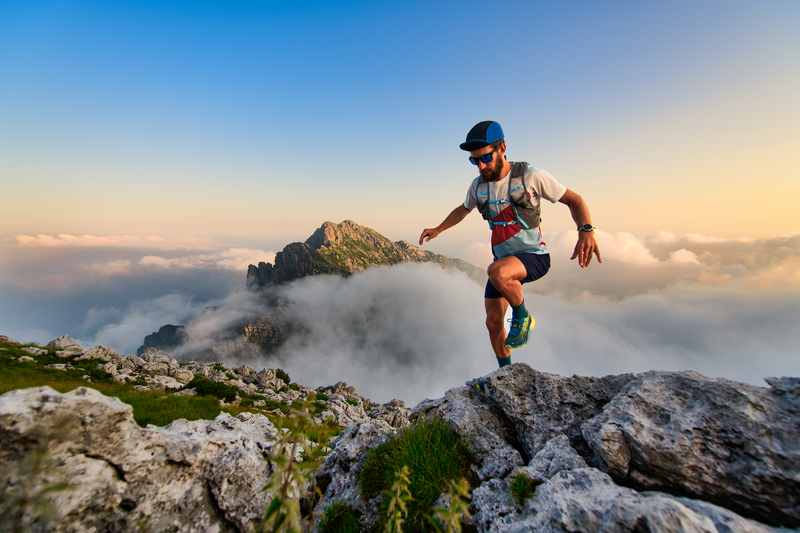
95% of researchers rate our articles as excellent or good
Learn more about the work of our research integrity team to safeguard the quality of each article we publish.
Find out more
REVIEW article
Front. Synaptic Neurosci. , 17 March 2022
Volume 14 - 2022 | https://doi.org/10.3389/fnsyn.2022.829354
This article is part of the Research Topic Molecular Nanomachines of the Presynaptic Terminal, Volume II View all 12 articles
Brain synapses pose special challenges on the quality control of their protein machineries as they are far away from the neuronal soma, display a high potential for plastic adaptation and have a high energy demand to fulfill their physiological tasks. This applies in particular to the presynaptic part where neurotransmitter is released from synaptic vesicles, which in turn have to be recycled and refilled in a complex membrane trafficking cycle. Pathways to remove outdated and damaged proteins include the ubiquitin-proteasome system acting in the cytoplasm as well as membrane-associated endolysosomal and the autophagy systems. Here we focus on the latter systems and review what is known about the spatial organization of autophagy and endolysomal processes within the presynapse. We provide an inventory of which components of these degradative systems were found to be present in presynaptic boutons and where they might be anchored to the presynaptic apparatus. We identify three presynaptic structures reported to interact with known constituents of membrane-based protein-degradation pathways and therefore may serve as docking stations. These are (i) scaffolding proteins of the cytomatrix at the active zone, such as Bassoon or Clarinet, (ii) the endocytic machinery localized mainly at the peri-active zone, and (iii) synaptic vesicles. Finally, we sketch scenarios, how presynaptic autophagic cargos are tagged and recruited and which cellular mechanisms may govern membrane-associated protein turnover in the presynapse.
Brain synapses can have long lifetimes (e.g., Holtmaat et al., 2005; Qiao et al., 2016) and display an enormous potential for plasticity (e.g., Citri and Malenka, 2008; Yang and Calakos, 2013). They also have a very high energy demand to maintain their functions (e.g., Harris et al., 2012), a situation that poses additional metabolic stress on synaptic protein components and requires an efficient management of proteostasis. This applies in particular to the presynaptic compartment with its apparatus for regulated neurotransmitter release, which rapidly and efficiently recycles releasable neurotransmitter-filled synaptic vesicles (SVs). Biosynthesis of presynaptic components occurs predominantly in the neuronal soma, where they are packaged into specific precursor organelles and are actively transported along the axon to presynaptic sites (for a review see Rizalar et al., 2021). The lifespan of presynaptic proteins varies with half-lives ranging from a few hours to several days (Hakim et al., 2016; Fornasiero et al., 2018; Cohen and Ziv, 2019), which is very short compared to the lifespan of neurons and synapses. Hence, presynaptic proteins must be continuously replaced in a specific and highly coordinated manner.
Three main systems are in place to mediate this turnover, i.e., the ubiquitin-proteasome system (UPS) acting in the cytoplasm (Rinetti and Schweizer, 2010; Lazarevic et al., 2011; Waites et al., 2013; Cohen and Ziv, 2017; Soykan et al., 2021), and the endolysosomal pathway and autophagy-related processes acting via degradative membranous organelles (Azarnia Tehran et al., 2018; Jin et al., 2018; Boecker and Holzbaur, 2019; Kuijpers et al., 2020; Lieberman and Sulzer, 2020; Andres-Alonso et al., 2021; Soykan et al., 2021; Figure 1A). Various autophagic pathways exist in parallel. These comprise macroautophagy, which can act in bulk or selective modes (including ER-phagy, aggrephagy and mitophagy) as well as chaperone-mediated autophagy (CMA) and microautophagy (Stavoe and Holzbaur, 2019). In this review, we will focus mainly on presynaptic macroautophagy, but will also consider constituents of other pathways of membrane-associated protein turnover. Macroautophagy (from here on referred to as autophagy) starts with the formation of a phagophore at a phagophore assembly site (PAS) and the recruitment of membranes from various sources via ATG9-containing vesicles (Figure 1B; Dikic and Elazar, 2018). Recruitment of cargo into the autophagosome is mediated via specific receptors/adaptors that bind ATG8-like proteins, which are anchored to the phagophore membrane via conjugation to phosphatidylethanolamine (e.g., Deng et al., 2017; Dikic and Elazar, 2018; Gatica et al., 2018). One major way of determining cargoes for autophagy is the conjugation of poly-ubiquitin chains, but there are also selective modes of autophagy that function independently of ubiquitination (Khaminets et al., 2016). On the other hand, ubiquitination is also involved in the tagging of proteins for proteasomal and endolysosomal degradation. This is achieved via several hundreds of E3-ubiquitin ligases encoded by mammalian genomes and makes them important surveyors of the various pathways of proteostasis (Wang and Le, 2019). In this regard, we will also address the question of which E3 ligases may mediate aspects of presynaptic autophagy.
Figure 1. Major pathways of cellular proteostasis. (A) Major degradative pathways at the presynapse include proteasomal degradation, endo-lysosomal degradation and different types of autophagy. (B) Schematic representation of autophagosome formation and cargo recruitment into autophagosomes via autophagy receptors such as p62/SQMTS1 or ATL3. CMA, chaperone-mediated autophagy; ER, endoplasmic reticulum; PAS, phagophore assembly site (also designated as pre-autophagosomal structure); SVs, synaptic vesicles.
In their axonal compartment, neurons entertain a steady process of basal autophagy (Figure 1B). Phagophore formation during basal autophagy is largely restricted to distal axons where autophagosomes are constitutively generated and then retrogradely transported toward the cell soma in a Dynein-dependent manner to fuse with lysosomes (Cheng et al., 2015; Maday and Holzbaur, 2016). Autophagy occurs at a basal level in all cells, but it can be up-regulated during stress, starvation, or infection. In neurons, however, conflicting reports have been published on whether neurons are sensitive (Boland et al., 2008; Nixon et al., 2008; Young et al., 2009; Alirezaei et al., 2010; Rubinsztein and Nixon, 2010; Hernandez et al., 2012; Catanese et al., 2018) or insensitive (Mizushima et al., 2004; Tsvetkov et al., 2010; Maday and Holzbaur, 2016; Kulkarni et al., 2020) to nutrient deprivation and/or mTOR inhibition. Likewise, the ordered recruitment of assembly factors for phagophore formation and maturation has been studied in much less detail for neuronal autophagy, but seems to be reminiscent of what has been found in non-neuronal cells (Maday and Holzbaur, 2014). The continuous formation of autophagosomes under steady-state conditions of neuronal signaling has led to the notion that autophagy is involved in homeostatic processes of proteostasis, particularly in axons. In fact, the primary membrane donor for the biogenesis of autophagosomes in distal axons is the endoplasmic reticulum (ER; Maday and Holzbaur, 2014). In very recent work, it was shown that the ER is also the main substrate for neuronal autophagy and that ER-phagy is important maintaining the integrity of axonal ER calcium stores and calcium release through ryanodine receptors (Kuijpers et al., 2021). In this contribution to the review series on ‘Molecular Nanomachines of the Presynaptic Terminal’, we will first examine which constituents of autophagic processes, as well as other interacting membrane-associated pathways of protein turnover, have been detected in presynaptic compartments to date, and how are they organized to fulfill their functions.
To assess the presence of components of the autophagic and the endolysosomal protein degradation systems as well as major elements contributing to chaperone-mediated autophagy (CMA) in brain synapses, we assembled, based on the relevant literature, a list of proteins contributing to membrane-based degradation pathways. More than 90 proteins and protein complexes were identified (Table 1), which were inspected for their localization in synapses and, in particular, in presynaptic compartments by examining whether they are included in relevant databases, i.e., the SynProt database of synaptic proteins1 (Pielot et al., 2012), and the synaptic gene ontology database SynGO, an expert-curated knowledge base of synaptic proteins2 (Koopmans et al., 2019). In addition, we checked whether their presence in the presynapse has been reported otherwise. One important source was a study on the so-called hidden proteome of SVs that identified dozens of SV-resident and SV-visitor proteins of the autophagic and endolysosomal pathways (Taoufiq et al., 2020). About 50% of the proteins listed in Table 1 are present in the SynProt databases, which include proteins identified in synaptic proteome studies (SynProt classics) and in studies on the proteomes of presynaptic compartments (PreProt). About one third of the proteins/protein complexes are found in SynGO, which provides a much higher and expert-evaluated resolution with respect to the compartmental localization of the identified proteins. In essence, we could assign many of the entries included in Table 1 to the presynaptic compartment. A closer inspection of these proteins allowed us to draw conclusions on the major presynaptic structures involved in the anchoring for the autophagic machinery. These include the cytomatrix at the active zone (CAZ) of neurotransmitter release, represented by the CAZ scaffolding protein Bassoon, the endocytic machinery mainly localized peripherally to the active zone (AZ), the peri-AZ, and SVs (Figure 2A). Many of the identified proteins that are included in SynGO can be functionally assigned to presynaptic biological processes related to the SV cycle and SV endocytosis (Figure 2B).
Figure 2. Sunburst plots of gene enrichment analyses for autophagy-related genes/proteins included in SynGO (Koopmans et al., 2019). Significantly enriched cellular components (A) and biological processes (B) are indicated by color code. The top-level terms of the Gene Ontology (GO) term tree are represented by the inner circle; the second level of the term tree is denoted by the innermost ring and so on. Presynaptic structures (A) and processes, like synaptic vesicle (SV) cycle and SV endocytosis (B) are significantly over-represented. Note, autophagy-related terms were not annotated in the database. Database entries for cellular components and for biological processes were considered as indicated in Table 1.
The AZ of neurotransmitter release is characterized by a prominent electron-dense meshwork of proteins, the CAZ, that organizes the regulated fusion of SVs with the presynaptic cell membrane (Garner et al., 2000; Gundelfinger and Fejtova, 2012; Sudhof, 2012; Ackermann et al., 2015). Two related multidomain proteins, Bassoon and Piccolo, serve among others as major scaffolding proteins of the CAZ (Gundelfinger et al., 2016). Knock-down of these two proteins has severe effects on the maintenance and integrity of neuronal synapses, which is in part mediated by their interaction with the E3 ubiquitin ligase Siah1 (Waites et al., 2013). More recent studies have shown that while Piccolo has important functions in SV retrieval (Ackermann et al., 2019), Bassoon contributes important binding sites for components of the proteosomal and the autophagic proteostasis pathways. Thus, Bassoon binds, in addition to Siah1, also the proteasomal subunit PSMB4 (alias β7) and thereby controls proteasome assembly (Montenegro-Venegas et al., 2021) as well as the autophagy protein ATG5, part of an E3-like protein ligase, and thus negatively regulates presynaptic autophagy (Okerlund et al., 2017). As will be detailed below, knockout of Bassoon causes increased ubiquitination of various presynaptic proteins, including various SV proteins, and enhances presynaptic autophagy (Hoffmann-Conaway et al., 2020) as well as proteasome activity (Montenegro-Venegas et al., 2021). Interestingly, the E3 ubiquitin ligase Parkin, an enzyme that is also involved in mitophagy and has been implicated in early onset Parkinsonism (Kitada et al., 1998; Dikic and Elazar, 2018), seems to antagonize Bassoon in this function (Montenegro-Venegas et al., 2020). How exactly Parkin acts in this context is unclear, as to date no physical interaction between Parkin and Bassoon has been detected. Interestingly, Parkin ubiquitinates constituents of the endocytic systems in the presynapse, including Synaptojanin-1, Endophilin-A and Dynamin-1 (Cao et al., 2014; Soukup et al., 2018). This could set the framework for the search for relevant functional interactions between Bassoon and the endocytic machinery to control induction of autophagy in the presynapse.
Overall, the findings discussed above suggest that Bassoon is a negative regulator of presynaptic autophagy and the UPS in the presynapse, and as such may act as an anchoring and control point of these two protein turnover pathways. It should be noted, in this context, that Bassoon itself, in contrast to Piccolo, is subject to autophagic degradation upon nutrient limitation in primary neuronal cultures (Catanese et al., 2018). This suggests that Bassoon may be highly feed-back regulated by the processes that it controls.
Interestingly, in C. elegans, another active zone protein, Clarinet, was recently reported to regulate presynaptic autophagy (Xuan et al., 2017, 2021). Clarinet is a large AZ protein that occurs in three different isoforms of about 9,000, 3,000, and 1,000 amino acids in length, that is required for proper synapse function. All three isoforms share a PDZ and a C2 domain similar to mammalian Piccolo, and Clarinet’s long and short isoforms are supposed to organize SV recruitment and clustering at the presynaptic AZ (Xuan et al., 2017). An elegant follow-up study by the same lab, uploaded recently onto the bioRxiv server, demonstrates that the long Clarinet isoform controls presynaptic autophagy by regulating ATG9 trafficking at the peri-active endocytic zone (Xuan et al., 2021). These observations further support the concept that large scaffolding proteins of presynaptic AZs couple exo- and endocytic zones at neurotransmitter release sites (Gundelfinger et al., 2003; Haucke et al., 2011) and can have essential roles in organizing presynaptic autophagy processes.
A tight linkage between autophagic and endocytic factors has been suggested previously (Soukup and Verstreken, 2017; Vijayan and Verstreken, 2017; Azarnia Tehran et al., 2018). Here, we discuss proteins involved in endocytic processes in neurons that have also been implicated in membranous organelle-based protein turnover as listed in Table 1. Endophilin-A/EndoA, a BAR-domain protein that is able to sense and modify membrane curvature, is crucially involved in Clathrin-dependent and -independent retrieval of membranes following the fusion SVs with the presynaptic AZ (Milosevic et al., 2011; Boucrot et al., 2015; Watanabe et al., 2018). More recent studies also suggest a function for Endophilins A in exocytosis of neurosecretory vesicles (Gowrisankaran et al., 2020) and the coordination of exo- and endocytic processes in presynapses (Kroll et al., 2019). Compensatory endocytosis associated with neurotransmitter release occurs primarily next to the AZ at the so-called peri-AZ (Haucke et al., 2011; Cano and Tabares, 2016). Two studies, one in Drosophila (Soukup et al., 2016) and the other one in mice (Murdoch et al., 2016) have identified Endophilin-A also as major player in generating initiation sites for autophagy. The former study showed that phosphorylation of the BAR domain of Endophilin-A by the leucine-rich repeat S/T protein kinase LRRK2 leads to membrane deformation putatively opening entry sites for the autophagy-related protein ATG3. ATG3 in turn can conjugate LC3/ATG8 to phosphatidyl-ethanolamine in the membrane, promoting progression of autophagosome formation (Soukup and Verstreken, 2017). Utilizing mutants for all three EndoA genes in the mouse genome, the second study revealed that partial or complete Endophilin-A-deficiency leads to age-dependent neurodegenerative changes in the brain and up-regulation of the E3 ubiquitin ligase FBXO32. Endophilins-A are essential for autophagosome formation and their proper interplay with FBXO32 coordinates the balance between autophagosomal and UPS-mediated protein turnover and maintains neuronal health (Murdoch et al., 2016). However, to date, FBXO32 has not yet been detected in the presynapse.
Synaptojanins are lipid phosphatases acting on phosphatidylinositols. In particular, the brain-enriched isoform Synaptojanin-1 is recruited to Endophilin-A complexes and, in the SV cycle, is required for Clathrin uncoating after endocytosis (Cremona et al., 1999; Verstreken et al., 2003). More recently, studies on the Drosophila larval neuromuscular junction revealed that an inactivating mutation in the SAC1 domain, one of the two enzymatically active lipid phosphatase domains of Synaptojanin, causes accumulation of lipid-binding protein ATG18a on nascent autophagosomes (Vanhauwaert et al., 2017). Interestingly, this mutation does not interfere with SV cycling. Similarly, accumulation of WIPI2, the mammalian ortholog of ATG18a, occurs in neurons derived from induced pluripotent stem cells from a human patient with the same mutation (Vanhauwaert et al., 2017).
The heterooligomeric AP-2 complex is also involved in Clathrin-mediated endocytosis during SV recycling, where it can act at the cell membrane or at a bulk-endocytosed membrane compartment (McMahon and Boucrot, 2011; Kononenko et al., 2014). In addition, AP-2 in cooperation with CALM/PICALM has been implicated in autophagic degradation of the C-terminal fragment of the amyloid precursor protein (APP), thus contributing to the clearance of the Alzheimer-related Aβ peptide (Tian et al., 2013). APP is a cell adhesion molecule that has been detected as a constituent of the presynaptic AZ (Lassek et al., 2013). Moreover, AP-2 can directly interact with ATG9A, a multispan transmembrane protein delivering membrane material for phagophore formation and extension (Imai et al., 2016; Dikic and Elazar, 2018). This interaction appears essential for the trafficking of ATG9A through the recycling endosome and making it available for the autophagy process (Imai et al., 2016). Similarly, delivery of ATG16L1 and in turn ATG12-ATG5 to the phagophore depends on Clathrin and AP-2 (Ravikumar et al., 2010), indicating a key role for AP-2 in autophagy initiation and progression.
Recent studies have revealed that AP-2, in conjunction with the RapGTPase-activating protein (RapGAP) SIPA1L2, serve unexpected roles in the transport of autophagosomes that contain actively signaling BDNF-activated TrkB receptors (Kononenko et al., 2017; Andres-Alonso et al., 2019). AP2 and SIPA1L2 link the TrkB receptor to a Dynein motor for retrograde trafficking via a direct interaction with Snapin, a component of the BLOC-1 complex (see below). Interestingly, SIPA1L2 concurrently associates via LC3 to Rab7-positive amphisomes and binding to LC3 promotes RapGAP activity. Endosomes fuse with autophagosomes to form amphisomes, and this step is required for the degradation of some proteins and the overall function of autophagy and the endosomal system (Cheng et al., 2015). Amphisomes are transient intermediate organelles that in non-neuronal cells rapidly enter a lysosomal-degradative pathway. However, in distal axons mature lysosomes are rare and therefore TrkB-LC3-SIPA1L2-AP2-carrying amphisomes have a longer lifespan. They thus can traffic retrogradely along axons and visit presynaptic boutons. Intriguingly, motility and signaling of amphisomes are controlled by SIPA1L2, whose RapGAP activity reduces the trafficking velocity near boutons (Andres-Alonso et al., 2019). Collectively, these data suggest that retrograde transport of BDNF/TrkB in neuronal amphisomes is involved in plasticity-relevant local signaling at presynaptic boutons and this process seems tightly coupled to autophagy.
LRRK2 is a crucial enzyme with roles in intracellular membrane trafficking, including functions in the SV cycle, autophagy and the endolysosomal system (Taylor and Alessi, 2020; Piccoli and Volta, 2021). VPS35 is the cargo binding component of the retromer complex, which can serve multiple functions in synapses (Brodin and Shupliakov, 2018). VPS35 is an upstream regulator of LRRK2 and, as LRRK2, it is linked to various neurodegenerative diseases including Parkinson’s disease. Inoshita et al. (2017) studied the functional interaction of these two proteins at the Drosophila larval neuromuscular junction. Here, they could be localized peripherally to the AZ and inside presynaptic boutons, where they are essential for proper SV retrieval (Inoshita et al., 2017). VPS35 constructs carrying a Parkinson’s disease-associated mutation were unable to complement this endocytotic function in VPS35 null mutants. Tight interaction of the Rab7-LRRK2 pathway and the retromer complex was also observed in fly and rat neurons, where overexpression of wildtype, but not mutant, VPS35 protein was able to rescue Parkinson-related sorting defects (MacLeod et al., 2013).
Proteins discussed in this section, i.e., Endophilin-A, Synaptojanin, AP-2, CALM/PICALM, LRRK2 and VPS35, are all associated with endocytic functions within the SV cycle (cf. Table 1). Evidently, they also contribute to the delivery of key components into membrane-based protein degradation processes. However, the spatial organization of this latter machinery and the question of where within the boutons phagophore assembly is initiated remains to be clarified. Similarly, the crosstalk between SV recycling and autophagic and endocytic pathways needs further attention (see also Overhoff et al., 2021).
A link between SV cycling and membrane trafficking processes controlling proteostasis is also indicated by the finding that constituents of autophagy and endolysosomal degradation pathways are associated with SV protein preparations (Takamori et al., 2006; Boyken et al., 2013; Taoufiq et al., 2020). These are also listed in Table 1 and include, e.g., small GTPases like Rab7, Rab11a, Rab12, Rab24, Rab26, Rab35, Rab37, Rab39a, Arf6 and the ADP-ribosylation factor-like GTPase Arl8, the SNARE proteins SNAP-29, Stx17 and VAMP7, constituents of the phosphatidylinositol 3-kinase class III (PIK3C3) complex (Vps34, Vps15, Beclin-1, CISD2, Uvrag), as well as other autophagy-related proteins, i.e., ATG2a, ATG9A, ATG16L1, LC3/ATG8, Tecpr1, and autophagy the receptors WDFY3/ALFY, components of the TRAPP complex and various others. Altogether, Taoufiq et al. (2020) identified ∼40 autophagy-related proteins within the hidden SV proteome, eleven of them in the SV-resident repertoire, while the others were defined as SV-visitors by the authors (Figure 3).
Figure 3. Autophagy-related proteins detected in the hidden proteome of synaptic vesicles (SV) (Taoufiq et al., 2020). Proteins of the SV-resident repertoire are indicated in different shades of orange; proteins defined by the authors as SV-visitors are gray-shaded. Note, the large active zone scaffolding protein Bassoon may be anchored to SVs via N-terminal myristoylation (Dresbach et al., 2003).
Of particular interest is the presence of ATG9 in SVs (Boyken et al., 2013; Taoufiq et al., 2020), a lipid scramblase that is involved in early steps of phagophore formation (Rao et al., 2016; Guardia et al., 2020; Maeda et al., 2020; Matoba and Noda, 2020; Matoba et al., 2020; Orii et al., 2021). Here, it may play a central role in organizing presynaptic autophagy in cooperation with the endocytic machinery (see below).
Rab26 is associated with a subset of recycled SVs, directing them for autophagic degradation (Binotti et al., 2015). This is modulated by the Pleckstrin homology containing family member 5 (PLEKHG5), which acts as guanine exchange factor (GEF) for Rab26. In motoneurons, the lack of PLEKHG5 leads to defective axon growth and impaired SV autophagy, a mechanism that may underlie motoneuron disease (Lüningschrör et al., 2017; Lüningschrör and Sendtner, 2018).
The presence of Arl8 in SV preparations is interesting as this protein has been characterized as a factor associated with anterogradely transported degradative lysosomes (Farias et al., 2017; Farfel-Becker et al., 2019). Vukoja et al. (2018) have proposed that SV precursors may share lysosomal membrane markers, but are distinct from bona fide lysosomes. Another, more recent study reported that Arl8 is involved exclusively in the anterograde transport of lysosomes (De Pace et al., 2020). In addition, Arl8 is involved in controlling fusion of autophagosomes with lysosomes (see Lieberman and Sulzer, 2020), where it may contribute to SV turnover via axonal autolysosomes.
SNAP-29 is regarded as an integral protein of vesicular membranes in the molecular SV model (Takamori et al., 2006; Taoufiq et al., 2020). In addition, SNAP-29, together with VAMP8, are considered as late endosomal/lysosomal SNAREs involved in the fusion with autophagosomes upon forming a SNARE complex with autophagosomal SNARE Syntaxin 17 (Itakura et al., 2012; Hill and Colon-Ramos, 2020).
In addition, multiple constituents of the endocytic machinery and the AZ, such as AP-2 complex, Bassoon, CALM, Endophilin-A, Endophilin-B1, Synaptojanin and VPS35, are associated with SV preparations (Table 1). Further proteins detected in SV preparations include the lysosomal-associated membrane protein LAMP1, the E3-ubiquitin ligase Parkin, Vps33B, a protein associated with late endosomes, as well as the Annexin A7. These proteins are mainly considered as visitor proteins associated with a subset of SVs (Figure 3). In many cases, however, their (pre-)synaptic localization has been reported utilizing immunocytochemistry.
A number of additional proteins with known functions in autophagic and/or endolysosomal degradative pathways could be localized to presynaptic structures as extracted from published literature (Table 1; SynGO database). Among them are:
- Atlastin2/3, which are involved in initiation of phagophore formation by guiding the Unc51-like kinase (ULK1) complex to the ER (Liu et al., 2021).
- Multiple GABARAPs that, as LC3, belong to the family of ATG8-like proteins that can be conjugated to phosphatidylethanolamine and are required for phagophore extension and closure (e.g., Martens and Fracchiolla, 2020).
- ATG5 that together with ATG12 and ATG16L forms an E3-like complex for the conjugation of ATG8/LC3 family members to phosphatidylethanolamine (Chang et al., 2021).
- Vps34, the catalytic kinase subunit of the lipid kinase PIK3C3-Complex 1, acting in phagophore nucleation (Dikic and Elazar, 2018; Chang et al., 2021).
- Sorting nexin 4 (SNX4)/ATG24B, a PI3P-binding protein controlling the recycling of ATG9A for reuse in phagophore formation (Ravussin et al., 2021).
- The cargo adaptors p62/SQSTM1 and Huntingtin (Deng et al., 2017).
- Snapin, a subunit of the BLOC-1 complex, which functions as a motor adaptor that coordinates retrograde transport and late endosomal-lysosomal trafficking (Cai et al., 2010). Moreover, Dynein-Snapin-mediated retrograde transport was reported to promote clearance of presynaptic mitophagosomes (Han et al., 2020).
- TBC1D24/skywalker, a GTPase-activating protein acting on Rab35 in the endolysosomal pathway (Fernandes et al., 2014; Wang et al., 2017) as well as on the small GTPase Arf6, which antagonizes Rab35 in SV recycling from early endosomes (Sheehan et al., 2016; Sheehan and Waites, 2019).
- Arf6, which in addition has been reported as a regulator of autophagosome formation by controlling phosphatidylinositol 4,5-bisphosphate (PIP2) generation and in turn phospholipase D activity (Moreau et al., 2012) and to rescue aberrant autophagosome formation in Synaptojanin-1-deficient in zebrafish cone photoreceptors (George et al., 2016). This includes Arf6 into the list of players in endocytosis-associated initiation of autophagy.
- Components of the HOPS (Homotypic fusion and vacuole protein sorting) complex involved in the fusion of late endosomal and lysosomal compartments (Jiang et al., 2014; van der Beek et al., 2019).
- Reticulon-3 (RTN3), which is involved in ER-phagy (Beese et al., 2019; Stavoe et al., 2019).
- HSC70, a cytosolic chaperonin of the HSP70 family that is involved in microautophagy and chaperone-mediated autophagy (CMA) (Kaushik and Cuervo, 2018), which is known to contribute significantly to the regulation of synaptic protein levels (Uytterhoeven et al., 2015).
Many of the autophagy-related proteins associated with the presynaptic endocytic machinery, the SV proteome, or other presynaptic structures are phospholipid-binding and -modifying proteins. These include, e.g., various subunits of PI3 kinases, PI phosphatases synaptojanin and OCRL, the lipid scramblase ATG9, lipid transfer protein ATG2a, as well as various other phospholipid binding proteins (Table 1). This indicates the significance of the metabolism of phospholipids in general and phosphoinositides in particular for the proper performance of presynaptic autophagy-related processes (for a detailed review see Marat and Haucke, 2016; Palamiuc et al., 2020).
Based on this inventory of autophagy-related proteins present at the presynapse (Figure 4), we can now start discussing potential cellular mechanisms of membrane-associated protein turnover in this compartment.
Figure 4. Inventory of autophagy-related proteins detected in presynaptic boutons and their relevant sites of action (for details see text and Table 1). Bsn, Bassoon; CAZ, cytomatrix at the active zone; EE, early endosome; ER, endoplasmic reticulum; LE/MVB, late endosome/multi-vesicular body; Lys, lysosome; PAS, phagophore assembly site/pre-autophagosomal structure; Pclo, Piccolo; RE, recycling endosome; SE, sorting endosome; and Bulk indicates bulk endocytosis.
A central feature of autophagic function is the recruitment and degradation of aged and damaged proteins and membranous organelles. As in most forms of autophagy in different cells and organisms, presynaptic boutons seem to use the ubiquitination system to tag proteins not only for proteasomal degradation, but also in many instances for their removal by autophagy. This versatile system is capable of attaching specific ubiquitin chains to substrates, and this ubiquitination coding plays an essential role in recruitment to autophagic structures (Deng et al., 2017; Kwon and Ciechanover, 2017). The process of substrate ubiquitination requires the regulated activation of several key component of this tagging system, including the ubiquitin activating enzyme E1, the ubiquitin conjugating enzymes E2 and the E3 adaptor/ligases (Nandi et al., 2006; Ding and Shen, 2008). While there is only one E1 enzyme in mammalian cells, there are tens of E2s and hundreds of E3s (Li et al., 2008; Kawabe and Stegmuller, 2021). Of these, E3s are posited to provide substrate specificity, while the E2s define the type of poly-ubiquitin chain (Kwon and Ciechanover, 2017). These poly-ubiquitin determinants are then recognized by a variety of adaptor proteins that mediate the recruitment of tagged cargos into specific degradation pathways. For example, during autophagy the p62/SQSTM1 adaptor protein is capable of recognizing K63-poly-ubiquitined proteins for their engulfment into autophagic organelles (Linares et al., 2013).
Fundamental questions related to presynaptic autophagy are: which of the many E2 and E3 ligases contribute to presynaptic proteostasis? Further, when are they used and how are they regulated? A number of presynaptic E3 ligases (e.g., Scrapper, FBXO45) have been identified that trigger the removal/destruction of AZ proteins including RIM1, Munc13 or Munc18 via the proteasome (Yao et al., 2007; Tada et al., 2010; Martin et al., 2014). Less is known about which E3s regulate the removal of integral SV proteins. As mentioned above, studies of boutons lacking the presynaptic AZ proteins Piccolo and Bassoon have led to the identification of two E3s, Siah1 and Parkin, linked to the removal of SV proteins via the autophagy degradative system (Waites et al., 2013; Okerlund et al., 2017; Hoffmann-Conaway et al., 2020). Mechanistically, these AZ proteins seem to act as negative regulators of these enzymes. This is best exemplified in studies of Bassoon knockout mice, where the total pool of SVs per bouton is dramatically reduced, which can be restored by knocking down the expression of either Siah1 or Parkin (Hoffmann-Conaway et al., 2020), indicating that Bassoon normally counteracts their actions (Figure 5). For Siah1 this is supported by experiments showing that it directly binds the zinc finger domains in Bassoon, which inhibits Siah1 ubiquitination activity (Waites et al., 2013).
Figure 5. Scenario for the regulation of presynaptic autophagy. Autophagy within presynaptic boutons appears to be locally regulated and mediated via the convergence of two major facets of autophagy. (1) Local tagging of aged and/or damaged proteins/organelles by the ubiquitination system. The active zone protein Bassoon is one regulator of this process by scaffolding E3 ligases such as Siah1 and Parkin. Bassoon can also control the induction of phagophore formation by inhibiting the activity of ATG5 and proteasome function via its binding to Psmb4 proteasomal subunit. (2) The formation of phagophore membranes, which requires an interplay between numerous proteins essential for the regulated conjugation of ATG8/LC3 to membranes containing the integral membrane protein ATG9. Many of these proteins are part of the hidden proteome of SVs (see Figure 3) and thus available for the rapid production of these membranes. This aspect of autophagy seems to be coupled to synaptic activity and the sorting and recycling of SV proteins through early endosomes. While not well understood, this compartment is well positioned to not only sort healthy ensembles of proteins regenerating functional SVs, but also damaged ubiquitinated proteins for engulfment into newly forming phagophores. This latter step requires the small GTPase Rab26 and its guanine exchange factor PLEKHG5, as well as autophagy adaptor proteins, like p62/SQSTM1, which binds both poly-ubiquitin chains and ATG8s. The inserted electron micrograph demonstrates the uptake of entire SV-like structures (asterisk) into autophagic vacuoles (arrowhead) within a presynapse (taken from Hoffmann-Conaway et al., 2020; size bar, 50 nm). Finally, the sorting/recycling endosomes also appears to function in the regeneration of SV-like membranes that carry ATG9, providing autophagic support for boutons in subsequent rounds of neurotransmitter release.
Hints to the substrates of these enzymes come from proteomic studies on synaptosomes isolated from the cortex of Bassoon-deficient mice (Hoffmann-Conaway et al., 2020). These studies identified a dramatic increase in ubiquitinated peptides from SV proteins and proteins involved in SV fusion with the presynaptic membrane, including SNAP25, Synaptotagmin 1, SV2, V-ATPase, VAMP2 and Syntaxin1b. Moreover, autophagy appears to be the major degradative pathway employed, as autophagic vacuoles, but not multi-vesicular bodies, accumulated in these boutons, and inhibiting autophagy restores SV pool size (Hoffmann-Conaway et al., 2020). Intriguingly, this study also found that the Ubiquitin-conjugating enzyme UBE2N was hyper-ubiquitinated. This is highly relevant, as UBE2N is an ubiquitin conjugating enzyme directly involved in creating K63-poly-ubiquitin chains (David et al., 2010), which can tag proteins for degradation via autophagy (Linares et al., 2013). UBE2N can also cooperate with both Siah1 and Parkin (Markson et al., 2009; Fiesel et al., 2014; Geisler et al., 2014). Another E3 ligase, HERC1, was proposed to dysregulate presynaptic pathways and to potentially affect SV autophagy, yet the mode of action is unclear (Montes-Fernandez et al., 2020). This highlights a general challenge for the field.
Given the plethora of known E3 ligases, it will be critical to define which of these are selectively involved in presynaptic autophagy, versus, for example, proteasomal and/or endolysosomal systems. Given the ability of these E3 enzymes to bind various E2s, this will be a daunting, but important challenge. Equally important will be the identification of their associated substrates. In the studies on synapses lacking Bassoon a poignant subset of SV proteins became hyper-ubiquitinated, but to what end? In another study, only a subset of SV proteins was selectively degraded by the endolysosomal system during high synaptic activity conditions (Sheehan et al., 2016), begging the question of which E2s and E3s direct these pathways of destruction.
While it is attractive to consider that there is a high specificity in the clearance systems for particular aspects of presynaptic proteostasis, it is far more likely that there is high flexibility and crosstalk between the different systems, including autophagy, endolysosomal or proteasomal degradation systems, that participate in the removal of different subsets of presynaptic proteins or organelles, like SVs, as was demonstrated for the degradation/turnover of other cellular systems. For example, ATG5-deficient neurons can survive and still display SV recycling (Negrete-Hurtado et al., 2020). Actually, they rather accumulate ER in axons and increase excitatory neurotransmission (Kuijpers et al., 2021) indicating flexibility and compensatory potential in the system.
From a functional perspective, there are several schools of thought: (i) that presynaptic autophagy primarily operates on a slow time scale for the basal removal of aging and/or defective proteins and membranous organelles, (ii) that it is directly involved in the destruction of SVs during high activity, and/or (iii) that it responds to eliminate proteins/membranes following acute and chronic stress or insults.
A key to solving some of these issues lies in how fast and with what specificity presynaptic autophagy can operate. Utilizing the light-activated free-radical generating protein SuperNova (SN) tethered to different SV proteins, it was recently shown that through the acute damage of these proteins, presynaptic autophagy could be induced in minutes and to depend on ubiquitination (Hoffmann et al., 2019). Here, the clearance mechanisms operated with high-specificity, directing the destruction of the SN-tagged protein (SN-synaptophysin or SN-synaptotagmin) to autophagy, but leaving other SV proteins in action. Intriguingly, such acute ROS-mediated damage had real-time effects on synaptic transmission only when autophagy was simultaneously inhibited with drugs or ATG5 knockdown (Hoffmann et al., 2019). One can thus conclude that the presynaptic autophagy machinery is capable of operating in real-time and can be highly selective, removing only damaged and ubiquitinated proteins ensuring the health and functionality of presynaptic boutons. Consistent with studies on boutons lacking Bassoon, these studies highlight the role of the ubiquitination system in tagging proteins for their elimination by autophagy. They also illustrate that the generation of ubiquitin-tagged damaged proteins is in and of itself a key driver of presynaptic autophagy. Potential cellular mechanisms associated with the sorting and delivery of these tagged proteins into newly formed phagophore membranes are discussed below. A point of consideration is the observation that, in boutons lacking Bassoon, autophagophore organelles contain seemingly intact SVs (Figure 5), indicating that whole SVs can also become cargos for autophagic destruction. From a ubiquitination perspective, this could be a consequence of the dramatic increase in the ubiquitination of many SV proteins due to the loss of Bassoon and the activation of Siah1 and Parkin, which then decorate the surface of SVs making them attractive as a cargo for engulfment (Hoffmann-Conaway et al., 2020).
The ability of presynaptic boutons to engage the autophagy degradative system in real-time to maintain its functionality, raise fundamental questions of how this is temporally and spatial achieved. At its most basic level, the ability of autophagy to operate in a spatially restricted manner requires the machinery to be present. Clearly there are two major arms to this process: (i) the sensing and tagging of misfolded or damaged proteins by ubiquitination, and (ii) the formation of autophagophore membranes capable of engulfing these cargos. As we have seen, some of the enzymes involved in the ligation of ubiquitin are scaffolded to components of the AZ cytoskeletal matrix, e.g., Bassoon and Piccolo (Waites et al., 2013; Okerlund et al., 2017; Hoffmann-Conaway et al., 2020).
Interestingly, many of the proteins critical for autophagophore formation are associated either with the perisynaptic endocytic zone, SVs or scaffold proteins of the AZ (Figure 4). Hints to how these might be linked together come from observations in C. elegans and Drosophila showing that there are hotspots of autophagosome formation in close proximity to or directly within presynaptic terminals (Soukup et al., 2016; Stavoe et al., 2016; Neisch et al., 2017; Vanhauwaert et al., 2017). Also, in mammals, many, but not all, constituents of the autophagic machinery have been detected reliably in synaptic boutons (Table 1). For example, of the Unc51-like kinases required for initiation of phagophore formation, only ULK3 has been detected in synaptosomes together with other components of the ULK complex, i.e., FIP100 or ATG101 (Taoufiq et al., 2020). This seems consistent with the observation that ULK1 and ULK2 are not essential for constitutive autophagy in the young murine brain (Wang et al., 2018) and hint to a function of ULK3 in (pre-)synaptic autophagy. Moreover, constituents of the PI3-Kinase C3 complex, including Vps34 and Beclin-1, have been found associated with SV preparations (Figure 3; Taoufiq et al., 2020). Further, there is compelling evidence that components of SV recycling interact with autophagy-related proteins (George et al., 2016; Soukup and Verstreken, 2017; Vanhauwaert et al., 2017), and core components of autophagosomes, including the membrane-delivering protein ATG9, are present in presynaptic boutons (Boyken et al., 2013; Stavoe et al., 2016). This suggests that the local biogenesis of autophagosomes could start at endocytic zones, following SV endocytosis or shortly thereafter when SV proteins pass through early and recycling endosome membranes, where quality control process sort out corrupted proteins.
Actually, membrane trafficking and protein sorting within boutons may be critical for autophagosome formation. Thus, the ATG9 is not only an integral component of the SV membrane (Boyken et al., 2013; Taoufiq et al., 2020), but is also the only transmembrane protein in the core machinery for autophagy (Lang et al., 2000; Noda et al., 2000; Young et al., 2006). A recent study deposited on the bioRxiv server actually suggests that SVs also directly may donate lipids to autophagosomes (Yang et al., 2020; Xuan et al., 2021). These authors propose that ATG9 is transported in vesicles generated at the trans-Golgi network to the presynaptic membrane, which undergo exo-endocytosis prior to accumulation at the peri-AZ and/or autophagosome formation. Mutations that prevent exocytosis (e.g., in UNC13, UNC18 genes) or endocytosis (e.g., in AP1, AP2, or SDPN-1/Syndapin 1 genes) negatively interfere with activity-dependent synaptic autophagy associated with the SV cycle (Imai et al., 2016; Yang et al., 2020; Xuan et al., 2021). Taken together the data suggest that membrane trafficking of ATG9 could couple the SV cycle to activity-dependent presynaptic autophagy. However, as mentioned above, SVs can also be recruited directly into autophagophores vi Rab26 and PLEKHG5 (Binotti et al., 2015; Lüningschrör et al., 2017; Figure 5).
How does neuronal activity and nutrient depletion affect presynaptic autophagy? Starvation/nutrient depletion usually induces autophagy via mTOR signaling (Bockaert and Marin, 2015). As stated above, it remains controversial whether and how strongly starvation affects presynaptic autophagy. At Drosophila larval NMJs, both starvation and increased neuronal activity induces ATG8 accumulation in presynaptic boutons (Soukup et al., 2016; Vanhauwaert et al., 2017). Similarly, mTOR inhibition by rapamycin induces autophagy at dopaminergic release sites and is associated with decreased SV numbers and evoked dopamine release (Hernandez et al., 2012). Furthermore, Catanese et al. (2018) report disorganized SV pools and rapid degradation of Bassoon upon nutrient limitation in rodent primary neuronal cultures. Re-supplementation of glucose can restore SV pools, but does not rescue Bassoon levels. In contrast, there are multiple studies reporting no or only minor effects on presynaptic autophagy (Mizushima et al., 2004; Maday and Holzbaur, 2016). The relative resistance of some neurons to autophagy induction in the presynaptic/axonal compartment by starvation/nutrient depletion may have various reasons that should be tested in future: one may be that presynaptic mTOR signaling is dominated by mTORC2 (McCabe et al., 2020), which is not linked to starvation-induced autophagy; another one could be that presynaptic autophagy (partly) depends on ULK3 (see above), while amino acid starvation-induced autophagy is controlled by ULK1/2 (Cheong et al., 2011) in a subset of neurons.
There is strong evidence for neuronal activity being a major factor regulating presynaptic autophagy. Thus, it is known that neuronal activity, and the recycling of SVs carrying key autophagic molecules, might contribute to the assembly of autophagosomes within boutons. Consistently, KCl stimulation has been shown to induce axonal autophagy and enhances retrograde autophagy flux in hippocampal primary neurons (Wang et al., 2015). Of note, activity can also engage the endolysosomal/ESCRT system to degrade subsets of SV protein (Sheehan et al., 2016).
At the organismal level, various studies imply that autophagy has a major impact on learning and memory (Shehata and Inokuchi, 2014; Liang and Sigrist, 2018; Hwang et al., 2019; Liang, 2019). However, in most cases it is impossible to assign these effects to neurons or even neuronal compartments where the relevant autophagic effect on memory formation is expressed. A particular case of presynaptic autophagic dysfunction seems to be the tambaleante (tbl) mouse, where the HERC1 E3 ubiquitin ligase is mutated. Among other phenotypes these mice display poor performance hippocampus-dependent learning including novel-object recognition, T-maze and Morris water maze tests (Montes-Fernandez et al., 2020; Perez-Villegas et al., 2020). In fruit flies, a tight association between age-dependent memory impairment and structural changes in presynaptic AZs has been observed. Both structural changes and memory decline could be counteracted by treatment with the autophagy enhancer spermidine (Gupta et al., 2016; Liang and Sigrist, 2018). Finally, conditional knockout of the presynaptic AZ protein Bassoon only in excitatory neurons of the murine forebrain, which is supposed to cause enhanced autophagy exclusively in glutamatergic terminals, causes improved memory performance in dentate gyrus-dependent learning tasks, such as contextual fear memory or spatial pattern separation (Annamneedi et al., 2018). This memory improvement was associated with the maintenance of juvenile synaptic plasticity at relevant performant path to dentate gyrus synapses. These examples support the view that well-functioning presynaptic autophagy is a prerequisite for maintaining brain synapses plastic and healthy.
An important unresolved question is how basal, activity-dependent and protein damage induced autophagy relate to each other? One potential answer is they are unrelated. A more parsimonious answer is they are intimately linked playing complementary and sometimes redundant roles. Moreover, the transition between them may primarily relate to the rate of flux and the pathways activated. Given the available data and emerging concepts in the field, we can conceive the following scenario for ubiquitination-dependent modes of protein turnover. It begins with a few knowns. First, that productive autophagy requires the generation of poly-ubiquitin tagged cargos (Figure 5), which can occur during use and natural aging of SVs and their associated proteins or through their acute damage, e.g., by ROS or nitrosylation among others. This could also occur during development, e.g., during synaptic pruning and/or trophic factor withdrawal via the E3 ligase MYCBP2/RPM-1/Highwire (Grill et al., 2016; Crawley et al., 2019). In principle, all this would be mediated by ubiquitin ligases, only a few of which are currently known. Second, that the degradation of these cargos requires the generation of phagophore membranes, which through adaptor proteins such as p62/SQSTM1, recruit the ubiquitinated cargos (Figure 5). This in turn requires the presence and activation of the machinery required to create the phagophore membrane. While there is a clear interdependency of these two arms of autophagy, as disruption of either blocks presynaptic autophagy, it is unclear how this is coordinated, regulated and/or what sensors are used. An attractive focal point for the generation of phagophore membranes and the sorting of ubiquitin-tagged cargos within presynaptic boutons are the early and sorting/recycling endosomal compartments, which maybe be used during basal, activity-dependent and protein damage induced autophagy. Here, it is well appreciated that these membranes operate as quality control stations, working to regenerate functional SVs with the correct complement of proteins. As such, it is easy to imagine that ubiquitin tagged proteins destined for destruction would be sorted into autophagic cargo vesicles at this point. Similarly, it would be a natural site for sorting the autophagy machinery (ATG9 among others), normally associated with SV-like vesicles, into precursor phagophore membranes that then mature into autophagosomes decorated with ATG8-like molecules (LC3, GABARAPs). In the event that there is little cargo, ATG9 would mostly be recycled back into the existing pool of SV-like membranes. Given the complexity of membrane trafficking, it would be important to entertain that many membranes could be used to generate autophagosomes and/or carry ubiquitinated cargos, including the plasma membrane, other endosomal compartments and/or the ER and potentially also SVs.
It is well documented that accurate performance of all types of autophagy as well as the endolysosomal system are of utmost importance for cellular health. This applies particularly to neurons and their synapses with their extremely long lifespans. All major brain disorders are associated with failures of these clearance systems, either as causes or consequences of the pathology. In particular, neurodegenerative disorders, such as Parkinson’s Disease or Alzheimer’s can be directly linked with autophagy failures, though physiological aging is also associated with slow exhaustion of clearing systems. We have largely ignored this aspect in the present article and focused mainly on the molecular inventory of the presynapse, because disease-related issues have been the subject of numerous review articles during recent years (e.g., Rubinsztein, 2006; Mizushima et al., 2008; Bezprozvanny and Hiesinger, 2013; Michel et al., 2016; Deng et al., 2017; Menzies et al., 2017; Dikic and Elazar, 2018; Liang and Sigrist, 2018; Soukup et al., 2018; Lee and Kim, 2019; Malik et al., 2019; Pan et al., 2019; Giovedi et al., 2020; Tomoda et al., 2020). However, it is very obvious that there are multiple gaps in our knowledge about disease mechanisms. Therefore, a detailed understanding of the molecular organization of (pre-)synaptic protein turnover and clearance systems at the nanoscale is a major future challenge for the field.
Additional questions worth consideration include: Do clearance systems operate in a similar manner at all synapses? Do autophagic events occur near/within boutons or more remotely? On what time scales does autophagy operate? Which ubiquitin tagging systems (E2, E3 enzymes) are utilized? How many and when are the different lipidated ATG8-like conjugates used? Are different Unc-51-like kinase employed under specific conditions? What autophagy receptors/cargo adaptors are tapped and at which synapses? What ubiquitin-independent mechanisms are in operation?
Further questions concern the sorting and routing of damaged proteins. Where does the sorting take place? Which organelles contribute membranes to the phagophore? Do SVs contribute to phagophore formation? Can SVs even act as a starting point for phagophore assembly? How can clearance pathways compensate for each other and is this controlled and regulated?
Finally, there are interesting recent observations that liquid phase separation might also play a role in PAS formation and the initiation of autophagy (Fujioka et al., 2020; Hollenstein and Kraft, 2020). For example, evidence from yeast indicates that the endocytic protein Ede1 (the yeast homolog of p115/Uso1) can mediate the formation endocytic protein condensates (ENDs) that then enter an autophagic degradation path (Wilfling et al., 2020). Another recent study revealed that condensed liquid droplets, formed from the AZ proteins RIM, RIM-binding protein and ELKS/CAST2 can tether SVs in vitro (Wu et al., 2021). The authors hypothesize that similar processes play a role in organelle biogenesis and autophagy. It will thus be interesting to see whether phase separation indeed triggers autophagy at the perisynaptic endocytic zone.
EG, CG, and MK wrote the article. AK designed and prepared the figures. RP provided bioinformatic and database analyses including Figure 2. All authors contributed to the conceptualization and the discussion of the content.
EG, AK, CG, and MK are supported by the Deutsche Forschungsgemeinschaft (DFG, FOR 5228 Syntophagy RP03 and RP06; and RTG 2413 SynAge TP04 and TP08). In addition, AK was supported by a LIN Special Project; RP by the state Saxony-Anhalt and the EU-EFRE program (ZS/2016/04/78113); CG was supported by the German Center for Neurodegenerative Diseases; the DFG CRC958 and the German Excellence Strategy (EXC-2049-390688087); and MK by grants from the Leibniz SAW program (SynERCa and SyMetAge).
The authors declare that the research was conducted in the absence of any commercial or financial relationships that could be construed as a potential conflict of interest.
All claims expressed in this article are solely those of the authors and do not necessarily represent those of their affiliated organizations, or those of the publisher, the editors and the reviewers. Any product that may be evaluated in this article, or claim that may be made by its manufacturer, is not guaranteed or endorsed by the publisher.
AZ, active zone; BAR domain, Bin-Amphiphysin-Rvs domain; CMA, chaperone-mediated autophagy; NMJ, neuromuscular junction; PAS, pre-autophagosomal structure (also: phagophore assembly site); Peri-AZ, periactive zone, = main endocytic zone; SV, synaptic vesicle; SN, SuperNova; S/T, Serine/Threonine; UPS, Ubiquitin-Proteasome-System.
Ackermann, F., Schink, K. O., Bruns, C., Izsvak, Z., Hamra, F. K., Rosenmund, C., et al. (2019). Critical role for Piccolo in synaptic vesicle retrieval. Elife 8:e46629. doi: 10.7554/eLife.46629
Ackermann, F., Waites, C. L., and Garner, C. C. (2015). Presynaptic active zones in invertebrates and vertebrates. EMBO Rep. 16, 923–938. doi: 10.15252/embr.201540434
Alers, S., Loffler, A. S., Wesselborg, S., and Stork, B. (2012). The incredible ULKs. Cell Commun. Signal. 10:7. doi: 10.1186/1478-811X-10-7
Alirezaei, M., Kemball, C. C., Flynn, C. T., Wood, M. R., Whitton, J. L., and Kiosses, W. B. (2010). Short-term fasting induces profound neuronal autophagy. Autophagy 6, 702–710. doi: 10.4161/auto.6.6.12376
Andres-Alonso, M., Ammar, M. R., Butnaru, I., Gomes, G. M., Acuna Sanhueza, G., Raman, R., et al. (2019). SIPA1L2 controls trafficking and local signaling of TrkB-containing amphisomes at presynaptic terminals. Nat. Commun. 10:5448. doi: 10.1038/s41467-019-13224-z
Andres-Alonso, M., Kreutz, M. R., and Karpova, A. (2021). Autophagy and the endolysosomal system in presynaptic function. Cell Mol. Life Sci. 78, 2621–2639. doi: 10.1007/s00018-020-03722-5
Annamneedi, A., Caliskan, G., Muller, S., Montag, D., Budinger, E., Angenstein, F., et al. (2018). Ablation of the presynaptic organizer Bassoon in excitatory neurons retards dentate gyrus maturation and enhances learning performance. Brain Struct. Funct. 223, 3423–3445. doi: 10.1007/s00429-018-1692-3
Arias, E., Koga, H., Diaz, A., Mocholi, E., Patel, B., and Cuervo, A. M. (2015). Lysosomal mTORC2/PHLPP1/Akt Regulate Chaperone-Mediated Autophagy. Mol. Cell 59, 270–284. doi: 10.1016/j.molcel.2015.05.030
Azarnia Tehran, D., Kuijpers, M., and Haucke, V. (2018). Presynaptic endocytic factors in autophagy and neurodegeneration. Curr. Opin. Neurobiol. 48, 153–159. doi: 10.1016/j.conb.2017.12.018
Bakula, D., Muller, A. J., Zuleger, T., Takacs, Z., Franz-Wachtel, M., Thost, A. K., et al. (2017). WIPI3 and WIPI4 beta-propellers are scaffolds for LKB1-AMPK-TSC signalling circuits in the control of autophagy. Nat. Commun. 8:15637. doi: 10.1038/ncomms15637
Beese, C. J., Brynjolfsdottir, S. H., and Frankel, L. B. (2019). Selective Autophagy of the Protein Homeostasis Machinery: ribophagy, Proteaphagy and ER-Phagy. Front. Cell Dev. Biol. 7:373. doi: 10.3389/fcell.2019.00373
Behrends, C., Sowa, M. E., Gygi, S. P., and Harper, J. W. (2010). Network organization of the human autophagy system. Nature 466, 68–76. doi: 10.1038/nature09204
Bezprozvanny, I., and Hiesinger, P. R. (2013). The synaptic maintenance problem: membrane recycling, Ca2+ homeostasis and late onset degeneration. Mol. Neurodegener. 8:23. doi: 10.1186/1750-1326-8-23
Binotti, B., Jahn, R., and Chua, J. J. (2016). Functions of Rab Proteins at Presynaptic Sites. Cells 5:7. doi: 10.3390/cells5010007
Binotti, B., Pavlos, N. J., Riedel, D., Wenzel, D., Vorbruggen, G., Schalk, A. M., et al. (2015). The GTPase Rab26 links synaptic vesicles to the autophagy pathway. Elife 4:e05597. doi: 10.7554/eLife.05597
Bockaert, J., and Marin, P. (2015). mTOR in Brain Physiology and Pathologies. Physiol. Rev. 95, 1157–1187. doi: 10.1152/physrev.00038.2014
Boecker, C. A., and Holzbaur, E. L. (2019). Vesicular degradation pathways in neurons: at the crossroads of autophagy and endo-lysosomal degradation. Curr. Opin. Neurobiol. 57, 94–101. doi: 10.1016/j.conb.2019.01.005
Boland, B., Kumar, A., Lee, S., Platt, F. M., Wegiel, J., Yu, W. H., et al. (2008). Autophagy induction and autophagosome clearance in neurons: relationship to autophagic pathology in Alzheimer’s disease. J. Neurosci. 28, 6926–6937. doi: 10.1523/JNEUROSCI.0800-08.2008
Borchers, A. C., Langemeyer, L., and Ungermann, C. (2021). Who’s in control? Principles of Rab GTPase activation in endolysosomal membrane trafficking and beyond. J. Cell Biol. 220:e202105120. doi: 10.1083/jcb.202105120
Boucrot, E., Ferreira, A. P., Almeida-Souza, L., Debard, S., Vallis, Y., Howard, G., et al. (2015). Endophilin marks and controls a clathrin-independent endocytic pathway. Nature 517, 460–465. doi: 10.1038/nature14067
Boyken, J., Gronborg, M., Riedel, D., Urlaub, H., Jahn, R., and Chua, J. J. (2013). Molecular profiling of synaptic vesicle docking sites reveals novel proteins but few differences between glutamatergic and GABAergic synapses. Neuron 78, 285–297. doi: 10.1016/j.neuron.2013.02.027
Brodin, L., and Shupliakov, O. (2018). Retromer in Synaptic Function and Pathology. Front. Synaptic Neurosci. 10:37. doi: 10.3389/fnsyn.2018.00037
Cai, C. Z., Yang, C., Zhuang, X. X., Yuan, N. N., Wu, M. Y., Tan, J. Q., et al. (2021). NRBF2 is a RAB7 effector required for autophagosome maturation and mediates the association of APP-CTFs with active form of RAB7 for degradation. Autophagy 17, 1112–1130. doi: 10.1080/15548627.2020.1760623
Cai, Q., Lu, L., Tian, J. H., Zhu, Y. B., Qiao, H., and Sheng, Z. H. (2010). Snapin-regulated late endosomal transport is critical for efficient autophagy-lysosomal function in neurons. Neuron 68, 73–86. doi: 10.1016/j.neuron.2010.09.022
Cano, R., and Tabares, L. (2016). The Active and Periactive Zone Organization and the Functional Properties of Small and Large Synapses. Front. Synaptic Neurosci. 8:12. doi: 10.3389/fnsyn.2016.00012
Cao, M., Milosevic, I., Giovedi, S., and De Camilli, P. (2014). Upregulation of Parkin in endophilin mutant mice. J. Neurosci. 34, 16544–16549. doi: 10.1523/JNEUROSCI.1710-14.2014
Cason, S. E., Carman, P. J., Van Duyne, C., Goldsmith, J., Dominguez, R., and Holzbaur, E. L. F. (2021). Sequential dynein effectors regulate axonal autophagosome motility in a maturation-dependent pathway. J. Cell Biol. 220:e202010179. doi: 10.1083/jcb.202010179
Catanese, A., Garrido, D., Walther, P., Roselli, F., and Boeckers, T. M. (2018). Nutrient limitation affects presynaptic structures through dissociable Bassoon autophagic degradation and impaired vesicle release. J. Cereb. Blood Flow Metab. 38, 1924–1939. doi: 10.1177/0271678X18786356
Chang, C., Jensen, L. E., and Hurley, J. H. (2021). Autophagosome biogenesis comes out of the black box. Nat. Cell Biol. 23, 450–456. doi: 10.1038/s41556-021-00669-y
Chang, N. C., Nguyen, M., Germain, M., and Shore, G. C. (2010). Antagonism of Beclin 1-dependent autophagy by BCL-2 at the endoplasmic reticulum requires NAF-1. EMBO J. 29, 606–618. doi: 10.1038/emboj.2009.369
Chen, D., Fan, W., Lu, Y., Ding, X., Chen, S., and Zhong, Q. (2012). A mammalian autophagosome maturation mechanism mediated by TECPR1 and the Atg12-Atg5 conjugate. Mol. Cell 45, 629–641. doi: 10.1016/j.molcel.2011.12.036
Chen, S., Mari, M., Parashar, S., Liu, D., Cui, Y., Reggiori, F., et al. (2020). Vps13 is required for the packaging of the ER into autophagosomes during ER-phagy. Proc. Natl. Acad. Sci. U. S. A. 117, 18530–18539. doi: 10.1073/pnas.2008923117
Cheng, X. T., Zhou, B., Lin, M. Y., Cai, Q., and Sheng, Z. H. (2015). Axonal autophagosomes recruit dynein for retrograde transport through fusion with late endosomes. J. Cell Biol. 209, 377–386. doi: 10.1083/jcb.201412046
Cheong, H., Lindsten, T., Wu, J., Lu, C., and Thompson, C. B. (2011). Ammonia-induced autophagy is independent of ULK1/ULK2 kinases. Proc. Natl. Acad. Sci. U. S. A. 108, 11121–11126. doi: 10.1073/pnas.1107969108
Citri, A., and Malenka, R. C. (2008). Synaptic plasticity: multiple forms, functions, and mechanisms. Neuropsychopharmacology 33, 18–41. doi: 10.1038/sj.npp.1301559
Cohen, L. D., and Ziv, N. E. (2017). Recent insights on principles of synaptic protein degradation. F1000Res. 6:675. doi: 10.12688/f1000research.10599.1
Cohen, L. D., and Ziv, N. E. (2019). Neuronal and synaptic protein lifetimes. Curr. Opin. Neurobiol. 57, 9–16. doi: 10.1016/j.conb.2018.12.007
Crawley, O., Opperman, K. J., Desbois, M., Adrados, I., Borgen, M. A., Giles, A. C., et al. (2019). Autophagy is inhibited by ubiquitin ligase activity in the nervous system. Nat. Commun. 10:5017. doi: 10.1038/s41467-019-12804-3
Cremona, O., Di Paolo, G., Wenk, M. R., Luthi, A., Kim, W. T., Takei, K., et al. (1999). Essential role of phosphoinositide metabolism in synaptic vesicle recycling. Cell 99, 179–188. doi: 10.1016/s0092-8674(00)81649-9
David, Y., Ziv, T., Admon, A., and Navon, A. (2010). The E2 ubiquitin-conjugating enzymes direct polyubiquitination to preferred lysines. J. Biol. Chem. 285, 8595–8604. doi: 10.1074/jbc.M109.089003
De Gregorio, C., Delgado, R., Ibacache, A., Sierralta, J., and Couve, A. (2017). Drosophila Atlastin in motor neurons is required for locomotion and presynaptic function. J. Cell Sci. 130, 3507–3516. doi: 10.1242/jcs.201657
De Leo, M. G., Staiano, L., Vicinanza, M., Luciani, A., Carissimo, A., Mutarelli, M., et al. (2016). Autophagosome-lysosome fusion triggers a lysosomal response mediated by TLR9 and controlled by OCRL. Nat. Cell Biol. 18, 839–850. doi: 10.1038/ncb3386
De Pace, R., Britt, D. J., Mercurio, J., Foster, A. M., Djavaherian, L., Hoffmann, V., et al. (2020). Synaptic Vesicle Precursors and Lysosomes Are Transported by Different Mechanisms in the Axon of Mammalian Neurons. Cell Rep. 31:107775. doi: 10.1016/j.celrep.2020.107775
Deng, Z., Purtell, K., Lachance, V., Wold, M. S., Chen, S., and Yue, Z. (2017). Autophagy Receptors and Neurodegenerative Diseases. Trends Cell Biol. 27, 491–504. doi: 10.1016/j.tcb.2017.01.001
Diao, J., Liu, R., Rong, Y., Zhao, M., Zhang, J., Lai, Y., et al. (2015). ATG14 promotes membrane tethering and fusion of autophagosomes to endolysosomes. Nature 520, 563–566. doi: 10.1038/nature14147
Dikic, I., and Elazar, Z. (2018). Mechanism and medical implications of mammalian autophagy. Nat. Rev. Mol. Cell Biol. 19, 349–364. doi: 10.1038/s41580-018-0003-4
Ding, M., and Shen, K. (2008). The role of the ubiquitin proteasome system in synapse remodeling and neurodegenerative diseases. Bioessays 30, 1075–1083. doi: 10.1002/bies.20843
Dresbach, T., Hempelmann, A., Spilker, C., tom Dieck, S., Altrock, W. D., Zuschratter, W., et al. (2003). Functional regions of the presynaptic cytomatrix protein bassoon: significance for synaptic targeting and cytomatrix anchoring. Mol. Cell Neurosci. 23, 279–291. doi: 10.1016/s1044743103000150
Farfel-Becker, T., Roney, J. C., Cheng, X. T., Li, S., Cuddy, S. R., and Sheng, Z. H. (2019). Neuronal Soma-Derived Degradative Lysosomes Are Continuously Delivered to Distal Axons to Maintain Local Degradation Capacity. Cell Rep. 28, 51–64e54. doi: 10.1016/j.celrep.2019.06.013
Farias, G. G., Guardia, C. M., De Pace, R., Britt, D. J., and Bonifacino, J. S. (2017). BORC/kinesin-1 ensemble drives polarized transport of lysosomes into the axon. Proc. Natl. Acad. Sci. U. S. A. 114, E2955–E2964. doi: 10.1073/pnas.1616363114
Fernandes, A. C., Uytterhoeven, V., Kuenen, S., Wang, Y. C., Slabbaert, J. R., Swerts, J., et al. (2014). Reduced synaptic vesicle protein degradation at lysosomes curbs TBC1D24/sky-induced neurodegeneration. J. Cell Biol. 207, 453–462. doi: 10.1083/jcb.201406026
Fiesel, F. C., Moussaud-Lamodiere, E. L., Ando, M., and Springer, W. (2014). A specific subset of E2 ubiquitin-conjugating enzymes regulate Parkin activation and mitophagy differently. J. Cell Sci. 127, 3488–3504. doi: 10.1242/jcs.147520
Fimia, G. M., Stoykova, A., Romagnoli, A., Giunta, L., Di Bartolomeo, S., Nardacci, R., et al. (2007). Ambra1 regulates autophagy and development of the nervous system. Nature 447, 1121–1125. doi: 10.1038/nature05925
Fornasiero, E. F., Mandad, S., Wildhagen, H., Alevra, M., Rammner, B., Keihani, S., et al. (2018). Precisely measured protein lifetimes in the mouse brain reveal differences across tissues and subcellular fractions. Nat. Commun. 9:4230. doi: 10.1038/s41467-018-06519-0
Fujioka, Y., Alam, J. M., Noshiro, D., Mouri, K., Ando, T., Okada, Y., et al. (2020). Phase separation organizes the site of autophagosome formation. Nature 578, 301–305. doi: 10.1038/s41586-020-1977-6
Garner, C. C., Kindler, S., and Gundelfinger, E. D. (2000). Molecular determinants of presynaptic active zones. Curr. Opin. Neurobiol. 10, 321–327. doi: 10.1016/s0959-4388(00)00093-3
Gatica, D., Lahiri, V., and Klionsky, D. J. (2018). Cargo recognition and degradation by selective autophagy. Nat. Cell Biol. 20, 233–242. doi: 10.1038/s41556-018-0037-z
Geisler, S., Vollmer, S., Golombek, S., and Kahle, P. J. (2014). The ubiquitin-conjugating enzymes UBE2N, UBE2L3 and UBE2D2/3 are essential for Parkin-dependent mitophagy. J. Cell Sci. 127, 3280–3293. doi: 10.1242/jcs.146035
George, A. A., Hayden, S., Stanton, G. R., and Brockerhoff, S. E. (2016). Arf6 and the 5’phosphatase of synaptojanin 1 regulate autophagy in cone photoreceptors. Bioessays 38, S119–S135. doi: 10.1002/bies.201670913
Giovedi, S., Ravanelli, M. M., Parisi, B., Bettegazzi, B., and Guarnieri, F. C. (2020). Dysfunctional Autophagy and Endolysosomal System in Neurodegenerative Diseases: relevance and Therapeutic Options. Front. Cell Neurosci. 14:602116. doi: 10.3389/fncel.2020.602116
Gowrisankaran, S., Houy, S., Del Castillo, J. G. P., Steubler, V., Gelker, M., Kroll, J., et al. (2020). Endophilin-A coordinates priming and fusion of neurosecretory vesicles via intersectin. Nat. Commun. 11:1266. doi: 10.1038/s41467-020-14993-8
Grill, B., Murphey, R. K., and Borgen, M. A. (2016). The PHR proteins: intracellular signaling hubs in neuronal development and axon degeneration. Neural Dev. 11:8. doi: 10.1186/s13064-016-0063-0
Grumati, P., Dikic, I., and Stolz, A. (2018). ER-phagy at a glance. J. Cell Sci. 131:jcs217364. doi: 10.1242/jcs.217364
Guardia, C. M., Jain, A., Mattera, R., Friefeld, A., Li, Y., and Bonifacino, J. S. (2021). RUSC2 and WDR47 oppositely regulate kinesin-1-dependent distribution of ATG9A to the cell periphery. Mol. Biol. Cell 32:ar25. doi: 10.1091/mbc.E21-06-0295
Guardia, C. M., Tan, X. F., Lian, T., Rana, M. S., Zhou, W., Christenson, E. T., et al. (2020). Structure of Human ATG9A, the Only Transmembrane Protein of the Core Autophagy Machinery. Cell Rep. 31:107837. doi: 10.1016/j.celrep.2020.107837
Gundelfinger, E. D., and Fejtova, A. (2012). Molecular organization and plasticity of the cytomatrix at the active zone. Curr. Opin. Neurobiol. 22, 423–430. doi: 10.1016/j.conb.2011.10.005
Gundelfinger, E. D., Kessels, M. M., and Qualmann, B. (2003). Temporal and spatial coordination of exocytosis and endocytosis. Nat. Rev. Mol. Cell Biol. 4, 127–139. doi: 10.1038/nrm1016
Gundelfinger, E. D., Reissner, C., and Garner, C. C. (2016). Role of Bassoon and Piccolo in Assembly and Molecular Organization of the Active Zone. Front. Synaptic Neurosci. 7:19. doi: 10.3389/fnsyn.2015.00019
Gupta, V. K., Pech, U., Bhukel, A., Fulterer, A., Ender, A., Mauermann, S. F., et al. (2016). Spermidine Suppresses Age-Associated Memory Impairment by Preventing Adverse Increase of Presynaptic Active Zone Size and Release. PLoS Biol. 14:e1002563. doi: 10.1371/journal.pbio.1002563
Hakim, V., Cohen, L. D., Zuchman, R., Ziv, T., and Ziv, N. E. (2016). The effects of proteasomal inhibition on synaptic proteostasis. EMBO J. 35, 2238–2262. doi: 10.15252/embj.201593594
Han, S., Jeong, Y. Y., Sheshadri, P., Su, X., and Cai, Q. (2020). Mitophagy regulates integrity of mitochondria at synapses and is critical for synaptic maintenance. EMBO Rep. 21:e49801. doi: 10.15252/embr.201949801
Harris, J. J., Jolivet, R., and Attwell, D. (2012). Synaptic energy use and supply. Neuron 75, 762–777. doi: 10.1016/j.neuron.2012.08.019
Haucke, V., Neher, E., and Sigrist, S. J. (2011). Protein scaffolds in the coupling of synaptic exocytosis and endocytosis. Nat. Rev. Neurosci. 12, 127–138. doi: 10.1038/nrn2948
Hernandez, D., Torres, C. A., Setlik, W., Cebrian, C., Mosharov, E. V., Tang, G., et al. (2012). Regulation of presynaptic neurotransmission by macroautophagy. Neuron 74, 277–284. doi: 10.1016/j.neuron.2012.02.020
Hill, S. E., and Colon-Ramos, D. A. (2019). A specific ATG-4 isoform is required for autophagic maturation and clearance in C. elegans neurons. Autophagy 15, 1840–1842. doi: 10.1080/15548627.2019.1632123
Hill, S. E., and Colon-Ramos, D. A. (2020). The Journey of the Synaptic Autophagosome: a Cell Biological Perspective. Neuron 105, 961–973. doi: 10.1016/j.neuron.2020.01.018
Hill, S. E., Kauffman, K. J., Krout, M., Richmond, J. E., Melia, T. J., and Colon-Ramos, D. A. (2019). Maturation and Clearance of Autophagosomes in Neurons Depends on a Specific Cysteine Protease Isoform, ATG-4.2. Dev. Cell 49, 251–266.e8. doi: 10.1016/j.devcel.2019.02.013
Hoffmann, S., Orlando, M., Andrzejak, E., Bruns, C., Trimbuch, T., Rosenmund, C., et al. (2019). Light-Activated ROS Production Induces Synaptic Autophagy. J. Neurosci. 39, 2163–2183. doi: 10.1523/JNEUROSCI.1317-18.2019
Hoffmann-Conaway, S., Brockmann, M. M., Schneider, K., Annamneedi, A., Rahman, K. A., Bruns, C., et al. (2020). Parkin contributes to synaptic vesicle autophagy in Bassoon-deficient mice. Elife 9:e56590. doi: 10.7554/eLife.56590
Hollenstein, D. M., and Kraft, C. (2020). Autophagosomes are formed at a distinct cellular structure. Curr. Opin. Cell Biol. 65, 50–57. doi: 10.1016/j.ceb.2020.02.012
Holtmaat, A. J., Trachtenberg, J. T., Wilbrecht, L., Shepherd, G. M., Zhang, X., Knott, G. W., et al. (2005). Transient and persistent dendritic spines in the neocortex in vivo. Neuron 45, 279–291. doi: 10.1016/j.neuron.2005.01.003
Hwang, J. Y., Yan, J., and Zukin, R. S. (2019). Autophagy and synaptic plasticity: epigenetic regulation. Curr. Opin. Neurobiol. 59, 207–212. doi: 10.1016/j.conb.2019.09.010
Hyttinen, J. M., Niittykoski, M., Salminen, A., and Kaarniranta, K. (2013). Maturation of autophagosomes and endosomes: a key role for Rab7. Biochim. Biophys. Acta 1833, 503–510. doi: 10.1016/j.bbamcr.2012.11.018
Imai, K., Hao, F., Fujita, N., Tsuji, Y., Oe, Y., Araki, Y., et al. (2016). Atg9A trafficking through the recycling endosomes is required for autophagosome formation. J. Cell Sci. 129, 3781–3791. doi: 10.1242/jcs.196196
Inaguma, Y., Ito, H., Iwamoto, I., Matsumoto, A., Yamagata, T., Tabata, H., et al. (2016). Morphological characterization of Class III phosphoinositide 3-kinase during mouse brain development. Med. Mol. Morphol. 49, 28–33. doi: 10.1007/s00795-015-0116-1
Inoshita, T., Arano, T., Hosaka, Y., Meng, H., Umezaki, Y., Kosugi, S., et al. (2017). Vps35 in cooperation with LRRK2 regulates synaptic vesicle endocytosis through the endosomal pathway in Drosophila. Hum. Mol. Genet. 26, 2933–2948. doi: 10.1093/hmg/ddx179
Issa, A. R., Sun, J., Petitgas, C., Mesquita, A., Dulac, A., Robin, M., et al. (2018). The lysosomal membrane protein LAMP2A promotes autophagic flux and prevents SNCA-induced Parkinson disease-like symptoms in the Drosophila brain. Autophagy 14, 1898–1910. doi: 10.1080/15548627.2018.1491489
Itakura, E., Kishi-Itakura, C., and Mizushima, N. (2012). The hairpin-type tail-anchored SNARE syntaxin 17 targets to autophagosomes for fusion with endosomes/lysosomes. Cell 151, 1256–1269. doi: 10.1016/j.cell.2012.11.001
Jaber, N., Mohd-Naim, N., Wang, Z., DeLeon, J. L., Kim, S., Zhong, H., et al. (2016). Vps34 regulates Rab7 and late endocytic trafficking through recruitment of the GTPase-activating protein Armus. J. Cell Sci. 129, 4424–4435. doi: 10.1242/jcs.192260
Ji, C., Tang, M., Zeidler, C., Hohfeld, J., and Johnson, G. V. (2019). BAG3 and SYNPO (synaptopodin) facilitate phospho-MAPT/Tau degradation via autophagy in neuronal processes. Autophagy 15, 1199–1213. doi: 10.1080/15548627.2019.1580096
Jiang, P., Nishimura, T., Sakamaki, Y., Itakura, E., Hatta, T., Natsume, T., et al. (2014). The HOPS complex mediates autophagosome-lysosome fusion through interaction with syntaxin 17. Mol. Biol. Cell 25, 1327–1337. doi: 10.1091/mbc.E13-08-0447
Jin, E. J., Kiral, F. R., Ozel, M. N., Burchardt, L. S., Osterland, M., Epstein, D., et al. (2018). Live Observation of Two Parallel Membrane Degradation Pathways at Axon Terminals. Curr. Biol. 28, 1027–1038.e4. doi: 10.1016/j.cub.2018.02.032
Kannan, M., Bayam, E., Wagner, C., Rinaldi, B., Kretz, P. F., Tilly, P., et al. (2017). WD40-repeat 47, a microtubule-associated protein, is essential for brain development and autophagy. Proc. Natl. Acad. Sci. U. S. A. 114, E9308–E9317. doi: 10.1073/pnas.1713625114
Kaushik, S., and Cuervo, A. M. (2018). The coming of age of chaperone-mediated autophagy. Nat. Rev. Mol. Cell Biol. 19, 365–381. doi: 10.1038/s41580-018-0001-6
Kawabe, H., and Stegmuller, J. (2021). The role of E3 ubiquitin ligases in synapse function in the healthy and diseased brain. Mol. Cell Neurosci. 112:103602. doi: 10.1016/j.mcn.2021.103602
Khaminets, A., Behl, C., and Dikic, I. (2016). Ubiquitin-Dependent And Independent Signals In Selective Autophagy. Trends Cell Biol. 26, 6–16. doi: 10.1016/j.tcb.2015.08.010
Khaminets, A., Heinrich, T., Mari, M., Grumati, P., Huebner, A. K., Akutsu, M., et al. (2015). Regulation of endoplasmic reticulum turnover by selective autophagy. Nature 522, 354–358. doi: 10.1038/nature14498
Kirkin, V., Lamark, T., Sou, Y. S., Bjorkoy, G., Nunn, J. L., Bruun, J. A., et al. (2009). A role for NBR1 in autophagosomal degradation of ubiquitinated substrates. Mol. Cell 33, 505–516. doi: 10.1016/j.molcel.2009.01.020
Kitada, T., Asakawa, S., Hattori, N., Matsumine, H., Yamamura, Y., Minoshima, S., et al. (1998). Mutations in the parkin gene cause autosomal recessive juvenile parkinsonism. Nature 392, 605–608. doi: 10.1038/33416
Kohrs, F. E., Daumann, I. M., Pavlovic, B., Jin, E. J., Kiral, F. R., Lin, S. C., et al. (2021). Systematic functional analysis of rab GTPases reveals limits of neuronal robustness to environmental challenges in flies. Elife 10:e59594. doi: 10.7554/eLife.59594
Komatsu, M., Wang, Q. J., Holstein, G. R., Friedrich, V. L. Jr., Iwata, J., Kominami, E., et al. (2007). Essential role for autophagy protein Atg7 in the maintenance of axonal homeostasis and the prevention of axonal degeneration. Proc. Natl. Acad. Sci. U. S. A. 104, 14489–14494. doi: 10.1073/pnas.0701311104
Kononenko, N. L., Classen, G. A., Kuijpers, M., Puchkov, D., Maritzen, T., Tempes, A., et al. (2017). Retrograde transport of TrkB-containing autophagosomes via the adaptor AP-2 mediates neuronal complexity and prevents neurodegeneration. Nat. Commun. 8:14819. doi: 10.1038/ncomms14819
Kononenko, N. L., Puchkov, D., Classen, G. A., Walter, A. M., Pechstein, A., Sawade, L., et al. (2014). Clathrin/AP-2 mediate synaptic vesicle reformation from endosome-like vacuoles but are not essential for membrane retrieval at central synapses. Neuron 82, 981–988. doi: 10.1016/j.neuron.2014.05.007
Koopmans, F., van Nierop, P., Andres-Alonso, M., Byrnes, A., Cijsouw, T., Coba, M. P., et al. (2019). SynGO: an Evidence-Based, Expert-Curated Knowledge Base for the Synapse. Neuron 103, 217–234.e4. doi: 10.1016/j.neuron.2019.05.002
Kroll, J., Jaime Tobon, L. M., Vogl, C., Neef, J., Kondratiuk, I., Konig, M., et al. (2019). Endophilin-A regulates presynaptic Ca2+ influx and synaptic vesicle recycling in auditory hair cells. EMBO J. 38:e100116. doi: 10.15252/embj.2018100116
Kuijpers, M., Azarnia Tehran, D., Haucke, V., and Soykan, T. (2020). The axonal endolysosomal and autophagic systems. J. Neurochem. 158, 589–602. doi: 10.1111/jnc.15287
Kuijpers, M., Kochlamazashvili, G., Stumpf, A., Puchkov, D., Swaminathan, A., Lucht, M. T., et al. (2021). Neuronal Autophagy Regulates Presynaptic Neurotransmission by Controlling the Axonal Endoplasmic Reticulum. Neuron 109, 299–313.e9. doi: 10.1016/j.neuron.2020.10.005
Kulkarni, A., Dong, A., Kulkarni, V. V., Chen, J., Laxton, O., Anand, A., et al. (2020). Differential regulation of autophagy during metabolic stress in astrocytes and neurons. Autophagy 16, 1651–1667. doi: 10.1080/15548627.2019.1703354
Kwon, Y. T., and Ciechanover, A. (2017). The Ubiquitin Code in the Ubiquitin-Proteasome System and Autophagy. Trends Biochem. Sci. 42, 873–886. doi: 10.1016/j.tibs.2017.09.002
Lamb, C. A., Nuhlen, S., Judith, D., Frith, D., Snijders, A. P., Behrends, C., et al. (2016). TBC1D14 regulates autophagy via the TRAPP complex and ATG9 traffic. EMBO J. 35, 281–301. doi: 10.15252/embj.201592695
Lang, T., Reiche, S., Straub, M., Bredschneider, M., and Thumm, M. (2000). Autophagy and the cvt pathway both depend on AUT9. J. Bacteriol. 182, 2125–2133. doi: 10.1128/JB.182.8.2125-2133.2000
Lassek, M., Weingarten, J., Einsfelder, U., Brendel, P., Muller, U., and Volknandt, W. (2013). Amyloid precursor proteins are constituents of the presynaptic active zone. J. Neurochem. 127, 48–56. doi: 10.1111/jnc.12358
Lazarevic, V., Schone, C., Heine, M., Gundelfinger, E. D., and Fejtova, A. (2011). Extensive Remodeling of the Presynaptic Cytomatrix upon Homeostatic Adaptation to Network Activity Silencing. J. Neurosci. 31, 10189–10200. doi: 10.1523/JNEUROSCI.2088-11.2011
Lee, E. J., and Tournier, C. (2011). The requirement of uncoordinated 51-like kinase 1 (ULK1) and ULK2 in the regulation of autophagy. Autophagy 7, 689–695. doi: 10.4161/auto.7.7.15450
Lee, W., and Kim, S. H. (2019). Autophagy at synapses in neurodegenerative diseases. Arch. Pharm. Res. 42, 407–415. doi: 10.1007/s12272-019-01148-7
Li, W., Bengtson, M. H., Ulbrich, A., Matsuda, A., Reddy, V. A., Orth, A., et al. (2008). Genome-wide and functional annotation of human E3 ubiquitin ligases identifies MULAN, a mitochondrial E3 that regulates the organelle’s dynamics and signaling. PLoS One 3:e1487. doi: 10.1371/journal.pone.0001487
Liang, Y. (2019). Emerging Concepts and Functions of Autophagy as a Regulator of Synaptic Components and Plasticity. Cells 8:34. doi: 10.3390/cells8010034
Liang, Y., and Sigrist, S. (2018). Autophagy and proteostasis in the control of synapse aging and disease. Curr. Opin. Neurobiol. 48, 113–121. doi: 10.1016/j.conb.2017.12.006
Lieberman, O. J., and Sulzer, D. (2020). The Synaptic Autophagy Cycle. J. Mol. Biol. 432, 2589–2604. doi: 10.1016/j.jmb.2019.12.028
Lin, B. C., Higgins, N. R., Phung, T. H., and Monteiro, M. J. (2021). UBQLN proteins in health and disease with a focus on UBQLN2 in ALS/FTD. FEBS J. [Online ahead of print] doi: 10.1111/febs.16129
Linares, J. F., Duran, A., Yajima, T., Pasparakis, M., Moscat, J., and Diaz-Meco, M. T. (2013). K63 polyubiquitination and activation of mTOR by the p62-TRAF6 complex in nutrient-activated cells. Mol. Cell 51, 283–296. doi: 10.1016/j.molcel.2013.06.020
Liu, N., Zhao, H., Zhao, Y. G., Hu, J., and Zhang, H. (2021). Atlastin 2/3 regulate ER targeting of the ULK1 complex to initiate autophagy. J. Cell Biol. 220:e202012091. doi: 10.1083/jcb.202012091
Lüningschrör, P., Binotti, B., Dombert, B., Heimann, P., Perez-Lara, A., Slotta, C., et al. (2017). Plekhg5-regulated autophagy of synaptic vesicles reveals a pathogenic mechanism in motoneuron disease. Nat. Commun. 8:678. doi: 10.1038/s41467-017-00689-z
Lüningschrör, P., and Sendtner, M. (2018). Autophagy in the presynaptic compartment. Curr. Opin. Neurobiol. 51, 80–85. doi: 10.1016/j.conb.2018.02.023
MacLeod, D. A., Rhinn, H., Kuwahara, T., Zolin, A., Di Paolo, G., McCabe, B. D., et al. (2013). RAB7L1 interacts with LRRK2 to modify intraneuronal protein sorting and Parkinson’s disease risk. Neuron 77, 425–439. doi: 10.1016/j.neuron.2012.11.033
Maday, S., and Holzbaur, E. L. (2014). Autophagosome biogenesis in primary neurons follows an ordered and spatially regulated pathway. Dev. Cell 30, 71–85. doi: 10.1016/j.devcel.2014.06.001
Maday, S., and Holzbaur, E. L. (2016). Compartment-Specific Regulation of Autophagy in Primary Neurons. J. Neurosci. 36, 5933–5945. doi: 10.1523/JNEUROSCI.4401-15.2016
Maeda, S., Yamamoto, H., Kinch, L. N., Garza, C. M., Takahashi, S., Otomo, C., et al. (2020). Structure, lipid scrambling activity and role in autophagosome formation of ATG9A. Nat. Struct. Mol. Biol. 27, 1194–1201. doi: 10.1038/s41594-020-00520-2
Malik, B. R., Maddison, D. C., Smith, G. A., and Peters, O. M. (2019). Autophagic and endo-lysosomal dysfunction in neurodegenerative disease. Mol. Brain 12:100. doi: 10.1186/s13041-019-0504-x
Marat, A. L., and Haucke, V. (2016). Phosphatidylinositol 3-phosphates-at the interface between cell signalling and membrane traffic. EMBO J. 35, 561–579. doi: 10.15252/embj.201593564
Markson, G., Kiel, C., Hyde, R., Brown, S., Charalabous, P., Bremm, A., et al. (2009). Analysis of the human E2 ubiquitin conjugating enzyme protein interaction network. Genome Res. 19, 1905–1911. doi: 10.1101/gr.093963.109
Martens, S., and Fracchiolla, D. (2020). Activation and targeting of ATG8 protein lipidation. Cell Discov. 6:23. doi: 10.1038/s41421-020-0155-1
Martin, S., Papadopulos, A., Tomatis, V. M., Sierecki, E., Malintan, N. T., Gormal, R. S., et al. (2014). Increased polyubiquitination and proteasomal degradation of a Munc18-1 disease-linked mutant causes temperature-sensitive defect in exocytosis. Cell Rep. 9, 206–218. doi: 10.1016/j.celrep.2014.08.059
Matoba, K., Kotani, T., Tsutsumi, A., Tsuji, T., Mori, T., Noshiro, D., et al. (2020). Atg9 is a lipid scramblase that mediates autophagosomal membrane expansion. Nat. Struct. Mol. Biol. 27, 1185–1193. doi: 10.1038/s41594-020-00518-w
Matoba, K., and Noda, N. N. (2020). Secret of Atg9: lipid scramblase activity drives de novo autophagosome biogenesis. Cell Death Differ. 27, 3386–3388. doi: 10.1038/s41418-020-00663-1
Matsui, T., and Fukuda, M. (2013). Rab12 regulates mTORC1 activity and autophagy through controlling the degradation of amino-acid transporter PAT4. EMBO Rep. 14, 450–457. doi: 10.1038/embor.2013.32
McCabe, M. P., Cullen, E. R., Barrows, C. M., Shore, A. N., Tooke, K. I., Laprade, K. A., et al. (2020). Genetic inactivation of mTORC1 or mTORC2 in neurons reveals distinct functions in glutamatergic synaptic transmission. Elife 9:e51440. doi: 10.7554/eLife.51440
McMahon, H. T., and Boucrot, E. (2011). Molecular mechanism and physiological functions of clathrin-mediated endocytosis. Nat. Rev. Mol. Cell Biol. 12, 517–533. doi: 10.1038/nrm3151
Menzies, F. M., Fleming, A., Caricasole, A., Bento, C. F., Andrews, S. P., Ashkenazi, A., et al. (2017). Autophagy and Neurodegeneration: pathogenic Mechanisms and Therapeutic Opportunities. Neuron 93, 1015–1034. doi: 10.1016/j.neuron.2017.01.022
Mercer, T. J., and Tooze, S. A. (2021). The ingenious ULKs: expanding the repertoire of the ULK complex with phosphoproteomics. Autophagy 17, 4491–4493. doi: 10.1080/15548627.2021.1968615
Michel, P. P., Hirsch, E. C., and Hunot, S. (2016). Understanding Dopaminergic Cell Death Pathways in Parkinson Disease. Neuron 90, 675–691. doi: 10.1016/j.neuron.2016.03.038
Milosevic, I., Giovedi, S., Lou, X., Raimondi, A., Collesi, C., Shen, H., et al. (2011). Recruitment of endophilin to clathrin-coated pit necks is required for efficient vesicle uncoating after fission. Neuron 72, 587–601. doi: 10.1016/j.neuron.2011.08.029
Minowa-Nozawa, A., Nozawa, T., Okamoto-Furuta, K., Kohda, H., and Nakagawa, I. (2017). Rab35 GTPase recruits NDP52 to autophagy targets. EMBO J. 36, 2790–2807. doi: 10.15252/embj.201796463
Mizushima, N., Levine, B., Cuervo, A. M., and Klionsky, D. J. (2008). Autophagy fights disease through cellular self-digestion. Nature 451, 1069–1075. doi: 10.1038/nature06639
Mizushima, N., Yamamoto, A., Matsui, M., Yoshimori, T., and Ohsumi, Y. (2004). In vivo analysis of autophagy in response to nutrient starvation using transgenic mice expressing a fluorescent autophagosome marker. Mol. Biol. Cell 15, 1101–1111. doi: 10.1091/mbc.e03-09-0704
Montenegro-Venegas, C., Annamneedi, A., Hoffmann-Conaway, S., Gundelfinger, E. D., and Garner, C. C. (2020). BSN (bassoon) and PRKN/parkin in concert control presynaptic vesicle autophagy. Autophagy 16, 1732–1733. doi: 10.1080/15548627.2020.1801259
Montenegro-Venegas, C., Fienko, S., Anni, D., Pina-Fernandez, E., Frischknecht, R., and Fejtova, A. (2021). Bassoon inhibits proteasome activity via interaction with PSMB4. Cell Mol. Life Sci. 78, 1545–1563. doi: 10.1007/s00018-020-03590-z
Montes-Fernandez, M. A., Perez-Villegas, E. M., Garcia-Gonzalo, F. R., Pedrazza, L., Rosa, J. L., de Toledo, G. A., et al. (2020). The HERC1 ubiquitin ligase regulates presynaptic membrane dynamics of central synapses. Sci. Rep. 10:12057. doi: 10.1038/s41598-020-68970-8
Moreau, K., Ravikumar, B., Puri, C., and Rubinsztein, D. C. (2012). Arf6 promotes autophagosome formation via effects on phosphatidylinositol 4,5-bisphosphate and phospholipase D. J. Cell Biol. 196, 483–496. doi: 10.1083/jcb.201110114
Mouatt-Prigent, A., Muriel, M. P., Gu, W. J., El Hachimi, K. H., Lucking, C. B., Brice, A., et al. (2004). Ultrastructural localization of parkin in the rat brainstem, thalamus and basal ganglia. J. Neural Transm. 111, 1209–1218. doi: 10.1007/s00702-004-0144-9
Murdoch, J. D., Rostosky, C. M., Gowrisankaran, S., Arora, A. S., Soukup, S. F., Vidal, R., et al. (2016). Endophilin-A Deficiency Induces the Foxo3a-Fbxo32 Network in the Brain and Causes Dysregulation of Autophagy and the Ubiquitin-Proteasome System. Cell Rep. 17, 1071–1086. doi: 10.1016/j.celrep.2016.09.058
Nandi, D., Tahiliani, P., Kumar, A., and Chandu, D. (2006). The ubiquitin-proteasome system. J. Biosci 31, 137–155. doi: 10.1007/BF02705243
Negrete-Hurtado, A., Overhoff, M., Bera, S., De Bruyckere, E., Schatzmuller, K., Kye, M. J., et al. (2020). Autophagy lipidation machinery regulates axonal microtubule dynamics but is dispensable for survival of mammalian neurons. Nat. Commun. 11:1535. doi: 10.1038/s41467-020-15287-9
Neisch, A. L., Neufeld, T. P., and Hays, T. S. (2017). A STRIPAK complex mediates axonal transport of autophagosomes and dense core vesicles through PP2A regulation. J. Cell Biol. 216, 441–461. doi: 10.1083/jcb.201606082
Nixon, R. A., Yang, D. S., and Lee, J. H. (2008). Neurodegenerative lysosomal disorders: a continuum from development to late age. Autophagy 4, 590–599. doi: 10.4161/auto.6259
Noda, T., Kim, J., Huang, W. P., Baba, M., Tokunaga, C., Ohsumi, Y., et al. (2000). Apg9p/Cvt7p is an integral membrane protein required for transport vesicle formation in the Cvt and autophagy pathways. J. Cell Biol. 148, 465–480. doi: 10.1083/jcb.148.3.465
Okerlund, N. D., Schneider, K., Leal-Ortiz, S., Montenegro-Venegas, C., Kim, S. A., Garner, L. C., et al. (2017). Bassoon Controls Presynaptic Autophagy through Atg5. Neuron 93, 897–913.e7. doi: 10.1016/j.neuron.2017.01.026
Orii, M., Tsuji, T., Ogasawara, Y., and Fujimoto, T. (2021). Transmembrane phospholipid translocation mediated by Atg9 is involved in autophagosome formation. J. Cell Biol. 220:e202009194. doi: 10.1083/jcb.202009194
Overhoff, M., De Bruyckere, E., and Kononenko, N. L. (2021). Mechanisms of neuronal survival safeguarded by endocytosis and autophagy. J. Neurochem. 157, 263–296. doi: 10.1111/jnc.15194
Palamiuc, L., Ravi, A., and Emerling, B. M. (2020). Phosphoinositides in autophagy: current roles and future insights. FEBS J. 287, 222–238. doi: 10.1111/febs.15127
Pan, P. Y., Zhu, Y., Shen, Y., and Yue, Z. (2019). Crosstalk between presynaptic trafficking and autophagy in Parkinson’s disease. Neurobiol. Dis. 122, 64–71. doi: 10.1016/j.nbd.2018.04.020
Perez-Villegas, E. M., Perez-Rodriguez, M., Negrete-Diaz, J. V., Ruiz, R., Rosa, J. L., de Toledo, G. A., et al. (2020). HERC1 Ubiquitin Ligase Is Required for Hippocampal Learning and Memory. Front. Neuroanat. 14:592797. doi: 10.3389/fnana.2020.592797
Piccoli, G., and Volta, M. (2021). LRRK2 along the Golgi and lysosome connection: a jamming situation. Biochem. Soc. Trans. 49, 2063–2072. doi: 10.1042/BST20201146
Pielot, R., Smalla, K. H., Muller, A., Landgraf, P., Lehmann, A. C., Eisenschmidt, E., et al. (2012). SynProt: a Database for Proteins of Detergent-Resistant Synaptic Protein Preparations. Front. Synaptic Neurosci. 4:1. doi: 10.3389/fnsyn.2012.00001
Puri, C., Vicinanza, M., and Rubinsztein, D. C. (2018). Phagophores evolve from recycling endosomes. Autophagy 14, 1475–1477. doi: 10.1080/15548627.2018.1482148
Pyo, J. O., Jang, M. H., Kwon, Y. K., Lee, H. J., Jun, J. I., Woo, H. N., et al. (2005). Essential roles of Atg5 and FADD in autophagic cell death: dissection of autophagic cell death into vacuole formation and cell death. J. Biol. Chem. 280, 20722–20729. doi: 10.1074/jbc.M413934200
Qiao, Q., Ma, L., Li, W., Tsai, J. W., Yang, G., and Gan, W. B. (2016). Long-term stability of axonal boutons in the mouse barrel cortex. Dev. Neurobiol. 76, 252–261. doi: 10.1002/dneu.22311
Rao, Y., Perna, M. G., Hofmann, B., Beier, V., and Wollert, T. (2016). The Atg1-kinase complex tethers Atg9-vesicles to initiate autophagy. Nat. Commun. 7:10338. doi: 10.1038/ncomms10338
Ravikumar, B., Moreau, K., Jahreiss, L., Puri, C., and Rubinsztein, D. C. (2010). Plasma membrane contributes to the formation of pre-autophagosomal structures. Nat. Cell Biol. 12, 747–757. doi: 10.1038/ncb2078
Ravussin, A., Brech, A., Tooze, S. A., and Stenmark, H. (2021). The phosphatidylinositol 3-phosphate-binding protein SNX4 controls ATG9A recycling and autophagy. J. Cell Sci. 134:jcs250670. doi: 10.1242/jcs.250670
Rinetti, G. V., and Schweizer, F. E. (2010). Ubiquitination acutely regulates presynaptic neurotransmitter release in mammalian neurons. J. Neurosci. 30, 3157–3166. doi: 10.1523/JNEUROSCI.3712-09.2010
Rizalar, F. S., Roosen, D. A., and Haucke, V. (2021). A Presynaptic Perspective on Transport and Assembly Mechanisms for Synapse Formation. Neuron 109, 27–41. doi: 10.1016/j.neuron.2020.09.038
Rubinsztein, D. C. (2006). The roles of intracellular protein-degradation pathways in neurodegeneration. Nature 443, 780–786. doi: 10.1038/nature05291
Rubinsztein, D. C., and Nixon, R. A. (2010). Rapamycin induces autophagic flux in neurons. Proc. Natl. Acad. Sci. U. S. A. 107:E181. doi: 10.1073/pnas.1014633107
Saudou, F., and Humbert, S. (2016). The Biology of Huntingtin. Neuron 89, 910–926. doi: 10.1016/j.neuron.2016.02.003
Sawa-Makarska, J., Baumann, V., Coudevylle, N., von Bulow, S., Nogellova, V., Abert, C., et al. (2020). Reconstitution of autophagosome nucleation defines Atg9 vesicles as seeds for membrane formation. Science 369:eaaz7714. doi: 10.1126/science.aaz7714
Seto, S., Sugaya, K., Tsujimura, K., Nagata, T., Horii, T., and Koide, Y. (2013). Rab39a interacts with phosphatidylinositol 3-kinase and negatively regulates autophagy induced by lipopolysaccharide stimulation in macrophages. PLoS One 8:e83324. doi: 10.1371/journal.pone.0083324
Sheehan, P., and Waites, C. L. (2019). Coordination of synaptic vesicle trafficking and turnover by the Rab35 signaling network. Small GTPases 10, 54–63. doi: 10.1080/21541248.2016.1270392
Sheehan, P., Zhu, M., Beskow, A., Vollmer, C., and Waites, C. L. (2016). Activity-Dependent Degradation of Synaptic Vesicle Proteins Requires Rab35 and the ESCRT Pathway. J. Neurosci. 36, 8668–8686. doi: 10.1523/JNEUROSCI.0725-16.2016
Shehata, M., and Inokuchi, K. (2014). Does autophagy work in synaptic plasticity and memory? Rev. Neurosci. 25, 543–557. doi: 10.1515/revneuro-2014-0002
Shen, Z. Q., Huang, Y. L., Teng, Y. C., Wang, T. W., Kao, C. H., Yeh, C. H., et al. (2021). CISD2 maintains cellular homeostasis. Biochim. Biophys. Acta Mol. Cell Res. 1868:118954. doi: 10.1016/j.bbamcr.2021.118954
Sheng, Y., Song, Y., Li, Z., Wang, Y., Lin, H., Cheng, H., et al. (2018). RAB37 interacts directly with ATG5 and promotes autophagosome formation via regulating ATG5-12-16 complex assembly. Cell Death Differ. 25, 918–934. doi: 10.1038/s41418-017-0023-1
Soukup, S. F., Kuenen, S., Vanhauwaert, R., Manetsberger, J., Hernandez-Diaz, S., Swerts, J., et al. (2016). A LRRK2-Dependent EndophilinA Phosphoswitch Is Critical for Macroautophagy at Presynaptic Terminals. Neuron 92, 829–844. doi: 10.1016/j.neuron.2016.09.037
Soukup, S. F., Vanhauwaert, R., and Verstreken, P. (2018). Parkinson’s disease: convergence on synaptic homeostasis. EMBO J. 37:e98960. doi: 10.15252/embj.201898960
Soukup, S. F., and Verstreken, P. (2017). EndoA/Endophilin-A creates docking stations for autophagic proteins at synapses. Autophagy 13, 971–972. doi: 10.1080/15548627.2017.1286440
Soykan, T., Haucke, V., and Kuijpers, M. (2021). Mechanism of synaptic protein turnover and its regulation by neuronal activity. Curr. Opin. Neurobiol. 69, 76–83. doi: 10.1016/j.conb.2021.02.006
Stavoe, A. K., Gopal, P. P., Gubas, A., Tooze, S. A., and Holzbaur, E. L. (2019). Expression of WIPI2B counteracts age-related decline in autophagosome biogenesis in neurons. Elife 8:e44219. doi: 10.7554/eLife.44219
Stavoe, A. K., Hill, S. E., Hall, D. H., and Colon-Ramos, D. A. (2016). KIF1A/UNC-104 Transports ATG-9 to Regulate Neurodevelopment and Autophagy at Synapses. Dev. Cell 38, 171–185. doi: 10.1016/j.devcel.2016.06.012
Stavoe, A. K. H., and Holzbaur, E. L. F. (2018). Axonal autophagy: mini-review for autophagy in the CNS. Neurosci. Lett. 697, 17–23. doi: 10.1016/j.neulet.2018.03.025
Stavoe, A. K. H., and Holzbaur, E. L. F. (2019). Autophagy in Neurons. Annu. Rev. Cell Dev. Biol. 35, 477–500. doi: 10.1146/annurev-cellbio-100818-125242
Sturner, E., and Behl, C. (2017). The Role of the Multifunctional BAG3 Protein in Cellular Protein Quality Control and in Disease. Front. Mol. Neurosci. 10:177. doi: 10.3389/fnmol.2017.00177
Sudhof, T. C. (2012). The presynaptic active zone. Neuron 75, 11–25. doi: 10.1016/j.neuron.2012.06.012
Sumitomo, A., Yukitake, H., Hirai, K., Horike, K., Ueta, K., Chung, Y., et al. (2018). Ulk2 controls cortical excitatory-inhibitory balance via autophagic regulation of p62 and GABAA receptor trafficking in pyramidal neurons. Hum. Mol. Genet. 27, 3165–3176. doi: 10.1093/hmg/ddy219
Tada, H., Okano, H. J., Takagi, H., Shibata, S., Yao, I., Matsumoto, M., et al. (2010). Fbxo45, a novel ubiquitin ligase, regulates synaptic activity. J. Biol Chem. 285, 3840–3849. doi: 10.1074/jbc.M109.046284
Takahashi, Y., Meyerkord, C. L., Hori, T., Runkle, K., Fox, T. E., Kester, M., et al. (2011). Bif-1 regulates Atg9 trafficking by mediating the fission of Golgi membranes during autophagy. Autophagy 7, 61–73. doi: 10.4161/auto.7.1.14015
Takamori, S., Holt, M., Stenius, K., Lemke, E. A., Gronborg, M., Riedel, D., et al. (2006). Molecular anatomy of a trafficking organelle. Cell 127, 831–846. doi: 10.1016/j.cell.2006.10.030
Tang, G., Gudsnuk, K., Kuo, S. H., Cotrina, M. L., Rosoklija, G., Sosunov, A., et al. (2014). Loss of mTOR-dependent macroautophagy causes autistic-like synaptic pruning deficits. Neuron 83, 1131–1143. doi: 10.1016/j.neuron.2014.07.040
Taoufiq, Z., Ninov, M., Villar-Briones, A., Wang, H. Y., Sasaki, T., Roy, M. C., et al. (2020). Hidden proteome of synaptic vesicles in the mammalian brain. Proc. Natl. Acad. Sci. U. S. A. 117, 33586–33596. doi: 10.1073/pnas.2011870117
Taylor, M., and Alessi, D. R. (2020). Advances in elucidating the function of leucine-rich repeat protein kinase-2 in normal cells and Parkinson’s disease. Curr. Opin. Cell Biol. 63, 102–113. doi: 10.1016/j.ceb.2020.01.001
Tian, X., Teng, J., and Chen, J. (2021). New insights regarding SNARE proteins in autophagosome-lysosome fusion. Autophagy 17, 2680–2688. doi: 10.1080/15548627.2020.1823124
Tian, Y., Chang, J. C., Fan, E. Y., Flajolet, M., and Greengard, P. (2013). Adaptor complex AP2/PICALM, through interaction with LC3, targets Alzheimer’s APP-CTF for terminal degradation via autophagy. Proc. Natl. Acad. Sci. U. S. A. 110, 17071–17076. doi: 10.1073/pnas.1315110110
Tian, Y., Chang, J. C., Greengard, P., and Flajolet, M. (2014). The convergence of endosomal and autophagosomal pathways: implications for APP-CTF degradation. Autophagy 10, 694–696. doi: 10.4161/auto.27802
Tomoda, T., Yang, K., and Sawa, A. (2020). Neuronal Autophagy in Synaptic Functions and Psychiatric Disorders. Biol. Psychiatry 87, 787–796. doi: 10.1016/j.biopsych.2019.07.018
Toyofuku, T., Morimoto, K., Sasawatari, S., and Kumanogoh, A. (2015). Leucine-Rich Repeat Kinase 1 Regulates Autophagy through Turning On TBC1D2-Dependent Rab7 Inactivation. Mol. Cell Biol. 35, 3044–3058. doi: 10.1128/MCB.00085-15
Tsvetkov, A. S., Miller, J., Arrasate, M., Wong, J. S., Pleiss, M. A., and Finkbeiner, S. (2010). A small-molecule scaffold induces autophagy in primary neurons and protects against toxicity in a Huntington disease model. Proc. Natl. Acad. Sci. U. S. A. 107, 16982–16987. doi: 10.1073/pnas.1004498107
Uytterhoeven, V., Lauwers, E., Maes, I., Miskiewicz, K., Melo, M. N., Swerts, J., et al. (2015). Hsc70-4 Deforms Membranes to Promote Synaptic Protein Turnover by Endosomal Microautophagy. Neuron 88, 735–748. doi: 10.1016/j.neuron.2015.10.012
van der Beek, J., Jonker, C., van der Welle, R., Liv, N., and Klumperman, J. (2019). CORVET, CHEVI and HOPS - multisubunit tethers of the endo-lysosomal system in health and disease. J. Cell Sci. 132:jcs189134. doi: 10.1242/jcs.189134
Vanhauwaert, R., Kuenen, S., Masius, R., Bademosi, A., Manetsberger, J., Schoovaerts, N., et al. (2017). The SAC1 domain in synaptojanin is required for autophagosome maturation at presynaptic terminals. EMBO J. 36, 1392–1411. doi: 10.15252/embj.201695773
Verderio, C., Cagnoli, C., Bergami, M., Francolini, M., Schenk, U., Colombo, A., et al. (2012). TI-VAMP/VAMP7 is the SNARE of secretory lysosomes contributing to ATP secretion from astrocytes. Biol. Cell 104, 213–228. doi: 10.1111/boc.201100070
Verstreken, P., Koh, T. W., Schulze, K. L., Zhai, R. G., Hiesinger, P. R., Zhou, Y., et al. (2003). Synaptojanin is recruited by endophilin to promote synaptic vesicle uncoating. Neuron 40, 733–748. doi: 10.1016/s0896-6273(03)00644-5
Vijayan, V., and Verstreken, P. (2017). Autophagy in the presynaptic compartment in health and disease. J. Cell Biol. 216, 1895–1906. doi: 10.1083/jcb.201611113
Vukoja, A., Rey, U., Petzoldt, A. G., Ott, C., Vollweiter, D., Quentin, C., et al. (2018). Presynaptic Biogenesis Requires Axonal Transport of Lysosome-Related Vesicles. Neuron 99, 1216–1232.e7. doi: 10.1016/j.neuron.2018.08.004
Waites, C. L., Leal-Ortiz, S. A., Okerlund, N., Dalke, H., Fejtova, A., Altrock, W. D., et al. (2013). Bassoon and Piccolo maintain synapse integrity by regulating protein ubiquitination and degradation. EMBO J. 32, 954–969. doi: 10.1038/emboj.2013.27
Wan, H., Wang, Q., Chen, X., Zeng, Q., Shao, Y., Fang, H., et al. (2020). WDR45 contributes to neurodegeneration through regulation of ER homeostasis and neuronal death. Autophagy 16, 531–547. doi: 10.1080/15548627.2019.1630224
Wang, B., Iyengar, R., Li-Harms, X., Joo, J. H., Wright, C., Lavado, A., et al. (2018). The autophagy-inducing kinases, ULK1 and ULK2, regulate axon guidance in the developing mouse forebrain via a noncanonical pathway. Autophagy 14, 796–811. doi: 10.1080/15548627.2017.1386820
Wang, T., Martin, S., Papadopulos, A., Harper, C. B., Mavlyutov, T. A., Niranjan, D., et al. (2015). Control of autophagosome axonal retrograde flux by presynaptic activity unveiled using botulinum neurotoxin type a. J. Neurosci. 35, 6179–6194. doi: 10.1523/JNEUROSCI.3757-14.2015
Wang, Y., and Le, W. D. (2019). Autophagy and Ubiquitin-Proteasome System. Adv. Exp. Med. Biol. 1206, 527–550. doi: 10.1007/978-981-15-0602-4_25
Wang, Y. C., Lauwers, E., and Verstreken, P. (2017). Presynaptic protein homeostasis and neuronal function. Curr. Opin. Genet. Dev. 44, 38–46. doi: 10.1016/j.gde.2017.01.015
Watanabe, S., Mamer, L. E., Raychaudhuri, S., Luvsanjav, D., Eisen, J., Trimbuch, T., et al. (2018). Synaptojanin and Endophilin Mediate Neck Formation during Ultrafast Endocytosis. Neuron 98, 1184–1197e1186. doi: 10.1016/j.neuron.2018.06.005
Wei, F., and Duan, Y. (2019). Crosstalk between Autophagy and Nanomaterials: internalization, Activation, Termination. Adv. Biosyst. 3:e1800259. doi: 10.1002/adbi.201800259
Weingarten, J., Lassek, M., Mueller, B. F., Rohmer, M., Lunger, I., Baeumlisberger, D., et al. (2014). The proteome of the presynaptic active zone from mouse brain. Mol. Cell Neurosci. 59, 106–118. doi: 10.1016/j.mcn.2014.02.003
Wetzel, L., Blanchard, S., Rama, S., Beier, V., Kaufmann, A., and Wollert, T. (2020). TECPR1 promotes aggrephagy by direct recruitment of LC3C autophagosomes to lysosomes. Nat. Commun. 11:2993. doi: 10.1038/s41467-020-16689-5
Whang, M. I., Tavares, R. M., Benjamin, D. I., Kattah, M. G., Advincula, R., Nomura, D. K., et al. (2017). The Ubiquitin Binding Protein TAX1BP1 Mediates Autophagasome Induction and the Metabolic Transition of Activated T Cells. Immunity 46, 405–420. doi: 10.1016/j.immuni.2017.02.018
Wilfling, F., Lee, C. W., Erdmann, P. S., Zheng, Y., Sherpa, D., Jentsch, S., et al. (2020). A Selective Autophagy Pathway for Phase-Separated Endocytic Protein Deposits. Mol. Cell 80, 764–778.e7. doi: 10.1016/j.molcel.2020.10.030
Wojnacki, J., and Galli, T. (2018). A new actin-binding domain glues autophagy together. J. Biol. Chem. 293, 4575–4576. doi: 10.1074/jbc.H118.002041
Wojnacki, J., Nola, S., Bun, P., Cholley, B., Filippini, F., Presse, M. T., et al. (2021). Role of VAMP7-dependent secretion of reticulon 3 in neurite growth. Cell Rep. 35:109006. doi: 10.1016/j.celrep.2021.109006
Wu, X., Ganzella, M., Zhou, J., Zhu, S., Jahn, R., and Zhang, M. (2021). Vesicle Tethering on the Surface of Phase-Separated Active Zone Condensates. Mol. Cell 81, 13–24.e7. doi: 10.1016/j.molcel.2020.10.029
Xing, R., Zhou, H., Jian, Y., Li, L., Wang, M., Liu, N., et al. (2021). The Rab7 effector WDR91 promotes autophagy-lysosome degradation in neurons by regulating lysosome fusion. J. Cell Biol. 220:e202007061. doi: 10.1083/jcb.202007061
Xu, J., Fotouhi, M., and McPherson, P. S. (2015). Phosphorylation of the exchange factor DENND3 by ULK in response to starvation activates Rab12 and induces autophagy. EMBO Rep. 16, 709–718. doi: 10.15252/embr.201440006
Xu, J., Kozlov, G., McPherson, P. S., and Gehring, K. (2018). A PH-like domain of the Rab12 guanine nucleotide exchange factor DENND3 binds actin and is required for autophagy. J. Biol. Chem. 293, 4566–4574. doi: 10.1074/jbc.RA117.001446
Xuan, Z., Manning, L., Nelson, J., Richmond, J. E., Colon-Ramos, D. A., Shen, K., et al. (2017). Clarinet (CLA-1), a novel active zone protein required for synaptic vesicle clustering and release. Elife 6:e29276. doi: 10.7554/eLife.29276
Xuan, Z., Yang, S., Hill, S. E., Clark, B., Manning, L., and Colón-Ramos, D. A. (2021). The active zone protein Clarinet regulates ATG-9 trafficking at synapses and presynaptic autophagy. bioRxiv [Preprint]. doi: 10.1101/2021.08.19.457026
Yang, S., Park, D., Manning, L., Hill, S. E., Cao, M., Xuan, Z., et al. (2020). Presynaptic autophagy is coupled to the synaptic vesicle cycle via ATG-9. bioRxiv [Preprint]. doi: 10.1101/2020.12.28.424508
Yang, Y., and Calakos, N. (2013). Presynaptic long-term plasticity. Front. Synaptic Neurosci. 5:8. doi: 10.3389/fnsyn.2013.00008
Yao, I., Takagi, H., Ageta, H., Kahyo, T., Sato, S., Hatanaka, K., et al. (2007). SCRAPPER-dependent ubiquitination of active zone protein RIM1 regulates synaptic vesicle release. Cell 130, 943–957. doi: 10.1016/j.cell.2007.06.052
Yla-Anttila, P., Mikkonen, E., Happonen, K. E., Holland, P., Ueno, T., Simonsen, A., et al. (2015). RAB24 facilitates clearance of autophagic compartments during basal conditions. Autophagy 11, 1833–1848. doi: 10.1080/15548627.2015.1086522
Young, A. R., Chan, E. Y., Hu, X. W., Kochl, R., Crawshaw, S. G., High, S., et al. (2006). Starvation and ULK1-dependent cycling of mammalian Atg9 between the TGN and endosomes. J. Cell Sci. 119, 3888–3900. doi: 10.1242/jcs.03172
Keywords: autophagy, endolysosomal system, active zone (AZ), Bassoon, endocytic zone, synaptic vesicle (SV), amphisome, presynaptic proteostasis
Citation: Gundelfinger ED, Karpova A, Pielot R, Garner CC and Kreutz MR (2022) Organization of Presynaptic Autophagy-Related Processes. Front. Synaptic Neurosci. 14:829354. doi: 10.3389/fnsyn.2022.829354
Received: 05 December 2021; Accepted: 04 January 2022;
Published: 17 March 2022.
Edited by:
Lucia Tabares, Seville University, SpainReviewed by:
Oleg Shupliakov, Karolinska Institutet, SwedenCopyright © 2022 Gundelfinger, Karpova, Pielot, Garner and Kreutz. This is an open-access article distributed under the terms of the Creative Commons Attribution License (CC BY). The use, distribution or reproduction in other forums is permitted, provided the original author(s) and the copyright owner(s) are credited and that the original publication in this journal is cited, in accordance with accepted academic practice. No use, distribution or reproduction is permitted which does not comply with these terms.
*Correspondence: Eckart D. Gundelfinger, ZWd1bmRlbGZAbGluLW1hZ2RlYnVyZy5kZQ==
†ORCID: Eckart D. Gundelfinger, orcid.org/0000-0001-9377-7414; Anna Karpova, orcid.org/0000-0001-7423-4764; Rainer Pielot, orcid.org/0000-0002-9681-3318; Craig C. Garner, orcid.org/0000-0003-1970-5417; Michael R. Kreutz, orcid.org/0000-0003-0575-6950
Disclaimer: All claims expressed in this article are solely those of the authors and do not necessarily represent those of their affiliated organizations, or those of the publisher, the editors and the reviewers. Any product that may be evaluated in this article or claim that may be made by its manufacturer is not guaranteed or endorsed by the publisher.
Research integrity at Frontiers
Learn more about the work of our research integrity team to safeguard the quality of each article we publish.