- 1Department of Pharmacology and Clinical Pharmacology, Belgorod State National Research University, Belgorod, Russia
- 2Department of Human Anatomy and Histology, I.M. Sechenov First Moscow State Medical University (Sechenov University), Moscow, Russia
- 3Pathophysiology Department, Kursk State Medical University, Kursk, Russia
- 4Research Institute of General Pathology, Kursk State Medical University, Kursk, Russia
- 5Research Institute of Experimental Medicine, Kursk State Medical University, Kursk, Russia
- 6Laboratory of Public Health Indicators Analysis and Health Digitalization, Moscow Institute of Physics and Technology, Dolgoprudny, Russia
- 7Scientific Center of Biomedical Technologies, Federal Medical and Biological Agency of Russia, Moscow, Russia
- 8Laboratory of Genomic Research, Research Institute for Genetic and Molecular Epidemiology, Kursk State Medical University, Kursk, Russia
- 9Department of Biology, Medical Genetics and Ecology, Kursk State Medical University, Kursk, Russia
As many proteins prioritize functionality over constancy of structure, a proteome is the shortest stave in the Liebig's barrel of cell sustainability. In this regard, both prokaryotes and eukaryotes possess abundant machinery supporting the quality of the proteome in healthy and stressful conditions. This machinery, namely chaperones, assists in folding, refolding, and the utilization of client proteins. The functions of chaperones are especially important for brain cells, which are highly sophisticated in terms of structural and functional organization. Molecular chaperones are known to exert beneficial effects in many brain diseases including one of the most threatening and widespread brain pathologies, ischemic stroke. However, whether and how they exert the antioxidant defense in stroke remains unclear. Herein, we discuss the chaperones shown to fight oxidative stress and the mechanisms of their antioxidant action. In ischemic stroke, during intense production of free radicals, molecular chaperones preserve the proteome by interacting with oxidized proteins, regulating imbalanced mitochondrial function, and directly fighting oxidative stress. For instance, cells recruit Hsp60 and Hsp70 to provide proper folding of newly synthesized proteins—these factors are required for early ischemic response and to refold damaged polypeptides. Additionally, Hsp70 upregulates some dedicated antioxidant pathways such as FOXO3 signaling. Small HSPs decrease oxidative stress via attenuation of mitochondrial function through their involvement in the regulation of Nrf- (Hsp22), Akt and Hippo (Hsp27) signaling pathways as well as mitophagy (Hsp27, Hsp22). A similar function has also been proposed for the Sigma-1 receptor, contributing to the regulation of mitochondrial function. Some chaperones can prevent excessive formation of reactive oxygen species whereas Hsp90 is suggested to be responsible for pro-oxidant effects in ischemic stroke. Finally, heat-resistant obscure proteins (Hero) are able to shield client proteins, thus preventing their possible over oxidation.
1 Introduction
The global burden inflicted by ischemic stroke is dramatic: the annual number of new cases [7,630,803 in 2019 according to the World Stroke Organization (Feigin et al., 2022)] is close to the population of Hong Kong. Similarly, the number of living people who have had at least one ischemic stroke for the mentioned year [77,192,498 (Feigin et al., 2022)] approaches the population of Germany.
Since an acute occlusion of large blood vessels is an unlikely event before the end of the peak of the reproductive period (Putaala et al., 2009), natural selection is mostly “blind” to ischemic stroke and organisms have yet to evolve reliable strategies to fight this disease. However, brain cells recruit essential evolutionarily conservative mechanisms in ischemia to respond to a severe shortage of oxygen (Leu et al., 2019). First, these mechanisms imply a quick switch to anaerobic pathways of ATP production and a decrease in energy demand. At the same time, the cell engages systems preserving structural integrity threatened by the accumulation of H+, Ca2+, and excessive production of free radicals (Lee et al., 2000).
Since the proteome is the key driver of every cellular process (including cell repair), one of the primary tasks during ischemia is to maintain the integrity of synthesized proteins as far as possible. Molecular assistants contributing to maintaining the integrity of proteins both in physiological and pathological conditions are called chaperones. According to current knowledge, the chaperome, a general term to define the unique steady-state composition of chaperones and their regulators in a given cell type (Wang et al., 2006b), comprises more than 300 proteins (Brehme et al., 2014), forming several rich families and superfamilies according to some of their members' features and functions. The most studied of them, heat shock proteins (HSPs), have a long history from their discovery in the 1960s to the first clinical trials of HSP-regulating drugs in the early 2010s (Porter et al., 2010) and further (Venediktov et al., 2023).
Although frequently discussed as such, many chaperones are not classified as HSPs. For instance, calnexin (Hammond et al., 1994) or the recently discovered family of Hero proteins (Tsuboyama et al., 2020) do not belong to HSPs. Moreover, classically, chaperones are considered to provide homeostasis of the proteome exclusively. However, some authors use the term “lipid chaperones” to refer to molecules selectively assisting in lipid trafficking (Furuhashi et al., 2011). Similarly, a group of metallochaperones exists facilitating intracellular transport of metal ions to other proteins (Rosenzweig, 2002).
However, by virtue of an immense diversity of various molecules called chaperones we will focus only on the “original” chaperones: those that have multiple protein substrates (Soti et al., 2005). In this review, we summarize their roles in the inner defense of the proteome against oxidative stress during ischemic stroke, focusing on the folding and refolding of substrates and the regulation of free radical production. We also outline the rationale, approaches and difficulties of chaperone-based therapy of ischemic stroke, considering their benefit-risk ratio and efficiency in reperfusion phase.
2 Outline of cellular damage during ischemic stroke
A hypoxic cell produces excessive amounts of lactate, which decreases pH. In order to decrease acidosis, a cell facilitates Na+/H+ exchange resulting in Na+-overload. According to the coupled exchanger theory (Allen and Xiao, 2003), excessive levels of Na+, in turn, decrease the driving force for Ca2+ efflux (O'Donnell and Bickler, 1994; Maulik et al., 2002). The latter is promoted by acute deficiency of nucleoside triphosphates impairing the continuous functioning of ion pumps, which regulate Ca2+ levels. The imbalanced concentration of cations causes neuronal depolarization and the release of glutamate, which cannot be taken up by hypoxic astrocytes and further aggravates Ca2+ influx. Ca2+ is known to stimulate the tricarboxylic acid (TCA) cycle and oxidative phosphorylation (McCormack and Denton, 1993; Groten and MacVicar, 2022). Accumulation of Ca2+ provokes cell damage by several mechanisms including activation of proteases and mitochondrial impairment with enhanced metabolic flux and the opening of mitochondrial permeability transition pore (mPTP) (O-Uchi et al., 2014; Tajeddine, 2016).
Although it may seem paradoxical and there have been long-lasting debates about it, hypoxia stimulates a dramatic increase of reactive oxygen species (ROS) production (Hernansanz-Agustín et al., 2014). Under physiological conditions, as much as 0.2%−2% of the electrons leak out of the ETC and interact with oxygen to produce superoxide () or hydrogen peroxide (H2O2) (Turrens, 2003; Cadenas and Davies, 2000; Zhao et al., 2019). This leakage gives rise to some basic level of ROS which is considered to play an important role in healthy cell signaling (Cobley et al., 2018). During acute hypoxia, precise functioning of ETC is imbalanced leading to oxidative stress (Hernansanz-Agustín et al., 2014). A total of 11 sites of ROS generation have been found in mammalian cells (Brand, 2016) but of those complexes I is suggested to be the main source of ROS during acute ischemia (Hernansanz-Agustín et al., 2017).
Thus, Ca2+-induced enhancement of TCA and oxidative phosphorylation rate in the absence of O2 favors electron leakage from the respiratory chain, generating superoxide and hydrogen peroxide (Semenza, 2011; Yan et al., 2006). Superoxide, in turn, can be dismutated into hydrogen peroxide with further breakdown by catalases and peroxidases or transformation into hydroxyl radicals (•OH). Not only are H2O2 and •OH highly toxic themselves but they also launch a chain reaction producing lipid peroxides (Gaschler and Stockwell, 2017), hypochlorous acid (HOCl) (Bushueva et al., 2015; Winterbourn et al., 2016), and reactive nitrogen by uncoupling NO synthase (Toma et al., 2021).
Especially important source of ROS in ischemic stroke is the NADPH oxidase (NOX) enzyme family. NOX enzymes are integral to the ETC in the plasma membrane and are widely distributed throughout brain tissue (Zhang et al., 2016). These enzymes generate free radicals by transferring electrons to molecular oxygen, producing a range of secondary reactive species (Vermot et al., 2021). This process plays a crucial role in energy homeostasis and ROS-dependent cellular signaling under both normal and pathological conditions (Brown and Griendling, 2009). In ischemic stroke models, several pharmacological and reverse genetic studies have confirmed that NOX enzymes contribute to the aggravation of the pathology (Zhang et al., 2016).
Additionally, ischemia leads to the increased production of nitric oxide (NO) from endothelial nitric oxide synthase (eNOS), which under normal conditions helps maintain vascular tone and neuroprotection. However, during ischemia, increased NO production can interact with ROS, specifically superoxide, to form peroxynitrite (ONOO−), a highly reactive nitrogen species that causes significant oxidative and nitrative damage to proteins, lipids, and DNA.
When overproduced, free radicals inflict oxidative damage to proteins as well as lipids and DNA (Halliwell and Gutteridge, 2015; Reichmann et al., 2018; Caldeira et al., 2014). This oxidative damage causes oxidative modifications of proteins such as cysteine, methionine or tyrosine oxidation, lysine glycoxidation (Kehm et al., 2021), S-nitrosation (Finelli, 2020), self-crosslinking of proteins (Li et al., 2013) (Figure 1), and crosslinking of proteins to DNA (Groehler et al., 2018).
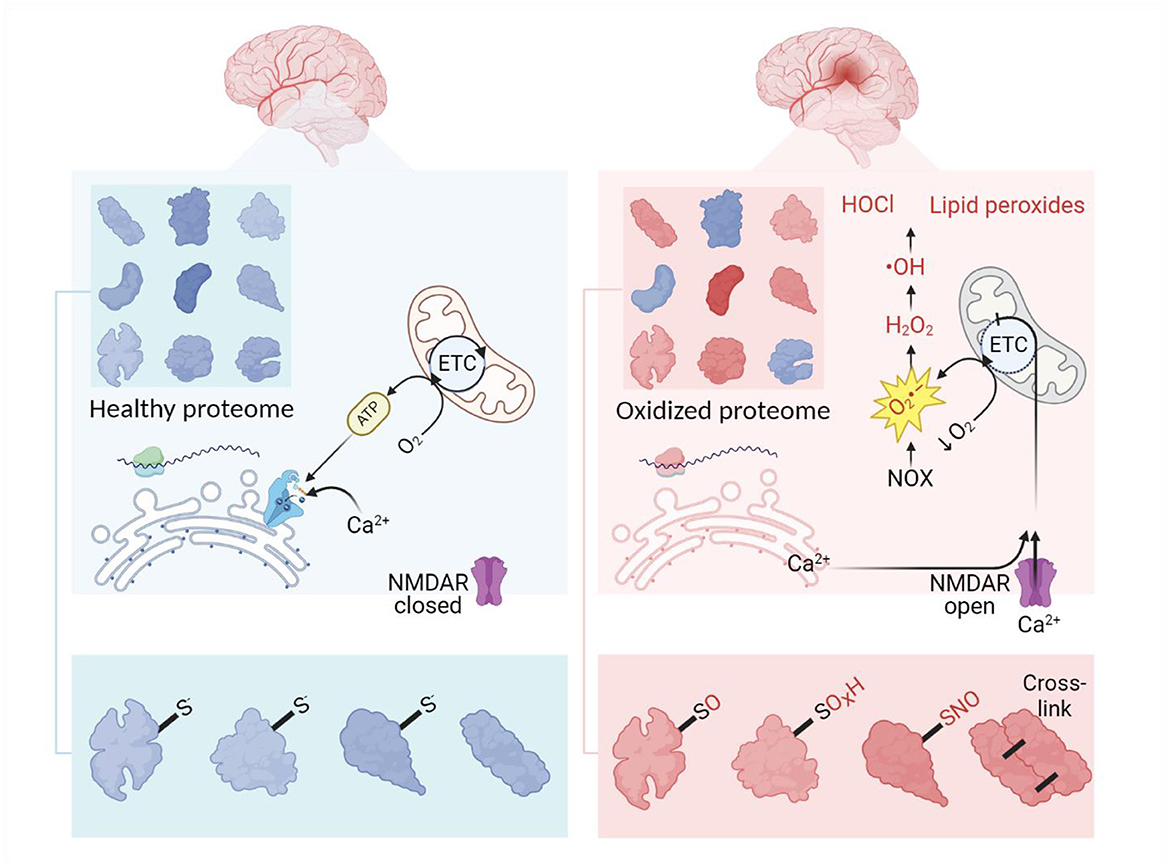
Figure 1. Outlines of oxidative stress and proteome overoxidation in ischemic stroke. Normally, the healthy proteome is supported by balanced translation, modification, and utilization of proteins in homeostatic conditions. At the site of ischemia, a decrease in oxygen provokes mitochondria to release excessive superoxide launching the cascade of ROS production. This process is further enhanced by imbalanced Ca2+ as a result of ATP depletion, glutamate excitotoxicity, and increased levels of protons. Developing oxidative stress leads to overoxidation of the proteome, thus complicating the functioning of the proteins. Oxidative modifications of the proteome usually imply the addition of O, OH, O2H, O3H and NO groups to sulfur-containing amino residues. ETC, electron transport chain. Created in https://BioRender.com.
In acute ischemia damaged proteins cannot be recovered by protein turnover and gene expression regulation because the protein translation capacity is limited, the phenomenon called translation arrest (Martín de la Vega et al., 2001). As well as other cell types, facing critical level of proteome oxidation, neurons seek to avoid necrosis inevitably followed by subsequent alteration of neighboring cells (Buttke and Sandstrom, 1994; Reichmann et al., 2018). Hypoxia is known to provoke neuronal apoptosis (Banasiak et al., 2000), ferroptosis (Lan et al., 2020; Yuan et al., 2023; Sanguigno et al., 2023), and autophagy-mediated neuronal death (Shi et al., 2012; Ginet et al., 2014). However, the fact that neurons are implicated in topologically sophisticated circuits and networks makes programmed cell death a barely acceptable scenario. In this regard, one of the most important adaptations is to mobilize antioxidant defenses such as the glutathione system, ROS scavenging (interception of reactive species prior to their reaction with cellular components) (Polonikov et al., 2012, 2022), and antioxidant enzymes (Bushueva et al., 2021; Vialykh et al., 2012), breaking down free radicals.
Cells have evolved several key molecular pathways orchestrating the oxidative stress response. The most important of them rely on Nuclear factor erythroid 2-related factors 1 and 2 (Nrf1 and Nrf2) as well as Forkhead box O (FOXO) transcription factors. While Nrf1 is primarily involved in maintaining cellular homeostasis under basal non-stress conditions (Hu et al., 2022a), Nrf2 is a major regulator of the cellular antioxidant response and activates one of the most important molecular cascades orchestrating antioxidative response in the ischemic brain (Wang et al., 2022b). It induces the expression of a wide range of antioxidant and detoxifying enzymes, such as heme oxygenase-1 (HO-1), glutathione S-transferases, and NAD(P)H oxidoreductase 1 (NQO1) (He et al., 2020). In turn, FOXO transcription factors, such as FOXO3, are also activated under oxidative stress conditions and promote the expression of antioxidant enzymes like superoxide dismutase (SOD) and catalase, DNA repair enzymes, and autophagy-related genes (Morris et al., 2015). This helps to mitigate oxidative damage, repair DNA, and remove damaged proteins or organelles. FOXO3 was shown to exert numerous beneficial effects in stroke (Omorou et al., 2023). Notably, both Nrf2 and FOXO3 are regulated by YAP and TAZ, the components of the Hippo signaling pathway which plays a crucial role in regulating cellular responses to stress, including oxidative stress. It primarily functions to control cell growth, survival, and apoptosis, and its activity can influence how cells respond to oxidative damage (Amanda et al., 2024).
However, the capacity of endogenous antioxidants is limited, and in critical ischemia, a majority of proteins are subjected to chemical stress. At this point cells engage molecular chaperones which exhibit a critical role in protecting proteins from excessive oxidation and aiding in the recovery of damaged proteins during ischemia, thereby helping to preserve cell viability.
3 Chaperones in ATP deficiency during ischemic stroke
During ischemic stroke, the loss of blood supply leads to a dramatic reduction in ATP levels. In the ischemic core, ATP is often completely depleted, resulting in inevitable cell death. In the surrounding penumbra, ATP levels are reduced to approximately 30%−50% of normal levels (Liu et al., 2010a; Salaudeen et al., 2024). In these peripheral areas, ATP depletion does not reach critical thresholds, allowing cells to maintain basic functions despite the oxidative stress and other damaging factors they face.
To protect the proteome under low-energy conditions, cells activate chaperones. Although the thermodynamic imbalance worsens under ATP deficiency, cells prioritize the use of available ATP to maintain chaperone function. Chaperones, such as Hsp100, Hsp90, Hsp70, and Hsp60, act as foldases (ATP-dependent enzymes that function according to Michaelis-Menten kinetics) to assist in protein folding and prevent aggregation (Goloubinoff et al., 2018). Foldases are believed to offer a broad range of protective functions, particularly under stress conditions. However, under acutely compromised metabolic states, holdases (ATP-independent chaperones) take over. These holdases, including Hsp27, α-crystallin, and Hero, protect client proteins by non-covalently binding exposed hydrophobic regions, thus preventing improper interactions and aggregation with other proteins (Suss and Reichmann, 2015; Voth and Jakob, 2017; Mitra et al., 2022).
Taking together, one may assume that ATP-independent chaperones such as Hsp27, α-crystallin, and Hero are likely to play a more crucial role in areas of severe ATP depletion and during the acute phase of ischemic stroke. In contrast, ATP-dependent chaperones are essential in regions with less severe ATP loss and are critical for recovery in the later stages of stroke (Figure 2).
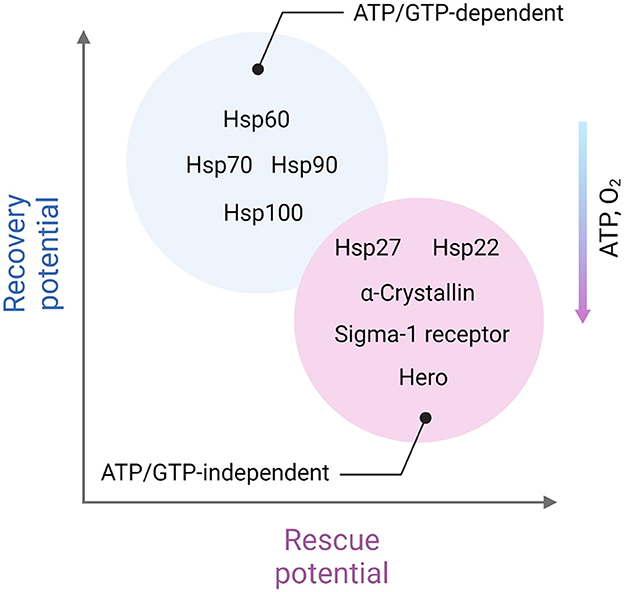
Figure 2. Distinct role of ATP-dependent and ATP-independent chaperones in ischemic stroke. ATP-independent chaperones, including Hsp22, Hsp27, α-crystallin, Sigma-1 receptor, and Hero, are especially vital in areas experiencing severe ATP depletion, closer to the ischemic core and in the acute phase of stroke. These chaperones function without the need for energy input from ATP, making them well-suited to stabilize and protect cellular proteins under energy-deficient conditions. They help prevent protein aggregation by binding to exposed hydrophobic regions of partially unfolded proteins, minimizing cellular damage when energy resources are critically low. On the other hand, ATP-dependent chaperones, which require ATP to assist in protein folding and repair, play a more prominent role in regions with moderate ATP depletion. These chaperones, such as Hsp60, Hsp70, Hsp90, and Hsp100 are particularly important during the recovery phases following the initial stroke event. As ATP levels gradually restore, ATP-dependent chaperones can actively refold and repair damaged proteins, contributing to cellular recovery and enhancing resilience in the affected areas. This complementary activity of ATP-independent and ATP-dependent chaperones reflects a dynamic, phase-specific chaperone response aimed at protecting and restoring the proteome in different stages of stroke-induced cellular stress. Created in https://BioRender.com.
4 Chaperones against overoxidation
Proteins appear to be significantly more susceptible to oxidative damage in their unfolded states than in their native states, explained by the more open position of side chains (Rollins and Dill, 2014; Zou et al., 2014; Santra et al., 2018; Klyosova and Yu, 2022). Despite the low number of polysomes and many mRNAs being actively translated by just a single ribosome, neurons still have a fairly high translational load, with many mRNAs disseminated throughout different cellular compartments (Biever et al., 2020). Even under the ischemia-induced translation arrest, the cell continues the translation of proteins that constitute the “stress response module” (including chaperones) (Niforou et al., 2014; DeGracia, 2017). For instance, it was shown that nearly half of the detected proteome was altered following stroke. In a murine model of ischemic stroke, affected regions were characterized by dramatically changed proteome patterns, and this response appeared in the early stages of the pathology (Gu et al., 2021). Of those upregulated proteins the chaperones can be detected in the very early stages after the beginning of ischemia (Sun et al., 2015). Moreover, some chaperones may contribute to these reactive changes of the proteome (Guo et al., 2018).
Interestingly, apart from its function in suppressing oxidative damage, many chaperones have been shown to sense overoxidation (Kumar et al., 2021). The latter is due to reversible modifications in redox-sensitive amino acid side chains (e.g. Cys, Met, and His) or metal centers (Kumsta and Jakob, 2009; Ulrich et al., 2021).
During oxidative stress, chaperones also eliminate irreversibly damaged proteins to protect the cell from abnormal polypeptides and allow reassembly of multiprotein complexes (Kiffin et al., 2004). For instance, chaperones are recruited to release oxidized subunits from ribosomes allowing them to be replaced with undamaged (unoxidized) ones (Yang et al., 2023). Oxidative stress stimulates chaperone-mediated autophagy (CMA) by upregulating lysosomal-associated membrane protein 2 (LAMP2A) and heat shock 70 kDa protein 8 (also designated Hsc70)—the CMA effectors—and also makes proteins more easily degradable by the CMA pathway via oxidative modifications (Le et al., 2022; Stricher et al., 2013). CMA is a mechanism known to rescue proteostasis imbalanced by oxidative stress in aging (Zhang and Cuervo, 2008; Schneider et al., 2015). Moreover, it has been reported that via degrading Kelch-like ECH-associated protein 1 (Keap1) CMA activates Nrf2 pathway (Zhang et al., 2004; Zhu et al., 2022b).
Additional important function of CMA is regulation of mitophagy and mitochondrial fragmentation (Nie et al., 2021), thus lowering ROS (Lei et al., 2021). There is another chaperone assisted molecular mechanism of scavenging the damaged proteins, known as Chaperone-assisted selective autophagy (CASA). CASA refers to a broader class of chaperone-mediated pathways that involve the selective targeting of specific protein aggregates or misfolded proteins to autophagosomes for degradation. This involves the classical autophagy machinery. Whereas CMA is primarily dependent on the Hsc70 and its cochaperone Hsp40, CASA involves Hsp70 and Hsp90 (Tedesco et al., 2023).
Mitochondrial chaperones have also been shown to be essential in supporting the stability of electron transport chain components and mitochondrial structure (Herrmann et al., 1994; Bahr et al., 2022; Vishwanathan and D'Silva, 2022; Adriaenssens et al., 2023). During oxidative stress, some chaperones decrease the activity of the complexes II and IV (Sciacovelli et al., 2013; Yoshida et al., 2013; Guzzo et al., 2014) reducing electron leakage and antagonizing the opening of mPTP (Penna et al., 2018).
5 Heat shock proteins
HSPs were first discovered in the early 1960s as the factors upregulated in Drosophila melanogaster salivary glands after exposure to heat, hence their name. Subsequently, according to their molecular weight, key HSP actors were divided into five major classes: Hsp60, Hsp70, Hsp90, Hsp110, and the small Hsp (Lindquist and Craig, 1988; Macario, 2007). The primary function of HSPs is assistance in the folding, assembling, and addressing of newly synthesized proteins and in refolding (Gupta et al., 2020) or degradation (Fernández-Fernández et al., 2017) of damaged ones.
Different classes of HSPs utilize various strategies to interact with target proteins and regulate their structural changes and assembly. ATP-independent HSPs are holdases or kinetic traps to bind a substrate or an intermediate agent, thus facilitating protein folding and preventing protein aggregation (Mitra et al., 2022). ATP-dependent HSPs, on the other hand, utilize sophisticated dimensional properties—such as the barrel-like Anfinsen cage structure of Hsp60 for sequestered folding of target proteins or modular clamps of Hsp70 and small Hsp—to protect hydrophobic structures in their targets (Bascos and Landry, 2019).
Under normal conditions, HSPs make up an enormous 5%−10% of the total cellular protein content (Hartl et al., 2011). Perhaps unsurprisingly, no single cellular process avoids these master regulators of proteostasis (Tucker et al., 2009; Kashyap et al., 2014). Apart from their reparative machinery, they regulate various molecular pathways of signaling transduction (Silveira et al., 2023). A large body of evidence shows that HSPs are important links contributing to the risk (Xu et al., 2012), course, and outcomes of ischemic stroke (Mohammadi et al., 2018). For example, in the foci of ischemia, Hsp70 is markedly upregulated (Sharp et al., 2000), and an artificial increase in its expression was shown to significantly reduce ischemic damage (Giffard and Yenari, 2004; Demyanenko et al., 2021). Moreover, the autoimmune response against HSPs, found in patients after single or repeated stroke, has been proposed as a link contributing to atherosclerosis plaque formation in cerebral arteries (Banecka-Majkutewicz et al., 2014; Yakovenko et al., 2015). Triggering an immune response against HSPs present on the vascular endothelium may lead to endothelial damage, potentially contributing to the formation of atherosclerotic plaques. This immune response is believed to be induced by cross-reactivity between human and bacterial HSPs following exposure to bacterial infections (Banecka-Majkutewicz et al., 2014).
In their excellent review, Szyller and Bil-Lula (2021) summarize the immense role of HSPs in oxidative and nitrosative stress during ischemia and reperfusion injury mostly in the focus of cardiology (Szyller and Bil-Lula, 2021). Hereinafter we provide a brief description of the main biochemical properties of HSPs (Table 1) and discuss these chaperones with a precise focus on brain ischemic injury.
5.1 Hsp70
70-kDa heat shock proteins (Hsp70s) subfamily is abundant and ubiquitous molecular chaperones involved in multiple processes such as assistance in the assembly, folding, refolding as well as membrane translocation of client proteins, and control of substrate degradation and activity of various downstream pathways (Mayer and Bukau, 2005; Fernández-Fernández et al., 2017). Hsp70s are ATP-dependent chaperones and require the cooperation with cochaperones and members of other subfamilies of HSPs (e.g., Hsp40, Hsp100, Hsp90) to perform some of the listed functions. The mechanisms by which Hsp70s assist nascent polypeptides folding and damaged/misfolded proteins refolding apparently rely on transient association of their substrate binding domain with short hydrophobic peptide segments within their substrate proteins (Mayer and Bukau, 2005; Xu, 2018). Notably, Hsp70 interacts with a wide variety of client proteins without having exclusive substrates (Murphy, 2013).
The Hsp70 subfamily occupies a central position in cellular proteostasis (Fernández-Fernández et al., 2017) and neuroprotection (Yenari et al., 1999; Bobkova et al., 2014, 2015; Evgen'ev et al., 2017, 2019; Zatsepina et al., 2021; Belenichev et al., 2023; Demyanenko et al., 2024). Some of Hsp70 polymorphisms were described as preventive in ischemic stroke in humans (Kobzeva et al., 2023), probably due to the reduction of thrombosis (Allende et al., 2016). In various rodent models, Hsp70 upregulation has been repeatedly reported to have attenuative effects on stroke (Kim et al., 2018, 2020; Wang et al., 2019; Chi et al., 2014a,b). Moreover, many studies have shown a reduction of oxidative stress to be an important component of its action (Guo et al., 2018; Kesaraju et al., 2014). For example, Hsp70 family members have been suggested to suppress ROS-induced apoptosis. The suppression may be mediated by the inactivation of pro-apoptotic enzymes (Venediktov et al., 2023) or via the downregulation of positive regulator of apoptosis STI1 (da Fonseca et al., 2021; Beraldo et al., 2013).
Plasma exosomes packed with Hsp70 were shown to protect cells against ischemia-reperfusion injury via suppression of ROS and upregulation of SOD (Jiang et al., 2020). Some studies suggest that Hsp70 is able to directly upregulate transcriptional factors mediating antioxidative defense. For example, Guo and colleagues reported that, during stroke, Hsp70 upregulates FOXO3 (Guo et al., 2018). It is known that FOXO3 activation exert numerous beneficial effects in stroke including that FOXO3 signaling pathway activation inhibits oxidative stress-mediated cell death through activation of autophagy (Deng et al., 2023). However, Vinokurov and colleagues have reported that in in vivo model of Parkinson's disease induced by the complex 1 uncoupling agent rotenone, despite on protecting neurons and astrocytes against cell death, exogenous Hsp70 upregulated ROS production (Vinokurov et al., 2024). This finding may be related to partial stimulation of the innate immunity response by virtue of use a non-exosome packed form of Hsp70. Even though, the authors reported successful penetration of Hsp70 into the cells and its distribution in mitochondria, one may expect that some fraction of Hsp70 activated the innate immune response cascades (see below in the section HSPs and Glia).
Some of the Hsp70 family members are integrated in mitochondria and contribute to mitochondrial quality control system. For example, mitochondrial Hsp70 (mortalin) performs two specific roles: as both a chaperone and stress-survival factor, it assists in protein quality control by (re)folding or degrading non-functional proteins as well as in controlling the mitochondrial fragmentation and inflammatory response (Havalová et al., 2021; Zhao et al., 2022) (Figure 3). It has been shown that Mortalin-knockdown murine preadipocyte cells display mitochondrial dysfunction, increased mitochondrial fragmentation, cytosolic mtDNA release and proinflammatory response. Mortalin overexpression increased cell viability, decreased ROS production, preserved mitochondrial membrane potential and ATP levels in primary astrocytes deprived of glucose or oxygen/glucose (Voloboueva et al., 2008). However, Wen et al. have reported that in ischemic brain injury, it was rather the inhibition of mortalin that led to beneficial effects. In their study, inhibition of mortalin was able to effectively ameliorate mitochondrial calcium overload and preserve mitochondrial function in both in vivo and in vitro stroke models (Wen et al., 2022). Future studies should address the specific roles of mortalin in the regulation of oxidative stress and cells' viability in ischemic stroke.
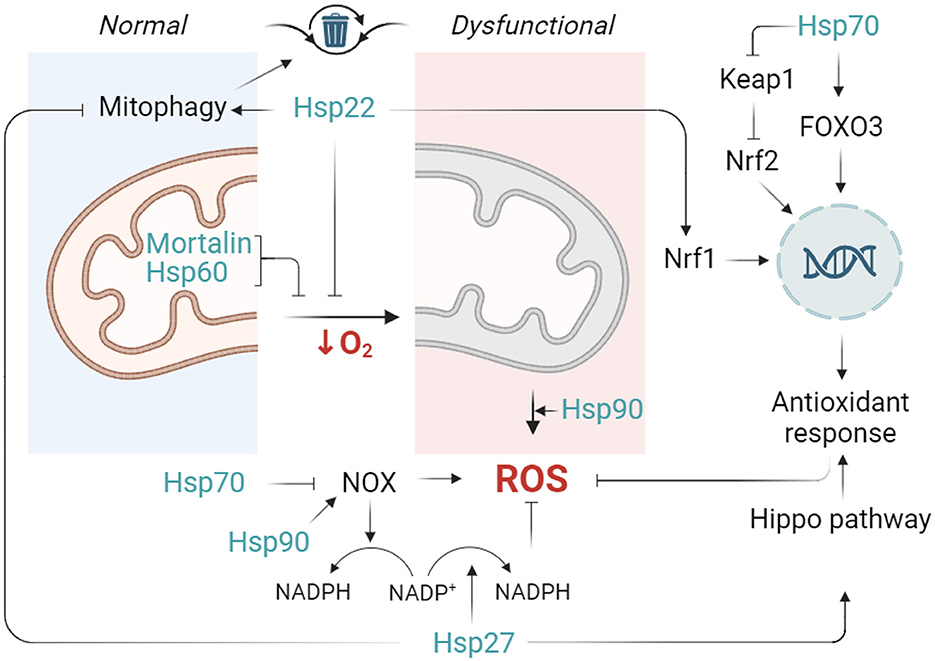
Figure 3. Outlines of the contribution of HSPs to controlling oxidative stress during ischemia. Normal functioning of mitochondria implies moderate leakage of electrons and production of reactive oxygen species (ROS). However, facing a lack of oxygen, mitochondria produce excessive ROS and enter a dysfunctional state. In this scenario, Hsp22 limits mitochondrial activity, thus preventing ROS production. In parallel, mitochondrial Hsp70 (mortalin) and mitochondrial Hsp60 attenuate mitochondrial dysfunction via their chaperone-dependent protection of mitochondrial integrity and ETC. Hsp70 stimulates FOXO3 transcription factor activates Nuclear factor erythroid 2-related factor 2 (Nrf2) pathway and inhibits NOX activity while Hsp22 activates Nuclear factor erythroid 2-related factor 1 (Nrf1). Nrf1 and Nrf2 are both transcription factors known for their roles in regulating the expression of antioxidant and detoxifying enzymes Finally, Hsp27 is able to launch a cellular antioxidant response (such as an increase in superoxide dismutase and glutathione production) through Hippo pathway modulation (and probably other not yet revealed mechanisms), whereas Hsp90 appears to favor ROS production (partly through NOX enzymes activity upregulation). Created in https://BioRender.com.
5.2 Hsp90
Hsp90s are five ubiquitous molecular chaperones (HSPC1-5, Kampinga et al., 2009) exerting a wide range of processes which, apart from assistance in protein maturation, degradation, and trafficking, include DNA repair, cell cycle control, cell survival, hormone, and other signaling pathways (Jackson, 2012). Hsp90 has multiple “clients” among which kinases, transcription factors, steroid hormone receptors, and E3 ubiquitin ligases (Schopf et al., 2017). Hsp90 perform their folding activity through a complex process called Hsp90 chaperone cycle where they closely interact with other chaperones. For example, while executing folding, Hsp90 binds the client proteins harbored by the Hsp70/Hsp40 protein complex to start its action (Murphy et al., 2001).
To simplify, in stroke, Hsp90 may be considered to display cellular activity opposite to Hsp70. In this regard, Hsp90 is suggested to exert harmful effects in cerebral ischemic injury (Qi et al., 2015). For example, Hsp90 has been shown to facilitate the pathways of cellular death caused by oxidative damage (Selim and Ratan, 2004) and to activate Acyl-CoA synthetase long-chain family member 4 (ACSL4)—the factor permitting ferroptosis—driven by accumulation of lipid hydroperoxides in ischemic stroke (Miao et al., 2022). In oxidative stress, Hsp90 also promotes the activity of RIP-1 kinase, the main regulator of necroptosis. Notably, the RIP-1-induced cascade additionally stimulates ROS formation, thereby generating a vicious circle of Hsp90-mediated oxidative stress (Zhou et al., 2021). However, another report provided by Wang et al. showed that bardoxolone derivatives drive Hsp90 to perform dephosphorylation of RIP-1 kinase while avoiding necroptosis (Wang et al., 2021a). Noteworthy, in contrast to its stimulative effect on ferroptosis and necroptosis, Hsp90 is a negative regulator of apoptosis. Binding proapoptotic factors Apaf-1, Ask-1, Akt, AIF, and endonuclease G, Hsp90 prevents apoptosis (Sumi and Ghosh, 2022).
In human cerebral microvascular endothelial cells (hCMEC/D3), inhibitors of Hsp90 reduced hydrogen-peroxide-induced ROS generation, suggesting Hsp90 increases free radical production (Uddin et al., 2021). Moreover, Hsp90 up-regulates the functioning of NOX enzymes in ischemic stroke, thereby enhancing generation of ROS (Zhang et al., 2021). For instance, pharmacological and genetic inhibition of Hsp90 directly reduced Nox5-derived superoxide without secondarily affecting downstream signaling events (Chen et al., 2011). Interestingly, Hsp70 and Hsp90 have opposing effects on NOX regulation: while Hsp90 binds to NOX and regulates its stability, these actions are counteracted by Hsp70 (Chen et al., 2012).
Additionally, Hsp90 has been shown to directly interact with Na+/H+ exchanger (NHE)1 (Odunewu-Aderibigbe and Fliegel, 2017a). (NHE)1 is one of the most known triggers of oxidative damage in the cells during hypoxia. Reducing intracellular acidification, it leads to an increase of Na+, followed by activation of Na+/Ca2+ exchanger and subsequent Ca2+ overload. As discussed in Section 2, decompensated increase of Ca2+ leads to oxidative stress. The growing body of evidence shows that NHE1 is deleterious in stroke (Li et al., 2019; Metwally et al., 2023). At the same time, the Hsp90 inhibitor 17-AAG decreased NHE1 activity and NHE1 phosphorylation, suggesting that Hsp90 is a positive regulator of NHE1 (Odunewu-Aderibigbe and Fliegel, 2017b).
Finally, Hsp90 plays a major role in the activation of iNOS and eNOS (Luo et al., 2011; Förstermann and Sessa, 2012), which likely contributes to the aggravation of nitrosative stress in stroke. Taken together, while Hsp90 appears to be a driver of stroke pathology, its anti-apoptotic function and crucial role in orchestrating CASA suggest that it may also exert beneficial effects during stroke recovery.
5.3 Hsp60
Hsp60, also referred to as chaperonin 60 (Cpn60), is a foldase typically functioning inside mitochondria together with its co-chaperone Hsp10 to maintain protein homeostasis in ATP- or GTP-dependent manner (Okamoto et al., 2017; Caruso Bavisotto et al., 2020). Apart from its canonical functions in mitochondria, Hsp60 participates in various processes within different cellular compartments being involved in inflammation, cell replication, and other events in health and disease (Macario and Conway de Macario, 2005). For instance, inside mitochondria Hsp60 assists the folding and trafficking of other proteins and prevents mitochondrial protein degradation, but in the cytosol, it can regulate apoptosis (Marino Gammazza et al., 2017; Chaudhuri (Chattopadhyay) and Rashid, 2019).
Hsp60 is considered one of the most important chaperones in sustaining oxidative stress and preserving mitochondrial integrity (Singh et al., 2024). It has been reported to stay upregulated for hours after acute cerebral ischemia (Izaki et al., 2001), but approximately 12–24 h after stroke onset, Hsp60 levels decrease (Kim and Lee, 2007). These temporal dynamics of Hsp60 are suggested to be related to its downregulation by rising iNOS/NO/STAT3, one of the crucial pathways regulating hypoxic response (Kim and Lee, 2007). In hypoxic brain, the role of Hsp60 appears controversial. On the one hand, Hsp60 is beneficial for protein refolding after the harmful impact of ROS. For instance, Cabiscol and colleagues showed that Hsp60 is upregulated in oxidative damage to provide the refolding of iron and sulfur-containing enzymes (Cabiscol et al., 2002). Sheng et al. revealed a negative correlation between the lesion volume and Hsp60 levels in the 1st h after the stroke (Sheng et al., 2011). Importantly, Hsp60 stabilizes respiratory complex I in ETC, and its silencing leads to an increase in ROS levels (Tang et al., 2016). On the other hand, it has been reported that in oxidative stress Hsp60 is responsible for mediating pro-inflammatory signals in astrocytes when it is recognized by innate immune receptors (Liyanagamage and Martinus, 2020). Notably, in vitro studies in the serum of ischemic stroke patients have also demonstrated that blocking of Hsp60 prevents inflammatory activation (Brea et al., 2011).
Interestingly, in our previous study, we observed an association between variations in HSPD1, which encodes the Hsp60 family member, and the risk of ischemic stroke, but this association was found exclusively in smokers. This finding further supports the role of Hsp60 in the molecular mechanisms regulating both hypoxia and oxidative stress (Stetskaya et al., 2024).
5.4 Hsp27
Hsp27 (HspB1) is constitutively present in cytosol and consists of the N-terminal domain, the α-crystallin domain, and the C-terminal domain. Its chaperone functions do not require ATP but take place due to formation of stable dimers that can further multimerize (Stetler et al., 2009). These multimers provide protein quality control by preventing the formation of protein aggregates, such as for actin filaments, and by directing oxidized proteins along the proteasomal degradation pathway (Arrigo, 2001). In addition, Hsp27 displays pronounced anti-apoptotic activity by downregulation of caspase-3 and caspase-9, the release of cytochrome c, and the inhibition of ASK1 (Stetler et al., 2009; Lanneau et al., 2007). Moreover, Hsp27 has been shown to downregulate the proapoptotic protein Bim binding to the 3′UTR of bim mRNA (Dávila et al., 2014).
Hsp27 belongs to chaperones exhibiting eminent neuroprotective activity in various neurological pathologies (Akbar et al., 2003; Shimura et al., 2004; Abisambra et al., 2010) including brain hypoxia and stroke (Stetler et al., 2008; Badin et al., 2006, 2009; Liu et al., 2010b; Tucker et al., 2011; Teramoto et al., 2013; Yu et al., 2013; Leak et al., 2013; Zhan et al., 2017; Shimada et al., 2018; Behdarvandy et al., 2020). Moreover, clinical data indicate marked dynamic changes of Hsp27 levels in patients with acute ischemic stroke (Gruden et al., 2013), suggesting its essential role in hypoxic injury.
Antioxidant role of Hsp27 was repeatedly demonstrated in various models (Vidyasagar et al., 2012; Lin et al., 2016; Önay Uçar et al., 2023). During oxidative stress, Hsp27 was shown to raise the intracellular concentration of the endogenous antioxidant glutathione (Mehlen et al., 1996). Additionally, Hsp27 knockdown reduced the expressions of SOD and catalase (Wang et al., 2022a). Little is known about molecular pathways mediating the upregulation of antioxidant factors by Hsp27. However, some studies suggest the putative role of Hsp27-induced Akt activation (Liu et al., 2007). Moreover, changes in glutathione content may be related to direct interaction between Hsp27 and the enzymes controlling glutathione exchange. Indeed, Lie and coll. demonstrated that Hsp27 overexpression promoted the formation of the complex between Hsp27 and oxidized peroxiredoxin 1 while activating glutathione reductase and thioredoxin reductase in H9c2 cells exposed to hydrogen peroxide (Liu et al., 2019). Additionally, in this study Hsp27 was shown to modulate the Hippo signaling pathway inducing dephosphorylation of MST1 (Liu et al., 2019).
Interestingly, Hsp27 has also been shown to be directly implemented in the regulation of metabolic control of oxidative stress in stroke. In their work on a stroke model in rats Imahori et al. (2017) reported that in hypoxia glucose 6-phosphate dehydrogenase activity in the pentose phosphate pathway (PPP) may be activated via Hsp27 phosphorylation by ATM-kinase. Moreover, pharmacological inactivation of Hsp27 phosphorylation significantly reduced the activity of PPP and resulted in 2-fold increase in infarct size 24-h after reperfusion following 90-min middle cerebral artery occlusion (Yamamoto et al., 2018). Given its essential role in the formation of NADPH, PPP is known as one of the core mechanisms downregulating the oxidative stress under hypoxia (Perl et al., 2011). Thus, direct crosstalk of Hsp27 with PPP indicates its substantial antioxidant role in reducing ischemic damage.
Additionally, one of the putative mechanisms for Hsp27-mediated neuroprotection is the recently discovered role of Hsp27 in suppressing lethal mitophagy by regulation of ceramide synthases and preventing ceramide accumulation in cells (Boyd et al., 2023).
5.5 Hsp22
Regardless of the high neuronal expression of small HSPs (Kirbach and Golenhofen, 2011; Bartelt-Kirbach et al., 2017), to date, few studies have described their exact role in the course and outcomes of ischemic brain injury. However, Hsp22 has been shown to directly correlate with the level of mitophagy in mice with middle cerebral artery occlusion and in murine N2A cell cultures subjected to oxygen-glucose deprivation or reoxygenation (Li et al., 2018) as well as in other ischemia models (Cheng et al., 2023). In terms of response to overoxidation, mitophagy is considered an important mechanism contributing to the suppression of oxidative stress (Shao et al., 2020). Increased Hsp22 expression also increased mitochondrial membrane potential and reduced oxidative stress in hippocampal cells of diabetes mellitus mice (Chang et al., 2022).
Hsp22, has been shown to prevent ischemic injury in vivo in gerbils and to attenuate oxidative-stress-induced hippocampal neuronal cell death through mitochondrial signaling (Jo et al., 2017). In a rat model of subarachnoid-hemorrhage-induced early brain injury, the exogenous Hsp22 maintained neurological function and reduced brain edema and mitochondrial apoptosis. Furthermore, these effects have been shown to be associated with Nrf1-induced mitochondrial biogenesis and reduction of oxidative stress (Fan et al., 2021).
Thus, the current advance portrays the small HSPs as important players in neuronal injury during stroke. Moreover, the mechanism underlying their contribution appears to be closely related to the regulation of mitochondrial function (Zhu et al., 2015; Fouché et al., 2023). The summarized contribution of HSPs in the regulation of oxidative stress is presented in Figure 3.
5.6 HSPs and glial cells
Glial cells, consisting of astrocytes (~20%−40%), microglia (~10%), and oligodendrocytes (~40%−60%), make up about half of the brain's cellular composition (Verkhratsky and Butt, 2018). Given its crucial biological role, glia is considered a dramatically important player in stroke (Jadhav et al., 2023). During the 1st min of stroke glial cells become activated for metabolic cooperation with neurons (Bonvento and Bolaños, 2021), redistribution of cerebral blood flow (Christie et al., 2023), clearance of tissue debris (Jia et al., 2022), and release of neuroprotective molecules (Xie and Liu, 2023), including HSPs (Guzhova et al., 2001; Taylor et al., 2007).
However, glial cells also act as drivers of inflammatory alteration followed by generation of free radicals. A big body of evidence shows that both astrocytes and microglia are rapidly and strongly activated after stroke, generating large amounts of ROS via mitochondrial and NOX pathways. In its turn, free radicals lead to the inflammatory activation of glia exacerbating tissue damage (Zhu et al., 2022a).
Interestingly, HSPs were reported to largely contribute to astrocytes activation in stroke (Barreto et al., 2012). However, heat shock response or Hsp70 alone have been suggested to suppress microglial/brain macrophage activation and astroglia-inducible nitric-oxide synthase expression by decreasing NF-kB activation (Feinstein et al., 1996; Heneka et al., 2000). Align with the last, Hsp70 has been shown to downregulate the expression of pro-inflammatory genes in hypoxia exposed murine astrocytes (Kim et al., 2015). Overexpression of Hsp70 in astrocytes also reduced oxidative stress and rescued glutathione levels in the cells exposed to hydrogen peroxide (Xu and Giffard, 1997).
In contrast, Hsp73 has been suggested to mediate NF-κB and NLRP3 inflammasome activation of astrocytes in ischemic injury (Mi et al., 2021). Similarly, Hsp90 can bind the NLRP3 inflammasome to stabilize its activity (Piippo et al., 2018) leading to NLRP3-induced microglia pyroptosis (Lin et al., 2024). Pyroptosis is a pro-inflammatory form of programmed cell death mostly characteristic for microbial infections (Cookson and Brennan, 2001) but also detected in other conditions including stroke (Chen et al., 2018).
Hsp60 as a ligand for TREM2 receptor on microglial plasma membrane mediates microglial release of pro-inflammatory cytokines, thereby enhancing oxidative stress in neurons (Stefano et al., 2009). Moreover, Hsp60 is required for phosphorylation and nuclear localization of NF-κB after stimulation by the pro-inflammatory cytokine IL-1β (Swaroop et al., 2018).
Notably, the biological effects of HSPs on inflammatory cascades markedly vary depending on their localization: extracellular or intracellular. Being predominantly intracellular molecules, secreted HSPs were suggested to act as damage-associated molecular patterns (DAMPs), “danger signals” activating the innate immune response cascades. Extracellular Hsp70 has been shown to display cytokine regulating activity (chaperokine) stimulating sterile inflammation and exaggerating already existing immune response by engaging TLR2 and TLR4 receptors (Asea et al., 2002; Vabulas et al., 2002a,b; Tsan and Gao, 2004; Hulina et al., 2018).
Later data have debated their role as DAMPs suggesting HSPs were rather “DAMPERs” (Chen et al., 2011; Chen and Nuñez, 2011; Broere et al., 2011; van Eden et al., 2012), but still, much evidence links extracellular Hsp70 with immune response (Zininga et al., 2018). Due to the antagonistic nature of Hsp70 according to its location, the ratio between extra- and intracellular Hsp70 fractions (Heck index) has been proposed as a marker of inflammatory status (Kim et al., 2015; Krause et al., 2015; Costa-Bebexr et al., 2022; Njemini et al., 2004) (Figure 4).
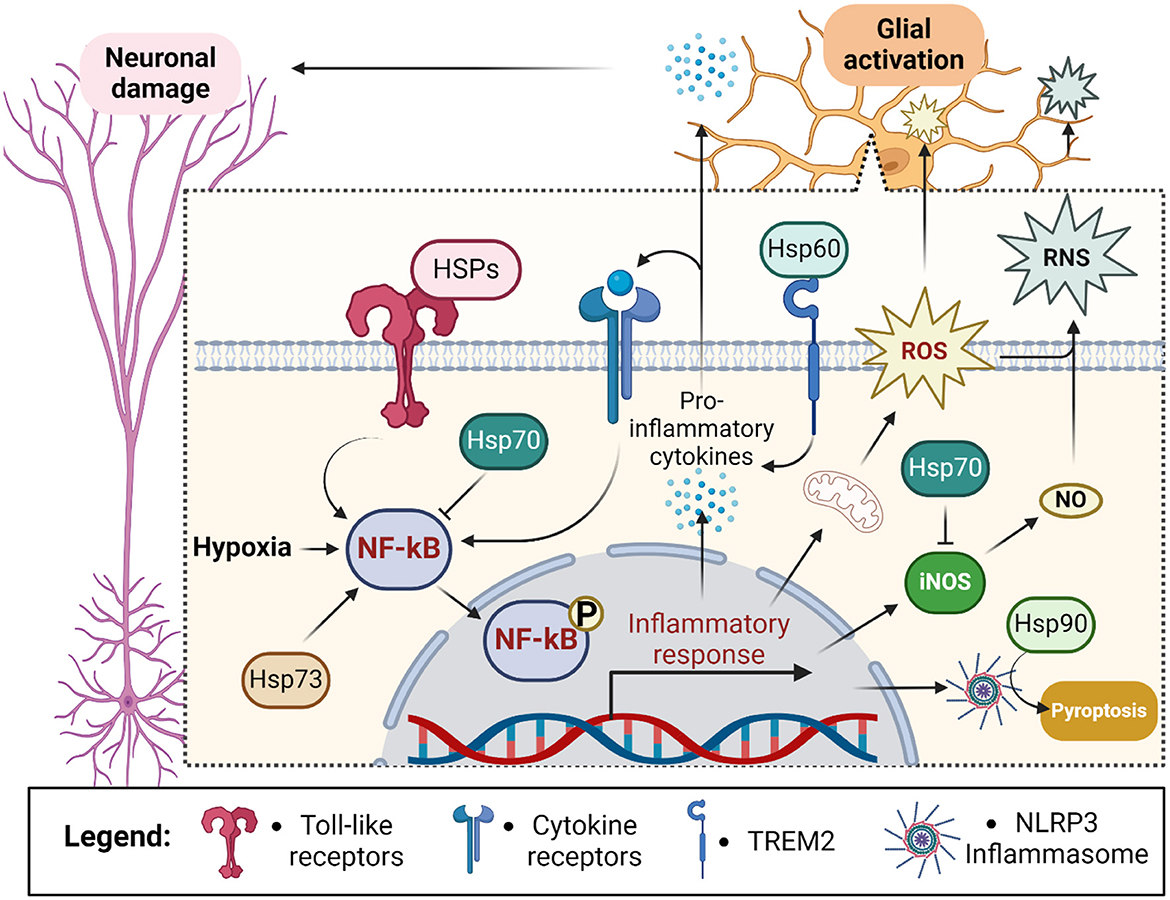
Figure 4. Outline of chaperones' contribution to the glial response during ischemic stroke. Hypoxia stimulates neuroinflammation through the activation of NF-kB in glial cells. NF-kB leads to the assembly of NLRP3 inflammasome and secretion of pro-inflammatory cytokines sending inflammatory stimuli to neurons and glial cells themselves. Glial activation also exacerbates hypoxia-induced reactive oxygen species (ROS) production and leads to the generation of reactive nitrogen species (RNS) via inducible nitric oxide synthase (iNOS)-dependent release of nitric oxide (NO) (Zhu et al., 2022a). Altogether it leads to activation of pyroptosis and damage of proximal neurons. Intracellularly localized Hsp70 prevents activation of NF-kB and iNOS thus suppressing ROS and RNS generation. In contrast, extracellularly localized heat shock proteins (HSPs) activate NF-kB via toll-like receptor signaling and TREM2 (Hsp60). Additionally, intracellular Hsp73 activates NF-kB whereas intracellular Hsp90 stabilizes NLRP3 leading to inflammatory activation and pyroptosis. Created in https://BioRender.com.
5.7 Hero
In 2020, Tsuboyama and colleagues discovered another family of small chaperones: “Hero proteins.” The authors described six heat-resistant proteins that display strong protective activity against different proteins exposed to damaging factors (Tsuboyama et al., 2020; Morimoto et al., 2022; Tan et al., 2023). Moreover, some of the Hero proteins were shown to decrease the deposition of human TDP-43-positive aggregates both in the eyes of transgenic Drosophila and human induced pluripotent stem (iPS)-derived motor neurons. These proteins were named based on their molecular weights (7, 9, 11, 13, 20, and 45 kDa) as Hero-7, Hero-9, Hero-11, Hero-13, Hero-20, and Hero-45. Although they mostly belong to distinct functional clusters, a common hallmark of these proteins is their remarkable thermostability, hydrophilicity, and disordered structure. Additionally, Hero proteins exhibit unusually high charges: Hero-45, Hero-7, and Hero-11 are highly positively charged, whereas Hero-9, Hero-20, and Hero-13 are highly negatively charged. Their protein-defensive properties are thought to arise from their ability to shield target molecules or function as chaperone holdases, a role facilitated by their flexible structure and unusually high charges. Our group has explored a potential link between Hero proteins and ischemic stroke, revealing that genetic variations in genes encoding Hero protein members are associated with both the risk of ischemic stroke (Kobzeva et al., 2022; Belykh et al., 2023; Shilenok et al., 2023, 2024a,b) and its outcomes (unpublished data).
For instance, we identified that polymorphisms in the Hero- (C11orf58) and Hero- (C19orf53) genes are significant risk factors for ischemic stroke (IS). Bioinformatics analysis revealed that the SNPs of C11orf58 and C19orf53 are implicated in the molecular mechanisms underlying IS, particularly through their involvement in regulating redox homeostasis, inflammation, apoptosis, as well as the response to hypoxia and oxidative stress (Shilenok et al., 2024a,b). Furthermore, bioinformatics analysis using the STRING database (https://string-db.org/) indicated that Hero- and its primary functional partners are associated with six biological processes, primarily related to ATP synthesis. These processes include: ≪mitochondrial respiratory chain complex I assembly≫ (GO:0032981), ≪mitochondrial electron transport, NADH to ubiquinone≫ (GO:0006120); ≪proton motive force-driven mitochondrial ATP synthesis≫ (GO:0042776); proteostasis (≪proteasome-mediated ubiquitin-dependent protein catabolic process≫ (GO:0043161); ≪nucleobase-containing compound biosynthetic process≫ (GO:0034654); and metabolic pathways ≪nitrogen compound metabolic process≫ (GO:0006807) (Shilenok et al., 2024b).
In a separate study, we found that a mutation reducing the expression of Hero-7 (SERF2) is also associated with an increased risk of stroke (Belykh et al., 2023). However, there is no conclusive evidence linking SERF2 to the pathobiology of stroke, apart from its involvement in the atherothrombotic component. The neurological function of Hero-7 was examined by Stroo et al. (2023), who demonstrated that mice with brain-specific deletion of SERF2 were viable, exhibited delayed embryonic development, but showed no major behavioral or cognitive abnormalities. These mice were more prone to developing amyloid-beta (Aβ) deposits, and the composition of these deposits was structurally distinct (Stroo et al., 2023). Interestingly, this finding contradicted previous studies, which suggested that SERF1A and SERF2 proteins accelerate the aggregation of multiple amyloidogenic proteins in vitro and in C. elegans (Falsone et al., 2012; Yoshimura et al., 2017; Pras et al., 2021; Balasubramaniam et al., 2018). Nevertheless, RNA sequencing data from Serf2–/– mice in the study by Stroo et al. (2023) did not reveal significant alterations in cellular pathways typically associated with stroke. Moreover, when we analyzed the available data using bioinformatics tools, there was no strong evidence supporting a role for Hero-7 in oxidative stress, hypoxia response, cell death, or neuroinflammation (Supplementary Table 1).
Another Hero-protein, BEX3, has been identified as a gene with high expression in the brain, particularly in neurons. BEX3 is known to play a role in neuronal apoptosis (Sidhar and Giri, 2017; Figure 5) and neurodegeneration (Park et al., 2000; Yi et al., 2003). Using genetic knockdown animal models, Navas-Pérez et al. (2020) demonstrated that reduced expression of BEX3 results in abnormal brain structures and impaired cognitive function. Furthermore, BEX3 has been shown to directly participate in reactive oxygen species (ROS) sensing in cells. While excessive ROS can induce oxidative stress, their persistent depletion leads to a condition known as reductive stress (Manford et al., 2020). Cells detect reductive stress through the FNIP1 protein (Manford et al., 2020), and BEX3 plays a crucial role in preventing the degradation of FNIP1 (Manford et al., 2021). In their study, Manford et al. (2021) demonstrated that overexpression of BEX3 causes the re-localization of stabilized NRF2 from the nucleus to perinuclear regions. Overall, the high neuronal expression of BEX3, its regulation of apoptosis, and its chaperone function may contribute to its role in ischemic stroke.
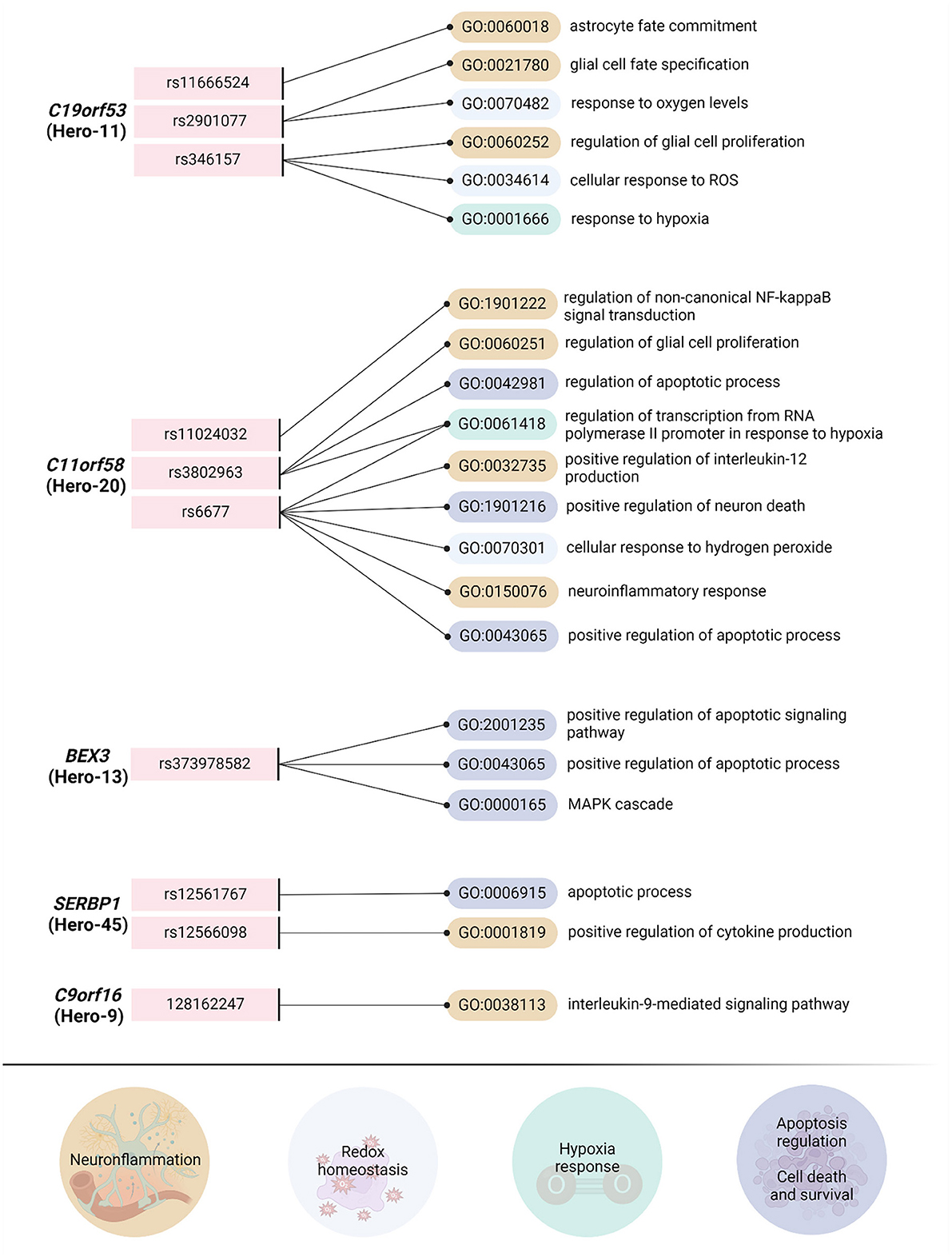
Figure 5. The analysis of overrepresented biological processes based on transcription factors associated with Hero-genetic polymorphisms. Using the Gene Ontology online tool (http://geneontology.org/, accessed on October 23, 2024), we identified overrepresented biological processes directly related to the pathogenesis of ischemic stroke (IS) by analyzing the involvement of transcription factors associated with reference and SNP alleles. For this analysis, we focused on the most frequent single nucleotide polymorphisms (SNPs) or, when available, tagging SNPs (TagSNPs). From the list of biological processes linked to transcription factors associated with the reference and SNP alleles, we selected only those directly relevant to the pathobiology of ischemic stroke. As a result, we classified the biological processes into four groups: “Neuroinflammation,” “Redox Homeostasis,” “Hypoxia Response,” and “Apoptosis Regulation/Cell Death and Survival.” Importantly, we intentionally excluded processes related to neuronal proliferation, migration, and differentiation. The raw data for BEX3, C9orf16, and SERF2 are provided in the Supplementary File. Data for SERBP1, C11orf58 and C19orf53 are presented in Shilenok et al. (2023), Shilenok et al. (2024a), and Shilenok et al. (2024b), respectively. Created in https://BioRender.com.
Finally, albeit we identified an association between the Hero-45 (SERBP1) polymorphism and ischemic stroke, data regarding its role in the response to brain injury are limited. However, its involvement in glioblastoma (Kosti et al., 2020), its presence in stress granules under oxidative stress conditions (Lee et al., 2014), and its strong suppression of TDP-43-induced pathology in the Drosophila eye system (Tsuboyama et al., 2020) suggest that SERBP1 may play a role in cellular responses to brain pathology. Additionally, our bioinformatics analysis revealed that representative single nucleotide polymorphisms (SNPs) of Hero-45 affect the binding of transcription factors involved in apoptosis regulation and cytokine production (Figure 5). This suggests that Hero-45 is involved in these molecular pathways.
5.8 Sigma-1 receptor (σ1R)
The Sigma-1 receptor (σ1R) is a molecular chaperone expressed in both neurons and glial cells, primarily localized to the mitochondria-associated endoplasmic reticulum (ER) membranes and plasma membranes (Hayashi and Su, 2005). As a chaperone, σ1R plays a vital role in proteome quality control by facilitating the degradation of misfolded proteins through the ER-associated degradation pathway linked to the ubiquitin-proteasome system (Miki et al., 2014). It is also involved in regulating cellular responses to oxidative stress, where, upon activation, it promotes antioxidant defenses, thereby helping to reduce cellular damage by mitigating oxidative stress (Chu and Ruoho, 2016).
σ1R enhances cellular resilience by interacting with and stabilizing antioxidant proteins, which is especially important under conditions of elevated oxidative stress, such as ischemia. In addition to managing oxidative stress, σ1R contributes to calcium homeostasis and has shown protective effects against both ischemic and non-ischemic brain injury in various animal models (Allahtavakoli and Jarrott, 2011; Wang et al., 2021b; Hong et al., 2015; Orciani et al., 2023; Vagnerova et al., 2006; Ruscher et al., 2011, 2012; Zhang et al., 2017, 2023; Song et al., 2023; Ajmo et al., 2006; Shi et al., 2021). These studies indicate that positive regulation of σ1R helps to attenuate brain damage across a broad spectrum of pathological conditions.
On a molecular level, σ1R enhances antioxidant defenses by interacting with key transcription factors like Nrf2 (Lasbleiz et al., 2022; Li et al., 2024), which regulate the expression of antioxidant proteins, such as SOD, catalase and heme oxygenase-1. σ1R also stabilizes structural proteins, including those associated with the cytoskeleton and cell membranes, helping to maintain cellular integrity under stress conditions. For example, σ1R has been observed to bind with ankyrins and other cytoskeletal components, thereby supporting cell membrane stability and resilience (Hayashi and Su, 2001; Su and Hayashi, 2001).
In the context of ischemic stroke, σ1R plays a critical role in modulating the unfolded protein response (UPR) (Hayashi and Su, 2007; Mori et al., 2013). The UPR is a compensatory mechanism activated to manage the accumulation of misfolded proteins under cellular stress (Hetz, 2012). Enhancing σ1R expression in cells has been shown to mitigate ER stress, while reductions in σ1R levels are associated with increased apoptosis (Hayashi and Su, 2007). Furthermore, σ1R exhibits mitoprotective properties, reduces neuroinflammation, and dampens excitotoxicity, providing further neuroprotection in ischemic conditions (Nguyen et al., 2017; Lu et al., 2012; Wegleiter et al., 2014; Rodríguez-Muñoz et al., 2018).
Interestingly, the role of σ1R in oxidative stress modulation can vary depending on the physiological context. While some studies suggest that σ1R activation promotes antioxidant defenses (Pal et al., 2012), others indicate that the absence of σ1R can also lead to enhanced antioxidant responses. For example, primary neuron-glia cultures obtained from mice with σ1R knockout displayed enhanced Nrf2 antioxidant defense especially when the cells were under stressful conditions (Weng et al., 2017). Goguadze et al. (2019) propose that σ1R may stimulate oxidative stress under normal physiological conditions but provides protection against excessive oxidative damage in pathological states.
The summarized contribution of σ1R and other chaperones to providing proteome quality control during ischemic stroke is pictured in Figure 6.
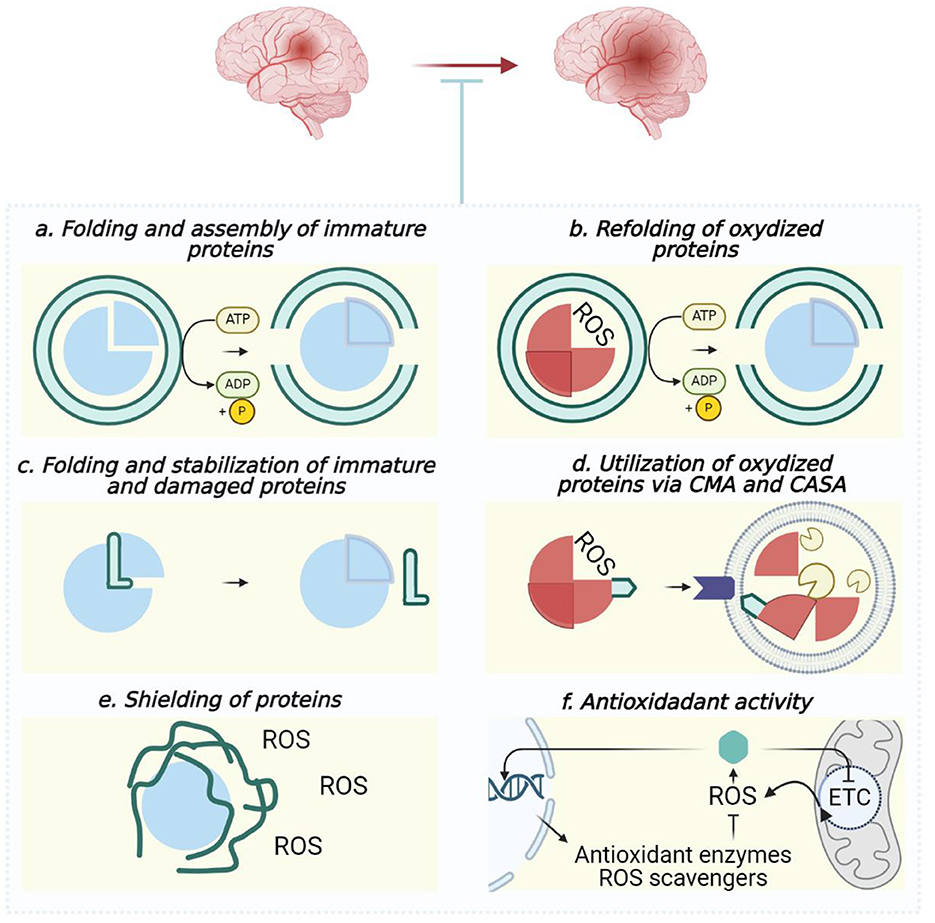
Figure 6. Outline of chaperones' contribution to maintaining proteome quality during ischemic stroke. In normal conditions (A, C) and to cope with overoxidation threats (A–F), chaperones co-interact with client proteins as foldases (A, B) or holdases (C), facilitate autophagic utilization of damaged polypeptides (D), shield client proteins to scavenge ROS (E) as well as provide ROS-sensing and activation of ROS-defense (F). Blue circles, undamaged proteins; red circles, damaged proteins; green objects interacting with blue or red circles, chaperones; round lipid envelope with yellow “pac-mans” inside, lysosome. (A, B) Hsp60, Hsp70, Hsp90, and Hsp100; (C) Hsp22, Hsp27, α-Crystallin, Sigma-1 receptor, and Hero-proteins; (D) Hsc70 (CMA), Hsp70, and Hsp90 (CASA); (E) Hero-proteins; (F) Hsp22, Hsp27, and Hsp70. Created in https://BioRender.com.
6 Perspectives
Molecular chaperones are a powerful system maintaining proteome quality control in either healthy or stressed cells. Accordingly, the idea of attenuating neuronal damage via this tool is attractive, although somewhat mechanistic. Despite being mostly anecdotal, myriad pharmacological strategies based on interventions upregulating or delivering various components of chaperone machinery in stroke have already been discussed (Kim et al., 2018; Shao et al., 2019). Nevertheless, many years of research experience and promising results in animal studies of chaperone-based approaches have not yet been successfully translated into clinical practice.
This may reflect that each chaperone is involved in numerous molecular pathways sophisticatedly organized and interconnected together. Thus, any intervention targeting or delivering chaperones is potentially associated to the risk of unbalancing signaling networks.
To date, dozens of clinical trials have investigated heat shock proteins (HSPs), with the majority focusing on cancer therapies, particularly Hsp90 inhibitors such as 17-AAG, XL888, and AT13387. Many trials have also targeted neurodegenerative diseases, aiming to pharmacologically upregulate Hsp70 (see our previous review, Venediktov et al., 2023). For example, an ongoing trial is assessing the efficacy of Arimoclomol in ALS (Benatar et al., 2024). Additionally, some studies have explored the role of HSPs in atherosclerosis (e.g., NCT04787770). The substantial body of clinical research on HSP modulators lays a solid foundation for their potential future application in ischemic stroke. Although dedicated studies on HSPs in stroke patients have not yet been conducted, the existing studies will provide valuable insights into the pharmacodynamics and potential side effects of these modulators.
In principle, as well as other molecules of a protein nature and predominantly cytoplasm-sited activity, chaperones may scarcely be used as a treatment option administered directly. There were successful attempts to utilize chaperones coupled with TAT, the leading sequence from human immunodeficiency virus, or with other cell-penetrating peptides to increase their cellular uptake (Komarova et al., 2015; Hino et al., 2023). This strategy was implemented with some chaperones and showed beneficial effects in models of neuronal pathologies (Wheeler et al., 2003; Lai et al., 2005; Kim et al., 2008), albeit the data were limited by in vitro research.
Some small chemicals such as Arimoclomol (Kieran et al., 2004; Cudkowicz et al., 2008; Kalmar et al., 2008), Colchicine (Mandrioli et al., 2019), Phencyclidine (Sharp et al., 1994; Narita et al., 1997), Quercetin (Chander et al., 2014), 17-dimethyl aminoethylamino-17-demethoxygeldanamycin hydrochloride (17-DMAG) (Qi et al., 2014; Zhang et al., 2021; Hu et al., 2023b), and Geldamycin (Xu et al., 2003; Shen et al., 2005; Manaenko et al., 2010, 2011) shown to regulate the activity of various chaperones with beneficial effects in diseases of CNS, can be considered as agents for the intervention in stroke or the post-stroke recovery. However, there is a lack of drugs specifically targeting any chaperone, and the aforementioned molecules are highly unspecific, suggesting that chaperones belong to “undruggable” or “under-drugged” targets (Chan and Tsourkas, 2024). Fortunately, drug design technologies led to the discovery of selective chaperone inhibitors (Ambrose et al., 2023; Hu et al., 2023a) and, hence, may be considered promising candidates to study as ischemic stroke therapeutics.
Effective pharmacological interventions selectively regulating a desired chaperone also might implement gene therapeutic tools, such as lipid nanoparticles, antisense oligonucleotides, siRNA and, especially, adeno-associated viruses. Some previous works have reported successful amelioration of CNS damage (Luecke et al., 2023; Moloney et al., 2014; Fink et al., 1997; Yenari et al., 1998) including ischemia (Chi et al., 2014a,b; Guo et al., 2018; Kesaraju et al., 2014; Badin et al., 2009; Kelly et al., 2002; Hoehn et al., 2001; Wen et al., 2022) via utilization of such approaches. Moreover, recent advances in gene therapeutic approaches enable the precise delivery of molecular constructions to desired organelles, e.g.; to the most important source of ROS: mitochondria (Soldatov et al., 2022). Further focus should obviously be on preclinical testing these approaches in more detail.
Particular interest might be given to the use of chaperones in the reperfusion phase of stroke. Nowadays, interventions restoring the blood flow are considered a golden standard in patients with early ischemic stroke (Hurford et al., 2020). The recanalization may be achieved due to intravenous administration of clog-busting drugs (Wardlaw et al., 2014), endovascular thromboectomy (Goyal et al., 2015), sonothrombolysis (Li et al., 2020) or their combination (Saver et al., 2015; Bracard et al., 2016). However, if a recurrent blood flow takes place, this phase—reperfusion—is known to exacerbate the oxidative stress (Hossmann, 2009; Sun et al., 2018) causing the second phase of alteration. This repeated burst of ROS orchestrates post-reperfusion inflammatory activation, leukocyte-endothelial adhesion, and a breach of the blood-brain barrier (Mandalaneni et al., 2024). Therefore, the treatments which have been proposed to reduce the reperfusion injury mostly imply the antioxidant therapy (Wood and Gladwin, 2007; Wang et al., 2006a).
However, some chaperones exhibit controversial biological effects that vary from positive to negative across various research. Moreover, many chaperones act as a double-edged sword causing different adverse effects, which limits the application of chaperone-based strategies in clinical practice. For instance, since they are secreted extracellularly, HSPs act as damage-associated molecular patterns (DAMPs), activating the innate immune system response (Lu and Eguchi, 2020). Moreover, HSPs are key players in promoting proliferation, differentiation, invasion, and metastasis, which also gives cause for concern in case of their pharmacological upregulation (Calderwood and Murshid, 2017).
Hsp90, Hsp70, and Hsp27 content tends to elevate in tumors (Calderwood and Gong, 2016) increasing the risk of cancer (Dai et al., 2007; Garrido et al., 2006; Vanhooren et al., 2008). In this respect, stroke appears to be among the most appropriate diseases to which to apply chaperone-based interventions. Since there is only a narrow window in which to intervene with therapy, the risk of adverse effects is potentially lower than during long-term treatment; for example, in the context of neurodegenerative disorders.
In light of the latest discoveries, we can expect upcoming breakthroughs involving the application of chaperones in ischemic stroke treatment. In particular, the recently discovered family of Hero proteins possess features that make them excellent candidates for the therapy of stroke: low molecular weight, high stability, minimal immunogenicity, and energy-independent activity.
7 Limitations
• This work was based on the narrative literature review discussing biological insights and did not imply following the classical principles of meta-analysis or critical reviews such as assessing of statistical power and strict inclusion/exclusion criteria.
• The authors admit that the manuscript does not discuss the bulk part of the chaperome members: given the immense diversity of chaperones, only the most studied and classical subfamilies and members were reviewed. However, the potential contributions of other chaperone families that may also play significant roles.
• The actual pathogenesis of oxidative damage in stroke is constituted of myriads of links, and some of them were missing or not paid itemized description within the article. To generalize, the authors deliberately did not discuss multifaceted significance of oxidative damage in different stages of ischemic stroke, the roles of concomitant pathology etc.
• All theoretical justifications given in this article require experimental approval.
Author contributions
VS: Conceptualization, Formal analysis, Supervision, Visualization, Writing – original draft, Writing – review & editing. AV: Writing – original draft, Writing – review & editing. AB: Writing – review & editing. GP: Writing – review & editing. MN: Writing – review & editing. NO: Writing – review & editing. NK: Writing – review & editing. OB: Conceptualization, Formal analysis, Supervision, Writing – original draft, Writing – review & editing.
Funding
The author(s) declare financial support was received for the research, authorship, and/or publication of this article. The study was supported by the Russian Science Foundation (No. 22-15-00288, https://rscf.ru/en/project/22-15-00288/).
Acknowledgments
The authors assert that no AI-based technologies were used to generate any content in this article. However, in some sections the authors did use ChatGPT-4 (OpenAI) to identify potential grammar mistakes. If any were found, they were manually corrected.
Conflict of interest
The authors declare that the research was conducted in the absence of any commercial or financial relationships that could be construed as a potential conflict of interest.
Publisher's note
All claims expressed in this article are solely those of the authors and do not necessarily represent those of their affiliated organizations, or those of the publisher, the editors and the reviewers. Any product that may be evaluated in this article, or claim that may be made by its manufacturer, is not guaranteed or endorsed by the publisher.
Supplementary material
The Supplementary Material for this article can be found online at: https://www.frontiersin.org/articles/10.3389/fnmol.2024.1513084/full#supplementary-material
References
Abisambra, J. F., Blair, L. J., Hill, S. E., Jones, J. R., Kraft, C., Rogers, J., et al. (2010). Phosphorylation dynamics regulate hsp27-mediated rescue of neuronal plasticity deficits in tau transgenic mice. J. Neurosci. 30, 15374–15382. doi: 10.1523/JNEUROSCI.3155-10.2010
Adriaenssens, E., Asselbergh, B., Rivera-Mejías, P., Bervoets, S., Vendredy, L., De Winter, V., et al. (2023). Small heat shock proteins operate as molecular chaperones in the mitochondrial intermembrane space. Nat. Cell Biol. 25, 467–480. doi: 10.1038/s41556-022-01074-9
Ajmo, C. Jr, Vernon, D., Collier, L., Pennypacker, K., and Cuevas, J. (2006). Sigma Receptor activation reduces infarct size at 24 hours after permanent middle cerebral artery occlusion in rats. CNR 3, 89–98. doi: 10.2174/156720206776875849
Akbar, M. T., Lundberg, A. M. C., Liu, K., Vidyadaran, S., Wells, K. E., Dolatshad, H., et al. (2003). The neuroprotective effects of heat shock protein 27 overexpression in transgenic animals against kainate-induced seizures and hippocampal cell death. J. Biol. Chem. 278, 19956–19965. doi: 10.1074/jbc.M207073200
Allahtavakoli, M., and Jarrott, B. (2011). Sigma-1 receptor ligand PRE-084 reduced infarct volume, neurological deficits, pro-inflammatory cytokines and enhanced anti-inflammatory cytokines after embolic stroke in rats. Brain Res. Bull. 85, 219–224. doi: 10.1016/j.brainresbull.2011.03.019
Allen, D. G., and Xiao, X. H. (2003). Role of the cardiac Na+/H+ exchanger during ischemia and reperfusion. Cardiovasc. Res. 57, 934–941. doi: 10.1016/S0008-6363(02)00836-2
Allende, M., Molina, E., Guruceaga, E., Tamayo, I., González-Porras, J. R., Gonzalez-López, T. J., et al. (2016). Hsp70 protects from stroke in atrial fibrillation patients by preventing thrombosis without increased bleeding risk. Cardiovasc. Res. 110, 309–318. doi: 10.1093/cvr/cvw049
Amanda, B., Pragasta, R., Cakrasana, H., Mustika, A., Faizah, Z., and Oceandy, D. (2024). The hippo signaling pathway, reactive oxygen species production, and oxidative stress: a two-way traffic regulation. Cells 13:1868. doi: 10.3390/cells13221868
Ambrose, A. J., Sivinski, J., Zerio, C. J., Zhu, X., Godek, J., Kumirov, V. K., et al. (2023). Discovery and development of a selective inhibitor of the ER resident chaperone Grp78. J. Med. Chem. 66, 677–694. doi: 10.1021/acs.jmedchem.2c01631
Androvitsanea, A., Stylianou, K., Drosataki, E., and Petrakis, I. (2021). The pathophysiological role of heat shock response in autoimmunity: a literature review. Cells. 10:2626. doi: 10.3390/cells10102626
Arrigo, A. P. (2001). Hsp27: novel regulator of intracellular redox state. IUBMB Life. 52, 303–307. doi: 10.1080/152165401317291156
Asea, A., Rehli, M., Kabingu, E., Boch, J. A., Baré, O., Auron, P. E., et al. (2002). Novel signal transduction pathway utilized by extracellular HSP70. Role of toll-like receptor (TLR) 2 and TLR4. J. Biol. Chem. 277, 15028–15034. doi: 10.1074/jbc.M200497200
Badin, R. A., Lythgoe, M. F., Van Der Weerd, L., Thomas, D. L., Gadian, D. G., and Latchman, D. S. (2006). Neuroprotective effects of virally delivered HSPs in experimental stroke. J. Cereb. Blood Flow Metab. 26, 371–381. doi: 10.1038/sj.jcbfm.9600190
Badin, R. A., Modo, M., Cheetham, M., Thomas, D. L., Gadian, D. G., Latchman, D. S., et al. (2009). Protective effect of post-ischaemic viral delivery of heat shock proteins in vivo. J. Cereb. Blood Flow Metab. 29, 254–263. doi: 10.1038/jcbfm.2008.106
Bahr, T., Katuri, J., Liang, T., and Bai, Y. (2022). Mitochondrial chaperones in human health and disease. Free Radic. Biol. Med. 179, 363–374. doi: 10.1016/j.freeradbiomed.2021.11.015
Balasubramaniam, M., Ayyadevara, S., and Shmookler Reis, R. J. (2018). Structural insights into pro-aggregation effects of C. elegans CRAM-1 and its human ortholog SERF2. Sci. Rep. 8:14891. doi: 10.1038/s41598-018-33143-1
Banasiak, K. J., Xia, Y., and Haddad, G. G. (2000). Mechanisms underlying hypoxia-induced neuronal apoptosis. Progr. Neurobiol. 62, 215–249. doi: 10.1016/S0301-0082(00)00011-3
Banecka-Majkutewicz, Z., Grabowski, M., Kadziński, L., Papkov, A., Wegrzyn, A., and Banecki, B. (2014). Increased levels of antibodies against heat shock proteins in stroke patients. Acta Biochim. Pol. 61, 379–383. doi: 10.18388/abp.2014_1910
Barreto, G. E., White, R. E., Xu, L., Palm, C. J., and Giffard, R. G. (2012). Effects of heat shock protein 72 (Hsp72) on evolution of astrocyte activation following stroke in the mouse. Exp. Neurol. 238, 284–296. doi: 10.1016/j.expneurol.2012.08.015
Bartelt-Kirbach, B., Slowik, A., Beyer, C., and Golenhofen, N. (2017). Upregulation and phosphorylation of HspB1/Hsp25 and HspB5/αB-crystallin after transient middle cerebral artery occlusion in rats. Cell Stress Chaper. 22, 653–663. doi: 10.1007/s12192-017-0794-9
Bascos, N. A. D., and Landry, S. J. (2019). A history of molecular chaperone structures in the protein data bank. IJMS 20:6195. doi: 10.3390/ijms20246195
Behdarvandy, M., Karimian, M., Atlasi, M. A., and Azami Tameh, A. (2020). Heat shock protein 27 as a neuroprotective biomarker and a suitable target for stem cell therapy and pharmacotherapy in ischemic stroke. Cell Biol. Int. 44, 356–367. doi: 10.1002/cbin.11237
Belenichev, I. F., Aliyeva, O. G., Popazova, O. O., and Bukhtiyarova, N. V. (2023). Involvement of heat shock proteins HSP70 in the mechanisms of endogenous neuroprotection: the prospect of using HSP70 modulators. Front. Cell. Neurosci. 17:1131683. doi: 10.3389/fncel.2023.1131683
Belykh, A. E., Soldatov, V. O., Stetskaya, T. A., Kobzeva, K. A., Soldatova, M. O., Polonikov, A. V., et al. (2023). Polymorphism of SERF2, the gene encoding a heat-resistant obscure (Hero) protein with chaperone activity, is a novel link in ischemic stroke. IBRO Neurosci. Rep. 14, 453–461. doi: 10.1016/j.ibneur.2023.05.004
Benatar, M., Hansen, T., Rom, D., Geist, M. A., Blaettler, T., Camu, W., et al. (2024). Safety and efficacy of arimoclomol in patients with early amyotrophic lateral sclerosis (ORARIALS-01): a randomised, double-blind, placebo-controlled, multicentre, phase 3 trial. Lancet Neurol. 23, 687–699. doi: 10.1016/S1474-4422(24)00134-0
Beraldo, F. H., Soares, I. N., Goncalves, D. F., Fan, J., Thomas, A. A., Santos, T. G., et al. (2013). Stress-inducible phosphoprotein 1 has unique cochaperone activity during development and regulates cellular response to ischemia via the prion protein. FASEB J.27, 3594–3607. doi: 10.1096/fj.13-232280
Biever, A., Glock, C., Tushev, G., Ciirdaeva, E., Dalmay, T., Langer, J. D., et al. (2020). Monosomes actively translate synaptic mRNAs in neuronal processes. Science 367:eaay4991. doi: 10.1126/science.aay4991
Bilog, A. D., Smulders, L., Oliverio, R., Labanieh, C., Zapanta, J., Stahelin, R. V., et al. (2019). Membrane localization of HspA1A, a stress inducible 70-kDa heat-shock protein, depends on its interaction with intracellular phosphatidylserine. Biomolecules 9:152. doi: 10.3390/biom9040152
Bobkova, N. V., Evgen'ev, M., Garbuz, D. G., Kulikov, A. M., Morozov, A., Samokhin, A., et al. (2015). Exogenous Hsp70 delays senescence and improves cognitive function in aging mice. Proc. Natl. Acad. Sci. U.S.A. 112, 16006–16011. doi: 10.1073/pnas.1516131112
Bobkova, N. V., Garbuz, D. G., Nesterova, I., Medvinskaya, N., Samokhin, A., Alexandrova, I., et al. (2014). Therapeutic effect of exogenous Hsp70 in mouse models of Alzheimer's disease. J. Alzheimers Dis. 38, 425–435. doi: 10.3233/JAD-130779
Bonvento, G., and Bolaños, J. P. (2021). Astrocyte-neuron metabolic cooperation shapes brain activity. Cell Metab. 33, 1546–1564. doi: 10.1016/j.cmet.2021.07.006
Boyd, R. A., Majumder, S., Stiban, J., Mavodza, G., Straus, A. J., Kempelingaiah, S. K., et al. (2023). The heat shock protein Hsp27 controls mitochondrial function by modulating ceramide generation. Cell Rep. 42:113081. doi: 10.1016/j.celrep.2023.113081
Bracard, S., Ducrocq, X., Mas, J. L., Soudant, M., Oppenheim, C., Moulin, T., et al. (2016). Mechanical thrombectomy after intravenous alteplase versus alteplase alone after stroke (THRACE): a randomised controlled trial. Lancet Neurol. 15, 1138–1147. doi: 10.1016/S1474-4422(16)30177-6
Brand, M. D. (2016). Mitochondrial generation of superoxide and hydrogen peroxide as the source of mitochondrial redox signaling. Free Radic. Biol. Med. 100, 14–31. doi: 10.1016/j.freeradbiomed.2016.04.001
Brea, D., Blanco, M., Ramos-Cabrer, P., Moldes, O., Arias, S., Pérez-Mato, M., et al. (2011). Toll-like receptors 2 and 4 in ischemic stroke: outcome and therapeutic values. J. Cereb. Blood Flow Metab. 31, 1424–1431. doi: 10.1038/jcbfm.2010.231
Brehme, M., Voisine, C., Rolland, T., Wachi, S., Soper, J. H., Zhu, Y., et al. (2014). A chaperome subnetwork safeguards proteostasis in aging and neurodegenerative disease. Cell Rep. 9, 1135–1150. doi: 10.1016/j.celrep.2014.09.042
Broere, F., van der Zee, R., and van Eden, W. (2011). Heat shock proteins are no DAMPs, rather 'DAMPERs'. Nat. Rev. Immunol. 11:565. doi: 10.1038/nri2873-c1
Brown, D. I., and Griendling, K. K. (2009). Nox proteins in signal transduction. Free Rad. Biol. Med. 47, 1239–1253. doi: 10.1016/j.freeradbiomed.2009.07.023
Bushueva, O., Barysheva, E., Markov, A., Belykh, A., Koroleva, I., Churkin, E., et al. (2021). DNA hypomethylation of the MPO gene in peripheral blood leukocytes is associated with cerebral stroke in the acute phase. J. Mol. Neurosci. 71, 1914–1932. doi: 10.1007/s12031-021-01840-8
Bushueva, O., Solodilova, M., Ivanov, V., and Polonikov, A. (2015). Gender-specific protective effect of the 463G. A polymorphism of myeloperoxidase gene against the risk of essential hypertension in Russians. J. Am. Soc. Hypert. 9, 902–906. doi: 10.1016/j.jash.2015.08.006
Buttke, T. M., and Sandstrom, P. A. (1994). Oxidative stress as a mediator of apoptosis. Immunol. Today. 15, 7–10. doi: 10.1016/0167-5699(94)90018-3
Cabiscol, E., Belli, G., Tamarit, J., Echave, P., Herrero, E., and Ros, J. (2002). Mitochondrial Hsp60, resistance to oxidative stress, and the labile iron pool are closely connected in saccharomyces cerevisiae. J. Biol. Chem. 277, 44531–44538. doi: 10.1074/jbc.M206525200
Cadenas, E., and Davies, K. J. (2000). Mitochondrial free radical generation, oxidative stress, and aging. Free Radic. Biol. Med. 29, 222–230. doi: 10.1016/S0891-5849(00)00317-8
Caldeira, M. V., Salazar, I. L., Curcio, M., Canzoniero, L. M. T., and Duarte, C. B. (2014). Role of the ubiquitin–proteasome system in brain ischemia: friend or foe? Prog. Neurobiol. 112, 50–69. doi: 10.1016/j.pneurobio.2013.10.003
Calderwood, S. K., and Gong, J. (2016). Heat shock proteins promote cancer: it's a protection racket. Trends Biochem. Sci. 41, 311–323. doi: 10.1016/j.tibs.2016.01.003
Calderwood, S. K., and Murshid, A. (2017). Molecular chaperone accumulation in cancer and decrease in Alzheimer's disease: the potential roles of HSF1. Front. Neurosci. 11, 192. doi: 10.3389/fnins.2017.00192
Caruso Bavisotto, C., Alberti, G., Vitale, A. M., Paladino, L., Campanella, C., Rappa, F., et al. (2020). Hsp60 post-translational modifications: functional and pathological consequences. Front. Mol. Biosci. 7:95. doi: 10.3389/fmolb.2020.00095
Chan, A., and Tsourkas, A. (2024). Intracellular protein delivery: approaches, challenges, and clinical applications. BME Front. 5:0035. doi: 10.34133/bmef.0035
Chander, K., Vaibhav, K., Ejaz, A.hmed, Md., Javed, H., Tabassum, R., Khan, A., et al. (2014). Quercetin mitigates lead acetate-induced behavioral and histological alterations via suppression of oxidative stress, Hsp-70, Bak and upregulation of Bcl-2. Food Chem. Toxicol. 68, 297–306. doi: 10.1016/j.fct.2014.02.012
Chang, Y., Wu, Y., Jiang, X., Zhu, J., Wang, C., Ma, R., et al. (2022). HSPB8 overexpression ameliorates cognitive impairment in diabetic mice via inhibiting NLRP3 inflammation activation. Oxid. Med. Cell. Longev. 2022, 1–15. doi: 10.1155/2022/9251835
Chaudhuri (Chattopadhyay), P., and Rashid, N. (2019). “HSP60 as modulators of apoptosis,” in Heat Shock Protein 60 in Human Diseases and Disorders. Heat Shock Proteins, eds. A. Asea, P. Kaur (Cham: Springer). doi: 10.1007/978-3-030-23154-5_4
Chen, F., Pandey, D., Chadli, A., Catravas, J. D., Chen, T., and Fulton, D. J. (2011). Hsp90 regulates NADPH oxidase activity and is necessary for superoxide but not hydrogen peroxide production. Antioxid. Redox Signal. 14, 2107–2119. doi: 10.1089/ars.2010.3669
Chen, F., Yu, Y., Qian, J., Wang, Y., Cheng, B., Dimitropoulou, C., et al. (2012). Opposing actions of heat shock protein 90 and 70 regulate nicotinamide adenine dinucleotide phosphate oxidase stability and reactive oxygen species production. Arterioscler. Thromb. Vasc. Biol. 32, 2989–2999. doi: 10.1161/ATVBAHA.112.300361
Chen, G., and Nuñez, G. (2011). Are heat shock proteins DAMPs? Nat. Rev. Immunol. 11:565. doi: 10.1038/nri2873-c2
Chen, S., Mei, S., Luo, Y., Wu, H., Zhang, J., and Zhu, J. (2018). Gasdermin family: a promising therapeutic target for stroke. Transl. Stroke Res. 9, 555–563. doi: 10.1007/s12975-018-0666-3
Cheng, J., Ji, M., Jing, H., and Lin, H. (2023). DUSP12 ameliorates myocardial ischemia–reperfusion injury through HSPB8-induced mitophagy. J. Biochem. Mol. Toxicol. 37:e23310. doi: 10.1002/jbt.23310
Chi, W., Meng, F., Li, Y., Li, P., Wang, G., Cheng, H., et al. (2014b). Impact of microRNA-134 on neural cell survival against ischemic injury in primary cultured neuronal cells and mouse brain with ischemic stroke by targeting HSPA12B. Brain Res. 1592, 22–33. doi: 10.1016/j.brainres.2014.09.072
Chi, W., Meng, F., Li, Y., Wang, Q., Wang, G., Han, S., et al. (2014a). Downregulation of miRNA-134 protects neural cells against ischemic injury in N2A cells and mouse brain with ischemic stroke by targeting HSPA12B. Neuroscience 277, 111–122. doi: 10.1016/j.neuroscience.2014.06.062
Christie, I. N., Theparambil, S. M., Braga, A., Doronin, M., Hosford, P. S., Brazhe, A., et al. (2023). Astrocytes produce nitric oxide via nitrite reduction in mitochondria to regulate cerebral blood flow during brain hypoxia. Cell Rep. 42:113514. doi: 10.1016/j.celrep.2023.113514
Chu, U. B., and Ruoho, A. E. (2016). Biochemical pharmacology of the sigma-1 receptor. Mol. Pharmacol. 89, 142–153. doi: 10.1124/mol.115.101170
Cobley, J. N., Fiorello, M. L., and Bailey, D. M. (2018). 13 reasons why the brain is susceptible to oxidative stress. Redox Biol. 15, 490–503. doi: 10.1016/j.redox.2018.01.008
Cookson, B. T., and Brennan, M. A. (2001). Pro-inflammatory programmed cell death. Trends Microbiol. 9, 113–114. doi: 10.1016/S0966-842X(00)01936-3
Costa-Bebexr, L. C., Hirsch, G. E., Heck, T. G., and Ludwig, M. S. (2022). Chaperone duality: the role of extracellular and intracellular HSP70 as a biomarker of endothelial dysfunction in the development of atherosclerosis. Arch. Physiol. Biochem. 128, 1016–1023. doi: 10.1080/13813455.2020.1745850
Cudkowicz, M. E., Shefner, J. M., Simpson, E., Grasso, D., Yu, H., Zhang, H., et al. (2008). Arimoclomol at dosages up to 300 mg/day is well tolerated and safe in amyotrophic lateral sclerosis. Muscle Nerve 38, 837–844. doi: 10.1002/mus.21059
da Fonseca, A. C. C., Matias, D., Geraldo, L. H. M., Leser, F. S., Pagnoncelli, I., Garcia, C., et al. (2021). The multiple functions of the co-chaperone stress inducible protein 1. Cytokine Growth Factor Rev. 57, 73–84. doi: 10.1016/j.cytogfr.2020.06.003
Dai, C., Whitesell, L., Rogers, A. B., and Lindquist, S. (2007). Heat shock factor 1 is a powerful multifaceted modifier of carcinogenesis. Cell 130, 1005–1018. doi: 10.1016/j.cell.2007.07.020
Dávila, D., Jiménez-Mateos, E. M., Mooney, C. M., Velasco, G., Henshall, D. C., and Prehn, J. H. (2014). Hsp27 binding to the 3′UTR of bim mRNA prevents neuronal death during oxidative stress-induced injury: a novel cytoprotective mechanism. Mol. Biol. Cell. 25, 3413–3423. doi: 10.1091/mbc.e13-08-0495
DeGracia, D. J. (2017). Regulation of mRNA following brain ischemia and reperfusion: mRNA regulation after brain ischemia and reperfusion. WIREs RNA 8:e1415. doi: 10.1002/wrna.1415
Demyanenko, S., Nikul, V., Rodkin, S., Davletshin, A., Evgen'ev, M. B., and Garbuz, D. G. (2021). Exogenous recombinant Hsp70 mediates neuroprotection after photothrombotic stroke. Cell Stress Chaperones 26, 103–114. doi: 10.1007/s12192-020-01159-0
Demyanenko, S. V., Kalyuzhnaya, Y. N., Bachurin, S. S., Khaitin, A. M., Kunitsyna, A. E., Batalshchikova, S. A., et al. (2024). Exogenous Hsp70 exerts neuroprotective effects in peripheral nerve rupture model. Exp. Neurol. 373:114670. doi: 10.1016/j.expneurol.2023.114670
Deng, A., Ma, L., Ji, Q., Xing, J., Qin, J., Zhou, X., et al. (2023). Activation of the Akt/FoxO3 signaling pathway enhances oxidative stress-induced autophagy and alleviates brain damage in a rat model of ischemic stroke. Can. J. Physiol. Pharmacol. 101, 18–26. doi: 10.1139/cjpp-2022-0341
Esfahanian, N., Knoblich, C. D., Bowman, G. A., and Rezvani, K. (2023). Mortalin: Protein partners, biological impacts, pathological roles, and therapeutic opportunities. Front. Cell Dev. Biol. 11:1028519. doi: 10.3389/fcell.2023.1028519
Evgen'ev, M., Bobkova, N., Krasnov, G., Garbuz, D., Funikov, S., Kudryavtseva, A., et al. (2019). The effect of human HSP70 administration on a mouse model of Alzheimer's disease strongly depends on transgenicity and age. J. Alzheimers Dis. 67, 1391–1404. doi: 10.3233/JAD-180987
Evgen'ev, M. B., Krasnov, G. S., Nesterova, I. V., Garbuz, D. G., Karpov, V. L., Morozov, A. V., et al. (2017). Molecular mechanisms underlying neuroprotective effect of intranasal administration of human hsp70 in mouse model of Alzheimer's disease. J. Alzheimers Dis. 59, 1415–1426. doi: 10.3233/JAD-170398
Falsone, S. F., Meyer, N. H., Schrank, E., Leitinger, G., Pham, C. L. L., Fodero-Tavoletti, M. T., et al. (2012). SERF protein is a direct modifier of amyloid fiber assembly. Cell Rep. 2, 358–371. doi: 10.1016/j.celrep.2012.06.012
Fan, H., Ding, R., Liu, W., Zhang, X., Li, R., Wei, B., et al. (2021). Heat shock protein 22 modulates NRF1/TFAM-dependent mitochondrial biogenesis and DRP1-sparked mitochondrial apoptosis through AMPK-PGC1α signaling pathway to alleviate the early brain injury of subarachnoid hemorrhage in rats. Redox Biol. 40:101856. doi: 10.1016/j.redox.2021.101856
Feigin, V. L., Brainin, M., Norrving, B., Martins, S., Sacco, R. L., Hacke, W., et al. (2022). World stroke organization (WSO): global stroke fact sheet 2022. Int. J. Stroke 17, 18–29. doi: 10.1177/17474930211065917
Feinstein, D. L., Galea, E., Aquino, D. A., Li, G. C., Xu, H., and Reis, D. J. (1996). Heat shock protein 70 suppresses astroglial-inducible nitric-oxide synthase expression by decreasing NFkappaB activation. J. Biol. Chem. 271, 17724–17732. doi: 10.1074/jbc.271.30.17724
Fernández-Fernández, M. R., Gragera, M., Ochoa-Ibarrola, L., Quintana-Gallardo, L., and Valpuesta, J. M. (2017). Hsp70 - a master regulator in protein degradation. FEBS Lett. 591, 2648–2660. doi: 10.1002/1873-3468.12751
Finelli, M. J. (2020). Redox post-translational modifications of protein thiols in brain aging and neurodegenerative conditions—focus on s-nitrosation. Front. Aging Neurosci. 12:254. doi: 10.3389/fnagi.2020.00254
Fink, S. L., Chang, L. K., Ho, D. Y., and Sapolsky, R. M. (1997). Defective herpes simplex virus vectors expressing the rat brain stress-inducible heat shock protein 72 protect cultured neurons from severe heat shock. J. Neurochem. 68, 961–969. doi: 10.1046/j.1471-4159.1997.68030961.x
Flachbartová, Z., and Kovacech, B. (2013). Mortalin – a multipotent chaperone regulating cellular processes ranging from viral infection to neurodegeneration. Acta Virol. 57, 3–15. doi: 10.4149/av_2013_01_3
Förstermann, U., and Sessa, W. C. (2012). Nitric oxide synthases: regulation and function. Eur. Heart J. 33, 829–837. doi: 10.1093/eurheartj/ehr304
Fouché, B., Turner, S., Gorham, R., Stephenson, E. J., Gutbier, S., Elson, J. L., et al. (2023). A novel mitochondria-targeting iron chelator neuroprotects multimodally via HIF-1 modulation against a mitochondrial toxin in a dopaminergic cell model of Parkinson's disease. Mol. Neurobiol. 60, 749–767. doi: 10.1007/s12035-022-03107-8
Furuhashi, M., Ishimura, S., Ota, H., and Miura, T. (2011). Lipid chaperones and metabolic inflammation. Int. J. Inflam. 2011, 1–12. doi: 10.4061/2011/642612
Garrido, C., Brunet, M., Didelot, C., Zermati, Y., Schmitt, E., and Kroemer, G. (2006). Heat shock proteins 27 and 70: anti-apoptotic proteins with tumorigenic properties. Cell Cycle 5, 2592–2601. doi: 10.4161/cc.5.22.3448
Gaschler, M. M., and Stockwell, B. R. (2017). Lipid peroxidation in cell death. Biochem. Biophys. Res. Commun. 482, 419–425. doi: 10.1016/j.bbrc.2016.10.086
Giffard, R. G., and Yenari, M. A. (2004). Many mechanisms for Hsp70 protection from cerebral ischemia. J. Neurosurg. Anesthesiol. 16, 53–61. doi: 10.1097/00008506-200401000-00010
Ginet, V., Spiehlmann, A., Rummel, C., Rudinskiy, N., Grishchuk, Y., Luthi-Carter, R., et al. (2014). Involvement of autophagy in hypoxic-excitotoxic neuronal death. Autophagy 10, 846–860. doi: 10.4161/auto.28264
Goguadze, N., Zhuravliova, E., Morin, D., Mikeladze, D., and Maurice, T. (2019). Sigma-1 receptor agonists induce oxidative stress in mitochondria and enhance complex i activity in physiological condition but protect against pathological oxidative stress. Neurotox. Res. 35, 1–18. doi: 10.1007/s12640-017-9838-2
Goloubinoff, P., Sassi, A. S., Fauvet, B., Barducci, A., and De Los Rios, P. (2018). Chaperones convert the energy from ATP into the nonequilibrium stabilization of native proteins. Nat. Chem. Biol. 14, 388–395. doi: 10.1038/s41589-018-0013-8
Goyal, M., Demchuk, A. M., Menon, B. K., Eesa, M., Rempel, J. L., Thornton, J., et al. (2015). Randomized assessment of rapid endovascular treatment of ischemic stroke. N. Engl. J. Med. 372, 1019–1030. doi: 10.1056/NEJMoa1414905
Groehler, A., Kren, S., Li, Q., Robledo-Villafane, M., Schmidt, J., Garry, M., et al. (2018). Oxidative cross-linking of proteins to DNA following ischemia-reperfusion injury. Free Radic. Biol. Med. 120, 89–101. doi: 10.1016/j.freeradbiomed.2018.03.010
Groten, C. J., and MacVicar, B. A. (2022). Mitochondrial Ca2+ uptake by the MCU facilitates pyramidal neuron excitability and metabolism during action potential firing. Commun. Biol. 5:900. doi: 10.1038/s42003-022-03848-1
Gruden, G., Barutta, F., Catto, I., Bosco, G., Caprioli, M. G., Pinach, S., et al. (2013). Serum levels of heat shock protein 27 in patients with acute ischemic stroke. Cell Stress Chaperones 18, 531–533. doi: 10.1007/s12192-013-0403-5
Gu, R.-F., Fang, T., Nelson, A., Gyoneva, S., Gao, B., Hedde, J., et al. (2021). Proteomic characterization of the dynamics of ischemic stroke in mice. J. Proteome Res. 20, 3689–3700. doi: 10.1021/acs.jproteome.1c00259
Guo, D., Ma, J., Li, T., and Yan, L. (2018). Up-regulation of miR-122 protects against neuronal cell death in ischemic stroke through the heat shock protein 70-dependent NF-κB pathway by targeting FOXO3. Exp. Cell Res. 369, 34–42. doi: 10.1016/j.yexcr.2018.04.027
Gupta, A., Bansal, A., and Hashimoto-Torii, K. (2020). HSP70 and HSP90 in neurodegenerative diseases. Neurosci. Lett. 716:134678. doi: 10.1016/j.neulet.2019.134678
Guzhova, I., Kislyakova, K., Moskaliova, O., Fridlanskaya, I., Tytell, M., Cheetham, M., et al. (2001). In vitro studies show that Hsp70 can be released by glia and that exogenous Hsp70 can enhance neuronal stress tolerance. Brain Res. 914, 66–73. doi: 10.1016/S0006-8993(01)02774-3
Guzzo, G., Sciacovelli, M., Bernardi, P., and Rasola, A. (2014). Inhibition of succinate dehydrogenase by the mitochondrial chaperone TRAP1 has anti-oxidant and anti-apoptotic effects on tumor cells. Oncotarget 5, 11897–11908. doi: 10.18632/oncotarget.2472
Halliwell, B., and Gutteridge, J. M. C. (2015). Free Radicals in Biology and Medicine. Fifth edition. Oxford, United Kingdom: Oxford University Press. doi: 10.1093/acprof:oso/9780198717478.001.0001
Hammond, C., Braakman, I., and Helenius, A. (1994). Role of N-linked oligosaccharide recognition, glucose trimming, and calnexin in glycoprotein folding and quality control. Proc. Natl. Acad. Sci. U.S.A. 91, 913–917. doi: 10.1073/pnas.91.3.913
Hartl, F. U. (1991). Heat shock proteins in protein folding and membrane translocation. Semin. Immunol. 3, 5–16.
Hartl, F. U., Bracher, A., and Hayer-Hartl, M. (2011). Molecular chaperones in protein folding and proteostasis. Nature 475, 324–332. doi: 10.1038/nature10317
Havalová, H., Ondrovičová, G., Keresztesová, B., Bauer, J. A., Pevala, V., Kutejová, E., et al. (2021). Mitochondrial HSP70 chaperone system—the influence of post-translational modifications and involvement in human diseases. IJMS 22:8077. doi: 10.3390/ijms22158077
Hayashi, T., and Su, T.- P. (2005). The sigma receptor: evolution of the concept in neuropsychopharmacology. CN 3, 267–280. doi: 10.2174/157015905774322516
Hayashi, T., and Su, T.-P. (2007). Sigma-1 receptor chaperones at the ER- mitochondrion interface regulate Ca2+ signaling and cell survival. Cell 131, 596–610. doi: 10.1016/j.cell.2007.08.036
Hayashi, T., and Su, T. P. (2001). Regulating ankyrin dynamics: roles of sigma-1 receptors. Proc. Natl. Acad. Sci. USA. 98, 491–496. doi: 10.1073/pnas.98.2.491
He, F., Ru, X., and Wen, T. (2020). NRF2, a transcription factor for stress response and beyond. Int. J. Mol. Sci. 21:4777. doi: 10.3390/ijms21134777
Heneka, M. T., Sharp, A., Klockgether, T., Gavrilyuk, V., and Feinstein, D. L. (2000). The heat shock response inhibits NF-kappaB activation, nitric oxide synthase type 2 expression, and macrophage/microglial activation in brain. J. Cereb. Blood Flow Metab. 20, 800–811. doi: 10.1097/00004647-200005000-00006
Hernansanz-Agustín, P., Izquierdo-Álvarez, A., Sánchez-Gómez, F. J., Ramos, E., Villa-Piña, T., Lamas, S., et al. (2014). Acute hypoxia produces a superoxide burst in cells. Free Radic. Biol. Med. 71, 146–156. doi: 10.1016/j.freeradbiomed.2014.03.011
Hernansanz-Agustín, P., Ramos, E., Navarro, E., Parada, E., Sánchez-López, N., Peláez-Aguado, L., et al. (2017). Mitochondrial complex I deactivation is related to superoxide production in acute hypoxia. Redox Biol. 12, 1040–1051. doi: 10.1016/j.redox.2017.04.025
Herrmann, J. M., Stuart, R. A., Craig, E. A., and Neupert, W. (1994). Mitochondrial heat shock protein 70, a molecular chaperone for proteins encoded by mitochondrial DNA. J. Cell Biol. 127, 893–902. doi: 10.1083/jcb.127.4.893
Hetz, C. (2012). The unfolded protein response: controlling cell fate decisions under ER stress and beyond. Nat. Rev. Mol. Cell Biol. 13, 89–102. doi: 10.1038/nrm3270
Hino, C., Chan, G., Jordaan, G., Chang, S. S., Saunders, J. T., Bashir, M. T., et al. (2023). Cellular protection from H2O2 toxicity by Fv-Hsp70: protection via catalase and gamma-glutamyl-cysteine synthase. Cell Stress Chaperones 28, 429–439. doi: 10.1007/s12192-023-01349-6
Hoehn, B., Ringer, T. M., Xu, L., Giffard, R. G., Sapolsky, R. M., Steinberg, G. K., et al. (2001). Overexpression of HSP72 after induction of experimental stroke protects neurons from ischemic damage. J. Cereb. Blood Flow Metab. 21, 1303–1309. doi: 10.1097/00004647-200111000-00006
Hong, J., Sha, S., Zhou, L., Wang, C., Yin, J., and Chen, L. (2015). Sigma-1 receptor deficiency reduces MPTP-induced parkinsonism and death of dopaminergic neurons. Cell Death Dis. 6, e1832–e1832. doi: 10.1038/cddis.2015.194
Hossmann, K.-A. (2009). Pathophysiological basis of translational stroke research. Folia Neuropathol. 47, 213–227.
Hu, C., Yang, J., Qi, Z., Wu, H., Wang, B., Zou, F., et al. (2022a). Heat shock proteins: Biological functions, pathological roles, and therapeutic opportunities. Med. Comm. 3:e161. doi: 10.1002/mco2.161
Hu, D., Mo, X., Luo, J., Wang, F., Huang, C., Xie, H., et al. (2023b). 17-DMAG ameliorates neuroinflammation and BBB disruption via SOX5 mediated PI3K/Akt pathway after intracerebral hemorrhage in rats. Int. Immunopharmacol. 123:110698. doi: 10.1016/j.intimp.2023.110698
Hu, L., Sun, C., Kidd, J. M., Han, J., Fang, X., Li, H., et al. (2023a). A first-in-class inhibitor of Hsp110 molecular chaperones of pathogenic fungi. Nat. Commun. 14:2745. doi: 10.1038/s41467-023-38220-2
Hu, S., Feng, J., Wang, M., Wufuer, R., Liu, K., Zhang, Z., et al. (2022b). Nrf1 is an indispensable redox-determining factor for mitochondrial homeostasis by integrating multi-hierarchical regulatory networks. Redox Biol. 57:102470. doi: 10.1016/j.redox.2022.102470
Hulina, A., Grdić Rajković, M., Jakšić Despot, D., Jelić, D., Dojder, A., Cepelak, I., et al. (2018). Extracellular Hsp70 induces inflammation and modulates LPS/LTA-stimulated inflammatory response in THP-1 cells. Cell Stress Chaper. 23, 373–384. doi: 10.1007/s12192-017-0847-0
Hurford, R., Sekhar, A., Hughes, T. A. T., and Muir, K. W. (2020). Diagnosis and management of acute ischaemic stroke. Pract. Neurol. 20, 304–316. doi: 10.1136/practneurol-2020-002557
Imahori, T., Hosoda, K., Nakai, T., Yamamoto, Y., Irino, Y., Shinohara, M., et al. (2017). Combined metabolic and transcriptional profiling identifies pentose phosphate pathway activation by HSP27 phosphorylation during cerebral ischemia. Neuroscience 349, 1–16. doi: 10.1016/j.neuroscience.2017.02.036
Izaki, K., Kinouchi, H., Watanabe, K., Owada, Y., Okubo, A., Itoh, H., et al. (2001). Induction of mitochondrial heat shock protein 60 and 10 mRNAs following transient focal cerebral ischemia in the rat. Brain Res. Mol. Brain Res. 88, 14–25. doi: 10.1016/S0169-328X(01)00012-2
Jackson, S. E. (2012). “Hsp90: structure and function,” in Molecular Chaperones, ed. S. Jackson (Berlin, Heidelberg: Springer), 155–240. doi: 10.1007/128_2012_356
Jadhav, P., Karande, M., Sarkar, A., Sahu, S., Sarmah, D., Datta, A., et al. (2023). Glial cells response in stroke. Cell. Mol. Neurobiol. 43, 99–113. doi: 10.1007/s10571-021-01183-3
Jia, J., Yang, L., Chen, Y., Zheng, L., Chen, Y., Xu, Y., et al. (2022). The role of microglial phagocytosis in ischemic stroke. Front. Immunol. 12:790201. doi: 10.3389/fimmu.2021.790201
Jiang, Y., He, R., Shi, Y., Liang, J., and Zhao, L. (2020). Plasma exosomes protect against cerebral ischemia/reperfusion injury via exosomal HSP70 mediated suppression of ROS. Life Sci. 256:117987. doi: 10.1016/j.lfs.2020.117987
Jo, H. S., Kim, D. W., Shin, M. J., Cho, S. B., Park, J. H., Lee, C. H., et al. (2017). Tat-HSP22 inhibits oxidative stress-induced hippocampal neuronal cell death by regulation of the mitochondrial pathway. Mol. Brain 10:1. doi: 10.1186/s13041-016-0281-8
Kalmar, B., Novoselov, S., Gray, A., Cheetham, M. E., Margulis, B., and Greensmith, L. (2008). Late-stage treatment with arimoclomol delays disease progression and prevents protein aggregation in the SOD1 G93A mouse model of ALS. J. Neurochem. 107, 339–350. doi: 10.1111/j.1471-4159.2008.05595.x
Kampinga, H. H., Hageman, J., Vos, M. J., Kubota, H., Tanguay, R. M., Bruford, E. A., et al. (2009). Guidelines for the nomenclature of the human heat shock proteins. Cell Stress Chaperones. 14, 105–111. doi: 10.1007/s12192-008-0068-7
Kashyap, B., Pegorsch, L., Frey, R. A., Sun, C., Shelden, E. A., and Stenkamp, D. L. (2014). Eye-specific gene expression following embryonic ethanol exposure in zebrafish: roles for heat shock factor 1. Reprod. Toxicol. 43, 111–124. doi: 10.1016/j.reprotox.2013.12.002
Kehm, R., Baldensperger, T., Raupbach, J., and Höhn, A. (2021). Protein oxidation – Formation mechanisms, detection and relevance as biomarkers in human diseases. Redox Biol. 42:101901. doi: 10.1016/j.redox.2021.101901
Kelly, S., Zhang, Z. J., Zhao, H., Xu, L., Giffard, R. G., Sapolsky, R. M., et al. (2002). Gene transfer of HSP72 protects cornu ammonis 1 region of the hippocampus neurons from global ischemia: Influence of Bcl-2. Ann. Neurol. 52, 160–167. doi: 10.1002/ana.10264
Kesaraju, S., Nayak, G., Prentice, H. M., and Milton, S. L. (2014). Upregulation of Hsp72 mediates anoxia/reoxygenation neuroprotection in the freshwater turtle via modulation of ROS. Brain Res. 1582, 247–256. doi: 10.1016/j.brainres.2014.07.044
Kieran, D., Kalmar, B., Dick, J. R. T., Riddoch-Contreras, J., Burnstock, G., and Greensmith, L. (2004). Treatment with arimoclomol, a coinducer of heat shock proteins, delays disease progression in ALS mice. Nat. Med. 10, 402–405. doi: 10.1038/nm1021
Kiffin, R., Christian, C., Knecht, E., and Cuervo, A. M. (2004). Activation of chaperone-mediated autophagy during oxidative stress. MBoC 15, 4829–4840. doi: 10.1091/mbc.e04-06-0477
Kim, J. Y., Han, Y., Lee, J. E., and Yenari, M. A. (2018). The 70-kDa heat shock protein (Hsp70) as a therapeutic target for stroke. Expert Opin. Ther. Targets 22, 191–199. doi: 10.1080/14728222.2018.1439477
Kim, J. Y., Kim, J. W., and Yenari, M. A. (2020). Heat shock protein signaling in brain ischemia and injury. Neurosci. Lett. 715:134642. doi: 10.1016/j.neulet.2019.134642
Kim, J. Y., Yenari, M. A., and Lee, J. E. (2015). Regulation of inflammatory transcription factors by heat shock protein 70 in primary cultured astrocytes exposed to oxygen-glucose deprivation. Neuroscience 286, 272–280. doi: 10.1016/j.neuroscience.2014.11.057
Kim, S.-A., Chang, S., Yoon, J.-H., and Ahn, S.-G. (2008). TAT-Hsp40 inhibits oxidative stress-mediated cytotoxicity via the inhibition of Hsp70 ubiquitination. FEBS Lett. 582, 734–740. doi: 10.1016/j.febslet.2008.01.053
Kim, S.-W., and Lee, J.-K. (2007). NO-induced downregulation of HSP10 and HSP60 expression in the postischemic brain. J. Neurosci. Res. 85, 1252–1259. doi: 10.1002/jnr.21236
Kirbach, B. B., and Golenhofen, N. (2011). Differential expression and induction of small heat shock proteins in rat brain and cultured hippocampal neurons. J. Neurosci. Res. 89, 162–175. doi: 10.1002/jnr.22536
Klyosova, E., and Yu. (2022). Genetic variation of ERN1 and susceptibility to type 2 diabetes. RR. B. 8, 268–277. doi: 10.18413/2658-6533-2022-8-3-0-1
Kobzeva, K. A., Shilenok, I. V., Belykh, A. E., Gurtovoy, D. E., Bobyleva, L. A., Krapiva, A. B., et al. (2022). C9orf16 (BBLN) gene, encoding a member of Hero proteins, is a novel marker in ischemic stroke risk. RR. B. 8, 278–292. doi: 10.18413/2658-6533-2022-8-3-0-2
Kobzeva, K. A., Soldatova, M. O., Stetskaya, T. A., Soldatov, V. O., Deykin, A. V., Freidin, M. B., et al. (2023). Association between HSPA8 gene variants and ischemic stroke: a pilot study providing additional evidence for the role of heat shock proteins in disease pathogenesis. Genes 14:1171. doi: 10.3390/genes14061171
Komarova, E. Y., Meshalkina, D. A., Aksenov, N. D., Pchelin, I. M., Martynova, E., Margulis, B. A., et al. (2015). The discovery of Hsp70 domain with cell-penetrating activity. Cell Stress Chaper. 20, 343–354. doi: 10.1007/s12192-014-0554-z
Kosti, A., de Araujo, P. R., Li, W. Q., Guardia, G. D. A., Chiou, J., Yi, C., et al. (2020). The RNA-binding protein SERBP1 functions as a novel oncogenic factor in glioblastoma by bridging cancer metabolism and epigenetic regulation. Genome Biol. 21:195. doi: 10.1186/s13059-020-02115-y
Krause, M., Heck, T. G., Bittencourt, A., Scomazzon, S. P., Newsholme, P., Curi, R., et al. (2015). The chaperone balance hypothesis: the importance of the extracellular to intracellular HSP70 ratio to inflammation-driven type 2 diabetes, the effect of exercise, and the implications for clinical management. Med. Inflamm. 2015:249205. doi: 10.1155/2015/249205
Kumar, S., Moniruzzaman, M., Chakraborty, A., Sarbajna, A., and Chakraborty, S. B. (2021). Crosstalk between heat shock proteins, NRF2, NF-κB and different endogenous antioxidants during lead-induced hepatotoxicity in Puntius ticto. Aquat. Toxicol. 233:105771. doi: 10.1016/j.aquatox.2021.105771
Kumsta, C., and Jakob, U. (2009). Redox-regulated chaperones. Biochemistry 48, 4666–4676. doi: 10.1021/bi9003556
Lai, Y., Du, L., Dunsmore, K. E., Jenkins, L. W., Wong, H. R., and Clark, R. S. B. (2005). Selectively increasing inducible heat shock protein 70 via TAT-protein transduction protects neurons from nitrosative stress and excitotoxicity. J. Neurochem. 94, 360–366. doi: 10.1111/j.1471-4159.2005.03212.x
Lan, B., Ge, J. W., Cheng, S. W., Zheng, X. L., Liao, J., He, C., et al. (2020). Extract of Naotaifang, a compound Chinese herbal medicine, protects neuron ferroptosis induced by acute cerebral ischemia in rats. J. Integr. Med. 18, 344–350. doi: 10.1016/j.joim.2020.01.008
Lanneau, D., de Thonel, A., Maurel, S., Didelot, C., and Garrido, C. (2007). Apoptosis versus cell differentiation: role of heat shock proteins HSP90, HSP70 and HSP27. Prion. 1, 53–60. doi: 10.4161/pri.1.1.4059
Lasbleiz, C., Peyrel, A., Tarot, P., Sarniguet, J., Crouzier, L., Cubedo, N., et al. (2022). Sigma-1 receptor agonist PRE-084 confers protection against TAR DNA-binding protein-43 toxicity through NRF2 signaling. Redox Biol. 58, 102542. doi: 10.1016/j.redox.2022.102542
Le, S., Fu, X., Pang, M., Zhou, Y., Yin, G., Zhang, J., et al. (2022). The antioxidative role of chaperone-mediated autophagy as a downstream regulator of oxidative stress in human diseases. Technol. Cancer Res. Treat. 21:153303382211141. doi: 10.1177/15330338221114178
Leak, R. K., Zhang, L., Stetler, R. A., Weng, Z., Li, P., Atkins, G. B., et al. (2013). HSP27 protects the blood-brain barrier against ischemia-induced loss of integrity. CNSNDDT 12, 325–337. doi: 10.2174/1871527311312030006
Lee, J. M., Grabb, M. C., Zipfel, G. J., and Choi, D. W. (2000). Brain tissue responses to ischemia. J. Clin. Invest. 106, 723–731. doi: 10.1172/JCI11003
Lee, Y. J., Wei, H. M., Chen, L. Y., and Li, C. (2014). Localization of SERBP1 in stress granules and nucleoli. FEBS J.281, 352–364. doi: 10.1111/febs.12606
Lei, L., Yang, S., Lu, X., Zhang, Y., and Li, T. (2021). Research progress on the mechanism of mitochondrial autophagy in cerebral stroke. Front. Aging Neurosci. 13:698601. doi: 10.3389/fnagi.2021.698601
Leu, T., Schützhold, V., Fandrey, J., and Ferenz, K. B. (2019). When the brain yearns for oxygen. Neurosignals. 27, 50–61. doi: 10.33594/000000199
Li, F., Tan, J., Zhou, F., Hu, Z., and Yang, B. (2018). Heat shock protein B8 (HSPB8) reduces oxygen-glucose deprivation/reperfusion injury via the induction of mitophagy. Cell. Physiol. Biochem. 48, 1492–1504. doi: 10.1159/000492259
Li, W., Ward, R., Dong, G., Ergul, A., and O'Connor, P. (2019). Neurovascular protection in voltage-gated proton channel Hv1 knock-out rats after ischemic stroke: interaction with Na+/H+ exchanger-1 antagonism. Physiol. Rep. 7:e14142. doi: 10.14814/phy2.14142
Li, X., Du, H., Song, Z., Wang, H., Tan, Z., Xiao, M., et al. (2020). Efficacy and safety of sonothrombolysis in patients with acute ischemic stroke: a systematic review and meta-analysis. J. Neurol. Sci. 416:116998. doi: 10.1016/j.jns.2020.116998
Li, X., Fang, P., Mai, J., Choi, E. T., Wang, H., and Yang, X. (2013). Targeting mitochondrial reactive oxygen species as novel therapy for inflammatory diseases and cancers. J. Hematol. Oncol. 6:19. doi: 10.1186/1756-8722-6-19
Li, Z., Zhou, J., Cui, S., Hu, S., Li, B., Liu, X., et al. (2024). Activation of sigma-1 receptor ameliorates sepsis-induced myocardial injury by mediating the Nrf2/HO1 signaling pathway to attenuate mitochondrial oxidative stress. Int. Immunopharmacol. 127:111382. doi: 10.1016/j.intimp.2023.111382
Lin, Q. C., Wang, J., Wang, X. L., Pan, C., Jin, S. W., Char, S., et al. (2024). Hippocampal HDAC6 promotes POCD by regulating NLRP3-induced microglia pyroptosis via HSP90/HSP70 in aged mice. Biochimica et biophysica acta. Molec. Basis Dis. 1870:167137. doi: 10.1016/j.bbadis.2024.167137
Lin, S., Wang, Y., Zhang, X., Kong, Q., Li, C., Li, Y., et al. (2016). HSP27 alleviates cardiac aging in mice via a mechanism involving antioxidation and mitophagy activation. Oxid. Med. Cell. Longev. 2016, 1–13. doi: 10.1155/2016/2586706
Lindquist, S., and Craig, E. A. (1988). The heat-shock proteins. Annu. Rev. Genet. 22, 631–677. doi: 10.1146/annurev.ge.22.120188.003215
Liu, L., Zhang, X. J., Jiang, S. R., Ding, Z. N., Ding, G. X., Huang, J., et al. (2007). Heat shock protein 27 regulates oxidative stress-induced apoptosis in cardiomyocytes: mechanisms via reactive oxygen species generation and Akt activation. Chin. Med. J. 120, 2271–2277. doi: 10.1097/00029330-200712020-00023
Liu, S., Levine, S. R., and Winn, H. R. (2010a). Targeting ischemic penumbra: part I – from pathophysiology to therapeutic strategy. J. Exp. Stroke Transl. Med. 3, 47–55. doi: 10.6030/1939-067X-3.1.47
Liu, S.-P., Ding, D.-C., Wang, H.-J., Su, C.-Y., Lin, S.-Z., Li, H., et al. (2010b). Nonsenescent Hsp27-Upregulated MSCs implantation promotes neuroplasticity in stroke model. Cell Transplant. 19, 1261–1279. doi: 10.3727/096368910X507204
Liu, X., Liu, K., Li, C., Cai, J., Huang, L., Chen, H., et al. (2019). Heat-shock protein B1 upholds the cytoplasm reduced state to inhibit activation of the hippo pathway in H9c2 cells. J. Cell. Physiol. 234, 5117–5133. doi: 10.1002/jcp.27322
Liyanagamage, D. S. N. K., and Martinus, R. D. (2020). Role of mitochondrial stress protein HSP60 in diabetes-induced neuroinflammation. Mediators Inflamm. 2020, 1–8. doi: 10.1155/2020/8073516
Lu, C.-W., Lin, T.-Y., Wang, C.-C., and Wang, S.-J. (2012). σ-1 receptor agonist SKF10047 inhibits glutamate release in rat cerebral cortex nerve endings. J. Pharmacol. Exp. Ther. 341, 532–542. doi: 10.1124/jpet.111.191189
Lu, Y., and Eguchi, T. (2020). “HSP stimulation on macrophages and dendritic cells activates innate immune system,” in Heat Shock Proteins in Inflammatory Diseases, eds. A.A.A. Asea and P. Kaur (Cham: Springer International Publishing), 53–67. doi: 10.1007/7515_2020_26
Luecke, I. W., Lin, G., Santarriaga, S., Scaglione, K. M., and Ebert, A. D. (2023). Viral vector gene delivery of the novel chaperone protein SRCP1 to modify insoluble protein in in vitro and in vivo models of ALS. Gene Ther. 30, 528–533. doi: 10.1038/s41434-021-00276-4
Luo, S., Wang, T., Qin, H., Lei, H., and Xia, Y. (2011). Obligatory role of heat shock protein 90 in INOS induction. Am. J. Physiol. Physiol. 301, C227–C233. doi: 10.1152/ajpcell.00493.2010
Macario, A. J. L. (2007). Molecular chaperones: multiple functions, pathologies, and potential applications. Front. Biosci. 12:2588. doi: 10.2741/2257
Macario, A. J. L., and Conway de Macario, E. (2005). Sick chaperones, cellular stress, and disease. N. Engl. J. Med. 353, 1489–1501. doi: 10.1056/NEJMra050111
Manaenko, A., Fathali, N., Chen, H., Suzuki, H., Williams, S., Zhang, J. H., et al. (2010). Heat shock protein 70 upregulation by geldanamycin reduces brain injury in a mouse model of intracerebral hemorrhage. Neurochem. Int. 57, 844–850. doi: 10.1016/j.neuint.2010.09.001
Manaenko, A., Fathali, N., Williams, S., Lekic, T., Zhang, J. H., and Tang, J. (2011). “Geldanamycin reduced brain injury in mouse model of intracerebral hemorrhage,” in Intracerebral Hemorrhage Research, eds. J. Zhang and A. Colohan (Vienna: Springer Vienna), 161–165. doi: 10.1007/978-3-7091-0693-8_27
Mandalaneni, K., Rayi, A., and Jillella, D. V. (2024). “Stroke reperfusion injury,” in StatPearls, Treasure Island (FL): StatPearls Publishing.
Mandrioli, J., Crippa, V., Cereda, C., Bonetto, V., Zucchi, E., Gessani, A., et al. (2019). Proteostasis and ALS: protocol for a phase II, randomised, double-blind, placebo-controlled, multicentre clinical trial for colchicine in ALS (Co-ALS). BMJ Open 9:e028486. doi: 10.1136/bmjopen-2018-028486
Manford, A. G., Mena, E. L., Shih, K. Y., Gee, C. L., McMinimy, R., Martínez-González, B., et al. (2021). Structural basis and regulation of the reductive stress response. Cell 184, 5375–5390. doi: 10.1016/j.cell.2021.09.002
Manford, A. G., Rodriguez-Perez, F., Shih, K. Y., Shi, Z., Berdan, C. A., Choe, M., et al. (2020). A cellular mechanism to detect and alleviate reductive stress. Cell 183, 46–61. doi: 10.1016/j.cell.2020.08.034
Marino Gammazza, A., Caruso Bavisotto, C., David, S., Barone, R., Rappa, F., Campanella, C., et al. (2017). HSP60 is a ubiquitous player in the physiological and pathogenic interactions between the chaperoning and the immune systems. Curr. Immunol. Rev. 13, 44–55. doi: 10.2174/1573395513666170412170540
Martín de la Vega, C., Burda, J., Nemethova, M., Quevedo, C., Alcázar, A., Martín, M. E., et al. (2001). Possible mechanisms involved in the down-regulation of translation during transient global ischaemia in the rat brain. Biochem. J.357, 819–826. doi: 10.1042/bj3570819
Maulik, D., Ashraf, Q. M., Mishra, O. P., and Delivoria-Papadopoulos, M. (2002). Effect of hypoxia on calcium influx and calcium/calmodulin-dependent kinase activity in cortical neuronal nuclei of the guinea pig fetus during development. Am. J. Obstet. Gynecol. 186, 658–662. doi: 10.1067/mob.2002.122392
Mayer, M. P., and Bukau, B. (2005). Hsp70 chaperones: cellular functions and molecular mechanism. Cell. Mol. Life Sci. 62, 670–684. doi: 10.1007/s00018-004-4464-6
McCormack, J. G., and Denton, R. M. (1993). Mitochondrial Ca2+ transport and the role of intramitochondrial Ca2+ in the regulation of energy metabolism. Dev. Neurosci. 15, 165–173. doi: 10.1159/000111332
Mehlen, P., Kretz-Remy, C., Préville, X., and Arrigo, A. P. (1996). Human hsp27, Drosophila hsp27 and human alphaB-crystallin expression-mediated increase in glutathione is essential for the protective activity of these proteins against TNFalpha-induced cell death. EMBO J. 15, 2695–2706. doi: 10.1002/j.1460-2075.1996.tb00630.x
Metwally, S. A. H., Paruchuri, S. S., Yu, L., Capuk, O., Pennock, N., Sun, D., et al. (2023). Pharmacological inhibition of NHE1 protein increases white matter resilience and neurofunctional recovery after ischemic stroke. Int. J. Mol. Sci. 24:13289. doi: 10.3390/ijms241713289
Mi, J., Yang, Y., Yao, H., Huan, Z., Xu, C., Ren, Z., et al. (2021). Inhibition of heat shock protein family A member 8 attenuates spinal cord ischemia-reperfusion injury via astrocyte NF-κB/NLRP3 inflammasome pathway: HSPA8 inhibition protects spinal ischemia-reperfusion injury. J. Neuroinflammation. 18, 170. doi: 10.1186/s12974-021-02220-0
Miao, Z., Tian, W., Ye, Y., Gu, W., Bao, Z., Xu, L., et al. (2022). Hsp90 induces Acsl4-dependent glioma ferroptosis via dephosphorylating Ser637 at Drp1. Cell Death Dis. 13, 548. doi: 10.1038/s41419-022-04997-1
Miki, Y., Mori, F., Kon, T., Tanji, K., Toyoshima, Y., Yoshida, M., et al. (2014). Accumulation of the sigma-1 receptor is common to neuronal nuclear inclusions in various neurodegenerative diseases. Neuropathology 34, 148–158. doi: 10.1111/neup.12080
Mitra, R., Wu, K., Lee, C., and Bardwell, J. C. A. (2022). ATP-independent chaperones. Annu. Rev. Biophys. 51, 409–429. doi: 10.1146/annurev-biophys-090121-082906
Mohammadi, F., Nezafat, N., Negahdaripour, M., Dabbagh, F., Haghghi, A. B., Kianpour, S., et al. (2018). Neuroprotective effects of heat shock protein70. CNSNDDT 17, 736–742. doi: 10.2174/1871527317666180827111152
Moloney, T. C., Hyland, R., O'Toole, D., Paucard, A., Kirik, D., O'Doherty, A., et al. (2014). Heat shock protein 70 reduces α-synuclein-induced predegenerative neuronal dystrophy in the α-synuclein viral gene transfer rat model of Parkinson's disease. CNS Neurosci. Ther. 20, 50–58. doi: 10.1111/cns.12200
Mori, T., Hayashi, T., Hayashi, E., and Su, T.-P. (2013). Sigma-1 Receptor Chaperone at the ER-mitochondrion interface mediates the mitochondrion-ER-nucleus signaling for cellular survival. PLoS ONE 8:e76941. doi: 10.1371/journal.pone.0076941
Morimoto, E., Tsuboyama, K., and Tomari, Y. (2022). Fusion with heat-resistant obscure (Hero) proteins have the potential to improve the molecular property of recombinant proteins. PLoS ONE 17:e0270097. doi: 10.1371/journal.pone.0270097
Morris, B. J., Willcox, D. C., Donlon, T. A., and Willcox, B. J. (2015). FOXO3: a major gene for human longevity – a mini-review. Gerontology 61, 515–525. doi: 10.1159/000375235
Murphy, M. E. (2013). The HSP70 family and cancer. Carcinogenesis 34, 1181–1188. doi: 10.1093/carcin/bgt111
Murphy, P. J. M., Kanelakis, K. C., Galigniana, M. D., Morishima, Y., and Pratt, W. B. (2001). Stoichiometry, abundance, and functional significance of the hsp90/hsp70-based multiprotein chaperone machinery in reticulocyte lysate. J. Biol. Chem. 276, 30092–30098. doi: 10.1074/jbc.M103773200
Narita, N., Hashimoto, K., Tomitaka, S., Minabe, Y., and Yamazaki, K. (1997). YM90K, a selective-amino-3-hydroxy5-methyl-4-isoxazole propionate (AMPA) receptor antagonist, prevents induction of heat shock protein HSP−70 and hsp−70 mRNA in rat retrosplenial cortex by phencyclidine. Addict. Biol. 2, 47–56. doi: 10.1080/13556219772859
Navas-Pérez, E., Vicente-García, C., Mirra, S., Burguera, D., Fernàndez-Castillo, N., Ferrán, J. L., et al. (2020). Characterization of a eutherian gene cluster generated after transposon domestication identifies Bex3 as relevant for advanced neurological functions. Genome Biol. 21:267. doi: 10.1186/s13059-020-02172-3
Nguyen, L., Lucke-Wold, B. P., Mookerjee, S., Kaushal, N., and Matsumoto, R. R. (2017). “Sigma-1 receptors and neurodegenerative diseases: towards a hypothesis of sigma-1 receptors as amplifiers of neurodegeneration and neuroprotection,” in Sigma Receptors: Their Role in Disease and as Therapeutic Targets, eds. S. B. Smith and T.-P. Su (Cham: Springer International Publishing), 133–152. doi: 10.1007/978-3-319-50174-1_10
Nie, T., Tao, K., Zhu, L., Huang, L., Hu, S., Yang, R., et al. (2021). Chaperone-mediated autophagy controls the turnover of E3 ubiquitin ligase MARCHF5 and regulates mitochondrial dynamics. Autophagy 17, 2923–2938. doi: 10.1080/15548627.2020.1848128
Niforou, K., Cheimonidou, C., and Trougakos, I. P. (2014). Molecular chaperones and proteostasis regulation during redox imbalance. Redox Biol. 2, 323–332. doi: 10.1016/j.redox.2014.01.017
Njemini, R., Demanet, C., and Mets, T. (2004). Inflammatory status as an important determinant of heat shock protein 70 serum concentrations during aging. Biogerontology 5, 31–38. doi: 10.1023/B:BGEN.0000017684.15626.29
O'Donnell, B. R., and Bickler, P. E. (1994). Influence of pH on calcium influx during hypoxia in rat cortical brain slices. Stroke 25, 171–177. doi: 10.1161/01.STR.25.1.171
Odunewu-Aderibigbe, A., and Fliegel, L. (2017a). Heat shock proteins and the Na+/H+ exchanger. Channels 11, 380–382. doi: 10.1080/19336950.2017.1347001
Odunewu-Aderibigbe, A., and Fliegel, L. (2017b). Protein mediated regulation of the NHE1 isoform of the Na+/H+ exchanger in renal cells. A regulatory role of Hsp90 and AKT kinase. Cell. Sign. 36, 145–153. doi: 10.1016/j.cellsig.2017.05.003
Okamoto, T., Yamamoto, H., Kudo, I., Matsumoto, K., Odaka, M., Grave, E., et al. (2017). HSP60 possesses a GTPase activity and mediates protein folding with HSP10. Sci. Rep. 7:16931. doi: 10.1038/s41598-017-17167-7
Omorou, M., Huang, Y., Gao, M., Mu, C., Xu, W., Han, Y., et al. (2023). The forkhead box O3 (FOXO3): a key player in the regulation of ischemia and reperfusion injury. Cell. Mol. Life Sci. 80:102. doi: 10.1007/s00018-023-04755-2
Önay Uçar, E., Sengelen, A., and Mertoglu Kamali, E. (2023). Hsp27, Hsp60, Hsp70, or Hsp90 depletion enhances the antitumor effects of resveratrol via oxidative and ER stress response in human glioblastoma cells. Biochem. Pharmacol. 208:115409. doi: 10.1016/j.bcp.2022.115409
Orciani, C., Do Carmo, S., Foret, M. K., Hall, H., Bonomo, Q., Lavagna, A., et al. (2023). Early treatment with an M1 and sigma-1 receptor agonist prevents cognitive decline in a transgenic rat model displaying Alzheimer-like amyloid pathology. Neurobiol. Aging 132, 220–232. doi: 10.1016/j.neurobiolaging.2023.09.010
O-Uchi, J., Ryu, S.-Y., Jhun, B. S., Hurst, S., and Sheu, S.-S. (2014). Mitochondrial ion channels/transporters as sensors and regulators of cellular redox signaling. Antioxid. Redox Signal. 21, 987–1006. doi: 10.1089/ars.2013.5681
Pal, A., Fontanilla, D., Gopalakrishnan, A., Chae, Y.-K., Markley, J. L., and Ruoho, A. E. (2012). The sigma-1 receptor protects against cellular oxidative stress and activates antioxidant response elements. Eur. J. Pharmacol. 682, 12–20. doi: 10.1016/j.ejphar.2012.01.030
Park, J. A., Lee, J. Y., Sato, T. A., and Koh, J. Y. (2000). Co–induction of p75NTR and p75NTR–associated death executor in neurons after zinc exposure in cortical culture or transient ischemia in the rat. J. Neurosci. 20, 9096–9103. doi: 10.1523/JNEUROSCI.20-24-09096.2000
Penna, C., Sorge, M., Femminò, S., Pagliaro, P., and Brancaccio, M. (2018). Redox aspects of chaperones in cardiac function. Front. Physiol. 9:216. doi: 10.3389/fphys.2018.00216
Perl, A., Hanczko, R., Telarico, T., Oaks, Z., and Landas, S. (2011). Oxidative stress, inflammation and carcinogenesis are controlled through the pentose phosphate pathway by transaldolase. Trends Mol. Med. 17, 395–403. doi: 10.1016/j.molmed.2011.01.014
Piippo, N., Korhonen, E., Hytti, M., Skottman, H., Kinnunen, K., Josifovska, N., et al. (2018). Hsp90 inhibition as a means to inhibit activation of the NLRP3 inflammasome. Sci. Rep. 8:6720. doi: 10.1038/s41598-018-25123-2
Polonikov, A., Bocharova, I., Azarova, I., Klyosova, E., Bykanova, M., Bushueva, O., et al. (2022). The impact of genetic polymorphisms in glutamate-cysteine ligase, a key enzyme of glutathione biosynthesis, on ischemic stroke risk and brain infarct size. Life (Basel) 12:602. doi: 10.3390/life12040602
Polonikov, A., Vialykh, E., Vasil'eva, O., Bulgakova, I., Bushueva, O., Illig, T., et al. (2012). Genetic variation in glutathione S-transferase genes and risk of nonfatal cerebral stroke in patients suffering from essential hypertension. J. Molec. Neurosci. 47, 511–513. doi: 10.1007/s12031-012-9764-y
Porter, J. R., Fritz, C. C., and Depew, K. M. (2010). Discovery and development of Hsp90 inhibitors: a promising pathway for cancer therapy. Curr. Opin. Chem. Biol. 14, 412–420. doi: 10.1016/j.cbpa.2010.03.019
Pras, A., Houben, B., Aprile, F. A., Seinstra, R., Gallardo, R., Janssen, L., et al. (2021). The cellular modifier MOAG-4/SERF drives amyloid formation through charge complementation. EMBO J. 40:e107568. doi: 10.15252/embj.2020107568
Putaala, J., Metso, A. J., Metso, T. M., Konkola, N., Kraemer, Y., Haapaniemi, E., et al. (2009). Analysis of 1008 consecutive patients aged 15 to 49 with first-ever ischemic stroke: the Helsinki young stroke registry. Stroke 40, 1195–1203. doi: 10.1161/STROKEAHA.108.529883
Qi, J., Han, X., Liu, H. T., Chen, T., Zhang, J. L., Yang, P., et al. (2014). 17-Dimethylaminoethylamino-17-demethoxygeldanamycin attenuates inflammatory responses in experimental stroke. Biol. Pharm. Bull. 37, 1713–1718. doi: 10.1248/bpb.b14-00208
Qi, J., Liu, Y., Yang, P., Chen, T., Liu, X. Z., Yin, Y., et al. (2015). Heat shock protein 90 inhibition by 17-Dimethylaminoethylamino-17-demethoxygeldanamycin protects blood-brain barrier integrity in cerebral ischemic stroke. Am. J. Transl. Res. 7, 1826–1837.
Reichmann, D., Voth, W., and Jakob, U. (2018). Maintaining a healthy proteome during oxidative stress. Mol. Cell 69, 203–213. doi: 10.1016/j.molcel.2017.12.021
Rodríguez-Muñoz, M., Onetti, Y., Cortés-Montero, E., Garzón, J., and Sánchez-Blázquez, P. (2018). Cannabidiol enhances morphine antinociception, diminishes NMDA-mediated seizures and reduces stroke damage via the sigma 1 receptor. Mol. Brain 11:51. doi: 10.1186/s13041-018-0395-2
Rollins, G. C., and Dill, K. A. (2014). General mechanism of two-state protein folding kinetics. J. Am. Chem. Soc. 136, 11420–11427. doi: 10.1021/ja5049434
Rosenzweig, A. C. (2002). Metallochaperones. Chem. Biol. 9, 673–677. doi: 10.1016/S1074-5521(02)00156-4
Ruscher, K., Inácio, A. R., Valind, K., Rowshan Ravan, A., Kuric, E., and Wieloch, T. (2012). Effects of the sigma-1 receptor agonist 1-(3,4-dimethoxyphenethyl)-4-(3-phenylpropyl)-piperazine dihydro-chloride on inflammation after stroke. PLoS ONE 7:e45118. doi: 10.1371/journal.pone.0045118
Ruscher, K., Shamloo, M., Rickhag, M., Ladunga, I., Soriano, L., Gisselsson, L., et al. (2011). The sigma-1 receptor enhances brain plasticity and functional recovery after experimental stroke. Brain 134, 732–746. doi: 10.1093/brain/awq367
Rutledge, B. S., Choy, W.-Y., and Duennwald, M. L. (2022). Folding or holding? — Hsp70 and Hsp90 chaperoning of misfolded proteins in neurodegenerative disease. J. Biol. Chem. 298:101905. doi: 10.1016/j.jbc.2022.101905
Salaudeen, M. A., Bello, N., Danraka, R. N., and Ammani, M. L. (2024). Understanding the pathophysiology of ischemic stroke: the basis of current therapies and opportunity for new ones. Biomolecules 14:305. doi: 10.3390/biom14030305
Sanguigno, L., Guida, N., Anzilotti, S., Cuomo, O., Mascolo, L., Serani, A., et al. (2023). Stroke by inducing HDAC9-dependent deacetylation of HIF-1 and Sp1, promotes TfR1 transcription and GPX4 reduction, thus determining ferroptotic neuronal death. Int. J. Biol. Sci. 19, 2695–2710. doi: 10.7150/ijbs.80735
Santra, M., Dill, K. A., and de Graff, A. M. R. (2018). How do chaperones protect a cell's proteins from oxidative damage? Cell Syst. 6, 743–751. doi: 10.1016/j.cels.2018.05.001
Saver, J. L., Goyal, M., Bonafe, A., Diener, H.-C., Levy, E. I., Pereira, V. M., et al. (2015). Stent-retriever thrombectomy after intravenous t-PA vs. t-PA alone in stroke. N. Engl. J. Med. 372, 2285–2295. doi: 10.1056/NEJMoa1415061
Schneider, J. L., Villarroya, J., Diaz-Carretero, A., Patel, B., Urbanska, A. M., Thi, M. M., et al. (2015). Loss of hepatic chaperone-mediated autophagy accelerates proteostasis failure in aging. Aging Cell 14, 249–264. doi: 10.1111/acel.12310
Schopf, F., Biebl, M., and Buchner, J. (2017). The HSP90 chaperone machinery. Nat. Rev. Mol. Cell Biol. 18, 345–360. doi: 10.1038/nrm.2017.20
Sciacovelli, M., Guzzo, G., Morello, V., Frezza, C., Zheng, L., Nannini, N., et al. (2013). The mitochondrial chaperone TRAP1 promotes neoplastic growth by inhibiting succinate dehydrogenase. Cell Metab. 17, 988–999. doi: 10.1016/j.cmet.2013.04.019
Selim, M. H., and Ratan, R. R. (2004). The role of iron neurotoxicity in ischemic stroke. Ageing Res. Rev. 3, 345–353. doi: 10.1016/j.arr.2004.04.001
Semenza, G. L. (2011). Hypoxia-inducible factor 1: Regulator of mitochondrial metabolism and mediator of ischemic preconditioning. Biochim. Biophys. Acta 1813, 1263–1268. doi: 10.1016/j.bbamcr.2010.08.006
Shao, A., Zhou, Y., Yao, Y., Zhang, W., Zhang, J., and Deng, Y. (2019). The role and therapeutic potential of heat shock proteins in haemorrhagic stroke. J. Cell. Mol. Med. 23, 5846–5858. doi: 10.1111/jcmm.14479
Shao, Z., Dou, S., Zhu, J., Wang, H., Xu, D., Wang, C., et al. (2020). The role of mitophagy in ischemic stroke. Front. Neurol. 11:608610. doi: 10.3389/fneur.2020.608610
Sharp, F. R., Butman, M., Koistinaho, J., Aardalen, K., Nakki, R., Massa, S. M., et al. (1994). Phencyclidine induction of the hsp70 stress gene in injured pyramidal neurons is mediated via multiple receptors and voltage gated calcium channels. Neuroscience 62, 1079–1092. doi: 10.1016/0306-4522(94)90345-X
Sharp, F. R., Lu, A., Tang, Y., and Millhorn, D. E. (2000). Multiple molecular penumbras after focal cerebral ischemia. J. Cereb. Blood Flow Metab. 20, 1011–1032. doi: 10.1097/00004647-200007000-00001
Shen, H.-Y., He, J.-C., Wang, Y., Huang, Q.-Y., and Chen, J.-F. (2005). Geldanamycin induces heat shock protein 70 and protects against MPTP-induced dopaminergic neurotoxicity in mice. J. Biol. Chem. 280, 39962–39969. doi: 10.1074/jbc.M505524200
Sheng, R., Zhang, L., Han, R., Gao, B., Liu, X., and Qin, Z. (2011). Combined prostaglandin E1 and lithium exert potent neuroprotection in a rat model of cerebral ischemia. Acta Pharmacol. Sin. 32, 303–310. doi: 10.1038/aps.2010.211
Shi, J.-J., Jiang, Q.-H., Zhang, T.-N., Sun, H., Shi, W.-W., Gunosewoyo, H., et al. (2021). Sigma-1 receptor agonist TS-157 improves motor functional recovery by promoting neurite outgrowth and pERK in rats with focal cerebral ischemia. Molecules 26:1212. doi: 10.3390/molecules26051212
Shi, R., Weng, J., Zhao, L., Li, X. M., Gao, T. M., and Kong, J. (2012). Excessive autophagy contributes to neuron death in cerebral ischemia. CNS Neurosci. Therap. 18, 250–260. doi: 10.1111/j.1755-5949.2012.00295.x
Shilenok, I., Kobzeva, K., Deykin, A., Pokrovsky, V., Patrakhanov, E., and Bushueva, O. (2024b). Obesity and environmental risk factors significantly modify the association between ischemic stroke and the hero chaperone C19orf53. Life 14:1158. doi: 10.3390/life14091158
Shilenok, I., Kobzeva, K., Soldatov, V., Deykin, A., and Bushueva, O. (2024a). C11orf58 (Hero20) gene polymorphism: contribution to ischemic stroke risk and interactions with other heat-resistant obscure chaperones. Biomedicines 12:2603. doi: 10.3390/biomedicines12112603
Shilenok, I., Kobzeva, K., Stetskaya, T., Freidin, M., Soldatova, M., Deykin, A., et al. (2023). SERPINE1 mRNA binding protein 1 is associated with ischemic stroke risk: a comprehensive molecular–genetic and bioinformatics analysis of SERBP1 SNPs. IJMS 24:8716. doi: 10.3390/ijms24108716
Shimada, Y., Shimura, H., Tanaka, R., Yamashiro, K., Koike, M., Uchiyama, Y., et al. (2018). Phosphorylated recombinant HSP27 protects the brain and attenuates blood-brain barrier disruption following stroke in mice receiving intravenous tissue-plasminogen activator. PLoS ONE 13:e0198039. doi: 10.1371/journal.pone.0198039
Shimura, H., Miura-Shimura, Y., and Kosik, K. S. (2004). Binding of tau to heat shock protein 27 leads to decreased concentration of hyperphosphorylated tau and enhanced cell survival. J. Biol. Chem. 279, 17957–17962. doi: 10.1074/jbc.M400351200
Sidhar, H., and Giri, R. (2017). Induction of Bex genes by curcumin is associated with apoptosis and activation of p53 in N2a neuroblastoma cells. Sci. Rep. 7:41420. doi: 10.1038/srep41420
Silveira, M. A. D., Khadangi, F., Mersaoui, S. Y., Naik, D., Masson, J.-Y., and Bilodeau, S. (2023). HSP70 mediates a crosstalk between the estrogen and the heat shock response pathways. J. Biol. Chem. 299:102872. doi: 10.1016/j.jbc.2023.102872
Singh, M. K., Shin, Y., Han, S., Ha, J., Tiwari, P. K., Kim, S. S., et al. (2024). Molecular chaperonin HSP60: current understanding and future prospects. Int. J. Mol. Sci. 25:5483. doi: 10.3390/ijms25105483
Soldatov, V. O., Kubekina, M. V., Skorkina, M., Yu Belykh, A. E., Egorova, T. V., et al. (2022). Current advances in gene therapy of mitochondrial diseases. J. Transl. Med. 20:562. doi: 10.1186/s12967-022-03685-0
Soltys, B. J., and Gupta, R. S. (1997). Cell surface localization of the 60 kDa heat shock chaperonin protein (hsp60) in mammalian cells. Cell Biol. Int. 21, 315–320. doi: 10.1006/cbir.1997.0144
Song, W., Yao, Y., Zhang, H., Hao, X., Zhou, L., Song, Z., et al. (2023). Sigma-1 receptor activation improves oligodendrogenesis and promotes white-matter integrity after stroke in mice with diabetic mellitus. Molecules 28:390. doi: 10.3390/molecules28010390
Soti, C., Pál, C., Papp, B., and Csermely, P. (2005). Molecular chaperones as regulatory elements of cellular networks. Curr. Opin. Cell Biol. 17, 210–215. doi: 10.1016/j.ceb.2005.02.012
Stefano, L., Racchetti, G., Bianco, F., Passini, N., Gupta, R. S., Panina Bordignon, P., et al. (2009). The surface-exposed chaperone, Hsp60, is an agonist of the microglial TREM2 receptor. J. Neurochem. 110, 284–294. doi: 10.1111/j.1471-4159.2009.06130.x
Stetler, R. A., Cao, G., Gao, Y., Zhang, F., Wang, S., Weng, Z., et al. (2008). Hsp27 Protects against ischemic brain injury via attenuation of a novel stress-response cascade upstream of mitochondrial cell death signaling. J. Neurosci. 28, 13038–13055. doi: 10.1523/JNEUROSCI.4407-08.2008
Stetler, R. A., Gao, Y., Signore, A. P., Cao, G., and Chen, J. (2009). HSP27: mechanisms of cellular protection against neuronal injury. Curr. Mol. Med. 9, 863–872. doi: 10.2174/156652409789105561
Stetskaya, T. A., Kobzeva, K. A., Zaytsev, S. M., Shilenok, I. V., Komkova, G. V., Goryainova, N. V., et al. (2024). HSPD1 gene polymorphism is associated with an increased risk of ischemic stroke in smokers. Res. Results Biomed. 10, 175–186. doi: 10.18413/2658-6533-2024-10-2-0-1
Stricher, F., Macri, C., Ruff, M., and Muller, S. (2013). HSPA8/HSC70 chaperone protein: structure, function, and chemical targeting. Autophagy 9, 1937–1954. doi: 10.4161/auto.26448
Stroo, E., Janssen, L., Sin, O., Hogewerf, W., Koster, M., Harkema, L., et al. (2023). Deletion of SERF2 in mice delays embryonic development and alters amyloid deposit structure in the brain. Life Sci. Alliance 6:e202201730. doi: 10.26508/lsa.202201730
Su, T. P., and Hayashi, T. (2001). Cocaine affects the dynamics of cytoskeletal proteins via sigma-(1) receptors. Trends Pharmacol. Sci. 22, 456–458. doi: 10.1016/S0165-6147(00)01740-5
Sumi, M. P., and Ghosh, A. (2022). Hsp90 in human diseases: molecular mechanisms to therapeutic approaches. Cells 11:976. doi: 10.3390/cells11060976
Sun, M.-S., Jin, H., Sun, X., Huang, S., Zhang, F.-L., Guo, Z.-N., et al. (2018). Free radical damage in ischemia-reperfusion injury: an obstacle in acute ischemic stroke after revascularization therapy. Oxid. Med. Cell. Longev. 2018, 1–17. doi: 10.1155/2018/3804979
Sun, X., Crawford, R., Liu, C., Luo, T., and Hu, B. (2015). Development-dependent regulation of molecular chaperones after hypoxia-ischemia. Neurobiol. Dis. 82, 123–131. doi: 10.1016/j.nbd.2015.06.001
Suss, O., and Reichmann, D. (2015). Protein plasticity underlines activation and function of ATP-independent chaperones. Front. Mol. Biosci. 2:43. doi: 10.3389/fmolb.2015.00043
Swaroop, S., Mahadevan, A., Shankar, S. K., Adlakha, Y. K., and Basu, A. (2018). HSP60 critically regulates endogenous IL-1beta production in activated microglia by stimulating NLRP3 inflammasome pathway. J. Neuroinflamm. 15:177. doi: 10.1186/s12974-018-1214-5
Szyller, J., and Bil-Lula, I. (2021). Heat shock proteins in oxidative stress and ischemia/reperfusion injury and benefits from physical exercises: a review to the current knowledge. Oxid. Med. Cell. Longev. 2021, 1–12. doi: 10.1155/2021/6678457
Tajeddine, N. (2016). How do reactive oxygen species and calcium trigger mitochondrial membrane permeabilisation? Biochim. Biophys. Acta 1860, 1079–1088. doi: 10.1016/j.bbagen.2016.02.013
Tan, C., Niitsu, A., and Sugita, Y. (2023). Highly charged proteins and their repulsive interactions antagonize biomolecular condensation. JACS Au 3, 834–848. doi: 10.1021/jacsau.2c00646
Tang, H., Li, J., Liu, X., Wang, G., Luo, M., and Deng, H. (2016). Down-regulation of HSP60 suppresses the proliferation of glioblastoma cells via the ROS/AMPK/mTOR pathway. Sci. Rep. 6:28388. doi: 10.1038/srep28388
Taylor, A. R., Robinson, M. B., Gifondorwa, D. J., Tytell, M., and Milligan, C. E. (2007). Regulation of heat shock protein 70 release in astrocytes: role of signaling kinases. Dev. Neurobiol. 67, 1815–1829. doi: 10.1002/dneu.20559
Tedesco, B., Vendredy, L., Timmerman, V., and Poletti, A. (2023). The chaperone-assisted selective autophagy complex dynamics and dysfunctions. Autophagy 19, 1619–1641. doi: 10.1080/15548627.2022.2160564
Teramoto, S., Shimura, H., Tanaka, R., Shimada, Y., Miyamoto, N., Arai, H., et al. (2013). Human-derived physiological heat shock protein 27 complex protects brain after focal cerebral ischemia in mice. PLoS ONE 8:e66001. doi: 10.1371/journal.pone.0066001
Toma, C., De Cillà, S., Palumbo, A., Garhwal, D. P., and Grossini, E. (2021). Oxidative and nitrosative stress in age-related macular degeneration: a review of their role in different stages of disease. Antioxidants (Basel) 10:653. doi: 10.3390/antiox10050653
Tsan, M. F., and Gao, B. (2004). Cytokine function of heat shock proteins. Am. J. Physiol. Cell Physiol. 286, C739–744. doi: 10.1152/ajpcell.00364.2003
Tsuboyama, K., Osaki, T., Matsuura-Suzuki, E., Kozuka-Hata, H., Okada, Y., Oyama, M., et al. (2020). A widespread family of heat-resistant obscure (Hero) proteins protect against protein instability and aggregation. PLoS Biol. 18:e3000632. doi: 10.1371/journal.pbio.3000632
Tucker, N. R., Middleton, R. C., Le, Q. P., and Shelden, E. A. (2011). HSF1 is essential for the resistance of zebrafish eye and brain tissues to hypoxia/reperfusion injury. PLoS ONE 6:e22268. doi: 10.1371/journal.pone.0022268
Tucker, N. R., Ustyugov, A., Bryantsev, A. L., Konkel, M. E., and Shelden E.ric., A. (2009). Hsp27 is persistently expressed in zebrafish skeletal and cardiac muscle tissues but dispensable for their morphogenesis. Cell Stress Chaperones 14, 521–533. doi: 10.1007/s12192-009-0105-1
Turrens, J. F. (2003). Mitochondrial formation of reactive oxygen species. J. Physiol. 552, 335–344. doi: 10.1113/jphysiol.2003.049478
Uddin, M. A., Akhter, M. S., Kubra, K.-T., Whitaker, K. E., Shipley, S. L., Smith, L. M., et al. (2021). Hsp90 inhibition protects the brain microvascular endothelium against oxidative stress. Brain Disord. 1:100001. doi: 10.1016/j.dscb.2020.100001
Ulrich, K., Schwappach, B., and Jakob, U. (2021). Thiol-based switching mechanisms of stress-sensing chaperones. Biol. Chem. 402, 239–252. doi: 10.1515/hsz-2020-0262
Vabulas, R. M., Ahmad-Nejad, P., Ghose, S., Kirschning, C. J., Issels, R. D., and Wagner, H. (2002a). HSP70 as endogenous stimulus of the Toll/interleukin-1 receptor signal pathway. J. Biol. Chem. 277, 15107–15112. doi: 10.1074/jbc.M111204200
Vabulas, R. M., Wagner, H., and Schild, H. (2002b). Heat shock proteins as ligands of toll-like receptors. Curr. Top. Microbiol. Immunol. 270, 169–184. doi: 10.1007/978-3-642-59430-4_11
Vagnerova, K., Hurn, P. D., Bhardwaj, A., and Kirsch, J. R. (2006). Sigma 1 receptor agonists act as neuroprotective drugs through inhibition of inducible nitric oxide synthase. Anesth. Analg. 103, 430–434. doi: 10.1213/01.ane.0000226133.85114.91
van Eden, W., Spiering, R., Broere, F., and van der Zee, R. (2012). A case of mistaken identity: HSPs are no DAMPs but DAMPERs. Cell Stress Chaper. 17, 281–292. doi: 10.1007/s12192-011-0311-5
Vanhooren, V., Liu, X.-E., Desmyter, L., Fan, Y.-D., Vanwalleghem, L., Van Molle, W., et al. (2008). Over-expression of heat shock protein 70 in mice is associated with growth retardation, tumor formation, and early death. Rejuven. Res. 11, 1013–1020. doi: 10.1089/rej.2008.0783
Venediktov, A. A., Bushueva, O. Y., Kudryavtseva, V. A., Kuzmin, E. A., Moiseeva, A. V., Baldycheva, A., et al. (2023). Closest horizons of Hsp70 engagement to manage neurodegeneration. Front. Mol. Neurosci. 16:1230436. doi: 10.3389/fnmol.2023.1230436
Verkhratsky, A., and Butt, A. M. (2018). The history of the decline and fall of the glial numbers legend. Neuroglia 1, 188–192. doi: 10.3390/neuroglia1010013
Vermot, A., Petit-Härtlein, I., Smith, S. M. E., and Fieschi, F. (2021). NADPH Oxidases (NOX): an overview from discovery, molecular mechanisms to physiology and pathology. Antioxidants 10:890. doi: 10.3390/antiox10060890
Vialykh, E. K., Solidolova, M. A., OIu, B., Bulgakova, I. V., and Polonikov, A. V. (2012). Catalase gene polymorphism is associated with increased risk of cerebral stroke in hypertensive patients. Zhurnal nevrologii i psikhiatrii imeni SS Korsakova. 112, 3–7.
Vidyasagar, A., Wilson, N. A., and Djamali, A. (2012). Heat shock protein 27 (HSP27): biomarker of disease and therapeutic target. Fibrogenesis Tissue Repair 5:7. doi: 10.1186/1755-1536-5-7
Vinokurov, A. Y., Palalov, A. A., Kritskaya, K. A., Demyanenko, S. V., Garbuz, D. G., Evgen'ev, M. B., et al. (2024). Cell-Permeable HSP70 protects neurons and astrocytes against cell death in the rotenone-induced and familial models of Parkinson's disease. Mol. Neurobiol. 61, 7785–7795. doi: 10.1007/s12035-024-04077-9
Vishwanathan, V., and D'Silva, P. (2022). Loss of function of mtHsp70 chaperone variants leads to mitochondrial dysfunction in congenital sideroblastic anemia. Front. Cell Dev. Biol. 10:847045. doi: 10.3389/fcell.2022.847045
Voloboueva, L. A., Duan, M., Ouyang, Y., Emery, J. F., Stoy, C., and Giffard, R. G. (2008). Overexpression of mitochondrial Hsp70/Hsp75 protects astrocytes against ischemic injury in vitro. J. Cerebr Blood Flow Metabol. 28, 1009–1016. doi: 10.1038/sj.jcbfm.9600600
Voth, W., and Jakob, U. (2017). Stress-activated chaperones: a first line of defense. Trends Biochem. Sci. 42, 899–913. doi: 10.1016/j.tibs.2017.08.006
Wadhwa, R., Takano, S., Kaur, K., Aida, S., Yaguchi, T., Kaul, Z., et al. (2005). Identification and characterization of molecular interactions between mortalin/mtHsp70 and HSP60. Biochem. J. 391, 185–190. doi: 10.1042/BJ20050861
Wang, L., Zhang, X., Xiong, X., Zhu, H., Chen, R., Zhang, S., et al. (2022b). Nrf2 regulates oxidative stress and its role in cerebral ischemic stroke. Antioxidants 11:2377. doi: 10.3390/antiox11122377
Wang, M., Wan, C., He, T., Han, C., Zhu, K., Waddington, J. L., et al. (2021b). Sigma-1 receptor regulates mitophagy in dopaminergic neurons and contributes to dopaminergic protection. Neuropharmacology 196:108360. doi: 10.1016/j.neuropharm.2020.108360
Wang, Q., Tompkins, K. D., Simonyi, A., Korthuis, R. J., Sun, A. Y., and Sun, G. Y. (2006a). Apocynin protects against global cerebral ischemia–reperfusion-induced oxidative stress and injury in the gerbil hippocampus. Brain Res. 1090, 182–189. doi: 10.1016/j.brainres.2006.03.060
Wang, X., Venable, J., LaPointe, P., Hutt, D. M., Koulov, A. V., Coppinger, J., et al. (2006b). Hsp90 cochaperone aha1 downregulation rescues misfolding of CFTR in cystic fibrosis. Cell 127, 803–815. doi: 10.1016/j.cell.2006.09.043
Wang, Y., Liu, Y., Sun, K., Wei, Y., Fu, L., Hou, Z., et al. (2019). The differential neuroprotection of HSP70-hom gene single nucleotide polymorphisms: in vitro (neuronal hypoxic injury model) and in vivo (rat MCAO model) studies. Gene 710, 354–362. doi: 10.1016/j.gene.2019.05.059
Wang, Y., Ma, H., Huang, J., Yao, Z., Yu, J., Zhang, W., et al. (2021a). Discovery of bardoxolone derivatives as novel orally active necroptosis inhibitors. Eur. J. Med. Chem. 212:113030. doi: 10.1016/j.ejmech.2020.113030
Wang, Z.-Y., Li, A., Huang, X., Bai, G.-L., Jiang, Y.-X., Li, R.-L., et al. (2022a). HSP27 protects skin from ultraviolet b -induced photodamage by regulating autophagy and reactive oxygen species production. Front. Cell Dev. Biol. 10:852244. doi: 10.3389/fcell.2022.852244
Wardlaw, J. M., Murray, V., Berge, E., and Del Zoppo, G. J. (2014). Thrombolysis for acute ischaemic stroke. Cochrane Database Syst. Rev. 2016:CD000213. doi: 10.1002/14651858.CD000213.pub3
Wegleiter, K., Hermann, M., Posod, A., Wechselberger, K., Stanika, R. I., Obermair, G. J., et al. (2014). The sigma-1 receptor agonist 4-phenyl-1-(4-phenylbutyl) piperidine (PPBP) protects against newborn excitotoxic brain injury by stabilizing the mitochondrial membrane potential in vitro and inhibiting microglial activation in vivo. Exp. Neurol. 261, 501–509. doi: 10.1016/j.expneurol.2014.07.022
Wen, B., Xu, K., Huang, R., Jiang, T., Wang, J., Chen, J., et al. (2022). Preserving mitochondrial function by inhibiting GRP75 ameliorates neuron injury under ischemic stroke. Mol. Med. Rep. 25:165. doi: 10.3892/mmr.2022.12681
Weng, T.-Y., Hung, D. T., Su, T.-P., and Tsai, S.-Y. A. (2017). Loss of sigma-1 receptor chaperone promotes astrocytosis and enhances the Nrf2 antioxidant defense. Oxid. Med. Cell. Longev. 2017, 1–14. doi: 10.1155/2017/4582135
Wheeler, D. S., Dunsmore, K. E., and Wong, H. R. (2003). Intracellular delivery of HSP70 using HIV-1 Tat protein transduction domain. Biochem. Biophys. Res. Commun. 301, 54–59. doi: 10.1016/S0006-291X(02)02986-8
Winterbourn, C. C., Kettle, A. J., and Hampton, M. B. (2016). Reactive oxygen species and neutrophil function. Annu. Rev. Biochem. 85, 765–792. doi: 10.1146/annurev-biochem-060815-014442
Woehrer, S. L., Aronica, L., Suhren, J. H., Busch, C. J., Noto, T., and Mochizuki, K. (2015). A tetrahymena Hsp90 co-chaperone promotes siRNA loading by ATP-dependent and ATP-independent mechanisms. EMBO J. 34, 559–577. doi: 10.15252/embj.201490062
Wood, K. C., and Gladwin, M. T. (2007). The hydrogen highway to reperfusion therapy. Nat. Med. 13, 673–674. doi: 10.1038/nm0607-673
Xie, X., and Liu, J. (2023). New role of astrocytes in neuroprotective mechanisms after ischemic stroke. Novo papel dos astrócitos nos mecanismos neuroprotetores após acidente vascular cerebral isquêmico. Arq. Neuropsiquiatr. 81, 748–755. doi: 10.1055/s-0043-1770352
Xu, H. (2018). Cochaperones enable Hsp70 to use ATP energy to stabilize native proteins out of the folding equilibrium. Sci. Rep. 8:13213. doi: 10.1038/s41598-018-31641-w
Xu, L., and Giffard, R. G. (1997). HSP70 protects murine astrocytes from glucose deprivation injury. Neurosci. Lett. 224, 9–12. doi: 10.1016/S0304-3940(97)13444-9
Xu, L., Ouyang, Y.-B., and Giffard, R. G. (2003). Geldanamycin reduces necrotic and apoptotic injury due to oxygen-glucose deprivation in astrocytes. Neurol. Res. 25, 697–700. doi: 10.1179/016164103101202183
Xu, Q., Metzler, B., Jahangiri, M., and Mandal, K. (2012). Molecular chaperones and heat shock proteins in atherosclerosis. Am. J. Physiol. Heart. Circ. Physiol. 302, H506–H514. doi: 10.1152/ajpheart.00646.2011
Yakovenko, L. F., Smalyuk, Y. V., Kapustyan, L. M., Chornyy, S. A., Pogrebnaya, A. P., Granich, V. N., et al. (2015). [Anti-Hsp60 antibodies in arterial hypertension different degree of severity]. Lik. Sprava. 3, 43–52. doi: 10.31640/LS-2015-(3-4)-06
Yamamoto, Y., Hosoda, K., Imahori, T., Tanaka, J., Matsuo, K., Nakai, T., et al. (2018). Pentose phosphate pathway activation via HSP27 phosphorylation by ATM kinase: a putative endogenous antioxidant defense mechanism during cerebral ischemia-reperfusion. Brain Res. 1687, 82–94. doi: 10.1016/j.brainres.2018.03.001
Yan, Y., Wei, C. L., Zhang, W. R., Cheng, H. P., and Liu, J. (2006). Cross-talk between calcium and reactive oxygen species signaling. Acta Pharmacol. Sin. 27, 821–826. doi: 10.1111/j.1745-7254.2006.00390.x
Yang, Y.-M., Jung, Y., Abegg, D., Adibekian, A., Carroll, K. S., and Karbstein, K. (2023). Chaperone-directed ribosome repair after oxidative damage. Mol. Cell 83, 1527–1537.e5. doi: 10.1016/j.molcel.2023.03.030
Yenari, M. A., Fink, S. L., Sun, G. H., Chang, L. K., Patel, M. K., Kunis, D. M., et al. (1998). Gene therapy with HSP72 is neuroprotective in rat models of stroke and epilepsy. Ann. Neurol. 44, 584–591. doi: 10.1002/ana.410440403
Yenari, M. A., Giffard, R. G., Sapolsky, R. M., and Steinberg, G. K. (1999). The neuroprotective potential of heat shock protein 70 (HSP70). Mol. Med. Today 5, 525–531. doi: 10.1016/S1357-4310(99)01599-3
Yi, J. S., Lee, S. K., Sato, T. A., and Koh, J. Y. (2003). Co-induction of p75(NTR) and the associated death executor NADE in degenerating hippocampal neurons after kainate-induced seizures in the rat. Neurosci. Lett. 347, 126–130. doi: 10.1016/S0304-3940(03)00656-6
Yoshida, S., Tsutsumi, S., Muhlebach, G., Sourbier, C., Lee, M.-J., Lee, S., et al. (2013). Molecular chaperone TRAP1 regulates a metabolic switch between mitochondrial respiration and aerobic glycolysis. Proc. Natl. Acad. Sci. U.S.A. 110, E1604–E1612. doi: 10.1073/pnas.1220659110
Yoshimura, Y., Holmberg, M. A., Kukic, P., Andersen, C. B., Mata-Cabana, A., Falsone, S. F., et al. (2017). MOAG-4 promotes the aggregation of α-synuclein by competing with self-protective electrostatic interactions. J. Biol. Chem. 292, 8269–8278. doi: 10.1074/jbc.M116.764886
Yu, S., Tajiri, N., Franzese, N., Franzblau, M., Bae, E., Platt, S., et al. (2013). Stem cell-like dog placenta cells afford neuroprotection against ischemic stroke model via heat shock protein upregulation. PLoS ONE 8:e76329. doi: 10.1371/journal.pone.0076329
Yuan, Z., Zhou, X., Zou, Y., Zhang, B., Jian, Y., Wu, Q., et al. (2023). Hypoxia aggravates neuron ferroptosis in early brain injury following subarachnoid hemorrhage via NCOA4-meditated ferritinophagy. Antioxidants 12:2097. doi: 10.3390/antiox12122097
Zatsepina, O. G., Evgen'ev, M. B., and Garbuz, D. G. (2021). Role of a heat shock transcription factor and the major heat shock protein hsp70 in memory formation and neuroprotection. Cells 10:1638. doi: 10.3390/cells10071638
Zhan, L., Liu, L., Li, K., Wu, B., Liu, D., Liang, D., et al. (2017). Neuroprotection of hypoxic postconditioning against global cerebral ischemia through influencing posttranslational regulations of heat shock protein 27 in adult rats. Brain Pathol. 27, 822–838. doi: 10.1111/bpa.12472
Zhang, C., and Cuervo, A. M. (2008). Restoration of chaperone-mediated autophagy in aging liver improves cellular maintenance and hepatic function. Nat. Med. 14, 959–965. doi: 10.1038/nm.1851
Zhang, D. D., Lo, S. C., Cross, J. V., Templeton, D. J., and Hannink, M. (2004). Keap1 is a redox-regulated substrate adaptor protein for a Cul3-dependent ubiquitin ligase complex. Mol. Cell. Biol. 24, 10941–10953. doi: 10.1128/MCB.24.24.10941-10953.2004
Zhang, G., Li, Q., Tao, W., Qin, P., Chen, J., Yang, H., et al. (2023). Sigma-1 receptor-regulated efferocytosis by infiltrating circulating macrophages/microglial cells protects against neuronal impairments and promotes functional recovery in cerebral ischemic stroke. Theranostics 13, 543–559. doi: 10.7150/thno.77088
Zhang, J., Wang, K., Qi, J., Cao, X., and Wang, F. (2021). The Hsp90 inhibitor 17-DMAG attenuates hyperglycemia-enhanced hemorrhagic transformation in experimental stroke. Biomed Res. Int. 2021, 1–7. doi: 10.1155/2021/5047501
Zhang, L., Wu, J., Duan, X., Tian, X., Shen, H., Sun, Q., et al. (2016). NADPH oxidase: a potential target for treatment of stroke. Oxid. Med. Cell. Longev. 2016:5026984. doi: 10.1155/2016/5026984
Zhang, X., Wu, F., Jiao, Y., Tang, T., Yang, L., Lu, C., et al. (2017). An increase of sigma-1 receptor in the penumbra neuron after acute ischemic stroke. J. Stroke Cerebrovasc. Dis. 26, 1981–1987. doi: 10.1016/j.jstrokecerebrovasdis.2017.06.013
Zhao, Q., Luo, T., Gao, F., Fu, Y., Li, B., Shao, X., et al. (2022). GRP75 regulates mitochondrial-supercomplex turnover to modulate insulin sensitivity. Diabetes 71, 233–248. doi: 10.2337/db21-0173
Zhao, R. Z., Jiang, S., Zhang, L., and Yu, Z. B. (2019). Mitochondrial electron transport chain, ROS generation and uncoupling (Review). Int. J. Mol. Med. 44, 3–15. doi: 10.3892/ijmm.2019.4188
Zhou, Y., Liao, J., Mei, Z., Liu, X., and Ge, J. (2021). Insight into crosstalk between ferroptosis and necroptosis: novel therapeutics in ischemic stroke. Oxid. Med. Cell. Longev. 2021, 1–19. doi: 10.1155/2021/9991001
Zhu, G., Wang, X., Chen, L., Lenahan, C., Fu, Z., Fang, Y., et al. (2022a). Crosstalk between the oxidative stress and glia cells after stroke: from mechanism to therapies. Front. Immunol. 13:852416. doi: 10.3389/fimmu.2022.852416
Zhu, L., He, S., Huang, L., Ren, D., Nie, T., Tao, K., et al. (2022b). Chaperone-mediated autophagy degrades Keap1 and promotes Nrf2-mediated antioxidative response. Aging Cell 21:e13616. doi: 10.1111/acel.13616
Zhu, Z., Li, R., Stricker, R., and Reiser, G. (2015). Extracellular α-crystallin protects astrocytes from cell death through activation of MAPK, PI3K/Akt signaling pathway and blockade of ROS release from mitochondria. Brain Res. 1620, 17–28. doi: 10.1016/j.brainres.2015.05.011
Zininga, T., Ramatsui, L., and Shonhai, A. (2018). Heat shock proteins as immunomodulants. Molecules 23:2846. doi: 10.3390/molecules23112846
Keywords: chaperones, stroke, HSP, ROS, hero-proteins
Citation: Soldatov V, Venediktov A, Belykh A, Piavchenko G, Naimzada MD, Ogneva N, Kartashkina N and Bushueva O (2024) Chaperones vs. oxidative stress in the pathobiology of ischemic stroke. Front. Mol. Neurosci. 17:1513084. doi: 10.3389/fnmol.2024.1513084
Received: 17 October 2024; Accepted: 20 November 2024;
Published: 11 December 2024.
Edited by:
Serena Carra, University of Modena and Reggio Emilia, ItalyCopyright © 2024 Soldatov, Venediktov, Belykh, Piavchenko, Naimzada, Ogneva, Kartashkina and Bushueva. This is an open-access article distributed under the terms of the Creative Commons Attribution License (CC BY). The use, distribution or reproduction in other forums is permitted, provided the original author(s) and the copyright owner(s) are credited and that the original publication in this journal is cited, in accordance with accepted academic practice. No use, distribution or reproduction is permitted which does not comply with these terms.
*Correspondence: Vladislav Soldatov, emlua2ZpbmdlcnNAZ21haWwuY29t; Olga Bushueva, b2xnYS5idXNodWV2YUBpbmJveC5ydQ==