- Department of Human Genetics, Emory University School of Medicine, Atlanta, GA, United States
Alzheimer’s disease (AD) is a complex neurodegenerative disorder and the most common form of dementia. There are two main types of AD: familial and sporadic. Familial AD is linked to mutations in amyloid precursor protein (APP), presenilin-1 (PSEN1), and presenilin-2 (PSEN2). On the other hand, sporadic AD is the more common form of the disease and has genetic, epigenetic, and environmental components that influence disease onset and progression. Investigating the epigenetic mechanisms associated with AD is essential for increasing understanding of pathology and identifying biomarkers for diagnosis and treatment. Chemical covalent modifications on DNA and RNA can epigenetically regulate gene expression at transcriptional and post-transcriptional levels and play protective or pathological roles in AD and other neurodegenerative diseases.
1 Introduction
Alzheimer’s disease (AD) is a progressive neurodegenerative disorder characterized by cognitive decline and memory deficits. AD accounts for 60–80% of all dementia cases and is estimated to affect 13.8 million people in the United States by 2050 (Alzheimer’s Association, 2023). Despite the prevalence of AD, a definitive diagnosis is only possible through postmortem histological evaluation indicating the presence of amyloid-beta (Aβ) plaques, neurofibrillary tangles (NFTs), and neuronal atrophy (Small and Gandy, 2006).
Aβ peptides are derived from the cleavage of amyloid precursor protein (APP) by β- or γ-secretase, resulting in the generation and aggregation of insoluble extracellular plaques and soluble oligomers that interfere with neuronal signaling, morphology, and function (Li et al., 2009; Zhang H. et al., 2015; Chen et al., 2017). Intracellularly, hyperphosphorylated isoforms (p-tau) of the microtubule-associated protein tau (MAPT) accumulate in NFTs and interact with other key AD proteins such as ubiquitin and apolipoprotein E (Drummond et al., 2020). P-tau oligomers contribute to neurodegeneration by disturbing microtubule dynamics, neuronal maturation, calcium (Ca2+) homeostasis, and organelle trafficking (Alonso et al., 2006; Swanson et al., 2017). Aβ aggregation has been shown to induce p-tau redistribution from axons to cell bodies and dendrites in hippocampal rat neuronal cultures, resulting in loss of dendritic spines, mitochondria, and microtubules and an increase in Aβ plaques (Zempel et al., 2010; Chen et al., 2021). This supports the amyloid cascade theory, suggesting that Aβ aggregation precedes and exacerbates p-tau pathology in AD. However, two FDA-approved immunotherapies targeting Aβ, aducanumab and lecanemab, show modest reductions in Aβ levels and cognitive decline in some patients with mild cognitive impairment (MCI) or early AD and a 30% risk of brain swelling or bleeding (Brockmann et al., 2023). Therefore, other mechanisms must contribute to AD development and progression. Alternate theories in the field include the tau hypothesis, where p-tau drives amyloidogenic APP cleavage and is transferred between neurons in a “prion-like” manner, and the oxidative stress hypothesis that postulates that dysregulation of reactive oxygen species causes a cytotoxic cascade resulting in neurodegeneration (Tardivel et al., 2016; Roy et al., 2023). Therefore, it is essential to investigate additional mechanisms and pathways that contribute to the development and progression of AD (Figure 1) and identify the critical therapeutic window for effective treatment.
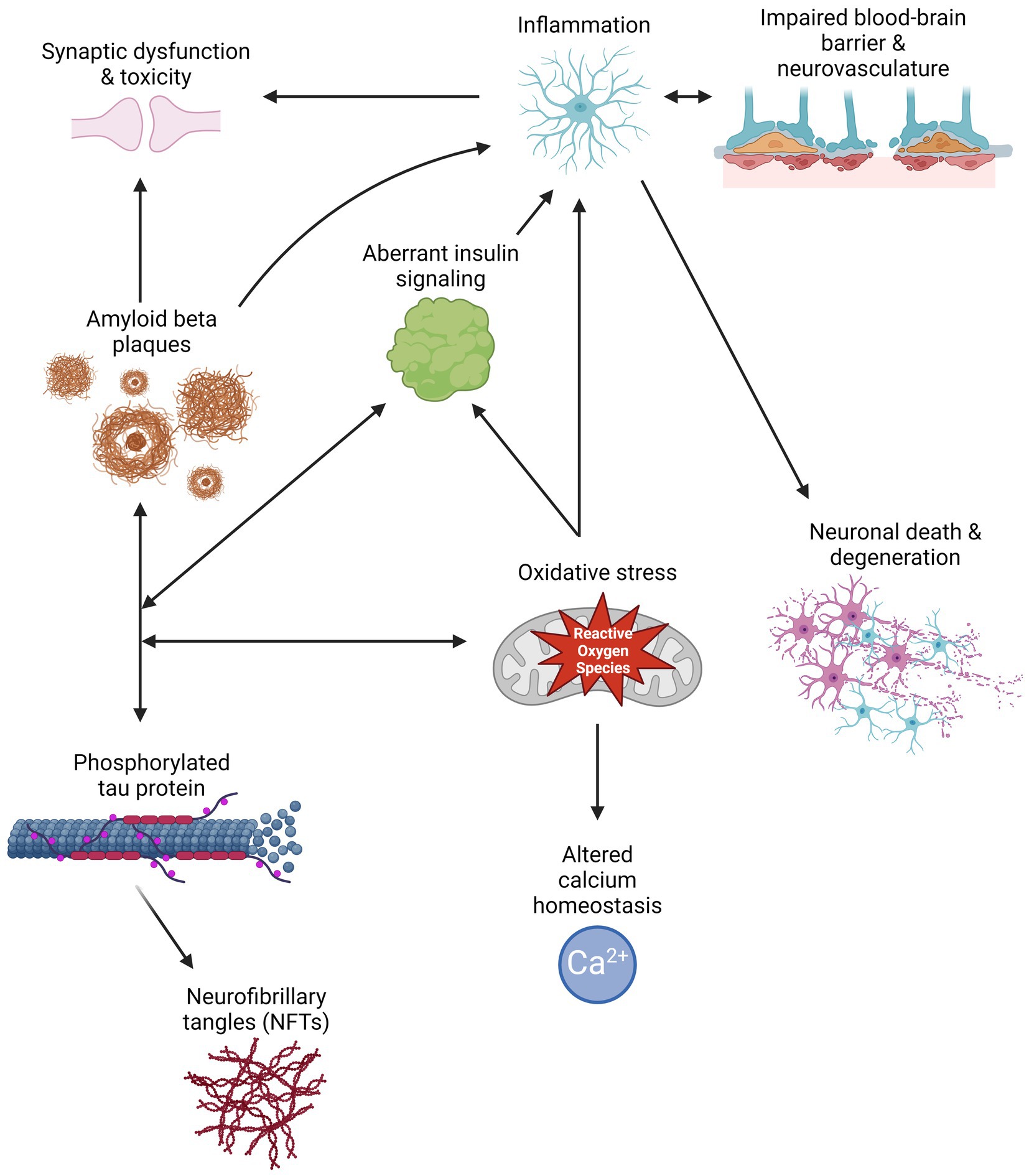
Figure 1. Schematic of multiple pathways that contribute to Alzheimer’s disease pathology, and how their interactions can exacerbate symptomology and progression. Created with BioRender.com.
AD is a complex disorder caused by genetic alterations in key genes, with an estimated heritability of 58–79% (Gatz et al., 2006). AD is subdivided into two types based on its genetic basis: early-onset Familial AD (EOAD) and late-onset sporadic AD (LOAD) (DeTure and Dickson, 2019). EOAD is a rare autosomal dominant form of the disease affecting adults younger than 65 years old caused by mutations in the amyloid precursor protein (APP), presenilin 1 (PSEN1), and presenilin 2 (PSEN2) genes (Hsu et al., 2018). PSEN1 and PSEN2 encode the γ-secretase proteolytic subunit responsible for Aβ cleavage, with PSEN1 mutations being the most common in EOAD (Xiao et al., 2021). Conversely, LOAD is the most common form of AD in adults over 65 years of age and is highly polygenic and multifactorial (Jansen et al., 2019; Kunkle et al., 2019). Factors contributing to the development of LOAD include aging, APOE isoforms, stress, changes in the gut microbiome, and comorbidities such as cardiovascular diseases (Leszek et al., 2020; Jin et al., 2021; Nagu et al., 2021; Raulin et al., 2022). While historically, most efforts have focused on the genetic basis of AD, it is becoming increasingly appreciated that epigenetic mechanisms could play pivotal roles in AD pathogenesis, even at the preclinical stage (Kuehner et al., 2021).
Epigenetics studies stable, heritable changes in gene expression without modification of the underlying DNA sequence. Epigenetic processes usually involve chemical covalent modifications, such as methylation, in histone proteins, DNA and coding or non-coding RNA molecules, that affect gene expression at both transcriptional and post-transcriptional levels. Dynamic epigenetic regulation occurs spatially and temporally, allowing for neurogenesis and neuronal patterning during neurodevelopment and neuroplasticity throughout adulthood (Yao et al., 2016). Aberrant epigenetic alterations induced by environmental stimuli such as radiation, oxygen radicals, and the aging process result in the ectopic repression or overexpression of critical genes that contribute to disease etiology. These epigenetic alterations have been associated with the development of pathologies, including cancer, chronic neuropathic pain, and neurodegenerative diseases such as AD (Takeshima and Ushijima, 2019; Ghosh and Pan, 2022). However, the mechanisms that result in the accumulation of epigenetic modifications and how their dysregulation in the central nervous system contributes to the onset and development of AD remains largely unknown. Moreover, identifying epigenetic biomarkers for the early diagnosis and treatment of AD is essential to increase the quality of life of patients and their caregivers. Here, we systematically summarize current knowledge and emerging roles of transcriptional and post-transcriptional epigenetic mechanisms in DNA and RNA associated with AD development and progression. We also highlight their potential as biomarkers for diagnosis and treatment. This review focuses on DNA modifications including DNA cytosine methylation and hydroxymethylation, DNA adenine methylation, RNA adenine methylation, tRNA modifications, long non-coding RNAs, microRNAs, and circular RNAs. Nonetheless, other DNA and RNA modifications not described in this review have been shown to or have the potential to influence AD pathology. Other reviews have explored the roles of histone modifications in AD and other neurological disorders, such as Park et al. (2022), Santana et al. (2023), and Qin et al. (2024).
2 DNA modifications
2.1 DNA cytosine methylation (5-methylcytosine, 5mC) and hydroxymethylation (5-hydroxymethylcytosine, 5hmC)
The most predominant DNA modifications occur on the cytosine residue. 5-methylcytosine (5mC) describes the addition of a methyl group to the fifth carbon position of cytosine, usually at CpG dinucleotides, by a group of DNA methyltransferases (DNMTs) in the mammalian genome. The addition of 5mC is associated with transcriptional repression at promoters and enhancers, resulting in cell and tissue-specific gene expression (Robertson and Wolffe, 2000). In 2009, it was discovered that 5mC is oxidized into 5-hydroxymethylcytosine (5hmC) by Ten-eleven translocation (TET) enzymes, resulting in a stable and independent epigenetic mark in DNA at gene bodies and enhancers that promotes critical gene expression during development (Tahiliani et al., 2009; He et al., 2021).
DNA methylation and hydroxymethylation are enriched in the brain and dynamically regulated in neurodevelopment (Kriaucionis and Heintz, 2009; Lister et al., 2013). Its dynamics play important roles in modulating neurogenesis, synaptic transmission, learning, and memory (Hahn et al., 2013; Meadows et al., 2015; Bayraktar et al., 2020; Zocher et al., 2021). Postmortem analysis of human brain tissue of embryos, young adults, and older adults, identified a shift from 5mC to 5hmC in the 5′ flanking region of PSEN1 associated with aging (Monti et al., 2020). Accordingly, loss of function of Dnmts or Tets result in embryonic lethality and impaired neurogenesis (Jin and Robertson, 2013; Kang et al., 2015).
Epigenetic clocks are algorithms used to estimate the age of an organism, tissue, or cells based on methylation changes at specific sites in the genome (Kabacik et al., 2022). Accelerated aging, defined as the difference between chronological and epigenetic clock age, can serve as a biomarker for functional aging and aid in age of onset predictions for neurodegenerative diseases. Several epigenetic clocks suggest that AD patients present accelerated epigenetic aging correlating with lifestyle risk factors, amyloid load, neurofibrillary tangles, and declines in memory and cognition (Levine et al., 2015; McCartney et al., 2018; Grodstein et al., 2021). A 4-year longitudinal study of blood DNA revealed that AD patients exhibit DNA methylation alterations in dementia-associated genes before their clinical diagnosis (Pérez et al., 2022). Furthermore, genome-wide analyses of postmortem human brains from the Religious Orders Study (ROS) and Memory and Aging Project (MAP) have identified whole brain and region-specific differentially methylated sites associated with known and novel AD risk loci related to cell adhesion, calcium homeostasis, and the immune system, key processes that are dysregulated in AD (De Jager et al., 2014; Semick et al., 2019). These changes are recapitulated in in vitro and in vivo animal models (Leparulo et al., 2022). AD forebrain organoids present global 5hmC alterations in known neurodevelopmental and AD risk genes (Kuehner et al., 2021). Similarly, 5xFAD mice with partial loss of Tet1 exhibit exacerbated symptom severity and aberrant 5mC and 5hmC expression at key genes, including TET3, DNMT3A, and Methyl-CpG binding protein 2 (MECP2) (Armstrong et al., 2023).
AD is caused by a combination of genetic, environmental, and lifestyle factors that impact age-related changes in the brain. Exposure to heavy metals or air pollutants, diets low in antioxidants, physical inactivity, comorbidities, and social factors can increase the risk of AD (Chen et al., 2016; Cacciottolo et al., 2017; Sinyor et al., 2020). Chronic stress and depression are known risk factors for the development of AD and accelerated aging (Wallensten et al., 2023). Mouse models of chronic stress recapitulate depression-like behaviors and exhibit global 5mC and 5hmC cortical alterations. In two separate studies, differentially hydroxymethylated regions were enriched for gene ontology terms associated with neurogenesis and synaptic signaling (Cheng et al., 2018; Kuehner et al., 2023). Notably, hypoxia-inducible factors (HIFs) Hif3 and Hif1α, known to play neuroprotective roles, were found to be associated with stress-induced methylation changes. Indeed, AD patients are reported to present lower Hif1α levels associated with neurovascular and calcium homeostasis dysfunctions that further exacerbate neuroinflammation, oxidative stress, and p-tau and Aβ depositions (Ashok et al., 2017; Mitroshina et al., 2021). DNA methylation and hydroxymethylation dynamics play important roles in AD-related pathways, and their contributions to disease onset and progression require further exploration to determine their potential as therapeutic targets.
2.2 DNA adenine methylation (N6-methyladenine, 6mA)
DNA N6-methyladenine (6mA) is a DNA modification on the sixth position of adenine that was thought to only be present in prokaryotes until recent technological advances enabled its identification and quantification in eukaryotes (Greer et al., 2015; Zhang G. et al., 2015; Wu et al., 2016). 6mA is thought to be placed on the DNA by N-6 adenine-specific DNA methyltransferase 1 (N6AMT1 or DAMT-1) and removed by demethylase Alkb homolog 1 histone h2a dioxygenase (ALKBH1); and appears to be enriched at exonic regions, intronic regions, and intergenic regions, as well as at retrotransposons including long interspersed nuclear elements (LINEs) (Wu et al., 2016; Yao et al., 2017; Zhang M. et al., 2020; Feng et al., 2024).
Recent studies suggest that 6mA plays a role in various stress responses. In Caenorhabditis elegans, 6mA is deposited at mitochondrial stress and heat stress response genes, and interacts with histone modifications to modulate the transgenerational inheritance of mitochondrial stress and hormetic heat stress adaptations (Ma C. et al., 2019; Wan et al., 2021). Similarly, 6mA has been shown to be enriched in human mitochondrial DNA (mtDNA), increases under hypoxic stress, and is associated with mtDNA gene expression repression via disruption of transcription factor A mitochondria (TFAM) binding (Hao et al., 2020). In a chronic stress mouse model, genome-wide 6mA accumulation and decreased intragenic 6mA levels negatively correlated with the upregulation of genes associated with neuronal development and function, and differentially 6mA regions overlapped with genes associated with neuropsychiatric disorders (Yao et al., 2017). Moreover, pregnant rats exposed to the brominated flame retardant α-hexabromocyclododecane gave birth to pups with motor and learning deficits that exhibited a trend toward decreased 6mA levels in the cerebellum (Fernandes et al., 2021). Similarly, neonatal rat exposure to predator odor stress was associated with altered 6mA levels in the amygdala, and sex-specific differences in 5-Hydroxytryptamine Receptor 2A (Htr2A) promoter repression and anxiety-like behavior (Kigar et al., 2017). These studies provide further evidence that 6mA plays a role in neurodevelopment. ALKBH1 knockdown mice exhibit impaired axon regeneration and the significant downregulation of 5 key neurodevelopment genes: Inhibitor of DNA Binding 1 (Id1), Ephrin A1 (Efna1), Neuritin 1 (Nrn1), Autophagy related 9B (ATG9B), and Complement C1q like 4 (C1QL4), as well as a trend toward downregulation for 14 others genes including APOE (Li et al., 2021). In accordance with these results, a preliminary study suggests that blood leukocyte 6mA levels are significantly lower in AD (Lv et al., 2022). The innate immune system plays important roles in AD through its response to pathogens, endogenous molecules such as Aβ, and debris released by necrotic cell death that contribute to pathogenic neuroinflammation (Takeuchi and Akira, 2010). The activation of murine and human cerebrospinal fluid (CSF)-derived macrophages was shown to be enhanced by the recognition of synthetic cytosolic dsDNA Gm6ATC and GTm6AC motifs, while recognition of the Cm6ATG motif attenuated macrophage activation, indicating that 6mA may modulate immune and inflammatory processes in a sequence- and context-specific manner (Balzarolo et al., 2021). Further investigation of 6mA dynamics in AD is warranted.
3 RNA modifications
More than 150 biochemical modifications have been identified in coding and non-coding RNAs (Boccaletto et al., 2022). This review focuses on exploring the contributions of RNA adenine methylation (N6-methyladenosine, m6A) and tRNA modifications in Alzheimer’s disease pathogenesis.
3.1 RNA adenine methylation (N6-methyladenosine, m6A)
RNA N6-methyladenosine (m6A) is the most abundant RNA modification, consisting of the addition of a methyl group to the sixth position of adenosine by the m6A methyltransferase complex formed by methyltransferase-like 3 (METTL3) and methyltransferase-like 14 (METTL14). m6A is removed from RNA by Fat mass obesity-associated (FTO) and alkB homolog 5 (ALKBH5) and recognized by several nuclear and cytoplasmic m6A-binding proteins, including YTHDF1/2/3 and YTHDC1/2 (Yang et al., 2018).
m6A is highly enriched in the brain, and its increased deposition is associated with adult neurogenesis, memory, learning, and BDNF and dopaminergic signaling pathways (Meyer et al., 2012; Hess et al., 2013; Li et al., 2017). Recently, m6A was also found to be dynamically regulated by extracellular signal-regulated kinase (ERK)/mitogen-activated protein kinase (MAPK) signaling. The ERK/MAPK pathway is involved in cell proliferation, differentiation, stress response, and neuroinflammation (Albert-Gascó et al., 2020; Guo et al., 2020). Activation of ERK in mouse embryonic stem cells (mESCs) increases METTL3 phosphorylation, leading to increased METTL3 deubiquination by ubiquitin specific peptidase 5 (USP5), which in turn increases METTL3 stability and m6A deposition (Sun et al., 2020). Moreover, m6A deposition on 3’UTRs is required for the localization of mRNAs, particularly calcium/calmodulin-dependent protein kinase II (CAMK2) and microtubule-associated protein 2 (MAP2), from the soma to neurites for proper synaptic and growth cone function in hippocampal neurons (Flamand and Meyer, 2022). CAMK2A is a gene known to play roles in synaptic plasticity, memory, and calcium homeostasis, and its dysregulation has been linked to AD susceptibility and pathology (Ghosh and Giese, 2015; Fang et al., 2019). MAP2 plays a key role in neurite and synapse growth and function, and its aberrant phosphorylation is associated with neuropsychiatric and neurodegenerative disorders (Grubisha et al., 2021; DeGiosio et al., 2022). Dysregulation of MAP2 is associated with tauopathies, given that MAP2 shares sequences homology with tau and can inhibit its aggregation by binding to the ends of the tau fibrils (Zhang and Dong, 2012; Holden et al., 2023).
Alterations in m6A are associated with AD pathology and progression. In C57BL/6 J mice, m6A levels decreased postnatally and increased during the aging process, resulting in tissue-specific regulation of mRNA expression and splicing. Furthermore, 5xFAD mice display decreased m6A levels in the 3’UTRs of AD-associated transcripts that correlate with decreased protein expression, despite mRNA levels remaining unchanged (Shafik et al., 2021). APP/PSEN1 mice exhibit increased m6A deposition and METTL3 activation associated with synaptic growth and function (Han et al., 2020). Conversely, AD postmortem human brains exhibit cell type-specific m6A dysregulation, with pyramidal neurons containing lower m6A and METTL3 levels associated with memory deficits, neuronal death, and neurite degeneration (Zhao et al., 2021). These results are further supported by Parkinson’s disease rats and cell model studies, where global m6A downregulation was associated with increased dopaminergic neuron death (Chen et al., 2019). Interestingly, AD glia cells exhibited increased m6A deposition, but these findings require further study (Zhao et al., 2021). METTL3 was also identified as the only m6A reader differentially activated in MCI patients (Zhao et al., 2021). In a mouse model of chronic restraint stress, physical exercise resulted in increased levels of m6A deposition by METTL3 at sites associated with synapse-related pathways in the medial prefrontal ortex, resulting in higher resilience against the effects of stress, as measured by behavioral testing. Furthermore, decreased levels of serum SAM levels in both patients with major depressive disorder (MDD) and in mice were associated with reduced resilience, suggesting that methyl donor S-adenosyl methionine (SAM) could be used as a biomarker and target for the treatment of anxiety, a risk factor for AD (Yan et al., 2022). In an Aβ-induced mouse model, loss of METTL3 in monocyte-derived macrophages decreased DNMT3A mRNA via YTHDF1 and increased macrophage recruitment, resulting in higher rates of Aβ clearance, decreased α-tubulin acetylation, and improved learning and memory scores (Yin et al., 2023). Taken together, these studies suggest that m6A plays a role in the early development and progression of AD.
3.2 tRNA modifications
Transfer RNAs (tRNAs) are stable RNAs involved in translation that recognize mRNA codons and provide the corresponding amino acids. tRNAs are modified post-transcriptionally as part of their maturation process, enhancing tRNA stability, translation efficiency, translation rate, and anticodon-codon specificity (Helm and Alfonzo, 2014; Agris et al., 2018). In eukaryotes, each tRNA molecule averages 13 modifications, which are most commonly found at the anticodon stem-loop at wobble position 34 and adjacent to the 3′ anticodon at position 37 (Dunin-Horkawicz et al., 2006; Jackman and Alfonzo, 2013). tRNA modifications also allow tRNAs to respond quickly to cellular stress, modulating translation to increase the production of key stress response proteins (Damon et al., 2015; Endres et al., 2015; Zhong et al., 2015). Consequently, dysregulation of tRNA modifications is associated with neurodevelopmental disorders, neurodegeneration, and brain tumorigenesis (Ishimura et al., 2014; Nagayoshi et al., 2021; Zhang et al., 2021).
Recent studies have begun to associate cytosolic and mitochondrial tRNA modifications with AD pathophysiology. The posterior cingulate cortex (PCC) is a key metabolic region affected in AD, and hypometabolism and gray matter loss in the PCC have been associated with disease progression (Scheltens et al., 2018; Lee et al., 2020). Astrocyte transcriptome analysis of human postmortem PCC samples identified 226 differentially expressed genes, including the mitochondrial-encoded gene tRNA methyltransferase 61 homolog B (TRMT61B) (Sekar et al., 2015). TRMT61B catalyzes the N1-methyladenine (m1A) modification, which is associated with tRNA folding and stability (Chujo and Suzuki, 2012; Bar-Yaacov et al., 2016). In both the 5xFAD mouse cortex and transgenic Drosophila, cytosolic and mitochondrial m1A hypomethylation and decreased expression of tRNA methyltransferases tRNA methyltransferase 10 homolog C (TRM10C), hydroxysteroid 17-beta dehydrogenase 10 (HSD17B10), and tRNA methyltransferase 61 homolog A (TRMT61A) resulted in increased AD phenotypic severity (Shafik et al., 2022). Additionally, aberrant methylation at mitochondrial tRNA position 9 (m1A9) in the cerebellum of AD patients may also disrupt the cloverleaf structure during tRNA folding and further contribute to mitochondrial dysfunction in neurodegeneration (Silzer et al., 2020; Xiong et al., 2023).
Recently, several tRNA modifying enzymes affecting the wobble position were found to be differentially expressed throughout the human AD brain. Among these were NOP2/Sun RNA methyltransferase 2 (NSUN2), adenosine deaminase tRNA specific 2 and 3 (ADAT2/3), alkB homolog 8 (ALKBH8), and the acetyltransferase elongator complex subunits 1, 3, and 6 (ELP1/3/6). The elongator complex has been reported to play roles in neurodevelopment and neurological disorders, such as amyotrophic lateral sclerosis (ALS), intellectual developmental disorders, neuropathies, and dysautonomias (Simpson et al., 2009; Cohen et al., 2015; Bento-Abreu et al., 2018; Gaik et al., 2022). Notably, ELP3 expression decreases in response to aggregated Aβ across several AD models, resulting in hypomodifications that contribute to tRNA instability and impaired translation (Pereira et al., 2024). Another tRNA modifying enzyme that may contribute to AD pathogenesis and progression is cytoplasmic cysteinyl-tRNA synthetase (CARS). In APP/PS1 mice and SH-SY5Y neuronal cells, increased CARS expression correlated with AD severity and localized to Aβ plaques, exacerbating memory deficits and inflammatory microglial activation (Qi et al., 2024). Ongoing studies into the functions of tRNA modifications and their modifying enzymes will increase our understanding of their role in AD pathology, neurodegeneration, and age-related diseases.
4 Other regulatory RNAs
Regulatory RNAs constitute a wide range of non-coding RNAs that interact with DNA, RNA, and proteins in numerous cellular pathways, and may serve as biomarkers and therapeutic targets for Alzheimer’s disease (Figure 2).
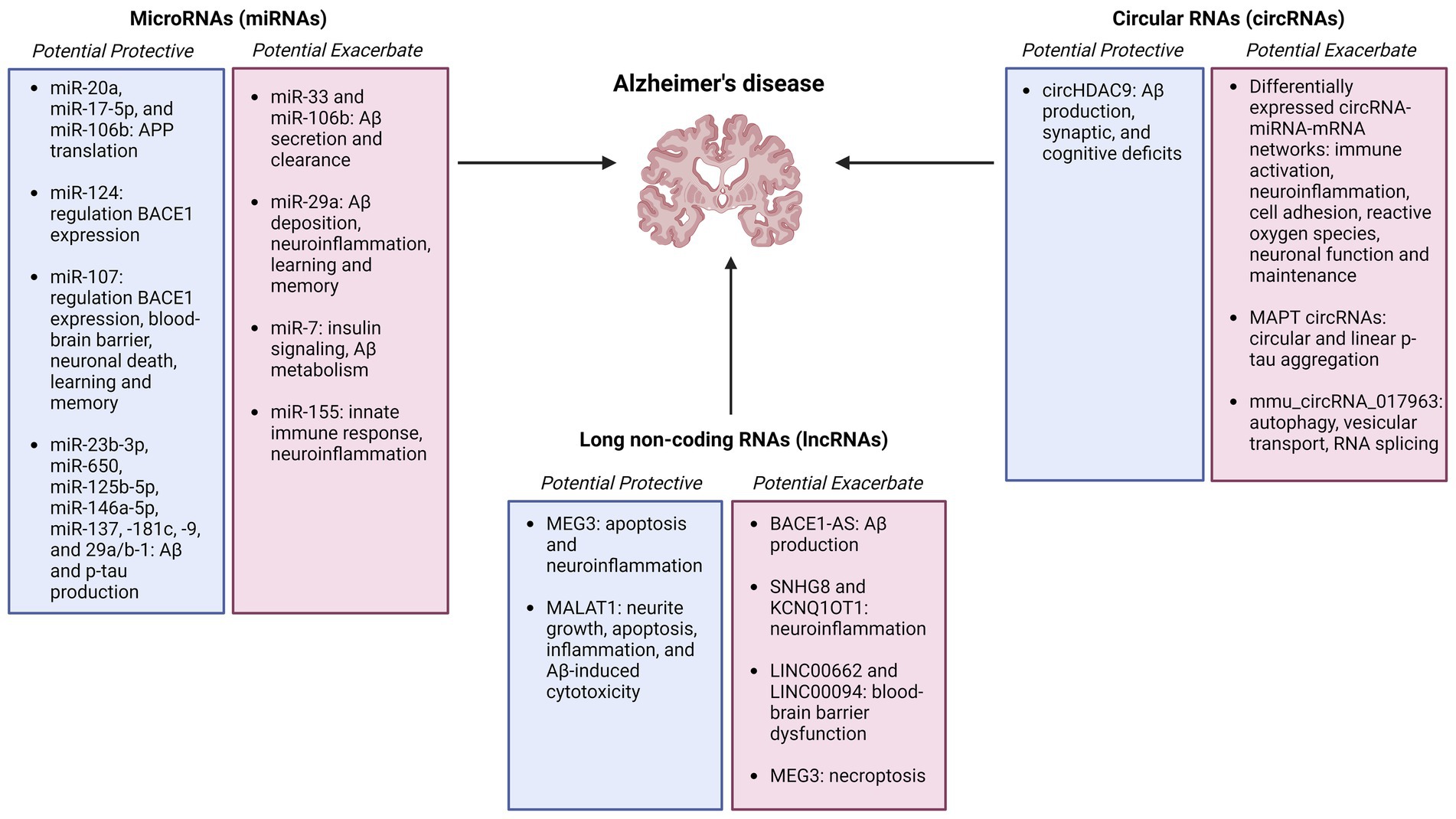
Figure 2. Summary figure of non-coding RNAs (microRNAs, circular RNAs, and long non-coding RNAs) identified as potentially protective or exacerbating in Alzheimer’s disease onset, pathology, and progression Created with BioRender.com.
4.1 Long non-coding RNAs
Long noncoding RNAs (lncRNAs) are over 200 nucleotides long and do not encode a protein product. lncRNAs regulate gene and protein expression in both cis and trans by forming complexes with DNA, RNA, and RNA binding proteins (RBPs) in tissue-specific and ubiquitous manners (Lee, 2012; Jiang et al., 2016). lncRNAs play transcriptional roles in chromatin architecture and RNA polymerase II activity, post-transcriptional roles in splicing and translation, and post-translational roles as protein scaffolds (Romero-Barrios et al., 2018; Hou et al., 2019; Karakas and Ozpolat, 2021; Johnsson et al., 2022). Approximately 40% of currently identified lncRNAs are specifically expressed in the brain, and many have been implicated in neuropathological processes via annotation of the GENCODE human gene catalog and experimental validation in rodent models, including synaptic plasticity, neuroinflammation, blood–brain barrier (BBB) permeability, and neuropathic pain (Derrien et al., 2012; Zhao et al., 2013).
β-secretase 1 (BACE1) is a key enzyme in the cleavage of APP into amyloidogenic Aβ. BACE1 expression is modulated by the lncRNA BACE1-AS derived from the antisense transcript. In the presence of Aβ plaques, BACE1-AS is upregulated and increases BACE1 protein expression by functioning as a competing endogenous RNA with miRNAs known to regulate BACE1. This creates a positive feedback loop that further drives Aβ production and AD pathology that has been validated in vitro and in vivo across several human and mouse tissues and cell lines (Faghihi et al., 2008; Zeng et al., 2019).
One critical lncRNA involved in neuroinflammation and BBB permeability is small nucleolar RNA host gene 8 (SNHG8). In ischemic stroke models, SNHG8 contributes to neuronal apoptosis, microglial activation, and neuroinflammation by sponging miR-449c-5p and miR-425-5p, resulting in the downstream regulation of sirtuin 1 (SIRT1) (Tian et al., 2021; Zhang et al., 2022). SIRT1 is a Nicotinamide adenine dinucleotide (NAD+)-dependent deacetylase enzyme involved in a wide range of AD-associated pathways, including Aβ and tau metabolism, neuronal development, memory, and inflammation (Min et al., 2010; Wang et al., 2013; Corpas et al., 2017). Moreover, in human AD, mouse, and in vitro tauopathy models expressing mutant MAPT, increased p-tau levels resulted in decreased SNHG8 expression, increased stress granule formation, and elevated cytotoxic granule-associated RNA binding protein TIA1 (Bhagat et al., 2023). Other dysregulated lncRNAs contributing to BBB permeability in AD via interactions with RBPs include LINC00662 and LINC00094 (Zhu et al., 2019; Liu et al., 2020). Additionally, KCNQ1OT1 has been shown to increase neuroinflammation and neuronal death in microglial HMC3 cells by sponging miR-30e-3p, resulting in increased expression of the NLR family pyrin domain containing 3 (NLRP3) inflammasome (Song et al., 2021).
Neuronal death is a key pathological hallmark of AD. The lncRNA maternally expressed 3 (MEG3) is upregulated in AD patients, and a human xenograft model suggests that MEG3 expression is sufficient to induce necroptosis (Balusu et al., 2023). Programmed inflammatory cell death is hypothesized to exacerbate AD progression by promoting microglial activation, synaptic loss, and BBB dysfunction (Zhang et al., 2023). Conversely, an AD Aβ25-35 microinjection rat model study suggests that MEG3 overexpression exerts a neuroprotective role by inhibiting apoptosis and inflammation through a MEG3-miR-93-Proteinase kinase B (PI3K/AKT) pathway (Yi et al., 2019). Metastasis associated lung adenocarcinoma transcript 1 (MALAT1) confers a neuroprotective role in AD by promoting neurite outgrowth and inhibiting apoptosis, inflammation, and Aβ1-42 induced cytotoxicity by interacting with miR-125, -200a, -26a, -26b, and their downstream targets Prostaglandin-endoperoxide Synthase 2 (PTGS2), Cyclin-dependent Kinase 5 (CDK5), Forkhead Box Q1 (FOXQ1), and Receptor Tyrosine Kinase (RTK) EphA2 (Ma P. et al., 2019). Further investigation into the roles of MEG3 and MALAT3 in AD pathology as possible therapeutic targets is warranted.
4.2 MicroRNAs
MicroRNAs (miRNAs) are single-stranded non-coding RNAs approximately 22 nucleotides long. miRNAs are transcribed from DNA into primary miRNAs (pri-miRNAs) and further processed into precursor miRNAs (pre-miRNAs) and mature miRNAs by DROSHA and Dicer complexes, respectively. miRNAs can regulate gene expression via translational repression or mRNA degradation and have been implicated in AD pathogenesis (O’Brien et al., 2018).
Aβ production and stability are under the regulation of multiple miRNAs. In human HeLa cells, miR-20a, miR-17-5p, and miR-106b modulate APP translation by binding to the 3’UTR without affecting mRNA levels. Despite all three miRNAs exhibiting significantly decreased levels during mouse embryonic stem cell development and differentiation, miR-106b showed the most significant decrease in human AD brains (Hébert et al., 2009). Additionally, overexpression of miR-106b in both mouse neuroblastoma N2A and human hepatocyte HepG2 cells promotes Aβ secretion and impairs its clearance by targeting the 3’UTR of the ATP-binding cassette transporter A1 (ABCA1) and suppressing its expression (Kim et al., 2012). ABCA1 is required to maintain APOE levels and lipidation, and its dysregulation has been implicated in AD and other inflammatory diseases (Wahrle et al., 2004; Lewandowski et al., 2022). In 5xFAD mice, miR-29a loss of function improved AD-associated deficits in learning and memory, Aβ deposition, and neuroinflammatory markers such as activated astrocytes and microglia. The predicted downstream targets of miR-29a, plexin-A1 (Plxna1), and WD Repeat and FYVE Domain Containing 1 (Wdfy1) have been associated with neuroinflammation, axon guidance, and neurogenesis in mice and rats (Mei et al., 2023).
Insulin is essential for glutamatergic signaling, BBB integrity, vasodilation, neuronal proliferation, differentiation, cognition, and memory (Åberg et al., 2003; Hallschmid et al., 2007; Sawallisch et al., 2009; Heni et al., 2011; Irvine et al., 2011; Kuwabara et al., 2011; Chirivella et al., 2017; Pomytkin et al., 2019; Fetterly et al., 2021; Wang et al., 2022; Nguyen et al., 2023). Changes to insulin signaling, particularly desensitization to insulin, result in chronic inflammation and are a known risk factor for AD across human and rodent in vitro and in vivo models (Baker et al., 2011; Blázquez et al., 2014; Craft et al., 2017). miR-7 is elevated in the cortex of AD patients and regulates critical insulin and Aβ metabolic genes, including ABCA1, insulin receptor (INSR), insulin receptor substrate 2 (IRS-2), insulin-degrading enzyme (IDE), and liver x receptor (LXR) (Fernández-de Frutos et al., 2019). Furthermore, miR-7 has been shown to play roles in neuroinflammatory pathways in astrocytes and mice (Dong et al., 2016; Yue et al., 2020). Another pro-inflammatory miRNA known to modulate the innate immune response is miR-155 (Hu et al., 2022). In 12-month 3xTg AD mice, upregulation of miR-155 correlated with increased neuroinflammatory markers and intracellular Aβ accumulation. This could create a positive feedback loop, as Aβ can activate the c-Jun N-terminal Kinase (JNK) signaling pathway, resulting in additional miR-155 overexpression and downregulation of its target suppressor of cytokine signaling 1 (SOCS-1) (Guedes et al., 2014).
miRNAs can also play protective roles in AD. miR-124 and miR-107 levels are significantly decreased in AD, resulting in the upregulation of BACE1 mRNA levels (Wang et al., 2008; An et al., 2018). In human brain microvascular endothelial cell (EC) co-cultures modeling the BBB, miR-107 overexpression stimulated tight junction-related proteins to repair BBB permeability (Liu et al., 2016). Additionally, miR-107 mimic administration in C57BL/6J mice alleviated behavioral and pathological symptoms of AD, including pyramidal neuronal loss and deficits in spatial memory and hippocampal long-term potentiation (Shu et al., 2018). Similarly, reductions of miR-23b-3p levels in APP/PSEN1 mice resulted in increased p-tau and neuronal death via impaired inhibition of its target glycogen synthase kinase-3β (GSK-3β). Other miRNAs downregulated in early AD that may inhibit Aβ and p-tau production include miR-23b-3p, miR-125b-5p, miR-146a-5p, miR-137, miR-181c, miR-9, and miR-29a/b-1 (Geekiyanage and Chan, 2011; Jiang et al., 2022; Yashooa and Nabi, 2022). For instance, targeting elevated miR-29a levels in 5xFAD mice with an AAV miR-29a sponge results in decreased miR-29a expression, Aβ deposition, and expression of neuroinflammatory markers, as well as improvements in learning and memory (Mei et al., 2023). Another potential therapeutic target for AD is miR-650, which has been found to be significantly increased in the human AD cortex and targets the key AD genes APOE, PSEN1, and CDK5. Overexpression of miR-650 in APP/PSEN1 mice results in the decreased levels of CDK5 and the downstream inhibition of Aβ and neuronal loss (Lin et al., 2023). Further investigations into the roles of miRNAs as protective or exacerbating factors in AD will aid in the understanding of the disease.
4.3 Circular RNAs
Circular RNAs (circRNAs) are single-stranded, covalently closed loops formed by non-canonical pre-mRNA back splicing (Salzman et al., 2012). They are stable, conserved, and widely expressed in neuronal tissues (Jeck et al., 2013; Ashwal-Fluss et al., 2014). CircRNAs most commonly function as miRNA sponges, where a circRNA binds in a sequence-specific manner to a miRNA or miRNA family, preventing its binding to downstream target genes and proteins (Hansen et al., 2013). CircRNAs accumulate with age in the brain depending on the host gene expression in cell type- and region-specific manners (Gruner et al., 2016; Chen et al., 2018). In AD patient samples from the Knight Alzheimer Disease Research Center (ADRC) and the Mount Sinai Brain Bank (MSBB), circRNA expression precluded AD symptoms, correlated with diagnosis and symptom severity, and associated with genes and miRNAs involved in AD-related pathways across several brain regions (Dube et al., 2019; Lo et al., 2020). Per these findings, the MAPT locus can generate two circRNAs, exon 12 → 7 and 12 → 10, resulting in the continuous rolling circle translation of tau proteins due to the lack of a stop codon and adenine deaminase acting on RNA (ADAR) activation, promoting circular and linear tau protein aggregation (Welden et al., 2022).
AD mouse models recapitulate the circRNA dysregulation implicated in AD postmortem patient samples. Senescence Accelerated Mouse-Prone 8 (SAMP8) mice are utilized to study age-related cognitive decline and recapitulate cognitive and behavioral AD phenotypes. SAMP8 8- and 10-month mice exhibit hippocampal circRNA dysregulation that increases with age and may be associated with AD progression and severity. One significantly dysregulated circRNA, mmu_circRNA_017963, was predicted to sponge mmu_miR_7033-3p and affect downstream AD signaling pathways, including autophagy, vesicular transport, and RNA splicing (Huang et al., 2018). In APP/PSEN1 mice, circHDAC9 sponges aberrantly elevated miR-138, resulting in increased levels of its target SIRT1 and amelioration of Aβ production, synaptic, and cognitive deficits (Lu et al., 2019). Furthermore, Tg2576 and APP/PSEN1 mice exhibit differentially expressed circRNA-miRNA-mRNA networks associated with AD pathology, including upregulation of immune and inflammatory activation, cellular adhesion, and reactive oxygen species; and downregulation of synapse and dendrite function and maintenance (Lee et al., 2019; Ma N. et al., 2019).
5 Discussion
Epigenetic alterations of DNA and RNA methylation, acetylation, and the many non-coding RNA species provide another dimension to the investigation of the pathological mechanisms underlying AD onset and progression.
Not discussed in this review are the numerous post-translational modifications of histone proteins that alter chromatin accessibility and protein interactions, including methylation, acetylation, phosphorylation, ubiquitination, and SUMOnylation among others. Briefly, histone modifications and histone-modifying enzymes that catalyze their addition or removal, such as histone acetylatylases (HATs) and histone deacetylases (HDACs), have been implicated in AD-related processes such as impaired neurogenesis, memory deficits, and decreased neuronal survival (Park et al., 2022; Santana et al., 2023). Recent developments using HDAC inhibitors to treat cancers, ALS, and other neurological diseases show promising results in ameliorating symptoms and highlight the necessity of exploring these new approaches to treat AD (Li et al., 2020; Bondarev et al., 2021).
This review focused on the emerging roles of RNA adenine methylation (m6A), tRNA modifications, lncRNAs, miRNAs, and circRNAs. However, several other RNA modifications and species have been implicated in age-related diseases, including AD. A meta-analysis of human brain datasets identified RNA editing events in transcripts for several protein-coding genes associated with AD, including synaptotagmin 11 (SYT11) and ORAI calcium release-activated calcium modulator 2 (ORAI2) (Ma et al., 2021). Synaptotagmins interact with APP to promote Aβ production, and ORAI2 is involved in calcium homeostasis. ORAI2 overexpression has been associated with dysregulated extracellular store-operated calcium entry (SOCE), synaptic plasticity, neuronal spine morphology, and increased Aβ42 accumulation (Gautam et al., 2015; Huang et al., 2020; Scremin et al., 2020). Recently, altered small RNA modification profiles have also been associated with AD, offering a potential avenue of investigation (Zhang X. et al., 2020). An additional RNA species emerging as a potential biomarker for AD are small non-coding tRNA fragments (tRFs). tRFs are generated from precursor and mature tRNA constitutively or in response to cellular stress (Yamasaki et al., 2009; Elkordy et al., 2018). A recent study of human AD samples found increased levels of tRFs in the hippocampus, including two Trf5-ProAGG isoforms that warrant further study to determine their possible correlation with aging and AD severity (Wu et al., 2021).
Another epigenetic mechanism associated with AD that needs further study is transposable elements (TE). TEs are non-coding regulatory DNA sequences that move their position in the genome via “copy-paste” mechanisms as retrotransposons that “cut-paste” in the genome as DNA transposons. TEs are associated with aging, cellular senescence, and neurodegenerative diseases. Phosphorylated tau levels elevate TE transcription in human and D. melanogaster brains, resulting in increased neuroinflammation via retrotransposon-derived double-stranded RNAs (dsRNAs) (Guo et al., 2018; Ochoa et al., 2023). Additionally, the silencing of PIWI-interacting RNAs (piRNAs) leads to the transcription of silenced TEs, and contributes to their dysregulation in LOAD and tauopathies (Sun et al., 2018; Macciardi et al., 2022). Future studies should continue exploring the role of TEs-associated mechanisms in aging and neurodegeneration.
Author contributions
PM-F: Writing – original draft, Writing – review & editing. PJ: Writing – review & editing. BY: Writing – review & editing.
Funding
The author(s) declare that financial support was received for the research, authorship, and/or publication of this article. This work was supported by the National Institutes of Health (NIH) grants R01MH117122, R01AG062577, R01AG064786, R01NS118819, and R01AG078937 to BY. The content is solely the authors’ responsibility and does not necessarily represent the official views of the National Institutes of Health.
Conflict of interest
The authors declare that the research was conducted in the absence of any commercial or financial relationships that could be construed as a potential conflict of interest.
Publisher’s note
All claims expressed in this article are solely those of the authors and do not necessarily represent those of their affiliated organizations, or those of the publisher, the editors and the reviewers. Any product that may be evaluated in this article, or claim that may be made by its manufacturer, is not guaranteed or endorsed by the publisher.
References
Åberg, M. A. I., Åberg, N. D., Palmer, T. D., Alborn, A.-M., Carlsson-Skwirut, C., Bang, P., et al. (2003). IGF-I has a direct proliferative effect in adult hippocampal progenitor cells. Mol. Cell. Neurosci. 24, 23–40. doi: 10.1016/S1044-7431(03)00082-4
Agris, P. F., Eruysal, E. R., Narendran, A., Väre, V. Y. P., Vangaveti, S., and Ranganathan, S. V. (2018). Celebrating wobble decoding: half a century and still much is new. RNA Biol. 15, 537–553. doi: 10.1080/15476286.2017.1356562
Albert-Gascó, H., Ros-Bernal, F., Castillo-Gómez, E., and Olucha-Bordonau, F. E. (2020). MAP/ERK signaling in developing cognitive and emotional function and its effect on pathological and neurodegenerative processes. Int. J. Mol. Sci. 21:4471. doi: 10.3390/ijms21124471
Alonso, A. C., Li, B., Grundke-Iqbal, I., and Iqbal, K. (2006). Polymerization of hyperphosphorylated tau into filaments eliminates its inhibitory activity. Proc. Natl. Acad. Sci. 103, 8864–8869. doi: 10.1073/pnas.0603214103
Alzheimer’s Association (2023). 2023 Alzheimer’s disease facts and figures. Alzheimers Dement. 19, 1598–1695. doi: 10.1002/alz.13016
An, F., Gong, G., Wang, Y., Bian, M., Yu, L., and Wei, C. (2018). Correction: MiR-124 acts as a target for Alzheimer’s disease by regulating BACE1. Oncotarget 9, –24871. doi: 10.18632/oncotarget.25461
Armstrong, M. J., Jin, Y., Vattathil, S. M., Huang, Y., Schroeder, J. P., Bennet, D. A., et al. (2023). Role of TET1-mediated epigenetic modulation in Alzheimer’s disease. Neurobiol. Dis. 185:106257. doi: 10.1016/j.nbd.2023.106257
Ashok, B. S., Ajith, T. A., and Sivanesan, S. (2017). Hypoxia-inducible factors as neuroprotective agent in Alzheimer’s disease. Clin. Exp. Pharmacol. Physiol. 44, 327–334. doi: 10.1111/1440-1681.12717
Ashwal-Fluss, R., Meyer, M., Pamudurti, N. R., Ivanov, A., Bartok, O., Hanan, M., et al. (2014). circRNA biogenesis competes with pre-mRNA splicing. Mol. Cell 56, 55–66. doi: 10.1016/j.molcel.2014.08.019
Baker, L. D., Cross, D. J., Minoshima, S., Belongia, D., Watson, G. S., and Craft, S. (2011). Insulin resistance and Alzheimer-like reductions in regional cerebral glucose metabolism for cognitively Normal adults with prediabetes or early type 2 diabetes. Arch. Neurol. 68, 51–57. doi: 10.1001/archneurol.2010.225
Balusu, S., Horré, K., Thrupp, N., Craessaerts, K., Snellinx, A., Serneels, L., et al. (2023). MEG3 activates necroptosis in human neuron xenografts modeling Alzheimer’s disease. Science 381, 1176–1182. doi: 10.1126/science.abp9556
Balzarolo, M., Engels, S., de Jong, A. J., Franke, K., van den Berg, T. K., Gulen, M. F., et al. (2021). m6A methylation potentiates cytosolic dsDNA recognition in a sequence-specific manner. Open Biol. 11:210030. doi: 10.1098/rsob.210030
Bar-Yaacov, D., Frumkin, I., Yashiro, Y., Chujo, T., Ishigami, Y., Chemla, Y., et al. (2016). Mitochondrial 16S rRNA is methylated by tRNA methyltransferase TRMT61B in all vertebrates. PLoS Biol. 14:e1002557. doi: 10.1371/journal.pbio.1002557
Bayraktar, G., Yuanxiang, P., Confettura, A. D., Gomes, G. M., Raza, S. A., Stork, O., et al. (2020). Synaptic control of DNA methylation involves activity-dependent degradation of DNMT3A1 in the nucleus. Neuropsychopharmacology 45, 2120–2130. doi: 10.1038/s41386-020-0780-2
Bento-Abreu, A., Jager, G., Swinnen, B., Rué, L., Hendrickx, S., Jones, A., et al. (2018). Elongator subunit 3 (ELP3) modifies ALS through tRNA modification. Hum. Mol. Genet. 27, 1276–1289. doi: 10.1093/hmg/ddy043
Bhagat, R., Minaya, M. A., Renganathan, A., Mehra, M., Marsh, J., Martinez, R., et al. (2023). Long non-coding RNA SNHG8 drives stress granule formation in tauopathies. Mol. Psychiatry 28, 4889–4901. doi: 10.1038/s41380-023-02237-2
Blázquez, E., Velázquez, E., Hurtado-Carneiro, V., and Ruiz-Albusac, J. M. (2014). Insulin in the brain: its pathophysiological implications for states related with central insulin resistance, type 2 diabetes and Alzheimer’s disease. Front. Endocrinol. 5:161. doi: 10.3389/fendo.2014.00161
Boccaletto, P., Stefaniak, F., Ray, A., Cappannini, A., Mukherjee, S., Purta, E., et al. (2022). MODOMICS: a database of RNA modification pathways. 2021 update. Nucleic Acids Res. 50, D231–D235. doi: 10.1093/nar/gkab1083
Bondarev, A. D., Attwood, M. M., Jonsson, J., Chubarev, V. N., Tarasov, V. V., and Schiöth, H. B. (2021). Recent developments of HDAC inhibitors: emerging indications and novel molecules. Brit. J. Clin. Pharma 87, 4577–4597. doi: 10.1111/bcp.14889
Brockmann, R., Nixon, J., Love, B. L., and Yunusa, I. (2023). Impacts of FDA approval and Medicare restriction on antiamyloid therapies for Alzheimer’s disease: patient outcomes, healthcare costs, and drug development. Lancet Reg. Health Am. 20:100467. doi: 10.1016/j.lana.2023.100467
Cacciottolo, M., Wang, X., Driscoll, I., Woodward, N., Saffari, A., Reyes, J., et al. (2017). Particulate air pollutants, APOE alleles and their contributions to cognitive impairment in older women and to amyloidogenesis in experimental models. Transl. Psychiatry 7, –e1022. doi: 10.1038/tp.2016.280
Chen, G., Xu, T., Yan, Y., Zhou, Y., Jiang, Y., Melcher, K., et al. (2017). Amyloid beta: structure, biology and structure-based therapeutic development. Acta Pharmacol. Sin. 38, 1205–1235. doi: 10.1038/aps.2017.28
Chen, B. J., Yang, B., and Janitz, M. (2018). Region-specific expression of circular RNAs in the mouse brain. Neurosci. Lett. 666, 44–47. doi: 10.1016/j.neulet.2017.12.022
Chen, X., Yu, C., Guo, M., Zheng, X., Ali, S., Huang, H., et al. (2019). Down-regulation of m6A mRNA methylation is involved in dopaminergic neuronal death. ACS Chem. Neurosci. 10, 2355–2363. doi: 10.1021/acschemneuro.8b00657
Chen, W.-W., Zhang, X., and Huang, W.-J. (2016). Role of physical exercise in Alzheimer’s disease (review). Biomed. Rep. 4, 403–407. doi: 10.3892/br.2016.607
Chen, Y., Zhao, S., Fan, Z., Li, Z., Zhu, Y., Shen, T., et al. (2021). Metformin attenuates plaque-associated tau pathology and reduces amyloid-β burden in APP/PS1 mice. Alzheimers Res. Ther. 13:40. doi: 10.1186/s13195-020-00761-9
Cheng, Y., Sun, M., Chen, L., Li, Y., Lin, L., Yao, B., et al. (2018). Ten-eleven translocation proteins modulate the response to environmental stress in mice. Cell Rep. 25, 3194–3203.e4. doi: 10.1016/j.celrep.2018.11.061
Chirivella, L., Kirstein, M., Ferrón, S. R., Domingo-Muelas, A., Durupt, F. C., Acosta-Umanzor, C., et al. (2017). Cyclin-dependent kinase 4 regulates adult neural stem cell proliferation and differentiation in response to insulin. Stem Cells 35, 2403–2416. doi: 10.1002/stem.2694
Chujo, T., and Suzuki, T. (2012). Trmt61B is a methyltransferase responsible for 1-methyladenosine at position 58 of human mitochondrial tRNAs. RNA 18, 2269–2276. doi: 10.1261/rna.035600.112
Cohen, J. S., Srivastava, S., Farwell, K. D., Lu, H.-M., Zeng, W., Lu, H., et al. (2015). ELP2 is a novel gene implicated in neurodevelopmental disabilities. Am. J. Med. Genet. A 167, 1391–1395. doi: 10.1002/ajmg.a.36935
Corpas, R., Revilla, S., Ursulet, S., Castro-Freire, M., Kaliman, P., Petegnief, V., et al. (2017). SIRT1 overexpression in mouse Hippocampus induces cognitive enhancement through Proteostatic and neurotrophic mechanisms. Mol. Neurobiol. 54, 5604–5619. doi: 10.1007/s12035-016-0087-9
Craft, S., Claxton, A., Baker, L. D., Hanson, A. J., Cholerton, B., Trittschuh, E. H., et al. (2017). Effects of regular and long-acting insulin on cognition and Alzheimer’s disease biomarkers: a pilot clinical trial. J. Alzheimers Dis. 57, 1325–1334. doi: 10.3233/JAD-161256
Damon, J. R., Pincus, D., and Ploegh, H. L. (2015). tRNA thiolation links translation to stress responses in Saccharomyces cerevisiae. MBoC 26, 270–282. doi: 10.1091/mbc.E14-06-1145
De Jager, P. L., Srivastava, G., Lunnon, K., Burgess, J., Schalkwyk, L. C., Yu, L., et al. (2014). Alzheimer’s disease: early alterations in brain DNA methylation at ANK1, BIN1, RHBDF2 and other loci. Nat. Neurosci. 17, 1156–1163. doi: 10.1038/nn.3786
DeGiosio, R. A., Grubisha, M. J., MacDonald, M. L., McKinney, B. C., Camacho, C. J., and Sweet, R. A. (2022). More than a marker: potential pathogenic functions of MAP2. Front. Mol. Neurosci. 15:974890. doi: 10.3389/fnmol.2022.974890
Derrien, T., Johnson, R., Bussotti, G., Tanzer, A., Djebali, S., Tilgner, H., et al. (2012). The GENCODE v7 catalog of human long noncoding RNAs: analysis of their gene structure, evolution, and expression. Genome Res. 22, 1775–1789. doi: 10.1101/gr.132159.111
DeTure, M. A., and Dickson, D. W. (2019). The neuropathological diagnosis of Alzheimer’s disease. Mol. Neurodegener. 14:32. doi: 10.1186/s13024-019-0333-5
Dong, Y.-F., Chen, Z.-Z., Zhao, Z., Yang, D.-D., Yan, H., Ji, J., et al. (2016). Potential role of microRNA-7 in the anti-neuroinflammation effects of nicorandil in astrocytes induced by oxygen-glucose deprivation. J. Neuroinflammation 13:60. doi: 10.1186/s12974-016-0527-5
Drummond, E., Pires, G., MacMurray, C., Askenazi, M., Nayak, S., Bourdon, M., et al. (2020). Phosphorylated tau interactome in the human Alzheimer’s disease brain. Brain 143, 2803–2817. doi: 10.1093/brain/awaa223
Dube, U., Del-Aguila, J. L., Li, Z., Budde, J. P., Jiang, S., Hsu, S., et al. (2019). An atlas of cortical circular RNA expression in Alzheimer disease brains demonstrates clinical and pathological associations. Nat. Neurosci. 22, 1903–1912. doi: 10.1038/s41593-019-0501-5
Dunin-Horkawicz, S., Czerwoniec, A., Gajda, M. J., Feder, M., Grosjean, H., and Bujnicki, J. M. (2006). MODOMICS: a database of RNA modification pathways. Nucleic Acids Res. 34, D145–D149. doi: 10.1093/nar/gkj084
Elkordy, A., Mishima, E., Niizuma, K., Akiyama, Y., Fujimura, M., Tominaga, T., et al. (2018). Stress-induced tRNA cleavage and tiRNA generation in rat neuronal PC12 cells. J. Neurochem. 146, 560–569. doi: 10.1111/jnc.14321
Endres, L., Dedon, P. C., and Begley, T. J. (2015). Codon-biased translation can be regulated by wobble-base tRNA modification systems during cellular stress responses. RNA Biol. 12, 603–614. doi: 10.1080/15476286.2015.1031947
Faghihi, M. A., Modarresi, F., Khalil, A. M., Wood, D. E., Sahagan, B. G., Morgan, T. E., et al. (2008). Expression of a noncoding RNA is elevated in Alzheimer’s disease and drives rapid feed-forward regulation of β-secretase. Nat. Med. 14, 723–730. doi: 10.1038/nm1784
Fang, X., Tang, W., Yang, F., Lu, W., Cai, J., Ni, J., et al. (2019). A comprehensive analysis of the CaMK2A gene and Susceptibility to Alzheimer’s disease in the Han Chinese population. Front. Aging Neurosci. 11:84. doi: 10.3389/fnagi.2019.00084
Feng, X., Cui, X., Zhang, L.-S., Ye, C., Wang, P., Zhong, Y., et al. (2024). Sequencing of N6-methyl-deoxyadenosine at single-base resolution across the mammalian genome. Mol. Cell 84, 596–610.e6. doi: 10.1016/j.molcel.2023.12.021
Fernandes, S., Grova, N., Roth, S., Duca, R.-C., Godderis, L., Guebels, P., et al. (2021). N 6 -Methyladenine in eukaryotic DNA: tissue distribution, early embryo development, and neuronal toxicity. Front. Genet. 12:657171. doi: 10.3389/fgene.2021.657171
Fernández-de Frutos, M., Galán-Chilet, I., Goedeke, L., Kim, B., Pardo-Marqués, V., Pérez-García, A., et al. (2019). MicroRNA 7 impairs insulin signaling and regulates Aβ levels through posttranscriptional regulation of the insulin receptor substrate 2, insulin receptor, insulin-degrading enzyme, and liver X receptor pathway. Mol. Cell. Biol. 39, e00170–e00119. doi: 10.1128/MCB.00170-19
Fetterly, T. L., Oginsky, M. F., Nieto, A. M., Alonso-Caraballo, Y., Santana-Rodriguez, Z., and Ferrario, C. R. (2021). Insulin Bidirectionally alters NAc glutamatergic transmission: interactions between insulin receptor activation, endogenous opioids, and glutamate release. J. Neurosci. 41, 2360–2372. doi: 10.1523/JNEUROSCI.3216-18.2021
Flamand, M. N., and Meyer, K. D. (2022). m6A and YTHDF proteins contribute to the localization of select neuronal mRNAs. Nucleic Acids Res. 50, 4464–4483. doi: 10.1093/nar/gkac251
Gaik, M., Kojic, M., Stegeman, M. R., Öncü-Öner, T., Kościelniak, A., Jones, A., et al. (2022). Functional divergence of the two Elongator subcomplexes during neurodevelopment. EMBO Mol. Med. 14:e15608. doi: 10.15252/emmm.202115608
Gatz, M., Reynolds, C. A., Fratiglioni, L., Johansson, B., Mortimer, J. A., Berg, S., et al. (2006). Role of genes and environments for explaining Alzheimer disease. Arch. Gen. Psychiatry 63, 168–174. doi: 10.1001/archpsyc.63.2.168
Gautam, V., D’Avanzo, C., Berezovska, O., Tanzi, R. E., and Kovacs, D. M. (2015). Synaptotagmins interact with APP and promote Aβ generation. Mol. Neurodegener. 10:31. doi: 10.1186/s13024-015-0028-5
Geekiyanage, H., and Chan, C. (2011). MicroRNA-137/181c regulates serine Palmitoyltransferase and in turn amyloid β, novel targets in sporadic Alzheimer’s disease. J. Neurosci. 31, 14820–14830. doi: 10.1523/JNEUROSCI.3883-11.2011
Ghosh, A., and Giese, K. P. (2015). Calcium/calmodulin-dependent kinase II and Alzheimer’s disease. Mol. Brain 8:78. doi: 10.1186/s13041-015-0166-2
Ghosh, K., and Pan, H.-L. (2022). Epigenetic mechanisms of neural plasticity in chronic neuropathic pain. ACS Chem. Neurosci. 13, 432–441. doi: 10.1021/acschemneuro.1c00841
Greer, E. L., Blanco, M. A., Gu, L., Sendinc, E., Liu, J., Aristizábal-Corrales, D., et al. (2015). DNA methylation on N6-adenine in C. elegans. Cell 161, 868–878. doi: 10.1016/j.cell.2015.04.005
Grodstein, F., Lemos, B., Yu, L., Klein, H.-U., Iatrou, A., Buchman, A. S., et al. (2021). The association of epigenetic clocks in brain tissue with brain pathologies and common aging phenotypes. Neurobiol. Dis. 157:105428. doi: 10.1016/j.nbd.2021.105428
Grubisha, M. J., Sun, X., MacDonald, M. L., Garver, M., Sun, Z., Paris, K. A., et al. (2021). MAP2 is differentially phosphorylated in schizophrenia, altering its function. Mol. Psychiatry 26, 5371–5388. doi: 10.1038/s41380-021-01034-z
Gruner, H., Cortés-López, M., Cooper, D. A., Bauer, M., and Miura, P. (2016). CircRNA accumulation in the aging mouse brain. Sci. Rep. 6:38907. doi: 10.1038/srep38907
Guedes, J. R., Custódia, C. M., Silva, R. J., de Almeida, L. P., Pedroso de Lima, M. C., and Cardoso, A. L. (2014). Early miR-155 upregulation contributes to neuroinflammation in Alzheimer’s disease triple transgenic mouse model. Hum. Mol. Genet. 23, 6286–6301. doi: 10.1093/hmg/ddu348
Guo, C., Jeong, H.-H., Hsieh, Y.-C., Klein, H.-U., Bennett, D. A., Jager, P. L. D., et al. (2018). Tau activates transposable elements in Alzheimer’s disease. Cell Rep. 23, 2874–2880. doi: 10.1016/j.celrep.2018.05.004
Guo, Y.-J., Pan, W.-W., Liu, S.-B., Shen, Z.-F., Xu, Y., and Hu, L.-L. (2020). ERK/MAPK signalling pathway and tumorigenesis (review). Exp. Ther. Med. 19, 1997–2007. doi: 10.3892/etm.2020.8454
Hahn, M. A., Qiu, R., Wu, X., Li, A. X., Zhang, H., Wang, J., et al. (2013). Dynamics of 5-Hydroxymethylcytosine and chromatin Marks in mammalian neurogenesis. Cell Rep. 3, 291–300. doi: 10.1016/j.celrep.2013.01.011
Hallschmid, M., Benedict, C., Born, J., and Kern, W. (2007). Targeting metabolic and cognitive pathways of the CNS by intranasal insulin administration. Expert Opin. Drug Deliv. 4, 319–322. doi: 10.1517/17425247.4.4.319
Han, M., Liu, Z., Xu, Y., Liu, X., Wang, D., Li, F., et al. (2020). Abnormality of m6A mRNA methylation is involved in Alzheimer’s disease. Front. Neurosci. 14:98. doi: 10.3389/fnins.2020.00098
Hansen, T. B., Jensen, T. I., Clausen, B. H., Bramsen, J. B., Finsen, B., Damgaard, C. K., et al. (2013). Natural RNA circles function as efficient microRNA sponges. Nature 495, 384–388. doi: 10.1038/nature11993
Hao, Z., Wu, T., Cui, X., Zhu, P., Tan, C., Dou, X., et al. (2020). N6-deoxyadenosine methylation in mammalian mitochondrial DNA. Mol. Cell 78, 382–395.e8. doi: 10.1016/j.molcel.2020.02.018
He, B., Zhang, C., Zhang, X., Fan, Y., Zeng, H., Liu, J., et al. (2021). Tissue-specific 5-hydroxymethylcytosine landscape of the human genome. Nat. Commun. 12:4249. doi: 10.1038/s41467-021-24425-w
Hébert, S. S., Horré, K., Nicolaï, L., Bergmans, B., Papadopoulou, A. S., Delacourte, A., et al. (2009). MicroRNA regulation of Alzheimer’s amyloid precursor protein expression. Neurobiol. Dis. 33, 422–428. doi: 10.1016/j.nbd.2008.11.009
Helm, M., and Alfonzo, J. D. (2014). Post-transcriptional RNA modifications: playing metabolic games in a cell’s chemical legoland. Chem. Biol. 21, 174–185. doi: 10.1016/j.chembiol.2013.10.015
Heni, M., Hennige, A. M., Peter, A., Siegel-Axel, D., Ordelheide, A. M., Krebs, N., et al. (2011). Insulin promotes glycogen storage and cell proliferation in primary human astrocytes. PLoS One 6:e21594. doi: 10.1371/journal.pone.0021594
Hess, M. E., Hess, S., Meyer, K. D., Verhagen, L. A. W., Koch, L., Brönneke, H. S., et al. (2013). The fat mass and obesity associated gene (Fto) regulates activity of the dopaminergic midbrain circuitry. Nat. Neurosci. 16, 1042–1048. doi: 10.1038/nn.3449
Holden, M. R., Krzesinski, B. J., Weismiller, H. A., Shady, J. R., and Margittai, M. (2023). MAP2 caps tau fibrils and inhibits aggregation. J. Biol. Chem. 299:104891. doi: 10.1016/j.jbc.2023.104891
Hou, Y., Zhang, R., and Sun, X. (2019). Enhancer LncRNAs influence chromatin interactions in different ways. Front. Genet. 10:936. doi: 10.3389/fgene.2019.00936
Hsu, S., Gordon, B. A., Hornbeck, R., Norton, J. B., Levitch, D., Louden, A., et al. (2018). Discovery and validation of autosomal dominant Alzheimer’s disease mutations. Alzheimers Res. Ther. 10:67. doi: 10.1186/s13195-018-0392-9
Hu, J., Huang, S., Liu, X., Zhang, Y., Wei, S., and Hu, X. (2022). miR-155: An important role in inflammation response. Journal of immunology. Research 2022:e7437281. doi: 10.1155/2022/7437281
Huang, J.-L., Qin, M.-C., Zhou, Y., Xu, Z.-H., Yang, S., Zhang, F., et al. (2018). Comprehensive analysis of differentially expressed profiles of Alzheimer’s disease associated circular RNAs in an Alzheimer’s disease mouse model. Aging 10, 253–265. doi: 10.18632/aging.101387
Huang, A. S., Tong, B. C. K., Wu, A. J., Chen, X., Sreenivasmurthy, S. G., Zhu, Z., et al. (2020). Rectifying attenuated store-operated calcium entry as a therapeutic approach for Alzheimer’s disease. Curr. Alzheimer Res. 17, 1072–1087. doi: 10.2174/1567205018666210119150613
Irvine, E. E., Drinkwater, L., Radwanska, K., Al-Qassab, H., Smith, M. A., O’Brien, M., et al. (2011). Insulin receptor substrate 2 is a negative regulator of memory formation. Learn. Mem. 18, 375–383. doi: 10.1101/lm.2111311
Ishimura, R., Nagy, G., Dotu, I., Zhou, H., Yang, X.-L., Schimmel, P., et al. (2014). Ribosome stalling induced by mutation of a CNS-specific tRNA causes neurodegeneration. Science 345, 455–459. doi: 10.1126/science.1249749
Jackman, J. E., and Alfonzo, J. D. (2013). Transfer RNA modifications: nature’s combinatorial chemistry playground. WIREs RNA 4, 35–48. doi: 10.1002/wrna.1144
Jansen, I. E., Savage, J. E., Watanabe, K., Bryois, J., Williams, D. M., Steinberg, S., et al. (2019). Genome-wide meta-analysis identifies new loci and functional pathways influencing Alzheimer’s disease risk. Nat. Genet. 51, 404–413. doi: 10.1038/s41588-018-0311-9
Jeck, W. R., Sorrentino, J. A., Wang, K., Slevin, M. K., Burd, C. E., Liu, J., et al. (2013). Circular RNAs are abundant, conserved, and associated with ALU repeats. RNA 19, 141–157. doi: 10.1261/rna.035667.112
Jiang, C., Li, Y., Zhao, Z., Lu, J., Chen, H., Ding, N., et al. (2016). Identifying and functionally characterizing tissue-specific and ubiquitously expressed human lncRNAs. Oncotarget 7, 7120–7133. doi: 10.18632/oncotarget.6859
Jiang, H., Liu, J., Guo, S., Zeng, L., Cai, Z., Zhang, J., et al. (2022). miR-23b-3p rescues cognition in Alzheimer’s disease by reducing tau phosphorylation and apoptosis via GSK-3β signaling pathways. Mol. Ther. Nucleic Acids 28, 539–557. doi: 10.1016/j.omtn.2022.04.008
Jin, B., and Robertson, K. D. (2013). DNA methyltransferases (DNMTs), DNA damage repair, and Cancer. Adv. Exp. Med. Biol. 754, 3–29. doi: 10.1007/978-1-4419-9967-2_1
Jin, W.-N., Shi, K., He, W., Sun, J.-H., Van Kaer, L., Shi, F.-D., et al. (2021). Neuroblast senescence in the aged brain augments natural killer cell cytotoxicity leading to impaired neurogenesis and cognition. Nat. Neurosci. 24, 61–73. doi: 10.1038/s41593-020-00745-w
Johnsson, P., Ziegenhain, C., Hartmanis, L., Hendriks, G.-J., Hagemann-Jensen, M., Reinius, B., et al. (2022). Transcriptional kinetics and molecular functions of long noncoding RNAs. Nat. Genet. 54, 306–317. doi: 10.1038/s41588-022-01014-1
Kabacik, S., Lowe, D., Fransen, L., Leonard, M., Ang, S.-L., Whiteman, C., et al. (2022). The relationship between epigenetic age and the hallmarks of aging in human cells. Nat. Aging 2, 484–493. doi: 10.1038/s43587-022-00220-0
Kang, J., Lienhard, M., Pastor, W. A., Chawla, A., Novotny, M., Tsagaratou, A., et al. (2015). Simultaneous deletion of the methylcytosine oxidases Tet1 and Tet3 increases transcriptome variability in early embryogenesis. Proc. Natl. Acad. Sci. USA 112, E4236–E4245. doi: 10.1073/pnas.1510510112
Karakas, D., and Ozpolat, B. (2021). The role of LncRNAs in translation. Non-Coding RNA 7:16. doi: 10.3390/ncrna7010016
Kigar, S. L., Chang, L., Guerrero, C. R., Sehring, J. R., Cuarenta, A., Parker, L. L., et al. (2017). N 6 -methyladenine is an epigenetic marker of mammalian early life stress. Sci. Rep. 7:18078. doi: 10.1038/s41598-017-18414-7
Kim, J., Yoon, H., Ramírez, C. M., Lee, S.-M., Hoe, H. S., Fernández-Hernando, C., et al. (2012). miR-106b impairs cholesterol efflux and increases Aβ levels by repressing ABCA1 expression. Exp. Neurol. 235, 476–483. doi: 10.1016/j.expneurol.2011.11.010
Kriaucionis, S., and Heintz, N. (2009). The nuclear DNA Base 5-Hydroxymethylcytosine is present in Purkinje neurons and the brain. Science 324, 929–930. doi: 10.1126/science.1169786
Kuehner, J. N., Chen, J., Bruggeman, E. C., Wang, F., Li, Y., Xu, C., et al. (2021). 5-hydroxymethylcytosine is dynamically regulated during forebrain organoid development and aberrantly altered in Alzheimer’s disease. Cell Rep. 35:109042. doi: 10.1016/j.celrep.2021.109042
Kuehner, J. N., Walia, N. R., Seong, R., Li, Y., Martinez-Feduchi, P., and Yao, B. (2023). Social defeat stress induces genome-wide 5mC and 5hmC alterations in the mouse brain. G3 (Bethesda) 13, 13:jkad114. doi: 10.1093/g3journal/jkad114
Kunkle, B. W., Grenier-Boley, B., Sims, R., Bis, J. C., Damotte, V., Naj, A. C., et al. (2019). Genetic meta-analysis of diagnosed Alzheimer’s disease identifies new risk loci and implicates Aβ, tau, immunity and lipid processing. Nat. Genet. 51, 414–430. doi: 10.1038/s41588-019-0358-2
Kuwabara, T., Kagalwala, M. N., Onuma, Y., Ito, Y., Warashina, M., Terashima, K., et al. (2011). Insulin biosynthesis in neuronal progenitors derived from adult hippocampus and the olfactory bulb. EMBO Mol. Med. 3, 742–754. doi: 10.1002/emmm.201100177
Lee, J. T. (2012). Epigenetic regulation by long noncoding RNAs. Science 338, 1435–1439. doi: 10.1126/science.1231776
Lee, P.-L., Chou, K.-H., Chung, C.-P., Lai, T.-H., Zhou, J. H., Wang, P.-N., et al. (2020). Posterior cingulate cortex network predicts Alzheimer’s disease progression. Front. Aging Neurosci. 12:608667. doi: 10.3389/fnagi.2020.608667
Lee, W.-J., Moon, J., Jeon, D., Shin, Y.-W., Yoo, J.-S., Park, D.-K., et al. (2019). Possible epigenetic regulatory effect of dysregulated circular RNAs in Alzheimer’s disease model. Sci. Rep. 9:11956. doi: 10.1038/s41598-019-48471-z
Leparulo, A., Bisio, M., Redolfi, N., Pozzan, T., Vassanelli, S., and Fasolato, C. (2022). Accelerated aging characterizes the early stage of Alzheimer’s disease. Cells 11:238. doi: 10.3390/cells11020238
Leszek, J., Mikhaylenko, E. V., Belousov, D. M., Koutsouraki, E., Szczechowiak, K., Kobusiak-Prokopowicz, M., et al. (2020). The links between cardiovascular diseases and Alzheimer’s disease. Curr. Neuropharmacol. 19, 152–169. doi: 10.2174/1570159X18666200729093724
Levine, M. E., Lu, A. T., Bennett, D. A., and Horvath, S. (2015). Epigenetic age of the pre-frontal cortex is associated with neuritic plaques, amyloid load, and Alzheimer’s disease related cognitive functioning. Aging 7, 1198–1211. doi: 10.18632/aging.100864
Lewandowski, C. T., Laham, M. S., and Thatcher, G. R. J. (2022). Remembering your a, B, C’s: Alzheimer’s disease and ABCA1. Acta Pharm. Sin. B 12, 995–1018. doi: 10.1016/j.apsb.2022.01.011
Li, S., Hong, S., Shepardson, N. E., Walsh, D. M., Shankar, G. M., and Selkoe, D. (2009). Soluble oligomers of amyloid β protein facilitate hippocampal long-term depression by disrupting neuronal glutamate uptake. Neuron 62, 788–801. doi: 10.1016/j.neuron.2009.05.012
Li, Q., Qian, C., Feng, H., Lin, T., Zhu, Q., Huang, Y., et al. (2021). N6-methyladenine DNA demethylase ALKBH1 regulates mammalian axon regeneration. Neurosci. Bull. 37, 809–814. doi: 10.1007/s12264-021-00671-2
Li, G., Tian, Y., and Zhu, W.-G. (2020). The roles of histone deacetylases and their inhibitors in Cancer therapy. Front. Cell Dev. Biol. 8:576946. doi: 10.3389/fcell.2020.576946
Li, L., Zang, L., Zhang, F., Chen, J., Shen, H., Shu, L., et al. (2017). Fat mass and obesity-associated (FTO) protein regulates adult neurogenesis. Hum. Mol. Genet. 26, 2398–2411. doi: 10.1093/hmg/ddx128
Lin, L., Liu, X., Cheng, X., Li, Y., Gearing, M., Levey, A., et al. (2023). MicroRNA-650 regulates the pathogenesis of Alzheimer’s disease through targeting cyclin-dependent kinase 5. Mol. Neurobiol. 60, 2426–2441. doi: 10.1007/s12035-023-03224-y
Lister, R., Mukamel, E. A., Nery, J. R., Urich, M., Puddifoot, C. A., Johnson, N. D., et al. (2013). Global epigenomic reconfiguration during mammalian brain development. Science 341:1237905. doi: 10.1126/science.1237905
Liu, W., Cai, H., Lin, M., Zhu, L., Gao, L., Zhong, R., et al. (2016). MicroRNA-107 prevents amyloid-beta induced blood-brain barrier disruption and endothelial cell dysfunction by targeting Endophilin-1. Exp. Cell Res. 343, 248–257. doi: 10.1016/j.yexcr.2016.03.026
Liu, Q., Zhu, L., Liu, X., Zheng, J., Liu, Y., Ruan, X., et al. (2020). TRA2A-induced upregulation of LINC00662 regulates blood-brain barrier permeability by affecting ELK4 mRNA stability in Alzheimer’s microenvironment. RNA Biol. 17, 1293–1308. doi: 10.1080/15476286.2020.1756055
Lo, I., Hill, J., Vilhjálmsson, B. J., and Kjems, J. (2020). Linking the association between circRNAs and Alzheimer’s disease progression by multi-tissue circular RNA characterization. RNA Biol. 17, 1789–1797. doi: 10.1080/15476286.2020.1783487
Lu, Y., Tan, L., and Wang, X. (2019). Circular HDAC9/microRNA-138/Sirtuin-1 pathway mediates synaptic and amyloid precursor protein processing deficits in Alzheimer’s disease. Neurosci. Bull. 35, 877–888. doi: 10.1007/s12264-019-00361-0
Lv, S., Zhou, X., Li, Y.-M., Yang, T., Zhang, S.-J., Wang, Y., et al. (2022). N6-methyladenine-modified DNA was decreased in Alzheimer’s disease patients. World J. Clin. Cases 10, 448–457. doi: 10.12998/wjcc.v10.i2.448
Ma, Y., Dammer, E. B., Felsky, D., Duong, D. M., Klein, H.-U., White, C. C., et al. (2021). Atlas of RNA editing events affecting protein expression in aged and Alzheimer’s disease human brain tissue. Nat. Commun. 12:7035. doi: 10.1038/s41467-021-27204-9
Ma, P., Li, Y., Zhang, W., Fang, F., Sun, J., Liu, M., et al. (2019). Long non-coding RNA MALAT1 inhibits neuron apoptosis and Neuroinflammation while stimulates neurite outgrowth and its correlation with MiR-125b mediates PTGS2, CDK5 and FOXQ1 in Alzheimer’s disease. Curr. Alzheimer Res. 16, 596–612. doi: 10.2174/1567205016666190725130134
Ma, C., Niu, R., Huang, T., Shao, L.-W., Peng, Y., Ding, W., et al. (2019). N6-methyldeoxyadenine is a transgenerational epigenetic signal for mitochondrial stress adaptation. Nat. Cell Biol. 21, 319–327. doi: 10.1038/s41556-018-0238-5
Ma, N., Pan, J., Ye, X., Yu, B., Zhang, W., and Wan, J. (2019). Whole-transcriptome analysis of APP/PS1 mouse brain and identification of circRNA-miRNA-mRNA networks to investigate AD pathogenesis. Mol. Ther. Nucleic Acids 18, 1049–1062. doi: 10.1016/j.omtn.2019.10.030
Macciardi, F., Giulia Bacalini, M., Miramontes, R., Boattini, A., Taccioli, C., Modenini, G., et al. (2022). A retrotransposon storm marks clinical phenoconversion to late-onset Alzheimer’s disease. GeroScience 44, 1525–1550. doi: 10.1007/s11357-022-00580-w
McCartney, D. L., Stevenson, A. J., Walker, R. M., Gibson, J., Morris, S. W., Campbell, A., et al. (2018). Investigating the relationship between DNA methylation age acceleration and risk factors for Alzheimer’s disease. Alzheimer’s Dement. 10, 429–437. doi: 10.1016/j.dadm.2018.05.006
Meadows, J. P., Guzman-Karlsson, M. C., Phillips, S., Holleman, C., Posey, J. L., Day, J. J., et al. (2015). DNA methylation regulates neuronal glutamatergic synaptic scaling. Sci. Signal. 8:ra61. doi: 10.1126/scisignal.aab0715
Mei Z., Liu J. Schroeder, J. P. Weinshener D., Duong D. M., Seyfried N. T., et al. (2023). Lowering hippocampal miR-29a expression slows cognitive decline and reduces beta-amyloid deposition in 5×FAD mice. Mol. Neurobiol. doi: 10.1007/s12035-023-03791-0
Meyer, K. D., Saletore, Y., Zumbo, P., Elemento, O., Mason, C. E., and Jaffrey, S. R. (2012). Comprehensive analysis of mRNA methylation reveals enrichment in 3′ UTRs and near stop codons. Cell 149, 1635–1646. doi: 10.1016/j.cell.2012.05.003
Min, S.-W., Cho, S.-H., Zhou, Y., Schroeder, S., Haroutunian, V., Seeley, W. W., et al. (2010). Acetylation of tau inhibits its degradation and contributes to Tauopathy. Neuron 67, 953–966. doi: 10.1016/j.neuron.2010.08.044
Mitroshina, E. V., Savyuk, M. O., Ponimaskin, E., and Vedunova, M. V. (2021). Hypoxia-inducible factor (HIF) in ischemic stroke and neurodegenerative disease. Front. Cell Dev. Biol. 9:703084. doi: 10.3389/fcell.2021.703084
Monti, N., Cavallaro, R. A., Stoccoro, A., Nicolia, V., Scarpa, S., Kovacs, G. G., et al. (2020). CpG and non-CpG Presenilin1 methylation pattern in course of neurodevelopment and neurodegeneration is associated with gene expression in human and murine brain. Epigenetics 15, 781–799. doi: 10.1080/15592294.2020.1722917
Nagayoshi, Y., Chujo, T., Hirata, S., Nakatsuka, H., Chen, C.-W., Takakura, M., et al. (2021). Loss of Ftsj1 perturbs codon-specific translation efficiency in the brain and is associated with X-linked intellectual disability. Sci. Adv. 7:eabf3072. doi: 10.1126/sciadv.abf3072
Nagu, P., Parashar, A., Behl, T., and Mehta, V. (2021). Gut microbiota composition and epigenetic molecular changes connected to the pathogenesis of Alzheimer’s disease. J. Mol. Neurosci. 71, 1436–1455. doi: 10.1007/s12031-021-01829-3
Nguyen, V., Thomas, P., Pemberton, S., Babin, A., Noonan, C., Weaver, R., et al. (2023). Central nervous system insulin signaling can influence the rate of insulin influx into brain. Fluids Barriers CNS 20:28. doi: 10.1186/s12987-023-00431-6
O’Brien, J., Hayder, H., Zayed, Y., and Peng, C. (2018). Overview of MicroRNA biogenesis, mechanisms of actions, and circulation. Front. Endocrinol. 9:402. doi: 10.3389/fendo.2018.00402
Ochoa, E., Ramirez, P., Gonzalez, E., De Mange, J., Ray, W. J., Bieniek, K. F., et al. (2023). Pathogenic tau–induced transposable element–derived dsRNA drives neuroinflammation. Sci. Adv. 9:eabq5423. doi: 10.1126/sciadv.abq5423
Park, J., Lee, K., Kim, K., and Yi, S.-J. (2022). The role of histone modifications: from neurodevelopment to neurodiseases. Sig. Transduct. Target Ther. 7, 217–223. doi: 10.1038/s41392-022-01078-9
Pereira, M., Ribeiro, D. R., Berg, M., Tsai, A. P., Dong, C., Nho, K., et al. (2024). Amyloid pathology reduces ELP3 expression and tRNA modifications leading to impaired proteostasis. Biochim. Biophys. Acta Mol. basis Dis. 1870:166857. doi: 10.1016/j.bbadis.2023.166857
Pérez, R. F., Alba-Linares, J. J., Tejedor, J. R., Fernández, A. F., Calero, M., Román-Domínguez, A., et al. (2022). Blood DNA methylation patterns in older adults with evolving dementia. J. Gerontol. Ser. A 77, 1743–1749. doi: 10.1093/gerona/glac068
Pomytkin, I., Krasil’nikova, I., Bakaeva, Z., Surin, A., and Pinelis, V. (2019). Excitotoxic glutamate causes neuronal insulin resistance by inhibiting insulin receptor/Akt/mTOR pathway. Mol. Brain 12:112. doi: 10.1186/s13041-019-0533-5
Qi, X.-H., Chen, P., Wang, Y.-J., Zhou, Z.-P., Liu, X.-C., Fang, H., et al. (2024). Increased cysteinyl-tRNA synthetase drives neuroinflammation in Alzheimer’s disease. Transl. Neurodegenerat. 13:3. doi: 10.1186/s40035-023-00394-6
Qin, Y., Yang, P., He, W., Li, D., Zeng, L., Li, J., et al. (2024). Novel histone post-translational modifications in Alzheimer’s disease: current advances and implications. Clin. Epigenetics 16:39. doi: 10.1186/s13148-024-01650-w
Raulin, A.-C., Doss, S. V., Trottier, Z. A., Ikezu, T. C., Bu, G., and Liu, C.-C. (2022). ApoE in Alzheimer’s disease: pathophysiology and therapeutic strategies. Mol. Neurodegener. 17:72. doi: 10.1186/s13024-022-00574-4
Robertson, K. D., and Wolffe, A. P. (2000). DNA methylation in health and disease. Nat. Rev. Genet. 1, 11–19. doi: 10.1038/35049533
Romero-Barrios, N., Legascue, M. F., Benhamed, M., Ariel, F., and Crespi, M. (2018). Splicing regulation by long noncoding RNAs. Nucleic Acids Res. 46, 2169–2184. doi: 10.1093/nar/gky095
Roy, R. G., Mandal, P. K., and Maroon, J. C. (2023). Oxidative stress occurs prior to amyloid Aβ plaque formation and tau phosphorylation in Alzheimer’s disease: role of glutathione and metal ions. ACS Chem. Neurosci. 14, 2944–2954. doi: 10.1021/acschemneuro.3c00486
Salzman, J., Gawad, C., Wang, P. L., Lacayo, N., and Brown, P. O. (2012). Circular RNAs are the predominant transcript isoform from hundreds of human genes in diverse cell types. PLoS One 7:e30733. doi: 10.1371/journal.pone.0030733
Santana, D. A., Smith, M. A. C., and Chen, E. S. (2023). Histone modifications in Alzheimer’s disease. Genes 14:347. doi: 10.3390/genes14020347
Sawallisch, C., Berhörster, K., Disanza, A., Mantoani, S., Kintscher, M., Stoenica, L., et al. (2009). The insulin receptor substrate of 53 kDa (IRSp53) limits hippocampal synaptic plasticity *. J. Biol. Chem. 284, 9225–9236. doi: 10.1074/jbc.M808425200
Scheltens, N. M. E., van der Weijden, K., Adriaanse, S. M., van Assema, D., Oomen, P. P., Krudop, W. A., et al. (2018). Hypometabolism of the posterior cingulate cortex is not restricted to Alzheimer’s disease. Neuroimage Clin. 19, 625–632. doi: 10.1016/j.nicl.2018.05.024
Scremin, E., Agostini, M., Leparulo, A., Pozzan, T., Greotti, E., and Fasolato, C. (2020). ORAI2 Down-regulation potentiates SOCE and decreases Aβ42 accumulation in human Neuroglioma cells. Int. J. Mol. Sci. 21:5288. doi: 10.3390/ijms21155288
Sekar, S., McDonald, J., Cuyugan, L., Aldrich, J., Kurdoglu, A., Adkins, J., et al. (2015). Alzheimer’s disease is associated with altered expression of genes involved in immune response and mitochondrial processes in astrocytes. Neurobiol. Aging 36, 583–591. doi: 10.1016/j.neurobiolaging.2014.09.027
Semick, S. A., Bharadwaj, R. A., Collado-Torres, L., Tao, R., Shin, J. H., Deep-Soboslay, A., et al. (2019). Integrated DNA methylation and gene expression profiling across multiple brain regions implicate novel genes in Alzheimer’s disease. Acta Neuropathol. 137, 557–569. doi: 10.1007/s00401-019-01966-5
Shafik, A. M., Zhang, F., Guo, Z., Dai, Q., Pajdzik, K., Li, Y., et al. (2021). N6-methyladenosine dynamics in neurodevelopment and aging, and its potential role in Alzheimer’s disease. Genome Biol. 22:17. doi: 10.1186/s13059-020-02249-z
Shafik, A. M., Zhou, H., Lim, J., Dickinson, B., and Jin, P. (2022). Dysregulated mitochondrial and cytosolic tRNA m1A methylation in Alzheimer’s disease. Hum. Mol. Genet. 31, 1673–1680. doi: 10.1093/hmg/ddab357
Shu, B., Zhang, X., Du, G., Fu, Q., and Huang, L. (2018). MicroRNA-107 prevents amyloid-β-induced neurotoxicity and memory impairment in mice. Int. J. Mol. Med. 41, 1665–1672. doi: 10.3892/ijmm.2017.3339
Silzer, T. K., Pathak, G. A., and Phillips, N. R. (2020). Mitochondrial tRNA methylation in Alzheimer’s disease and progressive supranuclear palsy. BMC Med. Genet. 13:71. doi: 10.1186/s12920-020-0727-9
Simpson, C. L., Lemmens, R., Miskiewicz, K., Broom, W. J., Hansen, V. K., van Vught, P. W. J., et al. (2009). Variants of the elongator protein 3 (ELP3) gene are associated with motor neuron degeneration. Hum. Mol. Genet. 18, 472–481. doi: 10.1093/hmg/ddn375
Sinyor, B., Mineo, J., and Ochner, C. (2020). Alzheimer’s disease, inflammation, and the role of antioxidants. J. Alzheimer’s Dis. Rep. 4, 175–183. doi: 10.3233/ADR-200171
Small, S. A., and Gandy, S. (2006). Sorting through the cell biology of Alzheimer’s disease: intracellular pathways to pathogenesis. Neuron 52, 15–31. doi: 10.1016/j.neuron.2006.09.001
Song, A., Yang, Y., He, H., Sun, J., Chang, Q., and Xue, Q. (2021). Inhibition of long non-coding RNA KCNQ1OT1 attenuates Neuroinflammation and neuronal apoptosis through regulating NLRP3 expression via sponging miR-30e-3p. JIR 14, 1731–1742. doi: 10.2147/JIR.S291274
Sun, W., Samimi, H., Gamez, M., Zare, H., and Frost, B. (2018). Pathogenic tau-induced piRNA depletion promotes neuronal death through transposable element dysregulation in neurodegenerative tauopathies. Nat. Neurosci. 21, 1038–1048. doi: 10.1038/s41593-018-0194-1
Sun, H.-L., Zhu, A. C., Gao, Y., Terajima, H., Fei, Q., Liu, S., et al. (2020). Stabilization of ERK-phosphorylated METTL3 by USP5 increases m6A methylation. Mol. Cell 80, 633–647.e7. doi: 10.1016/j.molcel.2020.10.026
Swanson, E., Breckenridge, L., McMahon, L., Som, S., McConnell, I., and Bloom, G. S. (2017). Extracellular tau oligomers induce invasion of endogenous tau into the Somatodendritic compartment and axonal transport dysfunction. J. Alzheimers Dis. 58, 803–820. doi: 10.3233/JAD-170168
Tahiliani, M., Koh, K. P., Shen, Y., Pastor, W. A., Bandukwala, H., Brudno, Y., et al. (2009). Conversion of 5-Methylcytosine to 5-Hydroxymethylcytosine in mammalian DNA by MLL partner TET1. Science 324, 930–935. doi: 10.1126/science.1170116
Takeshima, H., and Ushijima, T. (2019). Accumulation of genetic and epigenetic alterations in normal cells and cancer risk. Npj Precis. Oncol. 3, 7–8. doi: 10.1038/s41698-019-0079-0
Takeuchi, O., and Akira, S. (2010). Pattern recognition receptors and inflammation. Cell 140, 805–820. doi: 10.1016/j.cell.2010.01.022
Tardivel, M., Bégard, S., Bousset, L., Dujardin, S., Coens, A., Melki, R., et al. (2016). Tunneling nanotube (TNT)-mediated neuron-to neuron transfer of pathological tau protein assemblies. Acta Neuropathol. Commun. 4:117. doi: 10.1186/s40478-016-0386-4
Tian, J., Liu, Y., Wang, Z., Zhang, S., Yang, Y., Zhu, Y., et al. (2021). LncRNA Snhg8 attenuates microglial inflammation response and blood–brain barrier damage in ischemic stroke through regulating miR-425-5p mediated SIRT1/NF-κB signaling. J. Biochem. Mol. Toxicol. 35:e22724. doi: 10.1002/jbt.22724
Wahrle, S. E., Jiang, H., Parsadanian, M., Legleiter, J., Han, X., Fryer, J. D., et al. (2004). ABCA1 is required for Normal central nervous system ApoE levels and for Lipidation of astrocyte-secreted apoE *. J. Biol. Chem. 279, 40987–40993. doi: 10.1074/jbc.M407963200
Wallensten, J., Ljunggren, G., Nager, A., Wachtler, C., Bogdanovic, N., Petrovic, P., et al. (2023). Stress, depression, and risk of dementia – a cohort study in the total population between 18 and 65 years old in region Stockholm. Alzheimers Res. Ther. 15:161. doi: 10.1186/s13195-023-01308-4
Wan, Q.-L., Meng, X., Dai, W., Luo, Z., Wang, C., Fu, X., et al. (2021). N6-methyldeoxyadenine and histone methylation mediate transgenerational survival advantages induced by hormetic heat stress. Sci. Adv. 7, 7:eabc3026. doi: 10.1126/sciadv.abc3026
Wang, R., Li, J. J., Diao, S., Kwak, Y.-D., Liu, L., Zhi, L., et al. (2013). Metabolic stress modulates Alzheimer’s β-secretase gene transcription via SIRT1-PPARγ-PGC-1 in neurons. Cell Metab. 17, 685–694. doi: 10.1016/j.cmet.2013.03.016
Wang, W.-X., Rajeev, B. W., Stromberg, A. J., Ren, N., Tang, G., Huang, Q., et al. (2008). The expression of MicroRNA miR-107 decreases early in Alzheimer’s disease and may accelerate disease progression through regulation of β-site amyloid precursor protein-cleaving enzyme 1. J. Neurosci. 28, 1213–1223. doi: 10.1523/JNEUROSCI.5065-07.2008
Wang, Z., Tang, X., Swaminathan, S. K., Kandimalla, K. K., and Kalari, K. R. (2022). Mapping the dynamics of insulin-responsive pathways in the blood–brain barrier endothelium using time-series transcriptomics data. NPJ Syst. Biol. Appl. 8, 8, 1–10. doi: 10.1038/s41540-022-00235-8
Welden, J. R., Margvelani, G., Arizaca Maquera, K. A., Gudlavalleti, B., Miranda Sardón, S. C., Campos, A. R., et al. (2022). RNA editing of microtubule-associated protein tau circular RNAs promotes their translation and tau tangle formation. Nucleic Acids Res. 50, 12979–12996. doi: 10.1093/nar/gkac1129
Wu, W., Lee, I., Spratt, H., Fang, X., and Bao, X. (2021). tRNA-derived fragments in Alzheimer’s disease: implications for new disease biomarkers and neuropathological mechanisms. J. Alzheimers Dis. 79, 793–806. doi: 10.3233/JAD-200917
Wu, T. P., Wang, T., Seetin, M. G., Lai, Y., Zhu, S., Lin, K., et al. (2016). DNA methylation on N(6)-adenine in mammalian embryonic stem cells. Nature 532, 329–333. doi: 10.1038/nature17640
Xiao, X., Liu, H., Liu, X., Zhang, W., Zhang, S., and Jiao, B. (2021). APP, PSEN1, and PSEN2 variants in Alzheimer’s disease: systematic re-evaluation according to ACMG guidelines. Front. Aging Neurosci. 13:695808. doi: 10.3389/fnagi.2021.695808
Xiong, W., Zhao, Y., Wei, Z., Li, C., Zhao, R., Ge, J., et al. (2023). N1-methyladenosine formation, gene regulation, biological functions, and clinical relevance. Mol. Ther. 31, 308–330. doi: 10.1016/j.ymthe.2022.10.015
Yamasaki, S., Ivanov, P., Hu, G., and Anderson, P. (2009). Angiogenin cleaves tRNA and promotes stress-induced translational repression. J. Cell Biol. 185, 35–42. doi: 10.1083/jcb.200811106
Yan, L., Wei, J., Yang, F., Wang, M., Wang, S., Cheng, T., et al. (2022). Physical exercise prevented stress-induced anxiety via improving brain RNA methylation. Adv. Sci. 9:e2105731. doi: 10.1002/advs.202105731
Yang, Y., Hsu, P. J., Chen, Y.-S., and Yang, Y.-G. (2018). Dynamic transcriptomic m6A decoration: writers, erasers, readers and functions in RNA metabolism. Cell Res. 28, 616–624. doi: 10.1038/s41422-018-0040-8
Yao, B., Cheng, Y., Wang, Z., Li, Y., Chen, L., Huang, L., et al. (2017). DNA N6-methyladenine is dynamically regulated in the mouse brain following environmental stress. Nat. Commun. 8. doi: 10.1038/s41467-017-01195-y
Yao, B., Christian, K. M., He, C., Jin, P., Ming, G.-L., and Song, H. (2016). Epigenetic mechanisms in neurogenesis. Nat. Rev. Neurosci. 17, 537–549. doi: 10.1038/nrn.2016.70
Yashooa, R. K., and Nabi, A. Q. (2022). The miR-146a-5p and miR-125b-5p levels as biomarkers for early prediction of Alzheimer’s disease. Hum. Gene Ther. 34:201129. doi: 10.1016/j.humgen.2022.201129
Yi, J., Chen, B., Yao, X., Lei, Y., Ou, F., and Huang, F. (2019). Upregulation of the lncRNA MEG3 improves cognitive impairment, alleviates neuronal damage, and inhibits activation of astrocytes in hippocampus tissues in Alzheimer’s disease through inactivating the PI3K/Akt signaling pathway. J. Cell. Biochem. 120, 18053–18065. doi: 10.1002/jcb.29108
Yin, H., Ju, Z., Zheng, M., Zhang, X., Zuo, W., Wang, Y., et al. (2023). Loss of the m6A methyltransferase METTL3 in monocyte-derived macrophages ameliorates Alzheimer’s disease pathology in mice. PLoS Biol. 21:e3002017. doi: 10.1371/journal.pbio.3002017
Yue, D., Zhao, J., Chen, H., Guo, M., Chen, C., Zhou, Y., et al. (2020). MicroRNA-7, synergizes with RORα, negatively controls the pathology of brain tissue inflammation. J. Neuroinflammation 17:28. doi: 10.1186/s12974-020-1710-2
Zempel, H., Thies, E., Mandelkow, E., and Mandelkow, E.-M. (2010). Aβ oligomers cause localized Ca2+ elevation, Missorting of endogenous tau into dendrites, tau phosphorylation, and destruction of microtubules and spines. J. Neurosci. 30, 11938–11950. doi: 10.1523/JNEUROSCI.2357-10.2010
Zeng, T., Ni, H., Yu, Y., Zhang, M., Wu, M., Wang, Q., et al. (2019). BACE1-AS prevents BACE1 mRNA degradation through the sequestration of BACE1-targeting miRNAs. J. Chem. Neuroanat. 98, 87–96. doi: 10.1016/j.jchemneu.2019.04.001
Zhang, J., and Dong, X.-P. (2012). Dysfunction of microtubule-associated proteins of MAP2/tau family in prion disease. Prion 6, 334–338. doi: 10.4161/pri.20677
Zhang, G., Huang, H., Liu, D., Cheng, Y., Liu, X., Zhang, W., et al. (2015). N6-Methyladenine DNA modification in Drosophila. Cell 161, 893–906. doi: 10.1016/j.cell.2015.04.018
Zhang, D. Y., Ming, G., and Song, H. (2021). PUS7: a targetable epitranscriptomic regulator of glioblastoma growth. Trends Pharmacol. Sci. 42, 976–978. doi: 10.1016/j.tips.2021.10.002
Zhang, D., Pan, N., Jiang, C., and Hao, M. (2022). LncRNA SNHG8 sponges miR-449c-5p and regulates the SIRT1/FoxO1 pathway to affect microglia activation and blood-brain barrier permeability in ischemic stroke. J. Leukoc. Biol. 111, 953–966. doi: 10.1002/JLB.1A0421-217RR
Zhang, R., Song, Y., and Su, X. (2023). Necroptosis and Alzheimer’s disease: pathogenic mechanisms and therapeutic opportunities. J. Alzheimers Dis. 94, S367–S386. doi: 10.3233/JAD-220809
Zhang, X., Trebak, F., Souza, L. A. C., Shi, J., Zhou, T., Kehoe, P. G., et al. (2020). Small RNA modifications in Alzheimer’s disease. Neurobiol. Dis. 145:105058. doi: 10.1016/j.nbd.2020.105058
Zhang, H., Wu, L., Pchitskaya, E., Zakharova, O., Saito, T., Saido, T., et al. (2015). Neuronal store-operated calcium entry and mushroom spine loss in amyloid precursor protein Knock-in mouse model of Alzheimer’s disease. J. Neurosci. 35, 13275–13286. doi: 10.1523/JNEUROSCI.1034-15.2015
Zhang, M., Yang, S., Nelakanti, R., Zhao, W., Liu, G., Li, Z., et al. (2020). Mammalian ALKBH1 serves as an N 6 -mA demethylase of unpairing DNA. Cell Res. 30, 197–210. doi: 10.1038/s41422-019-0237-5
Zhao, X., Tang, Z., Zhang, H., Atianjoh, F. E., Zhao, J.-Y., Liang, L., et al. (2013). A long noncoding RNA contributes to neuropathic pain by silencing Kcna2 in primary afferent neurons. Nat. Neurosci. 16, 1024–1031. doi: 10.1038/nn.3438
Zhao, F., Xu, Y., Gao, S., Qin, L., Austria, Q., Siedlak, S. L., et al. (2021). METTL3-dependent RNA m6A dysregulation contributes to neurodegeneration in Alzheimer’s disease through aberrant cell cycle events. Mol. Neurodegener. 16:70. doi: 10.1186/s13024-021-00484-x
Zhong, J., Xiao, C., Gu, W., Du, G., Sun, X., He, Q.-Y., et al. (2015). Transfer RNAs mediate the rapid adaptation of Escherichia coli to oxidative stress. PLoS Genet. 11:e1005302. doi: 10.1371/journal.pgen.1005302
Zhu, L., Lin, M., Ma, J., Liu, W., Gao, L., Wei, S., et al. (2019). The role of LINC00094/miR-224-5p (miR-497-5p)/Endophilin-1 axis in Memantine mediated protective effects on blood-brain barrier in AD microenvironment. J. Cell. Mol. Med. 23, 3280–3292. doi: 10.1111/jcmm.14214
Keywords: Alzheimer’s disease, epigenetics, DNA methylation, non-coding RNAs, lncRNAs, miRNAs, circRNAs, RNA modifications
Citation: Martinez-Feduchi P, Jin P and Yao B (2024) Epigenetic modifications of DNA and RNA in Alzheimer’s disease. Front. Mol. Neurosci. 17:1398026. doi: 10.3389/fnmol.2024.1398026
Edited by:
João Laranjinha, University of Coimbra, PortugalReviewed by:
Federico V. Pallardó, University of Valencia, SpainGiuseppe Federighi, University of Pisa, Italy
Copyright © 2024 Martinez-Feduchi, Jin and Yao. This is an open-access article distributed under the terms of the Creative Commons Attribution License (CC BY). The use, distribution or reproduction in other forums is permitted, provided the original author(s) and the copyright owner(s) are credited and that the original publication in this journal is cited, in accordance with accepted academic practice. No use, distribution or reproduction is permitted which does not comply with these terms.
*Correspondence: Bing Yao, YmluZy55YW9AZW1vcnkuZWR1
†ORCID: Paula Martinez-Feduchi, https://orcid.org/0000-0002-5350-7468