- 1Grupo de Neurociencias y Muerte Celular, Facultad de Medicina e Instituto de Genética, Bogota, Colombia
- 2Grupo de Química de Productos Naturales Vegetales Bioactivos, Facultad de Ciencias, Universidad Nacional de Colombia—Sede Bogotá, Bogota, Colombia
Abnormal production of reactive oxygen species (ROS) has been implicated in the physiopathology of neuronal cell death. Increased ROS levels are associated with exacerbated peptide aggregation, inflammation, and mitochondrial dysfunction, which facilitate the triggering of specific cell death pathways. Antioxidant molecules are potentially useful in the amelioration of neurodegeneration. In this regard, natural products are an invaluable source of antioxidants. Therefore, we investigate the antioxidant and neuroprotective activities of four Colombian angiosperm extracts. Antioxidant activity was evaluated by phytochemical assays using TLC techniques with Dragendorff reagent, ninhydrin, and chloranil in dioxane, NH3, Fast Blue, and FeCl3, together with bioautography using DPPH and β-carotene. In vitro neuroprotective activity, cell death, and ROS accumulation were evaluated by MTT and flow cytometry in the SH-SY5Y cell line exposed to paraquat and C2-ceramide. We found that Zanthoxylum rhoifolium Lam, Zanthoxylum martinicense, Nectandra membranacea, and Nectandra reticulata extracts have antioxidant activity higher than quercetin under a β-carotene bleaching assay and protect SH-SY5Y cells against paraquat and C2-ceramide associated with a reduction in ROS. In conclusion, these extracts have a strong neuroprotective potential, and the precise mechanism requires more evaluation.
1 Introduction
The study of reactive oxygen species (ROS) is important because they are involved in many cellular processes, including cell cycle regulation, phagocytosis, and enzymatic activation, and have been implicated in neurodegenerative diseases (Di Meo et al., 2016). An imbalance between the production and scavenging of ROS can lead to the generation of oxidative stress that can damage DNA, lipids, and proteins (Verbon et al., 2012), leading to cell death. Antioxidant enzymatic enzymes, such as superoxide dismutase (SOD), glutathione peroxidases (GPX), glutaredoxins, thioredoxins, and catalases, act as free radical scavengers (Manoharan et al., 2016)
In Alzheimer’s disease (AD) models, it has been shown that the increase in ROS levels causes different pathogenic events and leads to alterations in the mitochondrial electron transport chain (Zhao and Zhao, 2013), leading to a vicious cycle of oxidative damage that generates more ROS (Zhang et al., 2023). Recently, ROS can also stimulate the production and accumulation of amyloid-beta (Aβ) peptides and phosphorylation of the tau protein, which are the major hallmarks of AD pathology (Holubiec et al., 2022). Consequently, the accumulation of these peptides induces a positive feedback loop where Aβ increases oxidative stress-inducing lipid peroxidation, protein oxidation, and DNA oxidation (Gandhi and Abramov, 2012), and reduces antioxidant potential (Zhao and Zhao, 2013; Kim et al., 2015).
Similarly, in PD models, oxidative stress is associated with mitochondrial dysfunction, impaired protein degradation systems, and dopaminergic neuronal death (Duan et al., 2023). An important physiopathological characteristic of PD is the inhibition of the complex I of the electron transport chain, which increases ROS levels and further reduces the activity of these complexes. In addition, the imbalance in ROS can produce mutations in the mitochondrial DNA. These changes are finally associated with the induction of apoptosis of dopaminergic neurons (Swerdlow et al., 1996; Lin and Beal, 2006; Hauser and Hastings, 2013; Blesa et al., 2015; Pallikonda et al., 2019). In accordance with this, targeting oxidative stress and enhancing antioxidant defenses have emerged as potential therapeutic approaches for mitigating neurodegenerative pathology by modulating ROS levels, restoring redox balance, and preserving neuronal function.
Cellular and animal models treated with molecules that induce ROS accumulation at toxic levels have been used to study ROS pathways. In these regards, most cellular and animal models of ROS are based on the toxic effect of inhibition of mitochondrial complex I, including the 1-methyl-4-phenyl-1,2,3,4-tetrahydropyridine (MPTP) and paraquat (PQ) exposure models, which increase ROS, induce mitochondrial dysfunction, and subsequently lead to dopaminergic cell death (William Langston, 1985; Nisticò et al., 2011). On the other hand, ceramides, a group of signaling lipids, are implicated in the generation of ROS by the induction of mitochondrial dysfunction that promotes the production of free radicals that induce cell death related to the progression of neurodegeneration in different contexts (Pan et al., 2023). Ceramides can also cause inhibition of the phosphoinositide 3-kinase/protein kinase B (PI3K/AKT) cell survival pathway and, therefore, have been used as an in vitro model of neuronal death (Arboleda et al., 2009; Chaurasia and Summers, 2015; Jazvinšćak Jembrek et al., 2015). As is well known, the SH-SY5Y neuroblastoma cells have been used in neurobiology because they have phenotype-like catecholaminergic neurons, are grown easily, and can be differentiated with retinoic acid (Kovalevich and Langford, 2013). SH-SY5Y cells give us an approximation of the neuronal response to an abnormal ROS concentration.
Historically, natural products have been the main sources of molecules with potential therapeutic applications due to the abundance of diverse molecules that can have multiple cellular targets (Atanasov et al., 2021). It is possible to isolate small molecules with structural and functional features that are difficult to obtain by biochemical synthesis (Zhao and Zhao, 2013), including molecules like curcumin (isolated from Curcuma longa L), quercetin (isolated from Dimorphandra mollis), berberine (isolated from Berberis aquifolium Pursh), rivastigmine (isolated from Physostigma venenosum Balf. F), and galantamine (isolated from Galanthus woronowii Losinsk). All these molecules have important antioxidant activity and other bioactive functions, including anti-inflammation Aβ aggregation inhibition, and acetylcholinesterase (AChE) inhibition (Marco-Contelles et al., 2006; Cai et al., 2016; Dighe et al., 2019; Voulgaropoulou et al., 2019).
The genus Zanthoxylum has been shown to have in vitro antioxidant effects and the ability to inhibit acetylcholinesterase in AD models (Plazas et al., 2018). According to ethnobotanical records, this genus is known to be distributed worldwide and is mainly used in Asia, Africa, and the Americas as an antidote to snake venom, analgesics, bactericides, and fungicides, among others (Okagu et al., 2021). The genus Nectandra can be found in the neotropics, and it has been reported to be used for antifungal, anti-inflammatory, antimalarial, analgesic, treating ulcers, and febrifuge purposes, among others (Macías-Villamizar et al., 2015). Furthermore, their potential as a biological source of bioactive molecules stems from the rich composition of flavonoid, polyphenolic, and alkaloid metabolites in both genera.
In the present work, we have demonstrated the antioxidant activity of extracts from several Colombian plants. Using bioautography, we found that plant extracts from Zanthoxylum rhoifolium Lam, Zanthoxylum martinicense, Nectandra membranacea, and Nectandra reticulata have strong antioxidant activity. We also showed that these extracts 1) have a protective effect on the β-carotene peroxidation to a higher level than quercetin and 2) increase the survival of cells exposed to paraquat and ceramide. This suggests that the extracts analyzed have a strong antioxidant activity that has neuroprotective capacity.
2 Materials and methods
2.1 Plant material and preparation of the extracts
Rutaceae and Lauracea species were collected from different regions of Colombia (Table 1). To obtain the ethanolic extracts, plant material was separated in parts, dried at room temperature, macerated using 96% ethanol, and then concentrated under a vacuum at 40°C. For alkaloid extraction, the classical acid–base methodology was used (Grycová et al., 2007; Doncheva et al., 2015). In brief, the ethanol-extracted plant was evaporated under reduced pressure at 35–40°C. Then, 3 g of each dried residue was resuspended with 10 mL of 2% HCl solution three times. The acidic phases were filtered, and the pH was adjusted to 10 with 10% NaOH. The extraction was performed with CHCl3 to obtain the alkaloid extracts in CHCl3. Finally, the solvent was evaporated, and the residual aqueous phases were neutralized and lyophilized (Table 1).
2.2 Phytochemical qualitative analysis of extracts by TLC and TLC bioautography
The extracts were seeded on silica gel 60 F254 chromatofolds (ref. 1055540001, Merck, United States) eluted with CHCl3-MeOH mixture (9:1) (solvents AR grade). Once the TLC development protocol was ready and dry, some chromoplates were stained with NH3, FeCl3, ninhydrin, Fast Blue, and Dragendorff reagents to identify what type of secondary metabolite was present. Other groups of chromoplates were used to evaluate the preliminary antioxidant activity. The developed chromoplates were dried and sprayed with 0.2% 2,2-diphenyl-1-picrylhydrazyl (DPPH) in methanol or a 0.05% solution of β-carotene in chloroform (β-carotene bleaching assay), following the protocol adapted from Takao et al. (1994) and Miller (1971) and described in detail previously by Plazas et al. (2018), Miller (1971), Takao et al. (1994), and Plazas et al. (2018).
2.3 Quantification of total phenols using the Folin–Ciocalteu reagent
To quantify total phenols, 5 mg of extract was dissolved in 1 mL of 95% methanol and then filtered using a 0.22-μm membrane (solution 1). Then, 50 μL of solution A was mixed with 200 μL of 10% Folin–Ciocalteu reagent, shaken for 1 min, allowed to stand for 1 min more; then, 800 μL of 700 mM of sodium carbonate (solution B) was added. It was incubated for 1 h at room temperature. A 200 μL aliquot of solution B was transferred to a 96-well plate, and the absorbance at 620 nm was read.
2.4 Quantification of antioxidant capacity using β-carotene
The quantification of antioxidant capacity was performed by adapting Miller’s method (Miller and Rice-Evans, 1996). This experiment measures the antioxidant capacity of extracts by quantifying the change in color of the carotene solution when this solution is oxidated by linoleic acid (yellow-uncolored). First, we prepared an emulsion with a 500 μL aliquot of 0.02% β-carotene in CHCl3 solution. The solvent was removed by rota-evaporation, and 10 mg of linoleic acid (0.9 mg/mL), 100 mg of tween-20, and 20 mL of distilled water were added. Vigorous shaking was necessary to homogenize the emulsion. Second, 230 μL of the emulsion and 20 mL of the extract (5 μg/mL) were added to a 96-well plate. The assay was read at 450–490 nm every 6 s to 180 min at 50°C. Physostigmine was used as a positive standard.
2.5 Cell culture
The human neuroblastoma SH-SY5Y cell line was obtained from the ATCC (CRL-2266, American Type Culture Collection, MD, United States). Cells were cultured in Dulbecco modified Eagle's Minimal Essential Medium (DMEM) and F12 with 10% serum fetal bovine (SFB) and 1% penicillin/streptomycin (100 units penicillin/mL; 100 µg streptomycin/ml; P/S) at 37°C in a humidified atmosphere of 5% CO2. To evaluate cytotoxicity, 10,000 cells were seeded per well in a 96-well plate in DMEM F12 containing 10 mM retinoic acid, with 1% SFB and 1% P/S. The cells were allowed to differentiate for 3 days.
2.6 MTT assay
MTT is a colorimetric method to determine cell viability indirectly by a reduction reaction made by complex II of viable mitochondria of 3-(4,5-dimethylthiazol-2-yl)-2,5-diphenyltetrazole bromide (MTT), which is yellow, in the formazan salt (E, Z)-5-(4,5-dimethylthiazol-2-yl)-1,3-diphenylformazan, which is purplish-blue. The SH-SY5Y cells were incubated for 24 h with a 100 μL dilution of the plant extracts, which were added in quadruplicate at concentrations of 25, 75, 125, and 250 mg/mL in the cell maintenance medium (1% P/S and 2% SFB in DMEM F12). Then, 10 μL of the MTT solution (5 mg/mL in PBS) was added for 4 h, the medium was removed, and 100 μL of cell lysis buffer (40% v/v of dimethylformamide, 5% v/v glacial acetic acid, and 16% w/v SDS in H2Odd) was added to each well. The plate was shaken in an orbital shaker at a speed of 65 rpm for 15 min, and absorbance was read at 540 nm on a plate reader (TekanSunrise™, Austria) (Table 2).
2.7 Flow cytometry
To approach the mechanisms of neuroprotection, flow cytometry was performed to follow ROS accumulation and the cell death mechanism using the Muse Cell Analyzer platform (Muse-Guava, Luminex). In brief, 100,000 cells per well were seeded in 12-well plates in a cell culture medium and exposed to different extract concentrations for 1 h or 6 h. Later, paraquat (PQ) or ceramide (C2) was added to the cells for 23 h. Then, 150 μL of 0.5% trypsin-EDTA solution (Gibco—ref. 15,400–054) was added for 3 min, followed by inactivation with 350 μL of 10% FBS in DMEM F12. Two dilutions were made from this mixture: 1) the first was adjusted to 1 × 105–5 × 105 cells per mL for staining with Annexin-V, following the standardized steps of the Muse™ Annexin V Dead Cell kit (Millipore Ref. MCH100105); 2) the second was adjusted to 1 × 106–5 × 107 cells per mL and stained following the product recommendation Muse® Oxidative Stress Kit (Millipore Ref. MCH100111) (Guava Muse Cell Analyzer, Luminex).
2.8 Statistical analysis
The statistical analysis was performed using Graph Pad Prism software version 6 (Graph Pad Software Inc.). All quantification values in this study are expressed as the mean ± standard error of the mean (SEM). In vitro experiments were evaluated using at least three independent assays. For statistical significance between multiple groups, the parametric data were analyzed using a multivariable 2-way or a 1-way ANOVA, followed by Tukey’s post hoc test for comparisons between several independent groups. Levels of significance were *p < 0.05, **p < 0.01, ***p < 0.001, and ****p < 0.0001.
3 Results
3.1 Determination of working concentration of plant extracts
Four Colombian plant extracts were selected to be evaluated by different approaches for their antioxidant capacity. The selected extracts were Z. rhoifolium Lam (code 1), Z. martinicense (code 2), N. membranacea (code 3), and N. reticulata (code 4). As a preliminary assay, we determined the optimal working concentration of each extract that did not affect the cell survival of SH-SY5Y cells by MTT. We found that 25 μg/mL was the working concentration for all extracts (Table 2).
3.2 Presence of free radical scavenging and antioxidant capacity of plant extracts
Free radical scavenging capacity was evaluated using the DPPH and β-carotene bleaching assays by bioautography. The DPPH assay is a stable and sensitive method to determine the presence of antioxidant activity in plant extracts. We found inhibition zones from Rf 0.1 to 1 (Table 3), meaning that all extracts have free radical scavenging capacity (Figure 1A; Table 3). The β-carotene bleaching assay is used to evaluate the potential inhibition of the lipid peroxidation of β-carotene exposed to UV light. We found that the bioautography assay showed dots in the TLC for all the extracts, so the extracts will act as lipid peroxidation protectors in a biological environment (Figure 1B).
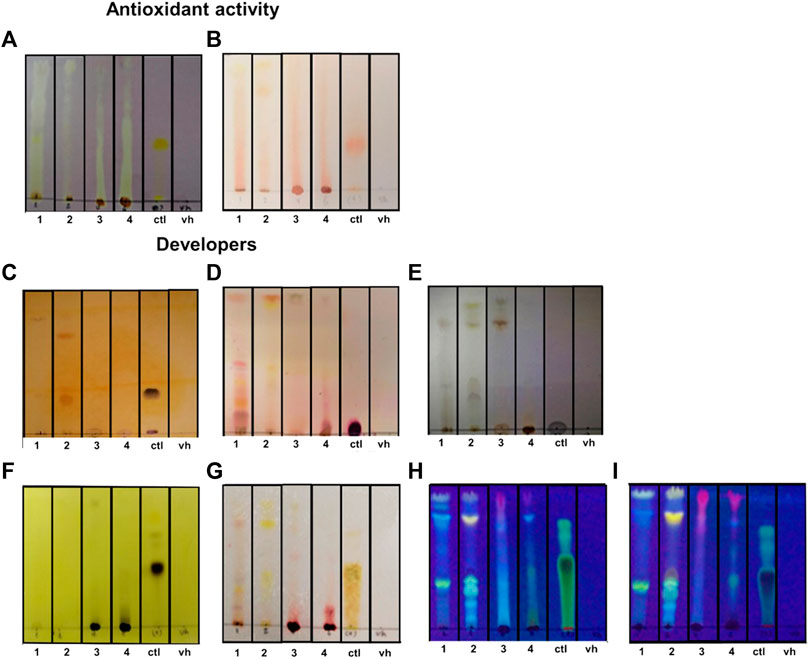
FIGURE 1. Antioxidant capacity of extracts and fractions. (A, B) DPPH bioautography (A) and β-carotene bioautography (B) to evaluate the antioxidant activity. (C) Chromatoplate developed with Dragendorff reagent to determine alkaloids. (D) Chromatoplate developed with ninhydrin to determine amino acids. (E) Chromatoplate developed with chloranil in dioxane to determine primary and secondary amines. (F) Chromatoplate developed with FeCl3 to determine the presence of phenolic molecules. (G) Chromatoplate developed with Fast Blue to determine the presence of a flavonoid. (H, I) Chromatoplates developed without NH3 vapor (H) and with NH3 vapor (I) at 365 nm to see the possible presence of flavonoids. UV development codes, 1: Zanthoxylum rhoifolium Lam., 2: Zanthoxylum martinicense, 3: Nectandra membranacea, 4: Nectandra reticulata. ctl: control (quercetin for A, B, F, G, H, and I; berberine for C; L-tryptophan for D and E); and vh: blank or solvent vehicle.
Normally, the antioxidant activity is related to the presence of phenols and flavonoids, but some studies suggest that alkaloids also have antioxidant activity (Plazas et al., 2018). Because we used two methods of extraction (alkaloidal and ethanolic), we decided to stain the chromoplates with specific reagents to the alkaloids, amino acids, primary and secondary amines, phenols, and flavonoids; these analyses were a preliminary study of the composition of the substances responsible for the antioxidant capacity of the extracts (Figure 1). To confirm this, we first used Dragendorff staining in the chromoplates. We found that only extract codes 1 (Rf 0.9) and 2 (Rf 0.8, 0.3) have dark spots similar to the control, which means they have alkaloids in their composition (Figure 1C; Table 3). Because the structures of alkaloids have nitrogenated groups, we decided to evaluate the presence of primary and secondary amines (chloranil in dioxane) and amino acids (ninhydrin) in the extracts to corroborate the Dragendorff stain. We found that extract codes 1 (retention factor (Rf) 0.9) and 2 (Rf 0.8, 0.3) probably have primary and secondary amines that could be part of the alkaloid structure (Figure 1E; Table 3). Additionally, extract code 1 had a positive spot for amino acids (Figure 1D), but these spots were not in the Rfs shown in the Dragendorff staining (0.5, 0.1).
We also evaluate the presence of phenols and flavonoids in the extracts. We applied the ferric chloride test to corroborate the presence of a phenol. We found black spots like the positive control in extract codes 3 and 4 (Figure 1F; Table 3). To determine the presence of flavonoids, we used the Fast Blue test. We found reddish spots in extract codes 3 and 4 (Figure 1G; Table 3). In addition, when comparing chromoplates before (Figure 1H) and after NH3 vapor (Figure 1I), we corroborated the presence of a phenol in the extracts. We found an increase in the intensity color or changes in color after NH3 exposure when the chromoplates were measured at 365 nm (Table 3). Together, these results indicate the presence of flavonoids in the extracts (Mabry et al., 1970; Tenorio, 2016).
3.3 Determination of total phenols
To confirm the presence of phenols and corroborate the presence of flavonoids as principal antioxidant secondary metabolites in the extracts, we evaluated the total phenol concentration in samples using the Folin–Ciocalteu assay. We found that the extracts of N. membranacea (code 3) and N. reticulata (code 4) had greater concentrations of phenols (4.593 μg/mL and 3.390 μg/mL, respectively) (Figure 2). These suggest that the antioxidant activity could be explained by the presence of the phenol and confirm the results observed in the chromoplates developed with FeCl3 (Figure 1F).
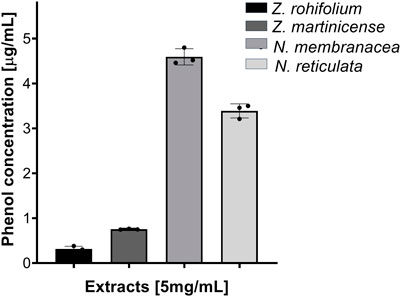
FIGURE 2. Quantification of total phenols. Extracts evaluated at 5,000 μg/mL, dissolved in MeOH. Reading at 620 nm.
3.4 Antioxidant and protective activity of β-carotene
To confirm the antioxidant potential and to evaluate the protective effect of extracts over time, we analyzed the kinetics of the β-carotene bleaching assay. We determine that the β-carotene present in the vehicle was fully oxidated after 160 min; however, when β-carotene was mixed with an extract or quercetin, the percentage of β-carotene was never less than 35% (Figure 3A), reflecting antioxidant activity. At 150 min, we found that all extracts protected β-carotene against oxidation (F (5,12) = 163.6; p < 0.001) (Figure 3B). Interestingly, the post hoc analysis showed that the extracts of Z. rhoifolium Lam (p = 0.0233), N. membranacea (p < 0.001), and N. reticulata (p < 0.001) exceed the effect of quercetin, a flavonoid that has been established as a powerful antioxidant widely used in the phytopharmaceutical industry (Figure 3B). These results again corroborate that the extracts could be considered potent antioxidant agents, especially N. reticulata, which presents the highest antioxidant effect, almost blocking the oxidizing effects (Figure 3A).
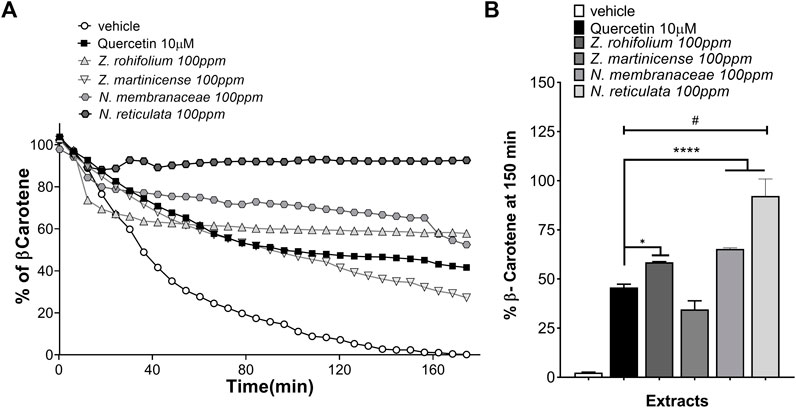
FIGURE 3. Antioxidant and protective activities of β-carotene. (A) Quantification of the percentage of β-carotene over 180 min, adding extracts of Zanthoxylum rhoifolium Lam, Zanthoxylum martinicense, Nectandra membranacea, and Nectandra reticulata, the solution used as vehicle and quercetin used as the positive control. (B) Quantification of the percentage of β-carotene using the extracts at 150 min; the graphic shows the mean and standard error of the mean (SEM). Statistical analysis performed by one-way ANOVA, followed by a multiple comparisons test of Tukey, where significant differences are shown: *(p < 0.05); ****(p < 0.0001), in relation to quercetin; #(p < 0.0001), in relation to the vehicle.
3.5 Extracts decrease the toxic effect of PQ on SH-SY5Y cells
We wanted to know if the antioxidant activity seen in the previous results could have a protective effect over SH-SY5Y cells exposed to toxic concentrations of PQ, which induces oxidative stress (Figure 4). As expected, we found that PQ triggers cellular death in a dose-dependent manner, with cells having a less-than-30% survival rate with 1 mM PQ (Figure 4). To investigate the protective effect of extracts against the induction of new free radicals, we used pretreatments of extracts of 1 h or 6 h before exposure to PQ.
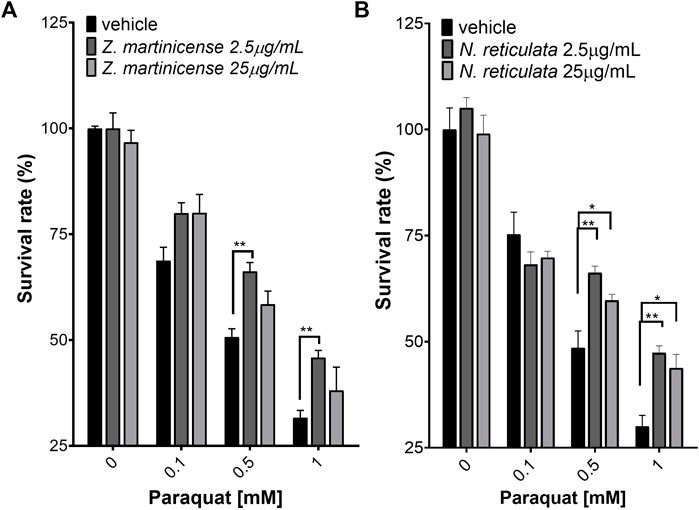
FIGURE 4. Neuroprotection assay evaluated by the variation of mitochondrial activity (MTT). (A) SHSY5Y cells pretreated for 1 h with the alkaloidal fraction (chloroform) of Zanthoxylum martinicense and exposed to PQ for 23 h. (B) SH-SY5Y cells pretreated for 1 h with Nectandra reticulata extract and exposed to PQ for 23 h. The graphic shows the mean and standard error of the mean (SEM). Statistical analysis performed by two-way ANOVA, followed by a multiple-comparison test of Tukey, where significant differences are shown as follows: *(p < 0.05); **(p < 0.01); ***(p < 0.001); ****(p < 0.0001).
When the cells were pretreated with extracts for 1 h, only the extract of Z. martinicense (Figure 4A) and N. reticulata (Figure 4B) had a protective effect against different PQ concentrations. The extract of Z. martinicense reduced the toxic effect of PQ, preventing the decrease in cell viability compared to cells that only had the vehicle and were exposed to the toxin (F (2, 36) = 11.30; p = 0.0002) (Figure 4A). The pretreatment with a concentration of 25 μg/mL of the extract of Z. martinicense exposed to 0.1 mM PQ significant increase the viability of cells (t (36) = 2.622; p = 0.025). Interestingly, a smaller concentration of this extract (2.5 μg/mL) significantly increased the viability of cells exposed to 0.1 mM (t (36) = 2.597; p < 0.05), 0.5 mM (t (36) = 3.605; p < 0.001), and 1 mM (t (36) = 3.295; p < 0.001) concentrations of PQ (Figure 4A).
Likewise, the extract of N. reticulata also decreased the toxic effect of PQ on cell viability (F (2, 36) = 6.328; p = 0.0044) (Figure 4B). We found that both 2.5 and 25 μg/mL concentrations of extract reduced the 0.5 mM and 1 mM PQ effect. The post hoc test indicated that this extract at 2.5 μg/mL presented a protective effect against 0.5 and 1 mM PQ (t (36) = 3.801; p = 0.0011; t (36) = 3.728; p = 0.0013). Additionally, pretreatment of N. reticulata at 25 μg/mL also decreased the effect of 0.5 mM and 1 mM PQ on cell viability (t (36) = 2.407; p = 0.0422; t (36) = 2.957; p = 0.0109) (Figure 4B).
When the extracts were used as pretreatment for 6 h, Z. martinicense and N. reticulata extracts again showed a protective effect, and additionally, the extract of Z. rhoifolium had protective effects on SH-SY5Y cell survival (Figure 5). The treatment with the extract of Z. rhoifolium (25 μg/mL) only reduced the cytotoxic effect of PQ (1 mM) in cell culture (F (2, 9) = 32.2; p < 0.0001) (Figure 5A). The treatment with 2.5 μg/mL Z. martinicense significantly reduced the cytotoxic effect of PQ (F (2, 36) = 12.60; p < 0.0001) at 0.1, 0.5, and 1 mM PQ (p = 0.0036, p = 0.0019, and p = 0.053) (Figure 5B). Finally, the extract of N. reticulata at 25 μg/mL reduced the cytotoxic effect of PQ (F (2, 36) = 7.215; p = 0.0023) at 0.01, 0.5, and 1 mM PQ (p = 0.0061, p = 0.0387, and p < 0.0305). Moreover, 2.5 μg/mL of N. reticulata extract had a protective effect on cell viability against 1 mM PQ (p = 0.0169) (Figure 5C).
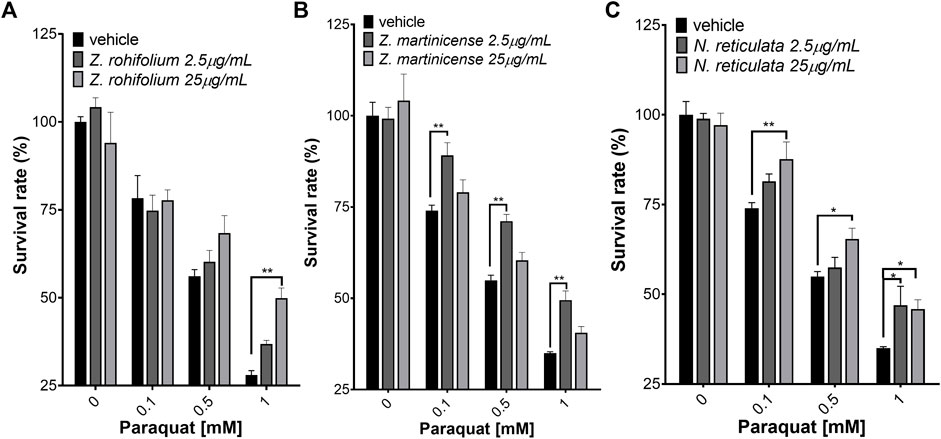
FIGURE 5. Neuroprotection assay assessed by MTT. (A) SH-SY5Y cells were pretreated for 6 h with Zanthoxylum rhoifolium extract and exposed to PQ for 23 h. (B) SH-SY5Y cells pretreated for 6 h with Zanthoxylum martinicense extract and exposed to PQ for 23 h. (C) SH-SY5Y cells pretreated for 6 h with Nectandra reticulata extract and exposed to PQ for 23 h. The figure shows the mean and standard error of the mean (SEM). Statistical analysis performed by two-way ANOVA, followed by a multiple comparison test of Tukey, where significant differences are shown as follows: *(p < 0.05), **(p < 0.01), ***(p < 0.001), and ****(p < 0.0001).
To verify the cellular protective effects observed by MTT, we performed flow cytometry to directly evaluate the cellular viability by labeling with Annexin V and 7AAD. Additionally, the dihydroethidium marker was used to stain intracellular ROS by flow cytometry and evaluate the relative change in the free radical with or without extracts. The concentrations of PQ, C2, and extracts used for this experiment were the concentrations that yielded the best results in the neuroprotection assay (Figure 6). The untreated SH-SY5Y cells show 99.38% living cells (Figure 6A) with a low basal level of ROS (17.91%) (Figure 7A). When the cells were treated with PQ, the percentage of living cells decreased in a concentration-dependent manner: 86.76% living cells at 0.1 mM PQ, 54.19% living cells at 0.5 mM PQ, and 27.70% living cells at 1 mM PQ (Figures 6B–D). Evaluation of ROS levels showed that cells treated with PQ increased the ROS levels in a concentration-dependent manner: 0.1 mM PQ: 32.61% ROS; 0.5 mM PQ: 50.58% ROS; and 1 mM PQ: 82.98% ROS (Figures 7B–D).
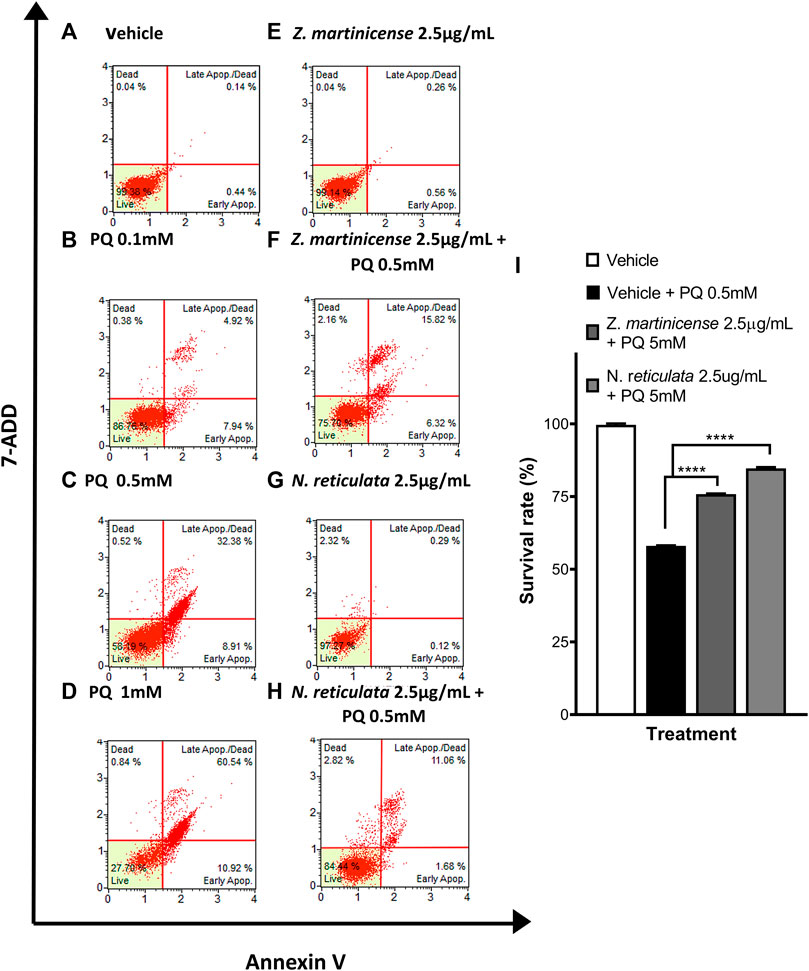
FIGURE 6. Evaluation of the neuroprotective effect of Zanthoxylum martinicense and Nectandra reticulata by flow cytometry. (A–H) Histograms of live, apoptotic cells (stained with annexin V) and necrotic cells (stained with 7-AAD). (A) Control cells cultured with the vehicle. (B) Cells exposed to PQ 0.1 mM for 23 h. (C) Cells exposed to PQ 0.5 mM for 23 h. (D) Cells exposed to PQ 1 mM for 23 h. (E) Cells exposed to the extract of Zanthoxylum martinicense 2.5 μg/mL for 24 h. (F) Cells pretreated with the extract of Zanthoxylum martinicense 2.5 μg/mL for 1 h and PQ 0.5 mM for 23 h. (G) Cells exposed to Nectandra reticulata 2.5 μg/mL extract for 24 h. (H) Cells pretreated with the extract of Nectandra reticulata 2.5 μg/mL extract for 1 h and PQ 0.5 mM for 23 h. (I) Bar chart of the percentage of live SH-SY5Y cells determined using annexin V and 7-AAD. The graphic shows the mean and standard error of the mean (SEM). Statistical analysis performed by one-way ANOVA, followed by a multiple comparison test of Tukey, where significant differences are shown: *(p < 0.05), **(p < 0.01), ***(p < 0.001), and ****(p < 0.0001).
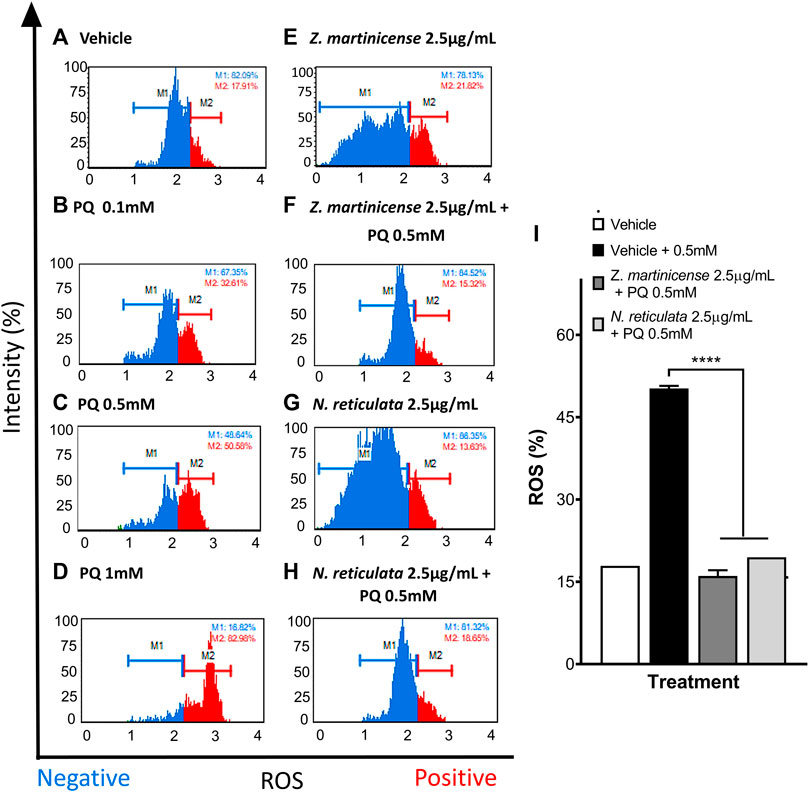
FIGURE 7. Evaluation of the antioxidant effect of Zanthoxylum martinicense and Nectandra reticulata by flow cytometry. (A–H) Histograms of SH-SY5Y cells labeled with dihydroethidium to evaluate the presence (red) or absence (blue) of ROS in cells. (A) Control cells cultured with the vehicle. (B) Cells exposed to PQ 0.1 mM for 23 h. (C) Cells exposed to PQ 0.5 mM for 23 h. (D) Cells exposed to PQ 1 mM for 23 h. (E) Cells exposed to the extract of Zanthoxylum martinicense 2.5 μg/mL for 24 h. (F) Cells pretreated for 1 h with the extract of Zanthoxylum martinicense 2.5 μg/mL and PQ 0.5 mM for 23 h. (G) Cells exposed to the extract of Nectandra reticulata 2.5 μg/mL for 24 h. (H) Cells pretreated for 1 h with the extract of Nectandra reticulata 2.5 μg/mL, followed by PQ 0.5 mM for 23 h. (I) Bar diagram of the percentage of SH-SY5Y cells with positive ROS levels identified by labeling ROS with ethidium bromide. The graphic shows the mean and standard error of the mean (SEM). Statistical analysis performed by one-way ANOVA, followed by a multiple comparison test of Tukey where significant differences are shown: *(p < 0.05), **(p < 0.01), ***(p < 0.001), and ****(p < 0.0001).
Treatment of SH-SY5Y cells with 2.5 μg/mL of plant extracts did not affect their viability. We found values of living cells close to 99%, and in all cases, the percentage of basal ROS levels was close to untreated cells (Figures 6E,G; Figures 7E,G). Interestingly, the pretreatment with plant extracts induced a neuroprotective effect in the SH-SY5Y cells (F (2, 3) = 454; p < 0.0001) and decreased ROS levels (F (3, 4) = 783.3; p < 0.0001) upon PQ exposure (Figure 6; Figure 7). Pretreatment with 2.5 μg/mL of Zanthoxylum martinicense extract significantly reduced the cytotoxic effect of PQ (58.05% vs. 75.85%; q (3) = 62.45; p < 0.0001) (Figures 6F,I) and decreased the ROS level of cells exposed to 0.5 mM PQ (50.24% vs. 16.06%; q (3) = 36; p < 0.0001) (Figures 7F,I). Pretreatment with 2.5 μg/mL of N. reticulata extracts significantly increased cell viability (58.05% vs. 84.72%; q (3) = 93.56; p < 0.0001) (Figures 6H,I) and decreased ROS levels (50.24% vs. 19.48%; q (3) = 32.4; p < 0.0001) upon 0.5 mM PQ exposure (Figures 7H,I).
Because the Z. rhoifolium plant extract showed neuroprotection after 6 h of pretreatment, we decided to evaluate cell death and ROS levels in SH-SY5Y cells by flow cytometry. We found that 25 μg/mL of extract did not affect the cell viability (98.72% of a living cell) (Figure 8C). When cells were exposed to 1 mM PQ, the percentage of living cells decreased to 27.79% (Figure 8B), and 6 h of pretreatment with the extract increased the cell viability (28.95% vs. 69.88%; F (2, 3) = 133; p < 0.0001; q (3) = 29.76; p = 0.002) upon 1 mM PQ exposure (Figures 8B,D,E). We found that cells exposed to PQ have a high level of ROS (83.09%) (Figure 9B), and 6 h of pretreatment with the extract induced a significant decrease in ROS levels (22.41%; F (2, 3) = 803.7; p < 0.0001). Remarkably, treatment with 25 μg/mL of Z. rhoifolium extract by itself increased ROS levels (30.99%) (Figure 9C), without affecting cell viability (98.72%) (Figure 8C).
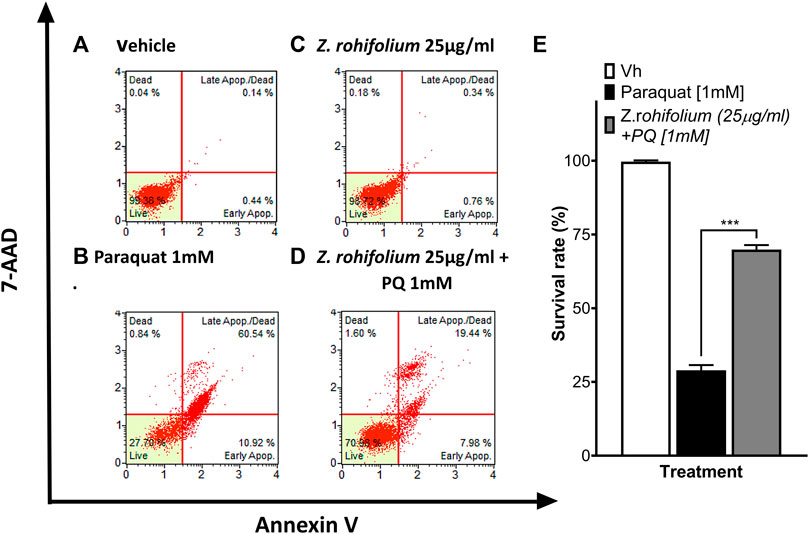
FIGURE 8. Evaluation of the neuroprotective effect of Z. rhoifolium by flow cytometry. (A–D) Histograms of SH-SY5Y live cells, apoptotic cells (stained with annexin V), and necrotic cells (stained with 7-AAD). (A) Control cells cultured with the vehicle. (B) Cells exposed to PQ 1 mM for 23 h. (C) Cells exposed to the extract of Zanthoxylum rhoifolium 25 μg/mL for 29 h. (D) Cells pretreated with the extract of Zanthoxylum rhoifolium 25 μg/mL for 1 h and exposed to PQ 1 mM for 23 h. (E). Bar chart of the percentage of live SH-SY5Y cells determined by analysis using annexin V and 7-AAD. The graphic shows the mean and standard error of the mean (SEM). Statistical analysis performed by one-way ANOVA, followed by a multiple comparison test of Tukey, where significant differences are shown: *(p < 0.05), **(p < 0.01), ***(p < 0.001), and ****(p < 0.0001).
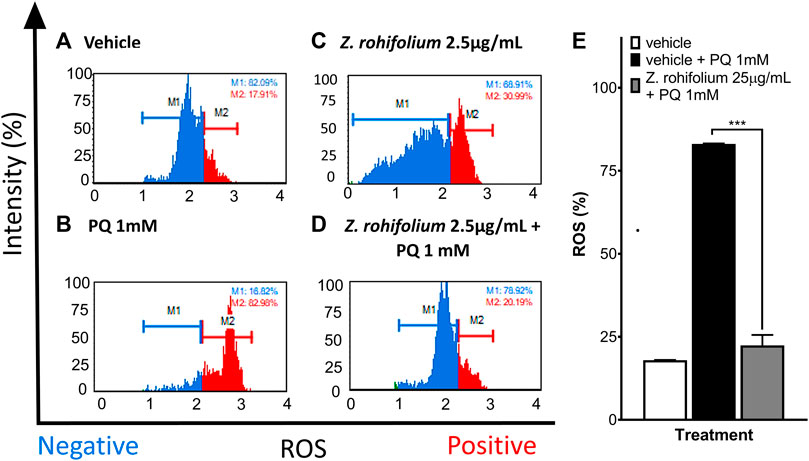
FIGURE 9. Evaluation of the antioxidant effect of Zanthoxylum rhoifolium by flow cytometry. (A–D) Histograms of SH-SY5Y cells labeled with dihydroethidium to evaluate the presence or absence of ROS in the cells. (A) Control cells cultured with the vehicle. (B) Cells exposed to PQ 1 mM for 23 h. (C) Cells exposed to the extract of Zanthoxylum rhoifolium 25 μg/mL for 29 h. (D) Cells pretreated with the extract of Zanthoxylum rhoifolium 25 μg/mL for 1 h and exposed to PQ 1 mM for 23 h. (E) Bar diagram of the percentage of SH-SY5Y cells with positive ROS levels identified by labeling ROS with ethidium bromide. The graphic shows the mean and standard error of the mean (SEM). Statistical analysis performed by one-way ANOVA, followed by a multiple comparison test of Tukey, where significant differences are shown: *(p < 0.05), **(p < 0.01), ***(p < 0.001), and ****(p < 0.0001).
3.6 Extracts decrease the toxic effect of C2-ceramide on SH-SY5Y cells
C2-ceramide (C2) induced the generation of ROS mediated by alteration of membrane phospholipids, disruption of mitochondria, and inactivation of intracellular signaling pathways, including PI3K/AKT and MAPK. To evaluate the effect of the plant extracts against C2, we used MMT assays and flow cytometry to measure cell death. We found that C2 decreases cell survival in a dose-dependent manner (Figure 10). However, pretreatment for 6 h with Z. rhoifolium (F (3, 32) = 24.76 p < 0.0001) and N. reticulata (F (3, 32) = 13.15; p < 0.0001) extracts induced a neuroprotective effect (Figure 10). We found that pretreatment with 0.25 μg/mL (t (32) = 6.442; p < 0.0001) and 2.5 μg/mL of Z. rhoifolium extract (t (32) = 5.573; p < 0.0001) protected cells against the toxic effect of 10 μM of C2 (44.82% living cells in C2; 77.39% living cells at 0.25 μg/mL + C2; and 72.99% living cells at 2.5 μg/mL + C2) (Figure 10A). Additionally, similar results were found with N. reticulata extract. Pretreatment with 0.25 μg/mL (t (32) = 3.584; p = 0.0033) and 2.5 μg/mL of N. reticulata (t (32) = 3.040; p = 0.0140) extract protected cells against the toxic effect of 10 μM of C2 (43.94% living cells in C2; 65.72% living cells at 0.25 μg/mL + C2; and 62.41% living cells at 2.5 μg/mL + C2) (Figure 10B).
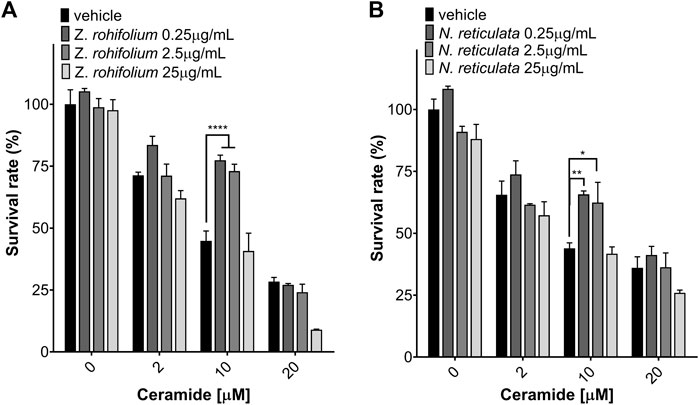
FIGURE 10. Neuroprotection assay of Zanthoxylum rhoifolium and Nectandra reticulata against C2-ceramide evaluated using MTT. (A) SH-SY5Y cells were pretreated for 6 h with Zanthoxylum rhoifolium extract and exposed to C2-ceramide for 23 h. (B) SH-SY5Y cells were pretreated for 6 h with the Nectandra reticulata extract and exposed to C2-ceramide for 23 h. The graphic shows the mean and standard error of the mean (SEM). Statistical analysis performed by two-way ANOVA, followed by a multiple comparisons test of Tukey where significant differences are shown as follows: *(p < 0.05); **(p < 0.01); ***(p < 0.001); ****(p < 0.0001).
Cell death and ROS levels in SH-SY5Y cells were evaluated by flow cytometry upon C2 exposure. C2 induced a dose-dependent cell death effect (2 μM C2: 74.35% living cells; 10 μM C2: 29.91% living cells; 20 μM C2: 3.15% living cells) (Figures 11B–D). Treating SH-SY5Y cells with 0.25 μg/mL of Z. rhoifolium or N. reticulata extract did not affect the viability of the cells (98.72% and 99.38% living cells) (Figures 11E,G). Pretreatment for 6 h with the plant extracts induced a neuroprotective effect upon C2 exposure (F (2, 3) = 639.2; p = 0.0001). Pretreatment with 0.5 ug/mL of Z. rhoifolium and N. reticulata extracts protected the SH-SY5Y cells against 10 μM of C2 (30.02% vs. 47.02%; q (3) = 23.72; p = 0.0003; 30.02% vs. 55.12%; q (3) = 35.03; p = 0.0001, respectively) (Figures 11F,H,I). Together, the results showed that the extracts were a potent cellular protector against C2 exposure.
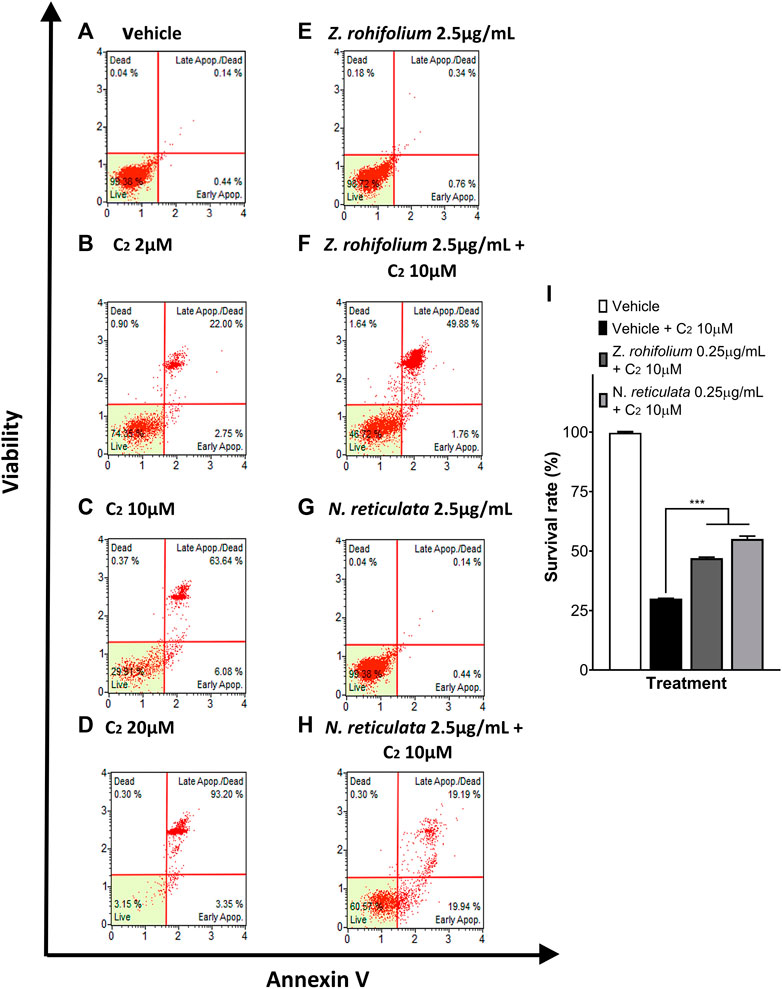
FIGURE 11. Evaluation of the neuroprotective capacity of Zanthoxylum rhoifolium and Nectandra reticulata extracts against C2-ceramide. (A–H) Histograms of live, apoptotic SH-SY5Y cells stained with annexin V and necrotic cells stained with 7-AAD. (A) Control cells cultured with the vehicle. (B) Cells exposed to C2 2 μM for 23 h. (C) Cells exposed to C2 10 μM for 23 h. (D) Cells exposed to C2 20 μM for 23 h. (E) SH-SY5Y cells treated for 29 h with the extract of Zanthoxylum rhoifolium 2.5 μg/mL. (F) SH-SY5Y cells pretreated for 1 h with the extract of Zanthoxylum rhoifolium 2.5 μg/mL and exposed to C2 10 μM for 23 h. (G) SH-SY5Y cells pretreated for 29 h with an extract of Nectandra reticulata 2.5 μg/mL. (H) SH-SY5Y cells pretreated for 1 h with an extract of Nectandra reticulata 2.5 μg/mL and exposed to C2 10 μM for 23 h. Bar chart of the percentage of live SH-SY5Y cells determined by analysis using annexin V and 7-AAD. Statistical analysis performed by two-way ANOVA, followed by a multiple comparison test of Tukey where significant differences are shown as follows: *(p < 0.05), **(p < 0.01), ***(p < 0.001), and ****(p < 0.0001).
4 Discussion
Extracts derived from diverse Colombian plants evaluated in the present paper have an important antioxidant and neuroprotective activity against PQ and C2, two toxins that are widely described to be involved in the physiopathology of various neurodegenerative diseases (Fukushima et al., 2002; Arboleda et al., 2009; Chaurasia and Summers, 2015; Jazvinšćak Jembrek et al., 2015; Yan et al., 2016; Cruciani-Guglielmacci et al., 2017; Islam et al., 2019). We proposed that the beneficial activities of these extracts could be attributed to three potential types of biomolecules: alkaloids, phenols, and flavonoids (Mabry et al., 1970). We found that the extracts with the best results were N. membranacea and N. reticulata.
The Fast Blue assay showed the presence of reddish spots in the chromoplate for the extracts N. membranacea and N. reticulata. These spots developed as the result of the formation of a red complex for the diazonium salt because of the presence of phenols in the extract. Additionally, we used NH3 vapors to confirm the presence of phenols. Because the ammonia interacting with phenols increases their electron density, we can see an increase in the intensity of a color change read at 365 nm (Mabry et al., 1970; Lock Sing de Ugaz, 1997; Tenorio, 2016). We again found that the extracts were associated with phenols and flavonoids. In addition, a relationship was found between the results of the FeCl3 (Figure 1F) and the quantification of total phenols by a Folin–Ciocalteu assay (Figure 2). On both assays, the extracts of N. membranacea and N. reticulata had a higher concentration of phenols that could be directly associated with the antioxidant effect observed in the bioautographies. These results confirm our hypothesis that secondary metabolites of the flavonoid type give the antioxidant characteristic to these extracts. Previous research had demonstrated that the genus Nectandra is rich in metabolites, such as polyphenols, flavonoids, and alkaloids, that confer analgesic, anti-inflammatory, and antioxidant activities (Le Quesne et al., 1980; Filho et al., 2004; Oliveira De Melo et al., 2006; Moreno et al., 2011; Ponci et al., 2015; Espitia Corredor et al., 2016). Additionally, some investigations suggested that some polyphenols have biological activities, such as transcription factors agonists (LXR agonist), BACE inhibition, and anti Aβ aggregation activity, that give the extracts from Nectandra potential action against AD (Park et al., 2004; Ondeyka et al., 2005; Wang and Du, 2009; Paula et al., 2019).
The antioxidant activity of Z. rhoifolium and Z. martinicense extracts could be explained by the presence of some alkaloids because they were obtained from the alkaloidal isolation before starting with the analysis. However, we found dark spots in the chromoplates of these extracts when they were developed with the Dragendorff reagent. Additionally, the chromoplates of these extracts showed spots when they were developed with ninhydrin. Interestingly, most of the ninhydrin spots in the chromoplates match the spots developed by the Dragendorff reagent (see Rfs in Table 3). Moreover, recent research has shown that Colombian Zanthoxylum alkaloidal extracts have antioxidant capacity provided by alkaloid molecules (Plazas et al., 2018). Furthermore, these extracts showed low concentrations of phenols (<0.8 μg/mL). This is because the presence of flavonoids in the alkaloid fractions is very unlikely, but some alkaloids that could also have an antioxidant effect in this type of extract have been reported (Gülçin et al., 2010; Rehman and Khan, 2017). Therefore, the genus Zanthoxylum is rich in alkaloids as fundamental metabolites with a variety of antibacterial, antifungal, antiviral, anti-inflammatory, antioxidant, cardiovascular protectant, and anti-cancer activities (Ethur et al., 1997; Nhiem et al., 2020). Some alkaloids, including dihydronitidine, 6-oxynitidine, magnoflorine, bocconoline, nitidine, chelerythrine, and skimmianine, were isolated from Z. rhoifolium. (Ethur et al., 1997; Blanco-Ayala et al., 2014; Tavares et al., 2014; Plazas et al., 2018; Pallikonda et al., 2019; Nhiem et al., 2020). 6-Oxynitidine has been associated with anti-inflammatory activity, and skimmianine has strong AChE inhibitor activity (Tavares et al., 2014; Pallikonda et al., 2019). This neuroprotective effect of alkaloids could explain that the present results are related to their antioxidant capacity and additional cell survival properties.
Exposure of SH-SY5Y cells, a neuroblastoma cell line, to PQ decreased their viability and increased ROS levels. However, a short pretreatment (1 h) of the cell with the extracts of N. membranacea or N. reticulata demonstrated a neuroprotective effect upon toxin exposure, which was related to a decrease in cellular ROS levels. Although the mechanisms for neuroprotection were not studied in the present paper, we suggest that it could be due to a direct antioxidant activity over harmful ROS that can induce lipid peroxidation, mitochondrial damage, and DNA mutation. Additionally, the bioactive extracts could induce post-transcriptional changes in proteins or molecules associated with the cellular response to increase free radical levels and therefore reduce their harmful effects on mitochondrial function and key cellular components. A longer pretreatment (6 h) with the extracts of Z. rhoifolium and N. reticulata also demonstrated a neuroprotective effect against PQ. In this case, we propose that these protections may be conferred, in part, by genomic regulation, increasing the transcription of genes related to free radical scavenging enzymes (catalase, superoxide dismutase, and glutathione peroxidase), pro-survival, and anti-apoptotic proteins that would confer resistance to the cells upon toxin exposure (Figure 12).
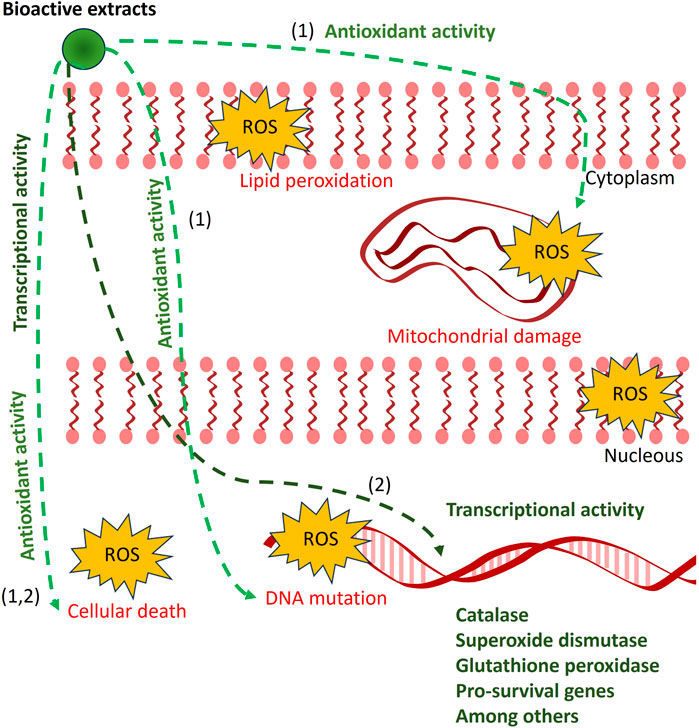
FIGURE 12. Proposed model of the antioxidant effect from bioactive extracts. Abnormal increases in ROS (red arrow) cause cellular death associated with lipid peroxidation, mitochondrial damage, and DNA mutation, among others. The bioactive extracts from Zanthoxylum rhoifolium, Zanthoxylum martinicense, Nectandra membranacea, and Nectandra reticulata reduced ROS levels (black and green arrows) and increased cell viability. We proposed two alternative models for the neuroprotective function of plant extracts depending on pretreatment duration: (1) in short treatments (1 h), the bioactive molecules could act directly as direct antioxidant agents against increased free ROS present in different cellular components such as membranes, mitochondria, and nuclei; and (2) in longer pretreatments (6 h), the bioactive molecules could directly or indirectly regulate gene transcriptional activity, increasing the expression of genes associated with antioxidant or pro-survival effects.
To test the additional cellular protective effect of the extracts, we exposed the cell to C2, which is a potent cellular toxin that activates different signaling pathways conducive to cell death and increases free radical generation. We found that only a longer pretreatment (6 h) with Z. rhoifolium and N. reticulata extracts showed a neuroprotective effect against toxic concentrations of C2. In this case, the extracts most probably regulate gene transcription toward a cell phenotype that confers resistance to diverse cellular insults and favors cell survival. Finally, recent findings of our laboratory suggest that longer treatment with these plant extracts induced diverse changes in gene expression toward a neuroprotective phenotype than non-treated conditions (unpublished data). However, additional studies are required to support this hypothesis.
5 Conclusion
Colombian plant extracts from Z. rhoifolium, Z. martinicense, N. membranacea, and N. reticulata have important antioxidant and neuroprotectant activities against known ROS-producing toxins and cell death triggers such as PQ and C2. The main metabolites associated with this activity are probably flavonoids and alkaloids, molecules with extensive research confirming their antioxidant potential, and could be used to reduce the deleterious effects of ROS in neurodegeneration.
Data availability statement
The original contributions presented in the study are included in the article/Supplementary material, further inquiries can be directed to the corresponding author.
Author contributions
AB-R: investigation, formal analysis, data curation, validation, and writing—original draft. JM-C: formal analysis, data curation, and writing—original draft. LC: writing—review and editing, and resources. GA: resources, visualization, and writing—review and editing. MA: visualization, methodology, resources, and writing—review and editing. AS-H: conceptualization, methodology, funding acquisition, project administration, visualization, and writing—review and editing. All authors contributed to the article and approved the submitted version.
Funding
This work was supported by the Ministerio de Ciencia, Tecnología e Innovación, Colombia (MinCiencias) [grant numbers 734-2018 and 727-2018].
Acknowledgments
The authors thank the Ministerio de Ciencia, Tecnología e Innovación, Colombia, for financial support and Universidad Nacional de Colombia - sede Bogotá.
Conflict of interest
The author GA declared that they were an editorial board member of Frontiers, at the time of submission. This had no impact on the peer review process and the final decision.
The authors declare that the research was conducted in the absence of any commercial or financial relationships that could be construed as a potential conflict of interest.
Publisher’s note
All claims expressed in this article are solely those of the authors and do not necessarily represent those of their affiliated organizations, or those of the publisher, the editors, and the reviewers. Any product that may be evaluated in this article, or claim that may be made by its manufacturer, is not guaranteed or endorsed by the publisher.
References
Arboleda, G., Morales, L. C., Benítez, B., and Arboleda, H. (2009). Regulation of ceramide-induced neuronal death: cell metabolism meets neurodegeneration. Brain Res. Rev. 59 (2), 333–346. doi:10.1016/J.BRAINRESREV.2008.10.001
Atanasov, A. G., Zotchev, S. B., Dirsch, V. M., Orhan, I. E., Banach, M., Rollinger, J. M., et al. (2021). Natural products in drug discovery: advances and opportunities. Nat. Rev. Drug Discov. 20 (3), 200–216. doi:10.1038/s41573-020-00114-z
Blanco-Ayala, T., Andérica-Romero, A. C., and Pedraza-Chaverri, J. (2014). New insights into antioxidant strategies against paraquat toxicity. Free Radic. Res. 48 (6), 623–640. doi:10.3109/10715762.2014.899694
Blesa, J., Trigo-Damas, I., Quiroga-Varela, A., and Jackson-Lewis, V. R. (2015). Oxidative stress and Parkinson’s disease. Front. Neuroanat. 9, 91. doi:10.3389/FNANA.2015.00091
Cai, Z., Wang, C., and Yang, W. (2016). Role of berberine in Alzheimer&rsquo;s disease. Neuropsychiatric Dis. Treat. 12, 2509–2520. doi:10.2147/NDT.S114846
Chaurasia, B., and Summers, S. A. (2015). Ceramides - lipotoxic inducers of metabolic disorders. Trends Endocrinol. Metabolism TEM 26 (10), 538–550. doi:10.1016/J.TEM.2015.07.006
Cruciani-Guglielmacci, C., López, M., Campana, M., and le Stunff, H. (2017). Brain ceramide metabolism in the control of energy balance. Front. Physiology 8, 787. doi:10.3389/fphys.2017.00787
Di Meo, S., Reed, T. T., Venditti, P., and Victor, V. M. (2016). Role of ROS and RNS sources in physiological and pathological conditions. Oxidative Med. Cell. Longev. 2016, 1–44. doi:10.1155/2016/1245049
Dighe, S. N., De la Mora, E., Chan, S., Kantham, S., McColl, G., Miles, J. A., et al. (2019). Rivastigmine and metabolite analogues with putative Alzheimer’s disease-modifying properties in a Caenorhabditis elegans model. Commun. Chem. 2 (1), 35–14. doi:10.1038/s42004-019-0133-4
Doncheva, T., Solongo, A., Kostova, N., Gerelt-Od, Y., Selenge, D., and Philipov, S. (2015). Leptopyrine, new alkaloid from Leptopyrum fumarioides L. (Ranunculaceae). Nat. Prod. Res. 29 (9), 853–856. doi:10.1080/14786419.2014.991322
Duan, W., Liu, C., Zhou, J., Yu, Q., Duan, Y., Zhang, T., et al. (2023). Upregulation of mitochondrial calcium uniporter contributes to paraquat-induced neuropathology linked to Parkinson’s disease via imbalanced OPA1 processing. J. Hazard. Mater. 453, 131369. doi:10.1016/J.JHAZMAT.2023.131369
Espitia Corredor, J. A., Cuca Suárez, L. E., and Guerrero Pabón, M. F. (2016). Assesment of platelet antiaggregant activity of A fraction from an ethanolic extract of the bark of Nectandra amazonum nees. Vitae 23 (2), 119–123. doi:10.17533/udea.vitae.v23n2a04
Ethur, E. M., Ribeiro, H. B., Machado, E. C., Zanatta, N., and Morel, A. F. (1997). Benzophenanthridine alkaloids from Zanthoxylum rhoifolium. Phytochemistry 46, 1443–1446. doi:10.1016/s0031-9422(97)00498-6
Filho, A. A. da S., Silva, M. L. A. e., Carvalho, J. C. T., and Bastos, J. K. (2004). Evaluation of analgesic and anti-inflammatory activities of Nectandra megapotamica (Lauraceae) in mice and rats. J. Pharm. Pharmacol. 56 (9), 1179–1184. doi:10.1211/0022357044058
Fukushima, T., Tanaka, K., Lim, H., and Moriyama, M. (2002). Mechanism of cytotoxicity of paraquat. Environ. Health Prev. Med. 7 (3), 89–94. doi:10.1265/ehpm.2002.89
Gandhi, S., and Abramov, A. Y. (2012). Mechanism of oxidative stress in neurodegeneration. Oxidative Med. Cell. Longev. 2012, 1–11. doi:10.1155/2012/428010
Grycová, L., Dostál, J., and Marek, R. (2007). Quaternary protoberberine alkaloids. Phytochemistry 68 (2), 150–175. doi:10.1016/j.phytochem.2006.10.004
Gülçin, I., Elias, R., Gepdiremen, A., Chea, A., and Topal, F. (2010). Antioxidant activity of bisbenzylisoquinoline alkaloids from stephania rotunda: cepharanthine and fangchinoline. J. Enzyme Inhibition Med. Chem. 25 (1), 44–53. doi:10.3109/14756360902932792
Hauser, D. N., and Hastings, T. G. (2013). Mitochondrial dysfunction and oxidative stress in Parkinson’s disease and monogenic parkinsonism. Neurobiol. Dis. 51, 35–42. doi:10.1016/J.NBD.2012.10.011
Holubiec, M. I., Gellert, M., and Hanschmann, E. M. (2022). Redox signaling and metabolism in Alzheimer’s disease. Front. Aging Neurosci. 14, 1003721. doi:10.3389/FNAGI.2022.1003721
Islam, B. ul, Jabir, N. R., and Tabrez, S. (2019). The role of mitochondrial defects and oxidative stress in Alzheimer’s disease. J. Drug Target. 27 (9), 932–942. doi:10.1080/1061186X.2019.1584808
Jazvinšćak Jembrek, M., Hof, P. R., and Šimić, G. (2015). Ceramides in alzheimer’s disease: key mediators of neuronal apoptosis induced by oxidative stress and AβAccumulation. Oxidative Med. Cell. Longev. 2015, 1–17. doi:10.1155/2015/346783
Kim, G. H., Kim, J. E., Rhie, S. J., and Yoon, S. (2015). The role of oxidative stress in neurodegenerative diseases. Exp. Neurobiol. 24 (4), 325–340. doi:10.5607/EN.2015.24.4.325
Kovalevich, J., and Langford, D. (2013). Considerations for the use of SH-SY5Y neuroblastoma cells in neurobiology. Methods Mol. Biol. Clift. N.J.) 1078, 9–21. doi:10.1007/978-1-62703-640-5_2
Le Quesne, P. W., Larrahondo, J. E., and Raffauf, R. F. (1980). Antitumor plants. X. Constituents of Nectandra rigida. J. Nat. Prod. 43 (3), 353–359. doi:10.1021/NP50009A006
Lin, M. T., and Beal, M. F. (2006). Mitochondrial dysfunction and oxidative stress in neurodegenerative diseases. Nature 443(7113), 787–795. doi:10.1038/nature05292
Lock Sing de Ugaz, O. (1997). Colorantes naturales. Available at: https://repositorio.pucp.edu.pe/index/handle/123456789/181503.
Mabry, T. J., Markham, K. R., and Thomas, M. B. (1970). The ultraviolet spectra of flavones and flavonols. Syst. Identif. Flavonoids 1970, 41–164. doi:10.1007/978-3-642-88458-0_5
Macías-Villamizar, V. E., Cuca-Suárez, L. E., and Coy-Barrera, E. D. (2015). Genus Nectandra: phytochemistry and biological activity. Bol. Latinoam. Del Caribe Plantas Med. Aromáticas 14 (4), 317–342.
Manoharan, S., Guillemin, G. J., Abiramasundari, R. S., Essa, M. M., Akbar, M., and Akbar, M. D. (2016). The role of reactive oxygen species in the pathogenesis of alzheimer’s disease, Parkinson’s disease, and huntington’s disease: A mini review. Oxidative Med. Cell. Longev. 2016, 1–15. doi:10.1155/2016/8590578
Marco-Contelles, J., do Carmo Carreiras, M., Rodríguez, C., Villarroya, M., and García, A. G. (2006). Synthesis and pharmacology of galantamine. Chem. Rev. 106 (1), 116–133. doi:10.1021/CR040415T
Miller, H. E. (1971). A simplified method for the evaluation of antioxidants. J. Am. Oil Chem. Soc. 48 (2), 91. doi:10.1007/bf02635693
Miller, N. J., and Rice-Evans, C. A. (1996). Spectrophotometric determination of antioxidant activity. Redox Rep. Commun. Free Radic. Res. 2 (3), 161–171. doi:10.1080/13510002.1996.11747044
Moreno, S., Carvalho, J., Nascimento, A. L., Pereira, M. J. S., Caldas, L., and Bernardo-Filho, M. (2011). Effects of a Nectandra membranacea extract on labeling of blood constituents with technetium-99m and on the morphology of red blood cells. World Acad. Sci. Eng. Technol. Int. J. Biol. Biomol. Agric. Food Biotechnol. Eng. 2011.
Nhiem, N. X., Quan, P. M., and Van, N. T. H. (2020). Alkaloids and their pharmacology effects from <em>Zanthoxylum</em> genus. Bioact. Compd. Nutraceutical Funct. Food Good Hum. Health 2019. doi:10.5772/INTECHOPEN.91685
Nisticò, R., Mehdawy, B., Piccirilli, S., and Mercuri, N. (2011). Paraquat-and rotenone-induced models of Parkinson’s disease. Int. J. Immunopathol. Pharmacol. 24 (2), 313–322. doi:10.1177/039463201102400205
Okagu, I. U., Ndefo, J. C., Aham, E. C., and Udenigwe, C. C. (2021). Zanthoxylum species: A comprehensive review of traditional uses, phytochemistry, pharmacological and nutraceutical applications. Molecules 26 (13), 4023. doi:10.3390/MOLECULES26134023
Oliveira De Melo, J., Truiti, M. D. C. T., Muscará, M. N., Bolonheis, S. M., Dantas, J. A., Caparroz-Assef, S. M., et al. (2006). Anti-inflammatory activity of crude extract and fractions of Nectandra falcifolia leaves. Biol. Pharm. Bull. 29 (11), 2241–2245. doi:10.1248/BPB.29.2241
Ondeyka, J. G., Jayasuriya, H., Herath, K. B., Guan, Z., Schulman, M., Collado, J., et al. (2005). Steroidal and triterpenoidal fungal metabolites as ligands of liver X receptors. J. Antibiotics 58 (9), 559–565. doi:10.1038/ja.2005.76
Pallikonda, G., Hsieh, C. Y., Su, H. L., and Hsieh, J. C. (2019). A combined pathway for the synthesis of nitidine family alkaloids. Res. Chem. Intermed. 45 (11), 5399–5407. doi:10.1007/s11164-019-03977-z
Pan, X., Dutta, D., Lu, S., and Bellen, H. J. (2023). Sphingolipids in neurodegenerative diseases. Front. Neurosci. 17, 1137893. doi:10.3389/FNINS.2023.1137893
Park, I. H., Jeon, S. Y., Lee, H. J., Kim, S. I., and Song, K. S. (2004). A beta-secretase (BACE1) inhibitor hispidin from the mycelial cultures of Phellinus linteus. Planta Medica 70 (2), 143–146. doi:10.1055/S-2004-815491
Paula, P. C., Maria, S. G. A., Luis, C. H., and Patricia, C. G. G. (2019). Preventive effect of quercetin in a triple transgenic alzheimer’s disease mice model. Molecules 24(12), 2287. doi:10.3390/MOLECULES24122287
Plazas, E. A., Avila, M. C., Delgado, W. A., Patino, O. J., and Cuca, L. E. (2018). In vitro antioxidant and anticholinesterase activities of Colombian plants as potential neuroprotective agents. Res. J. Med. Plants 12 (1), 9–18. doi:10.3923/RJMP.2018.9.18
Ponci, V., Figueiredo, C. R., Massaoka, M. H., De Farias, C. F., Matsuo, A. L., Sartorelli, P., et al. (2015). Neolignans from Nectandra megapotamica (lauraceae) display in vitro cytotoxic activity and induce apoptosis in leukemia cells. Molecules 20, 12757–12768. doi:10.3390/MOLECULES200712757
Rehman, S., and Khan, H. (2017). Advances in antioxidant potential of natural alkaloids. Curr. Bioact. Compd. 13 (2), 101–108. doi:10.2174/1573407212666160614075157
Swerdlow, R. H., Parks, J. K., Miller, S. W., Tuttle, J. B., Trimmer, P. A., Sheehan, J. P., et al. (1996). Origin and functional consequences of the complex I defect in Parkinson’s disease. Ann. Neurology 40 (4), 663–671. doi:10.1002/ANA.410400417
Takao, T., Kitatani, F., Watanabe, N., Yagi, A., and Sakata, K. (1994). A simple screening method for antioxidants and isolation of several antioxidants produced by marine bacteria from fish and shellfish. Biosci. Biotechnol. Biochem. 58 (10), 1780–1783. doi:10.1271/BBB.58.1780
Tavares, D. L. C., Zanon, G., Weber, A. D., Neto, A. T., Mostardeiro, C. P., Da Cruz, I. B. M., et al. (2014). Structure-activity relationship of benzophenanthridine alkaloids from Zanthoxylum rhoifolium having antimicrobial activity. Plos One 9 (5), e97000. doi:10.1371/JOURNAL.PONE.0097000
Tenorio, M. (2016). Flavonoids extracted from orange peelings tangelo (Citrus reticulata x Citrus paradisi) and their application as a natural antioxidant in sacha inchi (Plukenetia volubilis) vegetable oil. Sci. Agropecu. 7, 419–431. doi:10.17268/SCI.AGROPECU.2016.04.07
Verbon, E. H., Post, J. A., and Boonstra, J. (2012). The influence of reactive oxygen species on cell cycle progression in mammalian cells. Gene 511 (1), 1–6. doi:10.1016/J.GENE.2012.08.038
Voulgaropoulou, S. D., van Amelsvoort, T. A. M. J., Prickaerts, J., and Vingerhoets, C. (2019). The effect of curcumin on cognition in alzheimer’s disease and healthy aging: A systematic review of pre-clinical and clinical studies. Brain Res. 1725, 146476. doi:10.1016/J.BRAINRES.2019.146476
Wang, Y. H., and Du, G. H. (2009). Ginsenoside Rg1 inhibits β-secretase activity in vitro and protects against Aβ-induced cytotoxicity in PC12 cells. J. Asian Nat. Prod. Res. 11(7), 604–612. doi:10.1080/10286020902843152
William Langston, J. (1985). MPTP and Parkinson’s disease. Trends Neurosci. 8 (C), 79–83. doi:10.1016/0166-2236(85)90031-1
Yan, D., Zhang, Y., Liu, L., and Yan, H. (2016). Pesticide exposure and risk of alzheimer’s disease: A systematic review and meta-analysis. Sci. Rep. 6, 32222. doi:10.1038/SREP32222
Zhang, X., Wang, L., Li, B., Shi, J., Xu, J., and Yuan, M. (2023). Targeting mitochondrial dysfunction in neurodegenerative diseases: expanding the therapeutic approaches by plant-derived natural products. Pharmaceuticals 16 (2), 277. doi:10.3390/PH16020277
Keywords: Zanthoxylum, Nectandra, reactive oxygen species, antioxidative effect, neuroprotection, plant extract
Citation: Bustos-Rangel A, Muñoz-Cabrera J, Cuca L, Arboleda G, Ávila Murillo M and Sandoval-Hernández AG (2023) Neuroprotective and antioxidant activities of Colombian plants against paraquat and C2-ceramide exposure in SH-SY5Y cells. Front. Nat. Produc. 2:1169182. doi: 10.3389/fntpr.2023.1169182
Received: 19 February 2023; Accepted: 24 July 2023;
Published: 11 August 2023.
Edited by:
Mohamed L. Ashour, Ain Shams University, EgyptReviewed by:
Benita Wiatrak, Wroclaw Medical University, PolandDoaa A. Ghareeb, Alexandria University, Egypt
Copyright © 2023 Bustos-Rangel, Muñoz-Cabrera, Cuca, Arboleda, Ávila Murillo and Sandoval-Hernández. This is an open-access article distributed under the terms of the Creative Commons Attribution License (CC BY). The use, distribution or reproduction in other forums is permitted, provided the original author(s) and the copyright owner(s) are credited and that the original publication in this journal is cited, in accordance with accepted academic practice. No use, distribution or reproduction is permitted which does not comply with these terms.
*Correspondence: Adrián G. Sandoval-Hernández, YWdzYW5kb3ZhbGhAdW5hbC5lZHUuY28=