJ. Two distinct populations of exosomes are released from LIM1863 colon carcinoma cell-derived organoids. Mol Cell Proteomics. (2013a) 12:587–98. doi: 10.1074/mcp.M112.021303
134. Holleman J, Marchese A. The ubiquitin ligase deltex-3l regulates endosomal sorting of the G protein-coupled receptor CXCR4. Mol Biol Cell. (2014) 25:1892–904. doi: 10.1091/mbc.E13-10-0612
135. Van Niel G, Charrin S, Simoes S, Romao M, Rochin L, Saftig P, et al. The tetraspanin CD63 regulates ESCRT-independent and -dependent endosomal sorting during melanogenesis. Dev Cell. (2011) 21:708–21. doi: 10.1016/j.devcel.2011.08.019
136. Gibbings DJ, Ciaudo C, Erhardt M, Voinnet O. Multivesicular bodies associate with components of miRNA effector complexes and modulate miRNA activity. Nat Cell Biol. (2009) 11:1143–9. doi: 10.1038/ncb1929
137. Villarroya-Beltri C, Gutierrez-Vazquez C, Sanchez-Cabo F, Perez-Hernandez D, Vazquez J, Martin-Cofreces N, et al. Sumoylated hnRNPA2B1 controls the sorting of miRNAs into exosomes through binding to specific motifs. Nat Commun. (2013) 4:2980. doi: 10.1038/ncomms3980
138. Shurtleff MJ, Temoche-Diaz MM, Karfilis KV, Ri S, Schekman R. Y-box protein 1 is required to sort microRNAs into exosomes in cells and in a cell-free reaction. Elife. (2016) 5:e19276. doi: 10.7554/eLife.19276
139. Jaiswal R, Luk F, Gong J, Mathys JM, Grau GE, Bebawy M. Microparticle conferred microRNA profiles–implications in the transfer and dominance of cancer traits. Mol Cancer. (2012b) 11:37. doi: 10.1186/1476-4598-11-37
140. Eldh M, Ekstrom K, Valadi H, Sjostrand M, Olsson B, Jernas M, et al. Exosomes communicate protective messages during oxidative stress; possible role of exosomal shuttle RNA. PLoS ONE. (2010) 5:e15353. doi: 10.1371/journal.pone.0015353
141. Smith JA, Leonardi T, Huang B, Iraci N, Vega B, Pluchino S. Extracellular vesicles and their synthetic analogues in aging and age-associated brain diseases. Biogerontology. (2015) 16:147–85. doi: 10.1007/s10522-014-9510-7
142. Pegtel DM, Cosmopoulos K, Thorley-Lawson DA, Van Eijndhoven MA, Hopmans ES, Lindenberg JL, et al. Functional delivery of viral miRNAs via exosomes. Proc Natl Acad Sci USA. (2010) 107:6328–33. doi: 10.1073/pnas.0914843107
143. Carayon K, Chaoui K, Ronzier E, Lazar I, Bertrand-Michel J, Roques V, et al. Proteolipidic composition of exosomes changes during reticulocyte maturation. J Biol Chem. (2011) 286:34426–39. doi: 10.1074/jbc.M111.257444
144. Yu X, Harris SL, Levine AJ. The regulation of exosome secretion: a novel function of the p53 protein. Cancer Res. (2006) 66:4795–801. doi: 10.1158/0008-5472.can-05-4579
145. De Jong OG, Verhaar MC, Chen Y, Vader P, Gremmels H, Posthuma G, et al. Cellular stress conditions are reflected in the protein and RNA content of endothelial cell-derived exosomes. J Extracell Vesicles. (2012) 1:18396. doi: 10.3402/jev.v1i0.18396
146. Kucharzewska P, Christianson HC, Welch JE, Svensson KJ, Fredlund E, Ringner M, et al. Exosomes reflect the hypoxic status of glioma cells and mediate hypoxia-dependent activation of vascular cells during tumor development. Proc Natl Acad Sci USA. (2013) 110:7312–7. doi: 10.1073/pnas.1220998110
147. Demory Beckler M, Higginbotham JN, Franklin JL, Ham AJ, Halvey PJ, Imasuen IE, et al. Proteomic analysis of exosomes from mutant KRAS colon cancer cells identifies intercellular transfer of mutant KRAS. Mol Cell Proteomics. (2013) 12:343–55. doi: 10.1074/mcp.M112.022806
148. Tauro BJ, Mathias RA, Greening DW, Gopal SK, Ji H, Kapp EA, et al. Oncogenic H-ras reprograms Madin-Darby canine kidney (MDCK) cell-derived exosomal proteins following epithelial-mesenchymal transition. Mol Cell Proteomics. (2013b) 12:2148–59. doi: 10.1074/mcp.M112.027086
149. Boles JC, Williams JC, Hollingsworth RM, Wang JG, Glover SL, Owens AP III, et al. Anthracycline treatment of the human monocytic leukemia cell line THP-1 increases phosphatidylserine exposure and tissue factor activity. Thromb Res. (2012) 129:197–203. doi: 10.1016/j.thromres.2011.06.022
150. Amorim M, Fernandes G, Oliveira P, Martins-De-Souza D, Dias-Neto E, Nunes D. The overexpression of a single oncogene (ERBB2/HER2) alters the proteomic landscape of extracellular vesicles. Proteomics. (2014) 14:1472–9. doi: 10.1002/pmic.201300485
151. Bebawy M, Combes V, Lee E, Jaiswal R, Gong J, Bonhoure A, et al. Membrane microparticles mediate transfer of p-glycoprotein to drug sensitive cancer cells. Leukemia. (2009) 23:1643–9. doi: 10.1038/leu.2009.76
152. Jaiswal R, Luk F, Dalla PV, Grau GE, Bebawy M. Breast cancer-derived microparticles display tissue selectivity in the transfer of resistance proteins to cells. PLoS ONE. (2013) 8:e61515. doi: 10.1371/journal.pone.0061515
153. Lee H, Groot M, Pinilla-Vera M, Fredenburgh LE, Jin Y. Identification of miRNA-rich vesicles in bronchoalveolar lavage fluid: insights into the function and heterogeneity of extracellular vesicles. J Control Rel. (2018) 294:43–52. doi: 10.1016/j.jconrel.2018.12.008
154. Brill A, Dashevsky O, Rivo J, Gozal Y, Varon D. Platelet-derived microparticles induce angiogenesis and stimulate post-ischemic revascularization. Cardiovasc Res. (2005) 67:30–8. doi: 10.1016/j.cardiores.2005.04.007
155. Peinado H, Aleckovic M, Lavotshkin S, Matei I, Costa-Silva B, Moreno-Bueno G, et al. Melanoma exosomes educate bone marrow progenitor cells toward a pro-metastatic phenotype through MET. Nat Med. (2012) 18:883–91. doi: 10.1038/nm.2753
156. Minciacchi VR, Freeman MR, Di Vizio D. Extracellular vesicles in cancer: exosomes, microvesicles and the emerging role of large oncosomes. Semin Cell Dev Biol. (2015a) 40:41–51. doi: 10.1016/j.semcdb.2015.02.010
157. Gabriel K, Ingram A, Austin R, Kapoor A, Tang D, Majeed F, et al. Regulation of the tumor suppressor PTEN through exosomes: a diagnostic potential for prostate cancer. PLoS ONE. (2013) 8:e70047. doi: 10.1371/journal.pone.0070047
158. Baran J, Baj-Krzyworzeka M, Weglarczyk K, Szatanek R, Zembala M, Barbasz J, et al. Circulating tumour-derived microvesicles in plasma of gastric cancer patients. Cancer Immunol Immunother. (2010) 59:841–50. doi: 10.1007/s00262-009-0808-2
159. Feng Q, Zhang C, Lum D, Druso JE, Blank B, Wilson KF, et al. A class of extracellular vesicles from breast cancer cells activates VEGF receptors and tumour angiogenesis. Nat Commun. (2017) 8:14450. doi: 10.1038/ncomms14450
160. Del Conde I, Shrimpton CN, Thiagarajan P, Lopez JA. Tissue-factor-bearing microvesicles arise from lipid rafts and fuse with activated platelets to initiate coagulation. Blood. (2005) 106:1604–11. doi: 10.1182/blood-2004-03-1095
161. Morelli AE, Larregina AT, Shufesky WJ, Sullivan ML, Stolz DB, Papworth GD, et al. Endocytosis, intracellular sorting, and processing of exosomes by dendritic cells. Blood. (2004) 104:3257–66. doi: 10.1182/blood-2004-03-0824
162. Bastos-Amador P, Perez-Cabezas B, Izquierdo-Useros N, Puertas MC, Martinez-Picado J, Pujol-Borrell R, et al. Capture of cell-derived microvesicles (exosomes and apoptotic bodies) by human plasmacytoid dendritic cells. J Leukoc Biol. (2012) 91:751–8. doi: 10.1189/jlb.0111054
163. Svensson KJ, Christianson HC, Wittrup A, Bourseau-Guilmain E, Lindqvist E, Svensson LM, et al. Exosome uptake depends on ERK1/2-heat shock protein 27 signaling and lipid Raft-mediated endocytosis negatively regulated by caveolin-1. J Biol Chem. (2013) 288:17713–24. doi: 10.1074/jbc.M112.445403
164. Feng D, Zhao WL, Ye YY, Bai XC, Liu RQ, Chang LF, et al. Cellular internalization of exosomes occurs through phagocytosis. Traffic. (2010) 11:675–87. doi: 10.1111/j.1600-0854.2010.01041.x
165. Escrevente C, Keller S, Altevogt P, Costa J. Interaction and uptake of exosomes by ovarian cancer cells. BMC Cancer. (2011) 11:108. doi: 10.1186/1471-2407-11-108
166. Fitzner D, Schnaars M, Van Rossum D, Krishnamoorthy G, Dibaj P, Bakhti M, et al. Selective transfer of exosomes from oligodendrocytes to microglia by macropinocytosis. J Cell Sci. (2011) 124:447–58. doi: 10.1242/jcs.074088
167. Sedger LM, McDermott MF. TNF and TNF-receptors: From mediators of cell death and inflammation to therapeutic giants - past, present and future. Cytokine Growth Factor Rev. (2014) 25:453–72. doi: 10.1016/j.cytogfr.2014.07.016
168. Prada I, Meldolesi J. Binding and fusion of extracellular vesicles to the plasma membrane of their cell targets. Int J Mol Sci. (2016) 17:E1296. doi: 10.3390/ijms17081296
169. Rana S, Yue S, Stadel D, Zoller M. Toward tailored exosomes: the exosomal tetraspanin web contributes to target cell selection. Int J Biochem Cell Biol. (2012) 44:1574–84. doi: 10.1016/j.biocel.2012.06.018
170. Barres C, Blanc L, Bette-Bobillo P, Andre S, Mamoun R, Gabius HJ, et al. Galectin-5 is bound onto the surface of rat reticulocyte exosomes and modulates vesicle uptake by macrophages. Blood. (2010) 115:696–705. doi: 10.1182/blood-2009-07-231449
171. Raposo G, Stoorvogel W. Extracellular vesicles: exosomes, microvesicles, and friends. J Cell Biol. (2013) 200:373–83. doi: 10.1083/jcb.201211138
172. Christianson HC, Svensson KJ, Van Kuppevelt TH, Li JP, Belting M. Cancer cell exosomes depend on cell-surface heparan sulfate proteoglycans for their internalization and functional activity. Proc Natl Acad Sci USA. (2013) 110:17380–5. doi: 10.1073/pnas.1304266110
173. Maliniemi P, Vincendeau M, Mayer J, Frank O, Hahtola S, Karenko L, et al. Expression of human endogenous retrovirus-w including syncytin-1 in cutaneous T-cell lymphoma. PLoS ONE. (2013) 8:e76281. doi: 10.1371/journal.pone.0076281
174. Hoshino A, Costa-Silva B, Shen TL, Rodrigues G, Hashimoto A, Tesic Mark M, et al. Tumour exosome integrins determine organotropic metastasis. Nature. (2015) 527:329–35. doi: 10.1038/nature15756
175. Rak J. Microparticles in cancer. Semin Thromb Hemost. (2010) 36:888–906. doi: 10.1055/s-0030-1267043
176. Jaiswal R, Johnson MS, Pokharel D, Krishnan SR, Bebawy M. Microparticles shed from multidrug resistant breast cancer cells provide a parallel survival pathway through immune evasion. BMC Cancer. (2017) 17:104. doi: 10.1186/s12885-017-3102-2
177. Bergsmedh A, Szeles A, Henriksson M, Bratt A, Folkman MJ, Spetz AL, et al. Horizontal transfer of oncogenes by uptake of apoptotic bodies. Proc Natl Acad Sci USA. (2001) 98:6407–11. doi: 10.1073/pnas.101129998
178. Provencher DM, Gallagher CJ, Parulekar WR, Ledermann JA, Armstrong DK, Brundage M, et al. OV21/PETROC: a randomized Gynecologic Cancer Intergroup phase II study of intraperitoneal versus intravenous chemotherapy following neoadjuvant chemotherapy and optimal debulking surgery in epithelial ovarian cancer. Ann Oncol. (2018) 29:431–8. doi: 10.1093/annonc/mdx754
179. Bluff JE, Brown NJ, Reed MW, Staton CA. Tissue factor, angiogenesis and tumour progression. Breast Cancer Res. (2008) 10:204. doi: 10.1186/bcr1871
180. Yu JL, May L, Lhotak V, Shahrzad S, Shirasawa S, Weitz JI, et al. Oncogenic events regulate tissue factor expression in colorectal cancer cells: implications for tumor progression and angiogenesis. Blood. (2005) 105:1734–41. doi: 10.1182/blood-2004-05-2042
181. Lima LG, Leal AC, Vargas G, Porto-Carreiro I, Monteiro RQ. Intercellular transfer of tissue factor via the uptake of tumor-derived microvesicles. Thromb Res. (2013) 132:450–6. doi: 10.1016/j.thromres.2013.07.026
182. Yu JL, Rak JW. Shedding of tissue factor (TF)-containing microparticles rather than alternatively spliced TF is the main source of TF activity released from human cancer cells. J Thromb Haemost. (2004) 2:2065–7. doi: 10.1111/j.1538-7836.2004.00972.x
183. Geddings JE, Mackman N. Tumor-derived tissue factor-positive microparticles and venous thrombosis in cancer patients. Blood. (2013) 122:1873–80. doi: 10.1182/blood-2013-04-460139
184. Thaler J, Koder S, Kornek G, Pabinger I, Ay C. Microparticle-associated tissue factor activity in patients with metastatic pancreatic cancer and its effect on fibrin clot formation. Transl Res. (2014) 163:145–50. doi: 10.1016/j.trsl.2013.06.009
185. Qu JL, Qu XJ, Zhao MF, Teng YE, Zhang Y, Hou KZ, et al. Gastric cancer exosomes promote tumour cell proliferation through PI3K/Akt and MAPK/ERK activation. Dig Liver Dis. (2009) 41:875–80. doi: 10.1016/j.dld.2009.04.006
186. Song X, Ding Y, Liu G, Yang X, Zhao R, Zhang Y, et al. Cancer cell-derived exosomes induce mitogen-activated Protein Kinase-dependent monocyte survival by transport of functional receptor Tyrosine Kinases. J Biol Chem. (2016) 291:8453–64. doi: 10.1074/jbc.M116.716316
187. Marton A, Vizler C, Kusz E, Temesfoi V, Szathmary Z, Nagy K, et al. Melanoma cell-derived exosomes alter macrophage and dendritic cell functions in vitro. Immunol Lett. (2012) 148:34–8. doi: 10.1016/j.imlet.2012.07.006
188. Chow A, Zhou W, Liu L, Fong MY, Champer J, Van Haute D, et al. Macrophage immunomodulation by breast cancer-derived exosomes requires Toll-like receptor 2-mediated activation of NF-kappaB. Sci Rep. (2014) 4:5750. doi: 10.1038/srep05750
189. Wu L, Zhang X, Zhang B, Shi H, Yuan X, Sun Y, et al. Exosomes derived from gastric cancer cells activate NF-κB pathway in macrophages to promote cancer progression. Tumour Biol. (2016) 37:12169–80. doi: 10.1007/s13277-016-5071-5
190. Li X, Wang S, Zhu R, Li H, Han Q, Zhao RC. Lung tumor exosomes induce a pro-inflammatory phenotype in mesenchymal stem cells via NFκB-TLR signaling pathway. J Hematol Oncol. (2016) 9:42. doi: 10.1186/s13045-016-0269-y
191. Valenti R, Huber V, Filipazzi P, Pilla L, Sovena G, Villa A, et al. Human tumor-released microvesicles promote the differentiation of myeloid cells with transforming growth factor-beta-mediated suppressive activity on T lymphocytes. Cancer Res. (2006) 66:9290–8. doi: 10.1158/0008-5472.can-06-1819
192. Koppler B, Cohen C, Schlondorff D, Mack M. Differential mechanisms of microparticle transfer toB cells and monocytes: anti-inflammatory propertiesof microparticles. Eur J Immunol. (2006) 36:648–60. doi: 10.1002/eji.200535435
193. Zhuang X, Xiang X, Grizzle W, Sun D, Zhang S, Axtell RC, et al. Treatment of brain inflammatory diseases by delivering exosome encapsulated anti-inflammatory drugs from the nasal region to the brain. Mol Ther. (2011) 19:1769–79. doi: 10.1038/mt.2011.164
194. Gupta SC, Patchva S, Koh W, Aggarwal BB. Discovery of curcumin, a component of golden spice, and its miraculous biological activities. Clin Exp Pharmacol Physiol. (2012) 39:283–99. doi: 10.1111/j.1440-1681.2011.05648.x
195. Wilken R, Veena MS, Wang MB, Srivatsan ES. Curcumin: a review of anti-cancer properties and therapeutic activity in head and neck squamous cell carcinoma. Mol Cancer. (2011) 10:12. doi: 10.1186/1476-4598-10-12
196. Vyas A, Dandawate P, Padhye S, Ahmad A, Sarkar F. Perspectives on new synthetic curcumin analogs and their potential anticancer properties. Curr Pharm Des. (2013) 19:2047–69. doi: 10.2174/1381612811319110007
197. Taverna S, Giallombardo M, Pucci M, Flugy A, Manno M, Raccosta S, et al. Curcumin inhibits in vitro and in vivo chronic myelogenous leukemia cells growth: a possible role for exosomal disposal of miR-21. Oncotarget. (2015) 6:21918–33. doi: 10.18632/oncotarget.4204
198. Lu JF, Luk F, Gong J, Jaiswal R, Grau GE, Bebawy M. Microparticles mediate MRP1 intercellular transfer and the re-templating of intrinsic resistance pathways. Pharmacol Res. (2013) 76:77–83. doi: 10.1016/j.phrs.2013.07.009
199. Ifergan I, Goler-Baron V, Assaraf YG. Riboflavin concentration within ABCG2-rich extracellular vesicles is a novel marker for multidrug resistance in malignant cells. Biochem Biophys Res Commun. (2009) 380:5–10. doi: 10.1016/j.bbrc.2008.12.168
200. Aung T, Chapuy B, Vogel D, Wenzel D, Oppermann M, Lahmann M, et al. Exosomal evasion of humoral immunotherapy in aggressive B-cell lymphoma modulated by ATP-binding cassette transporter A3. Proc Natl Acad Sci USA. (2011) 108:15336–41. doi: 10.1073/pnas.1102855108
201. Dong Y, Pan Q, Jiang L, Chen Z, Zhang F, Liu Y, et al. Tumor endothelial expression of p-glycoprotein upon microvesicular transfer of TrpC5 derived from adriamycin-resistant breast cancer cells. Biochem Biophys Res Commun. (2014) 446:85–90. doi: 10.1016/j.bbrc.2014.02.076
202. Gong J, Jaiswal R, Mathys JM, Combes V, Grau GE, Bebawy M. Microparticles and their emerging role in cancer multidrug resistance. Cancer Treat Rev. (2012) 38:226–34. doi: 10.1016/j.ctrv.2011.06.005
203. Takahashi K, Yan IK, Wood J, Haga H, Patel T. Involvement of extracellular vesicle long noncoding RNA (linc-VLDLR) in tumor cell responses to chemotherapy. Mol Cancer Res. (2014) 12:1377–87. doi: 10.1158/1541-7786.mcr-13-0636
204. Gong J, Luk F, Jaiswal R, George AM, Grau GE, Bebawy M. Microparticle drug sequestration provides a parallel pathway in the acquisition of cancer drug resistance. Eur J Pharmacol. (2013) 721:116–25. doi: 10.1016/j.ejphar.2013.09.044
205. Shedden K, Xie XT, Chandaroy P, Chang YT, Rosania GR. Expulsion of small molecules in vesicles shed by cancer cells: association with gene expression and chemosensitivity profiles. Cancer Res. (2003) 63:4331–7.
206. Battke C, Ruiss R, Welsch U, Wimberger P, Lang S, Jochum S, et al. Tumour exosomes inhibit binding of tumour-reactive antibodies to tumour cells and reduce ADCC. Cancer Immunol Immunother. (2011) 60:639–48. doi: 10.1007/s00262-011-0979-5
207. Szczepanski MJ, Szajnik M, Welsh A, Whiteside TL, Boyiadzis M. Blast-derived microvesicles in sera from patients with acute myeloid leukemia suppress natural killer cell function via membrane-associated transforming growth factor-beta1. Haematologica. (2011) 96:1302–9. doi: 10.3324/haematol.2010.039743
208. Rong L, Li R, Li S, Luo R. Immunosuppression of breast cancer cells mediated by transforming growth factor-beta in exosomes from cancer cells. Oncol Lett. (2016) 11:500–4. doi: 10.3892/ol.2015.3841
209. Zhang HG, Liu C, Su K, Yu S, Zhang L, Zhang S, et al. A membrane form of TNF-alpha presented by exosomes delays T cell activation-induced cell death. J Immunol. (2006) 176:7385–93. doi: 10.4049/jimmunol.176.12.7385
210. Andreola G, Rivoltini L, Castelli C, Huber V, Perego P, Deho P, et al. Induction of lymphocyte apoptosis by tumor cell secretion of FasL-bearing microvesicles. J Exp Med. (2002) 195:1303–16. doi: 10.1084/jem.20011624
211. Hedlund M, Nagaeva O, Kargl D, Baranov V, Mincheva-Nilsson L. Thermal- and oxidative stress causes enhanced release of NKG2D ligand-bearing immunosuppressive exosomes in leukemia/lymphoma T and B cells. PLoS ONE. (2011) 6:e16899. doi: 10.1371/journal.pone.0016899
212. Hong CS, Muller L, Boyiadzis M, Whiteside TL. Isolation and characterization of CD34+ blast-derived exosomes in acute myeloid leukemia. PLoS ONE. (2014) 9:e103310. doi: 10.1371/journal.pone.0103310
213. Lundholm M, Schroder M, Nagaeva O, Baranov V, Widmark A, Mincheva-Nilsson L, et al. Prostate tumor-derived exosomes down-regulate NKG2D expression on natural killer cells and CD8+ T cells: mechanism of immune evasion. PLoS ONE. (2014) 9:e108925. doi: 10.1371/journal.pone.0108925
214. Berchem G, Noman MZ, Bosseler M, Paggetti J, Baconnais S, Le Cam E, et al. Hypoxic tumor-derived microvesicles negatively regulate NK cell function by a mechanism involving TGF-beta and miR23a transfer. Oncoimmunology. (2016) 5:e1062968. doi: 10.1080/2162402x.2015.1062968
215. Su MW, Yu SL, Lin WC, Tsai CH, Chen PH, Lee YL. Smoking-related microRNAs and mRNAs in human peripheral blood mononuclear cells. Toxicol Appl Pharmacol. (2016b) 305:169–75. doi: 10.1016/j.taap.2016.06.020
216. Dai S, Wan T, Wang B, Zhou X, Xiu F, Chen T, et al. More efficient induction of HLA-A*0201-restricted and carcinoembryonic antigen (CEA)-specific CTL response by immunization with exosomes prepared from heat-stressed CEA-positive tumor cells. Clin Cancer Res. (2005) 11:7554–63. doi: 10.1158/1078-0432.ccr-05-0810
217. Yang C, Kim SH, Bianco NR, Robbins PD. Tumor-derived exosomes confer antigen-specific immunosuppression in a murine delayed-type hypersensitivity model. PLoS ONE. (2011) 6:e22517. doi: 10.1371/journal.pone.0022517
218. Yang C, Ruffner MA, Kim SH, Robbins PD. Plasma-derived MHC class II+ exosomes from tumor-bearing mice suppress tumor antigen-specific immune responses. Eur J Immunol. (2012) 42:1778–84. doi: 10.1002/eji.201141978
219. Szajnik M, Czystowska M, Szczepanski MJ, Mandapathil M, Whiteside TL. Tumor-derived microvesicles induce, expand and up-regulate biological activities of human regulatory T cells (Treg). PLoS ONE. (2010) 5:e11469. doi: 10.1371/journal.pone.0011469
220. Mrizak D, Martin N, Barjon C, Jimenez-Pailhes AS, Mustapha R, Niki T, et al. Effect of nasopharyngeal carcinoma-derived exosomes on human regulatory T cells. J Natl Cancer Inst. (2015) 107:363. doi: 10.1093/jnci/dju363
221. Wen SW, Sceneay J, Lima LG, Wong CS, Becker M, Krumeich S, et al. The biodistribution and immune suppressive effects of breast cancer-derived exosomes. Cancer Res. (2016) 76:6816–27. doi: 10.1158/0008-5472.can-16-0868
222. Domenis R, Cesselli D, Toffoletto B, Bourkoula E, Caponnetto F, Manini I, et al. Systemic T cells immunosuppression of glioma stem cell-derived exosomes is mediated by monocytic myeloid-derived suppressor cells. PLoS ONE. (2017) 12:e0169932. doi: 10.1371/journal.pone.0169932
223. Baj-Krzyworzeka M, Mytar B, Szatanek R, Surmiak M, Weglarczyk K, Baran J, et al. Colorectal cancer-derived microvesicles modulate differentiation of human monocytes to macrophages. J Transl Med. (2016) 14:36. doi: 10.1186/s12967-016-0789-9
224. De Vrij J, Maas SL, Kwappenberg KM, Schnoor R, Kleijn A, Dekker L, et al. Glioblastoma-derived extracellular vesicles modify the phenotype of monocytic cells. Int J Cancer. (2015) 137:1630–42. doi: 10.1002/ijc.29521
225. Su MJ, Aldawsari H, Amiji M. Pancreatic cancer cell exosome-mediated macrophage reprogramming and the role of microRNAs 155 and 125b2 transfection using nanoparticle delivery systems. Sci Rep. (2016a) 6:30110. doi: 10.1038/srep30110
226. Ying X, Wu Q, Wu X, Zhu Q, Wang X, Jiang L, et al. Epithelial ovarian cancer-secreted exosomal miR-222-3p induces polarization of tumor-associated macrophages. Oncotarget. (2016) 7:43076–87. doi: 10.18632/oncotarget.9246
227. Quail DF, Joyce JA. Microenvironmental regulation of tumor progression and metastasis. Nat Med. (2013) 19:1423–37. doi: 10.1038/nm.3394
228. Carmeliet P, Jain RK. Molecular mechanisms and clinical applications of angiogenesis. Nature. (2011) 473:298–307. doi: 10.1038/nature10144
229. Marcola M, Rodrigues CE. Endothelial progenitor cells in tumor angiogenesis: another brick in the wall. Stem Cells Int. (2015) 2015:832649. doi: 10.1155/2015/832649
230. Al-Nedawi K, Meehan B, Kerbel RS, Allison AC, Rak J. Endothelial expression of autocrine VEGF upon the uptake of tumor-derived microvesicles containing oncogenic EGFR. Proc Natl Acad Sci USA. (2009a) 106:3794–9. doi: 10.1073/pnas.0804543106
231. Kosaka N, Iguchi H, Hagiwara K, Yoshioka Y, Takeshita F, Ochiya T. Neutral sphingomyelinase 2 (nSMase2)-dependent exosomal transfer of angiogenic microRNAs regulate cancer cell metastasis. J Biol Chem. (2013) 288:10849–59. doi: 10.1074/jbc.M112.446831
232. Tadokoro H, Umezu T, Ohyashiki K, Hirano T, Ohyashiki J. Exosomes derived from hypoxic leukemia cells enhance tube formation in endothelial cells. J Biol Chem. (2013) 288:34343–51. doi: 10.1074/jbc.M113.480822
233. Choi D, Montermini L, Kim DK, Meehan B, Roth FP, Rak J. The impact of oncogenic EGFRvIII on the proteome of extracellular vesicles released from glioblastoma cells. Mol Cell Proteomics. (2018) 17:1948–64. doi: 10.1074/mcp.RA118.000644
234. Kim CW, Lee HM, Lee TH, Kang C, Kleinman HK, Gho YS. Extracellular membrane vesicles from tumor cells promote angiogenesis via sphingomyelin. Cancer Res. (2002) 62:6312–7.
235. Gesierich S, Berezovskiy I, Ryschich E, Zoller M. Systemic induction of the angiogenesis switch by the tetraspanin D6.1A/CO-029. Cancer Res. (2006) 66:7083–94. doi: 10.1158/0008-5472.can-06-0391
236. Meckes DGJr, Gunawardena HP, Dekroon RM, Heaton PR, Edwards RH, Ozgur S, et al. Modulation of B-cell exosome proteins by gamma herpesvirus infection. Proc Natl Acad Sci USA. (2013) 110:E2925–2933. doi: 10.1073/pnas.1303906110
237. Yu J, May L, Milsom C, Anderson GM, Weitz JI, Luyendyk JP, et al. Contribution of host-derived tissue factor to tumor neovascularization. Arterioscler Thromb Vasc Biol. (2008) 28:1975–81. doi: 10.1161/atvbaha.108.175083
238. Janowska-Wieczorek A, Wysoczynski M, Kijowski J, Marquez-Curtis L, Machalinski B, Ratajczak J, et al. Microvesicles derived from activated platelets induce metastasis and angiogenesis in lung cancer. Int J Cancer. (2005) 113:752–60. doi: 10.1002/ijc.20657
239. Taverna S, Flugy A, Saieva L, Kohn EC, Santoro A, Meraviglia S, et al. Role of exosomes released by chronic myelogenous leukemia cells in angiogenesis. Int J Cancer. (2012) 130:2033–43. doi: 10.1002/ijc.26217
240. Zagrean AM, Hermann DM, Opris I, Zagrean L, Popa-Wagner A. Multicellular crosstalk between exosomes and the neurovascular Unit after cerebral ischemia. Therapeutic implications. Front Neurosci. (2018) 12:811. doi: 10.3389/fnins.2018.00811
241. Castellana D, Zobairi F, Martinez MC, Panaro MA, Mitolo V, Freyssinet JM, et al. Membrane microvesicles as actors in the establishment of a favorable prostatic tumoral niche: a role for activated fibroblasts and CX3CL1-CX3CR1 axis. Cancer Res. (2009) 69:785–93. doi: 10.1158/0008-5472.can-08-1946
242. Ginestra A, La Placa MD, Saladino F, Cassara D, Nagase H, Vittorelli ML. The amount and proteolytic content of vesicles shed by human cancer cell lines correlates with their in vitro invasiveness. Anticancer Res. (1998) 18:3433–7.
243. Gong J, Luk F, Jaiswal R, Bebawy M. Microparticles mediate the intercellular regulation of microRNA-503 and proline-rich tyrosine kinase 2 to alter the migration and invasion capacity of breast cancer cells. Front Oncol. (2014) 4:220. doi: 10.3389/fonc.2014.00220
244. Higginbotham JN, Demory Beckler M, Gephart JD, Franklin JL, Bogatcheva G, Kremers GJ, et al. Amphiregulin exosomes increase cancer cell invasion. Curr Biol. (2011) 21:779–86. doi: 10.1016/j.cub.2011.03.043
245. Singh R, Pochampally R, Watabe K, Lu Z, Mo YY. Exosome-mediated transfer of miR-10b promotes cell invasion in breast cancer. Mol Cancer. (2014) 13:256. doi: 10.1186/1476-4598-13-256
246. Chowdhury R, Webber JP, Gurney M, Mason MD, Tabi Z, Clayton A. Cancer exosomes trigger mesenchymal stem cell differentiation into pro-angiogenic and pro-invasive myofibroblasts. Oncotarget. (2015) 6:715–31. doi: 10.18632/oncotarget.2711
247. Ostenfeld MS, Jeppesen DK, Laurberg JR, Boysen AT, Bramsen JB, Primdal-Bengtson B, et al. Cellular disposal of miR23b by RAB27-dependent exosome release is linked to acquisition of metastatic properties. Cancer Res. (2014) 74:5758–71. doi: 10.1158/0008-5472.can-13-3512
248. Wang T, Gilkes DM, Takano N, Xiang L, Luo W, Bishop CJ, et al. Hypoxia-inducible factors and RAB22A mediate formation of microvesicles that stimulate breast cancer invasion and metastasis. Proc Natl Acad Sci USA. (2014) 111:E3234–42. doi: 10.1073/pnas.1410041111
249. Jung T, Castellana D, Klingbeil P, Cuesta Hernandez I, Vitacolonna M, Orlicky DJ, et al. CD44v6 dependence of premetastatic niche preparation by exosomes. Neoplasia. (2009) 11:1093–105. doi: 10.1593/neo.09822
250. Rana S, Malinowska K, Zoller M. Exosomal tumor microRNA modulates premetastatic organ cells. Neoplasia. (2013) 15:281–95. doi: 10.1593/neo.122010
251. Tominaga N, Kosaka N, Ono M, Katsuda T, Yoshioka Y, Tamura K, et al. Brain metastatic cancer cells release microRNA-181c-containing extracellular vesicles capable of destructing blood-brain barrier. Nat Commun. (2015) 6:6716. doi: 10.1038/ncomms7716
252. Liu Y, Xiang X, Zhuang X, Zhang S, Liu C, Cheng Z, et al. Contribution of MyD88 to the tumor exosome-mediated induction of myeloid derived suppressor cells. Am J Pathol. (2010) 176:2490–9. doi: 10.2353/ajpath.2010.090777
253. Deng Z, Cheng Z, Xiang X, Yan J, Zhuang X, Liu C, et al. Tumor cell cross talk with tumor-associated leukocytes leads to induction of tumor exosomal fibronectin and promotes tumor progression. Am J Pathol. (2012) 180:390–8. doi: 10.1016/j.ajpath.2011.09.023
254. Peinado H, Zhang H, Matei IR, Costa-Silva B, Hoshino A, Rodrigues G, et al. Pre-metastatic niches: organ-specific homes for metastases. Nat Rev Cancer. (2017) 17:302–17. doi: 10.1038/nrc.2017.6
255. Lu X, Kang Y. Organotropism of breast cancer metastasis. J Mammary Gland Biol Neoplasia. (2007) 12:153–62. doi: 10.1007/s10911-007-9047-3
256. Cox TR, Rumney RM, Schoof EM, Perryman L, Hoye AM, Agrawal A, et al. The hypoxic cancer secretome induces pre-metastatic bone lesions through lysyl oxidase. Nature. (2015) 522:106–10. doi: 10.1038/nature14492
257. Liu Y, Cao X. Organotropic metastasis: role of tumor exosomes. Cell Research. (2016) 26:149–50. doi: 10.1038/cr.2015.153
258. Luga V, Zhang L, Viloria-Petit AM, Ogunjimi AA, Inanlou MR, Chiu E, et al. Exosomes mediate stromal mobilization of autocrine Wnt-PCP signaling in breast cancer cell migration. Cell. (2012) 151:1542–56. doi: 10.1016/j.cell.2012.11.024
259. Kaplan RN, Riba RD, Zacharoulis S, Bramley AH, Vincent L, Costa C, et al. VEGFR1-positive haematopoietic bone marrow progenitors initiate the pre-metastatic niche. Nature. (2005) 438:820–7. doi: 10.1038/nature04186
260. Hiratsuka S, Watanabe A, Aburatani H, Maru Y. Tumour-mediated upregulation of chemoattractants and recruitment of myeloid cells predetermines lung metastasis. Nat Cell Biol. (2006) 8:1369–75. doi: 10.1038/ncb1507
261. Erler JT, Bennewith KL, Cox TR, Lang G, Bird D, Koong A, et al. Hypoxia-induced lysyl oxidase is a critical mediator of bone marrow cell recruitment to form the premetastatic niche. Cancer Cell. (2009) 15:35–44. doi: 10.1016/j.ccr.2008.11.012
262. Grange C, Tapparo M, Collino F, Vitillo L, Damasco C, Deregibus MC, et al. Microvesicles released from human renal cancer stem cells stimulate angiogenesis and formation of lung premetastatic niche. Cancer Res. (2011) 71:5346–56. doi: 10.1158/0008-5472.can-11-0241
263. Chin AR, Wang SE. Cancer tills the premetastatic field: Mechanistic basis and clinical Implications. Clin Cancer Res. (2016) 22:3725–33. doi: 10.1158/1078-0432.ccr-16-0028
264. Hood JL, San RS, Wickline SA. Exosomes released by melanoma cells prepare sentinel lymph nodes for tumor metastasis. Cancer Res. (2011) 71:3792–801. doi: 10.1158/0008-5472.can-10-4455
265. Costa-Silva B, Aiello NM, Ocean AJ, Singh S, Zhang H, Thakur BK, et al. Pancreatic cancer exosomes initiate pre-metastatic niche formation in the liver. Nat Cell Biol. (2015) 17:816–26. doi: 10.1038/ncb3169
266. Fong MY, Zhou W, Liu L, Alontaga AY, Chandra M, Ashby J, et al. Breast-cancer-secreted miR-122 reprograms glucose metabolism in premetastatic niche to promote metastasis. Nat Cell Biol. (2015) 17:183–94. doi: 10.1038/ncb3094
267. Le MT, Hamar P, Guo C, Basar E, Perdigao-Henriques R, Balaj L, et al. miR-200-containing extracellular vesicles promote breast cancer cell metastasis. J Clin Invest. (2014) 124:5109–28. doi: 10.1172/jci75695
268. Aguado BA, Bushnell GG, Rao SS, Jeruss JS, Shea LD. Engineering the pre-metastatic niche. Nat Biomed Eng. (2017) 1:0077. doi: 10.1038/s41551-017-0077
269. Marleau AM, Chen CS, Joyce JA, Tullis RH. Exosome removal as a therapeutic adjuvant in cancer. J Transl Med. (2012) 10:134. doi: 10.1186/1479-5876-10-134
270. Lima LG, Chammas R, Monteiro RQ, Moreira ME, Barcinski MA. Tumor-derived microvesicles modulate the establishment of metastatic melanoma in a phosphatidylserine-dependent manner. Cancer Lett. (2009) 283:168–75. doi: 10.1016/j.canlet.2009.03.041
271. Combes V, Latham SL, Wen B, Allison AC, Grau GE. Diannexin down-modulates TNF-induced endothelial microparticle release by blocking membrane budding process. Int J Innov Med Health Sci. (2016) 7:1–11.
272. Cai Z, Yang F, Yu L, Yu Z, Jiang L, Wang Q, et al. Activated T cell exosomes promote tumor invasion via Fas signaling pathway. J Immunol. (2012) 188:5954–61. doi: 10.4049/jimmunol.1103466
273. Mitchell PS, Parkin RK, Kroh EM, Fritz BR, Wyman SK, Pogosova-Agadjanyan EL, et al. Circulating microRNAs as stable blood-based markers for cancer detection. Proc Natl Acad Sci USA. (2008) 105:10513–8. doi: 10.1073/pnas.0804549105
274. Tavoosidana G, Ronquist G, Darmanis S, Yan J, Carlsson L, Wu D, et al. Multiple recognition assay reveals prostasomes as promising plasma biomarkers for prostate cancer. Proc Natl Acad Sci USA. (2011) 108:8809–14. doi: 10.1073/pnas.1019330108
275. Overbye A, Skotland T, Koehler CJ, Thiede B, Seierstad T, Berge V, et al. Identification of prostate cancer biomarkers in urinary exosomes. Oncotarget. (2015) 6:30357–76. doi: 10.18632/oncotarget.4851
276. Vardaki I, Ceder S, Rutishauser D, Baltatzis G, Foukakis T, Panaretakis T. Periostin is identified as a putative metastatic marker in breast cancer-derived exosomes. Oncotarget. (2016) 7:74966–78. doi: 10.18632/oncotarget.11663
277. Silvers CR, Liu YR, Wu CH, Miyamoto H, Messing EM, Lee YF. Identification of extracellular vesicle-borne periostin as a feature of muscle-invasive bladder cancer. Oncotarget. (2016) 7:23335–45. doi: 10.18632/oncotarget.8024
278. Melo SA, Luecke LB, Kahlert C, Fernandez AF, Gammon ST, Kaye J, et al. Glypican-1 identifies cancer exosomes and detects early pancreatic cancer. Nature. (2015) 23:177–82. doi: 10.1038/nature14581
279. Kahlert C, Melo SA, Protopopov A, Tang J, Seth S, Koch M, et al. Identification of double-stranded genomic DNA spanning all chromosomes with mutated KRAS and p53 DNA in the serum exosomes of patients with pancreatic cancer. J Biol Chem. (2014) 289:3869–75. doi: 10.1074/jbc.C113.532267
280. Ahadi A, Khoury S, Losseva M, Tran N. A comparative analysis of lncRNAs in prostate cancer exosomes and their parental cell lines. Genom Data. (2016) 9:7–9. doi: 10.1016/j.gdata.2016.05.010
281. Valadi H, Ekstrom K, Bossios A, Sjostrand M, Lee JJ, Lotvall JO. Exosome-mediated transfer of mRNAs and microRNAs is a novel mechanism of genetic exchange between cells. Nat Cell Biol. (2007) 9:654–9. doi: 10.1038/ncb1596
282. Pascucci L, Cocce V, Bonomi A, Ami D, Ceccarelli P, Ciusani E, et al. Paclitaxel is incorporated by mesenchymal stromal cells and released in exosomes that inhibit in vitro tumor growth: a new approach for drug delivery. J Control Release. (2014) 192:262–70. doi: 10.1016/j.jconrel.2014.07.042
283. Tang K, Zhang Y, Zhang H, Xu P, Liu J, Ma J, et al. Delivery of chemotherapeutic drugs in tumour cell-derived microparticles. Nat Commun. (2012) 3:1282. doi: 10.1038/ncomms2282
284. Jang SC, Kim OY, Yoon CM, Choi DS, Roh TY, Park J, et al. Bioinspired exosome-mimetic nanovesicles for targeted delivery of chemotherapeutics to malignant tumors. ACS Nano. (2013) 7:7698–710. doi: 10.1021/nn402232g
285. Tian Y, Li S, Song J, Ji T, Zhu M, Anderson GJ, et al. A doxorubicin delivery platform using engineered natural membrane vesicle exosomes for targeted tumor therapy. Biomaterials. (2014) 35:2383–90. doi: 10.1016/j.biomaterials.2013.11.083
286. Munagala R, Aqil F, Jeyabalan J, Gupta RC. Bovine milk-derived exosomes for drug delivery. Cancer Lett. (2016) 371:48–61. doi: 10.1016/j.canlet.2015.10.020
287. Aqil F, Kausar H, Agrawal AK, Jeyabalan J, Kyakulaga AH, Munagala R, et al. Exosomal formulation enhances therapeutic response of celastrol against lung cancer. Exp Mol Pathol. (2016) 101:12–21. doi: 10.1016/j.yexmp.2016.05.013
288. Sun D, Zhuang X, Xiang X, Liu Y, Zhang S, Liu C, et al. A novel nanoparticle drug delivery system: the anti-inflammatory activity of curcumin is enhanced when encapsulated in exosomes. Mol Ther. (2010) 18:1606–14. doi: 10.1038/mt.2010.105
289. Li X, Tsibouklis J, Weng T, Zhang B, Yin G, Feng G, et al. Nano carriers for drug transport across the blood-brain barrier. J Drug Target. (2017) 25:17–28. doi: 10.1080/1061186x.2016.1184272
290. Kosaka N, Iguchi H, Yoshioka Y, Hagiwara K, Takeshita F, Ochiya T. Competitive interactions of cancer cells and normal cells via secretory microRNAs. J Biol Chem. (2012) 287:1397–405. doi: 10.1074/jbc.M111.288662
291. Ohno S, Takanashi M, Sudo K, Ueda S, Ishikawa A, Matsuyama N, et al. Systemically injected exosomes targeted to EGFR deliver antitumor microRNA to breast cancer cells. Mol Ther. (2013) 21:185–91. doi: 10.1038/mt.2012.180
292. Katakowski M, Buller B, Zheng X, Lu Y, Rogers T, Osobamiro O, et al. Exosomes from marrow stromal cells expressing miR-146b inhibit glioma growth. Cancer Lett. (2013) 335:201–4. doi: 10.1016/j.canlet.2013.02.019
293. Shimbo K, Miyaki S, Ishitobi H, Kato Y, Kubo T, Shimose S, et al. Exosome-formed synthetic microRNA-143 is transferred to osteosarcoma cells and inhibits their migration. Biochem Biophys Res Commun. (2014) 445:381–7. doi: 10.1016/j.bbrc.2014.02.007
294. Alvarez-Erviti L, Seow Y, Yin H, Betts C, Lakhal S, Wood MJ. Delivery of siRNA to the mouse brain by systemic injection of targeted exosomes. Nat Biotechnol. (2011) 29:341–5. doi: 10.1038/nbt.1807
295. Shtam TA, Kovalev RA, Varfolomeeva EY, Makarov EM, Kil YV, Filatov MV. Exosomes are natural carriers of exogenous siRNA to human cells in vitro. Cell Commun Signal. (2013) 11:88. doi: 10.1186/1478-811x-11-88
296. Lunavat TR, Jang SC, Nilsson L, Park HT, Repiska G, Lasser C, et al. RNAi delivery by exosome-mimetic nanovesicles - Implications for targeting c-Myc in cancer. Biomaterials. (2016) 102:231–8. doi: 10.1016/j.biomaterials.2016.06.024
297. Greco KA, Franzen CA, Foreman KE, Flanigan RC, Kuo PC, Gupta GN. PLK-1 silencing in bladder cancer by siRNA delivered with exosomes. Urology. (2016) 91:241.e241–247. doi: 10.1016/j.urology.2016.01.028
298. Mizrak A, Bolukbasi MF, Ozdener GB, Brenner GJ, Madlener S, Erkan EP, et al. Genetically engineered microvesicles carrying suicide mRNA/protein inhibit schwannoma tumor growth. Mol Ther. (2013) 21:101–8. doi: 10.1038/mt.2012.161
299. Mahaweni NM, Kaijen-Lambers ME, Dekkers J, Aerts JG, Hegmans JP. Tumour-derived exosomes as antigen delivery carriers in dendritic cell-based immunotherapy for malignant mesothelioma. J Extracell Vesicles. (2013) 2:22492. doi: 10.3402/jev.v2i0.22492
300. Cho JA, Yeo DJ, Son HY, Kim HW, Jung DS, Ko JK, et al. Exosomes: a new delivery system for tumor antigens in cancer immunotherapy. Int J Cancer. (2005) 114:613–22. doi: 10.1002/ijc.20757
301. Delcayre A, Estelles A, Sperinde J, Roulon T, Paz P, Aguilar B, et al. Exosome display technology: applications to the development of new diagnostics and therapeutics. Blood Cells Mol Dis. (2005) 35:158–68. doi: 10.1016/j.bcmd.2005.07.003
302. Otzen DE, Blans K, Wang H, Gilbert GE, Rasmussen JT. Lactadherin binds to phosphatidylserine-containing vesicles in a two-step mechanism sensitive to vesicle size and composition. Biochim Biophys Acta. (2012) 1818:1019–27. doi: 10.1016/j.bbamem.2011.08.032
303. Hartman ZC, Wei J, Glass OK, Guo H, Lei G, Yang XY, et al. Increasing vaccine potency through exosome antigen targeting. Vaccine. (2011) 29:9361–7. doi: 10.1016/j.vaccine.2011.09.133
304. Rountree RB, Mandl SJ, Nachtwey JM, Dalpozzo K, Do L, Lombardo JR, et al. Exosome targeting of tumor antigens expressed by cancer vaccines can improve antigen immunogenicity and therapeutic efficacy. Cancer Res. (2011) 71:5235–44. doi: 10.1158/0008-5472.can-10-4076
305. Zhang H, Huang B. Tumor cell-derived microparticles: a new form of cancer vaccine. Oncoimmunology. (2015) 4:e1017704. doi: 10.1080/2162402x.2015.1017704
306. Escudier B, Dorval T, Chaput N, Andre F, Caby MP, Novault S, et al. Vaccination of metastatic melanoma patients with autologous dendritic cell (DC) derived-exosomes: results of thefirst phase I clinical trial. J Transl Med. (2005) 3:10. doi: 10.1186/1479-5876-3-10
307. Wu K, Xing F, Wu SY, Watabe K. Extracellular vesicles as emerging targets in cancer: Recent development from bench to bedside. Biochim Biophys Acta Rev Cancer. (2017) 1868:538–63. doi: 10.1016/j.bbcan.2017.10.001
308. Xu R, Rai A, Chen M, Suwakulsiri W, Greening DW, Simpson RJ. Extracellular vesicles in cancer - implications for future improvements in cancer care. Nat Rev Clin Oncol. (2018) 15:617–38. doi: 10.1038/s41571-018-0036-9
309. Maas SL, De Vrij J, Van Der Vlist EJ, Geragousian B, Van Bloois L, Mastrobattista E, et al. Possibilities and limitations of current technologies for quantification of biological extracellular vesicles and synthetic mimics. J Control Release. (2015) 200:87–96. doi: 10.1016/j.jconrel.2014.12.041
310. Valkonen S, Van Der Pol E, Boing A, Yuana Y, Yliperttula M, Nieuwland R, et al. Biological reference materials for extracellular vesicle studies. Eur J Pharm Sci. (2017) 98:4–16. doi: 10.1016/j.ejps.2016.09.008
311. Baek R, Sondergaard EK, Varming K, Jorgensen MM. The impact of various preanalytical treatments on the phenotype of small extracellular vesicles in blood analyzed by protein microarray. J Immunol Methods. (2016) 438:11–20. doi: 10.1016/j.jim.2016.08.007
312. Taylor DD, Shah S. Methods of isolating extracellular vesicles impact down-stream analyses of their cargoes. Methods. (2015) 87:3–10. doi: 10.1016/j.ymeth.2015.02.019
313. Momen-Heravi F, Balaj L, Alian S, Trachtenberg AJ, Hochberg FH, Skog J, et al. Impact of biofluid viscosity on size and sedimentation efficiency of the isolated microvesicles. Front Physiol. (2012) 3:162. doi: 10.3389/fphys.2012.00162
314. Xu R, Greening DW, Rai A, Ji H, Simpson RJ. Highly-purified exosomes and shed microvesicles isolated from the human colon cancer cell line LIM1863 by sequential centrifugal ultrafiltration are biochemically and functionally distinct. Methods. (2015) 87:11–25. doi: 10.1016/j.ymeth.2015.04.008
315. Lobb RJ, Becker M, Wen SW, Wong CS, Wiegmans AP, Leimgruber A, et al. Optimized exosome isolation protocol for cell culture supernatant and human plasma. J Extracell Vesicles. (2015) 4:27031. doi: 10.3402/jev.v4.27031
316. Rekker K, Saare M, Roost AM, Kubo AL, Zarovni N, Chiesi A, et al. Comparison of serum exosome isolation methods for microRNA profiling. Clin Biochem. (2014) 47:135–8. doi: 10.1016/j.clinbiochem.2013.10.020
317. Abramowicz A, Marczak L, Wojakowska A, Zapotoczny S, Whiteside TL, Widlak P, et al. Harmonization of exosome isolation from culture supernatants for optimized proteomics analysis. PLoS ONE. (2018) 13:e0205496. doi: 10.1371/journal.pone.0205496
318. Mathivanan S, Lim JW, Tauro BJ, Ji H, Moritz RL, Simpson RJ. Proteomics analysis of A33 immunoaffinity-purified exosomes released from the human colon tumor cell line LIM1215 reveals a tissue-specific protein signature. Mol Cell Proteomics. (2010) 9:197–208. doi: 10.1074/mcp.M900152-MCP200
319. Tauro BJ, Greening DW, Mathias RA, Ji H, Mathivanan S, Scott AM, et al. Comparison of ultracentrifugation, density gradient separation, and immunoaffinity capture methods for isolating human colon cancer cell line LIM1863-derived exosomes. Methods. (2012) 56:293–304. doi: 10.1016/j.ymeth.2012.01.002
320. Fang S, Tian H, Li X, Jin D, Li X, Kong J, et al. Clinical application of a microfluidic chip for immunocapture and quantification of circulating exosomes to assist breast cancer diagnosis and molecular classification. PLoS ONE. (2017) 12:e0175050. doi: 10.1371/journal.pone.0175050
321. Chen Y, Ma Y, Li N, Wang H, Chen B, Liang Z, et al. Efficacy and long-term longitudinal follow-up of bone marrow mesenchymal cell transplantation therapy in a diabetic patient with recurrent lower limb bullosis diabeticorum. Stem Cell Res Ther. (2018) 9:99. doi: 10.1186/s13287-018-0854-9
322. Im H, Shao H, Park YI, Peterson VM, Castro CM, Weissleder R, et al. Label-free detection and molecular profiling of exosomes with a nano-plasmonic sensor. Nat Biotechnol. (2014) 32:490–5. doi: 10.1038/nbt.2886
323. Lane RE, Korbie D, Anderson W, Vaidyanathan R, Trau M. Analysis of exosome purification methods using a model liposome system and tunable-resistive pulse sensing. Sci Rep. (2015) 5:7639. doi: 10.1038/srep07639
324. Liang K, Liu F, Fan J, Sun D, Liu C, Lyon CJ, et al. Nanoplasmonic quantification of tumour-derived extracellular vesicles in plasma microsamples for diagnosis and treatment monitoring. Nat Biomed Eng. (2017) 1:0021. doi: 10.1038/s41551-016-0021
325. Zitvogel L, Regnault A, Lozier A, Wolfers J, Flament C, Tenza D, et al. Eradication of established murine tumors using a novel cell-free vaccine: dendritic cell-derived exosomes. Nat Med. (1998) 4:594–600.
326. Besse B, Charrier M, Lapierre V, Dansin E, Lantz O, Planchard D, et al. Dendritic cell-derived exosomes as maintenance immunotherapy after first line chemotherapy in NSCLC. Oncoimmunology. (2016) 5:e1071008. doi: 10.1080/2162402x.2015.1071008
327. Harris DA, Patel SH, Gucek M, Hendrix A, Westbroek W, Taraska JW. Exosomes released from breast cancer carcinomas stimulate cell movement. PLoS ONE. (2015) 10:117495. doi: 10.1371/journal.pone.0117495
328. Zomer A, Maynard C, Verweij FJ, Kamermans A, Schäfer R, Beerling E, et al. In vivo imaging reveals extracellular vesicle-mediated phenocopying of metastatic behavior. Cell. (2015) 161:1046–57. doi: 10.1016/j.cell.2015.04.042
329. Ju S, Mu J, Dokland T, Zhuang X, Wang Q, Jiang H, et al. Grape exosome-like nanoparticles induce intestinal stem cells and protect mice from DSS-induced colitis. Mol Ther. (2013) 21:1345–57. doi: 10.1038/mt.2013.64
330. Wang AS, Kleinerman R, Armstrong AW, Fitzmaurice S, Pascucci A, Awasthi S, et al. Set-back versus buried vertical mattress suturing: results of a randomized blinded trial. J Am Acad Dermatol. (2015) 72:674–80. doi: 10.1016/j.jaad.2014.07.018
331. Luan X, Sansanaphongpricha K, Myers I, Chen H, Yuan H, Sun D. Engineering exosomes as refined biological nanoplatforms for drug delivery. Acta Pharmacol Sin. (2017) 38:754–63. doi: 10.1038/aps.2017.12
332. Kim MS, Haney MJ, Zhao Y, Mahajan V, Deygen I, Klyachko NL, et al. Development of exosome-encapsulated paclitaxel to overcome MDR in cancer cells. Nanomedicine. (2016) 12:655–64. doi: 10.1016/j.nano.2015.10.012
Keywords: cancer, cancer immunosuppression, cancer vaccine, exosome, extracellular vesicles, microparticle, pre-metastatic niche
Citation: Jaiswal R and Sedger LM (2019) Intercellular Vesicular Transfer by Exosomes, Microparticles and Oncosomes - Implications for Cancer Biology and Treatments. Front. Oncol. 9:125. doi: 10.3389/fonc.2019.00125
Received: 07 November 2018; Accepted: 12 February 2019;
Published: 06 March 2019.
Edited by:
Andreas Pircher, Innsbruck Medical University, AustriaReviewed by:
Lucas Treps, Vesalius Research Center, BelgiumYu Fujita, Jikei University School of Medicine, Japan
Copyright © 2019 Jaiswal and Sedger. This is an open-access article distributed under the terms of the Creative Commons Attribution License (CC BY). The use, distribution or reproduction in other forums is permitted, provided the original author(s) and the copyright owner(s) are credited and that the original publication in this journal is cited, in accordance with accepted academic practice. No use, distribution or reproduction is permitted which does not comply with these terms.
*Correspondence: Lisa M. Sedger, bGlzYS5zZWRnZXJAdXRzLmVkdS5hdQ==