- Department of Neurobiology and Anatomy, Drexel University College of Medicine, Philadelphia, PA, United States
Anxiety disorders are the most common class of mental illness in the U.S., affecting 40 million individuals annually. Anxiety is an adaptive response to a stressful or unpredictable life event. Though evolutionarily thought to aid in survival, excess intensity or duration of anxiogenic response can lead to a plethora of adverse symptoms and cognitive dysfunction. A wealth of data has implicated the medial prefrontal cortex (mPFC) in the regulation of anxiety. Norepinephrine (NE) is a crucial neuromodulator of arousal and vigilance believed to be responsible for many of the symptoms of anxiety disorders. NE is synthesized in the locus coeruleus (LC), which sends major noradrenergic inputs to the mPFC. Given the unique properties of LC-mPFC connections and the heterogeneous subpopulation of prefrontal neurons known to be involved in regulating anxiety-like behaviors, NE likely modulates PFC function in a cell-type and circuit-specific manner. In working memory and stress response, NE follows an inverted-U model, where an overly high or low release of NE is associated with sub-optimal neural functioning. In contrast, based on current literature review of the individual contributions of NE and the PFC in anxiety disorders, we propose a model of NE level- and adrenergic receptor-dependent, circuit-specific NE-PFC modulation of anxiety disorders. Further, the advent of new techniques to measure NE in the PFC with unprecedented spatial and temporal resolution will significantly help us understand how NE modulates PFC function in anxiety disorders.
Introduction
Anxiety is defined as the anticipation of future threat (American Psychiatric Association [APA], 2013). This physiological and psychological response is thought to be a normal, healthy, adaptive response to aid in survival in an ever-changing world. However, persistent, disruptive, and exacerbated anxiety can become debilitating through threat-generalization to non-threatening situations, producing a constant state of heightened arousal. Pathological anxiety disorders are separated into three main categories: obsessive-compulsive and related disorders, trauma- and stressor-related disorders, and generalized anxiety disorders (American Psychiatric Association [APA], 2013). Although these disorders vary in their etiology, in all cases, the resulting cognitive and behavioral deficits significantly impair normal functioning. Not only do disorders of this nature affect an individual’s performance at school/work, relationships, and self-esteem, but they also lead to significant economic and personal burdens (Bereza et al., 2009; Mondin et al., 2013; Pagotto et al., 2015). Anxiety disorders have a lifetime prevalence of 28% (Kessler et al., 2005), affecting about 40 million individuals in the United States of America and 970 million worldwide. Despite the commonality of these disorders, generalized anxiety disorder (GAD) is one of the least successfully treated psychiatric disorders (Li et al., 2020), and progress toward anxiolytic drug discovery has been slow (Griebel and Holmes, 2013). The treatment gaps in GAD and other anxiety disorders result from our limited understanding of the biological mechanisms by which anxiety symptoms emerge or how these mechanisms are altered by current treatments (Li et al., 2020).
It is increasingly recognized that cognitive deficits underlie various symptoms associated with stress-related psychiatric illnesses, such as anxiety (Beck, 2005; Moran, 2016). A frontal brain structure heavily involved in cognitive functioning is the prefrontal cortex (PFC). This brain region exerts top-down control over behavior, thought, and emotion (Datta and Arnsten, 2019). Lesions of the PFC produce symptoms such as poor judgment, increased distractibility and hyperactivity, poor attentional regulation, and disorganized behavior (Arnsten, 1998), similar to the symptoms seen in anxiety disorders. This suggests the PFC may be implicated in the pathophysiology of anxiety (Kenwood et al., 2022).
One neurotransmitter that is thought to play an extensive role in both anxiety and modulation of PFC function is norepinephrine (NE). The locus coeruleus (LC), a brainstem structure, provides the primary source of NE to the mammalian neocortex (Chandler et al., 2014b; Poe et al., 2020; Breton-Provencher et al., 2021; Ross and Van Bockstaele, 2021; see Figures 1, 2). Cortical projections from the LC are heterogeneous, with distinct biochemical and electrophysiological properties (Chandler and Waterhouse, 2012; Chandler et al., 2014a; Morris et al., 2020). Further, these minimally divergent projection neurons coordinate their molecular phenotypes and physiological profiles to the operation of their specific terminal fields, governing varying levels of NE release. For example, the LC projects to the PFC with much denser NE varicosities compared to other cortical regions such as sensory and motor cortices (Agster et al., 2013). This unique arrangement makes sense in terms of behavioral significance, since the LC exhibits more robust modulatory actions (such as greater NE release) in prefrontal decision-making circuits compared to circuits related to motor movement.
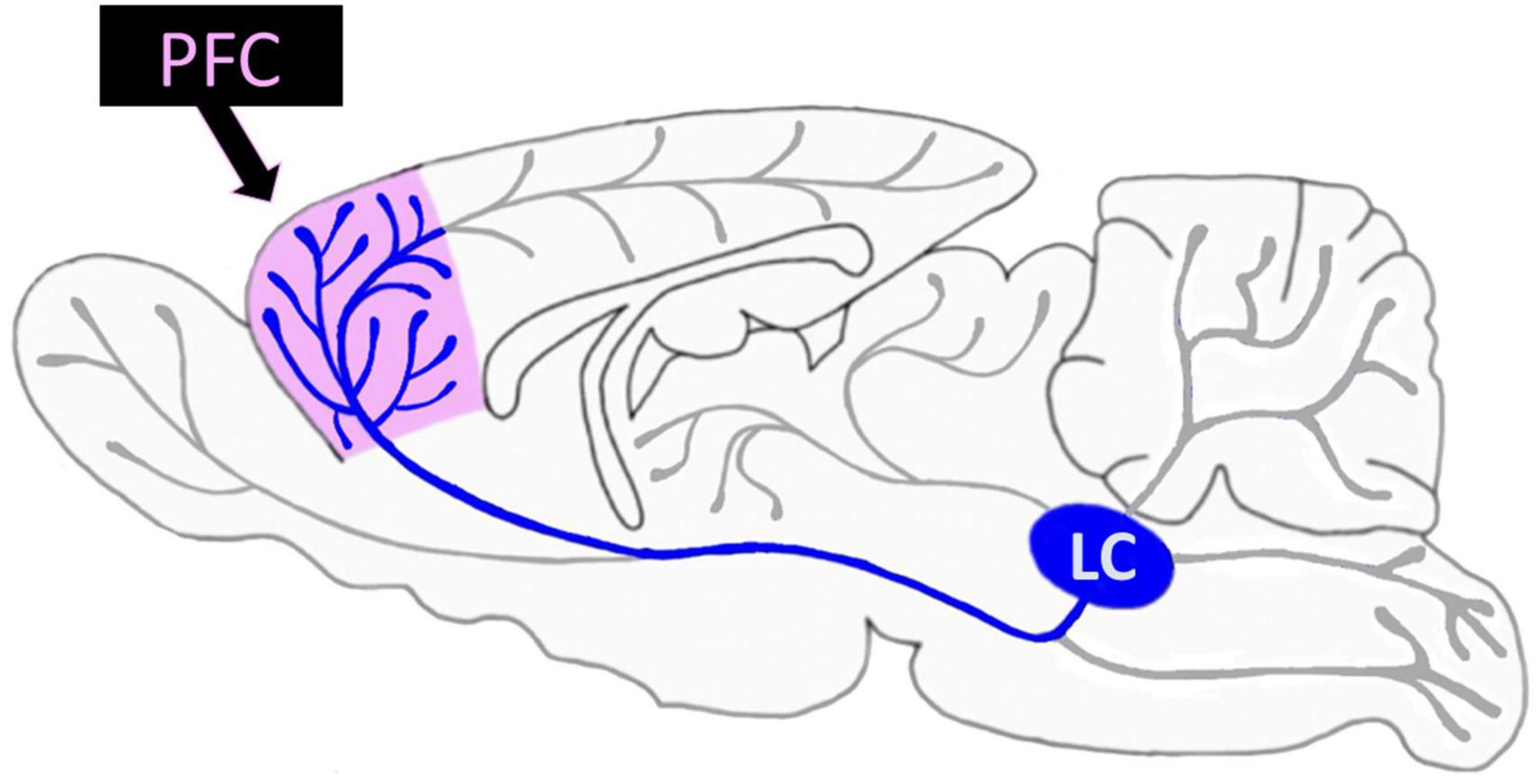
Figure 1. Rodent locus coeruleus (LC) projections are widespread but especially dense in the prefrontal cortex (PFC). The LC projects widely across the rodent brain (gray) including the PFC (pink); LC-PFC projections are particularly dense (blue) compared to other LC projections.
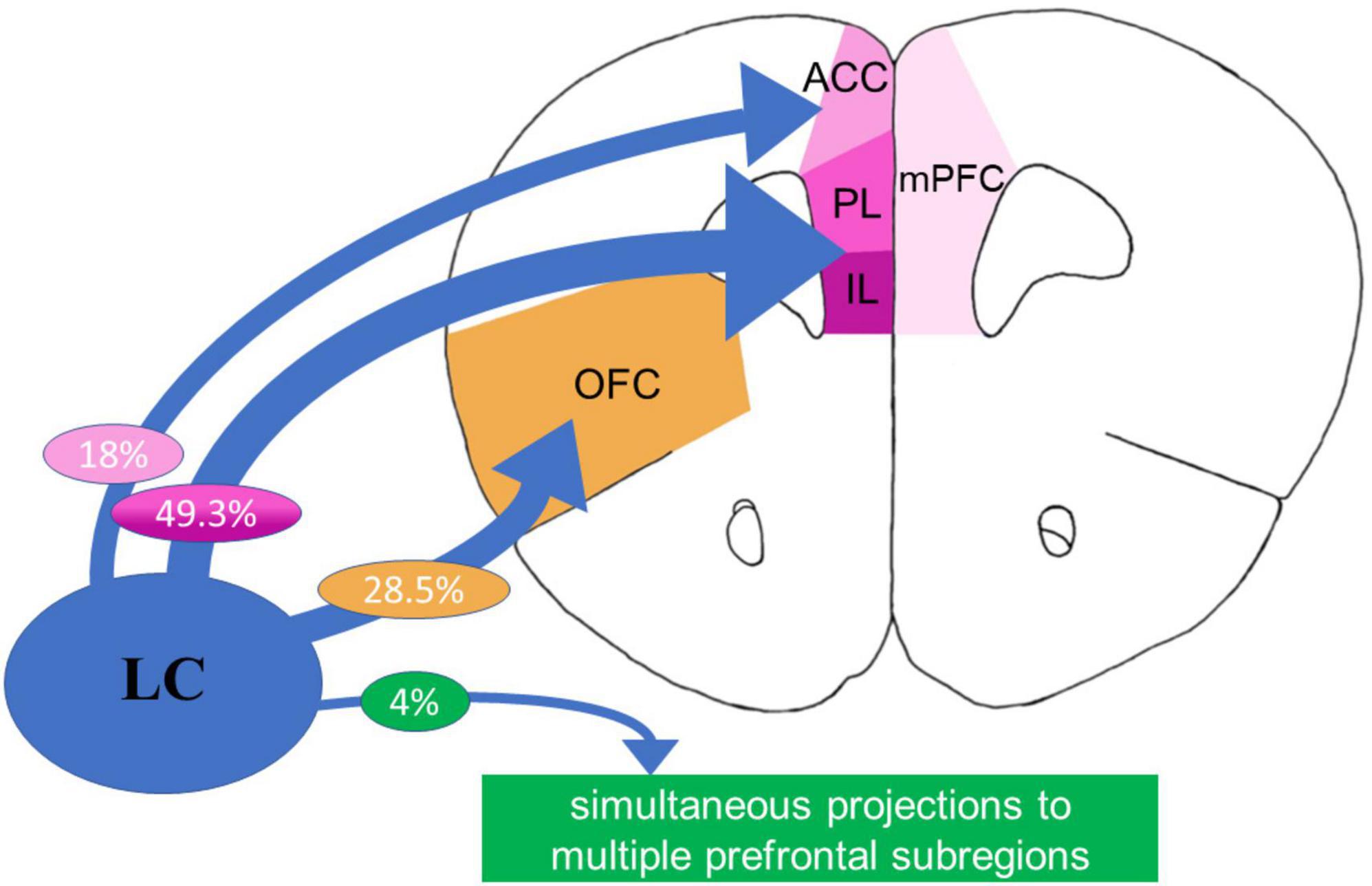
Figure 2. The rodent prefrontal cortex (PFC) receives dense projections from the locus coeruleus (LC). Subregions of the rat medial prefrontal cortex (mPFC) receive dense innervation from the LC, with significantly higher amount of norepinephrine (NE) varicosities (Agster et al., 2013) and high percentage of LC projections to the prelimbic (PL)/infralimbic (IL) regions compared to other cortical regions, and relatively low (4%) overlap (Chandler et al., 2014a).
Recent research has indicated that both NE and the PFC are extensively involved in anxiety etiology through distinctly different cell-type, microcircuit-, and macrocircuit-level modulation (Goddard et al., 2010). However, how NE modulation in the PFC coordinates action to optimize PFC function for appropriate attention, cognition, and behavior, and how this may go awry in pathological anxiety states remains unclear. This review summarizes current rodent, primate, and human literature regarding the neurobiology of LC-NE-PFC regulation. Further, we will bridge the work between what is known about LC-NE modulation in pathological anxiety and what is known about prefrontal regulation of pathological anxiety. We hope to shed light on the many remaining unknowns, which may be important for improving the therapeutic arsenal for the management of anxiety disorders.
LC-NE system modulates PFC neural activity
As introduced above, the PFC receives uniquely dense innervation from the LC, surpassing the degree of NE varicosities in other crucial brain sensory regions, including, motor, and thalamic regions (Agster et al., 2013; Figures 1, 2). It is proposed that activation of the LC and subsequent NE release terminates the brain’s resting state and commences a brain-state adjustment to orchestrate attention (Corbetta et al., 2008; Sestieri et al., 2011; Tang et al., 2012; Buckner, 2013), facilitate task-relevant behaviors, and help optimize task performance (Aston-Jones and Cohen, 2005). Investigators found that both within and between trials, LC neuron depolarization occurs before forebrain neural activity and is related to cognition (Bouret and Sara, 2005). LC-released NE has a robust effect on the functional integrity of the PFC. As LC-NE neuronal firing rate is associated with the attentional state, it has long been appreciated that NE significantly affects various attentional processes governed by the PFC. NE modulates cortical function during vigilance, attention, arousal, and stress (Aston-Jones et al., 1991; Berridge et al., 1993; Berridge and Waterhouse, 2003; Morilak et al., 2005). Specifically, noradrenergic signaling in the PFC is essential for cognitive changes associated with each of these states (Aston-Jones et al., 2000) and plays a modulatory role in the higher order functioning required to adapt to the demands of a changing or stressful environment (Lapiz and Morilak, 2006; Bondi et al., 2010; Arnsten, 2015).
Though differential innervation of the mPFC subregions (Chandler et al., 2013; Cerpa et al., 2019) and functional disassociations in these subregions (Cerpa et al., 2019) is recognized, the distinct modulatory effects of NE on the ACC, PL and IL prefrontal subregions remain barely studied. Nevertheless, some reported data indicate potential differential modulatory effects of NE on the PFC in a subregion-specific manner. For example, a recent study investigating changes in the norepinephrine transporter (NET) and dopamine-beta-hydroxylase (DBH) density in functionally distinct subregions of the PFC, including IL, PL, ACC, and OFC in adolescent rats found that NET, but not DBH, changed across adolescence in a regionally selective manner. The PL and the OFC showed higher levels of NET at early adolescence (Bradshaw et al., 2016). Additionally, infusion of methylphenidate, an NET inhibitor, into the ACC and IL, but not PL and OFC, inhibited social play (Achterberg et al., 2015), indicating NE-mediated region-specific inhibitory effects (Achterberg et al., 2015). In addition, the mPFC output to subcortical brain areas is known to control different cognitive, social, and emotional processing. Beyond these studies, however, it remains unknown whether and how NE may play unique modulatory roles in distinct subregions and cell types of the PFC. Also, despite the known effects of NE on general PFC-dependent cognition and attention and its interactions with stress, the spatiotemporal dynamic of adrenergic modulation of PFC-dependent behavior remains elusive (Breton-Provencher and Sur, 2019; Breton-Provencher et al., 2021, 2022). Thus, more research is needed to understand the differential and subregion-specific PFC-NE mechanisms associated with anxiety-like behaviors. We will first examine what is known about NE modulation at the molecular level in the PFC, how this affects behaviors, and what remains to be explored.
Laminar and synaptic distribution of adrenergic receptors
Norepinephrine modulates neural activity through various types of adrenergic receptors (ARs, Box 1). All subdivisions of the PFC contain cells expressing one or more variations of α- or β-adrenergic receptors and subtypes. The various AR receptors have been identified in both excitatory and inhibitory PFC neurons across numerous cortical layers pre- and post-synaptically (Tables 1, 2).
BOX 1 Noradrenergic receptor overview. |
Noradrenergic responsivity is mediated by three adrenergic receptors (ARs) in the brain: α1, α2, and β adrenergic receptors. Each family of these different G-protein-coupled receptors plays a distinct, often opposing, role in the brain based on their intrinsic signaling pathways. |
α1 receptors (consisting of three sub-types: α1A, α1B, and α1D) display anatomic and functional differences throughout the PFC depending on the receptor subtype. α1 receptors signal via the Gq-protein coupled receptor cascade, where they are coupled to the PKC signaling pathway. PKC signaling is mediated through activation of phospholipase CàDAG pathway, generating DAG and IP3. IP3 stimulates the release of intracellular Ca2+. Previous research has shown post-synaptic α1 receptor activation in the PFC may disengage optimal prefrontal functioning, as shown through impaired working-memory performance. |
α2 receptors (consisting of three sub-types: α2A, α2B, and α2D) signal through the Gi-protein coupled receptor cascade. Of the three subtypes, α2A is overwhelmingly predominant in the PFC. Following activation of α2 receptors, cAMP production is inhibited, which in turn, inhibits PKA and prevents phosphorylation of downstream proteins. In addition, inhibition of cAMP production reduces cAMPmediated opening of K+ channels and inhibits HCN channels. Closure of HCN channels on PFC dendritic spines suppresses isolated excitatory inputs and enhances responses to coherent bursts of synaptic activity, resulting in increased synaptic efficacy between communicating neurons. Additional activation of α2A receptors colocalized with HCN channels participate in signal enhancement and consequent improvements in the network “signal-to-noise” ratio through Gi-mediated inhibition of cAMP. Previous research has shown post-synaptic α2 receptor activation in the PFC may engage prefrontal functioning, as shown through enhanced working-memory performance. Contrastingly, presynaptic α2 noradrenergic receptors serve as autoreceptors and participate in a noradrenergic negative feedback mechanism to promote the closure of Ca2+ channels on NE axons, eventually inhibiting NE release in the synapse. |
β receptors (consisting of three sub-types: β1, β2, and β3) signal through the Gs-protein coupled receptor cascade. Following activation of β receptors, adenylyl cyclase initiates a cAMP-dependent protein kinase A (PKA) activation, resulting in the phosphorylation of Ca2+ channels and an increase in Ca2+ influx, thus, exciting pre-synaptic neurons and enhancing NE release in the synapse. Both pre- and post-synaptic β-ARs in layer V/VI mPFC pyramidal neurons enhance excitatory neurotransmission, though effects of these receptors, especially post-synaptically, have yet to be specifically studied in other distinct mPFC layers. |
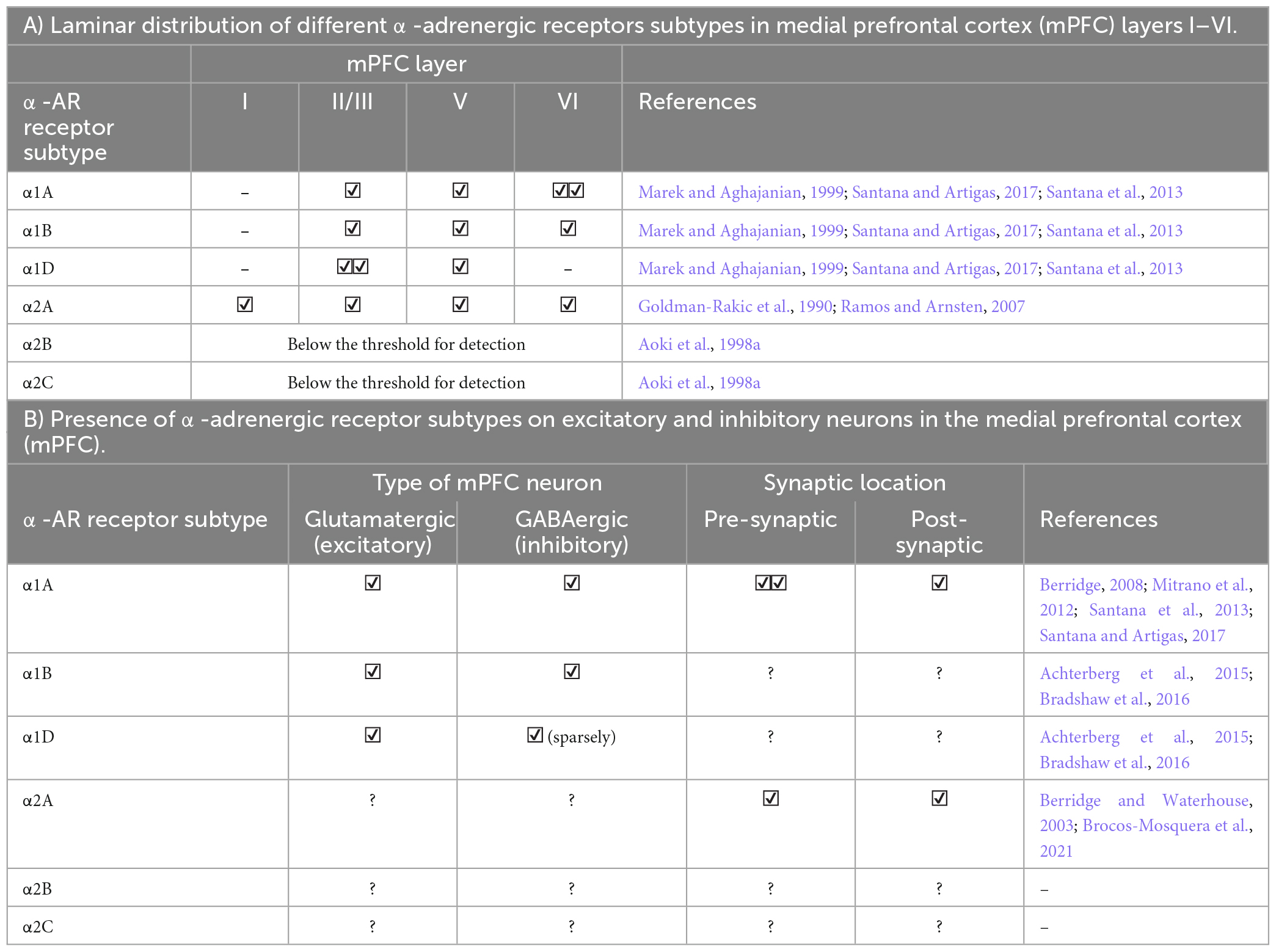
Table 1A. Laminar and cellular distribution of α-adrenergic receptors (AR) in the medial prefrontal cortex (mPFC).
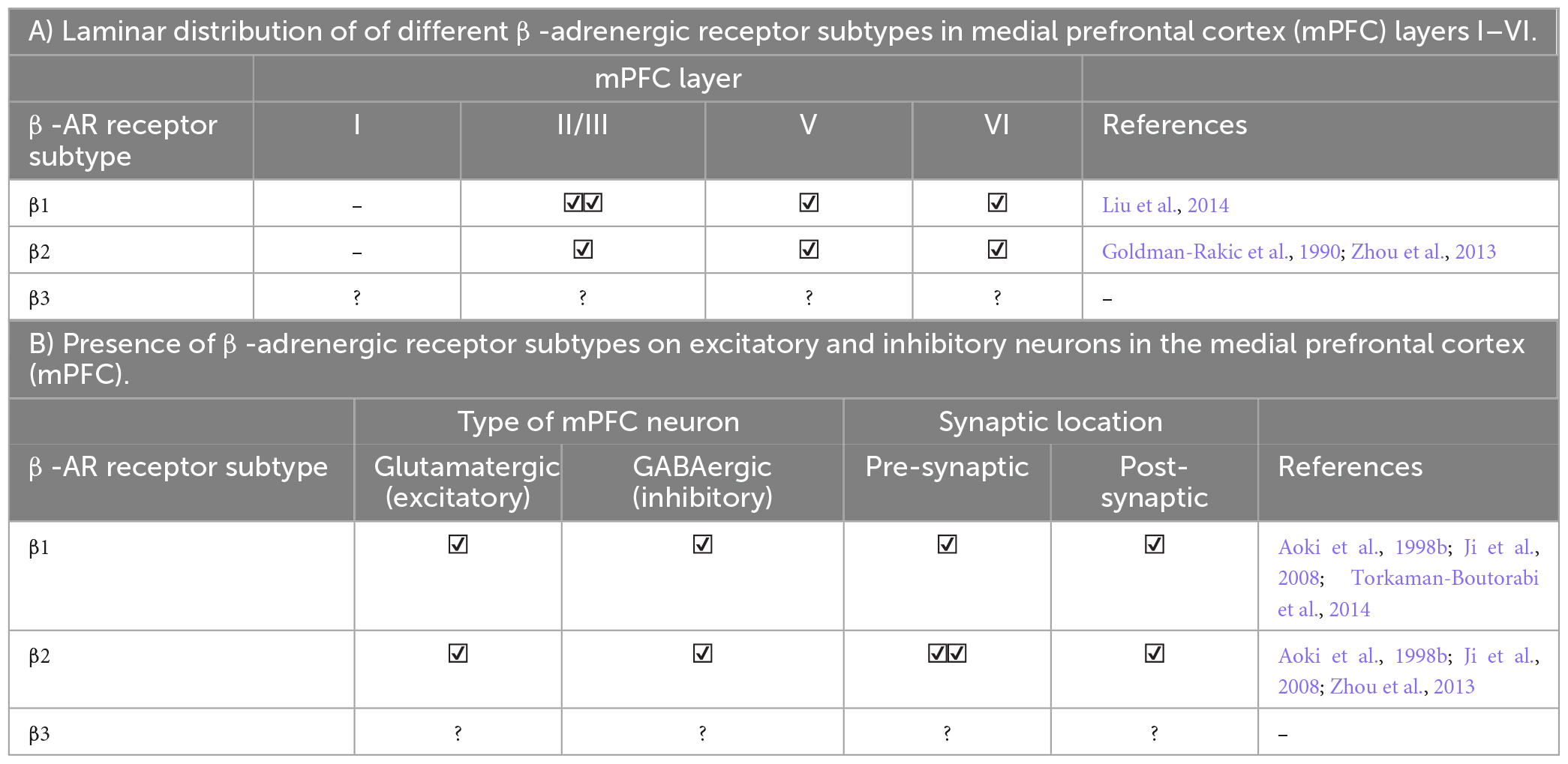
Table 2A. Laminar and cellular distribution of β-adrenergic receptors in the medial prefrontal cortex (mPFC).
Behavioral implications of adrenergic receptors
The focus of NE-related neuroscience research in recent decades has centered on understanding how activation or inhibition of these adrenergic receptors may affect different behaviors and their clinical implications in the treatment of various neurological disorders. The functionality of neuromodulators, including NE, follow an inverted-U model (Figures 3, 4; Arnsten, 2011; Cools and Arnsten, 2022). Under normal conditions, NE provides essential regulation of the PFC to keep neurons in an “awakened” state where they can effectively process and exchange information with one another. When conditions vary from “normal,” i.e., hypo- or hyper-arousal, NE-prefrontal dynamics also change. This dose-specific model demonstrates that during the moderate release of NE, PFC functioning is strengthened and sculpted to optimize function based on environmental demands; this results in alert phenotypes with optimal working memory, cognition, and attentional control. Conversely, in situations where NE release is either too sparse or too intense, a hindrance to PFC functioning occurs, and behavioral impairments arise (Arnsten, 2009, 2011; Chandler et al., 2014b; Xing et al., 2016; Northoff and Tumati, 2019; Ross and Van Bockstaele, 2021). For example, too little NE results in drowsiness and hypo-vigilance; contrastingly, too much NE elicits symptoms such as hyperarousal and anxiety. Furthermore, the varied release of NE into the PFC can cause differential receptor activation and consequent control of decision-making, arousal, and attention. Thus, the inverted-U model provides a basis for understanding how varying amounts of NE release influences prefrontal top-down control over other brain regions. Given the association between anxiety and excessive NE release, here we focus more on each adrenergic receptor subtype and its role in hyperarousal; hypoarousal, the other end of the inverted-U, is also briefly discussed (Figure 4).
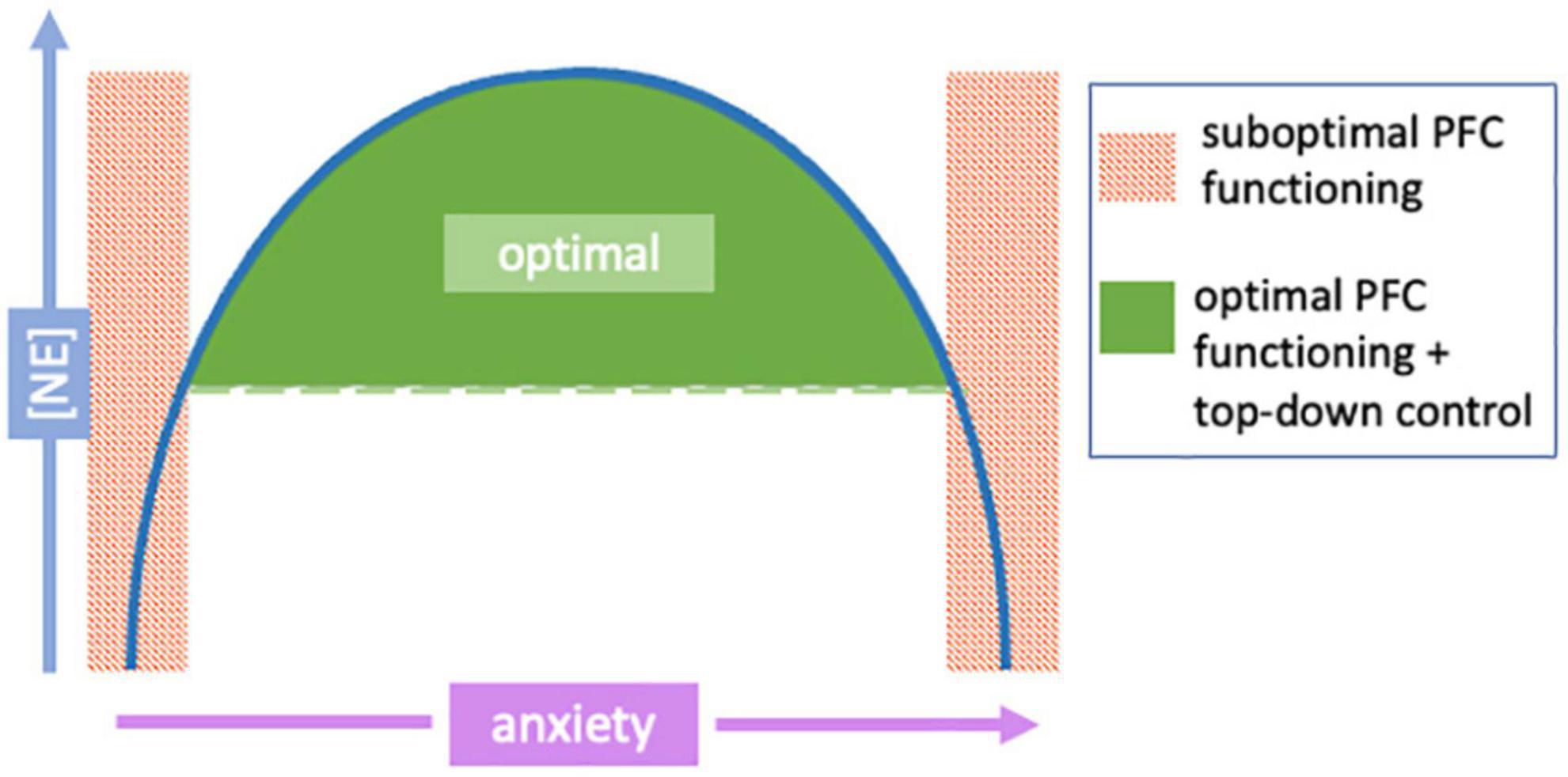
Figure 3. The relationship between norepinephrine (NE) levels and anxiety follows an inverted-U shaped correlation. The relationship of NE release and anxiety levels follows an inverted-U shape. Green areas indicate moderate and manageable arousal/anxiety levels as a result of moderate NE release; this area of a “happy medium” allows continued optimal prefrontal cortex (PFC) functioning and top-down control (and thus, normal behavior). Red shaded areas show areas of either hyper- or hypo-NE release, causing the PFC to be taken “offline” causing loss of necessary regulatory control over other brain regions (impaired PFC-dependent functions). Modified from Arnsten (2011).
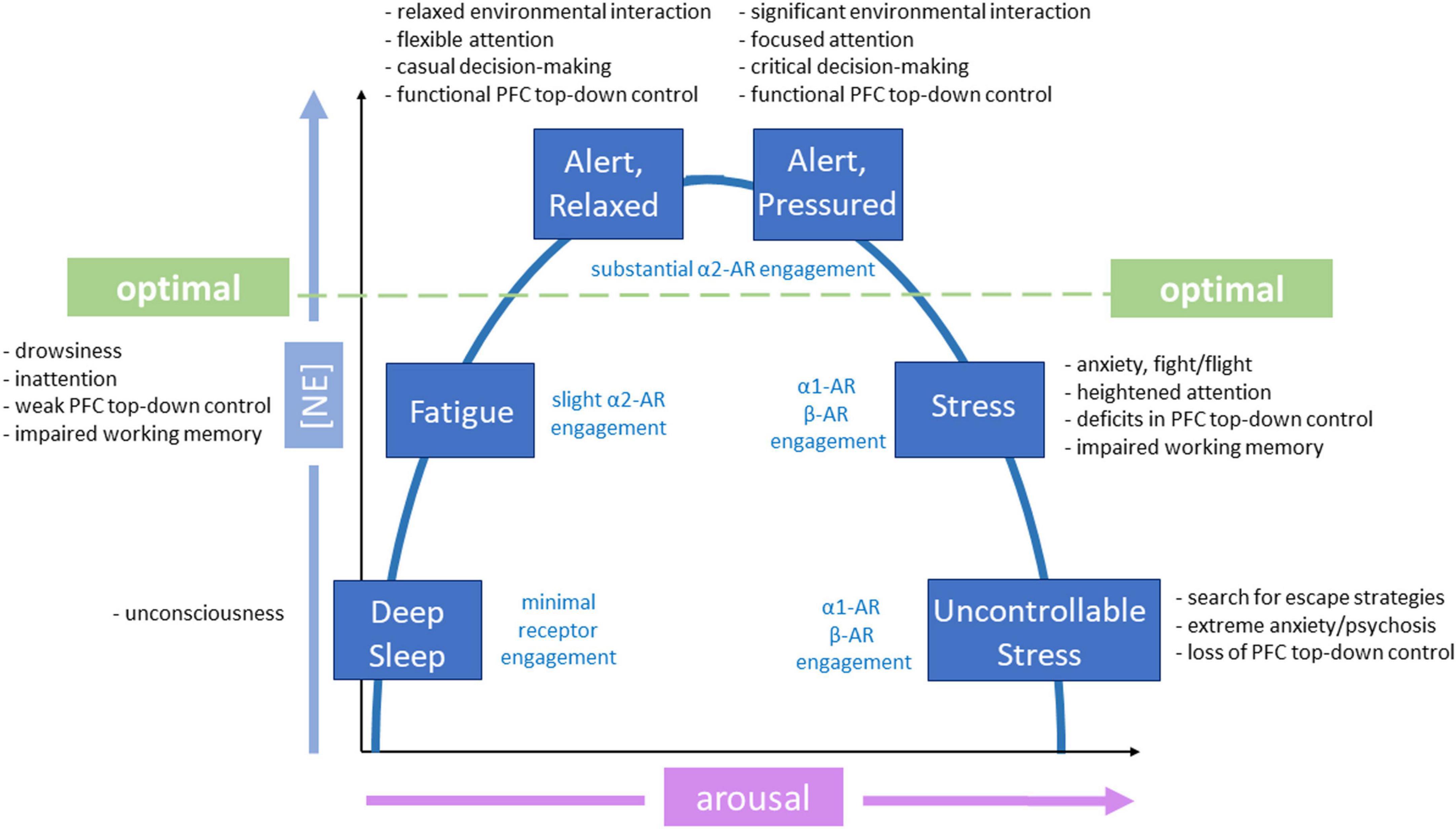
Figure 4. Inverted-U functionality of different adrenergic receptors in behaviors. Inverted-U model of norepinephrine (NE) modulation relates to activation of various levels and types of adrenergic receptors and phenotypes. Different adrenergic receptors are activated depending on level of arousal. The green dotted line indicates the threshold for optimal prefrontal functioning, influenced by substantial α2-adrenergic receptor (AR) engagement. Phenotypic manifestations associated with each state and level of NE release are also provided. Modified from Cools and Arnsten (2022).
Hyperarousal: NE overload
α1 adrenergic receptors
Excessive NE release, as would occur during an environmental stressor, activates lower affinity α1 adrenergic receptors (Arnsten et al., 1998; Arnsten, 2009; Datta et al., 2019). Bulk activation of these receptors depletes functional connectivity to more regulatory parts of the brain involved in executive function (such as the PFC), while enhancing connections to brain regions involved in emotional processing. This impairs higher-order functional abilities of the PFC, such as working memory and attention, and shifts the brain from a state of top-down control (PFC-mediated, thoughtful control) to bottom-up control (salience-driven, impulsive control that is mediated by subcortical structures). Specifically, α1-AR activation releases Ca2+ from intracellular stores through the PLC-PKC pathway (Ramos and Arnsten, 2007). Once this system is activated and the animal is aroused, it is difficult to deactivate this system, as it engages physiological processes that are designed to directly aid in the survival of an organism (Moran, 2016). Resulting arousal acts in a positive feedback loop, as NE neurons change their firing rate by arousal state (Arnsten, 2009). Thus, as α1 receptors are activated in the PFC, arousal state increases, excessive NE release is prolonged, and α1 receptors continue to be activated. Activation of α1 receptors is accompanied by intermediate levels of both tonic and phasic firing in the LC (Atzori et al., 2016).
Activation of prefrontal α1-ARs via phenylephrine, an α1 agonist, resulted in impaired working memory performance in spatial alternation tasks in rats. This effect was rescued through administration of the α1 antagonist, urapidil (Arnsten et al., 1999; Birnbaum et al., 1999). Pharmacological studies utilize α1 receptor blockers, such as prazosin, to treat hyper-arousal related symptoms of post-traumatic stress disorder (PTSD) (Arnsten, 2007). In addition, the neuroleptic α1 blocker, clozapine, prevented stress-induced impairments of cognitive functioning in rodents and non-human primates (Arnsten, 1998), further connecting the overactivation of these receptors to hyperarousal. The anxiogenic effects of α1-AR activation and anxiolytic effects of α1-AR blockade support the idea that activation of these receptors promote hyperarousal states that may lead to prefrontal dysfunction.
α2 adrenergic receptors
The α2-AR agonist guanfacine enhances prefrontal cortical functions in rats, monkeys, and human beings and ameliorates prefrontal cortical deficits in patients with ADHD (Franowicz et al., 2002; Carr et al., 2007; Wang et al., 2007). Blockade of α2 receptors in the primate PFC erodes delay-related firing and instigates a variety of symptoms of ADHD, including limited impulse control and impaired working memory, leading to increased distractibility (Arnsten, 2007, 2009; Gamo et al., 2010; Ross and Van Bockstaele, 2021). Over-induced NE release facilitates the engagement of α1 receptors and reduces the beneficial cognitive control provided by α2-AR activation (Arnsten, 2000, 2009). It is likely that under conditions of hyperarousal and excessive release of NE, α2 receptors completely lose their beneficiary effect on prefrontal function and are overpowered by the activation of α1 and β receptors.
Psychostimulants such as amphetamine and methylphenidate are given in low doses to enhance the release and prevent the reuptake of NE in the PFC (Berridge et al., 2006). These drugs given in small doses emphasize the fine line of NE between beneficial α2 stimulation and detrimental α2 receptor inactivation (Arnsten, 2007).
β adrenergic receptors
Excessive NE release engages β-ARs. This activation is associated with fight-or-flight response, life-or-death decision-making, high limbic activation, and likely impairment of PFC functioning. Massive engagement of cortical and subcortical β-ARs results in deficits in working memory and favors impulsive and autonomic sympathetic responses (Bouret and Sara, 2005; Hains and Arnsten, 2008). Hyperarousal and high β-AR engagement are accompanied by maximum levels of LC tonic firing and low levels of phasic firing (Atzori et al., 2016). Though intermediate levels of LC tonic firing can be helpful for normal attentional functioning, high tonic firing has been associated with anxious states (Morris et al., 2020), as well as distress and neurodegeneration (Atzori et al., 2016). Supporting this claim, β agonists induced anxiogenic effects in rodents (Hecht et al., 2014). Further, β activation impairs fear extinction (Atzori et al., 2016) and remote footshock-induced memory (Fan et al., 2022), which may lead to a more dramatic and persistent anxiogenic response upon bulk activation. Though research surrounding specific β-AR subtype modulation in the PFC is sparse, a recent study demonstrated β2 optogenetic activation in the mPFC resulted anxiogenic responses in the OFT and EZM. These effects were attenuated through miRNA knockout of β2 mPFC pyramidal cell receptors (Lei et al., 2022).
The use of β blockers rescues attenuation dopaminergic modulatory effects following acute restraint stress (Chang and Grace, 2013). Specifically, administration of propranolol, a β-AR antagonist, restored DA function through reversal of stress-induced attenuation of VTA dopamine neuron population activity. Moreover, β antagonism has ben shown to prevent the development of anxiety-like behaviors in mice (Gorman and Dunn, 1993) and humans (Jefferson, 1974) through modulation of anxiety-related somatic responses (Hayes and Schulz, 1987). In several other studies, administering β blockers decreased the biochemical and behavioral effects of social stress (Wohleb et al., 2011), restraint stress (Tamburella et al., 2010), and shock-probe defensive burying response (Bondi et al., 2007). In addition, administration of the β1 antagonist, betaxolol, improved working memory in both rats and monkeys, suggesting blockage of these receptors improves prefrontal cognitive functioning (Ramos et al., 2005). The anxiolytic effects of the β blockers support the notion that these receptors likely contribute to hyperarousal following excessive NE release.
Hypoarousal: insufficient NE
α1 adrenergic receptors
Generally, α1 receptors activate neurons to promote wakefulness and sustain neuronal activity. Insufficient stimulation of these receptors through inadequate NE release is less likely to be detrimental to cognition, as in the case of overstimulation, but likely induces inactivity and fatigue (Atzori et al., 2016). Additionally, given the lower binding affinity of α1 receptors compared to α2-ARs (Ramos and Arnsten, 2007), it is possible that very low levels of NE do not engage α1-ARs to cause detrimental behavioral phenotypes.
α2 adrenergic receptors
Since α2-ARs play a crucial role in optimal PFC functioning, insufficient activation of α2 receptors primarily impacts cognition and attention. Constant hypoactivation of α2 receptors may lead to impaired prefrontal, subcortical, and motor functioning, representing a depressive state. In human postmortem studies of patients diagnosed with major depressive disorder (MDD), increased α2-agonist ligand binding was observed at α2-adrenegic autoreceptors on NE neuronal cell bodies (García-Sevilla et al., 1999; Hamon and Blier, 2013). Consistently, postmortem analyses of the PFC of MDD-diagnosed suicide victims showed increases in mRNA levels of presynaptic inhibitory α2 autoreceptors (Escribá et al., 2004). Moreover, low stimulation of postsynaptic prefrontal α2 receptors induces symptoms such as cognitive impairment, inattention, and drowsiness (Blier and Briley, 2011). These findings suggest that insufficient levels of noradrenergic neurotransmission may contribute to depression etiology.
β adrenergic receptors
Insufficient activation of β-ARs, given their role in anxiogenesis, may not be as detrimental to prefrontal functioning as hypoactivation of other adrenergic receptors. Interestingly, down-regulation of beta adrenergic expression has been observed with antidepressant treatment (Stahl, 1992). Further, given that β-ARs have the lowest binding affinity of all noradrenergic receptor subtypes (Ramos and Arnsten, 2007), it is possible that lack of engagement of these receptors does not induce problematic or observable phenotypes.
Prefrontal circuit-level top-down modulation of anxiety
The execution of anxiety-related behaviors involves detection of environmental stimuli through sensory systems, assignment of emotional value to these cues via subcortical structures, and execution of behavior based on this information via cortical modulation. The PFC is thought to coordinate situational evaluation and corresponding behavioral outcomes through its extensive connections with other regions of the brain, including the amygdala, bed nucleus stria terminalis (BNST), insula, striatum, lateral septum, and the paraventricular thalamic nucleus (PVT), among others in underlying anxiety circuits (Calhoon and Tye, 2015; Mack et al., 2022; Figure 5). Elucidating distinct neural circuit dynamics involved in PFC control of maladaptive behaviors in pathological anxiety can provide insight into the neural pathology underlying dysfunction and may provide an avenue for future circuit-based treatments. Circuit-level modulation is often conserved in translation from mouse to human (Calhoon and Tye, 2015; Poe et al., 2020; Anastasiades and Carter, 2021), allowing for experimentation with animal models that offer clinical applications within this sector of modern neuroscience.
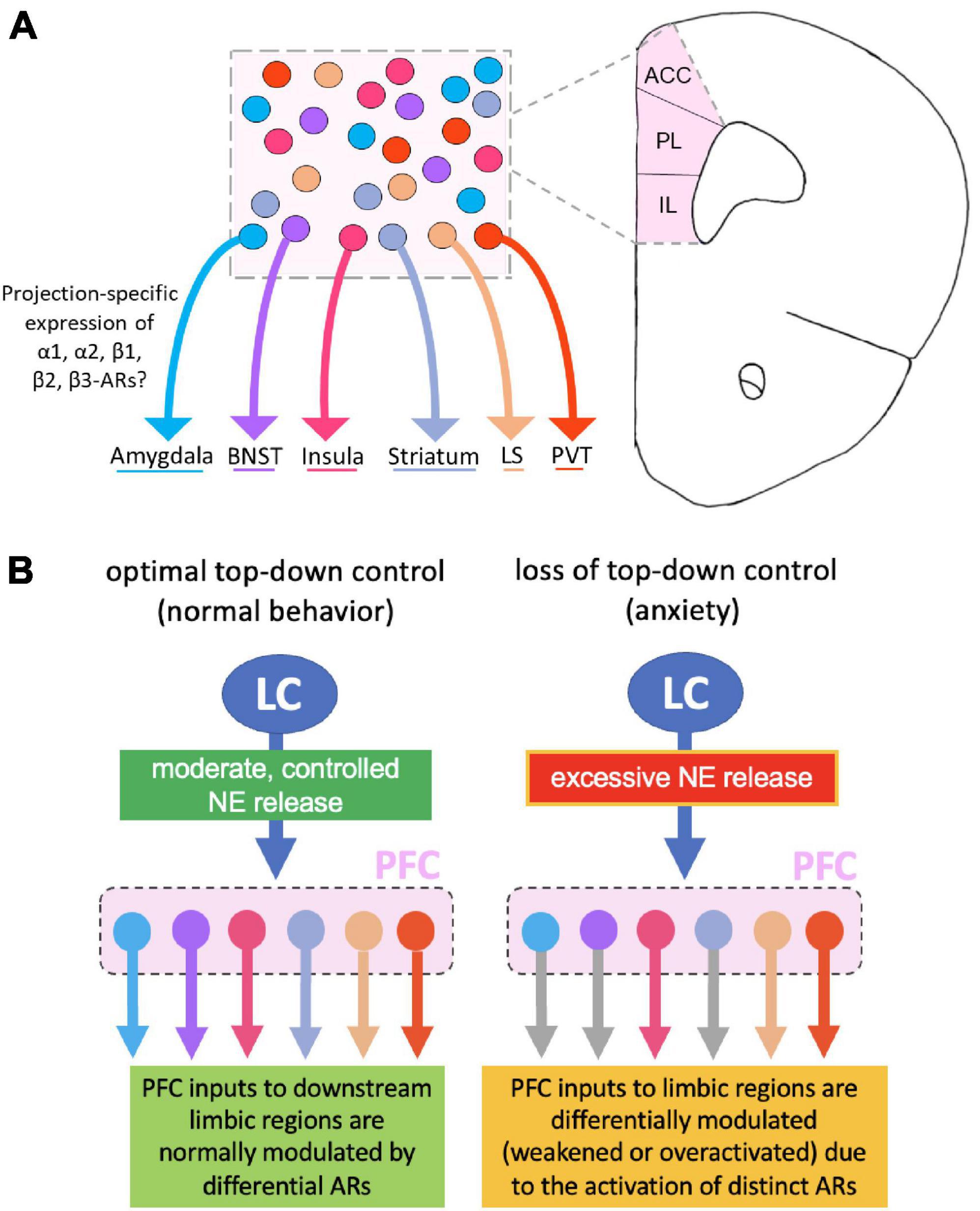
Figure 5. Norepinephrine (NE) modulation of distinct prefrontal cortical neurons that project to different anxiety-related subcortical regions. (A) The prefrontal cortex (PFC) sends dense projections to numerous brain regions implicated in anxiety, yet there is currently no research on which NE receptor subtypes exist on these output pathways. (B) Left panel: Potential circuit-based mechanisms by which optimal locus coeruleus (LC)-mediated NE release in the PFC modulates different PFC output circuits through activation of differential adrenergic receptors (ARs). Right panel: Excessive NE release weakens or over activates PFC outputs to the downstream limbic regions, depending on projection-specific activation of distinct AR subtypes. This in turn results in a shift of dynamic balance among the different circuitries, leading to a loss of prefrontal top-down control and the production of an anxiety-like phenotype.
Given what is known about the impact of varying levels of NE on prefrontal function, it is likely that these modulatory effects alter the activity of PFC projection neurons regulating downstream anxiety-related brain regions. To fully understand how NE modulates top-down prefrontal control, a better understanding of NE modulation of distinct PFC connections is necessary. Though very little is known about NE actions on unique PFC circuits, understanding the important prefrontal circuits involved in anxiolytic responses provides a direction for targeted investigation of how NE specifically modulates these pathways and affects consequent anxiety-related behaviors. In this section, we emphasize what is currently known about PFC, pathological anxiety and avoidance behavior through distinct PFC top-down connections to anxiety-relevant brain regions. This provides a basis for our later proposed model that integrates NE into the prefrontal top-down modulation of anxiety.
PFC-amygdala
The amygdala is one of the most highly studied brain regions regarding mood and anxiety disorders, and has long been investigated for its direct role in regulating sustained anxiety symptoms (Tye et al., 2011; Felix-Ortiz et al., 2013, 2016; Allsop et al., 2014; Felix-Ortiz and Tye, 2014; Calhoon and Tye, 2015). This brain region plays a significant role in emotional processing, cognitive evaluation of emotional stimuli, and emotional learning through its intricate connections to cortical and subcortical regions (Janak and Tye, 2015; Giovanniello et al., 2020). The amygdala is made up of several subnuclei [basolateral amygdala (BLA), central amygdala (CeA), centromedial amygdala (CeM), and basomedial amygdala (BMA), among others] (Marek et al., 2019), whose contribution to anxiety differs depending on the subregion (Treit et al., 1993; Möller et al., 1997; Moreira et al., 2007). The PFC sends projections to various subregions of the amygdala, including the BLA and CeA (Coley et al., 2021). Activation of the entire PFC produced a reduction in amygdala activity, demonstrating the inhibitory effect of PFC projections to the amygdala (Quirk et al., 2003). Moreover, specific effects of prefrontal regulation of the amygdala often vary by PFC subregion.
Both the prelimbic (PL) and infralimbic (IL) subregions of the rodent PFC project to BLA and CeA subregions. In a study by Adhikari et al. (2015), activation of the IL projections to the BMA resulted in reduced anxiety-like behaviors and physiological responses. In contrast to the anxiolytic effects of IL inputs to the amygdala, connections from the PL subregion are thought to drive fear expression (Pendyam et al., 2013; Marek et al., 2019) and promote anxiety (Kim et al., 2011). Haikonen et al. (2022) recently combined viral tracing and electrophysiological techniques to examine the effects of maternal separation (MS) on mPFC-to-BLA connectivity and function in young (P14-21) rats. Prolonged MS as an early-life stressor in young rodents is thought to induce emotional and behavioral abnormalities in adulthood, including increased anxiety-like behavior (Kestering-Ferreira et al., 2021). Interestingly, mice that underwent this MS-induced anxiogenic protocol demonstrated increased prefrontal inputs to BLA GABAergic interneurons and a transient increase in the strength of feed-forward inhibition in the BLA during development. The enhanced GABAergic inhibition raises the induction threshold of long-term potentiation and associates with lower functional synchronization within prefrontal-amygdala networks in vivo. These changes are sex-dependent, with the parameters detected in male but not female rats, who were also resistant to MS-dependent changes in anxiety-like behaviors (Haikonen et al., 2022).
In human studies of clinical anxiety disorders, consistent hyperactivation of the amygdala was observed (Etkin and Wager, 2007; Boehme et al., 2014). In fact, in early human connectivity analyses, subjects with more anxious temperaments had reduced vmPFC-amygdala coupling when presented with aversive stimuli (Pezawas et al., 2005). Interestingly, though varying from mouse studies, the dlPFC (dorsolateral PFC, analogous to rodent PL) exerted a significant inhibitory influence on the right amygdala that was absent in patients with GAD (Dong et al., 2019). Further, in patients with SAD (social anxiety disorder), decreased activity in PFC was also observed (Martin et al., 2010), which may explain some of the cognitive deficit-related symptoms observed in anxiety disorders. It is posited that overactivation of the amygdala observed in anxiety disorders is driven by the loss of prefrontal top-down control. This claim is supported by a recent study that found that stronger vmPFC-amygdala connectivity predicted lower anxiety levels (Kim and Whalen, 2009; Kim et al., 2011). Further, strength of dlPFC-amygdala connections were also correlated with anxiety levels, with the least anxious individuals having the most robust connections (Etkin et al., 2009). Given this evidence, it is likely that the loss of PFC-regulated top-down control is implicated in amygdala-mediated anxiogenic responses.
Hypoactivation of the PFC may lead to hyper-responsivity of the amygdala to even non-threatening environmental cues, triggering full-scale responses often observed in PTSD (Whalen et al., 1998), panic disorder (Kent and Rauch, 2003), and other anxiety disorders. This idea is displayed in mouse models, as mice with limited prefrontal-amygdala interaction displayed significant threat-generalization (Charney et al., 2017). Altogether, the evidence summarized here supports an association between PFC-amygdala circuitry and anxiety. Despite this, it remains unknown whether and how NE may modulate PFC projections to the amygdala under both normal and pathological conditions. While it has been shown that NE release in the BLA promotes anxiety-like behavior (McCall et al., 2017), it remains unclear how NE release in the PFC may act on BLA-projecting neurons. Given the inverse relationship between PFC-amygdala connectivity and anxiety, it is plausible that excessive NE release disrupts functionality of this circuit through loss of prefrontal top-down control.
PFC-BNST
Though the bed nucleus stria terminalis (BNST) is a relatively small brain region, it can be divided into 18 heterogeneous subregions (Robinson et al., 2019). These subregions are seemingly distinct and, at times, opposing in functionality (Jennings et al., 2013; Kim et al., 2013). Previous rodent studies have demonstrated direct input from the PFC to the BNST (Dong et al., 2001; Vertes, 2004; Radley and Sawchenko, 2011; Radley et al., 2013; Glangetas et al., 2017; Johnson et al., 2019) that is particularly dense in IL-avBNST circuits but is also present between the PL and avBNST regions (Johnson et al., 2019). Rodent studies investigating vmPFC-BNST and ACC-BNST (anterior cingulate cortex-BNST) connections in anxiety-like behaviors found that animals exposed to shock demonstrated decreased connectivity in both circuits (Alvarez et al., 2011). This finding supports a relationship between anxiety-like behaviors and loss of PFC top-down modulation of the BNST in rodents.
Moreover, silencing of PL inputs to the avBNST with optogenetics resulted in anxiogenic behavioral phenotypes, including increased immobility and elevated hormonal stress responses during shock-probe burying and tail suspension tests (Radley et al., 2009; Johnson et al., 2019). These results suggest a functional role of PL inputs to the avBNST in reducing anxiety-like behavior. Contrastingly, in a backward conditioning paradigm, IL inputs to the BNST are activated by unpredictable threats (Goode et al., 2019). This may have translational applications to anxiety- and other stress-related disorders, where threats are often inexplicit and unpredictable.
Evidence of prefrontal innervations to the BNST also exist in humans (Dong et al., 2001; Vertes, 2004; Motzkin et al., 2015). Though not PFC-BNST circuit specific, meta-analyses of neuroimaging studies have demonstrated heightened activation of the BNST while subjects awaited aversive cues (Clauss et al., 2015). Further, a correlation between level of BNST activation and arousal during exposure to unpredictable shocks was observed (Alvarez et al., 2011). In patients with PTSD, heightened sensitivity to stimuli, a hallmark of anxiety disorders, is associated with increased BNST activation. Moreover, increased BNST activity was observed in patients with GAD when compared to healthy controls (Yassa et al., 2012). Thus, there are several lines of evidence that support BNST-based modulation of hypervigilance and hyperarousal. It is possible that in the presence of a stressful or aversive event, BNST activation modulates a sustained, or continuous, anxious state. This heightened state may be exaggerated in anxious individuals (Yassa et al., 2012), resulting in some of the physical and emotional symptoms exhibited in anxiety disorders.
Given what is known about the anatomical and functional heterogeneity of PFC subregions, varying efferent connections from different cortical subregions to the BNST likely control different aspects of anxiety-like behavior (Jennings et al., 2013; Kim et al., 2013). Though similar anxiety-inducing experiments have not been conducted in humans due to ethical and technical limitations, considering the homology that exists between the rodent and human brain, similar anxiety-specific changes in PFC-BNST circuitry are likely present. The studies discussed above provide a robust connection between PFC-BNST modulation and anxiety disorders, but the downstream circuitry, cell-type specificity, and subregion modulation of these symptoms remain elusive. However, increased activity of the BNST and decreases in PFC-BNST connection during anxiety-like behavior supports the notion that anxiety may be due to loss of PFC top-down control to the BNST.
PFC-insula
The insula plays an essential role in emotional experience and subjective feelings (Calder et al., 2000; Borg et al., 2013), making it an essential node in the anxiety network. Given its extensive connections with the amygdala and PFC (Paulus, 2008; Simmons et al., 2008; Engel et al., 2009; Freese and Amaral, 2009), the insula has been consistently implicated in the etiology of anxiety disorders (Damsa et al., 2009). This brain structure is divided into rostral agranular insular cortex (raIC); gustory insular cortex (gIC); primary interoceptive posterior insular cortex (pIC), and caudal insular cortex (cIC) (Bruce et al., 2012). Chemogenetic experiments have revealed insular subregions have distinct and often opposing roles in anxiety response. For example, rostral regions play an anxiogenic role (as raIC inactivation increased exploratory behavior and activation decreased these behaviors), whereas caudal regions produce anxiolytic responses in rodents (cIC inactivation decreased exploration and cIC activation promoted exploratory behavior, indicative of decreased anxiety). Conversely, activation of raIC and gIC induced opposite anxiogenic effects, confirming prior results (Bruce et al., 2012).
In a human study by van Tol et al. (2012), subjects were given a word-encoding task (where subjects were presented with positive, negative, or neutral words) and found that negative words had greater insular activation in patients with anxiety disorders compared to healthy controls. Moreover, healthy patients with greater trait anxiety levels had proportional increases in insular activation (Stein et al., 2007), showing anxiety-like symptoms recruit activation of the insula. In healthy subjects, increased levels of trait anxiety consequently resulted in increased insular activation (Engel et al., 2009). Similarly, the degree of insular (right middle insula) activation in women diagnosed with PTSD was greater than in trauma-exposed controls (Lanius et al., 2007; Lindauer et al., 2008; Strigo et al., 2010). This pattern of heightened insular activation in patients with PSTD was further observed when subjects were exposed to emotional, trauma-unrelated stimuli (Simmons et al., 2008; Fonzo et al., 2010). Patients with anxiety disorders may constantly entertain exaggerated interoceptive cues generated by the overactivated insula, which could increase anxiety symptoms and lead to further elevation of insular activity (Stein et al., 2007).
Hyperactivation of various brain regions in anxiety disorders is thought to be attributed to loss of top-down control via vPFC hypoactivation (Bruce et al., 2012). Though PFC-IC circuit-specific research is limited, hypoactivation of the PFC is related to emotional control in patients with PTSD (Etkin and Wager, 2007). Further, decreased ACC volumes were positively correlated to the presence of PTSD symptoms (Kasai et al., 2008). This evidence combined with increased insular activity in these disorders supports the idea that the relationship between increased insular activation and anxiety is due to a loss of PFC top-down control to the insula. With simultaneous increased insular activation and loss of top-down control from the PFC, this circuit may serve in the development or exacerbation of anxiety disorders.
PFC-striatum
The striatum is a complex brain region that contributes to a plethora of behavioral processing implicated in anxiety disorders, including attention, motivation, and learning (Lago et al., 2017). Prior studies investigating the role of the striatum in anxiety disorders often focus on the ventral striatum for its role in processing affect (Cardinal et al., 2002; Christakou et al., 2004; Schott et al., 2008) but the dorsomedial (dmS) striatum has been and found to influence other aspects involved in anxiety disorders, including decision making (Balleine et al., 2007), avoidance behavior (Aupperle and Paulus, 2010; Aupperle et al., 2015; LeBlanc et al., 2020), and action initiation (Porter et al., 2015). Interestingly, deep brain stimulation of the striatum in rodents (Rodriguez-Romaguera et al., 2012) and humans (Rauch et al., 2006; Denys et al., 2010) has shown that activation of this brain region results in a reduction in anxiety-related symptoms.
In rodents, an especially relevant input to the dmS is the dorsomedial PFC (Sesack et al., 1989; Gabbott et al., 2005). The role of this dmPFC-dmS circuit has previously been demonstrated in decision-making under conflict, a trait that is often disrupted in anxiety disorders (Friedman et al., 2015). A recent study reported that greater activity in dmPFC-dmS projection neurons was observed during open arm occupancy compared to that of the closed arms in the elevated plus maze (EPM); this effect was not observed in other dmPFC circuits, such as the dmPFC-amygdalar projection neurons. Further, stimulation of the dmPFC-dmS pathway increased open arm exploration, showing an increased drive to approach and decreased anxiety-like behavior (Loewke et al., 2020). Inhibition of this circuit decreased open-arm exploration, illustrating the involvement of this pathway in anxiety-related avoidance. These findings provide evidence supporting the control of dmPFC-dmS circuitry in regulating anxiety-like behavior in the EPM and elevated zero maze (EZM) (Loewke et al., 2020). Moreover, these results support the model of prefrontal top-down control over defensive action, such as avoidance. Investigators have posed that corticostriatal circuitry may integrate previous learning contingencies and the behavioral state of the organism to integrate signals and select subsequent appropriate behavioral responses. This hypothesis is supported by the cortical processing of aversive experience; it is reasonable to conclude that this circuit plays a key role in the generation of defensive response via prefrontal-striatal projection neurons (Kirouac, 2021).
Ventral and dorsomedial regions of the striatum receive prominent PFC afferents (Cisler and Koster, 2010; Liljeholm and O’Doherty, 2012; Calhoon and Tye, 2015; Howland et al., 2022). The ventral striatum is known to be involved in learning and motivation (Dayan and Balleine, 2002; O’Doherty et al., 2004; Cauda et al., 2011; Porter et al., 2015). Motivation is often thought of in the context of addiction (Martin et al., 2002; Chambers et al., 2003), but can be included in anxiety research when reframed to the context of a motivation to avoid danger, or risk avoidance (Lago et al., 2017). This is particularly relevant as individuals diagnosed with anxiety disorders tend to possess abnormally high risk-avoidance levels, which could be attributed to ventral striatal dysfunction (Lago et al., 2017). Regarding circuit-level connectivity, the ventral striatum, which includes the nucleus accumbens (NAc) shell and core, receives input from orbitofrontal and anterior cingulate cortices in humans (Heimer et al., 2007; Ernst and Fudge, 2009; Bolstad et al., 2013; Porter et al., 2015). The ventral striatum, especially NAc, receives dense excitatory afferents from the PFC. NAc volume appears to be a predictor of anxiety symptoms following treatment (Burkhouse et al., 2020), while NAc deep brain stimulation decreases ratings of depression and anxiety (Bewernick et al., 2010). Many noradrenergic dopamine-beta-hydroxylase immunoreactive (DBH-ir) fibers were found in the shell but few were in the core regions (Berridge et al., 1997). A further study indicated that the primary source of NE afferents to the shell of NAc is from the A2 region, with lesser contribution from the A1 and LC (Delfs et al., 1998). Thus, LC-mediated NE release may influence NAc activity through PFC projection neurons. However, how PFC-NAc pathway is modulated by NE and which receptor subtypes are involved in the regulation of anxiety-like behavior remain to be determined.
PFC-PVT
The paraventricular thalamus (PVT) is a midline thalamic structure that integrates information from the motor, limbic, and cortical circuits in the brain (Sesack et al., 1989; Vertes, 2004; Gao et al., 2020, 2023; Iglesias and Flagel, 2021; Penzo and Gao, 2021). The PVT is often separated anatomically and functionally into two subregions: the anterior PVT (aPVT) and posterior PVT (pPVT) (Li and Kirouac, 2012; Kirouac, 2015). Robust sources of input to this brain region include the IL and PL cortices (Li and Kirouac, 2012; Kirouac, 2015), as indicated through retrograde (Krout et al., 2002; Otake et al., 2002) and anterograde (Hurley et al., 1991; Canteras and Swanson, 1992; Vertes, 2004) studies in rodents. The PVT has been known to be a key node in the emotional processing network (Barson et al., 2020) and mediates behavioral responses to stress.
The aPVT receives information from the IL concerning motivational state and arousal. The pPVT receives input from both PL and IL subregions of the PFC, which is thought to communicate information about salient emotional stimuli (Otis et al., 2017, 2019).
Due to the known involvement of the PVT and the PFC in fear, anxiety, and arousal, this circuit may work to modulate behavioral responses to aversive and/or threatening stimuli, though more work is needed to confirm this hypothesis. Although gaps in knowledge surrounding PFC-PVT circuitry do exist, the dense connections between these regions and the behaviors they are known to regulate suggest a likely top-down influence of the PFC on the PVT. While NE signaling in the PVT has been shown to influence cellular responses to stress (Beas et al., 2018), an interesting avenue of future research will be determining how NE release in the PFC alters activity between the PFC and the PVT, and how this may relate to stress and pathological anxiety.
PFC-lateral septum
The lateral septum (LS) is a forebrain region implicated in various behaviors, including feeding, rewards, sociability, and fear. Alongside these functions, the LS has long been involved in the control of stress responses and anxiety (Sheehan et al., 2004). This brain region was once described as a homogenous structure, but has now been recognized as a heterogeneous region with different subregions, cell types, and microcircuits (Risold and Swanson, 1997). The LS can be characterized into four major subregions, dorsolateral septum (dlLS), dorsomedial septum (dmLS), ventrolateral septum (vlLS), and ventromedial septum (vmLS), each exhibiting differential effects on anxiety based on their unique afferent and efferent connections (Rizzi-Wise and Wang, 2021). For example, the dorsal LS is implicated in promoting anxiety (Thomas et al., 2013), while the activation of ventral LS regions reduces anxiety (Parfitt et al., 2017) and fear (Parfitt et al., 2017; Besnard et al., 2020). Phenotypes that arise from vLS activation suggest this region plays a role in suppressing negative affect (Rizzi-Wise and Wang, 2021), thus blunting the psychological severity of stressors (Sheehan et al., 2004). On the other hand, lesions to the LS produce “septal rage” or over-reactivity to stimuli and excessive fear response (Albert and Chew, 1980). Similarly, inhibition of individual LS neurons increases anxiety (Sheehan et al., 2004).
There have been significantly fewer studies investigating PFC inputs to the LS. The IL subregion of the PFC sends dense projections to the intermediate parts of the LS, moderate inputs to the dorsal LS, and sparse inputs to the ventral LS (Hurley et al., 1991; Canteras and Swanson, 1992; Vertes, 2004). One of the only studies investigating PFC modulation of the LS found that optogenetic activation of PFC terminals in the LS had overall anxiogenic effects, as shown by increased open arm avoidance and decreased open arm entry probability in the EPM, as well as increased freezing and decreased time spent in the center of the arena in the open field test (OFT) (Chen et al., 2021). Further, opto- and chemo-genetic inhibition of the IL-LSe pathway produces anxiolytic effects, as observed through decreased open arm avoidance, increased probability of open arm entry, and increased center occupancy in the OFT (Chen et al., 2021). These findings identify the LS as a key target of IL to enhance anxiety-related behavioral responses, suggesting a direct, local IL-LS synaptic connection to modulate anxiety and fear (Chen et al., 2021). However, this finding seems inconsistent with the idea of top-down prefrontal control of the proper behavioral response. Further studies in a IL-LS subcircuit- and cell-type-specific manner would provide novel insight into the role of IL-LS pathway in anxiety-like behaviors (Besnard and Leroy, 2022).
A model of NE modulation of PFC top-down control
Despite the well-known sensitivity of the PFC to changes in the LC-NE system (Arnsten, 1998), it remains almost completely unexplored how NE release differentially influences each of the PFC circuits mentioned above. Especially, if these distinct PFC projections express different adrenergic receptors and whether they are differentially modulated by LC activity and its released NE in the PFC (Figure 5). Nonetheless, some evidence suggests NE regulates distinct PFC populations. For example, adrenergic modulation shifts the dynamic properties of corticopontine (CPn) but not commissural (COM) neurons and increases the excitability of CPn neurons significantly more than COM neurons (Dembrow et al., 2010), indicating subcircuit-specific neuromodulation in the PFC. These findings describe some of the functional consequences of selective neuromodulation on behavioral states during goal-directed behavior (Dembrow and Johnston, 2014). Evidence of differential effects of NE signaling on varying subcircuit-specific PFC modulation, though limited, inspires the idea that other prefrontal circuits are uniquely modulated by NE in the PFC. Combining our knowledge of PFC anxiety-related circuits with the molecular and behavioral framework of general NE modulation in the PFC, we propose a model of adrenergic influence on prefrontal top-down control of anxiety (Figure 5).
In this model, we posit that controlled NE release in the PFC maintains optimal functioning of the PFC, eliciting control over other more emotionally-related limbic regions involved in anxiety (eg., amygdala, BNST, insula, striatum, PVT). We pose that this prefrontal top-down control integrates limbic responses with conscious planning and decision-making to elicit appropriate behavioral responses (Figure 5). Conversely, conditions of excessive NE release, evoked by unpredictable environmental threats or other perceived psychological stressors (such as those observed in anxiety disorders), may either weaken or overactivate PFC projections to these anxiety-related brain regions. Given that excessive stimulation of ARs inhibits cognitive functioning (Arnsten, 1998) and may take the PFC “offline,” it is likely that the loss of top-down control over some or all of these aforementioned brain regions would shift brain states to a mode of subcortical modulation and thus play a vital role in the generation of anxiety (Figure 5).
However, this model has yet to be tested directly, and understanding exactly which PFC circuits are impacted by excessive NE release is of great interest from a preclinical and clinical perspective. Altogether, there is a need for more research to elucidate the impact of NE on specific PFC circuits known to be involved in pathological anxiety.
It is also noteworthy to consider the reciprocal connections between the PFC and each or some of these brain regions, and how these regions may individually impact optimal prefrontal functioning in the context of NE signaling. While it remains unknown which AR receptors are expressed on specific PFC output populations, it is also unclear whether afferent inputs to the PFC from other anxiety-related brain regions (e.g., ventral hippocampus) also express AR receptors, which could further contribute to substantial modulation of PFC activity following NE release. Although PFC projection pathways have been the focus of this review, the influence of NE on distinct afferent inputs proves to be a critical area of research in circuit- and systems-based neuroscience. More work is needed to understand whether and how PFC inputs and outputs are uniquely engaged by NE to regulate anxiety-like behaviors.
Finally, it should be noted that given the reciprocal descending pathways from the PFC to the LC, any dynamic activity changes in the mPFC could also have a feedback effect on the LC neuronal activity. However, there are limited studies investigating prefrontal-LC projections, making the speculation of how these projections may affect anxiety-like behavior challenging. Nevertheless, it was reported that electrical stimulation of the PFC in anesthetized rats activated the LC through NMDA and non-NMDA mechanisms (Jodo and Aston-Jones, 1997). Given the PFC’s role in attention via NE modulation (Arnsten, 1998, 2011; Berridge and Waterhouse, 2003Morris et al., 2020), presumably it is possible that low level’s of NE in the PFC can induce activation of the LC, which increases NE release to an optimal level to regulate sustained attention and decision-making. In contrast, a high level of NE release in the PFC, as would occur during stress or anxiety-evoking situations, can disengage optimal prefrontal functioning. Therefore, it is unlikely these prefrontal projections to the LC provide negative-feedback signals to provide top-down control to the LC to inhibit NE release during anxiety-like behavior. Even so, more research is needed on the neurochemical and behavioral effects of this descending pathway on anxiety-like behaviors.
Future directions
Preclinical experiments suggest that all NE receptor subtypes participate in anxiety-like processes. Given the extensive use of pharmacological agents that target NE receptors to treat pathological anxiety and the clear relationship between PFC dysfunction in the clinical population, revealing the intricacies of NE receptor signaling in modulation of PFC circuit-level control of anxiety is crucial.
Overall, evidence supports a decisive role for NE and distinct PFC circuits in driving anxiety-related behaviors. Significant progress has been made in the last two decades in investigating the LC-NE system’s direct influence on anxiety and other aversive behaviors. Despite these advances, more work is needed to bridge the gap between NE signaling and PFC circuit function in anxiety by revealing the precise circuit-level effects of NE release in the PFC. Although the field has yet to directly investigate NE-PFC influences on anxiety etiology, asking questions from a combinatorial standpoint of molecular signaling in conjunction with known circuit-based regulation of behavior, as in our proposed model, is now possible due to recent technological advances. For instance, particularly in rodents, new tools have provided extraordinary temporal and spatial resolution to investigate causal functions of neural circuits and have already yielded impressive results identifying discrete PFC circuits mediating specific anxious behaviors, including approach-avoidance, social deficits, and fear. Our model, though speculatory, can begin to be directly tested using tools such as the GRAB-NE biosensor to detect endogenous NE release in the brain, offering an unprecedented opportunity to uncover the temporal dynamics of NE signaling in the PFC and its resulting effects on behavior (Feng et al., 2019). These dynamics can be fine-tuned even further using the Cre-Lox system to restrict NE biosensor expression to specific PFC circuits and excitatory versus inhibitory PFC cell types. In addition, increasingly advanced computational data analysis, such as deep learning to analyze micro-behaviors, may reveal additional anxiety-like behaviors in rodents that were previously overlooked by manual scoring. Moreover, retrograde tracing studies to visualize exactly where NE-modulated PFC-projection neurons are located, in conjunction with fluorescent in situ hybridization (FISH) for specific NE receptor subtypes will further pry into the unknown details of NE receptor expression on distinct PFC-circuits. With vigorous investigation using these new tools, we can begin to address our proposed model and many other outstanding questions that remain (see Box 2–Outstanding questions). Implementing a combination of these new and improved techniques is needed to truly uncover the precise dynamics of the LC-NE-PFC’s role in anxiety, which is an exciting destination in the future of neuroscience research.
BOX 2 Outstanding questions. |
1. NE is known to modulate decision making and cognitive functions in the PFC, but the circuit-level mechanisms have been unexplored. Revealing which specific cell types and circuitry are affected by NE release in the PFC is an important and interesting avenue of future research |
2. Sex differences are known to play a role in NE signaling and receptor expression in the PFC and related circuitry. Whether and how these sex differences contribute to the susceptibility or etiology of pathological anxiety still remains unknown. |
3. PFC-NE plays a role in the functionality of the PFC and its ability to provide top-down control, yet the precise projection neurons and interneurons involved remains elusive. |
4. The presence of α1 and α2 receptors have been identified in both excitatory and GABAergic neurons in the PFC. However, it is unknown if these receptors are colocalized on the same cells or if cells are constricted to receptor subtype specificity. Moreover, which adrenergic receptor subtypes are expressed on various PFC projection neurons, especially those efferent pathways known to be involved in the modulation of anxiety. |
5. It is still unknown whether and how the development of pathological anxiety disorders alters NE release, adrenergic receptor expression, and/or sensitivity in PFC neurons. |
In addition, though sex-differences in anxiety etiology is not discussed in this review, this should not be overlooked. The US National Institute of Mental Health reports that the lifetime prevalence of anxiety disorders is two to three times higher in women than in men (Yonkers et al., 2003; Grant et al., 2005; Kessler et al., 2006, 2009, 2012; Tolin and Foa, 2006; Leach et al., 2008) and women demonstrate distinctly lower treatment efficacy (Donner and Lowry, 2013). Whether and how sex hormones converge with NE signaling in the PFC to guide behavior, and how this may potentially mediate sex differences in pathological anxiety is another intriguing and important line of future research. Despite some recent progress, there is still a substantial delay in the conceptual idea that the field must study both males and females to effectively investigate and treat disorders across sexes. A future of inclusive data collection generates hope for filling the gap in knowledge involving the female brain and developing improved, comprehensive treatments for anxiety and other psychiatric disorders.
It is interesting to speculate that a particular neural circuit dysfunction could be casually involved in multiple psychiatric diseases, including anxiety. Further, given the substantial rate of co-morbidity and shared symptomology among various mental illnesses, the identification of distinct neurobiological mechanisms underlying these diseases is one of the most pressing needs and invigorating avenues of research into psychiatric disorders. Treating psychiatric disorders that disrupt these complex, intertwined neural systems may require a broad, circuit-level approach. A shift in how we consider the underpinnings of anxiety—such that the brain works in a circuit-dependent manner, where changes (including neuromodulatory influences) in each subregion affect the next—promises to remodel how anxiety disorders are treated.
Current technological advances for neuroscience experiments, particularly in rodents, have provided exceptional temporal and spatial resolution to investigate causal functions of neural circuits mediating specific anxious behaviors, including approach-avoidance, social deficits, and fear. Combinatorial approaches with increasingly advanced computational data analysis, such as deep learning to analyze micro-behaviors, will directly aid researchers in answering these critical questions. In particular, the recent advent of biosensors to detect endogenous NE release in the brain offers an unprecedented opportunity to uncover the temporal dynamics of NE signaling in the PFC and its resulting effects on the behavior. In particular, using the Cre-Lox system to restrict NE biosensor expression to specific PFC circuits and cell types, we can begin to address some of the outstanding questions that remain (Box 2). Implementing a combination of these new and improved techniques is needed to truly uncover the precise dynamics of the LC-NE-PFC’s role in anxiety, which is an exciting destination in the future of neuroscience research.
Author contributions
NB, NM, and W-JG wrote the manuscript. All authors contributed to the article and approved the submitted version.
Funding
This study was supported by NIH R21MH121836, R21MH129989, R01MH131053, and Pennsylvania Commonwealth 4100085747 (CURE 2016) to W-JG.
Acknowledgments
We thank Jake D. Clarin and Leigh Taylor Flynn for their comments on the manuscript.
Conflict of interest
The authors declare that the research was conducted in the absence of any commercial or financial relationships that could be construed as a potential conflict of interest.
Publisher’s note
All claims expressed in this article are solely those of the authors and do not necessarily represent those of their affiliated organizations, or those of the publisher, the editors and the reviewers. Any product that may be evaluated in this article, or claim that may be made by its manufacturer, is not guaranteed or endorsed by the publisher.
Abbreviations
adBNST, anterodorsal bed nucleus stria terminalis; ADHD, attention deficit hyperactivity disorder; aPVT, anterior paraventricular thalamus; avBNST, anteroventral BNST; AR, adrenergic receptor; BLA, basolateral amygdala; BMA, basomedial amygdala; BNST, bed nucleus stria terminalis; cAMP, cyclic AMP (adenosine monophosphate); CeA, central amygdala; CeM, centromedial amygdala; cIC, caudal insular cortex; COM, commissural; CPn, corticopontine; DA, dopamine; DBH, dopamine beta hydroxylase; dlLS, dorsolateral lateral septum; dlPFC, dorsolateral prefrontal cortex; dmLS, dorsomedial lateral septum; dmS, dorsomedial striatum; DREADDS, designer receptors exclusively activated by designer drugs; EPM, elevated plus maze; EZM, elevated zero maze; GAD, generalized anxiety disorder; gIC, gustatory insular cortex; GRAB-NE, G-protein -coupled receptor activation based NE sensor; IL, infralimbic; LC, locus coeruleus; LS, lateral septum; MDD, major depressive disorder; NE, norepinephrine; NET, norepinephrine transporter; NMDA, N-methyl -D-aspartate; OCD, obsessive compulsive disorder; OFT, open field test; ovBNST, oval bed nucleus stria terminalis; PFC, prefrontal cortex; pIC, primary interoceptive posterior insular cortex; PL, prelimbic; pPVT, posterior paraventricular thalamus; PTSD, post-traumatic stress disorder; PVT, paraventricular thalamus; raIC, rostral agranular insular cortex; SAD, social anxiety disorder; vmPFC, ventromedial prefrontal cortex; vlLS, ventrolateral lateral septum.
References
Achterberg, E., van Kerkhof, L., Damsteegt, R., Trezza, V., and Vanderschuren, L. (2015). Methylphenidate and atomoxetine inhibit social play behavior through prefrontal and subcortical limbic mechanisms in rats. J. Neurosci. 35, 161–169. doi: 10.1523/JNEUROSCI.2945-14.2015
Adhikari, A., Lerner, T., Finkelstein, J., Pak, S., Jennings, J., Davidson, T., et al. (2015). Basomedial amygdala mediates top-down control of anxiety and fear. Nature 527, 179–185. doi: 10.1038/nature15698
Agster, K., Mejias-Aponte, C., Clark, B., and Waterhouse, B. (2013). Evidence for a regional specificity in the density and distribution of noradrenergic varicosities in rat cortex. J. Comp. Neurol. 521, 2195–2197. doi: 10.1002/cne.23270
Albert, D., and Chew, G. (1980). The septal forebrain and the inhibitory modulation of attack and defense in the rat. A review. Behav. Neural Biol. 30, 357–388. doi: 10.1016/s0163-1047(80)91247-9
Allsop, S., Vander Weele, C., Wichmann, R., and Tye, K. (2014). Optogenetic insights on the relationship between anxiety-related behaviors and social deficits. Front. Behav. Neurosci. 8:241. doi: 10.3389/fnbeh.2014.00241
Alvarez, R., Chen, G., Bodurka, J., Kaplan, R., and Grillon, C. (2011). Phasic and sustained fear in humans elicits distinct patterns of brain activity. Neuroimage 55, 389–400.
American Psychiatric Association [APA] (2013). Diagnostic and statistical manual of mental disorders, 5th Edn. Washington, DC: American Psychiatric Association Publishing.
Anastasiades, P., and Carter, A. (2021). Circuit organization of the rodent medial prefrontal cortex. Trends Neurosci. 44, 550–563. doi: 10.1016/j.tins.2021.03.006
Aoki, C., Venkatesan, C., Go, C., Forman, R., and Kurose, H. (1998a). Cellular and subcellular sites for noradrenergic action in the monkey dorsolateral prefrontal cortex as revealed by the immunocytochemical localization of noradrenergic receptors and axons. Cereb. Cortex 8, 269–277. doi: 10.1093/cercor/8.3.269
Aoki, C., Venkatesan, C., and Kurose, H. (1998b). Noradrenergic modulation of the prefrontal cortex as revealed by electron microscopic immunocytochemistry. Adv. Pharmacol. 42, 777–780. doi: 10.1016/s1054-3589(08)60862-5
Arnsten, A. (1998). Catecholamine modulation of prefrontal cortical cognitive function. Trends Cogn. Sci. 2, 436–447. doi: 10.1016/s1364-6613(98)01240-6
Arnsten, A. (2000). Through the looking glass: Differential noradenergic modulation of prefrontal cortical function. Neural Plast. 7, 133–146. doi: 10.1155/NP.2000.133
Arnsten, A. (2007). Catecholamine and second messenger influences on prefrontal cortical networks of “representational knowledge”: A rational bridge between genetics and the symptoms of mental illness. Cereb. Cortex 17(Suppl. 1), i6–i15. doi: 10.1093/cercor/bhm033
Arnsten, A. (2009). Stress signalling pathways that impair prefrontal cortex structure and function. Nat. Rev. Neurosci. 10, 410–422. doi: 10.1038/nrn2648
Arnsten, A. (2011). Catecholamine influences on dorsolateral prefrontal cortical networks. Biol. Psychiatry 69, e89–e99. doi: 10.1016/j.biopsych.2011.01.027
Arnsten, A. (2015). Stress weakens prefrontal networks: Molecular insults to higher cognition. Nat. Neurosci. 18, 1376–1385. doi: 10.1038/nn.4087
Arnsten, A., Mathew, R., Ubriani, R., Taylor, J., and Li, B. (1999). Alpha-1 noradrenergic receptor stimulation impairs prefrontal cortical cognitive function. Biol. Psychiatry 45, 26–31. doi: 10.1016/s0006-3223(98)00296-0
Arnsten, A., Steere, J., Jentsch, D., and Li, B. (1998). Noradrenergic influences on prefrontal cortical cognitive function: Opposing actions at postjunctional alpha 1 versus alpha 2-adrenergic receptors. Adv. Pharmacol. 42, 764–767. doi: 10.1016/s1054-3589(08)60859-5
Aston-Jones, G., Chiang, C., and Alexinsky, T. (1991). Discharge of noradrenergic locus coeruleus neurons in behaving rats and monkeys suggests a role in vigilance. Prog. Brain Res. 88, 501–520. doi: 10.1016/s0079-6123(08)63830-3
Aston-Jones, G., and Cohen, J. (2005). Adaptive gain and the role of the locus coeruleus-norepinephrine system in optimal performance. J. Comp. Neurol. 493, 99–110. doi: 10.1002/cne.20723
Aston-Jones, G., Rajkowski, J., and Cohen, J. (2000). Locus coeruleus and regulation of behavioral flexibility and attention. Prog. Brain Res. 126, 165–182. doi: 10.1016/S0079-6123(00)26013-5
Atzori, M., Cuevas-Olguin, R., Esquivel-Rendon, E., Garcia-Oscos, F., Salgado-Delgado, R., Saderi, N., et al. (2016). Locus ceruleus norepinephrine release: A central regulator of CNS spatio-temporal activation? Front. Synaptic Neurosci. 8:25. doi: 10.3389/fnsyn.2016.00025
Aupperle, R., Melrose, A., Francisco, A., Paulus, M., and Stein, M. (2015). Neural substrates of approach-avoidance conflict decision-making. Hum. Brain Mapp. 36, 449–462. doi: 10.1002/hbm.22639
Aupperle, R., and Paulus, M. (2010). Neural systems underlying approach and avoidance in anxiety disorders. Dialogues Clin. Neurosci. 12, 517–531. doi: 10.31887/DCNS.2010.12.4/raupperle
Balleine, B., Delgado, M., and Hikosaka, O. (2007). The role of the dorsal striatum in reward and decision-making. J. Neurosci. 27, 8161–8165. doi: 10.1523/JNEUROSCI.1554-07.2007
Barson, J., Mack, N., and Gao, W. (2020). The paraventricular nucleus of the thalamus is an important node in the emotional processing network. Front. Behav. Neurosci. 14:598469. doi: 10.3389/fnbeh.2020.598469
Beas, B., Wright, B., Skirzewski, M., Leng, Y., Hyun, J., Koita, O., et al. (2018). The locus coeruleus drives disinhibition in the midline thalamus via a dopaminergic mechanism. Nat. Neurosci. 21, 963–973. doi: 10.1038/s41593-018-0167-4
Beck, A. (2005). The current state of cognitive therapy: A 40-year retrospective. Arch. Gen. Psychiatry 62, 953–959. doi: 10.1001/archpsyc.62.9.953
Bereza, B., Machado, M., and Einarson, T. (2009). Systematic review and quality assessment of economic evaluations and quality-of-life studies related to generalized anxiety disorder. Clin. Ther. 31, 1279–1308. doi: 10.1016/j.clinthera.2009.06.004
Berridge, C. (2008). Noradrenergic modulation of arousal. Brain Res. Rev. 58, 1–17. doi: 10.1016/j.brainresrev.2007.10.013
Berridge, C., Devilbiss, D., Andrzejewski, M., Arnsten, A., Kelley, A., Schmeichel, B., et al. (2006). Methylphenidate preferentially increases catecholamine neurotransmission within the prefrontal cortex at low doses that enhance cognitive function. Biol. Psychiatry 60, 1111–1120. doi: 10.1016/j.biopsych.2006.04.022
Berridge, C., Page, M., Valentino, R., and Foote, S. (1993). Effects of locus coeruleus inactivation on electroencephalographic activity in neocortex and hippocampus. Neuroscience 55, 381–393. doi: 10.1016/0306-4522(93)90507-c
Berridge, C., Stratford, T., Foote, S., and Kelley, A. (1997). Distribution of dopamine beta-hydroxylase-like immunoreactive fibers within the shell subregion of the nucleus accumbens. Synapse 27, 230–241.
Berridge, C., and Waterhouse, B. (2003). The locus coeruleus-noradrenergic system: Modulation of behavioral state and state-dependent cognitive processes. Brain Res. Brain Res. Rev. 42, 33–84. doi: 10.1016/s0165-0173(03)00143-7
Besnard, A., and Leroy, F. (2022). Top-down regulation of motivated behaviors via lateral septum sub-circuits. Mol. Psychiatry 27, 3119–3128. doi: 10.1038/s41380-022-01599-3
Besnard, A., Miller, S., and Sahay, A. (2020). Distinct dorsal and ventral hippocampal CA3 outputs govern contextual fear discrimination. Cell Rep. 30, 2360–2373.e5. doi: 10.1016/j.celrep.2020.01.055
Bewernick, B., Hurlemann, R., Matusch, A., Kayser, S., Grubert, C., Hadrysiewicz, B., et al. (2010). Nucleus accumbens deep brain stimulation decreases ratings of depression and anxiety in treatment-resistant depression. Biol. Psychiatry 67, 110–116. doi: 10.1016/j.biopsych.2009.09.013
Birnbaum, S., Gobeske, K., Auerbach, J., Taylor, J., and Arnsten, A. F. (1999). A role for norepinephrine in stress-induced cognitive deficits: Alpha-1-adrenoceptor mediation in the prefrontal cortex. Biol. Psychiatry 46, 1266–1274. doi: 10.1016/s0006-3223(99)00138-9
Blier, P., and Briley, M. (2011). The noradrenergic symptom cluster: Clinical expression and neuropharmacology. Neuropsychiatr. Dis. Treat. 7(Suppl. 1), 15–20. doi: 10.2147/NDT.S19613
Boehme, S., Ritter, V., Tefikow, S., Stangier, U., Strauss, B., Miltner, W., et al. (2014). Brain activation during anticipatory anxiety in social anxiety disorder. Soc. Cogn. Affect. Neurosci. 9, 1413–1418. doi: 10.1093/scan/nst129
Bolstad, I., Andreassen, O., Reckless, G., Sigvartsen, N., Server, A., and Jensen, J. (2013). Aversive event anticipation affects connectivity between the ventral striatum and the orbitofrontal cortex in an fMRI avoidance task. PLoS One 8:e68494. doi: 10.1371/journal.pone.0068494
Bondi, C., Barrera, G., Lapiz, M., Bedard, T., Mahan, A., and Morilak, D. (2007). Noradrenergic facilitation of shock-probe defensive burying in lateral septum of rats, and modulation by chronic treatment with desipramine. Prog. Neuropsychopharmacol. Biol. Psychiatry 31, 482–495. doi: 10.1016/j.pnpbp.2006.11.015
Bondi, C., Jett, J., and Morilak, D. (2010). Beneficial effects of desipramine on cognitive function of chronically stressed rats are mediated by alpha1-adrenergic receptors in medial prefrontal cortex. Prog. Neuropsychopharmacol. Biol. Psychiatry 34, 913–923. doi: 10.1016/j.pnpbp.2010.04.016
Borg, C., Bedoin, N., Peyron, R., Bogey, S., Laurent, B., and Thomas-Antérion, C. (2013). Impaired emotional processing in a patient with a left posterior insula-SII lesion. Neurocase 19, 592–603. doi: 10.1080/13554794.2012.713491
Bouret, S., and Sara, S. (2005). Network reset: A simplified overarching theory of locus coeruleus noradrenaline function. Trends Neurosci. 28, 574–582. doi: 10.1016/j.tins.2005.09.002
Bradshaw, S., Agster, K., Waterhouse, B., and McGaughy, J. (2016). Age-related changes in prefrontal norepinephrine transporter density: The basis for improved cognitive flexibility after low doses of atomoxetine in adolescent rats. Brain Res. 1641(Pt B), 245–257. doi: 10.1016/j.brainres.2016.01.001
Breton-Provencher, V., Drummond, G., Feng, J., Li, Y., and Sur, M. (2022). Spatiotemporal dynamics of noradrenaline during learned behaviour. Nature 606, 732–738. doi: 10.1038/s41586-022-04782-2
Breton-Provencher, V., Drummond, G., and Sur, M. (2021). Locus coeruleus norepinephrine in learned behavior: Anatomical modularity and spatiotemporal integration in targets. Front. Neural Circuits 15:638007. doi: 10.3389/fncir.2021.638007
Breton-Provencher, V., and Sur, M. (2019). Active control of arousal by a locus coeruleus GABAergic circuit. Nat. Neurosci. 22, 218–228. doi: 10.1038/s41593-018-0305-z
Brocos-Mosquera, I., Gabilondo, A., Diez-Alarcia, R., Muguruza, C., Erdozain, A., Meana, J., et al. (2021). α2A- and α2C-adrenoceptor expression and functionality in postmortem prefrontal cortex of schizophrenia subjects. Eur. Neuropsychopharmacol. 52, 3–11. doi: 10.1016/j.euroneuro.2021.05.012
Bruce, S., Buchholz, K., Brown, W., Yan, L., Durbin, A., and Sheline, Y. (2012). Altered emotional interference processing in the amygdala and insula in women with post-traumatic stress disorder. Neuroimage Clin. 2, 43–49. doi: 10.1016/j.nicl.2012.11.003
Buckner, R. (2013). The brain’s default network: Origins and implications for the study of psychosis. Dialogues Clin. Neurosci. 15, 351–358. doi: 10.31887/DCNS.2013.15.3/rbuckner
Burkhouse, K., Jagan Jimmy, Defelice, N., Klumpp, H., Ajilore, O., Hosseini, B., et al. (2020). Nucleus accumbens volume as a predictor of anxiety symptom improvement following CBT and SSRI treatment in two independent samples. Neuropsychopharmacology 45, 561–569. doi: 10.1038/s41386-019-0575-5
Calder, A., Keane, J., Manes, F., Antoun, N., and Young, A. (2000). Impaired recognition and experience of disgust following brain injury. Nat. Neurosci. 3, 1077–1078. doi: 10.1038/80586
Calhoon, G., and Tye, K. (2015). Resolving the neural circuits of anxiety. Nat. Neurosci. 18, 1394–1404. doi: 10.1038/nn.4101
Canteras, N., and Swanson, L. (1992). Projections of the ventral subiculum to the amygdala, septum, and hypothalamus: A PHAL anterograde tract-tracing study in the rat. J. Comp. Neurol. 324, 180–194. doi: 10.1002/cne.903240204
Cardinal, R., Parkinson, J., Hall, J., and Everitt, B. (2002). Emotion and motivation: The role of the amygdala, ventral striatum, and prefrontal cortex. Neurosci. Biobehav. Rev. 26, 321–352. doi: 10.1016/s0149-7634(02)00007-6
Carr, D., Andrews, G., Glen, W., and Lavin, A. (2007). alpha2-Noradrenergic receptors activation enhances excitability and synaptic integration in rat prefrontal cortex pyramidal neurons via inhibition of HCN currents. J. Physiol. 584(Pt 2), 437–450. doi: 10.1113/jphysiol.2007.141671
Cauda, F., Cavanna, A., D’agata, F., Sacco, K., Duca, S., and Geminiani, G. (2011). Functional connectivity and coactivation of the nucleus accumbens: A combined functional connectivity and structure-based meta-analysis. J. Cogn. Neurosci. 23, 2864–2877. doi: 10.1162/jocn.2011.21624
Cerpa, J., Marchand, A., and Coutureau, E. (2019). Distinct regional patterns in noradrenergic innervation of the rat prefrontal cortex. J. Chem. Neuroanat. 96, 102–109. doi: 10.1016/j.jchemneu.2019.01.002
Chambers, R., Taylor, J., and Potenza, M. (2003). Developmental neurocircuitry of motivation in adolescence: A critical period of addiction vulnerability. Am. J. Psychiatry 160, 1041–1052. doi: 10.1176/appi.ajp.160.6.1041
Chandler, D., Gao, W., and Waterhouse, B. (2014a). Heterogeneous organization of the locus coeruleus projections to prefrontal and motor cortices. Proc. Natl. Acad. Sci. U.S.A. 111, 6816–6821. doi: 10.1073/pnas.1320827111
Chandler, D., Lamperski, C., and Waterhouse, B. (2013). Identification and distribution of projections from monoaminergic and cholinergic nuclei to functionally differentiated subregions of prefrontal cortex. Brain Res. 1522, 38–58. doi: 10.1016/j.brainres.2013.04.057
Chandler, D., and Waterhouse, B. (2012). Evidence for broad versus segregated projections from cholinergic and noradrenergic nuclei to functionally and anatomically discrete subregions of prefrontal cortex. Front. Behav. Neurosci. 6:20. doi: 10.3389/fnbeh.2012.00020
Chandler, D., Waterhouse, B., and Gao, W. (2014b). New perspectives on catecholaminergic regulation of executive circuits: Evidence for independent modulation of prefrontal functions by midbrain dopaminergic and noradrenergic neurons. Front. Neural Circuits 8:53. doi: 10.3389/fncir.2014.00053
Chang, C., and Grace, A. (2013). Amygdala β-noradrenergic receptors modulate delayed downregulation of dopamine activity following restraint. J. Neurosci. 33, 1441–1450. doi: 10.1523/JNEUROSCI.2420-12.2013
Charney, D. S., Nestler, E. J., Sklar, P., and Buxbaum, J. D. (2017). Charney and Nestler’s neurobiology of mental illness, 5th Edn. Oxford: Oxford University Press.
Chen, Y., Wu, J., Hu, N., Zhuang, J., Li, W., Zhang, S., et al. (2021). Distinct projections from the infralimbic cortex exert opposing effects in modulating anxiety and fear. J. Clin. Invest. 131:e145692. doi: 10.1172/JCI145692
Christakou, A., Robbins, T., and Everitt, B. (2004). Prefrontal cortical-ventral striatal interactions involved in affective modulation of attentional performance: Implications for corticostriatal circuit function. J. Neurosci. 24, 773–780. doi: 10.1523/JNEUROSCI.0949-03.2004
Cisler, J., and Koster, E. (2010). Mechanisms of attentional biases towards threat in anxiety disorders: An integrative review. Clin. Psychol. Rev. 30, 203–216. doi: 10.1016/j.cpr.2009.11.003
Clauss, J., Avery, S., and Blackford, J. (2015). The nature of individual differences in inhibited temperament and risk for psychiatric disease: A review and meta-analysis. Prog. Neurobiol. 127–128, 23–45. doi: 10.1016/j.pneurobio.2015.03.001
Coley, A., Padilla-Coreano, N., Patel, R., and Tye, K. (2021). Valence processing in the PFC: Reconciling circuit-level and systems-level views. Int. Rev. Neurobiol. 158, 171–212. doi: 10.1016/bs.irn.2020.12.002
Cools, R., and Arnsten, A. (2022). Neuromodulation of prefrontal cortex cognitive function in primates: The powerful roles of monoamines and acetylcholine. Neuropsychopharmacology 47, 309–328. doi: 10.1038/s41386-021-01100-8
Corbetta, M., Patel, G., and Shulman, G. (2008). The reorienting system of the human brain: From environment to theory of mind. Neuron 58, 306–324. doi: 10.1016/j.neuron.2008.04.017
Damsa, C., Kosel, M., and Moussally, J. (2009). Current status of brain imaging in anxiety disorders. Curr. Opin. Psychiatry 22, 96–110. doi: 10.1097/YCO.0b013e328319bd10
Datta, D., and Arnsten, A. (2019). Loss of prefrontal cortical higher cognition with uncontrollable stress: Molecular mechanisms, changes with age, and relevance to treatment. Brain Sci. 9:113. doi: 10.3390/brainsci9050113
Datta, D., Yang, S., Galvin, V., Solder, J., Luo, F., Morozov, Y., et al. (2019). Noradrenergic α1-adrenoceptor actions in the primate dorsolateral prefrontal cortex. J. Neurosci. 39, 2722–2734. doi: 10.1523/JNEUROSCI.2472-18.2019
Dayan, P., and Balleine, B. (2002). Reward, motivation, and reinforcement learning. Neuron 36, 285–298. doi: 10.1016/s0896-6273(02)00963-7
Delfs, J., Zhu, Y., Druhan, J., and Aston-Jones, G. (1998). Origin of noradrenergic afferents to the shell subregion of the nucleus accumbens: Anterograde and retrograde tract-tracing studies in the rat. Brain Res. 806, 127–140. doi: 10.1016/s0006-8993(98)00672-6
Dembrow, N., Chitwood, R., and Johnston, D. (2010). Projection-specific neuromodulation of medial prefrontal cortex neurons. J. Neurosci. 30, 16922–16937. doi: 10.1523/JNEUROSCI.3644-10.2010
Dembrow, N., and Johnston, D. (2014). Subcircuit-specific neuromodulation in the prefrontal cortex. Front. Neural Circuits 8:54. doi: 10.3389/fncir.2014.00054
Denys, D., Mantione, M., Figee, M., van den Munckhof, P., Koerselman, F., Westenberg, H., et al. (2010). Deep brain stimulation of the nucleus accumbens for treatment-refractory obsessive-compulsive disorder. Arch. Gen. Psychiatry 67, 1061–1068. doi: 10.1001/archgenpsychiatry.2010.122
Dong, H., Petrovich, G., and Swanson, L. (2001). Topography of projections from amygdala to bed nuclei of the stria terminalis. Brain Res. Brain Res. Rev. 38, 192–246. doi: 10.1016/s0165-0173(01)00079-0
Dong, M., Xia, L., Lu, M., Li, C., Xu, K., and Zhang, L. (2019). A failed top-down control from the prefrontal cortex to the amygdala in generalized anxiety disorder: Evidence from resting-state fMRI with granger causality analysis. Neurosci. Lett. 707:134314. doi: 10.1016/j.neulet.2019.134314
Donner, N., and Lowry, C. (2013). Sex differences in anxiety and emotional behavior. Pflugers Arch. 465, 601–626. doi: 10.1007/s00424-013-1271-7
Engel, K., Bandelow, B., Gruber, O., and Wedekind, D. (2009). Neuroimaging in anxiety disorders. J. Neural Transm 116, 703–716. doi: 10.1007/s00702-008-0077-9
Ernst, M., and Fudge, J. (2009). A developmental neurobiological model of motivated behavior: Anatomy, connectivity and ontogeny of the triadic nodes. Neurosci. Biobehav. Rev. 33, 367–382. doi: 10.1016/j.neubiorev.2008.10.009
Escribá, P., Ozaita, A., and García-Sevilla, J. (2004). Increased mRNA expression of alpha2A-adrenoceptors, serotonin receptors and mu-opioid receptors in the brains of suicide victims. Neuropsychopharmacology 29, 1512–1521. doi: 10.1038/sj.npp.1300459
Etkin, A., Prater, K., Schatzberg, A., Menon, V., and Greicius, M. (2009). Disrupted amygdalar subregion functional connectivity and evidence of a compensatory network in generalized anxiety disorder. Arch. Gen. Psychiatry 66, 1361–1372. doi: 10.1001/archgenpsychiatry.2009.104
Etkin, A., and Wager, T. (2007). Functional neuroimaging of anxiety: A meta-analysis of emotional processing in PTSD, social anxiety disorder, and specific phobia. Am. J. Psychiatry 164, 1476–1488. doi: 10.1176/appi.ajp.2007.07030504
Fan, X., Song, J., Ma, C., Lv, Y., Wang, F., Ma, L., et al. (2022). Noradrenergic signaling mediates cortical early tagging and storage of remote memory. Nat. Commun. 13:7623. doi: 10.1038/s41467-022-35342-x
Felix-Ortiz, A., Beyeler, A., Seo, C., Leppla, C., Wildes, C., and Tye, K. (2013). BLA to vHPC inputs modulate anxiety-related behaviors. Neuron 79, 658–664. doi: 10.1016/j.neuron.2013.06.016
Felix-Ortiz, A., Burgos-Robles, A., Bhagat, N., Leppla, C., and Tye, K. (2016). Bidirectional modulation of anxiety-related and social behaviors by amygdala projections to the medial prefrontal cortex. Neuroscience 321, 197–209. doi: 10.1016/j.neuroscience.2015.07.041
Felix-Ortiz, A., and Tye, K. (2014). Amygdala inputs to the ventral hippocampus bidirectionally modulate social behavior. J. Neurosci. 34, 586–595. doi: 10.1523/JNEUROSCI.4257-13.2014
Feng, J., Zhang, C., Lischinsky, J., Jing, M., Zhou, J., Wang, H., et al. (2019). A genetically encoded fluorescent sensor for rapid and specific in vivo detection of norepinephrine. Neuron 102, 745–761.e8. doi: 10.1016/j.neuron.2019.02.037
Fonzo, G., Simmons, A., Thorp, S., Norman, S., Paulus, M., and Stein, M. (2010). Exaggerated and disconnected insular-amygdalar blood oxygenation level-dependent response to threat-related emotional faces in women with intimate-partner violence posttraumatic stress disorder. Biol. Psychiatry 68, 433–441. doi: 10.1016/j.biopsych.2010.04.028
Franowicz, J., Kessler, L., Borja, C., Kobilka, B., Limbird, L., and Arnsten, A. (2002). Mutation of the alpha2A-adrenoceptor impairs working memory performance and annuls cognitive enhancement by guanfacine. J. Neurosci. 22, 8771–8777. doi: 10.1523/JNEUROSCI.22-19-08771.2002
Freese, J. L., and Amaral, D. G. (2009). “Neuroanatomy of the primate amygdala,” in The human amygdala, eds P. J. Whalen and E. A. Phelps (New York, NY: The Guilford Press), 3–42.
Friedman, A., Homma, D., Gibb, L., Amemori, K., Rubin, S., Hood, A., et al. (2015). A corticostriatal path targeting striosomes controls decision-making under conflict. Cell 161, 1320–1333. doi: 10.1016/j.cell.2015.04.049
Gabbott, P., Warner, T., Jays, P., Salway, P., and Busby, S. (2005). Prefrontal cortex in the rat: Projections to subcortical autonomic, motor, and limbic centers. J. Comp. Neurol. 492, 145–177. doi: 10.1002/cne.20738
Gamo, N., Wang, M., and Arnsten, A. (2010). Methylphenidate and atomoxetine enhance prefrontal function through α2-adrenergic and dopamine D1 receptors. J. Am. Acad. Child Adolesc. Psychiatry 49, 1011–1023. doi: 10.1016/j.jaac.2010.06.015
Gao, C., Gohel, C., Leng, Y., Ma, J., Goldman, D., Levine, A., et al. (2023). Molecular and spatial profiling of the paraventricular nucleus of the thalamus. eLife 12:e81818. doi: 10.7554/eLife.81818
Gao, C., Leng, Y., Ma, J., Rooke, V., Rodriguez-Gonzalez, S., Ramakrishnan, C., et al. (2020). Two genetically, anatomically and functionally distinct cell types segregate across anteroposterior axis of paraventricular thalamus. Nat. Neurosci. 23, 217–228. doi: 10.1038/s41593-019-0572-3
García-Sevilla, J., Escribá, P., Ozaita, A., La Harpe, R., Walzer, C., Eytan, A., et al. (1999). Up-regulation of immunolabeled alpha2A-adrenoceptors, Gi coupling proteins, and regulatory receptor kinases in the prefrontal cortex of depressed suicides. J. Neurochem. 72, 282–291. doi: 10.1046/j.1471-4159.1999.0720282.x
Giovanniello, J., Yu, K., Furlan, A., Nachtrab, G., Sharma, R., Chen, X., et al. (2020). A central amygdala-globus pallidus circuit conveys unconditioned stimulus-related information and controls fear learning. J. Neurosci. 40, 9043–9054. doi: 10.1523/JNEUROSCI.2090-20.2020
Glangetas, C., Massi, L., Fois, G., Jalabert, M., Girard, D., Diana, M., et al. (2017). NMDA-receptor-dependent plasticity in the bed nucleus of the stria terminalis triggers long-term anxiolysis. Nat. Commun. 8:14456. doi: 10.1038/ncomms14456
Goddard, A., Ball, S., Martinez, J., Robinson, M., Yang, C., Russell, J., et al. (2010). Current perspectives of the roles of the central norepinephrine system in anxiety and depression. Depress Anxiety 27, 339–350. doi: 10.1002/da.20642
Goldman-Rakic, P., Lidow, M., and Gallager, D. (1990). Overlap of dopaminergic, adrenergic, and serotoninergic receptors and complementarity of their subtypes in primate prefrontal cortex. J. Neurosci. 10, 2125–2138. doi: 10.1523/JNEUROSCI.10-07-02125.1990
Goode, T., Ressler, R., Acca, G., Miles, O., and Maren, S. (2019). Bed nucleus of the stria terminalis regulates fear to unpredictable threat signals. eLife 8:e46525. doi: 10.7554/eLife.46525
Gorman, A., and Dunn, A. (1993). Beta-adrenergic receptors are involved in stress-related behavioral changes. Pharmacol. Biochem. Behav. 45, 1–7. doi: 10.1016/0091-3057(93)90078-8
Grant, B., Hasin, D., Stinson, F., Dawson, D., June Ruan, W., Goldstein, R., et al. (2005). Prevalence, correlates, co-morbidity, and comparative disability of DSM-IV generalized anxiety disorder in the USA: Results from the national epidemiologic survey on alcohol and related conditions. Psychol. Med. 35, 1747–1759. doi: 10.1017/S0033291705006069
Griebel, G., and Holmes, A. (2013). 50 years of hurdles and hope in anxiolytic drug discovery. Nat. Rev. Drug. Discov. 12, 667–687. doi: 10.1038/nrd4075
Haikonen, J., Englund, J., Amarilla, S., Kharybina, Z., Shintyapina, A., Kegler, K., et al. (2022). Aberrant cortical projections to amygdala GABAergic neurons contribute to developmental circuit dysfunction following early life stress. iScience 26:105724. doi: 10.1016/j.isci.2022.105724
Hains, A., and Arnsten, A. (2008). Molecular mechanisms of stress-induced prefrontal cortical impairment: Implications for mental illness. Learn. Mem. 15, 551–564. doi: 10.1101/lm.921708
Hamon, M., and Blier, P. (2013). Monoamine neurocircuitry in depression and strategies for new treatments. Prog. Neuropsychopharmacol. Biol. Psychiatry 45, 54–63. doi: 10.1016/j.pnpbp.2013.04.009
Hayes, P., and Schulz, S. (1987). Beta-blockers in anxiety disorders. J. Affect. Disord. 13, 119–130. doi: 10.1016/0165-0327(87)90017-6
Hecht, P., Will, M., Schachtman, T., Welby, L., and Beversdorf, D. (2014). Beta-adrenergic antagonist effects on a novel cognitive flexibility task in rodents. Behav. Brain Res. 260, 148–154. doi: 10.1016/j.bbr.2013.11.041
Heimer, L., Van Hoesen, G., Trimble, M., and Zahm, D. (2007). Anatomy of neuropsychiatry, 1st Edn. Cambridge, MA: Academic Press.
Howland, J., Ito, R., Lapish, C., and Villaruel, F. (2022). The rodent medial prefrontal cortex and associated circuits in orchestrating adaptive behavior under variable demands. Neurosci. Biobehav. Rev. 135:104569. doi: 10.1016/j.neubiorev.2022.104569
Hurley, K., Herbert, H., Moga, M., and Saper, C. (1991). Efferent projections of the infralimbic cortex of the rat. J. Comp. Neurol. 308, 249–276. doi: 10.1002/cne.903080210
Iglesias, A., and Flagel, S. (2021). The paraventricular thalamus as a critical node of motivated behavior via the hypothalamic-thalamic-striatal circuit. Front. Integr. Neurosci. 15:706713. doi: 10.3389/fnint.2021.706713
Janak, P., and Tye, K. (2015). From circuits to behaviour in the amygdala. Nature 517, 284–292. doi: 10.1038/nature14188
Jefferson, J. (1974). Beta-adrenergic receptor blocking drugs in psychiatry. Arch. Gen. Psychiatry 31, 681–691. doi: 10.1001/archpsyc.1974.01760170071012
Jennings, J., Sparta, D., Stamatakis, A., Ung, R., Pleil, K., Kash, T., et al. (2013). Distinct extended amygdala circuits for divergent motivational states. Nature 496, 224–228. doi: 10.1038/nature12041
Ji, X., Cao, X., Zhang, C., Feng, Z., Zhang, X., Ma, L., et al. (2008). Pre- and postsynaptic beta-adrenergic activation enhances excitatory synaptic transmission in layer V/VI pyramidal neurons of the medial prefrontal cortex of rats. Cereb. Cortex 18, 1506–1520. doi: 10.1093/cercor/bhm177
Jodo, E., and Aston-Jones, G. (1997). Activation of locus coeruleus by prefrontal cortex is mediated by excitatory amino acid inputs. Brain Res. 768, 327–332. doi: 10.1016/s0006-8993(97)00703-8
Johnson, S., Emmons, E., Lingg, R., Anderson, R., Romig-Martin, S., LaLumiere, R., et al. (2019). Prefrontal-bed nucleus circuit modulation of a passive coping response set. J. Neurosci. 39, 1405–1419. doi: 10.1523/JNEUROSCI.1421-18.2018
Kasai, K., Yamasue, H., Gilbertson, M., Shenton, M., Rauch, S., and Pitman, R. (2008). Evidence for acquired pregenual anterior cingulate gray matter loss from a twin study of combat-related posttraumatic stress disorder. Biol. Psychiatry 63, 550–556. doi: 10.1016/j.biopsych.2007.06.022
Kent, J., and Rauch, S. (2003). Neurocircuitry of anxiety disorders. Curr Psychiatry Rep. 5, 266–273. doi: 10.1007/s11920-003-0055-8
Kenwood, M., Kalin, N., and Barbas, H. (2022). The prefrontal cortex, pathological anxiety, and anxiety disorders. Neuropsychopharmacology 47, 260–275. doi: 10.1038/s41386-021-01109-z
Kessler, R., Aguilar-Gaxiola, S., Alonso, J., Chatterji, S., Lee, S., Ormel, J., et al. (2009). The global burden of mental disorders: An update from the WHO world mental health (WMH) surveys. Epidemiol. Psichiatr. Soc. 18, 23–33. doi: 10.1017/s1121189x00001421
Kessler, R., Berglund, P., Demler, O., Jin, R., Merikangas, K., and Walters, E. (2005). Lifetime prevalence and age-of-onset distributions of DSM-IV disorders in the national comorbidity survey replication. Arch. Gen. Psychiatry 62, 593–602. doi: 10.1001/archpsyc.62.6.593
Kessler, R., Chiu, W., Jin, R., Ruscio, A., Shear, K., and Walters, E. (2006). The epidemiology of panic attacks, panic disorder, and agoraphobia in the national comorbidity survey replication. Arch. Gen. Psychiatry 63, 415–424. doi: 10.1001/archpsyc.63.4.415
Kessler, R., Petukhova, M., Sampson, N., Zaslavsky, A., and Wittchen, H. - (2012). Twelve-month and lifetime prevalence and lifetime morbid risk of anxiety and mood disorders in the United States. Int. J. Methods Psychiatr. Res. 21, 169–184. doi: 10.1002/mpr.1359
Kestering-Ferreira, E., Tractenberg, S., Lumertz, F., Orso, R., Creutzberg, K., Wearick-Silva, L., et al. (2021). Long-term effects of maternal separation on anxiety-like behavior and neuroendocrine parameters in adult Balb/c mice. Chronic Stress 5:24705470211067181. doi: 10.1177/24705470211067181
Kim, M., Loucks, R., Palmer, A., Brown, A., Solomon, K., Marchante, A., et al. (2011). The structural and functional connectivity of the amygdala: From normal emotion to pathological anxiety. Behav. Brain Res. 223, 403–410. doi: 10.1016/j.bbr.2011.04.025
Kim, M., and Whalen, P. (2009). The structural integrity of an amygdala-prefrontal pathway predicts trait anxiety. J. Neurosci. 29, 11614–11618. doi: 10.1523/JNEUROSCI.2335-09.2009
Kim, S., Adhikari, A., Lee, S., Marshel, J., Kim, C., Mallory, C., et al. (2013). Diverging neural pathways assemble a behavioural state from separable features in anxiety. Nature 496, 219–223. doi: 10.1038/nature12018
Kirouac, G. (2015). Placing the paraventricular nucleus of the thalamus within the brain circuits that control behavior. Neurosci. Biobehav. Rev. 56, 315–329. doi: 10.1016/j.neubiorev.2015.08.005
Kirouac, G. (2021). The paraventricular nucleus of the thalamus as an integrating and relay node in the brain anxiety network. Front. Behav. Neurosci. 15:627633. doi: 10.3389/fnbeh.2021.627633
Krout, K., Belzer, R., and Loewy, A. (2002). Brainstem projections to midline and intralaminar thalamic nuclei of the rat. J. Comp. Neurol. 448, 53–101. doi: 10.1002/cne.10236
Lago, T., Davis, A., Grillon, C., and Ernst, M. (2017). Striatum on the anxiety map: Small detours into adolescence. Brain Res. 1654(Pt B), 177–184. doi: 10.1016/j.brainres.2016.06.006
Lanius, R., Frewen, P., Girotti, M., Neufeld, R., Stevens, T., and Densmore, M. (2007). Neural correlates of trauma script-imagery in posttraumatic stress disorder with and without comorbid major depression: A functional MRI investigation. Psychiatry Res. 155, 45–56. doi: 10.1016/j.pscychresns.2006.11.006
Lapiz, M., and Morilak, D. (2006). Noradrenergic modulation of cognitive function in rat medial prefrontal cortex as measured by attentional set shifting capability. Neuroscience 137, 1039–1049. doi: 10.1016/j.neuroscience.2005.09.031
Leach, L., Christensen, H., Mackinnon, A., Windsor, T., and Butterworth, P. (2008). Gender differences in depression and anxiety across the adult lifespan: The role of psychosocial mediators. Soc. Psychiatry Psychiatr. Epidemiol. 43, 983–998. doi: 10.1007/s00127-008-0388-z
LeBlanc, K., London, T., Szczot, I., Bocarsly, M., Friend, D., Nguyen, K., et al. (2020). Striatopallidal neurons control avoidance behavior in exploratory tasks. Mol. Psychiatry 25, 491–505. doi: 10.1038/s41380-018-0051-3
Lei, Z., Lam, Y., Li, C., Fu, Z., Ramkrishnan, A., Liu, S., et al. (2022). β2-adrenoceptors in the medial prefrontal cortex excitatory neurons regulate anxiety-like behavior in mice. Int. J. Mol. Sci. 23:5578. doi: 10.3390/ijms23105578
Li, J., Zhong, Y., Ma, Z., Wu, Y., Pang, M., Wang, C., et al. (2020). Emotion reactivity-related brain network analysis in generalized anxiety disorder: A task fMRI study. BMC Psychiatry 20:429. doi: 10.1186/s12888-020-02831-6
Li, S., and Kirouac, G. (2012). Sources of inputs to the anterior and posterior aspects of the paraventricular nucleus of the thalamus. Brain Struct. Funct. 217, 257–273. doi: 10.1007/s00429-011-0360-7
Liljeholm, M., and O’Doherty, J. (2012). Contributions of the striatum to learning, motivation, and performance: An associative account. Trends Cogn. Sci. 16, 467–475. doi: 10.1016/j.tics.2012.07.007
Lindauer, R., Booij, J., Habraken, J., van Meijel, E., Uylings, H., Olff, M., et al. (2008). Effects of psychotherapy on regional cerebral blood flow during trauma imagery in patients with post-traumatic stress disorder: A randomized clinical trial. Psychol. Med. 38, 543–554. doi: 10.1017/S0033291707001432
Liu, Y., Liang, X., Ren, W., and Li, B. (2014). Expression of β1- and β2-adrenoceptors in different subtypes of interneurons in the medial prefrontal cortex of mice. Neuroscience 257, 149–157. doi: 10.1016/j.neuroscience.2013.10.078
Loewke, A. C., Minerva, A. R., Nelson, A. B., Kreitzer, A. C., and Gunaydin, L. A. (2020). Fronto-striatal projections regulate approach-avoidance conflict. bioRxiv [Preprint] doi: 10.1101/2020.03.05.979708
Mack, N., Deng, S., Yang, S., Shu, Y., and Gao, W. (2022). Prefrontal cortical control of anxiety: Recent advances. Neuroscientist doi: 10.1177/10738584211069071 [Epub ahead of print].
Marek, G., and Aghajanian, G. (1999). 5-HT2A receptor or alpha1-adrenoceptor activation induces excitatory postsynaptic currents in layer V pyramidal cells of the medial prefrontal cortex. Eur. J. Pharmacol. 367, 197–206. doi: 10.1016/s0014-2999(98)00945-5
Marek, R., Sun, Y., and Sah, P. (2019). Neural circuits for a top-down control of fear and extinction. Psychopharmacology 236, 313–320. doi: 10.1007/s00213-018-5033-2
Martin, C., Kelly, T., Rayens, M., Brogli, B., Brenzel, A., Smith, W., et al. (2002). Sensation seeking, puberty, and nicotine, alcohol, and marijuana use in adolescence. J. Am. Acad. Child Adolesc. Psychiatry 41, 1495–1502. doi: 10.1097/00004583-200212000-00022
Martin, E., Ressler, K., Binder, E., and Nemeroff, C. (2010). The neurobiology of anxiety disorders: Brain imaging, genetics, and psychoneuroendocrinology. Clin. Lab. Med. 30, 865–891. doi: 10.1016/j.cll.2010.07.006
McCall, J., Siuda, E., Bhatti, D., Lawson, L., McElligott, Z., Stuber, G., et al. (2017). Locus coeruleus to basolateral amygdala noradrenergic projections promote anxiety-like behavior. eLife 6:e18247. doi: 10.7554/eLife.18247
Mitrano, D., Schroeder, J., Smith, Y., Cortright, J., Bubula, N., Vezina, P., et al. (2012). α-1 Adrenergic receptors are localized on presynaptic elements in the nucleus accumbens and regulate mesolimbic dopamine transmission. Neuropsychopharmacology 37, 2161–2172. doi: 10.1038/npp.2012.68
Möller, C., Wiklund, L., Sommer, W., Thorsell, A., and Heilig, M. (1997). Decreased experimental anxiety and voluntary ethanol consumption in rats following central but not basolateral amygdala lesions. Brain Res. 760, 94–101. doi: 10.1016/s0006-8993(97)00308-9
Mondin, T., Konradt, C., Cardoso Tde, A., Quevedo Lde, A., Jansen, K., Mattos, L., et al. (2013). Anxiety disorders in young people: A population-based study. Braz. J. Psychiatry 35, 347–352. doi: 10.1590/1516-4446-2013-1155
Moran, T. (2016). Anxiety and working memory capacity: A meta-analysis and narrative review. Psychol. Bull. 142, 831–864. doi: 10.1037/bul0000051
Moreira, C., Masson, S., Carvalho, M., and Brandão, M. (2007). Exploratory behaviour of rats in the elevated plus-maze is differentially sensitive to inactivation of the basolateral and central amygdaloid nuclei. Brain Res. Bull. 71, 466–474. doi: 10.1016/j.brainresbull.2006.10.004
Morilak, D., Barrera, G., Echevarria, D., Garcia, A., Hernandez, A., Ma, S., et al. (2005). Role of brain norepinephrine in the behavioral response to stress. Prog. Neuropsychopharmacol. Biol. Psychiatry 29, 1214–1224. doi: 10.1016/j.pnpbp.2005.08.007
Morris, L., McCall, J., Charney, D., and Murrough, J. (2020). The role of the locus coeruleus in the generation of pathological anxiety. Brain Neurosci. Adv. 4:2398212820930321. doi: 10.1177/2398212820930321
Motzkin, J., Philippi, C., Oler, J., Kalin, N., Baskaya, M., and Koenigs, M. (2015). Ventromedial prefrontal cortex damage alters resting blood flow to the bed nucleus of stria terminalis. Cortex 64, 281–288. doi: 10.1016/j.cortex.2014.11.013
Northoff, G., and Tumati, S. (2019). “Average is good, extremes are bad” - Non-linear inverted U-shaped relationship between neural mechanisms and functionality of mental features. Neurosci. Biobehav. Rev. 104, 11–25. doi: 10.1016/j.neubiorev.2019.06.030
O’Doherty, J., Dayan, P., Schultz, J., Deichmann, R., Friston, K., and Dolan, R. (2004). Dissociable roles of ventral and dorsal striatum in instrumental conditioning. Science 304, 452–454. doi: 10.1126/science.1094285
Otake, K., Kin, K., and Nakamura, Y. (2002). Fos expression in afferents to the rat midline thalamus following immobilization stress. Neurosci. Res. 43, 269–282. doi: 10.1016/s0168-0102(02)00042-1
Otis, J., Namboodiri, V., Matan, A., Voets, E., Mohorn, E., Kosyk, O., et al. (2017). Prefrontal cortex output circuits guide reward seeking through divergent cue encoding. Nature 543, 103–107. doi: 10.1038/nature21376
Otis, J., Zhu, M., Namboodiri, V., Cook, C., Kosyk, O., Matan, A., et al. (2019). Paraventricular thalamus projection neurons integrate cortical and hypothalamic signals for cue-reward processing. Neuron 103, 423–431.e4.
Pagotto, L., Mendlowicz, M., Coutinho, E., Figueira, I., Luz, M., Araujo, A., et al. (2015). The impact of posttraumatic symptoms and comorbid mental disorders on the health-related quality of life in treatment-seeking PTSD patients. Compr. Psychiatry 58, 68–73. doi: 10.1016/j.comppsych.2015.01.002
Parfitt, G., Nguyen, R., Bang, J., Aqrabawi, A., Tran, M., Seo, D., et al. (2017). Bidirectional control of anxiety-related behaviors in mice: Role of inputs arising from the ventral hippocampus to the lateral septum and medial prefrontal cortex. Neuropsychopharmacology 42, 1715–1728. doi: 10.1038/npp.2017.56
Paulus, M. (2008). The role of neuroimaging for the diagnosis and treatment of anxiety disorders. Depress Anxiety 25, 348–356. doi: 10.1002/da.20499
Pendyam, S., Bravo-Rivera, C., Burgos-Robles, A., Sotres-Bayon, F., Quirk, G., and Nair, S. (2013). Fear signaling in the prelimbic-amygdala circuit: A computational modeling and recording study. J. Neurophysiol. 110, 844–861. doi: 10.1152/jn.00961.2012
Penzo, M., and Gao, C. (2021). The paraventricular nucleus of the thalamus: An integrative node underlying homeostatic behavior. Trends Neurosci. 44, 538–549. doi: 10.1016/j.tins.2021.03.001
Pezawas, L., Meyer-Lindenberg, A., Drabant, E., Verchinski, B., Munoz, K., Kolachana, B., et al. (2005). 5-HTTLPR polymorphism impacts human cingulate-amygdala interactions: A genetic susceptibility mechanism for depression. Nat. Neurosci. 8, 828–834. doi: 10.1038/nn1463
Poe, G., Foote, S., Eschenko, O., Johansen, J., Bouret, S., Aston-Jones, G., et al. (2020). Locus coeruleus: A new look at the blue spot. Nat. Rev. Neurosci. 21, 644–659. doi: 10.1038/s41583-020-0360-9
Porter, J., Roy, A., Benson, B., Carlisi, C., Collins, P., Leibenluft, E., et al. (2015). Age-related changes in the intrinsic functional connectivity of the human ventral vs. dorsal striatum from childhood to middle age. Dev. Cogn. Neurosci. 11, 83–95. doi: 10.1016/j.dcn.2014.08.011
Quirk, G., Likhtik, E., Pelletier, J., and Paré, D. (2003). Stimulation of medial prefrontal cortex decreases the responsiveness of central amygdala output neurons. J. Neurosci. 23, 8800–8807. doi: 10.1523/JNEUROSCI.23-25-08800.2003
Radley, J., Anderson, R., Hamilton, B., Alcock, J., and Romig-Martin, S. (2013). Chronic stress-induced alterations of dendritic spine subtypes predict functional decrements in an hypothalamo-pituitary-adrenal-inhibitory prefrontal circuit. J. Neurosci. 33, 14379–14391. doi: 10.1523/JNEUROSCI.0287-13.2013
Radley, J., Gosselink, K., and Sawchenko, P. (2009). A discrete GABAergic relay mediates medial prefrontal cortical inhibition of the neuroendocrine stress response. J. Neurosci. 29, 7330–7340. doi: 10.1523/JNEUROSCI.5924-08.2009
Radley, J., and Sawchenko, P. E. (2011). A common substrate for prefrontal and hippocampal inhibition of the neuroendocrine stress response. J. Neurosci. 31, 9683–9695. doi: 10.1523/JNEUROSCI.6040-10.2011
Ramos, B., and Arnsten, A. (2007). Adrenergic pharmacology and cognition: Focus on the prefrontal cortex. Pharmacol. Ther. 113, 523–536. doi: 10.1016/j.pharmthera.2006.11.006
Ramos, B., Colgan, L., Nou, E., Ovadia, S., Wilson, S., and Arnsten, A. (2005). The beta-1 adrenergic antagonist, betaxolol, improves working memory performance in rats and monkeys. Biol. Psychiatry 58, 894–900. doi: 10.1016/j.biopsych.2005.05.022
Rauch, S., Dougherty, D., Malone, D., Rezai, A., Friehs, G., Fischman, A., et al. (2006). A functional neuroimaging investigation of deep brain stimulation in patients with obsessive-compulsive disorder. J. Neurosurg. 104, 558–565. doi: 10.3171/jns.2006.104.4.558
Risold, P., and Swanson, L. (1997). Chemoarchitecture of the rat lateral septal nucleus. Brain Res. Brain Res. Rev. 24, 91–113. doi: 10.1016/s0165-0173(97)00008-8
Rizzi-Wise, C., and Wang, D. (2021). Putting together pieces of the lateral septum: Multifaceted functions and its neural pathways. eNeuro 8:ENEURO.0315-21.2021. doi: 10.1523/ENEURO.0315-21.2021
Robinson, O., Pike, A., Cornwell, B., and Grillon, C. (2019). The translational neural circuitry of anxiety. J. Neurol. Neurosurg. Psychiatry 90, 1353–1360. doi: 10.1136/jnnp-2019-321400
Rodriguez-Romaguera, J., Do Monte, F., and Quirk, G. (2012). Deep brain stimulation of the ventral striatum enhances extinction of conditioned fear. Proc. Natl. Acad. Sci. U.S.A. 109, 8764–8769. doi: 10.1073/pnas.1200782109
Ross, J., and Van Bockstaele, E. (2021). The locus coeruleus- norepinephrine system in stress and arousal: Unraveling historical, current, and future perspectives. Front. Psychiatry 11:601519. doi: 10.3389/fpsyt.2020.601519
Santana, N., and Artigas, F. (2017). Laminar and cellular distribution of monoamine receptors in rat medial prefrontal cortex. Front. Neuroanat. 11:87. doi: 10.3389/fnana.2017.00087
Santana, N., Mengod, G., and Artigas, F. (2013). Expression of α(1)-adrenergic receptors in rat prefrontal cortex: Cellular co-localization with 5-HT(2A) receptors. Int. J. Neuropsychopharmacol. 16, 1139–1151. doi: 10.1017/S1461145712001083
Schott, B., Minuzzi, L., Krebs, R., Elmenhorst, D., Lang, M., Winz, O., et al. (2008). Mesolimbic functional magnetic resonance imaging activations during reward anticipation correlate with reward-related ventral striatal dopamine release. J. Neurosci. 28, 14311–14319. doi: 10.1523/JNEUROSCI.2058-08.2008
Sesack, S., Deutch, A., Roth, R., and Bunney, B. (1989). Topographical organization of the efferent projections of the medial prefrontal cortex in the rat: An anterograde tract-tracing study with Phaseolus vulgaris leucoagglutinin. J. Comp. Neurol. 290, 213–242. doi: 10.1002/cne.902900205
Sestieri, C., Corbetta, M., Romani, G., and Shulman, G. (2011). Episodic memory retrieval, parietal cortex, and the default mode network: Functional and topographic analyses. J. Neurosci. 31, 4407–4420. doi: 10.1523/JNEUROSCI.3335-10.2011
Sheehan, T., Chambers, R., and Russell, D. (2004). Regulation of affect by the lateral septum: Implications for neuropsychiatry. Brain Res. Brain Res. Rev. 46, 71–117. doi: 10.1016/j.brainresrev.2004.04.009
Simmons, A., Paulus, M., Thorp, S., Matthews, S., Norman, S., and Stein, M. (2008). Functional activation and neural networks in women with posttraumatic stress disorder related to intimate partner violence. Biol. Psychiatry 64, 681–690. doi: 10.1016/j.biopsych.2008.05.027
Stahl, S. (1992). Neuroendocrine markers of serotonin responsivity in depression. Prog. Neuropsychopharmacol. Biol. Psychiatry 16, 655–659. doi: 10.1016/0278-5846(92)90022-7
Stein, M., Simmons, A., Feinstein, J., and Paulus, M. (2007). Increased amygdala and insula activation during emotion processing in anxiety-prone subjects. Am. J. Psychiatry 164, 318–327. doi: 10.1176/ajp.2007.164.2.318
Strigo, I., Matthews, S., and Simmons, A. (2010). Right anterior insula hypoactivity during anticipation of homeostatic shifts in major depressive disorder. Psychosom. Med. 72, 316–323. doi: 10.1097/PSY.0b013e3181d07873
Tamburella, A., Micale, V., Leggio, G., and Drago, F. (2010). The beta3 adrenoceptor agonist, amibegron (SR58611A) counteracts stress-induced behavioral and neurochemical changes. Eur. Neuropsychopharmacol. 20, 704–713. doi: 10.1016/j.euroneuro.2010.04.006
Tang, Y., Rothbart, M., and Posner, M. (2012). Neural correlates of establishing, maintaining, and switching brain states. Trends Cogn. Sci. 16, 330–337. doi: 10.1016/j.tics.2012.05.001
Thomas, E., Burock, D., Knudsen, K., Deterding, E., and Yadin, E. (2013). Single unit activity in the lateral septum and central nucleus of the amygdala in the elevated plus-maze: A model of exposure therapy? Neurosci. Lett. 548, 269–274. doi: 10.1016/j.neulet.2013.05.078
Tolin, D., and Foa, E. (2006). Sex differences in trauma and posttraumatic stress disorder: A quantitative review of 25 years of research. Psychol. Bull. 132, 959–992. doi: 10.1037/0033-2909.132.6.959
Torkaman-Boutorabi, A., Hashemi-Hezaveh, S., Sheidadoust, H., and Zarrindast, M. (2014). The possible role of medial prefrontal cortex beta-1-adrenoceptors in morphine-induced amnesia. Pharmacology 93, 272–277. doi: 10.1159/000362282
Treit, D., Pesold, C., and Rotzinger, S. (1993). Dissociating the anti-fear effects of septal and amygdaloid lesions using two pharmacologically validated models of rat anxiety. Behav. Neurosci. 107, 770–785. doi: 10.1037//0735-7044.107.5.770
Tye, K., Prakash, R., Kim, S., Fenno, L., Grosenick, L., Zarabi, H., et al. (2011). Amygdala circuitry mediating reversible and bidirectional control of anxiety. Nature 471, 358–362. doi: 10.1038/nature09820
van Tol, M., Demenescu, L., van der Wee, N., Kortekaas, R., Marjan, M. A., Boer, J., et al. (2012). Functional magnetic resonance imaging correlates of emotional word encoding and recognition in depression and anxiety disorders. Biol. Psychiatry 71, 593–602. doi: 10.1016/j.biopsych.2011.11.016
Vertes, R. (2004). Differential projections of the infralimbic and prelimbic cortex in the rat. Synapse 51, 32–58. doi: 10.1002/syn.10279
Wang, M., Ramos, B., Paspalas, C., Shu, Y., Simen, A., Duque, A., et al. (2007). Alpha2A-adrenoceptors strengthen working memory networks by inhibiting cAMP-HCN channel signaling in prefrontal cortex. Cell 129, 397–410. doi: 10.1016/j.cell.2007.03.015
Whalen, P., Bush, G., McNally, R., Wilhelm, S., McInerney, S., Jenike, M., et al. (1998). The emotional counting Stroop paradigm: A functional magnetic resonance imaging probe of the anterior cingulate affective division. Biol. Psychiatry 44, 1219–1228. doi: 10.1016/s0006-3223(98)00251-0
Wohleb, E., Hanke, M., Corona, A., Powell, N., Stiner, L., Bailey, M., et al. (2011). β-Adrenergic receptor antagonism prevents anxiety-like behavior and microglial reactivity induced by repeated social defeat. J. Neurosci. 31, 6277–6288. doi: 10.1523/JNEUROSCI.0450-11.2011
Xing, B., Li, Y., and Gao, W. (2016). Norepinephrine versus dopamine and their interaction in modulating synaptic function in the prefrontal cortex. Brain Res. 1641(Pt B), 217–233. doi: 10.1016/j.brainres.2016.01.005
Yassa, M., Hazlett, R., Stark, C., and Hoehn-Saric, R. (2012). Functional MRI of the amygdala and bed nucleus of the stria terminalis during conditions of uncertainty in generalized anxiety disorder. J. Psychiatr. Res. 46, 1045–1052. doi: 10.1016/j.jpsychires.2012.04.013
Yonkers, K., Bruce, S., Dyck, I., and Keller, M. (2003). Chronicity, relapse, and illness–course of panic disorder, social phobia, and generalized anxiety disorder: Findings in men and women from 8 years of follow-up. Depress Anxiety 17, 173–179. doi: 10.1002/da.10106
Keywords: prefrontal cortex, locus coeruleus, anxiety, norepinephrine, noradrenaline, noradrenergic receptors, stress
Citation: Bouras NN, Mack NR and Gao W-J (2023) Prefrontal modulation of anxiety through a lens of noradrenergic signaling. Front. Syst. Neurosci. 17:1173326. doi: 10.3389/fnsys.2023.1173326
Received: 24 February 2023; Accepted: 30 March 2023;
Published: 17 April 2023.
Edited by:
Bernat Kocsis, Harvard Medical School, United StatesReviewed by:
Heinrich S. Gompf, University of Massachusetts Medical School, United StatesDaniel Osorio-Gómez, National Autonomous University of Mexico, Mexico
Copyright © 2023 Bouras, Mack and Gao. This is an open-access article distributed under the terms of the Creative Commons Attribution License (CC BY). The use, distribution or reproduction in other forums is permitted, provided the original author(s) and the copyright owner(s) are credited and that the original publication in this journal is cited, in accordance with accepted academic practice. No use, distribution or reproduction is permitted which does not comply with these terms.
*Correspondence: Wen-Jun Gao, d2czOEBkcmV4ZWwuZWR1; Nancy R. Mack, bnJtNjRAZHJhZ29ucy5kcmV4ZWwuZWR1