- 1Department of Pharmacology and Experimental Therapeutics, Boston University School of Medicine, Boston, MA, United States
- 2Molecular and Translational Medicine, Department of Medicine, Boston University School of Medicine, Boston, MA, United States
- 3Department of Psychiatry, University of Pittsburgh School of Medicine, Pittsburgh, PA, United States
- 4Department of Cell Biology, University of Pittsburgh School of Medicine, Pittsburgh, PA, United States
- 5Center for Systems Neuroscience, Boston University, Boston, MA, United States
Despite the prevalence of opioid misuse, opioids remain the frontline treatment regimen for severe pain. However, opioid safety is hampered by side-effects such as analgesic tolerance, reduced analgesia to neuropathic pain, physical dependence, or reward. These side effects promote development of opioid use disorders and ultimately cause overdose deaths due to opioid-induced respiratory depression. The intertwined nature of signaling via μ-opioid receptors (MOR), the primary target of prescription opioids, with signaling pathways responsible for opioid side-effects presents important challenges. Therefore, a critical objective is to uncouple cellular and molecular mechanisms that selectively modulate analgesia from those that mediate side-effects. One such mechanism could be the transactivation of receptor tyrosine kinases (RTKs) via MOR. Notably, MOR-mediated side-effects can be uncoupled from analgesia signaling via targeting RTK family receptors, highlighting physiological relevance of MOR-RTKs crosstalk. This review focuses on the current state of knowledge surrounding the basic pharmacology of RTKs and bidirectional regulation of MOR signaling, as well as how MOR-RTK signaling may modulate undesirable effects of chronic opioid use, including opioid analgesic tolerance, reduced analgesia to neuropathic pain, physical dependence, and reward. Further research is needed to better understand RTK-MOR transactivation signaling pathways, and to determine if RTKs are a plausible therapeutic target for mitigating opioid side effects.
Introduction
The opioid epidemic has reached unprecedented proportions globally. In the United States alone, overdoses caused by opioids have claimed the lives of over hundreds of thousands of people, with rates of lethal overdoses expected to double in the next five years (Holland et al., 2021; Pickard and Lee, 2021). Prescription opioids are major contributors to the current opioid crisis, despite serving as the mainstay treatment for severe and chronic pain. Safe use of opioids is hampered by potentially severe side-effects including respiratory depression and the development of dependence and addiction (Benyamin et al., 2008; Pattinson, 2008; Henry et al., 2015; Hayhurst and Durieux, 2016; Algera et al., 2019). Emergence of these side-effects is promoted by escalating doses of opioids in chronic pain patients to mitigate the development of analgesic tolerance (Collett, 1998; Benyamin et al., 2008; Henry et al., 2015). High opioid doses are also necessary in neuropathic pain patients to overcome the minimal analgesic efficacy of current opioid-based therapies (Przewlocki and Przewlocka, 2001; Balayssac et al., 2009; Donica et al., 2014; Puig et al., 2020b). Such chronically high opioid doses promote physical dependence, causing deleterious physiological symptoms upon opioid withdrawal (Azolosa et al., 1994; Epstein et al., 2006; Burma et al., 2017), and ultimately prevents the discontinuation of opioid treatment. As a result, patients are forced to choose between effective pain treatments and the risk of physical dependence and/or addiction. With high doses, patients also risk developing respiratory depression (decreased respiration), the main cause of overdoses death (Pattinson, 2008; Algera et al., 2019). Opioid addiction has resulted in severe social and steep economic costs of hundreds of billions of dollars annually (The Council of Economic Advisers, 2017) and spurred a growing effort on finding new strategies to treat pain effectively and safely. One focus is toward finding a safe and “ideal” analgesic drug that would be free of addiction potentiating side-effects and have a low lethality. Unfortunately, to date, no safer alternative with equal analgesic efficacy to opioids has been found (Stuart et al., 2018). Many other proposed strategies involve reducing opioid dosage by locally targeting injured tissue (and limit central penetration), or reducing opioid prescriptions including establishing multimodal pain treatment regimens (as opposed to opioid monotherapy), opioid prescription monitoring, and restricted prescribing guidelines (Saloner et al., 2018; Mir et al., 2019; Franz et al., 2021). Yet this has not been enough. Therefore, it is imperative to continue efforts toward preserving long-term opioid analgesia, while mitigating side-effects. To this end, a better understanding of the molecular mechanisms underlying opioid signaling is needed.
Opioid receptors currently characterized include μ-opioid receptor (MOR), κ-opioid receptor (KOR), δ-opioid receptor (DOR), and opioid receptor like-1 (ORL1). These opioid receptors (ORs) belong to the class A (rhodopsin family) family of G protein-coupled receptors (GPCRs) which are coupled to inhibitory Gαi/o G proteins. These GPCRs function to reduce neuronal excitability primarily by increasing potassium conductance and inhibiting voltage-gated calcium channels (Al-Hasani and Bruchas, 2011). Prescription opioids specifically modulate analgesia through MOR (Matthes et al., 1996; Loh et al., 1998), which is concentrated in structures essential for conductance of pain-related signaling including peripheral sensory neurons, spinal cord, brainstem and central brain nuclei (Mansour et al., 1994a,b, 1995a,1995b; Basbaum et al., 2009; Scherrer et al., 2009). Activation of MOR expressed on pain processing neurons via endogenous (e.g., endorphin) or exogenous (e.g., morphine or fentanyl) opioids directly inhibits these cells’ activity and controls analgesia (Al-Hasani and Bruchas, 2011).
Mechanisms of opioid analgesic tolerance and side-effects are still poorly understood (Adhikary and Williams, 2022). Traditionally, tolerance was thought to occur via the direct modulation of MOR signaling and trafficking (Williams et al., 2013). More recent evidence suggests that MOR-mediated side-effects can be uncoupled from analgesia, suggesting distinct signaling pathways for opioid-induced side effects versus analgesia (Puig and Gutstein, 2017; Paul et al., 2021). Separable pathways suggests that specific therapeutic strategies can be developed to selectively target side-effects without altering analgesia. This is further complicated by the fact that, apart from observational clinical studies, in contrast to animal experiments, practically no rigorously controlled clinical trials have unequivocally demonstrated pharmacodynamic tolerance to opioids in human patients (Collett, 1998; Henry et al., 2015), hampering the clinical translatability of earlier preclinical models.
Though the precise mechanisms for the above opioid-related signaling pathways remain to be determined, important clues have emerged which involve the receptor tyrosine kinase (RTK) family (Wang et al., 2012). More specifically, RTK signaling selectively regulates analgesic tolerance to MOR selective agonists (Puig et al., 2020a,b). Emerging evidence also suggests that RTKs could be involved in reduced opioid analgesia against neuropathic pain (Donica et al., 2014; Puig et al., 2020b), physical dependence (Rezamohammadi et al., 2020; Dorval et al., 2022), and reward (Koo et al., 2014; Fetterly et al., 2021). Together, these studies suggest that targeting opioid side-effects with RTK inhibitors could constitute a promising strategy to improve opioid safety. This review summarizes current knowledge about signaling interactions and crosstalk between MORs and RTKs. Furthermore, we discuss the implications of these mechanisms in opioid-mediated side-effects, with a focus on tolerance, reduced neuropathic pain analgesia, physical dependence, and reward. Finally, we discuss the potential clinical use of RTK inhibitors. Though RTK inhibitors are FDA-approved cancer chemotherapy drugs (Karaman et al., 2008; Gialeli et al., 2014; Roskoski, 2018), we present the possibility that these medications can be repurposed as a novel therapy for chronic pain and to improve opioid safety.
Overview of mu-opioid receptor signaling
Brief overview of mu opioid receptor signaling transduction pathways
As a canonical GPCR, MOR recruits Gαi/o G proteins upon stimulation. These inhibitory G proteins are composed of a monomeric αi/o subunit and a dimeric Gβγ complex and are characterized by their sensitivity to pertussis toxin (Connor and Christie, 1999). At rest, the G proteins exist as an inactive Gα/βγ heterotrimeric complex that is GDP-bound. However, upon receptor activation by opioid ligands, changes in receptor conformation lead to the dissociation of Gα and Gβγ subunits via GDP/GTP exchange, which triggers intracellular signaling through downstream signaling effectors (Figure 1A). Canonical signaling pathways of Gαi/o include inhibition of adenylyl cyclase (AC), the enzyme responsible for production of cyclic adenosine monophosphate (cAMP)—a critical second messenger of ORs. The resulting decrease in intracellular cAMP diminishes activity of protein kinase A (PKA) and PKA-dependent processes including activation of the C-AMP Response Element-binding protein (CREB) transcription factor. Gαi/o signaling also positively regulates the activity of G protein-gated inwardly rectifying potassium (GIRK) channels, causing cellular hyperpolarization (Navarro et al., 1996). In parallel, Gβγ negatively regulates Ca2+ currents via inhibition of P/Q-type, N-type, or L-type Ca2+ channels, further contributing to overall inhibition of cellular activity (for review see: (Al-Hasani and Bruchas, 2011; Williams et al., 2013)). To illustrate, in pain circuitry, release of Gβγ subunits in presynaptic neurons results in inhibition of N-type Ca2+ channels for negatively modulating neurotransmitter release, while Gβγ subunits in postsynaptic neurons activate GIRKs, preventing neuronal depolarization (Chieng and Christie, 1994; Zamponi et al., 1997). Together, these mechanisms activated by MOR agonists result in analgesia via modulation of neuronal transmission in circuits conveying nociception. Following G protein signal transduction, G protein receptor kinases (GRKs) are recruited for phosphorylation of MOR on 11 potential phosphorylation sites present on the carboxyl terminal domain of the receptor, including serine (S), threonine (T), and tyrosine (Y) residues (Doll et al., 2011; Lau et al., 2011). Several GRKs (e.g., GRK2, GRK3, GRK5, GRK6) selectively phosphorylate different MOR phosphorylation sites, modulating signal transduction in a ligand and context-dependent manner (Lemel et al., 2020). Of note, other kinases, such as protein kinase C (PKC) or calcium/calmodulin-dependent protein kinase II (CaMKII), also phosphorylate MOR on selective phosphorylation sites in a ligand-dependent manner (Kelly et al., 2008). Additionally, MOR phosphorylation initiates receptor desensitization via receptor recruitment of β-arrestin2 (Figure 1B; Whistler and Von Zastrow, 1998; Martini and Whistler, 2007). This activates clathrin-mediated endocytosis of the MOR-β-arrestin2 complex, resulting in MOR internalization and recycling which terminates receptor signaling at the plasma membrane. The MOR-β-arrestin2 complex also recruits specific transduction signal proteins including kinases such as src, phosphoinositide 3-kinases (PI3K), or Mitogen-Activated Protein Kinases (MAPK), including extracellular signal-regulated kinases 1 and 2 (ERK 1 and 2), or c-Jun N- terminal Kinases (JNK) 1–3 (Pierce et al., 2001) (for full review of pathways see Williams et al., 2013; Jean-Charles et al., 2017). Finally, MOR signaling can be terminated by degradation via ubiquitination pathways (Chaturvedi et al., 2001; Petäjä-Repo et al., 2001).
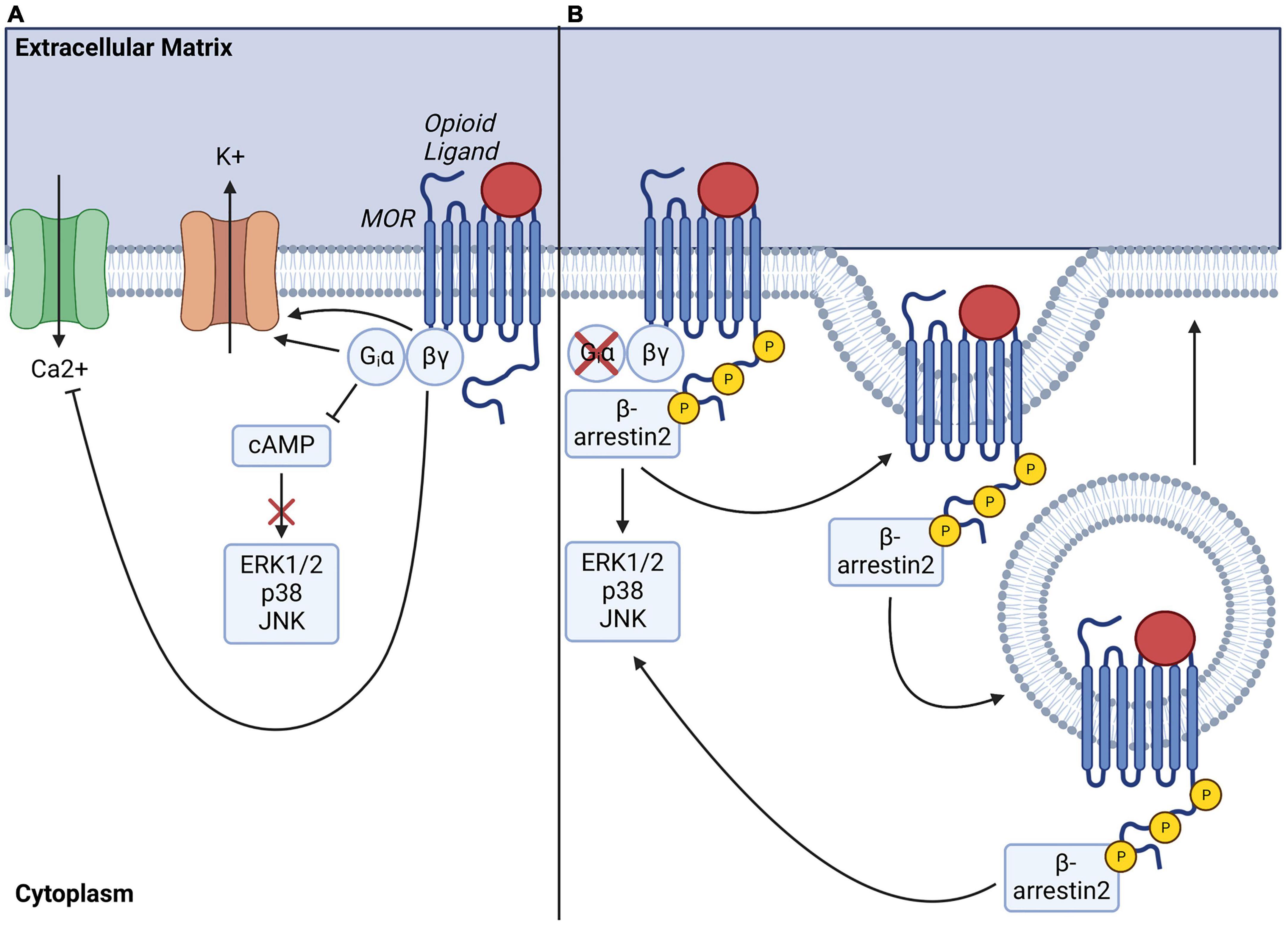
Figure 1. Mu-opioid receptor (MOR) signaling transduction pathways, internalization, and recycling. (A) Ligand-activation of MOR activates Gαi/o-induced inhibition of adenylate cyclase, resulting in decreased intracellular cAMP levels and depleted downstream signaling. Gαi/o also serves to activate G protein gated inwardly rectifying potassium channels, leading to efflux of potassium ions while βγ heterodimers simultaneously perturb calcium influx by inhibiting voltage gated calcium channels, overall inhibiting intracellular signaling and cellular activity. (B) Ligand-activation of MOR also eventually leads to phosphorylation of MOR c-terminal tail by G protein receptor kinases (GRKs), which enables docking of β-arrestin2 and initiates MOR endocytosis for further receptor degradation or recycling. Note that recruitment of β-arrestin2 can also drive activation of downstream signaling effectors, including ERK, p38 or JNK pathways.
Proposed mechanisms of opioid-mediated side-effects
Mu-opioid receptor signaling is essential for opioids to induce analgesia and their side-effects, as global deletion of the gene encoding MOR (Oprm1) completely blocks opioid analgesia, reward, and physical dependence in rodents (Matthes et al., 1996; Loh et al., 1998). Indeed, most signaling pathways downstream of MOR are critical for the development and maintenance of opioid side-effects (Al-Hasani and Bruchas, 2011; Williams et al., 2013; Allouche et al., 2014; Zhou et al., 2021). Historically, mechanisms explaining side-effects of morphine were generalized to all MOR ligands, however, it has been difficult to find a single unifying mechanism that could explain side-effect profiles shared by all MOR agonists (Raehal and Bohn, 2011; Raehal et al., 2011; Whistler, 2012). This is likely since MOR ligands differ in their potencies, pharmacokinetics, and receptor internalization. Such drug-specific differences may also lead to varying recruitment of signaling effectors and pathways (Duttaroy and Yoburn, 1995; Keith et al., 1996, 1998; Trafton et al., 2000; Bohn et al., 2004; Kenakin, 2011; Posa et al., 2016; Schmid et al., 2017). Relatedly, different MOR ligands can stabilize the receptor in distinct conformations unique to each drug. As a result, different ligands can preferentially activate distinct signaling cascades that are biased toward either G protein versus β-arrestin2 pathways (Alvarez et al., 2002; Kenakin, 2011). Biased signaling downstream of MOR was proposed to drive the distinction between opioid side-effects and analgesia. In such a model, β-arrestin2 signaling preferentially mediates opioid-induced side effects while G protein signaling preferentially mediates the analgesic properties of these drugs (Bohn et al., 1999, 2000, 2002, 2003, 2004; Raehal and Bohn, 2011; Schmid et al., 2017). Consequently, much research has focused on identifying opioid ligands with higher intrinsic efficacy for stimulating G protein signaling downstream of MOR, while not triggering activation of the β-arrestin2 pathway (Soergel et al., 2014; Manglik et al., 2016). Although several G protein-biased compounds provide efficacious analgesia (Singla et al., 2019; Viscusi et al., 2019), adverse effects remain (Hill et al., 2018; Conibear and Kelly, 2019). Additionally, despite different signaling bias, all prescription opioids cause side-effects such as tolerance. G protein-biased MOR ligands thus cannot fully explain the mechanisms responsible for analgesia versus side-effects (Gillis et al., 2020).
Ultimately, a major thrust of opioid research is to uncouple the signaling mechanisms that selectively regulate analgesia from mechanisms that regulate undesired side-effects. New approaches proposing mechanisms that may not involve traditional canonical MOR signaling pathways may be key in addressing this issue. We propose that RTK signaling may be a common signaling pathway recruited downstream of MOR by all opioid agonists beyond their signaling bias. Here, we will present evidence suggesting that RTK signaling selectively modulates opioid side-effects but not analgesia. Therefore, we hypothesize that targeting RTKs offers a novel strategy to prevent and/or treat opioid side-effects without altering analgesia a critical objective for the field.
Overview of receptor tyrosine kinase signaling
Receptor tyrosine kinases are a subclass of tyrosine kinases expressed at the cell surface which respond with high affinity to selective soluble polypeptide growth factors, cytokines, and hormones. RTKs constitute 20 sub-families (Robinson et al., 2000), including the ErbB family comprising the epidermal growth factor receptor (EGFR), platelet-derived growth factor receptor family (PDGFR), vascular-endothelial growth factor receptor family (VEGFR), tropomyosin receptor family (Trk), fibroblast growth factor receptor family (FGFR), ephrin receptor family (EphR), and insulin receptor family (IR) (Table 1). Structurally, RTKs are composed of single transmembrane glycoproteins, with the N-terminal extracellular domain containing the ligand-binding sequence, and the C-terminal intracellular domain containing multiple tyrosine residues which form the protein kinase catalytic core of these receptors (Du and Lovly, 2018; Figure 2). Ligand activation of RTKs elicits non-covalent oligomerization of monomeric RTKs and promotes formation of homo- or heterodimers. This process leads to trans-autophosphorylation (Honegger et al., 1989; Favelyukis et al., 2001) of key tyrosine residues on the interacting receptors. This activates downstream signaling via recruitment of selective docking proteins possessing Src homology-2 (SH2) and phosphotyrosine-binding (PTB) domains (Pawson, 2004); SH2 and PTB-domain-containing proteins include insulin receptor substrate-1 (IRS1), Grb2-associated binder (Gab1), and FGFR substrate 2 (FRS2α/FRS2β). These downstream proteins, lacking intrinsic kinase activity, serve as scaffolds to organize signaling complexes and trigger intracellular signaling cascades. Most docking proteins like Gab1 can be recruited by multiple RTKs. However, some are specific to a subset of receptors. For example, FRS2α and FRS2β are only involved in FGFR-, and Trk-mediated signaling (Schlessinger, 2000). This confers activation of specific signaling pathways by different subsets of RTKs and possibly enables signaling specificity. Pathways activated following docking protein recruitment include phospholipase Cγ (PLCγ), phosphoinositide 3-kinases (PI3K), mitogen-activated protein kinase/p38 (MAPK/p38), Ras-GTPase-activating protein (Ras-GAP), Janus kinase/signal transducer and activator of transcription (JAK/STAT), proto-oncogene c-Src, or focal adhesion kinase (FAK) signaling cascades (for a review see: (Lemmon and Schlessinger, 2010; Du and Lovly, 2018).
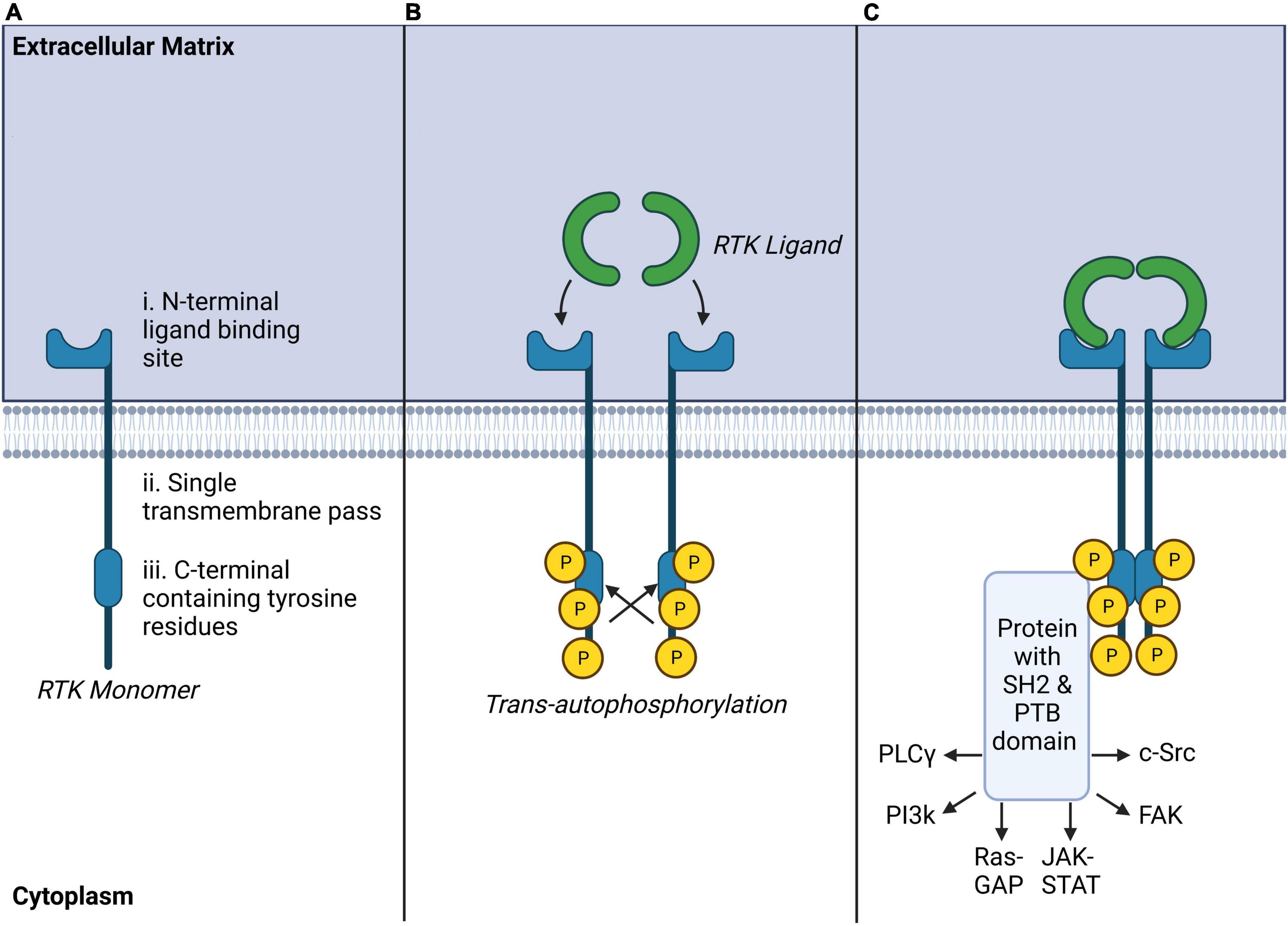
Figure 2. Receptor tyrosine kinase (RTK) structure, ligand binding and autophosphorylation, and common downstream signaling pathways. (A) RTK monomers are single transmembrane crossing peptides with extracellular ligand binding sites and tyrosine-rich intracellular effector regions. (B) RTK ligands bind as homo or heterodimers to RTKs inducing trans-autophosphorylation of opposing intracellular tyrosine residues. (C) Ligand-bound RTKs typically recruit protein complexes with SH2 and PTB domains which may activate a number of secondary intracellular messengers known to modulate other transmembrane receptors, intracellular signaling, or transcriptional regulation.
Historically, RTK signaling pathways were found to be involved in cell proliferation, differentiation, migration, or metabolic changes (Lemmon and Schlessinger, 2010), and were also associated with cancer development (Du and Lovly, 2018). Most, if not all, RTK signaling effectors are also activated by opioid receptors. Numerous protein kinases including, ERK, JNK, p38, PKC, AKT, and CaMKII are utilized by both MOR and RTKs (Lemmon and Schlessinger, 2010; Williams et al., 2013). The ability of multiple receptors to concurrently activate signaling effectors raises the possibility of complex crosstalk between these receptors or even receptor cross-activation by the same molecule. Importantly, nearly all downstream pathways utilized by RTK receptors including MAP kinase cascades (Mckay and Morrison, 2007), PI3K (Haglund et al., 2007), PKC (Heckman and Wade, 2018), Akt (Choudhary et al., 2009), or ubiquitination (Haglund et al., 2003) play roles in opioid signaling in analgesia, tolerance, and dependence. It remains unclear, however, how these pathways pertain to opioid behaviors and side-effects (Mouledous et al., 2007; Chen et al., 2008a; Macey et al., 2009; Wang et al., 2009; Gregus et al., 2010). These discrepancies could be related to cellular context and, most importantly, they may involve modulation of signaling via differential engagement of RTK signaling in response to specific opioids.
Mu-opioid receptors-receptor tyrosine kinases crosstalk
General mechanisms of G protein-coupled receptors-receptor tyrosine kinases transactivation
Crosstalk between GPCRs and RTKs can amplify signaling pathways downstream of one or both receptors in a process known as GPCR-RTK transactivation (Daub et al., 1996). This mechanism enables the integration of signal transduction between GPCRs and RTK signaling networks at signaling hubs shared between the respective receptor signaling pathways (Ragunathrao et al., 2019). Two major pathways of GPCR-RTK transactivation have been identified which involve either extracellular RTK ligand release (ligand-dependent) or intracellular recruitment of signaling effectors such as phosphotyrosine kinases (ligand-independent) (for review see Wetzker and Bohmer, 2003; Figure 3.
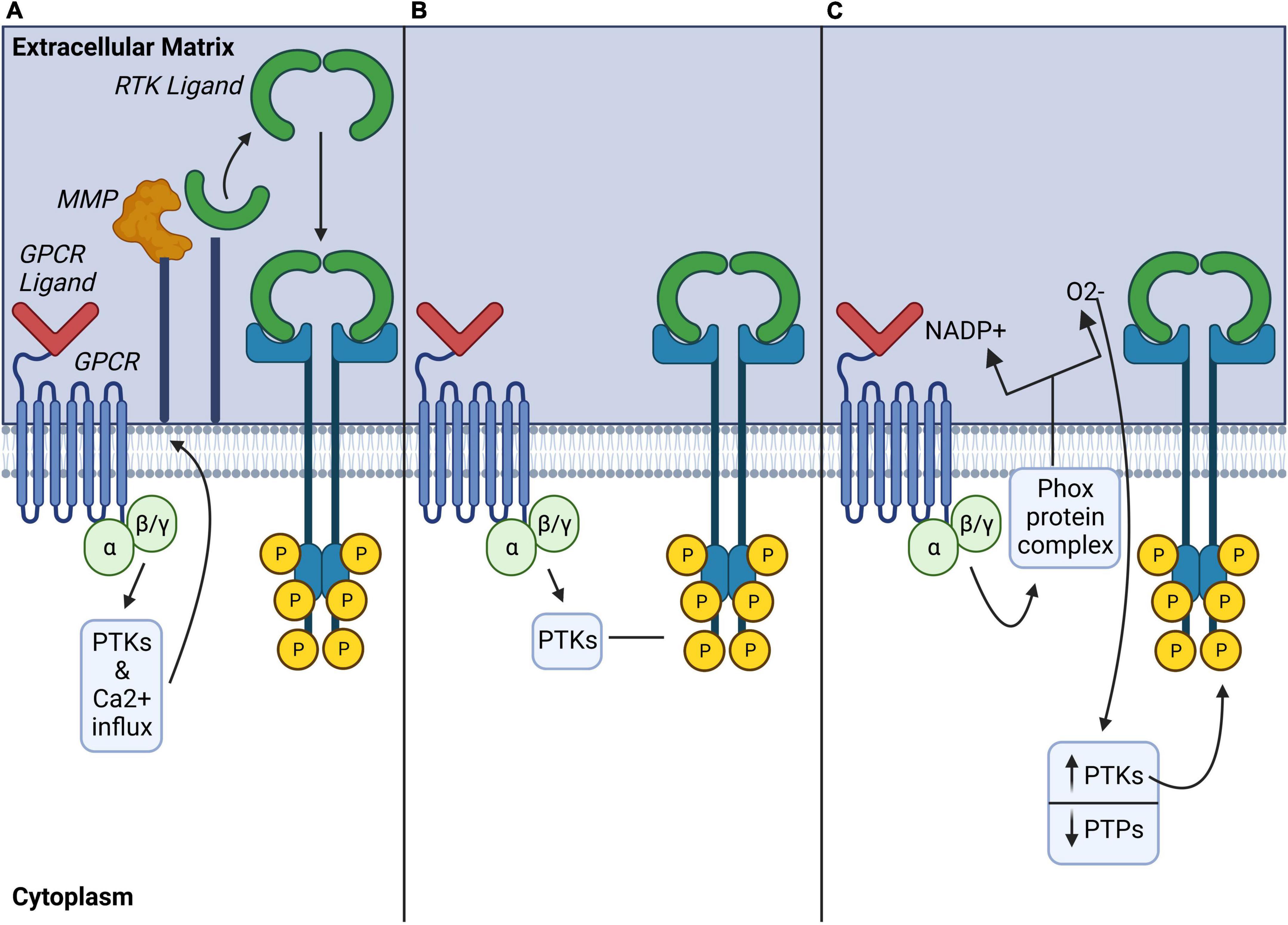
Figure 3. Ligand-dependent, ligand-independent, and atypical mechanisms of GPCR modulation of RTKs. (A) Ligand-dependent transactivation: Activated GPCRs induce a variety of downstream signaling pathways including activation of phospho-tyrosine kinases (PTKs), or increase of the influx of Ca2+, which activates matrix metalloproteinases (MMP) to cleave cell membrane-bound RTK ligands. (B) Ligand-independent transactivation: Activated GPCRs may also recruit intracellular PTKs to directly phosphorylate tyrosine residues on the intracellular domain of RTKs and induce their activation in a ligand-independent manner. (C) Atypical transactivation: Phox protein complexes activated by GPCRs generate reactive oxygen species which modulate phospho-tyrosine kinases (PTK) and phosphotyrosine phosphatases (PTP) activity to promote phosphorylation of intracellular RTK tyrosine residues. GPCR, G protein coupled receptor; MMP, matrix metalloproteinase; PTK, phosphotyrosine-kinase; PTP, phosphotyrosine phosphatases.
Ligand-dependent transactivation requires GPCR activation of matrix regulatory proteins such as membrane-bound matrix metalloproteinases (MMPs) or A Disintegrin and Metalloproteases (ADAMs) which contribute to the shedding of ligands. Several different MMPs or ADAMs are involved in the proteolytic ectodomain shedding of membrane bound RTK ligands from the extracellular matrix (ECM), which in turn, may transactivate several different RTKs (Cattaneo et al., 2014). This has been mostly described for EGFR as MMPs can cleave the heparin binding EGFR (Hb-EGF) to activate EGFR (Kilpatrick and Hill, 2021). Though the precise mechanisms of GPCR-mediated activation of MMPs or ADAMS are not fully understood, studies have implicated kinases such as c-src and PKC or calcium influx as activators of these proteases Figure 3A, for review, see Cattaneo et al. (2014). Notably, GPCR effectors like Gβγ (Overland and Insel, 2015) and β-arrestin2 (Noma et al., 2007; Oligny-Longpré et al., 2012).
Ligand-independent transactivation pathways involve complex intracellular signaling cascades which recruit kinases like Src or PI3K (Di Liberto et al., 2019) to phosphorylate selective tyrosine residues on RTKs (Figure 3B). This mode of GPCR-RTK transactivation can also require association of the two receptors via protein complex formation (for review see Wetzker and Bohmer, 2003). Of importance, GPCR-RTK heterodimerization may completely change GPCR signal transduction mechanisms and even promote a switch in the associated G protein. This is of particular interest because MOR signals via pertussis-toxin-insensitive stimulatory Gαs proteins following chronic morphine exposure or neuropathic pain (Chakrabarti et al., 2005, 2010; Chakrabarti and Gintzler, 2007; Tsai et al., 2009). Therefore, involvement of RTKs in G protein switching downstream of MOR is a possibility that remains to be investigated.
Other ligand-independent transactivation involves atypical mechanisms of GPCR-RTK crosstalk via reactive oxygen species (ROS), such as nitric oxide (NO) (Figure 3C). ROS production by GPCRs could block protein-tyrosine phosphatases, activate phosphotyrosine kinases and modulate phosphorylation of RTK tyrosine residues (Cattaneo et al., 2014). Such a mechanism may be particularly relevant to opioid actions since ROS modulate MOR-mediated behaviors in rodents (Doyle et al., 2013). This therefore raises the possibility that RTKs could be involved in these ROS-mediated signaling pathways.
Mu-opioid receptors-receptor tyrosine kinases transactivation in vitro
Most in vitro studies investigating RTK transactivation by MORs have focused on EGFR or PDGFRβ. In immortalized cell lines transfected with MOR, acute treatment with selective MOR agonists such as [D-Ala(2),MePhe(4),Gly-ol(5)]enkephalin (DAMGO) or morphine resulted in transactivation of EGFR (Belcheva et al., 2001, 2003, 2005; Phamduong et al., 2014) or PDGFRβ (Weber et al., 2013) as shown by phosphorylation of these RTKs. Interestingly, transactivation of RTKs by MOR activates downstream effector signaling at levels comparable to activation to direct activation of the RTKs themselves (Belcheva et al., 2001; Weber et al., 2013; Phamduong et al., 2014), and MOR-RTK transactivation can be abolished by pre-treatment with selective RTK inhibitors (Belcheva et al., 2001; Chen et al., 2008b; Weber et al., 2013). In cultured cells, mechanisms of MOR-EGFR and MOR-PDGFRβ transactivation were shown to require release of EGF (Belcheva et al., 2001, 2003, 2005; Phamduong et al., 2014) or of PDGF-B (Wang et al., 2012; Weber et al., 2013), respectively. Consistent with mechanisms of ligand-dependent GPCR-RTK transactivation, MOR-EGFR and MOR-PDGFRβ transactivation also require MMP activity (Belcheva et al., 2001). MMP activation by MOR may involve calmodulin (CaM), a Ca2+ sensor and binding protein. In resting conditions, CaM prevents MMP activity at the plasma membrane (PM) in HEK293 cells (Belcheva et al., 2001). Acute treatment with MOR agonist DAMGO promotes CaM translocation from the plasma membrane (PM) to MOR intracellular domains, lifting CaM inhibition on MMP via mechanisms involving activation of phospholipase C (PLC) and PKCε signaling (Belcheva et al., 2001, 2005; Miyatake et al., 2009; Figure 4A).
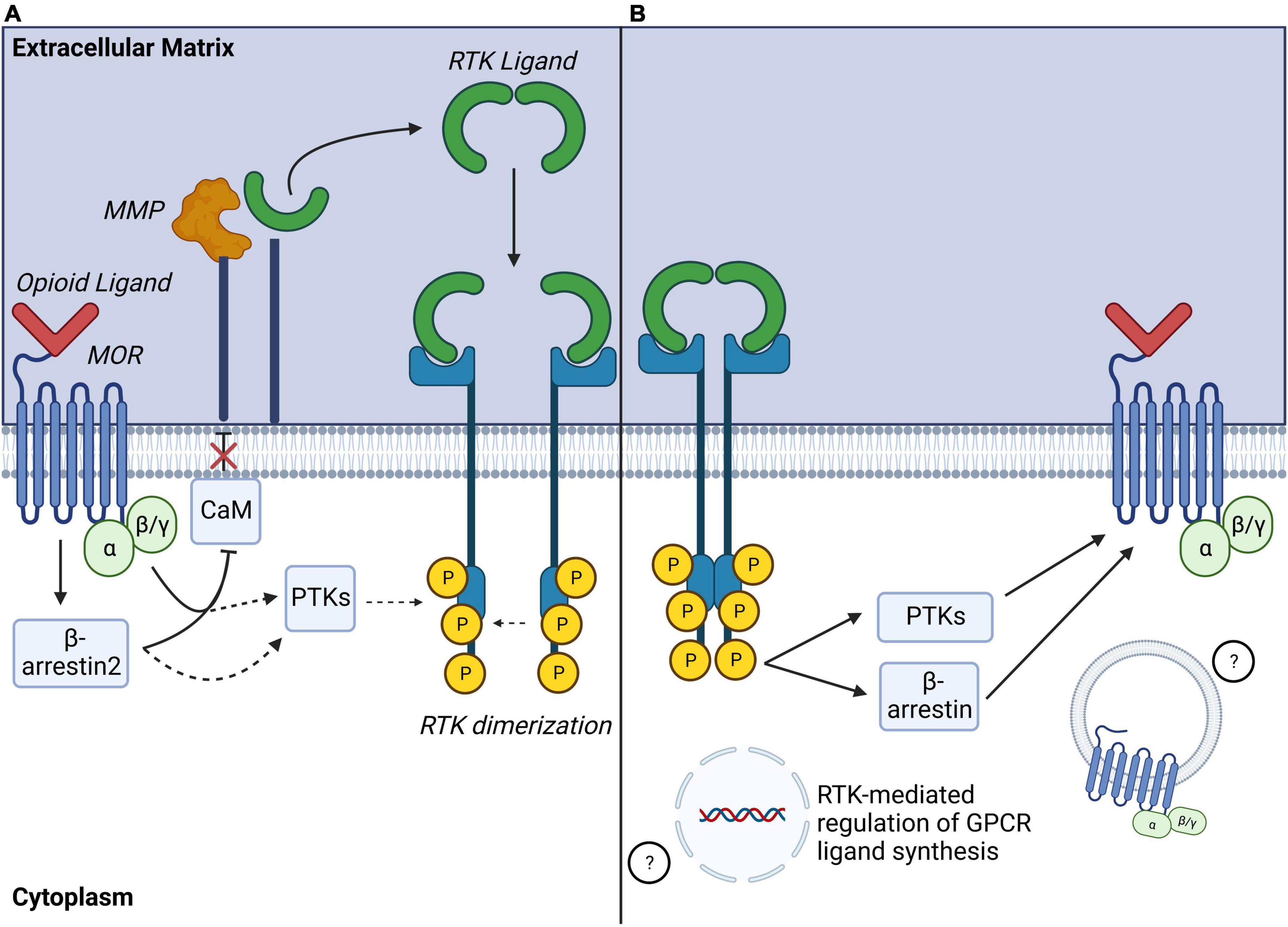
Figure 4. Identified mechanisms of MOR-RTK crosstalk. (A) Identified mechanisms of MOR-RTK transactivation: Activated MOR can induce MMP activation via mechanisms including disinhibition Calmodulin (CaM), leading to ligand shedding and ligand-dependent RTK activation. Other ligand-independent mechanisms may involve recruitment of intracellular phosphotyrosine kinases (PTKs) to phosphorylate RTK tyrosine residues. G protein and β-arrestin2 signaling may also be involved in MOR-RTK transactivation. (B) Identified mechanisms of RTK modulation of MOR signaling: Phosphorylated RTK may modulate activation of GPCR via a recruitment of PTKs, or β-arrestins activity. RTK activation may also lead to altered GPCR ligand gene expression or MOR internalization. MOR, mu-opioid receptor; MMP, matrix metalloproteinase; RTK, receptor tyrosine kinase; GPCR, G protein coupled receptor.
Other signaling effectors of MOR are involved in MOR-RTK transactivation. MOR-EGFR transactivation requires both Gαi/o and β-arrestin2. Indeed, opioid-mediated EGFR phosphorylation can be attenuated via pertussis toxin or by siRNA-mediated β-arrestin2 silencing in cultured rat astrocytes (Miyatake et al., 2009). In addition to canonical MOR transduction pathways, other common signaling effectors between GPCRs and RTKs can take part in MOR-RTK transactivation. PI3K inhibitors abolish EGFR activation by DAMGO-activated MORs in cultured rat astrocytes, suggesting involvement of this kinase in MOR-RTK transactivation (Belcheva et al., 2005). Similarly, JNK inhibitors block MOR-PDGFRβ transactivation in rat spinal neurons (Li et al., 2020). Together, these studies indicate that MOR-RTK transactivation likely involves a complex network of converging signaling pathways (Figure 4A). It is important to note that most studies of mechanisms of MOR-RTK transactivation have employed acute MOR agonist treatments. However, longer MOR agonism may have different effects on RTK activity (Belcheva et al., 2003; Miyatake et al., 2009). Over hours, longer term treatment with MOR agonists DAMGO, enkephalin, or morphine induces EGFR phosphorylation as well as both downregulation and decreased ERK phosphorylation. These mechanisms are β-arrestin2- and Gαi/o-dependent and not observed with acute opioid treatments on the order of minutes, suggesting that acute versus longer-term events cause temporally distinct effects on signaling (Belcheva et al., 2003; Miyatake et al., 2009). Because most opioid-mediated side-effects occur after long-term opioid treatment, further studies to understand the specific alterations of MOR-RTK transactivation mechanisms by long-term opioid MOR stimulation are still needed.
Intriguingly, mechanisms of MOR-EGFR transactivation identified in vitro in immortalized cell lines do not differ between opioids with different ability to internalize MOR (Belcheva et al., 2001). Belcheva and colleagues (Belcheva et al., 2001) found that EGFR was phosphorylated by MOR whether it was activated by morphine (low internalizing (Sternini et al., 1996), DAMGO (highly internalizing synthetic opioid peptide (Keith et al., 1998) or endomorphin (highly internalizing endogenous opioid peptide (Mcconalogue et al., 1999). In addition, mechanisms of MOR-EGFR transactivation by these agonists all required similar mechanisms of CaM recruitment and PKC signaling, although they had been characterized as opioids with different signaling bias toward G protein and β-arrestin recruitment (Schmid et al., 2017). Together this implies that RTK transactivation mechanisms may be independent from MOR-ligands bias.
Receptor tyrosine kinases transactivation of mu-opioid receptors
In addition to modulation of RTK signaling by GPCRs, RTKs can also modulate GPCR-mediated signaling, suggesting that the relationship between GPCRs and RTKs is reciprocal (Delcourt et al., 2007; Figure 4B). General mechanisms of GPCR transactivation by RTKs or “GPCR highjacking” (Delcourt et al., 2007) can involve recruitment of GPCR signaling effectors like GRKs (García-Sáinz et al., 2010; Sun et al., 2018), β-arrestins (Dalle et al., 2001; Povsic et al., 2003; Hupfeld and Olefsky, 2007) or activation of RTK downstream kinases including PI3K (Molina-Munþoz et al., 2006), Akt, or c-Src (Baltensperger et al., 1996; Doronin et al., 2002; Gavi et al., 2007). These mechanisms either require physical interactions between GPCRs and RTKs or transcriptional regulation of GPCR ligand synthesis (Delcourt et al., 2007). Relevant to this review, accumulating studies show that RTK signaling influences MOR signal transduction by modulation of phosphorylation. In cultured Xenopus laevi oocytes co-transfected with MOR and the insulin receptor (IR), pretreatment with insulin potentiated DAMGO-activated GIRK inward currents via MAPK signaling and possible dephosphorylation of MOR tyrosine residues, Y-106, or Y-166. Thus, indirectly demonstrating that IR signaling modulates MOR-signaling efficacy (Mclaughlin and Chavkin, 2001). In contrast, concomitant activation of MOR by DAMGO and activation of EGFR by EGF in HEK293 cells promotes MOR phosphorylation on Y-166 in a src-dependent manner, resulting in negative regulation of MOR-G protein coupling (Clayton et al., 2010). This suggests that regulation of MOR phosphorylation by opioids may be modulated by RTK-dependent activity. In separate studies, EGFR activation by EGF caused recruitment and translocation of G-coupled protein receptor kinase 2 (GRK-2) to the plasma membrane where it phosphorylated MOR on Serine-residues 363 and 375 (S-363, S-375), and Threonine-residue-370 (T-370), and enabled DAMGO-mediated MOR internalization (Chen et al., 2008b). Together, these studies highlight that RTKs can modulate MOR phosphorylation, signaling and internalization.
Involvement of receptor tyrosine kinase signaling opioid-mediated behaviors
While much of the literature has focused on MOR-RTK transactivation in vitro, this phenomenon is also relevant physiologically in vivo, particularly in the development and maintenance of deleterious opioid side-effects caused by MOR agonists. Reviewed here is evidence that several RTKs (Table 1) play major roles in mediating opioid side-effects such as analgesic tolerance, resistance of neuropathic pain to opioid analgesia, physical dependence or reward.
Receptor tyrosine kinase signaling and opioid analgesic tolerance
In the clinic, opioid analgesic tolerance is defined by a gradual loss of analgesic efficacy to a fixed dose of an opioid. As a result, escalation of opioid doses occurs over time to maintain analgesic benefit (Henry et al., 2015; Hayhurst and Durieux, 2016). MOR signaling is essential in the mechanisms of tolerance (Williams et al., 2013; Adhikary and Williams, 2022) and MOR is expressed in structures strongly implicated in tolerance and pain mechanisms including dorsal root ganglia (DRG) neurons and neurons of the spinal cord substantia gelatinosa (Mansour et al., 1988, 1995a; Scherrer et al., 2009; Corder et al., 2017; Puig and Gutstein, 2017). RTKs are similarly expressed alongside MOR in the spinal cord and DRG, including PDGFRβ (Sasahara et al., 1991; Eccleston et al., 1993), EGFR (Werner et al., 1988; Huerta et al., 1996), VEGFR2 (Spliet et al., 2004; Herrera et al., 2009), or Ephrin type-B receptor 1 (EphRB1) (Liu et al., 2011). Putative roles for RTK signaling in opioid tolerance were first shown in mice with global deletion of EphRB1 (Liu et al., 2011) as they failed to develop tolerance to spinal morphine administration. Similarly, we and others found that systemic or intrathecal co-administration of morphine alongside RTK inhibition via inhibitors of PDGFRβ (Wang et al., 2012; Li et al., 2020; Puig et al., 2020a), EGFR (Puig et al., 2020b), or VEGFR-2 (Lopez-Bellido et al., 2019) completely blocked tolerance. Together, these studies show that spinal RTK signaling is essential in morphine tolerance development. In addition, supraspinal inhibition of the RTK, FGFR, via intracerebroventricular (i.c.v.) injection also blocks tolerance to morphine injected subcutaneously (Fujita-Hamabe et al., 2011). Thus, other supraspinal structures of the pain circuitry may additionally contribute to RTK-mediated tolerance behaviors.
Precluding spinal signaling from one RTK at a time is sufficient to fully ablate tolerance. This apparent signaling redundancy raises the possibilities that: (1) spinal RTKs may work in parallel to transduce complex signaling cascades that specifically mediate tolerance and (2) that all signaling cascades recruited by RTKs are essential for tolerance. Interestingly, RTKs including EGFR and PDGFR-β were shown to heterodimerize in vitro (Habib et al., 1998; Saito et al., 2001). Heterodimerization could also happen in vivo, and co-transactivation of several spinal RTKs by MOR may be involved in mechanisms of tolerance. However, inhibition of RTKs individually alters tolerance in different ways depending on the RTK. For example, PDGFRβ inhibition only masks the expression of morphine tolerance (Wang et al., 2012), while EGFR inhibition completely blocks its development (Puig et al., 2020b). Importantly, these results have been reproduced by several independently conducted studies, highlighting the robustness of these findings (Wang et al., 2012; Li et al., 2020; Puig et al., 2020a).
In addition, we found that PDGFRβ inhibition blocks tolerance to several opioid analgesics used in the clinic including fentanyl, sufentanil, hydromorphone, and oxycodone (Puig et al., 2020a). Interestingly, these opioids have profoundly different pharmacokinetic and pharmacodynamic properties and have different signaling bias (Keith et al., 1998; Schmid et al., 2017). These findings show functional dissociation between MOR endocytosis, ligand signaling bias and tolerance, challenging the long-held hypotheses that mechanisms of MOR internalization (Whistler et al., 1999; Finn and Whistler, 2001) or of recruitment of β-arrestin2 (Bohn et al., 2000, 2004) are at the core of tolerance signaling. Instead, it suggests that PDGFRβ signaling could be a core mediator of opioid analgesic tolerance (Puig et al., 2020a). This is further supported by the fact that tolerance occurs independently of opioid-induced MOR internalization, and PDGFRβ inhibition does not modify levels of internalization while preventing tolerance (Puig et al., 2020a).
The precise RTK signaling pathways activated by opioid-stimulated MOR that mediate tolerance remain completely unknown. However, a recent in vivo study suggested that they could involve JNK signaling downstream of PDGFRβ (Li et al., 2020). Mechanisms of MOR-RTK transactivation in the spinal cord to mediate tolerance are similarly unclear. However, they seem to involve RTK ligand-dependent signaling pathways (Liu et al., 2011; Wang et al., 2012). Therefore, MOR may recruit RTK signaling in either an autocrine or a paracrine manner and RTKs may not necessarily need to be co-expressed with MOR. In addition, RTKs that have been involved in tolerance are closely phylogenetically related (Brunet et al., 2016). Indeed, VEGFR-2 and PDGFRβ share a direct ancestor gene, the RTK PDGF/VEGF receptor (Pvr) (Lopez-Bellido et al., 2019). This implies that involvement of RTKs in opioid tolerance could be a phylogenetically conserved function.
Receptor tyrosine kinase signaling and reduced opioid analgesia to neuropathic pain
Neuropathic pain results from lesions or diseases of the somatosensory system that lead to a combination of inflammation and nerve compression (Dworkin et al., 2003, 2010). NP can also result from nerve damage as a consequence of prolonged chemotherapy (Murnion, 2018; Finnerup et al., 2021). Due to a lack of better alternatives, opioids are commonly used for NP (Quasthoff and Hartung, 2002; Chong and Bajwa, 2003; Lynch et al., 2004; Balayssac et al., 2009). Nevertheless, opioids are not very effective in treating NP as several clinical studies have shown that, despite high opioid dosage, NP could not be alleviated following opioid administration (Chaparro et al., 2012; Cooper et al., 2017). Although combination therapy between opioids and gabapentin, a blocker of voltage-gated calcium channels, proved effective, it has been associated with severely debilitating side-effects including nausea, constipation, and vomiting (Chaparro et al., 2012). Therefore, new therapeutic strategies and targets are needed for ameliorating NP.
NP can be modeled in rodents by inducing nerve injury on spinal nerves via spinal nerve ligation (SNL) (Kim and Chung, 1992) or chronic contraction injury (CCI) of the sciatic nerve (Bennett and Xie, 1988). In these models, low doses of opioids fail to produce analgesia. Several studies have established the impact of signaling from different RTKs in neuropathic pain development and maintenance in rodents including signaling by PDGFRα (Narita et al., 2005), FLT3 (Rivat et al., 2018), EphRB1 (Han et al., 2008), EGFR (Martin et al., 2017), or TrkA (Ugolini et al., 2007). In addition, a clinical study demonstrated that targeted inhibition of EGFR significantly reduces pain in male and female NP patients (Kersten et al., 2019). Groundbreaking recent discoveries also established direct involvement of RTK signaling in NP resistance to opioid analgesia. Pharmacological inhibition of either PDGFRβ (Donica et al., 2014) or EGFR (Puig et al., 2020b) restores analgesia to a dose of morphine previously ineffective on mechanical allodynia caused by SNL. This shows that PDGFRβ or EGFR inhibition is sufficient to restore morphine analgesic properties that were abolished by alterations caused by nerve injury. Importantly, administration of the same doses of PDGFRβ or EGFR inhibitors alone does not have any analgesic effect. In fact, we estimated that the dose of EGFR inhibitor used to restore morphine analgesia is ∼20-fold lower than the dose previously needed to induce analgesia (Nair and Jacob, 2016; Puig et al., 2020b). These results emphasize that RTK inhibitors restore morphine-mediated analgesia rather than causing analgesia by themselves. This indicates that recruitment of PDGFRβ and EGFR signaling by nerve injury activates signaling pathways that may block opioid analgesic signaling during NP. Moreover, these mechanisms resemble findings in the context of opioid tolerance (Wang et al., 2012; Puig et al., 2020a,b), and imply that convergent mechanisms between opioid tolerance and NP involve RTK signaling. Based on these observations, it was speculated that injured nerves release growth factors such as PDGF-B to activate PDGFRβ signaling and induce morphine-resistant states (Donica et al., 2014). It has also been proposed that this endogenous PDGF-B release by injured nerves, is similar to the release of PDGF-B in response to opioid administration, leading to activation of MOR to mediate tolerance (Wang et al., 2012). In conclusion, RTK signaling mediating opioid analgesic resistance may form a mechanistic link between neuropathic pain development and opioid tolerance (Mao et al., 1995; Mayer et al., 1999; Joseph et al., 2010; Donica et al., 2014; Puig et al., 2020b).
Receptor tyrosine kinase signaling and opioid dependence
There is a complex bidirectional relationship between RTK gene expression and opioid dependence. Dorval and colleagues showed that mice that overexpress FGF21, an FGFR ligand (FGF21-Tg mice, 50-fold overexpression), have a reduced preference to morphine in a conditioned place preference paradigm (Dorval et al., 2022). Further, naloxone-precipitated physical dependence behavior, (i.e., number of vertical jumps post-naloxone injection) is depressed in FGF21-Tg mice compared to wildtype littermates, suggesting that acute morphine physical dependence is regulated by FGF21 activity. Interestingly, morphine analgesia and tolerance development were not altered in FGF21-Tg mice, showing that FGF21 plays a role in opioid dependence but not in analgesia or tolerance. These findings are consistent with previous studies showing that oxycodone self-administration is associated with elevated striatal fgf2, fgfr2, and fgfr3mRNA levels during incubation of oxycodone seeking (Blackwood et al., 2019). Furthermore, these changes in FGF receptor gene expression are associated with elevated c-fos mRNA expression in the dorsal striatum, and elevated junB mRNA levels in these same regions. Given that the striatum is an important region of the reward circuitry, Blackwood and collaborators (Blackwood et al., 2019) hypothesized that incubation of oxycodone seeking, a behavior correlated with future dependence, is mediated at least in-part by FGF2-dependent signaling. However, the mechanisms of FGF receptor-driven opioid dependence remain unknown.
Other studies have also indicated that brain-derived neurotrophic factor (BDNF), as well as its receptor, tropomyosin receptor kinase B (TrkB), may also play integral roles in opioid dependence and withdrawal development. BDNF, TrkB, IGF1, and IFG1R mRNA levels were found to be elevated in rodents frontal cortex in a model of physical dependence to morphine. In addition, BDNF was upregulated in hippocampus and midbrain (Peregud et al., 2016). Recruitment of BDNF-TrkB signaling by MOR during exposure to opioids or during withdrawal may be mediated via mechanisms of atypical GPCR-RTK transactivation involving a ROS, nitric oxide (NO) (as illustrated in Figure 3C). Withdrawal associated elevation of BDNF and TrkB and their respective receptors is markedly lower in animals pretreated with the nitric oxide synthase (NOS) inhibitor L-NG-nitroarginine methyl ester (L-NAME). L-NAME-treated animals also exhibited depressed amounts of phosphorylated TrkB following abstinence from morphine (Peregud et al., 2016). Confirming the role of TrkB signaling in withdrawal behaviors, a recent study showed that rats pre-treated with ANA-12, a TrkB antagonist, displayed greater drug dependence and significantly more spontaneous withdrawal behaviors after a chronic treatment with morphine. Furthermore, BDNF levels in the cerebrospinal fluid (CSF) of ANA-12 treated animals are depressed during morphine dependence, and elevated during withdrawal (Rezamohammadi et al., 2020). Together these studies show that FGFR and TrkB activation may have protective effects against physical dependence, highlighting that this should be carefully considered in the process of testing RTK targeting therapies to treat opioid side-effects.
One hallmark of chronic opioid use which occurs upon opioid withdrawal is opioid-induced hyperalgesia (OIH). Of interest, Ephrin receptors, the most prominent subfamily of RTKs, which are commonly associated with neuron-neuron and neuron-glia interactions have been implicated in OIH. In a rat model of remifentanil-induced hyperalgesia, remifentanil-induced decrease of mechanical and thermal pain threshold has been correlated with elevated spinal Fos protein levels. Interestingly, these effects were reversed by inhibition of either EphB ligand (via EphB1-Fc) or the NMDA receptor (NMDAR) (via MK801) (Xia et al., 2014). Further, intrathecal injection of ephrinB/EphB agonist, was sufficient to induce significant hyperalgesia in a NMDAR-dependent manner (Xia et al., 2014), showing that activation of ephrinB/EphB pathways are sufficient to mediate OIH development via NMDAR (Xia et al., 2014). Importantly, other RTKs are also known to be involved in NMDAR-mediated OIH, including BDNF-TrkB signaling in the spinal dorsal horn. Notably, previous work demonstrated that morphine-induced hyperalgesia occurs because of MOR-dependent BDNF release leading to a downregulation of K+/Cl– co-transporter (KCC2) in rat spinal lamina neurons (Ferrini et al., 2013). The resulting Cl– dysequilibrium serves as a driver of hyperalgesia which is reversible by inhibition of BDNF-TrkB or via prevention of KCC2 downregulation (Ferrini et al., 2017). Further, this reversible anion transport dysfunction induces a dampening of GABAergic and glycinergic spinal signaling and elevated NMDAR activity (Li et al., 2016).
Receptor tyrosine kinase signaling and opioid reward
RTK signaling and opioid reward involve midbrain dopamine neurons in the ventral tegmental area (VTA) which project to the nucleus accumbens (NAc) in the striatum. In the context of opioids, morphine promotes activation of striatal D1 receptor (D1R)-expressing MSNs which increase reward behaviors and decreases dopamine D2 receptor-expressing (D2R) MSNs which promote aversion. TrkB is expressed in both D1R+ and D2R+ MSNs (Freeman et al., 2003; Baydyuk et al., 2011) and most evidence about involvement of RTK signaling in opioid reward derives from work analyzing TrkB and morphine administration. Indeed, there is decreased conditioned place preference (CPP) for morphine when the selective TrkB antagonist, ANA-12, is injected into the NAc of rats (Jorjani et al., 2021). However, it was also shown that selective knockout of TrkB from D1R+ MSNs of the NAc in mice, enhances morphine CPP while knockout of D2R+ MSNs produces no change (Koo et al., 2014). Moreover, knocking out TrkB in the VTA produces a similar effect as TrkB knockout in D1R+ MSNs in the NAc with enhanced morphine CPP (Koo et al., 2014). Overall, these findings suggest that TrkB-based RTK signaling in D1R+ versus D2R+ MSNs mediates opposing actions that together modulate opioid-induced behaviors and that these actions are dependent on striatal dopamine release from projections of midbrain dopaminergic neurons.
IR signaling has also been implicated in opioid reward. In the hippocampus and hypothalamus, morphine induces IR phosphorylation in wildtype, but not MOR knockout mice, suggesting that MORs are able to transactivate IRs in these structures (Li et al., 2003). Additionally, given the important role of glutamatergic neurotransmission in drug reward (Britt et al., 2012), the increases in presynaptic glutamate release in the NAc in response to IR activation (Fetterly et al., 2021) may provide a further RTK-mediated mechanism for opioid actions. In contrast, insulin growth factor like receptor (IGFR) activation decreases presynaptic glutamate release in the same neuronal population, demonstrating differential effects depending on the RTK. Additional involvement of IR in opioid reward is supported by work showing that prolonged morphine-activated MORs in vitro can cause desensitization of IR signaling to Akt and ERK cascades (Li et al., 2003), both of which have been implicated in reward (Shi et al., 2014; Zamora-Martinez and Edwards, 2014). Inhibition of ERK in the NAc shell prevents development of morphine CPP (Xu et al., 2012). While, in a separate study, Russo and colleagues showed that downregulation of Akt and IR subunit 2, an essential component of functional IR signaling, in the VTA results in reward tolerance as shown by decreased CPP behaviors over time (Russo et al., 2007). Together, these results further reinforce the involvement of IRs in modulation of MOR-mediated reward signaling within the NAc. Further studies to establish if this could be generalized to other opioids remain necessary. For example, PDGFRβ is also expressed in brain regions involved in reward and addiction such as mPFC, NAc and dStr (Balayssac et al., 2009; Bor et al., 2017) and PDGFRβ levels were shown to be altered in the striatum and midbrain of rodents with disrupted dopaminergic signaling, a central component for reward signaling (Masuo et al., 2004). Overall, this work suggests RTKs could be promising, yet understudied, candidates to mitigate morphine reward, especially IRs.
Clinical implications
Treating human disease with RTK-targeted therapies is an established standard of care as a therapeutic strategy for cancer. Indeed, RTK inhibitors serve as the gold standard treatment of malignancies (Savage and Antman, 2002; Elisei et al., 2013; Sim et al., 2018). These medications are being explored for putative efficacy in other, non-oncological conditions. Specifically, EGFR and PDGFR inhibitors have recently received attention for their clinical therapeutic potential against pain. Several case reports have described analgesic effects by EGFR inhibitors in patients with severe pain (Kersten and Cameron, 2012; Kersten et al., 2015). Patients with either cancer pain (Moryl et al., 2006; Macey et al., 2009) or different types of neuropathic pain (Kersten et al., 2015) were treated with EGFR inhibitors which significantly improved their pain score after a few days. Most interestingly, in a clinical study led by Kersten and collaborators (Kersten et al., 2013), half the patients who experienced immediate pain relief following administration of the EGFR inhibitor cetuximab also decreased their required opioid doses. The authors concluded that cetuximab reversed opioid tolerance. Similarly, PDGFR-β inhibitor imatinib induced analgesia in cancer patients (Stankovic Stojanovic et al., 2011; Kutlar, 2013). Based on the promise of these recent clinical studies and case reports, it is possible that the improved pain relief observed with RTK inhibitors is due to the reversal of pre-existing opioid tolerance. We propose that combined treatment with opioids and RTK inhibitors may decouple the intertwined pathways mediating analgesia and tolerance.
Conclusion
Uncoupling analgesia from undesirable effects of opioids by RTK inhibitors could therefore enable patients to maintain opioid efficacy at smaller doses, mitigating the risk of side-effects associated with chronic opioid use. Importantly, in rodents, efficacious doses to mitigate opioid tolerance appear to be significantly lower than those required to treat cancers. This holds the promise that RTK doses required for effective prevention of opioid side-effects in humans should not have a deleterious impact that could outweigh advantages of RTK inhibitors. Nevertheless, more work is clearly needed to better understand how RTK inhibitors work in the context of tolerance. It is still unknown if RTK inhibitors could be used to treat opioid withdrawal symptoms or prevent rewarding properties of opioids in humans. It is imperative that future studies assess the power of concurrent opioid-RTK inhibitors treatments both in the clinic and in pre-clinical models of pain. If successful, RTK inhibitors may represent a promising new class of drugs to treat pain more safely in conjunction with opioids and therefore positively impact the lives of millions living with chronic pain.
Author contributions
MG, NS, and BW contributed equally by generating a first draft of this manuscript. LP, ZF, and RL contributed to the writing of this manuscript. SP initiated the manuscript, supervised collection of cited references, and contributed to first and final drafts. All authors contributed to the article and approved the submitted version.
Funding
SP was supported by National Institutes of Health NIDA R21DA051636. ZF and RL were supported by the National Institutes of Health NIDA R21DA052419 and NIAAA R21AA028800. RL was also supported by NHLBI R01HL150432 and NIDA R33DA041872. ZF was also supported by NIA R21AG068607, NIDDK R01DK124219, the Commonwealth of Pennsylvania Formula Fund (PA-HEALT), and the Department of Defense (PR210207 and PR192466).
Acknowledgments
We acknowledge the contribution of NIH NIDA, NHLBI, NIA, NIDDK Commonwealth of Pennsylvania Formula Fund and the Department of Defense to this manuscript by the support of the salary of authors of this manuscript (SP, LP, ZF, and RL).
Conflict of interest
The authors declare that the research was conducted in the absence of any commercial or financial relationships that could be construed as a potential conflict of interest.
Publisher’s note
All claims expressed in this article are solely those of the authors and do not necessarily represent those of their affiliated organizations, or those of the publisher, the editors and the reviewers. Any product that may be evaluated in this article, or claim that may be made by its manufacturer, is not guaranteed or endorsed by the publisher.
References
Adhikary, S., and Williams, J. T. (2022). Cellular tolerance induced by chronic opioids in the central nervous system. Front. Syst. Neurosci. 16:937126. doi: 10.3389/fnsys.2022.937126
Algera, M. H., Kamp, J., Van Der Schrier, R., Van Velzen, M., Niesters, M., Aarts, L., et al. (2019). Opioid-induced respiratory depression in humans: A review of pharmacokinetic-pharmacodynamic modelling of reversal. Br. J. Anaesth. 122, e168–e179. doi: 10.1016/j.bja.2018.12.023
Al-Hasani, R., and Bruchas, M. R. (2011). Molecular mechanisms of opioid receptor-dependent signaling and behavior. Anesthesiology 115, 1363–1381. doi: 10.1097/ALN.0b013e318238bba6
Allouche, S., Noble, F., and Marie, N. (2014). Opioid receptor desensitization: Mechanisms and its link to tolerance. Front. Pharmacol. 5:280. doi: 10.3389/fphar.2014.00280
Alvarez, V. A., Arttamangkul, S., Dang, V., Salem, A., Whistler, J. L., Von Zastrow, M., et al. (2002). Mu-opioid receptors: Ligand-dependent activation of potassium conductance, desensitization, and internalization. J. Neurosci. 22, 5769–5776. doi: 10.1523/JNEUROSCI.22-13-05769.2002
Azolosa, J. L., Stitzer, M. L., and Greenwald, M. K. (1994). Opioid physical dependence development: Effects of single versus repeated morphine pretreatments and of subjects’ opioid exposure history. Psychopharmacology (Berl) 114, 71–80. doi: 10.1007/BF02245446
Balayssac, D., Cayre, A., Ling, B., Maublant, J., Penault-Llorca, F., Eschalier, A., et al. (2009). Increase in morphine antinociceptive activity by a P-glycoprotein inhibitor in cisplatin-induced neuropathy. Neurosci. Lett. 465, 108–112. doi: 10.1016/j.neulet.2009.09.003
Baltensperger, K., Karoor, V., Paul, H., Ruoho, A., Czech, M. P., and Malbon, C. C. (1996). The β-adrenergic receptor is a substrate for the insulin receptor tyrosine kinase. J. Biol. Chem. 271, 1061–1064. doi: 10.1074/jbc.271.2.1061
Basbaum, A. I., Bautista, D. M., Scherrer, G., and Julius, D. (2009). Cellular and molecular mechanisms of pain. Cell 139, 267–284. doi: 10.1016/j.cell.2009.09.028
Baydyuk, M., Russell, T., Liao, G. Y., Zang, K., An, J. J., Reichardt, L. F., et al. (2011). TrkB receptor controls striatal formation by regulating the number of newborn striatal neurons. Proc. Natl. Acad. Sci. U.S.A. 108, 1669–1674. doi: 10.1073/pnas.1004744108
Belcheva, M. M., Clark, A. L., Haas, P. D., Serna, J. S., Hahn, J. W., Kiss, A., et al. (2005). Mu and kappa opioid receptors activate ERK/MAPK via different protein kinase C isoforms and secondary messengers in astrocytes. J. Biol. Chem. 280, 27662–27669. doi: 10.1074/jbc.M502593200
Belcheva, M. M., Szucs, M., Wang, D., Sadee, W., and Coscia, C. J. (2001). Mu-opioid receptor-mediated ERK activation involves calmodulin-dependent epidermal growth factor receptor transactivation. J. Biol. Chem. 276, 33847–33853. doi: 10.1074/jbc.M101535200
Belcheva, M. M., Tan, Y., Heaton, V. M., Clark, A. L., and Coscia, C. J. (2003). Mu opioid transactivation and down-regulation of the epidermal growth factor receptor in astrocytes: Implications for mitogen-activated protein kinase signaling. Mol. Pharmacol. 64, 1391–1401. doi: 10.1124/mol.64.6.1391
Bennett, G. J., and Xie, Y.-K. (1988). A peripheral mononeuropathy in rat that produces disorders of pain sensation like those seen in man. Pain 33, 87–107. doi: 10.1016/0304-3959(88)90209-6
Benyamin, R., Trescot, A. M., Datta, S., Buenaventura, R., Adlaka, R., Sehgal, N., et al. (2008). Opioid complications and side effects. Pain Physician 11, S105–S120. doi: 10.36076/ppj.2008/11/S105
Blackwood, C. A., Leary, M., Salisbury, A., Mccoy, M. T., and Cadet, J. L. (2019). Escalated oxycodone self-administration causes differential striatal mRNA expression of FGFs and IEGs following abstinence-associated incubation of oxycodone craving. Neuroscience 415, 173–183. doi: 10.1016/j.neuroscience.2019.07.030
Bohn, L. M., Dykstra, L. A., Lefkowitz, R. J., Caron, M. G., and Barak, L. S. (2004). Relative opioid efficacy is determined by the complements of the G protein-coupled receptor desensitization machinery. Mol. Pharmacol. 66, 106–112. doi: 10.1124/mol.66.1.106
Bohn, L. M., Gainetdinov, R. R., Lin, F. T., Lefkowitz, R. J., and Caron, M. G. (2000). Mu-opioid receptor desensitization by beta-arrestin-2 determines morphine tolerance but not dependence. Nature 408, 720–723. doi: 10.1038/35047086
Bohn, L. M., Gainetdinov, R. R., Sotnikova, T. D., Medvedev, I. O., Lefkowitz, R. J., Dykstra, L. A., et al. (2003). Enhanced rewarding properties of morphine, but not cocaine, in beta(arrestin)-2 knock-out mice. J. Neurosci. 23, 10265–10273. doi: 10.1523/JNEUROSCI.23-32-10265.2003
Bohn, L. M., Lefkowitz, R. J., and Caron, M. G. (2002). Differential mechanisms of morphine antinociceptive tolerance revealed in (beta)arrestin-2 knock-out mice. J. Neurosci. 22, 10494–10500. doi: 10.1523/JNEUROSCI.22-23-10494.2002
Bohn, L. M., Lefkowitz, R. J., Gainetdinov, R. R., Peppel, K., Caron, M. G., and Lin, F. T. (1999). Enhanced morphine analgesia in mice lacking beta-arrestin 2. Science 286, 2495–2498. doi: 10.1126/science.286.5449.2495
Bor, A., Nishijo, M., Nishimaru, H., Nakamura, T., Tran, N. N., Van Le, Q., et al. (2017). Effects of high fat diet and perinatal dioxin exposure on development of body size and expression of platelet-derived growth factor receptor beta in the rat brain. J. Integr. Neurosci. 16, 453–470. doi: 10.3233/JIN-170025
Britt, J. P., Benaliouad, F., Mcdevitt, R. A., Stuber, G. D., Wise, R. A., and Bonci, A. (2012). Synaptic and behavioral profile of multiple glutamatergic inputs to the nucleus accumbens. Neuron 76, 790–803. doi: 10.1016/j.neuron.2012.09.040
Brunet, F. G., Volff, J. N., and Schartl, M. (2016). Whole genome duplications shaped the receptor tyrosine kinase repertoire of jawed vertebrates. Genome Biol. Evol. 8, 1600–1613. doi: 10.1093/gbe/evw103
Burma, N. E., Kwok, C. H., and Trang, T. (2017). Therapies and mechanisms of opioid withdrawal. Pain Manag. 7, 455–459. doi: 10.2217/pmt-2017-0028
Cattaneo, F., Guerra, G., Parisi, M., De Marinis, M., Tafuri, D., Cinelli, M., et al. (2014). Cell-surface receptors transactivation mediated by g protein-coupled receptors. Int. J. Mol. Sci. 15, 19700–19728. doi: 10.3390/ijms151119700
Chakrabarti, S., and Gintzler, A. R. (2007). Phosphorylation of Gαs influences its association with the μ-opioid receptor and is modulated by long-term morphine exposure. Mol. Pharmacol. 72, 753–760. doi: 10.1124/mol.107.036145
Chakrabarti, S., Chang, A., and Gintzler, A. R. (2010). Subcellular localization of μ-opioid receptor GS signaling. J. Pharmacol. Exp. Therap. 333, 193–200. doi: 10.1124/jpet.109.165142
Chakrabarti, S., Regec, A., and Gintzler, A. R. (2005). Biochemical demonstration of mu-opioid receptor association with Gsα: Enhancement following morphine exposure. Mol. Brain Res. 135, 217–224. doi: 10.1016/j.molbrainres.2004.12.016
Chaparro, L. E., Wiffen, P. J., Moore, R. A., and Gilron, I. (2012). Combination pharmacotherapy for the treatment of neuropathic pain in adults. Cochrane Database Syst. Rev. 12:CD008943. doi: 10.1002/14651858.CD008943.pub2
Chaturvedi, K., Bandari, P., Chinen, N., and Howells, R. D. (2001). Proteasome involvement in agonist-induced down-regulation of μ and δ opioid receptors. J. Biol. Chem. 276, 12345–12355. doi: 10.1074/jbc.M008054200
Chen, Y., Geis, C., and Sommer, C. (2008a). Activation of TRPV1 contributes to morphine tolerance: Involvement of the mitogen-activated protein kinase signaling pathway. J. Neurosci. 28, 5836–5845. doi: 10.1523/JNEUROSCI.4170-07.2008
Chen, Y., Long, H., Wu, Z., Jiang, X., and Ma, L. (2008b). EGF transregulates opioid receptors through Egfr-mediated Grk2 phosphorylation and activation. Mol. Biol. Cell 19, 2973–2983. doi: 10.1091/mbc.e07-10-1058
Chieng, B., and Christie, M. (1994). Hyperpolarization by opioids acting on μ−receptors of a sub-population of rat periaqueductal gray neurones in vitro. Br. J. Pharmacol. 113, 121–128. doi: 10.1111/j.1476-5381.1994.tb16183.x
Chong, M. S., and Bajwa, Z. H. (2003). Diagnosis and treatment of neuropathic pain. J. Pain Symptom Manag. 25, S4–S11. doi: 10.1016/S0885-3924(03)00064-2
Choudhary, C., Olsen, J. V., Brandts, C., Cox, J., Reddy, P. N., Böhmer, F. D., et al. (2009). Mislocalized activation of oncogenic RTKs switches downstream signaling outcomes. Mol. Cell 36, 326–339. doi: 10.1016/j.molcel.2009.09.019
Clayton, C. C., Bruchas, M. R., Lee, M. L., and Chavkin, C. (2010). Phosphorylation of the mu-opioid receptor at tyrosine 166 (Tyr3.51) in the DRY motif reduces agonist efficacy. Mol. Pharmacol. 77, 339–347. doi: 10.1124/mol.109.060558
Collett, B. J. (1998). Opioid tolerance: The clinical perspective. Br. J. Anaesth. 81, 58–68. doi: 10.1093/bja/81.1.58
Conibear, A. E., and Kelly, E. (2019). A biased view of μ-opioid receptors? Mol. Pharmacol. 96, 542–549. doi: 10.1124/mol.119.115956
Connor, M., and Christie, M. J. (1999). Opioid receptor signalling mechanisms. Clin. Exp. Pharmacol. Physiol. 26, 493–499. doi: 10.1046/j.1440-1681.1999.03049.x
Cooper, T. E., Chen, J., Wiffen, P. J., Derry, S., Carr, D. B., Aldington, D., et al. (2017). Morphine for chronic neuropathic pain in adults. Cochrane Database Syst. Rev. 5:CD011669. doi: 10.1002/14651858.CD011669.pub2
Corder, G., Tawfik, V. L., Wang, D., Sypek, E. I., Low, S. A., Dickinson, J. R., et al. (2017). Loss of mu opioid receptor signaling in nociceptors, but not microglia, abrogates morphine tolerance without disrupting analgesia. Nat. Med. 23, 164–173. doi: 10.1038/nm.4262
Dalle, S., Ricketts, W., Imamura, T., Vollenweider, P., and Olefsky, J. M. (2001). Insulin and insulin-like growth factor I receptors utilize different G protein signaling components. J. Biol. Chem. 276, 15688–15695. doi: 10.1074/jbc.M010884200
Daub, H., Ulrich Weiss, F., Wallasch, C., and Ullrich, A. (1996). Role of transactivation of the EGF receptor in signalling by G-protein-coupled receptors. Nature 379, 557–560. doi: 10.1038/379557a0
Delcourt, N., Bockaert, J., and Marin, P. (2007). Gpcr-jacking: From a new route in RTK signalling to a new concept in GPCR activation. Trends Pharmacol. Sci. 28, 602–607. doi: 10.1016/j.tips.2007.09.007
Di Liberto, V., Mudò, G., and Belluardo, N. (2019). Crosstalk between receptor tyrosine kinases (Rtks) and G protein-coupled receptors (GPCR) in the brain: Focus on heteroreceptor complexes and related functional neurotrophic effects. Neuropharmacology 152, 67–77. doi: 10.1016/j.neuropharm.2018.11.018
Doll, C., Konietzko, J., Pöll, F., Koch, T., Höllt, V., and Schulz, S. (2011). Agonist-selective patterns of μ-opioid receptor phosphorylation revealed by phosphosite-specific antibodies. Br. J. Pharmacol. 164, 298–307. doi: 10.1111/j.1476-5381.2011.01382.x
Donica, C. L., Cui, Y., Shi, S., and Gutstein, H. B. (2014). Platelet-derived growth factor receptor-beta antagonism restores morphine analgesic potency against neuropathic pain. PLoS One 9:e97105. doi: 10.1371/journal.pone.0097105
Doronin, S., Shumay, E., Wang, H.-Y., and Malbon, C. C. (2002). Akt mediates sequestration of the β2-adrenergic receptor in response to insulin. J. Biol. Chem. 277, 15124–15131. doi: 10.1074/jbc.M108771200
Dorval, L., Knapp, B. I., Majekodunmi, O. A., Eliseeva, S., and Bidlack, J. M. (2022). Mice with high Fgf21 serum levels had a reduced preference for morphine and an attenuated development of acute antinociceptive tolerance and physical dependence. Neuropharmacology 202:108858. doi: 10.1016/j.neuropharm.2021.108858
Doyle, T., Esposito, E., Bryant, L., Cuzzocrea, S., and Salvemini, D. (2013). Nadph-oxidase 2 activation promotes opioid-induced antinociceptive tolerance in mice. Neuroscience 241, 1–9. doi: 10.1016/j.neuroscience.2013.02.042
Du, Z., and Lovly, C. M. (2018). Mechanisms of receptor tyrosine kinase activation in cancer. Mol. Cancer 17:58. doi: 10.1186/s12943-018-0782-4
Duttaroy, A., and Yoburn, B. C. (1995). The effect of intrinsic efficacy on opioid tolerance. Anesthesiology 82, 1226–1236. doi: 10.1097/00000542-199505000-00018
Dworkin, R. H., Backonja, M., Rowbotham, M. C., Allen, R. R., Argoff, C. R., Bennett, G. J., et al. (2003). Advances in neuropathic pain: Diagnosis, mechanisms, and treatment recommendations. Arch. Neurol. 60, 1524–1534. doi: 10.1001/archneur.60.11.1524
Dworkin, R. H., O’connor, A. B., Audette, J., Baron, R., Gourlay, G. K., Haanpää, M. L., et al. (2010). Recommendations for the pharmacological management of neuropathic pain: An overview and literature update. Mayo Clin. Proc. 85, S3–S14. doi: 10.4065/mcp.2009.0649
Eccleston, P. A., Funa, K., and Heldin, C. H. (1993). Expression of platelet-derived growth factor (PDGF) and PDGF alpha- and beta-receptors in the peripheral nervous system: An analysis of sciatic nerve and dorsal root ganglia. Dev. Biol. 155, 459–470. doi: 10.1006/dbio.1993.1044
Elisei, R., Schlumberger, M. J., Muller, S. P., Schoffski, P., Brose, M. S., Shah, M. H., et al. (2013). Cabozantinib in progressive medullary thyroid cancer. J. Clin. Oncol. 31, 3639–3646. doi: 10.1200/JCO.2012.48.4659
Epstein, D. H., Preston, K. L., and Jasinski, D. R. (2006). Abuse liability, behavioral pharmacology, and physical-dependence potential of opioids in humans and laboratory animals: Lessons from tramadol. Biol. Psychol. 73, 90–99. doi: 10.1016/j.biopsycho.2006.01.010
Favelyukis, S., Till, J. H., Hubbard, S. R., and Miller, W. T. (2001). Structure and autoregulation of the insulin-like growth factor 1 receptor kinase. Nat. Struct. Biol. 8, 1058–1063. doi: 10.1038/nsb721
Ferrini, F., Lorenzo, L. E., Godin, A. G., Quang, M. L., and De Koninck, Y. (2017). Enhancing Kcc2 function counteracts morphine-induced hyperalgesia. Sci. Rep. 7:3870. doi: 10.1038/s41598-017-04209-3
Ferrini, F., Trang, T., Mattioli, T. A., Laffray, S., Del’guidice, T., Lorenzo, L. E., et al. (2013). Morphine hyperalgesia gated through microglia-mediated disruption of neuronal Cl(–) homeostasis. Nat. Neurosci. 16, 183–192. doi: 10.1038/nn.3295
Fetterly, T. L., Oginsky, M. F., Nieto, A. M., Alonso-Caraballo, Y., Santana-Rodriguez, Z., and Ferrario, C. R. (2021). Insulin bidirectionally alters NAc glutamatergic transmission: Interactions between insulin receptor activation, endogenous opioids, and glutamate release. J. Neurosci. 41, 2360–2372. doi: 10.1523/JNEUROSCI.3216-18.2021
Finn, A. K., and Whistler, J. L. (2001). Endocytosis of the mu opioid receptor reduces tolerance and a cellular hallmark of opiate withdrawal. Neuron 32, 829–839. doi: 10.1016/S0896-6273(01)00517-7
Finnerup, N. B., Kuner, R., and Jensen, T. S. (2021). Neuropathic pain: From mechanisms to treatment. Physiol. Rev. 101, 259–301. doi: 10.1152/physrev.00045.2019
Franz, B., Cronin, C. E., and Pagan, J. A. (2021). What strategies are hospitals adopting to address the opioid epidemic? Evidence from a national sample of nonprofit hospitals. Public Health Rep. 136, 228–238. doi: 10.1177/0033354920968805
Freeman, A. Y., Soghomonian, J. J., and Pierce, R. C. (2003). Tyrosine kinase B and C receptors in the neostriatum and nucleus accumbens are co-localized in enkephalin-positive and enkephalin-negative neuronal profiles and their expression is influenced by cocaine. Neuroscience 117, 147–156. doi: 10.1016/S0306-4522(02)00802-3
Fujita-Hamabe, W., Nakamoto, K., and Tokuyama, S. (2011). Involvement of NCAM and FGF receptor signaling in the development of analgesic tolerance to morphine. Eur. J. Pharmacol. 672, 77–82. doi: 10.1016/j.ejphar.2011.04.029
García-Sáinz, J. A., Romero-Ávila, M. T., and Del Carmen Medina, L. (2010). Dissecting how receptor tyrosine kinases modulate G protein-coupled receptor function. Eur. J. Pharmacol. 648, 1–5. doi: 10.1016/j.ejphar.2010.08.049
Gavi, S., Yin, D., Shumay, E., Wang, H.-Y., and Malbon, C. C. (2007). Insulin-like growth factor-I provokes functional antagonism and internalization of β1-adrenergic receptors. Endocrinology 148, 2653–2662. doi: 10.1210/en.2006-1569
Gialeli, C., Nikitovic, D., Kletsas, D., Theocharis, A. D., Tzanakakis, G. N., and Karamanos, N. K. (2014). PDGF/PDGFR signaling and targeting in cancer growth and progression: Focus on tumor microenvironment and cancer-associated fibroblasts. Curr. Pharm. Des. 20, 2843–2848. doi: 10.2174/13816128113199990592
Gillis, A., Gondin, A. B., Kliewer, A., Sanchez, J., Lim, H. D., Alamein, C., et al. (2020). Low intrinsic efficacy for G protein activation can explain the improved side effect profiles of new opioid agonists. Sci. Signal. 13:eaaz3140. doi: 10.1126/scisignal.aaz3140
Gregus, A. M., Inra, C. N., Giordano, T. P. III, Costa, A. C. S., Rajadhyaksha, A. M., and Inturrisi, C. E. (2010). Spinal mediators that may contribute selectively to antinociceptive tolerance but not other effects of morphine as revealed by deletion of GluR5. Neuroscience 169, 475–487. doi: 10.1016/j.neuroscience.2010.03.051
Habib, A. A., Hognason, T., Ren, J., Stefansson, K., and Ratan, R. R. (1998). The epidermal growth factor receptor associates with and recruits phosphatidylinositol 3-kinase to the platelet-derived growth factor beta receptor. J. Biol. Chem. 273, 6885–6891. doi: 10.1074/jbc.273.12.6885
Haglund, K., Rusten, T. E., and Stenmark, H. (2007). Aberrant receptor signaling and trafficking as mechanisms in oncogenesis. Crit. Rev. Oncog. 13, 39–74. doi: 10.1615/CritRevOncog.v13.i1.20
Haglund, K., Sigismund, S., Polo, S., Szymkiewicz, I., Di Fiore, P. P., and Dikic, I. (2003). Multiple monoubiquitination of Rtks is sufficient for their endocytosis and degradation. Nat. Cell Biol. 5, 461–466. doi: 10.1038/ncb983
Han, Y., Song, X.-S., Liu, W.-T., Henkemeyer, M., and Song, X.-J. (2008). Targeted mutation of EphB1 receptor prevents development of neuropathic hyperalgesia and physical dependence on morphine in mice. Mol. Pain 4:60. doi: 10.1186/1744-8069-4-60
Hayhurst, C. J., and Durieux, M. E. (2016). Differential opioid tolerance and opioid-induced hyperalgesia: A clinical reality. Anesthesiology 124, 483–488. doi: 10.1097/ALN.0000000000000963
Heckman, C. A., and Wade, J. G. (2018). Protein kinase C: Its role in RTK processing. Trends Cancer Res. 13, 1–28.
Henry, S. G., Wilsey, B. L., Melnikow, J., and Iosif, A. M. (2015). Dose escalation during the first year of long-term opioid therapy for chronic pain. Pain Med. 16, 733–744. doi: 10.1111/pme.12634
Herrera, J. J., Nesic, O., and Narayana, P. A. (2009). Reduced vascular endothelial growth factor expression in contusive spinal cord injury. J. Neurotrauma. 26, 995–1003. doi: 10.1089/neu.2008.0779
Hill, R., Disney, A., Conibear, A., Sutcliffe, K., Dewey, W., Husbands, S., et al. (2018). The novel μ−opioid receptor agonist PZM21 depresses respiration and induces tolerance to antinociception. Br. J. Pharmacol. 175, 2653–2661. doi: 10.1111/bph.14224
Holland, K. M., Depadilla, L., Gervin, D. W., Parker, E. M., and Wright, M. (2021). The evolution of the overdose epidemic and CDC’s research response: A commentary. Drugs Context 10. doi: 10.7573/dic.2021-8-2
Honegger, A., Kris, R., Ullrich, A., and Schlessinger, J. (1989). Evidence that autophosphorylation of solubilized receptors for epidermal growth factor is mediated by intermolecular cross-phosphorylation. Proc. Natl Acad. Sci. U.S.A. 86, 925–929. doi: 10.1073/pnas.86.3.925
Huerta, J. J., Diaz-Trelles, R., Naves, F. J., Llamosas, M. M., Del Valle, M. E., and Vega, J. A. (1996). Epidermal growth factor receptor in adult human dorsal root ganglia. Anat. Embryol. (Berl) 194, 253–257. doi: 10.1007/BF00187136
Hupfeld, C. J., and Olefsky, J. M. (2007). Regulation of receptor tyrosine kinase signaling by GRKs and β-arrestins. Annu. Rev. Physiol. 69, 561–577. doi: 10.1146/annurev.physiol.69.022405.154626
Jean-Charles, P.-Y., Kaur, S., and Shenoy, S. K. (2017). GPCR signaling via β-arrestin-dependent mechanisms. J. Cardiovasc. Pharmacol. 70:142. doi: 10.1097/FJC.0000000000000482
Jorjani, H., Joneidi, M., Vafaei, A. A., Rashidy-Pour, A., Sameni, H., Bandegi, A. R., et al. (2021). Microinjection of the BDNF receptor antagonist ANA-12 into the nucleus accumbens and medial-prefrontal cortex attenuates morphine-induced reward memory, and alterations of BDNF levels and apoptotic cells in rats. Pharmacol. Biochem. Behav. 201:173111. doi: 10.1016/j.pbb.2021.173111
Joseph, E. K., Reichling, D. B., and Levine, J. D. (2010). Shared mechanisms for opioid tolerance and a transition to chronic pain. J. Neurosci. 30, 4660–4666. doi: 10.1523/JNEUROSCI.5530-09.2010
Karaman, M. W., Herrgard, S., Treiber, D. K., Gallant, P., Atteridge, C. E., Campbell, B. T., et al. (2008). A quantitative analysis of kinase inhibitor selectivity. Nat Biotechnol 26, 127–132. doi: 10.1038/nbt1358
Keith, D. E., Anton, B., Murray, S. R., Zaki, P. A., Chu, P. C., Lissin, D. V., et al. (1998). mu-Opioid receptor internalization: Opiate drugs have differential effects on a conserved endocytic mechanism in vitro and in the mammalian brain. Mol. Pharmacol. 53, 377–384. doi: 10.1124/mol.53.3.377
Keith, D. E., Murray, S. R., Zaki, P. A., Chu, P. C., Lissin, D. V., Kang, L., et al. (1996). Morphine activates opioid receptors without causing their rapid internalization. J. Biol. Chem. 271, 19021–19024. doi: 10.1074/jbc.271.32.19021
Kelly, E., Bailey, C. P., and Henderson, G. (2008). Agonist-selective mechanisms of Gpcr desensitization. Br. J. Pharmacol. 153, S379–S388. doi: 10.1038/sj.bjp.0707604
Kenakin, T. (2011). Functional selectivity and biased receptor signaling. J. Pharmacol. Exp. Ther. 336, 296–302. doi: 10.1124/jpet.110.173948
Kersten, C., and Cameron, M. G. (2012). Cetuximab alleviates neuropathic pain despite tumour progression. BMJ Case Rep. 2012:bcr1220115374. doi: 10.1136/bcr.12.2011.5374
Kersten, C., Cameron, M. G., and Mjaland, S. (2013). Epithelial growth factor receptor (EGFR)-inhibition for relief of neuropathic pain-A case series. Scand. J. Pain 4, 3–7. doi: 10.1016/j.sjpain.2012.11.011
Kersten, C., Cameron, M. G., Bailey, A. G., Fallon, M. T., Laird, B. J., Paterson, V., et al. (2019). Relief of neuropathic pain through epidermal growth factor receptor inhibition: A randomized proof-of-concept trial. Pain Med. 20, 2495–2505. doi: 10.1093/pm/pnz101
Kersten, C., Cameron, M. G., Laird, B., and Mjaland, S. (2015). Epidermal growth factor receptor-inhibition (Egfr-I) in the treatment of neuropathic pain. Br. J. Anaesth. 115, 761–767. doi: 10.1093/bja/aev326
Kilpatrick, L. E., and Hill, S. J. (2021). Transactivation of G protein-coupled receptors (GPCRS) and receptor tyrosine kinases (RTKs): Recent insights using luminescence and fluorescence technologies. Curr. Opin. Endocr. Metab. Res. 16, 102–112. doi: 10.1016/j.coemr.2020.10.003
Kim, S. H., and Chung, J. M. (1992). An experimental model for peripheral neuropathy produced by segmental spinal nerve ligation in the rat. Pain 50, 355–363. doi: 10.1016/0304-3959(92)90041-9
Koo, J. W., Lobo, M. K., Chaudhury, D., Labonte, B., Friedman, A., Heller, E., et al. (2014). Loss of BDNF signaling in D1R-expressing NAc neurons enhances morphine reward by reducing GABA inhibition. Neuropsychopharmacology 39, 2646–2653. doi: 10.1038/npp.2014.118
Koo, J. W., Mazei-Robison, M. S., Chaudhury, D., Juarez, B., Laplant, Q., Ferguson, D., et al. (2012). BDNF is a negative modulator of morphine action. Science 338, 124–128. doi: 10.1126/science.1222265
Kutlar, A. (2013). Glee-ful for sickle cell pain? Blood 122, 1846–1847. doi: 10.1182/blood-2013-07-510982
Lau, E. K., Trester-Zedlitz, M., Trinidad, J. C., Kotowski, S. J., Krutchinsky, A. N., Burlingame, A. L., et al. (2011). Quantitative encoding of the effect of a partial agonist on individual opioid receptors by multisite phosphorylation and threshold detection. Sci. Signal. 4:ra52. doi: 10.1126/scisignal.2001748
Lemel, L., Lane, J. R., and Canals, M. (2020). GRKs as key modulators of opioid receptor function. Cells 9:2400. doi: 10.3390/cells9112400
Lemmon, M. A., and Schlessinger, J. (2010). Cell signaling by receptor tyrosine kinases. Cell 141, 1117–1134. doi: 10.1016/j.cell.2010.06.011
Li, L., Chen, S. R., Chen, H., Wen, L., Hittelman, W. N., Xie, J. D., et al. (2016). Chloride homeostasis critically regulates synaptic NMDA receptor activity in neuropathic pain. Cell Rep. 15, 1376–1383. doi: 10.1016/j.celrep.2016.04.039
Li, Y., Eitan, S., Wu, J., Evans, C. J., Kieffer, B., Sun, X., et al. (2003). Morphine induces desensitization of insulin receptor signaling. Mol. Cell Biol. 23, 6255–6266. doi: 10.1128/MCB.23.17.6255-6266.2003
Li, Z., Jia, X., Peng, X., and Gao, F. (2020). The Interaction between spinal PDGFRbeta and mu opioid receptor in the activation of microglia in morphine-tolerant rats. J. Pain Res. 13, 1803–1810. doi: 10.2147/JPR.S255221
Liu, S., Liu, W. T., Liu, Y. P., Dong, H. L., Henkemeyer, M., Xiong, L. Z., et al. (2011). Blocking EphB1 receptor forward signaling in spinal cord relieves bone cancer pain and rescues analgesic effect of morphine treatment in rodents. Cancer Res. 71, 4392–4402. doi: 10.1158/0008-5472.CAN-10-3870
Loh, H. H., Liu, H. C., Cavalli, A., Yang, W., Chen, Y. F., and Wei, L. N. (1998). Mu opioid receptor knockout in mice: Effects on ligand-induced analgesia and morphine lethality. Brain Res. Mol. Brain Res. 54, 321–326. doi: 10.1016/S0169-328X(97)00353-7
Lopez-Bellido, R., Puig, S., Huang, P. J., Tsai, C. R., Turner, H. N., Galko, M. J., et al. (2019). Growth factor signaling regulates mechanical nociception in flies and vertebrates. J. Neurosci. 39, 6012–6030. doi: 10.1523/JNEUROSCI.2950-18.2019
Lynch, J. J. III, Wade, C. L., Zhong, C. M., Mikusa, J. P., and Honore, P. (2004). Attenuation of mechanical allodynia by clinically utilized drugs in a rat chemotherapy-induced neuropathic pain model. Pain 110, 56–63. doi: 10.1016/j.pain.2004.03.010
Macey, T. A., Bobeck, E. N., Hegarty, D. M., Aicher, S. A., Ingram, S. L., and Morgan, M. M. (2009). Extracellular signal-regulated kinase 1/2 activation counteracts morphine tolerance in the periaqueductal gray of the rat. J. Pharmacol. Exp. Therap. 331, 412–418. doi: 10.1124/jpet.109.152157
Manglik, A., Lin, H., Aryal, D. K., Mccorvy, J. D., Dengler, D., Corder, G., et al. (2016). Structure-based discovery of opioid analgesics with reduced side effects. Nature 537, 185–190. doi: 10.1038/nature19112
Mansour, A., Fox, C. A., Burke, S., Akil, H., and Watson, S. J. (1995a). Immunohistochemical localization of the cloned mu opioid receptor in the rat CNS. J. Chem. Neuroanat. 8, 283–305. doi: 10.1016/0891-0618(95)00055-C
Mansour, A., Watson, S. J., and Akil, H. (1995b). Opioid receptors: Past, present and future. Trends Neurosci. 18, 69–70. doi: 10.1016/0166-2236(95)80024-V
Mansour, A., Fox, C. A., Burke, S., Meng, F., Thompson, R. C., Akil, H., et al. (1994a). Mu, delta, and kappa opioid receptor mRNA expression in the rat CNS: An in situ hybridization study. J. Comp. Neurol. 350, 412–438. doi: 10.1002/cne.903500307
Mansour, A., Fox, C. A., Thompson, R. C., Akil, H., and Watson, S. J. (1994b). mu-Opioid receptor mRNA expression in the rat CNS: Comparison to mu-receptor binding. Brain Res. 643, 245–265. doi: 10.1016/0006-8993(94)90031-0
Mansour, A., Khachaturian, H., Lewis, M. E., Akil, H., and Watson, S. J. (1988). Anatomy of CNS opioid receptors. Trends Neurosci. 11, 308–314. doi: 10.1016/0166-2236(88)90093-8
Mao, J., Price, D. D., and Mayer, D. J. (1995). Mechanisms of hyperalgesia and morphine tolerance: A current view of their possible interactions. Pain 62, 259–274. doi: 10.1016/0304-3959(95)00073-2
Martin, L. J., Smith, S. B., Khoutorsky, A., Magnussen, C. A., Samoshkin, A., Sorge, R. E., et al. (2017). Epiregulin and EGFR interactions are involved in pain processing. J. Clin. Invest. 127, 3353–3366. doi: 10.1172/JCI87406
Martini, L., and Whistler, J. L. (2007). The role of mu opioid receptor desensitization and endocytosis in morphine tolerance and dependence. Curr.Opin. Neurobiol. 17, 556–564. doi: 10.1016/j.conb.2007.10.004
Masuo, Y., Morita, M., Oka, S., and Ishido, M. (2004). Motor hyperactivity caused by a deficit in dopaminergic neurons and the effects of endocrine disruptors: A study inspired by the physiological roles of PACAP in the brain. Regul. Pept. 123, 225–234. doi: 10.1016/j.regpep.2004.05.010
Matthes, H. W., Maldonado, R., Simonin, F., Valverde, O., Slowe, S., Kitchen, I., et al. (1996). Loss of morphine-induced analgesia, reward effect and withdrawal symptoms in mice lacking the mu-opioid-receptor gene. Nature 383, 819–823. doi: 10.1038/383819a0
Mayer, D. J., Mao, J., Holt, J., and Price, D. D. (1999). Cellular mechanisms of neuropathic pain, morphine tolerance, and their interactions. Proc. Natl. Acad. Sci. U.S.A. 96, 7731–7736. doi: 10.1073/pnas.96.14.7731
Mcconalogue, K., Grady, E. F., Minnis, J., Balestra, B., Tonini, M., Brecha, N. C., et al. (1999). Activation and internalization of the mu-opioid receptor by the newly discovered endogenous agonists, endomorphin-1 and endomorphin-2. Neuroscience 90, 1051–1059. doi: 10.1016/S0306-4522(98)00514-4
Mckay, M., and Morrison, D. (2007). Integrating signals from RTKs to ERK/MAPK. Oncogene 26, 3113–3121. doi: 10.1038/sj.onc.1210394
Mclaughlin, J. P., and Chavkin, C. (2001). Tyrosine phosphorylation of the mu-opioid receptor regulates agonist intrinsic efficacy. Mol. Pharmacol. 59, 1360–1368. doi: 10.1124/mol.59.6.1360
Mir, H. R., Miller, A. N., Obremskey, W. T., Jahangir, A. A., and Hsu, J. R. (2019). Confronting the opioid crisis: Practical pain management and strategies: AOA 2018 critical issues symposium. J. Bone Joint Surg. Am. 101:e126. doi: 10.2106/JBJS.19.00285
Miyatake, M., Rubinstein, T. J., Mclennan, G. P., Belcheva, M. M., and Coscia, C. J. (2009). Inhibition of EGF-induced ERK/Map kinase-mediated astrocyte proliferation by mu opioids: Integration of G protein and beta-arrestin 2-dependent pathways. J. Neurochem. 110, 662–674. doi: 10.1111/j.1471-4159.2009.06156.x
Molina-Munþoz, T., Romero-Aìvila, M. A. T., and Garciìa-Saìinz, J. A. (2006). Insulin-like growth factor-I induces α1B-adrenergic receptor phosphorylation through Gβγ and epidermal growth factor receptor transactivation. Mol. Endocrinol. 20, 2773–2783. doi: 10.1210/me.2006-0090
Moryl, N., Obbens, E. A., Ozigbo, O. H., and Kris, M. G. (2006). Analgesic effect of gefitinib in the treatment of non-small cell lung cancer. J. Support Oncol. 4:111.
Mouledous, L., Diaz, M. F., and Gutstein, H. B. (2007). Extracellular signal-regulated kinase (ERK) inhibition does not prevent the development or expression of tolerance to and dependence on morphine in the mouse. Pharmacol. Biochem. Behav. 88, 39–46. doi: 10.1016/j.pbb.2007.07.002
Murnion, B. P. (2018). Neuropathic pain: Current definition and review of drug treatment. Aust. Prescr. 41:60. doi: 10.18773/austprescr.2018.022
Nair, A. B., and Jacob, S. (2016). A simple practice guide for dose conversion between animals and human. J. Basic Clin. Pharm. 7:27. doi: 10.4103/0976-0105.177703
Narita, M., Usui, A., Narita, M., Niikura, K., Nozaki, H., Khotib, J., et al. (2005). Protease-activated receptor-1 and platelet-derived growth factor in spinal cord neurons are implicated in neuropathic pain after nerve injury. J. Neurosci. 25, 10000–10009. doi: 10.1523/JNEUROSCI.2507-05.2005
Navarro, B., Kennedy, M. E., Velimirović, B., Bhat, D., Peterson, A. S., and Clapham, D. E. (1996). Nonselective and Gβγ-insensitive weaver K+ channels. Science 272, 1950–1953. doi: 10.1126/science.272.5270.1950
Noma, T., Lemaire, A., Prasad, S. V. N., Barki-Harrington, L., Tilley, D. G., Chen, J., et al. (2007). β-arrestin–mediated β 1-adrenergic receptor transactivation of the Egfr confers cardioprotection. J. Clin. Invest. 117, 2445–2458. doi: 10.1172/JCI31901
Oligny-Longpré, G., Corbani, M., Zhou, J., Hogue, M., Guillon, G., and Bouvier, M. (2012). Engagement of β-arrestin by transactivated insulin-like growth factor receptor is needed for V2 vasopressin receptor-stimulated ERK1/2 activation. Proc. Natl Acad. Sci. U.S.A. 109, E1028–E1037. doi: 10.1073/pnas.1112422109
Overland, A. C., and Insel, P. A. (2015). Heterotrimeric G proteins directly regulate Mmp14/membrane type-1 matrix metalloprotease: A novel mechanism for GPCR-EGFR transactivation. J. Biol. Chem. 290, 9941–9947. doi: 10.1074/jbc.C115.647073
Pattinson, K. T. (2008). Opioids and the control of respiration. Br. J. Anaesth. 100, 747–758. doi: 10.1093/bja/aen094
Paul, A. K., Smith, C. M., Rahmatullah, M., Nissapatorn, V., Wilairatana, P., Spetea, M., et al. (2021). Opioid analgesia and opioid-induced adverse effects: A review. Pharmaceuticals (Basel) 14:1091. doi: 10.3390/ph14111091
Pawson, T. (2004). Specificity in signal transduction: From phosphotyrosine-Sh2 domain interactions to complex cellular systems. Cell 116, 191–203. doi: 10.1016/S0092-8674(03)01077-8
Peregud, D. I., Yakovlev, A. A., Stepanichev, M. Y., Onufriev, M. V., Panchenko, L. F., and Gulyaeva, N. V. (2016). Expression of BDNF and TRKB phosphorylation in the rat frontal cortex during morphine withdrawal are no dependent. Cell Mol. Neurobiol. 36, 839–849. doi: 10.1007/s10571-015-0267-6
Petäjä-Repo, U. E., Hogue, M., Laperriere, A., Bhalla, S., Walker, P., and Bouvier, M. (2001). Newly synthesized human δ opioid receptors retained in the endoplasmic reticulum are retrotranslocated to the cytosol, deglycosylated, ubiquitinated, and degraded by the proteasome. J. Biol. Chem. 276, 4416–4423. doi: 10.1074/jbc.M007151200
Phamduong, E., Rathore, M. K., Crews, N. R., D’angelo, A. S., Leinweber, A. L., Kappera, P., et al. (2014). Acute and chronic mu opioids differentially regulate thrombospondins 1 and 2 isoforms in astrocytes. ACS Chem. Neurosci. 5, 106–114. doi: 10.1021/cn400172n
Pickard, A. S., and Lee, T. A. (2021). Combating the opioid epidemic in the United States. Drugs Context 10. doi: 10.7573/dic.2021-10-7
Pierce, K. L., Luttrell, L. M., and Lefkowitz, R. J. (2001). New mechanisms in heptahelical receptor signaling to mitogen activated protein kinase cascades. Oncogene 20, 1532–1539. doi: 10.1038/sj.onc.1204184
Posa, L., Accarie, A., Noble, F., and Marie, N. (2016). Methadone reverses analgesic tolerance induced by morphine pretreatment. Int. J. Neuropsychopharmacol. 19:yv108. doi: 10.1093/ijnp/pyv108
Povsic, T. J., Kohout, T. A., and Lefkowitz, R. J. (2003). β-Arrestin1 mediates insulin-like growth factor 1 (Igf-1) activation of phosphatidylinositol 3-kinase (Pi3K) and anti-apoptosis. J. Biol. Chem. 278, 51334–51339. doi: 10.1074/jbc.M309968200
Przewlocki, R., and Przewlocka, B. (2001). Opioids in chronic pain. Eur. J. Pharmacol. 429, 79–91. doi: 10.1016/S0014-2999(01)01308-5
Puig, S., and Gutstein, H. B. (2017). Opioids: Keeping the good, eliminating the bad. Nat. Med. 23, 272–273. doi: 10.1038/nm.4277
Puig, S., Donica, C. L., and Gutstein, H. B. (2020b). EGFR Signaling causes morphine tolerance and mechanical sensitization in rats. eNeuro 7. doi: 10.1523/ENEURO.0460-18.2020
Puig, S., Barker, K. E., Szott, S. R., Kann, P. T., Morris, J. S., and Gutstein, H. B. (2020a). Spinal opioid tolerance depends upon platelet-derived growth factor receptor-beta signaling, not mu-opioid receptor internalization. Mol. Pharmacol. 98, 487–496. doi: 10.1124/mol.120.119552
Quasthoff, S., and Hartung, H. P. (2002). Chemotherapy-induced peripheral neuropathy. J. Neurol. 249, 9–17. doi: 10.1007/PL00007853
Raehal, K. M., and Bohn, L. M. (2011). The role of beta-arrestin2 in the severity of antinociceptive tolerance and physical dependence induced by different opioid pain therapeutics. Neuropharmacology 60, 58–65. doi: 10.1016/j.neuropharm.2010.08.003
Raehal, K. M., Schmid, C. L., Groer, C. E., and Bohn, L. M. (2011). Functional selectivity at the mu-opioid receptor: Implications for understanding opioid analgesia and tolerance. Pharmacol. Rev. 63, 1001–1019. doi: 10.1124/pr.111.004598
Ragunathrao, V. A. B., Anwar, M., Akhter, M. Z., Chavez, A., Natarajan, V., Lakshmikanthan, S., et al. (2019). Sphingosine-1-phosphate receptor 1 activity promotes tumor growth by amplifying Vegf-Vegfr2 angiogenic signaling. Cell Rep. 29, 3472–3487.e4. doi: 10.1016/j.celrep.2019.11.036
Rezamohammadi, F., Rahmani, M., Ghanbari, A., Khaleghian, A., and Miladi-Gorji, H. (2020). Bdnf receptor antagonism during the induction of morphine dependence exacerbates the severity of physical dependence and ameliorates psychological dependence in rats. Neurosci. Lett. 737:135332. doi: 10.1016/j.neulet.2020.135332
Rivat, C., Sar, C., Mechaly, I., Leyris, J.-P., Diouloufet, L., Sonrier, C., et al. (2018). Inhibition of neuronal Flt3 receptor tyrosine kinase alleviates peripheral neuropathic pain in mice. Nat. Commun. 9, 1–13. doi: 10.1038/s41467-018-03496-2
Robinson, D. R., Wu, Y.-M., and Lin, S.-F. (2000). The protein tyrosine kinase family of the human genome. Oncogene 19, 5548–5557. doi: 10.1038/sj.onc.1203957
Roskoski, R. Jr. (2018). The role of small molecule platelet-derived growth factor receptor (PDGFR) inhibitors in the treatment of neoplastic disorders. Pharmacol. Res. 129, 65–83. doi: 10.1016/j.phrs.2018.01.021
Russo, S. J., Bolanos, C. A., Theobald, D. E., Decarolis, N. A., Renthal, W., Kumar, A., et al. (2007). Irs2-Akt pathway in midbrain dopamine neurons regulates behavioral and cellular responses to opiates. Nat. Neurosci. 10, 93–99. doi: 10.1038/nn1812
Saito, Y., Haendeler, J., Hojo, Y., Yamamoto, K., and Berk, B. C. (2001). Receptor heterodimerization: Essential mechanism for platelet-derived growth factor-induced epidermal growth factor receptor transactivation. Mol. Cell Biol. 21, 6387–6394. doi: 10.1128/MCB.21.19.6387-6394.2001
Saloner, B., Mcginty, E. E., Beletsky, L., Bluthenthal, R., Beyrer, C., Botticelli, M., et al. (2018). A public health strategy for the opioid crisis. Public Health Rep. 133, 24S–34S. doi: 10.1177/0033354918793627
Sasahara, M., Fries, J. W., Raines, E. W., Gown, A. M., Westrum, L. E., Frosch, M. P., et al. (1991). PDGF B-chain in neurons of the central nervous system, posterior pituitary, and in a transgenic model. Cell 64, 217–227. doi: 10.1016/0092-8674(91)90223-L
Savage, D. G., and Antman, K. H. (2002). Imatinib mesylate–a new oral targeted therapy. N. Engl. J. Med. 346, 683–693. doi: 10.1056/NEJMra013339
Scherrer, G., Imamachi, N., Cao, Y. Q., Contet, C., Mennicken, F., O’donnell, D., et al. (2009). Dissociation of the opioid receptor mechanisms that control mechanical and heat pain. Cell 137, 1148–1159. doi: 10.1016/j.cell.2009.04.019
Schlessinger, J. (2000). New roles for Src kinases in control of cell survival and angiogenesis. Cell 100, 293–296. doi: 10.1016/S0092-8674(00)80664-9
Schmid, C. L., Kennedy, N. M., Ross, N. C., Lovell, K. M., Yue, Z., Morgenweck, J., et al. (2017). Bias factor and therapeutic window correlate to predict safer opioid analgesics. Cell 171, 1165–1175.e13. doi: 10.1016/j.cell.2017.10.035
Shi, X., Miller, J. S., Harper, L. J., Poole, R. L., Gould, T. J., and Unterwald, E. M. (2014). Reactivation of cocaine reward memory engages the AKT/Gsk3/MTOR signaling pathway and can be disrupted by Gsk3 inhibition. Psychopharmacology 231, 3109–3118. doi: 10.1007/s00213-014-3491-8
Sim, E. H., Yang, I. A., Wood-Baker, R., Bowman, R. V., and Fong, K. M. (2018). Gefitinib for advanced non-small cell lung cancer. Cochrane Database Syst. Rev. 1:Cd006847. doi: 10.1002/14651858.CD006847.pub2
Singla, N. K., Skobieranda, F., Soergel, D. G., Salamea, M., Burt, D. A., Demitrack, M. A., et al. (2019). Apollo-2: A randomized, placebo and active-controlled phase iii study investigating oliceridine (Trv 130), a G protein–biased ligand at the μ−opioid receptor, for management of moderate to severe acute pain following abdominoplasty. Pain Pract. 19, 715–731. doi: 10.1111/papr.12801
Soergel, D. G., Subach, R. A., Burnham, N., Lark, M. W., James, I. E., Sadler, B. M., et al. (2014). Biased agonism of the μ-opioid receptor by Trv130 increases analgesia and reduces on-target adverse effects versus morphine: A randomized, double-blind, placebo-controlled, crossover study in healthy volunteers. Pain 155, 1829–1835. doi: 10.1016/j.pain.2014.06.011
Spliet, W. G., Aronica, E., Ramkema, M., Witmer, A. N., Schlingemann, R. O., De Jong, J. M., et al. (2004). Immunohistochemical localization of vascular endothelial growth factor receptors–1, –2 and –3 in human spinal cord: Altered expression in amyotrophic lateral sclerosis. Neuropathol. Appl. Neurobiol. 30, 351–359. doi: 10.1111/j.1365-2990.2003.00543.x
Stankovic Stojanovic, K., Thioliere, B., Garandeau, E., Lecomte, I., Bachmeyer, C., and Lionnet, F. (2011). Chronic myeloid leukaemia and sickle cell disease: Could imatinib prevent vaso-occlusive crisis? Br. J. Haematol. 155, 271–272. doi: 10.1111/j.1365-2141.2011.08670.x
Sternini, C., Spann, M., Anton, B., Keith, D. E. Jr., Bunnett, N. W., Von Zastrow, M., et al. (1996). Agonist-selective endocytosis of mu opioid receptor by neurons in vivo. Proc Natl Acad Sci U.S.A. 93, 9241–9246. doi: 10.1073/pnas.93.17.9241
Stuart, G. L., Shorey, R. C., France, C. R., Macfie, J., Bell, K., Fortner, K. B., et al. (2018). Empirical studies addressing the opioid epidemic: An urgent call for research. Subst. Abuse 12:1178221818784294. doi: 10.1177/1178221818784294
Sun, N., Zhang, X., Guo, S., Le, H. T., Zhang, X., and Kim, K.-M. (2018). Molecular mechanisms involved in epidermal growth factor receptor-mediated inhibition of dopamine D3 receptor signaling. Biochim. Biophys. Acta 1865, 1187–1200. doi: 10.1016/j.bbamcr.2018.06.001
The Council of Economic Advisers (2017). The underestimated cost of the opioid crisis. Washington, DC: The Council of Economic Advisers.
Trafton, J. A., Abbadie, C., Marek, K., and Basbaum, A. I. (2000). Postsynaptic signaling via the [mu]-opioid receptor: Responses of dorsal horn neurons to exogenous opioids and noxious stimulation. J. Neurosci. 20, 8578–8584. doi: 10.1523/JNEUROSCI.20-23-08578.2000
Tsai, R.-Y., Tai, Y.-H., Tzeng, J.-I., Cherng, C.-H., Yeh, C.-C., and Wong, C.-S. (2009). Ultra-low dose naloxone restores the antinociceptive effect of morphine in pertussis toxin-treated rats by reversing the coupling of μ-opioid receptors from Gs-protein to coupling to Gi-protein. Neuroscience 164, 435–443. doi: 10.1016/j.neuroscience.2009.08.015
Ugolini, G., Marinelli, S., Covaceuszach, S., Cattaneo, A., and Pavone, F. (2007). The function neutralizing anti-TrkA antibody Mnac13 reduces inflammatory and neuropathic pain. Proc. Natl Acad. Sci. U.S.A. 104, 2985–2990. doi: 10.1073/pnas.0611253104
Viscusi, E. R., Skobieranda, F., Soergel, D. G., Cook, E., Burt, D. A., and Singla, N. (2019). Apollo-1: A randomized placebo and active-controlled phase Iii study investigating oliceridine (Trv130), a G protein-biased ligand at the μ-opioid receptor, for management of moderate-to-severe acute pain following bunionectomy. J. Pain Res. 12:927. doi: 10.2147/JPR.S171013
Wang, Y., Barker, K., Shi, S., Diaz, M., Mo, B., and Gutstein, H. B. (2012). Blockade of PDGFR-beta activation eliminates morphine analgesic tolerance. Nat. Med. 18, 385–387. doi: 10.1038/nm.2633
Wang, Z., Ma, W., Chabot, J.-G., and Quirion, R. (2009). Cell-type specific activation of p38 and Erk mediates calcitonin gene-related peptide involvement in tolerance to morphine-induced analgesia. FASEB J. 23, 2576–2586. doi: 10.1096/fj.08-128348
Weber, M. L., Chen, C., Li, Y., Farooqui, M., Nguyen, J., Poonawala, T., et al. (2013). Morphine stimulates platelet-derived growth factor receptor-beta signalling in mesangial cells in vitro and transgenic sickle mouse kidney in vivo. Br. J. Anaesth. 111, 1004–1012. doi: 10.1093/bja/aet221
Werner, M. H., Nanney, L. B., Stoscheck, C. M., and King, L. E. (1988). Localization of immunoreactive epidermal growth factor receptors in human nervous system. J. Histochem. Cytochem. 36, 81–86. doi: 10.1177/36.1.3275713
Wetzker, R., and Bohmer, F. D. (2003). Transactivation joins multiple tracks to the ERK/MAPK cascade. Nat. Rev. Mol. Cell Biol. 4, 651–657. doi: 10.1038/nrm1173
Whistler, J. L. (2012). Examining the role of mu opioid receptor endocytosis in the beneficial and side-effects of prolonged opioid use: From a symposium on new concepts in mu-opioid pharmacology. Drug Alcohol Depend. 121, 189–204. doi: 10.1016/j.drugalcdep.2011.10.031
Whistler, J. L., and Von Zastrow, M. (1998). Morphine-activated opioid receptors elude desensitization by β-arrestin. Proc. Natl. Acad. Sci. U.S.A. 95, 9914–9919. doi: 10.1073/pnas.95.17.9914
Whistler, J. L., Chuang, H. H., Chu, P., Jan, L. Y., and Von Zastrow, M. (1999). Functional dissociation of mu opioid receptor signaling and endocytosis: Implications for the biology of opiate tolerance and addiction. Neuron 23, 737–746. doi: 10.1016/S0896-6273(01)80032-5
Williams, J. T., Ingram, S. L., Henderson, G., Chavkin, C., Von Zastrow, M., Schulz, S., et al. (2013). Regulation of mu-opioid receptors: Desensitization, phosphorylation, internalization, and tolerance. Pharmacol. Rev. 65, 223–254. doi: 10.1124/pr.112.005942
Xia, W. S., Peng, Y. N., Tang, L. H., Jiang, L. S., Yu, L. N., Zhou, X. L., et al. (2014). Spinal ephrinB/EphB signalling contributed to remifentanil-induced hyperalgesia via NMDA receptor. Eur. J. Pain 18, 1231–1239. doi: 10.1002/j.1532-2149.2014.00478.x
Xu, Y., Lv, X.-F., Cui, C.-L., Ge, F.-F., Li, Y.-J., and Zhang, H.-L. (2012). Essential role of Nr2B-containing NMDA receptor–ERK pathway in nucleus accumbens shell in morphine-associated contextual memory. Brain Res. Bull. 89, 22–30. doi: 10.1016/j.brainresbull.2012.06.012
Yang, Y., Sun, Y., Hu, R., Yan, J., Wang, Z., Li, W, et al. (2021). Morphine promotes microglial activation by upregulating the EGFR/ERK signaling pathway. PLoS One 9:e0256870. doi: 10.1371/journal.pone.0256870
Zamora-Martinez, E. R., and Edwards, S. (2014). Neuronal extracellular signal-regulated kinase (ERK) activity as marker and mediator of alcohol and opioid dependence. Front. Integr. Neurosci. 8:24. doi: 10.3389/fnint.2014.00024
Zamponi, G. W., Bourinet, E., Nelson, D., Nargeot, J., and Snutch, T. P. (1997). Crosstalk between G proteins and protein kinase C mediated by the calcium channel α1 subunit. Nature 385, 442–446. doi: 10.1038/385442a0
Zhao, H., Wu, G., and Cao, X. (2013). Egfr dependent subcellular communication was responsible for morphine mediated Ac superactivation. Cell Signal. 25, 417–428. doi: 10.1016/j.cellsig.2012.10.016
Keywords: mu-opioid receptor, opioid signaling, pain, tolerance, neuropathic pain, physical dependence, reward, receptor tyrosine kinase
Citation: Gamble MC, Williams BR, Singh N, Posa L, Freyberg Z, Logan RW and Puig S (2022) Mu-opioid receptor and receptor tyrosine kinase crosstalk: Implications in mechanisms of opioid tolerance, reduced analgesia to neuropathic pain, dependence, and reward. Front. Syst. Neurosci. 16:1059089. doi: 10.3389/fnsys.2022.1059089
Received: 30 September 2022; Accepted: 31 October 2022;
Published: 01 December 2022.
Edited by:
Nicolas Massaly, Washington University in St. Louis, United StatesReviewed by:
Mariana Spetea, University of Innsbruck, AustriaMelih Özgür Celik, Charité Universitätsmedizin Berlin, Germany
Christoph Stein, Charité – Universitätsmedizin Berlin, Germany, in collaboration with reviewer MÖC
Copyright © 2022 Gamble, Williams, Singh, Posa, Freyberg, Logan and Puig. This is an open-access article distributed under the terms of the Creative Commons Attribution License (CC BY). The use, distribution or reproduction in other forums is permitted, provided the original author(s) and the copyright owner(s) are credited and that the original publication in this journal is cited, in accordance with accepted academic practice. No use, distribution or reproduction is permitted which does not comply with these terms.
*Correspondence: Stephanie Puig, c3B1aWdAYnUuZWR1
†These authors have contributed equally to this work and share first authorship