- 1Department of Pharmacology and Toxicology, Institute of Pharmacy and CMBI, University of Innsbruck, Innsbruck, Austria
- 2Department of Pharmaceutical Sciences, Research Group Experimental Pharmacology, Center for Neurosciences (C4N), Vrije Universiteit Brussel, Brussels, Belgium
Ghrelin is a peptide hormone released by specialized X/A cells in the stomach and activated by acylation. Following its secretion, it binds to ghrelin receptors in the periphery to regulate energy balance, but it also acts on the central nervous system where it induces a potent orexigenic effect. Several types of stressors have been shown to stimulate ghrelin release in rodents, including nutritional stressors like food deprivation, but also physical and psychological stressors such as foot shocks, social defeat, forced immobilization or chronic unpredictable mild stress. The mechanism through which these stressors drive ghrelin release from the stomach lining remains unknown and, to date, the resulting consequences of ghrelin release for stress coping remain poorly understood. Indeed, ghrelin has been proposed to act as a stress hormone that reduces fear, anxiety- and depression-like behaviors in rodents but some studies suggest that ghrelin may - in contrast - promote such behaviors. In this review, we aim to provide a comprehensive overview of the literature on the role of the ghrelin system in stress coping. We discuss whether ghrelin release is more than a byproduct of disrupted energy homeostasis following stress exposure. Furthermore, we explore the notion that ghrelin receptor signaling in the brain may have effects independent of circulating ghrelin and in what way this might influence stress coping in rodents. Finally, we examine how the ghrelin system could be utilized as a therapeutic avenue in stress-related psychiatric disorders (with a focus on anxiety- and trauma-related disorders), for example to develop novel biomarkers for a better diagnosis or new interventions to tackle relapse or treatment resistance in patients.
The Ghrelin System
Ghrelin
Ghrelin is a stomach-derived peptide hormone that is tonically released into the blood stream with peak concentrations in response to a negative energy balance (Kojima et al., 1999; Kojima and Kangawa, 2005). The 28-amino-acid hormone is primarily produced by X/A-like oxyntic gland cells in the gastric mucosa from its precursor pre-proghrelin (117 amino acids) (Kojima et al., 1999; Date et al., 2000). In addition, ghrelin expression has also been described in other organs such as the pancreas, kidneys, lungs, heart and placenta (Mori et al., 2000; Gualillo et al., 2001; Gnanapavan et al., 2002; Iglesias et al., 2004; Kageyama et al., 2005).
Ghrelin O-acyltransferase (GOAT) is the enzyme present in the endoplasmatic reticulum of ghrelin-producing cells that ensures the post-translational acylation of desacyl ghrelin (DAG) at the serine-3 residue, resulting in the formation of acyl ghrelin (AG) (Gutierrez et al., 2008). Both, DAG and AG, are released into the blood stream where AG is rapidly converted to DAG (De Vriese et al., 2004), resulting in an AG/DAG ratio of 0.1–0.4 in plasma compared to 2.5 in stomach tissue (Hassouna et al., 2014). While DAG has received less attention in literature, an increasing amount of evidence indicates that it is not only a byproduct of AG synthesis but may act as a separate hormone that modulates or even opposes the effects of AG (for review see Delhanty et al., 2014). For example, DAG was shown to impair the feeding response to peripherally injected AG (Fernandez et al., 2016) and its actions on anxiety and other stress-related behaviors may also be distinct from those of AG (Stark et al., 2016; Mahbod et al., 2018). In this review, we aim to analyze the effects of AG and DAG separately and use the term ‘ghrelin’ whenever it was not possible to make a clear distinction or acylation status was not specified by the authors.
Ghrelin Receptors (GHSR)
The N-terminus of AG binds with high affinity to the growth hormone secretagogue receptor (GHSR) (Willesen et al., 1999; Cowley et al., 2003), of which two different transcripts have been identified: GHSR-1a and GHSR-1b (McKee et al., 1997). The GHSR-1a, also known as ghrelin receptor (and hereafter simply referred to as ‘GHSR’), is a Gq-protein coupled receptor comprising seven transmembrane α-helical domains to which constitutive activity has been ascribed (Holst et al., 2003). The GHSR-1b form, a truncated splice variant, contains five transmembrane domains and while its physiological role remains poorly understood, it may negatively influence GHSR function by hindering conformational changes (Leung et al., 2007). AG is the main known high-affinity agonist for the GHSR, while (in physiological concentrations) DAG does not appear to act on the GHSR (Bednarek et al., 2000). A recent study indicates that the octanoyl chain is essential for the pharmacological action of AG and that DAG, while it might bind to the GHSR with very low affinity, is unlikely to stabilize an active GHSR conformation (Ferre et al., 2019). Several binding studies propose the existence of a separate receptor specific for DAG in different tissues and also the brain (e.g., Lear et al., 2010; Togliatto et al., 2010; Fernandez et al., 2016). However, such a receptor has not yet been identified (for review see Callaghan and Furness, 2014).
The GHSR is expressed in several organs such as the brain, the anterior pituitary, adrenal and thyroid glands, pancreas and the heart (Guan et al., 1997; Gnanapavan et al., 2002). Within the brain, GHSR expression has been described in several areas including the arcuate nucleus (ARC) and other subregions of the hypothalamus, the ventral tegmental area (VTA), the nucleus tractus solitarius (NTS), the hippocampus and the amygdala (Zigman et al., 2006; Mani et al., 2014). The function the GHSR has in those brain areas and whether they are accessible for circulating ghrelin is not yet fully understood (Perello et al., 2018). Thus, it is essential to note that the GHSR is constitutively active, signaling at 50% of its maximal activity in the absence of its ligand (Holst et al., 2003; Petersen et al., 2009). It is very likely that the function of the ghrelin/GHSR system is not solely dependent on the binding of AG to its receptor, but that also changes in GHSR expression levels might have pervasive biological effects. Furthermore, there is evidence that the GHSR can form heterodimers with various other receptors like for example those for dopamine, serotonin or oxytocin (Schellekens et al., 2013b; Kern et al., 2015; Wallace Fitzsimons et al., 2019), which affects downstream signaling and receptor trafficking (for review see Schellekens et al., 2013a; Abizaid and Hougland, 2020). Recent findings also suggest that the liver-expressed antimicrobial peptide 2 (LEAP2) acts as an endogenous antagonist/inverse agonist of the GHSR that modulates ghrelin function in response to the feeding status (Ge et al., 2018).
The Ghrelin System and Energy Homeostasis
Ghrelin is most widely known for its orexigenic effects. Following its release into the bloodstream, AG can diffuse passively through fenestrated capillaries of the median eminence into the ARC (Schaeffer et al., 2013) where it binds to GHSR that are expressed abundantly on neuropeptide Y/agouti-related peptide (NPY/AGRP) neurons (Figure 1) (Willesen et al., 1999; Cowley et al., 2003). Activation of these hypothalamic neurons by AG potently increases food intake and regulates homeostatic feeding behavior in order to maintain energy balance (Kamegai et al., 2000; Nakazato et al., 2001). Indeed, re-expression of GHSR in AGRP neurons of GHSR KO mice restores, albeit incompletely, the effect of systemic administration of AG on food intake (Wang et al., 2014). In addition, ghrelin also plays an important role in reward-based, hedonic eating behaviors (as reviewed in Perello and Zigman, 2012; Al Massadi et al., 2019), which are very likely mediated by GHSR on dopaminergic neurons of the VTA (Cornejo et al., 2020).
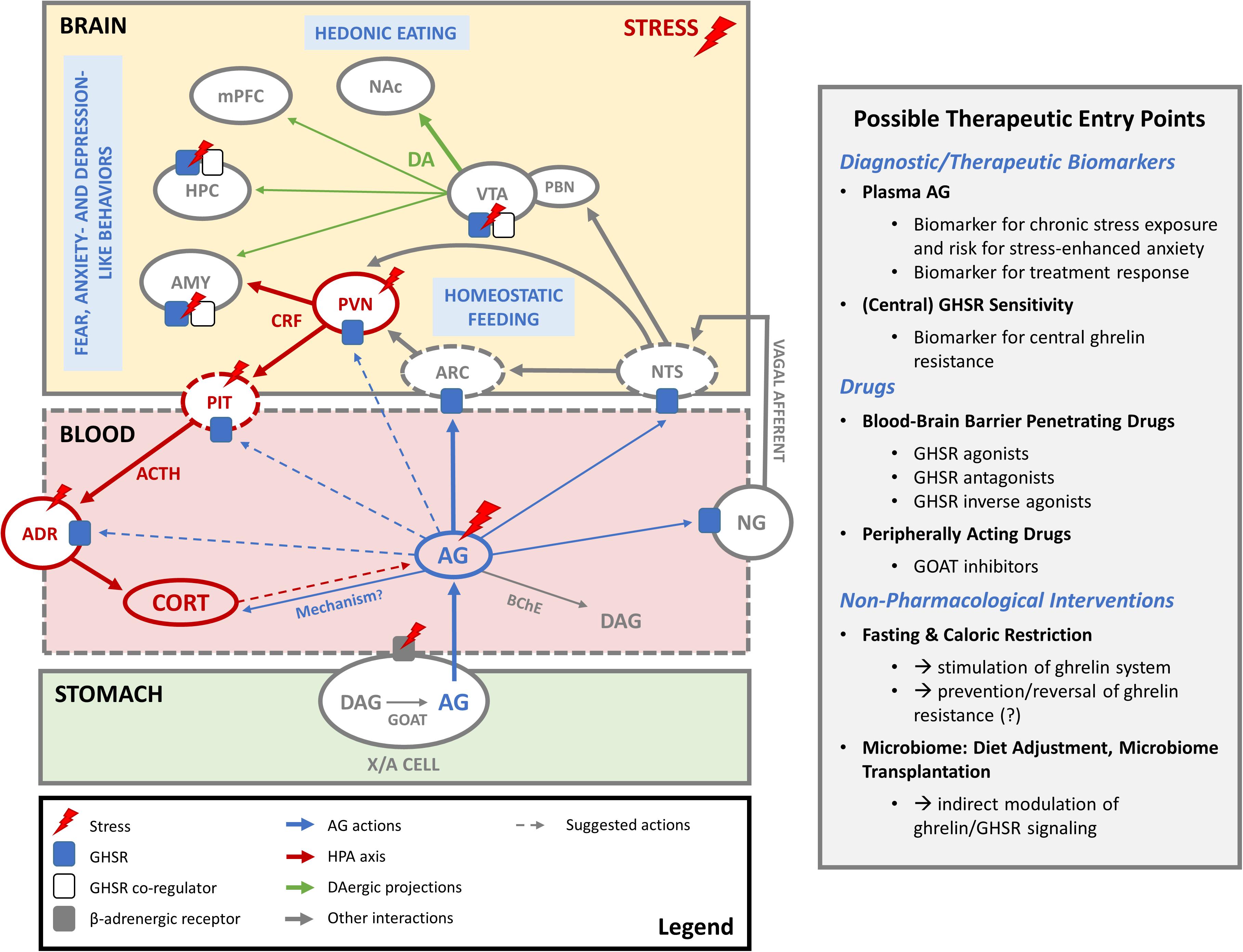
Figure 1. The ghrelin system in stress coping. In periods of hunger and stress, AG is produced by acylation of DAG by GOAT in gastric X/A cells and released into the blood stream. Before it is degraded to DAG, it acts on GHSR in the arcuate nucleus of the hypothalamus (ARC) to regulate homeostatic feeding. Increased AG levels during stress also seem to amplify HPA axis activation and induce corticosterone (CORT) release. The exact mechanism is not clear and could involve indirect activation of CRF-producing neurons in the PVN by inhibition of the local GABAergic tone, modulation of ACTH production in the pituitary (PIT) or direct effects on the adrenal cortex (ADR). AG may also indirectly influence the HPA axis via GHSR in the nodose ganglion (NG) of the vagal nerve or in the nucleus tractus solitarius (NTS). Conversely, whether HPA axis activity during stress has an impact on ghrelin release remains a subject of debate. Elevated AG levels following stress may also be secondary to activation of the sympathetic nervous system and beta receptors on ghrelin-producing X/A cells. Whether AG can pass the blood-brain barrier to act on stress-relevant brain areas where GHSR are expressed is not clear. However, constitutive activity of central GHSR may be regulated via changes in GHSR expression levels or formation of heterodimers with other receptors (e.g., dopamine, serotonin) as co-regulators. Such mechanisms might play a role in stress-related changes in feeding and fear, anxiety- and depression like behaviors, possibly via GHSR in the ventral tegmental area (VTA), hippocampus (HPC) and amygdala (AMY). Also mesocorticolimbic dopaminergic projections (DA) of the VTA to nucleus accumbens (NAc), medial prefrontal cortex (mPFC), HPC and AMY may play a role here. Abbreviations: AG, acyl ghrelin; DAG, desacyl ghrelin; GOAT, ghrelin O-acyltransferase; BChE, butyryl cholinesterase; ARC, arcuate nucleus of the hypothalamus; PVN, paraventricular nucleus of the hypothalamus; CRF, corticotropin releasing factor; PIT, pituitary gland; ACTH, adrenocorticotropic hormone; CORT, corticosterone/cortisol; ADR, adrenal cortex; NTS, nucleus tractus solitarius; NG, nodose ganglion; VTA, ventral tegmental area; PBN, parabrachial nucleus; DA, dopamine; NAc, nucleus accumbens; mPFC, medial prefrontal cortex; HPC, hippocampus; AMY, amygdala.
In humans, plasma ghrelin levels are typically increased under low-calorie conditions before meals and fall back to baseline levels after food consumption (Cummings, 2006). Also in rodents, ghrelin plasma levels rise during fasting periods and follow a diurnal rhythm peaking shortly after onset of the dark period, which is the major feeding time for rodents (Murakami et al., 2002; Bodosi et al., 2004). Peripherally circulating ghrelin has various effects on the gastrointestinal tract: it modulates intestinal motility, gastric emptying and gastric acid secretion as well as insulin release from the pancreas (for review see Kojima and Kangawa, 2005).
Overall, the role of ghrelin in driving food intake and energy homeostasis seems well established: it communicates with the brain and the gastrointestinal tract to promote feeding and ensure efficient energy storage and metabolism, lowering the risk of hypoglycemia (reviewed comprehensively by Yanagi et al., 2018). Importantly, while ghrelin signaling stimulates food intake, the effects of a loss of ghrelin signaling on feeding behavior are more subtle if detectable at all (as reviewed in Uchida et al., 2013). Nevertheless, ghrelin appears critical in the regulation of systemic glucose homeostasis and ensures survival after prolonged starvation (Goldstein et al., 2011; McFarlane et al., 2014), potentially through GHSR signaling in the nodose ganglion (NG) of the vagal nerve and the NTS (Scott et al., 2012; Okada et al., 2018) (Figure 1). Projections of the NTS to the parabrachial nucleus (Roman et al., 2016) and to the ARC (Aklan et al., 2020) were shown to regulate food intake in anorectic or hypoglycemic conditions, but it remains poorly understood how the ghrelin system modulates activity in these neurochemically distinct pathways. The effect of ghrelin on glucose homeostasis also involves the release of growth hormone in response to low blood glucose if body fat stores are depleted, which in turn increases glucose release from the liver and contributes to stabilization of blood glucose levels (Goldstein et al., 2011). Moreover, ghrelin modulates pancreatic insulin secretion and regulates its peripheral actions, although the exact mechanisms involved are still a matter of debate (as reviewed by Gray et al., 2019). Interestingly, it has been proposed that a decline in ghrelin system activity may play a role in age-dependent impairment of energy homeostasis and associated disease patterns like reduced appetite, obesity, diabetes or hepatic steatosis, but also cardiovascular dysfunction and neurodegenerative diseases (for review see Stoyanova, 2014; Yin and Zhang, 2016; Amitani et al., 2017).
Ghrelin/GHSR Interactions With the HPA Axis
Beyond its well-established role as a mediator of feeding behavior, the ghrelin system has also been implicated in many other functions. Notably, it has been proposed as a regulator of stress responses, as its function seems to be closely entwined with that of the hypothalamic-pituitary-adrenal (HPA) axis (for review see Spencer et al., 2015), as illustrated in Figure 1.
Several studies have demonstrated that systemic or central administration of ghrelin increases the plasma concentration of corticosterone in rodents or cortisol in humans (Asakawa et al., 2001a; Stevanovic et al., 2007; Lambert et al., 2011; Cabral et al., 2012, 2016; Jensen et al., 2016). The effects of ghrelin on plasma adrenocorticotropic hormone (ACTH), however, appear inconsistent. One study reported elevated ACTH and an increased volume of ACTH-producing cells in the pituitary following central ghrelin administration (Stevanovic et al., 2007), but another study found no influence of ghrelin on plasma ACTH levels (Jensen et al., 2016). In line with the notion that the ghrelin system may increase activity of the HPA axis, a blunted release of ACTH and corticosterone after exposure to a brief period of immobilization stress was previously observed in ghrelin KO mice (Spencer et al., 2012). Similarly, one study showed lower corticosterone levels in GHSR KO compared to wild type mice in a chronic social defeat stress (CSDS) paradigm (Chuang et al., 2011). In contrast to the aforementioned studies, a more recent publication reported higher corticosterone release after acute restraint stress in non-handled ghrelin KO mice versus wild type controls (Mahbod et al., 2018). Moreover, more elevated plasma corticosterone levels have also been observed in GHSR KO mice following acute and chronic stress paradigms (Patterson et al., 2013a; Mahbod et al., 2018). Interestingly, administration of DAG increased plasma corticosterone levels in non-stressful conditions in ghrelin KO mice, but attenuated corticosterone release during acute stress exposure (Stark et al., 2016), suggesting that the effects of DAG on HPA axis activity may depend on prior stress exposure.
Taken together, the influence of the ghrelin system on HPA axis activity is not fully resolved yet and the underlying mechanisms remain poorly understood. Cabral and colleagues demonstrated that peripheral AG administration induces c-Fos expression not only in the ARC but also in corticotropin releasing factor (CRF)-producing cells in the paraventricular nucleus (PVN) of the hypothalamus (Cabral et al., 2012). These cells, however, do not express GHSR, so in a further study the authors suggested that AG may indirectly activate CRF neurons in the PVN via inhibition of the local GABAergic tone (Cabral et al., 2012, 2016), which was confirmed by a more recent electrophysiological study (Dos-Santos et al., 2018). Ghrelin may also influence the HPA axis through other mechanisms (Figure 1). Intravenous administration of ghrelin appears to inhibit vagal afferents (Asakawa et al., 2001b; Date et al., 2006) and stimulate vagal efferents (Fujitsuka et al., 2011). The influence of the ghrelin system on activity of the vagal nerve remains controversial (Arnold et al., 2006) and may involve activation of GHSR in the NG of the vagal nerve or central mechanisms with activation of GHSR in the NTS (Bansal et al., 2012; Swartz et al., 2014; Su et al., 2019). Further elucidation of the interaction of the ghrelin system with the vagus nerve may be relevant in this context, given that vagal nerve stimulation influences HPA axis activity in rodents (De Herdt et al., 2009) and humans (Warren et al., 2019). Moreover, ghrelin may also have an impact on glucocorticoid production through direct effects on the adrenal gland (Rucinski et al., 2009).
Conversely, how activity in the HPA axis may influence the ghrelin system also remains subject of debate (Figure 1). Administration of an ACTH analog was shown to increase plasma ghrelin levels in humans by increasing cortisol levels (Azzam et al., 2017). On the other hand, chronic immobilization stress increased plasma AG levels in adrenalectomized rats (Meyer et al., 2014), indicating that stress can drive AG release independently of glucocorticoid production. Interestingly, a recent study demonstrated that elevated AG levels following chronic stress exposure could be reversed by the beta receptor antagonist atenolol (Gupta et al., 2019). This hydrophilic beta receptor antagonist does not readily cross the blood-brain barrier, suggesting that stress-induced elevations in plasma AG may be secondary to activation of the sympathetic nervous system.
The notion that ghrelin is released upon stress exposure and mediates at least some behavioral adaptations to stress, which we review and discuss in the following two sections, supports the idea of ghrelin as a novel stress hormone, acting in concert with but also partially independent of the central HPA axis.
The Role of the Ghrelin System in Stress-Related Behaviors in Rodents
Effects of Stress on Plasma Ghrelin Levels in Rodents
There is ample evidence showing that plasma ghrelin levels are affected by various acute and chronic stressors, as summarized in Table 1. Increased total ghrelin levels were reported following acute water avoidance stress in rats (Kristenssson et al., 2006, Kristenssson et al., 2007) or maternal separation in mice (Schmidt et al., 2006), while elevated DAG but not AG was observed in plasma of rats following a short immobilization stress episode (Nahata et al., 2014). A number of publications also showed no changes in AG or total ghrelin levels after a single exposure to foot shocks (Harmatz et al., 2016; Rostamkhani et al., 2016) or immobilization stress in rats (Gul et al., 2017). Importantly, nutritional status may also play a role, given that acute stressors such as novelty stress (Saegusa et al., 2011) or a single injection of lipopolysaccharide (LPS) (Stengel et al., 2010) led to a decrease in plasma AG alone or both, DAG and AG, when blood samples were collected in food-deprived conditions.
In subchronic stress paradigms like exposure of rats to a flooded cage, elevated AG levels were found (Ochi et al., 2008). Also socially isolated male but not female mice showed increased plasma AG in fasted conditions (Yamada et al., 2015). However, no changes or even decreases in serum ghrelin levels were reported following subchronic social stress or social isolation in rats in a fasted state (Izadi et al., 2018). The effects of stress exposure on plasma ghrelin levels may be dependent on developmental stage and sex, given that subchronic stress induced by limited nesting material seemed to affect plasma ghrelin levels differently in male and female mice, with minor alterations in male pups versus pronounced changes in serum DAG in female pups (Yam et al., 2017).
Regarding chronic stressors, a number of studies reported increased plasma AG levels following CSDS in mice (Lutter et al., 2008; Chuang et al., 2011; Patterson et al., 2013a; Guo et al., 2019; Han et al., 2019). Only one study in mice found decreased total ghrelin levels following CSDS (Razzoli et al., 2015), however, the decrease in total plasma ghrelin was not observed when the food intake was restricted throughout the CSDS paradigm. Similarly, chronic mild unpredictable stress (CUMS) protocols were shown to induce elevated total serum ghrelin levels in rats (Lopez Lopez et al., 2018) and plasma AG levels in mice (Patterson et al., 2010; Huang et al., 2017). Also chronic immobilization stress increased ghrelin or AG levels in rats (Elbassuoni, 2014; Meyer et al., 2014). A recent study showed that elevated plasma AG levels following chronic immobilization stress in adolescent rats persisted up to four months after stress exposure (Yousufzai et al., 2018). Only one study reported decreased total ghrelin levels following repeated exposure to foot shocks (Rostamkhani et al., 2016). In summary, while literature on the effects of acute or subchronic stress exposure on plasma ghrelin levels is conflicting, chronic exposure to stress relatively consistently increases plasma AG levels.
Effects of Stress on Food Intake and Body Weight in Rodents and the Possible Role of Ghrelin and GHSR Signaling
Exposure to acute and chronic stressors impacts on food intake and body weight in rodents and the ghrelin system has been proposed a role in stress-related feeding behaviors, which, however, is not fully established yet. In Table 2 we give an extensive, although certainly not exhaustive, overview of the effects of different acute and chronic stress paradigms on food intake and body weight.
Acute stressors such as a single session of social defeat (Meerlo et al., 1996), immobilization stress (Gul et al., 2017), novelty stress (Saegusa et al., 2011; Matsumoto et al., 2017) but also LPS injection (Stengel et al., 2010) were mostly found to reduce food intake. While only a few of these studies measured body weight, they reported a decrease following exposure to an acute stressor (Meerlo et al., 1996; Gul et al., 2017).
Also in subchronic and chronic stress paradigms food intake and body weight are typically negatively affected. For example, repeated immobilization stress decreased food intake (Jeong et al., 2013; Jiang and Eiden, 2016) and in parallel decreased (Li et al., 2015; Jiang and Eiden, 2016; Mograbi et al., 2020) or had no effect on body weight (Jeong et al., 2013). Also exposure to a flooded cage for five days decreased body weight (Ochi et al., 2008) and, similarly, social isolation for a week (Izadi et al., 2018) or chronic administration of foot shocks (Rostamkhani et al., 2016) reduced food intake and body weight. Early life stress induced by limited access to bedding and nesting material for a week led to attenuated weight gain during stress exposure in male and female pups, which was compensated by an increased weight gain after termination of the stress exposure (Yam et al., 2017). As an exception, only one study found increased food intake and body weight gain during immobilization stress over a period of three weeks, which was more pronounced in male than female rats (Elbassuoni, 2014).
Exposure to CUMS, which utilizes a battery of different stressors over a time course of several weeks, relatively consistently led to decreases in food intake (Patterson et al., 2010; Yao et al., 2016; He et al., 2018; Yan et al., 2018) and body weight in mice and rats (Forbes et al., 1996; Harris et al., 1997; Patterson et al., 2010; Lopez Lopez et al., 2018; Simas et al., 2018; Yan et al., 2018; Li et al., 2019; Yun et al., 2020). A few studies reported decreases in body weight or body weight gain, while food intake was not significantly altered (Harris et al., 1997; Lopez Lopez et al., 2018; Simas et al., 2018). Only one study found an increase in daily chow consumption and no differences in body weight between CUMS and non-CUMS mice after eight weeks of stress exposure (Huang et al., 2017). Interestingly, Simas et al. reported a decreased food intake in mice exposed to CUMS compared to non-stressed mice when only a high-fat diet (HFD) was provided, but not when fed a standard diet (Simas et al., 2018). This suggests that the rewarding properties of calorically dense food are abolished during CUMS. In line with this notion, several of the previously mentioned studies also evaluated sucrose or saccharine preference. Some found no differences (Forbes et al., 1996; Harris et al., 1997; Yun et al., 2020), but in several studies CUMS exposure led to reduced sucrose or saccharine intake (Yao et al., 2016; Huang et al., 2017; He et al., 2018; Yan et al., 2018), which may reflect stress-induced anhedonia.
On the other hand, CSDS in mice quite robustly led to increases in food intake (Lutter et al., 2008; Patterson et al., 2013a, b; Razzoli et al., 2015), associated with an increase in body weight in some studies (Patterson et al., 2013a; Razzoli et al., 2015) but not in others (Lutter et al., 2008; Patterson et al., 2013b). Chuang and colleagues showed that conditioned place preference (CPP) for HFD was significantly increased in CSDS-exposed mice, which was associated with a higher intake of HFD chow and a greater weight gain over the course of the CPP paradigm (Chuang et al., 2011). However, in contrast to these studies in mice, subchronic and chronic social defeat paradigms in rats resulted in decreases in daily food intake (Berton et al., 1998) and body weight (Berton et al., 1998; Fanous et al., 2011; Patki et al., 2013).
Some of the aforementioned studies also investigated the role of the GHSR in stress-related changes in feeding behavior and found that both increases (CSDS) or decreases (CUMS) in food intake and body weight gain were abolished in GHSR KO mice (Lutter et al., 2008; Patterson et al., 2010, 2013a). Moreover, increased CPP for and higher intake of HFD and an associated weight gain after CSDS exposure were not present in GHSR KO mice (Chuang et al., 2011), indicating a central role of ghrelin signaling in these stress-induced changes. The increased CPP, however, could be reinstated by selective re-expression of GHSR in tyrosine hydroxylase-positive neurons, which the authors concluded are very likely involved in mediating stress-induced food reward. Interestingly, a recent study used re-expression of GHSR in dopamine transporter (DAT)-positive neurons of GHSR KO mice to confirm that GHSR signaling in dopaminergic neurons controls appetitive and consummatory behaviors toward HFD in ad libitum fed mice (Cornejo et al., 2020). Indeed, it has been proposed previously that the ghrelin system may play an important role in stress-induced food reward behavior and act as a critical modulator at the interface of homeostatic control of appetite and food reward during stress, promoting hyperphagia and hedonic intake of calorically dense ‘comfort foods’ (Schellekens et al., 2012).
Even though stress paradigms typically increase AG levels and in some instances increase feeding, paradoxically, exposure to stress may also be associated with a decrease in food intake and body weight. During acute stress exposure, it is likely that the orexigenic effects of high plasma AG are overcome by other factors, such as stress-induced changes in CRF signaling in the brain (Jochman et al., 2005; Haque et al., 2013; Dunn et al., 2015; Mogami et al., 2016). Chronic stress, on the other hand, might induce central ghrelin resistance, associated with lower GHSR expression in particular brain areas (Harmatz et al., 2016), which then may further contribute to reduced food intake and anhedonia-like behaviors in a GHSR-dependent manner, but irrespective of high circulating AG concentrations. This may apply for physical stressors like foot shocks, immobilization stress or various stressors used during CUMS which are typically associated with reduced food intake and decreases in body weight. The same hypothesis, however, does not seem to hold true for CSDS in mice, where high plasma AG is mostly associated with increases in food intake and where GHSR signaling in dopaminergic neurons has been proposed to mediate stress-induced food reward as a coping mechanism (Chuang et al., 2011). The observation that CSDS increases food intake and/or body weight specifically in mice but not in rats could be attributed to species differences in social dominance behavior (Kondrakiewicz et al., 2019). The question remains what impact circulating ghrelin and central GHSR signaling may have on other stress-related behaviors in rodents.
Role of Circulating Ghrelin in Fear, Anxiety- and Depression-Like Behaviors
While some studies propose that ghrelin reduces fear, anxiety- and depression-like behaviors in rodents, others actually suggest an opposite role. One early study showed that central administration of ghrelin antisense DNA had anxiolytic and antidepressant-like effects and reduced the retrieval of conditioned fear in unstressed rats (Kanehisa et al., 2006). Similarly, Spencer and colleagues found reduced anxiety-like behaviors in ghrelin KO mice under baseline conditions, but increased anxiety scores following acute immobilization stress (Spencer et al., 2012). However, a more recent study also found higher anxiety-like behavior in ghrelin KO mice under non-stressed conditions (Mahbod et al., 2018). A genetic deletion of GOAT and thus attenuated AG levels (Zhao et al., 2010) reduced anxiety-like behavior prior to stress exposure (Mahbod et al., 2018) or was anxiogenic regardless of stress conditions (Stark et al., 2016).
Pharmacological studies investigating the effects of AG and other GHSR ligands have also delivered contradictory results. Systemic or central injection of a single dose of AG has been shown to induce anxiety- and depression-like behaviors in mice and rats that were not previously subjected to stress (Asakawa et al., 2001a; Carlini et al., 2002, 2004; Currie et al., 2012, 2014; Brockway et al., 2016; Jackson et al., 2019). Similarly, central infusion of ghrelin for one month increased anxiety- and depression-like behavior in rats (Hansson et al., 2011). In contrast, a few other studies reported anxiolytic and antidepressant-like effects of a single systemic dose of AG in non-stressed mice (Lutter et al., 2008; Jensen et al., 2016). In a recent publication, the administration of AG into the dorsal raphe nucleus was anxiolytic in an inhibitory avoidance test but facilitated learned escape behavior in rats (Cavalcante et al., 2019).
In studies where AG is administered systemically, it should generally be considered that it can be converted to DAG (De Vriese et al., 2004). This could be relevant, given that DAG was found to have anxiogenic effects in ghrelin KO mice which were not previously subjected to stress (Stark et al., 2016). Moreover, the effects of exogenous AG administration may also depend on feeding state. An injection of ghrelin into the amygdala had no significant effect in fed mice, but was anxiolytic when no food was available (Alvarez-Crespo et al., 2012), suggesting that AG may promote foraging and risk-taking in response to a negative energy balance.
When focusing on the effects of exogenously administered ghrelin following stress exposure, an anxiolytic effect appears more evident. Peripherally administered AG attenuated anxiety-like behavior and stress responses after exposure to air puffs or immobilization stress (Jensen et al., 2016). Interestingly, DAG also produced anxiolytic-like effects following stress exposure in ghrelin KO mice (Stark et al., 2016). Moreover, anxiety- and depression-like behaviors induced by CUMS were at least partly rescued by administration of ghrelin or the ghrelin agonist GHRP-6 (Huang et al., 2017, 2019). A recent study demonstrated that intra-hippocampal administration of ghrelin fully and intraperitoneal injection partly normalized stress-enhanced anxiety- and depression-like phenotypes in a CSDS paradigm (Han et al., 2019). However, another study found that reduced social interaction following CSDS exposure could not be rescued by subcutaneous administration of AG or ghrelin agonist GHRP-2 (Gupta et al., 2019).
A number of studies also investigated how GHSR ligands affect fear conditioning and extinction. Administration of the GHSR agonist MK0677, systemically or directly into the basolateral amygdala, around the time of fear conditioning reduced contextual and cued fear memory strength in unstressed rats, while the GHSR antagonist D-Lys3 increased it (Harmatz et al., 2016). An earlier study of the same group, however, showed that repeated systemic or intra-amygdalar injection of MK0677 led to an enhancement of cued fear memory strength (Meyer et al., 2014). In rats that were subjected to chronic immobilization stress, stress-induced increases in cued fear were abolished when the GHSR antagonist D-Lys3-GHRP-6 was administered daily before stress exposure (Meyer et al., 2014). In their 2016 study, this group reported that increased cued fear in chronically stress-exposed rats was associated with reduced binding of fluorescently labeled ghrelin in the basolateral amygdala, which they defined as central ghrelin resistance (Harmatz et al., 2016). Therefore, the authors hypothesized that a stress-induced elevation of AG levels and prolonged GHSR activation may lead to impaired GHSR signaling in the brain via downregulation of GHSR expression (Harmatz et al., 2016). Interestingly, infusion of MK0677 into the lateral amygdala was also shown to improve cued fear extinction in non-stressed mice (Huang et al., 2016). However, in our recently published study, we did not find significant effects of systemic or intra-VTA administration of MK0677 on cued fear expression or extinction in non-stressed mice (Pierre et al., 2020). Importantly, as the GHSR is constitutively active in the absence of ghrelin (Holst et al., 2003; Petersen et al., 2009), it is therefore important to consider the effects of central GHSR signaling independently of circulating AG or exogenous administration of an GHSR agonist.
Role of GHSR Signaling in Fear, Anxiety- and Depression-Like Behaviors
Under non-stressed conditions, no or only marginal effects on fear, anxiety- and depression like behaviors were observed in GHSR KO mice compared to wild type controls (Mahbod et al., 2018; Guo et al., 2019; Lu et al., 2019; Pierre et al., 2019). Nevertheless, one study found anxiolytic and antidepressant-like effects following overexpression of GHSR in the basolateral amygdala in non-stressed mice (Jensen et al., 2016). Another study reported reduced fear memory retention after contextual fear conditioning in unstressed GHSR KO mice (Albarran-Zeckler et al., 2012), but this observation was not confirmed in a more recent study (Hornsby et al., 2016).
Clearer evidence for behavioral effects in mice lacking the GHSR was obtained following stress exposure, where most studies reported impaired stress coping. For example, mild stressors like air puffs induced an exacerbated avoidance response in GHSR KO mice (Jensen et al., 2016). Similarly, CSDS resulted in more pronounced depression-like behavior in GHSR KO mice, as assessed by a social avoidance test (Lutter et al., 2008; Chuang et al., 2011). A local knockdown of the GHSR in the hippocampus also aggravated anxiety- and depression-like behaviors in mice following exposure to a CSDS or CUMS paradigm (Han et al., 2019; Huang et al., 2019), pointing to a protective role of the GHSR during chronic stress.
Effects of Caloric Restriction on Fear, Anxiety- and Depression-Like Behaviors
Caloric restriction and food deprivation paradigms typically increase plasma ghrelin levels and were also used in several studies to manipulate the endogenous ghrelin system and thus indirectly study its effects on fear, anxiety- and depression-related behaviors in rodents. An initial study demonstrated anxiolytic and antidepressant-like effects of caloric restriction that were abolished in GHSR KO mice (Lutter et al., 2008). In a later publication, the same group showed that the neuroprotective agent P7C3 restored the antidepressant-like effect of caloric restriction in GHSR KO mice, potentially by increasing hippocampal neurogenesis (Walker et al., 2015). This suggests that the effects of caloric restriction on depression-like behaviors may depend on GHSR signaling in the hippocampus and ensuing neurogenesis. Recently, a study reported that acute food deprivation for 24 h and chronic caloric restriction had anxiolytic- and antidepressant-like effects in wild type mice, which were abolished after re-feeding (Lu et al., 2019). In GHSR-KO mice, however, acute food deprivation enhanced, while only chronic caloric restriction reduced anxiety- and depression-like responses. This led the authors to conclude that the positive effect of acute but not chronic fasting was GHSR-dependent (Lu et al., 2019). The finding that the anxiolytic-like action of 24 h food deprivation in wild type mice was neutralized by administration of a GHSR antagonist further supported this conclusion (Lu et al., 2019).
In fear conditioning paradigms, caloric restriction and overnight fasting before extinction training were shown to enhance fear extinction and suppress return of fear in mice (Riddle et al., 2013; Huang et al., 2016; Verma et al., 2016), an effect that was abolished by administration of the GHSR antagonist D-Lys3 into the lateral amygdala (Huang et al., 2016). Interestingly, also in humans spontaneous recovery and reinstatement of fear were reduced after an overnight fast, which was correlated with ghrelin plasma levels (Shi et al., 2018). However, we could not confirm the extinction-promoting effect of overnight fasting using a weak extinction protocol and also found no difference between wild type and GHSR KO mice (Pierre et al., 2020). Thus, while manipulation of GHSR signaling may be an interesting avenue to curb fear expression or promote fear extinction, it appears that the experimental conditions under which such effects can be observed remain uncertain.
Summary, Shortcomings and Future Directions of Rodent Studies Investigating the Role of the Ghrelin System in Stress-Related Behaviors
The often mixed findings of rodent studies make it challenging to draw a general conclusion about the role of ghrelin and GHSR in stress-related behaviors. Possible reasons for these inconsistencies are diverse: the use of different mouse or rat strains, different age or sex of the animals, varying breeding, handling or habituation conditions, different time of day for testing and use of diverse behavioral and stress paradigms. The feeding status of the animals during testing may be of particular importance, as administration of exogenous ghrelin or GHSR agonists may confound the readout of behavioral assays by the instatement of hunger.
In summary, published literature suggests that the ghrelin system plays a different role in individuals depending on the amount and quality of previous stress exposure. This seems most obvious for GHSR signaling, which does not appear to affect fear, anxiety- and depression-like behaviors under non-stressed conditions but may exert mostly protective effects during chronic stress. A similar trend can be observed in pharmacological studies using GSHR ligands, where, without prior stress exposure, GHSR agonists predominantly promote anxiety- and depression-like behaviors, but seem to reduce chronic stress-related phenotypes. Also, caloric restriction may have beneficial effects on fear, anxiety- and depression-like behaviors in unstressed rodents through activation of GHSR. Nevertheless, the response of rodents to the potentially stressful nature of food deprivation might additionally challenge stress coping mechanisms and, together with differences in fasting procedures, contribute to inconsistencies in literature.
It has been proposed that prolonged elevation of plasma AG levels during chronic stress induces a central ghrelin resistance with reduced GHSR expression (Harmatz et al., 2016), which would impede ligand-dependent as well as ligand-independent GHSR signaling and thereby eliminate its protective role during stress. In the face of central ghrelin resistance, a rescue of stress-related phenotypes by AG or GHSR agonists as well as caloric restriction, which elevates endogenous ghrelin levels, seems unlikely. Nevertheless, many studies suggest beneficial effects of GHSR agonism and fasting on fear, anxiety-, and depression-like behaviors.
Only a few studies have considered the role of DAG in stress coping, although it constitutes the majority of total circulating plasma ghrelin and may have behavioral effects that are distinct from those of AG. This applies not only for feeding behaviors (Fernandez et al., 2016) but also for stress-related behaviors (Stark et al., 2016). This may be relevant during chronic stress and associated central ghrelin resistance, as AG can be rapidly converted to DAG (De Vriese et al., 2004), which appears to not act via the GHSR (Bednarek et al., 2000; Ferre et al., 2019). Thus, the reported beneficial effects of exogenous AG administration during chronic stress paradigms in rodents may be attributed to DAG rather than AG action. In this context, it also can be noted that the way of reporting plasma ghrelin levels has not been consistent in existing literature. Studies often report either only total or AG levels or do not specify acylation status at all, thereby hindering the overview of ghrelin alterations in baseline versus stressed conditions.
One more point to consider is that the GHSR may have functions independent from binding of AG, which is of particular importance since accessibility of many GHSR-expressing brain areas for the ghrelin peptide is not resolved yet (Perello et al., 2018). While the endogenous peptide LEAP2 has been shown to act as an antagonist/inverse agonist of the GHSR (Ge et al., 2018), it remains unknown to what extent it influences GHSR activity in different brain areas. Because of its high constitutive activity (Holst et al., 2003; Petersen et al., 2009) also changes in GHSR expression levels will accordingly alter activation of downstream signaling pathways as well as a possible formation of heterodimers with other receptors, such as receptors for dopamine and serotonin (Schellekens et al., 2013b; Kern et al., 2015). Given that heterodimer formation influences receptor signaling and trafficking (for review see Schellekens et al., 2013a; Abizaid and Hougland, 2020), this could serve to fine-tune GHSR signaling in response to certain neurotransmitters and, conversely, GHSR signaling would also affect these neurotransmitter systems. These complex (inter)actions of the GHSR could further result in individual differences, where an impairment of GHSR signaling occurs in stress-susceptible but not stress-resilient individuals, in which GHSR agonism still would have beneficial effects. Therefore, it might be useful to identify such individual differences in GHSR sensitivity and investigate which factors contribute to constitutive GHSR signaling.
Furthermore, this requires a clear distinction between findings from studies manipulating peptide hormone levels or using GHSR ligands (e.g., ghrelin KO mice, GOAT KO mice, pharmacological studies) versus studies conducted in GHSR KO mice. For a comprehensive dissection of the role of GHSR signaling in distinct neuronal populations, the development of new biomolecular tools like GHSR-Cre mice is indispensable in order to perform targeted pharmaco- and optogenetic manipulations and selectively employ new viral techniques. Such novel genetic and viral tools could also be used to create conditional knockouts of GHSR, GOAT or ghrelin. It cannot be excluded that in conventional germ-line knockouts compensatory mechanisms are at play that disguise the loss-of-function effect, thereby confounding results and leading to mixed findings and wrong conclusions.
The Ghrelin System as a Novel Therapeutic Avenue in Stress-Related Psychiatric Disorders?
A Need for New Treatment Strategies: Ghrelin as a Vantage Point?
In this review, we aimed to provide an overview of the current literature on the role of ghrelin system in stress coping. We summarized findings on fear, anxiety- and depression-like behaviors in rodent models and in this final chapter, we want to discuss the possible therapeutic utility of the ghrelin system in stress-related psychiatric disorders, with a focus on anxiety disorders and post-traumatic stress disorder (PTSD). They are the most common cause of psychiatric illness, with an estimated lifetime prevalence of up to 30% (Kessler et al., 2005a, 2012) and women being affected almost twice as often as men (Baxter et al., 2013). Besides reducing the quality of life of patients and their families dramatically, they cause an immense socioeconomic burden in modern western societies (Wittchen et al., 2011; Olesen et al., 2012). However, a lack of major advances in psychopharmacotherapy over the past three decades as well as limited validity of current symptom-based diagnostic manuals (Lang et al., 2016) leave anxiety disorders and PTSD poorly diagnosed and undertreated (Craske et al., 2017). Moreover, a large number of anxiety patients do not show long-term benefit from currently available treatments and fail to achieve full remission or often relapse with time (Hofmann and Smits, 2008; Bandelow et al., 2015; Koek et al., 2016; Craske et al., 2017). Currently, there are also no biomarkers for the measurement of treatment response available (Craske et al., 2017). Thus, there is an urgent need for the development of novel strategies to augment existing diagnostic and treatment options to improve their efficiency and tackle under- and misdiagnosis as well as treatment resistance (Singewald et al., 2015; Sartori and Singewald, 2019).
There is a growing interest in the involvement and therapeutic potential of the ghrelin system in psychiatric diseases. Positioned at the interface between feeding circuitry, metabolism and the HPA axis, its dysregulation could not only potentially influence food intake and energy homeostasis but also stress vulnerability. This involvement of the ghrelin system in different physiological processes has sparked a discussion about its role in a variety of pathological conditions like obesity and eating disorders, but also addictive disorders as well as anxiety disorders, PTSD and mood disorders (for review see Labarthe et al., 2014; Wittekind and Kluge, 2015; Bali and Jaggi, 2016; Stievenard et al., 2016; Jiao et al., 2017; Shi et al., 2017). The potential role of the ghrelin system is further underscored by the notion that mood-, anxiety- and trauma-related disorders are often associated with metabolic dysregulation and also show a high level of comorbidity with obesity or eating disorders (Scott et al., 2008; Ng et al., 2013; Mason et al., 2014; Meng and D’Arcy, 2015; Aaseth et al., 2019). It is very likely that comorbid diseases share a common neurobiological pathology, which could be of use to gain a better understanding of underlying disease mechanisms and to identify potential therapeutic targets.
(Dysregulation of) the Ghrelin System in Stress-Related Psychiatric Disorders
In humans, acute exposure to stressors is associated with elevated plasma ghrelin concentrations. In healthy individuals but also in obese patients with binge-eating disorder, total plasma ghrelin levels were elevated in those individuals who also had a high cortisol response to a Trier social stress test (TSST) (Rouach et al., 2007). Similarly, two studies with only female participants found elevated plasma cortisol and AG levels in healthy women (Raspopow et al., 2010) and higher saliva ghrelin but not cortisol levels in women suffering from bulimia nervosa (Monteleone et al., 2012) after stress exposure in a TSST. Other studies with only male participants reported no changes or, after alcohol consumption, even decreased AG levels following a TSST (Zimmermann et al., 2007) and no changes in total plasma ghrelin levels before and after a forced arithmetic task in a cohort of lean and obese men (Lambert et al., 2011). Taken together, these studies strongly suggest that the effects of acute stress exposure on plasma ghrelin levels may be dependent on sex. While the effects of chronic stress exposure have not been investigated explicitly in humans, one translational study reported elevated AG in teenagers 4.5 years after experiencing a severe traumatic event and proposed high plasma AG levels as a long-lasting biomarker for chronic stress exposure and a perpetuating risk factor for stress-enhanced fear (Yousufzai et al., 2018).
In line with the notion that alterations of the ghrelin system may be a biomarker for stress exposure, several other studies have investigated possible dysregulations of the ghrelin system in stress-related psychiatric disorders such as anxiety disorders, PTSD or major depressive disorder (MDD). The Gln90Le polymorphism in the pre-proghrelin gene has been linked to an increased risk of panic disorder (Hansson et al., 2013), while, in contrast, the Leu72Met variant of the pre-proghrelin gene was not associated with panic disorder (Nakashima et al., 2008) but with symptom severity in PTSD (Li et al., 2018) and MDD (Nakashima et al., 2008). A recent study reported elevated plasma ghrelin levels in children with a diagnosis of an anxiety disorder, which was more pronounced in girls than boys (Ozmen et al., 2019). Interestingly, ghrelin levels, serum cortisol and anxiety scores together with body mass index were more reduced in obese children following a combined dietary and mindfulness intervention in comparison to a control group that only underwent a dietary intervention (Lopez-Alarcon et al., 2020). However, in female patients diagnosed with anorexia nervosa or obesity, ghrelin levels were not correlated with depression- and anxiety-like symptoms independently of weight and body fat (Lawson et al., 2012). Numerous studies investigated plasma ghrelin levels in patients with MDD, which is often comorbid with anxiety- and trauma-related disorders and associated with stress-induced changes in food intake (Kessler, 1997, Kessler et al., 2005b; Kendler et al., 1999; Grant et al., 2013). They found increased fasting AG (Kurt et al., 2007; Algul and Ozcelik, 2018) and total ghrelin (Ozsoy et al., 2014), but also decreased fasting AG and DAG (Barim et al., 2009) or no changes in fasting AG or total ghrelin levels at all (Schanze et al., 2008; Kluge et al., 2010; Gimenez-Palop et al., 2012; Matsuo et al., 2012). Two of these studies showed a positive correlation of eating behavior with total ghrelin levels in MDD patients (Schanze et al., 2008; Matsuo et al., 2012), while another one reported lower AG levels in depressed patients with increased appetite (Simmons et al., 2020), indicating that plasma AG may not be good predictor for appetite or food intake in depressed patients.
The effect of treatment on ghrelin plasma levels in stress-related psychiatric disorders (mainly MDD) has also been examined. One study that found reduced plasma AG and DAG when comparing untreated MDD patients with healthy controls reported no effect of citalopram on ghrelin levels (Barim et al., 2009). Maprotiline treatment in male lean MDD patients, on the other hand, resulted in mildly increased plasma ghrelin levels (Pinar et al., 2008). In a mixed patient group suffering from bipolar disorder or MDD, serum AG levels were elevated compared to controls and decreased after electroconvulsive therapy (Kurt et al., 2007). A similar decrease in total ghrelin levels was reported in another study where patients received electroconvulsive therapy and/or different antidepressant drugs (Ozsoy et al., 2014). Interestingly, one study found, that therapy resistance in MDD and panic disorder was correlated with elevated AG (Ishitobi et al., 2012) and the effect of lithium augmentation treatment in treatment-resistant MDD could be characterized by a slight decrease in plasma AG in responders compared to an increase in non-responders (Ricken et al., 2017).
The conflicting findings in these studies probably result from confounding factors like nutritional status and time of day at sample collection, gender, age, body mass index, comorbid disorders and drug treatments, such as the type of antidepressant. Moreover, not all studies distinguished between AG and DAG and only measured total ghrelin levels or did not specify acylation status. Overall, it remains inconclusive whether genetic variants in the ghrelin system are causally involved in stress-related psychiatric disorders and whether altered ghrelin plasma levels are a predisposing factor for or just a byproduct of these diseases. Elevated plasma AG could indicate prior chronic stress exposure (Yousufzai et al., 2018), which in turn may enhance the risk for mental health disorders. Nevertheless, plasma AG appears to correlate with treatment resistance, suggesting more than mere association. Moreover, experiments in rodents indicate that stress-induced activation of the ghrelin system not only influences feeding but may also have a direct impact on fear, anxiety- and depression-like behaviors.
Therapeutic Potential of Targeting the Ghrelin System in Stress-Related Psychiatric Disorders
Despite the role of the ghrelin system in stress-related psychiatric disorders is not fully resolved yet, it provides a new and interesting vantage point in the search for more efficient diagnostic and therapeutic strategies (also see Figure 1). It remains unknown whether a modulation of the ghrelin system could be beneficial in the treatment of stress-related psychiatric disorders, however multiple pharmacological tools to do so have become available over the last two decades (McGovern et al., 2016; Lee et al., 2020; Moose et al., 2020). This includes GHSR agonists and antagonists as well as inverse agonists and GOAT inhibitors, although no authorized medicine is commercially available to date. The pharmacological characterization of many ligands has only just begun and it will be of great importance to consider their central penetrance and possible signaling bias and further elucidate downstream signaling in order to allow targeted predictive modeling and the design of future ghrelin-based therapies (Ramirez et al., 2019; Howick et al., 2020). To our knowledge, there are currently no studies published that directly investigated the effect of ghrelin-targeted interventions in stress-related psychiatric conditions. One study in healthy human participants found enhanced extinction memory retention and reduced return of fear following an overnight fast, which was negatively correlated with ghrelin plasma levels (Shi et al., 2018). Whether fasting may improve exposure-based psychotherapy outcomes in patients with anxiety disorders or PTSD and whether this would be associated with changes in plasma AG concentrations or brain GHSR function remains to be established.
As a biomarker, high plasma AG could indicate prior stress exposure (Yousufzai et al., 2018), which in turn may enhance the risk for stress-related psychiatric disorders. While plasma AG concentrations were not consistently associated with disease, they may predict treatment resistance (Ishitobi et al., 2012; Ricken et al., 2017). In several studies, a decrease in plasma AG or total ghrelin levels during therapy was also associated with treatment response (Kurt et al., 2007; Ozsoy et al., 2014; Ricken et al., 2017; Lopez-Alarcon et al., 2020). A lack of changes in plasma AG concentrations may thus reflect decreased sensitivity of the GHSR and dysregulation of its feedback system. In light of our previous discussion that GHSR in the brain may act partly independent of circulating AG, this highlights the therapeutic potential for the development of diagnostic tools to study brain GHSR function in order to aid further stratification of patients for an optimization of treatment strategies.
It also should be kept in mind that potentially beneficial effects associated with activation of the ghrelin system, as observed in some rodent experiments, may be lost in patients with impaired GHSR function (Harmatz et al., 2016). To this end, a precise definition of the phenomenon of central ghrelin resistance and corresponding neurobiological correlates is indispensable. Present literature merely suggests an impaired feeding response following ghrelin (agonist) administration, associated with reduced c-Fos expression in the ARC (Briggs et al., 2010) and a reduction of AG binding (Harmatz et al., 2016) as markers for disrupted GHSR function. In addition, it is presently unclear whether fasting or other interventions could rapidly reverse central ghrelin resistance. A loss of GHSR function in hypothalamic NPY/AgRP neurons as a result of diet-induced obesity could be rescued by caloric restriction (Briggs et al., 2010, 2013), but so far no study investigated whether this can be extrapolated to stress-induced central ghrelin resistance in brain regions beyond the hypothalamus. Instead of treatment, also a prevention of central ghrelin resistance could be considered. For example, a study in rats showed that administration of a GHSR antagonist during a chronic immobilization paradigm prevented the emergence of stress-enhanced fear (Meyer et al., 2014), while the same paradigm without GHSR antagonist administration led to reduced GHSR expression in the basolateral amygdala, which hampered the attenuation of stress-enhanced fear memories by a GHSR agonist (Harmatz et al., 2016). Therefore, preservation of GHSR function during chronic stress may keep the ghrelin system targetable for treatment interventions.
Also indirect targeting of the ghrelin system by other approaches may be of relevance in stress-related psychiatric disorders, for example via the gut microbiome (Schalla and Stengel, 2020). Changes in diet and gut microbiota composition have been linked to changes in plasma ghrelin levels in rodents and humans (for review see Lach et al., 2018). Moreover, it has been proposed that short-chain fatty acids produced by gastrointestinal microbiota may compete with octanoate for binding at the GOAT and interfere with the acylation of ghrelin (Heiman and Greenway, 2016). A recent study showed that different microbiota-derived metabolites, amongst them also short-chain fatty acids, may also directly attenuate GHSR signaling (Torres-Fuentes et al., 2019). Interestingly, one clinical study demonstrated that the use of dietary capsaicin altered gut microbiota composition and reduced ghrelin levels in healthy subjects (Kang et al., 2016). This is of particular interest, given that elevated plasma AG may reflect a higher risk for the development of stress-related psychiatric conditions (Yousufzai et al., 2018). Overall, this highlights the possibility of utilizing the gut microbiome to influence ghrelin signaling and thereby modulate the gut-brain axis in stress, although the underlying mechanisms how gut microbiota interact with the ghrelin system are not fully resolved yet.
We believe that the therapeutic potential of ghrelin-targeted interventions in stress-related psychiatric disorders is just starting to be explored. Especially a better understanding of the interactions of ghrelin with the HPA axis, the role of DAG versus AG and factors that control constitutive GHSR activity or lead to ghrelin resistance in the brain may uncover novel diagnostic and therapeutic avenues that have not been considered so far.
Interaction of Ghrelin With Neurotransmitter Systems as a Gateway to the Treatment of Psychiatric Disorders
Abundant evidence demonstrates interaction of the ghrelin system with different neurotransmitters, neuromodulators and their receptors, including monoamines, neuropeptides and endocannabinoids (Brunetti et al., 2002; Skibicka et al., 2012b; Edwards and Abizaid, 2016; Hedegaard and Holst, 2020). One possibility how the ghrelin system may affect behaviors relevant for psychiatric disorders is through its effect on the limbic system. These effects may result from direct activation of GHSR in different limbic areas like hippocampus or amygdala but may also be the consequence of activation of GHSR in neurotransmitter systems projecting to these limbic areas. In this regard, the effect of ghrelin signaling on the release of noradrenalin, serotonin and dopamine merits further discussion. Only few studies have investigated the effect of ghrelin on noradrenalin and serotonin release. One study found that peripheral ghrelin may activate the noradrenergic pathway from the hindbrain to the hypothalamus (Date et al., 2006) and another study reported that food deprivation and central administration of ghrelin augmented the increase of noradrenalin release in the PVN following foot shock stress in rats (Kawakami et al., 2008). Concerning ghrelin’s effects on serotonin signaling, one study showed that ghrelin postsynaptically depolarized serotonin neurons in rat brain slices containing the dorsal raphe nucleus (Ogaya et al., 2011), while a second study found that ghrelin reduced the firing rate of dorsal raphe neurons in brain slices prepared from rats chronically treated with ghrelin (Hansson et al., 2011). Interestingly, acute central administration of ghrelin in mice was shown to increase serotonin turnover and the expression of serotonin-related genes in the amygdala (Hansson et al., 2014). While these studies demonstrate that ghrelin influences serotonin and noradrenaline signaling, the direct implications for fear, anxiety- and mood-related behaviors remain unclear.
Because the interaction of the ghrelin with the dopamine system is studied more extensively, we will further highlight certain aspects of this interaction and, as an example, discuss possible therapeutic relevance. Ghrelin was shown to modulate the spontaneous firing frequency of mesolimbic dopaminergic neurons in the VTA (Abizaid et al., 2006) and trigger dopamine release in the nucleus accumbens (NAc), predominantly in the shell region (Jerlhag et al., 2007; Jerlhag, 2008; Quarta et al., 2009; Cone et al., 2014). Associated with this rise of extracellular dopamine levels in the NAc following systemic or intra-VTA administration of ghrelin, stimulation of locomotor activity and conditioned place preference was observed (Jerlhag et al., 2007; Jerlhag, 2008). GHSR antagonism or a ghrelin KO, on the other hand, attenuated alcohol-, cocaine-, amphetamine- or morphine-induced locomotor stimulation and CPP and abolished the associated increase of accumbal dopamine release (Jerlhag et al., 2010; Jerlhag et al., 2011; Engel et al., 2015). In paradigms offering a free choice between standard chow and rewarding (palatable) food, ghrelin in the VTA stimulated hedonic feeding, but not homeostatic food intake (Egecioglu et al., 2010; Skibicka et al., 2011, 2013; Schele et al., 2016). The mechanism via which ghrelin entrains the midbrain dopaminergic system, however, is not fully resolved yet. Recently, it was shown that ghrelin-binding cells are present in most subnuclei of the VTA, but not in the NAc (Cornejo et al., 2018). Moreover, Cornejo and colleagues demonstrated that centrally but not peripherally injected ghrelin recruits specific subsets of dopaminergic neurons in the parabrachial nucleus and GABAergic neurons in the interfascicular nucleus of the VTA (Cornejo et al., 2018). As for many other brain areas where the GHSR is expressed, there is no clear evidence whether peripherally circulating ghrelin reaches the VTA and directly acts on midbrain dopaminergic neurons (Perello et al., 2018). Also indirect mechanisms of activation (e.g., via orexigenic neurons in the lateral hypothalamus) have yet to be elucidated (Quarta and Smolders, 2014; Al Massadi et al., 2019). Furthermore, it has been proposed that (hippocampal) dopamine signaling is dependent on heterodimerization between dopamine D1 (DRD1) and GHS receptors, regulating DRD1-induced hippocampal synaptic plasticity and learning (Kern et al., 2015).
The role of ghrelin signaling on mesolimbic dopamine was previously reviewed extensively in the context of food reward (Skibicka and Dickson, 2011; Perello and Zigman, 2012; Skibicka et al., 2012a; Liu and Borgland, 2015; Al Massadi et al., 2019). However, these actions of ghrelin may also be relevant for stress-related psychiatric disorders. A possible role in mood disorders seems likely, given that ghrelin has been proposed to mediate CSDS-induced food reward (Chuang et al., 2011) and protect against depressive-like symptoms following CSDS in mice (Lutter et al., 2008). This is further corroborated by the observation that hyperphagic depressed patients showed a greater change in AG in response to a meal, which was associated with increased activity in the VTA (Cerit et al., 2019). As such, ghrelin signaling on mesolimbic dopamine neurons may indeed constitute the interface of stress, mood and food reward (Schellekens et al., 2012). With respect to anxiety disorders and PTSD, the abundant expression of the GHSR and GHSR mRNA on/in midbrain dopamine neurons of the VTA (Zigman et al., 2006; Mani et al., 2014) is particularly interesting (Figure 1), as accumulating evidence suggests midbrain dopaminergic signaling as an important player in fear extinction (Abraham et al., 2014; Kalisch et al., 2019). The extinction of fear is the driving mechanism behind the reduction of fear responses and very basis of exposure-based cognitive behavioral therapy (Milad and Quirk, 2012), which is first-line in the treatment of anxiety- and trauma-related disorders (Craske, 2010; Craske et al., 2017). Also translationally, this paradigm is very appealing as it is easy to model in the laboratory in rodent models as well as humans (Milad and Quirk, 2012; Singewald and Holmes, 2019). Moreover, impaired fear extinction was suggested as a relevant endophenotype for anxiety disorders and PTSD (Singewald and Holmes, 2019). Recently, it has been proposed that during extinction learning midbrain dopaminergic neurons encode a quasi-appetitive reward prediction error, signaling the omission of the aversive unconditioned stimulus as a ‘better-than-expected’ outcome (Luo et al., 2018; Salinas-Hernandez et al., 2018; Cai et al., 2020). Besides this temporally restricted role in extinction acquisition, dopamine was also suggested to play a key role in the consolidation phase in a human fMRI study (Gerlicher et al., 2018). In a collaborative study, our lab has previously shown that enhancing dopaminergic signaling by L-DOPA treatment helps the formation of long-lasting extinction memories and prevents return of fear in extinction-competent mice and healthy humans (Haaker et al., 2013). Moreover, we were able to show that L-DOPA treatment can rescue deficient fear extinction in a mouse model for refractory anxiety disorders (Whittle et al., 2016).
Despite this clear evidence of interaction of the two systems, very few studies have investigated the possible benefits of targeting the ghrelin-dopamine axis in stress-related psychiatric disorders. We were able to show recently, that administration of the ghrelin agonist MK0677 directly into the VTA induces dopamine release in the NAc but also in amygdala and medial prefrontal cortex, which are important parts of fear and anxiety circuitries (Pierre et al., 2020). But despite increasing dopamine release in VTA projection areas, in our hands, MK0677 did not influence fear acquisition or extinction and extinction memory retrieval in unstressed mice. One explanation could be that the achieved rise in dopamine may not have been sufficient or not timed adequately, which may be enhanced by optimizing ghrelin agonists, dosage and ways of administration. Since the elucidation of the exact role of dopamine during fear extinction has only begun, advances in this field could help to utilize the ghrelin-dopamine axis in a more targeted manner (e.g., timing, individual differences) in future studies.
Conclusion and Future Directions
Although the body of literature about the ghrelin system is growing rapidly, a great amount of questions regarding its effect on brain function remain unanswered. This complicates the evaluation of pathological relevance and therapeutic utility of ghrelin and GHSR in stress-related psychiatric disorders. To this end, a better understanding of the role of the ghrelin system in stress coping and its interplay with the HPA axis will be important. Further studies will be necessary to characterize brain GHSR signaling beyond the action of AG on its receptor, as DAG and ligand-independent GHSR activity have received little attention in existing literature. Moreover, the phenomenon of central ghrelin resistance in chronic stress needs to be studied more intensely in order to establish associated neurobiological correlates. The notion that ghrelin modulates fear, anxiety- and depression-like behaviors as a stress hormone seems to arise mostly from rodent experiments, while evidence from human studies is sparse and inconclusive. Nevertheless, it appears evident that ghrelin is much more than a ‘hunger hormone’ and represents an interesting target for novel interventions in stress-related psychiatric disorders that will become more accessible once its dynamics in the context of stress are better understood.
Author Contributions
EMF and DDB conceptualized the review and performed literature research. EMF wrote the manuscript with contributions and supervision from DDB. DDB and NS reviewed and revised all sections. All authors read and approved the final manuscript.
Funding
This work was supported by the Austrian Science Fund (FWF, I2433-B26 and DKW-1206-B18) and the Fund for Scientific Research Flanders (FWO-G001816N and FWO-11ZL616N) and the Strategic Research Programme of the Vrije Universiteit Brussel (SRP49).
Conflict of Interest
The authors declare that the research was conducted in the absence of any commercial or financial relationships that could be construed as a potential conflict of interest.
Abbreviations
ACTH, adrenocorticotropic hormone; AG, acyl ghrelin; AGRP, agouti-related peptide; ARC, arcuate nucleus; CPP, conditioned place preference; CRF, corticotropin-releasing factor; CSDS, chronic social defeat stress; CUMS, chronic mild unpredictable stress; DAG, desacyl ghrelin; GABA, gamma aminobutyric acid; GHSR, ghrelin receptor (growth hormone secretagogue receptor); GOAT, ghrelin-O-acyltransferase; HFD, high-fat diet; HPA, hypothalamic-pituitary-adrenal; KO, knockout; LEAP2, liver-expressed antimicrobial peptide 2; LPS, lipopolysaccharide; MDD, major depressive disorder; NAc, nucleus accumbens; NG, nodose ganglion; NPY, neuropeptide Y; NTS, nucleus tractus solitarius; PTSD, post-traumatic stress disorder; PVN, paraventricular nucleus; TSST, Trier social stress test; VTA, ventral tegmental area.
References
Aaseth, J., Roer, G. E., Lien, L., and Bjorklund, G. (2019). Is there a relationship between PTSD and complicated obesity? A review of the literature. Biomed. Pharmacother. 117:108834. doi: 10.1016/j.biopha.2019.108834
Abizaid, A., and Hougland, J. L. (2020). Ghrelin signaling: GOAT and GHS-R1a take a LEAP in complexity. Trends Endocrinol. Metab. 31, 107–117. doi: 10.1016/j.tem.2019.09.006
Abizaid, A., Liu, Z. W., Andrews, Z. B., Shanabrough, M., Borok, E., Elsworth, J. D., et al. (2006). Ghrelin modulates the activity and synaptic input organization of midbrain dopamine neurons while promoting appetite. J. Clin. Invest. 116, 3229–3239. doi: 10.1172/jci29867
Abraham, A. D., Neve, K. A., and Lattal, K. M. (2014). Dopamine and extinction: a convergence of theory with fear and reward circuitry. Neurobiol. Learn. Mem. 108, 65–77. doi: 10.1016/j.nlm.2013.11.007
Aklan, I., Sayar Atasoy, N., Yavuz, Y., Ates, T., Coban, I., Koksalar, F., et al. (2020). NTS catecholamine neurons mediate hypoglycemic hunger via medial hypothalamic feeding pathways. Cell Metab. 31, 313–326.e5. doi: 10.1016/j.cmet.2019.11.016
Al Massadi, O., Nogueiras, R., Dieguez, C., and Girault, J. A. (2019). Ghrelin and food reward. Neuropharmacology 148, 131–138. doi: 10.1016/j.neuropharm.2019.01.001
Albarran-Zeckler, R. G., Brantley, A. F., and Smith, R. G. (2012). Growth hormone secretagogue receptor (GHS-R1a) knockout mice exhibit improved spatial memory and deficits in contextual memory. Behav. Brain Res. 232, 13–19. doi: 10.1016/j.bbr.2012.03.012
Algul, S., and Ozcelik, O. (2018). Evaluating the levels of nesfatin-1 and ghrelin hormones in patients with moderate and severe major depressive disorders. Psychiatry Investig. 15, 214–218. doi: 10.30773/pi.2017.05.24
Alvarez-Crespo, M., Skibicka, K. P., Farkas, I., Molnar, C. S., Egecioglu, E., Hrabovszky, E., et al. (2012). The amygdala as a neurobiological target for ghrelin in rats: neuroanatomical, electrophysiological and behavioral evidence. PLoS One 7:e46321. doi: 10.1371/journal.pone.0046321
Amitani, M., Amitani, H., Cheng, K. C., Kairupan, T. S., Sameshima, N., Shimoshikiryo, I., et al. (2017). The role of ghrelin and ghrelin signaling in aging. Int. J. Mol. Sci. 18:1511. doi: 10.3390/ijms18071511
Arnold, M., Mura, A., Langhans, W., and Geary, N. (2006). Gut vagal afferents are not necessary for the eating-stimulatory effect of intraperitoneally injected ghrelin in the rat. J. Neurosci. 26, 11052–11060. doi: 10.1523/jneurosci.2606-06.2006
Asakawa, A., Inui, A., Kaga, T., Yuzuriha, H., Nagata, T., Fujimiya, M., et al. (2001a). A role of ghrelin in neuroendocrine and behavioral responses to stress in mice. Neuroendocrinology 74, 143–147. doi: 10.1159/000054680
Asakawa, A., Inui, A., Kaga, T., Yuzuriha, H., Nagata, T., Ueno, N., et al. (2001b). Ghrelin is an appetite-stimulatory signal from stomach with structural resemblance to motilin. Gastroenterology 120, 337–345. doi: 10.1053/gast.2001.22158
Azzam, I., Gilad, S., Limor, R., Stern, N., and Greenman, Y. (2017). Ghrelin stimulation by hypothalamic-pituitary-adrenal axis activation depends on increasing cortisol levels. Endocr. Connect. 6, 847–855. doi: 10.1530/EC-17-0212
Bali, A., and Jaggi, A. S. (2016). An integrative review on role and mechanisms of ghrelin in stress, anxiety and depression. Curr. Drug Targets 17, 495–507. doi: 10.2174/1389450116666150518095650
Bandelow, B., Reitt, M., Rover, C., Michaelis, S., Gorlich, Y., and Wedekind, D. (2015). Efficacy of treatments for anxiety disorders: a meta-analysis. Int. Clin. Psychopharmacol. 30, 183–192. doi: 10.1097/yic.0000000000000078
Bansal, V., Ryu, S. Y., Lopez, N., Allexan, S., Krzyzaniak, M., Eliceiri, B., et al. (2012). Vagal stimulation modulates inflammation through a ghrelin mediated mechanism in traumatic brain injury. Inflammation 35, 214–220. doi: 10.1007/s10753-011-9307-7
Barim, A. O., Aydin, S., Colak, R., Dag, E., Deniz, O., and Sahin, I. (2009). Ghrelin, paraoxonase and arylesterase levels in depressive patients before and after citalopram treatment. Clin. Biochem. 42, 1076–1081. doi: 10.1016/j.clinbiochem.2009.02.020
Baxter, A. J., Scott, K. M., Vos, T., and Whiteford, H. A. (2013). Global prevalence of anxiety disorders: a systematic review and meta-regression. Psychol. Med. 43, 897–910. doi: 10.1017/s003329171200147x
Bednarek, M. A., Feighner, S. D., Pong, S. S., Mckee, K. K., Hreniuk, D. L., Silva, M. V., et al. (2000). Structure-function studies on the new growth hormone-releasing peptide, ghrelin: minimal sequence of ghrelin necessary for activation of growth hormone secretagogue receptor 1a. J. Med. Chem. 43, 4370–4376. doi: 10.1021/jm0001727
Berton, O., Aguerre, S., Sarrieau, A., Mormede, P., and Chaouloff, F. (1998). Differential effects of social stress on central serotonergic activity and emotional reactivity in Lewis and spontaneously hypertensive rats. Neuroscience 82, 147–159. doi: 10.1016/s0306-4522(97)00282-0
Bodosi, B., Gardi, J., Hajdu, I., Szentirmai, E., Obal, F. Jr., and Krueger, J. M. (2004). Rhythms of ghrelin, leptin, and sleep in rats: effects of the normal diurnal cycle, restricted feeding, and sleep deprivation. Am. J. Physiol. Regul. Integr. Comp. Physiol. 287, R1071–R1079. doi: 10.1152/ajpregu.00294.2004
Briggs, D. I., Enriori, P. J., Lemus, M. B., Cowley, M. A., and Andrews, Z. B. (2010). Diet-induced obesity causes ghrelin resistance in arcuate NPY/AgRP neurons. Endocrinology 151, 4745–4755. doi: 10.1210/en.2010-0556
Briggs, D. I., Lockie, S. H., Wu, Q., Lemus, M. B., Stark, R., and Andrews, Z. B. (2013). Calorie-restricted weight loss reverses high-fat diet-induced ghrelin resistance, which contributes to rebound weight gain in a ghrelin-dependent manner. Endocrinology 154, 709–717. doi: 10.1210/en.2012-1421
Brockway, E. T., Krater, K. R., Selva, J. A., Wauson, S. E., and Currie, P. J. (2016). Impact of [d-Lys(3)]-GHRP-6 and feeding status on hypothalamic ghrelin-induced stress activation. Peptides 79, 95–102. doi: 10.1016/j.peptides.2016.03.013
Brunetti, L., Recinella, L., Orlando, G., Michelotto, B., Di Nisio, C., and Vacca, M. (2002). Effects of ghrelin and amylin on dopamine, norepinephrine and serotonin release in the hypothalamus. Eur. J. Pharmacol. 454, 189–192. doi: 10.1016/s0014-2999(02)02552-9
Cabral, A., Portiansky, E., Sanchez-Jaramillo, E., Zigman, J. M., and Perello, M. (2016). Ghrelin activates hypophysiotropic corticotropin-releasing factor neurons independently of the arcuate nucleus. Psychoneuroendocrinology 67, 27–39. doi: 10.1016/j.psyneuen.2016.01.027
Cabral, A., Suescun, O., Zigman, J. M., and Perello, M. (2012). Ghrelin indirectly activates hypophysiotropic CRF neurons in rodents. PLoS One 7:e31462. doi: 10.1371/journal.pone.0031462
Cai, L. X., Pizano, K., Gundersen, G. W., Hayes, C. L., Fleming, W. T., Holt, S., et al. (2020). Distinct signals in medial and lateral VTA dopamine neurons modulate fear extinction at different times. eLife 9:e54936. doi: 10.7554/eLife.54936
Callaghan, B., and Furness, J. B. (2014). Novel and conventional receptors for ghrelin, desacyl-ghrelin, and pharmacologically related compounds. Pharmacol. Rev. 66, 984–1001. doi: 10.1124/pr.113.008433
Carlini, V. P., Monzon, M. E., Varas, M. M., Cragnolini, A. B., Schioth, H. B., Scimonelli, T. N., et al. (2002). Ghrelin increases anxiety-like behavior and memory retention in rats. Biochem. Biophys. Res. Commun. 299, 739–743. doi: 10.1016/s0006-291x(02)02740-7
Carlini, V. P., Varas, M. M., Cragnolini, A. B., Schioth, H. B., Scimonelli, T. N., and De Barioglio, S. R. (2004). Differential role of the hippocampus, amygdala, and dorsal raphe nucleus in regulating feeding, memory, and anxiety-like behavioral responses to ghrelin. Biochem. Biophys. Res. Commun. 313, 635–641. doi: 10.1016/j.bbrc.2003.11.150
Cavalcante, D. P., Turones, L. C., Camargo-Silva, G., Santana, J. S., Colugnati, D. B., Pansani, A. P., et al. (2019). Role of dorsal raphe nucleus GHS-R1a receptors in the regulation of inhibitory avoidance and escape behaviors in rats. Behav. Brain Res. 365, 178–184. doi: 10.1016/j.bbr.2019.03.017
Cerit, H., Christensen, K., Moondra, P., Klibanski, A., Goldstein, J. M., and Holsen, L. M. (2019). Divergent associations between ghrelin and neural responsivity to palatable food in hyperphagic and hypophagic depression. J. Affect. Disord. 242, 29–38. doi: 10.1016/j.jad.2018.07.088
Chuang, J. C., Perello, M., Sakata, I., Osborne-Lawrence, S., Savitt, J. M., Lutter, M., et al. (2011). Ghrelin mediates stress-induced food-reward behavior in mice. J. Clin. Invest. 121, 2684–2692. doi: 10.1172/jci57660
Cone, J. J., Mccutcheon, J. E., and Roitman, M. F. (2014). Ghrelin acts as an interface between physiological state and phasic dopamine signaling. J. Neurosci. 34, 4905–4913. doi: 10.1523/jneurosci.4404-13.2014
Cornejo, M. P., Barrile, F., Cassano, D., Aguggia, J. P., Garcia Romero, G., Reynaldo, M., et al. (2020). Growth hormone secretagogue receptor in dopamine neurons controls appetitive and consummatory behaviors towards high-fat diet in ad-libitum fed mice. Psychoneuroendocrinology 119:104718. doi: 10.1016/j.psyneuen.2020.104718
Cornejo, M. P., Barrile, F., De Francesco, P. N., Portiansky, E. L., Reynaldo, M., and Perello, M. (2018). Ghrelin recruits specific subsets of dopamine and gaba neurons of different ventral tegmental area sub-nuclei. Neuroscience 392, 107–120. doi: 10.1016/j.neuroscience.2018.09.027
Cowley, M. A., Smith, R. G., Diano, S., Tschop, M., Pronchuk, N., Grove, K. L., et al. (2003). The distribution and mechanism of action of ghrelin in the CNS demonstrates a novel hypothalamic circuit regulating energy homeostasis. Neuron 37, 649–661. doi: 10.1016/s0896-6273(03)00063-1
Craske, M. G. (2010). Cognitive–Behavioral Therapy. Washington, DC: American Psychological Association.
Craske, M. G., Stein, M. B., Eley, T. C., Milad, M. R., Holmes, A., Rapee, R. M., et al. (2017). Anxiety disorders. Nat. Rev. Dis. Primers 3:17024.
Cummings, D. E. (2006). Ghrelin and the short- and long-term regulation of appetite and body weight. Physiol. Behav. 89, 71–84. doi: 10.1016/j.physbeh.2006.05.022
Currie, P. J., Khelemsky, R., Rigsbee, E. M., Dono, L. M., Coiro, C. D., Chapman, C. D., et al. (2012). Ghrelin is an orexigenic peptide and elicits anxiety-like behaviors following administration into discrete regions of the hypothalamus. Behav. Brain Res. 226, 96–105. doi: 10.1016/j.bbr.2011.08.037
Currie, P. J., Schuette, L. M., Wauson, S. E., Voss, W. N., and Angeles, M. J. (2014). Activation of urocortin 1 and ghrelin signaling in the basolateral amygdala induces anxiogenesis. Neuroreport 25, 60–64.
Date, Y., Kojima, M., Hosoda, H., Sawaguchi, A., Mondal, M. S., Suganuma, T., et al. (2000). Ghrelin, a novel growth hormone-releasing acylated peptide, is synthesized in a distinct endocrine cell type in the gastrointestinal tracts of rats and humans. Endocrinology 141, 4255–4261. doi: 10.1210/endo.141.11.7757
Date, Y., Shimbara, T., Koda, S., Toshinai, K., Ida, T., Murakami, N., et al. (2006). Peripheral ghrelin transmits orexigenic signals through the noradrenergic pathway from the hindbrain to the hypothalamus. Cell Metab. 4, 323–331. doi: 10.1016/j.cmet.2006.09.004
De Herdt, V., Puimege, L., De Waele, J., Raedt, R., Wyckhuys, T., El Tahry, R., et al. (2009). Increased rat serum corticosterone suggests immunomodulation by stimulation of the vagal nerve. J. Neuroimmunol. 212, 102–105. doi: 10.1016/j.jneuroim.2009.04.013
De Vriese, C., Gregoire, F., Lema-Kisoka, R., Waelbroeck, M., Robberecht, P., and Delporte, C. (2004). Ghrelin degradation by serum and tissue homogenates: identification of the cleavage sites. Endocrinology 145, 4997–5005. doi: 10.1210/en.2004-0569
Delhanty, P. J., Neggers, S. J., and Van Der Lely, A. J. (2014). Should we consider des-acyl ghrelin as a separate hormone and if so, what does it do? Front. Horm. Res. 42, 163–174. doi: 10.1159/000358345
Dos-Santos, R. C., Grover, H. M., Reis, L. C., Ferguson, A. V., and Mecawi, A. S. (2018). Electrophysiological effects of ghrelin in the hypothalamic paraventricular nucleus neurons. Front. Cell. Neurosci. 12:275. doi: 10.3389/fncel.2018.00275
Dunn, I. C., Wilson, P. W., D’eath, R. B., and Boswell, T. (2015). Hypothalamic agouti-related peptide mRNA is elevated during natural and stress-induced anorexia. J. Neuroendocrinol. 27, 681–691. doi: 10.1111/jne.12295
Edwards, A., and Abizaid, A. (2016). Driving the need to feed: insight into the collaborative interaction between ghrelin and endocannabinoid systems in modulating brain reward systems. Neurosci. Biobehav. Rev. 66, 33–53. doi: 10.1016/j.neubiorev.2016.03.032
Egecioglu, E., Jerlhag, E., Salome, N., Skibicka, K. P., Haage, D., Bohlooly, Y. M., et al. (2010). Ghrelin increases intake of rewarding food in rodents. Addict. Biol. 15, 304–311. doi: 10.1111/j.1369-1600.2010.00216.x
Elbassuoni, E. A. (2014). Gender differences in ghrelin response to chronic immobilization stress in rats: possible role of estrogen. Gen. Physiol. Biophys. 33, 111–120. doi: 10.4149/gpb_2013061
Engel, J. A., Nylander, I., and Jerlhag, E. (2015). A ghrelin receptor (GHS-R1A) antagonist attenuates the rewarding properties of morphine and increases opioid peptide levels in reward areas in mice. Eur. Neuropsychopharmacol. 25, 2364–2371. doi: 10.1016/j.euroneuro.2015.10.004
Fanous, S., Terwilliger, E. F., Hammer, R. P. Jr., and Nikulina, E. M. (2011). Viral depletion of VTA BDNF in rats modulates social behavior, consequences of intermittent social defeat stress, and long-term weight regulation. Neurosci. Lett. 502, 192–196. doi: 10.1016/j.neulet.2011.07.043
Fernandez, G., Cabral, A., Cornejo, M. P., De Francesco, P. N., Garcia-Romero, G., Reynaldo, M., et al. (2016). Des-acyl ghrelin directly targets the arcuate nucleus in a ghrelin-receptor independent manner and impairs the orexigenic effect of ghrelin. J. Neuroendocrinol. 28:12349.
Ferre, G., Louet, M., Saurel, O., Delort, B., Czaplicki, G., M’kadmi, C., et al. (2019). Structure and dynamics of G protein-coupled receptor-bound ghrelin reveal the critical role of the octanoyl chain. Proc. Natl. Acad. Sci. U.S.A. 116, 17525–17530. doi: 10.1073/pnas.1905105116
Forbes, N. F., Stewart, C. A., Matthews, K., and Reid, I. C. (1996). Chronic mild stress and sucrose consumption: validity as a model of depression. Physiol. Behav. 60, 1481–1484. doi: 10.1016/s0031-9384(96)00305-8
Fujitsuka, N., Asakawa, A., Uezono, Y., Minami, K., Yamaguchi, T., Niijima, A., et al. (2011). Potentiation of ghrelin signaling attenuates cancer anorexia-cachexia and prolongs survival. Transl. Psychiatry 1:e23. doi: 10.1038/tp.2011.25
Ge, X., Yang, H., Bednarek, M. A., Galon-Tilleman, H., Chen, P., Chen, M., et al. (2018). LEAP2 is an endogenous antagonist of the ghrelin receptor. Cell Metab. 27, 461–469.e6.
Gerlicher, A. M. V., Tuscher, O., and Kalisch, R. (2018). Dopamine-dependent prefrontal reactivations explain long-term benefit of fear extinction. Nat. Commun. 9:4294.
Gimenez-Palop, O., Coronas, R., Cobo, J., Gallart, L., Barbero, J. D., Parra, I., et al. (2012). Fasting plasma peptide YY concentrations are increased in patients with major depression who associate weight loss. J. Endocrinol. Invest. 35, 645–648.
Gnanapavan, S., Kola, B., Bustin, S. A., Morris, D. G., Mcgee, P., Fairclough, P., et al. (2002). The tissue distribution of the mRNA of ghrelin and subtypes of its receptor, GHS-R, in humans. J. Clin. Endocrinol. Metab. 87:2988. doi: 10.1210/jcem.87.6.8739
Goldstein, J. L., Zhao, T. J., Li, R. L., Sherbet, D. P., Liang, G., and Brown, M. S. (2011). Surviving starvation: essential role of the ghrelin-growth hormone axis. Cold Spring Harb. Symp. Quant. Biol. 76, 121–127. doi: 10.1101/sqb.2011.76.010447
Grant, F., Guille, C., and Sen, S. (2013). Well-being and the risk of depression under stress. PLoS One 8:e67395. doi: 10.1371/journal.pone.0067395
Gray, S. M., Page, L. C., and Tong, J. (2019). Ghrelin regulation of glucose metabolism. J. Neuroendocrinol. 31:e12705.
Gualillo, O., Caminos, J., Blanco, M., Garcia-Caballero, T., Kojima, M., Kangawa, K., et al. (2001). Ghrelin, a novel placental-derived hormone. Endocrinology 142, 788–794. doi: 10.1210/endo.142.2.7987
Guan, X. M., Yu, H., Palyha, O. C., Mckee, K. K., Feighner, S. D., Sirinathsinghji, D. J., et al. (1997). Distribution of mRNA encoding the growth hormone secretagogue receptor in brain and peripheral tissues. Brain Res. Mol. Brain Res. 48, 23–29. doi: 10.1016/s0169-328x(97)00071-5
Gul, S., Saleem, D., Haleem, M. A., and Haleem, D. J. (2017). Inhibition of hormonal and behavioral effects of stress by tryptophan in rats. Nutr. Neurosci. 22, 409–417. doi: 10.1080/1028415x.2017.1395551
Guo, L., Niu, M., Yang, J., Li, L., Liu, S., Sun, Y., et al. (2019). GHS-R1a deficiency alleviates depression-related behaviors after chronic social defeat stress. Front. Neurosci. 13:364. doi: 10.3389/fnins.2019.00364
Gupta, D., Chuang, J. C., Mani, B. K., Shankar, K., Rodriguez, J. A., Osborne-Lawrence, S., et al. (2019). beta1-adrenergic receptors mediate plasma acyl-ghrelin elevation and depressive-like behavior induced by chronic psychosocial stress. Neuropsychopharmacology 44, 1319–1327. doi: 10.1038/s41386-019-0334-7
Gutierrez, J. A., Solenberg, P. J., Perkins, D. R., Willency, J. A., Knierman, M. D., Jin, Z., et al. (2008). Ghrelin octanoylation mediated by an orphan lipid transferase. Proc. Natl. Acad. Sci. U.S.A. 105, 6320–6325. doi: 10.1073/pnas.0800708105
Haaker, J., Gaburro, S., Sah, A., Gartmann, N., Lonsdorf, T. B., Meier, K., et al. (2013). Single dose of L-dopa makes extinction memories context-independent and prevents the return of fear. Proc. Natl. Acad. Sci. U.S.A. 110, E2428–E2436.
Han, Q. Q., Huang, H. J., Wang, Y. L., Yang, L., Pilot, A., Zhu, X. C., et al. (2019). Ghrelin exhibited antidepressant and anxiolytic effect via the p38-MAPK signaling pathway in hippocampus. Prog. Neuropsychopharmacol. Biol. Psychiatry 93, 11–20. doi: 10.1016/j.pnpbp.2019.02.013
Hansson, C., Alvarez-Crespo, M., Taube, M., Skibicka, K. P., Schmidt, L., Karlsson-Lindahl, L., et al. (2014). Influence of ghrelin on the central serotonergic signaling system in mice. Neuropharmacology 79, 498–505. doi: 10.1016/j.neuropharm.2013.12.012
Hansson, C., Annerbrink, K., Nilsson, S., Bah, J., Olsson, M., Allgulander, C., et al. (2013). A possible association between panic disorder and a polymorphism in the preproghrelingene. Psychiatry Res. 206, 22–25. doi: 10.1016/j.psychres.2012.09.051
Hansson, C., Haage, D., Taube, M., Egecioglu, E., Salome, N., and Dickson, S. L. (2011). Central administration of ghrelin alters emotional responses in rats: behavioural, electrophysiological and molecular evidence. Neuroscience 180, 201–211. doi: 10.1016/j.neuroscience.2011.02.002
Haque, Z., Akbar, N., Yasmin, F., Haleem, M. A., and Haleem, D. J. (2013). Inhibition of immobilization stress-induced anorexia, behavioral deficits, and plasma corticosterone secretion by injected leptin in rats. Stress 16, 353–362. doi: 10.3109/10253890.2012.736047
Harmatz, E. S., Stone, L., Lim, S. H., Lee, G., Mcgrath, A., Gisabella, B., et al. (2016). Central ghrelin resistance permits the overconsolidation of fear memory. Biol. Psychiatry 81, 1003–1013. doi: 10.1016/j.biopsych.2016.11.009
Harris, R. B., Zhou, J., Youngblood, B. D., Smagin, G. N., and Ryan, D. H. (1997). Failure to change exploration or saccharin preference in rats exposed to chronic mild stress. Physiol. Behav. 63, 91–100. doi: 10.1016/s0031-9384(97)00425-3
Hassouna, R., Zizzari, P., Tomasetto, C., Veldhuis, J. D., Fiquet, O., Labarthe, A., et al. (2014). An early reduction in GH peak amplitude in preproghrelin-deficient male mice has a minor impact on linear growth. Endocrinology 155, 3561–3571. doi: 10.1210/en.2014-1126
He, D., Sai, X., Wang, N., Li, X., Wang, L., and Xu, Y. (2018). Camellia euphlebia exerts its antidepressant-like effect via modulation of the hypothalamic-pituitary-adrenal axis and brain monoaminergic systems. Metab. Brain Dis. 33, 301–312. doi: 10.1007/s11011-017-0167-1
Hedegaard, M. A., and Holst, B. (2020). The complex signaling pathways of the ghrelin receptor. Endocrinology 161:bqaa020.
Heiman, M. L., and Greenway, F. L. (2016). A healthy gastrointestinal microbiome is dependent on dietary diversity. Mol. Metab. 5, 317–320. doi: 10.1016/j.molmet.2016.02.005
Hofmann, S. G., and Smits, J. A. (2008). Cognitive-behavioral therapy for adult anxiety disorders: a meta-analysis of randomized placebo-controlled trials. J. Clin. Psychiatry 69, 621–632. doi: 10.4088/jcp.v69n0415
Holst, B., Cygankiewicz, A., Jensen, T. H., Ankersen, M., and Schwartz, T. W. (2003). High constitutive signaling of the ghrelin receptor–identification of a potent inverse agonist. Mol. Endocrinol. 17, 2201–2210. doi: 10.1210/me.2003-0069
Hornsby, A. K., Redhead, Y. T., Rees, D. J., Ratcliff, M. S., Reichenbach, A., Wells, T., et al. (2016). Short-term calorie restriction enhances adult hippocampal neurogenesis and remote fear memory in a Ghsr-dependent manner. Psychoneuroendocrinology 63, 198–207. doi: 10.1016/j.psyneuen.2015.09.023
Howick, K., Chruscicka, B., Felice, D., Ramirez, V. T., Van Leuven, L., Pietra, C., et al. (2020). Behavioural characterization of ghrelin ligands, anamorelin and HM01: appetite and reward-motivated effects in rodents. Neuropharmacology 168:108011. doi: 10.1016/j.neuropharm.2020.108011
Huang, C. C., Chou, D., Yeh, C. M., and Hsu, K. S. (2016). Acute food deprivation enhances fear extinction but inhibits long-term depression in the lateral amygdala via ghrelin signaling. Neuropharmacology 101, 36–45. doi: 10.1016/j.neuropharm.2015.09.018
Huang, H. J., Chen, X. R., Han, Q. Q., Wang, J., Pilot, A., Yu, R., et al. (2019). The protective effects of Ghrelin/GHSR on hippocampal neurogenesis in CUMS mice. Neuropharmacology 155, 31–43. doi: 10.1016/j.neuropharm.2019.05.013
Huang, H. J., Zhu, X. C., Han, Q. Q., Wang, Y. L., Yue, N., Wang, J., et al. (2017). Ghrelin alleviates anxiety- and depression-like behaviors induced by chronic unpredictable mild stress in rodents. Behav. Brain Res. 326, 33–43. doi: 10.1016/j.bbr.2017.02.040
Iglesias, M. J., Pineiro, R., Blanco, M., Gallego, R., Dieguez, C., Gualillo, O., et al. (2004). Growth hormone releasing peptide (ghrelin) is synthesized and secreted by cardiomyocytes. Cardiovasc. Res. 62, 481–488. doi: 10.1016/j.cardiores.2004.01.024
Ishitobi, Y., Kohno, K., Kanehisa, M., Inoue, A., Imanaga, J., Maruyama, Y., et al. (2012). Serum ghrelin levels and the effects of antidepressants in major depressive disorder and panic disorder. Neuropsychobiology 66, 185–192. doi: 10.1159/000339948
Izadi, M. S., Radahmadi, M., Ghasemi, M., and Rayatpour, A. (2018). Effects of isolation and social subchronic stresses on food intake and levels of leptin, ghrelin, and glucose in male rats. Adv. Biomed. Res. 7:118. doi: 10.4103/abr.abr_28_18
Jackson, T. M., Ostrowski, T. D., and Middlemas, D. S. (2019). Intracerebroventricular ghrelin administration increases depressive-like behavior in male juvenile rats. Front. Behav. Neurosci. 13:77. doi: 10.3389/fnbeh.2019.00077
Jensen, M., Ratner, C., Rudenko, O., Christiansen, S. H., Skov, L. J., Hundahl, C., et al. (2016). Anxiolytic-like effects of increased ghrelin receptor signaling in the amygdala. Int. J. Neuropsychopharmacol. 19:pyv123. doi: 10.1093/ijnp/pyv123
Jeong, J. Y., Lee, D. H., and Kang, S. S. (2013). Effects of chronic restraint stress on body weight, food intake, and hypothalamic gene expressions in mice. Endocrinol. Metab. 28, 288–296. doi: 10.3803/EnM.2013.28.4.288
Jerlhag, E. (2008). Systemic administration of ghrelin induces conditioned place preference and stimulates accumbal dopamine. Addict. Biol. 13, 358–363. doi: 10.1111/j.1369-1600.2008.00125.x
Jerlhag, E., Egecioglu, E., Dickson, S. L., Douhan, A., Svensson, L., and Engel, J. A. (2007). Ghrelin administration into tegmental areas stimulates locomotor activity and increases extracellular concentration of dopamine in the nucleus accumbens. Addict. Biol. 12, 6–16. doi: 10.1111/j.1369-1600.2006.00041.x
Jerlhag, E., Egecioglu, E., Dickson, S. L., and Engel, J. A. (2010). Ghrelin receptor antagonism attenuates cocaine- and amphetamine-induced locomotor stimulation, accumbal dopamine release, and conditioned place preference. Psychopharmacology 211, 415–422. doi: 10.1007/s00213-010-1907-7
Jerlhag, E., Landgren, S., Egecioglu, E., Dickson, S. L., and Engel, J. A. (2011). The alcohol-induced locomotor stimulation and accumbal dopamine release is suppressed in ghrelin knockout mice. Alcohol 45, 341–347. doi: 10.1016/j.alcohol.2010.10.002
Jiang, S. Z., and Eiden, L. E. (2016). Activation of the HPA axis and depression of feeding behavior induced by restraint stress are separately regulated by PACAPergic neurotransmission in the mouse. Stress 19, 374–382. doi: 10.1080/10253890.2016.1174851
Jiao, Q., Du, X., Li, Y., Gong, B., Shi, L., Tang, T., et al. (2017). The neurological effects of ghrelin in brain diseases: beyond metabolic functions. Neurosci. Biobehav. Rev. 73, 98–111. doi: 10.1016/j.neubiorev.2016.12.010
Jochman, K. A., Newman, S. M., Kalin, N. H., and Bakshi, V. P. (2005). Corticotropin-releasing factor-1 receptors in the basolateral amygdala mediate stress-induced anorexia. Behav. Neurosci. 119, 1448–1458. doi: 10.1037/0735-7044.119.6.1448
Kageyama, H., Funahashi, H., Hirayama, M., Takenoya, F., Kita, T., Kato, S., et al. (2005). Morphological analysis of ghrelin and its receptor distribution in the rat pancreas. Regul. Pept. 126, 67–71. doi: 10.1016/j.regpep.2004.08.031
Kalisch, R., Gerlicher, A. M. V., and Duvarci, S. (2019). A dopaminergic basis for fear extinction. Trends Cogn. Sci. 23, 274–277. doi: 10.1016/j.tics.2019.01.013
Kamegai, J., Tamura, H., Shimizu, T., Ishii, S., Sugihara, H., and Wakabayashi, I. (2000). Central effect of ghrelin, an endogenous growth hormone secretagogue, on hypothalamic peptide gene expression. Endocrinology 141, 4797–4800. doi: 10.1210/endo.141.12.7920
Kanehisa, M., Akiyoshi, J., Kitaichi, T., Matsushita, H., Tanaka, E., Kodama, K., et al. (2006). Administration of antisense DNA for ghrelin causes an antidepressant and anxiolytic response in rats. Prog. Neuropsychopharmacol. Biol. Psychiatry 30, 1403–1407. doi: 10.1016/j.pnpbp.2006.05.005
Kang, C., Zhang, Y., Zhu, X., Liu, K., Wang, X., Chen, M., et al. (2016). Healthy subjects differentially respond to dietary capsaicin correlating with specific gut enterotypes. J. Clin. Endocrinol. Metab. 101, 4681–4689. doi: 10.1210/jc.2016-2786
Kawakami, A., Okada, N., Rokkaku, K., Honda, K., Ishibashi, S., and Onaka, T. (2008). Leptin inhibits and ghrelin augments hypothalamic noradrenaline release after stress. Stress 11, 363–369. doi: 10.1080/10253890701820257
Kendler, K. S., Karkowski, L. M., and Prescott, C. A. (1999). Causal relationship between stressful life events and the onset of major depression. Am. J. Psychiatry 156, 837–841. doi: 10.1176/ajp.156.6.837
Kern, A., Mavrikaki, M., Ullrich, C., Albarran-Zeckler, R., Brantley, A. F., and Smith, R. G. (2015). Hippocampal Dopamine/DRD1 signaling dependent on the ghrelin receptor. Cell 163, 1176–1190. doi: 10.1016/j.cell.2015.10.062
Kessler, R. C. (1997). The effects of stressful life events on depression. Annu. Rev. Psychol. 48, 191–214. doi: 10.1146/annurev.psych.48.1.191
Kessler, R. C., Berglund, P., Demler, O., Jin, R., Merikangas, K. R., and Walters, E. E. (2005a). Lifetime prevalence and age-of-onset distributions of DSM-IV disorders in the National Comorbidity Survey Replication. Arch. Gen. Psychiatry 62, 593–602. doi: 10.1001/archpsyc.62.6.593
Kessler, R. C., Chiu, W. T., Demler, O., Merikangas, K. R., and Walters, E. E. (2005b). Prevalence, severity, and comorbidity of 12-month DSM-IV disorders in the National Comorbidity Survey Replication. Arch. Gen. Psychiatry 62, 617–627. doi: 10.1001/archpsyc.62.6.617
Kessler, R. C., Petukhova, M., Sampson, N. A., Zaslavsky, A. M., and Wittchen, H. U. (2012). Twelve-month and lifetime prevalence and lifetime morbid risk of anxiety and mood disorders in the United States. Int. J. Methods Psychiatr. Res. 21, 169–184. doi: 10.1002/mpr.1359
Kluge, M., Gazea, M., Schussler, P., Genzel, L., Dresler, M., Kleyer, S., et al. (2010). Ghrelin increases slow wave sleep and stage 2 sleep and decreases stage 1 sleep and REM sleep in elderly men but does not affect sleep in elderly women. Psychoneuroendocrinology 35, 297–304. doi: 10.1016/j.psyneuen.2009.07.007
Koek, R. J., Schwartz, H. N., Scully, S., Langevin, J. P., Spangler, S., Korotinsky, A., et al. (2016). Treatment-refractory posttraumatic stress disorder (TRPTSD): a review and framework for the future. Prog. Neuropsychopharmacol. Biol. Psychiatry 70, 170–218. doi: 10.1016/j.pnpbp.2016.01.015
Kojima, M., Hosoda, H., Date, Y., Nakazato, M., Matsuo, H., and Kangawa, K. (1999). Ghrelin is a growth-hormone-releasing acylated peptide from stomach. Nature 402, 656–660. doi: 10.1038/45230
Kojima, M., and Kangawa, K. (2005). Ghrelin: structure and function. Physiol. Rev. 85, 495–522. doi: 10.1152/physrev.00012.2004
Kondrakiewicz, K., Kostecki, M., Szadzinska, W., and Knapska, E. (2019). Ecological validity of social interaction tests in rats and mice. Genes Brain Behav. 18:e12525. doi: 10.1111/gbb.12525
Kristenssson, E., Sundqvist, M., Hakanson, R., and Lindstrom, E. (2007). High gastrin cell activity and low ghrelin cell activity in high-anxiety Wistar Kyoto rats. J. Endocrinol. 193, 245–250. doi: 10.1677/JOE-07-0028
Kristenssson, E., Sundqvist, M., Astin, M., Kjerling, M., Mattsson, H., Dornonville De La Cour, C., et al. (2006). Acute psychological stress raises plasma ghrelin in the rat. Regul. Pept. 134, 114–117. doi: 10.1016/j.regpep.2006.02.003
Kurt, E., Guler, O., Serteser, M., Cansel, N., Ozbulut, O., Altinbas, K., et al. (2007). The effects of electroconvulsive therapy on ghrelin, leptin and cholesterol levels in patients with mood disorders. Neurosci. Lett. 426, 49–53. doi: 10.1016/j.neulet.2007.08.018
Labarthe, A., Fiquet, O., Hassouna, R., Zizzari, P., Lanfumey, L., Ramoz, N., et al. (2014). Ghrelin-derived peptides: A link between appetite/reward, GH axis, and psychiatric disorders? Front. Endocrinol. 5:163. doi: 10.3389/fendo.2014.00163
Lach, G., Schellekens, H., Dinan, T. G., and Cryan, J. F. (2018). Anxiety, depression, and the microbiome: a role for gut peptides. Neurotherapeutics 15, 36–59. doi: 10.1007/s13311-017-0585-0
Lambert, E., Lambert, G., Ika-Sari, C., Dawood, T., Lee, K., Chopra, R., et al. (2011). Ghrelin modulates sympathetic nervous system activity and stress response in lean and overweight men. Hypertension 58, 43–50. doi: 10.1161/HYPERTENSIONAHA.111.171025
Lang, P. J., Mcteague, L. M., and Bradley, M. M. (2016). RDoC, DSM, and the reflex physiology of fear: a biodimensional analysis of the anxiety disorders spectrum. Psychophysiology 53, 336–347. doi: 10.1111/psyp.12462
Lawson, E. A., Miller, K. K., Blum, J. I., Meenaghan, E., Misra, M., Eddy, K. T., et al. (2012). Leptin levels are associated with decreased depressive symptoms in women across the weight spectrum, independent of body fat. Clin. Endocrinol. 76, 520–525. doi: 10.1111/j.1365-2265.2011.04182.x
Lear, P. V., Iglesias, M. J., Feijoo-Bandin, S., Rodriguez-Penas, D., Mosquera-Leal, A., Garcia-Rua, V., et al. (2010). Des-acyl ghrelin has specific binding sites and different metabolic effects from ghrelin in cardiomyocytes. Endocrinology 151, 3286–3298. doi: 10.1210/en.2009-1205
Lee, M. R., Tapocik, J. D., Ghareeb, M., Schwandt, M. L., Dias, A. A., Le, A. N., et al. (2020). The novel ghrelin receptor inverse agonist PF-5190457 administered with alcohol: preclinical safety experiments and a phase 1b human laboratory study. Mol. Psychiatry 25, 461–475. doi: 10.1038/s41380-018-0064-y
Leung, P. K., Chow, K. B., Lau, P. N., Chu, K. M., Chan, C. B., Cheng, C. H., et al. (2007). The truncated ghrelin receptor polypeptide (GHS-R1b) acts as a dominant-negative mutant of the ghrelin receptor. Cell. Signal. 19, 1011–1022. doi: 10.1016/j.cellsig.2006.11.011
Li, B., Xu, Y., Pan, D., Xiao, Q., Gao, Q., Chen, X., et al. (2015). Effect of immobilization stress on the appetite and stomach ghrelin expression in maternal mice. Int. J. Clin. Exp. Pathol. 8, 15993–15999.
Li, G., Zhang, K., Wang, L., Cao, C., Fang, R., Liu, P., et al. (2018). The preliminary investigation of orexigenic hormone gene polymorphisms on posttraumatic stress disorder symptoms. Psychoneuroendocrinology 100, 131–136. doi: 10.1016/j.psyneuen.2018.09.042
Li, H., Wang, P., Huang, L., Li, P., and Zhang, D. (2019). Effects of regulating gut microbiota on the serotonin metabolism in the chronic unpredictable mild stress rat model. Neurogastroenterol. Motil. 31:e13677. doi: 10.1111/nmo.13677
Liu, S., and Borgland, S. L. (2015). Regulation of the mesolimbic dopamine circuit by feeding peptides. Neuroscience 289, 19–42. doi: 10.1016/j.neuroscience.2014.12.046
Lopez Lopez, A. L., Escobar Villanueva, M. C., Brianza Padilla, M., Bonilla Jaime, H., and Alarcon Aguilar, F. J. (2018). Chronic unpredictable mild stress progressively disturbs glucose metabolism and appetite hormones in rats. Acta Endocrinol. 14, 16–23. doi: 10.4183/aeb.2018.16
Lopez-Alarcon, M., Zurita-Cruz, J. N., Torres-Rodriguez, A., Bedia-Mejia, K., Perez-Guemez, M., Jaramillo-Villanueva, L., et al. (2020). Mindfulness affects stress, ghrelin, and BMI of obese children: a clinical trial. Endocr. Connect. 9, 163–172. doi: 10.1530/EC-19-0461
Lu, Y., Niu, M., Qiu, X., Cao, H., Xing, B., Sun, Y., et al. (2019). Acute but not chronic calorie restriction defends against stress-related anxiety and despair in a GHS-R1a-dependent manner. Neuroscience 412, 94–104. doi: 10.1016/j.neuroscience.2019.05.067
Luo, R., Uematsu, A., Weitemier, A., Aquili, L., Koivumaa, J., Mchugh, T. J., et al. (2018). A dopaminergic switch for fear to safety transitions. Nat. Commun. 9:2483. doi: 10.1038/s41467-018-04784-7
Lutter, M., Sakata, I., Osborne-Lawrence, S., Rovinsky, S. A., Anderson, J. G., Jung, S., et al. (2008). The orexigenic hormone ghrelin defends against depressive symptoms of chronic stress. Nat. Neurosci. 11, 752–753. doi: 10.1038/nn.2139
Mahbod, P., Smith, E. P., Fitzgerald, M. E., Morano, R. L., Packard, B. A., Ghosal, S., et al. (2018). Desacyl Ghrelin decreases anxiety-like behavior in male mice. Endocrinology 159, 388–399. doi: 10.1210/en.2017-00540
Mani, B. K., Walker, A. K., Lopez Soto, E. J., Raingo, J., Lee, C. E., Perello, M., et al. (2014). Neuroanatomical characterization of a growth hormone secretagogue receptor-green fluorescent protein reporter mouse. J. Comp. Neurol. 522, 3644–3666. doi: 10.1002/cne.23627
Mason, S. M., Flint, A. J., Roberts, A. L., Agnew-Blais, J., Koenen, K. C., and Rich-Edwards, J. W. (2014). Posttraumatic stress disorder symptoms and food addiction in women by timing and type of trauma exposure. JAMA Psychiatry 71, 1271–1278. doi: 10.1001/jamapsychiatry.2014.1208
Matsumoto, C., Yamada, C., Sadakane, C., Nahata, M., Hattori, T., and Takeda, H. (2017). Psychological stress in aged female mice causes acute hypophagia independent of central serotonin 2C receptor activation. PLoS One 12:e0187937. doi: 10.1371/journal.pone.0187937
Matsuo, K., Nakano, M., Nakashima, M., Watanuki, T., Egashira, K., Matsubara, T., et al. (2012). Neural correlates of plasma acylated ghrelin level in individuals with major depressive disorder. Brain Res. 1473, 185–192. doi: 10.1016/j.brainres.2012.07.027
McFarlane, M. R., Brown, M. S., Goldstein, J. L., and Zhao, T. J. (2014). Induced ablation of ghrelin cells in adult mice does not decrease food intake, body weight, or response to high-fat diet. Cell Metab. 20, 54–60. doi: 10.1016/j.cmet.2014.04.007
McGovern, K. R., Darling, J. E., and Hougland, J. L. (2016). Progress in small molecule and biologic therapeutics targeting Ghrelin signaling. Mini Rev. Med. Chem. 16, 465–480. doi: 10.2174/1389557515666150722101329
McKee, K. K., Palyha, O. C., Feighner, S. D., Hreniuk, D. L., Tan, C. P., Phillips, M. S., et al. (1997). Molecular analysis of rat pituitary and hypothalamic growth hormone secretagogue receptors. Mol. Endocrinol. 11, 415–423. doi: 10.1210/mend.11.4.9908
Meerlo, P., Overkamp, G. J., Daan, S., Van Den Hoofdakker, R. H., and Koolhaas, J. M. (1996). Changes in behaviour and body weight following a single or double social defeat in rats. Stress 1, 21–32. doi: 10.3109/10253899609001093
Meng, X., and D’Arcy, C. (2015). Comorbidity between lifetime eating problems and mood and anxiety disorders: results from the Canadian Community Health Survey of Mental Health and Well-being. Eur. Eat Disord. Rev. 23, 156–162. doi: 10.1002/erv.2347
Meyer, R. M., Burgos-Robles, A., Liu, E., Correia, S. S., and Goosens, K. A. (2014). A ghrelin-growth hormone axis drives stress-induced vulnerability to enhanced fear. Mol. Psychiatry 19, 1284–1294. doi: 10.1038/mp.2013.135
Milad, M. R., and Quirk, G. J. (2012). Fear extinction as a model for translational neuroscience: ten years of progress. Annu. Rev. Psychol. 63, 129–151. doi: 10.1146/annurev.psych.121208.131631
Mogami, S., Sadakane, C., Nahata, M., Mizuhara, Y., Yamada, C., Hattori, T., et al. (2016). CRF receptor 1 antagonism and brain distribution of active components contribute to the ameliorative effect of rikkunshito on stress-induced anorexia. Sci. Rep. 6:27516. doi: 10.1038/srep27516
Mograbi, K. M., Suchecki, D., Da Silva, S. G., Covolan, L., and Hamani, C. (2020). Chronic unpredictable restraint stress increases hippocampal pro-inflammatory cytokines and decreases motivated behavior in rats. Stress 23, 427–436. doi: 10.1080/10253890.2020.1712355
Monteleone, P., Tortorella, A., Scognamiglio, P., Serino, I., Monteleone, A. M., and Maj, M. (2012). The acute salivary ghrelin response to a psychosocial stress is enhanced in symptomatic patients with bulimia nervosa: a pilot study. Neuropsychobiology 66, 230–236. doi: 10.1159/000341877
Moose, J. E., Leets, K. A., Mate, N. A., Chisholm, J. D., and Hougland, J. L. (2020). An overview of ghrelin O-acyltransferase inhibitors: a literature and patent review for 2010-2019. Expert Opin. Ther. Pat. doi: 10.1080/13543776.2020.1776263 [Epub ahead of print].
Mori, K., Yoshimoto, A., Takaya, K., Hosoda, K., Ariyasu, H., Yahata, K., et al. (2000). Kidney produces a novel acylated peptide, ghrelin. FEBS Lett. 486, 213–216. doi: 10.1016/S0014-5793(00)02308-5
Murakami, N., Hayashida, T., Kuroiwa, T., Nakahara, K., Ida, T., Mondal, M. S., et al. (2002). Role for central ghrelin in food intake and secretion profile of stomach ghrelin in rats. J. Endocrinol. 174, 283–288. doi: 10.1677/joe.0.1740283
Nahata, M., Saegusa, Y., Sadakane, C., Yamada, C., Nakagawa, K., Okubo, N., et al. (2014). Administration of exogenous acylated ghrelin or rikkunshito, an endogenous ghrelin enhancer, improves the decrease in postprandial gastric motility in an acute restraint stress mouse model. Neurogastroenterol. Motil. 26, 821–831. doi: 10.1111/nmo.12336
Nakashima, K., Akiyoshi, J., Hatano, K., Hanada, H., Tanaka, Y., Tsuru, J., et al. (2008). Ghrelin gene polymorphism is associated with depression, but not panic disorder. Psychiatr. Genet. 18:257. doi: 10.1097/YPG.0b013e328306c979
Nakazato, M., Murakami, N., Date, Y., Kojima, M., Matsuo, H., Kangawa, K., et al. (2001). A role for ghrelin in the central regulation of feeding. Nature 409, 194–198. doi: 10.1038/35051587
Ng, I. S., Cheung, K. C., and Chou, K. L. (2013). Correlates of eating disorder in middle-aged and older adults: evidence from 2007 British National Psychiatric Morbidity Survey. J. Aging Health 25, 1106–1120. doi: 10.1177/0898264313494798
Ochi, M., Tominaga, K., Tanaka, F., Tanigawa, T., Shiba, M., Watanabe, T., et al. (2008). Effect of chronic stress on gastric emptying and plasma ghrelin levels in rats. Life Sci. 82, 862–868. doi: 10.1016/j.lfs.2008.01.020
Ogaya, M., Kim, J., and Sasaki, K. (2011). Ghrelin postsynaptically depolarizes dorsal raphe neurons in rats in vitro. Peptides 32, 1606–1616. doi: 10.1016/j.peptides.2011.07.001
Okada, T., Waise, T. M. Z., Toshinai, K., Mita, Y., Sakoda, H., and Nakazato, M. (2018). Analysis of peripheral ghrelin signaling via the vagus nerve in ghrelin receptor-restored GHSR-null mice. Neurosci. Lett. 681, 50–55. doi: 10.1016/j.neulet.2018.05.035
Olesen, J., Gustavsson, A., Svensson, M., Wittchen, H. U., Jonsson, B., Group, C. S., et al. (2012). The economic cost of brain disorders in Europe. Eur. J. Neurol. 19, 155–162. doi: 10.1111/j.1468-1331.2011.03590.x
Ozmen, S., Seker, A., and Demirci, E. (2019). Ghrelin and leptin levels in children with anxiety disorders. J. Pediatr. Endocrinol. Metab. 32, 1043–1047. doi: 10.1515/jpem-2019-0229
Ozsoy, S., Besirli, A., Abdulrezzak, U., and Basturk, M. (2014). Serum ghrelin and leptin levels in patients with depression and the effects of treatment. Psychiatry Investig. 11, 167–172. doi: 10.1515/jpem-2019-0229
Patki, G., Solanki, N., Atrooz, F., Allam, F., and Salim, S. (2013). Depression, anxiety-like behavior and memory impairment are associated with increased oxidative stress and inflammation in a rat model of social stress. Brain Res. 1539, 73–86. doi: 10.1016/j.brainres.2013.09.033
Patterson, Z. R., Ducharme, R., Anisman, H., and Abizaid, A. (2010). Altered metabolic and neurochemical responses to chronic unpredictable stressors in ghrelin receptor-deficient mice. Eur. J. Neurosci. 32, 632–639. doi: 10.1111/j.1460-9568.2010.07310.x
Patterson, Z. R., Khazall, R., Mackay, H., Anisman, H., and Abizaid, A. (2013a). Central ghrelin signaling mediates the metabolic response of C57BL/6 male mice to chronic social defeat stress. Endocrinology 154, 1080–1091. doi: 10.1210/en.2012-1834
Patterson, Z. R., Parno, T., Isaacs, A. M., and Abizaid, A. (2013b). Interruption of ghrelin signaling in the PVN increases high-fat diet intake and body weight in stressed and non-stressed C57BL6J male mice. Front. Neurosci. 7:167. doi: 10.3389/fnins.2013.00167
Perello, M., Cabral, A., Cornejo, M. P., De Francesco, P. N., Fernandez, G., and Uriarte, M. (2018). Brain accessibility delineates the central effects of circulating ghrelin. J. Neuroendocrinol. 31:e12677. doi: 10.1111/jne.12677
Perello, M., and Zigman, J. M. (2012). The role of ghrelin in reward-based eating. Biol. Psychiatry 72, 347–353. doi: 10.1016/j.biopsych.2012.02.016
Petersen, P. S., Woldbye, D. P., Madsen, A. N., Egerod, K. L., Jin, C., Lang, M., et al. (2009). In vivo characterization of high Basal signaling from the ghrelin receptor. Endocrinology 150, 4920–4930. doi: 10.1210/en.2008-1638
Pierre, A., Regin, Y., Van Schuerbeek, A., Fritz, E. M., Muylle, K., Beckers, T., et al. (2019). Effects of disrupted ghrelin receptor function on fear processing, anxiety and saccharin preference in mice. Psychoneuroendocrinology 110:104430. doi: 10.1016/j.psyneuen.2019.104430
Pierre, A., Van Schuerbeek, A., Allaoui, W., Van Laere, S., Singewald, N., Van Eeckhaut, A., et al. (2020). Effects of ghrelin receptor activation on forebrain dopamine release, conditioned fear and fear extinction in C57BL/6J mice. J. Neurochem. 154:e14996. doi: 10.1111/jnc.14996
Pinar, M., Gulsun, M., Tasci, I., Erdil, A., Bolu, E., Acikel, C., et al. (2008). Maprotiline induced weight gain in depressive disorder: changes in circulating ghrelin and adiponectin levels and insulin sensitivity. Prog. Neuropsychopharmacol. Biol. Psychiatry 32, 135–139. doi: 10.1016/j.pnpbp.2007.07.028
Quarta, D., Di Francesco, C., Melotto, S., Mangiarini, L., Heidbreder, C., and Hedou, G. (2009). Systemic administration of ghrelin increases extracellular dopamine in the shell but not the core subdivision of the nucleus accumbens. Neurochem. Int. 54, 89–94. doi: 10.1016/j.neuint.2008.12.006
Quarta, D., and Smolders, I. (2014). Rewarding, reinforcing and incentive salient events involve orexigenic hypothalamic neuropeptides regulating mesolimbic dopaminergic neurotransmission. Eur. J. Pharm. Sci. 57, 2–10. doi: 10.1016/j.ejps.2014.01.008
Ramirez, V. T., Van Oeffelen, W., Torres-Fuentes, C., Chruscicka, B., Druelle, C., Golubeva, A. V., et al. (2019). Differential functional selectivity and downstream signaling bias of ghrelin receptor antagonists and inverse agonists. FASEB J. 33, 518–531. doi: 10.1096/fj.201800655R
Raspopow, K., Abizaid, A., Matheson, K., and Anisman, H. (2010). Psychosocial stressor effects on cortisol and ghrelin in emotional and non-emotional eaters: influence of anger and shame. Horm. Behav. 58, 677–684. doi: 10.1016/j.yhbeh.2010.06.003
Razzoli, M., Sanghez, V., and Bartolomucci, A. (2015). Chronic subordination stress induces hyperphagia and disrupts eating behavior in mice modeling binge-eating-like disorder. Front. Nutr. 1:30. doi: 10.3389/fnut.2014.00030
Ricken, R., Bopp, S., Schlattmann, P., Himmerich, H., Bschor, T., Richter, C., et al. (2017). Ghrelin serum concentrations are associated with treatment response during lithium augmentation of antidepressants. Int. J. Neuropsychopharmacol. 20, 692–697. doi: 10.1093/ijnp/pyw082
Riddle, M. C., Mckenna, M. C., Yoon, Y. J., Pattwell, S. S., Santos, P. M., Casey, B. J., et al. (2013). Caloric restriction enhances fear extinction learning in mice. Neuropsychopharmacology 38, 930–937. doi: 10.1038/npp.2012.268
Roman, C. W., Derkach, V. A., and Palmiter, R. D. (2016). Genetically and functionally defined NTS to PBN brain circuits mediating anorexia. Nat. Commun. 7:11905. doi: 10.1038/ncomms11905
Rostamkhani, F., Zardooz, H., Goshadrou, F., Baveisi, M., and Hedayati, M. (2016). Stress increased ghrelin secretion from pancreatic isolated islets in male rats. Gen. Physiol. Biophys. 35, 109–117.
Rouach, V., Bloch, M., Rosenberg, N., Gilad, S., Limor, R., Stern, N., et al. (2007). The acute ghrelin response to a psychological stress challenge does not predict the post-stress urge to eat. Psychoneuroendocrinology 32, 693–702. doi: 10.1016/j.psyneuen.2007.04.010
Rucinski, M., Ziolkowska, A., Tyczewska, M., and Malendowicz, L. K. (2009). Expression of prepro-ghrelin and related receptor genes in the rat adrenal gland and evidences that ghrelin exerts a potent stimulating effect on corticosterone secretion by cultured rat adrenocortical cells. Peptides 30, 1448–1455. doi: 10.1016/j.peptides.2009.04.016
Saegusa, Y., Takeda, H., Muto, S., Nakagawa, K., Ohnishi, S., Sadakane, C., et al. (2011). Decreased plasma ghrelin contributes to anorexia following novelty stress. Am. J. Physiol. Endocrinol. Metab. 301, E685–E696. doi: 10.1152/ajpendo.00121.2011
Salinas-Hernandez, X. I., Vogel, P., Betz, S., Kalisch, R., Sigurdsson, T., and Duvarci, S. (2018). Dopamine neurons drive fear extinction learning by signaling the omission of expected aversive outcomes. eLife 7:e38818. doi: 10.7554/eLife.38818.024
Sartori, S. B., and Singewald, N. (2019). Novel pharmacological targets in drug development for the treatment of anxiety and anxiety-related disorders. Pharmacol. Ther. 204:107402. doi: 10.1016/j.pharmthera.2019.107402
Schaeffer, M., Langlet, F., Lafont, C., Molino, F., Hodson, D. J., Roux, T., et al. (2013). Rapid sensing of circulating ghrelin by hypothalamic appetite-modifying neurons. Proc. Natl. Acad. Sci. U.S.A. 110, 1512–1517. doi: 10.1073/pnas.1212137110
Schalla, M. A., and Stengel, A. (2020). Effects of microbiome changes on endocrine ghrelin signaling - A systematic review. Peptides 133:170388. doi: 10.1016/j.peptides.2020.170388
Schanze, A., Reulbach, U., Scheuchenzuber, M., Groschl, M., Kornhuber, J., and Kraus, T. (2008). Ghrelin and eating disturbances in psychiatric disorders. Neuropsychobiology 57, 126–130. doi: 10.1159/000138915
Schele, E., Bake, T., Rabasa, C., and Dickson, S. L. (2016). Centrally administered ghrelin acutely influences food choice in rodents. PLoS One 11:e0149456. doi: 10.1371/journal.pone.0149456
Schellekens, H., Dinan, T. G., and Cryan, J. F. (2013a). Taking two to tango: a role for ghrelin receptor heterodimerization in stress and reward. Front. Neurosci. 7:148. doi: 10.3389/fnins.2013.00148
Schellekens, H., Finger, B. C., Dinan, T. G., and Cryan, J. F. (2012). Ghrelin signalling and obesity: at the interface of stress, mood and food reward. Pharmacol. Ther. 135, 316–326. doi: 10.1016/j.pharmthera.2012.06.004
Schellekens, H., Van Oeffelen, W. E., Dinan, T. G., and Cryan, J. F. (2013b). Promiscuous dimerization of the growth hormone secretagogue receptor (GHS-R1a) attenuates ghrelin-mediated signaling. J. Biol. Chem. 288, 181–191. doi: 10.1074/jbc.M112.382473
Schmidt, M. V., Levine, S., Alam, S., Harbich, D., Sterlemann, V., Ganea, K., et al. (2006). Metabolic signals modulate hypothalamic-pituitary-adrenal axis activation during maternal separation of the neonatal mouse. J. Neuroendocrinol. 18, 865–874. doi: 10.1111/j.1365-2826.2006.01482.x
Scott, K. M., Mcgee, M. A., Wells, J. E., and Oakley Browne, M. A. (2008). Obesity and mental disorders in the adult general population. J. Psychosom. Res. 64, 97–105. doi: 10.1016/j.jpsychores.2007.09.006
Scott, M. M., Perello, M., Chuang, J. C., Sakata, I., Gautron, L., Lee, C. E., et al. (2012). Hindbrain ghrelin receptor signaling is sufficient to maintain fasting glucose. PLoS One 7:e44089. doi: 10.1371/journal.pone.0044089
Shi, L., Deng, J., Chen, S., Que, J., Sun, Y., Wang, Z., et al. (2018). Fasting enhances extinction retention and prevents the return of fear in humans. Transl. Psychiatry 8:214. doi: 10.1038/s41398-018-0260-1
Shi, L., Du, X., Jiang, H., and Xie, J. (2017). Ghrelin and neurodegenerative disorders-a review. Mol. Neurobiol. 54, 1144–1155. doi: 10.1007/s12035-016-9729-1
Simas, B. B., Nunes, E. A., Crestani, C. C., and Speretta, G. F. (2018). Cardiovascular and metabolic consequences of the association between chronic stress and high-fat diet in rats. Stress 21, 247–256. doi: 10.1080/10253890.2018.1437413
Simmons, W. K., Burrows, K., Avery, J. A., Kerr, K. L., Taylor, A., Bodurka, J., et al. (2020). Appetite changes reveal depression subgroups with distinct endocrine, metabolic, and immune states. Mol. Psychiatry 25, 1457–1468. doi: 10.1038/s41380-018-0093-6
Singewald, N., and Holmes, A. (2019). Rodent models of impaired fear extinction. Psychopharmacology 236, 21–32. doi: 10.1007/s00213-018-5054-x
Singewald, N., Schmuckermair, C., Whittle, N., Holmes, A., and Ressler, K. J. (2015). Pharmacology of cognitive enhancers for exposure-based therapy of fear, anxiety and trauma-related disorders. Pharmacol. Ther. 149, 150–190. doi: 10.1016/j.pharmthera.2014.12.004
Skibicka, K. P., and Dickson, S. L. (2011). Ghrelin and food reward: the story of potential underlying substrates. Peptides 32, 2265–2273. doi: 10.1016/j.peptides.2011.05.016
Skibicka, K. P., Hansson, C., Alvarez-Crespo, M., Friberg, P. A., and Dickson, S. L. (2011). Ghrelin directly targets the ventral tegmental area to increase food motivation. Neuroscience 180, 129–137. doi: 10.1016/j.neuroscience.2011.02.016
Skibicka, K. P., Hansson, C., Egecioglu, E., and Dickson, S. L. (2012a). Role of ghrelin in food reward: impact of ghrelin on sucrose self-administration and mesolimbic dopamine and acetylcholine receptor gene expression. Addict. Biol. 17, 95–107. doi: 10.1111/j.1369-1600.2010.00294.x
Skibicka, K. P., Shirazi, R. H., Hansson, C., and Dickson, S. L. (2012b). Ghrelin interacts with neuropeptide Y Y1 and opioid receptors to increase food reward. Endocrinology 153, 1194–1205. doi: 10.1210/en.2011-1606
Skibicka, K. P., Shirazi, R. H., Rabasa-Papio, C., Alvarez-Crespo, M., Neuber, C., Vogel, H., et al. (2013). Divergent circuitry underlying food reward and intake effects of ghrelin: dopaminergic VTA-accumbens projection mediates ghrelin’s effect on food reward but not food intake. Neuropharmacology 73, 274–283. doi: 10.1016/j.neuropharm.2013.06.004
Spencer, S. J., Emmerzaal, T. L., Kozicz, T., and Andrews, Z. B. (2015). Ghrelin’s role in the hypothalamic-pituitary-adrenal axis stress response: implications for mood disorders. Biol. Psychiatry 78, 19–27. doi: 10.1016/j.biopsych.2014.10.021
Spencer, S. J., Xu, L., Clarke, M. A., Lemus, M., Reichenbach, A., Geenen, B., et al. (2012). Ghrelin regulates the hypothalamic-pituitary-adrenal axis and restricts anxiety after acute stress. Biol. Psychiatry 72, 457–465. doi: 10.1016/j.biopsych.2012.03.010
Stark, R., Santos, V. V., Geenen, B., Cabral, A., Dinan, T., Bayliss, J. A., et al. (2016). Des-Acyl Ghrelin and Ghrelin O-Acyltransferase Regulate Hypothalamic-Pituitary-Adrenal Axis Activation and Anxiety in Response to Acute Stress. Endocrinology 157, 3946–3957. doi: 10.1210/en.2016-1306
Stengel, A., Goebel, M., Wang, L., Reeve, J. R. Jr., Tache, Y., and Lambrecht, N. W. G. (2010). Lipopolysaccharide differentially decreases plasma acyl and desacyl ghrelin levels in rats: potential role of the circulating ghrelin-acylating enzyme GOAT. Peptides 31, 1689–1696. doi: 10.1016/j.peptides.2010.06.015
Stevanovic, D., Milosevic, V., Starcevic, V. P., and Severs, W. B. (2007). The effect of centrally administered ghrelin on pituitary ACTH cells and circulating ACTH and corticosterone in rats. Life Sci. 80, 867–872. doi: 10.1016/j.lfs.2006.11.018
Stievenard, A., Mequinion, M., Andrews, Z. B., Destee, A., Chartier-Harlin, M. C., Viltart, O., et al. (2016). Is there a role for ghrelin in central dopaminergic systems? Focus on nigrostriatal and mesocorticolimbic pathways. Neurosci. Biobehav. Rev. 73, 255–275. doi: 10.1016/j.neubiorev.2016.11.021
Stoyanova, I. (2014). Ghrelin: a link between ageing, metabolism and neurodegenerative disorders. Neurobiol. Dis. 72(Pt A), 72–83. doi: 10.1016/j.nbd.2014.08.026
Su, M., Yan, M., and Gong, Y. (2019). Ghrelin fiber projections from the hypothalamic arcuate nucleus into the dorsal vagal complex and the regulation of glycolipid metabolism. Neuropeptides 78:101972. doi: 10.1016/j.npep.2019.101972
Swartz, E. M., Browning, K. N., Travagli, R. A., and Holmes, G. M. (2014). Ghrelin increases vagally mediated gastric activity by central sites of action. Neurogastroenterol. Motil. 26, 272–282. doi: 10.1111/nmo.12261
Togliatto, G., Trombetta, A., Dentelli, P., Baragli, A., Rosso, A., Granata, R., et al. (2010). Unacylated ghrelin rescues endothelial progenitor cell function in individuals with type 2 diabetes. Diabetes 59, 1016–1025. doi: 10.2337/db09-0858
Torres-Fuentes, C., Golubeva, A. V., Zhdanov, A. V., Wallace, S., Arboleya, S., Papkovsky, D. B., et al. (2019). Short-chain fatty acids and microbiota metabolites attenuate ghrelin receptor signaling. FASEB J. 33, 13546–13559. doi: 10.1096/fj.201901433R
Uchida, A., Zigman, J. M., and Perello, M. (2013). Ghrelin and eating behavior: evidence and insights from genetically-modified mouse models. Front. Neurosci. 7:121. doi: 10.3389/fnins.2013.00121
Verma, D., Wood, J., Lach, G., Herzog, H., Sperk, G., and Tasan, R. (2016). Hunger promotes fear extinction by activation of an amygdala microcircuit. Neuropsychopharmacology 41, 431–439. doi: 10.1038/npp.2015.163
Walker, A. K., Rivera, P. D., Wang, Q., Chuang, J. C., Tran, S., Osborne-Lawrence, S., et al. (2015). The P7C3 class of neuroprotective compounds exerts antidepressant efficacy in mice by increasing hippocampal neurogenesis. Mol. Psychiatry 20, 500–508. doi: 10.1038/mp.2014.34
Wallace Fitzsimons, S. E., Chruscicka, B., Druelle, C., Stamou, P., Nally, K., Dinan, T. G., et al. (2019). A ghrelin receptor and oxytocin receptor heterocomplex impairs oxytocin mediated signalling. Neuropharmacology 152, 90–101. doi: 10.1016/j.neuropharm.2018.12.022
Wang, Q., Liu, C., Uchida, A., Chuang, J. C., Walker, A., Liu, T., et al. (2014). Arcuate AgRP neurons mediate orexigenic and glucoregulatory actions of ghrelin. Mol. Metab. 3, 64–72. doi: 10.1016/j.molmet.2013.10.001
Warren, C. M., Tona, K. D., Ouwerkerk, L., Van Paridon, J., Poletiek, F., Van Steenbergen, H., et al. (2019). The neuromodulatory and hormonal effects of transcutaneous vagus nerve stimulation as evidenced by salivary alpha amylase, salivary cortisol, pupil diameter, and the P3 event-related potential. Brain Stimul. 12, 635–642. doi: 10.1016/j.brs.2018.12.224
Whittle, N., Maurer, V., Murphy, C., Rainer, J., Bindreither, D., Hauschild, M., et al. (2016). Enhancing dopaminergic signaling and histone acetylation promotes long-term rescue of deficient fear extinction. Transl. Psychiatry 6:e974. doi: 10.1038/tp.2016.231
Willesen, M. G., Kristensen, P., and Romer, J. (1999). Co-localization of growth hormone secretagogue receptor and NPY mRNA in the arcuate nucleus of the rat. Neuroendocrinology 70, 306–316. doi: 10.1159/000054491
Wittchen, H. U., Jacobi, F., Rehm, J., Gustavsson, A., Svensson, M., Jonsson, B., et al. (2011). The size and burden of mental disorders and other disorders of the brain in Europe 2010. Eur. Neuropsychopharmacol. 21, 655–679. doi: 10.1016/j.euroneuro.2011.07.018
Wittekind, D. A., and Kluge, M. (2015). Ghrelin in psychiatric disorders - A review. Psychoneuroendocrinology 52, 176–194. doi: 10.1016/j.psyneuen.2014.11.013
Yam, K. Y., Ruigrok, S. R., Ziko, I., De Luca, S. N., Lucassen, P. J., Spencer, S. J., et al. (2017). Ghrelin and hypothalamic NPY/AgRP expression in mice are affected by chronic early-life stress exposure in a sex-specific manner. Psychoneuroendocrinology 86, 73–77. doi: 10.1016/j.psyneuen.2017.09.006
Yamada, C., Saegusa, Y., Nahata, M., Sadakane, C., Hattori, T., and Takeda, H. (2015). Influence of aging and gender differences on feeding behavior and Ghrelin-related factors during social isolation in mice. PLoS One 10:e0140094. doi: 10.1371/journal.pone.0140094
Yan, Z., Jiao, H., Ding, X., Ma, Q., Li, X., Pan, Q., et al. (2018). Xiaoyaosan improves depressive-like behaviors in mice through regulating Apelin-APJ system in hypothalamus. Molecules 23:1073. doi: 10.3390/molecules23051073
Yanagi, S., Sato, T., Kangawa, K., and Nakazato, M. (2018). The Homeostatic Force of Ghrelin. Cell Metab. 27, 786–804. doi: 10.1016/j.cmet.2018.02.008
Yao, L., Chen, J., Chen, H., Xiang, D., Yang, C., Xiao, L., et al. (2016). Hypothalamic gastrin-releasing peptide receptor mediates an antidepressant-like effect in a mouse model of stress. Am. J. Transl. Res. 8, 3097–3105.
Yin, Y., and Zhang, W. (2016). The role of Ghrelin in senescence: a mini-review. Gerontology 62, 155–162. doi: 10.1159/000433533
Yousufzai, M., Harmatz, E. S., Shah, M., Malik, M. O., and Goosens, K. A. (2018). Ghrelin is a persistent biomarker for chronic stress exposure in adolescent rats and humans. Transl. Psychiatry 8:74. doi: 10.1038/s41398-018-0135-5
Yun, B., Yoo, J. Y., Park, M. R., Ryu, S., Lee, W. J., Choi, H. J., et al. (2020). Ingestion of Gouda cheese ameliorates the chronic unpredictable mild stress in mice. Food Sci. Anim. Resour. 40, 145–153. doi: 10.5851/kosfa.2019.e81
Zhao, T. J., Liang, G., Li, R. L., Xie, X., Sleeman, M. W., Murphy, A. J., et al. (2010). Ghrelin O-acyltransferase (GOAT) is essential for growth hormone-mediated survival of calorie-restricted mice. Proc. Natl. Acad. Sci. U.S.A. 107, 7467–7472. doi: 10.1073/pnas.1002271107
Zigman, J. M., Jones, J. E., Lee, C. E., Saper, C. B., and Elmquist, J. K. (2006). Expression of ghrelin receptor mRNA in the rat and the mouse brain. J. Comp. Neurol. 494, 528–548. doi: 10.1002/cne.20823
Keywords: ghrelin, GHSR, stress, anxiety disorders, PTSD, central ghrelin resistance, food intake
Citation: Fritz EM, Singewald N and De Bundel D (2020) The Good, the Bad and the Unknown Aspects of Ghrelin in Stress Coping and Stress-Related Psychiatric Disorders. Front. Synaptic Neurosci. 12:594484. doi: 10.3389/fnsyn.2020.594484
Received: 13 August 2020; Accepted: 14 September 2020;
Published: 27 October 2020.
Edited by:
Sigismund Huck, Medical University of Vienna, AustriaReviewed by:
Wulf Eckhard Haubensak, Research Institute of Molecular Pathology (IMP), AustriaHarriet Schellekens, University College Cork, Ireland
Copyright © 2020 Fritz, Singewald and De Bundel. This is an open-access article distributed under the terms of the Creative Commons Attribution License (CC BY). The use, distribution or reproduction in other forums is permitted, provided the original author(s) and the copyright owner(s) are credited and that the original publication in this journal is cited, in accordance with accepted academic practice. No use, distribution or reproduction is permitted which does not comply with these terms.
*Correspondence: Dimitri De Bundel, ZGltaXRyaS5kZS5idW5kZWxAdnViLmJl