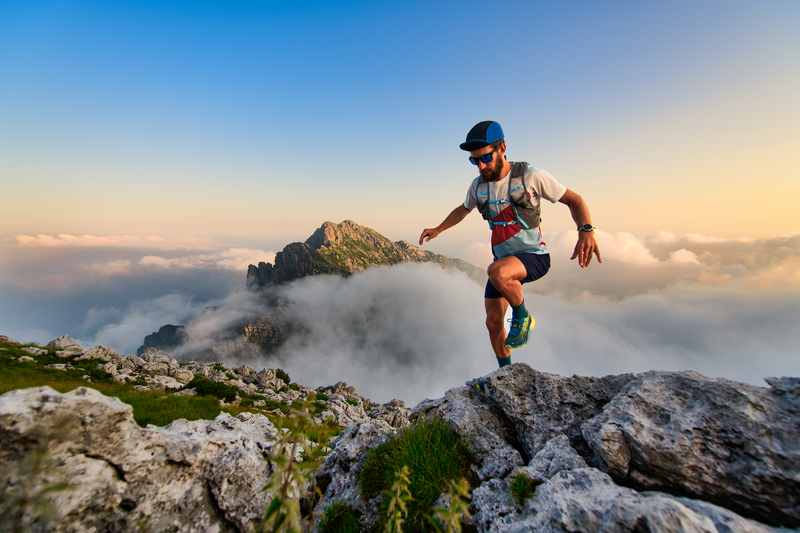
95% of researchers rate our articles as excellent or good
Learn more about the work of our research integrity team to safeguard the quality of each article we publish.
Find out more
REVIEW article
Front. Nat. Prod. , 21 September 2023
Sec. Biological Activities of Natural Products
Volume 2 - 2023 | https://doi.org/10.3389/fntpr.2023.1245869
Momilactones are defined as every lactone derived from rice husk. To date, these compounds can only be naturally found in rice (Oryza lineage) and moss (Pseudoleskeella papillosa and Hypnum plumaeforme). Their basic structure refers to the (9β-H)-pimarane skeleton bearing a γ-butyrolactone. In this review, the beneficial properties of momilactones, especially momilactones A (MA) and B (MB), including allelopathy, antimicrobials, antioxidants, anti-chronic diseases, anti-inflammation, and anticancer, are highlighted. Additionally, the signaling roles of momilactones in the response of host plants against environmental stresses are discussed, raising the question of whether momilactones act as novel phytohormones that require further comprehensive clarification. In another aspect, we come up with promising approaches to the future exploitation of these valuable compounds based on the current knowledge of their bioactivity mechanisms, biosynthetic pathways, and isolation protocols. However, momilactone biosynthetic processes remain elusive. Moreover, challenges in researching these compounds are mainly due to difficulties in isolation procedures and the lack of commercial sources, which have been least improved over the last half-century. Accordingly, we propose promising strategies to address these present problems and promote the effectiveness of further research, including: i) to improve the efficiency of momilactone extraction and isolation from plant sources; ii) to increase momilactone production applying synthetic models; iii) to generate crops that accumulate greater momilactone contents through genetic engineering approaches; iv) to discover new applications that combine momilactones with other prospective substances for enhancing targeted biological properties and preventing undesirable impacts; and v) to employ smart farming for optimizing the application of momilactones, monitoring their levels, and promoting sustainable agriculture. This paper may provide valuable insights for future strategies, focusing on the exploitation of momilactones for human health-beneficial purposes and the development of green agriculture aligned with sustainable development targets.
Momilactones were first discovered by Kato et al. (1973). The word “momi” means rice husk in Japanese. Accordingly, the term “momilactone” can be defined as every natural lactone derived from rice husks. Structurally, they contain a (9β-H)-pimarane skeleton bearing a γ-butyrolactone at the position of carbon-4,5,6 (Figure 1). Hitherto, seven molecules are acknowledged as momilactones, including momilactones A (MA), B (MB), C (MC), D (MD), E (ME), and F (MF) (Kato et al., 1973; Cartwright et al., 1980; Tsunakawa et al., 1976; Cho et al., 2015; Li et al., 2020). However, only compounds (1), (2), and (3) in Figure 1 were officially confirmed as MA, MB, and MC, respectively, since they have been detected in rice husks (Kato et al., 1973; Cartwright et al., 1980; Tsunakawa et al., 1976). On the other hand, MD (5) was isolated from rice roots (Cho et al., 2015). Compound (6) (ME) was mistakenly identified as a momilactone because its 19-nor-(9β-H)-pimarane structure is not linked to any lactone component. Besides, two compounds, (3) and (4), have been characterized as momilactone C (Tsunakawa et al., 1976; Liu et al., 2012). Of these, only compound (3) was isolated from rice husks (Tsunakawa et al., 1976). Compounds (4) (MC) and (7) (MF) were purified from moss species (Pseudoleskeella papillosa and Hypnum plumaeforme, respectively) (Liu et al., 2012; Li et al., 2020).
FIGURE 1. Momilactones and (9β-H)-pimarane skeleton linked to γ-butyrolactone structure. *Based on literature, compounds (3) and (4) have been characterized as momilactone C. †Momilactone E is a mistaken classification.
Momilactones, especially MA and MB, were initially indicated as potent allelochemicals and phytoalexins in rice and moss (Fukuta et al., 2007; Kato-Noguchi et al., 2013). In a later report, these compounds were found to play a more critical role in rice tolerance to drought and salinity stresses than in weed resistance (Xuan et al., 2016). Other studies implied that MA and MB accumulation increased in responses of rice and moss against adverse conditions, including ultraviolet (UV) exposure, heavy metals, nutrient deficit, and cantharidin (Kato-Noguchi, 2009; Kato-Noguchi and Kobayashi, 2009; Kato-Noguchi, 2011b). Recently, momilactones have been reported as valuable compounds with multiple beneficial properties, consisting of antioxidant (Fukuta et al., 2007; Quan et al., 2019a), antimicrobial (Fukuta et al., 2007), antidiabetic (Quan et al., 2019b; 2019c), anti-obesity (Quan et al., 2019c), anti-skin aging (Quan et al., 2019a), anti-ketosis (Kang et al., 2017), anti-inflammatory (Cho et al., 2015), and anticancer (for leukemia, lymphoma, multiple myeloma, colon and breast cancers) (Joung, 1992; Kim et al., 2007; Lee et al., 2008; Park et al., 2014; Anh et al., 2022a) properties. Based on that, a broad review on momilactone roles in plant responsive mechanisms against unfavorable conditions as well as their potential bioactivities for human health is presented in this work. Besides, their distribution in plant sources, release into the environment, and degradation in soil are extensively demonstrated. Additionally, momilactone biosynthesis in host plants and affecting factors are comprehensively discussed.
In another aspect, momilactones have exhibited significant potentials for multiple benefits, but further studies on these compounds and the development of practical implications have encountered difficulties due to their lack of commercial availability (Quan et al., 2019b). As far as we know, only one company named Biosynth Ltd. is currently providing MA at a very high price of 1,320 USD for 10 mg (Biosynth, 2023). In contrast, there has been no source offering MB yet. Additionally, the complication in their isolation and purification from plant sources is a significant challenge (Quan et al., 2019b). Only a relatively small number of research groups worldwide have reported successful processes for momilactone isolation (Quan et al., 2019b). Notably, there has been least improvement in isolation protocols of momilactones over the last five decades (Kato et al., 1973; Chung et al., 2005; Ahmad et al., 2019; Quan et al., 2019d). In this review, we describe a basic process for momilactone extraction and isolation that may help expand promising approaches for their exploitation from plant sources. Furthermore, potential strategies to promote further research on momilactones, including synthetic models, genetic engineering, and smart farming, are also deliberated in our study.
This research is conducted based on a comprehensive review of published reports concerning momilactones and their biological activities. Additionally, we focused on terms including distribution, release, degradation, biosynthesis, isolation methods, identification and quantification techniques, synthetic models, genetic engineering, and smart farming to explore and discuss the current status and promising approaches for exploiting the beneficial properties of momilactones. The search sources encompass studies and reports published between 1973 and 2023, which are indexed within Elsevier Databases, National Library of Medicine, and Web of Science.
Although relatively few studies on momilactones have been conducted due to the scarcity of material sources, evidence showing their valuable biological activities has been reported. Overviews of the biological activities of momilactones, especially allelopathic properties, were described in the studies of Kato-Noguchi (2023) and Serra et al. (2021). Those reports indicated that momilactones hold significant potential as allelochemicals, contributing to the development of sustainable weed management in the future (Serra et al., 2021; Kato-Noguchi, 2023). Conversely, the medicinal and pharmaceutical properties of momilactones were briefly introduced (Kato-Noguchi, 2023). In our research, we emphasize the potential of these compounds in treating certain chronic diseases and cancer. Of which, the activity of momilactones is compared with commonly used drugs. In addition, their action mechanisms are also discussed. Based on this information, the current achievements, limitations, and challenges are comprehensively pointed out. Moreover, we propose promising directions for the further development of momilactone-based medicines and products that benefit human health. The biological properties of momilactones, including allelopathy, medicinal, and pharmaceutical potentials, are summarized in Figure 2.
MA and MB were soon acknowledged as potent allelochemicals derived from rice. Particularly, they exhibited substantial allelopathic activity against indicator plants, especially problematic weeds (e.g., Echinochloa crus-galli, Monochoria vaginalis, Solidago altissima, etc.) (Fukuta et al., 2007; Kato-Noguchi et al., 2013; Quan et al., 2019d), as well as against blast fungus (Magnaporthe oryzae) (Cartwright et al., 1980; Zhao et al., 2018a) (Table 1). Besides, MA and MB exerted inhibitory effects on the mycelia growth of some fungi causing plant diseases, including Botrytis cinerea (gray mold) (Fukuta et al., 2007), Fusarium solani (root rot, damping-off, and wilt) (Fukuta et al., 2007), Colletrotrichum gloeosporioides (anthracnose) (Fukuta et al., 2007), Fusarium oxysporum (Fusarium wilt) (Fukuta et al., 2007), Phytophthora infestans (blight) (Li et al., 2020), Colletotrichum acutatum (anthracnose) (Li et al., 2020), Ustilago maydis (maize smut) (Li et al., 2020), and Pyricularia oryzae (rice blast) (Li et al., 2020) (Table 1). Generally, MB displays stronger allelopathic activity compared to MA (Fukuta et al., 2007). The mixture of MA and MB (MAB) (1:1, w/w) demonstrated a similar inhibitory effect to MB against the germination rate and seedling growth of E. crus-galli and S. altissima (Quan et al., 2019d). Significantly, MA and MB had a negligible effect on rice growth, as reported by Kato-Noguchi et al. (2013).
Although MA and MB have shown high effectiveness in suppressing problematic weeds, neither of them has been successfully developed as a natural herbicide. This situation may be due to the limitations in the exploitation of natural momilactones, which can only be isolated in small amounts from plant sources (Kato et al., 1973; Chung et al., 2005; Nozaki et al., 2007; Liu et al., 2012; Ahmad et al., 2019; Quan et al., 2019d; Li et al., 2020). On the other hand, their allelopathic mechanisms have received relatively little scrutiny. According to Fang et al. (2015), MA and MB were proven to inhibit the growth of E. crus-galli and Arabidopsis through related gene expression and transcriptional pathways. In particular, MA and MB may inhibit the germination of Arabidopsis by suppressing the degradation process of cruciferin and cruciferina, which are storage proteins that play an important role in the germination process as the initial source of nitrogen (Kato-Noguchi et al., 2013). Besides, momilactones can inhibit Arabidopsis seedling growth, with Arabidopsis hypocotyls being more sensitive to MA and MB than roots (Kato-Noguchi et al., 2012). MB was notably stronger than MA in suppressing Arabidopsis seedling growth (Kato-Noguchi et al., 2012). The allelopathic mechanism of MB against Arabidopsis may be explained through the regulation of relevant proteins to the suppression of metabolic turnover and production of intermediators for cell structure (Kato-Noguchi and Kitajima, 2015). In another aspect, the absorption of momilactones by receiver plants has also been unclear. A previous report indicated the absorptive ability of momilactones by Arabidopsis, recording a similar absorption level of MA and MB (Kato-Noguchi et al., 2012). However, the absorption of momilactones by other indicator plants, especially problematic weeds (e.g., E. crus-galli, M. vaginalis, etc.) should be deeply understood to develop them as novel herbicides.
In preceding studies, comprehensive investigations on momilactone accumulation and release in the presence of competitive weeds were conducted through laboratory bioassays and practical field conditions (Kato-Noguchi et al., 2003; Kong et al., 2006). Moreover, the release levels of momilactones into the soil were estimated to be sufficient for inhibiting neighboring plants (Kato-Noguchi et al., 2003; Kong et al., 2006). However, the interactions between momilactones and environmental factors in the practical ecosystem have been least elucidated. In fact, the contents of allelochemicals in soils may be negatively affected through degradation, oxidation, binding to other substances, as well as soil conditions, comprising electrical conductivity, osmotic pressure, microorganisms (bacteria and fungi), and adsorption to soil particles (Tharayil et al., 2008; Yoneyama and Natsume, 2013; Anh et al., 2021a). Kong et al. (2006) announced that momilactone quantities released from rice straw into the soil gradually decreased during the decomposition process, especially no momilactones were detected after 3 months. In other reports, momilactone exudation into the root rhizosphere was regulated under biotic and abiotic stresses (weeds, UV, heavy metals, nutrient deficit, and cantharidin) (Kato-Noguchi, 2009). On the other hand, momilactone accumulation in donor plants and their secretion into the soil may be altered by the growth stage, biomass, and density of competitive plants. Therefore, the release of momilactones into the soil and their interaction with environmental factors should be extensively elaborated.
In another aspect, an effective approach is to develop allelopathic mixtures that can contribute to the development of herbicides with multiple action modes (Anh et al., 2021a). Currently, numerous allelochemicals have been individually acknowledged (e.g., momilactones, phenolics, terpenoids, and fatty acids, etc.) for their potent inhibition against weeds. However, the allelopathic interactions among these compounds have been rarely explored (Anh et al., 2021a). Naturally, allelochemicals tend to be released from host plants in mixture forms instead of single compound. Moreover, several studies have proven that mixtures reveal more potent suppressing impacts against tested plants compared to individual compounds (Quan et al., 2019d). For example, ME and 7-ketostigmasterol individually exhibited negligible effects on S. altissima (Quan et al., 2019d). However, their mixture dramatically diminished S. altissima growth (Quan et al., 2019d). This phenomenon may arise from the reciprocal and synergistic interactions of various compounds within a mixture, resulting in an effective inhibitory effect (Albuquerque et al., 2011; Anh et al., 2021a). Additionally, the synergistic interactions among compounds at the molecular level can regulate the allelopathic properties of the mixture (Albuquerque et al., 2011). Therefore, the combined application of momilactones and other promising allelopathic substances (e.g., phenolics, terpenoids, and fatty acids, etc.) is highly recommended to establish and explore their allelopathic effects and mechanisms (Anh et al., 2021a). Moreover, the impacts of practical conditions (e.g., recipient plant growth periods, density, uptakes, and environmental factors, etc.) on the activity of allelopathic mixtures should be thoroughly considered (Anh et al., 2021a). Furthermore, the dose-effectiveness of allelopathic mixtures is also an integral part of developing novel natural herbicides with high efficiency (Anh et al., 2021a).
In the report of Cho et al. (2015), MA, MD, and ME reduced LPS-stimulated production of nitric oxide (NO), with required concentrations for 50% inhibition (IC50) being 1.7, 46.5, and 30.3 μM, respectively. On the other hand, MA exhibited potent cytotoxicity against RAW264.7 cells (IC50 = 9.6 μM). Meanwhile, MD and ME revealed slight inhibitory effects on RAW264.7 cell growth (IC50 of over 100 μM). Cho et al. (2015) indicated that MA, MD, and ME weakened inflammatory responses in macrophages by inhibiting NO production. Through RT-PCR and Western blot analyses, it was observed that MA might decrease the excessive production of NO by downregulating the expression of inducible nitric oxide synthase (iNOS). However, the mechanisms underlying the reduction of NO production by MD and ME were not elucidated.
Studies have reported that the cytotoxicity of momilactones is time- and dose-dependent (Joung, 1992; Anh et al., 2022a). Via cell viability (MTT) assay, it was established that MA and MB displayed potent cytotoxicity on the murine P388 leukemia cells with IC50 of 2.71 and 0.21 μM, respectively (Table 2) (Lee et al., 2008). In the research of Lee et al. (2008), MB suppressed the cell viability of human myeloblastic leukemia (HL-60) and mouse mastocytoma (p815) cell lines at concentrations higher than 6.00 μM. Meanwhile, MB inhibited human leukemic T cells (Jurkat) and basophilic leukemia (RBL-2H3) at concentrations lower than 1.60 µM (Table 2). Notably, MB induced Jurkat cell death by promoting its apoptotic process through the caspase and mitochondrial pathways (Lee et al., 2008). In other studies, MB suppressed colon cancer cells, including HT-29 and SW620 (IC50 values were less than 1.00 µM), as evaluated by MTT test. Additionally, the cytotoxicity of MB was clarified applying lactate dehydrogenase (LDH) and colony-forming ability assays (Kim et al., 2007). MB also suppressed human monocytic leukemia cell line U937 (IC50 of less than 3.03 µM in MTT assay) by stimulating apoptosis and arresting the cell cycle at G1 phase through a decrease in pRB phosphorylation and the upregulation of the CDK inhibitor p21Waf1/Cip1 (Park et al., 2014). Joung (1992) indicated that MB can inhibit breast cancer cells, including T-47D (IC50 = 51.52 µM) and MCF-7 (IC50 not determined), by increasing hypoxia-induced apoptosis. Recently, MB and the mixture of MA and MB (MAB) (1:1, w/w) have shown preventive effects on multiple types of human blood cancer cell lines, comprising acute promyelocytic leukemia (HL-60), chronic myeloid leukemia (Meg-01 and K562), mantle cell lymphoma (Mino), multiple myeloma (U266 and KMS-11) (Table 2) (Anh et al., 2022a). Generally, MB and MAB had a similar level of cytotoxic ability (IC50 around 5 µM) against these mentioned cancer cell lines, which is much stronger than MA (Anh et al., 2022a). Notably, an ideal anticancer candidate should have IC50 equal to or lower than 5 μM, suggesting that MB and MAB may be promising anti-blood cancer agents. According to Anh et al. (2022a) MB and MAB can suppress HL-60 and U266 cells by promoting the apoptotic process through the activation of phosphorylated p-38 in the mitogen-activated protein kinase (MAPK) pathway and the involved proteins (BCL-2 and caspase-3) in the mitochondrial pathway. Additionally, MB and MAB arrested the HL-60 cell cycle by activating p-38 and disrupting the CDK1 and cyclin B1 complex (Anh et al., 2022a). Importantly, MB and MAB revealed a close or stronger cytotoxicity against blood cancer cell lines compared to well-known drugs, including doxorubicin, all-trans retinoic acid (ATRA), and arsenic trioxide (ATO) (Anh et al., 2022a). Moreover, momilactones displayed a negligible impact on the normal cell line MeT-5A (Table 2) (Anh et al., 2022a).
In previous research, MA (IC50 = 0.133 and 0.992 mg/mL) and MB (IC50 = 0.129 and 0.612 mg/mL) revealed potent suppression on key enzymes associated with type 2 diabetes (α-amylase and α-glucosidase) (Quan et al., 2019c). Notably, MA and MB presented a stronger suppressing effect against α-glucosidase than the well-known drug acarbose (IC50 = 2.549 mg/mL) (Quan et al., 2019c). Besides, MA and MB showed inhibitory activity on an important enzyme (trypsin) linked to type 2 diabetes and obesity with IC50 values of 0.922 and 0.884 mg/mL, respectively (Table 2). These effects were more potent than those of the standard suppressor caffeic acid (IC50 = 7.310 mg/mL) (Quan et al., 2019c). In another study, momilactones exhibited potential for preventing skin wrinkles and freckles by inhibiting related enzymes, including pancreatic elastase and tyrosinase, as determined through in vitro bioassays (Table 2) (Quan et al., 2019a). Remarkably, MB and the mixture of MA and MB (MAB) (1:1, w/w) at a concentration of 2 mg/mL inhibited tyrosinase by 13% and 20%, respectively. This activity was comparable to that of the standard inhibitor, vanillin, which showed a 13% inhibition at a concentration of 2 mg/mL. For the antiketotic property, MB suppressed the expression of 3-hydroxy-3-methylglutaryl-CoA synthase-2 (HMGCS2) and regulated the angiopoietin-like-3-lipoprotein lipase (ANGPTL3-LPL) pathway (Kang et al., 2017).
In the study of Fukuta et al. (2007), MA (EC50 = 783.9 μg) and MB (EC50 = 790.7 μg) exhibited antiradical activity through 2,2-diphenyl-1-picrylhydrazyl (DPPH) assay. Besides, MA, MB, and their mixture MAB (1:1, v/v) presented scavenging properties against 2,2′-azinobis-(3-ethylbenzothiazoline-6-sulfonic acid) (ABTS) radical cations with IC50 values of 2.84, 1.28, and 0.32 mg/mL, respectively (Table 2) (Quan et al., 2019a). Meanwhile, MA (LPI = 75%) displayed a stronger inhibition on lipid peroxidation than MB (LPI = 62%) (Table 2) (Quan et al., 2019a).
In addition to the suppressing activities against harmful fungi (M. oryzae, B. cinerea, F. solani, C. gloeosporioides, F. oxysporum, P. oryzae, P. infestans, C. acutatum, and U. maydis) causing plant diseases, as described in the previous section, MA and MB revealed antibacterial properties against 4 common strains which cause problems in human digestion, leading to diarrhea and gastroenteritis (Fukuta et al., 2007; Li et al., 2020). Especially, MB exhibited notable growth inhibition against Pseudomonas ovalis, Bacillus cereus, and Bacillus pumilus, surpassing the potency of MA. Meanwhile, the inhibitory activity of MB was close to that of MA against Escherichia coli (Fukuta et al., 2007).
Momilactones, particularly MA and MB, have recently garnered attention for their promising medicinal and pharmaceutical properties. Notably, a correlation among these health problems has been indicated, which may eventually cause an increase in morbidity and mortality (Collins, 1999). On the other hand, the application of anti-cancer medicines (e.g., doxorubicin) might lead to oxidative stress by generating free radicals (Thorn et al., 2011), and a high risk of getting other chronic disorders such as diabetes, obesity, and skin diseases (Libby et al., 2009). An established approach to deal with these problems has been to use antioxidant agents for treating chronic disorders. Notwithstanding, most clinical examinations on patients have yielded unfavorable outcomes, potentially attributable to the lack of concurrent efficacy of potent antioxidant compounds in preventing different targeted diseases (Biswas, 2016). Alternatively, the utilization of non-selective antioxidant agents may exacerbate undesired symptoms (Biswas, 2016). Thus, momilactones, with potentials for preventing oxidative stress, chronic diseases, and cancer, stand as excellent candidates for further steps in developing effective treatment for patients complicated with these disorders (Anh et al., 2022a). However, almost all explorations regarding the pharmacological activities of momilactones have thus far been confined to in vitro approaches. To develop them for medical and pharmaceutical purposes as well as practical applications, multiple rigorous confirmation steps are necessary. Specifically, the bioaccessibility and bioavailability of natural compounds can be influenced by human digestion, necessitating scrutiny in forthcoming research (Un et al., 2022). Furthermore, the safety of these substances must undergo careful consideration and comprehensive assessment (Teschke and Xuan, 2020). Notably, determining effective doses is crucial to harness their most potent beneficial properties while avoiding potential risks (e.g., neurotoxicity and hepatotoxicity) (Quan et al., 2020). Accordingly, in vivo tests are imperative to validate their potential, paving the way for prospective and appropriate clinical trials.
In another consideration, the interactions between compounds may hold greater significance than their individual effects, which could enhance therapy efficiency via increasing multiple mechanism targets, reducing negative side effects, and overcoming drug resistance (Sanz et al., 2019; Anh et al., 2020a; 2020b; Jaaks et al., 2022; Rajkumar, 2022). In the research of Quan et al. (2019a), the mixture of MA and MB showed stronger antioxidant ability than single ones. Therefore, the combined use of momilactones with other bioactive compounds for treating human diseases is a promising approach that should be highly motivated in future investigations. Beyond momilactones, 47 momilactone-like molecules were listed in the review of Zhao et al. (2018a), implying a promising source to explore their benefits for human use. Notably, 13 momilactone-like compounds have revealed cytotoxic properties against human cancer cell lines, comprising melanoma (MDA-MB-435) (Monday Onakpa et al., 2014; Zhao et al., 2015a; 2015b), colorectal adenocarcinoma epithelial (HT-29) (Monday Onakpa et al., 2014), breast adenocarcinoma epithelial (MDA-MB-231) (Zhao et al., 2015a; 2015b), ovarian adenocarcinoma epithelial (OVCAR3) (Zhao et al., 2015a; 2015b), and ovarian cancer epithelial (A2780) (Adou et al., 2005) cell lines. Significantly, humirianthenolide C, 2β-humirianthenolide C, and humirianthol exhibited potent cytotoxic activities against tested tumors with IC50 values below 5 µM (Adou et al., 2005; Zhao et al., 2015a). The results suggest that these compounds are promising sources for human health benefits with individual applications as well as in combinations.
Principally, a thorough understanding of the distribution of momilactones in plant sources will lead to effective approaches and strategies for their beneficial exploitation. Previously, momilactones were commonly found in cultivated rice (O. sativa L.) varieties. In the years 1973 and 1976, MA, MB, and MC were first discovered in rice husks (O. sativa L. var. Koshihikari) (Kato et al., 1973; Tsunakawa et al., 1976). In 2015, MD and ME were introduced for the first time, which were isolated from rice roots (O. sativa L. var. Chucheongbyeo) by Cho et al. (2015). However, ME was mistakenly classified, as explained in the introduction part (Figure 1). During the last few decades, momilactones have predominantly been detected in husks, leaves, and roots of various rice (O. sativa) cultivars. Recently, with improved techniques for detection and quantification, momilactones can be also found in different rice (O. sativa) organs (e.g., grains and brans) (Quan et al., 2019b). Chung et al. (2006) indicated that momilactones had higher amounts in Korean varieties, varieties with awns and late maturing time. Meanwhile, MA and MB quantities in japonica rice leaves were found to be higher than those in indica rice (Kariya et al., 2020). Lee et al. (1999) reported that momilactone contents in rice straws (O. sativa L. var. Haresugata) were increased to the highest levels at the heading stage and subsequently decreased.
Alongside cultivated rice, momilactones have also been detected in wild rice (Kariya et al., 2020). Specifically, species with AA genome (e.g., O. sativa, Oryza rufipogon, Oryza barthii, Oryza glaberrima, Oryza glumaepatula, and Oryza meridionalis) and BB genome (e.g., Oryza punctata) were reported to accumulate momilactones, whereas FF genome species (e.g., Oryza brachyantha) did not exhibit this accumulation. Notably, AA and BB genome species have a close phylogenetic distance, which are far from FF genome species (Kariya et al., 2020). Although the health-beneficial effects of MB are generally considered stronger than those of MA, most previous studies indicated lower levels of MB compared to MA in rice organs (Chung et al., 2006; Quan et al., 2019b; 2019c). For example, the content of MA was approximately twice that of MB in Koshihikari white grains (Quan et al., 2019b). In contrast, a recent investigation revealed that Koshihikari germinated brown rice showed a significantly higher level of MB than MA (Hasan et al., 2023), suggesting a potential source for MB exploitation that needs further investigation across different rice varieties. This finding may lead to proper and effective strategies in developing rice production and enhancing the consumption value of brown rice and rice-based products that benefit human health.
In moss (H. plumaeforme), MA and MB have been detected in aerial parts since 2007 (Nozaki et al., 2007). In 2020, MA, MB, and MF were purified from whole plant of H. plumaeforme (Li et al., 2020). Meanwhile, MA and MC have been found in aerial parts of another moss species (P. papillosa) (Liu et al., 2012).
Principally, the release of momilactones into the environment is a crucial research aspect pertaining to their role as allelochemicals contributing to the allelopathic activities of host plants. This aspect has been clearly demonstrated in numerous studies over several decades and comprehensively assembled and discussed in the report of Kato-Noguchi (2023), particularly focusing on rice plants. Based on these findings, the secretion of momilactones into the surrounding environment is emphasized in our work, as illustrated in Figure 3 for easier visualization. Furthermore, the exudation of momilactones from moss species is also debated in this study, which is in line with the release pattern of momilactones from rice plants under the influences of weed invasion and other unfavorable conditions. Despite the distinct genetic backgrounds of these two plant species, they exhibited similar response mechanisms to biotic and abiotic stresses regarding momilactone accumulation and release. This implies that momilactones might be important molecules playing a role in the general defense system of certain plant species. Further comprehensive studies should be conducted to validate this proposition. Especially, the release mechanism of momilactones from host plants under adverse conditions is a noteworthy topic that requires extensive elucidation.
FIGURE 3. Momilactone B released by rice plants during their growth duration and decomposition process. Values in the rectangle, hexagon, and oval boxes indicate the momilactone B contents in rice shoots (µg/g fresh weight), roots (µg/g fresh weight), and root exudations into the soil (µg/plant/day), respectively.
In the research of Kato-Noguchi and Ino (2003), MB was secreted by rice seedlings at a sufficient phytotoxic level (1.8 nM/seedling), which inhibited cress (Lepidium sativum) in Petri dish. Additionally, the exudated levels of MB from seedlings were strongly correlated with the endogenous MB contents in rice seedlings. Therefore, the exudation of MB may depend on the endogenous production of MB in rice seedlings (Kato-Noguchi and Ino, 2005). In another report, 80-day-old rice plants exuded MB into their root rhizosphere at a concentration of 2.1 µg/plant/day, which was sufficient to suppress the growth of neighboring plants (Kato-Noguchi and Ino, 2004). Furthermore, Kato-Noguchi et al. (2003) indicated that MB was released during rice growth and development. The release levels rapidly increased to the maximal concentration in the flowering stage (2.1 µg/plant/day) and then sharply reduced (Figure 3). In a previous report, Kato-Noguchi et al. (2008) found that the endogenous content of MA was greater than that of MB, whereas the release of MB was higher than that of MA. Accordingly, Kato-Noguchi et al. (2008) hypothesized that MB was more selectively released into the root rhizosphere than MA. On average, an individual rice plant can release approximately 100 μg of MB into the surrounding environment throughout its growth duration (Kato-Noguchi et al., 2003). Theoretically, at a density of 100 rice plants/m2, MB was estimated to be released into the soil with a content of 10 mg/m2, which is sufficient to inhibit neighboring plants (Kato-Noguchi et al., 2003). According to Kong et al. (2006), MB was secreted into the soil by allelopathic rice varieties (PI312777 and Huagan-1) with a sufficient phytotoxic level at the early growth stage and achieved the highest concentration at the 5-leaf stage before dramatically reducing. Meanwhile, the non-allelopathic rice variety (Huajingxian) negligibly released MB into the root rhizosphere during its whole life cycle (Kong et al., 2006). Remarkably, the secreted content of MB by allelopathic rice was significantly enhanced (over 3 times higher) when they were grown with barnyardgrass (E. crus-galli) (Kong et al., 2006). However, this phenomenon was not observed in non-allelopathic rice grown in coculture with barnyardgrass (Kong et al., 2006). The results suggest that MB may significantly contribute to the rice defense system in the rhizosphere to compete with the invasion of neighboring plants (Kato-Noguchi et al., 2003). In another report, the allelopathic ability of rice plants may be increased through enhanced secretion of momilactones when rice was exposed to environmental stresses including UV, heavy metals, and cantharidin (Kato-Noguchi, 2009). In addition to root exudation, momilactones can be released into the soil through the decomposing process of rice straw (Figure 3), which inhibited surrounding plants (Kong et al., 2006).
Besides rice plants, moss (H. plumaeforme) can also secrete momilactones into the surrounding environment (Kato-Noguchi, 2011b). Interestingly, the endogenous amount of MA in H. plumaeforme was higher than that of MB; however, MB was released with a higher concentration than MA (Kato-Noguchi, 2011b). This suggests that MB is preferentially secreted by H. plumaeforme compared to MA, which aligns with the release propensity of momilactones in rice (Kato-Noguchi, 2011b). Furthermore, similar to rice, momilactones exudated from moss also increased under environmental stresses (UV, heavy metals, and cantharidin), leading to enhanced allelopathic activity (Kato-Noguchi, 2009). Accordingly, despite the phylogenetic distance between rice and moss, they may have a similar defense mechanism involving momilactone accumulation and release (Kato-Noguchi, 2011b).
To develop natural compounds as novel herbicides, understanding their degradation mechanisms is indispensable. Compound degradation involves processes of breakdown and transformation in the environment that influence their inherent activity, thereby being a significant reason for failures in practical applications. While the potential of momilactones for weed management has been extensively demonstrated, their degradation in the environment has not been thoroughly researched until now. Only one study in 2006 reported the levels of momilactones in the soil during different durations of rice straw decomposition (Figure 3). Specifically, momilactone amounts gradually reduced during this process (decreasing by over half from day 30 to day 60 for MB). Especially, MB was not detected in the soil after 90 days of decomposition (Kong et al., 2006). The study of Kong et al. (2006) implied that MB might undergo degradation in the soil within 90 days; however, its degradation mechanism remained unclear. Several factors can affect the degradation of natural compounds, consisting of temperature, pH, oxygen availability, enzyme catalysis, and microbial activity (Zhao et al., 2018b). Particularly, microorganisms (e.g., bacteria and fungi) play a crucial role in the degradation process through enzymatic activities, breaking down the compounds (Zhao et al., 2018b). Enzymatic reactions, such as oxidation and hydroxylation, can modify the structure of the compounds, resulting in their degradation (Zhao et al., 2018b). Therefore, these aspects are potential research directions to elucidate the degradation mechanisms of momilactones, which can significantly contribute to effective approaches and strategies for the practical implications of momilactones in weed management.
To effectively exploit target natural products, serious and thorough consideration should be given to their extraction and isolation protocols (Anh et al., 2023). Although momilactones have been found in different rice organs, only minor amounts of these compounds can be isolated (Kato et al., 1973; Chung et al., 2005; Ahmad et al., 2019; Quan et al., 2019d). Moreover, very few reports about momilactone isolation and purification have been published. In general, rice husks were commonly used as raw materials, which were extracted using organic solvents before being loaded onto a chromatography column (CC) filled with silica gel (SiO2) in the isolation process (Kato et al., 1973; Chung et al., 2005; Ahmad et al., 2019; Quan et al., 2019d). In 1973, MA and MB were purified for the first time from 200 kg of rice husks using a single CC with solvents of CHCl2 and EtOH to obtain yields of 150 mg and 100 mg, respectively (Kato et al., 1973). In a later report, Tsunakawa et al. (1976) successfully isolated MC with a minor amount of 3 mg from 300 kg of rice husks in 1976, but the isolation procedure was not described (Tsunakawa et al., 1976). After 32 years from the first discovery, a modified isolation protocol using repeated CC with a solvent system of hexane, EtOAc, MeOH, and methylene chloride was developed to achieve momilactones from rice husks with 18.75 and 20-fold greater yields for MA and MB, respectively (Chung et al., 2005). Since then, there has been no improved method to attain higher amounts of purified momilactones from plant sources (Table 3) (Kato et al., 1973; Chung et al., 2005; Nozaki et al., 2007; Liu et al., 2012; Ahmad et al., 2019; Quan et al., 2019d; Li et al., 2020). In the study of Cho et al. (2015), various fractions from dried rice roots (8.35 kg) were subjected to separate CC eluted with CHCl3/MeOH (45:1, v/v), CHCl3/MeOH (35:1, v/v), and hexane/EtOAc (8:1, v/v) to yield MA (35 mg), MD (2.7 mg), and ME (4.5 mg), respectively (Table 3). On the other hand, ME was mistakenly classified, as explained in the introduction part (Figure 1).
In the moss species H. plumaeforme, MA and MB were isolated from the aerial parts with amounts of 8.40 and 4.20 μg/g DW, respectively (Nozaki et al., 2007). In 2020, Li et al. (2020) purified MA, MB, and MF from the whole plant of H. plumaeforme with quantities of 2.13, 3.09, and 1.00 μg/g DW, respectively. In another moss species P. papillosa, MA and MC (11.52 and 72.02 μg/g DW) were obtained from the aerial parts (Table 3) (Liu et al., 2012).
Since momilactones exhibit significant biological benefits for humans (Figure 2), their isolation methods should be developed with increased efficiency in further studies to support the exploitation of these compounds from natural sources. In this section, we provide a basic process for momilactone isolation and purification (Figure 4). Methods for identification, characterization, and quantification of these compounds are also described. Furthermore, achievements, existing limitations, and future perspectives are debated.
FIGURE 4. Isolation process (assembled based on protocols described by Quan et al. (2019c) and Ahmad et al. (2019)) and identification methods for momilactones.
Sample preparation is a vital step in the isolation and purification processes of natural compounds (Anh et al., 2021a). Adequate sample preparation can enhance sample stability and extraction efficiency. Furthermore, careful preparation can help eliminate or minimize contaminants, supporting chemical analysis and subsequent isolation procedures (Palanisamy et al., 2020). The samples should be dried to reduce moisture content, as high moisture can lead to spoilage and microbial hydrolysis (Anh et al., 2023). In previous studies, Koshihikari rice husks were dried by sunlight for 1 week upon collection (Quan et al., 2019c). After that, the samples were meticulously sieved to eliminate impurities (Quan et al., 2019c). The samples were further dried in an oven at 50°C for 3 days (Quan et al., 2019c). Subsequently, the dried rice husks should be ground into powder to achieve homogeneity, facilitating easier and faster extraction using organic solvents. This physical processing aids in partially breaking down the cutin layer and cell wall, allowing for a swift release of compounds into the extraction solvent (Minh et al., 2018; Anh et al., 2023).
In the extraction steps for momilactones, the classical solid–liquid solvent extraction method (immersing dried rice or moss samples in organic solvents) has been commonly applied (Kato et al., 1973; Chung et al., 2005; Nozaki et al., 2007; Liu et al., 2012; Ahmad et al., 2019; Quan et al., 2019d; Li et al., 2020). Of which, methanol has been the most widely used solvent for this step (Chung et al., 2005; Ahmad et al., 2019; Quan et al., 2019d). Besides, aqueous methanol, acetone, and aqueous ethanol can also be employed (Nozaki et al., 2007; Liu et al., 2012; Li et al., 2020). While this conventional extraction method is simple, safe, and cost-effective (Garcia-Salas et al., 2010), it also has limitations such as time consumption, the need for large volumes of harmful solvents, and low efficiency (Garcia-Salas et al., 2010). Recently, only two studies aimed to improve the extraction process for momilactones (Minh et al., 2018; Ahmad et al., 2019). In particular, Minh et al. (2018) established a simple and optimized process for extracting MA and MB from rice husks by combining EtOAc, MeOH, distilled water, temperature, and pressure. The results demonstrated that rice husks treated with heat (100°C) and extracted using a combination of EtOAc and MeOH yielded the highest quantities of MA and MB (Minh et al., 2018). Besides, MB was obtained with larger amounts compared to MA (Minh et al., 2018). The application of pressure also enhanced the yields of MA and MB, resulting in respective 5- and 15-fold increases in purified amounts (Minh et al., 2018). In the study of Ahmad et al. (2019), different techniques for momilactone extraction, including percolation, agitation with heat, sonication, and Soxhlet, were examined, which screened various solvent systems, comprising EtOAc, acetone, acetonitrile, MeOH, and MeOH:water (8:2, v/v). Accordingly, the maximum extraction efficiency was achieved when applying a mixture of MeOH and MeOH:water in Soxhlet extraction, surpassing other methods (Ahmad et al., 2019). Besides, the applied processes of defatting under low temperature (Ahmad et al., 2019; Quan et al., 2019c), mixing with activated charcoal, filtering with celite beds (Ahmad et al., 2019), and Sep-Pak C18 cartridges (Quan et al., 2019d) during the treatment of crude extracts can aid in removing unwanted components, leading to higher yields and purer momilactones. Furthermore, advanced methods including microwave-assisted extraction (MAE), ultrasound-assisted extraction (UAE), supercritical CO2 extraction (SC-CO2), pressurized fluid extraction (PFE), and enzyme-assisted extraction (EAE) have shown high effectiveness in extracting natural compounds from plant sources (Aires, 2017). These techniques should be examined in further investigations for momilactone extraction to establish effective methods that help overcome the disadvantages of classical protocols and enhance efficiency.
In the next step of momilactone isolation, the obtained crude extracts can be dissolved in water and fractionated using liquid–liquid phase separation with different solvents (e.g., hexane and EtOAc) (Table 3; Figure 4). Subsequently, fractions containing momilactones can be collected for further isolation and purification processes. The most common method for momilactone isolation is column chromatography (Kato et al., 1973; Chung et al., 2005; Nozaki et al., 2007; Liu et al., 2012; Ahmad et al., 2019; Quan et al., 2019d; Li et al., 2020), which can separate compounds based on their adsorption (Palanisamy et al., 2020).
To support the isolation process, widely applied methods for the analysis of momilactones in obtained extracts and fractions include thin-layer chromatography (TLC), high-performance liquid chromatography (HPLC), gas chromatography-mass spectrometry (GC-MS), high resolution-mass spectrometry (HR-MS), electron ionization-mass spectroscopy (EI-MS), fast atom bombardment-mass spectrometry (FAB-MS), infrared spectroscopy (IS), electrospray ionization-mass spectrometry (ESI-MS), Fourier transform-infrared spectroscopy (FT-IR), high resolution-electrospray ionization-mass spectrometry (HR-ESI-MS), high-performance liquid chromatography-photo diode array-mass spectrometry (HPLC-PDA-MS), nuclear magnetic resonance (NMR), and X-ray crystallization (Table 3).
Among the aforementioned methods, TLC, with the advantages of simplicity, time- and cost-effectiveness, has been widely applied for momilactone identification in obtained fractions. As described by Quan et al. (2019b), TLC analysis for momilactones can be conducted on Merck TLC silica gel 60 plates with a layer thickness of 175–225 μm. MA and MB standards were used as references (Quan et al., 2019b). The fractions containing MA and MB were spotted onto the TLC plate and run alongside a mobile phase of CHCl3:MeOH (9.5:0.5, v/v) for 2.4 min (Quan et al., 2019b). The TLC plate was then stained with 1% vanillin-sulfuric acid in pure EtOH and dried in an oven at 100°C for 2 min. The separation of MA and MB was visually observed (Quan et al., 2019b) (Figure 5A). The retention factor (Rf) values were also calculated for the confirmation of MA and MB, based on the ratio of the traveled distance of the MA or MB spot to the traveled distance of the solvent on the TLC plate (Quan et al., 2019b).
FIGURE 5. Identification and characterization of momilactones applying (A) thin-layer chromatography (TLC) (Quan et al., 2019b), (B) high-performance liquid chromatography (HPLC) (Quan et al., 2019b), (C) gas chromatography-mass spectrometry (GC-MS) (Quan et al., 2019c), (D) electrospray ionization-mass spectrometry (ESI-MS) (Hasan et al., 2023), and (E) 1H- and 13C-nuclear magnetic resonance (NMR) techniques (Hasan et al., 2023). S, standard; MA, momilactone A; MB, momilactone B.
HPLC analysis is another effective approach for identifying momilactones. In which, isolated momilactones can be dissolved in MeOH and subjected to an HPLC system with a mobile phase consisting of 0.1% trifluoroacetic acid in 70% acetonitrile (Quan et al., 2019b). The analysis is operated with a detector set at 210 nm and a flow rate of 0.4 mL/min over 30 min (Quan et al., 2019b). As a result, isolated MA and MB are detected with retention times (RT) of 17.03 and 14.06 min, respectively, in comparison to standards (Figure 5B) (Quan et al., 2019b).
For molecular mass characterization, isolated MA and MB can be confirmed by GC-MS method, as presented in the study of Quan et al. (2019c). In which, MA and MB were identified with molecular ions at 314 and 330 m/z, respectively (Figure 5C) (Quan et al., 2019c). Additionally, the molecular masses of MA and MB can be confirmed using ESI-MS in a positive mode, as described by Hasan et al. (2023). According to the results, MA and MB were characterized with m/z = 315.19470 and 331.19006 [M + H]+, respectively (Figure 5D) (Hasan et al., 2023). The data obtained from GC-MS and ESI-MS were compared to literature and standards to confirm the isolated MA and MB (Quan et al., 2019c; Hasan et al., 2023).
The structures of isolated momilactones can be determined applying NMR method, which has been included in numerous studies. For instance, the 1H- and 13C NMR spectra of MA and MB (in deuterated chloroform, CDCl3) were acquired on an NMR spectrometer at 600 and 151 MHz, respectively (Hasan et al., 2023). Examples of the 1H- and 13C NMR spectra of MA and MB are presented in Figure 5E. The NMR spectra of isolated MA and MB were confirmed by comparing to literature (Hasan et al., 2023).
Hitherto, several techniques have been developed for quantifying momilactones in plant samples. Among these, HPLC has been commonly applied, being a central focus of development. However, due to the minor amounts of momilactones present in the plant sources, their detection and quantification have faced numerous limitations and challenges. To determine momilactone profiles in plant extracts using HPLC, the extraction process demands multiple complex steps to purify the extract and eliminate unwanted and interfering components (Kato-Noguchi and Kobayashi, 2009). Most studies have quantified momilactones in husks, leaves, and roots (Lee et al., 1999; Xuan et al., 2016; Minh et al., 2018; Quan et al., 2019d). Specifically, these methods employed columns with lengths ranging from 150 to 200 mm and an isocratic mobile phase (e.g., 0.1% trifluoroacetic acid in 70% aqueous acetonitrile) with a flow rate ranging from 0.4 to 0.8 mL/min. The procedures required a total run time of around 1 h per sample (Table 4) (Kato-Noguchi and Kobayashi, 2009; Minh et al., 2018), leading to time and solvent consumption. Recently, an improved ultra-performance liquid chromatography-electrospray ionization-mass spectrometry (UPLC-ESI-MS) method has been generated applying a 50 mm column alongside a gradient mobile phase consisting of 0.1% trifluoroacetic acid in water and 0.1% trifluoroacetic acid in acetonitrile with a flow rate of 0.3 mL/min, which reduced the total run time to 15.1 min for each sample (Quan et al., 2019a). This method did not require complicated extraction steps (Quan et al., 2019c; Anh et al., 2022b; Hasan et al., 2023; Jesmin et al., 2023). Notably, the protocol has demonstrated effectiveness and high detection sensitivity in identifying and quantifying momilactones in extract samples with low concentrations and complex mixtures containing various substances (Quan et al., 2019c; Anh et al., 2022b; Hasan et al., 2023; Jesmin et al., 2023). Furthermore, by examining a blank sample (e.g., pure MeOH) after measurements of each sample, no contamination and interfering chemicals were recorded, resulting in high analysis accuracy (Quan et al., 2019c; Anh et al., 2022b; Hasan et al., 2023; Jesmin et al., 2023). One of the breakthroughs of this method is its first-time detection and quantification of MA and MB in rice grains and brans, despite their presence in minor amounts (Quan et al., 2019c). By applying this protocol, MA were found with the highest quantity in rice husks (16.44 μg/g DW), followed by rice roots (8.06 μg/g DW), brans (6.65 μg/g DW), leaves (4.28 μg/g DW), and grains (2.07 μg/g DW) (Quan et al., 2019b). While MB were determined with the highest content in rice leaves (12.73 μg/g DW), followed by husks (9.24 μg/g DW), brans (6.24 μg/g DW), roots (5.69 μg/g DW), and grains (1.06 μg/g DW) (Quan et al., 2019b). An example of UPLC-ESI-MS analysis for confirmation and quantification of MA and MB in germinated brown rice samples is presented in Figure 6 (Hasan et al., 2023), in which peak detection and areas are shown.
FIGURE 6. UPLC-ESI-MS chromatograms of (A) standard momilactones A (MA) and B (MB), and (B) MA and MB detected in germinated brown rice sample (Hasan et al., 2023). RT, retention time; AA, peak areas in automatic peak detection mode.
In identification and quantification methods for natural compounds using HPLC techniques, the values of limit of detection (LOD) and limit of quantification (LOQ) are also important parameters, indicating the specific accuracy and reliability of the methods. Quan et al. (2019d) explained that there are several principal factors that contribute to enhancing the efficiency of HPLC analysis. For instance, the utilization of a Sep-Pak C18 cartridge in preparing extract samples can aid in the removal of unwanted or interfering substances, thereby yielding purer MA and MB. In addition, adjusting the conditions of the stationary and mobile phases and the flow rate can also affect the sensitivity of HPLC system. Particularly, employing a 150 mm column with an isocratic mobile phase (0.1% TFA in 70% aqueous acetonitrile) at a flow rate of 0.5 mL/min over 25 min demonstrated highly effective LOD (0.05 ng/mL) and LOQ (0.14 ng/mL) values for the detection and quantification of MA (Quan et al., 2019d). On the other hand, the UPLC-ESI-MS method, utilizing a 50 mm column with a gradient mobile phase (0.1% trifluoroacetic acid in water and 0.1% trifluoroacetic acid in acetonitrile) at a flow rate of 0.3 mL/min over 15.1 min, showed impressive sensitivity in detecting and quantifying MB with LOD and LOQ of 0.27 and 0.83 ng/mL, respectively (Quan et al., 2019a).
In preceding studies, the momilactone biosynthetic pathway (MBP) was extensively discussed (Nguyen and Dang, 2021; Serra et al., 2021; Kato-Noguchi, 2023). Specifically, Kato-Noguchi (2023) and Serra et al. (2021) predominantly focused on the MBP in rice and its association with allelopathic activity. On the other hand, Nguyen and Dang (2021) highlighted and deliberated the achievements obtained in unraveling the MBP over the past five decades since the first discovery of momilactones, outlining limitations and proposing promising strategies to further clarify the remaining gaps. For our study, understanding the MBP is pivotal which may enable effective approaches to enhance momilactone production in plant sources, thereby facilitating the exploitation of these compounds. Accordingly, we delve into the MBP along with the relevant enzymes and their specific functions in the formation of momilactones from precursors (Figure 7). Notably, we discuss the MBP across various plant species such as rice and moss. Factors and environmental conditions influencing momilactone biosynthesis are also presented in our research (Figure 7). Based on that, momilactones produced by the host plant may be regulated through the adjustment of external conditions. Furthermore, research on improving plant tolerance to stresses through the MBP is considered and proposed.
FIGURE 7. Biosynthesis of momilactones (aggregated based on pathways demonstrated by Zhao et al. (2018a) and Shimura et al. (2007)) and affecting factors. OsCPS4, gene encoding syn-copalyl diphosphate synthase-like; OsKSL4, gene encoding syn-pimara-7,15-diene synthase-like; CYP99A2, cytochrome P450 99A2-like; CYP99A3, gene encoding 9-beta-pimara-7,15-diene oxidase-like; OsMAS, gene encoding momilactone A synthase-like; OsMAS2, gene encoding momilactone A synthase-like.
In rice (O. sativa), momilactone biosynthetic genes (MBGs) have been located in the 168-kb region of the fourth chromosome (Figure 6), which includes OsCPS4, OsKSL4, CYP99A2, CYP99A3, and OsMAS genes (Wang et al., 2011; NCBI, 2023). These genes encode the enzymes consisting of syn-copalyl diphosphate synthase-like, syn-pimara-7,15-diene synthase-like, cytochrome P450 99A2-like, 9-beta-pimara-7,15-diene oxidase-like, and momilactone A synthase-like, respectively (Wang et al., 2011; NCBI, 2023). Particularly, the roles of CYP99A2 and CY99A3 in the MBP were confirmed in previous studies (Shimura et al., 2007; Wang et al., 2011). In which, CYP99A2 was found to have less involvement in this pathway compared to CYP99A3. These two enzymes act on the oxidation of the carbon-19 (C19) methyl group of (9β-H)-pimara-7,15-diene to form (9β-H)-pimara-7,15-dien-19-oic acid (Shimura et al., 2007), a predicted precursor of MC and ME (Zhao et al., 2018a). Subsequently, the lactone ring in the 3β-hydroxy-(9β-H)-pimara-7,15-dien-19,6β-olide structure is generated from (9β-H)-pimara-7,15-dien-19-oic acid via hydroxylation at the C3β- and C6β-positions with the catalytic activities of P450 genes CYP701A8 and CYP76M8 (Wang et al., 2012). In the formation of MA, two short-chain alcohol dehydrogenases/reductases (SDRs) catalyze the oxidation of 3β-hydroxy-(9β-H)-pimara-7,15-dien-19,6β-olide to produce the keto group of MA at the C3-position (Shimura et al., 2007; Kitaoka et al., 2016). Based on that, these two SDRs have been defined as momilactone synthase (MS) (Shimura et al., 2007; Kitaoka et al., 2016). For MB biosynthesis, hydroxylation at the C20-position and hemiketal ring closure are required (Zhang and Peters, 2020); however, the enzymes and related genes involved in this process have not been characterized yet.
A similar situation has been found in the momilactone biosynthetic gene cluster (MBGC) of other species within the Poaceae family, which is correlated with their close phylogenetic relationships (Miyamoto et al., 2016). For instance, O. punctata has been found to accumulate momilactones and show an analogous MBGC to that of O. sativa (Miyamoto et al., 2016). On the other hand, O. brachyantha and Leersia perrieri, which have more phylogenetic distance, neither produce momilactones nor contain the relevant biosynthetic genes (Miyamoto et al., 2016). Miyamoto et al. (2016) concluded that the MBGC evolved within the Oryza lineage. Remarkably, E. crus-galli, with much more phylogenetic distance from Oryza lineage, also possesses an orthologous MBGC (Guo et al., 2017). Phylogenetic analysis indicated that the MBGC of E. crus-galli is more consistent with that of Oryza than with other closely related plant species (Guo et al., 2017). However, the MBGC of E. crus-galli contains single copies of the CYP99A and MS families with some modifications in gene order (Guo et al., 2017). Additionally, E. crus-galli has not been reported to produce endogenous momilactones. This suggests that no momilactone relevant biosynthetic gene is self-sufficient.
Another species that both contains an MBGC and produces momilactones is C. plumiforme (moss) (Mao et al., 2020). Based on the genome sequence, the previously reported diterpene cyclase CpDTC was located (Okada et al., 2016), revealing that the neighboring region contains two specific CYPs (CYP75B and CYP707C subfamilies) and an SDR. Remarkably, CYP75B plays a role in C19 oxidase activity, similar to the Poaceae CYP99A (Okada et al., 2016). Besides, the C. plumiforme SDR was reported to have the same catalytic activity as the Poaceae MS (Okada et al., 2016). On the other hand, CYP707C was found to act as a C3β-hydroxylase (Okada et al., 2016). Accordingly, the two separately evolved MBGCs in rice and moss exhibited exactly the same relevant enzymatic activities. This leads to questions about these analogies despite a distinct evolutionary distance that requires deeper clarification.
Since the complete steps of the catalytic processes in momilactone biosynthesis have been unclear, especially the conversion from MA to MB. de La Peña and Sattely (2021) developed an effective approach by screening Nicotiana benthamiana genes, which clarified the entire pathway for momilactone biosynthesis, resulting in a yield over 10 times higher than that observed in rice.
Momilactones were first identified as rice allelochemicals. Accordingly, Kato-Noguchi (2011a) indicated that the MB content in rice seedlings grown with barnyardgrass was 6.9 times higher than that of rice plants grown independently. Besides, momilactone proliferation was recorded in rice response to pathogen attack (Umemura et al., 2003), UV (Kato-Noguchi, 2009; Anh et al., 2022b), heavy metals (Kato-Noguchi, 2009), salinity (Xuan et al., 2016; Huong et al., 2020; Xuan et al., 2022; Jesmin et al., 2023), drought (Xuan et al., 2016), chilling (Anh et al., 2022b), nutrient deficit (Kato-Noguchi, 2009), cantharidin (Kato-Noguchi, 2009), and jasmonic acid (JA) (Kato-Noguchi, 2009). These changes in rice momilactone contents can be explained through the expression of relevant genes in the biosynthetic pathway (Bajsa-Hirschel et al., 2020). For example, the expression of momilactone biosynthetic genes (MBGs), especially OsMAS and OsMAS2, was significantly elevated in the presence of banryardgrass (Bajsa-Hirschel et al., 2020). In another study, the production of momilactones in rice was enhanced under UV irradiation, consistent with the upregulated expression of MBGs (Anh et al., 2022b). Conversely, the accumulation of momilactones and expression of MBGs decreased under chilling effects (Anh et al., 2022b). Nevertheless, the impact of other adverse conditions (e.g., salinity, drought, heavy metals, nutrient deficit, etc.) on MBGs has not yet been elucidated.
In moss, momilactone accumulation also increased when exposed to barnyardgrass, heavy metals, and cantharidin (Kato-Noguchi and Kobayashi, 2009; Kato-Noguchi, 2011b). Momilactone contents in moss under the influence of biotic and abiotic stresses may also be regulated through the expression of related biosynthetic genes. For example, HpDTC1, which encodes a diterpene cyclase involved in the conversion of geranylgeranyl diphosphate (GGDP) to syn-pimara-7,15-diene in moss, showed an upregulation in expression when moss was subjected to stresses (Okada et al., 2016).
Momilactones are also known as labdane-related diterpenoids, sharing the same initiator (GGDP) with gibberellic acid (GA), a phytohormone, in the biosynthetic pathway. In particular, these compounds are formed through consecutive catalysis by two distinct diterpene synthases and cyclases (Miyamoto et al., 2016). Notably, GA has been identified as a major contributor to plant resistance against abiotic stresses, including cold, salt, osmotic, and submergence conditions (Colebrook et al., 2014). Meanwhile, momilactones have been proven to be responsive compounds in rice and moss plants against environmental stresses, including weed invasion (Kato-Noguchi, 2011b; 2011a; Bajsa-Hirschel et al., 2020), pathogen attack (Umemura et al., 2003), UV (Kato-Noguchi, 2009; Anh et al., 2022b), heavy metals (Kato-Noguchi, 2009), salinity (Xuan et al., 2016; Huong et al., 2020; Xuan et al., 2022; Jesmin et al., 2023), drought (Xuan et al., 2016), chilling (Anh et al., 2022b), nutrient deficit (Kato-Noguchi, 2009), and cantharidin (Kato-Noguchi, 2009).
The production of momilactone may be motivated by jasmonic acid (JA)-dependent and independent signaling pathways (Kato-Noguchi, 2023). For instance, after CuCl2 application, the involvement of JA biosynthesis inhibitors, comprising quinacrine, nordihydroguaiaretic acid, and salicylhydroxamic acid, prevented MA accumulation in rice (Rakwal et al., 1996). However, the subsequent addition of exogenous JA resulted in the proliferation of endogenous MA in rice (Rakwal et al., 1996), indicating that JA stimulated MA biosynthesis. Apart from JA, the exogenous application of salicylic acid (SA) also promoted momilactone accumulation in rice, though the exact mechanism remains unclear (Daw et al., 2008). Notably, JA and SA are signaling phytohormones that trigger plant defense responses, consisting of various defensive reactions to safeguard the plant (Zhao et al., 2017; Howe et al., 2018; Nguyen et al., 2022). In another report, Xuan et al. (2016) indicated that MA and MB were involved in rice response to abiotic stresses, including drought and salinity, rather than weed resistance. The accumulation of MA and MB in rice was also increased by the exogenous application of fulvic acid and magnesium sulfate (MgSO4) during salinity conditions, in line with rice’s elevated tolerance to salt stress (Xuan et al., 2022; Jesmin et al., 2023). These pieces of evidence suggest that momilactones might play essential roles in the plant’s defense system against adverse environments. However, their actual contribution remains elusive. Remarkably, Anh et al. (2022b) suggested that momilactones might not directly contribute to the antioxidant response of rice against UV and chilling stresses. Instead, a concomitant biosynthesis between momilactones and some acknowledged stress-resistant phenolics was documented in UV- and chilling-exposed rice plants (Anh et al., 2022b). In another research, the biosynthesis of momilactones was consistent with that of GA in rice cocultured with barnyardgrass (Bajsa-Hirschel et al., 2020). Consequently, we hypothesize that momilactones may act as signaling molecules in rice physiological response to unfavorable conditions.
Another consideration is that although moss MBGC differs from rice MBGC in terms of gene sequences and orders, they may share a similar response mechanism against environmental stresses with respect to momilactone biosynthesis and relevant gene expressions, as described in the previous section. Therefore, a proposed question arises: may momilactones play a role as novel phytohormones in rice and moss? This requires comprehensive validation in future studies. On the other hand, the potential signaling mechanisms of momilactones and their relationship with resistance-associated compounds (e.g., phytohormones: GA, SA, abscisic acid, ethylene, and JA) in rice and moss under stresses, as well as functional enzymes (e.g., antioxidant enzymes and DNA damage repair enzymes), may be promising fields of study that need further scrutinization.
In the exploitation of momilactones, synthetic models offer a promising solution given the limitations and challenges faced in isolating momilactones from natural sources. Over the past five decades, considerable effort has been dedicated to the artificial synthesis of the fundamental core structure of momilactones, known as the 9β-H pimarane skeleton (Zhang et al., 2022). However, a handful of studies have been conducted on the total synthesis of momilactones. Notably, several promising intermediate compounds for MA biosynthesis, comprising (±)-4,4-Dinor-(9β-H)-pimara-7,15-diene (Sichererroetman et al., 1984; Sicherer-Roetman et al., 2010), (9β-H)-Pimara-7,15-diene (Jansen et al., 1989; Chu and Coates, 1992), and 3β-hydroxy-(9β-H)-pimara-7,15-diene (Yajima et al., 2011), have been successfully generated requiring simple processes. However, there has been no report on the conversion of these intermediators to MA. On the other hand, in 2002, a complete procedure for MA synthesis was established (Germain and Deslongchamps, 2002). This procedure was complex, involving 9 steps to generate an intermediate compound, namely 95b, followed by an additional 14 steps to convert 95b to MA, yielding a moderate output of 40%–50% (Germain and Deslongchamps, 2002). Meanwhile, the synthetic methods for MB and other momilactones have not been reported. The unique trans-syn tricyclic skeleton and the high functionalization in momilactone rings can be listed as major challenges in synthesizing these diterpene lactones (Zhao et al., 2018a). Moreover, other limitations in these synthetic processes include intricate steps, high cost, and potential environmental unfriendliness (Zhao et al., 2018a).
From another perspective, synthesis and structural modifications hold promise in enhancing the target biological activities and stability of candidate compounds (Macias et al., 1999; Macias et al., 2007). Notably, in-depth understanding of momilactone structures and their biological activities may lead to effective strategies for structurally modifying these compounds. For instance, the biological activities of MB are generally more potent than those of MA, wherein, the differences in their structures, such as the presence of a hydroxyl group at the C3-position in MB, could be a crucial determinant (Quan et al., 2019b). Furthermore, the exploration of functional groups within momilactones is pivotal for developing novel synthesized derivatives with stronger activities, heightened stability, and better economic efficiency (Quan et al., 2019b).
Another advanced technology is genetic engineering, which can support the exploitation of momilactones. Particularly, induced gene expression, metabolic engineering, and genetic modification may be potential approaches to enhance the endogenous biosynthesis of momilactones and associated biological activities in plant sources (Bourgaud et al., 2001). As a proof, the overexpression of transcriptional factors such as OsTGAP1 (Okada, 2011), CIPK15 (Kurusu et al., 2010), ACDR1 (Kim et al., 2009), RAC1 GTPAse (Ono et al., 2001), SBP (Sawada et al., 2004), and Spl18 mutant (Mori et al., 2007) caused the proliferation of momilactones in rice (O. sativa). Moreover, increased accumulation of MA associated with enhanced resistance of rice against Magnaporthe grisea (in ACDR1 and SBP overexpression lines and Spl18 mutant lines) (Sawada et al., 2004; Mori et al., 2007; Kim et al., 2009) and Xanthomonas oryzae (in RAC1 and SBP overexpression lines) (Ono et al., 2001; Sawada et al., 2004). Other studies demonstrated that N-methyl-N-nitrosourea mutation led to elevated accumulation of MA and MB in mutant rice lines (Kakar et al., 2019). Therefore, an extensive comprehending of their biosynthetic outputs via enzymatic catalysis and relevant gene expression can help exploit a higher amount of target momilactones and related beneficial properties from host plants (Anh et al., 2021a).
To date, based on the rapid development of advanced techniques, momilactone biosynthetic genes (MBGs) have been progressively identified (NCBI, 2023), and their functions have been confirmed through knockdown assays (Shimura et al., 2007; Wang et al., 2011). Notably, a database of major genes in the momilactone biosynthetic pathway (MBP) can be openly accessed online via NCBI GenBank (NCBI, 2023). This database contains exhaustive information, consisting of genomic context, genomic regions, transcripts, products, etc. Moreover, useful tools for sequence analysis and primer design can be applied through the same system that supports genetic engineering studies (NCBI, 2023). Additionally, open sources of such data have been increasingly diversified. The insightful integration of these available resources and information technologies (IT), such as artificial intelligence (AI) and machine learning, is expected to create breakthroughs in establishing strategies for improving plant sources with greater production of momilactones.
In contrast, there remain uncharacterized enzymes in the MBP that need further clarification. Especially, the major enzymes involved in MB biosynthesis have been still elusive, even though the compound has been known for half a century. On the other hand, numerous MBGs are clustered in the genome. However, genes outside this cluster are also important but are more complicated to identify (Nguyen and Dang, 2021). A deeper understanding of the MBP and the functions of relevant enzymes and genes may contribute to expanding research on the endogenous proliferation of momilactones in plant sources. Particularly, genetic modification protocols, such as marker-assisted selection (MAS) and marker-assisted backcrossing (MABC), can improve host plants with a higher accumulation of target compounds (Anh et al., 2021a). On the contrary, the presence of multiple MBGs may lead to complications in breeding programs. Even if these genes can be successfully incorporated into the genetic background of targeted plants, their adaptation (gene expression) to the environment cannot be guaranteed (Anh et al., 2021a). Consequently, practical results may be unpredictable. Moreover, the potential negative influence on plant growth or tissues in terms of improving the endogenous accumulation of momilactones is still unknown. Thus, further comprehensive studies should be conducted to validate this paradigm.
Smart farming (SF), involving the application of advanced technologies to agricultural practices, has the potential to revolutionize sustainable agricultural production (Dhanaraju et al., 2022). Following the objective of improving the efficiency of momilactone exploitation by increasing their supply from plant sources and artificial synthesis, SF can be viewed as a broader strategic direction. The current knowledge of momilactones and the expected expansion of research on them may offer a valuable repository for integrating these compounds into SF using artificial intelligence (AI) and the Internet of Things (IoT). In this context, SF may bring numerous benefits in monitoring momilactone levels, optimizing their application, and enhancing overall agricultural sustainability. For example, the environmental factors influencing momilactone biosynthesis can be monitored and controlled through tools such as drones and satellites, which utilize remote sensing and monitoring techniques. By which, real-time data on environmental conditions (e.g., temperature, humidity, light intensity, soil conditions, etc.) can be collected (Dhanaraju et al., 2022) that may support farmers to create optimal conditions for crop growth with desired levels of momilactone production. Furthermore, precision agriculture techniques can play a vital role in optimizing the target application of momilactones. Specifically, variable rate technologies, including sensor-based systems (e.g., soil moisture sensors and plant health monitoring devices), can provide real-time information on crop conditions (Dhanaraju et al., 2022), which help farmers apply momilactones at varying rates based on specific crop growth stages and needs, thereby reducing waste and minimizing the environmental impact of excessive inputs. Sensor-based systems can also early detect and measure the presence of momilactones (deficiencies or excesses) in soil, water, and plant tissues, enabling timely interventions to ensure optimal crop health and growth. However, applying and integrating SF technologies into existing agricultural systems may require significant investment, infrastructure development, and training for farmers. On the contrary, addressing challenges and advancing the wider adoption of SF technologies will be crucial for their successful implementation across various agricultural scenarios.
To date, momilactones have shown valuable biological benefits, including allelopathic, antioxidant, antimicrobial, anti-chronic disease, anti-inflammatory, and anticancer properties. Additionally, momilactones may function as signaling phytohormones in the defense system of rice and moss against environmental stresses, which presents a promising avenue for future research. However, the applications of momilactones in practical contexts have encountered difficulties due to their limited commercial availability and challenges in isolation and purification. While several teams have successfully purified momilactones from rice and moss, the isolation yields were low, indicating the need for improvement in forthcoming studies.
Recently, advanced technologies have been enthusiastically improved, contributing to the effective exploitation of momilactones from natural sources or their synthesis from potential intermediates. Notably, the biosynthesis of momilactones can be induced by regulating genetic factors in host plants. Nevertheless, there have been no breeding programs applying genetic engineering methods to generate plant sources with a greater accumulation of these valuable compounds. Additionally, certain synthetic models for momilactones have been successfully established. Nonetheless, specific steps in both natural and artificial production of momilactones, especially for the potent bioactive compound MB, remain unclear. From another perspective, momilactone-like compounds with potential biological properties have been recently reported, suggesting an abundant source that could contribute to various beneficial purposes. Significantly, combined applications of momilactones, momilactone-like molecules, and commercial drugs should be comprehensively investigated since this may increase desirable effects.
In summary, the insights provided by this review may give rise to promising strategies, which can be a premise for the future exploitation of momilactones to develop novel herbicides as well as human health-beneficial products. Furthermore, the expected outcomes resulting from the expansion of research on momilactones could contribute to a valuable platform for integrating these compounds into smart farming practices, aligning with sustainable agricultural principles. This approach has the potential to optimize and effectively leverage the benefits of momilactones, minimizing unnecessary usage, and reducing environmental impacts.
TDX and TDK contributed to conception and design of the study. LHA organized the database and wrote the first draft of the manuscript. TDX, TDK, and LHA wrote sections of the manuscript. All authors contributed to the article and approved the submitted version.
We would like to thank Nguyen Van Quan (Graduate School of Advanced Science and Engineering, Hiroshima University, Japan) for his valuable discussion and suggestions during the review writing.
The authors declare that the research was conducted in the absence of any commercial or financial relationships that could be construed as a potential conflict of interest.
All claims expressed in this article are solely those of the authors and do not necessarily represent those of their affiliated organizations, or those of the publisher, the editors and the reviewers. Any product that may be evaluated in this article, or claim that may be made by its manufacturer, is not guaranteed or endorsed by the publisher.
Adou, E., Williams, R. B., Schilling, J. K., Malone, S., Meyer, J., Wisse, J. H., et al. (2005). Cytotoxic diterpenoids from two lianas from the Suriname rainforest. Bioorg. Med. Chem. 13 (21), 6009–6014. doi:10.1016/j.bmc.2005.07.026
Ahmad, A., Xuan, T. D., Minh, T. N., Siddiqui, N. A., and Quan, N. V. (2019). Comparative extraction and simple isolation improvement techniques of active constituents’ momilactone A and B from rice husks of Oryza sativa by HPLC analysis and column chromatography. Saudi Pharm. J. 27 (1), 17–24. doi:10.1016/j.jsps.2018.07.014
Aires, A. (2017). “Phenolics in foods: extraction, analysis and measurements,” in Phenolic compounds - natural sources, importance and applications. Editors M. Soto-Hernandez, M. Palma-Tenango, and R. Garcia-Mateos (London: InTech). doi:10.5772/66889
Albuquerque, M. B., Santos, R. C., Lima, L. M., Filho, P. de A. M., Nogueira, R. J. M. C., Câmara, C. A. G., et al. (2011). Allelopathy, an alternative tool to improve cropping systems. A review. A Rev. Agron. sustain. Dev. 31 (2), 379–395. doi:10.1051/agro/2010031
Anh, L. H., Quan, N. V., Nghia, L. T., and Xuan, T. D. (2021a). Phenolic allelochemicals: achievements, limitations, and prospective approaches in weed management. Weed Biol. Manag. 21 (2), 37–67. doi:10.1111/wbm.12230
Anh, L. H., Lam, V. Q., Takami, A., Khanh, T. D., Quan, N. V., and Xuan, T. D. (2022a). Cytotoxic mechanism of momilactones A and B against acute promyelocytic leukemia and multiple myeloma cell lines. Cancers 14 (19), 4848. doi:10.3390/cancers14194848
Anh, L. H., Quan, N. V., Lam, V. Q., Iuchi, Y., Takami, A., Teschke, R., et al. (2020b). Antioxidant, anti-tyrosinase, anti-α-amylase, and cytotoxic potentials of the invasive weed Andropogon virginicus. Plants 10 (1), 69. doi:10.3390/plants10010069
Anh, L. H., Quan, N. V., Lam, V. Q., Takami, A., Khanh, T. D., and Xuan, T. D. (2022b). Rice momilactones and phenolics: expression of relevant biosynthetic genes in response to uv and chilling stresses. Agronomy 12 (8), 1731. doi:10.3390/agronomy12081731
Anh, L. H., Quan, N. V., Lam, V. Q., Takami, A., and Xuan, T. D. (2021b). Anti-blood cancer property of momilactones A and B from rice husks. [2021 nishihon, chugoku-shikoku, and kansai branch joint meeting of the Japan society for bioscience, Biotechnology, and agrochemistry].
Anh, L. H., Xuan, T. D., Thuy, N. T. D., Quan, N. V., and Trang, L. T. (2020a). Antioxidant and α-amylase inhibitory activities and phytocompounds of Clausena indica Fruits. Medicines 7 (3), 10. doi:10.3390/medicines7030010
Anh, N. T. H., Anh, L. H., Mai, N. P., Quan, N. V., and Xuan, T. D. (2023). Identification and isolation techniques for plant growth inhibitors in rice. Separations 10 (2), 105. doi:10.3390/separations10020105
Bajsa-Hirschel, J., Pan, Z., and Duke, S. O. (2020). Rice momilactone gene cluster: transcriptional response to barnyard grass (echinochloa crus-galli). Mol. Biol. Rep. 47 (2), 1507–1512. doi:10.1007/s11033-019-05205-8
Biosynth, (2023). Momilactone A. Available at: https://www.biosynth.com/p/XM165579/51415-07-7-momilactone-a (Accessed August 1, 2023).
Biswas, S. K. (2016). Does the interdependence between oxidative stress and inflammation explain the antioxidant paradox? Oxid. Med. Cell. Longev. 2016, 1–9. doi:10.1155/2016/5698931
Bourgaud, F., Gravot, A., Milesi, S., and Gontier, E. (2001). Production of plant secondary metabolites: A historical perspective. Plant Sci. 161 (5), 839–851. doi:10.1016/S0168-9452(01)00490-3
Cartwright, D. W., Langcake, P., and Ride, J. P. (1980). Phytoalexin production in rice and its enhancement by a dichlorocyclopropane fungicide. Physiol. Plant Pathol. 17 (3), 259–IN2. doi:10.1016/S0048-4059(80)80019-1
Cho, J.-G., Cha, B.-J., Lee, S. M., Shrestha, S., Jeong, R.-H., Lee, D. S., et al. (2015). Diterpenes from the roots of Oryza sativa L. and their inhibition activity on NO production in LPS-stimulated RAW264.7 macrophages. Chem. Biodivers. 12 (9), 1356–1364. doi:10.1002/cbdv.201400239
Chu, M., and Coates, R. M. (1992). Partial synthesis of 9,10-syn-diterpenes via tosylhydrazone reduction: (-)-(9.beta.)-pimara-7,15-diene and (-)-(9.beta.)-isopimaradiene. J. Org. Chem. 57, 4590–4597. doi:10.1021/jo00043a013
Chung, I.-M., Hahn, S.-J., and Ahmad, A. (2005). Confirmation of potential herbicidal agents in hulls of rice, Oryza sativa. J. Chem. Ecol. 31 (6), 1339–1352. doi:10.1007/s10886-005-5290-5
Chung, I. M., Kim, J. T., and Kim, S.-H. (2006). Evaluation of allelopathic potential and quantification of momilactone A, B from rice hull extracts and assessment of inhibitory bioactivity on paddy field weeds. J. Agric. Food Chem. 54 (7), 2527–2536. doi:10.1021/jf052796x
Colebrook, E. H., Thomas, S. G., Phillips, A. L., and Hedden, P. (2014). The role of gibberellin signalling in plant responses to abiotic stress. J. Exp. Biol. 217 (1), 67–75. doi:10.1242/jeb.089938
Collins, T. (1999). “Acute and chronic inflammation,” in Pathologic basis of disease. Editors T. Cotran, R. S. Kumar, and V. Collins (Philadelphia: WB Saunders), 50–88.
Daw, B. D., Zhang, L. H., and Wang, Z. Z. (2008). Salicylic acid enhances antifungal resistance to Magnaporthe grisea in rice plants. Aust. Plant Pathol. 37, 637–644. doi:10.1071/AP08054
de La Peña, R., and Sattely, E. S. (2021). Rerouting plant terpene biosynthesis enables momilactone pathway elucidation. Nat. Chem. Biol. 17 (2), 205–212. doi:10.1038/s41589-020-00669-3
Dhanaraju, M., Chenniappan, P., Ramalingam, K., Pazhanivelan, S., and Kaliaperumal, R. (2022). Smart farming: internet of things (iot)-based sustainable agriculture. Agriculture 12 (10), 1745. doi:10.3390/agriculture12101745
Fang, C., Li, Y., Li, C., Li, B., Ren, Y., Zheng, H., et al. (2015). Identification and comparative analysis of microRNAs in barnyardgrass (Echinochloa crus-galli) in response to rice allelopathy. Plant Cell. Environ. 38 (7), 1368–1381. doi:10.1111/pce.12492
Fukuta, M., Xuan, T. D., Deba, F., Tawata, S., Khanh, T. D., and Chung, I. M. (2007). Comparative efficacies in vitro of antibacterial, fungicidal, antioxidant, and herbicidal activities of momilatones A and B. J. Plant Interact. 2 (4), 245–251. doi:10.1080/17429140701713811
Garcia-Salas, P., Morales-Soto, A., Segura-Carretero, A., and Fernández-Gutiérrez, A. (2010). Phenolic-compound-extraction systems for fruit and vegetable samples. Molecules 15 (12), 8813–8826. doi:10.3390/molecules15128813
Germain, J., and Deslongchamps, P. (2002). Total synthesis of (±)-momilactone A. J. Org. Chem. 67 (15), 5269–5278. doi:10.1021/jo025873l
Guo, L., Qiu, J., Ye, C., Jin, G., Mao, L., Zhang, H., et al. (2017). Echinochloa crus-galli genome analysis provides insight into its adaptation and invasiveness as a weed. Nat. Commun. 8 (1), 1031. doi:10.1038/s41467-017-01067-5
Hasan, M., Quan, N. V., Anh, L. H., Khanh, T. D., and Xuan, T. D. (2023). Salinity treatments promote the accumulations of momilactones and phenolic compounds in germinated brown rice. Foods 12 (13), 2501. doi:10.3390/foods12132501
Howe, G. A., Major, I. T., and Koo, A. J. (2018). Modularity in jasmonate signaling for multistress resilience. Ann. Rev. Plant Biol. 69, 387–415. doi:10.1146/annurev-arplant-042817-040047
Huong, C. T., Anh, T. T. T., Dat, T. D., Khanh, T. D., and Xuan, T. D. (2020). Uniparental inheritance of salinity tolerance and beneficial phytochemicals in rice. Agronomy 10 (7), 1032. doi:10.3390/agronomy10071032
Jaaks, P., Coker, E. A., Vis, D. J., Edwards, O., Carpenter, E. F., Leto, S. M., et al. (2022). Effective drug combinations in breast, colon and pancreatic cancer cells. Nature 603 (7899), 166–173. doi:10.1038/s41586-022-04437-2
Jansen, B. J. M., Schepers, G. C., and de Groot, A. (1989). The stereoselective synthesis of (±)-9βH-pimara-7,19-diene. Tetrahedron 45 (9), 2773–2776. doi:10.1016/S0040-4020(01)80107-1
Jesmin, A., Anh, L. H., Mai, N. P., Khanh, T. D., and Xuan, T. D. (2023). Fulvic acid improves salinity tolerance of rice seedlings: evidence from phenotypic performance, relevant phenolic acids, and momilactones. Plants 12 (12), 2359. doi:10.3390/plants12122359
Joung, Y. H., Lim, E. J., Kim, M. S., Lim, S. D., Yoon, S. Y., Lim, Y. C., et al. (1992). Enhancement of hypoxia-induced apoptosis of human breast cancer cells via STAT5b by momilactone B. Int. J. Oncol. 33 (3), 477–484. doi:10.3892/ijo_00000030
Kakar, K., Xuan, T. D., Quan, N. V., Wafa, I. K., Tran, H.-D., Khanh, T. D., et al. (2019). Efficacy of N-methyl-N-nitrosourea mutation on physicochemical properties, phytochemicals, and momilactones A and B in rice. Sustainability 11 (23), 6862. doi:10.3390/su11236862
Kang, D. Y., Nipin, S. P., Darvin, P., Joung, Y. H., Byun, H. J., Do, C. H., et al. (2017). Momilactone B inhibits ketosis in vitro by regulating the ANGPTL3-LPL pathway and inhibiting HMGCS2. Anim. Biotechnol. 28 (3), 189–197. doi:10.1080/10495398.2016.1252769
Kariya, K., Ube, N., Ueno, M., Teraishi, M., Okumoto, Y., Mori, N., et al. (2020). Natural variation of diterpenoid phytoalexins in cultivated and wild rice species. Phytochemistry 180, 112518. doi:10.1016/j.phytochem.2020.112518
Kato, T., Kabuto, C., Sasaki, N., Tsunagawa, M., Aizawa, H., Fujita, K., et al. (1973). Momilactones, growth inhibitors from rice, Oryza sativa L. Tetrahedron. Lett. 14 (39), 3861–3864. doi:10.1016/S0040-4039(01)87058-1
Kato-Noguchi, H., Ino, T., Sata, N., and Yamamura, S. (2002). Isolation and identification of a potent allelopathic substance in rice root exudates. Physiol. Plant. 115 (3), 401–405. doi:10.1034/j.1399-3054.2002.1150310.x
Kato-Noguchi, H., and Ino, T. (2003). Rice seedlings release momilactone B into the environment. Phytochemistry 63 (5), 551–554. doi:10.1016/S0031-9422(03)00194-8
Kato-Noguchi, H., Ino, T., and Ichii, M. (2003). Changes in release level of momilactone B into the environment from rice throughout its life cycle. Funct. Plant Biol. 30 (9), 995. doi:10.1071/FP03110
Kato-Noguchi, H., and Ino, T. (2004). Release level of momilactone B from rice plants. Plant Prod. Sci. 7 (2), 189–190. doi:10.1626/pps.7.189
Kato-Noguchi, H., and Ino, T. (2005). Concentration and release level of momilactone B in the seedlings of eight rice cultivars. J. Plant Physiol. 162 (9), 965–969. doi:10.1016/j.jplph.2005.02.007
Kato-Noguchi, H., Ota, K., and Ino, T. (2008). Release of momilactone A and B from rice plants into the rhizosphere and its bioactivities. Allelopathy J. 22 (2), 321–328.
Kato-Noguchi, H., and Kobayashi, K. (2009). Jasmonic acid, protein phosphatase inhibitor, metals and UV-irradiation increased momilactone A and B concentrations in the moss Hypnum plumaeforme. J. Plant Physiol. 166 (10), 1118–1122. doi:10.1016/j.jplph.2008.12.012
Kato-Noguchi, H. (2009). Stress-induced allelopathic activity and momilactone B in rice. Plant Growth Regul. 59 (2), 153–158. doi:10.1007/s10725-009-9398-4
Kato-Noguchi, H. (2011a). Barnyard grass-induced rice allelopathy and momilactone B. J. Plant Physiol. 168 (10), 1016–1020. doi:10.1016/j.jplph.2010.12.021
Kato-Noguchi, H. (2011b). Convergent or parallel molecular evolution of momilactone A and B: potent allelochemicals, momilactones have been found only in rice and the moss hypnum plumaeforme. J. Plant Physiol. 168 (13), 1511–1516. doi:10.1016/j.jplph.2011.03.014
Kato-Noguchi, H., Ota, K., and Kujime, H. (2012). Absorption of momilactone A and B by Arabidopsis thaliana L. and the growth inhibitory effects. J. Plant Physiol. 169 (15), 1471–1476. doi:10.1016/j.jplph.2012.05.022
Kato-Noguchi, H., Ota, K., Kujime, H., and Ogawa, M. (2013). Effects of momilactone on the protein expression in Arabidopsis germination. Weed Biol. Manag. 13 (1), 19–23. doi:10.1111/wbm.12005
Kato-Noguchi, H., and Kitajima, S. (2015). Momilactone sensitive proteins in Arabidopsis thaliana. Nat. Prod. Commun. 10 (5), 1934578X1501000–732. doi:10.1177/1934578X1501000508
Kato-Noguchi, H. (2023). Defensive molecules momilactones A and B: function, biosynthesis, induction and occurrence. Toxins 15 (4), 241. doi:10.3390/toxins15040241
Kim, J.-A., Cho, K., Singh, R., Jung, Y.-H., Jeong, S.-H., Kim, S.-H., et al. (2009). Rice OsACDR1 (Oryza sativa accelerated cell death and resistance 1) is a potential positive regulator of fungal disease resistance. Mol. Cells 28 (5), 431–439. doi:10.1007/s10059-009-0161-5
Kim, S.-J., Park, H.-R., Park, E., and Lee, S.-C. (2007). Cytotoxic and antitumor activity of momilactone B from rice hulls. J. Agric. Food Chem. 55 (5), 1702–1706. doi:10.1021/jf062020b
Kitaoka, N., Wu, Y., Zi, J., and Peters, R. J. (2016). Investigating inducible short-chain alcohol dehydrogenases/reductases clarifies rice oryzalexin biosynthesis. Plant J. 88 (2), 271–279. doi:10.1111/tpj.13249
Kong, C. H., Li, H. B., Hu, F., Xu, X. H., and Wang, P. (2006). Allelochemicals released by rice roots and residues in soil. Plant Soil 288 (1–2), 47–56. doi:10.1007/s11104-006-9033-3
Kurusu, T., Hamada, J., Nokajima, H., Kitagawa, Y., Kiyoduka, M., Takahashi, A., et al. (2010). Regulation of microbe-associated molecular pattern-induced hypersensitive cell death, phytoalexin production, and defense gene expression by calcineurin B-like protein-interacting protein kinases, OsCIPK14/15, in rice cultured cells. Plant Physiol. 153 (2), 678–692. doi:10.1104/pp.109.151852
Lee, C. W., Yoneyama, K., Takeuchi, Y., Konnai, M., Tamogami, S., and Kodama, O. (1999). Momilactones A and B in rice straw harvested at different growth stages. Biosci. Biotechnol. Biochem. 63 (7), 1318–1320. doi:10.1271/bbb.63.1318
Lee, S. C., Chung, I.-M., Jin, Y. J., Song, Y. S., Seo, S. Y., Park, B. S., et al. (2008). Momilactone B, an allelochemical of rice hulls, induces apoptosis on human lymphoma cells (Jurkat) in a micromolar concentration. Nutr. Cancer 60 (4), 542–551. doi:10.1080/01635580801927445
Li, J., Wie, L., Chen, C., Liu, D., Gu, Y., Duan-Mu, J., et al. (2020). Bioactive constituents from the bryophyta Hypnum plumaeforme. Chem. Biodivers. 17 (12), e2000552. doi:10.1002/cbdv.202000552
Libby, G., Donnelly, L. A., Donnan, P. T., Alessi, D. R., Morris, A. D., and Evans, J. M. M. (2009). New users of metformin are at low risk of incident cancer. Diabetes Care 32 (9), 1620–1625. doi:10.2337/dc08-2175
Liu, N., Wang, S., and Lou, H. (2012). A new pimarane-type diterpenoid from moss Pseudoleskeella papillosa (Lindb.) Kindb. Acta Pharm. Sin. B 2 (3), 256–259. doi:10.1016/j.apsb.2012.03.003
Macías, F. A., Galindo, J. C. G., Molinillo, J. M. G., Castellano, D., Velasco, R. F., and Chinchilla, D. (1999). Developing new herbicide models from allelochemicals. Pestic. Sci. 55 (6), 662–665. doi:10.1002/(sici)1096-9063(199906)55:6<662::aid-ps3>3.0.co;2-q
Macías, F. A., Molinillo, J. M. G., Varela, R. M., and Galindo, J. C. G. (2007). Allelopathy - a natural alternative for weed control. Pest Manag. Sci. 63 (4), 327–348. doi:10.1002/ps.1342
Mao, L., Kawaide, H., Higuchi, T., Chen, M., Miyamoto, K., Hirata, Y., et al. (2020). Genomic evidence for convergent evolution of gene clusters for momilactone biosynthesis in land plants. PNAS 117 (22), 12472–12480. doi:10.1073/pnas.1914373117
Minh, T. N., Xuan, T. D., Ahmad, A., Elzaawely, A. A., Teschke, R., and Van, T. M. (2018). Momilactones A and B: optimization of yields from isolation and purification. Separations 5 (2), 28. doi:10.3390/separations5020028
Miyamoto, K., Fujita, M., Shenton, M. R., Akashi, S., Sugawara, C., Sakai, A., et al. (2016). Evolutionary trajectory of phytoalexin biosynthetic gene clusters in rice. Plant J. 87 (3), 293–304. doi:10.1111/tpj.13200
Mori, M., Tomita, C., Sugimoto, K., Hasegawa, M., Hayashi, N., Dubouzet, J. G., et al. (2007). Isolation and molecular characterization of a Spotted leaf 18 mutant by modified activation-tagging in rice. Plant Mol. Biol. 63 (6), 847–860. doi:10.1007/s11103-006-9130-y
National Center for Biotechnology Information (NCBI) (2023). National center for Biotechnology information (NCBI). Available at: https://www.ncbi.nlm.nih.gov (Accessed March 12, 2023).
Nguyen, T.-D., and Dang, T.-T. T. (2021). Demystifying the momilactone pathway. Nat. Chem. Biol. 17 (2), 126–128. doi:10.1038/s41589-020-00707-0
Nguyen, T. H., Goossens, A., and Lacchini, E. (2022). Jasmonate: A hormone of primary importance for plant metabolism. Curr. Opin. Plant Biol. 67, 102197. doi:10.1016/j.pbi.2022.102197
Nozaki, H., Hayashi, K., Nishimura, N., Kawaide, H., Matsuo, A., and Takaoka, D. (2007). Momilactone A and B as allelochemicals from moss Hypnum plumaeforme: first occurrence in bryophytes. Biosci. Biotechnol. Biochem. 71 (12), 3127–3130. doi:10.1271/bbb.70625
Okada, K., Kawaide, H., Miyamoto, K., Miyazaki, S., Kainuma, R., Kimura, H., et al. (2016). HpDTC1, a stress-inducible bifunctional diterpene cyclase involved in momilactone biosynthesis, functions in chemical defence in the moss Hypnum plumaeforme. Sci. Rep. 6 (1), 25316. doi:10.1038/srep25316
Okada, K. (2011). The biosynthesis of isoprenoids and the mechanisms regulating it in plants. Biosci. Biotechnol. Biochem. 75 (7), 1219–1225. doi:10.1271/bbb.110228
Onakpa, M. M., Zhao, M., Gödecke, T., Chen, W.-L., Che, C.-T., Santarsiero, B. D., et al. (2014). Cytotoxic (9βH)-pimarane and (9βH)-17-norpimarane diterpenes from the tuber of Icacina trichantha. Chem. Biodivers. 11 (12), 1914–1922. doi:10.1002/cbdv.201400151
Ono, E., Wong, H. L., Kawasaki, T., Hasegawa, M., Kodama, O., and Shimamoto, K. (2001). Essential role of the small GTPase Rac in disease resistance of rice. PNAS 98 (2), 759–764. doi:10.1073/pnas.98.2.759
Palanisamy, C. P., Gunasekaran, V. P., Dominic, S., and Xuan, T. D. (2020). “Phenolic allelochemicals from crops and weed management,” in Plant phenolics in sustainable agriculture. Editors R. Lone, R. Shuab, and A. N. Kamili (Singapore: Springer Singapore), 183–199. doi:10.1007/978-981-15-4890-1_8
Park, C., Jeong, N. Y., Kim, G.-Y., Han, M. H., Chung, I.-M., Kim, W.-J., et al. (2014). Momilactone B induces apoptosis and G1 arrest of the cell cycle in human monocytic leukemia U937 cells through downregulation of pRB phosphorylation and induction of the cyclin-dependent kinase inhibitor p21Waf1/Cip1. Oncol. Rep. 31 (4), 1653–1660. doi:10.3892/or.2014.3008
Quan, N. V., Thien, D. D., Khanh, T. D., Tran, H.-D., and Xuan, T. D. (2019a). Momilactones A, B, and tricin in rice grain and by-products are potential skin aging inhibitors. Foods 8 (12), 602. doi:10.3390/foods8120602
Quan, N. V., Tran, H.-D., Xuan, T. D., Ahmad, A., Dat, T. D., Khanh, T. D., et al. (2019b). Momilactones A and B are α-amylase and α-glucosidase inhibitors. Molecules 24 (3), 482. doi:10.3390/molecules24030482
Quan, N. V., Xuan, T. D., and Teschke, R. (2020). Potential hepatotoxins found in herbal medicinal products: A systematic review. Int. J. Mol. Sci. 21 (14), 5011. doi:10.3390/ijms21145011
Quan, N. V., Xuan, T. D., Tran, H.-D., Ahmad, A., Khanh, T. D., and Dat, T. D. (2019c). Contribution of momilactones A and B to diabetes inhibitory potential of rice bran: evidence from in vitro assays. Saudi Pharm. J. 27 (5), 643–649. doi:10.1016/j.jsps.2019.03.006
Quan, N. V., Xuan, T. D., Tran, H. D., and Thuy, N. T. D. (2019d). Inhibitory activities of momilactones A, B, E, and 7-Ketostigmasterol isolated from rice husk on paddy and invasiveweeds. Plants 8 (6), 159. doi:10.3390/plants8060159
Rajkumar, S. V. (2022). Multiple myeloma: 2022 update on diagnosis, risk stratification, and management. Am. J. Hematol. 97 (8), 1086–1107. doi:10.1002/ajh.26590
Rakwal, R., Tamogami, S., and Kodama, O. (1996). Role of jasmonic acid as a signaling molecule in copper chloride-elicited rice phytoalexin production. Biosci. Biotech. Biochem. 60 (6), 1046–1048. doi:10.1271/bbb.60.1046
Sanz, M. A., Fenaux, P., Tallman, M. S., Estey, E. H., Löwenberg, B., Naoe, T., et al. (2019). Management of acute promyelocytic leukemia: updated recommendations from an expert panel of the european leukemianet. Blood 133 (15), 1630–1643. doi:10.1182/blood-2019-01-894980
Sawada, K., Hasegawa, M., Tokuda, L., Kameyama, J., Kodama, O., Kohchi, T., et al. (2004). Enhanced resistance to blast fungus and bacterial blight in transgenic rice constitutively expressing OsSBP, a rice homologue of mammalian selenium-binding proteins. Biosci. Biotechnol. Biochem. 68 (4), 873–880. doi:10.1271/bbb.68.873
Serra, N. S., Shanmuganathan, R., and Becker, C. (2021). Allelopathy in rice: A story of momilactones, kin recognition, and weed management. J. Exp. Bot. 72 (11), 4022–4037. doi:10.1093/jxb/erab084
Shimura, K., Okada, A., Okada, K., Jikumaru, Y., Ko, K.-W., Toyomasu, T., et al. (2007). Identification of a biosynthetic gene cluster in rice for momilactones. J. Biol. Chem. 282 (47), 34013–34018. doi:10.1074/jbc.M703344200
Sicherer-Roetman, A., Jansen, B. J. M., and de Groot, A. (2010). Investigations into the total synthesis of momilactones. Stereoselective preparation of (±)-4,4-dinor-9βH-pimara-7,19-diene. Recl. Trav. Chim. 104 (7–8), 193–202. doi:10.1002/recl.19851040702
Sichererroetman, A., Jansen, B. J. M., and de Groot, A. (1984). Stereospecific preparation of (±)-4,4-dinor-9-beta-H-pimara-7,15-diene, a model for the total synthesis of momilactone type diterpenes. Tetrahedron Lett. 25, 2593–2596. doi:10.1016/s0040-4039(01)81239-9
Teschke, R., and Xuan, T. D. (2020). Active nature based ingredients for drug discovery with pivotal role of clinical efficacy: review and prospective. JMMC 8 (1), 4–18. doi:10.12970/2308-8044.2020.08.02
Tharayil, N., Bhowmik, P. C., and Xing, B. (2008). Bioavailability of allelochemicals as affected by companion compounds in soil matrices. J. Agric. Food Chem. 56 (10), 3706–3713. doi:10.1021/jf073310a
Thorn, C. F., Oshiro, C., Marsh, S., Hernandez-Boussard, T., McLeod, H., Klein, T. E., et al. (2011). Doxorubicin pathways. Pharmacogenet. Genom. 21 (7), 440–446. doi:10.1097/FPC.0b013e32833ffb56
Tsunakawa, M., Ohba, A., Sasaki, N., Kabuto, C., Kato, T., Kitahara, Y., et al. (1976). Momilactone-C, a minor constituent of growth inhibitors in rice husk. Chem. Lett. 5 (11), 1157–1158. doi:10.1246/cl.1976.1157
Umemura, K., Ogawa, N., Shimura, M., Koga, J., Usami, H., and Kono, T. (2003). Possible role of phytocassane, rice phytoalexin, in disease resistance of rice against the blast fungus Magnaporthe grisea. Biosci. Biotech. Biochem. 67 (4), 899–902. doi:10.1271/bbb.67.899
Un, S., Quan, N. V., Anh, L. H., Lam, V. Q., Takami, A., Khanh, T. D., et al. (2022). Effects of in vitro digestion on anti-α-amylase and cytotoxic potentials of Sargassum spp. Molecules 27 (7), 2307. doi:10.3390/molecules27072307
Wang, Q., Hillwig, M. L., Okada, K., Yamazaki, K., Wu, Y., Swaminathan, S., et al. (2012). Characterization of CYP76M5–8 indicates metabolic plasticity within a plant biosynthetic gene cluster. J. Biol. Chem. 287 (9), 6159–6168. doi:10.1074/jbc.M111.305599
Wang, Q., Hillwig, M. L., and Peters, R. J. (2011). CYP99A3: functional identification of a diterpene oxidase from the momilactone biosynthetic gene cluster in rice. Plant J. 65 (1), 87–95. doi:10.1111/j.1365-313X.2010.04408.x
Xuan, T. D., Huong, C. T., Quan, N. V., Anh, L. H., Khanh, T. D., and Rayee, R. (2022). Improvement of salinity tolerance in rice seedlings by exogenous magnesium sulfate application. Soil Syst. 6 (3), 69. doi:10.3390/soilsystems6030069
Xuan, T. D., Minh, T. N., Anh, L. H., and Khanh, T. D. (2016). Allelopathic momilactones A and B are implied in rice drought and salinity tolerance, not weed resistance. Agron. Sustain. Dev. 36 (3), 52. doi:10.1007/s13593-016-0383-9
Yajima, A., Toda, K., Okada, K., Yamane, H., Yamamoto, M., Hasegawa, M., et al. (2011). Stereocontrolled total synthesis of (±)-3β-hydroxy-9β-pimara-7,15-diene, a putative biosynthetic intermediate of momilactones. Tetrahedron Lett. 52 (25), 3212–3215. doi:10.1016/j.tetlet.2011.04.044
Yoneyama, K., and Natsume, M. (2013). “Allelochemicals for plant–plant and plant–microbe interactions,” in Reference module in chemistry, molecular sciences and chemical engineering (Amsterdam: Elsevier). doi:10.1016/B978-0-12-409547-2.02802-X
Zhang, J., and Peters, R. J. (2020). Why are momilactones always associated with biosynthetic gene clusters in plants? PNAS 117 (25), 13867–13869. doi:10.1073/pnas.2007934117
Zhang, Y., Li, M., Liu, Q., Huang, J., and Chen, Y. (2022). A synthetic view on momilactones and related 9β-H pimarane skeleton diterpenoids. Front. Chem. 10, 882404. doi:10.3389/fchem.2022.882404
Zhao, L., Wu, Q., and Ma, A. (2018b). Biodegradation of phenolic contaminants: current status and perspectives. IOP Conf. Ser. Earth Environ. Sci. 111, 012024. doi:10.1088/1755-1315/111/1/012024
Zhao, M., Cheng, J., Guo, B., Duan, J., and Che, C.-T. (2018a). Momilactone and related diterpenoids as potential agricultural chemicals. J. Agric. Food Chem. 66 (30), 7859–7872. doi:10.1021/acs.jafc.8b02602
Zhao, M., Onakpa, M. M., Chen, W.-L., Santarsiero, B. D., Swanson, S. M., Burdette, J. E., et al. (2015a). 17-norpimaranes and (9βH)-17-norpimaranes from the tuber of Icacina trichantha. J. Nat. Prod. 78 (4), 789–796. doi:10.1021/np5010328
Zhao, M., Onakpa, M. M., Santarsiero, B. D., Chen, W.-L., Szymulanska-Ramamurthy, K. M., Swanson, S. M., et al. (2015b). (9βH)-Pimaranes and derivatives from the tuber of Icacina trichantha. J. Nat. Prod. 78 (11), 2731–2737. doi:10.1021/acs.jnatprod.5b00688
Keywords: diterpenoids, momilactones, biological activity, isolation, synthesis, genetic engineering, pharmaceutical application, sustainable agricultural development
Citation: Anh LH, Khanh TD and Xuan TD (2023) Biological roles of momilactones: achievements, challenges, and promising approaches to exploit their beneficial properties. Front. Nat. Produc. 2:1245869. doi: 10.3389/fntpr.2023.1245869
Received: 23 June 2023; Accepted: 29 August 2023;
Published: 21 September 2023.
Edited by:
Mansour Sobeh, Mohammed VI Polytechnic University, MoroccoReviewed by:
Sharad Vats, Banasthali University, IndiaCopyright © 2023 Anh, Khanh and Xuan. This is an open-access article distributed under the terms of the Creative Commons Attribution License (CC BY). The use, distribution or reproduction in other forums is permitted, provided the original author(s) and the copyright owner(s) are credited and that the original publication in this journal is cited, in accordance with accepted academic practice. No use, distribution or reproduction is permitted which does not comply with these terms.
*Correspondence: Tran Dang Xuan, dGR4dWFuQGhpcm9zaGltYS11LmFjLmpw
Disclaimer: All claims expressed in this article are solely those of the authors and do not necessarily represent those of their affiliated organizations, or those of the publisher, the editors and the reviewers. Any product that may be evaluated in this article or claim that may be made by its manufacturer is not guaranteed or endorsed by the publisher.
Research integrity at Frontiers
Learn more about the work of our research integrity team to safeguard the quality of each article we publish.