- 1Center for Neurogenomics and Cognitive Research, Vrije Universiteit Amsterdam, Amsterdam, Netherlands
- 2Research Center Juelich, Institute of Neuroscience and Medicine, Jülich, Germany
- 3Department of Psychiatry, Psychotherapy, and Psychosomatics, RWTH Aachen University Hospital, Aachen, Germany
- 4Jülich-Aachen Research Alliance, Translational Brain Medicine (JARA Brain), Aachen, Germany
Information transfer between principal neurons in neocortex occurs through (glutamatergic) synaptic transmission. In this focussed review, we provide a detailed overview on the strength of synaptic neurotransmission between pairs of excitatory neurons in human and laboratory animals with a specific focus on data obtained using patch clamp electrophysiology. We reach two major conclusions: (1) the synaptic strength, measured as unitary excitatory postsynaptic potential (or uEPSP), is remarkably consistent across species, cortical regions, layers and/or cell-types (median 0.5 mV, interquartile range 0.4–1.0 mV) with most variability associated with the cell-type specific connection studied (min 0.1–max 1.4 mV), (2) synaptic function cannot be generalized across human and rodent, which we exemplify by discussing the differences in anatomical and functional properties of pyramidal-to-pyramidal connections within human and rodent cortical layers 2 and 3. With only a handful of studies available on synaptic transmission in human, it is obvious that much remains unknown to date. Uncovering the shared and divergent principles of synaptic transmission across species however, will almost certainly be a pivotal step toward understanding human cognitive ability and brain function in health and disease.
Excitatory (glutamatergic) synaptic transmission is the primary mode of communication between principal neurons in the neocortex and allows information transfer between synaptically connected neurons. Local inhibitory (GABAergic) synapses modulate (i.e., reduce or disinhibit) the activity of neighboring principal neurons but do not directly contribute to information transfer, at least in the adult (Rheims et al., 2008; Kirmse et al., 2015). The basic blueprint of the synapse typically includes the neurotransmitter release machinery of the presynaptic axon and the associated receptor complex on the postsynaptic dendrite. However, this simplified scheme is easily extended to a spectrum of synapse types, including (i) axo-axonic synapses (Somogyi, 1977; Gonchar et al., 2002; Schneider-Mizell et al., 2021), (ii) axo-somatic synapses (Borst and Soria van Hoeve, 2012; Kubota et al., 2016), (iii) dendro-dendritic synapses [(Woolf et al., 1991; Aghvami et al., 2022)], or (iii) the tripartite synapse with juxta-posed astrocytes [(Araque et al., 1999; Liu et al., 2023)]. Furthermore, a single postsynaptic spine can be occupied by multiple presynaptic terminals and contact points are found between axonal bouton and postsynaptic spine neck or dendritic shaft (Cano-Astorga et al., 2021). Because the synapse is the fundamental locus for communication between neurons, it is not surprising that synaptic dysfunction leads to a plethora of brain disorders.
Structural features of individual synapses are studied at maximal spatial resolution using electron microscopy (EM), which can also provide information on the parent neuronal cell type and the local microcircuit (Kasthuri et al., 2015; Schmidt et al., 2017; Loomba et al., 2022). EM typically provides quantitative insight into absolute synapse number or synapse density, which in turn are specific to the brain region or sub-region (e.g., cortical layer) under investigation (DeFelipe et al., 2002). Single dendrite or single spine analysis using EM further uncovered branch-specific spine densities which can be extrapolated to a cumulative number of total spines per neuron (Benavides-Piccione et al., 2013; Eyal et al., 2018). This total number of synapses per neuron is an estimate of the total number of excitatory inputs that an identified postsynaptic neuron may receive (10,000–30,000), which ultimately determines the complexity of input/output transformations. Studies focusing on synapse structure have shown that, in cortical microcircuits, spine shape (Benavides-Piccione et al., 2002; Ofer et al., 2022), spine density (Benavides-Piccione et al., 2002, 2013; Loomba et al., 2022), or total number of spines per neuron can be highly divergent between species. For example, the number of presynaptic vesicles is higher in humans compared to rodents (Yakoubi et al., 2019a,b), and postsynaptic densities are larger (Benavides-Piccione et al., 2002).
Dense (and/or saturated) reconstruction methods using EM have advanced the field of neuroanatomy by revealing not only synapse structure and local connectivity, but also by uncovering the wiring rules of local microcircuits across highly diverse brain regions for rapidly increasing volumes (Helmstaedter et al., 2013; Kasthuri et al., 2015; Motta et al., 2019; Shapson-Coe et al., 2021). However, the functional characterization of synaptic transmission remains necessary, to translate static snapshots of wiring principles into dynamic properties of information transfer. These types of experiments are typically performed by dual recordings of synaptically connected neurons (Qi et al., 2020). Synchronous recording of multiple neurons increases the mapping efficiency but is technically demanding (Lefort et al., 2009; Perin et al., 2011; Jiang et al., 2015; Seeman et al., 2018; Peng et al., 2019; Campagnola et al., 2022). This approach also complicates a precise reconstruction of axonal morphology of pre- and postsynaptic neurons due to the extensive overlap of thin axons with relatively large somatodendritic domains. Wiring diagrams have been mapped with particular focus on sensory cortices (Lefort et al., 2009; Cossell et al., 2015; Jiang et al., 2015; Markram et al., 2015; Campagnola et al., 2022). These efforts reveal a complex interplay between morphologically identified cell types, connectivity rates and synapse function (Brown and Hestrin, 2009; Lefort et al., 2009; Campagnola et al., 2022). With the advent of new technologies to define cell types by their transcriptomic profile (Hodge et al., 2019; Scala et al., 2019; Gouwens et al., 2020), it remains crucial to use a consistent and unambiguous procedure to identify neuronal cell types as part of the experimental design. This is particularly important in view of wiring diagrams in which neurons have a variety of postsynaptic partners, and presynaptic neurons tuning their synaptic properties to the cell-type specific identity of the post-synaptic target neuron (Lefort et al., 2009; Peng et al., 2019). In addition, pre-synaptic neurotransmitter release, mean PSP amplitude, short-term depression or facilitation, and recovery from synaptic depression can be highly specific for the connection under study (Campagnola et al., 2022). In short, with only a fraction of all possible cell-to-cell connections characterized in any species, it is too early to generalize synaptic function for highly heterogeneous populations of neuronal cell types.
Fundamental properties of synaptic function include synaptic strength (excitatory/inhibitory postsynaptic potential or EPSP/IPSP, in mV, Figure 1 and Table 1), the dynamics during repetitive stimulation (facilitation/depression, paired pulse ratio), EPSP/IPSP decay kinetics (in ms), ionotropic receptor composition at the postsynaptic membrane, recovery from synaptic depression (τ, in ms) or long-term plasticity (LTP/LTD) rules. We are only beginning to understand how synaptic transmission may vary across species. In this focused review, we aim to summarize recent data on (excitatory) synaptic transmission in rodent and human cortical microcircuits. We compile data on unitary synaptic connections that have been mapped using whole-cell patch clamp electrophysiology. Most of this data comes from synaptic connections between pairs of pyramidal cells in layers 2/3 in which subtypes of pyramidal cells are typically pooled (Figure 1 and Table 1). We also include information on additional cortical layers, and where possible, provide information on specific pre- and postsynaptic cell type (i.e., L4 spiny stellate vs. L4 star pyramid or L6 corticocortical vs. L6 corticothalamic pyramidal cell, Figure 1 and Table 2). Note that in the majority of these connectivity studies, by convention, an extracellular calcium concentration of 2 mM (∼1.7 mM free Ca2+) was used; only a small subset used a calcium concentration of 1.3 mM with a minority of studies using concentrations of 1.8 mM, 2.5 mM, or 3.0 mM.
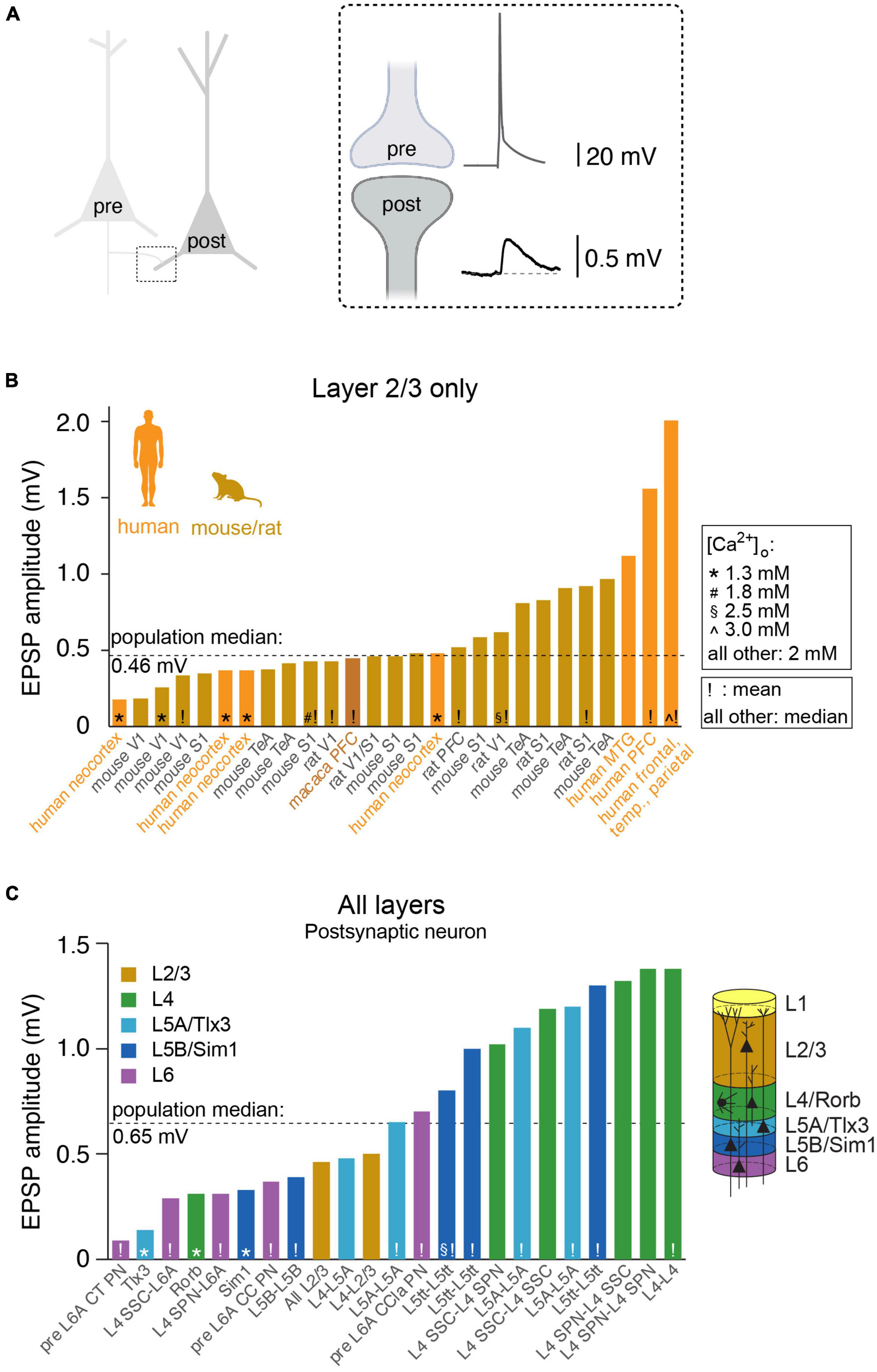
Figure 1. Synaptic strength between pairs of excitatory neurons across species, brain regions, cortical layers and/or cell-types. (A) Cartoon illustrating the synaptic connection between the axon of the presynaptic neuron and the dendrite of the postsynaptic neuron. Inset: the action potential in the presynaptic axon triggers neurotransmitter release, evoking a (unitary) excitatory postsynaptic potential (uEPSP) in dendritic spine of the postsynaptic neuron. (B) Overview of published uEPSP amplitudes (in mV) for connections exclusively within rodent layer 2/3 or human layer 2 and 3. Symbols indicate external calcium concentration (* 1.3 mM, # 1.8 mM, 2.5 mM, ∧ 3.0 mM, all other 2.0 mM), exclamation mark indicate that uEPSP amplitude was computed as average (all other: median). Abbreviations: V1: (primary) visual cortex, S1: (primary) somatosensory cortex, TeA: temporal association cortex, PFC: prefrontal cortex, MTG: middle temporal gyrus. (C) Analogous to B but for connections between or within additional cortical layers, with particular focus on rodent literature. CT, cortico-thalamic; CC, cortico-cortical; CCla, cortico-claustrum; PN, pyramidal neuron; SPN, star pyramidal neuron; SSC, spiny stellate cell; L5tt, layer 5 thick tufted pyramidal neuron.
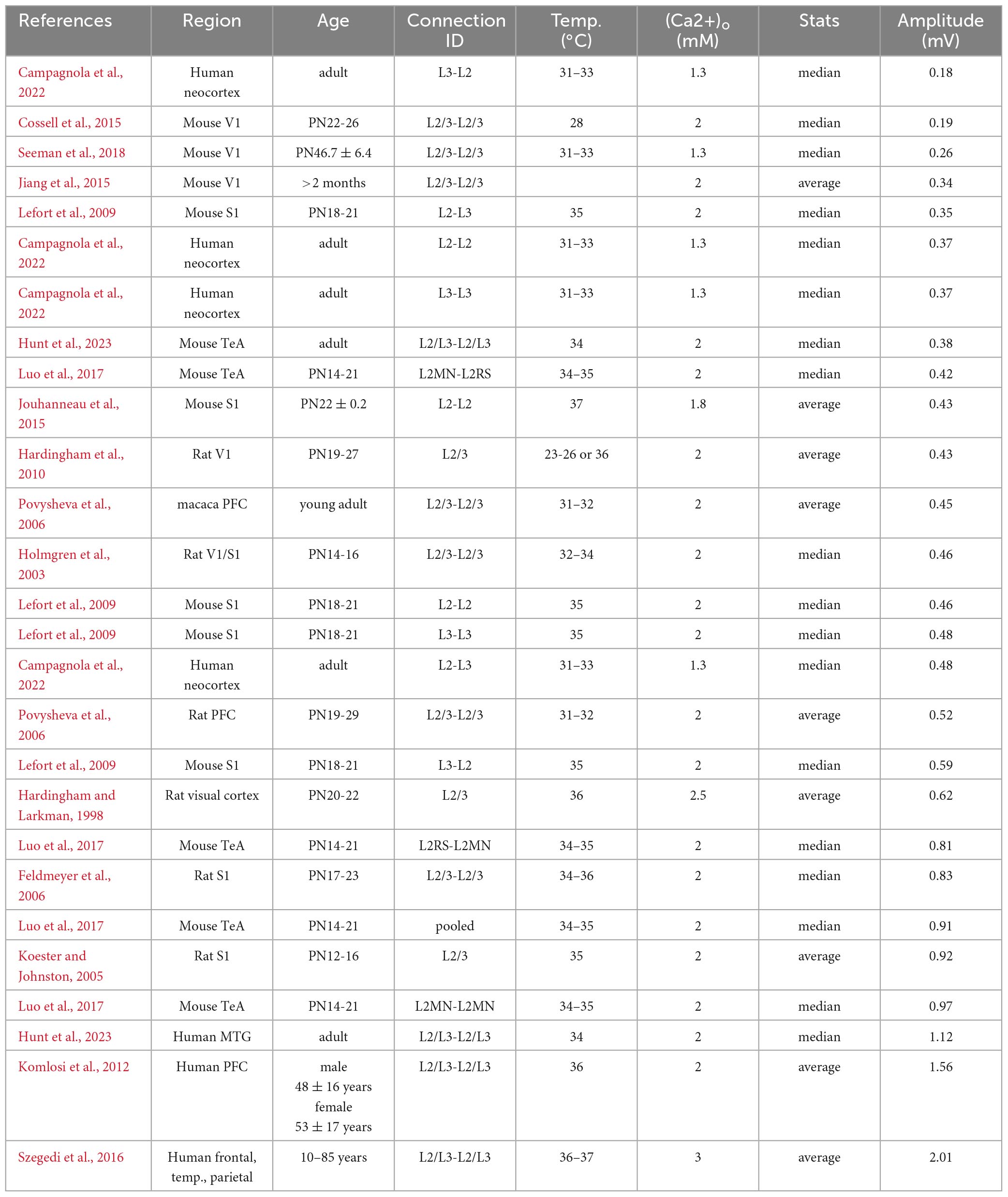
Table 1. uEPSP amplitude (in mV) for excitatory, pyramidal-to-pyramidal connections in cortical layer 2/3 across species.
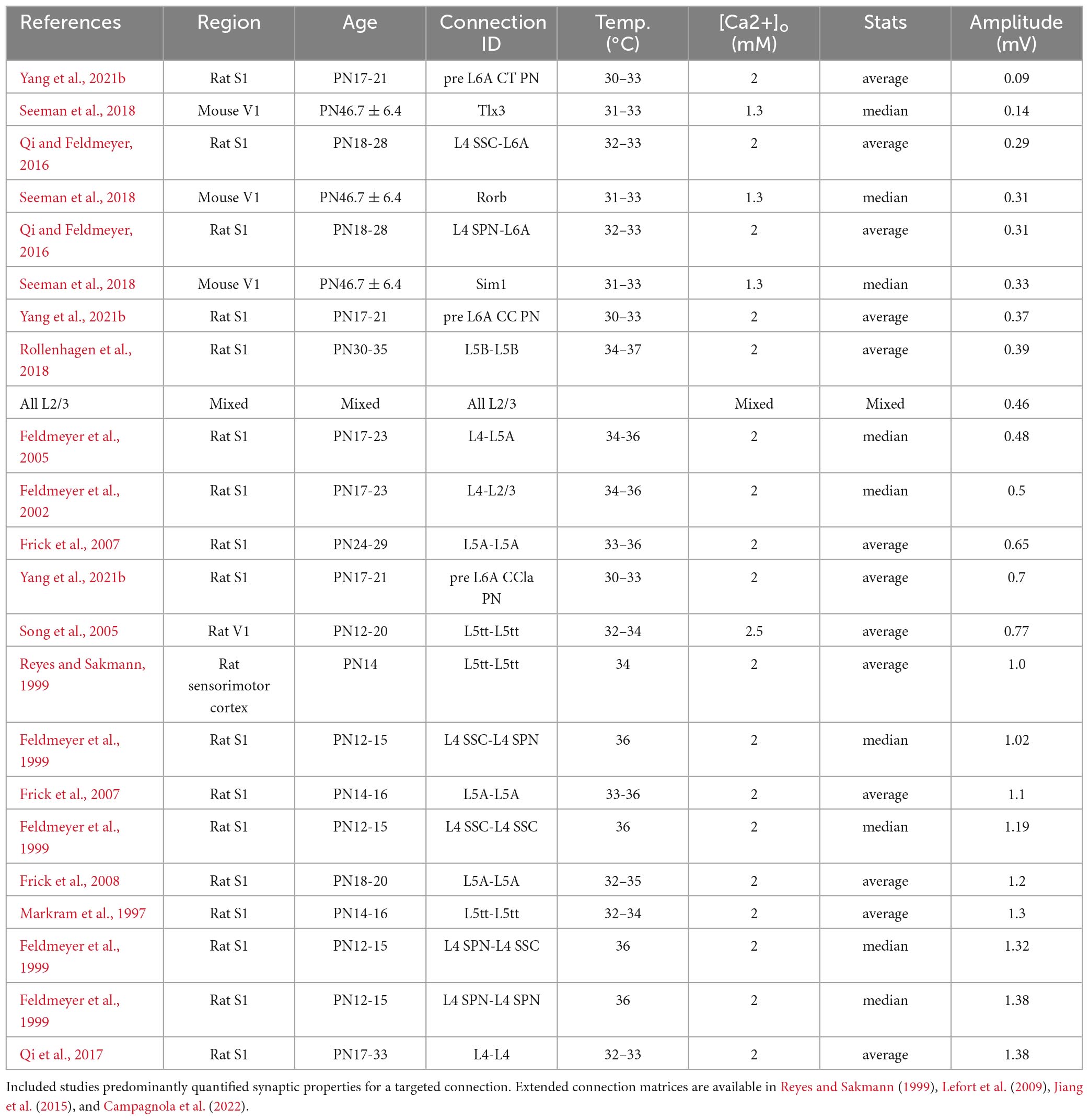
Table 2. uEPSP amplitude in mV for excitatory connections between identified cell-types and/or layers in neocortex.
A recent study has shown that the total extracellular Ca2+ in human cerebral spinal fluid can be as low as 1.2 mM (∼1.0 mM free Ca2+ (Forsberg et al., 2019). Since the Ca2+ concentration has been shown to affect neuronal excitability, presynaptic release probability and short-term synaptic plasticity (Molnar et al., 2016; Forsberg et al., 2019), it is crucial to incorporate this parameter into the comparison of synaptic strength across different studies. Recording temperature also affects synaptic release probability (hence: uEPSP amplitude), failure rate, reliability (Hardingham and Larkman, 1998), or synaptic plasticity (Klyachko and Stevens, 2006) and the overview thus provides both Ca2+ concentration and recording temperature at which the synaptic strength was quantified.
Perhaps the best-studied neuronal microcircuits are the primary somatosensory (S1) and primary visual (V1) cortices (Reyes and Sakmann, 1999; Lefort et al., 2009; Feldmeyer et al., 2013; Jiang et al., 2015; Markram et al., 2015; Seeman et al., 2018; Campagnola et al., 2022). Depending on the specific connection between excitatory cell types within or across layers, the EPSP amplitude at the soma can vary considerably (Figure 1 and Table 2). For example, the EPSP amplitude for the unitary connection between presynaptic L6A cortico-thalamic pyramidal neurons and a L6A excitatory cell type as postsynaptic target (in S1) is 0.09 mV (Yang et al., 2021b). A connection with a much larger mean unitary EPSP (uEPSP) amplitude is between L4 excitatory neurons (i.e., 1.38 mV) (Qi et al., 2017) and multiple EPSP amplitude values have been reported to fall within this range (Figure 1 and Tables 1, 2). The unitary synaptic connection between pyramidal neurons in cortical layers 2 and 3 has been characterized in several studies. They show substantial differences in the mean or median uEPSP amplitude for L2/3 pyramidal-to-pyramidal cell connections across different cortical regions (PFC, TeA, S1, V1, MTG) and species (mouse, rat, macaque, human; total range: 0.18–2.01 mV, Figure 1 and Table 1). Experimental or analytical conditions such as developmental stage (i.e., juvenile, adolescent, adult), external Ca2+ (1.3, 1.8, 2.0, 2.5 or 3.0 mM), temperature or reported mean/median could certainly influence the reported uEPSP amplitude, but it is also likely that the synaptic strength is dependent on the connection established between different L2/3 pyramidal cell types (Deitcher et al., 2017; Hodge et al., 2019; Berg et al., 2021; Hunt et al., 2023). Pyramidal-to-interneuron or interneuron-to-pyramidal cell connections are typically stronger (Molnar et al., 2016; Wilbers et al., 2023), but here we focus on synaptic transmission between pairs of excitatory neurons. In this context, it is important to emphasize that amplitude distributions are typically skewed with the majority of connections showing small amplitudes and a long tail of stronger connections (Feldmeyer et al., 1999; Holmgren et al., 2003; Song et al., 2005; Cossell et al., 2015; Seeman et al., 2018; Hunt et al., 2023). We would therefore argue that the median (and 1st-3rd interquartile ranges) should be consistently reported as it is more representative for the skewed population data. Ideally, these population statistics are also supplemented with the full range (min/max) of the population data as the extremes of the lognormal distribution may have biologically relevant functions (Szegedi et al., 2016, 2017). Synaptic connections with small mean uEPSP amplitudes may also fall below the detection power [Seeman et al., 2018; Supplementary Figure 14 in Campagnola et al. (2022) and Supplementary Figure 3 in Qi and Feldmeyer (2016)], especially when using a limited number of sweeps to probe for the presence of a connection. The combination of small amplitude connections and low detection power increases the false negative rate, underestimates the true connectivity, and may lead to an overestimation of mean EPSP amplitude of a given synaptic connection type. It is therefore perhaps not surprising that connections with a small uEPSP amplitude may be missed by electrophysiological recordings from the soma [but see Yang et al. (2021b)], but are reliably detected using EM techniques (Loomba et al., 2022). However, the use of EM techniques has also limitations when studying wiring diagrams because the axonal arbors occupy much larger volumes relative to the tissue blocks currently processed for EM examination (Oberlaender et al., 2011; Narayanan et al., 2015; Winnubst et al., 2019). Therefore, cross-scale techniques including correlated anatomical and physiological measurements are still urgently needed to generate a comprehensive, functional wiring diagram for microcircuits of interest.
For a given connection type, population distributions of uEPSP amplitude can thus show a pronounced skew with a subset of unitary connections being particularly strong and even sufficiently large to evoke AP firing (Feldmeyer et al., 1999, 2006; Holmgren et al., 2003; Silver et al., 2003; Song et al., 2005; Frick et al., 2008; Lefort et al., 2009). These exceptionally strong excitatory connections are attractive to study because in vivo recordings from primary somatosensory and primary visual cortices have shown that a small subset of excitatory neurons show a particularly strong response to sensory stimulation, while the majority of neurons respond with only a subthreshold depolarization or not all, [e.g., (Brecht et al., 2003; de Kock et al., 2007; Kerr et al., 2007; O’Connor et al., 2010; Crochet et al., 2011; Barth and Poulet, 2012; Cossell et al., 2015; Barz et al., 2021)]; these neurons can be referred to as ‘high responders’. A systematic analysis of the properties of these ‘high responder’ neurons and the impact of large uEPSPs is still lacking and as such a matter of debate. It would be interesting to determine whether particularly strong intracortical synapses go hand in hand with reliable sensory representation in vivo (Yassin et al., 2010).
For neurons with dendrites that are electrically relatively compact [e.g., rodent neurons, (Beaulieu-Laroche et al., 2018)], detection power may not be a major issue as distal synapses can still have a profound impact on the somatic membrane potential. However, cortical neurons of human brain have much longer dendrites as well as increased branching (Mohan et al., 2015). The outcome of these extended morphologies is a huge capacitance load on electrical signals traveling from distal regions to the soma resulting in electrically isolated subcompartments (Eyal et al., 2014; Beaulieu-Laroche et al., 2018; Gidon et al., 2020). Therefore, the risk of false negative connections increases substantially when probing human cortical circuits (Seeman et al., 2018). The increased path length from dendrite to soma and potentially increased dendritic attenuation opens the possibility of evolutionary adaptation of human neurons. For example, one neurophysiological property that may compensate for this, is active dendritic electrogenesis, which has been extensively documented for somatosensory L5 thick tufted neurons (Larkum et al., 1999, 2022) and to some extent in pyramidal cells in layer 6 and superficial cortical layers (Ledergerber and Larkum, 2010, 2012). Depending on the type of synaptic input, dendritic electrogenesis will initiate voltage-dependent Ca2+ spikes in the apical dendrites or NMDA spikes in the basal dendrites and the apical tuft dendrites; these serve to amplify synaptic signals and ensure proper propagation to the soma (Larkum et al., 2022). Alternatively, it was suggested that human L2/L3 neurons may have a lower membrane capacitance compared to rodents (human: 0.5 μF/cm2, rodent: 1.0 μF/cm2, [(Eyal et al., 2016), but see Gooch et al. (2022)]. This potential adaptation could be specific to L2/L3 neurons (Beaulieu-Laroche et al., 2018) and translates to enhanced synaptic charge-transfer from dendrites to soma and ultimately similar EPSP amplitudes across species (Figure 1 and Table 1). Given the extended morphologies and increased path length of human neurons, it is even more important to understand the active properties of human dendrites in order to understand the passive and active propagation of synaptic inputs and ultimately neuronal input/output transformations (Gidon et al., 2020; Beaulieu-Laroche et al., 2021; Kalmbach et al., 2021; Testa-Silva et al., 2022).
Synaptic connections (and thus uEPSP amplitudes) are also affected by neuromodulatory transmitters such as acetylcholine and monoamines, by neuropeptides, and by many hormones all of which act by activating or deactivating ionotropic ion channels and/or G protein-coupled receptors (GPCRs). The release of these neuromodulators is dependent on circadian rhythm, behavioral state and age. Examples of neuromodulator receptors that cause an increase or decrease in the synaptic release probability include muscarinic and nicotinic acetylcholine receptors, as well as several types of serotonin and adenosine receptors [for reviews see Puig and Gulledge (2011), Radnikow and Feldmeyer (2018), Yang et al. (2021a)]. In addition, the released neurotransmitter may diffuse out of the synaptic cleft (‘spill over’) and affect the synaptic release probability by acting on G protein-coupled receptors (such as metabotropic glutamate receptors or GABAB receptors) in the perisynaptic membrane of the same or neighbouring presynaptic terminals (Kullmann and Asztely, 1998; Uchida et al., 2012; Wild et al., 2015). These neuromodulators are present in the cerebrospinal fluid at low micromolar concentrations and therefore affect synaptic release even in the absence of pharmacological intervention. The discovery of divergent gene expression of a major neuromodulatory receptor system between human and mouse (i.e., serotonin, (Hodge et al., 2019), calls for a detailed characterization of the interplay between baseline synaptic transmission in humans and the impact of the major neuromodulator systems.
Synaptic connections in the human neocortex
To date, only a small number of studies exist that have examined synaptic transmission at autapses (Yin et al., 2018) or between pairs of excitatory neurons in human brain (Komlosi et al., 2012; Szegedi et al., 2016; Seeman et al., 2018; Peng et al., 2019; Campagnola et al., 2022; Hunt et al., 2023). The availability of human brain tissue is typically a by-product of neurosurgical resection of epileptic foci or tumors. This provides a window of opportunity to study synaptic transmission and quantify how neurons with overall larger morphologies, extended dendrites and most likely many more synaptic inputs than rodents deal with synaptic inputs. With only a handful of studies available, it is too premature to generalize, but the emerging data show common and divergent properties of synaptic transmission across species.
Measured at the soma, uEPSP amplitude for pyramidal-to-pyramidal cell connections in layer 2/3 across species is remarkably consistent (median ALL 0.46 mV, mouse: 0.43 mV, rat: 0.57 mV, macaque: 0.45 mV, human: 0.48 mV, Figure 1 and Table 1). This suggests that obvious differences in the principles of brain organization or neuronal architecture do not affect the fundamental unit of synaptic information transfer. Examples of these differences in organizational principles across species include brain mass [mouse 0.417 g, human 1,508 g, (Herculano-Houzel, 2009), the number of cortical neurons (mouse: 2 billion, human: 16 billion, (Herculano-Houzel, 2009), estimated spine count on an individual L2/3 pyramidal neuron (mouse: 10,000, human: 30,000, (Eyal et al., 2018) or total dendritic length (mouse: 5.3 mm, human: 14.5 mm, (Mohan et al., 2015)]. These are just a few example differences, but can easily be extended to dendritic path length, synapse density, and many more (Benavides-Piccione et al., 2002; Yakoubi et al., 2019a,b; Loomba et al., 2022). This certainly does not mean that everything is equal. A consistent finding now reported across two independent sites is that excitatory transmission is stronger in L2/L3 pyramidal-to-pyramidal connections for human compared to L2/3 in mouse (Campagnola et al., 2022; Hunt et al., 2023), which could be (in part) explained by increased contribution of NMDA receptor activation during unitary synaptic transmission in humans but not mice. Alternatively, increased synaptic strength can be the outcome of a difference in presynaptic release probability (Hunt et al., 2023). A second consistent finding is that recovery from synaptic depression is faster in humans compared to mice (Testa-Silva et al., 2014; Campagnola et al., 2022; Hunt et al., 2023). These differences have implications for the cellular information transfer, signal flow within neuronal microcircuits and thus ultimately cognition and mental ability (Goriounova et al., 2018).
Conclusion and outlook
The synapse is the fundamental building block of neuronal microcircuits across species. The anatomical features (Benavides-Piccione et al., 2002), functional properties (Seeman et al., 2018; Campagnola et al., 2022; Hunt et al., 2023), and plasticity dynamics (Testa-Silva et al., 2014) differ when comparing pyramidal-to-pyramidal cell connections in cortical L2/3 in human and mouse. Comparable differences in anatomy (Yakoubi et al., 2019a,b) or physiology (Molnar et al., 2016) have also been described in deeper layers or pyramidal-to-interneuron connections, respectively, suggesting a spectrum of human-specific adaptations in synaptic transmission. As we are only beginning to see the tip of the iceberg and much remains unknown about synaptic transmission in human (and non-human primates), it will be critical to continue efforts to study synaptic transmission in the human brain between identified cell types. This will accelerate efforts to build realistic biophysical models of individual neurons (Eyal et al., 2018; Hunt et al., 2023), microcircuit simulations (Markram et al., 2015; Joglekar et al., 2018) and ultimately facilitate a comprehensive understanding of human cognitive abilities in health and disease.
Author contributions
CK: Conceptualization, Funding acquisition, Writing—original draft, Writing—review and editing. DF: Conceptualization, Funding acquisition, Writing—original draft, Writing–review and editing.
Funding
The author(s) declare financial support was received for the research, authorship, and/or publication of this article. This work was supported by the National Institute of Mental Health (BICAN) grant UM1MH130981-01 (CK) and by The Dutch Research Council (NWO) Open Competition (ENW-M2) grant nr OCENW.M20.285 (CK), the Helmholtz Society (DF) and European Union’s Horizon 2020 Framework Programme for Research and Innovation under the Framework Partnership Agreement No. 650003 (HBP FPA) (DF).
Conflict of interest
The authors declare that the research was conducted in the absence of any commercial or financial relationships that could be construed as a potential conflict of interest.
The author(s) declared that they were an editorial board member of Frontiers, at the time of submission. This had no impact on the peer review process and the final decision.
Publisher’s note
All claims expressed in this article are solely those of the authors and do not necessarily represent those of their affiliated organizations, or those of the publisher, the editors and the reviewers. Any product that may be evaluated in this article, or claim that may be made by its manufacturer, is not guaranteed or endorsed by the publisher.
References
Aghvami, S. S., Kubota, Y., and Egger, V. (2022). anatomical and functional connectivity at the dendrodendritic reciprocal mitral cell-granule cell synapse: Impact on recurrent and lateral inhibition. Front. Neural Circuits 16:933201. doi: 10.3389/fncir.2022.933201
Araque, A., Parpura, V., Sanzgiri, R. P., and Haydon, P. G. (1999). Tripartite synapses: Glia, the unacknowledged partner. Trends Neurosci. 22, 208–215. doi: 10.1016/s0166-2236(98)01349-6
Barth, A. L., and Poulet, J. F. (2012). Experimental evidence for sparse firing in the neocortex. Trends Neurosci. 35, 345–355.
Barz, C. S., Garderes, P. M., Ganea, D. A., Reischauer, S., Feldmeyer, D., and Haiss, F. (2021). Functional and structural properties of highly responsive somatosensory neurons in mouse barrel cortex. Cereb. Cortex 31, 4533–4553.
Beaulieu-Laroche, L., Brown, N. J., Hansen, M., Toloza, E. H. S., Sharma, J., Williams, Z. M., et al. (2021). Allometric rules for mammalian cortical layer 5 neuron biophysics. Nature 600, 274–278. doi: 10.1038/s41586-021-04072-3
Beaulieu-Laroche, L., Toloza, E. H. S., van der Goes, M. S., Lafourcade, M., Barnagian, D., Williams, Z. M., et al. (2018). Enhanced dendritic compartmentalization in human cortical neurons. Cell 175, 643–651 e14. doi: 10.1016/j.cell.2018.08.045
Benavides-Piccione, R., Ballesteros-Yanez, I., DeFelipe, J., and Yuste, R. (2002). Cortical area and species differences in dendritic spine morphology. J. Neurocytol. 31, 337–346. doi: 10.1023/a:1024134312173
Benavides-Piccione, R., Fernaud-Espinosa, I., Robles, V., Yuste, R., and DeFelipe, J. (2013). Age-based comparison of human dendritic spine structure using complete three-dimensional reconstructions. Cereb. Cortex 23, 1798–1810. doi: 10.1093/cercor/bhs154
Berg, J., Sorensen, S. A., Ting, J. T., Miller, J. A., Chartrand, T., Buchin, A., et al. (2021). Human neocortical expansion involves glutamatergic neuron diversification. Nature 598, 151–158. doi: 10.1038/s41586-021-03813-8
Borst, J. G., and Soria van Hoeve, J. (2012). The calyx of Held synapse: From model synapse to auditory relay. Annu. Rev. Physiol. 74, 199–224. doi: 10.1146/annurev-physiol-020911-153236
Brecht, M., Roth, A., and Sakmann, B. (2003). Dynamic receptive fields of reconstructed pyramidal cells in layers 3 and 2 of rat somatosensory barrel cortex. J. Physiol. 553(Pt 1), 243–265.
Brown, S. P., and Hestrin, S. (2009). Intracortical circuits of pyramidal neurons reflect their long-range axonal targets. Nature 457, 1133–1136.
Campagnola, L., Seeman, S. C., Chartrand, T., Kim, L., Hoggarth, A., Gamlin, C., et al. (2022). Local connectivity and synaptic dynamics in mouse and human neocortex. Science 375:eabj5861. doi: 10.1126/science.abj5861
Cano-Astorga, N., DeFelipe, J., and Alonso-Nanclares, L. (2021). Three-dimensional synaptic organization of layer III of the human temporal neocortex. Cereb. Cortex 31, 4742–4764. doi: 10.1093/cercor/bhab120
Cossell, L., Iacaruso, M. F., Muir, D. R., Houlton, R., Sader, E. N., Ko, H., et al. (2015). Functional organization of excitatory synaptic strength in primary visual cortex. Nature 518, 399–403. doi: 10.1038/nature14182
Crochet, S., Poulet, J. F., Kremer, Y., and Petersen, C. C. (2011). Synaptic mechanisms underlying sparse coding of active touch. Neuron 69, 1160–1175.
de Kock, C. P., Bruno, R. M., Spors, H., and Sakmann, B. (2007). Layer and cell type specific suprathreshold stimulus representation in primary somatosensory cortex. J. Physiol. 581, 139–154.
DeFelipe, J., Alonso-Nanclares, L., and Arellano, J. I. (2002). Microstructure of the neocortex: Comparative aspects. J. Neurocytol. 31, 299–316.
Deitcher, Y., Eyal, G., Kanari, L., Verhoog, M. B., Atenekeng Kahou, G. A., Mansvelder, H. D., et al. (2017). Comprehensive morpho-electrotonic analysis shows 2 distinct classes of L2 and L3 pyramidal neurons in human temporal cortex. Cereb. Cortex 27, 5398–5414. doi: 10.1093/cercor/bhx226
Eyal, G., Mansvelder, H. D., de Kock, C. P., and Segev, I. (2014). Dendrites impact the encoding capabilities of the axon. J. Neurosci. 34, 8063–8071. doi: 10.1523/JNEUROSCI.5431-13.2014
Eyal, G., Verhoog, M. B., Testa-Silva, G., Deitcher, Y., Benavides-Piccione, R., DeFelipe, J., et al. (2018). Human cortical pyramidal neurons: From spines to spikes via models. Front. Cell. Neurosci. 12:181. doi: 10.3389/fncel.2018.00181
Eyal, G., Verhoog, M. B., Testa-Silva, G., Deitcher, Y., Lodder, J. C., Benavides-Piccione, R., et al. (2016). Unique membrane properties and enhanced signal processing in human neocortical neurons. Elife 5:e16553. doi: 10.7554/eLife.16553
Feldmeyer, D., Brecht, M., Helmchen, F., Petersen, C. C., Poulet, J. F., Staiger, J. F., et al. (2013). Barrel cortex function. Prog. Neurobiol. 103, 3–27. doi: 10.1016/j.pneurobio.2012.11.002
Feldmeyer, D., Egger, V., Lubke, J., and Sakmann, B. (1999). Reliable synaptic connections between pairs of excitatory layer 4 neurones within a single ‘barrel’ of developing rat somatosensory cortex. J. Physiol. 521(Pt 1), 169–190.
Feldmeyer, D., Lubke, J., and Sakmann, B. (2006). Efficacy and connectivity of intracolumnar pairs of layer 2/3 pyramidal cells in the barrel cortex of juvenile rats. J. Physiol. 575(Pt 2), 583–602. doi: 10.1113/jphysiol.2006.105106
Feldmeyer, D., Lubke, J., Silver, R. A., and Sakmann, B. (2002). Synaptic connections between layer 4 spiny neurone-layer 2/3 pyramidal cell pairs in juvenile rat barrel cortex: Physiology and anatomy of interlaminar signalling within a cortical column. J. Physiol. 538(Pt 3), 803–822.
Feldmeyer, D., Roth, A., and Sakmann, B. (2005). Monosynaptic connections between pairs of spiny stellate cells in layer 4 and pyramidal cells in layer 5A indicate that lemniscal and paralemniscal afferent pathways converge in the infragranular somatosensory cortex. J. Neurosci. 25, 3423–3431.
Forsberg, M., Seth, H., Bjorefeldt, A., Lyckenvik, T., Andersson, M., Wasling, P., et al. (2019). Ionized calcium in human cerebrospinal fluid and its influence on intrinsic and synaptic excitability of hippocampal pyramidal neurons in the rat. J. Neurochem. 149, 452–470. doi: 10.1111/jnc.14693
Frick, A., Feldmeyer, D., and Sakmann, B. (2007). Postnatal development of synaptic transmission in local networks of L5A pyramidal neurons in rat somatosensory cortex. J. Physiol. 585(Pt 1), 103–116. doi: 10.1113/jphysiol.2007.141788
Frick, A., Feldmeyer, D., Helmstaedter, M., and Sakmann, B. (2008). Monosynaptic connections between pairs of L5A pyramidal neurons in columns of juvenile rat somatosensory cortex. Cereb. Cortex 18, 397–406. doi: 10.1093/cercor/bhm074
Gidon, A., Zolnik, T. A., Fidzinski, P., Bolduan, F., Papoutsi, A., Poirazi, P., et al. (2020). Dendritic action potentials and computation in human layer 2/3 cortical neurons. Science 367, 83–87. doi: 10.1126/science.aax6239
Gonchar, Y., Turney, S., Price, J. L., and Burkhalter, A. (2002). Axo-axonic synapses formed by somatostatin-expressing GABAergic neurons in rat and monkey visual cortex. J. Comp. Neurol. 443, 1–14. doi: 10.1002/cne.1425
Gooch, H. M., Bluett, T., Perumal, M. B., Vo, H. D., Fletcher, L. N., Papacostas, J., et al. (2022). High-fidelity dendritic sodium spike generation in human layer 2/3 neocortical pyramidal neurons. Cell Rep. 41:111500. doi: 10.1016/j.celrep.2022.111500
Goriounova, N. A., Heyer, D. B., Wilbers, R., Verhoog, M. B., Giugliano, M., Verbist, C., et al. (2018). Large and fast human pyramidal neurons associate with intelligence. Elife 7:e41714. doi: 10.7554/eLife.41714
Gouwens, N. W., Sorensen, S. A., Baftizadeh, F., Budzillo, A., Lee, B. R., Jarsky, T., et al. (2020). Integrated morphoelectric and transcriptomic classification of cortical GABAergic cells. Cell 183, 935–953 e19. doi: 10.1016/j.cell.2020.09.057
Hardingham, N. R., and Larkman, A. U. (1998). Rapid report: The reliability of excitatory synaptic transmission in slices of rat visual cortex in vitro is temperature dependent. J. Physiol. 507 (Pt 1), 249–256. doi: 10.1111/j.1469-7793.1998.249bu.x
Hardingham, N. R., Read, J. C., Trevelyan, A. J., Nelson, J. C., Jack, J. J., and Bannister, N. J. (2010). Quantal analysis reveals a functional correlation between presynaptic and postsynaptic efficacy in excitatory connections from rat neocortex. J. Neurosci. 30, 1441–1451. doi: 10.1523/JNEUROSCI.3244-09.2010
Helmstaedter, M., Briggman, K. L., Turaga, S. C., Jain, V., Seung, H. S., and Denk, W. (2013). Connectomic reconstruction of the inner plexiform layer in the mouse retina. Nature 500, 168–174. doi: 10.1038/nature12346
Herculano-Houzel, S. (2009). The human brain in numbers: A linearly scaled-up primate brain. Front. Hum. Neurosci. 3:31. doi: 10.3389/neuro.09.031.2009
Hodge, R. D., Bakken, T. E., Miller, J. A., Smith, K. A., Barkan, E. R., Graybuck, L. T., et al. (2019). Conserved cell types with divergent features in human versus mouse cortex. Nature 573, 61–68. doi: 10.1038/s41586-019-1506-7
Holmgren, C., Harkany, T., Svennenfors, B., and Zilberter, Y. (2003). Pyramidal cell communication within local networks in layer 2/3 of rat neocortex. J. Physiol. 551(Pt 1), 139–153. doi: 10.1113/jphysiol.2003.044784
Hunt, S., Leibner, Y., Mertens, E. J., Barros-Zulaica, N., Kanari, L., Heistek, T. S., et al. (2023). Strong and reliable synaptic communication between pyramidal neurons in adult human cerebral cortex. Cereb. Cortex 33, 2857–2878. doi: 10.1093/cercor/bhac246
Jiang, X., Shen, S., Cadwell, C. R., Berens, P., Sinz, F., Ecker, A. S., et al. (2015). Principles of connectivity among morphologically defined cell types in adult neocortex. Science 350:aac9462. doi: 10.1126/science.aac9462
Joglekar, M. R., Mejias, J. F., Yang, G. R., and Wang, X. J. (2018). Inter-areal balanced amplification enhances signal propagation in a large-scale circuit model of the primate cortex. Neuron 98, 222–234.e8. doi: 10.1016/j.neuron.2018.02.031
Jouhanneau, J. S., Kremkow, J., Dorrn, A. L., and Poulet, J. F. (2015). In vivo monosynaptic excitatory transmission between layer 2 cortical pyramidal neurons. Cell Rep. 13, 2098–2106. doi: 10.1016/j.celrep.2015.11.011
Kalmbach, B. E., Hodge, R. D., Jorstad, N. L., Owen, S., de Frates, R., Yanny, A. M., et al. (2021). Signature morpho-electric, transcriptomic, and dendritic properties of human layer 5 neocortical pyramidal neurons. Neuron 109, 2914–2927 e15. doi: 10.1016/j.neuron.2021.08.030
Kasthuri, N., Hayworth, K. J., Berger, D. R., Schalek, R. L., Conchello, J. A., Knowles-Barley, S., et al. (2015). Saturated reconstruction of a volume of neocortex. Cell 162, 648–661. doi: 10.1016/j.cell.2015.06.054
Kerr, J. N., de Kock, C. P., Greenberg, D. S., Bruno, R. M., Sakmann, B., and Helmchen, F. (2007). Spatial organization of neuronal population responses in layer 2/3 of rat barrel cortex. J. Neurosci. 27, 13316–13328.
Kirmse, K., Kummer, M., Kovalchuk, Y., Witte, O. W., Garaschuk, O., and Holthoff, K. (2015). GABA depolarizes immature neurons and inhibits network activity in the neonatal neocortex in vivo. Nat. Commun. 6:7750. doi: 10.1038/ncomms8750
Klyachko, V. A., and Stevens, C. F. (2006). Temperature-dependent shift of balance among the components of short-term plasticity in hippocampal synapses. J. Neurosci. 26, 6945–6957. doi: 10.1523/JNEUROSCI.1382-06.2006
Koester, H. J., and Johnston, D. (2005). Target cell-dependent normalization of transmitter release at neocortical synapses. Science 308, 863–866. doi: 10.1126/science.1100815
Komlosi, G., Molnar, G., Rozsa, M., Olah, S., Barzo, P., and Tamas, G. (2012). Fluoxetine (prozac) and serotonin act on excitatory synaptic transmission to suppress single layer 2/3 pyramidal neuron-triggered cell assemblies in the human prefrontal cortex. J. Neurosci. 32, 16369–16378. doi: 10.1523/JNEUROSCI.2618-12.2012
Kubota, Y., Karube, F., Nomura, M., and Kawaguchi, Y. (2016). The diversity of cortical inhibitory synapses. Front. Neural Circuits 10:27. doi: 10.3389/fncir.2016.00027
Kullmann, D. M., and Asztely, F. (1998). Extrasynaptic glutamate spillover in the hippocampus: Evidence and implications. Trends Neurosci. 21, 8–14. doi: 10.1016/s0166-2236(97)01150-8
Larkum, M. E., Kaiser, K. M., and Sakmann, B. (1999). Calcium electrogenesis in distal apical dendrites of layer 5 pyramidal cells at a critical frequency of back-propagating action potentials. Proc. Natl. Acad. Sci. U.S.A. 96, 14600–14604.
Larkum, M. E., Wu, J., Duverdin, S. A., and Gidon, A. (2022). The guide to dendritic spikes of the mammalian cortex in vitro and in vivo. Neuroscience 489, 15–33. doi: 10.1016/j.neuroscience.2022.02.009
Ledergerber, D., and Larkum, M. E. (2010). Properties of layer 6 pyramidal neuron apical dendrites. J. Neurosci. 30, 13031–13044.
Ledergerber, D., and Larkum, M. E. (2012). The time window for generation of dendritic spikes by coincidence of action potentials and EPSPs is layer specific in somatosensory cortex. PLoS One 7:e33146. doi: 10.1371/journal.pone.0033146
Lefort, S., Tomm, C., Floyd Sarria, J. C., and Petersen, C. C. (2009). The excitatory neuronal network of the C2 barrel column in mouse primary somatosensory cortex. Neuron 61, 301–316.
Liu, Y., Shen, X., Zhang, Y., Zheng, X., Cepeda, C., Wang, Y., et al. (2023). Interactions of glial cells with neuronal synapses, from astrocytes to microglia and oligodendrocyte lineage cells. Glia 71, 1383–1401. doi: 10.1002/glia.24343
Loomba, S., Straehle, J., Gangadharan, V., Heike, N., Khalifa, A., Motta, A., et al. (2022). Connectomic comparison of mouse and human cortex. Science 377:eabo0924. doi: 10.1126/science.abo0924
Luo, H., Hasegawa, K., Liu, M., and Song, W. J. (2017). Comparison of the upper marginal neurons of cortical layer 2 with layer 2/3 pyramidal neurons in mouse temporal cortex. Front. Neuroanat. 11:115. doi: 10.3389/fnana.2017.00115
Markram, H., Lubke, J., Frotscher, M., Roth, A., and Sakmann, B. (1997). Physiology and anatomy of synaptic connections between thick tufted pyramidal neurones in the developing rat neocortex. J. Physiol. 500(Pt 2), 409–440.
Markram, H., Muller, E., Ramaswamy, S., Reimann, M. W., Abdellah, M., Sanchez, C. A., et al. (2015). Reconstruction and simulation of neocortical microcircuitry. Cell 163, 456–492. doi: 10.1016/j.cell.2015.09.029
Mohan, H., Verhoog, M. B., Doreswamy, K. K., Eyal, G., Aardse, R., Lodder, B. N., et al. (2015). Dendritic and axonal architecture of individual pyramidal neurons across layers of adult human neocortex. Cereb. Cortex 25, 4839–4853. doi: 10.1093/cercor/bhv188
Molnar, G., Rozsa, M., Baka, J., Holderith, N., Barzo, P., Nusser, Z., et al. (2016). Human pyramidal to interneuron synapses are mediated by multi-vesicular release and multiple docked vesicles. Elife 5:e18167. doi: 10.7554/eLife.18167
Motta, A., Berning, M., Boergens, K. M., Staffler, B., Beining, M., Loomba, S., et al. (2019). Dense connectomic reconstruction in layer 4 of the somatosensory cortex. Science 366:eaay3134. doi: 10.1126/science.aay3134
Narayanan, R. T., Egger, R., Johnson, A. S., Mansvelder, H. D., Sakmann, B., de Kock, C. P., et al. (2015). Beyond columnar organization: Cell type- and target layer-specific principles of horizontal axon projection patterns in rat vibrissal cortex. Cereb. Cortex 25, 4450–4468. doi: 10.1093/cercor/bhv053
Oberlaender, M., Boudewijns, Z. S., Kleele, T., Mansvelder, H. D., Sakmann, B., and de Kock, C. P. (2011). Three-dimensional axon morphologies of individual layer 5 neurons indicate cell type-specific intracortical pathways for whisker motion and touch. Proc. Natl. Acad. Sci. U.S.A. 108, 4188–4193.
O’Connor, D. H., Clack, N. G., Huber, D., Komiyama, T., Myers, E. W., and Svoboda, K. (2010). Vibrissa-based object localization in head-fixed mice. J. Neurosci. 30, 1947–1967.
Ofer, N., Benavides-Piccione, R., DeFelipe, J., and Yuste, R. (2022). Structural analysis of human and mouse dendritic spines reveals a morphological continuum and differences across ages and species. eNeuro 9. doi: 10.1523/ENEURO.0039-22.2022
Peng, Y., Mittermaier, F. X., Planert, H., Schneider, U. C., Alle, H., and Geiger, J. R. P. (2019). High-throughput microcircuit analysis of individual human brains through next-generation multineuron patch-clamp. Elife 8:e48178. doi: 10.7554/eLife.48178
Perin, R., Berger, T. K., and Markram, H. (2011). A synaptic organizing principle for cortical neuronal groups. Proc. Natl. Acad. Sci. U.S.A. 108, 5419–5424.
Povysheva, N. V., Gonzalez-Burgos, G., Zaitsev, A. V., Kroner, S., Barrionuevo, G., Lewis, D. A., et al. (2006). Properties of excitatory synaptic responses in fast-spiking interneurons and pyramidal cells from monkey and rat prefrontal cortex. Cereb. Cortex 16, 541–552. doi: 10.1093/cercor/bhj002
Puig, M. V., and Gulledge, A. T. (2011). Serotonin and prefrontal cortex function: Neurons, networks, and circuits. Mol. Neurobiol. 44, 449–464. doi: 10.1007/s12035-011-8214-0
Qi, G., and Feldmeyer, D. (2016). Dendritic target region-specific formation of synapses between excitatory layer 4 neurons and layer 6 pyramidal cells. Cereb. Cortex 26, 1569–1579. doi: 10.1093/cercor/bhu334
Qi, G., van Aerde, K., Abel, T., and Feldmeyer, D. (2017). Adenosine differentially modulates synaptic transmission of excitatory and inhibitory microcircuits in layer 4 of rat barrel cortex. Cereb. Cortex 27, 4411–4422. doi: 10.1093/cercor/bhw243
Qi, G., Yang, D., Ding, C., and Feldmeyer, D. (2020). Unveiling the synaptic function and structure using paired recordings from synaptically coupled neurons. Front. Synaptic Neurosci. 12:5. doi: 10.3389/fnsyn.2020.00005
Radnikow, G., and Feldmeyer, D. (2018). Layer- and cell type-specific modulation of excitatory neuronal activity in the neocortex. Front. Neuroanat. 12:1. doi: 10.3389/fnana.2018.00001
Reyes, A., and Sakmann, B. (1999). Developmental switch in the short-term modification of unitary EPSPs evoked in layer 2/3 and layer 5 pyramidal neurons of rat neocortex. J. Neurosci. 19, 3827–3835.
Rheims, S., Minlebaev, M., Ivanov, A., Represa, A., Khazipov, R., Holmes, G. L., et al. (2008). Excitatory GABA in rodent developing neocortex in vitro. J. Neurophysiol. 100, 609–619. doi: 10.1152/jn.90402.2008
Rollenhagen, A., Ohana, O., Satzler, K., Hilgetag, C. C., Kuhl, D., and Lubke, J. H. R. (2018). Structural properties of synaptic transmission and temporal dynamics at excitatory layer 5B synapses in the adult rat somatosensory cortex. Front. Synaptic Neurosci. 10:24. doi: 10.3389/fnsyn.2018.00024
Scala, F., Kobak, D., Shan, S., Bernaerts, Y., Laturnus, S., Cadwell, C. R., et al. (2019). Layer 4 of mouse neocortex differs in cell types and circuit organization between sensory areas. Nat. Commun. 10:4174. doi: 10.1038/s41467-019-12058-z
Schmidt, H., Gour, A., Straehle, J., Boergens, K. M., Brecht, M., and Helmstaedter, M. (2017). Axonal synapse sorting in medial entorhinal cortex. Nature 549, 469–475. doi: 10.1038/nature24005
Schneider-Mizell, C. M., Bodor, A. L., Collman, F., Brittain, D., Bleckert, A., Dorkenwald, S., et al. (2021). Structure and function of axo-axonic inhibition. Elife 10:e73783. doi: 10.7554/eLife.73783
Seeman, S. C., Campagnola, L., Davoudian, P. A., Hoggarth, A., Hage, T. A., Bosma-Moody, A., et al. (2018). Sparse recurrent excitatory connectivity in the microcircuit of the adult mouse and human cortex. Elife 7:e37349. doi: 10.7554/eLife.37349
Shapson-Coe, A., Januszewski, M., Berger, D. R., Pope, A., Wu, Y., Blakely, T., et al. (2021). A connectomic study of a petascale fragment of human cerebral cortex. BioRxiv [Preprint] doi: 10.1101/2021.05.29.446289
Silver, R. A., Lubke, J., Sakmann, B., and Feldmeyer, D. (2003). High-probability uniquantal transmission at excitatory synapses in barrel cortex. Science 302, 1981–1984.
Somogyi, P. (1977). A specific ‘axo-axonal’ interneuron in the visual cortex of the rat. Brain Res. 136, 345–350. doi: 10.1016/0006-8993(77)90808-3
Song, S., Sjostrom, P. J., Reigl, M., Nelson, S., and Chklovskii, D. B. (2005). Highly nonrandom features of synaptic connectivity in local cortical circuits. PLoS Biol. 3:e68. doi: 10.1371/journal.pbio.0030068
Szegedi, V., Molnar, G., Paizs, M., Csakvari, E., Barzo, P., Tamas, G., et al. (2017). High-precision fast-spiking basket cell discharges during complex events in the human neocortex. eNeuro 4. doi: 10.1523/ENEURO.0260-17.2017
Szegedi, V., Paizs, M., Csakvari, E., Molnar, G., Barzo, P., Tamas, G., et al. (2016). Plasticity in single axon glutamatergic connection to GABAergic interneurons regulates complex events in the human neocortex. PLoS Biol. 14:e2000237. doi: 10.1371/journal.pbio.2000237
Testa-Silva, G., Rosier, M., Honnuraiah, S., Guzulaitis, R., Megias, A. M., French, C., et al. (2022). High synaptic threshold for dendritic NMDA spike generation in human layer 2/3 pyramidal neurons. Cell Rep. 41:111787. doi: 10.1016/j.celrep.2022.111787
Testa-Silva, G., Verhoog, M. B., Linaro, D., de Kock, C. P., Baayen, J. C., Meredith, R. M., et al. (2014). High bandwidth synaptic communication and frequency tracking in human neocortex. PLoS Biol. 12:e1002007. doi: 10.1371/journal.pbio.1002007
Uchida, T., Fukuda, S., and Kamiya, H. (2012). Heterosynaptic enhancement of the excitability of hippocampal mossy fibers by long-range spill-over of glutamate. Hippocampus 22, 222–229. doi: 10.1002/hipo.20885
Wilbers, R., Galakhova, A. A., Driessens, S. L. W., Heistek, T. S., Metodieva, V. D., Hagemann, J., et al. (2023). Structural and functional specializations of human fast spiking neurons support fast cortical signaling. BioRxiv [Preprint] doi: 10.1101/2022.11.29.518193
Wild, A. R., Bollands, M., Morris, P. G., and Jones, S. (2015). Mechanisms regulating spill-over of synaptic glutamate to extrasynaptic NMDA receptors in mouse substantia nigra dopaminergic neurons. Eur. J. Neurosci. 42, 2633–2643. doi: 10.1111/ejn.13075
Winnubst, J., Bas, E., Ferreira, T. A., Wu, Z., Economo, M. N., Edson, P., et al. (2019). Reconstruction of 1,000 projection neurons reveals new cell types and organization of long-range connectivity in the mouse brain. Cell 179, 268–281 e13. doi: 10.1016/j.cell.2019.07.042
Woolf, T. B., Shepherd, G. M., and Greer, C. A. (1991). Serial reconstructions of granule cell spines in the mammalian olfactory bulb. Synapse 7, 181–192. doi: 10.1002/syn.890070303
Yakoubi, R., Rollenhagen, A., von Lehe, M., Miller, D., Walkenfort, B., Hasenberg, M., et al. (2019a). Ultrastructural heterogeneity of layer 4 excitatory synaptic boutons in the adult human temporal lobe neocortex. Elife 8:e48373. doi: 10.7554/eLife.48373
Yakoubi, R., Rollenhagen, A., von Lehe, M., Shao, Y., Satzler, K., and Lubke, J. H. R. (2019b). Quantitative three-dimensional reconstructions of excitatory synaptic boutons in layer 5 of the adult human temporal lobe neocortex: A fine-scale electron microscopic analysis. Cereb. Cortex 29, 2797–2814. doi: 10.1093/cercor/bhy146
Yang, D., Ding, C., Qi, G., and Feldmeyer, D. (2021a). Cholinergic and adenosinergic modulation of synaptic release. Neuroscience 456, 114–130. doi: 10.1016/j.neuroscience.2020.06.006
Yang, D., Qi, G., Ding, C., and Feldmeyer, D. (2021b). Layer 6A pyramidal cell subtypes form synaptic microcircuits with distinct functional and structural properties. Cereb. Cortex 32, 2095–2111. doi: 10.1093/cercor/bhab340
Yassin, L., Benedetti, B. L., Jouhanneau, J. S., Wen, J. A., Poulet, J. F., and Barth, A. L. (2010). An embedded subnetwork of highly active neurons in the neocortex. Neuron 68, 1043–1050. doi: 10.1016/j.neuron.2010.11.029
Keywords: excitatory neurotransmission, rodent, primate, human, synapse, EPSP, neocortex
Citation: de Kock CPJ and Feldmeyer D (2023) Shared and divergent principles of synaptic transmission between cortical excitatory neurons in rodent and human brain. Front. Synaptic Neurosci. 15:1274383. doi: 10.3389/fnsyn.2023.1274383
Received: 08 August 2023; Accepted: 21 August 2023;
Published: 05 September 2023.
Edited by:
Karri P. Lamsa, University of Szeged, HungaryReviewed by:
Viktor Szegedi, Hungarian Centre of Excellence for Molecular Medicine (HCEMM), HungaryEric Hanse, University of Gothenburg, Sweden
Copyright © 2023 de Kock and Feldmeyer. This is an open-access article distributed under the terms of the Creative Commons Attribution License (CC BY). The use, distribution or reproduction in other forums is permitted, provided the original author(s) and the copyright owner(s) are credited and that the original publication in this journal is cited, in accordance with accepted academic practice. No use, distribution or reproduction is permitted which does not comply with these terms.
*Correspondence: Christiaan P. J. de Kock, Y2tvY2tAZmFsdy52dS5ubA==; Dirk Feldmeyer, ZC5mZWxkbWV5ZXJAZnotanVlbGljaC5kZQ==