- Department of Physiology, University of Prishtina, Prishtina, Kosovo
An increasing amount of research has recently strengthened the case for the existence of iron dysmetabolism in prostate cancer. It is characterized with a wide array of differential expression of iron-related proteins compared to normal cells. These proteins control iron entry, cellular iron distribution but also iron exit from prostate cells. Iron dysmetabolism is not an exclusive feature of prostate cancer cells, but it is observed in other cells of the tumor microenvironment. Disrupting the machinery that secures iron for prostate cancer cells can retard tumor growth and its invasive potential. This review unveils the current understanding of the ways that prostate cancer cells secure iron in the tumor milieu and how can we exploit this knowledge for therapeutic purposes.
Introduction
Cancer cells are known for their voracious appetites for different metabolites and nutrients in order to satisfy their metabolic needs (1). Creating an environment that suits these needs means that cancer cells have to adapt manifold; they have to be able to secure energy for their metabolism in hypoxic conditions, but also they should be able to increase the transport of metabolites and micronutrients, even by “stealing” from their “neighborhood” (1). Eventually, this strategy proves successful for cancer cells, because they will invade transport fluids (blood, lymph) and spread across the body. One of the micronutrients that provides cancer cells the ability to grow and survive is iron. Its importance has been observed when cancer cells are introduced in iron-rich and iron-starving conditions. Iron-rich supply helps cancer cell growth, while iron starvation retards their growth (2, 3). But, cancer cells face a problem due to their high cellular iron supply; iron overload can cause an increase in reactive oxygen species, which are harmful for cell structures. Cancer cells have developed mechanisms that protect them against oxidative damage. These mechanisms are used by different cancers, including prostate cancer (PCa) cells. The increased activity of enzymes with antioxidant properties ensures a proper environment for cancer cells, where they can use high levels of iron without the accompanying side effect of oxidative damage (4–6). Thus, only very high levels of cellular iron can be detrimental for cancer cells (7).
Iron is used by cancer cells for important biochemical reactions, such as DNA synthesis, mitochondrial metabolism, tumor proliferation through increased angiogenesis, and metastasis (8). In PCa, iron is also important for tumor proliferation. Similar to other cancers, PCa cells need iron for their survival, including the use of iron for the activity of enzymes that control androgen receptor (AR) transcriptional activity, which is a known promoter of PCa (9). What is more, iron is needed by PCa cells to “reorganize” the intracellular enzymatic activity in order to increase energy production and extracellular matrix degradation (10, 11). Clinical data suggest that, in PCa, there is an increased iron sequestration in cancer cells, while in normal cells adjacent to PCa cells iron levels are low (12, 13).
Iron Entry in PCa Cells
Transferrin receptors (TFRs) are major routes of transferrin-bound iron (TBI) into cells (14). TFR is responsible for endocytosing TBI and releasing it in intracellular compartments (14). Inside endosomes created during this process iron is reduced and finally released in cytoplasm via divalent metal transporter 1 (DMT1) (14). PCa cells need more TFRs to increase their iron uptake, which is why we observe upregulation of these receptors in this cancer (15–19). But, studies with cultured PCa cells have shown that iron can enter PCa cells even when TFR is blocked (10). PCa models show that TFR gene (TFRC) is a downstream target of the prostatic oncogene MYC (18, 19), while TFRC expression has been used successfully to detect precancerous prostate lesions with transferrin-based PET imaging (19). The dysregulation of TFR expression is not the only anomaly detected during TBI uptake in PCa cells. Endocytosed TFR is not localized in perinuclear sites in PCa (as it happens in non-tumorous prostate cells), but is rather distributed diffusely in the cytoplasm and cellular extensions (17). This could indicate that intracellular TFR traffic is another strategy used by PCa cells to redistribute iron for their metabolic needs (17). TFR has also been linked with AR gene expression, which is the main promoter of PCa. This link is at least partially mediated via the activity of vacuolar ATPase (V-ATPase), which is crucial in maintaining low endosomal pH (20–22). Low endosomal pH ensures the release of iron from transferrin, but when V-ATPase is inhibited less iron will be released from the cytoplasm (20). Low intracellular iron conditions increase the stability of hypoxia-inducible factor 1 alpha (HIF-1α), which in turn downregulates AR expression. This is important since AR downregulation retards PCa cell growth. Therefore, V-ATPase suppression has been proposed as an important target in PCa because it can inhibit tumor growth in different cancer lines, even in those resistant to androgen ablation (21). But, the activity of currently used compounds for suppression of V-ATPase is not cell specific which is why they are responsible for serious side effects observed in human patients (23).
Other important molecules studied in PCa are six-transmembrane epithelial antigen of prostate (STEAP) proteins. They are expressed in prostate tissue, where they serve numerous physiologic functions. STEAP2 is one of the most interesting proteins of the STEAP family known for its iron reductive properties (24). It is predominantly expressed in prostate tissue, located in the plasma membrane, but also in the intracellular vesicular structures (25). STEAP2 is frequently upregulated during prostatic malignancy, and importantly, its expression is in relation with Gleason score (24–26). Knockdown of STEAP2 significantly suppresses the proliferation of prostatic cancer, though current data suggest that this effect occurs probably due to STEAP2 ability to induce enzymatic activity that results in degradation of extracellular matrix (24, 27). The ability of STEAP2 to influence iron metabolism in PCa has not been studied, though the role of STEAP2 in iron metabolism has been observed in erythroid cells, choroid plexus, and gastrointestinal tract (28). On the other hand, intracellular six transmembrane prostate protein 2 (STAMP2, also known as STEAP4), which is another protein with metalloreductase activity, has been linked with PCa growth through dysregulation of iron metabolism (29, 30). Furthermore, it has to be noted that STAMP2 activity as a metalloreductase is more prominent compared to other STAMPs (31). Also, STAMP2 is regulated by AR, and similarly to STEAP2, its expression is linked with Gleason score (30). In other cancers, STAMP2 is involved in iron transport to mitochondria, but this action of STAMP2 has not been studied in PCa (32).
Intracellular Iron Protein Machinery in PCa
The most important intracellular regulators of iron metabolism are iron responsive element-binding proteins (IRPs). IRPs regulate the expression of iron import and export proteins (14). In low iron concentrations IRPs stimulate TFR1 upregulation and ferritin downregulation which ensures increased availability of iron for cellular needs (33). Although IRPs can modulate FPN activity as well, the existence of IRP-insensitive isoforms of FPN and of other more potent modulators of FPN make IRPs action on FPN modest compared to its actions on TFR1 and ferritin (34). Indeed, this observation has been confirmed in cancer cells as well (35).
In PCa IRP2 is upregulated to ensure proper amount of iron entry in PCa cells (16) (Figure 1). This effect occurs due to TFR1 upregulation and ferritin downregulation caused by IRP2 (16). The importance of IRP2 in PCa is observed during IRP2 knockout; downregulation of IRP2 significantly reduces proliferation of PCa cells in a similar fashion to iron chelation (16). On the other hand, loss of IRP1 does not seem to have profound implications on PCa iron metabolism and tumor growth (16). IRP2 dysregulation seems to be the norm in other cancers as well, where the consequences of IRP2 overexpression are similar to PCa (3, 36, 37). It is still not known what is the cause behind overexpression of IRP2 in PCa, but oncogenes are supposed to be potential culprits (16, 35).
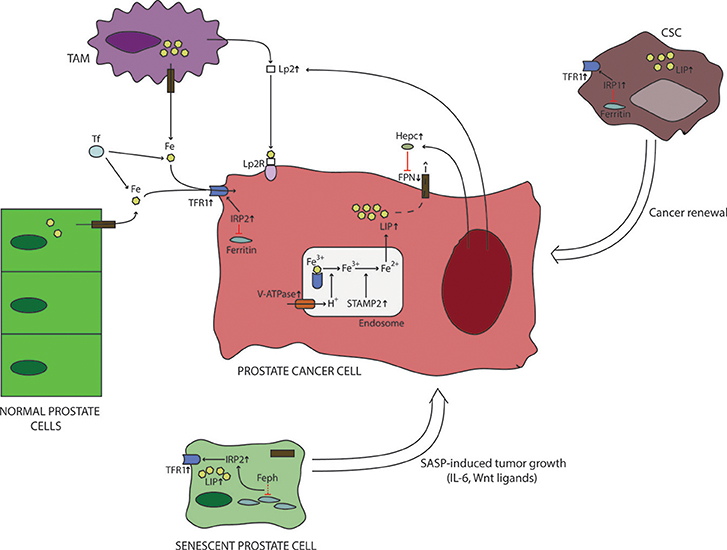
Figure 1. Iron metabolism in prostate cancer microenvironment. Prostate cancer cells are characterized with a differential expression of iron-related proteins compared to normal cells. These changes include upregulation of iron-import proteins (TFR1), overexpression of intracellular regulators of iron metabolism (IRP2), downregulation of iron export (FPN). In addition, recent data suggest that prostate cancer cells increase their labile iron pool by increasing the activity of hydrogen pumps and ferrireductases, which causes increased release of iron from endosomes. These actions will further increase labile iron pool. In addition, prostate cancer cells interact with their surroundings to increase iron delivery. This is believed to be done by stimulating TAMs to produce Lp2, which then binds iron and delivers it to cancer cells. Also, PCa cells secrete hepcidin locally to reduce their iron export through FPN downregulation and Lp2 to increase iron supply. Iron dymetabolism is also a feature of CSCs and sensescent prostate cells, whose numbers are increased in cancer. It is believed that iron dysregulation observed in CSCs is important for their survival and renewal of cancer cells. On the other hand, senescent prostate cells are known to secrete different molecules, some of which can directly influence the production of iron-related proteins by prostate cancer cells. CSC, cancer stem cell; Feph, ferritinophagy; FPN, ferroportin; Hepc, hepcidin; IL-6, interleukin-6; IRP, iron responsive element-binding protein; LIP, labile iron pool; Lp2R, lipocalin 2 receptor; SASP, senescence-associated secretory phenotype; STAMP2, six transmembrane prostate protein 2; TAM, tumor-associated macrophage; TFR1, transferrin receptor 1; V-ATPase, vacuolar ATPase; Wnt, wingless/integrated pathway.
Iron Export in PCa Cells
Ferroportin (FPN) is the only known cellular iron exporter. In many cancers its activity is downregulated, which increases iron pool in cancer cells (38–40). Similarly, FPN dowregulation has also been observed in PCa (41–44). By stimulating FPN activity we can curb the growth and proliferation of PCa (41–45). This occurs due to cellular iron deprivation caused by increased activity of FPN. Furthermore, consistent FPN overexpression overrules compensatory mechanisms used by PCa cells to replete iron depots via increase in iron import (16, 44). More importantly, FPN overexpression reduces tumor proliferation in different types of PCa's, and irrespective of androgen sensitivity (44).
FPN expression is regulated by different stimuli. It is the main target protein of hepcidin, which induces FPN degradation (46). So, it is no surprise to find that local hepcidin is upregulated in cancer cells, including PCa (47–49). Prostatic hepcidin is regulated via interleukin 6 (IL6), similar to systemic (liver) hepcidin, but non-cannonical pathways, such as wingless/integrated (Wnt) pathway, sclerostin domain-containing protein 1 (SOSTDC1) and bone morphogenetic protein 4/7 (BMP4/7) are also responsible for PCa hepcidin expression, which suggests a differential control of hepcidin expression in PCa compared to liver hepcidin expression (47) (Table 1). Further evidence of involvement of hepcidin in PCa pathophysiology is its relation with prostate-specific antigen (PSA). In PCa cells with high hepcidin expression there exists a positive correlation between hepcidin and PSA expression, while markers of tumor proliferation and survival are significantly increased in PCa cells with hepcidin upregulation (48). In experiments with cultured PCa cells loss of hepcidin expression significantly reduces the proliferative ability of cancer cells, while addition of hepcidin increases the proliferative ability of cancer cell progressively (49). All these data support the notion that PCa growth can be retarded by influencing iron export through FPN. It is still not known how do other regulators of FPN expression, such as inflammation, assert their influence on FPN activity in PCa. The rationale exists, since inflammation has been shown to promote cellular iron accumulation in different cells, but also it can promote tumor proliferation in PCa (34, 50–52).
Another field of study with many unanswered questions is the relation of mitochondrial iron metabolism with PCa pathogenesis. The gene responsible for coding mitoferrin, which is a mitochondrial iron transporter, is upregulated in PCa patients, but the importance of mitoferrin in PCa cells has still not been elucidated despite its proposed role in cancer (53–56).
Tumor-Associated Macrophages (TAMs) and Cancer Stem Cells (CSCs) in PCa; New Players Influenced by Iron Metabolism in the Complex PCa Tumor Environment
An often overlooked player in the tumor microenvironment are TAMs. They are leukocytes that promote tumor growth in different cancers, including PCa (57–61). Density of macrophages is associated with poor prognosis and more aggressive behavior of PCa (57–59). In PCa, TAM activity is influenced by chemical signals from tumor cells (59). One such signal could be the induction of lipocalin 2 (Lp2) secretion (62), which is why increased Lp2 from TAMs has been proposed as another strategy from cancer cells to provide additional iron for their needs (63). Lp2 is a protein which has a strong binding capacity for iron chelating molecules such as siderophores (64). It is produced by neutrophils, macrophages, epithelial cells but also by tumor cells (62–64). In-vitro and in-vivo data with breast cancer models show that iron-loaded Lp2 produced by TAMs can increase the iron pool of tumor cells, and consequently, influence their growth (64, 65). The importance of Lp2 in PCa iron metabolism is further enhanced by its frequently observed overexpression in PCa which is related with cancer proliferation (66–69). Furthermore, serum Lp2 has been found in high levels in patients with PCa (69). Still, the details of the TAM-Lip2 connection with PCa cell iron metabolism have not been studied and should be a subject of future studies.
In terms of their actions in tumor microenvironment macrophages have been generally divided into cytotoxic (M1 macrophages) and tumor promoting phenotype (M2 macrophages) (58). One of the most important population of leukocytes in cancer including PCa are M2 macrophages. Studies have unraveled the iron-release phenotype of these macrophages, which would support the proliferation of neighboring cancer cells (58, 59, 70–72). In addition, macrophages treated with iron chelators reduce the growth of tumors and their metastatic ability by changing their iron releasing phenotype into an iron sequestring one (71, 72). Similarly, in PCa, iron-laded macrophages exhibit infiltrative behavior and predict low response to iron chelation therapy (73).
Dysregulation of the iron metabolism is not an exclusive feature of cancer cells in PCa. Recently, it has been observed in CSCs of PCa as well (74). This is an important observation, because CSCs as precursors of cancer cells have been linked with the replenishment of cancer cell numbers, metastasis and resistance to cancer therapy (75). In models with cultured CSCs, iron supplementation helps cellular growth, while iron chelation retards it (76–78). More importantly, iron chelation has the benefit of having the impact on stemness markers of CSCs even when standard chemotherapy fails (76). In PCa, CSC labile iron pool is increased due to upregulation of iron import proteins. The intracellular regulators of iron metabolism in PCa CSCs show distinct features; IRP1 is upregulated in both mRNA and protein levels due to inefficient Fe-S cluster assembly in mitochondria, while IRP2 is not (74). It is interesting that while IRP2 is subject of regulatory control by iron levels in CSCs, the same does not occur in differentiated cancer cells (3, 16, 36, 74, 79). This suggests a possible scenario for progression of iron dysmetabolism in PCa; it involves IRP2 overexpression in differentiated cancer cells as an important moment when a full blown loss of the regulatory mechanisms occurs in cancer cells, which would create a vicious cycle of iron overload and resultant progressive proliferation of cancer cells. This might have important clinical implications for the treatment of PCa, and in other cancers as well.
Iron Dysmetabolism is a Characteristic of Aging Prostate Cells?
It is well-known that PCa is a disease of the old age, and similarly, prostate cell dysmetabolism could be an important pathogenic feature of aging prostate cells. It is known that the increased number of senescent cells is associated with age-related diseases (80). Recent data suggest that prostate senescent cells suffer from an increased iron load due to changes in the expression of proteins that regulate cellular iron transport (81). Iron dysregulation observed in experimental models with senescent prostate epithelial cells is intriguing and strikingly similar to PCa cells; it is characterized with TFR1, IRP2, ferritin upregulation, while FPN though upregulated, is mostly localized intracellularly, which means that it cannot participate in iron export (81). The reason behind these changes in senescent cells occur due to impaired ferritinophagy, which means that increased iron is sequestered into ferritin. This would signal senescent cells that there is a lack of intracellular iron, which in response increase iron import through TFR1 upregulation. Chemical induction of ferritinophagy reverses this process (81). Future studies should investigate potential disturbances of the process of ferritinophagy and its role in PCa.
Senescent cells accumulate in cancer and contribute to the pool of cancer cells by releasing chemicals that promote tumor growth (82). Some of these chemicals, such as Wnt ligands and IL-6, are known as upregulators of hepcidin in PCa cells (47, 83). On the other hand, it remains to be seen if the observed iron phenotype of prostate senescent cells affects its secretome and subsequent tumor growth.
Systemic Iron Metabolism and PCa
Clinical studies suggest that homozygote carriers of hemochromatosis mutations are not especially in risk for PCa (84, 85). Iron has also been ruled out as an important occupational risk factor which could influence PCa occurrence (86, 87). Similarly, dietary iron intake does not seem to increase the risk for PCa, though there are doubts that iron intake might be associated with aggressive forms of PCa, especially in men with low intake of antioxidant foods (88, 89). On the other hand, data from a Dutch cohort with PCa patients showed that the combined intake of oxidants (iron) and antioxidants was not related with risk of advanced PCa (90). The Dutch cohort registered heme iron intake, while CARET cohort registered total iron intake, which might have been higher compared to their European counterparts. In any case, the relevance of Dutch cohort compared to CARET cohort is strengthened by a much higher number of patients included in the Dutch study (88, 90). Also, the associations between high iron intake/low antioxidant intake and aggressive PCa observed by CARET were not strong not just due to a smaller number of patients but also due to the fact that the associated positive trend was not statistically significant. Finally, other factors might have influenced results from CARET cohort; PCa patients from CARET cohort had higher body mass index (BMI), which is important, since studies suggest that BMI values indicative of obesity are related with higher Gleason score (or more aggressive PCa) (91, 92). It is interesting to notice that blood donors and blood recipients are not significantly protected or at risk from PCa, which further strengthens the case that systemic iron overload is not a significant factor for PCa risk (93, 94).
Other serum markers of iron metabolism have been studied in PCa, albeit in studies with small number of patients. For example, high levels of sTFR and hepcidin have been observed in these patients (95–97). Increased levels of sTFR are to be expected, considering the reactive TFR upregulation in erythroid cells due to anemia (often found in patients with PCa) and the increased expression of TFR in PCa cells. But, larger studies with different group of patients characterized according to age, inflammatory status, anemia status, local TFR, sTFR, and their correlations should give more answers concerning the importance of sTFR in PCa. On the other hand, one small study has revealed that serum hepcidin is increased in patients with bone metastasis, and that the increase in serum hepcidin is partly related to cytokine production, such as IL6, which is a known upregulator of hepcidin (97). In any case, these studies are rare and little conclusions can be taken out of them.
More contradictory data come from studies relating ferritin levels and its association with PCa. High and low levels of serum ferritin have been associated with PCa risk, while others have suggested no such association (96, 98–100). The differences in patient numbers and selection between the studies might have been the reason behind these discrepancies. Kuvibidilla et al. study, which reported presence of low levels of serum ferritin in PCa patients, included a small number of patients (100). In addition, the statistical significance was borderline and most of the patients did not have advanced PCa. It has to be mentioned that normal range of serum ferritin is very wide, therefore measurements of serum ferritin in a small number of patients, is subjected to false positive or negative results (101–103). Chua et al. study (which reported no association between serum ferritin and PCa risk) included a relatively low number of patients with PCa, and also did not have any information about the cancer stage of the patients (99). In one of the largest studies (2002 patients) of this kind done by Wang et al., serum ferritin was shown to be a predictor of PCa risk (98). Close examination of the Wang et al. study reveals that the differences in median values for serum ferritin though significant are still small. But, when the patients were grouped according to increased levels of ferritin, the statistical differences were increased, which means that the biggest odds ratio for PCa risk was detected with serum ferritin levels above 400 ng/ml. Also, this study revealed that the diagnostic sensitivity of serum ferritin in PCa was highest in patients with age >65 and serum ferritin >400 ng/ml. Finally, the study revealed that higher serum ferritin values were associated with higher Gleason score, and higher serum ferritin was associated with high PCa tissue ferritin. This is in-line with other studies that suggest that high serum ferritin is most likely observed in advanced PCa (96, 104). Wang et al. study has revealed that high serum ferritin is related with PCa risk, but only in a subset of patients with advanced PCa, and especially older patients. The increased serum ferritin in PCa could occur from different sources; cancer tissue, cytokines, damaged cells (105, 106).
Treating PCa by Modulating Iron Metabolism
The strategy to treat PCa by modulating tumor iron metabolism has already been subject of research. Iron chelators, first introduced in the 1960s, have been tested as anticancer drugs for 30 years (107, 108). In PCa, the use of experimental treatment with iron chelators goes back to 1995. In the study done by Kovar et al. deferoxamine (DFO) reduced tumor growth by 35 and 38%, respectively, depending on the tumor cell lines used (109). DFO efficacy was increased with co-treatment with anti-TFR antibodies. Soon after, first human trial was realized with a small number of patients suffering from hormone-refractory PCa (110). The use of DFO for 8 h did not yield any significant results in disease control. DFO failure in human trials occurred probably due to the drugs difficulty penetrating and accumulating in PCa cells when used in a systemic form (108). Deferiprone is another iron-chelator that has been used to treat PCa in cultured medium. It has shown antitumor activity in different PCa cell lines, by influencing tumor energy production, cell migration and tumor volume, but these results have not been validated in human trials with PCa patients (73, 111). Newer iron chelators have also shown antitumor properties but they have been only tested in cultured cells (112, 113). It has to be mentioned that iron chelation has its drawbacks, such as toxicity, patient compliance, induction of reactive increase in iron import proteins, lower efficacy with higher density of iron laded TAMs in tumors (73, 76, 107, 114).
Natural iron chelators have also been used for their antitumor properties. Curcumin, which is the active compound of the spice called turmeric, can suppress PCa growth. This action is attributed to curcumins ability to chelate iron, which is why PCa cells increase IRP activity and the expression of TFR1 in response to curcumin treatment (115, 116). Reactive response from tumor cells to curcumin actions can help them adapt to iron deprivation. This is probably the reason why TFR1 blockade in addition to curcumin supplementation improves the suppressive effect of this compound in PCa (116). It could be that the additional effect of curcumin in PCa is mediated by its inhibitory effect on hepcidin expression, which has already been observed in hepatocytes, but it has not been studied in PCa (117–119). Other compounds with iron chelating properties have been used in PCa cultured cells, such as epigallocatechin gallate (EGCG) (120). EGCG can retard tumor growth in PCa, but also suppresses PSA and AR expression (121–124). The effect of EGCG on PCa iron metabolism has yet to be studied but we know from its use in neurons that it can increase FPN activity and reduce hepcidin expression (125, 126). Adding to the evidence is the observation that frequent green tea use (EGCG is the main component of the green tea) has been associated with lower incidence of PCa (127). On the other hand, the effect of supplementation with different polyphenols, including EGCG and curcumin has been studied in a randomized trial with PCa patients. This trial has shown that supplementation which includes EGCG and turmeric can significantly reduce PSA levels in short-term (128). Unfortunately, this trial did not examine direct objective evidence (prostate biopsy, MRI) that would link more clearly supplementation with EGCG and curcumin with PCa progression. Use of natural compounds with the ability to modulate the iron metabolism in PCa cells has many challenges before they can be used as new drugs in treatment of PCa. One of the major challenges is the low bioavailability of the natural compounds (129, 130). Nanotechnology seems to come in handy when one wants to increase compound bioavailability. Sanna et al. developed nanoparticles encapsulating EGCG, which were able to increase the time to full degradation from 1 to 24 h (130). Another important property of these nanocarriers is the ability to have a high specificity for PCa cells. This was done by providing nanoparticles with ligands that are able to bind to one of the most frequently observed antigen in PCa cells, such as prostate specific membrane antigen (PSMA) (130). The use of nanoparticles induces the inhibition of cancer cell viability more potently compared to “normal” EGCG, in both, in-vitro and in-vivo experiments (130). Similar results were achieved with curcumin loaded nanoparticles (131, 132). In any case, it is pertinent for future studies to evaluate the concrete mechanistic model of action of EGCG and curcumin in PCa iron metabolism.
Recent data suggest that the antineoplastic arsenal of iron therapy in PCa is wide and with different available options. In addition to the already established antitumorous effect of local TFR1 blockade (109, 133), emerging data has revealed that local iron dysmetabolism can also be tackled by local suppression of hepcidin, IRP2, V-ATPase, STAMP2, and stimulation of FPN activity (16, 20, 30, 41, 42, 47) (Table 2). These results further strengthen the case for cellular iron deprivation therapy as a viable option in treatment of PCa.
Conclusion
Iron dysmetabolism is a feature of different cancer cells. Accumulating data suggest a similar scenario for PCa cells as well. The picture that has unfolded shows how PCa cells are able to manipulate iron metabolism for their purposes. The main changes include the overexpression of iron import proteins (TFR1), intracellular regulators of iron import (IRP2), proton pumps that cause intracellular iron release, and ferrireductases such as STAMP2. In addition, the decrease of iron export (through FPN) is also an important strategy used by PCa cells to secure abundant iron for their needs. But, the full picture of the contribution of iron transport proteins remains unsolved. For example, the contribution of DMT1 in PCa is unknown, with data from tumor initiating cells showing no specific role of this protein in the iron transport of these cells (74). Another unexplained issue is the role of non-transferrin bound iron (NTBI) in PCa, especially during systemic iron load. On the other hand, the emerging role of zinc transporter proteins in the transport of NTBI in different cells does not seem to be translated in PCa cells, because most of these proteins are downregulated in prostate tumor cells, which probably serves as a protective strategy of prostate tumor cells against intracellular zinc accumulation (14, 134). The unknown role of DMT1 and probably irrelevant contribution of zinc transporters in iron transport begs the question how do PCa cells secure iron even when TFR is blocked? (11).
Recently, studies have revealed the increased complexity of the iron dysmetabolism in PCa cells, which indicates that cancer cell proliferation is related with the iron phenotype of other cells of the tumor microenvironment, such as TAMs and CSCs. TAMs are influenced directly by tumor cells and they show an iron-releasing phenotype which suits cancer cells. We do not know exactly how do TAMs influence the iron pool of PCa cells, but FPN and Lp2 seem to be obvious suspects. Furthermore, the study of iron dysmetabolism in TAMs has not been accompanied by examination of the role of inflammatory signaling in shaping iron metabolism of PCa cells, which should be a focus of future studies. This is important considering that inflammatory signals are known as upregulators of systemic and prostatic hepcidin.
Apart from PCa cells, TAMs and CSCs, other cells of the prostate tissue are important to understand temporal changes in iron metabolism in PCa. For example, senescent prostate epithelial cells show striking similarities with PCa cells in terms of the changes in the expression of iron-related proteins. This is important because senescent cells accumulate in cancer and contribute to the pool of cancer cells by releasing chemicals that promote tumor growth. Still, the role of the iron phenotype of prostate senescent cells in affecting tumor growth in PCa has yet to be observed considering the lack of studies in this area of research.
What about adjacent normal prostate cells, how do they behave in PCa microenvironment? Interestingly, in-vivo data show that normal cells adjacent to PCa cells suffer from iron deficiency, which may suggest that iron-hacking strategy could be one of the mechanisms used by prostate tumor cells to secure abundant iron (12, 13). How could this action occur is still unknown, though the logical target could be FPN of normal cells.
Clinical data with human patients show that iron dysmetabolism found in PCa cells is generally a local phenomenon not related to changes in systemic iron metabolism (90, 98). Details from these studies suggest that systemic iron load might be associated with PCa in a subset of older patients with high levels of ferritin, though it is not known if high iron load in these cases is of primary importance or it has a secondary role by aggravating the already dysregulated iron metabolism found in PCa cells. Bigger studies with a significant number of patients with different stages of PCa should explain important questions in this respect by examining the role of systemic hepcidin, sTFR, and other markers of global iron metabolism.
Finally, the importance of iron dysmetabolism for the survival of PCa cells has been tested in cultured cells. Results show that growth of PCa cells is suppressed when compounds that can affect iron metabolism of cancer cells accumulate in PCa cells. These results have been obtained with iron chelators, inhibitors of TFR1, IRP2, hepcidin, V-ATPase, STAMP2, but also with stimulators of FPN activity. But, the translation of these results from in-vitro conditions to human trials is associated with many challenges, which as recent data suggest, might be curbed with the use of nanocarriers that increase compound bioavailability and enable their cell specific delivery.
Author Contributions
The author confirms being the sole contributor of this work and has approved it for publication.
Conflict of Interest Statement
The author declares that the research was conducted in the absence of any commercial or financial relationships that could be construed as a potential conflict of interest.
References
1. DeBerardinis RJ, Chandel NS. Fundamentals of cancer metabolism. Sci Adv. (2016) 2:e1600200. doi: 10.1126/sciadv.1600200
2. Fu D, Richardson DR. Iron chelation and regulation of the cell cycle: 2 mechanisms of posttranscriptional regulation of the universal cyclin-dependent kinase inhibitor p21CIP1/WAF1 by iron depletion. Blood (2007) 110:752–61. doi: 10.1182/blood-2007-03-076737
3. Khiroya H, Moore JS, Ahmad N, Kay J, Woolnough K, Langman G, et al. IRP2 as a potential modulator of cell proliferation, apoptosis and prognosis in nonsmall cell lung cancer. Eur Respir J. (2017) 49:1600711. doi: 10.1183/13993003.00711-2016
4. Wang J, Xiao Q, Chen X, Tong S, Sun J, Lv R, et al. LanCL1 protects prostate cancer cells from oxidative stress via suppression of JNK pathway. Cell Death Dis. (2018) 9:197. doi: 10.1038/s41419-017-0207-0
5. Suchaoin W, Chanvorachote P. Caveolin-1 attenuates hydrogen peroxide-induced oxidative damage to lung carcinoma cells. Anticancer Res. (2012) 32:483–90.
6. Qu Y, Wang J, Ray PS, Guo H, Huang J, Shin-Sim M, et al. Thioredoxin-like 2 regulates human cancer cell growth and metastasis via redox homeostasis and NF-κB signaling. J Clin Invest. (2011) 121:212–25. doi: 10.1172/JCI43144
7. Li Z, Tanaka H, Galiano F, Glass J. Anticancer activity of the iron facilitator LS081. J Exp Clin Cancer Res. (2011) 30:34. doi: 10.1186/1756-9966-30-34
8. Richardson DR, Kalinowski DS, Lau S, Jansson PJ, Lovejoy DB. Cancer cell iron metabolism and the development of potent iron chelators as anti-tumour agents. Biochim Biophys Acta (2009) 1790:702–17. doi: 10.1016/j.bbagen.2008.04.003
9. Xiang Y, Zhu Z, Han G, Ye X, Xu B, Peng Z, et al. JARID1B is a histone H3 lysine 4 demethylase up-regulated in prostate cancer. Proc Natl Acad Sci USA. (2007) 104:19226–31. doi: 10.1073/pnas.0700735104
10. Juang H-H. Modulation of mitochondrial aconitase on the bioenergy of human prostate carcinoma cells. Mol Genet Metab. (2004) 81:244–52. doi: 10.1016/j.ymgme.2003.12.009
11. Ornstein DL, Zacharski LR. Iron stimulates urokinase plasminogen activator expression and activates NF-kappa B in human prostate cancer cells. Nutr Cancer (2007) 58:115–26. doi: 10.1080/01635580701308265
12. Guntupalli JNR, Padala S, Gummuluri AVRM, Muktineni RK, Byreddy SR, Sreerama L, et al. Trace elemental analysis of normal, benign hypertrophic and cancerous tissues of the prostate gland using the particle-induced X-ray emission technique. Eur J Cancer Prev. (2007) 16:108–15. doi: 10.1097/01.cej.0000228409.75976.b6
13. Sarafanov AG, Todorov TI, Centeno JA, Macias V, Gao W, Liang W-M, et al. Prostate cancer outcome and tissue levels of metal ions. Prostate (2011) 71:1231–8. doi: 10.1002/pros.21339
14. Bogdan AR, Miyazawa M, Hashimoto K, Tsuji Y. Regulators of iron homeostasis: new players in metabolism, cell death, and disease. Trends Biochem Sci. (2016) 41:274–86. doi: 10.1016/j.tibs.2015.11.012
15. Keer HN, Kozlowski JM, Tsai YC, Lee C, McEwan RN, Grayhack JT. Elevated transferrin receptor content in human prostate cancer cell lines assessed in vitro and in vivo. J Urol. (1990) 143:381–5. doi: 10.1016/S0022-5347(17)39970-6
16. Deng Z, Manz DH, Torti SV, Torti FM. Iron-responsive element-binding protein 2 plays an essential role in regulating prostate cancer cell growth. Oncotarget (2017) 8:82231–43. doi: 10.18632/oncotarget.19288
17. Johnson IRD, Parkinson-Lawrence EJ, Shandala T, Weigert R, Butler LM, Brooks DA. Altered endosome biogenesis in prostate cancer has biomarker potential. Mol Cancer Res. (2014) 12:1851–62. doi: 10.1158/1541-7786.MCR-14-0074
18. Ellwood-Yen K, Graeber TG, Wongvipat J, Iruela-Arispe ML, Zhang J, Matusik R, et al. Myc-driven murine prostate cancer shares molecular features with human prostate tumors. Cancer Cell (2003) 4:223–38. doi: 10.1016/S1535-6108(03)00197-1
19. Holland JP, Evans MJ, Rice SL, Wongvipat J, Sawyers CL, Lewis JS. Annotating MYC status with 89Zr-transferrin imaging. Nat Med. (2012) 18:1586–91. doi: 10.1038/nm.2935
20. Licon-Munoz Y, Fordyce CA, Hayek SR, Parra KJ. V-ATPase-dependent repression of androgen receptor in prostate cancer cells. Oncotarget (2018) 9:28921–34. doi: 10.18632/oncotarget.25641
21. Michel V, Licon-Munoz Y, Trujillo K, Bisoffi M, Parra KJ. Inhibitors of vacuolar ATPase proton pumps inhibit human prostate cancer cell invasion and prostate-specific antigen expression and secretion. Int J Cancer (2013) 132:E1–10. doi: 10.1002/ijc.27811
22. Smith GA, Howell GJ, Phillips C, Muench SP, Ponnambalam S, Harrison MA. Extracellular and luminal pH regulation by vacuolar H+-ATPase isoform expression and targeting to the plasma membrane and endosomes. J Biol Chem. (2016) 291:8500–15. doi: 10.1074/jbc.M116.723395
23. Huss M, Wieczorek H. Inhibitors of V-ATPases: old and new players. J Exp Biol. (2009) 212:341–6. doi: 10.1242/jeb.024067
24. Burnell SEA, Spencer-Harty S, Howarth S, Bodger O, Kynaston H, Morgan C, et al. STEAP2 knockdown reduces the invasive potential of prostate cancer cells. Sci Rep. (2018) 8:6252. doi: 10.1038/s41598-018-24655-x
25. Korkmaz KS, Elbi C, Korkmaz CG, Loda M, Hager GL, Saatcioglu F. Molecular cloning and characterization of STAMP1, a highly prostate-specific Six transmembrane protein that is overexpressed in prostate cancer. J Biol Chem. (2002) 277:36689–96. doi: 10.1074/jbc.M202414200
26. Porkka KP, Helenius MA, Visakorpi T. Cloning and characterization of a novel six-transmembrane protein STEAP2, expressed in normal and malignant prostate. Lab Invest. (2002) 82:1573–82. doi: 10.1097/01.LAB.0000038554.26102.C6
27. Wang L, Jin Y, Arnoldussen YJ, Jonson I, Qu S, Maelandsmo GM, et al. STAMP1 is both a proliferative and an antiapoptotic factor in prostate cancer. Cancer Res. (2010) 70:5818–28. doi: 10.1158/0008-5472.CAN-09-4697
28. Gomes IM, Maia CJ, Santos CR. STEAP proteins: from structure to applications in cancer therapy. Mol Cancer Res. (2012) 10:573–87. doi: 10.1158/1541-7786.MCR-11-0281
29. Korkmaz CG, Korkmaz KS, Kurys P, Elbi C, Wang L, Klokk TI, et al. Molecular cloning and characterization of STAMP2, an androgen-regulated six transmembrane protein that is overexpressed in prostate cancer. Oncogene (2005) 24:4934–45. doi: 10.1038/sj.onc.1208677
30. Jin Y, Wang L, Qu S, Sheng X, Kristian A, Mælandsmo GM, et al. STAMP2 increases oxidative stress and is critical for prostate cancer. EMBO Mol Med. (2015) 7:315–31. doi: 10.15252/emmm.201404181
31. Sikkeland J, Sheng X, Jin Y, Saatcioglu F. STAMPing at the crossroads of normal physiology and disease states. Mol Cell Endocrinol. (2016) 425:26–36. doi: 10.1016/j.mce.2016.02.013
32. Xue X, Bredell BX, Anderson ER, Martin A, Mays C, Nagao-Kitamoto H, et al. Quantitative proteomics identifies STEAP4 as a critical regulator of mitochondrial dysfunction linking inflammation and colon cancer. Proc Natl Acad Sci USA. (2017) 114:E9608–17. doi: 10.1073/pnas.1712946114
33. Anderson GJ, Frazer DM. Current understanding of iron homeostasis. Am J Clin Nutr. (2017) 106:1559S−66S. doi: 10.3945/ajcn.117.155804
34. Drakesmith H, Nemeth E, Ganz T. Ironing out ferroportin. Cell Metab. (2015) 22:777–87. doi: 10.1016/j.cmet.2015.09.006
35. Maffettone C, Chen G, Drozdov I, Ouzounis C, Pantopoulos K. Tumorigenic properties of iron regulatory protein 2 (IRP2) mediated by its specific 73-amino acids insert. PLoS ONE (2010) 5:e10163. doi: 10.1371/journal.pone.0010163
36. Wang W, Deng Z, Hatcher H, Miller LD, Di X, Tesfay L, et al. IRP2 regulates breast tumor growth. Cancer Res. (2014) 74:497–507. doi: 10.1158/0008-5472.CAN-13-1224
37. Horniblow RD, Bedford M, Hollingworth R, Evans S, Sutton E, Lal N, et al. BRAF mutations are associated with increased iron regulatory protein-2 expression in colorectal tumorigenesis. Cancer Sci. (2017) 108:1135–43. doi: 10.1111/cas.13234
38. Pinnix ZK, Miller LD, Wang W, D'Agostino R, Kute T, Willingham MC, et al. Ferroportin and iron regulation in breast cancer progression and prognosis. Sci Transl Med. (2010) 2:43ra56. doi: 10.1126/scisignal.3001127
39. Toshiyama R, Konno M, Eguchi H, Asai A, Noda T, Koseki J, et al. Association of iron metabolic enzyme hepcidin expression levels with the prognosis of patients with pancreatic cancer. Oncol Lett. (2018) 15:8125–33. doi: 10.3892/ol.2018.8357
40. Gu Z, Wang H, Xia J, Yang Y, Jin Z, Xu H, et al. Decreased ferroportin promotes myeloma cell growth and osteoclast differentiation. Cancer Res. (2015) 75:2211–21. doi: 10.1158/0008-5472.CAN-14-3804
41. Chen Y, Zhang Z, Yang K, Du J, Xu Y, Liu S. Myeloid zinc-finger 1 (MZF-1) suppresses prostate tumor growth through enforcing ferroportin-conducted iron egress. Oncogene (2015) 34:3839–47. doi: 10.1038/onc.2014.310
42. Xue D, Zhou C, Shi Y, Lu H, Xu R, He X. Nuclear transcription factor Nrf2 suppresses prostate cancer cells growth and migration through upregulating ferroportin. Oncotarget (2016) 7:78804–12. doi: 10.18632/oncotarget.12860
43. Xue D, Zhou CX, Shi YB, Lu H, He XZ. Decreased expression of ferroportin in prostate cancer. Oncol Lett. (2015) 10:913–6. doi: 10.3892/ol.2015.3363
44. Deng Z, Manz DH, Torti SV, Torti FM. Effects of ferroportin-mediated iron depletion in cells representative of different histological subtypess of prostate cancer. Antioxid Redox Signal (2017). doi: 10.1089/ars.2017.7023. [Epub ahead of print]
45. Jiang X, Zhang C, Qi S, Guo S, Chen Y, Du E, et al. Elevated expression of ZNF217 promotes prostate cancer growth by restraining ferroportin-conducted iron egress. Oncotarget (2016) 7:84893–906. doi: 10.18632/oncotarget.12753
46. Ganz T, Nemeth E. Hepcidin and iron homeostasis. Biochim Biophys Acta (2012) 1823:1434–43. doi: 10.1016/j.bbamcr.2012.01.014
47. Tesfay L, Clausen KA, Kim JW, Hegde P, Wang X, Miller LD, et al. Hepcidin regulation in prostate and its disruption in prostate cancer. Cancer Res. (2015) 75:2254–63. doi: 10.1158/0008-5472.CAN-14-2465
48. Wang F, Liu A, Bai R, Zhang B, Jin Y, Guo W, et al. Hepcidin and iron metabolism in the pathogenesis of prostate cancer. J BUON (2017) 22:1328–32.
49. Zhao B, Li R, Cheng G, Li Z, Zhang Z, Li J, et al. Role of hepcidin and iron metabolism in the onset of prostate cancer. Oncol Lett. (2018) 15:9953–8. doi: 10.3892/ol.2018.8544
50. Zhang Z, Hou L, Song JL, Song N, Sun YJ, Lin X, et al. Pro-inflammatory cytokine-mediated ferroportin down-regulation contributes to the nigral iron accumulation in lipopolysaccharide-induced Parkinsonian models. Neuroscience (2014) 257:20–30. doi: 10.1016/j.neuroscience.2013.09.037
51. Jain S, Suklabaidya S, Das B, Raghav SK, Batra SK, Senapati S. TLR4 activation by lipopolysaccharide confers survival advantage to growth factor deprived prostate cancer cells. Prostate (2015) 75:1020–33. doi: 10.1002/pros.22983
52. Xu P, Cai F, Liu X, Guo L. Sesamin inhibits lipopolysaccharide-induced proliferation and invasion through the p38-MAPK and NF-κB signaling pathways in prostate cancer cells. Oncol Rep. (2015) 33:3117–23. doi: 10.3892/or.2015.3888
53. Hsiao C-P, Wang D, Kaushal A, Saligan L. Mitochondria-related gene expression changes are associated with fatigue in patients with nonmetastatic prostate cancer receiving external beam radiation therapy. Cancer Nurs. (2013) 36:189–97. doi: 10.1097/NCC.0b013e318263f514
54. Hsiao C-P, Wang D, Kaushal A, Chen MK, Saligan L. Differential expression of genes related to mitochondrial biogenesis and bioenergetics in fatigued prostate cancer men receiving external beam radiation therapy. J Pain Symptom Manage. (2014) 48:1080–90. doi: 10.1016/j.jpainsymman.2014.03.010
55. Lytovchenko O, Kunji ERS. Expression and putative role of mitochondrial transport proteins in cancer. Biochim Biophys Acta Bioenerg. (2017) 1858:641–54. doi: 10.1016/j.bbabio.2017.03.006
56. Paul BT, Manz DH, Torti FM, Torti S V. Mitochondria and iron: current questions. Expert Rev Hematol. (2017) 10:65–79. doi: 10.1080/17474086.2016.1268047
57. Maolake A, Izumi K, Shigehara K, Natsagdorj A, Iwamoto H, Kadomoto S, et al. Tumor-associated macrophages promote prostate cancer migration through activation of the CCL22-CCR4 axis. Oncotarget (2017) 8:9739–51. doi: 10.18632/oncotarget.14185
58. Lanciotti M, Masieri L, Raspollini MR, Minervini A, Mari A, Comito G, et al. The role of M1 and M2 macrophages in prostate cancer in relation to extracapsular tumor extension and biochemical recurrence after radical prostatectomy. Biomed Res Int. (2014) 2014:1–6. doi: 10.1155/2014/486798
59. Lundholm M, Hägglöf C, Wikberg ML, Stattin P, Egevad L, Bergh A, et al. Secreted factors from colorectal and prostate cancer cells skew the immune response in opposite directions. Sci Rep. (2015) 5:15651. doi: 10.1038/srep15651
60. Fujii T, Shimada K, Asai O, Tanaka N, Fujimoto K, Hirao K, et al. Immunohistochemical analysis of inflammatory cells in benign and precancerous lesions and carcinoma of the prostate. Pathobiology (2013) 80:119–26. doi: 10.1159/000342396
61. Nonomura N, Takayama H, Nakayama M, Nakai Y, Kawashima A, Mukai M, et al. Infiltration of tumour-associated macrophages in prostate biopsy specimens is predictive of disease progression after hormonal therapy for prostate cancer. BJU Int. (2011) 107:1918–22. doi: 10.1111/j.1464-410X.2010.09804.x
62. Jung M, Ören B, Mora J, Mertens C, Dziumbla S, Popp R, et al. Lipocalin 2 from macrophages stimulated by tumor cell–derived sphingosine 1-phosphate promotes lymphangiogenesis and tumor metastasis. Sci Signal (2016) 9:ra64. doi: 10.1126/scisignal.aaf3241
63. Jung M, Weigert A, Mertens C, Rehwald C, Brüne B. Iron handling in tumor-associated macrophages-is there a new role for lipocalin-2? Front Immunol. (2017) 8:1171. doi: 10.3389/fimmu.2017.01171
64. Mertens C, Mora J, Ören B, Grein S, Winslow S, Scholich K, et al. Macrophage-derived lipocalin-2 transports iron in the tumor microenvironment. Oncoimmunology (2018) 7:e1408751. doi: 10.1080/2162402X.2017.1408751
65. Duan X, He K, Li J, Cheng M, Song H, Liu J, et al. Tumor associated macrophages deliver iron to tumor cells via Lcn2. Int J Physiol Pathophysiol Pharmacol. (2018) 10:105–14.
66. Mahadevan NR, Rodvold J, Almanza G, Pérez AF, Wheeler MC, Zanetti M. ER stress drives Lipocalin 2 upregulation in prostate cancer cells in an NF-κB-dependent manner. BMC Cancer (2011) 11:229. doi: 10.1186/1471-2407-11-229
67. Tung MC, Hsieh SC, Yang SF, Cheng CW, Tsai RT, Wang SC, et al. Knockdown of lipocalin-2 suppresses the growth and invasion of prostate cancer cells. Prostate (2013) 73:1281–90. doi: 10.1002/pros.22670
68. Ding G, Fang J, Tong S, Qu L, Jiang H, Ding Q, et al. Over-expression of lipocalin 2 promotes cell migration and invasion through activating ERK signaling to increase SLUG expression in prostate cancer. Prostate (2015) 75:957–68. doi: 10.1002/pros.22978
69. Ding G, Wang J, Feng C, Jiang H, Xu J, Ding Q. Lipocalin 2 over-expression facilitates progress of castration-resistant prostate cancer via improving androgen receptor transcriptional activity. Oncotarget (2016) 7:64309–17. doi: 10.18632/oncotarget.11790
70. Corna G, Campana L, Pignatti E, Castiglioni A, Tagliafico E, Bosurgi L, et al. Polarization dictates iron handling by inflammatory and alternatively activated macrophages. Haematologica (2010) 95:1814–22. doi: 10.3324/haematol.2010.023879
71. Recalcati S, Locati M, Marini A, Santambrogio P, Zaninotto F, De Pizzol M, et al. Differential regulation of iron homeostasis during human macrophage polarized activation. Eur J Immunol. (2010) 40:824–35. doi: 10.1002/eji.200939889
72. Mertens C, Akam EA, Rehwald C, Brüne B, Tomat E, Jung M. Intracellular iron chelation modulates the macrophage iron phenotype with consequences on tumor progression. PLoS ONE (2016) 11:e0166164. doi: 10.1371/journal.pone.0166164
73. Leftin A, Zhao H, Turkekul M, de Stanchina E, Manova K, Koutcher JA. Iron deposition is associated with differential macrophage infiltration and therapeutic response to iron chelation in prostate cancer. Sci Rep. (2017) 7:11632. doi: 10.1038/s41598-017-11899-2
74. Rychtarcikova Z, Lettlova S, Tomkova V, Korenkova V, Langerova L, Simonova E, et al. Tumor-initiating cells of breast and prostate origin show alterations in the expression of genes related to iron metabolism. Oncotarget (2017) 8:6376–98. doi: 10.18632/oncotarget.14093
75. Batlle E, Clevers H. Cancer stem cells revisited. Nat Med. (2017) 23:1124–34. doi: 10.1038/nm.4409
76. Ninomiya T, Ohara T, Noma K, Katsura Y, Katsube R, Kashima H, et al. Iron depletion is a novel therapeutic strategy to target cancer stem cells. Oncotarget (2017) 8:98405–16. doi: 10.18632/oncotarget.21846
77. Chanvorachote P, Luanpitpong S. Iron induces cancer stem cells and aggressive phenotypes in human lung cancer cells. Am J Physiol Physiol. (2016) 310:C728–39. doi: 10.1152/ajpcell.00322.2015
78. Fryknäs M, Zhang X, Bremberg U, Senkowski W, Olofsson MH, Brandt P, et al. Iron chelators target both proliferating and quiescent cancer cells. Sci Rep. (2016) 6:38343. doi: 10.1038/srep38343
79. Mai TT, Hamaï A, Hienzsch A, Cañeque T, Müller S, Wicinski J, et al. Salinomycin kills cancer stem cells by sequestering iron in lysosomes. Nat Chem. (2017) 9:1025–33. doi: 10.1038/nchem.2778
80. van Deursen JM. The role of senescent cells in ageing. Nature (2014) 509:439–46. doi: 10.1038/nature13193
81. Masaldan S, Clatworthy SAS, Gamell C, Meggyesy PM, Rigopoulos A-T, Haupt S, et al. Iron accumulation in senescent cells is coupled with impaired ferritinophagy and inhibition of ferroptosis. Redox Biol. (2018) 14:100–15. doi: 10.1016/j.redox.2017.08.015
82. Schosserer M, Grillari J, Breitenbach M. The dual role of cellular senescence in developing tumors and their response to cancer therapy. Front Oncol. (2017) 7:278. doi: 10.3389/fonc.2017.00278
83. Coppé J-P, Desprez P-Y, Krtolica A, Campisi J. The senescence-associated secretory phenotype: the dark side of tumor suppression. Annu Rev Pathol Mech Dis. (2010) 5:99–118. doi: 10.1146/annurev-pathol-121808-102144
84. Osborne NJ, Gurrin LC, Allen KJ, Constantine CC, Delatycki MB, McLaren CE, et al. HFE C282Y homozygotes are at increased risk of breast and colorectal cancer. Hepatology (2010) 51:1311–8. doi: 10.1002/hep.23448
85. Syrjäkoski K, Fredriksson H, Ikonen T, Kuukasjärvi T, Autio V, Matikainen MP, et al. Hemochromatosis gene mutations among Finnish male breast and prostate cancer patients. Int J Cancer (2006) 118:518–20. doi: 10.1002/ijc.21331
86. Dickhut S, Urfer W, Reich S, Bandel T, Bremicker K-D, Neugebauer W, et al. Occupational risk factors for prostate cancer in an area of former coal, iron, and steel industries in Germany. Part 1: results from a study performed in the 1980s. J Toxicol Environ Heal Part A (2016) 79:1125–9. doi: 10.1080/15287394.2016.1219605
87. Krech S, Selinski S, Bürger H, Hengstler JG, Jedrusik P, Hodzic J, et al. Occupational risk factors for prostate cancer in an area of former coal, iron, and steel industries in Germany. Part 2: results from a study performed in the 1990s. J Toxicol Environ Heal Part A (2016) 79:1130–5. doi: 10.1080/15287394.2016.1219603
88. Choi J-Y, Neuhouser ML, Barnett MJ, Hong C-C, Kristal AR, Thornquist MD, et al. Iron intake, oxidative stress-related genes (MnSOD and MPO) and prostate cancer risk in CARET cohort. Carcinogenesis (2008) 29:964–70. doi: 10.1093/carcin/bgn056
89. Jakszyn PG, Allen NE, Lujan-Barroso L, Gonzalez CA, Key TJ, Fonseca-Nunes A, et al. Nitrosamines and heme iron and risk of prostate cancer in the European prospective investigation into cancer and nutrition. Cancer Epidemiol Biomarkers Prev. (2012) 21:547–51. doi: 10.1158/1055-9965.EPI-11-1181
90. Geybels MS, Verhage BAJ, van Schooten FJ, van den Brandt PA. Measures of combined antioxidant and pro-oxidant exposures and risk of overall and advanced stage prostate cancer. Ann Epidemiol. (2012) 22:814–20. doi: 10.1016/j.annepidem.2012.07.010
91. Yamoah K, Zeigler-Johnson CM, Jeffers A, Malkowicz B, Spangler E, Park JY, et al. The impact of body mass index on treatment outcomes for patients with low-intermediate risk prostate cancer. BMC Cancer (2016) 16:557. doi: 10.1186/s12885-016-2572-y
92. Haque R, Van Den Eeden SK, Wallner LP, Richert-Boe K, Kallakury B, Wang R, et al. Association of body mass index and prostate cancer mortality. Obes Res Clin Pract. (2014) 8:e374–81. doi: 10.1016/j.orcp.2013.06.002
93. Edgren G, Tran TN, Hjalgrim H, Rostgaard K, Shanwell A, Titlestad K, et al. Improving health profile of blood donors as a consequence of transfusion safety efforts. Transfusion (2007) 47:2017–24. doi: 10.1111/j.1537-2995.2007.01425.x
94. Hjalgrim H, Edgren G, Rostgaard K, Reilly M, Tran TN, Titlestad KE, et al. Cancer Incidence in blood transfusion recipients. JNCI J Natl Cancer Inst. (2007) 99:1864–74. doi: 10.1093/jnci/djm248
95. Kuvibidila S, Gauthier T, Warrier RP, Rayford W. Increased levels of serum transferrin receptor and serum transferrin receptor/log ferritin ratios in men with prostate cancer and the implications for body-iron stores. J Lab Clin Med. (2004) 144:176–82. doi: 10.1016/j.lab.2004.03.017
96. Beshara S, Letocha H, Linde T, Wikström B, Sandhagen B, Nilsson S, et al. Anemia associated with advanced prostatic adenocarcinoma: effects of recombinant human erythropoietin. Prostate (1997) 31:153–60.
97. Tanno T, Rabel A, Alleyne M, Lee YT, Dahut WL, Gulley JL, et al. Hepcidin, anaemia, and prostate cancer. BJU Int. (2011) 107:678–9. doi: 10.1111/j.1464-410X.2011.10108.x
98. Wang X, An P, Zeng J, Liu X, Wang B, Fang X, et al. Serum ferritin in combination with prostate-specific antigen improves predictive accuracy for prostate cancer. Oncotarget (2017) 8:17862–72. doi: 10.18632/oncotarget.14977
99. Chua AC, Knuiman MW, Trinder D, Divitini ML, Olynyk JK. Higher concentrations of serum iron and transferrin saturation but not serum ferritin are associated with cancer outcomes. Am J Clin Nutr. (2016) 104:736–42. doi: 10.3945/ajcn.115.129411
100. Kuvibidila SR, Gauthier T, Rayford W. Serum ferritin levels and transferrin saturation in men with prostate cancer. J Natl Med Assoc. (2004) 96:641–649.
101. Lipschitz DA, Cook JD, Finch CA. A Clinical evaluation of serum ferritin as an index of iron stores. N Engl J Med. (1974) 290:1213–6. doi: 10.1056/NEJM197405302902201
102. Walters GO, Miller FM, Worwood M. Serum ferritin concentration and iron stores in normal subjects. J Clin Pathol. (1973) 26:770–2. doi: 10.1136/jcp.26.10.770
103. Cook JD, Lipschitz DA, Miles LEM, Finch CA. Serum ferritin as a measure of iron stores in normal subjects. Am J Clin Nutr. (1974) 27:681–7. doi: 10.1093/ajcn/27.7.681
104. Lee MH, Means RT. Extremely elevated serum ferritin levels in a university hospital: Associated diseases and clinical significance. Am J Med. (1995) 98:566–71. doi: 10.1016/S0002-9343(99)80015-1
105. Wang W, Knovich MA, Coffman LG, Torti FM, Torti S V. Serum ferritin: past, present and future. Biochim Biophys Acta Gen Subj. (2010) 1800:760–9. doi: 10.1016/j.bbagen.2010.03.011
106. Kell DB, Pretorius E. Serum ferritin is an important inflammatory disease marker, as it is mainly a leakage product from damaged cells. Metallomics (2014) 6:748–73. doi: 10.1039/C3MT00347G
107. Cappellini MD, Pattoneri P. Oral iron chelators. Annu Rev Med. (2009) 60:25–38. doi: 10.1146/annurev.med.60.041807.123243
108. Bedford MR, Ford SJ, Horniblow RD, Iqbal TH, Tselepis C. Iron chelation in the treatment of cancer: a new role for deferasirox? J Clin Pharmacol. (2013) 53:885–91. doi: 10.1002/jcph.113
109. Kovar J, Naumann PW, Stewart BC, Kemp JD. Differing sensitivity of non-hematopoietic human tumors to synergistic anti-transferrin receptor monoclonal antibodies and deferoxamine in vitro. Pathobiology (1995) 63:65–70. doi: 10.1159/000163935
110. Dreicer R, Kemp JD, Stegink LD, Cardillo T, Davis CS, Forest PK, et al. A phase II trial of deferoxamine in patients with hormone-refractory metastatic prostate cancer. Cancer Invest. (1997) 15:311–7. doi: 10.3109/07357909709039731
111. Simões RV, Veeraperumal S, Serganova IS, Kruchevsky N, Varshavsky J, Blasberg RG, et al. Inhibition of prostate cancer proliferation by Deferiprone. NMR Biomed. (2017) 30:e3712. doi: 10.1002/nbm.3712
112. Dixon KM, Lui GYL, Kovacevic Z, Zhang D, Yao M, Chen Z, et al. Dp44mT targets the AKT, TGF-β and ERK pathways via the metastasis suppressor NDRG1 in normal prostate epithelial cells and prostate cancer cells. Br J Cancer (2013) 108:409–19. doi: 10.1038/bjc.2012.582
113. Tian J, Peehl DM, Zheng W, Knox SJ. Anti-tumor and radiosensitization activities of the iron chelator HDp44mT are mediated by effects on intracellular redox status. Cancer Lett. (2010) 298:231–7. doi: 10.1016/j.canlet.2010.07.010
114. Zhang CY, Lu J, Tsourkas A. Iron chelator-based amplification strategy for improved targeting of transferrin receptor with SPIO. Cancer Biol Ther. (2008) 7:889–95. doi: 10.4161/cbt.7.6.5893
115. Yang J, Wang C, Zhang Z, Chen X, Jia Y, Wang B, et al. Curcumin inhibits the survival and metastasis of prostate cancer cells via the Notch-1 signaling pathway. APMIS (2017) 125:134–40. doi: 10.1111/apm.12650
116. Yang C, Hu Z, Wang Z, Liu S, Zeng X, Wang Y, et al. Transferrin receptor monoclonal antibody exacerbates curcumin-mediated apoptotic effect in castration resistant prostate cancer cells. (2016) 9:5306–12.
117. Jiao Y, Wilkinson J, Di X, Wang W, Hatcher H, Kock ND, et al. Curcumin, a cancer chemopreventive and chemotherapeutic agent, is a biologically active iron chelator. Blood (2009) 113:462–9. doi: 10.1182/blood-2008-05-155952
118. Chin D, Huebbe P, Frank J, Rimbach G, Pallauf K. Curcumin may impair iron status when fed to mice for six months. Redox Biol. (2014) 2:563–9. doi: 10.1016/j.redox.2014.01.018
119. Lainé F, Laviolle B, Bardou-Jacquet E, Fatih N, Jezequel C, Collet N, et al. Curcuma decreases serum hepcidin levels in healthy volunteers: a placebo-controlled, randomized, double-blind, cross-over study. Fundam Clin Pharmacol. (2017) 31:567–73. doi: 10.1111/fcp.12288
120. Thomas R, Kim MH. Epigallocatechin gallate inhibits HIF-1α degradation in prostate cancer cells. Biochem Biophys Res Commun. (2005) 334:543–8. doi: 10.1016/j.bbrc.2005.06.114
121. Liao S, Umekita Y, Guo J, Kokontis JM, Hiipakka RA. Growth inhibition and regression of human prostate and breast tumors in athymic mice by tea epigallocatechin gallate. Cancer Lett. (1995) 96:239–43. doi: 10.1016/0304-3835(95)03948-V
122. Sartor L, Pezzato E, Donà M, Dell'Aica I, Calabrese F, Morini M, et al. Prostate carcinoma and green tea: (–)epigallocatechin-3-gallate inhibits inflammation-triggered MMP-2 activation and invasion in murine TRAMP model. Int J Cancer (2004) 112:823–9. doi: 10.1002/ijc.20496
123. Chuu C-P, Chen R-Y, Kokontis JM, Hiipakka RA, Liao S. Suppression of androgen receptor signaling and prostate specific antigen expression by (-)-epigallocatechin-3-gallate in different progression stages of LNCaP prostate cancer cells. Cancer Lett. (2009) 275:86–92. doi: 10.1016/j.canlet.2008.10.001
124. Siddiqui IA, Zaman N, Aziz MH, Reagan-Shaw SR, Sarfaraz S, Adhami VM, et al. Inhibition of CWR22Rν1 tumor growth and PSA secretion in athymic nude mice by green and black teas. Carcinogenesis (2006) 27:833–9. doi: 10.1093/carcin/bgi323
125. Chen D, Kanthasamy AG, Reddy MB. EGCG protects against 6-OHDA-induced neurotoxicity in a cell culture model. Parkinsons Dis. (2015) 2015:1–10. doi: 10.1155/2015/843906
126. Xu Q, Langley M, Kanthasamy AG, Reddy MB. Epigallocatechin gallate has a neurorescue effect in a mouse model of parkinson disease. J Nutr. (2017) 147:1926–31. doi: 10.3945/jn.117.255034
127. Guo Y, Zhi F, Chen P, Zhao K, Xiang H, Mao Q, et al. Green tea and the risk of prostate cancer. Medicine (2017) 96:e6426. doi: 10.1097/MD.0000000000006426
128. Thomas R, Williams M, Sharma H, Chaudry A, Bellamy P. A double-blind, placebo-controlled randomised trial evaluating the effect of a polyphenol-rich whole food supplement on PSA progression in men with prostate cancer–the U.K. NCRN Pomi-T study. Prostate Cancer Prostatic Dis. (2014) 17:180–6. doi: 10.1038/pcan.2014.6
129. Burgos-Morón E, Calderón-Montaño JM, Salvador J, Robles A, López-Lázaro M. The dark side of curcumin. Int J Cancer (2010) 126:1771–5. doi: 10.1002/ijc.24967
130. Sanna V, Singh CK, Jashari R, Adhami VM, Chamcheu JC, Rady I, et al. Targeted nanoparticles encapsulating (-)-epigallocatechin-3-gallate for prostate cancer prevention and therapy. Sci Rep. (2017) 7:41573. doi: 10.1038/srep41573
131. Yallapu MM, Khan S, Maher DM, Ebeling MC, Sundram V, Chauhan N, et al. Anti-cancer activity of curcumin loaded nanoparticles in prostate cancer. Biomaterials (2014) 35:8635–48. doi: 10.1016/j.biomaterials.2014.06.040
132. Yan J, Wang Y, Zhang X, Liu S, Tian C, Wang H. Targeted nanomedicine for prostate cancer therapy: docetaxel and curcumin co-encapsulated lipid–polymer hybrid nanoparticles for the enhanced anti-tumor activity in vitro and in vivo. Drug Deliv. (2016) 23:1757–62. doi: 10.3109/10717544.2015.1069423
133. Donat SM, Powell CT, Israeli RS, Fair WR, Heston WD. Reversal by transferrin of growth-inhibitory effect of suramin on hormone-refractory human prostate cancer cells. J Natl Cancer Inst. (1995) 87:41–6. doi: 10.1093/jnci/87.1.41
Keywords: prostate cancer, iron metabolism, transferrin receptor 1, iron responsive element-binding protein 2, ferroportin, hepcidin, tumor associated macrophages, cancer stem cells
Citation: Vela D (2018) Iron Metabolism in Prostate Cancer; From Basic Science to New Therapeutic Strategies. Front. Oncol. 8:547. doi: 10.3389/fonc.2018.00547
Received: 23 September 2018; Accepted: 05 November 2018;
Published: 27 November 2018.
Edited by:
Justin Lathia, Cleveland Clinic Lerner College of Medicine, United StatesReviewed by:
Daniel Edward Frigo, University of Texas MD Anderson Cancer Center, United StatesJames Connor, Penn State Milton S. Hershey Medical Center, United States
Copyright © 2018 Vela. This is an open-access article distributed under the terms of the Creative Commons Attribution License (CC BY). The use, distribution or reproduction in other forums is permitted, provided the original author(s) and the copyright owner(s) are credited and that the original publication in this journal is cited, in accordance with accepted academic practice. No use, distribution or reproduction is permitted which does not comply with these terms.
*Correspondence: Driton Vela, ZHJpdG9uLnZlbGFAdW5pLXByLmVkdQ==