- 1Department of Biology, Pennsylvania State University, University Park, PA, United States
- 2Center for the Molecular Investigation of Neurological Disorders (CMIND), The Huck Institutes of the Life Sciences, Pennsylvania State University, University Park, PA, United States
Long-term memories are not stored in a stable state but must be flexible and dynamic to maintain relevance in response to new information. Existing memories are thought to be updated through the process of reconsolidation, in which memory retrieval initiates destabilization and updating to incorporate new information. Memory updating is impaired in old age, yet little is known about the mechanisms that go awry. One potential mechanism is the repressive histone deacetylase 3 (HDAC3), which is a powerful negative regulator of memory formation that contributes to age-related impairments in memory formation. Here, we tested whether HDAC3 also contributes to age-related impairments in memory updating using the Objects in Updated Locations (OUL) paradigm. We show that blocking HDAC3 immediately after updating with the pharmacological inhibitor RGFP966 ameliorated age-related impairments in memory updating in 18-m.o. male mice. Surprisingly, we found that post-update HDAC3 inhibition in young (3-m.o.) male mice had no effect on memory updating but instead impaired memory for the original information, suggesting that the original and updated information may compete for expression at test and HDAC3 helps regulate which information is expressed. To test this idea, we next assessed whether HDAC3 inhibition would improve memory updating in young male mice given a weak, subthreshold update. Consistent with our hypothesis, we found that HDAC3 blockade strengthened the subthreshold update without impairing memory for the original information, enabling balanced expression of the original and updated information. Together, this research suggests that HDAC3 may contribute to age-related impairments in memory updating and may regulate the strength of a memory update in young mice, shifting the balance between the original and updated information at test.
Introduction
The ability to learn is critical for survival across species. While research has heavily explored the mechanisms of initial memory formation, we know far less about how existing memories are modified with new information. The ability to modify or update existing memories, however, is critical to ensure accurate behavioral responses. Further, memory updating is impaired in old age, contributing to age-related cognitive impairments (Barense et al., 2002; Schoenbaum et al., 2002; Bizon et al., 2012; Kwapis et al., 2020). Despite its fundamental importance to survival and everyday functioning, we have yet to understand the molecular processes that support memory updating and we know even less about how these mechanisms change in old age. Understanding the mechanisms supporting memory updating may shed light on potential therapeutics for memory deficits observed in natural aging and psychiatric disorders.
A newly formed memory must first undergo consolidation to stabilize into long-term memory, a process that requires transcription and translation. During consolidation, a memory is initially labile, susceptible to disruption by a number of amnesic agents, including inhibitors of protein or mRNA synthesis (Davis and Squire, 1984; Nader et al., 2000; Igaz et al., 2002; Duvarci et al., 2008). Once consolidated, however, memories are resistant to disruption by these same amnesic agents. A second period of lability can be induced by memory retrieval, initiating a process termed reconsolidation. As with consolidation, memory reconsolidation requires transcription and translation for successful restabilization, a process that creates an approximately 6-h “reconsolidation-window” during which the memory is again susceptible to disruption (Nader et al., 2000; Monfils et al., 2009; Monfils and Holmes, 2018). Accumulating evidence demonstrates that the retrieval trial must contain some new information to initiate reconsolidation; when only familiar information is presented at retrieval, the existing memory remains stable and is not susceptible to amnesic agents (Morris et al., 2006; Sevenster et al., 2012, 2013, 2014; De Oliveira Alvares et al., 2013; Díaz-Mataix et al., 2013; Jarome et al., 2015; Kwapis et al., 2017b, 2020). Thus, one purpose of this reconsolidation process may be to allow existing memories to be modified or updated in response to new, relevant information. Age-related disruption of the reconsolidation process could therefore contribute to reduced memory updating and flexibility in old age.
Epigenetic mechanisms are well-positioned to play an important role in memory updating and contribute to age-related impairments in this process. Epigenetic mechanisms, which modify gene expression without changing the DNA sequence itself, dynamically regulate transcription during memory consolidation (Monsey et al., 2011; Alaghband et al., 2017; Kwapis et al., 2017a; Barchiesi et al., 2022) and reconsolidation (Da Silva et al., 2008; Lattal and Wood, 2013; Gräff et al., 2014). In particular, histone acetylation is a key epigenetic mechanism that is critical for memory consolidation and reconsolidation (Bredy and Barad, 2008; Bannister and Kouzarides, 2011; Lubin et al., 2011; Maddox and Schafe, 2011; Xhemalce et al., 2011; Felsenfeld, 2014; Gräff et al., 2014). Histone acetylation enables a permissive state that promotes gene expression (Grunstein, 1997; Strahl and Allis, 2000) and enhances memory formation (Peixoto and Abel, 2013; Villain et al., 2016; Ramzan et al., 2020). Histone acetylation is dynamically regulated through the competing actions of two classes of enzymes: histone acetyltransferases (HATs), which promote acetylation, and histone deacetyltransferases (HDACs), which reduce acetylation (Wolffe, 1996; Wapenaar and Dekker, 2016). HDAC3, the most highly expressed Class I HDAC in the brain, is an especially potent negative regulator of memory consolidation across several different types of memory, including fear conditioning (Kwapis et al., 2017a), fear extinction (Alaghband et al., 2017), object location memory (McQuown et al., 2011; Malvaez et al., 2013; Kwapis et al., 2019), object recognition memory (McQuown et al., 2011), cocaine-context place preference consolidation (Rogge et al., 2013), auditory reward learning (Bieszczad et al., 2015), and cocaine place preference extinction (Malvaez et al., 2013). Typically, pharmacological or genetic disruption of HDAC3 around the time of learning transforms a weak learning event into one that establishes a strong and lasting memory, suggesting HDAC3 limits memory strength and persistence during consolidation. Deletion or disruption of HDAC3 in the dorsal hippocampus of old mice is also sufficient to ameliorate age-related impairments in object location memory, indicating HDAC3 also plays a role in age-related impairments in memory consolidation (Kwapis et al., 2018). Although HDAC3 plays a clear role in initial memory consolidation, whether it plays a similar role in reconsolidation-dependent memory updating is currently unknown. Further, it is also unclear whether HDAC3 also contributes to age-related impairments in memory updating.
To better understand the role of HDAC3 in memory updating in both young and old animals, we used our recently developed spatial memory updating paradigm, the objects in updated locations (OUL) task. OUL leverages a mouse’s innate attraction to novelty to determine how a memory update incorporates into an existing object location memory. Importantly, OUL can assess both the original memory and the updated information in a single, non-stressful test session. In addition, OUL depends on the dorsal hippocampus, a brain region critical for memory and affected early in aging (Bach et al., 1999; Kwapis et al., 2018; Aziz et al., 2019). OUL is therefore ideal for understanding both the mechanisms that support memory updating and the mechanisms that contribute to age-related updating deficits. Here, using the pharmacological HDAC3 inhibitor RGFP966 (Malvaez et al., 2013; Bieszczad et al., 2015; Phan et al., 2017; Shang et al., 2019), we tested the role of HDAC3 in memory updating in young and old male mice. Our results show that HDAC3 inhibition after memory updating enhanced memory for the update in old male mice but, to our surprise, impaired memory for the original information in young male mice. A follow-up study then determined that the original and updated information compete for expression, with HDAC3 inhibition shifting which information is expressed at test. Overall, our studies suggest that HDAC3 negatively regulates reconsolidation-dependent memory updating in young and old male mice and contributes to memory updating impairments in old male mice.
Methods
Animals
Adult (3-month-old) male C57BL/6 J mice were obtained from Jackson Laboratories (JAX), and old (18-20-month-old) male C57BL/6 J mice were obtained from the National Institute on Aging (NIA) Aged Rodent Colony. We specifically chose 18-20-m.o. mice, as this is when we and others have previously observed deficits in memory and similar behaviors (Bach et al., 1999; Weber et al., 2015; Shoji et al., 2016; Kwapis et al., 2018; Ederer et al., 2022; Hendrickx et al., 2022; Brito et al., 2023), including age-related impairments in OUL (Kwapis et al., 2020). All animals were maintained in a temperature (68–79°C) and humidity-controlled (30–70%) environment under a 12-h light/dark cycle (lights on at 7A, lights off at 7P). Behavior was conducted during the light phase, when we observe peak spatial memory performance (Urban et al., 2021; Bellfy et al., 2023; Brunswick et al., 2023). Mice had access to food and water ad libitum. All experiments were conducted according to US National Institutes of Health guidelines for animal care and use and were approved by the Institutional Animal Care and Use Committee of Pennsylvania State University. All animals were group-housed with four animals per cage.
RGFP966 preparation
To selectively block HDAC3, we used the pharmacological HDAC3 inhibitor RGFP966 (Malvaez et al., 2013; Bieszczad et al., 2015; Shang et al., 2019), which inhibits HDAC3 at an IC50 of 0.08 μM but does not inhibit other HDACs up to 15 μM. RGFP966 rapidly penetrates the blood–brain barrier (within 30 min) and improves multiple forms of memory with a single post-training injection (Malvaez et al., 2013; Bieszczad et al., 2015; Hitchcock et al., 2019; Shang et al., 2019), making it an excellent tool for investigating the role of HDAC3 in memory updating. We chose RGFP966 over non-specific HDAC inhibitors (e.g., Cl-994, VPA) in order to specifically test the role of HDAC3 in memory updating. Finally, although a new benzamide class inhibitor of HDAC3, PT3, shows great promise in selectively blocking HDAC3 to improve memory (Pulya et al., 2021), far less is known about its effects on memory compared to RGFP966 and it is unknown if a single post-training injection of PT3 is sufficient to improve memory. Therefore, we chose to use RGFP966 to determine if acute post-update manipulation of HDAC3 affects memory updating in our OUL paradigm.
A solution containing 30% HP-ß-CD and 100 mM Na-acetate was made in sterile water, brought to a pH of 5.4 with HCl, and sterilized with a 0.22 μm filter. The sterilized HP-ß-CD solution was then used to make the 1% DMSO vehicle. Finally, a stock solution of 10 mg/mL RGFP966 in DMSO was diluted to 0.1 mg/mL with the sterile HP-ß-CD solution for systemic subcutaneous injections of 10 mg/kg. We chose this dose as it has been shown to cross the blood–brain barrier at sufficient concentrations (Malvaez et al., 2013) and improves memory in multiple tasks (Malvaez et al., 2013; Bieszczad et al., 2015; Shang et al., 2019). To block HDAC3 during the ~6-h reconsolidation window without affecting the experience of the update itself (i.e., without the drug present during updating), we injected RGFP966 immediately after the update session. It takes approximately 30 min for RGFP966 to reach the brain (Malvaez et al., 2013), where it remains detectable for up to 4 h (Bieszczad et al., 2015), ensuring peak effects of RGFP966 would occur during this ~6-h reconsolidation window.
Objects in updated locations (OUL) paradigm
The Objects in Updated Location task was conducted similarly to that previously described (Wright et al., 2020). Briefly, animals were handled for 4 days for 2 mins/day and habituated to the context for six consecutive days for 5 min per day, with two handling days overlapping with habituation days. Animals were scruffed and weighed following habituation sessions 4 days prior to training to get accustomed to being scruffed for injections. Animals were then trained with two identical objects (200 mL tall form graduated beakers filled with cement) placed in specific locations (A1 and A2) for 10 min, either for one or three consecutive days, with 24 h separating each session. Next, 24 h later, mice were given an update session, in which one of the identical objects was moved to a new location (A3). Animals were allowed to explore for 5 min (1 min for the subthreshold update experiment) to learn this updated location. Immediately after the update session, mice were given subcutaneous injections of either RGFP966 or vehicle. Finally, 24 h later, mice were given a retention test to assess memory for both the original and the updated object locations. At test, four identical objects were presented in specific locations within this familiar context: two objects in the original locations (A1 and A2), one object in the updated location (A3), and one object in a completely novel location (A4) (Figures 1–3). Memory for the original training location was tested by comparing exploration of novel location A4 to the original locations A1 and A2. Memory for the update was assessed by comparing exploration of the novel location A4 to the updated location A3.
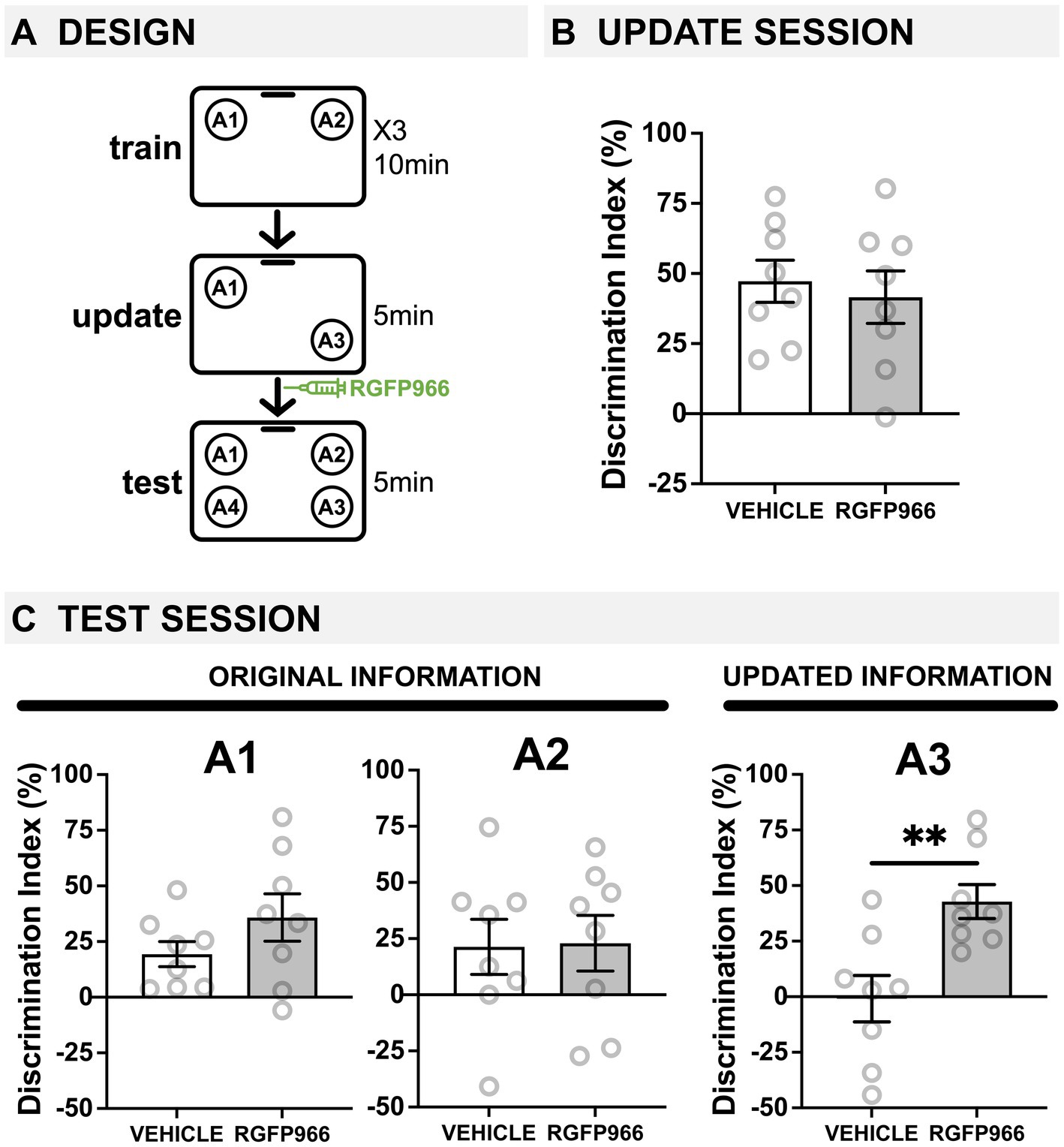
Figure 1. Age-related deficits in memory updating are ameliorated by blocking HDAC3 immediately after updating. (A) Experimental design using the OUL paradigm with 18-20-m.o. male mice. Mice were injected with the HDAC3 inhibitor RGFP966 immediately after the update session. (B) Update session behavior (pre-injection). No differences were observed during updating, before RGFP966 injection. (C) Test session behavior. For locations A1 and A2, no differences were observed between RGFP966-treated and vehicle-treated animals suggesting post-update RGFP966 injections did not impact memory for the original information. For the updated location A3, however, RGFP966 injections improved memory, with RGFP966 mice showing significantly higher DIs for A3 compared to vehicle controls. (*p < 0.05, **p < 0.01, ***p < 0.001).
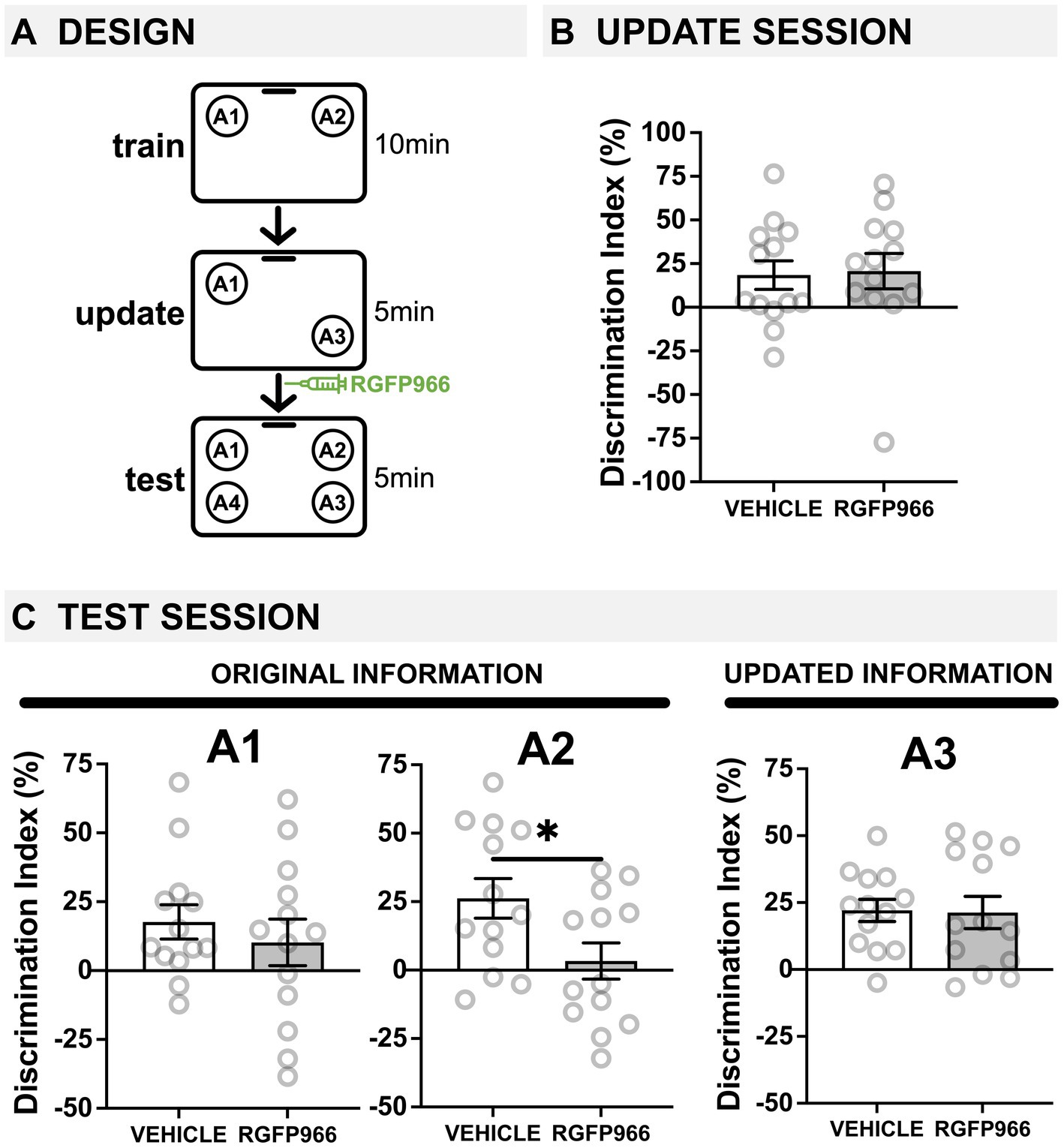
Figure 2. Post-update HDAC3 inhibition with RGFP966 impairs the original memory in young mice. (A) Experimental design using the OUL paradigm with young male mice. (B) Update session behavior (pre-injection). No differences were observed during updating, before RGFP966 injection. (C) Test session behavior. For locations A1 and A3, no differences were observed between RGFP966-treated and vehicle-treated animals. For the original location A2, however, post-update RGFP966 injections significantly impaired memory, with RGFP966 mice showing significantly lower DIs for A2 compared to vehicle controls. (*p < 0.05, **p < 0.01, ***p < 0.001).
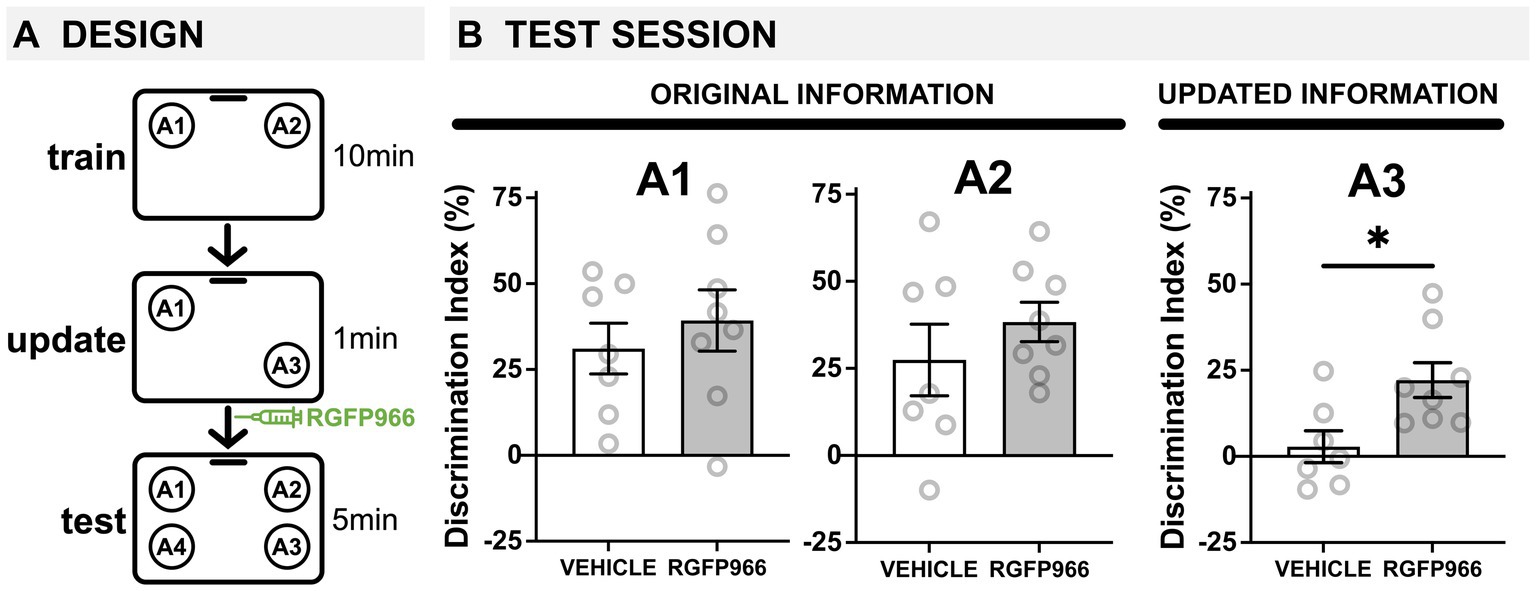
Figure 3. HDAC3 blockade transforms a weak update into a strong update in young mice. (A) Experimental design using the OUL paradigm with a subthreshold updating time of 1 min with young male mice. (B) Test session behavior. No differences were observed between RGFP966-treated and vehicle-treated animals for A1 or A2, suggesting post-update RGFP966 injections did not impact memory for the original information. For A3, the subthreshold update was insufficient to drive memory updating in vehicle mice. In RGFP966 mice, however, this subthreshold update drove memory updating, with RGFP966 mice showing significantly higher DIs for A3 compared to vehicle controls. (*p < 0.05, **p < 0.01, ***p < 0.001).
Data analysis and statistics
Behavior videos were collected using Ethovision (Noldus, Leesburg, VA). Habituation data (movement distance and speed) was calculated within the Ethovision software for all behavioral experiments; a reduction in movement and velocity was used to indicate successful habituation to the context (see Supplementary Figures S1–S3). Behavioral videos were manually scored by experimenters blinded to the experimental groups using Deepethogram (Bohnslav et al., 2021) or Object Task Timer (UC Irvine). Deepethogram and Object Task methods were validated within and between experimenters. The criteria for behavioral scoring followed previously described criteria (Vogel-Ciernia and Wood, 2014; Wright et al., 2020). Briefly, exploration was scored when the mouse’s nose was within 1 cm and directly pointed at the object and we do not count rearing, jumping, biting, bumping, grooming, or other repetitive behaviors near the objects. For each session, we calculated a Discrimination Index (DI): DI = (tnovel – tfamiliar) / (tnovel + tfamiliar) x 100%. For the test session, three different DIs were calculated for objects A1, A2, and A3 (familiar) compared to the novel location A4. When both objects were equally novel or familiar (e.g., training), the ‘novel’ and ‘familiar’ locations were randomly chosen and counterbalanced between conditions. Statistical analyses were conducted using GraphPad Prism (RRID:SCR_002798). Any mouse with a Discrimination Index % (DI) more than two standard deviations away from the mean, that failed to explore one of the objects even briefly, or with an exploration time of less than 2 s during testing for both aged (experiment 1: n = 4/20) and young (experiment 2: n = 4/30) experiments or 1 s for the subthreshold update session (to ensure some exploration during the shortened session time; experiment 3: n = 9/24) were removed from all behavioral analyses. DI data from each group in each experimental session (training, updating, testing) was tested for normality using the Shapiro–Wilk test. All data were normally distributed with one exception: the training DI for the vehicle group in experiment 3 (Supplementary Figure S3B). In this instance, group differences were tested with a nonparametric Mann–Whitney test. For all other graphs, group differences were analyzed with parametric Student’s t-tests or 2-way ANOVAs and Sidak’s multiple comparison post hoc tests. For each group, to determine if each DI at test was significantly different from a value of 0, indicating no preference for either location, we used one-sample t-tests comparing each group to the hypothetical value of 0. For the non-normally distributed vehicle group training data in Supplementary Figure S3B, we used the nonparametric Wilcoxon Signed Rank test to compare the group value to 0. In all experiments, an α-value of 0.05 was required for significance. Error bars in all figures indicate SEM.
Results
HDAC3 blockade ameliorates age-related impairments in memory updating
We first aimed to test whether HDAC3 inhibition could ameliorate age-related deficits in memory updating. We previously demonstrated that 18-month-old mice show impaired memory updating in OUL even when the original memory is intact (Kwapis et al., 2020). Here, to test the role of HDAC3, we used the selective pharmacological inhibitor RGFP966 (Malvaez et al., 2013; Bieszczad et al., 2015) to specifically block HDAC3 during the post-update reconsolidation period in 18-20-month-old male mice (Figure 1A). During habituation, all mice showed similar decreases in movement across each session (Supplementary Figure S1A), indicating normal habituation. Next, mice were given 3 days of training, in which they were exposed to two identical objects in specific locations (A1 and A2) in this familiar context. We observed low DIs during each of the 3 days of training, with no significant difference between groups any day of training, demonstrating there was no strong preference for object location A1 or A2 before learning (Supplementary Figure S1B; two-tailed t-tests: day 1: t(14) = 0.524, p > 0.05; day 2: t(14) = 0.771, p > 0.05; day 3: t(14) = 0.65, p > 0.05).
Next, mice were given a memory update, in which one object was moved to a new location (A3). During this update session, all mice showed intact memory for the original training information and preferentially explored the moved location (A3) over the familiar location (A1). Specifically, aged mice in both groups showed a DI significantly higher than zero (Figure 1B; one-sample t-tests compared to 0: vehicle: t(7) = 6.311, p = 0.0004; RGFP966: t(7) = 4.432, p = 0.0030), and these groups were not significantly different from each other before receiving any injection (two-tailed t-test t(14) = 0.4728, p = 0.644). Therefore, 18-month-old mice successfully learned the original object locations with a 3-day training protocol, and all mice performed similarly before injection.
Immediately after updating, mice were given systemic injections of vehicle or RGFP966 (s.c., 10 mg/kg) to block HDAC3 during reconsolidation and were tested the following day. At test, all mice were exposed to four identical objects: three in previously exposed locations (A1, A2, and A3) and one in a completely novel location (A4), which was used to assess memory for each of the familiar locations. Old mice given post-update RGFP966 showed normal memory for the original training locations and improved memory for the updated location compared to vehicle controls. Specifically, we found that 18-m.o. male mice injected with either RGFP966 or vehicle showed intact memory for original location A1, as both groups preferentially explored novel location A4 over familiar location A1 (Figure 1C; one-sample t-tests compared to 0: vehicle: t(7) = 3.417, p = 0.0112, RGFP966: t(7) = 3.360, p = 0.0121). For location A2, similar to what we have previously observed (Kwapis et al., 2020), old mice show weak memory (DI close to zero), possibly due to the longer retention interval (48 h) between training and testing for location A2. Although both vehicle and RGFP966 mice showed a positive DI, neither group was statistically different from 0 (one-sample t-test compared to 0: vehicle: t(7) = 1.737, p = 0.1260; RGFP966: t(7) = 1.848, p = 0.1071). For both of the original locations A1 and A2, however, there were no significant group differences in DI, indicating that the groups showed similar memory for the original information regardless of drug treatment (two-tailed t-tests: A1: t(14) = 1.362, p = 0.195; A2: t(14) = 0.0916, p = 0.928). For the updated location A3, however, only RGFP966 mice showed intact memory, preferring to explore novel location A4 over update location A3 (Figure 1C; RGFP966: one-sample t-test compared to 0: t(7) = 5.599, p = 0.0008); vehicle-injected mice showed equal preference for locations A3 and A4 (vehicle: one-sample t-test compared to 0: t(7) = 0.08333, p = 0.9359), indicating no observable memory for the update, as we have previously observed in 18-m.o. mice (Kwapis et al., 2020). Further, RGFP966-injected mice had a significantly higher DI for A3 than vehicle mice (two-tailed t-test: t(14) = 3.374, p = 0.0045), indicating that RGFP966 enhanced memory for the updated information compared to controls. No differences in exploration time were observed between groups at test (Supplementary Figure S1C; two-tailed t-test: t(14) = 1.047, p = 0.313). Together, these results demonstrate that blocking HDAC3 with RGFP966 immediately after an update can ameliorate age-related impairments in memory updating without affecting the original memory.
Post-update HDAC3 blockade in young male mice impairs the original information
Next, we wanted to see if post-update RGFP966 had any effect on young mice. As young mice successfully learn both the training and the updated information (Kwapis et al., 2020; Wright et al., 2020; Huff et al., 2024), we expected to observe no effect of post-update RGFP966. Here, young male mice given one day of training were given a 5-min memory update followed by systemic injections of RGFP966 or vehicle (Figure 2A). All mice were tested the following day. During habituation, all young mice showed similar decreases in movement across days (Supplementary Figure S2A), indicating normal habituation. We also observed low DIs during training, demonstrating there was no strong preference for object location A1 or A2 before learning (Supplementary Figure S2B; one-sample t-tests compared to 0: vehicle: t(12) = 0.7250, p = 0.4823; RGFP966: t(12) = 0.6431, p = 0.5323; two-tailed t-test comparing vehicle and RGFP966: t(24) = 0.06; p = 0.95).
During the update session, both vehicle and RGFP966 mice showed similar memory for the original training information (Figure 2B; two-tailed t-test: t(24) = 0.1717, p = 0.8651). Interestingly, only animals destined to be injected with vehicle showed a DI significantly higher than zero (one-sample t-tests compared to 0: vehicle: t(12) = 2.252, p = 0.0438), although RGFP animals showed a positive DI that was non-significantly higher than zero (RGFP966: t(12) = 2.042, p = 0.0637). Therefore, young (3-m.o.) male mice showed weak memory for the original object locations with a single training session and mice destined to receive RGFP966 or vehicle performed similarly before injection.
Immediately after the update session, mice received systemic injections of either vehicle or RGFP966 (s.c., 10 mg/kg) to block HDAC3 during reconsolidation and were tested the following day. At test, young male mice given post-update RGFP966 showed normal memory updating but impaired memory for the original object locations. For location A1, we found that only young mice injected with vehicle showed a DI significantly higher than zero (Figure 2C; one-sample t-tests compared to 0: vehicle: t(12) = 2.847, p = 0.0147, RGFP966: t(12) = 1.209, p = 0.2500), but we observed no significant difference between the two drug conditions (Figure 2; two-tailed t-test: t(24) = 0.7085, p = 0.4855). This indicates that both groups remembered the original location A1 at test, but mice injected with RGFP966 showed slightly reduced memory for the A1 location compared to vehicle controls. Unexpectedly, however, we found that post-update RGFP966 significantly impaired memory for the original location A2. While vehicle mice showed a significant preference for location A2 at test (one-sample t-test compared to 0: t(12) = 3.626, p = 0.0035), RGFP966-injected mice failed to show a DI significantly higher than zero (one-sample t-test compared to 0: t(12) = 0.4979, p = 0.6276). Further, RGFP966-injected mice showed a significantly lower DI for A2 than vehicle controls (two-tailed t-test: t(24) = 2.339, p = 0.0280), indicating that post-update RGFP966 in young mice actually impairs memory for the original location A2. For location A3, both vehicle- and RGFP966-injected mice showed similarly intact memory; both groups had DIs significantly greater than zero (one-sample t-tests compared to 0: vehicle: t(12) = 5.338, p = 0.0002; RGFP966: t(12) = 3.547, p = 0.0040) and were not significantly different from each other (two-tailed t-test: t(24) = 0.2080, p = 0.9149), indicating successful updating. Mice injected with RGFP966 showed significantly higher total exploration than vehicle-injected mice (Supplementary Figure S2B; two-tailed t-test: t(24) = 2.509, p = 0.0193), possibly reflecting this group’s impaired ability to detect that locations A2 and A1 were familiar and did not need to be explored. Therefore, in young male mice that successfully update memory in OUL, blocking HDAC3 immediately after the update impairs memory for the original location A2.
HDAC3 blockade transforms a subthreshold update into a robust memory update
We were surprised to find that blocking HDAC3 after updating actually impaired memory for the original information in young mice. One possible explanation for this effect is that the original and updated information compete for expression at test, so that in old mice, blocking HDAC3 stabilizes the weak update memory to enable its expression at test. In young mice that already successfully update, blocking HDAC3 after updating could stabilize and strengthen the memory update at the expense of the original training information, enabling the memory update to outcompete the original information so that only the updated information is properly expressed at test. To test this hypothesis, we next assessed whether blocking HDAC3 after a weak ‘subthreshold’ update would still be capable of repressing the original training information. To this end, we ran an identical experiment, except that we shortened the update session from 5 min to 1 min. By reducing the update session, we created a weak update that was not capable of driving successful updating on its own in young mice, essentially mimicking the impaired updating we typically observe in old mice.
During habituation, all mice showed similar decreases in movement across each session (Supplementary Figure S3A), indicating normal habituation. We also observed low DIs during training, with no significant differences between groups, demonstrating there was no strong preference for object location A1 or A2 before learning (Supplementary Figure S3B; Vehicle group failed Shapiro–Wilk test of normality (W = 0.755, p = 0.014); Wilcoxon signed-rank test (nonparametric) comparing Vehicle to 0: W = 0.0; p > 0.99; one-sample t-test comparing RGFP966 to 0: t(7) = 0.686, p = 0.515; Mann–Whitney U Test (nonparametric) comparing Vehicle to RGFP966: U = 27, p = 0.95). The following day, mice were all given a brief, 1-min update session. Since this update session is so brief, mice show very low exploration times that cannot be accurately used to calculate a DI. However, young male mice injected with vehicle or RGFP966 showed similar investigation times, not significantly different from each other (Supplementary Figure S3C; two-tailed t-test: t(13) = 0.235, p = 0.818), suggesting similar exploration levels during the update.
As before, immediately after this weak update session, young male mice were given systemic injections of vehicle or RGFP966 (s.c. 10 mg/kg) and were tested the following day (Figure 3A). At test, we found that the post-update HDAC3 blockade was capable of transforming a subthreshold update into a robust update in young male mice without impairing the original memory. Specifically, we found that 3-m.o. mice injected with either RGFP966 or vehicle showed intact memory for original training locations A1 and A2 (Figure 3B; one-sample t-tests compared to 0: A1 vehicle: t(6) = 4.203, p = 0.00575, A1 RGFP966: t(7) = 4.414, p = 0.0031; A2 vehicle: t(6) = 2.666, p = 0.0372, A2 RGFP966: t(7) = 6.802, p = 0.0003; two-tailed t-tests comparing vehicle and RGFP966: A1: t(13) = 0.696, p = 0.499; A2: t(13) = 0.963, p = 0.353) indicating all animals successfully recalled the original object location memory. In contrast, for the updated location A3, only RGFP966 mice showed intact memory, preferring to explore novel location A4 over update location A3 (Figure 3B; one-sample t-test compared to 0: t(8) = 4.395, p = 0.0032). Vehicle mice showed equal preference for locations A3 and A4 (Figure 3B; one-sample t-test compared to 0: t(6) = 0.6058, p = 0.5669), indicating no observable memory for the update. This confirms that the 1-min update was not sufficient to support memory updating in young control mice. Consistent with this, RGFP966 mice showed significantly higher DIs for location A3 than vehicle mice (Figure 3B; two-tailed t-test: t(13) = 2.788, p = 0.0154) with no differences in exploration time between groups (Supplementary Figure S3C; two-tailed t-test: t(13) = 1.443, p = 0.173). Together, this suggests that blocking HDAC3 can strengthen a subthreshold update in young male mice without impairing memory for the original training locations. Overall, this work is consistent with the idea that the original and updated information compete for expression at test, with HDAC3 inhibition shifting this balance to change which information is expressed at test.
Discussion
Overall, our results show that blocking the repressive histone deacetylase HDAC3 can improve memory updating in old male mice. In contrast, in young male mice, we found that blocking HDAC3 after updating impairs the original memory, possibly because it creates an intensely strong update memory that can outcompete the original memory for expression at test. Together, this suggests that competition exists between the original and the updated information when a memory is updated and HDAC3 can shift the balance between which information is expressed at test. In old mice that typically show weak memory updating, RGFP966 enhances the memory update, enabling it to compete for expression with the stronger original memory. In young mice that already show robust memory updating, HDAC3 further strengthens the memory update so that it can outcompete the original information. Finally, when young mice are given a subthreshold update that alone is insufficient to support memory updating, HDAC3 can transform this weak update into one that drives robust memory updating that is expressed at test along with the original memory. Overall, this suggests that HDAC3 regulates the strength of the memory update and contributes to age-related impairments in memory updating.
Perhaps our most surprising finding was that in young male mice, inhibiting HDAC3 following updating actually impaired the original memory. Enhancing the memory update by blocking HDAC3 may, therefore, come at the expense of the original training information. Only A2 was absent from the update session, suggesting that the information presented before HDAC3 inhibition becomes strengthened, whereas absent information is weakened. Inhibiting HDAC3 did not universally weaken the original information, as memory for A1 remained intact but weakened any information that was not presented during the update (A2). We observed good memory for the updated information in young animals regardless of the drug treatment. While HDAC3 inhibition did not further enhance memory for A3, the updated A3 information may have reached a ceiling for expressing behavioral enhancements in this paradigm. In the following experiments, a subthreshold update helped us to determine that this is indeed the case, as enhancing weakly updated information does not affect the original A2 information.
One interesting observation is that young mice given the HDAC3 inhibitor following a full update showed significantly more total object exploration than vehicle-treated animals, with a similar trend also observed in the subthreshold update experiment (Supplementary Figures S2C, S3C). While our DI calculation controls for investigation (DIs are calculated as a percentage of total exploration time), this may suggest that RGFP966-treated animals explore the objects more when they are unable to clearly detect which locations are familiar, either because the original memory was weakened by drug treatment or because the update was exceedingly brief. Indeed, movement was normal in all animals during the habituation sessions, suggesting that this difference in test exploration did not stem from an inability to move or explore normally, but rather a difference in memory for the original or updated information.
We hypothesized that competition between the original and updated information was responsible for weaker memory for the original A2 location. To test this idea, we tried weakening the update in young male mice to effectively mimic the unsuccessful updating we initially observed in old mice. Specifically, we tested the effects of HDAC3 inhibition following a subthreshold update in young mice. We found that a one-minute update session was insufficient for updating unless combined with HDAC3 inhibition (Figure 3). Importantly, when RGFP966 was given after this subthreshold update, memory for the original information (A2) was unaffected (Figure 3), whereas the same inhibitor given after a full update impaired memory for the original information. This indicates that HDAC3 inhibition can manipulate the balance between the original and updated information; blocking HDAC3 strengthened the update information, creating either an intensely robust memory that outcompeted the original information or transforming a weak update into one capable of competing for expression with the original memory.
Our work, therefore, indicates that the repressive histone deacetylase HDAC3 plays a key role in reconsolidation-based memory updating, and inhibiting HDAC3 can improve memory updating in old mice. This is consistent with the well-documented role of HDAC3 in regulating memory strength and persistence across paradigms (McQuown et al., 2011; McQuown and Wood, 2011; Malvaez et al., 2013; Rogge et al., 2013; Alaghband et al., 2017; Kwapis et al., 2017a; Shu et al., 2018; Hervera et al., 2019; Hitchcock et al., 2019; Kwapis et al., 2019; Campbell et al., 2021; Keiser et al., 2021). This is a key finding, considering the lack of information about the specific roles of epigenetic mechanisms in memory reconsolidation or memory updating. Past work using less selective HAT or HDAC inhibitors has demonstrated impairment or enhancement of reconsolidation in fear conditioning, respectively, with an emerging role for Class I HDACs (including HDACs 1, 2, 3, and 8; Bredy and Barad, 2008; Maddox and Schafe, 2011; Maddox et al., 2013a,b; Gräff et al., 2014). Inhibitors specific to Class I HDACs (including VPA and Cl-994) are sufficient to improve reconsolidation (Bredy and Barad, 2008; Gräff et al., 2014). Here, our work indicates that HDAC3 plays a crucial role in modulating the strength of reconsolidation-dependent memory updating in both young and old mice. In addition to work showing a key role for DNA methyltransferase activity in reconsolidation, (Maddox et al., 2013b, 2014) it is becoming increasingly clear that a range of epigenetic mechanisms may be critical for successful memory reconsolidation and, possibly, memory updating.
As the OUL paradigm is heavily hippocampus-dependent, the effects observed in the current study may not being universal to all memory updating tasks, especially those that depend less on the hippocampus. A number of other updating tasks exist, including fear-related tasks (Kwapis et al., 2017b; Ferrara et al., 2019; Popik et al., 2023), object recognition memory (Gonzalez et al., 2019), Y maze updating (Winters et al., 2011), and water maze updating tasks (De Jaeger et al., 2014) and understanding the role of HDAC3 in other paradigms will be important to gain a holistic understanding of its role in memory updating more broadly. OUL has the unique ability to simultaneously test memory for the updated and original information, making it ideal for testing memory competition. Whether similar effects will be observable in these other updating paradigms is an outstanding question that should be the focus of future research.
Although the OUL memory updating paradigm is new, a number of labs have used this task to better understand how memory updating works. Previous work has confirmed that OUL drives memory updating rather than the formation of a new memory. First, blocking protein synthesis within the dorsal hippocampus after the update session impairs memory for both the original training information and the update, suggesting the original memory is destabilized in response to the updated location (Kwapis et al., 2020). Second, using Arc catFISH, we have previously confirmed that the update session preferentially reactivates the neuronal ensemble supporting the original object location memory (Kwapis et al., 2020). OUL therefore, appears to drive reconsolidation-based updating in the original neuronal ensemble. How, then, does the original and the updated information compete for expression if there is a single population of neurons supporting both memories? Although speculative, we have two possible explanations for this phenomenon. First, although the original and updated memories clearly share a common population of cells, there is never 100% overlap between the cells activated by the original information and the updated information, even in a No Update condition (Kwapis et al., 2020). Thus, even when the original population is preferentially re-engaged during updating, there is an opportunity for new cells to be integrated into the ensemble, possibly allowing for dynamic memory updating, flexibility, and even ensemble drift or reorganization (Sweis et al., 2021). HDAC3 inhibition may allow more of these ‘new’ cells to be incorporated into the ensemble, enabling memory updating in situations in which it otherwise fails (i.e., in old animals or after subthreshold updating) or overpowering the original memory when updating is already strongly learned. A second possibility is that even when the same neuronal population encodes an original memory and an update, changes in the synaptic connections and the weight of those connections might support these different pieces of information. Clearly an animal that has learned the update behaves differently than an animal not given the update, despite both groups showing a similar, shared neuronal ensemble (Kwapis et al., 2020), suggesting that this information is encoded at another level, such as the synaptic or even the molecular level. Future work should systematically test these possibilities.
The current experiments show evidence for HDAC3-mediated memory competition among original and updated object location memories. To better understand what HDAC3 is doing during memory updating, future studies should focus on identifying which genes are mediated by HDAC3 during updating and determine if these genes are identical to or different from the genes required for initial memory consolidation. Determining how these transcriptional profiles change in old age when memory updating is impaired will also be critical. In addition, it will be important for future experiments to test middle age timepoints to identify when these deficits in memory updating emerge across the lifespan and to determine if age-related deficits in memory updating can be prevented with early intervention. Finally, the current experiments were all done in male mice, and subsequent studies will need to add female mice to determine if these mechanisms are similar in both sexes. While our lab has just started to use the OUL paradigm with female mice, the molecular mechanisms by which memories compete may differ in females.
Overall, our findings show that HDAC3 regulates the competition between an original memory and a memory update and suggest that HDAC3 may contribute to age-related impairments in memory updating. Our findings demonstrate that epigenetic mechanisms play a key role in memory updating and suggest that OUL is poised to help identify the molecular, cellular, and circuit-level mechanisms that support hippocampal memory updating in rodents.
Data availability statement
The raw data supporting the conclusions of this article will be made available by the authors, without undue reservation.
Ethics statement
The animal study was approved by Pennsylvania State University Institutional Animal Care and Use Committee. The study was conducted in accordance with the local legislation and institutional requirements.
Author contributions
CS: Conceptualization, Formal analysis, Investigation, Methodology, Writing – original draft. LB: Investigation, Writing – review & editing. DW: Investigation, Writing – review & editing. SB: Investigation, Writing – review & editing. MU: Investigation, Writing – review & editing. CB: Investigation, Writing – review & editing. GS: Investigation, Writing – review & editing. JK: Conceptualization, Funding acquisition, Supervision, Writing – review & editing.
Funding
The author(s) declare that financial support was received for the research, authorship, and/or publication of this article. This research was funded by NIH grant R01AG074041, the Hevolution/AFAR New Investigator Award in Aging Biology and Geroscience Research, and start-up funds from the Eberly College of Science and Department of Biology at Pennsylvania State University (JK).
Conflict of interest
The authors declare that the research was conducted in the absence of any commercial or financial relationships that could be construed as a potential conflict of interest.
The author(s) declared that they were an editorial board member of Frontiers, at the time of submission. This had no impact on the peer review process and the final decision.
Publisher’s note
All claims expressed in this article are solely those of the authors and do not necessarily represent those of their affiliated organizations, or those of the publisher, the editors and the reviewers. Any product that may be evaluated in this article, or claim that may be made by its manufacturer, is not guaranteed or endorsed by the publisher.
Supplementary material
The Supplementary material for this article can be found online at: https://www.frontiersin.org/articles/10.3389/fnmol.2024.1429880/full#supplementary-material
References
Alaghband, Y., Kwapis, J. L., López, A. J., White, A. O., Aimiuwu, O. V., Al-Kachak, A., et al. (2017). Distinct roles for the deacetylase domain of HDAC3 in the hippocampus and medial prefrontal cortex in the formation and extinction of memory. Neurobiol. Learn. Mem. 145, 94–104. doi: 10.1016/j.nlm.2017.09.001
Aziz, W., Kraev, I., Mizuno, K., Kirby, A., Fang, T., Rupawala, H., et al. (2019). Multi-input synapses, but not LTP-strengthened synapses, correlate with hippocampal memory storage in aged mice. Curr. Biol. 29, 3600–3610.e4. doi: 10.1016/j.cub.2019.08.064
Bach, M. E., Barad, M., Son, H., Zhuo, M., Lu, Y.-F., Shih, R., et al. (1999). Age-related defects in spatial memory are correlated with defects in the late phase of hippocampal long-term potentiation in vitro and are attenuated by drugs that enhance the cAMP signaling pathway. Proc. Natl. Acad. Sci. 96, 5280–5285. doi: 10.1073/pnas.96.9.5280
Bannister, A. J., and Kouzarides, T. (2011). Regulation of chromatin by histone modifications. Cell Res. 21, 381–395. doi: 10.1038/cr.2011.22
Barchiesi, R., Chanthongdee, K., Petrella, M., Xu, L., Söderholm, S., Domi, E., et al. (2022). An epigenetic mechanism for over-consolidation of fear memories. Mol. Psychiatry 27, 4893–4904. doi: 10.1038/s41380-022-01758-6
Barense, M. D., Fox, M. T., and Baxter, M. G. (2002). Aged rats are impaired on an attentional set-shifting task sensitive to medial frontal cortex damage in young rats. Learn. Mem. 9, 191–201. doi: 10.1101/lm.48602
Bellfy, L., Smies, C. W., Bernhardt, A. R., Bodinayake, K. K., Sebastian, A., Stuart, E. M., et al. (2023). The clock gene Per1 may exert diurnal control over hippocampal memory consolidation. Neuropsychopharmacology 48, 1789–1797. doi: 10.1038/s41386-023-01616-1
Bieszczad, K. M., Bechay, K., Rusche, J. R., Jacques, V., Kudugunti, S., Miao, W., et al. (2015). Histone deacetylase inhibition via RGFP966 releases the brakes on sensory cortical plasticity and the specificity of memory formation. J. Neurosci. 35, 13124–13132. doi: 10.1523/JNEUROSCI.0914-15.2015
Bizon, J. L., Foster, T. C., Alexander, G. E., and Glisky, E. L. (2012). Characterizing cognitive aging of working memory and executive function in animal models. Front. Aging Neurosci. 4:19. doi: 10.3389/fnagi.2012.00019
Bohnslav, J. P., Wimalasena, N. K., Clausing, K. J., Dai, Y. Y., Yarmolinsky, D. A., Cruz, T., et al. (2021). DeepEthogram, a machine learning pipeline for supervised behavior classification from raw pixels. eLife 10:e63377. doi: 10.7554/eLife.63377
Bredy, T. W., and Barad, M. (2008). The histone deacetylase inhibitor valproic acid enhances acquisition, extinction, and reconsolidation of conditioned fear. Learn. Mem. 15, 39–45. doi: 10.1101/lm.801108
Brito, D. V. C., Esteves, F., Rajado, A. T., Silva, N., ALFA score Consortium, Andrade, RApolónio, J., et al. (2023). Assessing cognitive decline in the aging brain: lessons from rodent and human studies. Npj Aging 9:23. doi: 10.1038/s41514-023-00120-6
Brunswick, C. A., Baldwin, D. J., Bodinayake, K. K., McKenna, A. R., Lo, C.-Y., Bellfy, L., et al. (2023). The clock gene Per1 is necessary in the retrosplenial cortex—but not in the suprachiasmatic nucleus—for incidental learning in young and aging male mice. Neurobiol. Aging 126, 77–90. doi: 10.1016/j.neurobiolaging.2023.02.009
Campbell, R. R., Kramár, E. A., Pham, L., Beardwood, J. H., Augustynski, A. S., López, A. J., et al. (2021). HDAC3 activity within the nucleus Accumbens regulates cocaine-induced plasticity and behavior in a cell-type-specific manner. J. Neurosci. 41, 2814–2827. doi: 10.1523/JNEUROSCI.2829-20.2021
Da Silva, W. C., Bonini, J. S., Bevilaqua, L. R. M., Medina, J. H., Izquierdo, I., and Cammarota, M. (2008). Inhibition of mRNA synthesis in the hippocampus impairs consolidation and reconsolidation of spatial memory. Hippocampus 18, 29–39. doi: 10.1002/hipo.20362
Davis, H. P., and Squire, L. R. (1984). Protein synthesis and memory: a review. Psychol. Bull. 96, 518–559. doi: 10.1037/0033-2909.96.3.518
De Jaeger, X., Courtey, J., Brus, M., Artinian, J., Villain, H., Bacquié, E., et al. (2014). Characterization of spatial memory reconsolidation. Learn. Mem. 21, 316–324. doi: 10.1101/lm.033415.113
De Oliveira Alvares, L., Crestani, A. P., Cassini, L. F., Haubrich, J., Santana, F., and Quillfeldt, J. A. (2013). Reactivation enables memory updating, precision-keeping and strengthening: exploring the possible biological roles of reconsolidation. Neuroscience 244, 42–48. doi: 10.1016/j.neuroscience.2013.04.005
Díaz-Mataix, L., Ruiz Martinez, R. C., Schafe, G. E., LeDoux, J. E., and Doyère, V. (2013). Detection of a temporal error triggers reconsolidation of amygdala-dependent memories. Curr. Biol. 23, 467–472. doi: 10.1016/j.cub.2013.01.053
Duvarci, S., Nader, K., and LeDoux, J. E. (2008). De novo mRNA synthesis is required for both consolidation and reconsolidation of fear memories in the amygdala. Learn. Mem. 15, 747–755. doi: 10.1101/lm.1027208
Ederer, M.-L., Günther, M., Best, L., Lindner, J., Kaleta, C., Witte, O. W., et al. (2022). Voluntary wheel running in old C57BL/6 mice reduces age-related inflammation in the Colon but not in the brain. Cells 11:566. doi: 10.3390/cells11030566
Felsenfeld, G. (2014). A brief history of epigenetics. Cold Spring Harb. Perspect. Biol. 6:a018200. doi: 10.1101/cshperspect.a018200
Ferrara, N. C., Jarome, T. J., Cullen, P. K., Orsi, S. A., Kwapis, J. L., Trask, S., et al. (2019). GluR2 endocytosis-dependent protein degradation in the amygdala mediates memory updating. Sci. Rep. 9:5180. doi: 10.1038/s41598-019-41526-1
Gonzalez, M. C., Rossato, J. I., Radiske, A., Pádua Reis, M., and Cammarota, M. (2019). Recognition memory reconsolidation requires hippocampal Zif268. Sci. Rep. 9:16620. doi: 10.1038/s41598-019-53005-8
Gräff, J., Joseph, N. F., Horn, M. E., Samiei, A., Meng, J., Seo, J., et al. (2014). Epigenetic priming of memory updating during reconsolidation to attenuate remote fear memories. Cell 156, 261–276. doi: 10.1016/j.cell.2013.12.020
Grunstein, M. (1997). Histone acetylation in chromatin structure and transcription. Nature 389, 349–352. doi: 10.1038/38664
Hendrickx, J. O., De Moudt, S., Calus, E., De Deyn, P. P., Van Dam, D., and De Meyer, G. R. Y. (2022). Age-related cognitive decline in spatial learning and memory of C57BL/6J mice. Behav. Brain Res. 418:113649. doi: 10.1016/j.bbr.2021.113649
Hervera, A., Zhou, L., Palmisano, I., McLachlan, E., Kong, G., Hutson, T. H., et al. (2019). PP4-dependent HDAC3 dephosphorylation discriminates between axonal regeneration and regenerative failure. EMBO J. 38:e101032. doi: 10.15252/embj.2018101032
Hitchcock, L. N., Raybuck, J. D., Wood, M. A., and Lattal, K. M. (2019). Effects of a histone deacetylase 3 inhibitor on extinction and reinstatement of cocaine self-administration in rats. Psychopharmacology 236, 517–529. doi: 10.1007/s00213-018-5122-2
Huff, A. E., O’Neill, O. S., Messer, W. S., and Winters, B. D. (2024). Muscarinic receptor activation promotes destabilization and updating of object location memories in mice. Behav. Brain Res. 461:114847. doi: 10.1016/j.bbr.2024.114847
Igaz, L. M., Vianna, M. R. M., Medina, J. H., and Izquierdo, I. (2002). Two time periods of hippocampal mRNA synthesis are required for memory consolidation of fear-motivated learning. J. Neurosci. 22, 6781–6789. doi: 10.1523/JNEUROSCI.22-15-06781.2002
Jarome, T. J., Ferrara, N. C., Kwapis, J. L., and Helmstetter, F. J. (2015). Contextual information drives the reconsolidation-dependent updating of retrieved fear memories. Neuropsychopharmacology 40, 3044–3052. doi: 10.1038/npp.2015.161
Keiser, A. A., Kramár, E. A., Dong, T., Shanur, S., Pirodan, M., Ru, N., et al. (2021). Systemic HDAC3 inhibition ameliorates impairments in synaptic plasticity caused by simulated galactic cosmic radiation exposure in male mice. Neurobiol. Learn. Mem. 178:107367. doi: 10.1016/j.nlm.2020.107367
Kwapis, J. L., Alaghband, Y., Keiser, A. A., Dong, T. N., Michael, C. M., Rhee, D., et al. (2020). Aging mice show impaired memory updating in the novel OUL updating paradigm. Neuropsychopharmacology 45, 337–346. doi: 10.1038/s41386-019-0438-0
Kwapis, J. L., Alaghband, Y., Kramár, E. A., López, A. J., Vogel Ciernia, A., White, A. O., et al. (2018). Epigenetic regulation of the circadian gene Per1 contributes to age-related changes in hippocampal memory. Nat. Commun. 9:3323. doi: 10.1038/s41467-018-05868-0
Kwapis, J. L., Alaghband, Y., López, A. J., Long, J. M., Li, X., Shu, G., et al. (2019). HDAC3-mediated repression of the Nr4a family contributes to age-related impairments in Long-term memory. J. Neurosci. 39, 4999–5009. doi: 10.1523/JNEUROSCI.2799-18.2019
Kwapis, J. L., Alaghband, Y., López, A. J., White, A. O., Campbell, R. R., Dang, R. T., et al. (2017a). Context and auditory fear are differentially regulated by HDAC3 activity in the lateral and basal subnuclei of the amygdala. Neuropsychopharmacology 42, 1284–1294. doi: 10.1038/npp.2016.274
Kwapis, J. L., Jarome, T. J., Ferrara, N. C., and Helmstetter, F. J. (2017b). Updating procedures can reorganize the neural circuit supporting a fear memory. Neuropsychopharmacology 42, 1688–1697. doi: 10.1038/npp.2017.23
Lattal, K. M., and Wood, M. A. (2013). Epigenetics and persistent memory: implications for reconsolidation and silent extinction beyond the zero. Nat. Neurosci. 16, 124–129. doi: 10.1038/nn.3302
Lubin, F. D., Gupta, S., Parrish, R. R., Grissom, N. M., and Davis, R. L. (2011). Epigenetic mechanisms: critical contributors to Long-term memory formation. Neuroscientist 17, 616–632. doi: 10.1177/1073858410386967
Maddox, S. A., and Schafe, G. E. (2011). Epigenetic alterations in the lateral amygdala are required for reconsolidation of a Pavlovian fear memory. Learn. Mem. 18, 579–593. doi: 10.1101/lm.2243411
Maddox, S. A., Watts, C. S., Doyère, V., and Schafe, G. E. (2013a). A naturally-occurring histone acetyltransferase inhibitor derived from Garcinia indica impairs newly acquired and reactivated fear memories. PLoS One 8:e54463. doi: 10.1371/journal.pone.0054463
Maddox, S. A., Watts, C. S., and Schafe, G. E. (2013b). P300/CBP histone acetyltransferase activity is required for newly acquired and reactivated fear memories in the lateral amygdala. Learn. Mem. 20, 109–119. doi: 10.1101/lm.029157.112
Maddox, S. A., Watts, C. S., and Schafe, G. E. (2014). DNA methyltransferase activity is required for memory-related neural plasticity in the lateral amygdala. Neurobiol. Learn. Mem. 107, 93–100. doi: 10.1016/j.nlm.2013.11.008
Malvaez, M., McQuown, S. C., Rogge, G. A., Astarabadi, M., Jacques, V., Carreiro, S., et al. (2013). HDAC3-selective inhibitor enhances extinction of cocaine-seeking behavior in a persistent manner. Proc. Natl. Acad. Sci. 110, 2647–2652. doi: 10.1073/pnas.1213364110
McQuown, S. C., Barrett, R. M., Matheos, D. P., Post, R. J., Rogge, G. A., Alenghat, T., et al. (2011). HDAC3 is a critical negative regulator of Long-term memory formation. J. Neurosci. 31, 764–774. doi: 10.1523/JNEUROSCI.5052-10.2011
McQuown, S. C., and Wood, M. A. (2011). HDAC3 and the molecular brake pad hypothesis. Neurobiol. Learn. Mem. 96, 27–34. doi: 10.1016/j.nlm.2011.04.005
Monfils, M. H., Cowansage, K. K., Klann, E., and LeDoux, J. (2009). Extinction-reconsolidation boundaries: key to persistent attenuation of fear memories. Science 324, 951–955. doi: 10.1126/science.1167975
Monfils, M. H., and Holmes, E. A. (2018). Memory boundaries: opening a window inspired by reconsolidation to treat anxiety, trauma-related, and addiction disorders. Lancet Psychiatry 5, 1032–1042. doi: 10.1016/S2215-0366(18)30270-0
Monsey, M. S., Ota, K. T., Akingbade, I. F., Hong, E. S., and Schafe, G. E. (2011). Epigenetic alterations are critical for fear memory consolidation and synaptic plasticity in the lateral amygdala. PLoS One 6:e19958. doi: 10.1371/journal.pone.0019958
Morris, R. G. M., Inglis, J., Ainge, J. A., Olverman, H. J., Tulloch, J., Dudai, Y., et al. (2006). Memory reconsolidation: sensitivity of spatial memory to inhibition of protein synthesis in dorsal Hippocampus during encoding and retrieval. Neuron 50, 479–489. doi: 10.1016/j.neuron.2006.04.012
Nader, K., Schafe, G. E., and Le Doux, J. E. (2000). Fear memories require protein synthesis in the amygdala for reconsolidation after retrieval. Nature 406, 722–726. doi: 10.1038/35021052
Peixoto, L., and Abel, T. (2013). The role of histone acetylation in memory formation and cognitive impairments. Neuropsychopharmacology 38, 62–76. doi: 10.1038/npp.2012.86
Phan, M. L., Gergues, M. M., Mahidadia, S., Jimenez-Castillo, J., Vicario, D. S., and Bieszczad, K. M. (2017). HDAC3 inhibitor RGFP966 modulates neuronal memory for vocal communication signals in a songbird model. Front. Syst. Neurosci. 11:65. doi: 10.3389/fnsys.2017.00065
Popik, B., Guerra, K. T. K., Luft, J. G., Fernandes, H. S., and De Oliveira Alvares, L. (2023). Characterization of deconditioning-update on fear memory attenuation. Neurobiol. Learn. Mem. 202:107763. doi: 10.1016/j.nlm.2023.107763
Pulya, S., Mahale, A., Bobde, Y., Routholla, G., Patel, T., Swati, B., et al. (2021). PT3: a novel Benzamide class histone deacetylase 3 inhibitor improves learning and memory in novel object recognition mouse model. ACS Chem. Neurosci. 12, 883–892. doi: 10.1021/acschemneuro.0c00721
Ramzan, F., Creighton, S. D., Hall, M., Baumbach, J., Wahdan, M., Poulson, S. J., et al. (2020). Sex-specific effects of the histone variant H2A.Z on fear memory, stress-enhanced fear learning and hypersensitivity to pain. Sci. Rep. 10:14331. doi: 10.1038/s41598-020-71229-x
Rogge, G. A., Singh, H., Dang, R., and Wood, M. A. (2013). HDAC3 is a negative regulator of cocaine-context-associated memory formation. J. Neurosci. 33, 6623–6632. doi: 10.1523/JNEUROSCI.4472-12.2013
Schoenbaum, G., Nugent, S., Saddoris, M. P., and Gallagher, M. (2002). Teaching old rats new tricks: age-related impairments in olfactory reversal learning. Neurobiol. Aging 23, 555–564. doi: 10.1016/S0197-4580(01)00343-8
Sevenster, D., Beckers, T., and Kindt, M. (2012). Retrieval per se is not sufficient to trigger reconsolidation of human fear memory. Neurobiol. Learn. Mem. 97, 338–345. doi: 10.1016/j.nlm.2012.01.009
Sevenster, D., Beckers, T., and Kindt, M. (2013). Prediction error governs pharmacologically induced amnesia for learned fear. Science 339, 830–833. doi: 10.1126/science.1231357
Sevenster, D., Beckers, T., and Kindt, M. (2014). Prediction error demarcates the transition from retrieval, to reconsolidation, to new learning. Learn. Mem. 21, 580–584. doi: 10.1101/lm.035493.114
Shang, A., Bylipudi, S., and Bieszczad, K. M. (2019). Inhibition of histone deacetylase 3 via RGFP966 facilitates cortical plasticity underlying unusually accurate auditory associative cue memory for excitatory and inhibitory cue-reward associations. Behav. Brain Res. 356, 453–469. doi: 10.1016/j.bbr.2018.05.036
Shoji, H., Takao, K., Hattori, S., and Miyakawa, T. (2016). Age-related changes in behavior in C57BL/6J mice from young adulthood to middle age. Mol. Brain 9:11. doi: 10.1186/s13041-016-0191-9
Shu, G., Kramár, E. A., López, A. J., Huynh, G., Wood, M. A., and Kwapis, J. L. (2018). Deleting HDAC3 rescues long-term memory impairments induced by disruption of the neuron-specific chromatin remodeling subunit BAF53b. Learn. Mem. 25, 109–114. doi: 10.1101/lm.046920.117
Strahl, B. D., and Allis, C. D. (2000). The language of covalent histone modifications. Nature 403, 41–45. doi: 10.1038/47412
Sweis, B. M., Mau, W., Rabinowitz, S., and Cai, D. J. (2021). Dynamic and heterogeneous neural ensembles contribute to a memory engram. Curr. Opin. Neurobiol. 67, 199–206. doi: 10.1016/j.conb.2020.11.017
Urban, M. W., Lo, C., Bodinayake, K. K., Brunswick, C. A., Murakami, S., Heimann, A. C., et al. (2021). The circadian clock gene Per1 modulates context fear memory formation within the retrosplenial cortex in a sex-specific manner. Neurobiol. Learn. Mem. 185:107535. doi: 10.1016/j.nlm.2021.107535
Villain, H., Florian, C., and Roullet, P. (2016). HDAC inhibition promotes both initial consolidation and reconsolidation of spatial memory in mice. Sci. Rep. 6:27015. doi: 10.1038/srep27015
Vogel-Ciernia, A., and Wood, M. A. (2014). Examining object location and object recognition memory in mice. Curr. Protoc. Neurosci. 69, 8.3111–8.3117. doi: 10.1002/0471142301.ns0831s69
Wapenaar, H., and Dekker, F. J. (2016). Histone acetyltransferases: challenges in targeting bi-substrate enzymes. Clin. Epigenetics 8:59. doi: 10.1186/s13148-016-0225-2
Weber, M., Wu, T., Hanson, J. E., Alam, N. M., Solanoy, H., Ngu, H., et al. (2015). Cognitive deficits, changes in synaptic function, and brain pathology in a mouse model of Normal aging. Eneuro 2:ENEURO.0047-15.2015. doi: 10.1523/ENEURO.0047-15.2015
Winters, B. D., Tucci, M. C., Jacklin, D. L., Reid, J. M., and Newsome, J. (2011). On the dynamic nature of the engram: evidence for circuit-level reorganization of object memory traces following reactivation. J. Neurosci. 31, 17719–17728. doi: 10.1523/JNEUROSCI.2968-11.2011
Wolffe, A. P. (1996). Histone deacetylase: a regulator of transcription. Science 272:371. doi: 10.1126/science.272.5260.371
Wright, D. S., Bodinayake, K. K., and Kwapis, J. L. (2020). Investigating memory updating in mice using the objects in updated locations task. Curr. Protoc. Neurosci. 91:e87. doi: 10.1002/cpns.87
Keywords: histone deacetylase 3 (HDAC3), memory updating, reconsolidation, objects in updated locations (OUL), object location memory (OLM), RGFP966, aging, memory
Citation: Smies CW, Bellfy L, Wright DS, Bennetts SG, Urban MW, Brunswick CA, Shu G and Kwapis JL (2024) Pharmacological HDAC3 inhibition alters memory updating in young and old male mice. Front. Mol. Neurosci. 17:1429880. doi: 10.3389/fnmol.2024.1429880
Edited by:
Declan McKernan, University of Galway, IrelandReviewed by:
Timothy J. Jarome, Virginia Tech, United StatesRoger Marek, The University of Queensland, Australia
Copyright © 2024 Smies, Bellfy, Wright, Bennetts, Urban, Brunswick, Shu and Kwapis. This is an open-access article distributed under the terms of the Creative Commons Attribution License (CC BY). The use, distribution or reproduction in other forums is permitted, provided the original author(s) and the copyright owner(s) are credited and that the original publication in this journal is cited, in accordance with accepted academic practice. No use, distribution or reproduction is permitted which does not comply with these terms.
*Correspondence: Janine L. Kwapis, amxrODU1QHBzdS5lZHU=