- MCD Biology, University of Colorado Boulder, Boulder, CO, United States
Synaptic vesicle release is regulated by upwards of 30 proteins at the fusion complex alone, but disruptions in any one of these components can have devastating consequences for neuronal communication. Aberrant molecular responses to calcium signaling at the pre-synaptic terminal dramatically affect vesicle trafficking, docking, fusion, and release. At the organismal level, this is reflected in disorders such as epilepsy, depression, and neurodegeneration. Among the myriad pre-synaptic proteins, perhaps the most functionally mysterious is synaptophysin (SYP). On its own, this vesicular transmembrane protein has been proposed to function as a calcium sensor, a cholesterol-binding protein, and to form ion channels across the phospholipid bilayer. The downstream effects of these functions are largely unknown. The physiological relevance of SYP is readily apparent in its interaction with synaptobrevin (VAMP2), an integral element of the neuronal SNARE complex. SNAREs, soluble NSF attachment protein receptors, comprise a family of proteins essential for vesicle fusion. The complex formed by SYP and VAMP2 is thought to be involved in both trafficking to the pre-synaptic membrane as well as regulation of SNARE complex formation. Recent structural observations specifically implicate the SYP/VAMP2 complex in anchoring the SNARE assembly at the pre-synaptic membrane prior to vesicle fusion. Thus, the SYP/VAMP2 complex appears vital to the form and function of neuronal exocytotic machinery.
Introduction
Communication between neurons is a fundamental process of the nervous system. Regulated neurotransmitter release contributes to everything from memory consolidation to mood regulation (Jurado et al., 2013; Kandel et al., 2014; Metzger et al., 2017). Aberrant synaptic release is associated with numerous neurological disorders, and the molecular mechanisms underlying this process are elaborate (Roselli and Caroni, 2015; Körber and Kuner, 2016; Ramos-Miguel et al., 2018). The general role of vesicle and target SNAREs, v-SNAREs and t-SNAREs respectively, in vesicle fusion at the pre-synaptic membrane has been widely studied, but some of the individual components of this pathway are more nebulous (Karmakar et al., 2019). Specifically, SYP, while prolific at most pre-synaptic terminals, has no well-defined role within the synaptic architecture (Marqueze-Pouey et al., 1991). Putative functions of the vesicle membrane protein SYP include vesicular ion channel activity, vesicle endocytosis, synaptobrevin trafficking during SNARE assembly, and the kiss-and-run archetype of dense-core vesicle fusion (Gincel and Shoshan-Barmatz, 2002; Kwon and Chapman, 2011; Harper et al., 2017; Chang et al., 2021). Probing these functions has proved difficult, however, due to the compensatory nature of various physin family proteins (Janz et al., 1999). SYP’s interaction with VAMP2 is of particular interest; the two proteins form a complex thought to contribute to the characteristic speed and reactivity of synaptic vesicle release (Adams et al., 2015). Recent advances have further illuminated the individual and cooperative roles of SYP and VAMP2 in synaptic vesicle regulation. Recent advances have further illuminated the individual and cooperative roles of SYP and VAMP2 in synaptic vesicle regulation as well as the interaction network between other key players in vesicle fusion (Figure 1, Table 1; Szklarczyk et al., 2019).
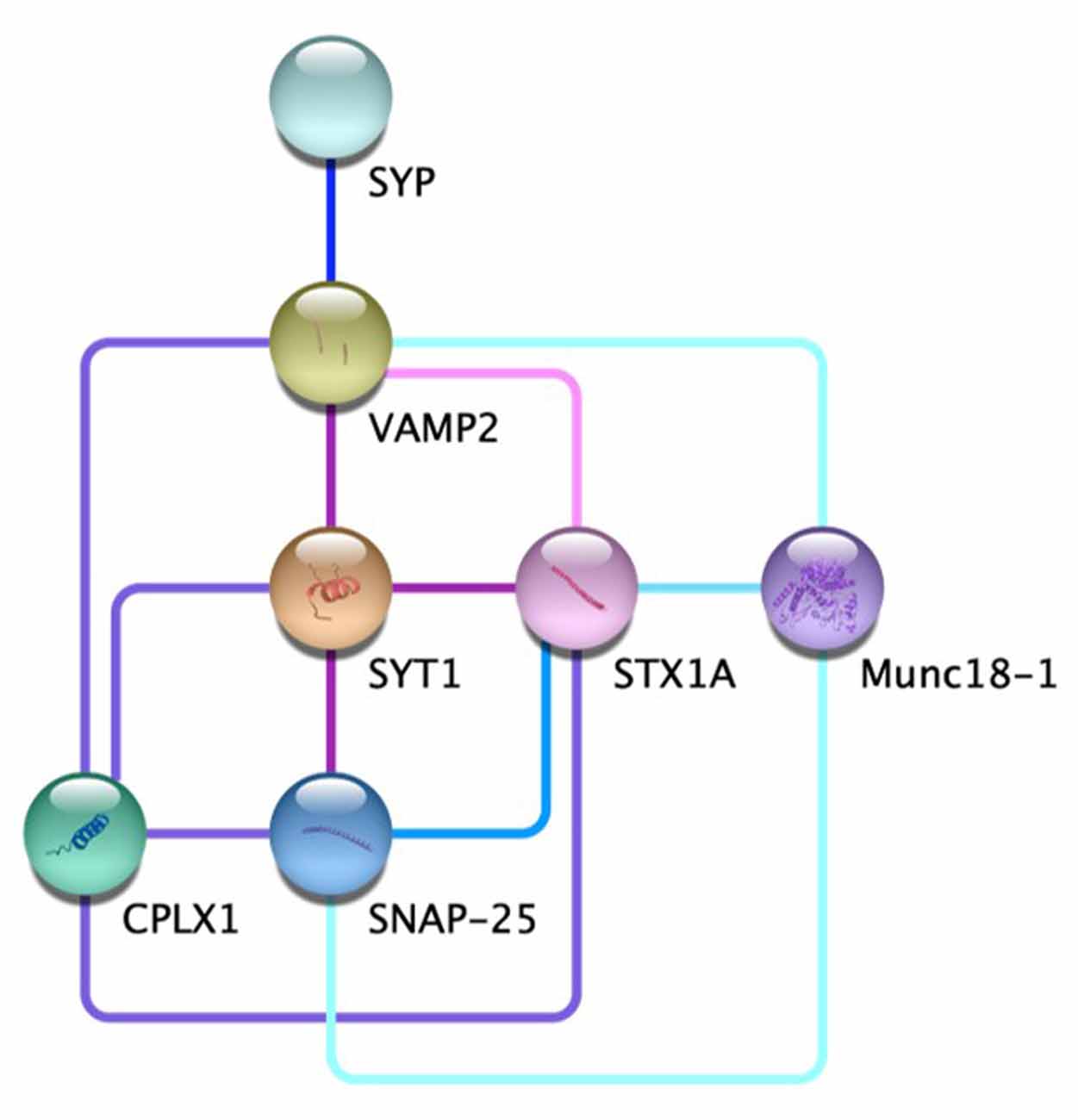
Figure 1. The protein-protein interaction network at the chemical synapse. Direct evidence for the edges displayed for VAMP2-STX1A (Far et al., 1995; Hazzard et al., 1999); VAMP2-SYT1-SNAP25
(Söllner et al., 1993a,b; Chapman et al., 1995; Zhang et al., 2002); VAMP2-SYT1- SYTX1A-CPLX1
(Pabst et al., 2000; Bracher et al., 2002; Chen et al., 2002; Zhou et al., 2017); SYP-VAMP2
(Edelmann et al., 1995; Felkl and Leube, 2008; Gordon et al., 2011; Adams et al., 2015); STX1A-SNAP25
(McMahon and Südhof, 1995; Fasshauer et al., 1997); STX1A-Munc18-1
(Hata et al., 1993; Misura et al., 2000; Zilly et al., 2006; Rickman et al., 2007); VAMP2-SNAP25-Munc18-1
(Carr et al., 1999; Dulubova et al., 2007).
Synaptophysin
Despite its prevalence at the pre-synaptic terminal, synaptophysin’s role in vesicular neurotransmission is highly speculative. Synaptophysin (SYP) forms a transmembrane structure on synaptic vesicles similar to canonical gap junctions and mechanosensitive ion channels (Arthur and Stowell, 2007). This structure is concordant with the idea that SYP forms functional ion channels within membranes (Yin et al., 2002). Indeed, SYP multimers reconstituted in phospholipid bilayers are selective for cations and show a preference for potassium (Gincel and Shoshan-Barmatz, 2002). Though past studies saw no change in ion channel activity in response to fluctuation in Ca2+ concentration, SYP is known to bind cytoplasmic calcium (Rehm et al., 1986). The importance of calcium-dependent exocytosis in neurotransmitter release is widely accepted, but the specific function of SYP-Ca2+ binding is unclear (Karmakar et al., 2019).
In addition to its association with Ca2+, SYP readily binds cholesterol in the plasma membrane. This binding is necessary for the initial formation of synaptic vesicles (Thiele et al., 2000). Further roles for cholesterol in the function of SYP, including regulation of synaptic plasticity and the interaction of synaptophysin with synaptobrevin, have been suggested, but the precise physiology underlying this relationship remains elusive (Mitter et al., 2003; Ya et al., 2013).
Synaptobrevin
VAMP2, syntaxin, and SNAP-25 form the core assembly of SNARE proteins (Brunger, 2005). The collaborative functions of these and other SNARE-associated proteins are necessary for Ca2+-dependent neurotransmitter release at presynaptic terminals (Weber et al., 1998). VAMP2 knockout mice exhibit profoundly reduced rates of vesicle fusion, though fusion is not abrogated entirely (Schoch et al., 2001). While VAMP2 alone may not be necessary for fusion overall, its absence profoundly affects the rate of neurotransmission. Additionally, VAMP2/VAMP3 double knockout results in complete termination of presynaptic vesicle fusion, reinforcing the significance of synaptobrevin and its structural homologs (Borisovska et al., 2005).
VAMP2 may also be required for the maintenance of the readily releasable pool (RRP). Specifically, VAMP2 appears to be associated with the “fast endocytosis” necessary for quick Ca2+ signaling. At terminals with depleted RRPs, the rate of vesicle recycling is significantly impacted by the absence of VAMP2 (Deák et al., 2004). “Slow endocytosis” is also VAMP2 dependent, but appears to rely on the activity of additional SNAREs syntaxin and SNAP-25 (Xu et al., 2013a). This dual role of VAMP2 heavily implicates it in the cycle of exo- and endocytosis required to maintain the RRP.
SYP/VAMP2 Complex
Exocytosis
While Ca2+ induced exocytosis is widely recognized as the basis of neurotransmitter release, the molecular architecture underlying this process is a point of contention (Berridge, 1998; Neher and Sakaba, 2008; Williams and Smith, 2018). The neuronal SNARE fusion complex at the pre-synaptic plasma membrane results from the assembly of numerous fusion proteins, including syntaxin, SNAP-25, and synaptobrevin (VAMP2; Brunger, 2005). Recent cryoelectron tomographic evidence points to a conserved, symmetric distribution of proteins at primed active zones. While the identity of these proteins is unknown, it is clear that their assembly is dependent on the upstream function of SNARE and SNARE-interacting molecules (Radhakrishnan et al., 2021).
One such complex is formed by SYP and VAMP2. This hexameric complex is thought to provide a template for the assembly of proteins at primed active zones, thereby controlling exocytosis through regulation of VAMP2 binding (Edelmann et al., 1995). The SYP/VAMP2 complex, assembled prior to docking and priming, is functionally suited for this purpose: the early assembly of SYP/VAMP2 facilitates a quick temporal response necessary for Ca2+-mediated exocytosis (Adams et al., 2015). Interestingly, there is evidence that SYP is specifically involved in the negative regulation of VAMP2-syntaxin binding, further supporting the importance of synaptophysin in temporal control of SNARE formation (Raja et al., 2019). SYP mutations that affect VAMP2 trafficking are associated with neurodevelopmental disorders, and understanding the molecular sequence of events underlying SYP/VAMP2-mediated trafficking is vital for addressing public health concerns (Harper et al., 2017; John et al., 2021).
Endocytosis
In addition to neurotransmitter release, vesicular recycling at the synaptic cleft is also thought to be influenced by the SYP/VAMP2 complex. Synaptophysin itself is crucial to the maintenance of synaptic vesicle endocytosis; SYP loss-of-function mutations result in severely reduced recycling rates (Kwon and Chapman, 2011). During vesicle recycling, SYP appears to be required for the reuptake of VAMP2 into the pre-synaptic bouton, as well as maintaining appropriate levels of VAMP2 at the pre-synapse (Gordon et al., 2011; Kokotos et al., 2019). Additionally, this relationship is heavily reliant upon the ratio of SYP to VAMP2; disruptions in the physiological balance of the two proteins results in drastically reduced trafficking of VAMP2 back to pre-synaptic vesicles (Gordon et al., 2016).
Endocytosis is primarily dependent on SYP’s cytoplasmic C-terminus, which is necessary for the efficient recovery of VAMP2 (Harper et al., 2021). While the direct molecular basis of SYP/VAMP2 binding is becoming clearer, there are several extrinsic factors that contribute to the complex’s role in overall synaptic function. A particularly interesting property of the SYP/VAMP2 complex is its dependence on cholesterol, with assembly preferentially occurring in high cholesterol environments (Mitter et al., 2003; Hussain et al., 2019). With further investigation, external components like cholesterol may reveal functional links to some puzzling physiologies. One example is the predilection of synapses for recently synthesized vesicle proteins. Synaptophysin exits the recycling pool relatively quickly following production and is replaced by freshly-synthesized molecules; how this ties into the balance of SYP/VAMP2 is unknown (Truckenbrodt et al., 2018).
Contextual Roles
Many integral pre-synaptic functions remain ambiguously defined at the molecular level. The calcium ion is a key player in vesicular docking and fusion; Ca2+ concentration is directly related to the rate of vesicle recruitment and release (Neher and Sakaba, 2008). The direct role of Ca2+ within the pre-synaptic terminal is highly contextual and depends on local signal strength as well as the type of synapse (excitatory vs. inhibitory; Schneggenburger and Neher, 2005; Williams and Smith, 2018). Despite this physiological range, many Ca2+ sensors have been identified at the pre-synaptic terminal. Regulation of the readily releasable pool (RRP) is one process that relies upon such Ca2+ sensors. The RRP links Ca2+ flux to synaptic strength and release probability (Thanawala and Regehr, 2013). In addition, Munc18-1 has been established as a key physiological regulator of cognate SNARE activation (Shen et al., 2007). Munc18-1 contributes to the maintenance of the SNARE complex in the presence of destabilizing factors. This kind of synapse-specific Ca2+ response could be important in the molecular regulation of atypical synaptic release.
In addition to traditional synapses, which use a single primary neurotransmitter, so-called dual-release terminals are found in many pathways throughout the brain and are capable of producing and releasing two primary neurotransmitters, such as glutamate and GABA (Vaaga et al., 2014; Root et al., 2018). These terminals release GABA and glutamate at a specific ratio, and disruptions of this ratio are implicated in major depressive disorder and addiction (Shabel et al., 2014; Root et al., 2020). Despite the prevalence and associated pathologies of dual-release neurons, the pre-synaptic machinery regulating most release properties is unknown.
A major connection between dual-release physiology and Ca2+-mediated vesicular release may be found in the SYP/VAMP2 complex and associated superstructures. A central Ca2+ sensor, synaptotagmin (syt), is responsible for the induction of synchronous events at the pre-synaptic membrane through regulation of vSNARE activity via its C2B domain (Chang et al., 2018). Syt has recently been shown to form a ring-like structure upon which SNAREs are assembled (Rothman et al., 2017). Specifically, the C2AB domain of syt “clamps” the SNARE assembly at the synaptic vesicle (Figure 2). This conceptually allows docking of the vesicle at the plasma membrane with the syt ring attached, followed by Ca2+-mediated disassembly of the syt ring upon action potential stimulation and subsequent fusion of vSNAREs to the membrane. This framework could contribute to the previously described speed of SNARE complex assembly, vesicle docking, and fusion. The physiological role of syt extends beyond a simple scaffold, as demonstrated in its ability to negatively regulate the attachment of vSNAREs to their plasma membrane-bound tSNARE targets at low intracellular Ca2+ concentrations (Wang et al., 2014). Interestingly, it appears that the syt “ring” acts as a vesicle “landing gear,” guiding a pre-synaptic vesicle to specific active zones determined by the concentration of PIP2 (Zhu et al., 2021). The specificity of this docking might elucidate the basis of ratio-based dual-release. If the two neurotransmitters of a dual-release terminal are packaged in separate vesicles, an idea that has recently been contested, then one of the logical methods for regulating the ratio of release is via differential trafficking to the pre-synaptic membrane (Wang et al., 2020; Kim et al., 2021). The interaction of syt with the vesicular SYP/VAMP2 complex provides a structural basis for distinct trafficking pathways, but further study is needed to define the precise mechanism. Understanding how the v-SNARE pantheon contributes to dual-release physiology is essential for refining signaling pathways, understanding mood regulation, and discovering targeted treatments for associated disorders. The key to this understanding will come by obtaining high-resolution structure data of the SYP/VAMP complex so that mutational disruption of the complex can be explored functionally. Methods such as cryo-electron tomography and super-resolution microscopy will be vital next steps in analyzing pre-synaptic structures at a sufficient resolution. Questions regarding the spatial arrangement, temporal dynamics, and association kinetics of SNARE-associated complexes are crucial yet remain unanswered.
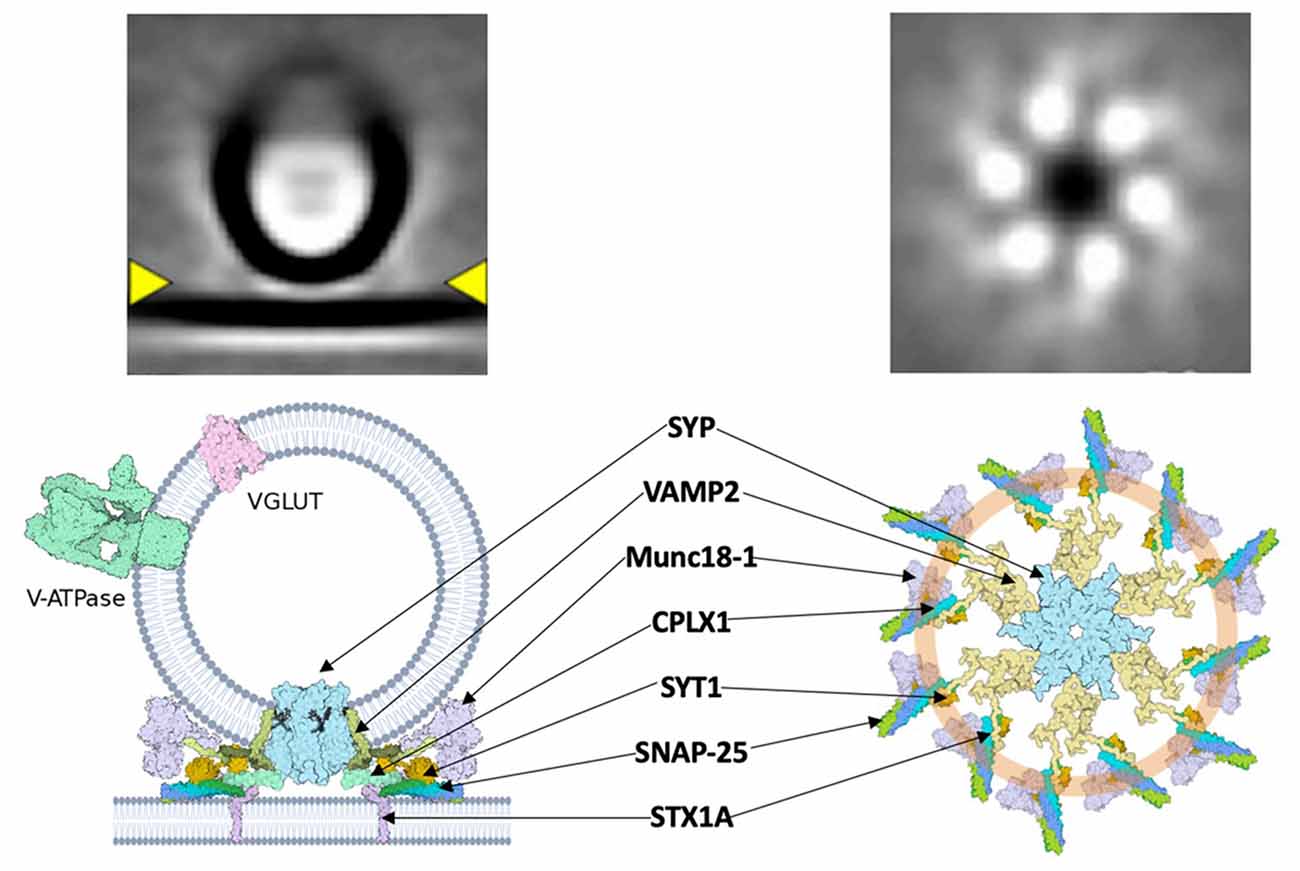
Figure 2. A hypothetical synaptic fusion nanomachine comprised of a multimeric assembly organized by synaptophysin. Top, cryo-ET averages of docked synaptic vesicles (Radhakrishnan et al., 2021). Bottom, proposed fusion assembly based upon the prior synaptophysin/synaptobrevin complex (Adams et al., 2015) and the buttressed ring model (Rothman et al., 2017).
Author Contributions
DW and MS wrote the manuscript and approved it for publication. All authors contributed to the article and approved the submitted version.
Funding
This work was supported by the NIH/CU Molecular Biophysics Program and NIH Biophysics Training Grant T32 GM-065103 to DW, and the MCDB Neurodegenerative Disease Fund to MS.
Conflict of Interest
The authors declare that the research was conducted in the absence of any commercial or financial relationships that could be construed as a potential conflict of interest.
Publisher’s Note
All claims expressed in this article are solely those of the authors and do not necessarily represent those of their affiliated organizations, or those of the publisher, the editors and the reviewers. Any product that may be evaluated in this article, or claim that may be made by its manufacturer, is not guaranteed or endorsed by the publisher.
Acknowledgments
We thank J. Shen for helpful comments.
References
Adams, D. J., Arthur, C. P., and Stowell, M. H. B. (2015). Architecture of the synaptophysin/synaptobrevin complex: structural evidence for an entropic clustering function at the synapse. Sci. Rep. 5:13659. doi: 10.1038/srep13659
Arthur, C. P., and Stowell, M. H. B. (2007). Structure of synaptophysin: a hexameric MARVEL domain channel protein. Structure 15, 707–714. doi: 10.1016/j.str.2007.04.011
Berridge, M. J. (1998). Neuronal Calcium Signaling. Neuron 21, 13–26. doi: 10.1016/s0896-6273(00)80510-3
Borisovska, M., Zhao, Y., Tsytsyura, Y., Glyvuk, N., Takamori, S., Matti, U., et al. (2005). v-SNAREs control exocytosis of vesicles from priming to fusion. EMBO J. 24, 2114–2126. doi: 10.1038/sj.emboj.7600696
Bracher, A., Kadlec, J., Betz, H., and Weissenhorn, W. (2002). X-ray structure of a neuronal complexin-snare complex from squid. J. Biol. Chem. 277, 26517–26523. doi: 10.1074/jbc.M203460200
Brunger, A. T. (2005). Structure and function of SNARE and SNARE-interacting proteins. Q. Rev. Biophys. 38, 1–47. doi: 10.1017/S0033583505004051
Brunger, A. T., Leitz, J., Zhou, Q., Choi, U. B., and Lai, Y. (2018). Ca2+-triggered synaptic vesicle fusion initiated by release of inhibition. Trends Cell Biol. 28, 631–645. doi: 10.1016/j.tcb.2018.03.004
Carr, C. M., Grote, E., Munson, M., Hughson, F. M., and Novick, P. J. (1999). Sec1p binds to SNARE complexes and concentrates at sites of secretion. J. Cell Biol. 146, 333–344. doi: 10.1083/jcb.146.2.333
Chang, C.-W., Hsiao, Y.-T., and Jackson, M. B. (2021). Synaptophysin regulates fusion pores and exocytosis mode in chromaffin cells. J. Neurosci. 41, 3563–3578. doi: 10.1523/JNEUROSCI.2833-20.2021
Chang, S., Trimbuch, T., and Rosenmund, C. (2018). Synaptotagmin-1 drives synchronous Ca2+-triggered fusion by C2B-domain-mediated synaptic-vesicle-membrane attachment. Nat. Neurosci. 21, 33–40. doi: 10.1038/s41593-017-0037-5
Chapman, E. R., Hanson, P. I., An, S., and Jahn, R. (1995). Ca2+ regulates the interaction between synaptotagmin and syntaxin 1. J. Biol. Chem. 270, 23667–23671. doi: 10.1074/jbc.270.40.23667
Chen, X., Tomchick, D. R., Kovrigin, E., Araç, D., Machius, M., Südhof, T. C., et al. (2002). Three-dimensional structure of the complexin/SNARE complex. Neuron 33, 397–409. doi: 10.1016/s0896-6273(02)00583-4
Deák, F., Schoch, S., Liu, X., Südhof, T. C., and Kavalali, E. T. (2004). Synaptobrevin is essential for fast synaptic-vesicle endocytosis. Nat. Cell Biol. 6, 1102–1108. doi: 10.1038/ncb1185
Deák, F., Xu, Y., Chang, W.-P., Dulubova, I., Khvotchev, M., Liu, X., et al. (2009). Munc18-1 binding to the neuronal SNARE complex controls synaptic vesicle priming. J. Cell Biol. 184, 751–764. doi: 10.1083/jcb.200812026
Dulubova, I., Khvotchev, M., Liu, S., Huryeva, I., Südhof, T. C., and Rizo, J. (2007). Munc18-1 binds directly to the neuronal SNARE complex. Proc. Natl. Acad. Sci. USA 104, 2697–2702. doi: 10.1073/pnas.0611318104
Edelmann, L., Hanson, P., Chapman, E., and Jahn, R. (1995). Synaptobrevin binding to synaptophysin: a potential mechanism for controlling the exocytotic fusion machine. EMBO J. 14, 224–231.
Far, O. E., Charvin, N., Leveque, C., Martin-Moutot, N., Takahashi, M., and Seagar, M. J. (1995). Interaction of a synaptobrevin (VAMP)-syntaxin complex with presynaptic calcium channels. FEBS Lett. 361, 101–105. doi: 10.1016/0014-5793(95)00156-4
Fasshauer, D., Bruns, D., Shen, B., Jahn, R., and Brünger, A. T. (1997). A structural change occurs upon binding of syntaxin to SNAP-25. J. Biol. Chem. 272, 4582–4590. doi: 10.1074/jbc.272.7.4582
Felkl, M., and Leube, R. E. (2008). Interaction assays in yeast and cultured cells confirm known and identify novel partners of the synaptic vesicle protein synaptophysin. Neuroscience 156, 344–352. doi: 10.1016/j.neuroscience.2008.07.033
Fernàndez-castillo, N., Cormand, B., Roncero, C., Sánchez-Mora, C., Grau-Lopez, L., Gonzalvo, B., et al. (2012). Candidate pathway association study in cocaine dependence: The control of neurotransmitter release. World J. Biol. Psychiatry 13, 126–134. doi: 10.3109/15622975.2010.551406
Gincel, D., and Shoshan-Barmatz, V. (2002). The synaptic vesicle protein synaptophysin: purification and characterization of its channel activity. Biophys. J. 83, 3223–3229. doi: 10.1016/S0006-3495(02)75324-1
Gordon, S. L., Harper, C. B., Smillie, K. J., and Cousin, M. A. (2016). A fine balance of synaptophysin levels underlies efficient retrieval of synaptobrevin II to synaptic vesicles. PLoS One 11:e0149457. doi: 10.1371/journal.pone.0149457
Gordon, S. L., Leube, R. E., and Cousin, M. A. (2011). Synaptophysin is required for synaptobrevin retrieval during synaptic vesicle endocytosis. J. Neurosci. 31, 14032–14036. doi: 10.1523/JNEUROSCI.3162-11.2011
Hamdan, F. F., Piton, A., Gauthier, J., Lortie, A., Dubeau, F., Dobrzeniecka, S., et al. (2009). De novo STXBP1 mutations in mental retardation and nonsyndromic epilepsy. Ann. Neurol. 65, 748–753. doi: 10.1002/ana.21625
Harper, C. B., Blumrich, E.-M., and Cousin, M. A. (2021). Synaptophysin controls synaptobrevin-II retrieval via a cryptic C-terminal interaction site. J. Biol. Chem. 296:100266. doi: 10.1016/j.jbc.2021.100266
Harper, C. B., Mancini, G. M. S., van Slegtenhorst, M., and Cousin, M. A. (2017). Altered synaptobrevin-II trafficking in neurons expressing a synaptophysin mutation associated with a severe neurodevelopmental disorder. Neurobiol. Dis. 108, 298–306. doi: 10.1016/j.nbd.2017.08.021
Hata, Y., Slaughter, C. A., and Südhof, T. C. (1993). Synaptic vesicle fusion complex contains unc-18 homologue bound to syntaxin. Nature 366, 347–351. doi: 10.1038/366347a0
Hazzard, J., Südhof, T. C., and Rizo, J. (1999). NMR analysis of the structure of synaptobrevin and of its interaction with syntaxin. J. Biomol. NMR 14, 203–207. doi: 10.1023/a:1008382027065
Honer, W. G., Ramos-Miguel, A., Alamri, J., Sawada, K., Barr, A. M., Schneider, J. A., et al. (2019). The synaptic pathology of cognitive life. Dialogues Clin. Neurosci. 21, 271–279. doi: 10.31887/DCNS.2019.21.3/whoner
Hussain, G., Wang, J., Rasul, A., Anwar, H., Imran, A., Qasim, M., et al. (2019). Role of cholesterol and sphingolipids in brain development and neurological diseases. Lipids Health Dis. 18:26. doi: 10.1186/s12944-019-0965-z
Janz, R., Südhof, T. C., Hammer, R. E., Unni, V., Siegelbaum, S. A., and Bolshakov, V. Y. (1999). Essential roles in synaptic plasticity for synaptogyrin I and synaptophysin I. Neuron 24, 687–700. doi: 10.1016/s0896-6273(00)81122-8
John, A., Ng-Cordell, E., Hanna, N., Brkic, D., and Baker, K. (2021). The neurodevelopmental spectrum of synaptic vesicle cycling disorders. J. Neurochem. 157, 208–228. doi: 10.1111/jnc.15135
Jurado, S., Goswami, D., Zhang, Y., Molina, A. J. M., Südhof, T. C., and Malenka, R. C. (2013). LTP requires a unique postsynaptic SNARE fusion machinery. Neuron 77, 542–558. doi: 10.1016/j.neuron.2012.11.029
Kandel, E. R., Dudai, Y., and Mayford, M. R. (2014). The molecular and systems biology of memory. Cell 157, 163–186. doi: 10.1016/j.cell.2014.03.001
Karim, S., Essenberg, R. C., Dillwith, J. W., Tucker, J. S., Bowman, A. S., and Sauer, J. R. (2002). Identification of SNARE and cell trafficking regulatory proteins in the salivary glands of the lone star tick, Amblyomma americanum (L.). Insect Biochem. Mol. Biol. 32, 1711–1721. doi: 10.1016/s0965-1748(02)00111-x
Karmakar, S., Sharma, L. G., Roy, A., Patel, A., and Pandey, L. M. (2019). Neuronal SNARE complex: a protein folding system with intricate protein-protein interactions and its common neuropathological hallmark, SNAP25. Neurochem. Int. 122, 196–207. doi: 10.1016/j.neuint.2018.12.001
Kataoka, M., Yamamori, S., Suzuki, E., Watanabe, S., Sato, T., Miyaoka, H., et al. (2011). A single amino acid mutation in SNAP-25 induces anxiety-related behavior in mouse. PLoS One 6:e25158. doi: 10.1371/journal.pone.0025158
Kim, S., Wallace, M., El-Rifai, M., Knudsen, A., and Sabatini, B. (2021). Biophysical demonstration of co-packaging of glutamate and GABA in individual synaptic vesicles in the central nervous system. bioRxiv [Preprint]. doi: 10.1101/2021.03.23.436594
Kokotos, A. C., Harper, C. B., Marland, J. R. K., Smillie, K. J., Cousin, M. A., and Gordon, S. L. (2019). Synaptophysin sustains presynaptic performance by preserving vesicular synaptobrevin-II levels. J. Neurochem. 151, 28–37. doi: 10.1111/jnc.14797
Körber, C., and Kuner, T. (2016). Molecular machines regulating the release probability of synaptic vesicles at the active zone. Front. Synaptic Neurosci. 8:5. doi: 10.3389/fnsyn.2016.00005
Kwon, S. E., and Chapman, E. R. (2011). Synaptophysin regulates the kinetics of synaptic vesicle endocytosis in central neurons. Neuron 70, 847–854. doi: 10.1016/j.neuron.2011.04.001
Liang, B., and Tamm, L. K. (2018). Solution NMR of SNAREs, complexin and α-synuclein in association with membrane-mimetics. Prog. Nucl. Magn. Reson. Spectrosc. 105, 41–53. doi: 10.1016/j.pnmrs.2018.02.001
Ma, C., Li, W., Xu, Y., and Rizo, J. (2011). Munc13 mediates the transition from the closed syntaxin-Munc18 complex to the SNARE complex. Nat. Struct. Mol. Biol. 18, 542–549. doi: 10.1038/nsmb.2047
Marqueze-Pouey, B., Wisden, W., Malosio, M., and Betz, H. (1991). Differential expression of synaptophysin and synaptoporin mRNAs in the postnatal rat central nervous system. J. Neurosci. 11, 3388–3397. doi: 10.1523/JNEUROSCI.11-11-03388.1991
McMahon, H. T., and Südhof, T. C. (1995). Synaptic core complex of synaptobrevin, syntaxin and SNAP25 forms high affinity-SNAP binding site. J. Biol. Chem. 270, 2213–2217. doi: 10.1074/jbc.270.5.2213
Metzger, M., Bueno, D., and Lima, L. B. (2017). The lateral habenula and the serotonergic system. Pharmacol. Biochem. Behav. 162, 22–28. doi: 10.1016/j.pbb.2017.05.007
Misura, K. M., Scheller, R. H., and Weis, W. I. (2000). Three-dimensional structure of the neuronal-Sec1-syntaxin 1a complex. Nature 404, 355–362. doi: 10.1038/35006120
Mitter, D., Reisinger, C., Hinz, B., Hollmann, S., Yelamanchili, S. V., Treiber-Held, S., et al. (2003). The synaptophysin/synaptobrevin interaction critically depends on the cholesterol content. J. Neurochem. 84, 35–42. doi: 10.1046/j.1471-4159.2003.01258.x
Neher, E., and Sakaba, T. (2008). Multiple roles of calcium ions in the regulation of neurotransmitter release. Neuron 59, 861–872. doi: 10.1016/j.neuron.2008.08.019
Ostenson, C.-G., Gaisano, H., Sheu, L., Tibell, A., and Bartfai, T. (2006). Impaired gene and protein expression of exocytotic soluble N-ethylmaleimide attachment protein receptor complex proteins in pancreatic islets of type 2 diabetic patients. Diabetes 55, 435–440. doi: 10.2337/diabetes.55.02.06.db04-1575
Pabst, S., Hazzard, J. W., Antonin, W., Südhof, T. C., Jahn, R., Rizo, J., et al. (2000). Selective interaction of complexin with the neuronal snare complex: determination of the binding regions. J. Biol. Chem. 275, 19808–19818. doi: 10.1074/jbc.M002571200
Radhakrishnan, A., Li, X., Grushin, K., Krishnakumar, S. S., Liu, J., and Rothman, J. E. (2021). Symmetrical arrangement of proteins under release-ready vesicles in presynaptic terminals. Proc. Natl. Acad. Sci. USA 118:e2024029118. doi: 10.1073/pnas.2024029118
Raja, M. K., Preobraschenski, J., Del Olmo-Cabrera, S., Martinez-Turrillas, R., Jahn, R., Perez-Otano, I., et al. (2019). Elevated synaptic vesicle release probability in synaptophysin/gyrin family quadruple knockouts. eLife 8:e40744. doi: 10.7554/eLife.40744
Ramos-Miguel, A., Jones, A. A., Sawada, K., Barr, A. M., Bayer, T. A., Falkai, P., et al. (2018). Frontotemporal dysregulation of the SNARE protein interactome is associated with faster cognitive decline in old age. Neurobiol. Dis. 114, 31–44. doi: 10.1016/j.nbd.2018.02.006
Rehm, H., Wiedenmann, B., and Betz, H. (1986). Molecular characterization of synaptophysin, a major calcium-binding protein of the synaptic vesicle membrane. EMBO J. 5, 535–541.
Rickman, C., Medine, C. N., Bergmann, A., and Duncan, R. R. (2007). Functionally and spatially distinct modes of munc18-syntaxin 1 interaction. J. Biol. Chem. 282, 12097–12103. doi: 10.1074/jbc.M700227200
Root, D. H., Barker, D. J., Estrin, D. J., Miranda-Barrientos, J. A., Liu, B., Zhang, S., et al. (2020). Distinct signaling by ventral tegmental area glutamate, gaba and combinatorial glutamate-gaba neurons in motivated behavior. Cell Rep. 32:108094. doi: 10.1016/j.celrep.2020.108094
Root, D. H., Zhang, S., Barker, D. J., Miranda-Barrientos, J., Liu, B., Wang, H.-L., et al. (2018). Selective brain distribution and distinctive synaptic architecture of dual glutamatergic-GABAergic neurons. Cell Rep. 23, 3465–3479. doi: 10.1016/j.celrep.2018.05.063
Roselli, F., and Caroni, P. (2015). From intrinsic firing properties to selective neuronal vulnerability in neurodegenerative diseases. Neuron 85, 901–910. doi: 10.1016/j.neuron.2014.12.063
Rothman, J. E., Krishnakumar, S. S., Grushin, K., and Pincet, F. (2017). Hypothesis—buttressed rings assemble, clamp and release SNAREpins for synaptic transmission. FEBS Lett. 591, 3459–3480. doi: 10.1002/1873-3468.12874
Salpietro, V., Malintan, N. T., Llano-Rivas, I., Spaeth, C. G., Efthymiou, S., Striano, P., et al. (2019). Mutations in the neuronal vesicular SNARE VAMP2 affect synaptic membrane fusion and impair human neurodevelopment. Am. J. Hum. Genet. 104, 721–730. doi: 10.1016/j.ajhg.2019.02.016
Schneggenburger, R., and Neher, E. (2005). Presynaptic calcium and control of vesicle fusion. Curr. Opin. Neurobiol. 15, 266–274. doi: 10.1016/j.conb.2005.05.006
Schoch, S., Deák, F., Königstorfer, A., Mozhayeva, M., Sara, Y., Südhof, T. C., et al. (2001). SNARE function analyzed in synaptobrevin/VAMP knockout mice. Science 294, 1117–1122. doi: 10.1126/science.1064335
Sclip, A., Bacaj, T., Giam, L. R., and Südhof, T. C. (2016). Extended synaptotagmin (ESyt) triple knock-out mice are viable and fertile without obvious endoplasmic reticulum dysfunction. PLoS One 11:e0158295. doi: 10.1371/journal.pone.0158295
Shabel, S. J., Proulx, C. D., Piriz, J., and Malinow, R. (2014). Mood regulation. GABA/glutamate co-release controls habenula output and is modified by antidepressant treatment. Science 345, 1494–1498. doi: 10.1126/science.1250469
Shen, J., Tareste, D. C., Paumet, F., Rothman, J. E., and Melia, T. J. (2007). Selective activation of cognate SNAREpins by Sec1/Munc18 proteins. Cell 128, 183–195. doi: 10.1016/j.cell.2006.12.016
Söllner, T., Bennett, M. K., Whiteheart, S. W., Scheller, R. H., and Rothman, J. E. (1993b). A protein assembly-disassembly pathway in vitro that may correspond to sequential steps of synaptic vesicle docking, activation and fusion. Cell 75, 409–418. doi: 10.1016/0092-8674(93)90376-2
Söllner, T., Whiteheart, S. W., Brunner, M., Erdjument-Bromage, H., Geromanos, S., Tempst, P., et al. (1993a). SNAP receptors implicated in vesicle targeting and fusion. Nature 362, 318–324. doi: 10.1038/362318a0
Stepien, K. P., Prinslow, E. A., and Rizo, J. (2019). Munc18-1 is crucial to overcome the inhibition of synaptic vesicle fusion by αSNAP. Nat. Commun. 10:4326. doi: 10.1038/s41467-019-12188-4
Szklarczyk, D., Gable, A. L., Lyon, D., Junge, A., Wyder, S., Huerta-Cepas, J., et al. (2019). STRING v11: protein-protein association networks with increased coverage, supporting functional discovery in genome-wide experimental datasets. Nucleic Acids Res. 47, D607–D613. doi: 10.1093/nar/gky1131
Thanawala, M. S., and Regehr, W. G. (2013). Presynaptic calcium influx controls neurotransmitter release in part by regulating the effective size of the readily releasable pool. J. Neurosci. 33, 4625–4633. doi: 10.1523/JNEUROSCI.4031-12.2013
Thiele, C., Hannah, M. J., Fahrenholz, F., and Huttner, W. B. (2000). Cholesterol binds to synaptophysin and is required for biogenesis of synaptic vesicles. Nat. Cell Biol. 2, 42–49. doi: 10.1038/71366
Thomas, S. G., Takahashi, M., Neill, J. D., and Clarke, I. J. (1998). Components of the neuronal exocytotic machinery in the anterior pituitary of the ovariectomised ewe and the effects of oestrogen in gonadotropes as studied with confocal microscopy. Neuroendocrinology 67, 244–259. doi: 10.1159/000054320
Trimbuch, T., Xu, J., Flaherty, D., Tomchick, D. R., Rizo, J., and Rosenmund, C. (2014). Re-examining how complexin inhibits neurotransmitter release. eLife 3:e02391. doi: 10.7554/eLife.02391
Truckenbrodt, S., Viplav, A., Jähne, S., Vogts, A., Denker, A., Wildhagen, H., et al. (2018). Newly produced synaptic vesicle proteins are preferentially used in synaptic transmission. EMBO J. 37:e98044. doi: 10.15252/embj.201798044
Vaaga, C. E., Borisovska, M., and Westbrook, G. L. (2014). Dual-transmitter neurons: functional implications of co-release and co-transmission. Curr. Opin. Neurobiol. 29, 25–32. doi: 10.1016/j.conb.2014.04.010
Wang, C., Tu, J., Zhang, S., Cai, B., Liu, Z., Hou, S., et al. (2020). Different regions of synaptic vesicle membrane regulate VAMP2 conformation for the SNARE assembly. Nat. Commun. 11:1531. doi: 10.1038/s41467-020-15270-4
Wang, J., Bello, O., Auclair, S. M., Wang, J., Coleman, J., Pincet, F., et al. (2014). Calcium sensitive ring-like oligomers formed by synaptotagmin. Proc. Natl. Acad. Sci. U S A 111, 13966–13971. doi: 10.1073/pnas.1415849111
Weber, T., Zemelman, B. V., McNew, J. A., Westermann, B., Gmachl, M., Parlati, F., et al. (1998). SNAREpins: minimal machinery for membrane fusion. Cell 92, 759–772. doi: 10.1016/s0092-8674(00)81404-x
Williams, C. L., and Smith, S. M. (2018). Calcium dependence of spontaneous neurotransmitter release. J. Neurosci. Res. 96, 335–347. doi: 10.1002/jnr.24116
Wrackmeyer, U., Kaldrack, J., Jüttner, R., Pannasch, U., Gimber, N., Freiberg, F., et al. (2019). The cell adhesion protein CAR is a negative regulator of synaptic transmission. Sci. Rep. 9:6768. doi: 10.1038/s41598-019-43150-5
Xu, J., Brewer, K. D., Perez-Castillejos, R., and Rizo, J. (2013b). Subtle interplay between synaptotagmin and complexin binding to the SNARE complex. J. Mol. Biol. 425, 3461–3475. doi: 10.1016/j.jmb.2013.07.001
Xu, J., Luo, F., Zhang, Z., Xue, L., Wu, X.-S., Chiang, H.-C., et al. (2013a). SNARE proteins synaptobrevin, SNAP-25 and syntaxin are involved in rapid and slow endocytosis at synapses. Cell Rep. 3, 1414–1421. doi: 10.1016/j.celrep.2013.03.010
Xue, M., Ma, C., Craig, T. K., Rosenmund, C., and Rizo, J. (2008). The Janus-faced nature of the C(2)B domain is fundamental for synaptotagmin-1 function. Nat. Struct. Mol. Biol. 15, 1160–1168. doi: 10.1038/nsmb.1508
Ya, B.-L., Liu, W.-Y., Ge, F., Zhang, Y.-X., Zhu, B.-L., and Bai, B. (2013). Dietary cholesterol alters memory and synaptic structural plasticity in young rat brain. Neurol. Sci. 34, 1355–1365. doi: 10.1007/s10072-012-1241-4
Yan, M.-L., Zhang, S., Zhao, H.-M., Xia, S.-N., Jin, Z., Xu, Y., et al. (2020). MicroRNA-153 impairs presynaptic plasticity by blocking vesicle release following chronic brain hypoperfusion. Cell Commun. Signal. 18:57. doi: 10.1186/s12964-020-00551-8
Yim, Y. Y., Zurawski, Z., and Hamm, H. (2018). GPCR regulation of secretion. Pharmacol. Ther. 192, 124–140. doi: 10.1016/j.pharmthera.2018.07.005
Yin, Y., Dayanithi, G., and Lemos, J. R. (2002). Ca2+-regulated, neurosecretory granule channel involved in release from neurohypophysial terminals. J. Physiol. 539, 409–418. doi: 10.1113/jphysiol.2001.012943
Zhang, X., Kim-Miller, M. J., Fukuda, M., Kowalchyk, J. A., and Martin, T. F. J. (2002). Ca2+-dependent synaptotagmin binding to SNAP-25 is essential for Ca2+-triggered exocytosis. Neuron 34, 599–611. doi: 10.1016/s0896-6273(02)00671-2
Zhou, J., Liu, Z., Yu, J., Han, X., Fan, S., Shao, W., et al. (2015). Quantitative proteomic analysis reveals molecular adaptations in the hippocampal synaptic active zone of chronic mild stress-unsusceptible rats. Int. J. Neuropsychopharmacol. 19:pyv100. doi: 10.1093/ijnp/pyv100
Zhou, Q., Zhou, P., Wang, A. L., Wu, D., Zhao, M., Südhof, T. C., et al. (2017). The primed SNARE-complexin-synaptotagmin complex for neuronal exocytosis. Nature 548, 420–425. doi: 10.1038/nature23484
Zhu, J., McDargh, Z. A., Li, F., Krishnakumar, S., Rothman, J. E., and O’Shaughnessy, B. (2021). Synaptotagmin rings as high sensitivity regulators of synaptic vesicle docking and fusion. bioRxiv [Preprint]. doi: 10.1101/2021.03.12.435193
Keywords: synaptic fusion, fusion machinery, supercomplex, synaptobrevin, synaptophysin (SYP)
Citation: White DN and Stowell MHB (2021) Room for Two: The Synaptophysin/Synaptobrevin Complex. Front. Synaptic Neurosci. 13:740318. doi: 10.3389/fnsyn.2021.740318
Received: 12 July 2021; Accepted: 18 August 2021;
Published: 20 September 2021.
Edited by:
Lucia Tabares, Sevilla University, SpainReviewed by:
Janet E. Richmond, University of Illinois at Chicago, United StatesLuís F. Ribeiro, VIB & KU Leuven Center for Brain & Disease Research, Belgium
Copyright © 2021 White and Stowell. This is an open-access article distributed under the terms of the Creative Commons Attribution License (CC BY). The use, distribution or reproduction in other forums is permitted, provided the original author(s) and the copyright owner(s) are credited and that the original publication in this journal is cited, in accordance with accepted academic practice. No use, distribution or reproduction is permitted which does not comply with these terms.
*Correspondence: Dustin N. White, ZHVzdGluLm4ud2hpdGVAY29sb3JhZG8uZWR1; Michael H. B. Stowell, c3Rvd2VsbG1AY29sb3JhZG8uZWR1