- Department of Kinesiology, McMaster University, Hamilton, ON, Canada
Aerobic exercise facilitates neuroplasticity and has been linked to improvements in cognitive and motor function. Transcranial magnetic stimulation (TMS) is a non-invasive technique that can be used to quantify changes in neurophysiology induced by exercise. The present review summarizes the single- and paired-pulse TMS paradigms that can be used to probe exercise-induced neuroplasticity, the optimal stimulation parameters and the current understanding of the neurophysiology underlying each paradigm. Further, this review amalgamates previous research exploring the modulation of these paradigms with exercise-induced neuroplasticity in healthy and clinical populations and highlights important considerations for future TMS-exercise research.
Introduction
Exercise-induced neuroplasticity refers to the change in the nervous system that occurs following an acute or chronic bout of exercise. These neuroplastic effects of exercise are exerted at the molecular, cellular and structural levels of the nervous system, which accumulate to induce changes in brain function (El-Sayes et al., 2019a). Transcranial magnetic stimulation (TMS) is a non-invasive technique that can be used to quantify subtle changes in brain excitation and inhibition that accompany exercise.
Transcranial magnetic stimulation produces a magnetic field that depolarizes superficial pyramidal neurons within the cortex. When delivered to the primary motor cortex (M1), TMS trans-synaptically activates corticospinal output neurons, evoking a descending corticospinal volley that results in a motor response within the target muscle (Hallett, 2007). This muscle response, known as the motor-evoked potential (MEP), is then quantified with surface electromyography (EMG) (Figure 1). There are numerous TMS paradigms that can be used to study different aspects of neurophysiology including cortical excitability, motor neuron recruitment and, indirectly, neurotransmitter receptor function. As such, TMS provides a unique opportunity to non-invasively probe the neuronal mechanisms that underpin exercise-induced neuroplasticity.
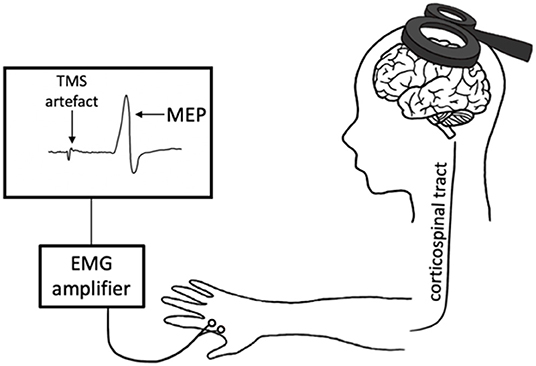
Figure 1. Transcranial magnetic stimulation (TMS) schematic. An electric current is generated in the TMS coil, producing a magnetic field perpendicular to the coil. This magnetic field passes through the scalp, inducing a perpendicular secondary electric field within the cortex. This electric current depolarizes the cortical neurons in M1, evoking a descending volley along the corticospinal tract and subsequently a motor-evoked potential (MEP) in the target muscle. This response is measured with electromyography (EMG).
The goal of this review is to guide users on the application of TMS for evaluating the neurophysiological mechanisms underlying exercise-induced neuroplasticity and to provide a comprehensive review of the literature that have used TMS to evaluate exercise-induced neuroplasticity. Specifically, we will discuss single- and paired-pulse TMS paradigms that are valuable for quantifying neurophysiological changes that accompany aerobic exercise. We will also discuss the use of these techniques in previous research that explored exercise-induced neuroplasticity in healthy and clinical populations. Within the context of this review, acute exercise refers to a single session of exercise while chronic exercise refers to multiple sessions of exercise or aerobic training.
Single-Pulse TMS Paradigms
Epidural recordings have provided insight into the neural populations activated by a single pulse of TMS when delivered to M1. TMS depolarizes superficial cortical layers, leading to trans-synaptic activation of the pyramidal output neurons and evoking a series of descending volleys known as indirect waves (I-waves) that are labeled in order of appearance (I1–I4) (Di Lazzaro et al., 2012). Only at very high intensities is TMS able to directly activate deeper pyramidal output neurons in M1, evoking a direct wave (D-wave) in the epidural space (Di Lazzaro et al., 2012). Modulation of I-waves provides valuable insight into the physiology underlying TMS paradigms, as discussed below. Table 1 shows how single-pulse TMS paradigms reportedly change following aerobic exercise.
Motor Threshold
Resting motor threshold (RMT) refers to the lowest intensity of TMS that evokes a MEP. RMT represents excitation of the lowest threshold neurons within M1 and is therefore an assessment of baseline cortical excitability. It is increased by drugs that block voltage-gated Na+ channels (Ziemann et al., 1996b; Chen et al., 1997; Boroojerdi et al., 2001; Sommer et al., 2012; Lang et al., 2013), suggesting that RMT reflects axonal excitability. For example, a low RMT suggests a lower threshold for activation of neurons within M1, or greater cortical excitability.
Several approaches exist to quantify RMT. There is the traditional Rossini-Rothwell method proposed by Rossini et al. (1994) and later revised (Rothwell et al., 1999) that uses a systematic approach to estimate RMT. This approach may require up to ~50 trials to accurately determine RMT (Mills and Nithi, 1997; Mishory et al., 2004). Alternatively, more automated approaches can be used to estimate RMT with similar accuracy and reliability, but with fewer trials required. For example, the adaptive threshold-hunting method based on maximum likelihood parameter estimation by sequential testing (ML-PEST) predicts the TMS intensity that yields a 50% probability of evoking an MEP of at least 50 μV (Awiszus, 2003). This approach requires only 20 trials to reliably estimate RMT (Ah Sen et al., 2017). In addition, a Bayesian adaptive method has been shown to require as little as 7 trials to estimate RMT (Qi et al., 2011).
Active motor threshold (AMT) refers to the lowest intensity of TMS that evokes a MEP of at least 200 μV with a probability of 50%, while the target muscle is isometrically contracted at 10–20% of the maximum voluntary contraction (MVC) (Groppa et al., 2012). The AMT is typically lower than RMT since descending commands for voluntary contraction partially activate the upper and lower motor neurons of the corticospinal tract. Therefore, less TMS intensity is needed to bring the corticospinal tract to threshold and evoke a MEP. Similar to RMT, AMT can be estimated with systematic or automated approaches, where automated approaches require fewer trials for accurate estimation (Ah Sen et al., 2017).
Previous research has shown that an acute bout of aerobic exercise does not change RMT (Smith et al., 2014; Lulic et al., 2017; Yamazaki et al., 2019; El-Sayes et al., 2020; Morris et al., 2020; Nicolini et al., 2020) or AMT (Lulic et al., 2017; Nicolini et al., 2020). Similarly, in chronic stroke, a single session of either high-intensity interval exercise (HIIE) or moderate intensity continuous exercise (MICE) does not alter RMT (Abraha et al., 2018), while HIIE reduces AMT but MICE does not (Boyne et al., 2019). Eight weeks of aerobic training reduced RMT in healthy controls (Moscatelli et al., 2020), while 12-weeks of aerobic cycling did not change RMT in older adults (McGregor et al., 2018) and 4-weeks of treadmill training increased RMT in Parkinson's disease (PD) (Yang et al., 2013). This may suggest that acute aerobic exercise is not potent enough to influence the lowest threshold neurons within M1, while chronic aerobic exercise is. Although the literature suggests that RMT and AMT are typically unchanged following acute aerobic exercise, these results may reflect the specific and age-range of the participants tested. We therefore advise research to measure RMT and AMT in the event that changes in cortical excitability following exercise occur within the specific population tested. Further, acquisition of these metrics will still be required to adjust stimulation intensities for single- and paired-pulse TMS paradigms as described below.
Motor-Evoked Potentials
It is common to report the peak-to-peak MEP amplitude derived from a single TMS intensity. However, MEP recruitment curves capture the changing relationship between TMS intensity and MEP amplitude, providing a more comprehensive understanding of the effects of exercise. As TMS intensity is increased, MEP amplitude increases in a sigmoidal fashion (Devanne et al., 1997) (Figure 2A). The x-intercept of the curve reflects RMT, the rising slope reflects the rate of upper and lower motor neuron recruitment, the plateau at higher TMS intensities reflects recruitment of most motor neurons within the corticospinal tract, and the area under the MEP recruitment curve reflects corticospinal excitability. The slope of the MEP recruitment curve is also positively correlated with glutamate levels in M1 as assessed via magnetic resonance spectroscopy (MRS) (Stagg et al., 2011). Therefore, MEP recruitment curves provide unique information about motor neuron recruitment and glutamatergic neurotransmission compared to a single MEP. To construct a MEP recruitment curve, MEPs are obtained as a function of RMT or maximum stimulator output (MSO) (Boroojerdi et al., 2001), where the average peak-to-peak amplitude of minimum 5 MEPs (Cavaleri et al., 2017) is obtained in increments of 10% RMT or 10% MSO. For example, previous TMS exercise studies have acquired MEP recruitment curves using intensities ranging from 90 to 200% RMT in increments of 10% RMT (Mang et al., 2014; Singh et al., 2014; Smith et al., 2014; Lulic et al., 2017; Neva et al., 2017; Stavrinos and Coxon, 2017; El-Sayes et al., 2019b, 2020; MacDonald et al., 2019; Walsh et al., 2019; Brown et al., 2020; Nicolini et al., 2020). Studies have also obtained MEP recruitment curves during active contraction of 10% MVC and using intensities ranging from 90 to 200% AMT (Lulic et al., 2017; Nicolini et al., 2019, 2020).
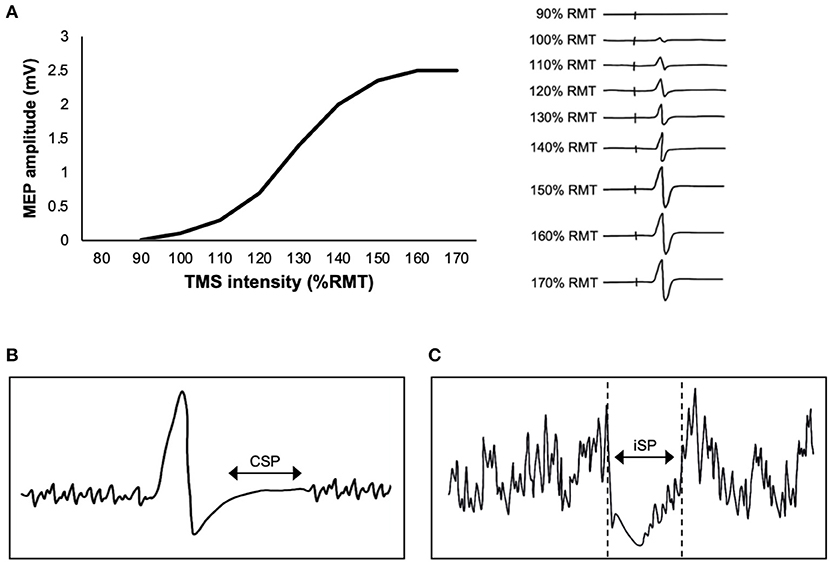
Figure 2. Single-pulse transcranial magnetic stimulation (TMS) paradigms. (A) Graph (left) shows an example of a motor-evoked potential (MEP) recruitment curve, plotting the relationship between TMS intensity and MEP amplitude. The individual MEPs (right) increase in amplitude as the TMS intensity is increased, reflecting recruitment of motor neurons within the corticospinal tract. (B) Schematic of the contralateral silent period (CSP), where EMG activity is suppressed immediately following a TMS pulse delivered to M1 during contraction of the target muscle (a muscle contralateral to TMS). (C) Schematic of the ipsilateral silent period (iSP), where EMG activity is suppressed immediately following a TMS pulse delivered to M1 during contraction of a muscle that is ipsilateral to TMS.
The effects of acute aerobic exercise on corticospinal excitability in healthy individuals are mixed. Studies have reported either an increase (Lulic et al., 2017; El-Sayes et al., 2019b; MacDonald et al., 2019; Opie and Semmler, 2019; Nicolini et al., 2020) or no change (McDonnell et al., 2013; Mang et al., 2014, 2016; Singh et al., 2014, 2016; Smith et al., 2014, 2018; Mooney et al., 2016; Neva et al., 2017; Stavrinos and Coxon, 2017; Walsh et al., 2019; Yamazaki et al., 2019; Andrews et al., 2020; Brown et al., 2020; El-Sayes et al., 2020; Morris et al., 2020) in corticospinal excitability following a single session of exercise. In these studies, corticospinal excitability was assessed with either single-pulse MEPs (McDonnell et al., 2013; Mang et al., 2016; Mooney et al., 2016; Singh et al., 2016; Smith et al., 2018; Opie and Semmler, 2019; Yamazaki et al., 2019; Andrews et al., 2020; Morris et al., 2020) or MEP recruitment curves (Mang et al., 2014; Singh et al., 2014; Smith et al., 2014; Lulic et al., 2017; Neva et al., 2017; Stavrinos and Coxon, 2017; El-Sayes et al., 2019b, 2020; MacDonald et al., 2019; Walsh et al., 2019; Brown et al., 2020; Nicolini et al., 2020). Further, studies have shown that an acute bout of aerobic exercise increases the area under the MEP recruitment curve when obtained during rest only but not active conditions (Lulic et al., 2017; Nicolini et al., 2020).
These studies assessing the effects of acute aerobic exercise used a range of exercise intensities (high, moderate, low), exercise patterns (interval, continuous) and cardiorespiratory fitness or physical activity inclusion criteria (sedentary, moderately active, highly active). However, the relationship between corticospinal excitability and these factors are unclear. One study showed that aerobic fitness was not related to the magnitude of corticospinal excitability change following exercise (MacDonald et al., 2019). Further, it was suggested that only highly-active participants showed an increase in corticospinal excitability following acute aerobic exercise (El-Sayes et al., 2020), however, our recent study showed an increase in corticospinal excitability within sedentary participants (Nicolini et al., 2020). Alternatively, an interaction between fitness and exercise intensity may exist such that higher intensity exercise is required to induce neuroplasticity in sedentary individuals. For example, several studies show no change in MEPs within low-fit individuals with moderate (Lulic et al., 2017; El-Sayes et al., 2020) and high (Stavrinos and Coxon, 2017; El-Sayes et al., 2020) intensity exercise. However, Nicolini et al. (2020) found an increase in MEPs within sedentary males when exercised at a high workload of 105–125% peak power output (Wpeak). This is in contrast to El-Sayes et al. (2020) where a lower workload of ~69% Wpeak was used in the HIIE protocol.
In stroke, one session of low-intensity cycling did not change MEPs within the first dorsal interosseous (FDI) muscle (Murdoch et al., 2016). However, one session of HIIE does increase MEPs in the extensor carpi radialis (ECR) muscle when TMS is delivered to the lesioned hemisphere, but not the non-lesioned hemisphere (Li et al., 2019). Further, one session of HIIE also increases MEPs in the non-paretic tibialis anterior (TA) muscle, but decreases MEPs in the paretic TA (Madhavan et al., 2016). Overall, these results show that high-intensity but not low-intensity exercise is sufficient to modulate corticospinal excitability in stroke. However, the direction of corticospinal excitability changes following a single session of HIIE is mixed. Therefore, further research will be needed to determine whether HIIE is capable of increasing corticospinal output to paretic musculature. Further, these results were acquired immediately following the exercise session, and it is unknown how long changes in corticospinal excitability persist.
The effects of chronic aerobic exercise on corticospinal excitability in healthy individuals is also mixed. Moscatelli et al. (2020) showed that MEP amplitude increases in untrained males following 12 weeks of moderate intensity aerobic training, and Nicolini et al. (2019) showed no change in MEP recruitment curves following 6 weeks of high-intensity interval training (HIIT) in sedentary males. At present, it is unknown whether females would exhibit a change in corticospinal excitability following long-term aerobic training, or if moderate-highly active individuals would respond to long-term aerobic training. In incomplete spinal cord injury (SCI), 3–5 months of aerobic treadmill training increased corticospinal excitability (Thomas and Gorassini, 2005). Specifically, there was an increase in the plateau of the MEP recruitment curve, MEPmax, and MEPs at the half-way point of the MEP recruitment curve. This suggests that chronic aerobic exercise induces short-term plasticity that may contribute to restoring the motor output in SCI.
Contralateral Silent Period (CSP)
Transcranial magnetic stimulation delivered to M1 during voluntary activation produces a period of EMG suppression directly following the MEP (Figure 2B). The length of this period is referred to as the contralateral silent period (CSP) (Cantello et al., 1992). The CSP is typically 100–300 ms in length (Säisänen et al., 2008), and reflects a combination of both intracortical and spinal mechanisms (Škarabot et al., 2019). The H-reflex is suppressed within the first 50–80 ms of the CSP (Ziemann et al., 1993). Further, the CSP is only >100 ms in length when evoked by TMS delivered to M1 but ~50 ms when evoked by electrical stimulation of the cervicomedullary junction (Inghilleri et al., 1993). These findings suggest that the earlier portion of the CSP (<80 ms) is mediated by spinal mechanisms, while the later portion (>80 ms) is cortically mediated.
The CSP is observed when the participant maintains isometric contraction of the contralateral target muscle at intensities ranging from 10% (Goodall et al., 2018) to 100% (Mira et al., 2017) of the MVC. However, CSP tends to shorten with greater contraction intensities (Matsugi, 2019). Further, the CSP tends to lengthen with higher TMS intensities until a plateau occurs at very high intensities (~>75% MSO) (Kimiskidis et al., 2005). This suggests that the inhibitory drive of voluntary activation saturates at high enough stimulation intensities. Previous TMS exercise studies have evoked CSP with a TMS intensity of 130% RMT and contraction for 20% MVC (Neva et al., 2017), or with a TMS intensity adjusted to evoked a silent period of 175 ms during 10% MVC (Mooney et al., 2016). Other research has also used the TMS intensity that evokes a 1 mV MEP at rest (Tremblay et al., 2011; Locke et al., 2020).
The CSP is lengthened by gamma-aminobutryic acid (GABA) reuptake inhibitors (Werhahn et al., 1999; Pierantozzi et al., 2004a). It has been hypothesized that CSP is modulated by GABAB receptor activity (Ziemann et al., 2015). Although multiple studies have reported no effect of GABAB receptor agonists on CSP length (Inghilleri et al., 1996; Ziemann et al., 1996b; McDonnell et al., 2006), those that have shown an increase in CSP delivered baclofen intrathecally, bypassing the blood brain barrier that baclofen does not readily cross (Siebner et al., 1998; Stetkarova and Kofler, 2013). Alternatively, oral administration of zolpidem, a positive allosteric modulator of the GABAA receptor, lengthens the CSP (Mohammadi et al., 2006). Therefore, the data suggests that CSP may indirectly reflect both GABAA and GABAB receptor activity.
The CSP is either reduced (Neva et al., 2017) or unchanged (Mooney et al., 2016) following acute aerobic exercise in healthy individuals. In stroke, one session of HIIE and MICE does not change CSP length (Boyne et al., 2019). The effects of chronic aerobic exercise on CSP in healthy individuals has yet to be investigated. However, 4–8 weeks of treadmill training lengthens CSP in PD (Fisher et al., 2008; Yang et al., 2013) and 3–5 months of treadmill training lengthens CSP in SCI (Thomas and Gorassini, 2005). This suggests that long-term aerobic training is needed to consistently induce a change in GABAergic neurotransmission within M1.
Ipsilateral Silent Period (iSP)
The ipsilateral silent period (iSP) is an assessment of transcallosal inhibition, whereby a suprathreshold pulse of TMS delivered to M1 induces interruption of EMG activity in an ipsilateral muscle (Figure 2C). For example, Neva et al. (2017) delivered TMS at 150% RMT and observed interruption of EMG activity in the ipsilateral abductor pollicis brevis (APB) that was isometrically contracted at 50% MVC. The duration of the iSP is ~30 ms (Perez and Cohen, 2009; Neva et al., 2017). The iSP is thought to be cortically-mediated, as it does not lead to reduction in the H-reflex (Wassermann et al., 1991). To our knowledge, only one study has investigated the effects of acute aerobic exercise on iSP. Neva et al. (2017) found that an acute bout of MICE reduced iSP bilaterally. Only one study has assessed the effects of chronic aerobic exercise on iSP. McGregor et al. (2018) showed that 12 weeks of aerobic cycling increased the duration of the iSP in older adults. Therefore, both acute and chronic aerobic exercise may modulate the excitability of transcallosal fibers.
Paired-Pulse TMS Paradigms
Transcranial magnetic stimulation can be used to study inhibitory and excitatory mechanisms within the cortex. These techniques involve paired-pulse paradigms, whereby a conditioning stimulus (CS) delivered prior to a test stimulus (TS) modulates the resulting MEP. Parameters including the stimulation intensities and interstimulus intervals (ISIs) can be adjusted to determine the direction of MEP modulation. Modulation of the MEP is then expressed as a ratio of the MEP produced by the CS-TS pair to that produced by the TS alone. A ratio above one denotes facilitation of the MEP, while a ratio below one denotes inhibition of the MEP. Table 1 shows paired-pulse TMS paradigms are reported to change following aerobic exercise.
Short-Interval Intracortical Inhibition (SICI)
Short-interval intracortical inhibition (SICI) is one such paired-pulse paradigm that describes inhibition of the MEP (Figure 3A). Short-interval intracortical inhibition is observed when two TMS pulses are delivered in quick succession, with an ISI between 1 and 6 ms (Kujirai et al., 1993). The ISI of 1 ms may reflect the axonal refractory period (Chan et al., 2002; Fisher et al., 2002) while SICI at ~2–3 ms reflects GABAergic neurotransmission (Di Lazzaro et al., 2005a). It is thought to be cortically mediated, as epidural recordings show that SICI reduces late I3 waves (Di Lazzaro et al., 1998) and does not modulate MEPs evoked by transcranial electric stimulation (TES), which directly activates pyramidal output neurons (Kujirai et al., 1993).
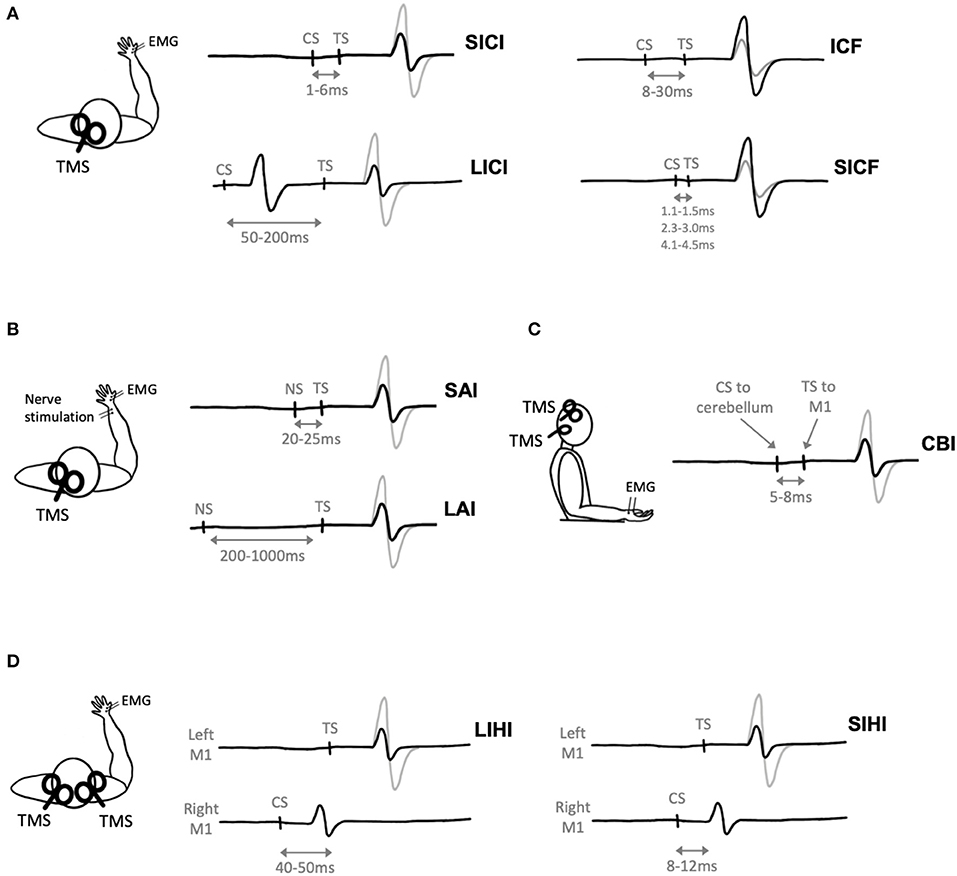
Figure 3. Paired-pulse transcranial magnetic stimulation (TMS) paradigms. Gray electromyography (EMG) traces represent trials where a single TMS pulse is delivered (i.e., test stimulus, TS), black traces represent trials where a conditioning stimulus (CS) and TS are delivered. (A) Two TMS pulses delivered in quick succession to M1 evokes short-interval intracortical inhibition (SICI), long-interval intracortical inhibition (LICI), intracortical facilitation (ICF), or short-interval intracortical facilitation (SICF) depending on the interstimulus interval (ISI) between the CS and TS. (B) Nerve stimulation (NS) delivered prior to TMS evoked short-latency afferent inhibition (SAI) or long-latency afferent inhibition (LAI) depending on the ISI between the NS and TS. (C) Cerebellar inhibition (CBI) is observed when the CS is delivered to the cerebellum 5ms prior to the TS delivered to M1. (D) A TMS pulse delivered to the right hemisphere (CS) prior to a pulse delivered to the left hemisphere (TS) evokes short-interval interhemispheric inhibition (SIHI) or long-interval interhemispheric inhibition (LIHI) depending on the ISI between the CS and TS.
To measure SICI, the first pulse (i.e., CS) is delivered at a sub-threshold intensity while the second pulse (i.e., TS) is delivered at a supra-threshold intensity. Previous TMS exercise studies have evoked SICI using CS intensities of 80–90% AMT (Smith et al., 2014; Mooney et al., 2016; Lulic et al., 2017; Stavrinos and Coxon, 2017; El-Sayes et al., 2019b; Nicolini et al., 2019, 2020; Yamazaki et al., 2019) or 70–90% RMT (Singh et al., 2014; Opie and Semmler, 2019; Morris et al., 2020) and TS intensities of 120% RMT (Singh et al., 2014; Morris et al., 2020) or adjusted to evoked a 1 mV MEP (Smith et al., 2014; Mooney et al., 2016; Lulic et al., 2017; Stavrinos and Coxon, 2017; El-Sayes et al., 2019b; Nicolini et al., 2019, 2020; Opie and Semmler, 2019; Yamazaki et al., 2019; Andrews et al., 2020).
Short-interval intracortical inhibition is mainly thought to indirectly reflect activity of the GABAA receptor. It is upregulated by benzodiazepines, positive allosteric modulators of the GABAA receptor (Di Lazzaro et al., 2005a, 2007), and may specifically involve GABAA receptors containing the α2 or α3-subunits (Di Lazzaro et al., 2007; Teo et al., 2009). Short-interval intracortical inhibition is also reduced by the GABAB agonist baclofen (McDonnell et al., 2006), although other studies show no effect (Ziemann et al., 1996b; McDonnell et al., 2007). Further, SICI is modulated by dopamine (DA) as DA agonists increase SICI (Ziemann et al., 1996a, 1997; Korchounov et al., 2007) and DA antagonists decrease SICI (Ziemann et al., 1997), and by noradrenaline (NA) as NA agonists decrease SICI (Ilić et al., 2003; Gilbert et al., 2006). Overall, the pharmacology of SICI suggests that this measure may reflect the activity of inhibitory interneurons expressing GABAA receptors, under the control of pre-synaptic GABAB-receptor mediated inhibition and modulated by DA and NA.
Modulation of SICI with exercise may indicate changes in GABAA receptor activity or the excitability of intracortical interneurons underlying SICI. In healthy controls, acute aerobic exercise either decreases (Singh et al., 2014; Smith et al., 2014; Lulic et al., 2017; Stavrinos and Coxon, 2017; El-Sayes et al., 2019b; Opie and Semmler, 2019; Yamazaki et al., 2019) or does not change SICI (Mooney et al., 2016; Opie and Semmler, 2019; Andrews et al., 2020; Morris et al., 2020; Nicolini et al., 2020). Notably, changes in SICI have only been shown when acquired with ISIs of 2–3 ms (Singh et al., 2014; Smith et al., 2014; Lulic et al., 2017; El-Sayes et al., 2019b; Opie and Semmler, 2019; Yamazaki et al., 2019) whereas SICI acquired with an ISI of 1 ms does not change with exercise (Mooney et al., 2016; Stavrinos and Coxon, 2017). This suggests that exercise may modulate GABAA receptor neurotransmission rather than axonal refractory periods.
In regards to chronic exercise, 6 weeks of HIIT does not change SICI in sedentary healthy males (Nicolini et al., 2019). Further, a single session of aerobic exercise is not sufficient to change SICI in the upper limb of individuals with stroke (Murdoch et al., 2016; Abraha et al., 2018; Li et al., 2019). However, 4 weeks of treadmill training increased lower-limb SICI in PD (Yang et al., 2013). This may suggest that prolonged aerobic exercise is required to modulate intracortical inhibition within clinical populations.
Intracortical Facilitation (ICF)
A subthreshold CS followed by a suprathreshold TS with an ISI ranging from 8 to 30 ms evokes intracortical facilitation (ICF) (Kujirai et al., 1993) (Figure 3A). Previous TMS exercise studies have evoked ICF using CS intensities of 80–90% AMT (Smith et al., 2014; Mooney et al., 2016; Lulic et al., 2017; Stavrinos and Coxon, 2017; El-Sayes et al., 2019b; Nicolini et al., 2019, 2020; Yamazaki et al., 2019) or 70–90% RMT (Singh et al., 2014; Opie and Semmler, 2019; Morris et al., 2020) and TS intensities of 120% RMT (Singh et al., 2014; Morris et al., 2020) or adjusted to evoked a 1 mV MEP (Smith et al., 2014; Mooney et al., 2016; Lulic et al., 2017; Stavrinos and Coxon, 2017; El-Sayes et al., 2019b; Nicolini et al., 2019, 2020; Opie and Semmler, 2019; Yamazaki et al., 2019; Andrews et al., 2020). The physiological mechanisms of ICF are less well understood, as ICF does not modulate epidural recordings of I-waves (Di Lazzaro et al., 2006). ICF is reduced by N-methyl-D-aspartate (NMDA) receptor antagonists (Ziemann et al., 1998a) and benzodiazepines, positive allosteric modulators of the GABAA receptor (Inghilleri et al., 1996; Ziemann et al., 1996c), but is increased by NA agonists (Moll et al., 2003; Gilbert et al., 2006).
Changes in ICF with exercise may indirectly reflect a change in glutamatergic neurotransmission with M1. In healthy individuals, multiple studies have shown no change in ICF following an acute bout of aerobic exercise (Yamazaki et al., 2019; Andrews et al., 2020; Nicolini et al., 2020) while others show ICF increased (Singh et al., 2014; Morris et al., 2020) and another revealed a decrease (Lulic et al., 2017). Further, a single session of HIIE or MICE does not change ICF in chronic stroke (Abraha et al., 2018). However, 6 weeks of HIIT reduces ICF in sedentary males (Nicolini et al., 2019). Interestingly, only ICF at the ISI of 10 ms has been shown to decrease with exercise (Lulic et al., 2017; Nicolini et al., 2019), while only ICF at the ISI of 12 ms increases with exercise (Singh et al., 2014; Morris et al., 2020). Therefore, we recommend that future studies acquire SICI and ICF at a range of ISIs in an input-output curve fashion to assess the time course of MEP modulation (Kujirai et al., 1993), as the neural mechanisms underlying these measures may differ at distinct ISIs.
Long-Interval Intracortical Inhibition (LICI)
Long-interval intracortical inhibition (LICI) is observed following a suprathreshold CS and suprathreshold TS with an ISI of 50–200 ms (Valls-Solé et al., 1992) (Figure 3A). Previous TMS exercise studies have evoked LICI using CS and TS intensities of 120% RMT (Singh et al., 2014; Yamazaki et al., 2019; Morris et al., 2020), adjusted to evoke a 1 mV MEP (Stavrinos and Coxon, 2017; Yamazaki et al., 2019; Andrews et al., 2020), or adjusted to evoke a CSP of 175 ms (Mooney et al., 2016). Long-interval intracortical inhibition reduces late I-waves, suggesting that this phenomenon is of cortical origin (Nakamura et al., 1997; Chen et al., 1999; Di Lazzaro et al., 2002). Further, LICI is increased by the GABAB receptor baclofen (McDonnell et al., 2006) and GABA reuptake inhibitors tiagabine (Werhahn et al., 1999) and vigabatrin (Pierantozzi et al., 2004b). However, benzodiazepines do not modulate LICI (Teo et al., 2009). Therefore, LICI may indirectly reflect activation of GABAB receptors.
A change in LICI with exercise-induced neuroplasticity may indicate that exercise modulates activity of the GABAB receptor or neural mechanisms underlying LICI. In healthy individuals, only one study has shown a decrease in LICI following aerobic exercise (Mooney et al., 2016), while majority of studies show no change in LICI (Singh et al., 2014; Smith et al., 2014; Stavrinos and Coxon, 2017; Yamazaki et al., 2019; Andrews et al., 2020; Morris et al., 2020). Alternatively, 4 weeks of treadmill training increases LICI in PD (Yang et al., 2013). Mooney et al. (2016) acquired LICI at longer ISIs of 125, 175, and 200 ms, whereas others used shorter ISIs of 100–120 ms (Yang et al., 2013; Singh et al., 2014; Smith et al., 2014; Stavrinos and Coxon, 2017; Yamazaki et al., 2019; Andrews et al., 2020; Morris et al., 2020). Therefore, these results may suggest that the neural mechanisms underlying LICI at shorter (<120 ms) and longer (>120 ms) ISIs are distinct.
Short-Interval Intracortical Facilitation (SICF)
Short-interval intracortical facilitation (SICF) occurs following a suprathreshold CS paired with a perithreshold or subthreshold TS at discrete ISIs of 1.1–1.5, 2.3–3.0, and 4.1–4.5 ms (Ziemann et al., 1998b) (Figure 3A). Previous TMS exercise studies have evoked SICF with a CS intensity of 90% RMT and TS intensity adjusted to evoke a 1 mV MEP (Lulic et al., 2017; Neva et al., 2017; Yamazaki et al., 2019).
Short-interval intracortical facilitation facilitates I-waves recorded from the epidural space, suggesting it is exerted intracortically (Di Lazzaro et al., 1999b). It is reduced by drugs that enhance inhibitory neurotransmission including lorazepam, vigabatrin and phenobarbital (Ziemann et al., 1998c). However, SICF may not reflect GABAB receptor activity as it is not modulated by baclofen (Ziemann et al., 1998c). Further, SICF is not modulated by drugs enhancing excitatory neurotransmission including sodium channel blockers and NMDA receptor antagonists (Ziemann et al., 1998c).
Modulation of SICF with exercise would indicate a change in the excitability of I-wave generating interneurons. An acute bout of aerobic exercise has been shown to either increase (Neva et al., 2017) or not change (Lulic et al., 2017; Yamazaki et al., 2019) SICF. At present, it is unknown whether long-term training modulates SICF, or if SICF is modulated following acute or chronic aerobic exercise in clinical populations.
Afferent Inhibition
Afferent inhibition refers to the suppression of MEPs when a conditioning electrical stimulus is applied to a peripheral nerve prior to TMS delivered to M1 (Figure 3B). Short-latency afferent inhibition (SAI) is observed when peripheral stimulation precedes TMS by 20–25 ms, while long-latency afferent inhibition (LAI) is observed at interstimulus intervals of 200–1,000 ms. Both SAI and LAI can be observed within muscles of the hand following stimulation of cutaneous digital nerves or the mixed ulnar or median nerves at the wrist. Previous TMS exercise studies have evoked SAI and LAI using TS intensities of 1 mV MEP (Yamazaki et al., 2019; Brown et al., 2020) or 120% RMT (Yamazaki et al., 2019), and nerve stimulation intensities corresponding to 3x sensory threshold (Yamazaki et al., 2019) or motor threshold (Brown et al., 2020).
It is well-known that SAI reflects cholinergic activity as it is reduced by the muscarinic antagonist scopolamine (Di Lazzaro et al., 2000) and increased by acetylcholinesterase inhibitors (Di Lazzaro et al., 2005c). In addition, SAI is reduced by lorazepam and zolpidem but not diazepam, suggesting that SAI reflects activity of GABAA receptors containing the α1-subunit (Di Lazzaro et al., 2005b, 2007; Turco et al., 2018). However, SAI is not modulated by the GABAB receptor agonist baclofen (Turco et al., 2018). Finally, NA reuptake inhibitor reboxetine reduces SAI (Kuo et al., 2017), potentially through the inhibition of acetylcholine release (Vizi and Pasztor, 1981). This pharmacological evidence may suggest that SAI is reflective of GABAergic neurotransmission controlled by cholinergic activity. Similar to SAI, LAI is also reduced by lorazepam but not baclofen, suggesting that it reflects GABAA not GABAB receptor activity (Turco et al., 2018).
Modulation of SAI and/or LAI with exercise may indicate changes in sensorimotor integration or excitability of sensorimotor pathways. Only two studies have used afferent inhibition to assess exercise-induce neuroplasticity. First, Yamazaki et al. (2019) showed that low-intensity pedaling reduced SAI within the exercised TA muscles and non-exercised FDI muscle. Second, Brown et al. (2020) showed that moderate-intensity cycling increased LAI, while SAI was unchanged. Further study replication would be needed to fully elucidate the effects of exercise-induced neuroplasticity on afferent inhibition.
Cerebellar Inhibition (CBI)
Cerebellar inhibition (CBI) is observed when a TMS pulse delivered to the cerebellum inhibits the MEP evoked by TMS delivered to M1 (Figure 3C). The ISI used to measure CBI is 5–8 ms (Daskalakis et al., 2004; Rothwell, 2011). When delivered to the cerebellum, it has been postulated that TMS activates Purkinje cells, leading to inhibition of M1 via the dentate nucleus (Ugawa et al., 1991; Tremblay et al., 2016). Previously, CBI has been used to investigate the cerebellum's role in motor learning (Jayaram et al., 2011; Schlerf et al., 2012; Baarbé et al., 2014; Spampinato and Celnik, 2018). Importantly, as the CS is delivered to the cerebellum, this will lead to activation of neck muscles (Demirtas-Tatlidede et al., 2011) and potentially discomfort to the participant (Fernandez et al., 2018).
Cerebellar inhibition is typically acquired with TS intensities of 1 mV MEP (Daskalakis et al., 2004; Baarbé et al., 2014) and CS intensities at or just below the maximum tolerated intensity, a value that varies according to TMS coil manufacturers (Spampinato et al., 2020). Alternatively, Baarbé et al. (2014) found that CS intensities evoking 50% inhibition of the MEP (termed CBI50) is a sensitive method to detect neuroplastic changes in cerebellar-M1 connectivity with motor learning.
To our knowledge, only one previous study has assessed CBI in the context of exercise. This study evoked CBI using a CS intensity of 100 or 120% RMT and a TS intensity of 1 mV MEP (Mang et al., 2016). A single session of high-intensity cycling reduced CBI in healthy participants (Mang et al., 2016). This suggests that exercise induced a change in the excitability of cerebellar-M1 networks. Disinhibition of the cerebellum (i.e., a reduction in CBI) is observed following motor learning (Jayaram et al., 2011; Schlerf et al., 2012; Baarbé et al., 2014). Therefore, a reduction in CBI following exercise may prime the central nervous system for subsequent motor learning, as suggested elsewhere (Mang et al., 2013, 2016). It is unknown whether long-term training also changes CBI, or if exercise changes CBI in clinical populations.
Interhemispheric Inhibition (IHI)
Interhemispheric inhibition (IHI) reflects the excitability of transcallosal pathways between bilateral motor cortices. Interhemispheric inhibition can be measured when a suprathreshold CS is delivered to M1 prior to a suprathreshold TS delivered to the opposite M1 (Figure 3D). Short-interval IHI (SIHI) is observed at ISIs of 8–12 ms and long-interval IHI (LIHI) is observed at ISIs of 40–50 ms (Ni et al., 2009). The CS and TS intensities are typically adjusted to a 1 mV MEP (Daskalakis et al., 2002; Kukaswadia et al., 2005). Previous work shows that SIHI reduces I2 and I3 waves (Di Lazzaro et al., 1999a), while the I-wave origin of LIHI has yet to be investigated.
Both baclofen (Irlbacher et al., 2007) and lorazepam (Sommer et al., 2012) increase LIHI, but not midazolam (Irlbacher et al., 2007). Further, SIHI is not modulated by baclofen (Irlbacher et al., 2007; Florian et al., 2008) or the benzodiazepines midazolam (Irlbacher et al., 2007) and diazepam (Florian et al., 2008), but is reduced by carbamazepine (Sommer et al., 2012). This suggests that LIHI reflects GABAB and GABAA receptor activity, while SIHI is reflective of voltage-gated sodium channels. Therefore, SIHI and LIHI may act through distinct neuronal populations.
Interhemispheric inhibition is thought to be important for suppressing unwanted mirror movements (Duque et al., 2005, 2007) and bimanual control (Nelson et al., 2009). Recently, we showed that reduced IHI with muscle contraction is correlated with territorial expansion of that muscle's representation within M1 (Turco et al., 2019). This suggests that IHI may be an important mechanism that modulates M1 organization. To our knowledge, no studies have assessed effects of acute aerobic exercise on IHI, while only one has assessed the effects of chronic aerobic exercise. McGregor et al. (2018) found no change in LIHI following 12 weeks of aerobic cycling in older adults. At present, it is unknown if chronic exercise modulates LIHI in young adults or if chronic exercise modulates SIHI.
Important Considerations for TMS-Exercise Research
Changes in skin temperature and hydration occur (i.e., perspiration) during aerobic exercise, and these effects can reduce adherence and alter the placement of EMG electrodes (i.e., slippage). Yamazaki et al. (2019) found an increase in skin temperature overlaying the FDI muscle after a session of exercise, but did not assess whether changes in SICI and SAI with exercise were related to changes in skin temperature. Next, perspiration attenuates the surface EMG signal (Abdoli-Eramaki et al., 2012), and can induce shifts in the electrode position that alters the EMG signal (Garcia et al., 2017; Merletti and Muceli, 2019). One solution is to measure the maximal M-wave (Mmax) before and after exercise. The Mmax represents the recruitment of all motor units innervating a given muscle and is obtained by orthodromic activation of motor efferents following peripheral nerve stimulation given during muscle relaxation. Acute aerobic exercise is not anticipated to change Mmax since acute exercise-induced effects are likely to be mediated by changes in the central nervous system. For example, most studies have reported no change in Mmax following an acute bout of exercise (Neva et al., 2017; El-Sayes et al., 2019b; Walsh et al., 2019; Brown et al., 2020; Nicolini et al., 2020). However, there are instances where acute aerobic exercise reduces Mmax (McDonnell et al., 2013). This reduction is likely to relate to changes in the conductivity that can occur with shifts in electrode position related to perspiration. Therefore, one approach is to obtain measures of Mmax before and following exercise, and confirm that Mmax has not change. If Mmax has changed, another approach is to normalize MEP amplitude to Mmax as performed elsewhere (McDonnell et al., 2013; Neva et al., 2017; El-Sayes et al., 2019b; Nicolini et al., 2019, 2020; Walsh et al., 2019; Brown et al., 2020).
An important consideration when using TMS paradigms to assess exercise-induced neuroplasticity is the origin of plasticity. While epidural recordings and I-wave modulation provide insight into the spinal vs. cortical origin of TMS measures, it cannot be ruled out that changes in spinal excitability underly the neuroplastic effects induced by exercise. To disentangle spinal vs. cortical locations of neuroplasticity, studies may choose to measure spinal reflexes in addition to TMS measures. For example, H-reflexes and F-waves provide information about spinal motor neuron excitability (McNeil et al., 2013). Overall, using a combination of techniques probing cortical and spinal neural pathways provides greater insight into the mechanisms of exercise-induced neuroplasticity.
Fitness and/or physical activity levels may influence the propensity for exercise-induced neuroplasticity. Lulic et al. (2017) found that acute aerobic exercise only increased MEPs in the high physically active group, but not in the low physically active group as categorized by the International Physical Activity Questionnaire (IPAQ). However, MacDonald et al. (2019) found no correlation between corticospinal excitability change and aerobic fitness as assessed by peak oxygen uptake (VO2max). These results suggest that physical activity, but not necessarily cardiorespiratory fitness, is an important consideration for exercise-induced neuroplasticity. Nevertheless, both of these variables would aid in the interpretation of neurophysiological changes following exercise. Therefore, physical activity and/or fitness levels should be well-defined in future studies.
Another important consideration for TMS-exercise research is the timing of post-exercise assessments to determine the duration of neuroplasticity. A number of TMS exercise studies have implemented multiple post-exercise assessments of TMS measures (Singh et al., 2014; Smith et al., 2014; Mooney et al., 2016; Neva et al., 2017; MacDonald et al., 2019; Walsh et al., 2019; Yamazaki et al., 2019; Brown et al., 2020). For example, Mooney et al. (2016) found that LICI decreased 10 and 20 min after acute exercise but returned to baseline levels 30 min after exercise. This demonstrates that an acute bout of aerobic exercise does not induce neuroplasticity for an extended period of time. Further, multiple post-intervention assessments may capture delayed changes in neurophysiology. For example Smith et al. (2014) only found a decrease in SICI post 15 min and not immediately post the exercise. Therefore, future studies should carefully consider the number and timing of post-exercise TMS assessments to capture delayed neuroplastic effects and the duration of neuroplastic effects.
Future research should consider investigating exercise-induced neuroplasticity as a function of participant demographics. One study has showed that both males and females demonstrate increased MEP recruitment curves and reduced SICI after one session of aerobic exercise, regardless of menstrual cycle phase (El-Sayes et al., 2019b). However, the influence of biological sex and ovarian hormones on chronic aerobic exercise effects are unknown. Further, it is unknown if neuroplasticity can be induced by exercise within different age groups. With the exception of McGregor et al. (2018), all studies within Table 1 recruited participants with mean ages of 20–35 years. McGregor et al. (2018) showed that chronic aerobic exercise can increase iSP in older adults, but does not change LIHI. Further, McGregor et al. (2013) showed that the iSP is longer is physically fit compared to sedentary middle-aged adults. Harasym et al. (2020) reported no difference in single- (RMT, AMT, MEP recruitment curve) and paired-pulse (SICI, ICF, SAI, LAI, SIHI, LIHI) TMS measures between sedentary and active postmenopausal women. However, it is unknown if an acute bout of aerobic exercise is capable of inducing neuroplasticity within older participants. In addition, studies should consider acquiring paired-pulse TMS measures with different coil directions. First, compared to the more commonly used posterior-anterior current (PA), the anterior-posterior (AP) current activates different neuronal populations (Ni et al., 2011), providing insight into the I-wave generating interneurons that may be modulated following exercise. Second, the AP current evokes stronger SICI in older compared to younger adults, while the PA current evokes similar SICI between the two (Sale et al., 2016). Therefore, when investigating exercise-induced neuroplasticity across age groups, acquiring data in various current directions may lead to differing results.
Finally, future research may want to consider implementing reliability analyses within experimental designs. TMS measures of corticospinal excitability are subject to variability from multiple sources including, but not limited to, circadian factors (Lang et al., 2011; Bocquillon et al., 2017), variations in coil positioning (Koski et al., 2005; de Goede et al., 2018), and participant attention and fatigue (Stefan et al., 2004; Kotb et al., 2005; Kreuzer et al., 2011). The majority of studies reviewed herein employed a no-exercise control condition to confirm that were no significant changes in TMS measures due to the passage of time (McDonnell et al., 2013; Mang et al., 2014, 2016; Mooney et al., 2016; Stavrinos and Coxon, 2017; Smith et al., 2018; Opie and Semmler, 2019; Yamazaki et al., 2019; Andrews et al., 2020; Brown et al., 2020; Morris et al., 2020; Nicolini et al., 2020). However, future studies may wish to use these no-exercise conditions as an opportunity to determine the reliability of TMS assessments and increase the validity of their findings. For example, relative reliability assessments such as the intraclass correlation coefficient (ICC) would be useful in determining the test-retest reliability of a measurement (Koo and Li, 2016) and absolute reliability metrics such as the Standard Error of Measurement (SEM) can be computed to quantify measurement error (Weir, 2005). Further, the Smallest Detectable Change (SDC), defined as minimum change in a measurement that is beyond measurement error and is considered to be a “real” change (Weir, 2005; Beaulieu et al., 2017), can be calculated at the individual (SDCindividual) and group level (SDCgroup) (Schambra et al., 2015). Specifically, the SDCgroup of a measure can be calculated from the no-exercise condition and be used as a complement to hypothesis testing (Schambra et al., 2015). As an example, a study may assess the change in RMT following 20 min of aerobic cycling (exercise condition) or 20 min of rest (control condition). Calculations from the data within the control condition reveal that SDCindividual is 10% MSO and SDCgroup is 3% MSO. This indicates that an individual would need to exhibit a change in RMT >10% MSO in the exercise condition to confidently conclude that said individual showed real physiological change. In contrast, the group-averaged mean would need to exhibit a change in RMT greater than only 3% MSO in the exercise condition to confidently conclude a “real” change exceeding measurement error. Overall, reporting these metrics of reliability alongside means comparisons can increase confidence that exercise is or is not inducing real neuroplastic change in the nervous system.
Conclusion
This review aimed to summarize the physiology and methodology of single- and paired-pulse TMS paradigms that can be used to study exercise-induced neuroplasticity, and to highlight the previous research that has studied how these measures change following aerobic exercise. Future studies using TMS to investigate exercise-induced neuroplasticity should take into consideration important variables that could influence data interpretation including changes in electrode conductance and muscle contractility with exercise, spinal contributions to neuroplasticity, physical fitness, age, reliability, and the timing of TMS assessments.
Author Contributions
CVT conceived, wrote, edited, and finalized manuscript. AJN conceived, edited, and finalized manuscript.
Funding
This work was funded through the Canada Research Chairs program to AJN and funding from the Natural Sciences and Engineering Research Council of Canada to AJN.
Conflict of Interest
The authors declare that the research was conducted in the absence of any commercial or financial relationships that could be construed as a potential conflict of interest.
Abbreviations
AMT, active motor threshold; AP, anterior-posterior; APB, abductor pollicis brevis; CBI, cerebellar inhibition; CBI50, conditioning stimulus intensity resulting in 50% inhibition of the MEP; CS, conditioning stimulus; CSP, contralateral silent period; DA, dopamine; D-wave, direct wave; ECR, extensor carpi radialis; EMG, electromyography; FDI, first dorsal interosseous; GABA, gamma-aminobutyric acid; HICE, high-intensity continuous exercise; HIIE, high-intensity interval exercise; HIIT, high-intensity interval training; HRmax, maximal heart rate; HRR, heart rate reserve; ICF, intracortical facilitation; IHI, interhemispheric inhibition; IPAQ, International Physical Activity Questionnaire; ISI, interstimulus interval; iSP, ipsilateral silent period; I-wave, indirect wave; LAI, long-latency afferent inhibition; LICE, low-intensity continuous exercise; LICI, long-interval intracortical inhibition; LIHI, long-interval interhemispheric inhibition; M1, primary motor cortex; MEP, motor-evoked potential; MEPmax, maximum MEP amplitude; MIAT, moderate-intensity aerobic training; MICE, moderate-intensity continuous exercise; MIIE, moderate-intensity interval exercise; ML-PEST, maximum likelihood parameter estimation by sequential testing; Mmax, maximal M-wave; MRS, magnetic resonance spectroscopy; MSO, maximum stimulator output; MT, motor threshold; MTI, maximum-tolerated intensity; MVC, maximum voluntary contraction; NA, noradrenaline; NMDA, N-methyl-D-aspartate; PA, posterior-anterior; PD, Parkinson's disease; RMT, resting motor threshold; RPE, rating of perceived exertion; SAI, short-latency afferent inhibition; SCI, spinal cord injury; SICF, short-interval intracortical facilitation; SICI, short-interval intracortical inhibition; SIHI, short-interval interhemispheric inhibition; SNAP, sensory nerve action potential; ST, sensory threshold; TA, tibialis anterior; TES, transcranial electric stimulation; TMS, transcranial magnetic stimulation; TS, test stimulus; VO2max, peak oxygen uptake; Wpeak, peak power output.
References
Abdoli-Eramaki, M., Damecour, C., Christenson, J., and Stevenson, J. (2012). The effect of perspiration on the sEMG amplitude and power spectrum. J. Electromyogr. Kinesiol. 22, 908–913. doi: 10.1016/j.jelekin.2012.04.009
Abraha, B., Chaves, A. R., Kelly, L. P., Wallack, E. M., Wadden, K. P., McCarthy, J., et al. (2018). A bout of high intensity interval training lengthened nerve conduction latency to the non-exercised affected limb in chronic stroke. Front. Physiol. 9:827. doi: 10.3389/fphys.2018.00827
Ah Sen, C. B., Fassett, H. J., El-Sayes, J., Turco, C. V., Hameer, M. M., and Nelson, A. J. (2017). Active and resting motor threshold are efficiently obtained with adaptive threshold hunting. PLoS ONE 12:e0186007. doi: 10.1371/journal.pone.0186007
Andrews, S. C., Curtin, D., Hawi, Z., Wongtrakun, J., Stout, J. C., and Coxon, J. P. (2020). Intensity matters: high-intensity interval exercise enhances motor cortex plasticity more than moderate exercise. Cereb. Cortex 30, 101–112. doi: 10.1093/cercor/bhz075
Awiszus, F. (2003). TMS and threshold hunting. Suppl. Clin. Neurophysiol. 56, 13–23. doi: 10.1016/S1567-424X(09)70205-3
Baarbé, J., Yielder, P., Daligadu, J., Behbahani, H., Haavik, H., and Murphy, B. (2014). A novel protocol to investigate motor training-induced plasticity and sensorimotor integration in the cerebellum and motor cortex. J. Neurophysiol. 111, 715–721. doi: 10.1152/jn.00661.2013
Beaulieu, L. D., Flamand, V. H., Massé-Alarie, H., and Schneider, C. (2017). Reliability and minimal detectable change of transcranial magnetic stimulation outcomes in healthy adults: a systematic review. Brain Stimul. 10, 196–213. doi: 10.1016/j.brs.2016.12.008
Bocquillon, P., Charley-Monaca, C., Houdayer, E., Marques, A., Kwiatkowski, A., Derambure, P., et al. (2017). Reduced afferent-induced facilitation of primary motor cortex excitability in restless legs syndrome. Sleep Med. 30, 31–35. doi: 10.1016/j.sleep.2016.03.007
Boroojerdi, B., Battaglia, F., Muellbacher, W., and Cohen, L. G. (2001). Mechanisms influencing stimulus-response properties of the human corticospinal system. Clin. Neurophysiol. 112, 931–937. doi: 10.1016/S1388-2457(01)00523-5
Boyne, P., Meyrose, C., Westover, J., Whitesel, D., Hatter, K., Reisman, D. S., et al. (2019). Exercise intensity affects acute neurotrophic and neurophysiological responses poststroke. J. Appl. Physiol. 126, 431–443. doi: 10.1152/japplphysiol.00594.2018
Brown, K. E., Neva, J. L., Mang, C. S., Chau, B., Chiu, L. K., Francisco, B. A., et al. (2020). The influence of an acute bout of moderate-intensity cycling exercise on sensorimotor integration. Eur. J. Neurosci. 52, 4779–4790. doi: 10.1111/EJN.14909/v2/response1
Cantello, R., Gianelli, M., Civardi, C., and Mutani, R. (1992). Magnetic brain stimulation: the silent period after the motor evoked potential. Neurology 42, 1951–1959. doi: 10.1212/WNL.42.10.1951
Cavaleri, R., Schabrun, S. M., and Chipchase, L. S. (2017). The number of stimuli required to reliably assess corticomotor excitability and primary motor cortical representations using transcranial magnetic stimulation (TMS): a systematic review and meta-analysis. Syst. Rev. 6:48. doi: 10.1186/s13643-017-0440-8
Chan, J. H. L., Lin, C. S. Y., Pierrot-Deseilligny, E., and Burke, D. (2002). Excitability changes in human peripheral nerve axons in a paradigm mimicking paired-pulse transcranial magnetic stimulation. J. Physiol. 542, 951–961. doi: 10.1113/jphysiol.2002.018937
Chen, R., Lozano, A. M., and Ashby, P. (1999). Mechanism of the silent period following transcranial magnetic stimulation. Evidence from epidural recordings. Exp. Brain Res. 128, 539–542. doi: 10.1007/s002210050878
Chen, R., Samii, A., Caños, M., Wassermann, E. M., and Hallett, M. (1997). Effects of phenytoin on cortical excitability in humans. Neurology 49, 881–883. doi: 10.1212/WNL.49.3.881
Daskalakis, Z. J., Christensen, B. K., Fitzgerald, P. B., Roshan, L., and Chen, R. (2002). The mechanisms of interhemispheric inhibition in the human motor cortex. J. Physiol. 543, 317–326. doi: 10.1113/jphysiol.2002.017673
Daskalakis, Z. J., Paradiso, G. O., Christensen, B. K., Fitzgerald, P. B., Gunraj, C., and Chen, R. (2004). Exploring the connectivity between the cerebellum and motor cortex in humans. J. Physiol. 557, 689–700. doi: 10.1113/jphysiol.2003.059808
de Goede, A. A., ter Braack, E. M., and van Putten, M. J. A. M. (2018). Accurate coil positioning is important for single and paired pulse TMS on the subject level. Brain Topogr. 31, 917–930. doi: 10.1007/s10548-018-0655-6
Demirtas-Tatlidede, A., Freitas, C., Pascual-Leone, A., and Schmahmann, J. D. (2011). Modulatory effects of theta burst stimulation on cerebellar nonsomatic functions. Cerebellum 10, 495–503. doi: 10.1007/s12311-010-0230-5
Devanne, H., Lavoie, B. A., and Capaday, C. (1997). Input-output properties and gain changes in the human corticospinal pathway. Exp. Brain Res. 114, 329–338. doi: 10.1007/PL00005641
Di Lazzaro, V., Oliviero, A., Mazzone, P., Pilato, F., Saturno, E., Insola, A., et al. (2002). Direct demonstration of long latency cortico-cortical inhibition in normal subjects and in a patient with vascular parkinsonism. Clin. Neurophysiol. 113, 1673–1679. doi: 10.1016/S1388-2457(02)00264-X
Di Lazzaro, V., Oliviero, A., Profice, P., Insola, A., Mazzone, P., Tonali, P., et al. (1999a). Direct demonstration of interhemispheric inhibition of the human motor cortex produced by transcranial magnetic stimulation. Exp. Brain Res. 124, 520–524. doi: 10.1007/s002210050648
Di Lazzaro, V., Oliviero, A., Profice, P., Pennisi, M., Di Giovanni, S., Zito, G., et al. (2000). Muscarinic receptor blockade has differential effects on the excitability of intracortical circuits in the human motor cortex. Exp. Brain Res. 135, 455–461. doi: 10.1007/s002210000543
Di Lazzaro, V., Oliviero, A., Saturno, E., Dileone, M., Pilato, F., Nardone, R., et al. (2005a). Effects of lorazepam on short latency afferent inhibition and short latency intracortical inhibition in humans. J. Physiol. 564, 661–668. doi: 10.1113/jphysiol.2004.061747
Di Lazzaro, V., Pilato, F., Dileone, M., Profice, P., Ranieri, F., Ricci, V., et al. (2007). Segregating two inhibitory circuits in human motor cortex at the level of GABAA receptor subtypes: a TMS study. Clin. Neurophysiol. 118, 2207–2214. doi: 10.1016/j.clinph.2007.07.005
Di Lazzaro, V., Pilato, F., Dileone, M., Tonali, P., and Ziemann, U. (2005b). Dissociated effects of diazepam and lorazepam on short-latency afferent inhibition. J. Physiol. 569, 315–323. doi: 10.1113/jphysiol.2005.092155
Di Lazzaro, V., Pilato, F., Oliviero, A., Dileone, M., Saturno, E., Mazzone, P., et al. (2006). Origin of facilitation of motor-evoked potentials after paired magnetic stimulation: direct recording of epidural activity in conscious humans. J. Neurophysiol. 96, 1765–1771. doi: 10.1152/jn.00360.2006
Di Lazzaro, V., Pilato, F., Saturno, E., Oliviero, A., Dileone, M., Mazzone, P., et al. (2005c). Theta-burst repetitive transcranial magnetic stimulation suppresses specific excitatory circuits in the human motor cortex. J. Physiol. 565, 945–950. doi: 10.1113/jphysiol.2005.087288
Di Lazzaro, V., Profice, P., Ranieri, F., Capone, F., Dileone, M., Oliviero, A., et al. (2012). I-wave origin and modulation. Brain Stimul. 5, 512–525. doi: 10.1016/j.brs.2011.07.008
Di Lazzaro, V., Restuccia, D., Oliviero, A., Profice, P., Ferrara, L., Insola, A., et al. (1998). Magnetic transcranial stimulation at intensities below active motor threshold activates intracortical inhibitory circuits. Exp. Brain Res. 119, 265–268. doi: 10.1007/s002210050341
Di Lazzaro, V., Rothwell, J. C., Oliviero, A., Profice, P., Insola, A., Mazzone, P., et al. (1999b). Intracortical origin of the short latency facilitation produced by pairs of threshold magnetic stimuli applied to human motor cortex. Exp. Brain Res. 129, 494–499. doi: 10.1007/s002210050919
Duque, J., Mazzocchio, R., Dambrosia, J., Murase, N., Olivier, E., and Cohen, L. G. (2005). Kinematically specific interhemispheric inhibition operating in the process of generation of a voluntary movement. Cereb. Cortex 15, 588–593. doi: 10.1093/cercor/bhh160
Duque, J., Murase, N., Celnik, P., Hummel, F., Harris-Love, M., Mazzocchio, R., et al. (2007). Intermanual differences in movement-related interhemispheric inhibition. J. Cogn. Neurosci. 19, 204–213. doi: 10.1162/jocn.2007.19.2.204
El-Sayes, J., Harasym, D., Turco, C. V., Locke, M. B., and Nelson, A. J. (2019a). Exercise-induced neuroplasticity: a mechanistic model and prospects for promoting plasticity. Neuroscientist 25, 65–85. doi: 10.1177/1073858418771538
El-Sayes, J., Turco, C. V., Skelly, L. E., Locke, M. B., Gibala, M. J., and Nelson, A. J. (2020). Acute high-intensity and moderate-intensity interval exercise do not change corticospinal excitability in low fit, young adults. PLoS ONE 15:e0227581. doi: 10.1371/journal.pone.0227581
El-Sayes, J., Turco, C. V., Skelly, L. E., Nicolini, C., Fahnestock, M., Gibala, M. J., et al. (2019b). The effects of biological sex and ovarian hormones on exercise-induced neuroplasticity. Neuroscience 410, 29–40. doi: 10.1016/j.neuroscience.2019.04.054
Fernandez, L., Major, B. P., Teo, W. P., Byrne, L. K., and Enticott, P. G. (2018). The impact of stimulation intensity and coil type on reliability and tolerability of cerebellar brain inhibition (CBI) via dual-coil TMS. Cerebellum 17, 540–549. doi: 10.1007/s12311-018-0942-5
Fisher, B. E., Wu, A. D., Salem, G. J., Song, J., Lin, C. H., (Janice), Yip, J., et al. (2008). The effect of exercise training in improving motor performance and corticomotor excitability in people with early Parkinson's disease. Arch. Phys. Med. Rehabil. 89, 1221–1229. doi: 10.1016/j.apmr.2008.01.013
Fisher, R. J., Nakamura, Y., Bestmann, S., Rothwell, J. C., and Bostock, H. (2002). Two phases of intracortical inhibition revealed by transcranial magnetic threshold tracking. Exp. Brain Res. 143, 240–248. doi: 10.1007/s00221-001-0988-2
Florian, J., Müller-Dahlhaus, M., Liu, Y., and Ziemann, U. (2008). Inhibitory circuits and the nature of their interactions in the human motor cortex a pharmacological TMS study. J. Physiol. 586, 495–514. doi: 10.1113/jphysiol.2007.142059
Garcia, M. A. C., Souza, V. H., and Vargas, C. D. (2017). Can the recording of motor potentials evoked by transcranial magnetic stimulation be optimized? Front. Hum. Neurosci. 11:413. doi: 10.3389/fnhum.2017.00413
Gilbert, D. L., Ridel, K. R., Sallee, F. R., Zhang, J., Lipps, T. D., and Wassermann, E. M. (2006). Comparison of the inhibitory and excitatory effects of ADHD medications methylphenidate and atomoxetine on motor cortex. Neuropsychopharmacology 31, 442–449. doi: 10.1038/sj.npp.1300806
Goodall, S., Howatson, G., and Thomas, K. (2018). Modulation of specific inhibitory networks in fatigued locomotor muscles of healthy males. Exp. Brain Res. 236, 463–473. doi: 10.1007/s00221-017-5142-x
Groppa, S., Oliviero, A., Eisen, A., Quartarone, A., Cohen, L. G., Mall, V., et al. (2012). A practical guide to diagnostic transcranial magnetic stimulation: report of an IFCN committee. Clin. Neurophysiol. 123, 858–882. doi: 10.1016/j.clinph.2012.01.010
Hallett, M. (2007). Transcranial magnetic stimulation: a primer. Neuron 55, 187–199. doi: 10.1016/j.neuron.2007.06.026
Harasym, D., Turco, C. V., Nicolini, C., Toepp, S. L., Jenkins, E. M., Gibala, M. J., et al. (2020). Fitness level influences white matter microstructure in postmenopausal women. Front. Aging Neurosci. 12:129. doi: 10.3389/fnagi.2020.00129
Ilić, T. V., Korchounov, A., and Ziemann, U. (2003). Methylphenidate facilitates and disinhibits the motor cortex in intact humans. Neuroreport 14, 773–776. doi: 10.1097/00001756-200304150-00023
Inghilleri, M., Berardelli, A., Cruccu, G., and Manfredi, M. (1993). Silent period evoked by transcranial stimulation of the human cortex and cervicomedullary junction. J. Physiol. 466, 521–534.
Inghilleri, M., Berardelli, A., Marchetti, P., and Manfredi, M. (1996). Effects of diazepam, baclofen and thiopental on the silent period evoked by transcranial magnetic stimulation in humans. Exp. Brain Res. 109, 467–472. doi: 10.1007/BF00229631
Irlbacher, K., Brocke, J., Mechow, J. v., and Brandt, S. A. (2007). Effects of GABAA and GABAB agonists on interhemispheric inhibition in man. Clin. Neurophysiol. 118, 308–316. doi: 10.1016/j.clinph.2006.09.023
Jayaram, G., Galea, J. M., Bastian, A. J., and Celnik, P. (2011). Human locomotor adaptive learning is proportional to depression of cerebellar excitability. Cereb. Cortex 21, 1901–1909. doi: 10.1093/cercor/bhq263
Kimiskidis, V. K., Papagiannopoulos, S., Sotirakoglou, K., Kazis, D. A., Kazis, A., and Mills, K. R. (2005). Silent period to transcranial magnetic stimulation: construction and properties of stimulus-response curves in healthy volunteers. Exp. Brain Res. 163, 21–31. doi: 10.1007/s00221-004-2134-4
Koo, T. K., and Li, M. Y. (2016). A guideline of selecting and reporting intraclass correlation coefficients for reliability research. J. Chiropr. Med. 15, 155–163. doi: 10.1016/j.jcm.2016.02.012
Korchounov, A., Ilić, T. V., and Ziemann, U. (2007). TMS-assisted neurophysiological profiling of the dopamine receptor agonist cabergoline in human motor cortex. J. Neural Transm. 114, 223–229. doi: 10.1007/s00702-006-0523-5
Koski, L., Schrader, L. M., Wu, A. D., and Stern, J. M. (2005). Normative data on changes in transcranial magnetic stimulation measures over a ten hour period. Clin. Neurophysiol. 116, 2099–2109. doi: 10.1016/j.clinph.2005.06.006
Kotb, M. A., Mima, T., Ueki, Y., Begum, T., Khafagi, A. T., Fukuyama, H., et al. (2005). Effect of spatial attention on human sensorimotor integration studied by transcranial magnetic stimulation. Clin. Neurophysiol. 116, 1195–1200. doi: 10.1016/j.clinph.2004.12.006
Kreuzer, P., Langguth, B., Popp, R., Raster, R., Busch, V., Frank, E., et al. (2011). Reduced intra-cortical inhibition after sleep deprivation: a transcranial magnetic stimulation study. Neurosci. Lett. 493, 63–66. doi: 10.1016/j.neulet.2011.02.044
Kujirai, T., Caramia, M. D., Rothwell, J. C., Day, B. L., Thompson, P. D., Ferbert, A., et al. (1993). Corticocortical inhibition in human motor cortex. J. Physiol. 471, 501–519. doi: 10.1113/jphysiol.1993.sp019912
Kukaswadia, S., Wagle-Shukla, A., Morgante, F., Gunraj, C., and Chen, R. (2005). Interactions between long latency afferent inhibition and interhemispheric inhibitions in the human motor cortex. J. Physiol. 563, 915–924. doi: 10.1113/jphysiol.2004.080010
Kuo, H.-I., Paulus, W., Batsikadze, G., Jamil, A., Kuo, M.-F., and Nitsche, M. A. (2017). Acute and chronic noradrenergic effects on cortical excitability in healthy humans. Int. J. Neuropsychopharmacol. 20, 634–643. doi: 10.1016/j.brs.2017.01.430
Lang, N., Rothkegel, H., Peckolt, H., and Deuschl, G. (2013). Effects of lacosamide and carbamazepine on human motor cortex excitability: a double-blind, placebo-controlled transcranial magnetic stimulation study. Seizure 22, 726–730. doi: 10.1016/j.seizure.2013.05.010
Lang, N., Rothkegel, H., Reiber, H., Hasan, A., Sueske, E., Tergau, F., et al. (2011). Circadian modulation of GABA-mediated cortical inhibition. Cereb. Cortex 21, 2299–2306. doi: 10.1093/cercor/bhr003
Li, X., Charalambous, C. C., Reisman, D. S., and Morton, S. M. (2019). A short bout of high-intensity exercise alters ipsilesional motor cortical excitability post-stroke. Top. Stroke Rehabil. 26, 405–411. doi: 10.1101/530857
Locke, M. B., Toepp, S. L., Turco, C. V., Harasym, D. H., Rathbone, M. P., Noseworthy, M. D., et al. (2020). Altered motor system function in post-concussion syndrome as assessed via transcranial magnetic stimulation. Clin. Neurophysiol. Pract. 5, 157–164. doi: 10.1016/j.cnp.2020.07.004
Lulic, T., El-Sayes, J., Fassett, H. J., and Nelson, A. J. (2017). Physical activity levels determine exercise-induced changes in brain excitability. PLoS ONE 12:e0173672. doi: 10.1371/journal.pone.0173672
MacDonald, M. M., Khan, H., Kraeutner, S. N., Usai, F., Rogers, E. A., Kimmerly, D. S., et al. (2019). Intensity of acute aerobic exercise but not aerobic fitness impacts on corticospinal excitability. Appl. Physiol. Nutr. Metab. 44, 869–878. doi: 10.1139/apnm-2018-0643
Madhavan, S., Stinear, J. W., and Kanekar, N. (2016). Effects of a single session of high intensity interval treadmill training on corticomotor excitability following stroke: implications for therapy. Neural Plast. 2016:168414. doi: 10.1155/2016/1686414
Mang, C. S., Brown, K. E., Neva, J. L., Snow, N. J., Campbell, K. L., and Boyd, L. A. (2016). Promoting motor cortical plasticity with acute aerobic exercise: a role for cerebellar circuits. Neural Plast. 2016:6797928. doi: 10.1155/2016/6797928
Mang, C. S., Campbell, K. L., Ross, C. J. D., and Boyd, L. A. (2013). Promoting neuroplasticity for motor rehabilitation after stroke: considering the effects of aerobic exercise and genetic variation on brain-derived neurotrophic factor. Phys. Ther. 93, 1707–1716. doi: 10.2522/ptj.20130053
Mang, C. S., Snow, N. J., Campbell, K. L., Ross, C. J. D., and Boyd, L. A. (2014). A single bout of high-intensity aerobic exercise facilitates response to paired associative stimulation and promotes sequence-specific implicit motor learning. J. Appl. Physiol. 117, 1325–1336. doi: 10.1152/japplphysiol.00498.2014
Matsugi, A. (2019). Changes in the cortical silent period during force control. Somatosens. Mot. Res. 36, 8–13. doi: 10.1080/08990220.2018.1563536
McDonnell, M. N., Buckley, J. D., Opie, G. M., Ridding, M. C., and Semmler, J. G. (2013). A single bout of aerobic exercise promotes motor cortical neuroplasticity. J. Appl. Physiol. 114, 1174–1182. doi: 10.1152/japplphysiol.01378.2012
McDonnell, M. N., Orekhov, Y., and Ziemann, U. (2006). The role of GABAB receptors in intracortical inhibition in the human motor cortex. Exp. Brain Res. 173, 86–93. doi: 10.1007/s00221-006-0365-2
McDonnell, M. N., Orekhov, Y., and Ziemann, U. (2007). Suppression of LTP-like plasticity in human motor cortex by the GABA B receptor agonist baclofen. Exp. Brain Res. 180, 181–186. doi: 10.1007/s00221-006-0849-0
McGregor, K. M., Crosson, B., Mammino, K., Omar, J., García, P. S., and Nocera, J. R. (2018). Influences of 12-week physical activity interventions on TMS measures of cortical network inhibition and upper extremity motor performance in older adults-A feasibility study. Front. Aging Neurosci. 9:422. doi: 10.3389/fnagi.2017.00422
McGregor, K. M., Nocera, J. R., Sudhyadhom, A., Patten, C., Manini, T. M., Kleim, J. A., et al. (2013). Effects of aerobic fitness on aging-related changes of interhemispheric inhibition and motor performance. Front. Aging Neurosci. 5:66. doi: 10.3389/fnagi.2013.00066
McNeil, C. J., Butler, J. E., Taylor, J. L., and Gandevia, S. C. (2013). Testing the excitability of human motoneurones. Front. Hum. Neurosci. 7:152. doi: 10.3389/fnhum.2013.00152
Merletti, R., and Muceli, S. (2019). Tutorial. Surface EMG detection in space and time: best practices. J. Electromyogr. Kinesiol. 49:102363. doi: 10.1016/j.jelekin.2019.102363
Mills, K. R., and Nithi, K. A. (1997). Corticomotor threshold to magnetic stimulation: normal values and repeatability. Muscle Nerve 20, 570–576. doi: 10.1002/(SICI)1097-4598(199705)20:5<570::AID-MUS5>3.0.CO;2-6
Mira, J., Lapole, T., Souron, R., Messonnier, L., Millet, G. Y., and Rupp, T. (2017). Cortical voluntary activation testing methodology impacts central fatigue. Eur. J. Appl. Physiol. 117, 1845–1857. doi: 10.1007/s00421-017-3678-x
Mishory, A., Molnar, C., Koola, J., Li, X., Kozel, F. A., Myrick, H., et al. (2004). The Maximum-Likelihood Strategy for determining transcranial magnetic stimulation motor threshold, using parameter estimation by sequential testing is faster than conventional methods with similar precision. J. ECT 20, 160–165. doi: 10.1097/00124509-200409000-00007
Mohammadi, B., Krampfl, K., Petri, S., Bogdanova, D., Kossev, A., Bufler, J., et al. (2006). Selective and nonselective benzodiazepine agonists have different effects on motor cortex excitability. Muscle Nerve 33, 778–784. doi: 10.1002/mus.20531
Moll, G. H., Heinrich, H., and Rothenberger, A. (2003). Methylphenidate and intracortical excitability: opposite effects in healthy subjects and attention-deficit hyperactivity disorder. Acta Psychiatr. Scand. 107, 69–72. doi: 10.1034/j.1600-0447.2003.02114.x
Mooney, R. A., Coxon, J. P., Cirillo, J., Glenny, H., Gant, N., and Byblow, W. D. (2016). Acute aerobic exercise modulates primary motor cortex inhibition. Exp. Brain Res. 234, 3669–3676. doi: 10.1007/s00221-016-4767-5
Morris, T. P., Fried, P. J., Macone, J., Stillman, A., Gomes-Osman, J., Costa-Miserachs, D., et al. (2020). Light aerobic exercise modulates executive function and cortical excitability. Eur. J. Neurosci. 51, 1723–1734. doi: 10.1111/ejn.14593
Moscatelli, F., Messina, G., Valenzano, A., Triggiani, A. I., Sessa, F., Carotenuto, M., et al. (2020). 'Effects of twelve weeks' aerobic training on motor cortex excitability. J. Sports Med. Phys. Fitness 60, 1383–1389. doi: 10.23736/S0022-4707.20.10677-7
Murdoch, K., Buckley, J. D., and McDonnell, M. N. (2016). The effect of aerobic exercise on neuroplasticity within the motor cortex following stroke. PLoS ONE 11:e0152377. doi: 10.1371/journal.pone.0152377
Nakamura, H., Kitagawa, H., Kawaguchi, Y., and Tsuji, H. (1997). Intracortical facilitation and inhibition after transcranial magnetic stimulation in conscious humans. J. Physiol. 498, 817–823. doi: 10.1113/jphysiol.1997.sp021905
Nelson, A. J., Hoque, T., Gunraj, C., Ni, Z., and Chen, R. (2009). Bi-directional interhemispheric inhibition during unimanual sustained contractions. BMC Neurosci. 10:31. doi: 10.1186/1471-2202-10-31
Neva, J. L., Brown, K. E., Mang, C. S., Francisco, B. A., and Boyd, L. A. (2017). An acute bout of exercise modulates both intracortical and interhemispheric excitability. Eur. J. Neurosci. 45, 1343–1355. doi: 10.1111/ejn.13569
Ni, Z., Charab, S., Gunraj, C., Nelson, A. J., Udupa, K., Yeh, I.-J., et al. (2011). Transcranial magnetic stimulation in different current directions activates separate cortical circuits. J. Neurophysiol. 105, 749–756. doi: 10.1152/jn.00640.2010
Ni, Z., Gunraj, C., Nelson, A. J., Yeh, I. J., Castillo, G., Hoque, T., et al. (2009). Two phases of interhemispheric inhibition between motor related cortical areas and the primary motor cortex in human. Cereb. Cortex 19, 1654–1665. doi: 10.1093/cercor/bhn201
Nicolini, C., Michalski, B., Toepp, S. L., Turco, C. V., D'Hoine, T., Harasym, D., et al. (2020). A single bout of high-intensity interval exercise increases corticospinal excitability, brain-derived neurotrophic factor, and uncarboxylated osteolcalcin in sedentary, healthy males. Neuroscience 437, 242–255. doi: 10.1016/j.neuroscience.2020.03.042
Nicolini, C., Toepp, S., Harasym, D., Michalski, B., Fahnestock, M., Gibala, M., et al. (2019). No changes in corticospinal excitability, biochemical markers and working memory after six weeks of high-intensity interval training in sedentary males. Physiol. Rep. 7:e14140. doi: 10.14814/phy2.14140
Opie, G., and Semmler, J. (2019). Acute exercise at different intensities influences corticomotor excitabiity and performance of a ballistic thumb training task. Neuroscience 412, 29–39. doi: 10.1016/j.neuroscience.2019.05.049
Perez, M. A., and Cohen, L. G. (2009). Interhemispheric inhibition between primary motor cortices: what have we learned? J. Physiol. 587, 725–726. doi: 10.1113/jphysiol.2008.166926
Pierantozzi, M., Grazia Marciani, M., Giuseppina Palmieri, M., Brusa, L., Galati, S., Donatella Caramia, M., et al. (2004a). Effect of Vigabatrin on motor responses to transcranial magnetic stimulation: an effective tool to investigate in vivo GABAergic cortical inhibition in humans. Brain Res. 1028, 1–8. doi: 10.1016/j.brainres.2004.06.009
Pierantozzi, M., Panella, M., Palmieri, M. G., Koch, G., Giordano, A., Marciani, M. G., et al. (2004b). Different TMS patterns of intracortical inhibition in early onset Alzheimer dementia and frontotemporal dementia. Clin. Neurophysiol. 115, 2410–2418. doi: 10.1016/j.clinph.2004.04.022
Qi, F., Wu, A. D., and Schweighofer, N. (2011). Fast estimation of transcranial magnetic stimulation motor threshold. Brain Stimul. 4, 50–57. doi: 10.1016/j.brs.2010.06.002
Rossini, P. M., Barker, A. T., Berardelli, A., Caramia, M. D., Caruso, G., Cracco, R. Q., et al. (1994). Non-invasive electrical and magnetic stimulation of the brain, spinal cord and roots: basic principles and procedures for routine clinical application. Report of an IFCN committee. Electroencephalogr. Clin. Neurophysiol. 91, 79–92. doi: 10.1016/0013-4694(94)90029-9
Rothwell, J. C. (2011). Using transcranial magnetic stimulation methods to probe connectivity between motor areas of the brain. Hum. Mov. Sci. 30, 906–915. doi: 10.1016/j.humov.2010.07.007
Rothwell, J. C., Hallett, M., Berardelli, A., Eisen, A., Rossini, P., and Paulus, W. (1999). Magnetic stimulation: motor evoked potentials. the international federation of clinical neurophysiology. Electroencephalogr. Clin. Neurophysiol. Suppl. 52, 97–103. Available at: https://pubmed.ncbi.nlm.nih.gov/10590980/ (accessed March 3, 2021).
Säisänen, L., Pirinen, E., Teitti, S., Könönen, M., Julkunen, P., Määttä, S., et al. (2008). Factors influencing cortical silent period: optimized stimulus location, intensity and muscle contraction. J. Neurosci. Methods 169, 231–238. doi: 10.1016/j.jneumeth.2007.12.005
Sale, M. V., Lavender, A. P., Opie, G. M., Nordstrom, M. A., and Semmler, J. G. (2016). Increased intracortical inhibition in elderly adults with anterior-posterior current flow: a TMS study. Clin. Neurophysiol. 127, 635–640. doi: 10.1016/j.clinph.2015.04.062
Schambra, H. M., Ogden, R. T., Martínez-Hernández, I. E., Lin, X., Chang, Y. B., Rahman, A., et al. (2015). The reliability of repeated TMS measures in older adults and in patients with subacute and chronic stroke. Front. Cell. Neurosci. 9:335. doi: 10.3389/fncel.2015.00335
Schlerf, J. E., Galea, J. M., Bastian, A. J., and Celnik, P. A. (2012). Dynamic modulation of cerebellar excitability for abrupt, but not gradual, visuomotor adaptation. J. Neurosci. 32, 11610–11617. doi: 10.1523/JNEUROSCI.1609-12.2012
Siebner, H. R., Dressnandt, J., Auer, C., and Conrad, B. (1998). Continuous intrathecal baclofen infusions induced a marked increase of the transcranially evoked silent period in a patient with generalized dystonia. Muscle Nerve 21, 1209–1212. doi: 10.1002/(SICI)1097-4598(199809)21:9<1209::AID-MUS15>3.0.CO;2-M
Singh, A. M., Duncan, R. E., Neva, J. L., and Staines, W. R. (2014). Aerobic exercise modulates intracortical inhibition and facilitation in a nonexercised upper limb muscle. BMC Sports Sci. Med. Rehabil. 6:23. doi: 10.1186/2052-1847-6-23
Singh, A. M., Neva, J. L., and Staines, W. R. (2016). Aerobic exercise enhances neural correlates of motor skill learning. Behav. Brain Res. 301, 19–26. doi: 10.1016/j.bbr.2015.12.020
Škarabot, J., Mesquita, R. N. O., Brownstein, C. G., and Ansdell, P. (2019). Myths and Methodologies: how loud is the story told by the transcranial magnetic stimulation-evoked silent period? Exp. Physiol. 104, 635–642. doi: 10.1113/EP087557
Smith, A. E., Goldsworthy, M. R., Garside, T., Wood, F. M., and Ridding, M. C. (2014). The influence of a single bout of aerobic exercise on short-interval intracortical excitability. Exp. Brain Res. 232, 1875–1882. doi: 10.1007/s00221-014-3879-z
Smith, A. E., Goldsworthy, M. R., Wood, F. M., Olds, T. S., Garside, T., and Ridding, M. C. (2018). High-intensity aerobic exercise blocks the facilitation of iTBS-induced plasticity in the human motor cortex. Neuroscience 373, 1–6. doi: 10.1016/j.neuroscience.2017.12.034
Sommer, M., Gileles, E., Knappmeyer, K., Rothkegel, H., Polania, R., and Paulus, W. (2012). Carbamazepine reduces short-interval interhemispheric inhibition in healthy humans. Clin. Neurophysiol. 123, 351–357. doi: 10.1016/j.clinph.2011.07.027
Spampinato, D., and Celnik, P. (2018). Deconstructing skill learning and its physiological mechanisms. Cortex 104, 90–102. doi: 10.1016/j.cortex.2018.03.017
Spampinato, D., Ibáñez, J., Spanoudakis, M., Hammond, P., and Rothwell, J. C. (2020). Cerebellar transcranial magnetic stimulation: the role of coil type from distinct manufacturers. Brain Stimul. 13, 153–156. doi: 10.1016/j.brs.2019.09.005
Stagg, C. J., Bestmann, S., Constantinescu, A. O., Moreno, L. M., Allman, C., Mekle, R., et al. (2011). Relationship between physiological measures of excitability and levels of glutamate and GABA in the human motor cortex. J. Physiol. 589, 5845–5855. doi: 10.1113/jphysiol.2011.216978
Stavrinos, E. L., and Coxon, J. P. (2017). High-intensity interval exercise promotes motor cortex disinhibition and early motor skill consolidation. J. Cogn. Neurosci. 29, 593–604. doi: 10.1162/jocn_a_01078
Stefan, K., Wycislo, M., and Classen, J. (2004). Modulation of associative human motor cortical plasticity by attention. J. Neurophysiol. 92, 66–72. doi: 10.1152/jn.00383.2003
Stetkarova, I., and Kofler, M. (2013). Differential effect of baclofen on cortical and spinal inhibitory circuits. Clin. Neurophysiol. 124, 339–345. doi: 10.1016/j.clinph.2012.07.005
Teo, J. T. H., Terranova, C., Swayne, O., Greenwood, R. J., and Rothwell, J. C. (2009). Differing effects of intracortical circuits on plasticity. Exp. Brain Res. 193, 555–563. doi: 10.1007/s00221-008-1658-4
Thomas, S. L., and Gorassini, M. A. (2005). Increases in corticospinal tract function by treadmill training after incomplete spinal cord injury. J. Neurophysiol. 94, 2844–2855. doi: 10.1152/jn.00532.2005
Tremblay, S., Austin, D., Hannah, R., and Rothwell, J. C. (2016). Non-invasive brain stimulation as a tool to study cerebellar-M1 interactions in humans. Cereb. Ataxias 3:19. doi: 10.1186/s40673-016-0057-z
Tremblay, S., de Beaumont, L., Lassonde, M., and Théoret, H. (2011). Evidence for the specificity of intracortical inhibitory dysfunction in asymptomatic concussed athletes. J. Neurotrauma 28, 493–502. doi: 10.1089/neu.2010.1615
Turco, C. V., El-Sayes, J., Locke, M. B., Chen, R., Baker, S., and Nelson, A. J. (2018). Effects of lorazepam and baclofen on short- and long-latency afferent inhibition. J. Physiol. 596, 5267–5280. doi: 10.1113/JP276710
Turco, C. V., Fassett, H. J., Locke, M. B., El-Sayes, J., and Nelson, A. J. (2019). Parallel modulation of interhemispheric inhibition and the size of a cortical hand muscle representation during active contraction. J. Neurophysiol. 122, 368–377. doi: 10.1152/jn.00030.2019
Ugawa, Y., Day, B. L., Rothwell, J. C., Thompson, P. D., Merton, P. A., and Marsden, C. D. (1991). Modulation of motor cortical excitability by electrical stimulation over the cerebellum in man. J. Physiol. 441, 57–72. doi: 10.1113/jphysiol.1991.sp018738
Valls-Solé, J., Pascual-Leone, A., Wassermann, E. M., and Hallett, M. (1992). Human motor evoked responses to paired transcranial magnetic stimuli. Electroencephalogr. Clin. Neurophysiol. Evoked Potentials 85, 355–364. doi: 10.1016/0168-5597(92)90048-G
Vizi, E. S., and Pasztor, E. (1981). Release of acetylcholine from isolated human cortical slices: inhibitory effect of norepinephrine and phenytoin. Exp. Neurol. 73, 144–153. doi: 10.1016/0014-4886(81)90051-0
Walsh, J. A., Stapley, P. J., Shemmell, J. B. H., Lepers, R., and McAndrew, D. J. (2019). Global corticospinal excitability as assessed in a non-exercised upper limb muscle compared between concentric and eccentric modes of leg cycling. Sci. Rep. 9:19212. doi: 10.1038/s41598-019-55858-5
Wassermann, E. M., Fuhr, P., Cohen, L. G., and Hallett, M. (1991). Effects of transcranial magnetic stimulation on ipsilateral muscles. Neurology 41, 1795–1799. doi: 10.1212/WNL.41.11.1795
Weir, J. (2005). Quantifying test-retest reliability using the intraclass correlation coefficient. J. Strength Cond. Res. 19, 231–240. doi: 10.1519/00124278-200502000-00038
Werhahn, K. J., Kunesch, E., Noachtar, S., Benecke, R., and Classen, J. (1999). Differential effects on motorcortical inhibition induced by blockade of GABA uptake in humans. J. Physiol. 517, 591–597. doi: 10.1111/j.1469-7793.1999.0591t.x
Yamazaki, Y., Sato, D., Yamashiro, K., Nakano, S., Onishi, H., and Maruyama, A. (2019). Acute low-intensity aerobic exercise modulates intracortical inhibitory and excitatory circuits in an exercised and a non-exercised muscle in the primary motor cortex. Front. Physiol. 10:1361. doi: 10.3389/fphys.2019.01361
Yang, Y. R., Tseng, C. Y., Chiou, S. Y., Liao, K. K., Cheng, S. J., Lai, K. L., et al. (2013). Combination of rTMS and treadmill training modulates corticomotor inhibition and improves walking in parkinson disease: a randomized trial. Neurorehabil. Neural Repair 27, 79–86. doi: 10.1177/1545968312451915
Ziemann, U., Bruns, D., and Paulus, W. (1996a). Enhancement of human motor cortex inhibition by the dopamine receptor agonist pergolide: Evidence from transcranial magnetic stimulation. Neurosci. Lett. 208, 187–190. doi: 10.1016/0304-3940(96)12575-1
Ziemann, U., Chen, R., Cohen, L. G., and Hallett, M. (1998a). Dextromethorphan decreases the excitability of the human motor cortex. Neurology 51, 1320–1324. doi: 10.1212/WNL.51.5.1320
Ziemann, U., Lönnecker, S., Steinhoff, B. J., and Paulus, W. (1996b). Effects of antiepileptic drugs on motor cortex excitability in humans: a transcranial magnetic stimulation study. Ann. Neurol. 40, 367–378. doi: 10.1002/ana.410400306
Ziemann, U., Lönnecker, S., Steinhoff, B. J., and Paulus, W. (1996c). The effect of lorazepam on the motor cortical excitability in man. Exp. Brain Res. 109, 127–135. doi: 10.1007/BF00228633
Ziemann, U., Netz, J., Szelényi, A., and Hömberg, V. (1993). Spinal and supraspinal mechanisms contribute to the silent period in the contracting soleus muscle after transcranial magnetic stimulation of human motor cortex. Neurosci. Lett. 156, 167–171. doi: 10.1016/0304-3940(93)90464-V
Ziemann, U., Reis, J., Schwenkreis, P., Rosanova, M., Strafella, A., Badawy, R., et al. (2015). TMS and drugs revisited 2014. Clin. Neurophysiol. 126, 1847–1868. doi: 10.1016/j.clinph.2014.08.028
Ziemann, U., Tergau, F., Bruns, D., Baudewig, J., and Paulus, W. (1997). Changes in human motor cortex excitability induced by dopaminergic and anti-dopaminergic drugs. Electroencephalogr. Clin. Neurophysiol. - Electromyogr. Mot. Control 105, 430–437. doi: 10.1016/S0924-980X(97)00050-7
Ziemann, U., Tergau, F., Wassermann, E. M., Wischer, S., Hildebrandt, J., and Paulus, W. (1998b). Demonstration of facilitatory I wave interaction in the human motor cortex by paired transcranial magnetic stimulation. J. Physiol. 511, 181–190. doi: 10.1111/j.1469-7793.1998.181bi.x
Ziemann, U., Tergau, F., Wischer, S., Hildebrandt, J., and Paulus, W. (1998c). Pharmacological control of facilitatory I-wave interaction in the human motor cortex. A paired transcranial magnetic stimulation study. Electroencephalogr. Clin. Neurophysiol. - Electromyogr. Mot. Control 109, 321–330. doi: 10.1016/S0924-980X(98)00023-X
Keywords: TMS, neurophysiology, aerobic exercise, corticospinal excitability, SICI
Citation: Turco CV and Nelson AJ (2021) Transcranial Magnetic Stimulation to Assess Exercise-Induced Neuroplasticity. Front. Neuroergon. 2:679033. doi: 10.3389/fnrgo.2021.679033
Received: 10 March 2021; Accepted: 06 May 2021;
Published: 31 May 2021.
Edited by:
Wei-Peng Teo, Nanyang Technological University, SingaporeReviewed by:
Jason L. Neva, University of Montreal, CanadaAndrew Philip Lavender, Federation University Australia, Australia
Copyright © 2021 Turco and Nelson. This is an open-access article distributed under the terms of the Creative Commons Attribution License (CC BY). The use, distribution or reproduction in other forums is permitted, provided the original author(s) and the copyright owner(s) are credited and that the original publication in this journal is cited, in accordance with accepted academic practice. No use, distribution or reproduction is permitted which does not comply with these terms.
*Correspondence: Aimee J. Nelson, nelsonaj@mcmaster.ca