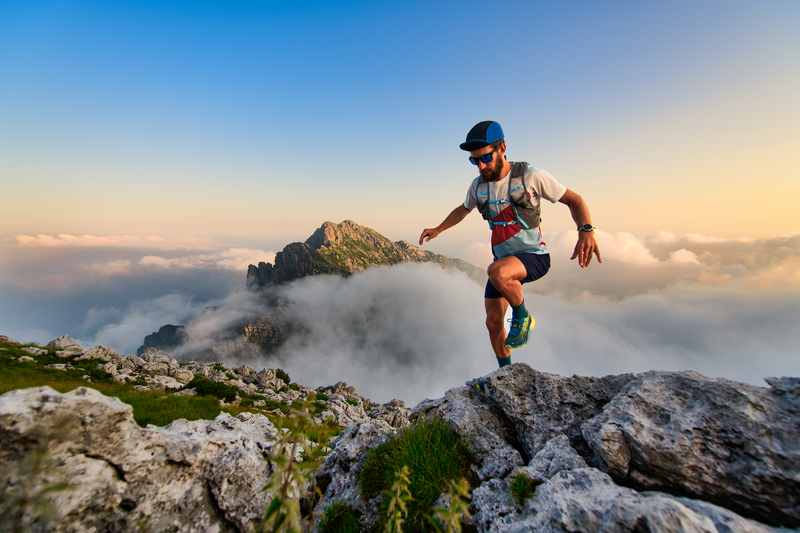
95% of researchers rate our articles as excellent or good
Learn more about the work of our research integrity team to safeguard the quality of each article we publish.
Find out more
ORIGINAL RESEARCH article
Front. Syst. Neurosci. , 01 February 2012
Volume 6 - 2012 | https://doi.org/10.3389/fnsys.2012.00002
This article is part of the Research Topic Ringing ears: the neuroscience of tinnitus View all 23 articles
The pathophysiology underlying tinnitus, a hearing disorder characterized by the chronic perception of phantom sound, has been related to aberrant plastic reorganization of the central auditory system. More specifically, tinnitus is thought to involve changes in the tonotopic representation of sound. In the present study we used high-resolution functional magnetic resonance imaging to determine tonotopic maps in the auditory cortex of 20 patients with tinnitus but otherwise near-normal hearing, and compared these to equivalent outcomes from 20 healthy controls with matched hearing thresholds. Using a dedicated experimental paradigm and data-driven analysis techniques, multiple tonotopic gradients could be robustly distinguished in both hemispheres, arranged in a pattern consistent with previous findings. Yet, maps were not found to significantly differ between the two groups in any way. In particular, we found no evidence for an overrepresentation of high sound frequencies, matching the tinnitus pitch. A significant difference in evoked response magnitude was found near the low-frequency tonotopic endpoint on the lateral extreme of left Heschl’s gyrus. Our results suggest that macroscopic tonotopic reorganization in the auditory cortex is not required for the emergence of tinnitus, and is not typical for tinnitus that accompanies normal hearing to mild hearing loss.
Subjective tinnitus is a prevalent and presently incurable hearing disorder that is characterized by the perception of sound in the absence of an identifiable sound source. Many people have experienced ephemeral episodes of tinnitus at some point in their life without permanent consequences. However, persisting tinnitus may have a debilitating effect on an individual’s state and functioning, leading many chronic tinnitus patients to seek medical attention.
In spite of a growingly useful body of behavioral and neurophysiological research in humans and animals, the pathophysiological mechanism that causes tinnitus still remains to be elucidated (Baguley, 2002; Eggermont, 2007a; Møller, 2007a; Rauschecker et al., 2010; Roberts et al., 2010; Kaltenbach, 2011). An important clue lies in the observation that the perceived tinnitus pitch often coincides with frequency regions in which hearing thresholds are found to be elevated. It is still debated whether tinnitus is most closely associated with the frequency range that covers the hearing loss (Noreña et al., 2002; Roberts et al., 2008) or with the edge-frequency where the audiogram is steepest (König et al., 2006; Moore and Vinay, 2010), but in either case the existence of the association suggests some causal relationship.
Tinnitus is not generated in the ear itself. Presumably, hearing loss results in the sensory deprivation of neural assemblies that are tuned to the affected frequencies, providing an incentive for plasticity to occur. In an effort to upregulate their reduced activity back to normal levels, neurons may change the strength of existing connections or develop new connections. If, through homeostatic plasticity (Burrone and Murthy, 2003), all excitatory synapses are strengthened or all inhibitory synapses are weakened in unison, then neurons may become more susceptible to be activated in response to low incoming levels of spontaneous activity already (Schaette and Kempter, 2006; Noreña, 2011). Alternatively, if inputs from unaffected frequencies are strengthened (or newly grown) and inputs from frequencies with hearing loss are suppressed (or pruned altogether), then a large body of neurons may end up responding to the same limited amount of sensory input, thus enhancing neural synchronicity across the population (Eggermont, 2007b). In the healthy auditory system, spontaneous activity is ubiquitously present, but it tends to be relatively weak and incoherent. Elevated levels of neural activity and synchronicity normally only occur in the presence of a driving stimulus, i.e., a sound source. Therefore, if the spontaneous activity or synchronicity is elevated as a result of functional changes that are induced by hearing loss, this can be perceived as the presence of a phantom sound percept in the absence of a true sound source (Dominguez et al., 2006; Chrostowski et al., 2011).
Research in animals as well as humans supports the theory that tinnitus is a side effect of plastic reorganization in the central auditory system. Some studies suggest that homeostatic mechanisms play a dominant role. In rats that developed behavioral signs of high-frequency tinnitus after exposure to loud noise, down-regulation of inhibitory synapses was observed in neurons that were tuned to high frequencies (Yang et al., 2011). In humans with tinnitus, auditory brainstem responses that originated from the periphery were found to be reduced, but those from more central levels had recovered to normal levels, suggesting an increase in neural gain (Schaette and McAlpine, 2011). At the same time, abnormalities in the tonotopic organization of the auditory cortex have been observed that are consistent with an enlarged representation of sensory input from edge-frequency regions. In various animal studies, neurons that were expected to be tuned to sound frequencies coinciding with the tinnitus pitch on the basis of their location in the tonotopic representation were found to display shifts in characteristic frequency (Rajan and Irvine, 1998; Noreña et al., 2003; Stolzberg et al., 2011). Signs of tinnitus disappeared when the representation was restored, suggesting that the map reorganization is responsible for the tinnitus (Engineer et al., 2011). In humans, magnetoencephalography was used to show deviant spatial source localization of frequency-dependent responses in tinnitus patients as compared to controls (Mühlnickel et al., 1998; Wienbruch et al., 2006). Finally, a number of therapeutical strategies that specifically aim to reestablish the tonotopic representation have been reported to be successful in suppressing tinnitus in humans (Herraiz et al., 2007; Pineda et al., 2008), providing indirect evidence for the importance of tonotopic reorganizations in tinnitus.
Unfortunately, the tonotopic organization in humans is still poorly understood even for normal-hearing subjects. Many studies have consistently shown that low sound frequencies are represented in the distal end of Heschl’s gyrus, anterolateral to the high frequencies, which are represented in the proximal root of Heschl’s gyrus (Romani et al., 1982; Lauter et al., 1985; Pan-tev et al., 1989; Wessinger et al., 1997). However, this ignores the existence of multiple functional fields in auditory cortex, many of which may display distinct tonotopic maps. In the last decade, research has started to differentiate between multiple abutting representations in much more detail. Initial results were groundbreaking but appeared somewhat contradictory (Formisano et al., 2003; Talavage et al., 2004). In the last couple of years, however, various studies were published that were in excellent agreement (Woods et al., 2009; Humphries et al., 2010; Da Costa et al., 2011; Langers and van Dijk, 2011; Striem-Amit et al., 2011). Encouraged by these developments, the present study was set up to map the tonotopic representation in tinnitus patients in similar detail. Because we were interested in effects related to tinnitus specifically, we extended our own recent findings to include patients with tinnitus but otherwise normal hearing.
Twenty healthy controls and 20 chronic subjective tinnitus patients participated in this functional magnetic resonance imaging (fMRI) study on the basis of written informed consent, in approved accordance with the requirements of the medical ethical committee at the University Medical Center Groningen in the Netherlands. Subjects were recruited from the hospital’s tinnitus outpatient clinic (for the patient group) as well as from advertisements in various media (for the control and patient groups). They reported no history of neurological or psychiatric disorders. The patients were not undergoing tinnitus treatment at the time of the study. Table 1 summarizes the subjects’ gender, handedness, and age.
Except for the presence of tinnitus in the patient group, all subjects were selected to have normal or near-normal hearing up to 8 kHz. Thresholds were determined in a frequency range of 0.25-16 kHz by means of pure-tone audiometry. Furthermore, subjects performed the adaptive categorical loudness scaling (ACALOS) procedure (Brand and Hohmann, 2002). These tests were carried out for the left and right ears separately, but because no notable differences were found results were averaged over both ears.
To characterize the participants’ self-reported complaints, all subjects filled out the 14-item hyperacusis questionnaire, relating to the attentional, social, and emotional aspects of auditory hyper-sensitivity (Khalfa et al., 2002). In addition, all subjects completed the 27-item short symptom checklist that screens for psychiatric symptoms in patients with somatic complaints, and that contains subscales for symptoms of depression, dysthymia, vegetativeness, agoraphobia, sociophobia, and mistrust (Hardt and Gerbershagen, 2001). The tinnitus patients also filled out questionnaires related to their tinnitus, including the Tinnitus Handicap Inventory (THI) that measures tinnitus severity in daily life (Newman et al., 1996), the Tinnitus Reaction Questionnaire (TRQ) that assesses the psychological distress associated with tinnitus (Wilson et al., 1991), and the Tinnitus Coping Style Questionnaire (TCSQ) that quantifies effective as well as maladaptive coping strategies (Budd and Pugh, 1996). All questionnaires were translated into Dutch, and outcome measures were linearly rescaled to obtain a uniform range from 0 to 100.
Tinnitus patients were furthermore asked where they perceived their tinnitus (lateralized toward the left or right ear, or centrally), and whether it was steady or pulsatile. Finally, all patients performed a modified tinnitus spectrum test (Noreña et al., 2002; Roberts et al., 2008). First, some example sounds were played and they were asked which type of sound resembled their tinnitus best: a tonal sound (pure tone), or a ringing or hissing sound (filtered noise with 0.04 or 0.15 octave bandwidths, respectively). Next, the chosen sound was repeatedly played at several center frequencies, and subjects were asked to indicate the subjective “likeness” of the presented sound compared to their tinnitus using a visual analog scale.
During the imaging session, subjects were placed supinely in the bore of a 3.0-T MR system (Philips Intera, Best, the Netherlands), which was equipped with an 8-channel phased-array (SENSE) transmit/receive head coil. The functional imaging session included three 8-min runs, each consisting of a dynamic series of 40 identical high-resolution T2*-sensitive gradient-echo echo-planar imaging (EPI) volume acquisitions (TR 12.0 s; TA 2.0 s; TE 22 ms; FA 90° matrix 128 × 128 × 40; resolution 1.5 mm × 1.5 mm × 1.5 mm; interleaved slice order, no slice gap). A sparse, clustered-volume sequence was employed to avoid interference from acoustic scanner noise (Edmister et al., 1999; Hall et al., 1999). The acquisition volume was positioned in an oblique axial orientation, tilted forward parallel to the Sylvian fissure, and approximately centered on the superior temporal sulci. Additional preparation scans were used to achieve stable image contrast and to trigger the start of stimulus delivery, but these were not included into the analysis. The scanner coolant pump and fan were turned off during imaging to diminish ambient noise levels.
To control their attentional state, subjects performed an engaging visual/emotional task that comprised 40 trials of 12-s duration per run (Langers and van Dijk, 2011). During the first 5 s of each trial, a fixation cross was presented on a screen. During the next 5 s, a picture was shown that was randomly selected out of 300 images from the International Affective Picture System (Lang et al., 2008). Subjects were instructed to empathize with the depicted scene, and decide whether the picture’s affective valence was positive, negative, or neutral. During the final 2 s, coinciding with the EPI acquisitions, subjects could respond by pressing any of three touch buttons on a handheld device. Before the scanning session, the task was clearly explained and demonstrated, and subjects were given the opportunity to practice.
To exclude that the tinnitus percept might have been masked by ambient noise during the scanning session, all patients were asked to rate their tinnitus at various moments before, between, and after the imaging runs on a scale from 0 to 10, where 0 signified that the tinnitus was absent and 10 signified that the tinnitus was maximal. Responses varied but never equaled 0 (the lowest rating encountered was 2) and also never systematically decreased during the session (contrariwise, according to some subjects it systematically increased due to accruing fatigue and stress).
During the functional runs, sound was presented by means of MR-compatible electrodynamic headphones(MR ConfonGmbH, Magdeburg, Germany; Baumgart et al., 1998) that were connected to a standard PC with soundcard. Underneath the headset, subjects wore foam ear plugs to further dampen the acoustic noise produced by the scanner. Subjects were informed beforehand that the presented sound stimuli were irrelevant for the purpose of the visual/emotional task. During the first 10 s of each trial, while the MR-scanner was inactive, a sequence of 50 identical 100-ms tone stimuli was presented at a rate of 5 Hz. The fundamental frequency f0 of the tones remained the same within a trial, and equaled f0 = 0.25, 0.50, 1.00, 2.00, 4.00, or 8.00 kHz. On top of a constant fundamental, each tone stimulus contained a first overtone that quickly decayed with an e-folding time τ= 25 ms. A windowing function A(t) was used to impose 5-ms linear rise and fall times. The corresponding waveform w(t) is given by the equation w(t) = A(t)· [sin(2π · f0 · t) + 1/2 · e-t/τ · sin(2π · 2f0 · t)]. An additional silent waveform [ w(t) = 0] was included in the set of stimuli.
All waveforms were digitized and saved as 16-bit 44.1-kHz data files, scaled at two levels that differed by a factor 10 in amplitude. As a result, the louder set of stimuli was precisely 20 dB louder than the softer set of stimuli. The stimuli were played at the same level for all subjects; the corresponding audible intensities were calibrated in a separate session, by determining audiometric thresholds to the presented tone stimuli inside the scanner environment, and comparing those to the corresponding standard audiometric thresholds. For example: if the loud 2-kHz stimulus needed to be attenuated by 40 dB to reach the threshold for that stimulus as determined inside the scanner, and if the standard audiometric threshold at 2 kHz was 5 dB HL, then the loud 2-kHz stimulus was inferred to have been presented at 45 dB HL, and the corresponding soft stimulus at 25 dB HL.
The stimulus frequencies and intensity levels were randomly varied across trials, in an order that differed across runs and subjects, and that was unrelated to the affective valence of the task-related pictures.
During data processing, we used Matlab (The MathWorks Inc., Natick, MA, USA), supplemented with processing routines from the SPM8b software package (Wellcome Department of Imaging Neuroscience, http://www.fil.ion.ucl.ac.uk/spm/).
Contrast differences between odd and even slices due to the interleaved slice order were eliminated by interpolating between pairs of adjacent slices, shifting the imaging grid over half the slice thickness. Next, the functional imaging volumes were corrected for motion effects using 3-D rigid body transformations. The anatomical images were coregistered to the functional volumes, and all images were normalized into Montreal Neurological Institute (MNI) stereotaxic space. Images were moderately smoothed using an isotropic 5-mm full-width at half-maximum Gaussian kernel, and resampled to a 2-mm isotropic resolution. A logarithmic transformation was carried out in order to naturally express all derived voxel signal measures in units of percentage signal change relative to the mean. (Given the small relative magnitude of the hemodynamic signal, a truncated Taylor series expansion of the transformed signal ŝ(t) = 100 . ln(s(t)) gives rise to Δŝ(t) = 100.Δs(t)/S, indicating that the absolute signal change in Δŝ(t) equals the relative signal change in Δs(t) expressed as a percentage of its mean S.)
Mass-univariate general linear regression models (GLMs) were constructed and assessed for each subject, including: (i) two regressors modeling the reported affective valences (positive or negative, relative to neutral); (ii) twelve regressors modeling the sound stimulus conditions (6 frequencies × 2 intensity levels, relative to silence); (iii) translation and rotation parameters in the x-, y- and z-direction, modeling residual motion effects; and (iv) a third-degree polynomial for each run, modeling baseline and drift effects. The estimated sound-evoked response amplitudes were entered into a group-level mixed effects analysis. On a voxel-by-voxel basis, the significance of the response to sound was assessed by means of an omnibus F-test, including the coefficients of all 12 sound-related regressors equally. A region of interest (ROI) was defined comprising the supra-threshold voxels. The 7517 voxels (i.e., 60 cm3) that remained formed two coherent clusters of approximately equal size, located bilaterally in the superior temporal lobes that contain auditory cortex (see Results). For every subject and for all six stimulus frequencies, the activation levels of these voxels in response to stimuli of a uniform intensity level of 40 dB HL were estimated by linearly interpolating the sound-evoked activity between the two intensities that were presented. The resulting activation levels were collected in an aggregate 300680 × 6 matrix B (40 subjects × 7517 voxels, 6 frequencies).
From this aggregate activation matrix, two principal components were extracted. Thus, B = x1 ⊗ f1 + X2 ⊗ f2 + ∊, where x1 and x2 are 300680-element vectors containing spatial response maps (masked by the ROI and aggregated across subjects), f1 and f2 are 6-element vectors containing the corresponding frequency response profiles, and e is a matrix containing the residuals that were minimized in least-squares sense. Because the magnitude of the outer products in the decomposition are well-defined, but the magnitudes of the maps or profiles individually are not, the frequency response profiles f1 and f2 were constrained to unitroot-mean-square amplitude. As a result, the spatial response maps x1 and x2 are expressed in common fMRI units of percentage signal change. The aggregate spatial response maps x1 and x2 were partitioned into 40 maps corresponding with individual subjects. From these, average maps were computed for each of the two subject groups.
For the purpose of bootstrap permutation testing, all 40 subjects were repeatedly subdivided into two random subgroups of 20 subjects each, and analyzed. On the basis of 1000 such repetitions, null-distributions were derived that were used to estimate the statistical significance of any differences between the groups of healthy controls and tinnitus patients.
Figure 1A displays the subjects’ hearing thresholds as a function of frequency. Except above 8 kHz, where some hearing loss occurred, average thresholds were normal. Subject groups were well matched with regard to hearing loss. At all frequencies except 4 kHz, the thresholds did not significantly differ; only at 4 kHz, the patients’ thresholds were worse by 7 dB on average (nominal p = 0.003). Table 1 includes the mean thresholds across all frequencies at which stimuli were presented in this study, i.e., the octave frequencies from 0.25 to 8.00 kHz. These did not differ significantly between groups. It also lists the dynamic intensity range that corresponded with a loudness ranging from the minimum to the maximum score according to the ACALOS test (i.e., 0-50, corresponding with labels “inaudible” to “too loud”). The intensity range was significantly reduced in tinnitus patients, indicating a diminished tolerance for loud sounds. This finding was confirmed by the hyperacusis questionnaire, which also revealed a significantly reduced self-reported tolerance to sound. According to the symptom checklist, patients showed significantly more depressive and dysthymic signs. With respect to the other sub-scales, the patients also scored worse, but differences remained insignificant. With regard to the specifically tinnitus-related questionnaires, patients showed a wide range of self-reported levels of tinnitus complaints, varying from very mild to very severe. The tinnitus severity according to the THI, the tinnitus-related distress according to the TRQ, and the maladaptive coping level according to the TCSQ were all strongly correlated (pairwise R > 0.85). The effective coping level according to the TCSQ did not correlate appreciably with any of these three measures (pairwise |R| < 0.05). Finally, Table 1 tabulates the occurrence of various tinnitus characteristics. Overall, the tinnitus percept was most prevalently perceived centrally in the head, steady over time, and with a high-frequency tone-like character. Figure 1B displays the obtained tinnitus spectra, which on average showed a monotonous increase as a function of frequency.
FIGURE 1. (A) Hearing thresholds were measured at frequencies from 0.25 to 16.00 kHz. Results were averaged over both ears, and shown by means of boxplots (showing inter-quartile ranges). Stimuli were presented at all octave frequencies from 0.25 to 8.00 kHz at two different intensity levels that differed by 20 dB. The light gray bars indicate the approximate presentation levels. In the analysis, the sound-evoked activation levels were interpolated to a uniform intensity level of 40 dB HL, indicated by the dark gray line. (B) Patients performed a tinnitus spectrum test in which they indicated the subjective “likeness” to their tinnitus percept of a range of sound stimuli with varying center frequencies. The majority of subjects showed high-frequency tinnitus (solid; likeness increasing with frequency); one subject showed a low-frequency tinnitus (dashed; likeness decreasing with frequency); two subjects showed a spectrum that could not be classified as high- or low-frequency (dotted; with a peak or a dip at intermediate frequencies).
The sound-evoked activation according to a family-wise error (FWE) corrected group-level omnibus F-test is shown in Figure 2. Figure 2A shows all significant voxels, assessed across all 40 subjects as a group. A minimum cluster size of 100 voxels was imposed in order to exclude small sub-cortical activation foci. The resulting two extensive activation clusters in the bilateral auditory cortices were used as a ROI. In the lower bar plot, the mean activation levels in this ROI for each of the six frequencies and each of the two subject groups are plotted. Results were linearly interpolated between the two employed stimulus intensities to estimate the activation levels that would be obtained at uniform stimulus intensity levels of 40 dB HL across all frequencies. For instance, the 2-kHz stimuli were presented at approximately 30 and 50 dB HL (see Figure 1A), and the two corresponding activations were therefore averaged; at 4 kHz, however, the presented stimulus intensities were approximately 26 and 46 dB HL, and therefore the corresponding activations were weighted at 0.3:0.7. The resulting profiles showed the largest activation levels below 1 kHz, and a gradual decline in activation toward the highest frequency of 8 kHz. No systematic differences between controls and patients were apparent.
FIGURE 2. (A) Overall activation to all sound stimuli in the controls and patients combined (thresholded at p < 0.05, FWE-corrected, and minimum cluster size of 100 voxels) occurred in the bilateral auditory cortices. Below the glass brain display, the bar plot shows the activation to various frequencies (interpolated to 40 dB HL) for both subject groups separately. Error bars indicate standard errors of the mean across subjects. (B) Testing for any differences between groups in the frequency-dependent sound-evoked activation profile (thresholded at p < 0.05, FWE-corrected, and minimum cluster size of 20 voxels) revealed one cluster in left lateral Heschl’s gyrus. The bar plot shows the mean response levels for this subset of voxels.
Figure 2B shows all locally significant differences in activation between the 20 healthy controls on the one hand and the 20 tinnitus patients on the other hand. Results were thresholded at a confidence level of p < 0.05 (FWE-corrected) and a minimum cluster size of 20 voxels. One cluster reached significance, located in the most lateral aspect of the Heschl’s gyrus in the left hemisphere, peaking at coordinates (x, y, z) = (-62, 0, 0). The average response profile of these voxels, shown in the bar plot, showed relatively weak activation in the controls and relatively strong activation in the patients. This qualitative difference was found at all frequencies, but it was quantitatively most prominent for the low frequencies.
In Figure 3A, the mean activation levels (expressed as a percentage signal change, interpolated to 40 dB HL) across all six stimulus frequencies are mapped for the two subject groups separately. Obviously, in both groups the overall activation tended to decline as the stimulus frequency increased. Additionally, a gradual transition in the activation pattern was visible. At the lowest frequencies, fMRI activation tended to peak in a region centered on lateral Heschl’s gyrus. At the highest frequencies, the activation cluster appeared to have broken up into two clusters, one on the rostral bank of medial Heschl’s gyrus, bordering the central sulcus, and one on its caudal bank, bordering the planum temporale. For intermediate frequencies, intermediate patterns were observed. These trends occurred similarly in both hemispheres and in both subject groups.
FIGURE 3. (A) Mean intensity projections of the activation to all sound stimuli (interpolated to 40 dB HL) in the controls and patients separately. (B) A principal component decomposition of the frequency-dependent response profiles across all voxels and all subjects resulted in a first component that summarized the general activation levels, and a second component that reflected the frequency-selectivity that differed between voxels. (C) For various mixtures of the first and second principal components’ frequency response profiles, one may obtain response behaviors that range from low- to high-frequency tuning as the ratio of the coefficients x2/x1 increases from negative to positive values. (D) Spatial maps of the ratio x2/x1 reveal the tonotopic organization of the auditory cortices. (E) By color-coding the gradient direction of the maps in (D), multiple parallel strips of cortex are distinguishable.
In order to summarize these activation maps more concisely, principal component analysis was employed. Figure 3B shows the first (left) and second (right) principal component’s spatial response maps x1,2 (averaged across the controls or patients separately), together with their corresponding frequency response profiles f1,2 (which apply to both groups equally). Unsurprisingly, for the first principal component, the spatial map well summarized the typical activation pattern that was already observed in Figure 3A, and the shape of the frequency profile well resembled the ROI average in Figure 2A. More interestingly, the second principal component summarized how voxels primarily deviated from that typical behavior. The frequency profile showed a monotonous and gradual increase from negative values (at low frequencies) to positive values (at high frequencies). In the spatial map, positive coefficients were encountered bilaterally on the rostral and caudal banks of medial Heschl’s gyrus, and negative values were found on its lateral crest. In combination, this means that the medial endpoints shown in blue tended to respond more strongly to high-frequency stimuli and less strongly to low-frequency stimuli, as compared to the average behavior of all voxels. Contrariwise, in the lateral endpoint shown in red, responses were stronger in response to low-frequency stimuli and weaker in response to high-frequency stimuli. This reflects two tonotopic representations on the rostral and caudal banks of Heschl’s gyrus. Comparing the second component’s spatial maps, these representations existed in a highly similar form for both subject groups.
Because the frequency profile for an individual voxel, as estimated by a combination of the profiles shown in Figure 3B, depends on the relative contribution of the first and the second principal component in that voxel (contained in x1 and x2, respectively), the ratio x2/x1 was calculated for each voxel. Figure 3C illustrates the shape of various mixtures of frequency profiles that may be obtained for a number of different ratios. The profile shifts from low to high frequencies as the ratio increases from negative to positive values. Therefore, the ratio x2/x1 may serve to quantify a voxel’s frequency tuning, where low/negative values indicate low-frequency tuning and high/positive values indicate high-frequency tuning.
Figure 3D displays the resulting ratio map. Qualitatively, the obtained pattern was very similar to that of the second component alone, but results were more pronounced toward the edges of the cluster, where responses were weakest. Moreover, a secondary low-frequency endpoint was more clearly found to exist in lateral planum temporale, posterior to the other low-frequency endpoint in lateral Heschl’s gyrus. Again, both groups showed highly similar results overall. Perhaps the most striking difference was observed in the left lateral Heschl’s gyrus, where the healthy controls showed highly pronounced low-frequency responses (dark red) whereas the tinnitus patients showed only moderately pronounced low-frequency responses (orange red). This is related to the fact that in this vicinity the first component’s spatial map was weaker in the controls than in the patients (see Figure 3B; further corroborated by Figure 2B), whereas the second component’s spatial map still showed similar magnitudes.
Finally, the direction of the gradient in the transverse maps of Figure 3D is color-coded in Figure 3E. In both hemispheres, a series of “strips” of auditory cortex could be distinguished, tentatively corresponding with distinct functional fields in auditory cortex. Fields were aligned more or less parallel to the axis of Heschl’s gyrus. On the rostral bank of Heschl’s gyrus, the low-to-high tonotopic gradient was homogeneously oriented in an anteromedial direction. Toward the caudal bank of Heschl’s gyrus, it showed a sharp transition to another homogeneous region where the gradient was oriented in the posterior direction. Further caudally, on the planum temporale, another gradient reversal was visible, followed possibly by yet another reversal near the temporoparietal junction. Again, comparable results were obtained for both groups.
In an effort to elucidate potential differences regarding the tonotopic organization in the two groups, we plotted the strength of the first component (x1) versus that of the second component (x2) in Figure 4A. Each voxel contributes one data point. Results are shown separately for the mean maps derived from the controls (left) and patients (right). In Figure 4B these data were transformed into a representation where the ratio x2/x1, reflecting frequency tuning, was plotted on the horizontal axis, and the value x1, reflecting the overall activation level, was plotted on the vertical axis. This once more shows that the strongest responses (i.e., high x1) occurred for voxels that were tuned to the lower frequencies (i.e., negative x2/x1). Some differences were visible in the shape of the data cloud of both groups, but the overall distributions were rather similar. To statistically quantify and assess the differences between both groups, we compared these outcomes in various ways. First, in Figure 4C, the value x1 (top) and the ratio x2/x1 (bottom) are plotted for one group versus the other. In other words, the response level and frequency tuning, respectively, of a particular voxel in one group is compared to that of the same voxel (i.e., at the same location in stereotaxic space) in the other group. The resulting scatter plots can both be seen to cluster along the diagonal. Any difference in excitability or any shift in frequency tuning would have been visible as a systematic deviation of the data cloud from the diagonal, but apart from stochastic variations hardly any such deviations were observed. Second, in Figure 4D we compared the marginal distributions of the scatter plots in Figure 4B by plotting the histograms of the value x1 (top) and the ratio x2/x1 (bottom). These plots also include the median and the surrounding 95% confidence intervals for these histograms, obtained by repeatedly sampling 20 random subjects (dashed lines). The overall shape of the distributions differed little, and did not significantly differ from that obtained from any random group. Third, in Figure 4E, we plotted the cumulative density function (cdf) of the value x1 (top) and the ratio x2/x1 (bottom) for one group versus the other, as is commonly done in the Kolmogorov-Smirnov test for statistical equality of distributions. If the groups show identical distributions, the result is a straight line along the diagonal. Again, the bootstrapped median and 95% confidence interval are shown by dashed lines. The obtained curves did not significantly deviate from the diagonal anywhere.
FIGURE 4. (A) The coefficients in the first and second components’ spatial response maps (see Figure 3B) were plotted against each other. Each data point corresponds with one voxel. The diagonal lines show where the ratio x2/x1 remains constant. (B) Transforming the representation in (A), the ratio of the first and second components’ coefficients was plotted against the coefficient of the first component. The vertical coordinate of a voxel’s data point reflects its sound-evoked activation level, and the horizontal coordinate reflects its frequency tuning. (C) A plot of the sound-evoked activation level (top) and frequency tuning (bottom), as quantified by the value x1 and ratio x2/x1, respectively, comparing healthy controls and tinnitus patients. (D)The probability density function (pdf) of the value x1 (top) and ratio x2/x1 (bottom) is plotted in the form of a histogram. Dashed lines indicate the median and 95% confidence intervals as obtained from a bootstrap procedure. (E)The corresponding cumulative density function (cdf) in both groups are plotted against each other. Again, dashed lines indicate the bootstrap median and 95% confidence intervals.
In Figure 5A, the second principal component’s spatial map (x2) is shown for each subject individually. (For individual subjects we avoided the ratio x2/x1 because it resulted in ill-defined values for a large abundance of weakly activated voxels due to “division by zero” divergences when x1 ≈ 0.0.) Per group, subjects were sorted in order of decreasing Pearson correlation (R) with the average map over all 40 subjects. In both groups, some subjects were highly representative of the mean (R > 0.5), whereas some others were not at all (R ≈ 0.0). Still, the median correlations (R = 0.44 for controls; R = 0.43 for patients) were substantial and did not appreciably differ between groups.
FIGURE 5. (A) Individual spatial response maps of the second principal component x2 for all subjects in the two groups, arranged in order of decreasing similarity with the mean map of all subjects (as quantified by Pearson correlations R). (B) The detailed but complex representations in (A) were projected onto a two-dimensional feature space that still captured a maximal amount of variance by means of principal component analysis. Each subject is represented by a colored symbol at coordinates that reflect the loading on the two features.The axes are labeled with images that display the spatial response maps corresponding with the dimensions of the feature space, as are various mixtures along diagonals in between. The further from the origin, the more pronounced a feature is represented in a subject.
Because it is hard to oversee differences between subjects in these map representations, we reduced the dimensional complexity of the data by projecting them onto a two-dimensional “feature space” that was obtained by means of principal component analysis, thus ensuring that a maximal amount of variance was retained (a procedure conceptually analogous to multidimensional scaling). In Figure 5B, the loadings of the individual subjects are plotted. The axes are labeled with images that show several mixtures of features that are represented in the various directions. The upward vertical axis, which explains the largest amount of signal power in the maps, well depicts the typical tonotopic layout that was already observed in Figure 3B (the downward vertical axis depicts negative loadings, where high- and low-frequency endpoints are therefore reversed). The rightward horizontal axis shows an overall representation of high frequencies (cyan colors) and the leftward horizontal axis shows an overall representation of low frequencies (yellow colors). The further from the origin, the more pronounced these features were represented in individuals. Subjects were typically located in the segments between the 11 o’clock and 2 o’clock positions, and therefore mostly showed a rather similar tonotopic map, with a slight over- or underrepresentation of higher or lower frequencies. The centroids of the two groups were located close to each other, especially when compared with the spread across individuals, indicating that the groups did not significantly differ with respect to these features. Although it is questionable whether these data can be formally assumed to be normally distributed, an F-test indeed confirmed that the groups did not differ significantly (p = 0.90).
Finally, to exclude that the obtained outcomes regarding tonotopic maps were dominated by the (stronger) low-frequency responses to such a degree that differences in the (weaker) high-frequency responses were rendered undetectable, we repeated the principal component decomposition and all subsequently described statistical analyses on the basis of only the half of the response data that concerned the 2-, 4-, and 8-kHz stimuli. Again, none of the tests resulted in significant (or nearly significant) outcomes, similarly indicating that the mean tonotopic maps were highly comparable in both groups.
In this study, we determined tonotopic representations in the bilateral human auditory cortices. The current stimulus and acquisition paradigm was identical to that in a previous publication (Langers and van Dijk, 2011). There, we reported in more detail on the current control group only, and demonstrated that the employed experimental setup may be used to robustly extract tonotopic maps. For the purpose of the present paper, the subject group was extended to include tinnitus patients that were matched with respect to hearing loss. Our goal was to test the hypothesis that tinnitus results from an abnormal tonotopic organization of the auditory cortex. We did not find supporting evidence for any such reorganization.
In spite of the fact that we well reproduced our previous findings in the control group, the analyses that we currently performed differed from our earlier report in two respects that are worth noting.
Firstly, instead of detailing the activation in response to the louder and softer set of sound stimuli separately, we interpolated our data to uniform intensity levels of 40 dB HL. The reason for this was that, unlike in our previous report, we currently did not mean to study the spread of activation that increasingly occurs at higher intensity levels. Because sound-evoked activation in primary auditory cortex as measured by fMRI increases more or less linearly with the stimulus intensity level in normal hearing subjects (Hall et al., 2001; Brechmann et al., 2002; Langers et al., 2007), the employed interpolation enabled us to largely account for differences in the presentation level across stimulus frequencies. We still observed a decline of the overall activation level as a function of frequency. This may partly be attributed to the small amount of hearing loss at the highest presentation frequencies.
Secondly, instead of simply averaging the response data across subjects for the purpose of principal component decomposition, we concatenated all data to obtain a single data matrix (B). The resulting data reduction method has been analogously incorporated in independent component analyses (Svensén et al., 2002). In contrast to our previous report, the present approach does not assume spatial response characteristics (including tonotopic representations) to be identical across subjects or groups. Instead, for each principal component, individual spatial response maps were obtained that were still identically interpretable across subjects due to the fact that they shared the same frequency response profile. This allowed us to statistically test for differences between the two subject groups in a data-driven but unbiased manner.
Using a conventional linear regression model, we found significantly stronger activation in the patients than in the controls in the vicinity of the low-frequency endpoint of the tonotopic map in left lateral Heschl’s gyrus (see Figure 2B). Note that the low-frequency preference of this cortical region does not agree with the typical high-pitched tinnitus percept. However, the lateral extreme of Heschl’s gyrus has also been proposed to subserve pitch processing in humans (Patterson et al., 2002; Penagos et al., 2004; Puschmann et al., 2010). Although high and low pitches may be processed in different ways (Oxenham et al., 2004) and the precise role of this area remains obscure (Hall and Plack, 2009; Barker et al., 2011), this interpretation suggests that the abnormal activity in tinnitus patients may be related to pitch extraction. Ongoing anomalous activity in such a center can easily be conceived to induce the percept of a continuous tone-like sound. The observed activity can be argued to result from tinnitus, since the ongoing presence of a tone-like phantom percept that is generated lower in the auditory pathway may well induce abnormal activity in an area that is dedicated to the assessment of pitch. Alternatively, this hyperactivity may be construed to underlie the cause of tinnitus itself. In either case, one expects elevated activity during silence in the presence of tinnitus, which would diminish rather than enlarge the contrast with the activation that occurs during the perception of true sound. Yet, at the same time, an intrinsically hyperexcitable pitch processing center may respond excessively to true sound as well. The latter effect would be consistent with our findings.
Interestingly, due to the superficial location of this area in the brain, it forms an accessible target for non-invasive therapeutic interventions. Our finding may therefore help explain the reported success of some recently developed transcranial magnetic stimulation (TMS) therapies that target left auditory cortex (Burger et al., 2011; Chung et al., 2012).
Hyperexcitability might also clarify why tinnitus is commonly accompanied by hyperacusis (Baguley, 2003; Møller, 2007b). Our patient group showed evidence for hyperacusis, both objectively in the form of a reduced dynamic range of audible but tolerable sound intensities, and subjectively on the basis of self-reported complaints in a hyperacusis questionnaire. It has been forwarded that hyperacusis forms a confound in fMRI and abnormal activity levels that are ascribed to tinnitus might actually be due to hyperacusis, particularly for the subcortical auditory nuclei (Gu et al., 2010). In the present study, we found only limited elevations in cortical sound-evoked response levels, since activation was not significantly different between groups in most of the auditory cortex. This suggests that hyperacusis did not play a dominant role, possibly as a result of the low sound presentation levels that were employed. However, this equality can also be argued to result from two opposing effects that happen to cancel each other: an increase in activation related to hyperacusis (due to hyperexcitability), and a concomitant decrease in activation due to tinnitus itself (for instance due to elevated levels of activity during baseline already). Because we are unable to disentangle these two subtle effects, their possible extent and magnitude presently remains speculative.
Apart from the aforementioned significant focal difference, we found remarkably few discrepancies between the control subjects and the tinnitus patients. In particular, the tonotopic maps that were extracted from both groups looked highly similar at first glance. Various detailed comparisons across voxels (Figure 4) and across subjects (Figure 5) subsequently indeed confirmed that any differences that did occur between the two groups could be completely ascribed to chance. We therefore found no evidence for macroscopic tonotopic reorganization in tinnitus patients. In particular, we found no signs of a systematic over-representation of the moderate to high sound frequencies that corresponded with the tinnitus pitch itself or its spectral edge. Nevertheless, a number of practical limitations should be kept in mind.
A first important limitation is that the fact that our data did not result in the rejection of the null-hypothesis should not be interpreted as proof that the null-hypothesis is true. If tonotopic reorganization takes place in a subtle form (for instance if it induces only a weak shift in the measurable best frequencies, or if it is confined to a small subregion of auditory cortex only), then the statistical power of our study may have been inadequate and our methodology insufficiently sensitive to detect it. However, the cortical tonotopic reorganizations that have been observed in animal studies are far from subtle, as response characteristics have been found to show changes that are immediately obvious and that extend over multiple octaves in the tonotopic map (Stolzberg et al., 2011; Yang et al., 2011). Although it is difficult to formulate and test a precise alternative hypothesis that would correspond with these animal data, the comparison in Figure 3D does not indicate any such large-scale reorganizations.
Moreover, our analyses were able to detect differences between the tonotopic organizations in individuals, as becomes clear from Figure 5. We do not attribute this variability to inaccuracies in the measurement method alone (i.e., “noise”). Within subjects, the detected tonotopic representations were still organized into large-scale patterns that exceeded the inherent resolution of the data. Therefore, we believe that the observed inter-subject variations reflect actual differences in the individual cortical organization. Then our inability to distinguish between healthy controls and tinnitus patients on the basis of their tonotopic organization is not a limitation of our paradigm, but an inherent characteristic of these populations instead. Although perhaps differences between the means of the two groups might have turned out significant if substantially larger numbers of subjects had been considered, these would then still have been clinically insignificant: the macroscopic tonotopic organization cannot serve as a practical criterion to objectify tinnitus in individuals.
A second limitation is related to the nature of the measurements that we performed. Functional MRI relies on hemodynamic changes in the volume and oxygenation of the brain’s local blood supply that occur as a result of variations in the tissue’s metabolic demand. These variations have been shown to be related to neuronal firing as well as synaptic events like the release and reuptake of excitatory and inhibitory neurotransmitters (Logothetis et al., 2001; Logothetis, 2008). However, importantly, fMRI has a limited temporal and spatial resolution. Functional MRI may be completely insensitive to the synchronicity of neuronal discharges, and the measured signal at best forms a correlate of the integrated activity over a large collection of neurons. If tonotopic reorganization is expressed only as a change in synchronicity or as a change in the fine-grained distribution of neural activity across microscopic cortical columns, for instance, then this will have remained undetected in this study. Other techniques (like electro- and magnetoencephalography, or electrophysiological recordings) may prove sensitive to some of these changes (Mühlnickel et al., 1998; Wienbruch et al., 2006), but these have so far been less successful in mapping the detailed cortical tonotopy in humans either because of their limited ability to distinguish activity from numerous sites simultaneously or because of their invasive nature.
A third limitation of this study may be related to the subject group. We deliberately included tinnitus patients with normal hearing thresholds over most of the range of audible frequencies in order to be able to attribute any detectable deviations specifically to the presence of tinnitus but not hearing loss. Although near-normal hearing is not entirely uncommon, tinnitus patients typically show substantially elevated thresholds at moderate frequencies already. Even in tinnitus patients with allegedly normal hearing thresholds, high-frequency hearing loss may occur that has been missed due to the fact that standard audiometry often does not extend beyond 8 kHz. Of particular importance in the comparison with previous results from animal studies, tinnitus in animals is often induced by acoustic trauma and can be accompanied by hearing loss in excess of 40 dB (Noreña et al., 2003; Yang et al., 2011). In contrast, for sufficiently small damage to the peripheral hearing organ, the balance between excitation and inhibition was shown not to change, suggesting that tonotopic maps are not required to shift under such circumstances (Rajan, 2001). Therefore, this study may inadvertently have considered a special subgroup in which tonotopic reorganization plays no role (Barnea et al., 1990), whereas this mechanism may still remain important in the patient population as a whole. Or, perhaps tonotopic reorganizations in these patients occurred only in areas tuned to frequencies beyond 8 kHz, where some hearing loss was observed, but which exceeded the highest presentation frequency in this study.
Still, except for a relative lack of hearing loss, this subpopulation of patients is surprisingly typical. This may be argued for our subjects in particular based on the data in Table 1 , but it has been independently pointed out for a much larger subject group previously (Sanchez et al., 2005). On average, tinnitus patients with normal hearing thresholds are younger (likely causing better thresholds) and less burdened (likely as a result of better thresholds) than the tinnitus population as a whole, but the perceived acoustic attributes of their tinnitus percept are remarkably similar. This raises the possibility that perhaps these patients have a type of hearing loss that conventional tone-audiometry is insensitive to. For instance, substantial loss of neurons in the cochlear nerve (especially neurons with high thresholds and low spontaneous activity) and even loss of inner hair cells may remain unnoticed if, in contrast to dead regions, such losses occur sparsely spread across a range of audible frequencies. Alternatively, the plastic changes may have been induced by temporary hearing loss that later recovered without reverting the tonotopic map back to normal. In conclusion, even though peripheral damage was not proven in our subject group, except at extreme frequencies, it is still conceivable that similar mechanisms may have occurred at the central level, resulting in tinnitus (Weisz et al., 2006; Kujawa and Liberman, 2009; Schaette and McAlpine, 2011).
We conclude by comparing our findings with models that attribute tinnitus to cortical plasticity, either homeostatic plasticity through upregulation of central gain or tonotopic plasticity through shifts in characteristic frequency. Figure 6A shows a highly simplified diagram of how sound information is normally transmitted from the periphery to the auditory cortex along numerous frequency channels in parallel. Of course, in reality complex signal transformations take place in several intermediate processing stages that comprise cross-channel integration of afferent as well as efferent information, and we do not mean to claim that these cannot play a role in tinnitus, but for the sake of clarity these intricacies will be presently overlooked.
FIGURE 6. (A) A simplified diagram showing transmission of acoustic information from the peripheral to the central auditory system across multiple parallel channels.The colors represent frequency tuning. (B) After complete high-frequency hearing loss (top panel), sensory deprivation leads to permanently reduced activity in the affected channels (symbolized by dotted lines and open circles). Following homeostatic upregulation (middle panel), neurons with increased gain (indicated with plusses) respond even to low levels of spontaneous activity. Alternatively, following tonotopic reorganization (bottom panel), large numbers of neurons receive input from the edge-frequency region. (C) In the present study, high-frequency hearing thresholds were largely normal, suggesting that sufficient hair cells and neurons in the affected frequency regions remained intact. Homeostatic upregulation or tonotopic reorganization would then be limited to the interspersed deprived neurons only, but would still lead to elevated activity or enhanced synchronicity, respectively, resulting in the presence of a tinnitus percept.
The top panel in Figure 6B illustrates what may happen after complete loss of hearing at high frequencies. Various channels are deprived from sensory input and their activity will permanently drop (indicated by the dotted lines and open circles). This provides a strong incentive for plasticity to occur, as depicted in the lower panels. Homeostatic upregulation may reinstate a normal rate of activity by increasing the gain in the affected channels. The model predicts that this induces elevated levels of spontaneous activity, which would be perceived as a high-pitched tinnitus. Alternatively, tonotopic reorganization may entice the high-frequency channels to start responding to input from the nearest frequency regions that still retain normal input, i.e., from the edge-frequency region. Thus, high-frequency regions in the central auditory system become massively tuned to a limited amount of sensory input that originates from a small tonotopic region in the periphery. This induces enhanced synchronicity across the high-frequency region, which could be similarly perceived as a high-pitched tinnitus. The fact that we found normal tonotopic representations in tinnitus patients would at first sight contradict the model that involves tonotopic reorganization.
However, our patients showed normal thresholds in much of the high-frequency range, suggesting that sensory deprivation may have been partial, and some fraction of neurons with intact input may have survived throughout the tonotopic axis. These may suffice in order to detect the presence of sound (explaining the normal thresholds), but there would still be loss of input such that plastic reorganization can occur (explaining the tinnitus). This situation is schematically depicted in Figure 6C. The model involving homeostatic upregulation would predict that elevated levels of spontaneous activity still occur for the deprived subpopulation of neurons, similarly leading to tinnitus as in the condition with complete loss. The model involving tonotopic reorganization might now predict negligible shifts in characteristic frequency, because neurons can still obtain input from intact nearby channels. Depending on the scale at which losses are clustered this might lead to a slightly coarser, more granular representation, but the macroscopic tonotopic organization would survive. Still, large numbers of neurons would be excited by a small number of inputs, thus increasing neural synchronicity, again leading to tinnitus. Following this argument, even though we exclude that large-scale macroscopic tonotopic reorganization is required for tinnitus to arise, our results can still be brought into agreement with a model based on tonotopic reorganization. However, it should be realized then that abnormalities can be limited to microscopic dimensions, and the resulting enhanced synchronicity is a more direct correlate of tinnitus than the reorganization itself.
Finally, we note that our data also showed little evidence for homeostatic changes in central gain. As far as elevated levels of excitability were observed, they occurred in low-frequency regions in the left hemisphere. This is hard to reconcile with the prediction that neural gain would be upregulated in the high-frequency regions where the tinnitus pitch was found. In contrast, in high frequency regions, no significant elevations in activity were found. However, for this model as well, our findings might be explained to some degree if the elevation in spontaneous activity would happen to equal the elevation in evoked activity. Because fMRI is sensitive only to the contrast between these conditions, such an overall effect might remain unobservable.
In conclusion, our findings strongly suggest that macroscopic tonotopic reorganization is not required for tinnitus to arise, at least in patients with normal hearing or mild hearing loss only. Although this observation can be reconciled with prevailing models regarding the pathophysiology of tinnitus in the central auditory system, it sheds a new and subtle light on how these mechanisms may naturally take shape. We plan to extend this study to subjects with hearing loss, both with and without tinnitus, in an effort to further unravel tinnitus pathophysiology in humans.
The authors declare that the research was conducted in the absence of any commercial or financial relationships that could be construed as a potential conflict of interest.
The author Dave R. M. Langers was funded by VENI research grant 016.096.011 from the Netherlands organization for scientific research (NWO) and the Netherlands organization for health research and development (ZonMw). Further financial support wasprovided bythe HeinsiusHoubolt Foundation. We thank Eline Deenen for the audiometric evaluation of all subjects.
Barker, D., Plack, C. J., and Hall, D. A. (2011). Reexamining the evidence for a pitch-sensitive region: a human fMRI study using iterated ripple noise. Cereb. Cortex. doi: 10.1093/cercor/bhr065 [Epub ahead of print].
Barnea, G., Attias, J., Gold, S., and Shahar, A. (1990). Tinnitus with normal hearing sensitivity: extended high-frequency audiometry and auditory-nerve brain-stem-evoked responses. Audiology 29, 36-45.
Baumgart, F., Kaulisch, T., Tempelmann, C., Gaschler-Markefski, B., Tegeler, C., Schindler, F., Stiller, D., and Scheich, H. (1998). Electrodynamic headphones and woofers for application in magnetic resonance imaging scanners. Med. Phys. 25, 2068-2070.
Brand, T., and Hohmann, V. (2002). An adaptive procedure for categorical loudness scaling. J. Acoust. Soc. Am. 112, 1597-1604.
Brechmann, A., Baumgart, F., and Scheich, H. (2002). Sound-level-dependent representation of frequency modulations in human auditory cortex: a low-noise fMRI study. J. Neurophysiol. 87, 423-433.
Budd, R. J., and Pugh, R. (1996). Tinnitus coping style and its relationship to tinnitus severity and emotional distress. J. Psychosom. Res. 41, 327-335.
Burger, J., Frank, E., Kreuzer, P., Kleinjung, T., Vielsmeier, V., Landgrebe, M., Hajak, G., and Langguth, B. (2011). Transcranial magnetic stimulation for the treatment of tinnitus: 4-year follow-up in treatment responders a retrospective analysis. Brain Stimul. 4, 222-227.
Burrone, J., and Murthy, V. N. (2003). Synaptic gain control and homeostasis. Curr. Opin. Neurobiol. 13, 560-567.
Chrostowski, M., Yang, L., Wilson, H. R., Bruce, I. C., and Becker, S. (2011). Can homeostatic plasticity in deafferented primary auditory cortex lead to travelling waves of excitation? J. Comput. Neurosci. 30, 279-299.
Chung, H.-K., Tsai, C.-H., Lin, Y.-C., Chen, J.-M., Tsou, Y.-A., Wang, C.-Y., Lin, C.-D., Jeng, F.-C., Chung, J.-G., and Tsai, M.-H. (2012). Effectiveness of theta-burst repetitive transcranial magnetic stimulation for treating chronic tinnitus. Audiol. Neurootol. 17, 112-120.
Da Costa, S., van der Zwaag, W., Marques, J. P., Frackowiak, R. S. J., Clarke, S., and Saenz, M. (2011). Human primary auditory cortex follows the shape of Heschl’s gyrus. J. Neurosci. 31, 14067-14075.
Dominguez, M., Becker, S., Bruce, I., and Read, H. (2006). A spiking neuron model of cortical correlates of sensorineural hearing loss: spontaneous firing, synchrony, and tinnitus. Neural Comput. 18, 2942-2958.
Edmister, W. B., Talavage, T. M., Ledden, P. J., and Weisskoff, R. M. (1999). Improved auditory cortex imaging using clustered volume acquisitions. Hum. Brain Mapp. 7, 89-97.
Eggermont, J. J. (2007b). Correlated neural activity as the driving force for functional changes in auditory cortex. Hear. Res. 229, 69-80.
Engineer, N. D., Riley, J. R., Seale, J. D., Vrana, W. A., Shetake, J. A., Sudanagunta, S. P., Borland, M. S., and Kilgard, M. P. (2011). Reversing pathological neural activity using targeted plasticity. Nature 470, 101-104.
Formisano, E., Kim, D. S., Di Salle, F., van de Moortele, P. F., Ugurbil, K., and Goebel, R. (2003). Mirror-symmetric tonotopic maps in human primary auditory cortex. Neuron 40, 859-869.
Gu, J. W., Halpin, C. F., Nam, E.-C., Levine, R. A., and Melcher, J. R. (2010). Tinnitus, diminished sound-level tolerance, and elevated auditory activity in humans with clinically normal hearing sensitivity. J. Neurophysiol. 104, 3361-3370.
Hall, D. A., Haggard, M. P., Akeroyd, M. A., Palmer, A. R., Summerfield, A. Q., Elliott, M. R., Gurney, E. M., and Bowtell, R. W. (1999). “Sparse” temporal sampling in auditory fMRI. Hum. Brain Mapp. 7, 213-223.
Hall, D. A., Haggard, M. P., Summerfield, A. Q.,Akeroyd, M. A., Palmer, A. R., and Bowtell, R. W. (2001). Functional magnetic resonance imaging measurements of sound-level encoding in the absence of background scanner noise. J. Acoust. Soc. Am. 109, 1559-1570.
Hall, D. A., and Plack, C. J. (2009). Pitch processing sites in the human auditory brain. Cereb. Cortex 19, 576-585.
Hardt, J., and Gerbershagen, H. U. (2001). Cross-validation of the SCL-27: a short psychometric screening instrument for chronic pain patients. Eur. J. Pain 5, 187-197.
Herraiz, C., Diges, I., and Cobo, P. (2007). Auditory discrimination therapy (ADT) for tinnitus management. Prog. Brain Res. 166, 467-471.
Humphries, C., Liebenthal, E., and Binder, J. R. (2010). Tonotopic organization of human auditory cortex. Neuroimage 50, 1202-1211.
Khalfa, S., Dubal, S., Veuillet, E., Perez-Diaz, F., Jouvent, R., and Collet, L. (2002). Psychometric normalization of a hyperacusis questionnaire. ORL J. Otorhinolaryngol. Relat. Spec. 64, 436-442.
König, O., Schaette, R., Kempter, R., and Gross, M. (2006). Course of hearing loss and occurrence of tinnitus. Hear. Res. 221, 59-64.
Kujawa, S. G., and Liberman, M. C. (2009). Adding insult to injury: cochlear nerve degeneration after “temporary” noise-induced hearing loss. J. Neurosci. 29, 14077-14085.
Lang, P. J., Bradley, M. M., and Cuthbert, B. N. (2008). International Affective Picture System (IAPS): Affective Ratings of Pictures and Instruction Manual. Technical Report A-8. Gainesville, FL: University of Florida.
Langers, D. R. M., and van Dijk, P. (2011). Mapping the tonotopic organization in human auditory cortex with minimally salient acoustic stimulation. Cereb. Cortex. doi: 10.1093/cercor/bhr282 [Epub ahead of print].
Langers, D. R. M., van Dijk, P., Schoenmaker, E. S., and Backes, W. H. (2007). fMRI activation in relation to sound intensity and loudness. Neuroimage 35, 709-718.
Lauter, J. L., Herscovitch, P., Formby, C., and Raichle, M. E. (1985). Tonotopic organization in human auditory cortex revealed by positron emission tomography. Hear. Res. 20, 199-205.
Logothetis, N. K., Pauls, J., Augath, M., Trinath, T., and Oeltermann, A. (2001). Neurophysiological investigation of the basis of the fMRI signal. Nature 412, 150-157.
Moore, B. C. J., and Vinay, S. N. (2010). The relationship between tinnitus pitch and the edge frequency of the audiogram in individuals with hearing impairment and tonal tinnitus. Hear. Res.. 261, 51-56.
Mühlnickel, W., Elbert, T., Taub, E., and Flor, H. (1998). Reorganization of auditory cortex in tinnitus. Proc. Natl. Acad. Sci. U.S.A. 95, 10340- 10343.
Newman, C. W., Jacobson, G. P., and Spitzer, J. B. (1996). Development of the Tinnitus Handicap Inventory. Arch. Otolaryngol. Head Neck Surg. 122, 143-148.
Noreña, A., Micheyl, C., Chéry-Croze, S., and Collet, L. (2002). Psychoacoustic characterization of the tinnitus spectrum: implications for the underlying mechanisms of tinnitus. Audiol. Neurootol. 7, 358-369.
Noreña, A. J. (2011). An integrative model of tinnitus based on a central gain controlling neural sensitivity. Neurosci. Biobehav. Rev. 35, 1089-1109.
Noreña, A. J., Tomita, M., and Eggermont, J. J. (2003). Neural changes in cat auditory cortex after a transient pure-tone trauma. J. Neurophysiol. 90, 2387-2401.
Oxenham, A. J., Bernstein, J. G. W., and Penagos, H. (2004). Correct tonotopic representation is necessary for complex pitch perception. Proc. Natl. Acad. Sci. U.S.A. 101, 1421-1425.
Pantev, C., Hoke, M., Lütkenhöner, B., and Lehnertz, K. (1989). Tonotopic organization of the auditory cortex: pitch versus frequency representation. Science 246, 486-488.
Patterson, R. D., Uppenkamp, S., Johnsrude, I. S., and Griffiths, T. D. (2002). The processing of temporal pitch and melody information in auditory cortex. Neuron 36, 767-776.
Penagos, H., Melcher, J. R., and Oxenham, A. J. (2004). A neural representation of pitch salience in nonprimary human auditory cortex revealed with functional magnetic resonance imaging. J. Neurosci. 24, 6810-6815.
Pineda, J. A., Moore, F. R., and Viirre, E. (2008). Tinnitus treatment with customized sounds. Int. Tinnitus J. 14, 17-25.
Puschmann, S., Uppenkamp, S., Kollmeier, B., and Thiel, C. M. (2010). Dichotic pitch activates pitch processing centre in Heschl’s gyrus. Neuroimage 49, 1641-1649.
Rajan, R. (2001). Plasticity of excitation and inhibition in the receptive field of primary auditory cortical neurons after limited receptor organ damage. Cereb. Cortex 11, 171-182.
Rajan, R., and Irvine, D. R. (1998). Neuronal responses across cortical field A1 in plasticity induced by peripheral auditory organ damage. Audiol. Neurootol. 3, 123-144.
Rauschecker, J. P., Leaver, A. M., and Mühlau, M. (2010). Tuning out the noise: limbicauditory interactions in tinnitus. Neuron 66, 819-826.
Roberts, L. E., Eggermont, J. J., Caspary, D. M., Shore, S. E., Melcher, J. R., and Kaltenbach, J. A. (2010). Ringing ears: the neuroscience of tinnitus. J. Neurosci. 30, 14972-14979.
Roberts, L. E., Moffat, G., Baumann, M., Ward, L. M., and Bosnyak, D. J. (2008). Residual inhibition functions overlap tinnitus spectra and the region of auditory threshold shift. J. Assoc. Res. Otolaryngol. 9, 417-435.
Romani, G. L., Williamson, S. J., and Kaufman, L. (1982). Tonotopic organization of the human auditory cortex. Science 216, 1339-1340.
Sanchez, T. G., de Medeiros, I. R. T., Levy, C. P. D., da Rosa Oiti-cica Ramalho, J., and Bento, R. F. (2005). Tinnitus in normally hearing patients: clinical aspects and repercussions. Braz. J. Otorhinolaryngol. 71, 427-431.
Schaette, R., and Kempter, R. (2006). Development of tinnitus-related neuronal hyperactivity through homeostatic plasticity after hearing loss: a computational model. Eur. J. Neurosci. 23, 3124-3138.
Schaette, R., and McAlpine, D. (2011). Tinnitus with a normal audiogram: physiological evidence for hidden hearing loss and computational model. J. Neurosci. 31, 13452-13457.
Stolzberg, D., Chen, G.-D., Allman, B. L., and Salvi, R. J. (2011). Salicylate-induced peripheral auditory changes and tonotopic reorganization of auditory cortex. Neuro-science 180, 157-164.
Striem-Amit, E., Hertz, U., and Amedi, A. (2011). Extensive cochleotopic mapping of human auditory cortical fields obtained with phaseencoding fMRI. PLoS ONE 6, e17832. doi: 10.1371/journal.pone. 0017832.
Svensén, M., Kruggel, F., and Benali, H. (2002). ICA of fMRI group study data. Neuroimage 16, 551-563.
Talavage, T. M., Sereno, M. I., Melcher, J. R., Ledden, P. J., Rosen, B. R., and Dale, A. M. (2004). Tonotopic organization in human auditory cortex revealed by progressions of frequency sensitivity. J. Neurophysiol. 91, 1282-1296.
Weisz, N., Hartmann, T., Dohrmann, K., Schlee, W., and Norena, A. (2006). High-frequency tinnitus without hearing loss does not mean absence of deafferentation. Hear. Res. 222, 108-114.
Wessinger, C. M., Buonocore, M. H., Kussmaul, C. L., and Mangun, G. R. (1997). Tonotopy in human auditory cortex examined with functional magnetic resonance imaging. Hum. Brain Mapp. 5, 18-25.
Wienbruch, C., Paul, I., Weisz, N., Elbert, T., and Roberts, L. E. (2006). Frequency organization of the 40Hz auditory steady-state response in normal hearing and in tinnitus. Neuroimage 33, 180-194.
Wilson, P. H., Henry, J., Bowen, M., and Haralambous, G. (1991). Tinnitus Reaction Questionnaire: psychometric properties of a measure of distress associated with tinnitus. J. Speech Hear. Res. 34, 197-201.
Woods, D. L., Stecker, G. C., Rinne, T., Herron, T. J., Cate, A. D., Yund, E. W., Liao, I., and Kang, X. (2009). Functional maps of human auditory cortex: effectsof acoustic features and attention. PLoS ONE 4, e5183. doi: 10.1371/journal.pone.0005183.
Keywords: functional magnetic resonance imaging, auditory cortex, humans, tinnitus, tonotopy
Citation: Langers DRM, de Kleine E and van Dijk P (2012) Tinnitus does not require macroscopic tonotopic map reorganization. Front. Syst. Neurosci. 6:2. doi: 10.3389/fnsys.2012.00002
Received: 20 December 2011; Accepted: 16 January 2012;
Published online: 01 February 2012.
Edited by:
Jos J. Eggermont, University of Calgary, CanadaCopyright: © 2012 Langers, de Kleine and van Dijk. This is an openaccess article distributed under the terms of the Creative Commons Attribution Non Commercial License, which permits non-commercial use, distribution, and reproduction in other forums, provided the original authors and source are credited.
*Correspondence:Dave R. M. Langers, Department of Otorhinolaryngology/Head and Neck Surgery, University Medical Center Groningen, University of Groningen, P.O. Box 30.001, 9700 RB Groningen, Netherlands. e-mail:ZC5yLm0ubGFuZ2Vyc0B1bWNnLm5s
Disclaimer: All claims expressed in this article are solely those of the authors and do not necessarily represent those of their affiliated organizations, or those of the publisher, the editors and the reviewers. Any product that may be evaluated in this article or claim that may be made by its manufacturer is not guaranteed or endorsed by the publisher.
Research integrity at Frontiers
Learn more about the work of our research integrity team to safeguard the quality of each article we publish.