- 1Australian Nuclear Science and Technology Organisation, Sydney, NSW, Australia
- 2Centre for Medical Radiation Physics, University of Wollongong, Wollongong, NSW, Australia
Introduction: Current fission-based methods of 99Mo production use targets that are based on high output without taking into consideration the amount of radioactive waste produced. We examine the idea of using a low enriched target (<20%) to reduce the amount of nuclear and chemical wastes produced by the manufacture of 99Mo via fission of uranium-based targets.
Methods: MCNP6.2 was used to model targets of 20%, 10%, 7%, 3%, and 1% enrichment for 99Mo output and sustainability.
Results and Discussion: The 1% enriched target at the lowest density of 0.2 g/cm3 of UO2 was found to have the highest sustainability score at 6 days irradiation but it had a low 99Mo output. On the other hand, the highest output target was found to be 20% enriched with a density of 8.0 g UO2/cm3 with an irradiation time of 20 days. Target security and safeguards concerns were modelled and found to be of minimal concern.
1 Introduction
The nuclear industry is currently at a crossroads due to growing concerns regarding environmental, social, and governance (ESG) factors, which require changes including lower carbon emissions for electricity generation. While nuclear power has a much lower carbon emission profile compared to coal, its drawbacks and the main point of contention preventing its widespread acceptance is nuclear waste, which requires long-term storage and management (Morningstar Equity Research, 2017). Nuclear waste can be grouped into different classes based on its radioactivity (high, intermediate, and low) and composition (solid, liquid, and gaseous), each of which require different treatment and storage methods. The main options for handling radioactive waste are based on either concentrating and containing it in long-term facilities, diluting it to acceptable levels that are not harmful to the environment and then dispersing it, or a mixture of the two, which involves first containment to decay and then releasing into the environment once an acceptable level has been reached (Baisden & Choppin, 2007).
Nuclear waste occurs through various stages of the nuclear fuel cycle and results from the enrichment of uranium above its naturally found state. Nuclear waste storage is a global problem, with countries including Belgium, Canada, China, Finland, France, Germany, India, Japan, Russia, South Korea, Spain, Sweden, Switzerland, and the United Kingdom all listed as currently working on high-level nuclear waste repositories. High and intermediate solid nuclear waste requires safe storage for long periods and must remain isolated from the biosphere for thousands to hundreds of thousands of years depending on the waste composition. This long timeframe is a challenging issue that spans many generations; as a result, countries are finding it difficult to execute plans for building storage facilities. Technical issues in developing safe nuclear waste storage facilities include site safety including minimising the average and maximum dose to workers, economic considerations, security against theft or malicious damage, and geological aspects of sites. In addition to the technical challenges, the political challenges include polarisation between pro- or anti-nuclear agents; the lack of procedures and public involvement; the lack of integrated approaches combining technical, procedural, and political issues; political paralysis due to confusing responsibilities and the ongoing struggle regarding decisions around future energy mixes. The challenges continue to increase due to delays, as nuclear waste continues to accumulate due to the public’s lack of trust in the nuclear waste industry and a legacy of mishandling of waste from weapons facilities in the US (Rosa et al., 2010; Johnson et al., 2017; Hocke & Renn, 2019). Changing regulations and standards can also increase the difficulty of securing waste storage facilities, as shown in the Yucca Mountain repository, which was intended to be built in the US and has still not been completed. One significant issue with the site was a changing performance standard. At the time of design, there was no US Environmental Protection Agency performance standard, which resulted in the site being designed to last 10,000 years. Since 2008, a standard has been created that now requires the site to be designed to last 1,000,000 years, which significantly changed the scope of the project and led to further delays (Ewing and von Hippel, 2009).
Another source of long-term nuclear waste besides nuclear power is the 99Mo production industry, which currently mainly uses low-enriched uranium targets for the manufacture of the essential radioisotope (National Academies of Science, 2016). The present study aimed to reduce the amount of long-term nuclear waste requiring storage from the production of 99Mo by defining the uranium target sustainability in a novel way and examining the theoretical possibility of target redesign that could be used in existing processes to reduce solid and liquid radioactive wastes.
2 Nuclear security, non-proliferation, and radiopharmaceutical production
Although the main contributor to the nuclear waste issue is spent fuel in the nuclear power industry, the radiopharmaceutical industry can also aim to reduce the world’s nuclear waste burden by decreasing the amounts of nuclear waste byproducts of medical radioisotope production. 99mTc is the most widely used radioisotope and is the daughter of 99Mo, which is produced mainly by the fission of uranium. The applications of 99mTc include the diagnosis of diseases in various organs, with around 50% of its applications in nuclear cardiology (myocardial perfusion imaging). Other uses are for bone, brain, lung, liver, and kidney imaging (National Academies of Science, 2016). Although other methods of production do not involve the use of uranium, regular supply shortages mean that uranium-based manufacturing of 99Mo will likely continue for some time (Cutler & Schwarz, 2014). Fission-based 99Mo manufacturing generally requires the use of targets that contain high enriched uranium (HEU), with enrichment levels >20% 235U; however, due to nuclear security concerns, 99Mo manufacturers and research reactors have been transitioning to low-enriched uranium (LEU) targets containing <20% 235U (OECD, 2012; von Hippel & Kahn, 2006). Whilst LEU targets have a lower nuclear security risk than HEU targets, the trade-off of lower enrichment levels is the increased amount of plutonium produced when the target is irradiated due to the larger quantities of 238U present in the target, which produce Pu by neutron capture. The enrichment level of just less than 20% was chosen by the Reduced Enrichment for Research and Test Reactors (RERTR) as the optimal enrichment level to minimise target proliferation potential and strategic value. Strategic value is complex and depends on the sophistication levels of the potential proliferator. For example, natural uranium targets are of similar strategic value assuming basic nuclear capability (only uranium extraction) but are much more unattractive due to the highest levels of Pu build-up for advanced proliferators (uranium and plutonium extraction), even though the 235U levels were the minimum compared to higher levels of enriched targets (Glaser, 2005). Non-proliferation is a complex issue, with the benefits of medical isotope production weighed against the potential to use nuclear material for harm. HEU may be more dangerous than Pu as 50 kg of HEU is needed to create a harmful device, whereas the high level of spontaneous neutron emission in Pu restricts the level of harm in a device to less than one-thousandth of that in an HEU device (von Hippel and Kahn, 2006). To put matters into perspective, as of 2015 the world’s stockpile of weapon-grade material was an estimated 238 tonnes of Pu and 1330 tonnes of HEU, enough for an estimated 80,000 nuclear weapons (Albright and Kelleher-Vergantini, 2015). One possible method of reducing the proliferation risk with existing nuclear material inventories includes converting the purified material into a form that meets the spent fuel standard, which involves mixing inventories with highly radioactive nuclear material to simulate current spent fuel. This makes the material much harder to steal due to the lethal radioactive doses and high mass of the final product. Another method for reducing the chances of theft is to immobilise the material along with a highly radioactive material into a corrosion-resistant ceramic matrix (Ringwood et al., 1981; Lyman, 2014). The trade-off of increasing non-proliferation is the increased production of nuclear waste, which creates another long-term problem and additional costs to the industry.
3 99Mo supply and radioactive waste
Ensuring a continuous supply of 99Mo to the world is an extremely complex process involving target manufacturers, irradiators, processors, the radiopharmaceutical industry, governments, and organisations. To facilitate a continuous supply, the High-Level Group on the Security of Supply of Medical Radioisotopes (HLG-MR) was established in 2009. Some of the challenges affecting supply included the aged facilities used by current manufacturers, the uneconomical transition from HEU to LEU for producers, and the challenges of developing alternative technologies due to economic, technical hurdles and difficulties in transporting enriched uranium, and the current cost structure. These challenges mean that 99Mo is uneconomical without government subsidies. One of the recommendations of the HLG-MR was for governments to encourage the development of non-HEU alternative technologies to increase manufacturing diversity (Nuclear Energy Agency and Megascience, 2011).
The current supply chain contains many inefficiencies due to regulations and logistics that lead to product waste; therefore, 99Mo suppliers must oversupply the market to absorb these losses (Ballinger, 2010). Whilst it makes sense for 99Mo producers who use uranium fission-based methods of 99Mo production to focus on the maximum output of product per unit of time, i.e., GBq/week, to meet customer demands and maintain an economically sound business, this in conjunction with the trend toward LEU-based manufacturing targets logically lead to a trend of increasing volumes of radioactive waste as the target volume must increase to maintain the same amount of 235U compared to an HEU target. Approximately five times more radioactive waste is produced using LEU instead of HEU targets, with a corresponding five-fold reduction in 99Mo production by these targets (Nuclear Energy Agency and Megascience, 2011; Hassan and Ryu, 2015). The HLG-MR estimates that the switch to LEU targets may impact 99Mo yield by 0%–50%, depending on the manufacturer, due to the lower amount of 235U in the targets and the burdens of increased operational and waste storage costs. One method currently under development is the use of higher-density LEU targets to increase the total 235U in the LEU targets, thus offsetting the loss in production from the transition from HEU targets (Nuclear Energy Agency and Megascience, 2011; OECD, 2012). Since the predominant method of production is to dissolve these targets, larger volumes of both solid and liquid waste are produced. Moreover, no 99Mo manufacturers are currently using a recovery process to reuse the uranium waste (Stassen and Suthiram, 2015). The liquid waste requires further processing to transform it into a solid state through either cementation or Synroc for long-term storage (Carter et al., 2009). The HLG-MR believes that the increased costs associated with LEU-produced 99Mo are not factored into 99Mo pricing, which is detrimental to producers (OECD., 2019). All these factors are likely to lead to increased cost of production, as LEU-based 99Mo will be more expensive than HEU-produced 99Mo, leading to the price increase recommendations by the HLG-MR (OECD, 2012). These price increases have been difficult to implement as the current supply chain structure causes barriers to the price increases required by the industry to reduce or eliminate the need for government subsidy. One barrier that is hard to change is the highly regulated enriched uranium targets that must meet stringent safeguards controls, with much of the market being supplied by a single target fabricator (OECD., 2019).
One method to reduce the amount of liquid waste is the use of a target that does not require destruction to remove the 99Mo. What if, instead of the aim to produce the maximum amount of output per unit time, the aim was instead to maximise the output per gram of 235U burned up? This would potentially allow 99Mo producers to reduce the amount of uranium nuclear waste produced per run and become more sustainable, potentially leading to lower overall production costs when considering the whole production cycle, including waste management. There would be a possibility to use natural uranium to produce 99Mo, which would eliminate the wastes associated with uranium enrichment and reduce the safeguard costs for fabricating and transporting such targets. The potential downside of increased Pu production due to this type of target will also require investigation and assessment.
A simplistic way to describe the current methods of production is based on the following formula, which gives the amount of saleable product from a scheduling point of view:
Achieving a high output from a target design perspective requires consideration of the following factors: uranium density, mass, flux at the irradiated position, reactivity worth, heat flux, total heat, and accident analysis. When considering uranium density and mass to produce a high output, it is logical to pack as much 235U into the target as possible to ensure the maximum number of total fissions per unit of time. In this case, the 235U is in a state of saturation as significantly greater quantities are present in the target than will ever fission in a short time in a reactor.
An argument for the proposed alternative “lower waste” method could look like this:
99Mo target efficiency (
An important point is that the target efficiency is not absolute and will behave differently under different irradiation conditions such as flux and irradiation time; thus, the target efficiency must be optimised for the typical irradiation conditions it will experience. A 99Mo target efficiency used in isolation would lead to poor target design if it were hypothetically taken to its extreme. A target of 100 atoms of 235U would be highly efficient given the extremely high probability of all 100 atoms fissioning to produce approximately six atoms of 99Mo, which is of no use to a 99Mo producer used to dealing with activities in the GBq range. Therefore, an efficient target would minimise the amount of 235U needed to produce the required activity of 99Mo to satisfy customer demand and also minimises waste. However, a highly efficient target does not consider the additional requirement of 99Mo producers for a certain production output needed for customer demands. So, taking our example one step further, a target that minimises both the amount of 235U burned up and considers the need for 99Mo total output (
Thus, a target with a high
Hence, a target with high 99Mo
This paper continued work previously reported by Raposio et al. (2021) and investigated sustainability using MCNP6.2 (Werner et al., 2018) modelling of a theoretical target to determine the parameters for maximum 99Mo target sustainability.
This study configured a target to maximise a sustainability index
where
The sustainability index
where
Since a target with high “sustainability” with respect to 235U is expected to have a higher burnup and, thus, produce a greater amount of Pu, Pu build-up in the target will be also assessed. As 239Pu has a low bare critical mass (10.4 kg), this isotope will be monitored along with 238Pu, which, with its high decay heat, adds to non-proliferation if it comprises 12% or more of the mass of the total Pu in the target (Peryoga et al., 2005).
4 Results
This study used the target dimensions described by Raposio et al. (2021). The following variables were used in MCNP6.2 to model 235U burnup in grams, GBq of 99Mo produced, and Pu build-up:
UO2 densities in target (g/cm3): .2–8 g/cm3 in .2 g/cm3 intervals.
Irradiation time (days): 2, 3, 4, 5, 6, 7, 8, 9, 10, 15, and 20.
Target enrichment (% 235U/238U): 1, 3, 7, 10, and 20.
CINDER90 was used during MCNP6.2 modelling to determine the amount of fission products present at set irradiation times and to determine 99Mo output, 235U burned, and Pu build-up.
The ENDF/B-VI.5 data library was used for the simulations.
A total of 5,000 initial neutrons were selected for all simulations, which showed uncertainties of 1.18% for k-eff and 12% for flux tallies (García-Herranz et al., 2009).
4.1 Total output
The current focus in target design is to maximise output; thus, high-density targets have been proposed to obtain the maximum amount of 99Mo in the shortest amount of time (Nazaré, 1984; Lee et al., 1997). To understand how these targets are intended to work, a simulation was performed of an LEU UO2-based target, which maximised the output and disregarded other factors such as sustainability.
The99Mo target total output in GBq versus UO2 density in g/cm3 and versus irradiation time for varying target enrichment levels was plotted (Figures 1–5).
4.2 Sustainability score
For the same target configuration as in Figures 1–5, the sustainability score was plotted for all five enrichment types (Figures 6–10). Next, to examine the effects of self-shielding, the output versus enrichment for an example target of 2 g/cm3 density irradiation for 6 days of irradiation was plotted (Figure 11).
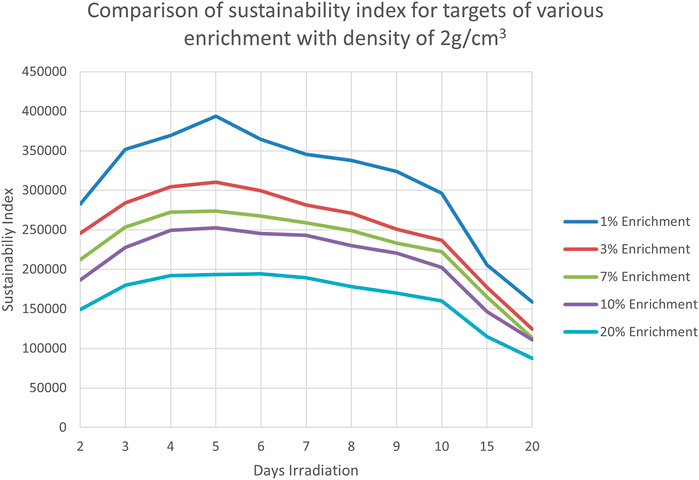
FIGURE 11. Comparison of sustainability index for targets of various enrichment with density of 2 g/cm3.
4.3 Plutonium build-up
For the same target configuration as in Figures 1–5, the plutonium build-up was plotted for all five enrichment types. The 239Pu build-up was normalised to a 100,000 GBq production run to compare the values on a relative basis. The number of 100,000 GBq production runs required to achieve 1 bare critical mass (BCM) of 239Pu is given in Figure 12, where 1 BCM is the mass required to create a nuclear weapon.
5 Discussion
As shown in Figures 1–5, the maximum output occurs at the “top-right” of the graph, which is the highest UO2 density and longest irradiation time. The more enriched the target, the greater the 99Mo output. It makes economic sense that existing target designs would aim for this type of configuration as it maximises output for 99Mo producers and assists in meeting customer demands.
The sustainability score is highest at the “top-left” of the figures, which is opposite to the results from the maximum output (see Figures 6–10). Moreover, short (<4 days) and long (>7 days) target irradiation times lead to large drops in target sustainability. As Figure 10 shows, the optimal ranges for target sustainability score are irradiation times of 4–7 days, with the peak sustainability occurring in the middle of this range (5 days).
As expected, the 20% enriched target showed the lowest sustainability index and the highest 99Mo output. This makes sense as a current target design based on maximising output for 99Mo producers. Thus, the current target design based on maximum output has a relatively low sustainability score. Comparison of results between output and sustainability for the same target shows very different outcomes. The highest sustainability score of 399925 was obtained for 6 days irradiation with a density of .2 g UO2/cm3 for a 1% enriched UO2 target. This is to be expected, as this type of target would have a much lower 235U self-shielding effect compared to a higher-density, more enriched target. This score gave a total output of 407 GBq, which is relatively low and could be a concern when using the sustainability score. In contrast, the highest total output was 88208 GBq for 20 days of irradiation and a density of 8.0 g UO2/cm3 with 20% enriched UO2, which gave a sustainability score of 40419. Hence, the 99Mo output was 217 times higher for a target that was approximately 10 times less sustainable as it uses 752 times more 235U when comparing the target with the highest output to the target with the highest sustainability.
Figure 12 highlights the loss in output as enrichment increases. Enrichments of 1%–3% showed no losses, whereas losses due to self-shielding increased by greater margins for 7%, 10%, and 20% enriched targets.
Figure 13 shows that the 1% enriched target produced more 239Pu compared to those of higher enrichment, as expected. However, from a practical aspect, the 1% target still requires >80,000 production runs with 7 days of irradiation worth of targets to create 1 BCM, which is a low proliferation concern due to the difficulty of obtaining and extracting 239Pu from over 80,000 targets.
6 Conclusion
When considering the full life cycle of 99Mo production, the target sustainability must be considered during target design. A high sustainability score means the use of less fissile uranium and therefore less waste at the end of the process, with the prospect of using natural uranium, which also eliminates enrichment waste. The trade-off with a high sustainability score is a lower total output, which is a negative outcome for producers. There is some light at the end of the tunnel for the sustainability argument, however, as it took 390 times as much 235U to produce only 174 times as much 99Mo output when comparing high output versus high sustainability targets. This was attributed to the effects of self-shielding that occur as the amount of 235U increases in a target of the same volume. This suggests that, with regards to sustainability, a promising area for further study is the use of low-density and higher-volume targets to potentially increase the total amount of 235U in the target to levels comparable to those of the high output targets, whilst maintaining a higher sustainability score than a smaller volume higher density target due to reduced self-shielding effects. A potential downside to a higher volume target is that research reactors have limited space, which limits the target volume and, therefore, output. Also, a target with very low enrichment would contain a low amount of 235U but a much greater 238U component, which would lead to waste, which also requires consideration in the overall waste amount. With regards to non-proliferation a lower enriched target would be even less of a security risk to proliferators targeting 235U; however, as the aforementioned results demonstrated, the amount of 239Pu increases with output as target enrichment decreases, which may be a non-proliferation concern. Further examination showed that the actual number of targets required for this increased risk is quite significant and not practical for a proliferator even when using a 1% enriched target; thus, the non-proliferation risk is minimal.
Data availability statement
The raw data supporting the conclusion of this article will be made available by the authors, without undue reservation.
Author contributions
GB—reviewing of MCNP data. AR and GT—reviewing and supervision. JB-W—reviewing of MCNP data.
Conflict of interest
The authors declare that the research was conducted in the absence of any commercial or financial relationships that could be construed as a potential conflict of interest.
Publisher’s note
All claims expressed in this article are solely those of the authors and do not necessarily represent those of their affiliated organizations, or those of the publisher, the editors, and the reviewers. Any product that may be evaluated in this article, or claim that may be made by its manufacturer, is not guaranteed or endorsed by the publisher.
References
Albright, D., and Kelleher-Vergantini, S. (2015). Plutonium and highly enriched uranium 2015 military highly enriched uranium and plutonium stocks in acknowledged nuclear weapon states a end of 2014.
Baisden, P. A., and Choppin, G. R. (2007). “Nuclear waste management and the nuclear fuel cycle,” in Encyclopaedia of life support systems (EOLSS). Editor Sándor Nagy.
Ballinger, J. R. (2010). 99Mo shortage in nuclear medicine: Crisis or challenge? J. Label. Compd. Radiopharm. 53 (4), 167–168. doi:10.1002/jlcr.1743
Carter, M. L., Li, H., Zhang, Y., Vance, E. R., and Mitchell, D. R. G. (2009). Titanate ceramics for immobilisation of uranium-rich radioactive wastes arising from 99Mo production. J. Nucl. Mater. 384 (3), 322–326. doi:10.1016/j.jnucmat.2008.12.042
Cutler, C. S., and Schwarz, S. W. (2014). Diversification in the supply chain of 99Mo ensures a future for 99mTc. J. Nucl. Med. 55 (7), 1208–1213. doi:10.2967/jnumed.113.131953
Ewing, R. C., and von Hippel, F. N. (2009). Nuclear waste management in the United States - starting over. Science 325 (5937), 151–152. doi:10.1126/science.1174594
García-Herranz, N., Cabellos, O., and Sanz, J. (2009). Assessment of the MCNP-ACAB code system for burnup credit analyses.
Hassan, M. ul, and Ryu, H. J. (2015). Radioactive waste issues related to production of fission-based 99Mo by using low enriched uranium (LEU). J. Nucl. Fuel Cycle Waste Technol. 13 (2), 155–161. doi:10.7733/jnfcwt.2015.13.2.155
Hocke, P., and Renn, O. (2019). Concerned public and the paralysis of decision-making: Nuclear waste management policy in Germany. J. Risk Res. 12, 921–940. doi:10.1080/13669870903126382
Johnson, B., Newman, A., and King, J. (2017). Optimizing high-level nuclear waste disposal within a deep geologic repository. Ann. Operations Res. 253 (2), 733–755. doi:10.1007/s10479-016-2194-4
Lee, D. B., Kim, K. H., and Kim, C. K. (1997). Thermal compatibility studies of unirradiated U-Mo alloys dispersed in aluminum. J. Nucl. Mater. 250, 79–82. doi:10.1016/s0022-3115(97)00252-3
Lyman, E. S. (2014). Excess plutonium disposition: The failure of MOX and the promise of its alternatives. www.ucsusa.org/our-work/nuclear-.
National Academies of Science (2016). “Molybdenum-99 for medical imaging,” in Molybdenum-99 for medical imaging (Washington, DC: National Academies Press). doi:10.17226/23563
Nazaré, S. (1984). Low enrichment dispersion fuels for research and test reactors. J. Nucl. Mater. 124, 14–24. doi:10.1016/0022-3115(84)90005-9
Nuclear Energy Agency, & Megascience (2011). The supply of medical radioisotopes : The path to reliability. Paris: OECD Publishing.
OECD (2012). The supply of medical radioisotopes market impacts of converting to low-enriched uranium targets for medical isotope production. Paris: OECD Publishing.
Peryoga, Y., Sagara, H., Saito, M., and Ezoubtchenko, A. (2005). Inherent protection of plutonium by doping minor actinide in thermal neutron spectra. J. Nucl. Sci. Technol. 42 (5), 442–450. doi:10.1080/18811248.2005.9726412
Raposio, R., Braoudakis, G., Rosenfeld, A., and Thorogood, G. J. (2021). Modelling of reusable target materials for the production of fission produced 99Mo using MCNP6.2 and CINDER90. Appl. Radiat. Isotopes 176, 109827. doi:10.1016/j.apradiso.2021.109827
Ringwood, A. E., Oversby, V. M., Kesson, S. E., Sinclair, W., Ware, N., Hibberson, W., et al. (1981). Immobilization of high-level nuclear reactor wastes in synroc: A current appraisal. Nucl. Chem. Waste Manag. 2 (4), 287–305. doi:10.1016/0191-815X(81)90055-3
Rosa, E. A., Tuler, S. P., Fischhoff, B., Webler, T., Friedman, S. M., Sclove, R. E., et al. (2010). Nuclear waste: Knowledge waste? Science 329 (5993), 762–763. doi:10.1126/science.1193205
Stassen, L., and Suthiram, J. (2015). Initial development of an alkaline process for recovery of uranium from 99Mo production process waste residue. J. Radioanalytical Nucl. Chem. 305 (1), 41–50. doi:10.1007/s10967-015-3974-z
von Hippel, F. N., and Kahn, L. H. (2006). Feasibility of eliminating the use of highly enriched uranium in the production of medical radioisotopes. Sci. Glob. Secur. 14 (2–3), 151–162. doi:10.1080/08929880600993071
Keywords: 99Mo production, uranium target, nuclear waste, MCNP modelling, sustainable manufacturing and design
Citation: Raposio R, Braoudakis G, Rosenfeld A, Thorogood G and Bedwell-Wilson J (2023) Investigating an alternative sustainable low-enriched uranium target for the manufacture of 99Mo using MCNP6.2 modelling with CINDER90. Front. Nucl. Eng. 1:978948. doi: 10.3389/fnuen.2022.978948
Received: 27 June 2022; Accepted: 19 December 2022;
Published: 10 January 2023.
Edited by:
Timothy Gerald Lach, Oak Ridge National Laboratory (DOE), United StatesReviewed by:
Hoai-Nam Tran, Duy Tan University, VietnamStefan Neumeier, Julich Research Center, Helmholtz Association of German Research Centres (HZ), Germany
Copyright © 2023 Raposio, Braoudakis, Rosenfeld, Thorogood and Bedwell-Wilson. This is an open-access article distributed under the terms of the Creative Commons Attribution License (CC BY). The use, distribution or reproduction in other forums is permitted, provided the original author(s) and the copyright owner(s) are credited and that the original publication in this journal is cited, in accordance with accepted academic practice. No use, distribution or reproduction is permitted which does not comply with these terms.
*Correspondence: Robert Raposio, rra@ansto.gov.au
†These authors share first authorship