- 1Department of Bioactive Material Sciences, Jeonbuk National University, Jeonju, Republic of Korea
- 2Department of Molecular Biology, and Research Center of Bioactive Materials, Jeonbuk National University, Jeonju, Republic of Korea
- 3Division of Life Sciences, Jeonbuk National University, Jeonju, Republic of Korea
cAMP response element-binding protein (CREB) is a ubiquitously expressed nuclear transcription factor, which can be constitutively activated regardless of external stimuli or be inducibly activated by external factors such as stressors, hormones, neurotransmitters, and growth factors. However, CREB controls diverse biological processes including cell growth, differentiation, proliferation, survival, apoptosis in a cell-type-specific manner. The diverse functions of CREB appear to be due to CREB-mediated differential gene expression that depends on cAMP response elements and multi-faceted regulation of CREB activity. Indeed, the transcriptional activity of CREB is controlled at several levels including alternative splicing, post-translational modification, dimerization, specific transcriptional co-activators, non-coding small RNAs, and epigenetic regulation. In this review, we present versatile regulatory modes of CREB family transcription factors and discuss their functional consequences.
1 Introduction
cAMP response element-binding protein (CREB) is a nuclear transcription factor that contain a conserved basic region/leucine zipper (bZIP) domain and involved in a wide range of biological processes, precisely in learning, memory, stress response and addiction (Hai and Hartman, 2001; Chowdhury et al., 2023). The protein sequences, regulatory consequences, or even cellular and biological roles of CREB repeatedly conserved over the species, which was first identified in 1987 as a cAMP-responsive transcription factor that controls the somatostatin gene (Montminy et al., 1986, 1990; Montminy and Bilezikjian, 1987; Yamamoto et al., 1988). The regulatory mode of CREB is a complex multi-step process, involving signal activation, signal transduction, protein kinase activation, CREB phosphorylation, dimerization, DNA binding, and transcriptional regulation. This complexity permits explicit regulation and fine-tuning of gene expression.
In response to a variety of extracellular stimuli the activated kinases, such as protein kinase A (PKA), Ca2+/calmodulin-dependent protein kinases (CaMKs), mitogen- and stress-activated protein kinases (MSKs), and ribosomal protein S6 kinases (RSKs), phosphorylate the multiple phosphorylation sites in the kinase-inducible domain (KID), leading to either activation or inactivation of CREB. Here, the physiological stimuli that activate or inhibit CREB include hormones (insulin), BDNF, EGF, neurotransmitters (dopamine and glutamate), intracellular second messengers (cAMP and Ca2+), neural activity, sensory stimuli (light and smell), and different types of stressors (exercise, toxins, and UV radiation) (Wang et al., 2018; Imoto et al., 2020; Narasimhamurthy et al., 2022; Chowdhury et al., 2023; Nayar et al., 2024). The detailed CREB-mediated signaling pathway and its functional roles have been well reviewed elsewhere (Manning et al., 2017; Chowdhury et al., 2023). The conserved basic leucine zipper domain mediates dimerization to form a homodimer or heterodimer (Gonzalez et al., 1989; Meyer et al., 1993). The active dimer of the CREB/activating transcription factor (ATF) family binds to the conserved sequences (5′-TGACGT(C/G) A-3′ or 5′-CGTCA-3′ in human and 5′-GTGAC-GT(A/C) (A/G)-3′ in Drosophila) in the promoter regions of target genes, named as cis-acting cAMP responsive elements (CREs) and subsequently CREB recruits co-activators like CREB binding protein (CBP) or p300, leading to additional recruitment of the RNA polymerase complex and the initiation of transcription (Deutsch et al., 1988; Roesler et al., 1988; Chrivia et al., 1993).
Experimental studies demonstrate that CREB is involved in cell growth through regulation of cyclin D1 and c-myc, cell differentiation through GATA-1/GATA-2 and NRF-2, cell survival through Bcl-2, Bcl-xL, and MCL-1 as well as cell metabolism through glucokinase and fatty acid synthase (Wilson et al., 1996; Blobel et al., 1998; Wang et al., 1999; Katoh et al., 2001; Boulon et al., 2002; Jiang et al., 2008). Therefore, dysregulation of CREB alters expression levels of target genes, affecting many different aspects of cellular functions, development and balanced physiology.
In this review we first address the functional properties of CREB structural components and functional differences of distinguished splice isoforms. We also discuss how kinase-mediated phosphorylation or post-translational modification of CREB alters its binding affinity, dimerization property, and recruitment of co-activators, and finally we summarize how these complexes promote or suppress the transcription of target genes (Table 1) to exert biological functions.
2 Structural components
Mammals have been identified with more than 20 proteins named with the CREB/ATF prefix (Cui et al., 2021), while Drosophila melanogaster has two CREB proteins termed dCREBA and dCREBB/CREBB-17A (Yin et al., 1995a,b; Andrew et al., 1997). The dCREBB/CREBB-17A is more homologous to mammalian CREB and cAMP responsive element modulator (CREM) proteins, whereas dCREBA is far less conserved (Eisenhardt et al., 2003; Shanware et al., 2010). These conserved CREB proteins share common structural domains, and each of those domains is capable of regulating transcription (Figure 1). The DNA-binding and dimerization domains at the C-terminus of CREB protein are named bZIP domains (Figure 1). Multiple phosphorylation sequences are known as KID domains (Bartsch et al., 1998; Luo et al., 2012), and glutamine rich 1 (Q1) and 2 (Q2) domains are considered to be constitutive active domains (CADs) of CREB proteins (Sandoval et al., 2009). Functionally, the bZIP domain facilitates binding and dimerization, while stimulus-dependent phosphorylation of the KID domain initiates co-activator interaction. Q1 and Q2 domains interact with the basal transcriptional machinery, facilitating CRE-driven expression that is independent of stimulus (Hoeffler et al., 1988; Gonzalez and Montminy, 1989; Hai et al., 1989; Foulkes et al., 1991). The alignment of multiple CREB/ATF-1 or CREM family proteins with numerous studies suggests that CREB, as an activator, has a domain organization of Q1-KID-Q2-bZIP with consistent exon usage (Figure 1; Lalli et al., 1996; Zhang D. et al., 2022).
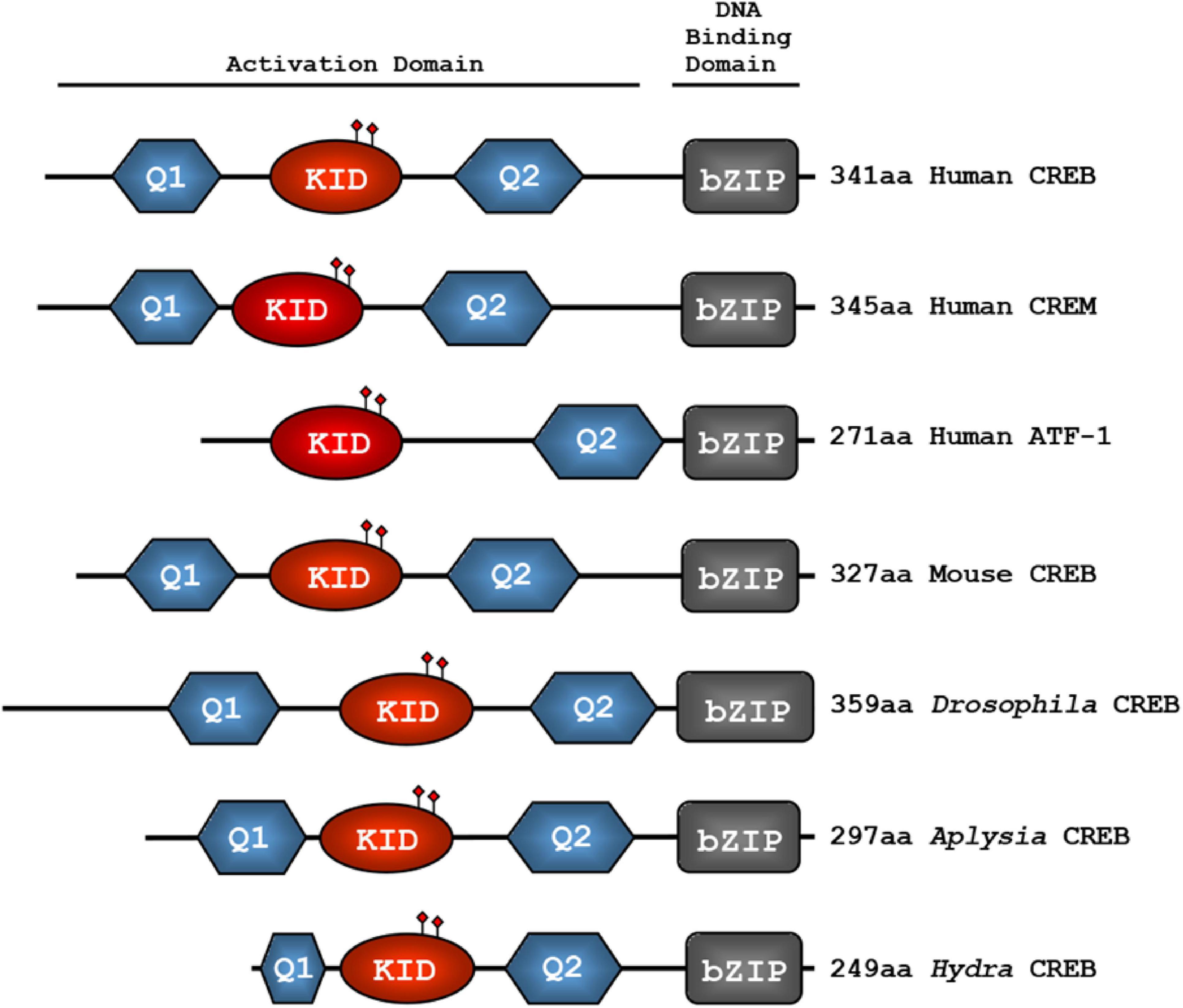
Figure 1. Evolutionarily conserved domains of CREB family transcription factors. The glutamine-rich domains (Q1 and Q2), the kinase-inducible domain (KID), and the basic region/leucine zipper domain (bZIP) are conserved from hydra to human. The KID domain contains several functional phosphorylation sites which are shown in closed diamond shape. The basic region mediates the binding to CRE sequences, whereas the leucine zipper domain induces dimerization. The Q1-KID-Q2 domains together provide transcriptional activation function.
The repressor isoforms lack the Q (Q1 or Q2) and KID domains, for examples: inducible cAMP early repressor (ICER) and cAMP responsive element modulator (S-CREM) are the repressor isoforms of human CREB and CREM, respectively (Walker et al., 1994; Borlikova and Endo, 2009). Interestingly, in Drosophila, both activator and repressor isoforms share the Q1-KID-Q2-bZIP domains, except for a few exons (presence or absence of exon 2, 4, or 6) (Figure 2; Girardet et al., 1996; Poels et al., 2004). On the basis of the sequence orientation of CREB genes, four major functional domains from the N terminal to C terminal and nuclear localization signaling peptide are described below:
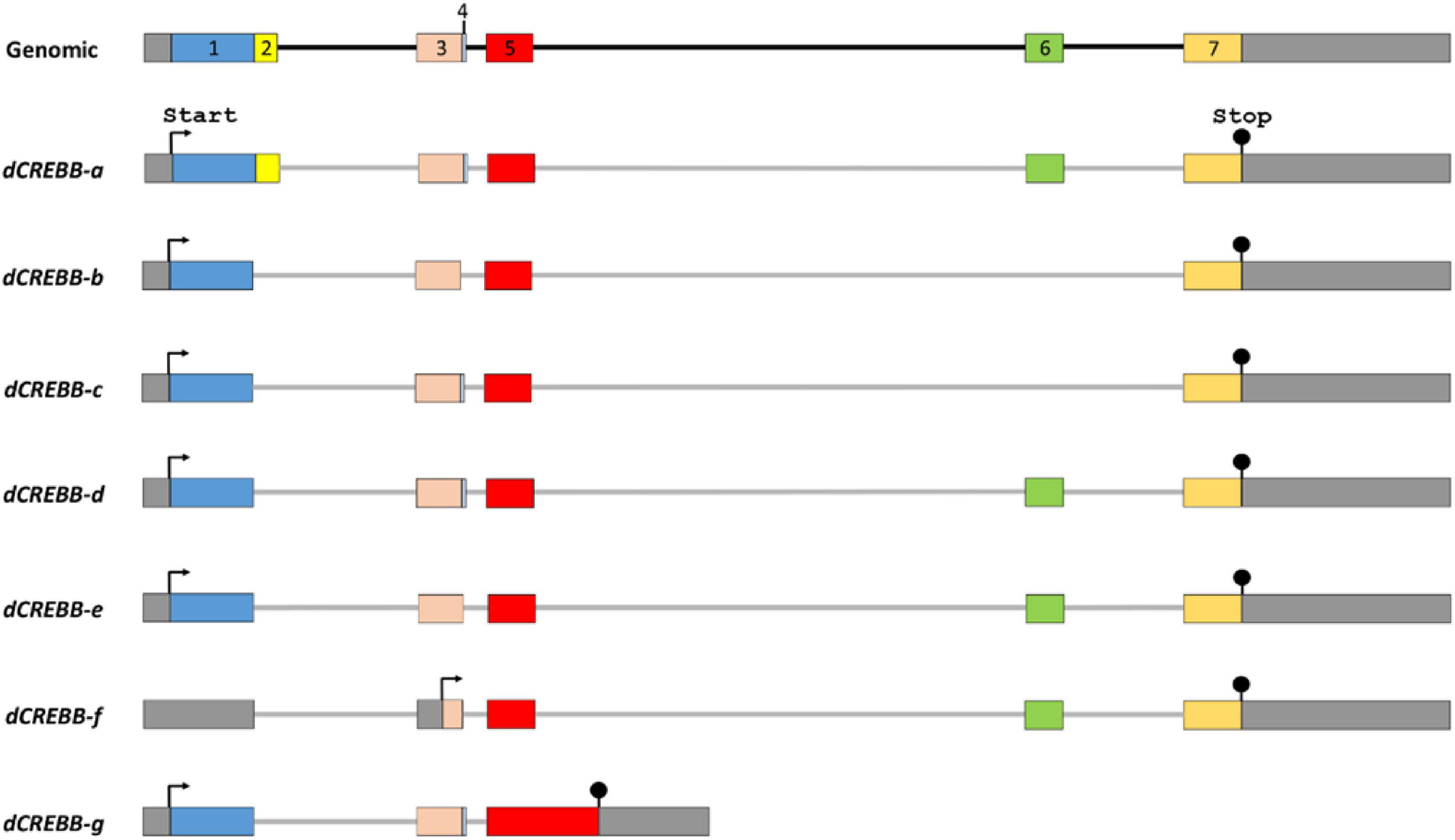
Figure 2. Alternative splicing of the dCREBB gene. Alternative splicing produces at least 7 different protein isoforms from a single dCREBB gene in Drosophila. The protein dCREBB-a contains Q1, Q2, KID, and bZIP domains, whereas the isoform dCREBB-b lacks Q2 domain. Each arrow indicates the start codon (ATG). Stop codon (TAA) is marked by “Stop.”
Glutamine rich 1 (Q1) domain−the Q1 domain is required for transactivation. Intensive studies demonstrate that Q1 has no direct role in the recruitment of co-activators or RNA polymerase II initiation complex (Mayr et al., 2001). However, collectively Q1 and Q2 domains interact with TATA binding protein-associated factor II 135 (TAFII135) and CCAAT/enhancer binding proteins (C/EBPs) to stimulate overall transcription (Horikoshi et al., 1988; Felinski and Quinn, 1999; Chen et al., 2003).
Kinase inducible domain (KID)−contains multiple phosphorylation sites and modulates transcription upon context-dependent phosphorylation by several kinases (Figure 3; Kwok et al., 1994). Specifically, phosphorylation of Ser-133 in the KID domain of CREB increases its binding affinity for the kinase-inducible domain interacting (KIX) domain of the co-activator CREB-binding protein (CBP). The active interaction between phospho-KID and KIX triggers the transcription machinery to bind to CREs, and modulates the transcription of genes to exert biological functions, such as circadian rhythm regulation, and long-term memory (Eckner et al., 1994; Parker et al., 1996; Radhakrishnan et al., 1997; Zor et al., 2002).
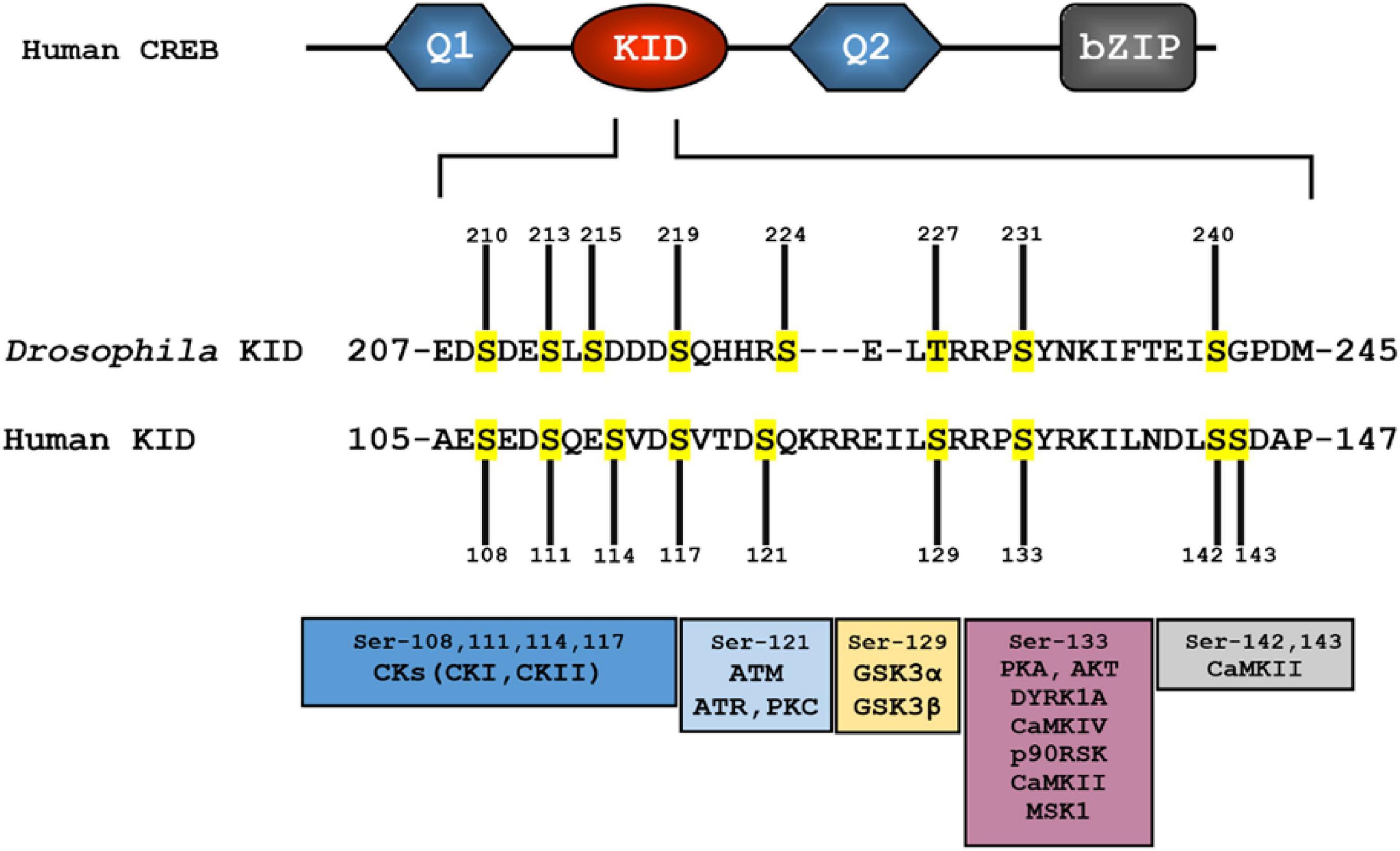
Figure 3. Several phosphorylation sites (in yellow) observed in the human kinase-inducible domain (KID) appear to be conserved in the Drosophila KID. Multiple protein kinases that can phosphorylate specific serine residue(s) are shown in the bottom. Homologous protein kinases to some of human protein kinases are also found in Drosophila (see Table 2).
Glutamine rich 2 (Q2) domain−is responsible for recognizing and binding to the canonical CRE, as well as binding to the RNA polymerase II initiation complex. This constitutive activation domain (CAD) interacts with and recruits the promoter recognition factor TFIID/TAFII130 (Ferreri et al., 1994; Altarejos and Montminy, 2011). Both KID and Q2 domains, collectively known as transactivation domain, are required for basal transcription, and trigger CRE-driven gene expression independently of external stimuli (Zor et al., 2002; Conkright et al., 2003b). Mutations in Q2 impair the overall basal transcriptional activity of CREB (Brindle et al., 1993; Ferreri et al., 1994). Collectively, the two glutamine rich domains Q1 and Q2 are essential for basal transactivation activity (Martinez-Yamout et al., 2023).
Basic/leucine zipper domain (bZIP)−is required for dimerization and binding to the consensus CRE region (Montminy et al., 1986; Delegeane et al., 1987; Hardy and Shenk, 1988; Yamamoto et al., 1988; Hurst et al., 1990; Sassone-Corsi, 1998). Site-directed mutational analysis indicates that this leucine zipper domain is critical for the formation of CREB protein homodimer and transcriptional activity (Behr and Weinbauer, 2000; Craig et al., 2001; Poels and Vanden Broeck, 2004). The binding affinity of CREB to DNA can be modulated by Mg2+ ions; an increase in Mg2+ concentration leads to a 2-fold increase in the binding of phosphorylated CREB to the CRE-binding site, whereas Mg2+ ions facilitate bZIP’s affinity for CRE by more than 25-fold and potentiate gene expression (Schumacher et al., 2000; Moll et al., 2002). Interstingly a recent study demotsrate that CREB basic region and leucine zipper can fold separately and undergoes a clear conformational change upon binding to different types of DNA elements (specific-half or full CRE and nonspecific sequences (Bentley et al., 2023). At the same time Q1-KID-Q2 affects the bZIP conformational landscape and subsequently modulates DNA binding, dimerization, and overall CREB transcriptional functionality (Bentley et al., 2023).
Nuclear localization signal (NLS) peptide−is a short peptide (RRKKK) located in between the basic region and leucine zipper domain of CREB protein (Figures 2, 3), and this NLS peptide facilitates the cytoplasmic CREB proteins to enter the nucleus. Therefore, contextual subcellular localization acts as a molecular switch on the transcriptional activity of CREB (Arnould et al., 2002; Hou et al., 2019). Under pathophysiological condition, epigenetic mechanisms and post-translational modifications, such as phosphorylation and SUMOylation, alter the subcellular distribution of CREB or even in mitochondrial matrix (Waeber and Habener, 1991; Acin-Perez et al., 2009; De Rasmo et al., 2009; Steven and Seliger, 2016; Lu et al., 2021).
CREB family transcription factors consist of multiple domains that play a critical role in regulating diverse cellular functions. Given that precise inter-domain interactions are generally required for the normal function of a multi-domain protein (Xia et al., 2023), the multiple domains of CREB are suggested to cooperatively control CREB-dependent gene expression via distinct domain-domain interactions.
3 Alternative splicing
The splicing of pre-mRNA is a fundamental process in eukaryotic gene expression. Alternative splicing, which contributes greatly to protein diversity, plays an instrumental role in regulating the activity of many transcription factors. Multi-exonic gene CREB undergoes extensive splicing in many animals like Aplysia, Drosophila, and human (Figure 2; Ruppert et al., 1992; Meyer et al., 1993; Yin et al., 1995b). CREB family genes produce multiple spliced isoforms that either enhance or repress gene expression, mediating modulatory function in different biological contexts (Bartsch et al., 1998; Sassone-Corsi, 1998; Blöcher et al., 2003, 2005; Poels et al., 2004; Sadamoto et al., 2010). In Drosophila, CREB proteins are identified as dCREBA and dCREBB (Yin et al., 1995a,b; Andrew et al., 1997; Rose et al., 1997). To date, dCREBB has been identified as 10 transcripts and 10 polypeptides (7 distinct); notable spliced isoforms include dCREBB-a, -b, -c, -d, -e, -f, and -g (Figure 3; Yin et al., 1994, 1995a; 1995b). Several studies confirmed that some isoforms showed remarkable functional differences. A gain-of-function study using CREBB-a and CREBB-b demonstrated that CREBB-a improves olfactory memory, while CREBB-b impairs it (Yin et al., 1994, 1995b). Accordingly, two distinct isoforms may function as a transcriptional activator or repressor (Yin et al., 1994, 1995b; Perazzona et al., 2004), while the specific function of other isoforms in a cellular context remains to be discovered.
The other CREB family protein, dCREBA is required for normal embryonic development (dorso-ventral patterning) and encodes only one type of protein isoform, which is less conserved to mammalian CREB, but shows high conservation to mammalian CREB3L1 (Smolik et al., 1992; Rose et al., 1997). However, recent studies on dCREBA confirm that it promotes protein synthesis-dependent long-term memory (LTM) formation (Lin et al., 2021). Apart from Drosophila, studies confirmed that in Aplysia, at least three different CREB isoforms function in a competitive manner in LTM formation through synaptic facilitation (Upadhya et al., 2004; Mohamed et al., 2005; Liu et al., 2008; Liu and Aguilera, 2009). In addition, Lymnaea CREB1 (Figure 2), a homologue of mammalian CREB, enhanced synaptic facilitation and produced activator and repressor isoforms whose levels of expression changed in a contextual/learning-dependent manner (Sadamoto et al., 2004, 2010, 2011). In a broader sense alternative splicing appears to increase the functional diversity of proteins from a single gene, which should be associated with the gain of new macromolecular interactomes as well as the evolutionary new traits (Wright et al., 2022). Therefore, alternatively spliced CREB gene variants, such as repressor or activator not only functionally exert different biological function, but also possess a fine-tuning regulation over transcriptional activity by facilitating or inhibiting the function of other isoforms (Lin et al., 2021).
4 Differential phosphorylation of CREB
CREB proteins contain a conserved cluster of kinase sites within the KID domain (Figures 1, 3) (Trinh et al., 2013; Thiel et al., 2021). A large number of studies have been conducted in the KID domain to define phosphorylation sites and to determine the physiological stimuli and protein kinases that induce phosphorylation. Also, one intriguing question is in what context, or how, phosphorylation modulates CREB transcriptional activity in a biological process (Gau et al., 2002; Kornhauser et al., 2002). So far, 14 phosphorylation sites observed in human KID include residues S89, S98, T100, S108, S111, S114, S117, T119, S121, S129, S133, S142, S143, and S156 (Figure 3 and Table 2; Sun et al., 1994; Shanware et al., 2007).
CREB is unavoidably phosphorylated in response to a broad spectrum of physiological stimuli that activate a variety of signaling cascades, including the Ras/ERK, PI3K/Akt, Ca2+, nitric oxide, and p38 MAPK signaling pathways (Figure 3; Xing et al., 1998; Arthur et al., 2004; Riccio et al., 2006). Many protein kinases found in diverse signaling pathways have been shown to modulate the CREB activity. These CREB upstream protein kinases include PKA, mitogen-activated protein kinases (MEKs), phosphoinositide 3 kinases (PI3K), protein kinase B (PKB)/AKT, protein kinase C (PKC), Ca2+/calmodulin-dependent protein kinase II (CaMKII), CaMKIV, RSK2, casein kinases I & II (CKI & CKII), ataxia-telangiectasia mutant kinase (ATM), HIK, mitogen and stress-activated protein kinase 1 (MSK1) and MSK2, SGK, TSSK5, and Dyrk1/MNB (Brindle et al., 1993; Enslen et al., 1995; Gubina et al., 2001; Wu and McMurray, 2001; Miyamoto, 2006; Yamashima, 2012). CaMKIV quickly phosphorylates CREB in a transient manner, while signaling from the MEK/ERK pathway induces a slower, but long-lasting phosphorylation of CREB (Impey and Goodman, 2001; Deisseroth and Tsien, 2002). RSK2 enhances robust CREB-dependent transcriptional response. In the case of MSKs, they mediate the late and prolonged phosphorylation of CREB (Xing et al., 1996; Seternes et al., 1999; Misra et al., 2002; Zhu et al., 2019), even though they are unable to enhance recruitment of CBP/p300. MSK-induced CREB phosphorylation downstream of MAPK signaling pathway is required for CREB-dependent gene expression (Naqvi et al., 2014). In addition, forskolin has been able to induce CREB-mediated transcription by consequential activation of adenylyl cyclase and PKA (Seternes et al., 1999; Misra and Pizzo, 2005).
Several reports also indicate that CREB phosphorylation can be promoted by multiple signaling molecules including N-methyl-D-aspartate (NMDA)-type glutamate receptors (Hardingham et al., 2001, 2002; Ghiani et al., 2007), cytosolic tyrosine kinase c-Src (Zhao et al., 2003), fibroblast growth factor receptor 1 (FGFR1) (Stachowiak et al., 2003; Hu et al., 2004; Fang et al., 2005), and estrogen receptors (Lazennec et al., 2001; Szego et al., 2006; Sharma et al., 2007). Major phosphorylation sites within the KID domain can be classified as follows:
Casein kinase site: In mammals and Drosophila, KID has multiple serine residues thought to be targets for casein kinases (CKs). For human CREB, CK sites 108, 111, 114, and 117 are homologous to residues 210, 213, 215, and 219, respectively, found in Drosophila dCREBB (Figure 3). However, human CREB possesses an extra CK site at residue 121. Studies have suggested CKs-mediated phosphorylation exerts functions in the nervous system (Blanquet, 2000; Horiuchi et al., 2004). Although it is reported that phosphorylation of the CK sites prevents DNA binding, while dephosphorylation allows favorable CREB-CRE binding, in that circumstance the exact consequence of phosphorylation for CREB activation remains to be determined (Lee et al., 1990; Armstrong et al., 1997; Pinna, 2002; Kim et al., 2016).
Glycogen synthase kinase 3 site: Ser-129 in human and Ser-227 in Drosophila are believed to be a substrate for glycogen synthase kinase 3 (GSK3) (Fiol et al., 1994). GSK3β-mediated phosphorylation of only Ser-129 leads to inactivation of CREB (Wang et al., 1994) through reducing the DNA binding activity (Figure 3; Bullock and Habener, 1998; Grimes and Jope, 2001a,b). However, in vitro experiments demonstrate that secondary phosphorylation by GSK-3 right after the phosphorylation of PKA plays a vital role for cAMP control of CREB (Fiol et al., 1994; Beurel et al., 2015).
Protein kinase A site: The mammalian Ser-133 residue is homologous to the Drosophila dCREBB Ser-231, which is critical for nuclear localization and transcriptional activity (Riabowol et al., 1988). A serine to alanine (S133A) mutation at this site does not affect binding to CRE sites of target genes, but results in impaired transcription (Figure 3; Du and Montminy, 1998; Shaywitz and Greenberg, 1999; Sakamoto and Frank, 2009). Typically, Ser-133 is phosphorylated by PKA to stabilize the alpha–helix domain of CREB, and allows for recruitment of the CREB co-activators CBP and p300 to induce transcription of target genes, either in homodimer or heterodimer form (Richards et al., 1996; Parker et al., 1998; Fimia et al., 1999; Jean-Rene et al., 2000; Naqvi et al., 2014). Similarly, Ser-133 residue is also phosphorylated by several CaMKs, even before being activated by calcium and calmodulin (Enslen et al., 1994; Matthews et al., 1994; Upadhya et al., 2004; Sakagami et al., 2005; Miyamoto, 2006; Yan et al., 2016).
CaMKIV site: Multiple studies have demonstrated that CaMKIV mediates the early phase of Ser-133 or dCREBB Ser-231 phosphorylation and enhances transcription. Interestingly, CaMKIV also phosphorylates the CREB co-activator CBP at Ser-301, which is considered to be a major target of CaMKIV phosphorylation, both in vitro and in vivo (Girardet et al., 1996).
CaMKII site: CaMKII can phosphorylate human CREB at Ser-133 (dCREBB Ser-231) and a second site, Ser-142 (dCREBB Ser-240) (Sun et al., 1994; Liu et al., 2013a). Phosphorylation of Ser-142 by CaMKII inhibits the dimerization of CREB and induces dissociation of CREB from the CRE (Parker et al., 1998; Wu and McMurray, 2001). Therefore, subsequent phosphorylation at Ser-133 and Ser-142 by CaMKII blocks the transactivation by inhibiting CBP protein interactions (Wu and McMurray, 2001; Deisseroth and Tsien, 2002). Interestingly, molecular manipulation of Ser-142 to alanine (S142A) increases CREB activity, which is induced by CaMKII- and CaMKIV-mediated phosphorylation, and also enhances intracellular Ca2+ accumulation, elevating CREB activity (Sun et al., 1994).
In contrast to other phosphorylation sites, Ser-133 is recurrently phosphorylated, followed by other serine, tyrosine, and threonine residues in the kinase inducible domain (KID), exerting a constructive influence on transcription (Quinn and Granner, 1990). However, there is considerable evidence that other types of CREB phosphorylation patterns can lead to the opposite effect on its transcriptional activity. CKs and ATM-mediated phosphorylation at serine residues 108, 111, 114, 117, and 121 inhibits CREB-mediated transcription by resisting co-activator recruitment and DNA binding, and even interfering with chromatin occupancy (Table 2; Shanware et al., 2007, 2009; Trinh et al., 2013; Kim et al., 2016).
Under rheostat conditions, membrane depolarization with high K+ level facilitates the influx of Ca2+, which induces CREB phosphorylation at Ser-133 and two additional sites, Ser-142 and Ser-143, promoting the activation of CREB (Kornhauser et al., 2002). Interestingly, phosphorylation of Ser-142 by CaMKII inhibits CaMKII-induced CREB activation, whereas the dual phosphorylation of CREB at Ser-142 and Ser-143 interferes with protein-protein interaction between CREB and CBP, raising the possibility that Ca2+ influx-mediated CREB activation in neurons may be independent of a CBP-based mechanism (Sun et al., 1994; Kreusser and Backs, 2014; Atsumi et al., 2024).
Apart from the regulation of physiological functionality, phosphorylation is also responsible for compartmental gating of CREB in different location in the cell. Even though no mitochondrial targeting sequence is present in CREB, Ser-133 phosphorylated form of CREB was found in inner mitochondrial compartment of adult rat brain (Fernandez-Silva et al., 1997; Cammarota et al., 1999). The phospho-CREB (Ser-133) forms a TOM (translocase of the outer membrane) complex with mtHSP70, and is transported into the mitochondrial matrix. Subsequently CREB binds to the CRE-like sequence in the non-coding region of the mitochondrial DNA (mtDNA) or D-loop of mtDNA (Lee et al., 2005; Marinov et al., 2014) and enhances the mitochondrial gene expression, such as ND2, ND5, and ND6 (Lee and Wei, 2005; Ryu et al., 2005). Interestingly any irregularities of CREB-mediated mitochondrial gene expression were shown to lead to neurodegenerative disorders (Lee et al., 2005).
In summary, it is well established that the phosphorylation of Ser-133 by PKA lead to nuclear localization of CREB and promotes the recruitment of co-activator CBP to initiate CREB dependent transcription. Other kinases, such as CaMKII, CaMKIV, RSK, GSK-3, MSK, and MAPK, phosphorylate Ser-133 and/or other neighboring serine residue(s), which affect overall transcriptional activity through the modulation of nuclear gating, DNA binding, dimerization, and recruitment of co-activators (Table 2). Taken together, differential and combinatorial phosphorylation of CREB appears to provide a versatile regulatory mechanism that plays essential roles in diverse biological processes (Deisseroth et al., 1996; Yang et al., 2004a; Restivo et al., 2009; Steven et al., 2020).
5 Homodimerization and heterodimerization
The basic and leucine zipper domains of mammalian CREB and dCREBB show a remarkably high similarity in their amino acid sequences. Approximately 78% of amino acids in leucine zippers and 92 % in putative DNA-binding domains of dCREBB and mammalian CREB, are identical (Hai et al., 1989; Pu and Struhl, 1991). CREB family proteins are organized into distinct domains that form active homodimer and heterodimer to assist interaction with the CREs, co-activators, and the basal transcriptional factors, thereby initiating robust transcription (Figure 1; Montminy, 1997). Functionally, the leucine zipper domain is responsible for dimer formation of CREB (Dwarki et al., 1990; Gui et al., 2012). Each mutation of three leucine residues L1, L2, and L3 in the leucine zipper domain shows significantly reduced DNA binding activity of CREB proteins, whereas the mutation of leucine residue L4 does not affect dimer formation and DNA binding (Loriaux et al., 1993, 1994).
Therefore, the dimer formation of CREB protein is more likely required for binding to CRE sites. In contrast, some mutations, which are introduced into the basic region, affect DNA binding activity drastically, but not dimerization of CREB (Dwarki et al., 1990). CRE binding proteins are structurally and functionally very similar, so they can dimerize with the activator protein 1 (AP-1) family members, such as the Fos, Jun, and ATF protein subfamilies (Hoeffler et al., 1988; Hai and Curran, 1991; Li and Green, 1996; Wu et al., 1998). For dimerization, CRE length plays significant roles. Studies have shown that the dimerization tendency of CREB family proteins is stronger at half CRE, due to higher competition with the CREB/ATF family at the complete CRE sequence. However, homodimers and heterodimers of ATF1 and CREM possess higher stability with full CRE binding than half CRE binding (Muchardt et al., 1990; Shaywitz and Greenberg, 1999).
Other bZIP family transcription factors, such as ATF2 or ATF3, can form heterodimers with c-Jun and c-Fos, but not with CREB or ATF1 (Hai and Curran, 1991; Vlahopoulos et al., 2008). Comparative study suggests that c-Jun/c-Fos and c-Jun/ATF2 & ATF3 heterodimers exhibit a higher affinity for full CRE sequences, but not at half CRE (Glick et al., 2016). It is mentionable that CREB protein fails to heterodimerize with Jun and Fos proteins (Benbrook and Jones, 1990; Jansen et al., 1997). Among the divergence in dimerization, the CREB/CREB homodimer exerts the longest half-life (10–20 minutes) with greater stability and potentiates the active transcriptional activity (Dworkin and Mantamadiotis, 2010). In addition to increasing or decreasing DNA binding affinity, different combinations of dimerization can also create diverse binding specificities, exerting their dual roles in activation and repression. For an example, human CREB5 protein, part of the CREB family, binds to CRE specifically with c-Jun or CRE-BP1 as a homodimer or heterodimer to function as a CRE-dependent trans-activator and act as an oncogene or biological marker in multiple cancers, particularly glioma (Wu et al., 2024). These clarify why different types of homodimers and heterodimers possess differential transcriptional and biological activity (Greschik et al., 1999; Vinson et al., 2006; Mulero et al., 2017).
6 Transcriptional co-activators
CREB-mediated activation of gene transcription depends on the recruitments of specific transcriptional co-activators, such as CREB-regulated transcriptional co-activators (CRTCs) (Iourgenko et al., 2003) and CREB-binding protein (CBP)/p300 (Altarejos and Montminy, 2011; Smith et al., 2021). The phosphorylation and dephosphorylation of CREB serve as a complex balance mechanism for co-activators recruitments and overall CREB transcriptional activity (Amelio et al., 2007; Ravnskjaer et al., 2013). Compared to wild-type CREB, mutant CREB[S133A] showed reduced capacity of CBP recruitment and compromised CREB activity in mice, even though it binds and occupies CRE sites (Gonzalez and Montminy, 1989). Surprisingly, mutant CREB[Y134F] act as a constitutive activator, which stimulate Ser133-dependent recruitment of CBP/p300 and overall transcription (Keyong et al., 2000).
Apart from CBP, CRTCs are activated through dephosphorylation and nuclear entry, subsequently form a transcriptional complex with CREB, CBP NONO, and KAT2B to promote the expression of CREB target genes (Conkright et al., 2003a; Ravnskjaer et al., 2007; He et al., 2009). Recruitment of CRTCs to the promotor enhances the association of CREB with the TFIID subunit TAFII130, rather than affecting the DNA binding activity of CREB (Conkright et al., 2003a).
The functional role of transcriptional co-activator CRTCs and CBP is tightly associated with cAMP and CREB activity (Wood et al., 2005, 2006; Talukdar and Chatterji, 2023). Phospho-KID of CREB interacts with the KIX domain of CBP, which is critical for the regulation of LTM and circadian activity (Chatterjee et al., 2020), whereas CBP was shown to improve cognitive impairments in an Alzheimer’s disease mouse model by increasing BDNF level and CREB activation (Wood et al., 2005; Nagahara et al., 2009; Creighton et al., 2022). CRTC1 regulates leptin-dependent glucose metabolism in diabetic state, CRTC2 endorses glucagon-induced gluconeogenic program in the liver, and CRTC3 controls lipid metabolism (Lv et al., 2016; Qiao et al., 2021). Moreover, these metabolic functions of CRTCs are actively correlated with CREB activity (Mair et al., 2011; Cui et al., 2021; Yoon et al., 2021).
7 Epigenetic, post-transcriptional, and post-translational control of CREB
The CREB regulon (set of CREB target genes) (Table 1) contains one or more consensus CRE sites in their cis-regulatory elements, which are involved in CREB-mediated regulation (Impey et al., 2004). A more extensive scan of the complete genome for CREB binding patterns revealed 1,349 mouse and 1,663 human CREB interacting sites in which the overexpression of 5,000 putative genes was reported upon CREB activation (Zhang et al., 2005). The list of presumed CREB target genes increases day-by-day, and includes genes that are involved in synaptic transmission, immune regulation, cell cycle & survival, metabolic pathways, and signal transduction (Zafra et al., 1992; Shieh et al., 1998; Tao et al., 1998; Mayr et al., 2001; Lonze et al., 2002).
In recent years, bioinformatics methods have been combined with microarrays and chromatin immunoprecipitation (ChIP)-based chromatin occupancy analysis (including ChIP-on-chip and SACO-serial analyses of chromatin occupancy), by which CRE and CREB-regulated gene expression was being studied across a variety of genomic regions (Fass et al., 2003; McClung and Nestler, 2003; Ghia et al., 2004; Zhang et al., 2005). However, the expression pattern analysis with a few CREB-dependent genes provide strong evidence to refute the idea that the expression of target genes is largely regulated by CRE and the phosphorylated CREB proteins (Zhang et al., 2005). Rather, CREB-mediated differential gene expression and functional regulation is controlled by diverse molecular mechanisms including epigenetic modifications, post-translational modifications, such as phosphorylation, methylation, SUMOylation, ubiquitination, glycosylation, and post-transcriptional modifications including microRNAs (miRNAs), and long non-coding RNA molecules (Lamarre-Vincent and Hsieh-Wilson, 2003; Pigazzi et al., 2009; Kaleem et al., 2011; Tan et al., 2012a; Liu et al., 2013b; Chen et al., 2014; Lin et al., 2014; Bordonaro and Lazarova, 2015; Deng et al., 2016; Noguchi et al., 2016; Wang et al., 2023).
Interestingly, CpG methylation of CRE promoters (TGACGTCA-containing CpG dinucleotides) both weakens the specific factor binding, and impairs transcriptional activity, eventually suppressing context-related gene expression (Iguchi-Ariga and Schaffner, 1989; Mancini et al., 1999; Iannello et al., 2000). In addition, HEK293T cells were examined for CREB binding affinity to CREs in both methylated and unmethylated states, and concluded that CREB binding within the genome depends on DNA methylation state in a tissue-specific way and the phosphorylation state of CREB (Jean-Rene et al., 2000; Keyong et al., 2000; Hiroshi et al., 2001) and also that binding to the CRE are not the primary regulators of target gene activation (Zhang et al., 2005). The transcriptional activity and targeted gene expression of CREB require the conscription of other transcriptional apparatus CBP/p300 (Arias et al., 1994; Kwok et al., 1994), CRTC (Iourgenko et al., 2003; He et al., 2009), and TAFII4 to the promoter site (Ferreri et al., 1994; Felinski and Quinn, 1999). CREB-binding protein CBP (CBP, also known as Nejire in Drosophila) and p300 both stand for histone intrinsic acetyltransferase (HAT) linked with transcriptional activators to the basal transcriptional apparatus (Gerritsen et al., 1997; Shankaranarayanan et al., 2001; Lu et al., 2002).
In particular, CBP acetylates the histone H3 at Lys-27, and non-histone protein CREB at three different lysine residues Lys-91, Lys-96, and Lys-136 (Paz et al., 2014; Shaukat et al., 2021). In combination, acetylation of histone H3 at lysine 27 alters the local chromatin environment and/or structure to promote DNA-binding, thereby enhancing CREB-CRE or CREB-CBP-mediated rapid gene expression (Bannister and Kouzarides, 1996; Barral et al., 2014; Li et al., 2015; Atsumi et al., 2024). Acetylation of three different lysine residues in CREB appears to enhance its interaction with both the CRE and CBP, promoting transcription of CREB-responsive genes, such as BDNF. Unexpectedly, mutations of three putative acetylation sites in CREB, which decreased interaction with the CRE or CBP, noticeably activated the gene expression of a cAMP-responsive element-dependent reporter (Lu et al., 2003; Bordonaro and Lazarova, 2015; Attar and Kurdistani, 2017; Guo et al., 2018; Akinsiku et al., 2021). As epigenetic regulation of chromatin accessibility modulates the CREB-dependent transcription, further study is needed to address the regulatory role of CREB acetylation (Kim and Kaang, 2017).
Ubiquitination and SUMO-modification can also affect the quality rheostat of CREB expression, target gene activation, and overall CREB function (Jeoung et al., 2022). Notably, hyperphosphorylated CREB can be subjected to ubiquitination and subsequent proteasomal degradation (Mu et al., 2011). SUMOylation in many cases inhibits the transcriptional activity by modifying subcellular compartmentalization and/or protein-protein interactions of transcriptional activators (Girdwood et al., 2004; Johnson, 2004). CREB contains three SUMO-modification motifs, such as EKSE (residues 154–157), RKRE (residues 284–287), and KKKE (residues 303–306) (Johnson, 2004; Du et al., 2008). Site-directed mutagenesis analysis showed that the K304R mutation in CREB diminishes the SUMO-modification and also prevents its nuclear localization (Comerford et al., 2003; Ryan et al., 2010). Intriguingly, CREB SUMOylation can be controlled by SUMOylation of AKT. Through the BDNF-TrkB signaling, AKT is phosphorylated at T380 and S473, and also SUMOylated at K276, enhancing its stability and kinase activity. Translocated active AKT directly phosphorylates SUMO1 at T76 and makes SUMO1 stable enough to SUMOylate nuclear proteins, such as CREB (Lin et al., 2016). Furthermore, there is a delicate crosstalk between phosphorylation and SUMOylation of CREB (Jeoung et al., 2022). Suppression of SUMOylation promotes the CREB phosphorylation, while preventing phosphorylation antagonizes the SUMOylation of CREB (Chen et al., 2014). Spatial memory training was shown to upregulate the expression of protein inhibitor of activated STAT1 (PIAS1) through phosphorylated CREB in the rat hippocampal CA1 region and then the SUMO E3 ligase PIAS1 then increased SUMOylation of CREB. As a summary, CREB phosphorylation seems to be an early event that sets off long-term memory formation, whereas later-induced CREB SUMOylation appears to be involved in maintaining long-term memory (Chen et al., 2014).
O-glycosylation of proteins is a potent, inducible post-translational alteration that exerts parallel effects, as like the phosphorylation’s event (Love and Hanover, 2005; Hart et al., 2007; Rexach et al., 2008, 2012). Due to the abundance of O-linked N-acetylglucosamine (GlcNAc) glycosylation observed in the brain, it is presumed to be a modulator of CREB activity and functionality (Khidekel et al., 2004; Vosseller et al., 2006). An in vivo study in the mammalian brain found the O-GlcNAc glycosylation of residues 256-261 (Ser-260 and Thr-256, -259, and -261) of the CREB Q2 domain, which impairs more than 50% of basal association with TAFII130/135 (Saluja et al., 1998). In vitro transcription studies showed that O-GlcNAc glycosylation significantly reduced the transcriptional activity of CREB, leading to downregulation of the basal expression of CREB target genes, such as wnt2 and c-Fos (Rexach et al., 2008). These findings suggest that glycosylation acts like a constant repressor of CREB and deviates overall cellular function (Zachara and Hart, 2002; Khidekel et al., 2003; Zhang N. et al., 2022).
CREB has been reported as the direct target of several miRNAs, such as miR-1224, miR-128, miR-134, miR-144, miR-34b, miR-23a, miR-200b, and miR-301 (Tan et al., 2012a; Noguchi et al., 2016; Liu et al., 2020; Yang et al., 2021). In most of the case miRNAs negatively modulate the CREB expression and CREB-mediated signaling pathways through interaction with 3′-UTR of CREB (Steven and Seliger, 2016). Interestingly, CREB was also reported to regulate the expression of certain miR-9 and miR-373 that are involved in different type of cancer/tumor growth (Tan et al., 2012a,b; Zhang et al., 2013). The miR-466f-3p upregulation via CREB activation is associated with spatial learning (Wang I. F. et al., 2021, 2022).
In summary, it is obvious that the activation of CREB by phosphorylation and the presence of CREB response elements (CREs) are necessary, but not sufficient for the control of CREB-mediated target gene expression. A recent surprising report demonstrates that a dephospho-mimic mutant, S133A-CREB, can interact with CRTC family co-activators, leading to enhanced mesenchymal gene expression in mouse lens epithelial cells (LECs) (Zhang et al., 2023). This finding necessitates a re-evaluation of the role of phosphorylation-dependent CREB activation. However, in addition to phosphorylation-dependent regulation, epigenetic changes, non-coding small RNAs and post-translational modifications all cooperates with the transcriptional regulation of CREB to induce the selective expression of its target genes in different cell types.
8 Conclusion and standpoints
Given that the various cellular functions mediated by the CREB family transcription factors require CREB-induced expression of different combination of target genes, how differential gene expression by CREB is achieved is an intriguing question. Here, we present several regulatory modes of CREB family transcription factors that are associated with their pleiotropic functions in the nervous system as well as in various non-neuronal tissues. First, the extensively spliced CREB isoforms are classified as activators or repressors on the basis of functionality, correlating with the structural domain organization. Second, differential and combinatorial phosphorylation of CREB through the KID domain plays an essential role in modulating the transcriptional activity of CREB. A large number of protein kinases that were known to phosphorylate the KID domain function as major mediators of multiple signaling pathways activated in response to different types of physiological stimuli. This explains how several signaling pathways coupled with different cellular functions converge on and integrate with the CREB transcription factors. Third, homodimerization and heterodimerization of CREB proteins provide another regulatory mechanism underlying their differential transcriptional activity. Fourth, distinct transcriptional co-activators, such as CRTCs and CBP, are required for CREB-mediated gene expression. Fifth, epigenetic modifications, such as DNA methylation and histone acetylation, are also used to CREB- or CBP-dependent gene expression. Sixth, several miRNAs were shown to directly target and downregulate CREB genes and interestingly certain miRNA genes were reported to be regulated by CREB, suggesting a potential feedback loop. Lastly, in addition to phosphorylation, ubiquitination, SUMO-modification, and O-glycosylation contribute to the modulation of CREB expression, activity, and target gene expression.
Although great progress has been made in understanding the regulatory mechanisms of the CREB family transcription factors, there are still many unanswered questions. For example, what are the differences in the functions of each CREB isoform in neuronal and non-neuronal cells? Several CREB isoforms across species have been implicated in neuronal memory, but their specific roles as activators or repressors are unclear. Additionally, non-neuronal variants like human testicular htCREB and mitochondrial mitoCREB exist. However, the structural and functional differences between these isoforms remain poorly understood. Further research is needed to clarify these distinctions.
Differential and combinatorial phosphorylation of CREB through the KID domain plays an essential role in modulating the transcriptional activity of CREB. While cAMP and PKA were considered as the key modulator of CREB’s transcriptional activity, recent advancement confirms the involvement of another kinases and stimuli. It’s obvious that CREB phosphorylation appears much more intricate and precise regulatory mechanism than we expect.
Another interesting question is what effect the phosphorylation code has on other post-translational modifications, such as ubiquitination, SUMO-modification, and O-glycosylation. In general, differential gene expression in different types of cells can be regulated by modular and pleiotropic enhancers, distinct combinations of transcription factors, and epigenetic modifications. In this respect, additional researches are needed on other transcription factors and cofactors that work in cooperation with CREB, the CREB enhancer modularity, and epigenetic changes that are influenced by CREB-associated physiological stimuli. Since dysregulated CREB signaling are associated with a wide range of neuronal and non-neuronal diseases, the identification of CREB target genes directly responsible for the onset of these diseases will be of great help in developing their treatments.
Author contributions
MC: Conceptualization, Data curation, Writing−review and editing, Formal analysis, Investigation, Resources, Visualization, Writing−original draft. MH: Data curation, Formal analysis, Investigation, Writing−review and editing. JL: Data curation, Writing−review and editing, Supervision, Validation. SJ: Data curation, Supervision, Validation, Writing−review and editing, Conceptualization, Funding acquisition.
Funding
The author(s) declare financial support was received for the research, authorship, and/or publication of the article. This work was supported by a National Research Foundation (NRF) grant funded by the Korea government (Ministry of Science and ICT) (2021R1I1A3059555 and 2018R1A2B6008037).
Acknowledgments
We thank Dr. Jungeun An for her careful reading and thoughtful comments on our manuscript.
Conflict of interest
The authors declare that the research was conducted in the absence of any commercial or financial relationships that could be construed as a potential conflict of interest.
Publisher’s note
All claims expressed in this article are solely those of the authors and do not necessarily represent those of their affiliated organizations, or those of the publisher, the editors and the reviewers. Any product that may be evaluated in this article, or claim that may be made by its manufacturer, is not guaranteed or endorsed by the publisher.
References
Acin-Perez, R., Salazar, E., Brosel, S., Yang, H., Schon, E. A., and Manfredi, G. (2009). Modulation of mitochondrial protein phosphorylation by soluble adenylyl cyclase ameliorates cytochrome oxidase defects. EMBO Mol. Med. 1, 392–406.
Akinsiku, O. E., Soremekun, O. S., and Soliman, M. E. S. (2021). Update and potential opportunities in CBP [Cyclic adenosine monophosphate (cAMP) response element-binding protein (CREB)-binding protein] research using computational techniques. Protein J. 40, 19–27. doi: 10.1007/s10930-020-09951-8
Allada, R., White, N. E., So, W. V., Hall, J. C., and Rosbash, M. (1998). A mutant Drosophila homolog of mammalian Clock disrupts circadian rhythms and transcription of period and timeless. Cell 93, 791–804. doi: 10.1016/s0092-8674(00)81440-3
Altarejos, J. Y., and Montminy, M. (2011). CREB and the CRTC co-activators: Sensors for hormonal and metabolic signals. Nat. Rev. 12, 141–151. doi: 10.1038/nrm3072
Amelio, A. L., Miraglia, L. J., Conkright, J. J., Mercer, B. A., Batalov, S., Cavett, V., et al. (2007). A coactivator trap identifies NONO (p54nrb) as a component of the cAMP-signaling pathway. Proc. Natl. Acad. Sci. U.S.A. 104, 20314–20319. doi: 10.1073/pnas.0707999105
Andrew, D. J., Baig, A., Bhanot, P., Smolik, S. M., and Henderson, K. D. (1997). The Drosophila dCREB-A gene is required for dorsal/ventral patterning of the larval cuticle. Development 124, 181–193. doi: 10.1242/dev.124.1.181
Arias, J., Alberts, A. S., Brindle, P., Claret, F. X., Smeal, T., Karin, M., et al. (1994). Activation of cAMP and mitogen responsive genes relies on a common nuclear factor. Nature 370, 226–229. doi: 10.1038/370226a0
Armstrong, S. A., Barry, D. A., Leggett, R. W., and Mueller, C. R. (1997). Casein kinase II-mediated phosphorylation of the C terminus of Sp1 decreases its DNA binding activity. J. Biol. Chem. 272, 13489–13495. doi: 10.1074/jbc.272.21.13489
Arnould, T., Vankoningsloo, S., Renard, P., Houbion, A., Ninane, N., Demazy, C., et al. (2002). CREB activation induced by mitochondrial dysfunction is a new signaling pathway that impairs cell proliferation. EMBO J. 21, 53–63. doi: 10.1093/emboj/21.1.53
Arthur, J. S. C., Fong, A. L., Dwyer, J. M., Davare, M., Reese, E., Obrietan, K., et al. (2004). Mitogen- and stress-activated protein kinase 1 mediates cAMP response element-binding protein phosphorylation and activation by neurotrophins. J. Neurosci. 24, 4324–4332. doi: 10.1523/JNEUROSCI.5227-03.2004
Atsumi, Y., Iwata, R., Kimura, H., Vanderhaeghen, P., Yamamoto, N., and Sugo, N. (2024). Repetitive CREB-DNA interactions at gene loci predetermined by CBP induce activity-dependent gene expression in human cortical neurons. Cell Rep. 43:113576. doi: 10.1016/j.celrep.2023.113576
Attar, N., and Kurdistani, S. K. (2017). Exploitation of EP300 and CREBBP lysine acetyltransferases by cancer. Cold Spring Harb. Perspect. Med. 7:6534. doi: 10.1101/cshperspect.a026534
Bannister, A. J., and Kouzarides, T. (1996). The CBP co-activator is a histone acetyltransferase. Nature 384, 641–643. doi: 10.1038/384641a0
Barral, S., Reitz, C., Small, S. A., and Mayeux, R. (2014). Genetic variants in a ‘cAMP element binding protein’ (CREB)-dependent histone acetylation pathway influence memory performance in cognitively healthy elderly individuals. Neurobiol. Aging 35:2881.e2887–2881.e2810. doi: 10.1016/j.neurobiolaging.2014.06.024
Bartsch, D., Casadio, A., Karl, K. A., Serodio, P., and Kandel, E. R. (1998). CREB1 encodes a nuclear activator, a repressor, and a cytoplasmic modulator that form a regulatory unit critical for long-term facilitation. Cell 95, 211–223. doi: 10.1016/s0092-8674(00)81752-3
Behr, R., and Weinbauer, G. F. (2000). CREM activator and repressor isoforms in human testis: Sequence variations and inaccurate splicing during impaired spermatogenesis. Mol. Hum. Reprod. 6, 967–972. doi: 10.1093/molehr/6.11.967
Benbrook, D. M., and Jones, N. C. (1990). Heterodimer formation between CREB and JUN proteins. Oncogene 5, 295–302.
Bentley, E. P., Scholl, D., Wright, P. E., and Deniz, A. A. (2023). Coupling of binding and differential subdomain folding of the intrinsically disordered transcription factor CREB. FEBS Lett. 597, 917–932. doi: 10.1002/1873-3468.14554
Beurel, E., Grieco, S. F., and Jope, R. S. (2015). Glycogen synthase kinase-3 (GSK3): Regulation, actions, and diseases. Pharmacol. Ther. 148, 114–131. doi: 10.1016/j.pharmthera.2014.11.016
Blanquet, P. R. (2000). Casein kinase 2 as a potentially important enzyme in the nervous system. Prog. Neurobiol. 60, 211–246. doi: 10.1016/s0301-0082(99)00026-x
Blobel, G. A., Nakajima, T., Eckner, R., Montminy, M., and Orkin, S. H. (1998). CREB-binding protein cooperates with transcription factor GATA-1 and is required for erythroid differentiation. Proc. Natl. Acad. Sci. U.S.A. 95, 2061–2066. doi: 10.1073/pnas.95.5.2061
Blöcher, S., Behr, R., Weinbauer, G. F., Bergmann, M., and Steger, K. (2003). Different CREM-isoform gene expression between equine and human normal and impaired spermatogenesis. Theriogenology 60, 1357–1369. doi: 10.1016/s0093-691x(03)00142-0
Blöcher, S., Fink, L., Bohle, R. M., Bergmann, M., and Steger, K. (2005). CREM activator and repressor isoform expression in human male germ cells. Int. J. Androl. 28, 215–223. doi: 10.1111/j.1365-2605.2005.00532.x
Bordonaro, M., and Lazarova, D. L. (2015). CREB-binding protein, p300, butyrate, and Wnt signaling in colorectal cancer. World J. Gastroenterol. 21, 8238–8248. doi: 10.3748/wjg.v21.i27.8238
Borlikova, G., and Endo, S. (2009). Inducible cAMP early repressor (ICER) and brain functions. Mol. Neurobiol. 40, 73–86. doi: 10.1007/s12035-009-8072-1
Boulon, S., Dantonel, J.-C., Binet, V., Vié, A., Blanchard, J.-M., Hipskind, R. A., et al. (2002). Oct-1 potentiates CREB-driven cyclin D1 promoter activation via a phospho-CREB- and CREB binding protein-independent mechanism. Mol. Cell. Biol. 22, 7769–7779. doi: 10.1128/MCB.22.22.7769-7779.2002
Brindle, P., Linke, S., and Montminy, M. (1993). Protein-kinase-A-dependent activator in transcription factor CREB reveals new role for CREM repressors. Nature 364, 821–824. doi: 10.1038/364821A0
Brindle, P., Nakajima, T., and Montminy, M. (1995). Multiple protein kinase A-regulated events are required for transcriptional induction by cAMP. Proc. Natl. Acad. Sci. U.S.A. 92, 10521–10525. doi: 10.1073/pnas.92.23.10521
Bullock, B. P., and Habener, J. F. (1998). Phosphorylation of the cAMP response element binding protein CREB by cAMP-dependent protein kinase A and glycogen synthase kinase-3 alters DNA-binding affinity, conformation, and increases net charge. Biochemistry 37, 3795–3809. doi: 10.1021/bi970982t
Cammarota, M., Paratcha, G., Bevilaqua, L. R., Levi de Stein, M., Lopez, M., Pellegrino de Iraldi, A., et al. (1999). Cyclic AMP-responsive element binding protein in brain mitochondria. J. Neurochem. 72, 2272–2277.
Chatterjee, S., Angelakos, C. C., Bahl, E., Hawk, J. D., Gaine, M. E., Poplawski, S. G., et al. (2020). The CBP KIX domain regulates long-term memory and circadian activity. BMC Biol. 18:155–155. doi: 10.1186/s12915-020-00886-1
Chen, Y., Zhuang, S., Cassenaer, S., Casteel, D. E., Gudi, T., Boss, G. R., et al. (2003). Synergism between calcium and cyclic GMP in cyclic AMP response element-dependent transcriptional regulation requires cooperation between CREB and C/EBP-beta. Mol. Cell Biol. 23, 4066–4082.
Chen, Y.-C., Hsu, W.-L., Ma, Y.-L., Tai, D. J. C., and Lee, E. H. Y. (2014). CREB SUMOylation by the E3 ligase PIAS1 enhances spatial memory. J. Neurosci. 34, 9574–9589. doi: 10.1523/JNEUROSCI.4302-13.2014
Chowdhury, M. A. R., An, J., and Jeong, S. (2023). The pleiotropic face of CREB family transcription factors. Mol. Cells 46, 399–413. doi: 10.14348/molcells.2023.2193
Chrivia, J. C., Kwok, R. P., Lamb, N., Hagiwara, M., Montminy, M. R., and Goodman, R. H. (1993). Phosphorylated CREB binds specifically to the nuclear protein CBP. Nature 365, 855–859. doi: 10.1038/365855a0
Citri, Y., Colot, H. V., Jacquier, A. C., Yu, Q., Hall, J. C., Baltimore, D., et al. (1987). A family of unusually spliced biologically active transcripts encoded by a Drosophila clock gene. Nature 326, 42–47. doi: 10.1038/326042a0
Comerford, K. M., Leonard, M. O., Karhausen, J., Carey, R., Colgan, S. P., and Taylor, C. T. (2003). Small ubiquitin-related modifier-1 modification mediates resolution of CREB-dependent responses to hypoxia. Proc. Natl. Acad. Sci. U.S.A. 100, 986–991. doi: 10.1073/pnas.0337412100
Conkright, M. D., Guzmán, E., Flechner, L., Su, A. I., Hogenesch, J. B., and Montminy, M. (2003b). Genome-wide analysis of CREB target genes reveals a core promoter requirement for cAMP responsiveness. Mol. Cell 11, 1101–1108. doi: 10.1016/s1097-2765(03)00134-5
Conkright, M. D., Canettieri, G., Screaton, R., Guzman, E., Miraglia, L., Hogenesch, J. B., et al. (2003a). TORCs: Transducers of regulated CREB activity. Mol. Cell 12, 413–423. doi: 10.1016/J.MOLCEL.2003.08.013
Craig, J. C., Schumacher, M. A., Mansoor, S. E., Farrens, D. L., Brennan, R. G., and Goodman, R. H. (2001). Consensus and variant cAMP-regulated enhancers have distinct CREB-binding properties. J. Biol. Chem. 276, 11719–11728. doi: 10.1074/jbc.M010263200
Creighton, S. D., Jardine, K. H., Desimone, A., Zmetana, M., Castellano, S., Milite, C., et al. (2022). Age-dependent attenuation of spatial memory deficits by the histone acetyltransferase p300/CBP-associated factor (PCAF) in 3xTG Alzheimer’s disease mice. Learn. Mem. 29, 71–76. doi: 10.1101/lm.053536.121
Cui, A., Ding, D., and Li, Y. (2021). Regulation of hepatic metabolism and cell growth by the ATF/CREB family of transcription factors. Diabetes 70, 653–664. doi: 10.2337/dbi20-0006
Darlington, T. K., Wager-Smith, K., Ceriani, M. F., Staknis, D., Gekakis, N., Steeves, T. D., et al. (1998). Closing the circadian loop: CLOCK-induced transcription of its own inhibitors per and tim. Science 280, 1599–1603. doi: 10.1126/science.280.5369.1599
Dash, P. K., Karl, K. A., Colicos, M. A., Prywes, R., and Kandel, E. R. (1991). cAMP response element-binding protein is activated by Ca2+/calmodulin- as well as cAMP-dependent protein kinase. Proc. Natl. Acad. Sci. U.S.A. 88, 5061–5065. doi: 10.1073/pnas.88.11.5061
De Cesare, D., Jacquot, S., Hanauer, A., and Sassone-Corsi, P. (1998). Rsk-2 activity is necessary for epidermal growth factor-induced phosphorylation of CREB protein and transcription of c-fos gene. Proc. Natl. Acad. Sci. U.S.A. 95, 12202–12207. doi: 10.1073/pnas.95.21.12202
De Rasmo, D., Signorile, A., Roca, E., and Papa, S. (2009). cAMP response element-binding protein (CREB) is imported into mitochondria and promotes protein synthesis. FEBS J. 276, 4325–4333. doi: 10.1111/j.1742-4658.2009.07133.x
Deak, M., Clifton, A. D., Lucocq, L. M., and Alessi, D. R. (1998). Mitogen- and stress-activated protein kinase-1 (MSK1) is directly activated by MAPK and SAPK2/p38, and may mediate activation of CREB. EMBO J. 17, 4426–4441. doi: 10.1093/emboj/17.15.4426
Deisseroth, K., and Tsien, R. W. (2002). Dynamic multiphosphorylation passwords for activity-dependent gene expression. Neuron 34, 179–182. doi: 10.1016/S0896-6273(02)00664-5
Deisseroth, K., Bito, H., and Tsien, R. W. (1996). Signaling from synapse to nucleus: Postsynaptic CREB phosphorylation during multiple forms of hippocampal synaptic plasticity. Neuron 16, 89–101. doi: 10.1016/s0896-6273(00)80026-4
Delegeane, A. M., Ferland, L. H., and Mellon, P. L. (1987). Tissue-specific enhancer of the human glycoprotein hormone alpha-subunit gene: Dependence on cyclic AMP-inducible elements. Mol. Cell. Biol. 7, 3994–4002. doi: 10.1128/mcb.7.11.3994-4002.1987
Deng, X., Deng, L., Wang, P., Cheng, C., and Xu, K. (2016). Post-translational modification of CREB-1 decreases collagen I expression by inhibiting the TGF-β1 signaling pathway in rat hepatic stellate cells. Mol. Med. Rep. 14, 5751–5759. doi: 10.3892/mmr.2016.5926
Deutsch, P. J., Hoeffler, J. P., Jameson, J. L., Lin, J. C., and Habener, J. F. (1988). Structural determinants for transcriptional activation by cAMP-responsive DNA elements. J. Biol. Chem. 263, 18466–18472.
Dodson, G. E., and Tibbetts, R. S. (2006). DNA replication stress-induced phosphorylation of cyclic AMP response element-binding protein mediated by ATM. J. Biol. Chem. 281, 1692–1697. doi: 10.1074/jbc.M509577200
Dooley, K. A., Bennett, M. K., and Osborne, T. F. (1999). A critical role for cAMP response element-binding protein (CREB) as a Co-activator in sterol-regulated transcription of 3-hydroxy-3-methylglutaryl coenzyme A synthase promoter. J. Biol. Chem. 274, 5285–5291. doi: 10.1074/jbc.274.9.5285
Du, J. X., Bialkowska, A. B., McConnell, B. B., and Yang, V. W. (2008). SUMOylation regulates nuclear localization of Krüppel-like factor 5. J. Biol. Chem. 283, 31991–32002.
Du, K., and Montminy, M. (1998). CREB is a regulatory target for the protein kinase Akt/PKB. J. Biol. Chem. 273, 32377–32379. doi: 10.1074/jbc.273.49.32377
Dubnau, J., Chiang, A.-S., Grady, L., Barditch, J., Gossweiler, S., McNeil, J., et al. (2003). The staufen/pumilio pathway is involved in Drosophila long-term memory. Curr. Biol. 13, 286–296. doi: 10.1016/s0960-9822(03)00064-2
Dwarki, V. J., Montminy, M., and Verma, I. M. (1990). Both the basic region and the ‘leucine zipper’ domain of the cyclic AMP response element binding (CREB) protein are essential for transcriptional activation. EMBO J. 9, 225–232. doi: 10.1002/j.1460-2075.1990.tb08099.x
Dworkin, S., and Mantamadiotis, T. (2010). Targeting CREB signalling in neurogenesis. Expert Opin. Ther. Targets 14, 869–879. doi: 10.1517/14728222.2010.501332
Eckner, R., Ewen, M. E., Newsome, D., Gerdes, M., DeCaprio, J. A., Lawrence, J. B., et al. (1994). Molecular cloning and functional analysis of the adenovirus E1A-associated 300-kD protein (p300) reveals a protein with properties of a transcriptional adaptor. Genes Dev. 8, 869–884. doi: 10.1101/gad.8.8.869
Eisenhardt, D., Friedrich, A., Stollhoff, N., Müller, U., Kress, H., and Menzel, R. (2003). The AmCREB gene is an ortholog of the mammalian CREB/CREM family of transcription factors and encodes several splice variants in the honeybee brain. Insect Mol. Biol. 12, 373–382. doi: 10.1046/j.1365-2583.2003.00421.x
El Jamali, A., Freund, C., Rechner, C., Scheidereit, C., Dietz, R., and Bergmann, M. W. (2004). Reoxygenation after severe hypoxia induces cardiomyocyte hypertrophy in vitro: Activation of CREB downstream of GSK3beta. FASEB J. 18, 1096–1098. doi: 10.1096/fj.03-1054fje
Enslen, H., Sun, P., Brickey, D., Soderling, S. H., Klamo, E., and Soderling, T. R. (1994). Characterization of Ca2+/calmodulin-dependent protein kinase IV. Role in transcriptional regulation. J. Biol. Chem. 269, 15520–15527.
Enslen, H., Tokumitsu, H., and Soderling, T. R. (1995). Phosphorylation of CREB by CaM-kinase IV activated by CaM-kinase IV kinase. Biochem. Biophys. Res. Commun. 207, 1038–1043. doi: 10.1006/bbrc.1995.1289
Eresh, S., Riese, J., Jackson, D. B., Bohmann, D., and Bienz, M. (1997). A CREB-binding site as a target for decapentaplegic signalling during Drosophila endoderm induction. EMBO J. 16, 2014–2022. doi: 10.1093/emboj/16.8.2014
Fang, X., Stachowiak, E. K., Dunham-Ems, S. M., Klejbor, I., and Stachowiak, M. K. (2005). Control of CREB-binding protein signaling by nuclear fibroblast growth factor receptor-1: A novel mechanism of gene regulation. J. Biol. Chem. 280, 28451–28462. doi: 10.1074/jbc.M504400200
Fass, D. M., Butler, J. E. F., and Goodman, R. H. (2003). Deacetylase activity is required for cAMP activation of a subset of CREB target genes. J. Biol. Chem. 278, 43014–43019. doi: 10.1074/jbc.M305905200
Felinski, E. A., and Quinn, P. G. (1999). The CREB constitutive activation domain interacts with TATA-binding protein-associated factor 110 (TAF110) through specific hydrophobic residues in one of the three subdomains required for both activation and TAF110 binding*. J. Biol. Chem. 274, 11672–11678. doi: 10.1074/jbc.274.17.11672
Fernandez-Silva, P., Martinez-Azorin, F., Micol, V., and Attardi, G. (1997). The human mitochondrial transcription termination factor (mTERF) is a multizipper protein but binds to DNA as a monomer, with evidence pointing to intramolecular leucine zipper interactions. EMBO J. 16, 1066–1079.
Ferreri, K., Gill, G., and Montminy, M. (1994). The cAMP-regulated transcription factor CREB interacts with a component of the TFIID complex. Proc. Natl. Acad. Sci. U.S.A. 91, 1210–1213. doi: 10.1073/pnas.91.4.1210
Fimia, G. M., De Cesare, D., and Sassone-Corsi, P. (1999). CBP-independent activation of CREM and CREB by the LIM-only protein ACT. Nature 398, 165–169. doi: 10.1038/18237
Fiol, C. J., Williams, J. S., Chou, C. H., Wang, Q. M., Roach, P. J., and Andrisani, O. M. (1994). A secondary phosphorylation of CREB341 at Ser129 is required for the cAMP-mediated control of gene expression. A role for glycogen synthase kinase-3 in the control of gene expression. J. Biol. Chem. 269, 32187–32193.
Foulkes, N. S., Borrelli, E., and Sassone-Corsi, P. (1991). CREM gene: Use of alternative DNA-binding domains generates multiple antagonists of cAMP-induced transcription. Cell 64, 739–749. doi: 10.1016/0092-8674(91)90503-q
Gau, D., Lemberger, T., von Gall, C., Kretz, O., Le Minh, N., Gass, P., et al. (2002). Phosphorylation of CREB Ser142 regulates light-induced phase shifts of the circadian clock. Neuron 34, 245–253. doi: 10.1016/s0896-6273(02)00656-6
Gerritsen, M. E., Williams, A. J., Neish, A. S., Moore, S., Shi, Y., and Collins, T. (1997). CREB-binding protein/p300 are transcriptional coactivators of p65. Proc. Natl. Acad. Sci. U.S.A. 94, 2927–2932. doi: 10.1073/pnas.94.7.2927
Ghia, E., Royce, T. E., Paul, B., Rebecca, M., Rinn, J. L., Kenneth, N. F., et al. (2004). CREB Binds to Multiple Loci on Human Chromosome 22. Mol. Cell. Biol. 24, 3804–3814. doi: 10.1128/MCB.24.9.3804-3814.2004
Ghiani, C. A., Beltran-Parrazal, L., Sforza, D. M., Malvar, J. S., Seksenyan, A., Cole, R., et al. (2007). Genetic program of neuronal differentiation and growth induced by specific activation of NMDA receptors. Neurochem. Res. 32, 363–376. doi: 10.1007/s11064-006-9213-9
Girardet, C., Walker, W. H., and Habener, J. F. (1996). An alternatively spliced polycistronic mRNA encoding cyclic adenosine 3′,5′-monophosphate (cAMP)-responsive transcription factor CREB (cAMP response element-binding protein) in human testis extinguishes expression of an internally translated inhibitor CR. Mol. Endocrinol. 10, 879–891. doi: 10.1210/mend.10.7.8813728
Girdwood, D. W. H., Tatham, M. H., and Hay, R. T. (2004). SUMO and transcriptional regulation. Semin. Cell Dev. Biol. 15, 201–210. doi: 10.1016/j.semcdb.2003.12.001
Glick, Y., Orenstein, Y., Chen, D., Avrahami, D., Zor, T., Shamir, R., et al. (2016). Integrated microfluidic approach for quantitative high-throughput measurements of transcription factor binding affinities. Nucleic Acids Res. 44:e51. doi: 10.1093/nar/gkv1327
Gonzalez, G. A., and Montminy, M. R. (1989). Cyclic AMP stimulates somatostatin gene transcription by phosphorylation of CREB at serine 133. Cell 59, 675–680. doi: 10.1016/0092-8674(89)90013-5
Gonzalez, G. A., Yamamoto, K. K., Fischer, W. H., Karr, D., Menzel, P., and Biggs, W. III, et al. (1989). A cluster of phosphorylation sites on the cyclic AMP-regulated nuclear factor CREB predicted by its sequence. Nature 337, 749–752. doi: 10.1038/337749a0
Greschik, H., Wurtz, J. M., Hublitz, P., Köhler, F., Moras, D., and Schüle, R. (1999). Characterization of the DNA-binding and dimerization properties of the nuclear orphan receptor germ cell nuclear factor. Mol. Cell. Biol. 19, 690–703. doi: 10.1128/MCB.19.1.690
Grimes, C. A., and Jope, R. S. (2001a). CREB DNA binding activity is inhibited by glycogen synthase kinase-3 beta and facilitated by lithium. J. Neurochem. 78, 1219–1232. doi: 10.1046/j.1471-4159.2001.00495.x
Grimes, C. A., and Jope, R. S. (2001b). The multifaceted roles of glycogen synthase kinase 3beta in cellular signaling. Prog. Neurobiol. 65, 391–426. doi: 10.1016/s0301-0082(01)00011-9
Gubina, E., Luo, X., Kwon, E., Sakamoto, K., Shi, Y. F., and Mufson, R. A. (2001). betac cytokine receptor-induced stimulation of cAMP response element binding protein phosphorylation requires protein kinase C in myeloid cells: A novel cytokine signal transduction cascade. J. Immunol. 167, 4303–4310. doi: 10.4049/jimmunol.167.8.4303
Gui, B., Han, X., Zhang, Y., Liang, J., Wang, D., Xuan, C., et al. (2012). Dimerization of ZIP promotes its transcriptional repressive function and biological activity. Int. J. Biochem. Cell Biol. 44, 886–895. doi: 10.1016/j.biocel.2012.02.012
Guo, P., Chen, W., Li, H., Li, M., and Li, L. (2018). The Histone Acetylation Modifications of Breast Cancer and their Therapeutic Implications. Pathol. Oncol. Res. 24, 807–813. doi: 10.1007/s12253-018-0433-5
Hai, T. W., Liu, F., Coukos, W. J., and Green, M. R. (1989). Transcription factor ATF cDNA clones: An extensive family of leucine zipper proteins able to selectively form DNA-binding heterodimers. Genes Dev. 3, 2083–2090. doi: 10.1101/gad.3.12b.2083
Hai, T., and Curran, T. (1991). Cross-family dimerization of transcription factors Fos/Jun and ATF/CREB alters DNA binding specificity. Proc. Natl. Acad. Sci. U.S.A. 88, 3720–3724. doi: 10.1073/pnas.88.9.3720
Hai, T., and Hartman, M. G. (2001). The molecular biology and nomenclature of the activating transcription factor/cAMP responsive element binding family of transcription factors: Activating transcription factor proteins and homeostasis. Gene 273, 1–11. doi: 10.1016/s0378-1119(01)00551-0
Hansen, T. V. O., Rehfeld, J. F., and Nielsen, F. C. (2004). GSK-3beta reduces cAMP-induced cholecystokinin gene expression by inhibiting CREB binding. Neuroreport 15, 841–845. doi: 10.1097/00001756-200404090-00021
Hardingham, G. E., Arnold, F. J., and Bading, H. (2001). A calcium microdomain near NMDA receptors: On switch for ERK-dependent synapse-to-nucleus communication. Nat. Neurosci. 4, 565–566. doi: 10.1038/88380
Hardingham, G. E., Fukunaga, Y., and Bading, H. (2002). Extrasynaptic NMDARs oppose synaptic NMDARs by triggering CREB shut-off and cell death pathways. Nat. Neurosci. 5, 405–414. doi: 10.1038/nn835
Hardy, S., and Shenk, T. (1988). Adenoviral control regions activated by E1A and the cAMP response element bind to the same factor. Proc. Natl. Acad. Sci. U.S.A. 85, 4171–4175. doi: 10.1073/pnas.85.12.4171
Hart, G. W., Housley, M. P., and Slawson, C. (2007). Cycling of O-linked beta-N-acetylglucosamine on nucleocytoplasmic proteins. Nature 446, 1017–1022. doi: 10.1038/nature05815
He, L., Sabet, A., Djedjos, S., Miller, R., Sun, X., Hussain, M. A., et al. (2009). Metformin and insulin suppress hepatic gluconeogenesis through phosphorylation of CREB binding protein. Cell 137, 635–646. doi: 10.1016/j.cell.2009.03.016
Helfrich-Förster, C. (2000). Differential control of morning and evening components in the activity rhythm of Drosophila melanogaster–sex-specific differences suggest a different quality of activity. J. Biol. Rhythms 15, 135–154. doi: 10.1177/074873040001500208
Hiroshi, A., Buyung, S., Ernesto, G., Keyong, D., and Irwin, D. (2001). Chromatin-dependent cooperativity between constitutive and inducible activation domains in CREB. Mol. Cell. Biol. 21:2001. doi: 10.1128/MCB.21.23.7892-7900.2001
Hoeffler, J. P., Meyer, T. E., Yun, Y., Jameson, J. L., and Habener, J. F. (1988). Cyclic AMP-responsive DNA-binding protein: Structure based on a cloned placental cDNA. Science 242, 1430–1433. doi: 10.1126/science.2974179
Horikoshi, M., Hai, T., Lin, Y. S., Green, M. R., and Roeder, R. G. (1988). Transcription factor ATF interacts with the TATA factor to facilitate establishment of a preinitiation complex. Cell 54, 1033–1042. doi: 10.1016/0092-8674(88)90118-3
Horiuchi, J., Jiang, W., Zhou, H., Wu, P., and Yin, J. C. P. (2004). Phosphorylation of conserved casein kinase sites regulates cAMP-response element-binding protein DNA binding in Drosophila. J. Biol. Chem. 279, 12117–12125. doi: 10.1074/jbc.M212839200
Hou, L., Li, B., Ding, D., Kang, L., and Wang, X. (2019). CREB-B acts as a key mediator of NPF/NO pathway involved in phase-related locomotor plasticity in locusts. PLoS Genet. 15:e1008176. doi: 10.1371/journal.pgen.1008176
Hu, Y., Fang, X., Dunham, S. M., Prada, C., Stachowiak, E. K., and Stachowiak, M. K. (2004). 90-kDa ribosomal S6 kinase is a direct target for the nuclear fibroblast growth factor receptor 1 (FGFR1): Role in FGFR1 signaling. J. Biol. Chem. 279, 29325–29335. doi: 10.1074/jbc.M311144200
Hurst, H. C., Masson, N., Jones, N. C., and Lee, K. A. (1990). The cellular transcription factor CREB corresponds to activating transcription factor 47 (ATF-47) and forms complexes with a group of polypeptides related to ATF-43. Mol. Cell. Biol. 10, 6192–6203. doi: 10.1128/mcb.10.12.6192-6203.1990
Iannello, R. C., Gould, J. A., Young, J. C., Giudice, A., Medcalf, R., and Kola, I. (2000). Methylation-dependent silencing of the testis-specific pdha-2 basal promoter occurs through selective targeting of an activating transcription factor/cAMP-responsive element-binding site *. J. Biol. Chem. 275, 19603–19608. doi: 10.1074/jbc.M001867200
Iguchi-Ariga, S. M., and Schaffner, W. (1989). CpG methylation of the cAMP-responsive enhancer/promoter sequence TGACGTCA abolishes specific factor binding as well as transcriptional activation. Genes Dev. 3, 612–619. doi: 10.1101/gad.3.5.612
Imoto, T., Minoshima, M., Yokoyama, T., Emery, B. P., Bull, S. D., Bito, H., et al. (2020). A photodeactivatable antagonist for controlling CREB-dependent gene expression. ACS Cent. Sci. 6, 1813–1818. doi: 10.1021/acscentsci.0c00736
Impey, S., and Goodman, R. H. (2001). CREB signaling–timing is everything. Sci. STKE 2001:e1. doi: 10.1126/stke.2001.82.pe1
Impey, S., McCorkle, S. R., Cha-Molstad, H., Dwyer, J. M., Yochum, G. S., Boss, J. M., et al. (2004). Defining the CREB regulon: A genome-wide analysis of transcription factor regulatory regions. Cell 119, 1041–1054. doi: 10.1016/j.cell.2004.10.032
Iourgenko, V., Zhang, W., Mickanin, C., Daly, I., Jiang, C., Hexham, J. M., et al. (2003). Identification of a family of cAMP response element-binding protein coactivators by genome-scale functional analysis in mammalian cells. Proc. Natl. Acad. Sci. U.S.A. 100, 12147–12152. doi: 10.1073/pnas.1932773100
Jackson, F. R., Bargiello, T. A., Yun, S. H., and Young, M. W. (1986). Product of per locus of Drosophila shares homology with proteoglycans. Nature 320, 185–188. doi: 10.1038/320185a0
Jansen, E., Ayoubi, T. A., Meulemans, S. M., and Van de Ven, W. J. (1997). Cell type-specific protein-DNA interactions at the cAMP response elements of the prohormone convertase 1 promoter. Evidence for additional transactivators distinct from CREB/ATF family members. J. Biol. Chem. 272, 2500–2508. doi: 10.1074/jbc.272.4.2500
Jean-Rene, C., Notis, J. C., Qinghong, Z., Ngan, V., Craig, J. C., Fass, D. M., et al. (2000). Recruitment of CREB binding protein is sufficient for CREB-mediated gene activation. Mol. Cell. Biol. 20, 1546–1552. doi: 10.1128/MCB.20.5.1546-1552.2000
Jeoung, S. W., Park, H. S., Ryoo, Z. Y., Cho, D. H., Lee, H. S., and Ryu, H. Y. (2022). SUMOylation and major depressive disorder. Int. J. Mol. Sci. 23:8023. doi: 10.3390/ijms23148023
Jiang, H., Liu, L., Yang, S., Tomomi, T., and Toru, N. (2008). CREB-binding proteins (CBP) as a transcriptional coactivator of GATA-2. Sci. China Ser. C Life Sci. 51, 191–198. doi: 10.1007/s11427-008-0038-4
Johnson, E. S. (2004). Protein modification by SUMO. Annu. Rev. Biochem. 73, 355–382. doi: 10.1146/annurev.biochem.73.011303.074118
Kaleem, A., Hoessli, D. C., Haq, I. U., Walker-Nasir, E., Butt, A., Iqbal, Z., et al. (2011). CREB in long-term potentiation in hippocampus: Role of post-translational modifications-studies In silico. J. Cell Biochem. 112, 138–146.
Kandel, E. R. (2012). The molecular biology of memory: cAMP, PKA, CRE, CREB-1, CREB-2, and CPEB. Mol. Brain 5:14. doi: 10.1186/1756-6606-5-14
Kanellopoulos, A. K., Semelidou, O., Kotini, A. G., Anezaki, M., and Skoulakis, E. M. C. (2012). Learning and memory deficits consequent to reduction of the fragile X mental retardation protein result from metabotropic glutamate receptor-mediated inhibition of cAMP signaling in Drosophila. J. Neurosci. 32, 13111–13124. doi: 10.1523/JNEUROSCI.1347-12.2012
Katoh, Y., Itoh, K., Yoshida, E., Miyagishi, M., Fukamizu, A., and Yamamoto, M. (2001). Two domains of Nrf2 cooperatively bind CBP, a CREB binding protein, and synergistically activate transcription. Genes Cells 6, 857–868. doi: 10.1046/j.1365-2443.2001.00469.x
Keyong, D., Hiroshi, A., Jhala, U. S., Wagner, B. L., and Marc, M. (2000). Characterization of a CREB gain-of-function mutant with constitutive transcriptional activity in vivo. Mol. Cell. Biol. 20, 4320–4327. doi: 10.1128/MCB.20.12.4320-4327.2000
Khidekel, N., Arndt, S., Lamarre-Vincent, N., Lippert, A., Poulin-Kerstien, K. G., Ramakrishnan, B., et al. (2003). A chemoenzymatic approach toward the rapid and sensitive detection of O-GlcNAc posttranslational modifications. J. Am. Chem. Soc. 125, 16162–16163. doi: 10.1021/ja038545r
Khidekel, N., Ficarro, S. B., Peters, E. C., and Hsieh-Wilson, L. C. (2004). Exploring the O-GlcNAc proteome: Direct identification of O-GlcNAc-modified proteins from the brain. Proc. Natl. Acad. Sci. U.S.A. 101, 13132–13137. doi: 10.1073/pnas.0403471101
Kim, S. H., Trinh, A. T., Larsen, M. C., Mastrocola, A. S., Jefcoate, C. R., Bushel, P. R., et al. (2016). Tunable regulation of CREB DNA binding activity couples genotoxic stress response and metabolism. Nucleic Acids Res. 44, 9667–9680. doi: 10.1093/nar/gkw643
Kim, S., and Kaang, B.-K. (2017). Epigenetic regulation and chromatin remodeling in learning and memory. Exp. Mol. Med. 49:e281. doi: 10.1038/emm.2016.140
Kloss, B., Price, J. L., Saez, L., Blau, J., Rothenfluh, A., Wesley, C. S., et al. (1998). The Drosophila clock gene double-time encodes a protein closely related to human casein kinase Iepsilon. Cell 94, 97–107. doi: 10.1016/s0092-8674(00)81225-8
Kornhauser, J. M., Cowan, C. W., Shaywitz, A. J., Dolmetsch, R. E., Griffith, E. C., Hu, L. S., et al. (2002). CREB transcriptional activity in neurons is regulated by multiple, calcium-specific phosphorylation events. Neuron 34, 221–233. doi: 10.1016/s0896-6273(02)00655-4
Kreusser, M. M., and Backs, J. (2014). Integrated mechanisms of CaMKII-dependent ventricular remodeling. Front. Pharmacol. 5:36–36. doi: 10.3389/fphar.2014.00036
Kwok, R. P. S., Lundblad, J. R., Chrivia, J. C., Richards, J. P., Bächinger, H. P., Brennan, R. G., et al. (1994). Nuclear protein CBP is a coactivator for the transcription factor CREB. Nature 370, 223–226. doi: 10.1038/370223a0
Lalli, E., Lee, J. S., Lamas, M., Tamai, K., Zazopoulos, E., Nantel, F., et al. (1996). The nuclear response to cAMP: Role of transcription factor CREM. Philos. Trans. R. Soc. Lond. Ser. B Biol. Sci. 351, 201–209. doi: 10.1098/rstb.1996.0017
Lamarre-Vincent, N., and Hsieh-Wilson, L. C. (2003). Dynamic glycosylation of the transcription factor CREB: A potential role in gene regulation. J. Am. Chem. Soc. 125, 6612–6613. doi: 10.1021/ja028200t
Lazennec, G., Thomas, J. A., and Katzenellenbogen, B. S. (2001). Involvement of cyclic AMP response element binding protein (CREB) and estrogen receptor phosphorylation in the synergistic activation of the estrogen receptor by estradiol and protein kinase activators. J. Steroid Biochem. Mol. Biol. 77, 193–203. doi: 10.1016/s0960-0760(01)00060-7
Lee, C. Q., Yun, Y. D., Hoeffler, J. P., and Habener, J. F. (1990). Cyclic-AMP-responsive transcriptional activation of CREB-327 involves interdependent phosphorylated subdomains. EMBO J. 9, 4455–4465. doi: 10.1002/j.1460-2075.1990.tb07896.x
Lee, H. C., and Wei, Y. H. (2005). Mitochondrial biogenesis and mitochondrial DNA maintenance of mammalian cells under oxidative stress. Int. J. Biochem. Cell Biol. 37, 822–834.
Lee, J., Kim, C. H., Simon, D. K., Aminova, L. R., Andreyev, A. Y., Kushnareva, Y. E., et al. (2005). Mitochondrial cyclic AMP response element-binding protein (CREB) mediates mitochondrial gene expression and neuronal survival. J. Biol. Chem. 280, 40398–40401.
Li, X. Y., and Green, M. R. (1996). Intramolecular inhibition of activating transcription factor-2 function by its DNA-binding domain. Genes Dev. 10, 517–527. doi: 10.1101/gad.10.5.517
Li, X., Zhang, B., Wu, Q., Ci, X., Zhao, R., Zhang, Z., et al. (2015). Interruption of KLF5 acetylation converts its function from tumor suppressor to tumor promoter in prostate cancer cells. Int. J. Cancer 136, 536–546. doi: 10.1002/ijc.29028
Lin, C. H., Liu, S. Y., and Lee, E. H. Y. (2016). SUMO modification of Akt regulates global SUMOylation and substrate SUMOylation specificity through Akt phosphorylation of Ubc9 and SUMO1. Oncogene 35, 595–607. doi: 10.1038/onc.2015.115
Lin, H.-W., Chen, C.-C., de Belle, J. S., Tully, T., and Chiang, A.-S. (2021). CREBA and CREBB in two identified neurons gate long-term memory formation in Drosophila. Proc. Natl. Acad. Sci. U.S.A. 118:e2100624118. doi: 10.1073/pnas.2100624118
Lin, X. P., Feng, L., Xie, C. G., Chen, D. B., Pei, Z., Liang, X. L., et al. (2014). Valproic acid attenuates the suppression of acetyl histone H3 and CREB activity in an inducible cell model of Machado-Joseph disease. Int. J. Dev. Neurosci. 38, 17–22. doi: 10.1016/j.ijdevneu.2014.07.004
Liu, Y., and Aguilera, G. (2009). Cyclic AMP inducible early repressor mediates the termination of corticotropin releasing hormone transcription in hypothalamic neurons. Cell. Mol. Neurobiol. 29, 1275–1281. doi: 10.1007/s10571-009-9423-1
Liu, Y., Kamitakahara, A., Kim, A. J., and Aguilera, G. (2008). Cyclic adenosine 3′,5′-monophosphate responsive element binding protein phosphorylation is required but not sufficient for activation of corticotropin-releasing hormone transcription. Endocrinology 149, 3512–3520. doi: 10.1210/en.2008-0052
Liu, Y., Sun, L.-Y., Singer, D. V., Ginnan, R., and Singer, H. A. (2013a). CaMKIIδ-dependent inhibition of cAMP-response element-binding protein activity in vascular smooth muscle. J. Biol. Chem. 288, 33519–33529. doi: 10.1074/jbc.M113.490870
Liu, Y., Zhao, Z., Yang, F., Gao, Y., Song, J., and Wan, Y. (2013b). microRNA-181a is involved in insulin-like growth factor-1-mediated regulation of the transcription factor CREB1. J. Neurochem. 126, 771–780. doi: 10.1111/jnc.12370
Liu, Z. Y., Lu, M., Liu, J., Wang, Z. N., Wang, W. W., Li, Y., et al. (2020). MicroRNA-144 regulates angiotensin II-induced cardiac fibroblast activation by targeting CREB. Exp. Ther. Med. 20, 2113–2121. doi: 10.3892/etm.2020.8901
Lonze, B. E., Riccio, A., Cohen, S., and Ginty, D. D. (2002). Apoptosis, axonal growth defects, and degeneration of peripheral neurons in mice lacking CREB. Neuron 34, 371–385. doi: 10.1016/s0896-6273(02)00686-4
Loriaux, M. M., Brennan, R. G., and Goodman, R. H. (1994). Modulatory function of CREB.CREM alpha heterodimers depends upon CREM alpha phosphorylation. J. Biol. Chem. 269, 28839–28843. doi: 10.1016/S0021-9258(19)61983-6
Loriaux, M. M., Rehfuss, R. P., Brennan, R. G., and Goodman, R. H. (1993). Engineered leucine zippers show that hemiphosphorylated CREB complexes are transcriptionally active. Proc. Natl. Acad. Sci. U.S.A. 90, 9046–9050. doi: 10.1073/pnas.90.19.9046
Love, D. C., and Hanover, J. A. (2005). The hexosamine signaling pathway: Deciphering the “O-GlcNAc code”. Sci. STKE 2005:re13. doi: 10.1126/stke.3122005re13
Lu, H., Pise-Masison, C. A., Fletcher, T. M., Schiltz, R. L., Nagaich, A. K., Radonovich, M., et al. (2002). Acetylation of nucleosomal histones by p300 facilitates transcription from tax-responsive human T-cell leukemia virus type 1 chromatin template. Mol. Cell. Biol. 22, 4450–4462. doi: 10.1128/MCB.22.13.4450-4462.2002
Lu, J., Wu, T., Zhang, B., Liu, S., Song, W., Qiao, J., et al. (2021). Types of nuclear localization signals and mechanisms of protein import into the nucleus. Cell Commun. Signal. 19, 60–60. doi: 10.1186/s12964-021-00741-y
Lu, Q., Hutchins, A. E., Doyle, C. M., Lundblad, J. R., and Kwok, R. P. S. (2003). Acetylation of cAMP-responsive element-binding protein (CREB) by CREB-binding protein enhances CREB-dependent transcription. J. Biol. Chem. 278, 15727–15734. doi: 10.1074/jbc.M300546200
Luo, Q., Viste, K., Urday-Zaa, J. C., Senthil Kumar, G., Tsai, W.-W., Talai, A., et al. (2012). Mechanism of CREB recognition and coactivation by the CREB-regulated transcriptional coactivator CRTC2. Proc. Natl. Acad. Sci. U.S.A. 109, 20865–20870. doi: 10.1073/pnas.1219028109
Lv, S., Qiu, X., Li, J., Li, W., Zhang, C., Zhang, Z.-N., et al. (2016). Suppression of CRTC2-mediated hepatic gluconeogenesis by TRAF6 contributes to hypoglycemia in septic shock. Cell Discov. 2:16046. doi: 10.1038/celldisc.2016.46
Mair, W., Morantte, I., Rodrigues, A. P. C., Manning, G., Montminy, M., Shaw, R. J., et al. (2011). Lifespan extension induced by AMPK and calcineurin is mediated by CRTC-1 and CREB. Nature 470, 404–408. doi: 10.1038/nature09706
Mancini, D. N., Singh, S. M., Archer, T. K., and Rodenhiser, D. I. (1999). Site-specific DNA methylation in the neurofibromatosis (NF1) promoter interferes with binding of CREB and SP1 transcription factors. Oncogene 18, 4108–4119. doi: 10.1038/sj.onc.1202764
Manning, C. E., Williams, E. S., and Robison, A. J. (2017). Reward network immediate early gene expression in mood disorders. Front. Behav. Neurosci. 11:77. doi: 10.3389/fnbeh.2017.00077
Marinov, G. K., Wang, Y. E., Chan, D., and Wold, B. J. (2014). Evidence for site-specific occupancy of the mitochondrial genome by nuclear transcription factors. PLoS One 9:e84713. doi: 10.1371/journal.pone.0084713
Martin, M., Rehani, K., Jope, R. S., and Michalek, S. M. (2005). Toll-like receptor-mediated cytokine production is differentially regulated by glycogen synthase kinase 3. Nat. Immunol. 6, 777–784. doi: 10.1038/ni1221
Martinez-Yamout, M. A., Nasir, I., Shnitkind, S., Ellis, J. P., Berlow, R. B., Kroon, G., et al. (2023). Glutamine-rich regions of the disordered CREB transactivation domain mediate dynamic intra- and intermolecular interactions. Proc. Natl. Acad. Sci. U.S.A. 120:e2313835120. doi: 10.1073/pnas.2313835120
Matthews, R. P., Guthrie, C. R., Wailes, L. M., Zhao, X., Means, A. R., and McKnight, G. S. (1994). Calcium/calmodulin-dependent protein kinase types II and IV differentially regulate CREB-dependent gene expression. Mol. Cell. Biol. 14, 6107–6116. doi: 10.1128/mcb.14.9.6107-6116.1994
Mayr, B. M., Canettieri, G., and Montminy, M. R. (2001). Distinct effects of cAMP and mitogenic signals on CREB-binding protein recruitment impart specificity to target gene activation via CREB. Proc. Natl. Acad. Sci. U.S.A. 98, 10936–10941. doi: 10.1073/pnas.191152098
McClung, C. A., and Nestler, E. J. (2003). Regulation of gene expression and cocaine reward by CREB and DeltaFosB. Nat. Neurosci. 6, 1208–1215. doi: 10.1038/nn1143
Meyer, T. E., Waeber, G., Lin, J., Beckmann, W., and Habener, J. F. (1993). The promoter of the gene encoding 3′,5′-cyclic adenosine monophosphate (cAMP) response element binding protein contains cAMP response elements: Evidence for positive autoregulation of gene transcription. Endocrinology 132, 770–780. doi: 10.1210/endo.132.2.8381074
Misra, U. K., and Pizzo, S. V. (2005). Coordinate regulation of forskolin-induced cellular proliferation in macrophages by protein kinase A/cAMP-response element-binding protein (CREB) and Epac1-Rap1 signaling: Effects of silencing CREB gene expression on Akt activation. J. Biol. Chem. 280, 38276–38289. doi: 10.1074/jbc.M507332200
Misra, U. K., Gonzalez-Gronow, M., Gawdi, G., Hart, J. P., Johnson, C. E., and Pizzo, S. V. (2002). The role of Grp 78 in alpha 2-macroglobulin-induced signal transduction. Evidence from RNA interference that the low density lipoprotein receptor-related protein is associated with, but not necessary for, GRP 78-mediated signal transduction. J. Biol. Chem. 277, 42082–42087. doi: 10.1074/jbc.M206174200
Miyamoto, E. (2006). Molecular mechanism of neuronal plasticity: Induction and maintenance of long-term potentiation in the hippocampus. J. Pharmacol. Sci. 100, 433–442. doi: 10.1254/jphs.cpj06007x
Miyashita, T., Oda, Y., Horiuchi, J., Yin, J. C. P., Morimoto, T., and Saitoe, M. (2012). Mg(2+) block of Drosophila NMDA receptors is required for long-term memory formation and CREB-dependent gene expression. Neuron 74, 887–898. doi: 10.1016/j.neuron.2012.03.039
Mohamed, H. A., Yao, W., Fioravante, D., Smolen, P. D., and Byrne, J. H. (2005). cAMP-response elements in Aplysia creb1, creb2, and Ap-uch promoters: Implications for feedback loops modulating long term memory. J. Biol. Chem. 280, 27035–27043. doi: 10.1074/jbc.M502541200
Moll, J. R., Acharya, A., Gal, J., Mir, A. A., and Vinson, C. (2002). Magnesium is required for specific DNA binding of the CREB B-ZIP domain. Nucleic Acids Res. 30, 1240–1246. doi: 10.1093/nar/30.5.1240
Montminy, M. (1997). Transcriptional regulation by cyclic AMP. Annu. Rev. Biochem. 66, 807–822. doi: 10.1146/annurev.biochem.66.1.807
Montminy, M. R., and Bilezikjian, L. M. (1987). Binding of a nuclear protein to the cyclic-AMP response element of the somatostatin gene. Nature 328, 175–178. doi: 10.1038/328175A0
Montminy, M. R., Gonzalez, G. A., and Yamamoto, K. K. (1990). Regulation of cAMP-inducible genes by CREB. Trends Neurosci. 13, 184–188. doi: 10.1016/0166-2236(90)90045-c
Montminy, M. R., Sevarino, K. A., Wagner, J. A., Mandel, G., and Goodman, R. H. (1986). Identification of a cyclic-AMP-responsive element within the rat somatostatin gene. Proc. Natl. Acad. Sci. U.S.A. 83, 6682–6686. doi: 10.1073/pnas.83.18.6682
Montminy, M., Brindle, P., Arias, J., Ferreri, K., and Armstrong, R. (1996). Regulation of somatostatin gene transcription by cyclic adenosine monophosphate. Metab. Clin. Exp. 45, 4–7. doi: 10.1016/s0026-0495(96)90068-2
Mu, Y., Yu, Y., Yue, X., Musarat, I., Gong, R., Zhu, C., et al. (2011). The X protein of HBV induces HIV-1 long terminal repeat transcription by enhancing the binding of C/EBPβ and CREB1/2 regulatory proteins to the long terminal repeat of HIV-1. Virus Res. 156, 81–90. doi: 10.1016/j.virusres.2011.01.001
Muchardt, C., Li, C., Kornuc, M., and Gaynor, R. (1990). CREB regulation of cellular cyclic AMP-responsive and adenovirus early promoters. J. Virol. 64, 4296–4305. doi: 10.1128/JVI.64.9.4296-4305.1990
Mulero, M. C., Huang, D.-B., Nguyen, H. T., Wang, V. Y.-F., Li, Y., Biswas, T., et al. (2017). DNA-binding affinity and transcriptional activity of the RelA homodimer of nuclear factor κB are not correlated. J. Biol. Chem. 292, 18821–18830. doi: 10.1074/jbc.M117.813980
Nagahara, A. H., Merrill, D. A., Coppola, G., Tsukada, S., Schroeder, B. E., Shaked, G. M., et al. (2009). Neuroprotective effects of brain-derived neurotrophic factor in rodent and primate models of Alzheimer’s disease. Nat. Med. 15, 331–337. doi: 10.1038/nm.1912
Naidoo, N., Ferber, M., Galante, R. J., McShane, B., Hu, J. H., Zimmerman, J., et al. (2012). Role of Homer proteins in the maintenance of sleep-wake states. PLoS One 7:e35174. doi: 10.1371/journal.pone.0035174
Naqvi, S., Martin, K. J., and Arthur, J. S. C. (2014). CREB phosphorylation at Ser133 regulates transcription via distinct mechanisms downstream of cAMP and MAPK signalling. Biochem. J. 458, 469–479. doi: 10.1042/BJ20131115
Narasimhamurthy, R. K., Andrade, D., and Mumbrekar, K. D. (2022). Modulation of CREB and its associated upstream signaling pathways in pesticide-induced neurotoxicity. Mol. Cell Biochem. 477, 2581–2593. doi: 10.1007/s11010-022-04472-7
Nayar, J. C., Abboud, M., and Dixon, K. M. (2024). Cyclic AMP-regulatory element-binding protein: A novel UV-targeted transcription factor in skin cancer. Photochem. Photobiol. Sci. 23, 1209–1215. doi: 10.1007/s43630-024-00578-7
Noguchi, S., Kumazaki, M., Mori, T., Baba, K., Okuda, M., Mizuno, T., et al. (2016). Analysis of microRNA-203 function in CREB/MITF/RAB27a pathway: Comparison between canine and human melanoma cells. Vet. Comp. Oncol. 14, 384–394. doi: 10.1111/vco.12118
Parker, D., Ferreri, K., Nakajima, T., LaMorte, V. J., Evans, R., Koerber, S. C., et al. (1996). Phosphorylation of CREB at Ser-133 induces complex formation with CREB-binding protein via a direct mechanism. Mol. Cell. Biol. 16, 694–703. doi: 10.1128/MCB.16.2.694
Parker, D., Jhala, U. S., Radhakrishnan, I., Yaffe, M. B., Reyes, C., Shulman, A. I., et al. (1998). Analysis of an activator:coactivator complex reveals an essential role for secondary structure in transcriptional activation. Mol. Cell 2, 353–359. doi: 10.1016/s1097-2765(00)80279-8
Paz, J. C., Park, S., Phillips, N., Matsumura, S., Tsai, W.-W., Kasper, L., et al. (2014). Combinatorial regulation of a signal-dependent activator by phosphorylation and acetylation. Proc. Natl. Acad. Sci. U.S.A. 111, 17116–17121. doi: 10.1073/pnas.1420389111
Perazzona, B., Isabel, G., Preat, T., and Davis, R. L. (2004). The role of cAMP response element-binding protein in Drosophila long-term memory. J. Neurosci. 24, 8823–8828. doi: 10.1523/JNEUROSCI.4542-03.2004
Pigazzi, M., Manara, E., Baron, E., and Basso, G. (2009). miR-34b targets cyclic AMP-responsive element binding protein in acute myeloid leukemia. Cancer Res. 69, 2471–2478. doi: 10.1158/0008-5472.CAN-08-3404
Pinna, L. A. (2002). Protein kinase CK2: A challenge to canons. J. Cell Sci. 115, 3873–3878. doi: 10.1242/jcs.00074
Poels, J., and Vanden Broeck, J. (2004). Insect basic leucine zipper proteins and their role in cyclic AMP-dependent regulation of gene expression. Int. Rev. Cytol. 241, 277–309. doi: 10.1016/S0074-7696(04)41005-5
Poels, J., Franssens, V., Van Loy, T., Martinez, A., Suner, M.-M., Dunbar, S. J., et al. (2004). Isoforms of cyclic AMP response element binding proteins in Drosophila S2 cells. Biochem. Biophys. Res. Commun. 320, 318–324. doi: 10.1016/j.bbrc.2004.05.165
Price, J. L., Blau, J., Rothenfluh, A., Abodeely, M., Kloss, B., and Young, M. W. (1998). Double-time is a novel Drosophila clock gene that regulates PERIOD protein accumulation. Cell 94, 83–95. doi: 10.1016/s0092-8674(00)81224-6
Pu, W. T., and Struhl, K. (1991). The leucine zipper symmetrically positions the adjacent basic regions for specific DNA binding. Proc. Natl. Acad. Sci. U.S.A. 88, 6901–6905. doi: 10.1073/pnas.88.16.6901
Qiao, A., Zhou, J., Xu, S., Ma, W., Boriboun, C., Kim, T., et al. (2021). Sam68 promotes hepatic gluconeogenesis via CRTC2. Nat. Commun. 12:3340. doi: 10.1038/s41467-021-23624-9
Quinn, P. G., and Granner, D. K. (1990). Cyclic AMP-dependent protein kinase regulates transcription of the phosphoenolpyruvate carboxykinase gene but not binding of nuclear factors to the cyclic AMP regulatory element. Mol. Cell. Biol. 10, 3357–3364. doi: 10.1128/mcb.10.7.3357-3364.1990
Radhakrishnan, I., Pérez-Alvarado, G. C., Parker, D., Dyson, H. J., Montminy, M. R., and Wright, P. E. (1997). Solution structure of the KIX domain of CBP bound to the transactivation domain of CREB: A model for activator:coactivator interactions. Cell 91, 741–752. doi: 10.1016/s0092-8674(00)80463-8
Ravnskjaer, K., Hogan, M. F., Lackey, D., Tora, L., Dent, S. Y. R., Olefsky, J., et al. (2013). Glucagon regulates gluconeogenesis through KAT2B- and WDR5-mediated epigenetic effects. J. Clin. Invest. 123, 4318–4328. doi: 10.1172/JCI69035
Ravnskjaer, K., Kester, H., Liu, Y., Zhang, X., Lee, D., and Yates, J. R. III, et al. (2007). Cooperative interactions between CBP and TORC2 confer selectivity to CREB target gene expression. EMBO J. 26, 2880–2889. doi: 10.1038/sj.emboj.7601715
Reddy, P., Zehring, W. A., Wheeler, D. A., Pirrotta, V., Hadfield, C., Hall, J. C., et al. (1984). Molecular analysis of the period locus in Drosophila melanogaster and identification of a transcript involved in biological rhythms. Cell 38, 701–710. doi: 10.1016/0092-8674(84)90265-4
Restivo, L., Tafi, E., Ammassari-Teule, M., and Marie, H. (2009). Viral-mediated expression of a constitutively active form of CREB in hippocampal neurons increases memory. Hippocampus 19, 228–234. doi: 10.1002/hipo.20527
Rexach, J. E., Clark, P. M., and Hsieh-Wilson, L. C. (2008). Chemical approaches to understanding O-GlcNAc glycosylation in the brain. Nat. Chem. Biol. 4, 97–106. doi: 10.1038/nchembio.68
Rexach, J. E., Clark, P. M., Mason, D. E., Neve, R. L., Peters, E. C., and Hsieh-Wilson, L. C. (2012). Dynamic O-GlcNAc modification regulates CREB-mediated gene expression and memory formation. Nat. Chem. Biol. 8, 253–261. doi: 10.1038/nchembio.770
Riabowol, K. T., Fink, J. S., Gilman, M. Z., Walsh, D. A., Goodman, R. H., and Feramisco, J. R. (1988). The catalytic subunit of cAMP-dependent protein kinase induces expression of genes containing cAMP-responsive enhancer elements. Nature 336, 83–86. doi: 10.1038/336083A0
Riccio, A., Alvania, R. S., Lonze, B. E., Ramanan, N., Kim, T., Huang, Y., et al. (2006). A nitric oxide signaling pathway controls CREB-mediated gene expression in neurons. Mol. Cell 21, 283–294. doi: 10.1016/j.molcel.2005.12.006
Richards, J. P., Bächinger, H. P., Goodman, R. H., and Brennan, R. G. (1996). Analysis of the structural properties of cAMP-responsive element-binding protein (CREB) and phosphorylated CREB. J. Biol. Chem. 271, 13716–13723. doi: 10.1074/jbc.271.23.13716
Roesler, W. J., Vandenbark, G. R., and Hanson, R. W. (1988). Cyclic AMP and the induction of eukaryotic gene transcription. J. Biol. Chem. 263, 9063–9066.
Rose, R. E., Gallaher, N. M., Andrew, D. J., Goodman, R. H., and Smolik, S. M. (1997). The CRE-binding protein dCREB-A is required for Drosophila embryonic development. Genetics 146, 595–606. doi: 10.1093/genetics/146.2.595
Ruppert, S., Cole, T. J., Boshart, M., Schmid, E., and Schütz, G. (1992). Multiple mRNA isoforms of the transcription activator protein CREB: Generation by alternative splicing and specific expression in primary spermatocytes. EMBO J. 11, 1503–1512. doi: 10.1002/j.1460-2075.1992.tb05195.x
Rutila, J. E., Suri, V., Le, M., So, W. V., Rosbash, M., and Hall, J. C. (1998). CYCLE is a second bHLH-PAS clock protein essential for circadian rhythmicity and transcription of Drosophila period and timeless. Cell 93, 805–814. doi: 10.1016/s0092-8674(00)81441-5
Ryan, C. M., Kindle, K. B., Collins, H. M., and Heery, D. M. (2010). SUMOylation regulates the nuclear mobility of CREB binding protein and its association with nuclear bodies in live cells. Biochem. Biophys. Res. Commun. 391, 1136–1141. doi: 10.1016/j.bbrc.2009.12.040
Ryu, H., Lee, J., Impey, S., Ratan, R. R., and Ferrante, R. J. (2005). Antioxidants modulate mitochondrial PKA and increase CREB binding to D-loop DNA of the mitochondrial genome in neurons. Proc. Natl. Acad. Sci. U.S.A. 102, 13915–13920.
Sadamoto, H., Kitahashi, T., Fujito, Y., and Ito, E. (2010). Learning-dependent gene expression of CREB1 isoforms in the molluscan brain. Front. Behav. Neurosci. 4:25. doi: 10.3389/fnbeh.2010.00025
Sadamoto, H., Saito, K., Muto, H., Kinjo, M., and Ito, E. (2011). Direct observation of dimerization between different CREB1 isoforms in a living cell. PLoS One 6:e20285. doi: 10.1371/journal.pone.0020285
Sadamoto, H., Sato, H., Kobayashi, S., Murakami, J., Aonuma, H., Ando, H., et al. (2004). CREB in the pond snail Lymnaea stagnalis: Cloning, gene expression, and function in identifiable neurons of the central nervous system. J. Neurobiol. 58, 455–466. doi: 10.1002/neu.10296
Saeki, K., Yuo, A., and Takaku, F. (1999). Cell-cycle-regulated phosphorylation of cAMP response element-binding protein: Identification of novel phosphorylation sites. Biochem. J. 1, 49–54.
Sakagami, H., Kamata, A., Nishimura, H., Kasahara, J., Owada, Y., Takeuchi, Y., et al. (2005). Prominent expression and activity-dependent nuclear translocation of Ca2+/calmodulin-dependent protein kinase Idelta in hippocampal neurons. Eur. J. Neurosci. 22, 2697–2707. doi: 10.1111/j.1460-9568.2005.04463.x
Sakamoto, K. M., and Frank, D. A. (2009). CREB in the pathophysiology of cancer: Implications for targeting transcription factors for cancer therapy. Clin. Cancer Res. 15, 2583–2583. doi: 10.1158/1078-0432.CCR-08-1137
Saluja, D., Vassallo, M. F., and Tanese, N. (1998). Distinct subdomains of human TAFII130 are required for interactions with glutamine-rich transcriptional activators. Mol. Cell. Biol. 18, 5734–5743. doi: 10.1128/MCB.18.10.5734
Sandoval, S., Pigazzi, M., and Sakamoto, K. M. (2009). CREB: A key regulator of normal and neoplastic hematopoiesis. Adv. Hematol. 2009:634292. doi: 10.1155/2009/634292
Sassone-Corsi, P. (1998). Coupling gene expression to cAMP signalling: Role of CREB and CREM. Int. J. Biochem. Cell Biol. 30, 27–38. doi: 10.1016/s1357-2725(97)00093-9
Sawamura, N., Ando, T., Maruyama, Y., Fujimuro, M., Mochizuki, H., Honjo, K., et al. (2008). Nuclear DISC1 regulates CRE-mediated gene transcription and sleep homeostasis in the fruit fly. Mol. Psychiatry 1069, 1138–1148. doi: 10.1038/mp.2008.101
Schumacher, M. A., Goodman, R. H., and Brennan, R. G. (2000). The structure of a CREB bZIP.somatostatin CRE complex reveals the basis for selective dimerization and divalent cation-enhanced DNA binding. J. Biol. Chem. 275, 35242–35247. doi: 10.1074/jbc.M007293200
Schuster, C. M., Davis, G. W., Fetter, R. D., and Goodman, C. S. (1996). Genetic dissection of structural and functional components of synaptic plasticity. II. Fasciclin II controls presynaptic structural plasticity. Neuron 17, 655–667. doi: 10.1016/s0896-6273(00)80198-1
Seternes, O. M., Johansen, B., and Moens, U. (1999). A dominant role for the Raf-MEK pathway in forskolin, 12-O-tetradecanoyl-phorbol acetate, and platelet-derived growth factor-induced CREB (cAMP-responsive element-binding protein) activation, uncoupled from serine 133 phosphorylation in NIH 3T3 cells. Mol. Endocrinol. 13, 1071–1083. doi: 10.1210/mend.13.7.0293
Shankaranarayanan, P., Chaitidis, P., Kühn, H., and Nigam, S. (2001). Acetylation by histone acetyltransferase CREB-binding protein/p300 of STAT6 is required for transcriptional activation of the 15-lipoxygenase-1 gene. J. Biol. Chem. 276, 42753–42760. doi: 10.1074/jbc.M102626200
Shanware, N. P., Trinh, A. T., Williams, L. M., and Tibbetts, R. S. (2007). Coregulated ataxia telangiectasia-mutated and casein kinase sites modulate cAMP-response element-binding protein-coactivator interactions in response to DNA damage. J. Biol. Chem. 282, 6283–6291. doi: 10.1074/jbc.M610674200
Shanware, N. P., Williams, L. M., Bowler, M. J., and Tibbetts, R. S. (2009). Non-specific in vivo inhibition of CK1 by the pyridinyl imidazole p38 inhibitors SB 203580 and SB 202190. BMB Rep. 42, 142–147. doi: 10.5483/bmbrep.2009.42.3.142
Shanware, N. P., Zhan, L., Hutchinson, J. A., Kim, S. H., Williams, L. M., and Tibbetts, R. S. (2010). Conserved and distinct modes of CREB/ATF transcription factor regulation by PP2A/B56gamma and genotoxic stress. PLoS One 5:e12173–e12173. doi: 10.1371/journal.pone.0012173
Sharma, K., Mehra, R. D., Dhar, P., and Vij, U. (2007). Chronic exposure to estrogen and tamoxifen regulates synaptophysin and phosphorylated cAMP response element-binding (CREB) protein expression in CA1 of ovariectomized rat hippocampus. Brain Res. 1132, 10–19. doi: 10.1016/j.brainres.2006.11.027
Shaukat, A., Khan, M. H. F., Ahmad, H., Umer, Z., and Tariq, M. (2021). Interplay between BALL and CREB binding protein maintains H3K27 acetylation on active genes in Drosophila. Front. Cell Dev. Biol. 9:740866. doi: 10.3389/fcell.2021.740866
Shaywitz, A. J., and Greenberg, M. E. (1999). CREB: A stimulus-induced transcription factor activated by a diverse array of extracellular signals. Annu. Rev. Biochem. 68, 821–861. doi: 10.1146/annurev.biochem.68.1.821
Sheng, M., Thompson, M. A., and Greenberg, M. E. (1991). CREB: A Ca(2+)-regulated transcription factor phosphorylated by calmodulin-dependent kinases. Science 252, 1427–1430. doi: 10.1126/science.1646483
Shi, Y., Venkataraman, S. L., Dodson, G. E., Mabb, A. M., LeBlanc, S., and Tibbetts, R. S. (2004). Direct regulation of CREB transcriptional activity by ATM in response to genotoxic stress. Proc. Natl. Acad. Sci. U.S.A. 101, 5898–5903. doi: 10.1073/pnas.0307718101
Shieh, P. B., Hu, S. C., Bobb, K., Timmusk, T., and Ghosh, A. (1998). Identification of a signaling pathway involved in calcium regulation of BDNF expression. Neuron 20, 727–740. doi: 10.1016/s0896-6273(00)81011-9
Shnitkind, S., Martinez-Yamout, M. A., Dyson, H. J., and Wright, P. E. (2018). Structural basis for graded inhibition of CREB:DNA interactions by multisite phosphorylation. Biochemistry 57, 6964–6972. doi: 10.1021/acs.biochem.8b01092
Smith, L. I. F., Zhao, Z., Walker, J., Lightman, S., and Spiga, F. (2021). Activation and expression of endogenous CREB-regulated transcription coactivators (CRTC) 1, 2 and 3 in the rat adrenal gland. J. Neuroendocrinol. 33:e12920. doi: 10.1111/jne.12920
Smolik, S. M., Rose, R. E., and Goodman, R. H. (1992). A cyclic AMP-responsive element-binding transcriptional activator in Drosophila melanogaster, dCREB-A, is a member of the leucine zipper family. Mol. Cell. Biol. 12, 4123–4131. doi: 10.1128/mcb.12.9.4123-4131.1992
Stachowiak, E. K., Fang, X., Myers, J., Dunham, S., and Stachowiak, M. K. (2003). cAMP-induced differentiation of human neuronal progenitor cells is mediated by nuclear fibroblast growth factor receptor-1 (FGFR1). J. Neurochem. 84, 1296–1312. doi: 10.1046/j.1471-4159.2003.01624.x
Steven, A., and Seliger, B. (2016). Control of CREB expression in tumors: From molecular mechanisms and signal transduction pathways to therapeutic target. Oncotarget 7, 35454–35465. doi: 10.18632/oncotarget.7721
Steven, A., Friedrich, M., Jank, P., Heimer, N., Budczies, J., Denkert, C., et al. (2020). What turns CREB on? And off? And why does it matter? Cell. Mol. Life Sci. 77, 4049–4067. doi: 10.1007/s00018-020-03525-8
Sun, P., Enslen, H., Myung, P. S., and Maurer, R. A. (1994). Differential activation of CREB by Ca2+/calmodulin-dependent protein kinases type II and type IV involves phosphorylation of a site that negatively regulates activity. Genes Dev. 8, 2527–2539. doi: 10.1101/gad.8.21.2527
Sun, P., Lou, L., and Maurer, R. A. (1996). Regulation of activating transcription factor-1 and the cAMP response element-binding protein by Ca2+/calmodulin-dependent protein kinases type I, II, and IV. J. Biol. Chem. 271, 3066–3073. doi: 10.1074/jbc.271.6.3066
Szego, E. M., Barabás, K., Balog, J., Szilágyi, N., Korach, K. S., Juhász, G., et al. (2006). Estrogen induces estrogen receptor alpha-dependent cAMP response element-binding protein phosphorylation via mitogen activated protein kinase pathway in basal forebrain cholinergic neurons in vivo. J. Neurosci. 26, 4104–4110. doi: 10.1523/JNEUROSCI.0222-06.2006
Talukdar, P. D., and Chatterji, U. (2023). Transcriptional co-activators: Emerging roles in signaling pathways and potential therapeutic targets for diseases. Signal Transd. Target. Ther. 8:427. doi: 10.1038/s41392-023-01651-w
Tan, X., Wang, S., Yang, B., Zhu, L., Yin, B., Chao, T., et al. (2012a). The CREB-miR-9 Negative feedback minicircuitry coordinates the migration and proliferation of glioma cells. PLoS One 7:e49570. doi: 10.1371/journal.pone.0049570
Tan, X., Wang, S., Zhu, L., Wu, C., Yin, B., Zhao, J., et al. (2012b). cAMP response element-binding protein promotes gliomagenesis by modulating the expression of oncogenic microRNA-23a. Proc. Natl. Acad. Sci. U.S.A. 109, 15805–15810. doi: 10.1073/pnas.1207787109
Tao, X., Finkbeiner, S., Arnold, D. B., Shaywitz, A. J., and Greenberg, M. E. (1998). Ca2+ influx regulates BDNF transcription by a CREB family transcription factor-dependent mechanism. Neuron 20, 709–726. doi: 10.1016/s0896-6273(00)81010-7
Tejedor, F. J., and Hämmerle, B. (2011). MNB/DYRK1A as a multiple regulator of neuronal development. FEBS J. 278, 223–235. doi: 10.1111/j.1742-4658.2010.07954.x
Thiel, G., Schmidt, T., and Rössler, O. G. (2021). Ca(2+) Microdomains, Calcineurin and the regulation of gene transcription. Cells 10:875. doi: 10.3390/cells10040875
Thüringer, F., Cohen, S. M., and Bienz, M. (1993). Dissection of an indirect autoregulatory response of a homeotic Drosophila gene. EMBO J. 12, 2419–2430. doi: 10.1002/j.1460-2075.1993.tb05896.x
Trinh, A. T., Kim, S. H., Chang, H.-Y., Mastrocola, A. S., and Tibbetts, R. S. (2013). Cyclin-dependent kinase 1-dependent phosphorylation of cAMP response element-binding protein decreases chromatin occupancy. J. Biol. Chem. 288, 23765–23775. doi: 10.1074/jbc.M113.464057
Tyson, D. R., Swarthout, J. T., Jefcoat, S. C., and Partridge, N. C. (2002). PTH induction of transcriptional activity of the cAMP response element-binding protein requires the serine 129 site and glycogen synthase kinase-3 activity, but not casein kinase II sites. Endocrinology 143, 674–682. doi: 10.1210/endo.143.2.8626
Upadhya, S. C., Smith, T. K., and Hegde, A. N. (2004). Ubiquitin-proteasome-mediated CREB repressor degradation during induction of long-term facilitation. J. Neurochem. 91, 210–219. doi: 10.1111/j.1471-4159.2004.02707.x
Venkatesh, T. V., Park, M., Ocorr, K., Nemaceck, J., Golden, K., Wemple, M., et al. (2000). Cardiac enhancer activity of the homeobox gene tinman depends on CREB consensus binding sites in Drosophila. Genesis 26, 55–66.
Vinson, C., Acharya, A., and Taparowsky, E. J. (2006). Deciphering B-ZIP transcription factor interactions in vitro and in vivo. Biochim. Biophys. Acta 1759, 4–12. doi: 10.1016/j.bbaexp.2005.12.005
Vlahopoulos, S. A., Logotheti, S., Mikas, D., Giarika, A., Gorgoulis, V., and Zoumpourlis, V. (2008). The role of ATF-2 in oncogenesis. BioEssays 30, 314–327. doi: 10.1002/bies.20734
Vosseller, K., Trinidad, J. C., Chalkley, R. J., Specht, C. G., Thalhammer, A., Lynn, A. J., et al. (2006). O-linked N-acetylglucosamine proteomics of postsynaptic density preparations using lectin weak affinity chromatography and mass spectrometry. Mol. Cell. Proteomics 5, 923–934. doi: 10.1074/mcp.T500040-MCP200
Waeber, G., and Habener, J. F. (1991). Nuclear translocation and DNA recognition signals colocalized within the bZIP domain of cyclic adenosine 3′,5′-monophosphate response element-binding protein CREB. Mol. Endocrinol. 5, 1431–1438. doi: 10.1210/mend-5-10-1431
Walker, W. H., Sanborn, B. M., and Habener, J. F. (1994). An isoform of transcription factor CREM expressed during spermatogenesis lacks the phosphorylation domain and represses cAMP-induced transcription. Proc. Natl. Acad. Sci. U.S.A. 91, 12423–12427. doi: 10.1073/pnas.91.26.12423
Wang, H., Xu, J., Lazarovici, P., Quirion, R., and Zheng, W. (2018). cAMP response element-binding protein (CREB): A possible signaling molecule link in the pathophysiology of schizophrenia. Front. Mol. Neurosci. 11:255. doi: 10.3389/fnmol.2018.00255
Wang, I. F., Ho, P. C., and Tsai, K. A.-O. (2022). MicroRNAs in learning and memory and their impact on alzheimer’s disease. Biomedicines 10:1856. doi: 10.3390/biomedicines10081856
Wang, I. F., Wang, Y., Yang, Y.-H., Huang, G.-J., Tsai, K.-J., and Shen, C.-K. J. (2021). Activation of a hippocampal CREB-pCREB-miRNA-MEF2 axis modulates individual variation of spatial learning and memory capability. Cell Rep. 36:109477. doi: 10.1016/j.celrep.2021.109477
Wang, T., Wiater, E., Zhang, X., Thomas, J. B., and Montminy, M. (2021). Crtc modulates fasting programs associated with 1-C metabolism and inhibition of insulin signaling. Proc. Natl. Acad. Sci. U.S.A. 118:e2024865118. doi: 10.1073/pnas.2024865118
Wang, J. M., Chao, J. R., Chen, W., Kuo, M. L., Yen, J. J., and Yang-Yen, H. F. (1999). The antiapoptotic gene mcl-1 is up-regulated by the phosphatidylinositol 3-kinase/Akt signaling pathway through a transcription factor complex containing CREB. Mol. Cell. Biol. 19, 6195–6206. doi: 10.1128/MCB.19.9.6195
Wang, Q. M., Fiol, C. J., DePaoli-Roach, A. A., and Roach, P. J. (1994). Glycogen synthase kinase-3 beta is a dual specificity kinase differentially regulated by tyrosine and serine/threonine phosphorylation. J. Biol. Chem. 269, 14566–14574.
Wang, Y., Ghezzi, A., Yin, J. C. P., and Atkinson, N. S. (2009). CREB regulation of BK channel gene expression underlies rapid drug tolerance. Genes Brain behav. 8, 369–376. doi: 10.1111/j.1601-183X.2009.00479.x
Wang, Y., Hu, J., Wu, S., Fleishman, J. S., Li, Y., Xu, Y., et al. (2023). Targeting epigenetic and posttranslational modifications regulating ferroptosis for the treatment of diseases. Signal Transd. Target. Ther. 8:449. doi: 10.1038/s41392-023-01720-0
Wiggin, G. R., Soloaga, A., Foster, J. M., Murray-Tait, V., Cohen, P., and Arthur, J. S. C. (2002). MSK1 and MSK2 are required for the mitogen- and stress-induced phosphorylation of CREB and ATF1 in fibroblasts. Mol. Cell. Biol. 22, 2871–2881. doi: 10.1128/MCB.22.8.2871-2881.2002
Wilson, B. E., Mochon, E., and Boxer, L. M. (1996). Induction of bcl-2 expression by phosphorylated CREB proteins during B-cell activation and rescue from apoptosis. Mol. Cell. Biol. 16, 5546–5556. doi: 10.1128/MCB.16.10.5546
Wood, M. A., Attner, M. A., Oliveira, A. M. M., Brindle, P. K., and Abel, T. (2006). A transcription factor-binding domain of the coactivator CBP is essential for long-term memory and the expression of specific target genes. Learn. Mem. 13, 609–617. doi: 10.1101/lm.213906
Wood, M. A., Kaplan, M. P., Park, A., Blanchard, E. J., Oliveira, A. M. M., Lombardi, T. L., et al. (2005). Transgenic mice expressing a truncated form of CREB-binding protein (CBP) exhibit deficits in hippocampal synaptic plasticity and memory storage. Learn. Mem. 12, 111–119. doi: 10.1101/lm.86605
Wright, C. J., Smith, C. W. J., and Jiggins, C. D. (2022). Alternative splicing as a source of phenotypic diversity. Nat. Rev. Genet. 23, 697–710. doi: 10.1038/s41576-022-00514-4
Wu, X., and McMurray, C. T. (2001). Calmodulin kinase II attenuation of gene transcription by preventing cAMP response element-binding protein (CREB) dimerization and binding of the CREB-binding protein. J. Biol. Chem. 276, 1735–1741. doi: 10.1074/jbc.M006727200
Wu, X., Spiro, C., Owen, W. G., and McMurray, C. T. (1998). cAMP response element-binding protein monomers cooperatively assemble to form dimers on DNA. J. Biol. Chem. 273, 20820–20827. doi: 10.1074/jbc.273.33.20820
Wu, Z., Wang, X., Wu, H., Du, S., Wang, Z., Xie, S., et al. (2024). Identification of CREB5 as a prognostic and immunotherapeutic biomarker in glioma through multi-omics pan-cancer analysis. Comput. Biol. Med. 173:108307. doi: 10.1016/j.compbiomed.2024.108307
Xia, Y., Zhao, K., Liu, D., Zhou, X., and Zhang, G. (2023). Multi-domain and complex protein structure prediction using inter-domain interactions from deep learning. Commun. Biol. 6:221. doi: 10.1038/s42003-023-05610-7
Xing, J., Ginty, D. D., and Greenberg, M. E. (1996). Coupling of the RAS-MAPK pathway to gene activation by RSK2, a growth factor-regulated CREB kinase. Science 273, 959–963. doi: 10.1126/science.273.5277.959
Xing, J., Kornhauser, J. M., Xia, Z., Thiele, E. A., and Greenberg, M. E. (1998). Nerve growth factor activates extracellular signal-regulated kinase and p38 mitogen-activated protein kinase pathways to stimulate CREB serine 133 phosphorylation. Mol. Cell. Biol. 18, 1946–1955. doi: 10.1128/MCB.18.4.1946
Yamamoto, K. K., Gonzalez, G. A., Biggs, W. H., and Montminy, M. R. (1988). Phosphorylation-induced binding and transcriptional efficacy of nuclear factor CREB. Nature 334, 494–498. doi: 10.1038/334494A0
Yamashima, T. (2012). ‘PUFA-GPR40-CREB signaling’ hypothesis for the adult primate neurogenesis. Prog. Lipid Res. 51, 221–231. doi: 10.1016/j.plipres.2012.02.001
Yan, X., Liu, J., Ye, Z., Huang, J., He, F., Xiao, W., et al. (2016). CaMKII-mediated CREB phosphorylation is involved in Ca2+-induced BDNF mRNA transcription and neurite outgrowth promoted by electrical stimulation. PLoS One 11:e0162784.
Yang, E. J., Ahn, Y. S., and Chung, K. C. (2001). Protein kinase Dyrk1 activates cAMP response element-binding protein during neuronal differentiation in hippocampal progenitor cells*. J. Biol. Chem. 276, 39819–39824. doi: 10.1074/jbc.M104091200
Yang, E. J., Yoon, J.-H., and Chung, K. C. (2004a). Bruton’s tyrosine kinase phosphorylates cAMP-responsive element-binding protein at serine 133 during neuronal differentiation in immortalized hippocampal progenitor cells. J. Biol. Chem. 279, 1827–1837. doi: 10.1074/jbc.M308722200
Yang, E. J., Yoon, J.-H., Min, D. S., and Chung, K. C. (2004b). LIM kinase 1 activates cAMP-responsive element-binding protein during the neuronal differentiation of immortalized hippocampal progenitor cells *. J. Biol. Chem. 279, 8903–8910. doi: 10.1074/jbc.M311913200
Yang, S., Jiang, W., Yang, W., Yang, C., Yang, X., Chen, K., et al. (2021). Epigenetically modulated miR-1224 suppresses the proliferation of HCC through CREB-mediated activation of YAP signaling pathway. Mol. Ther. 23, 944–958. doi: 10.1016/j.omtn.2021.01.008
Yin, J. C., Wallach, J. S., Wilder, E. L., Klingensmith, J., Dang, D., Perrimon, N., et al. (1995a). A Drosophila CREB/CREM homolog encodes multiple isoforms, including a cyclic AMP-dependent protein kinase-responsive transcriptional activator and antagonist. Mol. Cell. Biol. 15, 5123–5130. doi: 10.1128/MCB.15.9.5123
Yin, J. C., Del Vecchio, M., Zhou, H., and Tully, T. (1995b). CREB as a memory modulator: Induced expression of andCREB2 activator isoform enhances long-term memory in Drosophila. Cell 81, 107–115. doi: 10.1016/0092-8674(95)90375-5
Yin, J. C., Wallach, J. S., Del Vecchio, M., Wilder, E. L., Zhou, H., Quinn, W. G., et al. (1994). Induction of a dominant negative CREB transgene specifically blocks long-term memory in Drosophila. Cell 79, 49–58. doi: 10.1016/0092-8674(94)90399-9
Yoon, Y.-S., Liu, W., Van de Velde, S., Matsumura, S., Wiater, E., Huang, L., et al. (2021). Activation of the adipocyte CREB/CRTC pathway in obesity. Commun. Biol. 4, 1214–1214. doi: 10.1038/s42003-021-02735-5
Zachara, N. E., and Hart, G. W. (2002). The emerging significance of O-GlcNAc in cellular regulation. Chem. Rev. 102, 431–438. doi: 10.1021/cr000406u
Zafra, F., Lindholm, D., Castrén, E., Hartikka, J., and Thoenen, H. (1992). Regulation of brain-derived neurotrophic factor and nerve growth factor mRNA in primary cultures of hippocampal neurons and astrocytes. J. Neurosci. 12, 4793–4799. doi: 10.1523/JNEUROSCI.12-12-04793.1992
Zhang, D., Zhang, Q., Wang, L., Li, J., Hao, W., Sun, Y., et al. (2022). Alternative splicing isoforms of porcine CREB are differentially involved in transcriptional transactivation. Genes 13:1304. doi: 10.3390/genes13081304
Zhang, N., Shi, L., and Wang, Y. (2022). CREB-associated glycosylation and function in human disease. Adv. Clin. Exp. Med. 31, 1289–1297. doi: 10.17219/acem/151026
Zhang, L., Hu, X., Xiao, Y., Bai, Y., Fu, J., Wang, Y., et al. (2023). S133A-CREB Acts as a novel transcription factor to regulate EMT in lens epithelial cells. Invest. Ophthalmol. Vis. Sci. 64, 1947–1947.
Zhang, X., Odom, D. T., Koo, S.-H., Conkright, M. D., Canettieri, G., Best, J., et al. (2005). Genome-wide analysis of cAMP-response element binding protein occupancy, phosphorylation, and target gene activation in human tissues. Proc. Natl. Acad. Sci. U.S.A. 102, 4459–4464. doi: 10.1073/pnas.0501076102
Zhang, Y., Yang, J., Cui, X., Chen, Y., Zhu, V. F., Hagan, J. P., et al. (2013). A novel epigenetic CREB-miR-373 axis mediates ZIP4-induced pancreatic cancer growth. EMBO Mol. Med. 5, 1322–1334. doi: 10.1002/emmm.201302507
Zhao, W.-Q., Alkon, D. L., and Ma, W. (2003). c-Src protein tyrosine kinase activity is required for muscarinic receptor-mediated DNA synthesis and neurogenesis via ERK1/2 and c-AMP-responsive element-binding protein signaling in neural precursor cells. J. Neurosci. Res. 72, 334–342. doi: 10.1002/jnr.10591
Zhu, G., Liu, Y., Zhi, Y., Jin, Y., Li, J., Shi, W., et al. (2019). PKA- and Ca(2+)-dependent p38 MAPK/CREB activation protects against manganese-mediated neuronal apoptosis. Toxicol. Lett. 309, 10–19. doi: 10.1016/j.toxlet.2019.04.004
Keywords: cAMP responsive element, CREB, alternative splicing, transcriptional co-activator, post-transcriptional modification, post-translational modification, epigenetic modification
Citation: Chowdhury MAR, Haq MM, Lee JH and Jeong S (2024) Multi-faceted regulation of CREB family transcription factors. Front. Mol. Neurosci. 17:1408949. doi: 10.3389/fnmol.2024.1408949
Received: 04 April 2024; Accepted: 12 July 2024;
Published: 06 August 2024.
Edited by:
Bo Zhao, Indiana University Bloomington, United StatesReviewed by:
Derek J. C. Tai, Harvard Medical School, United StatesMatteo Spinelli, University College London, United Kingdom
Copyright © 2024 Chowdhury, Haq, Lee and Jeong. This is an open-access article distributed under the terms of the Creative Commons Attribution License (CC BY). The use, distribution or reproduction in other forums is permitted, provided the original author(s) and the copyright owner(s) are credited and that the original publication in this journal is cited, in accordance with accepted academic practice. No use, distribution or reproduction is permitted which does not comply with these terms.
*Correspondence: Sangyun Jeong, c2plb25nNEBqYm51LmFjLmty