- Department of Neurosurgery, University Hospitals Case Medical Center, Case Western Reserve University, Cleveland, OH, USA
Traumatic brain injury (TBI) is the leading cause of death and disability in individuals below age 45, and five million Americans live with chronic disability as a result. Mild TBI (mTBI), defined as TBI in the absence of major imaging or histopathological defects, is responsible for a majority of cases. Despite the lack of overt morphological defects, victims of mTBI frequently suffer lasting cognitive deficits, memory difficulties, and behavioral disturbances. There is increasing evidence that cognitive and memory dysfunction is related to subtle physiological changes that occur in the hippocampus, and these impact both the phenotype of deficits observed and subsequent recovery. Therapeutic modulation of physiological activity by means of medications commonly used for other indications or brain stimulation may represent novel treatment approaches. This review summarizes the present body of knowledge regarding neurophysiologic changes that occur in the hippocampus after mTBI, as well as potential targets for therapeutic modulation of neurologic activity.
Introduction
Every year, one out of every 200 people worldwide will suffer a traumatic brain injury (TBI), including 1.7 million people in the United States alone, with an annual associated cost of approximately $76.5 billion (Faul et al., 2010). It is estimated that 5.3 million Americans live with long-term disability as a result of their injury (Centers for Disease Control and Prevention [CDC], 2013). Young men and the elderly are particularly vulnerable because of an increased incidence of trauma and falls, respectively (Susman et al., 2002). TBI is also prevalent in the military, with approximately 20% of all returning deployed American troops suffering some form of brain injury from a blast exposure (Terrio et al., 2009), the vast majority of these being mild injuries.
Mild TBI (mTBI), sometimes termed concussion, accounts for 75% of all head injuries (Faul et al., 2010). Symptoms include headache, sleep impairment, memory deficit, difficulty concentrating (Levin et al., 1987), and an increased incidence of affective disorders such as depression and anxiety (Park et al., 2008). These clinical problems are persistent and severe in 10% of cases, lasting for months to years, sometimes indefinitely (Greig et al., 2014). Due to the striking prevalence and disruptive clinical manifestations of mTBI, research has focused on elucidating the pathophysiological mechanisms underlying primary and secondary brain injury in order to develop therapies that may alter or prevent these processes. More recently, changes in neural circuitry underlying many of the clinical manifestations of mTBI, especially memory and affective disorders, has been studied in an attempt to understand mechanisms of disruption and how modulation of these networks may be used to therapeutic advantage. In order to determine how novel neuromodulation treatments might be able to improve TBI-associated deficits, it is important to establish how individual neurons and subpopulations of neurons contribute to network activity and how these networks respond to injury. This review will discuss neurophysiological changes in the hippocampus associated with mTBI, and ways in which therapeutic neuromodulation might be used to reverse these deficits.
Biochemical and Genetic Changes in the Hippocampus Following TBI
Neurological injury that occurs after a traumatic insult can result from two different mechanisms: primary and secondary injury. Primary injury refers to the mechanical forces of shearing and compression at the time of impact, while secondary injury involves the subsequent cascade of pathological events that occurs minutes to days after the insult (Greve and Zink, 2009). Such secondary processes include hypoxia/ischemia, edema, raised intracranial pressure, excitotoxity through glutamate release, calcium dysregulation, cytoskeletal proteolysis, free radical oxidative damage, altered synaptic physiology, cytokine release causing inflammation, and ultimately neuronal cell death (Choi et al., 1987; Raghupathi, 2004; Maas et al., 2008; Barkhoudarian et al., 2011; Greig et al., 2014; Merlo et al., 2014). These secondary injury mechanisms are especially important for therapeutic consideration as they can theoretically be altered or inhibited with the aim of preventing injury or protecting brain tissue that has the potential to recover.
There are several commonalities between the biochemical cascades involved in secondary mTBI injury mechanisms and those found in degenerative central nervous system (CNS) diseases such as Parkinson’s disease (PD; Eakin and Miller, 2012; van Bregt et al., 2012), Alzheimer’s disease (AD), Huntington’s disease (HD), and amyotrophic lateral sclerosis (Daneshvar et al., 2011). As a result, pathways that have been extensively characterized in these diseases can be extrapolated and applied to analogous pathways involved in TBI in order to better understand the mechanisms of injury and potential novel therapeutic approaches. This has important implications for diagnosis: a complex mathematical model of semantic predications was recently used to search almost 100,000 citations of neural injury networks and produced a list of 17 potential biomarkers that may indicate mTBI, with glutamate, glucose, and lactate being the most common (Cairelli et al., 2015). In combination with other compounds, such as the apolipoprotein E-4 allele which adversely influences recovery following brain injury (Lawrence et al., 2015), these biomarkers may aid in the early diagnosis of mTBI, allowing the prompt administration of neuroprotective drugs or neuromodulatory therapies.
Glutamate is particularly important, as this excitatory neurotransmitter is known to play a strong role in secondary injury mechanisms throughout the brain. Microdialysis studies, in both rodents (Folkersma et al., 2011) and humans (Chamoun et al., 2010), have shown that glutamate concentrations increase in the acute phase following injury, whereas magnetic resonance spectroscopy (MRS) studies have shown a persistent decrease in glutamate concentrations following injury. The reason for this discrepancy is that microdialysis measures extracellular glutamate, while MRS measures both intra and extracellular glutamate (Guerriero et al., 2015).
Specifically in the hippocampus, glutamate decreases following moderate to severe temporal cortex injury, as measured by in vivo proton MRS in a direct cortical injury model in rodents (Harris et al., 2012). Although the hippocampus was considered perilesional tissue in this experiment, the temporal neocortex, i.e., the lesional tissue, also showed a decrease in glutamate in the acute phase. Human MRS studies also in the acute phase following concussion, however, showed no changes in hippocampal glutamate concentrations, despite finding decreased glutamate levels in the motor cortex (Henry et al., 2010). A reason for this discrepancy may be that the human study was in patients with mTBI, whereas the rodent study looked at moderate to severe TBI.
Another important compound altered in the hippocampus after TBI is the neurotransmitter gamma-aminobutyric acid (GABA). The expression of various GABA receptor subunits is changed, causing reductions of inhibitory postsynaptic currents and increases in excitatory post-synaptic currents after TBI in rodents (Hunt et al., 2011; Almeida-Suhett et al., 2015; Drexel et al., 2015). As a result, deficits occur in long-term potentiation, which can translate into cognitive impairment. Similarly, up and down-regulation of other proteins can lead to impairments in hippocampal synaptic transmission, including those involved in the extracellular signal-regulated kinase (ERK) pathways (Atkins et al., 2009), and the soluble N-ethylmaleimide-sensitive factor attachment protein receptor (SNARE) complex (Carlson et al., 2016).
The regulation of gene transcription affects not only the creation of proteins involved in neurotransmission after injury, but also the synthesis of neurons themselves. Because TBI is associated with hippocampal neuronal damage and death, it is important to develop therapeutic strategies to either protect immature neurons or enhance neurogenesis in the hippocampus after injury. Methods shown to drive neurogenesis post injury include forced overexpression of insulin-like growth factor 1 (IGF-1; Carlson et al., 2014), and the transplantation of synthetic human progenitor cells into the hippocampus after TBI (Blaya et al., 2015). Interestingly, TBI severity has been found to correlate with the degree of post-traumatic neurogenesis in rat hippocampus. Whereas mTBI had no effect on neurogenesis, moderate TBI promoted neural stem cell proliferation without increasing neurogenesis, and severe TBI increased neurogenesis (Wang et al., 2015a). A summary of the biochemical and genetic changes following TBI in rodent hippocampus can be found in Table 1.
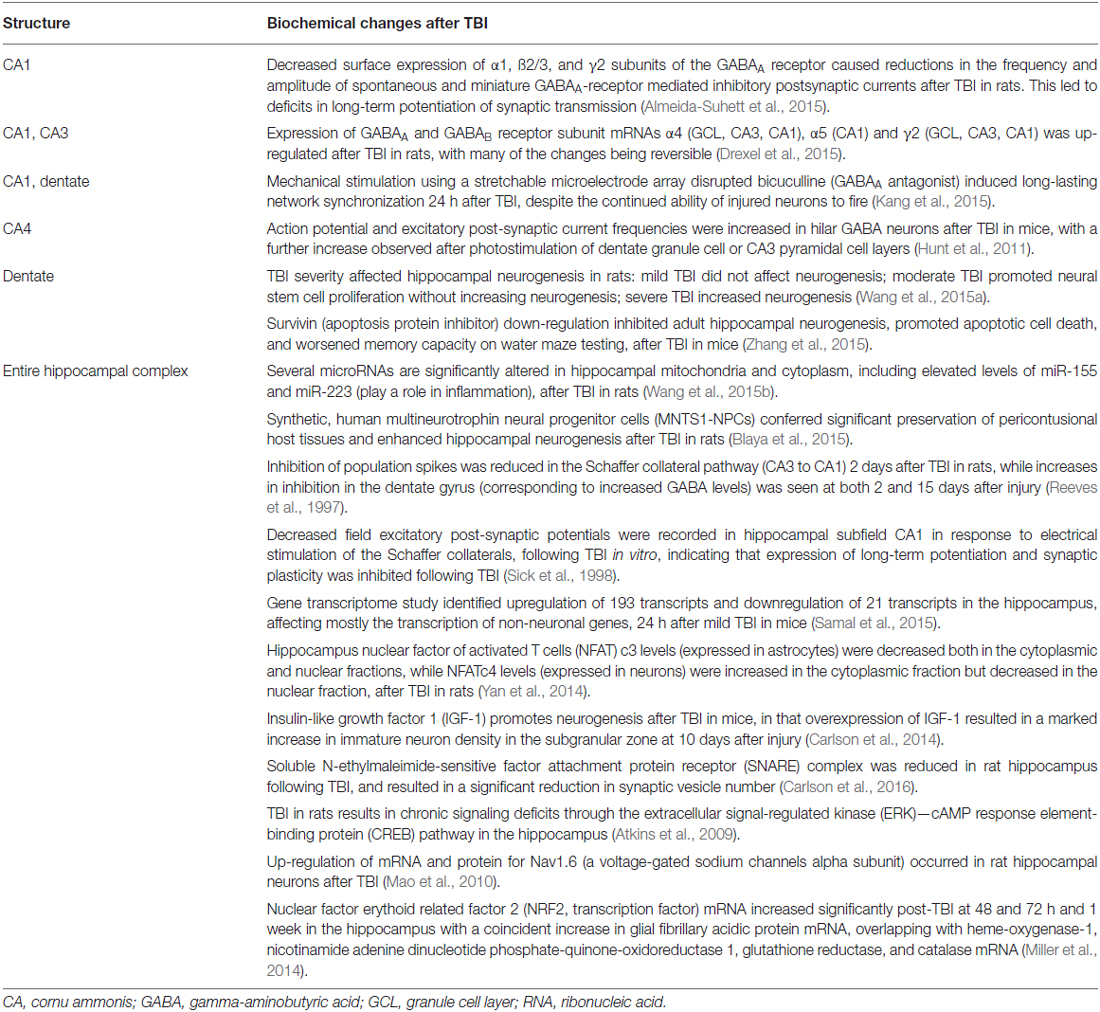
Table 1. Biochemical changes in rodent hippocampus following TBI, with effects categorized by subsection of the hippocampal complex.
Hippocampus Biochemical Pathways and Neuroprotective Agents in mTBI
Since mechanisms of secondary injury after mTBI are similar to other diseases, it is likely that drugs that are already in use for other indications may have efficacy for mTBI as well. One example of such a mechanism is the glucagon-like peptide-1 (GLP-1) signaling pathway. GLP-1 was initially described based on its role in the pancreatic regulation of insulin, but receptors are also found in the CNS and changes in this pathway have been implicated in a number of neurodegenerative diseases (Li et al., 2009, 2010, 2012; Martin et al., 2009; Salcedo et al., 2012). Through coupling to a cAMP second messenger pathway, activation of GLP-1 receptors elicits neurotrophic actions such as neuritic growth while protecting neurons against various insults (Perry et al., 2002). As a result, there have been a number of investigations into modulation of this pathway to treat neurological disease.
Exendin-4 is a synthetic GLP-1 receptor agonist currently approved as a drug for the treatment of hyperglycemia in type-2 diabetes that crosses the blood brain barrier (Kastin and Akerstrom, 2003). When administered after mTBI, this neuroprotective agent has been shown to prevent changes in rodent hippocampal gene expression following mTBI. Many of these genes protected by Exendin-4 are also associated with memory loss in AD (which has been associated with TBI), and administration ameliorated memory deficits after injury (Tweedie et al., 2013). Additional rodent studies using markers of cell death have shown that Exedin-4 improved hydrogen peroxide mediated oxidative stress and glutamate toxicity in neuronal cultures in vitro. In vivo, using markers of cognitive function, the drug ameliorated mTBI-induced deficits in novel object recognition and maze testing, both when administered prior to and after the insult (Eakin et al., 2013; Rachmany et al., 2013). Therefore, data is emerging to support a shift in indication for the use of Exedin-4 from an antihyperglycemic medication to a potential agent to minimize secondary injury after mTBI.
Another agent with potential efficacy for secondary injury after mTBI is N-Acetyl-cystein (NAC), which is currently used as a mucolytic agent and an antidote to acetaminophen overdose. NAC has been shown to have antioxidant and neurovascular-protective effects after mTBI (Ellis et al., 1991; Hicdonmez et al., 2006; Chen et al., 2008) and has been demonstrated to reverse behavioral deficits in mTBI rodents when administered 30–60 min after injury (Eakin et al., 2014). Specifically in the hippocampus, NAC was found to decrease levels of cytosolic-free Ca2+ and reactive oxygen species, reduce apoptosis, and lower caspase-3 and -9 neuronal activities, following TBI in rats (Naziroğlu et al., 2014). In humans, NAC has been shown in a randomized double-blind trial to improve auditory, vestibular, and cognitive functional sequelae after blast-induced mTBI in military personnel (Hoffer et al., 2013). Although the mechanism of action in the trauma setting has yet to be elucidated, the 40 year safety profile and ease of administration of the medication makes it an attractive option for widespread use.
Numerous other compounds have been found to exert effects in the hippocampus following injury. As summarized in Table 2, different substances affect different subsections of the hippocampal complex. Although all these investigations were performed in rodents, several of these biochemical compounds may prove to have beneficial effects in humans suffering from cognitive and memory impairments following TBI.
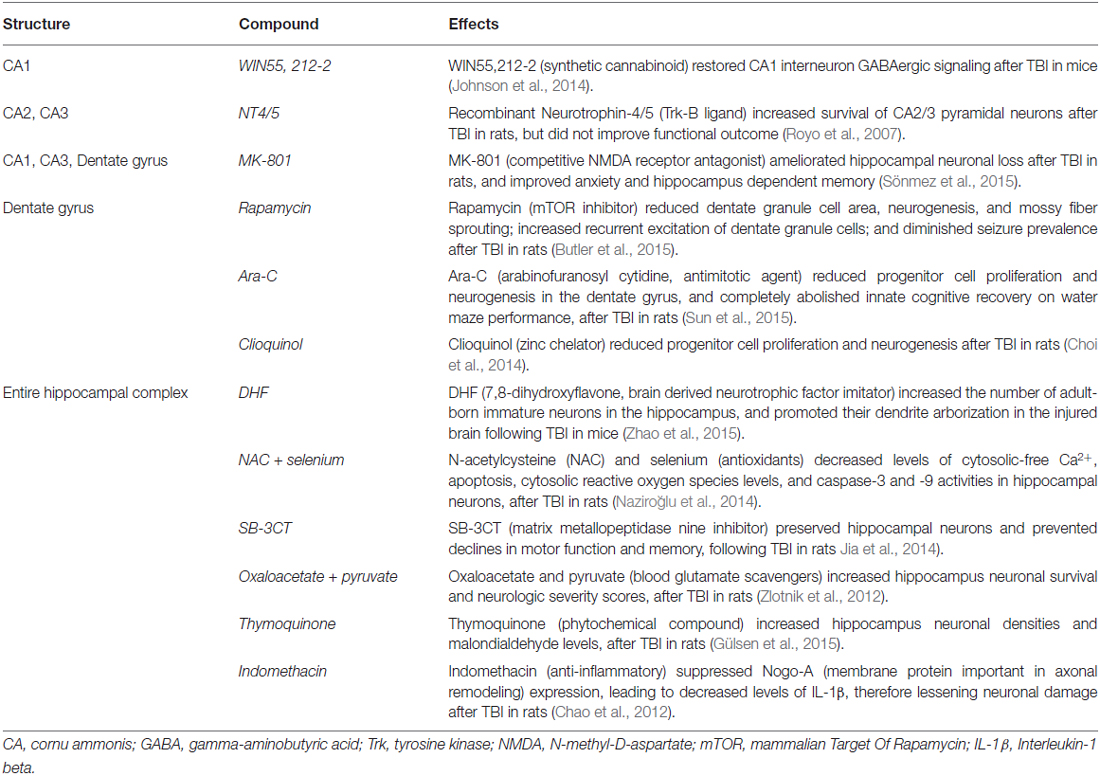
Table 2. Biochemical compounds investigated in rodent hippocampus following TBI, with effects categorized by subsection of the hippocampal complex.
Hippocampal Circuitry and Neurophysiologic Changes After mTBI
In addition to biochemical changes after injury, changes in electrical neural activity also play a role in the sequelae of TBI. The high prevalence of memory deficits after injury point to a relative vulnerability of the hippocampus and mesial temporal structures as compared to the rest of the brain (Hamm et al., 1993; Finset et al., 1999; Comper et al., 2005), and electrophysiologic studies have shown that changes occur in hippocampal circuit excitability after TBI (Witgen et al., 2005; Ortiz and Gutiérrez, 2015). In addition, moderate and severe TBI is associated with neuronal cell loss in several hippocampal regions (Lowenstein et al., 1992) as well as alterations in cellular homeostasis (D’Ambrosio et al., 1999) and dysregulated synaptic transmission (Toth et al., 1997; D’Ambrosio et al., 1998) in this region. On the other hand, mTBI has been associated with more subtle changes in firing patterns that are present even in the absence of morphological changes.
Studies comparing control rats to those who had undergone lateral fluid percussion induced mTBI found changes in working memory in the absence of differences in histology or neuronal loss (Eakin and Miller, 2012). Single unit recordings from cornu ammonis 1 (CA1) and CA3 subfields during a working memory task showed no differences in firing rates or spike characteristics between the two groups, but rats exposed to mTBI were found to have significantly fewer cells with spatiotemporal activity, and this correlated with task performance. Moreover, in a similar experiment looking at the burst characteristics of hippocampal cells during an object exploration task, memory deficits were found to be associated with decreased burst activity of certain subsets of neurons within the pyramidal cell layer (Munyon et al., 2014). Therefore, functional impairment may stem from an alteration in the activity of certain subpopulations of cells within the hippocampus.
These changes in neuronal firing patterns within the hippocampus can persist after TBI and correlate with changes in cognitive function (Witgen et al., 2005). In the analysis of local field potentials after injury, hippocampal broadband power decreased (Paterno et al., 2015), and specifically disruption of theta rhythm (4–7 Hz) has been implicated in the pathophysiology of memory loss (Winson, 1978; Fedor et al., 2010). It has been theorized that using electrical stimulation to artificially counteract and reverse these disrupted firing patterns within the hippocampus and other structures may regulate cellular and network processes and restore functional memory circuits (Diamond et al., 1988; Nakao et al., 2003; Shirvalkar et al., 2010; Tabansky et al., 2014; Hanell et al., 2015). Accordingly, theta burst stimulation (200 Hz in 50 ms trains, five trains per second; 60 mA biphasic pulses) delivered to the fornix of rats with mTBI improved deficits in learning and memory, whereas non-oscillatory low or high frequency stimulation did not (Sweet et al., 2014). In a related manner, theta band stimulation of the medial septal nucleus of mTBI rats resulted in a transient increase in hippocampal theta activity and improved spatial working memory (Lee et al., 2013), whereas 100 Hz gamma stimulation did not (Lee et al., 2015).
In humans, theta burst stimulation of the fornix has been studied in patients undergoing stereo-electroencephalography evaluation for drug-resistant epilepsy, and was shown to produce evoked potentials in the ipsilateral hippocampus. This resulted in significant and reversible improvements in immediate and delayed performance on a visual-spatial memory task (Miller et al., 2015). Temporally patterned stimulation paradigms may therefore present novel neuromodulatory strategies in the treatment of post-traumatic memory loss and cognitive deficits. Potential targets include the hippocampus, its associated white matter tracts, as well as the medial prefrontal cortex. The dysfunction of the latter is thought to play a role in the failure of emotion regulation after mTBI through top down amygdalar regulation (van der Horn et al., 2016), a mechanism that may result in persistent post-concussive symptoms.
Imaging in mTBI
Recent advances in imaging have allowed for in-depth structural analysis of brain regions both acutely after concussive injury and in the chronic phase. This is important because the ability to identify abnormalities that may previously have been missed is the first step in developing modalities to treat these phenomena, or in the least decide which patients require treatment. Although no single imaging modality has yet proven to be sufficient for all patients with TBI due to the heterogeneity of the condition (Amyot et al., 2015), each method offers its own advantages.
Conventional magnetic resonance imaging (MRI) and computed tomography (CT) are typically negative in mTBI patients with persistent symptoms of post-concussive syndrome. However, compared to controls, mTBI patients were found to have lower cortical thickness of the right temporal lobe and left insula, and increasing number of mTBIs was associated with lower cortical thickness in more brain areas, including bilateral insula, right middle temporal gyrus, and right entorhinal area (List et al., 2015). These findings are worrisome because if further deterioration of these structures occur as part of the aging process, subtle cognitive symptoms may progress to clinical dementia earlier than in age-matched controls. Diffusion tensor imaging is another technique that has recently emerged as a powerful adjunct to conventional MRI. It enables the measurement of fractional anisotropy, a value that indicates the degree of restriction experienced by water molecules diffusing along white matter fibers. Such a tool offers a noninvasive glimpse into the neurophysiology of these tracts and may allow detection of demyelination and axonal damage that would otherwise be missed on a conventional MRI. Indeed, fractional anisotropy was significantly reduced in the right superior longitudinal fasciculus of patients with mTBI and normal MRI as compared with controls, showing that diffusion tensor imaging is more sensitive than standard imaging in this population (Adam et al., 2015). Another study showed similar but subtler findings, as only those with mTBI who had abnormalities on conventional MRI had white matter changes on diffusion tensor imaging (Panenka et al., 2015).
Although increasing abnormality on post-injury CT and MRI correlate with lower neuropsychological performance (Bigler et al., 2015), diffusion tensor imaging has also shown promise in predicting neuropsychological outcomes following mTBI in patients with normal CT and MRI. Immediately following injury and 6 months post-trauma, patients with mTBI showed significant differences in fractional anisotropy and radial diffusivity as compared to controls, specifically in the corona radiata, anterior limb of internal capsule, cingulum, superior longitudinal fasciculus, optic radiation, and genu of corpus callosum, changes which demonstrated associations with neuropsychological outcomes across both time points (Veeramuthu et al., 2015). Another study has shown significantly reduced fractional anisotropy in the internal capsule of both TBI and depression patients (Maller et al., 2010), further underscoring the potential role for diffusion tensor imaging as a noninvasive biomarker useful in the prognostication of post-concussive symptoms.
MRS is another noninvasive technique that measures metabolic changes within brain tissue. In combination with vascular imaging techniques such as transcranial Doppler ultrasound, these modalities have begun to shed light on metabolic processes and the control and regulation of cerebral blood flow following mTBI (Len and Neary, 2011). In the hippocampus, N-acetyl aspartate to choline and N-acetyl aspartate to creatine ratios were decreased in patients with mTBI in comparison to control subjects (Hetherington et al., 2014). Similar changes were found following mTBI in the thalamus, with a reduction of N-acetyl aspartate to creatine and choline to creatine ratios, findings that are classically associated with ischemia (Sours et al., 2015). Of interest, these results correlated with self-reported sensory symptoms, leading the authors to speculate that novel interventions could target these changes in the hopes of treating patients with persistent post-concussive symptoms, as well as use the absence of such imaging features as an indicator to help predict safe return to work.
Magnetoencephalography is an imaging modality largely restricted to the study of epilepsy, but may prove useful as a sensitive detector of local field potentials in brain areas affected by mTBI. A recent innovative study looked at the tracking of eye movements as a marker for sustained attention over time and internal anticipatory control, measurements that are impaired in chronic mTBI while the patients were undergoing magnetoencephalography (Diwakar et al., 2015). Compared to controls, mTBI patients demonstrated impaired eye tracking that was concurrent with abnormal beta activity, which was suppressed in the right parietal cortex and enhanced in the left caudate and frontal-temporal areas. The clinical significance of these findings is yet to be seen, but this is a strong example of novel imaging methods being used in new and original ways to better understand the neurophysiology of injured neural circuits, methods that may alter the manner in which mTBI is diagnosed and treated in the future.
Future Directions
Direct modulation of neural activity via deep brain stimulation (DBS) currently does not play a direct role in the setting mTBI, but it does carry promise in the treatment of post-concussive affective disorders, trauma-induced tremor, and neurodegenerative disorders. DBS is an effective treatment for certain patients with severe major depression (Lozano et al., 2012) and obsessive-compulsive disorder (Kisely et al., 2014), but has yet to be investigated in the treatment of these disorders following brain injury. Likewise, PD often responds well to DBS of the subthalamic nucleus or globus pallidus internus (Okun, 2012), but data is lacking in the setting of trauma-induced Parkinson’s. However, tremor following severe brain injury has been shown in small studies to respond to both thalamic and pallidal DBS (Issar et al., 2013; Carvalho et al., 2014).
If neurostimulation augments improvements in cognitive deficits after brain injury, memory disorders may present other potential applications for DBS (Shin et al., 2014). Forniceal DBS in a mouse model of Rett syndrome restored in vivo hippocampal long-term potentiation and neurogenesis, and rescued contextual fear memory and spatial learning (Hao et al., 2015). In humans, the fornix and nucleus basalis of Meynert are being investigated as possible DBS targets for improvement of memory in the treatment of AD (Sankar et al., 2014; Suthana and Fried, 2014). Although these strategies seem promising, they will likely be tested for efficacy and safety in the setting of severe TBI, prior to being applied in patients with mTBI.
Less invasive neuromodulation options for the treatment of mTBI include transcranial magnetic stimulation (TMS) and transcranial direct current stimulation (TDCS). TMS uses a surface coil to create a low frequency electric current that is delivered to the surface of the brain, while TDCS uses electrodes placed directly on the scalp. There is evidence in severe TBI that TMS may aid in motor recovery, aphasia, visuospatial neglect (Castel-Lacanal et al., 2014), and cognitive function (Polanowska and Seniów, 2010), but high frequencies are contraindicated due to the risk of seizures, especially in the setting of trauma as the injured brain is already predisposed to epileptiform activity. TDCS uses lower currents and therefore has a lower risk of seizures, but subsequently may also have less clinical effect, as current studies show mixed results in the treatment of post-concussive symptoms (Kang et al., 2012; Leśniak et al., 2014; Ulam et al., 2015). TMS is currently the most extensively studied brain stimulation modality in TBI, but most interventions have been non-targeted and focused on the chronic phase of recovery after the injury (Li et al., 2015). As can be expected, better clinical outcomes occur when these neuromodulatory approaches are coupled with cognitive therapy and neurorehabilitation (Chantsoulis et al., 2015; Page et al., 2015).
Finally, nanotechnology and brain-computer interfaces may present new opportunities. By using a computer to bridge the gap between the brain and peripheral nerves in the setting of an injured spinal cord, brain-computer interfaces are “a form of extended embodiment that become integrated into the individual’s conception of himself as an autonomous agent” (Glannon, 2014). Such systems are still in the experimental stage, but offer hope to those patients with severe motor deficits. Similarly, carbon nanotubes are being used to synthesize nanoelectrode arrays that permit real time monitoring and modulation of electrochemical events at the neural and synaptic level (Andrews, 2007), and may offer a glimpse into the future of the field of neuromodulation. As with DBS, however, these modalities will likely be reserved for patients with severe TBI until efficacy, safety, and economic feasibility can be established.
Conclusion
Despite the high incidence and prevalence of mTBI, only recently have the pathophysiologic mechanisms begun to emerge. Understanding the biochemical signaling pathways involved in the propagation of secondary injury mechanisms has led to the discovery of novel uses for medications currently used for other indications. Biochemical markers and advances in imaging are allowing for earlier diagnosis of concussions, and developments in the area of DBS for the treatment of memory and affective disorders are creating new avenues for the management of post-concussive syndrome. Ultimately, these advances in neuromodulation strive to improve outcomes in mTBI and substantially impact the lives of patients and families suffering from this condition.
Author Contributions
FG, JP, JS and JPM wrote and edited the manuscript.
Conflict of Interest Statement
The authors declare that the research was conducted in the absence of any commercial or financial relationships that could be construed as a potential conflict of interest.
References
Adam, O., Mac Donald, C. L., Rivet, D., Ritter, J., May, T., Barefield, M., et al. (2015). Clinical and imaging assessment of acute combat mild traumatic brain injury in Afghanistan. Neurology 85, 219–227. doi: 10.1212/WNL.0000000000001758
Almeida-Suhett, C. P., Prager, E. M., Pidoplichko, V., Figueiredo, T. H., Marini, A. M., Li, Z., et al. (2015). GABAergic interneuronal loss and reduced inhibitory synaptic transmission in the hippocampal CA1 region after mild traumatic brain injury. Exp. Neurol. 273, 11–23. doi: 10.1016/j.expneurol.2015.07.028
Amyot, F., Arciniegas, D. B., Brazaitis, M. P., Curley, K. C., Diaz-Arrastia, R., Gandjbakhche, A., et al. (2015). A review of the effectiveness of neuroimaging modalities for the detection of traumatic brain injury. J. Neurotrauma 32, 1693–1721. doi: 10.1089/neu.2013.3306
Andrews, R. J. (2007). Neuroprotection at the nanolevel–Part II: nanodevices for neuromodulation—deep brain stimulation and spinal cord injury. Ann. N Y Acad. Sci. 1122, 185–196. doi: 10.1196/annals.1403.013
Atkins, C. M., Falo, M. C., Alonso, O. F., Bramlett, H. M., and Dietrich, W. D. (2009). Deficits in ERK and CREB activation in the hippocampus after traumatic brain injury. Neurosci. Lett. 459, 52–56. doi: 10.1016/j.neulet.2009.04.064
Barkhoudarian, G., Hovda, D. A., and Giza, C. C. (2011). The molecular pathophysiology of concussive brain injury. Clin. Sports Med. 30, 33–48. doi: 10.1016/j.csm.2010.09.001
Bigler, E. D., Jantz, P. B., Farrer, T. J., Abildskov, T. J., Dennis, M., Gerhardt, C. A., et al. (2015). Day of injury CT and late MRI findings: cognitive outcome in a paediatric sample with complicated mild traumatic brain injury. Brain Inj. 29, 1062–1070. doi: 10.3109/02699052.2015.1011234
Blaya, M. O., Tsoulfas, P., Bramlett, H. M., and Dietrich, W. D. (2015). Neural progenitor cell transplantation promotes neuroprotection, enhances hippocampal neurogenesis and improves cognitive outcomes after traumatic brain injury. Exp. Neurol. 264, 67–81. doi: 10.1016/j.expneurol.2014.11.014
Butler, C. R., Boychuk, J. A., and Smith, B. N. (2015). Effects of rapamycin treatment on neurogenesis and synaptic reorganization in the dentate gyrus after controlled cortical impact injury in mice. Front. Syst. Neurosci. 9:163. doi: 10.3389/fnsys.2015.00163
Cairelli, M. J., Fiszman, M., Zhang, H., and Rindflesch, T. C. (2015). Networks of neuroinjury semantic predications to identify biomarkers for mild traumatic brain injury. J. Biomed. Semantics 6:25. doi: 10.1186/s13326-015-0022-4
Carlson, S. W., Madathil, S. K., Sama, D. M., Gao, X., Chen, J., and Saatman, K. E. (2014). Conditional overexpression of insulin-like growth factor-1 enhances hippocampal neurogenesis and restores immature neuron dendritic processes after traumatic brain injury. J. Neuropathol. Exp. Neurol. 73, 734–746. doi: 10.1097/NEN.0000000000000092
Carlson, S. W., Yan, H., Ma, M., Li, Y., Henchir, J., and Dixon, C. E. (2016). Traumatic brain injury impairs soluble N-ethylmaleimide-sensitive factor attachment protein receptor complex formation and alters synaptic vesicle distribution in the hippocampus. J. Neurotrauma 33, 113–121. doi: 10.1089/neu.2014.3839
Carvalho, K. S., Sukul, V. V., Bookland, M. J., Koch, S. A., and Connolly, P. J. (2014). Deep brain stimulation of the globus pallidus suppresses post-traumatic dystonic tremor. J. Clin. Neurosci. 21, 153–155. doi: 10.1016/j.jocn.2013.02.009
Castel-Lacanal, E., Tarri, M., Loubinoux, I., Gasq, D., de Boissezon, X., Marque, P., et al. (2014). Transcranial magnetic stimulation in brain injury. Ann. Fr. Anesth. Reanim. 33, 83–87. doi: 10.1016/j.annfar.2013.11.006
Centers for Disease Control and Prevention [CDC]. (2013). CDC grand rounds: reducing severe traumatic brain injury in the United States. MMWR Morb. Mortal. Wkly. Rep. 62, 549–552.
Chamoun, R., Suki, D., Gopinath, S. P., Goodman, J. C., and Robertson, C. (2010). Role of extracellular glutamate measured by cerebral microdialysis in severe traumatic brain injury. J. Neurosurg. 113, 564–570. doi: 10.3171/2009.12.JNS09689
Chantsoulis, M., Mirski, A., Rasmus, A., Kropotov, J. D., and Pachalska, M. (2015). Neuropsychological rehabilitation for traumatic brain injury patients. Ann. Agric. Environ. Med. 22, 368–379. doi: 10.5604/12321966.1152097
Chao, P. K., Lu, K. T., Jhu, J. Y., Wo, Y. Y., Huang, T. C., Ro, L. S., et al. (2012). Indomethacin protects rats from neuronal damage induced by traumatic brain injury and suppresses hippocampal IL-1beta release through the inhibition of Nogo-A expression. J. Neuroinflammation 9:121. doi: 10.1186/1742-2094-9-121
Chen, G., Shi, J., Hu, Z., and Hang, C. (2008). Inhibitory effect on cerebral inflammatory response following traumatic brain injury in rats: a potential neuroprotective mechanism of N-acetylcysteine. Mediators Inflamm. 2008:716458. doi: 10.1155/2008/716458
Choi, B. Y., Kim, J. H., Kim, H. J., Lee, B. E., Kim, I. Y., Sohn, M., et al. (2014). Zinc chelation reduces traumatic brain injury-induced neurogenesis in the subgranular zone of the hippocampal dentate gyrus. J. Trace. Elem. Med. Biol. 28, 474–481. doi: 10.1016/j.jtemb.2014.07.007
Choi, D. W., Maulucci-Gedde, M., and Kriegstein, A. R. (1987). Glutamate neurotoxicity in cortical cell culture. J. Neurosci. 7, 357–368.
Comper, P., Bisschop, S. M., Carnide, N., and Tricco, A. (2005). A systematic review of treatments for mild traumatic brain injury. Brain Inj. 19, 863–880. doi: 10.1080/02699050400025042
D’Ambrosio, R., Maris, D. O., Grady, M. S., Winn, H. R., and Janigro, D. (1998). Selective loss of hippocampal long-term potentiation, but not depression, following fluid percussion injury. Brain Res. 786, 64–79. doi: 10.1016/s0006-8993(97)01412-1
D’Ambrosio, R., Maris, D. O., Grady, M. S., Winn, H. R., and Janigro, D. (1999). Impaired K(+) homeostasis and altered electrophysiological properties of post-traumatic hippocampal glia. J. Neurosci. 19, 8152–8162.
Daneshvar, D. H., Riley, D. O., Nowinski, C. J., Mckee, A. C., Stern, R. A., and Cantu, R. C. (2011). Long-term consequences: effects on normal development profile after concussion. Phys. Med. Rehabil. Clin. N Am. 22, 683–700. doi: 10.1016/j.pmr.2011.08.009
Diamond, D. M., Dunwiddie, T. V., and Rose, G. M. (1988). Characteristics of hippocampal primed burst potentiation in vitro and in the awake rat. J. Neurosci. 8, 4079–4088.
Diwakar, M., Harrington, D. L., Maruta, J., Ghajar, J., El-Gabalawy, F., Muzzatti, L., et al. (2015). Filling in the gaps: anticipatory control of eye movements in chronic mild traumatic brain injury. Neuroimage Clin. 8, 210–223. doi: 10.1016/j.nicl.2015.04.011
Drexel, M., Puhakka, N., Kirchmair, E., Hörtnagl, H., Pitkänen, A., and Sperk, G. (2015). Expression of GABA receptor subunits in the hippocampus and thalamus after experimental traumatic brain injury. Neuropharmacology 88, 122–133. doi: 10.1016/j.neuropharm.2014.08.023
Eakin, K., Baratz-Goldstein, R., Pick, C. G., Zindel, O., Balaban, C. D., Hoffer, M. E., et al. (2014). Efficacy of N-acetyl cysteine in traumatic brain injury. PLoS One 9:e90617. doi: 10.1371/journal.pone.0090617
Eakin, K., Li, Y., Chiang, Y. H., Hoffer, B. J., Rosenheim, H., Greig, N. H., et al. (2013). Exendin-4 ameliorates traumatic brain injury-induced cognitive impairment in rats. PLoS One 8:e82016. doi: 10.1371/journal.pone.0082016
Eakin, K., and Miller, J. P. (2012). Mild traumatic brain injury is associated with impaired hippocampal spatiotemporal representation in the absence of histological changes. J. Neurotrauma 29, 1180–1187. doi: 10.1089/neu.2011.2192
Ellis, E. F., Dodson, L. Y., and Police, R. J. (1991). Restoration of cerebrovascular responsiveness to hyperventilation by the oxygen radical scavenger n-acetylcysteine following experimental traumatic brain injury. J. Neurosurg. 75, 774–779. doi: 10.3171/jns.1991.75.5.0774
Faul, M. X. L., Wald, M., and Coronado, V. (2010). Traumatic Brain Injury in the United States: Emergency Department Visits, Hospitalizations and Deaths, 2002–2006. Atlanta, GA: Centers for Disease Control and Prevention.
Fedor, M., Berman, R. F., Muizelaar, J. P., and Lyeth, B. G. (2010). Hippocampal theta dysfunction after lateral fluid percussion injury. J. Neurotrauma 27, 1605–1615. doi: 10.1089/neu.2010.1370
Finset, A., Anke, A. W., Hofft, E., Roaldsen, K. S., Pillgram-Larsen, J., and Stanghelle, J. K. (1999). Cognitive performance in multiple trauma patients 3 years after injury. Psychosom. Med. 61, 576–583. doi: 10.1097/00006842-199907000-00024
Folkersma, H., Foster Dingley, J. C., van Berckel, B. N., Rozemuller, A., Boellaard, R., Huisman, M. C., et al. (2011). Increased cerebral (R)-[(11)C]PK11195 uptake and glutamate release in a rat model of traumatic brain injury: a longitudinal pilot study. J. Neuroinflammation 8:67. doi: 10.1186/1742-2094-8-67
Glannon, W. (2014). Neuromodulation, agency and autonomy. Brain Topogr. 27, 46–54. doi: 10.1007/s10548-012-0269-3
Greig, N. H., Tweedie, D., Rachmany, L., Li, Y., Rubovitch, V., Schreiber, S., et al. (2014). Incretin mimetics as pharmacologic tools to elucidate and as a new drug strategy to treat traumatic brain injury. Alzheimers Dement. 10, S62–S75. doi: 10.1016/j.jalz.2013.12.011
Greve, M. W., and Zink, B. J. (2009). Pathophysiology of traumatic brain injury. Mt. Sinai J. Med. 76, 97–104. doi: 10.1002/msj.20104
Guerriero, R. M., Giza, C. C., and Rotenberg, A. (2015). Glutamate and GABA imbalance following traumatic brain injury. Curr. Neurol. Neurosci. Rep. 15:27. doi: 10.1007/s11910-015-0545-1
Gülsen, I., Ak, H., Çölçimen, N., Alp, H. H., Akyol, M. E., Demir, I., et al. (2015). Neuroprotective effects of thymoquinone on the hippocampus in a rat model of traumatic brain injury. World Neurosurg. doi: 10.1016/j.wneu.2015.09.052 [Epub ahead of print].
Hamm, R. J., Lyeth, B. G., Jenkins, L. W., O’Dell, D. M., and Pike, B. R. (1993). Selective cognitive impairment following traumatic brain injury in rats. Behav. Brain Res. 59, 169–173. doi: 10.1016/0166-4328(93)90164-l
Hanell, A., Greer, J. E., and Jacobs, K. M. (2015). Increased network excitability due to altered synaptic inputs to neocortical layer v intact and axotomized pyramidal neurons after mild traumatic brain injury. J. Neurotrauma 32, 1590–1598. doi: 10.1089/neu.2014.3592
Hao, S., Tang, B., Wu, Z., Ure, K., Sun, Y., Tao, H., et al. (2015). Forniceal deep brain stimulation rescues hippocampal memory in Rett syndrome mice. Nature 526, 430–434. doi: 10.1038/nature15694
Harris, J. L., Yeh, H. W., Choi, I. Y., Lee, P., Berman, N. E., Swerdlow, R. H., et al. (2012). Altered neurochemical profile after traumatic brain injury: (1)H-MRS biomarkers of pathological mechanisms. J. Cereb. Blood Flow Metab. 32, 2122–2134. doi: 10.1038/jcbfm.2012.114
Henry, L. C., Tremblay, S., Boulanger, Y., Ellemberg, D., and Lassonde, M. (2010). Neurometabolic changes in the acute phase after sports concussions correlate with symptom severity. J. Neurotrauma 27, 65–76. doi: 10.1089/neu.2009.0962
Hetherington, H. P., Hamid, H., Kulas, J., Ling, G., Bandak, F., de Lanerolle, N. C., et al. (2014). MRSI of the medial temporal lobe at 7 T in explosive blast mild traumatic brain injury. Magn. Reson. Med. 71, 1358–1367. doi: 10.1002/mrm.24814
Hicdonmez, T., Kanter, M., Tiryaki, M., Parsak, T., and Cobanoglu, S. (2006). Neuroprotective effects of N-acetylcysteine on experimental closed head trauma in rats. Neurochem. Res. 31, 473–481. doi: 10.1007/s11064-006-9040-z
Hoffer, M. E., Balaban, C., Slade, M. D., Tsao, J. W., and Hoffer, B. (2013). Amelioration of acute sequelae of blast induced mild traumatic brain injury by N-acetyl cysteine: a double-blind, placebo controlled study. PLoS One 8:e54163. doi: 10.1371/journal.pone.0054163
Hunt, R. F., Scheff, S. W., and Smith, B. N. (2011). Synaptic reorganization of inhibitory hilar interneuron circuitry after traumatic brain injury in mice. J. Neurosci. 31, 6880–6890. doi: 10.1523/JNEUROSCI.0032-11.2011
Issar, N. M., Hedera, P., Phibbs, F. T., Konrad, P. E., and Neimat, J. S. (2013). Treating post-traumatic tremor with deep brain stimulation: report of five cases. Parkinsonism Relat. Disord. 19, 1100–1105. doi: 10.1016/j.parkreldis.2013.07.022
Jia, F., Yin, Y. H., Gao, G. Y., Wang, Y., Cen, L., and Jiang, J. Y. (2014). MMP-9 inhibitor SB-3CT attenuates behavioral impairments and hippocampal loss after traumatic brain injury in rat. J. Neurotrauma 31, 1225–1234. doi: 10.1089/neu.2013.3230
Johnson, B. N., Palmer, C. P., Bourgeois, E. B., Elkind, J. A., Putnam, B. J., and Cohen, A. S. (2014). Augmented inhibition from cannabinoid-sensitive interneurons diminishes CA1 output after traumatic brain injury. Front. Cell. Neurosci. 8:435. doi: 10.3389/fncel.2014.00435
Kang, W. H., Cao, W., Graudejus, O., Patel, T. P., Wagner, S., Meaney, D. F., et al. (2015). Alterations in hippocampal network activity after in vitro traumatic brain injury. J. Neurotrauma 32, 1011–1019. doi: 10.1089/neu.2014.3667
Kang, E. K., Kim, D. Y., and Paik, N. J. (2012). Transcranial direct current stimulation of the left prefrontal cortex improves attention in patients with traumatic brain injury: a pilot study. J. Rehabil. Med. 44, 346–350. doi: 10.2340/16501977-0947
Kastin, A. J., and Akerstrom, V. (2003). Entry of exendin-4 into brain is rapid but may be limited at high doses. Int. J. Obes. Relat. Metab. Disord. 27, 313–318. doi: 10.1038/sj.ijo.0802206
Kisely, S., Hall, K., Siskind, D., Frater, J., Olson, S., and Crompton, D. (2014). Deep brain stimulation for obsessive-compulsive disorder: a systematic review and meta-analysis. Psychol. Med. 44, 3533–3542. doi: 10.1017/s0033291714000981
Lawrence, D. W., Comper, P., Hutchison, M. G., and Sharma, B. (2015). The role of apolipoprotein E episilon (epsilon)-4 allele on outcome following traumatic brain injury: a systematic review. Brain Inj. 29, 1018–1031. doi: 10.3109/02699052.2015.1005131
Lee, D. J., Gurkoff, G. G., Izadi, A., Berman, R. F., Ekstrom, A. D., Muizelaar, J. P., et al. (2013). Medial septal nucleus theta frequency deep brain stimulation improves spatial working memory after traumatic brain injury. J. Neurotrauma 30, 131–139. doi: 10.1089/neu.2012.2646
Lee, D. J., Gurkoff, G. G., Izadi, A., Seidl, S. E., Echeverri, A., Melnik, M., et al. (2015). Septohippocampal neuromodulation improves cognition after traumatic brain injury. J. Neurotrauma 32, 1822–1832. doi: 10.1089/neu.2014.3744
Len, T. K., and Neary, J. P. (2011). Cerebrovascular pathophysiology following mild traumatic brain injury. Clin. Physiol. Funct. Imaging 31, 85–93. doi: 10.1111/j.1475-097X.2010.00990.x
Leśniak, M., Polanowska, K., Seniów, J., and Czlonkowska, A. (2014). Effects of repeated anodal tDCS coupled with cognitive training for patients with severe traumatic brain injury: a pilot randomized controlled trial. J. Head Trauma Rehabil. 29, E20–E29. doi: 10.1097/HTR.0b013e318292a4c2
Levin, H. S., Mattis, S., Ruff, R. M., Eisenberg, H. M., Marshall, L. F., Tabaddor, K., et al. (1987). Neurobehavioral outcome following minor head injury: a three-center study. J. Neurosurg. 66, 234–243. doi: 10.3171/jns.1987.66.2.0234
Li, Y., Chigurupati, S., Holloway, H. W., Mughal, M., Tweedie, D., Bruestle, D. A., et al. (2012). Exendin-4 ameliorates motor neuron degeneration in cellular and animal models of amyotrophic lateral sclerosis. PLoS One 7:e32008. doi: 10.1371/journal.pone.0032008
Li, H., Lee, C. H., Yoo, K. Y., Choi, J. H., Park, O. K., Yan, B. C., et al. (2010). Chronic treatment of exendin-4 affects cell proliferation and neuroblast differentiation in the adult mouse hippocampal dentate gyrus. Neurosci. Lett. 486, 38–42. doi: 10.1016/j.neulet.2010.09.040
Li, Y., Perry, T., Kindy, M. S., Harvey, B. K., Tweedie, D., Holloway, H. W., et al. (2009). GLP-1 receptor stimulation preserves primary cortical and dopaminergic neurons in cellular and rodent models of stroke and Parkinsonism. Proc. Natl. Acad. Sci. U S A 106, 1285–1290. doi: 10.1073/pnas.0806720106
Li, S., Zaninotto, A. L., Neville, I. S., Paiva, W. S., Nunn, D., and Fregni, F. (2015). Clinical utility of brain stimulation modalities following traumatic brain injury: current evidence. Neuropsychiatr. Dis. Treat. 11, 1573–1586. doi: 10.2147/NDT.s65816
List, J., Ott, S., Bukowski, M., Lindenberg, R., and Floel, A. (2015). Cognitive function and brain structure after recurrent mild traumatic brain injuries in young-to-middle-aged adults. Front. Hum. Neurosci. 9:228. doi: 10.3389/fnhum.2015.00228
Lowenstein, D. H., Thomas, M. J., Smith, D. H., and McIntosh, T. K. (1992). Selective vulnerability of dentate hilar neurons following traumatic brain injury: a potential mechanistic link between head trauma and disorders of the hippocampus. J. Neurosci. 12, 4846–4853.
Lozano, A. M., Giacobbe, P., Hamani, C., Rizvi, S. J., Kennedy, S. H., Kolivakis, T. T., et al. (2012). A multicenter pilot study of subcallosal cingulate area deep brain stimulation for treatment-resistant depression. J. Neurosurg. 116, 315–322. doi: 10.3171/2011.10.JNS102122
Maas, A. I., Stocchetti, N., and Bullock, R. (2008). Moderate and severe traumatic brain injury in adults. Lancet Neurol. 7, 728–741. doi: 10.1016/s1474-4422(08)70164-9
Maller, J. J., Thomson, R. H., Lewis, P. M., Rose, S. E., Pannek, K., and Fitzgerald, P. B. (2010). Traumatic brain injury, major depression, and diffusion tensor imaging: making connections. Brain Res. Rev. 64, 213–240. doi: 10.1016/j.brainresrev.2010.04.003
Mao, Q., Jia, F., Zhang, X. H., Qiu, Y. M., Ge, J. W., Bao, W. J., et al. (2010). The up-regulation of voltage-gated sodium channel Nav1.6 expression following fluid percussion traumatic brain injury in rats. Neurosurgery 66, 1134–1139; discussion 1139. doi: 10.1227/01.neu.0000369612.31946.a2
Martin, B., Golden, E., Carlson, O. D., Pistell, P., Zhou, J., Kim, W., et al. (2009). Exendin-4 improves glycemic control, ameliorates brain and pancreatic pathologies and extends survival in a mouse model of Huntington’s disease. Diabetes 58, 318–328. doi: 10.2337/db08-0799
Merlo, L., Cimino, F., Angileri, F. F., La Torre, D., Conti, A., Cardali, S. M., et al. (2014). Alteration in synaptic junction proteins following traumatic brain injury. J. Neurotrauma 31, 1375–1385. doi: 10.1089/neu.2014.3385
Miller, J. P., Sweet, J. A., Bailey, C. M., Munyon, C. N., Luders, H. O., and Fastenau, P. S. (2015). Visual-spatial memory may be enhanced with theta burst deep brain stimulation of the fornix: a preliminary investigation with four cases. Brain 138, 1833–1842. doi: 10.1093/brain/awv095
Miller, D. M., Wang, J. A., Buchanan, A. K., and Hall, E. D. (2014). Temporal and spatial dynamics of nrf2-antioxidant response elements mediated gene targets in cortex and hippocampus after controlled cortical impact traumatic brain injury in mice. J. Neurotrauma 31, 1194–1201. doi: 10.1089/neu.2013.3218
Munyon, C., Eakin, K. C., Sweet, J. A., and Miller, J. P. (2014). Decreased bursting and novel object-specific cell firing in the hippocampus after mild traumatic brain injury. Brain Res. 1582, 220–226. doi: 10.1016/j.brainres.2014.07.036
Nakao, K., Ikegaya, Y., Yamada, M. K., Nishiyama, N., and Matsuki, N. (2003). Fimbrial control of bidirectional synaptic plasticity of medial perforant path-dentate transmission. Synapse 47, 163–168. doi: 10.1002/syn.10168
Naziroğlu, M., Senol, N., Ghazizadeh, V., and Yürüker, V. (2014). Neuroprotection induced by N-acetylcysteine and selenium against traumatic brain injury-induced apoptosis and calcium entry in hippocampus of rat. Cell. Mol. Neurobiol. 34, 895–903. doi: 10.1007/s10571-014-0069-2
Okun, M. S. (2012). Deep-brain stimulation for Parkinson’s disease. N. Engl. J. Med. 367, 1529–1538. doi: 10.1056/NEJMct1208070
Ortiz, F., and Gutiérrez, R. (2015). Entorhinal cortex lesions result in adenosine-sensitive high frequency oscillations in the hippocampus. Exp. Neurol. 271, 319–328. doi: 10.1016/j.expneurol.2015.06.009
Page, S. J., Cunningham, D. A., Plow, E., and Blazak, B. (2015). It takes two: noninvasive brain stimulation combined with neurorehabilitation. Arch. Phys. Med. Rehabil. 96, S89–S93. doi: 10.1016/j.apmr.2014.09.019
Panenka, W. J., Lange, R. T., Bouix, S., Shewchuk, J. R., Heran, M. K., Brubacher, J. R., et al. (2015). Neuropsychological outcome and diffusion tensor imaging in complicated versus uncomplicated mild traumatic brain injury. PLoS One 10:e0122746. doi: 10.1371/journal.pone.0122746
Park, E., Bell, J. D., and Baker, A. J. (2008). Traumatic brain injury: can the consequences be stopped? CMAJ 178, 1163–1170. doi: 10.1503/cmaj.080282
Paterno, R., Metheny, H., Xiong, G., Elkind, J., and Cohen, A. S. (2015). Mild traumatic brain injury decreases broadband power in area CA1. J. Neurotrauma doi: 10.1089/neu.2015.4107 [Epub ahead of print].
Perry, T., Lahiri, D. K., Chen, D., Zhou, J., Shaw, K. T., Egan, J. M., et al. (2002). A novel neurotrophic property of glucagon-like peptide 1: a promoter of nerve growth factor-mediated differentiation in PC12 cells. J. Pharmacol. Exp. Ther. 300, 958–966. doi: 10.1124/jpet.300.3.958
Polanowska, K., and Seniów, J. (2010). [Influence of transcranial direct current stimulation on cognitive functioning of patients with brain injury]. Neurol. Neurochir. Pol. 44, 580–590. doi: 10.1016/S0028-3843(14)60156-0
Rachmany, L., Tweedie, D., Li, Y., Rubovitch, V., Holloway, H. W., Miller, J., et al. (2013). Exendin-4 induced glucagon-like peptide-1 receptor activation reverses behavioral impairments of mild traumatic brain injury in mice. Age (Dordr.) 35, 1621–1636. doi: 10.1007/s11357-012-9464-0
Raghupathi, R. (2004). Cell death mechanisms following traumatic brain injury. Brain Pathol. 14, 215–222. doi: 10.1111/j.1750-3639.2004.tb00056.x
Reeves, T. M., Lyeth, B. G., Phillips, L. L., Hamm, R. J., and Povlishock, J. T. (1997). The effects of traumatic brain injury on inhibition in the hippocampus and dentate gyrus. Brain Res. 757, 119–132. doi: 10.1016/s0006-8993(97)00170-4
Royo, N. C., LeBold, D., Magge, S. N., Chen, I., Hauspurg, A., Cohen, A. S., et al. (2007). Neurotrophin-mediated neuroprotection of hippocampal neurons following traumatic brain injury is not associated with acute recovery of hippocampal function. Neuroscience 148, 359–370. doi: 10.1016/j.neuroscience.2007.06.014
Salcedo, I., Tweedie, D., Li, Y., and Greig, N. H. (2012). Neuroprotective and neurotrophic actions of glucagon-like peptide-1: an emerging opportunity to treat neurodegenerative and cerebrovascular disorders. Br. J. Pharmacol. 166, 1586–1599. doi: 10.1111/j.1476-5381.2012.01971.x
Samal, B. B., Waites, C. K., Almeida-Suhett, C., Li, Z., Marini, A. M., Samal, N. R., et al. (2015). Acute response of the hippocampal transcriptome following mild traumatic brain injury after controlled cortical impact in the rat. J. Mol. Neurosci. 57, 282–303. doi: 10.1007/s12031-015-0626-2
Sankar, T., Lipsman, N., and Lozano, A. M. (2014). Deep brain stimulation for disorders of memory and cognition. Neurotherapeutics 11, 527–534. doi: 10.1007/s13311-014-0275-0
Shin, S. S., Dixon, C. E., Okonkwo, D. O., and Richardson, R. M. (2014). Neurostimulation for traumatic brain injury. J. Neurosurg. 121, 1219–1231. doi: 10.3171/2014.7.JNS131826
Shirvalkar, P. R., Rapp, P. R., and Shapiro, M. L. (2010). Bidirectional changes to hippocampal theta-gamma comodulation predict memory for recent spatial episodes. Proc. Natl. Acad. Sci. U S A 107, 7054–7059. doi: 10.1073/pnas.0911184107
Sick, T. J., Pérez-Pinzón, M. A., and Feng, Z. Z. (1998). Impaired expression of long-term potentiation in hippocampal slices 4 and 48 h following mild fluid-percussion brain injury in vivo. Brain Res. 785, 287–292. doi: 10.1016/s0006-8993(97)01418-2
Sönmez, A., Sayin, O., Gürgen, S. G., and Çalişir, M. (2015). Neuroprotective effects of MK-801 against traumatic brain injury in immature rats. Neurosci. Lett. 597, 137–142. doi: 10.1016/j.neulet.2015.05.001
Sours, C., George, E. O., Zhuo, J., Roys, S., and Gullapalli, R. P. (2015). Hyper-connectivity of the thalamus during early stages following mild traumatic brain injury. Brain Imaging Behav. 9, 550–563. doi: 10.1007/s11682-015-9424-2
Sun, D., Daniels, T. E., Rolfe, A., Waters, M., and Hamm, R. (2015). Inhibition of injury-induced cell proliferation in the dentate gyrus of the hippocampus impairs spontaneous cognitive recovery after traumatic brain injury. J. Neurotrauma 32, 495–505. doi: 10.1089/neu.2014.3545
Susman, M., DiRusso, S. M., Sullivan, T., Risucci, D., Nealon, P., Cuff, S., et al. (2002). Traumatic brain injury in the elderly: increased mortality and worse functional outcome at discharge despite lower injury severity. J. Trauma 53, 219–224. doi: 10.1097/00005373-200208000-00004
Suthana, N., and Fried, I. (2014). Deep brain stimulation for enhancement of learning and memory. Neuroimage 85, 996–1002. doi: 10.1016/j.neuroimage.2013.07.066
Sweet, J. A., Eakin, K. C., Munyon, C. N., and Miller, J. P. (2014). Improved learning and memory with theta-burst stimulation of the fornix in rat model of traumatic brain injury. Hippocampus 24, 1592–1600. doi: 10.1002/hipo.22338
Tabansky, I., Quinkert, A. W., Rahman, N., Muller, S. Z., Lofgren, J., Rudling, J., et al. (2014). Temporally-patterned deep brain stimulation in a mouse model of multiple traumatic brain injury. Behav. Brain Res. 273, 123–132. doi: 10.1016/j.bbr.2014.07.026
Terrio, H., Brenner, L. A., Ivins, B. J., Cho, J. M., Helmick, K., Schwab, K., et al. (2009). Traumatic brain injury screening: preliminary findings in a US Army Brigade Combat Team. J. Head Trauma Rehabil. 24, 14–23. doi: 10.1097/htr.0b013e31819581d8
Toth, Z., Hollrigel, G. S., Gorcs, T., and Soltesz, I. (1997). Instantaneous perturbation of dentate interneuronal networks by a pressure wave-transient delivered to the neocortex. J. Neurosci. 17, 8106–8117.
Tweedie, D., Rachmany, L., Rubovitch, V., Lehrmann, E., Zhang, Y., Becker, K. G., et al. (2013). Exendin-4, a glucagon-like peptide-1 receptor agonist prevents mTBI-induced changes in hippocampus gene expression and memory deficits in mice. Exp. Neurol. 239, 170–182. doi: 10.1016/j.expneurol.2012.10.001
Ulam, F., Shelton, C., Richards, L., Davis, L., Hunter, B., Fregni, F., et al. (2015). Cumulative effects of transcranial direct current stimulation on EEG oscillations and attention/working memory during subacute neurorehabilitation of traumatic brain injury. Clin. Neurophysiol. 126, 486–496. doi: 10.1016/j.clinph.2014.05.015
van Bregt, D. R., Thomas, T. C., Hinzman, J. M., Cao, T., Liu, M., Bing, G., et al. (2012). Substantia nigra vulnerability after a single moderate diffuse brain injury in the rat. Exp. Neurol. 234, 8–19. doi: 10.1016/j.expneurol.2011.12.003
van der Horn, H. J., Liemburg, E. J., Aleman, A., Spikman, J. M., and van der Naalt, J. (2016). Brain networks subserving emotion regulation and adaptation after mild traumatic brain injury. J. Neurotrauma 33, 1–9. doi: 10.1089/neu.2015.3905
Veeramuthu, V., Narayanan, V., Kuo, T. L., Delano-Wood, L., Chinna, K., Bondi, M. W., et al. (2015). Diffusion tensor imaging parameters in mild traumatic brain injury and its correlation with early neuropsychological impairment: a longitudinal study. J. Neurotrauma 32, 1497–1509. doi: 10.1089/neu.2014.3750
Wang, X., Gao, X., Michalski, S., Zhao, S., and Chen, J. (2015a). Traumatic brain injury severity affects neurogenesis in adult mouse hippocampus. J. Neurotrauma doi: 10.1089/neu.2015.4097 [Epub ahead of print].
Wang, W. X., Visavadiya, N. P., Pandya, J. D., Nelson, P. T., Sullivan, P. G., and Springer, J. E. (2015b). Mitochondria-associated microRNAs in rat hippocampus following traumatic brain injury. Exp. Neurol. 265, 84–93. doi: 10.1016/j.expneurol.2014.12.018
Winson, J. (1978). Loss of hippocampal theta rhythm results in spatial memory deficit in the rat. Science 201, 160–163. doi: 10.1126/science.663646
Witgen, B. M., Lifshitz, J., Smith, M. L., Schwarzbach, E., Liang, S. L., Grady, M. S., et al. (2005). Regional hippocampal alteration associated with cognitive deficit following experimental brain injury: a systems, network and cellular evaluation. Neuroscience 133, 1–15. doi: 10.1016/j.neuroscience.2005.01.052
Yan, H. Q., Shin, S. S., Ma, X., Li, Y., and Dixon, C. E. (2014). Differential effect of traumatic brain injury on the nuclear factor of activated T Cells C3 and C4 isoforms in the rat hippocampus. Brain Res. 1548, 63–72. doi: 10.1016/j.brainres.2013.12.028
Zhang, Z., Wang, H., Jin, Z., Cai, X., Gao, N., Cui, X., et al. (2015). Downregulation of survivin regulates adult hippocampal neurogenesis and apoptosis and inhibits spatial learning and memory following traumatic brain injury. Neuroscience 300, 219–228. doi: 10.1016/j.neuroscience.2015.05.025
Zhao, S., Yu, A., Wang, X., Gao, X., and Chen, J. (2015). Post-injury treatment of 7,8-dihydroxyflavone promotes neurogenesis in the hippocampus of the adult mouse. J. Neurotrauma doi: 10.1089/neu.2015.4036 [Epub ahead of print].
Zlotnik, A., Sinelnikov, I., Gruenbaum, B. F., Gruenbaum, S. E., Dubilet, M., Dubilet, E., et al. (2012). Effect of glutamate and blood glutamate scavengers oxaloacetate and pyruvate on neurological outcome and pathohistology of the hippocampus after traumatic brain injury in rats. Anesthesiology 116, 73–83. doi: 10.1097/aln.0b013e31823d7731
Keywords: hippocampus, mild traumatic brain injury, neurophysiology, neuromodulation, cognitive dysfunction, drug repositioning
Citation: Girgis F, Pace J, Sweet J and Miller JP (2016) Hippocampal Neurophysiologic Changes after Mild Traumatic Brain Injury and Potential Neuromodulation Treatment Approaches. Front. Syst. Neurosci. 10:8. doi: 10.3389/fnsys.2016.00008
Received: 18 November 2015; Accepted: 25 January 2016;
Published: 09 February 2016.
Edited by:
Akiva Cohen, University of Pennsylvania & Research Institute of Children’s Hospital of Philadelphia, USACopyright © 2016 Girgis, Pace, Sweet and Miller. This is an open-access article distributed under the terms of the Creative Commons Attribution License (CC BY). The use, distribution and reproduction in other forums is permitted, provided the original author(s) or licensor are credited and that the original publication in this journal is cited, in accordance with accepted academic practice. No use, distribution or reproduction is permitted which does not comply with these terms.
*Correspondence: Jonathan P. Miller, am9uYXRoYW4ubWlsbGVyQHVoaG9zcGl0YWxzLm9yZw==