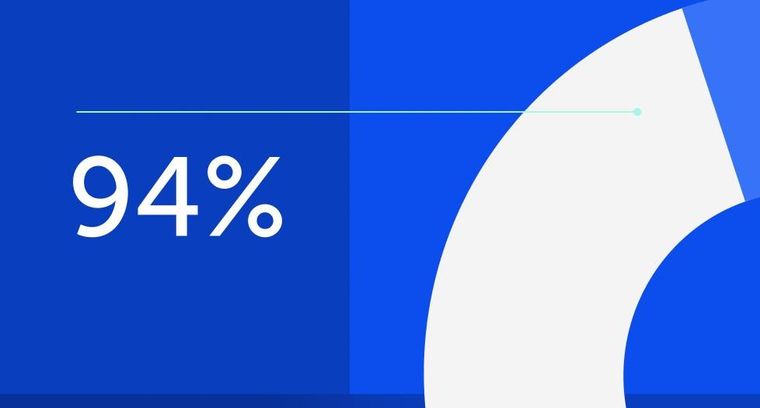
94% of researchers rate our articles as excellent or good
Learn more about the work of our research integrity team to safeguard the quality of each article we publish.
Find out more
REVIEW article
Front. Mol. Neurosci., 18 July 2024
Sec. Brain Disease Mechanisms
Volume 17 - 2024 | https://doi.org/10.3389/fnmol.2024.1415567
The gut-brain axis (GBA) plays a dominant role in maintaining homeostasis as well as contributes to mental health maintenance. The pathways that underpin the axis expand from macroscopic interactions with the nervous system, to the molecular signals that include microbial metabolites, tight junction protein expression, or cytokines released during inflammation. The dysfunctional GBA has been repeatedly linked to the occurrence of anxiety- and depressive-like behaviors development. The importance of the inflammatory aspects of the altered GBA has recently been highlighted in the literature. Here we summarize current reports on GBA signaling which involves the immune response within the intestinal and blood-brain barrier (BBB). We also emphasize the effect of stress response on altering barriers' permeability, and the therapeutic potential of microbiota restoration by probiotic administration or microbiota transplantation, based on the latest animal studies. Most research performed on various stress models showed an association between anxiety- and depressive-like behaviors, dysbiosis of gut microbiota, and disruption of intestinal permeability with simultaneous changes in BBB integrity. It could be postulated that under stress conditions impaired communication across BBB may therefore represent a significant mechanism allowing the gut microbiota to affect brain functions.
The bidirectional communication network between the central nervous system (CNS) and the gastrointestinal tract, known as the gut-brain axis (GBA) was found to play a substantial role in the etiopathogenesis of many diseases, including neurodegenerative diseases, and mood disorders. The GBA involves the integration of gut functions (including immune activity or intestinal permeability) with the emotional and cognitive centers of the brain (Carabotti et al., 2015). The existence of complex gut-brain communication is supported by several animal and human studies, although the underlying mechanisms are not fully elucidated. Research in this field is focused on neural (Cryan et al., 2019), neuroendocrine (Kasarello et al., 2023), immune (Rutsch et al., 2020), and metabolic pathways (Ahmed et al., 2022). These consist of autonomic (parasympathetic) nervous system signaling via the vagal nerve, enteric nervous system, innate and adaptive immune responses, neurotransmitters (or false neurotransmitters known as neurotransmitter-like compounds) or short chain fatty acids (SCFAs) being also metabolites or products of microbial functioning (Cryan et al., 2019).
Recently, it was proposed that besides the critical role of the gut microbiota as a component potentially influencing all these neuroimmune-endocrine pathways, it can affect the integrity of the blood-brain barrier (BBB), by changing barrier permeability and dysregulating tight junctions (TJs) (Braniste et al., 2014; Margolis et al., 2021). Impaired communication across the BBB may therefore represent a significant mechanism allowing the gut microbiota to affect brain functions, for example under stress conditions.
The GBA remains under the continuous influence of environmental factors, from early life through the entire lifespan, also during acute or chronic stress conditions (De Palma et al., 2015; Golubeva et al., 2015; Brzozowski et al., 2016). The role of immune signaling in neuronal development has also been thoroughly studied (Kipnis et al., 2004). Moreover, it was determined that presence of immune T cells, mediated partially by gut microbiota, has a vital role in microglia maturation (Pasciuto et al., 2020). Even short-term exposure to stress can induce dysbiosis. This term refers to alterations in microbiome composition, which leads to disturbed homeostasis, metabolic, and functional changes as the consequence of shifts in bacteria species residing in the gut (Levy et al., 2017). Gut dysbiosis is associated with the progress or worsening of mood disorders caused by various aspects of GBA functioning, such as activation of immune signaling during inflammatory processes. It is now well established that CNS disorders as well as diseases directly associated with the gut have strong stress-based pathogenesis (Holmqvist et al., 2014; Gao et al., 2018). Importantly, experimental alteration of gut microbiota influences stress responsiveness, anxiety- and depressive-like behavior, and activates the hypothalamic-pituitary-adrenal (HPA) axis (De Palma et al., 2014; Golubeva et al., 2015; Ergang et al., 2021). Significant shifts in gut microbiota composition reported in animal models of CNS diseases related to early life stress, such as maternal separation (De Palma et al., 2015), chronic restraint stress (CRS) (Gubert et al., 2020) or chronic unpredictable mild stress (CUMS) (Zhang et al., 2023) were linked with alterations in microbiota-related metabolites and immune signaling pathways suggesting that these systems may be important in stress-related conditions including depression.
Therefore, the aim of this review paper is to summarize findings linking the gut microbiota and brain health with special emphasis on inflammation and immune signaling pathways. We highlight current studies on inflammatory aspects of altered GBA functioning and deliver insights on its association with neuroinflammation and mood disorder development. So far, many authors have developed the concept of GBA, which undoubtedly contributed to increasing awareness of its role in systemic homeostasis (Carabotti et al., 2015; Cryan et al., 2019; Rutsch et al., 2020; Margolis et al., 2021). However, one of the main threads that distinguishes this work is the focus on the aspect of fluctuations within the BBB. We discuss the impact of metabolites generated by gut microbes on the BBB integrity, the involvement of stress exposure in intestinal barrier leakiness, and further changes in BBB permeability. Finally, we summarize the data from animal studies employing the therapeutic potential of manipulating the gut microbiota through probiotic, synbiotic, or fecal microbiota transplantation in the context of CNS disorders.
Apart from molecular mechanisms engaged in GBA signaling, this axis also stands for physical barriers surrounding the gastrointestinal tract and CNS (Figure 1).
Figure 1. Barriers within the GBA- a structural overview. The IB is composed of epithelial cells with goblet, enterochromaffin and enteroendocrine cells in between. The IgA, defensins and glycoproteins are main components of mucosal layer. Dendritic cells and macrophages reside in the intestinal lamina propria. The vascular barriers are both composed of endothelial cells, pericytes and glial cells. Each vascular unit shows the most highly expressed junctional proteins. Created with BioRender.com.
The lumen of gastrointestinal tract is separated from the blood circuitry by an intestinal barrier (IB) and the transport into blood vessels is controlled by the gut-vascular barrier (GVB) (Di Tommaso et al., 2023). The CNS is covered by two barriers interconnecting the blood and the brain, the BBB, and the blood-cerebrospinal fluid barrier, which involves choroid plexus epithelium (Kim et al., 2021; Hall and Bendtsen, 2023). Starting from the gut lumen surface, the IB is composed of the mucus-producing goblet cells, positioned between the epithelial cells (Suzuki, 2020). The crypt mucosal coverage, which is composed of secretory IgA, defensins and glycoproteins, and lamina propria with residing immune cells protects the gut wall from harmful pathogens (Suzuki, 2020; Kim et al., 2021). The epithelial layer has a similar function to the BBB endothelial layer. Both the epithelial and endothelial cells allow the passage of required molecules via either paracellular (IB) or transcellular (IB, BBB) active and vesicular transport. The paracellular passage in BBB is a symptom of disturbed homeostasis (Bauer et al., 2014; Haddad-Tóvolli et al., 2017). The vascular barriers, GVB and BBB are similar in structure. Both the gut-vascular unit and neurovascular unit consist of an endothelial layer wrapped in pericytes along with either enteric glial cells (GVB) or astrocytes (BBB) (Kadry et al., 2020; Di Tommaso et al., 2023). The endothelial cells are located on the luminal surface and are tightened by TJs, which modulate the barrier permeability thereby mediating signaling pathways (Bauer et al., 2014). The major difference between GVB and BBB is the eight times larger molecule flux allowed by GVB (Scalise et al., 2021). The reason for such distinction is associated with the gut functionality that involves nutrient absorption. Though the TJs and adherens junctions (AJs) expression vary among different cell types, claudins have been established to be the key components for changing barriers' permeability (Banks and Erickson, 2010; Scalise et al., 2021), claudin-5 being the most abundant throughout the BBB and in lymphatic endothelial cells of small and large intestine (Greene et al., 2019). Indeed, there are examples of expression similarity such as claudin-1, claudin-12, ZO-1, ZO-2 (TJs) or VE-cadherin and α- or β-catenin (AJs), both in the brain and in the gut (Scalise et al., 2021). The BBB anatomy enables the ionic and metabolic exchange homeostasis, proper neurotransmitter uptake and signaling mediation, and the whole barrier integrity, while the TJs and AJs between adjacent cells restrict paracellular transport and protect the brain parenchyma from unwanted hydrophilic particles passage (Scalise et al., 2021; Park and Im, 2022). In turn, the GVB adjusts its permeability to the current state of the intestinal environment and allows the passage of needed molecules while maintaining protection from adverse molecules.
Non-specific innate immunity is built on granulocytes, monocytes, macrophages, dendritic and mast cells as well as natural killer cells. These cells present the pattern-recognition receptor protein family, which includes Toll-like and NOD-like receptors (TLRs, NLRs), and act via inflammasome—an oligomeric protein complex. An inflammasome distinguishes pathogen/microbial/damage-associated molecular patterns present on the pathogenic cell surface, from host cell antigens (Ghilas et al., 2022). The recognition of specific pathogen-associated molecular patterns is associated with inflammasome mobilization, proinflammatory cytokines, IL-1β, and IL-18 release into the bloodstream, along with pyroptosis induction (Cryan et al., 2019; Taguchi and Mukai, 2019; Sahoo, 2020). The most studied transmembrane Toll-like protein is TLR4, which is proven to interact with bacteria-derived lipopolysaccharide (LPS) which triggers microglia—the most abundant immune cells residing in the brain. Apart from glial cells, the perivascular macrophages are the only endogenous immune cells in the CNS (Prinz and Priller, 2017). The microbiota-microglia reaction might be the cause of neuroinflammation and BBB disruption (Banks and Erickson, 2010). On the other hand, in homeostatic condition highly specific adaptive immune response evolves throughout the host's lifespan and includes T and B lymphocytes. In a recent study, the CD4+ and CD8+ T lymphocytes were observed in the brain and accessed brain parenchyma, leading to BBB altered permeability, and neuroinflammation (Park and Im, 2022).
Some unresolved questions remain as to the mechanism(s) of communication between the gut microbiota and the brain, but three major pathways have been established as involved in the signal transmission within the immune system in the GBA: the systemic, cellular, and neuronal pathways.
The systemic pathway, also known as the humoral pathway, focuses on the effect of local alterations either in the brain or in the gut, on the overall state of homeostasis. The altered IB and GVB permeability results in the inflammatory factors flux, bacterial translocation, and other triggers that cause the immune response, into the bloodstream (Kinashi and Hase, 2021; Mou et al., 2022). Persistent systemic chronic inflammation might affect the BBB structure, leading to its disruption, which facilitates access to the brain parenchyma (Rochfort et al., 2014). The glial cells react by pro-inflammatory (IL-1β, IL-18, IL-6, TNF-α) or anti-inflammatory (IL-10, IL-4, TGF-β) cytokines release, depending on the current state of the immune response (Nagyoszi et al., 2015). Recent animal studies showed elevated levels of TNF-α in rats (Chen et al., 2019), and IL-1β in nestin-Cre mice (Wong et al., 2019) in inflammation-induced BBB disruption models. IL-6 and IL-18 were found to promote hippocampal stem cell apoptosis and induce further inflammation in NZB/W-F1 lupus-prone mice (Nikolopoulos et al., 2023). A recent study performed on CD-1 mice confirmed the augmentation of CCL2 and CCL5 chemokines during inflammation and pointed out that it is the CCL2 and CCL5 ligands might have the ability to cross an intact BBB (Quaranta et al., 2023). In a mice model of intracerebral hemorrhage, Lin et al. (2023) showed that LPS ip. injection enhanced the activity of the CCL5 signaling pathway which was associated with increased permeability of the BBB.
The systemic pathway is linked with the HPA axis. As a result of peripheral (intestinal) inflammation, the HPA axis might be activated, resulting in the release of glucocorticoids (GCs) (Misiak et al., 2020). The impact of GCs overproduction on the alterations in microbiota composition was proven in animal studies (Petrosus et al., 2018; Couch et al., 2023). It is suggested that because of HPA axis-induced microbiota perturbations, the inflammatory response is activated via Th17 lymphocytes, which are also a part of the cellular pathway (Sun C. Y. et al., 2023).
The cellular pathway is focused on intestinal immune cells function. In an afferent way, intestinal immune cells can migrate into the CNS to promote or inhibit neuroinflammation. The efferent signaling might start from the stress-induced microbiota alteration, leading to immune response activation, immune cells mobilization and translocation to the brain parenchyma, worsening the already existent neuroinflammation (Rochfort et al., 2014). The cells engaged in intestinal immune response are myeloid cells, such as macrophages and dendritic cells, residing in lamina propria, responsible for promoting proinflammatory cytokines release (Ghilas et al., 2022). Myeloid cells also mediate Th17 responses employing the Th17 lymphocytes, a subset of CD4+ T cells. Moreover, Foxp3+RORγt+ regulatory T (Treg) cells of intestinal origin, were found to express similar gene patterns to Th17 cells, and so, were extensively studied regarding their properties. The either proinflammatory or suppressive phenotype of these cells, was proven to be dependent on the cytokine milieu, TGF-βand the type of myeloid cells that partake in T cell priming (Zhou et al., 2008; Sun C. Y. et al., 2023). In a study by Yang et al. (2016) the suppressive phenotype of Foxp3+RORγt+ Treg cells in a colitis mouse model was confirmed. -Pro-inflammatory cytokines overexpression, especially IL-1, IL-6, and IL-23 was determined to participate in the initiation of autoimmune processes (Yasuda et al., 2019). In a healthy host, gut-derived interferon γ positive natural killer (NK) cells (IFNγ+ NK) were proven to promote TRAIL+ and LAMP1+, anti-inflammatory astrocytes, responsible for neuroimmune regulation via T cell apoptosis (Sanmarco et al., 2021). Additionally, naïve B cells differentiate into IgA-producing plasma cells after an antigen encounter (Rojas et al., 2019; Fitzpatrick et al., 2020; Keppler et al., 2021). The migration pattern of these cells has not been specified yet, nonetheless, they have been repeatedly found in the BBB, playing a vital role in preventing pathogens from entering the brain parenchyma (Rojas et al., 2019; Jacobson et al., 2021). In a recent study, Brioschi et al. (2021) determined the presence of B cells in diverse development stages in mouse meninges, through which the B cells might be recruited. The lymphoid B cells were also closely linked with enteric glia and innate immunity, mediated by neurotrophic factors (Ibiza et al., 2016).
The neuronal pathway is associated with the autonomic nervous system and the enteric nervous system (ENS). In homeostasis enteric glial cells interact with intrinsic sensory neurons, interneurons and motor neurons, and satellite glia, located in the dorsal root ganglia (Morales-Soto and Gulbransen, 2019; Fung and Vanden Berghe, 2020; Dowling et al., 2022). During inflammation, increased S100β protein expression in enteric glia was proven to upregulate iNOS expression in T cells, macrophages, and dendritic cells (Esposito et al., 2007). Enterochromaffin cells are responsible for 5-HT (5-hydroxytryptamine) production (Reigstad et al., 2015; Rao and Gershon, 2016; Malinova et al., 2018). Enteroendocrine cells modulate signaling between enteric glial cells and GVB (Dowling et al., 2022), release cholecystokinin or YY peptide (Hayashi et al., 2023), and partake in microbial metabolites conversion such as tryptophan, and further, kynurenine (Ye et al., 2021; Zhao et al., 2023). The ENS detects bacteria-derived LPS via highly abundant TLRs, especially TLR2 and TLR4 (Hyland and Cryan, 2016).
Emerging evidence suggests that gut dysbiosis and microbiota-derived metabolites significantly impact the GBA (Dalile et al., 2019). Currently, most of the research is conducted on SCFAs: acetate, propionate, and butyrate. In microbiota-depleted mice, the enteric neurons and glia loss were observed, which was then restored by SCFAs (Vicentini et al., 2021). SCFAs were also determined to mediate microglia maturation in germ-free (GF) mice (Erny et al., 2015, 2021).
SCFAs are products of fiber fermentation by gut microbiota (Martin-Gallausiaux et al., 2021; Tan et al., 2023). Therefore, in a fiber deficiency mouse model, altered cognition, hippocampal synaptic loss, and impaired brain aging were established (Shi et al., 2021). A study on butyrate and a high-fiber diet confirmed the amelioration of aging-associated neuroinflammation in mice (Matt et al., 2018). Dietary enrichment with fiber resulted in restrained inflammation (Caetano-Silva et al., 2023). In aging mice, whose microglia shifted to pro-inflammatory phenotype, dietary fiber was found to reverse this effect by increasing the SCFAs levels, thereby lowering the inflammatory features (Vailati-Riboni et al., 2022). This phenomenon was also confirmed in a microglial cell culture (Wenzel et al., 2020). An acetate shortage in type 1 diabetes (T1D) mouse model was proven to enhance cognitive impairment and aggravate hippocampal synaptophysin (SYP) expression, associated with synaptic plasticity (Zheng et al., 2021). Bacteria-derived propionate was confirmed to improve neuroregeneration (Serger et al., 2022). Based on the poststroke recovery study conducted on the murine model, SFCAs functioned as the direct mediators of T-cell functioning and microglia activation (Sadler et al., 2020).
The impairment of the BBB is one of the features associated with neuroinflammation in addition to the widely described activation of glial cells (microglia and astrocytes). The role of microglial activation in the neuroinflammation process and its interaction with BBB was studied in a mouse model of chronic systemic inflammation (MRL/lpr mice). Using in vivo imaging, the authors showed that microglial activation starts with glial migration to the vessel to protect the intact BBB. As the inflammation progresses, the expression of phagocytic markers (AIF-1, CD68) increases, leading to a microglial phenotype shift. Then, the AIF-1+ CD68+ microglial cells damage the BBB components, and the permeability increases which causes widespread neuroinflammation (Haruwaka et al., 2019). Chronic intestinal inflammation was found to induce dysbiosis in the gut and subsequently become systemic over time (Thevaranjan et al., 2017). As in this chronic inflammation state, a persistent microglia mobilization was observed, along with the immune cells flux which resulted in neurotoxicity and damage to the brain (Cowan and Petri, 2018; Guo et al., 2022; Brandl and Reindl, 2023). Thus, microglia-induced neural cytotoxicity, of gut microbiota origin as well, can lead to neurodegenerative and mood disorders development (Megur et al., 2020; Xia et al., 2023).
Regarding the association between neuroinflammation and altered BBB integrity, several studies focused on the potential role of SCFAs in BBB permeability. In a study by Li et al. (2023), mice with an induced sepsis-associated encephalopathy were treated with SCFA supplementation. It was confirmed that the SCFAs caused an increased expression of ZO-1 and occludin expression in the intestine and BBB. In research on GF (germ free) and SPF (specific pathogen-free) mice, authors observed the lower expression of occludin and claudin-5 in the frontal cortex, striatum, and hippocampus in both sexes of GF mice, suggesting an association between lack of microbiota and increased BBB permeability (Braniste et al., 2014). In LPS-exposed cerebromicrovascular endothelial cell culture, ZO-1 and occludin expression increased after SCFA treatment in comparison with the LPS-exposed but not treated one (Li et al., 2023). It was also determined that restoration of gut microbiota and microbial metabolites, such as SCFAs, alters TJs expression and BBB permeability (Braniste et al., 2014). The modulatory effects of SCFAs on BBB integrity were confirmed in the in vitro study employing a human endothelium cell line (hCMEC/D3), showing that propionate protected the BBB from inflammation by inhibition of TLR-specific pathways as well as from oxidative stress via NRF2 signaling (Hoyles et al., 2018).
Chronic stress is widely acknowledged as a predisposing or precipitating factor in neuropsychiatric diseases (Becker et al., 2007). There is a clear relationship between disturbances induced by stressful stimuli, especially long-lasting, and cognitive deficits in rodent models of affective disorders (Knapman et al., 2010). Chronic stress activates the HPA axis and sympathetic nervous system, stimulating the release of catecholamines and GCs (Misiak et al., 2020) that alter the integrity of BBB. Changes in BBB integrity were associated with the promotion of depression-like behaviors in male mice (Dion-Albert et al., 2022), indicating a link between neurovascular pathology and stress vulnerability. Stress-susceptible mice following chronic social defeat stress showed depressive-like behaviors which were associated with altered BBB integrity through a decline in claudin-5 expression in the nucleus accumbens (Menard et al., 2017; Dudek et al., 2020). Additionally, the loss of tight junction proteins promoted increased peripheral IL-6 passage across BBB and monocyte accumulation which participated in the development of depression (Menard et al., 2017). Concomitant increases in intestinal permeability may intensify these effects (Braniste et al., 2014). Stress-induced impairments of intestinal barrier function (e.g., changes in TJs protein expression) contribute to increased intestinal permeability and the movement of antigens and factors, such as LPS, from the gut lumen into the circulation, which exacerbate the immune response (Dinan and Cryan, 2012; Yang et al., 2023). Recently, chronic stress inducing a downregulation of intestinal and hippocampal expression of α-actin, claudin-1, claudin-5, occludin, and ZO-1 was observed along with altered microbial diversity in the gut (Geng et al., 2020; Chi et al., 2021). Compromised IB integrity was accompanied by seral declined levels of 5-HT, GABA, and increased levels of endotoxin, IL-6, and TNF-α as well as increased IL-6, NF-κB, iNOS, and NGAL in the intestine (Chi et al., 2021). In turn, disrupted BBB correlated with increased norepinephrine levels in the prefrontal cortex (PFC), hippocampus, and amygdala of female SPF mice (Geng et al., 2020). Consequences of peripheral inflammation, such as disruption of BBB integrity (de Vries et al., 1996) and stimulation of neuroinflammation (Geng et al., 2018), have been associated with cognitive impairments and mood disturbances.
The role of stress exposure in GBA dysregulation is widely explored in the literature. However, the influence of stress response on intestinal barrier leakiness, and further changes in BBB permeability were investigated only in a few experimental studies (summarized in Table 1). Most research performed in various stress models shows that functional changes (anxiety- and depressive-like behaviors) correlated with dysbiosis of the GBA, and IB disruption with an enhanced peripheral inflammatory response was associated with changes in BBB permeability and neuroinflammation. The impact of stress on GBA functions in the context of BBB changes is most commonly described in chronic stress paradigms (Liang et al., 2015; Bharwani et al., 2017; Nie et al., 2019; Yang et al., 2021; Jiang et al., 2023; Kitaoka et al., 2023), especially by employing social stressors (chronic social stress, or CUMS). CUMS-induced behavioral impairments were associated with the downregulation of intestinal TJs, upregulation of intestinal inflammatory factors followed by altered microbial diversity, and an increase in cortical and hippocampal microglia activation (Ait-Belgnaoui et al., 2014; He et al., 2024).
Additionally, Westfall et al. (2021a) showing activation of microglia and elevated cortical expression of chemokines and adhesion molecules (ICAM, VCAM) with simultaneous changes in ileal immune regulation, confirmed that stress-related behavioral impairments may depend in such way on GBA dysregulation. In the study by Rincel et al. (2019) stressed mice (multifactorial early-life adversity consisting of prenatal LPS injections, chronic maternal separation, and exposure to CUMS during lactation) showed gut dysbiosis in both sexes, with differences in behavioral impairments. In males, authors reported social deficits, while in females—increased anxiety- and compulsive-like behaviors. Sex-specific variations were also observed in response to single prolonged stress (PTSD, post-traumatic stress disorder model) in male and female rats. These differences extend beyond behavioral and physiological changes to the gut microbiota and its metabolites (Tanelian et al., 2024). The results confirm the importance of gender in the behavioral response to chronic stress and suggest that it should be considered in experimental studies regarding stress and the GBA. The identification of sex-specific alterations in gut microbiota composition, functionality, and metabolites might be important for the development of sex-specific therapeutic interventions for those vulnerable to stress-induced psychopathologies.
In the CRS model, which is widely used to recapitulate depression phenotypes in rodents, the presence of anxiety- and depressive-like behavior was connected either with the central or peripheral inflammation, including downregulation of TJs, enhanced expression of proinflammatory factors, and increased IB permeability (Deng et al., 2021; Yang et al., 2021; Jiang et al., 2023). In the study by Yang et al. (2021) CRS mice manifested elevated hippocampal levels of proinflammatory cytokines with a simultaneous decline of BDNF (brain-derived neurotrophic factor) and 5-HT, dopamine, noradrenaline, their corresponding metabolites levels, as well as gut microbiota dysbiosis, which was linked with depressive-like behavior. Notably, depressive behaviors, the altered neurotransmitter metabolism, and microbiota dysbiosis observed in CRS mice can be restored by dexamethasone administration, underscoring the key role of inflammation in gut dysbiosis in stress-induced depressive behaviors (Yang et al., 2021). In a study by Deng et al. (2021) exposure to CRS affected kynurenine pathway components in the intestinal tract and the brain structures. Downregulated ZO-1 expression caused IB disruption which resulted in enhanced inflammatory cell infiltration of intestinal crypts. Following CRS, the elevation of IL-6 levels in the proximal and distal colon, and the increase of IL-1β only in the distal section were observed in stressed females. Greater sensitivity to stress observed in females was associated with anxiety-like behavior which correlated with the abundance of specific gut microbes, increased protein levels of IL-1β, IL-6, and gene expression of ZO-1 in the hippocampus, probably because of peripheral inflammation (Jiang et al., 2023).
Signaling pathways of NLRP3 (nucleotide oligomerization domain-like receptor family, pyrin domain containing 3) inflammasome might be involved in GBA regulation in stress conditions since its inhibition has been associated with decreased BBB permeability (Bellut et al., 2021), ameliorated cognitive impairment (Zhu et al., 2021), or reprogramming of microglial phagocytic phenotype (Jia et al., 2022). Yi et al. (2021) observed a decrease in cecal butyrate, and an increase in IL-1β, IL-6, and serum TNF-α levels and activation or inhibition of central NLRP3 inflammasomes because of exposure to high- or low-temperature stress, respectively. CUMS-exposed rats showed enhanced NLRP3 activity, along with a decrease in ZO-1 and occludin expression in the colon as well as in the prefrontal cortex and hippocampus (Huang et al., 2023). Additionally, it was proposed that intestinal epithelial NLRs might be the novel modulators of the GBA. NOD1/NOD2 knockout (KO) mice, following exposure to acute psychological stress linked with increased permeability in the ileum and colon, showed increased susceptibility to HPA axis hyperactivation, cognitive impairments, anxiety- and depressive-like behaviors. These results were associated with the altered 5-HT signaling in the hippocampus, suggesting a link between 5-HT and NLR (Pusceddu et al., 2019). Following chronic cold stress, a decrease in dopamine signaling pathway-related metabolites levels was associated with increased intestinal barrier permeability and increased release of immune response mediators. Moreover, stressed mice also manifested depressive-like behaviors (Sun C. Y. et al., 2023; Sun L. et al., 2023).
As the intestinal microbiota is highly susceptible to environmental fluctuations, dysbiosis restoration treatments were proposed as a strategy to alleviate behavioral impairments. Such treatments may modify gut microbiota and levels of its metabolites thus mediating the therapeutic action in stress-induced brain dysfunctions. The beneficial impacts of the oral prebiotic (Jiang et al., 2023), probiotic (Burokas et al., 2017; De Santa et al., 2024), and/or synbiotic (Westfall et al., 2021a,b) applications on stress-induced alterations were confirmed in a few experimental studies. The application of specific prebiotic types might moderate inflammation in the hippocampus and colon, and thereby improve stress-induced depressive- and anxiety-like behaviors (Jiang et al., 2023). Moreover, the treatment with probiotics was found to downregulate stress-induced CORT (corticosterone) release and proinflammatory cytokine levels, increase acetate and propionate amounts in the intestine, and significantly alleviate depressive- and anxiety-like behaviors in mice (Burokas et al., 2017). In a study by Westfall et al. (2021a,b) mice were subjected to synbiotic treatment (L. plantarum, B. longum) after chronic unpredictable stress exposure. The synbiotic application weakened neuroinflammation in the hippocampus and prefrontal cortex and alleviated peripheral inflammation via downregulation of proinflammatory cytokines, TLR4, and NLRP3 expression. It also decreased the Th17/Treg ratio and subsequently the IL-17/IL-10 ratio in serum, and improved depressive- and anxiety-like behaviors. In a study by De Santa et al. (2024), the multi-strain probiotic treatment reversed depressive-like, and anxiety-like behaviors induced by the maternal separation, and normalized neuroinflammation by restoring gut microbiota. Additionally, the probiotic treatment also significantly affected the production of SCFA and the level of butyrate.
Microbiota-induced inflammation was proven to be completely reversed by microbial restoration via fecal microbiota transplant (FMT). The goal of FMT involves direct transfer of fecal microbiota from a healthy donor to the GI of a recipient to treat the disease by restoring the phylogenetic diversity and microbiota of a healthy person. In a few animal models of the Alzheimer's disease, FMT was shown to be effective not only in restoring gut microbiota but also in ameliorating cognitive impairments, and downregulating Aβ plaques accumulation (Sun et al., 2019; Kim et al., 2020; Elangovan et al., 2022; Hang et al., 2022). The FMT effectiveness was also determined in Parkinson's disease animal models via alleviating LPS-induced neuroinflammation (Sun et al., 2018; Zhao et al., 2021). Currently, there is a growing emphasis on the studies that use FMT approaches to study the role of stress-related microbiota composition in behavioral changes. A link was also recently demonstrated between disease-related microbiota and behavior where FMT from depressed patients to microbiota-depleted rodents increased anhedonia and anxiety-like behaviors (Kelly et al., 2016; Liu et al., 2020). Some studies employed FMT from control and stressed animals (donors) (Marcondes Ávila et al., 2020; Sharma et al., 2020; Rao et al., 2021; Huang et al., 2023; He et al., 2024) confirming the vital role of FMT in regulating brain inflammation, and behavioral changes in the chronic stress paradigms. He et al. (2024) employed FMT from stress-resilient and stress-susceptible mice to CUMS-exposed ones. Besides the significant behavioral improvement, the FMT from stress-resilient mice regulated CX3CL1 and CD200 mediators, contributing to efficient communication between microglia and neurons. In a study by Li et al. (2019), the authors determined worsened behavioral deficits because of an FMT from CUMS-treated donors. Additionally, recipient mice exhibited increased IDO-1, TNF-α, and IFN-γ levels in the hippocampus. Marcondes Ávila et al. (2020) confirmed that chronic mild stress (CMS) aggravated behavioral impairments like the effects of FMT from stressed donors to non-stressed recipients. FMT from stress-exposed rats contributed to elevated IL-6 and TNF-α hippocampal expression. On the other hand, the downregulation of proinflammatory cytokines and behavioral development was established as a result of FMT treatment from healthy donors.
Several studies have been exploring neuroimmune pathways involved in the regulation of the GBA in animal models of diseases, but bidirectional communication remains not fully explained. Most of the research performed in various disease models shows the involvement of the activation of the NLRP3 inflammasome complex. A few studies report the intestinal barrier's altered permeability manifested by the changes in TJs protein expression and further increased levels of cytokines and chemokines. Since behavioral impairments resulting from stress exposure are accompanied by exacerbated inflammation, special attention should be drawn to the BBB permeability. A couple of studies pointed out that stress-induced anxiety- and depressive-like behaviors correlated with dysbiosis of gut microbiota, and disruption of intestinal permeability with simultaneous changes observed in the hippocampus and prefrontal cortex. Namely, authors observed involvement of neuroinflammation processes (microglial activation, increased expression of NLRP3 and pro-inflammatory cytokines), alterations in levels of neurotransmitters, and decreased expression of ZO-1, occludin, and claudins. It could be postulated that impaired communication across BBB may therefore represent a significant mechanism allowing the gut microbiota to affect brain functions for example under stress conditions (Figure 2).
Figure 2. Overview of GBA fluctuations during inflammatory processes under stress stimuli. As for bidirectional communication, it has been proven so far that the leaky intestinal barrier affects the blood-brain barrier's permeability via multiple proinflammatory agents migrating within the blood circuitry, and contrariwise. Inflammation of either intestinal or brain origin, results in the NLRP3 inflammasome activation, and decreased expression of tight junction proteins and related barrier's disruption. Peripheral inflammation may activate phagocytic microglia phenotype, causing neuroinflammation. Then, neuroinflammation alters microbial diversity, thereby influencing the production and release of SCFAs, LPS, and other microbial metabolites. Created with BioRender.com.
Understanding the molecular mechanisms underlying stress susceptibility seems to be crucial to the identification of novel pharmacological treatments for gut-brain disorders. Currently, scientists acknowledge the significance of changes in gut microbiota composition in mood disorders. However, using animal models to evaluate the modifications of gut microbiota (probiotics, prebiotics, FMT) requires paying special attention to the experimental design. Despite the growing use of FMT in clinical and animal studies, accompanied by scientifically proven efficiency showing anti-inflammatory properties and changes in behavior, alterations in the BBB have not been addressed so far. It remains an open question whether animal models should be used as adequate research tools to study modifications of gut microbiota under stress conditions. Therefore, drawing direct conclusions about the role of BBB integrity in the beneficial role of modification of gut microbiota is not fully possible, and it should be considered in future studies.
JM: Investigation, Visualization, Writing – original draft. AM: Supervision, Writing – review & editing. MN-C: Conceptualization, Project administration, Supervision, Writing – original draft, Writing – review & editing.
The author(s) declare that financial support was received for the research, authorship, and/or publication of this article. This work was supported by the statutory grant: AWF/NF/ZB1/2024/from the Academy of Physical Education, Katowice, Poland and from Polish National Science Center under Grant 2022/47/B/NZ7/02135.
The authors declare that the research was conducted in the absence of any commercial or financial relationships that could be construed as a potential conflict of interest.
All claims expressed in this article are solely those of the authors and do not necessarily represent those of their affiliated organizations, or those of the publisher, the editors and the reviewers. Any product that may be evaluated in this article, or claim that may be made by its manufacturer, is not guaranteed or endorsed by the publisher.
5-HT, 5-hydroxytryptamine; AJs, adherens junctions; BBB, blood-brain barrier; BDNF, brain-derived neurotrophic factor; CMS, chronic mild stress; CNS, central nervous system; CORT, corticosterone; CRS, chronic restraint stress; CUMS, chronic mild stress; ENS, enteric nervous system; FMT, fecal microbiota transplantation; GBA, gut-brain axis; GCs, glucocorticoids; GF, germ free; GVB, gut-vascular barrier; HPA, hypothalamic-pituitary-adrenal axis; IB, intestinal barrier; KO, knockout; LPS, lipopolysaccharide; NK, natural killer; NLR, NOD-like receptor; NLRP3, nucleotide oligomerization domain-like receptor family, pyrin domain containing 3; PFC, prefrontal cortex; PTSD, post-traumatic stress disorder; SCFAs, short-chain fatty acids; SPF, specific pathogen-free; SYP, synaptophysin; T1D, type 1 diabetes; TJs, tight junctions; TLR, Toll-like receptor.
Ahmed, H., Leyrolle, Q., Koistinen, V., Kärkkäinen, O., Layé, S., Delzenne, N., et al. (2022). Microbiota-derived metabolites as drivers of gut-brain communication. Gut Microbes 14, 2102878. doi: 10.1080/19490976.2022.2102878
Ait-Belgnaoui, A., Colom, A., Braniste, V., Ramalho, L., Marrot, A., Cartier, C., et al. (2014). Probiotic gut effect prevents the chronic psychological stress-induced brain activity abnormality in mice. Neurogastroenterol. Motility 26, 510–520. doi: 10.1111/nmo.12295
Banks, W. A., and Erickson, M. A. (2010). The blood-brain barrier and immune function and dysfunction. Neurobiol. Dis. 37, 26–32. doi: 10.1016/j.nbd.2009.07.031
Bauer, H. C., Krizbai, I. A., Bauer, H., and Traweger, A. (2014). “You Shall Not Pass”-tight junctions of the blood brain barrier. Front. Neurosci. 8:392. doi: 10.3389/fnins.2014.00392
Becker, J. B., Monteggia, L. M., Perrot-Sinal, T. S., Romeo, R. D., Taylor, J. R., Yehuda, R., et al. (2007). Stress and disease: is being female a predisposing factor? J Neurosci. 27, 11851–11855. doi: 10.1523/JNEUROSCI.3565-07.2007
Bellut, M., Papp, L., Bieber, M., Kraft, P., Stoll, G., and Schuhmann, M. K. (2021). NLPR3 inflammasome inhibition alleviates hypoxic endothelial cell death in vitro and protects blood-brain barrier integrity in murine stroke. Cell Death Dis. 13, 20. doi: 10.1038/s41419-021-04379-z
Bharwani, A., Mian, M. F., Surette, M. G., Bienenstock, J., and Forsythe, P. (2017). Oral treatment with Lactobacillus rhamnosus attenuates behavioural deficits and immune changes in chronic social stress. BMC Med. 15:7. doi: 10.1186/s12916-016-0771-7
Brandl, S., and Reindl, M. (2023). Blood-brain barrier breakdown in neuroinflammation: current in vitro models. Int. J. Mol. Sci. 24:12699. doi: 10.3390/ijms241612699
Braniste, V., Al-Asmakh, M., Kowal, C., Anuar, F., Abbaspour, A., Tóth, M., et al. (2014). The gut microbiota influences blood-brain barrier permeability in mice. Sci. Transl. Med. 6, 263ra158. doi: 10.1126/scitranslmed.3009759
Brioschi, S., Wang, W. L., Peng, V., Wang, M., Shchukina, I., Greenberg, Z. J., et al. (2021). Heterogeneity of meningeal B cells reveals a lymphopoietic niche at the CNS borders. Science 373:eabf9277. doi: 10.1126/science.abf9277
Brzozowski, B., Mazur-Bialy, A., Pajdo, R., Kwiecien, S., Bilski, J., Zwolinska-Wcislo, M., et al. (2016). Mechanisms by which Stress Affects the Experimental and Clinical Inflammatory Bowel Disease (IBD): Role of Brain-Gut Axis. Curr. Neuropharmacol. 14, 892–900. doi: 10.2174/1570159X14666160404124127
Burokas, A., Arboleya, S., Moloney, R. D., Peterson, V. L., Murphy, K., Clarke, G., et al. (2017). Targeting the microbiota-gut-brain axis: prebiotics have anxiolytic and antidepressant-like effects and reverse the impact of chronic stress in mice. Biol. Psychiatry 82, 472–487. doi: 10.1016/j.biopsych.2016.12.031
Caetano-Silva, M. E., Rund, L., Hutchinson, N. T., Woods, J. A., Steelman, A. J., and Johnson, R. W. (2023). Inhibition of inflammatory microglia by dietary fiber and short-chain fatty acids. Sci. Rep. 13:2819. doi: 10.1038/s41598-022-27086-x
Carabotti, M., Scirocco, A., Maselli, M. A., and Severi, C. (2015). The gut-brain axis: interactions between enteric microbiota, central and enteric nervous systems. Ann. Gastroenterol. 28, 203–209.
Chen, A. Q., Fang, Z., Chen, X. L., Yang, S., Zhou, Y. F., Mao, L., et al. (2019). Microglia-derived TNF-α mediates endothelial necroptosis aggravating blood brain-barrier disruption after ischemic stroke. Cell Death Dis. 10, 487. doi: 10.1038/s41419-019-1716-9
Chi, H., Cao, W., Zhang, M., Su, D., Yang, H., Li, Z., et al. (2021). Environmental noise stress disturbs commensal microbiota homeostasis and induces oxi-inflammmation and AD-like neuropathology through epithelial barrier disruption in the EOAD mouse model. J. Neuroinflammation 18:9. doi: 10.1186/s12974-020-02053-3
Couch, C. E., Neal, W. T., Herron, C. L., Kent, M. L., Schreck, C. B., and Peterson, J. T. (2023). Gut microbiome composition associates with corticosteroid treatment, morbidity, and senescence in Chinook salmon (Oncorhynchus tshawytscha). Sci. Rep. 13, 2567. doi: 10.1038/s41598-023-29663-0
Cowan, M., and Petri, W. A. Jr (2018). Microglia: immune regulators of neurodevelopment. Front. Immunol. 9, 2576. doi: 10.3389/fimmu.2018.02576
Cryan, J. F., O'Riordan, K. J., Cowan, C. S. M., Sandhu, K. V., Bastiaanssen, T. F. S., Boehme, M., et al. (2019). The microbiota-gut-brain axis. Physiol. Rev. 99, 1877–2013. doi: 10.1152/physrev.00018.2018
Dalile, B., Van Oudenhove, L., Vervliet, B., and Verbeke, K. (2019). The role of short-chain fatty acids in microbiota-gut-brain communication. Nature reviews. Gastroenterol. Hepatol. 16, 461–478. doi: 10.1038/s41575-019-0157-3
De Palma, G., Blennerhassett, P., Lu, J., Deng, Y., Park, A. J., Green, W., et al. (2015). Microbiota and host determinants of behavioural phenotype in maternally separated mice. Nat. Commun. 6:7735. doi: 10.1038/ncomms8735
De Palma, G., Collins, S. M., Bercik, P., and Verdu, E. F. (2014). The microbiota-gut-brain axis in gastrointestinal disorders: stressed bugs, stressed brain or both?. J. Physiol. 592, 2989–2997. doi: 10.1113/jphysiol.2014.273995
De Santa, F., Strimpakos, G., Marchetti, N., Gargari, G., Torcinaro, A., Arioli, S., et al. (2024). Effect of a multi-strain probiotic mixture consumption on anxiety and depression symptoms induced in adult mice by postnatal maternal separation. Microbiome 12, 29. doi: 10.1186/s40168-024-01752-w
de Vries, H. E., Blom-Roosemalen, M. C., van Oosten, M., de Boer, A. G., van Berkel, T. J., Breimer, D. D., et al. (1996). The influence of cytokines on the integrity of the blood-brain barrier in vitro. J. Neuroimmunol. 64, 37–43. doi: 10.1016/0165-5728(95)00148-4
Deng, Y., Zhou, M., Wang, J., Yao, J., Yu, J., Liu, W., et al. (2021). Involvement of the microbiota-gut-brain axis in chronic restraint stress: disturbances of the kynurenine metabolic pathway in both the gut and brain. Gut Microbes 13, 1–16. doi: 10.1080/19490976.2020.1869501
Di Tommaso, N., Santopaolo, F., Gasbarrini, A., and Ponziani, F. R. (2023). The gut-vascular barrier as a new protagonist in intestinal and extraintestinal diseases. Int. J. Mol. Sci. 24, 1470. doi: 10.3390/ijms24021470
Dinan, T. G., and Cryan, J. F. (2012). Regulation of the stress response by the gut microbiota: implications for psychoneuroendocrinology. Psychoneuroendocrinology 37, 1369–1378. doi: 10.1016/j.psyneuen.2012.03.007
Dion-Albert, L., Cadoret, A., Doney, E., Kaufmann, F. N., Dudek, K. A., Daigle, B., et al. (2022). Vascular and blood-brain barrier-related changes underlie stress responses and resilience in female mice and depression in human tissue. Nat. Commun. 13, 164. doi: 10.1038/s41467-021-27604-x
Dowling, L. R., Strazzari, M. R., Keely, S., and Kaiko, G. E. (2022). Enteric nervous system and intestinal epithelial regulation of the gut-brain axis. J. Allergy Clin. Immunol. 150, 513–522. doi: 10.1016/j.jaci.2022.07.015
Dudek, K. A., Dion-Albert, L., Lebel, M., LeClair, K., Labrecque, S., Tuck, E., et al. (2020). Molecular adaptations of the blood-brain barrier promote stress resilience vs. depression. Proc. Nat. Acad. Sci. U. S. A. 117, 3326–3336. doi: 10.1073/pnas.1914655117
Elangovan, S., Borody, T. J., and Holsinger, R. M. D. (2022). Fecal microbiota transplantation reduces pathology and improves cognition in a mouse model of Alzheimer's disease. Cells 12, 119. doi: 10.3390/cells12010119
Ergang, P., Vagnerová, K., Hermanová, P., Vodička, M., Jágr, M., Šrutková, D., et al. (2021). The gut microbiota affects corticosterone production in the murine small intestine. Int. J. Mol. Sci. 22, 4229. doi: 10.3390/ijms22084229
Erny, D., Dokalis, N., Mez,ö, C., Castoldi, A., Mossad, O., Staszewski, O., et al. (2021). Microbiota-derived acetate enables the metabolic fitness of the brain innate immune system during health and disease. Cell Metabol. 33, 2260–2276.e7. doi: 10.1016/j.cmet.2021.10.010
Erny, D., Hrabě de Angelis, A. L., Jaitin, D., Wieghofer, P., Staszewski, O., David, E., et al. (2015). Host microbiota constantly control maturation and function of microglia in the CNS. Nat. Neurosci. 18, 965–977. doi: 10.1038/nn.4030
Esposito, G., Cirillo, C., Sarnelli, G., De Filippis, D., D'Armiento, F. P., Rocco, A., et al. (2007). Enteric glial-derived S100B protein stimulates nitric oxide production in celiac disease. Gastroenterology 133, 918–925. doi: 10.1053/j.gastro.2007.06.009
Fitzpatrick, Z., Frazer, G., Ferro, A., Clare, S., Bouladoux, N., Ferdinand, J., et al. (2020). Gut-educated IgA plasma cells defend the meningeal venous sinuses. Nature 587, 472–476. doi: 10.1038/s41586-020-2886-4
Fung, C., and Vanden Berghe, P. (2020). Functional circuits and signal processing in the enteric nervous system. Cell. Mol. Life Sci. 77, 4505–4522. doi: 10.1007/s00018-020-03543-6
Gao, X., Cao, Q., Cheng, Y., Zhao, D., Wang, Z., Yang, H., et al. (2018). Chronic stress promotes colitis by disturbing the gut microbiota and triggering immune system response. Proc. Natl. Acad. Sci. USA. 115, E2960–E2969. doi: 10.1073/pnas.1720696115
Geng, J., Wang, L., Zhang, L., Qin, C., Song, Y., Ma, Y., et al. (2018). Blood-brain barrier disruption induced cognitive impairment is associated with increase of inflammatory cytokine. Front. Aging Neurosci. 10, 129. doi: 10.3389/fnagi.2018.00129
Geng, S., Yang, L., Cheng, F., Zhang, Z., Li, J., Liu, W., et al. (2020). Gut microbiota are associated with psychological stress-induced defections in intestinal and blood-brain barriers. Front. Microbiol. 10, 3067. doi: 10.3389/fmicb.2019.03067
Ghilas, S., O'Keefe, R., Mielke, L. A., Raghu, D., Buchert, M., and Ernst, M. (2022). Crosstalk between epithelium, myeloid and innate lymphoid cells during gut homeostasis and disease. Front. Immunol. 13:944982. doi: 10.3389/fimmu.2022.944982
Golubeva, A. V., Crampton, S., Desbonnet, L., Edge, D., O'Sullivan, O., Lomasney, K. W., et al. (2015). Prenatal stress-induced alterations in major physiological systems correlate with gut microbiota composition in adulthood. Psychoneuroendocrinology 60, 58–74. doi: 10.1016/j.psyneuen.2015.06.002
Greene, C., Hanley, N., and Campbell, M. (2019). Claudin-5: gatekeeper of neurological function. Fluids Barriers CNS 16, 3. doi: 10.1186/s12987-019-0123-z
Gubert, C., Kong, G., Renoir, T., and Hannan, A. J. (2020). Exercise, diet and stress as modulators of gut microbiota: Implications for neurodegenerative diseases. Neurobiol. Dis. 134, 104621. doi: 10.1016/j.nbd.2019.104621
Guo, S., Wang, H., and Yin, Y. (2022). Microglia polarization from M1 to M2 in neurodegenerative diseases. Front. Aging Neurosci. 14:815347. doi: 10.3389/fnagi.2022.815347
Haddad-Tóvolli, R., Dragano, N. R. V., Ramalho, A. F. S., and Velloso, L. A. (2017). Development and function of the blood-brain barrier in the context of metabolic control. Front. Neurosci. 11:224. doi: 10.3389/fnins.2017.00224
Hall, V., and Bendtsen, K. M. S. (2023). Getting closer to modeling the gut-brain axis using induced pluripotent stem cells. Front. Cell Dev. Biol. 11:1146062. doi: 10.3389/fcell.2023.1146062
Hang, Z., Cai, S., Lei, T., Zhang, X., Xiao, Z., Wang, D., et al. (2022). Transfer of tumor-bearing mice intestinal flora can ameliorate cognition in alzheimer's disease mice. J. Alzheimer's Dis. 86, 1287–1300. doi: 10.3233/JAD-215495
Haruwaka, K., Ikegami, A., Tachibana, Y., Ohno, N., Konishi, H., Hashimoto, A., et al. (2019). Dual microglia effects on blood brain barrier permeability induced by systemic inflammation. Nat. Commun. 10, 5816. doi: 10.1038/s41467-019-13812-z
Hayashi, M., Kaye, J. A., Douglas, E. R., Joshi, N. R., Gribble, F. M., Reimann, F., et al. (2023). Enteroendocrine cell lineages that differentially control feeding and gut motility. Elife 12:e78512. doi: 10.7554/eLife.78512.sa2
He, H., He, H., Mo, L., Yuan, Q., Xiao, C., Ma, Q., et al. (2024). Gut microbiota regulate stress resistance by influencing microglia-neuron interactions in the hippocampus. Brain, Behav. Immunity - Health 36:100729. doi: 10.1016/j.bbih.2024.100729
Holmqvist, S., Chutna, O., Bousset, L., Aldrin-Kirk, P., Li, W., Björklund, T., et al. (2014). Direct evidence of Parkinson pathology spread from the gastrointestinal tract to the brain in rats. Acta Neuropathol. 128, 805–820. doi: 10.1007/s00401-014-1343-6
Hoyles, L., Snelling, T., Umlai, U. K., Nicholson, J. K., Carding, S. R., Glen, R. C., et al. (2018). Microbiome-host systems interactions: protective effects of propionate upon the blood-brain barrier. Microbiome 6:55. doi: 10.1186/s40168-018-0439-y
Huang, L., Ma, Z., Ze, X., Zhao, X., Zhang, M., Lv, X., et al. (2023). Gut microbiota decreased inflammation induced by chronic unpredictable mild stress through affecting NLRP3 inflammasome. Front. Cell. Infect. Microbiol. 13, 1189008. doi: 10.3389/fcimb.2023.1189008
Hyland, N. P., and Cryan, J. F. (2016). Microbe-host interactions: Influence of the gut microbiota on the enteric nervous system. Dev. Biol. 417, 182–187. doi: 10.1016/j.ydbio.2016.06.027
Ibiza, S., García-Cassani, B., Ribeiro, H., Carvalho, T., Almeida, L., Marques, R., et al. (2016). Glial-cell-derived neuroregulators control type 3 innate lymphoid cells and gut defence. Nature 535, 440–443. doi: 10.1038/nature18644
Jacobson, A., Yang, D., Vella, M., and Chiu, I. M. (2021). The intestinal neuro-immune axis: crosstalk between neurons, immune cells, and microbes. Mucosal Immunol. 14, 555–565. doi: 10.1038/s41385-020-00368-1
Jia, H., Qi, X., Fu, L., Wu, H., Shang, J., Qu, M., et al. (2022). NLRP3 inflammasome inhibitor ameliorates ischemic stroke by reprogramming the phenotype of microglia/macrophage in a murine model of distal middle cerebral artery occlusion. Neuropathology 42, 181–189. doi: 10.1111/neup.12802
Jiang, J., Fu, Y., Tang, A., Gao, X., Zhang, D., Shen, Y., et al. (2023). Sex difference in prebiotics on gut and blood-brain barrier dysfunction underlying stress-induced anxiety and depression. CNS Neurosci. Therapeut. 29, 115–128. doi: 10.1111/cns.14091
Kadry, H., Noorani, B., and Cucullo, L. (2020). A blood-brain barrier overview on structure, function, impairment, and biomarkers of integrity. Fluids Barriers CNS 17:69. doi: 10.1186/s12987-020-00230-3
Kasarello, K., Cudnoch-Jedrzejewska, A., and Czarzasta, K. (2023). Communication of gut microbiota and brain via immune and neuroendocrine signaling. Front. Microbiol. 14:1118529. doi: 10.3389/fmicb.2023.1118529
Kelly, J. R., Borre, Y., O' Brien, C., Patterson, E., El Aidy, S., Deane, J., et al. (2016). Transferring the blues: depression-associated gut microbiota induces neurobehavioural changes in the rat. J. Psychiatr. Res. 82, 109–118. doi: 10.1016/j.jpsychires.2016.07.019
Keppler, S. J., Goess, M. C., and Heinze, J. M. (2021). The wanderings of gut-derived IgA plasma cells: impact on systemic immune responses. Front. Immunol. 12:670290. doi: 10.3389/fimmu.2021.670290
Kim, M. H., Kim, D., and Sung, J. H. (2021). A Gut-Brain Axis-on-a-Chip for studying transport across epithelial and endothelial barriers. J. Indust. Eng. Chem. 101, 126–134. doi: 10.1016/j.jiec.2021.06.021
Kim, M. S., Kim, Y., Choi, H., Kim, W., Park, S., Lee, D., et al. (2020). Transfer of a healthy microbiota reduces amyloid and tau pathology in an Alzheimer's disease animal model. Gut 69, 283–294. doi: 10.1136/gutjnl-2018-317431
Kinashi, Y., and Hase, K. (2021). Partners in leaky gut syndrome: intestinal dysbiosis and autoimmunity. Front. Immunol. 12:673708. doi: 10.3389/fimmu.2021.673708
Kipnis, J., Cohen, H., Cardon, M., Ziv, Y., and Schwartz, M. (2004). T cell deficiency leads to cognitive dysfunction: implications for therapeutic vaccination for schizophrenia and other psychiatric conditions. Proc. Natl. Acad. Sci. USA. 101, 8180–8185. doi: 10.1073/pnas.0402268101
Kitaoka, S., Tomohiro, A., Ukeshima, S., Liu, K., Wake, H., Kimura, S. H., et al. (2023). Repeated social defeat stress induces HMGB1 nuclear export in prefrontal neurons, leading to social avoidance in mice. Cells 12, 1789. doi: 10.3390/cells12131789
Knapman, A., Heinzmann, J. M., Hellweg, R., Holsboer, F., Landgraf, R., and Touma, C. (2010). Increased stress reactivity is associated with cognitive deficits and decreased hippocampal brain-derived neurotrophic factor in a mouse model of affective disorders. J. Psychiatr. Res. 44, 566–575. doi: 10.1016/j.jpsychires.2009.11.014
Levy, M., Kolodziejczyk, A. A., Thaiss, C. A., and Elinav, E. (2017). Dysbiosis and the immune system. Nat. Rev. Immunol. 17, 219–232. doi: 10.1038/nri.2017.7
Li, N., Wang, Q., Wang, Y., Sun, A., Lin, Y., Jin, Y., et al. (2019). Fecal microbiota transplantation from chronic unpredictable mild stress mice donors affects anxiety-like and depression-like behavior in recipient mice via the gut microbiota-inflammation-brain axis. Stress 22, 592–602. doi: 10.1080/10253890.2019.1617267
Li, Z., Zhang, F., Sun, M., Liu, J., Zhao, L., Liu, S., et al. (2023). The modulatory effects of gut microbes and metabolites on blood-brain barrier integrity and brain function in sepsis-associated encephalopathy. PeerJ 11:e15122. doi: 10.7717/peerj.15122
Liang, S., Wang, T., Hu, X., Luo, J., Li, W., Wu, X., et al. (2015). Administration of Lactobacillus helveticus NS8 improves behavioral, cognitive, and biochemical aberrations caused by chronic restraint stress. Neuroscience 310, 561–577. doi: 10.1016/j.neuroscience.2015.09.033
Lin, J., Xu, Y., Guo, P., Chen, Y. J., Zhou, J., Xia, M., et al. (2023). CCL5/CCR5-mediated peripheral inflammation exacerbates blood–brain barrier disruption after intracerebral hemorrhage in mice. J. Transl. Med. 21, 196. doi: 10.1186/s12967-023-04044-3
Liu, S., Guo, R., Liu, F., Yuan, Q., Yu, Y., and Ren, F. (2020). Gut microbiota regulates depression-like behavior in rats through the neuroendocrine-immune-mitochondrial pathway. Neuropsychiatr. Dis. Treat. 16, 859–869. doi: 10.2147/NDT.S243551
Malinova, T. S., Dijkstra, C. D., and de Vries, H. E. (2018). Serotonin: A mediator of the gut-brain axis in multiple sclerosis. Multiple Sclerosis 24, 1144–1150. doi: 10.1177/1352458517739975
Marcondes Ávila, P. R., Fiorot, M., Michels, M., Dominguini, D., Abatti, M., Vieira, A., et al. (2020). Effects of microbiota transplantation and the role of the vagus nerve in gut-brain axis in animals subjected to chronic mild stress. J. Affect. Disord. 277, 410–416. doi: 10.1016/j.jad.2020.08.013
Margolis, K. G., Cryan, J. F., and Mayer, E. A. (2021). The microbiota-gut-brain axis: from motility to mood. Gastroenterology 160, 1486–1501. doi: 10.1053/j.gastro.2020.10.066
Martin-Gallausiaux, C., Marinelli, L., Blottière, H. M., Larraufie, P., and Lapaque, N. (2021). SCFA: mechanisms and functional importance in the gut. Proc. Nutr. Soc. 80, 37–49. doi: 10.1017/S0029665120006916
Matt, S. M., Allen, J. M., Lawson, M. A., Mailing, L. J., Woods, J. A., and Johnson, R. W. (2018). Butyrate and Dietary Soluble Fiber Improve Neuroinflammation Associated With Aging in Mice. Front. Immunol. 9:1832. doi: 10.3389/fimmu.2018.01832
Megur, A., Baltriukiene, D., Bukelskiene, V., and Burokas, A. (2020). The microbiota-gut-brain axis and alzheimer's disease: neuroinflammation is to blame?. Nutrients 13:37. doi: 10.3390/nu13010037
Menard, C., Pfau, M. L., Hodes, G. E., Kana, V., Wang, V. X., Bouchard, S., et al. (2017). Social stress induces neurovascular pathology promoting depression. Nat. Neurosci. 20, 1752–1760. doi: 10.1038/s41593-017-0010-3
Misiak, B., Łoniewski, I., Marlicz, W., Frydecka, D., Szulc, A., Rudzki, L., et al. (2020). The HPA axis dysregulation in severe mental illness: Can we shift the blame to gut microbiota? Prog. Neuro-Psychopharmacol. Biol. Psychiatry 102:109951. doi: 10.1016/j.pnpbp.2020.109951
Morales-Soto, W., and Gulbransen, B. D. (2019). Enteric glia: a new player in abdominal pain. Cell. Mol. Gastroenterol. 7, 433–445. doi: 10.1016/j.jcmgh.2018.11.005
Mou, Y., Du, Y., Zhou, L., Yue, J., Hu, X., Liu, Y., et al. (2022). Gut microbiota interact with the brain through systemic chronic inflammation: implications on neuroinflammation, neurodegeneration, and aging. Front. Immunol. 13:796288. doi: 10.3389/fimmu.2022.796288
Nagyoszi, P., Nyúl-Tóth, Á., Fazakas, C., Wilhelm, I., Kozma, M., Molnár, J., et al. (2015). Regulation of NOD-like receptors and inflammasome activation in cerebral endothelial cells. J. Neurochem. 135, 551–564. doi: 10.1111/jnc.13197
Nie, X., Kitaoka, S., Shinohara, M., Kakizuka, A., Narumiya, S., and Furuyashiki, T. (2019). Roles of Toll-like receptor 2/4, monoacylglycerol lipase, and cyclooxygenase in social defeat stress-induced prostaglandin E2 synthesis in the brain and their behavioral relevance. Sci. Rep. 9, 17548. doi: 10.1038/s41598-019-54082-5
Nikolopoulos, D., Manolakou, T., Polissidis, A., Filia, A., Bertsias, G., Koutmani, Y., et al. (2023). Microglia activation in the presence of intact blood-brain barrier and disruption of hippocampal neurogenesis via IL-6 and IL-18 mediate early diffuse neuropsychiatric lupus. Ann. Rheum. Dis. 82, 646–657. doi: 10.1136/ard-2022-223506
Park, J. C., and Im, S. H. (2022). The gut-immune-brain axis in neurodevelopment and neurological disorders. Microbiome research reports 1, 23. doi: 10.20517/mrr.2022.11
Pasciuto, E., Burton, O. T., Roca, C. P., Lagou, V., Rajan, W. D., Theys, T., et al. (2020). Microglia require CD4 T cells to complete the fetal-to-adult transition. Cell 182, 625–640.e24. doi: 10.1016/j.cell.2020.06.026
Petrosus, E., Silva, E. B., Lay, D. Jr, and Eicher, S. D. (2018). Effects of orally administered cortisol and norepinephrine on weanling piglet gut microbial populations and Salmonella passage. J. Anim. Sci. 96, 4543–4551. doi: 10.1093/jas/sky312
Prinz, M., and Priller, J. (2017). The role of peripheral immune cells in the CNS in steady state and disease. Nat. Neurosci. 20, 136–144. doi: 10.1038/nn.4475
Pusceddu, M. M., Barboza, M., Keogh, C. E., Schneider, M., Stokes, P., Sladek, J. A., et al. (2019). Nod-like receptors are critical for gut-brain axis signalling in mice. J. Physiol. 597, 5777–5797. doi: 10.1113/JP278640
Quaranta, D. V., Weaver, R. R., Baumann, K. K., Fujimoto, T., Williams, L. M., Kim, H. C., et al. (2023). Transport of the proinflammatory chemokines C-C motif chemokine ligand 2 (MCP-1) and C-C motif chemokine ligand 5 (RANTES) across the intact mouse blood-brain barrier is inhibited by heparin and eprodisate and increased with systemic inflammation. J. Pharmacol. Exp. Ther. 384, 205–223. doi: 10.1124/jpet.122.001380
Rao, J., Qiao, Y., Xie, R., Lin, L., Jiang, J., Wang, C., et al. (2021). Fecal microbiota transplantation ameliorates stress-induced depression-like behaviors associated with the inhibition of glial and NLRP3 inflammasome in rat brain. J. Psychiatr. Res. 137, 147–157. doi: 10.1016/j.jpsychires.2021.02.057
Rao, M., and Gershon, M. D. (2016). The bowel and beyond: the enteric nervous system in neurological disorders. Nature reviews. Gastroenterol. Hepatol. 13, 517–528. doi: 10.1038/nrgastro.2016.107
Reigstad, C. S., Salmonson, C. E., Rainey, J. F. 3rd, Szurszewski, J. H., Linden, D. R., Sonnenburg, J. L., Farrugia, G., et al. (2015). Gut microbes promote colonic serotonin production through an effect of short-chain fatty acids on enterochromaffin cells. FASEB J. 29, 1395–1403. doi: 10.1096/fj.14-259598
Rincel, M., Aubert, P., Chevalier, J., Grohard, P. A., Basso, L., Monchaux de Oliveira, C., et al. (2019). Multi-hit early life adversity affects gut microbiota, brain and behavior in a sex-dependent manner. Brain Behav. Immun. 80, 179–192. doi: 10.1016/j.bbi.2019.03.006
Rochfort, K. D., Collins, L. E., Murphy, R. P., and Cummins, P. M. (2014). Downregulation of blood-brain barrier phenotype by proinflammatory cytokines involves NADPH oxidase-dependent ROS generation: consequences for interendothelial adherens and tight junctions. PLoS ONE 9:e101815. doi: 10.1371/journal.pone.0101815
Rojas, O. L., Pröbstel, A. K., Porfilio, E. A., Wang, A. A., Charabati, M., Sun, T., et al. (2019). Recirculating intestinal IgA-producing cells regulate neuroinflammation via IL-10. Cell 176, 610–624.e18. doi: 10.1016/j.cell.2018.11.035
Rutsch, A., Kantsjö, J. B., and Ronchi, F. (2020). The gut-brain axis: how microbiota and host inflammasome influence brain physiology and pathology. Front. Immunol. 11:604179. doi: 10.3389/fimmu.2020.604179
Sadler, R., Cramer, J. V., Heindl, S., Kostidis, S., Betz, D., Zuurbier, K. R., et al. (2020). Short-chain fatty acids improve poststroke recovery via immunological mechanisms. J. Neurosci. 40, 1162–1173. doi: 10.1523/JNEUROSCI.1359-19.2019
Sahoo, B. R. (2020). Structure of fish Toll-like receptors (TLR) and NOD-like receptors (NLR). Int. J. Biol. Macromol. 161, 1602–1617. doi: 10.1016/j.ijbiomac.2020.07.293
Sanmarco, L. M., Wheeler, M. A., Gutiérrez-Vázquez, C., Polonio, C. M., Linnerbauer, M., Pinho-Ribeiro, F. A., et al. (2021). Gut-licensed IFNγ+ NK cells drive LAMP1+TRAIL+ anti-inflammatory astrocytes. Nature 590, 473–479. doi: 10.1038/s41586-020-03116-4
Scalise, A. A., Kakogiannos, N., Zanardi, F., Iannelli, F., and Giannotta, M. (2021). The blood-brain and gut-vascular barriers: from the perspective of claudins. Tissue Barriers 9:1926190. doi: 10.1080/21688370.2021.1926190
Serger, E., Luengo-Gutierrez, L., Chadwick, J. S., Kong, G., Zhou, L., Crawford, G., et al. (2022). The gut metabolite indole-3 propionate promotes nerve regeneration and repair. Nature 607, 585–592. doi: 10.1038/s41586-022-04884-x
Sharma, R. K., Oliveira, A. C., Yang, T., Karas, M. M., Li, J., Lobaton, G. O., et al. (2020). Gut pathology and its rescue by ACE2 (Angiotensin-Converting Enzyme 2) in hypoxia-induced pulmonary hypertension. Hypertension 76, 206–216. doi: 10.1161/HYPERTENSIONAHA.120.14931
Shi, H., Ge, X., Ma, X., Zheng, M., Cui, X., Pan, W., et al. (2021). A fiber-deprived diet causes cognitive impairment and hippocampal microglia-mediated synaptic loss through the gut microbiota and metabolites. Microbiome 9:223. doi: 10.1186/s40168-021-01172-0
Sun, C. Y., Yang, N., Zheng, Z. L., Liu, D., and Xu, Q. L. (2023). T helper 17 (Th17) cell responses to the gut microbiota in human diseases. Biomed. Pharmacoth. 161:114483. doi: 10.1016/j.biopha.2023.114483
Sun, J., Xu, J., Ling, Y., Wang, F., Gong, T., Yang, C., et al. (2019). Fecal microbiota transplantation alleviated Alzheimer's disease-like pathogenesis in APP/PS1 transgenic mice. Transl. Psychiatry 9, 189. doi: 10.1038/s41398-019-0525-3
Sun, L., Wang, X., Zou, Y., He, Y., Liang, C., Li, J., et al. (2023). Cold stress induces colitis-like phenotypes in mice by altering gut microbiota and metabolites. Front. Microbiol. 14, 1134246. doi: 10.3389/fmicb.2023.1134246
Sun, M. F., Zhu, Y. L., Zhou, Z. L., Jia, X. B., Xu, Y. D., Yang, Q., et al. (2018). Neuroprotective effects of fecal microbiota transplantation on MPTP-induced Parkinson's disease mice: Gut microbiota, glial reaction and TLR4/TNF-α signaling pathway. Brain Behav. Immun. 70, 48–60. doi: 10.1016/j.bbi.2018.02.005
Suzuki, T. (2020). Regulation of the intestinal barrier by nutrients: the role of tight junctions. Anim. Sci. J. 91:e13357. doi: 10.1111/asj.13357
Taguchi, T., and Mukai, K. (2019). Innate immunity signalling and membrane trafficking. Curr. Opin. Cell Biol. 59, 1–7. doi: 10.1016/j.ceb.2019.02.002
Tan, J. K., Macia, L., and Mackay, C. R. (2023). Dietary fiber and SCFAs in the regulation of mucosal immunity. J. Allergy Clin. Immunol. 151, 361–370. doi: 10.1016/j.jaci.2022.11.007
Tanelian, A., Nankova, B., Miari, M., and Sabban, E. L. (2024). Microbial composition, functionality, and stress resilience or susceptibility: unraveling sex-specific patterns. Biol. Sex Differ. 15, 20. doi: 10.1186/s13293-024-00590-7
Thevaranjan, N., Puchta, A., Schulz, C., Naidoo, A., Szamosi, J. C., Verschoor, C. P., et al. (2017). Age-associated microbial dysbiosis promotes intestinal permeability, systemic inflammation, and macrophage dysfunction. Cell Host Microbe 21, 455–466.e4. doi: 10.1016/j.chom.2017.03.002
Vailati-Riboni, M., Rund, L., Caetano-Silva, M. E., Hutchinson, N. T., Wang, S. S., Soto-Díaz, K., et al. (2022). Dietary fiber as a counterbalance to age-related microglial cell dysfunction. Front. Nutrit. 9:835824. doi: 10.3389/fnut.2022.835824
Vicentini, F. A., Keenan, C. M., Wallace, L. E., Woods, C., Cavin, J. B., Flockton, A. R., et al. (2021). Intestinal microbiota shapes gut physiology and regulates enteric neurons and glia. Microbiome 9, 210. doi: 10.1186/s40168-021-01165-z
Wenzel, T. J., Gates, E. J., Ranger, A. L., and Klegeris, A. (2020). Short-chain fatty acids (SCFAs) alone or in combination regulate select immune functions of microglia-like cells. Mol. Cell. Neurosci. 105:103493. doi: 10.1016/j.mcn.2020.103493
Westfall, S., Caracci, F., Estill, M., Frolinger, T., Shen, L., and Pasinetti, G. M. (2021a). Chronic stress-induced depression and anxiety priming modulated by gut-brain-axis immunity. Front. Immunol. 12:670500. doi: 10.3389/fimmu.2021.670500
Westfall, S., Caracci, F., Zhao, D., Wu, Q. L., Frolinger, T., Simon, J., et al. (2021b). Microbiota metabolites modulate the T helper 17 to regulatory T cell (Th17/Treg) imbalance promoting resilience to stress-induced anxiety- and depressive-like behaviors. Brain Behav. Immun. 91, 350–368. doi: 10.1016/j.bbi.2020.10.013
Wong, R., Lénárt, N., Hill, L., Toms, L., Coutts, G., Martinecz, B., et al. (2019). Interleukin-1 mediates ischaemic brain injury via distinct actions on endothelial cells and cholinergic neurons. Brain Behav. Immun. 76, 126–138. doi: 10.1016/j.bbi.2018.11.012
Xia, Y., Xiao, Y., Wang, Z. H., Liu, X., Alam, A. M., Haran, J. P., et al. (2023). Bacteroides Fragilis in the gut microbiomes of Alzheimer's disease activates microglia and triggers pathogenesis in neuronal C/EBPβ transgenic mice. Nat. Commun. 14:5471. doi: 10.1038/s41467-023-41283-w
Yang, B. H., Hagemann, S., Mamareli, P., Lauer, U., Hoffmann, U., Beckstette, M., et al. (2016). Foxp3(+) T cells expressing RORγt represent a stable regulatory T-cell effector lineage with enhanced suppressive capacity during intestinal inflammation. Mucosal Immunol. 9, 444–457. doi: 10.1038/mi.2015.74
Yang, D. F., Huang, W. C., Wu, C. W., Huang, C. Y., Yang, Y. S. H., and Tung, Y. T. (2023). Acute sleep deprivation exacerbates systemic inflammation and psychiatry disorders through gut microbiota dysbiosis and disruption of circadian rhythms. Microbiol. Res. 268, 127292. doi: 10.1016/j.micres.2022.127292
Yang, H. L., Li, M. M., Zhou, M. F., Xu, H. S., Huan, F., Liu, N., et al. (2021). Links between gut dysbiosis and neurotransmitter disturbance in chronic restraint stress-induced depressive behaviours: the role of inflammation. Inflammation 44, 2448–2462. doi: 10.1007/s10753-021-01514-y
Yasuda, K., Takeuchi, Y., and Hirota, K. (2019). The pathogenicity of Th17 cells in autoimmune diseases. Semin. Immunopathol. 41, 283–297. doi: 10.1007/s00281-019-00733-8
Ye, L., Bae, M., Cassilly, C. D., Jabba, S. V., Thorpe, D. W., Martin, A. M., et al. (2021). Enteroendocrine cells sense bacterial tryptophan catabolites to activate enteric and vagal neuronal pathways. Cell Host Microbe 29, 179–196.e9. doi: 10.1016/j.chom.2020.11.011
Yi, W., Cheng, J., Wei, Q., Pan, R., Song, S., He, Y., et al. (2021). Effect of temperature stress on gut-brain axis in mice: Regulation of intestinal microbiome and central NLRP3 inflammasomes. Sci. Total Environ. 772, 144568. doi: 10.1016/j.scitotenv.2020.144568
Zhang, M., Li, A., Yang, Q., Li, J., Zheng, L., Wang, G., et al. (2023). Matrine alleviates depressive-like behaviors via modulating microbiota-gut-brain axis in CUMS-induced mice. J. Transl. Med. 21, 145. doi: 10.1186/s12967-023-03993-z
Zhao, L. P., Wu, J., Quan, W., Zhou, Y., Hong, H., Niu, G. Y., et al. (2023). DSS-induced colitis activates the kynurenine pathway in serum and brain by affecting IDO-1 and gut microbiota. Front. Immunol. 13, 1089200. doi: 10.3389/fimmu.2022.1089200
Zhao, Z., Ning, J., Bao, X. Q., Shang, M., Ma, J., Li, G., et al. (2021). Fecal microbiota transplantation protects rotenone-induced Parkinson's disease mice via suppressing inflammation mediated by the lipopolysaccharide-TLR4 signaling pathway through the microbiota-gut-brain axis. Microbiome 9, 226. doi: 10.1186/s40168-021-01107-9
Zheng, H., Xu, P., Jiang, Q., Xu, Q., Zheng, Y., Yan, J., et al. (2021). Depletion of acetate-producing bacteria from the gut microbiota facilitates cognitive impairment through the gut-brain neural mechanism in diabetic mice. Microbiome 9:145. doi: 10.1186/s40168-021-01088-9
Zhou, L., Lopes, J. E., Chong, M. M., Ivanov, I. I., Min, R., Victora, G. D., et al. (2008). TGF-beta-induced Foxp3 inhibits T(H)17 cell differentiation by antagonizing RORgammat function. Nature 453, 236–240. doi: 10.1038/nature06878
Keywords: gut-brain axis, blood-brain barrier, tight junctions, stress, inflammation, mood disorders
Citation: Morys J, Małecki A and Nowacka-Chmielewska M (2024) Stress and the gut-brain axis: an inflammatory perspective. Front. Mol. Neurosci. 17:1415567. doi: 10.3389/fnmol.2024.1415567
Received: 10 April 2024; Accepted: 24 June 2024;
Published: 18 July 2024.
Edited by:
Jefferson Antônio Leite, Johannes Gutenberg-University Mainz, GermanyReviewed by:
Eduardo Duarte-Silva, Northwestern University, United StatesCopyright © 2024 Morys, Małecki and Nowacka-Chmielewska. This is an open-access article distributed under the terms of the Creative Commons Attribution License (CC BY). The use, distribution or reproduction in other forums is permitted, provided the original author(s) and the copyright owner(s) are credited and that the original publication in this journal is cited, in accordance with accepted academic practice. No use, distribution or reproduction is permitted which does not comply with these terms.
*Correspondence: Marta Nowacka-Chmielewska, bS5ub3dhY2thQGF3Zi5rYXRvd2ljZS5wbA==
Disclaimer: All claims expressed in this article are solely those of the authors and do not necessarily represent those of their affiliated organizations, or those of the publisher, the editors and the reviewers. Any product that may be evaluated in this article or claim that may be made by its manufacturer is not guaranteed or endorsed by the publisher.
Research integrity at Frontiers
Learn more about the work of our research integrity team to safeguard the quality of each article we publish.