- 1Institute of Biology, Humboldt University of Berlin, Berlin, Germany
- 2Institute of Computer Science, University of Tartu, Tartu, Estonia
- 3School of Law, University of Tartu, Tartu, Estonia
- 4Neurocure Center for Excellence, Charité Universitätsmedizin Berlin, Berlin, Germany
One fundamental feature of consciousness is that the contents of consciousness depend on the state of consciousness. Here, we propose an answer to why this is so: both the state and the contents of consciousness depend on the activity of cortical layer 5 pyramidal (L5p) neurons. These neurons affect both cortical and thalamic processing, hence coupling the cortico-cortical and thalamo-cortical loops with each other. Functionally this coupling corresponds to the coupling between the state and the contents of consciousness. Together the cortico-cortical and thalamo-cortical loops form a thalamo-cortical broadcasting system, where the L5p cells are the central elements. This perspective makes one quite specific prediction: cortical processing that does not include L5p neurons will be unconscious. More generally, the present perspective suggests that L5p neurons have a central role in the mechanisms underlying consciousness.
Introduction
Each of us can be fully conscious, have a dream, be in deep sleep or be anesthetized. These are typical states of consciousness. On the other hand, we can be conscious of a dog, a paper, coconut taste, itch etc. These are examples of the contents of consciousness (from among a world of different possibilities). Unfortunately for consciousness research, the state of consciousness is mostly studied separately from the contents (Bachmann and Hudetz, 2014). The research done on the state of consciousness mainly revolves around the thalamus and thalamocortical interactions (reviews: Laureys, 2005; Alkire et al., 2008; Schiff, 2010). On the other hand, the search for the correlates of the contents of consciousness is mostly focused on cortical processing (reviews: Rees et al., 2002; Dehaene and Changeux, 2011; Koch et al., 2016).
However, one basic fact about consciousness is that the state of consciousness can never be dissociated from the contents of consciousness. One cannot be conscious of the coconut taste while being in an unconscious state. And the other way around: in typical healthy subjects, one cannot be in a conscious state while not being conscious of anything at all1. In other words, contents of consciousness and states of consciousness make up an integrated whole. Studying one while disregarding the other can only provide half of an answer.
The intertwinement of state and contents of consciousness is a well-known issue in consciousness research (e.g., Hohwy, 2009; Bachmann, 2012; Bachmann and Hudetz, 2014; Mashour and Hudetz, 2017). However, it is currently unclear why contents and state of consciousness have to be so tightly coupled in the first place. In this work, we highlight a clear neurobiological correlate for this intertwinement. Namely, cortical layer 5 pyramidal (L5p) neurons participate in both the thalamo-cortical and cortico-cortical loops and couple these two loops in a unique way, hence functionally coupling the state and contents of consciousness (Figure 1). This view reconciles the two traditions of consciousness research: the tradition of studying the state of consciousness with the focus on the thalamo-cortical system and the tradition of investigating the contents of consciousness with a particular emphasis on the cortico-cortical processing. We will first very briefly review these two traditions and then explain how L5p neurons couple the activity patterns of the thalamo-cortical and cortico-cortical loops.
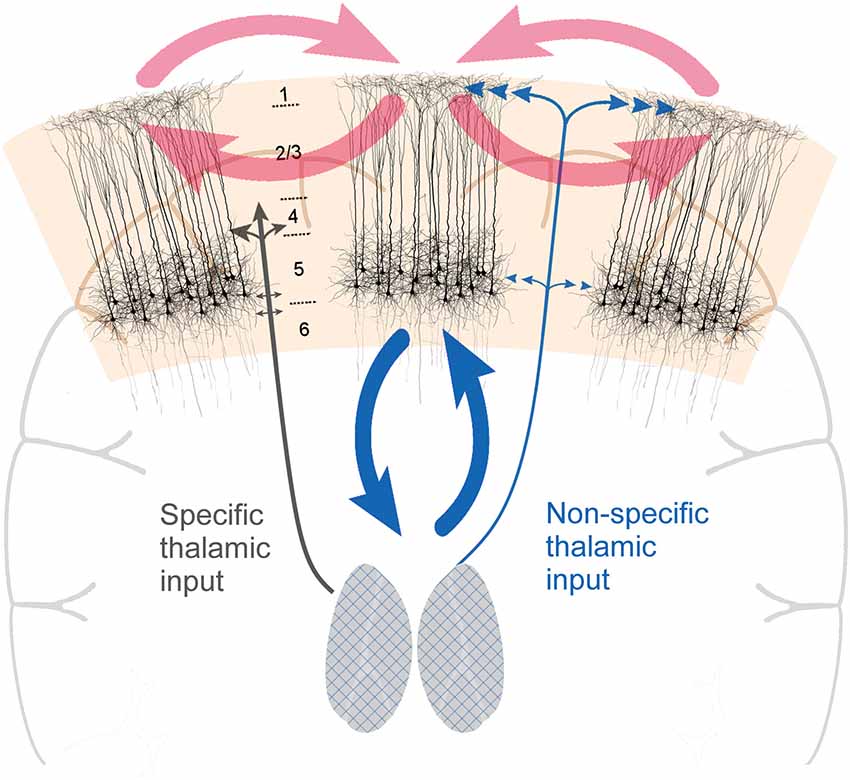
Figure 1. Cortical layer 5 pyramidal (L5p) neurons (the black-colored neurons on the image) play a central role in both cortico-cortical and thalamo-cortical loops. By being central to both loops, they effectively couple them, functionally coupling the state and contents of consciousness. Two types of thalamic projections are highlighted: specific (SP) and nonspecific (NSP) types of projections that have different cortical projection patterns. The grids on the thalami are illustrative of the fact that thalamocortical neurons with NSP types of projections can be found in different parts of the thalamus.
Thalamo-Cortical Loop and the State of Consciousness
Conscious experience alternates between being present and not being present, with intermediate states between full absence of conscious mentation and full presence of clear, vivid and temporally seamless subjective experience (e.g., sleep, vegetative state, minimally conscious state, hypnagogic state, fully conscious state—with varying levels of clarity or degree of experience across different states of alertness).
The idea that thalamus plays a key role in controlling the states of consciousness is not new. According to the prevailing views of the mid-20th century thalamus is part of the reticulo-thalamic system with two general types of thalamo-cortical pathways: (1) the so-called specific pathways (SP) which function as the carriers of afferent information to the cortex [the prime example is the lateral geniculate nucleus (LGN) for vision]; and (2) the so-called non-specific pathways (NSP) that have no direct role in transmitting information to cortex, but are capable of modulating the state of the cortex (Figure 1; Moruzzi and Magoun, 1949; Jung, 1958; Magoun, 1958; Riklan and Levita, 1969; Doty, 1970; Purpura, 1970; Somjen, 1972; Brooks and Jung, 1973; Brazier, 1977; Hassler, 1978; Smirnov et al., 1978; Singer, 1979; Livingstone and Hubel, 1980; Steriade, 1981a; Mesulam, 1985; Newman, 1995a). Historically, these two types of pathways were first distinguished based on the identity of the thalamic nucleus they originated from (LGN would be an example of SP nuclei, the intralaminar thalamic nuclei are classic examples of NSP nuclei). However, it later turned out that the projections are more intermingled (e.g., Jones, 2012; Clascá et al., 2016), hence it is too simplified to refer to SP and NSP nuclei. In this manuscript when we refer to NSP thalamus we have in mind these thalamic cells that project to the superficial and deep layers of the cortex (see also Figure 1). Such thalamocortical cells mainly reside in the classic NSP nuclei, but there is much diversity among them2. There are several types of evidence demonstrating that especially the NSP thalamus is directly involved in controlling the state of consciousness.
First, alternation of sleep and wakefulness depends on NSP; for example, intralaminar thalamocortical neurons increase their firing rate about 10 s before EEG desynchronization in natural transitions from slow-wave sleep to waking or active sleep (Steriade, 1981b). At sleep onset thalamic deactivation is observed first, followed by cortical changes (Magnin et al., 2010). Recently, Honjoh et al. (2018) showed that stimulation of the NSP ventromedial (VM) thalamic nucleus awoke mice from NREM sleep and anesthesia and caused EEG activation in the high-frequency band.
In addition, virtually all general anesthetics, despite their differences in neurobiological effects elsewhere, have common target in the NSP thalamus (Alkire et al., 2000, 2008). If the NSP thalamus of the anesthetized experimental animals is electrically stimulated, desynchronization of EEG occurs with activity patterns typical to the awake brain (Bremer, 1935; Moruzzi and Magoun, 1949; Brazier, 1977; Munk et al., 1996). These results are corroborated by the fact that injuries and tumors localized in the NSP thalamus often cause absence of consciousness in patients despite the fact that the SP system has remained relatively intact (e.g., Riklan and Levita, 1969; Penfield, 1975; Kinney et al., 1994; Bogen, 1995a,b; Newman, 1995b). Taken together, these findings show that thalamus is in an optimal position to modulate and control the state and level of consciousness (but see Alkire et al., 2008; Hudetz, 2012; Mashour, 2014; for counter-arguments suggesting that the effects in thalamus are only secondary to those in cortex).
Based on this evidence, thalamo-cortical theories of consciousness were introduced (e.g., Purpura, 1970; Hassler, 1978; Bachmann, 1984, 1989, 1999; Bogen, 1995a,b; Newman, 1995a; Llinás, 1996; LaBerge, 1997; Purpura and Schiff, 1997; Tononi and Edelman, 1998; Ward, 2011). As the name suggests, a thalamocortical theory of consciousness proposes that conscious experience is based on the interactions between thalamic nuclei and areas of cortex. According to these theories, there is no specific area of the cortex that is related to consciousness: it is the interplay with the thalamus that matters.
Cortico-Cortical Loop and the Contents of Consciousness
Since the turn of the century, many leading theories of consciousness have emphasized the relationship between consciousness and cortical processing. For example, although the neural global workspace theory (Dehaene and Naccache, 2001; Dehaene and Changeux, 2011) includes thalamocortical processing, it has a minor role compared to the cortical frontoparietal broadcasting system (Dehaene and Changeux, 2011). In addition, the theory that consciousness is related to cortical recurrent processing is purely cortico-centric (Lamme, 2003, 2010). As a final example, the higher order thought theory of consciousness claims that consciousness is tightly linked to computations that crucially depend on the prefrontal cortex (PFC; Lau and Rosenthal, 2011).
Perhaps this change of perspective from thalamus to cortex was driven by methodological advances: by combining appropriate experimental approaches with microelectrode recordings and functional magnetic resonance imaging (fMRI) much evidence could be gathered about the role of cortical areas in consciousness. For example, it was shown that during binocular rivalry the firing of cells in primary visual cortex was not modulated by conscious experience of the monkey (Leopold and Logothetis, 1996). In contrast, the firing of neurons in inferior temporal cortex was stronger when the preferred stimulus was consciously perceived (Sheinberg and Logothetis, 1997). This result was celebrated as paving the way for understanding the neural correlates of the contents of consciousness. For instance, in the enthusiastic commentary accompanying the first of the above-mentioned articles, Francis Crick wrote about the problem of consciousness that “with a little luck, we may glimpse the outline of the solution before the end of the century” (Crick, 1996).
In 1998, as one of the first seminal studies about the neural markers of the contents of consciousness with human neuroimaging, Lumer et al. (1998) used binocular rivalry and fMRI to demonstrate that the activity of the frontoparietal networks is involved in the changes of the content of consciousness (i.e., the dominating image in rivalry). In a later study using visual masking (Dehaene et al., 2001) it was revealed with fMRI that visible words elicited a strong activation of the frontoparietal network that was not observed when the same words were made invisible through masking. Based on these findings the neural global workspace theory (Dehaene and Naccache, 2001; Dehaene and Changeux, 2011) was established and became one of the most influential theories of consciousness.
Hence, the experimental techniques combined with psychophysical paradigms had opened up new a venues for studying the contents of consciousness. Consciousness research became dominated by studies done on healthy humans and the contents of consciousness. This research has generated many interesting findings (reviewed in Rees et al., 2002; Dehaene and Changeux, 2011; Koch et al., 2016) and many controversies (Aru et al., 2012; De Graaf et al., 2012, for a recent debate see for example Boly et al., 2017; Odegaard et al., 2017). However, this focus on the contents of consciousness in humans has left unanswered the question why these contents of consciousness are dependent on the state of consciousness. We would like to demonstrate next how thinking about this issue might also lead us closer to understanding the neurobiological mechanisms of consciousness in general.
State and Content of Consciousness Interact in Layer 5 Pyramidal Neurons
Why are the contents of consciousness dependent on the state of consciousness? In a nutshell, we claim that thalamo-cortical and cortico-cortical processes mechanistically interact at the level of cortical L5p cells, functionally corresponding to the intertwinement of the state and contents of consciousness. Our view, thus, has the potential to reconcile the thalamo-cortical and cortico-cortical theories of consciousness.
From the inspection of the thalamocortical system, it becomes evident that thalamo-cortical and cortico-cortical loops uniquely intersect at the level of L5p neurons (Figure 1). L5p cells participate in both loops by receiving input from and sending output to both thalamus and cortex (Sherman and Guillery, 2001; Jones, 2012; Harris and Shepherd, 2015). There are at least two subpopulations of L5p cells and it might be that some of them (L5A neurons, in upper layer 5) are mainly involved in cortico-cortical loops whereas others (L5B neurons, in deeper layer 5) mainly participate in the loop with the NSP thalamic nuclei (Larsen et al., 2008; Kawaguchi, 2017; Takahashi et al., under revision). Thus, as a population, L5p neurons affect both thalamo-cortical and cortico-cortical processing. It has long been suspected that these cells also have a central role in the neural mechanisms underlying consciousness (Crick and Koch, 1992; Angel, 1993; Llinás and Ribary, 2001; LaBerge, 2006; Meyer, 2015).
Cortical L5p neurons have two functionally distinct sites of integration, one in the soma (somatic compartment) and one near the top of their apical trunk (apical compartment; e.g., Larkum, 2013). This segregation is interesting as the input to the basal dendrites and the apical tuft are known to be both functionally and anatomically quite distinct (Larkum, 2013). In particular, the apical tuft receives diverse input from higher cortical areas and NSP thalamic nuclei whereas the basal dendrites receive more specific feedforward information from lower cortical areas (and, in sensory cortex, from thalamic relay nuclei; Llinás and Ribary, 2001; Jones, 2012; Larkum, 2013).
If basal and apical compartments are depolarized at around the same time, the probability that the neuron fires a burst of action potentials is greatly increased (e.g., Larkum et al., 1999; Larkum, 2013). These bursts have a profound effect on both cortical and thalamic processing, as a burst makes it more likely that the spiking of a neuron contributes to learning (Lisman, 1997) and behavior (Takahashi et al., 2016). The NSP thalamic nuclei have diffuse projection patterns across the entire cortex, hence a burst firing of a few cortical columns could reach the whole thalamo-cortical system. For example, it is known that the secondary somatosensory cortex (S2) can be activated by L5p neurons in S1 through the NSP thalamus (Theyel et al., 2010). After chemically deactivating the respective thalamic nucleus, the activation of S2 was eliminated, suggesting that this trans-thalamic pathway is a key component for cortical activity propagation (Theyel et al., 2010). Similarly, there is a circuit from L5p cells of S1 through the NSP thalamus to the primary motor cortex (M1; Mo and Sherman, 2019). Thus, the NSP thalamus is the basis for a thalamo-cortical broadcasting system and L5p cells are the central elements of it.
The direct relationship between L5p cell activity and the state of consciousness has been described in Murayama and Larkum (2009). These authors performed fiberoptic Ca2+ imaging of the apical dendrites in L5p cells. They compared the effect of different states of consciousness on the activity of the apical compartment while delivering brief air-puffs to the hindlimb of the animal. The apical dendritic response to hindlimb stimulation was 4-fold stronger in the quiet awake state than in the anesthetized state. However, when the animal moved its hindlimb in response to the air-puff, the apical dendritic response was an astonishing 14-fold stronger than under anesthesia. This increase in the Ca2+ activity mostly stemmed from a prolonged duration of the response: when the animal moved its hindlimb, the apical dendritic response lasted for several seconds. This result shows that the awake state has an enormous impact on the activity of L5p apical dendrites (Phillips et al., 2018). Moreover, while the authors did not interpret their findings in this fashion, it is also possible to see the interaction between the state and contents of consciousness in these results. Namely, one way to interpret the behavior of the animals is that when they reacted to the air-puff with a movement, they perceived it, whereas when they did not move in reaction to the air-puff then they did not. Under this scenario, the massive increase in the duration of the activity of L5p apical dendrites is reflecting the interaction between the state and the contents of consciousness.
There is more evidence showing that L5p neurons are tightly linked to the contents of perception. Takahashi et al. (2016) found that manipulation of apical dendritic activity of L5p cells affects the behavioral report of the animal. In this experiment awake behaving mice learned to detect weak whisker stimuli of different magnitudes i.e., sometimes at, sometimes below, sometimes above the detection threshold. This allowed the researchers to delineate psychometric curves for whisker stimulation detection and to correlate these curves with neurometric curves. To monitor the activity of the apical tuft dendrites the authors performed fast-scanning two-photon Ca2+ imaging. They observed that the Ca2+ signal of apical dendrites of L5p cells was well correlated with the behavior of the animal. In addition to aligning with the psychometric curves, the apical dendritic signals could predict the behavioral hits and misses of threshold stimuli. Most importantly, directly modulating the dendritic activity through pharmacological intervention or optogenetics had a measurable influence on the detection behavior of the animal by shifting the psychometric curve. Optogenetic enhancement of apical dendritic activity also led to false alarms. In other words, activating the apical compartment caused the animal to lick as if a whisker stimulus would have been present. A bold but highly plausible interpretation would be that the animal experienced an illusory stimulus, just like humans have been shown to do when expecting a stimulus given some context (Aru and Bachmann, 2017; Aru et al., 2018).
Interestingly, in the follow-up experiments, it was learned that the positive relationship between dendritic activity and animal behavior is largely constrained to the pyramidal cells in layer 5B that project to subcortical regions including non-specific thalamic nuclei (Takahashi et al., under revision). On the other hand, layer 5A cells with denser corticocortical projections were not so tightly correlated with the perceptual report of the animal (Takahashi et al., under revision). Hence, these findings support the conclusion that L5p cells, especially those projecting to the thalamus, are vital for conscious perception.
Non-Conscious Contents in a Conscious State
A framework that proposes how the state and content of consciousness interact in the brain has to give answers to two questions. First, why are state and content so tightly bound in the brain? This question was answered in the first part of this work: we claim that the intertwinement of state and contents of consciousness arises at the level of single L5p neurons because they hold a central position in both thalamo-cortical and cortico-cortical loops. Second, the theory has to explain the existence of unconscious processing of contents: if state and content are bound in the brain, then how can some processing of the contents be unconscious? This is the question we turn to next.
Mental information processing can proceed also in its subliminal mode, meaning that not all sensory signals that are veridically encoded and adequately responded to are consciously experienced (for reviews, see Tulving et al., 1982; Kim and Blake, 2005; Mitchell, 2006; Kouider and Dehaene, 2007; Riener, 2017). Only part of the signals carrying contents become conscious. In fact, consciousness only has access to very specific levels of representation. Other levels are firmly locked away from it. For example, we do not have access to the processes that segregate our surrounding environment into discrete objects. How border ownership is calculated remains elusive to consciousness—we only experience the result of it. Similarly, as we speak or write in sentences we have no conscious experience of how our brain constructs them, i.e., chooses the words and puts them in the right order with correct grammar. Hence, conscious experience is restricted to certain contents and computations.
The present theory offers a natural way to understand this: all subcortical and cortical processing that does not involve L5p neurons will remain non-conscious. For example, motor control is based on the computations of basal ganglia and cerebellum, which are detached from the loop of consciousness. Both basal ganglia and the cerebellum have connections to the thalamus and cortex, which means that the outputs of the processes happening there can be incorporated into consciousness, while the computations themselves taking place within the cerebellum and basal ganglia remain unconscious (Tononi, 2004).
However, non-conscious processing also happens in cortex. According to the present view, if the processing does not involve L5p cells or the apical-dendritic modulation of these cells remains insufficient, this processing will not reach the NSP thalamus and will not be conscious. In other words, we make the strong prediction that cortical processing in itself, when not integrated with the NSP thalamic nuclei via L5p neurons, is not conscious. In particular, feedforward cortical processing, where information is mainly flowing within the cortical superficial layers bypassing thalamocortical neurons, is non-conscious.
Thalamo-Cortical Broadcasting in Action
As a closing argument, we wish to demonstrate how the presently proposed view can explain one central phenomenon often used in consciousness research. It is well known that conscious experience has a limited temporal resolution, meaning that if the presentation of different stimuli occurs too rapidly then some fail to be consciously experienced. This is evidenced in empirical phenomena like backward masking (for review, see Bachmann and Francis, 2013) and attentional blink (for review, see Martens and Wyble, 2010). Even when an identical stimulus is switched on and off intermittently at very high frequency, the multiplicity of stimulus replications will not be consciously perceived and the stimuli will fuse into a continuous experience (Watson, 1986). This characteristic is tightly related to the previous one: if conscious perception is based on the processing within the thalamocortical loop, then it is hard for conscious perception to resolve anything that happens faster than the processing time of this loop. In other words, we claim that the temporal resolution of conscious experience stems from the propagation time between the L5p neurons, NSP thalamus and higher cortical areas.
In backward masking, two stimuli are presented in quick succession. Under certain conditions, the second stimulus (“the mask”) can completely abolish the first (“the target”) from consciousness (Bachmann, 1984, 1994; Bachmann, 2000). Thus, although the target is presented 50–100 ms before the mask, it is not consciously perceived. The present theory explains this phenomenon in the following way. When the first stimulus, the target, activates L5p neurons, it initiates the thalamocortical broadcasting loop. However, by the time this activity propagates from the L5p neurons to the NSP thalamus and back to the apical dendrites of L5p neurons, the second stimulus, the mask, has taken over early cortical representation and now “steals the fire” which was started by the target. The target starts the loop, but the mask benefits from it.
The Future of the Present Hypothesis
The present conjecture can be directly tested in the rodent model, where it is possible to directly manipulate the different components of the loops depicted in Figure 1. For example, it is possible to specifically affect the different compartments of L5p neurons (e.g., Takahashi et al., 2016; Suzuki and Larkum, 2017). Which elements of the L5p neurons are most affected by the changes in the state of consciousness? It has been suggested that anesthesia especially affects the apical dendritic compartment (Phillips et al., 2018), but there are several other possibilities too (Meyer, 2015). It is also possible to specifically target the NSP thalamus (Honjoh et al., 2018) and to combine this with sensory stimulation. Future studies using the modern technological toolkit will directly test the merit of the present proposal. In this sense, the present ideas are clearly preliminary and will need to be refined, but the goal here was to propose that future work on the mechanisms of consciousness should specifically target the L5p cells.
This work will also inform the measures and theories of consciousness. In healthy subjects, it has been possible to show that in deep sleep (Massimini et al., 2005) and anesthesia (Ferrarelli et al., 2010) there is a breakdown of effective connectivity. These studies used a combination of high-density EEG with transcranial magnetic stimulation (TMS). By perturbing the cortex focally with TMS during conscious wakefulness the global deterministic brain response reflects high effective connectivity, i.e., the interactions between brain regions are invariably complex and extend over space and time. When the same stimulation is performed during NREM sleep, however, a qualitatively different pattern emerges. Now the brain’s response is dominated by bistability and even if the local bistable response to perturbation is very strong, it fails to engage the rest of the brain in complex interaction (Massimini et al., 2005; Ferrarelli et al., 2010).
These two reactivity patterns can be formally quantified by the perturbational complexity index (PCI; Casali et al., 2013)—arguably the most successful neuronal measure of conscious state so far. PCI is inspired by integrated information theory of consciousness (IIT; Tononi, 2004; Tononi et al., 2016) and aims to capture both segregation and integration of neural processing in one measure. It can differentiate between the conscious state and unconscious state, generalizing to different types of anesthesia, deep sleep and disorders of consciousness (Casali et al., 2013). Based on the present hypothesis we predict that the central reason for why information integration breaks down in the unconscious state is to be found on the level of L5p neurons and their interactions with the thalamo-cortical system. Hence, we hope that the present work can lead to a better understanding of the neurobiological mechanisms underlying the measures of consciousness.
Limitations
The hypothesis presented in this work has several limitations mainly related to the anatomy of the cortico-thalamo-cortical circuits. First, not much is known about human L5p cells. Recent work (Beaulieu-Laroche et al., 2018) has demonstrated that dendritic integration is functionally more segregated in human as compared to rat L5p neurons. Most importantly for the current purposes, virtually nothing is known about the projection patterns of human L5p neurons. We do not know whether similarly to the rodent there are two classes of L5p neurons that project either to other cortical areas or NSP thalamus (for rodent data see Larsen et al., 2008; Kawaguchi, 2017; Takahashi et al., under revision). Most likely there are even more than simply these two classes. This brings us to the second problem: if there are distinct classes of L5p neurons that participate in cortico-cortical and cortico-thalamo-cortical loops, then is it even meaningful to suggest that there is an intersection of these loops? Which of these cell types (e.g., L5A or L5B pyramidal cells) are then crucially related to consciousness? If we would have to pick one specific type of neuron, then it would be the thick-tufted L5B neurons, which are the major output neurons of the cortex, project heavily to NSP thalamus and have also been historically related to consciousness. Even if it would turn out that these cells do not send long-range cortico-cortical projections, it seems that they do receive such long-range cortico-cortical projections, especially on their apical dendrites (Cauller et al., 1998; Feldmeyer, 2012; Lee et al., 2013). We note that the direct evidence for this last claim is scarce, but we hope that the next years will bring clarity about this issue.
Third, the thalamic projection patterns as presented here are necessarily simplified. For clarity and simplicity, we mainly adhered to the classic distinction between SP and NSP thalamus (as in functional terms this distinction has not been invalidated). However, this distinction is only part of the story, with no clear consensus on how to classify thalamo-cortical projections (Jones, 2012 advocates the distinction of thalamic core vs. matrix cells; however, this distinction also seems too simplistic, see Clascá et al., 2016).
Conclusion
The contents of consciousness depend on the state of consciousness. Here, we argued that there is a clear neurobiological reason for this intertwinement: L5p cells are in the center of both cortico-cortical and thalamo-cortical loops, hence functionally coupling the state and contents of consciousness. The main message going forward is that given the advances in understanding the neural computations, more attention should be given to the relationship between consciousness and the L5p cells. After all, Santiago Ramón y Cajal called them the “psychic cells.”
Data Availability
No datasets were generated or analyzed for this study.
Author Contributions
JA and TB wrote the initial version of the manuscript. All authors read and commented on the manuscript.
Funding
This work was supported by Grants IUT20-40 and PUT1476 from the Estonian Ministry of Education and Research (Eesti Teadusagentuur). JA was also supported by the European Union’s Horizon 2020 Research and Innovation Programme under the Marie Skłodowska-Curie Grant Agreement No. 799411. MS was supported by German Research Foundation EXC 257. ML was supported by the European Union’s Horizon 2020 research and innovation program and Euratom research and training program 2014-2018 under Grant Agreement No. 670118. This project has received funding from the European Union’s Horizon 2020 Framework Programme for Research and Innovation under the Specific Grant Agreement No. 720270 (Human Brain Project SGA1 and SGA2) and from Deutsche Forschungsgemeinschaft (Grant No. LA 3442/3-1 and Grant No. LA 3442/5-1).
Conflict of Interest Statement
The authors declare that the research was conducted in the absence of any commercial or financial relationships that could be construed as a potential conflict of interest.
Acknowledgments
We are indebted to Timothy Zolnik for reading and commenting a draft of this article. We thank Prof. Igor Timofeev for kind assistance with the publication process.
Footnotes
- ^ Here one might want to suggest that during some forms of meditation people can be conscious without being conscious of anything, but even if one agrees with this claim, the length of the necessary training demonstrates how hard it is to separate the state and the contents of consciousness. Furthermore, it is possible to say that the “emptiness” of conscious experience itself is a content (according to set theory, an empty set is a subset of all sets).
- ^ We note that even this division is a simplification, as there also exist thalamocortical projections that have the specific projection pattern (i.e., To layer 4) in one cortical target area, while having a non-specific projection pattern (i.e., To layers 1 and 5/6) in another (the so-called multi-specific thalamocortical projection cells, see Clascá et al., 2016).
References
Alkire, M. T., Haier, R. J., and Fallon, J. H. (2000). Toward a unified theory of narcosis: brain imaging evidence for a thalamocortical switch as the neurophysiologic basis of anesthetic-induced unconsciousness. Conscious. Cogn. 9, 370–386. doi: 10.1006/ccog.1999.0423
Alkire, M. T., Hudetz, A. G., and Tononi, G. (2008). Consciousness and anesthesia. Science 322, 876–880. doi: 10.1126/science.1149213
Angel, A. (1993). Central neuronal pathways and the process of anaesthesia. Br. J. Anaesth. 71, 148–163. doi: 10.1093/bja/71.1.148
Aru, J., and Bachmann, T. (2017). Expectation creates something out of nothing: the role of attention in iconic memory reconsidered. Conscious. Cogn. 53, 203–210. doi: 10.1016/j.concog.2017.06.017
Aru, J., Bachmann, T., Singer, W., and Melloni, L. (2012). Distilling the neural correlates of consciousness. Neurosci. Biobehav. Rev. 36, 737–746. doi: 10.1016/j.neubiorev.2011.12.003
Aru, J., Tulver, K., and Bachmann, T. (2018). It’s all in your head: expectations create illusory perception in a dual-task setup. Conscious. Cogn. 65, 197–208. doi: 10.1016/j.concog.2018.09.001
Bachmann, T. (1984). The process of perceptual retouch: nonspecific afferent activation dynamics in explaining visual masking. Percept. Psychophys. 35, 69–84. doi: 10.3758/bf03205926
Bachmann, T. (1989). Psychophysiological Bases of Visual Masking (in Russian). Tartu: Tartu University Press.
Bachmann, T. (1994). Psychophysiology of Visual Masking. The Fine Structure of Conscious Experience. Commack, NY: Nova Science Publishers, Inc.
Bachmann, T. (1999). “Twelve spatiotemporal phenomena and one explanation,” in Cognitive Contributions to the Perception of Spatial and Temporal Events, eds G. Aschersleben, T. Bachmann and J. Müsseler (Amsterdam: Elsevier/North-Holland), 173–206.
Bachmann, T. (2000). Microgenetic Approach to the Conscious Mind. Amsterdam/Philadelphia: John Benjamins.
Bachmann, T. (2012). How to begin to overcome the ambiguity present in differentiation between contents and levels of consciousness? Front. Psychol. 3:82. doi: 10.3389/fpsyg.2012.00082
Bachmann, T., and Francis, G. (2013). Visual Masking: Studying Perception, Attention, and Consciousness. San Diego, CA: Academic Press.
Bachmann, T., and Hudetz, A. G. (2014). It is time to combine the two main traditions in the research on the neural correlates of consciousness: C = L × D. Front. Psychol. 5:940. doi: 10.3389/fpsyg.2014.00940
Beaulieu-Laroche, L., Toloza, E. H., van der Goes, M. S., Lafourcade, M., Barnagian, D., Williams, Z. M., et al. (2018). Enhanced dendritic compartmentalization in human cortical neurons. Cell 175, 643–651. doi: 10.1016/j.cell.2018.08.045
Bogen, J. E. (1995a). On the neurophysiology of consciousness: I. An overview. Conscious. Cogn. 4, 52–62. doi: 10.1006/ccog.1995.1003
Bogen, J. E. (1995b). On the neurophysiology of consciousness: Part II. Constraining the semantic problem. Conscious. Cogn. 4, 137–158. doi: 10.1006/ccog.1995.1020
Boly, M., Massimini, M., Tsuchiya, N., Postle, B. R., Koch, C., and Tononi, G. (2017). Are the neural correlates of consciousness in the front or in the back of the cerebral cortex? Clinical and neuroimaging evidence. Neuron 37, 9603–9613. doi: 10.1523/jneurosci.3218-16.2017
Brooks, B., and Jung, R. (1973). “Neuronal physiology of the visual cortex,” in Handbook of Sensory Physiology. Central Processing of Visual Information. Part B. (Vol. VII/3), ed. R. Jung (New York, NY: Springer-Verlag), 325–440.
Casali, A. G., Gosseries, O., Rosanova, M., Boly, M., Sarasso, S., Casali, K. R., et al. (2013). A theoretically based index of consciousness independent of sensory processing and behavior. Sci. Transl. Med. 5, 198ra105–198ra105. doi: 10.1126/scitranslmed.3006294
Cauller, L. J., Clancy, B., and Connors, B. W. (1998). Backward cortical projections to primary somatosensory cortex in rats extend long horizontal axons in layer I. J. Comp. Neurol. 390, 297–310. doi: 10.1002/(sici)1096-9861(19980112)390:2<297::aid-cne11>3.3.co;2-0
Clascá, F., Porrero, C., Galazo, M. J., Rubio-Garrido, P., and Evangelio, M. (2016). “Anatomy and development of multispecific thalamocortical axons: implications for cortical dynamics and evolution,” in Axons and Brain Architecture, ed. S. R. Kathleen (Cambridge, MA: Academic Press), 69–92.
Crick, F. (1996). Visual perception: rivalry and consciousness. Nature 379, 485–486. doi: 10.1038/379485a0
Crick, F., and Koch, C. (1992). The problem of consciousness. Sci. Am. 267, 152–159. doi: 10.1038/scientificamerican0992-152
De Graaf, T. A., Hsieh, P. J., and Sack, A. T. (2012). The ‘correlates’ in neural correlates of consciousness. Neurosci. Biobehav. Rev. 36, 191–197. doi: 10.1016/j.neubiorev.2011.05.012
Dehaene, S., and Changeux, J. P. (2011). Experimental and theoretical approaches to conscious processing. Neuron 70, 200–227. doi: 10.1016/j.neuron.2011.03.018
Dehaene, S., and Naccache, L. (2001). Towards a cognitive neuroscience of consciousness: basic evidence and a workspace framework. Cognition 79, 1–37. doi: 10.1016/s0010-0277(00)00123-2
Dehaene, S., Naccache, L., Cohen, L., Le Bihan, D., Mangin, J. F., Poline, J. B., et al. (2001). Cerebral mechanisms of word masking and unconscious repetition priming. Nat. Neurosci. 4, 752–758. doi: 10.1038/89551
Doty, R. W. (1970). “Modulation of visual input by brain-stem system,” in Early Experience and Visual Information Processing in Perceptual and Reading Disorders, eds F. A. Young and D. B. Lindsley (Washington, DC: National Academy of Sciences), 143–150.
Feldmeyer, D. (2012). Excitatory neuronal connectivity in the barrel cortex. Front. Neuroanat. 6:24. doi: 10.3389/fnana.2012.00024
Ferrarelli, F., Massimini, M., Sarasso, S., Casali, A., Riedner, B. A., Angelini, G., et al. (2010). Breakdown in cortical effective connectivity during midazolam-induced loss of consciousness. Proc. Natl. Acad. Sci. U S A 107, 2681–2686. doi: 10.1073/pnas.0913008107
Harris, K. D., and Shepherd, G. M. (2015). The neocortical circuit: themes and variations. Nat. Neurosci. 18, 170–181. doi: 10.1038/nn.3917
Hassler, R. (1978). “Interaction of reticular activating system for vigilance and the truncothalamic and pallidal systems for directing awareness and attention under striatal control,” in Cerebral Correlates of Conscious Experience, eds P. A. Buser and A. Rougeul-Buser (Amsterdam: North-Holland), 111–129.
Hohwy, J. (2009). The neural correlates of consciousness: new experimental approaches needed? Conscious. Cogn. 18, 428–438. doi: 10.1016/j.concog.2009.02.006
Honjoh, S., Sasai, S., Schiereck, S. S., Nagai, H., Tononi, G., and Cirelli, C. (2018). Regulation of cortical activity and arousal by the matrix cells of the ventromedial thalamic nucleus. Nat. Commun. 9:2100. doi: 10.1038/s41467-018-04497-x
Hudetz, A. G. (2012). General anesthesia and human brain connectivity. Brain Connect. 2, 291–302. doi: 10.1089/brain.2012.0107
Jung, R. (1958). “Coordination of specific and nonspecific afferent impulses at single neurons of the visual cortex,” in Reticular Formation of the Brain, ed. H. H. Jasper (Boston, MA: Little, Brown and Co), 423–434.
Kawaguchi, Y. (2017). Pyramidal cell subtypes and their synaptic connections in layer 5 of rat frontal cortex. Cereb. Cortex 27, 5755–5771. doi: 10.1093/cercor/bhx252
Kim, C. Y., and Blake, R. (2005). Psychophysical magic: rendering the visible ‘invisible’. Trends Cogn. Sci. 9, 381–388. doi: 10.1016/j.tics.2005.06.012
Kinney, H. C., Korein, J., Panigrahy, A., Dikkes, P., and Goode, R. (1994). Neuropathological findings in the brain of Karen Ann Quinlan–the role of the thalamus in the persistent vegetative state. N. Engl. J. Med. 330, 1469–1475. doi: 10.1056/nejm199405263302101
Koch, C., Massimini, M., Boly, M., and Tononi, G. (2016). Neural correlates of consciousness: progress and problems. Nat. Rev. Neurosci. 17, 307–321. doi: 10.1038/nrn.2016.61
Kouider, S., and Dehaene, S. (2007). Levels of processing during non-conscious perception: a critical review of visual masking. Philos. Trans. R. Soc. Lond. B Biol. Sci. 362, 857–875. doi: 10.1098/rstb.2007.2093
LaBerge, D. (1997). Attention, awareness and the triangular circuit. Conscious. Cogn. 6, 149–181. doi: 10.1006/ccog.1997.0305
LaBerge, D. (2006). Apical dendrite activity in cognition and consciousness. Conscious. Cogn. 15, 235–257. doi: 10.1016/j.concog.2005.09.007
Lamme, V. A. F. (2003). Why visual attention and awareness are different. Trends Cogn. Sci. 7, 12–18. doi: 10.1016/s1364-6613(02)00013-x
Lamme, V. A. F. (2010). How neuroscience will change our view on consciousness. Cogn. Neurosci. 1, 204–220. doi: 10.1080/17588921003731586
Larkum, M. E. (2013). A cellular mechanism for cortical associations: an organizing principle for the cerebral cortex. Trends Neurosci. 36, 141–151. doi: 10.1016/j.tins.2012.11.006
Larkum, M. E., Zhu, J. J., and Sakmann, B. (1999). A new cellular mechanism for coupling inputs arriving at different cortical layers. Nature 398, 338–341. doi: 10.1038/18686
Larsen, D. D., Wickersham, I. R., and Callaway, E. M. (2008). Retrograde tracing with recombinant rabies virus reveals correlations between projection targets and dendritic architecture in layer 5 of mouse barrel cortex. Front. Neural Circuits 2:5. doi: 10.3389/neuro.04.005.2007
Lau, H., and Rosenthal, D. (2011). Empirical support for higher-order theories of conscious awareness. Trends Cogn. Sci. 15, 365–373. doi: 10.1016/j.tics.2011.05.009
Laureys, S. (2005). The neural correlate of (un) awareness: lessons from the vegetative state. Trends Cogn. Sci. 9, 556–559. doi: 10.1016/j.tics.2005.10.010
Lee, S., Kruglikov, I., Huang, Z. J., Fishell, G., and Rudy, B. (2013). A disinhibitory circuit mediates motor integration in the somatosensory cortex. Nat. Neurosci. 16, 1662–1670. doi: 10.1038/nn.3544
Leopold, D. A., and Logothetis, N. K. (1996). Activity changes in early visual cortex reflect monkeys’ percepts during binocular rivalry. Nature 379, 549–553. doi: 10.1038/379549a0
Lisman, J. E. (1997). Bursts as a unit of neural information: making unreliable synapses reliable. Trends Neurosci. 20, 38–43. doi: 10.1016/s0166-2236(96)10070-9
Livingstone, M. S., and Hubel, D. H. (1980). Evoked responses and spontaneous activity of cells in the visual cortex during waking and slow-wave sleep. ARVO 1980. Supplement to Investigative Ophtalmology and Visual Science, 223.
Llinás, R. (1996). “Content and context in the thalamocortical system: the basis for cognition,” in Paper Presented at Toward a Science of Consciousness 1996. “Tucson II,” International Conference, April 8-13, Tucson, Arizona, 8–13.
Llinás, R., and Ribary, U. (2001). Consciousness and the brain. The thalamocortical dialogue in health and disease. Ann. N. Y. Acad. Sci. 929, 166–175. doi: 10.1111/j.1749-6632.2001.tb05715.x
Lumer, E. D., Friston, K. J., and Rees, G. (1998). Neural correlates of perceptual rivalry in the human brain. Science 280, 1930–1934. doi: 10.1126/science.280.5371.1930
Magnin, M., Rey, M., Bastuji, H., Guillemant, P., Mauguiere, F., and Garcia-Larrea, L. (2010). Thalamic deactivation at sleep onset precedes that of the cerebral cortex in humans. Proc. Natl. Acad. Sci. U S A 107, 3829–3833. doi: 10.1073/pnas.0909710107
Martens, S., and Wyble, B. (2010). The attentional blink: past, present, and future of a blind spot in perceptual awareness. Neurosci. Biobehav. Rev. 34, 947–957. doi: 10.1016/j.neubiorev.2009.12.005
Mashour, G. A. (2014). Top-down mechanisms of anesthetic-induced unconsciousness. Front. Syst. Neurosci. 8:115. doi: 10.3389/fnsys.2014.00115
Mashour, G. A., and Hudetz, A. G. (2017). Bottom-up and top-down mechanisms of general anesthetics modulate different dimensions of consciousness. Front. Neural Circuits 11:44. doi: 10.3389/fncir.2017.00044
Massimini, M., Ferrarelli, F., Huber, R., Esser, S. K., Singh, H., and Tononi, G. (2005). Breakdown of cortical effective connectivity during sleep. Science 309, 2228–2232. doi: 10.1126/science.1117256
Meyer, K. (2015). The role of dendritic signaling in the anesthetic suppression of consciousness. Anesthesiology 122, 1415–1431. doi: 10.1097/ALN.0000000000000673
Mitchell, D. B. (2006). Nonconscious priming after 17 years: invulnerable implicit memory? Psychol. Sci. 17, 925–929. doi: 10.1111/j.1467-9280.2006.01805.x
Mo, C., and Sherman, S. M. (2019). A sensorimotor pathway via higher-order thalamus. J. Neurosci. 39, 692–704. doi: 10.1523/JNEUROSCI.1467-18.2018
Moruzzi, G., and Magoun, H. W. (1949). Brain stem reticular formation and activation of the EEG. Electroencephalogr. Clin. Neurophysiol. 1, 455–473. doi: 10.1016/0013-4694(49)90066-8
Munk, M. H. J., Roelfsema, P. R., König, P., Engel, A. K., and Singer, W. (1996). Role of reticular activation in the modulation of intracortical synchronization. Science 272, 271–274. doi: 10.1126/science.272.5259.271
Murayama, M., and Larkum, M. E. (2009). Enhanced dendritic activity in awake rats. Proc. Natl. Acad. Sci. U S A 106, 20482–20486. doi: 10.1073/pnas.0910379106
Newman, J. (1995a). Thalamic contributions to attention and consciousness. Conscious. Cogn. 4, 172–193. doi: 10.1006/ccog.1995.1024
Newman, J. (1995b). Reticular-thalamic activation of the cortex generates conscious contents. Behav. Brain Sci. 18, 691–692. doi: 10.1017/s0140525x00040577
Odegaard, B., Knight, R. T., and Lau, H. (2017). Should a few null findings falsify prefrontal theories of conscious perception? J. Neurosci. 37, 9593–9602. doi: 10.1523/JNEUROSCI.3217-16.2017
Penfield, W. (1975). The Mystery of the Mind: A Critical Study of Consciousness and the Human Brain. Toledo, OH: Princeton University Press.
Phillips, W. A., Bachmann, T., and Storm, J. F. (2018). Apical function in neocortical pyramidal cells: a common pathway by which general anesthetics can affect mental state. Front. Neural Circuits 12:50. doi: 10.3389/fncir.2018.00050
Purpura, D. P. (1970). “Operations and processes in thalamic and synaptically related neural subsystems,” in The Neurosciences. Second Study Program, ed. F. O. Schmitt (New York, NY: Rockefeller University Press), 458–470.
Purpura, K. P., and Schiff, N. D. (1997). The thalamic intralaminar nuclei: a role in visual awareness. Neuroscientist 3, 8–15. doi: 10.1177/107385849700300110
Rees, G., Kreiman, G., and Koch, C. (2002). Neural correlates of consciousness in humans. Nat. Rev. Neurosci. 3, 261–270. doi: 10.1038/nrn783
Riener, A. (2017). “Subliminal perception or ‘can we perceive and be influenced by stimuli that do not reach us on a conscious level?’,” in Emotions and Affect in Human Factors and Human-Computer Interaction, ed. M. Jeon (San Diego, CA: Elsevier Academic Press), 503–538.
Riklan, M., and Levita, E. (1969). Subcortical Correlates of Human Behavior. A Psychological Study of Thalamic and Basal Ganglia Surgery. Baltimore, MD: Williams and Wilkins.
Schiff, N. D. (2010). Recovery of consciousness after brain injury: a mesocircuit hypothesis. Trends Neurosci. 33, 1–9. doi: 10.1016/j.tins.2009.11.002
Sheinberg, D. L., and Logothetis, N. K. (1997). The role of temporal cortical areas in perceptual organization. Proc. Natl. Acad. Sci. U S A 94, 3408–3413. doi: 10.1073/pnas.94.7.3408
Singer, W. (1979). “Central core control of visual cortex functions,” in The Neurosciences. Fourth Study Program, eds F. O. Schmitt and F. G. Worden (Cambridge, MA: MIT), 1093–1110.
Smirnov, V. M., Muchnik, L. S., and Shandurina, A. N. (1978). “The outline and the functions of the deep structures of the brain (in Russian),” in Estestvennonauchnye osnovy psikhologii, eds A. A. Smirnov, A. R. Luria and V. D. Nebylitsyn (Moscow: Pedagogika), 76–108.
Somjen, G. (1972). Sensory Coding in the Mammalian Nervous System. New York, NY: Appleton-Century-Crofts.
Steriade, M. (1981a). “Mechanisms underlying cortical activation: neuronal organization and properties of the midbrain reticular core and intralaminar thalamic nuclei,” in Brain Mechanisms and Perceptual Awareness, eds O. Pompeiano and C. Ajmone Marsan (New York, NY: Raven), 327–377.
Steriade, M. (1981b). EEG desynchronisation is associated with cellular events that are prerequisites for active behavioral states. Behav. Brain Sci. 4, 489–492. doi: 10.1017/s0140525x00010037
Suzuki, M., and Larkum, M. E. (2017). Dendritic calcium spikes are clearly detectable at the cortical surface. Nat. Commun. 8:276. doi: 10.1038/s41467-017-00282-4
Takahashi, N., Ebner, C., Sigl-Glöckner, J., Nierwetberg, S. and Larkum, M. E. (under revision). Active dendritic currents gates descending cortical outputs in perception.
Takahashi, N., Oertner, T. G., Hegemann, P., and Larkum, M. E. (2016). Active cortical dendrites modulate perception. Science 354, 1587–1590. doi: 10.1126/science.aah6066
Theyel, B. B., Llano, D. A., and Sherman, S. M. (2010). The corticothalamocortical circuit drives higher-order cortex in the mouse. Nat. Neurosci. 13, 84–88. doi: 10.1038/nn.2449
Tononi, G. (2004). An information integration theory of consciousness. BMC Neurosci. 5:42. doi: 10.1186/1471-2202-5-42
Tononi, G., Boly, M., Massimini, M., and Koch, C. (2016). Integrated information theory: from consciousness to its physical substrate. Nat. Rev. Neurosci. 17, 450–461. doi: 10.1038/nrn.2016.44
Tononi, G., and Edelman, G. M. (1998). Consciousness and complexity. Science 282, 1846–1851. doi: 10.1126/science.282.5395.1846
Tulving, E., Schacter, D. L., and Stark, H. A. (1982). Priming effects in word-fragment completion are independent of recognition memory. J. Exp. Psychol. Learn. Mem. Cogn. 8, 336–342. doi: 10.1037/0278-7393.8.4.336
Ward, L. M. (2011). The thalamic dynamic core theory of conscious experience. Conscious. Cogn. 20, 464–486. doi: 10.1016/j.concog.2011.01.007
Keywords: consciousness, thalamus, pyramidal neurons, dendrites, unconscious processing, state of consciousness
Citation: Aru J, Suzuki M, Rutiku R, Larkum ME and Bachmann T (2019) Coupling the State and Contents of Consciousness. Front. Syst. Neurosci. 13:43. doi: 10.3389/fnsys.2019.00043
Received: 29 March 2019; Accepted: 13 August 2019;
Published: 30 August 2019.
Edited by:
Igor Timofeev, Laval University, CanadaReviewed by:
Francisco Clasca, Autonomous University of Madrid, SpainMarco Atzori, Universidad Autónoma de San Luis Potosí, Mexico
Copyright © 2019 Aru, Suzuki, Rutiku, Larkum and Bachmann. This is an open-access article distributed under the terms of the Creative Commons Attribution License (CC BY). The use, distribution or reproduction in other forums is permitted, provided the original author(s) and the copyright owner(s) are credited and that the original publication in this journal is cited, in accordance with accepted academic practice. No use, distribution or reproduction is permitted which does not comply with these terms.
*Correspondence: Jaan Aru, amFhbi5hcnVAZ21haWwuY29t