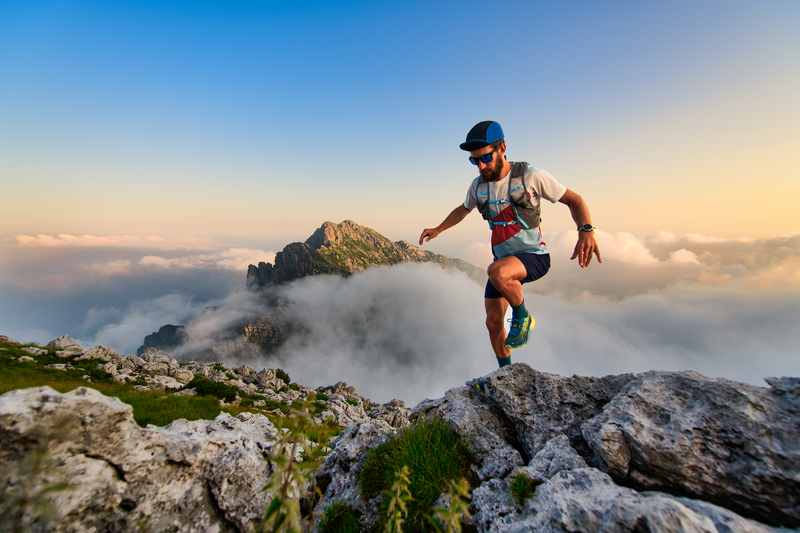
95% of researchers rate our articles as excellent or good
Learn more about the work of our research integrity team to safeguard the quality of each article we publish.
Find out more
REVIEW article
Front. Synaptic Neurosci. , 04 April 2022
Volume 14 - 2022 | https://doi.org/10.3389/fnsyn.2022.861215
This article is part of the Research Topic Molecular Nanomachines of the Presynaptic Terminal, Volume II View all 12 articles
Cyclic adenosine monophosphate (cAMP) is a crucial second messenger involved in both pre- and postsynaptic plasticity in many neuronal types across species. In the hippocampal mossy fiber (MF) synapse, cAMP mediates presynaptic long-term potentiation and depression. The main cAMP-dependent signaling pathway linked to MF synaptic plasticity acts via the activation of the protein kinase A (PKA) molecular cascade. Accordingly, various downstream putative synaptic PKA target proteins have been linked to cAMP-dependent MF synaptic plasticity, such as synapsin, rabphilin, synaptotagmin-12, RIM1a, tomosyn, and P/Q-type calcium channels. Regulating the expression of some of these proteins alters synaptic release probability and calcium channel clustering, resulting in short- and long-term changes to synaptic efficacy. However, despite decades of research, the exact molecular mechanisms by which cAMP and PKA exert their influences in MF terminals remain largely unknown. Here, we review current knowledge of different cAMP catalysts and potential downstream PKA-dependent molecular cascades, in addition to non-canonical cAMP-dependent but PKA-independent cascades, which might serve as alternative, compensatory or competing pathways to the canonical PKA cascade. Since several other central synapses share a similar form of presynaptic plasticity with the MF, a better description of the molecular mechanisms governing MF plasticity could be key to understanding the relationship between the transcriptional and computational levels across brain regions.
The molecular mechanisms of synaptic transmission have been intensely studied in recent decades, resulting in the functional characterization of many synaptic proteins involved in vesicle docking, priming, fusion and recycling. These includes the SNARE proteins, synaptotagmin, regulatory proteins, such as RAB3a, Munc13, Munc18, tomosyn, and active zone proteins, such as Piccolo, Bassoon and RIM1a, as well as several structural and endocytotic proteins (Dresbach et al., 2001; Südhof, 2013; Rizo, 2018; Chanaday et al., 2019). In many cases, the order of interactions involving these proteins, as well as their roles at different stages of the synaptic vesicle cycle, have been extensively described (Brunger et al., 2019). However, the exact contribution of these proteins to synaptic plasticity is still poorly understood. Several second messengers, such as calcium (Ca2+) and cyclic adenosine mono-phosphate (cAMP), are known to produce short- and long-term changes in vesicle release probability (Pr), the number of release sites, and the clustering of calcium channels at the presynaptic terminal. However, whereas the role of Ca2+ in regulating both pre- and postsynaptic processes has been described in detail (Zucker, 1999; Schneggenburger and Neher, 2005; Lisman et al., 2007; Kavalali, 2020) the identities of cAMP downstream effectors, especially in the presynaptic terminal, are still largely unknown.
cAMP is a ubiquitous second-messenger found in the three domains of life, namely Eukarya, Bacteria and Archaea (Gehring, 2010; Kalia et al., 2013). A direct link between cAMP, synaptic transmission and potentiation was first demonstrated in a seminal work showing that exposing sensory neurons of the sea mollusk Aplysia to cAMP molecules resulted in increased neurotransmitter release, in turn affecting a short-term memory process known as sensitization (Cedar et al., 1972; Klein and Kandel, 1980; Schacher et al., 1988; Kandel et al., 2000). Later studies extended the connection between cAMP and synaptic plasticity to other model organisms, such as Drosophila (Davis et al., 1998) and mice (Nguyen and Kandel, 1997; Wong et al., 1999), where cAMP exposure was also shown to lead to an increase in Pr (Ariel et al., 2013; Fukaya et al., 2021), vesicle redistribution (Orlando et al., 2021) and changes in Ca2+ channel clustering (Midorikawa and Sakaba, 2017).
Until recently, research into the molecular cascades activated by cAMP focused on a single downstream effector—protein kinase A (PKA) (Corbin and Krebs, 1969). PKA is a tetrameric enzyme consisting of two regulatory and two catalytic subunits (Bauman and Scott, 2002; Taylor et al., 2012). Binding of cAMP to the PKA complex results in detachment of the regulatory subunits from the catalytic subunits, thereby removing inhibition from the latter (Turnham and Scott, 2016; Mucignat-Caretta and Caretta, 2020). Subsequently, the catalytic subunits of PKA are able to phosphorylate a myriad of protein targets, thus modifying their functions (Waltereit and Weller, 2003; Kandel, 2012). In Aplysia, injection of the PKA catalytic subunits yielded similar effects to those observed following injection of cAMP alone (Castellucci et al., 1980), leading to a prevailing consensus that PKA is the principal, and perhaps sole downstream effector of cAMP. In the mammalian brain, the hippocampal mossy fiber (MF) synapse was found to be particularly prone to cAMP-mediated regulation of neurotransmitter release, as will be discussed in detail below.
The hippocampus is a central cortical structure in the mammalian brain known to mediate key mnemonic and cognitive functions. The hippocampus is classically divided into three unidirectional pathways, collectively known as the trisynaptic circuit. According to this notion of hippocampal information flow, neuronal activity originating in the adjacent entorhinal cortex (EC) is relayed via the perforant pathway (PP), primarily to the hippocampal dentate gyrus (DG), where it is processed and relayed to CA3 and CA2 pyramidal neurons via the mossy fibers (MF) pathway. From these regions, which are internally connected in an auto-associative network, the information is delivered almost exclusively to CA1 pyramidal neurons via Schaffer’s collaterals (SC), which finally redistribute the processed signals across cortical and sub-cortical regions (Figures 1A,B; Lieberman, 1965; Witter, 2007). The MF synapse, corresponding to the second synapse in this circuit, is generally considered to be an important locus for the formation, storage and retrieval of contextual and episodic memories in mammals (Lieberman, 1965; Neves et al., 2008; Lisman et al., 2017), through a computational process termed “pattern separation” (Leutgeb et al., 2007; Schmidt et al., 2012; Rolls, 2013).
Figure 1. Morphological and physiological properties of the hippocampal mossy fiber pathway. (A) Three-dimensional visualization of the anatomical location of the dorsal hippocampus in mouse brain. (B) Schematic representation of hippocampal sub-regions, with emphasis on the input and output to and from the DG. (C) Schematic representation of the unique anatomical and morphological structure of the MF-CA3 synapse. Insert: mossy fiber bouton (MFB, orange) and postsynaptic thorny excrescence (TE, light green). (D) A representative confocal image of the hippocampus following injection of AAV-DIO-EF1a-tdTomato into the DG of a Prox1-cre transgenic mouse (top). The images below show the MF tract at higher magnification (tdTomato, orange), following immunolabeling for VGluT1 (VGluT1, cyan), and demonstrate the size differences between the large MF terminals (white arrows) and the small S.R. terminals (magenta arrows). Scale bars represent 200, 10 and 2 μm for the top, right column and left column images, respectively. (E,F) MF-CA3 short-term plasticity demonstrated by measurements of paired-pulse (E) and a high-frequency burst (F) stimulation, delivered electrically to the DG while recording fEPSPs from the S.L. (G,H) MF-CA3 long-term plasticity demonstrated by measurements of FSK- (G) and tetanus- (H) induced potentiation, with subsequent application of DCG-IV, blocking synaptic transmission. (I). MF-CA3 long-term plasticity (LTD) following a prolonged low-frequency stimulation, with subsequent application of DCG-IV, blocking synaptic transmission. Images in (A) were adapted from the Allen institute’s Brain Explorer 2 (http://mouse.brain-map.org/static/brainexplorer).
The MF pathway is characterized by non-myelinated axons that originate in dentate gyrus granule cells (DGCs) and travel immediately above and below the CA3 Stratum Pyramidale (S.P.), forming the Stratum Lucidum (S.L.). There, each axon forms about a dozen enormous synapses (up to several micrometers in diameter (Rollenhagen et al., 2007), termed mossy fiber boutons (MFB), onto the large proximal dendritic spines of CA3 neurons termed thorny excrescences (TEs) (Amaral and Dent, 1981; Figures 1C,D). A single large MFB contains 25 active zones on average and harbors some 16,000 synaptic vesicles, of which only about 600 are located within 60 nm from the AZ and are considered part of the readily releasable pool of vesicles, while an additional 4,000 vesicles are found at a short distance of 200 nm from the AZ and are considered part of the recycling pool (Hallermann et al., 2003; Rollenhagen et al., 2007). Though it has been speculated that this organization might be essential for supporting the various plasticity processes taking place at the MFB (Rollenhagen et al., 2007), the reason for such extreme redundancy of synaptic vesicles remains unclear.
In contrast to its prominent size, the MF synapse is characterized by a very low basal Pr (Jonas et al., 1993) and as a result, following a single action potential (AP) elicits weak excitatory post-synaptic potentials (EPSPs) in CA3 neurons (Lysetskiy et al., 2005). However, following a short train of high-frequency APs, the accumulation of Ca2+ in the MF synapse produces a dramatic increase in Pr, manifested as robust short-term synaptic facilitation (Figures 1E,F). This facilitation, together with the strategic location of the synapse in proximity to the CA3 somata, and its multiple release sites, allow a single MF synapse to elicit APs in its postsynaptic target following a short train of APs (Henze et al., 2000; Evstratova and Tóth, 2014; Chamberland et al., 2018). This trait has led researchers to describe the MF synapse as a “conditional detonator” or a “high-pass filter,” due to the tendency of the synapse to selectively propagate high-frequency activity patterns (Pelkey and McBain, 2005; Vyleta et al., 2016).
While other hippocampal synapses have been shown to display primarily postsynaptic N-methyl-D-aspartate receptor (NMDAR)-dependent long-term potentiation (LTP) (Bliss and Collingridge, 2013), MF synapses are characterized by NMDAR-independent LTP (Zalutsky and Nicoll, 1990; Johnston et al., 1992; Huang et al., 1994; Salin et al., 1996b; Castillo, 2012). Long-term potentiation in the MF synapse (MF-LTP, Figure 1G) manifests as a long-term increase in the presynaptic Pr and is mediated by cAMP, evident by the robust potentiation observed also following application of the adenylyl cyclase (AC) agonist forskolin (FSK, Figure 1H; Weisskopf et al., 1994; Salin et al., 1996b; Villacres et al., 1998; Castillo, 2012). Using pharmacological tools that control cAMP levels, it was demonstrated that both cAMP and PKA are important for the induction and maintenance of MF-LTP (Huang et al., 1994; Weisskopf et al., 1994). These studies were followed by genetic perturbation of PKA subunits (Huang et al., 1995) that provided genetic evidence for the involvement of PKA in MF-LTP.
Like the MF-synapse, several other synapses in the mammalian brain display NMDAR independent presynaptic forms of LTP (Yang and Calakos, 2013). These include corticothalamic (Castro-Alamancos and Calcagnotto, 1999), thalamocortical (Andrade-Talavera et al., 2013), cortical interneuron (Chen et al., 2009; Sarihi et al., 2012) and subiculo-cortical synapses (Behr et al., 2009), as well as synapses of cerebellar parallel fibers (Salin et al., 1996a; Chen and Regehr, 1997; Bender et al., 2009), and several different inputs to the lateral amygdala (López de Armentia and Sah, 2007; Fourcaudot et al., 2008). However, due to vast morphological and molecular differences between these synapses and the MF, it remains to be determined exactly how similar are the molecular mechanisms that underlie their presynaptic LTP.
In addition to MF-LTP, which is induced by a short train of high-frequency activity, long-term depression in the MF synapse (MF-LTD) can also be elicited, by applying a prolonged (15 min) low-frequency stimulation (Figure 1I). Like MF-LTP, MF-LTD is also NMDAR-independent, however, it is mediated by a reduction in cAMP levels and is manifested as a decrease in Pr (Tzounopoulos et al., 1998; Kobayashi, 2010). MF-LTD induction can be blocked by the metabotropic glutamate receptor (mGluR) antagonist MCPG (Fitzjohn et al., 1998) or mGluR2/3 KO (Lyon et al., 2011). At the same time, application of the mGluR2/3 agonist DCG-IV completely blocks MF synaptic transmission synapses (Figures 1G–I), while having little to no effect on other hippocampal synapses (Yoshino et al., 1996; Kamiya and Ozawa, 1999). Since mGluR2/3 inhibits cAMP synthesis, it can be inferred that bidirectional changes in cAMP concentration control Pr at the MF synapse and can lead to LTP or LTD, depending on the direction of the change.
FSK is one of the main pharmacological tools for elevating cAMP levels that leads to synaptic potentiation. However, FSK affects both the presynaptic terminal and the postsynaptic cells, in addition to astrocytes, and is, therefore, not specific for the presynaptic terminal. New recently implemented tools, such as photoactivated adenylyl cyclase bPAC from the bacteria Beggiatoa (Stierl et al., 2011; Raffelberg et al., 2013) or pharmacogenetic tools, like Designer Receptors Exclusively Activated by Designer Drugs DREADDs (Roth, 2016; Campbell and Marchant, 2018), now enable control of cAMP levels with substantially better cellular, spatial and temporal precision than possible using pharmacological agents, such as FSK. Recently, photoactivation of a synaptically-translocated bPAC, termed SynaptoPAC, selectively in MF synapses, enabled cAMP synthesis with physiological kinetics and led to long-term changes in Pr, mimicking the effects of tetanus-induced LTP (Kees et al., 2021; Oldani et al., 2021). Such tools, together with new cAMP sensors (Odaka et al., 2014; Harada et al., 2017; Ohta et al., 2018; Linghu et al., 2020) will allow better understanding of the effects of cAMP on synaptic physiology at MF synapses.
MF synapses are characterized by additional forms of plasticity that should be briefly mentioned. Although MF-LTP is NMDA-independent, there are indications that presynaptic NMDA receptors support a different form of LTP in the MF synapse, which is dependent on Protein Kinase C (PKC) (Kwon and Castillo, 2008; Lituma et al., 2021). Furthermore, it should be noted that in addition to the large presynaptic MF terminal on pyramidal CA3 neurons, MF axons also innervate S.L. interneurons (SLINs) by small en-passant varicosities, also known as filopodial extensions (Acsády et al., 1998). These synapses on interneurons contribute to feed-forward inhibition and exhibit different forms of synaptic plasticity mediated by mGluR7 in a process only partially mediated by cAMP (Toth et al., 2000; Pelkey et al., 2008). MF-SLIN synapses also undergo cAMP-dependent plasticity changes, albeit in a unique way. These synapses reverse their polarity in an activity-dependent manner, with the internalization of mGluR7s serving as the switch between two states. In response to high frequency stimulation, naïve-state MF-IN synapses undergo cAMP-independent presynaptic LTD, whereas synapses that have internalized mGluR7s (LTD, resulting from previous high-frequency stimulation) undergo cAMP-dependent presynaptic LTP (Pelkey et al., 2008). This mechanism might allow initial bursts of APs to propagate uninhibited to CA3, yet prevents subsequent bursts from generating runaway excitation (Pelkey et al., 2008).
In addition, most previous research suggests that postsynaptic depolarization is not necessary for MF synapse LTP induction, although under certain conditions, such depolarization could still exert influence over presynaptic physiology (Castillo et al., 2012; Makani et al., 2021; Vandael et al., 2021). This implies that synchronous activation of pre- and postsynaptic neurons is not necessarily a prerequisite for this form of LTP, suggesting that MF synapse LTP is not Hebbian in nature. Together, these unique properties of the MF synapse are likely to support roles served by the hippocampus, such as the suggested role of MFs in spatial orientation through a mnemonic function known as pattern separation (Yassa and Stark, 2011; Schmidt et al., 2012; Rolls, 2013).
As in most other synapses, vesicle release at the MF terminal depends on Ca2+ influx through voltage-dependent calcium channels (VGCC), such as the P/Q-, N-, L-, and R-type calcium channels (Li et al., 2007; Vyleta and Jonas, 2014; Shin et al., 2018). In addition, the activation of presynaptic NMDA receptors during high frequency activity (Carta et al., 2018; Lituma et al., 2021) and release of Ca2+ from internal stores (Lauri et al., 2003; Scott and Rusakov, 2006) also contribute to Ca2+ dynamics at the MF synapse. These dynamic changes in presynaptic calcium drive MF short- and long-term synaptic plasticity (Castillo et al., 1994; Regehr et al., 1994; Kapur et al., 1998; Breustedt et al., 2003; Pelkey et al., 2006; Li et al., 2007; Vyleta and Jonas, 2014). In a mature MFB, it was demonstrated that synaptic vesicles are only loosely coupled to Ca2+ channels, allowing for a highly dynamic Pr range (Vyleta and Jonas, 2014; Böhme et al., 2018; Brockmann et al., 2019; Orlando et al., 2021). Changes in distances between calcium channels and the synaptic machinery, in channel density, and/or in concentrations of endogenous calcium buffers can enable rapid and dynamic modulation of synaptic strength, as discussed below.
In addition to its direct effects on vesicle fusion and neurotransmitter release, Ca2+ also exerts a powerful effect on MF synaptic strength through the Ca2+ sensor calmodulin and activation of AC, leading to increased levels of cAMP. Numerous studies have pointed to cAMP as the primary mediator of MF-LTP, given how the application of the potent AC agonist FSK or of cAMP analogs, such as Sp-8-CPT-cAMPs, elicit a strong and sustained increase in the basal Pr (Weisskopf et al., 1994; Lonart et al., 1998; Tzounopoulos et al., 1998; Wang et al., 2003; Kaeser-Woo et al., 2013; Hashimotodani et al., 2017). In the mammalian brain, ten different isoforms of the Adcy gene encode for ten distinct AC enzymes (AC1-10). These are classified primarily according to their main upstream activator (Hanoune and Defer, 2001) and are differentially distributed across brain regions and cell types (Sanabra and Mengod, 2011). In the mouse hippocampus, Adcy isoforms 1 and 2 are strongly and selectively expressed in the DG but not in CA3 neurons (Figures 2A,B), and while KO of Adcy8 was shown to affect MF synaptic plasticity (Wang et al., 2003), comparatively low mRNA levels in the DG (Figures 2A,B) suggest that further experiments are needed to validate these results. Of the two most abundant AC isoforms in the DG, AC1 is known to be activated by an increase in Ca2+ and its downstream effector calmodulin (Hanoune and Defer, 2001), whereas AC2 is largely Ca2+-insensitive and is instead activated by PKC and the Gq protein-associated βγ subunit complex (Hanoune and Defer, 2001; Willoughby and Cooper, 2007; Halls and Cooper, 2011). Alongside these basic properties, additional evidence further supports a central role for AC1 in the MF synapse. First, AC1 is inhibited by the Gi cascade, such as that associated with mGluR2/3, leading to a reduction in cAMP levels (Sadana and Dessauer, 2009), an effect which potentially underlies the complete silencing of MF synaptic transmission following application of DCG-IV. Furthermore, Adcy1 KO mice were found to exhibit impaired MF-LTP but not PP-LTP and while MF short-term plasticity and FSK-induced potentiation were not altered in Adcy1 KO mice (Villacres et al., 1998; Table 1), this effect could have still been mediated by other AC isoforms, being that FSK is not a selective AC1 agonist. Last, immunolabeling of AC1 in the hippocampus revealed it to be enriched in the hilus and mossy fibers (Conti et al., 2007), implying that AC1 is preferentially trafficked to MF synaptic domains.
Figure 2. Expression of AC isoforms across hippocampal sub-regions. (A) Distribution pattern of the ten AC isoforms in the hippocampus of adult mice following in situ hybridization (Lein et al., 2007). (B) A heat map showing the relative mRNA expression levels of Adcy isoforms 1–9 across different hippocampal sub-regions. fpkm—fragments per kilobase of transcript per million mapped reads (Cembrowski et al., 2016). (C) as in (B), a heat map showing relative Adcy isoform 1–9 mRNA expression levels for the main cell clusters across several central brain regions. The right-most column specifies for which cell clusters evidence supports the existence of presynaptic LTP (Saunders et al., 2018). Data in (A) were adapted from the Allen institutes ISH brain atlas (http://mouse.brain-map.org/), data in (B) were adapted from hipposeq.janelia.org/and data in (C) were adapted from Dropviz.org.
Together, these observations suggest that AC1 is the dominant isoform mediating Ca2+ dependent increases, or mGluR2/3-dependent decreases in cAMP levels, to control MF synaptic plasticity. Interestingly, the distribution of AC1 mRNA across brain regions reveals strong correlation between cell types in which Adcy1 is enriched, with such synapses having been previously shown to express a presynaptic form of LTP (Figure 2C). This correlation can potentially support the notion that shared molecular mechanisms, driven by up-stream AC1 activation, underlies presynaptic LTP across cell types. In the hippocampus, this conjuncture could apply to neurons of the CA2 sub-region, in which Adcy1 is also enriched. While previous studies suggested that CA2 neurons do not display any form of postsynaptic plasticity (Zhao et al., 2007), the nature of the presynaptic mechanisms at play in their synapses onto CA1 neurons, corresponding to their principal output (Kohara et al., 2014), have not yet been investigated. Further experiments are required to determine whether cAMP- and PKA-dependent presynaptic LTP can also be induced there, and if so, one can ask what sort of functional relevance this might have on information processing in this pathway.
In addition to the Ca2+-mediated activation of AC1, increased cAMP levels could also potentially arise from direct activation of AC2 and AC9 through the alpha subunit of Gs protein-coupled receptors (Hanoune and Defer, 2001), such as group 1 serotonin receptors (Hamblin et al., 1998), the beta sub-class of noradrenaline receptors (Johnson, 2006) and dopamine D1-like receptors (Girault and Greengard, 2004). In contrast, other Gi protein-coupled neuromodulator receptors, such as group 1 and 5 serotonin receptors, the alpha2 sub-class of noradrenergic receptors and the dopamine D2 receptor, would have an opposite effect on cAMP production, potentially supporting MF-LTD. It has previously been shown that neuromodulators can indeed exert effects on MF synaptic transmission in various manners (Yang and Calakos, 2013; Nozaki et al., 2016; Kobayashi et al., 2020). This contradictory mode of action of different receptors in response to the same ligand and their differential expression patterns across hippocampal sub-regions make it difficult to determine what would be the net effect of each receptor on synaptic transmission and plasticity. In addition, it has yet to be shown where these receptors are trafficked within the cells and whether they are enriched in presynaptic domains. Such knowledge is essential for determining whether neuromodulators could have a direct effect on neurotransmitter release at the MF-CA3 synapse, or whether the observed effects of neuromodulator application during recordings arise from changes in DGC somatic excitability or from various influences on CA3 postsynaptic domains. The use of specific cAMP sensors (Odaka et al., 2014; Harada et al., 2017; Ohta et al., 2018; Linghu et al., 2020) that can be targeted to the synapse will allow characterization of the spatiotemporal distribution of cMAP and can clarify the above debates.
As mentioned above, MF-LTP depends on cAMP levels, with this effect having largely been attributed to downstream activation of PKA. This assessment was further supported by the observation that LTP is absent following KO of the PKA catalytic subunit-encoding gene (Huang et al., 1995) and that application of KT5720, a selective blocker of the PKA catalytic subunit, prevents MF-LTP (Weisskopf et al., 1994). In addition, it was suggested that FSK-induced potentiation interacts with LTP, as the two processes were shown to mutually occlude one another (Weisskopf et al., 1994). These findings led to the suggestion that activation of PKA is an essential step in MF-LTP. Accordingly, research conducted in recent decades identified several putative synaptic PKA targets thought to be involved in cAMP-dependent synaptic plasticity at MF synapses. These include RAB3a-interacting molecule 1a (RIM1a) and rabphilin, both acting through the vesicular GTPase Ras-related protein Rab-3A (RAB3a) (Takai et al., 1996; Wang et al., 2001), as well as synapsin (Bykhovskaia, 2011), synaptotagmin-12 (Maximov et al., 2007), and tomosyn-1 (Ashery et al., 2009). The involvement of these proteins in cAMP-dependent plasticity was mainly studied by examining the effects of deletion or mutations in each of the encoding genes on short-term plasticity (STP), FSK-induced potentiation, LTP and LTD (Table 1 and Figure 3).
Figure 3. cAMP cascades and cAMP-dependent synaptic plasticity in the MF synapse. (A) Cascades of activation of cAMP, PKA, and Epac2 in the MF terminal. A train of action potentials arriving at the MF synapse triggers a calcium influx through voltage-dependent calcium channels and subsequent activation of CaM that activates cAMP synthesis by AC1. AC1 acts on PKA or Epac2 to up regulate synaptic transmission (dashed green arrows between vesicles). Application of FSK activates both AC1 and AC2 and elevates cAMP. AC2 is also activated by PKC following the activation by a G-coupled protein. Application of DCG-IV, an agonist to mGluR2/3, leads to inhibition of AC1. Green arrows: activation, red arrow: inhibition, blue arrows: application of pharmacological reagents. (B) Downstream PKA cascades and their relation to synaptic plasticity. PKA activity is dependent on the anchoring protein AKAP7, and is inhibited by the negative regulator PKAα. PKA, in turn, phosphorylates multiple proteins including synapsin, rabphilin, tomosyn-1, synaptotagmin12 (Syt12) and RIM1a. Of these, deletion of synapsin and rabphilin does not impair MF synaptic plasticity. Ablation of RAB3A, although not a direct PKA target, and RIM1a abolishes MF-LTP but does not affect STP and FSK-induced potentiation (FIP). Synaptotagmin-12 KO abolishes MF-LTP and impairs FSK-induced potentiation but do not affect STP, while deletion of tomosyn-1 abolishes MF-LTP, impairs FSK-induced potentiation and also affects STP. Epac2 manipulation also abolishes LTP and impairs FSK-induced potentiation. STP- short-term potentiation, LTP- long-term potentiation, FIP- FSK-induced potentiation. Orange arrow represent PKA-independent pathway, Green arrows shades represent different pathways.
Earlier efforts paid substantial attention to the small vesicular GTPase RAB3a, which is seemingly involved in vesicle mobilization and fusion (Geppert et al., 1997; Lonart and Südhof, 1998). While RAB3a is not considered a putative PKA target, it is known to be regulated by other PKA targets, such as RIM1a and rabphilin and has, therefore, been linked to the PKA-mediated cascade. RAB3a KO was shown to block LTP, impair LTD formation in MF synapse and led to memory impairment (D’Adamo et al., 2004), although neither STP nor FSK-induced potentiation were affected (Castillo et al., 1997). These effects were thought to be the result of an enhanced effect of Ca2+ on the secretory apparatus (Lonart et al., 1998), although the precise mechanism involved was not identified.
RIM1a is an active zone protein and putative PKA target (Wang et al., 1997; Castillo et al., 2002; Lonart et al., 2003). Similar to what was seen in the absence of RAB3a, KO of RIM1a also prevented MF-LTP but not FSK-induced potentiation or STP (Castillo et al., 2002; Figure 3 and Table 1), suggesting a direct link between PKA, RIM1a, RAB3a, and MF-LTP. However, this effect appears to be PKA-independent, as RIM1aS413A, an isoform that carries a point mutation preventing RIM1a phosphorylation by PKA, was found to have no impact on either short- or long-term plasticity, nor on behavior (Kaeser et al., 2008). Hence, despite the dependence of LTP on RIM1a, the link through PKA is less defined and suggests that PKA operates either through a different mechanism, or via yet another unidentified parallel pathway/s.
Rabphilin, another RAB3a effector, regulates vesicle priming through interactions with RAB3a and the SNARE protein SNAP-25, with the former occurring in a GTP-dependent manner (Tsuboi and Fukuda, 2005; Deák et al., 2006). Rabphilin was found to be phosphorylated following application of FSK in the MF but not in the SC synapse (Lonart and Südhof, 1998), highlighting that significant differences exist in the molecular mechanisms underlying synaptic plasticity between these different hippocampal regions. However, KO of the rph3a gene encoding for rabphilin did not change either short-term facilitation or MF-LTP at the MF terminal (Schlüter et al., 1999; Figure 3 and Table 1).
In addition to the RAB3a cascade, synapsin was considered as another candidate PKA downstream effector. Synapsins are among the most abundant synaptic proteins that are known to be phosphorylated by PKA, and are involved in both synaptic transmission and plasticity (De Camilli and Greengard, 1986; Gitler et al., 2004). Accordingly, synapsins were one of the first synaptic protein families to be examined as targets for cAMP and PKA-dependent plasticity at the MF. Synapsin binds synaptic vesicles and tethers them to the cytoskeleton, yet can release these vesicles following phosphorylation by PKA, enabling their translocation to the active zone in preparation for exocytosis (De Camilli et al., 1983; Spillane et al., 1995). A triple KO of all three synapsin isoform-encoding genes led to impaired plasticity in primary hippocampal neuronal cultures, with such changes being associated with PKA activity (Cheng et al., 2018). However, in the MF pathway of acute hippocampal slices, a double KO of the syn1 and syn2 genes, encoding the main isoforms expressed in CNS neurons, did not alter cAMP-dependent LTP or FSK-induced potentiation (Figure 3B and Table 1; Spillane et al., 1995). While further research is required to reconcile these contradicting results, these findings suggest that phosphorylation of synapsin1 and synapsin2 by PKA is unlikely to be required for cAMP-dependent MF synaptic plasticity.
More recent studies have examined other synaptic proteins thought to be involved in PKA-dependent LTP at the MF synapse. Synaptotagmin-12 differs from other synaptotagmins in that it does not bind Ca2+ (Wolfes and Dean, 2020). However, synaptotagmin-12 is phosphorylated by PKA and is involved in the regulation of synaptic vesicles fusion and Pr (Maximov et al., 2007). Synaptotagmin-12S97A mutant mice, in which the site of PKA-mediated phosphorylation was mutated, displayed impaired MF-LTP and FSK-induced potentiation, although their STP remained unaltered (Kaeser-Woo et al., 2013). Consequently, synaptotagmin-12 can be regarded as the first protein shown to affect both LTP and FSK-induced potentiation in a PKA-dependent manner.
Tomosyn is a PKA-dependent negative regulator of Pr and its over-expression leads to an inhibition of vesicle priming, while knockdown, knockout, or mutations in the tomosyn-1-encoding gene led to an enhancement of vesicle fusion (Yizhar et al., 2004; Chevlet et al., 2006; Gracheva et al., 2006; McEwen et al., 2006; Ben-Simon et al., 2015). Tomosyn-1, which was shown to be enriched in the MF pathway (Barak et al., 2010, 2013), can be phosphorylated by PKA at Ser-724 (Baba et al., 2005) and its acute down-regulation in the MF synapse leads to reductions in both LTP and FSK-induced potentiation (Ben-Simon et al., 2015). Interestingly, this manipulation also strongly reduced MF facilitation and STP. These effects are likely the result of an increase in the basal Pr following down-regulation of tomosyn-1, which also led to occlusion of both potentiation and facilitation. Although acute KD of tomosyn reduced both LTP and FSK-induced potentiation (Ben-Simon et al., 2015), a direct link between PKA-driven tomosyn-1 phosphorylation and synaptic plasticity has yet to be demonstrated.
Although FSK-induced potentiation and MF-LTP are thought to be interlinked and are driven by cAMP elevation (Weisskopf et al., 1994), deletion of most PKA-dependent synaptic proteins abolished only MF-LTP and not FSK-induced potentiation. This could suggest the presence of additional parallel cAMP-dependent pathways that are not fully PKA-dependent. It is also possible that high frequency stimulation-induced LTP and FSK affect downstream events with different cAMP-mediated dynamics, which would differently affect MF-LTP and FSK-induced potentiation. However, the same does not apply to the loss of synaptic proteins, such as of tomosyn-1 or synaptotagmin-12, which impaired both MF-LTP and FSK-induced potentiation (Kaeser-Woo et al., 2013; Ben-Simon et al., 2015). It is reasonable to assume that the impact of the loss of tomosyn-1 on MF-LTP and FSK-induced potentiation can be explained through an increase in basal Pr that is further translated into a reduction in synaptic potentiation. What is clear is that further studies are needed to characterize the exact contributions of these and other synaptic proteins and pathways to the cAMP dependent plasticity of the MF.
Additional proteins that interact with PKA, like PKAα and AKAP7, have also been shown to affect MF plasticity. PKAα is a PKA inhibitor whose activity decreases following synaptic stimulation, leading to a relief of PKA inhibition during neuronal activity. Moreover, blocking this pathway with anti-sense oligonucleotides to PKAα resulted in elimination of MF-LTP in the synapse (De Lecea et al., 1998). AKAP7 is a PKA-anchoring protein also essential for the function of PKA. Ablation of AKAP7 results in even more pronounced effects than those seen with PKAα inhibition, specifically, not only the elimination of MF-LTP but also various measurable behavioral deficits (Huang et al., 1995; Jones et al., 2016; Figure 3B and Table 1).
Recent results obtained with isolated MF terminals indicate that cAMP can directly affect Pr via regulation of Ca2+ signaling (Midorikawa and Sakaba, 2017; Fukaya et al., 2021). Applying cAMP to isolated MF terminals increased Pr, although the number of vesicles in the readily releasable pool and replenishment of this pool following depletion remained unchanged. It has been suggested that this increase in Pr is associated with changes in the physical coupling between of P/Q-type Ca2+ channels and readily releasable vesicles (Midorikawa and Sakaba, 2017), which can alter the synaptic properties at the terminal (Bucurenciu et al., 2008; Vyleta and Jonas, 2014). As such, following an action potential, Ca2+ concentrations near release sites are expected to be higher, thereby leading to synaptic potentiation. Such molecular rearrangements were recently shown to occur within 5–10 min following FSK application (Fukaya et al., 2021).
A recent paper showed that FSK-induced potentiation was associated with fast remodeling of MF synapse presynaptic ultrastructure. However, the distance between P/Q-type calcium channels and Munc13–1 as a proxy for the release machinery was not altered and was found to be 65 nm (Orlando et al., 2021). On the other hand, FSK-induced potentiation was associated with synaptic vesicle accumulation at active zones, increases in the numbers of docked and tethered vesicles and an overall increase in the number of active AZs. In addition, vesicles were more dispersed, possibly mediated by PKA phosphorylation of synapsin (Sihra et al., 1989; Pechstein et al., 2020), allowing for the mobilization of vesicles into the readily releasable vesicle pool. A similar increase in the number of docked vesicles was recently suggested to take place following high-frequency stimulation of MF synapses (Vandael et al., 2020) and was referred as a “pool engram,” enabling post-tetanic potentiation. Reorganization of active zone and changes in the recycling vesicle pool also occurs after FSK-induced LTP at CA3-CA1 synapses and might represent a basic mechanism of presynaptic LTP in central synaptic synapses (Rey et al., 2020) and in the Drosophila neuromuscular junction (Weyhersmüller et al., 2011). Such processes are likely to be mediated by AZ proteins, such as RIM1a that interacts with Munnc13–1, or Rab3A together with calcium channels (Betz et al., 2001; Schoch et al., 2002; Han et al., 2011; Kaeser et al., 2011; Eggermann et al., 2012), via RIM-BP2 that stabilizes Munc13-1 protein clusters at MF AZs channels (Brockmann et al., 2019), or via synapsin phosphorylation. However, as mentioned above, PKA-mediated phosphorylation of RIM1a was shown to have no effect on MF synaptic transmission (Kaeser et al., 2008), while synapsin KO had no effect on MF-LTP. Additional super-resolution microscopy methods that can inspect organizational changes at the 10–20 nm range can resolve how the release machinery, AZ and calcium channels are restructured following elevations in cAMP levels.
PKA is considered by many to be a master regulator of synaptic plasticity in the hippocampal MF synapse, as well as in many other synapses (Waltereit and Weller, 2003). Consequently, other than the intensive studies conducted on the involvement of PKA targets in MF-LTP, only few studies have considered other possible cAMP-dependent pathways. One such example is a study that linked Epac2, a member of a family of cAMP-dependent guanine nucleotide exchange proteins (GEFs), to synaptic plasticity at the MF synapse (Fernandes et al., 2015). The two cAMP-dependent GEFs, Epac1, and Epac2 (encoded by the Rapgef3 and Rapgef4 genes, respectively), were linked to several cAMP-dependent processes (Gloerich and Bos, 2010), and it was further suggested that these proteins are relevant to synaptic plasticity processes (De Rooij et al., 1998; Kawasaki et al., 1998; Fernandes et al., 2015). Epac was shown to activate a MAP-kinase called p38 (Figure 4) in cerebellar and hippocampal neurons and this was correlated with modulation of neuronal excitability (Ster et al., 2007, 2009). In a separate study, several experiments have demonstrated that blocking Epac2 signaling led to reduced levels of p38 phosphorylation (Emery et al., 2014). This suggests that Epac2 responds to cAMP by activating ERK and the small GTPase Rap, as well as its downstream kinase p38-MAPK, thereby bypassing the canonical PKA cascade (Figure 4). Epac2 plays a crucial role in MF-LTP, as KO of the encoding gene led to deficits in MF-LTP and impaired FSK-induced potentiation without affecting basal synaptic properties, as measured by changes in STP (Fernandes et al., 2015; Figure 3B and Table 1).
Figure 4. Model of molecular mechanisms of cAMP-dependent LTP. Three cAMP-dependent effectors, Epac, PKA, and Rapgef2, give rise to three parallel molecular pathways. Each of the three cAMP-dependent molecular pathways include a transcription factor that upon activation leads to long-term effects on gene regulation that support synaptic plasticity.
More recently, an additional member of the RAPGEF family, RapGEF2, was hypothesized to participate in neuronal and synaptic processes. Due to its unconventional cAMP-binding motif, RapGEF2 was long considered not to be a cAMP sensor (Liao et al., 1999; Kuiperij et al., 2003; Kannan et al., 2007). However, more recent studies have shown that a specific RAPGEF2 isoform expressed exclusively in neurons and endocrine cells, termed NCS-Rapgef2, can phosphorylate ERK in a cAMP-dependent manner in these cells (Emery et al., 2013; Figure 4). In neurons, this observed effect was modulated by activation of the dopamine receptor D1 (DRD1), potentially modulating postsynaptic sensitivity in response to dopamine release as well as in a dopamine receptor D1 (DRD1)-dependent manner (Jiang et al., 2017). In addition, KO of NCS-Rapgef2 in DRD1+ medium spiny neurons (MSN) of the nucleus accumbens was shown to have behavioral consequences, resulting in impairments in cocaine-induced locomotor sensitization (CILS) and in conditioned place preference (Jiang et al., 2021). Interestingly, CILS was previously shown to require ERK-dependent DRD1-MSN neuroplasticity (Ferguson et al., 2006; Girault et al., 2007), further supporting the contribution of ERK-dependent pathways and the possible involvement of NCS-RAPGEF2 in neuroplasticity. Still, the involvement of NCS-Rapgef2 in presynaptic plasticity has yet to demonstrated.
Based on current understanding, we can assume the existence of at least three main cAMP-dependent molecular pathways relevant to synaptic plasticity. These can be described according to the proteins directly activated by cAMP, and by the principal kinases or RAPGEFs downstream of these proteins, which execute many of the regulatory functions ascribed to their respective pathways. The three principal pathways are PKA → CREB, Epac → p38 and Rapgef2 → Erk (Figure 4). These pathways can work independently but can also interact and influence one another (Emery et al., 2014, 2016, 2017). Lastly, these molecular pathways can activate plasticity processes that differ in several aspects, such as the locus of plastic changes (e.g., pre- vs. postsynaptic plasticity), resistance to pharmacological compounds, and even associated behavioral processes (Morozov et al., 2003; Fernandes et al., 2015).
In summary, the presynaptic forms of LTP and LTD that transpire in the hippocampal MF-CA3 synapse are thought to depend on cAMP-PKA-dependent cascades. Various synaptic proteins have been linked to these cascades, and manipulations of several of these proteins, such as RAB3a, RIM1a, synaptotagmin-12, and tomosyn-1, are crucial for signaling along these cascades. Interestingly, manipulations of these proteins mostly impact MF-LTP formation and only in some cases, FSK-induced potentiation or STP. This indicates the strong possibility of the existence of several parallel cAMP-dependent cascades, which are still only partially understood. Mechanistically, elevation of cAMP in the MF synapse involves the rearrangement of synaptic vesicles near release sites and the clustering of calcium channels near the release machinery, which lead to synaptic potentiation. Adopting additional methods that can detect nanometric-level organizational changes in the AZ will help resolve how the release machinery is restructured following elevation in cAMP with more detail. Such changes in synaptic plasticity can be mediated via other PKA targets or via non-canonical PKA-independent but cAMP-dependent pathways, like those involving the RAPGEF family of proteins. Combinations of cell type-specific manipulation of specific proteins, along with synapse-specific manipulation of cAMP by either optogenetic or pharmacogenetic approaches, as well as the ability to measure cAMP levels in response to natural stimulation patterns, will allow a more detailed understanding of the relationship between cAMP and synaptic plasticity at the MF synapse. Such findings could potentially be extrapolated to other synapses, which bear similar physiological hallmarks, to expand our understanding of the links between cellular transcriptomics, synaptic physiology and behavior.
All authors contributed to discussion, writing, and editing the manuscript.
This work was supported by the Israel Science Foundation (ISF grant 2141/20) to UA.
The authors declare that the research was conducted in the absence of any commercial or financial relationships that could be construed as a potential conflict of interest.
All claims expressed in this article are solely those of the authors and do not necessarily represent those of their affiliated organizations, or those of the publisher, the editors and the reviewers. Any product that may be evaluated in this article, or claim that may be made by its manufacturer, is not guaranteed or endorsed by the publisher.
Acsády, L., Kamondi, A., Sík, A., Freund, T., and Buzsáki, G. (1998). GABAergic cells are the major postsynaptic targets of mossy fibers in the rat hippocampus. J. Neurosci. 18, 3386–3403. doi: 10.1523/jneurosci.18-09-03386.1998
Amaral, D. G., and Dent, J. A. (1981). Development of the mossy fibers of the dentate gyrus: I. A light and electron microscopic study of the mossy fibers and their expansions. J. Comp. Neurol. 195, 51–86. doi: 10.1002/cne.901950106
Andrade-Talavera, Y., Duque-Feria, P., Sihra, T. S., and Rodríguez-Moreno, A. (2013). Pre-synaptic kainate receptor-mediated facilitation of glutamate release involves PKA and Ca2+-calmodulin at thalamocortical synapses. J. Neurochem. 126, 565–578. doi: 10.1111/jnc.12310
Ariel, P., Hoppa, M. B., and Ryan, T. A. (2013). Intrinsic variability in Pv, RRP size, Ca2+ channel repertoire, and presynaptic potentiation in individual synaptic boutons. Front. Synaptic Neurosci. 4:9. doi: 10.3389/fnsyn.2012.00009
Ashery, U., Bielopolski, N., Barak, B., and Yizhar, O. (2009). Friends and foes in synaptic transmission: the role of tomosyn in vesicle priming. Trends Neurosci. 32, 275–282. doi: 10.1016/j.tins.2009.01.004
Baba, T., Sakisaka, T., Mochida, S., and Takai, Y. (2005). PKA-catalyzed phosphorylation of tomosyn and its implication in Ca2+-dependent exocytosis of neurotransmitter. J. Cell Biol. 170, 1113–1125. doi: 10.1083/jcb.200504055
Barak, B., Okun, E., Ben-Simon, Y., Lavi, A., Shapira, R., Madar, R., et al. (2013). Neuron-specific expression of tomosyn1 in the mouse hippocampal dentate gyrus impairs spatial learning and memory. Neuromolecular Med. 15, 351–363. doi: 10.1007/s12017-013-8223-4
Barak, B., Williams, A., Bielopolski, N., Gottfried, I., Okun, E., Brown, M. A., et al. (2010). Tomosyn expression pattern in the mouse hippocampus suggests both presynaptic and postsynaptic functions. Front. Neuroanat. 4:149. doi: 10.3389/fnana.2010.00149
Bauman, A. L., and Scott, J. D. (2002). Kinase- and phosphatase-anchoring proteins: harnessing the dynamic duo. Nat. Cell Biol. 4, E203–E206. doi: 10.1038/ncb0802-e203
Behr, J., Wozny, C., Fidzinski, P., and Schmitz, D. (2009). Synaptic plasticity in the subiculum. Prog. Neurobiol. 89, 334–342. doi: 10.1016/J.PNEUROBIO.2009.09.002
Bender, V. A., Pugh, J. R., and Jahr, C. E. (2009). Presynaptically expressed long-term potentiation increases multivesicular release at parallel fiber synapses. J. Neurosci. 29, 10974–10978. doi: 10.1523/JNEUROSCI.2123-09.2009
Ben-Simon, Y., Rodenas-Ruano, A., Alviña, K., Lam, A. D., Stuenkel, E. L., Castillo, P. E., et al. (2015). A Combined Optogenetic-Knockdown Strategy Reveals a Major Role of Tomosyn in Mossy Fiber Synaptic Plasticity. Cell Rep. 12, 396–404. doi: 10.1016/j.celrep.2015.06.037
Betz, A., Thakur, P., Junge, H. J., Ashery, U., Rhee, J. S., Scheuss, V., et al. (2001). Functional interaction of the active zone proteins Munc13-1 and RIM1 in synaptic vesicle priming. Neuron 30, 183–196. doi: 10.1016/S0896-6273(01)00272-0
Bliss, T. V. P., and Collingridge, G. L. (2013). Expression of NMDA receptor-dependent LTP in the hippocampus: bridging the divide. Mol. Brain 6:5. doi: 10.1186/1756-6606-6-5
Böhme, M. A., Grasskamp, A. T., and Walter, A. M. (2018). Regulation of synaptic release-site Ca2+ channel coupling as a mechanism to control release probability and short-term plasticity. FEBS Lett. 592, 3516–3531. doi: 10.1002/1873-3468.13188
Breustedt, J., Vogt, K. E., Miller, R. J., Nicoll, R. A., and Schmitz, D. (2003). α1E-Containing Ca2+ channels are involved in synaptic plasticity. Proc. Natl. Acad. Sci. U. S. A. 100, 12450–12455. doi: 10.1073/pnas.2035117100
Brockmann, M. M., Maglione, M., Willmes, C. G., Stumpf, A., Bouazza, B. A., Velasquez, L. M., et al. (2019). RIM-BP2 primes synaptic vesicles via recruitment of Munc13-1 at hippocampal mossy fiber synapses. Elife 8:e43243. doi: 10.7554/eLife.43243
Brunger, A. T., Choi, U. B., Lai, Y., Leitz, J., White, K. I., and Zhou, Q. (2019). The pre-synaptic fusion machinery. Curr. Opin. Struct. Biol. 54, 179–188. doi: 10.1016/j.sbi.2019.03.007
Bucurenciu, I., Kulik, A., Schwaller, B., Frotscher, M., and Jonas, P. (2008). Nanodomain coupling between Ca2+ channels and Ca2+ sensors promotes fast and efficient transmitter release at a cortical GABAergic synapse. Neuron 57, 536–545. doi: 10.1016/j.neuron.2007.12.026
Bykhovskaia, M. (2011). Synapsin regulation of vesicle organization and functional pools. Semin. Cell Dev. Biol. 22, 387–392. doi: 10.1016/J.SEMCDB.2011.07.003
Campbell, E. J., and Marchant, N. J. (2018). The use of chemogenetics in behavioural neuroscience: receptor variants, targeting approaches and caveats. Br. J. Pharmacol. 175, 994–1003. doi: 10.1111/bph.14146
Carta, M., Srikumar, B. N., Gorlewicz, A., Rebola, N., and Mulle, C. (2018). Activity-dependent control of NMDA receptor subunit composition at hippocampal mossy fibre synapses. J. Physiol. 596, 703–716. doi: 10.1113/JP275226
Castellucci, V. F., Kandel, E. R., and Schwartz, J. H. (1980). Intracellular injection of the catalytic subunit of cyclic AMP-dependent protein kinase simulates facilitation of transmitter release underlying behavioral sensitization in Aplysia. Proc. Natl. Acad. Sci. U. S. A. 77, 7492–7496. doi: 10.1073/pnas.77.12.7492
Castillo, P. E. (2012). Presynaptic LTP and LTD of excitatory and inhibitory synapses. Cold Spring Harb. Perspect. Biol. 4:a005728. doi: 10.1101/cshperspect.a005728
Castillo, P. E., Schoch, S., Schmitz, F., Sudhof, T. C., and Malenka, R. C. (2002). RIM1 alpha is required for presynaptic long-term potentiation. Nature 415, 327–330. doi: 10.1038/415327a
Castillo, P. E., Weisskopf, M. G., and Nicoll, R. A. (1994). The role of Ca2+ channels in hippocampal mossy fiber synaptic transmission and long-term potentiation. Neuron 12, 261–269. doi: 10.1016/0896-6273(94)90269-0
Castillo, P. E., Younts, T. J., Chávez, A. E., and Hashimotodani, Y. (2012). Endocannabinoid signaling and synaptic function. Neuron 76, 70–81. doi: 10.1016/J.NEURON.2012.09.020
Castillo, P. E. E., Janz, R., Südhof, T. C., Tzounopoulos, T., Malenka, R. C. C., Nicoll, R. A. A., et al. (1997). Rab3A is essential for mossy fibre long-term potentiation in the hippocampus. Nature 388, 590–593. doi: 10.1038/41574
Castro-Alamancos, M. A., and Calcagnotto, M. E. (1999). Presynaptic Long-Term Potentiation in Corticothalamic Synapses. J. Neurosci. 19, 9090–9097. doi: 10.1523/JNEUROSCI.19-20-09090.1999
Cedar, H., Kandel, E. R., and Schwartz, J. H. (1972). Cyclic Adenosine Monophosphate in the Nervous System of Aplysia californica?: I. Increased synthesis in response to synaptic stimulation. J. Gen. Physiol. 60, 558–569. doi: 10.1085/jgp.60.5.558
Cembrowski, M. S., Wang, L., Sugino, K., Shields, B. C., and Spruston, N. (2016). Hipposeq: a comprehensive RNA-seq database of gene expression in hippocampal principal neurons. eLife 5:e14997. doi: 10.7554/eLife.14997
Chamberland, S., Timofeeva, Y., Evstratova, A., Volynski, K., and Tóth, K. (2018). Action potential counting at giant mossy fiber terminals gates information transfer in the hippocampus. Proc. Natl. Acad. Sci. U. S. A. 115, 7434–7439. doi: 10.1073/PNAS.1720659115
Chanaday, N. L., Cousin, M. A., Milosevic, I., Watanabe, S., and Morgan, J. R. (2019). The synaptic vesicle cycle revisited: new insights into the modes and mechanisms. J. Neurosci. 39, 8209–8216. doi: 10.1523/JNEUROSCI.1158-19.2019
Chen, C., and Regehr, W. G. (1997). The mechanism of cAMP-mediated enhancement at a cerebellar synapse. J. Neurosci. 17, 8687–8694. doi: 10.1523/jneurosci.17-22-08687.1997
Chen, H.-X., Jiang, M., Akakin, D., and Roper, S. N. (2009). Long-Term Potentiation of Excitatory Synapses on Neocortical Somatostatin-Expressing Interneurons. J. Neurophysiol. 102, 3251–3259. doi: 10.1152/JN.00641.2009
Cheng, Q., Song, S. H., and Augustine, G. J. (2018). Molecular mechanisms of short-term plasticity: role of synapsin phosphorylation in augmentation and potentiation of spontaneous glutamate release. Front. Synaptic Neurosci. 10:33. doi: 10.3389/fnsyn.2018.00033
Chevlet, S., Bezzi, P., Ivarsson, R., Renström, E., Viertl, D., Kasas, S., et al. (2006). Tomosyn-1 is involved in a post-docking event required for pancreatic β-cell exocytosis. J. Cell Sci. 119, 2912–2920. doi: 10.1242/jcs.03037
Conti, A. C., Maas, J. W., Muglia, L. M., Dave, B. A., Vogt, S. K., Tran, T. T., et al. (2007). Distinct regional and subcellular localization of adenylyl cyclases type 1 and 8 in mouse brain. Neuroscience 146, 713–729. doi: 10.1016/J.NEUROSCIENCE.2007.01.045
Corbin, J. D., and Krebs, E. G. (1969). A cyclic AMP–stimulated protein kinase in adipose tissue. Biochem. Biophys. Res. Commun. 36, 328–336. doi: 10.1016/0006-291X(69)90334-9
D’Adamo, P., Wolfer, D. R., Kopp, C., Tobler, I., Toniolo, D., and Lipp, H. P. (2004). Mice deficient for the synaptic vesicle protein Rab3a show impaired spatial reversal learning and increased explorative activity but none of the behavioral changes shown by mice deficient for the Rab3a regulator Gdi1. Eur. J. Neurosci. 19, 1895–1905. doi: 10.1111/J.1460-9568.2004.03270.X
Davis, G. W., DiAntonio, A., Petersen, S. A., and Goodman, C. S. (1998). Postsynaptic PKA Controls Quantal Size and Reveals a Retrograde Signal that Regulates Presynaptic Transmitter Release in Drosophila. Neuron 20, 305–315. doi: 10.1016/S0896-6273(00)80458-4
López de Armentia, M. L., and Sah, P. (2007). Bidirectional synaptic plasticity at nociceptive afferents in the rat central amygdala. J. Physiol. 581, 961–970. doi: 10.1113/JPHYSIOL.2006.121822
De Camilli, P., Cameron, R., and Greengard, P. (1983). Synapsin I (protein I), a nerve terminal-specific phosphoprotein. I. Its general distribution in synapses of the central and peripheral nervous system demonstrated by immunofluorescence in frozen and plastic sections. J. Cell Biol. 96, 1337–1354. doi: 10.1083/jcb.96.5.1337
De Camilli, P., and Greengard, O. (1986). Synapsin I: a synaptic vesicle-associated neuronal phosphoprotein. Biochem. Pharmacol. 35, 4349–4357. doi: 10.1016/0006-2952(86)90747-1
De Lecea, L., Criado, J. R., Rivera, S., Wen, W., Soriano, E., Henriksen, S. J., et al. (1998). Endogenous protein kinase a inhibitor (PKIα) modulates synaptic activity. J. Neurosci. Res. 53, 269–278. doi: 10.1002/(SICI)1097-4547(19980801)53:3<269::AID-JNR1<3.0.CO;2-8
De Rooij, J., Zwartkruis, F. J. T., Verheijen, M. H. G., Cool, R. H., Nijman, S. M. B., Wittinghofer, A., et al. (1998). Epac is a Rap1 guanine-nucleotide-exchange factor directly activated by cyclic AMP. Nature 396, 474–477. doi: 10.1038/24884
Deák, F., Shin, O. H., Tang, J., Hanson, P., Ubach, J., Jahn, R., et al. (2006). Rabphilin regulates SNARE-dependent re-priming of synaptic vesicles for fusion. EMBO J. 25, 2856–2866. doi: 10.1038/sj.emboj.7601165
Dresbach, T., Qualmann, B., Kessels, M. M., Garner, C. C., and Gundelfinger, E. D. (2001). The presynaptic cytomatrix of brain synapses. Cell. Mol. Life Sci. 58, 94–116. doi: 10.1007/PL00000781
Eggermann, E., Bucurenciu, I., Goswami, S. P., and Jonas, P. (2012). Nanodomain coupling between Ca 2+ channels and sensors of exocytosis at fast mammalian synapses. Nat. Rev. Neurosci. 13, 7–21. doi: 10.1038/nrn3125
Emery, A. C., Eiden, M. V., and Eiden, L. E. (2014). Separate cyclic AMP sensors for neuritogenesis, growth arrest, and survival of neuroendocrine cells. J. Biol. Chem. 289, 10126–10139. doi: 10.1074/jbc.M113.529321
Emery, A. C., Eiden, M. V., and Eiden, L. E. (2016). Evaluating the Specificity and Potency of Activators and Inhibitors of the cAMP Sensors PKA, Epac2/Rapgef4, and NCS/Rapgef2 Using Cell-Based Assays for Activation of CREB, p38 MAPK, and ERK. FASEB J. 30, 1190.9–1190.9. doi: 10.1096/fasebj.30.1_supplement.1190.9
Emery, A. C., Eiden, M. V., Mustafa, T., and Eiden, L. E. (2013). Rapgef2 connects GPCR-mediated cAMP signals to ERK activation in neuronal and endocrine cells. Sci. Signal. 6:ra51. doi: 10.1126/scisignal.2003993
Emery, A. C., Xu, W., Eiden, M. V., and Eiden, L. E. (2017). Guanine nucleotide exchange factor Epac2-dependent activation of the GTP-binding protein Rap2A mediates cAMP-dependent growth arrest in neuroendocrine cells. J. Biol. Chem. 292, 12220–12231. doi: 10.1074/JBC.M117.790329
Evstratova, A., and Tóth, K. (2014). Information processing and synaptic plasticity at hippocampal mossy fiber terminals. Front. Cell. Neurosci. 8:28. doi: 10.3389/fncel.2014.00028
Ferguson, S. M., Fasano, S., Yang, P., Brambilla, R., and Robinson, T. E. (2006). Knockout of ERK1 enhances cocaine-evoked immediate early gene expression and behavioral plasticity. Neuropsychopharmacology 31, 2660–2668. doi: 10.1038/sj.npp.1301014
Fernandes, H. B., Riordan, S., Nomura, T., Remmers, C. L., Kraniotis, S., Marshall, J. J., et al. (2015). Epac2 mediates cAMP-dependent potentiation of neurotransmission in the hippocampus. J. Neurosci. 35, 6544–6553. doi: 10.1523/JNEUROSCI.0314-14.2015
Fitzjohn, S. M., Bortolotto, Z. A., Palmer, M. J., Doherty, A. J., Ornstein, P. L., Schoepp, D. D., et al. (1998). The potent mGlu receptor antagonist LY341495 identifies roles for both cloned and novel mGlu receptors in hippocampal synaptic plasticity. Neuropharmacology 37, 1445–1458. doi: 10.1016/S0028-3908(98)00145-2
Fourcaudot, E., Gambino, F., Humeau, Y., Casassus, G., Shaban, H., Poulain, B., et al. (2008). cAMP/PKA signaling and RIM1α mediate presynaptic LTP in the lateral amygdala. Proc. Natl. Acad. Sci. U. S. A. 105, 15130–15135. doi: 10.1073/pnas.0806938105
Fukaya, R., Maglione, M., Sigrist, S. J., and Sakaba, T. (2021). Rapid Ca2+ channel accumulation contributes to cAMP-mediated increase in transmission at hippocampal mossy fiber synapses. Proc. Natl. Acad. Sci. U. S. A. 118:e2016754118. doi: 10.1073/PNAS.2016754118
Gehring, C. (2010). Adenyl cyclases and cAMP in plant signaling - past and present. Cell Commun. Signal. 8:15. doi: 10.1186/1478-811X-8-15
Geppert, M., Goda, Y., Stevens, C. F., and Südhof, T. C. (1997). The small GTP-binding protein Rab3A regulates a late step in synaptic vesicle fusion. Nature 387, 810–814. doi: 10.1038/42954
Girault, J. A., and Greengard, P. (2004). The Neurobiology of Dopamine Signaling. Arch. Neurol. 61, 641–644. doi: 10.1001/archneur.61.5.641
Girault, J. A., Valjent, E., Caboche, J., and Hervé, D. (2007). ERK2: a logical AND gate critical for drug-induced plasticity? Curr. Opin. Pharmacol. 7, 77–85. doi: 10.1016/j.coph.2006.08.012
Gitler, D., Takagishi, Y., Feng, J., Ren, Y., Rodriguiz, R. M., Wetsel, W. C., et al. (2004). Different Presynaptic Roles of Synapsins at Excitatory and Inhibitory Synapses. J. Neurosci. 24, 11368–11380. doi: 10.1523/JNEUROSCI.3795-04.2004
Gloerich, M., and Bos, J. L. (2010). Epac: defining a New Mechanism for cAMP Action. Annu. Rev. Pharmacol. Toxicol. 50, 355–375. doi: 10.1146/annurev.pharmtox.010909.105714
Gracheva, E. O., Burdina, A. O., Holgado, A. M., Berthelot-Grosjean, M., Ackley, B. D., Hadwiger, G., et al. (2006). Tomosyn inhibits synaptic vesicle priming in Caenorhabditis elegans. PLoS Biol. 4, 1426–1437. doi: 10.1371/journal.pbio.0040261
Hallermann, S., Pawlu, C., Jonas, P., and Heckmann, M. (2003). A large pool of releasable vesicles in a cortical glutamatergic synapse. Proc. Natl. Acad. Sci. U. S. A. 100, 8975–8980. doi: 10.1073/pnas.1432836100
Halls, M. L., and Cooper, D. M. F. (2011). Regulation by Ca2+-signaling pathways of adenylyl cyclases. Cold Spring Harb. Perspect. Biol. 3:a004143. doi: 10.1101/CSHPERSPECT.A004143
Hamblin, M. W., Guthrie, C. R., Kohen, R., and Heidmann, D. E. A. (1998). Gs protein-coupled serotonin receptors: receptor isoforms and functional differences. Ann. N. Y. Acad. Sci. 861, 31–37. doi: 10.1111/j.1749-6632.1998.tb10170.x
Han, Y., Kaeser, P. S., Südhof, T. C., and Schneggenburger, R. (2011). RIM determines Ca2+ channel density and vesicle docking at the presynaptic active zone. Neuron 69, 304–316. doi: 10.1016/j.neuron.2010.12.014
Hanoune, J., and Defer, N. (2001). Regulation and role of adenylyl cyclase isoforms. Annu. Rev. Pharmacol. Toxicol. 41, 145–174. doi: 10.1146/ANNUREV.PHARMTOX.41.1.145
Harada, K., Ito, M., Wang, X., Tanaka, M., Wongso, D., Konno, A., et al. (2017). Red fluorescent protein-based cAMP indicator applicable to optogenetics and in vivo imaging. Sci. Rep. 7:7351. doi: 10.1038/s41598-017-07820-6
Hashimotodani, Y., Nasrallah, K., Jensen, K. R., Chávez, A. E., Carrera, D., and Castillo, P. E. (2017). LTP at Hilar Mossy Cell-Dentate Granule Cell Synapses Modulates Dentate Gyrus Output by Increasing Excitation/Inhibition Balance. Neuron 95, 928–943.e3. doi: 10.1016/J.NEURON.2017.07.028
Henze, D. A., Urban, N. N., and Barrionuevo, G. (2000). The multifarious hippocampal mossy fiber pathway: a review. Neuroscience 98, 407–427. doi: 10.1016/s0306-4522(00)00146-9
Huang, Y. Y., Kandel, E. R., Varshavsky, L., Brandon, E. P., Qi, M., Idzerda, R. L., et al. (1995). A genetic test of the effects of mutations in PKA on mossy fiber LTP and its relation to spatial and contextual learning. Cell 83, 1211–1222. doi: 10.1016/0092-8674(95)90146-9
Huang, Y. Y., Li, X. C., and Kandel, E. R. (1994). cAMP contributes to mossy fiber LTP by initiating both a covalently mediated early phase and macromolecular synthesis-dependent late phase. Cell 79, 69–79. doi: 10.1016/0092-8674(94)90401-4
Jiang, S. Z., Sweat, S., Dahlke, S. P., Loane, K., Drossel, G., Xu, W., et al. (2021). Cocaine-Dependent Acquisition of Locomotor Sensitization and Conditioned Place Preference Requires D1 Dopaminergic Signaling through a Cyclic AMP, NCS-Rapgef2, ERK, and Egr-1/Zif268 Pathway. J. Neurosci. 41, 711–725. doi: 10.1523/JNEUROSCI.1497-20.2020
Jiang, S. Z., Xu, W., Emery, A. C., Gerfen, C. R., Eiden, M. V., and Eiden, L. E. (2017). NCS-Rapgef2, the Protein Product of the Neuronal Rapgef2 Gene, Is a Specific Activator of D1 Dopamine Receptor-Dependent ERK Phosphorylation in Mouse Brain. eNeuro 4:ENEURO.248-ENEURO.217. doi: 10.1523/ENEURO.0248-17.2017
Johnson, M. (2006). Molecular mechanisms of β2-adrenergic receptor function, response, and regulation. J. Allergy Clin. Immunol. 117, 18–24. doi: 10.1016/j.jaci.2005.11.012
Johnston, D., Williams, S., Jaffe, D., and Gray, R. (1992). NMDA-receptor-independent long-term potentiation. Annu. Rev. Physiol. 54, 489–505. doi: 10.1146/annurev.ph.54.030192.002421
Jonas, P., Major, G., and Sakmann, B. (1993). Quantal components of unitary EPSCs at the mossy fibre synapse on CA3 pyramidal cells of rat hippocampus. J. Physiol. 472, 615–663. doi: 10.1113/jphysiol.1993.sp019965
Jones, B. W., Deem, J., Younts, T. J., Weisenhaus, M., Sanford, C. A., Slack, M. C., et al. (2016). Targeted deletion of AKAP7 in dentate granule cells impairs spatial discrimination. Elife 5:e20695. doi: 10.7554/ELIFE.20695
Kaeser, P. S., Deng, L., Wang, Y., Dulubova, I., Liu, X., Rizo, J., et al. (2011). RIM proteins tether Ca2+ channels to presynaptic active zones via a direct PDZ-domain interaction. Cell 144, 282–295. doi: 10.1016/j.cell.2010.12.029
Kaeser, P. S., Kwon, H. B., Blundell, J., Chevaleyre, V., Morishita, W., Malenka, R. C., et al. (2008). RIM1α phosphorylation at serine-413 by protein kinase A is not required for presynaptic long-term plasticity or learning. Proc. Natl. Acad. Sci. U. S. A. 105, 14680–14685. doi: 10.1073/pnas.0806679105
Kaeser-Woo, Y. J., Younts, T. J., Yang, X., Zhou, P., Wu, D., Castillo, P. E., et al. (2013). Synaptotagmin-12 phosphorylation by cAMP-dependent protein kinase is essential for hippocampal mossy fiber LTP. J. Neurosci. 33, 9769–9780. doi: 10.1523/JNEUROSCI.5814-12.2013
Kalia, D., Merey, G., Nakayama, S., Zheng, Y., Zhou, J., Luo, Y., et al. (2013). Nucleotide, c-di-GMP, c-di-AMP, cGMP, cAMP, (p)ppGpp signaling in bacteria and implications in pathogenesis. Chem. Soc. Rev. 42, 305–341. doi: 10.1039/C2CS35206K
Kamiya, H., and Ozawa, S. (1999). Dual mechanism for presynaptic modulation by axonal metabotropic glutamate receptor at the mouse mossy fibre-CA3 synapse. J. Physiol. 518, 497–506. doi: 10.1111/J.1469-7793.1999.0497P.X
Kandel, E. R. (2012). The molecular biology of memory: cAMP, PKA, CRE, CREB-1, CREB-2, and CPEB. Mol. Brain 5:14. doi: 10.1186/1756-6606-5-14
Kandel, E. R., Schwartz, J. H., Siegelbaum, S., and Jessell, T. M. (2000). Principles of neural science, 4th Edn. New York: McGraw-hill.
Kannan, N., Wu, J., Anand, G. S., Yooseph, S., Neuwald, A. F., Venter, J. C., et al. (2007). Evolution of allostery in the cyclic nucleotide binding module. Genome Biol. 8:R264. doi: 10.1186/gb-2007-8-12-r264
Kapur, A., Yeckel, M. F., Gray, R., and Johnston, D. (1998). L-type calcium channels are required for one form of hippocampal mossy fiber LTP. J. Neurophysiol. 79, 2181–2190. doi: 10.1152/jn.1998.79.4.2181
Kavalali, E. T. (2020). Neuronal Ca2+ signalling at rest and during spontaneous neurotransmission. J. Physiol. 598, 1649–1654. doi: 10.1113/JP276541
Kawasaki, H., Springett, G. M., Mochizuki, N., Toki, S., Nakaya, M., Matsuda, M., et al. (1998). A family of cAMP-binding proteins that directly activate Rap1. Science 282, 2275–2279. doi: 10.1126/science.282.5397.2275
Kees, A. L., Marneffe, C., and Mulle, C. (2021). Lighting up pre-synaptic potentiation: an Editorial for “SynaptoPAC, an optogenetic tool for induction of presynaptic plasticity” on page 324. J. Neurochem. 156, 270–272. doi: 10.1111/jnc.15236
Klein, M., and Kandel, E. R. (1980). Mechanism of calcium current modulation underlying presynaptic facilitation and behavioral sensitization in Aplysia. Proc. Natl. Acad. Sci. U. S. A. 77, 6912–6916. doi: 10.1073/pnas.77.11.6912
Kobayashi, K. (2010). Hippocampal Mossy Fiber Synaptic Transmission and Its Modulation. Vitam. Horm. 82, 65–85. doi: 10.1016/S0083-6729(10)82004-7
Kobayashi, K., Mikahara, Y., Murata, Y., Morita, D., Matsuura, S., Segi-Nishida, E., et al. (2020). Predominant Role of Serotonin at the Hippocampal Mossy Fiber Synapse with Redundant Monoaminergic Modulation. iScience 23:101025. doi: 10.1016/j.isci.2020.101025
Kohara, K., Pignatelli, M., Rivest, A. J., Jung, H. Y., Kitamura, T., Suh, J., et al. (2014). Cell type-specific genetic and optogenetic tools reveal hippocampal CA2 circuits. Nat. Neurosci. 17, 269–279. doi: 10.1038/nn.3614
Kuiperij, H. B., De Rooij, J., Rehmann, H., Van Triest, M., Wittinghofer, A., Bos, J. L., et al. (2003). Characterisation of PDZ-GEFs, a family of guanine nucleotide exchange factors specific for Rap1 and Rap2. Biochim. Biophys. Acta Mol. Cell Res. 1593, 141–149. doi: 10.1016/S0167-4889(02)00365-8
Kwon, H. B., and Castillo, P. E. (2008). Long-term potentiation selectively expressed by NMDA receptors at hippocampal mossy fiber synapses. Neuron 57, 108–120. doi: 10.1016/j.neuron.2007.11.024
Lauri, S. E., Bortolotto, Z. A., Nistico, R., Bleakman, D., Ornstein, P. L., Lodge, D., et al. (2003). A role for Ca2+ stores in kainate receptor-dependent synaptic facilitation and LTP at mossy fiber synapses in the hippocampus. Neuron 39, 327–341. doi: 10.1016/s0896-6273(03)00369-6
Lein, E. S., Hawrylycz, M. J., Ao, N., Ayres, M., Bensinger, A., Bernard, A., et al. (2007). Genome-wide atlas of gene expression in the adult mouse brain. Nature 445, 168–176. doi: 10.1038/nature05453
Leutgeb, J. K., Leutgeb, S., Moser, M. B., and Moser, E. I. (2007). Pattern separation in the dentate gyrus and CA3 of the hippocampus. Science 315, 961–966. doi: 10.1126/science.1135801
Li, L., Bischofberger, J., and Jonas, P. (2007). Differential gating and recruitment of P/Q-, N-, and R-type Ca2+ channels in hippocampal mossy fiber boutons. J. Neurosci. 27, 13420–13429. doi: 10.1523/JNEUROSCI.1709-07.2007
Liao, Y., Kariya, K. I., Hu, C. D., Shibatohge, M., Goshima, M., Okada, T., et al. (1999). RA-GEF, a novel Rap1A guanine nucleotide exchange factor containing a Ras/Rap1A-associating domain, is conserved between nematode and humans. J. Biol. Chem. 274, 37815–37820. doi: 10.1074/jbc.274.53.37815
Lieberman, L. R. (1965). The Boy Who Wouldn’t Say “Huh”: A Suggested Area For Research. J. Speech Hear. Disord. 30, 184–186. doi: 10.1044/jshd.3002.184
Linghu, C., Johnson, S. L., Valdes, P. A., Shemesh, O. A., Park, W. M., Park, D., et al. (2020). Spatial Multiplexing of Fluorescent Reporters for Imaging Signaling Network Dynamics. Cell 183, 1682–1698.e24. doi: 10.1016/j.cell.2020.10.035
Lisman, J., Buzsáki, G., Eichenbaum, H., Nadel, L., Rangananth, C., and Redish, A. D. (2017). Viewpoints: how the hippocampus contributes to memory, navigation and cognition. Nat. Neurosci. 20, 1434–1447. doi: 10.1038/NN.4661
Lisman, J. E., Raghavachari, S., and Tsien, R. W. (2007). The sequence of events that underlie quantal transmission at central glutamatergic synapses. Nat. Rev. Neurosci. 8, 597–609. doi: 10.1038/nrn2191
Lituma, P. J., Kwon, H. B., Alviña, K., Luján, R., and Castillo, P. E. (2021). Presynaptic nmda receptors facilitate short-term plasticity and bdnf release at hippocampal mossy fiber synapses. Elife 10:e66612. doi: 10.7554/eLife.66612
Lonart, G., Janz, R., Johnson, K. M., and Südhof, T. C. (1998). Mechanism of action of rab3A in mossy fiber LTP. Neuron 21, 1141–1150. doi: 10.1016/s0896-6273(00)80631-5
Lonart, G., Schoch, S., Kaeser, P. S., Larkin, C. J., Südhof, T. C., and Linden, D. J. (2003). Phosphorylation of RIM1α by PKA Triggers Presynaptic Long-Term Potentiation at Cerebellar Parallel Fiber Synapses. Cell 115, 49–60. doi: 10.1016/S0092-8674(03)00727-X
Lonart, G., and Südhof, T. C. (1998). Region-Specific Phosphorylation of Rabphilin in Mossy Fiber Nerve Terminals of the Hippocampus. J. Neurosci. 18, 634–640. doi: 10.1523/JNEUROSCI.18-02-00634.1998
Lyon, L., Borel, M., Carrión, M., Kew, J. N. C., Corti, C., Harrison, P. J., et al. (2011). Hippocampal mossy fiber long-term depression in Grm2/3 double knockout mice. Synapse 65, 945–954. doi: 10.1002/SYN.20923
Lysetskiy, M., Földy, C., and Soltesz, I. (2005). Long- and short-term plasticity at mossy fiber synapses on mossy cells in the rat dentate gyrus. Hippocampus 15, 691–696. doi: 10.1002/hipo.20096
Makani, S., Lutzu, S., Lituma, P. J., Hunt, D. L., and Castillo, P. E. (2021). Retrograde Suppression of Post-Tetanic Potentiation at the Mossy Fiber-CA3 Pyramidal Cell Synapse. eNeuro 8:ENEURO.0450-20.2021. doi: 10.1523/ENEURO.0450-20.2021
Maximov, A., Shin, O. H., Liu, X., and Südhof, T. C. (2007). Synaptotagmin-12, a synaptic vesicle phosphoprotein that modulates spontaneous neurotransmitter release. J. Cell Biol. 176, 113–124. doi: 10.1083/JCB.200607021
McEwen, J. M., Madison, J. M., Dybbs, M., and Kaplan, J. M. (2006). Antagonistic Regulation of Synaptic Vesicle Priming by Tomosyn and UNC-13. Neuron 51, 303–315. doi: 10.1016/j.neuron.2006.06.025
Midorikawa, M., and Sakaba, T. (2017). Kinetics of Releasable Synaptic Vesicles and Their Plastic Changes at Hippocampal Mossy Fiber Synapses. Neuron 96, 1033–1040e3. doi: 10.1016/j.neuron.2017.10.016
Morozov, A., Muzzio, I. A., Bourtchouladze, R., Van-Strien, N., Lapidus, K., Yin, D., et al. (2003). Rap1 Couples cAMP Signaling to a Distinct Pool of p42/44MAPK Regulating Excitability, Synaptic Plasticity, Learning, and Memory. Neuron 39, 309–325. doi: 10.1016/S0896-6273(03)00404-5
Mucignat-Caretta, C., and Caretta, A. (2020). Protein kinase a catalytic and regulatory subunits interact differently in various areas of mouse brain. Int. J. Mol. Sci. 21:3051. doi: 10.3390/ijms21093051
Neves, G., Cooke, S. F., and Bliss, T. V. (2008). Synaptic plasticity, memory and the hippocampus: a neural network approach to causality. Nat. Rev. Neurosci. 9, 65–75. doi: 10.1038/nrn2303
Nguyen, P. V., and Kandel, E. R. (1997). Brief theta-burst stimulation induces a transcription-dependent late phase of LTP requiring cAMP in area CA1 of the mouse hippocampus. Learn. Mem. 4, 230–243. doi: 10.1101/lm.4.2.230
Nozaki, K., Kubo, R., and Furukawa, Y. (2016). Serotonin modulates the excitatory synaptic transmission in the dentate granule cells. J. Neurophysiol. 115, 2997–3007. doi: 10.1152/jn.00064.2016
Odaka, H., Arai, S., Inoue, T., and Kitaguchi, T. (2014). Genetically-encoded yellow fluorescent cAMP indicator with an expanded dynamic range for dual-color imaging. PLoS One 9:e100252. doi: 10.1371/journal.pone.0100252
Ohta, Y., Furuta, T., Nagai, T., and Horikawa, K. (2018). Red fluorescent cAMP indicator with increased affinity and expanded dynamic range. Sci. Rep. 8:1866. doi: 10.1038/s41598-018-20251-1
Oldani, S., Moreno-Velasquez, L., Faiss, L., Stumpf, A., Rosenmund, C., Schmitz, D., et al. (2021). SynaptoPAC, an optogenetic tool for induction of presynaptic plasticity. J. Neurochem. 156, 324–336. doi: 10.1111/jnc.15210
Orlando, M., Dvorzhak, A., Bruentgens, F., Maglione, M., Rost, B. R., Sigrist, S. J., et al. (2021). Recruitment of release sites underlies chemical presynaptic potentiation at hippocampal mossy fiber boutons. PLoS Biol. 19:e3001149. doi: 10.1371/journal.pbio.3001149
Pechstein, A., Tomilin, N., Fredrich, K., Vorontsova, O., Sopova, E., Evergren, E., et al. (2020). Vesicle Clustering in a Living Synapse Depends on a Synapsin Region that Mediates Phase Separation. Cell Rep. 30, 2594–2602.e3. doi: 10.1016/j.celrep.2020.01.092
Pelkey, K. A., and McBain, C. J. (2005). How to Dismantle a Detonator Synapse. Neuron 45, 327–329. doi: 10.1016/j.neuron.2005.01.018
Pelkey, K. A., Topolnik, L., Lacaille, J. C., and McBain, C. J. (2006). Compartmentalized Ca2+ Channel Regulation at Divergent Mossy-Fiber Release Sites Underlies Target Cell-Dependent Plasticity. Neuron 52, 497–510. doi: 10.1016/j.neuron.2006.08.032
Pelkey, K. A., Topolnik, L., Yuan, X. Q., Lacaille, J. C., and McBain, C. J. (2008). State-dependent cAMP sensitivity of presynaptic function underlies metaplasticity in a hippocampal feedforward inhibitory circuit. Neuron 60, 980–987. doi: 10.1016/J.NEURON.2008.11.018
Raffelberg, S., Wang, L., Gao, S., Losi, A., Gärtner, W., and Nagel, G. (2013). A LOV-domain-mediated blue-light-activated adenylate (adenylyl) cyclase from the cyanobacterium Microcoleus chthonoplastes PCC 7420. Biochem. J. 455, 359–365. doi: 10.1042/BJ20130637
Regehr, W. G., Delaney, K. R., and Tank, D. W. (1994). The role of presynaptic calcium in short-term enhancement at the hippocampal mossy fiber synapse. J. Neurosci. 14, 523–537. doi: 10.1523/jneurosci.14-02-00523.1994
Rey, S., Marra, V., Smith, C., and Staras, K. (2020). Nanoscale Remodeling of Functional Synaptic Vesicle Pools in Hebbian Plasticity. Cell Rep. 30, 2006–2017.e3. doi: 10.1016/j.celrep.2020.01.051
Rizo, J. (2018). Mechanism of neurotransmitter release coming into focus. Protein Sci. 27, 1364–1391. doi: 10.1002/pro.3445
Rollenhagen, A., Sätzler, K., Rodríguez, E. P., Jonas, P., Frotscher, M., and Lübke, J. H. R. (2007). Structural determinants of transmission at large hippocampal mossy fiber synapses. J. Neurosci. 27, 10434–10444. doi: 10.1523/JNEUROSCI.1946-07.2007
Rolls, E. T. (2013). The mechanisms for pattern completion and pattern separation in the hippocampus. Front. Syst. Neurosci. 7:74. doi: 10.3389/FNSYS.2013.00074
Roth, B. L. (2016). DREADDs for Neuroscientists. Neuron 89, 683–694. doi: 10.1016/j.neuron.2016.01.040
Sadana, R., and Dessauer, C. W. (2009). Physiological roles for G protein-regulated adenylyl cyclase isoforms: insights from knockout and overexpression studies. Neurosignals. 17, 5–22. doi: 10.1159/000166277
Salin, P. A., Scanziani, M., Malenka, R. C., and Nicoll, R. A. (1996b). Distinct short-term plasticity at two excitatory synapses in the hippocampus. Proc. Natl. Acad. Sci. U. S. A. 93, 13304–13309. doi: 10.1073/PNAS.93.23.13304
Salin, P. A., Malenka, R. C., and Nicoll, R. A. (1996a). Cyclic AMP Mediates a Presynaptic Form of LTP at Cerebellar Parallel Fiber Synapses. Neuron 16, 797–803. doi: 10.1016/S0896-6273(00)80099-9
Sanabra, C., and Mengod, G. (2011). Neuroanatomical distribution and neurochemical characterization of cells expressing adenylyl cyclase isoforms in mouse and rat brain. J. Chem. Neuroanat. 41, 43–54. doi: 10.1016/j.jchemneu.2010.11.001
Sarihi, A., Mirnajafi-Zadeh, J., Jiang, B., Sohya, K., Safari, M. S., Arami, M. K., et al. (2012). Cell type-specific, presynaptic LTP of inhibitory synapses on fast-spiking GABAergic neurons in the mouse visual cortex. J. Neurosci. 32, 13189–13199. doi: 10.1523/JNEUROSCI.1386-12.2012
Saunders, A., Macosko, E. Z., Wysoker, A., Goldman, M., Krienen, F. M. de Rivera, H., et al. (2018). Molecular diversity and specializations among the cells of the adult mouse brain. Cell 174, 1015–1030.e16. doi: 10.1016/j.cell.2018.07.028
Schacher, S., Castellucci, V. F., and Kandel, E. R. (1988). cAMP Evokes Long-Term Facilitation in Aplysia Sensory Neurons That Requires New Protein Synthesis. Science 240, 1667–1669. doi: 10.1126/science.2454509
Schlüter, O. M., Schnell, E., Verhage, M., Tzonopoulos, T., Nicoll, R. A., Janz, R., et al. (1999). Rabphilin knock-out mice reveal that rabphilin is not required for rab3 function in regulating neurotransmitter release. J. Neurosci. 19, 5834–5846. doi: 10.1523/JNEUROSCI.19-14-05834.1999
Schmidt, B., Marrone, D. F., and Markus, E. J. (2012). Disambiguating the similar: the dentate gyrus and pattern separation. Behav. Brain Res. 226, 56–65. doi: 10.1016/J.BBR.2011.08.039
Schneggenburger, R., and Neher, E. (2005). Presynaptic calcium and control of vesicle fusion. Curr. Opin. Neurobiol. 15, 266–274. doi: 10.1016/j.conb.2005.05.006
Schoch, S., Castillo, P. E., Jo, T., Mukherjee, K., Geppert, M., Wang, Y., et al. (2002). RIM1alpha forms a protein scaffold for regulating neurotransmitter release at the active zone. Nature 415, 321–326. doi: 10.1038/415321a
Scott, R., and Rusakov, D. A. (2006). Main determinants of presynaptic Ca2+ dynamics at individual mossy fiber-CA3 pyramidal cell synapses. J. Neurosci. 26, 7071–7081. doi: 10.1523/JNEUROSCI.0946-06.2006
Shin, M. C., Nonaka, K., Yamaga, T., Wakita, M., Akaike, H., and Akaike, N. (2018). Calcium channel subtypes on glutamatergic mossy fiber terminals synapsing onto rat hippocampal CA3 neurons. J. Neurophysiol. 120, 1264–1273. doi: 10.1152/jn.00571.2017
Sihra, T. S., Wang, J. K. T., Gorelick, F. S., and Greengard, P. (1989). Translocation of synapsin I in response to depolarization of isolated nerve terminals. Proc. Natl. Acad. Sci. U. S. A. 86, 8108–8112. doi: 10.1073/pnas.86.20.8108
Spillane, D. M., Rosahl, T. W., Sudhof, T. C., and Malenka, R. C. (1995). Long-term potentiation in mice lacking synapsins. Neuropharmacology 34, 1573–1579. doi: 10.1016/0028-3908(95)00107-h
Ster, J., de Bock, F., Bertaso, F., Abitbol, K., Daniel, H., Bockaert, J., et al. (2009). Epac mediates PACAP-dependent long-term depression in the hippocampus. J. Physiol. 587, 101–113. doi: 10.1113/jphysiol.2008.157461
Ster, J., De Bock, F., Guérineau, N. C., Janossy, A., Barrère-Lemaire, S., Bos, J. L., et al. (2007). Exchange protein activated by cAMP (Epac) mediates cAMP activation of p38 MAPK and modulation of Ca2+-dependent K+ channels in cerebellar neurons. Proc. Natl. Acad. Sci. U. S. A. 104, 2519–2524. doi: 10.1073/pnas.0611031104
Stierl, M., Stumpf, P., Udwari, D., Gueta, R., Hagedorn, R., Losi, A., et al. (2011). Light modulation of cellular cAMP by a small bacterial photoactivated adenylyl cyclase, bPAC, of the soil bacterium Beggiatoa. J. Biol. Chem. 286, 1181–1188. doi: 10.1074/jbc.M110.185496
Südhof, T. C. (2013). Neurotransmitter release: the last millisecond in the life of a synaptic vesicle. Neuron 80, 675–690. doi: 10.1016/j.neuron.2013.10.022
Takai, Y., Sasaki, T., Shirataki, H., and Nakanishi, H. (1996). Rab3A small GTP-binding protein in Ca(2+)-dependent exocytosis. Genes Cells 1, 615–632. doi: 10.1046/J.1365-2443.1996.00257.X
Taylor, S. S., Ilouz, R., Zhang, P., and Kornev, A. P. (2012). Assembly of allosteric macromolecular switches: lessons from PKA. Nat. Rev. Mol. Cell Biol. 13, 646–658. doi: 10.1038/nrm3432
Toth, K., Suares, G., Lawrence, J. J., Philips-Tansey, E., and McBain, C. J. (2000). Differential mechanisms of transmission at three types of mossy fiber synapse. J. Neurosci. 20, 8279–8289. doi: 10.1523/jneurosci.20-22-08279.2000
Tsuboi, T., and Fukuda, M. (2005). The C2B domain of rabphilin directly interacts with SNAP-25 and regulates the docking step of dense core vesicle exocytosis in PC12 cells. J. Biol. Chem. 280, 39253–39259. doi: 10.1074/jbc.M507173200
Turnham, R. E., and Scott, J. D. (2016). Protein kinase A catalytic subunit isoform PRKACA, History, function and physiology. Gene 577, 101–108. doi: 10.1016/j.gene.2015.11.052
Tzounopoulos, T., Janz, R., Südhof, T. C., Nicoll, R. A., and Malenka, R. C. (1998). A role for cAMP in long-term depression at hippocampal mossy fiber synapses. Neuron 21, 837–845. doi: 10.1016/S0896-6273(00)80599-1
Vandael, D., Borges-Merjane, C., Zhang, X., and Jonas, P. (2020). Short-Term Plasticity at Hippocampal Mossy Fiber Synapses Is Induced by Natural Activity Patterns and Associated with Vesicle Pool Engram Formation. Neuron 107, 509–521.e7. doi: 10.1016/j.neuron.2020.05.013
Vandael, D., Okamoto, Y., and Jonas, P. (2021). Transsynaptic modulation of presynaptic short-term plasticity in hippocampal mossy fiber synapses. Nat. Commun. 12:2912. doi: 10.1038/S41467-021-23153-5
Villacres, E. C., Wong, S. T., Chavkin, C., and Storm, D. R. (1998). Type I Adenylyl Cyclase Mutant Mice Have Impaired Mossy Fiber Long-Term Potentiation. J. Neurosci. 18, 3186–3194. doi: 10.1523/JNEUROSCI.18-09-03186.1998
Vyleta, N. P., Borges-Merjane, C., and Jonas, P. (2016). Plasticity-dependent, full detonation at hippocampal mossy fiber–CA3 pyramidal neuron synapses. Elife 5:e17977. doi: 10.7554/eLife.17977
Vyleta, N. P., and Jonas, P. (2014). Loose coupling between Ca2+ channels and release sensors at a plastic hippocampal synapse. Science 343, 665–670. doi: 10.1126/SCIENCE.1244811
Waltereit, R., and Weller, M. (2003). Signaling from cAMP/PKA to MAPK and synaptic plasticity. Mol. Neurobiol. 27, 99–106. doi: 10.1385/MN:27:1:99
Wang, H., Pineda, V. V., Chan, G. C. K., Wong, S. T., Muglia, L. J., and Storm, D. R. (2003). Type 8 Adenylyl Cyclase Is Targeted to Excitatory Synapses and Required for Mossy Fiber Long-Term Potentiation. J. Neurosci. 23, 9710–9718. doi: 10.1523/JNEUROSCI.23-30-09710.2003
Wang, X., Hu, B., Zimmermann, B., and Kilimann, M. W. (2001). Rim1 and rabphilin-3 bind Rab3-GTP by composite determinants partially related through N-terminal alpha -helix motifs. J. Biol. Chem. 276, 32480–32488. doi: 10.1074/JBC.M103337200
Wang, Y., Okamoto, M., Schmitz, F., Hofmann, K., and Südhof, T. C. (1997). Rim is a putative Rab3 effector in regulating synaptic-vesicle fusion. Nature 388, 593–598. doi: 10.1038/41580
Weisskopf, M. G., Castillo, P. E., Zalutsky, R. A., and Nicoll, R. A. (1994). Mediation of hippocampal mossy fiber long-term potentiation by cyclic AMP. Science 265, 1878–1882. doi: 10.1126/science.7916482
Weyhersmüller, A., Hallermann, S., Wagner, N., and Eilers, J. (2011). Rapid active zone remodeling during synaptic plasticity. J. Neurosci. 31, 6041–6052. doi: 10.1523/JNEUROSCI.6698-10.2011
Willoughby, D., and Cooper, D. M. F. (2007). Organization and Ca2+ regulation of adenylyl cyclases in cAMP microdomains. Physiol. Rev. 87, 965–1010. doi: 10.1152/PHYSREV.00049.2006
Witter, M. P. (2007). The perforant path: projections from the entorhinal cortex to the dentate gyrus. Prog. Brain Res. 163, 43–61. doi: 10.1016/S0079-6123(07)63003-9
Wolfes, A. C., and Dean, C. (2020). The diversity of synaptotagmin isoforms. Curr. Opin. Neurobiol. 63, 198–209. doi: 10.1016/J.CONB.2020.04.006
Wong, S. T., Athos, J., Figueroa, X. A., Pineda, V. V., Schaefer, M. L., Chavkin, C. C., et al. (1999). Calcium-Stimulated Adenylyl Cyclase Activity Is Critical for Hippocampus-Dependent Long-Term Memory and Late Phase LTP. Neuron 23, 787–798. doi: 10.1016/S0896-6273(01)80036-2
Yang, Y., and Calakos, N. (2013). Presynaptic long-term plasticity. Front. Synaptic Neurosci. 5:8. doi: 10.3389/fnsyn.2013.00008
Yassa, M. A., and Stark, C. E. L. (2011). Pattern separation in the hippocampus. Trends Neurosci. 34, 515–525. doi: 10.1016/j.tins.2011.06.006
Yizhar, O., Matti, U., Melamed, R., Hagalili, Y., Bruns, D., Rettig, J., et al. (2004). Tomosyn inhibits priming of large dense-core vesicles in a calcium-dependent manner. Proc. Natl. Acad. Sci. U. S. A. 101, 2578–2583. doi: 10.1073/pnas.0308700100
Yoshino, M., Sawada, S., Yamamoto, C., and Kamiya, H. (1996). A metabotropic glutamate receptor agonist DCG-IV suppresses synaptic transmission at mossy fiber pathway of the guinea pig hippocampus. Neurosci. Lett. 207, 70–72. doi: 10.1016/0304-3940(96)12486-1
Zalutsky, R. A., and Nicoll, R. A. (1990). Comparison of two forms of long-term potentiation in single hippocampal neurons. Science 248, 1619–1624. doi: 10.1126/science.2114039
Zhao, M., Choi, Y. S., Obrietan, K., and Dudek, S. M. (2007). Synaptic plasticity (and the lack thereof) in hippocampal CA2 neurons. J. Neurosci. 27, 12025–12032. doi: 10.1523/JNEUROSCI.4094-07.2007
Keywords: cAMP, PKA, synaptic plasticity, mossy fiber synapse, LTP, forskolin-induced potentiation
Citation: Shahoha M, Cohen R, Ben-Simon Y and Ashery U (2022) cAMP-Dependent Synaptic Plasticity at the Hippocampal Mossy Fiber Terminal. Front. Synaptic Neurosci. 14:861215. doi: 10.3389/fnsyn.2022.861215
Received: 24 January 2022; Accepted: 23 February 2022;
Published: 04 April 2022.
Edited by:
Lucia Tabares, Seville University, SpainReviewed by:
Tommaso Patriarchi, University of Zurich, SwitzerlandCopyright © 2022 Shahoha, Cohen, Ben-Simon and Ashery. This is an open-access article distributed under the terms of the Creative Commons Attribution License (CC BY). The use, distribution or reproduction in other forums is permitted, provided the original author(s) and the copyright owner(s) are credited and that the original publication in this journal is cited, in accordance with accepted academic practice. No use, distribution or reproduction is permitted which does not comply with these terms.
*Correspondence: Yoav Ben-Simon, eW9hdi5iZW5zaW1vbkBtZWR1bml3aWVuLmFjLmF0; Uri Ashery, dXJpYXNoZXJ5QGdtYWlsLmNvbQ==
†These authors have contributed equally to this work
Disclaimer: All claims expressed in this article are solely those of the authors and do not necessarily represent those of their affiliated organizations, or those of the publisher, the editors and the reviewers. Any product that may be evaluated in this article or claim that may be made by its manufacturer is not guaranteed or endorsed by the publisher.
Research integrity at Frontiers
Learn more about the work of our research integrity team to safeguard the quality of each article we publish.