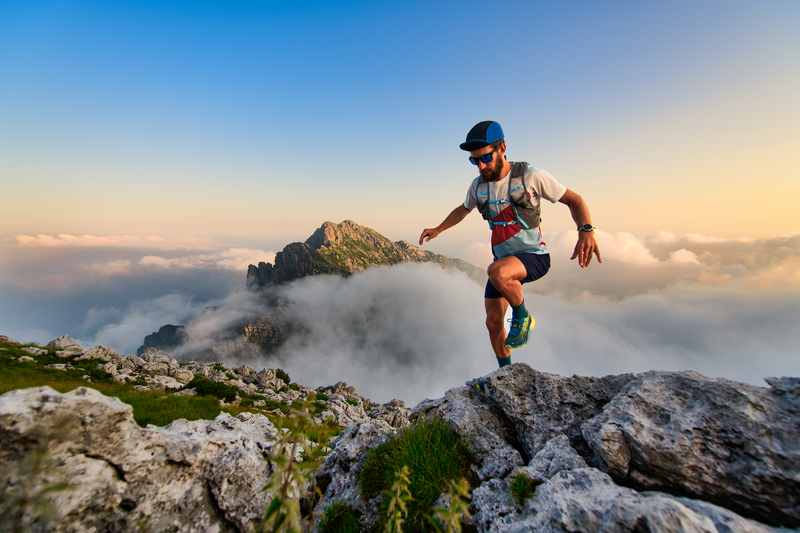
95% of researchers rate our articles as excellent or good
Learn more about the work of our research integrity team to safeguard the quality of each article we publish.
Find out more
ORIGINAL RESEARCH article
Front. Nucl. Eng. , 30 May 2023
Sec. Radioactive Waste Management
Volume 2 - 2023 | https://doi.org/10.3389/fnuen.2023.1188789
This article is part of the Research Topic Solubility Phenomena in The Context of Nuclear Waste Disposal View all 10 articles
Sorel phases are the binder phases of the magnesia building material (Sorel cement/concrete) and of special concern for the construction of long-term stable geotechnical barriers in repositories for radioactive waste in rock salt, as potentially occurring brines are expected to contain MgCl2. Sorel phases, in addition to Mg(OH)2, are equally important as pH buffers to minimize solubility and potential mobilization of radionuclides in brine systems. In order to obtain a detailed database of the relevant solid-liquid equilibria and the related pHm values of the equilibrium solutions, extensive experimental investigations were carried out. Solid phase formation was studied by suspending MgO and Mg(OH)2 in NaCl saturated MgCl2-solutions at 25°C. Mg(OH)2 and the 3-1-8 Sorel phase were identified as the stable solid phases, while the 5-1-8 Sorel phase is metastable. Equilibration at 40°C did not lead to any solid phase changes. Both OH− and H+ equilibrium concentrations were analyzed as a function of MgCl2 concentration at 25°C and 40°C. In addition to our already published solid-liquid equilibria for the ternary system Mg-Cl-OH-H2O (25°C–120°C), the equilibrium H+ concentrations (pHm) determined at 25°C, 40°C and 60°C are now reported. Analyzing these data together with known ion-interaction Pitzer coefficients, the solubility constants for Mg(OH)2 and the 3-1-8 phase at these three temperatures, for the metastable 5-1-8 phase at 25°C and for the 2-1-4 phase at 60°C have been consistently calculated.
Sorel phases are of particular importance for the construction of geotechnical barriers (plugs and sealing systems) in the host rock salt and the establishment of a favorable geochemical environment (i.e., by buffering of pHm and limiting carbonate concentrations) to minimize radionuclide transport processes via potential aqueous solutions.
Sorel phases [named after Stanislas Sorel, who was the first to describe the formation of a hardening material by the reaction of caustic magnesium oxide with magnesium chloride solution (Sorel & Dumas, 1867)] are the binder phases of the magnesia building material (Sorel cement or concrete) with the general composition xMg(OH)2∙yMgCl2∙zH2O (x-y-z phases). It has been empirically known for more than 100 years that Sorel cement does not corrode in salt (Unknown author, 1902). However, within the safety assessment of a repository, the long-term stability of the geotechnical barrier must be proven. This can be evidenced by analyzing the existing solubility equilibria between Sorel phases and salt solutions of the host rock. Depending on the ratio of MgO and the MgCl2 solution (and their concentration) in the building material formulation and the developing setting temperature, the 3-1-8, 5-1-8 and/or also 9-1-4 phase may be present when the setting reaction is complete. However, sufficient solubility equilibrium data of Sorel phases in NaCl-saturated solutions are not yet available in the literature.
In addition to the binder phase function, both Sorel phases and Mg(OH)2 can act as pH buffers of salt solutions (Bodine, 1976) as is realized in the Waste Isolation Pilot Plant (WIPP) in New Mexico with MgO as additive of backfill materials (Monastra & Grandstaff, 1999). In case of a brine access a weakly alkaline milieu is generated, providing favorable geochemical conditions for radionuclide retention. Therefore, in addition to the usual data describing solubility equilibria [Na+, Mg2+, Cl−, OH− solution concentrations in the presence of Mg(OH)2 and Sorel phases], the H+ concentrations are of particular interest.
In recent decades, the Sorel phases occurring in the basic ternary system Mg-Cl-OH-H2O- the 3-1-8, 5-1-8, 9-1-4, 2-1-4, 2-1-2, and 3-1-0 phases - have been extensively characterized (de Wolff & Walter-Lévy, 1949; Bianco, 1951; Walter-Lévy & Bianco, 1951; de Wolff & Walter-Lévy, 1953; de Wolff & Kortlandt, 1954; Cole & Demediuk, 1955; Demediuk et al., 1955; Bianco, 1958; Sugimoto et al., 2007; Dinnebier et al., 2010; Dinnebier et al., 2012; Bette et al., 2015). Solubility data are available in terms of OH− or H+ concentrations in MgCl2-containing solutions (ternary system Mg-Cl-OH-H2O) or in NaCl-MgCl2-containing mixed solutions (system Na-Mg-Cl-OH-H2O). For a conversion between concentration of free OH− and H+ the ionic product of water,
In addition to the free OH−, which is related to H+ via the Kw, in basic systems other hydroxide solution species [e.g., MgOH+ (Palmer & Wesolowski, 1997)] exist, which yield together with the free OH− the total OH− concentration.
A comprehensive data set is available for the ternary system Mg-Cl-OH-H2O from 25°C to 120°C by specifying the OH− solution concentrations (Pannach et al., 2017), including the evaluation of earlier data (20°C: D’Ans & Katz, 1941, 25°C; Robinson & Waggaman, 1909, 50°C; Nakayama, 1960, 100°C; Nakayama, 1959, 120°C; Dinnebier et al., 2010). Accordingly, in addition to Mg(OH)2, the four basic magnesium chloride hydrates (Sorel phases) 3-1-8, 9-1-4, 2-1-4, and 2-1-2 occur as thermodynamically stable solid phases depending on temperature and solution concentration (Figure 1). The 3-1-8 phase exists between 25°C and 80°C and was found to be metastable at 100°C. The 9-1-4, 2-1-4, and 2-1-2 phases occur at the higher temperatures or higher MgCl2 concentrations. In general, the hydroxide solution concentration in the system increases with temperature. For the 5-1-8 phase, only a temporary occurrence was observed during the solubility studies. It is therefore a metastable phase of the system (Feitknecht, 1926; Feitknecht & Held, 1944; Bianco, 1951; Cole & Demediuk, 1955; Newman, 1955; Bianco, 1958; Pannach et al., 2017).
FIGURE 1. Solubility data of the ternary system Mg-Cl-OH-H2O from 25°C to 120°C (Pannach et al., 2017).
For the NaCl-containing system Na-Mg-Cl-OH-H2O, a data set exists only for 20°C at not specified NaCl solution concentrations (D'Ans et al., 1955). Analogous to the ternary system, the stable solids are Mg(OH)2 in solutions with low MgCl2 concentrations, and the 3-1-8 phase at higher MgCl2 concentrations. The invariant point Mg(OH)2(s) + 3-1-8 phase was given by D'Ans et al., 1955 with 0.555 MgCl2 molal and 0.53 Mg(OH)2 mmolal. Metastable data points for Mg(OH)2 are also given. A comparison of these 20°C data from D'Ans et al., 1955 with those of the ternary system Mg-Cl-OH-H2O at 25°C from Pannach et al. (2017) in Figure 2 shows that in the presence of NaCl the stability range of the 3-1-8 phase extends towards lower MgCl2 concentrations.
FIGURE 2. Comparison of solubility data in the quaternary system Na-Mg-Cl-OH-H2O at 20°C (D'Ans et al., 1955) and the ternary system Mg-Cl-OH-H2O at 25°C (Pannach et al., 2017).
Molal H+ concentrations (pHm) were determined in the ternary system at 22°C ± 2°C by Altmaier et al. (2003) in the presence of Mg(OH)2 and the 3-1-8 phase; in the quaternary system Na-Mg-Cl-OH-H2O for Mg(OH)2 in solutions with very low NaCl concentration by Altmaier et al. (2003); Xiong (2008) and in almost NaCl-saturated solutions for the 5-1-8 phase (Xiong et al., 2010). Comparison of these data in Figure 3 shows that the pHm in the presence of Mg(OH)2 decreases more with increasing MgCl2 concentration (from about 10.7 to 8.9) than in the region of the 3-1-8 phase (from about 8.9 to 8.7) as shown in the comprehensive data set for the ternary system Mg-Cl-OH-H2O. In agreement with the “OH− based data set” (Figures 1, 2), the invariant point of Mg(OH)2 and the 3-1-8 phase is inferred to exist between 1.5 and 2.0 MgCl2 molal in the ternary system. In the presence of NaCl there are no data for > 0.1 MgCl2 molal for neither Mg(OH)2 nor the 3-1-8 phase available. The pHm values determined by Xiong et al. (2010) in the presence of the 5-1-8 phase in the MgCl2 concentration range from 0.5 to 2.0 molal in nearly NaCl-saturated solutions roughly compare to the trend of the 3-1-8 phase for the ternary system, but are shifted to a higher basicity.
FIGURE 3. Molal H+ concentrations (as -lg m (H+) = pHm) of the ternary Mg-Cl-OH-H2O and the quaternary Na-Mg-Cl-OH-H2O system at 25°C from literature. In the solutions containing very low MgCl2 of Altmaier et al. (2003), the NaCl concentrations vary from 0.01 to 5.0 molal. In the measurements of Xiong et al., 2010, an approximated saturation concentration of NaCl was adjusted.
With the determination of the H+ molalities, solubility constants were calculated by Altmaier and Xiong using an activity coefficient model for 25 °C corresponding to the reactions (2), (3) and (4) (see also chapter 3.3):
lgKS,25°C,Mg(OH)2 = 17.16 ± 0.1 from Mg-Cl-OH-H2O system Altmaier et al. (2003)
= 17.01 ± 0.1 from Na-Mg-Cl-OH-H2O system Altmaier et al. (2003)
= 17.05 ± 0.2 from Na-Mg-Cl-OH-H2O system Xiong (2008)
lgKS,25°C,3-1-8 = 26.15 ± 0.16 from Mg-Cl-OH-H2O system Altmaier et al. (2003)
lgKS,25°C,5-1-8 = 43.21 ± 0.33 from Na-Mg-Cl-OH-H2O system Xiong et al. (2010)
The solubility constant determined for Mg(OH)2 in both the Mg-Cl-OH-H2O and Na-Mg-Cl-OH-H2O system agree very well within the given error limits.
In this work, the studies on the solubility equilibria of the Sorel phases and Mg(OH)2 (brucite as mineral) were extended with the determination of the OH− and H+ solution concentrations in the system Na-Mg-Cl-OH-H2O (NaCl-saturated) at 25°C and 40°C. In addition to the already known H+ equilibrium concentrations in the ternary system Mg-Cl-OH-H2O at 25°C, measurements at 40°C and 60°C beside 25°C were presented together with the calculation of solubility constants for Mg(OH)2 and the Sorel phases at 25°C, 40°C, and 60°C.
Part II of the paper present the geochemical model developing on the basis of the experimental data.
MgCl2·6H2O, CAS No. 7791–18–6, (Fluka, purity > 99%, and Merck, ACS reagent, > 99%), Mg(OH)2, CAS No. 1309–42–8 (BioUltra, > 99%, from Fluka and M725 from Magnesia, 99%), MgO, CAS No. 1309–48–4 (M2923 from Magnesia, > 97%) and NaCl, CAS No. 7647–14–5 (from Merck, ACS reagent, ≥ 99.0%) were used for the investigations. The phase purity of all substances was checked by X-ray powder diffraction.
The magnesium chloride solutions were prepared by appropriate weighing of deionized water (CO2-free by boiling or flowing through with argon for 1 h) and MgCl2·6H2O. A suspension of NaCl ensured saturation in the respective MgCl2 solutions.
All solution concentrations are given in molal (molality m) with the unit mol·(kg H2O)−1 or mmolal = mmol·(kg H2O) −1.
For the preparation of the mixtures of solutions with solids in the quaternary system Na-Mg-Cl-OH-H2O at 25°C (sample Series 1 of TUBAF, Table 1), 1 g MgO was weighed into 250 mL PP wide-neck bottles with 200 g NaCl-saturated, n molal in MgCl2 each. The suspensions were annealed at 25°C ± 1°C and regularly shaken by hand every 1–3 days over a period of about 1 year.
TABLE 1. Set of samples used in Series 1. Sample compositions and analytical results after 349 days of equilibration at 25°C.
Another sample series (sample Series 2 of KIT-INE, Table 3) was prepared by weighing 300 mg of Mg(OH)2 (Fluka, BioUltra) into approximately 200 mL (240–270 g solution) of NaCl-saturated n molal MgCl2 solutions. The suspensions were stored in sealed vessels at 24°C ± 2°C in a glove box under argon atmosphere and also shaken regularly by hand. Over a period of 6 years, the development of the H+ solution concentrations were followed with a glass electrode (see chapter 2.4) and finally the solid phase was determined by X-ray.
The solids obtained after 1 year in sample series 1 [these were Mg(OH)2 and the 3-1-8 phase] were filtered off and re-suspended in new solutions of 200 g NaCl-saturated n molal MgCl2 solutions (partly in double approach) in 250 mL PP wide-neck bottles and equilibrated at 25°C for 131–144 days (sample Series 3a of TUBAF) and at 40°C for 32–84 days (sample Series 3b of TUBAF), see Table 4. In the case of the 40°C samples, the 2-1-4 Sorel phase (obtained in our previous studies on the ternary system Mg-Cl-OH-H2O (Pannach et al., 2017)) was also added. The temperature of the suspensions was controlled at 25°C ± 0.2°C and at 40°C ± 0.2°C in a water bath with continuous stirring using a Teflon magnetic stirrer. Subsequently, the solution and solid phases were separated using a micro glass fibre filter (Munktell, grade MGF = 0.7 µm).
In Pannach et al. (2017) we published our results on the determination of solubility equilibria in the ternary system Mg-Cl-OH-H2O at 25°C–120°C. In addition to the reported OH− solubility concentrations as a function of MgCl2 concentration, we also finally measured the H+ concentrations in the equilibrated suspensions at 25, 40°C and 60°C. These results are presented here as sample Series 4 (a-c) of TUBAF. The weights of the initial solution and solid components are given in Table 5 together with the equilibrium data. All suspensions were tempered in sealed 250 mL wide-necked PP bottles in water baths (25°C ± 0.2°C and 40°C ± 0.2°C, thermostat type EB, Julabo Labortechnik GmbH or 60°C ± 0.2 °C shaking water bath, company GFL, model 1,083) and shaken regularly by hand (25°C, 40°C) or automatically (60°C). Further experimental details can be found in Pannach et al. (2017).
Solutions: The Mg2+ concentration was analyzed by complexometric titration in NH3/NH4Cl buffered solutions (pH 9–10) with Erio T as indicator and 0.05 M Na-EDTA solution (Biedermann & Schwarzenbach, 1948) (relative analytical error ± 0.3%). The Cl− concentration was determined according to the method of Mohr (Schulze & Simon, 2009) in a Na2CO3/NaHCO3 buffered solution (pH 8–9) with K2CrO4 as indicator and 0.1 M AgNO3 solution (relative analytical error ± 0.2%). The total OH− concentrations were determined volumetrically by acid-base back titration (0.01 M HCl and 0.01 M NaOH solution) with potentiometric endpoint determination and a pH glass electrode (combination electrode, type ROSS, Thermo-Orion 8103BN, filling solution 3 M KCl). Reported results are the mean of double or triple titrations, where for each the sample solution of 10–20 g was directly weighed (relative analytical error ± 0.3%). The analyzed OH− concentrations are given as Mg(OH)2 concentrations in the following figures and tables. The Na+ concentration was determined with the flame photometer (type BWB-XP, BWB-XP Technologies) and from sample No. 26 (Table 3, sample series 3) by ion chromatography due to equipment failure of the flame photometer.
The H+ concentrations were determined potentiometrically according to the method of Altmaier et al. (2003) using glass electrodes calibrated to m (H+) at 25°C, 40°C and 60°C. At 25°C the potential measurements were carried out with a ROSS type pH glass electrode, Thermo-Orion 8103BN (combination electrode, filling solution 3 M KCl) - same for sample Series 2 (KIT-INE laboratory) and 3a and 4a (TUBAF laboratory). For the measurements at 40°C and 60°C (TUBAF sample Series 3b and 4b, 4c), a combination electrode with a temperature sensor of the type SenTix82 (company WTW, filling solution 3 M KCl solution) was used. Both pH electrodes were calibrated against standard pH buffer solutions (pH = 4–10, Riedel de Haën, Fisher Brand and Merck) at the corresponding temperatures. The pH value is defined in dilute aqueous solutions of ionic strength I < 0.1 molal by the activity of the H+ concentration. Measurements in aqueous solutions of higher ionic strength give to an operative pH value (pHexp), from which the H+ solution concentration is calculated using an empirical correction factor Am (Altmaier et al., 2003):
The correction factor Am depends on the solution composition, absolute concentration and temperature. This empirical relationship between A and a n molal salt solution of I > 0.1 molal is described by the following quadratic equations (in molal concentration units mi):
Na-Mg-Cl-OH-H2O system:
25°C (for sample Series 2 and 3a with NaCl and MgCl2 calibration solutions):
with ANaCl = −0.0988 + 0.1715 · mNaCl + 0.0013 · (mNaCl)2
40°C (for sample Series 3b with NaCl/MgCl2 mixing solutions):
Mg-Cl-OH-H2O system (for sample Series 4):
25°C (for Series 4a):
40°C (for Series 4b):
60°C (for Series 4c):
Using the correction factor Am and the measured pHexp values, the molal H+ concentrations of the solutions were determined with an accuracy of ± 0.05 pH units.
Solids: The solid phases (after filtration from the solution washed with cold (T < 5°C) deionized water and subsequently with cold (T < 5°C) ethanol to remove adhering wash water; finally dried at room temperature in an exsiccator) were identified by X-ray powder diffraction (Bruker D8 Discover with linear detector Vantec-1 at TUBAF or Bruker AXS D8 Advance at KIT-INE; each with Cu-Kα radiation). The samples were prepared as flat plates after grinding.
SEM images (exemplarily from the appropriately washed solids) were taken by TUBAF on gold-coated samples using a TESCAN Vega 5130 SB scanning electron microscope with a cathode voltage of 20 kV.
The development of the solid phase composition in the system Na-Mg-Cl-OH-H2O was followed at 25°C in NaCl saturated n molal MgCl2 solutions by suspending caustic MgO (sample Series 1 of TUBAF) as well as Mg(OH)2 (sample Series 2 of KIT-INE) with ≤ 1 g solid in 200 g solution.
MgO suspension in dilute MgCl2 solutions (0.2 molal) resulted in the formation of Mg(OH)2(s) beside the present NaCl. Mg(OH)2(s) was also formed in 0.5 mMgCl2 solution. Finally, after 349 days, a mixture of Mg(OH)2(s) and 3-1-8 phase was detected. The MgCl2 concentration was slightly increased to 0.56 molal (Table 1). In a 1.0 mMgCl2 solution, Mg(OH)2(s) was also identified within the first weeks and transformed into the 3-1-8 phase over months (see powder diffractograms taken within 1 year in Figure 4A). The SEM image (Figure 4C) shows the typical long needles of the 3-1-8 phase, between which particles of Mg(OH)2(s) are still visible in the “212-day sample” (Figure 4B).
FIGURE 4. (A) X-ray powder diffractograms of the solids obtained after different times of MgO suspension in NaCl-saturated 1.0 mMgCl2 solution (diff. No. 1–5); diff. No. 6: solid after re-suspension of the “349-day solid” (diff. No. 5) in new NaCl-saturated 1.0 mMgCl2 solution and further 131 days of suspension (= sample No. 18 of Series 3, Table 4); (B) SEM images of the solid after 212 days (diff. No. 4) and (C) after 349 days (diff. No. 5).
In 2 or 3 mMgCl2 solutions, the formation of the 5-1-8 phase in addition to Mg(OH)2 and/or the 3-1-8 phase was observed within the first few days (Figure 5). After a few weeks, only the 3-1-8 phase was present in addition to NaCl(s). In the concentrated MgCl2 solutions (4 and 5.5 mMgCl2, NaCl-saturated), the 3-1-8 phase could be identified as a solid phase after 3 days already. This time-dependent change of the solid phase composition in the differently concentrated MgCl2 solutions (NaCl-saturated) at 25°C ± 1°C is schematically summarized in Figure 6. All values of the initial solid-solution samples as well as the analyzed solution are given in Table 1.
FIGURE 5. X-ray powder diffractograms of the solids obtained after different times of MgO suspension in NaCl-saturated 2.0 mMgCl2 solution (diff. No. 1–5); diff. No. 6: solid after re-suspension of the “349-day solid” (diff. No. 5) in new NaCl-saturated 2.0 mMgCl2 solution and further 131 days of suspension (= sample No. 20 of Series 3, Table 4).
FIGURE 6. Time-dependent solid phase formation at 25°C starting from MgO suspension in the NaCl-saturated MgCl2 solutions (sample Series 1, Table 1). The solution concentrations subsequently analyzed are shown in Figure 9 (blue symbols).
In the case of the Mg(OH)2(s) suspension (sample Series 2), the development of the pHm was monitored over an even longer period of 2,394 days (6.5 years) (Figure 7). At the beginning of the measurements, three samples (the 0.5, 2.0 and 4.0 mMgCl2 suspensions, all NaCl-saturated) were selected, from which a few milligrams of solid phase were taken after 16 days to determine their composition. At this time, only the 3-1-8 phase was identified in the 4.0 mMgCl2 solution, whereas a mixture of Mg(OH)2(s) and 3-1-8 phase was found in the 2.0 mMgCl2 solution. In this solution, the Mg(OH)2(s) continued to transform into the 3-1-8 phase with time, as shown in the final diffractograms recorded after 2,394 days (6.5 years) in Figure 8. Only in the 0.5 mMgCl2 solution Mg(OH)2 could be detected as the remaining solid.
FIGURE 7. Time-dependent development of pHm in samples with initial Mg(OH)2(s) suspended in NaCl-saturated MgCl2 solutions (sample Series 2). Filled symbols indicate measured values with deviations from each other smaller than the analytical error of pHm = ± 0.05 (the beginning of gradually stabilizing values).
FIGURE 8. X-ray powder diffractograms of the solid phases after 2,394 days of suspension of Mg(OH)2(s) in NaCl-saturated MgCl2 solutions (sample Series 2, see Table 3).
The measured pHm show values (of the otherwise weakly acidic NaCl-MgCl2 solutions) that are buffered in the weakly basic range within the first 400 days. After a maximum value is reached, a decrease to a constant value is observed, indicating that the equilibrium state has been established. The difference between the intermediate maximum value and the equilibrium value was up to 0.2 pHm units in the solutions with lower MgCl2 concentrations, in the more concentrated MgCl2 solutions (3, 4 mMgCl2) it was significantly smaller. Within the analytical error of ± 0.05 pHm units, it can be assumed that equilibrium was reached in all suspensions after approximately 500 days. In general, higher pHm values can be observed in solutions of lower MgCl2 concentration (Figure 7). All analytical results obtained at the end of the measurements, after 2,394 days, are shown in Table 3 together with the initial data of this sample Series 2.
The results of the sample Series 1 and 2 show unanimously that, starting from MgO or Mg(OH)2(s) in MgCl2 solutions of medium concentration (0.5< mMgCl2 < 4), the approach to equilibrium needs longer periods of time (months—about 1 year), but is relatively quickly (hours - days) in 4 mMgCl2, where the equilibrium phase is 3-1-8 phase. Up to 0.5 mMgCl2, Mg(OH)2(s) is identified as the stable solid phase. In the case of sample Series 1, the intermediate occurrence of the 5-1-8 phase was also observed.
The stable solids, Mg(OH)2(s) and 3-1-8 phase, as well as their mixture (all being obtained from sample Series 1, where respective solids were filtered off), were again suspended as a new sample Series 3a in corresponding NaCl-MgCl2 solutions at 25°C and analyzed after 131–140 days. No further changes in the composition of the solid phases were observed. The analyzed OH− solution concentrations (for Series 1 in Table 1 and Series 3 in Table 4) are shown in Figure 9 as a function of MgCl2 concentration. The comparison in Figure 9 shows that the OH− solution concentrations of sample Series 1 are significantly higher than those of sample Series 3, despite their long-term suspension of almost 1 year (349 days). We have already observed such a behavior (remaining in the OH− supersaturated region) in our investigations on the solid-solution equilibria in the ternary system Mg-Cl-OH-H2O at 25°C (not at higher temperatures), using caustic MgO as the initial phase (Pannach et al., 2017). The causes seem to be structural defects (stacking faults in the H2O-Mg-OH polyhedral layer sequences) and amorphous fractions, especially of the 3-1-8 phase, which arise during its formation from highly OH− supersaturated solutions. These stacking fault (domains) cause a higher inner energy of the 3-1-8 lattice and are the reason for the slightly higher solubility. On contact or suspension in appropriate OH− free solution, they dissolve with recrystallisation (defect-free) until equilibrium is reached at low OH− solution concentrations according to sample Series 3. Still further, i.e., multiple recrystallisation tests did not lead to any further lowering of the OH− solution concentration. Therefore, in the following, only the OH− concentrations determined by means of sample Series 3 will be considered as equilibrium concentrations. They agree well with those of D'Ans et al., 1955 at unknown NaCl concentration (open black squares in Figure 9 (cf; Figure 2) up to ≤ 5 mMgCl2. A clear deviation to higher values can be seen in 5.0–5.5 mMgCl2 solution, suggesting still supersaturated states. The reported invariant point between Mg(OH)2(s) and 3-1-8 phase at a MgCl2 concentration of 0.555 molal and 0.53 Mg(OH)2 mmolal agrees very well with our values of 0.56 MgCl2 molal and 0.55 ± 0.03 Mg(OH)2 mmolal. Furthermore, D'Ans et al., 1955 still give metastable data points for Mg(OH)2(s) at higher MgCl2 concentrations (open circles in Figure 9).
FIGURE 9. Mg(OH)2 solution concentrations in the Na-Mg-Cl-OH-H2O system at 25°C, obtained with sample Series 1 after 349 days, blue symbols (suspension of caustic MgO as initial solid, supersaturation approach) and sample Series 3a after 131–144 days, full black symbols [equilibration of Mg(OH)2(s) and 3-1-8 phase as initial solids].
In addition to the samples equilibrated at 25°C, Mg(OH)2(s) and the 3-1-8 phase as well as the 2-1-4 phase were also suspended at 40°C in corresponding NaCl-saturated MgCl2 solutions for 32–84 days (sample Series 3b). Again, only Mg(OH)2(s) and the 3-1-8 phase remained as the stable solids at this temperature, the 2-1-4 phase converted either into Mg(OH)2(s) or the 3-1-8 phase as shown in Table 4. Compared to the 25°C data, higher OH− concentrations are analyzed at 40°C with a consistent trend towards increasing OH− concentrations with increasing mMgCl2. The highest values are reached at MgCl2 saturation. At 40°C its value is twice the one at 25°C. The invariant point between Mg(OH)2(s) and 3-1-8 phase (+NaCl) has shifted from 0.56 mMgCl2 at 25°C to about 1.1–1.3 mMgCl2 at 40 °C in the NaCl-saturated solutions (Figure 10). The corresponding OH− concentration has approximately tripled from about 0.0005 m Mg(OH)2 to 0.0013–0.0015 m.
FIGURE 10. Mg(OH)2 equilibrium concentrations in presence of the stable solids Mg(OH)2(s) and 3-1-8 phase (and MgCl2·6H2O at MgCl2-saturation) at NaCl saturation in the Na-Mg-Cl-OH-H2O system at 25°C and 40°C.
The pHm analyzed in sample Series 2 at 25°C and sample Series 3a and 3b at 25°C and 40°C respectively (Tables 3, 4) are presented in Figure 11. The 25°C values of Series 2 form a common isotherm with those determined for Series 3a. Obviously, equilibrium with respect to H+ solution concentrations had been reached with both sample series in the different laboratories. As no OH− concentrations were analyzed for sample Series 2 (KIT-INE), it is not possible to say whether equilibrium has also been reached for the OH− solution concentration in these suspensions over the long monitoring period of 6.5 years in comparison with sample Series 3a.
FIGURE 11. pHm in the system Na-Mg-Cl-OH-H2O at NaCl saturation and 25°C (filled black symbols: sample Series 2 and sample Series 3a) in comparison with literature values (open circle: Altmaier et al., 2003, blue; Xiong et al., 2010, cf; Figure 3) and 40°C (green symbols: sample Series 3b).
The pHm measured at 25°C are highest (H+ concentrations lowest) in the solutions with lowest MgCl2 concentrations in equilibrium with Mg(OH)2(s) (pHm = 9.57 in 0.2 mMgCl2 NaCl saturated solution, see all values in Tables 3, 4). In MgCl2-free NaCl-saturated solution, pHm still increases to 10.72, as shown by the data point from Altmaier et al. (2003) in Figure 11. At the invariant point of Mg(OH)2(s) + 3-1-8-phase + NaCl in 0.56 mMgCl2 solution, the pHm was determined to be 9.32. As the MgCl2 concentration increases, the pHm in the presence of 3-1-8 phase + NaCl(s) decreases only gradually over the wide concentration range to pHm = 8.7 in 5.5 mMgCl2 solution. Compared to the 25°C data of Xiong et al. (2010) for the 5-1-8 phase in the investigated range of 0.5≥ mMgCl2 ≤ 2 (NaCl saturated), the pHm are at higher values compared to both Mg(OH)2(s) and the 3-1-8 phase. This indicates a metastable occurrence of the 5-1-8 phase, which is consistent with our observations of solid phase formation in the system (see Section 3.1), showing the intermediate occurrence of the 5-1-8 phase.
The data curve shown in Figure 11 for the samples at 40°C runs nearly parallel to the respective curve for 25°C, shifted to lower pHm by about 0.6 units in the presence of Mg(OH)2(s) and about 0.4 units in the presence of the 3-1-8 phase (see also Table 4). With Mg(OH)2(s) as solid phase in 0.5 and 1 mMgCl2 solution, the pHm was measured as 8.72 and 8.64 respectively. In the presence of the 3-1-8 phase, the values again decrease only slightly with increasing mMgCl2. Between 4 < mMgCl2 < 5 a flat minimum occurs at pHm ∼ 8.3. At the invariant point of 3-1-8 phase + MgCl2·6H2O(s) + NaCl(s) the pHm is 8.4–8.5.
Beside the analyzed OH− and H+ solution concentration, also the NaCl concentrations of saturation determined in sample Series 3a are plotted for 25°C in Figure 12. As the data show, at least approximate NaCl saturation is approached with the initial compositions of sample Series 2 (Table 3) after equilibration in the Na-Mg-Cl-OH-H2O system. Compared to the OH− free, ternary Na-Mg-Cl-H2O system, the adjustment of the very low OH− saturation concentration causes within the scatter of the experimental data only a very slight decrease of the NaCl saturation concentration in the Na-Mg-Cl-OH-H2O system.
FIGURE 12. Analyzed NaCl saturation concentrations in the system Na-Mg-Cl-OH-H2O in presence of Mg(OH)2(s) resp. 3-1-8 phase (see Table 4, Series 3a) in compare to the OH− free, ternary Na-Mg-Cl-H2O system (literature data) at 25°C (Takegami, 1921, Keitel, 1923, Rode, 1947, Han et al., 2017).
In the study on the solubility equilibria in the ternary system Mg-Cl-OH-H2O from 25°C to 120°C (Pannach et al., 2017), the pHm in the samples equilibrated at 25°C, 40°C and 60°C were measured but not yet published. The results are given now in Table 4 (as sample Series 4a, b and c) together with all initial components. Figure 13 shows the pHm as a function of MgCl2 concentration for all three temperatures. The values for 40°C and 60°C are shifted to lower pHm by about 0.6–0.4 units. The intersection of the branches for Mg(OH)2(s) and 3-1-8 phase data indicate the invariant points, which shift from 1.7–1.8 mMgCl2 at 25°C to about 2 at 40°C and 2.5 at 60°C (as already observed by Pannach et al. (2017) on the basis of the “OH− isotherms”). At 60°C a further Sorel phase, the 2-1-4 phase, increases relative to the 3-1-8 phase near the MgCl2 saturation. Thus, we could measure also a pHm value in presence of this phase in 5.8 mMgCl2 solution.
FIGURE 13. pHm in the Mg-Cl-OH-H2O system at 25°C (after 1,172 or 1,417 days of equilibration), 40°C (after 1,885–2,148 days) and 60°C (after 1,081–1,830 days) in comparison with the literature (open black symbols: Altmaier et al., 2003).
Comparing the two systems at 25°C shows that the stability field of the 3-1-8 phase in NaCl-saturated solutions expands towards lower MgCl2 concentrations, as can be seen by the shift of the invariant point with Mg(OH)2(s) from mMgCl2 of 1.7–1.8 in NaCl-free to 0.56 in NaCl-saturated solution (Figures 14, 15).
FIGURE 14. Comparison of OH− solution concentrations in the systems Na-Mg-Cl-OH-H2O (black symbols) and Mg-Cl-OH-H2O (Pannach et al., 2017) (brown symbols) at 25°C.
FIGURE 15. Comparison of pHm in the Na-Mg-Cl-OH-H2O system (black symbols) and the Mg-Cl-OH-H2O system (brown symbols) at 25°C.
The OH− concentrations and thus the solubility of the 3-1-8 phase are lower in NaCl-saturated than in NaCl-free solutions. However, the OH− concentrations approach each other when reaching saturation concentration of MgCl2 (Figure 14).
With the invariant point at 0.56 mMgCl2, a MgO-based concrete geotechnical barrier consisting of 3-1-8 binder phase (besides inert aggregates) will be in equilibrium with NaCl-saturated solutions as soon as concentrations of MgCl2 as low as 0.56 mMgCl2 are present. For solutions not saturated with NaCl higher concentrations are needed to ensure stability of the binder phase against the solution. In NaCl-free solutions a minimum of 1.7–1.8 mMgCl2 is required.
As can be seen in Figure 15, Mg(OH)2(s) buffers a pHm range from 9.3 to 9.6 in NaCl-saturated solutions, and if they are free of MgCl2 up to 10.7. From 0.56 mMgCl2, a pHm of about 9.3 is established by the 3-1-8 phase, decreasing to pHm = 8.7 with further increase in MgCl2 concentration. This pHm range also is found in NaCl-free solutions in the presence of the 3-1-8 phase. An analogous situation would be found when comparing the 40°C data of both systems, however, shifted by 0.6–0.4 units to lower pHm values.
The solubility constants, lg KS, for Mg(OH)2(s) and the Sorel phases 3-1-8 and 2-1-4 were calculated from the molal equilibrium H+ concentrations in the NaCl-saturated MgCl2 solutions at 25°C and 40°C (Tables 3, 4) and in the MgCl2 solutions free of NaCl at 25°C, 40°C and 60°C (Table 5) according to Eqs 11–18. The calculation of lg KS was also possible for the metastable 5-1-8 phase by measuring the H+ concentration during its intermediate appearance in a 25 °C suspension (sample No. 46 in Table 5). The activity coefficients γi of Mg2+, H+ und Cl− and the water activity aW were calculated using the Pitzer data set of THEREDA for the hexary system of oceanic salts including acids and bases [THEREDA, Voigt (2020)].
Mg(OH)2:
3-1-8 phase:
2-1-4 phase:
5-1-8 phase:
All calculated lg KS values are given as mean values in Table 2 for each system and temperature (single values in Tables 3–5) compared with literature data where available. For Mg(OH)2(s) a mean value at 25°C of lg KS = −17.17 ± 0.03 is obtained for NaCl-saturated solutions (Tables 3, 4) and lg KS = −17.16 ± 0.08 for NaCl-free solutions (Table 5). The values are in a very good agreement with those of Altmaier et al. (2003) and Xiong (2008) within the given error range as can be seen in Table 2. There are some more studies on solubility constants for Mg(OH)2(s) discussed and evaluated by Altmaier et al. (2003), which agree within an overall mean value of lg KS,Mg(OH)2,25°C = −17.1 ± 0.2. For 40°C we determined lg KS,Mg(OH)2,40°C = −15.9 ± 0.2 from the two mean values, lg KS = −16.00 ± 0.06 from NaCl-saturated solutions (Table 4) and lg KS = −15.86 ± 0.09 from the NaCl-free solutions (Table 5). At 60°C a value of lg KS,Mg(OH)2,60°C = −14.92 ± 0.02 was calculated from the NaCl-free MgCl2 solutions (Table 5).
TABLE 2. Solubility constants (lg KS) for Mg(OH)2(s), and the Sorel phases 3-1-8, 5-1-8 und 2-1-4 in compare to available data from literature.
TABLE 3. Initial components and sample composition for sample Series 2 (KIT-INE). Solution composition after equilibration (6.5 years) and calculated solubility constants for the stable solids Mg(OH)2 and 3-1-8 phase in the Na-Mg-Cl-OH-H2O (NaCl-saturated) system at 25°C.
TABLE 4. Initial components and sample composition for sample Series 3 (TUBAF). Analyzed solution composition after equilibration at 25°C (131–144 days), at 40°C (32–84 days) and calculated solubility constants for the stable solids Mg(OH)2 and 3-1-8 phase in the Na-Mg-Cl-OH-H2O (NaCl-saturated) system.
TABLE 5. Initial components and sample composition for sample Series 4 (TUBAF). Analyzed solution composition after equilibration at 25°C (3 years), at 40°C (3 years) and 60°C (205–318 days) in the system Mg-Cl-OH-H2O. Calculated solubility constants for the stable solids Mg(OH)2, 3-1-8 and 2-1-4 phase and the metastable 5-1-8 phase.
For the 3-1-8 phase the obtained value of lg KS,3-1-8,25°C = −26.10 ± 0.13 agrees very well with Altmaier et al. (2003) (−26.15 ± 0.16) from measurements in MgCl2 solutions at 25°C. Our measurements in NaCl-saturated MgCl2 solutions (Tables 3, 4) yield a consistent value of lg KS,3-1-8,25°C = −26.16 ± 0.13. At 40°C, equally consistent values with lg KS,3-1-8,40°C = −24.88 ± 0.16 and −24.72 ± 0.04 were calculated from the NaCl-saturated (Table 4) and NaCl-free solutions (Table 5). From the measurements at 60°C in MgCl2 solutions a lg KS,3-1-8,60°C = −23.04 ± 0.11 results accordingly. For the 2-1-4 phase occurring at and above 60°C in concentrated MgCl2 solution, the solubility constant could only be determined from one sample (in 5.79 molal MgCl2-solution, Table 5) with lg KS,2-1-4,60°C = −32.95 ± 0.2.
Sorel phases are binder phases of the magnesia building material (Sorel cement/concrete) highly suitable for construction of geotechnical barriers in rock salt formations. Compared with classical concrete, materials based on Sorel phases possess the principal property of being stable against saline MgCl2 containing solutions. This specific feature represents a crucial prerequisite for the construction of geotechnical barriers in repositories for radioactive waste in rock salt, as potentially occurring brines are expected to contain MgCl2. In addition, to minimize solubility and possible mobilization of actinides in brines systems, the pH should preferably be buffered in the weakly alkaline range. Detailed understanding of relevant solid-liquid equilibria and related pHm data for the equilibrium solutions provide the scientific basis and information in the context of safety analysis.
In this work, the solid-liquid equilibria of the Sorel phases and Mg(OH)2(s) in the system Na-Mg-Cl-OH-H2O at saturation of NaCl were determined at 25°C and 40°C. Starting from the suspension of MgO (sample Series 1, ss 1) and Mg(OH)2(s) (sample Series 2, ss 2), the solid phase formation was followed at 25°C over a period of one (ss 1) and 6 years, respectively, including the determination of H+ solution concentrations, pHm, (ss 2) as a function of MgCl2 molality.
Mg(OH)2(s) and the 3-1-8 phase were identified as the stable solids in the system. Following their further equilibration at 25°C and 40°C (ss 3), the equilibrium OH− and H+ concentrations (pHm) were determined. Mg(OH)2(s) is the stable phase in the presence of NaCl-saturated solutions with low MgCl2 content (up to about 0.56 mMgCl2) and the 3-1-8 phase in the more concentrated MgCl2 solutions at 25°C. At 40°C, the invariant point shifts to 1–1.5 mMgCl2. The 5-1-8 Sorel phase was found to occur only intermediately during the study period of ss1 and is therefore only a metastable phase in the solid-solution system.
In addition to the already published solid-liquid equilibria for the ternary system Mg-Cl-OH-H2O (25°C–120°C) in Pannach et al. (2017), the pHm determined at 25°C, 40°C and 60°C are reported here. Using these and with known ion-interaction Pitzer coefficients, the solubility constants for Mg(OH)2(s) and the 3-1-8 phase could be calculated for 25°C, 40°C and 60°C. From the measurements in the ternary system it was also possible to calculate the solubility constant for the metastable 5-1-8 phase at 25°C and for the 2-1-4 phase at 60°C, which was found only at higher temperature in the ternary system at high MgCl2 concentrations.
On the basis of the solid-liquid equilibria determined in this work, it is now possible to predict the long-term stability of magnesia building material in contact with solutions in a saline environment on a significantly improved scientific level and with high robustness. The pHm values developing in equilibrium with the respective Sorel phases or Mg(OH)2(s) are identified as a function of the solution composition for the system Na-Mg-Cl-OH-H2O at NaCl saturation.
The data also allow an extension of the Pitzer dataset in THEREDA to calculate the solubility equilibria of Sorel phases and Mg(OH)2(s) in complex salt solutions of the hexary system of oceanic salts, which is presented in Part II of this work.
The original contributions presented in the study are included in the article/supplementary material, further inquiries can be directed to the corresponding author.
DF: initiator of the investigation, head of the research area, data evaluation and writer of the paper. MP: experimentator, analysis and data evaluation, preparation of figures and tables. IP: experimentator, analysis. VM: experimentator and data evaluation. MA: experimentator, analysis, date evaluation, and co-writer of the paper. WV: initiator of some measurements, advisor, and co-writer of the paper.
Open Access Funding by the Publication Fund of the TU Bergakademie Freiberg.
We would like to thank the former German Federal Ministry for Economic Affairs and Energy (BMWi) for financial support within the R&D project 02E10880 “Relationship between chemism and mechanical properties of the MgO building material” (2010–2014), and the Federal Company for the Disposal of Radioactive Waste (BGE) with the current research project TEKFuE-21-02-js “Sorel phases equilibria”.
The authors declare that the research was conducted in the absence of any commercial or financial relationships that could be construed as a potential conflict of interest.
All claims expressed in this article are solely those of the authors and do not necessarily represent those of their affiliated organizations, or those of the publisher, the editors and the reviewers. Any product that may be evaluated in this article, or claim that may be made by its manufacturer, is not guaranteed or endorsed by the publisher.
Altmaier, M., Metz, V., Neck, V., Müller, R., and Fanghänel, T. (2003). Solid-liquid equilibria of Mg(OH)2(cr) and Mg2(OH)3Cl·4H2O(cr) in the system Mg-Na-H-OH-Cl-H2O at 25°C. Geochim. Cosmochim. Acta 67 (19), 3595–3601. doi:10.1016/s0016-7037(03)00165-0
Bette, S., Dinnebier, R. E., Röder, C., and und Freyer, D. (2015). A solid solution series of atacamite type Ni2xMg2-2xCl(OH)3. J. Solid State Chem. 228, 131–140. doi:10.1016/j.jssc.2015.04.015
Bianco, Y. (1951). The formation of basic magnesium chlorides at 50-175°C by aqueous methods. C. R. Acad. Sci. 232, 1108–1110.
Biedermann, T. W., and Schwarzenbach, G. (1948). The “complexometric” titration of alkaline earths and some other metals with Eriochromschwarz. Chimia 2, 56–59.
Bodine, M. W. (1976). Magnesium hydroxychloride: A possible pH buffer in marine evaporite. Geology 4, 76–80. doi:10.1130/0091-7613(1976)4<76:mhappb>2.0.co;2
Cole, W. F., and Demediuk, T. (1955). X-ray, thermal and dehydration studies on magnesium oxychlorides. Austr. J. Chem. 8 (2), 234–251. doi:10.1071/ch9550234
D’Ans, J., Busse, W., and Freud, H. E. (1955). Über basische Magnesiumchloride. Kali Steinsalz 8, 3–7.
D’Ans, J., and Katz, W. (1941). Magnesiumhydroxyd-Löslichkeiten, pH-Zahlen und Pufferung im System H2O-MgCl2-Mg(OH)2. Kali Steinsalz 35, 37–41.
de Wolff, P. M., and Kortlandt, D. (1954). Crystal structure determination from an x-ray powder diffraction pattern of β-Mg2(OH)3Cl. Appl. Sci. Res. B3, 3, 400–408. doi:10.1007/bf02123918
de Wolff, P. M., and Walter-Lévy, L. (1949). Structures and formulas of some constituents of Sorel cement. C. R. Acad. Sci. 229, 1232–1234.
de Wolff, P. M., and Walter-Lévy, L. (1953). The crystal structure of Mg2(OH)3(Cl, Br)·4H2O. Acta Cryst. 6, 40–44. doi:10.1107/s0365110x53000089
Demediuk, T., Cole, W. F., and Hueber, V. H. (1955). Studies on magnesium and calcium oxychlorides. Austr. J. Chem. 8 (2), 215–233. doi:10.1071/ch9550215
Dinnebier, R. E., Freyer, D., Bette, S., and Oestreich, M. (2010). 9Mg(OH)2·MgCl2·4H2O, a high temperature phase of the magnesia binder system. Inorg. Chem. 49 (21), 9770–9776. doi:10.1021/ic1004566
Dinnebier, R. E., Oestreich, M., Bette, S., and Freyer, D. (2012). 2Mg(OH)2·MgCl2·2H2O and 2Mg(OH)2·MgCl2·4H2O, two high temperature phases of the magnesia cement system. Z. Anorg. Allg. Chem. 638 (3-4), 628–633. doi:10.1002/zaac.201100497
Feitknecht, W., and Held, F. (1944). Über die Hydroxychloride des Magnesiums. Helv. Chim. Acta 27, 1480–1495. doi:10.1002/hlca.194402701189
Feitknecht, W. (1926). Über das Verhalten von schwer löslichen Metalloxyden in den Lösungen ihrer Salze. Zur Kenntnis der Magnesiumoxyd-Zemente I. Helv. Chim. Acta 9, 1018–1049. doi:10.1002/hlca.192600901137
Han, H., Dong, O., Li, D., and Zeng, D. (2017). Phase diagram of the NaCl-MgCl2-H2O system at 25-75°C and its application for MgCl2·6H2O purification. Russ. J. Phys. Chem. 91 (7), 1255–1259. doi:10.1134/s0036024417070147
Monastra, V., and Grandstaff, D. E. (1999). Kinetics of MgO dissolution and buffering of fluids in the waste isolation Pilot plant (WIPP) repository. Mat. Res. Soc. Symp. Proc. 556, 625–632. doi:10.1557/proc-556-625
Nakayama, M. (1959). A new basic triple salt containing magnesium hydroxide Part II. The quaternary system KCl-MgCl2-Mg(OH)2-H2O at 100°. Bull. Agr. Chem. Soc. Jpn. 23(1), 46–48. doi:10.1271/bbb1924.23.46
Nakayama, M. (1960). A new basic triple salt containing magnesium hydroxide Part IV. The quinary system KCl-K2SO4-MgCl2-MgSO4-Mg(OH)2-H2O at 50°. Bull. Agr. Soc. Jpn. 24(4), 362–371. doi:10.1271/bbb1924.24.362
Newman, E. S. (1955). A study of the system magnesium oxide-magnesium chloride-water and the heat of formation of magnesium oxychloride. J. Res. Natl. Bur. Stand. 54 (6), 347–355. doi:10.6028/jres.054.039
Palmer, D. A., and Wesolowski, D. J. (1997). Potentiometric measurements of the first hydrolysis quotient of magnesium (II) to 250°C and 5 molal ionic strength (NaCl). J. Solut. Chem. 26 (2), 217–232. doi:10.1007/bf02767923
Pannach, M., Bette, S., and Freyer, D. (2017). Solubility equilibria in the system Mg(OH)2-MgCl2-H2O from 298 to 393 K. J. Chem. Ing. Data 62, 1384–1396. doi:10.1021/acs.jced.6b00928
Robinson, W. O., and Waggaman, W. H. (1909). Basic magnesium chlorides. J. Phys. Chem. 13, 673–678. doi:10.1021/j150108a002
Rode, T. (1947). Vapor pressure and solubility of the aqueous reversible system 2 NaCl + MgSO4 ↔ Na2SO4 + MgCl2, stable and metastable diagram. Izv. Sek. Fiz. Khim. Anal. Akad. Nauk. SSSR 15, 234–265.
Sugimoto, K., Dinnebier, R. E., and Schlecht, T. (2007). Structure determination of Mg3(OH)5Cl·4H2O (F5 phase) from laboratory powder diffraction data and its impact on the analysis of problematic magnesia floors. Acta Cryst. B63, 805–811. doi:10.1107/s0108768107046654
Takegami, S. (1921). A study of the reciprocal salt pairs: Na2Cl2+MgSO4 ↔ Na2SO4+MgCl2 at 25°C. Mem. Coll. Sci. Kyoto Imp. Univ. 4, 317–342.
THEREDA. Thermodynamic reference database. Release (2021). Available at: www.thereda.de.
Voigt, W. (2020). Hexary system of oceanic salts – polythermal pitzer dataset (numerical supplement). THEREDA-J 01 (02), 1–9.
Walter-Lévy, L., and Bianco, Y. (1951). Action of magnesia on magnesium chloride solutions at 100°. C. R. Acad. Sci. 232, 730 – 732.
Xiong, Y., Deng, H., Nemer, M., and Johnsen, S. (2010). Experimental determination of the solubility constant for magnesium chloride hydroxide hydrate (Mg3Cl(OH)5·4H2O, phase 5) at room temperature, and its importance to nuclear waste isolation in geological repositories in salt formations. Geochim. Cosmochim. Acta 74, 4605–4611. doi:10.1016/j.gca.2010.05.029
Keywords: solubility equilibrium, system Na-Mg-Cl-OH-H2O, system Mg-Cl-OH-H2O, magnesium oxychloride, Sorel phases, Mg(OH)2, solubility constant
Citation: Pannach M, Paschke I, Metz V, Altmaier M, Voigt W and Freyer D (2023) Solid-liquid equilibria of Sorel phases and Mg (OH)2 in the system Na-Mg-Cl-OH-H2O. Part I: experimental determination of OH− and H+ equilibrium concentrations and solubility constants at 25°C, 40°C, and 60°C. Front. Nucl. Eng. 2:1188789. doi: 10.3389/fnuen.2023.1188789
Received: 17 March 2023; Accepted: 24 April 2023;
Published: 30 May 2023.
Edited by:
Taishi Kobayashi, Kyoto University, JapanReviewed by:
Kenso Fujiwra, Japan Atomic Energy Agency, JapanCopyright © 2023 Pannach, Paschke, Metz, Altmaier, Voigt and Freyer. This is an open-access article distributed under the terms of the Creative Commons Attribution License (CC BY). The use, distribution or reproduction in other forums is permitted, provided the original author(s) and the copyright owner(s) are credited and that the original publication in this journal is cited, in accordance with accepted academic practice. No use, distribution or reproduction is permitted which does not comply with these terms.
*Correspondence: Daniela Freyer, RGFuaWVsYS5mcmV5ZXJAY2hlbWllLnR1LWZyZWliZXJnLmRl
Disclaimer: All claims expressed in this article are solely those of the authors and do not necessarily represent those of their affiliated organizations, or those of the publisher, the editors and the reviewers. Any product that may be evaluated in this article or claim that may be made by its manufacturer is not guaranteed or endorsed by the publisher.
Research integrity at Frontiers
Learn more about the work of our research integrity team to safeguard the quality of each article we publish.