- Laboratory of Theoretical and Applied Neuroscience and Graduate Program in Neuroscience and Education, University of Macedonia, Thessalonica, Greece
Soul in the Rind
In the past, the distance that cognitively separates humans from other mammalian species was explained by our possessing a soul that animals did not have. Ramón y Cajal (1933) reduced the difference between human and animal intelligence to the abundance and complexity of cortical association fibers, the number of which increases in proportion to the quantity of gray matter: “The large cerebra of the elephant, whale, ox, horse, etc., possess many projection cells but relatively scarce association cells.”
Today, the explanation for human intellect is that we possess a bigger telencephalon: the richness of mental life depends on the surface area of an expanded cerebral cortex, considered to be the seat of consciousness (de Duve, 2002).
Creaseless to Crinkled
The vertebrate cerebral cortex varies from the trilaminar reptilian to the hexalaminar mammalian form (Shepherd, 2011). The evolution from lissencephaly to gyrencephaly provided mammals with a means to accommodate more cerebral cortex within the confines of their cranial vault. The degree of cortical folding depends on the cortical surface, thickness, volume, and convolutedness (Hofman, 1985). An increase in the gyrification index (GI) correlates with the increase in brain mass in mammalian orders, including primates, cetaceans, carnivores, and ungulates (Pillay and Manger, 2007). Cetaceans are the most gyrencephalic mammals, regardless of brain mass, which is a finding explained by their post-terrestrial return to a marine environment (Manger et al., 2012).
The areal expansion and the gyration of the cortical surface commence prenatally in the developing human. Gyrated cortices feature multipotent basal radial glial cells that reside in the outer subventricular zone (Ghosh and Jessberger, 2013). During late developmental stages, asymmetrical cell divisions in the ventricular zone generate radial glial cells and intermediate progenitor cells; subsequently, the latter divide symmetrically in the subventricular zone to produce multiple types of neurons (Rakic, 1988). The evolution of this two-step pattern of neurogenesis is theorized to have played an important role in the amplification of cell numbers underlying the radial and tangential cortical expansion (Martínez-Cerdeño et al., 2006). Cytoskeletal rearrangements also appear to be crucial for the development of gyrated brains (Nielsen et al., 2010).
The folding of the cerebral cortex has been attributed to a relative increase in the expansion of the superficial layers relative to deep layers and to the dissipation of the in-plane mechanical forces generated by the tangential cortical surface expansion (Van Essen, 1997; Bayer and Altman, 2006; Mota and Herculano-Houzel, 2015; Ronan and Fletcher, 2015). Axonal growth and synapse formation happen along with gyrification, such that laminar and regional cytoarchitecture is intimately linked to cortical “connectomics” and thalamocortical projections (Karten, 2015).
The developmental and evolutionary mechanisms of cerebrocortical gyrification, and their malformations, have been investigated by means of neuroimaging and molecular genetic methods (Rash and Rakic, 2014). Certain genes involved in the process of cortical gyrification demonstrate altered transcriptional activity during the time-frame when convolutions appear (Nielsen et al., 2010).
The fact that there is a similar pattern in the gyration across members within a species, but a different pattern among species, indicates that cortical convolution is a genetically-programmed process (Nielsen et al., 2010). A technical way to address the question of gyrification is by genomic analyses before and after the appearance of gyration in diverse species; that is, by comparing the differential expression of identified genes between the lissencephalic embryonic stage and the primary-folded gyrencephalic stage, as Nielsen et al. (2010) did in the pig.
The DNA-associated protein Trnp1 regulates cortical expansion tangentially and radially. In mice, high levels of Trnp1 lead to cortical gyrification in an animal that is normally lissencephalic (Stahl et al., 2013). Another gene, ARHGAP11B, which is unique to humans, has been shown to promote basal progenitor cell generation in the subventricular zone and induce cerebrocortical gyrification after insertion into the mouse genome (Florio et al., 2015).
The GPR56 gene encodes a heterotrimeric G-binding protein-coupled receptor expressed in cortical progenitor cells and required for normal cortical development; the GPR56 protein functions in cell adhesion and guidance (Nielsen et al., 2010; Rash and Rakic, 2014). A 15-base pair deletion in the regulatory region of GPR56 was discovered in patients with familial seizures, mental disability, and bilateral cortical abnormalities in the frontal lobe around the Sylvian fissure, including Broca's area (Bae et al., 2014); in mice, GPR56 overexpression led to an increase of cortical progenitor proliferation and influenced gyral patterning.
Functional Attributes
The issue of functional localization in the human cerebral cortex has journeyed from neuroanatomical phenomenology to hypothesis-driven neuropsychology and back. Classical neuroanatomists considered brain structure and function as one; they studied morphology from a histophysiological perspective, not as a mere parcellation of neurons (Jakob, 1939). Cajal, Brodmann, Economo, Koskinas, and the Vogts worked on the premise that morphological diversity reflected functional specifications, leaving it to future physiological and clinical studies to attribute functional individualities to anatomical subdivisions (Bartels and Zeki, 2005; Jones, 2008). Koskinas (1931) put it succinctly: “As a general principle, each physiological function presupposes a corresponding anatomical basis. From the precise knowledge of the structure of the cerebral cortex we may expect to shed light on issues of the utmost importance, such as the relationship between mental attributes and brain structure.” Examples of cytoarchitectonic subdivisions that reflect functional differentiation were found in motor, somatosensory, and visual fields in the frontal, parietal, and occipital lobes.
Cortical cytoarchitecture and myeloarchitecture are inextricable from neuronal connections (van den Heuvel et al., 2015). In defining cortical areas, connectivity is key; the guiding principle of neuroanatomists that cortical areas form parts of connectional networks is now being adopted by the neuroimaging community, including the streams of intrinsic cortico-cortical connections, the re-entrant projections from the thalamus, and their ontogeny (Jones, 2008).
The traditional hypothesis-driven paradigm faces new challenges (Frackowiak and Markram, 2015). The “piece-meal” research style we are used to cannot offer a full understanding of brain function; instead, an integrated, multilevel explanation seems imperative, comprising all organizational aspects of the nervous system, from DNA to behavior, and the cooperation of such different levels with each other.
Advances in neuroimaging have led to new knowledge about brain organization at a systems level, “a macro-scale road map for understanding perception, action, and cognition” (Badre et al., 2015). Neuroimaging researchers, equipped with ever more powerful magnets, seem content with locating a gyrus that corresponds to a Brodmann numbered area and referring a particular behavioral action to that location (Jones, 2008). They revived the notion of “cognitive brain mapping” and purport to unveil physical representations of cognition in living cerebral tissue; the term “activation” (adjustable pseudocolors generated by software) became an ambiguous catchall term (Smith, 2010). Typically, an activation occurs when two experimental conditions produce statistically-significant differences in relative, normalized signal strength between two neighboring anatomical regions. In fMRI, mental functions are taken as localized in cortical sites (“functional segregation”), a notion reflecting the old view that conscious processes must have a seat in the brain, rather than connections.
There are further limitations in decoding the results of functional magnetic resonance imaging (fMRI) (Haynes, 2015). Information contained in single voxels or voxel ensembles cannot be directly correlated with information encoded in single neurons because the method relies on the magnetization level of blood as an indirect marker of activity in pools of thousands of neurons in a nonlinear hemodynamic response. Signals can appear in disparate brain areas, not connected anatomically, and with different signal-to-noise ratios. Moreover, a positive signal in a fMRI can overestimate the information available at the neuronal level, influenced by the pattern of blood vessel drainage; conversely, absence of information in the fMRI does not mean absence of information at the level of local neuron populations (Haynes, 2015).
An inherent limitation of fMRI is resolution (macro-level). If we consider that the key to any behavioral outcome is the activity at the synapse (micro-level), we realize that today's imaging cannot reveal the ultimate morphological or chemical happenings that lead to behavior. There is a strong argument that it is not merely helpful to understand the nano-scale organization of the brain for insight into its function; it is a requisite (Südhof, 2017); a molecular understanding of the brain further necessitates taking into account the incessant neural plasticity, the non-synaptic communication between neurons, and the role of glia.
Cortical functions are integrative. Their underlying network commonality transcends parcellation and connectivity, especially with the thalamus, and is therefore crucial in defining any cortical area. Even white matter imaging methods, such as diffusion-tensor and diffusion-spectrum imaging, do not reveal the synaptic terminations of axons in the gray matter of the cerebral hemispheres (Jones, 2008).
Nonetheless, imaging techniques make it possible to study human representational space noninvasively in unprecedented ways, provided they are interpreted cautiously. Sizeable experimental data have been gathered in the effort to link particular behaviors to specific anatomical loci in the human cerebral hemispheres. A function is attributed to a cerebral lobe, cortical gyrus, lobule, or cytoarchitectonic area (Vandenberghe et al., 2001; Zysset et al., 2003; Rivera et al., 2005; Kitada et al., 2009; Sestieri et al., 2010). Brain imaging studies have gone as far as associating individualist, conservative and radical political ideologies to cortical areas—medial prefrontal cortex and temporoparietal junction, dorsolateral prefrontal cortex, and ventral striatum and posterior cingulate cortex, respectively (Zamboni et al., 2009)—and associating cortical gyri with economic-political decisions or voting behavior (Xia et al., 2015).
The Discrepancy of Species
Pioneers of neuroanatomy, including Obersteiner, Flatau, Edinger, Retzius, Jakob, Ariëns-Kappers, Herrick, and Welker, placed emphasis on comparative neurology in their quest to understand the human brain in the context of growth, form, and function (Obersteiner, 1890; Retzius, 1896; Edinger, 1899; Flatau and Jacobsohn, 1899; Jakob and Onelli, 1913; Hofman and Johnson, 2011).
The tendency to ascribe a function to each lobule or gyrus of the human cerebral hemispheres comes in sharp contrast to the fact that, for the plethora of mammals with rich gyration patterns of the cerebral cortex (Figure 1), we have very little data that allow us to attribute specific functions to each anatomically-defined ensemble. What do all these gyri and cytoarchitectonic areas do? It would be a paradox to concur that natural selection produced human gyri for specific functional outcomes, while in other species the presence of numerous gyri just serves to fill the cranial cavity.
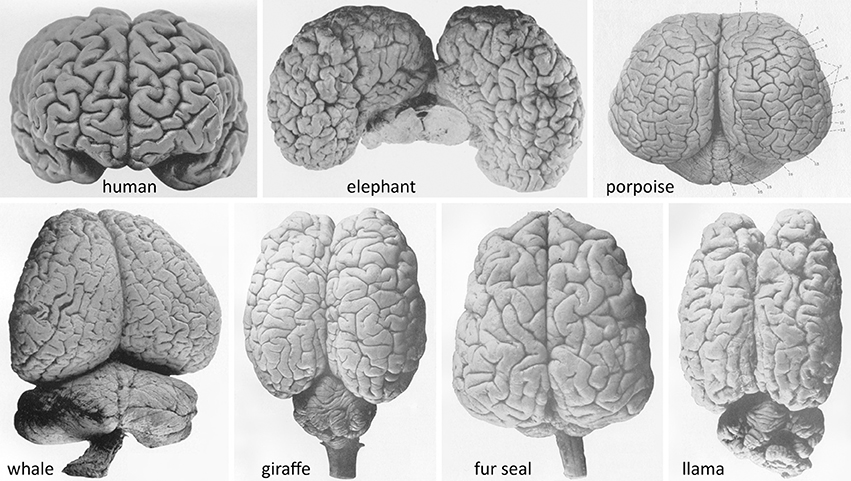
Figure 1. Brains of advanced gyrencephalic species depicted in classical neuroanatomical works (varying scales). (Upper): Adult human (Homo sapiens sapiens) (Retzius, 1896, plate 57/1); African elephant (Loxodonta africana) (Jakob and Onelli, 1913, plate 38/262); and common porpoise (Phocoena phocoena) (Flatau and Jacobsohn, 1899, plate 6/1). (Lower): Southern right whale (Eubalaena australis); giraffe (Giraffa camelopardalis); South American fur seal (Arctocephalus australis); and llama (Lama glama) (Jakob and Onelli, plates 37/254, 30/206, 36/242, 27/176, respectively). Modern photographs of brains of over 175 species are available online (NeuroscienceLibrary.org) at the National Museum of Health and Medicine of the Armed Forces Institute of Pathology, Washington, D.C., which houses several renowned neuroanatomical collections, including the University of Wisconsin–Madison Comparative Mammalian Brain Collection (Fobbs and Johnson, 2011).
The twentieth century in neuroscience has centered around a small number of models, such as mice, rats, cats, dogs, rabbits, and monkeys (so-called “classic laboratory animals”; Nielsen et al., 2010). The trend accelerated as genomic sequences and molecular genetic tools became available for specific species, leading to a “bottleneck.” It is now realized that the comparative study of species from different phyletic lineages can be useful for the formulation and critical testing of hypotheses (Brenowitz and Zakon, 2015). Evidently, mammalian species that have not yet been studied outnumber those studied. In an attempt to explain anatomical structure in tandem with functional specialization in complex brains, neuroscience research of the twenty-first century should establish a coherent denominator by extending research to a range of richly-gyrated, “exotic” animals that have not been studied extensively or at all. There are unique brain collections in comparative anatomy museums worldwide that remain unexploited (Iwaniuk, 2010). An ambitious plan is to generate a phylogenetic tree of functional cortical cartography; in other words, a comparative neurology that takes into account the fourth dimension—the macro-time scale of evolution (Triarhou, 2008). Only then can the blueprint of cortical gyration be understood in conjunction with its microstructure and integrative functional output.
Along that line, substantial progress is noted in the study of brain structure in gyrencephalic species beyond primates, such as the dromedary (Simon, 1965), llama (Welker et al., 1976), horse (Cozzi et al., 2014), hippopotamus (Butti et al., 2014), rhinoceros (Manger, 2011; Bhagwandin et al., 2017), elephant seal, and sea lion (Sawyer et al., 2016; Turner et al., 2017), not to mention the extensive literature on proboscidea (Dexler, 1907; Jakob, 1909; Janssen and Stephan, 1956; Haug, 1966; Cozzi et al., 2001; Shoshani et al., 2006; Jacobs et al., 2011; Herculano-Houzel et al., 2014) and cetacea (Tower, 1954; Haug, 1970; Walløe et al., 2010; Butti et al., 2011; Mortensen et al., 2014).
Common anatomical landmarks were documented across gyrencephalic species early in the scientific history of this topic (Flatau and Jacobsohn, 1899; Jakob and Onelli, 1913). The question emerges whether there are homologous areas, circuits, and pathways that mediate perceptual, visual, auditory, somatosensory, and other processes or, reversely, whether there are homologous behaviors, conceivably subserved by different cytoarchitectonic areas. Or is the assumption of homologies among species increasingly difficult to sustain, as Frackowiak and Markram (2015) reasoned? Beyond the basics, what is the morphofunctional nature of gyri? Which cytoarchitectonic areas are conserved in larger gyrated brains and which diverge? What is the layer distribution of neuronal types and how are they assembled into neocortical circuits? Histology, histochemistry, microelectrode recordings and biochemical analyses, which historically yielded landmark discoveries in neuroscience, should not be abandoned or underestimated as techniques for the future.
Computational methods of mapping and quantifying cortical area layout, such as the original comparative approach of Chaplin et al. (2013) in simian primates, are also meaningful in the quest to probe form and function in larger gyrated brains.
In the accounts of phylogenetic evolution, the occasional bias in the resolution of cladogram branches in favor of Homo sapiens was pinpointed (Sandvik, 2009). Perhaps the answers to the problems outlined above would help us to gain a broader understanding of neocortical gyration, and a less anthropocentric interpretation of neurobiology.
Author Contributions
The author confirms being the sole contributor of this work and approved it for publication.
Conflict of Interest Statement
The author declares that the research was conducted in the absence of any commercial or financial relationships that could be construed as a potential conflict of interest.
References
Badre, D., Frank, M. J., and Moore, C. I. (2015). Interactionist neuroscience. Neuron 88, 855–860. doi: 10.1016/j.neuron.2015.10.021
Bae, B. I., Tietjen, I., Atabay, K. D., Evrony, G. D., Johnson, M. B., Asare, E., et al. (2014). Evolutionarily dynamic alternative splicing of GPR56 regulates regional cerebral cortical patterning. Science 343, 764–768. doi: 10.1126/science.1244392
Bartels, A., and Zeki, S. (2005). The chronoarchitecture of the cerebral cortex. Philos. Trans. R. Soc. Lond. B Biol. Sci. 360, 733–750. doi: 10.1098/rstb.2005.1627
Bayer, S. A., and Altman, J. (2006). Atlas of Human Central Nervous System Development, Vol. 4: The Human Brain During the Late First Trimester. Boca Raton, FL: CRC Press/Taylor & Francis.
Bhagwandin, A., Haagensen, M., and Manger, P. R. (2017). The brain of the black (Diceros bicornis) and white (Ceratotherium simum) African rhinoceroses: morphology and volumetrics from magnetic resonance imaging. Front. Neuroanat. 11:74. doi: 10.3389/fnana.2017.00074
Brenowitz, E. A., and Zakon, H. H. (2015). Emerging from the bottleneck: benefits of the comparative approach to modern neuroscience. Trends Neurosci. 38, 273–278. doi: 10.1016/j.tins.2015.02.008
Butti, C., Ewan Fordyce, R., Ann Raghanti, M., Gu, X., Bonar, C. J., Wicinski, B. A., et al. (2014). The cerebral cortex of the pygmy hippopotamus, Hexaprotodon liberiensis (Cetartiodactyla, Hippopotamidae): MRI, cytoarchitecture, and neuronal morphology. Anat. Rec. 297, 670–700. doi: 10.1002/ar.22875
Butti, C., Raghanti, M. A., Sherwood, C. C., and Hof, P. R. (2011). The neocortex of cetaceans: cytoarchitecture and comparison with other aquatic and terrestrial species. Ann. N.Y. Acad. Sci. 1225, 47–58. doi: 10.1111/j.1749-6632.2011.05980.x
Chaplin, T. A., Yu, H. H., Soares, J. G., Gattass, R., and Rosa, M. G. (2013). A conserved pattern of differential expansion of cortical areas in simian primates. J. Neurosci. 33, 15120–15125. doi: 10.1523/JNEUROSCI.2909-13.2013
Cozzi, B., Povinelli, M., Ballarin, C., and Granato, A. (2014). The brain of the horse: weight and cephalization quotients. Brain Behav. Evol. 83, 9–16. doi: 10.1159/000356527
Cozzi, B., Spagnoli, S., and Bruno, L. (2001). An overview of the central nervous system of the elephant through a critical appraisal of the literature published in the XIX and XX centuries. Brain Res. Bull. 54, 219–227. doi: 10.1016/S0361-9230(00)00456-1
de Duve, C. (2002). Life Evolving: Molecules, Mind, and Meaning. New York, NY: Oxford University Press. 208, 210, 264.
Dexler, H. (1907). Zur Anatomie des Zentralnervensystems von Elephas indicus. Arb. Neurol. Inst. Wien. Univ. 15, 137–281.
Edinger, L. (1899). The Anatomy of the Central Nervous System of Man and of Vertebrates in General. Transl. by W. S. Hall, P. Holland, and E. P. Carlton. Philadelphia, PA: F. A. Davis.
Flatau, E., and Jacobsohn, L. (1899). Handbuch der Anatomie und Vergleichenden Anatomie des Centralnervensystems der Säugetiere: Makroskopischer Teil. Berlin: S. Karger.
Florio, M., Albert, M., Taverna, E., Namba, T., Brandl, H., Lewitus, E., et al. (2015). Human-specific gene ARHGAP11B promotes basal progenitor amplification and neocortex expansion. Science 347, 1465–1470. doi: 10.1126/science.aaa1975
Fobbs, A. J. Jr., and Johnson, J. I. (2011). Brain collections at the National Museum of Health and Medicine. Ann. N.Y. Acad. Sci. 1225(Suppl. 1), E20–E29. doi: 10.1111/j.1749-6632.2011.06036.x
Frackowiak, R., and Markram, H. (2015). The future of human cerebral cartography: a novel approach. Philos. Trans. R. Soc. Lond. B Biol. Sci. 370:20140171. doi: 10.1098/rstb.2014.0171
Ghosh, L., and Jessberger, S. (2013). Supersize me—new insights into cortical expansion and gyration of the mammalian brain. EMBO J. 32, 1793–1795. doi: 10.1038/emboj.2013.128
Haug, H. (1966). Zytoarchitektonische Untersuchungen an der Hirnrinde des Elefanten. Verh. Anat. Ges. 61, 331–337.
Haug, H. (1970). Der makroskopische Aufbau des Grosshirns. Qualitative und quantitative Untersuchungen an den Gehirnen des Menschen, der Delphinoideae und des Elefanten. Adv. Anat. Embryol. Cell Biol. 43, 3–70.
Haynes, J. D. (2015). A primer on pattern-based approaches to fMRI: principles, pitfalls, and perspectives. Neuron 87, 257–270. doi: 10.1016/j.neuron.2015.05.025
Herculano-Houzel, S., Avelino-de-Souza, K., Neves, K., Porfírio, J., Messeder, D., Mattos Feij,ó, L., et al. (2014). The elephant brain in numbers. Front. Neuroanat. 8:46. doi: 10.3389/fnana.2014.00046
Hofman, M. A. (1985). Size and shape of the cerebral cortex in mammals. I. The cortical surface. Brain Behav. Evol. 27, 28–40. doi: 10.1159/000118718
Hofman, M. A., and Johnson, J. I. (2011). The C. U. Ariëns Kappers brain collection. Ann. N.Y. Acad. Sci. 1225 (Suppl. 1), E64–E84. doi: 10.1111/j.1749-6632.2011.06010.x
Iwaniuk, A. N. (2010). Comparative brain collections are an indispensable resource for evolutionary neurobiology. Brain Behav. Evol. 76, 87–88. doi: 10.1159/000320214
Jacobs, B., Lubs, J., Hannan, M., Anderson, K., Butti, C., Sherwood, C. C., et al. (2011). Neuronal morphology in the African elephant (Loxodonta africana) neocortex. Brain Struct. Funct. 215, 273–298. doi: 10.1007/s00429-010-0288-3
Jakob, C. (1909). Autopsía cerebro craneana del elefante. Rev. Jardín Zool. B. Aires Época II 5, 30–33.
Jakob, C. (1939). El Cerebro Humano: Su Anatomía Sistemática y Topográfica (Folia Neurobiológica Argentina, Atlas I). Buenos Aires: Aniceto López.
Jakob, C., and Onelli, C. (1913). Atlas del Cerebro de los Mamíferos de la República Argentina. Estudios Anatómicos, Histológicos y Biológicos Comparados sobre la Evolución de los Hemisferios y de la Corteza Cerebral. Buenos Aires: Guillermo Kraft.
Janssen, P., and Stephan, H. (1956). Recherches sur le cerveau de l'éléphant d'Afrique (Loxodonta africana Blum). I. Introduction et considérations macroscopiques. Acta Neurol. Psychiatr. Belg. 56, 731–757.
Jones, E. G. (2008). Cortical maps and modern phrenology. Brain 131, 2227–2233. doi: 10.1093/brain/awn158
Karten, H. J. (2015). Vertebrate brains and evolutionary connectomics: on the origins of the mammalian “neocortex”. Philos. Trans. R. Soc. Lond. B Biol. Sci. 370:20150060. doi: 10.1098/rstb.2015.0060
Kitada, R., Johnsrude, I. S., Kochiyama, T., and Lederman, S. J. (2009). Functional specialization and convergence in the occipito-temporal cortex supporting haptic and visual identification of human faces and body parts: an fMRI study. J. Cogn. Neurosci. 21, 2027–2045. doi: 10.1162/jocn.2009.21115
Koskinas, G. N. (1931). Scientific Works Published in German: their Analyses and Principal Assessments by Eminent Scientists. Athens: Pyrsus Publishers.
Manger, P. R. (2011). Collectibles and collections for comparative and evolutionary neurobiological research in Africa. Ann. N.Y. Acad. Sci. 1225(Suppl. 1), E85–E93. doi: 10.1111/j.1749-6632.2010.05948.x
Manger, P. R., Prowse, M., Haagensen, M., and Hemingway, J. (2012). Quantitative analysis of neocortical gyrencephaly in African elephants (Loxodonta africana) and six species of cetaceans: comparison with other mammals. J. Comp. Neurol. 520, 2430–2439. doi: 10.1002/cne.23046
Martínez-Cerdeño, V., Noctor, S. C., and Kriegstein, A. R. (2006). The role of intermediate progenitor cells in the evolutionary expansion of the cerebral cortex. Cereb. Cortex 16(Suppl. 1), i152–i161. doi: 10.1093/cercor/bhk017
Mortensen, H. S., Pakkenberg, B., Dam, M., Dietz, R., Sonne, C., Mikkelsen, B., et al. (2014). Quantitative relationships in delphinid neocortex. Front. Neuroanat. 8:132. doi: 10.3389/fnana.2014.00132
Mota, B., and Herculano-Houzel, S. (2015). Cortical folding scales universally with surface area and thickness, not number of neurons. Science 349, 74–77. doi: 10.1126/science.aaa9101
Nielsen, K. B., Kruhøffer, M., Holm, I. E., Jørgensen, A. L., and Nielsen, A. L. (2010). Identification of genes differentially expressed in the embryonic pig cerebral cortex before and after appearance of gyration. BMC Res. Notes 3:127. doi: 10.1186/1756-0500-3-127
Obersteiner, H. (1890). The Anatomy of the Central Nervous Organs in Health and in Disease. Transl. by A. Hill. London: Charles Griffin.
Pillay, P., and Manger, P. R. (2007). Order-specific quantitative patterns of cortical gyrification. Eur. J. Neurosci. 25, 2705–2712. doi: 10.1111/j.1460-9568.2007.05524.x
Rakic, P. (1988). Specification of cerebral cortical areas. Science 241, 170–176. doi: 10.1126/science.3291116
Ramón y Cajal, S. (1933). Histology, 10th Edn. (revised by J. F. Tello-Muñoz, Transl. by M. Fernán-Núñez). Baltimore, MD: William Wood. 436.
Rash, B. G., and Rakic, P. (2014). Genetic resolutions of brain convolutions. Science 343, 744–745. doi: 10.1126/science.1250246
Retzius, G. (1896). Das Menschenhirn: Studien in der Makroskopischen Morphologie. Stockholm: P. A. Norstedt & Söner.
Rivera, S. M., Reiss, A. L., Eckert, M. A., and Menon, V. (2005). Developmental changes in mental arithmetic: evidence for increased functional specialization in the left inferior parietal cortex. Cereb. Cortex 15, 1779–1790. doi: 10.1093/cercor/bhi055
Ronan, L., and Fletcher, P. C. (2015). From genes to folds: a review of cortical gyrification theory. Brain Struct. Funct. 220, 2475–2483. doi: 10.1007/s00429-014-0961-z
Sandvik, H. (2009). Anthropocentricisms in cladograms. Biol. Philos. 24, 425–440. doi: 10.1007/s10539-007-9102-x
Sawyer, E. K., Turner, E. C., and Kaas, J. H. (2016). Somatosensory brainstem, thalamus, and cortex of the California sea lion (Zalophus californianus). J. Comp. Neurol. 524, 1957–1975. doi: 10.1002/cne.23984
Sestieri, C., Shulman, G. L., and Corbetta, M. (2010). Attention to memory and the environment: functional specialization and dynamic competition in human posterior parietal cortex. J. Neurosci. 30, 8445–8456. doi: 10.1523/JNEUROSCI.4719-09.2010
Shepherd, G. M. (2011). The microcircuit concept applied to cortical evolution: from three-layer to six-layer cortex. Front. Neuroanat. 5:30. doi: 10.3389/fnana.2011.00030
Shoshani, J., Kupsky, W. J., and Marchant, G. H. (2006). Elephant brain. Part I: gross morphology, functions, comparative anatomy, and evolution. Brain Res. Bull. 70, 124–157. doi: 10.1016/j.brainresbull.2006.03.016
Simon, E. (1965). Endocranium, Endokranialausguss und Gehirn beim einhöckerigen Kamel (Camelus dromedarius). Acta Anat. 60, 122–151. doi: 10.1159/000142639
Smith, D. F. (2010). Cognitive brain mapping for better or worse. Perspect. Biol. Med. 53, 321–329. doi: 10.1353/pbm.0.0165
Stahl, R., Walcher, T., De Juan Romero, C., Pilz, G. A., Cappello, S., Irmler, M., et al. (2013). Trnp1 regulates expansion and folding of the mammalian cerebral cortex by control of radial glial fate. Cell 153, 535–549. doi: 10.1016/j.cell.2013.03.027
Südhof, T. C. (2017). Molecular neuroscience in the 21st century: a personal perspective. Neuron 96, 536–541. doi: 10.1016/j.neuron.2017.10.005
Tower, D. B. (1954). Structural and functional organization of mammalian cerebral cortex; the correlation of neurone density with brain size; cortical neurone density in the fin whale (Balaenoptera physalus L.) with a note on the cortical neurone density in the Indian elephant. J. Comp. Neurol. 101, 19–51. doi: 10.1002/cne.901010103
Triarhou, L. C. (2008). “Understanding the brain in seven dimensions,” in Progress in Biological Psychology Research, ed G. A. Conti (Hauppauge, NY: Nova Science Publishers), 177–189.
Turner, E. C., Sawyer, E. K., and Kaas, J. H. (2017). Optic nerve, superior colliculus, visual thalamus, and primary visual cortex of the northern elephant seal (Mirounga angustirostris) and California sea lion (Zalophus californianus). J. Comp. Neurol. 525, 2109–2132. doi: 10.1002/cne.24188
van den Heuvel, M. P., Scholtens, L. H., Feldman Barrett, L., Hilgetag, C. C., and de Reus, M. A. (2015). Bridging cytoarchitectonics and connectomics in human cerebral cortex. J. Neurosci. 35, 13943–13948. doi: 10.1523/JNEUROSCI.2630-15.2015
Van Essen, D. C. (1997). A tension-based theory of morphogenesis and compact wiring in the central nervous system. Nature 385, 313–318. doi: 10.1038/385313a0
Vandenberghe, R., Gitelman, D. R., Parrish, T. B., and Mesulam, M. M. (2001). Functional specificity of superior parietal mediation of spatial shifting. Neuroimage 14, 661–673. doi: 10.1006/nimg.2001.0860
Walløe, S., Eriksen, N., Dabelsteen, T., and Pakkenberg, B. (2010). A neurological comparative study of the harp seal (Pagophilus groenlandicus) and harbor porpoise (Phocoena phocoena) brain. Anat. Rec. 293, 2129–2135. doi: 10.1002/ar.21295
Welker, W. I., Adrian, H. O., Lifschitz, W., Kaulen, R., Caviedes, E., and Gutman, W. (1976). Somatic sensory cortex of llama (Lama glama). Brain Behav. Evol. 13, 284–293. doi: 10.1159/000123816
Xia, C., Stolle, D., Gidengil, E., and Fellows, L. K. (2015). Lateral orbitofrontal cortex links social impressions to political choices. J. Neurosci. 35, 8507–8514. doi: 10.1523/JNEUROSCI.0526-15.2015
Zamboni, G., Gozzi, M., Krueger, F., Duhamel, J. R., Sirigu, A., and Grafman, J. (2009). Individualism, conservatism, and radicalism as criteria for processing political beliefs: a parametric fMRI study. Social Neurosci. 4, 367–383. doi: 10.1080/17470910902860308
Keywords: brain evolution, cerebral cortex, comparative neuroanatomy, cytoarchitectonics, ontophylogeny
Citation: Triarhou LC (2017) The Comparative Neurology of Neocortical Gyration and the Quest for Functional Specialization. Front. Syst. Neurosci. 11:96. doi: 10.3389/fnsys.2017.00096
Received: 21 November 2017; Accepted: 07 December 2017;
Published: 18 December 2017.
Edited by:
Mikhail Lebedev, Duke University, United StatesReviewed by:
Paul Manger, University of the Witwatersrand, South AfricaJoão G. Franca, Universidade Federal do Rio de Janeiro, Brazil
Copyright © 2017 Triarhou. This is an open-access article distributed under the terms of the Creative Commons Attribution License (CC BY). The use, distribution or reproduction in other forums is permitted, provided the original author(s) or licensor are credited and that the original publication in this journal is cited, in accordance with accepted academic practice. No use, distribution or reproduction is permitted which does not comply with these terms.
*Correspondence: Lazaros C. Triarhou, triarhou@uom.gr