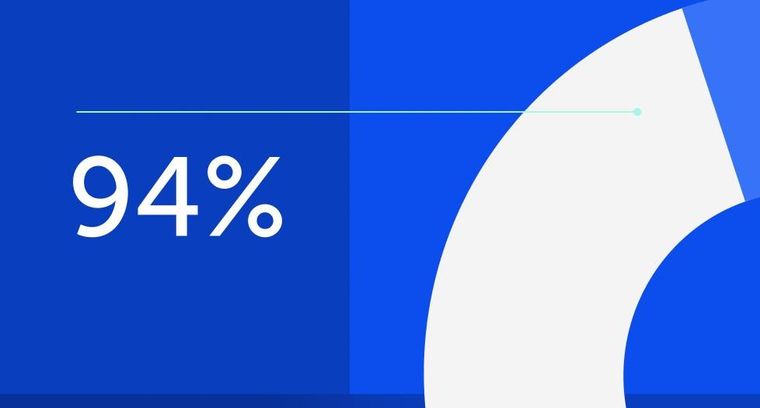
94% of researchers rate our articles as excellent or good
Learn more about the work of our research integrity team to safeguard the quality of each article we publish.
Find out more
ORIGINAL RESEARCH article
Front. Synaptic Neurosci., 04 January 2023
Volume 14 - 2022 | https://doi.org/10.3389/fnsyn.2022.1033743
This article is part of the Research TopicSynaptic Plasticity in the PeripheryView all 8 articles
Introduction: The ability of synapses to maintain physiological levels of evoked neurotransmission is essential for neuronal stability. A variety of perturbations can disrupt neurotransmission, but synapses often compensate for disruptions and work to stabilize activity levels, using forms of homeostatic synaptic plasticity. Presynaptic homeostatic potentiation (PHP) is one such mechanism. PHP is expressed at the Drosophila melanogaster larval neuromuscular junction (NMJ) synapse, as well as other NMJs. In PHP, presynaptic neurotransmitter release increases to offset the effects of impairing muscle transmitter receptors. Prior Drosophila work has studied PHP using different ways to perturb muscle receptor function—either acutely (using pharmacology) or chronically (using genetics). Some of our prior data suggested that cytoplasmic calcium signaling was important for expression of PHP after genetic impairment of glutamate receptors. Here we followed up on that observation.
Methods: We used a combination of transgenic Drosophila RNA interference and overexpression lines, along with NMJ electrophysiology, synapse imaging, and pharmacology to test if regulators of the calcium/calmodulin-dependent protein phosphatase calcineurin are necessary for the normal expression of PHP.
Results: We found that either pre- or postsynaptic dysregulation of a Drosophila gene regulating calcineurin, sarah (sra), blocks PHP. Tissue-specific manipulations showed that either increases or decreases in sra expression are detrimental to PHP. Additionally, pharmacologically and genetically induced forms of expression of PHP are functionally separable depending entirely upon which sra genetic manipulation is used. Surprisingly, dual-tissue pre- and postsynaptic sra knockdown or overexpression can ameliorate PHP blocks revealed in single-tissue experiments. Pharmacological and genetic inhibition of calcineurin corroborated this latter finding.
Discussion: Our results suggest tight calcineurin regulation is needed across multiple tissue types to stabilize peripheral synaptic outputs.
Normal synapse function permits a pre-determined physiological range of activity. Challenges to synapse function can force activity beyond setpoint limits. To maintain stability, synaptic and circuit level homeostatic mechanisms exist in vertebrates and invertebrates (Davis, 2006; Pozo and Goda, 2010; Marder, 2012; Frank, 2014a). Defects in homeostatic plasticity can impair the preservation of neuronal activity and may be associated with neurological conditions that display instabilities including ataxia, migraine, schizophrenia, Alzheimer’s disease, and epilepsy (Wondolowski and Dickman, 2013; Bliss et al., 2013; André et al., 2018). Synaptic homeostasis has also been implicated as being necessary for the manifestation of normal sleep behavior (Tononi and Cirelli, 2006; Kikuma et al., 2019). For these diverse behaviors or neurological disorders, the relevant synaptic activities and functional challenges operate on varying timescales, from minutes (e.g., perturbations that induce migraine) to years (e.g., conditions like Alzheimer’s Disease). Yet there are significant gaps in understanding how neuronal stability is maintained, across neural tissue types and over broad timescales.
We can address some of these gaps using model synapses, like the Drosophila melanogaster neuromuscular junction (NMJ). The Drosophila NMJ is a well-studied synapse (Menon et al., 2013). It is suitable for combining approaches in genetics, development, and electrophysiology (Jan and Jan, 1976). For homeostatic synaptic plasticity, the NMJ has been key for mechanistic discovery (Frank et al., 2020). One well-characterized form of homeostatic plasticity is called presynaptic homeostatic potentiation (PHP). In Drosophila, PHP is initiated when postsynaptic glutamate receptors are impaired, decreasing quantal size. The synapse detects this impairment, and a muscle-to-nerve signaling process drives increased presynaptic glutamate release. The prevailing model is that potentiated release happens after retrograde signaling triggers an increase in presynaptic calcium influx through CaV2 voltage-gated calcium channels (Frank et al., 2006, 2009; Müller and Davis, 2012; Younger et al., 2013; Wang et al., 2014). This increase in presynaptic calcium influx coincides with an increased readily releasable pool (RRP) of synaptic vesicles (Weyhersmüller et al., 2011; Müller et al., 2012, 2015; Harris et al., 2015). These modifications drive the ability of the motor neuron to deliver more glutamate to the cleft, offsetting the decreased quantal size.
PHP is studied using different kinds of manipulations. Acute inhibition of glutamate receptors causes PHP to be expressed rapidly, in minutes (Frank et al., 2006). By contrast, genetic manipulations last over extended developmental time (Petersen et al., 1997; Davis et al., 1998; Diantonio et al., 1999). Acute PHP can be induced and expressed by applying glutamate receptor inhibitors, like Philanthotoxin-433 (PhTx; Frank et al., 2006) or GYKI-53655 (Nair et al., 2021). Alternatively, glutamate receptors can be impaired genetically using a loss of function mutation in GluRIIASP16 (Petersen et al., 1997), RNAi-mediated knockdown of GluRIII (Brusich et al., 2015), or a variety of other manipulations that impair receptor expression throughout development (reviewed in Frank, 2014a). Each of these manipulations results in decreased quantal size, offset by increased quantal content, resulting in normal levels of evoked excitation.
In prior work, we reported that a signaling modality including Gq, Plc21C (Drosophila PLCβ homolog), IP3 receptors, and ryanodine receptors were necessary for the expression of PHP after genetic glutamate receptor loss, but not after acute pharmacological PhTx challenge (James et al., 2019). IP3 receptors and ryanodine receptors have dual pre- and postsynaptic functions by mediating the release of intracellular calcium from intracellular calcium stores (Philip et al., 2010; Kadamur and Ross, 2013). However, it is unknown which calcium-dependent molecules must be activated or regulated via the release of calcium from intracellular stores to promote homeostatic potentiation.
Calcineurin is a well conserved Ca2+/calmodulin-dependent protein phosphatase that is found in most mammalian tissues but is expressed at high levels in the brain, and it has been shown to regulate ion channel function and trafficking, apoptosis, gene regulation, receptor trafficking, neuronal plasticity, and immune processes (Rusnak and Mertz, 2000; Musson and Smit, 2011; Baumgärtel and Mansuy, 2012; Furman and Norris, 2014). Calcineurin is also regulated by a family of proteins known as regulators of calcineurin (RCAN1), which are the product of the Down Syndrome Critical Region 1 (DSCR1) gene (Harris et al., 2005; Park et al., 2009). There is evidence that suggests that RCAN family members inhibit calcineurin activity (Kingsbury and Cunningham, 2000; Lee et al., 2003). The Drosophila gene sarah (sra; also known as nebula) encodes the only known ortholog of DSCR1 in Drosophila (Chang et al., 2003). Sra/Nebula/DSCR1 (herein: Sra) has been shown to physically associate with the catalytic subunit of calcineurin (Takeo et al., 2006). Evidence suggests that Sra inhibits calcineurin, as calcineurin activity has been shown to be approximately 40% higher in sra mutants in comparison to controls or heterozygous mutants (Chang et al., 2003). sra gene function has been shown to be required for mitochondrial function (Chang and Min, 2005), female meiosis and egg activation (Horner et al., 2006; Takeo et al., 2006, 2012), sleep regulation (Nakai et al., 2011) and learning (Chang et al., 2003).
Here we report that either increases or decreases in sra gene expression—in either presynaptic neuron or postsynaptic muscle—result in PHP impairment. Using genetics, we found that different modes of PHP expression were functionally separable, as specific sra manipulations compromised the expression of PHP triggered by acute pharmacology or chronic genetic mutation. We found that tissue-specific manipulations of sra resulted in strong blocks of PHP. But surprisingly, dual tissue manipulations did not. Concurrent pre- and postsynaptic sra knockdown or overexpression led to weaker (or no) blocks of PHP compared to single tissue impairments. Consistent with this latter finding, global pharmacological inhibition of calcineurin via FK506 increases the homeostatic capacity of the NMJ following a genetic PHP challenge.
Taken together, our data highlight a novel idea for homeostasis at the synapse. Regulatory proteins like Sra are present in multiple synaptic tissues; if those factors are manipulated in tissue-specific ways, there can be profound defects in overall synapse stability. But conversely, global losses (or gains) of function may escape the most severe phenotypes. Our data set a framework for testing how individual molecules contribute to homeostatic synaptic plasticity from different synaptic tissues.
Drosophila melanogaster fruit flies were raised on a standard cornmeal and molasses medium prepared according to the recipe from the Bloomington Drosophila Stock Center (BDSC, Bloomington, IN, USA). Drosophila husbandry was performed according to standard practices (Greenspan, 2004). Larvae were raised at 25°C in humidity and light controlled (12 h light: 12 h dark) Percival DR-36VL incubators (Geneva Scientific, Williams Bay, WI, USA).
w1118 (Hazelrigg et al., 1984) was used as a non-transgenic wild type stock. The null GluRIIASP16 mutant was generated previously (Petersen et al., 1997). The UAS-GluRIII RNAi line that was used has been described previously (Brusich et al., 2015). GAL4 drivers that were used included the neuronal drivers elaV(C155)-GAL4 (Lin and Goodman, 1994) and Sca-GAL4 (Budnik et al., 1996) and the postsynaptic driver BG57-GAL4 (Budnik et al., 1996). The sra RNAi line that was used was TRiP.JF02557 (BDSC:27260; Shaw and Chang, 2013) and the sra overexpression line that was used was sraEY07182 (BDSC:15991; Lee et al., 2016). The sraMi06435 was used alone (BDSC:42393) and in conjunction with the deficiency line Df(3R)sbd104 (BDSC:1920). The CaNB RNAi line that was used was TRiP.JF02616 (BDSC:27307; Shaw and Chang, 2013).
Wandering third instar larvae were collected and dissected for NMJ analysis. Both control and experimental conditions were performed in parallel using identical conditions. Dissections and recordings were performed in a modified HL3 saline (70 mM NaCl, 5 mM KCl, 5 mM HEPES, 10 mM NaHCO3, 115 mM sucrose, 4.2 mM trehalose, 0.5 mM CaCl2 (unless otherwise noted), 10 mM MgCl2, pH 7.2 (Stewart et al., 1994). Neuromuscular junction sharp electrode recordings were performed on muscles 6/7 of abdominal segments A2, A3, and A4. Miniature excitatory postsynaptic potentials (mEPSPs) and excitatory postsynaptic potentials (EPSPs) were conducted as previously described (Brusich et al., 2015; Spring et al., 2016; Yeates et al., 2017; James et al., 2019; Mallik and Frank, 2022).
Uncorrected quantal content was calculated per NMJ by dividing the average EPSP by the average mEPSP. Quantal content was then corrected for non-linear summation (NLS quantal content) using the following calculation (Mclachlan and Martin, 1981):
Recordings were performed on an Olympus (Shinjuku City, Tokyo, Japan) BX51WI microscope using a 10× objective and acquired using an Axoclamp 900A amplifier, Digidata 1440A acquisition system, and pClamp10.7 (Molecular Devices, Sunnyvale, CA, USA) software. Data were analyzed using MiniAnalysis (Synaptosoft, Georgia, USA—now supplied by Bluecell, South Korea) and the Clampfit (Molecular Devices, Sunnyvale, CA, USA) programs. To generate mEPSP and EPSP traces for figures, (x, y) coordinates were pulled from the Clampfit program and imported into GraphPad Prism (GraphPad, San Diego, CA, USA) software. All traces display the recording that was at or closest to the calculated average of each specific condition.
Pharmacological agents were bath applied in recording saline at the final concentrations indicated in the text and figures. The agents included Philanthotoxin-433 (PhTx, Sigma-Aldrich, St. Louis, MO, USA), Cyclosporin A (Sigma-Aldrich), and FK506 (InvivoGen, San Diego, CA, USA).
Wandering third instar larvae were dissected on a Sylgard Petri plate in HL3 and fixed using Bouin’s fixative for 10 min or 4% paraformaldehyde in PBS for 30 min. Larvae were washed with PBS containing 0.2% Triton X-100 (PBST) for 40 min, blocked for an hour with 2.5% BSA, and incubated overnight with primary antibodies at 4°C. This was followed with another 40 min of washes, and incubation in secondary antibodies for 1.5 h at room temperature. The primary antibodies used were mouse anti-Synapsin (anti-Syn; 3C11) 1:50 (Developmental Studies Hybridoma Bank, Iowa City, IA); rabbit anti-Dlg 1:15,000 (Budnik et al., 1996); mouse anti-phosphorylated CaMKII (pCaMKII) 1:100 (MA1-047; Invitrogen, brand of Thermo Fisher Scientific, MA, USA); rabbit anti-GluRIII 1:150 (Marrus et al., 2004; Goel and Dickman, 2018). The following fluorophore conjugated secondary antibodies were also used (Jackson ImmunoResearch Laboratories, West Grove, PA, USA): goat anti-mouse 488 1:1,000 (DyLight) and goat anti–rabbit 549 1:2,000 (DyLight). Larval preparations were mounted in Vectashield (Vector Laboratories, Newark, CA, USA) and imaged at room temperature with a 700 Carl Zeiss, AG (Oberkochen, Germany) laser scanning confocal microscope equipped with 63×/1.4 NA oil immersion objective using separate channels with four laser lines (405, 488, 555, and 639 nm).
All quantifications were performed on muscle 6/7 segments A2 and A3. For NMJ growth analysis, anti-Dlg boutons were counted in muscle after verifying that there was a corresponding cluster of anti-Syn staining in motor neurons. For pCaMKII and GluRIII intensity measurements, a mask was created around the GluRIII channel to define the postsynaptic region and eliminate the background. Only pCaMKII signals within this mask at Ib postsynaptic densities were quantified. Images were prepared for publication using Adobe Illustrator.
Statistical significance was tested using either a Student’s T-Test to compare one experimental data set directly to a control data set or using a one-way ANOVA with a Tukey’s post-hoc test if multiple data sets were being compared. Specific p-value ranges used were as follows: *p < 0.05, **p < 0.01, and ***p < 0.001. All statistical analyses were conducted using GraphPad Prism software. Data were plotted in GraphPad Prism and final figures were compiled using Adobe Illustrator.
PHP is commonly assessed at the Drosophila NMJ using acute Philanthotoxin-433 (PhTx) application to test the rapid expression of PHP (Frank et al., 2006) or chronic genetic manipulations to test the expression of PHP throughout life, like the GluRIIASP16 the genetic null mutation (Petersen et al., 1997) or knockdown of GluRIII gene function by RNAi (Brusich et al., 2015). All of these manipulations result in decreased mEPSP amplitude while quantal content (corrected for non-linear summation, herein NLS quantal content) increases. Evoked excitation is maintained as a result. This indicates PHP mechanisms are working to sustain evoked potentials near normal levels.
Given our prior data suggesting that dysregulation of calcium stores disrupted the maintenance of PHP after genetic challenge (Brusich et al., 2015; James et al., 2019), we wanted to test whether combined pre- and postsynaptic sra loss-of-function conditions impaired PHP. To do this, we used a Drosophila line that contains both neuron and muscle GAL4 drivers to knockdown sra using RNAi and applied 20 μM PhTx to test rapid PHP expression. This sra RNAi line has been shown to knockdown the protein levels of Sarah (also known as Nebula) by about 60% (Shaw and Chang, 2013).
PhTx application decreased mEPSP amplitude for both driver control and sra RNAi conditions (Figure 1A; Frank et al., 2006). Evoked potentials were maintained for the driver control (Figure 1B) because of the compensatory significant increase in NLS quantal content (Figure 1C). Dual tissue knockdown of sra resulted in a mild decrease in evoked potentials following PhTx incubation. This might be consistent with a small defect in homeostatic plasticity, but there was still a significant increase in NLS quantal content compared to non-drugged controls (Figures 1A–D and Supplementary Table 1). At a minimum, this indicated partially intact acute PHP expression.
Dual pre and postsynaptic knockdown or overexpression of sra do not block PHP. (A–C) Quantification showing average mEPSP amplitude, EPSP amplitude, and NLS quantal content in pre + post-GAL4 control and pre + post-GAL4 driven sra RNAi. Black points show DMSO control synapses, green points show synapses treated with PhTx, and magenta points show animals chronically challenged with GluRIII RNAi. (D,H) Representative electrophysiological traces of EPSPs (above) and mEPSPs (below). Scale bars for all traces are y = 10 mV (1 mV), x = 20 ms (500 ms) for EPSPs (mEPSPs). (E–G) Quantification showing average mEPSP amplitude, EPSP amplitude, and NLS quantal content in pre + post-GAL4 control and pre + post-GAL4 driven sra overexpression (OE). Black points show DMSO control synapses, green points show synapses treated with PhTx, and magenta points show animals chronically challenged with GluRIII RNAi. *p < 0.05, **p < 0.01, ***p < 0.001 by Multiple Student’s T-test and corrected for multiple comparisons using the Holm-Sidak method. DMSO controls were compared to PhTx and GluRIII RNAi synapses for driver controls and separately for sra RNAi or OE. PHP, presynaptic homeostatic potentiation; mEPSPs, miniature excitatory postsynaptic potentials; EPSPs, excitatory postsynaptic potentials; NLS, non-linear summation.
To test for the maintenance of PHP, we first used a Drosophila line that contains both neuron and muscle GAL4 drivers, along with UAS-GluRIII RNAi to provide a genetic homeostatic challenge (Brusich et al., 2015). This UAS-GluRIII RNAi reagent is a good first test or screening tool to identify genes needed for the maintenance of PHP (Brusich et al., 2015). Again, while there were significant decreases in evoked amplitude in sra RNAi synapses challenged with either PhTx or GluRIII RNAi, there were still some compensatory increases in NLS quantal content (Figures 1A–D). Our interpretation of these data is that PHP mechanisms are partially (or largely) intact when sra function is knocked down.
We acquired reagents to test if simultaneous pre- and postsynaptic overexpression of sra resulted in impairment in forms of PHP. We used the sraEY07182 allele. This is a 5’ P-element insertion allele in the endogenous sra locus with UAS (Upstream Activating Sequence) binding sites for GAL4 transcriptional control upstream of the sra gene; this allows for conditional tissue-specific overexpression of sra. Overexpression of sra neuronally using the pan-neuronal driver, elaV, and sraEY07182 has been shown to result in transcript levels that were approximately twofold higher than controls (Lee et al., 2016).
For our electrophysiological experiments, there was a decrease in the EPSPs of neuron + muscle GAL4 ≫ sraEY07182 (“sra OE”—overexpression) + GluRIII RNAi synapses compared to neuron + muscle GAL4 ≫ sraEY07182 alone, and there was only a small—but not statistically significant—increase in NLS quantal content (Figures 1E–H and Supplementary Table 1). Our interpretation of these data is that the chronic expression of PHP is not fully intact. Additionally, there was no significant difference in the EPSPs of neuron + muscle GAL4 ≫ sraEY07182 overexpression animals alone or genetically identical overexpression animals challenged with PhTx (Figures 1E–H). Taken as a whole, concurrent overexpression of sra both pre-and postsynaptically does not appear to block homeostatic responses at the NMJ. If anything, phenotypes are subtle, indicating partial impairments of PHP.
We turned to mutant Drosophila stocks to examine loss-of-function alleles. We used the sraMi06435 transposon insertion to test if a global disruption of sra function would block PHP or show defective neurotransmission. We compared sraMi06435 alone to wild type and sraMi06435 over chromosomal deficiency (Df). We found no significant differences in EPSP amplitude or NLS quantal content between any groups (Figures 2A–C and Supplementary Table 2, indicating a normal level of baseline neurotransmission.
Global sra mutation does not preclude PHP but does show an evoked amplitude decrease in low calcium conditions. (A–C) Quantification showing average mEPSP, EPSP amplitude, and NLS quantal content in sraMi06345, sraMi06345/Df(3R)sbd104, and GluRIIASP16; sraMi06345/Df(3R)sbd104. (D,H) Representative electrophysiological traces of EPSPs (above) and mEPSPs (below). Scale bars for all traces are y = 10 mV (1 mV), x = 20 ms (500 ms) for EPSPs (mEPSPs). (E–G) Experiments conducted in low calcium (0.2 mM). Quantification showing average mEPSP, EPSP amplitude, and NLS quantal content in sraMi06345, sraMi06345/Df(3R)sbd104, and GluRIIASP16; sraMi06345/Df(3R)sbd104. Ordinary one-way ANOVAs were used to compare each genotype followed by a Tukey’s HSD test for multiple comparisons. *p < 0.05, ***p < 0.001.
To challenge the mutant NMJs and test for PHP, we used a GluRIIASP16 null mutant (Petersen et al., 1997) and combined it with the sraMi06435/Df allelic combination. The addition of the GluRIIASP16 mutation caused a significant decrease in mEPSP amplitude, as expected (Figure 2A). Yet our test for PHP expression was mixed. This is because, in the sra loss-of-function combination, the homeostatic challenge was coupled with a small, but not statistically significant decrease in EPSP amplitude (Figure 2B) and a small, but not significant increase in NLS quantal content (Figure 2C). If there were robust PHP for the sra mutants, one would predict an increase in quantal content in response to the homeostatic pressure of decreased mEPSPs. Conversely, if there were blocked PHP, one would expect a decrease in EPSP amplitude. Neither occurred.
Prior studies showed that mutants with intermediate PHP phenotypes can be sensitive to levels of calcium (Frank et al., 2006; Frank, 2014a, b; Genç and Davis, 2019). Therefore, we also examined sraMi06435 and sraMi06435/Df for defective neurotransmission in low calcium conditions (0.20 mM). Somewhat surprisingly, sraMi06435 and sraMi06435/Df showed decreased mEPSP amplitude and EPSP amplitude compared to wild type, and no increase in NLS quantal content (Figures 2E–G). Our sraMi06435 and sraMi06435/Df data lead us to the interpretation that PHP and NMJ functions are highly sensitive to low levels of calcium. This is a phenotype shared with some other homeostatic factors that govern presynaptic release (Frank et al., 2006; Frank, 2014b; Yeates and Frank, 2021).
After testing for dual-tissue functions for sra in PHP, we conducted single-tissue experiments. First, we used the UAS-sra RNAi transgene to knockdown sra expression in the muscle. We applied PhTx to animals that expressed UAS-sra RNAi in the muscle using the GAL4 driver, BG57-GAL4. Surprisingly, in contrast to the dual-tissue knockdown, postsynaptic knockdown of sra completely blocked the rapid expression of PHP, resulting in significantly decreased EPSP amplitudes and a failure to show a compensatory increase in NLS quantal content (Figures 3B,C and Supplementary Table 3). We tested that if the postsynaptic knockdown of sra would also block the chronic maintenance and expression of PHP. Knockdown of sra postsynaptically in a GluRIIASP16 background not only exhibited an expected decrease in mEPSP amplitude compared to non-GluRIIASP16 controls but also a marked decrease in EPSP amplitude (Figures 3E,F and Supplementary Table 3). There was a slight but significant increase in quantal content, which indicates partially intact PHP mechanisms (Figure 3G).
Acute expression of presynaptic homeostatic potentiation requires muscle expression of sra but maintenance does not. (A–C) Quantification showing average mEPSP amplitude, EPSP amplitude, and NLS quantal content in post-GAL4 control and post-GAL4 driven sra RNAi. Black points show DMSO control synapses, while green points show synapses treated with PhTx. (D,H) Representative electrophysiological traces of EPSPs (above) and mEPSPs (below). Scale bars for all traces are y = 10 mV (1 mV), x = 20 ms (500 ms) for EPSPs (mEPSPs). (E–G) Quantification showing average mEPSP amplitude, EPSP amplitude, and NLS quantal content in post-GAL4 control and post-GAL4 driven sra RNAi in both the presence and absence of GluRIIASP16. *p < 0.05, **p < 0.01, ***p < 0.001 by Student’s T-test vs. non-challenged genetic control.
Our results are consistent with the idea that the knockdown of sra postsynaptically induces a complete block when acutely challenged with pharmacology, but only a partial block when chronically challenged genetically. This suggests that the postsynaptic expression of sra may have a differential role in regulating two separable forms of PHP. We tested this idea further.
After examining postsynaptic sra knockdown, we did the opposite genetic perturbation: we examined the overexpression of sra postsynaptically. We found that applying PhTx to synapses with sra overexpressed postsynaptically reveals partially impaired rapid PHP expression (Figures 4A–D and Supplementary Table 4). There was no significant difference in EPSPs between unchallenged and challenged NMJs. There was a small numerical increase in NLS quantal content, but this increase did not achieve statistical significance (Figure 4C).
Maintenance of presynaptic homeostatic potentiation is impaired by muscle overexpression of sra but an acute expression is not. (A–C) Quantification showing average mEPSP amplitude, EPSP amplitude, and NLS quantal content in post-GAL4 control and post-GAL4 driven sra overexpression (OE). Black points show DMSO control synapses, while green points show synapses treated with PhTx. (D,H) Representative electrophysiological traces of EPSPs (above) and mEPSPs (below). Scale bars for all traces are y = 10 mV (1 mV), x = 20 ms (500 ms) for EPSPs (mEPSPs). (E–G) Quantification showing average mEPSP amplitude, EPSP amplitude, and NLS quantal content in post-GAL4 control and post-GAL4 driven sra OE in both the presence and absence of GluRIIASP16. *p < 0.05, **p < 0.01, ***p < 0.001 by Student’s T-test vs. non-challenged genetic control.
Next, we tested if postsynaptic overexpression of sra could block chronic expression of PHP using the sraEY07182 allele in a GluRIIASP16 background. Overexpression of sra postsynaptically yielded normal baseline EPSP amplitude (as in Figure 1), but this postsynaptic overexpression of sra in GluRIIASP16 null NMJ background triggered a significant decrease in EPSP amplitude along with a failure to increase NLS quantal content when compared to the unchallenged control (Figures 4E–H and Supplementary Table 4). In fact, the NLS quantal content was also significantly decreased in the GluRIIASP16 background, which is not a common phenotype. This is indicative of a strong block of chronically expressed PHP. Taken together, our data suggest that proper postsynaptic expression of sra is critical for the NMJ to respond to different kinds of PHP challenges.
Since the postsynaptic overexpression phenotype was the strongest, we checked if it correlated with NMJ developmental defects. In principle, synaptic developmental defects could explain the physiological phenotypes. We immunostained third instar larval Drosophila NMJs for presynaptic boutons to quantify their elaboration. We overexpressed sra postsynaptically alone and or in conjunction with GluRIIASP16. We visualized NMJ bouton development in synapse 6/7 muscle segments A2 and A3 by co-staining with anti-Synapsin (Syn, presynaptic vesicle marker) and anti-Discs Large (DLG, postsynaptic density marker).
We did not find marked differences in bouton number between any group for NMJ 6/7 within segment A2 or segment A3 (Figures 5A–G and Supplementary Table 5; segments analyzed separately due to documented differences in development). We quantified bouton number normalized per muscle area and found that postsynaptic overexpression of sra resulted in a significant increase compared to other genotypes (Figure 5H and Supplementary Table 5). This indicates that sra overexpression in the muscle may result in smaller muscles—but in any case, these unchallenged animals had intact physiology (Figure 4). These data indicate that postsynaptic overexpression of sra does not impair gross NMJ development, and therefore, a developmental defect is unlikely to be contributing towards the observed PHP block by electrophysiology. The caveat to this experiment is that more subtle developmental changes are possible on the level of active zones or glutamate receptor clusters. We decided to examine a marker known to correlate with PHP expression at a specific motor terminal.
Postsynaptic sra overexpression does not impair synapse development. (A–F) NMJs were co-stained with anti-DLG (magenta) and anti-Synapsin antibodies (green) to visualize synaptic boutons. (G) NMJ growth was assessed by bouton counting at abdominal segments A2 and A3, muscle 6/7, based on postsynaptic DLG staining and checking for presynaptic Synapsin. (H) Bouton counts were normalized per unit of muscle 6/7 area. Ordinary one-way ANOVAs were used to compare each genotype within each segment followed by a Tukey’s HSD test for multiple comparisons. *p < 0.05, **p < 0.01, ***p < 0.001. NMJ, neuromuscular junction; DLG, discs large.
To try to gain a more mechanistic understanding of how postsynaptic sra overexpression may be blocking the maintenance of PHP, we measured phosphorylated CaMKII (pCaMKII) levels and glutamate receptor signal intensity. Genetic glutamate receptor challenges normally result in decreased levels of pCaMKII immunofluorescence (Goel et al., 2017; Newman et al., 2017; Li et al., 2018; Kikuma et al., 2019; Perry et al., 2022), and this decrease correlates with the successful expression of PHP.
We measured pCaMKII intensity at Ib postsynaptic densities. We found a significant decrease in GluRIIASP16 animals compared to wild-type animals as has been shown previously (Figures 6A,B, and Supplementary Table 6). We note for this experiment that our muscle driver control lines also showed unexpected decreases in baseline pCaMKII and GluRIII staining compared to the wild type (Figures 6A,B). These effects are likely due to the genetic background of the driver, but they are important for subsequent comparison with sra overexpression.
Postsynaptic sra overexpression disrupts pCaMKII in Ib postsynaptic densities. (A) Maximum-intensity projections of pCaMKII (magenta) and GluRIII (green). Merged images of both channels are shown in the top row. Representative bouton maximum-intensity projections of pCaMKII (middle row) and GluRIII (bottom row) are shown as heat maps. (B) Quantification of pCaMKII intensity as a percentage relative to wild type boutons. Each point represents the intensity at an individual Ib postsynaptic density. (C) Quantification of GluRIII intensity as a percentage relative to wild type boutons. Ordinary one-way ANOVAs were used to compare each genotype followed by a Tukey’s HSD test for multiple comparisons. **p < 0.01, ***p < 0.001.
Surprisingly, when comparing overexpression of sra postsynaptically to overexpression of sra postsynaptically in GluRIIASP16 null NMJs, we not only failed to see a decrease in pCaMKII intensity, but we saw a significant increase (Figures 6A,B). Moreover, when examining GluRIII (also known as GluRIIC) intensity, we saw no significant difference between wild type and GluRIIASP16 (Figures 6A,C). However, we saw a significant increase in GluRIII intensity in sra postsynaptic overexpression in the GluRIIASP16 null background when compared to sra overexpression alone or wild type (Figures 6A,C). It is possible that the synapse (unsuccessfully) attempted to compensate for its electrophysiological deficits by trafficking in more glutamate receptors.
Because we saw strong tissue-specific phenotypes when genetically dysregulating sra in the muscle, we tested if the knockdown of sra presynaptically also affected PHP. Acute application of PhTx to NMJs with sra neuronal knockdown did not block Acute PHP expression as EPSP amplitudes were mostly maintained, and NLS quantal content was significantly increased between challenged and unchallenged animals (Figures 7A–D and Supplementary Table 7).
Maintenance of presynaptic homeostatic potentiation is impaired by neuronal knockdown of sra but acute expression is not. (A–C) Quantification showing average mEPSP amplitude, EPSP amplitude, and NLS quantal content in pre-GAL4 control and pre-GAL4 driven sra RNAi. Black points show DMSO control synapses, while green points show synapses treated with PhTx. (D,H) Representative electrophysiological traces of EPSPs (above) and mEPSPs (below). Scale bars for all traces are y = 10 mV (1 mV), x = 20 ms (500 ms) for EPSPs (mEPSPs). (E–G) Quantification showing average mEPSP amplitude, EPSP amplitude, and NLS quantal content in pre-GAL4 control and pre-GAL4 driven sra RNAi in both the presence and absence of GluRIIASP16. *p < 0.05, **p < 0.01, ***p < 0.001 by Student’s T-test vs. non-challenged genetic control.
To understand if decreasing sra expression presynaptically blocks the long-term expression of PHP, we turned to the GluRIIASP16 null mutant again. We found that decreased sra expression neuronally blocked chronic PHP because of decreased EPSP amplitude in GluRIIASP16, sra RNAi NMJs when compared to GluRIIASP16 or sra RNAi alone (Figures 7E–H and Supplementary Table 7). The GluRIIASP16, sra RNAi synapses also failed to show a significant increase in NLS quantal content compared to the unchallenged control (Figure 7G). This provides us with evidence that the knockdown of sra presynaptically blocks the long-term maintenance phase of PHP.
Finally, we tested if the presynaptic overexpression of sra affects PHP. After applying PhTx to NMJs with sra neuronal overexpression, we did not find any significant differences in evoked amplitude while getting a robust increase in NLS quantal content (Figures 8A–D and Supplementary Table 8).
Maintenance of presynaptic homeostatic potentiation is impaired by neuronal overexpression of sra but acute expression is not. (A–C) Quantification showing average mEPSP amplitude, EPSP amplitude, and NLS quantal content in pre-GAL4 control and pre-GAL4 driven sra overexpression (OE). Black points show DMSO control synapses, while green points show synapses treated with PhTx. (D,H) Representative electrophysiological traces of EPSPs (above) and mEPSPs (below). Scale bars for all traces are y = 10 mV (1 mV), x = 20 ms (500 ms) for EPSPs (mEPSPs). (E–G) Quantification showing average mEPSP amplitude, EPSP amplitude, and NLS quantal content in pre-GAL4 control and pre-GAL4 driven sra OE in both the presence and absence of GluRIIASP16. ***p < 0.001 by Student’s T-test vs. non-challenged genetic control.
Next, we attempted to test chronic PHP expression following neuronal sra overexpression in a GluRIIASP16 null background. There was a statistically significant decrease in the evoked amplitudes of elaV(C155)-Gal4 ≫ sraEY07182 synapses and a non-significant NLS quantal content increase (Figures 8E–H and Supplementary Table 8). Yet based on our controls, we cannot attribute those data to sra overexpression alone: this is because the neuronal driver in this experiment also seemed to contribute to the phenotype (Figures 8F–H).
We were puzzled why the single-tissue genetic manipulations of sra yielded stronger PHP inhibition. One possibility is that for instances where a molecule normally functions in multiple tissues, synapses could engage homeostatic systems to cope with a global loss (e.g., endogenous genetic mutation) of that molecule. In principle, challenging a synapse with single-tissue genetic manipulation could disrupt this process.
Our earlier experiments showed a strong chronic PHP block when sra was overexpressed postsynaptically (Figures 4E–H and Supplementary Table 4). Sra is a calcineurin inhibitor. Thus, overexpressing the sra gene (sra OE) postsynaptically should decrease calcineurin activity in muscle. If the above model is correct, then impairing calcineurin globally via a pharmacological calcineurin inhibitor could reverse the chronic PHP block.
FK506 (tacrolimus) is a calcineurin-inhibiting drug that binds to FK-binding protein l (Liu et al., 1991; Sigal and Dumont, 1992; Clardy, 1995). This immunophilin-drug binds to calcineurin and inhibits its protein phosphatase activity (Swanson et al., 1992; Clardy, 1995; Kuromi et al., 1997). There are notable phenotypes at synaptic preparations. For whole-cell patch clamp in rat cortex, the application of FK506 increased the frequency of spontaneous events (Victor et al., 1995). And the application of FK506 to Drosophila larval neuromuscular junctions has been reported to increase the endocytosis of synaptic vesicles while having no effect on the frequency of spontaneous events or the mean amplitude of evoked events (Kuromi et al., 1997), though the specificity of FK506 in impairing calcineurin at the Drosophila NMJ has not been thoroughly documented.
We repeated the experiment of overexpressing sra in the muscle, this time incubating NMJ preps with FK506. Larval preps were incubated with 50 μM FK506 for 15 min prior to electrophysiological recordings. We replicated our earlier finding that sra muscle overexpression challenged with the GluRIIASP16 null mutation showed decreased evoked amplitude and a marked decrease in NLS quantal content, instead of an increase (Figures 9B,C and Supplementary Table 9). We also repeated our driver controls, and we should note that in the case of this set of experiments, the driver also appeared to confer an impairment of PHP (Figures 9B,C and Supplementary Table 9)—though consistent with before (Figure 4), the effect was not nearly as severe as with sra muscle overexpression.
Pharmacological inhibition of calcineurin partially rescues the PHP deficit observed in chronically challenged postsynaptic overexpression of sra animals. (A–C) Quantification showing average mEPSP amplitude, EPSP amplitude, and NLS quantal content in post-GAL4 control and post-GAL4 driven sra overexpression (OE) in both the presence and absence of 50 μM FK506. Black points show DMSO control synapses while dark pink points represent 50 μM FK506. (D) Representative electrophysiological traces of EPSPs (above) and mEPSPs (below). Scale bars for all traces are y = 10 mV (1 mV), x = 20 ms (500 ms) for EPSPs (mEPSPs). Two-way ANOVAs were used to compare each genotype with and without the drug followed by a Tukey’s HSD test for multiple comparisons. *p < 0.05, **p < 0.01.
Genetically identical animals were incubated with FK506. We found that EPSP amplitude is significantly and specifically increased in the sra muscle overexpression challenged with the GluRIIASP16 null mutation—compared to non-drugged controls—and this is a result of increased NLS quantal content (Figures 9A–D and Supplementary Table 9). This experiment suggests that global inhibition of calcineurin across tissues can mitigate the chronic PHP block caused by single tissue sra manipulation. Alternatively, our results may point toward non-specific potentiation presynaptically, induced by FK506. This latter possibility did not seem likely due to the specificity of the effect on the sra overexpression line, but we conducted further tests.
We wondered if we could reverse other PHP defects by pharmacologically blocking calcineurin. We saw a block in chronic PHP following sra knockdown presynaptically (Figures 7E–H). We repeated this experiment while also including synapses incubated with FK506. As before, in the drug-free condition, EPSP amplitudes were deficient following sra presynaptic knockdown challenged via GluRIIASP16 and failed to increase NLS quantal content (Figures 10B,C). However, incubation with FK506 significantly increased EPSP amplitude in these animals, and this was accompanied by a robust increase in NLS quantal content (Figures 10A–D and Supplementary Table 10). This provides further support that inhibition of calcineurin in a non-tissue-specific pharmacological manner can reverse defects resulting from single tissue sra impairments and restore PHP.
Pharmacological inhibition of calcineurin rescues the PHP deficit observed in chronically challenged presynaptic knockdown of sra animals. (A–C) Quantification showing average mEPSP amplitude, EPSP amplitude, and NLS quantal content in pre-GAL4 control and pre-GAL4 driven sra knockdown in both the presence and absence of 50 μM FK506. Black points show DMSO control synapses while dark pink points represent 50 μM FK506. (D) Representative electrophysiological traces of EPSPs (above) and mEPSPs (below). Scale bars for all traces are y = 10 mV (1 mV), x = 20 ms (500 ms) for EPSPs (mEPSPs). Two-way ANOVAs were used to compare each genotype with and without the drug followed by a Tukey’s HSD test for multiple comparisons. *p < 0.05, ***p < 0.001.
We next tested if the pharmacological inhibition of calcineurin alone would also block PHP. We applied FK506 to GluRIIASP16 animals at either 10 μM or 50 μM concentrations. We saw no change in EPSP amplitude in wild-type animals with drug application at either concentration, but interestingly, in GluRIIASP16 animals, we saw an increase in EPSP amplitude and NLS quantal content following 50 μM FK506 incubation compared to non-drugged controls (Figure 11B and Supplementary Table 11). The 50 μM concentration also produced increases in mEPSP frequency in both wild type and GluRIIASP16 animals (Figure 11D and Supplementary Table 11). These data are consistent with our hypothesis that global inhibition of calcineurin may create scenarios at the NMJ that are favorable to the expression of PHP.
Maintenance of presynaptic homeostatic potentiation can be further potentiated by pharmacologically inhibiting calcineurin. (A–D) Quantification showing average mEPSP amplitude, EPSP amplitude, NLS quantal content, and mEPSP frequency in wild type and GluRIIASP16 animals. Black points show 10 μM DMSO control, light pink points show 10 μM FK506, gray points show 50 μM DMSO control, and dark pink points show 50 μM FK506. (E,F) Quantification of failure analysis experiment. 0.10 mM Ca2+ was used in this experiment. “% failures” represents the number of stimulation pulses that failed to elicit any discernable response out of the 100 total pulses per synapse. Black points represent DMSO control, while the dark pink points represent 50 μM FK506. (G–I) Quantification showing average mEPSP amplitude, EPSP amplitude, and NLS quantal content in wild type and GluRIIASP16 animals dissected in low calcium conditions (0.20 mM Ca2+). Black points represent DMSO control, while the dark pink points represent 50 μM FK506. *p < 0.05, **p < 0.01, ***p < 0.001 by Student’s T-test vs. non-drugged control.
We tested if FK506 increases release probability on its own. To do this, we conducted a failure analysis in very low calcium (0.14 mM; Petersen et al., 1997; Frank et al., 2006). In extremely low calcium, many presynaptic events fail to release vesicles and therefore fail to generate an EPSP. In these low calcium conditions, the probability of vesicle release is low (Del Castillo and Katz, 1954). Our failure analysis on wild-type and GluRIIASP16 animals with and without FK506 incubation showed that FK506 significantly decreases the number of failures compared to non-drugged controls (Figure 11E and Supplementary Table 11). Additionally, successful evoked events were significantly bigger in amplitude (on average) after FK506 incubation compared to non-drugged controls (Figure 11F). Taken together, these data suggest that FK506 increases release probability. This may be occurring because of calcineurin inhibition increasing presynaptic calcium either via influx from voltage-gated channels or efflux via intracellular stores.
As shown in our failure analysis experiment, FK506 can increase the EPSP amplitude in wild-type animals in low calcium (Figure 11F and Supplementary Table 11). While there were no changes at our standard calcium concentration of 0.5 mM (Figure 11B), it is possible that at our standard calcium concentration, we are having a “ceiling effect” where the application of FK506 may not be able to increase the EPSPs any further due to electromotive driving forces. To test this idea, we conducted an experiment in 0.20 mM Ca2+ in both wild type and GluRIIASP16 animals with and without FK506. As we predicted, the lower calcium concentration allowed us to detect an increase in evoked amplitude and NLS quantal content in both genotypes following incubation with FK506 (Figures 11H,I and Supplementary Table 11). This supports the idea that pharmacological calcineurin inhibition may be potentiating calcium levels to increase release probability.
Finally, we tested if the genetic knockdown of a calcineurin subunit led to similar blocks in PHP as observed with our sra manipulations. In Drosophila, there are three genes that encode the catalytic subunit, CN A (CaNA1, Pp2B-14D, and CaNA-14F), and two genes that encode the regulatory subunit, CN B (CaNB and CaNB2; Takeo et al., 2006). CaNA14-F and Pp2B-14D share 82% protein sequence identity and share approximately 62% identity with CaNA1, while CaNB and CaNB2 share 98% similarity in protein sequence (Tomita et al., 2011). Presynaptic knockdown of CaNB leads to an increased frequency of ectopic neuromuscular contacts, and CaNB knockouts have been shown to have significantly reduced sleep (Nakai et al., 2011; Vonhoff and Keshishian, 2017). Knockdown of CaNB reduces calcineurin activity, similar to sra overexpression (Shaw and Chang, 2013; Lee et al., 2016).
We tested whether combined pre- and postsynaptic CaNB conditions impaired PHP, like how we tested sra overexpression conditions (Figure 1). Dual tissue knockdown of CaNB resulted in a mild decrease in evoked potentials following PhTx incubation, and there was a slight but insignificant increase in NLS quantal content (Figures 12A–D and Supplementary Table 12). When testing the maintenance of PHP using UAS-GluRIII RNAi, dual tissue knockdown of CaNB did not impair evoked potentials and showed a robust increase in NLS quantal content. Taken together, these data suggest a weak effect of CaNB dual tissue knockdown on acute PHP expression and no effect on long-term PHP maintenance.
Dual pre- and postsynaptic knockdown of CaNB does not block PHP while knockdown in muscle alone precluded the maintenance of PHP. (A–C) Quantification showing average mEPSP amplitude, EPSP amplitude, and NLS quantal content in pre + post-GAL4 control and pre + post-GAL4 driven CaNB RNAi. Black points show DMSO control synapses, green points show synapses treated with PhTx, and magenta points show animals chronically challenged with GluRIII RNAi. (D) Representative electrophysiological traces of EPSPs (above) and mEPSPs (below). Scale bars for all traces are y = 10 mV (1 mV), x = 20 ms (500 ms) for EPSPs (mEPSPs). *p < 0.05, ***p < 0.001 by Multiple Student’s T-test and corrected for multiple comparisons using the Holm-Sidak method. DMSO controls were compared to PhTx and GluRIII RNAi synapses for driver controls and separately for CaNB RNAi.
In this study, we provide a framework for understanding how a protein residing in different synaptic tissues can contribute to Presynaptic Homeostatic Potentiation (PHP). The core finding is that a single gene encoding a calcineurin regulator can regulate PHP in multiple ways, in different tissues, and over various periods of time throughout the life of the synapse. Our work offers a way to consider how overall synaptic stability is maintained through different kinds of challenges to synapse function.
We show that concurrent neuronal and muscle knockdown or overexpression of sra, a gene encoding a calcineurin regulator, does not strongly impair synaptic homeostatic compensation mechanisms (Figure 1). Global genetic mutation of sra does not impair homeostasis either, but it does reveal that the mutant synapse is sensitive to low levels of extracellular calcium (Figure 2). Impairing sra in tissue-specific manners leads to numerous blocks in PHP.
In the muscle, postsynaptic knockdown of sra disrupts acute, PhTx-induced PHP (Figure 3) while postsynaptic overexpression strongly blocks expression of PHP induced by GluRIIA loss (Figure 4). We checked if developmental defects might be associated with that strong PHP impairment. Postsynaptic overexpression of sra does not alter gross NMJ development (Figure 5), but it does cause an increased amount of pCaMKII apposing type-Ib boutons in a GluRIIA mutant background (Figure 6).
For the neuron, we saw that presynaptic knockdown of sra blocks chronic PHP (Figure 7) and presynaptic overexpression also impairs it (Figure 8). Additionally, we were able to alleviate observed PHP blocks via FK506). We also showed that the maintenance of PHP can be further potentiated via FK506, especially when the system is crippled by low extracellular calcium (Figure 11). Finally, we demonstrated that concurrent neuronal and muscle knockdown of the calcineurin subunit CaNB led to a weak block in acute PHP (Figure 12).
The full complement of pre- and postsynaptic data is complex. One model is that the relative balance of calcineurin function at NMJ tissues may control homeostatic signaling capacity. It is possible that impairing sra either pre- or postsynaptically (separately) leads to a PHP block as it can disrupt the relative amounts of calcineurin activity between the two sides of the synapse. This idea could explain why a combined pre- and postsynaptic sra impairment (or a global pharmacological impairment of calcineurin) may not block PHP. Even though calcineurin activity is changed, the changes are reciprocal between both neuron and muscle.
To our knowledge, this type of idea (relative pre- or postsynaptic balance) is new to the field of homeostatic plasticity. But it is reminiscent of prior ideas that have emerged from studies of Hebbian plasticity. One example is the Bienenstock-Cooper-Munro (BCM) rule for visual cortex learning. The BCM rule dictates that either potentiation or depression can occur, but the direction of plasticity ultimately depends upon the level of postsynaptic activity after a presynaptic neuron fires (Bienenstock et al., 1982). By contrast, if the activity is globally impaired (e.g., by blocking glutamate receptors), no directional plasticity is possible (Bienenstock et al., 1982). In our case, we have the opposite kind of phenomenon. The block of glutamate receptors initiates a form of homeostatic plasticity. The expression of that plasticity can be blocked by tissue-specific manipulations to calcineurin—but not global manipulations.
Calcineurin regulation is the most notable function of sra. Decreased expression of sra increases calcineurin activity, while increased sra via overexpression is presumed to decrease it (Chang et al., 2003; Shaw and Chang, 2013). Both of these types of manipulations are relevant in the neuronal expression of PHP (Figures 7, 8).
Calcineurin has several presynaptic roles in various models that have been shown including affecting synaptic vesicle exocytosis and endocytosis, axon remodeling, and regulating neurotransmitter release (Tarasova et al., 2018). One target of presynaptic calcineurin is to dephosphorylate Synapsin 1 which can affect the mobilization of synaptic vesicles from the reserve pool to active zones (Jovanovic et al., 2001; Chi et al., 2003; Cesca et al., 2010). For those studies, opposing interactions between calcineurin and various kinases regulate vesicle mobilization. This may explain why neuronal sra overexpression impaired acute PHP because increased sra decreases calcineurin activity which may impair the mobilization of vesicles to active zones. Challenges to NMJ function (e.g., GluRIIASP16 or PhTx) consistently increase the number of vesicles that are released, and failure to mobilize enough vesicles in response to a challenge may lead to a failure for compensatory NLS quantal content increase and impair evoked release.
There are conflicting results on how the use of calcineurin inhibitors affects exocytosis. They have been shown to increase the rate of exocytosis, show no change at all, or suppress exocytosis (Yakel, 1997; Sim et al., 2003; Kumashiro et al., 2005). Specifically in the Drosophila NMJ, calcineurin inhibition via FK506 has been shown to increase the endocytosis of synaptic vesicles, while having no effect on the frequency of spontaneous events or the mean amplitude of evoked events (Kuromi et al., 1997). This is contrary to the results of the current study where we showed that mEPSP frequency is increased following FK506 incubation (Figure 11D). This difference may be explained by the differences in concentration of FK506 that was used as previously, only 5 μM and 10 μM were tested. We see no change in mEPSP frequency at 10 μM FK506, like in previous work as we only see the increase in spontaneous event frequency following 50 μM FK506. Alternatively, it is formally possible that FK506 could have some off-target effects at the NMJ that affect parameters like mini frequency. Some possible ER-localized off-targets that could do this are the ryanodine receptors (Ahern et al., 1994; Bultynck et al., 2000).
Calcineurin is believed to be a calcium-dependent regulator of endocytosis in both neuronal and non-neuronal secretory cells (Wu et al., 2014). Calcineurin inhibitors slow endocytosis in response to a small calcium influx but do not alter the speed of endocytosis in response to a large calcium influx. If calcineurin is inhibiting synaptic vesicle recycling, this may explain why we see a defect in chronic PHP following neuronal sra knockdown. Knockdown of sra leads to increased calcineurin activity, and therefore, increases inhibition of vesicular recycling. While this may not lead to abnormal neurotransmission in the absence of a challenge, the evoked amplitude may not be maintained when chronically challenged due to an increased need for vesicle recycling in response to the challenge.
Calcineurin may be affecting calcium influx as the targets of calcineurin in mouse NMJ are L-type calcium channels (Gaydukov et al., 2013). Specifically, it was shown that calcineurin downregulates neurotransmitter release by suppressing L-type calcium channels and calcium release from intracellular stores through ryanodine receptors. In the Drosophila NMJ, it has recently been shown that while L-type calcium channels are localized around active zones and contribute to AP-triggered calcium influx, they are dispensable for evoked release (Krick et al., 2021). However, it has not been shown if these channels are necessary for homeostatic signaling processes. Calcium influx increases in response to both acute and chronic challenges to the synapse (Müller and Davis, 2012). L-type calcium channels may be necessary for homeostatic compensation as they not only serve as an additional entry point of calcium into the presynaptic compartment to contribute to neurotransmitter release, but calcium entry through these channels also induces calcium release from intracellular stores. In the current study, presynaptic loss of sra could lead to increased calcineurin which may lead to increased inhibition of L-type calcium channels and not allow for a large enough compensatory increase in calcium influx necessary to respond to a homeostatic challenge.
We also showed muscle roles for Sarah at the NMJ. Postsynaptic sra knockdown impaired acute homeostatic signaling (Figure 3) while postsynaptic sra overexpression strongly blocked chronic homeostatic signaling (Figure 4).
It is known that postsynaptic calcineurin activation can lead to the dephosphorylation of AMPA receptors that can lead to their internalization (Lin et al., 2000; He et al., 2009; D’Amelio et al., 2011). Ionotropic glutamate receptor subunits that are localized to muscle in Drosophila are DGluRIIA and DGluRIIB, both of which are AMPA/kainite type receptors (Betz et al., 1993; Petersen et al., 1997). This presents a potential mechanism for how sra knockdown in the muscle blocks PHP- decreased sra expression increases calcineurin activity which may lead to increased internalization of postsynaptic glutamate receptors. A decrease in the amount of available glutamate receptors may be detrimental when glutamate receptors are already being challenged via philanthotoxin. However, we showed that the amount of GluRIII is increased in animals with postsynaptic overexpression of sra (Figure 6). Our data suggest that sra overexpression postsynaptically triggers a system to compensate for increased pCaMKII levels by trafficking in more glutamate receptors to Ib boutons.
Our study also showed an increase in pCaMKII at Ib postsynaptic densities following overexpression of sra postsynaptically in the chronically challenged GluRIIASP16 null background (Figure 6). Calcineurin is known to dephosphorylate I-1 which activates PPI to in turn dephosphorylate and inactivate CaMKII (Tarasova et al., 2018). In the Drosophila NMJ, pCaMKII levels have been found to be decreased following genetic glutamate receptor impairment (Goel et al., 2017; Newman et al., 2017; Li et al., 2018). Additionally, postsynaptic overexpression of the constitutively active phosphor-mimetic form of CaMKIIT287D blocks chronic PHP expression (Li et al., 2018). Chronic overexpression of sra in the muscle decreases calcineurin function, which may be leading to an increased amount of pCaMKII, contrary to what normally occurs in response to a homeostatic challenge. Our data provides correlative evidence that pCaMKII levels signal the PHP expression state, but mechanistically, it is unclear how decreased pCaMKII contributes to PHP. It has been shown that mutations that alter CaMKII can impair the elaboration of the postsynaptic subsynaptic reticulum (SSR) and in turn result in defective PHP (Koh et al., 1999; Koles et al., 2015). It will be interesting to test how Sarah may interact with the development of the SSR and more broadly how decreases in pCaMKII contribute to PHP.
It is likely that Sarah’s impact on PHP is at least partly through its role in calcineurin regulation. Dual-tissue knockdown of the calcineurin subunit CaNB partly impairs the acute phase of PHP (Figure 12). Calcineurin and CaMKII have been shown to act as a switch-like mechanism both for controlling the direction of calcium dependent growth cone tuning and for the regulation of cofilin activity and subsequently actin cytoskeletal reorganization (Wen et al., 2004; Zhao et al., 2012). It is possible that at the Drosophila NMJ, calcineurin and CaMKII act as a switch for regulating postsynaptic signaling that is necessary for PHP maintenance.
Previous work in rat cortex suggests that RCAN1 expression is upregulated following incubation with Aβ (Lloret et al., 2011). The authors proposed that RCAN1 proteins not only inhibit calcineurin but can also shift tau to a hyperphosphorylated state via inducing expression of GSK3β. This provides evidence that RCAN1 proteins are linked to Alzheimer’s and neurodegeneration. Like this work and human studies, Aβ42-expressing Drosophila show increased Sra levels in the brain (Lee et al., 2016). Genetic overexpression of sra in combination with Aβ42 impaired locomotor activity and lifespan compared to overexpression of sra alone. However, data from Drosophila suggest that the upregulation of sra can be neuroprotective in the presence of amyloid precursor protein (APP) by facilitating the transport of synaptic proteins and mitochondria (Shaw and Chang, 2013). These conflicting results regarding the overexpression of sra demonstrate that sra is involved with both APP and Aβ42 separate from its function of regulating calcineurin. It is unclear if sra is working through calcineurin, APP, Aβ42, or other molecules to contribute to both pre- and postsynaptic homeostatic signaling processes. This line of research may also lead to examining other open questions such as how defective homeostatic synaptic processes may contribute to neurodegeneration and other neural pathologies. It is known that most genes that are necessary for PHP are linked to neural disorders and pathologies such as autism spectrum disorders, schizophrenia, Fragile X Syndrome, migraine, and epilepsy (Wondolowski and Dickman, 2013; Frank et al., 2020).
Another idea for how the expression of sra may be regulating PHP is through its effects on mitochondrial function. Both increases and decreases in sra have been shown to result in decreased ATP levels, increased ROS production, decreased mitochondrial DNA in Drosophila heads, decreased mitochondrial size, and increased mitochondrial number (Chang and Min, 2005). This is independent of its function of regulating calcineurin as decreasing calcineurin activity in sra mutants did not rescue mitochondrial enzymatic activity. Recent work also indicates that Mitochondrial Complex I (MCI) is necessary for synapse function and plasticity at the Drosophila NMJ (Mallik and Frank, 2022). Genetic knockdown or pharmacological inhibition of MCI impaired neurotransmission, reduced NMJ growth, and altered NMJ morphology.
There is strong evidence to suggest that there are shared mechanisms underlying both the acute and chronic forms of PHP signaling as both forms display robust increases in readily releasable pool (RRP) size and CaV2-mediated calcium influx that result in increases in quantal content necessary for function PHP (Müller and Davis, 2012; Davis and Müller, 2015). Recent work has found that the acute and chronic forms of PHP are functionally separable (James et al., 2019). Our data support this idea by showing minimal overlap in a specific sra impairment in a specific tissue. For example, sra knockdown postsynaptically only blocked acute PHP while leaving the chronic phase intact; conversely, sra overexpression postsynaptically did not impair acute PHP while blocking chronic PHP. These data demonstrate that sra is tightly regulated as both too much and too little sra can disrupt PHP.
Our CaNB knockdown data also support the separation of the acute and chronic phases of PHP as global knockdown led to a weak impairment in acute PHP while leaving chronic PHP intact. These data support the separation of acute and chronic PHP as sra and CaNB expression are likely regulating different processes at different timepoints that integrate to produce functional PHP.
Other work has suggested that PHP pathways converge onto the same targets (Goel et al., 2017). While it is possible that discrete signaling pathways converge, it is more likely that there is some degree of convergence while also some amount of separate signaling systems. It will be important for future studies to test how the individual influences of molecules from distinct synaptic tissues underly the different phases of PHP to promote a fully functional PHP signaling system. Collectively, our data indicate that some situations where PHP is blocked in the short run are eventually resolved over developmental time if the synapse is given sufficient time to grow and implement an array of compensatory mechanisms at its disposal (Goel et al., 2019). Conversely there could be other situations where the synapse has a capacity for a quick response but fails to respond after long periods of continuous challenge.
The field of homeostatic synaptic plasticity faces a conundrum related to how PHP is executed. Distinct acute pharmacological (PhTx) and chronic genetic manipulations (GluRIIA loss) result in similar PHP expression mechanisms (Müller et al., 2012; Goel et al., 2017; Böhme et al., 2019). Yet—depending on the type of challenge used—important differences in PHP expression have been observed. This has given rise to different models. Some groups have proposed there exist differences in how the synapse executes PHP at phasic vs. tonic motor terminals (Newman et al., 2017; Genç and Davis, 2019; Aponte-santiago and Littleton, 2020; Sauvola et al., 2021), depending upon pharamacological vs. genetic challenge. Recent characterization of motor neuron-specific tools allows refinement of analyses, at least in the context of presynaptic terminals (Aponte-santiago et al., 2020; Wang et al., 2021; Han et al., 2022). For a second model, a recent study suggests that the type of pharmacological perturbation to glutamate receptors might control the eventual response (Nair et al., 2021). A third model is that short-term and long-term events that stabilize the NMJ are governed by genetically separable signaling systems (Brusich et al., 2015; Spring et al., 2016; Yeates et al., 2017; James et al., 2019). This latter idea is analogous to “early phase” and “late phase” events that trigger and consolidate Hebbian types of plasticity like LTP (Bosch et al., 2014), and it gives rise to terms like “acute PHP” and “chronic PHP, ” similar to what we have used here.
Importantly, these models are not mutually exclusive. Our data regarding calcineurin regulation via Sarah is consistent with any of them. Intriguingly, misexpression of Sarah in the postsynaptic muscle causes an increase in the levels of pCaMKII at boutons. By contrast, the downregulation of pCaMKII has been shown by other groups to be a sentinel of successful PHP expression apposing type-Ib boutons, specifically when genetically impairing glutamate receptors (Newman et al., 2017; Li et al., 2018). Whether or not such a mechanistic difference is dictated strictly spatially or also temporally is yet to be fully tested.
The original contributions presented in the study are included in the article/Supplementary material. Further inquiries can be directed to the corresponding author.
NSA and CAF designed the research, analyzed the data, and wrote the manuscript. NSA performed the research. Both authors contributed to the article and approved the submitted version.
This work was supported by grants from the National Institutes of Health/National Institute of Neurological Disorders and Stroke (NINDS) (NS085164 and NS130108) to CAF. This work was also supported by NIH/NINDS grant supplement to NSA with grant NS085164 (PI—CAF). Additional funds supporting parts of this work have been provided by the University of Iowa Carver College of Medicine (CCOM) and the PI’s home department within CCOM, Anatomy and Cell Biology.
We thank members of the Frank lab for helpful comments throughout this study. We thank the laboratories of Tina Tootle, Toshihiro Kitamoto, Pamela Geyer, and Lori Wallrath for helpful discussions. Additionally, we thank Drs. Samuel Young, Joshua Weiner, and Catherine Marcinkiewcz for helpful suggestions. We also thank the Bloomington Drosophila Resource Stock Center for several fly stocks detailed in the “Methods” section.
The authors declare that the research was conducted in the absence of any commercial or financial relationships that could be construed as a potential conflict of interest.
All claims expressed in this article are solely those of the authors and do not necessarily represent those of their affiliated organizations, or those of the publisher, the editors and the reviewers. Any product that may be evaluated in this article, or claim that may be made by its manufacturer, is not guaranteed or endorsed by the publisher.
The Supplementary Material for this article can be found online at: https://www.frontiersin.org/articles/10.3389/fnsyn.2022.1033743/full#supplementary-material.
SUPPLEMENTARY TABLES S1–S12 | Supplementary Tables S1–S4 and S7–S12 show raw electrophysiology data. Table S5 shows raw bouton counts and measurements for NMJ growth assessment. Table S6 shows raw pCaMKII and GluRIII intensity measurements.
Ahern, G. P., Junankar, P. R., and Dulhunty, A. F. (1994). Single channel activity of the ryanodine receptor calcium release channel is modulated by FK-506. FEBS Lett. 352, 369–374. doi: 10.1016/0014-5793(94)01001-3
André, E. A., Forcelli, P. A., and Pak, D. T. (2018). What goes up must come down: homeostatic synaptic plasticity strategies in neurological disease. Future Neurol. 13, 13–21. doi: 10.2217/fnl-2017-0028
Aponte-santiago, N. A., and Littleton, J. T. (2020). Synaptic properties and plasticity mechanisms of invertebrate tonic and phasic neurons. Front. Physiol. 11:611982. doi: 10.3389/fphys.2020.611982
Aponte-santiago, N. A., Ormerod, K. G., Akbergenova, Y., and Littleton, J. T. (2020). Synaptic plasticity induced by differential manipulation of tonic and phasic motoneurons in Drosophila. J. Neurosci. 40, 6270–6288. doi: 10.1523/JNEUROSCI.0925-20.2020
Baumgärtel, K., and Mansuy, I. M. (2012). Neural functions of calcineurin in synaptic plasticity and memory. Learn. Mem. 19, 375–384. doi: 10.1101/lm.027201.112
Betz, H., Schuster, C., Ultsch, A., and Schmitt, B. (1993). Molecular biology of ionotropic glutamate receptors in Drosophila melanogaster. Trends Pharmacol. Sci. 14, 428–431. doi: 10.1016/0165-6147(93)90177-l
Bienenstock, E. L., Cooper, L. N., and Munro, P. W. (1982). Theory for the development of neuron selectivity: orientation specificity and binocular interaction in visual cortex. J. Neurosci. 2, 32–48. doi: 10.1523/JNEUROSCI.02-01-00032.1982
Bliss, T. V. P., Collingridge, G. L., and Morris, R. G. M. (2013). Synaptic plasticity in health and disease: introduction and overview. Philos. Trans. R. Soc. Lond B Biol. Sci. 369:20130129. doi: 10.1098/rstb.2013.0129
Böhme, M. A., Mccarthy, A. W., Grasskamp, A. T., Beuschel, C. B., Goel, P., Jusyte, M., et al. (2019). Rapid active zone remodeling consolidates presynaptic potentiation. Nat. Commun. 10:1085. doi: 10.1038/s41467-019-08977-6
Bosch, M., Castro, J., Saneyoshi, T., Matsuno, H., Sur, M., and Hayashi, Y. (2014). Structural and molecular remodeling of dendritic spine substructures during long-term potentiation. Neuron 82, 444–459. doi: 10.1016/j.neuron.2014.03.021
Brusich, D. J., Spring, A. M., and Frank, C. A. (2015). A single-cross, RNA interference-based genetic tool for examining the long-term maintenance of homeostatic plasticity. Front. Cell. Neurosci. 9:107. doi: 10.3389/fncel.2015.00107
Budnik, V., Koh, Y. H., Guan, B., Hartmann, B., Hough, C., Woods, D., et al. (1996). Regulation of synapse structure and function by the Drosophila tumor suppressor gene dlg. Neuron 17, 627–640. doi: 10.1016/s0896-6273(00)80196-8
Bultynck, G., De Smet, P., Weidema, A. F., Ver Heyen, M., Maes, K., Callewaert, G., et al. (2000). Effects of the immunosuppressant FK506 on intracellular Ca2+ release and Ca2+ accumulation mechanisms. J. Physiol. 525, 681–693. doi: 10.1111/j.1469-7793.2000.t01-1-00681.x
Cesca, F., Baldelli, P., Valtorta, F., and Benfenati, F. (2010). The synapsins: key actors of synapse function and plasticity. Prog. Neurobiol. 91, 313–348. doi: 10.1016/j.pneurobio.2010.04.006
Chang, K. T., and Min, K. T. (2005). Drosophila melanogaster homolog of Down syndrome critical region 1 is critical for mitochondrial function. Nat. Neurosci. 8, 1577–1585. doi: 10.1038/nn1564
Chang, K. T., Shi, Y.-J., and Min, K.-T. (2003). The Drosophila homolog of Down’s syndrome critical region 1 gene regulates learning: implications for mental retardation. Proc. Natl. Acad. Sci. U S A 100, 15794–15799. doi: 10.1073/pnas.2536696100
Chi, P., Greengard, P., and Ryan, T. A. (2003). Synaptic vesicle mobilization is regulated by distinct synapsin I phosphorylation pathways at different frequencies. Neuron 38, 69–78. doi: 10.1016/s0896-6273(03)00151-x
Clardy, J. (1995). The chemistry of signal transduction. Proc. Natl. Acad. Sci. U S A 92, 56–61. doi: 10.1073/pnas.92.1.56
D’Amelio, M., Cavallucci, V., Middei, S., Marchetti, C., Pacioni, S., Ferri, A., et al. (2011). Caspase-3 triggers early synaptic dysfunction in a mouse model of Alzheimer’s disease. Nat. Neurosci. 14, 69–76. doi: 10.1038/nn.2709
Davis, G. W. (2006). Homeostatic control of neural activity: from phenomenology to molecular design. Annu. Rev. Neurosci. 29, 307–323. doi: 10.1146/annurev.neuro.28.061604.135751
Davis, G. W., Diantonio, A., Petersen, S. A., and Goodman, C. S. (1998). Postsynaptic PKA controls quantal size and reveals a retrograde signal that regulates presynaptic transmitter release in Drosophila. Neuron 20, 305–315. doi: 10.1016/s0896-6273(00)80458-4
Davis, G. W., and Müller, M. (2015). Homeostatic control of presynaptic neurotransmitter release. Annu. Rev. Physiol. 77, 251–270. doi: 10.1146/annurev-physiol-021014-071740
Del Castillo, J., and Katz, B. (1954). Quantal components of the end-plate potential. J. Physiol. 124, 560–573. doi: 10.1113/jphysiol.1954.sp005129
Diantonio, A., Petersen, S. A., Heckmann, M., and Goodman, C. S. (1999). Glutamate receptor expression regulates quantal size and quantal content at the Drosophila neuromuscular junction. J. Neurosci. 19, 3023–3032. doi: 10.1523/JNEUROSCI.19-08-03023.1999
Frank, C. A. (2014a). Homeostatic plasticity at the Drosophila neuromuscular junction. Neuropharmacology 78, 63–74. doi: 10.1016/j.neuropharm.2013.06.015
Frank, C. A. (2014b). How voltage-gated calcium channels gate forms of homeostatic synaptic plasticity. Front. Cell. Neurosci. 8:40. doi: 10.3389/fncel.2014.00040
Frank, C. A., James, T. D., and Müller, M. (2020). Homeostatic control of Drosophila neuromuscular junction function. Synapse 74:e22133. doi: 10.1002/syn.22133
Frank, C. A., Kennedy, M. J., Goold, C. P., Marek, K. W., and Davis, G. W. (2006). Mechanisms underlying the rapid induction and sustained expression of synaptic homeostasis. Neuron 52, 663–677. doi: 10.1016/j.neuron.2006.09.029
Frank, C. A., Pielage, J., and Davis, G. W. (2009). A presynaptic homeostatic signaling system composed of the Eph receptor, ephexin, Cdc42 and CaV2.1 calcium channels. Neuron 61, 556–569. doi: 10.1016/j.neuron.2008.12.028
Furman, J. L., and Norris, C. M. (2014). Calcineurin and glial signaling: neuroinflammation and beyond. J. Neuroinflammation 11:158. doi: 10.1186/s12974-014-0158-7
Gaydukov, A., Tarasova, E., and Balezina, O. (2013). Calcium-dependent phosphatase calcineurin downregulates evoked neurotransmitter release in neuromuscular junctions of mice. Neurochem. J. 7, 29–33. doi: 10.1134/S1819712413010030
Genç, Ö., and Davis, G. W. (2019). Target-wide induction and synapse type-specific robustness of presynaptic homeostasis. Curr. Biol. 29, 3863–3873.e2. doi: 10.1007/s11606-022-07891-w
Goel, P., and Dickman, D. (2018). Distinct homeostatic modulations stabilize reduced postsynaptic receptivity in response to presynaptic DLK signaling. Nat. Commun. 9:1856. doi: 10.1038/s41467-018-04270-0
Goel, P., Khan, M., Howard, S., Kim, G., Kiragasi, B., Kikuma, K., et al. (2019). A screen for synaptic growth mutants reveals mechanisms that stabilize synaptic strength. J. Neurosci. 39, 4051–4065. doi: 10.1523/JNEUROSCI.2601-18.2019
Goel, P., Li, X., and Dickman, D. (2017). Disparate postsynaptic induction mechanisms ultimately converge to drive the retrograde enhancement of presynaptic efficacy. Cell Rep. 21, 2339–2347. doi: 10.1016/j.celrep.2017.10.116
Greenspan, R. J. (2004). Fly Pushing: The Theory and Practice of Drosophila Genetics. Cold Spring Harbor, NY: Cold Spring Harbor Laboratory Press.
Han, Y., Chien, C., Goel, P., He, K., Pinales, C., Buser, C., et al. (2022). Botulinum neurotoxin accurately separates tonic vs. phasic transmission and reveals heterosynaptic plasticity rules in Drosophila. eLife 11:e77924. doi: 10.7554/eLife.77924
Harris, N., Braiser, D. J., Dickman, D. K., Fetter, R. D., Tong, A., and Davis, G. W. (2015). The innate immune receptor PGRP-LC controls presynaptic homeostatic plasticity. Neuron 88, 1157–1164. doi: 10.1016/j.neuron.2015.10.049
Harris, C. D., Ermak, G., and Davies, K. J. (2005). Multiple roles of the DSCR1 (Adapt78 or RCAN1) gene and its protein product calcipressin 1 (or RCAN1) in disease. Cell. Mol. Life Sci. 62, 2477–2486. doi: 10.1007/s00018-005-5085-4
Hazelrigg, T., Levis, R., and Rubin, G. M. (1984). Transformation of white locus DNA in Drosophila: dosage compensation, zeste interaction and position effects. Cell 36, 469–481. doi: 10.1016/0092-8674(84)90240-x
He, K., Song, L., Cummings, L. W., Goldman, J., Huganir, R. L., and Lee, H.-K. (2009). Stabilization of Ca2+-permeable AMPA receptors at perisynaptic sites by GluR1–S845 phosphorylation. Proc. Natl. Acad. Sci. U S A 106, 20033–20038. doi: 10.1073/pnas.0910338106
Horner, V. L., Czank, A., Jang, J. K., Singh, N., Williams, B. C., Puro, J., et al. (2006). The Drosophila calcipressin sarah is required for several aspects of egg activation. Curr. Biol. 16, 1441–1446. doi: 10.1016/j.cub.2006.06.024
James, T. D., Zwiefelhofer, D. J., and Frank, C. A. (2019). Maintenance of homeostatic plasticity at the Drosophila neuromuscular synapse requires continuous IP3-directed signaling. eLife 8:e39643. doi: 10.7554/eLife.39643
Jan, L. Y., and Jan, Y. N. (1976). Properties of the larval neuromuscular junction in Drosophila melanogaster. J. Physiol. 262, 189–214. doi: 10.1113/jphysiol.1976.sp011592
Jovanovic, J. N., Sihra, T. S., Nairn, A. C., Hemmings, H. C., Jr., Greengard, P., CzerniK, A. J., et al. (2001). Opposing changes in phosphorylation of specific sites in synapsin I during Ca2+-dependent glutamate release in isolated nerve terminals. J. Neurosci. 21, 7944–7953. doi: 10.1523/JNEUROSCI.21-20-07944.2001
Kadamur, G., and Ross, E. M. (2013). Mammalian phospholipase C. Annu. Rev. Physiol. 75, 127–154. doi: 10.1146/annurev-physiol-030212-183750
Kikuma, K., Li, X., Perry, S., Li, Q., Goel, P., Chen, C., et al. (2019). Cul3 and insomniac are required for rapid ubiquitination of postsynaptic targets and retrograde homeostatic signaling. Nat. Commun. 10:2998. doi: 10.1038/s41467-019-10992-6
Kingsbury, T. J., and Cunningham, K. W. (2000). A conserved family of calcineurin regulators. Genes Dev. 14, 1595–1604. doi: 10.1101/gad.14.13.1595
Koh, Y. H., Popova, E., Thomas, U., Griffith, L. C., and Budnik, V. (1999). Regulation of DLG localization at synapses by CaMKII-dependent phosphorylation. Cell 98, 353–363. doi: 10.1016/s0092-8674(00)81964-9
Koles, K., Messelaar, E. M., Feiger, Z., Yu, C. J., Frank, C. A., and Rodal, A. A. (2015). The EHD protein Past1 controls postsynaptic membrane elaboration and synaptic function. Mol. Biol. Cell 26, 3275–3288. doi: 10.1091/mbc.E15-02-0093
Krick, N., Ryglewski, S., Pichler, A., Bikbaev, A., Götz, T., Kobler, O., et al. (2021). Separation of presynaptic Cav2 and Cav1 channel function in synaptic vesicle exo- and endocytosis by the membrane anchored Ca2+ pump PMCA. Proc. Natl. Acad. Sci. U S A 118:e2106621118. doi: 10.1073/pnas.2106621118
Kumashiro, S., Lu, Y.-F., Tomizawa, K., Matsushita, M., Wei, F.-Y., and Matsui, H. (2005). Regulation of synaptic vesicle recycling by calcineurin in different vesicle pools. Neurosci. Res. 51, 435–443. doi: 10.1016/j.neures.2004.12.018
Kuromi, H., Yoshihara, M., and Kidokoro, Y. (1997). An inhibitory role of calcineurin in endocytosis of synaptic vesicles at nerve terminals of Drosophila larvae. Neurosci. Res. 27, 101–113. doi: 10.1016/s0168-0102(96)01132-7
Lee, S., Bang, S. M., Hong, Y. K., Lee, J. H., Jeong, H., Park, S. H., et al. (2016). The calcineurin inhibitor Sarah (Nebula) exacerbates Abeta42 phenotypes in a Drosophila model of Alzheimer’s disease. Dis. Model. Mech. 9, 295–306. doi: 10.1242/dmm.018069
Lee, J. I., Dhakal, B. K., Lee, J., Bandyopadhyay, J., Jeong, S. Y., Eom, S. H., et al. (2003). The caenorhabditis elegans homologue of Down Syndrome critical region 1, RCN-1, inhibits multiple functions of the phosphatase calcineurin. J. Mol. Biol. 328, 147–156. doi: 10.1016/s0022-2836(03)00237-7
Li, X., Goel, P., Chen, C., Angajala, V., Chen, X., and Dickman, D. K. (2018). Synapse-specific and compartmentalized expression of presynaptic homeostatic potentiation. eLife 7:e34338. doi: 10.7554/eLife.34338
Lin, D. M., and Goodman, C. S. (1994). Ectopic and increased expression of Fasciclin II alters motoneuron growth cone guidance. Neuron 13, 507–523. doi: 10.1016/0896-6273(94)90022-1
Lin, J. W., Ju, W., Foster, K., Lee, S. H., Ahmadian, G., Wyszynski, M., et al. (2000). Distinct molecular mechanisms and divergent endocytotic pathways of AMPA receptor internalization. Nat. Neurosci. 3, 1282–1290. doi: 10.1038/81814
Liu, J., Farmer, J. D., Jr., Lane, W. S., Friedman, J., Weissman, I., Schreiber, S. L., et al. (1991). Calcineurin is a common target of cyclophilin-cyclosporin A and FKBP-FK506 complexes. Cell 66, 807–815. doi: 10.1016/0092-8674(91)90124-h
Lloret, A., Badia, M. C., Giraldo, E., Ermak, G., Alonso, M. D., Pallardö, F. V., et al. (2011). Amyloid-β toxicity and tau hyperphosphorylation are linked via RCAN1 in Alzheimer’s disease. J. Alzheimers Dis. 27, 701–709. doi: 10.3233/JAD-2011-110890
Mallik, B., and Frank, C. A. (2022). Roles for mitochondrial complex I subunits in regulating synaptic transmission and growth. Front. Neurosci. 16:846425. doi: 10.3389/fnins.2022.846425
Marder, E. (2012). Neuromodulation of neuronal circuits: back to the future. Neuron 76, 1–11. doi: 10.1016/j.neuron.2012.09.010
Marrus, S. B., Portman, S. L., Allen, M. J., Moffat, K. G., and Diantonio, A. (2004). Differential localization of glutamate receptor subunits at the Drosophila neuromuscular junction. J. Neurosci. 24, 1406–1415. doi: 10.1523/JNEUROSCI.1527-05.2005
Mclachlan, E. M., and Martin, A. R. (1981). Non-linear summation of end-plate potentials in the frog and mouse. J. Physiol. 311, 307–324. doi: 10.1113/jphysiol.1981.sp013586
Menon, K. P., Carrillo, R. A., and Zinn, K. (2013). Development and plasticity of the Drosophila larval neuromuscular junction. Wiley Interdiscip. Rev. Dev. Biol. 2, 647–670. doi: 10.1002/wdev.108
Müller, M., and Davis, G. W. (2012). Transsynaptic control of presynaptic Ca2+ influx achieves homeostatic potentiation of neurotransmitter release. Curr. Biol. 22, 1102–1108. doi: 10.1016/j.cub.2012.04.018
Müller, M., Genç, Ö., and Davis, G. W. (2015). RIM-binding protein links synaptic homeostasis to the stabilization and replenishment of high release probability vesicles. Neuron 85, 1056–1069. doi: 10.1016/j.neuron.2015.01.024
Müller, M., Liu, K. S., Sigrist, S. J., and Davis, G. W. (2012). RIM controls homeostatic plasticity through modulation of the readily-releasable vesicle pool. J. Neurosci. 32, 16574–16585. doi: 10.1523/JNEUROSCI.0981-12.2012
Musson, R. E., and Smit, N. P. (2011). Regulatory mechanisms of calcineurin phosphatase activity. Curr. Med. Chem. 18, 301–315. doi: 10.2174/092986711794088407
Nair, A. G., Muttathukunnel, P., and Müller, M. (2021). Distinct molecular pathways govern presynaptic homeostatic plasticity. Cell Rep. 37:110105. doi: 10.1016/j.celrep.2021.110105
Nakai, Y., Horiuchi, J., Tsuda, M., Takeo, S., Akahori, S., Matsuo, T., et al. (2011). Calcineurin and its regulator sra/DSCR1 are essential for sleep in Drosophila. J. Neurosci. 31, 12759–12766. doi: 10.1523/JNEUROSCI.1337-11.2011
Newman, Z. L., Hoagland, A., Aghi, K., Worden, K., Levy, S. L., Son, J. H., et al. (2017). Input-specific plasticity and homeostasis at the Drosophila larval neuromuscular junction. Neuron 93, 1388–1404.e10. doi: 10.1016/j.neuron.2017.02.028
Park, J., Oh, Y., and Chung, K. C. (2009). Two key genes closely implicated with the neuropathological characteristics in Down syndrome: DYRK1A and RCAN1. BMB Rep. 42, 6–15. doi: 10.5483/bmbrep.2009.42.1.006
Perry, S., Han, Y., Qiu, C., Chien, C., Goel, P., Nishimura, S., et al. (2022). A glutamate receptor C-tail recruits CaMKII to suppress retrograde homeostatic signaling. Nat. Commun. 13:7656. doi: 10.1038/s41467-022-35417-9
Petersen, S. A., Fetter, R. D., Noordermeer, J. N., Goodman, C. S., and Diantonio, A. (1997). Genetic analysis of glutamate receptors in Drosophila reveals a retrograde signal regulating presynaptic transmitter release. Neuron 19, 1237–1248. doi: 10.1016/s0896-6273(00)80415-8
Philip, F., Kadamur, G., Silos, R. G., Woodson, J., and Ross, E. M. (2010). Synergistic activation of phospholipase C-beta3 by Galpha(q) and Gbetagamma describes a simple two-state coincidence detector. Curr. Biol. 20, 1327–1335. doi: 10.1016/j.cub.2010.06.013
Pozo, K., and Goda, Y. (2010). Unraveling mechanisms of homeostatic synaptic plasticity. Neuron 66, 337–351. doi: 10.1016/j.neuron.2010.04.028
Rusnak, F., and Mertz, P. (2000). Calcineurin: form and function. Physiol. Rev. 80, 1483–1521. doi: 10.1152/physrev.2000.80.4.1483
Sauvola, C. W., Akbergenova, Y., Cunningham, K. L., Aponte-Santiago, N. A., and Littleton, J. T. (2021). The decoy SNARE Tomosyn sets tonic versus phasic release properties and is required for homeostatic synaptic plasticity. eLife 10:e72841. doi: 10.7554/eLife.72841
Shaw, J. L., and Chang, K. T. (2013). Nebula/DSCR1 upregulation delays neurodegeneration and protects against app-induced axonal transport defects by restoring calcineurin and GSK-3β signaling. PLoS Genet. 9:e1003792. doi: 10.1371/journal.pgen.1003792
Sigal, N. H., and Dumont, F. J. (1992). Cyclosporin A, FK-506 and rapamycin: pharmacologic probes of lymphocyte signal transduction. Annu. Rev. Immunol. 10, 519–560. doi: 10.1146/annurev.iy.10.040192.002511
Sim, A. T., Baldwin, M. L., Rostas, J. A., Holst, J., and Ludowyke, R. I. (2003). The role of serine/threonine protein phosphatases in exocytosis. Biochem. J. 373, 641–659. doi: 10.1042/BJ20030484
Spring, A. M., Brusich, D. J., and Frank, C. A. (2016). C-terminal Src kinase gates homeostatic synaptic plasticity and regulates fasciclin II expression at the Drosophila neuromuscular junction. PLoS Genet. 12:e1005886. doi: 10.1371/journal.pgen.1005886
Stewart, B., Atwood, H., Renger, J., Wang, J., and Wu, C. (1994). Improved stability of Drosophila larval neuromuscular preparations in haemolymph-like physiological solutions. J. Comp. Physiol. A 175, 179–191. doi: 10.1007/BF00215114
Swanson, S. K., Born, T., Zydowsky, L. D., Cho, H., Chang, H. Y., Walsh, C. T., et al. (1992). Cyclosporin-mediated inhibition of bovine calcineurin by cyclophilins A and B. Proc. Natl. Acad. Sci. U S A, 89, 3741–3745. doi: 10.1073/pnas.89.9.3741
Takeo, S., Swanson, S. K., Nandanan, K., Nakai, Y., Aigaki, T., Washburn, M. P., et al. (2012). Shaggy/glycogen synthase kinase 3β and phosphorylation of Sarah/regulator of calcineurin are essential for completion of Drosophila female meiosis. Proc. Natl. Acad. Sci. U S A 109, 6382–6389. doi: 10.1073/pnas.1120367109
Takeo, S., Tsuda, M., Akahori, S., Matsuo, T., and Aigaki, T. (2006). The calcineurin regulator sra plays an essential role in female meiosis in Drosophila. Curr. Biol. 16, 1435–1440. doi: 10.1016/j.cub.2006.05.058
Tarasova, E. O., Gaydukov, A. E., and Balezina, O. P. (2018). Calcineurin and its role in synaptic transmission. Biochemistry (Mosc) 83, 674–689. doi: 10.1134/S0006297918060056
Tomita, J., Mitsuyoshi, M., Ueno, T., Aso, Y., Tanimoto, H., Nakai, Y., et al. (2011). Pan-Neuronal knockdown of calcineurin reduces sleep in the fruit fly, Drosophila melanogaster. J. Neurosci. 31, 13137–13146. doi: 10.1523/JNEUROSCI.5860-10.2011
Tononi, G., and Cirelli, C. (2006). Sleep function and synaptic homeostasis. Sleep Med. Rev. 10, 49–62. doi: 10.1016/j.smrv.2005.05.002
Victor, R. G., Thomas, G. D., Marban, E., and O’Rourke, B. (1995). Presynaptic modulation of cortical synaptic activity by calcineurin. Proc. Natl. Acad. Sci. U S A 92, 6269–6273. doi: 10.1073/pnas.92.14.6269
Vonhoff, F., and Keshishian, H. (2017). In vivo calcium signaling during synaptic refinement at the Drosophila neuromuscular junction. J. Neurosci. 37, 5511–5526. doi: 10.1523/JNEUROSCI.2922-16.2017
Wang, T., Hauswirth, A. G., Tong, A., Dickman, D. K., and Davis, G. W. (2014). Endostatin is a trans-synaptic signal for homeostatic synaptic plasticity. Neuron 83, 616–629. doi: 10.1016/j.neuron.2014.07.003
Wang, Y., Lobb-Rabe, M., Ashley, J., Anand, V., and Carrillo, R. A. (2021). Structural and functional synaptic plasticity induced by convergent synapse loss in the Drosophila neuromuscular circuit. J. Neurosci. 41, 1401–1417. doi: 10.1523/JNEUROSCI.1492-20.2020
Wen, Z., Guirland, C., Ming, G. L., and Zheng, J. Q. (2004). A CaMKII/calcineurin switch controls the direction of Ca2+-dependent growth cone guidance. Neuron 43, 835–846. doi: 10.1016/j.neuron.2004.08.037
Weyhersmüller, A., Hallermann, S., Wagner, N., and Eilers, J. (2011). Rapid active zone remodeling during synaptic plasticity. J. Neurosci. 31, 6041–6052. doi: 10.1523/JNEUROSCI.6698-10.2011
Wondolowski, J., and Dickman, D. (2013). Emerging links between homeostatic synaptic plasticity and neurological disease. Front. Cell. Neurosci. 7:223. doi: 10.3389/fncel.2013.00223
Wu, X.-S., Zhang, Z., Zhao, W.-D., Wang, D., Luo, F., and Wu, L.-G. (2014). Calcineurin is universally involved in vesicle endocytosis at neuronal and nonneuronal secretory cells. Cell Rep. 7, 982–988. doi: 10.1016/j.celrep.2014.04.020
Yakel, J. L. (1997). Calcineurin regulation of synaptic function: from ion channels to transmitter release and gene transcription. Trends Pharmacol. Sci. 18, 124–134. doi: 10.1016/s0165-6147(97)01046-8
Yeates, C. J., and Frank, C. A. (2021). Homeostatic depression shows heightened sensitivity to synaptic calcium. Front. Cell. Neurosci. 15:618393. doi: 10.3389/fncel.2021.618393
Yeates, C. J., Zwiefelhofer, D. J., and Frank, C. A. (2017). The maintenance of synaptic homeostasis at the Drosophila neuromuscular junction is reversible and sensitive to high temperature. eNeuro 4:ENEURO.0220-17.2017. doi: 10.1523/ENEURO.0220-17.2017
Younger, M. A., Müller, M., Tong, A., Pym, E. C., and Davis, G. W. (2013). A presynaptic ENaC channel drives homeostatic plasticity. Neuron 79, 1183–1196. doi: 10.1016/j.neuron.2013.06.048
Keywords: Drosophila melanogaster, NMJ–neuromuscular junction, neurotransmission, plasticity, synaptic homeostasis, synaptic dysfunction, calcineurin (CaN), Sarah
Citation: Armstrong NS and Frank CA (2023) The calcineurin regulator Sarah enables distinct forms of homeostatic plasticity at the Drosophila neuromuscular junction. Front. Synaptic Neurosci. 14:1033743. doi: 10.3389/fnsyn.2022.1033743
Received: 31 August 2022; Accepted: 05 December 2022;
Published: 04 January 2023.
Edited by:
David J. Adams, University of Wollongong, AustraliaReviewed by:
S. Sean Millard, The University of Queensland, AustraliaCopyright © 2023 Armstrong and Frank. This is an open-access article distributed under the terms of the Creative Commons Attribution License (CC BY). The use, distribution or reproduction in other forums is permitted, provided the original author(s) and the copyright owner(s) are credited and that the original publication in this journal is cited, in accordance with accepted academic practice. No use, distribution or reproduction is permitted which does not comply with these terms.
*Correspondence: C. Andrew Frank, YW5keS1mcmFua0B1aW93YS5lZHU=
Disclaimer: All claims expressed in this article are solely those of the authors and do not necessarily represent those of their affiliated organizations, or those of the publisher, the editors and the reviewers. Any product that may be evaluated in this article or claim that may be made by its manufacturer is not guaranteed or endorsed by the publisher.
Research integrity at Frontiers
Learn more about the work of our research integrity team to safeguard the quality of each article we publish.