- Translational Neuroscience Program, Department of Psychiatry, University of Pittsburgh, Pittsburgh, PA, United States
Developing novel treatments for alcohol use disorders (AUDs) is of paramount importance for improving patient outcomes and alleviating the suffering related to the disease. A better understanding of the molecular and neurocircuit mechanisms through which alcohol alters brain function will be instrumental in the rational development of new efficacious treatments. Clinical studies have consistently associated the prefrontal cortex (PFC) function with symptoms of AUDs. Population-level analyses have linked the PFC structure and function with heavy drinking and/or AUD diagnosis. Thus, targeting specific PFC cell types and neural circuits holds promise for the development of new treatments. Here, we overview the tremendous diversity in the form and function of inhibitory neuron subtypes within PFC and describe their therapeutic potential. We then summarize AUD population genetics studies, clinical neurophysiology findings, and translational neuroscience discoveries. This study collectively suggests that changes in fast transmission through PFC inhibitory microcircuits are a central component of the neurobiological effects of ethanol and the core symptoms of AUDs. Finally, we submit that there is a significant and timely need to examine sex as a biological variable and human postmortem brain tissue to maximize the efforts in translating findings to new clinical treatments.
Background
Alcohol use disorders (AUDs) present a tremendous burden on individuals suffering from the disease, their immediate and extended families, and society at large (Hasin et al., 2007; Whiteford et al., 2013; Grant et al., 2017). The United States Food and Drug Administration has approved disulfiram, naloxone, and acamprosate for AUD; however, these options are limited by insufficient efficacy, unsatisfactory side effects, and relatively low compliance (Jonas et al., 2014). Developing novel treatments is, therefore, of paramount importance for improving patient outcomes. Rational treatment development, in turn, will be driven by a better understanding of the molecular and neurocircuit mechanisms through which ethanol exposure alters brain function. This review summarizes historical findings and suggests that changes in fast transmission through the frontal cortex inhibitory microcircuits are a central component of ethanol's neurobiological effects. In addition, we highlight recent mechanistic studies in preclinical models that provide new avenues for breakthrough treatment approaches and argue there is a tremendous need to examine sex as a biological variable and a human postmortem brain tissue to maximize efforts in translating findings to new clinical treatments.
Prefrontal cortex inhibitory neurons as specific and selective targets
Excessive craving and motivation to drink, along with challenges in moderating consumption are key symptoms associated with AUDs. Clinical studies have consistently associated the prefrontal cortex (PFC) function with these behaviors and symptoms (Grusser et al., 2004; Koob, 2014; Courtney et al., 2016; Blaine et al., 2020; Yang et al., 2021). In addition, population-level analyses have linked the PFC structure and function with heavy drinking and/or AUD diagnosis (Medina et al., 2008; Sorg et al., 2012; Wang et al., 2016). Finally, several interventional studies have linked a direct stimulation of PFC areas with reductions in alcohol craving and drinking metrics (Boggio et al., 2008; Addolorato et al., 2017; Philip et al., 2020), providing exciting proof-of-concept for the potential of new tools to modulate PFC function as breakthrough treatments for AUDs. Together, these translational and clinical studies motivate continued basic neuroscience studies and postmortem studies to identify PFC cell populations that may be modulated as novel standalone or adjunct treatments for AUDs.
Several properties make gamma-aminobutyric acid (GABA) inhibitory neurons (INs) highly attractive targets for developing novel pharmacological treatments for AUD and other neuropsychiatric diseases (Box 1). In human PFC, the INs make up approximately 20% of all neurons. There are more than 20 IN subtypes, each displaying distinct transcriptional programs (Tasic et al., 2016, 2018; Tremblay et al., 2016; Krienen et al., 2020). Thus, it may be possible to specifically modulate transcriptionally unique IN subtypes without altering other INs or cell types, providing opportunities to develop treatment mechanisms with a low risk of deleterious side effects. Moreover, the tremendous diversity in the form and function of IN subtypes (Figure 1) confers them with a great potential for developing means to restore specific pathophysiological disruptions.
BOX 1. Assembly and function of GABAA receptors.
GABAA receptors are ligand-gated anion channels that generally hyperpolarize neurons by fluxing chloride ions (Mody and Pearce, 2004; Lobo and Harris, 2008). GABAA receptors are members of the Cys-loop superfamily and assemble as obligate pentamers. Several GABAA receptor subunits exist, generating a wide variety of combinations of functional channels in the mammalian CNS. There are six types of α subunits, three β, three γ, and one δ, ε, π, and θ. There are also three ρ subunits, but these generally assemble homopentamers and are classified as GABAC receptors. The most abundant GABAA receptor assemblies contain two α, two β, and one γ subunit. GABA binds on the interface between α and β subunits facilitating a structural rearrangement that allows for chloride ions to permeate the central pore.
The combinatorial nature of GABAA receptor assembly yields a great diversity in function. The subunit stoichiometry of a specific GABAA pentamer can affect its subcellular localization and biophysical properties, such as GABA affinity, desensitization/deactivation kinetics, and voltage rectification (Mody and Pearce, 2004; Olsen and Sieghart, 2009; Lee and Maguire, 2014). From this inherent diversity, GABAA subunits have garnered significant attention in translational studies aimed at developing selective approaches to modulate inhibitory circuit function. For example, α1-containing GABAA receptors regulate the sedative and amnestic effects of benzodiazepines, whereas their anxiolytic properties are mediated by alternative α isoforms (Rudolph et al., 1999; Kralic et al., 2002). Thus, subunit-selective GABAA receptor modulators have the potential to preserve the therapeutic effects of existing medications while mitigating undesirable side effects (Mohler, 2006).
GABAA receptor subunit isoforms display heterogeneous patterns of expression. α1, β1-3, and γ2 GABAA subunits are expressed widely throughout the brain (Wisden and Seeburg, 1992; Pirker et al., 2000; Hortnagl et al., 2013). α3-5, γ1, γ3, and δ subunit isoforms, in contrast, are expressed in distinct, restricted patterns throughout the forebrain. α6 is essentially restricted to the cerebellum. Genetic deletion experiments suggest that γ2 subunits are absolutely critical for broad synaptic inhibitory transmission (Gunther et al., 1995; Martenson et al., 2017), whereas the more abundant α or β isoforms are each dispensable or redundant to some extent (Homanics et al., 1997; Sur et al., 2001; Vicini et al., 2001). Synaptic receptors mediate phasic transmission, whereas certain GABAA receptor subunit combinations are enriched at extrasynaptic sites and facilitate tonic inhibitory currents (Belelli et al., 2009; Lee and Maguire, 2014). These extrasynaptic receptors commonly assemble with α5, γ2, and/or δ subunits, conferring them with a high affinity for GABA and slow desensitization, providing prolonged inhibition in response to low concentrations of GABA. Extrasynaptic receptors have been the subject of recent reviews covering their basic neurobiology (Lee and Maguire, 2014) as well as more focused analyses with respect to AUD (Weiner and Valenzuela, 2006; Lovinger and Homanics, 2007).
Even among synaptic GABAA receptors, the subunit composition has a major effect on subcellular localization. While α1 subunits are found in synapses across all subcellular domains (Nusser et al., 1996), other isoforms display restricted subcellular expression patterns. α5 subunits, for example, are primarily localized to inhibitory synapses in pyramidal cell dendrites (Magnin et al., 2019), where they receive GABA from SST-INs but not PV-INs (Schulz et al., 2018). In contrast, α2 subunits are expressed at higher levels postsynaptic to chandelier IN inputs onto the axon initial segment (Nusser et al., 1996) and in somatic synapses that preferentially receive inputs from CCK-INs (Nyiri et al., 2001). Together, these findings raise the possibility that subunit-selective GABAA receptor allosteric modulators could be developed to selectively modulate distinct microcircuits.
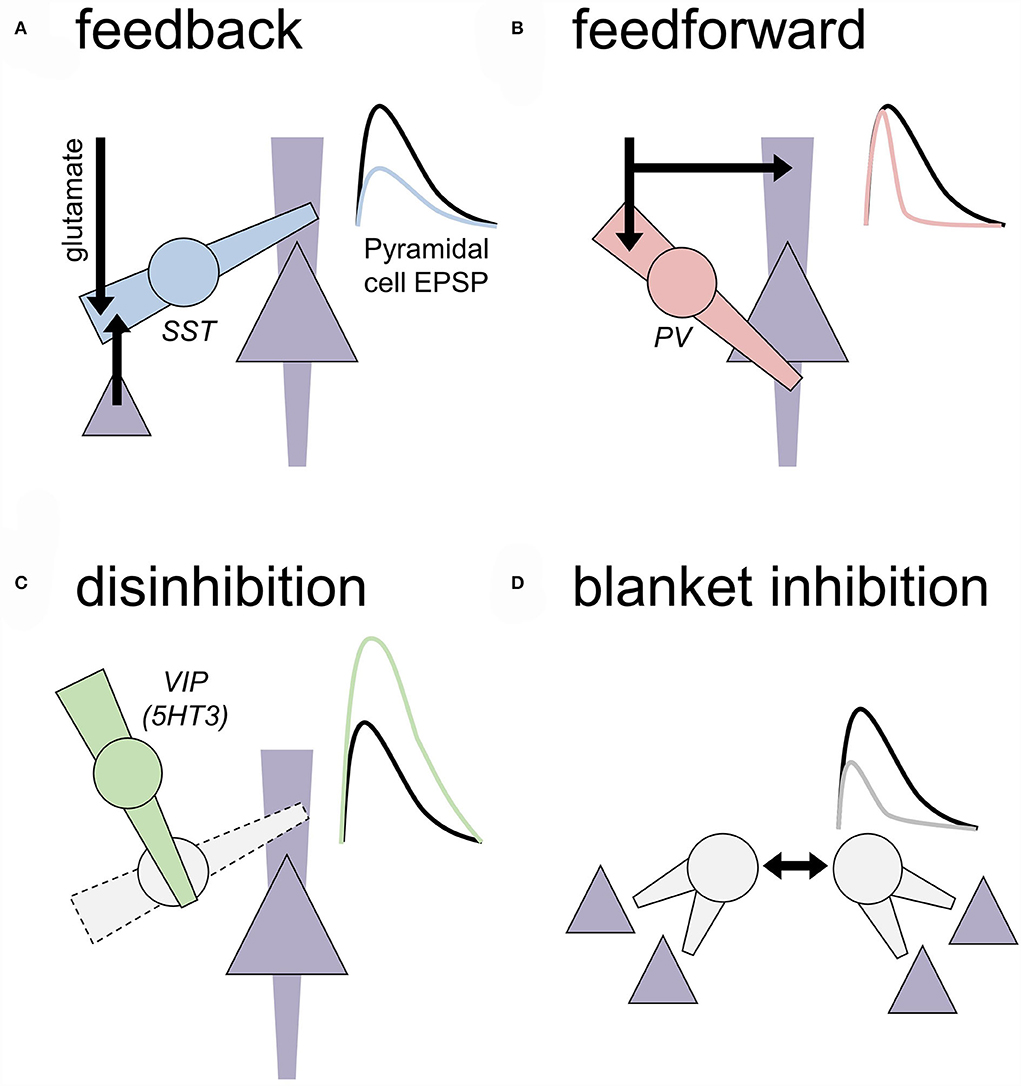
Figure 1. Motifs of local inhibition in the central nervous system. Generalized motifs of cortical inhibition are depicted that include cellular/subcellular targets and approximated effects on an excitatory postsynaptic potential (EPSP) recorded from a nearby pyramidal cell. To some extent, these inhibitory transmission motifs are present across all cortical layers, but some motifs are more prevalent in some layers based on where IN subtypes reside. (A) Feedback inhibition. A local inhibitory neuron (IN; circle) receives an excitatory transmission from long-range glutamate inputs or local competing ensembles or cortical columns (black arrows). Feedback INs regulate dendritic compartments of principal neurons (triangle) to decrease the amplitude of EPSPs. Cortical somatostatin (SST) INs represent a primary example of feedback INs. (B) Feedforward inhibition. A local IN and neighboring principal neuron receive coincidental excitatory input. Feedforward INs, often expressing parvalbumin (PV), are optimized to inhibit the nearby cell bodies rapidly and faithfully after receiving excitatory inputs. Through this process, feedforward INs restrict the window during which EPSPs may be converted into action potential firing. Feedforward INs can regulate dozens of related principal cells to recruit neuronal ensembles and generate oscillatory activity in many brain areas. (C) Disinhibition. INs can inhibit other INs to relieve inhibition and ultimately facilitate EPSPs on the principal neurons. Prime examples of this motif include SST-INs inhibiting PV-INs and vasoactive intestinal peptide (VIP) INs inhibiting SST-INs. (D) Blanket inhibition. Some INs subtypes help drive their activity to maintain wide networks of inhibition and low levels of excitatory drive throughout broad brain areas. For example, PV-INs form extensive gap junctions with other PV-INs and cholecystokinin INs can co-release glutamate to excite onto each other.
Adaptations in PFC inhibitory microcircuits are associated with changes in mood, decision-making, executive control, and symptom domains that are present in some individuals with an AUD. Interestingly, disruptions to inhibitory microcircuits have been observed in several comorbid brain diseases including affective disorders and schizophrenia (Lewis et al., 2005, 2012; Luscher et al., 2011; Fogaca and Duman, 2019), supporting the idea that IN pathophysiology may be a feature of AUDs as well. Nonetheless, our understanding of AUD-associated microcircuit disruptions in the human brain lags substantially behind that of other psychiatric diseases, and warrants postmortem studies with AUD patient population samples.
Mechanistic research focused on the PFC has gained traction since the early 1990's (Laubach et al., 2018) after it was shown that the primate dorsolateral prefrontal cortex (dlPFC) (Brodmann's areas (BA)9 and BA46) was a working memory hub (Funahashi et al., 1989; Williams and Goldman-Rakic, 1995). The findings from these studies were supported by those of contemporaneous human neuroimaging studies that implicated the dlPFC in higher-order cognitive processing, including working memory (Pardo et al., 1991; McCarthy et al., 1994). Although the rodent PFC was initially believed to be anatomically and functionally similar to the primate dlPFC, a substantial amount of data challenges this view (extensively reviewed in Laubach et al., 2018). Especially in mice, which do not have a granular layer 4 within the PFC, there is no equivalent to the primate BA9 and BA46, which is where most “PFC” studies have focused. In rodents, the focus is frequently on the medial PFC and its three main subregions: cingulate, prelimbic, and infralimbic. While these subregions are involved in working memory processes, affective behaviors, and motivation, the degree to which rodent PFC regions map onto primate and human homologs remain an area of significant debate.
Non-overlapping neocortical IN subpopulations
In general, INs within the rodent neocortex can be split into three non-overlapping classes. INs that arise from the medial ganglionic eminence express either the Ca2+-binding protein parvalbumin (PV) or the neuropeptide somatostatin (SST), whereas INs arising from the caudal ganglionic eminence (CGE) express the ionotropic serotonin receptor, 5HT3a subtype (Lee et al., 2010; Pfeffer et al., 2013; Tremblay et al., 2016; Lim et al., 2018). For the most part, these three major classes exist in humans. There are, however, important differences in the proportion of GABA neurons immunoreactive for subtype-specific markers between rodents and primates. In primates, approximately 50% of GABA neurons are calretinin (CR) immunoreactive, while approximately 20% are PV immunoreactive (Conde et al., 1994; Gabbott and Bacon, 1996; Krienen et al., 2020). In contrast to primates, CR neurons are not considered a canonical GABA subtype in rodents; instead, vasoactive intestinal peptide (VIP), which highly colocalizes with CR in primates, is frequently used to define a major group of INs in rodents. Approximately, 20% of GABA neurons in rodents are VIP positive, while nearly half are PV immunoreactive. Considering these IN subtypes that integrate within PFC microcircuits in distinct manners (refer to next section for details), this difference is expected to differentially impact the local and global network. Here, we provide an overview of each of these three major classes of INs based on rodent studies, pointing out important species differences where relevant, to orient the reader and aid in interpreting functional findings from the clinic.
Parvalbumin INs
In the cortex, the PV is mainly expressed in two morphologically unique GABAergic neurons, PV basket cells and PV chandelier cells (Celio, 1986; Kawaguchi et al., 1987; Kosaka et al., 1987; DeFelipe, 1993; Hu et al., 2014). Approximately, 80% of PV-INs are PV basket cells. In the rodent neocortex, PV basket cells mainly reside in the middle layers while most PV chandelier cells are located along the layer 1–2 boarder and in deep layers. PV-INs have been studied more extensively than other IN classes, in part due to their distinguishing firing properties including high firing frequency, rapid action potential kinetics, and minimal spike-firing adaptation (McCormick et al., 1985; Connors and Gutnick, 1990; Kawaguchi, 1993a,b; Kawaguchi and Kubota, 1997, 1998; Markram et al., 2004; Rainnie et al., 2006; Woodruff and Sah, 2007; Gittis et al., 2010; McGarry and Carter, 2016; O'Hare et al., 2017; Joffe et al., 2020; Unal et al., 2020). These distinctive intrinsic properties provided opportunities to examine PV-IN function nearly two decades before the advent of genetic tools to identify and modulate specific IN subpopulations. Throughout this review, the term, “PV-IN” will be used broadly when referring to PV basket cells including experiments that only classified neurons by their firing properties.
Several properties of PV-INs confide them with an ability to synchronize neural ensembles, ultimately coupling and strengthening the network activity (Cobb et al., 1995; Royer et al., 2012; Knoblich et al., 2019). PV-INs mainly innervate the perisomatic region (basket cells— proximal dendrites and soma; chandelier cells —axon initial segment) of principal neurons and other PV-INs (Hu et al., 2014). The perisomatic innervation and rapid signal propagation kinetics provide PV-INs with the ability to efficiently transmit feed-forward inhibition (Mallet et al., 2005) (Figure 1B). Approximately, half of the synapses onto PV-INs arise from local axon collaterals (Melchitzky and Lewis, 2003); the other half comes from long-range excitatory afferents, with only approximately 2% from the thalamus (Rotaru et al., 2005). Relative to principal cells, PV-IN dendrites display rapid, high-conductance excitatory postsynaptic currents (EPSCs) related to high glutamate release probability, GluA1/GluA4-containing α-amino-3-hydroxy-5-methyl-4-isoxazolepropionic acid (AMPA) receptors, and transmembrane AMPA receptor regulatory proteins (Box 2) (Geiger et al., 1995; Gittis et al., 2010, 2011; Tao et al., 2013; Lalanne et al., 2016). Uncommon assemblies of AMPA receptor subunits (Bowie and Mayer, 1995; Geiger et al., 1995) confer PV-INs with distinct synaptic plasticity rules and mechanisms (Sambandan et al., 2010; Szabo et al., 2012; Manz et al., 2020). In contrast, PV-INs display minimal postsynaptic NMDA receptor currents (Matta et al., 2013; McGarry and Carter, 2016; Bogart and O'Donnell, 2018), although presynaptic NMDA receptors do regulate PV-IN GABA release (Pafundo et al., 2018). At the PV-IN cell body, voltage-gated Kv3.1 potassium channels contribute to rapid and non-accommodating spiking (Chow et al., 1999; Rudy and McBain, 2001; Goldberg et al., 2005), which rarely fails and efficiently propagates electrical signals down the PV-IN axon (Doischer et al., 2008). Throughout the axon, the expression of the sodium/potassium ATPase (Anderson et al., 2010), hyperpolarization-activated cation channels (Roth and Hu, 2020), and other sodium channels (Hu et al., 2018) counters the metabolic demands of high frequency firing. At PV-IN presynaptic terminals, synaptotagmin 2 (Sommeijer and Levelt, 2012; Bouhours et al., 2017) and a small number of ω-conotoxin-sensitive P/Q type voltage-gated Ca2+ channels (Zaitsev et al., 2007; Bucurenciu et al., 2010; Rossignol et al., 2013) contribute to high-fidelity GABA release from the top of 10 release sites (Buhl et al., 1994; Kraushaar and Jonas, 2000), generating GABAergic inhibitory events with the rapid rise and decay kinetics (Gittis et al., 2010; Marlin and Carter, 2014; Unal et al., 2020). Thus, through specialized molecules in several subcellular compartments, PV-INs transmit feedforward inhibition of coincidental principal cell excitatory postsynaptic potentials within 5–10 ms, facilitating spike-timing-dependent synaptic plasticity (Tremblay et al., 2016).
BOX 2. Ionotropic glutamate receptor expression in INs.
Glutamate mediates fast excitatory transmission via cationotropic cell surface receptors (Mayer and Armstrong, 2004; Traynelis et al., 2010; Paoletti et al., 2013). Glutamate receptors assemble as obligate tetramers and can be split into three main types, AMPA receptors, kainate receptors, and NMDA receptors. For all three types, selective cationic pores form within the center of tetrameric assemblies and open when glutamate binds within large extracellular domains. AMPA and kainate receptors flux Na+ to depolarize membranes from the rest. In contrast, NMDA receptors do not effectively transmit currents at hyperpolarized membrane potentials but allow for Na+ and Ca2+ to enter during mild depolarization. Through these properties, NMDA receptors readily detect coincidental synaptic transmission and promote Ca2+-dependent plasticity.
The four AMPA receptor subunits of GluA1-4 exhibit slight differences in biophysical properties (Mayer and Armstrong, 2004; Traynelis et al., 2010). The RNA editing of the Gria2 transcript alters a key peptide residue in the GluA2 pore-forming region such that GluA2-containing AMPA receptors are selectively permeable to monovalent cations (Burnashev et al., 1992). Of note, AMPA receptors that lack GluA2 not only flux monovalent cations but also allow for Ca2+ ions to pass (Hollmann et al., 1991). AMPA receptors that contain GluA2 subunits are the most common assemblies in principal neuron synapses, whereas many types of inhibitory INs express AMPA receptors that lack GluA2. Variation in channel opening kinetics, glutamate affinity, and interactions with auxiliary proteins and intracellular polyamines also confer unique properties to distinct assemblies of AMPA receptor subunits enriched on INs (Soto et al., 2007; Ziff, 2007). Most types of INs also express kainate receptors, primarily those containing GluK1 and GluK2 subunits (Cauli et al., 2000; Pelkey et al., 2017; Huntley et al., 2020). Pre and postsynaptic kainate receptors regulate IN excitability, inhibitory transmission onto principal neurons, and network oscillations (Cossart et al., 1998; Frerking et al., 1998; Fisahn et al., 2004; Daw et al., 2010).
NMDA receptors must contain two GluN1 subunits that bind glycine or D-serine, and generally include two GluN2 subunits (Paoletti et al., 2013; Iacobucci and Popescu, 2017). The glutamate interacts with the GluN2 subunits, which exist in four isoforms produced from four genes (GluN2A-D, Grin2A-D). GluN2A and GluN2B are the most abundant. GluN2B-containing NMDA receptors display longer channel opening kinetics and a higher affinity for glutamate than their GluN2A-containing counterparts. These properties enhance channel conductance and make GluN2B an important signaling molecule under conditions with low glutamate concentrations, such as extrasynaptic domains and in nascent, silent synapses particularly important for long-term plasticity and adaptive responses to drugs of abuse (Kerchner and Nicoll, 2008; Dong, 2016). GluN2C and GluN2D subunits have even higher affinities for glutamate, slower desensitization kinetics, and transmit more current at hyperpolarized potentials (Paoletti et al., 2013; Iacobucci and Popescu, 2017). GluN2C and GluN2D display relatively restricted patterns of expression in adulthood, but these receptor subunits are notably enriched in cortical INs (Monyer et al., 1994; Perszyk et al., 2016; Hanson et al., 2019; Garst-Orozco et al., 2020).
NMDA receptor inhibition is an important component underlying some of the physiological effects of ethanol (Woodward, 2000; Lovinger and Roberto, 2013). Relative to other ionotropic receptors, ethanol preferentially inhibits charge transfer through NMDA receptors (Lovinger et al., 1989, 1990; Morrisett et al., 1991; Yaka et al., 2003; Hendricson et al., 2004), and potencies of alcohol for inhibiting NMDA receptors and intoxicating humans(Lovinger et al., 1989, 1990). More recent molecular studies have revealed that mutations in the modulatory extracellular N-terminal domain and transmembrane regions of multiple NMDA receptor subunits alter the inhibitory actions of ethanol (Ronald et al., 2001; Woodward and Smothers, 2003; Smothers et al., 2013; Smothers and Woodward, 2016). Specific mutations within the GluN1 transmembrane region also alter several behavioral effects of low-dose ethanol (den Hartog et al., 2013). Taken together, several lines of molecular, circuit-level, and behavioral studies suggest that direct interactions with NMDA receptors regulate some of the central effects of ethanol.
In addition to their specialized cellular properties, PV-INs are integrated at the circuit-level to promote concerted network activity. PV-INs express gap junction proteins, and a single PV-IN may be electrically connected with over 60 others (Katsumaru et al., 1988; Gibson et al., 1999; Fukuda and Kosaka, 2000, 2003; Muller et al., 2005; Fukuda et al., 2006; Woodruff and Sah, 2007). PV-INs also display extensive reciprocal GABAergic synapses that can readily undergo LTP (Galarreta and Hestrin, 2002; Sarihi et al., 2012; Pfeffer et al., 2013). PV-INs serve a key role in regulating γ oscillations, a high frequency range whose power increases during complex tasks that require high cognitive demand (Bartos et al., 2007; Gonzalez-Burgos et al., 2015). The PV-IN activity tracks closely to γ oscillations (Tukker et al., 2007), and PV-IN manipulations bidirectionally regulate the power of γ oscillations (Cardin et al., 2009; Sohal et al., 2009; Chen et al., 2017; Cho et al., 2020). Taken together, the molecular, cellular, and microcircuit-level properties establish PV-INs as key actuators controlling the gain within locally assembled neural networks.
Somatostatin INs
In the neocortex, SST-expressing neurons are exclusively GABAergic (Hendry et al., 1984a; Melchitzky and Lewis, 2008; Urban-Ciecko and Barth, 2016). Most SST-INs are low-threshold spiking cells, characterized by high input resistance, depolarized resting potential, low rheobase, and occasional firing upon hyperpolarization rebound (Kawaguchi and Kubota, 1997, 1998; Marlin and Carter, 2014; McGarry and Carter, 2016; Tremblay et al., 2016; Nigro et al., 2018; Joffe et al., 2020; Unal et al., 2020). The vast majority of SST-IN synaptic contacts are made with dendrites of non-GABAergic neurons (Katona et al., 1999; Muller et al., 2007; Melchitzky and Lewis, 2008). For example, a morphologically unique SST-IN, the Martinotti cell, primarily synapses on the most distal dendrites (in layer 1) of nearby pyramidal cells, providing feedback inhibition between competing ensembles or cortical columns (de Lima and Morrison, 1989; Kawaguchi and Kubota, 1997; Muller et al., 2007; Murayama et al., 2009; Marlin and Carter, 2014; Unal et al., 2020) (Figure 1A). Through their synapses onto pyramidal cell dendrites, SST-INs regulate Ca2+ signaling and NMDA receptor activation (Gentet et al., 2012; Lovett-Barron et al., 2012; Chiu et al., 2013; Marlin and Carter, 2014; Ali et al., 2020), and, conversely, NMDA receptor activation can strengthen SST-IN inputs to pyramidal cells through heterosynaptic LTP of GABAA transmission (Chiu et al., 2013; Horn and Nicoll, 2018). Furthermore, SST-IN synapses on principal cells are enriched with α5-containing GABAA receptors, which display outward rectification profiles comparable to NMDA receptors (Schulz et al., 2018). These α5-containing receptor currents provide shunting inhibition to attenuate NMDA receptor function, action potential back propagation, and spike-timing-dependent plasticity (Groen et al., 2014; Schulz et al., 2018). Together, the tight coordination with NMDA receptor signaling suggests that SST-INs are highly specialized for regulating plasticity at active, depolarized synapses while mitigating the liability for broad blanket inhibition. Consistent with these molecular studies, the SST-IN regulation of principal cell dendrites is critical for spine reorganization to support skill acquisition (Chen et al., 2015) and rapid antidepressant response (Ali et al., 2020). The preclinical literature broadly supports the idea that SST-INs are key substrates for filtering and guiding experience-dependent synaptic plasticity.
Somatostatin-inhibitory neurons are uniquely integrated into local circuits relative to other types of INs. Unlike the depressing transmission observed onto most types of INs, SST-INs display strongly facilitating excitatory input (Pouille and Scanziani, 2004; Silberberg and Markram, 2007; Xu et al., 2013; McGarry and Carter, 2016). The characteristic low probability of glutamate release onto SST-INs is guided in trans by the postsynaptic expression of extracellular leucine-rich repeat fibronectin containing 1 (Elfn1) protein (Sylwestrak and Ghosh, 2012). Transsynaptic modulation by Elfn1 subsequently promotes the constitutive activity of presynaptic mGlu7 receptors, thereby maintaining low glutamate release probability onto SST-INs (Shigemoto et al., 1996; Dunn et al., 2018; Stachniak et al., 2019). These facilitating and summating excitatory synapses, in combination with a high membrane resistance, allow SST-INs to respond to repeated stimulation from even just one presynaptic neuron (Kapfer et al., 2007; Silberberg and Markram, 2007). This “winner-take-all” cellular logic has been proposed as a primary means through which competing ensembles influence and filter each other (Tremblay et al., 2016). Accordingly, SST-INs inhibit pyramidal cells during high frequency barrage, while the influence of other IN subtypes wanes due to their depressing synapses (Beierlein et al., 2003; Gonzalez-Burgos et al., 2004; Kapfer et al., 2007; McGarry and Carter, 2016). High frequency stimulation can also induce the release of SST peptide (Dao et al., 2019), which can modulate microcircuit function through 5 subtypes of G protein-coupled receptors (Viollet et al., 2008; Yavorska and Wehr, 2016). In addition to their specialized responses to sustained stimulation, the spontaneous SST-IN activity in vivo can regulate pyramidal cells through metabotropic GABAB receptors (Gentet et al., 2012; Urban-Ciecko et al., 2015; Urban-Ciecko and Barth, 2016). Furthermore, the molecular logic guiding synaptic plasticity onto SST-INs contrasts sharply with that in pyramidal cells. SST-INs display anti-Hebbian long-term potentiation, whereby postsynaptic hyperpolarization can increase EPSCs in response to tetanic stimulation (Lamsa et al., 2007). The long-term potentiation (LTP) of SST-IN is induced through postsynaptic CP-AMPA receptors and mGlu1/mGlu5 receptors, although maintenance may occur at presynaptic sites (Oren et al., 2009; Le Duigou and Kullmann, 2011; Maksymetz et al., 2021; Joffe et al., 2022). Alterative splicing of Grm1 and several AMPA receptor genes may contribute to the distinct synaptic plasticity mechanisms that occur in SST-INs (Furlanis et al., 2019). Collectively, these findings suggest that plastic interactions between SST-INs and their local networks are critical for long-term changes in how microcircuits filter incoming information.
In addition to Martinotti cells, several other GABAergic subtypes express SST in the neocortex and hippocampus (Oliva et al., 2000; Ma et al., 2006; Hu et al., 2013; Mikulovic et al., 2015; Yavorska and Wehr, 2016). In fact, Yavorska and Wehr estimated that neocortical SST-INs can be further subdivided by form, function, and gene expression into potentially 100 subgroups (Yavorska and Wehr, 2016). In addition to morphological and physiological studies, the SST-IN subdivision has been motivated by the serendipitous, restricted expression of GFP in specific GABAergic cell types (Oliva et al., 2000; Ma et al., 2006). One GAD67-GFP line, termed X98, strictly labels CB-expressing Martinotti cells, while another line, X94, can be used to identify stuttering INs in layer 4 (Ma et al., 2006). Later studies discovered that SST-INs labeled in the X94 line, in stark contrast to Martinotti cells, project only within deep layers of the cortex and preferentially target PV-INs (Xu et al., 2013). While the rodent frontal cortex does not contain a layer 4 per se, similar SST-IN disinhibitory motifs have been proposed to exist there (Xu et al., 2019; Cummings and Clem, 2020), in the motor cortex (Zhang et al., 2016), and the visual cortex (Pfeffer et al., 2013). Furthermore, supporting the existence of distinct SST-IN subpopulations, comparative studies of genetically-defined INs often discover that SST-INs functionally segregate into at least two subtypes (Kvitsiani et al., 2013; Knoblich et al., 2019). Finally, several studies have shown that a relatively small number of SST-INs, primarily located in layer 6, co-express neuropeptide Y (NPY), neuronal nitric oxide synthase (nNOS), and the substance P receptor 1 (Tomioka et al., 2005), and send long-range inhibitory projections to the striatum and other extracortical areas (Rock et al., 2016).
5HT3-INs
The final major class of neocortical INs arises from the CGE. In rodents, but not primates, nearly all CGE-derived INs express the 5HT3a serotonin receptor (Lee et al., 2010; Krienen et al., 2020). 5HT3-INs are primarily localized within the supragranular layers of the neocortex and potentially represent the most diverse of the three non-overlapping classes of INs. Based on morphology, cells in this class have been described as basket cells, bipolar cells, multipolar cells, single (and some double) bouquet cells, and neurogliaform cells (Lim et al., 2018). It is important to note that in primates, some of these morphological features are shared with or restricted to other IN classes. For example, in primates, CR expression is often used as a broad classifier of CGE-derived neurons, and approximately 25% of CR-IN synapses target pyramidal neurons (Melchitzky and Lewis, 2008). Coordinated translational research spanning rodent and primate models will be important for understanding and reconciling species differences in CGE-derived IN physiology.
From a molecular standpoint, 5HT3-INs can be divided into two groups in the rodent neocortex, each containing transcriptionally unique cells, based on the expression of VIP) (Schuman et al., 2019). VIP-expressing INs are the largest and best-studied subgroup, comprising about 40% of INs in the superficial neocortex (Lee et al., 2010). VIP-INs can be split into two, generally, non-overlapping subpopulations based on the expression of cholecystokinin (CCK) or CR (Porter et al., 1998; He et al., 2016; Tasic et al., 2018). VIP/CCK-INs are basket cells closely related to other CCK-INs (Tasic et al., 2018). In contrast, VIP/CR-INs are bipolar cells whose terminals avoid pyramidal cells and instead target other IN subtypes, especially SST-INs (Lee et al., 2013; Pfeffer et al., 2013; Pi et al., 2013; Fu et al., 2015; Anastasiades et al., 2018) (Figure 1C).
Most 5HT3-INs that do not express VIP express LAMP5 (Tasic et al., 2016, 2018), a brain-specific membrane protein that regulates presynaptic neurotransmission (Tiveron et al., 2016). Interestingly, deep-layer LAMP5-INs are approximately 10-fold more abundant in primates than in rodents (Krienen et al., 2020), but their functional significance is unknown. 5HT3-INs that do not express VIP can be further subdivided into three non-overlapping subtypes [NDNF/NPY+, NDNF/NPY-, or α7 nACh receptor+], each with a characteristic electrophysiological signature (Schuman et al., 2019).
Glutamate synapses onto 5HT3-INs tend to exhibit large EPSCs from NMDA receptors and GluA2-containing CI (calcium-impermeable)-AMPA receptors (Szabo et al., 2012; Matta et al., 2013). With the important exception of CCK-INs, monosynaptic inputs from 5HT3-INs to pyramidal cells are biased toward apical dendrites even more than SST-INs (Marlin and Carter, 2014). In addition, a major function of 5HT3-INs is to relieve inhibition of principal neurons by quietening other IN subtypes (Tremblay et al., 2016). Many subtypes of 5HT3-INs are also characterized by high co-expression of neuropeptides (Vincent et al., 1982; Hendry et al., 1984a,b). The signaling and functions of VIP (White et al., 2010; Harmar et al., 2012), CCK (Rotzinger and Vaccarino, 2003), NPY (Robinson and Thiele, 2017; Thorsell and Mathe, 2017), and corticotropin-releasing factor (CRF) (Agoglia and Herman, 2018) peptides are complex, have been reviewed well, and will not be discussed here in detail. Instead, this review treats these neuropeptides as cell type-specific markers and describes how IN subclasses are specialized to regulate principal neurons through GABA transmission.
Electrophysiological studies in rodents have found that VIP-INs are functionally distinguished by high input resistance and adaptive action potentials (Lee et al., 2013; Marlin and Carter, 2014; Pronneke et al., 2015; Anastasiades et al., 2018; Schuman et al., 2019; Dudai et al., 2020). On the other hand, CCK-IN basket cells fire in a regular-spiking pattern, display long dendritic Ca2+ transients and release GABA in a bulky asynchronous manner (Kawaguchi and Kubota, 1998; Bartos and Elgueta, 2012; Tremblay et al., 2016). Neocortical CCK-INs transmit short-latency inhibitory postsynaptic currents that broadly inhibit multiple types of neurons, including pyramidal cells and other classes of INs (Nguyen et al., 2020). CCK-INs receive weak inhibition and depressing excitatory transmission, which, along with a long membrane time constant, allow for CCK-INs to summate information from separate afferents (Glickfeld and Scanziani, 2006). Therefore, like PV-INs, CCK-INs may detect and augment coincidental information to regulate the gain within local neural microcircuits.
Evidence from humans linking GABAergic circuit function with AUDs
Genetic studies
Many studies have detected relationships between AUD diagnosis and variation in genes involved with GABAA receptor function (Table 1). Over 20 studies have associated single nucleotide polymorphisms (SNPs) within the α2 GABAA receptor subunit gene, GABRA2, and either AUD diagnosis or the acute effects of ethanol (Covault et al., 2004; Edenberg et al., 2004; Haughey et al., 2008; Roh et al., 2011; Yang et al., 2017; Koulentaki and Kouroumalis, 2018). Consistent with these studies, distinct ethanol-related phenotypes have been observed in mice with genetically-altered ethanol-insensitive α2 subunits (Blednov et al., 2011) and those with complete Gabra2 genetic deletion (Dixon et al., 2012). Together, these studies suggest that potentiating GABAA receptors with α2 subunits, or the synapses from CCK-IN synapses that preferentially target their segments (Nusser et al., 1996; Nyiri et al., 2001), might confer some protection against the likelihood to develop AUD. Variation in GABRG1 has also been linked with AUD (Covault et al., 2008; Enoch et al., 2009). Murine studies suggest that Gabrg1 displays the restricted expression, again pointing to CCK-INs as a potential cellular target (Tasic et al., 2018). Associations between variation in GABRA6 and GABRG3 have also been made with alcohol dependence (Dick et al., 2004; Radel et al., 2005).
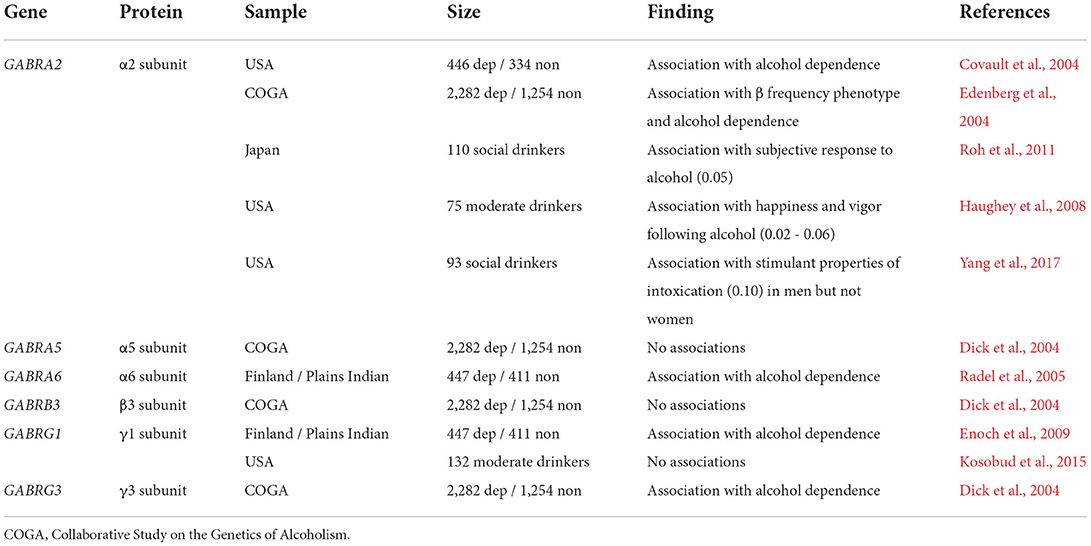
Table 1. Selected studies examining the association of GABAA receptor gene single nucleotide polymorphisms (SNPs) with drinking or AUD vulnerability.
Genome-wide association studies (GWAS) have also been used to identify targets related to AUDs. A recent GWAS study (Meyers et al., 2017) identified ZEB2, a transcription factor that guides PV-IN and SST-IN migration (McKinsey et al., 2013), among several candidate genes underlying AUD. In addition, an association has been detected between AUDs and SIX3 (Kranzler et al., 2019), another transcription factor important for neocortical development and interneuron maturation (Lagutin et al., 2003; Shi et al., 2021). In stark contrast to the litany of candidate gene studies, only two out of more than 20 contemporary genome-wide AUD association studies have identified a significant link with GABAA receptors (Koulentaki and Kouroumalis, 2018). This inconsistency suggests a weak or modest link between GABAA receptor function and AUD vulnerability. Nonetheless, the sheer volume of significant candidate gene studies, along with functional correlates, are consistent with a veritable association between GABRA2 function and AUD vulnerability. The association between GABAA receptor dysfunction and AUD is bolstered by imaging studies that revealed decreased benzodiazepine site availability across the cortex of patients with AUD (Abi-Dargham et al., 1998; Lingford-Hughes et al., 1998). The relatively weak effects observed in GWAS studies suggest that GABA receptor-related AUD phenotypes may be plastic and potentially overcome with the proper treatment. In addition, GWAS studies may not be sufficiently powered to detect genes from a highly comorbid and polygenic disease, such as AUDs. Nonetheless, while the genetic associations link inhibitory microcircuits with AUD diagnosis at the population level, the field is in need of post-mortem studies to investigate changes at the level of cytoarchitecture, protein expression, and/or transcript expression.
Neurophysiology studies
Given that inhibitory INs are crucial for maintaining oscillatory activity, resting electroencephalography (EEG) studies can provide an instantaneous window into microcircuit function in patient populations. The EEG studies offer many technical and practical advantages over laborious neuroimaging techniques. One important caveat, however, is that signals can only be recorded from superficial structures, essentially limiting human studies to the neocortex. Continuous EEG recordings are commonly decomposed into the following frequency bands (in Hz): δ (<3), theta (4–7), α (8–12), β (13–29), and γ (>30) (Rangaswamy and Porjesz, 2014). Distinct INs contribute to oscillatory activity across these ranges (Kuki et al., 2015; Chen et al., 2017); therefore, disease-associated variation within a specific band may implicate one or more specific IN subtypes.
Changes to band activity following alcohol administration, and differences related to family or individual history, have been commonly observed within the α and β frequency bands (reviewed by Porjesz and Begleiter, 2003). Low-to-moderate doses of alcohol generally increase slow α power, particularly in the frontal cortices (Lukas et al., 1986, 1989; Ehlers et al., 1989; Ehlers and Schuckit, 1991; Cohen et al., 1993). Alcohol-induced increases in slow α power are exacerbated in individuals with a family history of AUD and correlate with the feeling of euphoria and desire to drink (Pollock et al., 1983; Lukas et al., 1986; Kaplan et al., 1988; Cohen et al., 1993). Alcohol-induced changes in the fast α spectrum, however, have shown conflicting results, possibly related to the effects of other medications or significant genetic stratification across self-identified racial groups (Ehlers and Schuckit, 1991). More consistently, alcohol administration also increases β frequency power, especially in at-risk individuals (Ehlers and Schuckit, 1990; Stenberg et al., 1994).
In the absence of alcohol, AUD diagnosis is associated with decreased α power (Jones and Holmes, 1976; Coutin-Churchman et al., 2006), and this effect is more pronounced in patients who have relapsed (Saletu-Zyhlarz et al., 2004). Decreased α power has also been observed in individuals with a family history of AUD (Propping et al., 1981; Ehlers and Schuckit, 1991). In contrast, β power, which is increased by alcohol itself, is also elevated in moderate drinkers and abstinent patients with AUD (Ehlers and Schuckit, 1990; Stenberg et al., 1994; Costa and Bauer, 1997; Rangaswamy et al., 2002; Coutin-Churchman et al., 2006). In addition, increased β power is more pronounced in recovering patients who relapse (Saletu-Zyhlarz et al., 2004), and may be more predictive of relapse than either illness severity and depressive symptoms (Bauer, 2001). Furthermore, the β power phenotype in patients with AUD has been linked with genetic variation in a locus encompassing GABRA2, GABRA4, and GABRB1 (Porjesz et al., 2002; Edenberg et al., 2004) and also ZEB2, a transcription factor that regulates IN migration (McKinsey et al., 2013; Meyers et al., 2017). Together, these findings suggest that differences in frequency band power, particularly within the β range, may be considered an AUD endophenotype and leveraged as biomarkers for investigational treatments (Salvatore Curr Addict Rep 2015). EEG phenotypes have been observed in treatment-naïve and unmedicated patients (Bauer, 2001; Fein and Allen, 2005), but it is important to note that benzodiazepines and other medications may contribute to effects in broad patient populations with AUD. Another major caveat to neurophysiology studies in AUD has excluded female subjects so it is unclear how well these findings extend to all individuals. Thus, it is paramount that future studies assess sex as a biological variable.
While the consistent associations between EEG phenotypes and AUD diagnosis are striking, our mechanistic understanding of the IN populations underlying cortical EEG signatures remains limited. In adult mice, neocortical SST-IN activity can promote oscillations in the β frequency range (Chen et al., 2017). In contrast, PV-IN activity can suppress β power (Cho et al., 2015; Kuki et al., 2015) even though it promotes γ frequency activity (Gonzalez-Burgos et al., 2015). These findings suggest that SST-IN hyperactivity or PV-IN hypoactivity underlies increased β power and vulnerability to relapse in patients with abstinent AUD. For the moment, however, this hypothesis remains widely speculative without further preclinical studies. A relatively limited understanding of the basic microcircuits underpinning EEG power bands highlights the need for translational preclinical studies to provide context for clinical findings and to develop new testable hypotheses for treatment and biomarker development.
Mechanistic insight from preclinical studies
Ethanol modulates ionotropic GABA receptors
Due to its low molecular weight, hydrophilicity, and neutral charge, ethanol is readily distributed throughout the entire body and within all subcellular regions that contain water. These physiochemical properties confer ethanol with the ability to interact with a myriad of biological molecules. The use of stringent criteria regarding concentration-dependence, binding-site inhabitation, and genetic manipulation was recently suggested to define targets that underlie ethanol's anxiolytic, amnestic, sedative, and addictive properties (Abrahao et al., 2017). This literature will not be discussed here in detail, as ethanol's molecular targets have been reviewed by others (Harris et al., 2008; Trudell et al., 2014; Abrahao et al., 2017). While several specific findings have not been consistently replicated across laboratories, significant evidence supports the notion that ethanol induces adaptive behavioral effects by modulating fast neurotransmission within inhibitory microcircuits.
Ethanol modulates ionotropic receptors across the entire Cys-loop superfamily (Trudell et al., 2014), including glycine receptors (Perkins et al., 2010; Badanich et al., 2013), nicotinic receptors (Hendrickson et al., 2013; Gao et al., 2019), 5HT3a receptors (Parker et al., 1996; Lovinger, 1999), and, notoriously, GABAA receptors. The acute actions of ethanol on the biophysical properties of isolated GABAA receptors have been reviewed by others (Criswell and Breese, 2005; Weiner and Valenzuela, 2006; Lovinger and Homanics, 2007; Lobo and Harris, 2008). To describe this literature as controversial is a considerable understatement. Studies from isolated neurons have concluded that ethanol potentiates, does not affect, or inhibits currents elicited with exogenous GABA. In contrast, synaptic GABAA receptor currents are more consistently facilitated by ethanol, although many studies have implicated an increased GABA release and not a direct action with the GABAA receptor. Experiments conducted using recombinant expression systems raise additional questions about the physiological relevance of the direct interactions with GABAA receptors, as ethanol is generally inefficacious at concentrations below 50 mM (~0.24 mg/dl). One noteworthy exception lies within a subset of oocyte studies suggesting that physiologically relevant concentrations of ethanol potentiate the conductance of GABAA receptors containing α4, α6, and δ subunits (Sundstrom-Poromaa et al., 2002; Wallner et al., 2003; Wei et al., 2004; Bowen et al., 2015), but some conflicting results have also challenged whether this finding is widely reproducible (Borghese and Harris, 2007; Mehta et al., 2007). Despite the conflicting and inconclusive molecular literature, a litany of behavioral studies suggests that behavioral affects of ethanol occur through fast GABAergic transmission.
Convergent results from pharmacological and genetic studies indicate that interactions between ethanol and distinct pools of GABAA receptors are relevant for behavioral effects and disease outcomes. Ethanol shares behavioral effects with many compounds that positively modulate GABAA receptors, and conversely, GABAA receptor negative modulators block these same effects (Grant, 1994; Criswell and Breese, 2005; Weiner and Valenzuela, 2006). Studies using transgenic mice also suggest that specific GABAA receptor peptide residues are important for some behavioral effects of ethanol (Boehm et al., 2004; Kumar et al., 2009). Knock-in mice with ethanol-insensitive α1 subunits exhibit altered ethanol-induced anxiolysis and motor impairment (Werner et al., 2006). Similarly, the SNP in Gabra6 confers α6-containing GABAA receptors with enhanced sensitivity to ethanol and cerebellar motor impairing effects (Hanchar et al., 2005). In contrast, a similar mutation to α2 subunits does not affect anxiolysis or motor incoordination but alters volitional drinking, conditioned taste aversion, and ethanol's stimulant properties (Blednov et al., 2011; Newman et al., 2016). GABAA α4 and δ subunits also regulate the discrete effects of ethanol, including binge drinking, motor effects, and abstinence-induced affective disturbances (Melon et al., 2018; Darnieder et al., 2019). The expression and function of these extrasynaptic subunits are modulated by neuroactive steroids and female sex hormones (Sundstrom-Poromaa et al., 2002; Stell et al., 2003; Maguire et al., 2005; Abramian et al., 2014), stressing the need to continue assessing sex as a biological variable. Finally, mice with an introduced point of mutation that enhances channel conductance in the GABAA β1 subunit work harder to obtain ethanol and are more sensitive to intoxication (Anstee et al., 2013). These studies, along with many others not cited here, suggest that interactions between ethanol and specific GABAA receptors mediate specific adaptive behavioral responses, consistent with ethanol acting across a constellation of distinct inhibitory synapses.
Considering that GABAA receptor subunits vary with regard to their subcellular expression and inhibitory synapses in the mammalian forebrain arise from diverse groups of local GABAergic INs, the above findings suggest that distinct inhibitory microcircuits regulate the specific aspects of anxiolytic, sedative, and addictive properties of ethanol. The conclusion that ethanol exerts differential effects across GABAA receptor subpopulations in vivo, in the context of the consistently conflicting findings from reduced systems, underscores strong motivation to understand how acute and chronic ethanol modulates heterogeneous inhibitory microcircuits. Examining how ethanol alters the physiology and circuit function of defined IN subpopulations should help coalesce existing gaps within the preclinical literature and uncover differences in the cellular substrates that mediate anxiolytic, sedative, and addictive properties of ethanol.
Mechanisms of PFC GABAergic IN dysfunction in preclinical models
Acute ethanol administration
Studies examining how acute ethanol modulates neocortical GABAA receptor function have yielded mixed results. In response to exogenous GABA, ethanol facilitates hyperpolarizing currents in neocortical neurons in culture (Aguayo, 1990; Reynolds and Prasad, 1991), acute slices (Proctor et al., 1992; Soldo et al., 1998), and intact systems (Nestoros, 1980). In contrast, PFC synaptic GABAA receptor currents are unaffected by modest concentrations of ethanol (<50 mM) (Proctor et al., 1992; Marszalec et al., 1998; Criswell and Breese, 2005; Weitlauf and Woodward, 2008; Fleming et al., 2009). One potential mechanism contributing to this discrepancy is that ethanol may preferentially enhance extrasynaptic GABAA receptors. Indeed, acute ethanol can induce or modulate tonic currents in pyramidal cells within the prelimbic PFC and the orbitofrontal cortex (Carlson et al., 2016a; Centanni et al., 2017). These tonic currents are generally mediated by α5- and δ-containing GABAA receptors in the neocortex, but studies from the hippocampus have also suggested that ethanol can interact with α1- and δ-containing GABAA receptors (Glykys et al., 2007). In addition, studies in the orbitofrontal cortex have shown that ethanol decreases current-evoked spiking by modulating glycine receptors (Badanich et al., 2013; Nimitvilai et al., 2016). Glycine receptors are also present and active in the prelimbic PFC (Salling and Harrison, 2014), but whether acute ethanol modulates them in the subregion has not been reported. Taken together, these studies indicate that under some conditions, acute ethanol can enhance inhibitory currents in the frontal cortex. Changes to α5-mediated tonic currents, potentially driven by SST-IN GABA release, may therefore underlie ethanol-induced changes to decision-making and cognition. Future studies using cell type-specific optogenetics are warranted to test this hypothesis with exciting ramifications for treatment development.
Several lines of evidence indicate that systemic ethanol delivery modulates neocortical inhibitory microcircuits. Low-to-moderate doses of ethanol (0.375–1.5 g/kg) decrease the mean firing rate of PFC pyramidal cells in rats (Tu et al., 2007) while other studies have shown that ethanol (1 g/kg) acutely decreases PFC GABA levels (Carton et al., 2019). While these findings are seemingly in opposition, a potential explanation is that ethanol may disrupt pyramidal cell synchrony by impairing PV-IN function. Accordingly, 25–50 mM ethanol (comparable to maximum concentrations reached by 1–3 g/kg) disrupts PV-IN up-state activity in slice culture (Woodward and Pava, 2009). In addition to the effects on PV-INs, a recent in vivo mouse study found that low doses of ethanol (0.5–1 g/kg) increase calcium mobilization in SST-INs while high doses (2–3 g/kg) decrease calcium activity (Li et al., 2021). These bidirectional changes in SST-IN calcium activity were paralleled by complementary changes in pyramidal cell activity. Furthermore, high doses of ethanol (3.5–5 g/kg) increase α4 localization in the synaptic compartment of pyramidal cells (Liang et al., 2007; Kumar et al., 2009; Carlson et al., 2014; Bohnsack et al., 2018). Together, these findings suggest that decreased SST-IN activity and increased pyramidal cell activity may trigger a homeostatic increase in GABAA receptor function following ethanol exposure. Studies in slice culture have illustrated that these changes are dynamic, as α4 and δ subunit expression decrease following longer durations of ethanol exposure (Carlson et al., 2016b). These biphasic adaptations provide a potential mechanism contributing to acute ethanol tolerance (Liang et al., 2007; Gonzalez et al., 2012) and may have contributed to the significant variation observed in earlier studies.
Additional insight into the actions of ethanol on discrete IN types might be gleaned from studies in the hippocampus. In CA1 pyramidal cells, synaptic IPSCs evoked near the soma display enhanced sensitivity to ethanol (Weiner et al., 1997), raising the possibility that perisomatic PV-IN or CCK-IN synapses onto pyramidal cells may be especially sensitive to acute ethanol. On the other hand, spike-firing of spontaneously active INs located in the stratum lacunosum moleculare (potentially analogous to neocortical SST-INs and/or CCK-INs) is facilitated by 10–30 mM ethanol (comparable to maximum concentrations reached by 0.5–1 g/kg) (Yan et al., 2009). Ethanol has little effect on the intrinsic properties of quiescent INs, but in active INs, ethanol activates the pacemaker HCN channel current and increases spontaneous firing. Additional experiments in spontaneously firing INs within the stratum oriens (akin to SST-INs) revealed that concentrations of ethanol as low as 3 mM (less than maximum concentrations reached by 0.5 g/kg) enhance action potential frequency, again by facilitating HCN channel function (Yan et al., 2010). In contrast, low concentrations of ethanol (5–10 mM) may decrease the activity of some IN classes by attenuating kainate receptor transmission and induced spike-firing (Carta et al., 2003). Other functions of the kainate receptor were not disrupted, suggesting that substantial endogenous glutamate transmission is required for ethanol application to inhibit the IN activity. Thus, ethanol may preferentially exert some of its effects through inhibitory microcircuits with high glutamatergic tone. Collectively, these studies illustrate that acute ethanol induces heterogeneous responses to the synaptic and membrane physiology of distinct IN subclasses. In addition, the hippocampal and neocortical literature suggests that SST-INs may be the IN class with the greatest sensitivity to ethanol. Clearly, the presented compelling rationale supports revisiting effects of ethanol on the intrinsic properties of defined PFC INs using contemporary transgenic and optogenetic methodologies.
Moderate exposure and voluntary drinking
Adaptations to PFC microcircuitry have been observed in several binge drinking models (Figure 2A). Most rodent studies examining how voluntary drinking affects PFC inhibitory transmission have been conducted following intermittent two-bottle choice (2BC). In male rats, PFC Gabra5 and Gabrb1 expression each positively correlate with ethanol intake (Pickering et al., 2007), suggesting frontal cortex microcircuits, and tonic inhibition through α5 receptors may contribute to individual variation in the desire to drink. Consistent with this hypothesis, decreased GABRA5 expression has been observed in the PFC of patients with AUD, an effect that is exacerbated in female individuals (Janeczek et al., 2020). Studies have also implicated changes in PFC phasic inhibitory transmission following binge drinking. Decreased Gabra1 expression has been observed in the PFC of male rats that underwent intermittent 2BC (Bohnsack et al., 2018). Furthermore, Dao et al. (2021) recently found decreased sIPSC frequency following drinking in dark (DID) in mice, and 1-day abstinence from intermittent 2BC led to a trend decrease in sIPSC frequency and a prolonged sIPSC rise time in the pyramidal cells of male rats (Klenowski et al., 2016). These findings raise the possibility that drinking may alter the relative contributions of IN subpopulations to the inhibitory milieu (e.g., a decreased contribution of peri-somatic PV-IN activity to overall sIPSCs). Recently, Cannady et al. (2020) reported that 1-day abstinence from intermittent 2BC did not affect sIPSC amplitude or frequency in the anterior cingulate cortex of male mice. Kinetics parameters were not reported in these studies, precluding direct comparisons with some prior work in prelimbic PFC. Moreover, an important consideration for future experiments is that neocortical pyramidal cells can be differentiated into several subclasses based on projection target as well as the expression of GPCRs and other proteins. Thus, it is likely that disease-related experiences differentially alter the IN regulation of pyramidal cell subpopulations, as we have observed with respect to excitatory transmission (Joffe et al., 2021). Future studies will need to use a priori means to distinguish cell types to parse subtle or bidirectional changes to specific subpopulations. In addition, postmortem studies, in mixed-sex cohorts, should be conducted to assess whether changes in GABAA receptor protein are apparent in patient populations.
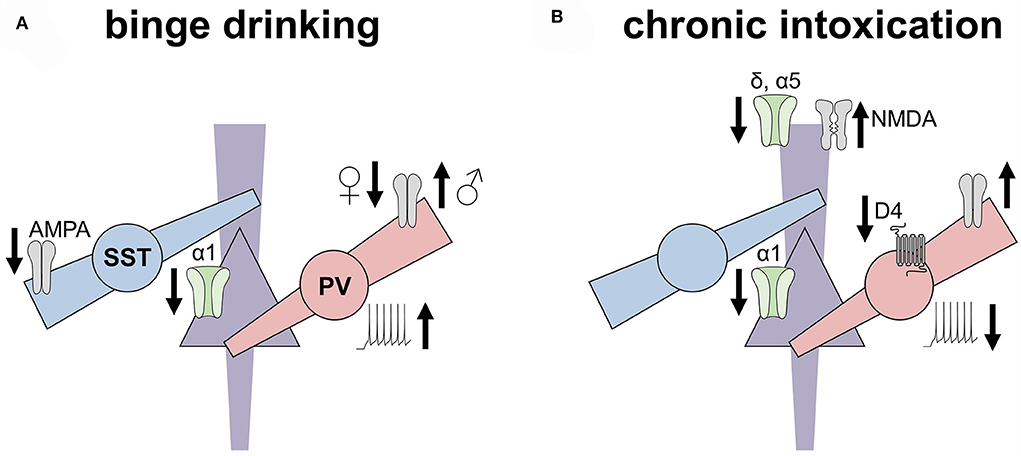
Figure 2. Ethanol experiences induced disparate adaptations to inhibitory microcircuits in the rodent prefrontal cortex. This non-exhaustive figure highlights key adaptations to the prefrontal cortex inhibitory microcircuits observed in rodent models of binge drinking (left) and dependence (right). (A) Excitatory drive onto somatostatin interneurons (SST-INs) is decreased following chronic voluntary drinking, along with a concomitant decrease in the expression of α1 GABAA subunits on pyramidal cells. In contrast, excitatory drive onto parvalbumin (PV) INs is decreased in female mice but increased in male mice following binge drinking. PV-INs from drinking mice also exhibit enhanced excitability. (B) After chronic intoxication or dependence, however, PV-INs display reduced excitability relative to controls, along with reduced regulation by dopamine receptors. Increased excitatory drive onto PV-INs of both female and male mice has also been observed following the induction of ethanol place preference but not place aversion. Pyramidal cells from dependent animals display reduced α1 and α5 GABA receptor subunits, the latter of which may relate to increased NMDA receptor function observed in these models.
Several lines of convergent evidence have related PFC PV-IN function with binge drinking. One-day abstinence from intermittent 2BC increases Fos expression in one-third of PFC INs in male rats (George et al., 2012), but it is unclear from those studies which class(es) of INs were involved. Contemporary studies leveraging transgenic tools are beginning to assess how ethanol experiences alter defined subtypes of PFC INs. A day after intermittent 2BC, we found that prelimbic PV-INs displayed enhanced excitability in mice of all sexes (Joffe et al., 2020). However, we observed a notable sex difference with regard to synaptic adaptations. After intermittent 2BC, PV-INs displayed diminished excitatory drive in female mice but enhanced postsynaptic AMPA receptor function in male mice. These adaptations to PV-INs in male mice provide two compelling mechanisms for the increased Fos expression observed in the prior male rat studies (i.e., increased excitability and/or synaptic drive). In addition, others have shown that genetic disruption of synaptic transmission onto cortical INs decreases voluntary drinking (Radke et al., 2017) and that PV-IN disinhibition increases binge drinking in male mice, but not female mice (Melon et al., 2018). Together, these studies support the hypothesis that increased activity of forebrain PV-INs contributes to drinking behaviors and that PFC PV-INs are involved in sex differences in top-down control.
Recent studies have begun to assess how drinking experiences modulate SST-IN physiology. In contrast to the findings from PV-INs, studies from our lab examining deep layer prelimbic SST-IN intrinsic properties in female mice or male mice found no differences associated with 1-day abstinence from intermittent 2BC (Joffe et al., 2020). Interestingly, in prelimbic layers 2/3, Dao et al. (2021) observed that one-day abstinence from DID was associated with decreased excitability of SST-INs. Together, these data suggest that there may be layer-specific or light phase-specific adaptations to SST-IN during early abstinence from voluntary drinking. An earlier study by (Dao et. al 2020) examined SST-IN physiology following extended withdrawal from continuous 2BC in female mice, finding that SST-INs in the abstinence group exhibited enhanced current-evoked spike firing, a finding directly opposite to their studies conducted in early abstinence (Dao et al., 2021). The discrepancy between these two recent studies is likely to be stemmed from differences in the length of abstinence or between intermittent vs. continuous ethanol exposure. Another important consideration is that the enhanced SST-IN excitability was observed in slices from mice subjected to a battery of depressive-like behaviors, including a forced swim test 90 min before tissue collection (Dao et al., 2020). Thus, abstinence from ethanol may prime SST-INs for intrinsic excitability plasticity following acute stress. Testing whether these adaptations mediate stress-induced relapse will be an important series of future studies. Considering PFC SST-INs have been widely implicated in MDD etiology (Rajkowska, 2000; Tripp et al., 2011), adaptations to stressful stimuli (Xu et al., 2019; Cummings and Clem, 2020; Joffe et al., 2022), and rapid antidepressant action (Gerhard et al., 2019; Ali et al., 2020), the SST-IN system represents an exciting target for ameliorating the negative affective symptoms related to AUD (Robinson and Thiele, 2020; Crowley and Joffe, 2022).
Convergent findings suggest that decreased excitatory drive onto SST-INs emerges in early abstinence from voluntary drinking (Joffe et al., 2020; Dao et al., 2021). We also found a subtle sex difference in deep layer SST-INs, as electrically evoked EPSCs from male mice, but not female mice, displayed a decreased paired-pulse ratio following intermittent 2BC (Joffe et al., 2020). One potential explanation underlying this unexpected adaptation is that the paired-pulse ratio in SST-INs may reflect postsynaptic features, such as interactions with transmembrane AMPA receptor regulatory proteins or the intracellular polyamine block of CP-AMPA receptors (Rozov and Burnashev, 1999; Soto et al., 2007). Intriguing support for that hypothesis lies in a recent study that discovered female mice express more Gria2 in PFC SST-INs than male mice (Gerhard et al., 2019). Thus, excitatory synapses onto SST-INs in female mice may not express CP-AMPA receptors to a greater degree, and receptor internalization following intermittent 2BC would not be expected to alter polyamine sensitivity. Future studies should explicitly examine AMPA receptor stoichiometry in SST-INs in all sexes, as these changes are likely to alter synaptic plasticity and the response to many potential SST-IN-directed treatments (Tomita et al., 2006). Sex differences in SST-IN-mediated disinhibition of other interneurons also appear to manifest following binge drinking, specifically in male individuals (Dao et al., 2021).
Despite the compelling molecular evidence indicating ethanol modulates 5HT3 receptor function (Lovinger and White, 1991; Lovinger, 1999), few studies have directly addressed how ethanol affects 5HT3-INs. The PFC transcript expression for the 5HT3 receptor (Htr3a) positively correlates with alcohol consumption in male rats (Pickering et al., 2007). In addition, the prefrontal cortex Htr3a expression positively correlates with reward learning following ethanol (0.5 g/kg) place conditioning (Xu et al., 2012). To the best of our knowledge, however, no studies have been published that directly assess whether acute exposure, voluntary drinking, or ethanol dependence alter the physiology of any subtype of PFC 5HT3-IN. Several studies, however, do implicate neuropeptides potentially released from PFC 5HT3-INs in the regulation of voluntary drinking. For instance, the site-specific delivery of NPY receptor or CRF receptor ligands can modulate binge drinking in the DID mouse model (Robinson et al., 2019a,b). Ethanol-naïve alcohol preferring P rats also exhibit lower levels of NPY immunoreactivity in the frontal cortex than controls, although NPY expression was not altered by ethanol exposure (Ehlers et al., 1998). Taken together, these studies provide compelling evidence for future studies to assess physiological adaptations across several genetically defined PFC IN subtypes in preclinical models of AUD. Discovering pharmacological agents to dampen PV-IN activity and/or boost excitatory drive onto SST-INs appear to provide particularly promising avenues toward attenuating symptoms associated with early abstinence.
High exposure and dependence
Several rodent studies have shown that high ethanol exposure differentially modulates inhibitory microcircuits across the frontal cortex areas (Figure 2B). An important caveat to this literature is that nearly all published PFC experiments in dependence models have been restricted to male subjects. Acute withdrawal from chronic intermittent ethanol (CIE), vaporized ethanol exposure for 16 h/day, 4 days/week, 1–4 weeks, is associated with decreased sIPSC frequency on pyramidal cells in the mouse infralimbic cortex (Pleil et al., 2015). Tetrodotoxin blocked this effect, indicating that early withdrawal disrupts active inhibitory microcircuits. Studies on the prelimbic cortex have yielded more conflicting results. In mice, acute withdrawal from CIE did not affect sIPSC parameters in studies from two laboratories (Trantham-Davidson et al., 2014; Pleil et al., 2015). On the other hand, acute withdrawal from chronic ethanol gavage decreased sIPSC frequency in the prelimbic cortex pyramidal cells in rats (Bohnsack et al., 2018; Hughes et al., 2019), and these adaptations have been corroborated by transcript and protein level changes (Devaud et al., 1997; Kumar et al., 2009; Bohnsack et al., 2018). The discrepancies in findings related to dependence effects on the prelimbic pyramidal cell function might be related to the route of ethanol administration and/or species; however, it is also possible that variation in the source of GABA during spontaneous recordings might have contributed to discrepancies across laboratories. Future studies using transgenic technologies to isolate outputs from specific IN classes will be important to reconcile discrepancies in the preclinical literature.
Studies directly targeting PFC INs suggest that ethanol dependence alters their physiology in a cell-autonomous manner. Trantham-Davidson and Chandler (2015) found that dopamine lost the ability to modulate PV-IN membrane physiology 1 week after withdrawal from CIE in male rats. In contrast, mGlu1 receptor potentiation retained the ability to modulate PV-INs, suggesting that CIE withdrawal precipitates differential changes to GPCR signaling cascades within INs. More recently, Hughes et al. (2020) used a viral-assisted approach to label INs in the rat PFC via the mDlx promotor (Dimidschstein et al., 2016). After 1 day of discontinuation from chronic ethanol gavage, INs were patched and functionally classified as “Fast-Spiking” (putative PV-INs) or “Martinotti” (putative SST-INs). Decreased PV-IN excitability was observed in cells from female and male ethanol-treated rats (Hughes et al., 2020). Similarly, we observed decreased excitability in fluorescently-identified PV-INs from transgenic mice following conditioned place preference to repeated ethanol injections (Ferranti et al., 2022). Interestingly, despite receiving identical ethanol exposure, PV-INs from mice that underwent conditioned place aversion were not different from controls, indicating that adaptations to PV-INs vary based on the learned experience. Based on these studies, manipulations that enhance PV-IN function may have the potential to remediate long-term physiological and behavioral changes associated with dependence. Additional studies need to be performed to better understand SST-IN and 5HT3-IN adaptations in models of high ethanol exposure.
Adolescent exposure
Across mammalian species, the PFC is one of the latest brain areas to fully mature, with the refinement of synaptic connections and improved cognitive functions not occurring until young adulthood (Kolk and Rakic, 2022). Understanding how drinking affects the adolescent brain is essential for modeling facets of AUD, as adolescents are more likely to binge drink than adults, and the early onset of drinking can increase the likelihood of an individual developing an AUD later in life (Hingson et al., 2006; Marshall, 2014).
Importantly, in preclinical studies, ethanol dependence during adolescence differentially affects PFC function relative to ethanol administered to adults (Trantham-Davidson et al., 2014, 2017; Barker et al., 2017). PV-INs in adult male rats exposed to 4 weeks of CIE during adolescence display decreased current-evoked firing relative to air controls (Trantham-Davidson et al., 2017). In addition, adolescent CIE decreases the sIPSC amplitude in adults and prevents the development of δ subunit tonic currents in male rats and female rats (Centanni et al., 2017). These effects were specific to deep layers, suggesting greater disruptions at synapses from PV-INs or SST-INs relative to 5HT3-INs. Results from several studies suggest that the tonic GABAA receptor current in PFC pyramidal cells is developmentally regulated. Minimal tonic current has been reported in the frontal cortex of relatively young rats and mice (3–6 weeks) (Drasbek and Jensen, 2006; Weitlauf and Woodward, 2008; Salling and Harrison, 2014; Centanni et al., 2017), whereas PFC pyramidal cells from adult animals (>8 weeks) display tonic currents mediated by receptors containing α5 and/or δ subunits (Lee and Maguire, 2014; Centanni et al., 2017). These findings suggest that ethanol dependence during adolescence can stunt or delay PFC maturation (Spear and Swartzwelder, 2014; Centanni et al., 2017). Consistent with this notion, adolescent 2BC disrupts the developmental trajectory of PFC pyramidal cell intrinsic properties (Salling et al., 2018). In the hippocampus, as well, decreased δ and α4 GABAA subunits have been observed following extended withdrawal from CIE delivery to adolescent male mice (Fleming et al., 2013; Centanni et al., 2014).
Conclusion and future directions
In this review, we outlined the basic, translational, and population-level studies that implicate PFC inhibitory microcircuit function in the acute effects of ethanol and the symptoms and development of AUDs. Significant research has described the effects of ethanol on isolated components of IN synaptic transmission, and there are convergent findings between preclinical research and human studies using postmortem samples and genetics. Nonetheless, we are just beginning to understand how long-term ethanol exposure generates adaptations to defined IN classes and cell types. Emerging preclinical findings suggest that PV-IN intrinsic properties display biphasic adaptations, such that modest ethanol exposure increases but prolonged the exposure and decreases the dependence of PV-IN excitability. In addition, excitatory drive onto PV-INs may represent an exciting potential substrate underlying sex differences in alcohol-seeking and AUD vulnerability. In contrast, decreased excitatory drive onto SST-INs has been observed in multiple laboratories in mouse studies using all sexes, but it remains less clear how SST-IN physiology may be altered in models of high ethanol exposure.
Recent advances in transgenic tools, fluorescent biosensors, and opto-/chemogenetics provide unprecedented opportunities to assess ethanol-induced adaptations to discrete cell types and circuits, paving the way for the development of new rationally designed treatments for AUDs. Nonetheless, translating molecular and circuit-specific discoveries into new life-saving therapies persists as a fundamental challenge in neuroscience. What realistic interventions can target discrete cortical microcircuits in patients? In 30 years, perhaps, cell type-specific expression of optogenetic proteins, chemogenetic actuators, or other exogenous bioactive molecules may become viable treatment options for psychiatric diseases. Indeed, continued work identifying and optimizing viral promotors that enable cell type-restricted expression following systemic delivery (Dimidschstein et al., 2016; Vormstein-Schneider et al., 2020) holds a great promise for minimizing the invasiveness of approaches that require gene therapy. In addition, pharmacogenetic systems, like drugs acutely restricted by tethering (Shields et al., 2017), may provide opportunities to modulate any number of endogenous receptors on discrete cell populations.
Despite the promise of these next-generation approaches, such treatments will offer no relief for individuals suffering from AUDs within the next decade. At this time, brain stimulation and conventional pharmacology approaches remain the only safe and readily deployable ways to modulate brain function in the clinic. Due to its fortuitous location near the surface of the skull, the PFC is amenable to stimulation-based treatments including transcranial magnetic stimulation and transcranial direct current stimulation (Salling and Martinez, 2016; Philip et al., 2020). In recent years, several exciting and well-controlled trials have demonstrated that focal PFC stimulation can attenuate changes in the corticostriatal function observed in AUD populations and can alleviate cravings during abstinence (Hanlon et al., 2018). These groundbreaking studies have demonstrated exciting proof-of-concept that targeting PFC function can provide new therapies for AUDs. While the cells and mechanisms through which PFC brain stimulation can reduce alcohol cravings remain unresolved, we propose that cortical INs represent compelling candidates. TMS modifies neural circuits through repetitive rhythmic stimulations thought to entrain oscillatory activities (Lin et al., 2021). In addition, the long-term efficacy of brain stimulation approaches is thought to be related to persistent effects on synaptic plasticity (Hanlon et al., 2018). As discussed in this review, INs play key roles in regulating the endogenous processes recruited by TMS; therefore, we believe modulating PFC IN activities may provide synergistic opportunities to enhance the efficacy of brain stimulation approaches. Future mechanistic studies assessing the physiological adaptations that occur to define IN subtypes following brain stimulation would provide a promising avenue toward treatment optimization and may help identify new paths to treatment altogether.
Conventional pharmacology offers alternative means of targeting cortical microcircuits in the clinic. GABAA receptor modulators have been used for decades to treat symptoms of acute alcohol withdrawal, anxiety, and sleep disturbances. Despite their utility and efficacy, these drugs are hampered by concerning side effects and their own liabilities for dependence. In general, drugs that minimize direct receptor agonism and that target more specific subunit combinations of GABAA receptors exhibit safer clinical profiles; however, improvements are still very much possible and desired from GABAA modulators currently in widespread use. Excitingly, the neuroactive steroid, brexanolone, a medication that works by potentiating δ-containing GABAA receptors (Walton and Maguire, 2019), was recently approved for postpartum depression and deserves some attention for the potential to craft a microcircuit-based treatment for AUDs. To this end, we believe continued mechanistic studies examining PFC INs are essential. Because IN subtypes display specialized transcriptomes relative to other cell types, precise efforts to identify receptors selectively expressed by INs may yield novel druggable targets with circuit-specific actions. For example, the M2 subtype of muscarinic receptor displays restricted expression within a subclass of SST-IN and other types of INs (Hajos et al., 1998; McDonald and Mascagni, 2011), and variation in the M2 gene CHRM2 predisposes individuals to AUD and is linked with the severity of illness (Wang et al., 2004; Jung et al., 2011). Based on this, the development of small molecule modulators of M2 receptors may represent a path to leverage endogenous neurobiological specificity toward circuit-specific therapies. The metabotropic glutamate receptor, subtype 1 represents another exemplar receptor whose preferential expression in SST-INs has the potential to be leveraged to develop microcircuit-specific pharmacological treatments (van Hooft et al., 2000; Maksymetz et al., 2021). In addition to receptors expressed by INs themselves, approaches that manipulate their targets may prove advantageous. Toward this goal, a variety of neuropeptide receptor systems (e.g., SST, VIP, CCK, and NPY) represent identified targets and relatively low-hanging fruit. Metabotropic and ionotropic receptors for GABA itself may also be targeted to generate circuit-specific treatments, although their relatively widespread expression, combined with the known side effect liability of existing GABAergic medications, seems to indicate that a pharmacogenetic approach may be required to selectively target these molecules.
Continued transcriptomics studies, in postmortem tissues and animal models, will be essential to identify endogenous molecules to target medication development. Now, more than ever, it is essential that parallel multiplex protein and in situ hybridization studies in human postmortem brain tissue should be conducted to assess whether phenomena described in rodents also occur in patient populations. Over the last 10 years, methodological advances (Sweet et al., 2010; Glausier et al., 2014, 2021; Fish et al., 2018) and the establishment of the NIH NeuroBioBank have created an incredible opportunity to learn more about AUDs by studying postmortem tissue. Floyd Bloom said, “The gains in brain are mainly in the stain.” Similar to the new James Webb Space Telescope which is going to allow scientists to explore uncharted galaxies, blackholes, and planetary systems, the ability to perform quantitative, highly multiplexed immunofluorescence imaging of human postmortem tissue from subjects with AUDs will undoubtedly provide new insights to the etiology and provide valuable information about potential drug targets. For example, quantitative fluorescence microscopy was recently used to show that the density of synapses arising from a unique chandelier GABA neuron subtype is higher in PFC layer 2 of subjects with schizophrenia relative to matched comparison subjects (Rocco et al., 2017). Moreover, studies of postmortem tissue are not limited to just immunohistochemistry and immunocytochemistry: it is possible to perform multiplex RNA in situ hybridization (Fish et al., 2018), proteomics (MacDonald et al., 2019), and genomic (e.g., RNAseq) (Arion et al., 2007; Enwright and Lewis, 2021) analyses at once. Importantly, the methods used to collect tissue are becoming standardized such that high quality tissue is readily available (Stan et al., 2006). In addition, the techniques being employed can be used to rapidly assess large cohorts so that potentially confounding comorbidities can be assessed. Rigorously examining PFC microcircuit function in parallel studies in preclinical models and postmortem tissues holds a great promise for the development of breakthrough treatments for AUDs.
Author contributions
MJ prepared the first draft. KF and MJ equally contributed to revising all subsequent drafts. Both authors contributed to the article and approved the submitted version.
Funding
This study was supported by the NIH (K99/R00AA027806 to MJ and MH096985 to KF).
Acknowledgments
We thank the members of the KF and MJ laboratories and the UPMC TNP for stimulating discussions.
Conflict of interest
The authors declare that the research was conducted in the absence of any commercial or financial relationships that could be construed as a potential conflict of interest.
Publisher's note
All claims expressed in this article are solely those of the authors and do not necessarily represent those of their affiliated organizations, or those of the publisher, the editors and the reviewers. Any product that may be evaluated in this article, or claim that may be made by its manufacturer, is not guaranteed or endorsed by the publisher.
References
Abi-Dargham, A., Krystal, J. H., Anjilvel, S., Scanley, B. E., Zoghbi, S., Baldwin, R. M., et al. (1998). Alterations of benzodiazepine receptors in type II alcoholic subjects measured with SPECT and [123I]iomazenil. Am. J. Psychiatry 155, 1550–1555. doi: 10.1176/ajp.155.11.1550
Abrahao, K. P., Salinas, A. G., and Lovinger, D. M. (2017). Alcohol and the brain: neuronal molecular targets, synapses, and circuits. Neuron 96, 1223–1238. doi: 10.1016/j.neuron.2017.10.032
Abramian, A. M., Comenencia-Ortiz, E., Modgil, A., Vien, T. N., Nakamura, Y., Moore, Y. E., et al. (2014). Neurosteroids promote phosphorylation and membrane insertion of extrasynaptic GABAA receptors. Proc. Natl. Acad. Sci. USA. 111, 7132–7137. doi: 10.1073/pnas.1403285111
Addolorato, G., Antonelli, M., Cocciolillo, F., Vassallo, G. A., Tarli, C., Sestito, L., et al. (2017). Deep transcranial magnetic stimulation of the dorsolateral prefrontal cortex in alcohol use disorder patients: effects on dopamine transporter availability and alcohol intake. Eur. Neuropsychopharmacol. 27, 450–461. doi: 10.1016/j.euroneuro.2017.03.008
Agoglia, A. E., and Herman, M. A. (2018). The center of the emotional universe: alcohol, stress, and CRF1 amygdala circuitry. Alcohol 72, 61–73. doi: 10.1016/j.alcohol.2018.03.009
Aguayo, L. G. (1990). Ethanol potentiates the GABAA-activated Cl- current in mouse hippocampal and cortical neurons. Eur. J. Pharmacol. 187, 127–130. doi: 10.1016/0014-2999(90)90349-B
Ali, F., Gerhard, D. M., Sweasy, K., Pothula, S., Pittenger, C., Duman, R. S., et al. (2020). Ketamine disinhibits dendrites and enhances calcium signals in prefrontal dendritic spines. Nat. Commun. 11, 72. doi: 10.1038/s41467-019-13809-8
Anastasiades, P. G., Boada, C., and Carter, A. G. (2018). Cell-type-specific D1 dopamine receptor modulation of projection neurons and interneurons in the prefrontal cortex. Cereb Cortex. 29, 3224–3242 doi: 10.1093/cercor/bhy299
Anderson, T. R., Huguenard, J. R., and Prince, D. A. (2010). Differential effects of Na+-K+ ATPase blockade on cortical layer V neurons. J. Physiol. 588, 4401–4414. doi: 10.1113/jphysiol.2010.191858
Anstee, Q. M., Knapp, S., Maguire, E. P., Hosie, A. M., Thomas, P., Mortensen, M., et al. (2013). Mutations in the Gabrb1 gene promote alcohol consumption through increased tonic inhibition. Nat. Commun. 4, 2816. doi: 10.1038/ncomms3816
Arion, D., Unger, T., Lewis, D. A., Levitt, P., and Mirnics, K. (2007). Molecular evidence for increased expression of genes related to immune and chaperone function in the prefrontal cortex in schizophrenia. Biol. Psychiatry 62, 711–721. doi: 10.1016/j.biopsych.2006.12.021
Badanich, K. A., Mulholland, P. J., Beckley, J. T., Trantham-Davidson, H., and Woodward, J. J. (2013). Ethanol reduces neuronal excitability of lateral orbitofrontal cortex neurons via a glycine receptor dependent mechanism. Neuropsychopharmacology 38, 1176–1188. doi: 10.1038/npp.2013.12
Barker, J. M., Bryant, K. G., Osborne, J. I., and Chandler, L. J. (2017). Age and sex interact to mediate the effects of intermittent, high-dose ethanol exposure on behavioral flexibility. Front. Pharmacol. 8, 450. doi: 10.3389/fphar.2017.00450
Bartos, M., and Elgueta, C. (2012). Functional characteristics of parvalbumin- and cholecystokinin-expressing basket cells. J. Physiol. 590, 669–681. doi: 10.1113/jphysiol.2011.226175
Bartos, M., Vida, I., and Jonas, P. (2007). Synaptic mechanisms of synchronized gamma oscillations in inhibitory interneuron networks. Nat. Rev. Neurosci. 8, 45–56. doi: 10.1038/nrn2044
Bauer, L. O. (2001). Predicting relapse to alcohol and drug abuse via quantitative electroencephalography. Neuropsychopharmacology 25, 332–340. doi: 10.1016/S0893-133X(01)00236-6
Beierlein, M., Gibson, J. R., and Connors, B. W. (2003). Two dynamically distinct inhibitory networks in layer 4 of the neocortex. J. Neurophysiol. 90, 2987–3000. doi: 10.1152/jn.00283.2003
Belelli, D., Harrison, N. L., Maguire, J., Macdonald, R. L., Walker, M. C., and Cope, D. W. (2009). Extrasynaptic GABAA receptors: form, pharmacology, and function. J. Neurosci. 29, 12757–12763. doi: 10.1523/JNEUROSCI.3340-09.2009
Blaine, S. K., Wemm, S., Fogelman, N., Lacadie, C., Seo, D., Scheinost, D., et al. (2020). Association of prefrontal-striatal functional pathology with alcohol abstinence days at treatment initiation and heavy drinking after treatment initiation. Am. J. Psychiatry 177, 1048–1059. doi: 10.1176/appi.ajp.2020.19070703
Blednov, Y. A., Borghese, C. M., McCracken, M. L., Benavidez, J. M., Geil, C. R., Osterndorff-Kahanek, E., et al. (2011). Loss of ethanol conditioned taste aversion and motor stimulation in knockin mice with ethanol-insensitive alpha2-containing GABA(A) receptors. J. Pharmacol. Exp. Ther. 336, 145–154. doi: 10.1124/jpet.110.171645
Boehm, S. L. 2nd, Ponomarev, I., Jennings, A. W., Whiting, P. J., Rosahl, T. W., Garrett, E. M., et al. (2004). gamma-Aminobutyric acid A receptor subunit mutant mice: new perspectives on alcohol actions. Biochem. Pharmacol. 68, 1581–1602. doi: 10.1016/j.bcp.2004.07.023
Bogart, L. J., and O'Donnell, P. (2018). Multiple long-range inputs evoke NMDA currents in prefrontal cortex fast-spiking interneurons. Neuropsychopharmacology 43, 2101–2108. doi: 10.1038/s41386-018-0029-5
Boggio, P. S., Sultani, N., Fecteau, S., Merabet, L., Mecca, T., Pascual-Leone, A., et al. (2008). Prefrontal cortex modulation using transcranial DC stimulation reduces alcohol craving: a double-blind, sham-controlled study. Drug Alcohol Depend. 92, 55–60. doi: 10.1016/j.drugalcdep.2007.06.011
Bohnsack, J. P., Hughes, B. A., O'Buckley, T. K., Edokpolor, K., Besheer, J., and Morrow, A. L. (2018). Histone deacetylases mediate GABAA receptor expression, physiology, and behavioral maladaptations in rat models of alcohol dependence. Neuropsychopharmacology 43, 1518–1529. doi: 10.1038/s41386-018-0034-8
Borghese, C. M., and Harris, R. A. (2007). Studies of ethanol actions on recombinant delta-containing gamma-aminobutyric acid type A receptors yield contradictory results. Alcohol 41, 155–162. doi: 10.1016/j.alcohol.2007.03.006
Bouhours, B., Gjoni, E., Kochubey, O., and Schneggenburger, R. (2017). Synaptotagmin2 (Syt2) drives fast release redundantly with Syt1 at the output synapses of parvalbumin-expressing inhibitory neurons. J. Neurosci. 37, 4604–4617. doi: 10.1523/JNEUROSCI.3736-16.2017
Bowen, M. T., Peters, S. T., Absalom, N., Chebib, M., Neumann, I. D., and McGregor, I. S. (2015). Oxytocin prevents ethanol actions at delta subunit-containing GABAA receptors and attenuates ethanol-induced motor impairment in rats. Proc. Natl. Acad. Sci. USA. 112, 3104–3109. doi: 10.1073/pnas.1416900112
Bowie, D., and Mayer, M. L. (1995). Inward rectification of both AMPA and kainate subtype glutamate receptors generated by polyamine-mediated ion channel block. Neuron 15, 453–462. doi: 10.1016/0896-6273(95)90049-7
Bucurenciu, I., Bischofberger, J., and Jonas, P. (2010). A small number of open Ca2+ channels trigger transmitter release at a central GABAergic synapse. Nat. Neurosci. 13, 19–21. doi: 10.1038/nn.2461
Buhl, E. H., Halasy, K., and Somogyi, P. (1994). Diverse sources of hippocampal unitary inhibitory postsynaptic potentials and the number of synaptic release sites. Nature 368, 823–828. doi: 10.1038/368823a0
Burnashev, N., Monyer, H., Seeburg, P. H., and Sakmann, B. (1992). Divalent ion permeability of AMPA receptor channels is dominated by the edited form of a single subunit. Neuron 8, 189–198. doi: 10.1016/0896-6273(92)90120-3
Cannady, R., Nimitvilai-Roberts, S., Jennings, S. D., Woodward, J. J., and Mulholland, P. J. (2020). Distinct region- and time-dependent functional cortical adaptations in C57BL/6J mice after short and prolonged alcohol drinking. eNeuro 7:ENEURO.0077–20.2020. doi: 10.1523/ENEURO.0077-20.2020
Cardin, J. A., Carlen, M., Meletis, K., Knoblich, U., Zhang, F., Deisseroth, K., et al. (2009). Driving fast-spiking cells induces gamma rhythm and controls sensory responses. Nature 459, 663–667. doi: 10.1038/nature08002
Carlson, S. L., Bohnsack, J. P., and Morrow, A. L. (2016b). Ethanol regulation of synaptic GABAA alpha4 receptors is prevented by protein kinase A activation. J. Pharmacol. Exp. Ther. 357, 10–16. doi: 10.1124/jpet.115.230417
Carlson, S. L., Bohnsack, J. P., Patel, V., and Morrow, A. L. (2016a). Regulation of extrasynaptic GABAA alpha4 receptors by ethanol-induced protein kinase A, but not protein kinase C activation in cultured rat cerebral cortical neurons. J. Pharmacol. Exp. Ther. 356, 148–156. doi: 10.1124/jpet.115.228056
Carlson, S. L., O'Buckley, T. K., Thomas, R., Thiele, T. E., and Morrow, A. L. (2014). Altered GABAA receptor expression and seizure threshold following acute ethanol challenge in mice lacking the RIIbeta subunit of PKA. Neurochem. Res. 39, 1079–1087. doi: 10.1007/s11064-013-1167-0
Carta, M., Partridge, L. D., Savage, D. D., and Valenzuela, C. F. (2003). Neurosteroid modulation of glutamate release in hippocampal neurons: lack of an effect of a chronic prenatal ethanol exposure paradigm. Alcohol. Clin. Exp. Res. 27, 1194–1198. doi: 10.1097/01.ALC.0000075828.50697.70
Carton, L., Auger, F., Kyheng, M., Petrault, M., Durieux, N., Allorge, D., et al. (2019). Dose-dependent metabolite changes after ethanol intoxication in rat prefrontal cortex using in vivo magnetic resonance spectroscopy. Sci. Rep. 9, 10682. doi: 10.1038/s41598-019-47187-4
Cauli, B., Porter, J. T., Tsuzuki, K., Lambolez, B., Rossier, J., Quenet, B., et al. (2000). Classification of fusiform neocortical interneurons based on unsupervised clustering. Proc. Natl. Acad. Sci. USA. 97, 6144–6149. doi: 10.1073/pnas.97.11.6144
Celio, M. R. (1986). Parvalbumin in most gamma-aminobutyric acid-containing neurons of the rat cerebral cortex. Science . 231, 995–997. doi: 10.1126/science.3945815
Centanni, S. W., Burnett, E. J., Trantham-Davidson, H., and Chandler, L. J. (2017). Loss of delta-GABAA receptor-mediated tonic currents in the adult prelimbic cortex following adolescent alcohol exposure. Addict. Biol. 22, 616–628. doi: 10.1111/adb.12353
Centanni, S. W., Teppen, T., Risher, M. L., Fleming, R. L., Moss, J. L., Acheson, S. K., et al. (2014). Adolescent alcohol exposure alters GABAA receptor subunit expression in adult hippocampus. Alcohol. Clin. Exp. Res. 38, 2800–2808. doi: 10.1111/acer.12562
Chen, G., Zhang, Y., Li, X., Zhao, X., Ye, Q., Lin, Y., et al. (2017). Distinct inhibitory circuits orchestrate cortical beta and gamma band oscillations. Neuron 96, 1403–1418 e6. doi: 10.1016/j.neuron.2017.11.033
Chen, S. X., Kim, A. N., Peters, A. J., and Komiyama, T. (2015). Subtype-specific plasticity of inhibitory circuits in motor cortex during motor learning. Nat. Neurosci. 18, 1109–1115. doi: 10.1038/nn.4049
Chiu, C. Q., Lur, G., Morse, T. M., Carnevale, N. T., Ellis-Davies, G. C., and Higley, M. J. (2013). Compartmentalization of GABAergic inhibition by dendritic spines. Science 340, 759–762. doi: 10.1126/science.1234274
Cho, K. K., Hoch, R., Lee, A. T., Patel, T., Rubenstein, J. L., and Sohal, V. S. (2015). Gamma rhythms link prefrontal interneuron dysfunction with cognitive inflexibility in Dlx5/6(+/-) mice. Neuron 85, 1332–1343. doi: 10.1016/j.neuron.2015.02.019
Cho, K. K. A., Davidson, T. J., Bouvier, G., Marshall, J. D., Schnitzer, M. J., and Sohal, V. S. (2020). Cross-hemispheric gamma synchrony between prefrontal parvalbumin interneurons supports behavioral adaptation during rule shift learning. Nat. Neurosci. 23, 892–902. doi: 10.1038/s41593-020-0647-1
Chow, A., Erisir, A., Farb, C., Nadal, M. S., Ozaita, A., Lau, D., et al. (1999). K(+) channel expression distinguishes subpopulations of parvalbumin- and somatostatin-containing neocortical interneurons. J. Neurosci. 19, 9332–9345. doi: 10.1523/JNEUROSCI.19-21-09332.1999
Cobb, S. R., Buhl, E. H., Halasy, K., Paulsen, O., and Somogyi, P. (1995). Synchronization of neuronal activity in hippocampus by individual GABAergic interneurons. Nature 378, 75–78. doi: 10.1038/378075a0
Cohen, H. L., Porjesz, B., and Begleiter, H. (1993). Ethanol-induced alterations in electroencephalographic activity in adult males. Neuropsychopharmacology 8, 365–370. doi: 10.1038/npp.1993.36
Conde, F., Lund, J. S., Jacobowitz, D. M., Baimbridge, K. G., and Lewis, D. A. (1994). Local circuit neurons immunoreactive for calretinin, calbindin D-28k or parvalbumin in monkey prefrontal cortex: distribution and morphology. J. Comp. Neurol. 341, 95–116. doi: 10.1002/cne.903410109
Connors, B. W., and Gutnick, M. J. (1990). Intrinsic firing patterns of diverse neocortical neurons. Trends Neurosci. 13, 99–104. doi: 10.1016/0166-2236(90)90185-D
Cossart, R., Esclapez, M., Hirsch, J. C., Bernard, C., and Ben-Ari, Y. (1998). GluR5 kainate receptor activation in interneurons increases tonic inhibition of pyramidal cells. Nat. Neurosci. 1, 470–478. doi: 10.1038/2185
Costa, L., and Bauer, L. (1997). Quantitative electroencephalographic differences associated with alcohol, cocaine, heroin and dual-substance dependence. Drug Alcohol Depend. 46, 87–93. doi: 10.1016/S0376-8716(97)00058-6
Courtney, K. E., Schacht, J. P., Hutchison, K., Roche, D. J., and Ray, L. A. (2016). Neural substrates of cue reactivity: association with treatment outcomes and relapse. Addict. Biol. 21, 3–22. doi: 10.1111/adb.12314
Coutin-Churchman, P., Moreno, R., Anez, Y., and Vergara, F. (2006). Clinical correlates of quantitative EEG alterations in alcoholic patients. Clin. Neurophysiol. 117, 740–751. doi: 10.1016/j.clinph.2005.12.021
Covault, J., Gelernter, J., Hesselbrock, V., Nellissery, M., and Kranzler, H. R. (2004). Allelic and haplotypic association of GABRA2 with alcohol dependence. Am. J. Med. Genet. B Neuropsychiatr. Genet. 129B, 104–109. doi: 10.1002/ajmg.b.30091
Covault, J., Gelernter, J., Jensen, K., Anton, R., and Kranzler, H. R. (2008). Markers in the 5'-region of GABRG1 associate to alcohol dependence and are in linkage disequilibrium with markers in the adjacent GABRA2 gene. Neuropsychopharmacology 33, 837–848. doi: 10.1038/sj.npp.1301456
Criswell, H. E., and Breese, G. R. (2005). A conceptualization of integrated actions of ethanol contributing to its GABAmimetic profile: a commentary. Neuropsychopharmacology 30, 1407–1425. doi: 10.1038/sj.npp.1300750
Crowley, N. A., and Joffe, M. E. (2022). Developing breakthrough psychiatric treatments by modulating G protein-coupled receptors on prefrontal cortex somatostatin interneurons. Neuropsychopharmacology 47, 389–390. doi: 10.1038/s41386-021-01119-x
Cummings, K. A., and Clem, R. L. (2020). Prefrontal somatostatin interneurons encode fear memory. Nat. Neurosci. 23, 61–74. doi: 10.1038/s41593-019-0552-7
Dao, N. C., Brockway, D. F., and Crowley, N. A. (2019). In vitro optogenetic characterization of neuropeptide release from prefrontal cortical somatostatin neurons. Neuroscience 419, 1–4. doi: 10.1016/j.neuroscience.2019.08.014
Dao, N. C., Brockway, D. F., Suresh Nair, M., Sicher, A. R., and Crowley, N. A. (2021). Somatostatin neurons control an alcohol binge drinking prelimbic microcircuit in mice. Neuropsychopharmacology. 46, 1906–1917.doi: 10.1038/s41386-021-01050-1
Dao, N. C., Suresh Nair, M., Magee, S. N., Moyer, J. B., Sendao, V., Brockway, D. F., et al. (2020). Forced abstinence from alcohol induces sex-specific depression-like behavioral and neural adaptations in somatostatin neurons in cortical and amygdalar regions. Front. Behav. Neurosci. 14, 86. doi: 10.3389/fnbeh.2020.00086
Darnieder, L. M., Melon, L. C., Do, T., Walton, N. L., Miczek, K. A., and Maguire, J. L. (2019). Female-specific decreases in alcohol binge-like drinking resulting from GABAA receptor delta-subunit knockdown in the VTA. Sci. Rep. 9, 8102. doi: 10.1038/s41598-019-44286-0
Daw, M. I., Pelkey, K. A., Chittajallu, R., and McBain, C. J. (2010). Presynaptic kainate receptor activation preserves asynchronous GABA release despite the reduction in synchronous release from hippocampal cholecystokinin interneurons. J. Neurosci. 30, 11202–11209. doi: 10.1523/JNEUROSCI.6334-09.2010
de Lima, A. D., and Morrison, J. H. (1989). Ultrastructural analysis of somatostatin-immunoreactive neurons and synapses in the temporal and occipital cortex of the macaque monkey. J. Comp. Neurol. 283, 212–227. doi: 10.1002/cne.902830205
DeFelipe, J. (1993). Neocortical neuronal diversity: chemical heterogeneity revealed by colocalization studies of classic neurotransmitters, neuropeptides, calcium-binding proteins, and cell surface molecules. Cereb. Cortex 3, 273–289. doi: 10.1093/cercor/3.4.273
den Hartog, C. R., Beckley, J. T., Smothers, T. C., Lench, D. H., Holseberg, Z. L., Fedarovich, H., et al. (2013). Alterations in ethanol-induced behaviors and consumption in knock-in mice expressing ethanol-resistant NMDA receptors. PLoS ONE 8, e80541. doi: 10.1371/journal.pone.0080541
Devaud, L. L., Fritschy, J. M., Sieghart, W., and Morrow, A. L. (1997). Bidirectional alterations of GABA(A) receptor subunit peptide levels in rat cortex during chronic ethanol consumption and withdrawal. J. Neurochem. 69, 126–130. doi: 10.1046/j.1471-4159.1997.69010126.x
Dick, D. M., Edenberg, H. J., Xuei, X., Goate, A., Kuperman, S., Schuckit, M., et al. (2004). Association of GABRG3 with alcohol dependence. Alcohol. Clin. Exp. Res. 28, 4–9. doi: 10.1097/01.ALC.0000108645.54345.98
Dimidschstein, J., Chen, Q., Tremblay, R., Rogers, S. L., Saldi, G. A., Guo, L., et al. (2016). A viral strategy for targeting and manipulating interneurons across vertebrate species. Nat. Neurosci. 19, 1743–1749. doi: 10.1038/nn.4430
Dixon, C. I., Walker, S. E., King, S. L., and Stephens, D. N. (2012). Deletion of the gabra2 gene results in hypersensitivity to the acute effects of ethanol but does not alter ethanol self administration. PLoS ONE 7, e47135. doi: 10.1371/journal.pone.0047135
Doischer, D., Hosp, J. A., Yanagawa, Y., Obata, K., Jonas, P., Vida, I., et al. (2008). Postnatal differentiation of basket cells from slow to fast signaling devices. J. Neurosci. 28, 12956–12968. doi: 10.1523/JNEUROSCI.2890-08.2008
Dong, Y. (2016). Silent synapse-based circuitry remodeling in drug addiction. Int. J. Neuropsychopharmacol. 19, pyv136. doi: 10.1093/ijnp/pyv136
Drasbek, K. R., and Jensen, K. (2006). THIP, a hypnotic and antinociceptive drug, enhances an extrasynaptic GABAA receptor-mediated conductance in mouse neocortex. Cereb. Cortex 16, 1134–1141. doi: 10.1093/cercor/bhj055
Dudai, A., Yayon, N., Lerner, V., Tasaka, G. I., Deitcher, Y., Gorfine, K., et al. (2020). Barrel cortex VIP/ChAT interneurons suppress sensory responses in vivo. PLoS Biol. 18, e3000613. doi: 10.1371/journal.pbio.3000613
Dunn, H. A., Patil, D. N., Cao, Y., Orlandi, C., and Martemyanov, K. A. (2018). Synaptic adhesion protein ELFN1 is a selective allosteric modulator of group III metabotropic glutamate receptors in trans. Proc. Natl. Acad. Sci. USA. 115, 5022–5027. doi: 10.1073/pnas.1722498115
Edenberg, H. J., Dick, D. M., Xuei, X., Tian, H., Almasy, L., Bauer, L. O., et al. (2004). Variations in GABRA2, encoding the alpha 2 subunit of the GABA(A) receptor, are associated with alcohol dependence and with brain oscillations. Am. J. Hum. Genet. 74, 705–714. doi: 10.1086/383283
Ehlers, C. L., Li, T. K., Lumeng, L., Hwang, B. H., Somes, C., Jimenez, P., et al. (1998). Neuropeptide Y levels in ethanol-naive alcohol-preferring and nonpreferring rats and in Wistar rats after ethanol exposure. Alcohol. Clin. Exp. Res. 22, 1778–1782. doi: 10.1111/j.1530-0277.1998.tb03979.x
Ehlers, C. L., and Schuckit, M. A. (1990). EEG fast frequency activity in the sons of alcoholics. Biol. Psychiatry 27, 631–641. doi: 10.1016/0006-3223(90)90531-6
Ehlers, C. L., and Schuckit, M. A. (1991). Evaluation of EEG alpha activity in sons of alcoholics. Neuropsychopharmacology 4, 199–205.
Ehlers, C. L., Wall, T. L., and Schuckit, M. A. (1989). EEG spectral characteristics following ethanol administration in young men. Electroencephalogr. Clin. Neurophysiol. 73, 179–187. doi: 10.1016/0013-4694(89)90118-1
Enoch, M. A., Hodgkinson, C. A., Yuan, Q., Albaugh, B., Virkkunen, M., and Goldman, D. (2009). GABRG1 and GABRA2 as independent predictors for alcoholism in two populations. Neuropsychopharmacology 34, 1245–1254. doi: 10.1038/npp.2008.171
Enwright, J. F., and Lewis, D. A. (2021). Similarities in cortical transcriptome alterations between schizophrenia and bipolar disorder are related to the presence of psychosis. Schizophr. Bull. 47, 1442–1451. doi: 10.1093/schbul/sbaa195
Fein, G., and Allen, J. (2005). EEG spectral changes in treatment-naive, actively drinking alcoholics. Alcohol. Clin. Exp. Res. 29, 538–546. doi: 10.1097/01.ALC.0000159107.08471.97
Ferranti, A. S., Johnson, K. A., Winder, D. G., Conn, P. J., and Joffe, M. E. (2022). Prefrontal cortex parvalbumin interneurons exhibit decreased excitability and potentiated synaptic strength after ethanol reward learning. Alcohol 101, 17–26. doi: 10.1016/j.alcohol.2022.02.003
Fisahn, A., Contractor, A., Traub, R. D., Buhl, E. H., Heinemann, S. F., and McBain, C. J. (2004). Distinct roles for the kainate receptor subunits GluR5 and GluR6 in kainate-induced hippocampal gamma oscillations. J. Neurosci. 24, 9658–9668. doi: 10.1523/JNEUROSCI.2973-04.2004
Fish, K. N., Rocco, B. R., and Lewis, D. A. (2018). Laminar distribution of subsets of GABAergic axon terminals in human prefrontal cortex. Front. Neuroanat. 12, 9. doi: 10.3389/fnana.2018.00009
Fleming, R. L., Li, Q., Risher, M. L., Sexton, H. G., Moore, S. D., Wilson, W. A., et al. (2013). Binge-pattern ethanol exposure during adolescence, but not adulthood, causes persistent changes in GABAA receptor-mediated tonic inhibition in dentate granule cells. Alcohol. Clin. Exp. Res. 37, 1154–1160. doi: 10.1111/acer.12087
Fleming, R. L., Manis, P. B., and Morrow, A. L. (2009). The effects of acute and chronic ethanol exposure on presynaptic and postsynaptic gamma-aminobutyric acid (GABA) neurotransmission in cultured cortical and hippocampal neurons. Alcohol 43, 603–618. doi: 10.1016/j.alcohol.2009.10.006
Fogaca, M. V., and Duman, R. S. (2019). Cortical GABAergic dysfunction in stress and depression: new insights for therapeutic interventions. Front. Cell. Neurosci. 13, 87. doi: 10.3389/fncel.2019.00087
Frerking, M., Malenka, R. C., and Nicoll, R. A. (1998). Synaptic activation of kainate receptors on hippocampal interneurons. Nat. Neurosci. 1, 479–486. doi: 10.1038/2194
Fu, Y., Kaneko, M., Tang, Y., Alvarez-Buylla, A., and Stryker, M. P. (2015). A cortical disinhibitory circuit for enhancing adult plasticity. Elife 4, e05558. doi: 10.7554/eLife.05558.010
Fukuda, T., and Kosaka, T. (2000). Gap junctions linking the dendritic network of GABAergic interneurons in the hippocampus. J. Neurosci. 20, 1519–1528. doi: 10.1523/JNEUROSCI.20-04-01519.2000
Fukuda, T., and Kosaka, T. (2003). Ultrastructural study of gap junctions between dendrites of parvalbumin-containing GABAergic neurons in various neocortical areas of the adult rat. Neuroscience 120, 5–20. doi: 10.1016/S0306-4522(03)00328-2
Fukuda, T., Kosaka, T., Singer, W., and Galuske, R. A. (2006). Gap junctions among dendrites of cortical GABAergic neurons establish a dense and widespread intercolumnar network. J. Neurosci. 26, 3434–3443. doi: 10.1523/JNEUROSCI.4076-05.2006
Funahashi, S., Bruce, C. J., and Goldman-Rakic, P. S. (1989). Mnemonic coding of visual space in the monkey's dorsolateral prefrontal cortex. J. Neurophysiol. 61, 331–349. doi: 10.1152/jn.1989.61.2.331
Furlanis, E., Traunmuller, L., Fucile, G., and Scheiffele, P. (2019). Landscape of ribosome-engaged transcript isoforms reveals extensive neuronal-cell-class-specific alternative splicing programs. Nat. Neurosci. 22, 1709–1717. doi: 10.1038/s41593-019-0465-5
Gabbott, P. L., and Bacon, S. J. (1996). Local circuit neurons in the medial prefrontal cortex (areas 24a,b,c, 25 and 32) in the monkey: I. Cell morphology and morphometrics. J. Comp. Neurol. 364, 567–608. doi: 10.1002/(SICI)1096-9861(19960122)364:4<567::AID-CNE1>3.0.CO;2-1
Galarreta, M., and Hestrin, S. (2002). Electrical and chemical synapses among parvalbumin fast-spiking GABAergic interneurons in adult mouse neocortex. Proc. Natl. Acad. Sci. USA. 99, 12438–12443. doi: 10.1073/pnas.192159599
Gao, F., Chen, D., Ma, X., Sudweeks, S., Yorgason, J. T., Gao, M., et al. (2019). Alpha6-containing nicotinic acetylcholine receptor is a highly sensitive target of alcohol. Neuropharmacology 149, 45–54. doi: 10.1016/j.neuropharm.2019.01.021
Garst-Orozco, J., Malik, R., Lanz, T. A., Weber, M. L., Xi, H., Arion, D., et al. (2020). GluN2D-mediated excitatory drive onto medial prefrontal cortical PV+ fast-spiking inhibitory interneurons. PLoS ONE 15, e0233895. doi: 10.1371/journal.pone.0233895
Geiger, J. R., Melcher, T., Koh, D. S., Sakmann, B., Seeburg, P. H., Jonas, P., et al. (1995). Relative abundance of subunit mRNAs determines gating and Ca2+ permeability of AMPA receptors in principal neurons and interneurons in rat CNS. Neuron 15, 193–204. doi: 10.1016/0896-6273(95)90076-4
Gentet, L. J., Kremer, Y., Taniguchi, H., Huang, Z. J., Staiger, J. F., and Petersen, C. C. (2012). Unique functional properties of somatostatin-expressing GABAergic neurons in mouse barrel cortex. Nat. Neurosci. 15, 607–612. doi: 10.1038/nn.3051
George, O., Sanders, C., Freiling, J., Grigoryan, E., Vu, S., Allen, C. D., et al. (2012). Recruitment of medial prefrontal cortex neurons during alcohol withdrawal predicts cognitive impairment and excessive alcohol drinking. Proc. Natl. Acad. Sci. USA. 109, 18156–18161. doi: 10.1073/pnas.1116523109
Gerhard, D. M., Pothula, S., Liu, R. J., Wu, M., Li, X. Y., Girgenti, M. J., et al. (2019). GABA interneurons are the cellular trigger for ketamine's rapid antidepressant actions. J. Clin. Invest. 130, 1336–1349. doi: 10.1172/JCI130808
Gibson, J. R., Beierlein, M., and Connors, B. W. (1999). Two networks of electrically coupled inhibitory neurons in neocortex. Nature 402, 75–79. doi: 10.1038/47035
Gittis, A. H., Leventhal, D. K., Fensterheim, B. A., Pettibone, J. R., Berke, J. D., and Kreitzer, A. C. (2011). Selective inhibition of striatal fast-spiking interneurons causes dyskinesias. J. Neurosci. 31, 15727–15731. doi: 10.1523/JNEUROSCI.3875-11.2011
Gittis, A. H., Nelson, A. B., Thwin, M. T., Palop, J. J., and Kreitzer, A. C. (2010). Distinct roles of GABAergic interneurons in the regulation of striatal output pathways. J. Neurosci. 30, 2223–2234. doi: 10.1523/JNEUROSCI.4870-09.2010
Glausier, J. R., Datta, D., Fish, K. N., Chung, D. W., Melchitzky, D. S., and Lewis, D. A. (2021). Laminar differences in the targeting of dendritic spines by cortical pyramidal neurons and interneurons in human dorsolateral prefrontal cortex. Neuroscience 452, 181–191. doi: 10.1016/j.neuroscience.2020.10.022
Glausier, J. R., Fish, K. N., and Lewis, D. A. (2014). Altered parvalbumin basket cell inputs in the dorsolateral prefrontal cortex of schizophrenia subjects. Mol. Psychiatry 19, 30–36. doi: 10.1038/mp.2013.152
Glickfeld, L. L., and Scanziani, M. (2006). Distinct timing in the activity of cannabinoid-sensitive and cannabinoid-insensitive basket cells. Nat. Neurosci. 9, 807–815. doi: 10.1038/nn1688
Glykys, J., Peng, Z., Chandra, D., Homanics, G. E., Houser, C. R., and Mody, I. (2007). A new naturally occurring GABA(A) receptor subunit partnership with high sensitivity to ethanol. Nat. Neurosci. 10, 40–48. doi: 10.1038/nn1813
Goldberg, E. M., Watanabe, S., Chang, S. Y., Joho, R. H., Huang, Z. J., Leonard, C. S., et al. (2005). Specific functions of synaptically localized potassium channels in synaptic transmission at the neocortical GABAergic fast-spiking cell synapse. J. Neurosci. 25, 5230–5235. doi: 10.1523/JNEUROSCI.0722-05.2005
Gonzalez, C., Moss, S. J., and Olsen, R. W. (2012). Ethanol promotes clathrin adaptor-mediated endocytosis via the intracellular domain of delta-containing GABAA receptors. J. Neurosci. 32, 17874–17881. doi: 10.1523/JNEUROSCI.2535-12.2012
Gonzalez-Burgos, G., Cho, R. Y., and Lewis, D. A. (2015). Alterations in cortical network oscillations and parvalbumin neurons in schizophrenia. Biol. Psychiatry 77, 1031–1040. doi: 10.1016/j.biopsych.2015.03.010
Gonzalez-Burgos, G., Krimer, L. S., Urban, N. N., Barrionuevo, G., and Lewis, D. A. (2004). Synaptic efficacy during repetitive activation of excitatory inputs in primate dorsolateral prefrontal cortex. Cereb. Cortex 14, 530–542. doi: 10.1093/cercor/bhh015
Grant, B. F., Chou, S. P., Saha, T. D., Pickering, R. P., Kerridge, B. T., Ruan, W. J., et al. (2017). Prevalence of 12-Month Alcohol Use, High-Risk Drinking, and DSM-IV Alcohol Use Disorder in the United States, 2001-2002 to 2012-2013: results from the national epidemiologic survey on alcohol and related conditions. JAMA Psychiatry 74, 911–923. doi: 10.1001/jamapsychiatry.2017.2161
Grant, K. A. (1994). Emerging neurochemical concepts in the actions of ethanol at ligand-gated ion channels. Behav. Pharmacol. 5, 383–404. doi: 10.1097/00008877-199408000-00003
Groen, M. R., Paulsen, O., Perez-Garci, E., Nevian, T., Wortel, J., Dekker, M. P., et al. (2014). Development of dendritic tonic GABAergic inhibition regulates excitability and plasticity in CA1 pyramidal neurons. J. Neurophysiol. 112, 287–299. doi: 10.1152/jn.00066.2014
Grusser, S. M., Wrase, J., Klein, S., Hermann, D., Smolka, M. N., Ruf, M., et al. (2004). Cue-induced activation of the striatum and medial prefrontal cortex is associated with subsequent relapse in abstinent alcoholics. Psychopharmacology (Berl) 175, 296–302. doi: 10.1007/s00213-004-1828-4
Gunther, U., Benson, J., Benke, D., Fritschy, J. M., Reyes, G., Knoflach, F., et al. (1995). Benzodiazepine-insensitive mice generated by targeted disruption of the gamma 2 subunit gene of gamma-aminobutyric acid type A receptors. Proc. Natl. Acad. Sci. USA. 92, 7749–7753. doi: 10.1073/pnas.92.17.7749
Hajos, N., Papp, E. C., Acsady, L., Levey, A. I., and Freund, T. F. (1998). Distinct interneuron types express m2 muscarinic receptor immunoreactivity on their dendrites or axon terminals in the hippocampus. Neuroscience 82, 355–376. doi: 10.1016/S0306-4522(97)00300-X
Hanchar, H. J., Dodson, P. D., Olsen, R. W., Otis, T. S., and Wallner, M. (2005). Alcohol-induced motor impairment caused by increased extrasynaptic GABA(A) receptor activity. Nat. Neurosci. 8, 339–345. doi: 10.1038/nn1398
Hanlon, C. A., Dowdle, L. T., and Henderson, J. S. (2018). Modulating neural circuits with transcranial magnetic stimulation: implications for addiction treatment development. Pharmacol. Rev. 70, 661–683. doi: 10.1124/pr.116.013649
Hanson, E., Armbruster, M., Lau, L. A., Sommer, M. E., Klaft, Z. J., Swanger, S. A., et al. (2019). Tonic Activation of GluN2C/GluN2D-Containing NMDA Receptors by ambient glutamate facilitates cortical interneuron maturation. J. Neurosci. 39, 3611–3626. doi: 10.1523/JNEUROSCI.1392-18.2019
Harmar, A. J., Fahrenkrug, J., Gozes, I., Laburthe, M., May, V., Pisegna, J. R., et al. (2012). Pharmacology and functions of receptors for vasoactive intestinal peptide and pituitary adenylate cyclase-activating polypeptide: IUPHAR review 1. Br. J. Pharmacol. 166, 4–17. doi: 10.1111/j.1476-5381.2012.01871.x
Harris, R. A., Trudell, J. R., and Mihic, S. J. (2008). Ethanol's molecular targets. Sci. Signal 1, re7. doi: 10.1126/scisignal.128re7
Hasin, D. S., Stinson, F. S., Ogburn, E., and Grant, B. F. (2007). Prevalence, correlates, disability, and comorbidity of DSM-IV alcohol abuse and dependence in the United States: results from the National Epidemiologic Survey on Alcohol and Related Conditions. Arch. Gen. Psychiatry 64, 830–842. doi: 10.1001/archpsyc.64.7.830
Haughey, H. M., Ray, L. A., Finan, P., Villanueva, R., Niculescu, M., and Hutchison, K. E. (2008). Human gamma-aminobutyric acid A receptor alpha2 gene moderates the acute effects of alcohol and brain mRNA expression. Genes Brain Behav. 7, 447–454. doi: 10.1111/j.1601-183X.2007.00369.x
He, M., Tucciarone, J., Lee, S., Nigro, M. J., Kim, Y., Levine, J. M., et al. (2016). Strategies and tools for combinatorial targeting of GABAergic neurons in mouse cerebral cortex. Neuron 91, 1228–1243. doi: 10.1016/j.neuron.2016.08.021
Hendrickson, L. M., Guildford, M. J., and Tapper, A. R. (2013). Neuronal nicotinic acetylcholine receptors: common molecular substrates of nicotine and alcohol dependence. Front. Psychiatry 4, 29. doi: 10.3389/fpsyt.2013.00029
Hendricson, A. W., Sibbald, J. R., and Morrisett, R. A. (2004). Ethanol alters the frequency, amplitude, and decay kinetics of Sr2+-supported, asynchronous NMDAR mEPSCs in rat hippocampal slices. J. Neurophysiol. 91, 2568–2577. doi: 10.1152/jn.00997.2003
Hendry, S. H., Jones, E. G., DeFelipe, J., Schmechel, D., Brandon, C., and Emson, P. C. (1984a). Neuropeptide-containing neurons of the cerebral cortex are also GABAergic. Proc. Natl. Acad. Sci. USA. 81, 6526–6530. doi: 10.1073/pnas.81.20.6526
Hendry, S. H., Jones, E. G., and Emson, P. C. (1984b). Morphology, distribution, and synaptic relations of somatostatin- and neuropeptide Y-immunoreactive neurons in rat and monkey neocortex. J. Neurosci. 4, 2497–2517. doi: 10.1523/JNEUROSCI.04-10-02497.1984
Hingson, R. W., Heeren, T., and Winter, M. R. (2006). Age at drinking onset and alcohol dependence: age at onset, duration, and severity. Arch. Pediatr. Adolesc. Med. 160, 739–746. doi: 10.1001/archpedi.160.7.739
Hollmann, M., Hartley, M., and Heinemann, S. (1991). Ca2+ permeability of KA-AMPA–gated glutamate receptor channels depends on subunit composition. Science 252, 851–853. doi: 10.1126/science.1709304
Homanics, G. E., DeLorey, T. M., Firestone, L. L., Quinlan, J. J., Handforth, A., Harrison, N. L., et al. (1997). Mice devoid of gamma-aminobutyrate type A receptor beta3 subunit have epilepsy, cleft palate, and hypersensitive behavior. Proc. Natl. Acad. Sci. USA. 94, 4143–4148. doi: 10.1073/pnas.94.8.4143
Horn, M. E., and Nicoll, R. A. (2018). Somatostatin and parvalbumin inhibitory synapses onto hippocampal pyramidal neurons are regulated by distinct mechanisms. Proc. Natl. Acad. Sci. USA. 115, 589–594. doi: 10.1073/pnas.1719523115
Hortnagl, H., Tasan, R. O., Wieselthaler, A., Kirchmair, E., Sieghart, W., and Sperk, G. (2013). Patterns of mRNA and protein expression for 12 GABAA receptor subunits in the mouse brain. Neuroscience 236, 345–372. doi: 10.1016/j.neuroscience.2013.01.008
Hu, H., Cavendish, J. Z., and Agmon, A. (2013). Not all that glitters is gold: off-target recombination in the somatostatin-IRES-Cre mouse line labels a subset of fast-spiking interneurons. Front. Neural Circuits 7, 195. doi: 10.3389/fncir.2013.00195
Hu, H., Gan, J., and Jonas, P. (2014). Interneurons. Fast-spiking, parvalbumin(+) GABAergic interneurons: from cellular design to microcircuit function. Science 345, 1255263. doi: 10.1126/science.1255263
Hu, H., Roth, F. C., Vandael, D., and Jonas, P. (2018). Complementary tuning of Na(+) and K(+) channel gating underlies fast and energy-efficient action potentials in GABAergic interneuron axons. Neuron 98, 156–165 e6. doi: 10.1016/j.neuron.2018.02.024
Hughes, B. A., Bohnsack, J. P., O'Buckley, T. K., Herman, M. A., and Morrow, A. L. (2019). Chronic ethanol exposure and withdrawal impair synaptic GABAA receptor-mediated neurotransmission in deep-layer prefrontal cortex. Alcohol. Clin. Exp. Res. 43, 822–832. doi: 10.1111/acer.14015
Hughes, B. A., Crofton, E. J., O'Buckley, T. K., Herman, M. A., and Morrow, A. L. (2020). Chronic ethanol exposure alters prelimbic prefrontal cortical Fast-Spiking and Martinotti interneuron function with differential sex specificity in rat brain. Neuropharmacology 162, 107805. doi: 10.1016/j.neuropharm.2019.107805
Huntley, M. A., Srinivasan, K., Friedman, B. A., Wang, T. M., Yee, A. X., Wang, Y., et al. (2020). Genome-wide analysis of differential gene expression and splicing in excitatory neurons and interneuron subtypes. J. Neurosci. 40, 958–973. doi: 10.1523/JNEUROSCI.1615-19.2019
Iacobucci, G. J., and Popescu, G. K. (2017). NMDA receptors: linking physiological output to biophysical operation. Nat. Rev. Neurosci. 18, 236–249. doi: 10.1038/nrn.2017.24
Janeczek, P., Colson, N., Dodd, P. R., and Lewohl, J. M. (2020). Sex differences in the expression of the alpha5 subunit of the GABAA receptor in alcoholics with and without cirrhosis of the liver. Alcohol. Clin. Exp. Res. 44, 423–434. doi: 10.1111/acer.14266
Joffe, M., Maksymetz, J., Luschinger, J., Dogra, S., Ferranti, A., Luessen, D., et al. (2022). Acute restraint stress redirects prefrontal cortex circuit function through mGlu5 receptor plasticity on somatostatin-expressing interneurons. Neuron. 110, 1068–1083.e5. doi: 10.1016/j.neuron.2021.12.027
Joffe, M. E., Winder, D. G., and Conn, P. J. (2020). Contrasting sex-dependent adaptations to synaptic physiology and membrane properties of prefrontal cortex interneuron subtypes in a mouse model of binge drinking. Neuropharmacology 178, 108126. doi: 10.1016/j.neuropharm.2020.108126
Joffe, M. E., Winder, D. G., and Conn, P. J. (2021). Increased synaptic strength and mGlu2/3 receptor plasticity on mouse prefrontal cortex intratelencephalic pyramidal cells following intermittent access to ethanol. Alcohol. Clin. Exp. Res. 45, 518–529. doi: 10.1111/acer.14546
Jonas, D. E., Amick, H. R., Feltner, C., Bobashev, G., Thomas, K., Wines, R., et al. (2014). Pharmacotherapy for adults with alcohol use disorders in outpatient settings: a systematic review and meta-analysis. JAMA 311, 1889–1900. doi: 10.1001/jama.2014.3628
Jones, F. W., and Holmes, D. S. (1976). Alcoholism, alpha production, and biofeedback. J. Consult. Clin. Psychol. 44, 224–228. doi: 10.1037/0022-006X.44.2.224
Jung, M. H., Park, B. L., Lee, B. C., Ro, Y., Park, R., Shin, H. D., et al. (2011). Association of CHRM2 polymorphisms with severity of alcohol dependence. Genes Brain Behav. 10, 253–256. doi: 10.1111/j.1601-183X.2010.00663.x
Kapfer, C., Glickfeld, L. L., Atallah, B. V., and Scanziani, M. (2007). Supralinear increase of recurrent inhibition during sparse activity in the somatosensory cortex. Nat. Neurosci. 10, 743–753. doi: 10.1038/nn1909
Kaplan, R. F., Hesselbrock, V. M., O'Connor, S., and DePalma, N. (1988). Behavioral and EEG responses to alcohol in nonalcoholic men with a family history of alcoholism. Prog. Neuropsychopharmacol. Biol. Psychiatry 12, 873–885. doi: 10.1016/0278-5846(88)90083-8
Katona, I., Acsady, L., and Freund, T. F. (1999). Postsynaptic targets of somatostatin-immunoreactive interneurons in the rat hippocampus. Neuroscience 88, 37–55. doi: 10.1016/S0306-4522(98)00302-9
Katsumaru, H., Kosaka, T., Heizmann, C. W., and Hama, K. (1988). Gap junctions on GABAergic neurons containing the calcium-binding protein parvalbumin in the rat hippocampus (CA1 region). Exp. Brain Res. 72, 363–370. doi: 10.1007/BF00250257
Kawaguchi, Y. (1993a). Groupings of nonpyramidal and pyramidal cells with specific physiological and morphological characteristics in rat frontal cortex. J. Neurophysiol. 69, 416–431. doi: 10.1152/jn.1993.69.2.416
Kawaguchi, Y. (1993b). Physiological, morphological, and histochemical characterization of three classes of interneurons in rat neostriatum. J. Neurosci. 13, 4908–4923. doi: 10.1523/JNEUROSCI.13-11-04908.1993
Kawaguchi, Y., Katsumaru, H., Kosaka, T., Heizmann, C. W., and Hama, K. (1987). Fast spiking cells in rat hippocampus (CA1 region) contain the calcium-binding protein parvalbumin. Brain Res. 416, 369–374. doi: 10.1016/0006-8993(87)90921-8
Kawaguchi, Y., and Kubota, Y. (1997). GABAergic cell subtypes and their synaptic connections in rat frontal cortex. Cereb. Cortex 7, 476–486. doi: 10.1093/cercor/7.6.476
Kawaguchi, Y., and Kubota, Y. (1998). Neurochemical features and synaptic connections of large physiologically-identified GABAergic cells in the rat frontal cortex. Neuroscience 85, 677–701. doi: 10.1016/S0306-4522(97)00685-4
Kerchner, G. A., and Nicoll, R. A. (2008). Silent synapses and the emergence of a postsynaptic mechanism for LTP. Nat. Rev. Neurosci. 9, 813–825. doi: 10.1038/nrn2501
Klenowski, P. M., Fogarty, M. J., Shariff, M., Belmer, A., Bellingham, M. C., and Bartlett, S. E. (2016). Increased synaptic excitation and abnormal dendritic structure of prefrontal cortex layer V pyramidal neurons following prolonged binge-like consumption of ethanol. eNeuro 3:ENEURO.0248–16.2016. doi: 10.1523/ENEURO.0248-16.2016
Knoblich, U., Huang, L., Zeng, H., and Li, L. (2019). Neuronal cell-subtype specificity of neural synchronization in mouse primary visual cortex. Nat. Commun. 10, 2533. doi: 10.1038/s41467-019-10498-1
Kolk, S. M., and Rakic, P. (2022). Development of prefrontal cortex. Neuropsychopharmacology 47, 41–57. doi: 10.1038/s41386-021-01137-9
Koob, G. F. (2014). Neurocircuitry of alcohol addiction: synthesis from animal models. Handb. Clin. Neurol. 125, 33–54. doi: 10.1016/B978-0-444-62619-6.00003-3
Kosaka, T., Katsumaru, H., Hama, K., Wu, J. Y., and Heizmann, C. W. (1987). GABAergic neurons containing the Ca2+-binding protein parvalbumin in the rat hippocampus and dentate gyrus. Brain Res. 419, 119–130. doi: 10.1016/0006-8993(87)90575-0
Kosobud, A. E., Wetherill, L., Plawecki, M. H., Kareken, D. A., Liang, T., Nurnberger, J. L., et al. (2015). Adaptation of subjective responses to alcohol is affected by an interaction of gabra2 genotype and recent drinking. Alcohol Clin Exp Res. 39, 1148–1157. doi: 10.1111/acer.12749
Koulentaki, M., and Kouroumalis, E. (2018). GABAA receptor polymorphisms in alcohol use disorder in the GWAS era. Psychopharmacology (Berl) 235, 1845–1865. doi: 10.1007/s00213-018-4918-4
Kralic, J. E., O'Buckley, T. K., Khisti, R. T., Hodge, C. W., Homanics, G. E., and Morrow, A. L. (2002). GABA(A) receptor alpha-1 subunit deletion alters receptor subtype assembly, pharmacological and behavioral responses to benzodiazepines and zolpidem. Neuropharmacology 43, 685–694. doi: 10.1016/S0028-3908(02)00174-0
Kranzler, H. R., Zhou, H., Kember, R. L., Vickers Smith, R., Justice, A. C., Damrauer, S., et al. (2019). Genome-wide association study of alcohol consumption and use disorder in 274,424 individuals from multiple populations. Nat. Commun. 10, 1499. doi: 10.1038/s41467-019-09480-8
Kraushaar, U., and Jonas, P. (2000). Efficacy and stability of quantal GABA release at a hippocampal interneuron-principal neuron synapse. J. Neurosci. 20, 5594–5607. doi: 10.1523/JNEUROSCI.20-15-05594.2000
Krienen, F. M., Goldman, M., Zhang, Q., C H Del Rosario, R., Florio, M., Machold, R., et al. (2020). Innovations present in the primate interneuron repertoire. Nature 586, 262–269. doi: 10.1038/s41586-020-2781-z
Kuki, T., Fujihara, K., Miwa, H., Tamamaki, N., Yanagawa, Y., and Mushiake, H. (2015). Contribution of parvalbumin and somatostatin-expressing GABAergic neurons to slow oscillations and the balance in beta-gamma oscillations across cortical layers. Front. Neural Circuits 9, 6. doi: 10.3389/fncir.2015.00006
Kumar, S., Porcu, P., Werner, D. F., Matthews, D. B., Diaz-Granados, J. L., Helfand, R. S., et al. (2009). The role of GABA(A) receptors in the acute and chronic effects of ethanol: a decade of progress. Psychopharmacology (Berl) 205, 529–564. doi: 10.1007/s00213-009-1562-z
Kvitsiani, D., Ranade, S., Hangya, B., Taniguchi, H., Huang, J. Z., and Kepecs, A. (2013). Distinct behavioural and network correlates of two interneuron types in prefrontal cortex. Nature 498, 363–366. doi: 10.1038/nature12176
Lagutin, O. V., Zhu, C. C., Kobayashi, D., Topczewski, J., Shimamura, K., Puelles, L., et al. (2003). Six3 repression of Wnt signaling in the anterior neuroectoderm is essential for vertebrate forebrain development. Genes Dev. 17, 368–379. doi: 10.1101/gad.1059403
Lalanne, T., Oyrer, J., Mancino, A., Gregor, E., Chung, A., Huynh, L., et al. (2016). Synapse-specific expression of calcium-permeable AMPA receptors in neocortical layer 5. J. Physiol. 594, 837–861. doi: 10.1113/JP271394
Lamsa, K. P., Heeroma, J. H., Somogyi, P., Rusakov, D. A., and Kullmann, D. M. (2007). Anti-Hebbian long-term potentiation in the hippocampal feedback inhibitory circuit. Science 315, 1262–1266. doi: 10.1126/science.1137450
Laubach, M., Amarante, L. M., Swanson, K., and White, S. R. (2018). What if anything, is rodent prefrontal cortex? eNeuro 5:ENEURO.0315–18.2018. doi: 10.31234/osf.io/c2a79
Le Duigou, C., and Kullmann, D. M. (2011). Group I mGluR agonist-evoked long-term potentiation in hippocampal oriens interneurons. J. Neurosci. 31, 5777–5781. doi: 10.1523/JNEUROSCI.6265-10.2011
Lee, S., Hjerling-Leffler, J., Zagha, E., Fishell, G., and Rudy, B. (2010). The largest group of superficial neocortical GABAergic interneurons expresses ionotropic serotonin receptors. J. Neurosci. 30, 16796–16808. doi: 10.1523/JNEUROSCI.1869-10.2010
Lee, S., Kruglikov, I., Huang, Z. J., Fishell, G., and Rudy, B. (2013). A disinhibitory circuit mediates motor integration in the somatosensory cortex. Nat. Neurosci. 16, 1662–1670. doi: 10.1038/nn.3544
Lee, V., and Maguire, J. (2014). The impact of tonic GABAA receptor-mediated inhibition on neuronal excitability varies across brain region and cell type. Front. Neural Circuits 8, 3. doi: 10.3389/fncir.2014.00003
Lewis, D. A., Curley, A. A., Glausier, J. R., and Volk, D. W. (2012). Cortical parvalbumin interneurons and cognitive dysfunction in schizophrenia. Trends Neurosci. 35, 57–67. doi: 10.1016/j.tins.2011.10.004
Lewis, D. A., Hashimoto, T., and Volk, D. W. (2005). Cortical inhibitory neurons and schizophrenia. Nat. Rev. Neurosci. 6, 312–324. doi: 10.1038/nrn1648
Li, M., Cabrera-Garcia, D., Salling, M. C., Au, E., Yang, G., and Harrison, N. L. (2021). Alcohol reduces the activity of somatostatin interneurons in the mouse prefrontal cortex: a neural basis for its disinhibitory effect? Neuropharmacology 188, 108501. doi: 10.1016/j.neuropharm.2021.108501
Liang, J., Suryanarayanan, A., Abriam, A., Snyder, B., Olsen, R. W., and Spigelman, I. (2007). Mechanisms of reversible GABAA receptor plasticity after ethanol intoxication. J. Neurosci. 27, 12367–12377. doi: 10.1523/JNEUROSCI.2786-07.2007
Lim, L., Mi, D., Llorca, A., and Marin, O. (2018). Development and Functional Diversification of Cortical Interneurons. Neuron 100, 294–313. doi: 10.1016/j.neuron.2018.10.009
Lin, Y. J., Shukla, L., Dugue, L., Valero-Cabre, A., and Carrasco, M. (2021). Transcranial magnetic stimulation entrains alpha oscillatory activity in occipital cortex. Sci. Rep. 11, 18562. doi: 10.1038/s41598-021-96849-9
Lingford-Hughes, A. R., Acton, P. D., Gacinovic, S., Suckling, J., Busatto, G. F., Boddington, S. J., et al. (1998). Reduced levels of GABA-benzodiazepine receptor in alcohol dependency in the absence of grey matter atrophy. Br. J. Psychiatry 173, 116–122. doi: 10.1192/bjp.173.2.116
Lobo, I. A., and Harris, R. A. (2008). GABA(A) receptors and alcohol. Pharmacol. Biochem. Behav. 90, 90–94. doi: 10.1016/j.pbb.2008.03.006
Lovett-Barron, M., Turi, G. F., Kaifosh, P., Lee, P. H., Bolze, F., Sun, X. H., et al. (2012). Regulation of neuronal input transformations by tunable dendritic inhibition. Nat. Neurosci. 15, 423–430, S1–3. doi: 10.1038/nn.3024
Lovinger, D. M. (1999). 5-HT3 receptors and the neural actions of alcohols: an increasingly exciting topic. Neurochem. Int. 35, 125–130. doi: 10.1016/S0197-0186(99)00054-6
Lovinger, D. M., and Homanics, G. E. (2007). Tonic for what ails us? high-affinity GABAA receptors and alcohol. Alcohol 41, 139–143. doi: 10.1016/j.alcohol.2007.03.008
Lovinger, D. M., and Roberto, M. (2013). Synaptic effects induced by alcohol. Curr. Top. Behav. Neurosci. 13, 31–86. doi: 10.1007/978-3-642-28720-6_143
Lovinger, D. M., and White, G. (1991). Ethanol potentiation of 5-hydroxytryptamine3 receptor-mediated ion current in neuroblastoma cells and isolated adult mammalian neurons. Mol. Pharmacol. 40, 263–270. doi: 10.1016/0304-3940(91)90192-V
Lovinger, D. M., White, G., and Weight, F. F. (1989). Ethanol inhibits NMDA-activated ion current in hippocampal neurons. Science 243, 1721–1724. doi: 10.1126/science.2467382
Lovinger, D. M., White, G., and Weight, F. F. (1990). NMDA receptor-mediated synaptic excitation selectively inhibited by ethanol in hippocampal slice from adult rat. J. Neurosci. 10, 1372–1379. doi: 10.1523/JNEUROSCI.10-04-01372.1990
Lukas, S. E., Mendelson, J. H., Benedikt, R. A., and Jones, B. (1986). EEG alpha activity increases during transient episodes of ethanol-induced euphoria. Pharmacol. Biochem. Behav. 25, 889–895. doi: 10.1016/0091-3057(86)90403-X
Lukas, S. E., Mendelson, J. H., Woods, B. T., Mello, N. K., and Teoh, S. K. (1989). Topographic distribution of EEG alpha activity during ethanol-induced intoxication in women. J. Stud. Alcohol 50, 176–185. doi: 10.15288/jsa.1989.50.176
Luscher, B., Shen, Q., and Sahir, N. (2011). The GABAergic deficit hypothesis of major depressive disorder. Mol. Psychiatry 16, 383–406. doi: 10.1038/mp.2010.120
Ma, Y., Hu, H., Berrebi, A. S., Mathers, P. H., and Agmon, A. (2006). Distinct subtypes of somatostatin-containing neocortical interneurons revealed in transgenic mice. J. Neurosci. 26, 5069–5082. doi: 10.1523/JNEUROSCI.0661-06.2006
MacDonald, M. L., Favo, D., Garver, M., Sun, Z., Arion, D., Ding, Y., et al. (2019). Laser capture microdissection-targeted mass spectrometry: a method for multiplexed protein quantification within individual layers of the cerebral cortex. Neuropsychopharmacology 44, 743–748. doi: 10.1038/s41386-018-0260-0
Magnin, E., Francavilla, R., Amalyan, S., Gervais, E., David, L. S., Luo, X., et al. (2019). Input-specific synaptic location and function of the alpha5 GABAA receptor subunit in the mouse CA1 hippocampal neurons. J. Neurosci. 39, 788–801. doi: 10.1523/JNEUROSCI.0567-18.2018
Maguire, J. L., Stell, B. M., Rafizadeh, M., and Mody, I. (2005). Ovarian cycle-linked changes in GABA(A) receptors mediating tonic inhibition alter seizure susceptibility and anxiety. Nat. Neurosci. 8, 797–804. doi: 10.1038/nn1469
Maksymetz, J., Byun, N. E., Luessen, D. J., Li, B., Barry, R. L., Gore, J. C., et al. (2021). mGlu1 potentiation enhances prelimbic somatostatin interneuron activity to rescue schizophrenia-like physiological and cognitive deficits. Cell Rep. 37, 109950. doi: 10.1016/j.celrep.2021.109950
Mallet, N., Le Moine, C., Charpier, S., and Gonon, F. (2005). Feedforward inhibition of projection neurons by fast-spiking GABA interneurons in the rat striatum in vivo. J. Neurosci. 25, 3857–3869. doi: 10.1523/JNEUROSCI.5027-04.2005
Manz, K. M., Ghose, D., Turner, B. D., Taylor, A., Becker, J., Grueter, C. A., et al. (2020). Calcium-permeable AMPA receptors promote endocannabinoid signaling at parvalbumin interneuron synapses in the nucleus accumbens core. Cell Rep. 32, 107971. doi: 10.1016/j.celrep.2020.107971
Markram, H., Toledo-Rodriguez, M., Wang, Y., Gupta, A., Silberberg, G., and Wu, C. (2004). Interneurons of the neocortical inhibitory system. Nat. Rev. Neurosci. 5, 793–807. doi: 10.1038/nrn1519
Marlin, J. J., and Carter, A. G. (2014). GABA-A receptor inhibition of local calcium signaling in spines and dendrites. J. Neurosci. 34, 15898–15911. doi: 10.1523/JNEUROSCI.0869-13.2014
Marshall, E. J. (2014). Adolescent alcohol use: risks and consequences. Alcohol Alcohol. 49, 160–164. doi: 10.1093/alcalc/agt180
Marszalec, W., Aistrup, G. L., and Narahashi, T. (1998). Ethanol modulation of excitatory and inhibitory synaptic interactions in cultured cortical neurons. Alcohol. Clin. Exp. Res. 22, 1516–1524. doi: 10.1111/j.1530-0277.1998.tb03944.x
Martenson, J. S., Yamasaki, T., Chaudhury, N. H., Albrecht, D., and Tomita, S. (2017). Assembly rules for GABAA receptor complexes in the brain. Elife 6. doi: 10.7554/eLife.27443
Matta, J. A., Pelkey, K. A., Craig, M. T., Chittajallu, R., Jeffries, B. W., and McBain, C. J. (2013). Developmental origin dictates interneuron AMPA and NMDA receptor subunit composition and plasticity. Nat. Neurosci. 16, 1032–1041. doi: 10.1038/nn.3459
Mayer, M. L., and Armstrong, N. (2004). Structure and function of glutamate receptor ion channels. Annu. Rev. Physiol. 66, 161–181. doi: 10.1146/annurev.physiol.66.050802.084104
McCarthy, G., Blamire, A. M., Puce, A., Nobre, A. C., Bloch, G., Hyder, F., et al. (1994). Functional magnetic resonance imaging of human prefrontal cortex activation during a spatial working memory task. Proc. Natl. Acad. Sci. USA. 91, 8690–8694. doi: 10.1073/pnas.91.18.8690
McCormick, D. A., Connors, B. W., Lighthall, J. W., and Prince, D. A. (1985). Comparative electrophysiology of pyramidal and sparsely spiny stellate neurons of the neocortex. J. Neurophysiol. 54, 782–806. doi: 10.1152/jn.1985.54.4.782
McDonald, A. J., and Mascagni, F. (2011). Neuronal localization of M2 muscarinic receptor immunoreactivity in the rat amygdala. Neuroscience 196, 49–65. doi: 10.1016/j.neuroscience.2011.08.032
McGarry, L. M., and Carter, A. G. (2016). Inhibitory gating of basolateral amygdala inputs to the prefrontal cortex. J. Neurosci. 36, 9391–9406. doi: 10.1523/JNEUROSCI.0874-16.2016
McKinsey, G. L., Lindtner, S., Trzcinski, B., Visel, A., Pennacchio, L. A., Huylebroeck, D., et al. (2013). Dlx1and2-dependent expression of Zfhx1b (Sip1, Zeb2) regulates the fate switch between cortical and striatal interneurons. Neuron 77, 83–98. doi: 10.1016/j.neuron.2012.11.035
Medina, K. L., McQueeny, T., Nagel, B. J., Hanson, K. L., Schweinsburg, A. D., and Tapert, S. F. (2008). Prefrontal cortex volumes in adolescents with alcohol use disorders: unique gender effects. Alcohol. Clin. Exp. Res. 32, 386–394. doi: 10.1111/j.1530-0277.2007.00602.x
Mehta, A. K., Marutha Ravindran, C. R., and Ticku, M. K. (2007). Low concentrations of ethanol do not affect radioligand binding to the delta-subunit-containing GABAA receptors in the rat brain. Brain Res. 1165, 15–20. doi: 10.1016/j.brainres.2007.06.051
Melchitzky, D. S., and Lewis, D. A. (2003). Pyramidal neuron local axon terminals in monkey prefrontal cortex: differential targeting of subclasses of GABA neurons. Cereb. Cortex 13, 452–460. doi: 10.1093/cercor/13.5.452
Melchitzky, D. S., and Lewis, D. A. (2008). Dendritic-targeting GABA neurons in monkey prefrontal cortex: comparison of somatostatin- and calretinin-immunoreactive axon terminals. Synapse 62, 456–465. doi: 10.1002/syn.20514
Melon, L. C., Nasman, J. T., John, A. S., Mbonu, K., and Maguire, J. L. (2018). Interneuronal delta-GABAA receptors regulate binge drinking and are necessary for the behavioral effects of early withdrawal. Neuropsychopharmacology. 44, 425–434 doi: 10.1038/s41386-018-0164-z
Meyers, J. L., Zhang, J., Wang, J. C., Su, J., Kuo, S. I., Kapoor, M., et al. (2017). An endophenotype approach to the genetics of alcohol dependence: a genome wide association study of fast beta EEG in families of African ancestry. Mol. Psychiatry 22, 1767–1775. doi: 10.1038/mp.2016.239
Mikulovic, S., Restrepo, C. E., Hilscher, M. M., Kullander, K., and Leao, R. N. (2015). Novel markers for OLM interneurons in the hippocampus. Front. Cell. Neurosci. 9, 201. doi: 10.3389/fncel.2015.00201
Mody, I., and Pearce, R. A. (2004). Diversity of inhibitory neurotransmission through GABA(A) receptors. Trends Neurosci. 27, 569–575. doi: 10.1016/j.tins.2004.07.002
Mohler, H. (2006). GABA(A) receptor diversity and pharmacology. Cell Tissue Res. 326, 505–516. doi: 10.1007/s00441-006-0284-3
Monyer, H., Burnashev, N., Laurie, D. J., Sakmann, B., and Seeburg, P. H. (1994). Developmental and regional expression in the rat brain and functional properties of four NMDA receptors. Neuron 12, 529–540. doi: 10.1016/0896-6273(94)90210-0
Morrisett, R. A., Martin, D., Oetting, T. A., Lewis, D. V., Wilson, W. A., and Swartzwelder, H. S. (1991). Ethanol and magnesium ions inhibit N-methyl-D-aspartate-mediated synaptic potentials in an interactive manner. Neuropharmacology 30, 1173–1178. doi: 10.1016/0028-3908(91)90162-5
Muller, J. F., Mascagni, F., and McDonald, A. J. (2005). Coupled networks of parvalbumin-immunoreactive interneurons in the rat basolateral amygdala. J. Neurosci. 25, 7366–7376. doi: 10.1523/JNEUROSCI.0899-05.2005
Muller, J. F., Mascagni, F., and McDonald, A. J. (2007). Postsynaptic targets of somatostatin-containing interneurons in the rat basolateral amygdala. J. Comp. Neurol. 500, 513–529. doi: 10.1002/cne.21185
Murayama, M., Perez-Garci, E., Nevian, T., Bock, T., Senn, W., and Larkum, M. E. (2009). Dendritic encoding of sensory stimuli controlled by deep cortical interneurons. Nature 457, 1137–1141. doi: 10.1038/nature07663
Nestoros, J. N. (1980). Ethanol selectively potentiates GABA-mediated inhibition of single feline cortical neurons. Life Sci. 26, 519–523. doi: 10.1016/0024-3205(80)90314-8
Newman, E. L., Gunner, G., Huynh, P., Gachette, D., Moss, S. J., Smart, T. G., et al. (2016). Effects of Gabra2 point mutations on alcohol intake: increased binge-like and blunted chronic drinking by mice. Alcohol. Clin. Exp. Res. 40, 2445–2455. doi: 10.1111/acer.13215
Nguyen, R., Venkatesan, S., Binko, M., Bang, J. Y., Cajanding, J. D., Briggs, C., et al. (2020). Cholecystokinin-expressing interneurons of the medial prefrontal cortex mediate working memory retrieval. J. Neurosci. 40, 2314–2331. doi: 10.1523/JNEUROSCI.1919-19.2020
Nigro, M. J., Hashikawa-Yamasaki, Y., and Rudy, B. (2018). Diversity and connectivity of layer 5 somatostatin-expressing interneurons in the mouse barrel cortex. J. Neurosci. 38, 1622–1633. doi: 10.1523/JNEUROSCI.2415-17.2017
Nimitvilai, S., Lopez, M. F., Mulholland, P. J., and Woodward, J. J. (2016). Chronic intermittent ethanol exposure enhances the excitability and synaptic plasticity of lateral orbitofrontal cortex neurons and induces a tolerance to the acute inhibitory actions of ethanol. Neuropsychopharmacology 41, 1112–1127. doi: 10.1038/npp.2015.250
Nusser, Z., Sieghart, W., Benke, D., Fritschy, J. M., and Somogyi, P. (1996). Differential synaptic localization of two major gamma-aminobutyric acid type A receptor alpha subunits on hippocampal pyramidal cells. Proc. Natl. Acad. Sci. USA. 93, 11939–11944. doi: 10.1073/pnas.93.21.11939
Nyiri, G., Freund, T. F., and Somogyi, P. (2001). Input-dependent synaptic targeting of alpha(2)-subunit-containing GABA(A) receptors in synapses of hippocampal pyramidal cells of the rat. Eur. J. Neurosci. 13, 428–442. doi: 10.1046/j.1460-9568.2001.01407.x
O'Hare, J. K., Li, H., Kim, N., Gaidis, E., Ade, K., Beck, J., et al. (2017). Striatal fast-spiking interneurons selectively modulate circuit output and are required for habitual behavior. Elife 6, e26231. doi: 10.7554/eLife.26231
Oliva, A. A. Jr., Jiang, M., Lam, T., Smith, K. L., and Swann, J. W. (2000). Novel hippocampal interneuronal subtypes identified using transgenic mice that express green fluorescent protein in GABAergic interneurons. J. Neurosci. 20, 3354–3368. doi: 10.1523/JNEUROSCI.20-09-03354.2000
Olsen, R. W., and Sieghart, W. (2009). GABA A receptors: subtypes provide diversity of function and pharmacology. Neuropharmacology 56, 141–148. doi: 10.1016/j.neuropharm.2008.07.045
Oren, I., Nissen, W., Kullmann, D. M., Somogyi, P., and Lamsa, K. P. (2009). Role of ionotropic glutamate receptors in long-term potentiation in rat hippocampal CA1 oriens-lacunosum moleculare interneurons. J. Neurosci. 29, 939–950. doi: 10.1523/JNEUROSCI.3251-08.2009
Pafundo, D. E., Miyamae, T., Lewis, D. A., and Gonzalez-Burgos, G. (2018). Presynaptic effects of N-Methyl-D-Aspartate receptors enhance parvalbumin cell-mediated inhibition of pyramidal cells in mouse prefrontal cortex. Biol. Psychiatry 84, 460–470. doi: 10.1016/j.biopsych.2018.01.018
Paoletti, P., Bellone, C., and Zhou, Q. (2013). NMDA receptor subunit diversity: impact on receptor properties, synaptic plasticity and disease. Nat. Rev. Neurosci. 14, 383–400. doi: 10.1038/nrn3504
Pardo, J. V., Fox, P. T., and Raichle, M. E. (1991). Localization of a human system for sustained attention by positron emission tomography. Nature 349, 61–64. doi: 10.1038/349061a0
Parker, R. M., Bentley, K. R., and Barnes, N. M. (1996). Allosteric modulation of 5-HT3 receptors: focus on alcohols and anaesthetic agents. Trends Pharmacol. Sci. 17, 95–99. doi: 10.1016/0165-6147(96)10003-1
Pelkey, K. A., Chittajallu, R., Craig, M. T., Tricoire, L., Wester, J. C., and McBain, C. J. (2017). Hippocampal GABAergic inhibitory interneurons. Physiol. Rev. 97, 1619–1747. doi: 10.1152/physrev.00007.2017
Perkins, D. I., Trudell, J. R., Crawford, D. K., Alkana, R. L., and Davies, D. L. (2010). Molecular targets and mechanisms for ethanol action in glycine receptors. Pharmacol. Ther. 127, 53–65. doi: 10.1016/j.pharmthera.2010.03.003
Perszyk, R. E., DiRaddo, J. O., Strong, K. L., Low, C. M., Ogden, K. K., Khatri, A., et al. (2016). GluN2D-Containing N-methyl-d-Aspartate receptors mediate synaptic transmission in hippocampal interneurons and regulate interneuron activity. Mol. Pharmacol. 90, 689–702. doi: 10.1124/mol.116.105130
Pfeffer, C. K., Xue, M., He, M., Huang, Z. J., and Scanziani, M. (2013). Inhibition of inhibition in visual cortex: the logic of connections between molecularly distinct interneurons. Nat. Neurosci. 16, 1068–1076. doi: 10.1038/nn.3446
Philip, N. S., Sorensen, D. O., McCalley, D. M., and Hanlon, C. A. (2020). Non-invasive brain stimulation for alcohol use disorders: state of the art and future directions. Neurotherapeutics 17, 116–126. doi: 10.1007/s13311-019-00780-x
Pi, H. J., Hangya, B., Kvitsiani, D., Sanders, J. I., Huang, Z. J., and Kepecs, A. (2013). Cortical interneurons that specialize in disinhibitory control. Nature 503, 521–524. doi: 10.1038/nature12676
Pickering, C., Avesson, L., Lindblom, J., Liljequist, S., and Schioth, H. B. (2007). Identification of neurotransmitter receptor genes involved in alcohol self-administration in the rat prefrontal cortex, hippocampus and amygdala. Prog. Neuropsychopharmacol. Biol. Psychiatry 31, 53–64. doi: 10.1016/j.pnpbp.2006.06.010
Pirker, S., Schwarzer, C., Wieselthaler, A., Sieghart, W., and Sperk, G. (2000). GABA(A) receptors: immunocytochemical distribution of 13 subunits in the adult rat brain. Neuroscience 101, 815–850. doi: 10.1016/S0306-4522(00)00442-5
Pleil, K. E., Lowery-Gionta, E. G., Crowley, N. A., Li, C., Marcinkiewcz, C. A., Rose, J. H., et al. (2015). Effects of chronic ethanol exposure on neuronal function in the prefrontal cortex and extended amygdala. Neuropharmacology 99, 735–749. doi: 10.1016/j.neuropharm.2015.06.017
Pollock, V. E., Volavka, J., Goodwin, D. W., Mednick, S. A., Gabrielli, W. F., Knop, J., et al. (1983). The EEG after alcohol administration in men at risk for alcoholism. Arch. Gen. Psychiatry 40, 857–861. doi: 10.1001/archpsyc.1983.01790070047006
Porjesz, B., Almasy, L., Edenberg, H. J., Wang, K., Chorlian, D. B., Foroud, T., et al. (2002). Linkage disequilibrium between the beta frequency of the human EEG and a GABAA receptor gene locus. Proc. Natl. Acad. Sci. USA. 99, 3729–3733. doi: 10.1073/pnas.052716399
Porjesz, B., and Begleiter, H. (2003). Alcoholism and human electrophysiology. Alcohol Res. Health 27, 153–160.
Porter, J. T., Cauli, B., Staiger, J. F., Lambolez, B., Rossier, J., and Audinat, E. (1998). Properties of bipolar VIPergic interneurons and their excitation by pyramidal neurons in the rat neocortex. Eur. J. Neurosci. 10, 3617–3628. doi: 10.1046/j.1460-9568.1998.00367.x
Pouille, F., and Scanziani, M. (2004). Routing of spike series by dynamic circuits in the hippocampus. Nature 429, 717–723. doi: 10.1038/nature02615
Proctor, W. R., Soldo, B. L., Allan, A. M., and Dunwiddie, T. V. (1992). Ethanol enhances synaptically evoked GABAA receptor-mediated responses in cerebral cortical neurons in rat brain slices. Brain Res. 595, 220–227. doi: 10.1016/0006-8993(92)91053-H
Pronneke, A., Scheuer, B., Wagener, R. J., Mock, M., Witte, M., and Staiger, J. F. (2015). Characterizing VIP neurons in the barrel cortex of VIPcre/tdTomato mice reveals layer-specific differences. Cereb. Cortex 25, 4854–4868. doi: 10.1093/cercor/bhv202
Propping, P., Kruger, J., and Mark, N. (1981). Genetic disposition to alcoholism. An EEG study in alcoholics and their relatives. Hum. Genet. 59, 51–59. doi: 10.1007/BF00278854
Radel, M., Vallejo, R. L., Iwata, N., Aragon, R., Long, J. C., Virkkunen, M., et al. (2005). Haplotype-based localization of an alcohol dependence gene to the 5q34 {gamma}-aminobutyric acid type A gene cluster. Arch. Gen. Psychiatry 62, 47–55. doi: 10.1001/archpsyc.62.1.47
Radke, A. K., Jury, N. J., Delpire, E., Nakazawa, K., and Holmes, A. (2017). Reduced ethanol drinking following selective cortical interneuron deletion of the GluN2B NMDA receptors subunit. Alcohol 58, 47–51. doi: 10.1016/j.alcohol.2016.07.005
Rainnie, D. G., Mania, I., Mascagni, F., and McDonald, A. J. (2006). Physiological and morphological characterization of parvalbumin-containing interneurons of the rat basolateral amygdala. J. Comp. Neurol. 498, 142–161. doi: 10.1002/cne.21049
Rajkowska, G. (2000). Postmortem studies in mood disorders indicate altered numbers of neurons and glial cells. Biol. Psychiatry 48, 766–777. doi: 10.1016/S0006-3223(00)00950-1
Rangaswamy, M., and Porjesz, B. (2014). Understanding alcohol use disorders with neuroelectrophysiology. Handb. Clin. Neurol. 125, 383–414. doi: 10.1016/B978-0-444-62619-6.00023-9
Rangaswamy, M., Porjesz, B., Chorlian, D. B., Wang, K., Jones, K. A., Bauer, L. O., et al. (2002). Beta power in the EEG of alcoholics. Biol. Psychiatry 52, 831–842. doi: 10.1016/S0006-3223(02)01362-8
Reynolds, J. N., and Prasad, A. (1991). Ethanol enhances GABAA receptor-activated chloride currents in chick cerebral cortical neurons. Brain Res. 564, 138–142. doi: 10.1016/0006-8993(91)91363-6
Robinson, S. L., Marrero, I. M., Perez-Heydrich, C. A., Sepulveda-Orengo, M. T., Reissner, K. J., and Thiele, T. E. (2019b). Medial prefrontal cortex neuropeptide Y modulates binge-like ethanol consumption in C57BL/6J mice. Neuropsychopharmacology 44, 1132–1140. doi: 10.1038/s41386-018-0310-7
Robinson, S. L., Perez-Heydrich, C. A., and Thiele, T. E. (2019a). Corticotropin releasing factor type 1 and 2 receptor signaling in the medial prefrontal cortex modulates binge-like ethanol consumption in C57BL/6J Mice. Brain Sci. 9, 171. doi: 10.3390/brainsci9070171
Robinson, S. L., and Thiele, T. E. (2017). The role of neuropeptide Y (NPY) in alcohol and drug abuse disorders. Int. Rev. Neurobiol. 136, 177–197. doi: 10.1016/bs.irn.2017.06.005
Robinson, S. L., and Thiele, T. E. (2020). A role for the neuropeptide somatostatin in the neurobiology of behaviors associated with substances abuse and affective disorders. Neuropharmacology 167, 107983. doi: 10.1016/j.neuropharm.2020.107983
Rocco, B. R., DeDionisio, A. M., Lewis, D. A., and Fish, K. N. (2017). Alterations in a unique class of cortical chandelier cell axon cartridges in schizophrenia. Biol. Psychiatry 82, 40–48. doi: 10.1016/j.biopsych.2016.09.018
Rock, C., Zurita, H., Wilson, C., and Apicella, A. J. (2016). An inhibitory corticostriatal pathway. Elife 5, e15890. doi: 10.7554/eLife.15890.009
Roh, S., Matsushita, S., Hara, S., Maesato, H., Matsui, T., Suzuki, G., et al. (2011). Role of GABRA2 in moderating subjective responses to alcohol. Alcohol. Clin. Exp. Res. 35, 400–407. doi: 10.1111/j.1530-0277.2010.01357.x
Ronald, K. M., Mirshahi, T., and Woodward, J. J. (2001). Ethanol inhibition of N-methyl-D-aspartate receptors is reduced by site-directed mutagenesis of a transmembrane domain phenylalanine residue. J. Biol. Chem. 276, 44729–44735. doi: 10.1074/jbc.M102800200
Rossignol, E., Kruglikov, I., van den Maagdenberg, A. M., Rudy, B., and Fishell, G. (2013). CaV 2.1 ablation in cortical interneurons selectively impairs fast-spiking basket cells and causes generalized seizures. Ann. Neurol. 74, 209–222. doi: 10.1002/ana.23913
Rotaru, D. C., Barrionuevo, G., and Sesack, S. R. (2005). Mediodorsal thalamic afferents to layer III of the rat prefrontal cortex: synaptic relationships to subclasses of interneurons. J. Comp. Neurol. 490, 220–238. doi: 10.1002/cne.20661
Roth, F. C., and Hu, H. (2020). An axon-specific expression of HCN channels catalyzes fast action potential signaling in GABAergic interneurons. Nat. Commun. 11, 2248. doi: 10.1038/s41467-020-15791-y
Rotzinger, S., and Vaccarino, F. J. (2003). Cholecystokinin receptor subtypes: role in the modulation of anxiety-related and reward-related behaviours in animal models. J. Psychiatry Neurosci. 28, 171–181.
Royer, S., Zemelman, B. V., Losonczy, A., Kim, J., Chance, F., Magee, J. C., et al. (2012). Control of timing, rate and bursts of hippocampal place cells by dendritic and somatic inhibition. Nat. Neurosci. 15, 769–775. doi: 10.1038/nn.3077
Rozov, A., and Burnashev, N. (1999). Polyamine-dependent facilitation of postsynaptic AMPA receptors counteracts paired-pulse depression. Nature 401, 594–598. doi: 10.1038/44151
Rudolph, U., Crestani, F., Benke, D., Brunig, I., Benson, J. A., Fritschy, J. M., et al. (1999). Benzodiazepine actions mediated by specific gamma-aminobutyric acid(A) receptor subtypes. Nature 401, 796–800. doi: 10.1038/44579
Rudy, B., and McBain, C. J. (2001). Kv3 channels: voltage-gated K+ channels designed for high-frequency repetitive firing. Trends Neurosci. 24, 517–526. doi: 10.1016/S0166-2236(00)01892-0
Saletu-Zyhlarz, G. M., Arnold, O., Anderer, P., Oberndorfer, S., Walter, H., Lesch, O. M., et al. (2004). Differences in brain function between relapsing and abstaining alcohol-dependent patients, evaluated by EEG mapping. Alcohol Alcohol. 39, 233–240. doi: 10.1093/alcalc/agh041
Salling, M. C., and Harrison, N. L. (2014). Strychnine-sensitive glycine receptors on pyramidal neurons in layers II/III of the mouse prefrontal cortex are tonically activated. J. Neurophysiol. 112, 1169–1178. doi: 10.1152/jn.00714.2013
Salling, M. C., Jane Skelly, M., Avegno, E., Regan, S., Zeric, T., Nichols, E., et al. (2018). Alcohol consumption during adolescence in a mouse model of binge drinking alters the intrinsic excitability and function of the prefrontal cortex through a reduction in the hyperpolarization-activated cation current. J. Neurosci. 38, 6207–6222 doi: 10.1523/JNEUROSCI.0550-18.2018
Salling, M. C., and Martinez, D. (2016). Brain stimulation in addiction. Neuropsychopharmacology 41, 2798–2809. doi: 10.1038/npp.2016.80
Sambandan, S., Sauer, J. F., Vida, I., and Bartos, M. (2010). Associative plasticity at excitatory synapses facilitates recruitment of fast-spiking interneurons in the dentate gyrus. J. Neurosci. 30, 11826–11837. doi: 10.1523/JNEUROSCI.2012-10.2010
Sarihi, A., Mirnajafi-Zadeh, J., Jiang, B., Sohya, K., Safari, M. S., Arami, M. K., et al. (2012). Cell type-specific, presynaptic LTP of inhibitory synapses on fast-spiking GABAergic neurons in the mouse visual cortex. J. Neurosci. 32, 13189–13199. doi: 10.1523/JNEUROSCI.1386-12.2012
Schulz, J. M., Knoflach, F., Hernandez, M. C., and Bischofberger, J. (2018). Dendrite-targeting interneurons control synaptic NMDA-receptor activation via nonlinear alpha5-GABAA receptors. Nat. Commun. 9, 3576. doi: 10.1038/s41467-018-06004-8
Schuman, B., Machold, R. P., Hashikawa, Y., Fuzik, J., Fishell, G. J., and Rudy, B. (2019). Four unique interneuron populations reside in neocortical layer 1. J. Neurosci. 39, 125–139. doi: 10.1523/JNEUROSCI.1613-18.2018
Shi, Y., Wang, M., Mi, D., Lu, T., Wang, B., Dong, H., et al. (2021). Mouse and human share conserved transcriptional programs for interneuron development. Science 374, eabj6641. doi: 10.1126/science.abj6641
Shields, B. C., Kahuno, E., Kim, C., Apostolides, P. F., Brown, J., Lindo, S., et al. (2017). Deconstructing behavioral neuropharmacology with cellular specificity. Science 356, eaaj2161. doi: 10.1126/science.aaj2161
Shigemoto, R., Kulik, A., Roberts, J. D., Ohishi, H., Nusser, Z., Kaneko, T., et al. (1996). Target-cell-specific concentration of a metabotropic glutamate receptor in the presynaptic active zone. Nature 381, 523–525. doi: 10.1038/381523a0
Silberberg, G., and Markram, H. (2007). Disynaptic inhibition between neocortical pyramidal cells mediated by Martinotti cells. Neuron 53, 735–746. doi: 10.1016/j.neuron.2007.02.012
Smothers, C. T., Jin, C., and Woodward, J. J. (2013). Deletion of the N-terminal domain alters the ethanol inhibition of N-methyl-D-aspartate receptors in a subunit-dependent manner. Alcohol. Clin. Exp. Res. 37, 1882–1890. doi: 10.1111/acer.12168
Smothers, C. T., and Woodward, J. J. (2016). Differential effects of TM4 tryptophan mutations on inhibition of N-methyl-d-aspartate receptors by ethanol and toluene. Alcohol 56, 15–19. doi: 10.1016/j.alcohol.2016.10.001
Sohal, V. S., Zhang, F., Yizhar, O., and Deisseroth, K. (2009). Parvalbumin neurons and gamma rhythms enhance cortical circuit performance. Nature 459, 698–702. doi: 10.1038/nature07991
Soldo, B. L., Proctor, W. R., and Dunwiddie, T. V. (1998). Ethanol selectively enhances the hyperpolarizing component of neocortical neuronal responses to locally applied GABA. Brain Res. 800, 187–197. doi: 10.1016/S0006-8993(98)00455-7
Sommeijer, J. P., and Levelt, C. N. (2012). Synaptotagmin-2 is a reliable marker for parvalbumin positive inhibitory boutons in the mouse visual cortex. PLoS ONE 7, e35323. doi: 10.1371/annotation/1c5484e5-41c0-44dc-8422-2dbd3a002f3b
Sorg, S. F., Taylor, M. J., Alhassoon, O. M., Gongvatana, A., Theilmann, R. J., Frank, L. R., et al. (2012). Frontal white matter integrity predictors of adult alcohol treatment outcome. Biol. Psychiatry 71, 262–268. doi: 10.1016/j.biopsych.2011.09.022
Soto, D., Coombs, I. D., Kelly, L., Farrant, M., and Cull-Candy, S. G. (2007). Stargazin attenuates intracellular polyamine block of calcium-permeable AMPA receptors. Nat. Neurosci. 10, 1260–1267. doi: 10.1038/nn1966
Spear, L. P., and Swartzwelder, H. S. (2014). Adolescent alcohol exposure and persistence of adolescent-typical phenotypes into adulthood: a mini-review. Neurosci. Biobehav. Rev. 45, 1–8. doi: 10.1016/j.neubiorev.2014.04.012
Stachniak, T. J., Sylwestrak, E. L., Scheiffele, P., Hall, B. J., and Ghosh, A. (2019). Elfn1-Induced constitutive activation of mGluR7 determines frequency-dependent recruitment of somatostatin interneurons. J. Neurosci. 39, 4461–4474. doi: 10.1523/JNEUROSCI.2276-18.2019
Stan, A. D., Ghose, S., Gao, X. M., Roberts, R. C., Lewis-Amezcua, K., Hatanpaa, K. J., et al. (2006). Human postmortem tissue: what quality markers matter? Brain Res. 1123, 1–11. doi: 10.1016/j.brainres.2006.09.025
Stell, B. M., Brickley, S. G., Tang, C. Y., Farrant, M., and Mody, I. (2003). Neuroactive steroids reduce neuronal excitability by selectively enhancing tonic inhibition mediated by delta subunit-containing GABAA receptors. Proc. Natl. Acad. Sci. USA. 100, 14439–14444. doi: 10.1073/pnas.2435457100
Stenberg, G., Sano, M., Rosen, I., and Ingvar, D. H. (1994). EEG topography of acute ethanol effects in resting and activated normals. J. Stud. Alcohol 55, 645–656. doi: 10.15288/jsa.1994.55.645
Sundstrom-Poromaa, I., Smith, D. H., Gong, Q. H., Sabado, T. N., Li, X., Light, A., et al. (2002). Hormonally regulated alpha(4)beta(2)delta GABA(A) receptors are a target for alcohol. Nat. Neurosci. 5, 721–722. doi: 10.1038/nn888
Sur, C., Wafford, K. A., Reynolds, D. S., Hadingham, K. L., Bromidge, F., Macaulay, A., et al. (2001). Loss of the major GABA(A) receptor subtype in the brain is not lethal in mice. J. Neurosci. 21, 3409–3418. doi: 10.1523/JNEUROSCI.21-10-03409.2001
Sweet, R. A., Fish, K. N., and Lewis, D. A. (2010). Mapping synaptic pathology within cerebral cortical circuits in subjects with schizophrenia. Front. Hum. Neurosci. 4, 44. doi: 10.3389/fnhum.2010.00044
Sylwestrak, E. L., and Ghosh, A. (2012). Elfn1 regulates target-specific release probability at CA1-interneuron synapses. Science 338, 536–540. doi: 10.1126/science.1222482
Szabo, A., Somogyi, J., Cauli, B., Lambolez, B., Somogyi, P., and Lamsa, K. P. (2012). Calcium-permeable AMPA receptors provide a common mechanism for LTP in glutamatergic synapses of distinct hippocampal interneuron types. J. Neurosci. 32, 6511–6516. doi: 10.1523/JNEUROSCI.0206-12.2012
Tao, Y., Chen, Y. J., Shen, C., Luo, Z., Bates, C. R., Lee, D., et al. (2013). Erbin interacts with TARP gamma-2 for surface expression of AMPA receptors in cortical interneurons. Nat. Neurosci. 16, 290–299. doi: 10.1038/nn.3320
Tasic, B., Menon, V., Nguyen, T. N., Kim, T. K., Jarsky, T., Yao, Z., et al. (2016). Adult mouse cortical cell taxonomy revealed by single cell transcriptomics. Nat. Neurosci. 19, 335–346. doi: 10.1038/nn.4216
Tasic, B., Yao, Z., Graybuck, L. T., Smith, K. A., Nguyen, T. N., Bertagnolli, D., et al. (2018). Shared and distinct transcriptomic cell types across neocortical areas. Nature 563, 72–78. doi: 10.1038/s41586-018-0654-5
Thorsell, A., and Mathe, A. A. (2017). Neuropeptide Y in alcohol addiction and affective disorders. Front. Endocrinol. (Lausanne) 8, 178. doi: 10.3389/fendo.2017.00178
Tiveron, M. C., Beurrier, C., Ceni, C., Andriambao, N., Combes, A., Koehl, M., et al. (2016). LAMP5 Fine-Tunes GABAergic synaptic transmission in defined circuits of the mouse brain. PLoS ONE 11, e0157052. doi: 10.1371/journal.pone.0157052
Tomioka, R., Okamoto, K., Furuta, T., Fujiyama, F., Iwasato, T., Yanagawa, Y., et al. (2005). Demonstration of long-range GABAergic connections distributed throughout the mouse neocortex. Eur. J. Neurosci. 21, 1587–1600. doi: 10.1111/j.1460-9568.2005.03989.x
Tomita, S., Sekiguchi, M., Wada, K., Nicoll, R. A., and Bredt, D. S. (2006). Stargazin controls the pharmacology of AMPA receptor potentiators. Proc. Natl. Acad. Sci. USA. 103, 10064–10067. doi: 10.1073/pnas.0603128103
Trantham-Davidson, H., Burnett, E. J., Gass, J. T., Lopez, M. F., Mulholland, P. J., Centanni, S. W., et al. (2014). Chronic alcohol disrupts dopamine receptor activity and the cognitive function of the medial prefrontal cortex. J. Neurosci. 34, 3706–3718. doi: 10.1523/JNEUROSCI.0623-13.2014
Trantham-Davidson, H., Centanni, S. W., Garr, S. C., New, N. N., Mulholland, P. J., Gass, J. T., et al. (2017). Binge-like alcohol exposure during adolescence disrupts dopaminergic neurotransmission in the adult prelimbic cortex. Neuropsychopharmacology 42, 1024–1036. doi: 10.1038/npp.2016.190
Trantham-Davidson, H., and Chandler, L. J. (2015). Alcohol-induced alterations in dopamine modulation of prefrontal activity. Alcohol 49, 773–779. doi: 10.1016/j.alcohol.2015.09.001
Traynelis, S. F., Wollmuth, L. P., McBain, C. J., Menniti, F. S., Vance, K. M., Ogden, K. K., et al. (2010). Glutamate receptor ion channels: structure, regulation, and function. Pharmacol. Rev. 62, 405–496. doi: 10.1124/pr.109.002451
Tremblay, R., Lee, S., and Rudy, B. (2016). GABAergic interneurons in the neocortex: from cellular properties to circuits. Neuron 91, 260–292. doi: 10.1016/j.neuron.2016.06.033
Tripp, A., Kota, R. S., Lewis, D. A., and Sibille, E. (2011). Reduced somatostatin in subgenual anterior cingulate cortex in major depression. Neurobiol. Dis. 42, 116–124. doi: 10.1016/j.nbd.2011.01.014
Trudell, J. R., Messing, R. O., Mayfield, J., and Harris, R. A. (2014). Alcohol dependence: molecular and behavioral evidence. Trends Pharmacol. Sci. 35, 317–323. doi: 10.1016/j.tips.2014.04.009
Tu, Y., Kroener, S., Abernathy, K., Lapish, C., Seamans, J., Chandler, L. J., et al. (2007). Ethanol inhibits persistent activity in prefrontal cortical neurons. J. Neurosci. 27, 4765–4775. doi: 10.1523/JNEUROSCI.5378-06.2007
Tukker, J. J., Fuentealba, P., Hartwich, K., Somogyi, P., and Klausberger, T. (2007). Cell type-specific tuning of hippocampal interneuron firing during gamma oscillations in vivo. J. Neurosci. 27, 8184–8189. doi: 10.1523/JNEUROSCI.1685-07.2007
Unal, C. T., Unal, B., and Bolton, M. M. (2020). Low-threshold spiking interneurons perform feedback inhibition in the lateral amygdala. Brain Struct. Funct. 225, 909–923. doi: 10.1007/s00429-020-02051-4
Urban-Ciecko, J., and Barth, A. L. (2016). Somatostatin-expressing neurons in cortical networks. Nat. Rev. Neurosci. 17, 401–409. doi: 10.1038/nrn.2016.53
Urban-Ciecko, J., Fanselow, E. E., and Barth, A. L. (2015). Neocortical somatostatin neurons reversibly silence excitatory transmission via GABAb receptors. Curr. Biol. 25, 722–731. doi: 10.1016/j.cub.2015.01.035
van Hooft, J. A., Giuffrida, R., Blatow, M., and Monyer, H. (2000). Differential expression of group I metabotropic glutamate receptors in functionally distinct hippocampal interneurons. J. Neurosci. 20, 3544–3551. doi: 10.1523/JNEUROSCI.20-10-03544.2000
Vicini, S., Ferguson, C., Prybylowski, K., Kralic, J., Morrow, A. L., and Homanics, G. E. (2001). GABA(A) receptor alpha1 subunit deletion prevents developmental changes of inhibitory synaptic currents in cerebellar neurons. J. Neurosci. 21, 3009–3016. doi: 10.1523/JNEUROSCI.21-09-03009.2001
Vincent, S. R., Johansson, O., Hokfelt, T., Meyerson, B., Sachs, C., Elde, R. P., et al. (1982). Neuropeptide coexistence in human cortical neurones. Nature 298, 65–67. doi: 10.1038/298065a0
Viollet, C., Lepousez, G., Loudes, C., Videau, C., Simon, A., and Epelbaum, J. (2008). Somatostatinergic systems in brain: networks and functions. Mol. Cell. Endocrinol. 286, 75–87. doi: 10.1016/j.mce.2007.09.007
Vormstein-Schneider, D., Lin, J. D., Pelkey, K. A., Chittajallu, R., Guo, B., Arias-Garcia, M. A., et al. (2020). Viral manipulation of functionally distinct interneurons in mice, non-human primates and humans. Nat. Neurosci. 23, 1629–1636. doi: 10.1038/s41593-020-0692-9
Wallner, M., Hanchar, H. J., and Olsen, R. W. (2003). Ethanol enhances alpha 4 beta 3 delta and alpha 6 beta 3 delta gamma-aminobutyric acid type A receptors at low concentrations known to affect humans. Proc. Natl. Acad. Sci. USA. 100, 15218–15223. doi: 10.1073/pnas.2435171100
Walton, N., and Maguire, J. (2019). Allopregnanolone-based treatments for postpartum depression: Why/how do they work? Neurobiol Stress 11, 100198. doi: 10.1016/j.ynstr.2019.100198
Wang, J., Fan, Y., Dong, Y., Ma, M., Ma, Y., Dong, Y., et al. (2016). Alterations in brain structure and functional connectivity in alcohol dependent patients and possible association with impulsivity. PLoS ONE 11, e0161956. doi: 10.1371/journal.pone.0161956
Wang, J. C., Hinrichs, A. L., Stock, H., Budde, J., Allen, R., Bertelsen, S., et al. (2004). Evidence of common and specific genetic effects: association of the muscarinic acetylcholine receptor M2 (CHRM2) gene with alcohol dependence and major depressive syndrome. Hum. Mol. Genet. 13, 1903–1911. doi: 10.1093/hmg/ddh194
Wei, W., Faria, L. C., and Mody, I. (2004). Low ethanol concentrations selectively augment the tonic inhibition mediated by delta subunit-containing GABAA receptors in hippocampal neurons. J. Neurosci. 24, 8379–8382. doi: 10.1523/JNEUROSCI.2040-04.2004
Weiner, J. L., Gu, C., and Dunwiddie, T. V. (1997). Differential ethanol sensitivity of subpopulations of GABAA synapses onto rat hippocampal CA1 pyramidal neurons. J. Neurophysiol. 77, 1306–1312. doi: 10.1152/jn.1997.77.3.1306
Weiner, J. L., and Valenzuela, C. F. (2006). Ethanol modulation of GABAergic transmission: the view from the slice. Pharmacol. Ther. 111, 533–554. doi: 10.1016/j.pharmthera.2005.11.002
Weitlauf, C., and Woodward, J. J. (2008). Ethanol selectively attenuates NMDAR-mediated synaptic transmission in the prefrontal cortex. Alcohol. Clin. Exp. Res. 32, 690–698. doi: 10.1111/j.1530-0277.2008.00625.x
Werner, D. F., Blednov, Y. A., Ariwodola, O. J., Silberman, Y., Logan, E., Berry, R. B., et al. (2006). Knockin mice with ethanol-insensitive alpha1-containing gamma-aminobutyric acid type A receptors display selective alterations in behavioral responses to ethanol. J. Pharmacol. Exp. Ther. 319, 219–227. doi: 10.1124/jpet.106.106161
White, C. M., Ji, S., Cai, H., Maudsley, S., and Martin, B. (2010). Therapeutic potential of vasoactive intestinal peptide and its receptors in neurological disorders. CNS Neurol. Disord. Drug Targets 9, 661–666. doi: 10.2174/187152710793361595
Whiteford, H. A., Degenhardt, L., Rehm, J., Baxter, A. J., Ferrari, A. J., Erskine, H. E., et al. (2013). Global burden of disease attributable to mental and substance use disorders: findings from the Global Burden of Disease Study 2010. Lancet 382, 1575–1586. doi: 10.1016/S0140-6736(13)61611-6
Williams, G. V., and Goldman-Rakic, P. S. (1995). Modulation of memory fields by dopamine D1 receptors in prefrontal cortex. Nature 376, 572–575. doi: 10.1038/376572a0
Wisden, W., and Seeburg, P. H. (1992). GABAA receptor channels: from subunits to functional entities. Curr. Opin. Neurobiol. 2, 263–269. doi: 10.1016/0959-4388(92)90113-Y
Woodruff, A. R., and Sah, P. (2007). Networks of parvalbumin-positive interneurons in the basolateral amygdala. J. Neurosci. 27, 553–563. doi: 10.1523/JNEUROSCI.3686-06.2007
Woodward, J. J. (2000). Ethanol and NMDA receptor signaling. Crit. Rev. Neurobiol. 14, 69–89. doi: 10.1615/CritRevNeurobiol.v14.i1.40
Woodward, J. J., and Pava, M. J. (2009). Effects of ethanol on persistent activity and up-States in excitatory and inhibitory neurons in prefrontal cortex. Alcohol. Clin. Exp. Res. 33, 2134–2140. doi: 10.1111/j.1530-0277.2009.01059.x
Woodward, J. J., and Smothers, C. (2003). Ethanol inhibition of recombinant NR1/2A receptors: effects of heavy metal chelators and a zinc-insensitive NR2A mutant. Alcohol 31, 71–76. doi: 10.1016/j.alcohol.2003.07.002
Xu, H., Jeong, H. Y., Tremblay, R., and Rudy, B. (2013). Neocortical somatostatin-expressing GABAergic interneurons disinhibit the thalamorecipient layer 4. Neuron 77, 155–167. doi: 10.1016/j.neuron.2012.11.004
Xu, H., Liu, L., Tian, Y., Wang, J., Li, J., Zheng, J., et al. (2019). A disinhibitory microcircuit mediates conditioned social fear in the prefrontal cortex. Neuron 102, 668–682 e5. doi: 10.1016/j.neuron.2019.02.026
Xu, Y., Liu, X., Zhang, X., Zhang, G., Zhang, R., Liu, T., et al. (2012). Histone acetylation of the htr3a gene in the prefrontal cortex of Wistar rats regulates ethanol-seeking behavior. Neural Regen Res 7, 1021–1028. doi: 10.3969/j.issn.1673-5374.2012.13.009
Yaka, R., Phamluong, K., and Ron, D. (2003). Scaffolding of Fyn kinase to the NMDA receptor determines brain region sensitivity to ethanol. J. Neurosci. 23, 3623–3632. doi: 10.1523/JNEUROSCI.23-09-03623.2003
Yan, H., Li, Q., Fleming, R., Madison, R. D., Wilson, W. A., and Swartzwelder, H. S. (2009). Developmental sensitivity of hippocampal interneurons to ethanol: involvement of the hyperpolarization-activated current, Ih. J. Neurophysiol. 101, 67–83. doi: 10.1152/jn.90557.2008
Yan, H., Li, Q., Madison, R., Wilson, W. A., and Swartzwelder, H. S. (2010). Differential sensitivity of hippocampal interneurons to ethanol in adolescent and adult rats. J. Pharmacol. Exp. Ther. 335, 51–60. doi: 10.1124/jpet.110.168450
Yang, B. Z., Arias, A. J., Feinn, R., Krystal, J. H., Gelernter, J., and Petrakis, I. L. (2017). GRIK1 and GABRA2 variants have distinct effects on the dose-related subjective response to intravenous alcohol in healthy social drinkers. Alcohol. Clin. Exp. Res. 41, 2025–2032. doi: 10.1111/acer.13516
Yang, X., Meng, Y. J., Tao, Y. J., Deng, R. H., Wang, H. Y., Li, X. J., et al. (2021). Functional connectivity of nucleus accumbens and medial prefrontal cortex with other brain regions during early-abstinence is associated with alcohol dependence and relapse: a resting-functional magnetic resonance imaging study. Front. Psychiatry 12, 609458. doi: 10.3389/fpsyt.2021.609458
Yavorska, I., and Wehr, M. (2016). Somatostatin-expressing inhibitory interneurons in cortical circuits. Front. Neural Circuits 10, 76. doi: 10.3389/fncir.2016.00076
Zaitsev, A. V., Povysheva, N. V., Lewis, D. A., and Krimer, L. S. (2007). P/Q-type, but not N-type, calcium channels mediate GABA release from fast-spiking interneurons to pyramidal cells in rat prefrontal cortex. J. Neurophysiol. 97, 3567–3573. doi: 10.1152/jn.01293.2006
Zhang, W., Zhang, L., Liang, B., Schroeder, D., Zhang, Z. W., Cox, G. A., et al. (2016). Hyperactive somatostatin interneurons contribute to excitotoxicity in neurodegenerative disorders. Nat. Neurosci. 19, 557–559. doi: 10.1038/nn.4257
Keywords: electrophysiology, addiction, drug abuse, preclinical models, interneurons, synaptic plasticity, GABA, glutamate
Citation: Fish KN and Joffe ME (2022) Targeting prefrontal cortex GABAergic microcircuits for the treatment of alcohol use disorder. Front. Synaptic Neurosci. 14:936911. doi: 10.3389/fnsyn.2022.936911
Received: 05 May 2022; Accepted: 27 July 2022;
Published: 29 August 2022.
Edited by:
Florence P. Varodayan, Binghamton University - SUNY, United StatesReviewed by:
Michael C. Salling, Louisiana State University, United StatesSam Centanni, Wake Forest University, United States
Copyright © 2022 Fish and Joffe. This is an open-access article distributed under the terms of the Creative Commons Attribution License (CC BY). The use, distribution or reproduction in other forums is permitted, provided the original author(s) and the copyright owner(s) are credited and that the original publication in this journal is cited, in accordance with accepted academic practice. No use, distribution or reproduction is permitted which does not comply with these terms.
*Correspondence: Max E. Joffe, am9mZmVtZUB1cG1jLmVkdQ==