- 1Department of Fist Clinical Medical College, Heilongjiang University of Chinese Medicine, Harbin, China
- 2First Affiliated Hospital, Heilongjiang University of Chinese Medicine, Harbin, China
The pathogenesis of epilepsy is related to the microbiota-gut-brain axis, but the mechanism has not been clarified. The microbiota-gut-brain axis is divided into the microbiota-gut-brain axis (upward pathways) and the brain-gut-microbiota axis (downward pathways) according to the direction of conduction. Gut microorganisms are involved in pathological and physiological processes in the human body and participate in epileptogenesis through neurological, immunological, endocrine, and metabolic pathways, as well as through the gut barrier and blood brain barrier mediated upward pathways. After epilepsy, the downward pathway mediated by the HPA axis and autonomic nerves triggers “leaky brain “and “leaky gut,” resulting in the formation of microbial structures and enterobacterial metabolites associated with epileptogenicity, re-initiating seizures via the upward pathway. Characteristic changes in microbial and metabolic pathways in the gut of epileptic patients provide new targets for clinical prevention and treatment of epilepsy through the upward pathway. Based on these changes, this review further redescribes the pathogenesis of epilepsy and provides a new direction for its prevention and treatment.
1 Introduction
Epilepsy (Wirrell et al., 2022) is an episodic neurological disorder in which nerve cells in the brain hypersynchronize abnormal discharges, causing recurrent and transient brain dysfunction. Approximately 50 million people worldwide are currently living with epilepsy, and approximately 4 million new cases are diagnosed each year (Leonardi et al., 2024). Frequent seizures and/or epileptiform discharges cause irreversible damage to the brain (Bastos and Cross, 2020), resulting in progressive dysfunction or degeneration of cognitive, linguistic, sensory, motor and behavioral aspects of the patient, imposing a heavy burden on the individual, family and society. Despite the proliferation of epilepsy treatments in recent years, such as surgery, ketogenic diet, and deep brain stimulation, the etiology of epilepsy in two-thirds of patients has not been thoroughly elucidated (Duan et al., 2022; Serino et al., 2019), especially with the uncontrollable seizures in 20–30% of epileptic seizures as well as the prevalence of behavioral toxicities of antiepileptic drugs; therefore, epilepsy pathogenesis, treatment, and prevention remain a focus of current research in the medical community.
In recent years, many studies have found that the pathogenesis of central nervous system (CNS) diseases may be related to the gut microbiota (GM) (Ma et al., 2019; Amlerova et al., 2021; Zhang et al., 2023; Liu et al., 2020; Aljeradat et al., 2024). The GM contributes to the development and progression of neurological diseases from the bottom up by producing metabolites, stimulating nerves, activating immune pathways, etc. (Guo et al., 2024; Dicks, 2022). The brain characteristically alters the structure of the GM after the onset of the disease through neurological and endocrine pathways from the top down (Abdel-Haq et al., 2019; Wang and Kasper, 2014; Singh et al., 2016). The signaling pathway between the GM and the brain that influences brain-gut interactions through neurological, endocrine, immune, and substance metabolism mechanisms is known as the “Microbiota-gut-brain (MGB axis) axis “(Mayer et al., 2022). The signaling mechanism from the GM to the brain is designated as the upward pathway, while the signaling mechanism from the brain to the GM is called the downward pathway. The gut barrier and the blood–brain barrier (BBB) play crucial roles in ensuring gut and brain homeostasis, blocking the intrusion of risk factors into the MGB axis, and preventing the development of neurological diseases (Cryan et al., 2019; Socala et al., 2021). In addition, favorable therapeutic effects have been shown in drug-resistant epilepsy by reconstructing the epileptic host GM (Table 1) (Chen et al., 2023). This paper explores the pathogenesis of epilepsy based on the MGB axis by outlining the upward and downward pathways, providing new ideas for clinical diagnosis, treatment, and prevention of epilepsy.
2 Upward pathway induction and prevention of epilepsy
Healthy GM plays an essential role in host nutrient metabolism, drug metabolism, maintenance of the structural integrity of the gut mucosal barrier, immune regulation and defense against pathogens, etc (Wang and Wang, 2016). GM dysregulation and gut dysfunction can increase the risk of seizures (Table 2), Zou et al. (2024) found that ciprofloxacin treatment by tube feeding to alter GM and its metabolites increased susceptibility to pentylenetetrazol-induced seizures in rats, with a significant increase in the number of seizures and the mean duration of each seizure, but with a decrease in susceptibility after fecal transplantation. Riazi et al. (2008) found that colitis rats were highly susceptible to pentylenetetrazol-induced seizures, and it was closely related to the severity of gut inflammation. GM is involved in the pathogenesis of epilepsy through the action of “common information molecules and their corresponding receptors,” “metabolite changes” between the nervous, endocrine and immune systems, leading to structural and functional changes in the brain (see Figure 1). The endocrine and immune pathways differ from the direct neural pathway in that GM communication with the brain must be screened by two natural signaling barriers (the gut barrier and the BBB). Since gut microbes can modulate the permeability of both structures, the signaling from the gut to the brain is highly variable, depending on the state of the host. The relevant mechanisms are summarized below.
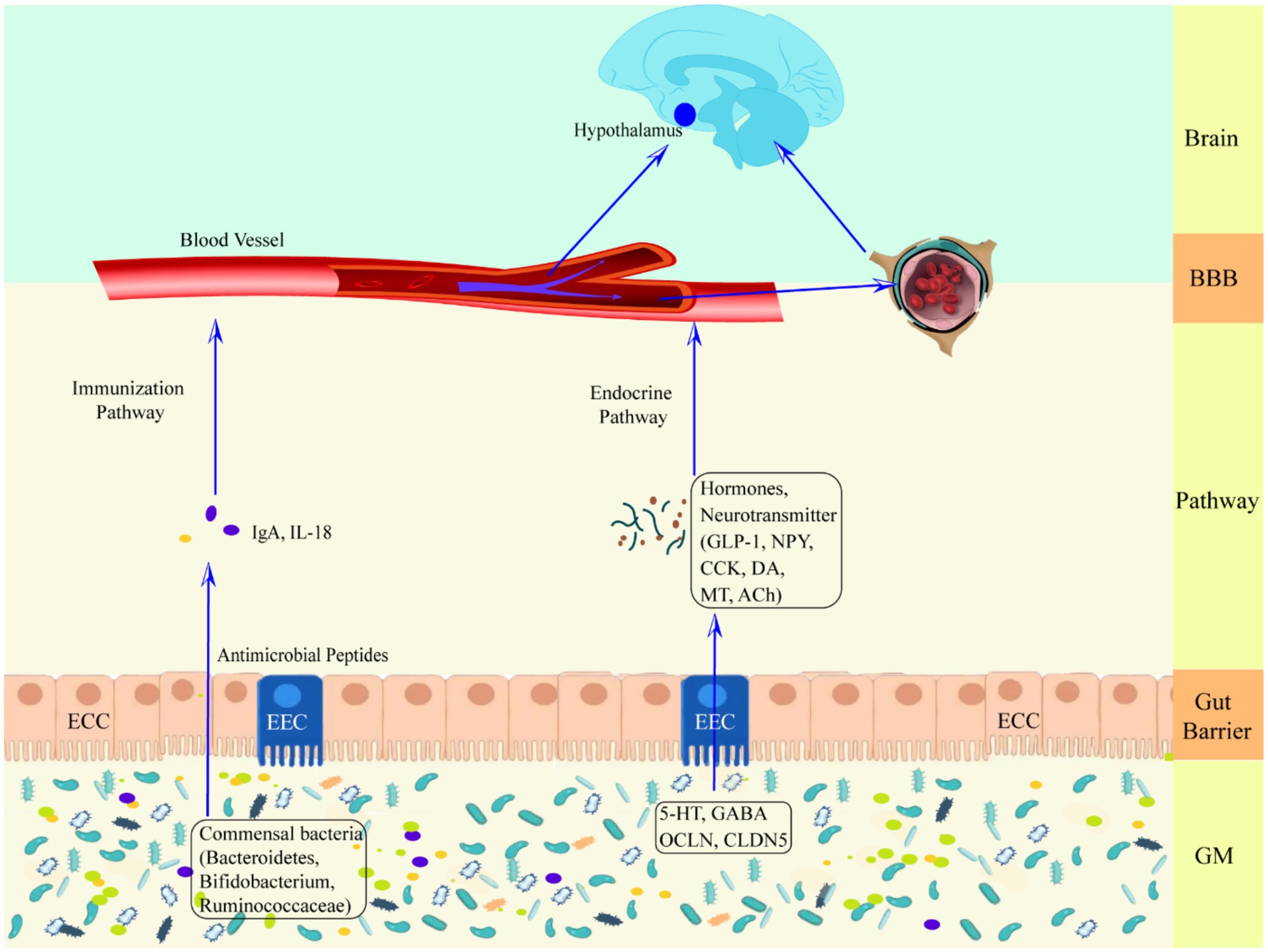
Figure 1. Schematic representation of the defense mechanism of the upward pathway “microbiota-gut-brain” through two barriers. Ach, acetylcholine; BBB, blood–brain barrier; CCK, cholecystokinin; CLDN5, claudin 5; DA, dopamine; ECC, enteroendocrine cell; EEC, enterochromaffin cell; GLP-1, glucagon-like peptide-1; GM, gut microbiota; HPA axis, hypothalamic–pituitary–adrenal axis; IgA, immunoglobulin A; IL-18, interleukin-18; MT, melatonin; NPY, neuropeptide Y; OCLN, occludin.
2.1 Neural pathways
A direct neural pathway exists between gut microbes and the brain that does not pass through a dynamic barrier and consists of neural pathways from the spinal enteric nervous system, autonomic nervous system, and vagus nervous system (Mayer et al., 2014; see Figure 2A).
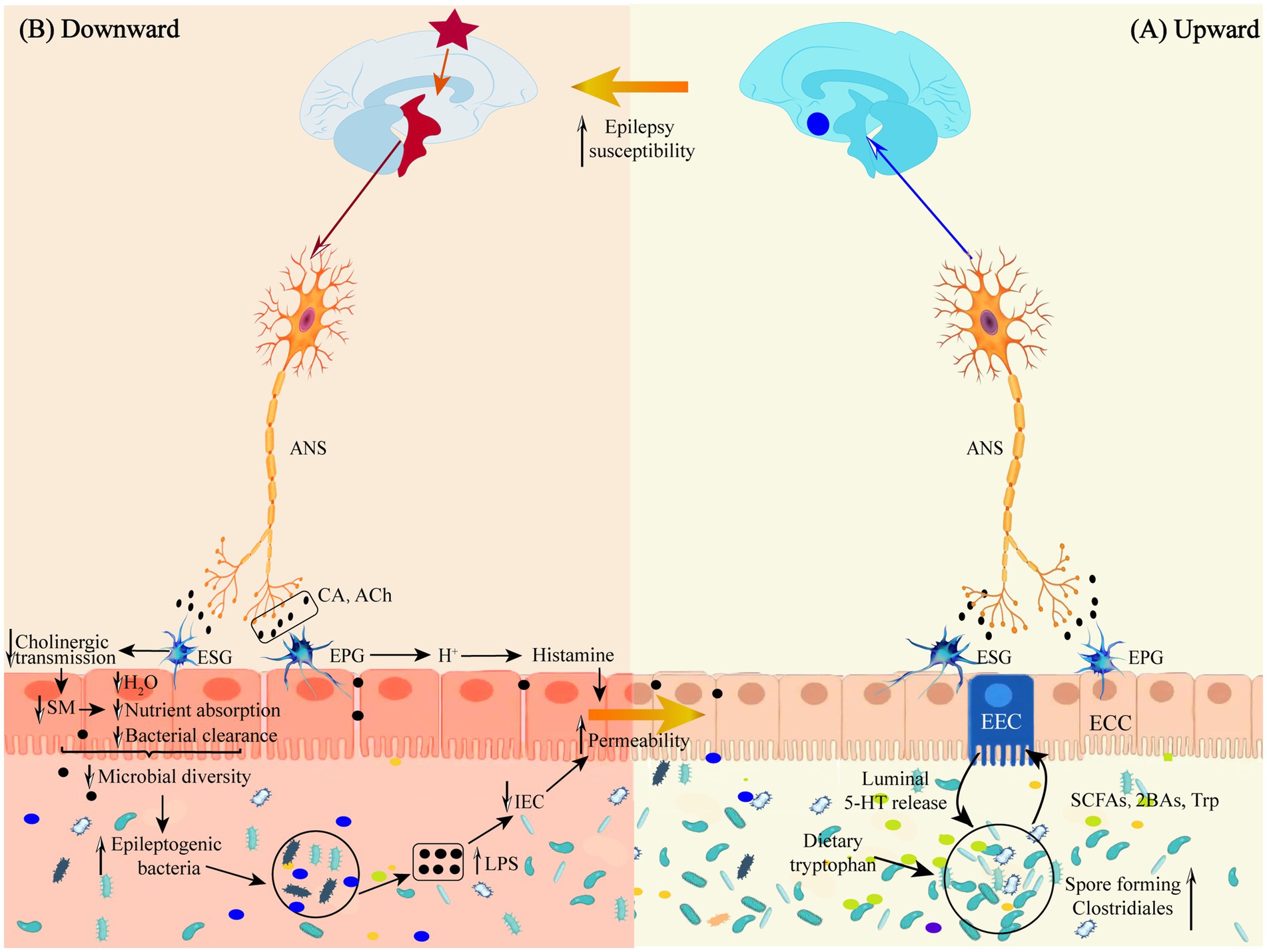
Figure 2. (A) Schematic representation of the defense mechanism of the “microbiota-gut-brain” direct neural pathway in the upward pathway. (B) Schematic diagram of the mechanism of injury in the downward pathway “epileptic brain-gut-microbiota” via the direct neural pathway. Ach, acetylcholine; ANS, autonomic nervous system; 2BAs, second bile acids; CA, catecholamine; ECC, enteroendocrine cell; EEC, enterochromaffin cell; EPG, Enteric Parasympathetic ganglion; ESG, Enteric sympathetic ganglion; IEC, intestinal epithelia cell; LPS, lipopolysaccharide; SCFAs, short-chain fatty acids; SM, smooth muscle; Trp, tryptophan.
The gut has a direct neural connection to the brain via the vagus nerve (Moradi et al., 2021), which does not cross the gut epithelium to interact directly with the GM but instead transmits gut information via enteroendocrine cell receptors that bind to synapses in the gut branches of the vagus nerve. Vagus nerve receptors transmit signals to the CNS by sensing regulatory gut peptides, inflammatory molecules, dietary components, and flora metabolites (Borre et al., 2014; Bravo et al., 2011). Thus, the vagus nerve may signal upward to induce an anti-inflammatory response early in gut infections and before circulating cytokines are elevated (Kaelberer et al., 2018; Wang and Powley, 2007; Siopi et al., 2023). Some GMs can directly activate neurons, such as Lactobacillus rhamnosus, B. fragilis, and polysaccharide A isolated from B. fragilis, which have all been shown to activate gut afferent neurons directly (Mao et al., 2013).
Goehler et al. (2005), in order to verify whether localized gut infection rapidly activates the vagus nerve, orally inoculated Campylobacter jejuni into a mouse model and found that c-fos expression was increased in the brainstem vagus sensory ganglia and primary sensory relay nuclei. In contrast, there was no increase in the level of pro-inflammatory cytokines in the circulatory system. This suggests that the GM interacts with enteroendocrine cell receptors by releasing neurotransmitters via the vagal synapses to transmit signals to neurons and rapidly modulate CNS nerve cell excitability. This finding provides theoretical solid support for the treatment of epilepsy and other neurological diseases by modulating GM. Vagus nerve stimulation has become an accepted treatment for epilepsy since it was first reported in 1988 (Attenello et al., 2015). Ressler and Mayberg (2007) found that electrical stimulation of vagal afferent fibers altered the concentrations of 5-HT, γ-aminobutyric acid (GABA), and glutamate in the brain, thereby reducing seizure duration, frequency, and severity.
The microbiota may also affect the CNS by altering adult hippocampal neurogenesis (AHN) (Lin et al., 2016). The adult hippocampus and lateral ventricles function to produce new neurons. The AHN has a vital role in learning and memory and that can influence the pathogenesis of many neurological disorder-related diseases and symptoms, such as epilepsy, depression, Alzheimer’s disease, and Parkinson’s disease. It was found (Atmaca et al., 2017) that there were differences in hippocampal neurons between germ-free (GF) and normal mice and that colonization of the colony after weaning did not alter the number of AHN, suggesting that microorganisms play an essential role in AHN early in life.
2.2 Endocrine pathway
There are more than 20 types of enteroendocrine cells in the gut, constituting the largest endocrine organ in the body (Gunawardene et al., 2011). The endocrine pathway is mediated by the enteroendocrine signaling pathway (Latorre et al., 2016) and the enterochromaffin cell signaling pathway (Xu et al., 2021). Enteroendocrine cells have chemosensory mechanisms that initiate appropriate functional responses through gut-brain and brain-gut interactions, some of which involve direct enteroendocrine cell-neuron connections through basal cytoplasmic processes (Psichas et al., 2015). The primary mechanism by which enteroendocrine cells communicate with nerves, either directly or indirectly, is through the release of peptides that act in the intestinal mucosa (Dockray, 2013; Furness et al., 2013). It plays a key role in the control of gastrointestinal secretion and peristalsis, regulation of food intake, postprandial blood glucose levels and metabolism (Latorre et al., 2016). Enterochromaffin cells are the most abundant subtype of colonic enteroendocrine cells, and although they make up less than 1% of intestinal epithelial cells, they produce and release 90% of the body’s serotonin, which is essential for intestinal motility, platelet function, immune response, and bone development (Karsenty and Yadav, 2011; Matthes and Bader, 2018). The enteroendocrine signaling pathway is mainly composed of enteroendocrine cells and enterochromaffin cells. Enteroendocrine cells receive stimuli and then influence CNS function in an endocrine and paracrine manner. Endocrine transmission is mainly mediated by enteroendocrine cells, which convert afferent signals into chemical stimuli by interacting with intestinal microbial metabolites, such as short chain fatty acids (SCFAs) (Tolhurst et al., 2012), secondary bile acids (Joyce and O’Malley, 2022), and tryptophan metabolites (O’Mahony et al., 2015), to induce the gut secretion of neurohormones, chemotransmitters, and other informational molecules and their effects on the hypothalamus and its related brain regions through the circulatory system (Lum et al., 2020). An essential component of endocrine transmission is the Hypothalamic–pituitary–adrenal (HPA) axis. When subjected to stress, the HAP axis releases cortisol, which regulates the activity of gut immune cells and the release of cytokines (Tetel et al., 2018). Moreover, cytokine release regulates the osmotic function and barrier function of the gut and the structure of GM (Mayer, 2000). At the same time, GM can also regulate the HPA axis to affect brain activity (Mangiola et al., 2016). Furlano et al. (2001) reported for the first time that symbiotic gut microorganisms are associated with the HPA axis, which is vital for learning and memory, and that disruption of the HPA axis can lead to hippocampal memory impairment. GF mice have reduced levels of brain-derived neurotrophic factor, N-methyl-D-aspartate (NMDA), and c-fos, which play a role in memory retention, cognition, and other brain functions (Bravo et al., 2011; Rusch et al., 2023).
Paracrine transmission is mainly mediated by the enterochromaffin signaling pathway, which consists mainly of enterochromaffin cells and GM. GM can produce a series of neurotransmitters beneficial or detrimental to the CNS (Tran and Mohajeri, 2021), according to changes in the gut milieu during digestion and metabolism. They also affect CNS activity by acting on the vagus nerve. For example, Lysinibacillus fusiformis produces SCFAs and secondary bile acids, which increase tryptophan utilization and stimulate enterochromaffin cells to synthesize human serotonin (5-Hydroxytryptamine, 5-HT) and release it into the circulation. When there is too much or too little 5-HT in the circulation, enterochromaffin cells communicate with central vagal afferent nerve fibers through synaptic connections between neuropod-like extensions and enteric vagal afferent nerve endings to regulate the synthesis and release of 5-HT and to maintain a stable level of 5-HT in the circulation (Ridaura and Belkaid, 2015). Some GM secrete neurotransmitters independently, such as γ-aminobutyric acid (GABA) secreted by Bifidobacterium, which can directly cross the gut barrier and the BBB to enter the CNS (Braga et al., 2024; Takanaga et al., 2001). When GM structure is altered, it may mediate pro-excitatory effects of peripheral inflammation by activating the immune system through the release of cytokines such as: tumor necrosis factor-alpha (TNF-α) and monocyte chemoattractant protein-1, chemokines and lipopolysaccharides (LPS) (Erny et al., 2015). It is also involved in epileptogenesis by regulating the excitatory and inhibitory neuronal balance through the production of neurotransmitters, especially glutamate, GABA and 5-HT (Paiva et al., 2024).
2.3 Immune pathway
The immune pathway is mediated by GM (Fung et al., 2017), which influences neurophysiological processes such as neurodevelopment, neurotransmission, CNS immune activation, and BBB integrity by regulating the development and function of CNS immune cells (microglia and astrocytes) (Liu et al., 2024; Ding et al., 2022). GM also induces inflammation in the brain by influencing the immune system of the gut mucosa and mediating peripheral immune responses, which can lead to brain damage and abnormal behavior (Mu et al., 2022).
Astrocytes and microglia also play an essential role in regulating immune responses in the CNS. Astrocytes are the most abundant glial cells in the brain and are involved in neurotransmitter recycling, immune response, and regulation of BBB integrity. Microglia are macrophages in the brain that promote inflammation and participate in immune monitoring (Aloisi, 2001). Interactions between astrocytes and microglia lead to pro-inflammatory cytokine production and increased BBB permeability, which allows infiltration of peripheral blood immune cells and cytokines into the CNS and leads to chronic neuroinflammation, which is involved in epilepsy pathogenesis (Moradi et al., 2021; Devinsky et al., 2013).
In addition to glial cells in the CNS, GM affects brain function through three main immune pathways (De Timary et al., 2017): Cytokines induced by GMs to be produced enter the circulatory system and enter the brain through the transporter system on the BBB, which has a direct effect on brain activity and function; Toll-like receptors (TLRs) are also expressed on macrophages cells in the circumventricular organs and choroid plexus, which respond to microbe-associated model molecules (MAMPs) of GM in the circulatory system and release cytokines. Since the circumventricular organs is outside the BBB, the released cytokines enter the brain by free diffusion and affect brain activity; IL-1 receptors expressed by perivascular macrophages and epithelial cells of small brain vessels can bind directly to IL-1 produced by GM in the circulatory system, generating prostaglandin E2 that regulates brain activity and function.
The immune mechanism plays a vital role in the onset and development of epilepsy, and the pathogenesis of some refractory epilepsy patients is closely related to the immune mechanism (Gozubatik-Celik et al., 2017; Maranduba et al., 2015). In a meta-analysis of epilepsy and systemic autoimmune diseases, Lin et al. (2016) found that patients with epilepsy had a more than 2.5-fold increased risk of developing autoimmune diseases, and patients with autoimmune diseases had a more than 2.5-fold increased risk of epilepsy, suggesting that epilepsy and autoimmune diseases often co-occur and that aberrant immune responses are involved in epilepsy pathogenesis. Atmaca et al. (2017) investigated the prevalence of neuronal autoantibodies in 22 patients with persistent status epilepticus of unknown cause. The study’s results showed that the overall prevalence of antibody-positive patients was 22.7% (5/22). This suggests that neuronal autoantibodies are involved in the pathogenesis of immunologic factors in epilepsy.
These studies demonstrate that GM can modulate host susceptibility to epileptic seizures through immune mechanisms. Therefore, immune modulation and GM reconstruction may be important strategies for treating epilepsy in the future.
2.4 Substance metabolism pathways
GM stimulates the body to produce a variety of neurotransmitters and modulators, cytokines, and metabolites (Bienenstock et al., 2015), such as 5-HT, dopamine, melatonin, GABA, histamine, acetylcholine, IL-1, and SCFAs, which not only have a direct effect on the enteric nervous system and the vagus nervous system, but also act on the enteroendocrine cells and affect the activities of the CNS through the endocrine and paracrine effects. For example, spore anaerobic bacteria can regulate the synthesis and secretion of 5-HT by enterochromaffin cells (Bauer et al., 1963). Disruption of this complex homeostatic relationship between the MGB axis affects the regulation of CNS functions such as mood, behavior, memory, and cognitive function and can lead to various CNS disorders (De Timary et al., 2017; Cerdo et al., 2017).
GM induces neurophysiological changes in the host by producing chemicals that bind to receptors inside and outside the gut (Manolios et al., 1988). Compared with specific pathogen free (SPF) animals, GF animals lacking GM colonization showed significant changes in neurobiochemical indicators and related behaviors. For example, GF mice had significantly lower serum levels of 5-HT, defective learning memory, and more “risk-taking behaviors.” After the colonization of the intestines of GF animals with the microbiota of normal animals, serum and colonic levels of 5-HT, adrenocorticotropic hormone, and corticosterone reached normal levels, suggesting that the HPA axis is a critical structure in GM regulation affecting the stress response and the development of the CNS (Furlano et al., 2001; Torrente et al., 2002).
SCFAs, including acetic acid, propionic acid, and butyric acid, are produced by GM through the fermentation of insoluble dietary fibers (Den Besten et al., 2013). SCFAs play essential roles in microglia maturation, enteric and brain nervous system, BBB permeability, and stress response through direct or indirect pathways, and all are closely related to epilepsy (de Caro et al., 2019). Propionic acid reduces seizure intensity and prolongs seizure latency by reducing mitochondrial damage, inhibiting hippocampal apoptosis, and ameliorating neurological deficits (Cheng et al., 2019). In animal models, SCFAs can affect neurodevelopmental and cognitive functions in animals with neurodegenerative diseases (Erny et al., 2015). Aloisi (2001) injected SCFAs into the blood of rats and showed that serum levels of propionic acid were significantly higher (p < 0.05) than in normal controls, and neurochemical changes such as neuroinflammation, increased oxidative stress and depletion of antioxidants were observed, resulting in mitochondrial dysfunction. Neural activation molecules serotonin, melatonin, histamine, acetylcholine, and other microbial sources also play a role in the MGB axis (Devinsky et al., 2013).
2.5 Relationship between double barrier in upward pathway and epilepsy
Epilepsy occurs as a result of disruption of brain homeostasis and overactivation of neurons by stimuli such as inflammatory factors. The gut barrier and the BBB protect brain homeostasis mainly in the MGB axis (Braniste et al., 2014). The integrity of both barriers is associated with GM. GM protects the integrity of the gut barrier (Martin et al., 2018) and maintains gut homeostasis (Hooper and Macpherson, 2010; Renz et al., 2011; Stecher and Hardt, 2011) by regulating cell growth, differentiation, and promoting the expression of occluding (OCLN) and claudin 5 (CLDN 5), so that peripheral inflammatory factors and neurotransmitters are unable to enter the bloodstream, thus maintaining the BBB integrity and ensuring the CNS homeostasis (Braniste et al., 2014).
2.5.1 Gut barrier
Based on composition and function, the gut barrier is categorized into biological, chemical, physical, and immune barriers (Mowat, 2003). Among them, gut barrier permeability is an indicator reflecting the integrity of the gut barrier (Usuda et al., 2021). The increase of some pathogenic GM and its metabolites, such as Fusobacteria, Verrucomicrobia, and Nitrospirae, induces inflammation, degrades mucins, and increases nitrite toxicity (Engevik et al., 2021; Mao et al., 2021), which further reduces the stability of the gut mucus layer and the integrity of the gut epithelium, and the increase in gut barrier permeability, triggering a “leaky gut” (Usuda et al., 2021). Signaling molecules, such as cytokines, chemokines and neurotransmitters, are transmitted from the gut lumen to the lamina propria or the blood circulation, increasing the permeability of the BBB and leading to “leaky brain” (Logsdon et al., 2018), triggering epilepsy.
2.5.1.1 Gut biobarrier and epilepsy
The gut is the most significant interface between the human body and the external environment, and the GM it contains forms a significant biological barrier for the body (Lavelle and Sokol, 2020). A healthy and intact biobarrier with anaerobic Bacteroidetes and Firmicutes accounting for more than 90% of the total number of bacterial species, with the remaining facultative bacilli being Proteobacteria and Actinobacteria, prevents the onset of neuropsychiatric disorders such as multiple sclerosis (iMSMS Consortium, 2022), autism (Xiong et al., 2016), Parkinson’s disease (Sampson et al., 2016) and epilepsy (Ding et al., 2022).
Chronic physical and psychological stress can lead to structural and functional changes in GM that predispose to epileptogenesis. When rats are subjected to chronic restraint stress, the number of pro-inflammatory flora associated with Lysinibacillus fusiformis, Helicobacter pylori, Peptococcaceae, Streptococcus, and Enterococcus faecalis is elevated, and susceptibility to epilepsy is increased. A small amount of electrical stimulation of the basolateral amygdala induces complete and sustained epileptic seizures in rats. Further, rat GM was transplanted into the gut of SPF rats, and SPF rats transplanted with GM had reduced thresholds for seizures induced by electrical stimulation, whereas SPF rats receiving GM from pseudo chronic restraint stress rats had seizure thresholds and seizure durations as in the control group (Medel-Matus et al., 2018).
A chronic lifestyle of a high-fat, high-sugar, high-carbon-water diet can induce obesity, leading to an increase in the abundance of the Firmicutes and a decrease in the abundance of the Bacteroidetes, which disrupts the GM structure and lowers the seizure threshold (Bell, 2015). Kang et al. (2015) found that high-fat diet-induced obese mice treated with kainic acid ignition exhibited more grade IV or V seizures on the behavioral Racine classification, a significant increase in the number of seizure spikes and slow waves and seizure duration on the electroencephalogram, and an increase in pathologic hippocampal neuronal death. However, the ketogenic diet (KD), which is high in fat, adequate in protein, and low in carbohydrates, has beneficial effects on many neurological disorders, including Alzheimer’s disease (Phillips et al., 2021), Parkinson’s disease (Jiang et al., 2023), and epilepsy (Pizzo et al., 2022). Ketones may confer neurologic protection and have antioxidant, anti-inflammatory, cellular, epigenetic, and gut microbiome modifying effects (Simeone et al., 2018; Zhang et al., 2018; Boison, 2017). It has been shown that children with refractory epilepsy have a reduced α-diversity of intestinal flora, a significant increase in the abundance of Bacteroides, and a decrease in the abundance of Firmicutes and Actinobacteria after treatment with KD, and that the increase in Bacteroides may be closely related to the antiepileptic effect of KD by modulating the secretion of IL-6 and IL-17 in dendritic cells (Zhang et al., 2018; Xie et al., 2017). The KD decreases the biodiversity of the gut microbiota and alters the proportions of specific microbiota (e.g., Akkermansia and Parabacteroides), which increases γ-glutamyl amino acids levels, increases epilepsy susceptibility and affects the levels of other gut microbe-derived metabolites, such as SCFAs, lactic acid (Xie et al., 2017; Prast-Nielsen, 2023; Gubert et al., 2020).
Antibiotic use is one of the significant factors contributing to GM dysregulation. Short-term administration of antibiotics significantly targets and alters GM composition, neurogenesis, apoptosis, and synaptic modifications (Ianiro et al., 2016; Jakobsson et al., 2010). Long-term high doses of both β-lactams (i.e., penicillins, cephalosporins, and carbapenems) and quinolones have been shown to inhibit GABA synthesis or block GABA receptors (Segev et al., 1988; Hantson et al., 1999). At the same time, taking carbapenems and quinolones can reduce the glutaminergic N-methyl-D-aspartate (NMDA) receptor agonist activity, which is more likely to stimulate excitatory transmission and lower the seizure threshold (Lode, 1999; Wanleenuwat et al., 2020; Schmuck et al., 1998).
2.5.1.2 Gut chemical barrier and epilepsy
The enterochemical barrier consists of a mucus layer and neurotransmitters metabolized by microorganisms. The mucus layer comprises gut epithelial cells with bacteriostatic substances secreted by the GM, which facilitate gastrointestinal transit and separate bacteria from epithelial cells in the gut to maintain gut homeostasis (Cai et al., 2020). When the mucus layer is disrupted, pathogenic microbiota attaches and invades the mucus layer, causing gut diseases (Kudelka et al., 2020; Song et al., 2023). Gut diseases will further aggravate the dysbiosis and increase the number of pathogenic bacteria, which will produce more LPS to induce epithelial cell damage and exacerbate the destruction of the mucus layer, thus triggering “leaky gut,” which will cause LPS to escape into the blood circulation and stimulate the synthesis of pro-inflammatory factors by glial cells of the CNS, such as interleukin-1β and tumor necrosis factor, and increase neuronal excitation, which will lead to a rise in neuronal excitability (Usuda et al., 2021; Mazarati et al., 2021). Johansson et al. (2014) found an impenetrable mucus layer in the gut of healthy people. At the same time, bacteria were detected on the gut epithelial cells of both animal models and patients with colitis.
2.5.1.3 Gut physical barrier and epilepsy
The gut physical barrier consists mainly of gut epithelial cells and intercellular tight junction proteins (Chelakkot et al., 2018). The gut epithelial cells not only form a physical barrier between microorganisms and the lamina propria, but also play an essential role in gut immunity by recognizing pathogenic bacteria and their components (Goto and Ivanov, 2013) (e.g., LPS). The epithelial cells are connected by tight junction proteins such as OCLN and CLDN 5. When the structure of gut epithelium is abnormal, or the intercellular junctions are disrupted, the physical barrier of the gut is impaired, and the gut mucosal permeability increases, which leads to the dysbiosis of the gut microbiota by bacterial translocation and causes diseases. Gut inflammation is associated with increased expression of anti-claudin 2 antibody (claudin-2) and decreased expression of tight junction protein (Weber et al., 2008). Alhasson et al. (2017) found that LPS-induced changes in GM led to intestinal damage, resulting in increased claudin-2 expression, decreased OCLN expression, increased intestinal mucosal permeability, and transfer of LPS from the intestine to the bloodstream. At the same time, the significant increase in TLR-4 expression and the increased expression of inflammatory bacteria such as Dorea spp., Proteus spp., Coprococcus spp., Ruminococcus spp., and Turibacter spp. led to an increase in the secretion of the inflammatory factor IL-1β and monocyte chemoattractant protein-1, and these toxins and inflammatory factors stimulated the BBB via the blood circulation, causing its endothelial cells to secrete proteases (e.g., matrix metalloproteinase), lipase, and carbohydrase into the extracellular matrix, disrupting BBB zonula occludens protein 1, OCLN, and CLDN 5, and further increasing the permeability of the BBB, which facilitates the entry of pathogens and inflammatory factors (e.g., LPS, Aβ, IL-1β, and IFN-γ) from the bloodstream into the brain parenchyma, which then induces neuroinflammation and ultimately causes seizures (Welcome, 2019).
2.5.1.4 Gut immune barrier and epilepsy
More than half of the body’s immune cells are in the gut mucosa (Tlaskalova-Hogenova et al., 2005). The gut immune barrier consists mainly of gut mucosal lymphoid tissue and is distributed within the gut or scattered in the gut epithelium and lamina propria. GM affects the number and distribution of immune cells (Gao et al., 2018). For example, GF mice have incompletely developed lymphoid structures, reduced levels of T and B cells, and reduced cytokine production (Bauer et al., 1963; Manolios et al., 1988); Autistic patients with gastrointestinal disorders had a higher number of infiltrating T cells as well as a higher distribution of CD19+ B cells in the duodenal, terminal ileum, and colon biopsy tissues compared to non-inflammatory controls (Furlano et al., 2001; Torrente et al., 2002; Ashwood et al., 2003). In addition, GM diversity is critical for microglia maturation and CNS function; for example, mice treated with sterility and antibiotics produce defects in microglia morphology and maturation, activation, and differentiation, leading to deficient immune responses to a wide range of pathogens, which are later restored to normal by colony transplantation (Erny et al., 2015). GM modulates innate immunity, adaptive immunity, and inflammatory mechanisms to control the development of epilepsy.
GM induces epilepsy through the innate immune pathway. Microglia are macrophages in the CNS that mediate innate immune responses (Ginhoux et al., 2013), promote inflammation, modulate neuronal activity, clear neurons and induce chronic seizures. Microglia of GF and antibiotic pretreated mice were morphologically and functionally immature and had defective immune responses to a wide range of pathogens, but immune responses returned to normal after recolonization with healthy flora (Erny et al., 2015). Peptidoglycan, which is mainly found in human GM, is an essential component of the bacterial cell wall and activates mainly microglia in GM-to-brain signaling (Spielbauer et al., 2024), promoting “leaky gut” and “leaky brain” through the induction of inflammatory cytokines (e.g., interleukin IL-1β, IL-18),” which allows the entry of gut-associated metabolites such as peptidoglycan to drive chronic inflammation in the CNS, leading to hippocampal sclerosis and the induction of temporal lobe epilepsy (Gales and Prayson, 2017).
GM induces epilepsy through the adaptive immune pathway.GM induces immune cells to produce cytokines that enter the brain through the gut barrier and the BBB and activate brain immune cells to participate in the immune response. T helper cell 17 (Th17) is a pro-inflammatory CD4+ T cell subset that is a key component of adaptive immunity (Chow and Mazmanian, 2009). Interleukin 17 (IL17) is a cytokine produced by Th17 cells and is regulated by specific GMs such as Bacteroides vulgatus group (Ivanov et al., 2008). It has been found that patients with epilepsy have higher levels of IL-17 in the cerebrospinal fluid and peripheral blood than controls, which is highly correlated with the frequency and severity of seizures (Han et al., 2020). Thus, GM influences epileptogenesis by mediating IL17 production from Th17. GM metabolites, such as SCFAs, influence immunoglobulin synthesis and secretion by regulating B lymphocyte differentiation (Honda and Littman, 2016; Kim et al., 2016). In the face of exogenous infections, the organism secretes IgA to protect intestinal epithelial cells from infection by commensal flora, and immunoscreens commensal flora to maintain intestinal immune homeostasis (Fagarasan et al., 2010; Sonnenberg et al., 2012); Meanwhile, GM and flora metabolites stimulation induces gut dendritic cells and primitive B cells to secrete protective IgA, which regulates the development and function of immune cells in the body (Yu et al., 2018). Deficiency of gut commensal flora down-regulates IgA and IgG expression and up-regulates IgE expression, increasing epilepsy susceptibility (Gensollen et al., 2016; McCoy et al., 2017).
GM mediates CNS inflammation to induce epilepsy (Li et al., 2021). GM is necessary for microglia maturation and astrocyte activation. Vascular endothelial growth factor-B and transforming growth factor-α promote pathogenic and inflammatory responses in astrocytes (Rothhammer et al., 2018). GM metabolizes dietary tryptophan into an aromatic hydrocarbon receptor agonist and interacts with microglia receptors to activate microglia, upregulate the expression of vascular endothelial growth factor-B and transform growth factor-alpha, and modulate the pathogenic response activity of astrocytes (Rothhammer et al., 2016). Meanwhile, inflammatory factors released by astrocytes increase microglia activity, including migration, phagocytosis, and synaptic modifications in apoptotic cells (Yang et al., 2020). Interactions between astrocytes and microglia result in increased inflammatory response, increased BBB permeability, and easier infiltration of peripheral blood immune cells and cytokines through the BBB into the CNS, causing chronic neuroinflammation to occur and increasing susceptibility to epilepsy (Moradi et al., 2021).
2.5.2 Blood–brain barrier
The blood–brain barrier is an active interface between the systemic circulation and the CNS that maintains homeostasis within the brain by blocking the entry of potentially toxic or harmful substances and regulating the transport of nutrients and the removal of metabolites.
GM has a key role in regulating blood–brain barrier permeability (Xie et al., 2023). Michel and Prat (2016) found lower expression of tight junction proteins and higher BBB permeability in GF mice compared to SPF mice. When transplanting GM from SPF mice or GM that can produce short-chain fatty acids, the expression of tight junction proteins is elevated and BBB permeability is normalized. Stress, inflammation or drugs disrupt the GM structure, and pathogenic bacteria such as Firmicutes, Clostridium and Proteusbacillus Vulgaris increase the LPS content of the gut, activating TLR-4 to release large amounts of cytokines and trigger “leaky gut,” which leads to the entry of cytokines into the blood circulation (Obrenovich, 2018); At the same time, under the influence of pathogenic bacteria, the expression of gut tight junction proteins decreases and gut permeability increases, which allows cytokines TNF-α and IL1-β to enter the body circulation (Ait-Belgnaoui et al., 2012), and cytokines entering the body circulation through the two pathways work together to disrupt the expression of vascular cell adhesion molecule 1 and intercellular adhesion molecule 1 in the BBB, which increases the permeability of the BBB, and induces “brain leakage,” cytokines and peripheral immune cells enter the brain inducing microglia and astrocytes to activate in large numbers, releasing cytokines, inducing neuroinflammation and contributing to epileptogenesis (Moradi et al., 2021). At the same time, the increased extracellular levels of potassium and glutamate due to the destruction of the BBB increase the excitability of the brain’s neurons, causing a sustained state of abnormal excitation in the brain’s neural network, leading to seizures (Thion et al., 2018).
3 Effects of epilepsy on GM via the downward pathway
The downward pathway mainly proceeds through the direct neural and neuroendocrine pathways (see Figure 3). The direct neural pathway is mediated by two branches of the autonomic nervous system. It induces changes in intestinal physiology by regulating intestinal functions such as intestinal motility, gastric acid secretion, gut barrier permeability, and mucosal immune responses, which regulate GM composition and activity (Bonaz et al., 2018). The CNS mediates the neuroendocrine pathway. It communicates directly with the GM by activating the HPA axis or releasing neurotransmitters from neurons, immune cells, and enterochromaffin cells, such as catecholamines, cytokines, and 5-HT (Martin et al., 2018).
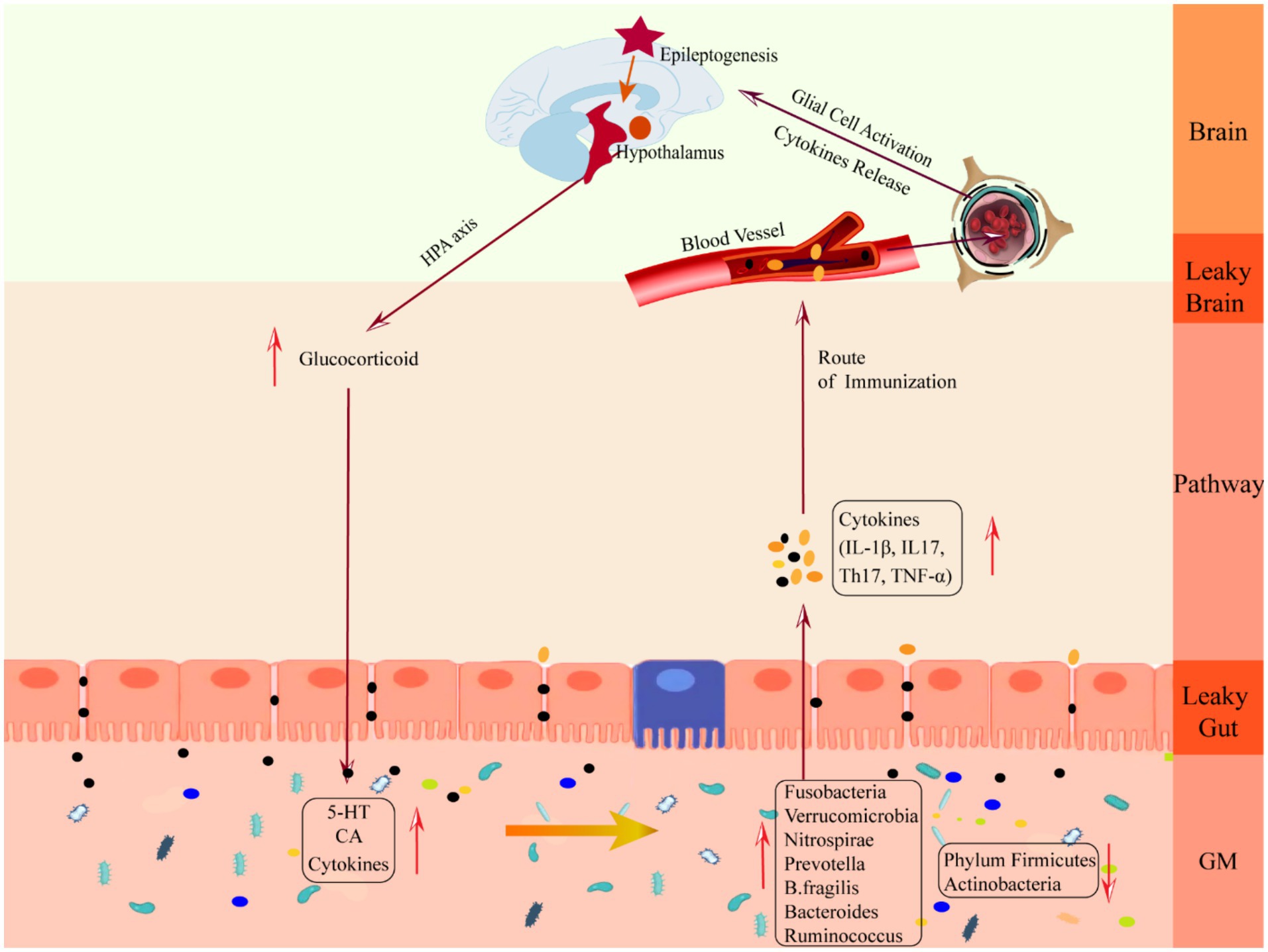
Figure 3. Schematic diagram of the mechanism of damage in the downward pathway “epileptic brain-gut-microbiota”. CA, catecholamine; GM, gut microbiota; IL-1β, Interleukin-1β; IL17, Interleukin 17, TH17, T helper 17; TNF-α, tumor necrosis factor-α.
Clinical studies have shown that patients with CNS injury are often accompanied by severe gastrointestinal dysfunction, mainly manifested by inhibition of gastrointestinal peristalsis and damage to the gastrointestinal mucosa (Iruthayarajah et al., 2018; Camara-Lemarroy et al., 2014), which leads to changes in the gut microenvironment, such as disturbances in the gut immune system (Huh and Veiga-Fernandes, 2020) and translocation of gut bacteria (Stanley et al., 2016), resulting in increased gut permeability and ultimately changes in the structure of the gut flora (Singh et al., 2016). At the same time, inflammatory factors produced by interactions between gut neurons and immune cells, as well as secretions and metabolites of the gut flora, can enter the circulation through the gut barrier, exacerbating peripheral inflammation and CNS damage (Durgan et al., 2019; Liu et al., 2019; Arneth, 2018).
3.1 Mechanisms by which epilepsy alters GM through the autonomic nervous system
Autonomic nerves regulate gut physiology, environment and GM composition and activity through the enteric nervous system to maintain host gut homeostasis (Rubio et al., 2023). Following epileptogenesis, sympathetic and parasympathetic nerves (vagus nerves) are activated in response to inflammatory factor stimulation, releasing neurotransmitters, such as catecholamines and acetylcholine, into the peripheral circulation, where they act on gut sympathetic and parasympathetic ganglia (see Figure 2B).
There are two subpopulations of gut sympathetic postganglionic nerves, a subpopulation consisting of vasoconstrictor neurons, secretory inhibitory neurons, and motor inhibitory neurons, which slow gastrointestinal secretion and motility by inhibiting cholinergic transmission and attenuating smooth muscle stimulation. Diminished gut motility decreases gut water content, nutrient absorption and bacterial clearance and increases gut transit time. Gut transport time is strongly correlated with microbial abundance and composition (Vandeputte et al., 2016), and increased gut transport time reduces bacterial biomass and diversity in the distal gut region (Tottey et al., 2017). Reduced bacterial clearance prevents the timely expulsion of harmful flora from the gut, allowing them to adhere to the surface of gut cells (Jänig, 2006); another subpopulation is involved in mucosal immunity by interacting with the GM, the gut mucosa. Epileptogenic bacteria stimulate the gut by releasing endotoxins or substances such as SCFAs and acetylcholine, which reduce gut blood flow, causing apoptosis of gut epithelial cells and reduction of cupped cells. Meanwhile, harmful bacteria attached to the surface of gut cells bind to molecules on the surface of gut epithelial cells, disrupting the expression of tight junction proteins and increasing the permeability of the gut mucosa (Mracsko et al., 2014; Shi et al., 2018).
Gastrointestinal parasympathetic nerves consist of the vagus and sacral parasympathetic nerves, and the vagus nerve directly stimulates the gastric wall cells to secrete acid. It indirectly stimulates the gastric sinus G-cells to release gastrin, which increases the concentration of H+ ions to promote the overproduction of histamine and increase gastrointestinal permeability (Li et al., 2020). At the same time, the vagus nerve increases gut barrier permeability via enteric nerves and/or cells (e.g., enteric neuroglia) (Bonaz, 2022). Increased gut barrier permeability triggers a “leaky gut,” which causes bacterial translocation and an inflammatory response, disrupting the gut environment and microbial survival conditions and altering the characteristics of the microflora (Elenkov et al., 2000).
3.2 Mechanisms by which epilepsy alters GM via the HPA axis
The HPA axis is regulated by the prefrontal cortex, hippocampus, and amygdala, which are at the core of the stress response and one of the key facilitators of epilepsy induction (Cano-Lopez and Gonzalez-Bono, 2019). When activated, neurons in the paraventricular nucleus of the hypothalamus synthesize and secrete adrenocorticotropin-releasing hormone (Ashwood et al., 2013), which causes the anterior pituitary gland to release adrenocorticotropic hormone through the pituitary portal system, which stimulates the adrenal cortex to synthesize and secrete glucocorticoid hormones and to enter the blood circulation, binds to the glucocorticoid receptor in the gut tract or in the brain to exert its effects, and acts on the negative feedback loop between the hypothalamus and the pituitary gland to regulate the secretion of the HPA axis (Smith and Vale, 2006). The brain can influence gut homeostasis by regulating changes in the HPA axis. GABA is an inhibitory neurotransmitter in the brain. When seizures occur, GABAergic control of hypothalamic paraventricular nucleus neurons, which are at the apex of HPA axis control, is impaired (O’Toole et al., 2014), the negative feedback loop is disrupted, and the HPA axis is overexcited, resulting in massive production and release of glucocorticoids, leading to increased cerebral excitability (Castro et al., 2012) and human cortisolism (Tetel et al., 2018), gut immune cells, gut epithelial cells and enteroendocrine cells are over-activated, and large amounts of epinephrine and norepinephrine stimulate the GM sensing mechanism, increasing the toxicity of several intestinal pathogens as well as non-pathogenic microorganisms, which collectively trigger an gut immune-inflammatory response and decrease gut barrier permeability. At the same time, excessive glucocorticoids increase gut barrier permeability (Moussaoui et al., 2014), reduce motility, and decrease mucus secretion (Da et al., 2014), which triggers “leaky gut,” which translocates harmful bacteria (Tena-Garitaonaindia et al., 2022) and breaks down the GM structure, triggering gastrointestinal disorders such as abdominal pain, colitis, or irritable bowel syndrome (Park et al., 2013), which is a mechanism of gastrointestinal dysfunction in epileptic patients. Mechanism of gastrointestinal dysfunction in patients with epilepsy.
4 Characterization of GM changes in patients with epilepsy
Differences in microbial composition exist between patients with epilepsy and healthy individuals. These differences are due to a variety of factors, such as inflammatory responses, altered neurotransmitters, and medication. These factors can affect the gut environment and microbial conditions and alter the characteristics of the microflora.
Patients with epilepsy have an abundance of harmful bacterial phyla such as Fusobacteria, Verrucomicrobia, and Nitrospirae in their gut, and a lower number of Firmicutes and Spirochaetes. There is a positive correlation between Fusobacteria and serum 2-Deoxy-D-ribose levels (Liang et al., 2020). 2-Deoxy-D-ribose together with thymidine phosphorylase and vascular endothelial growth factor inhibit tight junction proteins leading to the disruption of the BBB and causing inflammatory disorders of the CNS (Chapouly et al., 2015). Meanwhile, 2-Deoxy-D-ribose promotes seizures by inducing hippocampal neuronal apoptosis through oxidative stress (Formichi et al., 2009). Verrucomicrobia produces high abundance of SCFAs that degrade mucins and increase the permeability of the BBB and small intestinal epithelial barrier by altering OCLN and CLDN 5 expression (De la Cuesta-Zuluaga et al., 2017). Verrucomicrobia are positively correlated with the serum pro-inflammatory cytokine IFN-γ (Lin et al., 2019), and with altered permeability, bacterial translocation and neuroinflammatory substances are susceptible to enter the brain and induce epilepsy (Gao et al., 2017). Verrucomicrobia is involved in epileptogenesis by increasing glutamate and glutamine levels and decreasing serotonin levels (Sun et al., 2016). Nitrospirae increases nitrite toxicity, which ultimately leads to structural disruption of the BBB with increased permeability and triggers epilepsy. In patients with chronic inflammatory diseases, the abundance of Spirochaetes is much lower than in healthy individuals (Cao et al., 2018), suggesting that Spirochaetes plays a protective role in immune defense.
Patients with epilepsy have elevated levels of Prevotella. Prevotella can produce SCFAs and when in high abundance can lead to sustained production of IL-6 in the gut, triggering an inflammatory response, and neuroinflammation can lead to neuronal hyperexcitability, causing seizures (Sanz and Garcia-Gimeno, 2020). Prevotella is involved in seizures by affecting cytokine levels, but the mechanism of action needs to be further elucidated. B. fragilis is also closely associated with epilepsy. B. fragilis is an anaerobic rod-shaped Gram-negative bacterium that secretes pro-inflammatory substances, such as LPS and B. fragilis toxins, which cause an increase in the permeability of the BBB through E-calmodulin in epithelial cells, making it easier for microbial-derived neurotoxic substances to enter the brain and promote neuronal hyperexcitability that triggers epilepsy (Lukiw et al., 2021; Vezzani et al., 2016). Thus, B. fragilis and its epileptogenic metabolites induce a neuroinflammatory response by disrupting the BBB.
Epilepsy-associated microflora dysbiosis can also be characterized by changes in the abundance of certain genera. In epileptic patients the abundance of the genera Bacteroidetes and Ruminococcus is increased. Colonization of Ruminococcus in GF mice resulted in increased levels of the intestinal pro-inflammatory cell Th17, and activation of T17 increased the risk of autoimmune epilepsy (Han et al., 2020). Ruminococcus has been shown to be strongly associated with irritable bowel syndrome (Hall et al., 2017), Crohn’s disease (Nagayama et al., 2020) and generalized anxiety (Jiang et al., 2018), among others. Rumatococcus promote epilepsy progression by exacerbating inflammation and inducing intestinal mucosal degeneration (Clark and Mach, 2016). Furthermore, the gut microbiome of patients with drug-resistant epilepsy differs from that of patients with drug-sensitive epilepsy. Drug-resistant patients have increased alpha diversity and relative abundance of thick-walled phyla (Peng et al., 2018).
In conclusion, significant differences in GM composition were observed in patients with epilepsy compared to healthy individuals. Prevotella, Bacteroides, Fusobacterium, B. fragilis, Ruminococcus, and Nitrospirae are potential risk factors for seizures, and elevated levels of Clostridium and Verrucomicrobia can be used as a biomarker for the diagnosis of epilepsy (Dong et al., 2022). Characteristic changes in GM and metabolic pathways in epileptic patients suggest new targets for the treatment of epilepsy.
5 Summary and outlook
In summary, the present study reveals the association between GM and epilepsy based on the upward and downward pathways of the MGB axis. Gut dysfunction/disorders are closely associated with the susceptibility and pathogenesis of epilepsy, and dysregulation of GM leads to increased gut barrier and BBB permeability, which induces neuroinflammatory substances to enter the brain, leading to increased neuronal excitability and inducing epileptic seizures. Frequent seizures repeatedly activate intracranial immune response, excessive activation of the autonomic nervous system and HPA and other downward pathways, triggering “leaky brain “, causing inflammatory factors to be transported to the gut through the blood circulation, destroying the gut barrier and triggering “leaky gut,” leading to bacterial translocation; at the same time, gut sympathetic nerve excitation inhibits gastrointestinal motility, causing excessive accumulation of harmful bacteria and their metabolites, which in turn increases susceptibility to seizures through the upward pathway. At the same time, gut sympathetic nerve excitation inhibits gastrointestinal peristalsis, leading to excessive accumulation of harmful bacteria and their metabolites, which alters the structure and function of the GM, which in turn increases the susceptibility to seizures through the upward pathway. If not intervened, breaking the “positive feedback” MGB axis loop will increase the frequency and duration of seizures. There are differences in microbiome composition between epileptic patients and healthy individuals, and these differences are due to inflammatory responses, neurotransmitter alterations, medications, and other factors. Some specific GM may serve as gut biomarkers and potential therapeutic targets in patients with epilepsy. By regulating GM it may be possible to improve the symptoms and prognosis of patients with epilepsy. In conclusion, dysregulation of GM is a potential risk factor for causing epileptic seizures, and epileptogenic GM and its metabolites become biomarkers for diagnosing epilepsy after seizures; the characteristic changes in GM and metabolic pathways may provide new targets for epilepsy treatment.
In the future, the following aspects can be improved: (1) Further investigate the mechanism of action between GM and epilepsy, including the effect of microbial metabolites on neuronal excitability, and the regulation mechanism of gut barrier permeability. (2) Compare the microbial composition and functional changes in patients with different types of epilepsy, and search for biomarkers of specific microbiota to provide a basis for the diagnosis and treatment of epilepsy. (3) To explore the therapeutic effects of precise gut microbial agents, such as probiotics and prebiotics, on epilepsy, and to provide new strategies for the individualized treatment of epilepsy.
Author contributions
WY: Writing – original draft. HC: Writing – original draft. CW: Methodology, Supervision, Writing – review & editing. XW: Funding acquisition, Writing – review & editing. CY: Investigation, Writing – review & editing. WC: Conceptualization, Methodology, Supervision, Writing – review & editing.
Funding
The author(s) declare that financial support was received for the research, authorship, and/or publication of this article. This research was supported by the Heilongjiang Province Postdoctoral Special Funding Project, China (LBH-Z22282) and Heilongjiang University of Chinese Medicine “State Administration of Traditional Chinese Medicine High-Level Construction and Double First-Class” Discipline Development Support Fund, China (GJJGSPZDXK31004).
Acknowledgments
We would like to express our gratitude to all members for their valuable assistance and suggestions throughout the collection and analysis of our article.
Conflict of interest
The authors declare that the research was conducted in the absence of any commercial or financial relationships that could be construed as a potential conflict of interest.
Publisher’s note
All claims expressed in this article are solely those of the authors and do not necessarily represent those of their affiliated organizations, or those of the publisher, the editors and the reviewers. Any product that may be evaluated in this article, or claim that may be made by its manufacturer, is not guaranteed or endorsed by the publisher.
References
Abdel-Haq, R., Schlachetzki, J., Glass, C. K., and Mazmanian, S. K. (2019). Microbiome-microglia connections via the gut-brain axis. J. Exp. Med. 216, 41–59. doi: 10.1084/jem.20180794
Ait-Belgnaoui, A., Durand, H., Cartier, C., Chaumaz, G., Eutamene, H., Ferrier, L., et al. (2012). Prevention of gut leakiness by a probiotic treatment leads to attenuated hpa response to an acute psychological stress in rats. Psychoneuroendocrinology 37, 1885–1895. doi: 10.1016/j.psyneuen.2012.03.024
Alhasson, F., Das, S., Seth, R., Dattaroy, D., Chandrashekaran, V., Ryan, C. N., et al. (2017). Altered gut microbiome in a mouse model of gulf war illness causes neuroinflammation and intestinal injury via leaky gut and tlr4 activation. PLoS One 12:e0172914. doi: 10.1371/journal.pone.0172914
Aljeradat, B., Kumar, D., Abdulmuizz, S., Kundu, M., Almealawy, Y. F., Batarseh, D. R., et al. (2024). Neuromodulation and the gut-brain axis: therapeutic mechanisms and implications for gastrointestinal and neurological disorders. Pathophysiology 31, 244–268. doi: 10.3390/pathophysiology31020019
Amlerova, J., Sroubek, J., Angelucci, F., and Hort, J. (2021). Evidences for a role of gut microbiota in pathogenesis and management of epilepsy. Int. J. Mol. Sci. 22:22. doi: 10.3390/ijms22115576
Arneth, B. M. (2018). Gut-brain axis biochemical signalling from the gastrointestinal tract to the central nervous system: gut dysbiosis and altered brain function. Postgrad. Med. J. 94, 446–452. doi: 10.1136/postgradmedj-2017-135424
Ashwood, P., Anthony, A., Pellicer, A. A., Torrente, F., Walker-Smith, J. A., and Wakefield, A. J. (2003). Intestinal lymphocyte populations in children with regressive autism: evidence for extensive mucosal immunopathology. J. Clin. Immunol. 23, 504–517. doi: 10.1023/b:joci.0000010427.05143.bb
Ashwood, E. R., Bruns, D. E., Tietz, N. W., and Burtis, C. A. (2013). Tietz textbook of clinical chemistry and molecular diagnostics, vol. 2. Amsterdam, Netherlands: Elsevier Health Sciences.
Atmaca, M. M., Tuzun, E., Erdag, E., Bebek, N., Baykan, B., and Gurses, C. (2017). Investigation of anti-neuronal antibodies in status epilepticus of unknown etiology: a prospective study. Acta Neurol. Belg. 117, 841–848. doi: 10.1007/s13760-017-0796-5
Attenello, F., Amar, A. P., Liu, C., and Apuzzo, M. L. (2015). Theoretical basis of vagus nerve stimulation. Prog. Neurol. Surg. 29, 20–28. doi: 10.1159/000434652
Bastos, F., and Cross, J. H. (2020). Epilepsy. Handb. Clin. Neurol. 174, 137–158. doi: 10.1016/B978-0-444-64148-9.00011-9
Bauer, H., Horowitz, R. E., Levenson, S. M., and Popper, H. (1963). The response of the lymphatic tissue to the microbial flora. Studies on germfree mice. Am. J. Pathol. 42, 471–483.
Bell, D. S. (2015). Changes seen in gut bacteria content and distribution with obesity: causation or association? Postgrad. Med. 127, 863–868. doi: 10.1080/00325481.2015.1098519
Bienenstock, J., Kunze, W., and Forsythe, P. (2015). Microbiota and the gut-brain axis. Nutr. Rev. 73, 28–31. doi: 10.1093/nutrit/nuv019
Boison, D. (2017). New insights into the mechanisms of the ketogenic diet. Curr. Opin. Neurol. 30, 187–192. doi: 10.1097/WCO.0000000000000432
Bonaz, B. (2022). Anti-inflammatory effects of vagal nerve stimulation with a special attention to intestinal barrier dysfunction. Neurogastroenterol. Motil. 34:e14456. doi: 10.1111/nmo.14456
Bonaz, B., Bazin, T., and Pellissier, S. (2018). The vagus nerve at the interface of the microbiota-gut-brain axis. Front. Neurosci. 12:49. doi: 10.3389/fnins.2018.00049
Borre, Y. E., O'Keeffe, G. W., Clarke, G., Stanton, C., Dinan, T. G., and Cryan, J. F. (2014). Microbiota and neurodevelopmental windows: implications for brain disorders. Trends Mol. Med. 20, 509–518. doi: 10.1016/j.molmed.2014.05.002
Braga, J. D., Thongngam, M., and Kumrungsee, T. (2024). Gamma-aminobutyric acid as a potential postbiotic mediator in the gut-brain axis. NPJ Sci. Food 8:16. doi: 10.1038/s41538-024-00253-2
Braniste, V., Al-Asmakh, M., Kowal, C., Anuar, F., Abbaspour, A., Toth, M., et al. (2014). The gut microbiota influences blood-brain barrier permeability in mice. Sci. Transl. Med. 6:263ra158. doi: 10.1126/scitranslmed.3009759
Bravo, J. A., Forsythe, P., Chew, M. V., Escaravage, E., Savignac, H. M., Dinan, T. G., et al. (2011). Ingestion of lactobacillus strain regulates emotional behavior and central gaba receptor expression in a mouse via the vagus nerve. Proc. Natl. Acad. Sci. U. S. A. 108, 16050–16055. doi: 10.1073/pnas.1102999108
Cai, R., Cheng, C., Chen, J., Xu, X., Ding, C., and Gu, B. (2020). Interactions of commensal and pathogenic microorganisms with the mucus layer in the colon. Gut Microbes 11, 680–690. doi: 10.1080/19490976.2020.1735606
Camara-Lemarroy, C. R., Ibarra-Yruegas, B. E., and Gongora-Rivera, F. (2014). Gastrointestinal complications after ischemic stroke. J. Neurol. Sci. 346, 20–25. doi: 10.1016/j.jns.2014.08.027
Cano-Lopez, I., and Gonzalez-Bono, E. (2019). Cortisol levels and seizures in adults with epilepsy: a systematic review. Neurosci. Biobehav. Rev. 103, 216–229. doi: 10.1016/j.neubiorev.2019.05.023
Cao, Y., Qiao, M., Tian, Z., Yu, Y., Xu, B., Lao, W., et al. (2018). Comparative analyses of subgingival microbiome in chronic periodontitis patients with and without Iga nephropathy by high throughput 16s rrna sequencing. Cell. Physiol. Biochem. 47, 774–783. doi: 10.1159/000490029
Castro, O. W., Santos, V. R., Pun, R. Y., McKlveen, J. M., Batie, M., Holland, K. D., et al. (2012). Impact of corticosterone treatment on spontaneous seizure frequency and epileptiform activity in mice with chronic epilepsy. PLoS One 7:e46044. doi: 10.1371/journal.pone.0046044
Cerdo, T., Ruiz, A., Suarez, A., and Campoy, C. (2017). Probiotic, prebiotic, and brain development. Nutrients 9:9. doi: 10.3390/nu9111247
Chapouly, C., Tadesse, A. A., Horng, S., Castro, K., Zhang, J., Asp, L., et al. (2015). Astrocytic tymp and vegfa drive blood-brain barrier opening in inflammatory central nervous system lesions. Brain 138, 1548–1567. doi: 10.1093/brain/awv077
Chelakkot, C., Ghim, J., and Ryu, S. H. (2018). Mechanisms regulating intestinal barrier integrity and its pathological implications. Exp. Mol. Med. 50, 1–9. doi: 10.1038/s12276-018-0126-x
Chen, S., Jiao, Y., Han, C., Li, Y., Zou, W., and Liu, J. (2023). Drug-resistant epilepsy and gut-brain axis: an overview of a new strategy for treatment. Mol. Neurobiol. doi: 10.1007/s12035-023-03757-2
Cheng, Y., Mai, Q., Zeng, X., Wang, H., Xiao, Y., Tang, L., et al. (2019). Propionate relieves pentylenetetrazol-induced seizures, consequent mitochondrial disruption, neuron necrosis and neurological deficits in mice. Biochem. Pharmacol. 169:113607. doi: 10.1016/j.bcp.2019.08.009
Chow, J., and Mazmanian, S. K. (2009). Getting the bugs out of the immune system: do bacterial microbiota "fix" intestinal t cell responses? Cell Host Microbe 5, 8–12. doi: 10.1016/j.chom.2008.12.006
Clark, A., and Mach, N. (2016). Exercise-induced stress behavior, gut-microbiota-brain axis and diet: a systematic review for athletes. J. Int. Soc. Sports Nutr. 13:43. doi: 10.1186/s12970-016-0155-6
Cryan, J. F., O'Riordan, K. J., Cowan, C., Sandhu, K. V., Bastiaanssen, T., Boehme, M., et al. (2019). The microbiota-gut-brain axis. Physiol. Rev. 99, 1877–2013. doi: 10.1152/physrev.00018.2018
Da, S. S., Robbe-Masselot, C., Ait-Belgnaoui, A., Mancuso, A., Mercade-Loubiere, M., Salvador-Cartier, C., et al. (2014). Stress disrupts intestinal mucus barrier in rats via mucin o-glycosylation shift: prevention by a probiotic treatment. Am. J. Physiol. Gastrointest. Liver Physiol. 307, G420–G429. doi: 10.1152/ajpgi.00290.2013
de Caro, C., Iannone, L. F., Citraro, R., Striano, P., de Sarro, G., Constanti, A., et al. (2019). Can we 'seize' the gut microbiota to treat epilepsy? Neurosci. Biobehav. Rev. 107, 750–764. doi: 10.1016/j.neubiorev.2019.10.002
De la Cuesta-Zuluaga, J., Mueller, N. T., Corrales-Agudelo, V., Velasquez-Mejia, E. P., Carmona, J. A., Abad, J. M., et al. (2017). Metformin is associated with higher relative abundance of mucin-degrading akkermansia muciniphila and several short-chain fatty acid-producing microbiota in the gut. Diabetes Care 40, 54–62. doi: 10.2337/dc16-1324
De Timary, P., Starkel, P., Delzenne, N. M., and Leclercq, S. (2017). A role for the peripheral immune system in the development of alcohol use disorders? Neuropharmacology 122, 148–160. doi: 10.1016/j.neuropharm.2017.04.013
Den Besten, G., van Eunen, K., Groen, A. K., Venema, K., Reijngoud, D. J., and Bakker, B. M. (2013). The role of short-chain fatty acids in the interplay between diet, gut microbiota, and host energy metabolism. J. Lipid Res. 54, 2325–2340. doi: 10.1194/jlr.R036012
Devinsky, O., Vezzani, A., Najjar, S., De Lanerolle, N. C., and Rogawski, M. A. (2013). Glia and epilepsy: excitability and inflammation. Trends Neurosci. 36, 174–184. doi: 10.1016/j.tins.2012.11.008
Dicks, L. (2022). Gut Bacteria and neurotransmitters. Microorganisms 10:10. doi: 10.3390/microorganisms10091838
Ding, X., Zhou, J., Zhao, L., Chen, M., Wang, S., Zhang, M., et al. (2022). Intestinal flora composition determines microglia activation and improves epileptic episode progress. Front. Cell. Infect. Microbiol. 12:835217. doi: 10.3389/fcimb.2022.835217
Dockray, G. J. (2013). Enteroendocrine cell signalling via the vagus nerve. Curr. Opin. Pharmacol. 13, 954–958. doi: 10.1016/j.coph.2013.09.007
Dong, L., Zheng, Q., Cheng, Y., Zhou, M., Wang, M., Xu, J., et al. (2022). Gut microbial characteristics of adult patients with epilepsy. Front. Neurosci. 16:803538. doi: 10.3389/fnins.2022.803538
Duan, R., Li, H. M., Hu, W. B., Hong, C. G., Chen, M. L., Cao, J., et al. (2022). Recurrent de novo single point variant on the gene encoding na(+)/k(+) pump results in epilepsy. Prog. Neurobiol. 216:102310. doi: 10.1016/j.pneurobio.2022.102310
Durgan, D. J., Lee, J., McCullough, L. D., and Bryan, R. J. (2019). Examining the role of the microbiota-gut-brain axis in stroke. Stroke 50, 2270–2277. doi: 10.1161/STROKEAHA.119.025140
Elenkov, I. J., Wilder, R. L., Chrousos, G. P., and Vizi, E. S. (2000). The sympathetic nerve--an integrative interface between two supersystems: the brain and the immune system. Pharmacol. Rev. 52, 595–638
Engevik, M. A., Danhof, H. A., Ruan, W., Engevik, A. C., Chang-Graham, A. L., Engevik, K. A., et al. (2021). Fusobacterium nucleatum secretes outer membrane vesicles and promotes intestinal inflammation. MBio 12:12. doi: 10.1128/mBio.02706-20
Erny, D., Hrabe, D. A. A., Jaitin, D., Wieghofer, P., Staszewski, O., David, E., et al. (2015). Host microbiota constantly control maturation and function of microglia in the cns. Nat. Neurosci. 18, 965–977. doi: 10.1038/nn.4030
Fagarasan, S., Kawamoto, S., Kanagawa, O., and Suzuki, K. (2010). Adaptive immune regulation in the gut: t cell-dependent and t cell-independent Iga synthesis. Annu. Rev. Immunol. 28, 243–273. doi: 10.1146/annurev-immunol-030409-101314
Formichi, P., Radi, E., Battisti, C., Di Maio, G., Tarquini, E., Leonini, A., et al. (2009). Apoptosis in cadasil: an in vitro study of lymphocytes and fibroblasts from a cohort of italian patients. J. Cell. Physiol. 219, 494–502. doi: 10.1002/jcp.21695
Fung, T. C., Olson, C. A., and Hsiao, E. Y. (2017). Interactions between the microbiota, immune and nervous systems in health and disease. Nat. Neurosci. 20, 145–155. doi: 10.1038/nn.4476
Furlano, R. I., Anthony, A., Day, R., Brown, A., McGarvey, L., Thomson, M. A., et al. (2001). Colonic cd8 and gamma delta t-cell infiltration with epithelial damage in children with autism. J. Pediatr. 138, 366–372. doi: 10.1067/mpd.2001.111323
Furness, J. B., Rivera, L. R., Cho, H. J., Bravo, D. M., and Callaghan, B. (2013). The gut as a sensory organ. Nat. Rev. Gastroenterol. Hepatol. 10, 729–740. doi: 10.1038/nrgastro.2013.180
Gales, J. M., and Prayson, R. A. (2017). Chronic inflammation in refractory hippocampal sclerosis-related temporal lobe epilepsy. Ann. Diagn. Pathol. 30, 12–16. doi: 10.1016/j.anndiagpath.2017.05.009
Gao, F., Gao, Y., Zhang, S. J., Zhe, X., Meng, F. L., Qian, H., et al. (2017). Alteration of plasma cytokines in patients with active epilepsy. Acta Neurol. Scand. 135, 663–669. doi: 10.1111/ane.12665
Gao, J., Xu, K., Liu, H., Liu, G., Bai, M., Peng, C., et al. (2018). Impact of the gut microbiota on intestinal immunity mediated by tryptophan metabolism. Front. Cell. Infect. Microbiol. 8:13. doi: 10.3389/fcimb.2018.00013
Gensollen, T., Iyer, S. S., Kasper, D. L., and Blumberg, R. S. (2016). How colonization by microbiota in early life shapes the immune system. Science 352, 539–544. doi: 10.1126/science.aad9378
Ginhoux, F., Lim, S., Hoeffel, G., Low, D., and Huber, T. (2013). Origin and differentiation of microglia. Front. Cell. Neurosci. 7:45. doi: 10.3389/fncel.2013.00045
Goehler, L. E., Gaykema, R. P., Opitz, N., Reddaway, R., Badr, N., and Lyte, M. (2005). Activation in vagal afferents and central autonomic pathways: early responses to intestinal infection with Campylobacter jejuni. Brain Behav. Immun. 19, 334–344. doi: 10.1016/j.bbi.2004.09.002
Goto, Y., and Ivanov, I. I. (2013). Intestinal epithelial cells as mediators of the commensal-host immune crosstalk. Immunol. Cell Biol. 91, 204–214. doi: 10.1038/icb.2012.80
Gozubatik-Celik, G., Ozkara, C., Ulusoy, C., Gunduz, A., Delil, S., Yeni, N., et al. (2017). Anti-neuronal autoantibodies in both drug responsive and resistant focal seizures with unknown cause. Epilepsy Res. 135, 131–136. doi: 10.1016/j.eplepsyres.2017.06.008
Gubert, C., Kong, G., Renoir, T., and Hannan, A. J. (2020). Exercise, diet and stress as modulators of gut microbiota: implications for neurodegenerative diseases. Neurobiol. Dis. 134:104621. doi: 10.1016/j.nbd.2019.104621
Gunawardene, A. R., Corfe, B. M., and Staton, C. A. (2011). Classification and functions of enteroendocrine cells of the lower gastrointestinal tract. Int. J. Exp. Pathol. 92, 219–231. doi: 10.1111/j.1365-2613.2011.00767.x
Guo, H., Liu, X., Chen, T., Wang, X., and Zhang, X. (2024). Akkermansia muciniphila improves depressive-like symptoms by modulating the level of 5-ht neurotransmitters in the gut and brain of mice. Mol. Neurobiol. 61, 821–834. doi: 10.1007/s12035-023-03602-6
Hall, A. B., Yassour, M., Sauk, J., Garner, A., Jiang, X., Arthur, T., et al. (2017). A novel Ruminococcus gnavus clade enriched in inflammatory bowel disease patients. Genome Med. 9:103. doi: 10.1186/s13073-017-0490-5
Han, Y., Yang, L., Liu, X., Feng, Y., Pang, Z., and Lin, Y. (2020). Hmgb1/cxcl12-mediated immunity and th17 cells might underlie highly suspected autoimmune epilepsy in elderly individuals. Neuropsychiatr. Dis. Treat. 16, 1285–1293. doi: 10.2147/NDT.S242766
Hantson, P., Leonard, F., Maloteaux, J. M., and Mahieu, P. (1999). How epileptogenic are the recent antibiotics? Acta Clin. Belg. 54, 80–87. doi: 10.1080/17843286.1999.11754213
Honda, K., and Littman, D. R. (2016). The microbiota in adaptive immune homeostasis and disease. Nature 535, 75–84. doi: 10.1038/nature18848
Hooper, L. V., and Macpherson, A. J. (2010). Immune adaptations that maintain homeostasis with the intestinal microbiota. Nat. Rev. Immunol. 10, 159–169. doi: 10.1038/nri2710
Huh, J. R., and Veiga-Fernandes, H. (2020). Neuroimmune circuits in inter-organ communication. Nat. Rev. Immunol. 20, 217–228. doi: 10.1038/s41577-019-0247-z
Ianiro, G., Tilg, H., and Gasbarrini, A. (2016). Antibiotics as deep modulators of gut microbiota: between good and evil. Gut 65, 1906–1915. doi: 10.1136/gutjnl-2016-312297
iMSMS Consortium (2022). Gut microbiome of multiple sclerosis patients and paired household healthy controls reveal associations with disease risk and course. Cell 185, 3467–3486.e16. doi: 10.1016/j.cell.2022.08.021
Iruthayarajah, J., McIntyre, A., Mirkowski, M., Welch-West, P., Loh, E., and Teasell, R. (2018). Risk factors for dysphagia after a spinal cord injury: a systematic review and meta-analysis. Spinal Cord 56, 1116–1123. doi: 10.1038/s41393-018-0170-3
Ivanov, I. I., Frutos, R. L., Manel, N., Yoshinaga, K., Rifkin, D. B., Sartor, R. B., et al. (2008). Specific microbiota direct the differentiation of il-17-producing t-helper cells in the mucosa of the small intestine. Cell Host Microbe 4, 337–349. doi: 10.1016/j.chom.2008.09.009
Jakobsson, H. E., Jernberg, C., Andersson, A. F., Sjolund-Karlsson, M., Jansson, J. K., and Engstrand, L. (2010). Short-term antibiotic treatment has differing long-term impacts on the human throat and gut microbiome. PLoS One 5:e9836. doi: 10.1371/journal.pone.0009836
Jänig, W. (2006). The integrative action of the autonomic nervous system: neurobiology of homeostasis. Cambridge, UK: Cambridge University Press, 610.
Jiang, Z., Wang, X., Zhang, H., Yin, J., Zhao, P., Yin, Q., et al. (2023). Ketogenic diet protects mptp-induced mouse model of parkinson's disease via altering gut microbiota and metabolites. Medcomm (2020) 4:e268. doi: 10.1002/mco2.268
Jiang, H. Y., Zhang, X., Yu, Z. H., Zhang, Z., Deng, M., Zhao, J. H., et al. (2018). Altered gut microbiota profile in patients with generalized anxiety disorder. J. Psychiatr. Res. 104, 130–136. doi: 10.1016/j.jpsychires.2018.07.007
Johansson, M. E., Gustafsson, J. K., Holmen-Larsson, J., Jabbar, K. S., Xia, L., Xu, H., et al. (2014). Bacteria penetrate the normally impenetrable inner colon mucus layer in both murine colitis models and patients with ulcerative colitis. Gut 63, 281–291. doi: 10.1136/gutjnl-2012-303207
Joyce, S. A., and O’Malley, D. (2022). Bile acids, bioactive signalling molecules in interoceptive gut-to-brain communication. J. Physiol. 600, 2565–2578. doi: 10.1113/JP281727
Kaelberer, M. M., Buchanan, K. L., Klein, M. E., Barth, B. B., Montoya, M. M., Shen, X., et al. (2018). A gut-brain neural circuit for nutrient sensory transduction. Science 361:361. doi: 10.1126/science.aat5236
Kang, D. H., Heo, R. W., Yi, C. O., Kim, H., Choi, C. H., and Roh, G. S. (2015). High-fat diet-induced obesity exacerbates kainic acid-induced hippocampal cell death. BMC Neurosci. 16:72. doi: 10.1186/s12868-015-0202-2
Karsenty, G., and Yadav, V. K. (2011). Regulation of bone mass by serotonin: molecular biology and therapeutic implications. Annu. Rev. Med. 62, 323–331. doi: 10.1146/annurev-med-090710-133426
Kim, M., Qie, Y., Park, J., and Kim, C. H. (2016). Gut microbial metabolites fuel host antibody responses. Cell Host Microbe 20, 202–214. doi: 10.1016/j.chom.2016.07.001
Kudelka, M. R., Stowell, S. R., Cummings, R. D., and Neish, A. S. (2020). Intestinal epithelial glycosylation in homeostasis and gut microbiota interactions in ibd. Nat. Rev. Gastroenterol. Hepatol. 17, 597–617. doi: 10.1038/s41575-020-0331-7
Latorre, R., Sternini, C., De Giorgio, R., and Greenwood-Van, M. B. (2016). Enteroendocrine cells: a review of their role in brain-gut communication. Neurogastroenterol. Motil. 28, 620–630. doi: 10.1111/nmo.12754
Lavelle, A., and Sokol, H. (2020). Gut microbiota-derived metabolites as key actors in inflammatory bowel disease. Nat. Rev. Gastroenterol. Hepatol. 17, 223–237. doi: 10.1038/s41575-019-0258-z
Leonardi, M., Martelletti, P., Burstein, R., Fornari, A., Grazzi, L., Guekht, A., et al. (2024). The world health organization intersectoral global action plan on epilepsy and other neurological disorders and the headache revolution: from headache burden to a global action plan for headache disorders. J. Headache Pain 25:4. doi: 10.1186/s10194-023-01700-3
Li, Y., Yang, X., Zhang, J., Jiang, T., Zhang, Z., Wang, Z., et al. (2021). Ketogenic diets induced glucose intolerance and lipid accumulation in mice with alterations in gut microbiota and metabolites. MBio 12:12. doi: 10.1128/mBio.03601-20
Li, X. J., You, X. Y., Wang, C. Y., Li, X. L., Sheng, Y. Y., Zhuang, P. W., et al. (2020). Bidirectional brain-gut-microbiota axis in increased intestinal permeability induced by central nervous system injury. CNS Neurosci. Ther. 26, 783–790. doi: 10.1111/cns.13401
Liang, S., Mao, Y., Liao, M., Xu, Y., Chen, Y., Huang, X., et al. (2020). Gut microbiome associated with apc gene mutation in patients with intestinal adenomatous polyps. Int. J. Biol. Sci. 16, 135–146. doi: 10.7150/ijbs.37399
Lin, C. H., Chen, C. C., Chiang, H. L., Liou, J. M., Chang, C. M., Lu, T. P., et al. (2019). Altered gut microbiota and inflammatory cytokine responses in patients with parkinson's disease. J. Neuroinflammation 16:129. doi: 10.1186/s12974-019-1528-y
Lin, Z., Si, Q., and Xiaoyi, Z. (2016). Association between epilepsy and systemic autoimmune diseases: a meta-analysis. Seizure 41, 160–166. doi: 10.1016/j.seizure.2016.08.003
Liu, S., Gao, J., Zhu, M., Liu, K., and Zhang, H. L. (2020). Gut microbiota and dysbiosis in alzheimer's disease: implications for pathogenesis and treatment. Mol. Neurobiol. 57, 5026–5043. doi: 10.1007/s12035-020-02073-3
Liu, Y., Jia, N., Tang, C., Long, H., and Wang, J. (2024). Microglia in microbiota-gut-brain axis: a hub in epilepsy. Mol. Neurobiol. 61, 7109–7126. doi: 10.1007/s12035-024-04022-w
Liu, Q., Johnson, E. M., Lam, R. K., Wang, Q., Bo, Y. H., Wilson, E. N., et al. (2019). Peripheral trem1 responses to brain and intestinal immunogens amplify stroke severity. Nat. Immunol. 20, 1023–1034. doi: 10.1038/s41590-019-0421-2
Lode, H. (1999). Potential interactions of the extended-spectrum fluoroquinolones with the cns. Drug Saf. 21, 123–135. doi: 10.2165/00002018-199921020-00005
Logsdon, A. F., Erickson, M. A., Rhea, E. M., Salameh, T. S., and Banks, W. A. (2018). Gut reactions: how the blood-brain barrier connects the microbiome and the brain. Exp. Biol. Med. (Maywood) 243, 159–165. doi: 10.1177/1535370217743766
Lukiw, W. J., Arceneaux, L., Li, W., Bond, T., and Zhao, Y. (2021). Gastrointestinal (gi)-tract microbiome derived neurotoxins and their potential contribution to inflammatory neurodegeneration in alzheimer's disease (ad). J. Alzheimers Dis. Parkinsonism 11:525.
Lum, G. R., Olson, C. A., and Hsiao, E. Y. (2020). Emerging roles for the intestinal microbiome in epilepsy. Neurobiol. Dis. 135:104576. doi: 10.1016/j.nbd.2019.104576
Ma, Q., Xing, C., Long, W., Wang, H. Y., Liu, Q., and Wang, R. F. (2019). Impact of microbiota on central nervous system and neurological diseases: the gut-brain axis. J. Neuroinflammation 16:53. doi: 10.1186/s12974-019-1434-3
Mangiola, F., Ianiro, G., Franceschi, F., Fagiuoli, S., Gasbarrini, G., and Gasbarrini, A. (2016). Gut microbiota in autism and mood disorders. World J. Gastroenterol. 22, 361–368. doi: 10.3748/wjg.v22.i1.361
Manolios, N., Geczy, C. L., and Schrieber, L. (1988). High endothelial venule morphology and function are inducible in germ-free mice: a possible role for interferon-gamma. Cell. Immunol. 117, 136–151. doi: 10.1016/0008-8749(88)90083-4
Mao, Y. K., Kasper, D. L., Wang, B., Forsythe, P., Bienenstock, J., and Kunze, W. A. (2013). Bacteroides fragilis polysaccharide a is necessary and sufficient for acute activation of intestinal sensory neurons. Nat. Commun. 4:1465. doi: 10.1038/ncomms2478
Mao, T., Su, C. W., Ji, Q., Chen, C. Y., Wang, R., Vijaya, K. D., et al. (2021). Hyaluronan-induced alterations of the gut microbiome protects mice against Citrobacter rodentium infection and intestinal inflammation. Gut Microbes 13:1972757. doi: 10.1080/19490976.2021.1972757
Maranduba, C. M., De Castro, S. B., de Souza, G. T., Rossato, C., Da, G. F., Valente, M. A., et al. (2015). Intestinal microbiota as modulators of the immune system and neuroimmune system: impact on the host health and homeostasis. J Immunol Res 2015:931574. doi: 10.1155/2015/931574
Martin, C. R., Osadchiy, V., Kalani, A., and Mayer, E. A. (2018). The brain-gut-microbiome axis. Cell. Mol. Gastroenterol. Hepatol. 6, 133–148. doi: 10.1016/j.jcmgh.2018.04.003
Matthes, S., and Bader, M. (2018). Peripheral serotonin synthesis as a new drug target. Trends Pharmacol. Sci. 39, 560–572. doi: 10.1016/j.tips.2018.03.004
Mayer, E. A. (2000). The neurobiology of stress and gastrointestinal disease. Gut 47, 861–869. doi: 10.1136/gut.47.6.861
Mayer, E. A., Knight, R., Mazmanian, S. K., Cryan, J. F., and Tillisch, K. (2014). Gut microbes and the brain: paradigm shift in neuroscience. J. Neurosci. 34, 15490–15496. doi: 10.1523/JNEUROSCI.3299-14.2014
Mayer, E. A., Nance, K., and Chen, S. (2022). The gut-brain axis. Annu. Rev. Med. 73, 439–453. doi: 10.1146/annurev-med-042320-014032
Mazarati, A., Medel-Matus, J. S., Shin, D., Jacobs, J. P., and Sankar, R. (2021). Disruption of intestinal barrier and endotoxemia after traumatic brain injury: implications for post-traumatic epilepsy. Epilepsia 62, 1472–1481. doi: 10.1111/epi.16909
McCoy, K. D., Ronchi, F., and Geuking, M. B. (2017). Host-microbiota interactions and adaptive immunity. Immunol. Rev. 279, 63–69. doi: 10.1111/imr.12575
Medel-Matus, J. S., Shin, D., Dorfman, E., Sankar, R., and Mazarati, A. (2018). Facilitation of kindling epileptogenesis by chronic stress may be mediated by intestinal microbiome. Epilepsia Open 3, 290–294. doi: 10.1002/epi4.12114
Michel, L., and Prat, A. (2016). One more role for the gut: microbiota and blood brain barrier. Ann. Transl. Med. 4:15. doi: 10.3978/j.issn.2305-5839.2015.10.16
Moradi, K., Ashraf-Ganjouei, A., Tavolinejad, H., Bagheri, S., and Akhondzadeh, S. (2021). The interplay between gut microbiota and autism spectrum disorders: a focus on immunological pathways. Prog. Neuropsychopharmacol. Biol. Psychiatry 106:110091. doi: 10.1016/j.pnpbp.2020.110091
Moussaoui, N., Braniste, V., Ait-Belgnaoui, A., Gabanou, M., Sekkal, S., Olier, M., et al. (2014). Changes in intestinal glucocorticoid sensitivity in early life shape the risk of epithelial barrier defect in maternal-deprived rats. PLoS One 9:e88382. doi: 10.1371/journal.pone.0088382
Mowat, A. M. (2003). Anatomical basis of tolerance and immunity to intestinal antigens. Nat. Rev. Immunol. 3, 331–341. doi: 10.1038/nri1057
Mracsko, E., Liesz, A., Karcher, S., Zorn, M., Bari, F., and Veltkamp, R. (2014). Differential effects of sympathetic nervous system and hypothalamic-pituitary-adrenal axis on systemic immune cells after severe experimental stroke. Brain Behav. Immun. 41, 200–209. doi: 10.1016/j.bbi.2014.05.015
Mu, X., Zhang, X., Gao, H., Gao, L., Li, Q., and Zhao, C. (2022). Crosstalk between peripheral and the brain-resident immune components in epilepsy. J. Integr. Neurosci. 21:9. doi: 10.31083/j.jin2101009
Nagayama, M., Yano, T., Atarashi, K., Tanoue, T., Sekiya, M., Kobayashi, Y., et al. (2020). Th1 cell-inducing Escherichia coli strain identified from the small intestinal mucosa of patients with crohn's disease. Gut Microbes 12:1788898. doi: 10.1080/19490976.2020.1788898
O’Mahony, S. M., Clarke, G., Borre, Y. E., Dinan, T. G., and Cryan, J. F. (2015). Serotonin, tryptophan metabolism and the brain-gut-microbiome axis. Behav. Brain Res. 277, 32–48. doi: 10.1016/j.bbr.2014.07.027
O’Toole, K. K., Hooper, A., Wakefield, S., and Maguire, J. (2014). Seizure-induced disinhibition of the hpa axis increases seizure susceptibility. Epilepsy Res. 108, 29–43. doi: 10.1016/j.eplepsyres.2013.10.013
Obrenovich, M. (2018). Leaky gut, leaky brain? Microorganisms 6:107. doi: 10.3390/microorganisms6040107
Paiva, I., Maciel, L. M., Silva, R., Mendonca, I. P., Souza, J., and Peixoto, C. A. (2024). Prebiotics modulate the microbiota-gut-brain axis and ameliorate anxiety and depression-like behavior in hfd-fed mice. Food Res. Int. 182:114153. doi: 10.1016/j.foodres.2024.114153
Park, A. J., Collins, J., Blennerhassett, P. A., Ghia, J. E., Verdu, E. F., Bercik, P., et al. (2013). Altered colonic function and microbiota profile in a mouse model of chronic depression. Neurogastroenterol. Motil. 25:733. doi: 10.1111/nmo.12153
Peng, A., Qiu, X., Lai, W., Li, W., Zhang, L., Zhu, X., et al. (2018). Altered composition of the gut microbiome in patients with drug-resistant epilepsy. Epilepsy Res. 147, 102–107. doi: 10.1016/j.eplepsyres.2018.09.013
Phillips, M., Deprez, L. M., Mortimer, G., Murtagh, D., McCoy, S., Mylchreest, R., et al. (2021). Randomized crossover trial of a modified ketogenic diet in alzheimer's disease. Alzheimers Res. Ther. 13:51. doi: 10.1186/s13195-021-00783-x
Pizzo, F., Collotta, A. D., Di Nora, A., Costanza, G., Ruggieri, M., and Falsaperla, R. (2022). Ketogenic diet in pediatric seizures: a randomized controlled trial review and meta-analysis. Expert. Rev. Neurother. 22, 169–177. doi: 10.1080/14737175.2022.2030220
Prast-Nielsen, S. (2023). Fecal transfers from children on the ketogenic diet mimic the anti-seizure effect in mice. Cell Rep. 42:113570. doi: 10.1016/j.celrep.2023.113570
Psichas, A., Reimann, F., and Gribble, F. M. (2015). Gut chemosensing mechanisms. J. Clin. Invest. 125, 908–917. doi: 10.1172/JCI76309
Renz, H., Brandtzaeg, P., and Hornef, M. (2011). The impact of perinatal immune development on mucosal homeostasis and chronic inflammation. Nat. Rev. Immunol. 12, 9–23. doi: 10.1038/nri3112
Ressler, K. J., and Mayberg, H. S. (2007). Targeting abnormal neural circuits in mood and anxiety disorders: from the laboratory to the clinic. Nat. Neurosci. 10, 1116–1124. doi: 10.1038/nn1944
Riazi, K., Galic, M. A., Kuzmiski, J. B., Ho, W., Sharkey, K. A., and Pittman, Q. J. (2008). Microglial activation and tnfalpha production mediate altered cns excitability following peripheral inflammation. Proc. Natl. Acad. Sci. U. S. A. 105, 17151–17156. doi: 10.1073/pnas.0806682105
Ridaura, V., and Belkaid, Y. (2015). Gut microbiota: the link to your second brain. Cell 161, 193–194. doi: 10.1016/j.cell.2015.03.033
Rothhammer, V., Borucki, D. M., Tjon, E. C., Takenaka, M. C., Chao, C. C., Ardura-Fabregat, A., et al. (2018). Microglial control of astrocytes in response to microbial metabolites. Nature 557, 724–728. doi: 10.1038/s41586-018-0119-x
Rothhammer, V., Mascanfroni, I. D., Bunse, L., Takenaka, M. C., Kenison, J. E., Mayo, L., et al. (2016). Type i interferons and microbial metabolites of tryptophan modulate astrocyte activity and central nervous system inflammation via the aryl hydrocarbon receptor. Nat. Med. 22, 586–597. doi: 10.1038/nm.4106
Rubio, C., Ochoa, E., Gatica, F., Portilla, A., Vazquez, D., and Rubio-Osornio, M. (2023). The role of the vagus nerve in the microbiome and digestive system in relation to epilepsy. Curr. Med. Chem. doi: 10.2174/0109298673260479231010044020
Rusch, J. A., Layden, B. T., and Dugas, L. R. (2023). Signalling cognition: the gut microbiota and hypothalamic-pituitary-adrenal axis. Front Endocrinol (Lausanne). 14:1130689. doi: 10.3389/fendo.2023.1130689
Sampson, T. R., Debelius, J. W., Thron, T., Janssen, S., Shastri, G. G., Ilhan, Z. E., et al. (2016). Gut microbiota regulate motor deficits and neuroinflammation in a model of parkinson's disease. Cell 167, 1469–1480.e12. doi: 10.1016/j.cell.2016.11.018
Sanz, P., and Garcia-Gimeno, M. A. (2020). Reactive glia inflammatory signaling pathways and epilepsy. Int. J. Mol. Sci. 21:21. doi: 10.3390/ijms21114096
Schmuck, G., Schurmann, A., and Schluter, G. (1998). Determination of the excitatory potencies of fluoroquinolones in the central nervous system by an in vitro model. Antimicrob. Agents Chemother. 42, 1831–1836. doi: 10.1128/AAC.42.7.1831
Segev, S., Rehavi, M., and Rubinstein, E. (1988). Quinolones, theophylline, and diclofenac interactions with the gamma-aminobutyric acid receptor. Antimicrob. Agents Chemother. 32, 1624–1626. doi: 10.1128/AAC.32.11.1624
Serino, D., Santarone, M. E., Caputo, D., and Fusco, L. (2019). Febrile infection-related epilepsy syndrome (fires): prevalence, impact and management strategies. Neuropsychiatr. Dis. Treat. 15, 1897–1903. doi: 10.2147/NDT.S177803
Shi, K., Wood, K., Shi, F. D., Wang, X., and Liu, Q. (2018). Stroke-induced immunosuppression and poststroke infection. Stroke Vasc. Neurol. 3, 34–41. doi: 10.1136/svn-2017-000123
Simeone, T. A., Simeone, K. A., Stafstrom, C. E., and Rho, J. M. (2018). Do ketone bodies mediate the anti-seizure effects of the ketogenic diet? Neuropharmacology 133, 233–241. doi: 10.1016/j.neuropharm.2018.01.011
Singh, V., Roth, S., Llovera, G., Sadler, R., Garzetti, D., Stecher, B., et al. (2016). Microbiota dysbiosis controls the neuroinflammatory response after stroke. J. Neurosci. 36, 7428–7440. doi: 10.1523/JNEUROSCI.1114-16.2016
Siopi, E., Galerne, M., Rivagorda, M., Saha, S., Moigneu, C., Moriceau, S., et al. (2023). Gut microbiota changes require vagus nerve integrity to promote depressive-like behaviors in mice. Mol. Psychiatry 28, 3002–3012. doi: 10.1038/s41380-023-02071-6
Smith, S. M., and Vale, W. W. (2006). The role of the hypothalamic-pituitary-adrenal axis in neuroendocrine responses to stress. Dialogues Clin. Neurosci. 8, 383–395. doi: 10.31887/DCNS.2006.8.4/ssmith
Socala, K., Doboszewska, U., Szopa, A., Serefko, A., Wlodarczyk, M., Zielinska, A., et al. (2021). The role of microbiota-gut-brain axis in neuropsychiatric and neurological disorders. Pharmacol. Res. 172:105840. doi: 10.1016/j.phrs.2021.105840
Song, C., Chai, Z., Chen, S., Zhang, H., Zhang, X., and Zhou, Y. (2023). Intestinal mucus components and secretion mechanisms: what we do and do not know. Exp. Mol. Med. 55, 681–691. doi: 10.1038/s12276-023-00960-y
Sonnenberg, G. F., Monticelli, L. A., Alenghat, T., Fung, T. C., Hutnick, N. A., Kunisawa, J., et al. (2012). Innate lymphoid cells promote anatomical containment of lymphoid-resident commensal bacteria. Science 336, 1321–1325. doi: 10.1126/science.1222551
Spielbauer, J., Glotfelty, E., Sarlus, H., Harris, R. A., Heijtz, R. D., and Karlsson, T. E. (2024). Bacterial peptidoglycan signalling in microglia: activation by mdp via the nf-kappab/mapk pathway. Brain Behav. Immun. 121, 43–55. doi: 10.1016/j.bbi.2024.06.027
Stanley, D., Mason, L. J., Mackin, K. E., Srikhanta, Y. N., Lyras, D., Prakash, M. D., et al. (2016). Translocation and dissemination of commensal bacteria in post-stroke infection. Nat. Med. 22, 1277–1284. doi: 10.1038/nm.4194
Stecher, B., and Hardt, W. D. (2011). Mechanisms controlling pathogen colonization of the gut. Curr. Opin. Microbiol. 14, 82–91. doi: 10.1016/j.mib.2010.10.003
Sun, Y., Su, Y., and Zhu, W. (2016). Microbiome-metabolome responses in the cecum and colon of pig to a high resistant starch diet. Front. Microbiol. 7:779. doi: 10.3389/fmicb.2016.00779
Takanaga, H., Ohtsuki, S., Hosoya, K., and Terasaki, T. (2001). Gat2/bgt-1 as a system responsible for the transport of gamma-aminobutyric acid at the mouse blood-brain barrier. J. Cereb. Blood Flow Metab. 21, 1232–1239. doi: 10.1097/00004647-200110000-00012
Tena-Garitaonaindia, M., Arredondo-Amador, M., Mascaraque, C., Asensio, M., Marin, J., Martinez-Augustin, O., et al. (2022). Modulation of intestinal barrier function by glucocorticoids: lessons from preclinical models. Pharmacol. Res. 177:106056. doi: 10.1016/j.phrs.2022.106056
Tetel, M. J., de Vries, G. J., Melcangi, R. C., Panzica, G., and O'Mahony, S. M. (2018). Steroids, stress and the gut microbiome-brain axis. J. Neuroendocrinol. 30:30. doi: 10.1111/jne.12548
Thion, M. S., Low, D., Silvin, A., Chen, J., Grisel, P., Schulte-Schrepping, J., et al. (2018). Microbiome influences prenatal and adult microglia in a sex-specific manner. Cell 172, 500–516.e16. doi: 10.1016/j.cell.2017.11.042
Tlaskalova-Hogenova, H., Tuckova, L., Mestecky, J., Kolinska, J., Rossmann, P., Stepankova, R., et al. (2005). Interaction of mucosal microbiota with the innate immune system. Scand. J. Immunol. 62, 106–113. doi: 10.1111/j.1365-3083.2005.01618.x
Tolhurst, G., Heffron, H., Lam, Y. S., Parker, H. E., Habib, A. M., Diakogiannaki, E., et al. (2012). Short-chain fatty acids stimulate glucagon-like peptide-1 secretion via the g-protein-coupled receptor ffar2. Diabetes 61, 364–371. doi: 10.2337/db11-1019
Torrente, F., Ashwood, P., Day, R., Machado, N., Furlano, R. I., Anthony, A., et al. (2002). Small intestinal enteropathy with epithelial igg and complement deposition in children with regressive autism. Mol. Psychiatry 7:334. doi: 10.1038/sj.mp.4001079
Tottey, W., Feria-Gervasio, D., Gaci, N., Laillet, B., Pujos, E., Martin, J. F., et al. (2017). Colonic transit time is a driven force of the gut microbiota composition and metabolism: in vitro evidence. J. Neurogastroenterol. Motil. 23, 124–134. doi: 10.5056/jnm16042
Tran, S. M., and Mohajeri, M. H. (2021). The role of gut bacterial metabolites in brain development, aging and disease. Nutrients 13:13. doi: 10.3390/nu13030732
Usuda, H., Okamoto, T., and Wada, K. (2021). Leaky gut: effect of dietary fiber and fats on microbiome and intestinal barrier. Int. J. Mol. Sci. 22:22. doi: 10.3390/ijms22147613
Vandeputte, D., Falony, G., Vieira-Silva, S., Tito, R. Y., Joossens, M., and Raes, J. (2016). Stool consistency is strongly associated with gut microbiota richness and composition, enterotypes and bacterial growth rates. Gut 65, 57–62. doi: 10.1136/gutjnl-2015-309618
Vezzani, A., Fujinami, R. S., White, H. S., Preux, P. M., Blumcke, I., Sander, J. W., et al. (2016). Infections, inflammation and epilepsy. Acta Neuropathol. 131, 211–234. doi: 10.1007/s00401-015-1481-5
Wang, Y., and Kasper, L. H. (2014). The role of microbiome in central nervous system disorders. Brain Behav. Immun. 38, 1–12. doi: 10.1016/j.bbi.2013.12.015
Wang, F. B., and Powley, T. L. (2007). Vagal innervation of intestines: afferent pathways mapped with new en bloc horseradish peroxidase adaptation. Cell Tissue Res. 329, 221–230. doi: 10.1007/s00441-007-0413-7
Wang, H. X., and Wang, Y. P. (2016). Gut microbiota-brain axis. Chin. Med. J. 129, 2373–2380. doi: 10.4103/0366-6999.190667
Wanleenuwat, P., Suntharampillai, N., and Iwanowski, P. (2020). Antibiotic-induced epileptic seizures: mechanisms of action and clinical considerations. Seizure 81, 167–174. doi: 10.1016/j.seizure.2020.08.012
Weber, C. R., Nalle, S. C., Tretiakova, M., Rubin, D. T., and Turner, J. R. (2008). Claudin-1 and claudin-2 expression is elevated in inflammatory bowel disease and may contribute to early neoplastic transformation. Lab. Investig. 88, 1110–1120. doi: 10.1038/labinvest.2008.78
Welcome, M. O. (2019). Gut microbiota disorder, gut epithelial and blood-brain barrier dysfunctions in etiopathogenesis of dementia: molecular mechanisms and signaling pathways. NeuroMolecular Med. 21, 205–226. doi: 10.1007/s12017-019-08547-5
Wirrell, E. C., Nabbout, R., Scheffer, I. E., Alsaadi, T., Bogacz, A., French, J. A., et al. (2022). Methodology for classification and definition of epilepsy syndromes with list of syndromes: report of the ilae task force on nosology and definitions. Epilepsia 63, 1333–1348. doi: 10.1111/epi.17237
Xie, J., Bruggeman, A., De Nolf, C., Vandendriessche, C., Van Imschoot, G., Van Wonterghem, E., et al. (2023). Gut microbiota regulates blood-cerebrospinal fluid barrier function and abeta pathology. EMBO J. 42:e111515. doi: 10.15252/embj.2022111515
Xie, G., Zhou, Q., Qiu, C. Z., Dai, W. K., Wang, H. P., Li, Y. H., et al. (2017). Ketogenic diet poses a significant effect on imbalanced gut microbiota in infants with refractory epilepsy. World J. Gastroenterol. 23, 6164–6171. doi: 10.3748/wjg.v23.i33.6164
Xiong, X., Liu, D., Wang, Y., Zeng, T., and Peng, Y. (2016). Urinary 3-(3-hydroxyphenyl)-3-hydroxypropionic acid, 3-hydroxyphenylacetic acid, and 3-hydroxyhippuric acid are elevated in children with autism spectrum disorders. Biomed. Res. Int. 2016:9485412. doi: 10.1155/2016/9485412
Xu, X., Chen, R., Zhan, G., Wang, D., Tan, X., and Xu, H. (2021). Enterochromaffin cells: sentinels to gut microbiota in hyperalgesia? Front. Cell. Infect. Microbiol. 11:760076. doi: 10.3389/fcimb.2021.760076
Yang, L., Zhou, Y., Jia, H., Qi, Y., Tu, S., and Shao, A. (2020). Affective immunology: the crosstalk between microglia and astrocytes plays key role? Front. Immunol. 11:1818. doi: 10.3389/fimmu.2020.01818
Yu, Q., Jia, A., Li, Y., Bi, Y., and Liu, G. (2018). Microbiota regulate the development and function of the immune cells. Int. Rev. Immunol. 37, 79–89. doi: 10.1080/08830185.2018.1429428
Zhang, X., Tang, B., and Guo, J. (2023). Parkinson's disease and gut microbiota: from clinical to mechanistic and therapeutic studies. Transl. Neurodegener. 12:59. doi: 10.1186/s40035-023-00392-8
Zhang, Y., Zhou, S., Zhou, Y., Yu, L., Zhang, L., and Wang, Y. (2018). Altered gut microbiome composition in children with refractory epilepsy after ketogenic diet. Epilepsy Res. 145, 163–168. doi: 10.1016/j.eplepsyres.2018.06.015
Keywords: microbiota-gut-brain-axis, epilepsy, gut barrier, blood–brain barrier, pathological, physiological
Citation: Yang W, Cui H, Wang C, Wang X, Yan C and Cheng W (2024) A review of the pathogenesis of epilepsy based on the microbiota-gut-brain-axis theory. Front. Mol. Neurosci. 17:1454780. doi: 10.3389/fnmol.2024.1454780
Edited by:
Shaunik Sharma, University of Ottawa, CanadaReviewed by:
Kiran Veer Sandhu, University College Cork, IrelandVenkat Venkataraman, Rowan University School of Osteopathic Medicine, United States
Copyright © 2024 Yang, Cui, Wang, Wang, Yan and Cheng. This is an open-access article distributed under the terms of the Creative Commons Attribution License (CC BY). The use, distribution or reproduction in other forums is permitted, provided the original author(s) and the copyright owner(s) are credited and that the original publication in this journal is cited, in accordance with accepted academic practice. No use, distribution or reproduction is permitted which does not comply with these terms.
*Correspondence: Xuan Wang, wangxuan@hljucm.edu.cn; Weiping Cheng, chengweiping@hljucm.edu.cn