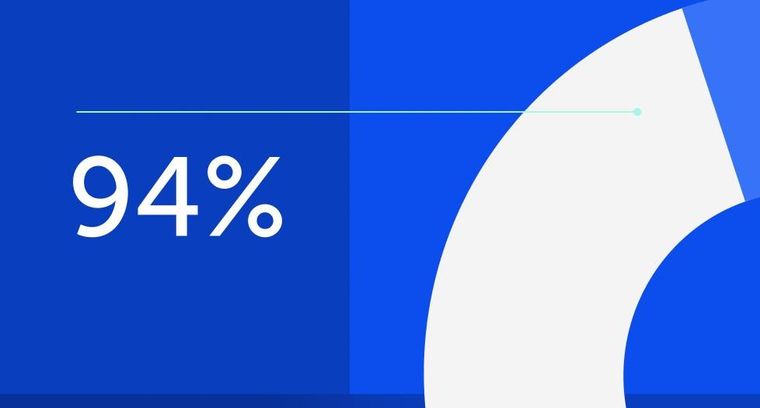
94% of researchers rate our articles as excellent or good
Learn more about the work of our research integrity team to safeguard the quality of each article we publish.
Find out more
REVIEW article
Front. Nat. Prod., 28 November 2022
Sec. Isolation and Purification
Volume 1 - 2022 | https://doi.org/10.3389/fntpr.2022.1043147
This article is part of the Research TopicFrom Chemistry to Therapeutics: Exploring the Universe of Cannabinoids and Related MeroterpenoidsView all 5 articles
Cannabinoids are gaining attention in many sectors, including the pharmaceutical, nutraceutical, and cosmetic sectors. To date, several conventional and alternative techniques have been applied for Cannabis sativa L (C. sativa L.) extraction at the industrial scale. The conventional methods are liquid solvent extraction, including polar and non-polar solvents such as ethanol, hexane, petroleum ether, and other solvent extraction. Pressurized gas extraction is another conventional method and comprises gaseous hydrocarbon extraction such as n-butane and n-propane, supercritical or subcritical carbon dioxide extraction, and extraction using a refrigerant gas such as 1,1,1,2-tetrafluoroethane (HFC 134a). Alternative extraction methods include microwave-assisted extraction (MAE), ultrasound-assisted extraction (UAE), hydrodynamic cavitation, and pulsed electric fields (PEF). This review thoroughly analyzes and compares the main extraction techniques and technologies at the industrial scale found in the patent literature. In addition, several aspects of the pretreatment of cannabis plant material and its influence on extraction are also discussed.
The methodology used to extract cannabinoids from cannabis plant matter must be carefully selected according to key considerations. Extraction yield (mass extracted vs. mass of plant material), extraction efficiency (extracted cannabinoids vs. cannabinoids present in the plant material), and extract quality are crucial factors, in addition to environmental, safety, and scalability considerations (Radoiu et al., 2020). The characteristics of the desired final product determine the required quality of the extract in terms of cannabinoid purity and the presence of additional compounds with combined synergistic effects, such as terpenes or flavonoids. In general, the extraction method must preserve the biological and pharmaceutical properties of the cannabinoids while providing an acceptable extraction yield (Baldino et al., 2020).
Overall, cannabinoid extraction techniques can be classified as conventional, such as liquid solvent extraction or pressurized gas extraction (including butane or subcritical or supercritical fluid extraction), and innovative or alternative, such as extraction with hot gas, ultrasonic-assisted extraction (UAE), microwave-assisted extraction (MAE), hydrodynamic cavitation, and pulsed electric field (PEF).
Among the main parameters affecting the extraction process, the most important are the solvent nature and affinity with the target compounds, temperature, pressure, mixing rate, solvent-to-vegetable matter ratio, contact time, and particle size of the plant material (pre-treatment) (Ramirez et al., 2019; Baldino et al., 2020). These parameters must be properly selected to improve the extraction yield while enabling the scalability of the process. Successfully scaling up an extraction process is difficult since many factors are intertwined and must be taken into account, including safety, analytical, chemical, and engineering considerations; the availability of the required substances; installation and operating costs and times; and environmental and legal restrictions such as waste disposal (Radoiu et al., 2020). Special considerations are also required for the selection of the extraction solvent and its impact on process scale-up. For example, an increased solvent-to-plant material ratio will enhance the driving forces for diffusion and increase the extraction rate. In contrast, lower solvent ratios reduce the time and energy required for solvent removal, recycling, or disposal volume, at the expense of the extraction yield.
Another factor to consider in solvent selection is solvent toxicity due to residual solvents in the final pharmaceutical/nutraceutical product. These solvents are not eliminated during manufacturing and their maximum concentrations in the final product are regulated by International Council for Harmonization (ICH) guidelines. These standards list the class to which each solvent belongs as well as the maximum concentration limit allowed for each residual solvent in the final pharmaceutical products (Table 1).
Class I solvents cannot be used due to their high toxicity. Class II solvents have lower toxicity, and their use must be limited to avoid possible adverse effects. Finally, class III solvents are the least toxic but should only be used as appropriate. For instance, ethanol, which is class III, is less toxic than hexane, which is class II. Thus, CO2, a pressurized gas that leaves no residue in the final product, appears to be the least toxic type of extraction if no co-solvents are used in the process.
Solvents can be classified as polar or non-polar. Ethanol is the most widely used polar solvent for cannabinoid extraction, while typical non-polar solvents include petroleum ether, propane, butane, hexane, and CO2. Due to the concentration gradient between the biomass and the solvent, cannabinoids diffuse from the glandular trichome head and dissolve in the liquid solvent during extraction. Diffusion is the rate-limiting step in extraction and determines the production output (Shuja, 2018). Therefore, the solubility and diffusivity of the cannabinoids in the solvent are crucial parameters affecting the process efficiency and selectivity. Solubility and diffusivity are strongly related to the nature of the extraction solvent, particle size of the plant matter, solvent-to-raw material ratio, extraction temperature, and maceration time (Radoiu et al., 2020).
The cannabinoid profile of the plant material must also be considered, since non-decarboxylated raw material contains the cannabinoids mainly in their acid forms, which are more soluble in polar solvents than neutral cannabinoids, whose solubility in polar solvents is very low. Therefore, selecting a solvent with appropriate polarity is essential to selectively dissolve the desired cannabinoids and avoid the co-extraction of unwanted substances, including nitrogenous compounds, amino acids, sugars, aldehydes, alcohols, ketones, flavonoids, glycosides, vitamins, pigments, and terpenes, among others (Whittle et al., 2008; Baldino et al., 2020). For example, non-polar solvents remove lipid-soluble material such as fats and waxes from the biomass, while polar solvents dissolve a variety of polar compounds present in the cannabis plant such as chlorophyll, terpenes, or alkaloids, which affect the quality of the final product in terms of aroma, flavor, and consistency, unless post-processing steps are performed to remove these impurities (Whittle et al., 2015). Moreover, low extraction selectivity decreases the relative concentrations of the desired cannabinoids in the final product.
However, even if all the above-mentioned extraction parameters are optimized, currently available techniques cannot dissolve all the cannabinoids present in the raw material; they can only extract a small fraction of the cannabinoids, and undesired compounds are often co-extracted due to the lack of efficiency and selectivity of these methods (Goldner, 2019). Therefore, to increase the solubility of cannabinoids in the solvent, pre-treatment steps to improve the accessibility of the cannabinoids are commonly reported in the patent literature. However, some pre-treatments can disrupt plant cells, which facilitate the release of undesired compounds. Therefore, the advantages and disadvantages of the pre-treatment processes must be carefully considered regarding their effects on extraction. The next sections analyze the pre-treatment of the cannabis plant material, including state-of-the-art technologies and challenges of the available extraction techniques.
Harvesting biomass is the first step of the global process, usually followed by treatment of the harvested biomass to make it suitable for extraction. Cannabis plant matter contains active chemicals such as cannabinoids, terpenes, and other phenolic compounds that are produced and stored in the trichomes, which are glands on the surface of the female plant’s buds, mainly in the flowers. Trichomes function as a protection from winds and fungal growth and as a defense mechanism against predators due to their bitter taste and strong smell (Tegen et al., 2019; Koumans, 2021). Glandular trichomes have a crystalline appearance; when handled, they release a resin rich in cannabinoids that can be extracted. However, minimal amounts of the resin are formed in non-glandular tissue, such as leaves, roots, or stems, which cannot be extracted by directly soaking intact plant material in a solvent (Fairbairn, 1972; Fairbairn and Liebmann, 1973; Andre et al., 2016). The accessibility of the trichomes can be increased by pre-treating the biomass, and the cannabinoids contained in non-glandular material can be extracted after pre-treatment consisting of air-drying and powdering (Fairbairn and Liebmann, 1973; Koumans, 2021). Obtaining plant material with the highest trichome concentration is crucial for the extraction of cannabinoids such as CBG, CBC, CBD, THC, and CBN (Dijkstra, 2019; Koumans, 2021).
Typical treatment of the harvested cannabis biomass includes physical processing to separate the cannabinoid-rich flowers and leaves from the stems, drying to a moisture content of 0–15 wt%, and other practices such as milling and sieving the plant material < 0.5 mm (Raber and Elzinga, 2017; Ko and Hughes, 2019; Ko, 2020). Biomass particle size affects extraction kinetics, as particles that are too small can produce channeling phenomena, clogging phenomena, and overpressure in liquid extraction, which negatively affect extraction performance. In contrast, too-large particles result in increased extraction times (Baldino et al., 2020). When the stems and roots of the cannabis plant are used as raw material, they are cut into small pieces before being placed into the extraction vessel. However, size reduction before extraction is not usually performed for flowers and leaves (Keller, 2018). In some cases, plant material is cut or chopped into pieces to facilitate drying to reduce the moisture present, which negatively affects extraction efficiency due to the higher total weight of humid material compared to dry material (Keller, 2018; Koumans, 2021).
Drying is a complex process and is difficult to optimize and implement at an industrial scale due to the requirement for long periods and large spaces. Moreover, the energy consumption during this practice affects the overall costs, and uniformly drying the plant material is a challenge that can affect the product quality (Challa et al., 2021). Cannabis plants can be naturally dried in a dark storage room with adequate temperature and humidity levels, a method known as “slow drying” (Hawes and Cohen, 2015; Challa et al., 2021). The plant material can be hung upside down or spread on drying screens, although the latter technique results in heterogeneous drying due to differences in bud sizes. However, natural drying requires an extended time (5–6 days) and generates large pieces of plant material. In addition, extended drying periods and uncontrolled environmental conditions can lead to mold growth because of incomplete drying (Challa et al., 2021). A common practice in the industry is to use dehumidifiers to dry cannabis plant material (Hawes and Cohen, 2015; Challa et al., 2021). To meet the challenge of scaling up the extraction processes and the drying step, oven drying, hot-air drying such as spray drying, and microwave drying have been used to speed the drying process and avoid long periods between harvesting and extraction. However, heat can alter bioactive compounds and evaporate volatile terpenes while microwaves may compromise the concentration of psychoactive cannabinoids (Mujumdar, 2006; Shuja, 2018; Rivas, 2019).
Freeze-drying may also facilitate the extraction of substances from cannabis plant material (Goldner, 2019). Freeze-drying or lyophilization is a sophisticated method that reduces the pieces of plant matter to a powder, which breaks the plant cells to release the active compounds. Since this process operates under vacuum at low temperatures, the loss of volatiles and the degradation of other valuable components is considerably reduced compared to other drying techniques, resulting in dried cannabis of higher quality that contains the desired cannabinoids and terpenoids (Mujumdar, 2006; Challa et al., 2021). However, the use of vacuums is expensive and requires high energy consumption. Thus, freeze-drying is more expensive than slow drying and costs 4–10 times more than hot-air drying such as spray drying (Challa et al., 2021). Consequently, its use is limited to high-value products such as cannabinoids. This method is described in patent US20190358278A1 (Goldner, 2019) to improve the efficiency of cannabinoid extraction with liquid or supercritical carbon dioxide. According to the invention, the biomass is placed into a freeze dryer and chilled to a freezing temperature that makes the moisture transition to ice crystals, with a subsequent expansion, thereby destroying microscopic structures of the plant matter and exposing cannabinoids that were difficult to extract. The temperature is preferably below the triple point of water to ensure the sublimation of the ice crystals. The result is a powder of cannabis plant matter of significantly smaller particle size than that produced by natural drying. The invention claims that the freeze-dried plant matter has relatively uniform particle size and higher surface area, which improve the access of the carbon dioxide during the extraction process (increasing the proportion of extracted cannabinoids) and the potential weight of biomass that can be used in the extraction vessel compared to the naturally dried biomass, with larger particle size.
Patent US20180099236A1 (Shuja, 2018) describes a method to accelerate the drying and extraction steps of target compounds from cannabis plants by applying pulsed electric fields (PEF). According to the authors, processing times are reduced compared to extraction without PEF pre-treatment, increasing the production and overcoming two important limitations of conventional drying/extraction techniques; namely, the preservation of thermally labile compounds and the reduction of the diffusion kinetics limitation of the solvent into the plant cells. The application of a rapid and intense electric pulse (in the range of 0.1–12 kJ/kg at 20–30 kV) to the plant material positioned between two electrodes causes electroporation or modification of the cell membrane within a millisecond, with the creation of nanopores that increase the diffusion coefficients to facilitate the migration of molecules into the external solvent. By precise tuning the electric field strength, number of pulses, duration, amplitude, and frequency, the nanopore size can be controlled to allow the selective release of molecules based on their atomic radius: small water molecules can be easily released, while large organic molecules are retained until they contact a solvent during extraction. Combining PEF with pressing (applied weight of 1–2 tons or pressure of 50–500 bar), water molecules can be rapidly removed through the nanopores to <10wt% water content without substantial loss in the available cannabinoid content. While drying by natural convection requires several days, the application of PEF at 6 kJ/kg and subsequent compression at 100 bar, followed by laying out the plant material on a tarp, the plant material can be dried in 2 h with a temperature increase of no more than a few degrees during the drying process, thus preserving thermally labile compounds. Extraction benefits from the nanopores, with accelerated solvent penetration into the plant and the selective removal of target compounds. According to the authors, combining PEF pretreatment with supercritical CO2 extraction in a separated stage allows extraction at 1,100–2000 psi (76–138 bar), such as 1,200 psi (83 bar) at lower temperatures, ideally 28–35°C, resulting in a THC-rich extract with low lipid content that requires less exhaustive post-processing steps for lipid removal. After 1–3 h, the extraction of compounds slows; to target CBD and any residual cannabinoids, the pressure can be increased to 1,500–2,500 psi (103–172 bar) at 20–60°C. As described in the invention, PEF pretreatment delays the requirement for a higher-pressure SFE stage. For comparison, the pressure and temperature conditions for supercritical CO2 extraction without PEF pretreatment usually range from 100 to 300 bar and from 35 to 60°C (Whittle et al., 2008; Eiroa et al., 2016a; Popp et al., 2019).
Nevertheless, the extraction of cannabinoids from intact fresh plant material that has not been pretreated is also reported in the patent literature when the co-extraction of volatile terpenes is desired (Koumans, 2021). Moreover, the extraction of fresh plant material is an energy-efficient process that does not require high temperatures during the drying step. Overall, the use of intact plant material not only minimizes the loss of volatile components but also avoids damaging the cell structures and, thus, the release of contaminants such as chlorophyll (Koumans, 2021). However, intact fresh plant material has a larger size than dried material; therefore, closed chambers or containers are needed to preserve the harvested material until extraction.
In some cases, the drying step can be simultaneously performed with the decarboxylation of the plant material in a single heating step or in a multi-step heating process to convert the acid cannabinoids into their neutral form (Flockhart et al., 2010). Decarboxylation can be avoided when the compounds of interest are cannabinoids in their acid forms. Decarboxylation is a function of time and temperature, which depend on the desired cannabinoid. In addition, decarboxylation conditions must be properly selected to minimize the thermal degradation of cannabinoids, particularly the degradation of Δ9-THC to cannabinol (CBN). Plant material can be decarboxylated before extraction when non-polar solvents such as hexane or supercritical CO2 are used since neutral cannabinoids show higher solubility in non-polar solvents due to their lower polarity compared to the acidic forms (Flockhart et al., 2010; Cid and Van Houten, 2015; Grijó et al., 2019; Baldino et al., 2020). Decarboxylation can also be carried out after extraction not only to avoid negative effects such as modification of the plant profile, combustion of the plant material, or terpene losses (Popek et al., 2019a; Nicola and Osmanoglou, 2019; Oroskar et al., 2019; Cipolletti et al., 2020) but also to avoid the use of large-scale ovens to perform decarboxylation. However, extraction yield is affected by the polarities of the solvent and the cannabinoids; thus, the extraction of a non-decarboxylated plant material (cannabinoids in their acidic forms) produces lower yields compared to the extraction of a decarboxylated plant material when using a non-polar solvent due to the lower polarity of neutral than acidic cannabinoids. Overall, cannabis plant material is subjected to pre-treatment depending on the nature of the extraction process and the desired final cannabinoid profile. The pre-treated (or not) biomass is then extracted to obtain the cannabinoids and remaining terpenes.
Liquid solvent extraction consists of the maceration of plant material containing cannabinoids in polar or non-polar organic solvents (Ramirez et al., 2019). The plant material is immersed and stirred for several hours or days in the solvent, generally at room temperature or colder. Several (2–3) extraction stages are typically performed to completely extract the target cannabinoids, increasing the use of solvent and energy.
After extraction, the solvent is commonly removed by evaporation at temperatures preferably < 60°C to produce a concentrated extract, since the presence of solvent residues compromises the quality of the product for its further application (Whittle et al., 2015). Very low solvent residues are allowed, especially for pharmaceutical purposes. For example, the pharmaceutical limits for residual solvents set by the ICH Guideline for drug products are 290 ppm for hexane, considered a solvent to be limited, and <5,000 ppm for ethanol, considered as lower risk to human health (International Council for Harmonisation, 2020). In addition to rotary evaporators or distillation units, the liquid solvent extraction technique usually comprises the use of a centrifuge or a filter unit to separate solid plant matter or impurities from the extract.
Despite the simplicity of the liquid solvent extraction technique, the disadvantages of this method should be considered, including the relatively high solvent consumption, which must be evaporated or separated from the biomass, the presence of solvent residues in the final extract, environmental concerns, and the co-extraction of unwanted products and their subsequent removal in downstream purification steps (Baldino et al., 2020; Radoiu et al., 2020). Nevertheless, liquid solvent extraction is performed when the convenience of simplicity outweighs these downsides. Table 2 summarizes the important features of liquid solvent extraction.
Since there exists no ideal selective solvent with no toxicity or flammability, the decision to use one solvent or another relies on the balance between their selectivity, toxicity, volatility (which determines the ease with which they are evaporated from the extract), and their flammability (which poses a risk for explosion), which compromise the scalability of the process. The performance of the most common liquid solvents used for cannabinoid extraction, namely, ethanol, liquid hydrocarbons such as hexane and petroleum ether, as well as other solvents (other organic compounds and mixtures, vegetable oils, etc.), is discussed and compared below to establish the most suitable solvent for a specific use according to their characteristics, advantages, and downsides.
Ethanol is one of the most widely used solvents for the extraction of cannabinoids (Ivanov, 2018a; Marshall and Campbell, 2019; Qu and Cui, 2020). Due to its polar nature and high solvent power, ethanol dissolves both cannabinoids and terpenoids as well as non-desired water-soluble molecules like chlorophyll, glycosides, sugars, and alkaloid salts that must be removed in a large number of post-processing steps to meet high purity specifications (Whittle et al., 2015; Ramirez et al., 2019). Although in less magnitude than non-polar solvents, ethanol also dissolves lipid material so that a winterization step may be required to remove waxes. Advantageously, the winterization step may not be as exhaustive as when using a non-polar solvent for extraction. Ethanol is a generally recognized as a safe (GRAS) solvent, safer than other solvents like butane, and its use does not require high pressures (Oroskar et al., 2019). Table 2 summarizes the most relevant facts included in this section.
Cold extraction; that is, extraction with ethanol at low temperatures (5°C to -80°C) is an advantageous modification that allows the extraction of cannabinoids and terpenoids while also minimizing the co-extraction of chlorophyll and other compounds such as triglycerides and waxes present in the plant (Ko and Hughes, 2019; Farokhi et al., 2020). However, extraction with cold ethanol increases the energy consumption to cool the extraction device. In general, ethanol extraction of bio-compounds is convenient in cases where minor solvent residues are allowed in the final product or at low temperatures in applications in which the terpenoid profile of the plant must be preserved (Splinter et al., 2019a).
Published methods for cannabinoid extraction using ethanol at room temperature include purification steps such as filtration and decolorization to remove unwanted compounds (Oroskar et al., 2019). For instance, the US patent publication Nº 10189762 B1 (Oroskar et al., 2019) describes a method to produce high-purity CBD products (>95% purity) on a commercial scale from dried hemp and cannabis leaves extracting the cannabinoids by soaking 150 Kg of plant material in ethanol (600 L) at room temperature and atmospheric pressure for 2 h with agitation. The mixture stands overnight for 8–12 h to form the first ethanol layer. Then, the ethanol containing the cannabinoids and other impurities is removed by decantation and the raw material is mixed again with ethanol (400 L) for 2 h with agitation, followed by decantation, and the remaining wet material is pressed, resulting in spent plant material and a third liquid decanted extract, which is combined with the first and second decanted ethanolic extracts. The total solvent-to-plant material ratio used for the extraction is 6.66 L/kg. The combined decanted extract contains cannabinoids including CBD, THC, CBDA, and THCA, as well as small particles, chlorophylls, color bodies, sugars and carbohydrates, lipids, plant waxes, and other impurities. After the first filtration step, the filtered crude extract comprises 3.4–3.7 wt% total cannabinoids in the liquid mixture. Then, the crude cannabinoid extract is subjected to a sequence of purification steps including decolorization, decarboxylation, dewaxing, a continuous simulated moving bed process, polishing, and crystallization in hexane at −20°C or less for 24–72 h, followed by recrystallization in hexane at room temperature for 24–72 h, to obtain high purity THC-free CBD crystals (above 99 wt%). The purification steps will be discussed further in the purification section.
Invention US10413843B2 (Ko and Hughes, 2019) describes an extraction process from cannabis plant material using ethanol at −45°C, considered the optimum extraction temperature. The extraction occurs in a column pressurized at 70–280 kPa (0.7–2.8 bar), while the selected pressure must be low enough to prevent clogging of the column. The plant material soaks for 5 min (when the temperature is below −40°C) or the ethanol is pumped continuously, using approximately 50 L of ethanol for every 5 kg of plant material (10 L/kg). The described filtration stage involves the successive addition, mixture, and filtration by charcoal, clay, and silica particles to remove fats, lipids, waxes, chlorophyll, heavy metals, and other undesirable compounds from the extracted crude oil and ethanol mixture. Although filtration is always required, this purification stage may be less exhaustive if extraction has been performed using chilled ethanol. In contrast, filtration is essential after extraction with ethanol at room temperature, to obtain an extract with low content of non-desired substances. Extraction using chilled ethanol is slightly less efficient with respect to extraction yield; however, the general process is more efficient since chilled ethanol minimizes the extraction of fats, waxes, and lipids; thus, exhaustive post-filtration steps are not needed. Although the amount and kind of impurities to be removed from the extract depend on the type of plant material, in general, room-temperature ethanol produces 15% more extract than chilled ethanol since the latter has reduced solvent power. The same company patented an extraction system using cold ethanol (US10646793B2 (Ko, 2020)). Similar to US10413843B2, the described system for the extraction of cannabinoids, such as CBD and THC, from cannabis plant material combines chilled ethanol and a centrifuge (soaking time of 5–20 min before spinning), followed by filtration, evaporation, decarboxylation, and distillation using a wiped-film apparatus. Ethanol is chilled to a preferred temperature of −45°C. The author states that more filtering is required for warmer temperatures, which means that additional treatment steps are needed to remove a very small number of impurities (waxes, lipids, and fats) produced even when chilled ethanol is used. These impurities present in the crude oil and ethanol mixture are removed by adsorbent media treatment (i.e., charcoal, clay) and filtration in a chilled state. The filtration system comprises a plurality of filter stages containing vertically oriented filters of decreasing pore size. The mixture of oil and ethanol is forced through the filters using a pressurized gas, such as nitrogen, at 70–210 kPa (0.7–2.1 bar). Following filtration, the ethanol is evaporated and the extract is decarboxylated and further purified by wipe film distillation. Overall, the invented system produces pure or nearly-pure distillate oil starting from 36 kg of raw plant material over 12 h, using a solvent-to-raw material ratio of approximately 40–50 L of ethanol for every 5 kg of cannabis material (8–10 L/kg).
Using cold ethanol (from −60°C to −40°C) for the large-scale extraction of cannabinoids such as CBD or THC is also described in patent US10493377B1 (Ferraro et al., 2019), which reports a system for continuous biomass extraction and centrifugation. According to the authors, the system provides constant and repeatable extraction results and includes biomass and solvent feed tanks, several extraction vessels, and a centrifuge coupled in-line. The process is arranged to operate in a continuous or quasi-continuous manner as multiple extraction and centrifugation steps take place simultaneously. As described, a first slurry is formed in the first vessel, when the first portion of biomass is combined with ethanol in a typical ratio of 1:5 (80 kg of biomass and 400 kg of ethanol) for 12 min, during which the second portion of biomass and solvent is sent to the second vessel to form a second slurry. The first slurry is centrifuged for 4 min to separate the first extract and waste discharges, followed by centrifugation of the second slurry, and so on. After centrifugation of each batch, the extract discharges may be subjected to further filtering or purification to eliminate the solvent and obtain the cannabinoid in a substantially pure form. The authors do not provide any extraction yield or efficiency data, as the patent is a mere description of the process technology.
Cold ethanol extraction is also described in patent US10717717B1 (Castillo, 2020), which reports a process to produce refined and decarboxylated cannabis extracts from freshly harvested, pre-frozen cannabis including the inflorescence, floral leaves, and small stems of flowering cannabis plants. The frozen plant material is pulverized by cryogenic grinding and transferred into filter bags (pore diameter 220 μm, nominal capacity of 3.78 L), weighing about 450 g. The authors recommend a temperature of 0–10°C for the cryogenic grinding to minimize decarboxylation and the loss of volatile compounds. Two filter-bags of pulverized material are placed into the extraction apparatus, a vertical cylindrical drum rotating at 40 rpm, and extracted for 10 min with 40 L of alcohol (ethanol or isopropyl alcohol, solvent-to-plant material ratio of 44 L/kg), that is preferably pre-chilled to −30°C to minimize the co-extraction of chlorophyll and lipid material. The alcohol extract is filtrated to remove undissolved plant material, producing a particulate “remainder fraction”, a colorless cake recovered from the surface of the filter, and a filtrate that may be subjected to decarboxylation. The remainder fraction contains plant waxes and lipids, carbohydrates, as well as very small particles of undissolved plant material, and can be added to therapeutic formulations producing an “entourage effect” with the active cannabis compounds. When extraction is performed at higher temperatures such as 0°C, a larger volume of the remainder fraction is formed with a green color, indicating increased co-extraction of chlorophyll and lipids. The alcohol is removed from the filtrate by rotary evaporation at 40°C, yielding 300 ml of extract for every two filter-bags of pulverized cannabis. The extract is then diluted with alcohol and water. According to the authors, the addition of water to the diluted extract inhibits the formation of CBN and the degradation of terpenes and cannabinoids, although no supporting information is provided. The diluted extract is refluxed at preferably 80°C for 2 h to promote the decarboxylation of THCA to Δ9-THC, followed by rotary evaporation of the diluents, yielding a refined, decarboxylated cannabis extract. Finally, the invention describes a method to enrich the refined cannabis extract with Δ8-THC, by mixing the extract with bleaching clay and activated charcoal and distilling at 157–220°C in a short-path distillation apparatus.
Another example of cold ethanol extraction to produce a purified cannabis extract is described in US20210008138A1/WO2019130201A1 (Eyal and Zeitouni, 2022), although the provided examples are laboratory-scale. The peculiarity of this invention is that after extraction with ethanol (containing 5–20 wt% of water) at frozen or room temperature with agitation for at least 0.5 min and <15–30 min, the extract is maintained at temperatures below zero for the addition of water (20–40 wt%) in a subsequent step, so that some cannabinoids, waxes, or colored impurities precipitate and are separated from the resulting extract by decantation, filtration, or centrifugation. The extract comprises ethanol, cannabinoids, water, terpenes, and some other impurities. The hydroalcoholic solvent comprising water and ethanol is then evaporated from the extract. The invention provides some laboratory examples of the extraction of 100 g of dried and ground cannabis buds with 1 kg of >95% ethanol (ratio of 12.7 L/kg) at −20°C and 25°C with agitation during 10 and 30 min, respectively. After precipitate removal, the filtrates were evaporated to remove ethanol and decarboxylated. Although the authors do not provide the extraction yield or the amount of extract, the best result in terms of cannabinoid content was obtained at −20°C with ethanol (>95%) for 10 min. Under these conditions, a light brown extract with 3% THC and 68% CBD was obtained after decarboxylation. For comparison, extraction at 25°C with >95% ethanol for 30 min produced a dark brown extract with 3% THC and 64% CBD after decarboxylation. These results suggest purer and less colored extracts (and, thus, fewer colored impurities) at lower temperatures and shorter contact times. These results are similar to those reported by Moreno-Sanz et al. (2020) for extraction with ethanol (three times: 1 + 0.75+0.75 L, 2.5 L of ethanol in total, ratio of 25 L/kg) at room temperature of 100 g of the CBD-rich Sara variety (CPVO 2015/0098, 7–10.5% total CBD), producing a 20.1% extraction yield and 67.14% CBD after decarboxylation of the extract. Another example shows the effect of water addition on CBD purity. After 30 min of extraction with ethanol (>95%) at 25°C with agitation, the solution is filtrated and water is added to the filtrate, forming a dark precipitate. After overnight refrigeration, the extract is filtered and the filtrate is evaporated and decarboxylated, producing a light brown extract with 3% THC and 88% CBD. The authors report that the cannabinoid content decreases from 7.92 g to 5.54 g after the addition of water, so that 30% of the cannabinoids co-precipitate with colored impurities.
Additionally, cold ethanol extraction can be used in combination with supercritical CO2 extraction to separately extract terpene and cannabinoid oils, as described in patent US10507404B2 (Tucker, 2019). The invention reports a method for producing a terpene-enhanced cannabinoid concentrate, in which terpene oil is extracted using supercritical CO2 (26–43°C, 1,000–1,300 psi [69–89 bar]) and the cannabinoid concentrate is extracted from the residual cannabis material (which is dried and cryogenically frozen) using cold ethanol extraction (<0°C). The ethanol is removed by vacuum distillation, producing an oil containing 50–90% cannabinoids (for example THCA, THC, THCV, CBDA, CBD, CBC, CBG, CBN), and up to 20% terpenes. The cannabinoid oil can be concentrated by distillation to separate the cannabinoid distillates from the residual contaminants, obtaining cannabinoid concentrations of 80–99.99%. Terpenes are then added back to the purified cannabinoid concentrate to enhance the flavor. Patent US9199960B2 (Ivanov, 2018b) describes a cannabinoid extraction method that involves initially freezing the plant material for 24 h, followed by decarboxylation, drying, and grinding of the plant material into a powder form, and soaking the dried plant powder in a solvent for at least 24 h at room temperature, where the solvent is ethanol or a mixture of isopropyl alcohol and hexane. The authors mentioned two examples of solvent-to-plant powder ratio: 31 g of ground plant matter in 300 ml of solvent (9.7 L/kg) and 13 g of ground plant matter in 100 ml of solvent (7.7 L/kg). The solvent/powder mixture is frozen for 12 h and subsequently shaken, heated to 80–90°C for 20–30 min, and then filtered to remove the spent plant material. Afterward, the cannabinoid extract is obtained after evaporation of the solvent. For example, 300 ml of filtered material yields 7.5–8 ml of the evaporated extract containing high concentrations of THC, CBD, CBN, and terpenes. The patented method is said to increase the yield of cannabinoid extraction, particularly CBD extraction, but given that no numeric result is provided, it can only be assumed that the extraction improvement is associated with initially freezing the plant material and the low extraction temperatures.
Despite being one of the most widely used extraction solvents, most patents found in the literature lack numeric data on the performance of ethanol extraction. Only a few examples can be found from which the extraction yield can be calculated. For instance, patent US10864458B2 (Roura, 2017) describes the extraction by maceration in ethanol at room temperature for 1 h (repeated two more times, 3 h in total) of several cannabis varieties, including Carma (CBGA as predominant), Aida (Community Plant Variety Office [CVPO] number 2016/0167, CBGA_predominant), Futura 75 (CBDA predominant) and Pilar (CVPO 2016/0115, CBDA predominant). The starting plant materials, (50 g) of the CBGA-rich varieties Carma and Aida, were non-decarboxylated and were macerated with ethanol-to-plant-material ratios of 29.8 and 29.4 L/kg, respectively, with extraction yields of 9.4 and 18%. The extracts were then subjected to maceration and crystallization in hexane, yielding CBGA crystals with 90–95% purity (>95% purity for Carma). The CBD-rich varieties Futura 75 (100 g, 22.5 L/kg) and Pilar (50 g, 29.8 L/kg) were decarboxylated before extraction, with extraction yields of 5.8 and 9.8%, respectively. The CBD extracts from Futura 75 and Pilar were subjected to maceration and crystallization in petroleum ether, yielding CBD crystals with >95% purity. The same company reported a 16.4% extraction yield for ethanol at room temperature in continuous mode (1 L/min, 25 + 25 L, 19.3 L/kg) with the non-decarboxylated CBDA-rich variety Goya (2018/0113, 10.39% of CBDA + CBD). The obtained extract had a total CBDA + CBD content of 52.7% (extraction efficiency 83.1% from the CBDA + CBD in the plant material). However, after decarboxylation of the extract and subsequent purification by crystallization and recrystallizations in petroleum ether, the purity of the obtained CBD crystals was <98%.
The extracts reported by Phytoplant Research in patent US10864458B2 (Roura, 2017) were first macerated and then crystallized in petroleum ether or hexane, or purified by centrifugal partition chromatography (CPC), yielding isolated cannabinoids with >95% purity without performing additional purification steps or molecular distillation. For comparison, the CBD crystals (>99 wt%) reported by patent US10189762B1 (Oroskar et al., 2019) were obtained after numerous purification steps including decolorization, decarboxylation, dewaxing, a continuous simulated moving bed process, polishing, and crystallization in hexane. Multi-step processes tend to be complex and time-consuming, and show decreased yield due to the loss of compounds. Therefore, simpler purification processes are preferred. Moreover, wipe film distillation after filtration with charcoal, clay, and silica was required to obtain 95% CBD oil from hemp, as reported in patent US10413843B2 (Ko and Hughes, 2019). In summary, only Phytoplant Research (patent US10207199B2) (Roura, 2017) has reported the simple and efficient production of >95% pure CBD crystals from extracts produced by ethanol extraction at room temperature.
Moreno-Sanz et al. (2020) reported ethanol extraction yields for other cannabis varieties, in which they described the extraction of 100 g of non-decarboxylated plant material at room temperature (three times in ethanol: 1 + 0.75+0.75 L, 2.5 L of ethanol in total, ratio of 25 L/kg). The authors reported a 28% extraction yield for the THC-rich variety Moniek (CPVO number 2016/0114, 15.5–22% THC), obtaining an extract with 49.52% THCA and 10.25% THC (58.31% THC after decarboxylation). The CBD-rich varieties Sara (CPVO 2015/0098, 7–10.5% total CBD) and Pilar (CVPO 2016/0115, 4.5–8.5% total CBD) produced extracts with 20.1% and 12% extraction yields and 67.14% and 61.31% CBD after decarboxylation, respectively. Comparing the results for the extraction with ethanol of the Pilar variety in this study (12% yield, 25 L/kg) and the patent US10207199B2 (9.8% yield, 29.8 L/kg) show that the higher extraction yield of 12% reported by Moreno-Sanz et al. was obtained from non-decarboxylated Pilar material, whereas the plant material was decarboxylated before extraction in the example described in the patent. Although the extraction was performed with a lower ethanol-to-plant-material ratio (25 L/kg), the higher extraction yield can be explained by the greater affinity between the polarities of the ethanol with the non-decarboxylated plant material, which contains cannabinoids in their acid and more polar forms, in contrast to the neutral cannabinoids contained in the decarboxylated material. Therefore, the results confirmed higher yields for polar solvents such as ethanol, starting from non-decarboxylated materials.
Moreno-Sanz et al. also reported the preparation and extraction yield of other cannabinoid extracts, including CBG and CBDV. An extract with 45.9% CBGA and 9.7% CBG (56.14% of CBG after decarboxylation) was obtained with an 8.2% yield from Juani (CPVO number 2016/0117, CBG + CBGV 3–6%). Likewise, CBDV (15.05% in the decarboxylated extract) was obtained from Theresa (CPVO 2016/0116, CBD + CBDV 6–11%) with an 8.8% extraction yield.
The results reported by Moreno-Sanz et al. (2020) can be compared to those for the extraction yield reported in patent US20200383893A1 (Ham et al., 2020) for the laboratory-scale extraction of non-decarboxylated, dried, and finely-cut cannabis leaves in ethanol (1 h in an ultrasonic processor at 40% power, then 24 h at room temperature, 20 L/kg). After evaporation, an extract with 8.73% CBDA + CBD was obtained, with a 16.6% yield. Although this yield is in the same range as that reported by Moreno-Sanz et al. (2020) with the varieties Sara (20.1%) and Pilar (12%), the extraction efficiency cannot be compared since patent US20200383893A1 does not provide data on the total CBD content of the cannabis leaves. In addition, the extraction described by Moreno-Sanz is carried out with leaves and flowers. Nevertheless, the CBD contents of the dry extracts obtained with Sara (32.87% CBDA, 34.5% CBD) and Pilar (61.6% CBDA, 8.71% CBD) reported by Moreno-Sanz et al. were considerably higher.
Generally, ethanol can be used as the extraction solvent for CBDA/CBD, THCA/THC and minor cannabinoids such as CBGA/CBG and CBDVA/CBDV, with extraction yields in the range of 5.8–28% and reported solvent-to-plant material ratios of 5–44.4 L/kg. Unfortunately, due to the lack of yield or efficiency data of the ethanol extractions described in the patented methods found in literature, their cannabinoid extraction performances cannot be compared. In general, cold ethanol extraction appears to improve the whole process by minimizing the co-extraction of unwanted compounds, although the yield is slightly lower than that for extraction at room temperature. Nevertheless, isolated cannabinoids of >95% purity can be obtained from ethanol extracts after purification steps such as crystallization, liquid-liquid chromatography, or distillation, regardless of whether the extraction was carried out at below zero or room temperature.
Hexane is a non-polar solvent commonly used to extract neutral and acidic cannabinoids, such as CBD, THC, CBG, CBGV, CBC, CBDA, Δ9-THCA, or CBGA, from plant material. Despite its good solvent power, hexane must be completely eliminated from the final product since in pharmaceutical applications its use is controlled due to its inherent toxicity. This is a considerable disadvantage compared to ethanol, which is considered to be of lower risk (International Council for Harmonisation, 2020). However, compared to polar solvents such as ethanol, hexane does not co-extract chlorophylls, even though it co-extracts waxes and lipid material due to its non-polar nature. Unlike other hydrocarbons such as butane or propane, which are gases at room temperature, hexane has a boiling point of 68.73°C at 1 atm (National Library of Medicine, 2021a); therefore, its removal requires an additional evaporation step. Although hexane is flammable and carries the risk of fire and explosion, which increases the investment required for safety procedures during operation, its hazard risk (NFPA Health Rating 0 and NFPA Fire Rating 3, meaning that can be ignited under almost all ambient temperature conditions) is less severe than that of butane (NFPA Health Rating 1 and NFPA Fire Rating 4, meaning that can cause significant irritation, rapidly or completely vaporize at atmospheric pressure and normal ambient temperature, and burn readily) (National Library of Medicine, 2021b). Table 2 summarizes relevant facts regarding hexane extraction.
While CBG, the most polar cannabinoid, can be obtained by extraction with ethanol followed by distillation, the tendency of ethanol to co-extract polar components such as chlorophyll and the requirement for a distillation unit for CBG separation are the main disadvantages to the use of hexane as the extraction solvent. Hexane has been used to prepare enriched CBG and CBC extracts and extract acid cannabinoids such as Δ9-THCA or CBDA, according to patent US7700368B2 (Flockhart et al., 2010), which describes the preparation of cannabinoid-rich extracts and substantially pure cannabinoids with hexane and subcritical CO2. Among the preferred liquid non-polar solvents, the invention includes lower C5-C12 straight-chain or branched-chain alkanes. Plant material is sometimes decarboxylated before extraction when the target compounds are neutral cannabinoids. For example, 100 g of a CBG or CBC chemovar is decarboxylated and then extracted twice with hexane at a solvent-to-plant material ratio of 15:1. According to the invention, hexane is preferably acidified with 0.1% (v/v) acetic acid to minimize ionization for the extraction of acid cannabinoids (THCA and CBDA). Although the use of non-polar solvents such as hexane may involve the co-extraction of waxy material that is usually removed in a winterization step, this step is not mentioned in the patent during the post-processing of the CBG, CBC, Δ9-THCA, and CBDA extracts, as opposed to the THC and THCV extracts obtained after subcritical CO2 extraction. Thus, if the extraction is performed with hexane, winterization is not necessary but is required if the extraction is performed with CO2. After extraction with hexane, the cannabinoid-rich extract is filtered and the hexane is removed by evaporation before purification by chromatography and crystallization. The purification steps yield THCA and CBDA crystals of 98% and 94% purity, respectively, as well as substantially pure solutions of CBDA (98.9%), CBG (99.9%), and CBC (99.6%). The invention does not include any extraction yield or efficiency data beyond reporting that 100 g of a specific cannabinoid chemovar (THC, CBD, CBG, or CBC) yields approximately 5 g of purified Δ9-THCA or CBDA, or 300 mg of CBG or CBC-enriched fractions.
Another example of CBGA and CBG extraction through the maceration of plant material with hexane at room temperature is reported in patent US10207199B2 from Phytoplant Research S.L. (Roura, 2017). According to the invention, CBGA extract can be obtained from 4 kg of plant material (Aida variety registered at the Community Plant Variety Office [CVPO], number 2016/0167, CBGA predominant) and 25 L of hexane for 1 hour, which is repeated twice more with 20 L each, for a total solvent/plant material ratio of 16.25 L/kg. Similarly, a CBG-rich extract can be obtained by the maceration of 3.65 kg of decarboxylated plant material (4 kg of the Aida variety decarboxylated at 150°C for 1 h) with 25 L of hexane for 1 hour, repeated twice more with 20 L of hexane, with a total solvent/plant material ratio of 17.8 L/kg. Maceration is followed by filtering, evaporation of the hexane, and subsequent hexane crystallization and recrystallization, yielding CBGA or CBG with >95% purity. In one example, CBG with 99.3% purity was obtained after washing with ethanol and evaporation of the mother liquors to yield a CBG oil that was then recrystallized with hexane.
Phytoplant Research S.L. described in invention (Roura, 2019) WO2019145552A1 methods to isolate cannabinoids of interest by crystallization and liquid-liquid chromatography, in which cannabinoid-rich extracts are obtained by three rounds of maceration with agitation of the plant material in a non-polar solvent at room temperature for 1 h. CBG and CBGV can be obtained by the maceration of 100 g of plant material (variety Juani, CPVO number 2016/0117) in hexane (1 L for 1 h and then two times with 0.75 L; that is, 2.5 L of hexane total for 100 g, 25 L/kg) with a 6% extraction yield. Moreover, THCA and THC can be obtained by the maceration of 100 g of plant material (variety Moniek, CPVO number 2016/0114) in hexane (25 L/kg, 3 h total) with a 26% extraction yield. THC and THCV (variety Raquel, CPVO number 2018/0114) can be obtained likewise in hexane (25 L/kg, 3 h total) with a 16% extraction yield. Moreno-Sanz et al. (2020) also reported similar extraction yields for hexane and ethanol for different plant varieties, except for CBG-rich varieties, namely, Aida, Octavia (CPVO number 2017/0148), and Juani, which showed slightly higher yields for ethanol compared to hexane (9.5 vs. 7.6% for Aida, 6.8 vs. 4.9% for Octavia, and 8.2 vs. 5.7% for Juani), possibly due to the higher polarity of the main cannabinoid, CBG. However, internal data from Phytoplant Research showed a yield of 10.27% from the extraction of non-decarboxylated Aida (5.7% CBGA + CBG) with hexane (three times, 45 + 30+15 min, 18.33 L/kg total), producing an extract with 39.12% CBGA + CBG, corresponding to a 70.48% recovery from the plant material. Although hexane is a non-polar solvent, THCA + THC extraction efficiencies above 95% (Phytoplant Research internal data) are possible for the extraction of non-decarboxylated Moniek (flowers and leaves, ca. 11% THCA + THC) using a hexane-plant material ratio of 14–15 L/kg, resulting in an extract with 57–59% THCA + THC and extraction yields of 18–27%.
Patent US20200039908A1 (Elsohly et al., 2021) provides a few examples from which yield and efficiency data can be obtained. The maceration at room temperature with hexane (24 h, 1 L hexane, three times, 10.9 L/kg of solvent/plant material ratio) of 274 g of air-dried and powdered buds of a high CBD variety (3.0% CBD) produces 16.6 g of decarboxylated extract (after evaporation of hexane and decarboxylation) with 40% CBD, resulting in 80.8% CBD recovery from the plant and 6.09% extraction yield, which is quite low compared to the yields in hexane reported by Moreno-Sanz et al. (2020) for the extraction of 100 g of plant material also at room temperature (three times in hexane: 1 + 0.75+0.75 L, 2.5 L of hexane in total, ratio of 25 L/kg), including an 18.7% yield for the Sara variety (2015/0098) and 11.7% yield for the Pilar variety (2016/0115), both with CBDA + CBD recoveries from plant material >95%. US20200039908A1 also reported high extraction efficiencies starting from larger amounts of plant material: 182.3 g of extract (52.2% CBD, 78.7% recovery, 6.08% extraction yield) from hexane maceration of 3 kg of plant material (4.03% CBD) in hexane (20 L two times, 13.33 L/kg ratio), while 4.16 kg of extract (52.9% THC) can be obtained from 31.126 kg (9.96% THC) macerated in hexane (140 L, 4.5 L/kg) for 12 h and subsequent decarboxylation, which represents a final recovery of 70.98%, and 13.36% extraction + decarboxylation yield. For comparison, Moreno-Sanz et al. (2020) reported extraction yields of 28.4% and 20% in hexane (three times, 3 h and 25 L/kg in total) at room temperature from 100 g of the THC-rich varieties Moniek (2016/0114, flowers 19–26% THC) and Magda (2017/0145, flowers THC 13–16.5%), respectively, whereas Phytoplant Research S.L. reported an extraction yield of 18.28% and THCA + THC recovery of 93.33% from Moniek (flowers and leaves, 11.67% THCA + THC) also at room temperature but with a lower hexane-plant material ratio (15.8 L/kg). In addition, Phytoplant Research used three rounds of extraction with a total duration of 1.5 h (45 + 30+15 min), which is considerably less than the 12 h reported in the invention US20200039908A1. Although increasing the solvent-to-plant material ratio seems to result in higher extraction yields, the cannabinoid content of the plant material has a greater influence so that higher extraction yields are produced from richer cannabinoid varieties. Nevertheless, independent of the solvent-plant material ratio, hexane can extract THCA + THC with >90% efficiency. THC (98% purity) can be prepared after purification of a hexane extract of C. sativa L. (Elsohly and Ross, 2000). Patent WO2000025127A1 reported the production of 105.8 g of extract (40.35% THC) with 10.58% extraction yield and 96.58% THC recovery from 1 kg of fine powdered plant material (4.42% THC) by maceration with hexane (6 L) in a percolator for 24 h at room temperature. After filtration, the macerate is re-extracted with 5 L of hexane at the same conditions. The total ratio of hexane to plant material is 11 L/kg. Compared to the results reported by Phytoplant Research S.L. (26.85% yield and >95% efficiency) for the extraction of a THC-rich variety (Moniek, flowers and leaves, 11.67% THCA + THC) at room temperature with a ratio of 14.3 L/kg, these results highlight the relationship between the three extraction parameters: higher cannabinoid content in the starting plant material and higher solvent-to-plant material ratio, generally result in increased extraction yield, while the extraction efficiency is more related to the solvent affinity for the cannabinoids.
Patent WO2013165251A1 reports yields of 33–40% (Cid and Van Houten, 2015), for the extraction of decarboxylated plant material (flowers) with hexane (ratio 1 g of cannabis per 10 ml of hexane, 10 L/kg), obtaining extracts with 55% (33.33% extraction yield from 1.8 kg of plant material) and 28% (40.65% extraction yield from 615 g of plant material) THC. In contrast to the former examples, these yields are higher than those reported by Moreno-Sanz et al. (28.4% yield, variety Moniek, three times with hexane, room temperature) for 25 L/kg. The differences in the extraction yield may be explained by the type of plant material used since the extraction yields of 33–40% reported in patent WO2013165251A1 are produced from decarboxylated flowers rich in neutral cannabinoids, whereas the starting plant material in Moreno-Sanz et al. consisted of non-decarboxylated flowers. Higher extraction yields are obtained from decarboxylated plant materials compared to starting from non-decarboxylated materials when a non-polar solvent is used for extraction. However, the comparison of the extraction yields is incomplete since WO2013165251A1 does not include the cannabinoid content of the plant material or the extraction conditions such as the temperature or extraction time.
Patent WO2019020738A1 (Cipolletti et al., 2020) describes a process to produce CBD from industrial hemp, where the extraction of CBDA and CBD takes place by keeping the hemp in contact with hexane at 10–25°C for at least 10 min. An extraction yield of 18% using 132 kg of hexane (201.6 L and then washing the filtered biomass with 6 L) can be achieved starting from 40 kg of micronized hemp (CBDA/CBD ratio of about 70/30) and stirring the hexane/hemp mixture (5.19 L/kg) for 4 h at 20°C in a steel-jacketed reactor, followed by the filtration and evaporation of hexane to produce 7.48 kg of extract containing 508.64 g of CBDA (6.8%) and 125.7 g of CBD (1.7%). the yield of this example (18% with a solvent/plant material ratio of 5.19 L/kg) is higher than that reported by US20200039908A1 (Elsohly et al., 2021), which was 6.08% yield for the extraction of 3 kg of plant material (4.03% CBD) with a solvent/plant material ratio of 13.33 L/kg without agitation. In turn, the 18% yield is similar to that reported by Moreno-Sanz et al. (2020) (18.7% yield with the variety Sara (2015/0098)) at room temperature but with a higher solvent/plant material ratio (25 L/kg), under constant stirring in a mini orbital shaker. The similar extraction yields with a lower solvent-to-plant material ratio may be a consequence of stirring the hexane/plant material mixture during extraction at the industrial scale, which improves the extraction performance and, thus, the yield. However, the reported extraction performances cannot be appropriately compared without the cannabinoid composition of the starting plant material, in addition to the differences in the scale of the extraction process.
WO2019020738A1 describes a similar process in the pilot-scale extraction of 150 kg of micronized hemp (CBDA/CBD ratio of about 90/10) under stirring with 700 L of hexane in a steel dryer filter for 1 h at room temperature. The suspension is then filtered and the biomass retained by the filter is washed twice with 450 L of hexane under stirring (1 h, room temperature, total solvent/plant material ratio of 10.7 L/kg). The filtered product of the whole process containing CBDA is collected. This process is repeated with an additional 150 kg of micronized hemp. The extract obtained after the two extraction steps is subjected to a similar process as described above for the separation of CBDA from CBD, producing 30.3 kg of CBD, which is then further purified by crystallization in hexane. Even though the yield of the whole process is 20.2%, the authors do not report the amount of extract obtained before the separation of CBDA; thus, the hexane extraction yield is unknown and cannot be compared to those of the other examples.
Hexane is effective for the extraction at room temperature and atmospheric pressure of CBD, THC, CBG, CBGV, CBC, and their acids. Hexane extraction of THC-rich varieties results in an extraction yield of 18–26% and >95% THCA + THC recovery from plant material, with a solvent-to-plant material ratio of 14–15 L/kg (Phytoplant Research S.L. internal data). Moreover, extraction yields as high as 40% were also reported starting from a decarboxylated THC-rich variety (solvent-to-plant material ratio of 10 L/kg), although the cannabinoid recovery from plant material is unknown (Cid and Van Houten, 2015). Regarding CBDA/CBD-rich varieties, extraction yields as high as 18% are reported for laboratory and pilot-scale under agitation, which seems to improve the extraction performance, with 5–25 L/kg of solvent/plant material ratio (Cipolletti et al., 2020; Moreno-Sanz et al., 2020). The extraction of minor cannabinoids such as THCV and CBGA/CBG has also been reported, with hexane yields as high as 16 and 10.27%, respectively, with 25 and 18 L/kg [40, Phytoplant Research S.L. internal data]. All the examples found in the literature using hexane as the extraction solvent report the production of isolated cannabinoids with >95% purity after purification steps such as crystallization, liquid-liquid chromatography, or distillation of the hexane extracts.
Petroleum ether is a petroleum fraction consisting of mainly aliphatic C5 to C11 hydrocarbons with a boiling point of 30–80°C and is one of the most volatile liquid hydrocarbon solvents. The composition of petroleum ether is typically 40–80% aliphatic hydrocarbons, 25–50% naphthenic hydrocarbons, 0–10% benzene, and 0–20% other aromatic hydrocarbons (Chemical book, 2021). Petroleum ether fractions with a boiling point of 40–60°C are composed mainly of n-pentane and 2-methylpentane and lesser amounts of iso-pentane, 2,2-and 2,3-dimethylpentane, and 3-methylpentane (Panreac Applichem, 2021a). Other commonly available fractions are those with boiling points of 50–70°C, composed mainly of 2- and 3-methyl-pentanes and n-hexane, and 60–80°C, which mainly contain 3-methylpentane and n-hexane (Panreac Applichem, 2021b; Panreac Applichem, 2021c). While its relatively low cost compared to other organic solvents makes petroleum ether an attractive non-polar extraction solvent, its main disadvantages are its flammability and the lack of consistency among suppliers and even between lots, which negatively affects process controllability. Thus, extraction with petroleum ether usually comprises high levels of impurities (Goodwin et al., 2009; Romano and Hazekamp, 2013). Therefore, it is important to obtain a certificate of analysis for this solvent before its use. Table 2 provides an overview of the most relevant items related to petroleum ether extraction.
A fraction with a long distillation range (i.e., 30–80°C) (compared to a shorter distillation range of 40–60°C), will be more difficult to recover and reuse due to the wide range of boiling temperatures of the fraction, and will usually contain high levels of impurities.
The efficiency of petroleum ether as a cannabinoid extraction solvent has been reported in the literature, although most of the studies are laboratory-scale. For example, Lehmann et al. (Lehmann and Brenneisen, 1992) obtained an amorphous powder of >99% pure THCA after extraction with petroleum ether acidified with 0.2% acetic acid and subsequent fractionation using alkali and diethyl ether, and purification by medium-pressure liquid chromatography. Fairbairn et al. (Fairbairn and Liebmann, 1973) studied the extraction of THC from fresh plant material, herbal cannabis, cannabis resin, and reefers, concluding that chloroform was a more efficient solvent than light petroleum (b.p. 60–80°) or ethanol. Smith et al. (Smith and Vaughan, 1977) reported that petroleum ether (b.p. 40–60°) was less efficient than ethanol, methanol, and chloroform in the extraction of neutral and acidic cannabinoids from cannabis resin, according to the relative efficiencies of the solvents tested, although the CBD and THC extraction efficiencies of petroleum ether were >90% and similar to those of the other solvents. However, chloroform is not desirable as an industrial extraction solvent due to its toxicity to humans (mainly related to anesthetic potency, liver injury, and suspicion of carcinogenic potential). Thus, its use is limited in pharmaceutical products by the ICH guideline, with a concentration limit of 60 ppm (National Research Council, 1984; International Council for Harmonisation, 2020).
However, several examples can be found in the patent literature. For instance, US10207199B2 from Phytoplant Research S.L. (Roura, 2019) describes the extraction of cannabinoids by incubating the plant material with a non-polar solvent consisting of petroleum ether, pentane, hexane, and heptane, and subsequent crystallization and recrystallization with a non-polar solvent (hexane, pentane, heptane, or petroleum ethers) to isolate the cannabinoids. CBD, CBDV, and CBDA are extracted by incubating the plant material (100 g) of the Theresa (CBD/CBDV, CPVO 2016/0116) or Sara (CBD/CBDA, CPVO 2015/0098) varieties at room temperature with petroleum ether (b.p. 40–60°) for 1 hour and then repeating the process twice (1 + 0.75+0.75 L, 25 L/kg in total), with extraction yields of 9.1 and 15%, respectively. After filtering the plant material and evaporating the petroleum ether, the cannabinoids of interest (CBD, CBDV. or CBDA) are isolated by crystallization with petroleum ether before or after a liquid-liquid chromatography step such as centrifugation partitioning chromatography (CPC) with >95% pure cannabinoids. Extraction examples from the same company using lower petroleum ether-plant material ratios showed no significant differences in extraction yields, which can be attributed to the solvent/plant material ratio. For example, the extraction of 2.52 kg of the non-decarboxylated variety Theresa (3.91% CBD/CBDV, three times: 45 + 30+15 min) at room temperature with 12.8 L/kg of petroleum ether produced an extract with 34.27% CBDA + CBD and 8.57% CBDVA + CBDV and extract 6.21% of extraction yield and 68% recovery of total CBD + CBDV from plant material. Although the extraction yield is slightly inferior to the reported in patent WO2019145552A1 (9.1%), the difference is attributed to lower cannabinoid content in the plant material (Theresa), resulting in a decreased extraction yield. Importantly, the extraction yield is not as relevant as the cannabinoid efficiency or recovery, which is related to the solvent affinity, which allows more selective extraction. This is especially true when the target products are purified cannabinoids since higher extraction yields do not equal higher purity extracts and a higher yield may be the result of extracting undesired compounds like chlorophylls such as in the case of ethanol. Extraction efficiencies of CBDA + CBD >95% have been obtained with 3 kg of the non-decarboxylated variety Goya (2018/0113, 8.29% CBDA + CBD, three times: 45 + 30+15 min) at room temperature with 16.10 L/kg of petroleum ether, producing an extract with 5.17% CBDA, 51.65% CBD, and 15.2% extraction yield (internal data of Phytoplant Research S.L.).
Currently, comparing the extraction capacity of different solvents is difficult, especially when the examples found in the literature are carried out under different extraction conditions such as temperature or solvent-to-plant material ratio, or use starting materials with diverse cannabinoid profiles. Non-decarboxylated Aida variety (3.35% CBGA + CBG) extracted using petroleum ether and hexane under the same conditions (continuous, 17 L/kg, room temperature) showed no significant differences in extraction yield (7.46% for petroleum ether, 8.58% for hexane), while the total CBGA + CBG recovery was 87.64% with petroleum ether and 96.89% for hexane (internal data of Phytoplant Research S.L.). Although the solubility of decarboxylated CBD and, thus, the extraction yield should be higher in organic solvents such as petroleum ether, n-hexane, or dichloromethane (see previous examples of reported extraction yields for non-decarboxylated CBD varieties: 8–20% with ethanol, 6–18% with hexane, and 9–15% with petroleum ether (Roura, 2019; Moreno-Sanz et al., 2020; Elsohly et al., 2021)), US10301242B2 (Zhang et al., 2019) describes the extraction of CBD from hemp using ethanol to overcome the drawbacks associated with other organic solvents such as toxicity, long-term environmental effects, and difficulty in completely removing solvent residues in the final product. As mentioned before, the use of organic solvents such as hexane in pharmaceutical products has a more stringent residual solvent limit (290 ppm) than ethanol (5,000 ppm) according to the ICH Guideline, while the same guideline lists petroleum ether as a solvent for which no adequate toxicological data is found (International Council for Harmonisation, 2020). Overall, the main downside of the use of petroleum ether as the extraction solvent appears to be its potential toxicity despite the absence of available toxicological information and its proven ability to produce CBD isolates of >95% purity. Nevertheless, if the final product complies with the residual solvent restrictions, there is no objection to their use as extraction solvents.
In addition to the commonly used solvents such as ethanol, n-butane, hexane, and petroleum ether, other organic compounds and mixtures have been reported in the patent literature for the extraction of cannabinoids from plant material. For example, WO patent 2016200438A1 (Ayres, 2016) reports a method combining cold temperatures (−25°C to −100°C) with a dual polar/non-polar solvent system (acetone and carbon dioxide as co-solvent, preferably at 50/50 w/w or v/v, 26 L/kg) at atmospheric pressure, for the selective extraction of cannabinoids, terpenes, and flavonoids at laboratory-scale while avoiding the co-extraction of waxes and resins. The extraction process is said to last 5–30 min under stirring. The authors reported an overall extraction yield of 18–20% with this procedure, with a cannabinoid purity > 90%. This process also reduces the flammability or explosion potential by operating at atmospheric pressure and cold temperatures in the presence of CO2, which removes the oxygen. Unfortunately, the extraction yield cannot be adequately compared since the cannabinoid content of the plant material was not clear in the report.
The patent literature also includes alternative extraction methods using organic solvents. For example, US20200165219A1 (Changoer et al., 2021) describes a method in which Δ9-THCA is extracted from cannabis flowers (100 g) using heptane (1.5 L, 15 L/kg) for 60 min at room temperature with stirring, then separation using a NaOH/NaCl solution adjusted to a pH of 13.2–13.4 to form a Δ9-THCA salt. The salt is extracted with tert-butyl methyl ether (MTBE) and converted back to Δ9-THCA using an acidic solution. After removing the solvent and aqueous residues, the extraction yield is 10–15%, with a Δ9-THCA recovery of 55–70% from cannabis flowers. The Δ9-THCA is then decarboxylated and extracted again with pentane, yielding crude Δ9-THC with 92–94% purity, which can be combined with a carrier for pharmaceutical applications. Although the authors claim that the patented invention improves the extraction of Δ9-THCA from cannabis flowers, Phytoplant Research S.L. obtained a significantly higher extraction yield (26%) and Δ9-THCA efficiency (94%) after a three-step extraction with hexane (45 + 45+30 min, 14 L/kg in total) at room temperature starting from flowers of the Moniek variety (internal data). In other words, a simpler and significantly less time-consuming procedure than the patented method described in US20200165219A1 provided better Δ9-THCA extraction from cannabis flowers.
Another process using heptane to obtain Δ9-THC is described in patent WO2009133376A1 (Bhatarah et al., 2009). The particularity of this method is that all the steps take place in the same non-polar solvent (heptane), without a solvent swap, including decarboxylation of Δ9-THCA into Δ9-THC in the presence of an aqueous base, NaOH 22 wt%. Extraction with heptane takes place three times (4–4.5 h, 1 h, 4 h) at room temperature under stirring and nitrogen atmosphere. The obtained Δ9-THC is washed with an aqueous solution to remove inorganic impurities, still in the same initial solvent. Further purification is carried out by charcoal filtration and reverse-phase column chromatography. The present invention did not provide an extraction yield; therefore, it can only qualitatively be compared to other inventions. In contrast to the previous methods relying on solvent swaps to remove impurities, this invention provides a method that is simpler, faster, and easier to scale-up, with fewer steps required to obtain the final product and reduced waste. Without providing numeric data, the authors claimed increased Δ9-THC yields compared to methods that convert Δ9-THCA into a salt, such as those described in patents US7592468B2 and US20200165219A1 (Goodwin et al., 2009; Changoer et al., 2021), in which Δ9-THC is not converted into an alkaline salt and, thus, is not extracted along with the Δ9-THCA salt, but rather discarded as an impurity.
Patent US20200023286A1 describes a continuous and scalable process using dimethyl ether (DME) as the extraction solvent (Melnichuk and Kelly, 2020). DME is an efficient solvent to extract polar and non-polar compounds (lipids, terpenes, flavonoids, cannabinoids, and alkaloids) from plant material, which is saturated with liquid DME in a closed vessel at sufficient pressure and low temperature to guarantee the liquid state of the solvent. After extraction is completed, depressurization causes the DME to vaporize, carrying with it the dissolved compounds. The vapor mixture is directed into a series of condensation vessels at successively lower pressures. The extracted compounds are separated in a distillation column into individual or grouped compounds with no residual solvent, which is removed at the end of the condensation sequence for discharge or recycling. However, safety precautions must be taken since DME is flammable. Unfortunately, the invention did not provide yield data for the cannabis extraction.
Patent US9950976B1 (Keller, 2018) reports a CBD extraction process and subsequent conversion to THC in which the cannabis plant material is mixed with dichloromethane (DCM) with stirring in a blending unit to form an initial extract, which is filtered and treated with activated carbon. The DCM is at least partially removed in a primary solvent exchange rotary evaporator unit wherein ethanol is added to the extract and then processed through a wax coalescing unit and filtered to remove waxes and lipids. The evaporated and defatted extract comprises 60–80 wt% of CBD in ethanol. The CBD can then be converted to THC in a conversion rotary reflux unit in the presence of an acidic component. The reflux extract is placed in a separator unit with the addition of DCM and water, the layers are separated, and the remaining organic effluent is treated with activated carbon and rotary evaporated to remove DCM while adding ethanol. The solvents are partially evaporated to produce an exchange reflux containing THC in ethanol, with 60–80 wt% THC, which is separated in a fractionation unit. According to the authors, the described method is a safe and economical process for obtaining a THC-rich product from industrial hemp. The process can be scaled to accommodate a feedstock input of about 115 kg of raw cannabis plant material every 8 hours, producing about 35–45 kg of THC product comprising approximately 60–80 wt% THC.
Another trend is the use of vegetable oils such as coconut, canola, sunflower, walnut, palm, or hemp oil for cannabinoid extraction, avoiding the use of toxic and hazardous hydrocarbon solvents in addition to the high pressures involved in the supercritical extraction with CO2, a technique that is further explained later. Thus, extraction with vegetable oils or lipids is considered to be solvent-free, safer, and more environmentally friendly compared to traditional extraction methods using butane or alcohols since it can be performed using certified organic solvents and a post-processing step for solvent removal is not needed as the cannabinoid product is diluted in oil (Barringer, 2017; Raber and Elzinga, 2017; Splinter et al., 2019a). Obtaining an oily cannabinoid product can be convenient since most commercialized CBD products are diluted in an oily medium like hemp seed oil (Koumans, 2021). Many examples of the use of vegetable oils have been reported in the patent literature. For example, invention WO2019207554A1 (Splinter et al., 2019a), which will be further discussed in the “Alternative extraction methods” section, describes a method for extracting cannabinoids from cannabis in a continuous flow microwave-assisted extractor at 25–75°C, using a carrier fluid suitable for inclusion in a final formulation, such as a polyunsaturated fatty acid (PUFA), safflower oil, canola oil, cottonseed oil, soybean oil, olive oil, mono, di and triglycerides, lecithin, limonene, essential oils, or fish oil, among others. The invention provides a table comparing different solvents for the extraction of THCA from cannabis biomass. With a solvent-to-solid ratio of 8 L/kg at 30°C, a solvent comprising pentane and a medium chain triglyceride (MCT oil) produces an extract with 3.5% THCA (96% recovery from plant material), whereas pentane alone yields an extract with 92% THCA (89% recovery from plant material), indicating that the use of MCT oil results in the co-extraction of other compounds and, thus, a less pure extract, despite the higher THCA recovery.
Patent US9808494B2 (Barringer, 2017) describes a lipid solvent cannabinoid extraction technique from cannabis in a packed column agitated with ultrasonic waves or by mechanical means to produce a cannabinoid-infused fat. In this case, the solvents are cocoa butter, sunflower oil, coconut oil, safflower oil, or canola oil. The extraction is performed at 0.2–1 bar and, for example, 60°C. The authors claim that the patented process requires a relatively short contact period between the cannabis material (2.7–7.4 kg of dried plant material containing 10% cannabinoids) and solvent (170 L, 23–63 L/kg) and can remove ≥90% of cannabinoids in 30–120 min. The output flow rate is 1–3 L/min and the solvent is separated by filtration. The resulting extract contains a cannabinoid concentration of 15 mg/ml using a single extraction chamber, or 33 mg/ml using two chambers in series.
Invention WO2019240581A1 (Koumans, 2021) describes a simple and low-cost process for cannabis extraction, resulting in an oil comprising cannabinoids and terpenes, using vegetable oils such as olive, grapeseed, safflower, canola, sunflower, and coconut oil, among others. Briefly, the process consists of contact with the oil, which flows continuously in a closed loop at atmospheric pressure, with the plant material contained in one or more steeping reservoirs (≤30 kg of plant material per reservoir), at a temperature of preferably 50–80°C. Thus, high levels of oil-soluble components are extracted from plant material with a minimum loss of volatile components. The plant material can be replaced periodically, preferably when ≥80% of the trichome material is dissolved, to increase the concentration of oil-soluble compounds in the oil, which translates to an extraction time of 0.5–3 h. The plant material is preferably intact to avoid the release and co-extraction of contaminants such as chlorophyll. The described closed loop system is designed to operate from micro-production up to 5000 L of oil, depending on the needs. According to the authors, one of the main advantages of this process is that the oil or concentrated extract can be directly used in physiological applications, suitable for oral or topical application. The obtained oil can have a CBD content of about 5 wt% by total weight and may be used as CBD oil without further processing, depending on the characteristics of the desired final product and its intended use.
Invention US10512856B1 (Jackson and Gildrien, 2019) describes a system that uses a heated carrier oil as the vehicle for extraction from the leaves, flowers, rhizomes, roots, and/or stems of the cannabis plant. The carrier oil is continuously mixed with plant material, at a ratio by weight of carrier oil to plant material between 0.5:1 and 2:1, and heated to 65.6–104.4°C in a press device, producing an oil mixture from which an extract containing CBDA, CBGA, CBD, CBG, CBN, THC, and/or THCA can be extracted. The carrier oil can be olive oil, a medium-chain triglyceride oil, palm oil, vitamin E oil, or hemp oil, among others. According to the authors, the described process involves no chemical solvents and a limited number of steps since many of them can be performed simultaneously by heating and/or mixing in the press device, which implies reduced necessary equipment. However, no extraction yield or efficiency data were provided.
Another process using a vegetable oil or lipid compositions, comprising canola oil, peanut oil, sunflower oil, avocado oil, grapeseed oil, walnut oil, or fish oil, an ionic liquid such as tributylmethylammonium methylsulfate, or an imidazolium salt such as 1-butyl-3-methylimidazolium chloride, as an extraction agent or carrier is described in invention US20180016203A1 (Raber and Elzinga, 2017). After extraction by mixing, stirring, and heating to preferably 50°C for 5–90 min, the enriched lipid solvent is separated from the plant matter residue, and subjected to distillation to volatilize the cannabinoids that can be further decarboxylated or concentrated at reduced pressure. The obtained final product can be a cannabinoid-rich fraction containing 65–75% THC, which can be subjected to another distillation at 165°C, obtaining a resin containing THC with a purity larger than 80%.
Pharmaceutical compositions or nutritional supplements comprising THCA, CBDA, or CBGA and a natural oil such as seed- or nut-based oils (i.e., flax seed, chia seed, hemp seed, sunflower seed, walnut oil, or pecan oil), can be obtained from cannabis extraction using a source of natural oil according to patent WO2018209425A1 (Carnahan, 2018). The method describes the combination and milling of the THCA-containing cannabis and the source of natural oil by mechanical extrusion in controlled temperature and pressure conditions, up to 80°C and 150 psi (10 bar) to extract THCA. The authors provided some examples of oil-to-plant material ratios, such as 90 wt% flax seed (containing 40% oil) to 10 wt% flowers (containing 10% THCA), or 10 kg of the carrier mixed with 1 kg of cannabis, resulting in 3 L of extracted oil containing THCA. The process can produce 100 ml/min or 220 L every 24 h of extracted oil. The resulting nutritional supplement is in the form of seed oil, protein powder, or paste/butter, containing 10 wt% essential fatty acids and 0.2 wt% THCA/THC and/or CBDA/CBD, or 30 mg/ml of THCA, the general standard concentration of THCA/THC medicines. According to the patent, cannabis flowers containing 10% THCA can produce a cannabis oil with 20 mg/ml THCA. In addition, cannabis flowers with 0.097% CBGA produce a cannabis oil containing 0.26 mg/ml CBGA. The relatively low temperatures used in this method avoid decarboxylation and preserve the terpenes and volatile aromatic oils. The method is claimed to be safer and faster than conventional extraction methods, and also environmentally friendly, precise, reliable, and efficient for the commercial production of medicinal and/or nutraceutical products. The invention, and generally the oil-based extraction techniques described in this section, are limited to specific applications such as producing cannabinoid formulations for pharmaceutical and nutritional products; however, unlike extraction techniques based on the use of organic solvents such as hexane or petroleum ether followed by crystallization or chromatography, the obtained product is not pure, with a cannabinoid concentration in the oil lower than that reported for other non-polar solvents such as hexane (30–80%) (Cid and Van Houten, 2015; Moreno-Sanz et al., 2020)). Moreover, among patents using vegetable oils as the extraction solvent, only US20180016203A1 (Raber and Elzinga, 2017) reported a concentration of 65–75% or >80% THC after distillation, which is considerably lower than the concentrations (>95%) reported after purification of ethanol, hexane, butane, or petroleum ether extracts, as described in previous sections. The formulations resulting from oil extraction cannot be further concentrated and the cannabinoid concentration is closely linked to the plant’s cannabinoid profile. In contrast, it is easier to adjust the concentration of a formulation from a purified product, which can be obtained after extraction with organic solvents described in previous sections, followed by further purification. In addition, as described in the referenced patents that used vegetable oil as an extraction solvent, the extraction temperature is 30–80°C, which represents a higher energy consumption compared to extraction at room temperatures with organic solvents such as hexane or petroleum ether. A summary of the most important aspects of oil extraction is presented in Table 3.
This section describes the use of pressurized gases, such as gaseous hydrocarbons (n-butane and n-propane), subcritical or supercritical CO2, and C1-4 fluorinated hydrocarbons (1,1,1,2-tetrafluoroethane HFC 134a), as the extraction solvent. Unlike liquid solvent extraction, the use of pressurized gases requires special pressurized equipment such as closed extraction vessels, collection reservoirs, or solvent recovery systems. As described in more detail in the next sections, the sophistication of this equipment, the severe extraction conditions, and the intrinsic characteristics of the pressurized solvents (e.g., butane is toxic and flammable) are the main drawbacks of using pressurized gases. However, the main advantage of using pressurized gases over liquid organic solvents at ambient temperatures is that, although the extraction occurs in the liquid phase, these solvents are easily purged at the end of the extraction by increasing temperature due to their lower boiling point, resulting in an almost complete recovery of the same. Thus, the extract may contain practically no traces of solvent residues (Oroskar et al., 2019). Table 4 provides an overview of the relevant aspects of different pressurized gas extraction techniques.
Hydrocarbons such as n-propane and n-butane are pressurized into a liquid state and used as solvents for the extraction of non-polar cannabinoids. The process is typically performed by passing the pressurized gas through the cannabis material contained in an extraction vessel. To avoid dragging the plant material, the biomass is retained by a mesh or filter at one end of the container (Oroskar et al., 2019).
Butane extraction is one of the most popular extraction methods due to its great versatility and potency, reaching cannabinoid concentrations of up to 90%. Butane extraction is the most used technique for the production of currently available cannabinoid concentrates, such as the popular “shatter”, a viscous substance with a high THC concentration (Oroskar et al., 2019; Radoiu et al., 2020).
Non-polar solvents like n-butane do not dissolve polar compounds such as chlorophyll, which is an advantage over ethanol extraction. Therefore, butane is an ideal solvent for selectively extracting neutral cannabinoids and terpenes. However, butane has a higher tendency to co-extract waxes that must be removed in further purification steps. In addition, the volatile and non-polar butane and propane have higher toxicity compared to polar solvents when inhaled since they are rapidly absorbed through the respiratory system and the blood-brain barrier due to their high lipophilicity; thus, they concentrate in lipid-rich organs such as the liver and the brain, causing effects on the nervous system (Sironi et al., 2016). Moreover, butane and propane are highly flammable; thus, safety facilities are required due to the risk of explosion. The difficulty in scaling up is another drawback to the use of hydrocarbon solvents for the extraction of cannabinoids. Hydrocarbon extraction is performed in batches and the scalability of the process is only feasible by the addition of multiple units (Radoiu et al., 2020). Moreover, the solvents used must be pure to prevent the presence of toxic hydrocarbons or heavy metals in the final product (Ayres, 2016; Keller, 2018).
Some examples of hydrocarbon extraction can be found in the patent literature. For example, US20200102283A1 (Dibble and Cole, 2020) describes a method of obtaining crystallized THCA (purity >95%) by first pouring butane (600 ml) at 10°C or colder for 5–10 min into an extraction column packed with optionally dried plant material (50 g–200 g; 3–12 L/kg) with 10% THCA + THC. This process can be repeated several times, obtaining an extract with 75% THC + THCA, an extraction yield of 12%, and 90% (THC + THCA) recovery from plant material. The obtained solvent extract is subjected to a cooling step to remove pigments and lignocellulosic impurities. THCA crystals with a purity >95% are then obtained by crystallization.
Another patent using a hydrocarbon-based solvent, such as butane, reports the extraction of cannabinoids from cannabis plant material but provided no data on its efficiency. The patent, US9789147B2 (Jones, 2017), describes in detail a closed-cycle extraction system that allows solved recovery. The butane contained in a solvent reservoir is chilled (−17°C to −78°C), condensed into the liquid phase, and then flows into an extraction chamber containing the plant material. Butane washes through the material, dissolving the extractable compounds, forming a solution that is collected in a collection reservoir, which is at a higher temperature to volatilize the solvent and remove it from the extracted compounds, leaving no residues. The resulting extract can be further purified, while the gaseous butane is returned to the solvent chamber and condensed. The process comprises a cold refinement chamber to solidify co-extracted waxes, which can be filtered from the extract along with solids and heavier oil components.
Invention US10888596B1 reports the use of propane as the preferred extraction solvent to produce extracts with high THC concentrations (high quality and purity) in the form of shatter, wax, or budder for dabbing or vaping (Hindi, 2021). According to the method, the starting material is frozen and dehydrated plant material, which is placed in a chamber with a chiller column (only to obtain a shatter product) so that the extraction occurs at −5 to −40°C to immobilize the lipids and water-soluble molecules within the plant material to make shatter. The gaseous solvent is pumped into the chamber and passed through a filter and the plant material at low pressure to maintain the solvent in a liquid state. The gaseous solvent is collected in a vessel and removed by applying heat so that the solvent is evaporated and pumped back into the gas holding tank. To produce a shatter product, the plant material “cookie” (a dry, crystalline, powdery round disc) is scraped from the chamber, powdered, and heated under vacuum at 21–43°C for 15 min to 48 h. Then, the powder is heated at 71–98°C under atmospheric pressure with a heat gun until a clear and transparent shatter product is obtained with 70–98% THCA/THC. To produce a wax product, the “cookie” is heated to 51–63°C to evaporate residual moisture and solvent gas. During the heating, the “cookie” is stirred until a waxy substance is obtained. Similarly, a budder product is produced by heating the “cookie” to 54–57°C to remove residual solvent or moisture. The “cookie” is stirred during heating to produce a budder product with a creamier texture. The authors did not provide any performance data such as extraction yield or efficiency. According to them, the final product, whether a shatter, wax, or budder, is free of chlorophyll and residual solvent. In addition to propane, other suitable solvents are butane and isobutane.
The lack of patents using gaseous hydrocarbons such as butane or propane as the extraction solvent is striking. To our knowledge, only patent US20200102283A1 (Dibble and Cole, 2020) has provided numerical data on the efficiency of butane extraction; thus, it is the only reference that can be used to compare the performance of butane to other solvents in the patent literature. While butane provides cannabinoid recovery and extraction yields in the same range as ethanol or hexane, the use of gaseous hydrocarbon solvents has several advantages; i.e., high cannabinoid concentrations, no solvent residues to remove the requirement for an evaporation step, and no co-extraction of chlorophylls compared with ethanol) Nevertheless, the co-extraction of waxes involves a mandatory winterization step when the target product is a cannabinoid isolate, whereas the intrinsic characteristics of the solvent such as toxicity and flammability, which implies the use of safety facilities, diminishes the desirability of gaseous hydrocarbons as extraction solvents.
Among extraction techniques, supercritical fluid extraction (SFE) has become one of the most widespread methods for the commercial production of cannabis extracts, for which supercritical carbon dioxide (SC- CO2) is the most common solvent. Generally, a supercritical extraction unit consists of a pressurizing device, a high-pressure extraction vessel, one or more separators, a solvent recovery system, and several heat exchangers (Chess and McCutcheon, 2017; Ramirez et al., 2019). The CO₂ is pressurized and heated under controlled pressure until it reaches a supercritical state. SC-CO2 is then pumped through the cannabis material in the extraction vessel. After extraction, the supercritical solution is usually pumped into a separation unit with separators in series, where extracted compounds with different solubilities are selectively condensed at reduced pressure conditions. After the CO2 is removed from the extract, it can be pumped and recycled to the pressurizing device.
The use of supercritical fluids has some advantages over conventional organic solvents, the most important of which is the ability to modify the solvent power (which is correlated to the fluid’s density) by varying the pressure and temperature conditions above the critical point of the fluid, which allows selective extraction (Perrotin-Brunel et al., 2010; Chess and McCutcheon, 2017). CO2 reaches its critical point at a relatively low temperature and pressure (31.1°C and 73.7 bar, respectively), allowing the extraction of thermally labile components and, thus, preserving the cannabinoid and terpenoid profiles of the plant (Rivas, 2019). In addition, CO2 is non-toxic, non-flammable, abundant in nature, inexpensive, can be recycled in industrial plants, and does not leave residues in the resulting extracts since the solvent is easily and efficiently removed by decreasing the pressure (Perrotin-Brunel et al., 2010; Omar et al., 2013; Ramirez et al., 2019; Radoiu et al., 2020). Therefore, the supercritical extraction technique is usually preferred for medicinal and pharmaceutical applications, which require high-quality products without toxic residues.
However, the extraction of cannabinoids is inefficient since polar compounds have low solubility in SC-CO2. Nevertheless, large amounts of waxy material are co-extracted, which must be removed in subsequent purification steps (i.e. winterization) that may compromise the cannabinoid concentration of the extract (Rivas, 2019). Moreover, supercritical conditions require high pressures and expensive equipment and it is considerably more dangerous to scale processes operating closer to ambient conditions. Therefore, economic and technical factors require consideration to assess the feasibility of supercritical CO2 extraction at the industrial scale (Keller, 2018; Radoiu et al., 2020). The sophisticated equipment and the requirement for a winterization step are disadvantages compared to liquid organic solvents such as ethanol and even hexane, which, despite being nonpolar, dissolve less lipid material at room temperature and atmospheric pressure than the pressurized gases such as butane or supercritical CO2. This is based on the few patents that have reported the necessity for a winterization step after hexane extraction, compared to most patents, which include a winterization step after butane and CO2 extraction, as described below.
Two approaches have been applied to overcome the low-solubility challenge of polar cannabinoids in SC-CO2. One option is to decarboxylate large amounts of the cannabis material before extraction to transform the polar acidic cannabinoids into their neutral non-polar forms, although this negatively affects the overall costs and may lead to the loss of heat-sensitive terpenes, in addition to requiring large ovens to perform this decarboxylation (Whittle et al., 2008; Flockhart et al., 2010; Ivanov, 2016). The other option to increase the solubility of polar cannabinoids in SC-CO2 is to add a co-solvent that modifies the density and, thus, the solvent power of SC-CO2 (Rovetto and Aieta, 2017; Vági et al., 2020). Ethanol is the most widely used co-solvent to increase the polarity of the solvent mixture, although other organic solvents such as methanol and ethyl acetate have also been reported (Barrie-Webster and Leornard, 2000; Gallo-Molina et al., 2019). Rovetto et al. (2017) reported that the addition of 5wt% ethanol as a modifier improved the overall extraction rate in the pilot plant-scale SC-CO2 extraction of THC from C. sativa L., while lowering the solvent to the feed ratio required to achieve high yields. Gallo-Molina et al. (2019) observed that the addition of 2–5% ethanol favored extraction yield from cannabis plants, while the THC recovery was slightly reduced compared to the same conditions using pure SC-CO2. The authors reported a maximum extraction yield of 26.36% at 33 MPa, 80°C and 5% EtOH with a 2.2% THC recovery from plant material (initially 7.04% THC in the cannabis plant), while the highest THC content in the extract (37.85%) was achieved at 33 MPa, 60°C, and 2% EtOH, with a 16.01% extraction yield and 5.38% THC recovery. The two main drawbacks are associated with the addition of ethanol. Namely, the co-extraction of undesired products due to the increase in solvent power, which leads to a reduced relative concentration of cannabinoids in the extract, and the condensation of ethanol along with the extracted compounds in the separator vessels at atmospheric pressure. However, the co-extraction of non-desired material can be avoided using a purification step such as winterization, which involves the solubilization of the extract in ethanol.
Above the critical temperature, the density and, thus, the solvating power of CO2 increases significantly. Despite the higher extraction yields, selectivity is severely affected since both more cannabinoids and non-desired compounds are solubilized, resulting in a complex extract in which the concentration of the target compound is diluted, which requires more exhaustive purification steps (Whittle et al., 2008). Hence, some authors have proposed extraction at subcritical conditions; that is, at temperatures and pressures below 31°C and 73 bar, respectively, so that CO₂ is in a liquid form. In addition, low temperatures not only preserve terpenes but also reduce the co-extraction of waxy material since its solubility decreases with the reduction of temperature.
The authors of patent US7344736B2 (Whittle et al., 2008) claimed that the greatest specific separation of cannabinoids is obtained under subcritical rather than supercritical conditions due to enhance extraction selectivity as a result of the low density of subcritical CO2. The described method comprises a previous decarboxylation step followed by batch extraction of 60 kg of plant material with 1,250 kg/h liquid CO2 under subcritical conditions at preferably 10°C and 60 bars. After 4 h, the resulting cannabinoid-rich extract only contains a reduced number of undesired compounds, such as waxes, which are easily removed in a winterization step with ethanol and an optional treatment with activated charcoal. In the subcritical conditions described above, an extract with 63.6 wt% of CBD can be achieved, with an extraction yield of 8.4 wt% and a recovery of 72.9% from the decarboxylated plant material (7.3 wt% CBD). Comparatively, switching to supercritical conditions (40°C and 100 bar) produces an extract with 54.4 wt% CBD (10.7 wt% yield) and a 79.5% recovery. Despite the modest advantage in terms of CBD recovery and yield, at supercritical conditions, the CBD content in the extract is reduced by approximately 10%. Moreover, the relatively low pressures and temperatures allow more economical processing in subcritical conditions. The invention also reports that the addition of a polar modifier such as 2 wt% of ethanol to supercritical CO2, increases both the recovery of the available CBD (91 wt%) and the concentration of CBD in the extract (64.6 wt%), with a total extraction yield of 10.3 wt%. Thus, the non-cannabinoid material present in the plant may be less polar than the target CBD, resulting in reduced co-extraction with increasing polarity. The same company published the extraction method operating at subcritical conditions in invention US8846409B2 (Flockhart et al., 2010), which describes the preparation of substantially pure cannabinoids starting from plant material. Particularly, the Δ9-THC and Δ9-THCV extracts are preferably obtained by the decarboxylation of the plant material, followed by liquid CO2 extraction under subcritical conditions, winterization, and treatment with activated charcoal, as described in US7344736B2. Unfortunately, no extraction yield or efficiency data were provided in US7344736B2 since it is more focused on the description of the purification procedure by column chromatography and the successive dissolution of the partially purified extract in methanol and pentane for the removal of insoluble material.
Overall, the cannabinoid and terpene ratio can be controlled by changing the operating temperature and pressure during SC-CO2 extraction or, as described in patent US10507404B2 (Tucker, 2019), supercritical CO2 extraction can be performed in combination with other extraction techniques such as cold ethanol extraction to obtain a blended extract of cannabinoids and terpenes to overcome the limited success in simultaneously extracting cannabinoids and preserving terpenes described in the prior art. As previously discussed in the ethanol extraction section, terpenes can be first extracted from ground and frozen cannabis material using supercritical CO2 (26–43°C, 1,000–1,300 psi, that is 69–89 bar), for 15 min to 6 h, to produce terpene oil (mixture of up to 99% terpenes and up to 15% cannabinoids) and hydrosols (containing 1–10% water-based terpenes, water, and up to 5% cannabinoids) in the collection vessel after evaporating the CO2. A cannabinoid concentrate (with 50–90% of cannabinoids and may contain up to 20% terpenes) can be extracted from the residual material with cold ethanol. A blended extract is obtained after purification and the addition of the CO2-extracted terpenes to the purified cannabinoid concentrate. Thus, the SC- CO2 extracts can be applied to obtain cannabis products that show a synergistic effect between cannabinoids and terpenes. According to the invention, the described process can be performed at any scale, from laboratory to industrial.
Patent US20200246406A1 (Speier, 2022) describes methods of obtaining an extract including cannabinoids, terpenoids, and/or flavonoids from dried or fresh cannabis plant material by fractional SC-CO2 extraction, which leads to a concentrate or an essential oil after solvent removal. The concentrate, including at least one of THC, CBD, CBN, CBG, CBC, CBL, or their acid forms, can be further purified or used directly in a pharmaceutical dosage form, such as a transdermal patch or an oral thin film, without further purification. The SC extraction can be performed multiple times (fractional SC extraction), in which at least one of the following conditions is varied: polarity and proticity (protic or aprotic) of the solvent system (sole CO2 or CO2 modified with ethanol, methanol, or hexane), temperature, or pressure. The fractional SC extraction process includes two extractions to separately obtain an extract enriched for non-THC cannabinoids at 1800 ± 400 psi (124 ± 27 bar) and 49 ± 15°C for 3 ± 1.5 h, and an extract enriched for THC at 3,000 ± 800 psi (206 ± 55 bar) and 49 ± 15°C for 6 ± 2.5 h. The THC extract contains at least 75–90 wt% THC and a lower concentration of non-THC cannabinoids. In turn, the extract enriched in non-THC cannabinoids contains at least 90 wt% of CBN/CBNA, CBD/CBDA, THCV, CBG/CBGA, CBC/CBCA, or CBL/CBLA, as well as a lower concentration of THC. The extract can be further purified by chromatography, crystallization, or distillation.
Patent US20140248379A1 (Mueller, 2020) describes a method for preparing an extract containing THC, CBD, and possibly their carboxylic acids, from ground, dried industrial hemp (Fedora 19 with 1.54% CBD, 0.25% Δ9-THC) with supercritical CO2 at preferably 60°C and 250 bars and a flow rate of 50–150 kg of CO2/kg of starting material (64–192 L/kg, considering the density of CO2 at the specified conditions). The separation vessels are filled with an adsorbent such as zeolitic molecular sieves and diatomaceous earth, to remove alkaloids, flavonoids, and chlorophylls from the extract. Monoterpenes and sesquiterpenes are separated from the extract in a separation vessel at 45°C and 60 bars. After separation of the CO2 in a separation vessel at subcritical conditions (50 bar and 20°C) and decarboxylation at 80°C for 2 h, the resulting primary extract from industrial hemp contains 58% CBD, 9.5% Δ9-THC, 0.1% CBN, 0.01% chlorophylls, 0.15% flavonoids, 0.001% alkaloids, and <0.035% monoterpenes and sesquiterpenes. The authors claim that the primary extract produced by this method shows high cannabinoid content and significantly lower terpene, chlorophyll, flavonoid, and alkaloid content without any further purification steps compared to prior-art extracts obtained with ethanol or hexane. The invention also describes additional steps such as winterization for wax removal, and further purification in a high-pressure column under supercritical conditions. However, compared to published ethanol and hexane extraction results, the overall cannabinoid content (67.5%: 58% CBD and 9.5% THC) obtained after decarboxylation of the extract is lower than that reported by Moreno-Sanz (2020) after ethanol and hexane extraction of the Sara variety (CBD 7–10.5%) and subsequent decarboxylation of the extract (67.14% CBD with ethanol and 83.7% CBD with hexane). Similar CBD concentrations have been reported from the Pilar variety (CBD 4.5–8.5%): 61.31% CBD with ethanol and 83.79% with hexane). Although the high CBD concentrations in the decarboxylated extract cannot be adequately compared since the starting materials of Moreno-Sanz et al. have greater cannabinoid content than the Fedora variety in US20140248379A1, the extraction results reported by Moreno-Sanz were obtained under milder conditions (room temperature, atmospheric pressure, 25 L/kg) than those reported for supercritical conditions (60°C, 250 bar) at a significantly high solvent-to-plant material ratio (64–192 L/kg).
CBD isolates can be prepared by supercritical CO2 extraction from industrial hemp material (<0.5% w/w of THC + THCA and CBD <5%) after purification by distillation, flash chromatography, and/or crystallization, according to WO2016153347A1 (Eiroa et al., 2016b). More particularly, the starting material of the invention is preferably industrial-hemp waste material. The attractiveness of industrial hemp waste as a CBD source is its availability as a waste product from various industries (textile, paper, plastics, etc.), which provides an economically viable process. According to the method, supercritical extraction occurs at 40–140°C and 100–400 bar. The resulting crude CBD extract is then decarboxylated. For example, after decarboxylation of the extract obtained by the batch extraction of 200 g of hemp material from industrial hemp waste pellets (60°C, 300 bars, 2 h, 9.5 kg/h CO2, that is 22.9 L in 2 h, 114.5 L of CO2/kg of hemp), a black resin is obtained containing 20% CBD, which is considerably lower than the CBD content of the extracts obtained with organic solvents such as ethanol and hexane, and even compared to other reported extractions with CO2 under subcritical and supercritical conditions using starting materials with higher CBD content than industrial hemp (Whittle et al., 2008; Moreno-Sanz et al., 2020). The extraction yield of the present invention is not mentioned, nor can it be calculated from the amount of extract obtained, which is also not provided. The resin is further purified by thin-film evaporation, winterization, flash chromatography, or crystallization to obtain a CBD isolate of 96–99.8% purity that can be used as an ingredient for pharmaceutical applications. Although the authors claimed that the process offers a high-purity CBD extract with good yields and high reproducibility, the production of CBD isolates from industrial hemp requires a distillation step to obtain 60% CBD (as reported in the patent) for further purification by flash chromatography or crystallization. According to the authors, an extract containing 20% CBD is impossible to crystallize directly without a distillation step due to the low concentration.
Similarly, patent US10561693B2 (Pertile and Black, 2020) describes a method of obtaining CBD oil by supercritical CO2 extraction (35°C, 127 bar, 4 h) of industrial hemp. According to the invention, approximately 65–70 pounds of liquid CO2 (29–31 kg, 37–39 L considering the solubility of CO2 at the specified conditions) are required to complete the extraction of 4.5 kg of plant material, corresponding to a solvent-to-plant material ratio of 8–9 L/kg. The extracted full-spectrum oil, containing the cannabinoids and other fatty materials and plant waste, is decarboxylated and further processed through winterization, distillation, and crystallization with a non-polar solvent to obtain a pure CBD oil without THC and its characteristic psychoactive effects. Although no numeric results are provided, the invention is similar to the previous one since it describes an intermediate distillation step to obtain pure CBD from industrial hemp before crystallization of the extract.
In addition to the cannabinoid extraction techniques per se, SC-CO2 extraction devices are also described in the patent literature, such as US9782691B2 (Chess and McCutcheon, 2017), which reports a supercritical or subcritical CO2 extraction and recovery system that utilizes a dual pumping system including a high-pressure pump to keep the CO2 in the liquid phase and a modified refrigerant recovery pump for the complete recovery of CO2 at the end of an extraction cycle. The recovery system recirculates the SC-CO2 in a continuous loop for maximum extraction efficiency. Subcritical CO2 extraction using the described apparatus would occur at 800–900 psi (55–62 bar), while the separators would be kept at 500 psi (34 bar). Patent WO2019043259A1 describes a pilot-scale supercritical extraction of cannabinoids from C. sativa L. (Popp et al., 2019). The extraction vessels (1 and 2 L) are operated at 15 MPa (150 bar) and 35°C, while the pressure in the separators (200 ml each) is reduced to 4 MPa (40 bar) and 30°C. CO2 is continuously recycled at a flow rate of 5 kg/h and can be pumped to up to 10 kg/h. The extract is partially collected every 40 min, with the whole procedure lasting approximately 30 h. The resulting extract is lyophilized and the cannabinoids are isolated by centrifugal partition chromatography (CPC) using a pH-zone refining method.
The lack of numeric data on the yield or efficiency of CO2 extraction makes it difficult to compare not only the performance of the different SC-CO2 extraction inventions but that between SC- CO2 extraction and other techniques such as solvent extraction. Among the patents found in the literature, only US7344736B2 (Whittle et al., 2008) provides numeric data, with extraction yields ranging from 8.4 to 10.7%, CBD purity after extraction of 54–64%, CBD recoveries or efficiencies of 72.9–79.5 using subcritical and supercritical CO2, respectively, and 91% efficiency with supercritical CO2 and 2% of ethanol as a modifier. If the extraction yields reported in patent US7344736B2 are representative of the CO2 extraction technique, it can be concluded that CO2 extraction does not exceed the yields reported using other solvents such as ethanol, hexane, and petroleum ether, not even at the severe extraction conditions of up to 60°C and 300 bars. In addition, the reported CO2-to-plant material ratios (up to 192 L/kg) in the patent literature are considerably higher than those reported for other solvents (5–44 L/kg for ethanol, 3–12L/kg for butane, 5–45 L/kg for hexane, 5–25L/kg for petroleum ether, and 8–63 L/kg for vegetable oils). Although SC-CO2 extraction offers considerable advantages such as the preservation of the cannabinoid and terpenoid plant profiles, no -CO2 residue in the extract, CO2 recyclability, and environmental and security benefits due to the lack of toxicity and flammability of CO2, SC-CO2 does not show a better performance in terms of extraction yield compared extraction with organic solvents, considering the long extraction times (3–6 h vs. minutes no more than 3 h for extraction with other solvents such as ethanol, hexane or butane), energy consumption, and the sophisticated equipment required to operate at elevated temperatures and pressures.
Solvent formulations comprising C1-4 fluorinated hydrocarbon or a C1-4 hydrofluorocarbon ether for extracting cannabinoids from cannabis biomass are reported in patent WO2019158505A1 (Nicola and Osmanoglou, 2019). The proposed method consists of contacting the biomass in a sealed column with a solvent formulation, preferably 1,1,1,2-tetrafluoroethane HFC 134a, at a <-5°C and a rate of 0.02–1 ml/min per gram of biomass. Extracting at cold temperatures prevents the solubilization of impurities and helps to provide high-purity cannabinoids from the biomass. The solvent formulation may include a modifier to adjust the solvating properties of HFC 134a, preferably 5 wt% dimethyl ether or 5 wt% n-butane. After extraction, the extract is separated and dissolved in a minimum volume of ethanol and can be further purified by winterization, although the method is sufficiently selective so that waxes are not extracted. In an example, 500 g of biomass is decarboxylated (5.9 wt% THC content) and extracted with HFC 134a (5 L/h) at -5°C for 2 h (20 L/kg). The extract is then dissolved in a minimum volume of ethanol. The extraction is then continued at 25–30°C for 4 h (40 L/kg, 60 L/kg in total). The second extract is also dissolved in ethanol. Afterward, the extracts are winterized at -20°C and filtered through a celite bed. The ethanol is removed by vacuum distillation. As might be expected, the extraction yield from non-decarboxylated biomass is lower (0.82%) at -5°C for 2 h than that obtained after a second extraction step at 25–30°C for 4 h (5.26%). Nevertheless, the THC purities are 95.3% and 84% for the first and second extracts, respectively, which confirms that lower temperatures minimize the extraction of impurities, resulting in lower yields but higher cannabinoid purity. When using 5 wt% dimethyl ether and 5 wt% butane as co-solvents, the yields are slightly increased for the first and second extracts (1.34 and 5.44% for DME and 1.42 and 5.62% for butane). The invention provides data on recovery and THC purity for the blended extracts (88.8% and 93.9% THC recovery for 5 wt% dimethyl ether and 5 wt% butane as co-solvent, respectively, with a THC purity of 77.3% and 78.7%, respectively). For comparison, an extraction yield of 12% with a 75% THC + THCA purity (90% THC + THCA recovery) was reported in patent US20200102283A1 (Dibble and Cole, 2020) using butane as the sole solvent, which are similar results but with a considerably higher yield. A similar example is provided for the extraction of a decarboxylated CBDA-rich biomass (250 g, 3.9 wt% CBDA) with HFC 134a (3 kg/h, that is 2.5 L/h for a density of approximately 1,200 kg/m3 (Gasservei 8, 2021)), collecting the first extract after 1 h at -6°C (10 L/kg) and a second extract after a further 6 h at 25°C (60 L/kg, 70 L/kg in total). Both extracts are dissolved in a minimum volume of ethanol and winterized as described in the other example. Again, greater purity is obtained at lower temperatures at the expense of the extraction yield since the first extract has a 1% yield, 26.67% CBD recovery, and 98.9% CBD purity, while the second extract has 3.88% yield, 65.65% CBD recovery, and 65.98% CBD purity. The invention provides a detailed description of the extraction apparatus, which includes a solvent storage vessel from which the liquid is pumped into the jacketed stainless-steel extraction column where the biomass is tightly packed, followed by an evaporation/extract collection vessel, a gas compressor, and a heat-exchanger to reliquefy the fluid before its return to the solvent storage vessel. An alternative apparatus is described, which includes three evaporation/collection vessels, another storage vessel, and a pipeline to supply the modifier solvent that is mixed with the liquified gas. Another alternative apparatus for use in continuous mode is also described, including 2–5 extraction columns with volumes of preferably between 2 and 50 L, two to five evaporation/collection vessels with a volume of between 2 L and 50L, a solvent recycling vessel with a volume of between 5 and 200 L, 2–4 compressors, and 2–4 liquid feed pumps, each with a capacity of between 50 and 1000 kg/h depending on the scale of the operation. The authors claim that the method is particularly efficient at extracting high-purity cannabinoids from biomass without requiring extensive downstream purification before their use as a botanical drug substance (BTS). Nevertheless, the described solvent-to-plant material ratio (60–70 kg/L) is considerably higher than that used in liquid solvent extractions using ethanol, hexane, or petroleum ether (up to 45 L/kg). In addition, extraction using HFC 134a is performed for 6–7 h, which is similar to that for extraction with CO2, but high compared to the times for liquid solvent extraction (up to 2–3 h).
While the residual solvent limit for HFC 134a is not mentioned in the ICH Guideline for drug products, HFC 134a refrigerant is not considered toxic or carcinogenic. No toxicity data are available on humans following exposure to HFC-134a, even though it is rapidly absorbed by inhalation and can be retained in the liver and the adrenal gland, as reported in studies on rats (National Research Council, 1996). Regarding its environmental impact, HFC-134a is considered a potent greenhouse gas with a global warming potential (United States Environmental Protection Agency, 2021).
Several patents disclose the use of a hot gas for the extraction of cannabinoids from plant material without the use of solvents at temperatures higher than those traditionally used in distillation. Generally, the heated gas is drawn through the cannabis matter, causing the volatilization of the cannabinoids, which are subsequently collected in a condenser and separated into fractions (Whittle et al., 2015; Nevitt, 2020). The reported “heated gases” in the literature include hot air (which can result in the oxidative degradation of chemical compounds), “non-oxidizing gases” (which cause less oxidation than air under equivalent process conditions) such as dry steam, and “reducing gases”, such as sulfur dioxide mixed with steam, carbon dioxide, and inert gases (nitrogen, helium, or argon). A reducing gas is preferred for the extraction of cannabinoids from cannabis biomass.
This technique usually combines decarboxylation and extraction in a single step since the temperatures used to volatilize the cannabinoids are sufficient to allow decarboxylation into their neutral forms (Whittle et al., 2015). Eliminating the need for an additional decarboxylation step is an advantage over techniques such as extraction with ethanol or SC- CO2, which require pre- or post-extraction steps to decarboxylate the cannabis plant material or the resulting extract.
For applications in which the presence of terpenes is not desired in the product, an extract free of terpenes can be easily obtained with hot gas extraction using a multi-step temperature profile. As they are more volatile than cannabinoids, terpenes can be removed at a lower temperature in a preliminary extraction step. The temperature can then be increased in discrete steps to volatilize and condense the cannabinoids as separate fractions, which is convenient for the production of oil compositions with particular chemical profiles. In addition, nonvolatile materials such as waxes are not volatilized during extraction; thus, an additional purification step such as “winterization” is not required to remove lipid material from the extract.
Since cannabinoids are directly distilled from plant matter, this technique eliminates the use of solvents and liquid distillation equipment. Moreover, this technique can provide medicinal compositions that are free of any residual solvents. In contrast, this technique requires expensive equipment and the use of a gas at elevated temperatures to cause the degradation of natural compounds by pyrolysis or oxidation. A summary of relevant points in the hot gas extraction of cannabinoids is shown in Table 5.
Some examples of hot gas extraction can be found in the patent literature. For instance, US patent US10195159B2 (Whittle et al., 2015) describes a process and the apparatus for preparing a cannabinoid-rich extract from cannabis biomass or a primary solvent extract using a heated gas, preferably an inert gas, dry steam, or a reducing gas produced in situ by the addition of sodium metabisulphite (10%) to a stream of heated steam. Sodium metabisulphite reacts with the moisture content of the plant material to produce sulfur dioxide, which provides an antioxidant environment. The authors claim that the separation of cannabinoids from the natural product can be done by continuous agitation of the product (typical load of 5 kg of cannabis, according to the description) in contact with a stream of a heated gas at a temperature >100°C (preferably 175–200°C for high CBD material and 130–175°C for high THC material) at or above atmospheric pressure, which is sufficient to simultaneously decarboxylate and volatilize the cannabinoids without causing pyrolysis of the starting material. The cannabinoids are volatilized in one or more stages at increasing temperatures, producing a vapor that is condensed and collected as separate cannabinoid-rich fractions of high purity. The authors claimed that the process exhibited high efficiency and selectivity compared to other methods of solvent extraction and can also be operated continuously for the large-scale commercial production of extracts. Moreover, since the cannabinoids are separated in fractions because of the extraction at several stages of increasing temperatures, minimal non-desired compounds are co-extracted in those high-purity fractions; thus, the produced extracts are suitable for direct formulation into pharmaceutical forms, in contrast to cannabis extracts prepared by extraction with ethanol or SC- CO2. For example, starting with 5 kg of plant material and using reducing steam at 150°C with sodium metabisulfite (10%), followed by nitrogen gas at 218°C, the condensed extract contains 98% THC (the raw material was a high-THC chemovar, where THC comprises 95% of the total cannabinoids) and 98% CBD (starting from a chemovar where CBD comprised 90% of the total cannabinoids). Although the extraction time was not specified for the described apparatus, the authors reported that 50 g of plant material can be charged and heated for approximately 15 min on a pilot-scale apparatus. Nevertheless, the described apparatus has a typical load of 5 kg of cannabis, considerably less than other reported load capacities for apparatuses using other solvents; e.g., 60 kg through CO2 extraction (Whittle et al., 2008), 40 Kg with hexane (Cipolletti et al., 2020), and 150 Kg with ethanol (Oroskar et al., 2019). Patent US10596486B2 (Nevitt, 2020) provides a similar system for the solvent-free distillation of plant material to extract terpenes and cannabinoids using a heated air flow or inert gas, while heated nitrogen is preferred when the temperature is up to the range of plant matter combustion. The described procedure can process 45–90 kg of dried plant material at a time and includes a heat air induction system, a heated chamber where the plant substrate is agitated and contacts a flow of heated air, a distillation tube, and one or more collecting flasks. Different compounds can be collected by controlling the operating temperature of the heated chamber, while the pressure is prevented from rising too far above atmospheric pressure. For example, terpenes (caryophyllene, humulene) and acid cannabinoids (THCA, CBDA, CBCA) are collected at a lower temperature range (104–140°C), while terpenes such as alpha-pinene, myrcene, limonene, linalool, and terpinolene, and cannabinoids such as CBN, CBD, Δ9-THC, and Δ8-TCH, are collected at a higher temperature range (155–198°C). The system can be adapted for industrial-scale use. The compositions (oils with particular chemical profiles) produced by the system are free of solvent residues and can be used in products such as tinctures, lotions, sprays, pills, and e-cigarettes.
US patent US10617974B2 (Thomas, 2020) describes a system comprising a gas-moving device, an extraction chamber, and a condensation surface or cooling chamber where a cannabinoid-rich oil is collected. The heated gas (at or near atmospheric pressure) consists of atmospheric air, an inert gas such as nitrogen, or a reducing gas such as CO2. The extraction chamber contains at least a heated surface at 143–221°C where plant material is exposed to the heated gas stream for a minimal period (15–135 s) to prevent degradation of the plant oils. The invention claims that by adjusting the temperature of the heated gas and/or the heated surfaces, a variety of plant oils can be isolated into substantially purified fractions. For example, to target the extraction of Δ9-THC, the temperature is kept near 157°C. For the extraction of CBD, a first extraction cycle is needed at 157°C to remove the Δ9-THC, followed by a second extraction cycle at 180°C to volatilize the CBD. To target the extraction of all cannabinoids with a volatilization temperature below that of THCV, the temperature is kept near 220°C. Unlike the previous inventions, in this case, the volatilized cannabinoids are then condensed in a condensation surface or a cooling chamber, where a collection solvent is sprayed to cool the gas stream. A large portion of the volatilized oils condenses on the surface of the cooling spray droplets, where they are captured in the collection solvent. The collection solvent is preferably ethanol or a mixture of ethanol and water. The described system includes an evaporation device (i.e., thin-film, wiped-film, or short-path evaporators) to separate the cannabinoid oils from the collection solvent. In this case, by collecting the cannabinoids in a collection solvent, the advantage of extraction with a heated gas; namely, to obtain a solvent-free extract without an additional solvent removal step, is lost compared to other solvent extraction techniques. However, in some forms of the invention, the described apparatus involves a centrifugal oil droplet separator, electrostatic collection surfaces, or a filter collection section to avoid the use of a collection solvent to separate the oil droplets from the gas stream.
A similar process is described in patent US10765965B1 (Sherwood et al., 2020), in which a solvent-less extraction method can be operated in batch or continuous mode, in laboratory or large scales to process multiple tons of biomass per day. The method starts with the physical treatment of the cannabis material (chopping, cutting, or grinding), followed by moisture removal by preheating the feedstock in a low-temperature evaporator to a first temperature of at least 110°C under atmospheric or sub-atmospheric pressure (0.07–0.5 bar) for 10–120 min. During this step, low-boiling volatile compounds such as mono- and sesquiterpenes are collected. The preheated feedstock is then subjected to a flow of a motive gas (ratio of 13–5300 L/kg of feedstock) and further heated to a second temperature of 120–200°C at sub-atmospheric pressure (0.07–0.5 bar) in an evaporation chamber for 20–200 min. The authors report simultaneous decarboxylation and extraction of cannabinoids in these conditions. The motive gas is warm or hot air or a non-oxidizing gas such as nitrogen, CO2 and superheated steam, or an inert gas such as helium or argon. The motive gas drives off volatile compounds with relatively high boiling points such as cannabinoids, forming a “pregnant motive gas” that also contains sesquiterpenes, steam, and non-condensable gases. The pregnant motive gas is then directed to a recovery unit and the collected volatile compounds are condensed to produce an extract. According to the authors, the method produces high-purity cannabinoid-rich fractions with >80 wt% and even 90 wt% total cannabinoids, with up to 80% CBD in the extract, exhibiting great efficiency (CBD removal of up to 90% from hemp) and selectivity since the resulting oil is substantially free of non-volatile chlorophyll, waxes, and lipid-soluble compounds generally present in extracts produced by solvent and CO2 extraction methods. Additionally, compared to solvent and supercritical CO2 extraction systems, the described method does not require specialized equipment or additional purification steps such as winterization. Moreover, the extract also has a “full-spectrum” quality, with similar ratios to the cannabinoids present in the feedstock.
The absence of any extraction yields in the patents described above is noteworthy. Only US10765965B1 (Sherwood et al., 2020) provides CBD extraction efficiency data (up to 90% from hemp, similar to that of conventional techniques). The more sophisticated equipment required by the hot gas extraction technique and the elevated operating temperatures results in higher costs compared to simpler extraction methods with liquid organic solvents (ethanol, hexane, or petroleum ether), which are usually performed at room temperature (no more than 50–80°C) and atmospheric pressure. However, compared to SC-CO2 extraction at temperatures up to 60°C and pressures up to 300 bar, the hot extraction technique, though expensive, benefits from not requiring expensive high-pressure equipment that also involves pressurizing costs. As mentioned before, some advantages of the hot gas technique are derived from carrying out the extraction without a solvent, which means that the resulting oils or compositions have no solvent residues and can be directly applied to formulations, and no waste management or additional steps are needed for solvent evaporation. Moreover, this technique offers no additional step for decarboxylation and minimal co-extraction of undesired compounds such as waxes or chlorophylls. However, like SC-CO2 extraction, the use of hot gas extraction is mainly relevant in applications where oil compositions with specified terpene and cannabinoid profiles are needed since the sophistication of the necessary equipment and the energy consumption required to increase the temperature do not outweigh its benefits.
Along with conventional techniques, some alternative extraction methods are becoming increasingly popular in the cannabinoid extraction industry, including microwave-assisted extraction (MAE), ultrasound-assisted extraction (UAE), hydrodynamic cavitation, and pulsed-electric field (PEF) (Mancosky, 2018; Ramirez et al., 2019; Radoiu et al., 2020). In general, these techniques disrupt plant cell walls; thus, they are especially suitable for extracting compounds located in the inner cell walls. However, in the case of cannabis, the cannabinoids are in the trichomes found on the surface of the plant, so the main advantage of these alternative techniques compared to solvent extraction is reduced. Moreover, breaking the cell walls also has the inconvenient effect of releasing undesired compounds such as chlorophyll, which is contained inside the chloroplasts of plant cells (Ramirez et al., 2019). A summary of the main aspects of the most important alternative techniques is shown in Table 6.
The main difference between microwave-assisted extraction (MAE) and conventional techniques is that the mass transfer in MAE is enhanced by pressure because of microwaves (Radoiu et al., 2020). When microwaves are applied to a biomass, the water contained in the cells selectively absorbs the microwave energy, causing instant increases in temperature and pressure within the cells, facilitating cell rupture and enhancing the rapid dissolution of compounds in the solvent (Chang et al., 2017). Microwave heating is instant, more uniform, and efficient compared to the use of heat in conventional techniques. Therefore, microwaves offer a great advantage since they allow precise control of the process, which results in improved product quality and cost-effectiveness. In addition, MAE can extract bioactive compounds with a higher recovery rate compared to conventional techniques, although this is not applicable to cannabis since cannabinoids are not located in the inner cell walls (Drinić et al., 2020). However, MAE requires expensive and sophisticated equipment that has a relatively short lifetime.
The diffusion of compounds from the plant cells in conventional extraction techniques is slow and reaches a saturation point, requiring high amounts of solvent and several extraction stages. In contrast, extraction with MAE is rapid and continues as long as energy is applied. Therefore, for cases in which desired compounds must be extracted from plant cells, solvent requirements and extraction times can be reduced by controlled modification of the MAE process parameters such as extraction temperature, microwave frequency, and power density, resulting in environmental preservation (Chang et al., 2017; Radoiu et al., 2020). However, the microwave power must be carefully dosed since the extraction yield increases until the optimal temperature is reached; beyond that point, the yield starts to decrease due to the thermal degradation of the compounds (Cavalloro et al., 2021). This technique has been commercially demonstrated at an industrial scale in Canada, as a continuous-flow microwave extractor has been operated for >5 years with inputs of 200 kg/h of cannabis biomass, and up to 95% recovery of active compounds. In this process, biomass is mixed with a solvent (i.e., ethanol, isopropyl alcohol, pentane, polyethylene glycol) to form a slurry (Radoiu et al., 2020). This slurry is continuously pumped into the MAE reactor and heated by microwaves of 915 MHz at a maximum of 75 kW. An MAE extractor operated in continuous flow at atmospheric pressure requires less time to process larger amounts of biomass compared to conventional extraction methods, with a higher extraction efficiency, product yield, and extract quality. For example, 100 kg of biomass (flow of 30 kg/h, 3.3 h) per run (two runs of 6.6 h in total) can be processed with 360 L/h of ethanol (12 L/kg) producing an extract with 61.4% THC (92.6% THC recovery in the extract) in the first run and 55.1% THC in the second run (93.4% THC recovery). For comparison, the large-scale CO2 extraction of 60 kg of cannabis biomass required 4 h with a solvent-biomass ratio of 94–132 L/kg under subcritical (10°C, 60 bar) and supercritical (40°C, 100 bar) conditions to recover less CBD (72.9–79.5%, 8.4–10.7% yield and 63.6–54.4% purity) (Whittle et al., 2008). Hexane extraction of 31 kg of biomass for 12 h produces less THC (70.98%, 13.36% yield, 52.9% purity). However, the extraction occurs at room temperature, with a hexane-biomass ratio of 4.5 L/kg (Elsohly et al., 2021), which is less than half the ratio used in the MAE example. Moreover, by doubling the hexane-biomass ratio to 11 L/kg, a THC recovery of 96.58% (10.58% yield, 40.35% purity) has been reported at room temperature, although the total extraction time was 48 h. Although not large-scale, Phytoplant Research S.L. (internal data) has obtained THCA + THC recovery of up to 95% when extracting 1.3 kg of the non-decarboxylated Moniek variety (11.43% THCA + THC) at room temperature with hexane (14.3 L/kg) in just 2 h (extraction yield of 26.85%), while >95% recovery of CBDA + CBD has been obtained by extracting 2.5 kg of the non-decarboxylated Goya variety (10.76% CBDA + CBD) with ethanol (15L/kg) in 1.5 h at room temperature (extraction yield of 15.17%). Despite the use of different chemotypes, comparison of these results shows that the benefit of MAE over solvent extraction techniques without auxiliary energy is not clear since similar cannabinoid recoveries are reported in less time for similar solvent consumption.
The patent literature includes some examples of microwave-assisted extraction. For instance, WO2019207554A1 (Splinter et al., 2019a), also discussed in the “Other solvent extraction”, reported a method of extracting active cannabinoids such as THCA/THC from cannabis biomass in a continuous-flow, microwave-assisted extractor. The apparatus is also described in detail in patent WO2019211794A1 (Popek et al., 2019b) from the same authors/company. In this process, a slurry is formed with biomass and a carrier fluid, which is a suitable solvent for inclusion in a final formulation, such as a medium-chain triglyceride (i.e., coconut oil), or a food-grade solvent. The slurry is transported to the extraction chamber coupled to a microwave generator, where it is exposed to microwaves and heated to 25–75°C with a contact time of 1–30 min and a microwave energy density of 0.1–10 kW/kg. The spent biomass is then separated from the carrier fluid containing the cannabinoids in a filtration unit. The invention provides results for the extraction using MCT or canola oil at different solvent-to-solid ratios (8–12 L/kg) and temperatures (30–60°C). These conditions produce an extract with 0.33–1.3% THCA purity, with a THCA recovery of 66–91%. The carrier fluid or MCT solvent containing the active compounds can be subjected to decarboxylation or incorporated into pharmaceutical, food, or cosmetic formulations. Again, the lack of data on the cannabinoid purity achieved with vegetable oils is striking, even though, compared to other patent results, MAE allows the extraction to take place in less time and uses a lower solvent-to-plant material ratio at a similar temperature than the extraction with vegetable oils without microwaves assistance (60°C, 30–120 min, 26–63 L/kg, >90% cannabinoid recovery) (Barringer, 2017).
This process is further described in patent WO2019211795A1 (Splinter et al., 2019b) by the same company, using an alcohol, alkane, or ketone as the extraction solvent, under identical conditions as specified above. The invention provides laboratory test data obtained for the microwave-assisted extraction of THC-type biomass using different solvents, such as ethanol, pentane, and polyethylene glycol. According to the authors, 4 g of biomass containing 4.1% THCA extracted with 32 ml of ethanol or polyethylene glycol (8 L/kg) resulted in 90% and 78% THCA recovery, respectively. The best recovery (93%) was obtained with pentane from a biomass with 5.3% THCA using a solvent-to-biomass ratio of 8 L/kg. After extraction, the solvent is evaporated in an evaporator chamber. According to the authors, the extraction of the target compounds can be maximized by adjusting the microwave parameters, the flow rate, or the chamber dimensions, while reducing the run time and co-extraction of non-desirable compounds. The authors claim that exposing the slurry to a microwave generator will produce high recovery percentages of cannabinoids in a short extraction time. For example, the invention provides data on CBDA and THCA recovery at different extractor temperatures and residence times (the time for which the slurry is exposed to microwave energy). At a residence time of 10 min, the recovery of CBDA is optimized to 90%. According to the provided data, increasing the extraction temperature from 23 to 27°C results in the enhancement of THCA recovery from 86 to 93%. Likewise, the CBDA recovery rises from 85 to 91% as the extractor temperature is increased from 40 to 60°C. The extraction procedure is followed by a final formulation step where a cannabinoid, such as THC, is diluted into an MCT carrier oil with a concentration of 72% THC. However, these examples do not provide the cannabinoid purity. As the temperature increases, more unwanted compounds may be extracted in addition to more cannabinoids. Moreover, the invention does not mention any control experiment without the use of the auxiliary energy to confirm that the recovery increase does not negatively affect the purity and that the use of MAE is indeed effective for the extraction of cannabinoids.
According to patent US10537592B2 (Kotra et al., 2020), microwave-assisted extraction can be combined with decarboxylation of the cannabinoids in a single step. The invention describes laboratory-scale methods of producing decarboxylated cannabis resins by simultaneous extraction and decarboxylation using microwaves and solvents such as ethanol. The method also comprises decarboxylation with microwaves before or after extraction. Microwave-assisted extraction and decarboxylation are performed by suspending cannabis plant material in a solvent with stirring or agitation and subjecting the mixture to microwaves of 2.45 GHz and a wavelength of about 1.22 × 108 nm in a sealed vessel at 100-200°C and 2–22 bar for 10–30 min, followed by filtration. According to the authors, the simultaneous extraction and decarboxylation must be performed in the microwave vessel under pressure to sustain the temperature over the required period to ensure complete decarboxylation without burning the plant material or evaporating the solvent. The invention claims that the decarboxylation of cannabinoids is complete by subjecting the extract to microwaves, or by simultaneous decarboxylation and microwave-assisted extraction, which optimizes the decarboxylation and cannabinoid recovery from the biomass and improves the consistency and reproducibility of the final product, which is formulations for pharmaceutical or natural health products comprising the decarboxylated resins. The decarboxylated cannabis resins can be also used directly as medicines, natural health products, or for recreational use.
Patent US10537592B2 provides results for laboratory tests at the gram scale, from which extraction yields can be calculated. Variable extraction yields ranging from 25 to 73% were reported, depending on the starting material and MAE extraction conditions such as temperature and time. For example, dried and crushed plant material (7.18% THC, 8.6% CBD) submerged in ethanol (10 L/kg) in a sealed vial and subjected to microwave-assisted extraction for 10 min at 100°C, produced a non-decarboxylated THCA extract with 32% yield. By increasing the temperature and processing time, the authors claim that 1 g of cannabis can be successfully extracted and decarboxylated with ethanol (10 L/kg) under microwave conditions yielding 27% (150°C, 30 min) or 23% (150°C, 20 min) extracts. In another example, the starting material is an SFE extract that is subjected to microwave-assisted decarboxylation with ethanol to produce a decarboxylated extract yield ranging from 25% (150°C, 10 min) to 73% (100°C, 30 min). According to the authors, the method can be scaled up with appropriate adjustments to the conditions. Moreover, the large-scale apparatus is published in patent WO2019119153A1 (Kotra et al., 2019). Although the authors claim that the high-volume extractor allows highly efficient extraction and decarboxylation in a single step, the process description indicates that the cannabis plant material is first mixed with ethanol (4.3 kg and 34 L, 8 L/kg) to produce a slurry/suspension that is stirred at room temperature for 4 h, during which the cannabinoids are extracted. Then, the solution or resin is filtrated and run through the continuous flow microwave apparatus for decarboxylation (135–200°C at 10–25 bar); thus, the extraction and the decarboxylation processes occur in different stages and the extraction step is not microwave-assisted.
Although microwave-assisted extraction of cannabis has been applied industrially, the inventions in the patent are only gram scale. The main challenge in scaling up microwave reactors is the limited depth of penetration of microwaves, which means that by increasing the scale or volume of the reactor, the energy generated by the microwaves is rapidly dissipated and only the outer layer of the reaction mixture is heated. Therefore, MAE cannot be scaled up by using large-stirred vessels; however, the use of continuous flow reactors would overcome this penetration depth limitation. Although industrial-scale microwave ovens are commercially available, the industrial use of MAE still had economic and technological limitations including the high equipment cost and operation expenditure compared to the relatively low lifetime of magnetrons (Radoiu et al., 2020). However, the economic and technical analysis is more complex since the application of MAE to cannabis allows precise extraction control but does not necessarily result in less solvent consumption or reduced extraction times than conventional techniques without auxiliary energy. Nonetheless, published studies comparing microwave to conventional heated reactors for biodiesel production indicate that although capital expenditures are high, the energy efficiency of microwave heating is >30% better than conventional heated processes (Priecel and Lopez-Sanchez, 2018).
Comparable to the cannabis extraction with vegetable oils, the final products of the MAE patents (extracts or resins containing relatively low cannabinoids concentrations), can be directly applied as medicines or natural health products, or used in pharmaceutical product formulations, without obtaining pure cannabinoids (>95%) by crystallization or chromatography, which are the most common next steps in organic solvent extraction.
Our thorough review of the patent literature suggests that microwave-assisted extraction has similar cannabinoid recovery from plant material compared to conventional techniques such as solvent extraction, although clear whether the effect of using auxiliary energy during extraction constitutes an advantage in terms of processing time and solvent-to-plant material ratios is not yet clear. Future studies should examine the economic and technological aspects of MAE scaling and conventional techniques so that cannabis producers have as much information as possible to implement the most appropriate technique for each application and intended final product.
Ultrasound-assisted extraction (UAE) generally improves extraction kinetics and mass transfer by using sound waves to break apart plant cell walls. Ultrasonication allows uniform mixing and reduces thermal gradients; thus, UAE is carried out at mild temperatures and requires shorter extraction times compared to conventional techniques (Omar et al., 2013; Baldino et al., 2020; De Vita et al., 2020). The application of sound waves causes a cavitation phenomenon, in which microbubbles or tiny localized hotspots form, which cause cell walls to swell and collapse, allowing the faster dissolution of target compounds into the solvent (Agarwal et al., 2018).
The key parameters affecting UAE efficiency are temperature, time, solvent nature, and ultrasound power and frequency (Agarwal et al., 2018). While cavitation is affected by the physical properties of the solvent (surface tension, viscosity, vapor pressure), time and temperature are directly correlated since temperature increases with time owing to the heat generated in the process (Wen et al., 2018). Therefore, extended sonication time is not desirable as it could increase the temperature and subsequent degradation of heat-sensitive compounds, in addition to increased energy and operating costs. Temperatures of 20–70°C, especially those below 30°C, benefit ultrasound-assisted extraction compared to non-sonicated methods due to the increased number of cavitation bubbles, solid-solvent contact area, and solvent diffusivity that traduces in better desorption and solubility of the compounds of interest (Chemat et al., 2017). However, these effects diminish at temperatures close to the boiling point of the solvent; therefore, increasing the UAE operating temperature provides no additional benefits. Ultrasound power affects the intensity of the ultrasonic waves that pass through the plant material-solvent mixture, which impacts the extraction efficiency. While the high power of sonication may result in an increased temperature and subsequent degradation of thermolabile compounds, some compounds may require high ultrasound power for their removal from the plant matrix and are dissolved into the solvent, which is not the case for cannabinoid extraction from the trichomes. Moreover, traces of metal can be identified inside the extracts if the ultrasonic probes are not ceramic.
In addition to short extraction times and reduced energy requirements, UAE is a simple, reliable, and inexpensive technique that does not require large amounts of solvent. Tuning the parameters affecting UAE can provide process controllability and reproducibility, resulting in improved quality of the final product. Since it does not require high temperatures, UAE can be performed in applications in which cannabinoid and terpene integrity and concentration must be preserved. Nevertheless, scaling the UAE process remains challenging (Baldino et al., 2020). Large-scale UAE designs require the optimization of equipment size and configuration to maximize the energy transfer to the fluid and reduce energy/electricity consumption of the ultrasonic bath and probe systems, which are the most common configurations used in the industry (Wen et al., 2018). The ultrasonic probe produces more energy than the conventional ultrasound bath, which traduces in shorter extraction times, although it also produces a sharp increase in the sample temperature during sonication and is restricted to a small processing volume. This problem can be overcome by using a continuous reactor that allows ultrasound to be concentrated in a smaller reactor volume while processing larger amounts of material, or an ultrasonic bath with a larger radiating surface and a stirring system.
The extraction of cannabinoids via UAE has been reported in the literature, with improved efficiency and shorter extraction times compared to those for conventional techniques; however, most of the studies have been laboratory-scale (Omar et al., 2013; Brighenti et al., 2017; Agarwal et al., 2018; De Vita et al., 2020). The patent literature includes several examples of ultrasound-assisted extraction. For example, the invention US20200063061A1 (Vanaman, 2021) describes a cannabis oil extraction apparatus including an ultrasound-assisted extraction unit that can be continuously operated. The extraction vessel incorporates a transducer to emit ultrasonic soundwaves through the mixture of plant material and solvent (e.g., ethanol) and is heated up to 104°C within a negative pressure environment (from 0.68 to −1.03 bar). The authors claim that UAE enhances extraction via cellular disruption, although in the case of cannabis, cannabinoids are contained in the trichomes (plant surface), and no data about efficiency, yield, or solvent-to-biomass ratio have been provided. The sonication amplitudes of the transducer are adapted to assist in breaking apart the plant cells. The transducer generates soundwaves between 5 kHz and 250 kHz or more and can alternate frequencies to provide full-spectrum emission to target a wide variety of plant parts with different characteristics such as density The extraction system can be configured for the complete end-to-end processing of plant material into refined cannabinoid oil extract products at custom concentrations, with further steps such as winterization, filtration, and short-path distillation.
Patent WO2020028992A1 (Farokhi et al., 2020) describes a method for the extraction of cannabinoids and terpenes from fresh or dried plant material with a cold organic solvent (ethanol, acetone, or ethyl acetate at 0 to −80°C, which uses ultrasound to rapidly liberate cannabinoids. Sonication in the open extraction vessel is achieved by alternating high and low-pressure cycles. According to the patent, the combination of cold temperatures and ultrasound power improves cannabis extraction runtime and efficiency. The invention gives some data on the relative extraction efficiency. The ultrasound-assisted extraction with cold acetone at −20°C for 10 min results in relative extraction efficiencies for THCA + THC, CBDA + CBD, and total cannabinoids of 92.6%, 87.1%, and 89.5%, respectively, with a total cannabinoid purity of 91.7%. For the same experimental conditions with acetone but without UAE, the relative extraction efficiencies were 96.8%, 81%, and 86.7%, respectively, with a purity of 84.5%. The patent provides comparative figures of cannabinoid efficiencies and purities for different extraction conditions, which demonstrated that, although the extraction efficiency of THCA + THC was negatively affected by UAE, extraction with acetone and ultrasound allowed the recovery of additional CBDA + CBD and total cannabinoids as well as higher cannabinoid purity at both room temperature and below zero temperatures. The cannabinoid purity obtained with ethanol is very similar and even slightly inferior when the extraction is assisted with ultrasound at both room and cold temperatures, presumably due to the co-extraction of other compounds such as terpenes. One of the provided figures showed that UAE increased terpene extraction when using ethanol or acetone as solvents. After extraction, the extract is subjected to decarboxylation (if the plant material was not decarboxylated before extraction), bleaching with clays, silica gel, activated carbon or diatomaceous Earth, to remove pigments, fatty acids, peroxides, etc., and winterization to remove waxes, so that the refined extract can be formulated with a pharmaceutically acceptable carrier, diluent, or excipient to provide compositions for oral, topical or transmucosal administration.
Another patented process and apparatus for extracting cannabinoids from cannabis plant material using UAE can be found in the invention US20160279183A1 (Hospodor and Rapp, 2021). In this process, the cannabis plant material is filled in a hopper connected to a sonic or ultrasonic transducer, while the solvent (hexane or heptane) is circulated through the hopper. Ultrasonic frequencies are applied to the saturated solvent. However, the authors do not specify the extraction conditions, explain the benefits of UAE, or give any efficiency data since the invention is a mere description of the extraction apparatus.
Patent US10301242B2 describes the ultrasonic extraction with ethanol in a method for CBD extraction from hemp (Zhang et al., 2019). According to the invention, ground and dried hemp is extracted 1–3 times with 30–100% (v/v) ethanol (solvent-to-biomass ratio of preferably 4–6 L/kg each time) by reflux extraction (0.5–3 h each time), ultrasonic extraction (0.1–1 h each time) and/or soaking extraction (0.5–5 h each time). The extract is then subjected to water precipitation to remove impurities, followed by centrifugation and the addition of 10–100% (v/v) ethanol to dissolve the precipitate, which is further purified by column chromatography and crystallization in ethanol to obtain pure CBD. The patent provides data on the CBD extraction efficiency (called the “extraction rate”) and purity of the final product after the purification process. The extraction process was performed under the same conditions (80% ethanol, two times, 5 L/kg each, 10 L/kg in total) by different preparation methods; i.e., ultrasonic (70 Hz, 0.5 h each time), reflux (1.5 each time), and soaking extraction (2.5 each time), with no substantial differences in the extraction efficiency (96.4–96.8%) and purity of the final product (97.4–99.5%). However, to achieve the same CBD efficiency, ultrasound-assisted extraction assisted with takes considerably less time compared to reflux and soaking extractions, even though the UA extraction described in the patent is preceded by additional steps such as water precipitation and centrifugation, which are not generally needed before conventional solvent extractions.
A thorough review of the patent literature is not sufficient to determine whether ultrasound-assisted extraction results in a significantly reduced extraction time compared to solvent extraction techniques with similar extraction efficiencies of up to 97% CBD and 93% THC recoveries, respectively. However, as none of the patents provide data on extraction yield, this parameter cannot be compared. UAE does not require harsh pressure or temperature conditions and can be used with food-grade solvents such as ethanol, with a solvent-to-biomass ratio of 10 L/kg, similar to the solvent consumption reported for MAE, although ratios of up to 25 L/kg have also been reported for UAE with acetone (Zhang et al., 2019; Farokhi et al., 2020). Ultrasound-assisted extraction produces refined cannabinoid extracts that can be formulated into pharmaceutical products, as well as pure CBD (>95%) after purification of the extract using common chromatography or crystallization techniques, as seen for conventional techniques. While UAE is considered a green technology with reduced environmental impact, further research is needed to develop its industrial application for cannabis extraction and demonstrate its economic viability over conventional techniques.
As discussed with MAE and UAE techniques, pressure fluctuations can enhance the extraction of diverse natural compounds from lignocellulosic material. Cavitation of the fluid can produce high-intensity shock waves that traduce in rapid and highly energetic pressure variations that penetrate the plant material, causing cell wall lysis and liberating desirable compounds other than cannabinoids, which are located in the trichomes. Although ultrasonic and hydrodynamic cavitation generate similar cavitation effects, the main difference between these methods is the cause of the cavitation phenomenon. While ultrasonic cavitation is caused by pressure variations of the ultrasonic waves passing through a fluid, hydrodynamic cavitation can occur in any turbulent fluid and is produced by the sudden change in velocity of a moving fluid, which results in a localized pressure drop resulting in the vaporization of the fluid and the formation of cavitation bubbles (Li et al., 2020). For example, a sudden change in velocity can occur when the fluid passes through a constriction such as an orifice plate, a venturi, or throttle valve (Panda et al., 2020). While ultrasonic cavitation relies on a source of vibrations, which limits its scalability, hydrodynamic cavitation is more energy efficient and easier to implement at scale since the restrictions of maximum or minimum processing flow depend on the ability of the pump to achieve the required pressures and guarantee a constant flow through the cavitation region.
Although hydrodynamic cavitation is a promising technology, the lack of patents or research studies on cannabis is striking. However, patent US10011804B2 describes a method and suitable apparatus for applying controlled cavitation technology for the extraction of CBD and THC from cannabis plants (Mancosky, 2018). The extraction method includes drying, grinding, or chopping the cannabis plant, and mixing the resulting plant pieces with a solvent to form a mixture. The solvent is carbon dioxide, alcohol, glycerin, propane, butane, or mixtures thereof. The mixture is circulated through a controlled cavitation reactor to liberate the cannabinoids, which are subsequently separated from the fluid. The intensity of the shock waves and the resulting pressure fluctuations are controlled by adjusting the rotation rate of the rotor in the cavitation reactor. The rotor surface has cavitation-inducing structures such as arrays of bores in its peripheral cylindrical surface, which generate the cavitation phenomena that allows a more selective extraction by simply liberating the cannabinoids from the trichomes or breaking down more difficult structures to release compounds that otherwise would not be liberated. According to the authors, the controlled cavitation technology for the extraction of CBD, THC, desirable oils, and other compounds, may be also used as a previous or post-treatment step in combination with traditional extraction methods to maximize the usually low extraction yields characteristic of these previous techniques. In addition, controlled cavitation can reverse hornification, defined as the adhesion of fiber surfaces to each other because of drying, which limits the accessibility of the capillary system of the plant for future extraction. The highly energetic pressure fluctuations induced by cavitation can force the solvent into the dried structures and reopen them to almost their original configuration before drying, increasing the extraction yield.
Overall, the patented controlled cavitation technology can be implemented at any commercial scale with good results, whereas a similar extraction technology such as UAE, is not as simple to scale up and reproduce on a commercial scale. The shock waves produced by cavitation events are more energetic than ultrasound waves, resulting in faster extractions with higher yields, higher efficiency, and lower cost. Nevertheless, the invention does provide data on yield or efficiency or even extraction conditions such as temperature, pressure, or solvent-to-biomass ratio. The report is just a description of the apparatus. Thus, its comparison to other extraction methods can only be made with assumptions based on qualitative aspects. Although the hydrodynamic cavitation technique offers great advantages such as scalability, and fast extractions with low solvent consumption, the equipment is more sophisticated than those used in conventional techniques such as solvent extraction. Since no data on the extraction yields and efficiencies have been reported, whether the advantages outweigh the greater sophistication of the equipment compared to other extraction methods remains unknown.
As introduced in the pre-treatment section, the application of pulsed electric fields (PEF) to plant material creates or expands existing nanopores within the cell membranes. This causes irreversible cell wall rupture or lysis through electroporation due to the movement of ions toward the cell membrane, producing an enhanced electric field that creates the pores. Generally, PEF in extraction processes facilitates the release of valuable compounds from plant material by increasing their bioavailability, resulting in a fast (from nanoseconds to milliseconds), environmentally friendly, and energy-efficient method with low-cost operation, which is easy to scale and perform in an industrial continuous process (Martínez et al., 2020). However, in the case of cannabis, in which the cannabinoids are located in the trichomes, this technique does not necessarily represent a significant advantage over conventional techniques. PEF is a non-thermal process that can be performed at cold temperatures, which minimizes the degradation of heat-sensitive compounds (Barba et al., 2015; Haji-Moradkhani et al., 2019). Compared to conventional techniques, PEF-assisted extraction has increased mass transfer, better extraction performance, and reduced conventional extraction parameters such as temperature and solvent concentration (Barba et al., 2015).
However, the limitations of PEF-assisted extraction include the high equipment cost and that the required power largely depends on the conductivity of the medium in which the biomass is diluted; therefore, the influence of the extraction solvent on the electrical conductivity of the biomass is an important factor that can affect the extraction performance. Moreover, a variety of non-desired electrochemical reactions can occur at the interface between the electrodes and the biomass solution, which can cause the release of gases and toxic chemicals (mainly H2, O2, H2O2, HCl, HClO), water electrolysis, changes in the fluid chemical properties (pH, electrical conductivity), and fouling of the electrode’s surface that can cause problems such as distortion of the local electric field or contamination of the treated material (Golberg et al., 2016). The extent of these unwanted reactions can be reduced by optimizing PET process parameters such as chamber design, electrode material, maximum voltage, specific energy input, polarity, and duration of the pulse, among others. Nevertheless, electrochemical reactions and the dissolution of electrode materials are, in the long run, unavoidable.
Despite being widely used in the food industry with commercial plants in the United States, Germany, and the Netherlands, the use of pulsed electric fields in the cannabis industry is generally limited to cannabis oil extraction at the laboratory scale (Haji-Moradkhani et al., 2019). The use of PEF for the extraction of cannabinoids from cannabis has only been reported in US10773184B2 (Held and Stanis, 2020). In particular, the invention describes a PEF treatment device configured for batch or continuous processing, in which a cannabis slurry is placed into a treatment zone for PEF application. Cannabis mechanical processing and PEF treatment can be performed separately or at the same time. When both steps occur simultaneously, the cannabis material is chopped, crushed, or pulverized by compressing or crushed as it passes through two rollers that function as electrodes applying the PEF. The slurry is then formed by soaking the crushed material in a liquid stream of water or a solvent consisting of hexane, butane, or, most preferably, ethanol, for 1–6 h. The liquid-to-plant material weight percent ratio within the slurry can vary from 10:90 to 90:10.
When performed separately, the processed slurry is inserted into the treatment zone of a PEF device. The slurry is continuously fed into the channel of the high-voltage electrode with a flow rate of up to 100,000 L/h, whereas the inlet temperature varies from 10 to 90°C. High-voltage pulsed signals are applied to the high-voltage electrode, creating a pulsed electric field in the treatment zone with a field strength of 500–5 kV/cm. By controlling the field strength of the PEF, optimal breakdown of the cell walls to a predetermined size can be achieved while minimizing energy consumption. Correspondingly, the frequency of the PEF is adjusted based on the measured flow rate of the slurry into or out of the treatment zone to apply an energy of 1–300 kJ/L. The pulse duration can vary from 0.1 μs to 10 ms. All those parameters can be optimized depending on, for example, the water or solvent content of the slurry entering the PEF treatment device, the temperature of said slurry, or its conductivity.
After PEF treatment, the product can be separated or isolated in specific compounds by post-processing in a separation unit that includes filtration, distillation, crystallization, or liquid-liquid extraction units, among others. The separation unit may consist of two distillation columns configured to create THC- and CBD-rich products containing 80–99.9 wt% THC or CBD, respectively. Unfortunately, the extent to which the extraction of cannabinoids is benefited by applying a pulsed electric field cannot be determined since no yield or efficiency data are available.
Similar to MAE, UAE, and hydrodynamic cavitation extractions, PEF-assisted extraction has great potential in the cannabis industry due to its low energy consumption, reduced environmental impact, and adaptability to processing capacities of thousands of liters per hour. However, further studies are required to determine whether its application to cannabinoid extraction leads to a significant decrease in processing time and solvent consumption over traditional extraction techniques. Thus, further pilot and industrial scale studies are also needed to evaluate the economic and environmental benefits of PEF-assisted extraction compared to conventional technologies. Additionally, the long-term performance of industrial PEF systems in continuous operation remains unknown.
Finally, this section discusses inventions described in the cannabis patent literature that describe in detail sophisticated extraction systems or processes, although few provide data on the extraction efficiency. This section may also help to show the whole picture of the available state-of-the-art technologies.
US10272360B2 (Lopa, 2019) describes an advanced extraction system for removing essential oils and phytochemicals (cannabinoids and terpenes) from cannabis without using a solvent. In contrast to methods that use a heated gas (see the “Hot gas extraction” section), this procedure uses a temperature <100°C to avoid heat-induced alteration or degradation of the extracted phytochemical composition. To reduce the volatilization temperature of the compounds, the extraction is carried out under vacuum. The system comprises a vacuum chamber configured to maintain the plant material under vacuum, an evacuation pump to create a vacuum sufficient to volatilize one phytochemical, and a collection chamber for phytochemical precipitation.
A continuous system to concentrate cannabinoids from cannabis plant material, such as CBD or THC, CBN, and CBG or CBC is described in detail in patent US20200155995A1/US10946329B2 (Kolesinski, 2021). The extraction system consists of heating the plant material, which is mechanically processed to increase its surface area by reducing the average particle size, to the volatilization temperature of a target product such as CBD (between 160°C and 180°C). This temperature can also cause decarboxylation of the cannabinoids to their neutral forms, eliminating the need for previous steps. The plant material is heated in a furnace or an open or closed chamber comprising resistive heater elements. An air flow directs the volatilized product onto a functionalized belt where it is adsorbed; the functionalized belt containing the target product is then contacted with a reagent that releases the target product by desorption. This contact can take place by immersing the functionalized belt in the releasing reagent or by spraying the releasing reagent over the functionalized belt. The releasing reagents include aqueous mixtures of organic solvents (acetonitrile, alcohols) or salt solutions. The belt is a polymer with functionalized surfaces that chemically interact with the target product, with high affinity and specificity. The belt includes a substrate made of synthetic organic, metallic, or fibrous materials such as non-woven and woven fibers, and membrane structures. The system can be operated continuously to process large amounts of raw plant material into concentrated cannabinoid solutions at an industrial scale. Although the patented method eliminates the use of liquid reagents to extract the target compounds from the plant material, it does not eliminate the use of liquid reagents, since the release of the target compound from the functionalized belt is generally produced by immersion of the functionalized belt into the releasing reagent.
Conveniently, the extraction techniques can be performed in combination to enhance their performance. For example, patent US10428040B2 (Dijkstra, 2019) describes processes that combine stripping and ethanol extraction to obtain an extract containing almost pure cannabinoids with high yields on a large scale. First, chopped cannabis plant pieces or a cannabis extract are dispersed in oil, preferably a vegetable oil such as soybean, rapeseed, corn germ, sunflower seed, or hemp seed oils. Vacuum stripping and ethanol extraction are then combined to isolate the cannabinoids from the oily suspension. Although they can be performed separately, it is preferred to combine both techniques since stripping allows the separation of the colored compounds, while ethanol generally co-extracts chlorophylls. Hence, the first step of the method involves vacuum stripping the dispersion at a temperature <250°C with a gaseous stripping medium (water vapor), whose required amount for extraction is proportional to the amount of processed oil. By gradually increasing the temperature of the water vapor, compounds will be stripped out of the dispersion at different temperatures depending on their volatility, providing several fractions that are condensed and collected. For example, the dispersion can be first heated to 100°C to strip light terpenes from the oil. Increasing the temperature to 150°C allows the stripping of a fraction containing heavier sesquiterpenes. This temperature also ensures the optimal decarboxylation of the cannabinoid acids in the dispersion. The temperature can then be increased to 200°C to strip further compounds that negatively affect taste, smell, and/or appearance. Finally, cannabinoid fractions containing one or more cannabinoids with high purity can be extracted from the dispersion by heating to about 240°C, which attracts attention since this temperature causes cannabinoid degradation. After stripping, the dispersion containing the remaining cannabinoids can be further extracted with a polar solvent (ethanol). The ethanol phase is then evaporated to obtain an evaporation residue containing the cannabinoids. The authors claim that the stripping step is preferably carried out in batches to allow the consecutive removal of the compounds by gradually heating the dispersion. This action can also be performed continuously by the simultaneous removal of all the volatile compounds and then using various condensers in series to selectively collect fractions at different temperatures. Stripping is a high-yield step to isolate cannabinoids from an initial extract containing 54 wt% cannabinoids, forming a distillate with 78 wt% cannabinoids and leaving a residual cannabinoid content as low as 0.5 wt% in the oil after being stripped. Moreover, the authors reported that ethanol can remove the cannabinoids from the oil with up to 96% efficiency while leaving behind waxes and triglyceride oils that were dissolved in the oil suspension. Depending on the desired characteristics of the final product, the cannabinoid fractions can be blended with terpene fractions to provide taste and smell.
Finally, patent US10822320B2 (Thomas and DePalo, 2020) describes a vaporization and condensation method that allows the simultaneous and fast purification and decarboxylation of cannabinoids such as CBDA, CBDVA, and THCA, without producing undesirable side products such as terpenes or flavonoids, or degradation products such as CBN. Briefly, the cannabinoid is rapidly vaporized and decarboxylated, and then is contacted with a heat sink (i.e., an aerosol or a foam with a large surface area) for condensation of the decarboxylated cannabinoid. The starting material is a relatively high concentration of cannabinoids, which comprises an extracted cannabis oil and a powder, wax, crystal, or suspension of solid particles or liquid droplets in a gas. The composition should have a high surface-area-to-volume ratio (>500/meter) to favor the vaporization and decarboxylation of a cannabinoid without vaporizing other molecules. Moreover, to reduce undesirable side reactions such as oxidation, pyrolysis, or isomerization of the cannabinoids, the cannabinoid should be condensed immediately after vaporization to minimize the time that the cannabinoid vapor is in contact with other heated molecules. For example, the composition can be ground hemp with a surface-area-to-volume ratio >500/meter and suspended in a heated gas phase such as water or ethanol vapor of at least 5% by volume. The energy can be applied by directing the composition along a heated path of 5–50 m or by contacting the composition with a heated gas or heated surface at a temperature of 190–250°C. The method allows the purification of a cannabinoid from a non-volatile molecule, both contained in a liquid or solid composition, by selectively vaporizing the cannabinoid molecule. In other words, the composition is heated above the boiling point of the volatile molecule and below the boiling point of the non-volatile molecule. The energy applied to vaporize the cannabinoid ranges from 1 to 100 kW per gram of the composition for 0.2–20 s. The vaporized cannabinoid in the gas phase is mechanically separated from suspended non-volatile molecules using a cyclone or filter. The cannabinoid is then condensed into a liquid distillate 1–5 s after the cannabinoid molecule is vaporized. The obtained product contains the condensed cannabinoid at a concentration of 55–99.9 wt% with a cannabinoid recovery >90%.
This section provided an overview of some cannabinoid extraction systems and procedures that, due to their characteristics and peculiarities, could not be included in the previous sections. Some of these inventions are performed without a liquid organic solvent through volatilization of the cannabinoid-containing biomass and subsequent collection of the condensed cannabinoids. In general, these processes are based on more advanced technologies than those used in conventional techniques such as solvent extraction. Some of these methods combine steps such as decarboxylation, which reduces the overall processing time. However, the high temperatures (up to 250°C) at which the processes described in these patents are carried out, as well as the sophistication of the equipment required, directly affect the price of the extraction process. As reflected throughout this review, cannabis producers must choose in each case the most appropriate technique based on an evaluation of its pros and cons, according to the characteristics and application of the desired product, and the material and economic resources available.
This review gathered information about existing extraction processes in the cannabis field, with a special focus on their industrial application and the patent literature. The demand is currently increasing for more green and efficient extraction techniques in terms of product yield, cannabinoid recovery from plant material, solvent consumption, extraction time, energy, and economics, while being easy to automate and scale up. This review thoroughly discussed and compared several extraction techniques, providing a brief description of the necessary equipment in each case as well as some yield and efficiency data that may shed light on their extraction performance.
To provide more detail, this review also included some of the most common pre-treatment methods of cannabis plant material used in industry, such as physical processing to separate different plant parts, adjustment of the particle size, and drying. However, the extraction from intact fresh plant material has also been reported in the patent literature, which preserves thermolabile compounds such as terpenes. As pre-treatment affects the time, cost, and energy demand of the overall process the advantages of pre-treatment must be carefully considered in each case in the context of profitability and benefits for the extraction. Moreover, the ideal extraction technique should minimize the requirement for a pre-treatment step to increase the efficiency of the overall process.
Among the reviewed extraction techniques, maceration of the cannabis plant material in a polar or non-polar liquid organic solvent is the most widely used due to its simplicity, with ethanol the preferred non-polar solvent, and petroleum ether and hexane the typical non-polar solvents. One disadvantage of liquid organic solvents is that they must be removed from the final product, usually by rotary evaporation, since very low solvent residues are allowed, especially for pharmaceutical purposes. After analyzing various examples in patents that use the solvents mentioned above, it is clear that solvent polarity is an important factor to selectively dissolve the cannabinoids while avoiding the co-extraction of unwanted compounds that must be removed in downstream purification steps.
Ethanol, a GRAS solvent, benefits from high solvent power, does not require high pressures to operate, and is safer than other organic solvents such as butane. However, the drawbacks related to the use of ethanol include the energy consumption for cooling the extraction device for extraction at cold temperatures and the co-extraction of chlorophylls (which can be minimized at cold temperatures without a significant decrease in the extraction yield compared to that of the extraction at room temperature) among other compounds that must be removed in post-processing steps such as filtration and decolorization using an adsorbent media (charcoal, clay, or silica particles). The results of our extensive literature review showed that the filtration step may be less exhaustive if the extraction is performed using cold ethanol and is essential after extraction with ethanol at room temperature. Overall, ethanol extraction is preferred when minor solvent residues are allowed in the final product or at cold temperatures when the terpenoid profile of the plant must be preserved.
Hexane has good solvent power and, unlike ethanol, does not co-extract chlorophylls due to its non-polar nature, even though it does co-extract waxes and lipid material. However, the use of hexane is limited in pharmaceutical applications due to its toxicity. Therefore, post-extraction steps are compulsory for the complete removal of hexane from the final product. Despite its flammability and risk of fire and explosion, the hazard risks of hexane are less severe than those for other flammable solvents such as butane.
Few studies and patents have described the use of petroleum ether as an extraction solvent, although it has been reported for the extraction at room temperature and atmospheric pressure of CBD-type varieties. Despite its attractiveness as a non-polar extraction solvent, its main downsides are the lack of consistency among suppliers/lots and its potential toxicity, although toxicological information is not available. However, as previously mentioned, petroleum ether and other potentially toxic solvents can be used as extraction solvents if the final product complies with residual solvent restrictions.
This review also addressed cannabinoid extraction using other liquid solvents, among which vegetable oils are the most popular method for producing cannabinoid formulations for pharmaceutical and nutritional products as the final product generally comprises the cannabinoid product diluted in oil (no need to remove the solvent in a post-processing step). However, the obtained cannabinoid concentration in oil is lower than those for other non-polar solvents (e.g., up to 80% of cannabinoids in hexane extracts).
The use of pressurized gases as the extraction solvent was another main section of this review. The major drawback associated with pressurized gases compared to liquid solvent extraction is the use of sophisticated equipment able to operate in severe extraction conditions, which implies high operation and maintenance costs. Moreover, gaseous hydrocarbons such as n-butane and n-propane are toxic and flammable, requiring the use of safety equipment. Nevertheless, one advantage over liquid solvent extraction is that the obtained extract contains practically no solvent residues since the pressurized gases can be removed and recovered at the end of the extraction by increasing the temperature due to their lower boiling point.
Butane is the most common solvent for extracting neutral cannabinoids and terpenes and producing cannabinoid concentrates since the butane extracts reach cannabinoid concentrations of up to 90%. While the obtained extracts are chlorophyll-free, a winterization step is required to remove co-extracted waxes. Among non-polar solvents, winterization is commonly reported in the patent literature as a post-extraction step in the case of butane or CO2, but not in the case of hexane, which seems to indicate that at room temperature and atmospheric pressure, hexane dissolves less lipid material compared to pressurized gasses.
As it is non-toxic and easily removed from the extracts, supercritical CO2 extraction is preferred for medicinal and pharmaceutical applications. Supercritical CO2 extraction is usually carried out in the presence of a polar co-solvent such as ethanol to increase the solubility of polar cannabinoids. The decarboxylation of large amounts of cannabis material before extraction to transform the polar cannabinoids into their neutral forms, more soluble in CO2 has also been reported. The downsides of this technique include the high operating temperatures and pressures, the expensive equipment, and, as mentioned above, the requirement of a winterization step to remove wax and lipids from the extracts.
Cannabis extraction using HFC 134a (1,1,1,2-tetrafluoroethane) refrigerant with or without a modifier, is described only in one patent with similar THC recoveries to that of butane, even though the extraction yield using HFC 134a is considerably lower. In addition, the use of this refrigerant not only implies more sophisticated equipment and, therefore, greater investment but the reported solvent-to-plant-material ratio and the extraction time are also much higher than those for extraction with liquid solvents.
Extraction with heated gases (hot air or non-oxidizing, reducing, or inert gases) at high temperatures and near-atmospheric pressure benefits from the absence of a solvent (no-solvent residues, no post-evaporation of the solvent), lack of volatilization of waxes, and no requirement for high-pressure equipment, making technique particularly relevant for producing oil compositions with specified terpene and cannabinoid profiles at the expense of high energy consumption and sophisticated equipment.
Furthermore, this review also evaluated the performance of alternative extraction methods showing increasing popularity in the cannabinoid extraction industry. The evaluation of MAE, UAE, hydrodynamic cavitation, and PEF technologies should consider that although studies have reported the presence of cannabinoids within cell walls, which can be disrupted by the application of auxiliary energies (microwaves, ultrasound, etc.) to release the cannabinoids and increase extraction performance, these findings cannot be true since cannabinoids are found in trichomes, in resins that lack cell walls; therefore, the use of auxiliary energies does not contribute to extraction performance (yield, solvent consumption, or time) as discussed in the previous corresponding sections of this review. Since auxiliary energies such as microwaving and sonication require high energy consumption and sophisticated equipment, which implies a high maintenance cost, the economic feasibility of the so-called alternative techniques over conventional techniques is yet to be demonstrated.
The techniques and solvents (except vegetable oils) in this review produce isolated cannabinoids with >95% purity after purification of the extract by crystallization, liquid-liquid chromatography, or distillation, regardless of the solvent polarity or extraction conditions. However, the reviewed MAE patents did not include these purification steps since the decarboxylated or winterized extracts are directly applied in pharmaceutical or nutraceutical formulations.
Unfortunately, the evaluation of all extraction techniques and solvents is difficult and incomplete since many of the reviewed patents lacked information on the extraction yield and efficiency. This coupled with the complexity of the processes themselves and multiple variables such as the cannabinoid profile of the plant material or the extraction conditions hinder comparisons of techniques. In the end, the characteristics of the desired cannabinoid product (purity, formulation, presence of terpenes or flavonoids with synergistic effects, etc.), conditioned on the specifications of the final application, will be the determining factor for selecting pre-treatment steps for cannabis plant material, the extraction method, or the required post-processing steps while considering technical and economic factors to ensure profitability.
In conclusion, consumer demand has created a strong and growing interest in the extraction and isolation of cannabinoid compounds for medicinal, pharmaceutical, and nutraceutical applications. The selected extraction method must preserve the cannabinoid biological and pharmaceutical properties while meeting the required specifications in terms of extraction yield, extraction efficiency, and extract quality, as well as environmental, safety, and scalability considerations. As the cannabis market is expected to continue growing, there remains much work to do in optimizing current extraction techniques for the industrial mass production of high-quality extracts while meeting environmental and safety standards.
CF-V, MG-V, JH, and VS contributed to the study conception and design. CL-O wrote the first draft of the manuscript. VS and CL-O wrote sections of the manuscript. All authors contributed to manuscript revision and review and approved the submitted version.
Authors VS, CF-V, MG-V, JH, and CL-O were employees of Phytoplant Research S.L.U.
All claims expressed in this article are solely those of the authors and do not necessarily represent those of their affiliated organizations, or those of the publisher, the editors, and the reviewers. Any product that may be evaluated in this article, or claim that may be made by its manufacturer, is not guaranteed or endorsed by the publisher.
Agarwal, C., Máthé, K., Hofmann, T., and Csóka, L. (2018). Ultrasound-assisted extraction of cannabinoids from Cannabis sativa L. optimized by response surface methodology. J. food Sci. 83 (3), 700–710. doi:10.1111/1750-3841.14075
Andre, C. M., Hausman, J. F., and Guerriero, G. (2016). Cannabis sativa: The plant of the thousand and one molecules. Front. Plant Sci. 7, 19. doi:10.3389/fpls.2016.00019
Ayres, D. (2016). WO2016200438A1 - process for extraction, separation and purification of cannabinoids, flavonoids and terpenes from cannabis.
Baldino, L., Scognamiglio, M., and Reverchon, E. (2020). Supercritical fluid technologies applied to the extraction of compounds of industrial interest from cannabis sativa L. and to their pharmaceutical formulations: A review. J. Supercrit. Fluids 165, 104960. doi:10.1016/j.supflu.2020.104960
Barba, F. J., Parniakov, O., Pereira, S. A., Wiktor, A., Grimi, N., Boussetta, N., et al. (2015). Current applications and new opportunities for the use of pulsed electric fields in food science and industry. Food Res. Int. 77, 773–798. doi:10.1016/j.foodres.2015.09.015
Barrie-Webster, G. R., and Leornard, P. (2000). Cannabinoid extraction method, 457. U.S Patent No. 09/490US6403126B1.
Barringer, I. (2017). Process for the extraction of cannabinoids from cannabinoids from cannabis usin`g lipids as an extraction solvent. Washington, DC: U.S. Patent and Trademark Office. U.S. Patent No. 9,808,494.
Bhatarah, P., McHattie, D., and Greenwood, A. K. (2009). WO2009133376A1 - production of delta 9 tetrahydrocannabinol.
Brighenti, V., Pellati, F., Steinbach, M., Maran, D., and Benvenuti, S. (2017). Development of a new extraction technique and HPLC method for the analysis of non-psychoactive cannabinoids in fibre-type Cannabis sativa L.(hemp). J. Pharm. Biomed. Analysis 143, 228–236. doi:10.1016/j.jpba.2017.05.049
Castillo, J. (2020). Active fraction from therapeutic cannabis plant extracts. Washington, DC: U.S. Patent and Trademark Office. U.S. Patent No. 10,717,717.
Cavalloro, V., Martino, E., Linciano, P., and Collina, S. (2021). “Microwave-assisted solid extraction from natural matrices,” in Microwave heating-electromagnetic fields causing thermal and non-thermal effects (London, United Kingdom: IntechOpen).
Challa, S. K. R., Misra, N. N., and Martynenko, A. (2021). Drying of cannabis—state of the practices and future needs. Dry. Technol. 39 (14), 2055–2064. doi:10.1080/07373937.2020.1752230
Chang, C. W., Yen, C. C., Wu, M. T., Hsu, M. C., and Wu, Y. T. (2017). Microwave-assisted extraction of cannabinoids in hemp nut using response surface methodology: Optimization and comparative study. Molecules 22 (11), 1894. doi:10.3390/molecules22111894
Changoer, L., Van Der Loo, C. H. M., and Keller, E. (2021). Process to extract and purify delta-9-tetrahydrocannabinol. Washington, DC: U.S. Patent and Trademark Office. U.S. Patent No. 11,091,455.
Chemat, F., Rombaut, N., Sicaire, A. G., Meullemiestre, A., Fabiano-Tixier, A. S., and Abert-Vian, M. (2017). Ultrasound assisted extraction of food and natural products. Mechanisms, techniques, combinations, protocols and applications. A review. Ultrason. sonochemistry 34, 540–560. doi:10.1016/j.ultsonch.2016.06.035
Chemical book (2021). Chemical book. Available at: https://www.chemicalbook.com/ChemicalProductProperty_EN_cb6248174.htm.
Chess, F. J., and McCutcheon, J. M. (2017). Closed loop supercritical and subcritical carbon dioxide extraction system for working with multiple compressed gases. Washington, DC: U.S. Patent and Trademark Office. U.S. Patent No. 9,782,691.
Cid, M. V. F., and Van Houten, D. (2015). Cannabis plant isolate comprising/\9-tetrahydrocannabinol and a method for preparing such an isolate, 417. U.S. Patent Application No. 14/398.
Cipolletti, G., Vagnoli, L., Matulli, M., Febbruari, B., and Chini, J. (2020). Method for the production of cannabinoids from types of industrial hemp, 311. U.S. Patent Application No. 16/634.
De Vita, D., Madia, V. N., Tudino, V., Saccoliti, F., De Leo, A., Messore, A., et al. (2020). Comparison of different methods for the extraction of cannabinoids from cannabis. Nat. Prod. Res. 34 (20), 2952–2958. doi:10.1080/14786419.2019.1601194
Dibble, C. J., and Cole, I. B. (2020). Methods for obtaining purified cannabis extracts and thca crystals, 080. U.S. Patent Application No. 16/686.
Dijkstra, A. J. (2019). Processes for the isolation of a cannabinoid extract and product from Cannabis plant material. Washington, DC: U.S. Patent and Trademark Office. U.S. Patent No. 10,428,040.
Drinić, Z., Vladić, J., Koren, A., Zeremski, T., Stojanov, N., Kiprovski, B., et al. (2020). Microwave-assisted extraction of cannabinoids and antioxidants from Cannabis sativa aerial parts and process modeling. J. Chem. Technol. Biotechnol. 95 (3), 831–839. doi:10.1002/jctb.6273
Eiroa, M. C. M., Van Houten, D., Fernandez, C. M. V., and Woerlee, G. F. (2016). WO2016153347A1 - cannabidiol isolate from industrial-hemp and use thereof in pharmaceutical and/or cosmetic preparations.
Eiroa, M. C. M., Van Houten, D., Fernandez, C. M. V., and Woerlee, G. G. (2016). WO2016153347A1 - cannabidiol isolate from industrial-hemp and use thereof in pharmaceutical and/or cosmetic.
Elsohly, M. A., Gul, W., Radwan, M. M., and Wanas, A. S. (2021). Isolation of pure cannabinoids from Cannabis. Washington, DC: U.S. Patent and Trademark Office. U.S. Patent No. 11,117,852.
Elsohly, M. A., and Ross, S. A. (2000). WO2000025127A1 Method of preparing delta-9-tetrahydrocannabinol l.
Eyal, A. M., and Zeitouni, D. B. (2022). Purified cannabis extracts and methods for production thereof. Washington, DC: U.S. Patent and Trademark Office. U.S. Patent No. 11,395,842.
Fairbairn, J. W., and Liebmann, J. A. (1973). The extraction and estimation of the cannabinoids in Cannabis sativa L. and its products. J. Pharm. Pharmacol. 25 (2), 150–155. doi:10.1111/j.2042-7158.1973.tb10609.x
Fairbairn, J. W. (1972). The trichomes and glands of Cannabis sativa L. U. N. Bull. Narcotics 24, 29–33.
Farokhi, F., St-Jean, P., and Villeneuve, E. (2020). WO2020028992A1 Cold extraction method for cannabinoids and terpenes from cannabis by organic solvents.
Ferraro, A., Edwards, B., and Ahrens, T. (2019). Biomass extraction and centrifugation systems and methods. Washington, DC: U.S. Patent and Trademark Office. U.S. Patent No. 10,493,377.
Flockhart, I., Wheatley, G. W., Dring, S., and Archer, L. (2010). Methods of purifying cannabinoids from plant material. Washington, DC: U.S. Patent and Trademark Office. La cita es del primero. U.S. Patent No. 7,700,368.
Gallo-Molina, A. C., Castro-Vargas, H. I., Garzón-Méndez, W. F., Ramírez, J. A. M., Monroy, Z. J. R., King, J. W., et al. (2019). Extraction, isolation and purification of tetrahydrocannabinol from the Cannabis sativa L. plant using supercritical fluid extraction and solid phase extraction. J. Supercrit. Fluids 146, 208–216. doi:10.1016/j.supflu.2019.01.020
Gasservei 8 (2021). Technical data sheet R134a. Available at: https://gas-servei.com/shop/docs/technical-data-sheet-r-134a-gas-servei.pdf.
Golberg, A., Sack, M., Teissie, J., Pataro, G., Pliquett, U., Saulis, G., et al. (2016). Energy-efficient biomass processing with pulsed electric fields for bioeconomy and sustainable development. Biotechnol. Biofuels 9 (1), 94–22. doi:10.1186/s13068-016-0508-z
Goldner, S. (2019). Freeze dry process. Washington, DC: U.S. Patent and Trademark Office. U.S. Patent No. 10,307,447.
Goodwin, N. J., Archer, N. J., Murray, C., Greenwood, A. K., and McHattie, D. (2009). Production of delta9 tetrahydrocannabinol. Washington, DC: U.S. Patent and Trademark Office. U.S. Patent No. 7,592,468.
Grijó, D. R., Osorio, I. A. V., and Cardozo-Filho, L. (2019). Supercritical extraction strategies using CO2 and ethanol to obtain cannabinoid compounds from Cannabis hybrid flowers. J. CO2 Util. 30, 241–248. doi:10.1016/j.jcou.2018.12.014
Haji-Moradkhani, A., Rezaei, R., and Moghimi, M. (2019). Optimization of pulsed electric field-assisted oil extraction from cannabis seeds. J. Food Process Eng. 42 (4), e13028. doi:10.1111/jfpe.13028
Ham, J., Kim, T., Ha, S., Chung, B. C., Lee, S., Pilju, C. H. O. I., et al. (2020). Method of isolating cannabidiol from cannabis plant and use thereof, 099. U.S. Patent Application No. 16/893.
Hawes, M. D., and Cohen, M. R. (2015). Method of drying cannabis materials, 286. U.S. Patent Application No. 14/050.
Held, J. S., and Stanis, K. (2020). Extraction of compounds from cannabis. Washington, DC: U.S. Patent and Trademark Office. U.S. Patent No. 10,773,184.
Hindi, J. (2021). Method of preparing Cannabis extracts. Washington, DC: U.S. Patent and Trademark Office. U.S. Patent No. 10,888,596.
Hospodor, A. D., and Rapp, R. J. (2021). Recycling cannabinoid extractor. Washington, DC: U.S. Patent and Trademark Office. U.S. Patent No. 11,071,761.
International Council for Harmonisation (2020). Q3C(R8) impurities: Guideline for residual solvents. Available: at: https://www.ich.org/page/quality-guidelines.
Ivanov, E. M. (2018). Method and apparatus for processing herbaceous plant materials including the cannabis plant, 911. U.S. Patent Application No. 15/575.
Ivanov, E. M. (2018). Purification and separation techniques for cannabinoids, 911. U.S. Patent Application No. 15/575.
Jackson, A. P., and Gildrien, J. A. (2019). Method of extracting one or more chemical extracts from a plant product. Washington, DC: U.S. Patent and Trademark Office. U.S. Patent No. 10,512,856.
Jones, A. (2017). Extraction devices, systems, and methods. Washington, DC: U.S. Patent and Trademark Office. U.S. Patent No. 9,789,147.
Keller, R. M. (2018). Cannabidiol extraction and conversion process. Washington, DC: U.S. Patent and Trademark Office. U.S. Patent No. 9,950,976.
Ko, R. D. (2020). Elevated multi-stage chilled filter system. Washington, DC: U.S. Patent and Trademark Office. U.S. Patent No. 10,646,793.
Ko, R. D., and Hughes, B. (2019). Cannabinoid extraction and distillation. Washington, DC: U.S. Patent and Trademark Office. U.S. Patent No. 10,413,843.
Kolesinski, H. S. (2021). System and methods for separating and purifying cannabinoids from plant material. Washington, DC: U.S. Patent and Trademark Office. U.S. Patent No. 10,946,329.
Kotra, L. P., Lewis, M. M., Wasilewski, E., and Grover, H. (2020). Decarboxylated cannabis resins, uses thereof and methods of making same. Washington, DC: U.S. Patent and Trademark Office. U.S. Patent No. 10,537,592.
Kotra, L. P., Sampson, P. B., Hurley, N., and Cheekati, G. (2019). Apparatus and method for extraction and decarboxylation of phytocannabinoids WO2019119153A1.
Koumans, F. J. R. (2021). Process for the extraction of oil soluble components from plant material, 250. U.S. Patent Application No. 17/252.
Lehmann, T., and Brenneisen, R. (1992). A new chromatographic method for the isolation of (−)-Δ9-(trans)-tetrahydrocannabinolic acid A. Phytochem. Anal. 3 (2), 88–90. doi:10.1002/pca.2800030210
Li, C., Yang, F., Huang, Y., Huang, C., Zhang, K., Yan, L., et al. (2020). Comparison of hydrodynamic and ultrasonic cavitation effects on soy protein isolate functionality. J. Food Eng. 265. 109697. doi:10.1016/j.jfoodeng.2019.109697
Lopa, F. A. (2019). Phytochemical extraction system and methods to extract phytochemicals from plants including plants of the family cannabaceae sensu stricto. Washington, DC: U.S. Patent and Trademark Office. U.S. Patent No. 10,272,360.
Mancosky, D. G. (2018). Method of extracting CBD, THC, and other compounds from cannabis using controlled cavitation. Washington, DC: U.S. Patent and Trademark Office. U.S. Patent No. 10,011,804.
Marshall, J. G., and Campbell, L. G. (2019). Extraction and purification of cannabinoid compounds, 547. U.S. Patent Application No. 16/201.
Martínez, J. M., Delso, C., Álvarez, I., and Raso, J. (2020). Pulsed electric field-assisted extraction of valuable compounds from microorganisms. Compr. Rev. food Sci. food Saf. 19 (2), 530–552. doi:10.1111/1541-4337.12512
Melnichuk, L. J., and Kelly, K. S. (2020). Method for continuous extraction and separation of useful compounds for plant or animal material. Washington, DCl: U.S. Patent and Trademark Office. U.S. Patent No. 10,625,176.
Moreno-Sanz, G., Ferreiro Vera, C., Sánchez-Carnerero, C., Nadal Roura, X., and Sánchez de Medina Baena, V. (2020). Biological activity of Cannabis sativa L. extracts critically depends on solvent polarity and decarboxylation. Separations 7 (4), 56. doi:10.3390/separations7040056
Mueller, A. (2020). Process for producing an extract containing tetrahydrocannabinol and cannabidiol from cannabis plant material, and cannabis extracts. Washington, DC: U.S. Patent and Trademark Office. U.S. Patent No. 10,870,632.
National Library of Medicine (2021). Butane. Available at: https://pubchem.ncbi.nlm.nih.gov/compound/butane.
National Library of Medicine (2021). n-HEXANE. Available at: https://pubchem.ncbi.nlm.nih.gov/compound/Hexane.
National Research Council (1984). Emergency and continuous exposure limits for selected airborne contaminants volume 2, 1. National Academies Press. (U.
National Research Council (1996). “Exposure guidance levels for hydrofluorocarbon-134a,” in Toxicity of alternatives to chlorofluorocarbons: HFC-134a and HCFC-123 (National Academies Press US).
Nevitt, L. P. (2020). Plant matter fractional distillation system using heated air induction into precisely heated chamber to extract a plant's organic compounds without use of solvents. Washington, DCs: U.S. Patent and Trademark Office. U.S. Patent No. 10,596,486.
Omar, J., Olivares, M., Alzaga, M., and Etxebarria, N. (2013). Optimisation and characterisation of marihuana extracts obtained by supercritical fluid extraction and focused ultrasound extraction and retention time locking GC-MS. J. Sep. Sci. 36 (8), 1397–1404. doi:10.1002/jssc.201201103
Oroskar, A. R., House, D. W., Edirisinghe, P. D., Oroskar, A. A., Faridedin, A. D. E. L., Chen, X., et al. (2019). Process for purification and separation of cannabinoids, from dried hemp and cannabis leaves. Washington, DC: U.S. Patent and Trademark Office. U.S. Patent No. 10,189,762.
Panda, D., Saharan, V. K., and Manickam, S. (2020). Controlled hydrodynamic cavitation: A review of recent advances and perspectives for greener processing. Processes 8 (2), 220. doi:10.3390/pr8020220
Panreac Applichem (2021). Safety datasheet panreac - petroleum ether 50-70°C (reag. Ph. Eur.) for analysis.
Perrotin-Brunel, H., Kroon, M. C., Van Roosmalen, M. J., Van Spronsen, J., Peters, C. J., and Witkamp, G. J. (2010). Solubility of non-psychoactive cannabinoids in supercritical carbon dioxide and comparison with psychoactive cannabinoids. J. Supercrit. Fluids 55 (2), 603–608. doi:10.1016/j.supflu.2010.09.011
Pertile, R., and Black, T. (2020). Cultivation, processing, and synthesis of cannabidiols. Washington, DC: U.S. Patent and Trademark Office. U.S. Patent No. 10,561,693.
Popek, T., Splinter, S., Kaur, H., and Bakowska-Barczak, A. (2019). WO2019211797A1 method of decarboxylating acidic cannabinoids in cannabis extract suspended within a carrier fluid.
Popek, T., Splinter, S., and Sloley, B. (2019). Extraction using a microwave assisted extractor WO2019211794A1.
Popp, J. R., Bonn, G., Stuppner, H., Skaltsounis, A. L., PetrakisAngelis, E. A. A., and Halabalaki, M. (2019). WO2019043259A1 Recovery of acidic cannabinoids from plant material.
Priecel, P., and Lopez-Sanchez, J. A. (2018). Advantages and limitations of microwave reactors: From chemical synthesis to the catalytic valorization of biobased chemicals. ACS Sustain. Chem. Eng. 7 (1), 3–21. doi:10.1021/acssuschemeng.8b03286
Qu, G., and Cui, M. (2020). Method for preparing high-purity cannabidiol. Washington, DC: U.S. Patent and Trademark Office. U.S. Patent No. 10,662,137.
Raber, J. C., and Elzinga, S. (2017). Solvent-free processing, system and methods. Washington, DC: U.S. Patent and Trademark Office. U.S. Patent No. 9,732,009.
Radoiu, M., Kaur, H., Bakowska-Barczak, A., and Splinter, S. (2020). Microwave-assisted industrial scale cannabis extraction. Technologies 8 (3), 45. doi:10.3390/technologies8030045
Ramirez, C. L., Fanovich, M. A., and Churio, M. S. (2019). Cannabinoids: Extraction methods, analysis, and physicochemical characterization. Stud. Nat. Prod. Chem. 61, 143–173. doi:10.1016/b978-0-444-64183-0.00004-x
Rivas, J. (2019). Method for preparation of pharmacologically-relevant compounds from botanical sources, 145. U.S. Patent Application No. 16/550.
Romano, L. L., and Hazekamp, A. (2013). Cannabis oil: Chemical evaluation of an upcoming cannabis-based medicine. Cannabinoids 1 (1), 1–11.
Roura, X. N. (2019). U.S. Patent No. 10,207,199. Washington, DC: U.S. Patent and Trademark Office.Methods of purifying cannabinoids using liquid-liquid chromatography
Roura, X. N. (2017). Methods of purifying cannabinoids, compositions and kits thereof. Washington, DC: U.S. Patent and Trademark Office. U.S. Patent No. 9,765,000.
Rovetto, L. J., and Aieta, N. V. (2017). Supercritical carbon dioxide extraction of cannabinoids from Cannabis sativa L. J. Supercrit. Fluids 129, 16–27. doi:10.1016/j.supflu.2017.03.014
Sherwood, R., Sherwood, S., Cullimore, R., and Mehos, G. (2020). Systems, methods, and equipment for chemical extraction. Washington, DC: U.S. Patent and Trademark Office. U.S. Patent No. 10,765,965.
Shuja, A. (2018). Rapid drying extraction targeting oil resin plant extracts. Washington, DC: U.S. Patent and Trademark Office. U.S. Patent No. 9,981,203.
Sironi, L., Amadasi, A., and Zoja, R. (2016). Recreational inhalation of butane and propane in adolescents: Two forensic cases of accidental death. Forensic Sci. Int. 266, e52–e58. doi:10.1016/j.forsciint.2016.05.028
Smith, R. N., and Vaughan, C. G. (1977). The decomposition of acidic and neutral cannabinoids in organic solvents. J. Pharm. Pharmacol. 29 (1), 286–290. doi:10.1111/j.2042-7158.1977.tb11313.x
Speier, G. J. (2022). Pharmaceutical composition and method of manufacturing. Washington, DC: U.S. Patent and Trademark Office. U.S. Patent No. 11,331,358.
Splinter, S., Popek, T., Kaur, H., and Bakowska-Barczak, A. (2019). Continuous flow microwave-assisted extraction of a cannabis biomass WO2019211795A1.
Splinter, S., Popek, T., Kaur, H., Bakowska-Barczak, A., and Sloley, B. (2019). WO2019207554A1 Extraction of compounds from cannabis biomass using food grade solvent.
Tegen, M. G., Joon, C. H. O., and Sutterlin, W. R. (2019). Processes for solvent extraction of cannabinoids, terpenes and flavonoids from biomass. Washington, DC: U.S. Patent and Trademark Office. U.S. Patent No. 10,413,845.
Thomas, C. R., and DePalo, M. M. (2020). Methods to purify cannabinoids. Washington, DC: U.S. Patent and Trademark Office. U.S. Patent No. 10,822,320.
Thomas, C. R. (2020). Method and apparatus for extracting botanical oils. Washington, DC: U.S. Patent and Trademark Office. U.S. Patent No. 10,617,974.
Tucker, G. (2019). Method for removing contaminants from cannabinoid distillates. Washington, DC: U.S. Patent and Trademark Office. U.S. Patent No. 10,507,404.
United States Environmental Protection Agency (2021). Refrigerant transition & environmental impacts. Available at: https://www.epa.gov/mvac/refrigerant-transition-environmental-impacts.
Vági, E., Balázs, M., Komoczi, A., Mihalovits, M., and Székely, E. (2020). Fractionation of phytocannabinoids from industrial hemp residues with high-pressure technologies. J. Supercrit. Fluids 164, 104898. doi:10.1016/j.supflu.2020.104898
Vanaman, B. (2021). Systems and methods of cannabis oil extraction. Washington, DC: U.S. Patent and Trademark Office. U.S. Patent No. 11,118,131.
Wen, C., Zhang, J., Zhang, H., Dzah, C. S., Zandile, M., Duan, Y., et al. (2018). Advances in ultrasound assisted extraction of bioactive compounds from cash crops–A review. Ultrason. sonochemistry 48, 538–549. doi:10.1016/j.ultsonch.2018.07.018
Whittle, B., Guy, G., Downs, D., and Pate, D. W. (2015). Processes and apparatus for extraction of active substances and enriched extracts from natural products. Washington, DC: U.S. Patent and Trademark Office. U.S. Patent No. 9,034,395.
Whittle, B., Hill, C. A., Flockhart, I. R., Downs, D. V., Gibson, P., and Wheatley, G. W. (2008). Extraction of pharmaceutically active components from plant materials. Washington, DC: U.S. Patent and Trademark Office. U.S. Patent No. 7,344,736.
Keywords: cannabinoids, extraction, efficiency, recovery, alternative extraction methods, conventional extraction methods
Citation: López-Olmos C, García-Valverde MT, Hidalgo J, Ferrerio-Vera C and Sánchez de Medina V (2022) Comprehensive comparison of industrial cannabinoid extraction techniques: Evaluation of the most relevant patents and studies at pilot scale. Front. Nat. Prod. 1:1043147. doi: 10.3389/fntpr.2022.1043147
Received: 13 September 2022; Accepted: 21 October 2022;
Published: 28 November 2022.
Edited by:
Orazio Taglialatela-Scafati, University of Naples Federico II, ItalyReviewed by:
Lucio Cardozo-Filho, State University of Maringá, BrazilCopyright © 2022 López-Olmos, García-Valverde, Hidalgo, Ferrerio-Vera and Sánchez de Medina. This is an open-access article distributed under the terms of the Creative Commons Attribution License (CC BY). The use, distribution or reproduction in other forums is permitted, provided the original author(s) and the copyright owner(s) are credited and that the original publication in this journal is cited, in accordance with accepted academic practice. No use, distribution or reproduction is permitted which does not comply with these terms.
*Correspondence: Verónica Sánchez de Medina, di5zYW5jaGV6QHBoeXRvcGxhbnQuZXM=
Disclaimer: All claims expressed in this article are solely those of the authors and do not necessarily represent those of their affiliated organizations, or those of the publisher, the editors and the reviewers. Any product that may be evaluated in this article or claim that may be made by its manufacturer is not guaranteed or endorsed by the publisher.
Research integrity at Frontiers
Learn more about the work of our research integrity team to safeguard the quality of each article we publish.