- 1 Departamento de Biologia Experimental, Faculdade de Medicina, Universidade do Porto, Porto, Portugal
- 2 Instituto de Biologia Molecular e Celular, Universidade do Porto, Porto, Portugal
- 3 Department of Neurobiology, Duke University Medical Center, Durham, NC, USA
- 4 Center for Neuroengineering, Duke University Medical Center, Durham, NC, USA
- 5 Department of Psychology and Neurosciences, Duke University Medical Center, Durham, NC, USA
- 6 Department of Biomedical Engineering, Duke University Medical Center, Durham, NC, USA
- 7 Edmond and Lily Safra International Institute of Neuroscience of Natal, Natal, Brazil
The insular cortex (IC) contains the primary sensory cortex for oral chemosensation including gustation, and its integrity is required for appropriate control of feeding behavior. However, it remains unknown whether the role of this brain area in food selection relies on the presence of peripheral taste input. Using multielectrode recordings, we found that the responses of populations of neurons in the IC of freely licking, sweet-blind Trpm5−/− mice are modulated by the rewarding postingestive effects of sucrose. FOS immunoreactivity analyses revealed that these responses are restricted to the dorsal insula. Furthermore, bilateral lesions in this area abolished taste-independent preferences for sucrose that can be conditioned in these Trpm5−/− animals while preserving their ability to detect sucrose. Overall, these findings demonstrate that, even in the absence of peripheral taste input, IC regulates food choices based on postingestive signals.
Introduction
The insula contains the primary gustatory cortex (GC), which encodes the oral chemosensory properties of food (Yamamoto et al., 1980; Cechetto and Saper, 1987; Ogawa et al., 1992; Hanamori et al., 1998b; Scott and Plata-Salaman, 1999; Katz et al., 2002; Rolls, 2006; Stapleton et al., 2006; Accolla et al., 2007). Furthermore, the insular cortex (IC), and particularly the GC, has been shown to participate in the regulation of feeding (Balleine and Dickinson, 2000; Cubero and Puerto, 2000; Stoeckel et al., 2008; Wagner et al., 2008). In rats, bilateral lesions of the GC reduce their ability to adequately modulate the incentive value of food outcomes, an effect that has been attributed to deficits in taste memory (Balleine and Dickinson, 2000). On the other hand, electrical stimulation of the IC induces robust flavor preferences, possibly due to modulation of the orosensory insular representation of that flavor (Cubero and Puerto, 2000). Thus, the involvement of the insula in the regulation of feeding has primarily been attributed to its role in processing oral chemosensory information.
However, a more integrative role for the insula in feeding is suggested by the fact that, in humans (Small et al., 2001), as well as in rats (de Araujo et al., 2006), insular neuronal responses to food are inhibited by postingestive satiation. In addition, the effects of electrical stimulation of the insula in eliciting flavor preferences has also been attributed to the “imitation” of neural patterns evoked by reinforcing visceral information (Cubero and Puerto, 2000).
The transient receptor potential M5 (TRPM5) channel (Perez et al., 2002) is required for peripheral transduction of sweet, bitter, and umami tastants (Zhang et al., 2003). Mice lacking functional TRPM5 channels (Trpm5−/−) have absent (Zhang et al., 2003) or vastly diminished (Damak et al., 2006) peripheral neural responses to sweet tastants and, in contrast to wild-type mice, do not show a preference for sweet tasting solutions in behavioral paradigms that are dependent on orosensory responses (Zhang et al., 2003; de Araujo et al., 2008). We and others have shown that, in Trpm5−/− animals, sucrose or glucose can be used to condition the development of spout preferences that are independent of orosensory input (de Araujo et al., 2008; Ren et al., 2010). Furthermore, in unconditioned Trpm5−/− mice, sucrose evoked dopamine release in the ventral striatum (de Araujo et al., 2008). These behavioral and neurochemical responses, considered as a measure of food reward, were shown to depend on processes driven by caloric postingestive feedback, since they were absent when sucralose, a non-caloric sweetener, was used in place of sucrose (de Araujo et al., 2008). For these reasons, Trpm5−/− mice are an ideal preparation to investigate a taste-independent role of the insula in the regulation of appetitive feeding. Here, we investigated the neural representation of the postingestive effects of sucrose in the dorsal IC of Trpm5−/− sweet-blind mice, and determined whether integrity of the dorsal IC is necessary for expression of appropriate food selection behaviors when no taste input is present.
Materials and Methods
Animals
A total of 33 male mice were used. At the time of the experiments, animals were 3–6 months old. They were all homozygous for a partial deletion of the Trpm5 gene (Trpm5−/−; Zhang et al., 2003) on a C57BL/6 background, and were bred from mice generously donated by C. S. Zuker (UCSD, San Diego, CA, USA). Genotype was confirmed by PCR amplification of the Trpm5 gene. Ten Trpm5−/− mice were implanted with microelectrode arrays for neural recordings. Eight others were used for FOS immunoreactivity. Fifteen animals were used in lesion experiments of the IC (eight with IC lesions and seven with sham operations). All procedures were approved by the Duke University Institutional Animal Care and Use Committee.
Stimuli
Sucrose solutions (0.8M; Sigma-Aldrich, USA) were prepared daily at room temperature in deionized water. Deionized water was also used as a baseline stimulus. Whenever the terms “sucrose” or “water” are used, we imply 0.8 M sucrose solutions or deionized water, respectively.
Behavioral Experiments
The behavioral component of all experiments involving licking was conducted in mouse-behavior chambers enclosed in a ventilated and sound attenuating cubicle (Med Associates Inc., St. Albans, VT, USA), as described previously (de Araujo et al., 2008). All experiments were conducted with naïve animals under a 20- to 22-h-long food and water deprivation schedule.
Conditioning to postingestive effects
A conditioning protocol that allows Trpm5−/− mice to manifest tastant-independent preferences for sucrose was adapted from previous experiments (de Araujo et al., 2008). This protocol assesses the ability of sweet-blind animals to develop a preference for drinking from a sipper that is located in a position of the behavioral cage associated with the availability of 0.8 M sucrose. Briefly (also see Table 1), after side-preference was determined for each animal in preliminary two-bottle tests where both sippers contained water, a pre-conditioning two-bottle sucrose vs. water preference test was conducted for 10 min. Thereafter, animals were exposed to a conditioning protocol, where 30 min ad libitum access to either water or sucrose was alternated for six consecutive days and, finally, to a post-conditioning two-bottle sucrose vs. water preference test conducted analogously to the pre-conditioning test. In all sessions, water sippers were located on the original bias side and sucrose sippers on the opposite side.
Two-bottle preference tests
In IC-lesioned and sham-operated animals, conditioned as described above, further sucrose vs. water two-bottle choice tests (10 min long) were conducted to verify taste-dependent preferences. To account for the effect of side-biases, mice were tested in each condition for four consecutive days with daily inversion of sucrose and water bottle positions (de Araujo et al., 2008), such that any consistent preference would depend on sensory factors, rather than a side-bias (de Araujo et al., 2008).
Preference measures
Two-bottle preference tests were analyzed by calculating the preference ratios (P) as P(Sipper 1) = n(Sipper 1)/[n(Sipper 1) + n(Sipper 2)] where n(.) denotes the total number of licks for a given stimulus during a session. Significance tests were based on one sample t-tests against 0.5, which is the reference value meaning indifference with respect to either sipper.
Water maze behavioral testing
In IC-lesioned and sham-operated animals, behavioral testing of spatial orientation was conducted in a Morris water maze (MWM) task, as described previously (Kee et al., 2007). Briefly, on each of six consecutive training days, mice received eight training trials divided in two blocks of four. At each trial they were placed in the water facing the wall in one of four possible different start locations (randomly chosen without substitution) and left to swim freely until they found the platform or 60 s had passed. Time to reach the platform was recorded in each trial and averaged across all trials for each animal in each day. One day after completion of training (day 7), spatial memory was tested in a probe trial where the platform was removed from the pool. The time spent searching in the correct quadrant of the maze (where the platform had been during training) was averaged across all animals and was compared to 15 s (one sample t-test), the time animals would be expected to swim in that quadrant if they were searching randomly.
FOS Protein Immunohistochemistry
Eight Trpm5−/− mice were habituated to drink water from a single sipper in daily 30 min sessions. Once stable licking rates were obtained, animals were exposed to either water (four animals) or 0.8 M sucrose (four animals) in a single 30 min long session. To avoid unspecific effects associated with licking and/or different volumes ingested, consumption of sucrose was yoked to that of water. Two hours after the start of behavioral sessions, animals were deeply anesthetized with 100 mg/kg pentobarbital and perfused through the left ventricle with a saline flush (100 mL) followed by 4% paraformaldehyde in phosphate buffered saline (PBS; pH, 7.4; 500 mL). Brains were post-fixed in the same fixative for 2 h, and then transferred to 30% sucrose with 0.02% sodium azide in PBS.
The fixed brains were then analyzed by a different experimenter that was blind to the treatment conditions. Free-floating serial 40 μm thick coronal sections of these brains were cut with a freezing-microtome and alternate sections were used for either FOS immunohistochemistry (Contreras et al., 2007; Kee et al., 2007) or thionin staining. Sections for immunohistochemistry were incubated in PBS with 1% H2O2 for 20 min, rinsed in PBS with 0.3% Triton-X100 (PBS–T), and then transferred to 10% normal swine serum (NSS) in PBS–T blocking solution for 2 h. Sections were then incubated in the primary antibody solution: rabbit anti FOS polyclonal antibody (Calbiochem, CA, USA) 1:10000 in PBS–T with 2% NSS. After 3 days at 4°C, sections were rinsed with PBS–T, incubated in 1:200 biotinylated swine anti-rabbit antibody for 1 h, rinsed with PBS–T, incubated for 1 h in 1:200 Vectastain ABC Elite kit (Vector Laboratories, CA, USA), rinsed with Tris buffer, and reacted for 3 min with a diaminobenzidine hydrochloride (DAB) solution containing 0.005% H2O2 in Tris buffer. After DAB staining, the sections were rinsed in PBS and serially mounted for counting of FOS-immunoreactive nuclei. IC boundaries were traced over the thionin-stained sections using the Paxinos and Franklin (2001) drawings as guidelines for regional boundaries, prior to counting of FOS-immunoreactive nuclei. Counting was performed manually using a microscope camera lucida and cells were counted bilaterally in each section.
Stereotaxic Surgery for IC Lesion, Sham Operations, and Implantation of Multielectrode Microarrays
Twenty-five Trpm5−/− mice were anesthetized using 5% halothane followed by intramuscular injection of xylazine (5 mg/kg) and ketamine (75 mg/kg). Supplemental doses were administered whenever necessary. Craniotomies measuring ∼1 mm2 were drilled at (AP = 0.9 mm, ML = ±3.1 mm) relative to bregma. In eight animals a cannula was slowly lowered to ∼2 mm below the brain surface to target the IC (Paxinos and Franklin, 2001) and 0.1 μL of a 20-mg/mL NMDA (N-methyl-D-aspartic acid; Sigma-Aldrich, USA) solution was manually injected into the IC (Corbit et al., 2002). After the injected solution had dispersed for 2 min, the needle was removed and the contralateral side of the brain was subjected to the same procedure. In seven animals, the same surgical methodologies were repeated but NMDA was not injected (sham operation). In 10 other animals, a multielectrode microarray (16 channels) was implanted into the same area (see Figure 5). The side of implantation was balanced between left and right hemispheres. After surgery, animals were given ∼1 week to recover before experimental testing was initiated.
Neuronal Recordings
Recordings of neural activity and timestamps of licking responses were performed simultaneously according to the procedure previously described (de Araujo et al., 2008). In two implanted animals, single-neuron activity could not be recorded. In the remaining eight mice, recordings were performed in each animal during a series of two or one-bottle tests, conducted in eight consecutive days (see Table 1). No assumption was made on the identity/stability of units recorded during different sessions.
Histological Confirmation of IC Lesion and Electrode Tips Placement
In all animals, we followed a previously described histological method to identify the location of lesions or microwire implantation (de Araujo et al., 2008). Brain slices from lesioned and sham-operated animals were examined with a light microscope, and compared. Placement and extent of lesion was assessed by location of cannula tract and areas of gliosis (Corbit et al., 2002). In two mice, the insula lesion was only correctly placed on one side, while in one implanted animal the microwires were implanted outside of the insula, in the striatum. Data from these animals were excluded from the analyses.
Electrophysiology Data Analysis
All neuronal data analyses were performed with custom software written in Matlab (R14, MathWorks, Inc.) or using the Nex software (Nex Technologies, TX, USA).
Peri-event histograms
Peri-event histograms (PEHs) were constructed for each unit-stimulus pair using the PEH function of the Nex software (Nex Technologies, TX, USA). PEHs show the conditional probability of observing a spike in a spike train at time t, on the condition that there is a reference event at time zero. The time axis was divided into bins and bin counts were normalized by the number of reference events. Analyses of responses in single cells were performed by constructing 1 s (±500 ms) “PEHs” with 5 ms bins, using licks to sucrose or water as defining events. Bin values were expressed as impulses per second (i.e., normalized bin counts × bin size in seconds), and a Gaussian filter (width = 3 bins) was applied to the resulting PEH. PEHs were constructed such that they would correspond to time intervals that occurred within licking clusters (see below).
Stimulus-specific single-neuron responses
Confidence limits for expected bin count in each PEH were calculated in the Nex software, using the assumption that the expected bin count has a Poisson distribution (Abeles, 1982). The count for each bin in a PEH was then compared to the respective confidence limits. A given unit was considered responsive to a stimulus when the values of at least three consecutive bins were outside the 95% confidence interval. Units that responded to only one of the two stimuli (water or sucrose) were considered to be “stimulus-specific,” while units that responded to neither or both stimuli were not considered “stimulus-specific.”
Mean population responses
For each neuron isolated during a given recording session, and for each licking cluster (see below) to sucrose, we first calculated the total number of spikes within this cluster normalized to cluster duration. These quantities were then averaged for each neuron across all clusters. Results were averaged across neurons, so that the resulting quantity was defined as the mean population firing rate response to sucrose, denoted FRSUC. For water, the quantity was analogously defined. Next, we defined the quantities
and
taken as a measure of the differential mean population response to water and sucrose for each pre- and post-conditioning session respectively. Finally, for each recorded animal, we defined the quantity
that represents the absolute value of the changes in the differential population responses to water and sucrose as a function of conditioning. These values were then correlated with a learning index (LI; see below for definition).
Learning index
The efficacy of the conditioning protocol was measured in each recorded animal by a quantity we denoted as the “LI.” This index provides a measure of the extent to which, after conditioning, Trpm5−/− animals increased their preference (P) for the sipper associated with sucrose. For each animal, LI was thus defined as LI = (PSucrose)POST − (PSucrose)PRE, where POST and PRE refer to post- and pre-conditioning test sessions respectively.
Determination of licking clusters
The analysis of licking patterns was performed as described previously (Davis and Smith, 1992; Gutierrez et al., 2006), i.e., pauses in licking longer than 0.5 s defined the end of a cluster. Cluster duration and average lick rates within clusters were used as controls for oromotor influences on neural activity.
Statistical Analyses
Unless otherwise stated, results from data analyses were expressed as mean ± SE of the mean. Analyses were performed with custom software written in Matlab (R14, MathWorks, Inc.) or with Prism (GraphPad, San Diego). Analyses were two-way or one-way ANOVAs (with Bonferroni Post hoc tests), paired or unpaired two-sample t-tests, or one sample t-tests. Bonferroni–Holm’s corrections for multiple comparisons (Holm, 1979) were performed when several independent tests were used for the same dataset. Correlation analyses were performed using Pearson’s product moment correlation and proportions were compared using Fisher’s exact tests. The Kolmogorov–Smirnov test was used to check the goodness of fit with the normal distribution for each measure of behavior or neuronal activity.
Results
In the Absence of Peripheral Taste Input, Neuronal Populations in IC Encode the Reinforcing Value of Sucrose Solutions
In 7 Trpm5−/− mice, a multielectrode microarray comprising 16 electrodes was implanted unilaterally into the dorsal IC, where the GC is found (Cechetto and Saper, 1987; Ogawa et al., 1990; Stapleton et al., 2006; Accolla et al., 2007). Electrophysiological recordings of IC neuronal ensembles (average 6.8 neurons per ensemble) were conducted in eight recording sessions per animal. These recordings were performed while the animals were exposed to a conditioning protocol where the expression of preferences for sucrose depends solely on taste-independent, postingestive effects (de Araujo et al., 2008; see Materials and Methods and Table 1 for protocol).
During conditioning sessions, Trpm5−/− mice consumed significantly more sucrose than water (Figure 1A), indicating that these animals were sensitive to the postingestive effects of sucrose. Sipper-preference patterns observed during the post-conditioning sucrose vs. water tests were also indicative that the sweet-blind mice were sensitive to the postingestive effects of sucrose. Indeed, the consumption of water was unchanged while sucrose consumption significantly increased in the post-conditioning relative to the pre-conditioning testing sessions (Figure 1B). Accordingly, sucrose preference was significantly higher in post-conditioning than in pre-conditioning testing sessions (Figure 1C).
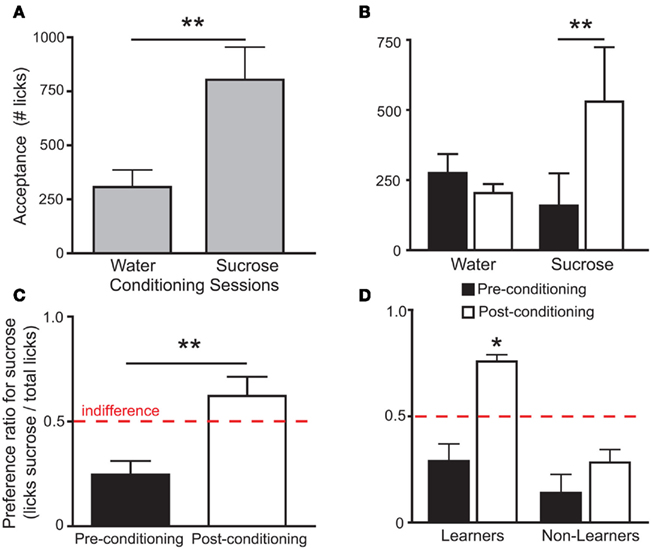
Figure 1. Behavioral responses to sucrose and water in Trpm5−/− mice before and after conditioning. In all figures, error bars indicate mean ± SEM. (A) In the Trpm5−/− animals where IC activity was recorded, average acceptance of sucrose during conditioning sessions (see Table 1) was higher than that of water (803 ± 152 and 308 ± 79 licks respectively; t6 = 5.1, **p < 0.003, paired two-sample t-test). (B) In the same animals, when acceptance of sucrose and water was compared in pre-conditioning and post-conditioning preference tests, a significant effect was found for session (pre- vs. post-conditioning, F1,12 = 4.9 p < 0.05) but not for tastant (water vs. sucrose, F1,12 = 0.5, p > 0.5). However, the two factors also interacted significantly (F1,12 = 10.7, p < 0.007; two-way repeated measures ANOVA) and, while the consumption of sucrose increased from the pre-conditioning to the post-conditioning preference tests (159 ± 115 and 529 ± 194 licks, respectively; t = 3.9, **p < 0.01) the consumption of water was unchanged (276 ± 67 and 204 ± 32 licks, respectively; t = 0.8, p > 0.05, Post hoc Bonferroni). (C) In accordance with the acceptance data, sucrose preference increased after conditioning (0.25 ± 0.06 to 0.62 ± 0.09; t6 = 5.3 **p < 0.002, paired two-sample t-test). Red dashed line corresponds to 0.5 indifference level. While sucrose preference was significantly lower from the indifference ratio of 0.5 in the pre-conditioning test (t6 = 3.9, p < 0.02), it was not in the post-conditioning test (t6 = 1.3, p > 0.2; one sample t-tests vs. 0.5 with Bonferroni–Holm’s correction for multiple comparisons). (D) In Learners (see text) preference for the sucrose sipper was not significantly different from 0.5 in the pre-conditioning session (preference ratio = 0.29 ± 0.08; t4 = 2.6, p > 0.05), but was significantly higher than 0.5 in the post-conditioning test session (0.76 ± 0.03; t4 = 5.3, *p < 0.05). In Non-Learners, preference in both pre and post-conditioning was low (0.14 ± 0.09 and 0.28 ± 0.05) and not significantly different from 0.5 (t1 = 4.1, p > 0.1 and t1 = 3.6, p > 0.1, respectively; one sample t-tests vs. 0.5 with Bonferroni–Holm’s correction for multiple comparisons – note that only two animals were Non-Learners which hinders statistical significance).
To assess the involvement of single IC neurons in the development of preferences for sucrose, we calculated in pre- and post-conditioning sessions the proportion of stimulus-specific neurons, i.e., neurons that responded selectively when the animal was licking for sucrose or for water (see Figure 2A and Materials and Methods). While the proportion of such neurons increased in the post-conditioning (23 out of 59 neurons, ∼39%) relative to the pre-conditioning sessions (14 out of 61 neurons, ∼23%), this difference was only borderline significant (p > 0.07; Fisher’s exact test). Nevertheless, we hypothesized that the variable individual propensities to sense the postingestive effects of sucrose were mirrored by the corresponding IC neural activity levels. Consequently, animals were divided according to their post-conditioning preference ratio for sucrose. Five mice displayed a preference ratio higher than 0.5 and were defined as “Learners,” while the remaining mice were classified as “Non-Learners” (Figure 1D). The proportions of stimulus-specific neurons in pre- and post-conditioning sessions were then compared separately for each of the two groups. In Learners, the stimulus-specific neurons occurred with greater frequency in post- relative to pre-conditioning sessions; an effect that was not observed in Non-Learners (Figure 2B).
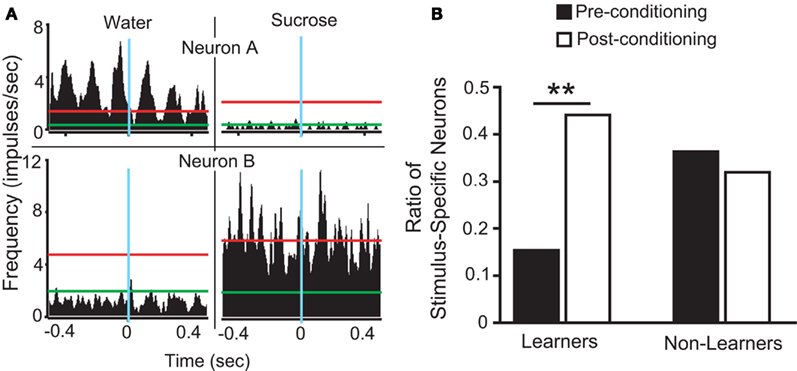
Figure 2. Stimulus selective IC responses to sucrose and water in Trpm5−/− mice before and after conditioning. (A) Each panel corresponds to a peri-event histogram centered on licks (blue line) for water (left panels) and sucrose (right panels). The green lines indicate baseline level of activity, and red lines define the 95% confidence interval, as defined in Section “Materials and Methods.” Neurons “A” and “B” are examples of stimulus-specific neurons, i.e., those neurons that responded only to water (neuron “A”) or to sucrose (neuron “B”). (B) The proportion of stimulus-specific neurons increased when post-conditioning sessions were compared to pre-conditioning sessions in Learners (15/34 vs. 6/39 neurons in post- vs. pre-conditioning respectively; **p < 0.01), but not in Non-Learners (8/25 vs. 8/22 in post- vs. pre-conditioning respectively; p > 0.7). This proportion did not differ significantly between the two groups during pre-conditioning sessions (p > 0.1; Fisher’s exact test).
Furthermore, for each animal, variation in the proportion of stimulus-specific neurons occurring in post-conditioning sessions (27, 33, 36, 36, 44, 50, and 75%) relative to pre-conditioning sessions (31, 9, 13, 44, 18, 17, and 33%) was calculated, showing that this difference (post-conditioning – pre-conditioning) was significantly different between Learners (29.9 ± 3.4%) and Non-Learners (−6.1 ± 2.6%; t5 = 6.1, p < 0.002, unpaired two-sample t-test).
To further analyze changes in IC responses from pre- to post-conditioning, for each of the preference-testing sessions we calculated the mean population firing rate while animals licked for either stimulus and subtracted the within-session neuronal population responses to water from those to sucrose. The absolute difference between the values thus obtained in pre- and post-conditioning sessions for each animal, denoted as “Δ Firing Rate” [ see Materials and Methods], represents the extent to which the relationship between sucrose and water population responses changed after conditioning. Each animal was also assigned a “LI,” [LI = (PSucrose)POST − (PSucrose)PRE, see Materials and Methods] that is a measure of the increase in sucrose preference during the post-conditioning relative to the pre-conditioning preference tests (see Figure 1C). As seen in Figure 3A, a significant positive correlation was found between ΔFR and the LI. Additionally, we verified that ΔFR differs significantly between Learners and Non-Learners (1.4 ± 0.2 and 0.09 ± 0.08 respectively; t5 = 3.7, p < 0.03, unpaired two-sample t-test).
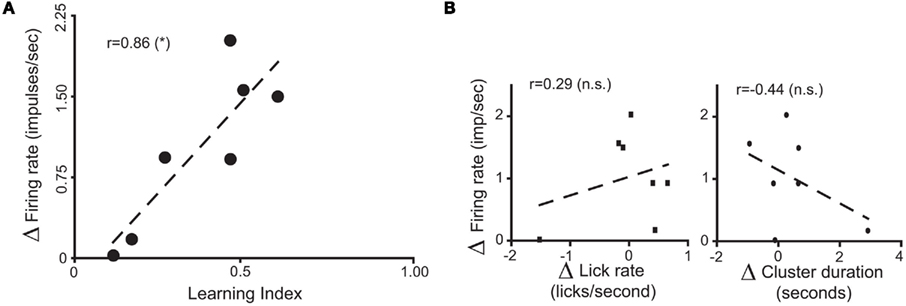
Figure 3. Population responses of IC neural ensembles before and after postingestive learning. (A) “Δ Firing Rate” or “Δ FR,” where represents the changes in the differential neural population responses to water and sucrose as a function of conditioning (POST and PRE refer to post- and pre-conditioning test sessions respectively). “learning index” (LI) was defined as LI = (PSucrose)POST − (PSucrose)PRE, and is a measure of the extent to which Trpm5−/− animals increased their preference (P) for the sipper associated with sucrose during conditioning (see Materials and Methods for further details). These values were calculated for each animal (each circle represents data from one animal) and a significant positive correlation (r = 0.86, *p < 0.02) was found between Δ FR and LI. (B) To show that the correlation between ΔFR and LI seen in (A) does not arise from learning-induced licking-related, oromotor behaviors, we tested if ΔFR would also correlate with changes in measures of oromotor activity before and after conditioning. To that point, after conditioning, neither Δ of lick rate (left panel) nor Δ of cluster duration (right panel) were correlated with ΔFR (r = 0.29 and r = −0.44 respectively; p > 0.3 for both), showing that they were not responsible for the learning-related changes in IC neural population responses seen in (A).
Concerning the relationship between ΔFR and LI, we were concerned that the variation in ΔFR could reflect learning-induced modifications in licking-related oromotor behaviors. To investigate this possibility, we tested if ΔFR would also correlate with changes in measures of oromotor activity, calculated analogously to the LI. Changes in lick frequency and licking cluster duration did not correlate with ΔFR (Figure 3B), thereby showing that these parameters cannot account for the relationship between ΔFR and the LI. In summary, these electrophysiological data showed that, in the absence of peripheral taste transduction events for sucrose, IC neuronal populations reflect the postingestive reinforcing value of sucrose solutions.
Postingestive Responses to Sucrose are Found Specifically in the Dorsal IC
The above electrophysiological results show postingestive-driven responses to sucrose in the dorsal insula, but do not provide information as to other IC locations where such responses could be found. To further investigate insular sites where postingestive reward may be represented, we measured patterns of FOS immunoreactivity in the IC of Trpm5−/− mice (Figure 4A) following the ingestion of water or 0.8 M sucrose solutions (n = 4 mice for each tastant). In these KO mice, the ingestion of the sucrose solution induced a significantly greater amount of FOS protein synthesis in IC neurons than did the ingestion of the same volume of water (Figure 4B). Importantly, this effect was restricted to the dorsal zones of the IC. No significant differences were found in either the ventral insula or the claustrum (Figure 4B). Because the consumption of sucrose was experimentally yoked to that of water (Figure 4C), these effects were not accounted for by unspecific visceral stimulation associated with differential volumes of ingestion (e.g., gastric distention, Cechetto and Saper, 1987) or oromotor-related factors (e.g., licking-dependent somatosensory stimulation, Stapleton et al., 2006). Thus, as ascertained by FOS measurements, the sensitivity of the IC to postingestive factors in Trpm5−/− mice is anatomically restricted to the dorsal zones.
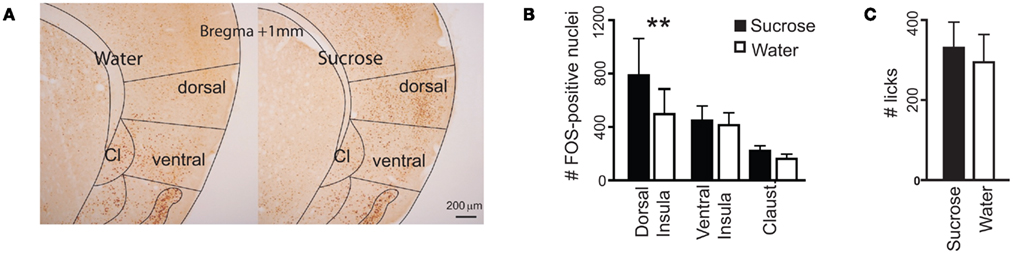
Figure 4. Consumption of sucrose-induced FOS protein expression in the dorsal insula of Trpm5−/− mice. (A) Coronal sections of the IC showing FOS expression. The number of FOS-positive nuclei, averaged across brain sections in each animal, was compared between animals following consumption of water or sucrose (examples in left and right panels respectively), in the dorsal and ventral insula and the claustrum (Cl), divided as shown. (B) The number of FOS-positive nuclei was not significantly different between the three brain areas (F2,9 = 2.3, p > 0.15), but was impacted by the consumption of sucrose (F1,9 = 5.9, p < 0.005) and the two factors interacted significantly (F2,9 = 5.9, p < 0.03, two-way repeated measures ANOVA). The effect of sucrose ingestion was significant in the dorsal insula (water – 497 ± 188, sucrose – 787 ± 276; t = 5, **p < 0.01) but not the ventral insula (water – 413 ± 93, sucrose – 447 ± 110; t = 0.6, p > 0.05) or the claustrum (water – 162 ± 35, sucrose – 221 ± 38; t = 1, p > 0.05; Post hoc Bonferroni). (C) Consumption of the two tastants was yoked (water: 295 ± 69 licks, sucrose: 331 ± 64; t6 = 0.4, p > 0.7, unpaired two-sample t-test).
The IC is Necessary for Trpm5−/− Mice to Express Side Preferences Conditioned by Sucrose
We next inquired whether, in the absence of peripheral taste signaling, the IC is required for the development of preferences for sucrose. Following either bilateral lesions targeting the dorsal IC, induced with 2 μg of N-methyl-D-aspartic acid, a glutamate receptor agonist (n = 6), or sham surgery (n = 7; Figure 5 and Materials and Methods), we analyzed the behavioral performance of Trpm5−/− mice in the conditioning protocol (Table 1). We found that bilateral lesions to IC produced no behavioral effects on either pre-conditioning preference tests (Figure 6A) or conditioning sessions (Figure 6B). However, during post-conditioning preference tests, sham-operated animals not only consumed significantly more sucrose than water (Figure 6C) but also developed a significant preference for sucrose (0.76 ± 0.07; t6 = 4, p < 0.02), whereas no such effects were observed in IC-lesioned animals (0.42 ± 0.1; t5 = 0.8, p > 0.4; one sample t-tests vs. 0.5 with Bonferroni–Holm’s correction for multiple comparisons; Figure 6D).
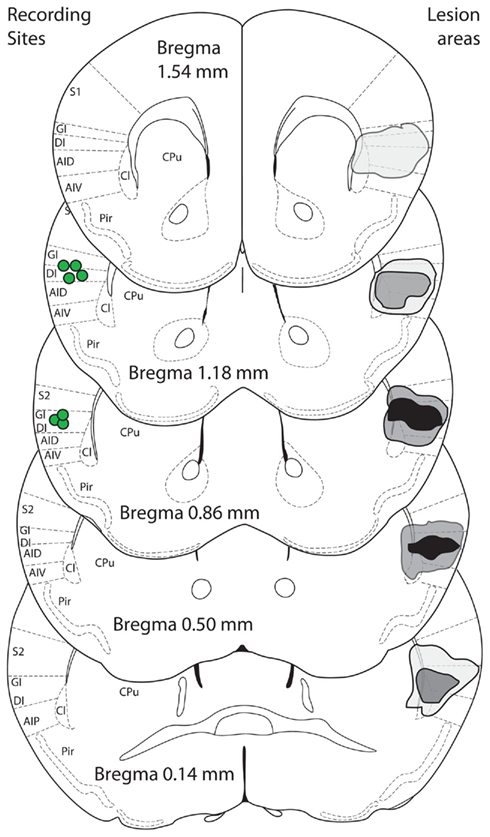
Figure 5. Bilateral IC lesions. Sucrose-induced changes of FOS expression were found in the same area of the insula (dorsal) from which neural activity was recorded. A bilateral lesion study was performed to target that same area. Schematic figures (modified from Paxinos and Franklin, 2001) show composite lesion areas on the right side of image, as assessed by microscopic examination for cannula tract and areas of gliosis. Black areas are where all animals were lesioned bilaterally, light gray when only one animal was lesioned unilaterally or bilaterally and dark gray for intermediate situations. The center of recording sites in animals with multielectrode arrays implanted is shown on the left side (green circles). S1/S2, somatosensory cortices; GI, granular insula; DI, dysgranular insula; AID/AIV/AIP, agranular insula; Cl, claustrum; Pir, piriform cortex; CPu, caudate/putamen.
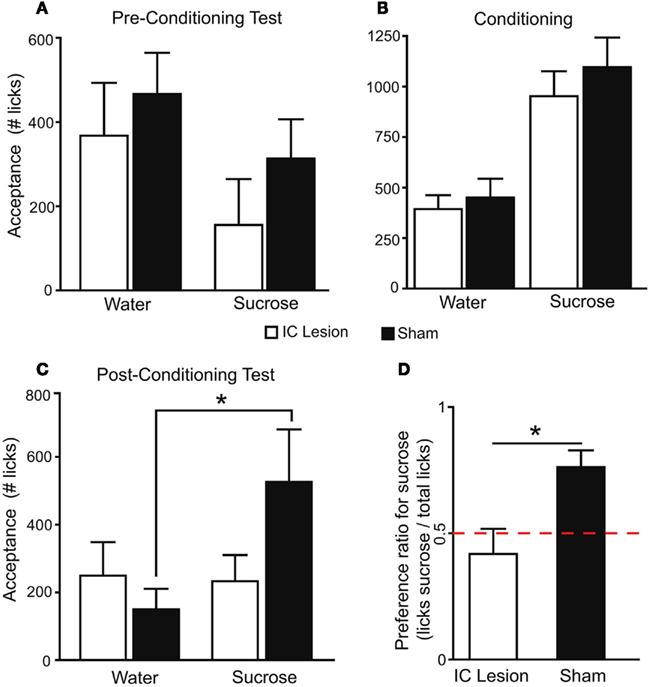
Figure 6. The IC is necessary for the expression of taste-independent preferences conditioned by postingestive reward.(A) In pre-conditioning sucrose vs. water two-bottle preference tests, since sucrose was presented on the side opposite to bias, mice consumed more water (lesion: 368 ± 125 licks; sham: 466 ± 98) than sucrose (lesion: 156 ± 109; sham: 313 ± 93; F1,11 = 11.3, p < 0.007). However, lesion status had no effect (F1,11 = 0.8, p > 0.37) and the two factors did not interact (F1,11 = 0.3, p > 0.59; two-way, repeated measures ANOVA). (B) During the conditioning protocol, animals consumed more sucrose (sham: 1096 ± 147; lesion: 952 ± 123) than water (451 ± 93 and 393 ± 69 licks respectively; F1,11 = 101.3, p < 0.0001) and no lesion-dependent effects were found (F1,11 = 0.4, p > 0.51 and F1,11 = 0.5, p > 0.48, respectively for lesion and interaction, two-way, repeated measures ANOVA). Thus, the two groups were equally able to detect the reinforcing postingestive effects of sucrose. (C) In post-conditioning tests, tastant had an effect on consumption (F1,11 = 6.6, p < 0.03) while lesion status did not (F1,11 = 0.5, p > 0.48). However, the two factors interacted significantly (F1,11 = 7.8, p < 0.02; two-way, repeated measures ANOVA). Data was then analyzed separately for each group. Sham-operated animals consumed more sucrose (526 ± 155 licks) than water (150 ± 61; t6 = 3.2, *p < 0.02), while in the lesion group consumption did not differ (233 ± 77 and 250 ± 99 respectively; t5 = 0.2, p > 0.8; paired two-sample t-tests). (D) Average preference for sucrose in the post-conditioning test differed significantly between groups (t11 = 3, *p < 0.02; unpaired two-sample t-test). Red dashed line corresponds to 0.5 indifference level.
These findings suggested a highly specific role for the dorsal IC in the development of taste-independent side preferences conditioned by sucrose. In fact, during conditioning, both sham-treated and lesioned animals exhibited more licking during sucrose than water sessions. It is important to note that, in these conditioning sessions, mice had access to a single bottle, delivering either water or sucrose in alternate days. Thus, they only needed to detect sucrose in order to consume it differentially relative to water. We therefore concluded that the dorsal IC is not necessary for detection of the postingestive value of sucrose. However, during the post-conditioning preference-testing session, both water and sucrose were present, and the animals had to choose between the two tastants. Since Trpm5−/− mice are taste-blind, in this last session sucrose and water were presented in the same positions as during the conditioning sessions (sucrose on the left and water on the right side or vice-versa), and animals had to use previously learned side cues to be able to demonstrate a preference for sucrose (de Araujo et al., 2008). During post-conditioning tests sham-treated mice, but not lesioned animals, displayed the expected higher preference for sucrose, suggesting that the dorsal IC was necessary for the association between sipper positions and the postingestive effects associated with the availability of sucrose. The importance of side cues for sham-treated animals to demonstrate a preference for sucrose was further confirmed in additional two-bottle sucrose vs. water preference tests, conducted across several days with alternation of bottle positions to eliminate side-bias. Under these conditions, preference for sucrose was not significant in either group (lesion: 0.57 ± 0.05, sham: 0.58 ± 0.06; respectively t5 = 1.3, and t6 = 1.2, p > 0.2 for both, one sample t-tests vs. 0.5) and did not differ between them (t11 = 0.1, p > 0.9; unpaired two-sample t-test). Thus, the preference for sucrose initially expressed by sham-operated animals was disrupted, confirming that the development of such preferences in Trpm5−/− mice is dependent on side associations while being independent of any particular orosensory cue (de Araujo et al., 2008).
An alternate explanation for the above results relates to possible differences in the duration of the experimental sessions. In fact, conditioning sessions were conducted for 30 min while preference sessions lasted only 10 min, raising the possibility that the differences in conditioning vs. preference sessions may result simply from differences in their duration. To test for this possibility, data for consumption during conditioning sessions was reanalyzed, considering only the first 10 min for each session. No significant differences were found between sham and IC-lesioned animals (Figure 7A). Further comparisons were performed considering each sucrose or water conditioning day separately (i.e., days 1, 3, and 5 for sucrose and 2, 4, and 6 for water), to verify if there were different trends in consumption across conditioning. Again, no significant differences were found between sham and IC-lesioned animals (Figures 7B,C), a finding that further supports a more fundamental effect of dorsal IC lesions during preference tests, as argued above.
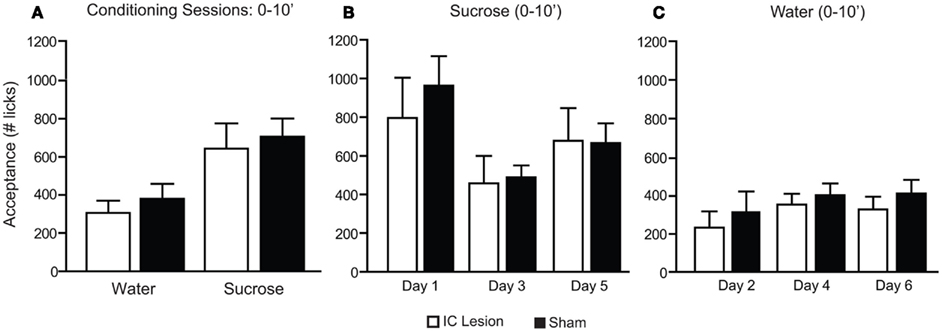
Figure 7. Consumption patterns of sucrose and water during the first 10 min of conditioning sessions in animals with bilateral IC or sham lesions. (A) During the initial 10 min (10′) of conditioning sessions, animals consumed more sucrose (sham: 706 ± 95; lesion: 644 ± 132) than water (379 ± 79 and 306 ± 65 licks respectively; F1,11 = 39.4, p < 0.0001) and no lesion-dependent effects were found (F1,11 = 0.3, p > 0.59 and F1,11 = 0.01, p > 0.91, respectively for lesion and interaction; two-way, repeated measures ANOVA). (B) When the initial 10′ of sucrose consumption was analyzed across sessions a significant effect was found for the comparison of conditioning day 1 (sham: 963 ± 152; lesion: 796 ± 208), day 3 (sham: 488 ± 62; lesion: 457 ± 144) and day 5 (sham: 666 ± 102; lesion: 678 ± 169; F2,11 = 7.2. p < 0.005) but no lesion-dependent effects were found (F1,11 = 0.2, p > 0.7 and F2,11 = 0.4, p > 0.68, respectively for lesion and interaction; two-way, repeated measures ANOVA). Reduced sucrose consumption across days could be potentially ascribed to learned satiety. (C) For water sessions a significant effect was also found for the comparison of conditioning day 2 (sham: 320 ± 108; lesion: 234 ± 85), day 4 (sham: 405 ± 62; lesion: 355 ± 57) and day 6 (sham: 414 ± 72; lesion: 330 ± 67; F2,11 = 5.6, p < 0.02) but no lesion-dependent effects were found (F1,11 = 0.5, p > 0.49 and F2,11 = 0.2, p > 0.83, respectively for lesion and interaction; two-way, repeated measures ANOVA).
From the above experiments it follows that IC lesions disrupt the development of side preferences conditioned by sucrose. To eliminate the possibility that this disruption was due to impairments in spatial cognition (Bermudez-Rattoni et al., 1991), we tested the same animals in a MWM protocol (Kee et al., 2007). Animals were trained in the MWM for 6 days, a period with comparable duration to that of the postingestive conditioning. On the sixth day, no differences were found between the two groups in time to reach a hidden platform (Figure 8A). Furthermore, on a probe test conducted on the seventh day, both groups retained information on the spatial location of the submerged platform (Figure 8B). Thus, we conclude that animals with IC lesion had conserved spatial orientation in the MWM, and that IC integrity, while not necessary for the detection of the postingestive properties of sucrose, is required for such factors to condition side preferences in a two-bottle test.
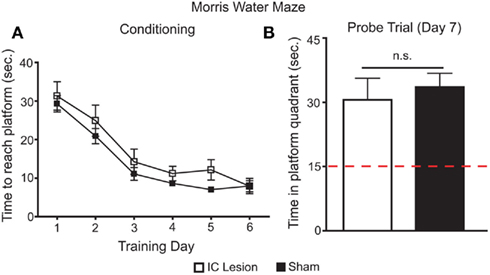
Figure 8. Effects of bilateral IC lesion on spatial orientation learning. (A) Sham-operated and IC-lesioned animals were trained, for 6 days, to find a hidden and submerged platform in a Morris water maze (MWM). During training, there was an overall main effect for lesioning (lesioned vs. control subjects, F1,55 = 5.2, p < 0.045), but both groups improved their performance across the six training days (F5,55 = 29.4, p < 0.0001) in a similar fashion (interaction – F5,55 = 0.3, p > 0.91; two-way repeated measures ANOVA) and reached equal levels of performance in the last day (lesion: 7.9 ± 1.4 s to reach platform; sham: 7.9 ± 2; t = 0.02, p > 0.05; Bonferroni Post hoc test). Thus, during training there were only small differences between sham and real lesioned animals. (B) On the seventh day, animals were tested for 60 s in a probe trial where the platform was removed from the maze. Here, both groups spent similar times searching for the platform in the correct quadrant of the maze (30.6 ± 5.1 and 33.6 ± 3.2 s respectively; t11 = 0.5, p > 0.6; unpaired two-sample t-test) and, in both cases, this search period was significantly higher than what would be expected if they were moving randomly (lesion – t5 = 3.1, p < 0.03; sham – t6 = 5.8, p < 0.003; one sample t-tests vs. 15 s with Bonferroni–Holm’s correction for multiple comparisons). Thus, the minor differences found in MWM during training do not seem to explain the deficits found in two-bottle tests (see Figures 6C,D) since both groups learned the spatial location of the submerged platform and IC-lesioned animals have seemingly normal spatial orientation memory in the MWM.
Discussion
In this study we have shown that the activity of neurons located in dorsal regions of the IC of sweet-blind Trpm5−/− mice display a heightened sensitivity to taste-independent postingestive effects produced by caloric sucrose solutions. In addition, we found that changes in IC neuronal activity elicited by these taste-independent postingestive effects were mostly restricted to more dorsal regions of the IC, where the gustatory aspect of the insula is located. Finally, we have shown that focal lesions to dorsal insular areas, that were ineffective in disrupting the sensitivity to taste-independent postingestive effects of sucrose, do disrupt the ability of mice to associate a particular sipper position with the postingestive effects produced by the sucrose solutions. Overall, our data supports the concept that even in the absence of taste transduction, dorsal parts of the IC are critical for the formation of associations between environmental cues and postingestive effects.
Chemosensory responses in gustatory insular neurons are broadly tuned to multiple taste qualities, including sweet (Katz et al., 2002; Stapleton et al., 2006, 2007). However, in the absence of taste input, it remained unknown as to whether the rewarding postingestive properties of sucrose were represented in the insula. Here we have described adaptations in IC responses as sweet-blind Trpm5−/− animals developed a preference for sucrose. This preference was established through a conditioning protocol that associates the contents of a particular sipper with its positive postingestive effects (de Araujo et al., 2008; Ren et al., 2010). In mice that developed a preference for sucrose, such neuronal adaptations occurred both at the single-neuron level, where they were expressed as an increased “discriminability” between sucrose and water responses (Figure 2B), and at the neural population level, where they were expressed as changes in the difference between population responses to sucrose and water as a function of the behavioral sensitivity (LI) to the conditioning protocol (Figure 3A). Using an immunohistochemical approach, we confirmed the occurrence of taste-independent IC responses to sucrose and, furthermore, showed that these responses were restricted to the dorsal subdivision of the insula (Figure 4). Finally, using excitotoxic lesions (Figure 5) to explore the functional relevance of IC responses to sucrose in the absence of peripheral sweet taste transduction, we found that, after conditioning, animals with bilateral IC lesions did not develop a preference for sipper positions associated with sucrose availability (Figures 6C,D). These results define a new dimension in the insular representation of sugars, ascribing new functions to the IC that go beyond oral chemosensory representation. In particular, they suggest a more fundamental role for the IC in food-reinforcement mechanisms that cannot be explained as arising from orosensory reward.
The Trpm5−/− mice used in this study have a well defined deficit in the transduction of sweet, bitter, and umami tastants (Zhang et al., 2003). Indeed, their peripheral neural and behavioral responses to sweet tastants are essentially abolished (Zhang et al., 2003; de Araujo et al., 2008; Oliveira-Maia et al., 2009; Ren et al., 2010). Furthermore, we have shown that, even after being conditioned to the postingestive effects of sucrose, these animals do not express a preference for this tastant when they are tested in paradigms that depend on the detection of orosensory cues (see text and de Araujo et al., 2008). Thus, even if any such orosensory cues exist (e.g., osmolarity, viscosity), in this experimental paradigm these KO animals do not use them to guide their behavior.
Insular cortex recordings performed in Trpm5−/− mice before (pre) and after (post) conditioning demonstrated that, between these two periods, adaptations occurred in the neural responses to sucrose and water (Figures 2B and 3A). We interpreted these adaptations in the IC as reflecting postingestive-dependent learning. Two main factors support this interpretation: first IC responses to the two tastants were significantly changed after conditioning, and second that these adaptations co-varied with behavioral indices of the degree to which each animal developed a preference for sucrose (Figures 2B and 3A). The possibility that, in Trpm5−/− mice, these IC responses could reflect orosensory factors is unlikely, not only because these animals do not exhibit behavioral and peripheral neural responses to sucrose (Zhang et al., 2003; de Araujo et al., 2008; Oliveira-Maia et al., 2009; Ren et al., 2010), but also because, as discussed above, both here and in a prior study (de Araujo et al., 2008), we have shown that, in Trpm5−/− mice, the expression of conditioned preferences for sucrose is independent of orosensory factors. Finally, interpretations purely based on oromotor factors such as lick rate and licking cluster duration were eliminated (Figure 3B).
Additional experiments were performed to confirm the relevance of IC responses for taste-independent postingestive learning. FOS immunostaining was used as an index of neuronal activation and confirmed the presence of IC neural responses to sucrose in Trpm5−/− mice (Figure 4). These responses were obtained in animals where consumption of sucrose was yoked to that of water (Figure 4C), eliminating the possibility that they result from increased volume consumption, with unspecific effects resulting, for example, from stomach distention (Cechetto and Saper, 1987). Critical confirmation that IC responses were related to postingestive learning came from IC lesion experiments demonstrating that the IC is necessary for Trpm5−/− mice to show a post-conditioning preference for sucrose (Figures 6C,D).
In contrast to these results, a prior study with rats failed to demonstrate behavioral effects of bilateral insular lesions in a flavor-nutrient conditioning task (Touzani and Sclafani, 2007). We note that, because we were testing taste-independent responses, these experiments were conducted only in Trpm5−/− mice. Thus, these results do not necessarily generalize to rats or possibly even to wild-type mice, even though the very localized expression pattern of Trpm5 mRNA (Perez et al., 2002) renders the latter possibility less likely. Several other factors may have contributed to the difference between the Touzani and Sclafani (2007) study and our results. In this regard, similar discrepancies have been described relative to the effects of IC lesions on flavor and taste aversion learning, and several reasons have been used to rationalize these inconsistent results. These include the nature of stimuli (taste vs. olfactory) and location of lesion (Kiefer et al., 1982; Mackey et al., 1986; Kiefer and Morrow, 1991; Yamamoto et al., 1995; Cubero et al., 1999; Fresquet et al., 2004; Inui et al., 2006; Roman et al., 2006). Relative to the effects of IC lesions on appetitive conditioning, Touzani and Sclafani (2007) performed experiments involving conditioning to a distinctive flavor, and the possibility remains that the presence of olfactory and/or taste cues may have influenced the ability of lesioned animals to form associations between the solutions and their nutritive value. Furthermore, their IC lesions were centered in the agranular, more ventral division of the rat insula (Touzani and Sclafani, 2007) which, according to our FOS expression analyses in mice, was not recruited by the postingestive–related effects produced by sucrose intake (see Figure 4).
In fact, the post-sucrose consumption increase in IC FOS immunostaining in Trpm5−/− mice was restricted to the dorsal subdivision of the insula, an area where electrophysiological measurements were also performed, and that was targeted in the IC lesion experiment (Figure 5). This area includes a more dorsal granular area, were visceral responses have been identified (Cechetto and Saper, 1987; Barnabi and Cechetto, 2001), and an immediately ventral dysgranular area, where taste responses have been reported in rats (Yamamoto et al., 1988; Lundy and Norgren, 2004). That said, the definition of such distinct functional subdivisions of the insula has been debated since single-neurons throughout the insula can respond to multiple sensory modalities, namely taste, visceral, and nociceptive stimuli (Cechetto and Saper, 1987; Hanamori et al., 1997, 1998a,b) and taste responsive neurons have also been described in the granular cortex (Ogawa et al., 1992; Accolla et al., 2007). Thus, the exact identities of the neural networks involved in the taste-independent postingestive responses in the dorsal IC that are described here remain to be elucidated.
As mentioned, the primary GC is located in the IC (Cechetto and Saper, 1987; Accolla et al., 2007) and, besides its role in encoding the chemosensory properties of tastants (Rolls, 2006; Stapleton et al., 2006; Accolla et al., 2007), it is required for associations to be formed between taste and malaise (Braun et al., 1972; Lorden, 1976; Yamamoto et al., 1980; Bermudez-Rattoni et al., 1991; Accolla and Carleton, 2008). Thus, in conditioned taste aversion (CTA) paradigms, pharmacological manipulations (Bermudez-Rattoni et al., 1991; Gutierrez et al., 1999), protein synthesis inhibition (Rosenblum et al., 1993) or irreversible lesions (Braun et al., 1972; Lorden, 1976; Yamamoto et al., 1980) in the GC disrupt the formation of a “memory trace” linking a conditioned taste cue to ensuing visceral malaise. Here we have identified two new functions for the IC in the integration of postingestive sensory information. First, we showed that the IC can represent positive postingestive outcomes related to the caloric value of a sucrose solution (Figures 2–4) and second, that this brain area plays a relevant role in the modulation of learned behavior toward positive postingestive outcomes, even in the absence of orosensory taste input (Figure 6).
While our results support the concept that postingestive reward is represented in the IC, it is important to mention that, in Trpm5−/− mice with bilateral IC lesions, unconditioned responses to sucrose were conserved. In fact, during conditioning sessions, both lesioned and sham-operated mice consumed more sucrose than water (Figures 6B and 7), showing that the IC is not necessary for detection of the reinforcing postingestive effects of sucrose. Clearly, other brain areas must participate in this process (de Araujo et al., 2008; Touzani et al., 2008; Oliveira-Maia et al., 2011). Nevertheless, the effects of IC lesion on postingestive dependent conditioning seem to reflect a deficit in the capacity to associate between postingestive effects and the side of the behavioral box where they were obtained (Figures 6C,D). Our results thus contribute additional evidence toward the view that a primary role of the IC in CTA (Braun et al., 1972; Lorden, 1976; Yamamoto et al., 1980; Accolla and Carleton, 2008), and possibly other taste-guided learning (Cubero and Puerto, 2000), is the association between stimuli (such as spout-side and postingestive reward).
A broad role for the insula in the representation of aversive outcomes, even in the absence of gustatory-related stimulation, has previously been extensively reported (Phillips et al., 1997; Ploghaus et al., 1999; O’Doherty et al., 2003; Wicker et al., 2003; Simmons et al., 2004; Singer et al., 2004; Contreras et al., 2007). In fact, aversion-related representation in the IC has been suggested as a potential explanation for the effects of insula lesions on addictive behaviors (Contreras et al., 2007; Naqvi et al., 2007) and affective decision-making (Clark et al., 2008). Insular representation of positive emotions has also been described, mostly in association with food-related stimuli (Small et al., 2001; de Araujo et al., 2006; Stoeckel et al., 2008; Wagner et al., 2008), but is not as well established (Jabbi et al., 2007). One study demonstrated that hypocretin transmission in the dorsal insula regulates the reinforcing effects of nicotine infusions (Hollander et al., 2008). Here we further show that the same area of the insula is involved in the representation of a positive outcome that, while being food-related, occurs independently of oral chemosensation.
In summary, we have shown that insular IC neurons are modulated during feeding and can exert control on feeding behaviors even when no oral chemosensory inputs are present. This novel finding could underlie the reorganization of neuronal representations of taste cues in the GC following changes in internal state (Buresova et al., 1979; Accolla and Carleton, 2008; Grossman et al., 2008). Additionally, our findings indicate that the IC performs previously unidentified functions in representing the rewarding postingestive consequences of consuming calorie-dense foods, possibly underlying the proposed involvement of the insula in pathological feeding behaviors (Stoeckel et al., 2008; Wagner et al., 2008).
Conflict of Interest Statement
The authors declare that the research was conducted in the absence of any commercial or financial relationships that could be construed as a potential conflict of interest.
Acknowledgments
We thank Teresa Maia, Pedro Nicolelis, Jim Meloy and Gary Lehew for technical support; Drs. Sidney Simon, Ranier Gutierrez, and Shih-Chieh Lin for invaluable discussions and suggestions, and Susan Halkiotis for review of the manuscript. This work was supported in part by funding from NIH R01DC001065 to Dr. Sidney Simon, the Duke School of Medicine Professorship in Neurosciences and NIH R01DE011451 to MALN, Philip Morris USA Inc and Philip Morris International to Dr. Sidney Simon and IEdeA, a GABBA fellowship from FCT and a post-doctoral grant from the AXA Research Fund to Albino J. Oliveira-Maia.
References
Abeles, M. (1982). Quantification, smoothing, and confidence limits for single-units histograms. J. Neurosci. Methods 5, 317–325.
Accolla, R., Bathellier, B., Petersen, C. C., and Carleton, A. (2007). Differential spatial representation of taste modalities in the rat gustatory cortex. J. Neurosci. 27, 1396–1404.
Accolla, R., and Carleton, A. (2008). Internal body state influences topographical plasticity of sensory representations in the rat gustatory cortex. Proc. Natl. Acad. Sci. U.S.A. 105, 4010–4015.
Balleine, B. W., and Dickinson, A. (2000). The effect of lesions of the insular cortex on instrumental conditioning: evidence for a role in incentive memory. J. Neurosci. 20, 8954–8964.
Barnabi, F., and Cechetto, D. F. (2001). Neurotransmitters in the thalamus relaying visceral input to the insular cortex in the rat. Am. J. Physiol. Regul. Integr. Comp. Physiol. 281, R1665–R1674.
Bermudez-Rattoni, F., Introini-Collison, I. B., and McGaugh, J. L. (1991). Reversible inactivation of the insular cortex by tetrodotoxin produces retrograde and anterograde amnesia for inhibitory avoidance and spatial learning. Proc. Natl. Acad. Sci. U.S.A. 88, 5379–5382.
Braun, J. J., Slick, T. B., and Lorden, J. F. (1972). Involvement of gustatory neocortex in the learning of taste aversions. Physiol. Behav. 9, 637–641.
Buresova, O., Aleksanyan, Z. A., and Bures, J. (1979). Electrophysiological analysis of retrieval of conditioned taste aversion in rats. Unit activity changes in critical brain regions. Physiol. Bohemoslov. 28, 525–536.
Cechetto, D. F., and Saper, C. B. (1987). Evidence for a viscerotopic sensory representation in the cortex and thalamus in the rat. J. Comp. Neurol. 262, 27–45.
Clark, L., Bechara, A., Damasio, H., Aitken, M. R., Sahakian, B. J., and Robbins, T. W. (2008). Differential effects of insular and ventromedial prefrontal cortex lesions on risky decision-making. Brain 131(Pt 5), 1311–1322.
Contreras, M., Ceric, F., and Torrealba, F. (2007). Inactivation of the interoceptive insula disrupts drug craving and malaise induced by lithium. Science 318, 655–658.
Corbit, L. H., Ostlund, S. B., and Balleine, B. W. (2002). Sensitivity to instrumental contingency degradation is mediated by the entorhinal cortex and its efferents via the dorsal hippocampus. J. Neurosci. 22, 10976–10984.
Cubero, I., and Puerto, A. (2000). Electrical stimulation of the insular cortex induces flavor-preferences in rats. Brain Res. 872, 134–140.
Cubero, I., Thiele, T. E., and Bernstein, I. L. (1999). Insular cortex lesions and taste aversion learning: effects of conditioning method and timing of lesion. Brain Res. 839, 323–330.
Damak, S., Rong, M., Yasumatsu, K., Kokrashvili, Z., Pérez, C. A., Shigemura, N., Yoshida, R., Mosinger, B. Jr., Glendinning, J. I., Ninomiya, Y., and Margolskee, R. F. (2006). Trpm5 null mice respond to bitter, sweet, and umami compounds. Chem. Senses 31, 253–264.
Davis, J. D., and Smith, G. P. (1992). Analysis of the microstructure of the rhythmic tongue movements of rats ingesting maltose and sucrose solutions. Behav. Neurosci. 106, 217–228.
de Araujo, I. E., Gutierrez, R., Oliveira-Maia, A. J., Pereira, A. Jr., Nicolelis, M. A. L., and Simon, S. A. (2006). Neural ensemble coding of satiety States. Neuron 51, 483–494.
de Araujo, I. E., Oliveira-Maia, A. J., Sotnikova, T. D., Gainetdinov, R. R., Caron, M. G., Nicolelis, M. A., and Simon, S. A. (2008). Food reward in the absence of taste receptor signaling. Neuron 57, 930–941.
Fresquet, N., Angst, M. J., and Sandner, G. (2004). Insular cortex lesions alter conditioned taste avoidance in rats differentially when using two methods of sucrose delivery. Behav. Brain Res. 153, 357–365.
Grossman, S. E., Fontanini, A., Wieskopf, J. S., and Katz, D. B. (2008). Learning-related plasticity of temporal coding in simultaneously recorded amygdala-cortical ensembles. J. Neurosci. 28, 2864–2873.
Gutierrez, H., Hernandez-Echeagaray, E., Ramírez-Amaya, V., and Bermúdez-Rattoni, F. (1999). Blockade of N-methyl-D-aspartate receptors in the insular cortex disrupts taste aversion and spatial memory formation. Neuroscience 89, 751–758.
Gutierrez, R., Carmena, J. M., Nicolelis, M. A., and Simon, S. A. (2006). Orbitofrontal ensemble activity monitors licking and distinguishes among natural rewards. J. Neurophysiol. 95, 119–133.
Hanamori, T., Kunitake, T., Kato, K., and Kannan, H. (1997). Convergence of oropharyngolaryngeal, baroreceptor and chemoreceptor afferents onto insular cortex neurons in rats. Chem. Senses 22, 399–406.
Hanamori, T., Kunitake, T., Kato, K., and Kannan, H. (1998a). Neurons in the posterior insular cortex are responsive to gustatory stimulation of the pharyngolarynx, baroreceptor and chemoreceptor stimulation, and tail pinch in rats. Brain Res. 785, 97–106.
Hanamori, T., Kunitake, T., Kato, K., and Kannan, H. (1998b). Responses of neurons in the insular cortex to gustatory, visceral, and nociceptive stimuli in rats. J. Neurophysiol. 79, 2535–2545.
Hollander, J. A., Lu, Q., Cameron, M. D., Kamenecka, T. M., and Kenny, P. J. (2008). Insular hypocretin transmission regulates nicotine reward. Proc. Natl. Acad. Sci. U.S.A. 105, 19480–19485.
Holm, S. (1979). A simple sequential rejective multiple test procedure. Scand. Stat. Theory Appl. 6, 65–70.
Inui, T., Shimura, T., and Yamamoto, T. (2006). Effects of brain lesions on taste-potentiated odor aversion in rats. Behav. Neurosci. 120, 590–599.
Jabbi, M., Swart, M., and Keysers, C. (2007). Empathy for positive and negative emotions in the gustatory cortex. Neuroimage 34, 1744–1753.
Katz, D. B., Simon, S. A., and Nicolelis, M. A. (2002). Taste-specific neuronal ensembles in the gustatory cortex of awake rats. J. Neurosci. 22, 1850–1857.
Kee, N., Teixeira, C. M., Wang, A. H., and Frankland, P. W. (2007). Imaging activation of adult-generated granule cells in spatial memory. Nat. Protoc. 2, 3033–3044.
Kiefer, S. W., and Morrow, N. S. (1991). Odor cue mediation of alcohol aversion learning in rats lacking gustatory neocortex. Behav. Neurosci. 105, 25–32.
Kiefer, S. W., Rusiniak, K. W., and Garcia, J. (1982). Flavor-illness aversions: gustatory neocortex ablations disrupt taste but not taste-potentiated odor cues. J. Comp. Physiol. Psychol. 96, 540–548.
Lorden, J. F. (1976). Effects of lesions of the gustatory necortex on taste aversion learning in the rat. J. Comp. Physiol. Psychol. 90, 665–679.
Lundy, R. F. Jr., and Norgren, R. (2004). “Gustatory system,” in The Rat Nervous System, ed. G. Paxinos (San Diego, CA: Elsevier, Academic Press), 891–921.
Mackey, W. B., Keller, J., and van der Kooy, D. (1986). Visceral cortex lesions block conditioned taste aversions induced by morphine. Pharmacol. Biochem. Behav. 24, 71–78.
Naqvi, N. H., Rudrauf, D., Damasio, H., and Bechara, A. (2007). Damage to the insula disrupts addiction to cigarette smoking. Science 315, 531–534.
O’Doherty, J., Critchley, H., Deichmann, R., and Dolan, R. J. (2003). Dissociating valence of outcome from behavioral control in human orbital and ventral prefrontal cortices. J. Neurosci. 23, 7931–7939.
Ogawa, H., Hasegawa, K., and Murayama, N. (1992). Difference in taste quality coding between two cortical taste areas, granular and dysgranular insular areas, in rats. Exp. Brain Res. 91, 415–424.
Ogawa, H., Ito, S., Murayama, N., and Hasegawa, K. (1990). Taste area in granular and dysgranular insular cortices in the rat identified by stimulation of the entire oral cavity. Neurosci. Res. 9, 196–201.
Oliveira-Maia, A. J., Roberts, C. D., Walker, Q. D., Luo, B., Kuhn, C., Simon, S. A., and Nicolelis, M. A. (2011). Intravascular food reward. PLoS ONE 6, e24992. doi:10.1371/journal.pone.0024992
Oliveira-Maia, A. J., Stapleton-Kotloski, J. R., Lyall, V., Phan, T. H., Mummalaneni, S., Melone, P., Desimone, J. A., Nicolelis, M. A., and Simon, S. A. (2009). Nicotine activates TRPM5-dependent and independent taste pathways. Proc. Natl. Acad. Sci. U.S.A. 106, 1596–1601.
Paxinos, G., and Franklin, K. B. J. (2001). The Mouse Brain in Stereotaxic Coordinates. San Diego: Academic Press.
Perez, C. A., Huang, L., Rong, M., Kozak, J. A., Preuss, A. K., Zhang, H., Max, M., and Margolskee, R. F. (2002). A transient receptor potential channel expressed in taste receptor cells. Nat. Neurosci. 5, 1169–1176.
Phillips, M. L., Young, A. W., Senior, C., Brammer, M., Andrew, C., Calder, A. J., Bullmore, E. T., Perrett, D. I., Rowland, D., Williams, S. C., Gray, J. A., and David, A. S. (1997). A specific neural substrate for perceiving facial expressions of disgust. Nature 389, 495–498.
Ploghaus, A., Tracey, I., Gati, J. S., Clare, S., Menon, R. S., Matthews, P. M., and Rawlins, J. N. (1999). Dissociating pain from its anticipation in the human brain. Science 284, 1979–1981.
Ren, X., Ferreira, J. G., Zhou, L., Shammah-Lagnado, S. J., Yeckel, C. W., and de Araujo, I. E. (2010). Nutrient selection in the absence of taste receptor signaling. J. Neurosci. 30, 8012–8023.
Rolls, E. T. (2006). Brain mechanisms underlying flavour and appetite. Philos. Trans. R. Soc. Lond. B Biol. Sci. 361, 1123–1136.
Roman, C., Nebieridze, N., Sastre, A., and Reilly, S. (2006). Effects of lesions of the bed nucleus of the stria terminalis, lateral hypothalamus, or insular cortex on conditioned taste aversion and conditioned odor aversion. Behav. Neurosci. 120, 1257–1267.
Rosenblum, K., Meiri, N., and Dudai, Y. (1993). Taste memory: the role of protein synthesis in gustatory cortex. Behav. Neural Biol. 59, 49–56.
Scott, T. R., and Plata-Salaman, C. R. (1999). Taste in the monkey cortex. Physiol. Behav. 67, 489–511.
Simmons, A., Matthews, S. C., Stein, M. B., and Paulus, M. P. (2004). Anticipation of emotionally aversive visual stimuli activates right insula. Neuroreport 15, 2261–2265.
Singer, T., Seymour, B., O’Doherty, J., Kaube, H., Dolan, R. J., and Frith, C. D. (2004). Empathy for pain involves the affective but not sensory components of pain. Science 303, 1157–1162.
Small, D. M., Zatorre, R. J., Dagher, A., Evans, A. C., and Jones-Gotman, M. (2001). Changes in brain activity related to eating chocolate: from pleasure to aversion. Brain 124(Pt 9), 1720–1733.
Stapleton, J. R., Lavine, M. L., Nicolelis, M. A., and Simon, S. A. (2007). Ensembles of gustatory cortical neurons anticipate and discriminate between tastants in a single lick. Front. Neurosci. 1:1. doi:10.3389/neuro.01/1.1.012.2007
Stapleton, J. R., Lavine, M. L., Wolpert, R. L., Nicolelis, M. A., and Simon, S. A. (2006). Rapid taste responses in the gustatory cortex during licking. J. Neurosci. 26, 4126–4138.
Stoeckel, L. E., Weller, R. E., Cook, E. W. III, Twieg, D. B., Knowlton, R. C., and Cox, J. E. (2008). Widespread reward-system activation in obese women in response to pictures of high-calorie foods. Neuroimage 41, 636–647.
Touzani, K., Bodnar, R., and Sclafani, A. (2008). Activation of dopamine D1-like receptors in nucleus accumbens is critical for the acquisition, but not the expression, of nutrient-conditioned flavor preferences in rats. Eur. J. Neurosci. 27, 1525–1533.
Touzani, K., and Sclafani, A. (2007). Insular cortex lesions fail to block flavor and taste preference learning in rats. Eur. J. Neurosci. 26, 1692–1700.
Wagner, A., Aizenstein, H., Mazurkewicz, L., Fudge, J., Frank, G. K., Putnam, K., Bailer, U. F., Fischer, L., and Kaye, W. H. (2008). Altered insula response to taste stimuli in individuals recovered from restricting-type anorexia nervosa. Neuropsychopharmacology 33, 513–523.
Wicker, B., Keysers, C., Plailly, J., Royet, J. P., Gallese, V., and Rizzolatti, G. (2003). Both of us disgusted in My insula: the common neural basis of seeing and feeling disgust. Neuron 40, 655–664.
Yamamoto, T., Fujimoto, Y., Shimura, T., and Sakai, N. (1995). Conditioned taste aversion in rats with excitotoxic brain lesions. Neurosci. Res. 22, 31–49.
Yamamoto, T., Matsuo, R., and Kawamura, Y. (1980). Localization of cortical gustatory area in rats and its role in taste discrimination. J. Neurophysiol. 44, 440–455.
Yamamoto, T., Matsuo, R., Kiyomitsu, Y., and Kitamura, R. (1988). Sensory inputs from the oral region to the cerebral cortex in behaving rats: an analysis of unit responses in cortical somatosensory and taste areas during ingestive behavior. J. Neurophysiol. 60, 1303–1321.
Keywords: insular cortex, gustatory cortex, Trpm5, taste, food preference, postingestive reward
Citation: Oliveira-Maia AJ, de Araujo IE, Monteiro C, Workman V, Galhardo V and Nicolelis MAL (2012) The insular cortex controls food preferences independently of taste receptor signaling. Front. Syst. Neurosci. 6:5. doi: 10.3389/fnsys.2012.00005
Received: 15 December 2011;
Accepted: 30 January 2012;
Published online: 01 March 2012.
Edited by:
Milagros Gallo, University of Granada, SpainReviewed by:
Christine E. Collins, Vanderbilt University, USAMilagros Gallo, University of Granada, Spain
Copyright: © 2012 Oliveira-Maia, de Araujo, Monteiro, Workman, Galhardo and Nicolelis. This is an open-access article distributed under the terms of the Creative Commons Attribution Non Commercial License, which permits non-commercial use, distribution, and reproduction in other forums, provided the original authors and source are credited.
*Correspondence: Ivan E. de Araujo, The John B. Pierce Laboratory and Yale University School of Medicine, 290 Congress Avenue, New Haven, CT 06519, USA. e-mail:aWFyYXVqb0BqYnBpZXJjZS5vcmc=
†Present address: Albino J. Oliveira-Maia, Champalimaud Neuroscience Program, Instituto Gulbenkian de Ciência, 2780-156 Oeiras, Portugal; Department of Psychiatry and Mental Health, Centro Hospitalar de Lisboa Ocidental, 1300-542 Lisboa, Portugal; Ivan E. de Araujo, The John B. Pierce Laboratory, New Haven, CT 06519, USA; Department of Psychiatry, Yale University School of Medicine, New Haven, CT 06520, USA.