- 1Department of Neuroscience, Max Delbrück Center for Molecular Medicine in the Helmholtz Association (MDC), Berlin, Germany
- 2Neuroscience Research Center, Charité-Universitätsmedizin, Berlin, Germany
Although we know a great deal about monosynaptic connectivity, transmission and integration in the mammalian nervous system from in vitro studies, very little is known in vivo. This is partly because it is technically difficult to evoke action potentials and simultaneously record small amplitude subthreshold responses in closely (<150 μm) located pairs of neurons. To address this, we have developed in vivo two-photon targeted multiple (2–4) whole-cell patch clamp recordings of nearby neurons in superficial cortical layers 1–3. Here, we describe a step-by-step guide to this approach in the anesthetized mouse primary somatosensory cortex, including: the design of the setup, surgery, preparation of pipettes, targeting and acquisition of multiple whole-cell recordings, as well as in vivo and post hoc histology. The procedure takes ~4 h from start of surgery to end of recording and allows examinations both into the electrophysiological features of unitary excitatory and inhibitory monosynaptic inputs during different brain states as well as the synaptic mechanisms of correlated neuronal activity.
Introduction
Monosynaptic transmission underpins action potential generation and the flow of information within neural circuits. Over the last decades, in vitro approaches have provided an enormous amount of data on the connectivity rates and the electrophysiological and anatomical properties of synaptic connections. More recently, a hybrid approach has been developed to link neuronal function, measured in vivo, with connectivity, measured in vitro (Ko et al., 2011; Cossell et al., 2015; Weiler et al., 2018). There is still, however, a large gap in our knowledge about monosynaptic transmission and the membrane potential (Vm) activity of connected neurons in vivo.
In vivo approaches to identify connected pairs of neurons in the mammalian nervous system have typically performed electrophysiological recordings of multiple single neurons and examined the average response of one neuron to spontaneously occurring action potentials in another neuron. “Spike triggered averaging” of cortical neurons has been performed both with multiple extracellular recordings (Reid and Alonso, 1995; Csicsvari et al., 1998; Swadlow and Gusev, 2002; Barthó et al., 2004; Fujisawa et al., 2008; English et al., 2017), a combination of extracellular and intracellular Vm recordings (Matsumura et al., 1996; Bruno and Sakmann, 2006; London et al., 2010; Yu and Ferster, 2013) and dual Vm recordings (Crochet et al., 2005). However, because it is not yet possible to record the activity of all neurons presynaptic to the cells of interest and cortical neurons can fire simultaneously, it is difficult to confirm whether correlated activity is the result of a direct synaptic connection between the two recorded neurons or input from a third, unrecorded neuron with similar firing dynamics. One approach to get around this problem is to have experimental control of action potential timing using single cell stimulation while simultaneously recording the evoked Vm response from a second neuron. While care has to be taken in concluding that any synaptic response is the result of a monosynaptic rather than polysynaptic input (Berry and Pentreath, 1976; Parker, 2010), this approach has been used in vivo to characterize the wiring and functional properties of synaptic connections in a number of non-mammalian species (Burrows, 1996; Parker, 2003; Poulet and Hedwig, 2006; Roberts et al., 2010) as well as in a more limited number of studies in mammals (Crochet et al., 2005; Jouhanneau et al., 2015, 2018; Pala and Petersen, 2015, 2018).
Mapping the synaptic properties and monosynaptic connectivity rates in neocortex has been a central aim of in vitro slice studies, with visually guided multiple whole-cell patch clamp Vm recordings being the method of choice (Edwards et al., 1989; Mason et al., 1991; Deuchars and Thomson, 1995; Geiger et al., 1997; Markram et al., 1997b; Feldmeyer et al., 2006; Debanne et al., 2008; Lefort et al., 2009; Yassin et al., 2010; Wozny and Williams, 2011; Wang et al., 2015; Feldmeyer and Radnikow, 2016; Lalanne et al., 2016). The whole-cell recording technique has also been adapted for use in vivo (Margrie et al., 2002; Petersen, 2017; Lee and Brecht, 2018), with more recent studies using dual, blind, whole-cell recordings to assess correlations of sub- and supra-threshold Vm activity between pairs of cortical neurons in awake mice (Poulet and Petersen, 2008; Gentet et al., 2010; Zhao et al., 2016; Arroyo et al., 2018). Dual Vm recordings provide a technical basis for testing for monosynaptic connectivity, but the likelihood of two cortical neurons being connected is low, dependent on cell type and negatively correlated with inter-somatic distance (Holmgren et al., 2003; Perin et al., 2011). Therefore, to identify connected pairs of cortical neurons in vivo, it would help to be able to record from nearby, genetically labeled neurons using visual control.
Here, we describe in detail an approach using in vivo two-photon microscopy to target whole-cell recordings to neighboring, fluorescently labeled layer 2/3 cortical neurons. We show that this technique can be used to evoke action potentials and isolate unitary excitatory and inhibitory postsynaptic potentials in postsynaptic neurons (Jouhanneau et al., 2015, 2018; Ferrarese et al., 2018). A troubleshooting table is provided (Table 1) and we go on to discuss potential improvements and future applications of this technique in assessing the link between monosynaptic transmission and cortical function.
Materials and Methods
The aim of this article is to provide a description of multiple, two-photon targeted whole-cell patch-clamp recordings to monitor monosynaptic connectivity in vivo. The procedure is described for an acute 1-day experiment in anesthetized mice. All experiments were performed according to protocols approved by the Berlin Animal Ethics committee (Landesamt für Gesundheit und Sociales, LAGeSo) and comply with the European animal welfare law.
Two-Photon Microscope
In vivo two-photon microscopy with galvanometric scanning (Femto2D, Femtonics) is used to visualize neurons and the whole-cell recording pipettes (Figure 1). The microscope is fixed to an air damped table (Tuned damping table RS 2000, Newport). While our microscope can only move in the vertical Z axis, the experimental equipment, including pipette manipulators and headstages and mouse holder, are mounted on a shifting table (V380FM-L, Luigs and Neumann) allowing horizontal movements in X and Y. Two, photomultiplier tubes (PMTs; GasAsP detectors, Hamamatsu) are used to detect light, one fitted with a 498–570 nm band pass filter and the second with a 598–700 nm band pass filter to enable detection of green and red fluorophores respectively. A CCD camera is coupled to the microscope and used at the start of the experiment to place the electrodes over the region of interest using a 4× objective (UPLFLN 4×, NA 0.13, W.D 17 mm, Olympus). Subsequently, a 40× water immersion objective with a long working distance (LUMPLFLN 40×W, NA 0.8, W.D 3.3 mm, Olympus) is used to target cell soma of interest in a field of view of 200 × 200 μm (0.84 μm per pixel). The tunable (680–1,080 nm), mode-locked Ti:Sapphire laser (Chameleon Ultra II, Coherent) is used to excite a wide range of fluorophores (e.g., GFP, Alexa 488, Alexa 594, tdTomato). The Pockel cell (E.O. Modulator, Conoptics) enables a fine control of the laser beam intensity. To avoid tissue damage during cellular two-photon imaging, we kept the laser power <20 mW under the objective as we observed that damage can occur while targeting neurons with a laser power >20 mW. The microscope is controlled by a Matlab (mathworks) based imaging data acquisition software (MES v4.0 software, Femtonics). Different combinations of pipette and cellular fluorophores can be used. For example, we used the red fluorophore Alexa 594 in the intracellular solution when using mice lines expressing GFP in neurons. Note that because of their different excitation spectra it is possible to use the red fluorophore Alexa 594 in the intracellular solution visible at 820 nm to target neurons expressing td-Tomato which are visible at 950 nm (Jouhanneau et al., 2018). For deeper recordings, soma-restricted expression of fluorescent proteins may help improve depth resolution by reducing neuropil fluorescence (Baker et al., 2016).
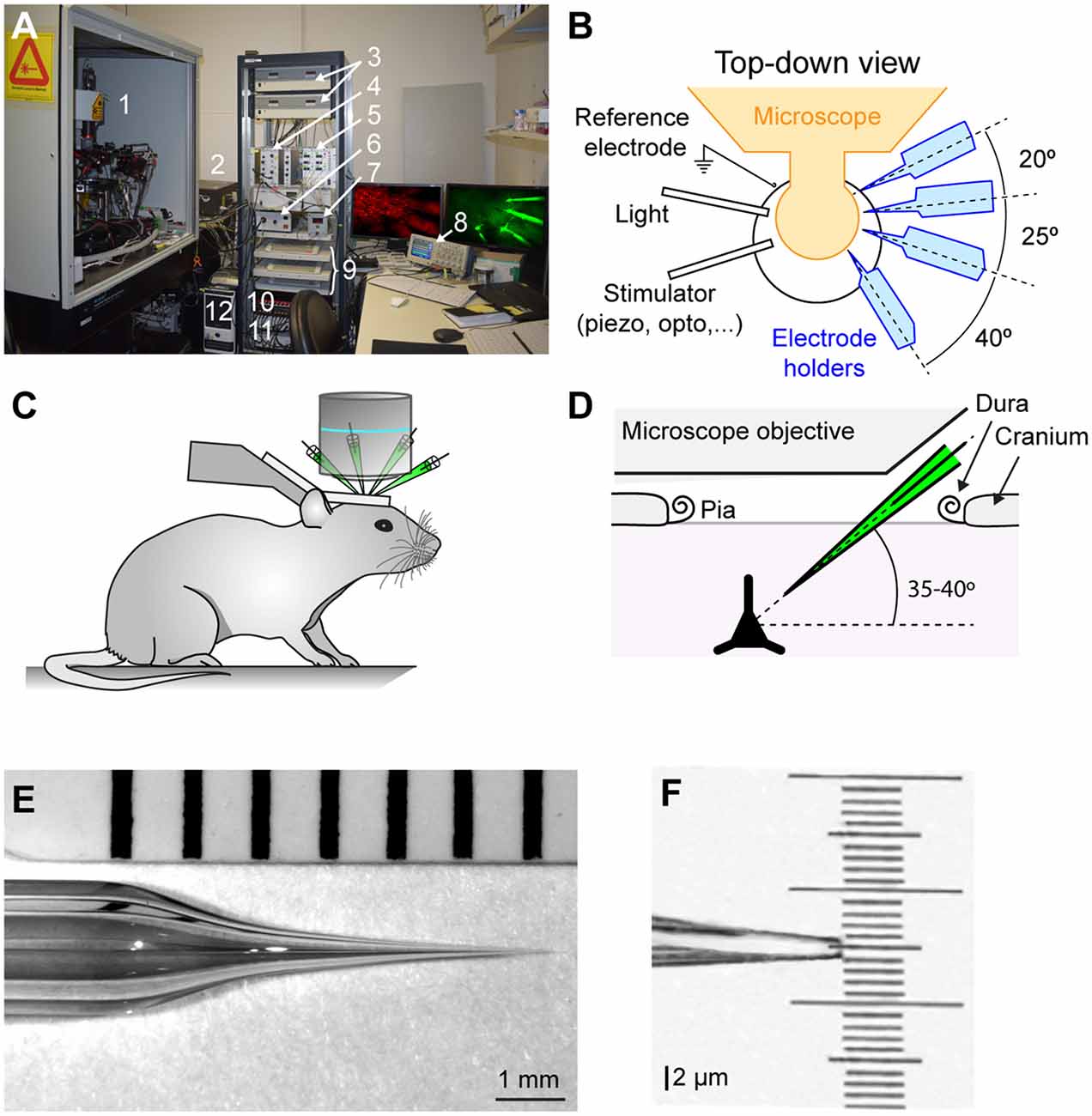
Figure 1. In vivo two-photon targeted multiple whole-cell patch-clamp setup. (A) Photograph of the setup showing: (1) two-photon microscope, micromanipulators and pre-amplifier fixed on an air-damped table; (2) Chameleon Ultra II laser; (3) Luigs and Neumann micromanipulator control units; (4) Sigmann Electronik air pressure controller; (5) Sigmann Electronik dual piezo amplifier; (6) light source to illuminate the preparation; (7) FHC temperature controller for anesthetized experiments; (8) Tektronix Oscilloscope; (9) Luigs and Neumman micromanipulator and shifting table control pads; (10) ITC-18 Heka analog to digital converter board; (11) Multiclamp 700B amplifier; and (12) data acquisition computer. (B) Schematic top-down view of recording area showing arrangement of electrode holders, light, reference electrode and somatosensory/optogenetic stimulator. Note that all the recording electrodes are on the same side for ease of targeting and to allow space on the contralateral side for stimulation devices. (C) Cartoon showing mouse position and head support. (D) Schematic showing the angle of pipettes defined by the X-axis used to allow access under the objective. (E) Photograph of the glass recording pipette showing optimal taper for in vivo two-photon targeted patch-clamp recording. (F) Photograph showing a zoom of the pipette tip from (E).
Mice
The technique works with both wild type mice and strains expressing fluorescent proteins in subsets of neurons. In this article, we used mice aged between P18 and P30 from C57bl6J, NEX-cre (Goebbels et al., 2006) × Ai9 (Madisen et al., 2010), fosGFP (Barth et al., 2004), GAD67-GFP (Tamamaki et al., 2003), PV-cre (Hippenmeyer et al., 2005) × Ai9, or SST-cre (Taniguchi et al., 2011) × Ai9. Mice were maintained on a 12 h light-dark cycle and had food and water ad libitum.
Surgery
To expose the brain for recordings, mice are first anesthetized with 1.5% isoflurane and show an absence of tail pinch reflex and whisker movements. Eye ointment (Bei trockenen Auge, Visidic) is used to protect the eyes and body temperature is maintained using a closed loop system with rectal probe and heating pad (DC Temperature controller, FHC). All tools are cleaned and dry heat sterilized using hot glass beads sterilizer (Steri 250, Keller, Fine Science Tools) prior to surgery. The head is shaved, skin removed and skull cleaned, if necessary, intrinsic optical imaging through the skull can be performed at this stage if functional localization of recording site is required. The connective tissue is carefully removed using forceps and micro-scissors (Fine science Tools) and the skull is cleaned using a microcurette (Fine science Tools) to remove any remaining tissue on the surface of the bone. A solution of 3% hydrogen peroxide (H2O2) can be applied for 30 s at this stage to help clean the exposed bone surface, however, the bone can also be cleaned by gently scraping the bone with a razor blade. Next, the skull is washed thoroughly with Ringer’s solution (in mM: 135 NaCl, 5 KCl, 5 HEPES, 1.8 CaCl2, and 1 MgCl2135 NaCl, 5 KCl, 5 HEPES, 1.8 CaCl2, and 1 MgCl2) and thoroughly dried. Avoiding the recording site, the exposed skull is then lightly scratched with a 25G syringe needle to create grooves in the skull. It is important to remove any remaining hairs or dirt at this stage to avoid possible infection. Next, glue (Loctite 401) is applied first at the edges of the exposed skull, to glue the skin to the bone, and then to the entire exposed skull surface avoiding the recording site. A lightweight metal head implant is then placed on the hemisphere contralateral to the recording site and covered with glue. To secure the head implant, dental cement is applied on top of the entire layer of glue. As the dental cement viscosity increases, a recording chamber with access for the recording pipettes can be modeled around the area of interest using a spatula. It is important to completely cover the head implant with glue and dental cement to avoid any possible contact between the Ringer’s solution and the metal of the head implant during the recording which can lead to electrical noise and voltage offsets.
Once hardened, the recording chamber is filled with Ringer’s solution. After a few minutes, the skull will become translucent and the blood vessels visible. Then, the Ringer’s solution should be removed to let the bone dry and a 500 μm diameter dental drill head (Komet, Brassler) operated by a dental drill (Success 40, Osada) is used to thin the skull over the recording site. The ideal craniotomy size is ~700 μm2 for anesthetized mice; note that a craniotomy exceeding 1 mm in diameter will impair recording stability (see Table 1). Drilling is stopped as soon as blood vessels become clearly visible through the bone. This corresponds to a bone thickness of ~50 μm (Papadopoulos et al., 2017). Bone dust is removed with wet tissue paper and the chamber is refilled with Ringer’s solution. A 30G syringe needle is used to pick away the final layer of bone with great care. Next, a durectomy is made using a smaller diameter needle (e.g., 29G), with a handmade small hook at the tip of the needle. Adjusting the angle of illumination of the craniotomy is key to visualizing the dura (~30°). The handmade hooked-tip of the needle is used to gently lift the dura away from the future spot of pipette insertion.
Whole-Cell Pipettes and Electrophysiological Equipment
We use a four-step pulling custom program on a Sutter puller (Model P-1000, Sutter instrument) with 2 mm diameter borosilicate capillaries (Hilgenberg) to pull 5–8 MΩ pipettes. The first two steps of the pulling program are identical and used to create a taper of ~6 mm, the third step is short and design to decrease the diameter of the capillary, and finally, the fourth step is used to create a tip of ~2 μm (Figures 1E,F). The taper is slightly longer than that typically used in vitro to avoid causing excess pressure on surrounding tissue and possible damage. Three to four pipettes are filled with intracellular solution containing, in mM: 135 potassium gluconate, 4 KCl, 10 HEPES, 10 phosphocreatine, 4 MgATP, 0.3 Na3GTP (adjusted to pH 7.3 with KOH), 25 μM Alexa-594 (Invitrogen) and 2 mg/ml biocytin. Pipettes are next fixed to a pipette holder (Molecular Devices) mounted on a LN Junior 3-axis (X, Y, and Z) micromanipulator with low drift and a long traverse path (up to 22 mm on the X-axis) where the X-axis is angled at 35–40° (Figure 1D; Luigs and Neumann). An Ag/AgCl ground electrode is next placed into the recording chamber filled with Ringer’s solution and electrophysiological signals are amplified using Axon Instruments amplifiers Multiclamp 700B (Molecular Devices). The analog signals recorded are filtered at 10 kHz and digitized at 20 kHz using the analog/digital converter ITC-18 board (Heka) and IgorPro (Wavemetrics) running on a Windows PC. For online visualization of the electrophysiological signal, we use an oscilloscope (Tektronix TDS2024C). To allow easier and faster access to the exposed brain and space for stimulators on the contralateral side, all pipettes are positioned on one side of the preparation (Figure 1B).
Multiple Two-Photon Targeted Whole-Cell Patch Clamp Recordings
As soon as the pipettes are inserted into the pipette holders, a 180–200 mbar positive pressure is applied via a syringe. A manual-seal-sucker (Sigmann Elektronik GmbH) manometer is used to monitor the pressure applied to all channels independently. All electrodes are moved into the Ringer’s solution in the recording chamber in voltage-clamp seal-test mode to monitor the pipette tip resistance on the oscilloscope.
Step 1: Positioning Above Brain (Figure 2A)
Using the low magnification 4× objective with green light illumination and the CCD camera, the pipettes are placed under positive pressure (~200 mbar) into the Ringer’s solution and then the tips are moved to within ~20–30 μm apart from each other and ~300 μm above the craniotomy. At this time, the pipette resistance is checked (5–8 MΩ; see Table 1). Then, by switching to the higher magnification 40× objective, the Ringer’s solution comes in contact with the objective and the pipettes tips are moved to the same focal plane. The coordinates of the pipette micromanipulator control pads are reset to zero. Next, we use the experimental stage micromanipulators (X and Y axis) and the objective focus (Z axis) to inspect the brain surface and find an entry point clear from large blood vessels, dirt or irregular surfaces. Clean entrance of the pipettes into the brain is critical for successful patching. The coordinates of the manipulator units controlling the stage are noted at the selected insertion point as a reference to help guide the movement of the pipette tips onto the brain surface. Next, the focus is moved back up to the pipette tips which are then carefully aligned. The focal plane is moved to the brain surface and the pipettes are lowered vertically one by one using a medium control sensitivity of the micromanipulator control pads (28 μm per handwheel rotation). As the pipettes are lowered, slight lateral movements are performed to help visualize the shadow of the tips. Because of the positive pressure applied to the pipette, as soon as the pipette gets into contact with the brain a clear depression can be seen on its surface which will coincide with a sudden increase in resistance of about ~20% of the peak value (as observed by a decrease in the current step amplitude on the oscilloscope). At this point, the pipette micromanipulator control pad values are reset to zero.
Step 2: Entering the Brain (Figure 2B)
Using the highest sensitivity speed on the micromanipulator (3 μm per handwheel rotation), the pipettes are slowly moved through the pia one-by-one. During insertion into the brain, the pipette resistance will gradually increase and then suddenly return to their initial value as they break throughout the pial surface. Then the pressure is reduced to 70–90 mbar. Next, two-photon imaging is used to move the pipette tips to −50 μm depth using the X-axis focus. Because of the positive pressure, the dye (Alexa-594) contained in the intracellular solution will diffuse into the neuropil and highlight blood vessels, cell soma and dendrites as dark “shadows” allowing targeting cells of interest even in wild type mice (Kitamura et al., 2008). Care should be taken during this step not to use high laser power as it may cause tissue damage (see Table 1).
Step 3: Targeting Cells of Interest (Figure 2C)
Having lowered the pressure to 70–90 mbar, the pipettes are then moved one-by-one to a depth of −150 to −200 μm (border of cortical layers 1 and 2) using the highest sensitivity movement setting. During pipette travel through the brain, great care is taken to avoid cells bodies and capillaries using both visual control from the two-photon imaging and the seal test pulse on the oscilloscope. In wild-type mice, without expression of fluorescent proteins, the contrast between the dye in the neuropil and the dark unlabeled cells, the shadow patching technique (Kitamura et al., 2008), can be used to target neurons of interest. With experience, the dendritic shape of the cell can help identify excitatory from inhibitory neurons. Lateral movement should be kept to a minimum with a maximum of 100 μm per pipette. Then the pressure is decreased to 30 mbar and the pipette micromanipulator controls are switched to a stepping mode (2 μm per step).
Step 4: Sealing (Figure 2D)
The final approach and seal is performed sequentially, one pipette at a time. The pipette voltage offset is set to 0 mV and the first pipette tip is lowered using 2 μm steps onto the cell membrane using the X-axis while watching the oscilloscope closely to observe changes in tip resistance. The sign of a good contact between the pipette tip and the neuron membrane is when the seal test pulse rapidly reduces in amplitude and fluctuates with a wave-like pattern. In contrast, an abrupt and sustained reduction in pulse amplitude (i.e., resistance increase) without fluctuations is typical of a contact with a capillary. Good contact can sometimes be visualized during two-photon scanning as a small, expanding, fluorescent dimple in the cell membrane. As soon as a good first contact has been observed, one or two further steps are made and the positive pressure immediately released followed by a transient negative pressure to optimize the seal. Vm holding voltage is immediately placed at −70 mV to help improve seal. Typically, this leads to a large reduction in the amplitude of the test pulse and the formation of a giga-seal, however light manual suction is sometimes required to improve the quality of the seal and/or transiently hyperpolarizing the cell to −100 mV. This procedure is then repeated with the other pipettes one after the other.
Step 5: Whole-Cell Configuration (Figure 2E)
When all the pipettes are sealed onto the targeted neurons, a brief and gentle suction is used to break the membrane and enter whole-cell configuration. With the whole-cell configuration established, we next slowly retract each pipette away from the cell body ~5 μm using the axis used for the final approach to the cell. All recordings are then switched to current clamp mode for Vm recordings.
Intracellular Current Injection
After allowing the cells to recover (~2–3 min), we next use intracellular current stimulation protocol to characterize their intrinsic properties. In our experiments, each neuron receives 500 ms square current injections ranging in the amplitude of −200, −100 pA, and then 50, 100, 150, 200 pA. This helps define rheobase, intrinsic excitability, and firing pattern of the recorded neurons. Next, hyperpolarizing current pulses of −100 pA, 200 ms duration, 200 ms interval, are applied for 30 s to determine the input resistance followed by 30 s without stimulation to record spontaneous sub- and supra-threshold activity. The access resistance should be <50 MΩ, high access resistance can make it difficult to inject sufficient current to evoke single spikes, filters action potential recordings and makes estimation of the Vm during current injection problematic. Next, we define the square current pulse amplitude necessary to drive the recorded neuron to fire a single action potential. We aim to use the smallest duration possible, usually between 10 and 15 ms of 100–400 pA amplitude, however higher amplitude and shorter duration pulses could be attempted. After establishing these parameters, we stimulated at 0.5 or 1 Hz (Figure 3C).
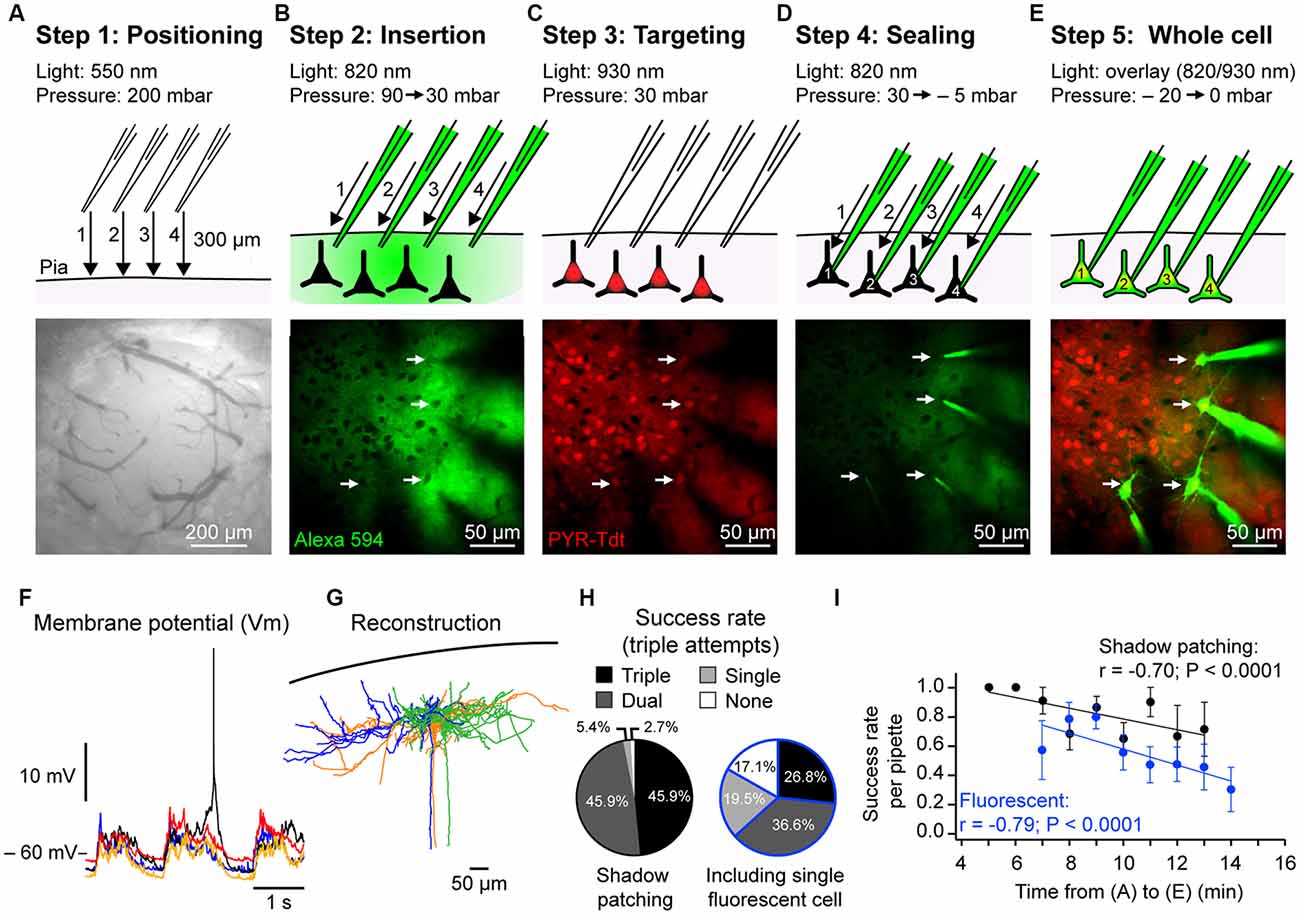
Figure 2. Two-photon targeted whole-cell patch-clamp procedure. (A) Step 1. Top: schematic showing position, movement direction (arrow) and movement order (1–4) of dye-filled (Alexa 594) recording pipettes from ~300 μm above the craniotomy to the brain surface, high positive pressure is maintained to avoid pipette tip clogging. Bottom: photograph taken using a CCD camera illuminated with green light showing example craniotomy used for anesthetized patching (~500 μm diameter) in which the dura has been removed. (B) Step 2. As in (A), but showing, top: the insertion of the pipettes through the pia under visual control (820 nm) using high positive pressure to ~−100 μm from pial surface. As soon as the pipettes enter the brain the pressure is reduced to 100 mbar and then 30 mbar when closer to the cell body positions. Bottom: in vivo two-photon image showing the position of four pipettes in the same focal plane near the cell bodies of interest. Positioning is performed sequentially, one pipette at a time. Cell bodies are revealed as dark shadows contrasting with the fluorescent signal of the extracellular dye. (C) Step 3. Top: the targeting phase where the excitation light wavelength is altered to visualize the cells of interest; in this case, excitatory glutamatergic neurons expressing the red fluorophore tdT (white arrows). Bottom: in vivo fluorescent image of pyramidal neurons (PYR) expressing tdT corresponding to the photo in (B). (D) Step 4. Top: the final sealing phase of the procedure. A recording pipette is pushed into the cell soma membrane and then, upon strong contact, the pressure is released to achieve a giga-seal. The cells are sealed sequentially under visual control. Bottom: in vivo image following sealing of all four pipettes. Note the reduction in background fluorescence during sealing because of the reduction in extracellular dye. (E) Step 5. Top: entering whole-cell configuration following seal-breaking by applying a transient negative pressure. As soon as the membrane patch is ruptured the dye within the pipettes will fill the neurons. Bottom: in vivo image of neurons filled with Alexa 594 (pseudo-colored) after the recording experiments. (F) Simultaneous example in vivo whole-cell Vm recordings of the four excitatory pyramidal neurons shown in (E) showing spontaneous activity with Up- and Down-states under urethane anesthesia. (G) Post hoc reconstruction of three biocytin-filled excitatory pyramidal neurons from a multiple whole-cell recording. (H) Proportion of triple, double, single and no recordings from trials using three pipettes in (left) wild type mice using the shadow patching method and (right) in mice expressing a cell type-specific fluorophore (PV-cre x Ai9 and SST-cre x Ai9). Data from fluorescent mice included at least one fluorescent neuron in the single/double/triple recordings. (I) Plots showing the negative correlation between the success rate of achieving a whole-cell patch clamp recording in wild type and fluorescent mice (same data as in (H)) and the time taken from phases (A) to (E) described above. Each dot represents the mean success rate for a 1 min time bin from 15 wild type mice (37 trials) and 18 mice expressing fluorescent proteins (SST-cre × Ai9 and PV-crex Ai9; 42 trials).
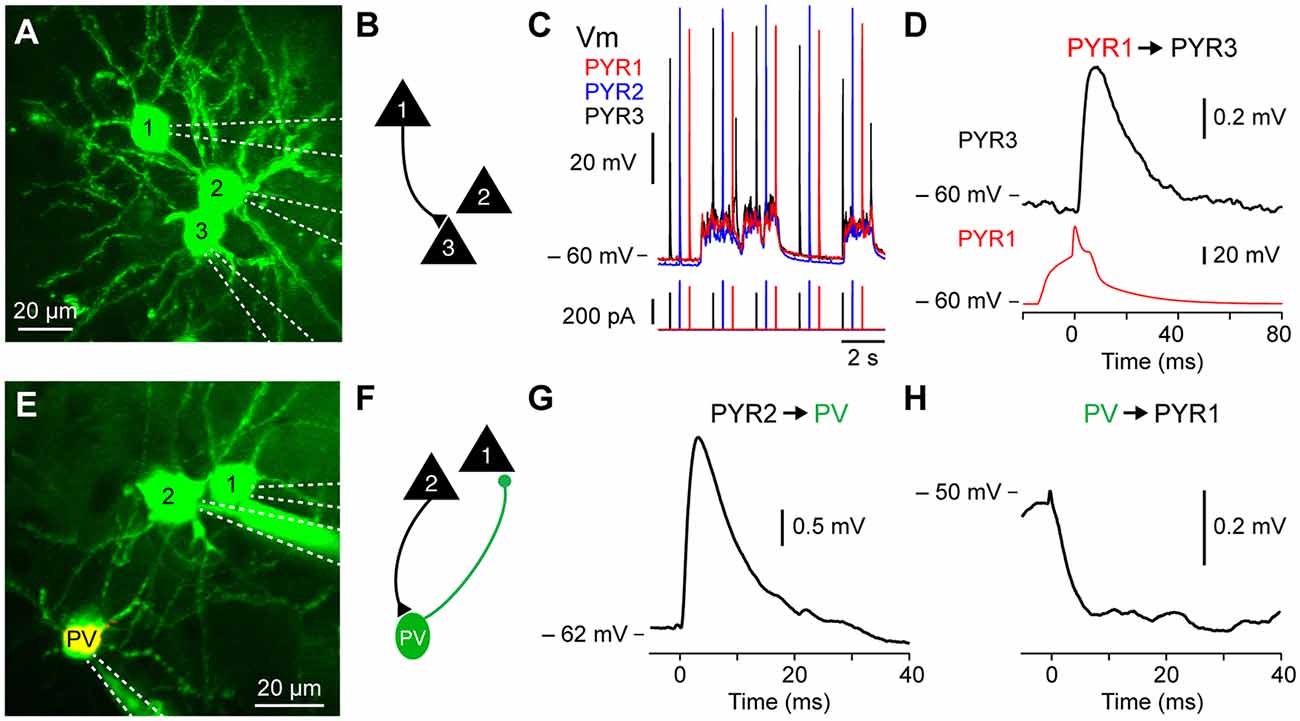
Figure 3. Excitatory and inhibitory monosynaptic connections in vivo. (A) In vivo two-photon fluorescent imaging of three excitatory pyramidal neurons filled with Alexa 594 pseudo-colored. (B) Connectivity diagram of experiment shown in (A) where PYR1 is connected to PYR3. (C) Vm fluctuation of the pyramidal neurons recoded in (A) showing the stimulation protocol consisting of brief current injection to evoke single action potentials in each neuron every 2 s. (D) Example monosynaptic excitatory connection from PYR1 to PYR3 shown in (A–C). (E) In vivo image of a triple recording including a PV-tdT expressing GABA-ergic inhibitory interneuron. (F) Connectivity diagram of experiment from the triple recording shown in (E) where an excitatory pyramidal neuron PYR2 is connected to PV-tdT expressing GABA-ergic inhibitory PV, while PV sends an inhibitory monosynaptic connection to the excitatory pyramidal neuron PYR1. (G) Excitatory monosynaptic connection from PYR2 to PV shown in (E,F). (H) Inhibitory monosynaptic connection from PV to PYR1 shown in (D).
There was no tonic current injection applied during the recording to avoid misestimation of the Vm due to possible changes in the access resistance during the recording. Recordings are terminated when the most hyperpolarized sections of the Downstate Vm are more depolarized than −50 mV. Due to differences in ionic concentration, valency and mobility between the intracellular and extracellular solution, a Liquid Junction Potential (LJP) will be established when the pipette enters the recording chamber (Barry and Lynch, 1991). The LJP can be ~10 mV and is complex to calculate accurately in vivo, therefore, to avoid miscalculation, we do not subtract the LJP from the recorded values.
Identifying a Connection
In anesthetized Downstates or during hyperpolarized phases of network activity in awake animals, even small amplitude (0.1–0.5 mV) monosynaptic connections can typically be observed by eye in single trials. However, online, running averages of the postsynaptic response to an evoked spike helps monitor the presence of a connection as well as the quality of the recording. To confirm the presence of a connection post hoc, we used a non-parametric two-tailed Wilcoxon signed rank test comparing trial-by-trial amplitude measurements of the connection with shuffled measurements. We also used a bootstrapping method in which we compared a randomly selected, with replacement, amplitude measurements from the individual trial responses with those from shuffled measurements of amplitude. Next, we calculated the mean response amplitude and the mean shuffled, noise amplitude from the bootstrapped distributions. To obtain the 95% confidence intervals, we then repeated this process 10,000 times (see Jouhanneau et al., 2015).
Anatomy: Live Fluorescent Two-Photon Imaging and Post Hoc Biocytin Staining
During a successful recording, the fluorophore Alexa 594 diffuses into the neurons and allows live visualization of the cell’s anatomy (Figures 2E, 3A,E). Stacks of scans at 820 nm wavelength separated by 2 μm can help identify the cell type using the somatic and dendritic anatomy as well as the presence of dendritic spines. However, for higher resolution, anatomical reconstruction mice are deeply anesthetized with an i.p. injection of urethane (2.5 g/kg, Sigma-Aldrich) before being transcardially perfused with cold Ringer’s solution and then by 4% paraformaldehyde solution (PFA, Roti-Histofix 4%, Roth). After perfusion, the brain is removed and placed in 4% PFA overnight at 4°C and then in phosphate buffer (Roti-CELL 10× PBS, Roth) and stored at 4°C until further processing.
Tangential slices of 100 μm are cut using a Leica VT1000 S vibratome and stored in phosphate buffer. Intracellular staining with biocytin is then revealed using a standard ABC kit (Vectastain Elite ABC-Peroxidase kit, Biozol), with diaminobenzidine (DAB, Vector lab) enhancement. Treated slices are mounted on glass slides using a gel mounting agent (Moviol, Sigma-Aldrich), sealed with nail polish and stored at 4°C. Reconstructions of the recorded neurons are performed using the software NeuroLucida (MicroBrightField; Figure 2G).
Success Rates
We next calculated the success rates of our approach during patching of layer 2 neurons (depth: −182.0 ± 2.5 μm; distance: 39.0 ± 1.8 μm) in 37 trials, each trial corresponding to one insertion of three pipettes into the brain, in 15 anesthetized wild-type mice (males, 22.0 ± 0.3 days-old) using the shadow patch method performed by a trained researcher. We calculated the number of times we were unsuccessful or obtained a single, dual or triple whole-cell recording. In 17/37 trials we obtained a triple recording, in 17/37 trials a dual, in 2/37 single and in 1/37 no recordings (Figure 2H). Thus in 92% of shadow patching trials using three pipettes, we obtained at least a dual recoding that would allow a connectivity test. We then repeated this analysis for attempted triple recordings (three pipettes) in mice expressing a fluorescently labeled indicator in a subset of GABA-ergic interneurons (PV-tdT or SST-tdT), where a successful recording trial had to include a least one fluorescently labeled neuron. In 11/41 trials from 18 mice, we obtained a triple recording, in 15/41 trials double, 8/41 single (i.e., one tdT labeled neuron recorded) and were unsuccessful in 7/41 attempts (Figure 2H). Thus, in 63% of trials with fluorescently labeled mice, we obtained at least a dual recording including one fluorescent GABA-ergic neuron to allow for a connectivity test.
During these experiments, we noticed that for trials that took longer it seemed harder to perform a successful whole-cell recording. From our triple recording dataset in Figure 2, we therefore systematically recorded the time to go from Step 1 (positioning above brain) to Step 5 (whole-cell recording). Plotting the time taken against the success rates of successfully patching one neuron showed a significant negative correlation and confirmed that reducing the time taken to patch improves success rates for patching (Figure 2I). In a different set of experiments where the recordings were not terminated prematurely and the Downstate Vm was ≤50 mV, we calculated a mean recording time of 15 ± 6 min (n = 143 cells) with a minimum recording time of 5 min and a maximum of 32 min.
In vivo Glutamatergic Excitatory Monosynaptic Inputs to Excitatory Pyramidal Neurons and GABA-ergic Inhibitory Interneurons
During slow-wave sleep and under anesthesia, the Vm of cortical neurons fluctuates between hyperpolarized, synaptically quiescent, Downstates and depolarized, synaptically active, Upstates (Steriade et al., 1993). We first examined synaptic transmission between excitatory pyramidal neurons in Downstates. In wild type mice, pyramidal neurons were targeted using their pyramidal shaped soma and apical trunk visible as shadows against the fluorescent extracellular space and after each successful recording, confirmed using Z-stack images to visualize the somatic and dendritic morphology (e.g., spines). Moreover, we used transgenic mice expressing fluorophore in excitatory pyramidal neurons (PYR) using offspring of the NEX-cre line crossed with the Ai9 reporter mouse to study PYR to PYR monosynaptic connectivity. To trigger spikes and measure synaptic transmission we depolarized each neuron with injection of a short depolarizing current 100–400 pA of 20–50 ms duration at 0.5 or 1 Hz to evoked a single action potential and used spike-triggered averages to look at the corresponding unitary excitatory postsynaptic potential (uEPSP) (Figures 3A–D). To study short term synaptic dynamics, multiple action potentials could be triggered by increasing the current duration number and time.
We went on to use the same approach to examine excitatory connections from PYRs to different subtypes of GABA-ergic inhibitory interneurons (INTs) including parvalbumin (PV), somatostatin (SST) and vasoactive intestinal polypeptide (VIP) expressing neurons (Figures 3E–G). The PV-cre, SST-cre and VIP-cre mice were crossed with the Ai9 reporter line to visualize the subpopulation of GABA-ergic neurons of interest. The approach to target recordings of interneurons is technically similar to that of excitatory neurons. However, in some cases, during the sealing step, the positive pressure was lower than usual (~10 mbar) in order to target small diameter neurons like VIP interneurons. For further details on inputs to PV and SST interneurons in vivo see Jouhanneau et al. (2018).
Using this approach, we found that barrel cortex layer 2 excitatory pyramidal neurons had a connectivity rate of 6.7% (Jouhanneau et al., 2015), while connections from excitatory pyramidal neuron to PV neurons was 44.4% and to SST neurons was 43.6% (Jouhanneau et al., 2018). For further details see Jouhanneau et al. (2015, 2018).
Inhibitory Monosynaptic Connections to PYRs and INTs in vivo
We next used multiple two-photon targeted patch-clamp recordings to examine inhibitory monosynaptic neurotransmission from GABA-ergic INTs to PYRs (Figures 3E,F,H). The connectivity rate from layer 2 barrel PV neurons to excitatory neurons was 60.6% and from SST neurons to excitatory neurons 47.1% (see Jouhanneau et al., 2018). The recording procedure is similar to that described above, but because interneurons often have a higher input resistance, small amplitude and shorter duration current steps are used to trigger single action potentials. Moreover, because of their hyperpolarized reversal potential, uIPSPs are more visible at more depolarized postsynaptic Vm values. This was evident in our recordings, where the amplitude of uIPSPs was larger in Upstates compared to Downstates (see Jouhanneau et al., 2018).
Discussion
Understanding the link between monosynaptic connectivity and the functional properties of cortical neurons is a key goal of neuroscience. Here, we have described an approach that allows Vm recordings of monosynaptically connected cortical neurons in vivo. The setup uses a standard in vivo two-photon microscope, whole-cell patch clamp amplifiers and motorized micromanipulators. With training, multiple whole-cell recordings of neurons in layer 2 can be performed with a success rate of forming a dual recording of ~90% and recording duration (~15 min) similar to single electrode, blind, in vivo patch clamp recordings. Perhaps the key indicator of patching success is an unhindered pipette entrance into the brain and rapid progress through the tissue (Figure 2). In Table 1, we have outlined a list of common problems with targeted patch-clamp recordings and possible solutions. Here, we discuss the key features, limitations and future perspectives for multiple, targeted in vivo whole-cell recordings.
Increasing the number of pipettes per trial helps test more possible connections with two pipettes allow the testing of two possible connections, three allowing six tests and four 12 tests. More pipettes provide an opportunity to not only to improve the changes of identifying a connection but also look at higher order connectivity motifs (Guzman et al., 2016; Peng et al., 2017). While we have successfully used four pipettes to obtain quadruple whole-cell recordings (Figures 2A–E), our data on success rates (Figures 2H,I) was taken from a series of experiments using three pipettes. In wildtype mice, during shadow patching, four pipettes or more could be a significant advantage to help increase the yield of recorded cells. However, for targeting fluorescently labeled subsets of neurons, the experimenter needs to weigh the advantage of using a fourth pipette against the extra time taken to insert four pipettes into the brain and target the labeled neurons.
A limitation of our approach is the use of anesthesia during the recording session. While multiple whole-cell recordings have been performed in awake animals (Poulet and Petersen, 2008; Gentet et al., 2010; Zhao et al., 2016; Arroyo et al., 2018), little data exists on monosynaptic transmission in awake animals (Jouhanneau et al., 2018; Pala and Petersen, 2018). The increased movement of the brain in awake animals not only limits the chances of forming a seal between the pipette and the cell membrane but also reduces the recording duration preventing longer-term plasticity protocols (e.g., spike timing dependent plasticity (Bell et al., 1997; Markram et al., 1997a). The use of agarose or glass cover slips on the brain surface helps reduce movement during imaging experiments and can be used for targeted whole-cell recordings. Moreover, having the mouse standing on a trackball or platform with suspension can help reduce the pressure exerted on the head during leg movements. Together, these approaches may help stabilize the brain for longer duration recordings both in anesthetized and awake mice.
The approach presented above focusses on recordings from superficial layer cortical neurons. Moreover, as with the vast majority of cortical slice work, the neurons targeted were closely positioned (<150 μm apart). It is important to examine synaptic transmission between deeper and more distant neurons, perhaps even in different cortical regions. Electrodes can easily be positioned to target different parts or depths of the brain, but both the depth and field of view are determined by the optical properties of the microscope. The combination of cell type-specific mouse lines (Gerfen et al., 2013; Harris et al., 2014; Daigle et al., 2018) with improved depth resolution two-photon microscopes (Papadopoulos et al., 2017) has provided optical access to granular and infragranular layers and may make multiple targeted recordings possible in deeper layers. Moreover, new two-photon microscope designs with larger fields of view could allow experimenters to examine neurons situated 1,000s of micrometers apart (Sofroniew et al., 2016; Stirman et al., 2016). Even with improved microscopes, however, the scattered fluorescence from the extracellular dye puffed out during patching remains a problem for accurate visualization of the pipette tip and targeted recordings. One possibility may be to use a coating material on the pipette tip to limit light scatter and improve contrast of the tips (Andrásfalvy et al., 2014).
Cortical excitatory neurons are sparsely connected and therefore a key limitation to the throughput of any connectivity study is to find and record from connected pairs. Both in vitro and in vivo studies are normally made blind to connectivity which can lead to many frustrating recordings from unconnected neurons. One way to address this is to increase the numbers of recording pipettes to allow the testing of more connections per recording session. This has been successfully implemented in vitro (Perin et al., 2011; Peng et al., 2017), but will require more challenging surgery and manipulation of the pipettes in vivo. Another approach could be to use transsynaptic tracing to visualize connected pairs prior to recording (Wickersham et al., 2007). So far, however, single cell initiated transsynaptic tracing has been used with sequential rather than simultaneous anatomical tracing (Vélez-Fort et al., 2018), or calcium imaging (Wertz et al., 2015) of presynaptic neurons. With the development of less toxic rabies viruses variants (Reardon et al., 2016; Ciabatti et al., 2017), however, this approach could now be attempted with simultaneous recordings from pre- and post-synaptic neurons.
Our approach allows a limited number of cells (2–4) to be tested for putative connections, but cortical neurons integrate synaptic inputs from thousands of presynaptic neurons. To investigate synaptic integration further, it will be important to be able to activate unitary monosynaptic inputs from more than one neuron with high temporal precision. The recent development of in vivo single cell optogenetic stimulation (Rickgauer et al., 2014; Packer et al., 2015), has provided a way to activate multiple single neurons with high resolution spatial and temporal patterns. A combination of this technique with in vivo whole-cell recordings to monitor small amplitude subthreshold synaptic inputs could provide an exciting method to investigate the integration of multiple unitary inputs in vivo.
An in vivo patch clamp recording session can be slow, especially when learning the technique or using multiple electrodes. In particular, the replacement of old pipettes with unused ones at each new recording attempt takes up valuable time. A recent study has circumvented this problem with the use of a commercially available detergent and rinsing procedure (Kolb et al., 2016). This allowed the reuse of the same pipettes with no degradation in signal fidelity both in vitro and in vivo. Robotic assistance to move the pipettes also may help speed up this process and has recently been implemented for the entire visualized patching process (Annecchino et al., 2017; Suk et al., 2017).
The whole-cell technique allows intracellular access to the recorded neurons and future work could make a more detailed anatomical and genetic characterization of the recorded neurons. For example using single-cell RNA sequencing (Jiang et al., 2015; Cadwell et al., 2016; Fuzik et al., 2016; Muñoz et al., 2017; Boldog et al., 2018) or higher resolution bright field (Feldmeyer et al., 2006) or electron microscopic (Fernández et al., 1996) anatomical analysis of the recorded synaptic connections.
The craniotomy and glass recording pipette exposes the brain and requires the use of extracellular Ringer’s solution as well as an intracellular solution. These solutions are made in the lab and therefore provide an access point for the application of extra- and intra-cellular (Palmer et al., 2014; Ferrarese et al., 2018), pharmacological agents in vivo. For example, we recently applied intracellular blockers of different ion channels via the intracellular solution to investigate their impact on synaptic integration during network activity (Ferrarese et al., 2018), and extracellular antagonist to monitor the impact of acetylcholine on monosynaptic excitatory transmission between PYR neurons and neighboring SST GABA-ergic neurons (Urban-Ciecko et al., 2018). Future work could, therefore, use specific pharmacological agents to help understand the ionic mechanisms of synaptic transmission in active, intact networks.
Conclusion
Two-photon targeted multiple-whole cell recordings provide a high resolution and cell-type specific way of identifying monosynaptically connected neurons in vivo. This approach will allow studies into the impact of network activity on synaptic transmission, the synaptic mechanisms underlying action potentials generation and link connectivity to functional responses at a millisecond time scale. Moreover, the possibility to record the Vm of both pre- and post-synaptic neurons provides a way to examine the synaptic basis of correlated spiking activity of cortical neurons.
Ethics Statement
All experiments were performed according to protocols approved by the Berlin Animal Ethics committee (Landesamt für Gesundheit und Sociales, LAGeSo) and comply with the European animal welfare law.
Author Contributions
J-SJ performed the experiments and analyzed the data. J-SJ and JP designed the study and wrote the manuscript.
Funding
This work was funded by the European Research Council (ERC-2015-CoG-682422, JP), the European Union (3x3 Dimaging 323945), the Deutsche Forschungsgemeinschaft (DFG, FOR 2143, SFB 1315, JP), the American National Institute of Health (NIH R01NS088958, JP), the Thyssen Foundation (JP), and the Helmholtz Society (JP).
Conflict of Interest Statement
The authors declare that the research was conducted in the absence of any commercial or financial relationships that could be construed as a potential conflict of interest.
Acknowledgments
We would like to thank Janett König, Charlene Memler and Femtonics for technical assistance, Mario Carta, Sylvain Crochet, Alison Barth and Evgeny Bobrov for comments on earlier version of the manuscript. We thank Poulet lab members for help and constructive comments.
References
Andrásfalvy, B. K., Galiñanes, G. L., Huber, D., Barbic, M., Macklin, J. J., Susumu, K., et al. (2014). Quantum dot-based multiphoton fluorescent pipettes for targeted neuronal electrophysiology. Nat. Methods 11, 1237–1241. doi: 10.1038/nmeth.3146
Annecchino, L. A., Morris, A. R., Copeland, C. S., Agabi, O. E., Chadderton, P., and Schultz, S. R. (2017). Robotic automation of in vivo two-photon targeted whole-cell patch-clamp electrophysiology. Neuron 95, 1048.e3–1055.e3. doi: 10.1016/j.neuron.2017.08.018
Arroyo, S., Bennett, C., and Hestrin, S. (2018). Correlaon of synaptic inputs in the visual cortex of awake, behaving mice. Neuron 99, 1289.e2–1301.e2. doi: 10.1016/j.neuron.2018.08.008
Baker, C. A., Elyada, Y. M., Parra, A., and Bolton, M. M. L. (2016). Cellular resolution circuit mapping with temporal-focused excitation of soma-targeted channelrhodopsin. Elife 5:e14193. doi: 10.7554/elife.14193
Barry, P. H., and Lynch, J. W. (1991). Liquid junction potentials and small cell effects in patch-clamp analysis. J. Membr. Biol. 121, 101–117. doi: 10.1007/bf01870526
Barth, A. L., Gerkin, R. C., and Dean, K. L. (2004). Alteration of neuronal firing properties after in vivo experience in a FosGFP transgenic mouse. J. Neurosci. 24, 6466–6475. doi: 10.1523/JNEUROSCI.4737-03.2004
Barthó, P., Hirase, H., Monconduit, L., Zugaro, M., Harris, K. D., and Buzsáki, G. (2004). Characterization of neocortical principal cells and interneurons by network interactions and extracellular features. J. Neurophysiol. 92, 600–608. doi: 10.1152/jn.01170.2003
Bell, C. C., Han, V. Z., Sugawara, Y., and Grant, K. (1997). Synaptic plasticity in a cerebellum-like structure depends on temporal order. Nature 387, 278–281. doi: 10.1038/387278a0
Berry, M. S., and Pentreath, V. W. (1976). Criteria for distinguishing between monosynaptic and polysynaptic transmission. Brain Res. 105, 1–20. doi: 10.1016/0006-8993(76)90919-7
Boldog, E., Bakken, T. E., Hodge, R. D., Novotny, M., Aevermann, B. D., Baka, J., et al. (2018). Transcriptomic and morphophysiological evidence for a specialized human cortical GABAergic cell type. Nat. Neurosci. 21, 1185–1195. doi: 10.1038/s41593-018-0205-2
Bruno, R. M., and Sakmann, B. (2006). Cortex is driven by weak but synchronously active thalamocortical synapses. Science 312, 1622–1627. doi: 10.1126/science.1124593
Cadwell, C. R., Palasantza, A., Jiang, X., Berens, P., Deng, Q., Yilmaz, M., et al. (2016). Electrophysiological, transcriptomic and morphologic profiling of single neurons using Patch-seq. Nat. Biotechnol. 34, 199–203. doi: 10.1038/nbt.3445
Ciabatti, E., González-Rueda, A., Mariotti, L., Morgese, F., and Tripodi, M. (2017). Life-long genetic and functional access to neural circuits using self-inactivating rabies virus. Cell 170, 382.e14–392.e14. doi: 10.1016/j.cell.2017.06.014
Cossell, L., Iacaruso, M. F., Muir, D. R., Houlton, R., Sader, E. N., Ko, H., et al. (2015). Functional organization of excitatory synaptic strength in primary visual cortex. Nature 518, 399–403. doi: 10.1038/nature14182
Crochet, S., Chauvette, S., Boucetta, S., and Timofeev, I. (2005). Modulation of synaptic transmission in neocortex by network activities. Eur. J. Neurosci. 21, 1030–1044. doi: 10.1111/j.1460-9568.2005.03932.x
Csicsvari, J., Hirase, H., Czurko, A., and Buzsaki, G. (1998). Reliability and state dependence of pyramidal cell-interneuron synapses in the hippocampus: an ensemble approach in the behaving rat. Neuron 21, 179–189. doi: 10.1016/s0896-6273(00)80525-5
Daigle, T. L., Madisen, L., Hage, T. A., Valley, M. T., Knoblich, U., Larsen, R. S., et al. (2018). A suite of transgenic driver and reporter mouse lines with enhanced brain-cell-type targeting and functionality. Cell 174, 465.e22–480.e22. doi: 10.1016/j.cell.2018.06.035
Debanne, D., Boudkkazi, S., Campanac, E., Cudmore, R. H., Giraud, P., Fronzaroli-Molinieres, L., et al. (2008). Paired-recordings from synaptically coupled cortical and hippocampal neurons in acute and cultured brain slices. Nat. Protoc. 3, 1559–1568. doi: 10.1038/nprot.2008.147
Deuchars, J., and Thomson, A. M. (1995). Innervation of burst firing spiny interneurons by pyramidal cells in deep layers of rat somatomotor cortex: paired intracellular recordings with biocytin filling. Neuroscience 69, 739–755. doi: 10.1016/0306-4522(95)00288-t
Edwards, F. A., Konnerth, A., Sakmann, B., and Takahashi, T. (1989). A thin slice preparation for patch clamp recordings from neurones of the mammalian central nervous system. Pflugers Arch. 414, 600–612. doi: 10.1007/bf00580998
English, D. F., McKenzie, S., Evans, T., Kim, K., Yoon, E., and Buzsáki, G. (2017). Pyramidal cell-interneuron circuit architecture and dynamics in hippocampal networks. Neuron 96, 505.e7–520.e7. doi: 10.1016/j.neuron.2017.09.033
Feldmeyer, D., Lübke, J., and Sakmann, B. (2006). Efficacy and connectivity of intracolumnar pairs of layer 2/3 pyramidal cells in the barrel cortex of juvenile rats. J. Physiol. 575, 583–602. doi: 10.1113/jphysiol.2006.105106
Feldmeyer, D., and Radnikow, G. (2016). “Paired recordings from synaptically coupled neurones in acute neocortical slices,” in Advanced Patch-Clamp Analysis for Neuroscientists, ed. A. Korngreen (New York, NY: Springer), 171–191.
Fernández, A., Radmilovich, M., Russo, R. E., Hounsgaard, J., and Trujillo-Cenóz, O. (1996). Monosynaptic connections between primary afferents and giant neurons in the turtle spinal dorsal horn. Exp. Brain Res. 108, 347–356. doi: 10.1007/bf00227258
Ferrarese, L., Jouhanneau, J.-S., Remme, M. W. H., Kremkow, J., Katona, G., Rózsa, B., et al. (2018). Dendrite-specific amplification of weak synaptic input during network activity in vivo. Cell Rep. 24, 3455.e5–3465.e5. doi: 10.1016/j.celrep.2018.08.088
Fujisawa, S., Amarasingham, A., Harrison, M. T., and Buzsáki, G. (2008). Behavior-dependent short-term assembly dynamics in the medial prefrontal cortex. Nat. Neurosci. 11, 823–833. doi: 10.1038/nn.2134
Fuzik, J., Zeisel, A., Máté, Z., Calvigioni, D., Yanagawa, Y., Szabó, G., et al. (2016). Integration of electrophysiological recordings with single-cell RNA-seq data identifies neuronal subtypes. Nat. Biotechnol. 34, 175–183. doi: 10.1038/nbt.3443
Geiger, J. R., Lübke, J., Roth, A., Frotscher, M., and Jonas, P. (1997). Submillisecond AMPA receptor-mediated signaling at a principal neuron-interneuron synapse. Neuron 18, 1009–1023. doi: 10.1016/s0896-6273(00)80339-6
Gentet, L. J., Avermann, M., Matyas, F., Staiger, J. F., and Petersen, C. C. H. (2010). Membrane potential dynamics of GABAergic neurons in the barrel cortex of behaving mice. Neuron 65, 422–435. doi: 10.1016/j.neuron.2010.01.006
Gerfen, C. R., Paletzki, R., and Heintz, N. (2013). GENSAT BAC cre-recombinase driver lines to study the functional organization of cerebral cortical and basal ganglia circuits. Neuron 80, 1368–1383. doi: 10.1016/j.neuron.2013.10.016
Goebbels, S., Bormuth, I., Bode, U., Hermanson, O., Schwab, M. H., and Nave, K.-A. (2006). Genetic targeting of principal neurons in neocortex and hippocampus of NEX-Cre mice. Genesis 44, 611–621. doi: 10.1002/dvg.20256
Guzman, S. J., Schlögl, A., Frotscher, M., and Jonas, P. (2016). Synaptic mechanisms of pattern completion in the hippocampal CA3 network. Science 353, 1117–1123. doi: 10.1126/science.aaf1836
Hamada, H., Takaori, M., Kimura, K., Fukui, A., and Fujita, Y. (1993). Changes in circulating blood volume following isoflurane or sevoflurane anesthesia. Pharmacol. Rep. 7, 316–324. doi: 10.1007/s0054030070316
Harris, J. A., Hirokawa, K. E., Sorensen, S. A., Gu, H., Mills, M., Ng, L. L., et al. (2014). Anatomical characterization of Cre driver mice for neural circuit mapping and manipulation. Front. Neural Circuits 8:76. doi: 10.3389/fncir.2014.00076
Hippenmeyer, S., Vrieseling, E., Sigrist, M., Portmann, T., Laengle, C., Ladle, D. R., et al. (2005). A developmental switch in the response of DRG neurons to ETS transcription factor signaling. PLoS Biol. 3:e159. doi: 10.1371/journal.pbio.0030159
Holmgren, C., Harkany, T., Svennenfors, B., and Zilberter, Y. (2003). Pyramidal cell communication within local networks in layer 2/3 of rat neocortex. J. Physiol. 551, 139–153. doi: 10.1113/jphysiol.2003.044784
Jiang, X., Shen, S., Cadwell, C. R., Berens, P., Sinz, F., Ecker, A. S., et al. (2015). Principles of connectivity among morphologically defined cell types in adult neocortex. Science 350:aac9462. doi: 10.1126/science.aac9462
Jouhanneau, J.-S., Kremkow, J., and Poulet, J. F. A. (2018). Single synaptic inputs drive high-precision action potentials in parvalbumin expressing GABA-ergic cortical neurons in vivo. Nat. Commun. 9:1540. doi: 10.1038/s41467-018-03995-2
Jouhanneau, J.-S., Kremkow, J., Dorrn, A. L., and Poulet, J. F. A. (2015). In vivo monosynaptic excitatory transmission between layer 2 cortical pyramidal neurons. Cell Rep. 13, 2098–2106. doi: 10.1016/j.celrep.2015.11.011
Kitamura, K., Judkewitz, B., Kano, M., Denk, W., and Häusser, M. (2008). Targeted patch-clamp recordings and single-cell electroporation of unlabeled neurons in vivo. Nat. Methods 5, 61–67. doi: 10.1038/nmeth1150
Ko, H., Hofer, S. B., Pichler, B., Buchanan, K. A., Sjöström, P. J., and Mrsic-Flogel, T. D. (2011). Functional specificity of local synaptic connections in neocortical networks. Nature 473, 87–91. doi: 10.1038/nature09880
Kolb, I., Stoy, W. A., Rousseau, E. B., Moody, O. A., Jenkins, A., and Forest, C. R. (2016). Cleaning patch-clamp pipettes for immediate reuse. Sci. Rep. 6:35001. doi: 10.1038/srep35001
Lalanne, T., Abrahamsson, T., and Sjöström, P. J. (2016). Using multiple whole-cell recordings to study spike-timing-dependent plasticity in acute neocortical slices. Cold Spring Harb. Protoc. 2016:pdb.prot091306. doi: 10.1101/pdb.prot091306
Lee, A. K., and Brecht, M. (2018). Elucidating neuronal mechanisms using intracellular recordings during behavior. Trends Neurosci. 41, 385–403. doi: 10.1016/j.tins.2018.03.014
Lefort, S., Tomm, C., Sarria, J. C. F., and Petersen, C. C. H. (2009). The excitatory neuronal networkof the C2 barrel columnin mouse primary somatosensory cortex. Neuron 61, 301–316. doi: 10.1016/j.neuron.2008.12.020
London, M., Roth, A., Beeren, L., Häusser, M., and Latham, P. E. (2010). Sensitivity to perturbations in vivo implies high noise and suggests rate coding in cortex. Nature 466, 123–127. doi: 10.1038/nature09086
Madisen, L., Zwingman, T. A., Sunkin, S. M., Oh, S. W., Zariwala, H. A., Gu, H., et al. (2010). A robust and high-throughput Cre reporting and characterization system for the whole mouse brain. Nat. Neurosci. 13, 133–140. doi: 10.1038/nn.2467
Margrie, T., Brecht, M., and Sakmann, B. (2002). In vivo, low-resistance, whole-cell recordings from neurons in the anaesthetized and awake mammalian brain. Pflugers Arch. 444, 491–498. doi: 10.1007/s00424-002-0831-z
Markram, H., Lübke, J., Frotscher, M., Roth, A., and Sakmann, B. (1997a). Physiology and anatomy of synaptic connections between thick tufted pyramidal neurones in the developing rat neocortex. J. Physiol. 500, 409–440. doi: 10.1113/jphysiol.1997.sp022031
Markram, H., Lübke, J., Frotscher, M., and Sakmann, B. (1997b). Regulation of synaptic efficacy by coincidence of postsynaptic APs and EPSPs. Science 275, 213–215. doi: 10.1126/science.275.5297.213
Mason, A., Nicoll, A., and Stratford, K. (1991). Synaptic transmission between individual pyramidal neurons of the rat visual cortex in vitro. J. Neurosci. 11, 72–84. doi: 10.1523/JNEUROSCI.11-01-00072.1991
Matsumura, M., Chen, D., Sawaguchi, T., Kubota, K., and Fetz, E. E. (1996). Synaptic interactions between primate precentral cortex neurons revealed by spike-triggered averaging of intracellular membrane potentials in vivo. J. Neurosci. 16, 7757–7767. doi: 10.1523/JNEUROSCI.16-23-07757.1996
Muñoz, W., Tremblay, R., Levenstein, D., and Rudy, B. (2017). Layer-specific modulation of neocortical dendritic inhibition during active wakefulness. Science 355, 954–959. doi: 10.1126/science.aag2599
Packer, A. M., Russell, L. E., Dalgleish, H. W. P., and Häusser, M. (2015). Simultaneous all-optical manipulation and recording of neural circuit activity with cellular resolution in vivo. Nat. Methods 12, 140–146. doi: 10.1038/nmeth.3217
Pala, A., and Petersen, C. C. H. (2015). In vivo measurement of cell-type-specific synaptic connectivity and synaptic transmission in layer 2/3 mouse barrel cortex. Neuron 85, 68–75. doi: 10.1016/j.neuron.2014.11.025
Pala, A., and Petersen, C. C. (2018). State-dependent cell-type-specific membrane potential dynamics and unitary synaptic inputs in awake mice. eLife 7:e35869. doi: 10.7554/elife.35869
Palmer, L. M., Shai, A. S., Reeve, J. E., Anderson, H. L., Paulsen, O., and Larkum, M. E. (2014). NMDA spikes enhance action potential generation during sensory input. Nat. Neurosci. 17, 383–390. doi: 10.1038/nn.3646
Papadopoulos, I. N., Jouhanneau, J.-S., Poulet, J. F. A., and Judkewitz, B. (2017). Scattering compensation by focus scanning holographic aberration probing (F-SHARP). Nat. Photonics 11, 116–123. doi: 10.1038/nphoton.2016.252
Parker, D. (2003). Variable properties in a single class of excitatory spinal synapse. J. Neurosci. 23, 3154–3163. doi: 10.1523/JNEUROSCI.23-08-03154.2003
Parker, D. (2010). Neuronal network analyses: premises, promises and uncertainties. Philos. Trans. R. Soc. B Biol. Sci. 365, 2315–2328. doi: 10.1098/rstb.2010.0043
Peng, Y., Barreda Tomás, F. J., Klisch, C., Vida, I., and Geiger, J. R. P. (2017). Layer-specific organization of local excitatory and inhibitory synaptic connectivity in the rat presubiculum. Cereb. Cortex 27, 2435–2452. doi: 10.1093/cercor/bhx049
Perin, R., Berger, T. K., and Markram, H. (2011). A synaptic organizing principle for cortical neuronal groups. Proc. Natl. Acad. Sci. U S A 108, 5419–5424. doi: 10.1073/pnas.1016051108
Petersen, C. C. H. (2017). Whole-cell recording of neuronal membrane potential during behavior. Neuron 95, 1266–1281. doi: 10.1016/j.neuron.2017.06.049
Poulet, J. F. A., and Hedwig, B. (2006). The cellular basis of a corollary discharge. Science 311, 518–522. doi: 10.1126/science.1120847
Poulet, J. F. A., and Petersen, C. C. H. (2008). Internal brain state regulates membrane potential synchrony in barrel cortex of behaving mice. Nature 454, 881–885. doi: 10.1038/nature07150
Reardon, T. R., Murray, A. J., Turi, G. F., Wirblich, C., Croce, K. R., Schnell, M. J., et al. (2016). Rabies virus CVS-N2c(ΔG) strain enhances retrograde synaptic transfer and neuronal viability. Neuron 89, 711–724. doi: 10.1016/j.neuron.2016.01.004
Reid, R. C., and Alonso, J. M. (1995). Specificity of monosynaptic connections from thalamus to visual cortex. Nature 378, 281–284. doi: 10.1038/378281a0
Rickgauer, J. P., Deisseroth, K., and Tank, D. W. (2014). Simultaneous cellular-resolution optical perturbation and imaging of place cell firing fields. Nat. Neurosci. 17, 1816–1824. doi: 10.1038/nn.3866
Roberts, A., Li, W.-C., and Soffe, S. R. (2010). How neurons generate behavior in a hatchling amphibian tadpole: an outline. Front. Behav. Neurosci. 4:16. doi: 10.3389/fnbeh.2010.00016
Sofroniew, N. J., Flickinger, D., King, J., and Svoboda, K. (2016). A large field of view two-photon mesoscope with subcellular resolution for in vivo imaging. eLife 5:e14472. doi: 10.7554/elife.14472
Steriade, M., Nuñez, A., and Amzica, F. (1993). A novel slow (<1 Hz) oscillation of neocortical neurons in vivo: depolarizing and hyperpolarizing components. J. Neurosci. Methods 13, 3252–3265. doi: 10.1523/JNEUROSCI.13-08-03252.1993
Stirman, J. N., Smith, I. T., Kudenov, M. W., and Smith, S. L. (2016). Wide field-of-view, multi-region, two-photon imaging of neuronal activity in the mammalian brain. Nat. Biotechnol. 34, 857–862. doi: 10.1038/nbt.3594
Suk, H.-J., van Welie, I., Kodandaramaiah, S. B., Allen, B., Forest, C. R., and Boyden, E. S. (2017). Closed-loop real-time imaging enables fully automated cell-targeted patch-clamp neural recording in vivo. Neuron 96, 244–245. doi: 10.1016/j.neuron.2017.09.012
Swadlow, H. A., and Gusev, A. G. (2002). Receptive-field construction in cortical inhibitory interneurons. Nat. Neurosci. 5, 403–404. doi: 10.1038/nn847
Tamamaki, N., Yanagawa, Y., Tomioka, R., Miyazaki, J.-I., Obata, K., and Kaneko, T. (2003). Green fluorescent protein expression and colocalization with calretinin, parvalbumin, and somatostatin in the GAD67-GFP knock-in mouse. J. Comp. Neurol. 467, 60–79. doi: 10.1002/cne.10905
Taniguchi, H., He, M., Wu, P., Kim, S., Paik, R., Sugino, K., et al. (2011). A resource of Cre driver lines for genetic targeting of GABAergic neurons in cerebral cortex. Neuron 71, 995–1013. doi: 10.1016/j.neuron.2011.07.026
Urban-Ciecko, J., Jouhanneau, J.-S., Myal, S. E., Poulet, J. F. A., and Barth, A. L. (2018). Precisely timed nicotinic activation drives SST inhibition in neocortical circuits. Neuron 97, 611.e5–625.e5. doi: 10.1016/j.neuron.2018.01.037
Vélez-Fort, M., Bracey, E. F., Keshavarzi, S., Rousseau, C. V., Cossell, L., Lenzi, S. C., et al. (2018). A circuit for integration of head- and visual-motion signals in layer 6 of mouse primary visual cortex. Neuron 98, 179.e6–191.e6. doi: 10.1016/j.neuron.2018.02.023
Wang, G., Wyskiel, D. R., Yang, W., Wang, Y., Milbern, L. C., Lalanne, T., et al. (2015). An optogenetics- and imaging-assisted simultaneous multiple patch-clamp recording system for decoding complex neural circuits. Nat. Protoc. 10, 397–412. doi: 10.1038/nprot.2015.019
Weiler, S., Bauer, J., Hübener, M., Bonhoeffer, T., Rose, T., and Scheuss, V. (2018). High-yield in vitro recordings from neurons functionally characterized in vivo. Nat. Protoc. 13, 1275–1293. doi: 10.1038/nprot.2018.026
Wertz, A., Trenholm, S., Yonehara, K., Hillier, D., Raics, Z., Leinweber, M., et al. (2015). Presynaptic Networks. Single-cell-initiated monosynaptic tracing reveals layer-specific cortical network modules. Science 349, 70–74. doi: 10.1126/science.aab1687
Wickersham, I. R., Lyon, D. C., Barnard, R. J. O., Mori, T., Finke, S., Conzelmann, K.-K., et al. (2007). Monosynaptic restriction of transsynaptic tracing from single, genetically targeted neurons. Neuron 53, 639–647. doi: 10.1016/j.neuron.2007.01.033
Wozny, C., and Williams, S. R. (2011). Specificity of synaptic connectivity between layer 1 inhibitory interneurons and layer 2/3 pyramidal neurons in the rat neocortex. Cereb. Cortex 21, 1818–1826. doi: 10.1093/cercor/bhq257
Yassin, L., Benedetti, B. L., Jouhanneau, J.-S., Wen, J. A., Poulet, J. F. A., and Barth, A. L. (2010). An embedded subnetwork of highly active neurons in the neocortex. Neuron 68, 1043–1050. doi: 10.1016/j.neuron.2010.11.029
Yu, J., and Ferster, D. (2013). Functional coupling from simple to complex cells in the visually driven cortical circuit. J. Neurosci. 33, 18855–18866. doi: 10.1523/JNEUROSCI.2665-13.2013
Keywords: cortex, synapse, whole-cell, two-photon microscopy, in vivo
Citation: Jouhanneau J-S and Poulet JFA (2019) Multiple Two-Photon Targeted Whole-Cell Patch-Clamp Recordings From Monosynaptically Connected Neurons in vivo. Front. Synaptic Neurosci. 11:15. doi: 10.3389/fnsyn.2019.00015
Received: 20 December 2018; Accepted: 23 April 2019;
Published: 16 May 2019.
Edited by:
Dirk Feldmeyer, Julich Research Centre, GermanyReviewed by:
Paul Chadderton, University of Bristol, United KingdomDominique Debanne, INSERM U1072 Neurobiologie des canaux Ioniques et de la Synapse, France
Szabolcs Oláh, University of Szeged, Hungary
Copyright © 2019 Jouhanneau and Poulet. This is an open-access article distributed under the terms of the Creative Commons Attribution License (CC BY). The use, distribution or reproduction in other forums is permitted, provided the original author(s) and the copyright owner(s) are credited and that the original publication in this journal is cited, in accordance with accepted academic practice. No use, distribution or reproduction is permitted which does not comply with these terms.
*Correspondence: James F. A. Poulet, amFtZXMucG91bGV0QG1kYy1iZXJsaW4uZGU=