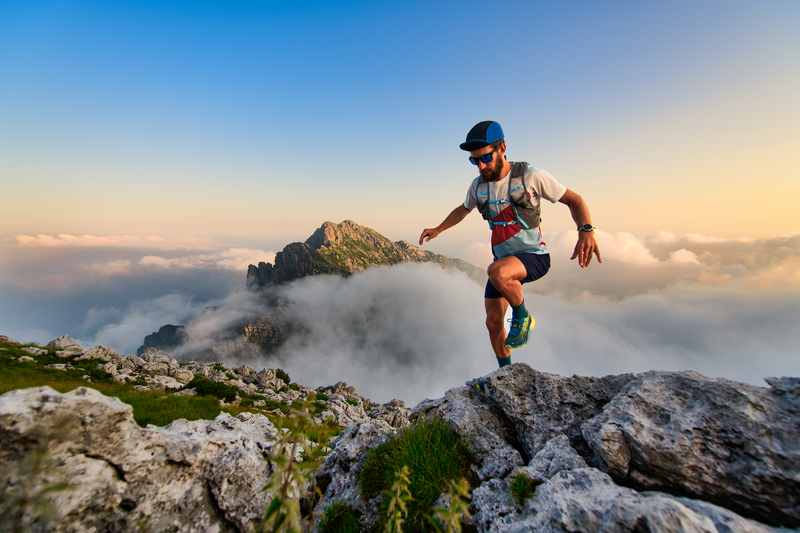
95% of researchers rate our articles as excellent or good
Learn more about the work of our research integrity team to safeguard the quality of each article we publish.
Find out more
ORIGINAL RESEARCH article
Front. Synaptic Neurosci. , 03 April 2024
Volume 16 - 2024 | https://doi.org/10.3389/fnsyn.2024.1322771
From fly to man, the Wingless (Wg)/Wnt signaling molecule is essential for both the stability and plasticity of the nervous system. The Drosophila neuromuscular junction (NMJ) has proven to be a useful system for deciphering the role of Wg in directing activity-dependent synaptic plasticity (ADSP), which, in the motoneuron, has been shown to be dependent on both the canonical and the noncanonical calcium Wg pathways. Here we show that the noncanonical planar cell polarity (PCP) pathway is an essential component of the Wg signaling system controlling plasticity at the motoneuron synapse. We present evidence that disturbing the PCP pathway leads to a perturbation in ADSP. We first show that a PCP-specific allele of disheveled (dsh) affects the de novo synaptic structures produced during ADSP. We then show that the Rho GTPases downstream of Dsh in the PCP pathway are also involved in regulating the morphological changes that take place after repeated stimulation. Finally, we show that Jun kinase is essential for this phenomenon, whereas we found no indication of the involvement of the transcription factor complex AP1 (Jun/Fos). This work shows the involvement of the neuronal PCP signaling pathway in supporting ADSP. Because we find that AP1 mutants can perform ADSP adequately, we hypothesize that, upon Wg activation, the Rho GTPases and Jun kinase are involved locally at the synapse, in instructing cytoskeletal dynamics responsible for the appearance of the morphological changes occurring during ADSP.
Synapses are the site of plastic events that shape their morphological and electrophysiological properties depending on their experiences (Shepherd, 2004; Holtmaat and Svoboda, 2009; Ho et al., 2011; Pereda, 2014; Harris and Littleton, 2015). One such plastic event is activity-dependent synaptic plasticity (ADSP), by which the synaptic efficacy can be increased or decreased, reflecting previous excitatory or inhibitory stimuli (Castillo, 2012; Lüscher and Malenka, 2012). This plasticity is thought to be the cellular correlate of learning and memory, and it is essential to improve our understanding of the molecules and molecular signals underlying this phenomenon. The secreted molecule Wg/Wnt regulates ADSP (Budnik and Salinas, 2011; Rosso et al., 2013). Indeed, activity-dependent mechanisms induce Wnt release that, in turn, induces plasticity signaling in hippocampal neurons (Chen et al., 2006; Tabatadze et al., 2014). This increase in Wnt secretion provokes structural alterations in dendritic arborizations (Yu and Malenka, 2003; Wayman et al., 2006; Ferrari et al., 2018) and spines (Ciani et al., 2011; Tabatadze et al., 2014; McLeod et al., 2018), promoting changes in synaptic strength and plasticity. Drosophila served as a model of choice to explore the instrumental role of Wg in directing ADSP (Budnik and Salinas, 2011; Bai and Suzuki, 2020). ADSP can be elicited at the NMJ when the preparation is submitted to a repeated stimulation protocol (Ataman et al., 2008; Piccioli and Littleton, 2014; Vasin et al., 2014, 2019; Maldonado-Díaz et al., 2021), similar to the one used on hippocampal neurons to elicit LTP (Wu et al., 2001). The changes associated with the NMJ experiencing ADSP are both electrophysiological and structural (Ataman et al., 2008; Alicea et al., 2017; Maldonado-Díaz et al., 2021). Live and confocal imaging, electron microscopy and tomography at the NMJ allowed for the observation of these structural changes (Ataman et al., 2008; Vasin et al., 2019). Two types of synaptic modifications appeared very quickly (within 1 h) after repeated stimulation: filipodia-like structures called synaptopods, and newly formed varicosities containing synaptic vesicles but devoid of postsynaptic structures, called ghost boutons (Figure 1A). These ghost boutons develop within 24 h into mature boutons by acquiring presynaptic active zone markers and postsynaptic glutamate receptors. Because they are easily identifiable, ghost boutons are used to assess ADSP. The Wg canonical pathway is essential for this plasticity (Ataman et al., 2008). Repeated stimulation of the NMJ promotes an increase in synaptic Wg that leads to rapid structural and electrophysiological changes (Ataman et al., 2008). In fact, Wg signaling regulates these changes bidirectionally; post-synaptically through nuclear import of its receptor, and pre-synaptically by the inhibition of GSK3ß/Sgg (Ataman et al., 2008). In addition, there is evidence that the calcium pathway is involved in this plasticity. In this pathway, an increase in intracellular calcium activates CaMKII and the transcription factor NFAT (Koles and Budnik, 2012). The neuronal expression of NFAT subunit A at the NMJ prevents the formation of ghost boutons upon stimulation (Freeman et al., 2011). In addition, synaptic CaMKII protein expression increases upon stimulation which correlates with de novo bouton formation (Nesler et al., 2016). However, to date the third pathway that responds to the Wg/Wnt signal, the planar cell polarity (PCP) pathway, has not yet been assessed for its role in regulating ADSP.
Figure 1. Dishevelled and its planar cell polarity domain regulate ADSP. (A) Schematic diagram representing a NMJ labeled with pre-synaptic (red) and post synaptic (green) markers. Upon stimulation, de novo synaptic boutons called “ghost boutons” are identifiable because they only show the pre-synaptic marker HRP. These structures, with time, will acquire post-synaptic differentiation. (B) Representative confocal photograph of an unstimulated control synapse showing HRP (presynaptic marker) and DLG (postsynaptic marker) immunoreactivity. (C) Representative confocal photograph of a stimulated control synapse. Arrowheads point to ghost boutons. (D) Representative confocal photograph of a stimulated synapse expressing dsh RNAi. (E) Schematic diagram illustrating the components of the Wg planar cell polarity pathway. (F–M) Quantification of the ghost bouton number in (F) unstimulated and (G) stimulated control and preparations expressing dsh RNAi constitutively; (H) unstimulated and (I) stimulated control and preparation carrying the suppressed conditional expression system at 18°C; (J) unstimulated and (K) stimulated control and preparation expressing dsh RNAi for 48 h prior to the stimulation (activated expression system at 29°C); (L) unstimulated and (M) stimulated control and dsh1 mutant preparations. Scale bar: 10 μm. (F,H) Mann–Whitney test. (G,I–M) Two-tailed, unpaired t-tests: *p < 0.05; **p < 0.01. Data are shown as scatter plots and means ± SEM.
Here we use the Drosophila NMJ model to ask whether the Wg PCP pathway (Figure 1E) is involved in ADSP. We use the appearance of de novo synaptic structures, ghost boutons, to quantify the extent of these changes. Because of their rapid development (90 min following stimulation), ghost boutons represent early pre-synaptic structures that are devoid of post-synaptic differentiation (Figure 1A). This characteristic has been widely used to easily identify ghost boutons at the NMJ, in order to quantify ADSP (Ataman et al., 2008; Fuentes-Medel et al., 2009; Freeman et al., 2011; Nesler et al., 2013, 2016; Piccioli and Littleton, 2014; Vasin et al., 2014, 2019; Alicea et al., 2017; Maldonado-Díaz et al., 2021). Our present work investigates the role of the PCP pathway in the production of these morphological synaptic changes. We find that several pathway members are involved in this process. Indeed, we first show that a PCP-specific dsh allele (Perrimon and Mahowald, 1987) is necessary for optimal ADSP. We then show that activity of the Rho1, Rac1, and Cdc42 GTPases can also influence ADSP. We then investigate the Jun kinase (JNK), an effector of these GTPases (Coso et al., 1995; Rosso et al., 2005) and show that its loss of function is detrimental to ADSP; however, the transcription factor AP1 (Fos/Jun), a target of JNK (Sanyal et al., 2002; Etter et al., 2005), is not necessary for this process. In most of our experiments, we use an inducible RNA expression system to selectively down- or up-regulate the activity of the members of the PCP pathway pre-synaptically only (post-synaptic expression is unchanged) and late in development (after the establishment of the NMJ). This allows us to conclude that the effects we observed are due to a change in the PCP pathway activity within the motoneuron and not a consequence of developmental perturbation. On the contrary, we argue that it reflects a need for the PCP pathway within the motoneuron to achieve optimal ADSP.
For experiments using the elavc155-gal4 insertion on the X chromosome, only females were used (in order to consider heterozygote animals). In the other experimental procedures, animals from both sexes were used. Flies were reared on standard fly food at 18°C for conditional experiments or at 25°C in all other cases. Our methods are consistent with standard husbandry and care. Our laboratory follows ethical practices while carrying out experiments as well as the cataloging of Drosophila. The fly strains used in this study include dsh1 [Bloomington Drosophila stock center (BDSC), stock #5298], and w1 (BDSC, stock #145) as a control. We used the Gal4/UAS system (Brand and Perrimon, 1993) to express dominant-negative or constitutively active genes in neurons. For this, we used the pan-neuronal driver elavc155-gal4 (BDSC, stock #458) whose expression starts early in embryogenesis (after neuroblast mitosis) in combination with UAS-dsh-RNAi (BDSC, stock #31306), UAS-rac1N17 (BDSC, stock #6292), UAS-rac1V12 (BDSC, stock #6291), UAS-rho1N19 (BDSC, stock #58818), UAS-rho1V12 (BDSC, stock #58817), UAS-cdc42N17(BDSC, stock #6288), UAS-cdc42V12 (BDSC, stock #4854), Tub-Gal80TS (BDSC, stock #7108), UAS-jnkDN (BDSC, stock #9311), UAS-fosDN (BDSC, stock #7215) and UAS-junDN (BDSC, stock #7218). The controls for these experiments were the driver elavc155-gal4/+. For the conditional experiments, we made a fly containing the elavc155-gal4 on the first chromosome and Tub-Gal80TS on the second chromosome. This fly expresses the thermosensitive inhibitor Gal80TS (McGuire et al., 2004) under the control of an ubiquitous tubulin (Tub) promoter. Larvae for the conditional experiments were reared at 18°C, where Gal80TS inhibits protein expression by binding to the Gal4 activator. Then, we shifted them to 29°C, inhibiting Gal80TS and therefore allowing the expression of specific proteins activated by Gal4. Considering the lethality and the axon pathfinding errors associated with the early expression of these transgenes, we decided to limit their expression to 48 h before performing the stimulation protocol. At this time, we minimize the effect of the transgenes’ expression on synapse development and growth while affecting their function for ADSP.
We dissected the body wall muscles from third instar larvae in hemolymph-like HL3 saline (70 mM NaCl, 10 mM NaHCO3, 115 mM sucrose, 5 mM trehalose, 5 mM HEPES, 10 mM MgCl2), leaving unharmed the CNS and the peripheral nerves innervating the body wall muscles. The protocol was carried out on partially intact and non-stretched preparations. The stimulation protocol that we used was adapted from Ataman et al. (2008) and is constituted of 5 stages that alternate stimulation and rest periods. In the first three stages the preparations were stimulated for 2 min followed by a 15 min rest period. The fourth stage is composed of a 4 min stimulation period followed by a 15 min rest while the fifth and last stage is composed of a 6 min stimulation period followed by a 14 min rest. The 5 stimulation periods were elicited using a potassium- and calcium-rich HL3 saline solution (90 mM KCl and 1.5 mM CaCl2), while during the five rest periods a HL3 saline containing 5 mM KCl and 0.1 mM CaCl2 was applied.
After stimulation, the preparations were fixed for 15 min at room temperature in a solution of 4% paraformaldehyde in PBS before being incubated overnight at 4°C within a primary (anti-Dlg) antibody solution (1:20; Budnik et al., 1996). The secondary antibody (1,300; Alexa Fluor 488-conjugated AffiniPure goat anti-mouse or anti-rabbit, Jackson ImmunoResearch) and Anti-Hrp (1,300; Cy3-conjugated AffiniPure goat anti-horseradish peroxidase, Jackson ImmunoResearch; Jan and Jan, 1982) were applied for 1 h at room temperature as previously described (Alicea et al., 2017; Maldonado-Díaz et al., 2021).
We treated control preparations in parallel with each experimental genotype to account for the potential variation in our experimental manipulations. The control preparations were designed to contain the experimental preparations’ genetic background (w1 for dsh1 or the corresponding gal4 driver for the different expression systems used). Control and experimental preparations were also reared at the same temperature and submitted to the same shift (18–29°C) when applicable. We identified a ghost bouton as a bouton that had a positive immunoreactivity to anti-HRP and negative immunoreactivity to anti-Dlg. In all the experiments, ghost boutons were counted on muscle 6/7 NMJs, in A3 segments of third instar larvae. To carry out these observations, we used a Nikon Eclipse 80i microscope at a magnification of 400X.
We first identified any outlier using the ROUT method with a Q = 0.1%. Then, we assessed whether the data fitted a normal distribution using the Shapiro–Wilk normality test. For data sets with n ≥ 12, parametric tests were run when the Shapiro–Wilk normality test showed p > 0.0001. For data sets with n < 12, parametric tests were run when the Shapiro–Wilk normality test showed p > 0.05. For cases of parametric statistics, we ran an ANOVA test—when there are three or more data sets. We applied a post hoc Dunnett’s correction test when multiple comparisons are carried out against a control value. When only two data sets were compared, we performed two-tailed, unpaired t-tests. For non-parametric tests and when comparing three or more data sets, we ran a Kruskal–Wallis test with a post hoc Dunn’s multiple comparison test. When comparing two data sets, the Mann-Witney test was used. GraphPad Prism 6 was used to apply these statistical treatments.
Previous studies showed that the secreted molecule Wg and its pre-synaptic receptor Fz2 controlled ADSP at the Drosophila NMJ (Ataman et al., 2008; Alicea et al., 2017). Because Disheveled (Dsh) is the cytoplasmic molecule making the link between the Wg receptor and its different effectors, it is often called the Wg hub (Gao and Chen, 2010). We asked whether Dsh loss of function could reduce ADSP and phenocopy the defects in ADSP observed in the wg and fz2 loss of function backgrounds (Ataman et al., 2008; Alicea et al., 2017). We used a well-described method to repeatedly stimulate the NMJ preparation in a way reminiscent of the stimulation protocol used on hippocampal neurons in culture (Wu et al., 2001). In these conditions, after a 90-min patterned repeated stimulation protocol, we observe the appearance of ghost boutons (see schematic diagram Figure 1A; Ataman et al., 2008) that show the neuronal membrane-specific marker revealed by the anti-HRP immunoreactivity (Jan and Jan, 1982), without the apposition of the post-synaptic marker Dlg (Disc large, the membrane-associated guanylate kinase homolog; Lahey et al., 1994; Budnik et al., 1996). These de-novo synaptic structures are noticeable when comparing unstimulated control synapses (Figure 1B) to stimulated control synapses (Figure 1C; arrowheads point to ghost boutons) and are easily quantifiable (Figures 1F–M).
Throughout this manuscript, we paid attention to the status of the synapse at rest (without stimulation) to ensure that there was no significant difference in the mean synaptic ghost bouton number between the studied genetic backgrounds. This way, we can infer that any observed differences after stimulation reflect a change in the synaptic answer to the activity-dependent process. In fact, unstimulated preparations across all the genotypes studied (Figures 1F,H,J,L) show no differences. We later ensured that our stimulation protocol was efficient in eliciting ghost boutons. Here, our unstimulated control preparations showed a mean of 0.36 ± 0.15 ghost boutons per synapse (Figure 1F, black circle, n = 11), and our stimulated controls showed 7.28 ± 0.69 ghost boutons (Figure 1G, black circle, n = 25), indicating an increase in ghost boutons comparable to previously published work (Ataman et al., 2008; Freeman et al., 2011; Nesler et al., 2013, 2016; Piccioli and Littleton, 2014; Vasin et al., 2014, 2019; Alicea et al., 2017; Lee et al., 2017; Maldonado-Díaz et al., 2021).
We then asked whether Dsh was necessary to perform ADSP. Because of the importance of Dsh in transducing the Wg signal, its null alleles (for example dshX788 and dshnYn234Y) are embryonic lethal and therefore do not allow the study of the larval NMJ (Perrimon and Mahowald, 1987; Klingensmith et al., 1994). To create a viable loss of function condition for dsh, we used RNA interference (RNAi; Piccin et al., 2001) and expressed it using the elav-Gal4 post-mitotic neuronal driver (Robinow and White, 1991). When these preparations were submitted to the patterned repeated stimulation protocol, they showed fewer ghost boutons (Figure 1G, red circles). The mean ghost bouton number in these preparations was 4.46 ± 2.48, a value less than the control value (p = 0.0026; n = 22), suggesting that Dsh is indeed involved in ADSP.
Although we used the postmitotic driver elav-Gal4 to initiate dsh loss of function, its expression starts early in development (end of embryogenesis). Therefore, the phenotype we observe could be caused by a detrimental event during synapse formation due to a lack of Dsh activity. To rule out this possibility, we first examined the NMJ and did not notice any phenotype affecting axon routing or synaptic growth. Secondly, we designed an inducible expression system to affect Dsh activity. This system involves the expression of 3 different transgenes within the studied animal: the elav-Gal4 driver, the effector UAS-dsh-RNAi, and the inhibitor Tub-Gal80TS. In this system, the temperature-sensitive (TS) Gal80TS protein is expressed ubiquitously (under the control of the tubulin promoter) and able to repress Gal4 at permissive temperature (18°C; McGuire et al., 2004). Indeed, at this temperature, the expression of dsh RNAi is shut off and we observe no difference between the controls and the animals containing dsh RNAi in both non-stimulated (Figure 1H) and stimulated (Figure 1I) preparations. At the restrictive temperature (29°C), the temperature-sensitive mutation carried by Gal80TS is revealed, producing a non-functional Gal80, which leads to the de-repression of the system, and the expression of dsh RNAi. In this condition, we can observe a loss of function phenotype when we elicit ADSP. When we derepressed the Gal4/UAS system for 48 h before performing the stimulus protocol, we did not observe any difference at rest between controls and dsh RNAi loss of function (the mean value of controls is 0.75 ± 0.25, n = 8, while it is 0.67 ± 0.19, n = 12 for dsh RNAi expressing animals; Figures 1B,J). After repeated patterned stimulations, we did observe differences between controls and preparations containing the dsh RNAi construct. While the mean value of ghost boutons is 9 ± 0.8 for controls (n = 9; Figures 1C,K), it is reduced in dsh RNAi animals (4.8 ± 1.07 ghost boutons; n = 10; p = 0.007; Figures 1D,K). This reduction, provoked by a loss of Dsh activity only 48 h before applying the repeated stimulation protocol, is comparable to the reduction observed when we inhibit Dsh function since the end of embryogenesis (Figure 1G). This suggests that the effect observed on ADSP is due to the requirement of Dsh function at the time of the stimulation challenge and not a consequence of perturbed development. It is important to note that we have previously shown that temperature itself can have limited yet significant effects on the apparition of ghost boutons (Maldonado-Díaz et al., 2021). This is why our work here will only compare the data emanating from animals that experienced identical temperatures during their development and experimental procedures.
Having established the importance of Dsh in transducing the signal responsible for ADSP, we asked whether the planar cell polarity pathway (PCP) was involved in this phenomenon. To do so, we took advantage of the previously studied and isolated dsh1 allele (Perrimon and Mahowald, 1987). This allele, often referred to as a PCP-specific allele, encodes a version of Dsh that selectively affects the transduction of the Wg signaling to the PCP pathway without altering the efficacy of the Wg signal toward the canonical or the calcium pathway (Axelrod et al., 1998; Boutros et al., 1998; Yanfeng et al., 2011). At rest, there is no difference between control and dsh1 animals (the mean value of controls and dsh1 animals are 0.54 ± 0.21, n = 13 and 0.55 ± 0.8, n = 14 respectively; Figure 1L). Nevertheless, after repeated patterned stimulation, the mean number of ghost boutons appearing at the synapse is reduced in dsh1 animals (4.32 ± 0.54, n = 25; red circles, Figure 1M) when compared to control (6.81 ± 0.83, n = 21; p = 0.017; black circles, Figure 1M). This result suggests that part of the Wg signal required to perform ADSP is transduced through the PCP pathway. To consolidate the idea that the Wg PCP pathway is an integral part of ADSP machinery, we decided to ask whether other members of this pathway (Figure 1E) could affect this plasticity.
Since Dsh transduces the Wg signal through several GTPases (Boutros et al., 1998; Fanto et al., 2000; Schlessinger et al., 2007), we decided to investigate the role of Rho1 in ADSP. Rho1 is a small GTPase that functions as a molecular switch cycling between an inactive GDP-bound form and an active GTP-bound form to mainly regulate the assembly and reorganization of the actin cytoskeleton controlling different cellular activities (Jaffe and Hall, 2005). In this way, Rho1 influences several biological processes such as embryogenesis, cell polarity, and cell division (Hariharan et al., 1995; Olson et al., 1995; Barrett et al., 1997; Strutt et al., 1997). Its role in neuronal development has also been studied; Rho activation can promote or inhibit neuronal growth cones as well as inhibit dendritic growth (Luo, 2000; Dickson, 2001; Auer et al., 2011). Reducing the activity of p190 RhoGAP, a negative regulator of Rho, provokes axonal guidance defects and decreases axon number in hippocampal cells (Brouns et al., 2001). In Drosophila mushroom bodies, the reduction of p190 RhoGAP promotes retraction of axonal branching, while its overexpression promotes axonal extension (Billuart et al., 2001). In addition, inhibition of neural activity in cortical neurons decreases active Rho levels while expression of constitutively active Rho increases axon branching by elimination and addition of branches (Ohnami et al., 2008).
Here we asked whether Rho1 was involved in ADSP at the NMJ. Because of Rho1’s multiple cellular functions its null mutations are lethal, so we therefore decided to express a dominant negative version (Rho1DN, see material and methods) and a constitutively active version (Rho1CA, see material and methods). We used the Gal4/Gal80TS inducible system described previously to bypass the potential effects of these constructs’ expression on axon pathfinding and neuronal growth. We first checked that there were no notable differences regarding the number of ghost boutons in unstimulated preparations across all the genotypes considered at both permissive temperature (controls showed 1.21 ± 0.27 ghost boutons; n = 19; Rho1DN 0.91 ± 0.28; n = 11; p = 0.57; Rho1CA 0.64 ± 0.2; n = 14; p = 0.2; Figure 2D) and restrictive temperature (control showed 1.6 ± 0.29 ghost boutons; n = 22; Rho1DN 1.14 ± 0.31; n = 14; p = 0.6; Rho1CA 1.82 ± 0.28; n = 22; p = 0.97; Figure 2F). We also asked whether the inhibition of the Rho1DN or Rho1CA expression by Gal80TS at 18°C was consistent with the observed results; we expected all the preparations to behave like controls if the expression was silenced efficiently. Indeed, the stimulated control preparations showed a mean number of ghost boutons of 6.5 ± 0.73 (Figure 2E; n = 10), a number not statistically different from the one observed in the stimulated preparations carrying the silenced dominant negative construct (7.75 ± 0.93; n = 12; p = 0.42) or the silenced constitutively active construct (5.9 ± 0.5; n = 10; p = 0.82). This suggests that the expression of these transgenes is efficiently suppressed by Gal80TS at 18°C or that it does not affect the mean ghost bouton number. Notably, we can confirm that our constructs worked effectively because the expression of either Rho1DN or Rho1CA at 25°C was lethal. We therefore shifted the temperature to 29°C 48 h before assessing ADSP. In this condition, we observed a mean number of ghost boutons of 11.14 ± 0.68 (Figure 2A,G; n = 35) in control animals, while animals expressing the dominant negative form of Rho1 presented a mean ghost bouton number of 12.10 ± 1.5 (n = 20), a value similar to control (Figure 2B,G; p > 0.99). This suggests that Rho1 activity is not required for the formation of the morphological synaptic structures associated with ADSP. In contrast, when we expressed the constitutively active version of Rho1 in neurons 48 h before repeated stimulation we could observe a decrease in the mean number of GB formed (Figure 2C,G; 5.6 ± 0.27; n = 15; p < 0.0001). These results show that the Rho1 GTPase activity can suppress part of the morphological synaptic response that is associated with activity-dependent synaptic plasticity. This is reminiscent of its role in neuronal morphogenesis and structural plasticity in which Rho1 signaling regulates repulsive axon guidance cues and axon or dendritic retraction (Luo, 2002).
Figure 2. The GTPase Rho1 can repress ADSP. (A) Representative confocal photograph of a stimulated control synapse showing HRP and DLG immunoreactivity and ghost bouton formation (arrowheads). (B) Representative confocal photograph of a stimulated synapse expressing a dominant negative (DN) form of the Rho1 GTPase. (C) Representative confocal photograph of a stimulated synapse expressing a constitutively active (CA) form of the Rho1 GTPase. (D–G) Quantification of mean ghost bouton number in (D) unstimulated and (E) stimulated preparations containing the repressed (18°C) conditional expression system to express Rho1DN or Rho1CA; (F) unstimulated and (G) stimulated preparations expressing (29°C) Rho1DN or Rho1CA 48 h prior to the stimulation. Scale bar: 10 μm. (D,G) Kruskal–Wallis with Dunn’s multiple comparison test. (E,F) ANOVA with Dunnett’s multiple comparisons test: ****p < 0.0001. Data are shown as scatter plots and means ± SEM.
We then investigated the role of another GTPase, Cdc42, known to be activated downstream of Dsh (Moriguchi et al., 1999; Schlessinger et al., 2007). We first constitutively expressed dominant negative (DN) or constitutively active (CA) versions of Cdc42 in post-mitotic neurons (elav-Gal4 driver). Under these conditions, we did not observe any differences in the mean number of ghost boutons in unstimulated and stimulated preparations (Figures 3D,E). This suggests that changing Cdc42 activity might have no consequences on the neuronal properties underlying ADSP or that these consequences might be compensated for during development. To distinguish between these two possibilities, we used the previously described inducible expression system in which expression of the altered Cdc42 constructs is switched on 48 h before carrying out the repeated stimulation protocol. As previously described, we confirmed again that the system was inhibited at 18°C (data not shown) before de-repressing the expression system 48 h prior to the repeated stimulation protocol. Following this activation, we observed no change in unstimulated preparations (Figure 3F). In contrast, after repeated stimulations we observed an increase in the mean number of ghost boutons when we expressed the dominant negative form of Cdc42 (compare 8.61 ± 0.46 in control to 11.23 ± 4.46 in cdc42DN; p = 0.009; Figures 3A,B,G). Interestingly, the expression of the constitutively active form of Cdc42 showed a decrease in the number of morphological changes associated with ADSP (average of ghost bouton is 5.83 ± 0.58 in cdc42CA; p = 0.002; Figures 3C,G). These results suggest that Cdc42 is involved in repressing the expression of ADSP.
Figure 3. The GTPase Cdc42 represses ADSP. (A) Representative confocal photograph of a stimulated control synapse showing HRP and DLG immunoreactivity and ghost bouton formation (arrowheads). (B) Representative confocal photograph of a stimulated synapse expressing a dominant negative (DN) form of the Cdc42 GTPase. (C) Representative confocal photograph of a stimulated synapse expressing a constitutively active (CA) form of the Cdc42 GTPase. (D–G) Quantification of mean ghost bouton number in (D) unstimulated and (E) stimulated control and preparations expressing Cdc42DN or Cdc42CA constitutively; (F) unstimulated and (G) stimulated control and preparations expressing Cdc42DN or Cdc42CA for 48 h prior to the stimulation. Scale bar: 10 μm. (D) Kruskal–Wallis with Dunn’s multiple comparison test. (E–G) ANOVA with Dunnett’s multiple comparisons test: **p < 0.01. Data are shown as scatter plots and mean ± SEM.
We tested a third GTPase activated by Dsh and the Wg signaling, Rac1 (Fanto et al., 2000; Bikkavilli et al., 2008; Li et al., 2016), for its potential role in shaping activity-dependent synaptic plasticity. As previously described, we used a temperature-driven conditional expression system to express Rac1 dominant negative (DN) or constitutively active (CA) versions. We first verified that no phenotypes were associated with Rac1 expression at low temperatures (18°C; data not shown). We then induced Rac1DN or Rac1CA expression 48 h before our experimental procedures. While we observed no differences in unstimulated preparations (Figure 4D), we did observe changes in the mean number of ghost boutons present at the Rac1DN- and Rac1CA- expressing synapses. When control synapses showed a mean number of ghost boutons of 9.62 ± 0.42 (dark circles; n = 65; Figure 4A), synapses expressing both Rac1 constructs showed an increase in the mean ghost bouton number. Indeed, Rac1DN expressing synapses showed 12.93 ± 1.2 ghost boutons (red circles; n = 30; p = 0.002; Figures 4B,E) and Rac1CA expressing synapses showed 14.2 ± 1.3 (blue circles; n = 18; p = 0.0009; Figures 4C,E). Although it seems counterintuitive that the expression of both the dominant negative and the constitutively active forms of Rac1 provokes the same phenotype it is reminiscent of phenotypes previously observed during axon pathfinding within neurons expressing Rac1DN or Rac1CA. Indeed, ISNb axons of motoneurons expressing either Rac1DN or Rac1CA during embryonic development showed arrested growth cones (Kaufmann et al., 1998). In addition, the expression of both Rac1DN and Rac1CA during embryonic axonal outgrowth causes increased axonal loss (Luo et al., 1994). Interestingly, that study showed that Rac1CA expression induced a stronger phenotype and an accumulation of F-actin that was not present in Rac1DN-expressing animals (Luo et al., 1994), suggesting that Rac1CA and Rac1DN expression led to the same phenotype by affecting different cytoskeletal processes. Interestingly, at the NMJ expression of Rac1DN (Park et al., 2022) and Rac1CA (Ball et al., 2010) both cause an increase of synaptic boutons. Here we show that changing Rac1 activity increases the morphological changes associated with activity-dependent synaptic plasticity. Taken together, these results show that the Rho family GTPases downstream of Dsh are involved in the morphological response associated with activity-dependent synaptic plasticity.
Figure 4. The GTPase Rac 1 can regulate ADSP. (A) Representative confocal photograph of a stimulated control synapse showing HRP and DLG immunoreactivity and ghost bouton formation (arrowheads). (B) Representative confocal photograph of a stimulated synapse expressing a dominant negative (DN) form of the Rac1 GTPase. (C) Representative confocal photograph of a stimulated synapse expressing a constitutively active (CA) form of the Rac1 GTPase. Quantification of mean ghost bouton number in (D) unstimulated and (E) stimulated control and preparations expressing Cdc42DN or Cdc42CA for 48 h prior to the stimulation. Scale bar: 10 μm. ANOVA with Dunnett’s multiple comparisons test: **p < 0.01; ***p < 0.001. Data shown as scatter plots and mean ± SEM.
One of the major PCP pathway effectors is Jun kinase (Jnk; Strutt et al., 1997; Boutros et al., 1998; Jenny, 2010). This kinase can be activated by the Rho GTPases and can in turn activate cytoskeletal effectors (Strutt et al., 1997; Fanto et al., 2000; Bikkavilli et al., 2008) and transcriptional regulators. The latter are composed of the transcription factors cJun and cFos which act as dimers known as AP1 (Curran and Franza, 1988; Karin et al., 1997). We expressed a dominant-negative form of Jun kinase (JnkDN) in a constitutive manner (Figure 5E) and used the temperature-driven conditional expression (48 h prior to repeated stimulations; Figures 5B,G). In both cases, we observed a significant decrease in ghost bouton formation. In the experiment in which we use constitutive expression, control preparations showed a mean of 6.82 ± 0.52 (Figure 5E) ghost boutons after stimulation, while the mean ghost bouton number of preparations with JnkDN constitutive expression was 2.47 ± 0.6 (Figure 5E; p < 0.0001). When we use the conditional expression system, the control synapses showed 8.97 ± 0.57 (Figures 5A,G) but only 5.63 ± 0.61 (Figures 5B,G; p = 0.0018) in preparations presenting JnkDN conditional expression. This shows that Jnk activity is required to fully achieve the morphological changes associated with ADSP.
Figure 5. Jun Kinase is required for optimal ADSP in an AP1-independent manner. (A) Representative confocal photograph of a stimulated control synapse showing HRP and DLG immunoreactivity and ghost bouton formation (arrowheads). (B) Representative confocal photograph of a stimulated synapse expressing a dominant negative (DN) form of Jun Kinase. (C) Representative confocal photograph of a stimulated synapse expressing a dominant negative (DN) form of c Jun. (D–G) Quantification of mean ghost bouton number in (D) unstimulated and (E) stimulated control and preparations constitutively expressing JnkDN or c JunDN or c FosDN; (F) unstimulated and (G) preparations expressing JnkDN or c JunDN or c FosDN for 48 h prior to the stimulation. Scale bar: 10 μm. (D,F,G) Kruskal–Wallis with Dunn’s multiple comparisons test (E) ANOVA with Dunnett’s multiple comparisons test: **p < 0.01; ****p < 0.0001. Data are shown as scatter plots and mean ± SEM.
We then logically asked whether the transcription factors cJun and cFos were involved in transducing the signal necessary for the ghost bouton formation after stimulation. To do so, we expressed dominant negative forms (Eresh et al., 1997) of these two constructs in a constitutive and conditional form (Figures 5C–G). We observed no difference between control preparations and preparations containing the dominant negative forms in unstimulated and stimulated procedures. When expressing the dominant negative constructs we noticed smaller synapses, a phenotype documented before (Ballard et al., 2014) that validates our expression system. This result suggests that the transcriptional control exercised by AP1 is not necessary for the morphological changes associated with activity-dependent synaptic plasticity. We favor the hypothesis that Jnk and the Rho GTPases act on cytoskeletal regulators to achieve these morphological changes.
Structural synaptic plasticity is a fundamental feature of the nervous system, in which Wg/Wnt signaling plays an essential role. Reduction of Wg and its receptor Fz2 impaired the formation of activity-dependent modifications of synaptic structure (Ataman et al., 2008; Alicea et al., 2017). Overexpression of GSK3β/Sgg, an important canonical pathway member, prevents ADSP at the Drosophila NMJ (Ataman et al., 2008). Upon stimulation, Wg protein release increases at the synapse (Ataman et al., 2008), which suggests an increased activation of Wg signaling pathways. Repeated simulation of the synapse also promotes an increase of CaMKII, and reducing synaptic CaMKII impairs the activity-dependent formation of de novo boutons (Nesler et al., 2016). This suggests some role of the Wnt/Ca2+ non-canonical pathway on activity-dependent synaptic plasticity.
Despite the existing interest in the Wnt/Wg PCP pathway, its role regarding processes of synaptic plasticity has not been extensively investigated. The Wg signal for polarity has been described extensively in several cellular environments (Maung and Jenny, 2011). In the developing eye, alteration of the PCP pathway provokes defects in the anterior–posterior and dorsal-ventral axes in the arrangement of ommatidia (Strutt et al., 1997; Boutros et al., 1998; Fanto et al., 2000; Adler, 2002; Strutt et al., 2013). In the Drosophila wing, the orientation of cellular hairs in the proximal-distal axis is impaired by defects of the PCP pathway (Strutt et al., 1997; Adler, 2002; Wu et al., 2013; Fagan et al., 2014). Neurons are one of the most morphologically polarized cell types, and mutations of Wnt/PCP pathway members like Dsh, Prickle, Strabismus, and Fz provoke axonal branching defects in mushroom bodies (MB) of Drosophila adult brain (Ng, 2012). Studies showed that Rac GTPase inactivation in mushroom bodies results in axonal branching defects (Ng et al., 2002). Wnt5, Fz, and Dsh act in concert with the small GTPase Rac1 to activate the actin assembly functions of dDAAM (dsh-associated activator of morphogenesis) necessary for the correct targeting of MB axons (Gombos et al., 2015). In addition, dDAAM regulates microtubule stability and synaptic growth at the Drosophila NMJ (Migh et al., 2018) while expression of RacDN (Park et al., 2022) and RacCA (Ball et al., 2010) promote synaptic overgrowth at the NMJ. Here, we have provided evidence that key molecules involved in the PCP pathway can affect the morphological modifications consequent to ADSP and are therefore necessary to achieve optimal activity-dependent synaptic plasticity. It is important to note that we never observed a complete ghost bouton elimination after affecting the PCP pathway. This makes sense since the other Wg pathways — the canonical pathway (Ataman et al., 2008; Alicea et al., 2017) and the calcium pathway (Freeman et al., 2011; Nesler et al., 2016)— are also necessary to achieve full ADSP. The contribution of the Wg PCP pathway to the formation of de novo synaptic structures after stimulation illustrates the redundancy and robustness of the Wg/Wnt signal in directing ADSP.
Our work revealed PCP pathway molecules exercising both activation and repression of ADSP. Indeed, the loss of function of dsh, either using RNAi or the PCP-specific allele dsh1, showed compromised plasticity. The same was true for the loss of function of jnk. This suggests that the role of these molecules is to promote activity. Activation and repression of ghost bouton formation was also achieved by modifying Rho GTPase activity. Expression of the constitutively active forms of the three GTPases we tested showed phenotypes, suggesting that these molecules can regulate ADSP. While Cdc42CA and Rho1CA expressing synapses show reduced plasticity, Rac1CA expression elicited an over plastic response. This antagonistic relationship between Rho1 and Rac1 has been documented previously. For example, Rac1 activation promotes neurite formation and outgrowth, but Rho1 activation leads to neurite retraction and suppresses neurite outgrowth (Luo, 2000; Lorenzetto et al., 2013). Similarly, proper dendritic spine morphogenesis requires balanced Rho GTPase activity regulation (Penzes and Cahill, 2012). Interestingly, Rac1 induces the formation and maintenance of dendritic spines, while Rho1 decreases spine formation by promoting spine pruning or retraction (Nakayama et al., 2000; Tashiro et al., 2000; Newey et al., 2005; Bolognin et al., 2014). Like for neurite and spine formation but also cell migration (Ridley, 2015; Xu et al., 2019), our work has the potential to illustrate the importance of the relative balance of the GTPases’ spatiotemporal activity in regulating cytoskeletal dynamics.
Indeed, our work provides an insight into the complexity of the Rho GTPases at the NMJ. It is intriguing that the expression of the dominant negative or constitutively active forms of cdc42 do not provide a phenotype when expressed since early embryogenesis. In contrast, both constructs’ expressions provoke a phenotype when expressed for 2 days before eliciting ADSP. This is reminiscent of the results obtained with Wg overexpression, where only a transient expression could influence synaptic proteins’ expression (Alicea et al., 2017). This perhaps illustrates that for Wg and its downstream GTPases, a briefer change of expression or activity likely better mimics the timescale relevant to ADSP than a constitutive expression. This result could be due to compensatory mechanisms between different GTPases. Indeed, the functional redundancy between Cdc42 and Rac1 is established. For example, Rac1 and Cdc42 both promote dendritic spine formation (Nakayama and Luo, 2000; Bolognin et al., 2014), cell extension (Nakayama et al., 2000; Swetman et al., 2002), and actin polymerization (Nobes and Hall, 1995; Lamarche et al., 1996). In addition, Cdc42 and Rac1 can be activated by the same molecular cascade (Buchsbaum, 2007) and there is evidence suggesting that depletion of Cdc42 in Hela cells can be compensated for by other GTPases to restore functional actin dynamic (Bonfim-Melo et al., 2018). Therefore, although there is evidence that GTPases functions are required at different times (Vadodaria et al., 2013), Rac1 could substitute Cdc42. The observed Cdc42 phenotypes indicate a role in repressing ADSP. Indeed, the dominant negative form is over plastic, while the constitutively active form is under plastic. This shows that in our system Cdc42 represses ghost bouton formation. This is surprising since most studies show that Cdc42 and the Rho GTPases in general promote membrane protrusion (Sadok and Marshall, 2014). Nevertheless, at the NMJ, cdc42 mutations provoke an overgrown synapse, suggesting that Cdc42 represses synaptic growth (Rodal et al., 2008) while expression of RacDN (Park et al., 2022), RacCA and RacOE (Ball et al., 2010) promotes synaptic growth. These studies might indicate a more complex interaction between the Rho GTPases.
While Rho1CA animals showed repressed ADSP, Rho1DN did not show any phenotype. It is important to note that we know that Rho1DN expression can decrease Rho function since its constitutive expression creates lethality. We can therefore hypothesize that the absence of phenotype in the Rho1DN animals might be due to compensation/redundancy from other GTPase activity. Alternatively, its function at the time at which we express its dominant negative form is perhaps not to repress ADSP. Indeed, Rho GTPases serve different functions at different times. For example, in hippocampal neurogenesis, Cdc42 promotes initial dendritic development and spine maturation, while Rac1 is essential for the late stages of dendritic growth and spine maturation (Vadodaria et al., 2013).
Finally, both the constitutive and dominant negative forms of Rac1 provoke over plasticity at the NMJ. Although surprising, this is not the first time that the same phenotype has been observed when expressing these two constructs. Expression of both constitutively active and dominant-negative forms of Rac1 causes axonal loss in Drosophila (Luo et al., 1994), and Rac1 can have a dual function regulating attractive and repulsive signals in axonal guidance (Fan et al., 2003). Taken together, these results give an image of an intricate and complex local role of the Rho GTPases in regulating ADSP induced at the synapse upon high neuronal activity.
We have shown that the transcriptional regulator AP1 is unlikely to be involved in ADSP. We favor the hypothesis that regulation of the cytoskeleton by the PCP pathway is involved in regulating this plasticity. The reorganization of the synaptic cytoskeleton has been extensively studied in spines and is linked to processes of activity-dependent plasticity and learning and memory (Repetto et al., 2014; Macgillavry et al., 2016; Cornelius et al., 2021). This process involves the reorganization of filamentous actin, actin regulators, and microtubules (Ka and Kim, 2016; Schätzle et al., 2018; Cornelius et al., 2021). In fact, we have previously isolated one such regulator, the actin regulator Cortactin (Weed et al., 2000; Weaver et al., 2001) and showed that it is a regulator of ADSP, and that its protein is upregulated under the control of Wg signaling (Alicea et al., 2017). Determining whether the PCP pathway is involved in this regulation will be a challenge for the future.
The raw data supporting the conclusions of this article will be made available by the authors, without undue reservation.
Ethical approval was not required for the study involving animals in accordance with the local legislation and institutional requirements because our laboratory follows the guidelines required by the NIH and NSF granting agencies and uses ethical practices when handling our model system animal, the fruit fly Drosophila melanogaster. Our lab follows a Standard Operating Procedure (SOP) manual that was validated by the University. This document refers to and/or dictates the rules and lab conduct regarding safety and animals handling. It describes how to handle Drosophila properly and ethically.
CD-C: Conceptualization, Formal Analysis, Investigation, Visualization, Writing – original draft, Writing – review & editing. MV: Investigation, Writing – review & editing. BM: Conceptualization, Funding acquisition, Supervision, Visualization, Writing – original draft, Writing – review & editing.
The author(s) declare financial support was received for the research, authorship, and/or publication of this article. This work was supported by the NIH NINDS-R21NS114774 to BM, the NSF HRD-1736019 grants to BM and MV and the NIH RISE R25GM061838 grant to CD-C. Confocal microscopy was supported by NIH NIGMS GM103642 and fly husbandry by NIH-RCMI 5U54MD007600.
We thank Jonathan Blagburn for his valuable comments on previous versions of this manuscript. Parts of our schematic diagrams (Figures 1A,E) were created with Biorender.com.
The authors declare that the research was conducted in the absence of any commercial or financial relationships that could be construed as a potential conflict of interest.
The author(s) declared that they were an editorial board member of Frontiers, at the time of submission. This had no impact on the peer review process and the final decision.
All claims expressed in this article are solely those of the authors and do not necessarily represent those of their affiliated organizations, or those of the publisher, the editors and the reviewers. Any product that may be evaluated in this article, or claim that may be made by its manufacturer, is not guaranteed or endorsed by the publisher.
Adler, P. N. (2002). Planar signaling and morphogenesis in Drosophila. Dev. Cell 2, 525–535. doi: 10.1016/s1534-5807(02)00176-4
Alicea, D., Perez, M., Maldonado, C., Dominicci-Cotto, C., and Marie, B. (2017). Cortactin is a regulator of activity-dependent synaptic plasticity controlled by Wingless. J. Neurosci. 37, 2203–2215. doi: 10.1523/JNEUROSCI.1375-16.2017
Ataman, B., Ashley, J., Gorczyca, M., Ramachandran, P., Fouquet, W., Sigrist, S. J., et al. (2008). Rapid activity-dependent modifications in synaptic structure and function require bidirectional Wnt signaling. Neuron 57, 705–718. doi: 10.1016/j.neuron.2008.01.026
Auer, M., Hausott, B., and Klimaschewski, L. (2011). Rho GTPases as regulators of morphological neuroplasticity. Ann. Anat. 193, 259–266. doi: 10.1016/j.aanat.2011.02.015
Axelrod, J. D., Miller, J. R., Shulman, J. M., Moon, R. T., and Perrimon, N. (1998). Differential recruitment of dishevelled provides signaling specificity in the planar cell polarity and Wingless signaling pathways. Genes Dev. 12, 2610–2622. doi: 10.1101/gad.12.16.2610
Bai, Y., and Suzuki, T. (2020). Activity-dependent synaptic plasticity in Drosophila melanogaster. Front. Physiol. 11:161. doi: 10.3389/fphys.2020.00161
Ballard, S. L., Miller, D. L., and Ganetzky, B. (2014). Retrograde neurotrophin signaling through Tollo regulates synaptic growth in Drosophila. J. Cell Biol. 204, 1157–1172. doi: 10.1083/JCB.201308115
Ball, R. W., Warren-Paquin, M., Tsurudome, K., Liao, E. H., Elazzouzi, F., Cavanagh, C., et al. (2010). Retrograde BMP signaling controls synaptic growth at the nmj by regulating trio expression in motor neurons. Neuron 66, 536–549. doi: 10.1016/j.neuron.2010.04.011
Barrett, K., Leptin, M., and Settleman, J. (1997). The rho GTPase and a putative RhoGEF mediate a signaling pathway for the cell shape changes in Drosophila gastrulation. Cell 91, 905–915. doi: 10.1016/S0092-8674(00)80482-1
Bikkavilli, R. K., Feigin, M. E., and Malbon, C. C. (2008). Gαo mediates WNT-JNK signaling through dishevelled 1 and 3, RhoA family members, and MEKK 1 and 4 in mammalian cells. J. Cell Sci. 121, 234–245. doi: 10.1242/jcs.021964
Billuart, P., Winter, C. G., Maresh, A., Zhao, X., and Luo, L. (2001). Regulating axon branch stability: the role of p190 RhoGAP in repressing a retraction signaling pathway. Cell 107, 195–207. doi: 10.1016/S0092-8674(01)00522-0
Bolognin, S., Lorenzetto, E., Diana, G., and Buffelli, M. (2014). The potential role of rho GTPases in Alzheimer’s disease pathogenesis. Mol. Neurobiol. 50, 406–422. doi: 10.1007/s12035-014-8637-5
Bonfim-Melo, A., Ferreira, É. R., and Mortara, R. A. (2018). Rac1/WAVE2 and Cdc42/N-WASP participation in actin-dependent host cell invasion by extracellular amastigotes of Trypanosoma cruzi. Front. microbiol. 9:360, 1–14. doi: 10.3389/fmicb.2018.00360
Boutros, M., Paricio, N., Strutt, D. I., and Mlodzik, M. (1998). Dishevelled activates JNK and discriminates between JNK pathways in planar polarity and Wingless signaling. Cell 94, 109–118. doi: 10.1016/S0092-8674(00)81226-X
Brand, A. H., and Perrimon, N. (1993). Targeted gene expression as a means of altering cell fates and generating dominant phenotypes. Development 118, 401–415. doi: 10.1242/dev.118.2.401
Brouns, M. R., Matheson, S. F., and Settleman, J. (2001). p190 RhoGAP is the principal Src substrate in brain and regulates axon outgrowth, guidance and fasciculation. Nat. Cell Biol. 3, 361–367. doi: 10.1038/35070042
Buchsbaum, R. J. (2007). Rho activation at a glance. J. Cell Sci. 120, 1149–1152. doi: 10.1242/jcs.03428
Budnik, V., Koh, Y. H., Guan, B., Hartmann, B., Hough, C., Woods, D., et al. (1996). Regulation of synapse structure and function by the Drosophila tumor suppressor gene dlg. Neuron 17, 627–640. doi: 10.1016/S0896-6273(00)80196-8
Budnik, V., and Salinas, P. C. (2011). Wnt signaling during synaptic development and plasticity. Curr. Opin. Neurobiol. 21, 151–159. doi: 10.1016/j.conb.2010.12.002
Castillo, P. E. (2012). Presynaptic LTP and LTD of excitatory and inhibitory synapses. Cold Spring Harb. Perspect. Biol. 4, 1–23. doi: 10.1101/cshperspect.a005728
Chen, J., Park, C. S., and Tang, S.-J. (2006). Activity-dependent synaptic Wnt release regulates hippocampal long term potentiation. J. Biol. Chem. 281, 11910–11916. doi: 10.1074/jbc.M511920200
Ciani, L., Boyle, K. A., Dickins, E., Sahores, M., Anane, D., Lopes, D. M., et al. (2011). Wnt7a signaling promotes dendritic spine growth and synaptic strength through ca 2+/calmodulin-dependent protein kinase II. Proc. Natl. Acad. Sci. U. S. A. 108, 10732–10737. doi: 10.1073/pnas.1018132108
Cornelius, J., Rottner, K., Korte, M., and Michaelsen-preusse, K. (2021). Cortactin contributes to activity-dependent modulation of spine actin dynamics and spatial memory formation. Cells 10, 1–13. doi: 10.3390/cells10071835
Coso, O. A., Chiariello, M., Yu, J. C., Teramoto, H., Crespo, P., Xu, N., et al. (1995). The small GTP-binding proteins Rac1 and Cdc42regulate the activity of the JNK/SAPK signaling pathway. Cell 81, 1137–1146. doi: 10.1016/S0092-8674(05)80018-2
Curran, T., and Franza, B. R. (1988). Fos and Jun: the AP-1 connection. Cell 55, 395–397. doi: 10.1016/0092-8674(88)90024-4
Dickson, B. J. (2001). Rho GTPases in growth cone guidance. Curr. Opin. Neurobiol. 11, 103–110. doi: 10.1016/s0959-4388(00)00180-x
Eresh, S., Riese, J., Jackson, D. B., Bohmann, D., and Bienz, M. (1997). A CREB-binding site as a target for decapentaplegic signalling during Drosophila endoderm induction. EMBO J. 16, 2014–2022. doi: 10.1093/emboj/16.8.2014
Etter, P. D., Narayanan, R., Navratilova, Z., Patel, C., Bohmann, D., Jasper, H., et al. (2005). Synaptic and genomic responses to JNK and AP-1 signaling in Drosophila neurons. BMC Neurosci. 6:39. doi: 10.1186/1471-2202-6-39
Fagan, J. K., Dollar, G., Lu, Q., Barnett, A., Jorge, J. P., Schlosser, A., et al. (2014). Combover/CG10732, a novel PCP effector for Drosophila wing hair formation. PLoS One 9:e107311. doi: 10.1371/JOURNAL.PONE.0107311
Fanto, M., Weber, U., Strutt, D. I., and Mlodzik, M. (2000). Nuclear signaling by Rac and rho GTPases is required in the establishment of epithelial planar polarity in the Drosophila eye. Curr. Biol. 10, 979–9S1. doi: 10.1016/S0960-9822(00)00645-X
Fan, X., Labrador, J. P., Hing, H., and Bashaw, G. J. (2003). Slit stimulation recruits dock and Pak to the roundabout receptor and increases Rac activity to regulate axon repulsion at the CNS midline. Neuron 40, 113–127. doi: 10.1016/S0896-6273(03)00591-9
Ferrari, M. E., Bernis, M. E., McLeod, F., Podpolny, M., Coullery, R. P., Casadei, I. M., et al. (2018). Wnt7b signalling through Frizzled-7 receptor promotes dendrite development by coactivating CaMKII and JNK. J. Cell Sci. 131, 1–14. doi: 10.1242/jcs.216101
Freeman, A., Franciscovich, A., Bowers, M., Sandstrom, D. J., and Sanyal, S. (2011). NFAT regulates pre-synaptic development and activity-dependent plasticity in Drosophila. Mol. Cell. Neurosci. 46, 535–547. doi: 10.1016/j.mcn.2010.12.010
Fuentes-Medel, Y., Logan, M. A., Ashley, J., Ataman, B., Budnik, V., and Freeman, M. R. (2009). Glia and muscle sculpt neuromuscular arbors by engulfing destabilized synaptic boutons and shed presynaptic debris. PLoS Biol. 7:e1000184. doi: 10.1371/journal.pbio.1000184
Gao, C., and Chen, Y. G. (2010). Dishevelled: the hub of Wnt signaling. Cell. Signal. 22, 717–727. doi: 10.1016/j.cellsig.2009.11.021
Gombos, R., Migh, E., Antal, O., Mukherjee, A., Jenny, A., and Mihaly, J. (2015). The Formin DAAM functions as molecular effector of the planar cell polarity pathway during axonal development in Drosophila. J. Neurosci. 35, 10154–10167. doi: 10.1523/JNEUROSCI.3708-14.2015
Hariharan, I. K., Hu, K. Q., Asha, H., Quintanilla, A., Ezzell, R. M., and Settleman, J. (1995). Characterization of rho GTPase family homologues in Drosophila melanogaster: overexpressing Rho1 in retinal cells causes a late developmental defect. EMBO J. 14, 292–302. doi: 10.1002/j.1460-2075.1995.tb07003.x
Harris, K. P., and Littleton, J. T. (2015). Transmission, development, and plasticity of synapses. Genetics 201, 345–375. doi: 10.1534/GENETICS.115.176529
Holtmaat, A., and Svoboda, K. (2009). Experience-dependent structural synaptic plasticity in the mammalian brain. Nat. Rev. Neurosci. 10, 647–658. doi: 10.1038/nrn2699
Ho, V. M., Lee, J. A., and Martin, K. C. (2011). The cell biology of synaptic plasticity. Science 334, 623–628. doi: 10.1126/science.1209236
Jaffe, A. B., and Hall, A. (2005). Rho GTPases: biochemistry and biology. Ann. Rev. Cell Develop. Biol. 21, 247–269. doi: 10.1146/annurev.cellbio.21.020604.150721
Jan, L. Y., and Jan, Y. N. (1982). Antibodies to horseradish peroxidase as specific neuronal markers in Drosophila and in grasshopper embryos. Proc. Natl. Acad. Sci. U. S. A. 79, 2700–2704. doi: 10.1073/pnas.79.8.2700
Jenny, A. (2010). Planar cell polarity signaling in the Drosophila eye. Curr. Topics Develop. Biol 93, 189–227. doi: 10.1016/B978-0-12-385044-7.00007-2
Ka, M., and Kim, W. Y. (2016). Microtubule-actin crosslinking factor 1 is required for dendritic Arborization and axon outgrowth in the developing brain. Mol. Neurobiol. 53, 6018–6032. doi: 10.1007/s12035-015-9508-4
Karin, M., Liu, Z. G., and Zandi, E. (1997). AP-1 function and regulation. Curr. Opin. Cell Biol. 9, 240–246. doi: 10.1016/S0955-0674(97)80068-3
Kaufmann, N., Wills, Z. P., and Van Vactor, D. (1998). Drosophila Rac1 controls motor axon guidance. Development 125, 453–461. doi: 10.1242/dev.125.3.453
Klingensmith, J., Nusse, R., and Perrimon, N. (1994). The Drosophila segment polarity gene dishevelled encodes a novel protein required for response to the Wingless signal. Genes Dev. 8, 118–130. doi: 10.1101/gad.8.1.118
Koles, K., and Budnik, V. (2012). Wnt signaling in neuromuscular junction development. Cold Spring Harb. Perspect. Biol. 4, 1–22. doi: 10.1101/cshperspect.a008045
Lahey, T., Gorczyca, M., Jia, X. X., and Budnik, V. (1994). The Drosophila tumor suppressor gene dlg is required for normal synaptic Bouton structure. Neuron 13, 823–835. doi: 10.1016/0896-6273(94)90249-6
Lamarche, N., Tapon, N., Stowers, L., Burbelo, P. D., Aspenström, P., Bridges, T., et al. (1996). Rac and Cdc42 induce actin polymerization and G1 cell cycle progression independently of p65PAK and the JNK/SAPK MAP kinase cascade. Cell 87, 519–529. doi: 10.1016/S0092-8674(00)81371-9
Lee, J. Y., Geng, J., Lee, J., Wang, A. R., and Chang, K. T. (2017). Activity-induced synaptic structural modifications by an activator of integrin signaling at the Drosophila neuromuscular junction. J. Neurosci. 37, 3246–3263. doi: 10.1523/JNEUROSCI.3128-16.2017
Li, X., Wang, Y., Wang, H., Liu, T., Guo, J., Yi, W., et al. (2016). Epithelia-derived Wingless regulates dendrite directional growth of drosophila ddaE neuron through the Fz-Fmi-Dsh-Rac1 pathway. Mol. Brain 9, 1–15. doi: 10.1186/S13041-016-0228-0
Lorenzetto, E., Ettorre, M., Pontelli, V., Bolomini-Vittori, M., Bolognin, S., Zorzan, S., et al. (2013). Rac1 selective activation improves retina ganglion cell survival and regeneration. PLoS One 8:e64350. doi: 10.1371/journal.pone.0064350
Luo, L. (2000). Rho GTPases in neuronal morphogenesis. Nat. Rev. Neurosci. 1, 173–180. doi: 10.1038/35044547
Luo, L. (2002). Actin cytoskeleton regulation in neuronal morphogenesis and structural plasticity. Annu. Rev. Cell Dev. Biol. 18, 601–635. doi: 10.1146/annurev.cellbio.18.031802.150501
Luo, L., Joyce Liao, Y., Jan, L. Y., and Jan, Y. N. (1994). Distinct morphogenetic functions of similar small GTPases: Drosophila Drac1 is involved in axonal outgrowth and myoblast fusion. Genes Dev. 8, 1787–1802. doi: 10.1101/gad.8.15.1787
Lüscher, C., and Malenka, R. C. (2012). NMDA receptor-dependent long-term potentiation and long-term depression (LTP/LTD). Cold Spring Harb. Perspect. Biol. 4, 1–15. doi: 10.1101/cshperspect.a005710
Macgillavry, H. D., Kerr, J. M., Kassner, J., Frost, N. A., and Blanpied, T. A. (2016). Shank-cortactin interactions control actin dynamics to maintain flexibility of neuronal spines and synapses. Eur. J. Neurosci. 43, 179–193. doi: 10.1111/ejn.13129
Maldonado-Díaz, C., Vazquez, M., and Marie, B. (2021). A comparison of three different methods of eliciting rapid activity-dependent synaptic plasticity at the Drosophila NMJ. PLoS One 16:e0260553. doi: 10.1371/journal.pone.0260553
Maung, S. M. T. W., and Jenny, A. (2011). Planar cell polarity in Drosophila. Organogenesis 7, 165–179. doi: 10.4161/ORG.7.3.18143
McGuire, S. E., Mao, Z., and Davis, R. L. (2004). Spatiotemporal gene expression targeting with the TARGET and gene-switch systems in Drosophila. Sci. STKE 2004, 1–10. doi: 10.1126/stke.2202004pl6
McLeod, F., Bossio, A., Marzo, A., Ciani, L., Sibilla, S., Hannan, S., et al. (2018). Wnt signaling mediates LTP-dependent spine plasticity and AMPAR localization through Frizzled-7 receptors. Cell Rep. 23, 1060–1071. doi: 10.1016/j.celrep.2018.03.119
Migh, E., Götz, T., Földi, I., Szikora, S., Gombos, R., Darula, Z., et al. (2018). Microtubule organization in presynaptic boutons relies on the formin DAAM. Development (Cambridge) 145, 1–13. doi: 10.1242/dev.158519
Moriguchi, T., Kawachi, K., Kamakura, S., Masuyama, N., Yamanaka, H., Matsumoto, K., et al. (1999). Distinct domains of mouse dishevelled are responsible for the c-Jun N-terminal kinase/stress-activated protein kinase activation and the Axis formation in vertebrates. J. Biol. Chem. 274, 30957–30962. doi: 10.1074/JBC.274.43.30957
Nakayama, A. Y., Harms, M. B., and Luo, L. (2000). Small GTPases Rac and rho in the maintenance of dendritic spines and branches in hippocampal pyramidal neurons. J. Neurosci. 20, 5329–5338. doi: 10.1523/jneurosci.20-14-05329.2000
Nakayama, A. Y., and Luo, L. (2000). Intracellular signalling pathways that regulate dendritic spine morphogenesis. Hippocampus 10, 582–586. doi: 10.1002/1098-1063(2000)10:5<582::AID-HIPO8>3.0.CO;2-K
Nesler, K. R., Sand, R. I., Symmes, B. A., Pradhan, S. J., Boin, N. G., Laun, A. E., et al. (2013). The miRNA pathway controls rapid changes in activity-dependent synaptic structure at the Drosophila melanogaster neuromuscular junction. PLoS One 8:e68385. doi: 10.1371/journal.pone.0068385
Nesler, K. R., Starke, E. L., Boin, N. G., Ritz, M., and Barbee, S. A. (2016). Presynaptic CamKII regulates activity-dependent axon terminal growth. Mol. Cell. Neurosci. 76, 33–41. doi: 10.1016/j.mcn.2016.08.007
Newey, S. E., Velamoor, V., Govek, E. E., and Van Aelst, L. (2005). Rho GTPases, dendritic structure, and mental retardation. J. Neurobiol. 64, 58–74. doi: 10.1002/neu.20153
Ng, J. (2012). Wnt/PCP proteins regulate stereotyped axon branch extension in Drosophila. Development 139, 165–177. doi: 10.1242/dev.068668
Ng, J., Nardine, T., Harms, M., Tzu, J., Goldstein, A., Sun, Y., et al. (2002). Rac GTPases control axon growth, guidance and branching. Nature 416, 442–447. doi: 10.1038/416442a
Nobes, C. D., and Hall, A. (1995). Rho, Rac, and Cdc42 GTPases regulate the assembly of multimolecular focal complexes associated with actin stress fibers, lamellipodia, and filopodia. Cell 81, 53–62. doi: 10.1016/0092-8674(95)90370-4
Ohnami, S., Endo, M., Hirai, S., Uesaka, N., Hatanaka, Y., Yamashita, T., et al. (2008). Role of RhoA in activity-dependent cortical axon branching. J. Neurosci. 28, 9117–9121. doi: 10.1523/JNEUROSCI.1731-08.2008
Olson, M. F., Ashworth, A., and Hall, A. (1995). An essential role for rho, Rac, and Cdc42 GTPases in cell cycle progression through G1. Science 269, 1270–1272. doi: 10.1126/science.7652575
Park, H. G., Kim, Y. D., Cho, E., Lu, T. Y., Yao, C. K., Lee, J., et al. (2022). Vav independently regulates synaptic growth and plasticity through distinct actin-based processes. J. Cell Biol. 221, 1–21. doi: 10.1083/jcb.202203048
Penzes, P., and Cahill, M. E. (2012). Deconstructing signal transduction pathways that regulate the actin cytoskeleton in dendritic spines. Cytoskeleton 69, 426–441. doi: 10.1002/cm.21015
Pereda, A. E. (2014). Electrical synapses and their functional interactions with chemical synapses. Nat. Rev. Neurosci. 15, 250–263. doi: 10.1038/nrn3708
Perrimon, N., and Mahowald, A. P. (1987). Multiple functions of segment polarity genes in Drosophila. Dev. Biol. 119, 587–600. doi: 10.1016/0012-1606(87)90061-3
Piccin, A., Salameh, A., Benna, C., Sandrelli, F., Mazzotta, G., Zordan, M., et al. (2001). Efficient and heritable functional knock-out of an adult phenotype in Drosophila using a GAL4-driven hairpin RNA incorporating a heterologous spacer. Nucleic Acids Res. 29:55e. doi: 10.1093/NAR/29.12.E55
Piccioli, Z. D., and Littleton, J. T. (2014). Retrograde BMP signaling modulates rapid activity-dependent synaptic growth via presynaptic lim kinase regulation of Cofilin. J. Neurosci. 34, 4371–4381. doi: 10.1523/JNEUROSCI.4943-13.2014
Repetto, D., Camera, P., Melani, R., Morello, N., Russo, I., Calcagno, E., et al. (2014). p140Cap regulates memory and synaptic plasticity through Src-mediated and citron-N-mediated actin reorganization. J. Neurosci. 34, 1542–1553. doi: 10.1523/JNEUROSCI.2341-13.2014
Ridley, A. J. (2015). Rho GTPase signalling in cell migration. Curr. Opinion Cell Biol. 36, 103–112. doi: 10.1016/j.ceb.2015.08.005
Robinow, S., and White, K. (1991). Characterization and spatial distribution of the ELAV protein during Drosophila melanogaster development. J. Neurobiol. 22, 443–461. doi: 10.1002/neu.480220503
Rodal, A. A., Motola-Barnes, R. N., and Littleton, J. T. (2008). Nervous wreck and Cdc42 cooperate to regulate endocytic actin assembly during synaptic growth. J. Neurosci. 28, 8316–8325. doi: 10.1523/JNEUROSCI.2304-08.2008
Rosso, S. B., Inestrosa, N. C., and Rosso, S. B. (2013). WNT signaling in neuronal maturation and synaptogenesis. Front. Cell. Neurosci. 7:103. doi: 10.3389/fncel.2013.00103
Rosso, S. B., Sussman, D., Wynshaw-Boris, A., and Salinas, P. C. (2005). Wnt signaling through Dishevelled, Rac and JNK regulates dendritic development. Nat. Neurosci. 8, 34–42. doi: 10.1038/nn1374
Sadok, A., and Marshall, C. J. (2014). Rho gtpases masters of cell migration. Small GTPases 5:e983878. doi: 10.4161/sgtp.29710
Sanyal, S., Sandstrom, D. J., Hoeffer, C. A., and Ramaswami, M. (2002). Ap-1 functions upstream of CREB to control synaptic plasticity in drosophila. Nature 416, 870–874. doi: 10.1038/416870a
Schätzle, P., Esteves da Silva, M., Tas, R. P., Katrukha, E. A., Hu, H. Y., Wierenga, C. J., et al. (2018). Activity-dependent actin remodeling at the base of dendritic spines promotes microtubule entry. Curr. Biol. 28, 2081–2093.e6. doi: 10.1016/j.cub.2018.05.004
Schlessinger, K., McManus, E. J., and Hall, A. (2007). Cdc42 and noncanonical Wnt signal transduction pathways cooperate to promote cell polarity. J. Cell Biol. 178, 355–361. doi: 10.1083/jcb.200701083
Shepherd, G. M. (ed.). (2004). “Introduction to synaptic circuits” in The synaptic Organization of the Brain. 5th ed. (New York: Oxford University Press).
Strutt, D. I., Weber, U., and Mlodzik, M. (1997). The role of RhoA in tissue polarity and frizzled signalling. Nature 387, 292–295. doi: 10.1038/387292a0
Strutt, H., Searle, E., Thomas-Macarthur, V., Brookfield, R., and Strutt, D. (2013). A Cul-3-BTB ubiquitylation pathway regulates junctional levels and asymmetry of core planar polarity proteins. Development 140, 1693–1702. doi: 10.1242/dev.089656
Swetman, C. A., Leverrier, Y., Garg, R., Gan, C. H. V., Ridley, A. J., Katz, D. R., et al. (2002). Extension, retraction and contraction in the formation of a dendritic cell dendrite: distinct roles for rho GTPases. Eur. J. Immunol. 32, 2074–2083. doi: 10.1002/1521-4141(200207)32:7<2074::AID-IMMU2074>3.0.CO;2-S
Tabatadze, N., Mcgonigal, R., Neve, R. L., and Routtenberg, A. (2014). Activity-dependent Wnt 7 dendritic targeting in hippocampal neurons: plasticity- and tagging-related retrograde signaling mechanism? Hippocampus 24, 455–465. doi: 10.1002/hipo.22239
Tashiro, A., Minden, A., and Yuste, R. (2000). Regulation of dendritic spine morphology by the rho family of small GTPases: antagonistic roles of Rac and Rho. Cereb. Cortex. 10, 927–938. doi: 10.1093/cercor/10.10.927
Vadodaria, K. C., Brakebusch, C., Suter, U., and Jessberger, S. (2013). Stage-specific functions of the small rho GTPases Cdc42 and Rac1 for adult hippocampal neurogenesis. J. Neurosci. 33, 1179–1189. doi: 10.1523/JNEUROSCI.2103-12.2013
Vasin, A., Sabeva, N., Torres, C., Phan, S., Bushong, E. A., Ellisman, M. H., et al. (2019). Two pathways for the activity-dependent growth and differentiation of synaptic boutons in drosophila. ENeuro 6, ENEURO.0060–ENEU19.2019. doi: 10.1523/ENEURO.0060-19.2019
Vasin, A., Zueva, L., Torrez, C., Volfson, D., Troy Littleton, J., and Bykhovskaia, M. (2014). Synapsin regulates activity-dependent outgrowth of synaptic boutons at the Drosophila neuromuscular junction. J. Neurosci. 34, 10554–10563. doi: 10.1523/JNEUROSCI.5074-13.2014
Wayman, G. A., Impey, S., Marks, D., Saneyoshi, T., Grant, W. F., Derkach, V., et al. (2006). Activity-dependent dendritic Arborization mediated by CaM-kinase I activation and enhanced CREB-dependent transcription of Wnt-2. Neuron 50, 897–909. doi: 10.1016/j.neuron.2006.05.008
Weaver, A. M., Karginov, A. V., Kinley, A. W., Weed, S. A., Li, Y., Parsons, J. T., et al. (2001). Cortactin promotes and stabilizes Arp2/3-induced actin filament network formation. Curr. Biol. 11, 370–374. doi: 10.1016/S0960-9822(01)00098-7
Weed, S. A., Karginov, A. V., Schafer, D. A., Weaver, A. M., Kinley, A. W., Cooper, J. A., et al. (2000). Cortactin localization to sites of actin assembly in Lamellipodia requires interactions with F-actin and the Arp2/3 complex. J. Cell Biol. 151, 29–40. doi: 10.1083/JCB.151.1.29
Wu, G. Y., Deisseroth, K., and Tsien, R. W. (2001). Spaced stimuli stabilize MAPK pathway activation and its effects on dendritic morphology. Nat. Neurosci. 4, 151–158. doi: 10.1038/83976
Wu, J., Roman, A. C., Carvajal-Gonzalez, J. M., and Mlodzik, M. (2013). Wg and Wnt4 provide long-range directional input to planar cell polarity orientation in Drosophila. Nat. Cell Biol. 15, 1045–1055. doi: 10.1038/ncb2806
Xu, Z., Chen, Y., and Chen, Y. (2019). Spatiotemporal regulation of rho GTPases in neuronal migration. Cells 8:568. doi: 10.3390/cells8060568
Yanfeng, W. A., Berhane, H., Mola, M., Singh, J., Jenny, A., and Mlodzik, M. (2011). Functional dissection of phosphorylation of disheveled in Drosophila. Dev. Biol. 360, 132–142. doi: 10.1016/j.ydbio.2011.09.017
Keywords: NMJ, disheveled, Rho1, Rac1, Cdc42, Jun kinase, AP1
Citation: Dominicci-Cotto C, Vazquez M and Marie B (2024) The Wingless planar cell polarity pathway is essential for optimal activity-dependent synaptic plasticity. Front. Synaptic Neurosci. 16:1322771. doi: 10.3389/fnsyn.2024.1322771
Received: 16 October 2023; Accepted: 18 March 2024;
Published: 03 April 2024.
Edited by:
Maria Bykhovskaia, Wayne State University, United StatesReviewed by:
C. Andrew Frank, The University of Iowa, United StatesCopyright © 2024 Dominicci-Cotto, Vazquez and Marie. This is an open-access article distributed under the terms of the Creative Commons Attribution License (CC BY). The use, distribution or reproduction in other forums is permitted, provided the original author(s) and the copyright owner(s) are credited and that the original publication in this journal is cited, in accordance with accepted academic practice. No use, distribution or reproduction is permitted which does not comply with these terms.
*Correspondence: Bruno Marie, YnJ1bm8ubWFyaWVAdXByLmVkdQ==
Disclaimer: All claims expressed in this article are solely those of the authors and do not necessarily represent those of their affiliated organizations, or those of the publisher, the editors and the reviewers. Any product that may be evaluated in this article or claim that may be made by its manufacturer is not guaranteed or endorsed by the publisher.
Research integrity at Frontiers
Learn more about the work of our research integrity team to safeguard the quality of each article we publish.