- Neuronal Signal Transduction, Max Planck Florida Institute for Neuroscience (MPFI), Jupiter, FL, United States
Dendritic spines are small protrusive structures on dendritic surfaces, and function as postsynaptic compartments for excitatory synapses. Plasticity of spine structure is associated with many forms of long-term neuronal plasticity, learning and memory. Inside these small dendritic compartments, biochemical states and protein-protein interactions are dynamically modulated by synaptic activity, leading to the regulation of protein synthesis and reorganization of cytoskeletal architecture. This in turn causes plasticity of structure and function of the spine. Technical advances in monitoring molecular behaviors in single dendritic spines have revealed that each signaling pathway is differently regulated across multiple spatiotemporal domains. The spatial pattern of signaling activity expands from a single spine to the nearby dendritic area, dendritic branch and the nucleus, regulating different cellular events at each spatial scale. Temporally, biochemical events are typically triggered by short Ca2+ pulses (~10–100 ms). However, these signals can then trigger activation of downstream protein cascades that can last from milliseconds to hours. Recent imaging studies provide many insights into the biochemical processes governing signaling events of molecular assemblies at different spatial localizations. Here, we highlight recent findings of signaling dynamics during synaptic plasticity and discuss their roles in long-term structural plasticity of dendritic spines.
Introduction
The dendritic spine is a small protrusive structure that typically houses a single excitatory postsynapse. The spine is made of a head (~0.01–1 fL) and a narrow neck (~0.1 μm in diameter) that connects the head and dendritic surface. This structure spatially restricts electrical and biochemical access from the spine head to the dendritic shaft (Svoboda et al., 1996; Bloodgood and Sabatini, 2005; Gulledge et al., 2012; Yuste, 2013). This rather unusual structure is maintained by a network of actin cytoskeleton (Hotulainen and Hoogenraad, 2010; Colgan and Yasuda, 2014). The actin network also acts as a scaffold for stably positioning channels, cell adhesion proteins and sub-spine structure such as endosomes and postsynaptic densities (PSD; Spence and Soderling, 2015).
While dendritic spines can be stable for months to years (Grutzendler et al., 2002; Trachtenberg et al., 2002), which perhaps is important for stable function of neuronal circuits, structural plasticity of dendritic spines are known to be correlated with circuit plasticity during learning (Trachtenberg et al., 2002; Yang et al., 2009, 2014; Hayashi-Takagi et al., 2015; Li et al., 2017). Importantly, models of long-term synaptic plasticity such as long-term potentiation (LTP) and long-term depression (LTD) also are associated with long-term enlargement and shrinkage of dendritic spines, respectively (Matsuzaki et al., 2004; Zhou et al., 2004). These forms of plasticity, termed structural LTP (sLTP) and structural LTD (sLTD), are thus perhaps the basis of long-term circuit reorganization during learning and memory (Kasai et al., 2010). Structural plasticity of dendritic spines is associated with molecular reorganization. For example, the actin cytoskeletal mesh, which maintains the spine structure, needs to be rearranged. In addition, PSD size and the number of glutamate receptors on the spine also changes (Makino and Malinow, 2009; Bosch et al., 2014; Meyer et al., 2014).
sLTP is perhaps the most studied form of spine structural plasticity. It has been shown that plasticity has several temporal phases with distinct sensitivity to pharmacological and genetic perturbations (Matsuzaki et al., 2004; Murakoshi et al., 2011). Immediately after induction (either by electrical stimulation or glutamate uncaging), spines undergo a rapid and large volume increase. This is called the transient phase, and the exact physiological role of this phase is unknown. The volume decreases over several minutes but stabilizes at a level higher than the original volume. This is called the sustained phase, and continues for more than an hour. This phase is associated with an increase in the postsynaptic sensitivity to glutamate (Matsuzaki et al., 2004; Harvey et al., 2008; Lee et al., 2009; Murakoshi et al., 2011). Depending on the conditions, the sustained phase can be protein-synthesis dependent (Nguyen and Kandel, 1997; Kelleher et al., 2004; Tanaka et al., 2008; Govindarajan et al., 2011).
The rapid and sustained structural remodeling of spines depends crucially on intracellular signaling networks to orchestrate posttranslational modifications and nascent protein synthesis. In this review article, we highlight recent findings demonstrating intracellular and extracellular molecular interactions regulating actin cytoskeleton as a structural basis of spine remodeling as well as implications of activity-dependent local translation for long-lasting synaptic plasticity.
Structural Regulation of Dendritic Spines
The principal architectural component of the spine is the actin cytoskeleton (Korobova and Svitkina, 2010). Long- and short-branched filamentous actin (F-actin) are connected through multiple actin-binding proteins (ABPs), forming a highly branched network (Hotulainen and Hoogenraad, 2010; Colgan and Yasuda, 2014). Therefore, dynamic remodeling of actin networks within dendritic spines is essential for activity-dependent structural changes of spines (Okamoto et al., 2004; Honkura et al., 2008; Frost et al., 2010).
F-actin is formed by polymerization of monomeric globular actin (G-actin). These two forms of actin undergo a cycle called tread-milling: ATP-bound G-actin is added to the fast-growing end (barbed end or plus end) and ADP-bound G-actin is dissociated from the other side (pointed end or minus end) of F-actin (Figure 1). The cycle of tread-milling in spines is fast: on average, most actin monomers in a filament are replaced every minute. However, it was found that a small population of actin near the base of the spine neck is much more stable (Honkura et al., 2008). This actin population remains in filaments for more than tens of minutes. The balance between actin polymerization and depolymerization plays a major role in structural plasticity of dendritic spines (Hotulainen and Hoogenraad, 2010). For example, during spine volume increases associated with sLTP, the balance is shifted toward actin polymerization, thereby elongating actin filaments and expanding the actin network (Okamoto et al., 2004; Honkura et al., 2008; Bosch et al., 2014).
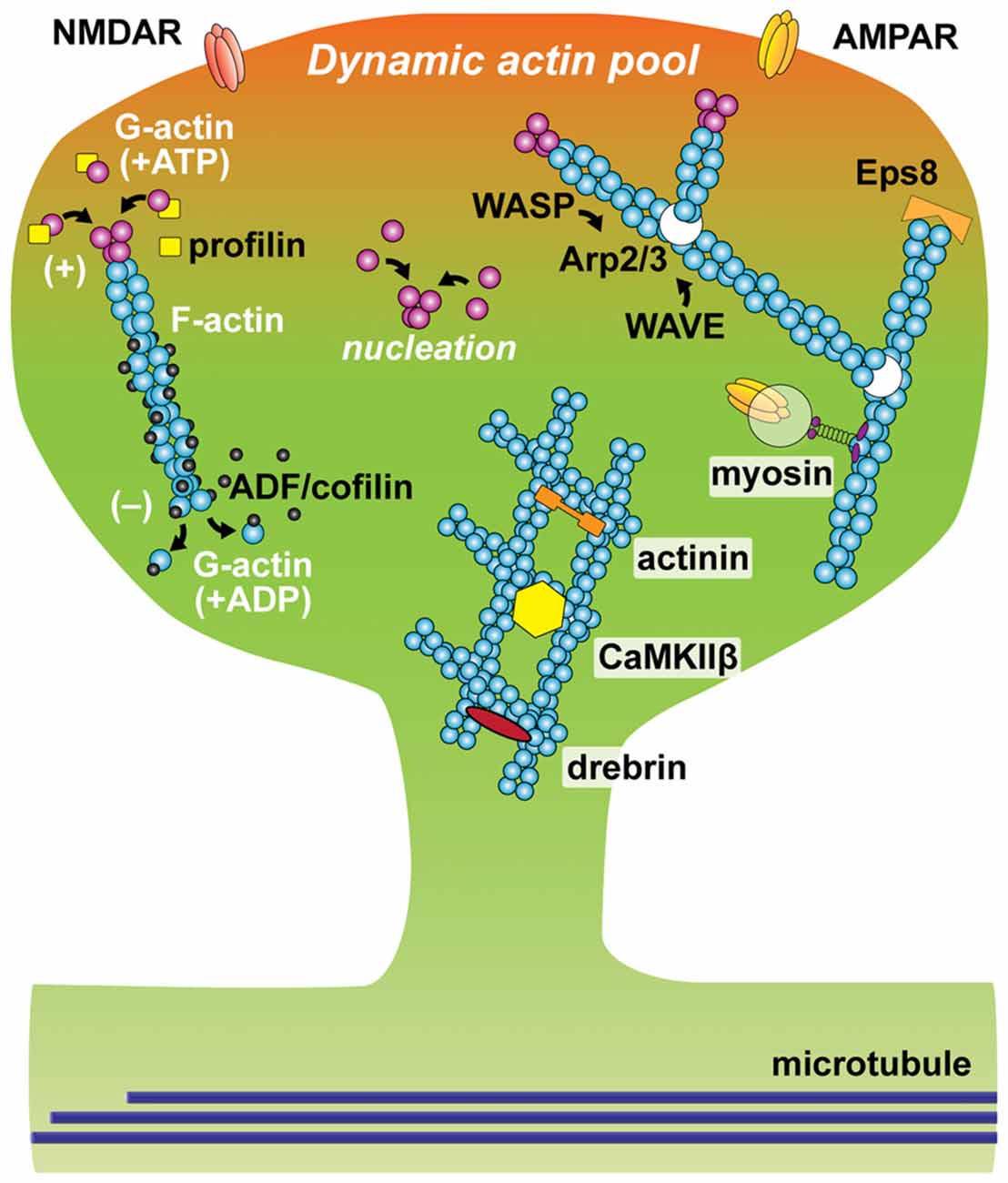
Figure 1. Schematic of actin and actin-binding proteins (ABPs) in a dendritic spine. Filamentous actin (F-actin) is formed by polymerization of globular actin (G-actin). The constant process of polymerization of ATP-bound G-actin (magenta oval) at barbed (plus) end and depolymerization of ADP-bound G-actin (cyan oval) at pointed (minus) end is called actin tread-milling. Profilin (yellow square) binds to monomeric G-actin and accelerates exchange of its nucleotide from ADP to ATP, as the result enhancing actin polymerization. ADF/cofilin (black oval) binds to ADP-bound actin and accelerates actin depolymerization at a low concentration. Arp2/3 complex (white oval) nucleates actin branching. The function of Arp2/3 complex is activated by Wiskott-Aldrich syndrome family protein (WASP) and inhibited by WASP family verprolin-homologous protein (WAVE). Epidermal growth factor receptor kinase substrate 8 (Eps8), binds to barbed-end and stabilizes actin filaments. Cross-linking proteins including actinin, CaMKIIβ and drebrin stabilize F-actin and form actin network. Active vesicular transport along F-actin is regulated by myosin.
Changes in the actin network are regulated by multiple ABPs (Figure 1). These proteins play roles in many different aspects of actin dynamics: actin polymerization, depolymerization, nucleation, branching, capping, cross-linking and trafficking. For example, the actin-related protein-2/3 (Arp2/3) complex nucleates the formation of actin filaments that branch off existing filaments at a specific angle (70 degrees). Thus, it is an important component for producing the mesh-like structure of actin filaments. It is activated and inhibited by members of the Wiskott-Aldrich syndrome family protein (WASP) and WASP family verprolin-homologous protein (WAVE), respectively (Soderling and Scott, 2006). Disruption of the Arp2/3 complex results in an increase in immature spines and abnormal behavior (Kim et al., 2013). Another ABP, profilin, plays an important role for actin polymerization by binding G-actin and accelerating the exchange of ADP to ATP, enhancing the rate of tread-milling (Ackermann and Matus, 2003; Neuhoff et al., 2005). At the plus-end of actin filaments, actin-capping proteins such as epidermal growth factor receptor kinase substrate 8 (Eps8), bind to and stabilize actin filaments (Menna et al., 2013). The function of Eps8 is downregulated by MAPK/ERK-dependent phosphorylation (Menna et al., 2009). Another important ABP for synaptic plasticity is ADF/cofilin (Zhou et al., 2004; Bosch et al., 2014; Rust, 2015). The action of ADF/cofilin family proteins is known to be concentration-dependent. While it induces depolymerization at the minus-end at a low concentration, ADF/cofilin can co-polymerize with actin filaments, stabilizing filamentous structure at a high concentration (Andrianantoandro and Pollard, 2006). LIM kinase (LIMK)-dependent phosphorylation inhibits its action. During LTP, ADF/cofilin shows biphasic phosphorylation: transient increase followed by decrease in the stimulated spine (Chen et al., 2007). This dynamic regulation of phosphorylation causes a persistent accumulation of ADF/cofilin at the neck of the stimulated spine (Chen et al., 2007; Bosch et al., 2014; Noguchi et al., 2016). During this process, ADF/cofilin appears to form the polymerized structure, stabilizing actin cytoskeleton for a long time (more than ~30 min). For formation and stabilization of complex actin networks, cross-linking proteins such as drebrin, α-actinin and Calcium/calmodulin-dependent protein kinase type II subunit β (CaMKIIβ) play a key role for bundling F-actin (Hotulainen and Hoogenraad, 2010; Kim et al., 2015). The transient exit and reentry of drebrin A in the spine head is thought to be important for spine remodeling (Bosch et al., 2014; Mizui et al., 2014; Shirao et al., 2017). In addition, myosin, a superfamily of ATP-driven motor proteins, regulates spine structure via its multiple functions including protein trafficking and contractile bundling of F-actin networks (Ryu et al., 2006; Correia et al., 2008; Wang Z. et al., 2008; Korobova and Svitkina, 2010). Thus, structural plasticity of dendritic spines requires spatiotemporal coordination of ABPs. While exact actin dynamics during synaptic plasticity remains elusive, it has been proposed that actin network becomes fluid in the initial phase of structural changes, perhaps due to the dissociation of ABPs, allowing actin to be reorganized, and then subsequently stabilized by the re-binding of ABPs (Okamoto et al., 2004; Kim et al., 2015).
Transient Ca2+ Elevation: The Trigger of Multiple Signaling Cascades for Spine Remodeling
Since regulation of actin cytoskeleton is tightly coupled with changes in spine structure, the signaling system connecting synaptic activity and various ABPs plays an important role in spine structural plasticity. Indeed, recent studies using molecular imaging, such as FRET-based fluorescence lifetime microscopy (FLIM) imaging have revealed the spatiotemporal dynamics of key signaling molecules regulating actin cytoskeleton in dendritic spines (Nishiyama and Yasuda, 2015).
Strong excitatory synaptic inputs induce postsynaptic Ca2+ elevation through NMDA receptors and/or voltage-gated Ca2+ channels (VGCCs) in the activated spine (Sabatini et al., 2002). Ca2+ elevation in spines triggers signaling cascades for long-term synaptic plasticity including LTP and sLTP. Ca2+ binds to calmodulin (CaM), a Ca2+-binding protein and Ca2+ bound CaM (Ca2+/CaM) subsequently activates Ca2+/CaM-dependent kinases and phosphatases such as CaMKII and calcineurin (CaN; Lee et al., 2009; Fujii et al., 2013; Chang et al., 2017). Traditionally, it has been considered that CaMKII and CaN are exclusively activated during postsynaptic activation and plays roles in LTP and LTD, respectively (Malenka and Bear, 2004). Consistent with this, it has been shown that CaMKII is activated during the induction of sLTP (Lee et al., 2009; Chang et al., 2017). Also, CaN was shown to be necessary for sLTD (Zhou et al., 2004; Oh et al., 2015). However, a recent study suggested that CaN is activated in LTP-inducing stimuli, as well as LTD-inducing stimuli (Fujii et al., 2013). Furthermore, it has been reported that CaMKII activity is required for inducing LTD as well as LTP (Coultrap et al., 2014; Goodell et al., 2017). Both CaMKII and CaN activity rapidly decay over a few seconds (Fujii et al., 2013; Chang et al., 2017).
It should be worth mentioning that CaMKII activation has been proposed to act as a biochemical memory lasting more than hours (Lisman et al., 2002). Upon Ca2+/CaM binding, active CaMKII can undergo autophosphorylation at Thr286 (for CaMKIIα or Thr287 for CaMKIIβ), which makes the kinase activity independent of Ca2+/CaM binding. This Ca2+/CaM-independent activation, which is often referred to as “autonomous” activity, could persist for a long time after Ca2+ decays. Indeed, it has been found that autophosphorylation at Thr286 persists for more than ~1 h after LTP induction (Barria et al., 1997). Furthermore, transgenic mice bearing a single point mutation at Thr286 (T286A) of CaMKIIα showed deficits in LTP and learning. However, pharmacological studies showed that, while inhibition of CaMKII during the induction inhibits the induction of LTP, inhibition after the induction of LTP does not reverse LTP, suggesting that CaMKII is required for induction, but not for the maintenance, of LTP (Buard et al., 2010). Similarly, CaMKII is found to be necessary for the formation, but not for the maintenance or retrieval, of amygdala-dependent fear memory (Buard et al., 2010). More recent study with optogenetic inhibitor of CaMKII further refined the temporal window of CaMKII action (Murakoshi et al., 2017). This study showed that CaMKII activation is necessary only for the first ~1 min of LTP induction. In addition, CaMKII activation in amygdala during the training (~3 min), but not after the training, is required for fear memory formation in the inhibitory avoidance task (Murakoshi et al., 2017). This apparent inconsistency between the early biochemical studies and the results from pharmacological and optogenetic inhibition could be because autophosphorylation does not correlate with CaMKII activation under some conditions (Lengyel et al., 2004).
During sLTP, short CaMKII activation is relayed to diverse downstream signaling molecules including small GTPase proteins. The activity of these downstream signals lasts more than tens of minutes, reorganizing actin cytoskeleton over this time period (Yasuda, 2017). The process of small GTPase signaling will be discussed in the “Rapid Structural Remodeling of the Spine” section.
Rapid Structural Remodeling of the Spine
Intracellular Signaling Networks for Rapid Cytoskeletal Restructuring
Cytoskeletal remodeling during structural plasticity of spines requires activation of small GTPase proteins (Harvey et al., 2008; Murakoshi et al., 2011; Bosch et al., 2014; Hedrick et al., 2016; Figure 2A). RhoA, Cdc42, Rac1 and Ras are all activated by CaMKII, and required for sLTP (Harvey et al., 2008; Murakoshi et al., 2011; Bosch et al., 2014; Hedrick et al., 2016). In addition, knockout of Rac1 and Cdc42 from excitatory neurons causes impaired LTP and memory formation (Haditsch et al., 2009; Kim et al., 2014). RhoA activation controls spine remodeling through the activation of downstream effectors such as Rho-associated protein kinase (ROCK). Activated ROCK phosphorylates LIMK, which further phosphorylate ADF/cofilin (Arber et al., 1998). Cdc42 and Rac1 promote actin polymerization through activating WASP and WAVE, respectively. The activated WASP and WAVE bind to and upregulate Arp2/3 complex, which induces actin nucleation and thus spine enlargement (Hlushchenko et al., 2016). Cdc42 and Rac1 also stabilizes actin cytoskeleton by inhibiting ADF/cofilin-mediated actin depolymerization through downstream effectors p21-activated kinase (PAK)-LIMK pathway and the PAK-phosphatases slingshot (SSH) pathway (Zhou et al., 2012; Bosch et al., 2014). Recent studies suggest that Copine-6, a Ca2+-binding molecule is another upstream regulator of the Rac1-PAK-LIMK pathway (Reinhard et al., 2016; Burk et al., 2018).
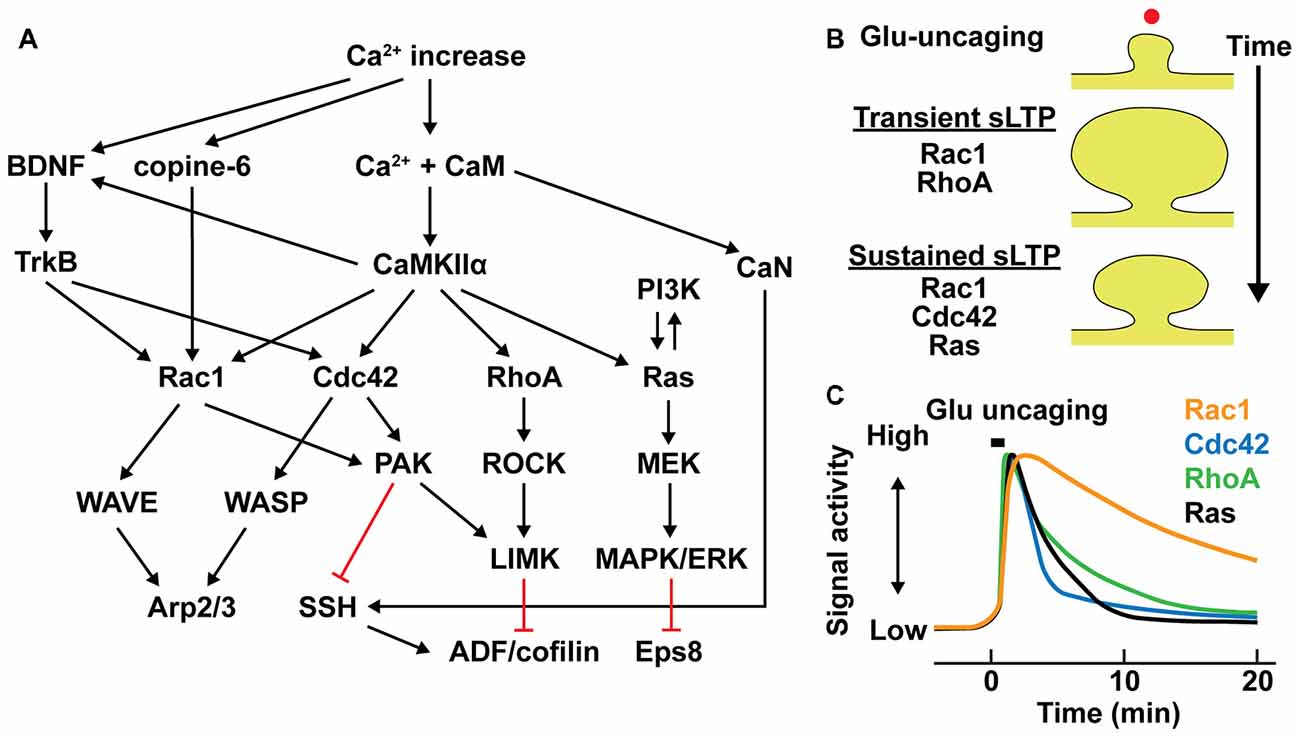
Figure 2. Intracellular signal regulation during structural long-term potentiation (LTP). (A) Signaling pathways controlling actin binding proteins (ABPs) in dendritic spines. Black arrows represent downstream activation and red lines represent downstream inhibition. (B) Different contributions of small GTPase activations in transient and sustained structural LTP (sLTP) in stimulated spines. Red dot represents a single spine stimulation by glutamate uncaging. (C) Schematic time course of small GTPase activation profiles in stimulated spines (Murakoshi et al., 2011; Oliveira and Yasuda, 2014; Hedrick et al., 2016).
The contributions of small GTPase activation to the transient and sustained phases of sLTP are distinctive. Activations of RhoA is relatively required for the transient phase of sLTP, while Cdc42 and Ras are required only for the sustained phase and Rac1 is required for both transient and sustained phases of sLTP (Harvey et al., 2008; Murakoshi et al., 2011; Oliveira and Yasuda, 2014; Hedrick et al., 2016; Figure 2B). Furthermore, they show different time courses of activity during sLTP. While activities of these GTPases remain elevated over 20 min, Rac1 displays prominently slower inactivation than those of RhoA, Cdc42 and Ras (Figure 2C). In addition, their spatial profiles are different: RhoA, Rac1 and Ras activities spread out from stimulated spine to the dendritic shaft and adjacent spines, whereas Cdc42 activity is restricted to the stimulated spine compartment (Harvey et al., 2008; Murakoshi et al., 2011; Oliveira and Yasuda, 2014; Hedrick et al., 2016; Figure 3). The spreading of Ras, RhoA and Rac1 facilitates sLTP in surrounding spines (Harvey et al., 2008; Hedrick et al., 2016; Hedrick and Yasuda, 2017). Although the regulatory mechanism of spatial constraint of Cdc42 activation is not clear, differential distributions of Cdc42 or interacting proteins such as Cdc42-specific GTPase activating protein (GAP) in dendritic shaft could limit the spreading of Cdc42 activity (Yasuda and Murakoshi, 2011; Yasuda, 2017).
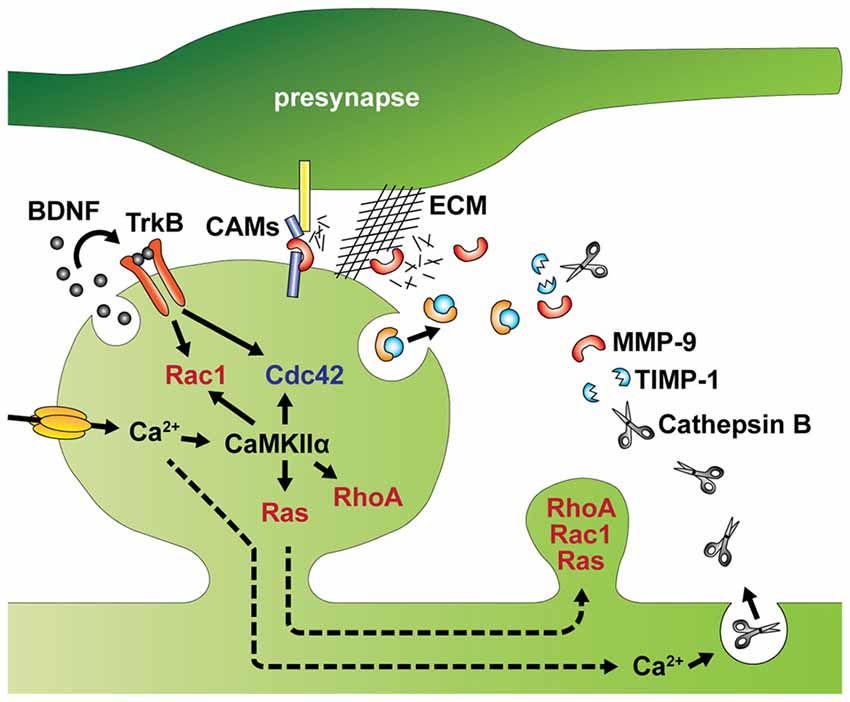
Figure 3. Intracellular and extracellular factors that regulate spine structural plasticity. Activity-dependent autocrine brain-derived neurotrophic factor (BDNF)-TrkB signaling activates Cdc42 and Rac1 in single spines. Ca2+ influx through NMDA receptors or voltage gated Ca2+ channels (VGCCs) activates CaMKIIα and its downstream signaling including Cdc42, Rac1, RhoA and Ras. Activation of Rac1 and Cdc42 are regulated by autocrine BDNF-TrkB signaling. Activities of RhoA, Rac1 and Ras spread to dendritic shaft and adjacent spines from stimulated spines. MMP-9 and TIMP-1 are also released from the postsynaptic cells. In addition, Ca2+ elevation induces lysosomal fusion with the plasma membrane, releasing Cathepsin B to outside of the cell. Extracellular Cathepsin B cleaves the tissue inhibitor of metalloproteinases-1 (TIMP-1), an endogenous inhibitor of the matrix metalloprotease 9 (MMP-9). Disinhibited MMP-9 cleaves cell adhesion molecules (CAMs) and the extracellular matrix (ECM), which facilitate structural remodeling of spines. Broken arrows represents signal spreading.
In addition to these rapid actin remodeling, another major cytoskeletal protein, microtubules also may undergo large structural changes. Microtubules are formed by polymerization of tubulin monomers (Nogales et al., 1998). Usually they are localized in the dendritic shafts and excluded from dendritic spines (Korobova and Svitkina, 2010). However, microtubule tips frequently enter dendritic spines transiently, in an activity-dependent manner (Hu et al., 2008; Jaworski et al., 2009; Merriam et al., 2011). Microtubule tip entry is correlated with spine enlargement, suggesting that this phenomenon may be important for spine structural plasticity (Jaworski et al., 2009; Merriam et al., 2011, 2013). Consistent with this, microtubule dynamics has been implicated in LTP and spine structural plasticity as well as maintenance of normal spine structure (Hu et al., 2008; Jaworski et al., 2009; Merriam et al., 2011, 2013). Microtubule tip entry recruit microtubule tip binding protein EB3, which is usually used to monitor microtubule tip, and EB-binding protein p140CAP, a regulator of Src kinase activity, into spines (Jaworski et al., 2009; Merriam et al., 2013). The entry of such protein complex may play an important role in regulating actin dynamics in dendritic spines and inducing spine structural plasticity (Dent, 2017).
Extracellular Factors Inducing Spine Remodeling
In addition to the intracellular regulation of spine structure, spine remodeling is also controlled by extracellular factors, such as autocrine signaling of brain-derived neurotrophic factor (BDNF; Harward et al., 2016; Hedrick et al., 2016) and proteolytic cleavage of extracellular matrix (ECM) and trans-synaptic cell adhesion molecules (CAMs; Sonderegger and Matsumoto-Miyai, 2014; Reinhard et al., 2015; Figure 3).
BDNF has long been deemed crucial to LTP (Minichiello, 2009). Recent studies have further demonstrated that BDNF can be released from dendritic spines during the induction of LTP to activate TrkB receptors in the same spine to regulate sLTP (Tanaka et al., 2008; Edelmann et al., 2015; Harward et al., 2016). Thus, BDNF acts as autocrine loop signaling through extracellular space of stimulated spines, which can subsequently regulate structural reorganization at the spine and adjacent spines via Rac1 and Cdc42 activities (Hedrick et al., 2016). The autocrine BDNF may play additional roles in protein synthesis (Tanaka et al., 2008), as BDNF can induce the local synthesis of several molecules including Arc, LIMK1 and CaMKIIα (Leal et al., 2014; Panja and Bramham, 2014).
Synaptic plasticity is also associated with restructuring of the extracellular space through proteolytic cleavage of the ECM and CAMs (Sonderegger and Matsumoto-Miyai, 2014; Reinhard et al., 2015). Among several endopeptidases that control synaptic functions, the matrix metalloprotease 9 (MMP-9) has been recently implicated in spine morphogenesis and synaptic plasticity (Wang X. et al., 2008; Sonderegger and Matsumoto-Miyai, 2014; Gorkiewicz et al., 2015; Magnowska et al., 2016). MMP-9 is released from dendritic spines, and cleaves components of the ECM including brevican, laminin and aggrecan, as well as CAMs such as N-cadherin and neuroligin-1 (Nagappan-Chettiar et al., 2017). In the visual cortex in adult mice, sensory experience increases MMP-9 activity, which mediates functional and morphological remodeling of synapses by digesting the ECM (Murase et al., 2017). Furthermore, NMDA receptor-mediated cleavage of intercellular adhesion molecule-5 (ICAM-5) via MMP appears to occur during LTP (Conant et al., 2010). Since ICAM-5 is known to inhibit spine maturation and enlargement by interacting with actin regulating proteins (Furutani et al., 2007), shedding of this molecule may play an important role in spine enlargement during LTP (Furutani et al., 2007; Conant et al., 2010). Interestingly, the proteolytic activity of MMP-9 is usually suppressed by tissue inhibitor of metalloproteinases-1 (TIMP-1), an endogenous inhibitor (Stawarski et al., 2014). This inhibition can be suppressed in an activity-dependent manner. Specifically, activity induces fusion of lysosomes with the plasma membrane releasing Cathepsin B extracellularly. Cathepsin B is then able to cleave TIMP-1. Thus, it appears that both MMP-9 and TIMP-1 are released from the postsynaptic cell to regulate spine structural plasticity (Padamsey et al., 2017).
In addition to the release of proteins, recent studies provide a novel concept of mRNA transmission between neurons via exosomal vesicles (EVs; Ashley et al., 2018; Pastuzyn et al., 2018). It has been known that the EV-mediated molecular transmissions occurs between neurons in an activity-dependent manner (Budnik et al., 2016). Interestingly, two studies identified that Arc protein self-assembles viral capsid, group-specific antigen (Gag)-like structure containing Arc mRNA. The EVs load this virus-like assembly and release them into extracellular space, received by other neurons (Ashley et al., 2018; Pastuzyn et al., 2018). During the transmission, mRNA is encapsulated by Arc protein and thus resistant to RNase. The received mRNA is translated, thereby producing Arc protein in the receiving neurons: even in Arc knockout neurons, application of the virus-like capsids can result in Arc expression (Pastuzyn et al., 2018). Since the expression of Arc, an immediate-early gene, controls functional and structural plasticity of dendritic spines (as discussed below), the EV-mediated transfer of Arc mRNA between cells might play important roles in various forms of synaptic plasticity (Pastuzyn et al., 2018).
Interestingly, Arc and viral Gag protein shares homologous DNA sequences, as well as structural similarity, indicating that Ty3/gypsy family of retrotransposon is presumably the ancestral origin of Arc (Campillos et al., 2006; Zhang et al., 2015). Arc and viral Gag share key functional features such as membrane binding (Barylko et al., 2018), self-oligomerization of capsid-like protein (Myrum et al., 2015; Ashley et al., 2018; Pastuzyn et al., 2018), RNA-binding and EV-mediated exosomal releases (Ashley et al., 2018; Pastuzyn et al., 2018; Shepherd, 2018). It is still elusive whether other proteins and mRNAs can be also transferred between neurons through similar mechanisms. However, human genome contains at least 103 Gag-like protein sequences including mitogen-activated protein kinase 1 (MAPK1) and retrotransposon-derived proteins PEG10 and PEG3. Interestingly, some of them are implicated in cellular development and apoptosis (Campillos et al., 2006). Therefore, these Gag-like proteins may take advantages of similar retrotransposon-like, intercellular communication systems for their physiological functions.
Protein Synthesis-Dependent Remodeling of the Spine
Some forms of sustained structural plasticity of dendritic spines require activity-dependent protein synthesis (Tanaka et al., 2008; Govindarajan et al., 2011; Bosch et al., 2014). Growing evidence has suggested that local translation near the stimulated spines occurs, and influences cytoskeletal remodeling during spine morphogenesis (Jung et al., 2014; Rangaraju et al., 2017). We next discuss molecular networks controlling local translation and its implications for spine structural plasticity.
Local Translations in Dendrites
Among 2550 mRNAs localized in dendrites and axons of hippocampal neurons (Cajigas et al., 2012), many of them, such as β-actin, Arc, PSD-95, GluA1, CaMKIIα and zipcode binding protein 1 (ZBP1), are relevant to synaptic plasticity. (Mayford et al., 1996; Tiruchinapalli et al., 2003; Sutton et al., 2006; Butko et al., 2012; Steward et al., 2015; Yoon et al., 2016). In particular, dendritic localization of CaMKIIα mRNA has been suggested to be important for LTP and learning (Miller et al., 2002). Translationally inhibited mRNAs make complexes called ribonucleoprotein (RNP) particles, a transport type RNA granule (RNG) that contains mRNA, RNA binding proteins (RBPs) and microRNA (miRNA/miR; Kiebler and Bassell, 2006; Darnell, 2013; Figure 4). RNPs are actively transported by motor proteins along the cytoskeleton (Bramham and Wells, 2007). The association of trans-acting RBPs on cis-acting elements in 3’-untranslated region (UTR) of mRNA is critical for mRNA transport and translational repression (Kanai et al., 2004; Hüttelmaier et al., 2005; Doyle and Kiebler, 2011; Darnell and Richter, 2012). Indeed, CaMKIIα mRNA lacking the 3’-UTR is not trafficked to the dendrite and impairs late-LTP and learning (Miller et al., 2002). “Unloading” of mRNA at the correct location is regulated by RBP phosphorylation, which causes mRNA dissociation and initiates the translation (Fernandez-Moya et al., 2014). In addition, inhibition of cap-dependent translation initiation during consolidation of aversive conditioning impairs the accumulation of polyribosomes in spines in the hippocampus (Ostroff et al., 2017). Thus, mRNA transport and cap-dependent translation may coincide specifically at activated spines during memory consolidation.
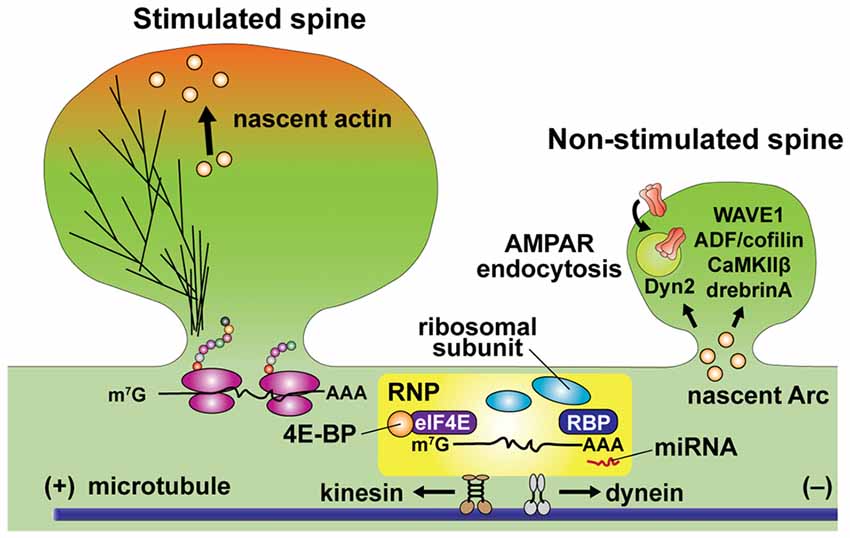
Figure 4. Schematic diagram of local translation in dendrites. mRNA is transported in the ribonucleoprotein (RNP) particle, which includes mRNA, translation initiation factors, ribosomal subunits, RNA binding proteins (RBPs) and microRNA (miRNA). Association with RNA binding proteins (RBPs) at 3’-UTR (representing as AAA) and eukaryotic translation initiation factor 4E (eIF4E) at 5’-cap (representing as m7G). RBP binding is critical for dendritic trafficking of mRNA, while repressing translation. Cap-dependent translation initiation is regulated by the interaction of mRNA with eIF4E binding protein (4E-BP). Kinesin and dynein actively transport RNP toward anterograde and retrograde directions along microtubules, respectively. Unloading of β-actin mRNA and translation initiation are simultaneously regulated at the base of stimulated spines. Newly synthesized β-actin translocates to the peripheral region of the stimulated spine head. Warm color depicts dynamic actin pools. Newly synthesized Arc preferentially increases in non-stimulated spines and changing cytoskeletal dynamics via interacting ABPs including WAVE1, ADF/cofilin, CaMKIIβ and drebrin A. Arc promotes AMPA receptor (AMPAR) endocytosis through interaction to dynamin-2 (Dyn2).
Recent advances in single mRNA imaging techniques have revealed a number of fine details about RNP transport in dendrites (Glock et al., 2017). Single-molecule fluorescence in situ hybridization (smFISH) revealed that, in dendrites, β-actin mRNA in RNA granules is sterically masked from the translation machinery as well as FISH probes, and unmasked by neuronal activity (Buxbaum et al., 2014). Live-cell imaging of RNA granules also became possible using a knock-in mouse line in which multiple MS2 binding site (MBS) stem loops are inserted at 3’-UTR of β-actin mRNA. By expressing MS2-GFP in these mice, endogenous mRNA can be fluorescently labeled. This technique has revealed that many of the RNPs in dendrites move in a bidirectional manner (Lionnet et al., 2011; Yoon et al., 2016), indicating that RNPs are transported along microtubules by both kinesin and dynein molecular motors (Kanai et al., 2004; Gagnon and Mowry, 2011). When sLTP is induced at a single spine with glutamate uncaging, mobile mRNA particles are stopped at the base of the stimulated spine, and newly synthesized actin appears at the tip of the stimulated spine (Buxbaum et al., 2015; Yoon et al., 2016). These events may correspond to mRNA unloading and translation near or in the stimulated spine by activity-dependent phosphorylation of RBPs in dendrites (Rangaraju et al., 2017).
Recently techniques to simultaneously monitor single mRNAs and newly translated polypeptides in intact cells have been developed (Chekulaeva and Landthaler, 2016; Morisaki et al., 2016; Wang et al., 2016; Wu et al., 2016; Yan et al., 2016). These techniques may further improve our knowledge about the regulation of local dendritic translation required for morphogenesis of dendritic spines.
Interactions Between Regulators of Protein Synthesis and That of Actin Cytoskeleton
The finding of activity-dependent β-actin translation near stimulated spines provides a direct link between protein synthesis and actin dynamics during spine morphogenesis (Yoon et al., 2016). Recent studies also suggest more intricate interactions between cytoskeletal proteins and synthesized proteins. One form of interaction is through the synthesis of actin regulating proteins. For example, it has been reported that oligophrenin-1 (OPHN1), a Rho GTPase activating protein (Rho-GAP), is rapidly upregulated by activity, regulating actin cytoskeleton during mGluR-dependent LTD (Nadif Kasri et al., 2011). In addition, RhoA appears to be locally synthesized in response to BDNF application or neuronal activity, and plays an important role in LTP (Briz et al., 2015). Interestingly, local translation of Arc, an activity-dependent immediate early gene, is also reported to regulate actin cytoskeleton and control spine morphology during LTP and LTD (Newpher et al., 2018). It has been known that newly synthesized Arc can interact with actin regulatory proteins such as WAVE1, ADF/cofilin, CaMKIIβ and drebrin A, regulating actin filaments (Messaoudi et al., 2007; Okuno et al., 2012; Zhang et al., 2015; Nair et al., 2017; Figure 4). Arc is also known to interact with dynamin-2 to promote endocytosis of AMPA receptor (AMPARs; Chowdhury et al., 2006; Newpher et al., 2018). The location of Arc synthesis and its regulation of actin cytoskeleton has been also studied extensively. It has been suggested that newly synthesized Arc is accumulated in non-stimulated spines and suppresses synaptic potentiation by decreasing surface expression of AMPA receptors (Okuno et al., 2012). This is in general consistent with the role of Arc expression in inhibiting LTP (Plath et al., 2006; Rial Verde et al., 2006).
Another interesting interaction between local translation and cytoskeletal remodeling involves the dual roles of cytoplasmic fragile X mental retardation protein (FMRP) interacting protein 1 (CYFIP1, Sra1). It has been demonstrated that CYFIP1 regulates spine remodeling both through promoting translation initiation and Arp2/3-mediated rapid actin nucleation (De Rubeis et al., 2013; Figure 5). CYFIP1 has been identified as a non-canonical eukaryotic translation initiation factor 4E (eIF4E) binding protein (4E-BP), which repress cap-dependent translation by forming a complex with FMRP and eIF4E (Napoli et al., 2008; Udagawa et al., 2012; Panja and Bramham, 2014). BDNF stimulation induces the dissociation of CYFIP1 from the FMRP-CYFIP1-eIF4E complex at synapses, which upregulates translation initiation of Arc, MAP1B and CaMKIIα (Napoli et al., 2008; De Rubeis and Bagni, 2011). The release of CYFIP1 occurs through the activity of Mnk1, a downstream molecule of MAPK/ERK signaling (Genheden et al., 2015; Bramham et al., 2016). In addition to the induction of translation initiation, the dissociated CYFIP1 can form a WAVE regulatory complex (WRC) that triggers Arp2/3-dependent actin nucleation with interacting Rac1 (Derivery et al., 2009; De Rubeis and Bagni, 2011). Indeed, either knockdown of Cyfip1 or mutations of interacting regions with eIF4E or WRC impairs structural maturation of dendritic spines (De Rubeis et al., 2013), suggesting that interplay of CYFIP1 controls both translation-dependent and -independent remodeling of the spine structure.
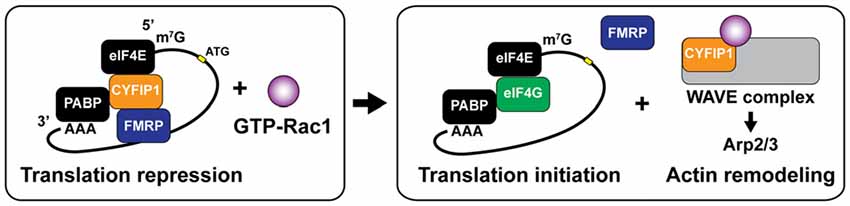
Figure 5. Dual roles ofcytoplasmic fragile X mental retardation protein (FMRP) interacting protein 1 (CYFIP1) for translation initiation and rapid actin remodeling. CYFIP1 makes FMRP-CYFIP1-eIF4E complex on mRNA and plays a role as a non-canonical eukaryotic initiation factor 4E (eIF4E) binding protein (4E-BP) which represses the translation initiation by interfering the association of eukaryotic initiation factor 4G (eIF4G). Binding of active form Rac1 (GTP-Rac1) dissociates CYFIP1 from the mRNA complex and initiates the translation. Dissociated CYFIP1 from mRNA complex forms WAVE complex, which promotes actin nucleation and branching via activating Arp2/3 complex. PABP, poly (A)-binding protein.
Finally, the mTOR complex 2 (mTORC2) pathway, a major pathway that regulates protein synthesis, is also found to regulate dynamics of actin cytoskeleton through Rac1 activation (Huang et al., 2013; Switon et al., 2017). Although the precise mechanism of mTORC2-mediated remodeling of actin cytoskeleton remains elusive, it has been proposed that the recruitment of Tiam1, a Rac1-specific guanine exchange factor (GEF), by Rictor, an essential component of mTORC2, regulates Rac1 activity and subsequent actin polymerization (Huang et al., 2013).
Local Translational Repression and Degradation
miRNAs are short (21–25 nucleotides) non-coding RNAs, which have important roles for specific translational control at synapses. miRNAs silence translation and induce degradation via making RNA-induced silencing complex (RISC) and binding corresponding sequences in 3’-UTR of mRNAs (Wahid et al., 2010). Since miRNAs have moderate specificity due to their short sequences, they can target a group of mRNAs. Many miRNA have been identified as regulators of actin related proteins and synaptic plasticity (Ryan et al., 2015). For example, miR-134 makes RISC with association of Argonaute (Ago) protein and represses translation of LIMK1 in dendritic spines of hippocampal neurons by NMDAR-dependent manner, decreasing the size of dendritic spines (Schratt et al., 2006; Rajgor et al., 2018). miR-132 is upregulated in an activity-dependent manner through the MAPK/ERK pathway, and represses translation of p250GAP, a brain-enriched Rho-GAP (Wayman et al., 2008). p250GAP is known to regulate spine morphology and is implicated in wide range of neuropathologies (Nakazawa et al., 2008; Impey et al., 2010; Qian et al., 2017). In dendrites, miR-138 represses acyl-protein thioesterase 1 (APT1), a depalmitoylation enzyme, and thus inhibits spine growth via increasing membrane localization of α13 subunits of G proteins (Gα13), which upregulates RhoA activity and presumably promotes actin depolymerization (Siegel et al., 2009).
Interestingly, miRNA maturation process appears to be localized near and in stimulated spines (Sambandan et al., 2017). The study by Sambandan et al. (2017) used a cleavage-inducible fluorescent sensor to measure the activity of Dicer and demonstrated that the maturation of miR-181a occurs in spines stimulated with glutamate uncaging. Thus, miRNAs may act locally to regulate activity-dependent tuning of the translation in stimulated spines. Moreover, the authors visualized newly synthesized CaMKIIα by using proximity ligation assay (PLA)-based technique (tom Dieck et al., 2015) and found that nascent CaMKIIα showed marked reduction in the area generated the mature miRNA (Sambandan et al., 2017). Thus, precise spatiotemporal maturation of miRNA regulates local translation of key signaling molecules in dendritic subcompartments. Taken together with the important role of miRNAs in regulating the actin cytoskeleton, miRNA-mediated translation repression and degradation may be a key regulatory system for structural plasticity of dendritic spines.
Concluding Remarks
Structural plasticity of dendritic spines is regulated by the reorganization of actin cytoskeleton through interaction with ABPs and their regulatory molecules. Ca2+ elevation in spines activates multiple signaling pathways that relay short Ca2+ signals into much longer signals. These signaling pathways have specific spatiotemporal patterns that orchestrate different aspect of dynamic cytoskeletal regulation, such as polymerization, depolymerization, nucleation, cross-linking and capping in the stimulated spines. In addition, activity-dependent signaling such as RNA trafficking and miRNAs regulate local protein translation of β-actin and actin regulatory proteins, providing an efficient local supply of the necessary material (Holt and Schuman, 2013; Rangaraju et al., 2017). The regulation of cytoskeletal elements and translational regulation appear to be coupled, providing an additional layer of regulation to structural plasticity (De Rubeis et al., 2013; Buxbaum et al., 2015; Yoon et al., 2016; Rangaraju et al., 2017; Sambandan et al., 2017).
Many neurological disorders, including autism spectrum disorders (ASDs), schizophrenia and Fragile X syndrome (FXS), are thought to be associated with dysregulation of cytoskeletal and translational signals (Buffington et al., 2014; Huber et al., 2015; Bhambhvani et al., 2017; Joensuu et al., 2018). These two signaling systems appear to be tightly coupled and important for regulating spine structural plasticity (De Rubeis et al., 2013; Hadziselimovic et al., 2014). Further studies of the interplay between the local regulation of cytoskeleton and translation during spine structural plasticity will be provide us with a better understanding of these diseases as well as basic understanding of spine structural plasticity.
Signal transduction regulating structure and function of dendritic spines are exceedingly complicated, and it will be long way to understand the whole signaling system. Due to the morphological complexity of neurons, the spatiotemporal dynamics of signaling play particularly important roles in neuronal plasticity. While quantitative measurements of more detailed signaling pathways will lead to a better understanding of overall signaling system, it would be critical to create theoretical frameworks that can integrate the spatiotemporal dynamics of many different signaling pathways (Brown et al., 2011). As we observe more sub-spine signal compartmentalization, the theory needs to include sub-spine structure and compartmentalization (Colgan and Yasuda, 2014). Finally, since most of our efforts of measuring signal transduction is still limited to in vitro models such as cultured neurons and brain slices, developments of systems to measure signaling activity in live animals with high spatiotemporal resolution would be necessary to connect biochemical events single dendrite with neuronal circuit plasticity in specific behavioral paradigm.
Author Contributions
Both authors listed have made a substantial, direct and intellectual contribution to the work, and approved it for publication.
Funding
This work was supported by National Institute of Mental Health grant number R01MH080047, R01MH111486 and National Institute of Neurological Disorders and Stroke grant number DP1NS096787.
Conflict of Interest Statement
The authors declare that the research was conducted in the absence of any commercial or financial relationships that could be construed as a potential conflict of interest.
Acknowledgments
We would like to thank Dr. Lesley A. Colgan for critical reading and the members of the Yasuda lab for discussion.
References
Ackermann, M., and Matus, A. (2003). Activity-induced targeting of profilin and stabilization of dendritic spine morphology. Nat. Neurosci. 6, 1194–1200. doi: 10.1038/nn1135
Andrianantoandro, E., and Pollard, T. D. (2006). Mechanism of actin filament turnover by severing and nucleation at different concentrations of ADF/cofilin. Mol. Cell 24, 13–23. doi: 10.1016/j.molcel.2006.08.006
Arber, S., Barbayannis, F. A., Hanser, H., Schneider, C., Stanyon, C. A., Bernard, O., et al. (1998). Regulation of actin dynamics through phosphorylation of cofilin by LIM-kinase. Nature 393, 805–809. doi: 10.1038/31729
Ashley, J., Cordy, B., Lucia, D., Fradkin, L. G., Budnik, V., and Thomson, T. (2018). Retrovirus-like Gag protein Arc1 binds RNA and traffics across synaptic boutons. Cell 172, 262.e11–274.e11. doi: 10.1016/j.cell.2017.12.022
Barria, A., Muller, D., Derkach, V., Griffith, L. C., and Soderling, T. R. (1997). Regulatory phosphorylation of AMPA-type glutamate receptors by CaM-KII during long-term potentiation. Science 276, 2042–2045. doi: 10.1126/science.276.5321.2042
Barylko, B., Wilkerson, J. R., Cavalier, S. H., Binns, D. D., James, N. G., Jameson, D. M., et al. (2018). Palmitoylation and membrane binding of Arc/Arg3.1: a potential role in synaptic depression. Biochemistry 57, 520–524. doi: 10.1021/acs.biochem.7b00959
Bhambhvani, H. P., Mueller, T. M., Simmons, M. S., and Meador-Woodruff, J. H. (2017). Actin polymerization is reduced in the anterior cingulate cortex of elderly patients with schizophrenia. Transl. Psychiatry 7:1278. doi: 10.1038/s41398-017-0045-y
Bloodgood, B. L., and Sabatini, B. L. (2005). Neuronal activity regulates diffusion across the neck of dendritic spines. Science 310, 866–869. doi: 10.1126/science.1114816
Bosch, M., Castro, J., Saneyoshi, T., Matsuno, H., Sur, M., and Hayashi, Y. (2014). Structural and molecular remodeling of dendritic spine substructures during long-term potentiation. Neuron 82, 444–459. doi: 10.1016/j.neuron.2014.03.021
Bramham, C. R., Jensen, K. B., and Proud, C. G. (2016). Tuning specific translation in cancer metastasis and synaptic memory: control at the MNK-eIF4E axis. Trends Biochem. Sci. 41, 847–858. doi: 10.1016/j.tibs.2016.07.008
Bramham, C. R., and Wells, D. G. (2007). Dendritic mRNA: transport, translation and function. Nat. Rev. Neurosci. 8, 776–789. doi: 10.1038/nrn2150
Briz, V., Zhu, G., Wang, Y., Liu, Y., Avetisyan, M., Bi, X., et al. (2015). Activity-dependent rapid local RhoA synthesis is required for hippocampal synaptic plasticity. J. Neurosci. 35, 2269–2282. doi: 10.1523/JNEUROSCI.2302-14.2015
Brown, S. A., Moraru, I. I., Schaff, J. C., and Loew, L. M. (2011). Virtual NEURON: a strategy for merged biochemical and electrophysiological modeling. J. Comput. Neurosci. 31, 385–400. doi: 10.1007/s10827-011-0317-0
Buard, I., Coultrap, S. J., Freund, R. K., Lee, Y.-S., Dell’Acqua, M. L., Silva, A. J., et al. (2010). CaMKII “autonomy” is required for initiating but not for maintaining neuronal long-term information storage. J. Neurosci. 30, 8214–8220. doi: 10.1523/JNEUROSCI.1469-10.2010
Budnik, V., Ruiz-Cañada, C., and Wendler, F. (2016). Extracellular vesicles round off communication in the nervous system. Nat. Rev. Neurosci. 17, 160–172. doi: 10.1038/nrn.2015.29
Buffington, S. A., Huang, W., and Costa-Mattioli, M. (2014). Translational control in synaptic plasticity and cognitive dysfunction. Annu. Rev. Neurosci. 37, 17–38. doi: 10.1146/annurev-neuro-071013-014100
Burk, K., Ramachandran, B., Ahmed, S., Hurtado-Zavala, J. I., Awasthi, A., Benito, E., et al. (2018). Regulation of dendritic spine morphology in hippocampal neurons by copine-6. Cereb. Cortex 28, 1087–1104. doi: 10.1093/cercor/bhx009
Butko, M. T., Yang, J., Geng, Y., Kim, H. J., Jeon, N. L., Shu, X., et al. (2012). Fluorescent and photo-oxidizing TimeSTAMP tags track protein fates in light and electron microscopy. Nat. Neurosci. 15, 1742–1751. doi: 10.1038/nn.3246
Buxbaum, A. R., Haimovich, G., and Singer, R. H. (2015). In the right place at the right time: visualizing and understanding mRNA localization. Nat. Rev. Mol. Cell Biol. 16, 95–109. doi: 10.1038/nrm3918
Buxbaum, A. R., Wu, B., and Singer, R. H. (2014). Single -actin mRNA detection in neurons reveals a mechanism for regulating its translatability. Science 343, 419–422. doi: 10.1126/science.1242939
Cajigas, I. J., Tushev, G., Will, T. J., Tom Dieck, S., Fuerst, N., and Schuman, E. M. (2012). The local transcriptome in the synaptic neuropil revealed by deep sequencing and high-resolution imaging. Neuron 74, 453–466. doi: 10.1016/j.neuron.2012.02.036
Campillos, M., Doerks, T., Shah, P. K., and Bork, P. (2006). Computational characterization of multiple Gag-like human proteins. Trends Genet. 22, 585–589. doi: 10.1016/j.tig.2006.09.006
Chang, J. Y., Parra-Bueno, P., Laviv, T., Szatmari, E. M., Lee, S. J. R., and Yasuda, R. (2017). CaMKII autophosphorylation is necessary for optimal integration of Ca2+ signals during LTP induction, but not maintenance. Neuron 94, 800.e4–808.e4. doi: 10.1016/j.neuron.2017.04.041
Chekulaeva, M., and Landthaler, M. (2016). Eyes on translation. Mol. Cell 63, 918–925. doi: 10.1016/j.molcel.2016.08.031
Chen, L. Y., Rex, C. S., Casale, M. S., Gall, C. M., and Lynch, G. (2007). Changes in synaptic morphology accompany actin signaling during LTP. J. Neurosci. 27, 5363–5372. doi: 10.1523/JNEUROSCI.0164-07.2007
Chowdhury, S., Shepherd, J. D., Okuno, H., Lyford, G., Petralia, R. S., Plath, N., et al. (2006). Arc/Arg3.1 interacts with the endocytic machinery to regulate AMPA receptor trafficking. Neuron 52, 445–459. doi: 10.1016/j.neuron.2006.08.033
Colgan, L. A., and Yasuda, R. (2014). Plasticity of dendritic spines: subcompartmentalization of signaling. Annu. Rev. Physiol. 76, 365–385. doi: 10.1146/annurev-physiol-021113-170400
Conant, K., Wang, Y., Szklarczyk, A., Dudak, A., Mattson, M. P., and Lim, S. T. (2010). Matrix metalloproteinase-dependent shedding of intercellular adhesion molecule-5 occurs with long-term potentiation. Neuroscience 166, 508–521. doi: 10.1016/j.neuroscience.2009.12.061
Correia, S. S., Bassani, S., Brown, T. C., Lisé, M. F., Backos, D. S., El-Husseini, A., et al. (2008). Motor protein-dependent transport of AMPA receptors into spines during long-term potentiation. Nat. Neurosci. 11, 457–466. doi: 10.1038/nn2063
Coultrap, S. J., Freund, R. K., O’Leary, H., Sanderson, J. L., Roche, K. W., Dell’Acqua, M. L., et al. (2014). Autonomous CaMKII mediates both LTP and LTD using a mechanism for differential substrate site selection. Cell Rep. 6, 431–437. doi: 10.1016/j.celrep.2014.01.005
Darnell, R. B. (2013). RNA protein interaction in neurons. Annu. Rev. Neurosci. 36, 243–270. doi: 10.1146/annurev-neuro-062912-114322
Darnell, J. C., and Richter, J. D. (2012). Cytoplasmic RNA-binding proteins and the control of complex brain function. Cold Spring Harb. Perspect. Biol. 4:a012344. doi: 10.1101/cshperspect.a012344
Dent, E. W. (2017). Of microtubules and memory: implications for microtubule dynamics in dendrites and spines. Mol. Biol. Cell 28, 1–8. doi: 10.1091/mbc.E15-11-0769
Derivery, E., Sousa, C., Gautier, J. J., Lombard, B., Loew, D., and Gautreau, A. (2009). The Arp2/3 activator WASH controls the fission of endosomes through a large multiprotein complex. Dev. Cell 17, 712–723. doi: 10.1016/j.devcel.2009.09.010
De Rubeis, S., and Bagni, C. (2011). Regulation of molecular pathways in the fragile X syndrome: insights into autism spectrum disorders. J. Neurodev. Disord. 3, 257–269. doi: 10.1007/s11689-011-9087-2
De Rubeis, S., Pasciuto, E., Li, K. W., Fernández, E., Di Marino, D., Buzzi, A., et al. (2013). CYFIP1 coordinates mRNA translation and cytoskeleton remodeling to ensure proper dendritic Spine formation. Neuron 79, 1169–1182. doi: 10.1016/j.neuron.2013.06.039
Doyle, M., and Kiebler, M. A. (2011). Mechanisms of dendritic mRNA transport and its role in synaptic tagging. EMBO J. 30, 3540–3552. doi: 10.1038/emboj.2011.278
Edelmann, E., Cepeda-Prado, E., Franck, M., Lichtenecker, P., Brigadski, T., and Leßmann, V. (2015). Theta burst firing recruits BDNF release and signaling in postsynaptic CA1 neurons in spike-timing-dependent LTP. Neuron 86, 1041–1054. doi: 10.1016/j.neuron.2015.04.007
Fernandez-Moya, S. M., Bauer, K. E., and Kiebler, M. A. (2014). Meet the players: local translation at the synapse. Front. Mol. Neurosci. 7:84. doi: 10.3389/fnmol.2014.00084
Frost, N. A., Shroff, H., Kong, H., Betzig, E., and Blanpied, T. A. (2010). Single-molecule discrimination of discrete perisynaptic and distributed sites of actin filament assembly within dendritic spines. Neuron 67, 86–99. doi: 10.1016/j.neuron.2010.05.026
Fujii, H., Inoue, M., Okuno, H., Sano, Y., Takemoto-Kimura, S., Kitamura, K., et al. (2013). Nonlinear decoding and asymmetric representation of neuronal input information by CaMKIIα and calcineurin. Cell Rep. 3, 978–987. doi: 10.1016/j.celrep.2013.03.033
Furutani, Y., Matsuno, H., Kawasaki, M., Sasaki, T., Mori, K., and Yoshihara, Y. (2007). Interaction between telencephalin and ERM family proteins mediates dendritic filopodia formation. J. Neurosci. 27, 8866–8876. doi: 10.1523/JNEUROSCI.1047-07.2007
Gagnon, J. A., and Mowry, K. L. (2011). Molecular motors: directing traffic during RNA localization. Crit. Rev. Biochem. Mol. Biol. 46, 229–239. doi: 10.3109/10409238.2011.572861
Genheden, M., Kenney, J. W., Johnston, H. E., Manousopoulou, A., Garbis, S. D., and Proud, C. G. (2015). BDNF stimulation of protein synthesis in cortical neurons requires the MAP kinase-interacting kinase MNK1. J. Neurosci. 35, 972–984. doi: 10.1523/JNEUROSCI.2641-14.2015
Glock, C., Heumüller, M., and Schuman, E. M. (2017). mRNA transport and local translation in neurons. Curr. Opin. Neurobiol. 45, 169–177. doi: 10.1016/j.conb.2017.05.005
Goodell, D. J., Zaegel, V., Coultrap, S. J., Hell, J. W., and Bayer, K. U. (2017). DAPK1 mediates LTD by making CaMKII/GluN2B binding LTP specific. Cell Rep. 19, 2231–2243. doi: 10.1016/j.celrep.2017.05.068
Gorkiewicz, T., Balcerzyk, M., Kaczmarek, L., and Knapska, E. (2015). Matrix metalloproteinase 9 (MMP-9) is indispensable for long term potentiation in the central and basal but not in the lateral nucleus of the amygdala. Front. Cell. Neurosci. 9:73. doi: 10.3389/fncel.2015.00073
Govindarajan, A., Israely, I., Huang, S. Y., and Tonegawa, S. (2011). The dendritic branch is the preferred integrative unit for protein synthesis-dependent LTP. Neuron 69, 132–146. doi: 10.1016/j.neuron.2010.12.008
Grutzendler, J., Kasthuri, N., and Gan, W. (2002). Long-term dendritic spine stability in the adult cortex. Nature 420, 812–816. doi: 10.1038/nature01276
Gulledge, A. T., Carnevale, N. T., and Stuart, G. J. (2012). Electrical advantages of dendritic spines. PLoS One 7:e36007. doi: 10.1371/journal.pone.0036007
Haditsch, U., Leone, D. P., Farinelli, M., Chrostek-Grashoff, A., Brakebusch, C., Mansuy, I. M., et al. (2009). A central role for the small GTPase Rac1 in hippocampal plasticity and spatial learning and memory. Mol. Cell. Neurosci. 41, 409–419. doi: 10.1016/j.mcn.2009.04.005
Hadziselimovic, N., Vukojevic, V., Peter, F., Milnik, A., Fastenrath, M., Fenyves, B. G., et al. (2014). Forgetting is regulated via musashi-mediated translational control of the Arp2/3 complex. Cell 156, 1153–1166. doi: 10.1016/j.cell.2014.01.054
Harvey, C. D., Yasuda, R., Zhong, H., and Svoboda, K. (2008). The spread of ras activity triggered by activation of a single dendritic spine. Science 321, 136–140. doi: 10.1126/science.1159675
Harward, S. C., Hedrick, N. G., Hall, C. E., Parra-Bueno, P., Milner, T. A., Pan, E., et al. (2016). Autocrine BDNF-TrkB signalling within a single dendritic spine. Nature 538, 99–103. doi: 10.1038/nature19766
Hayashi-Takagi, A., Yagishita, S., Nakamura, M., Shirai, F., Wu, Y. I., Loshbaugh, A. L., et al. (2015). Labelling and optical erasure of synaptic memory traces in the motor cortex. Nature 525, 333–338. doi: 10.1038/nature15257
Hedrick, N. G., Harward, S. C., Hall, C. E., Murakoshi, H., McNamara, J. O., and Yasuda, R. (2016). Rho GTPase complementation underlies BDNF-dependent homo- and heterosynaptic plasticity. Nature 538, 104–108. doi: 10.1038/nature19784
Hedrick, N. G., and Yasuda, R. (2017). Regulation of Rho GTPase proteins during spine structural plasticity for the control of local dendritic plasticity. Curr. Opin. Neurobiol. 45, 193–201. doi: 10.1016/j.conb.2017.06.002
Hlushchenko, I., Koskinen, M., and Hotulainen, P. (2016). Dendritic spine actin dynamics in neuronal maturation and synaptic plasticity. Cytoskeleton 73, 435–441. doi: 10.1002/cm.21280
Holt, C. E., and Schuman, E. M. (2013). The central dogma decentralized: new perspectives on RNA function and local translation in neurons. Neuron 80, 648–657. doi: 10.1016/j.neuron.2013.10.036
Honkura, N., Matsuzaki, M., Noguchi, J., Ellis-Davies, G. C. R., and Kasai, H. (2008). The subspine organization of actin fibers regulates the structure and plasticity of dendritic spines. Neuron 57, 719–729. doi: 10.1016/j.neuron.2008.01.013
Hotulainen, P., and Hoogenraad, C. C. (2010). Actin in dendritic spines: connecting dynamics to function. J. Cell Biol. 189, 619–629. doi: 10.1083/jcb.201003008
Hu, X., Viesselmann, C., Nam, S., Merriam, E., and Dent, E. W. (2008). Activity-dependent dynamic microtubule invasion of dendritic spines. J. Neurosci. 28, 13094–13105. doi: 10.1523/JNEUROSCI.3074-08.2008
Huang, W., Zhu, P. J., Zhang, S., Zhou, H., Stoica, L., Galiano, M., et al. (2013). MTORC2 controls actin polymerization required for consolidation of long-term memory. Nat. Neurosci. 16, 441–448. doi: 10.1038/nn.3351
Huber, K. M., Klann, E., Costa-Mattioli, M., and Zukin, R. S. (2015). Dysregulation of mammalian target of rapamycin signaling in mouse models of autism. J. Neurosci. 35, 13836–13842. doi: 10.1523/JNEUROSCI.2656-15.2015
Hüttelmaier, S., Zenklusen, D., Lederer, M., Dictenberg, J., Lorenz, M., Meng, X., et al. (2005). Spatial regulation of β-actin translation by Src-dependent phosphorylation of ZBP1. Nature 438, 512–515. doi: 10.1038/nature04115
Impey, S., Davare, M., Lasiek, A., Fortin, D., Ando, H., Varlamova, O., et al. (2010). An activity-induced microRNA controls dendritic spine formation by regulating Rac1-PAK signaling. Mol. Cell. Neurosci. 43, 146–156. doi: 10.1016/j.mcn.2009.10.005
Jaworski, J., Kapitein, L. C., Gouveia, S. M., Dortland, B. R., Wulf, P. S., Grigoriev, I., et al. (2009). Dynamic microtubules regulate dendritic spine morphology and synaptic plasticity. Neuron 61, 85–100. doi: 10.1016/j.neuron.2008.11.013
Joensuu, M., Lanoue, V., and Hotulainen, P. (2018). Dendritic spine actin cytoskeleton in autism spectrum disorder. Prog. Neuropsychopharmacol. Biol. Psychiatry 84, 362–381. doi: 10.1016/j.pnpbp.2017.08.023
Jung, H., Gkogkas, C. G., Sonenberg, N., and Holt, C. E. (2014). Remote control of gene function by local translation. Cell 157, 26–40. doi: 10.1016/j.cell.2014.03.005
Kanai, Y., Dohmae, N., and Hirokawa, N. (2004). Kinesin transports RNA: isolation and characterization of an RNA-transporting granule. Neuron 43, 513–525. doi: 10.1016/j.neuron.2004.07.022
Kasai, H., Fukuda, M., Watanabe, S., Hayashi-Takagi, A., and Noguchi, J. (2010). Structural dynamics of dendritic spines in memory and cognition. Trends Neurosci. 33, 121–129. doi: 10.1016/j.tins.2010.01.001
Kelleher, R. J., Govindarajan, A., Jung, H.-Y., Kang, H., and Tonegawa, S. (2004). Translational control by MAPK signaling in long-term synaptic plasticity and memory. Cell 116, 467–479. doi: 10.1016/s0092-8674(04)00115-1
Kiebler, M. A., and Bassell, G. J. (2006). Neuronal RNA granules: movers and makers. Neuron 51, 685–690. doi: 10.1016/j.neuron.2006.08.021
Kim, K., Lakhanpal, G., Lu, H. E., Khan, M., Suzuki, A., Kato-Hayashi, M., et al. (2015). A temporary gating of actin remodeling during synaptic plasticity consists of the interplay between the kinase and structural functions of CaMKII. Neuron 87, 813–826. doi: 10.1016/j.neuron.2015.07.023
Kim, I. H., Racz, B., Wang, H., Burianek, L., Weinberg, R., Yasuda, R., et al. (2013). Disruption of Arp2/3 results in asymmetric structural plasticity of dendritic spines and progressive synaptic and behavioral abnormalities. J. Neurosci. 33, 6081–6092. doi: 10.1523/JNEUROSCI.0035-13.2013
Kim, I. H., Wang, H., Soderling, S. H., and Yasuda, R. (2014). Loss of Cdc42 leads to defects in synaptic plasticity and remote memory recall. Elife 3:e02839. doi: 10.7554/eLife.02839
Korobova, F., and Svitkina, T. (2010). Molecular architecture of synaptic actin cytoskeleton in hippocampal neurons reveals a mechanism of dendritic spine morphogenesis. Mol. Biol. Cell 21, 165–176. doi: 10.1091/mbc.E09-07-0596
Leal, G., Comprido, D., and Duarte, C. B. (2014). BDNF-induced local protein synthesis and synaptic plasticity. Neuropharmacology 76, 639–656. doi: 10.1016/j.neuropharm.2013.04.005
Lee, S.-J. R., Escobedo-Lozoya, Y., Szatmari, E. M., and Yasuda, R. (2009). Activation of CaMKII in single dendritic spines during long-term potentiation. Nature 458, 299–304. doi: 10.1038/nature07842
Lengyel, I., Voss, K., Cammarota, M., Bradshaw, K., Brent, V., Murphy, K. P. S. J., et al. (2004). Autonomous activity of CaMKII is only transiently increased following the induction of long-term potentiation in the rat hippocampus. Eur. J. Neurosci. 20, 3063–3072. doi: 10.1111/j.1460-9568.2004.03748.x
Li, W., Ma, L., Yang, G., and Gan, W. B. (2017). REM sleep selectively prunes and maintains new synapses in development and learning. Nat. Neurosci. 20, 427–437. doi: 10.1038/nn.4479
Lionnet, T., Czaplinski, K., Darzacq, X., Shav-Tal, Y., Wells, A. L., Chao, J. A., et al. (2011). A transgenic mouse for in vivo detection of endogenous labeled mRNA. Nat. Methods 8, 165–170. doi: 10.1038/nmeth.1551
Lisman, J., Schulman, H., and Cline, H. (2002). The molecular basis of CaMKII function in synaptic and behavioural memory. Nat. Rev. Neurosci. 3, 175–190. doi: 10.1038/nrn753
Magnowska, M., Gorkiewicz, T., Suska, A., Wawrzyniak, M., Rutkowska-Wlodarczyk, I., Kaczmarek, L., et al. (2016). Transient ECM protease activity promotes synaptic plasticity. Sci. Rep. 6:27757. doi: 10.1038/srep27757
Makino, H., and Malinow, R. (2009). AMPA receptor incorporation into synapses during LTP: the role of lateral movement and exocytosis. Neuron 64, 381–390. doi: 10.1016/j.neuron.2009.08.035
Malenka, R. C., and Bear, M. F. (2004). LTP and LTD: an embarrassment of riches. Neuron 44, 5–21. doi: 10.1016/j.neuron.2004.09.012
Matsuzaki, M., Honkura, N., Ellis-Davies, G. C. R., and Kasai, H. (2004). Structural basis of long-term potentiation in single dendritic spines. Nature 429, 761–766. doi: 10.1038/nature02617
Mayford, M., Baranes, D., Podsypanina, K., and Kandel, E. R. (1996). The 3′-untranslated region of CaMKII is a cis-acting signal for the localization and translation of mRNA in dendrites. Proc. Natl. Acad. Sci. U S A 93, 13250–13255. doi: 10.1073/pnas.93.23.13250
Menna, E., Disanza, A., Cagnoli, C., Schenk, U., Gelsomino, G., Frittoli, E., et al. (2009). Eps8 regulates axonal filopodia in hippocampal neurons in response to brain-derived neurotrophic factor (BDNF). PLoS Biol. 7:e1000138. doi: 10.1371/journal.pbio.1000138
Menna, E., Zambetti, S., Morini, R., Donzelli, A., Disanza, A., Calvigioni, D., et al. (2013). Eps8 controls dendritic spine density and synaptic plasticity through its actin-capping activity. EMBO J. 32, 1730–1744. doi: 10.1038/emboj.2013.107
Merriam, E. B., Lumbard, D. C., Viesselmann, C., Ballweg, J., Stevenson, M., Pietila, L., et al. (2011). Dynamic microtubules promote synaptic NMDA receptor-dependent spine enlargement. PLoS One 6:e27688. doi: 10.1371/journal.pone.0027688
Merriam, E. B., Millette, M., Lumbard, D. C., Saengsawang, W., Fothergill, T., Hu, X., et al. (2013). Synaptic regulation of microtubule dynamics in dendritic spines by calcium, F-actin, and drebrin. J. Neurosci. 33, 16471–16482. doi: 10.1523/JNEUROSCI.0661-13.2013
Messaoudi, E., Kanhema, T., Soulé, J., Tiron, A., Dagyte, G., da Silva, B., et al. (2007). Sustained Arc/Arg3.1 synthesis controls long-term potentiation consolidation through regulation of local actin polymerization in the dentate gyrus in vivo. J. Neurosci. 27, 10445–10455. doi: 10.1523/JNEUROSCI.2883-07.2007
Meyer, D., Bonhoeffer, T., and Scheuss, V. (2014). Balance and stability of synaptic structures during synaptic plasticity. Neuron 82, 430–443. doi: 10.1016/j.neuron.2014.02.031
Miller, S., Yasuda, M., Coats, J. K., Jones, Y., Martone, M. E., and Mayford, M. (2002). Disruption of dendritic translation of CaMKIIα impairs stabilization of synaptic plasticity and memory consolidation. Neuron 36, 507–519. doi: 10.1016/s0896-6273(02)00978-9
Minichiello, L. (2009). TrkB signalling pathways in LTP and learning. Nat. Rev. Neurosci. 10, 850–860. doi: 10.1038/nrn2738
Mizui, T., Sekino, Y., Yamazaki, H., Ishizuka, Y., Takahashi, H., Kojima, N., et al. (2014). Myosin II ATPase activity mediates the long-term potentiation-induced exodus of stable F-actin bound by drebrin a from dendritic spines. PLoS One 9:e85367. doi: 10.1371/journal.pone.0085367
Morisaki, T., Lyon, K., DeLuca, K. F., DeLuca, J. G., English, B. P., Zhang, Z., et al. (2016). Real-time quantification of single RNA translation dynamics in living cells. Science 352, 1425–1429. doi: 10.1126/science.aaf0899
Murakoshi, H., Shin, M. E., Parra-Bueno, P., Szatmari, E. M., Shibata, A. C. E., and Yasuda, R. (2017). Kinetics of endogenous CaMKII required for synaptic plasticity revealed by optogenetic kinase inhibitor. Neuron 94:690. doi: 10.1016/j.neuron.2017.04.027
Murakoshi, H., Wang, H., and Yasuda, R. (2011). Local, persistent activation of Rho GTPases during plasticity of single dendritic spines. Nature 472, 100–104. doi: 10.1038/nature09823
Murase, S., Lantz, C. L., and Quinlan, E. M. (2017). Light reintroduction after dark exposure reactivates plasticity in adults via perisynaptic activation of MMP-9. Elife 6:e27345. doi: 10.7554/eLife.27345
Myrum, C., Baumann, A., Bustad, H. J., Flydal, M. I., Mariaule, V., Alvira, S., et al. (2015). Arc is a flexible modular protein capable of reversible self-oligomerization. Biochem. J. 468, 145–158. doi: 10.1042/BJ20141446
Nadif Kasri, N., Nakano-Kobayashi, A., and Van Aelst, L. (2011). Rapid synthesis of the X-linked mental retardation protein OPHN1 mediates mglur-dependent LTD through interaction with the endocytic machinery. Neuron 72, 300–315. doi: 10.1016/j.neuron.2011.09.001
Nagappan-Chettiar, S., Johnson-Venkatesh, E. M., and Umemori, H. (2017). Activity-dependent proteolytic cleavage of cell adhesion molecules regulates excitatory synaptic development and function. Neurosci. Res. 116, 60–69. doi: 10.1016/j.neures.2016.12.003
Nair, R. R., Patil, S., Tiron, A., Kanhema, T., Panja, D., Schiro, L., et al. (2017). Dynamic Arc SUMOylation and selective interaction with F-actin-binding protein drebrin A in LTP consolidation in vivo. Front. Synaptic Neurosci. 9:8. doi: 10.3389/fnsyn.2017.00008
Nakazawa, T., Kuriu, T., Tezuka, T., Umemori, H., Okabe, S., and Yamamoto, T. (2008). Regulation of dendritic spine morphology by an NMDA receptor-associated Rho GTPase-activating protein, p250GAP. J. Neurochem. 105, 1384–1393. doi: 10.1111/j.1471-4159.2008.05335.x
Napoli, I., Mercaldo, V., Boyl, P. P., Eleuteri, B., Zalfa, F., De Rubeis, S., et al. (2008). The fragile X syndrome protein represses activity-dependent translation through CYFIP1, a new 4E-BP. Cell 134, 1042–1054. doi: 10.1016/j.cell.2008.07.031
Neuhoff, H., Sassoè-Pognetto, M., Panzanelli, P., Maas, C., Witke, W., and Kneussel, M. (2005). The actin-binding protein profilin I is localized at synaptic sites in an activity-regulated manner. Eur. J. Neurosci. 21, 15–25. doi: 10.1111/j.1460-9568.2004.03814.x
Newpher, T. M., Harris, S., Pringle, J., Hamilton, C., and Soderling, S. (2018). Regulation of spine structural plasticity by Arc/Arg3.1. Semin. Cell Dev. Biol. 77, 25–32. doi: 10.1016/j.semcdb.2017.09.022
Nguyen, P. V., and Kandel, E. R. (1997). Brief theta-burst stimulation induces a transcription-dependent late phase of LTP requiring cAMP in area CA1 of the mouse hippocampus. Learn. Mem. 4, 230–243. doi: 10.1101/lm.4.2.230
Nishiyama, J., and Yasuda, R. (2015). Biochemical computation for spine structural plasticity. Neuron 87, 63–75. doi: 10.1016/j.neuron.2015.05.043
Nogales, E., Wolf, S. G., and Downing, K. H. (1998). Structure of the αβ tubulin dimer by electron crystallography. Nature 391, 199–203. doi: 10.1038/34465
Noguchi, J., Hayama, T., Watanabe, S., Ucar, H., Yagishita, S., Takahashi, N., et al. (2016). State-dependent diffusion of actin-depolymerizing factor/cofilin underlies the enlargement and shrinkage of dendritic spines. Sci. Rep. 6:32897. doi: 10.1038/srep32897
Oh, W. C., Parajuli, L. K., and Zito, K. (2015). Heterosynaptic structural plasticity on local dendritic segments of hippocampal CA1 neurons. Cell Rep. 10, 162–169. doi: 10.1016/j.celrep.2014.12.016
Okamoto, K. I., Nagai, T., Miyawaki, A., and Hayashi, Y. (2004). Rapid and persistent modulation of actin dynamics regulates postsynaptic reorganization underlying bidirectional plasticity. Nat. Neurosci. 7, 1104–1112. doi: 10.1038/nn1311
Okuno, H., Akashi, K., Ishii, Y., Yagishita-Kyo, N., Suzuki, K., Nonaka, M., et al. (2012). Inverse synaptic tagging of inactive synapses via dynamic interaction of Arc/Arg3.1 with CaMKIIβ. Cell 149, 886–898. doi: 10.1016/j.cell.2012.02.062
Oliveira, A. F., and Yasuda, R. (2014). Neurofibromin is the major ras inactivator in dendritic spines. J. Neurosci. 34, 776–783. doi: 10.1523/JNEUROSCI.3096-13.2014
Ostroff, L. E., Botsford, B., Gindina, S., Cowansage, K. K., LeDoux, J. E., Klann, E., et al. (2017). Accumulation of polyribosomes in dendritic spine heads, but not bases and necks, during memory consolidation depends on cap-dependent translation initiation. J. Neurosci. 37, 1862–1872. doi: 10.1523/JNEUROSCI.3301-16.2017
Padamsey, Z., McGuinness, L., Bardo, S. J., Reinhart, M., Tong, R., Hedegaard, A., et al. (2017). Activity-dependent exocytosis of lysosomes regulates the structural plasticity of dendritic spines. Neuron 93, 132–146. doi: 10.1016/j.neuron.2016.11.013
Panja, D., and Bramham, C. R. (2014). BDNF mechanisms in late LTP formation: a synthesis and breakdown. Neuropharmacology 76, 664–676. doi: 10.1016/j.neuropharm.2013.06.024
Pastuzyn, E. D., Day, C. E., Kearns, R. B., Kyrke-Smith, M., Taibi, A. V., McCormick, J., et al. (2018). The neuronal gene Arc encodes a repurposed retrotransposon Gag protein that mediates intercellular RNA transfer. Cell 172, 275.e18–288.e18. doi: 10.1016/j.cell.2017.12.024
Plath, N., Ohana, O., Dammermann, B., Errington, M. L., Schmitz, D., Gross, C., et al. (2006). Arc/Arg3.1 is essential for the consolidation of synaptic plasticity and memories. Neuron 52, 437–444. doi: 10.1016/j.neuron.2006.08.024
Qian, Y., Song, J., Ouyang, Y., Han, Q., Chen, W., Zhao, X., et al. (2017). Advances in roles of miR-132 in the nervous system. Front. Pharmacol. 8:770. doi: 10.3389/fphar.2017.00770
Rajgor, D., Sanderson, T. M., Amici, M., Collingridge, G. L., and Hanley, J. G. (2018). NMDAR-dependent Argonaute 2 phosphorylation regulates miRNA activity and dendritic spine plasticity. EMBO J. 44:e97943. doi: 10.15252/embj.201797943
Rangaraju, V., Tom Dieck, S., and Schuman, E. M. (2017). Local translation in neuronal compartments: how local is local? EMBO Rep. 18, 693–711. doi: 10.15252/embr.201744045
Reinhard, J. R., Kriz, A., Galic, M., Angliker, N., Rajalu, M., Vogt, K. E., et al. (2016). The calcium sensor Copine-6 regulates spine structural plasticity and learning and memory. Nat. Commun. 7:11613. doi: 10.1038/ncomms11613
Reinhard, S. M., Razak, K., and Ethell, I. M. (2015). A delicate balance: role of MMP-9 in brain development and pathophysiology of neurodevelopmental disorders. Front. Cell. Neurosci. 9:280. doi: 10.3389/fncel.2015.00280
Rial Verde, E. M., Lee-Osbourne, J., Worley, P. F., Malinow, R., and Cline, H. T. (2006). Increased expression of the immediate-early gene Arc/Arg3.1 reduces AMPA receptor-mediated synaptic transmission. Neuron 52, 461–474. doi: 10.1016/j.neuron.2006.09.031
Rust, M. B. (2015). ADF/cofilin: a crucial regulator of synapse physiology and behavior. Cell. Mol. Life Sci. 72, 3521–3529. doi: 10.1007/s00018-015-1941-z
Ryan, B., Joilin, G., and Williams, J. M. (2015). Plasticity-related microRNA and their potential contribution to the maintenance of long-term potentiation. Front. Mol. Neurosci. 8:4. doi: 10.3389/fnmol.2015.00004
Ryu, J., Liu, L., Wong, T. P., Wu, D. C., Burette, A., Weinberg, R., et al. (2006). A critical role for myosin IIB in dendritic spine morphology and synaptic function. Neuron 49, 175–182. doi: 10.1016/j.neuron.2005.12.017
Sabatini, B., Oertner, T. G., and Svoboda, K. (2002). The life cycle of Ca2+ ions in dendritic spines. Neuron 33, 439–452. doi: 10.1016/s0896-6273(02)00573-1
Sambandan, S., Akbalik, G., Kochen, L., Rinne, J., Kahlstatt, J., Glock, C., et al. (2017). Activity-dependent spatially localized miRNA maturation in neuronal dendrites. Science 355, 634–637. doi: 10.1126/science.aaf8995
Schratt, G. M., Tuebing, F., Nigh, E. A., Kane, C. G., Sabatini, M. E., Kiebler, M., et al. (2006). A brain-specific microRNA regulates dendritic spine development. Nature 439, 283–289. doi: 10.1038/nature04367
Shepherd, J. D. (2018). Arc - An endogenous neuronal retrovirus? Semin. Cell Dev. Biol. 77, 73–78. doi: 10.1016/j.semcdb.2017.09.029
Shirao, T., Hanamura, K., Koganezawa, N., Ishizuka, Y., Yamazaki, H., and Sekino, Y. (2017). The role of drebrin in neurons. J. Neurochem. 141, 819–834. doi: 10.1111/jnc.13988
Siegel, G., Obernosterer, G., Fiore, R., Oehmen, M., Bicker, S., Christensen, M., et al. (2009). A functional screen implicates microRNA-138-dependent regulation of the depalmitoylation enzyme APT1 in dendritic spine morphogenesis. Nat. Cell Biol. 11, 705–716. doi: 10.1038/ncb1876
Soderling, S. H., and Scott, J. D. (2006). WAVE signalling: from biochemistry to biology. Biochem. Soc. Trans. 34, 73–76. doi: 10.1042/bst0340073
Sonderegger, P., and Matsumoto-Miyai, K. (2014). Activity-controlled proteolytic cleavage at the synapse. Trends Neurosci. 37, 413–423. doi: 10.1016/j.tins.2014.05.007
Spence, E. F., and Soderling, S. H. (2015). Actin out: regulation of the synaptic cytoskeleton. J. Biol. Chem. 290, 28613–28622. doi: 10.1074/jbc.r115.655118
Stawarski, M., Stefaniuk, M., and Wlodarczyk, J. (2014). Matrix metalloproteinase-9 involvement in the structural plasticity of dendritic spines. Front. Neuroanat. 8:68. doi: 10.3389/fnana.2014.00068
Steward, O., Farris, S., Pirbhoy, P. S., Darnell, J., and Driesche, S. J. (2015). Localization and local translation of Arc/Arg3.1 mRNA at synapses: some observations and paradoxes. Front. Mol. Neurosci. 7:101. doi: 10.3389/fnmol.2014.00101
Sutton, M. A., Ito, H. T., Cressy, P., Kempf, C., Woo, J. C., and Schuman, E. M. (2006). Miniature neurotransmission stabilizes synaptic function via tonic suppression of local dendritic protein synthesis. Cell 125, 785–799. doi: 10.1016/j.cell.2006.03.040
Svoboda, K., Tank, D. W., and Denk, W. (1996). Direct measurement of coupling between dendritic spines and shafts. Science 272, 716–719. doi: 10.1126/science.272.5262.716
Switon, K., Kotulska, K., Janusz-Kaminska, A., Zmorzynska, J., and Jaworski, J. (2017). Molecular neurobiology of mTOR. Neuroscience 341, 112–153. doi: 10.1016/j.neuroscience.2016.11.017
Tanaka, J., Horiike, Y., Matsuzaki, M., Miyazaki, T., Ellis-Davies, G. C. R., and Kasai, H. (2008). Protein synthesis and neurotrophin-dependent structural plasticity of single dendritic spines. Science 319, 1683–1687. doi: 10.1126/science.1152864
Tiruchinapalli, D. M., Oleynikov, Y., Kelic, S., Shenoy, S. M., Hartley, A., Stanton, P. K., et al. (2003). Activity-dependent trafficking and dynamic localization of zipcode binding protein 1 and β-actin mRNA in dendrites and spines of hippocampal neurons. J. Neurosci. 23, 3251–3261. doi: 10.1523/jneurosci.23-08-03251.2003
tom Dieck, S., Kochen, L., Hanus, C., Heumüller, M., Bartnik, I., Nassim-Assir, B., et al. (2015). Direct visualization of newly synthesized target proteins in situ. Nat. Methods 12, 411–414. doi: 10.1038/nmeth.3319
Trachtenberg, J. T., Chen, B. E., Knott, G. W., Feng, G., Sanes, J. R., Welker, E., et al. (2002). Long-term in vivo imaging of experience-dependent synaptic plasticity in adult cortex. Nature 420, 788–794. doi: 10.1038/nature01273
Udagawa, T., Swanger, S. A., Takeuchi, K., Kim, J. H., Nalavadi, V., Shin, J., et al. (2012). Bidirectional control of mrna translation and synaptic plasticity by the cytoplasmic polyadenylation complex. Mol. Cell 47, 253–266. doi: 10.1016/j.molcel.2012.05.016
Wahid, F., Shehzad, A., Khan, T., and Kim, Y. Y. (2010). MicroRNAs: synthesis, mechanism, function and recent clinical trials. Biochim. Biophys. Acta 1803, 1231–1243. doi: 10.1016/j.bbamcr.2010.06.013
Wang, X., Bozdagi, O., Nikitczuk, J. S., Wei, Z., Zhou, Q., and Huntley, G. W. (2008). Extracellular proteolysis by matrix metalloproteinase-9 drives dendritic spine enlargement and long-term potentiation coordinately. Proc. Natl. Acad. Sci. U S A 105, 19520–19525. doi: 10.1073/pnas.0807248105
Wang, Z., Edwards, J. G., Riley, N., Provance, D. W., Karcher, R., Li, X., et al. (2008). Myosin Vb mobilizes recycling endosomes and AMPA receptors for postsynaptic plasticity. Cell 135, 535–548. doi: 10.1016/j.cell.2008.09.057
Wang, C., Han, B., Zhou, R., and Zhuang, X. (2016). Real-time imaging of translation on single mRNA transcripts in live cells. Cell 165, 990–1001. doi: 10.1016/j.cell.2016.04.040
Wayman, G. A., Davare, M., Ando, H., Fortin, D., Varlamova, O., Cheng, H.-Y. M., et al. (2008). An activity-regulated microRNA controls dendritic plasticity by down-regulating p250GAP. Proc. Natl. Acad. Sci. U S A 105, 9093–9098. doi: 10.1073/pnas.0803072105
Wu, B., Eliscovich, C., Yoon, Y. J., and Singer, R. H. (2016). Translation dynamics of single mRNAs in live cells and neurons. Science 352, 1430–1435. doi: 10.1126/science.aaf1084
Yan, X., Hoek, T. A., Vale, R. D., and Tanenbaum, M. E. (2016). Dynamics of translation of single mRNA molecules in vivo. Cell 165, 976–989. doi: 10.1016/j.cell.2016.04.034
Yang, G., Lai, C. S. W., Cichon, J., Ma, L., Li, W., and Gan, W.-B. (2014). Sleep promotes branch-specific formation of dendritic spines after learning. Science 344, 1173–1178. doi: 10.1126/science.1249098
Yang, G., Pan, F., and Gan, W.-B. (2009). Stably maintained dendritic spines are associated with lifelong memories. Nature 462, 920–924. doi: 10.1038/nature08577
Yasuda, R. (2017). Biophysics of biochemical signaling in dendritic spines: implications in synaptic plasticity. Biophys. J. 113, 2152–2159. doi: 10.1016/j.bpj.2017.07.029
Yasuda, R., and Murakoshi, H. (2011). The mechanisms underlying the spatial spreading of signaling activity. Curr. Opin. Neurobiol. 21, 313–321. doi: 10.1016/j.conb.2011.02.008
Yoon, Y. J., Wu, B., Buxbaum, A. R., Das, S., Tsai, A., English, B. P., et al. (2016). Glutamate-induced RNA localization and translation in neurons. Proc. Natl. Acad. Sci. U S A 113, E6877–E6886. doi: 10.1073/pnas.1614267113
Yuste, R. (2013). Electrical compartmentalization in dendritic spines. Annu. Rev. Neurosci. 36, 429–449. doi: 10.1146/annurev-neuro-062111-150455
Zhang, W., Wu, J., Ward, M. D., Yang, S., Chuang, Y. A., Xiao, M., et al. (2015). Structural basis of arc binding to synaptic proteins: implications for cognitive disease. Neuron 86, 490–500. doi: 10.1016/j.neuron.2015.03.030
Zhou, Q., Homma, K. J., and Poo, M. (2004). Shrinkage of dendritic spines associated with long-term depression of hippocampal synapses. Neuron 44, 749–757. doi: 10.1016/j.neuron.2004.11.011
Keywords: dendritic spine, calcium signaling, synaptic plasticity, actin cytoskeleton, CaMKII, small GTPase, FRET FLIM, LTP
Citation: Nakahata Y and Yasuda R (2018) Plasticity of Spine Structure: Local Signaling, Translation and Cytoskeletal Reorganization. Front. Synaptic Neurosci. 10:29. doi: 10.3389/fnsyn.2018.00029
Received: 30 April 2018; Accepted: 07 August 2018;
Published: 29 August 2018.
Edited by:
George Augustine, Nanyang Technological University, SingaporeReviewed by:
Toh Hean Ch’ng, Nanyang Technological University, SingaporeMarc Fivaz, University of Greenwich, United Kingdom
Copyright © 2018 Nakahata and Yasuda. This is an open-access article distributed under the terms of the Creative Commons Attribution License (CC BY). The use, distribution or reproduction in other forums is permitted, provided the original author(s) and the copyright owner(s) are credited and that the original publication in this journal is cited, in accordance with accepted academic practice. No use, distribution or reproduction is permitted which does not comply with these terms.
*Correspondence: Ryohei Yasuda, ryohei.yasuda@mpfi.org