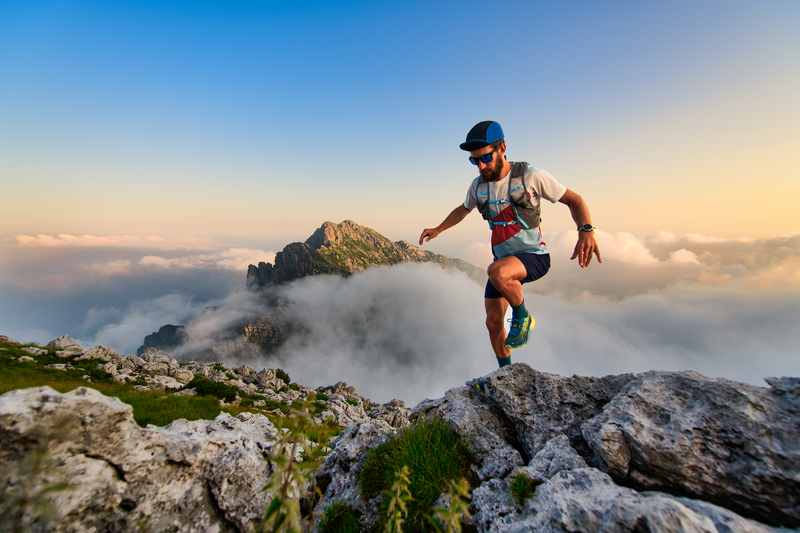
95% of researchers rate our articles as excellent or good
Learn more about the work of our research integrity team to safeguard the quality of each article we publish.
Find out more
REVIEW article
Front. Syst. Neurosci. , 22 March 2022
Volume 16 - 2022 | https://doi.org/10.3389/fnsys.2022.841869
This article is part of the Research Topic Feed-forward and Feed-back Processing in the Cerebral Cortex: Connectivity and Function View all 8 articles
The study of long-range GABAergic projections has traditionally been focused on those with subcortical origin. In the last few years, cortical GABAergic neurons have been shown to not only mediate local inhibition, but also extend long-range axons to remote cortical and subcortical areas. In this review, we delineate the different types of long-range GABAergic neurons (LRGNs) that have been reported to arise from the hippocampus and neocortex, paying attention to the anatomical and functional circuits they form to understand their role in behavior. Although cortical LRGNs are similar to their interneuron and subcortical counterparts, they comprise distinct populations that show specific patterns of cortico-cortical and cortico-fugal connectivity. Functionally, cortical LRGNs likely induce timed disinhibition in target regions to synchronize network activity. Thus, LRGNs are emerging as a new element of cortical output, acting in concert with long-range excitatory projections to shape brain function in health and disease.
Cortical inhibitory GABAergic neurons can act locally or remotely through short-range or long-range axons, respectively (Tamamaki and Tomioka, 2010; Caputi et al., 2013). They have been studied mainly with respect to their local synaptic interactions within the cortical microcircuitry (DeFelipe, 2002; Markram et al., 2004; Monyer and Markram, 2004). Although they represent only a small percentage of cortical GABAergic cells in rodents, cats and monkeys (Gonchar et al., 1995; Tomioka et al., 2005; Higo et al., 2007; Tomioka and Rockland, 2007), long-range GABAergic neurons (LRGNs; see list of abbreviations in Table 1) are emerging as key players in cortical function (Lee et al., 2014; Basu et al., 2016; Melzer et al., 2017).
Thus far, most of the work has focused on characterizing LRGNs, with a few papers having studied their impact on neural circuit function. In this review, we will describe the diverse anatomical circuits formed by LRGNs across different cortical regions. Whenever possible we will highlight the pre and postsynaptic cell types that compose the circuit, incorporating their morphological and functional characteristics. As a comparison, we will briefly introduce essential features of local inhibitory circuits in the cortex as well as classical non-cortical GABAergic projections. Cortical LRGNs are defined as GABA-producing neurons that extend their axons outside of their region of origin, e.g., the hippocampus (HPC) or neocortex, enabling communication between functionally distinct brain regions (Tamamaki and Tomioka, 2010; Caputi et al., 2013; Melzer and Monyer, 2020). According to this definition, cortical LRGNs comprise a heterogeneous population, showing differences in morphology, intrinsic membrane properties and expression of molecular markers. Despite such diversity, cortical LRGNs have in common the propensity to target GABAergic neurons (Toth et al., 1997; Melzer et al., 2012; Caputi et al., 2013; Melzer and Monyer, 2020), thereby mainly mediating long-range disinhibition. The impact of such GABA-GABA cell connectivity depends on the precise cellular subtypes targeted and the microcircuits in which they participate, adding to the complexity of circuit mechanisms that regulate brain function.
To understand how cortical LRGNs uniquely contribute to brain function and behavior, we will start by introducing key features of their better-known counterparts, namely cortical GABAergic interneurons (INs) and subcortical GABAergic projection neurons. Cortical LRGNs will be discussed depending on whether they originate from the HPC or the neocortex, two regions of intense research. Finally, we will summarize works that reveal LRGN modulation of behaviors and postulate general principles underlying LRGN function.
GABA-producing neurons constitute the primary cellular population that mediates inhibition in the cortex. Their postsynaptic targets are typically local neurons; thus, these GABAergic cells are commonly referred to as INs (DeFelipe, 2002; Markram et al., 2004; Monyer and Markram, 2004). Cortical GABAergic INs are highly diverse, identified by a range of neurochemical markers, firing patterns, morphological features and subcellular targets (Miles et al., 1996; Gonchar and Burkhalter, 1997; Petilla Interneuron Nomenclature Group [PING], Ascoli et al., 2008; Xu et al., 2010; Rudy et al., 2011; Tremblay et al., 2016). A popular GABAergic IN classification scheme is based on the non-overlapping co-expression of either parvalbumin (PV), somatostatin (SOM), or the ionotropic serotonin receptor 5-HT3A (5-HT3aR). PV-INs comprise ∼40% of cortical GABAergic neurons and target peri-somatic and axon initial segment subregions of pyramidal neurons to control their spiking output (Kvitsiani et al., 2013; Hu et al., 2014). SOM-INs make up ∼30% of cortical GABAergic neurons and mostly form synapses onto distal dendrites of principal neurons to regulate excitatory synaptic signaling (Wang et al., 2004; Murayama et al., 2009; Chiu et al., 2013). The remaining 5HT3aR-INs are less understood, consisting of a diverse group of mostly dendrite-targeting cells that includes INs expressing the vasoactive intestinal peptide (VIP) and cholecystokinin (CCK; Rudy et al., 2011; Tremblay et al., 2016). It is important to note each of these IN categories contains heterogeneous subpopulations of cells and ongoing efforts currently aim to classify them more precisely. Recent single-cell RNA sequencing studies reveal IN clusters that often correspond to previously defined cell types (Fishell and Rudy, 2011; Zeisel et al., 2015; Tasic et al., 2018), which can be further subdivided depending on a combination of morphological, electrophysiological and transcriptomic features into “met-types” (Gouwens et al., 2020). It remains unclear whether these transcriptionally defined met-types reflect core cellular entities or map onto a continuum of developmental and activity-dependent states.
At the cellular level, specialized GABAergic IN subtypes fine-tune the functional integration of input signals. Whereas PV-INs mediate feedforward inhibition, SOM-INs are responsible for feedback inhibition (Pouille and Scanziani, 2001; Kapfer et al., 2007; Silberberg and Markram, 2007). Although VIP-INs make inhibitory synapses on pyramidal neurons, they are thought to primarily inhibit other GABAergic cells to drive disinhibition of the cortex and HPC (Acsady et al., 1996; Lee et al., 2013; Pi et al., 2013). Notably, the different IN classes can be reciprocally interconnected (Cottam et al., 2013; Pfeffer et al., 2013), enabling bidirectional communication that adds to the computational power of microcircuit processing. The activity of specific GABAergic IN subtypes has been linked to distinct temporal patterns in the local circuit (Klausberger and Somogyi, 2008; Cardin, 2018), reflecting differential interactions of these INs with local excitatory networks (Caroni, 2015). For instance, PV-INs in the neocortex may entrain rhythmic activity in the gamma-range (Cardin et al., 2009; Sohal et al., 2009) to facilitate sensory and cognitive processing. In HPC, PV-INs fire at different time-points of a theta-oscillatory cycle and in spatial exploration tasks compared to those that express CCK (Freund, 2003; Klausberger et al., 2005), suggesting that even basket cells can be subdivided to support different aspects of exploratory behavior. As cortical LRGNs also express these neurochemical markers of GABAergic INs, it will be crucial to determine the extent to which the well-characterized properties of inhibitory neurons apply in the context of long-range inhibition.
The existence of long-range GABAergic projections in the brain has long been appreciated, especially from subcortical regions that comprise a substantial population of GABAergic neurons such as the striatum, amygdalar complex and brainstem. Although Purkinje cells of the cerebellum are classical long-range GABAergic projecting neurons, they have not been shown to be innervated by cortical LRGNs thus far and therefore are not included in this review. Subcortical GABAergic projections are briefly discussed here with the aim of contrasting their characteristics with the more recently appreciated cortical counterparts as well as to provide background to understand the potential impact of long-range cortical inhibition onto these regions. We refer to excellent reviews on the organization and function of subcortical GABAergic projections elsewhere (Calabresi et al., 2014; Gordon-Fennell and Stuber, 2021).
The striatum works as an integrating hub, where principal medium spiny neurons (MSNs) receive glutamatergic inputs from different cortical and thalamic areas, and in the case of the ventral portion, also from the ventral HPC and amygdala (Berendse and Groenewegen, 1990; Wall et al., 2013; Nelson and Kreitzer, 2014; Mandelbaum et al., 2019). Furthermore, the excitatory activity of MSNs is locally regulated by GABAergic and cholinergic INs (Tepper et al., 2008) and differentially modulated by dopaminergic inputs from the substantia nigra pars compacta and the ventral tegmental area (VTA) depending on the postsynaptic expression of type 1 (D1R-MSN) or type 2 (D2R-MSN) dopamine receptors (Le Moine and Bloch, 1995; Gerfen and Surmeier, 2011; Tritsch and Sabatini, 2012). MSNs send profuse GABAergic projections to neighboring basal ganglia nuclei (Kemp and Powell, 1971; Smith et al., 1998; Tepper et al., 2007) and have been extensively studied to understand their contribution to the generation of motor learning, habitual and motivated behaviors, mood and reward (Gerfen and Surmeier, 2011; Tritsch and Sabatini, 2012; Bariselli et al., 2019). By inhibiting the main GABAergic output nuclei of the basal ganglia to the thalamus (thalamic disinhibition), D1R-MSNs of the direct pathway facilitate motor behavior or the approach to a rewarding stimulus. In contrast, D2R-MSNs of the indirect pathway, acting through multiple stages within the basal ganglia, produce inhibition of the thalamus to suppress movements or to avoid non-rewarding stimuli (Beckstead and Cruz, 1986; Alexander and Crutcher, 1990; Hedreen and DeLong, 1991). Interestingly, the basal ganglia may also exert its effects independent of the thalamus as it also sends GABAergic projections directly to the neocortex (Jayaraman, 1980; Saunders et al., 2015; Sun Q. et al., 2019). In a mapping study using monosynaptic viral tracers, these particular long-range GABA neurons have been shown to target GABAergic INs (Sun Q. et al., 2019), indicating that the basal ganglia may control their own cortical feedback via disinhibition.
The amygdala is made up of a diverse collection of interconnected nuclei to regulate emotionally relevant behaviors (LeDoux, 2007; Janak and Tye, 2015). For example, the central nucleus of the amygdala (CeA) is a striatal-like GABAergic structure that projects to the hypothalamus and brainstem to initiate fear responses (Pare and Duvarci, 2012). Recent viral tracing work suggests that single neurons in the CeA send bifurcating projections to the medial prefrontal cortex (mPFC) and the ventrolateral periaqueductal gray (Sun Y. et al., 2019) to potentially dually regulate adaptive emotional responses. Interestingly, such GABAergic projections were also found to originate from the basolateral amygdala (BLA), a region thought to be cortical-like and predominately populated by glutamatergic neurons. This finding adds to the growing evidence that GABAergic cells in the BLA are not solely INs but can also send long-range axons to distal regions like the entorhinal cortex (EC; McDonald and Zaric, 2015) and basal forebrain (McDonald et al., 2012). Indeed, the entire amygdaloid complex may make GABAergic connections throughout the brain. Large intercalated cells of the amygdala, many of which are PV+, project to the perirhinal, entorhinal, and piriform cortex, synapsing selectively on GABAergic cells (Bienvenu et al., 2015). Furthermore, neurons in the bed nucleus of the stria terminalis, a heterogeneous extension of the amygdala, provide distal GABAergic inputs onto non-dopaminergic, likely GABAergic (Nair-Roberts et al., 2008), neurons in the VTA to promote active reward seeking (Jennings et al., 2013). Indeed, GABAergic INs in the VTA are preferred targets of inhibitory inputs from numerous subcortical regions, including the lateral hypothalamus (Nieh et al., 2015, 2016), medial preoptic area (McHenry et al., 2017) and the nucleus accumbens (NAcc; Bocklisch et al., 2013). A recent viral genetic mapping study revealed that whereas GABA INs in the VTA strongly connect to nearby dopaminergic neurons, distal GABAergic inputs preferentially target local GABA INs (Soden et al., 2020). Given that GABA cells in the VTA also receive excitatory inputs from diverse brain areas (Morales and Margolis, 2017; Beier et al., 2019; Bouarab et al., 2019), they likely act as key integrators of information about the external environment and internal state to control dopaminergic neuromodulation in motivated behavior.
In addition to mediating local inhibition, GABA neurons in the VTA also provide long-distance inhibition to several distinct brain regions, including the basal ganglia, amygdala, dorsal raphe nucleus, HPC and prefrontal cortex as revealed by retrograde tracing (Ntamati and Luscher, 2016; Bouarab et al., 2019; Breton et al., 2019). Interestingly, discrete subsets of these neurons target different brain structures, with little axonal collateralization, suggesting independent parallel circuits. Because they have mostly been identified anatomically and not characterized functionally (but see the section “Neurotransmitter Co-release in Local and Distant Inhibition”), how the distinct GABAergic projections from VTA subregions act in parallel to regulate different facets of reward and aversion remains to be determined.
Besides its better known endocrine function, the hypothalamus, at least the lateral portion (LH), is another important brain structure that sends GABAergic projections to distant regions like the basal forebrain (Cassidy et al., 2019), VTA (Nieh et al., 2015, 2016), and dorsal pons (Marino et al., 2020) to regulate motivated behaviors like feeding and social approach. Interestingly, optogenetic activation of inhibitory and excitatory inputs from LH to the VTA disinhibited and suppressed dopamine neurons, respectively, presumably with local VTA INs as common postsynaptic targets, thereby exerting opposing influences on social approach and interaction (Nieh et al., 2016). Studies employing similar strategies suggest that the brain circuitry underlying compulsive overeating may involve GABA-GABA connectivity between LH and a region medial to the locus coeruleus but not between LH and VTA (Marino et al., 2020). Taken together, GABA is a major neurotransmitter in subcortical long-range communication, often operating via disinhibition in downstream brain regions.
An essential characteristic of cortical areas is its organization in layers, which is thought to be crucial for amplifying and computing incoming signals. Here, we will consider as cortical structures the neocortex and the HPC (sometimes referred to as allocortex). Early evidence suggesting the existence of cortical LRGNs came from the observation of non-pyramidal retrogradely labeled somata in the cortex (Code and Winer, 1985; Totterdell and Hayes, 1987; Hughes and Peters, 1990). The advent of improved viral tracing techniques in transgenic mice to selectively monitor and manipulate GABAergic neurons enabled more precise interrogation of LRGN connectivity and functional impact.
In the context of cortical LRGNs, those originating from HPC are among the best described. As the HPC is well known for coordinating spatial and contextual information via its various subfields (Yassa and Stark, 2011; Lee et al., 2020), we will describe key features of LRGNs located in different subregions of the hippocampal circuit highlighting their connectivity with distal structures such as the medial septum (MS) and EC (Figure 1 and Table 2) that are important for navigation and contextual learning. For simplicity, hippocampal GABAergic innervations in remote subcortical regions such as the NAcc (Totterdell and Hayes, 1987) are not discussed below.
Figure 1. Long-range GABAergic neuron (LRGN) projections to and from the hippocampus. The HPC is reciprocally connected with the EC and MS through SOM-LRGNs (HPC outputs) and PV-LRGNs (HPC inputs). Even a single LRGN in the MS can project to both the EC and HPC. In parallel, LRGNs that are identified by markers other than PV and SOM (magenta), such as Reelin/NPY, in the HPC send axons to the RSC. Inhibitory projections from the HPC to subcortical structures have been described, such as the STR and the AMY. HPC-AMY LRGNs are diverse in their neurochemical expression, including SOM, PV and others. The HPC also sends nNOS + LRGNs to the HYP and the MS. Contralateral projections are not included here. AMY, amygdala; EC, entorhinal cortex; HYP, hypothalamus; HPC, hippocampus; MS, medial septum; RSC, retrosplenial cortex; STR, striatum; PV, parvalbumin, SOM, somatostatin; NPY: neuropeptide Y; nNOS, neuronal nitric oxide synthase.
Primary excitatory inputs to the HPC arrive in the molecular layer of the dentate gyrus (DG) from layer II/III of EC, a region with medial (MEC) and lateral (LEC) anatomical subdivisions (Amaral and Witter, 1989; Witter et al., 2017). These glutamatergic perforant paths depolarize the dendrites of principal granule cells (GC), whose soma are in the granular layer. In parallel, several hilar SOM + GABAergic cells mediate local inhibition as hilar-perforant path associated interneurons (HIPPs; Bakst et al., 1986; Halasy and Somogyi, 1993; Han et al., 1993) to modulate GC dendritic excitability. Notably, SOM + neurons in the hilus also send feedback inhibitory projections to the MEC as revealed in retrograde tracer studies with post-hoc immunohistochemistry (Ino et al., 1990; Melzer et al., 2012) to potentially control incoming perforant path activity. GABAergic cells in the hilus have also been shown to project to other distal regions such as the subiculum and MS (Jinno and Kosaka, 2002; Jinno et al., 2007). More recently, a new subclass of SOM + septal-projecting LRGNs in the hilus, called hilus-associated INs (HILs), has been shown to also send local axon collaterals to exert strong perisomatic inhibition over DG PV-INs (Yuan et al., 2017), and thus disinhibiting GCs and facilitating GC-CA3 communication. The impact of these HILs in setting MS activity is unclear, as they functionally target PV + GABAergic, cholinergic and glutamatergic neurons, as confirmed by optogenetic stimulation and post-hoc immunohistochemistry. Regardless, HILs may powerfully determine the output of the target cell in MS by establishing perisomatic inhibition as in the DG, unlike typical cortical SOM-INs. Given demonstrated reciprocal LRGN interconnectivity between the HPC and MS (Toth et al., 1993; Jinno et al., 2007; Takacs et al., 2008), HILs may also be reciprocally inhibited by these septal LRGNs. This possibility remains to be investigated. Moreover, individual GABAergic LRGNs in the MS can send projections to both the HPC and MEC, making synaptic contacts with different IN subtypes (Fuchs et al., 2016). These dually projecting LRGNs may help to coordinate the activity of these three areas: HPC, MS, and EC.
CA3 is the second stage of the trisynaptic circuit of the HPC and receives excitatory axon fibers from GCs of the DG (called mossy fibers) and direct glutamatergic inputs from layer II of the EC in stratum lacunosum moleculare (SLM). CA3 pyramidal neurons project their excitatory axons through Schaffer collaterals in the stratum radiatum (SR) to the CA1 area. CA1 pyramidal cells are the main output of the HPC, but also receive direct excitatory inputs from the EC (Amaral and Witter, 1989). Additionally, pyramidal cells interact with a plethora of different INs subtypes distributed across all layers of the CA region (Klausberger and Somogyi, 2008).
Although distributed LRGNs in CA3 have been found, not much information about them is available. A common feature of CA3-originating LRGNs is that they express SOM as a molecular marker but are heterogeneous, showing a diversity of co-expression with other markers (Zappone and Sloviter, 2001; Jinno and Kosaka, 2002; Jinno et al., 2007). The soma of MS-projecting LRGNs are located in all CA3 sublayers whereas those of LRGNs that project to the subiculum are restricted to SR/SLM (Jinno et al., 2007). The function of hippocampal GABAergic projections from CA3 is unknown. Notably, GABAergic cells in the CA3 have been shown to receive long-range inhibitory projections from PV + LRGNs in the MEC (Melzer et al., 2012) and MS (Freund and Antal, 1988). Combining anterograde labeling with immunohistochemistry, it was shown that GABAergic septo-hippocampal projections target SOM-, VIP- and CCK-INs in CA3 as well as other areas of the HPC (Gulyas et al., 1990). Stimulation of the septo-hippocampal connections triggers disinhibition of CA3 pyramidal cells (Toth et al., 1997) and alters network activity (Joshi et al., 2017; Unal et al., 2018; Salib et al., 2019). This may also apply for the entorhinal-hippocampal inhibitory afferents. For a better understanding of the CA3 long-range inhibitory connectivity, further research is needed.
As previously mentioned, CA1 serves as the final stage of the trisynaptic HPC circuit, moving highly processed information back to the EC, with a synaptic relay in the subiculum. In contrast to CA3, CA1 LRGNs that send axons to the MEC are located in the stratum oriens (SO) and SR, extending hundreds of microns horizontally throughout MEC layer I (Melzer et al., 2012). Whereas the targets of these long-range projections are a heterogeneous population of GABAergic cells identified electrophysiologically, CA1-MEC LRGNs are most frequently characterized by SOM expression (Jinno et al., 2007) as are a primary IN population in the SO that sends axons to the SLM (called O-LM cells). It remains to be determined whether O-LM INs may also be LRGNs in CA1. Moreover, GABAergic cells in SO of CA1 also project to the MS, but given the lack of tracer co-labeling, these cells are likely distinct from those projecting to the MEC. Because these CA1-MS GABAergic cells synapse onto local INs and remote MS GABA neurons, they likely serve double duty, simultaneously disinhibiting CA1 and MS (Gulyas et al., 2003). Interestingly, the dorsal and ventral HPC are also connected by these cells (Gulyas et al., 2003), potentially coordinating activity between the two portions of the HPC that play differential roles in memory and emotional processes, respectively (Fanselow and Dong, 2010).
Recently, using an intersectional viral transduction approach, GABAergic cells in the SO and stratum pyramidal (SP) have been discovered to also project to extrahippocampal regions such as the band of broca and the tenia tectum in the frontal cortex (Christenson Wick et al., 2019). Notably, these cells typically express the neuronal nitric oxide synthase (nNOS) and neuropeptide Y (NPY) but not other IN markers such as PV, SOM or VIP. Moreover, these nNOS + LRGNs also form GABAergic synapses onto local pyramidal neurons and INs in CA1, exhibiting similar inhibitory postsynaptic response characteristics irrespective of target cell type. Optogenetic activation of these nNOS + cells increases local field potential (LFP) power at the stimulated frequency within the HPC and frontal cortex individually as well as enhances LFP coherence between these two regions, suggesting that they coordinate both local network activity and inter-regional communication.
GABAergic cells in CA1 have been shown to project to the retrosplenial cortex (RSC; Jinno et al., 2007; Miyashita and Rockland, 2007), another cortical structure that is part of the navigation system. In contrast to the CA1-MEC LRGNs, these CA1-RSC LRGNs are principally located at the SR/SLM border and make functional synapses onto the apical dendritic tufts of layer V pyramidal neurons, to potentially modulate synaptic excitation from the anterior thalamic nuclei (Yamawaki et al., 2019). Interestingly, the SR/SLM border of CA1 appears to be a site of integration, receiving long-range inhibitory PV + axons from MEC and LEC (Melzer et al., 2012; Basu et al., 2016) and excitatory perforant path inputs from the EC. Indeed, LRGNs from LEC have been reported to target CCK + INs to facilitate the integration of perforant path and CA3 inputs in CA1 pyramidal neurons (Basu et al., 2016). In addition, CCK-INs located in SP and SR, as well as SOM-INs in SO, receive GABAergic projections from the MS (Gulyas et al., 1990), which are probably PV + (Freund and Antal, 1988). In brain slice electrophysiological recordings, synaptic terminals of PV + septal-hippocampal LRGNs display a lower probability of GABA release and reduced short-term synaptic depression that is frequency-dependent compared to that of hippocampal PV-INs (Yi et al., 2021), suggesting differential activity-dependent recruitment of local vs. distal inhibition in the HPC.
The HPC is a coordination center for several cortical and subcortical areas to drive behaviors such as navigation, learning and memory. Like glutamatergic connections, LRGNs also form a large interconnected inhibitory network linking HPC, EC, and MS. Given the importance of the MS in theta oscillations in MEC and HPC and spatial navigation (Mitchell et al., 1982; Hagan et al., 1988; McNaughton et al., 2006), particularly the GABAergic constituency (Dwyer et al., 2007; Pang et al., 2011), LRGNs from and to the HPC may contribute to shape such rhythmic activity and modulate exploratory behavior.
The neocortex is classified as a higher-order center, and it is involved in diverse processes such as perception, control of movement, emotional control, and cognitive function (Mountcastle, 1978; Harris and Shepherd, 2015; Squire et al., 2015). In the canonical circuit, principal pyramidal neurons that are preferentially located in infra-granular layers constitute the main output of the neocortex. As in other cortical regions, LRGNs are present in the neocortex, with their somas located preferentially in layers II/III and V/VI as well as white matter of sensory cortex (Gonchar et al., 1995; Fabri and Manzoni, 2004; Tomioka et al., 2005; Higo et al., 2007, 2009; Rock et al., 2018; Bertero et al., 2021), motor cortex (MC; Tomioka et al., 2005; Rock et al., 2016; Melzer et al., 2017), and associative cortex (Lee et al., 2014; Tomioka et al., 2015). When examined, LRGNs in the neocortex that project to subcortical areas (cortical-fugal) seem to arise from deep layers (Figure 2 and Table 2). In contrast, cortico-cortical LRGNs are more diverse, with soma that span both supra- and infra-pyramidal layers. Given that cortico-cortical projections may target higher or lower-ordered cortical regions (Figure 3 and Table 2), it is possible that this heterogeneity may be linked to bottom-up feedforward vs. top-down feedback functions.
Figure 2. Known cortico-fugal LRGNs. LRGNs in the neocortex project to a variety subcortical areas, some of which are not included here. For simplicity, we highlight those that have been functionally characterized or investigated in more detail. Corticofugal LRGNs are diverse in their molecular markers. The PFC sends PV-LRGN projections to the STR, and the molecular identity of LRGNs to the AMY remains unidentified. SOM+, PV+, and other LRGNs (magenta) in the OFC send axons to the STR, and those that project to the AMY and SN are unidentified (gray). SOM+, PV+, and VIP+ axons to the STR arise from the MC and AC, which also sends SOM+ and VIP+ projections to the AMY and COL. The SC and RSC contain PV-LRGNs that project to the STR. AC, auditory cortex; AMY, amygdala; COL, colliculus; MC, motor cortex; OFC, orbitofrontal cortex; PFC, prefrontal cortex; RSC, retrosplenial cortex; SC, somatosensory cortex; SN, substantia nigra, STR, striatum; PV, parvalbumin; SOM, somatostatin, and VIP, vasoactive intestinal peptide.
Figure 3. Long-range GABAergic neuron connections between neocortical regions. Ipsilateral connections are mostly dominated by SOM + LRGNs. The PFC sends and receives inhibitory projections from the MC, which also sends inhibitory axons to the SC, AC, and VC. The only reported ipsilateral PV+ connection originates from the MC and terminates in the SC. VC receives SOM + LRGN projections from the MC, SC, and RSC. The molecular identity of LRGNs from the OFC to the PFC, MC and SC remains to be determined. Inhibitory connections between contralateral ACs are PV+ and VIP+, whereas contralateral projections between VCs are PV+ only. The molecular identity of contralateral LRGNs between SCs is unknown. AC, auditory cortex; MC, motor cortex; OFC, orbitofrontal cortex; PFC, prefrontal cortex; RSC, retrosplenial cortex; SC, somatosensory cortex; STR, striatum; VC, visual cortex; PV, parvalbumin; SOM, somatostatin; VIP, vasoactive intestinal peptide. Dashed line refers to connections that remains to be confirmed.
The first cortico-fugal LRGNs discovered were PV neurons from somatosensory cortex (SC) and RSC that project to the caudal striatum (Jinno and Kosaka, 2004), showing scarce but consistent cell body labeling in layer V. Although these retrogradely identified cells have been shown to be GAD immunoreactive, their functional characterization (i.e., inhibition of postsynaptic striatal cells) is lacking.
Long-range GABAergic neurons in the motor cortex (MC) located in deep layers connect distal subcortical and cortical regions that influence movement behaviors (Tomioka et al., 2005; Rock et al., 2016; Melzer et al., 2017; Bertero et al., 2021). In primary motor cortex (M1), PV + and SOM + GABAergic cells project to the dorsal striatum. M1-LRGNs positive for PV (PV-M1-LRGNs) mainly inhibit D1R-MSNs of the direct pathway, and thus optogenetic activation of their terminals in the striatum increased the duration of immobility bouts (Melzer et al., 2017). On the other hand, M1-LRGNs positive for SOM (SOM-M1–LRGNs) primarily inhibit cholinergic interneurons, and their stimulation induced the opposite effect on locomotion. These results suggest that distinct LRGN subtypes located in the same cortical region can have differing impact on behavior depending on the identity of their targets. Interestingly, LRGNs in the secondary motor cortex (M2) that express SOM (SOM-M2-LRGNs) mainly inhibit striatal D1R-MSNs, and therefore their activation mimics that of PV-M1-LRGNs rather than of SOM-M1-LRGNs (Melzer et al., 2017). Importantly, activation of PV-M1-LRGNs and SOM-M2-LRGNs decrease locomotion by affecting different aspects of movement (i.e., duration of immobility vs. speed of mobility), consistent with the notion that M1 is more associated with motor execution while M2 is more related to motor planning. It will be important to study the precise conditions under which PV-M1-LRGNs and SOM-M2-LRGNs are activated to dissect their specific roles further.
More recently, through viral genetic means, VIP-LRGNs in M1 has been shown to innervate the striatum (Bertero et al., 2021). Their distribution across cortical layers and impact on circuit activity and behavior remains to be determined.
In the auditory cortex (AC), LRGNs corresponding to diverse neurochemical classes of cortical GABAergic neurons (e.g., PV, SOM, VIP) have been found, and they project to different subcortical regions. For example, as revealed by viral tracing, SOM + LRGNs projecting to the lateral amygdala (LA) were primarily located in layers V/VI of AC (Bertero et al., 2019), potentially co-mixing with cortico-amygdalar projecting pyramidal neurons (LeDoux et al., 1991) to finetune the emotional significance of auditory signals. Indeed, optogenetic activation of AC-LA LRGNs control the spiking of principal LA neurons (Bertero et al., 2019); however, how they modulate aversive and rewarding behavior has not been investigated. SOM + and PV + LRGNs in deep layers of the AC also project to striatum (Rock et al., 2016; Bertero et al., 2020). Although SOM + LRGNs directly inhibit MSNs, it is unknown whether DR1-MSNs or DR2-MSNs are preferential targets and whether these LRGNs make bifurcating projections to both the amygdala and striatum, as has been reported in subcortical regions and the HPC (Fuchs et al., 2016; Yuan et al., 2017). Intriguingly, the PV + LRGNs exhibit morphology and intrinsic electrophysiological properties similar to PV-INs (Bertero et al., 2020), raising the possibility that individual PV + GABAergic cells may send both local and distal axons.
LRGNs in the auditory cortex can also express VIP, innervating remote areas including the ipsilateral striatum, amygdala and medial geniculate body as well as the superior and inferior colliculus (Bertero et al., 2021). The functional relevance of auditory VIP + LRGNs is not known. VIP + GABAergic cells will likely emerge as a significant population of LRGNs as research in this field continues; for example, in the HPC, VIP + cells projecting to the subiculum are suppressed during locomotion-associated theta rhythms (Francavilla et al., 2018), suggesting that VIP + LRGNs are disengaged during movement to enable the flow of hippocampal-related information. More research is needed to determine whether VIP + LRGNs in the neocortex work similarly.
mPFC-LRGNs projecting to the NAcc are located in layer II/III and are either PV+ or VIP+ but not SOM+ as revealed in viral tracers in Cre-driver mouse lines (Lee et al., 2014). Although some of the PV-LRGNs display electrophysiological properties similar to PV-INs, not all of them are fast-spiking. Since in vivo activation of these inhibitory projections to NAcc elicits avoidance, mPFC-NAcc LRGNs may either directly inhibit D1R-MSNs or inhibit local GABAergic INs to disinhibit D2R-MSNs. However, the specific circuits that drive this avoidance behavior remain to be described. A caveat is the finding that LRGNs in the mPFC also project to other subcortical targets, including dorsal striatum, claustrum and amygdala (Lee et al., 2014). LRGNs also arise from other associative cortical regions. For example, GABAergic cells from the orbitofrontal cortex project to the caudate putamen, with retrogradely labeled soma displaying SOM and PV but not VIP immunoreactivity in mainly infragranular layers (Tomioka et al., 2015). These LRGNs in the orbitofrontal cortex make distributed inhibitory connections, targeting unidentified cell types in other subcortical (e.g., amygdala and substantia nigra) and cortical (e.g., MC, SC, and PFC) regions.
Long-range GABAergic neurons of sensory, motor and associative neocortex have been reported to connect to other neocortical areas in the ipsi- and contralateral hemisphere (Code and Winer, 1985; Buhl and Singer, 1989; Hughes and Peters, 1990; McDonald and Burkhalter, 1993; Gonchar et al., 1995; Fabri and Manzoni, 1996, 2004; Tomioka et al., 2005; Tamamaki and Tomioka, 2010; Rock et al., 2018). Injection of a retrograde tracer in SC labelled LRGNs in M1 and M2 (ipsilateral) with soma localized mainly in layers II/III and V/VI within the same hemisphere (Tomioka et al., 2005). In addition to being positive for GABA, the ipsilaterally projecting MC-SC LRGNs were immunoreactive to diverse markers but show a tendency for shorter and longer projecting LRGNs to express PV and SOM, respectively. Notably, M2 also sends long-range GABAergic afferents to primary and secondary visual cortex, suggesting that most, if not all, primary sensory cortical regions receive movement-related inhibitory feedback (Melzer et al., 2017). Moreover, retrograde tracer injections in M1 revealed reciprocal long-range inhibition from primary and secondary SC but not primary visual cortex (Tomioka et al., 2005). Given that both SC and MC show similar body representations (i.e., homunculus), it remains to be determined whether these SC-MC and MC-SC LRGNs are topographically linked. Although, ipsilateral cortico-cortical inhibitory connections in rodents show similar patterns of connectivity, layer localization and immunoreactivity in other species such as cats (Higo et al., 2007) and monkeys (Tomioka and Rockland, 2007), there is almost no information regarding the cellular targets or the circuit dynamic alterations they exert in brain function and behavior.
Although early research supported the existence of callosal-LRGNs (Code and Winer, 1985; Buhl and Singer, 1989; Hughes and Peters, 1990; Gonchar et al., 1995; Kimura and Baughman, 1997), it was not until 2017 that distal axons from PV-LRGNs in AC, MC and visual cortex to the contralateral counterpart were reported (Rock et al., 2018; Zurita et al., 2018); however, their cellular targets were not identified. Interestingly, callosal AC-LRGNs also send axon collaterals within the AC and inhibit local pyramidal neurons. Although not yet replicated in MC or visual cortex, it is possible that bifurcating callosal-LRGNs in general coordinate activity between bilateral neocortical regions of the same modality. Moreover, there is evidence that LRGNs show similar but not identical electrophysiological properties as their interneuron counterpart. For example, in the AC, callosal PV-LRGNs exhibited a higher expression of a subtype of voltage-sensitive potassium channel (i.e., Kv1.1) than PV-INs and thus were less excitable (Zurita et al., 2018). As callosal VIP-LRGNs was recently found in the AC (Bertero et al., 2021), it will be possible in the near future to determine whether these cells also send collaterals to inhibit GABAergic interneurons and mediate network disinhibition as cortical VIP-INs are known to do in superficial layers (Acsady et al., 1996; Lee et al., 2013; Pi et al., 2013). In order to dissect the circuit motif of callosal long-range inhibition and understand their impact on behavior, more work is needed.
A clue of LRGN function may come from developmental studies. According to carbocyanine tracing work, corticofugal LRGNs are among the earliest born cells in the murine cortical subplate, being most numerous perinatally and subsequently decreasing in number within the first postnatal week (Boon et al., 2019). Interestingly, contralateral GABAergic projections showed the opposite pattern, gradually increasing during the first postnatal week. Using BrdU birthdating techniques, nNOS + LRGNs in the HPC have also been shown to arise embryonically (Christenson Wick et al., 2019). It is unclear whether these GABA cells can already be distinguished by their long axonal phenotype shortly after birth and what roles they may play during embryonic development. Recent genetic fate mapping studies reveal a subpopulation of highly interconnected early born HUB neurons that may initially shape development of hippocampal networks (Bonifazi et al., 2009) and subsequently mediate long-range GABAergic communication between the HPC and MS in adulthood (Picardo et al., 2011; Villette et al., 2016). More longitudinal studies are needed to determine whether all cortical LRGNs originate from these pioneer HUB neurons and to understand their role in development.
As the list of cortical LRGNs grows, it is becoming clear that these cells often contain neuropeptides that can act as transmitters in addition to GABA. For example, GABAergic projections from the HPC to the MS are SOM+. HPC-amygdala LRGNs can express SOM, NPY and CCK (Lubkemann et al., 2015). Moreover, cortico-cortical LRGNs often co-express SOM, nNOS, or NPY (Tomioka et al., 2005; Higo et al., 2007; Tomioka and Rockland, 2007), whose activity may be enhanced during sleep (Kilduff et al., 2011). The contribution of these bioactive molecules to LRGN function has not been investigated. A possible interaction between GABA and neuropeptide transmission can be garnered from pharmacological work in subcortical regions. In the hypothalamus, GABA agonism in vivo can temper the ability of NPY to induce presynaptic secretion of the leutinizing hormone from arcuate nucleus neurons (Horvath et al., 2001). In the basal forebrain, SOM application suppresses GABA release onto cholinergic neurons (Momiyama and Zaborszky, 2006). Moreover, exogenous GABA has been shown to suppress SOM release in in vitro cortical preparations (Gemignani et al., 1994). Such findings suggest that neuropeptides and GABA may mutually finetune each other’s influences. However, because neuropeptide secretion from neurons occurs through a pathway distinct from vesicular GABA release, requiring slower dense core vesicles and stronger persistent depolarization (Edwards, 1998; van den Pol, 2012), the physiological conditions that simultaneously recruit GABAergic and peptidergic actions are unknown.
Co-release of multiple neurotransmitters has been shown from projecting subcortical GABAergic neurons. For example, a combination of retrograde tracing and optogenetic electrophysiological recordings show that VTA axons to the habenula and to DG are both GABAergic and glutamatergic (Root et al., 2014; Ntamati and Luscher, 2016). Despite dual release of GABA and glutamate, stimulation of the VTA-DG connection appears to be primarily inhibitory in function, reducing GC firing in vivo under anesthesia (Ntamati and Luscher, 2016). Moreover, monosynaptic connections from the supramammillary nucleus of the hypothalamus to GCs and GABAergic interneurons in the DG co-release glutamate and GABA (Hashimotodani et al., 2018). These mixed afferents alone are unable to drive GC firing but can facilitate the excitatory influence of the perforant pathway when co-stimulated. Even at the extensively studied glutamatergic mossy fiber synapse in the HPC, GABA and glutamate co-transmission has been suggested to occur transiently during early postnatal development (Walker et al., 2001; Gutierrez et al., 2003; Beltran et al., 2012), raising the possibility that neurotransmitter co-release may be dynamically regulated.
In the HPC, co-transmission of acetylcholine (ACh) and GABA from optogenetically activated septal fibers occurs via different vesicles that are regulated by distinct presynaptic calcium channels and elicits fast GABA-mediated hyperpolarization and slow ACh-mediated depolarization in CA1 pyramidal neurons (Takacs et al., 2018). Interestingly, the GABAergic component of this basal forebrain projection alone is sufficient to suppress sharp wave ripples and epileptiform activity in the HPC. In the frontal cortex, optogenetic activation of cholinergic projections from the basal ganglia triggers co-transmission of GABA and ACh, through separate vesicular pools, resulting in both inhibitory and excitatory postsynaptic influences in local INs, respectively (Saunders et al., 2015).
Neuropeptides do not have to be co-transmitted with GABA to modulate its function. In the hypothalamus, sustained postsynaptic depolarization induces somatodendritic secretion of CCK that subsequently triggers NO production and potentiates presynaptic GABA release (Crosby et al., 2015). Furthermore, in the cortex, SOM can be released following prolonged neural activity (Dao et al., 2019) and has been shown to reduce pyramidal cell excitability (Riedemann and Sutor, 2019) as well as suppress excitatory synaptic drive onto PV-INs (Song et al., 2020). Interestingly, stimulation of PV-INs and SOM-INs has opposing impact on astrocytic calcium signaling in the cortex, with SOM peptidergic activity playing a crucial role (Mariotti et al., 2018). Although these works were focused on cortical INs, the common ability of LRGNs to synthesize SOM as well as other neuropeptides (Paul et al., 2017) posits that peptidergic modulation may also be an important component of LRGN function.
There is a general agreement that behavior not only depends on the activity of one structure but also needs to be coordinated among several brain areas (Friston, 2003; Fries, 2015; Sporns and Betzel, 2016). In this context, the cerebral cortex acts as a hub to multiplex a great variety of processes, ranging from perception to cognition. Understanding how this highly interconnected structure can communicate within different cortical subregions and across subcortical areas is one of the main goals in neuroscience research. In addition to the canonical circuit of excitatory connections, it is becoming increasingly clear that long-range GABAergic inhibition constitutes a new element of the cortical output. Indeed, inhibitory projections may even leave their area of origin alongside long-range excitatory efferents (Rock et al., 2016; Bertero et al., 2019). Consistent with this idea, GABAergic innervations from the somatosensory cortex are intermingled with cortico-fugal glutamatergic ones in the striatum and thalamus (Jinno and Kosaka, 2004). Moreover, layer V pyramidal cells of the RSC have been shown to receive inhibitory projections from CA1 and excitatory inputs from the thalamus (Yamawaki et al., 2019). These examples highlight cortical LRGNs as critical components of feedforward and feedback circuits to counter excitation. As disruption of excitatory-inhibitory balance has been linked to psychiatric disorders like schizophrenia and autism (Marin, 2012; Gonzalez-Burgos et al., 2015), it would not be surprising to learn about contributions of cortical LRGNs in the pathophysiology of these disorders in the near future.
Several recent works support a role of LRGNs in brain function. For example, LEC-CA1 LRGNs have been shown to contribute to sensory processing (Basu et al., 2016). Using in vivo calcium imaging, Basu and colleagues demonstrate that the GABAergic axon terminals from the LEC onto CA1 interneurons are active during unimodal sensory stimulation (e.g., air, water, light or tone). Interestingly, during multimodal stimulation, calcium activity of these terminals further increased, suggesting that LEC-CA1 LRGNs integrate multisensory signals to provide contextual information. Indeed, inactivation of these LRGNs impairs learning by changing the specificity and context of formed memories but not memory storage per se. At the circuit level, this disinhibitory pathway can shape a form of synaptic plasticity that depends on the timing of inputs; whether this mechanism directly participates in contextual learning remains to be determined. However, it is noteworthy that this study revealed a role of LRGNs in synaptic plasticity that is considered to be a neural correlate of learning and memory (Malenka and Bear, 2004; Whitlock et al., 2006).
In the olfactory system, LRGNs from the cortex and basal forebrain have been suggested to exert inhibitory feedback onto subcortical sensory areas to regulate odor discrimination (Nunez-Parra et al., 2013; Mazo et al., 2020). In this context, LRGNs from the anterior olfactory cortex form synapses with mitral cells, tufted cells and deep-layer GABA INs of the olfactory bulb (OB). Inhibitory projections from the horizontal diagonal band of Broca (HDB) in the basal forebrain modulates OB granule cells, resulting in a net inhibition of both spontaneous and odor-evoked activity in local and output neurons. How these two distinct long-range inhibitory pathways interact to modulate odor perception is awaiting investigation. Notably, HDB-OB LRGNs are under tonic endocannabinoid (eCB) control, showing reduced probability of GABA release in basal conditions that can be alleviated by cannabinoid receptor antagonism (Zhou and Puche, 2021). As eCBs are key retrograde signals in activity-dependent synaptic plasticity, it is likely that OB granule cells can self-regulate the inhibition they receive from these LRGNs to fine-tune odor discrimination. It will be interesting to see whether LRGN inputs from the anterior olfactory cortex can be similarly regulated and whether neuromodulation of non-classical LRGNs can be applied to other brain regions as has been established for the classical subcortical counterparts like the MSNs (Gerfen and Surmeier, 2011; Tritsch and Sabatini, 2012).
Long-range GABAergic neurons in the PFC are anatomically connected to several subcortical areas, but their impact on network function and behavior is only beginning to be revealed. For example, activation of PFC-NAcc LRGNs induces avoidance in a real-time place preference task, suggesting that these inhibitory connections transmit aversive signals and modulate motivational valence (Lee et al., 2014). Interestingly, a more recent study in bioRxiv proposes a previously unknown direct inhibitory connection from the PFC to the HPC that modulates object exploration (Malik et al., 2021). This pathway is composed of diverse LRGNs that specifically inhibit VIP-INs of the CA1 area. As VIP-INs mediate disinhibition of pyramidal neurons, activation of PFC-HPC inhibitory projections leads to a “double disinhibitory long-range motif,” which in turn increases inhibition of hippocampal pyramidal cells and enhances gamma synchrony between the PFC and HPC during exploratory behavior. This is in agreement with the idea that LRGNs coordinate oscillatory activity between distal brain regions (Caputi et al., 2013; Melzer and Monyer, 2020), which facilitates communication and cognition (Engel et al., 2001; Fries, 2015; Abbas et al., 2018; Adaikkan and Tsai, 2020).
PFC-HPC LRGN activation also increases gamma and theta power within the HPC (Malik et al., 2021). Moreover, LRGN projections between the HPC and MS have been shown to play an essential role in hippocampal theta oscillations (Toth et al., 1997; Borhegyi et al., 2004; Jinno et al., 2007; Joshi et al., 2017; Katona et al., 2017; Unal et al., 2018). Such local rhythms likely emerge from the targeting of local GABAergic interneurons (Figure 4), as supported by theoretical models (Buzsaki et al., 2004; Buzsaki and Wang, 2012). Both PV- and SOM-INs are linked to gamma oscillations (Cardin et al., 2009; Sohal et al., 2009; Chen et al., 2017; Veit et al., 2017; Booker et al., 2020) and are phase-locked with theta cycles (Huh et al., 2016). Notably, the impact of local inhibition from the cortex on regional network activity is critically dependent on the target IN subtype. For example, cortical PV-INs are typically rapidly activated by extracortical inputs and exert fast and strong inhibition on principal cell output (Pouille and Scanziani, 2001; Petilla Interneuron Nomenclature Group [PING], Ascoli et al., 2008; Rudy et al., 2011). On the other hand, SOM-INs are generally recruited by local excitation and mainly target dendrites of pyramidal neurons, hence regulating synaptic integration and recurrent microcircuit activity (Silberberg and Markram, 2007; Murayama et al., 2009; Chiu et al., 2013). Moreover, VIP-INs are best known for inhibiting PV- and SOM-INs and thus primarily mediate disinhibition (Acsady et al., 1996; Lee et al., 2013; Pi et al., 2013), setting in place the “double disinhibitory long-range motif” described above (Malik et al., 2021). Taken together, it will be critical to identify the IN subtypes targeted by cortical LRGNs in the future to understand the final impact of distal inhibitory projections from the cortex.
Figure 4. Identified cellular targets of cortical LRGNs. Cortical LRGNs (from HPC or neocortex) project to distal subcortical or cortical areas (yellow box), establishing synapses with different cell subtypes. Some LRGNs (black circle) target excitatory neurons (white triangles) mediating direct long-range inhibition, but the main targets are different subtypes of GABAergic neurons (gray circle). If the postsynaptic targets are PV-INs or Fast Spiking (blue), the final effect of LRGN activity is to increase the output of principal cells in the distal region. By inhibiting dendrite-targeting CCK-INs (orange), LRGNs would potentiate synaptic gain and information flow. Recent non-peer review work reveals potential VIP + targets (dark yellow), which suggests that LRGNs may also act by reducing disinhibition. Moreover, corticofugal LRGNs can target other GABAergic cells (MSNs and diverse INs) and cholinergic INs (pink), whose final impact on circuit function may be multiplicative and affect neuromodulation. Interestingly, some LRGNs like HPC-MS* have diverse targets, thus likely have multiplexed functions. Additionally, LRGNs can locally target inhibitory or excitatory neurons, as HPC-MS LRGNs in DG and callosal PV-LRGNs in the AC, respectively; presumably coordinating both local and distal activity.
The function of cortical LRGNs is also related to their activation patterns. In the HPC, LRGNs originating from CA1 SO increase their firing during immobility (Katona et al., 2017; Francavilla et al., 2018), but the activity of those in CA1 SR are better coupled to exploration-linked theta oscillations (Jinno et al., 2007), suggesting that SO residing LRGNs act during inactive states whereas SR residing LRGNs work during locomotion (Melzer and Monyer, 2020). Notably, even the immobility-associated LRGN population in SO are heterogeneous, with some but not all cells showing activity that is phase-locked to sharp-wave ripples (Katona et al., 2017; Francavilla et al., 2018). Interestingly, these HPC ripples are linked to memory consolidation in sleep (Buzsaki, 2015; Oliva et al., 2020), potentially in coordination with cortical and subcortical regions (Cox et al., 2020; Norimoto et al., 2020; Skelin et al., 2021). Thus, it is possible that HPC LRGNs may serve to synchronize activity in the HPC as well as in distributed brain regions in memory processing.
Cortical LRGNs in general may function to mediate timed cross-regional dialogue. For example, using viral tracing and optogenetic stimulation, PV + LRGNs have been found to directly connect bilateral cortical regions including the AC, VC and MC (Rock et al., 2018). In AC, these LRGNs are excited by optogenetic stimulation of the auditory thalamus (i.e., medial geniculate body) and robustly inhibit local pyramidal neurons as well. Interestingly, optogenetic activation of undifferentiated callosal projections selectively suppresses and stimulates cortico-cortical and cortico-fugal pyramidal neurons in the AC, respectively (Rock and Apicella, 2015). Although a role for long-range excitation of local INs has been demonstrated in cortico-cortical inhibition, it remains unknown whether and how cortical LRGNs may contribute to the diverging neuronal responses to callosal activity. Regardless, taken together, these findings support the idea that callosal LRGNs may mediate local and interhemispheric feedforward inhibition, synchronizing neural activity in bilateral cortical regions to promote sensory perception and motor response. It is important to note that studies employing vesicular glutamate transporter 2 staining, a classical marker for thalamocortical afferents (Kaneko and Fujiyama, 2002), have suggested that PV + callosal LRGNs (Rock et al., 2018) but not SOM + LRGNs (Tomioka et al., 2005) in MC receive feedforward inputs from the thalamus. Moreover, a recent monosynaptic circuit mapping study suggests that SOM + AC-amygdala LRGNs are excitated by local pyramidal neurons that are innervated by BLA projections (Bertero et al., 2019), thereby forming a cortico-amygdalar loop. Whether synaptic inputs selectively target specific cortical LRGNs remains to be investigated. Such efforts would help us to understand the function of these numerous cortical LRGN types.
Although the presence of LRGNs in the cortex challenges the classical view of local inhibition, evidence of their ubiquitous existence and function continues to strengthen. Cortical LRGNs are not simply conventional non-cortical GABAergic projection neurons replicated in the cortex. Canonical subcortical long-range GABAergic projections are more numerous, and even in some cases they emanate from principal cells that express calcium-calmodulin kinase IIα rather than the classical GABA-producing cell markers observed in the cortex (Erondu and Kennedy, 1985; Tepper et al., 2007; Cook et al., 2018). Nevertheless, a model is emerging in which cortical and non-cortical LRGNs have in common a preference to target other GABAergic cells that likely mediate local inhibition.
Cortical LRGNs are not simply cortical INs with long axons, but instead may exhibit distinctive synaptic targeting patterns and kinetic properties. Therefore, although INs and LRGNs share some electrophysiological and molecular similarities, it is presumptuous to think that LRGNs act like their short-range counterparts. As discussed in this review, SOM + LRGNs can deviate from the dendrite-targeting phenotype of SOM-INs in the HPC (Yuan et al., 2017), cortical PV + LRGNs show electrophysiological properties distinct from PV-INs (Lee et al., 2014; Zurita et al., 2018; Yi et al., 2021) and VIP + LRGNs in the HPC target both INs and pyramidal neurons remotely (Francavilla et al., 2018). Moreover, cortical GABAergic cells can possess both short and long axons (Gulyas et al., 2003; Yuan et al., 2017; Rock et al., 2018; Christenson Wick et al., 2019), suggesting that cortical LRGNs may comprise a subpopulation of INs and mediate both local and distant inhibition. It remains to be investigated whether all LRGNs also make short-range synapses. The impact of such dual functionality is unclear but may be beneficial for optimally synchronizing activity between distant regions.
The functional impact of cortical LRGNs on behavior is beginning to be revealed. In this regard, in vivo electrophysiological recordings have made great strides. It will be important to determine the circumstances by which these LRGNs are recruited by the network and to assess the causal relationship of their activity to performance in specific tasks. The growing availability of activity-dependent reporters, viral tracers and transgenic mice will facilitate in vivo imaging and manipulation of labeled LRGNs throughout the cortex in live animals. In addition, many of the cellular targets of the different LRGNs remain unknown, as well as their pattern of innervation, the properties of the synapses they form and how these synapses are modulated by the great variety of neurochemicals present in the brain. The combination of tracing techniques and high throughput single cell-resolution imaging technology such as light-sheet microscopy will enable the discovery of novel circuits formed by LRGNs as well as propel electrophysiological interrogation of synaptic plasticity and neuromodulation in long-range GABAergic communication. If these long-range inhibitory synapses show activity-dependent plasticity, what is the implication for learning and memory? In addition to discovering new cortical LRGNs, the next steps in research need to focus on advancing our knowledge of the known cortical LRGNs with more detailed study of their function under normal and pathological conditions.
JU-P, CM-M, and CQC conceived of the general ideas presented in this review. JU-P and CM-M drove this work to completion, contributing equally to all sections. NS-G and APE contributed to the specific subsections of the written work, provided feedback, and helped with figure construction. CQC supervised the work. All authors made significant contributions to the information content and writing of this final manuscript.
This work was funded by grants from the Chilean National Research and Development Agency (ANID) to JU-P (Doctorate Fellowship 21192112), NS-G (Doctorate Fellowship 21202528), APE (Postdoctoral Fondecyt 3190843), and CQC (Regular Fondecyt 1171840 and Millenium Institute Program P09-022F and ACE210014).
The authors declare that the research was conducted in the absence of any commercial or financial relationships that could be construed as a potential conflict of interest.
All claims expressed in this article are solely those of the authors and do not necessarily represent those of their affiliated organizations, or those of the publisher, the editors and the reviewers. Any product that may be evaluated in this article, or claim that may be made by its manufacturer, is not guaranteed or endorsed by the publisher.
We thank fellow lab members and colleagues in the Universidad de Valparaíso for critical reading and helpful constructive comments.
Abbas, A. I., Sundiang, M. J. M., Henoch, B., Morton, M. P., Bolkan, S. S., Park, A. J., et al. (2018). Somatostatin interneurons facilitate hippocampal-prefrontal synchrony and prefrontal spatial encoding. Neuron 100, 926–939.e3. doi: 10.1016/j.neuron.2018.09.029
Acsady, L., Gorcs, T. J., and Freund, T. F. (1996). Different populations of vasoactive intestinal polypeptide-immunoreactive interneurons are specialized to control pyramidal cells or interneurons in the hippocampus. Neuroscience 73, 317–334. doi: 10.1016/0306-4522(95)00609-5
Adaikkan, C., and Tsai, L. H. (2020). Gamma entrainment: impact on neurocircuits, glia, and therapeutic opportunities. Trends Neurosci. 43, 24–41. doi: 10.1016/j.tins.2019.11.001
Alexander, G. E., and Crutcher, M. D. (1990). Functional architecture of basal ganglia circuits: neural substrates of parallel processing. Trends Neurosci. 13, 266–271.
Amaral, D. G., and Witter, M. P. (1989). The three-dimensional organization of the hippocampal formation: a review of anatomical data. Neuroscience 31, 571–591. doi: 10.1016/0306-4522(89)90424-7
Bakst, I., Avendano, C., Morrison, J. H., and Amaral, D. G. (1986). An experimental analysis of the origins of somatostatin-like immunoreactivity in the dentate gyrus of the rat. J. Neurosci. 6, 1452–1462. doi: 10.1523/JNEUROSCI.06-05-01452.1986
Bariselli, S., Fobbs, W. C., Creed, M. C., and Kravitz, A. V. (2019). A competitive model for striatal action selection. Brain Res. 1713, 70–79. doi: 10.1016/j.brainres.2018.10.009
Basu, J., Zaremba, J. D., Cheung, S. K., Hitti, F. L., Zemelman, B. V., Losonczy, A., et al. (2016). Gating of hippocampal activity, plasticity, and memory by entorhinal cortex long-range inhibition. Science 351:aaa5694.
Beckstead, R. M., and Cruz, C. J. (1986). Striatal axons to the globus pallidus, entopeduncular nucleus and substantia nigra come mainly from separate cell populations in cat. Neuroscience 19, 147–158. doi: 10.1016/0306-4522(86)90012-6
Beier, K. T., Gao, X. J., Xie, S., Deloach, K. E., Malenka, R. C., and Luo, L. (2019). Topological organization of ventral tegmental area connectivity revealed by viral-genetic dissection of input-output relations. Cell Rep. 26, 159–167.e6. doi: 10.1016/j.celrep.2018.12.040
Beltran, J. Q., Reyes, S., Perez-Guzman, J. A., Elias-Vinas, D., and Gutierrez, R. (2012). Dissociation of CA3 pyramidal cells with attached, functional, identified mossy fiber and interneuronal boutons for studying glutamatergic and GABAergic synaptic transmission. J. Neurosci. Methods 208, 155–160. doi: 10.1016/j.jneumeth.2012.05.012
Berendse, H. W., and Groenewegen, H. J. (1990). Organization of the thalamostriatal projections in the rat, with special emphasis on the ventral striatum. J. Comp. Neurol. 299, 187–228. doi: 10.1002/cne.902990206
Bertero, A., Feyen, P. L. C., Zurita, H., and Apicella, A. J. (2019). A non-canonical cortico-amygdala inhibitory loop. J. Neurosci. 39, 8424–8438. doi: 10.1523/JNEUROSCI.1515-19.2019
Bertero, A., Garcia, C., and Apicella, A. J. (2021). Corticofugal VIP Gabaergic projection neurons in the mouse auditory and motor cortex. Front. Neural Circuits 15:714780. doi: 10.3389/fncir.2021.714780
Bertero, A., Zurita, H., Normandin, M., and Apicella, A. J. (2020). Auditory long-range parvalbumin cortico-striatal neurons. Front. Neural Circuits 14:45. doi: 10.3389/fncir.2020.00045
Bienvenu, T. C., Busti, D., Micklem, B. R., Mansouri, M., Magill, P. J., Ferraguti, F., et al. (2015). Large intercalated neurons of amygdala relay noxious sensory information. J. Neurosci. 35, 2044–2057. doi: 10.1523/JNEUROSCI.1323-14.2015
Bocklisch, C., Pascoli, V., Wong, J. C., House, D. R., Yvon, C., De Roo, M., et al. (2013). Cocaine disinhibits dopamine neurons by potentiation of GABA transmission in the ventral tegmental area. Science 341, 1521–1525. doi: 10.1126/science.1237059
Bonifazi, P., Goldin, M., Picardo, M. A., Jorquera, I., Cattani, A., Bianconi, G., et al. (2009). GABAergic hub neurons orchestrate synchrony in developing hippocampal networks. Science 326, 1419–1424. doi: 10.1126/science.1175509
Booker, S. A., Harada, H., Elgueta, C., Bank, J., Bartos, M., Kulik, A., et al. (2020). Presynaptic GABAB receptors functionally uncouple somatostatin interneurons from the active hippocampal network. Elife 9:e51156. doi: 10.7554/eLife.51156
Boon, J., Clarke, E., Kessaris, N., Goffinet, A., Molnar, Z., and Hoerder-Suabedissen, A. (2019). Long-range projections from sparse populations of GABAergic neurons in murine subplate. J. Comp. Neurol. 527, 1610–1620. doi: 10.1002/cne.24592
Borhegyi, Z., Varga, V., Szilagyi, N., Fabo, D., and Freund, T. F. (2004). Phase segregation of medial septal GABAergic neurons during hippocampal theta activity. J. Neurosci. 24, 8470–8479. doi: 10.1523/JNEUROSCI.1413-04.2004
Bouarab, C., Thompson, B., and Polter, A. M. (2019). VTA GABA neurons at the interface of stress and reward. Front. Neural Circuits 13:78. doi: 10.3389/fncir.2019.00078
Breton, J. M., Charbit, A. R., Snyder, B. J., Fong, P. T. K., Dias, E. V., Himmels, P., et al. (2019). Relative contributions and mapping of ventral tegmental area dopamine and GABA neurons by projection target in the rat. J. Comp. Neurol. 527, 916–941. doi: 10.1002/cne.24572
Buhl, E. H., and Singer, W. (1989). The callosal projection in cat visual cortex as revealed by a combination of retrograde tracing and intracellular injection. Exp. Brain Res. 75, 470–476. doi: 10.1007/BF00249898
Buzsaki, G. (2015). Hippocampal sharp wave-ripple: a cognitive biomarker for episodic memory and planning. Hippocampus 25, 1073–188. doi: 10.1002/hipo.22488
Buzsaki, G., and Wang, X. J. (2012). Mechanisms of gamma oscillations. Annu. Rev. Neurosci. 35, 203–225. doi: 10.1146/annurev-neuro-062111-150444
Buzsaki, G., Geisler, C., Henze, D. A., and Wang, X. J. (2004). Interneuron diversity series: circuit complexity and axon wiring economy of cortical interneurons. Trends Neurosci. 27, 186–193. doi: 10.1016/j.tins.2004.02.007
Calabresi, P., Picconi, B., Tozzi, A., Ghiglieri, V., and Di Filippo, M. (2014). Direct and indirect pathways of basal ganglia: a critical reappraisal. Nat. Neurosci. 17, 1022–1030. doi: 10.1038/nn.3743
Caputi, A., Melzer, S., Michael, M., and Monyer, H. (2013). The long and short of GABAergic neurons. Curr. Opin. Neurobiol. 23, 179–186. doi: 10.1016/j.conb.2013.01.021
Cardin, J. A. (2018). Inhibitory interneurons regulate temporal precision and correlations in cortical circuits. Trends Neurosci. 41, 689–700. doi: 10.1016/j.tins.2018.07.015
Cardin, J. A., Carlen, M., Meletis, K., Knoblich, U., Zhang, F., Deisseroth, K., et al. (2009). Driving fast-spiking cells induces gamma rhythm and controls sensory responses. Nature 459, 663–667. doi: 10.1038/nature08002
Caroni, P. (2015). Inhibitory microcircuit modules in hippocampal learning. Curr. Opin. Neurobiol. 35, 66–73. doi: 10.1016/j.conb.2015.06.010
Cassidy, R. M., Lu, Y., Jere, M., Tian, J. B., Xu, Y., Mangieri, L. R., et al. (2019). A lateral hypothalamus to basal forebrain neurocircuit promotes feeding by suppressing responses to anxiogenic environmental cues. Sci. Adv. 5:eaav1640. doi: 10.1126/sciadv.aav1640
Chen, G., Zhang, Y., Li, X., Zhao, X., Ye, Q., Lin, Y., et al. (2017). Distinct inhibitory circuits orchestrate cortical beta and gamma band oscillations. Neuron 96, 1403–1418.e6. doi: 10.1016/j.neuron.2017.11.033
Chiu, C. Q., Lur, G., Morse, T. M., Carnevale, N. T., Ellis-Davies, G. C., and Higley, M. J. (2013). Compartmentalization of GABAergic inhibition by dendritic spines. Science 340, 759–762. doi: 10.1126/science.1234274
Christenson Wick, Z., Tetzlaff, M. R., and Krook-Magnuson, E. (2019). Novel long-range inhibitory nNOS-expressing hippocampal cells. Elife 8:e46816. doi: 10.7554/eLife.46816
Code, R. A., and Winer, J. A. (1985). Commissural neurons in layer III of cat primary auditory cortex (AI): pyramidal and non-pyramidal cell input. J. Comp. Neurol. 242, 485–510. doi: 10.1002/cne.902420404
Cook, S. G., Bourke, A. M., O’leary, H., Zaegel, V., Lasda, E., Mize-Berge, J., et al. (2018). Analysis of the CaMKIIalpha and beta splice-variant distribution among brain regions reveals isoform-specific differences in holoenzyme formation. Sci. Rep. 8:5448. doi: 10.1038/s41598-018-23779-4
Cottam, J. C., Smith, S. L., and Hausser, M. (2013). Target-specific effects of somatostatin-expressing interneurons on neocortical visual processing. J. Neurosci. 33, 19567–19578. doi: 10.1523/JNEUROSCI.2624-13.2013
Cox, R., Ruber, T., Staresina, B. P., and Fell, J. (2020). Sharp wave-ripples in human amygdala and their coordination with hippocampus during NREM sleep. Cereb. Cortex Commun. 1:tgaa051. doi: 10.1093/texcom/tgaa051
Crosby, K. M., Baimoukhametova, D. V., Bains, J. S., and Pittman, Q. J. (2015). Postsynaptic depolarization enhances GABA drive to dorsomedial hypothalamic neurons through somatodendritic cholecystokinin release. J. Neurosci. 35, 13160–13170. doi: 10.1523/JNEUROSCI.3123-14.2015
Dao, N. C., Brockway, D. F., and Crowley, N. A. (2019). In vitro optogenetic characterization of neuropeptide release from prefrontal cortical somatostatin neurons. Neuroscience 419, 1–4. doi: 10.1016/j.neuroscience.2019.08.014
DeFelipe, J. (2002). Cortical interneurons: from Cajal to 2001. Prog. Brain Res. 136, 215–238. doi: 10.1016/s0079-6123(02)36019-9
Dwyer, T. A., Servatius, R. J., and Pang, K. C. (2007). Noncholinergic lesions of the medial septum impair sequential learning of different spatial locations. J. Neurosci. 27, 299–303. doi: 10.1523/JNEUROSCI.4189-06.2007
Edwards, R. H. (1998). Neurotransmitter release: variations on a theme. Curr. Biol. 8, R883–R885. doi: 10.1016/s0960-9822(07)00551-9
Engel, A. K., Fries, P., and Singer, W. (2001). Dynamic predictions: oscillations and synchrony in top-down processing. Nat. Rev. Neurosci. 2, 704–716. doi: 10.1038/35094565
Erondu, N. E., and Kennedy, M. B. (1985). Regional distribution of type II Ca2+/calmodulin-dependent protein kinase in rat brain. J. Neurosci. 5, 3270–3277. doi: 10.1523/JNEUROSCI.05-12-03270.1985
Fabri, M., and Manzoni, T. (1996). Glutamate decarboxylase immunoreactivity in corticocortical projecting neurons of rat somatic sensory cortex. Neuroscience 72, 435–448. doi: 10.1016/0306-4522(95)00568-4
Fabri, M., and Manzoni, T. (2004). Glutamic acid decarboxylase immunoreactivity in callosal projecting neurons of cat and rat somatic sensory areas. Neuroscience 123, 557–566. doi: 10.1016/j.neuroscience.2003.09.011
Fanselow, M. S., and Dong, H. W. (2010). Are the dorsal and ventral hippocampus functionally distinct structures? Neuron 65, 7–19. doi: 10.1016/j.neuron.2009.11.031
Fishell, G., and Rudy, B. (2011). Mechanisms of inhibition within the telencephalon: “where the wild things are”. Annu. Rev. Neurosci. 34, 535–567. doi: 10.1146/annurev-neuro-061010-113717
Francavilla, R., Villette, V., Luo, X., Chamberland, S., Munoz-Pino, E., Camire, O., et al. (2018). Connectivity and network state-dependent recruitment of long-range VIP-GABAergic neurons in the mouse hippocampus. Nat. Commun. 9:5043. doi: 10.1038/s41467-018-07162-5
Freund, T. F. (2003). Interneuron diversity series: rhythm and mood in perisomatic inhibition. Trends Neurosci. 26, 489–495. doi: 10.1016/S0166-2236(03)00227-3
Freund, T. F., and Antal, M. (1988). GABA-containing neurons in the septum control inhibitory interneurons in the hippocampus. Nature 336, 170–173. doi: 10.1038/336170a0
Fries, P. (2015). Rhythms for cognition: communication through coherence. Neuron 88, 220–235. doi: 10.1016/j.neuron.2015.09.034
Friston, K. (2003). Learning and inference in the brain. Neural Netw. 16, 1325–1352. doi: 10.1016/j.neunet.2003.06.005
Fuchs, E. C., Neitz, A., Pinna, R., Melzer, S., Caputi, A., and Monyer, H. (2016). Local and distant input controlling excitation in layer II of the medial entorhinal cortex. Neuron 89, 194–208. doi: 10.1016/j.neuron.2015.11.029
Gemignani, A., Paudice, P., Bonanno, G., and Raiteri, M. (1994). Pharmacological discrimination between gamma-aminobutyric acid type B receptors regulating cholecystokinin and somatostatin release from rat neocortex synaptosomes. Mol. Pharmacol. 46, 558–562.
Gerfen, C. R., and Surmeier, D. J. (2011). Modulation of striatal projection systems by dopamine. Annu. Rev. Neurosci. 34, 441–466. doi: 10.1146/annurev-neuro-061010-113641
Gonchar, Y. A., Johnson, P. B., and Weinberg, R. J. (1995). GABA-immunopositive neurons in rat neocortex with contralateral projections to S-I. Brain Res. 697, 27–34. doi: 10.1016/0006-8993(95)00746-d
Gonchar, Y., and Burkhalter, A. (1997). Three distinct families of GABAergic neurons in rat visual cortex. Cereb. Cortex 7, 347–358. doi: 10.1093/cercor/7.4.347
Gonzalez-Burgos, G., Cho, R. Y., and Lewis, D. A. (2015). Alterations in cortical network oscillations and parvalbumin neurons in schizophrenia. Biol. Psychiatry 77, 1031–1040. doi: 10.1016/j.biopsych.2015.03.010
Gordon-Fennell, A., and Stuber, G. D. (2021). Illuminating subcortical GABAergic and glutamatergic circuits for reward and aversion. Neuropharmacology 198:108725. doi: 10.1016/j.neuropharm.2021.108725
Gouwens, N. W., Sorensen, S. A., Baftizadeh, F., Budzillo, A., Lee, B. R., Jarsky, T., et al. (2020). Integrated morphoelectric and transcriptomic classification of cortical GABAergic cells. Cell 183, 935–953.e19. doi: 10.1016/j.cell.2020.09.057
Gulyas, A. I., Gorcs, T. J., and Freund, T. F. (1990). Innervation of different peptide-containing neurons in the hippocampus by GABAergic septal afferents. Neuroscience 37, 31–44. doi: 10.1016/0306-4522(90)90189-b
Gulyas, A. I., Hajos, N., Katona, I., and Freund, T. F. (2003). Interneurons are the local targets of hippocampal inhibitory cells which project to the medial septum. Eur. J. Neurosci. 17, 1861–1872. doi: 10.1046/j.1460-9568.2003.02630.x
Gutierrez, R., Romo-Parra, H., Maqueda, J., Vivar, C., Ramirez, M., Morales, M. A., et al. (2003). Plasticity of the GABAergic phenotype of the “glutamatergic” granule cells of the rat dentate gyrus. J. Neurosci. 23, 5594–5598. doi: 10.1523/JNEUROSCI.23-13-05594.2003
Hagan, J. J., Salamone, J. D., Simpson, J., Iversen, S. D., and Morris, R. G. (1988). Place navigation in rats is impaired by lesions of medial septum and diagonal band but not nucleus basalis magnocellularis. Behav. Brain Res. 27, 9–20. doi: 10.1016/0166-4328(88)90105-2
Halasy, K., and Somogyi, P. (1993). Subdivisions in the multiple GABAergic innervation of granule cells in the dentate gyrus of the rat hippocampus. Eur. J. Neurosci. 5, 411–429. doi: 10.1111/j.1460-9568.1993.tb00508.x
Han, Z. S., Buhl, E. H., Lorinczi, Z., and Somogyi, P. (1993). A high degree of spatial selectivity in the axonal and dendritic domains of physiologically identified local-circuit neurons in the dentate gyrus of the rat hippocampus. Eur. J. Neurosci. 5, 395–410. doi: 10.1111/j.1460-9568.1993.tb00507.x
Harris, K. D., and Shepherd, G. M. (2015). The neocortical circuit: themes and variations. Nat. Neurosci. 18, 170–181. doi: 10.1038/nn.3917
Hashimotodani, Y., Karube, F., Yanagawa, Y., Fujiyama, F., and Kano, M. (2018). Supramammillary nucleus afferents to the dentate Gyrus co-release glutamate and GABA and potentiate granule cell output. Cell Rep. 25, 2704–2715.e4. doi: 10.1016/j.celrep.2018.11.016
Hedreen, J. C., and DeLong, M. R. (1991). Organization of striatopallidal, striatonigral, and nigrostriatal projections in the macaque. J. Comp. Neurol. 304, 569–595. doi: 10.1002/cne.903040406
Higo, S., Akashi, K., Sakimura, K., and Tamamaki, N. (2009). Subtypes of GABAergic neurons project axons in the neocortex. Front. Neuroanat. 3:25. doi: 10.3389/neuro.05.025.2009
Higo, S., Udaka, N., and Tamamaki, N. (2007). Long-range GABAergic projection neurons in the cat neocortex. J. Comp. Neurol. 503, 421–431. doi: 10.1002/cne.21395
Horvath, T. L., Pu, S., Dube, M. G., Diano, S., and Kalra, S. P. (2001). A GABA-neuropeptide Y (NPY) interplay in LH release. Peptides 22, 473–481. doi: 10.1016/s0196-9781(01)00343-6
Hu, H., Gan, J., and Jonas, P. (2014). Interneurons. Fast-spiking, parvalbumin(+) GABAergic interneurons: from cellular design to microcircuit function. Science 345:1255263. doi: 10.1126/science.1255263
Hughes, C. M., and Peters, A. (1990). Morphological evidence for callosally projecting nonpyramidal neurons in rat visual cortex. Anat. Embryol. (Berl) 182, 591–603. doi: 10.1007/BF00186466
Huh, C. Y., Amilhon, B., Ferguson, K. A., Manseau, F., Torres-Platas, S. G., Peach, J. P., et al. (2016). Excitatory inputs determine phase-locking strength and spike-timing of CA1 Stratum Oriens/Alveus Parvalbumin and Somatostatin interneurons during intrinsically generated hippocampal theta rhythm. J. Neurosci. 36, 6605–6622. doi: 10.1523/JNEUROSCI.3951-13.2016
Ino, T., Matsuzaki, S., Shinonaga, Y., Ohishi, H., Ogawa-Meguro, R., and Mizuno, N. (1990). Direct projections of non-pyramidal neurons of Ammon’s horn to the amygdala and the entorhinal cortex. Neurosci. Lett. 115, 161–166. doi: 10.1016/0304-3940(90)90448-i
Janak, P. H., and Tye, K. M. (2015). From circuits to behaviour in the amygdala. Nature 517, 284–292.
Jayaraman, A. (1980). Anatomical evidence for cortical projections from the striatum in the cat. Brain Res. 195, 29–36. doi: 10.1016/0006-8993(80)90863-x
Jennings, J. H., Sparta, D. R., Stamatakis, A. M., Ung, R. L., Pleil, K. E., Kash, T. L., et al. (2013). Distinct extended amygdala circuits for divergent motivational states. Nature 496, 224–228. doi: 10.1038/nature12041
Jinno, S., and Kosaka, T. (2002). Immunocytochemical characterization of hippocamposeptal projecting GABAergic nonprincipal neurons in the mouse brain: a retrograde labeling study. Brain Res. 945, 219–231. doi: 10.1016/s0006-8993(02)02804-4
Jinno, S., and Kosaka, T. (2004). Parvalbumin is expressed in glutamatergic and GABAergic corticostriatal pathway in mice. J. Comp. Neurol. 477, 188–201. doi: 10.1002/cne.20246
Jinno, S., Klausberger, T., Marton, L. F., Dalezios, Y., Roberts, J. D., Fuentealba, P., et al. (2007). Neuronal diversity in GABAergic long-range projections from the hippocampus. J. Neurosci. 27, 8790–8804. doi: 10.1523/JNEUROSCI.1847-07.2007
Joshi, A., Salib, M., Viney, T. J., Dupret, D., and Somogyi, P. (2017). Behavior-dependent activity and synaptic organization of Septo-hippocampal GABAergic neurons selectively targeting the hippocampal CA3 Area. Neuron 96, 1342–1357.e5. doi: 10.1016/j.neuron.2017.10.033
Kaneko, T., and Fujiyama, F. (2002). Complementary distribution of vesicular glutamate transporters in the central nervous system. Neurosci. Res. 42, 243–250. doi: 10.1016/s0168-0102(02)00009-3
Kapfer, C., Glickfeld, L. L., Atallah, B. V., and Scanziani, M. (2007). Supralinear increase of recurrent inhibition during sparse activity in the somatosensory cortex. Nat. Neurosci. 10, 743–753. doi: 10.1038/nn1909
Katona, L., Micklem, B., Borhegyi, Z., Swiejkowski, D. A., Valenti, O., Viney, T. J., et al. (2017). Behavior-dependent activity patterns of GABAergic long-range projecting neurons in the rat hippocampus. Hippocampus 27, 359–377. doi: 10.1002/hipo.22696
Kemp, J. M., and Powell, T. P. (1971). The connexions of the striatum and globus pallidus: synthesis and speculation. Philos. Trans. R. Soc. Lond. B Biol. Sci. 262, 441–457. doi: 10.1098/rstb.1971.0106
Kilduff, T. S., Cauli, B., and Gerashchenko, D. (2011). Activation of cortical interneurons during sleep: an anatomical link to homeostatic sleep regulation? Trends Neurosci. 34, 10–19. doi: 10.1016/j.tins.2010.09.005
Kimura, F., and Baughman, R. W. (1997). GABAergic transcallosal neurons in developing rat neocortex. Eur. J. Neurosci. 9, 1137–1143. doi: 10.1111/j.1460-9568.1997.tb01467.x
Klausberger, T., and Somogyi, P. (2008). Neuronal diversity and temporal dynamics: the unity of hippocampal circuit operations. Science 321, 53–57. doi: 10.1126/science.1149381
Klausberger, T., Marton, L. F., O’neill, J., Huck, J. H., Dalezios, Y., Fuentealba, P., et al. (2005). Complementary roles of cholecystokinin- and parvalbumin-expressing GABAergic neurons in hippocampal network oscillations. J. Neurosci. 25, 9782–9793. doi: 10.1523/JNEUROSCI.3269-05.2005
Kvitsiani, D., Ranade, S., Hangya, B., Taniguchi, H., Huang, J. Z., and Kepecs, A. (2013). Distinct behavioural and network correlates of two interneuron types in prefrontal cortex. Nature 498, 363–366. doi: 10.1038/nature12176
Le Moine, C., and Bloch, B. (1995). D1 and D2 dopamine receptor gene expression in the rat striatum: sensitive cRNA probes demonstrate prominent segregation of D1 and D2 mRNAs in distinct neuronal populations of the dorsal and ventral striatum. J. Comp. Neurol. 355, 418–426. doi: 10.1002/cne.903550308
LeDoux, J. E., Farb, C. R., and Romanski, L. M. (1991). Overlapping projections to the amygdala and striatum from auditory processing areas of the thalamus and cortex. Neurosci. Lett. 134, 139–144. doi: 10.1016/0304-3940(91)90526-y
Lee, A. T., Vogt, D., Rubenstein, J. L., and Sohal, V. S. (2014). A class of GABAergic neurons in the prefrontal cortex sends long-range projections to the nucleus accumbens and elicits acute avoidance behavior. J. Neurosci. 34, 11519–11525. doi: 10.1523/JNEUROSCI.1157-14.2014
Lee, H., Goodsmith, D., and Knierim, J. J. (2020). Parallel processing streams in the hippocampus. Curr. Opin. Neurobiol. 64, 127–134. doi: 10.1016/j.conb.2020.03.004
Lee, S., Kruglikov, I., Huang, Z. J., Fishell, G., and Rudy, B. (2013). A disinhibitory circuit mediates motor integration in the somatosensory cortex. Nat. Neurosci. 16, 1662–1670. doi: 10.1038/nn.3544
Lubkemann, R., Eberhardt, J., Rohl, F. W., Janitzky, K., Nullmeier, S., Stork, O., et al. (2015). Identification and characterization of GABAergic projection neurons from ventral hippocampus to amygdala. Brain Sci. 5, 299–317. doi: 10.3390/brainsci5030299
Malik, R., Li, Y., Schamiloglu, S., and Sohal, V. S. (2021). Top-down control of hippocampal signal-to-noise by prefrontal long-range inhibition. bioRxiv [Preprint]. doi: 10.1101/2021.03.01.433441
Mandelbaum, G., Taranda, J., Haynes, T. M., Hochbaum, D. R., Huang, K. W., Hyun, M., et al. (2019). Distinct cortical-thalamic-striatal circuits through the parafascicular nucleus. Neuron 102, 636–652.e7. doi: 10.1016/j.neuron.2019.02.035
Marin, O. (2012). Interneuron dysfunction in psychiatric disorders. Nat. Rev. Neurosci. 13, 107–120. doi: 10.1038/nrn3155
Marino, R. A. M., Mcdevitt, R. A., Gantz, S. C., Shen, H., Pignatelli, M., Xin, W., et al. (2020). Control of food approach and eating by a GABAergic projection from lateral hypothalamus to dorsal pons. Proc. Natl. Acad. Sci. U. S. A. 117, 8611–8615. doi: 10.1073/pnas.1909340117
Mariotti, L., Losi, G., Lia, A., Melone, M., Chiavegato, A., Gomez-Gonzalo, M., et al. (2018). Interneuron-specific signaling evokes distinctive somatostatin-mediated responses in adult cortical astrocytes. Nat. Commun. 9:82. doi: 10.1038/s41467-017-02642-6
Markram, H., Toledo-Rodriguez, M., Wang, Y., Gupta, A., Silberberg, G., and Wu, C. (2004). Interneurons of the neocortical inhibitory system. Nat. Rev. Neurosci. 5, 793–807. doi: 10.1038/nrn1519
Mazo, C., Saha, S., Nissant, A., Peroni, E., Lledo, P. M., and Lepousez, G. (2020). Long-range GABAergic projections contribute to cortical feedback control of sensory processing. bioRxiv [Preprint]. doi: 10.1101/2020.12.19.423599
McDonald, A. J., and Zaric, V. (2015). GABAergic somatostatin-immunoreactive neurons in the amygdala project to the entorhinal cortex. Neuroscience 290, 227–242. doi: 10.1016/j.neuroscience.2015.01.028
McDonald, A. J., Mascagni, F., and Zaric, V. (2012). Subpopulations of somatostatin-immunoreactive non-pyramidal neurons in the amygdala and adjacent external capsule project to the basal forebrain: evidence for the existence of GABAergic projection neurons in the cortical nuclei and basolateral nuclear complex. Front. Neural Circuits 6:46. doi: 10.3389/fncir.2012.00046
McDonald, C. T., and Burkhalter, A. (1993). Organization of long-range inhibitory connections with rat visual cortex. J. Neurosci. 13, 768–781. doi: 10.1523/JNEUROSCI.13-02-00768.1993
McHenry, J. A., Otis, J. M., Rossi, M. A., Robinson, J. E., Kosyk, O., Miller, N. W., et al. (2017). Hormonal gain control of a medial preoptic area social reward circuit. Nat. Neurosci. 20, 449–458.
McNaughton, N., Ruan, M., and Woodnorth, M. A. (2006). Restoring theta-like rhythmicity in rats restores initial learning in the Morris water maze. Hippocampus 16, 1102–1110. doi: 10.1002/hipo.20235
Melzer, S., and Monyer, H. (2020). Diversity and function of corticopetal and corticofugal GABAergic projection neurons. Nat. Rev. Neurosci. 21, 499–515. doi: 10.1038/s41583-020-0344-9
Melzer, S., Gil, M., Koser, D. E., Michael, M., Huang, K. W., and Monyer, H. (2017). Distinct Corticostriatal GABAergic neurons modulate striatal output neurons and motor activity. Cell Rep. 19, 1045–1055. doi: 10.1016/j.celrep.2017.04.024
Melzer, S., Michael, M., Caputi, A., Eliava, M., Fuchs, E. C., Whittington, M. A., et al. (2012). Long-range-projecting GABAergic neurons modulate inhibition in hippocampus and entorhinal cortex. Science 335, 1506–1510. doi: 10.1126/science.1217139
Miles, R., Toth, K., Gulyas, A. I., Hajos, N., and Freund, T. F. (1996). Differences between somatic and dendritic inhibition in the hippocampus. Neuron 16, 815–823. doi: 10.1016/s0896-6273(00)80101-4
Mitchell, S. J., Rawlins, J. N., Steward, O., and Olton, D. S. (1982). Medial septal area lesions disrupt theta rhythm and cholinergic staining in medial entorhinal cortex and produce impaired radial arm maze behavior in rats. J. Neurosci. 2, 292–302. doi: 10.1523/JNEUROSCI.02-03-00292.1982
Miyashita, T., and Rockland, K. S. (2007). GABAergic projections from the hippocampus to the retrosplenial cortex in the rat. Eur. J. Neurosci. 26, 1193–1204. doi: 10.1111/j.1460-9568.2007.05745.x
Momiyama, T., and Zaborszky, L. (2006). Somatostatin presynaptically inhibits both GABA and glutamate release onto rat basal forebrain cholinergic neurons. J. Neurophysiol. 96, 686–694. doi: 10.1152/jn.00507.2005
Monyer, H., and Markram, H. (2004). Interneuron diversity series: molecular and genetic tools to study GABAergic interneuron diversity and function. Trends Neurosci. 27, 90–97. doi: 10.1016/j.tins.2003.12.008
Morales, M., and Margolis, E. B. (2017). Ventral tegmental area: cellular heterogeneity, connectivity and behaviour. Nat. Rev. Neurosci. 18, 73–85. doi: 10.1038/nrn.2016.165
Mountcastle, V. B. (1978). Brain mechanisms for directed attention. J. R. Soc. Med. 71, 14–28. doi: 10.1177/014107687807100105
Murayama, M., Perez-Garci, E., Nevian, T., Bock, T., Senn, W., and Larkum, M. E. (2009). Dendritic encoding of sensory stimuli controlled by deep cortical interneurons. Nature 457, 1137–1141. doi: 10.1038/nature07663
Nair-Roberts, R. G., Chatelain-Badie, S. D., Benson, E., White-Cooper, H., Bolam, J. P., and Ungless, M. A. (2008). Stereological estimates of dopaminergic, GABAergic and glutamatergic neurons in the ventral tegmental area, substantia nigra and retrorubral field in the rat. Neuroscience 152, 1024–1031. doi: 10.1016/j.neuroscience.2008.01.046
Nelson, A. B., and Kreitzer, A. C. (2014). Reassessing models of basal ganglia function and dysfunction. Annu. Rev. Neurosci. 37, 117–135. doi: 10.1146/annurev-neuro-071013-013916
Nieh, E. H., Matthews, G. A., Allsop, S. A., Presbrey, K. N., Leppla, C. A., Wichmann, R., et al. (2015). Decoding neural circuits that control compulsive sucrose seeking. Cell 160, 528–541. doi: 10.1016/j.cell.2015.01.003
Nieh, E. H., Vander Weele, C. M., Matthews, G. A., Presbrey, K. N., Wichmann, R., Leppla, C. A., et al. (2016). Inhibitory input from the lateral hypothalamus to the ventral tegmental area disinhibits dopamine neurons and promotes behavioral activation. Neuron 90, 1286–1298. doi: 10.1016/j.neuron.2016.04.035
Norimoto, H., Fenk, L. A., Li, H. H., Tosches, M. A., Gallego-Flores, T., Hain, D., et al. (2020). A claustrum in reptiles and its role in slow-wave sleep. Nature 578, 413–418. doi: 10.1038/s41586-020-1993-6
Ntamati, N. R., and Luscher, C. (2016). VTA projection neurons releasing GABA and glutamate in the dentate gyrus. eNeuro 3:ENEURO.137-16.2016. doi: 10.1523/ENEURO.0137-16.2016
Nunez-Parra, A., Maurer, R. K., Krahe, K., Smith, R. S., and Araneda, R. C. (2013). Disruption of centrifugal inhibition to olfactory bulb granule cells impairs olfactory discrimination. Proc. Natl. Acad. Sci. U. S. A. 110, 14777–14782. doi: 10.1073/pnas.1310686110
Oliva, A., Fernandez-Ruiz, A., Leroy, F., and Siegelbaum, S. A. (2020). Hippocampal CA2 sharp-wave ripples reactivate and promote social memory. Nature 587, 264–269. doi: 10.1038/s41586-020-2758-y
Pang, K. C., Jiao, X., Sinha, S., Beck, K. D., and Servatius, R. J. (2011). Damage of GABAergic neurons in the medial septum impairs spatial working memory and extinction of active avoidance: effects on proactive interference. Hippocampus 21, 835–846. doi: 10.1002/hipo.20799
Pare, D., and Duvarci, S. (2012). Amygdala microcircuits mediating fear expression and extinction. Curr. Opin. Neurobiol. 22, 717–723. doi: 10.1016/j.conb.2012.02.014
Paul, A., Crow, M., Raudales, R., He, M., Gillis, J., and Huang, Z. J. (2017). Transcriptional architecture of synaptic communication delineates GABAergic neuron identity. Cell 171, 522–539.e20. doi: 10.1016/j.cell.2017.08.032
Petilla Interneuron Nomenclature Group [PING], Ascoli, G. A., Alonso-Nanclares, L., Anderson, S. A., Barrionuevo, G., Benavides-Piccione, R., et al. (2008). Petilla terminology: nomenclature of features of GABAergic interneurons of the cerebral cortex. Nat. Rev. Neurosci. 9, 557–568. doi: 10.1038/nrn2402
Pfeffer, C. K., Xue, M., He, M., Huang, Z. J., and Scanziani, M. (2013). Inhibition of inhibition in visual cortex: the logic of connections between molecularly distinct interneurons. Nat. Neurosci. 16, 1068–1076. doi: 10.1038/nn.3446
Pi, H. J., Hangya, B., Kvitsiani, D., Sanders, J. I., Huang, Z. J., and Kepecs, A. (2013). Cortical interneurons that specialize in disinhibitory control. Nature 503, 521–524. doi: 10.1038/nature12676
Picardo, M. A., Guigue, P., Bonifazi, P., Batista-Brito, R., Allene, C., Ribas, A., et al. (2011). Pioneer GABA cells comprise a subpopulation of hub neurons in the developing hippocampus. Neuron 71, 695–709. doi: 10.1016/j.neuron.2011.06.018
Pouille, F., and Scanziani, M. (2001). Enforcement of temporal fidelity in pyramidal cells by somatic feed-forward inhibition. Science 293, 1159–1163. doi: 10.1126/science.1060342
Riedemann, T., and Sutor, B. (2019). Long-lasting actions of somatostatin on pyramidal cell excitability in the mouse cingulate cortex. Neurosci. Lett. 698, 217–223. doi: 10.1016/j.neulet.2019.01.034
Rock, C., and Apicella, A. J. (2015). Callosal projections drive neuronal-specific responses in the mouse auditory cortex. J. Neurosci. 35, 6703–6713. doi: 10.1523/JNEUROSCI.5049-14.2015
Rock, C., Zurita, H., Lebby, S., Wilson, C. J., and Apicella, A. J. (2018). Cortical circuits of callosal GABAergic neurons. Cereb. Cortex 28, 1154–1167. doi: 10.1093/cercor/bhx025
Rock, C., Zurita, H., Wilson, C., and Apicella, A. J. (2016). An inhibitory corticostriatal pathway. Elife 5:e15890. doi: 10.7554/eLife.15890
Root, D. H., Mejias-Aponte, C. A., Zhang, S., Wang, H. L., Hoffman, A. F., Lupica, C. R., et al. (2014). Single rodent mesohabenular axons release glutamate and GABA. Nat. Neurosci. 17, 1543–1551. doi: 10.1038/nn.3823
Rudy, B., Fishell, G., Lee, S., and Hjerling-Leffler, J. (2011). Three groups of interneurons account for nearly 100% of neocortical GABAergic neurons. Dev. Neurobiol. 71, 45–61. doi: 10.1002/dneu.20853
Salib, M., Joshi, A., Katona, L., Howarth, M., Micklem, B. R., Somogyi, P., et al. (2019). GABAergic medial septal neurons with low-rhythmic firing innervating the dentate gyrus and hippocampal area CA3. J. Neurosci. 39, 4527–4549. doi: 10.1523/JNEUROSCI.3024-18.2019
Saunders, A., Oldenburg, I. A., Berezovskii, V. K., Johnson, C. A., Kingery, N. D., Elliott, H. L., et al. (2015). A direct GABAergic output from the basal ganglia to frontal cortex. Nature 521, 85–89. doi: 10.1038/nature14179
Silberberg, G., and Markram, H. (2007). Disynaptic inhibition between neocortical pyramidal cells mediated by Martinotti cells. Neuron 53, 735–746. doi: 10.1016/j.neuron.2007.02.012
Skelin, I., Zhang, H., Zheng, J., Ma, S., Mander, B. A., Kim Mcmanus, O., et al. (2021). Coupling between slow waves and sharp-wave ripples engages distributed neural activity during sleep in humans. Proc. Natl. Acad. Sci. U. S. A. 118:e2012075118. doi: 10.1073/pnas.2012075118
Smith, Y., Bevan, M. D., Shink, E., and Bolam, J. P. (1998). Microcircuitry of the direct and indirect pathways of the basal ganglia. Neuroscience 86, 353–387. doi: 10.1016/s0306-4522(98)00004-9
Soden, M. E., Chung, A. S., Cuevas, B., Resnick, J. M., Awatramani, R., and Zweifel, L. S. (2020). Anatomic resolution of neurotransmitter-specific projections to the VTA reveals diversity of GABAergic inputs. Nat. Neurosci. 23, 968–980. doi: 10.1038/s41593-020-0657-z
Sohal, V. S., Zhang, F., Yizhar, O., and Deisseroth, K. (2009). Parvalbumin neurons and gamma rhythms enhance cortical circuit performance. Nature 459, 698–702. doi: 10.1038/nature07991
Song, Y. H., Hwang, Y. S., Kim, K., Lee, H. R., Kim, J. H., Maclachlan, C., et al. (2020). Somatostatin enhances visual processing and perception by suppressing excitatory inputs to parvalbumin-positive interneurons in V1. Sci. Adv. 6:eaaz0517. doi: 10.1126/sciadv.aaz0517
Sporns, O., and Betzel, R. F. (2016). Modular brain networks. Annu. Rev. Psychol. 67, 613–640. doi: 10.1146/annurev-psych-122414-033634
Squire, L. R., Genzel, L., Wixted, J. T., and Morris, R. G. (2015). Memory consolidation. Cold Spring Harb. Perspect. Biol. 7:a021766.
Sun, Q., Li, X., Ren, M., Zhao, M., Zhong, Q., Ren, Y., et al. (2019). A whole-brain map of long-range inputs to GABAergic interneurons in the mouse medial prefrontal cortex. Nat. Neurosci. 22, 1357–1370. doi: 10.1038/s41593-019-0429-9
Sun, Y., Blanco-Centurion, C., Zou, B., Bendell, E., Shiromani, P. J., and Liu, M. (2019). Amygdala GABA Neurons Project To vlPAG And mPFC. IBRO Rep. 6, 132–136. doi: 10.1016/j.ibror.2019.03.001
Takacs, V. T., Cserep, C., Schlingloff, D., Posfai, B., Szonyi, A., Sos, K. E., et al. (2018). Co-transmission of acetylcholine and GABA regulates hippocampal states. Nat. Commun. 9:2848. doi: 10.1038/s41467-018-05136-1
Takacs, V. T., Freund, T. F., and Gulyas, A. I. (2008). Types and synaptic connections of hippocampal inhibitory neurons reciprocally connected with the medial septum. Eur. J. Neurosci. 28, 148–164. doi: 10.1111/j.1460-9568.2008.06319.x
Tamamaki, N., and Tomioka, R. (2010). Long-range GABAergic connections distributed throughout the neocortex and their possible function. Front. Neurosci. 4:202. doi: 10.3389/fnins.2010.00202
Tasic, B., Yao, Z., Graybuck, L. T., Smith, K. A., Nguyen, T. N., Bertagnolli, D., et al. (2018). Shared and distinct transcriptomic cell types across neocortical areas. Nature 563, 72–78. doi: 10.1038/s41586-018-0654-5
Tepper, J. M., Abercrombie, E. D., and Bolam, J. P. (2007). Basal ganglia macrocircuits. Prog. Brain Res. 160, 3–7. doi: 10.1016/s0079-6123(06)60001-0
Tepper, J. M., Wilson, C. J., and Koos, T. (2008). Feedforward and feedback inhibition in neostriatal GABAergic spiny neurons. Brain Res. Rev. 58, 272–281. doi: 10.1016/j.brainresrev.2007.10.008
Tomioka, R., and Rockland, K. S. (2007). Long-distance corticocortical GABAergic neurons in the adult monkey white and gray matter. J. Comp. Neurol. 505, 526–538. doi: 10.1002/cne.21504
Tomioka, R., Okamoto, K., Furuta, T., Fujiyama, F., Iwasato, T., Yanagawa, Y., et al. (2005). Demonstration of long-range GABAergic connections distributed throughout the mouse neocortex. Eur. J. Neurosci. 21, 1587–1600. doi: 10.1111/j.1460-9568.2005.03989.x
Tomioka, R., Sakimura, K., and Yanagawa, Y. (2015). Corticofugal GABAergic projection neurons in the mouse frontal cortex. Front. Neuroanat. 9:133. doi: 10.3389/fnana.2015.00133
Toth, K., Borhegyi, Z., and Freund, T. F. (1993). Postsynaptic targets of GABAergic hippocampal neurons in the medial septum-diagonal band of broca complex. J. Neurosci. 13, 3712–3724. doi: 10.1523/JNEUROSCI.13-09-03712.1993
Toth, K., Freund, T. F., and Miles, R. (1997). Disinhibition of rat hippocampal pyramidal cells by GABAergic afferents from the septum. J. Physiol. 500, 463–474. doi: 10.1113/jphysiol.1997.sp022033
Totterdell, S., and Hayes, L. (1987). Non-pyramidal hippocampal projection neurons: a light and electron microscopic study. J. Neurocytol. 16, 477–485. doi: 10.1007/BF01668502
Tremblay, R., Lee, S., and Rudy, B. (2016). GABAergic interneurons in the neocortex: from cellular properties to circuits. Neuron 91, 260–292. doi: 10.1016/j.neuron.2016.06.033
Tritsch, N. X., and Sabatini, B. L. (2012). Dopaminergic modulation of synaptic transmission in cortex and striatum. Neuron 76, 33–50. doi: 10.1016/j.neuron.2012.09.023
Unal, G., Crump, M. G., Viney, T. J., Eltes, T., Katona, L., Klausberger, T., et al. (2018). Spatio-temporal specialization of GABAergic septo-hippocampal neurons for rhythmic network activity. Brain Struct. Funct. 223, 2409–2432. doi: 10.1007/s00429-018-1626-0
van den Pol, A. N. (2012). Neuropeptide transmission in brain circuits. Neuron 76, 98–115. doi: 10.1016/j.neuron.2012.09.014
Veit, J., Hakim, R., Jadi, M. P., Sejnowski, T. J., and Adesnik, H. (2017). Cortical gamma band synchronization through somatostatin interneurons. Nat. Neurosci. 20, 951–959. doi: 10.1038/nn.4562
Villette, V., Guigue, P., Picardo, M. A., Sousa, V. H., Leprince, E., Lachamp, P., et al. (2016). Development of early-born gamma-Aminobutyric acid hub neurons in mouse hippocampus from embryogenesis to adulthood. J. Comp. Neurol. 524, 2440–2461. doi: 10.1002/cne.23961
Walker, M. C., Ruiz, A., and Kullmann, D. M. (2001). Monosynaptic GABAergic signaling from dentate to CA3 with a pharmacological and physiological profile typical of mossy fiber synapses. Neuron 29, 703–715. doi: 10.1016/s0896-6273(01)00245-8
Wall, N. R., De La Parra, M., Callaway, E. M., and Kreitzer, A. C. (2013). Differential innervation of direct- and indirect-pathway striatal projection neurons. Neuron 79, 347–360. doi: 10.1016/j.neuron.2013.05.014
Wang, Y., Toledo-Rodriguez, M., Gupta, A., Wu, C., Silberberg, G., Luo, J., et al. (2004). Anatomical, physiological and molecular properties of Martinotti cells in the somatosensory cortex of the juvenile rat. J. Physiol. 561, 65–90. doi: 10.1113/jphysiol.2004.073353
Whitlock, J. R., Heynen, A. J., Shuler, M. G., and Bear, M. F. (2006). Learning induces long-term potentiation in the hippocampus. Science 313, 1093–1097. doi: 10.1126/science.1128134
Witter, M. P., Doan, T. P., Jacobsen, B., Nilssen, E. S., and Ohara, S. (2017). Architecture of the entorhinal cortex A review of entorhinal anatomy in rodents with some comparative notes. Front. Syst. Neurosci. 11:46. doi: 10.3389/fnsys.2017.00046
Xu, X., Roby, K. D., and Callaway, E. M. (2010). Immunochemical characterization of inhibitory mouse cortical neurons: three chemically distinct classes of inhibitory cells. J. Comp. Neurol. 518, 389–404. doi: 10.1002/cne.22229
Yamawaki, N., Li, X., Lambot, L., Ren, L. Y., Radulovic, J., and Shepherd, G. M. G. (2019). Long-range inhibitory intersection of a retrosplenial thalamocortical circuit by apical tuft-targeting CA1 neurons. Nat. Neurosci. 22, 618–626. doi: 10.1038/s41593-019-0355-x
Yassa, M. A., and Stark, C. E. (2011). Pattern separation in the hippocampus. Trends Neurosci. 34, 515–525.
Yi, F., Garrett, T., Deisseroth, K., Haario, H., Stone, E., and Lawrence, J. J. (2021). Septohippocampal transmission from parvalbumin-positive neurons features rapid recovery from synaptic depression. Sci. Rep. 11:2117.
Yuan, M., Meyer, T., Benkowitz, C., Savanthrapadian, S., Ansel-Bollepalli, L., Foggetti, A., et al. (2017). Somatostatin-positive interneurons in the dentate gyrus of mice provide local- and long-range septal synaptic inhibition. Elife 6:e21105. doi: 10.7554/eLife.21105
Zappone, C. A., and Sloviter, R. S. (2001). Commissurally projecting inhibitory interneurons of the rat hippocampal dentate gyrus: a colocalization study of neuronal markers and the retrograde tracer Fluoro-gold. J. Comp. Neurol. 441, 324–344. doi: 10.1002/cne.1415
Zeisel, A., Munoz-Manchado, A. B., Codeluppi, S., Lonnerberg, P., La Manno, G., Jureus, A., et al. (2015). Brain structure. Cell types in the mouse cortex and hippocampus revealed by single-cell RNA-seq. Science 347, 1138–1142. doi: 10.1126/science.aaa1934
Zhou, F. W., and Puche, A. C. (2021). Short-term plasticity in cortical GABAergic synapses on olfactory bulb granule cells is modulated by endocannabinoids. Front. Cell Neurosci. 15:629052. doi: 10.3389/fncel.2021.629052
Keywords: long-range GABAergic axons, inhibitory connections, disinhibition, hippocampus, neocortex, cortico-cortical, corticofugal
Citation: Urrutia-Piñones J, Morales-Moraga C, Sanguinetti-González N, Escobar AP and Chiu CQ (2022) Long-Range GABAergic Projections of Cortical Origin in Brain Function. Front. Syst. Neurosci. 16:841869. doi: 10.3389/fnsys.2022.841869
Received: 22 December 2021; Accepted: 10 February 2022;
Published: 22 March 2022.
Edited by:
Edward Zagha, University of California, Riverside, United StatesReviewed by:
Alice Bertero, The University of Texas at San Antonio, United StatesCopyright © 2022 Urrutia-Piñones, Morales-Moraga, Sanguinetti-González, Escobar and Chiu. This is an open-access article distributed under the terms of the Creative Commons Attribution License (CC BY). The use, distribution or reproduction in other forums is permitted, provided the original author(s) and the copyright owner(s) are credited and that the original publication in this journal is cited, in accordance with accepted academic practice. No use, distribution or reproduction is permitted which does not comply with these terms.
*Correspondence: Chiayu Q. Chiu, Y2hpYXl1LmNoaXVAdXYuY2w=
†These authors have contributed equally to this work and share first authorship
Disclaimer: All claims expressed in this article are solely those of the authors and do not necessarily represent those of their affiliated organizations, or those of the publisher, the editors and the reviewers. Any product that may be evaluated in this article or claim that may be made by its manufacturer is not guaranteed or endorsed by the publisher.
Research integrity at Frontiers
Learn more about the work of our research integrity team to safeguard the quality of each article we publish.