- 1Instituto de Investigação e Inovação em Saúde (I3S), University of Porto, Porto, Portugal
- 2Institute of Molecular Pathology and Immunology of the University of Porto (IPATIMUP), Porto, Portugal
- 3Institute of Biomedical Sciences of Abel Salazar (ICBAS), University of Porto, Porto, Portugal
- 4Biochemistry and Molecular Biology Unit, Department of Biology, University of Girona, Girona, Spain
- 5Medical Faculty, University of Porto, Porto, Portugal
Gastrointestinal (GI) cancer is the most common group of malignancies and many of its types are among the most deadly. Various glycoconjugates have been used in clinical practice as serum biomarker for several GI tumors, however, with limited diagnose application. Despite the good accessibility by endoscopy of many GI organs, the lack of reliable serum biomarkers often leads to late diagnosis of malignancy and consequently low 5-year survival rates. Recent advances in analytical techniques have provided novel glycoproteomic and glycomic data and generated functional information and putative biomarker targets in oncology. Glycosylation alterations have been demonstrated in a series of glycoconjugates (glycoproteins, proteoglycans, and glycosphingolipids) that are involved in cancer cell adhesion, signaling, invasion, and metastasis formation. In this review, we present an overview on the major glycosylation alterations in GI cancer and the current serological biomarkers used in the clinical oncology setting. We further describe recent glycomic studies in GI cancer, namely gastric, colorectal, and pancreatic cancer. Moreover, we discuss the role of glycosylation as a modulator of the function of several key players in cancer cell biology. Finally, we address several state-of-the-art techniques currently applied in this field, such as glycomic and glycoproteomic analyses, the application of glycoengineered cell line models, microarray and proximity ligation assay, and imaging mass spectrometry, and provide an outlook to future perspectives and clinical applications.
Glycobiology in Cancer
The cells’ glycocalix constitutes an important interface with the extracellular milieu and plays critical roles in physiological and pathological conditions. This glycan-rich coating of the cells’ plasma membrane is composed by different classes of glycoconjugates, including glycoproteins, glycolipids, and proteoglycans, which participate in key regulatory events for cellular and organ homeostasis. Alterations in glycosylation can interfere with normal molecular functions such as cell–cell recognition, communication, and adhesion, leading to acquisition of malignant features. Moreover, the shedding of aberrant glycoconjugates, uniquely expressed by tumor cells, into circulation provides one valuable source of biomarkers for cancer diagnosis and prognosis (1).
Substantial advances in the frontiers of cancer glycobiology have been possible in the recent past due to the combination of novel tumor cell biology concepts with cutting-edge glycomic technologies. Specific glycosylation alterations have been identified in tumors and some of the molecular pathways underlying these modifications have been disclosed (2). In addition, aberrant glycoforms have been demonstrated to be molecularly associated with more aggressive cancer cell and tumor features, including increased migration, invasion, and metastization potential, providing novel targets for therapeutic intervention (3–5).
This review describes the recent progress in gastric cancer, colorectal cancer (CRC), and pancreatic ductal adenocarcinoma (PDAC) glycobiology and discusses the clinical value of aberrant glycosylation as a source of screening biomarkers and therapeutic targets. A comprehensive overview of the advances in glycomic and glycoproteomic tools is also provided and their possible applications for tumor glycan-profiling and discovery of novel targets for improving gastrointestinal (GI) tumors’ clinical management are discussed.
Glycosylation Alteration in Gastrointestinal Cancers
Despite the large amount of glycan epitopes that can be found in the human GI tract and the complex and manifold alterations of the glycosylation machinery during the process of carcinogenesis and cancer progression, the current knowledge makes it possible to group the most common glycan alterations. Expression of truncated simple O-glycans, changes in N-glycan branching, and increase in sialylation and fucosylation are three major N- and O-glycosylation events involved in GI cancer that will be described in detail (Figure 1). Furthermore, we give an overview on other common glycosylation alterations in cancer, such as changes in O-GlcNAcylation, modified glycosphingolipids, and glycosaminoglycans (GAGs) and proteoglycans.
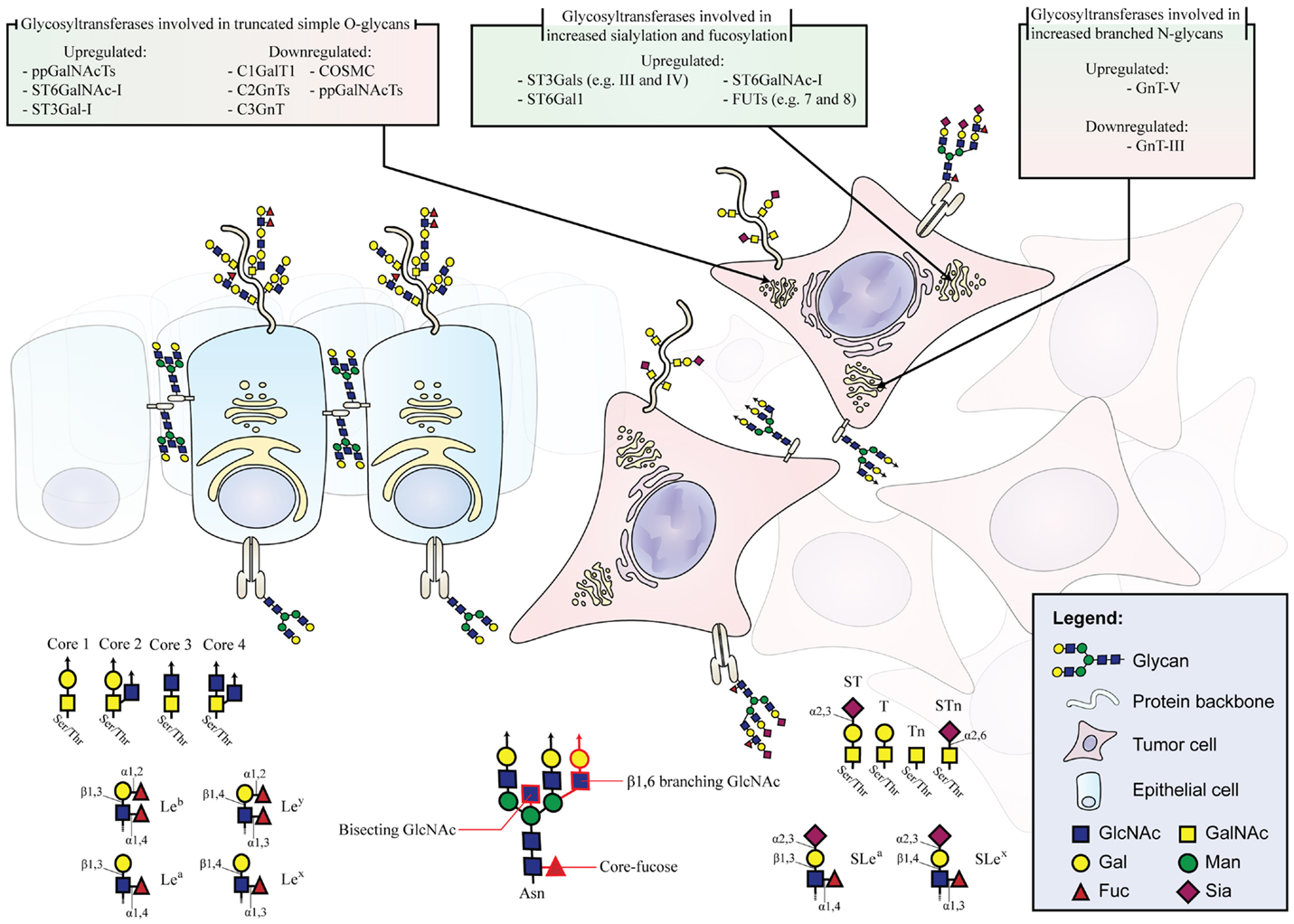
Figure 1. Schematic depiction of glycan alterations during malignant transformation. Healthy epithelium displaying cell polarity, organized glycoprotein localization, and normal glycosylation pattern. Malignant cells with misslocalized glycoproteins and altered expression of genes involved in glycosylation pathways leading to the aberrant expression of glycan moieties. The glycosyltransferases involved in glycan alterations during malignant transformation are listed.
Truncated Simple O-Glycans
One common feature observed in GI tumors is the overexpression and exposure of short, truncated O-glycans. Mucin-type O-glycans are found on most transmembrane and secreted proteins. A single O-glycan oligosaccharide chain can present more than 20 monosaccharide constituents (6). In malignancy, O-glycans are often shortened resulting in an increase of the monosaccharide Tn antigen (GalNAcα1-Ser/Thr), the disaccharide T antigen (also known as Thomsen–Friedenreich antigen or core 1 structure, Galβ1-3GalNAcα1-Ser/Thr) and their sialylated forms, STn (Neu5Acα2-6GalNAcα1-Ser/Thr), and ST (Neu5Acα2-3Galβ1-3GalNAcα1-Ser/Thr), respectively (Figure 1) (7, 8).
Polypeptide GalNAc transferases (ppGalNAcTs), which are the initiating enzymes of the mucin-type O-glycosylation (9, 10), show often altered expression in cancer (11–13). A total of 20 different ppGalNAcTs are known in human and their expression profile and subcellular localization determine O-glycosylation sites and densities (9, 14). In CRC, for example, the ppGalNAc-T3 is associated with tumor differentiation, disease aggressiveness, and prognosis (12). In gastric cancer, the expression of ppGalNAc-T6 is associated with venous invasion and the downregulation of ppGalNAc-T2 increases the cancer cell proliferation, adhesion, and invasion (11, 15).
In addition, enzymes competing for the same substrate can also induce expression of truncated glycans and exposure of protein epitopes that would be hidden otherwise. For instance, the relative enzymatic activities of C2GnT (N-acetylglucosaminyltransferase) and ST3Gal-I (sialyltransferase), two glycosyltransferases that compete for the same substrate, have been shown to determine the O-glycan structure in cancer cells (16).
STn is expressed in most GI carcinomas correlating with decreased cancer cell adhesion, increased cancer cell invasion, and poor prognosis of the patients (17–23). The terminal STn epitope is synthesized by the sialylation of Tn by the ST6GalNAc-I sialyltransferase (17, 18). In cancer, the formation of STn may occur due to ST6GalNAc-I upregulation, early sialylation caused by glycosyltransferase misslocalization in the secretory pathway, or the impairment of the elongation of the Tn antigen (14, 17, 18, 24).
In gastric cancer, expression of STn is a common feature associated with more malignant phenotypes. The overexpression of ST6GalNAc-I has been shown to induce migration and invasion in gastric carcinoma cells in vitro (20).
In this regard, another gene that can underlie the synthesis of truncated O-glycans is COSMC, which encodes for a C1GalT1 dedicated chaperone (25). The galactosyltransferase C1GalT1 is responsible for the elongation of the Tn antigen to form the core 1 structure also known as the T antigen. The absence of a functional COSMC entails the dysfunction of C1GalT1. In PDAC, it has been shown that hypermethylation of COSMC, and not somatic mutations, is the prevalent cause of truncated O-glycans (23). In addition, the downregulation of C1GalT1 in combination with the upregulation of ST6GalNAc-I has been associated with increased STn expression in CRC cell lines and epithelial cells derived from resected CRC tumor tissue (26). In contrary, the overexpression of C1GalT1 is associated with invasion, metastization, and poor survival in CRC. In C1GalT1 overexpressing CRC cells, the knockdown of C1GalT1 suppresses the malignant phenotype in vitro and in vivo (27). Increased levels of C2GnT, a glycosyltransferase responsible for the biosynthesis of core 2 structures, are also frequent in CRC (28). This enzyme has also a critical role in the biosynthesis of terminal sialylated Lewis antigens on O-glycans that will be further discussed in Section “Increased Sialylation and Fucosylation.”
Normal pancreas does not express the Tn antigen and its corresponding sialylated epitope STn (21). The Tn antigen is detected in 75–90% of PDACs and up to 67% in precursor lesions (24). The appearance of the STn in mucins, on the other hand, is a late event in PDAC disease progression (29). These truncated O-glycans are associated with cancer cell growth and tumor invasion in PDAC (23, 24). The situation is slightly different in CRC, where the overexpression of T antigen is associated with early events in cancer progression and both Tn and STn antigens are frequently overexpressed in advanced and poorly differentiated adenocarcinomas and also in mucinous carcinomas. Therefore, these antigens are considered useful markers for poor outcome (22).
Besides cores 1 and 2, O-glycans with cores 3 and 4 are also often expressed in normal GI epithelia, especially in colon, but are significantly decreased in malignancy due to downregulation of the core 3 and 4-synthetase (8, 30–32).
The de novo expression of truncated O-glycans in GI cancers is of avail in the search of specific cancer biomarkers. It leads to the expression of unique glycopeptide structures and, because of the small steric size of these truncated O-glycan moieties, to the exposure of protein regions that would otherwise be masked, and, therefore, not detected by specific antibodies (29, 33).
Branched N-Glycans
The biosynthesis and maturation of N-glycan structures is defined by a complex interplay of numerous glycosidases and glycosyltransferases in the endoplasmic reticulum and Golgi. Among N-glycan types, the complex N-glycans display the largest structural diversity. Two structural features of complex N-glycans are the β1,6-branching, catalyzed by the glycosyltransferase GnT-V, and the bisecting-GlcNAc, added by the glycosyltransferase GnT-III. GnT-V is known to be upregulated in gastric carcinoma (Figure 1) (34), leading to the increased branching of N-glycans and contributing to cancer cell invasion and metastases (35, 36). Analogically, normal colon epithelium presents high levels of bisecting-GlcNAc, due to high expression levels of GnT-III, which is associated with suppression of the tumor progression. However, during cancer progression, these bisecting structures are decreased (37) and it has been described a general increase of β1,6-branched in complex N-linked glycans that are also associated with tumor invasion and metastasis (38). Histochemical studies using specific lectins for the detection of β1,6-branched structures showed increased staining concomitant with tumor CRC staging (39), and an association with lymph node metastasis and decreased survival rates in CRC patients (40). GnT-V, the enzyme responsible for the synthesis of β1,6-branched N-glycans, is commonly upregulated in CRC correlating to the metastatic potential and consequently considered an important prognosis factor to detect poor CRC patients’ outcome (41). A recent study demonstrated that GnT-V levels modulate CRC stem cells and tumor formation by Wnt signaling (42). Increased extension of β1,6-branched complex N-glycans by long polymers of N-acetyllactosamine (LacNAc) due to upregulation of β3GnT8 has also been described in CRC cells (43).
Regarding PDAC progression, little has been described about bisecting structures, although the increase in highly branched N-glycans is well established. The number of tri- and tetra-antennary glycans is significantly increased in both pancreatic cancer cells and PDAC patients’ serum and correlate with cancer progression (44, 45).
Another mechanism leading to increased branching is the downregulation of GnT-III and the addition of bisecting-GlcNAc. Bisected structures cannot be further modified by GnT-V and, therefore, preclude the formation of branched N-glycans under healthy conditions.
The interplay of GnT-III and GnT-V modulates cell adhesion and migration in a gastric cancer context (46, 47). This has been shown to be particularly important for cell–cell and cell–matrix interactions in gastric cancer by altering the functionality of E-cadherin and integrins in malignant transformation. E-cadherin promotes adherence junction formation and, thus, maintains intercellular adhesion. E-cadherin is stabilized by bisected N-glycans delaying its endocytosis and turnover (47–49). Furthermore, bisecting-GlcNAc on E-cadherin is gastric tumor suppressive by downregulating signaling pathways involved in cell motility and the EMT process (50–54). Conversely, E-cadherin is dysregulated when glycosylated with branched N-glycans by GnT-V in the context of gastric cancer (34, 52, 53). GnT-V is commonly upregulated in gastric carcinomas contributing to cell invasion and metastases (35, 36). The overexpression of GnT-V leads to destabilization of adherence junctions, delocalization of E-cadherin into the cytoplasm, and mesenchymal appearance of the cells with increased metastatic capability (34, 52, 55).
Integrins convey adhesion to extracellular matrix components and are often altered in GI carcinomas. In gastric cancer, the modification of α3β1 integrin with branched N-glycans increases cell migration (56). The modification of α3β1 integrin with bisecting-GlcNAc has the opposite effect by inhibiting cell migration (56). Consistently, the overexpression of GnT-III resulted in the inhibition of α5β1 integrin-mediated cell migration and reduced binding to fibronectin due to a specific N-glycosylation site on the α5 integrin (57, 58).
Increased Sialylation and Fucosylation
Sialic acids are the largest and the only intrinsically negatively charged monosaccharides present in human glycosylation. As a terminal event, sialylation caps glycosylation chains usually resulting in exposed locations of the negative charge at the forefront of the oligosaccharides and first encounter point for adjacent glycans, proteins, and cells. Sialylation has, therefore, been shown to play important roles in modulating cellular recognition, cell adhesion, and cell signaling (59). Moreover, cancer cell sialylation patterns define sialic acid-binding lectins (Siglecs) interactions and modulate immune response (60, 61).
An increase in global sialylation, especially in α2,6- and α2,3-linked sialylation, owing to altered glycosyltransferases expression, has been closely associated with cancer and commonly described as one of the main modifications in GI cancers (62, 63). For example, ST6Gal-I, the enzyme that adds α2,6-linked sialic acid to lactosamine chains (Neu5Acα2,6Galβ1,4GlcNAc), is commonly overexpressed in GI cancers correlating with poor prognosis (59, 64, 65). Additionally, α2,3-sialyltransferases, such as ST3Gal-III and ST3Gal-IV, are often upregulated in the course of gastric cancer and PDAC progression leading to a more invasive and metastatic phenotypes of the cancer cells (65–69). Furthermore, sialylation, in particular α2,3 and α2,6-linked, can modulate the ECM adhesion and migration. Specifically, it has been described that while the overexpression of terminal α2,6-linked sialic acid leads to increased ECM adhesion, the overexpression of α2,3-linked terminal sialic acid epitopes in PDAC cancer cell lines results in a more migratory phenotype (70). Similarly, in gastric cancer cells, the overexpression of α2,3-linked terminal sialic acid epitopes causes a more invasive phenotype in vitro and in vivo (67).
The major α2,3-sialylated antigens associated with cancer are SLea and SLex (Figure 1). Although these structures can also be present in non-neoplastic cells, SLea and SLex have been demonstrated to be highly expressed in many malignant tissues, including GI tumors, both in glycoproteins and glycosphigolipids (71–74). SLex-increased expression levels are associated with advanced stages and have been correlated with poor survival in GI cancer patients (75–77). SLex is the well-known ligand for selectins (78). During inflammation, selectins mediate the initial attachment of leukocytes to the endothelium during the process of leukocyte extravasation. In cancer, SLex interactions with selectins favor metastasis by forming emboli of cancer cells and platelets and promoting their arrest on endothelia (77).
The overexpression of SLex in a gastric carcinoma cell line transfected with ST3GAL4 has shown to increase the cells invasive potential both in vitro and in vivo due to the activation of the oncogenic c-Met receptor tyrosine kinase (67). Moreover, overexpression of ST3GAL4 has been shown to result in RON receptor tyrosine kinase activation and co-expression of RON and SLex is observed in gastric tumors (79). This is of particular biological relevance since it has been described that RON activation contributes to tumor progression, angiogenesis, and therapy resistance and correlates with bad prognosis (80–84).
Sialylated Lewis epitopes are potential good markers for prognosis due to their high incidence of recurrence or presence in metastasis and correlation with the tumor stage. For example, a recent work described the increase of the SLex epitope on ceruloplasmin in PDAC. The increased ceruloplasmin with the SLex epitope in chronic pancreatitis was lower, suggesting good specificity for pancreatic malignancy (85). Moreover, studies using high-density antibody microarray also detected increased levels of SLex and SLea antigens on glycoproteins in serum or plasma of CRC patients (86).
Overexpression of the enzyme β-galactoside α2,6-sialyltransferase I (ST6Gal-I), especially in N-glycans and not in O-glycans, has been associated with CRC progression, increased invasion, and metastization and consequently poor prognosis in CRC patients (64, 87). Further studies taking into consideration the low levels of ST6Gal-I in healthy individuals and upregulation in CRC patients could lead to the development of new diagnostic and therapeutic targets.
Fucosylation is also an important modification involved in cancer and inflammation (88). The attachment of fucose to N-glycans, O-glycans, and glycolipids has been reported in cancer tissues, regulating different biological processes, and being also responsible for the increased expression of Lewis antigens (89) (and sialylated-Lewis antigens, as previously described). Different studies link the presence of fucosylated epitopes on specific glycoproteins with cancer. In particular, research performed on some acute phase proteins suggest the suitability of fucosylated epitopes for cancer management. It has been demonstrated that fucosylated alpha-fetoprotein (AFP) is more specific as a hepatocellular carcinoma biomarker than AFP itself. Nowadays fucosylated AFP (AFP-L3) is used for hepatocellular carcinoma risk assessment (90, 91). Acute phase proteins, such as AFP, are proteins synthesized by hepatocytes and have shown clinical value as markers for liver and pancreatic-related diseases. For example, haptoglobin and AGP have revealed an increase in fucosylated epitopes that could help to improve PDAC diagnosis (89, 92).
Increased activity in α1,3 and α1,4 fucosyltransferases (FUTs) was described in CRC patients, resulting in the synthesis of SLex and SLea epitopes, respectively (89). Particularly, FUT6 was more recently reported as the major regulator of SLex biosynthesis in CRC (93). Increased levels of fucosylation in plasma samples of CRC patients compared to normal controls were also described using methods for N-glycoproteomics analysis to identify plasma markers (94). In addition, increased levels of α1,2-FUT1 and FUT2, which add fucose to terminal galactose and are essential for the synthesis of Lewis Y and B antigens, were shown in CRC tumors (95). Alterations of FUT expression have also been described in the process of gastric carcinogenesis (96). In particular, the downregulation of FUT3 and FUT5 changes the Lewis antigens expression and reduces the adhesion capacities of gastric cancer cells (97). This is contrary to what is observed in gastric inflamed mucosa, where FUT3 is upregulated (98).
Increased core-fucosylation of N-glycans catalyzed by α1,6-FUT8 has been described in CRC patients and is associated with tumor aggressiveness (37, 99). The core-fucosylation of E-cadherin enhances the cellular adhesion of CRC cells (100). However, in gastric cancer, the decrease of core-fucosylation has been demonstrated to be a common event contributing to cancer cell proliferation (101).
Other Relevant Glycosylation Alterations
In addition to the mucin-type O-glycosylation, there are further forms of protein O-glycosylation, including the modification of nuclear and cytoplasmic proteins with O-linked β-N-acetylglucosamine (O-GlcNAc). Noteworthy, increased O-GlcNAcylation is a general feature of cancer and the modification of proteins with O-GlcNAc has been shown to play key regulatory roles in tumor cell signaling (102). The addition of O-GlcNAc to nuclear and cytosolic proteins is mediated by the O-GlcNAc transferase (OGT), whereas the enzyme O-GlcNAc-selective N-acetyl-beta-d-glucosaminidase (O-GlcNAcase) removes the O-GlcNAc, returning the protein to its basal state (103). O-GlcNAcylation has been shown to have extensive crosstalk with phosphorylation and to antagonize phosphorylation-mediated cell signaling (104).
In the pancreas, beta-cells are characterized by expressing high levels of the OGT enzyme. This allows these cells to dynamically respond to physiological increases in the extracellular glucose levels by converting glucose to UDP-GlcNAc, which is the OGT substrate, and therefore modulating intracellular O-linked protein glycosylation (105). In PDAC, hyper-O-GlcNAcylation has been associated with increased expression of the OGT enzyme and reduction of the O-Glc-NAcase glycosidase and has been demonstrated to block cancer cell apoptosis and to lead to the oncogenic activation of the NF-kB signaling pathway (106). Similarly, increased O-GlcNAcylation in colon has been demonstrated to contribute for the development of colitis and colitis-associated cancer by enhancing NF-kB-mediated signaling (107).
Along with aberrant protein glycosylation, cancer cells also display major glycosylation alterations on other classes of glycoconjugates, including the proteoglycans and the glycosphingolipids. Proteoglycans consist of a core protein with one or more covalently attached large GAG chains, and can be either located at the cell membrane or secreted. The syndecans are a family of transmembrane proteoglycans that carry heparan sulfate GAG chains and that can also be additionally modified with chondroitin sulfate chains (108). The heparan sulfate-rich proteoglycan syndecan-4, a critical partner of integrins for the establishment of focal adhesion complexes, has been shown to be upregulated in gastric mucosa in response to the oncogenic bacteria Helicobacter pylori. However, its functional role in the gastric carcinogenesis process remains to be disclosed (109, 110). Another syndecan family member, the syndecan-1, has been reported to be differently regulated and expressed in GI tumors. Loss of syndecan-1 expression has been described in gastric adenocarcinomas of higher stages (111), while in CRC and PDAC the expression of this proteoglycan has been shown to be upregulated, suggesting its possible application as a biomarker (112, 113).
Heparan sulfate GAG chains can also be carried by glypicans, a family of glycosylphosphatidylinositol (GPI)-anchored proteoglycans. Glypicans have been shown to bind a wide range of signaling molecules and to regulate the signaling of the Wnt, Hedgehog, fibroblast growth factor, and bone morphogenetic protein (BMP) pathways (114). Glypican-1 has been shown to be overexpressed in PDAC cell models and patient tumors (115). Moreover, the key role of glypican-1 in PDAC progression has been well documented using mouse models (116, 117). Recently, glypican-1 has been shown to be specifically expressed by cancer circulating exosomes and, therefore, to have potential to be used as a minimal-invasive diagnostic and screening tool to detect early PDAC stages (118).
The CD44 proteoglycan has also been on the focus of tumor biology research because the expression of specific splice variants is strongly associated with malignancy. Specifically, the exon v6-containing CD44 isoform (CD44v6) is highly expressed in premalignant and malignant gastric lesions (119). Modification of CD44v6 with STn was demonstrated in gastric mucosa and serum of cancer patients, indicating its potential as a biomarker for early diagnosis of gastric tumors (120). Different strategies aiming the impairment of CD44-dependent cancer cell migration have been proposed. The ceramide nanoliposome (CNL) was shown to induce anoikis and to limit metastasis by inducing lysosomal degradation of CD44 in PDAC cells (121).
Another glycosylation modification frequently observed in cancer is the altered sialylation of glycosphingolipids that can lead to the appearance of tumor-associated antigens. The human plasma membrane-associated sialidase NEU3, which catalyzes the removal of sialic acids from glycoproteins and glycolipids, is a key enzyme for ganglioside degradation. NEU3 has been shown to be overexpressed in many tumors, including CRC (122). Modulation of ganglioside expression by increased NEU3 activity has been proposed as a mechanism of protection against programed cell death and has, therefore, a critical implication in therapeutic strategies (123). Recently, NEU3 was demonstrated to regulate the Wnt signaling pathway, therefore contributing for the malignant transformation of CRC cells (124). These findings suggest NEU as a relevant target for diagnosis and therapy of CRC. During CRC progression, besides reduced expression of sialylated gangliosides, overall alteration in glycosphingolipids glycosylation includes increased fucosylation, decreased acetylation and sulfation, and reduced expression of globo-series glycans (125).
Glycan Cancer Biomarkers
Glycosylation changes on glycoconjugates either expressed on the cell surface or secreted by cancer cells are potential sources of cancer biomarkers. The overexpression of these altered glycosylated structures and the loss of polarity of carcinoma cells lead to the shedding of glycoconjugates with altered glycosylation into the circulation. Currently, several serological assays used in the clinics are based on the quantification of glycoconjugate levels in the serum of cancer patients. Most of these biomarkers have been useful for prognostic and monitoring purposes. These include well-established serological biomarkers, such as the CA15-3 assay, detecting mucin MUC1 glycoprotein used for breast cancer (126–130), the CA125 assay, which detects the circulating mucin MUC16 in ovarian cancer (131, 132), and the prostate-specific antigen (PSA), which is used to detect prostate diseases (133, 134).
Regarding GI cancer, one of the most used serological assay detects the SLea carbohydrate antigen. SLea is present on circulating glycolipids and glycoproteins and is detected by the CA19-9 assay. This serological assay is applied in patients with a previously established diagnosis of PDAC, CRC, gastric, or biliary cancers and used to monitor their clinical response to therapy (135–138).
Another important serological test used in the clinics for GI tumors is the carcinoembryonic antigen (CEA) assay, which detects the CEA glycoprotein produced by carcinoma cells. In GI cancer, CEA is expressed at high levels and shed into the bloodstream being useful for prognosis evaluation and follow-up of these patients (129, 137, 139, 140).
In general, most of these serological assays have primarily been useful for prognosis and patients’ monitoring applications. Unfortunately, some of these biomarkers can also be detected due to benign lesions or other factors, such as smoking, which has limited their use in cancer screening strategies for diagnostic purposes. Given the usually late diagnosis of GI cancer, highly specific serum markers for cancer detection and screening are highly needed. Recent developed strategies and advanced technologies are contributing to the definition of novel and more specific glycoconjugate targets. Several of these new targets are currently evaluated and hold potential for improving the cancer detection and early diagnosis.
Innovative Glycobiological Strategies
The difficulty of glycobiological research lies in the intrinsic complexity of glycosylation and its versatile conjugates. Whereas genomic and proteomic analysis made a leap forward by DNA sequencing and mass-spectrometric protein sequencing, respectively, that enabled the reading of a linear code with limited number of variabilities; for the more complex glycans, no comparable tool exists.
Nevertheless, the recent years have brought up many innovative approaches and methods that enable the unraveling of glycobiological challenges. With the development of glycoengineered cell strategies, glycan complexities have been reduced and the effects of specific glycan epitopes have been pinpointed. On the other hand, analytical methods and protocols for glycomic and glycoproteomic analyses have improved and new approaches, such as the adaptation of the array technology on glycans and lectins or novel antibody-based assays, have accelerated the acquisition of glycobiological knowledge. The following sections discuss several promising strategies in the glycobiology field.
Glycoengineered Cell Line Models
The characterization of the function of glycans in cancer has been a major challenge in the field due to technical difficulties related to the complexity and heterogeneity of glycans synthesized in eukaryotic cells.
Genetic engineered cell models have been developed to study the functions of specific glycan epitopes in cancer. Some of these models include the overexpression of glycosyltransferases, which has allowed the characterization of the biosynthesis and function of simple cancer-associated carbohydrate epitopes, such as Tn, STn, T, and ST (17, 18, 20, 141, 142). Similar strategies have used stably transfected cell lines with glycosyltransferases to characterize the function of branched glycan structures (52, 56) as well as terminal sialylated/fucosylated structures frequently overexpressed by cancer cells, as previously explained in Section “Increased Sialylation and Fucosylation” (67, 143, 144).
Another major challenge in the field was related to the identification of structures at individual glycosylation sites. Major efforts have been done in this discipline with the generation of site-specific mutants of important proteins in cancer. One example is the use of site-specific mutants of N-glycosylation sites of the human epidermal growth factor receptor. This strategy has allowed the demonstration that Asn-420-linked oligosaccharide chain in this receptor interferes with its activation in cancer cell lines (145). Another cell line model has addressed the role of E-cadherin N-glycosylation sites in gastric cancer (146–148). The use of E-cadherin constructs engineered to lack specific N-glycosylation sites has demonstrated the effect of specific N-glycosylation structures on cell adhesion (149, 150).
The recent use of genomic editing tools has allowed the development of isogenic cell systems that along with extensive application of mass spectrometry (MS) methods is utilized for high-throughput site-specific O-Glycosylation (O-GalNAc and O-Mannose) proteomics. These technologies have enabled the precise determination of protein O-glycosylation sites in cells (151, 152). These strategies have greatly evolved in the past years and are showing vast potential in the glycobiology field. One approach has used the zinc-finger nucleases targeting the knockout of COSMC gene and has been applied in several human cancer cell lines originated from different organs (152). These so-called SimpleCell models produce stable cells expressing homogeneous truncated O-glycosylation with Tn and/or STn O-glycans (24, 120, 151, 153).
These cell models have provided a source of unlimited material for isolation and identification of GalNAc O-glycopeptides from cell lysates or secretomes using lectin chromatography followed by advanced MS, enabling the identification of hundreds of unique O-glycoproteins and O-glycosylation sites in several cell line models from different tissues (120, 153, 154). In addition, this approach has provided a versatile method for the functional analysis of different ppGalNAc-Ts (153, 155). Furthermore, similar strategies have been applied targeting the O-mannose glycoproteome. To reduce the structural heterogeneity of O-mannosylation (O-Man), the nuclease-mediated gene editing of a human cell line was performed by zinc-finger nuclease targeting of the POMGNT1 gene. This gene encodes for the enzyme POMGnT1 that controls the first step in the elongation of O-Man glycans. The O-Man glycoproteome has been characterized using both chromatography and advanced MS (156).
The knowledge of O-glycosites in specific cancer cell types allows for the analysis of novel biological functions of glycosylation and for potential cancer cell-specific O-glycosites. This is particularly important given the complexity of O-glycosylation and that the various ppGalNAc-Ts that control the protein O-glycosylation sites may determine large variation at protein, cell, and tissue levels (9).
Glycomic Strategy
Glycomics is the study of all glycan structures of a given cell, tissue, or organism. The intrinsic complexity of glycan structures and their versatile conjugates render this field particularly challenging. Due to the constant advancement of analytical instruments and methods, the N- and O-glycomic characterization of cancer cell lines, tumors, and cancer patients’ body fluids has rendered possible. Still, there is no single ideal method for this analysis and, thus, today a large variety of analytical methods is available for the glycomic characterization, resulting from different combinations of initial sample preparation, derivatization, glycan separation, and detection. Each method bares advantages and disadvantages.
For the glycomic analysis of cells or tumors, the sample is usually homogenized and proteins are denatured, followed by the release of glycans (Figure 2A). The study of the glycans of serum or plasma is more challenging and requires often purification steps for glycoproteins prior to the release of their glycan structures. There are several methods to release glycans from the protein backbone to facilitate their characterization. The release of glycans is not a prerequisite as the analysis of whole glycopeptides is also possible (covered in Section “Glycoproteomic Strategy”). The most prominent technique to release N-glycans is by Peptide-N-Glycosidase F (PNGase F). The release of N-glycans via PNGase F is robust, fast, and efficient and is capable of liberating all types of human N-glycan structures. PNGase F-released glycans can be chemically labeled. On the other hand, no enzyme has so far been characterized that enables the efficient release of all types of O-glycans. For instance, the enzyme O-glycanase releases only core 1 O-glycans from their peptide backbone. Therefore, chemical techniques have to be utilized for whole O-glycomic analyses, such as reductive β-elimination.
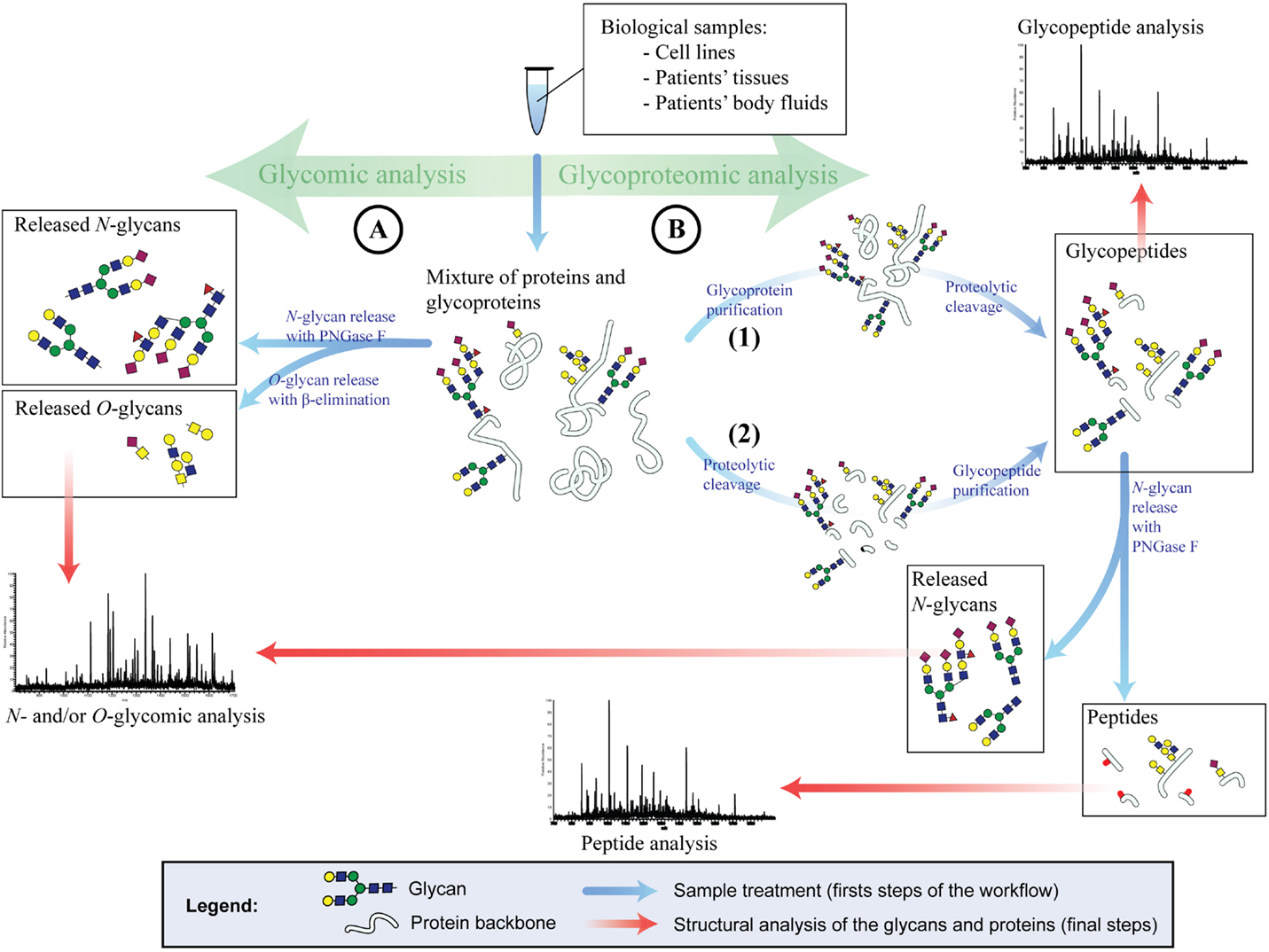
Figure 2. Glycomic and glycoproteomic strategies for glycan analysis. (A) Glycomic analysis. N- and O-glycans are PNGase F or chemically released, respectively. Once the glycans are released, they are structurally analyzed (usually by mass spectrometry). (B) Glycoproteomic analysis. The glycoproteins are either purified from a complex mixture and then enzymatically digested (1) or first digested and then purified (2). Method 1 will result in glycopeptides and non-glycosylated peptides originated solely from glycoproteins. Method 2 will result in glycopeptides only. These glycopeptides can be directly analyzed to generate site-specific glycan structures. Alternatively, the glycans of the glycopeptides can be released as described in (A) and the peptides are analyzed.
Released glycans can be analyzed after derivatization or in their native form (underivatized). The derivatization of glycans bares several advantages, such as adding fluorescent tags for the photometric detection or chemical modification of side groups to stabilize glycan constituents. Despite these advantages, it is preferred in some cases to work with the native glycan, avoiding several time-consuming preparation steps and sample losses.
Complex mixtures of glycans, as they arise from clinical samples or even cell lines are usually separated by chromatography or capillary electrophoresis [reviewed in Ref. (157)] and detected by fluorescence detector (FLD) or MS. The sensitive and quantitative fluorescence detection requires fluorescently tagged glycans, and gives on its own only limited structural information derived from chromatographic or electrophoretic retention times. MS, on the other hand, can be applied on both native and derivatized oligosaccharides and may yield detailed structural information of the glycans. Since the analytes are not consumed by FLD, a sequential setup with MS is possible and often advantageous.
Three successful glycomic workflows that have revealed in the past years several findings in GI cancer are porous graphitized carbon separation with electrospray ionization and tandem MS (PGC-ESI-MS/MS), hydrophilic interaction ultra/high performance liquid chromatography with FLD (HILIC-FLD-UPLC/HPLC), and matrix-assisted laser desorption/ionization MS (MALDI-MS).
Porous graphitized carbon separation with electrospray ionization and tandem MS is a workflow used for both N- and O-glycomic analyses. First, the N-glycans are liberated from the glycoproteins with PNGase F, followed by reductive β-elimination of the glycoproteins to release the remaining O-glycans. The N- and O-glycans are separated by liquid chromatography with a PGC column, which resolves most isomeric structures and complements, therefore, ideally the subsequent MS and MS/MS structural analysis. A recent glycomic study by PGC-ESI-MS/MS has described structural glycan alterations in CRC, including several unique glycans found solely in the tumor region and indicated a correlation between EGFR expression and sialylation in CRC (158). This method has lately been further utilized for the N- and O-glycomic characterization of CRC cell lines and tumors, revealing great O-glycomic differences between tumors and all tested cell line models (26).
Hydrophilic interaction ultra/high performance liquid chromatography with FLD is used for the quantitative profiling of N-glycans. The N-glycans are released by PNGase F and reductively aminated with a fluorophore. The labeled N-glycans are applied on a HILIC-ultra performance chromatography (UPLC), which separates the glycans according to their size and monosaccharide composition. The retention time can be converted to glucose units (GU) by comparing it with a dextran ladder, yielding reproducible results. Hence, due to the few sample preparation steps, the high recovery of the HILIC column, the quantitative detection via the fluorescent tag, and the possibility of multiplexing, this analysis can be applied for large-scale N-glycomic studies. The HILIC-FLD-UPLC N-glycomic analysis has recently been applied in a large-scale discovery study on serum of gastric cancer patients revealing an increase in certain SLex carrying N-glycan structures that correlated with disease progression. Furthermore, in this study other structures, such as bisected N-glycans, have been shown to decrease with disease progression (159, 160).
Recently, the combination of HILIC-FLD-UPLC and PGC-ESI-MS/MS has been used for N- and O-glycomic analysis of a gastric cancer cell line overexpressing the sialyltransferase ST3Gal-IV (79). This cell line has previously been shown to present a more invasive phenotype (67). The glycomic analysis revealed a broad range of cancer-associated alterations, such as decreased bisected and increased branched structures, truncation of O-glycans, and a shift from α2,6- to α2,3-sialylated N-glycans (79).
The use of MALDI-MS is another very successful approach of analyzing the N-glycome of clinical samples, such as body fluids. MALDI is based on a laser impulse that excites a solid matrix in which the analytes are embedded which in turn desorbs and ionizes the analytes for MS analysis. MALDI is relatively tolerant to salt and other contaminants, which allows uncomplicated sample preparation after the release of N-glycans. This method has recently been applied on the serum of gastric cancer patients and control groups and has been able to identify N-glycomic differences between the serum of gastric cancer patients and that of non-atrophic gastritis patients (161). In a large-scale study on sera of PDAC patients, a tendency toward higher branched and fucosylated N-glycans has been observed when compared to sera from healthy individuals. The major part of the significantly altered N-glycan structures were specifically increased in patients with distant metastases and the ratio of the quantity of two glycans has been proposed as a robust diagnostic marker for PDAC (162). Another recent study utilizing MALDI-MS has revealed in pancreatic cyst fluids, of which certain subtypes bare a high risk of undergoing malignant transformation, the hyperfucosylation of N-glycans (163).
A broad range of alterations in CRC tissues versus controls have been identified by sequential analyses of fluorescently tagged N-glycans by HILIC-FLD-HPLC and MALDI-MS. Additionally, multivariate statistical evaluation and further MS-based structure elucidation have been applied and revealed among others the decrease of bisected structures and the increase of glycans with sialylated lewis epitopes. Furthermore, abnormal core-fucosylated high mannose N-glycans have been uniquely found in cancer tissue (37).
Glycoproteomic Strategy
Glycoproteomics is the study of proteins that carry glycan modifications. It usually focuses on the identification and quantification of glycoproteins and the characterization of protein glycosylation sites. Given that most clinical cancer biomarkers are glycoproteins, this field is particularly promising for the identification of new biomarker targets in cancer. Biological samples, such as cell lines, tissues, and body fluids, can be analyzed. However, the glycoproteomic analysis of complex biological samples, such as tissues or sera, is analytically challenging due to the large complexity and vast dynamic range of concentrations of glycoproteins.
The glycoproteomic pipeline typically consists of numerous steps, such as glycoprotein or glycopeptide enrichment, isotopic labeling (optional), multidimensional protein or peptide separation, tandem mass-spectrometric analysis, and bioinformatic data interpretation (Figure 2B). In cancer, the vast majority of glycoproteomic findings are based on bottom-up analysis of peptides (“shotgun proteomics”). For this purpose, glycoproteins are proteolytically cleaved into glycopeptides before or after the enrichment step. The enrichment of glycoproteins or glycopeptides is a critical step of the glycoproteomic analysis. Even though this field is rapidly evolving, so far no method has been established that captures unbiased every glycoprotein or glycopeptide and enables full glycoproteomic coverage. Currently, most popular enrichment methods are based on lectins (164–166) or on hydrazide solid-phase extraction (167, 168) and sometimes applied in combination to increase the glycoproteomic coverage (168). Alternative strategies are boronic acid functionalized beads (169), size exclusion chromatography (170), hydrophilic interaction (171), and graphite powder micro column (172). Due to the difficulties of covering the whole glycoproteome many cancer studies pursue a different strategy of enriching specifically glycoproteins and glycopeptides carrying cancer-relevant glycan epitopes, such as sialic acids or sialylated Lewis epitopes. These methods are usually based on lectins [such as SNA, WGA, and MAL (173)], antibodies (159), enrichment by titanium dioxide (79, 174), or affinity purification of metabolic labeled glycoproteins (175–177). After the enrichment and proteolytic digestion (not necessarily in this order), glycopeptides may be deglycosylated and are multidimensional separated via chromatography and/or electrophoresis and analyzed by tandem MS. The deglycosylation is a requirement of some enrichment methods, such as hydrazide solid-phase extraction, but may be also applied for all N-glycoproteomic analysis. The PNGase F release of N-glycans leads to the conversion of the N-glycan carrying asparagine to aspartic acid and can, thus, be spotted on the peptide backbone by MS. For the generation of site-specific structural information of N- and O-glycans, whole glycopeptides are analyzed utilizing a combination of different MS fragmentation methods or collision energies that either fragment peptides or glycans (178–180). This strategy is being optimized in recent years and bares great potential for the discovery of new cancer biomarkers because it unravels site-specific glycan alterations in cancer.
Glycoproteomic analyses have been applied in GI cancer mainly for the identification of biomarkers, such diagnostic biomarkers or biomarkers for multidrug resistance in gastric cancer (181, 182). Glycoproteomics in combination with glycoengineered cell line models was in recent years able to increase the coverage of O-glycosylated proteins and to identify numerous novel O-glycosylation sites in gastric cancer and PDAC, generating several new potential biomarkers (24, 120).
Other Glycoanalytical Techniques
MS-based glycomic and glycoproteomic analyses require expensive equipments and a fair amount of expertise. MS-independent methods, such as glycoprotein, antibody-lectin-sandwich, and lectin arrays, are capable of rapid data acquisition of glycomic alterations in cancer samples. Glycan arrays, on the other hand, enable a screening for specificities of glycan-binding proteins, improving the data interpretation of antibody and lectin-based research. Regarding tumor biology, it is very relevant to determine not only the glycosylation modifications harbored by tumor cells but also to disclose the topographic distribution of these alterations within the tumor and adjacent tissue. Novel approaches for the identification of in situ glycan modification of specific proteins include proximity ligation assay (PLA) and imaging mass spectometry (IMS).
Arrays
The binding of biological molecules to solid matrixes was an idea first described by Chang in 1983 (183). This technology initially consisted of coating glass cover slips with different antibodies in close proximity forming a matrix-like array. Arrays recognize partners from large amounts of biological material using high-throughput screening miniaturized, multiplexed and parallel processing, and detection methods based on multiple probes covalently attached to a solid substrate. Depending on the molecule that is deposited on the surface, different microarrays exist. To analyze glycan-containing structures, the most common classification is glycan, glycoprotein, or lectin microarray, and also a variant of the latter called antibody-lectin sandwich array (Figure 3) (184). The advantages that the microarray technology offers are the small volume of sample required for the analyses, the high reproducibility, and the reduced cost and time to process many samples. Therefore, microarray platforms have been highlighted by its extensive application in the field of biomarker validation, where a large number of samples must be analyzed multiple times (185). Moreover, depending on the type of microarray assay performed, information about the glycan-linkage configuration can be obtained.
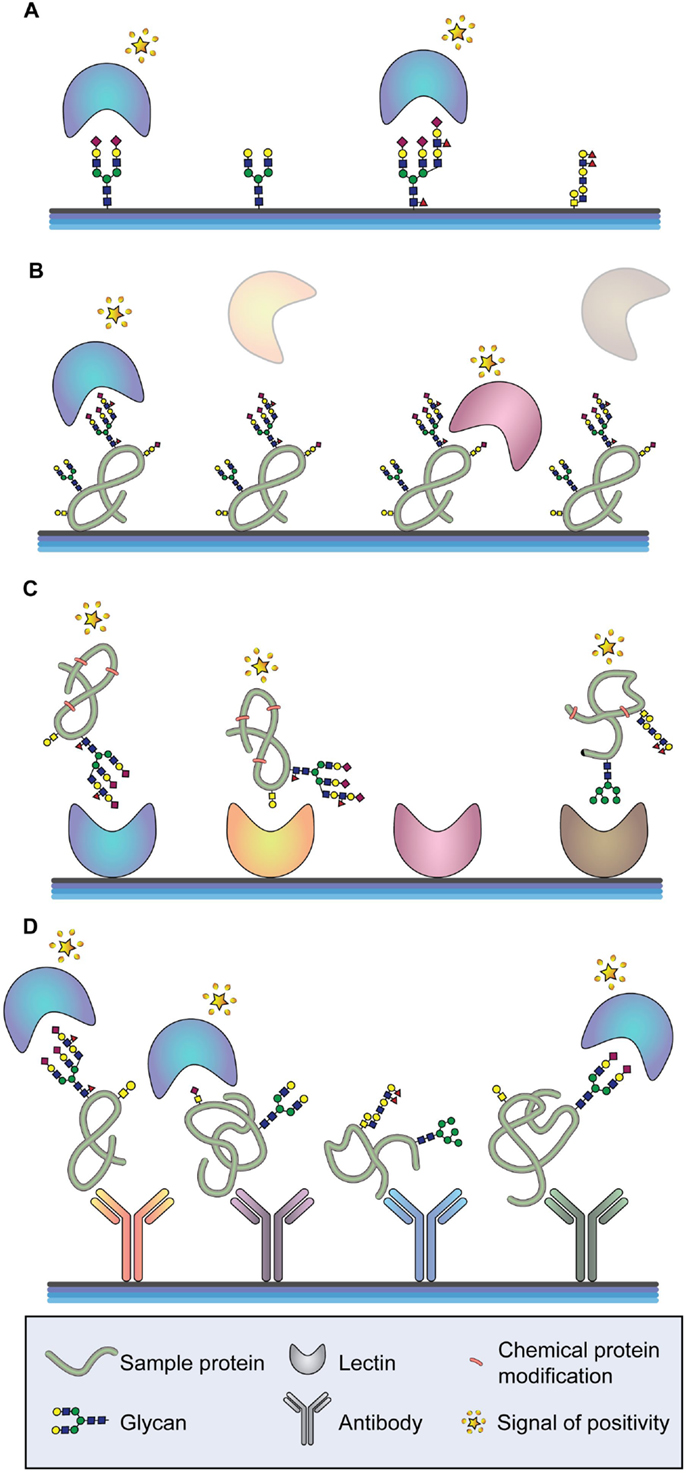
Figure 3. Arrays for glycan analysis. (A) Glycan arrays. Different glycan moieties are spotted onto the array to determine specificities of labeled glycan-binding molecules (typically lectins or antibodies). (B) Glycoprotein arrays. Glycoproteins are enriched from a sample and spotted onto the array. The glycosylation moieties of the spotted glycoproteins are determined by screening with different glycan-binding molecules. (C) Lectin arrays. A series of lectins with well-defined glycan-binding properties are spotted onto the array and different labeled proteins are tested. (D) Antibody-lectin sandwich arrays. Multiple antibodies for a series of proteins of interest are spotted onto the array, and the glycan epitopes of the captured proteins are probed using labeled lectins and glycan-binding antibodies.
Glycan microarrays are used mainly to characterize the binding specificities and affinities of proteins (mostly antibodies and lectins) toward glycans (Figure 3A) (186–188). However, they can also be applied for the screening of inhibitors of carbohydrate-mediated interactions and of sugar interactions of an entire organism, such as a whole cell or virus (185, 189, 190). Current available platforms consist of approximately 20,000 microspots of antigens reaching the capacity to include most known human microbial pathogens, autoantigens, and tumor-associated antigens (191–193). The diversity and scope of glycan arrays are continuously increasing allowing a better characterization of glycan-binding proteins but leading to more complex data. Different software tools are currently available for data interpretation (194–196). Glycan arrays present oligosaccharides that were either purified from a biological source or de novo synthesized. Regarding the latest ones, it is important to highlight recent works describing new methodologies that allow sialic acid (197, 198) and GAG synthesis (199).
As an alternative to the direct binding of glycans to the array surface, glycans can be presented on proteins or peptides that are attached to the array. A recent advancement in this approach is the coiled coil-based technology, which allows the presentation of the antigens at high densities while mimicking the in vivo orientation attached to a fiber-forming peptide. This platform showed increased sensitivity for the identification of antibodies against parasitic glycan antigens and might be adapted in the future for cancer diagnostic (200).
Glycoprotein microarrays are based on printing purified or enriched glycoproteins onto the slides and screening these proteins for glycan epitopes using different lectins or glycan-recognizing antibodies (Figure 3B). This approach is usually followed by analytical techniques to identify the spotted proteins and to verify the glycan epitopes found by the array analysis. A recently performed glycoprotein array analysis of lectin-enriched sera from PDAC patients, chronic pancreatitis patients (benign pancreatic disease), and healthy individuals has correctly clustered these three groups, being the PDAC group significantly different from the other two (201). In addition, the glycoprotein microarray may use synthesized peptides and recombinant protein fragments that have been in vitro glycosylated for the detection of human autoantibodies (202, 203).
Lectin microarrays, where different lectins are spotted onto the slide, enable a rapid and high-sensitivity profiling of glycan features found in complex samples, such as cells, tissues, body fluids, and synthetic glycans and their mimics (Figure 3C) (204, 205). Lectin arrays offer a general view of the glycan structures on a complex sample and integrate the information from all proteins with the disadvantage that no information about specific glycan changes of the respective protein constituents will be obtained (206). A recent work displayed different glycopatterns in gastric cancer compared to gastric ulcer applying Cy3-labeled proteins extracted from tissues to lectin microarrays (207). Another recent lectin array approach has identified differences in α2-macroglobulin glycosylation between healthy individuals and patients with CRC. The spotted serum purified α2-macroglobulin has displayed, among other changes, significant differences in the content of branched N-glycans and α2,6 sialylation (208).
A variant of the lectin array is the antibody-lectin sandwich array. Antibodies to known glycoproteins are spotted on a solid support, and complex glycoprotein samples, which can be crude or prefractionated, are bound to the microarray (Figure 3D) (184, 185). The glycosylation of the captured target proteins are then screened by labeled lectins and glycan-specific antibodies. Antibody-lectin sandwich arrays are highly effective for profiling variation in specific glycans on multiple target proteins. Performing this technology, specific glycoforms of MUC5AC and endorepellin glycoproteins in the cyst fluid of patients with precancerous pancreatic cysts have been found (209). In these assays, the glycoprotein nature of the antibodies must be considered and different approaches to prevent glycan recognition of the antibodies by the secondary antibody or lectin applied exist. The chemical derivatization of the glycans of the spotted antibodies also prevents their ability to be recognized by glycan-binding molecules, both antibodies and lectins. One efficient method to study glycans on individual proteins from complex mixtures uses chemically derivatized capture antibodies and tests the glycosylation of captured target proteins by lectins and glycan-binding antibodies. Applying this approach, cancer-associated glycan alterations on the proteins MUC1 and CEA in the serum of PDAC patients have been identified (210). Another strategy consists on producing recombinant antibodies in organisms that do not carry out post-translational modification, such as glycosylation. This approach has been performed for the detection of glycans linked to CEA by ELISA coating the microplate with recombinantly scFv expressed in Escherichia coli and using lectins as detection probes (211).
Regarding GI cancer research, arrays have been widely used to discover new biomarkers consisting of proteins bearing aberrant glycosylation that could lead to a more accurate diagnostic.
In situ Proximity Ligation Assay
The association of the glycan expression and location with clinical and molecular characteristics of cancer tissues has rendered possible by histochemistry techniques using glycan-binding antibodies or lectins (205). However, one major limitation of this technique is the lack of capacity to identify the proteins in situ on which these glycan motifs are localized. This limitation has been surpassed by the development of the in situ proximity ligation assay (PLA) (Figure 4) (212, 213).
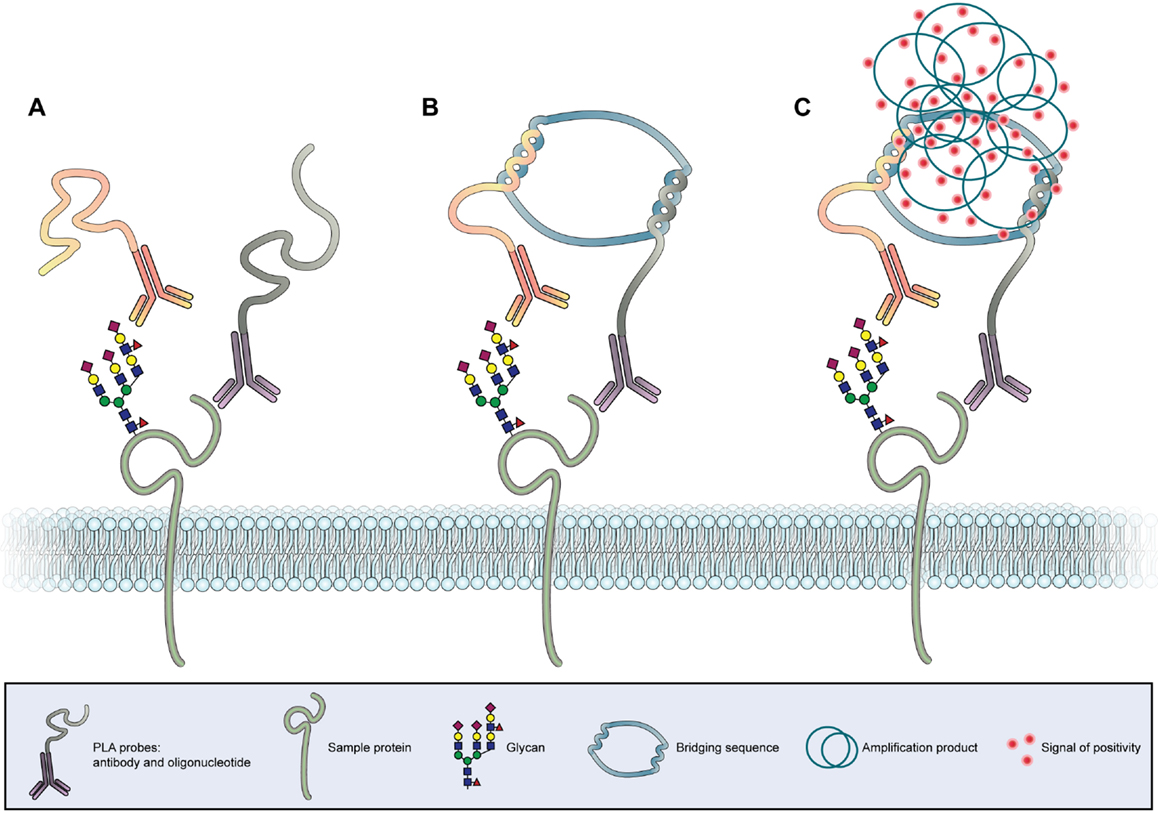
Figure 4. In situ proximity ligation assay. (A) Two antibodies, one specific for the protein backbone and another specific for a glycan epitope, are conjugated with two different oligonucleotide chains (PLA probes). (B) In case the antibodies bind to molecules in close proximity a bridging sequence links the two oligonucleotide sequences. (C) A subsequent polymerase induced amplification in combination with labeled nucleotides leads to the formation of a fluorescent or chromogenic signal at the co-expression site of the protein and glycan, allowing the in situ detection of the PLA signal.
This sensitive antibody-based method reveals the colocalization between specific proteins and specific glycan structures in tissues and cell samples. The identification of protein glycoforms is of utmost importance for the understanding of glycobiological cellular processes in cancer. PLA could also detect other post-translational modifications of proteins in tissue samples with subcellular resolution. The PLA technology is based on the binding of two specific PLA probes, each containing a unique oligonucleotide, to two targets of interest (Figure 4A). Antibodies, lectins, and other binding proteins can act as probes. A ligation solution, containing bridging oligonucleotides and a ligase, will hybridize the oligonucleotides of the PLA probes if they are in close molecular proximity to form a closed circle (Figure 4B). This closed nucleotide circle will be amplified by a DNA polymerase generating repeated copies of the circular DNA strands. Finally, fluorescent or chromogenic oligonucleotides hybridize to the amplification product and can be detected as individual spot by microscopy (Figure 4C) (213). The first study using this innovative PLA strategy applied to glycobiology has showed that the mucin MUC2 is a major carrier of the cancer-associated STn glycan antigen both in intestinal metaplasia and gastric carcinoma (214). The use of in situ PLA for the identification of a mucin glycosylation profile in cancer lesions is being extended, opening new opportunities for the development of novel diagnostic and prognostic markers. One recent study screened for tissue-specific aberrant mucin glycoforms in mucinous adenocarcinomas from different organs (stomach, ampulla of Vater, colon, lung, breast, and ovary). In GI tissues mucins carrying a set of truncated, simple O-glycans and sialylated Lewis antigens have been detected by this approach (215).
More recently, PLA has been used in combination with different glycoproteomics strategies to identify specific glycoforms as potential biomarkers in gastric cancer, leading to the identification of CD44v6/STn (120) and RON/SLex (79).
The PLA technique will further improve our understanding of specific protein glycosylation changes that occurs in cancer tissues and that could be applied in clinic as new markers for GI cancer progression (216).
Imaging Mass Spectrometry
Imaging mass spectrometry is a very novel and promising technology that was first developed in 1997 by Caprioli and colleagues for the analysis of proteins (217). This method is based on MALDI-MS and utilizes the laser ionization of a localized area for the two-dimensional screening of a tissue sample. IMS generates for each ionization point of the tissue a spectra that yields structural information and, thus, reveals the spatial distribution of analytes (Figure 5). Recently, this technique has been adapted for glycomic analysis and has allowed to create N-glycosylation maps of several different frozen tissue (218). Following, N-glycan IMS has been also applied on formalin-fixed paraffin-embedded tissue (219). The N-glycan IMS workflow consists of four steps. First, N-glycans are liberated by PNGase F incubation of the deparaffinized or thawed tissue slide. Second, a thin layer of MALDI matrix is sprayed on top of the tissue slide. Third, the slide is two-dimensionally screened by multiple MALDI-MS analysis. Lastly, each identified N-glycan structure can be computationally visualized on the tissue, generating an epitope map.
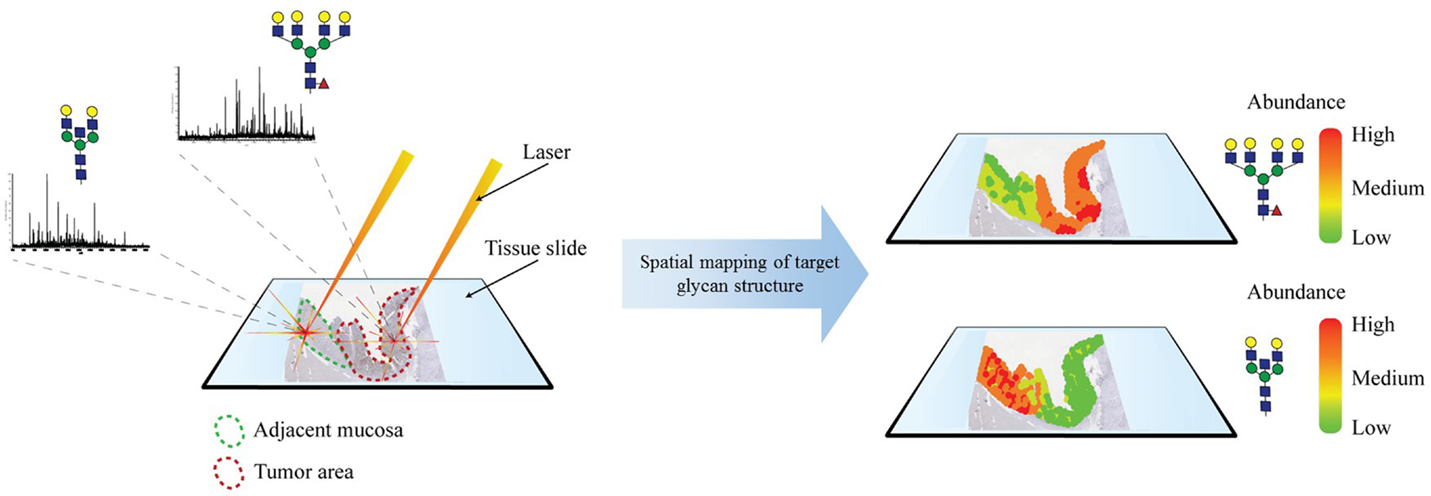
Figure 5. Imaging mass spectrometry. MALDI-based ionization generates an MS glycan spectrum of a selected spot of a tissue section. This process is repeated throughout the tissue. Identified glycan structures are mapped on the tissue and can be correlated with histopathological features.
IMS applied on hepatocellular carcinomas has shown to be capable of spatially defining glycan compositions and distinguishing malignant tissue from healthy tissue (220). Preliminary IMS results on other tumors, such as PDAC, have been able to differentiate between histopathological areas, such as fibroconnective tissue (220).
The IMS application on N-glycan analysis is still in its early stages of development, but bares enormous potential as a next-generation N-glycomic tumor characterization tool.
Future Perspectives and Clinical Applications
The recent advances in the glycomic and glycoproteomic fields are currently providing crucial information on the understanding of the role that glycans play in the biology of cells, tissues, and organisms, both in physiological and pathological conditions. However, many issues still remain to be understood, particularly in complex diseases, such as cancer. Advances in the glycobiology field could contribute to disclose key information regarding cancer biological properties, including the identification of prognostic and therapeutic response biomarkers.
In addition, the recent developments in this field could contribute to overcome the limitations of the current serological assays. The set of novel strategies presented in this review provide a clear view for future validation of potential biomarkers and points toward the translation of these strategies in the clinical setting.
Author Contributions
All authors have contributed to the conception, drafting, and revision of the manuscript. SM and MB designed the figures. All authors approved the review final form.
Conflict of Interest Statement
The authors declare that the research was conducted in the absence of any commercial or financial relationships that could be construed as a potential conflict of interest.
Funding
We acknowledge the support from the European Union, Seventh Framework Programme, Gastric Glyco Explorer initial training network: grant number 316929. IPATIMUP integrates the i3S Research Unit, which is partially supported by FCT, the Portuguese Foundation for Science and Technology. This work is funded by FEDER funds through the Operational Programme for Competitiveness Factors-COMPETE (FCOMP-01-0124-FEDER028188) and National Funds through the FCT-Foundation for Science and Technology, under the projects: PEst-C/SAU/LA0003/2013, PTDC/BBB-EBI/0786/2012, and PTDC/BBB-EBI/0567/2014. AM acknowledges the grant received from FCT, POPH (Programa Operacional Potencial Humano), and FSE (Fundo Social Europeu) (SFRH/BPD/75871/2011). MB acknowledges the University of Girona for pre-doctoral fellowship.
References
1. Reis CA, Osorio H, Silva L, Gomes C, David L. Alterations in glycosylation as biomarkers for cancer detection. J Clin Pathol (2010) 63(4):322–9. doi:10.1136/jcp.2009.071035
2. Pinho SS, Reis CA. Glycosylation in cancer: mechanisms and clinical implications. Nat Rev Cancer (2015) 15(9):540–55. doi:10.1038/nrc3982
3. Stowell SR, Ju T, Cummings RD. Protein glycosylation in cancer. Annu Rev Pathol (2015) 10:473–510. doi:10.1146/annurev-pathol-012414-040438
4. Rabinovich GA, Croci DO. Regulatory circuits mediated by lectin-glycan interactions in autoimmunity and cancer. Immunity (2012) 36(3):322–35. doi:10.1016/j.immuni.2012.03.004
5. Boligan KF, Mesa C, Fernandez LE, von Gunten S. Cancer intelligence acquired (CIA): tumor glycosylation and sialylation codes dismantling antitumor defense. Cell Mol Life Sci (2015) 72(7):1231–48. doi:10.1007/s00018-014-1799-5
6. Sangadala S, Bhat UR, Mendicino J. Quantitation and structures of oligosaccharide chains in human trachea mucin glycoproteins. Mol Cell Biochem (1992) 118(1):75–90. doi:10.1007/BF00249697
7. Kudelka MR, Ju T, Heimburg-Molinaro J, Cummings RD. Simple sugars to complex disease – mucin-type O-glycans in cancer. Adv Cancer Res (2015) 126:53–135. doi:10.1016/bs.acr.2014.11.002
8. Brockhausen I. Mucin-type O-glycans in human colon and breast cancer: glycodynamics and functions. EMBO Rep (2006) 7(6):599–604. doi:10.1038/sj.embor.7400705
9. Bennett EP, Mandel U, Clausen H, Gerken TA, Fritz TA, Tabak LA. Control of mucin-type O-glycosylation: a classification of the polypeptide GalNAc-transferase gene family. Glycobiology (2012) 22(6):736–56. doi:10.1093/glycob/cwr182
10. Clausen H, Bennett EP. A family of UDP-GalNAc: polypeptide N-acetylgalactosaminyl-transferases control the initiation of mucin-type O-linked glycosylation. Glycobiology (1996) 6(6):635–46. doi:10.1093/glycob/6.6.635
11. Gomes J, Marcos NT, Berois N, Osinaga E, Magalhaes A, Pinto-de-Sousa J, et al. Expression of UDP-N-acetyl-d-galactosamine: polypeptide N-acetylgalactosaminyltransferase-6 in gastric mucosa, intestinal metaplasia, and gastric carcinoma. J Histochem Cytochem (2009) 57(1):79–86. doi:10.1369/jhc.2008.952283
12. Shibao K, Izumi H, Nakayama Y, Ohta R, Nagata N, Nomoto M, et al. Expression of UDP-N-acetyl-alpha-d-galactosamine-polypeptide galNAc N-acetylgalactosaminyl transferase-3 in relation to differentiation and prognosis in patients with colorectal carcinoma. Cancer (2002) 94(7):1939–46. doi:10.1002/cncr.10423
13. Taniuchi K, Cerny RL, Tanouchi A, Kohno K, Kotani N, Honke K, et al. Overexpression of GalNAc-transferase GalNAc-T3 promotes pancreatic cancer cell growth. Oncogene (2011) 30(49):4843–54. doi:10.1038/onc.2011.194
14. Gill DJ, Clausen H, Bard F. Location, location, location: new insights into O-GalNAc protein glycosylation. Trends Cell Biol (2011) 21(3):149–58. doi:10.1016/j.tcb.2010.11.004
15. Hua D, Shen L, Xu L, Jiang Z, Zhou Y, Yue A, et al. Polypeptide N-acetylgalactosaminyltransferase 2 regulates cellular metastasis-associated behavior in gastric cancer. Int J Mol Med (2012) 30(6):1267–74. doi:10.3892/ijmm.2012.1130
16. Dalziel M, Whitehouse C, McFarlane I, Brockhausen I, Gschmeissner S, Schwientek T, et al. The relative activities of the C2GnT1 and ST3Gal-I glycosyltransferases determine O-glycan structure and expression of a tumor-associated epitope on MUC1. J Biol Chem (2001) 276(14):11007–15. doi:10.1074/jbc.M006523200
17. Marcos NT, Pinho S, Grandela C, Cruz A, Samyn-Petit B, Harduin-Lepers A, et al. Role of the human ST6GalNAc-I and ST6GalNAc-II in the synthesis of the cancer-associated sialyl-Tn antigen. Cancer Res (2004) 64(19):7050–7. doi:10.1158/0008-5472.CAN-04-1921
18. Marcos NT, Bennett EP, Gomes J, Magalhaes A, Gomes C, David L, et al. ST6GalNAc-I controls expression of sialyl-Tn antigen in gastrointestinal tissues. Front Biosci (Elite Ed) (2011) 3:1443–55.
19. David L, Nesland JM, Clausen H, Carneiro F, Sobrinho-Simoes M. Simple mucin-type carbohydrate antigens (Tn, sialosyl-Tn and T) in gastric mucosa, carcinomas and metastases. APMIS Suppl (1992) 27:162–72.
20. Pinho S, Marcos NT, Ferreira B, Carvalho AS, Oliveira MJ, Santos-Silva F, et al. Biological significance of cancer-associated sialyl-Tn antigen: modulation of malignant phenotype in gastric carcinoma cells. Cancer Lett (2007) 249(2):157–70. doi:10.1016/j.canlet.2006.08.010
21. Itzkowitz S, Kjeldsen T, Friera A, Hakomori S, Yang US, Kim YS. Expression of Tn, sialosyl Tn, and T antigens in human pancreas. Gastroenterology (1991) 100(6):1691–700.
22. Itzkowitz SH, Yuan M, Montgomery CK, Kjeldsen T, Takahashi HK, Bigbee WL, et al. Expression of Tn, sialosyl-Tn, and T antigens in human colon cancer. Cancer Res (1989) 49(1):197–204.
23. Radhakrishnan P, Dabelsteen S, Madsen FB, Francavilla C, Kopp KL, Steentoft C, et al. Immature truncated O-glycophenotype of cancer directly induces oncogenic features. Proc Natl Acad Sci U S A (2014) 111(39):E4066–75. doi:10.1073/pnas.1406619111
24. Hofmann BT, Schluter L, Lange P, Mercanoglu B, Ewald F, Folster A, et al. COSMC knockdown mediated aberrant O-glycosylation promotes oncogenic properties in pancreatic cancer. Mol Cancer (2015) 14:109. doi:10.1186/s12943-015-0386-1
25. Ju T, Cummings RD. A unique molecular chaperone Cosmc required for activity of the mammalian core 1 beta 3-galactosyltransferase. Proc Natl Acad Sci U S A (2002) 99(26):16613–8. doi:10.1073/pnas.262438199
26. Chik JH, Zhou J, Moh ES, Christopherson R, Clarke SJ, Molloy MP, et al. Comprehensive glycomics comparison between colon cancer cell cultures and tumours: implications for biomarker studies. J Proteomics (2014) 108:146–62. doi:10.1016/j.jprot.2014.05.002
27. Hung JS, Huang J, Lin YC, Huang MJ, Lee PH, Lai HS, et al. C1GALT1 overexpression promotes the invasive behavior of colon cancer cells through modifying O-glycosylation of FGFR2. Oncotarget (2014) 5(8):2096–106. doi:10.18632/oncotarget.1815
28. Shimodaira K, Nakayama J, Nakamura N, Hasebe O, Katsuyama T, Fukuda M. Carcinoma-associated expression of core 2 beta-1,6-N-acetylglucosaminyltransferase gene in human colorectal cancer: role of O-glycans in tumor progression. Cancer Res (1997) 57(23):5201–6.
29. Remmers N, Anderson JM, Linde EM, DiMaio DJ, Lazenby AJ, Wandall HH, et al. Aberrant expression of mucin core proteins and o-linked glycans associated with progression of pancreatic cancer. Clin Cancer Res (2013) 19(8):1981–93. doi:10.1158/1078-0432.CCR-12-2662
30. Iwai T, Kudo T, Kawamoto R, Kubota T, Togayachi A, Hiruma T, et al. Core 3 synthase is down-regulated in colon carcinoma and profoundly suppresses the metastatic potential of carcinoma cells. Proc Natl Acad Sci U S A (2005) 102(12):4572–7. doi:10.1073/pnas.0407983102
31. Rossez Y, Maes E, Lefebvre Darroman T, Gosset P, Ecobichon C, Joncquel Chevalier Curt M, et al. Almost all human gastric mucin O-glycans harbor blood group A, B or H antigens and are potential binding sites for Helicobacter pylori. Glycobiology (2012) 22(9):1193–206. doi:10.1093/glycob/cws072
32. Kenny DT, Skoog EC, Linden SK, Struwe WB, Rudd PM, Karlsson NG. Presence of terminal N-acetylgalactosaminebeta1-4N-acetylglucosamine residues on O-linked oligosaccharides from gastric MUC5AC: involvement in Helicobacter pylori colonization? Glycobiology (2012) 22(8):1077–85. doi:10.1093/glycob/cws076
33. Reis CA, David L, Seixas M, Burchell J, Sobrinho-Simoes M. Expression of fully and under-glycosylated forms of MUC1 mucin in gastric carcinoma. Int J Cancer (1998) 79(4):402–10. doi:10.1002/(SICI)1097-0215(19980821)79:4<402::AID-IJC16>3.0.CO;2-6
34. Tian H, Miyoshi E, Kawaguchi N, Shaker M, Ito Y, Taniguchi N, et al. The implication of N-acetylglucosaminyltransferase V expression in gastric cancer. Pathobiology (2008) 75(5):288–94. doi:10.1159/000151709
35. Granovsky M, Fata J, Pawling J, Muller WJ, Khokha R, Dennis JW. Suppression of tumor growth and metastasis in Mgat5-deficient mice. Nat Med (2000) 6(3):306–12. doi:10.1038/73163
36. Taniguchi N, Korekane H. Branched N-glycans and their implications for cell adhesion, signaling and clinical applications for cancer biomarkers and in therapeutics. BMB Rep (2011) 44(12):772–81. doi:10.5483/BMBRep.2011.44.12.772
37. Balog CI, Stavenhagen K, Fung WL, Koeleman CA, McDonnell LA, Verhoeven A, et al. N-glycosylation of colorectal cancer tissues: a liquid chromatography and mass spectrometry-based investigation. Mol Cell Proteomics (2012) 11(9):571–85. doi:10.1074/mcp.M111.011601
38. Dennis JW, Granovsky M, Warren CE. Glycoprotein glycosylation and cancer progression. Biochim Biophys Acta (1999) 1473(1):21–34. doi:10.1016/S0304-4165(99)00167-1
39. Fernandes B, Sagman U, Auger M, Demetrio M, Dennis JW. Beta 1-6 branched oligosaccharides as a marker of tumor progression in human breast and colon neoplasia. Cancer Res (1991) 51(2):718–23.
40. Seelentag WK, Li WP, Schmitz SF, Metzger U, Aeberhard P, Heitz PU, et al. Prognostic value of beta1,6-branched oligosaccharides in human colorectal carcinoma. Cancer Res (1998) 58(23):5559–64.
41. Murata K, Miyoshi E, Kameyama M, Ishikawa O, Kabuto T, Sasaki Y, et al. Expression of N-acetylglucosaminyltransferase V in colorectal cancer correlates with metastasis and poor prognosis. Clin Cancer Res (2000) 6(5):1772–7.
42. Guo H, Nagy T, Pierce M. Post-translational glycoprotein modifications regulate colon cancer stem cells and colon adenoma progression in Apc(min/+) mice through altered Wnt receptor signaling. J Biol Chem (2014) 289(45):31534–49. doi:10.1074/jbc.M114.602680
43. Ishida H, Togayachi A, Sakai T, Iwai T, Hiruma T, Sato T, et al. A novel beta1,3-N-acetylglucosaminyltransferase (beta3Gn-T8), which synthesizes poly-N-acetyllactosamine, is dramatically upregulated in colon cancer. FEBS Lett (2005) 579(1):71–8. doi:10.1016/j.febslet.2004.11.037
44. Park HM, Hwang MP, Kim YW, Kim KJ, Jin JM, Kim YH, et al. Mass spectrometry-based N-linked glycomic profiling as a means for tracking pancreatic cancer metastasis. Carbohydr Res (2015) 413:5–11. doi:10.1016/j.carres.2015.04.019
45. Zhao J, Qiu W, Simeone DM, Lubman DM. N-linked glycosylation profiling of pancreatic cancer serum using capillary liquid phase separation coupled with mass spectrometric analysis. J Proteome Res (2007) 6(3):1126–38. doi:10.1021/pr0604458
46. Gu J, Isaji T, Xu Q, Kariya Y, Gu W, Fukuda T, et al. Potential roles of N-glycosylation in cell adhesion. Glycoconj J (2012) 29(8–9):599–607. doi:10.1007/s10719-012-9386-1
47. Pinho SS, Reis CA, Paredes J, Magalhaes AM, Ferreira AC, Figueiredo J, et al. The role of N-acetylglucosaminyltransferase III and V in the post-transcriptional modifications of E-cadherin. Hum Mol Genet (2009) 18(14):2599–608. doi:10.1093/hmg/ddp194
48. Pinho SS, Osorio H, Nita-Lazar M, Gomes J, Lopes C, Gartner F, et al. Role of E-cadherin N-glycosylation profile in a mammary tumor model. Biochem Biophys Res Commun (2009) 379(4):1091–6. doi:10.1016/j.bbrc.2009.01.024
49. Gu J, Sato Y, Kariya Y, Isaji T, Taniguchi N, Fukuda T. A mutual regulation between cell-cell adhesion and N-glycosylation: implication of the bisecting GlcNAc for biological functions. J Proteome Res (2009) 8(2):431–5. doi:10.1021/pr800674g
50. Yoshimura M, Ihara Y, Matsuzawa Y, Taniguchi N. Aberrant glycosylation of E-cadherin enhances cell-cell binding to suppress metastasis. J Biol Chem (1996) 271(23):13811–5. doi:10.1074/jbc.271.23.13811
51. Kitada T, Miyoshi E, Noda K, Higashiyama S, Ihara H, Matsuura N, et al. The addition of bisecting N-acetylglucosamine residues to E-cadherin down-regulates the tyrosine phosphorylation of beta-catenin. J Biol Chem (2001) 276(1):475–80. doi:10.1074/jbc.M006689200
52. Pinho SS, Figueiredo J, Cabral J, Carvalho S, Dourado J, Magalhaes A, et al. E-cadherin and adherens-junctions stability in gastric carcinoma: functional implications of glycosyltransferases involving N-glycan branching biosynthesis, N-acetylglucosaminyltransferases III and V. Biochim Biophys Acta (2013) 1830(3):2690–700. doi:10.1016/j.bbagen.2012.10.021
53. Pinho SS, Oliveira P, Cabral J, Carvalho S, Huntsman D, Gartner F, et al. Loss and recovery of Mgat3 and GnT-III mediated E-cadherin N-glycosylation is a mechanism involved in epithelial-mesenchymal-epithelial transitions. PLoS One (2012) 7(3):e33191. doi:10.1371/journal.pone.0033191
54. Xu Q, Isaji T, Lu Y, Gu W, Kondo M, Fukuda T, et al. Roles of N-acetylglucosaminyltransferase III in epithelial-to-mesenchymal transition induced by transforming growth factor beta1 (TGF-beta1) in epithelial cell lines. J Biol Chem (2012) 287(20):16563–74. doi:10.1074/jbc.M111.262154
55. Ihara S, Miyoshi E, Ko JH, Murata K, Nakahara S, Honke K, et al. Prometastatic effect of N-acetylglucosaminyltransferase V is due to modification and stabilization of active matriptase by adding beta 1-6 GlcNAc branching. J Biol Chem (2002) 277(19):16960–7. doi:10.1074/jbc.M200673200
56. Zhao Y, Nakagawa T, Itoh S, Inamori K, Isaji T, Kariya Y, et al. N-acetylglucosaminyltransferase III antagonizes the effect of N-acetylglucosaminyltransferase V on alpha3beta1 integrin-mediated cell migration. J Biol Chem (2006) 281(43):32122–30. doi:10.1074/jbc.M607274200
57. Isaji T, Gu J, Nishiuchi R, Zhao Y, Takahashi M, Miyoshi E, et al. Introduction of bisecting GlcNAc into integrin alpha5beta1 reduces ligand binding and down-regulates cell adhesion and cell migration. J Biol Chem (2004) 279(19):19747–54. doi:10.1074/jbc.M311627200
58. Sato Y, Isaji T, Tajiri M, Yoshida-Yamamoto S, Yoshinaka T, Somehara T, et al. An N-glycosylation site on the beta-propeller domain of the integrin alpha5 subunit plays key roles in both its function and site-specific modification by beta1,4-N-acetylglucosaminyltransferase III. J Biol Chem (2009) 284(18):11873–81. doi:10.1074/jbc.M807660200
59. Dall’Olio F, Chiricolo M. Sialyltransferases in cancer. Glycoconj J (2001) 18(11–12):841–50. doi:10.1023/A:1022288022969
60. Jandus C, Boligan KF, Chijioke O, Liu H, Dahlhaus M, Demoulins T, et al. Interactions between Siglec-7/9 receptors and ligands influence NK cell-dependent tumor immunosurveillance. J Clin Invest (2014) 124(4):1810–20. doi:10.1172/JCI65899
61. Laubli H, Pearce OM, Schwarz F, Siddiqui SS, Deng L, Stanczak MA, et al. Engagement of myelomonocytic Siglecs by tumor-associated ligands modulates the innate immune response to cancer. Proc Natl Acad Sci U S A (2014) 111(39):14211–6. doi:10.1073/pnas.1409580111
62. Kim YJ, Varki A. Perspectives on the significance of altered glycosylation of glycoproteins in cancer. Glycoconj J (1997) 14(5):569–76. doi:10.1023/A:1018580324971
63. Dall’Olio F, Malagolini N, Trinchera M, Chiricolo M. Sialosignaling: sialyltransferases as engines of self-fueling loops in cancer progression. Biochim Biophys Acta (2014) 1840(9):2752–64. doi:10.1016/j.bbagen.2014.06.006
64. Lise M, Belluco C, Perera SP, Patel R, Thomas P, Ganguly A. Clinical correlations of alpha2,6-sialyltransferase expression in colorectal cancer patients. Hybridoma (2000) 19(4):281–6. doi:10.1089/027245700429828
65. Gretschel S, Haensch W, Schlag PM, Kemmner W. Clinical relevance of sialyltransferases ST6GAL-I and ST3GAL-III in gastric cancer. Oncology (2003) 65(2):139–45. doi:10.1159/000072339
66. Petretti T, Schulze B, Schlag PM, Kemmner W. Altered mRNA expression of glycosyltransferases in human gastric carcinomas. Biochim Biophys Acta (1999) 1428(2–3):209–18. doi:10.1016/S0304-4165(99)00080-X
67. Gomes C, Osorio H, Pinto MT, Campos D, Oliveira MJ, Reis CA. Expression of ST3GAL4 leads to SLe(x) expression and induces c-Met activation and an invasive phenotype in gastric carcinoma cells. PLoS One (2013) 8(6):e66737. doi:10.1371/journal.pone.0066737
68. Perez-Garay M, Arteta B, Llop E, Cobler L, Pages L, Ortiz R, et al. alpha2,3-sialyltransferase ST3Gal IV promotes migration and metastasis in pancreatic adenocarcinoma cells and tends to be highly expressed in pancreatic adenocarcinoma tissues. Int J Biochem Cell Biol (2013) 45(8):1748–57. doi:10.1016/j.biocel.2013.05.015
69. Perez-Garay M, Arteta B, Pages L, de Llorens R, de Bolos C, Vidal-Vanaclocha F, et al. alpha2,3-sialyltransferase ST3Gal III modulates pancreatic cancer cell motility and adhesion in vitro and enhances its metastatic potential in vivo. PLoS One (2010) 5(9):e12524. doi:10.1371/journal.pone.0012524
70. Bassaganas S, Perez-Garay M, Peracaula R. Cell surface sialic acid modulates extracellular matrix adhesion and migration in pancreatic adenocarcinoma cells. Pancreas (2014) 43(1):109–17. doi:10.1097/MPA.0b013e31829d9090
71. Nakagoe T, Fukushima K, Nanashima A, Sawai T, Tsuji T, Jibiki M, et al. Expression of Lewis(a), sialyl Lewis(a), Lewis(x) and sialyl Lewis(x) antigens as prognostic factors in patients with colorectal cancer. Can J Gastroenterol (2000) 14(9):753–60.
72. Satomura Y, Sawabu N, Takemori Y, Ohta H, Watanabe H, Okai T, et al. Expression of various sialylated carbohydrate antigens in malignant and nonmalignant pancreatic tissues. Pancreas (1991) 6(4):448–58. doi:10.1097/00006676-199107000-00012
73. Peracaula R, Tabares G, Lopez-Ferrer A, Brossmer R, de Bolos C, de Llorens R. Role of sialyltransferases involved in the biosynthesis of Lewis antigens in human pancreatic tumour cells. Glycoconj J (2005) 22(3):135–44. doi:10.1007/s10719-005-0734-2
74. Nakagoe T, Sawai T, Tsuji T, Jibiki MA, Nanashima A, Yamaguchi H, et al. Predictive factors for preoperative serum levels of sialy Lewis(x), sialyl Lewis(a) and sialyl Tn antigens in gastric cancer patients. Anticancer Res (2002) 22(1A):451–8.
75. Amado M, Carneiro F, Seixas M, Clausen H, Sobrinho-Simoes M. Dimeric sialyl-Le(x) expression in gastric carcinoma correlates with venous invasion and poor outcome. Gastroenterology (1998) 114(3):462–70. doi:10.1016/S0016-5085(98)70529-3
76. Baldus SE, Zirbes TK, Monig SP, Engel S, Monaca E, Rafiqpoor K, et al. Histopathological subtypes and prognosis of gastric cancer are correlated with the expression of mucin-associated sialylated antigens: sialosyl-Lewis(a), sialosyl-Lewis(x) and sialosyl-Tn. Tumour Biol (1998) 19(6):445–53. doi:10.1159/000030036
77. Nakamori S, Kameyama M, Imaoka S, Furukawa H, Ishikawa O, Sasaki Y, et al. Increased expression of sialyl Lewisx antigen correlates with poor survival in patients with colorectal carcinoma: clinicopathological and immunohistochemical study. Cancer Res (1993) 53(15):3632–7.
78. Rosen SD, Bertozzi CR. The selectins and their ligands. Curr Opin Cell Biol (1994) 6(5):663–73. doi:10.1016/0955-0674(94)90092-2
79. Mereiter S, Magalhaes A, Adamczyk B, Jin C, Almeida A, Drici L, et al. Glycomic analysis of gastric carcinoma cells discloses glycans as modulators of RON receptor tyrosine kinase activation in cancer. Biochim Biophys Acta (2015). doi:10.1016/j.bbagen.2015.12.016
80. Wang MH, Lee W, Luo YL, Weis MT, Yao HP. Altered expression of the RON receptor tyrosine kinase in various epithelial cancers and its contribution to tumourigenic phenotypes in thyroid cancer cells. J Pathol (2007) 213(4):402–11. doi:10.1002/path.2245
81. Thobe MN, Gurusamy D, Pathrose P, Waltz SE. The Ron receptor tyrosine kinase positively regulates angiogenic chemokine production in prostate cancer cells. Oncogene (2010) 29(2):214–26. doi:10.1038/onc.2009.331
82. Logan-Collins J, Thomas RM, Yu P, Jaquish D, Mose E, French R, et al. Silencing of RON receptor signaling promotes apoptosis and gemcitabine sensitivity in pancreatic cancers. Cancer Res (2010) 70(3):1130–40. doi:10.1158/0008-5472.CAN-09-0761
83. McClaine RJ, Marshall AM, Wagh PK, Waltz SE. Ron receptor tyrosine kinase activation confers resistance to tamoxifen in breast cancer cell lines. Neoplasia (2010) 12(8):650–8. doi:10.1593/neo.10476
84. Catenacci DV, Cervantes G, Yala S, Nelson EA, El-Hashani E, Kanteti R, et al. RON (MST1R) is a novel prognostic marker and therapeutic target for gastroesophageal adenocarcinoma. Cancer Biol Ther (2011) 12(1):9–46. doi:10.4161/cbt.12.1.15747
85. Balmana M, Sarrats A, Llop E, Barrabes S, Saldova R, Ferri MJ, et al. Identification of potential pancreatic cancer serum markers: increased sialyl-Lewis X on ceruloplasmin. Clin Chim Acta (2015) 442:56–62. doi:10.1016/j.cca.2015.01.007
86. Rho JH, Mead JR, Wright WS, Brenner DE, Stave JW, Gildersleeve JC, et al. Discovery of sialyl Lewis A and Lewis X modified protein cancer biomarkers using high density antibody arrays. J Proteomics (2014) 96:291–9. doi:10.1016/j.jprot.2013.10.030
87. Park JJ, Lee M. Increasing the alpha 2, 6 sialylation of glycoproteins may contribute to metastatic spread and therapeutic resistance in colorectal cancer. Gut Liver (2013) 7(6):629–41. doi:10.5009/gnl.2013.7.6.629
88. Miyoshi E, Moriwaki K, Terao N, Tan CC, Terao M, Nakagawa T, et al. Fucosylation is a promising target for cancer diagnosis and therapy. Biomolecules (2012) 2(1):34–45. doi:10.3390/biom2010034
89. Miyoshi E, Moriwaki K, Nakagawa T. Biological function of fucosylation in cancer biology. J Biochem (2008) 143(6):725–9. doi:10.1093/jb/mvn011
90. Breborowicz J, Mackiewicz A, Breborowicz D. Microheterogeneity of alpha-fetoprotein in patient serum as demonstrated by lectin affino-electrophoresis. Scand J Immunol (1981) 14(1):15–20. doi:10.1111/j.1365-3083.1981.tb00179.x
91. Wong RJ, Ahmed A, Gish RG. Elevated alpha-fetoprotein: differential diagnosis – hepatocellular carcinoma and other disorders. Clin Liver Dis (2015) 19(2):309–23. doi:10.1016/j.cld.2015.01.005
92. Balmana M, Gimenez E, Puerta A, Llop E, Figueras J, Fort E, et al. Increased alpha1-3 fucosylation of alpha-1-acid glycoprotein (AGP) in pancreatic cancer. J Proteomics (2015) 132:144–54. doi:10.1016/j.jprot.2015.11.006
93. Trinchera M, Malagolini N, Chiricolo M, Santini D, Minni F, Caretti A, et al. The biosynthesis of the selectin-ligand sialyl Lewis x in colorectal cancer tissues is regulated by fucosyltransferase VI and can be inhibited by an RNA interference-based approach. Int J Biochem Cell Biol (2011) 43(1):130–9. doi:10.1016/j.biocel.2010.10.004
94. Qiu Y, Patwa TH, Xu L, Shedden K, Misek DE, Tuck M, et al. Plasma glycoprotein profiling for colorectal cancer biomarker identification by lectin glycoarray and lectin blot. J Proteome Res (2008) 7(4):1693–703. doi:10.1021/pr700706s
95. Muinelo-Romay L, Gil-Martin E, Fernandez-Briera A. alpha(1,2)Fucosylation in human colorectal carcinoma. Oncol Lett (2010) 1(2):361–6. doi:10.3892/ol_00000064
96. Lopez-Ferrer A, de Bolos C, Barranco C, Garrido M, Isern J, Carlstedt I, et al. Role of fucosyltransferases in the association between apomucin and Lewis antigen expression in normal and malignant gastric epithelium. Gut (2000) 47(3):349–56. doi:10.1136/gut.47.3.349
97. Duell EJ, Bonet C, Munoz X, Lujan-Barroso L, Weiderpass E, Boutron-Ruault MC, et al. Variation at ABO histo-blood group and FUT loci and diffuse and intestinal gastric cancer risk in a European population. Int J Cancer (2015) 136(4):880–93. doi:10.1002/ijc.29034
98. Magalhaes A, Marcos-Pinto R, Nairn AV, Dela Rosa M, Ferreira RM, Junqueira-Neto S, et al. Helicobacter pylori chronic infection and mucosal inflammation switches the human gastric glycosylation pathways. Biochim Biophys Acta (2015) 1852(9):1928–39. doi:10.1016/j.bbadis.2015.07.001
99. Muinelo-Romay L, Vazquez-Martin C, Villar-Portela S, Cuevas E, Gil-Martin E, Fernandez-Briera A. Expression and enzyme activity of alpha(1,6)fucosyltransferase in human colorectal cancer. Int J Cancer (2008) 123(3):641–6. doi:10.1002/ijc.23521
100. Osumi D, Takahashi M, Miyoshi E, Yokoe S, Lee SH, Noda K, et al. Core fucosylation of E-cadherin enhances cell-cell adhesion in human colon carcinoma WiDr cells. Cancer Sci (2009) 100(5):888–95. doi:10.1111/j.1349-7006.2009.01125.x
101. Zhao YP, Xu XY, Fang M, Wang H, You Q, Yi CH, et al. Decreased core-fucosylation contributes to malignancy in gastric cancer. PLoS One (2014) 9(4):e94536. doi:10.1371/journal.pone.0094536
102. Ma Z, Vosseller K. Cancer metabolism and elevated O-GlcNAc in oncogenic signaling. J Biol Chem (2014) 289(50):34457–65. doi:10.1074/jbc.R114.577718
103. Fardini Y, Dehennaut V, Lefebvre T, Issad T. O-GlcNAcylation: a new cancer hallmark? Front Endocrinol (2013) 4:99. doi:10.3389/fendo.2013.00099
104. Hart GW, Slawson C, Ramirez-Correa G, Lagerlof O. Cross talk between O-GlcNAcylation and phosphorylation: roles in signaling, transcription, and chronic disease. Annu Rev Biochem (2011) 80:825–58. doi:10.1146/annurev-biochem-060608-102511
105. Konrad RJ, Kudlow JE. The role of O-linked protein glycosylation in beta-cell dysfunction. Int J Mol Med (2002) 10(5):535–9. doi:10.3892/ijmm.10.5.535
106. Ma Z, Vocadlo DJ, Vosseller K. Hyper-O-GlcNAcylation is anti-apoptotic and maintains constitutive NF-kappaB activity in pancreatic cancer cells. J Biol Chem (2013) 288(21):15121–30. doi:10.1074/jbc.M113.470047
107. Yang YR, Kim DH, Seo YK, Park D, Jang HJ, Choi SY, et al. Elevated O-GlcNAcylation promotes colonic inflammation and tumorigenesis by modulating NF-kappaB signaling. Oncotarget (2015) 6(14):12529–42. doi:10.18632/oncotarget.3725
108. Couchman JR, Gopal S, Lim HC, Norgaard S, Multhaupt HA. Syndecans: from peripheral coreceptors to mainstream regulators of cell behaviour. Int J Exp Pathol (2015) 96(1):1–10. doi:10.1111/iep.12112
109. Magalhaes A, Marcos NT, Carvalho AS, David L, Figueiredo C, Bastos J, et al. Helicobacter pylori cag pathogenicity island-positive strains induce syndecan-4 expression in gastric epithelial cells. FEMS Immunol Med Microbiol (2009) 56(3):223–32. doi:10.1111/j.1574-695X.2009.00569.x
110. Marcos NT, Magalhaes A, Ferreira B, Oliveira MJ, Carvalho AS, Mendes N, et al. Helicobacter pylori induces beta3GnT5 in human gastric cell lines, modulating expression of the SabA ligand sialyl-Lewis x. J Clin Invest (2008) 118(6):2325–36. doi:10.1172/JCI34324
111. Wiksten JP, Lundin J, Nordling S, Lundin M, Kokkola A, von Boguslawski K, et al. Epithelial and stromal syndecan-1 expression as predictor of outcome in patients with gastric cancer. Int J Cancer (2001) 95(1):1–6. doi:10.1002/1097-0215(20010120)95:1<1::AID-IJC1000>3.0.CO;2-5
112. Kim SY, Choi EJ, Yun JA, Jung ES, Oh ST, Kim JG, et al. Syndecan-1 expression is associated with tumor size and EGFR expression in colorectal carcinoma: a clinicopathological study of 230 cases. Int J Med Sci (2015) 12(2):92–9. doi:10.7150/ijms.10497
113. Conejo JR, Kleeff J, Koliopanos A, Matsuda K, Zhu ZW, Goecke H, et al. Syndecan-1 expression is up-regulated in pancreatic but not in other gastrointestinal cancers. Int J Cancer (2000) 88(1):12–20. doi:10.1002/1097-0215(20001001)88:1<12::AID-IJC3>3.0.CO;2-T
114. Filmus J, Capurro M, Rast J. Glypicans. Genome Biol (2008) 9(5):224. doi:10.1186/gb-2008-9-5-224
115. Kleeff J, Ishiwata T, Kumbasar A, Friess H, Buchler MW, Lander AD, et al. The cell-surface heparan sulfate proteoglycan glypican-1 regulates growth factor action in pancreatic carcinoma cells and is overexpressed in human pancreatic cancer. J Clin Invest (1998) 102(9):1662–73. doi:10.1172/JCI4105
116. Whipple CA, Young AL, Korc M. A KrasG12D-driven genetic mouse model of pancreatic cancer requires glypican-1 for efficient proliferation and angiogenesis. Oncogene (2012) 31(20):2535–44. doi:10.1038/onc.2011.430
117. Aikawa T, Whipple CA, Lopez ME, Gunn J, Young A, Lander AD, et al. Glypican-1 modulates the angiogenic and metastatic potential of human and mouse cancer cells. J Clin Invest (2008) 118(1):89–99. doi:10.1172/JCI32412
118. Melo SA, Luecke LB, Kahlert C, Fernandez AF, Gammon ST, Kaye J, et al. Glypican-1 identifies cancer exosomes and detects early pancreatic cancer. Nature (2015) 523(7559):177–82. doi:10.1038/nature14581
119. da Cunha CB, Oliveira C, Wen X, Gomes B, Sousa S, Suriano G, et al. De novo expression of CD44 variants in sporadic and hereditary gastric cancer. Lab Invest (2010) 90(11):1604–14. doi:10.1038/labinvest.2010.155
120. Campos D, Freitas D, Gomes J, Magalhaes A, Steentoft C, Gomes C, et al. Probing the O-glycoproteome of gastric cancer cell lines for biomarker discovery. Mol Cell Proteomics (2015) 14(6):1616–29. doi:10.1074/mcp.M114.046862
121. Haakenson JK, Khokhlatchev AV, Choi YJ, Linton SS, Zhang P, Zaki PM, et al. Lysosomal degradation of CD44 mediates ceramide nanoliposome-induced anoikis and diminished extravasation in metastatic carcinoma cells. J Biol Chem (2015) 290(13):8632–43. doi:10.1074/jbc.M114.609677
122. Miyagi T, Wada T, Yamaguchi K. Roles of plasma membrane-associated sialidase NEU3 in human cancers. Biochim Biophys Acta (2008) 1780(3):532–7. doi:10.1016/j.bbagen.2007.09.016
123. Kakugawa Y, Wada T, Yamaguchi K, Yamanami H, Ouchi K, Sato I, et al. Up-regulation of plasma membrane-associated ganglioside sialidase (Neu3) in human colon cancer and its involvement in apoptosis suppression. Proc Natl Acad Sci U S A (2002) 99(16):10718–23. doi:10.1073/pnas.152597199
124. Takahashi K, Hosono M, Sato I, Hata K, Wada T, Yamaguchi K, et al. Sialidase NEU3 contributes neoplastic potential on colon cancer cells as a key modulator of gangliosides by regulating Wnt signaling. Int J Cancer (2015) 137(7):1560–73. doi:10.1002/ijc.29527
125. Holst S, Stavenhagen K, Balog CI, Koeleman CA, McDonnell LM, Mayboroda OA, et al. Investigations on aberrant glycosylation of glycosphingolipids in colorectal cancer tissues using liquid chromatography and matrix-assisted laser desorption time-of-flight mass spectrometry (MALDI-TOF-MS). Mol Cell Proteomics (2013) 12(11):3081–93. doi:10.1074/mcp.M113.030387
126. Ebeling FG, Stieber P, Untch M, Nagel D, Konecny GE, Schmitt UM, et al. Serum CEA and CA 15-3 as prognostic factors in primary breast cancer. Br J Cancer (2002) 86(8):1217–22. doi:10.1038/sj.bjc.6600248
127. Harris L, Fritsche H, Mennel R, Norton L, Ravdin P, Taube S, et al. American Society of Clinical Oncology 2007 update of recommendations for the use of tumor markers in breast cancer. J Clin Oncol (2007) 25(33):5287–312. doi:10.1200/JCO.2007.14.2364
128. Kumpulainen EJ, Keskikuru RJ, Johansson RT. Serum tumor marker CA 15.3 and stage are the two most powerful predictors of survival in primary breast cancer. Breast Cancer Res Treat (2002) 76(2):95–102. doi:10.1023/A:1020514925143
129. Uehara M, Kinoshita T, Hojo T, Akashi-Tanaka S, Iwamoto E, Fukutomi T. Long-term prognostic study of carcinoembryonic antigen (CEA) and carbohydrate antigen 15-3 (CA 15-3) in breast cancer. Int J Clin Oncol (2008) 13(5):447–51. doi:10.1007/s10147-008-0773-3
130. Lauro S, Trasatti L, Bordin F, Lanzetta G, Bria E, Gelibter A, et al. Comparison of CEA, MCA, CA 15-3 and CA 27-29 in follow-up and monitoring therapeutic response in breast cancer patients. Anticancer Res (1999) 19(4C):3511–5.
131. Pauler DK, Menon U, McIntosh M, Symecko HL, Skates SJ, Jacobs IJ. Factors influencing serum CA125II levels in healthy postmenopausal women. Cancer Epidemiol Biomarkers Prev (2001) 10(5):489–93.
132. Gostout BS, Brewer MA. Guidelines for referral of the patient with an adnexal mass. Clin Obstet Gynecol (2006) 49(3):448–58. doi:10.1097/00003081-200609000-00005
133. Gilgunn S, Conroy PJ, Saldova R, Rudd PM, O’Kennedy RJ. Aberrant PSA glycosylation – a sweet predictor of prostate cancer. Nat Rev Urol (2013) 10(2):99–107. doi:10.1038/nrurol.2012.258
134. Saldova R, Fan Y, Fitzpatrick JM, Watson RW, Rudd PM. Core fucosylation and alpha2-3 sialylation in serum N-glycome is significantly increased in prostate cancer comparing to benign prostate hyperplasia. Glycobiology (2011) 21(2):195–205. doi:10.1093/glycob/cwq147
135. Safi F, Schlosser W, Kolb G, Beger HG. Diagnostic value of CA 19-9 in patients with pancreatic cancer and nonspecific gastrointestinal symptoms. J Gastrointest Surg (1997) 1(2):106–12. doi:10.1016/S1091-255X(97)80097-2
136. Locker GY, Hamilton S, Harris J, Jessup JM, Kemeny N, Macdonald JS, et al. ASCO 2006 update of recommendations for the use of tumor markers in gastrointestinal cancer. J Clin Oncol (2006) 24(33):5313–27. doi:10.1200/JCO.2006.08.2644
137. Duraker N, Celik AN. The prognostic significance of preoperative serum CA 19-9 in patients with resectable gastric carcinoma: comparison with CEA. J Surg Oncol (2001) 76(4):266–71. doi:10.1002/jso.1044
138. Shah UA, Saif MW. Tumor markers in pancreatic cancer: 2013. JOP (2013) 14(4):318–21. doi:10.6092/1590-8577/1653
139. Goldstein MJ, Mitchell EP. Carcinoembryonic antigen in the staging and follow-up of patients with colorectal cancer. Cancer Invest (2005) 23(4):338–51. doi:10.1081/CNV-58878
140. Steele SR, Chang GJ, Hendren S, Weiser M, Irani J, Buie WD, et al. Practice guideline for the surveillance of patients after curative treatment of colon and rectal cancer. Dis Colon Rectum (2015) 58(8):713–25. doi:10.1097/DCR.0000000000000410
141. Julien S, Adriaenssens E, Ottenberg K, Furlan A, Courtand G, Vercoutter-Edouart AS, et al. ST6GalNAc I expression in MDA-MB-231 breast cancer cells greatly modifies their O-glycosylation pattern and enhances their tumourigenicity. Glycobiology (2006) 16(1):54–64. doi:10.1093/glycob/cwj033
142. Julien S, Lagadec C, Krzewinski-Recchi MA, Courtand G, Le Bourhis X, Delannoy P. Stable expression of sialyl-Tn antigen in T47-D cells induces a decrease of cell adhesion and an increase of cell migration. Breast Cancer Res Treat (2005) 90(1):77–84. doi:10.1007/s10549-004-3137-3
143. Ma H, Zhou H, Li P, Song X, Miao X, Li Y, et al. Effect of ST3GAL 4 and FUT 7 on sialyl Lewis X synthesis and multidrug resistance in human acute myeloid leukemia. Biochim Biophys Acta (2014) 1842(9):1681–92. doi:10.1016/j.bbadis.2014.06.014
144. Yang XS, Liu S, Liu YJ, Liu JW, Liu TJ, Wang XQ, et al. Overexpression of fucosyltransferase IV promotes A431 cell proliferation through activating MAPK and PI3K/Akt signaling pathways. J Cell Physiol (2010) 225(2):612–9. doi:10.1002/jcp.22250
145. Tsuda T, Ikeda Y, Taniguchi N. The Asn-420-linked sugar chain in human epidermal growth factor receptor suppresses ligand-independent spontaneous oligomerization. Possible role of a specific sugar chain in controllable receptor activation. J Biol Chem (2000) 275(29):21988–94. doi:10.1074/jbc.M003400200
146. van Roy F, Berx G. The cell-cell adhesion molecule E-cadherin. Cell Mol Life Sci (2008) 65(23):3756–88. doi:10.1007/s00018-008-8281-1
147. Paredes J, Figueiredo J, Albergaria A, Oliveira P, Carvalho J, Ribeiro AS, et al. Epithelial E- and P-cadherins: role and clinical significance in cancer. Biochim Biophys Acta (2012) 1826(2):297–311. doi:10.1016/j.bbcan.2012.05.002
148. Oliveira C, Seruca R, Carneiro F. Genetics, pathology, and clinics of familial gastric cancer. Int J Surg Pathol (2006) 14(1):21–33. doi:10.1177/106689690601400105
149. Carvalho S, Catarino TA, Dias AM, Kato M, Almeida A, Hessling B, et al. Preventing E-cadherin aberrant N-glycosylation at Asn-554 improves its critical function in gastric cancer. Oncogene (2015). doi:10.1038/onc.2015.225
150. Pinho SS, Seruca R, Gartner F, Yamaguchi Y, Gu J, Taniguchi N, et al. Modulation of E-cadherin function and dysfunction by N-glycosylation. Cell Mol Life Sci (2011) 68(6):1011–20. doi:10.1007/s00018-010-0595-0
151. Steentoft C, Bennett EP, Schjoldager KT, Vakhrushev SY, Wandall HH, Clausen H. Precision genome editing: a small revolution for glycobiology. Glycobiology (2014) 24(8):663–80. doi:10.1093/glycob/cwu046
152. Steentoft C, Vakhrushev SY, Joshi HJ, Kong Y, Vester-Christensen MB, Schjoldager KT, et al. Precision mapping of the human O-GalNAc glycoproteome through SimpleCell technology. EMBO J (2013) 32(10):1478–88. doi:10.1038/emboj.2013.79
153. Schjoldager KT, Vakhrushev SY, Kong Y, Steentoft C, Nudelman AS, Pedersen NB, et al. Probing isoform-specific functions of polypeptide GalNAc-transferases using zinc finger nuclease glycoengineered SimpleCells. Proc Natl Acad Sci U S A (2012) 109(25):9893–8. doi:10.1073/pnas.1203563109
154. Vakhrushev SY, Steentoft C, Vester-Christensen MB, Bennett EP, Clausen H, Levery SB. Enhanced mass spectrometric mapping of the human GalNAc-type O-glycoproteome with SimpleCells. Mol Cell Proteomics (2013) 12(4):932–44. doi:10.1074/mcp.O112.021972
155. Schjoldager KT, Clausen H. Site-specific protein O-glycosylation modulates proprotein processing – deciphering specific functions of the large polypeptide GalNAc-transferase gene family. Biochim Biophys Acta (2012) 1820(12):2079–94. doi:10.1016/j.bbagen.2012.09.014
156. Vester-Christensen MB, Halim A, Joshi HJ, Steentoft C, Bennett EP, Levery SB, et al. Mining the O-mannose glycoproteome reveals cadherins as major O-mannosylated glycoproteins. Proc Natl Acad Sci U S A (2013) 110(52):21018–23. doi:10.1073/pnas.1313446110
157. Mechref Y. Analysis of glycans derived from glycoconjugates by capillary electrophoresis-mass spectrometry. Electrophoresis (2011) 32(24):3467–81. doi:10.1002/elps.201100342
158. Sethi MK, Kim H, Park CK, Baker MS, Paik YK, Packer NH, et al. In-depth N-glycome profiling of paired colorectal cancer and non-tumorigenic tissues reveals cancer-, stage- and EGFR-specific protein N-glycosylation. Glycobiology (2015) 25(10):1064–78. doi:10.1093/glycob/cwv042
159. Bones J, Byrne JC, O’Donoghue N, McManus C, Scaife C, Boissin H, et al. Glycomic and glycoproteomic analysis of serum from patients with stomach cancer reveals potential markers arising from host defense response mechanisms. J Proteome Res (2011) 10(3):1246–65. doi:10.1021/pr101036b
160. Bones J, Mittermayr S, O’Donoghue N, Guttman A, Rudd PM. Ultra performance liquid chromatographic profiling of serum N-glycans for fast and efficient identification of cancer associated alterations in glycosylation. Anal Chem (2010) 82(24):10208–15. doi:10.1021/ac102860w
161. Ozcan S, Barkauskas DA, Renee Ruhaak L, Torres J, Cooke CL, An HJ, et al. Serum glycan signatures of gastric cancer. Cancer Prev Res (Phila) (2014) 7(2):226–35. doi:10.1158/1940-6207.CAPR-13-0235
162. Nouso K, Amano M, Ito YM, Miyahara K, Morimoto Y, Kato H, et al. Clinical utility of high-throughput glycome analysis in patients with pancreatic cancer. J Gastroenterol (2013) 48(10):1171–9. doi:10.1007/s00535-012-0732-7
163. Mann BF, Goetz JA, House MG, Schmidt CM, Novotny MV. Glycomic and proteomic profiling of pancreatic cyst fluids identifies hyperfucosylated lactosamines on the N-linked glycans of overexpressed glycoproteins. Mol Cell Proteomics (2012) 11(7):M111015792. doi:10.1074/mcp.M111.015792
164. Kaji H, Saito H, Yamauchi Y, Shinkawa T, Taoka M, Hirabayashi J, et al. Lectin affinity capture, isotope-coded tagging and mass spectrometry to identify N-linked glycoproteins. Nat Biotechnol (2003) 21(6):667–72. doi:10.1038/nbt829
165. Yang Z, Hancock WS. Approach to the comprehensive analysis of glycoproteins isolated from human serum using a multi-lectin affinity column. J Chromatogr A (2004) 1053(1–2):79–88. doi:10.1016/S0021-9673(04)01433-5
166. Durham M, Regnier FE. Targeted glycoproteomics: serial lectin affinity chromatography in the selection of O-glycosylation sites on proteins from the human blood proteome. J Chromatogr A (2006) 1132(1–2):165–73. doi:10.1016/j.chroma.2006.07.070
167. Zhang H, Li XJ, Martin DB, Aebersold R. Identification and quantification of N-linked glycoproteins using hydrazide chemistry, stable isotope labeling and mass spectrometry. Nat Biotechnol (2003) 21(6):660–6. doi:10.1038/nbt827
168. Liu T, Qian WJ, Gritsenko MA, Camp DG II, Monroe ME, Moore RJ, et al. Human plasma N-glycoproteome analysis by immunoaffinity subtraction, hydrazide chemistry, and mass spectrometry. J Proteome Res (2005) 4(6):2070–80. doi:10.1021/pr0502065
169. Rawn JD, Lienhard GE. The binding of boronic acids to chymotrypsin. Biochemistry (1974) 13(15):3124–30. doi:10.1021/bi00712a019
170. Alvarez-Manilla G, Atwood J III, Guo Y, Warren NL, Orlando R, Pierce M. Tools for glycoproteomic analysis: size exclusion chromatography facilitates identification of tryptic glycopeptides with N-linked glycosylation sites. J Proteome Res (2006) 5(3):701–8. doi:10.1021/pr050275j
171. Hagglund P, Bunkenborg J, Elortza F, Jensen ON, Roepstorff P. A new strategy for identification of N-glycosylated proteins and unambiguous assignment of their glycosylation sites using HILIC enrichment and partial deglycosylation. J Proteome Res (2004) 3(3):556–66. doi:10.1021/pr034112b
172. Larsen MR, Hojrup P, Roepstorff P. Characterization of gel-separated glycoproteins using two-step proteolytic digestion combined with sequential microcolumns and mass spectrometry. Mol Cell Proteomics (2005) 4(2):107–19. doi:10.1074/mcp.M400068-MCP200
173. Zhao J, Simeone DM, Heidt D, Anderson MA, Lubman DM. Comparative serum glycoproteomics using lectin selected sialic acid glycoproteins with mass spectrometric analysis: application to pancreatic cancer serum. J Proteome Res (2006) 5(7):1792–802. doi:10.1021/pr060034r
174. Melo-Braga MN, Ibanez-Vea M, Larsen MR, Kulej K. Comprehensive protocol to simultaneously study protein phosphorylation, acetylation, and N-linked sialylated glycosylation. Methods Mol Biol (2015) 1295:275–92. doi:10.1007/978-1-4939-2550-6_21
175. Hang HC, Yu C, Kato DL, Bertozzi CR. A metabolic labeling approach toward proteomic analysis of mucin-type O-linked glycosylation. Proc Natl Acad Sci U S A (2003) 100(25):14846–51. doi:10.1073/pnas.2335201100
176. Laughlin ST, Bertozzi CR. Metabolic labeling of glycans with azido sugars and subsequent glycan-profiling and visualization via Staudinger ligation. Nat Protoc (2007) 2(11):2930–44. doi:10.1038/nprot.2007.422
177. Liang Y, Hua Q, Pan P, Yang J, Zhang Q. Development of a novel method to evaluate sialylation of glycoproteins and analysis of gp96 sialylation in Hela, SW1990 and A549 cell lines. Biol Res (2015) 48:52. doi:10.1186/s40659-015-0041-8
178. Wuhrer M, Catalina MI, Deelder AM, Hokke CH. Glycoproteomics based on tandem mass spectrometry of glycopeptides. J Chromatogr B Analyt Technol Biomed Life Sci (2007) 849(1–2):115–28. doi:10.1016/j.jchromb.2006.09.041
179. An HJ, Froehlich JW, Lebrilla CB. Determination of glycosylation sites and site-specific heterogeneity in glycoproteins. Curr Opin Chem Biol (2009) 13(4):421–6. doi:10.1016/j.cbpa.2009.07.022
180. Hinneburg H, Stavenhagen K, Schweiger-Hufnagel U, Pengelley S, Jabs W, Seeberger PH, et al. The art of destruction: optimizing collision energies in quadrupole-time of flight (Q-TOF) instruments for glycopeptide-based glycoproteomics. J Am Soc Mass Spectrom (2016) 27(3):507–19. doi:10.1007/s13361-015-1308-6
181. Gomes C, Almeida A, Ferreira JA, Silva L, Santos-Sousa H, Pinto-de-Sousa J, et al. Glycoproteomic analysis of serum from patients with gastric precancerous lesions. J Proteome Res (2013) 12(3):1454–66. doi:10.1021/pr301112x
182. Li K, Sun Z, Zheng J, Lu Y, Bian Y, Ye M, et al. In-depth research of multidrug resistance related cell surface glycoproteome in gastric cancer. J Proteomics (2013) 82:130–40. doi:10.1016/j.jprot.2013.02.021
183. Chang TW. Binding of cells to matrixes of distinct antibodies coated on solid surface. J Immunol Methods (1983) 65(1–2):217–23. doi:10.1016/0022-1759(83)90318-6
184. Rakus JF, Mahal LK. New technologies for glycomic analysis: toward a systematic understanding of the glycome. Annu Rev Anal Chem (Palo Alto Calif) (2011) 4:367–92. doi:10.1146/annurev-anchem-061010-113951
185. Patwa T, Li C, Simeone DM, Lubman DM. Glycoprotein analysis using protein microarrays and mass spectrometry. Mass Spectrom Rev (2010) 29(5):830–44. doi:10.1002/mas.20269
186. Gao C, Liu Y, Zhang H, Zhang Y, Fukuda MN, Palma AS, et al. Carbohydrate sequence of the prostate cancer-associated antigen F77 assigned by a mucin O-glycome designer array. J Biol Chem (2014) 289(23):16462–77. doi:10.1074/jbc.M114.558932
187. Blixt O, Head S, Mondala T, Scanlan C, Huflejt ME, Alvarez R, et al. Printed covalent glycan array for ligand profiling of diverse glycan binding proteins. Proc Natl Acad Sci U S A (2004) 101(49):17033–8. doi:10.1073/pnas.0407902101
188. Schneider C, Smith DF, Cummings RD, Boligan KF, Hamilton RG, Bochner BS, et al. The human IgG anti-carbohydrate repertoire exhibits a universal architecture and contains specificity for microbial attachment sites. Sci Transl Med (2015) 7(269):269ra1. doi:10.1126/scitranslmed.3010524
189. Horlacher T, Seeberger PH. Carbohydrate arrays as tools for research and diagnostics. Chem Soc Rev (2008) 37(7):1414–22. doi:10.1039/b708016f
190. Seeberger PH. Automated carbohydrate synthesis as platform to address fundamental aspects of glycobiology – current status and future challenges. Carbohydr Res (2008) 343(12):1889–96. doi:10.1016/j.carres.2008.05.023
191. Wang D, Liu S, Trummer BJ, Deng C, Wang A. Carbohydrate microarrays for the recognition of cross-reactive molecular markers of microbes and host cells. Nat Biotechnol (2002) 20(3):275–81. doi:10.1038/nbt0302-275
192. Liu Y, Palma AS, Feizi T. Carbohydrate microarrays: key developments in glycobiology. Biol Chem (2009) 390(7):647–56. doi:10.1515/BC.2009.071
193. Stevens J, Blixt O, Paulson JC, Wilson IA. Glycan microarray technologies: tools to survey host specificity of influenza viruses. Nat Rev Microbiol (2006) 4(11):857–64. doi:10.1038/nrmicro1530
194. Xuan P, Zhang Y, Tzeng TR, Wan XF, Luo F. A quantitative structure-activity relationship (QSAR) study on glycan array data to determine the specificities of glycan-binding proteins. Glycobiology (2012) 22(4):552–60. doi:10.1093/glycob/cwr163
195. Kletter D, Singh S, Bern M, Haab BB. Global comparisons of lectin-glycan interactions using a database of analyzed glycan array data. Mol Cell Proteomics (2013) 12(4):1026–35. doi:10.1074/mcp.M112.026641
196. Cholleti SR, Agravat S, Morris T, Saltz JH, Song X, Cummings RD, et al. Automated motif discovery from glycan array data. OMICS (2012) 16(10):497–512. doi:10.1089/omi.2012.0013
197. Fair RJ, Hahm HS, Seeberger PH. Combination of automated solid-phase and enzymatic oligosaccharide synthesis provides access to alpha(2,3)-sialylated glycans. Chem Commun (Camb) (2015) 51(28):6183–5. doi:10.1039/c5cc01368b
198. Lai CH, Hahm HS, Liang CF, Seeberger PH. Automated solid-phase synthesis of oligosaccharides containing sialic acids. Beilstein J Org Chem (2015) 11:617–21. doi:10.3762/bjoc.11.69
199. Liang CF, Hahm HS, Seeberger PH. Automated synthesis of chondroitin sulfate oligosaccharides. Methods Mol Biol (2015) 1229:3–10. doi:10.1007/978-1-4939-1714-3_1
200. Zacco E, Anish C, Martin CE, V Berlepsch H, Brandenburg E, Seeberger PH, et al. A self-assembling peptide scaffold for the multivalent presentation of antigens. Biomacromolecules (2015) 16(7):2188–97. doi:10.1021/acs.biomac.5b00572
201. Zhao J, Patwa TH, Qiu W, Shedden K, Hinderer R, Misek DE, et al. Glycoprotein microarrays with multi-lectin detection: unique lectin binding patterns as a tool for classifying normal, chronic pancreatitis and pancreatic cancer sera. J Proteome Res (2007) 6(5):1864–74. doi:10.1021/pr070062p
202. Pedersen JW, Blixt O, Bennett EP, Tarp MA, Dar I, Mandel U, et al. Seromic profiling of colorectal cancer patients with novel glycopeptide microarray. Int J Cancer (2011) 128(8):1860–71. doi:10.1002/ijc.25778
203. Burford B, Gentry-Maharaj A, Graham R, Allen D, Pedersen JW, Nudelman AS, et al. Autoantibodies to MUC1 glycopeptides cannot be used as a screening assay for early detection of breast, ovarian, lung or pancreatic cancer. Br J Cancer (2013) 108(10):2045–55. doi:10.1038/bjc.2013.214
204. Hirabayashi J, Yamada M, Kuno A, Tateno H. Lectin microarrays: concept, principle and applications. Chem Soc Rev (2013) 42(10):4443–58. doi:10.1039/c3cs35419a
205. Tang H, Hsueh P, Kletter D, Bern M, Haab B. The detection and discovery of glycan motifs in biological samples using lectins and antibodies: new methods and opportunities. Adv Cancer Res (2015) 126:167–202. doi:10.1016/bs.acr.2014.11.003
206. Yue T, Haab BB. Microarrays in glycoproteomics research. Clin Lab Med (2009) 29(1):15–29. doi:10.1016/j.cll.2009.01.001
207. Huang WL, Li YG, Lv YC, Guan XH, Ji HF, Chi BR. Use of lectin microarray to differentiate gastric cancer from gastric ulcer. World J Gastroenterol (2014) 20(18):5474–82. doi:10.3748/wjg.v20.i18.5474
208. Sunderic M, Sediva A, Robajac D, Miljus G, Gemeiner P, Nedic O, et al. Lectin-based protein microarray analysis of differences in serum alpha-2-macroglobulin glycosylation between patients with colorectal cancer and persons without cancer. Biotechnol Appl Biochem (2015). doi:10.1002/bab.1407
209. Cao Z, Maupin K, Curnutte B, Fallon B, Feasley CL, Brouhard E, et al. Specific glycoforms of MUC5AC and endorepellin accurately distinguish mucinous from nonmucinous pancreatic cysts. Mol Cell Proteomics (2013) 12(10):2724–34. doi:10.1074/mcp.M113.030700
210. Chen S, LaRoche T, Hamelinck D, Bergsma D, Brenner D, Simeone D, et al. Multiplexed analysis of glycan variation on native proteins captured by antibody microarrays. Nat Methods (2007) 4(5):437–44. doi:10.1038/nmeth1035
211. Kumada Y, Ohigashi Y, Emori Y, Imamura K, Omura Y, Kishimoto M. Improved lectin ELISA for glycosylation analysis of biomarkers using PS-tag-fused single-chain Fv. J Immunol Methods (2012) 385(1–2):15–22. doi:10.1016/j.jim.2012.07.021
212. Gustafsdottir SM, Schallmeiner E, Fredriksson S, Gullberg M, Soderberg O, Jarvius M, et al. Proximity ligation assays for sensitive and specific protein analyses. Anal Biochem (2005) 345(1):2–9. doi:10.1016/j.ab.2005.01.018
213. Soderberg O, Gullberg M, Jarvius M, Ridderstrale K, Leuchowius KJ, Jarvius J, et al. Direct observation of individual endogenous protein complexes in situ by proximity ligation. Nat Methods (2006) 3(12):995–1000. doi:10.1038/nmeth947
214. Conze T, Carvalho AS, Landegren U, Almeida R, Reis CA, David L, et al. MUC2 mucin is a major carrier of the cancer-associated sialyl-Tn antigen in intestinal metaplasia and gastric carcinomas. Glycobiology (2010) 20(2):199–206. doi:10.1093/glycob/cwp161
215. Pinto R, Carvalho AS, Conze T, Magalhaes A, Picco G, Burchell JM, et al. Identification of new cancer biomarkers based on aberrant mucin glycoforms by in situ proximity ligation. J Cell Mol Med (2012) 16(7):1474–84. doi:10.1111/j.1582-4934.2011.01436.x
216. Koos B, Kamali-Moghaddam M, David L, Sobrinho-Simoes M, Dimberg A, Nilsson M, et al. Next-generation pathology – surveillance of tumor microecology. J Mol Biol (2015) 427(11):2013–22. doi:10.1016/j.jmb.2015.02.017
217. Caprioli RM, Farmer TB, Gile J. Molecular imaging of biological samples: localization of peptides and proteins using MALDI-TOF MS. Anal Chem (1997) 69(23):4751–60. doi:10.1021/ac970888i
218. Powers TW, Jones EE, Betesh LR, Romano PR, Gao P, Copland JA, et al. Matrix assisted laser desorption ionization imaging mass spectrometry workflow for spatial profiling analysis of N-linked glycan expression in tissues. Anal Chem (2013) 85(20):9799–806. doi:10.1021/ac402108x
219. Powers TW, Neely BA, Shao Y, Tang H, Troyer DA, Mehta AS, et al. MALDI imaging mass spectrometry profiling of N-glycans in formalin-fixed paraffin embedded clinical tissue blocks and tissue microarrays. PLoS One (2014) 9(9):e106255. doi:10.1371/journal.pone.0106255
Keywords: gastric cancer, colorectal cancer, pancreatic cancer, glycomics, glycan biomarkers, microarray, proximity ligation assay, imaging mass spectrometry
Citation: Mereiter S, Balmaña M, Gomes J, Magalhães A and Reis CA (2016) Glycomic Approaches for the Discovery of Targets in Gastrointestinal Cancer. Front. Oncol. 6:55. doi: 10.3389/fonc.2016.00055
Received: 03 February 2016; Accepted: 24 February 2016;
Published: 09 March 2016
Edited by:
Leonardo Freire-de-Lima, Federal University of Rio de Janeiro, BrazilReviewed by:
Stephan Von Gunten, University of Bern, SwitzerlandAli Mobasheri, University of Surrey, UK
Copyright: © 2016 Mereiter, Balmaña, Gomes, Magalhães and Reis. This is an open-access article distributed under the terms of the Creative Commons Attribution License (CC BY). The use, distribution or reproduction in other forums is permitted, provided the original author(s) or licensor are credited and that the original publication in this journal is cited, in accordance with accepted academic practice. No use, distribution or reproduction is permitted which does not comply with these terms.
*Correspondence: Ana Magalhães, YW1hZ2FsaGFlcyYjeDAwMDQwO2lwYXRpbXVwLnB0;
Celso A. Reis, Y2Vsc29yJiN4MDAwNDA7aXBhdGltdXAucHQ=