- Department of Molecular Neurochemistry, Faculty of Health Sciences, Medical University of Lodz, Lodz, Poland
The complex nature of the retina demands well-organized signaling to uphold signal accuracy and avoid interference, a critical aspect in handling a variety of visual stimuli. A-kinase anchoring proteins (AKAPs), known for binding protein kinase A (PKA), contribute to the specificity and efficiency of retinal signaling. They play multifaceted roles in various retinal cell types, influencing photoreceptor sensitivity, neurotransmitter release in bipolar cells, and the integration of visual information in ganglion cells. AKAPs like AKAP79/150 and AKAP95 exhibit distinct subcellular localizations, impacting synaptic transmission and receptor sensitivity in photoreceptors and bipolar cells. Furthermore, AKAPs are involved in neuroprotective mechanisms and axonal degeneration, particularly in retinal ganglion cells. In particular, AKAP6 coordinates stress-specific signaling and promotes neuroprotection following optic nerve injury. As our review underscores the therapeutic potential of targeting AKAP signaling complexes for retinal neuroprotection and enhancement, it acknowledges challenges in developing selective drugs that target complex protein–protein interactions. Overall, this exploration of AKAPs provides valuable insights into the intricacies of retinal signaling, offering a foundation for understanding and potentially addressing retinal disorders.
1 Introduction
A-kinase anchoring proteins (AKAPs), named for their role in anchoring signaling molecules, are a diverse family of proteins that share a common feature – the protein kinase A-kinase binding domain (Dodge-Kafka et al., 2005). This domain facilitates the interaction between AKAPs and the regulatory subunits of protein kinase A (PKA), a central player in retinal signaling. This interaction provides a platform for the spatial localization of PKA and other signaling components, allowing for a highly regulated and localized response to extracellular stimuli. The world of AKAPs is characterized by its diversity, both in terms of structure and function. While the A-kinase binding domain is a unifying factor, AKAPs vary widely in their overall structures and associated domains. This diversity allows different AKAPs to engage in distinct protein–protein interactions and participate in various signaling pathways. Some AKAPs, for example, contain domains that interact with ion channels, receptors, or other enzymes, broadening their influence on cellular responses (Dodge-Kafka et al., 2005; Wild and Dell'Acqua, 2018).
Different AKAPs may target PKA to specific subcellular compartments, such as the plasma membrane, mitochondria, or the endoplasmic reticulum (Oliveria et al., 2007; Wild and Dell'Acqua, 2018, 2019; Boczek et al., 2021). This targeted localization ensures that PKA is strategically positioned to phosphorylate substrates in close proximity, optimizing the efficiency of signal transduction. By anchoring PKA and other signaling molecules to discrete locations, AKAPs contribute to the formation of microdomains where signaling events are confined, preventing crosstalk and ensuring that cellular responses are finely tuned and precisely regulated.
The interaction between AKAPs and PKA is central to the functional significance of AKAPs (Kapiloff et al., 1999; Dodge-Kafka et al., 2005). In resting conditions, PKA is bound to the regulatory subunits, maintaining the enzyme in an inactive state. When AKAPs bind to the regulatory subunits, a conformational change occurs, releasing the catalytic subunits and activating PKA. This dynamic regulation ensures that PKA is activated precisely when and where it is needed, contributing to the specificity of cellular responses. AKAP-mediated spatial compartmentalization not only enhances signaling specificity but also allows for dynamic regulation and crosstalk between different signaling pathways. AKAPs can integrate signals from multiple pathways by assembling diverse signaling components in the same microdomain. This dynamic regulation and crosstalk provide cells with the flexibility to respond to a variety of stimuli and adapt to changing environmental conditions.
2 The multifaceted roles of AKAPs in different retinal cell types
2.1 AKAPs in photoreceptor signaling
Photoreceptor cells, the frontline sensors of the retina, play a pivotal role in the conversion of light into neural signals, forming the foundation of visual perception. AKAPs anchor critical signaling molecules to specific subcellular compartments within photoreceptor cells. This spatial organization allows for localized and precise phosphorylation events, influencing the sensitivity of phototransduction pathways. AKAPs may play a role in modulating the activity of rhodopsin, the light-sensitive protein in rod photoreceptors. By localizing PKA and other effectors, AKAPs contribute to the regulation of rhodopsin phosphorylation, affecting its sensitivity to light and influencing the efficiency of phototransduction. They are also involved in the spatial organization of signaling components associated with light-adaptive mechanisms. This organization ensures that adaptive responses, such as changes in photoreceptor sensitivity, are efficiently initiated and coordinated in response to alterations in ambient light levels. By anchoring signaling molecules to specific microdomains in cone cells, AKAPs participate in the fine-tuning of phototransduction cascades, influencing color discrimination. Beyond their role in signaling, AKAPs may play a role in maintaining the function of cone photoreceptors (Baden et al., 2020).
AKAP79/150 is prominently localized to the synaptic terminals of photoreceptor cells, specifically in the ribbon synapses. This strategic positioning enables it to influence synaptic transmission by anchoring protein kinase A (PKA) and other signaling effectors. AKAP79/150 plays a crucial role in modulating the phosphorylation status of synaptic proteins, influencing neurotransmitter release. Its presence in photoreceptor terminals highlights its significance in shaping the initial stages of visual signal processing (Wang and Cortes, 2021).
AKAP450 is found in the inner segments of photoreceptor cells, adjacent to the connecting cilium. Its subcellular localization suggests a role in the regulation of processes occurring in this crucial region. The subcellular positioning of AKAP450 hints at its involvement in processes such as ciliogenesis. By influencing events in the inner segments, AKAP450 may contribute to the maintenance of photoreceptor structure and function (Subramanian and Sahoo, 2022).
2.2 AKAPs in bipolar cell signaling
In the complex landscape of retinal circuits, bipolar cells stand as pivotal intermediaries, translating signals from photoreceptors into meaningful neural information. AKAPs contribute to the fine-tuning of neurotransmitter release from bipolar cells (Bastola et al., 2023). By anchoring PKA and other effectors, AKAPs modulate the activity of calcium channels and vesicle release, influencing the strength and timing of synaptic transmission. AKAPs anchor signaling components in close proximity to receptors on bipolar cell dendrites. This spatial arrangement may influence the responsiveness of bipolar cells to signals received from photoreceptors, contributing to the modulation of receptor sensitivity. AKAPs also facilitate the integration of various signaling pathways. AKAP-mediated spatial organization contributes to the diversity of responses observed in different bipolar cell subtypes (Baden et al., 2020).
Dysregulation of AKAP-mediated signaling in bipolar cells may contribute to visual disorders affecting synaptic transmission. Investigating these mechanisms can provide insights into conditions, where altered signaling in bipolar cells plays a role, such as bipolar cell-associated retinal dystrophies. AKAP95 is an example of an anchoring protein found in bipolar cell dendrites, positioned near glutamate receptors. This subcellular localization allows AKAP95 to regulate receptor sensitivity and influence the responsiveness of bipolar cells to signals from photoreceptors. By tethering PKA and other effectors, AKAP95 contributes to the fine-tuning of receptor sensitivity, ensuring that bipolar cells can efficiently process and modulate signals before transmission to downstream neurons (Roa et al., 2021).
2.3 AKAPs in ganglion cells
AKAP150 is found in ganglion cells, positioning itself in the vicinity of ion channels and receptors crucial for signal transmission. This localization allows AKAP150 to influence the integration of signals within ganglion cells before they are transmitted to the brain. AKAP150 plays a key role in the integration of signals within ganglion cells, facilitating the convergence of inputs from various bipolar cells. Its presence near critical signaling components contributes to the orchestration of diverse visual information (Ostroveanu et al., 2007). Beyond their role in signaling, AKAPs may be involved in neuroprotective mechanisms within ganglion cells (Boczek et al., 2019; Boczek and Kapiloff, 2020; Bastola et al., 2023). Their roles in regulating cellular processes associated with survival highlight the potential of AKAPs as key players in preservation of ganglion cell function.
Recently, we have demonstrated that AKAP6-mediated signaling (Figure 1) in critical for survival of retinal ganglion cells (Boczek et al., 2019). By using genetically engineered delocalizing peptides, we confirmed that local cAMP/PKA pro-survival signaling within the AKAP6 signalosome is mediated by anchored phosphodiesterase 4D3 activity and enhanced ganglion cell survival can be achieved by increasing perinuclear cAMP concentration (Boczek et al., 2019). Furthermore, we have also developed a FRET-based technique to measure PKA signaling activity within AKAP6 signalosome that can be applied both in vitro and in vivo and help to monitor the changes in cAMP signaling following optic nerve injury. The expression of AKAPs in a particular cell type of retina, along with their binding partners, is summarized in Table 1.
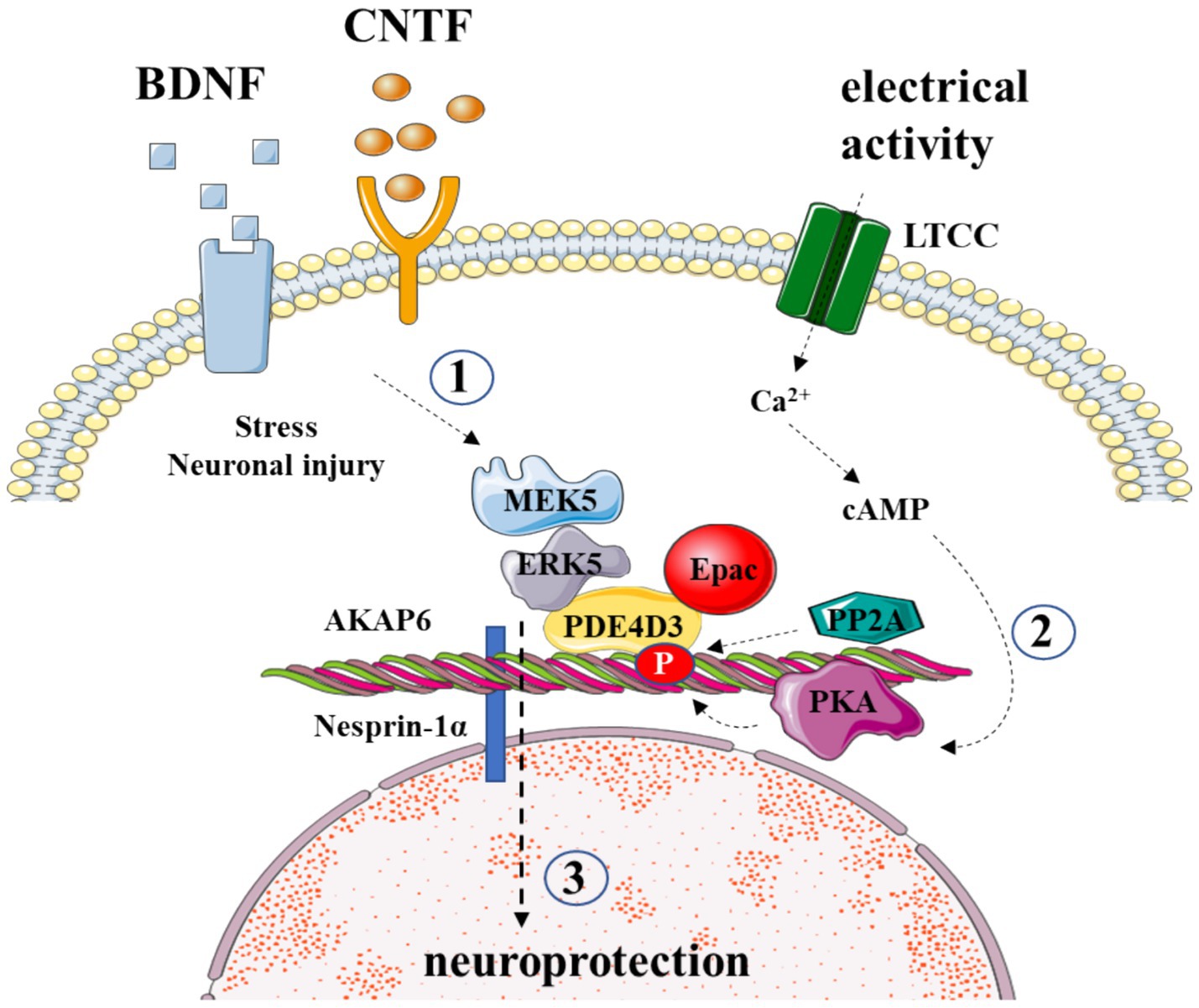
Figure 1. AKAP6 orchestrates the survival of retinal ganglion cells post-injury. (1) AKAP6 binds to PDE4D3, securing ERK5-MAPK pathway signaling elements, which respond to neurotrophic signaling; (2) AKAP6 tethers PKA, responsive to electrical activity; (3) the crosstalk of these pathways confers RGC survival after injury.
3 AKAP in axonal degeneration in the retina
The insufficient trophic signaling has long been implicated as the cause of CNS neuronal regeneration failure (Goldberg and Barres, 2000). Notably, the application of neurotrophic factors such as brain-derived neurotrophic factor (BDNF), glial-derived neurotrophic factor (GDNF), ciliary neurotrophic factor (CNTF), and fibroblast growth factor (FGF) can delay the death of retinal ganglion cells (RGCs) (Unoki and LaVail, 1994; Mo et al., 2002). Trophic signaling can be enhanced by electrical activity and cAMP elevations, providing neuroprotection (Corredor et al., 2012). The inhibition of PKA eliminates these protective effects, indicating a potential role for AKAP scaffolding (Goldberg et al., 2002). Recent research implicates muscle AKAP (mAKAP) in coordinating stress-specific signaling and axon growth in RGCs (Wang et al., 2015). mAKAP, with its three spectrin repeats, localizes to the outer nuclear membrane through interaction with the protein nesprin-1α. Two splice variants, mAKAPα and mAKAPβ, show tissue-specific expression patterns (Kapiloff et al., 1999; Dodge-Kafka et al., 2005). While mAKAPβ assembles signaling complexes in cardiomyocytes, mAKAPα, predominantly expressed in the brain, contains an additional anchoring site for 3-phosphoinositide-dependent kinase-1 (PDK1) (Michel et al., 2005).
Wang et al.’s study highlights the expression of mAKAPα in retinal ganglion cells and its crucial role in transducing pro-survival signaling induced by cAMP, BDNF, and CNTF. Knockout of mAKAPα hinders trophic signaling from promoting survival after optic nerve injury (Wang et al., 2015). Although the specific mechanisms of mAKAPα-directed neuroprotective signaling remain unclear, it is plausible that the scaffold facilitates crosstalk between cAMP and MAPK pathways. mAKAPα-anchored PKA and PP2A positively and negatively modulate PDE4D3 activity, respectively, controlling the local cAMP concentration at the ONM. Additionally, ERK5 indirectly binds to mAKAPα through association with PDE4D3. While further studies are required to elucidate the exact cross-talk mechanisms, it is evident that mAKAPα signaling plays a central role in the neuroprotective effects of neurotrophins following RGC damage. A more comprehensive understanding of these processes could pave the way for the development of neuroprotective interventions after neuronal injury and degeneration.
4 Targeting AKAPs as a therapeutic strategy in retinal degeneration
Disrupting AKAP signaling complexes to influence pathological signaling holds significant promise, particularly through the uncoupling of AKAP signaling components, allowing for targeted disruption within spatially confined nanodomains. With different AKAPs present in each cell type, each with distinct subcellular locations and functions (Kennedy and Scott, 2015), a key objective of future research is the development of agents that selectively target protein–protein interactions (PPIs) between specific AKAP isoforms and their binding partners. Currently, three classes of molecules—peptides, peptidomimetics, and small molecule inhibitors—are employed to disrupt AKAP-PPIs.
The initial tool developed to disrupt the RII-AKAP binding was a 24-amino acid peptide named Ht31, derived from the PKA binding domain of AKAP-Lbc (Carr et al., 1992). While Ht31 binds to all R subunit isoforms of PKA, it exhibits a higher affinity for RII and has been employed as a non-selective blocker of all AKAP-PKA interactions. Subsequent advancements have led to the development of more selective peptides with increased affinity for either RI or RII subunits. Notably, RIAD demonstrates a 1,000-fold higher affinity for RI, while superAKAP-IS exhibits a > 10,000-fold higher affinity for RII (Gold et al., 2006). Despite the utility of peptides in studying AKAP function in the nervous system by disrupting AKAP-PKA interactions, their instability within the cell and the potential for membrane retention, particularly with peptides like St-Ht31, place constraints on their therapeutic applicability (Kennedy and Scott, 2015).
Efforts have been made to improve the stability and permeability of peptides, leading to the emergence of peptidomimetics. This innovative category of inhibitors undergoes chemical modification with hydrocarbon positioned along the peptide backbone, accomplished by introducing non-natural amino acids into the peptide sequence, followed by chemical cross-linking (Wang et al., 2014). The result is a peptide with a structurally constrained α-helical configuration, termed a Stapled Anchoring Disruptor (STAD). Importantly, these peptidomimetics demonstrate significantly enhanced membrane permeability and intracellular stability (Kennedy and Scott, 2015; Hanold et al., 2017). Isoform-selective STADs have been effectively crafted to target the AKAP binding pocket on both the RI- and RII-subunit D/D domain (Wang et al., 2014). Although the current applicability of these proteins is constrained, this strategy is poised to inform the future development of compounds targeted at AKAP.
From a therapeutic perspective, small molecule inhibitors present several advantages compared to larger peptide molecules, such as heightened oral bioavailability, enhanced intracellular stability, and cost-effectiveness. Nonetheless, the design of small molecules targeting protein–protein interactions comes with added challenges, given the typically expansive and discontinuous surface area involved in these interactions, encompassing multiple essential residues (Calejo and Taskén, 2015). The inaugural small molecule inhibitor of PKA, FMP-API-1, was introduced, binding to a site external to the D/D domain and exerting allosteric inhibition of the AKAP-PKA interaction (Christian et al., 2011). Originating from high-throughput screening, this molecule disrupted the binding of both RI or RII to AKAP18δ, although the precise mechanism remains incompletely elucidated. The allosteric effects also prompted PKA activation, potentially through interaction with the auto-inhibitory domain. Consequently, further refinement of the compound is deemed necessary (Christian et al., 2011).
5 Conclusion
The continuous quest for innovative drug targets is focused on selectively modifying pathologically active signaling pathways with subcellular precision. The goal of this approach is to develop compounds with fewer off-target effects in comparison to those designed for broadly expressed signaling molecules such as kinases, phosphatases, ion channels, and receptors. AKAP-mediated signal compartmentalization is crucial for the nervous system, as it co-localizes signaling molecules within nanodomain compartments, orchestrating various critical processes known to be disrupted in injured retina. As a result, the acknowledgment of the therapeutic potential of targeting AKAP signaling complexes has gained momentum.
This review delves into the engagement of AKAPs in retinal functions. A growing body of evidence suggests that many insults leading to the degeneration of retinal ganglion cells could either be a consequence of AKAP dysfunction or have the potential to be alleviated through the modification of AKAP interactions. However, conclusive proof-of-principle studies utilizing genetic manipulations (e.g., knockout, knock-in, RNAi approaches) to disrupt AKAP signaling functions in animal disease models are essential to further validate AKAPs as viable therapeutic targets for retinal neuroprotection and neuroenhancement. A significant challenge in AKAP-centered drug discovery lies in the development of stable drugs capable of targeting complex protein–protein interactions between individual AKAPs and their binding partners with high selectivity. Despite efforts to generate peptides and small molecules for understanding the role of AKAP-anchored enzymes in neuronal physiology and disease, their therapeutic utility is currently limited.
Author contributions
JT: Conceptualization, Investigation, Software, Visualization, Writing – original draft, Writing – review & editing. JM: Conceptualization, Investigation, Resources, Visualization, Writing – original draft, Writing – review & editing. ML: Conceptualization, Data curation, Investigation, Resources, Software, Supervision, Visualization, Writing – original draft, Writing – review & editing. AK: Conceptualization, Investigation, Visualization, Writing – original draft, Writing – review & editing. TB: Conceptualization, Funding acquisition, Investigation, Project administration, Resources, Software, Visualization, Writing – original draft, Writing – review & editing.
Funding
The author(s) declare that no financial support was received for the research, authorship, and/or publication of this article.
Conflict of interest
The authors declare that the research was conducted in the absence of any commercial or financial relationships that could be construed as a potential conflict of interest.
Publisher's note
All claims expressed in this article are solely those of the authors and do not necessarily represent those of their affiliated organizations, or those of the publisher, the editors and the reviewers. Any product that may be evaluated in this article, or claim that may be made by its manufacturer, is not guaranteed or endorsed by the publisher.
References
Akileswaran, L., Taraska, J. W., Sayer, J. A., Gettemy, J. M., and Coghlan, V. M. (2001). A-kinase-anchoring protein AKAP95 is targeted to the nuclear matrix and associates with p68 RNA helicase. J. Biol. Chem. 276, 17448–17454. doi: 10.1074/jbc.M101171200
Arsenijevic, T., Degraef, C., Dumont, J. E., Roger, P. P., and Pirson, I. (2004). A novel partner for D-type cyclins: protein kinase A-anchoring protein AKAP95. Biochem. J. 378, 673–679. doi: 10.1042/BJ20031765
Arsenijevic, T., Degraef, C., Dumont, J. E., Roger, P. P., and Pirson, I. (2006). G1/S cyclins interact with regulatory subunit of PKA via A-kinase anchoring protein, AKAP95. Cell Cycle 5, 1217–1222. doi: 10.4161/cc.5.11.2802
Asirvatham, A. L., Galligan, S. G., Schillace, R. V., Davey, M. P., Vasta, V., Beavo, J. A., et al. (2004). A-kinase anchoring proteins interact with phosphodiesterases in T lymphocyte cell lines. J. Immunol. 173, 4806–4814. doi: 10.4049/jimmunol.173.8.4806
Baden, T., Euler, T., and Berens, P. (2020). Understanding the retinal basis of vision across species. Nat. Rev. Neurosci. 21, 5–20. doi: 10.1038/s41583-019-0242-1
Bastola, T., Perkins, G. A., Kim, K. Y., Choi, S., Kwon, J. W., Shen, Z., et al. (2023). Role of A-kinase anchoring protein 1 in retinal ganglion cells: neurodegeneration and neuroprotection. Cells 12:1539. doi: 10.3390/cells12111539
Boczek, T., Cameron, E. G., Yu, W., Xia, X., Shah, S. H., Castillo Chabeco, B., et al. (2019). Regulation of neuronal survival and axon growth by a perinuclear cAMP compartment. J. Neurosci. 39, 5466–5480. doi: 10.1523/JNEUROSCI.2752-18.2019
Boczek, T., and Kapiloff, M. S. (2020). Compartmentalization of local cAMP signaling in neuronal growth and survival. Neural Regen. Res. 15, 453–454. doi: 10.4103/1673-5374.266055
Boczek, T., Yu, Q., Zhu, Y., Dodge-Kafka, K. L., Goldberg, J. L., and Kapiloff, M. S. (2021). cAMP at perinuclear mAKAPα signalosomes is regulated by local ca. eNeuro 8:ENEURO.0298-20.2021. doi: 10.1523/ENEURO.0298-20.2021
Calejo, A. I., and Taskén, K. (2015). Targeting protein-protein interactions in complexes organized by a kinase anchoring proteins. Front. Pharmacol. 6:192. doi: 10.3389/fphar.2015.00192
Carr, D. W., Hausken, Z. E., Fraser, I. D., Stofko-Hahn, R. E., and Scott, J. D. (1992). Association of the type II cAMP-dependent protein kinase with a human thyroid RII-anchoring protein. Cloning and characterization of the RII-binding domain. J. Biol. Chem. 267, 13376–13382. doi: 10.1016/S0021-9258(18)42221-1
Christian, F., Szaszák, M., Friedl, S., Drewianka, S., Lorenz, D., Goncalves, A., et al. (2011). Small molecule AKAP-protein kinase a (PKA) interaction disruptors that activate PKA interfere with compartmentalized cAMP signaling in cardiac myocytes. J. Biol. Chem. 286, 9079–9096. doi: 10.1074/jbc.M110.160614
Coghlan, V. M., Perrino, B. A., Howard, M., Langeberg, L. K., Hicks, J. B., Gallatin, W. M., et al. (1995). Association of protein kinase a and protein phosphatase 2B with a common anchoring protein. Science 267, 108–111. doi: 10.1126/science.7528941
Colledge, M., Dean, R. A., Scott, G. K., Langeberg, L. K., Huganir, R. L., and Scott, J. D. (2000). Targeting of PKA to glutamate receptors through a MAGUK-AKAP complex. Neuron 27, 107–119. doi: 10.1016/s0896-6273(00)00013-1
Corredor, R. G., Trakhtenberg, E. F., Pita-Thomas, W., Jin, X., Hu, Y., and Goldberg, J. L. (2012). Soluble adenylyl cyclase activity is necessary for retinal ganglion cell survival and axon growth. J. Neurosci. 32, 7734–7744. doi: 10.1523/JNEUROSCI.5288-11.2012
Dirksen, E. H., Pinkse, M. W. H., Rijkers, D. T. S., Cloos, J., Liskamp, R. M. J., Slijper, M., et al. (2006). Investigating the dynamic nature of the interactions between nuclear proteins and histones upon DNA damage using an immobilized peptide chemical proteomics approach. J. Proteome Res. 5, 2380–2388. doi: 10.1021/pr060278b
Dodge, K. L., Khouangsathiene, S., Kapiloff, M. S., Mouton, R., Hill, E. V., Houslay, M. D., et al. (2001). mAKAP assembles a protein kinase a/PDE4 phosphodiesterase cAMP signaling module. EMBO J. 20, 1921–1930. doi: 10.1093/emboj/20.8.1921
Dodge-Kafka, K. L., Soughayer, J., Pare, G. C., Carlisle Michel, J. J., Langeberg, L. K., Kapiloff, M. S., et al. (2005). The protein kinase a anchoring protein mAKAP coordinates two integrated cAMP effector pathways. Nature 437, 574–578. doi: 10.1038/nature03966
Furusawa, M., Taira, T., Iguchi-Ariga, S. M., and Ariga, H. (2002). AMY-1 interacts with S-AKAP84 and AKAP95 in the cytoplasm and the nucleus, respectively, and inhibits cAMP-dependent protein kinase activity by preventing binding of its catalytic subunit to A-kinase-anchoring protein (AKAP) complex. J. Biol. Chem. 277, 50885–50892. doi: 10.1074/jbc.M206387200
Gao, T., Yatani, A., Dell'Acqua, M. L., Sako, H., Green, S. A., Dascal, N., et al. (1997). cAMP-dependent regulation of cardiac L-type Ca2+ channels requires membrane targeting of PKA and phosphorylation of channel subunits. Neuron 19, 185–196. doi: 10.1016/s0896-6273(00)80358-x
Gold, M. G., Lygren, B., Dokurno, P., Hoshi, N., McConnachie, G., Taskén, K., et al. (2006). Molecular basis of AKAP specificity for PKA regulatory subunits. Mol. Cell 24, 383–395. doi: 10.1016/j.molcel.2006.09.006
Goldberg, J. L., and Barres, B. A. (2000). The relationship between neuronal survival and regeneration. Annu. Rev. Neurosci. 23, 579–612. doi: 10.1146/annurev.neuro.23.1.579
Goldberg, J. L., Espinosa, J. S., Xu, Y., Davidson, N., Kovacs, G. T., and Barres, B. A. (2002). Retinal ganglion cells do not extend axons by default: promotion by neurotrophic signaling and electrical activity. Neuron 33, 689–702. doi: 10.1016/s0896-6273(02)00602-5
Hanold, L. E., Fulton, M. D., and Kennedy, E. J. (2017). Targeting kinase signaling pathways with constrained peptide scaffolds. Pharmacol. Ther. 173, 159–170. doi: 10.1016/j.pharmthera.2017.02.014
Hoshi, N., Zhang, J. S., Omaki, M., Takeuchi, T., Yokoyama, S., Wanaverbecq, N., et al. (2003). AKAP150 signaling complex promotes suppression of the M-current by muscarinic agonists. Nat. Neurosci. 6, 564–571. doi: 10.1038/nn1062
Jo, I., Ward, D. T., Baum, M. A., Scott, J. D., Coghlan, V. M., Hammond, T. G., et al. (2001). AQP2 is a substrate for endogenous PP2B activity within an inner medullary AKAP-signaling complex. Am. J. Physiol. Renal Physiol. 281, F958–F965. doi: 10.1152/ajprenal.2001.281.5.F958
Kamada, S., Kikkawa, U., Tsujimoto, Y., and Hunter, T. (2005). A-kinase-anchoring protein 95 functions as a potential carrier for the nuclear translocation of active caspase 3 through an enzyme-substrate-like association. Mol. Cell. Biol. 25, 9469–9477. doi: 10.1128/MCB.25.21.9469-9477.2005
Kapiloff, M. S., Jackson, N., and Airhart, N. (2001). mAKAP and the ryanodine receptor are part of a multi-component signaling complex on the cardiomyocyte nuclear envelope. J. Cell Sci. 114, 3167–3176. doi: 10.1242/jcs.114.17.3167
Kapiloff, M. S., Schillace, R. V., Westphal, A. M., and Scott, J. D. (1999). mAKAP: an A-kinase anchoring protein targeted to the nuclear membrane of differentiated myocytes. J. Cell Sci. 112, 2725–2736. doi: 10.1242/jcs.112.16.2725
Kennedy, E. J., and Scott, J. D. (2015). Selective disruption of the AKAP signaling complexes. Methods Mol. Biol. 1294, 137–150. doi: 10.1007/978-1-4939-2537-7_11
Klauck, T. M., Faux, M. C., Labudda, K., Langeberg, L. K., Jaken, S., and Scott, J. D. (1996). Coordination of three signaling enzymes by AKAP79, a mammalian scaffold protein. Science 271, 1589–1592. doi: 10.1126/science.271.5255.1589
Marx, S. O., Kurokawa, J., Reiken, S., Motoike, H., D'Armiento, J., Marks, A. R., et al. (2002). Requirement of a macromolecular signaling complex for beta adrenergic receptor modulation of the KCNQ1-KCNE1 potassium channel. Science 295, 496–499. doi: 10.1126/science.1066843
Marx, S. O., Reiken, S., Hisamatsu, Y., Jayaraman, T., Burkhoff, D., Rosemblit, N., et al. (2000). PKA phosphorylation dissociates FKBP12.6 from the calcium release channel (ryanodine receptor): defective regulation in failing hearts. Cell 101, 365–376. doi: 10.1016/s0092-8674(00)80847-8
McCartney, S., Little, B. M., Langeberg, L. K., and Scott, J. D. (1995). Cloning and characterization of A-kinase anchor protein 100 (AKAP100). A protein that targets A-kinase to the sarcoplasmic reticulum. J. Biol. Chem. 270, 9327–9333. doi: 10.1074/jbc.270.16.9327
Michel, J. J., Townley, I. K., Dodge-Kafka, K. L., Zhang, F., Kapiloff, M. S., and Scott, J. D. (2005). Spatial restriction of PDK1 activation cascades by anchoring to mAKAPalpha. Mol. Cell 20, 661–672. doi: 10.1016/j.molcel.2005.10.013
Mo, X., Yokoyama, A., Oshitari, T., Negishi, H., Dezawa, M., Mizota, A., et al. (2002). Rescue of axotomized retinal ganglion cells by BDNF gene electroporation in adult rats. Invest. Ophthalmol. Vis. Sci. 43, 2401–2405
Oliveria, S. F., Dell'Acqua, M. L., and Sather, W. A. (2007). AKAP79/150 anchoring of calcineurin controls neuronal L-type Ca2+ channel activity and nuclear signaling. Neuron 55, 261–275. doi: 10.1016/j.neuron.2007.06.032
Ostroveanu, A., Van der Zee, E. A., Dolga, A. M., Luiten, P. G., Eisel, U. L., and Nijholt, I. M. (2007). A-kinase anchoring protein 150 in the mouse brain is concentrated in areas involved in learning and memory. Brain Res. 1145, 97–107. doi: 10.1016/j.brainres.2007.01.117
Pare, G. C., Easlick, J. L., Mislow, J. M., McNally, E. M., and Kapiloff, M. S. (2005). Nesprin-1alpha contributes to the targeting of mAKAP to the cardiac myocyte nuclear envelope. Exp. Cell Res. 303, 388–399. doi: 10.1016/j.yexcr.2004.10.009
Roa, J. N., Ma, Y., Mikulski, Z., Xu, Q., Ilouz, R., Taylor, S. S., et al. (2021). Protein kinase a in human retina: differential localization of Cβ, Cα, RIIα, and RIIβ in photoreceptors highlights non-redundancy of protein kinase a subunits. Front. Mol. Neurosci. 14:782041. doi: 10.3389/fnmol.2021.782041
Schulze, D. H., Muqhal, M., Lederer, W. J., and Ruknudin, A. M. (2003). Sodium/calcium exchanger (NCX1) macromolecular complex. J. Biol. Chem. 278, 28849–28855. doi: 10.1074/jbc.M300754200
Shanks, R. A., Larocca, M. C., Berryman, M., Edwards, J. C., Urushidani, T., Navarre, J., et al. (2002). AKAP350 at the Golgi apparatus. II. Association of AKAP350 with a novel chloride intracellular channel (CLIC) family member. J. Biol. Chem. 277, 40973–40980. doi: 10.1074/jbc.M112277200
Sillibourne, J. E., Milne, D. M., Takahashi, M., Ono, Y., and Meek, D. W. (2002). Centrosomal anchoring of the protein kinase CK1delta mediated by attachment to the large, coiled-coil scaffolding protein CG-NAP/AKAP450. J. Mol. Biol. 322, 785–797. doi: 10.1016/s0022-2836(02)00857-4
Steen, R. L., Cubizolles, F., Le Guellec, K., and Collas, P. (2000). A kinase-anchoring protein (AKAP)95 recruits human chromosome-associated protein (hCAP)-D2/Eg7 for chromosome condensation in mitotic extract. J. Cell Biol. 149, 531–536. doi: 10.1083/jcb.149.3.531
Subramanian, R., and Sahoo, D. (2022). Boolean implication analysis of single-cell data predicts retinal cell type markers. BMC Bioinformatics 23:378. doi: 10.1186/s12859-022-04915-4
Takahashi, M., Mukai, H., Oishi, K., Isagawa, T., and Ono, Y. (2000). Association of immature hypophosphorylated protein kinase cepsilon with an anchoring protein CG-NAP. J. Biol. Chem. 275, 34592–34596. doi: 10.1074/jbc.M005285200
Takahashi, M., Shibata, H., Shimakawa, M., Miyamoto, M., Mukai, H., and Ono, Y. (1999). Characterization of a novel giant scaffolding protein, CG-NAP, that anchors multiple signaling enzymes to centrosome and the golgi apparatus. J. Biol. Chem. 274, 17267–17274. doi: 10.1074/jbc.274.24.17267
Takahashi, M., Yamagiwa, A., Nishimura, T., Mukai, H., and Ono, Y. (2002). Centrosomal proteins CG-NAP and kendrin provide microtubule nucleation sites by anchoring gamma-tubulin ring complex. Mol. Biol. Cell 13, 3235–3245. doi: 10.1091/mbc.e02-02-0112
Taskén, K. A., Collas, P., Kemmner, W. A., Witczak, O., Conti, M., and Taskén, K. (2001). Phosphodiesterase 4D and protein kinase a type II constitute a signaling unit in the centrosomal area. J. Biol. Chem. 276, 21999–22002. doi: 10.1074/jbc.C000911200
Tu, H., Tang, T. S., Wang, Z., and Bezprozvanny, I. (2004). Association of type 1 inositol 1,4,5-trisphosphate receptor with AKAP9 (Yotiao) and protein kinase a. J. Biol. Chem. 279, 19375–19382. doi: 10.1074/jbc.M313476200
Unoki, K., and LaVail, M. M. (1994). Protection of the rat retina from ischemic injury by brain-derived neurotrophic factor, ciliary neurotrophic factor, and basic fibroblast growth factor. Invest. Ophthalmol. Vis. Sci. 35, 907–915
Wang, Y., Cameron, E. G., Li, J., Stiles, T. L., Kritzer, M. D., Lodhavia, R., et al. (2015). Muscle A-kinase anchoring protein-α is an injury-specific signaling scaffold required for neurotrophic- and cyclic adenosine monophosphate-mediated survival. EBioMedicine 2, 1880–1887. doi: 10.1016/j.ebiom.2015.10.025
Wang, S., and Cortes, C. J. (2021). Interactions with PDZ proteins diversify voltage-gated calcium channel signaling. J. Neurosci. Res. 99, 332–348. doi: 10.1002/jnr.24650
Wang, Y., Ho, T. G., Bertinetti, D., Neddermann, M., Franz, E., Mo, G. C. H., et al. (2014). Isoform-selective disruption of AKAP-localized PKA using hydrocarbon stapled peptides. ACS Chem. Biol. 9, 635–642. doi: 10.1021/cb400900r
Westphal, R. S., Tavalin, S. J., Lin, J. W., Alto, N. M., Fraser, I. D. C., Langeberg, L. K., et al. (1999). Regulation of NMDA receptors by an associated phosphatase-kinase signaling complex. Science 285, 93–96. doi: 10.1126/science.285.5424.93
Wild, A. R., and Dell'Acqua, M. L. (2018). Potential for therapeutic targeting of AKAP signaling complexes in nervous system disorders. Pharmacol. Ther. 185, 99–121. doi: 10.1016/j.pharmthera.2017.12.004
Keywords: A-kinase anchoring proteins, visual system, retina, signaling compartmentalization, retinal ganglion cell
Citation: Tomczak J, Mackiewicz J, Lisek M, Kaluza A and Boczek T (2024) Exploring AKAPs in visual signaling. Front. Mol. Neurosci. 17:1412407. doi: 10.3389/fnmol.2024.1412407
Edited by:
Veronika Stoka, Institut Jožef Stefan (IJS), SloveniaReviewed by:
Kun-Che Chang, University of Pittsburgh, United StatesCopyright © 2024 Tomczak, Mackiewicz, Lisek, Kaluza and Boczek. This is an open-access article distributed under the terms of the Creative Commons Attribution License (CC BY). The use, distribution or reproduction in other forums is permitted, provided the original author(s) and the copyright owner(s) are credited and that the original publication in this journal is cited, in accordance with accepted academic practice. No use, distribution or reproduction is permitted which does not comply with these terms.
*Correspondence: Tomasz Boczek, dG9tYXN6LmJvY3pla0B1bWVkLmxvZHoucGw=