- 1Institute of Biotechnology, Faculty of Environment and Natural Sciences, Brandenburg University of Technology Cottbus-Senftenberg, Senftenberg, Germany
- 2German Center for Neurodegenerative Diseases (DZNE), Berlin, Germany
- 3Department of Neurology, Charité – Universitätsmedizin Berlin, Berlin, Germany
- 4Faculty of Health Sciences, Joint Faculty of the Brandenburg University of Technology Cottbus – Senftenberg, The Brandenburg Medical School Theodor Fontane and the University of Potsdam, Berlin, Germany
- 5PolyAn GmbH, Berlin, Germany
- 6Institute of Biochemistry and Biology, University of Potsdam, Potsdam, Germany
Neurodegenerative diseases (NDs) are characterized by abnormalities within neurons of the brain or spinal cord that gradually lose function, eventually leading to cell death. Upon examination of affected tissue, pathological changes reveal a loss of synapses, misfolded proteins, and activation of immune cells—all indicative of disease progression—before severe clinical symptoms become apparent. Early detection of NDs is crucial for potentially administering targeted medications that may delay disease advancement. Given their complex pathophysiological features and diverse clinical symptoms, there is a pressing need for sensitive and effective diagnostic methods for NDs. Biomarkers such as microRNAs (miRNAs) have been identified as potential tools for detecting these diseases. We explore the pivotal role of miRNAs in the context of NDs, focusing on Alzheimer’s disease, Parkinson’s disease, Multiple sclerosis, Huntington’s disease, and Amyotrophic Lateral Sclerosis. The review delves into the intricate relationship between aging and NDs, highlighting structural and functional alterations in the aging brain and their implications for disease development. It elucidates how miRNAs and RNA-binding proteins are implicated in the pathogenesis of NDs and underscores the importance of investigating their expression and function in aging. Significantly, miRNAs exert substantial influence on post-translational modifications (PTMs), impacting not just the nervous system but a wide array of tissues and cell types as well. Specific miRNAs have been found to target proteins involved in ubiquitination or de-ubiquitination processes, which play a significant role in regulating protein function and stability. We discuss the link between miRNA, PTM, and NDs. Additionally, the review discusses the significance of miRNAs as biomarkers for early disease detection, offering insights into diagnostic strategies.
1 Neurodegenerative disease
This review delves into the significance of microRNAs (miRNAs) involved in neurodegenerative diseases (NDs) in terms of biomarkers contributing to diagnostics. In general, NDs occur due to abnormalities in the neural networks, i.e., structural and functional loss of neurons because of severe damage (Agrawal and Biswas, 2015). Only symptomatic treatment is available for NDs; no drugs or medications can cure or prevent them (Grasso et al., 2014). In our study, we focused on the most prevalent and well-known NDs, such as Alzheimer’s disease (AD), Parkinson’s disease (PD), Multiple Sclerosis (MS), Huntington’s disease (HD), and Amyotrophic Lateral Sclerosis (ALS). Neurological disorders pose substantial global healthcare challenges, as they diminish quality of life and constitute a major health concern. Their prevalence, escalating healthcare costs, and growing incidence in aging populations contribute to this issue. The intricate nature of these conditions complicates diagnosis and treatment, often leading to misdiagnosis and delayed care – exacerbating symptoms and burdening patients and caregivers. To effectively address these challenges, a multifaceted strategy is required: enhancing access to care, investing in research for innovative diagnostic and therapeutic solutions, and raising public awareness about neurological disorders.
Aging is characterized by a gradual decline in decreased efficiency, and impaired tissue and organ function. The decrease in physical well-being increases the probability of death and vulnerability to a range of age-related diseases, including neurological disorders, osteoporosis, sarcopenia, cancer, and cardiovascular diseases (Santos and Lindner, 2017). The most frequent NDs are age-related diseases that cause memory and behavioral impairment (Tan et al., 2015). A United Nations report estimated that 1 in 11 cases of NDs occurred in people over the age of 65 years in 2019 and this would increase to an alarming number of 1 in 6 by the end of 2050 (Zheng and Chen, 2022). Researchers project that dementia cases in the developed world are expected to increase from 13.5 million in 2000 to 21.2 million by 2025. The projected figure is expected to rise to 36.7 million by 2050 (Zheng and Chen, 2022).
As the brain ages, it experiences progressive weight loss, reduced overall myelinated axon length, and diminished cortical volume resulting from neuronal atrophy predominantly affecting the frontal and temporal lobes. These changes are associated with aging-related alterations that may influence the development or progression of NDs, making aging the primary risk factor (Sowell et al., 2004; Hou et al., 2019). Disruption in protein balance, which is prominent in many age-related NDs, including AD and PD, is also widely detected throughout the normal aging process. Older people may show a more significant number of aggregates like β-amyloid (A-β), tau, and α-synuclein, even if they lack any noticeable cognitive problems or disease symptoms (Elobeid et al., 2016; Burdukiewicz et al., 2017). In older people, cognitive function declines, making them more susceptible to NDs (Hedden and Gabrieli, 2004). While metabolic dysfunction, neurite, and synaptic loss are observed in some NDs, such as AD and PD, the specific progression can vary among different conditions (Agrawal, 2020; Lamptey et al., 2022). The transition from metabolic disorders to the loss of neurites and synapses is not a universal feature of all NDs. A further consequence of NDs is that they spread to other brain regions and affect different types of cells (von Bernhardi, 2008). Overall cognitive decline can also lead to a decrease in quality of life as individuals with NDs become increasingly dependent on others for care and support. Additionally, these diseases are costly to treat, damaging healthcare systems. Thus, it is essential to focus on preventive measures to reduce the risk of cognitive decline.
Several studies have shown that neuronal problems often start 10–20 years before symptoms appear. As a result, it can sometimes be detected in its presymptomatic stage by using biomarkers such as serum neurofilament light chain (NfL), which detects neuronal damage before symptoms manifest (Gaetani et al., 2021; Piscopo et al., 2021). To treat these diseases effectively, new tools are needed to identify them as soon as possible, even before symptoms appear. Neurodegenerative diseases are diagnosed using magnetic resonance imaging (MRI), molecular diagnostics, biomarker analysis, and neuroimaging. Several types of dementia can be distinguished using structural MRI scans such as frontotemporal dementia (FTD), vascular dementia, and Lewy body dementia. Moreover, modern methods, such as positron emission tomography (PET) scans and cerebrospinal fluid (CSF) analysis, can also be used to detect NDs in their early stages (Sheinerman and Umansky, 2013; Agrawal and Biswas, 2015; Koikkalainen et al., 2016; Shusharina et al., 2023; Sharma et al., 2024). It has been shown that phosphorescence lifetime imaging microscopy (PLIM), fluorescence lifetime imaging microscopy (FLIM), fluorescence resonance energy transfer (FRET) (Ishikawa-Ankerhold et al., 2012; Jahn et al., 2015), and dot-blot (Subramanian et al., 2016) can improve diagnostics. In addition to measuring protein degradation, these techniques can detect CSF substances. Although these methods are less intrusive and costly, they are not ideal for initial screening (Apostolova et al., 2010; Fagan et al., 2011; Mori et al., 2012).
The study of NDs contributes to a better understanding of the general mechanisms of neurodegeneration. In the future, these findings can lead to the development of new biomarkers, diagnostic tools, and therapies. In this review, we will focus on molecular methods, specifically regarding the application of miRNAs as biomarkers for early disease detection. NDs can be diagnosed and detected using molecular diagnostics, crucial for accurately identifying and stratifying patients, which is vital for developing effective treatments and enhancing our understanding of these complex diseases. We also explore the aging process and its association with various molecular and cellular changes, including changes in miRNA expression and RNA-binding proteins (RBPs), both implicated in ND pathogenesis.
2 Biomarkers for neurodegenerative diseases
Biomarkers are traits that can be measured and used to show whether biological processes are objectively normal, whether a response to medication can be expected, or whether pathogenic processes are taking place so that the right treatments can be used. Figure 1 illustrates the applications of biomarkers at different disease stages. Molecular diagnostics can identify them using neuroimaging techniques such as PET, MRI, and nuclear magnetic resonance spectroscopy (NMRS) (Rachakonda et al., 2004; Agrawal and Biswas, 2015; Hussain et al., 2022). The combination of biomarkers and NMRS has demonstrated promise in molecular diagnostics alongside other techniques such as diffusion tensor imaging (DTI), functional MRI, and PET. NMRS is a quantitative imaging method that allows researchers to use specific neuronal metabolites as biomarkers to study real-time metabolic dysfunction and permanent neuronal damage. Researchers investigated whether NMRS could serve as a molecular imaging biomarker for in vivo diagnosis of PD and monitoring treatment efficacy (Ciurleo et al., 2014).
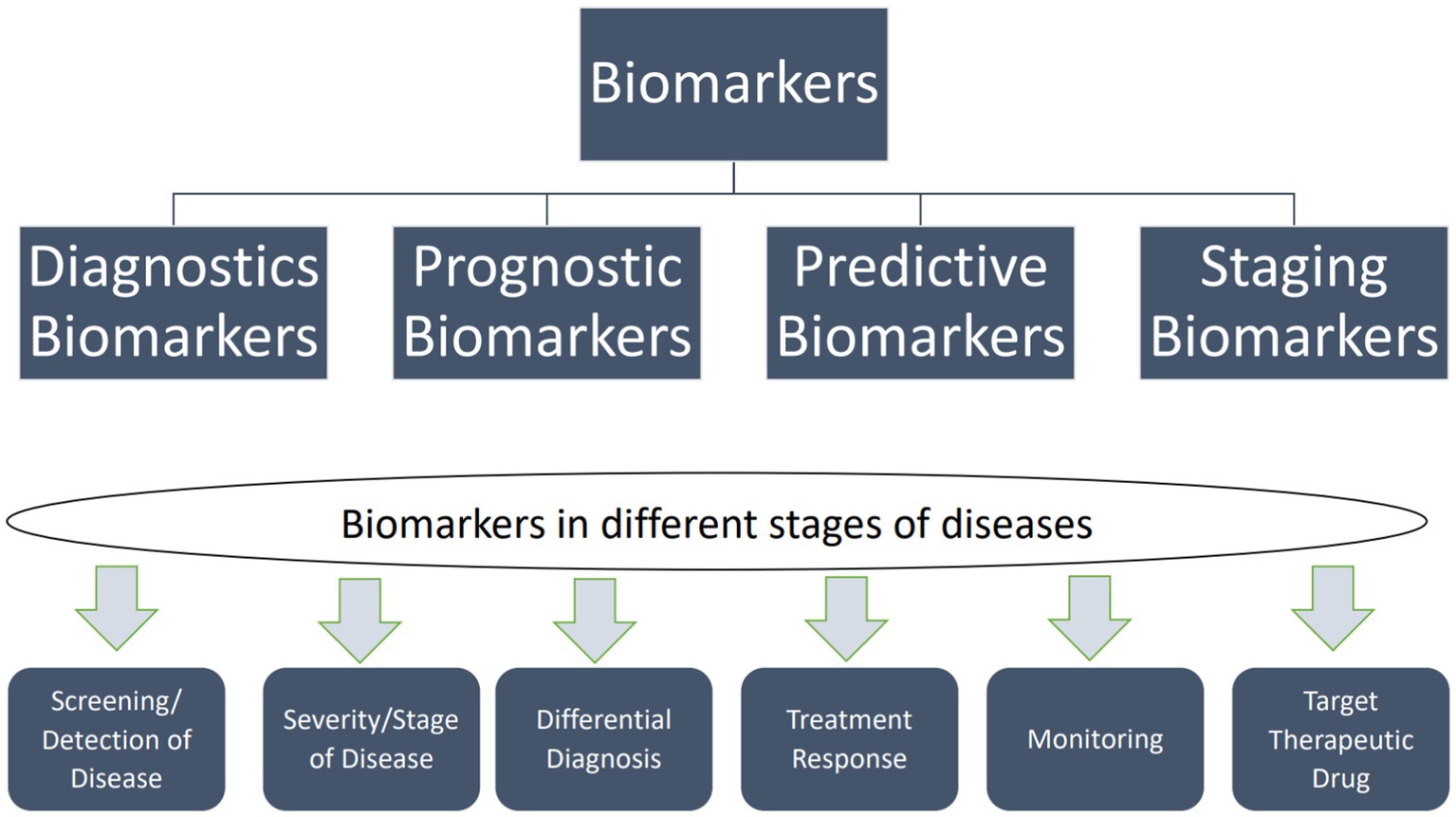
Figure 1. Types of biomarkers and their uses in different disease stages. Biomarkers can be categorized into two main types: exposure biomarkers and disease biomarkers. Exposure biomarkers are employed to predict the occurrence of a disease, while disease biomarkers are utilized for monitoring, screening, and diagnosing a disease. Moreover, predictive biomarkers can forecast an individual’s vulnerability to disease (Taj et al., 2020).
2.1 Fundamentals of microRNA
The sequencing of the human genome revealed a surprising finding. Only a relatively small part of the genetic information is coding for proteins. It is widely believed that a significant portion of the human genome, estimated at 90% by educated guesses, is non-coding (Santosh et al., 2015). Initially, it was assumed that the non-coding part was junk DNA. However, research has shown that non-coding DNA is constantly transcribed into non-coding RNA. These RNA molecules have specific physiological functions in cells (Santosh et al., 2015). Among the most critical classes of RNA are miRNAs and small interfering RNAs (siRNAs), which are typically 20 to 30 nucleotides in length, play a key role in regulating gene expression, and are implicated in diseases including NDs and cancer. While structurally and functionally similar, there are crucial differences between siRNAs and miRNAs. MicroRNAs are single-stranded, endogenously derived from non-coding RNA, while siRNAs are exogenous double-stranded RNA taken up by cells. Therefore, they have distinct properties that make them useful for different purposes.
Additionally, siRNAs are specific for a single target mRNA. At the same time, miRNAs can have multiple mRNA targets (exact numbers can vary widely depending on the particular miRNA and cellular context) due to their imperfect pairing. These differences contribute to their distinct roles in gene regulation and potential therapeutic applications (Mattick and Makunin, 2006; Esteller, 2011; Hombach and Kretz, 2016; Yang et al., 2016).
Mature miRNAs are made up of 21–25 nucleotide sequences. They are the end products of non-protein-coding genes and are known to be the most abundant class of RNA molecules (> 25,000 evolutionarily distinct miRNAs) in animals and plants (Chen, 2005; Grasso et al., 2014). MicroRNAs regulate 30% of human genes and are involved in the post-transcriptional regulation of gene expression linked to regulating many protein-coding genes (Li et al., 2023).
2.1.1 Biogenesis of microRNA
The biogenesis of miRNAs follows two pathways: (a) the intergenic (canonical processing) pathway and (b) the intronic (non-canonical) pathway (Bartel, 2004; MacFarlane and Murphy, 2010; Miyoshi et al., 2010; Ha and Kim, 2014; O’Brien et al., 2018).
The canonical miRNA biogenesis process (Intergenic miRNA) involves the two RNase III-type proteins, Drosha and Dicer. It begins with RNA-Polymerase II transcription of the primary miRNA-transcripts (pri-miRNAs). A microprocessor complex containing Drosha and DGCR8 (DiGeorge syndrome critical region 8) cleaves primary miRNA transcripts to produce miRNA precursor molecules (pre-miRNA). A pronounced hairpin structure characterizes pre-miRNAs. The pre-miRNAs are transported into the cytoplasm and cleaved by the RNase III enzyme Dicer into 20–23 nucleotides dsRNAs. The short-lived dsRNAs are unwound and then become single-stranded miRNAs. Mature miRNA is then incorporated into specific protein complexes, referred to as micro-ribonucleoprotein complexes (miRNPs) (Gregory et al., 2006; Beezhold et al., 2010; MacFarlane and Murphy, 2010; Wahid et al., 2010). Pre-miRNAs are transcribed into miRNAs within the nucleus by RNA polymerase II (Chen, 2005). Two RNA III enzymes then process this miRNA precursor (Wu et al., 2012). Drosha, DGCR8 (DiGeorge Critical Region 8), and the RNase III enzyme (RNASEN) form a protein complex after the first transcription. Figure 2 shows that a protein cooperating with Drosha and other helpers locates the primary miRNA and truncates its ends to generate the premature miRNA (Lee et al., 2003; Han et al., 2004). Exportin-5 is crucial for the pre-miRNA to be targeted and transported to the cytosol, where it is further processed via the GTP-binding nuclear protein Ran (RanGTP)-dependent pathway (Yi et al., 2003; Bohnsack et al., 2004). The Dicer complex, consisting of Argonaute RNA-induced silencing complex (RISC) catalytic component 2 (AGO2), Dicer (RNase), protein activator of the interferon-induced protein kinase (PACT), and transactivation response element RNA-Binding Protein (TRBP), transforms pre-miRNAs in the cytosol into mature miRNA duplexes composed of 22 nucleotides (Gregory et al., 2004; MacRae et al., 2008). Dicer is responsible for separating two types of miRNA, the passenger and guide strands. The guide strand communicates with AGO2 in RISC to find the target transcript (Kobayashi and Tomari, 2016).
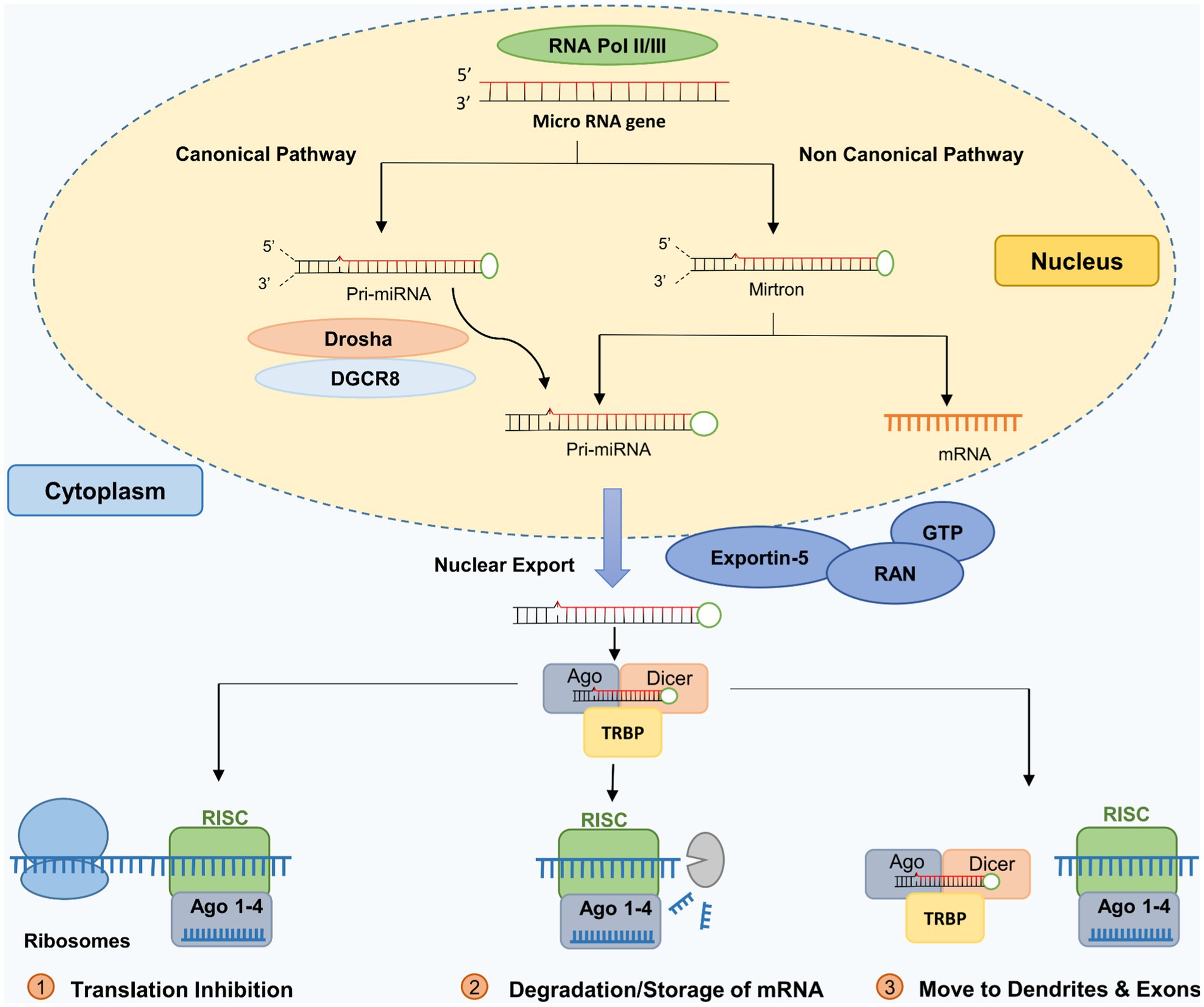
Figure 2. MicroRNA biosynthesis pathways. Adapted from Huang (2022) by biorender.com. For details, see (O’Carroll, D., et al., 2013; Maffioletti, E., et al., 2014).
Non-canonical processing (intronic pathways) refers to alternative pathways for miRNA biogenesis that do not involve Drosha or Dicer. In addition to Drosha/DGCR8 and Dicer, non-canonical miRNA biogenesis involves a variety of proteins that are an integral part of the conventional pathway, such as exportin 5, exportin 6, and AGO2 (MacFarlane and Murphy, 2010). Non-canonical processing pathways are less understood than the canonical pathway, and their roles in miRNA biogenesis and gene regulation are still being investigated. RNA-Polymerase II mediates intronic biogenesis. RNA-Polymerase II directly excises the pre-miRNA, the progenitor of miRNA, from introns.
2.1.2 Functions of microRNAs
More than 2,200 miRNA genes in the human genome are known, which regulate a significant portion of gene expression (Ardekani and Naeini, 2010; O’Brien et al., 2018). The precise mechanism of miRNA transcript positioning is not fully understood, but their location can influence miRNA expression in introns, non-protein-coding genes, exons, and proximity to other genes (O’Brien et al., 2018; Shang et al., 2023). It is reported that non-protein coding genes transcribe approximately 50% of the miRNAs expressed in the human genome and are coded in the introns of coding genes (Ardekani and Naeini, 2010). Human diseases develop and progress are impacted by miRNAs, which are also crucial for regulating drug metabolism and immune responses (Wang and Chen, 2021). MicroRNAs regulate cellular functions such as growth, development, differentiation, metabolism, and immune responses. They achieve this by binding to the corresponding target mRNA and inhibiting protein synthesis in the post-transcriptional phase (Johnston and Hobert, 2003; Ambros, 2004; Poy et al., 2004; Filipowicz, 2005; Hatfield et al., 2005; Zhao et al., 2005; O’Brien et al., 2018).
MicroRNAs play a crucial role in gene regulation through two main mechanisms: mRNA cleavage and translational repression. In the process of mRNA cleavage, miRNAs induce RISC-mediated degradation (cleavage) by binding to complementary regions within protein-coding mRNA sequences (Natarajan et al., 2013). This interaction results in the degradation or repression of target mRNA, impacting cellular processes such as cell division and differentiation (Wang, 2014). The key element in this mechanism is the seed region of the miRNA, a specific 6–8 nucleotide sequence that plays a pivotal role in gene silencing (Ying et al., 2008; O’Brien et al., 2018).
Translational repression involves miRNAs binding to the complementary region of the protein-coding sequence, which halts protein production without degrading the mRNA. This process is characterized by limited base-pairing between the miRNA and its target mRNA. MicroRNAs can bind to the 3′ untranslated regions (UTRs) of mRNAs (Bartel, 2004), inhibiting their translation and thereby regulating gene expression at the post-transcriptional level (Quévillon Huberdeau and Simard, 2019).
Therefore, while both mechanisms involve miRNA binding to specific regions of mRNA, they differ in their outcomes: mRNA cleavage leads to degradation or repression of the target mRNA, affecting gene expression at a broader level, whereas translational repression specifically inhibits protein production without altering mRNA stability. These distinct processes highlight the multifaceted role of miRNAs in fine-tuning gene expression for various cellular functions and regulatory pathways. By modulating these diverse mechanisms, miRNAs contribute to a wide range of biological processes, including development, differentiation, and disease progression.
2.1.2.1 Gene activation
Cell survival requires post-transcriptional regulation of gene expression by miRNAs and miRNPs (Vasudevan, 2012; Basak et al., 2016). MicroRNAs act as inhibitory regulators, repressing translation or enhancing target mRNA degradation. However, miRNAs may indirectly promote translation under specific circumstances or in certain cellular environments, although this is not their primary role. Additionally, miRNAs can lower the amount of expressed repressor proteins, which could cause translation to speed up for specific mRNA targets. It is significant to note that miRNAs regulate multiple mRNA expressions, and conversely, numerous miRNAs can target the same mRNA, contributing to complex regulatory networks within cells (Hashimoto et al., 2013).
3 MicroRNA as biomarker
MicroRNAs have gained attention as biomarkers in various scientific fields owing to their numerous desirable properties (Pabinger et al., 2014). The ideal biomarker must be particular, sensitive, and predictive (Lu et al., 2005). In body fluids, miRNAs are protected inside exosomes, microvesicles, apoptotic bodies, or protein complexes (bound to AGO2 or RISC). They exhibit stability in various conditions, including room temperature, boiling, multiple freeze-thawing cycles, pH fluctuations, and chemical or enzymatic fragmentation. MicroRNAs have a strong specificity and can be used with formalin-fixed, paraffin-embedded tissues (FFPE) and fresh, snap-frozen samples (Xi et al., 2007). This stability is essential for the clinical application of miRNA biomarkers because, in the context of pre-analytical procedures, it allows for easy transportation of samples to the clinical lab without needing special handling or immediate processing. Moreover, the pre-analytical phase is prone to less error, which is crucial for accurate and reliable results (Mitchell et al., 2008; Cortez et al., 2011; Mo et al., 2012).
Several body fluids, including blood, saliva, plasma, tears, and CSF, can measure miRNAs (Weber et al., 2010). A variety of techniques have been developed to profile miRNAs (Wang B. et al., 2011), including microarrays, sequencing, and quantitative reverse transcription PCR (RT-qPCR). Rio et al. (2010) purified the sample from total RNA using tri-reagents of acid phenol and guanidinium-thiocyanate, column filtration protocols, and chloroform from total RNA. It is widely accepted that adopting an appropriate normalized approach is critical to eliminate variances and improve the accuracy of miRNA quantification (Rio et al., 2010).
In general, there are no significant variations in the extraction of miRNAs from serum and venous plasma. However, sampling studies on humans have concluded that researchers should carefully select the blood collection procedure for specific miRNA biomarkers (Zhou et al., 2016). The detection of miRNAs molecules is difficult due to their inherent properties, such as their tiny size, low level, sequence homology across members, and tissue-or stage-specific expression (Wang J. et al., 2016; Ricci et al., 2018; Felekkis and Papaneophytou, 2020; Djebbi et al., 2021). Earlier research on miRNAs in the bloodstream failed to develop accurate biomarkers. Overlooking factors such as previous treatments, age, and gender contribute to this problem. Ensuring the reliability of both positive and negative results is challenging. Minimizing experimental or technical variations is crucial due to the minor differences in miRNA expression levels observed between healthy and sick persons. Researchers should carefully control the steps for miRNA identification, data processing, data normalization, and data optimization (Pabinger et al., 2014; Witwer, 2015). In this regard, a mix of exogenous and endogenous control miRNAs is recommended, as a single reference gene has proven unreliable (Schwarzenbach et al., 2015).
Evidence shows that miRNAs can be used as diagnostic biomarkers for various diseases. MicroRNAs have limited clinical applications due to inconsistencies in the literature, standard assays, and a lack of reproducibility (Precazzini et al., 2021; Ho et al., 2022). Additionally, selecting a suitable reference for miRNA quantification in biofluids is problematic. There is currently no method of analyzing circulating miRNAs without RNA extraction and purification, which may result in miRNA loss (Precazzini et al., 2021). Despite these challenges, circulating miRNAs have been established as promising non-invasive biomarkers for accurate diagnosis, prognosis of disease progression, and treatment responsiveness (Condrat et al., 2020). At least for cancer and other diseases, several miRNAs panels are already available for clinical use, and they have shown potential for early detection, subtype classification, and treatment strategy selection in cancer and other diseases (Wang R. et al., 2018; Tribolet et al., 2020). Another challenge is that miRNAs’ functionality can vary by disease stage, making them difficult to use as specific targets (Cao et al., 2016). Thus, circulating miRNAs as biomarkers still requires further research and multiple independent validation studies for clinical application.
3.1 MicroRNA in the brain
MicroRNA expression levels change during brain development, with different miRNAs expressed at different stages (Cho et al., 2019; Prodromidou and Matsas, 2019; Brennan and Henshall, 2020). High-throughput sequencing analyses show that the human brain expresses at least 550 miRNAs, a higher expression level than in other organs (Ardekani and Naeini, 2010; Chen and Qin, 2015).
MicroRNAs are crucial brain regulators and frequently show brain-specific expression patterns. MicroRNAs commonly co-express with their targets. They are essential in controlling various biological processes, such as synaptic plasticity and neurogenesis (Cao et al., 2016). The miR-134, miR-124, miR-79, miR-9, miR-137, and miR-132 play crucial roles in the growth and development of neurons, synapses, neuroplasticity, and dendrite spine formation (Bandiera et al., 2013; Sharma and Lu, 2018; Paul et al., 2020). A study demonstrated that miR-124 expression resulted in a substantial 50% decrease in dendritic spine formation (Garcia et al., 2021). Dysregulation of miRNAs has been observed in several NDs (Grasso et al., 2014), with common pathophysiological characteristics including neuroinflammation, protein aggregation, and mitochondrial dysfunction identified across these disorders. In vitro and in vivo model systems have been used to explore miRNA functions recently, and as a result, miRNA biomarkers, miRNA regulatory networks, and miRNA pathways have been discovered (Gascon and Gao, 2012; Mo, 2012; Juźwik et al., 2019).
3.2 MicroRNA in body fluids
Blood samples have various benefits when used in diagnostic procedures. Blood samples are less invasive to collect and more uncomplicated to store than CSF and tissue biopsies. Since miRNAs are stable in the blood, multiple blood samples can be taken to track the progress of the disease. This boosts the flexibility of the analysis (Ricci et al., 2018). In general, studies showed miRNAs with nominally significant blood–brain correlations. Some have been implicated as peripheral biomarkers of various psychiatric or brain-related disorders (Kos et al., 2022). The use of blood-based miRNAs as a biomarker for ND diagnostics is a topic of ongoing research. Dysregulated miRNAs in the blood have been proposed as potential biomarkers for diagnosing NDs (Mushtaq et al., 2016; Gentile et al., 2022). While miRNAs in the blood have the potential to serve as correlates of brain-based miRNA expression and as biomarkers for NDs, their utility is also influenced by various factors. Biomarkers in the CNS and the blood are regulated by the blood-CSF barrier, which means the same biomarker may be present in both biofluids (Gaetani et al., 2020; Gigase et al., 2023). In addition to nerve damage, neurological disorders often affect other organs and tissues, such as peripheral blood cells and degenerating muscles. In light of this, blood may be a valuable biofluid for discovering and validating ND biomarkers. MicroRNAs in the blood are a limited biomarker for NDs (Kos et al., 2022). MicroRNAs in the blood may indicate inflammatory status, responsiveness to pharmaceutical therapies, or other circumstances not directly connected to an ND. Therefore, they may function as confounding factors (Grasso et al., 2014; Spector et al., 2015; Vu and Bowser, 2017).
MicroRNAs found in the CSF can serve as sensitive indicators of brain changes (Cogswell et al., 2008; Rao et al., 2013) since they indicate both healthy and unhealthy conditions in the CNS. Immune cells, neurons, glial cells, and others secrete miRNAs into the CSF. Macrophages secrete miRNAs into the CSF as part of their physiological activities (Alexandrov et al., 2012; Mori et al., 2019). A study has shown that miRNA levels differ between AD patients with inflammation and non-inflammatory neurological conditions and those with FTD in the serum and cerebrospinal cortex. As compared to non-inflammatory controls, miR-26b (down) and miR-125b were found to be regulated in AD patients’ CSFs (Galimberti et al., 2014; Swarbrick et al., 2019; Noor Eddin et al., 2023).
4 MicroRNA biomarkers in neurodegenerative diseases
4.1 MicroRNA biomarkers in Alzheimer’s disease
Alzheimer’s disease is an ND that primarily affects older individuals and is becoming a global health concern due to the aging population (Ramachandran et al., 2021). According to statistics from 2020, nearly 55 million people worldwide suffer from AD (Weidner and Barbarino, 2019), and this number is expected to increase by 131.1 million by 2050 (Prince et al., 2015; Nagaraj et al., 2021). The main clinical signs of ADs include memory loss, executive dysfunction, difficulties carrying out routine activities, and visuospatial impairments. Early symptoms of AD include changes in cognitive function, memory loss, and disruptions in language and speech patterns (Tarawneh and Holtzman, 2012). Around 20 to 30% of individuals in the early stages of AD have notable symptoms of sadness and mood alterations, occurring before the first indication of memory decline (Zubenko et al., 2003). Patients in the later stages of AD have severe hallucinations, confusion, and a lack of self-sufficiency, finally dying due to respiratory infection or malnutrition (Kalia, 2003; Tarawneh and Holtzman, 2012). Cerebrovascular amyloidosis, inflammation, and substantial synaptic alterations (Dansokho and Heneka, 2018; Katsumoto et al., 2018) accompany the primary pathological symptoms of AD, which include amyloid beta plaques, gliosis, neurofibrillary tangles (NFTs), and neuronal loss (Itagaki et al., 1989; Terry et al., 1991; Iqbal and Grundke-Iqbal, 2002; Iqbal et al., 2016; Petrella et al., 2019).
In AD patients, specific brain areas show an accumulation of two abnormal protein structures, amyloid plaques and NFTs (aggregates of hyperphosphorylated tau protein in the brain) (Braak and Del Tredici, 2010), along with a loss of cell connections. Both amyloid plaques and NFTs are associated with normal aging; however, in AD patients, these two neuropathological biomarkers are abnormally abundant (Zetterberg and Blennow, 2006). The first source of amyloid plaques is an integral membrane protein, the so-called amyloid-beta precursor protein (APP). The three enzymes alpha (α), beta (β), and gamma (γ) -secretase are responsible for APP cleavage (Epis et al., 2012). First, α-secretase breaks the APP at a crucial point, which prevents amyloid plaques, as shown in Figure 3 (Schedin-Weiss et al., 2014). In contrast, an aspartyl protease called β-secretase, also known as β-site amyloid precursor protein-cleaving enzyme 1 (BACE-1), can attach to the APP cell membrane. The β-secretase cuts the APP protein at one end of the Aβ fragment (O’Brien and Wong, 2011; Simunkova et al., 2019). The remaining piece of APP, previously bound to the neuronal cell membrane, is cleaved by γ-secretase at the other end, resulting in the Aβ protein (MacLeod et al., 2015). Neurons typically produce Aβ protein, which they release into the extracellular space. In general, microglia and astrocytes break down the Aβ protein. However, excess extracellular release of Aβ leads to aggregates of different sizes, called oligomers (Epis et al., 2012; Guo et al., 2020).
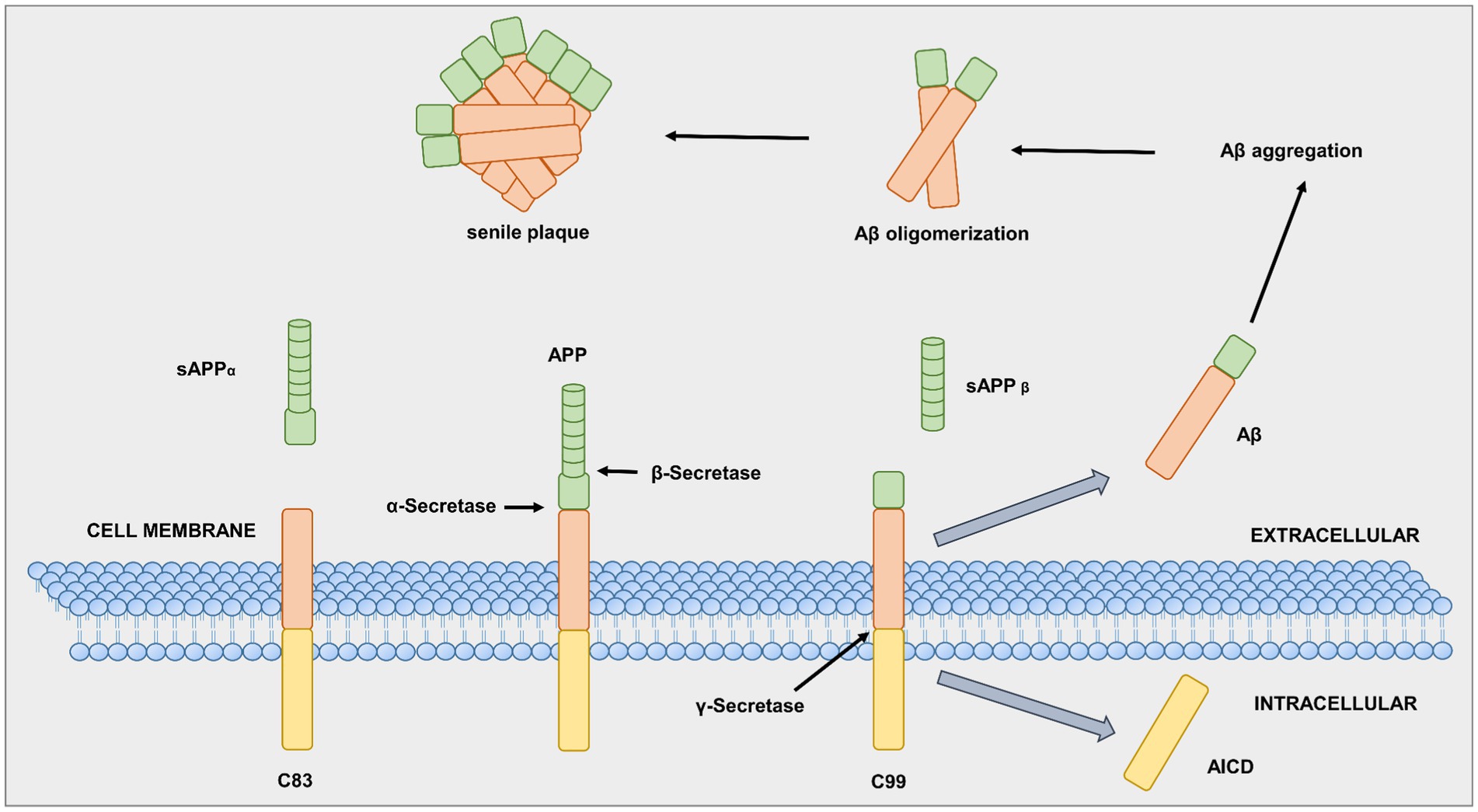
Figure 3. Alpha (α), beta (β), gamma (γ)-secretase in APP processing. Adapted from Gandy S. (2005).
Alzheimer’s disease is associated with tau protein, a primary component of NFTs (Kumar et al., 2018; Errico and Meyer-Luehmann, 2020). The tau protein is another abnormal protein structure that accumulates in AD and binds to microtubules in neurons, facilitating axon development. Despite being a natively unfolded protein, tau is highly soluble and does not tend to aggregate easily (Wang and Mandelkow, 2016). AD involves tau protein, the primary component of NFTs (Kumar et al., 2018; Errico and Meyer-Luehmann, 2020). It can undergo hyperphosphorylation in the brain (Iqbal et al., 2016), which leads to the detachment of tau from microtubules, instability of microtubules, self-aggregation, and the formation of neurofibrillary tangles. Several protein kinases and phosphatases regulate tau phosphorylation via phosphorylation-site-dependent pathways.
Furthermore, tau acetylation (Ac-Tau) is associated with tau aggregation, suggesting that Ac-Tau contributes to tau’s pathological conversion. Hence, targeting Ac-Tau may be a promising strategy to prevent tau aggregation and tauopathy progression. The combined effect of tau and Aβ may lead to neurodegeneration in AD. As a result, studies are underway to develop drugs that target Ac-Tau and potentially prevent or slow tauopathy progression. The synergistic interaction between tau and Aβ may cause neurodegeneration in AD (Iqbal et al., 2016; Guo et al., 2020).
In 2007, Schipper and colleagues published their first miRNA biomarker study for AD using microarray analysis. They found that the miRNA expression of peripheral blood mononuclear cells (PBMCs) was higher in AD patients than in healthy cells (Schipper et al., 2007). A 12-miRNA pattern distinguishing between AD patients and healthy blood cells has been identified with next-generation sequencing (NGS). It has 93% accuracy, 95% specificity, and 92% sensitivity (Leidinger et al., 2013).
Kumar et al. (2013) used nanoString technology to detect seven circulating miRNA signals in plasma to distinguish Alzheimer’s patients from healthy controls with 95% accuracy (Kumar et al., 2013). Cogswell and colleagues used RT-qPCR to identify a group of miRNAs only associated with AD. Their miRNA expression patterns in CSF were similar to those in AD patients’ brains (Cogswell et al., 2008). In their study, Garcia et al. (2021) reported the upregulation of miR-21-5p in CSF samples from mild cognitive impairment (MCI) patients who met the criteria for AD, compared to those without AD criteria. This upregulation was also observed in neurons, astrocytes, and microglia derived from induced pluripotent stem cells (iPSCs) of AD patients (Garcia et al., 2021).
Various miRNA-based markers have been identified, showing favorable accuracy, sensitivity, specificity, and cost-effectiveness (Sempere et al., 2004). Table 1 presents an overview of significant miRNAs proposed as AD biomarkers. These miRNAs are thought to be involved in AD pathogenesis and represent a valuable diagnostic tool for early AD diagnosis. Altogether, miRNAs can potentially be a powerful tool in AD diagnosis.
4.2 MicroRNA biomarkers in Parkinson’s disease
Parkinson’s disease (PD) is a standard ND that affects about 1% of individuals over the age of 60 (Tysnes and Storstein, 2017). It is associated with the severe loss of substantia nigra par compacta dopaminergic (DA) neurons. Its pathophysiology includes both hereditary and environmental risk factors. The clinical symptoms encompass motor dysfunction, such as bradykinesia, resting tremors, and postural instability. Autonomic dysfunction, such as constipation or erectile dysfunction, and depression can occur even before the onset of motor dysfunctions. Late-onset disease begins with detecting genetic mutations in the α-synuclein (SNCA) and leucine-rich repeat kinase 2 (LRRK2) genes. Additionally, mutations were identified in the Parkin (PARK2), PTEN-induced putative kinase 1 (PINK1), and oncogene DJ1 (DJ1) genes, which are associated with an early onset of disease (Coppedè, 2012).
Parkinson’s disease is diagnosed primarily based on clinical symptoms and neurological examinations (Zhang W.T. et al., 2022). However, this ND remains challenging to diagnose and treat because its symptoms may differ from one patient to the next and may overlap with other related conditions, called atypical Parkinsonian disorders. This is due to several complicating factors, including gene mutation, neurotrophic factor insufficiency, oxidative stress, excitotoxicity, immunological dysregulation, mitochondrial malfunction, and apoptosis (Angelova, 2021; Tolosa et al., 2021). As a result of the heterogeneity of the disease, reliable laboratory diagnostic tests are still lacking.
There is a widely recognized need for more diagnostic tools for PD. However, imaging techniques such as MRI and DaTscan of the brain and the unified Parkinson’s Hoehn-Yahr scale have been successfully used in PD diagnosis. Novel biomarkers are also urgently needed to address the shortcomings of the current diagnostic approach (Zhang W.T. et al., 2022). Therefore, miRNAs may be valuable biomarkers for PD (Roser et al., 2018; Chen et al., 2021). For example, miR-4639-5p has a sensitivity of 94% and a specificity of 85% in diagnosing PD (Chen et al., 2017), while miR-494 exhibits low diagnostic efficiency, with 61% sensitivity and 79% specificity (Li et al., 2021). However, the joint use of miR-124 and miR-494 has shown diagnostic efficacy in distinguishing PD patients from healthy controls. This approach achieved a sensitivity of 86% and a specificity of 85% (Li et al., 2021). Table 2 presents a compilation of miRNAs that can be used to diagnose PD.
Mouse models for PD have shown miR-124’s potential neuroprotective properties (Zhang F. et al., 2022). Parkinson’s disease mice treated with miR-124 showed improved motor deficits, reduced dopaminergic neuron loss, and reduced oxidative stress. It also targets Axin 1 (the protein encoded by the AXIN1 gene) and stimulates the Wnt/β-catenin signaling pathway, suppressing PD progression. Results indicate that miR-124 may be an effective treatment for PD (Kanagaraj et al., 2014; Zhang F. et al., 2022). Studies should focus on identifying strategies to increase miR-124 delivery to the brain and assessing the safety of miR-124 treatment in human subjects. Therefore, further research is needed to explore miR-124’s therapeutic potential.
4.3 MicroRNA biomarkers in amyotrophic lateral sclerosis
Amyotrophic lateral sclerosis is an incurable disease that gradually causes the loss of motor neurons in the brain and spinal cord (De Felice et al., 2012; Mead et al., 2023). Motor neurons are the only cells that degenerate and die in ALS, but there is evidence that a single cell type does not cause the disease. Non-neuronal cells in the environment, including microglia, astrocytes, muscle, and T cells, play a critical role in disease development (Vahsen et al., 2021)—motor neuron degeneration results in progressive weakening of the limbs, respiratory muscles, and bulbar muscles.
In the early stages of the disease, symptoms can vary depending on the extent of damage to motor neurons in the brain and spinal cord. Hyperreflexia and muscle cramps are upper motor neuron (UMN) injury symptoms. Lower motor neuron failure, on the other hand, results in generalized weakness, fasciculation, muscular atrophy, muscle cramps, and hyporeflexia (Brown and Al-Chalabi, 2017). Patients with ALS often have difficulty eating or swallowing and have slurred or nasal speech. About 25% of ALS patients demonstrate bulbar involvement, which is less common than limb involvement. Most patients have lower motor neuron (LMN) and UMN symptoms during the condition, caused by damage in the spinal and brainstem regions. Patients with ALS have a poor prognosis, and death generally occurs 2–4 years after the onset of symptoms owing to bulbar dysfunction and respiratory failure. However, in rare cases, patients with ALS may have a longer life expectancy (Phukan et al., 2007; Brown and Al-Chalabi, 2017; Grad et al., 2017; Ricci et al., 2018; Masrori and Van Damme, 2020; Zakharova and Abramova, 2021). Currently, there is no laboratory test available to diagnose ALS definitively. The diagnosis of ALS is typically established through clinical examinations, laboratory tests, and the exclusion of other conditions. The process of diagnosing ALS can be challenging, and it often involves a team of healthcare professionals, including neurologists. It is difficult to provide timely and effective treatment because it takes around a year from the initial symptoms to a diagnosis (Ricci et al., 2018; Štětkářová and Ehler, 2021).
Studies have proposed miRNAs as diagnostic marker candidates showing variable expression rates in ALS patients. It indicates that these miRNAs may regulate the development of diseases (De Felice et al., 2012; Freischmidt et al., 2013; Feneberg et al., 2014). The lists of miRNAs reported in ALS are mentioned in Table 3. For example, miR-155, a pro-inflammatory miRNA, is upregulated in spinal cord animal models and ALS patients. Interestingly, its inhibition has improved survival and motor function in experimental animals (Butovsky et al., 2012; Yu et al., 2013). Skeletal muscle exhibits elevated levels of miR-206 expression, known to regulate muscle development. It is downregulated in ALS patients and animal models, and its overexpression improves motor function in animal models (Williams et al., 2009). Other ALS-related miRNAs are miR-9, which regulates astrocyte function, and miR-124, which regulates neuronal differentiation and neurogenesis (Hawley et al., 2019; Zhao et al., 2021; Martinez and Peplow, 2022). Additionally, the miR-21, miR-146a, miR-132, and miR-124 have been implicated in the pathogenesis of ALS (Kovanda et al., 2018; Zhou F. et al., 2018; Barbosa et al., 2021; Bai and Bian, 2022; Gomes et al., 2022). Researchers have found that these miRNAs can change neuroinflammatory responses, suggesting they could be therapeutic targets (Ravnik-Glavač and Glavač, 2020; Martinez and Peplow, 2022). A study showed that miR-338-3p modulates glutamate transporter EAAT2 expression and that its downregulation may contribute to ALS (Rosenblum and Trotti, 2017; Sun et al., 2018; Tian et al., 2021).
In 2021, Dobrowolny and colleagues used absolute RT-qPCR quantification and next-generation sequencing to examine the levels of miRNA circulating in healthy individuals and ALS patients’ blood. They discovered a correlation between miR-151a-5p, miR-133a, and miR-206 levels in ALS patients and reduced function loss. Therefore, these miRNAs may help predict a person’s future performance. It is observed that miR-206 and miR-151a-5p expressions are elevated in the early stages of ALS, but expression declines in the subsequent moderate and severe stages (Dobrowolny et al., 2021). However, miR-199a-5p and miR-133a remained low throughout the disease. The findings suggest that miR-133a has diagnostic significance in the severe and moderate stages, whereas miR-199a-5p is essential in the early and terminal stages (Dobrowolny et al., 2021). Clinical researchers may be able to tailor treatment strategies based on miRNAs as prognostic indicators for ALS. Additionally, several miRNAs associated with disease severity and progression could be targeted to treat ALS (Dobrowolny et al., 2021).
In conclusion, studying the roles and functions of differentially expressed miRNAs in ALS might offer essential insights into the disease’s underlying processes and potential treatment targets. Therefore, miRNAs should be further investigated in ALS research.
4.4 MicroRNA biomarkers in Huntington’s disease
In 1872, the American physician George Huntington published the first clinical description of HD. This incurable autosomal dominant genetic disorder results in neuronal degeneration due to repeated replication of the cytosine-adenine-guanine (CAG) trinucleotide in the Huntingtin gene. Symptoms of HD typically appear between 39 ± 5 years of age, although they can appear in children (Jacobsen et al., 2010). They include uncontrollable excessive motor movements and cognitive or behavioral symptoms, generally discovered after the disease progresses. The unified HD rating, which provides an overall rating system based on motor, behavioral, mental, and functional assessments, can measure disease progression. In imaging studies, such as MRI or computed tomography (CT), the caudate nuclei may show evidence of atrophy early in the disease (Roos, 2010; Finkbeiner, 2011; Ghosh and Tabrizi, 2018).
Huntingtin gene was discovered on chromosome 4p16.3 in 1993 (MacDonald et al., 1993). The gene integrity is compromised by the repeated irregular expansion of the CAG triplet in the gene’s first exon. Normal brain development and function depend on translating the translated protein corresponding to the Htt allele, which consists of 6–35 CAG triplet repeats. In contrast, a CAG triplet expansion of 40 or more repeats indicates complete penetration, which indicates an abnormality. Alleles with 36–39 CAG repeats have low penetration. In contrast, CAG repeats ranging from 27 to 35 fall within the approved range and are known as intermediate or unstable alleles due to their ability to change size during reproduction (Southwell et al., 2015; Chen and Wolynes, 2017; Jarosińska and Rüdiger, 2021).
The mutant Huntingtin protein (mHTT) is produced by a mutation in the Htt gene due to a tremendous polyglutamine repeat that aggregates in the brain and forms toxic clumps (Coppen and Roos, 2017; Fodale et al., 2020). An estimated frequency of 5–10 per 100,000 of neurodegeneration results from mobility problems, cognitive impairment, and mental symptoms (Mestre et al., 2009; Roos, 2010). The mHTT protein can exist in monomeric and aggregated forms, and research has focused on developing antibodies against both forms for diagnostic and therapeutic purposes. Evidence shows that the soluble monomeric huntingtin protein and the aggregated huntingtin protein may also be toxic to neurons. A mouse model of HD demonstrated that antibodies against monomeric huntingtin protein prevented neuronal death (Southwell et al., 2015; Fodale et al., 2020; Tabrizi et al., 2020; Denis et al., 2023). According to these findings, HD can be treated by targeting the monomeric huntingtin protein. Nevertheless, more research is needed to understand better the roles of monomeric and aggregated huntingtin proteins in HD and develop effective treatments to treat these forms (Southwell et al., 2015). Further research is required to determine whether targeting monomeric huntingtin proteins can be a proper therapeutic strategy for HD.
The clinical symptoms of HD can be classified into three types: motor symptoms, mental challenges, and cognitive impairment (Tabrizi et al., 2020). The disease causes involuntary movements, such as chorea. There are several dyskinesias associated with Parkinson’s syndrome (Heo and Scott, 2017), including chorea-like symptoms, ataxia, dystonia, and Parkinson’s syndrome. Psychological symptoms such as anxiety and anger may occur before dyskinesia occurs in HD patients (Goh et al., 2018). Further, executive dysfunction is primarily responsible for HD patients’ cognitive impairment. According to some studies (Bayliss et al., 2019), cognitive impairment could worsen as CAGs multiply. MiRNAs regulate gene expression and contribute to HD pathogenesis. The dysregulation of miR-9 in individuals affected by HD has been associated with neuronal loss (Packer et al., 2008). MiR-124a controls neuronal differentiation and improves HD motor symptoms in mouse models (Lee et al., 2017; Martinez and Peplow, 2021). A list of miRNA biomarkers reported in the literature is mentioned in Table 4. These miRNA biomarkers can provide insight into the molecular mechanisms underlying HD and can be used to design therapeutic strategies for HD. As such, miRNAs are essential for understanding and managing HD.
4.5 MicroRNA biomarkers in multiple sclerosis
Multiple sclerosis is an autoimmune disease impacting the brain and spinal cord, resulting in chronic neurological impairment. A significant degree of physical disability results from demyelinating lesions that damage myelin and the axons of the CNS (Arneth and Kraus, 2022). Although the exact cause of MS is unknown, it is likely to be influenced by genetic and non-genetic factors, such as viral infections. Axons and myelin are permanently destroyed by an immune-mediated inflammatory process (Lawrence Steinman, 1996; O’Gorman et al., 2012; de Faria et al., 2013; Dobson and Giovannoni, 2019; Gao et al., 2021; Arneth and Kraus, 2022; Lamb, 2022).
Multiple sclerosis pathophysiology involves the formation of localized demyelination areas or plaques caused by the destruction of oligodendroglia cells that produce myelin (a fatty substance that protects nerve fibers and insulates them). MS pathophysiology is also marked by perivascular inflammation, further damaging the oligodendroglia cells and surrounding tissues. MS is classified as an autoimmune disease involving T-cells, where the immune system mistakenly attacks its healthy cells, including those in the CNS. MS can be divided into two main phases: relapsing–remitting and secondary progressive. The first phase is characterized by episodes of inflammation followed by periods of relative calm or remission, often affecting younger patients. The second phase, known as primary progressive MS, is marked by a steady deterioration of neurological function from the onset of the disease without distinct relapses or remissions. Secondary progressive MS occurs when there is progression after an initial period of relapsing–remitting disease (Lamb, 2022). MS is also characterized by perivenular infiltration of lymphocytes and macrophages. The prevalence of MS is rising globally, notably more prevalent in countries with temperate climates. Risk factors for MS include age, sex, race, vitamin D levels, smoking, childhood obesity, exposure to ultraviolet radiation (UVR), autoimmune diseases, genetic background, and Epstein–Barr virus (EBV) infection. Individuals with Northern European ancestry have a higher susceptibility to MS, while those of Asian, African, or Native American heritage have the least susceptibility (O’Gorman et al., 2012; Dobson and Giovannoni, 2019; Chacko et al., 2021).
Several studies have identified specific miRNAs that are differentially expressed in MS patients compared to healthy individuals, and some of these miRNAs have been associated with disease subtypes and clinical parameters. Specific miRNAs were associated with MRI-based phenotypes, suggesting a link to blood–brain barrier pathology (Pietrasik et al., 2021; Lamb, 2022). Table 5 presents a selection of miRNAs discovered in different body fluids. For instance, one study that involved four observational cohorts found that miR-484, miR-320a, miR-486-5p, miR-320c, and miR-140-5p expression is significantly different in MS patients than in healthy people (Regev et al., 2018). One study found that miR-92a-1 levels differed between relapsing–remitting MS (RRMS) patients and healthy controls and secondary progressive MS (SPMS) patients. Furthermore, this miRNA was associated with disease duration and the Expanded Disability Status Scale (EDSS) (Pietrasik et al., 2021).
A study by Helmond and colleagues analyzed an extensive group of MS patients using brain imaging (Gandhi et al., 2013). MicroRNAs were also associated with the EDSS and the duration of the disease (Gandhi et al., 2013). MicroRNAs were associated with various MRI-based phenotypes, indicating a possible relationship to the blood–brain barrier. The most reliable indicators of MRI subgroups were miR-22-3p, miR-345-5p, and miR-361-5p (Hemond et al., 2019).
MicroRNAs dysregulated expression in MS and association with disease parameters make them promising candidates for further investigation and development as MS biomarkers. Researchers have found that miRNAs can be used to distinguish between different types of MS and monitor their progression. Moreover, they emphasize the need for ongoing research to validate and further explore miRNAs as clinical biomarkers for MS. As such, miRNAs can be handy tools for diagnosing and treating the disease.
5 Role of microRNA in post translational modification of neurodegenerative diseases
5.1 Alzheimer disease
In the complex network of AD pathology, miRNAs function as regulatory elements within the post-translational modification (PTM), orchestrating molecular events that contribute to the onset and progression of the disease. These PTMs encompass a diverse array of processes, including acetylation, carbamylation, glycation, methylation, nitration, sumoylation, truncation, ubiquitination, and phosphorylation. Through the regulation of these pathways, miRNAs assume crucial positions in molding the molecular processes driving AD development and etiology (Singh et al., 2023). The miRNAs can influence tau protein expression in AD through multiple mechanisms like phosphorylation, splicing and acetylation. Tables 6, 7 scope key miRNAs involved in ADs pathology through their regulation of PTMs, driving disease progression and onset (Banzhaf-Strathmann et al., 2014; Salta et al., 2016; Sharma and Lu, 2018; Silvestro et al., 2019; Praticò, 2020; Lauretti et al., 2021; Park and Moon, 2022).
Carbamylation involves the covalent addition of carbamoyl groups to lysine residues, especially prevalent in lysine-rich proteins like tau, which is associated with AD (Guru Krishna Kumar et al., 2018). As a result, it accelerates the formation of Aβ in AD and contributes to the neurodegenerative process. A variety of miRNAs have been implicated in carbamylation effects through their interactions with histone deacetylases (HDACs), including miR-34c (Zovoilis et al., 2011), miR-134 (Gao et al., 2010), miR-206 (Williams et al., 2009), and miR-124 (Johnson et al., 2008). Dysregulation of miRNA (miRNA)-histone deacetylase (HDAC) networks has been implicated in the pathogenesis of various neurological disorders. This suggests their potential as therapeutic targets of neurodegenerative diseases.
In the complex interplay between Alzheimer’s and diabetes, individuals with diabetes are at an increased risk for AD, driven by processes such as glycation and the involvement of advanced glycation end-products (AGEs) (Dukic-Stefanovic et al., 2001). Protein structure is disrupted by glycation, leading to amyloid aggregation in AD. These proteins are affected, significantly contributing to the pathology of the disease (Ott et al., 1999; Xu et al., 2009). A study reported, that miR-142 affects neuroinflammation and AD risk by targeting the receptor for advanced glycation end product (RAGE) pathway (Zhang R. et al., 2018).
MicroRNAs play key roles in methylation processes, influencing DNA methylation patterns, as well as the activity of methyltransferases in epigenetic regulation (Shi et al., 2004). Studies found that miR-let-7a-3 methylation (Brueckner et al., 2007), and miR-125b upregulation (Pogue et al., 2010) are linked to AD pathology, emphasizing the intrinsic relationship between miRNAs and methylation. This suggests that miRNAs are important mediators of epigenetic modifications in AD pathogenesis.
Nitration occurs when a nitrogen species, nitryl, is added to a protein to cause the protein to misfold, which leads to oxidative stress, misfolded proteins, oxidative stress and cellular damage (Radi, 2013). It is regulated by miRNAs such as miR-132, miR-212, and miR-188. These miRNAs affect neuronal viability and synaptic function in AD by modulating the production of neuronal nitric oxide synthase (NOS1) and reducing Aβ-induced toxicity through inhibition of NOS1 expression (Wang et al., 2017; Chen M. et al., 2020).
Sumoylation, involving the non-covalent attachment of SUMO (Small Ubiquitin-like Modifier) proteins to target proteins, modifies amyloid beta (Aβ) production and tau protein function in AD (Li et al., 2003; Wilkinson and Henley, 2010). It regulates key proteins such as APP intracellular domain (AICD), APP, impacting Aβ levels and plaque formation. In AD, tau sumoylation plays a role in tau phosphorylation and degradation (Tao et al., 2017; Liu et al., 2021). Prenatal exposure to specific substances may disrupt sumoylation processes in a way that could contribute to Alzheimer’s disease. Linked to this condition are alterations in miRNA expression, such as increased miR-489 and decreased levels of miR-33, miR-19b, and miR-509 (Wnuk et al., 2019).
Truncation occurs when proteins are shortened by specific proteases or by mutations that end mRNA translation prematurely by cleaving at specific sites in the protein (Wirths and Zampar, 2019). MicroRNAs play an important regulatory role in the expression and processing of the APP, which is central to the pathogenesis of AD. Several miRNAs have been found to modulate the activity of enzymes involved in APP processing, such as BACE1, calpain, and caspases, thereby influencing the production of Aβ peptides. For example, miR-16, miR-338-5p, miR-485-5p, miR-107, and miR-186 play a critical role in controlling BACE-1 expression, which affects Aβ production in AD (Maoz et al., 2017; Zhao et al., 2017; Wang et al., 2019; Kou et al., 2020). Specific miRNAs, such as miR-298, have been shown to reduce the levels of APP, BACE1, and Aβ peptides (Aβ40 and Aβ42) in cell culture models (Chopra et al., 2021) Several studies suggest that miR-132 reduces truncated tau fragments by targeting Calpain 2 and Caspase 3 (El Fatimy et al., 2018). Other miRNAs such as miR-195, miR-135a, miR-135b, and miR-339–5p also modulate BACE-1 expression, implicating their involvement in AD pathology (De Strooper and Karran, 2016). While miRNAs are involved in modulating APP levels and the production of Aβ peptides, their direct role in amyloid APP truncation requires further investigation to establish a conclusive link.
Ubiquitination, a PTM essential for protein degradation and cellular proteostasis, is tightly regulated by miRNAs like miR-7, miR-9, miR-181c, and ciRS-7. These miRNAs target E3 ubiquitin ligases and deubiquitinases involved in the ubiquitin-proteasome system, influencing misfolded protein clearance and toxic aggregate formation in AD (Schonrock et al., 2010; Zhao et al., 2016; Shi et al., 2017; Cochran et al., 2020).
Acetylation of lysine residues in tau and Aβ peptides has been linked to the pathogenic accumulation of tau and Aβ aggregates, contributing to synaptic dysfunction (Mattson, 2010; Cohen T.J. et al., 2011; Dodson et al., 2013). MicroRNAs such as miR-9, miR-212, and miR-181c have been identified by researchers to modulate the regulation of SIRT1. This leads to changes in tau acetylation via deacetylase activity (Schonrock et al., 2012; Weinberg et al., 2015).
Phosphorylation, a decisive PTM in signal transduction and cellular regulation, is tightly regulated by miRNAs such as the miR-29 family members, miR-16, miR-338-5p, miR-107, and miR-186 (Che et al., 2014; Kim et al., 2016). These miRNAs modulate the expression of protein kinases and phosphatases implicated in tau hyperphosphorylation and Aβ production. MiRBase reports that miRNAs like miR-22-3p target specific proteins such as sirtuin 1 (SIRT1), cyclin-dependent kinase inhibitor 1 (p21), methyl-CpG-binding protein 2 (MeCP2), cyclin-dependent kinase inhibitor (CDK1), and phosphatase and tensin homolog (PTEN) (Praticò, 2020; Lauretti et al., 2021). PTEN inhibits the AKT (protein kinase B) signaling pathway, which plays a crucial role in various cellular processes such as cell survival, growth, and metabolism. This suppression leads to increased tau phosphorylation and aggregation. Another example is miR-132-3p, which specifically targets the 3’UTR of the tau protein and controls its phosphorylation and acetylation by targeting enzymes such as EP300, highlighting their role in modulating tau pathology in AD (El Fatimy et al., 2018). MiR-132-3p has been found to target the polypyrimidine tract binding protein 2 (PTBP2), which is involved in tau splicing. Dysregulation of miR-132-3p can thus affect tau splicing and isoform expression. Further, MeCP2, SIRT1, PTEN, and brain-derived neurotrophic factor (BDNF) enhance tau phosphorylation, acetylation, and splicing (Lauretti et al., 2021; Park and Moon, 2022).
Through the interaction with tau protein’s mRNA, miRNAs can induce degradation or inhibit translation, thereby modulating tau protein expression (Silvestro et al., 2019). MiR-132, for example, is a direct regulator of the tau protein. Its deletion may lead to aberrant tau metabolism, increased hyperphosphorylation, and aggregation (Salta et al., 2016). Additionally, miRNAs can also control tau protein expression by targeting other proteins involved in PTM or degradation pathways (Praticò, 2020). MiRNA-125b, for example, has been related to tau hyperphosphorylation and cognitive impairments in AD, presumably via the regulation of other target proteins (Banzhaf-Strathmann et al., 2014). Therefore, miRNAs are crucial for controlling tau expression and its pathological consequences.
The complex relationship between miRNAs and PTMs highlights their importance in AD pathogenesis, providing potential approaches for therapeutic intervention and biomarker discovery to combat this devastating neurodegenerative disorder.
5.2 Parkinson disease
Parkinson’s Disease is characterized by protein degradation, protein aggregation, and mitochondrial dysfunction caused by miRNA dysregulation (Lu et al., 2017). In PD, dysregulation of specific miRNAs has been implicated in the modulation of various protein PTMs, including phosphorylation, ubiquitination, acetylation, and sumoylation.
Phosphorylation of proteins such as α-synuclein, tau, and parkin is a hallmark of PD. Various miRNAs control the phosphorylation status of these proteins such as miR-153, miR-7, and miR-124 (Li S. et al., 2016). For instance, miR-153 targets α-synuclein mRNA, reducing its phosphorylation and aggregation, while miR-7 modulates tau protein phosphorylation (Nies et al., 2021). Moreover, miR-124 regulates ubiquitin E3 ligase parkin phosphorylation in PD-associated protein degradation pathways (Kang et al., 2017; Goh et al., 2019; Li et al., 2022).
In Parkinson’s disease, disruptions in ubiquitin-proteasome system (UPS) function have been strongly implicated in the development and progression of the condition. Research indicates that abnormalities in the ubiquitination process can lead to the accumulation of toxic protein aggregates, such as α-synuclein (Walden and Muqit, 2017; Behl et al., 2022; Buneeva and Medvedev, 2022). These protein aggregates can interfere with normal cellular processes, disrupt neuronal function, and ultimately contribute to the degeneration of dopaminergic neurons (Zheng et al., 2016). Evidence of UPS dysfunction in PD includes the presence of ubiquitin-immunopositive Lewy bodies in PD patients’ brains, as well as impaired proteasomal function observed in the substantia nigra of PD brains (Zheng et al., 2016). Multiple miRNAs, such as miR-34b/c, miR-7, and miR-205, target components of the UPS pathway, impacting protein ubiquitination and degradation. For instance, miR-34b/c targets parkin, resulting in its reduced expression and compromised ubiquitin ligase activity (Kabaria et al., 2015; Shlevkov et al., 2016; Jarome and Devulapalli, 2018; Nemeth et al., 2024).
Approximately 25% of miRNA-124 targets showed changed expression patterns in PD. This finding suggests that miRNAs may have multiple targets owing to their poor base pairing with mRNA 3’ UTR (Follert et al., 2014; Lu et al., 2017). In PD brains, miR-9 and miR-124 showed increased expression, and they are thought to regulate genes involved in apoptosis, oxidative stress, and inflammation (Follert et al., 2014; Lu et al., 2017). These results indicate that miRNAs have a significant role in PD pathophysiology. In Table 8, several brain-enriched miRNA families are summarized along with their potential roles in PD pathogenesis.
Unraveling the complex relationship between miRNAs and protein PTMs in PD pathogenesis offers therapeutic avenues. By targeting misregulated miRNAs or their downstream proteins involved in PTMs, there is a potential to restore protein homeostasis and mitigate neurodegeneration in PD patients. Therefore, strategies, including miRNA mimics, antagomirs, and small molecule inhibitors, are being explored to alter miRNA expression or activity.
5.3 Amyotrophic lateral sclerosis
Several neurodegenerative disorders, including ALS, are linked to RBP and PTMs. Researchers have identified PTMs, such as phosphorylation, acetylation, ubiquitination, and sumoylation, alter the function, localization, and stability of RBPs. It is suggested that PTMs play an essential role in RBP activities, stress granule dynamics, and the potential for targeting PTMs as a therapeutic intervention in ALS and other neurodegenerative conditions (Lee and Kemper, 2010; Choi and Kemper, 2013). The role of miRNAs in aging and age-related PTM changes has been described in a study examining the biological role and probable significance of m6A RNA methylation in aging and age-related disorders (Sun et al., 2022; Wu et al., 2022).
In ALS, miRNAs play a crucial role in phase transition by promoting or inhibiting the formation of multivalent contacts between phase-separating macromolecules and associating with or excluding other proteins and nucleic acids. These processes can promote or mitigate the proteinopathies that underlie neurodegeneration in ALS (Salem et al., 2022). For example, the PTM of the SOD1 protein, involved in familial cases of ALS, can contribute to disease progression. Changes in the deposition, location, maturation, and modification after translation of the SOD1 protein have been detected in post-mortem spinal cord samples from individuals with ALS (Peggion et al., 2022; Trist et al., 2022). These modifications were linked to instability and incorrect binding of enzymatically active SOD1 dimers, as well as changes to SOD1 modifications after protein synthesis and molecular chaperones that control SOD1 development (Peggion et al., 2022; Trist et al., 2022).
MicroRNAs have been identified as significant contributors to ALS pathogenesis through post-transcriptional gene regulation and interaction with ALS-related proteins. Several miRNAs have been proposed as potential biomarkers for ALS, and their dysregulation has been reported in ALS pathogenesis. Understanding these pathways can lead to novel approaches to ALS treatment and the development of practical diagnostic tools (Salem et al., 2022). Table 9 lists miRNAs involved in ALS pathogenesis. For example, miR-206 regulates myoblast development and maintains neuromuscular connections and synapses (Toivonen et al., 2014). Additionally, it inhibits muscle histone deacetylase 4 (HDAC4), influencing neuromuscular junction re-innervation (Williams et al., 2009). ALS patients exhibit a significant increase in miR-23 levels. It inhibits the translation of proliferator-activated receptor γ coactivator 1 α (PGC-1α) (a receptor activated by peroxisome proliferators and involved in mitochondria biosynthesis and functioning) (Russell et al., 2013). A miR-193b-3p controls the mechanistic target of rapamycin complex 1 (mTORC1) activity by targeting tuberous sclerosis 1 (TSC1), influencing cell survival and autophagy mechanisms (Li C. et al., 2017). The study found that miR-183b-5p targets RIP kinase 1 (RIPK) and programmed cell death 4 (PDCD4), indicating its role in programmed neuronal cell death (Li C. et al., 2017). A miR-155 is implicated in ALS neuroinflammation, and binding to SOCS1 mRNAs is linked to a rise in pro-inflammatory cytokines (Ceppi et al., 2009; O’Connell et al., 2010). These studies indicate that miRNAs play an essential role in ALS pathogenesis.
The research findings in the search support the significant impact of PTMs and miRNAs on ALS pathogenesis. Both PTMs and miRNAs are implicated in the dysregulation of proteins and gene expression associated with ALS, highlighting their potential as critical regulatory mechanisms and offering potential avenues for therapeutic intervention and diagnostic development. As a result, further research is needed into the role of PTMs and miRNAs in ALS.
5.4 Huntington diseases
Huntington’s Disease pathogenesis has been linked to protein PTMs, such as those of mHTT, a neuronal signaling protein, and other proteins involved in neuronal signaling (Lontay et al., 2020). As post-transcriptional regulators, miRNAs can indirectly influence these PTMs by regulating the expression of enzymes that carry out the modifications or proteins that target those modifications. In huntingtin protein, specific miRNAs can bind to the mRNA and inhibit it from being translated, thereby affecting levels of mHTT and PTMs that follow (Martinez and Peplow, 2021). Although it is not entirely understood what exactly miRNAs influence PTMs in HD, several miRNAs whose expression levels are altered, have been identified in patients with HD, including miR-1247-5p, miR-10b-5p, miR-615-3p, miR-196b-5p, and miR-196a-5p (Hoss et al., 2014; Guo et al., 2022). By overexpressing miR-196a and miR-155 in animal models of HD, mHTT mRNA, and protein levels decreased, indicating that these miRNAs could influence mHTT PTMs by regulating their expression (Martinez and Peplow, 2021). Table 10 provides a list of miRNAs associated with HD pathogenesis.
There is evidence that miRNAs such as miR-128 and miR-155 play a role in regulating phosphorylation events related to HD pathology. It is reported that miR-128 targets the mRNA of p35, a regulatory subunit of CDK5 (Guzman et al., 2018; Lian et al., 2018; Budi et al., 2023) Similarly, miR-155 targets the mRNA of glycogen synthase kinase 3 beta (GSK3β), a key kinase involved in mHTT phosphorylation and toxicity (Haramati et al., 2010; Shi et al., 2012; Varendi et al., 2014; Budi et al., 2023).
Impaired UPS function contributes to misfolded protein accumulation in HD. MicroRNAs such as miR-9 and miR-27a have been implicated in regulating UPS components, influencing protein ubiquitination and degradation. The miR-9 targets the mRNA of ubiquitin-conjugating enzyme E2I (UBE2I), while miR-27a targets the mRNA of ubiquitin-specific protease 25 (USP25), both involved in UPS-mediated protein degradation (Ma et al., 2010; Zhang et al., 2019; Dong and Cong, 2021; Zhu et al., 2021).
Acetylation of proteins by histone acetyltransferases (HATs) and HDACs plays a role in HD development. It has been associated with miRNAs that modulate acetylation processes, such as miR-22 and miR-124. The miR-22 targets the mRNA of HDAC4, a class II HDAC involved in mHTT-induced neurotoxicity, while miR-124 targets the mRNA of HAT1, a histone acetyltransferase implicated in transcriptional dysregulation in HD (Lee et al., 2011; Liu et al., 2020; Dong and Cong, 2021; Zhang et al., 2023).
MicroRNAs such as miR-132 and miR-146a have been implicated in modulating SUMOylation processes in HD. It is reported that miR-132 targets the mRNA of SUMO1, while miR-146a targets the mRNA of SUMOylation enzyme protein inhibitor of activated STAT1 (PIAS1), both of which regulate SUMOylation-mediated protein interactions and cellular homeostasis (Qian et al., 2017; Fan et al., 2020; Acuña et al., 2023).
In summary, studying miRNAs and abnormal proteins in HD is an active research area. This may provide a better understanding of disease mechanisms and therapeutic targets. It is critical to conduct further research on miRNAs and abnormal proteins to understand better their role and potential implications in Huntington’s disease. In addition, it is critical to develop effective biomarkers to monitor HD progression and treatment response.
5.5 Multiple sclerosis
Multiple Sclerosis is characterized by a significant involvement of miRNAs in pathophysiology, primarily affecting glial and peripheral immune cells (Gao et al., 2021). MicroRNAs mediate several cellular functions and developmental pathways, exhibiting various expression patterns. In addition to regulating T lymphocytes, they also play a role in MS development and progression (O’Brien et al., 2018; Michlewski and Cáceres, 2019; Soni and Biswas, 2021; Velázquez-Cruz et al., 2021). The dysregulation of miRNA can result in abnormal T lymphocyte function, potentially contributing to the autoimmune nature of MS. According to studies, miRNAs regulate T-cell-mediated immunity, an important aspect of MS pathophysiology (Wang and Liang, 2022). To develop new therapeutic strategies for MS, it is necessary to understand the role of miRNAs on T lymphocyte function.
Their effects on gene expression can explain the role of miRNAs in PTM. A critical role for miRNAs in MS is to modulate glial cells, another important player. We can gain a better understanding of immune-related disorders when we understand how miRNAs function in these processes. A majority of lesions (active and inactive), as well as normal-appearing white matter (NAWM), upregulate miR-155 (O’Brien et al., 2018; Michlewski and Cáceres, 2019; Soni and Biswas, 2021; Velázquez-Cruz et al., 2021). It regulates macrophage responses to inflammatory stimuli and impacts macrophage activity, which can indirectly influence PTM by modulating macrophage interactions with other proteins (Maciak et al., 2021; Zingale et al., 2022). MS patients have lower miR-320a expression and higher matrix metallopeptidase-9 (MMP-9) expression. MMP-9 disrupts the blood–brain barrier and degrades the essential myelin protein, contributing to the pathogenesis of MS. While MMP-9 is involved in protein degradation (a PTM-related process), miR-320a’s primary role is regulating gene expression (Aung et al., 2015). The miR-124 influences the activity of macrophages and microglial cells by targeting transcription factors (Ponomarev et al., 2011). MiR-338 is upregulated in NAWM and targets neurosteroid synthesis enzymes, potentially leading to PTM by altering the levels of specific molecules (Noorbakhsh et al., 2011; de Faria et al., 2013). MiR-23a plays a part in the differentiation of oligodendroglia and the myelination process, suggesting it plays a role in PTM by influencing processes related to myelination (Lin et al., 2013). The transformation of neural progenitor cells into oligodendrocytes promotes new neuron development. It reduces the formation of oligodendrocyte precursor cells, and peripheral myelin protein synthesis is suppressed by miR-9 regulation (Zhao et al., 2009; Coolen et al., 2013; Suzuki et al., 2020). MiR-27a has a crucial role in generating mature oligodendrocytes and influencing myelination. Its role in myelination suggests a potential role in PTM related to myelin proteins (Tripathi et al., 2019).
Although some of these miRNAs are not directly implicated in PTM, their regulatory effects on various cellular processes can indirectly affect PTM (Table 11). According to a study, search results provide more insight into the relationship between miRNAs and PTMs (Visser and Thomas, 2021). Micro RNAs may be candidates to control DNA damage response (DDR) enzyme levels and PTM. Histone PTM can also enhance or repress miRNA expression. The interplay between transcription factors and miRNAs can also profoundly impact gene expression, influencing PTM (Martinez and Walhout, 2009; Morales et al., 2017). As a result of these studies, cellular processes, including PTM, could be influenced by miRNAs via various regulatory mechanisms. This could provide insights into the intricate interactions between miRNAs and cellular processes.
6 MicroRNAs in aging
MicroRNAs regulate many genetic processes and biological pathways during aging. Their fluctuations in body fluids are associated with aging and age-related diseases such as NDs. In mammalian tissues, including the heart, brain, muscles, and bones, they have been demonstrated to regulate age-related processes and pathologies. Additionally, miRNAs regulate longevity in invertebrates through canonical aging pathways in invertebrates such as Drosophila and Caenorhabditis elegans (Santos and Lindner, 2017; Kinser and Pincus, 2020). Researchers demonstrated that mammalian brain miRNA composition changes with normal aging (Li N. et al., 2011; Inukai et al., 2012; Yin et al., 2015; Chen et al., 2019).
Further, miRNA expression changes are specific to certain brain regions (Persengiev et al., 2012). It is worth noting that the intricacies of normal brain aging remain complex and not yet fully understood. Consequently, the primary focus of miRNA research has been its role in age-related neuropathologies. Next, we highlight some evidence regarding the involvement of miRNAs in the brain’s aging.
• MiR-34 in Drosophila: It is upregulated induced by aging and is essential for brain health. Mutants of this miRNA show accelerated brain aging, while its upregulation extends lifespan and improves neurodegeneration (Liu et al., 2012).
• MiR-1000 in Drosophila: MiR-1000 plays a vital role in neuroprotection in aging by regulating glutamate transporters—mutants of miR-1000 experience shorter lifespans and neurodegeneration due to disrupted glutamate homeostasis (Verma et al., 2015).
• MiR-34 in Mammals: This miRNA is upregulated in the aging of AD patients. Its overexpression in the mouse hippocampus leads to memory impairment and decreased SIRT1 expression, a lifespan regulator. In mouse brain and blood, miR-34a levels increase with age and are inversely related to SIRT1 expression (Li et al., 2011b; Zovoilis et al., 2011).
• MicroRNAs and calorie restriction: Age-dependent miR-34a upregulation does not occur in calorically rested rodent brains. In calorie-restricted rodents, age increases the expression of the anti-apoptotic gene B-cell lymphoma 2 (BCL-2), targeted by miR-34a (Khanna et al., 2011).
• MiR-29 family: MiR-29a/b are upregulated with age in the mouse brain, contributing to microglia dysregulation and neuroinflammation, standard features of brain aging. These miRNAs directly suppress IGF-1 and CX3CL1 inhibitors of microglia activity (Fenn et al., 2013; Takeda and Tanabe, 2016; Ripa et al., 2017).
Studies have shown variations in miRNA expression patterns linked to NDs and prion-induced neurological conditions (Junn and Mouradian, 2012). The miRNA let-7, which targets the signaling of the nuclear receptor DAF-12 (abnormal dauer formation protein 12) and influences lifespan in worms (Junn and Mouradian, 2012), has been associated with AD. This relationship is made clear through genetic interactions observed in worms between let-7 and the homolog of APP known as APP-like-1 (apl-1). According to these findings, miRNA regulation may be involved in controlling Aβ peptide formation, even in non-mammalian organisms (Wang et al., 2008; Provost, 2010). In addition, miR-320 targets IGF-1 and IGF-1R in rats (Baek et al., 2008) and is upregulated in prion-induced neurological disorders (Saba et al., 2008). Table 12 lists dysregulated miRNAs for aging in human body fluids.
In conclusion, miRNA dysregulations in various aging-related disorders support the idea that aging is a fundamental factor in developing age-related diseases. MicroRNAs in body fluids fluctuate with age and age-related diseases. However, they could be used as potential biomarkers for aging, longevity, and monitoring the effectiveness of interventions to reduce the rate of aging. Investigations have examined changes in miRNA expression in various body fluids concerning age, including serum, plasma, and saliva (Smith-Vikos et al., 2016; Kinser and Pincus, 2020). A miRNA’s expression is regulated up or down with age, with some miRNAs playing a role in inflammation, cancer, cell cycle regulation, and DNA repair. A few studies have attempted to link circulatory miRNA profiles directly to human lifespan, showing associations between specific miRNAs and longevity (Caravia and López-Otín, 2015; Smith-Vikos et al., 2016; Kinser and Pincus, 2020; Zia et al., 2022). These studies have low overlap, and the hunt for a clear miRNA biomarker of aging remains due to research design, profiling methodologies, and individual backgrounds. Although miRNA expression patterns are inconsistent, they offer valuable insight into aging and its progression (Hooten et al., 2013; Olivieri et al., 2014; Sawada et al., 2014; Ameling et al., 2015; Machida et al., 2015; Zhang et al., 2015).
6.1 Summary and future perspectives
MicroRNA dysregulation has been extensively studied in NDs. Several studies have investigated miRNA dysregulation within specific NDs (Brites, 2020; Proshkina et al., 2020), while others examined miRNAs across multiple NDs (Brites, 2020; Proshkina et al., 2020). A study found that miR-155-5p, miR-146a-5p, and miR-223-3p were upregulated in patient specimens and animal models of 12 neurodegenerative diseases, such as AD, PD, HD, ALS, and MS, using Reporting Items for Systematic Reviews and Meta-Analyses (PRISMA). However, miR-132-3p, miR-21-5p, miR-29, miR-9-5p, and miR-124-3p exhibit mixed regulation (Juźwik et al., 2019). MiRNA dysregulation has been demonstrated in NDs, but questions remain regarding their suitability as diagnostic markers. MiRNAs can pose challenges when used as specific diagnostic markers due to their complex pathological characteristics and overlapping expression patterns between different NDs.
Our review delved into the landscape of miRNA biomarkers across various NDs, encompassing AD, PD, ALS, HD, and MS. Through meticulous examination of miRNA profiles across diverse sample sources, including serum, plasma, CSF, blood, and brain tissue, we aimed to elucidate unique miRNA signatures indicative of each disease’s pathogenesis. Our analysis unveiled a diverse array of dysregulated miRNAs across different sample types. For instance, in AD, miR-135a and miR-384 consistently exhibited upregulation (Table 1), whereas PD showcased downregulation of miR-146a-5p and miR-132-3p (Table 2). Additionally, ALS demonstrated consistent upregulation of miR-338-3p (Table 3), while HD exhibited upregulation of miR-34b (Table 4). Many miRNAs were upregulated in MS, including miR-223, miR-148b, miR-24, miR-34b, and miR-324-3p (Table 5). Moreover, several miRNAs displayed disease-specific dysregulation, potentially serving as diagnostic biomarkers or therapeutic targets.
Interestingly, miR-124 exhibited consistent downregulation across all diseases, while miR-146a and miR-223 had mixed regulation, suggesting that these genes play a crucial role in pathogenesis. An important point to emphasize is that a study by Mancuso et al. (2019) demonstrated that miRNAs can distinguish between several NDs, including AD and PD. According to them, miR-223 expression levels can be a discriminatory factor between these groups. This study has shown that miRNA dysregulation is associated with various NDs. For effective treatment and management strategies, it is crucial to identify disease-specific and shared changes in miRNA expression (Mancuso et al., 2019). However, despite promising results, it is necessary to conduct additional research to determine the accuracy and reliability of miRNAs for identifying various neurodegenerative diseases (Viswambharan et al., 2017; Wang and Zhang, 2020; Gentile et al., 2022; Nguyen et al., 2022; Li et al., 2023).
Overall, miRNA expression in NDs is influenced by many factors, including disease type and sample origin, producing a complex picture. The detailed analysis of our data reveals a diverse landscape of dysregulated miRNAs across different NDs. In our study, miRNA-9, miR-146a, and miR-181c emerged as prominent candidates with recurrent expression level changes across multiple diseases. In addition, miR-29a, miR-132, and miR-133b are consistently dysregulated, indicating ND pathogenesis. Our findings provide valuable insights into the complex mechanisms underlying NDs, despite the need for further investigation to clarify their precise roles in disease pathogenesis. The findings pave the way for future research projects examining miRNA-mediated molecular pathways in neurodegeneration.
It is challenging to accurately evaluate miRNA data due to the technical limitations of methods such as RT-qPCR and NGS (Huggett et al., 2013). These challenges can lead to inconsistent results, hindering miRNA expression assessment in NDs. Further complicating miRNA data interpretation in NDs is the lack of reliability and consistency in bioinformatics and biostatistical methods used to analyze miRNA expression data (Tellinghuisen and Spiess, 2019). Therefore, miRNA expression in NDs may not be consistent across studies.
MicroRNAs and PTMs in NDs are an emerging area of research. MicroRNAs influence PTMs indirectly by regulating the expression of enzymes that perform these modifications or proteins that target these modifications. MicroRNAs and PTMs enhance the regulatory mechanism for NDs. However, the specific impact of miRNAs on PTMs remains to be determined, and further research is needed. The technical challenge of measuring miRNA expression levels accurately in NDs is the need for specific and susceptible technologies, such as RT-qPCR and NGS. A further challenge in miRNA expression bioinformatics is identifying relevant miRNA targets, processing data, and normalizing it. Measurement and interpretation of miRNA expression data in the context of NDs require attention to these technical challenges.
In summary, miRNAs have shown great promise as diagnostic biomarkers for NDs. To advance this emerging field of research, it is critical to identify and characterize new miRNAs implicated in NDs. A critical need still exists for further research into how miRNAs influence various NDs, even though advancements in this field have been significant. However, further research is necessary to comprehend the complex regulatory roles of miRNAs fully. This is to overcome technical difficulties in measuring and analyzing them accurately. It is also to optimize their utility as diagnostic tools and treatment options within clinical contexts.
Author contributions
HA: Writing – original draft, Writing – review & editing, Conceptualization, Data curation, Formal analysis, Investigation, Methodology, Visualization. RR: Writing – review & editing. CG: Writing – review & editing. MK: Writing – review & editing. FD: Writing – review & editing. KH: Writing – review & editing. HP: Writing – review & editing. BH: Writing – review & editing. DR: Writing – review & editing. PS: Writing – review & editing, Conceptualization, Data curation, Formal analysis, Funding acquisition, Investigation, Methodology, Project administration, Resources, Software, Supervision, Validation, Visualization. SR: Conceptualization, Data curation, Formal analysis, Funding acquisition, Investigation, Methodology, Project administration, Resources, Software, Supervision, Validation, Visualization, Writing – review & editing.
Funding
The author(s) declare financial support was received for the research, authorship, and/or publication of this article. The work was funded by the Federal Ministry of Education and Research (Germany) in the framework of the RUBIN-NeuroMiR project (03RU1U051A) within the program “Innovation & Structural Change”.
Conflict of interest
FD is employed by PolyAn GmbH. SR is an advisor at GA Generic Assays GmbH.
The remaining authors declare that the research was conducted in the absence of any commercial or financial relationships that could be construed as a potential conflict of interest.
Publisher’s note
All claims expressed in this article are solely those of the authors and do not necessarily represent those of their affiliated organizations, or those of the publisher, the editors and the reviewers. Any product that may be evaluated in this article, or claim that may be made by its manufacturer, is not guaranteed or endorsed by the publisher.
References
Abolghasemi, M., Ashrafi, S. A., Asadi, M., Shanehbandi, D., Etehad, S. S., Poursaei, E., et al. (2022). Altered miR-10, miR-24a, miR-124, and miR-21 expression in peripheral blood mononuclear cells of patients with multiple Sclerosi. Res. Square. doi: 10.21203/rs.3.rs-1490169/v1
Absalon, S., Kochanek, D. M., Raghavan, V., and Krichevsky, A. M. (2013). MiR-26b, upregulated in Alzheimer’s disease, activates cell cycle entry, tau-phosphorylation, and apoptosis in Postmitotic neurons. J. Neurosci. 33, 14645–14659. doi: 10.1523/JNEUROSCI.1327-13.2013
Acuña, M. L., García-Morin, A., Orozco-Sepúlveda, R., Ontiveros, C., Flores, A., Diaz, A. V., et al. (2023). Alternative splicing of the SUMO1/2/3 transcripts affects cellular SUMOylation and produces functionally distinct SUMO protein isoforms. Sci. Rep. 13:2309. doi: 10.1038/s41598-023-29357-7
Agrawal, M. (2020). “Molecular basis of chronic neurodegeneration” in Clinical molecular medicine. ed. D. Kumar (Academic Press), 447–460.
Agrawal, M., and Biswas, A. (2015). Molecular diagnostics of neurodegenerative disorders. Front. Mol. Biosci. 2:54. doi: 10.3389/fmolb.2015.00054
Akhter, R., Shao, Y., Shaw, M., Formica, S., Khrestian, M., Leverenz, J. B., et al. (2018). Regulation of ADAM10 by miR-140-5p and potential relevance for Alzheimer’s disease. Neurobiol. Aging 63, 110–119. doi: 10.1016/j.neurobiolaging.2017.11.007
Alexandrov, P. N., Dua, P., Hill, J. M., Bhattacharjee, S., Zhao, Y., and Lukiw, W. J. (2012). micro RNA (miRNA) speciation in Alzheimer’s disease (AD) cerebrospinal fluid (CSF) and extracellular fluid (ECF). Int J Biochem Mol Biol 3, 365–373.
Alivernini, S., Gremese, E., McSharry, C., Tolusso, B., Ferraccioli, G., McInnes, I. B., et al. (2018). Micro RNA-155—at the critical Interface of innate and adaptive immunity in arthritis. Front. Immunol. 8:1932. doi: 10.3389/fimmu.2017.01932
Alvarez-Erviti, L., Seow, Y., Schapira, A. H., Rodriguez-Oroz, M. C., Obeso, J. A., and Cooper, J. M. (2013). Influence of microRNA deregulation on chaperone-mediated autophagy and α-synuclein pathology in Parkinson’s disease. Cell Death Dis. 4:e545. doi: 10.1038/cddis.2013.73
Ameling, S., Kacprowski, T., Chilukoti, R. K., Malsch, C., Liebscher, V., Suhre, K., et al. (2015). Associations of circulating plasma microRNAs with age, body mass index and sex in a population-based study. BMC Med. Genet. 8:61. doi: 10.1186/s12920-015-0136-7
Amoruso, A., Blonda, M., and Gironi, M. (2020). Immune and central nervous system-related miRNAs expression profiling in monocytes of multiple sclerosis patients. Sci. Rep. 10:6125. doi: 10.1038/s41598-020-63282-3
Angelova, P. R. (2021). Sources and triggers of oxidative damage in neurodegeneration. Free Radic. Biol. Med. 173, 52–63. doi: 10.1016/j.freeradbiomed.2021.07.003
Apostolova, L. G., Hwang, K. S., Andrawis, J. P., Green, A. E., Babakchanian, S., Morra, J. H., et al. (2010). 3D PIB and CSF biomarker associations with hippocampal atrophy in ADNI subjects. Neurobiol. Aging 31, 1284–1303. doi: 10.1016/j.neurobiolaging.2010.05.003
Ardekani, A. M., and Naeini, M. M. (2010). The role of MicroRNAs in human diseases. Avicenna J. Med. Biotechnol. 2, 161–179.
Arneth, B., and Kraus, J. (2022). Laboratory biomarkers of multiple sclerosis (MS). Clin. Biochem. 99, 1–8. doi: 10.1016/j.clinbiochem.2021.10.004
Augustin, R., Endres, K., Reinhardt, S., Kuhn, P.-H., Lichtenthaler, S. F., Hansen, J., et al. (2012). Computational identification and experimental validation of microRNAs binding to the Alzheimer-related gene ADAM10. BMC Med. Genet. 13:35. doi: 10.1186/1471-2350-13-35
Aung, L. L., Mouradian, M. M., Dhib-Jalbut, S., and Balashov, K. E. (2015). MMP-9 expression is increased in B lymphocytes during multiple sclerosis exacerbation and is regulated by microRNA-320a. J. Neuroimmunol. 278, 185–189. doi: 10.1016/j.jneuroim.2014.11.004
Baek, D., Villén, J., Shin, C., Camargo, F. D., Gygi, S. P., and Bartel, D. P. (2008). The impact of microRNAs on protein output. Nature 455, 64–71. doi: 10.1038/nature07242
Bai, X., and Bian, Z. (2022). MicroRNA-21 is a versatile regulator and potential treatment target in central nervous system disorders. Front. Mol. Neurosci. 15:842288. doi: 10.3389/fnmol.2022.842288
Bai, X., Tang, Y., Yu, M., Wu, L., Liu, F., Ni, J., et al. (2017). Downregulation of blood serum microRNA 29 family in patients with Parkinson’s disease. Sci. Rep. 7:5411. doi: 10.1038/s41598-017-03887-3
Bandiera, S., Cartault, F., Jannot, A.-S., Hatem, E., Girard, M., Rifai, L., et al. (2013). Genetic variations creating MicroRNA target sites in the FXN 3′-UTR affect Frataxin expression in Friedreich Ataxia. PLoS One 8:e54791. doi: 10.1371/journal.pone.0054791
Banzhaf-Strathmann, J., Benito, E., May, S., Arzberger, T., Tahirovic, S., Kretzschmar, H., et al. (2014). MicroRNA-125b induces tau hyperphosphorylation and cognitive deficits in Alzheimer’s disease. EMBO J. 33, 1667–1680. doi: 10.15252/embj.201387576
Barbosa, M., Gomes, C., Sequeira, C., Gonçalves-Ribeiro, J., Pina, C. C., Carvalho, L. A., et al. (2021). Recovery of depleted miR-146a in ALS cortical astrocytes reverts cell aberrancies and prevents paracrine pathogenicity on microglia and motor neurons. Front. Cell Dev. Biol. 9:634355. doi: 10.3389/fcell.2021.634355
Bartel, D. P. (2004). MicroRNAs: genomics, biogenesis, mechanism, and function. Cell 116, 281–297. doi: 10.1016/S0092-8674(04)00045-5
Basak, I., Patil, K. S., Alves, G., Larsen, J. P., and Møller, S. G. (2016). microRNAs as neuroregulators, biomarkers and therapeutic agents in neurodegenerative diseases. Cell. Mol. Life Sci. 73, 811–827. doi: 10.1007/s00018-015-2093-x
Bayliss, L., Galvez, V., Ochoa-Morales, A., Chávez-Oliveros, M., Rodríguez-Agudelo, Y., Delgado-García, G., et al. (2019). Theory of mind impairment in Huntington’s disease patients and their relatives. Arq. Neuropsiquiatr. 77, 574–578. doi: 10.1590/0004-282X20190092
Beezhold, K. J., Castranova, V., and Chen, F. (2010). Microprocessor of microRNAs: regulation and potential for therapeutic intervention. Mol. Cancer 9:134. doi: 10.1186/1476-4598-9-134
Behl, T., Kumar, S., Althafar, Z. M., Sehgal, A., Singh, S., Sharma, N., et al. (2022). Exploring the role of ubiquitin–proteasome system in Parkinson's disease. Mol. Neurobiol. 59, 4257–4273. doi: 10.1007/s12035-022-02851-1
Bekris, L. M., Lutz, F., Montine, T. J., Yu, C. E., Tsuang, D., Peskind, E. R., et al. (2013). MicroRNA in Alzheimer’s disease: An exploratory study in brain, cerebrospinal fluid and plasma. Biomarkers 18, 455–466. doi: 10.3109/1354750X.2013.814073
Benigni, M., Ricci, C., Jones, A. R., Giannini, F., Al-Chalabi, A., and Battistini, S. (2016). Identification of miRNAs as potential biomarkers in cerebrospinal fluid from amyotrophic lateral sclerosis patients. Neuro Mol. Med. 18, 551–560. doi: 10.1007/s12017-016-8396-8
Bohnsack, M. T., Czaplinski, K., and Gorlich, D. (2004). Exportin 5 is a RanGTP-dependent dsRNA-binding protein that mediates nuclear export of pre-miRNAs. RNA 10, 185–191. doi: 10.1261/rna.5167604
Boissonneault, V., Plante, I., Rivest, S., and Provost, P. (2009). MicroRNA-298 and MicroRNA-328 regulate expression of mouse β-amyloid precursor protein-converting enzyme 1 *. J. Biol. Chem. 284, 1971–1981. doi: 10.1074/jbc.M807530200
Botta-Orfila, T., Morató, X., Compta, Y., Lozano, J. J., Falgàs, N., Valldeoriola, F., et al. (2014). Identification of blood serum micro-RNAs associated with idiopathic and LRRK2 Parkinson’s disease. J. Neurosci. Res. 92, 1071–1077. doi: 10.1002/jnr.23377
Braak, H., and Del Tredici, K. (2010). “Neurofibrillary tangles” in Encyclopedia of movement disorders. eds. K. Kompoliti and L. V. Metman (Academic Press), 265–269.
Brennan, G. P., and Henshall, D. C. (2020). MicroRNAs as regulators of brain function and targets for treatment of epilepsy. Nat. Rev. Neurol. 16, 506–519. doi: 10.1038/s41582-020-0369-8
Brites, D. (2020). Regulatory function of microRNAs in microglia. Glia 68, 1631–1642. doi: 10.1002/glia.23846
Brown, R. H., and Al-Chalabi, A. (2017). Amyotrophic lateral sclerosis. N. Engl. J. Med. 377, 162–172. doi: 10.1056/NEJMra1603471
Brueckner, B., Stresemann, C., Kuner, R., Mund, C., Musch, T., Meister, M., et al. (2007). The human let-7a-3 locus contains an epigenetically regulated MicroRNA gene with oncogenic function. Cancer Res. 67, 1419–1423. doi: 10.1158/0008-5472.CAN-06-4074
Bucha, S., Mukhopadhyay, D., and Bhattacharyya, N. P. (2015). Regulation of mitochondrial morphology and cell cycle by microRNA-214 targeting Mitofusin2. Biochem. Biophys. Res. Commun. 465, 797–802. doi: 10.1016/j.bbrc.2015.08.090
Budi, H. S., Younus, L. A., Lafta, M. H., Parveen, S., Mohammad, H. J., Al-qaim, Z. H., et al. (2023). The role of miR-128 in cancer development, prevention, drug resistance, and immunotherapy. Front. Oncol. 12:1067974. doi: 10.3389/fonc.2022.1067974
Buneeva, O., and Medvedev, A. (2022). Atypical ubiquitination and Parkinson’s disease. Int. J. Mol. Sci. 23:3705. doi: 10.3390/ijms23073705
Burdukiewicz, M., Sobczyk, P., Rödiger, S., Duda-Madej, A., Mackiewicz, P., and Kotulska, M. (2017). Amyloidogenic motifs revealed by n-gram analysis. Sci. Rep. 7:12961. doi: 10.1038/s41598-017-13210-9
Burgos, K., Malenica, I., Metpally, R., Courtright, A., Rakela, B., Beach, T., et al. (2014). Profiles of extracellular miRNA in cerebrospinal fluid and serum from patients with Alzheimer’s and Parkinson’s diseases correlate with disease status and features of pathology. PLoS One 9:e94839. doi: 10.1371/journal.pone.0094839
Butovsky, O., Siddiqui, S., Gabriely, G., Lanser, A. J., Dake, B., Murugaiyan, G., et al. (2012). Modulating inflammatory monocytes with a unique microRNA gene signature ameliorates murine ALS. J. Clin. Invest. 122, 3063–3087. doi: 10.1172/JCI62636
Cao, D.-D., Li, L., and Chan, W.-Y. (2016). MicroRNAs: key regulators in the central nervous system and their implication in neurological diseases. Int. J. Mol. Sci. 17:842. doi: 10.3390/ijms17060842
Cao, X.-Y., Lu, J.-M., Zhao, Z.-Q., Li, M.-C., Lu, T., An, X.-S., et al. (2017). MicroRNA biomarkers of Parkinson’s disease in serum exosome-like microvesicles. Neurosci. Lett. 644, 94–99. doi: 10.1016/j.neulet.2017.02.045
Cao, L., Zhang, Y., Zhang, S., Jiang, T.-P., Chen, L., Liu, J., et al. (2018). MicroRNA-29b alleviates oxygen and glucose deprivation/reperfusion-induced injury via inhibition of the p53-dependent apoptosis pathway in N2a neuroblastoma cells. Exp. Ther. Med. 15, 67–74. doi: 10.3892/etm.2017.5410
Caravia, X. M., and López-Otín, C. (2015). “Regulatory roles of miRNAs in aging” in microRNA: Basic science: from molecular biology to clinical practice. ed. G. Santulli (Springer, Cham), 887, 213–230.
Cardo, L. F., Coto, E., de Mena, L., Ribacoba, R., Moris, G., Menéndez, M., et al. (2013). Profile of microRNAs in the plasma of Parkinson’s disease patients and healthy controls. J. Neurol. 260, 1420–1422. doi: 10.1007/s00415-013-6900-8
Cardo, L. F., Coto, E., Ribacoba, R., Menéndez, M., Moris, G., Suárez, E., et al. (2014). MiRNA profile in the substantia Nigra of Parkinson’s disease and healthy subjects. J. Mol. Neurosci. 54, 830–836. doi: 10.1007/s12031-014-0428-y
Catanesi, M., d’Angelo, M., Tupone, M. G., Benedetti, E., Giordano, A., Castelli, V., et al. (2020). MicroRNAs dysregulation and mitochondrial dysfunction in neurodegenerative diseases. Int. J. Mol. Sci. 21:5986. doi: 10.3390/ijms21175986
Ceppi, M., Pereira, P. M., Dunand-Sauthier, I., Barras, E., Reith, W., Santos, M. A., et al. (2009). MicroRNA-155 modulates the interleukin-1 signaling pathway in activated human monocyte-derived dendritic cells. Proc. Natl. Acad. Sci. USA 106, 2735–2740. doi: 10.1073/pnas.0811073106
Chacko, G., Patel, S., Galor, A., and Kumar, N. (2021). Heat exposure and multiple sclerosis—a regional and temporal analysis. Int. J. Environ. Res. Public Health 18:5962. doi: 10.3390/ijerph18115962
Chandiran, K., Lawlor, R., Pannuti, A., Perez, G. G., Srinivasan, J., Golde, T. E., et al. (2018). Notch1 primes CD4 T cells for T helper type I differentiation through its early effects on miR-29. Mol. Immunol. 99, 191–198. doi: 10.1016/j.molimm.2018.05.002
Chang, K.-H., Wu, Y.-R., and Chen, C.-M. (2017). Down-regulation of miR-9* in the peripheral leukocytes of Huntington’s disease patients. Orphanet J. Rare Dis. 12:185. doi: 10.1186/s13023-017-0742-x
Che, H., Sun, L.-H., Guo, F., Niu, H.-F., Su, X.-L., Bao, Y.-N., et al. (2014). Expression of amyloid-associated miRNAs in both the forebrain cortex and Hippocampus of middle-aged rat. Cell. Physiol. Biochem. 33, 11–22. doi: 10.1159/000356646
Chen, X. (2005). microRNA biogenesis and function in plants. FEBS Lett. 579, 5923–5931. doi: 10.1016/j.febslet.2005.07.071
Chen, C.-Y. A., Chang, J. T., Ho, Y.-F., and Shyu, A.-B. (2016). MiR-26 down-regulates TNF-α/NF-κB signalling and IL-6 expression by silencing HMGA1 and MALT1. Nucleic Acids Res. 44, 3772–3787. doi: 10.1093/nar/gkw205
Chen, Q., Deng, N., Lu, K., Liao, Q., Long, X., Gou, D., et al. (2021). Elevated plasma miR-133b and miR-221-3p as biomarkers for early Parkinson’s disease. Sci. Rep. 11:15268. doi: 10.1038/s41598-021-94734-z
Chen, Y., Gao, C., Sun, Q., Pan, H., Huang, P., Ding, J., et al. (2017). MicroRNA-4639 is a regulator of DJ-1 expression and a potential early diagnostic marker for Parkinson’s disease. Front. Aging Neurosci. 9:232. doi: 10.3389/fnagi.2017.00232
Chen, M., Li, L., Liu, C., and Song, L. (2020). Berberine attenuates Aβ-induced neuronal damage through regulating miR-188/NOS1 in Alzheimer’s disease. Mol. Cell. Biochem. 474, 285–294. doi: 10.1007/s11010-020-03852-1
Chen, W., and Qin, C. (2015). General hallmarks of microRNAs in brain evolution and development. RNA Biol. 12, 701–708. doi: 10.1080/15476286.2015.1048954
Chen, Y., Wei, Q., Chen, X., Li, C., Cao, B., Ou, R., et al. (2016). Aberration of miRNAs expression in leukocytes from sporadic amyotrophic lateral sclerosis. Front. Mol. Neurosci. 9:69. doi: 10.3389/fnmol.2016.00069
Chen, M., and Wolynes, P. G. (2017). Aggregation landscapes of huntingtin exon 1 protein fragments and the critical repeat length for the onset of Huntington’s disease. Proc. Natl. Acad. Sci. 114, 4406–4411. doi: 10.1073/pnas.1702237114
Chen, L., Yang, J., Lü, J., Cao, S., Zhao, Q., and Yu, Z. (2018). Identification of aberrant circulating miRNAs in Parkinson’s disease plasma samples. Brain Behav. 8:e00941. doi: 10.1002/brb3.941
Chen, Y., Zheng, J., Su, L., Chen, F., Zhu, R., Chen, X., et al. (2020). Increased salivary microRNAs that regulate DJ-1 gene expression as potential markers for Parkinson’s disease. Front. Aging Neurosci. 12:210. doi: 10.3389/fnagi.2020.00210
Chen, J., Zou, Q., Lv, D., Raza, M. A., Wang, X., Li, P., et al. (2019). Comprehensive transcriptional profiling of porcine brain aging. Gene 693, 1–9. doi: 10.1016/j.gene.2019.01.019
Cheng, P.-H., Li, C.-L., Chang, Y.-F., Tsai, S.-J., Lai, Y.-Y., Chan, A. W. S., et al. (2013). miR-196a ameliorates phenotypes of Huntington disease in cell, transgenic mouse, and induced pluripotent stem cell models. Am. J. Hum. Genet. 93, 306–312. doi: 10.1016/j.ajhg.2013.05.025
Cho, K. H. T., Xu, B., Blenkiron, C., and Fraser, M. (2019). Emerging roles of miRNAs in brain development and perinatal brain injury. Front. Physiol. 10:227. doi: 10.3389/fphys.2019.00227
Choi, S.-E., and Kemper, J. K. (2013). Regulation of SIRT1 by MicroRNAs. Mol. Cells 36, 385–392. doi: 10.1007/s10059-013-0297-1
Chopra, N., Wang, R., Maloney, B., Nho, K., Beck, J. S., Pourshafie, N., et al. (2021). MicroRNA-298 reduces levels of human amyloid-β precursor protein (APP), β-site APP-converting enzyme 1 (BACE1) and specific tau protein moieties. Mol. Psychiatry 26, 5636–5657. doi: 10.1038/s41380-019-0610-2
Chu, T., Shu, Y., Qu, Y., Gao, S., and Zhang, L. (2018). miR-26b inhibits total neurite outgrowth, promotes cells apoptosis and downregulates neprilysin in Alzheimer’s disease. Int. J. Clin. Exp. Pathol. 11, 3383–3390.
Ciurleo, R., Di Lorenzo, G., Bramanti, P., and Marino, S. (2014). Magnetic resonance spectroscopy: An in vivo molecular imaging biomarker for Parkinson’s disease? Biomed. Res. Int. 2014:e519816, 1–10. doi: 10.1155/2014/519816
Cochran, K. R., Gorospe, M., and De, S. (2020). Systematic identification of circularRNA in Alzheimer’s disease. FASEB J. 34:1. doi: 10.1096/fasebj.2020.34.s1.09341
Cogswell, J. P., Ward, J., Taylor, I. A., Waters, M., Shi, Y., Cannon, B., et al. (2008). Identification of miRNA changes in Alzheimer’s disease brain and CSF yields putative biomarkers and insights into disease pathways. J. Alzheimers Dis. 14, 27–41. doi: 10.3233/JAD-2008-14103
Cohen, T. J., Guo, J. L., Hurtado, D. E., Kwong, L. K., Mills, I. P., Trojanowski, J. Q., et al. (2011). The acetylation of tau inhibits its function and promotes pathological tau aggregation. Nat. Commun. 2:252. doi: 10.1038/ncomms1255
Cohen, J. E., Lee, P. R., Chen, S., Li, W., and Fields, R. D. (2011). MicroRNA regulation of homeostatic synaptic plasticity. Proc. Natl. Acad. Sci. USA 108, 11650–11655. doi: 10.1073/pnas.1017576108
Condrat, C. E., Thompson, D. C., Barbu, M. G., Bugnar, O. L., Boboc, A., Cretoiu, D., et al. (2020). miRNAs as biomarkers in disease: latest findings regarding their role in diagnosis and prognosis. Cells 9:276. doi: 10.3390/cells9020276
Coolen, M., Katz, S., and Bally-Cuif, L. (2013). miR-9: a versatile regulator of neurogenesis. Front. Cell. Neurosci. 7:220. doi: 10.3389/fncel.2013.00220
Coppedè, F. (2012). Genetics and epigenetics of Parkinson’s disease. Sci. World J. 2012:e489830. doi: 10.1100/2012/489830
Coppen, E. M., and Roos, R. A. C. (2017). Current pharmacological approaches to reduce chorea in Huntington’s disease. Drugs 77, 29–46. doi: 10.1007/s40265-016-0670-4
Cortez, M. A., Bueso-Ramos, C., Ferdin, J., Lopez-Berestein, G., Sood, A. K., and Calin, G. A. (2011). MicroRNAs in body fluids—the mix of hormones and biomarkers. Nature reviews. Clin. Oncol. 8, 467–477. doi: 10.1038/nrclinonc.2011.76
Cox, M. B., Cairns, M. J., Gandhi, K. S., Carroll, A. P., Moscovis, S., Stewart, G. J., et al. (2010). MicroRNAs miR-17 and miR-20a inhibit T cell activation genes and are under-expressed in MS whole blood. PLoS One 5:e12132. doi: 10.1371/journal.pone.0012132
Cressatti, M., Juwara, L., Galindez, J. M., Velly, A. M., Nkurunziza, E. S., Marier, S., et al. (2020). Salivary microR-153 and microR-223 levels as potential diagnostic biomarkers of idiopathic Parkinson’s disease. Mov. Disord. 35, 468–477. doi: 10.1002/mds.27935
Cui, J. G., Li, Y. Y., Zhao, Y., Bhattacharjee, S., and Lukiw, W. J. (2010). Differential regulation of Interleukin-1 receptor-associated Kinase-1 (IRAK-1) and IRAK-2 by MicroRNA-146a and NF-κB in stressed human Astroglial cells and in Alzheimer disease *. J. Biol. Chem. 285, 38951–38960. doi: 10.1074/jbc.M110.178848
Dansokho, C., and Heneka, M. T. (2018). Neuroinflammatory responses in Alzheimer’s disease. J. Neural Transm. 125, 771–779. doi: 10.1007/s00702-017-1831-7
Das, E., Jana, N. R., and Bhattacharyya, N. P. (2015). Delayed cell cycle progression in STHdhQ111/HdhQ111 cells, a cell model for Huntington’s disease mediated by microRNA-19a, microRNA-146a and microRNA-432. MicroRNA 4, 86–100. doi: 10.2174/2211536604666150713105606
de Andrade, H. M. T., de Albuquerque, M., Avansini, S. H., de Rocha, C., Dogini, D. B., Nucci, A., et al. (2016). MicroRNAs-424 and 206 are potential prognostic markers in spinal onset amyotrophic lateral sclerosis. J. Neurol. Sci. 368, 19–24. doi: 10.1016/j.jns.2016.06.046
de Faria, O., Moore, C., Kennedy, T., Antel, J., Bar-Or, A., and Dhaunchak, A. (2013). MicroRNA dysregulation in multiple sclerosis. Front. Genet. 3:311. doi: 10.3389/fgene.2012.00311
De Felice, B., Annunziata, A., Fiorentino, G., Borra, M., Biffali, E., Coppola, C., et al. (2014). miR-338-3p is over-expressed in blood, CFS, serum and spinal cord from sporadic amyotrophic lateral sclerosis patients. Neurogenetics 15, 243–253. doi: 10.1007/s10048-014-0420-2
De Felice, B., Guida, M., Guida, M., Coppola, C., De Mieri, G., and Cotrufo, R. (2012). A miRNA signature in leukocytes from sporadic amyotrophic lateral sclerosis. Gene 508, 35–40. doi: 10.1016/j.gene.2012.07.058
De Strooper, B., and Karran, E. (2016). The cellular phase of Alzheimer’s disease. Cell 164, 603–615. doi: 10.1016/j.cell.2015.12.056
Denis, H. L., Alpaugh, M., Alvarez, C. P., Fenyi, A., Barker, R. A., Chouinard, S., et al. (2023). Detection of antibodies against the huntingtin protein in human plasma. Cell. Mol. Life Sci. 80:45. doi: 10.1007/s00018-023-04687-x
Díez-Planelles, C., Sánchez-Lozano, P., Crespo, M. C., Gil-Zamorano, J., Ribacoba, R., González, N., et al. (2016). Circulating microRNAs in Huntington’s disease: emerging mediators in metabolic impairment. Pharmacol. Res. 108, 102–110. doi: 10.1016/j.phrs.2016.05.005
Ding, H., Huang, Z., Chen, M., Wang, C., Chen, X., Chen, J., et al. (2016). Identification of a panel of five serum miRNAs as a biomarker for Parkinson’s disease. Parkinsonism Relat. Disord. 22, 68–73. doi: 10.1016/j.parkreldis.2015.11.014
Djebbi, K., Bahri, M., Elaguech, M. A., Tian, R., Biao, S., Tlili, C., et al. (2021). “Recent advances in ultrasensitive miRNA biomarkers detection” in Advanced sensors for biomedical applications. Smart Sensors, Measurement and Instrumentation. eds. O. Kanoun and N. Derbel (Springer International Publishing), 137–164.
Dobrowolny, G., Martone, J., Lepore, E., Casola, I., Petrucci, A., Inghilleri, M., et al. (2021). A longitudinal study defined circulating microRNAs as reliable biomarkers for disease prognosis and progression in ALS human patients. Cell Death Discov. 7:4. doi: 10.1038/s41420-020-00397-6
Dobson, R., and Giovannoni, G. (2019). Multiple sclerosis – a review. Eur. J. Neurol. 26, 27–40. doi: 10.1111/ene.13819
Dodson, M. V., Jiang, Z., Du, M., and Hausman, G. J. (2013). Adipogenesis: it is not Just lipid that comprises adipose tissue. J. Genom. 1, 1–4. doi: 10.7150/jgen.3276
Dong, X., and Cong, S. (2021). MicroRNAs in Huntington’s disease: diagnostic biomarkers or therapeutic agents? Front. Cell. Neurosci. 15:705348. doi: 10.3389/fncel.2021.705348
Dong, H., Wang, C., Lu, S., Yu, C., Huang, L., Feng, W., et al. (2016). A panel of four decreased serum microRNAs as a novel biomarker for early Parkinson’s disease. Biomarkers 21, 129–137. doi: 10.3109/1354750X.2015.1118544
dos Santos, M. C. T., Barreto-Sanz, M. A., Correia, B. R. S., Bell, R., Widnall, C., Perez, L. T., et al. (2018). miRNA-based signatures in cerebrospinal fluid as potential diagnostic tools for early stage Parkinson’s disease. Oncotarget 9, 17455–17465. doi: 10.18632/oncotarget.24736
Doxakis, E. (2010). Post-transcriptional regulation of alpha-synuclein expression by mir-7 and mir-153. J. Biol. Chem. 285, 12726–12734. doi: 10.1074/jbc.M109.086827
Dukic-Stefanovic, S., Schinzel, R., Riederer, P., and Münch, G. (2001). AGES in brain ageing: AGE-inhibitors as neuroprotective and anti-dementia drugs? Biogerontology 2, 19–34. doi: 10.1023/A:1010052800347
El Fatimy, R., Li, S., Chen, Z., Mushannen, T., Gongala, S., Wei, Z., et al. (2018). MicroRNA-132 provides neuroprotection for tauopathies via multiple signaling pathways. Acta Neuropathol. 136, 537–555. doi: 10.1007/s00401-018-1880-5
Elobeid, A., Libard, S., Leino, M., Popova, S. N., and Alafuzoff, I. (2016). Altered proteins in the aging brain. J. Neuropathol. Exp. Neurol. 75, 316–325. doi: 10.1093/jnen/nlw002
Epis, R., Marcello, E., Gardoni, F., and Di Luca, M. (2012). Alpha, beta-and gamma-secretases in Alzheimer’s disease. Front. Biosci. S4, 1126–1150. doi: 10.2741/s322
Errico, P., and Meyer-Luehmann, M. (2020). Mechanisms of pathogenic tau and Aβ protein spreading in Alzheimer’s disease. Front. Aging Neurosci. 12:265. doi: 10.3389/fnagi.2020.00265
Eschenhagen, T., and Zimmermann, W. H. (2005). Engineering myocardial tissue. Circ. Res. 97, 1220–1231. doi: 10.1161/01.RES.0000196562.73231.7d
Eshkoor, S. A., Ghodsian, N., and Akhtari-Zavare, M. (2022). MicroRNAs influence and longevity. Egypt. J. Med. Hum. Genet. 23:105. doi: 10.1186/s43042-022-00316-7
Esteller, M. (2011). Non-coding RNAs in human disease. Nat. Rev. Genet. 12, 861–874. doi: 10.1038/nrg3074
Fagan, A. M., Shaw, L. M., Xiong, C., Vanderstichele, H., Mintun, M. A., Trojanowski, J. Q., et al. (2011). Comparison of analytical platforms for cerebrospinal fluid measures of β-amyloid 1-42, Total tau, and P-tau181 for identifying Alzheimer disease amyloid plaque pathology. Arch. Neurol. 68, 1137–1144. doi: 10.1001/archneurol.2011.105
Faghihi, M. A., Zhang, M., Huang, J., Modarresi, F., Van der Brug, M. P., Nalls, M. A., et al. (2010). Evidence for natural antisense transcript-mediated inhibition of microRNA function. Genome Biol. 11:R56. doi: 10.1186/gb-2010-11-5-r56
Fan, W., Liang, C., Ou, M., Zou, T., Sun, F., Zhou, H., et al. (2020). MicroRNA-146a is a wide-reaching neuroinflammatory regulator and potential treatment target in neurological diseases. Front. Mol. Neurosci. 13:90. doi: 10.3389/fnmol.2020.00090
Fang, M., Wang, J., Zhang, X., Geng, Y., Hu, Z., Rudd, J. A., et al. (2012). The miR-124 regulates the expression of BACE1/β-secretase correlated with cell death in Alzheimer’s disease. Toxicol. Lett. 209, 94–105. doi: 10.1016/j.toxlet.2011.11.032
Felekkis, K., and Papaneophytou, C. (2020). Challenges in using circulating Micro-RNAs as biomarkers for cardiovascular diseases. Int. J. Mol. Sci. 21:561. doi: 10.3390/ijms21020561
Feneberg, E., Steinacker, P., Lehnert, S., Schneider, A., Walther, P., Thal, D. R., et al. (2014). Limited role of free TDP-43 as a diagnostic tool in neurodegenerative diseases. Amyotr. Later. Scler. Frontot. Degen. 15, 351–356. doi: 10.3109/21678421.2014.905606
Fenn, A. M., Smith, K. M., Lovett-Racke, A. E., Guerau-de-Arellano, M., Whitacre, C. C., and Godbout, J. P. (2013). Increased miR-29b in the aged brain correlates with the reduction of IGF-1 and CX3CL1. Neurobiol. Aging 34, 2748–2758. doi: 10.1016/j.neurobiolaging.2013.06.007
Fenoglio, C., Ridolfi, E., Cantoni, C., De Riz, M., Bonsi, R., Serpente, M., et al. (2013). Decreased circulating miRNA levels in patients with primary progressive multiple sclerosis. Mult. Scler. J. 19, 1938–1942. doi: 10.1177/1352458513485654
Filipowicz, W. (2005). RNAi: the nuts and bolts of the RISC machine. Cell 122, 17–20. doi: 10.1016/j.cell.2005.06.023
Finkbeiner, S. (2011). Huntington’s disease. Cold Spring Harb. Perspect. Biol. 3:a007476. doi: 10.1101/cshperspect.a007476
Fodale, V., Pintauro, R., Daldin, M., Altobelli, R., Spiezia, M. C., Bisbocci, M., et al. (2020). Analysis of mutant and total huntingtin expression in Huntington’s disease murine models. Sci. Rep. 10:22137. doi: 10.1038/s41598-020-78790-5
Follert, P., Cremer, H., and Beclin, C. (2014). MicroRNAs in brain development and function: a matter of flexibility and stability. Front. Mol. Neurosci. 7:5. doi: 10.3389/fnmol.2014.00005
Fragkouli, A., and Doxakis, E. (2014). miR-7 and miR-153 protect neurons against MPP+-induced cell death via upregulation of mTOR pathway. Front. Cell. Neurosci. 8:182. doi: 10.3389/fncel.2014.00182
Freischmidt, A., Müller, K., Ludolph, A. C., and Weishaupt, J. H. (2013). Systemic dysregulation of TDP-43 binding microRNAs in amyotrophic lateral sclerosis. Acta Neuropathol. Commun. 1:42. doi: 10.1186/2051-5960-1-42
Freischmidt, A., Müller, K., Zondler, L., Weydt, P., Mayer, B., von Arnim, C. A. F., et al. (2015). Serum microRNAs in sporadic amyotrophic lateral sclerosis. Neurobiol. Aging 36, 2660.e15–2660.e20. doi: 10.1016/j.neurobiolaging.2015.06.003
Freischmidt, A., Müller, K., Zondler, L., Weydt, P., Volk, A. E., Božič, A. L., et al. (2014). Serum microRNAs in patients with genetic amyotrophic lateral sclerosis and pre-manifest mutation carriers. Brain 137, 2938–2950. doi: 10.1093/brain/awu249
Fu, M.-H., Li, C.-L., Lin, H.-L., Tsai, S.-J., Lai, Y.-Y., Chang, Y.-F., et al. (2015). The potential regulatory mechanisms of miR-196a in Huntington’s disease through Bioinformatic analyses. PLoS One 10:e0137637. doi: 10.1371/journal.pone.0137637
Gaetani, L., Paolini Paoletti, F., Bellomo, G., Mancini, A., Simoni, S., Di Filippo, M., et al. (2020). CSF and blood biomarkers in Neuroinflammatory and neurodegenerative diseases: implications for treatment. Trends Pharmacol. Sci. 41, 1023–1037. doi: 10.1016/j.tips.2020.09.011
Gaetani, L., Parnetti, L., Calabresi, P., and Di Filippo, M. (2021). Tracing neurological diseases in the Presymptomatic phase: insights from Neurofilament light chain. Front. Neurosci. 15:672954. doi: 10.3389/fnins.2021.672954
Galimberti, D., Villa, C., Fenoglio, C., Serpente, M., Ghezzi, L., Cioffi, S. M. G., et al. (2014). Circulating miRNAs as potential biomarkers in Alzheimer’s disease. J. Alzheimers Dis. 42, 1261–1267. doi: 10.3233/JAD-140756
Gandhi, R., Healy, B., Gholipour, T., Egorova, S., Musallam, A., Hussain, M. S., et al. (2013). Circulating MicroRNAs as biomarkers for disease staging in multiple sclerosis. Ann. Neurol. 73, 729–740. doi: 10.1002/ana.23880
Gandy, S. (2005). The role of cerebral amyloid beta accumulation in common forms of Alzheimer’s disease. Clin. Investig. 115, 1121–1129. doi: 10.1172/JCI25100
Gao, Y., Han, D., and Feng, J. (2021). MicroRNA in multiple sclerosis. Clin. Chim. Acta 516, 92–99. doi: 10.1016/j.cca.2021.01.020
Gao, H.-M., Jiang, J., Wilson, B., Zhang, W., Hong, J.-S., and Liu, B. (2002). Microglial activation-mediated delayed and progressive degeneration of rat nigral dopaminergic neurons: relevance to Parkinson’s disease. J. Neurochem. 81, 1285–1297. doi: 10.1046/j.1471-4159.2002.00928.x
Gao, J., Wang, W.-Y., Mao, Y.-W., Gräff, J., Guan, J.-S., Pan, L., et al. (2010). A novel pathway regulates memory and plasticity via SIRT1 and miR-134. Nature 466, 1105–1109. doi: 10.1038/nature09271
Garcia, G., Pinto, S., Cunha, M., Fernandes, A., Koistinaho, J., and Brites, D. (2021). Neuronal dynamics and miRNA signaling differ between SH-SY5Y APPSwe and PSEN1 mutant iPSC-derived AD models upon modulation with miR-124 mimic and inhibitor. Cells 10:2424. doi: 10.3390/cells10092424
Gascon, E., and Gao, F.-B. (2012). Cause or effect: misregulation of microRNA pathways in neurodegeneration. Front. Neurosci. 6:48. doi: 10.3389/fnins.2012.00048
Gaughwin, P. M., Ciesla, M., Lahiri, N., Tabrizi, S. J., Brundin, P., and Björkqvist, M. (2011). Hsa-miR-34b is a plasma-stable microRNA that is elevated in pre-manifest Huntington’s disease. Hum. Mol. Genet. 20, 2225–2237. doi: 10.1093/hmg/ddr111
Geekiyanage, H., and Chan, C. (2011). MicroRNA-137/181c regulates serine palmitoyltransferase and in turn amyloid β, novel targets in sporadic Alzheimer’s disease. J. Neurosci. 31, 14820–14830. doi: 10.1523/JNEUROSCI.3883-11.2011
Geekiyanage, H., Jicha, G. A., Nelson, P. T., and Chan, C. (2012). Blood serum miRNA: non-invasive biomarkers for Alzheimer’s disease. Exp. Neurol. 235, 491–496. doi: 10.1016/j.expneurol.2011.11.026
Gentile, G., Morello, G., La Cognata, V., Guarnaccia, M., Conforti, F. L., and Cavallaro, S. (2022). Dysregulated miRNAs as biomarkers and therapeutical targets in neurodegenerative diseases. J. Pers. Med. 12:770. doi: 10.3390/jpm12050770
Gerasymchuk, M., Cherkasova, V., Kovalchuk, O., and Kovalchuk, I. (2020). The role of microRNAs in organismal and skin aging. Int. J. Mol. Sci. 21:5281. doi: 10.3390/ijms21155281
Ghosh, R., and Tabrizi, S. J. (2018). “Clinical features of Huntington’s disease” in Polyglutamine Disorders. Advances in Experimental Medicine and Biology. eds. C. Nóbrega and L. Pereira de Almeida (Springer Cham), 1–28.
Gigase, F. A. J., Smith, E., Collins, B., Moore, K., Snijders, G. J. L. J., Katz, D., et al. (2023). The association between inflammatory markers in blood and cerebrospinal fluid: a systematic review and meta-analysis. Mol. Psychiatry 28, 1502–1515. doi: 10.1038/s41380-023-01976-6
Goh, S. Y., Chao, Y. X., Dheen, S. T., Tan, E.-K., and Tay, S. S.-W. (2019). Role of MicroRNAs in Parkinson’s disease. Int. J. Mol. Sci. 20:5649. doi: 10.3390/ijms20225649
Goh, A. M., Wibawa, P., Loi, S. M., Walterfang, M., Velakoulis, D., and Looi, J. C. (2018). Huntington’s disease: neuropsychiatric manifestations of Huntington’s disease. Australas. Psychiatry 26, 366–375. doi: 10.1177/1039856218791036
Gomes, C., Sequeira, C., Likhite, S., Dennys, C. N., Kolb, S. J., Shaw, P. J., et al. (2022). Neurotoxic astrocytes directly converted from sporadic and familial ALS patient fibroblasts reveal signature diversities and miR-146a Theragnostic potential in specific subtypes. Cells 11:1186. doi: 10.3390/cells11071186
Gong, G., An, F., Wang, Y., Bian, M., Yu, L.-J., and Wei, C. (2017). miR-15b represses BACE1 expression in sporadic Alzheimer’s disease. Oncotarget 8, 91551–91557. doi: 10.18632/oncotarget.21177
Gong, X., Huang, M., and Chen, L. (2022). Mechanism of miR-132-3p promoting Neuroinflammation and dopaminergic neurodegeneration in Parkinson’s disease. eNeuro 9:ENEURO.0393-21.2021. doi: 10.1523/ENEURO.0393-21.2021
Goodall, E. F., Heath, P. R., Bandmann, O., Kirby, J., and Shaw, P. J. (2013). Neuronal dark matter: the emerging role of microRNAs in neurodegeneration. Front. Cell. Neurosci. 7:178. doi: 10.3389/fncel.2013.00178
Grad, L. I., Rouleau, G. A., Ravits, J., and Cashman, N. R. (2017). Clinical Spectrum of amyotrophic lateral sclerosis (ALS). Cold Spring Harb. Perspect. Med. 7:a024117. doi: 10.1101/cshperspect.a024117
Grasso, M., Piscopo, P., Confaloni, A., and Denti, M. A. (2014). Circulating miRNAs as biomarkers for neurodegenerative disorders. Molecules 19, 6891–6910. doi: 10.3390/molecules19056891
Gregory, R. I., Chendrimada, T. P., and Shiekhattar, R. (2006). “MicroRNA biogenesis: isolation and characterization of the microprocessor complex” in MicroRNA protocols. ed. S.-Y. Ying (Humana Press), 33–47.
Gregory, R. I., Yan, K., Amuthan, G., Chendrimada, T., Doratotaj, B., Cooch, N., et al. (2004). The microprocessor complex mediates the genesis of microRNAs. Nature 432, 235–240. doi: 10.1038/nature03120
Gu, Q.-H., Yu, D., Hu, Z., Liu, X., Yang, Y., Luo, Y., et al. (2015). miR-26a and miR-384-5p are required for LTP maintenance and spine enlargement. Nat. Commun. 6:6789. doi: 10.1038/ncomms7789
Gui, Y., Liu, H., Zhang, L., Lv, W., and Hu, X. (2015). Altered microRNA profiles in cerebrospinal fluid exosome in Parkinson disease and Alzheimer disease. Oncotarget 6, 37043–37053. doi: 10.18632/oncotarget.6158
Guo, S., Yang, J., Jiang, B., Zhou, N., Ding, H., Zhou, G., et al. (2022). MicroRNA editing patterns in Huntington’s disease. Sci. Rep. 12:3173. doi: 10.1038/s41598-022-06970-6
Guo, T., Zhang, D., Zeng, Y., Huang, T. Y., Xu, H., and Zhao, Y. (2020). Molecular and cellular mechanisms underlying the pathogenesis of Alzheimer’s disease. Mol. Neurodegener. 15:40. doi: 10.1186/s13024-020-00391-7
Guru Krishna Kumar, V., Baweja, L., Ralhan, K., and Gupta, S. (2018). Carbamylation promotes amyloidogenesis and induces structural changes in tau-core hexapeptide fibrils. Biochim. Biophys. Acta Gen. Subj. 1862, 2590–2604. doi: 10.1016/j.bbagen.2018.07.030
Guzman, H., Sanders, K., Idica, A., Bochnakian, A., Jury, D., Daugaard, I., et al. (2018). miR-128 inhibits telomerase activity by targeting TERT mRNA. Oncotarget 9, 13244–13253. doi: 10.18632/oncotarget.24284
Ha, M., and Kim, V. N. (2014). Regulation of microRNA biogenesis. Nat. Rev. Mol. Cell Biol. 15, 509–524. doi: 10.1038/nrm3838
Haghikia, A., Haghikia, A., Hellwig, K., Baraniskin, A., Holzmann, A., Décard, B. F., et al. (2012). Regulated microRNAs in the CSF of patients with multiple sclerosis: a case-control study. Neurology 79, 2166–2170. doi: 10.1212/WNL.0b013e3182759621
Han, J., Lee, Y., Yeom, K.-H., Kim, Y.-K., Jin, H., and Kim, V. N. (2004). The Drosha-DGCR8 complex in primary microRNA processing. Genes Dev. 18, 3016–3027. doi: 10.1101/gad.1262504
Haramati, S., Chapnik, E., Sztainberg, Y., Eilam, R., Zwang, R., Gershoni, N., et al. (2010). miRNA malfunction causes spinal motor neuron disease. Proc. Natl. Acad. Sci. USA 107, 13111–13116. doi: 10.1073/pnas.1006151107
Hashimoto, Y., Akiyama, Y., and Yuasa, Y. (2013). Multiple-to-multiple relationships between MicroRNAs and target genes in gastric Cancer. PLoS One 8:e62589. doi: 10.1371/journal.pone.0062589
Hatfield, S. D., Shcherbata, H. R., Fischer, K. A., Nakahara, K., Carthew, R. W., and Ruohola-Baker, H. (2005). Stem cell division is regulated by the microRNA pathway. Nature 435, 974–978. doi: 10.1038/nature03816
Hawley, Z. C. E., Campos-Melo, D., and Strong, M. J. (2019). MiR-105 and miR-9 regulate the mRNA stability of neuronal intermediate filaments. Implications for the pathogenesis of amyotrophic lateral sclerosis (ALS). Brain Res. 1706, 93–100. doi: 10.1016/j.brainres.2018.10.032
He, Y., Liu, H., Jiang, L., Rui, B., Mei, J., and Xiao, H. (2019). miR-26 induces apoptosis and inhibits autophagy in non-small cell lung Cancer cells by suppressing TGF-β1-JNK signaling pathway. Front. Pharmacol. 9:1509. doi: 10.3389/fphar.2018.01509
Hébert, S. S., and De Strooper, B. (2007). Molecular biology. miRNAs in neurodegeneration. Science 317, 1179–1180. doi: 10.1126/science.1148530
Hébert, S. S., Horré, K., Nicolaï, L., Papadopoulou, A. S., Mandemakers, W., Silahtaroglu, A. N., et al. (2008). Loss of microRNA cluster miR-29a/b-1 in sporadic Alzheimer’s disease correlates with increased BACE1/β-secretase expression. Proc. Natl. Acad. Sci. USA 105, 6415–6420. doi: 10.1073/pnas.0710263105
Hébert, S. S., Papadopoulou, A. S., Smith, P., Galas, M.-C., Planel, E., Silahtaroglu, A. N., et al. (2010). Genetic ablation of dicer in adult forebrain neurons results in abnormal tau hyperphosphorylation and neurodegeneration. Hum. Mol. Genet. 19, 3959–3969. doi: 10.1093/hmg/ddq311
Hedden, T., and Gabrieli, J. D. E. (2004). Insights into the ageing mind: a view from cognitive neuroscience. Nat. Rev. Neurosci. 5, 87–96. doi: 10.1038/nrn1323
Helferich, A. M., Brockmann, S. J., Reinders, J., Deshpande, D., Holzmann, K., Brenner, D., et al. (2018). Dysregulation of a novel miR-1825/TBCB/TUBA4A pathway in sporadic and familial ALS. Cell. Mol. Life Sci. 75, 4301–4319. doi: 10.1007/s00018-018-2873-1
Hemond, C. C., Healy, B. C., Tauhid, S., Mazzola, M. A., Quintana, F. J., Gandhi, R., et al. (2019). MRI phenotypes in MS: longitudinal changes and miRNA signatures. Neurol. Neuroimmunol. Neuroinflam. 6:e530. doi: 10.1212/NXI.0000000000000530
Heo, Y.-A., and Scott, L. J. (2017). Deutetrabenazine: a review in chorea associated with Huntington’s disease. Drugs 77, 1857–1864. doi: 10.1007/s40265-017-0831-0
Ho, P. T. B., Clark, I. M., and Le, L. T. T. (2022). MicroRNA-based diagnosis and therapy. Int. J. Mol. Sci. 23:7167. doi: 10.3390/ijms23137167
Hombach, S., and Kretz, M. (2016). “Non-coding RNAs: classification, biology and functioning” in Non-coding RNAs in colorectal cancer. Advances in Experimental Medicine and Biology. eds. O. Slaby and G. A. Calin (Springer International Publishing), 937, 3–17.
Hooten, N. N., Fitzpatrick, M., Wood, W. H., De, S., Ejiogu, N., Zhang, Y., et al. (2013). Age-related changes in microRNA levels in serum. Aging 5, 725–740. doi: 10.18632/aging.100603
Hoss, A. G., Kartha, V. K., Dong, X., Latourelle, J. C., Dumitriu, A., Hadzi, T. C., et al. (2014). MicroRNAs located in the Hox gene clusters are implicated in Huntington’s disease pathogenesis. PLoS Genet. 10:e1004188. doi: 10.1371/journal.pgen.1004188
Hoss, A. G., Labadorf, A., Latourelle, J. C., Kartha, V. K., Hadzi, T. C., Gusella, J. F., et al. (2015a). miR-10b-5p expression in Huntington’s disease brain relates to age of onset and the extent of striatal involvement. BMC Med. Geno. 8:10. doi: 10.1186/s12920-015-0083-3
Hoss, A. G., Lagomarsino, V. N., Frank, S., Hadzi, T. C., Myers, R. H., and Latourelle, J. C. (2015b). Study of plasma-derived miRNAs mimic differences in Huntington’s disease brain. Mov. Disord. 30, 1961–1964. doi: 10.1002/mds.26457
Hou, Y., Dan, X., Babbar, M., Wei, Y., Hasselbalch, S. G., Croteau, D. L., et al. (2019). Ageing as a risk factor for neurodegenerative disease. Nature reviews. Neurology 15, 565–581. doi: 10.1038/s41582-019-0244-7
Huang, W., Feng, Y., Liang, J., Yu, H., Wang, C., Wang, B., et al. (2018). Loss of microRNA-128 promotes cardiomyocyte proliferation and heart regeneration. Nat. Commun. 9:700. doi: 10.1038/s41467-018-03019-z
Huang, E. (2022). miRNA processing mechanisms in the brain. Available at: https://app.biorender.com/biorender-templates/figures/all/t-62065c13d5d8d100a0125a1d-mirna-processing-mechanisms-in-the-brain
Huggett, J. F., Foy, C. A., Benes, V., Emslie, K., Garson, J. A., Haynes, R., et al. (2013). The digital MIQE guidelines: minimum information for publication of quantitative digital PCR experiments. Clin. Chem. 59, 892–902. doi: 10.1373/clinchem.2013.206375
Hussain, S., Mubeen, I., Ullah, N., Shah, S. S. U. D., Khan, B. A., Zahoor, M., et al. (2022). Modern diagnostic imaging technique applications and risk factors in the medical field: a review. Biomed. Res. Int. 2022:5164970. doi: 10.1155/2022/5164970
Inukai, S., de Lencastre, A., Turner, M., and Slack, F. (2012). Novel microRNAs differentially expressed during aging in the mouse brain. PLoS One 7:e40028. doi: 10.1371/journal.pone.0040028
Iqbal, K., and Grundke-Iqbal, I. (2002). Neurofibrillary pathology leads to synaptic loss and not the other way around in Alzheimer disease. J. Alzheimers Dis. 4, 235–238. doi: 10.3233/JAD-2002-4313
Iqbal, K., Liu, F., and Gong, C.-X. (2016). Tau and neurodegenerative disease: the story so far. Nat. Rev. Neurol. 12, 15–27. doi: 10.1038/nrneurol.2015.225
Ishikawa-Ankerhold, H. C., Ankerhold, R., and Drummen, G. P. C. (2012). Advanced fluorescence microscopy techniques—FRAP, FLIP, FLAP, FRET and FLIM. Molecules 17, 4047–4132. doi: 10.3390/molecules17044047
Itagaki, S., McGeer, P. L., Akiyama, H., Zhu, S., and Selkoe, D. (1989). Relationship of microglia and astrocytes to amyloid deposits of Alzheimer disease. J. Neuroimmunol. 24, 173–182. doi: 10.1016/0165-5728(89)90115-X
Jacobsen, J. C., Bawden, C. S., Rudiger, S. R., McLaughlan, C. J., Reid, S. J., Waldvogel, H. J., et al. (2010). An ovine transgenic Huntington’s disease model. Hum. Mol. Genet. 19, 1873–1882. doi: 10.1093/hmg/ddq063
Jahn, K., Buschmann, V., and Hille, C. (2015). Simultaneous fluorescence and phosphorescence lifetime imaging microscopy in living cells. Sci. Rep. 5:14334. doi: 10.1038/srep14334
Jarome, T. J., and Devulapalli, R. K. (2018). The ubiquitin-proteasome system and memory: moving beyond protein degradation. Neuroscientist 24, 639–651. doi: 10.1177/1073858418762317
Jarosińska, O. D., and Rüdiger, S. G. D. (2021). Molecular strategies to target protein aggregation in Huntington’s disease. Front. Mol. Biosci. 8:769184. doi: 10.3389/fmolb.2021.769184
Jegga, A. G., Schneider, L., Ouyang, X., and Zhang, J. (2011). Systems biology of the autophagy-lysosomal pathway. Autophagy 7, 477–489. doi: 10.4161/auto.7.5.14811
Jiang, Y., Xu, B., Chen, J., Sui, Y., Ren, L., Li, J., et al. (2018). Micro-RNA-137 inhibits tau hyperphosphorylation in Alzheimer’s disease and targets the CACNA1C gene in transgenic mice and human neuroblastoma SH-SY5Y cells. Med. Sci. Monit. 24, 5635–5644. doi: 10.12659/MSM.908765
Jiao, P., Wang, X.-P., Luoreng, Z.-M., Yang, J., Jia, L., Ma, Y., et al. (2021). miR-223: An effective regulator of immune cell differentiation and inflammation. Int. J. Biol. Sci. 17, 2308–2322. doi: 10.7150/ijbs.59876
Jin, F., Wang, Y., Li, M., Zhu, Y., Liang, H., Wang, C., et al. (2017). MiR-26 enhances chemosensitivity and promotes apoptosis of hepatocellular carcinoma cells through inhibiting autophagy. Cell Death Dis. 8:e2540. doi: 10.1038/cddis.2016.461
Johnson, R., and Buckley, N. J. (2009). Gene dysregulation in Huntington’s disease: REST, MicroRNAs and Beyond. Neuro Mol. Med. 11, 183–199. doi: 10.1007/s12017-009-8063-4
Johnson, R., Zuccato, C., Belyaev, N. D., Guest, D. J., Cattaneo, E., and Buckley, N. J. (2008). A microRNA-based gene dysregulation pathway in Huntington’s disease. Neurobiol. Dis. 29, 438–445. doi: 10.1016/j.nbd.2007.11.001
Johnston, R. J., and Hobert, O. (2003). A microRNA controlling left/right neuronal asymmetry in Caenorhabditis elegans. Nature 426, 845–849. doi: 10.1038/nature02255
Junn, E., and Mouradian, M. M. (2012). MicroRNAs in neurodegenerative diseases and their therapeutic potential. Pharmacol. Ther. 133, 142–150. doi: 10.1016/j.pharmthera.2011.10.002
Juźwik, C. A., Drake, S., Zhang, Y., Paradis-Isler, N., Sylvester, A., Amar-Zifkin, A., et al. (2019). microRNA dysregulation in neurodegenerative diseases: a systematic review. Prog. Neurobiol. 182:101664. doi: 10.1016/j.pneurobio.2019.101664
Kabaria, S., Choi, D. C., Chaudhuri, A. D., Mouradian, M. M., and Junn, E. (2015). Inhibition of miR-34b and miR-34c enhances α-synuclein expression in Parkinson’s disease. FEBS Lett. 589, 319–325. doi: 10.1016/j.febslet.2014.12.014
Kalia, M. (2003). Dysphagia and aspiration pneumonia in patients with Alzheimer’s disease. Metabolism 52, 36–38. doi: 10.1016/S0026-0495(03)00300-7
Kanagaraj, N., Beiping, H., Dheen, S. T., and Tay, S. S. W. (2014). Downregulation of miR-124 in MPTP-treated mouse model of Parkinson’s disease and MPP iodide-treated MN9D cells modulates the expression of the calpain/cdk5 pathway proteins. Neuroscience 272, 167–179. doi: 10.1016/j.neuroscience.2014.04.039
Kang, Q., Xiang, Y., Li, D., Liang, J., Zhang, X., Zhou, F., et al. (2017). MiR-124-3p attenuates hyperphosphorylation of tau protein-induced apoptosis via caveolin-1-PI3K/Akt/GSK3β pathway in N2a/APP695swe cells. Oncotarget 8, 24314–24326. doi: 10.18632/oncotarget.15149
Katsumoto, A., Takeuchi, H., Takahashi, K., and Tanaka, F. (2018). Microglia in Alzheimer’s disease: risk factors and inflammation. Front. Neurol. 9:978. doi: 10.3389/fneur.2018.00978
Keller, A., Leidinger, P., Lange, J., Borries, A., Schroers, H., Scheffler, M., et al. (2009). Multiple sclerosis: MicroRNA expression profiles accurately differentiate patients with relapsing-remitting disease from healthy controls. PLoS One 4:e7440. doi: 10.1371/journal.pone.0007440
Keller, A., Leidinger, P., Steinmeyer, F., Stähler, C., Franke, A., Hemmrich-Stanisak, G., et al. (2014). Comprehensive analysis of microRNA profiles in multiple sclerosis including next-generation sequencing. Mult. Scler. J. 20, 295–303. doi: 10.1177/1352458513496343
Khanna, A., Muthusamy, S., Liang, R., Sarojini, H., and Wang, E. (2011). Gain of survival signaling by down-regulation of three key miRNAs in brain of calorie-restricted mice. Aging 3, 223–236. doi: 10.18632/aging.100276
Khoo, S. K., Petillo, D., Kang, U. J., Resau, J. H., Berryhill, B., Linder, J., et al. (2012). Plasma-based circulating MicroRNA biomarkers for Parkinson’s disease. J. Parkinsons Dis. 2, 321–331. doi: 10.3233/JPD-012144
Kiko, T., Nakagawa, K., Tsuduki, T., Furukawa, K., Arai, H., and Miyazawa, T. (2014). MicroRNAs in plasma and cerebrospinal fluid as potential markers for Alzheimer’s disease. J. Alzheimers Dis. 39, 253–259. doi: 10.3233/JAD-130932
Kim, J., Inoue, K., Ishii, J., Vanti, W. B., Voronov, S. V., Murchison, E., et al. (2007). A MicroRNA feedback circuit in midbrain dopamine neurons. Science 317, 1220–1224. doi: 10.1126/science.1140481
Kim, K. Y., Kim, Y. R., Choi, K. W., Lee, M., Lee, S., Im, W., et al. (2020). Downregulated miR-18b-5p triggers apoptosis by inhibition of calcium signaling and neuronal cell differentiation in transgenic SOD1 (G93A) mice and SOD1 (G17S and G86S) ALS patients. Transl. Neurodegen. 9:23. doi: 10.1186/s40035-020-00203-4
Kim, J., Yoon, H., Chung, D., Brown, J. L., Belmonte, K. C., and Kim, J. (2016). miR-186 is decreased in aged brain and suppresses BACE1 expression. J. Neurochem. 137, 436–445. doi: 10.1111/jnc.13507
Kim, J., Yoon, H., Horie, T., Burchett, J. M., Restivo, J. L., Rotllan, N., et al. (2015). microRNA-33 regulates ApoE Lipidation and amyloid-β metabolism in the brain. J. Neurosci. Off. J. Soc. Neurosci. 35, 14717–14726. doi: 10.1523/JNEUROSCI.2053-15.2015
Kinser, H. E., and Pincus, Z. (2020). MicroRNAs as modulators of longevity and the aging process. Hum. Genet. 139, 291–308. doi: 10.1007/s00439-019-02046-0
Kobayashi, H., and Tomari, Y. (2016). RISC assembly: coordination between small RNAs and Argonaute proteins. Biochim. Biophys. Act 1859, 71–81. doi: 10.1016/j.bbagrm.2015.08.007
Koikkalainen, J., Rhodius-Meester, H., Tolonen, A., Barkhof, F., Tijms, B., Lemstra, A. W., et al. (2016). Differential diagnosis of neurodegenerative diseases using structural MRI data. Neuroimage 11, 435–449. doi: 10.1016/j.nicl.2016.02.019
Kos, M. Z., Puppala, S., Cruz, D., Neary, J. L., Kumar, A., Dalan, E., et al. (2022). Blood-based miRNA biomarkers as correlates of brain-based miRNA expression. Front. Mol. Neurosci. 15:817290. doi: 10.3389/fnmol.2022.817290
Kou, X., Chen, D., and Chen, N. (2020). The regulation of microRNAs in Alzheimer’s disease. Front. Neurol. 11:288. doi: 10.3389/fneur.2020.00288
Kovanda, A., Leonardis, L., Zidar, J., Koritnik, B., Dolenc-Groselj, L., Ristic Kovacic, S., et al. (2018). Differential expression of microRNAs and other small RNAs in muscle tissue of patients with ALS and healthy age-matched controls. Sci. Rep. 8:5609. doi: 10.1038/s41598-018-23139-2
Kumar, P., Dezso, Z., MacKenzie, C., Oestreicher, J., Agoulnik, S., Byrne, M., et al. (2013). Circulating miRNA biomarkers for Alzheimer’s disease. PLoS One 8:e69807. doi: 10.1371/journal.pone.0069807
Kumar, D., Ganeshpurkar, A., Kumar, D., Modi, G., Gupta, S. K., and Singh, S. K. (2018). Secretase inhibitors for the treatment of Alzheimer’s disease: long road ahead. Eur. J. Med. Chem. 148, 436–452. doi: 10.1016/j.ejmech.2018.02.035
Lamb, Y. N. (2022). Ocrelizumab: a review in multiple sclerosis. Drugs 82, 323–334. doi: 10.1007/s40265-022-01672-9
Lamptey, R. N. L., Chaulagain, B., Trivedi, R., Gothwal, A., Layek, B., and Singh, J. (2022). A review of the common neurodegenerative disorders: current therapeutic approaches and the potential role of Nanotherapeutics. Int. J. Mol. Sci. 23:1851. doi: 10.3390/ijms23031851
Langenfurth, A., Rinnenthal, J. L., Vinnakota, K., Prinz, V., Carlo, A.-S., Stadelmann, C., et al. (2014). Membrane-type 1 metalloproteinase is upregulated in microglia/brain macrophages in neurodegenerative and neuroinflammatory diseases. J. Neurosci. Res. 92, 275–286. doi: 10.1002/jnr.23288
Lauretti, E., Dincer, O., and Praticò, D. (2021). Regional and temporal miRNAs expression profile in a transgenic mouse model of tauopathy: implication for its pathogenesis. Mol. Psychiatry 26, 7020–7028. doi: 10.1038/s41380-020-0655-2
Lawrence Steinman, M. D. (1996). Multiple sclerosis: a coordinated immunological attack against myelin in the central nervous system. Cell 85, 299–302. doi: 10.1016/S0092-8674(00)81107-1
Lecca, D., Marangon, D., Coppolino, G. T., Méndez, A. M., Finardi, A., Costa, G. D., et al. (2016). MiR-125a-3p timely inhibits oligodendroglial maturation and is pathologically up-regulated in human multiple sclerosis. Sci. Rep. 6:34503. doi: 10.1038/srep34503
Lee, Y., Ahn, C., Han, J., Choi, H., Kim, J., Yim, J., et al. (2003). The nuclear RNase III Drosha initiates microRNA processing. Nature 425, 415–419. doi: 10.1038/nature01957
Lee, S.-T., Chu, K., Im, W.-S., Yoon, H.-J., Im, J.-Y., Park, J.-E., et al. (2011). Altered microRNA regulation in Huntington’s disease models. Exp. Neurol. 227, 172–179. doi: 10.1016/j.expneurol.2010.10.012
Lee, S.-T., Im, W., Ban, J.-J., Lee, M., Jung, K.-H., Lee, S. K., et al. (2017). Exosome-based delivery of miR-124 in a Huntington’s disease model. J. Mov. Disord. 10, 45–52. doi: 10.14802/jmd.16054
Lee, J., and Kemper, J. K. (2010). Controlling SIRT1 expression by microRNAs in health and metabolic disease. Aging 2, 527–534. doi: 10.18632/aging.100184
Lee, K., Kim, H., An, K., Kwon, O.-B., Park, S., Cha, J. H., et al. (2016). Replenishment of microRNA-188-5p restores the synaptic and cognitive deficits in 5XFAD mouse model of Alzheimer’s disease. Sci. Rep. 6:34433. doi: 10.1038/srep34433
Lehmann, S. M., Krüger, C., Park, B., Derkow, K., Rosenberger, K., Baumgart, J., et al. (2012). An unconventional role for miRNA: Let-7 activates toll-like receptor 7 and causes neurodegeneration. Nat. Neurosci. 15, 827–835. doi: 10.1038/nn.3113
Leidinger, P., Backes, C., Deutscher, S., Schmitt, K., Mueller, S. C., Frese, K., et al. (2013). A blood based 12-miRNA signature of Alzheimer disease patients. Genome Biol. 14:R78. doi: 10.1186/gb-2013-14-7-r78
Li, N., Bates, D. J., An, J., Terry, D. A., and Wang, E. (2011). Up-regulation of key microRNAs, and inverse down-regulation of their predicted oxidative phosphorylation target genes, during aging in mouse brain. Neurobiol. Aging 32, 944–955. doi: 10.1016/j.neurobiolaging.2009.04.020
Li, S., Bi, G., Han, S., and Huang, R. (2022). MicroRNAs play a role in Parkinson’s disease by regulating microglia function: from Pathogenetic involvement to therapeutic potential. Front. Mol. Neurosci. 14:744942. doi: 10.3389/fnmol.2021.744942
Li, C., Chen, Y., Chen, X., Wei, Q., Cao, B., and Shang, H. (2017). Downregulation of MicroRNA-193b-3p promotes autophagy and cell survival by targeting TSC1/mTOR signaling in NSC-34 cells. Front. Mol. Neurosci. 10:160. doi: 10.3389/fnmol.2017.00160
Li, J., Chen, W., Yi, Y., and Tong, Q. (2019a). miR-219-5p inhibits tau phosphorylation by targeting TTBK1 and GSK-3β in Alzheimer’s disease. J. Cell. Biochem. 120, 9936–9946. doi: 10.1002/jcb.28276
Li, J., Han, Y., Li, M., and Nie, C. (2019b). Curcumin promotes proliferation of adult neural stem cells and the birth of neurons in Alzheimer’s disease mice via notch signaling pathway. Cell. Reprogram. 21, 152–161. doi: 10.1089/cell.2018.0027
Li, X., Khanna, A., Li, N., and Wang, E. (2011b). Circulatory miR-34a as an RNA-based, noninvasive biomarker for brain aging. Aging 3, 985–1002. doi: 10.18632/aging.100371
Li, S., Lei, Z., and Sun, T. (2023). The role of microRNAs in neurodegenerative diseases: a review. Cell Biol. Toxicol. 39, 53–83. doi: 10.1007/s10565-022-09761-x
Li, H.-H., Lin, S.-L., Huang, C.-N., Lu, F.-J., Chiu, P.-Y., Huang, W.-N., et al. (2016). miR-302 attenuates amyloid-β-induced neurotoxicity through activation of Akt signaling. J. Alzheimers Dis. 50, 1083–1098. doi: 10.3233/JAD-150741
Li, S., Lv, X., Zhai, K., Xu, R., Zhang, Y., Zhao, S., et al. (2016). MicroRNA-7 inhibits neuronal apoptosis in a cellular Parkinson’s disease model by targeting Bax and Sirt2. Am. J. Transl. Res. 8, 993–1004.
Li, N., Pan, X., Zhang, J., Ma, A., Yang, S., Ma, J., et al. (2017). Plasma levels of miR-137 and miR-124 are associated with Parkinson’s disease but not with Parkinson’s disease with depression. Neurol. Sci. 38, 761–767. doi: 10.1007/s10072-017-2841-9
Li, J., and Wang, H. (2018). miR-15b reduces amyloid-β accumulation in SH-SY5Y cell line through targetting NF-κB signaling and BACE1. Biosci. Rep. 38:BSR20180051. doi: 10.1042/BSR20180051
Li, X., Wang, Z., Tan, L., Wang, Y., Lu, C., Chen, R., et al. (2017). Correcting miR92a-vGAT-mediated GABAergic dysfunctions rescues human tau-induced anxiety in mice. Mol. Ther. 25, 140–152. doi: 10.1016/j.ymthe.2016.10.010
Li, Y., Wang, H., Wang, S., Quon, D., Liu, Y.-W., and Cordell, B. (2003). Positive and negative regulation of APP amyloidogenesis by sumoylation. Proc. Natl. Acad. Sci. 100, 259–264. doi: 10.1073/pnas.0235361100
Li, J., Yin, Y., Wang, R., Zhang, P., Zhao, J., Liu, R., et al. (2021). Expressions and clinical significance of miR-124 and miR-494 in elderly patients with Parkinson disease. Chin. J. Behav. Med. Brain Sci. 30, 294–298. doi: 10.3760/cma.j.cn371468-20200828-01692
Li, Y., Zhou, D., Ren, Y., Zhang, Z., Guo, X., Ma, M., et al. (2018). Mir223 restrains autophagy and promotes CNS inflammation by targeting ATG16L1. Autophagy 15, 478–492. doi: 10.1080/15548627.2018.1522467
Lian, B., Yang, D., Liu, Y., Shi, G., Li, J., Yan, X., et al. (2018). miR-128 targets the SIRT1/ROS/DR5 pathway to sensitize colorectal Cancer to TRAIL-induced apoptosis. Cell. Physiol. Biochem. 49, 2151–2162. doi: 10.1159/000493818
Liguori, M., Nuzziello, N., Introna, A., Consiglio, A., Licciulli, F., D’Errico, E., et al. (2018). Dysregulation of MicroRNAs and target genes networks in peripheral blood of patients with sporadic amyotrophic lateral sclerosis. Front. Mol. Neurosci. 11:288. doi: 10.3389/fnmol.2018.00288
Lin, S.-T., Huang, Y., Zhang, L., Heng, M. Y., Ptácek, L. J., and Fu, Y.-H. (2013). MicroRNA-23a promotes myelination in the central nervous system. Proc. Natl. Acad. Sci. USA 110, 17468–17473. doi: 10.1073/pnas.1317182110
Lindberg, R. L. P., Hoffmann, F., Mehling, M., Kuhle, J., and Kappos, L. (2010). Altered expression of miR-17-5p in CD4+ lymphocytes of relapsing-remitting multiple sclerosis patients. Eur. J. Immunol. 40, 888–898. doi: 10.1002/eji.200940032
Liu, X., Feng, Z., Du, L., Huang, Y., Ge, J., Deng, Y., et al. (2020). The potential role of MicroRNA-124 in cerebral ischemia injury. Int. J. Mol. Sci. 21:120. doi: 10.3390/ijms21010120
Liu, X., He, F., Pang, R., Zhao, D., Qiu, W., Shan, K., et al. (2014). Interleukin-17 (IL-17)-induced MicroRNA 873 (miR-873) contributes to the pathogenesis of experimental autoimmune encephalomyelitis by targeting A20 ubiquitin-editing enzyme*. J. Biol. Chem. 289, 28971–28986. doi: 10.1074/jbc.M114.577429
Liu, Y.-C., Hsu, W.-L., Ma, Y.-L., and Lee, E. H. Y. (2021). Melatonin induction of APP intracellular domain 50 SUMOylation alleviates AD through enhanced transcriptional activation and Aβ degradation. Mol. Ther. 29, 376–395. doi: 10.1016/j.ymthe.2020.09.003
Liu, N., Landreh, M., Cao, K., Abe, M., Hendriks, G.-J., Kennerdell, J., et al. (2012). The microRNA miR-34 modulates aging and neurodegeneration in Drosophila. Nature 482, 519–523. doi: 10.1038/nature10810
Liu, W., Zhao, J., and Lu, G. (2016). miR-106b inhibits tau phosphorylation at Tyr18 by targeting Fyn in a model of Alzheimer’s disease. Biochem. Biophys. Res. Commun. 478, 852–857. doi: 10.1016/j.bbrc.2016.08.037
Long, J. M., Ray, B., and Lahiri, D. K. (2012). MicroRNA-153 physiologically inhibits expression of amyloid-β precursor protein in cultured human fetal brain cells and is dysregulated in a subset of Alzheimer disease patients *. J. Biol. Chem. 287, 31298–31310. doi: 10.1074/jbc.M112.366336
Long, J. M., Ray, B., and Lahiri, D. K. (2014). MicroRNA-339-5p down-regulates protein expression of β-site amyloid precursor protein-cleaving enzyme 1 (BACE1) in human primary brain cultures and is reduced in brain tissue specimens of Alzheimer disease subjects. J. Biol. Chem. 289, 5184–5198. doi: 10.1074/jbc.M113.518241
Long, X., Yao, X., Jiang, Q., Yang, Y., He, X., Tian, W., et al. (2020). Astrocyte-derived exosomes enriched with miR-873a-5p inhibit neuroinflammation via microglia phenotype modulation after traumatic brain injury. J. Neuroinflammation 17:89. doi: 10.1186/s12974-020-01761-0
Lontay, B., Kiss, A., Virág, L., and Tar, K. (2020). How do post-translational modifications influence the Pathomechanistic landscape of Huntington’s disease? A comprehensive review. Int. J. Mol. Sci. 21:4282. doi: 10.3390/ijms21124282
Lu, J., Getz, G., Miska, E. A., Alvarez-Saavedra, E., Lamb, J., Peck, D., et al. (2005). MicroRNA expression profiles classify human cancers. Nature 435, 834–838. doi: 10.1038/nature03702
Lu, J., Xu, Y., Quan, Z., Chen, Z., Sun, Z., and Qing, H. (2017). Dysregulated microRNAs in neural system: implication in pathogenesis and biomarker development in Parkinson’s disease. Neuroscience 365, 70–82. doi: 10.1016/j.neuroscience.2017.09.033
Lukiw, W. J. (2007). Micro-RNA speciation in fetal, adult and Alzheimer’s disease hippocampus. Neuro Rep. 18, 297–300. doi: 10.1097/WNR.0b013e3280148e8b
Lukiw, W. J., Zhao, Y., and Cui, J. G. (2008). An NF-κB-sensitive Micro RNA-146a-mediated inflammatory circuit in Alzheimer disease and in stressed human brain cells *. J. Biol. Chem. 283, 31315–31322. doi: 10.1074/jbc.M805371200
Lyu, G., Guan, Y., Zhang, C., Zong, L., Sun, L., Huang, X., et al. (2018). TGF-β signaling alters H4K20me3 status via miR-29 and contributes to cellular senescence and cardiac aging. Nat. Commun. 9:2560. doi: 10.1038/s41467-018-04994-z
Ma, W., Li, Y., Wang, C., Xu, F., Wang, M., and Liu, Y. (2016). Serum miR-221 serves as a biomarker for Parkinson’s disease. Cell Biochem. Funct. 34, 511–515. doi: 10.1002/cbf.3224
Ma, L., Young, J., Prabhala, H., Pan, E., Mestdagh, P., Muth, D., et al. (2010). miR-9, a MYC/MYCN-activated microRNA, regulates E-cadherin and cancer metastasis. Nat. Cell Biol. 12, 247–256. doi: 10.1038/ncb2024
Ma, X., Zhou, J., Zhong, Y., Jiang, L., Mu, P., Li, Y., et al. (2014). Expression, regulation and function of MicroRNAs in multiple sclerosis. Int. J. Med. Sci. 11, 810–818. doi: 10.7150/ijms.8647
MacDonald, M. E., Ambrose, C. M., Duyao, M. P., Myers, R. H., Lin, C., Srinidhi, L., et al. (1993). A novel gene containing a trinucleotide repeat that is expanded and unstable on Huntington’s disease chromosomes. Cell 72, 971–983. doi: 10.1016/0092-8674(93)90585-E
MacFarlane, L.-A., and Murphy, P. R. (2010). MicroRNA: biogenesis, function and role in Cancer. Curr. Genomics 11, 537–561. doi: 10.2174/138920210793175895
Machida, T., Tomofuji, T., Ekuni, D., Maruyama, T., Yoneda, T., Kawabata, Y., et al. (2015). MicroRNAs in salivary exosome as potential biomarkers of aging. Int. J. Mol. Sci. 16, 21294–21309. doi: 10.3390/ijms160921294
Maciak, K., Dziedzic, A., Miller, E., and Saluk-Bijak, J. (2021). miR-155 as an important regulator of multiple sclerosis pathogenesis. A review. Int. J. Mol. Sci. 22:4332. doi: 10.3390/ijms22094332
MacLeod, R., Hillert, E.-K., Cameron, R. T., and Baillie, G. S. (2015). The role and therapeutic targeting of α-, β-and γ-secretase in Alzheimer’s disease. Future Sci. OA 1:FSO11. doi: 10.4155/fso.15.9
MacRae, I. J., Ma, E., Zhou, M., Robinson, C. V., and Doudna, J. A. (2008). In vitro reconstitution of the human RISC-loading complex. Proc. Natl. Acad. Sci. USA 105, 512–517. doi: 10.1073/pnas.0710869105
Maffioletti, E., Tardito, D., Gennarelli, M., and Bocchio-Chiavetto, L. (2014). Micro spies from the brain to the periphery: new clues from studies on microRNAs in neuropsychiatric disorders. Front. Cell. Neurosci. 8:75. doi: 10.3389/fncel.2014.00075
Maimon, R., Ionescu, A., Bonnie, A., Sweetat, S., Wald-Altman, S., Inbar, S., et al. (2018). miR126-5p downregulation facilitates axon degeneration and NMJ disruption via a non-cell-autonomous mechanism in ALS. J. Neurosci. Off. J. Soc. Neurosci. 38, 5478–5494. doi: 10.1523/JNEUROSCI.3037-17.2018
Mancuso, R., Agostini, S., Hernis, A., Zanzottera, M., Bianchi, A., and Clerici, M. (2019). Circulatory miR-223-3p discriminates between Parkinson’s and Alzheimer’s patients. Sci. Rep. 9:9393. doi: 10.1038/s41598-019-45687-x
Mancuso, R., Hernis, A., Agostini, S., Rovaris, M., Caputo, D., and Clerici, M. (2015). MicroRNA-572 expression in multiple sclerosis patients with different patterns of clinical progression. J. Transl. Med. 13:148. doi: 10.1186/s12967-015-0504-2
Manzine, P. R., Pelucchi, S., Horst, M. A., Vale, F. A. C., Pavarini, S. C. I., Audano, M., et al. (2018). microRNA 221 targets ADAM10 mRNA and is downregulated in Alzheimer’s disease. J. Alzheimers Dis. 61, 113–123. doi: 10.3233/JAD-170592
Maoz, R., Garfinkel, B. P., and Soreq, H. (2017). “Alzheimer’s disease and ncRNAs” in Neuroepigenomics in aging and disease. Advances in Experimental Medicine and Biology. ed. R. Delgado-Morales (Springer International Publishing), 978, 337–361.
Marangon, D., Boda, E., Parolisi, R., Negri, C., Giorgi, C., Montarolo, F., et al. (2020). In vivo silencing of miR-125a-3p promotes myelin repair in models of white matter demyelination. Glia 68, 2001–2014. doi: 10.1002/glia.23819
Margis, R., Margis, R., and Rieder, C. R. M. (2011). Identification of blood microRNAs associated to Parkinsońs disease. J. Biotechnol. 152, 96–101. doi: 10.1016/j.jbiotec.2011.01.023
Marques, T. M., Kuiperij, H. B., Bruinsma, I. B., van Rumund, A., Aerts, M. B., Esselink, R. A. J., et al. (2017). MicroRNAs in cerebrospinal fluid as potential biomarkers for Parkinson’s disease and multiple system atrophy. Mol. Neurobiol. 54, 7736–7745. doi: 10.1007/s12035-016-0253-0
Martí, E., Pantano, L., Bañez-Coronel, M., Llorens, F., Miñones-Moyano, E., Porta, S., et al. (2010). A myriad of miRNA variants in control and Huntington’s disease brain regions detected by massively parallel sequencing. Nucleic Acids Res. 38, 7219–7235. doi: 10.1093/nar/gkq575
Martinez, B., and Peplow, P. V. (2021). Altered microRNA expression in animal models of Huntington’s disease and potential therapeutic strategies. Neural Regen. Res. 16, 2159–2169. doi: 10.4103/1673-5374.310673
Martinez, B., and Peplow, P. V. (2022). MicroRNA expression in animal models of amyotrophic lateral sclerosis and potential therapeutic approaches. Neural Regen. Res. 17:728. doi: 10.4103/1673-5374.322431
Martinez, N. J., and Walhout, A. J. M. (2009). The interplay between transcription factors and microRNAs in genome-scale regulatory networks. Bioessays 31, 435–445. doi: 10.1002/bies.200800212
Martins, M., Rosa, A., Guedes, L. C., Fonseca, B. V., Gotovac, K., Violante, S., et al. (2011). Convergence of miRNA expression profiling, α-synuclein interacton and GWAS in Parkinson’s disease. PLoS One 6:e25443. doi: 10.1371/journal.pone.0025443
Masrori, P., and Van Damme, P. (2020). Amyotrophic lateral sclerosis: a clinical review. Eur. J. Neurol. 27, 1918–1929. doi: 10.1111/ene.14393
Matamala, J. M., Arias-Carrasco, R., Sanchez, C., Uhrig, M., Bargsted, L., Matus, S., et al. (2018). Genome-wide circulating microRNA expression profiling reveals potential biomarkers for amyotrophic lateral sclerosis. Neurobiol. Aging 64, 123–138. doi: 10.1016/j.neurobiolaging.2017.12.020
Mattick, J. S., and Makunin, I. V. (2006). Non-coding RNA. Hum. Mol. Genet. 15, R17–R29. doi: 10.1093/hmg/ddl046
Mattson, M. P. (2010). Acetylation unleashes protein demons of dementia. Neuron 67, 900–902. doi: 10.1016/j.neuron.2010.09.010
McKeever, P. M., Schneider, R., Taghdiri, F., Weichert, A., Multani, N., Brown, R. A., et al. (2018). MicroRNA expression levels are altered in the cerebrospinal fluid of patients with Young-onset Alzheimer’s disease. Mol. Neurobiol. 55, 8826–8841. doi: 10.1007/s12035-018-1032-x
Mead, R. J., Shan, N., Reiser, H. J., Marshall, F., and Shaw, P. J. (2023). Amyotrophic lateral sclerosis: a neurodegenerative disorder poised for successful therapeutic translation. Nat. Rev. Drug Discov. 22, 185–212. doi: 10.1038/s41573-022-00612-2
Meder, B., Backes, C., Haas, J., Leidinger, P., Stähler, C., Großmann, T., et al. (2014). Influence of the confounding factors age and sex on MicroRNA profiles from peripheral blood. Clin. Chem. 60, 1200–1208. doi: 10.1373/clinchem.2014.224238
Mestre, T., Ferreira, J., Coelho, M. M., Rosa, M., and Sampaio, C. (2009). Therapeutic interventions for symptomatic treatment in Huntington’s disease. Cochrane Database Syst. Rev. 3:CD006456. doi: 10.1002/14651858.CD006456.pub2
Michlewski, G., and Cáceres, J. F. (2019). Post-transcriptional control of miRNA biogenesis. RNA 25, 1–16. doi: 10.1261/rna.068692.118
Miñones-Moyano, E., Porta, S., Escaramís, G., Rabionet, R., Iraola, S., Kagerbauer, B., et al. (2011). MicroRNA profiling of Parkinson’s disease brains identifies early downregulation of miR-34b/c which modulate mitochondrial function. Hum. Mol. Genet. 20, 3067–3078. doi: 10.1093/hmg/ddr210
Mitchell, P. S., Parkin, R. K., Kroh, E. M., Fritz, B. R., Wyman, S. K., Pogosova-Agadjanyan, E. L., et al. (2008). Circulating microRNAs as stable blood-based markers for cancer detection. Proc. Natl. Acad. Sci. USA 105, 10513–10518. doi: 10.1073/pnas.0804549105
Miyoshi, K., Miyoshi, T., and Siomi, H. (2010). Many ways to generate microRNA-like small RNAs: non-canonical pathways for microRNA production. Mol. Genet. Genom. 284, 95–103. doi: 10.1007/s00438-010-0556-1
Mo, Y.-Y. (2012). MicroRNA regulatory networks and human disease. Cell. Mol. Life Sci. 69, 3529–3531. doi: 10.1007/s00018-012-1123-1
Mo, M.-H., Chen, L., Fu, Y., Wang, W., and Fu, S. W. (2012). Cell-free circulating miRNA biomarkers in Cancer. J. Cancer 3, 432–448. doi: 10.7150/jca.4919
Mo, M., Xiao, Y., Huang, S., Cen, L., Chen, X., Zhang, L., et al. (2016). MicroRNA expressing profiles in A53T mutant alpha-synuclein transgenic mice and parkinsonian. Oncotarget 8, 15–28. doi: 10.18632/oncotarget.13905
Moncini, S., Lunghi, M., Valmadre, A., Grasso, M., Del Vescovo, V., Riva, P., et al. (2017). The miR-15/107 family of microRNA genes regulates CDK5R1/p35 with implications for Alzheimer’s disease pathogenesis. Mol. Neurobiol. 54, 4329–4342. doi: 10.1007/s12035-016-0002-4
Moore, C. S., Rao, V. T. S., Durafourt, B. A., Bedell, B. J., Ludwin, S. K., Bar-Or, A., et al. (2013). miR-155 as a multiple sclerosis-relevant regulator of myeloid cell polarization. Ann. Neurol. 74, 709–720. doi: 10.1002/ana.23967
Morales, S., Monzo, M., and Navarro, A. (2017). Epigenetic regulation mechanisms of microRNA expression. Biomol. Concepts 8, 203–212. doi: 10.1515/bmc-2017-0024
Mori, M. A., Ludwig, R. G., Garcia-Martin, R., Brandão, B. B., and Kahn, C. R. (2019). Extracellular miRNAs: From Biomarkers to Mediators of Physiology and Disease. Cell Metabol. 30, 656–673. doi: 10.1016/j.cmet.2019.07.011
Mori, T., Maeda, J., Shimada, H., Higuchi, M., Shinotoh, H., Ueno, S., et al. (2012). Molecular imaging of dementia. Psychogeriatrics 12, 106–114. doi: 10.1111/j.1479-8301.2012.00409.x
Morita, S., Horii, T., Kimura, M., Ochiya, T., Tajima, S., and Hatada, I. (2013). miR-29 represses the activities of DNA methyltransferases and DNA demethylases. Int. J. Mol. Sci. 14, 14647–14658. doi: 10.3390/ijms140714647
Morquette, B., Juźwik, C. A., Drake, S. S., Charabati, M., Zhang, Y., Lécuyer, M.-A., et al. (2019). MicroRNA-223 protects neurons from degeneration in experimental autoimmune encephalomyelitis. Brain J. Neurol. 142, 2979–2995. doi: 10.1093/brain/awz245
Mushtaq, G., Greig, N. H., Anwar, F., Zamzami, M. A., Choudhry, H., Shaik, M. M., et al. (2016). miRNAs as circulating biomarkers for Alzheimer’s disease and Parkinson’s disease. Med. Chem. 12, 217–225. doi: 10.2174/1573406411666151030112140
Nagaraj, S., Laskowska-Kaszub, K., Dębski, K. J., Wojsiat, J., Dąbrowski, M., Gabryelewicz, T., et al. (2017). Profile of 6 microRNA in blood plasma distinguish early stage Alzheimer’s disease patients from non-demented subjects. Oncotarget 8, 16122–16143. doi: 10.18632/oncotarget.15109
Nagaraj, S., Want, A., Laskowska-Kaszub, K., Fesiuk, A., Vaz, S., Logarinho, E., et al. (2021). Candidate Alzheimer’s disease biomarker miR-483-5p lowers TAU phosphorylation by direct ERK1/2 repression. Int. J. Mol. Sci. 22:3653. doi: 10.3390/ijms22073653
Natarajan, S. K., Smith, M. A., Wehrkamp, C. J., Mohr, A. M., and Mott, J. L. (2013). MicroRNA function in human diseases. Med. Epigenet. 1, 106–115. doi: 10.1159/000356447
Nelson, P. T., and Wang, W.-X. (2010). MiR-107 is reduced in Alzheimer’s disease brain neocortex: validation study. J. Alzheimers Dis. 21, 75–79. doi: 10.3233/JAD-2010-091603
Nemeth, K., Bayraktar, R., Ferracin, M., and Calin, G. A. (2024). Non-coding RNAs in disease: from mechanisms to therapeutics. Nat. Rev. Genet. 25, 211–232. doi: 10.1038/s41576-023-00662-1
Nguyen, T. P. N., Kumar, M., Fedele, E., Bonanno, G., and Bonifacino, T. (2022). MicroRNA alteration, application as biomarkers, and therapeutic approaches in neurodegenerative diseases. Int. J. Mol. Sci. 23:4718. doi: 10.3390/ijms23094718
Nies, Y. H., Mohamad Najib, N. H., Lim, W. L., Kamaruzzaman, M. A., Yahaya, M. F., and Teoh, S. L. (2021). MicroRNA dysregulation in Parkinson’s disease: a narrative review. Front. Neurosci. 15:660379. doi: 10.3389/fnins.2021.660379
Noor Eddin, A., Hamsho, K., Adi, G., Al-Rimawi, M., Alfuwais, M., Abdul Rab, S., et al. (2023). Cerebrospinal fluid microRNAs as potential biomarkers in Alzheimer’s disease. Front. Aging Neurosci. 15:1210191. doi: 10.3389/fnagi.2023.1210191
Noorbakhsh, F., Ellestad, K. K., Maingat, F., Warren, K. G., Han, M. H., Steinman, L., et al. (2011). Impaired neurosteroid synthesis in multiple sclerosis. Brain J. Neurol. 134, 2703–2721. doi: 10.1093/brain/awr200
O’Brien, J., Hayder, H., Zayed, Y., and Peng, C. (2018). Overview of MicroRNA biogenesis, mechanisms of actions, and circulation. Front. Endocrinol. 9:402. doi: 10.3389/fendo.2018.00402
O’Brien, R. J., and Wong, P. C. (2011). Amyloid precursor protein processing and Alzheimer’s disease. Annu. Rev. Neurosci. 34, 185–204. doi: 10.1146/annurev-neuro-061010-113613
O’Carroll, D., and Schaefer, A. (2013). General principals of mirna biogenesis and regulation in the brain. Neuropsychophar. 38, 39–54. doi: 10.1038/npp.2012.87
O’Connell, R. M., Kahn, D., Gibson, W. S. J., Round, J. L., Scholz, R. L., Chaudhuri, A. A., et al. (2010). MicroRNA-155 promotes autoimmune inflammation by enhancing inflammatory T cell development. Immunity 33, 607–619. doi: 10.1016/j.immuni.2010.09.009
O’Gorman, C., Lucas, R., and Taylor, B. (2012). Environmental risk factors for multiple sclerosis: a review with a focus on molecular mechanisms. Int. J. Mol. Sci. 13, 11718–11752. doi: 10.3390/ijms130911718
Ogino, M., Ichimura, M., Nakano, N., Minami, A., Kitagishi, Y., and Matsuda, S. (2016). Roles of PTEN with DNA repair in Parkinson’s disease. Int. J. Mol. Sci. 17:954. doi: 10.3390/ijms17060954
Olivieri, F., Bonafè, M., Spazzafumo, L., Gobbi, M., Prattichizzo, F., Recchioni, R., et al. (2014). Age-and glycemia-related miR-126-3p levels in plasma and endothelial cells. Aging 6, 771–786. doi: 10.18632/aging.100693
Otaegui, D., Baranzini, S. E., Armañanzas, R., Calvo, B., Muñoz-Culla, M., Khankhanian, P., et al. (2009). Differential Micro RNA expression in PBMC from multiple sclerosis patients. PLoS One 4:e6309. doi: 10.1371/journal.pone.0006309
Ott, A., Stolk, R. P., van Harskamp, F., Pols, H. A. P., Hofman, A., and Breteler, M. M. B. (1999). Diabetes mellitus and the risk of dementia. Neurology 53:1937. doi: 10.1212/WNL.53.9.1937
Ozdilek, B., and Demircan, B. (2021). Serum microRNA expression levels in Turkish patients with Parkinson’s disease. Int. J. Neurosci. 131, 1181–1189. doi: 10.1080/00207454.2020.1784165
Pabinger, S., Rödiger, S., Kriegner, A., Vierlinger, K., and Weinhäusel, A. (2014). A survey of tools for the analysis of quantitative PCR (qPCR) data. Biomol. Detect. Quantif. 1, 23–33. doi: 10.1016/j.bdq.2014.08.002
Packer, A. N., Xing, Y., Harper, S. Q., Jones, L., and Davidson, B. L. (2008). The bifunctional microRNA miR-9/miR-9* regulates REST and CoREST and is downregulated in Huntington’s disease. J. Neurosci. 28, 14341–14346. doi: 10.1523/JNEUROSCI.2390-08.2008
Parisi, C., Arisi, I., D’Ambrosi, N., Storti, A. E., Brandi, R., D’Onofrio, M., et al. (2013). Dysregulated microRNAs in amyotrophic lateral sclerosis microglia modulate genes linked to neuroinflammation. Cell Death Dis. 4:e959. doi: 10.1038/cddis.2013.491
Parisi, C., Napoli, G., Amadio, S., Spalloni, A., Apolloni, S., Longone, P., et al. (2016). MicroRNA-125b regulates microglia activation and motor neuron death in ALS. Cell Death Differ. 23, 531–541. doi: 10.1038/cdd.2015.153
Park, J. H., and Moon, J. (2022). Conserved 3′ UTR of severe acute respiratory syndrome coronavirus 2: potential therapeutic targets. Front. Genet. 13:893141. doi: 10.3389/fgene.2022.893141
Paul, S., Bravo Vázquez, L. A., Pérez Uribe, S., Roxana Reyes-Pérez, P., and Sharma, A. (2020). Current status of microRNA-based therapeutic approaches in neurodegenerative disorders. Cells 9:1698. doi: 10.3390/cells9071698
Peggion, C., Scalcon, V., Massimino, M. L., Nies, K., Lopreiato, R., Rigobello, M. P., et al. (2022). SOD1 in ALS: taking stock in pathogenic mechanisms and the role of glial and muscle cells. Antioxidants 11:614. doi: 10.3390/antiox11040614
Perdaens, O., Dang, H. A., D’Auria, L., and Pesch, V.van. (2020). CSF microRNAs discriminate MS activity and share similarity to other neuroinflammatory disorders. Neurol. Neuroimmunol. Neuroinflamm. 7:673. doi: 10.1212/NXI.0000000000000673
Persengiev, S. P., Kondova, I. I., and Bontrop, R. E. (2012). The impact of MicroRNAs on brain aging and neurodegeneration. Curr. Gerontol. Geriatr. Res. 2012:e359369. doi: 10.1155/2012/359369
Petrella, C., Di Certo, M. G., Barbato, C., Gabanella, F., Ralli, M., Greco, A., et al. (2019). Neuropeptides in Alzheimer’s disease: an update. Curr. Alzheimer Res. 16, 544–558. doi: 10.2174/1567205016666190503152555
Phukan, J., Pender, N. P., and Hardiman, O. (2007). Cognitive impairment in amyotrophic lateral sclerosis. Lancet Neurol. 6, 994–1003. doi: 10.1016/S1474-4422(07)70265-X
Pietrasik, S., Dziedzic, A., Miller, E., Starosta, M., and Saluk-Bijak, J. (2021). Circulating miRNAs as potential biomarkers distinguishing relapsing–remitting from secondary progressive multiple sclerosis. A review. Int. J. Mol. Sci. 22:11887. doi: 10.3390/ijms222111887
Piscopo, P., Bellenghi, M., Manzini, V., Crestini, A., Pontecorvi, G., Corbo, M., et al. (2021). A sex perspective in neurodegenerative diseases: microRNAs as possible peripheral biomarkers. Int. J. Mol. Sci. 22:4423. doi: 10.3390/ijms22094423
Pogue, A. I., Cui, J. G., Li, Y. Y., Zhao, Y., Culicchia, F., and Lukiw, W. J. (2010). Micro RNA-125b (miRNA-125b) function in astrogliosis and glial cell proliferation. Neurosci. Lett. 476, 18–22. doi: 10.1016/j.neulet.2010.03.054
Ponomarev, E. D., Veremeyko, T., Barteneva, N., Krichevsky, A. M., and Weiner, H. L. (2011). MicroRNA-124 promotes microglia quiescence and suppresses EAE by deactivating macrophages via the C/EBP-α-PU.1 pathway. Nat. Med. 17, 64–70. doi: 10.1038/nm.2266
Poy, M. N., Eliasson, L., Krutzfeldt, J., Kuwajima, S., Ma, X., MacDonald, P. E., et al. (2004). A pancreatic islet-specific microRNA regulates insulin secretion. Nature 432, 226–230. doi: 10.1038/nature03076
Praticò, D. (2020). The functional role of microRNAs in the pathogenesis of Tauopathy. Cells 9:2262. doi: 10.3390/cells9102262
Precazzini, F., Detassis, S., Imperatori, A. S., Denti, M. A., and Campomenosi, P. (2021). Measurements methods for the development of microRNA-based tests for cancer diagnosis. Int. J. Mol. Sci. 22:1176. doi: 10.3390/ijms22031176
Prince, M. J., Wimo, A., Guerchet, M. M., Ali, G. C., Wu, Y.-T., and Prina, M. (2015). World Alzheimer report 2015- the global impact of dementia: an analysis of prevalence, incidence, cost and trends. Available at: https://kclpure.kcl.ac.uk/portal/en/publications/world-alzheimer-report-2015--the-global-impact-of-dementia(ae525fda-1938-4892-8daa-a2222a672254).html
Prodromidou, K., and Matsas, R. (2019). Species-specific miRNAs in human brain development and disease. Front. Cell. Neurosci. 13:559. doi: 10.3389/fncel.2019.00559
Proshkina, E., Solovev, I., Koval, L., and Moskalev, A. (2020). The critical impacts of small RNA biogenesis proteins on aging, longevity and age-related diseases. Ageing Res. Rev. 62:101087. doi: 10.1016/j.arr.2020.101087
Provost, P. (2010). MicroRNAs as a molecular basis for mental retardation, Alzheimer’s and prion diseases. Brain Res. 1338, 58–66. doi: 10.1016/j.brainres.2010.03.069
Pusic, A. D., and Kraig, R. P. (2014). Youth and environmental enrichment generate serum exosomes containing miR-219 that promote CNS myelination. Glia 62, 284–299. doi: 10.1002/glia.22606
Qian, Y., Song, J., Ouyang, Y., Han, Q., Chen, W., Zhao, X., et al. (2017). Advances in roles of miR-132 in the nervous system. Front. Pharmacol. 8:770. doi: 10.3389/fphar.2017.00770
Qin, L.-X., Tan, J.-Q., Zhang, H.-N., Tang, J.-G., Jiang, B., Shen, X.-M., et al. (2021). Preliminary study of hsa-mir-626 change in the cerebrospinal fluid in Parkinson’s disease. Neurol. India 69, 115–118. doi: 10.4103/0028-3886.310102
Quévillon Huberdeau, M., and Simard, M. J. (2019). A guide to microRNA-mediated gene silencing. FEBS J. 286, 642–652. doi: 10.1111/febs.14666
Rachakonda, V., Pan, T. H., and Le, W. D. (2004). Biomarkers of neurodegenerative disorders: How good are they? Cell Res. 14, 349–358. doi: 10.1038/sj.cr.7290235
Radi, R. (2013). Protein tyrosine nitration: biochemical mechanisms and structural basis of functional effects. Acc. Chem. Res. 46, 550–559. doi: 10.1021/ar300234c
Raheja, R., Regev, K., Healy, B. C., Mazzola, M. A., Beynon, V., Von Glehn, F., et al. (2018). Correlating serum micrornas and clinical parameters in amyotrophic lateral sclerosis. Muscle Nerve 58, 261–269. doi: 10.1002/mus.26106
Ramachandran, A. K., Das, S., Joseph, A., Gurupur Gautham, S., Alex, A. T., and Mudgal, J. (2021). Neurodegenerative pathways in Alzheimer’s disease: a review. Curr. Neuropharmacol. 19, 679–692. doi: 10.2174/1570159X18666200807130637
Rao, P., Benito, E., and Fischer, A. (2013). MicroRNAs as biomarkers for CNS disease. Front. Mol. Neurosci. 6:39. doi: 10.3389/fnmol.2013.00039
Ravnik-Glavač, M., and Glavač, D. (2020). Circulating RNAs as potential biomarkers in amyotrophic lateral sclerosis. Int. J. Mol. Sci. 21:1714. doi: 10.3390/ijms21051714
Reed, E. R., Latourelle, J. C., Bockholt, J. H., Bregu, J., Smock, J., Paulsen, J. S., et al. (2018). MicroRNAs in CSF as prodromal biomarkers for Huntington disease in the PREDICT-HD study. Neurology 90, e264–e272. doi: 10.1212/WNL.0000000000004844
Regev, K., Healy, B. C., Paul, A., Diaz-Cruz, C., Mazzola, M. A., Raheja, R., et al. (2018). Identification of MS-specific serum miRNAs in an international multicenter study. Neurol. Neuroimmunol. Neuroinflam. 5:e491. doi: 10.1212/NXI.0000000000000491
Ricci, C., Marzocchi, C., and Battistini, S. (2018). MicroRNAs as biomarkers in amyotrophic lateral sclerosis. Cells 7:219. doi: 10.3390/cells7110219
Rio, D. C., Ares, M., Hannon, G. J., and Nilsen, T. W. (2010, 2010). Purification of RNA using TRIzol (TRI reagent). Cold Spring Harb. Protoc. 6:pdb.prot5439. doi: 10.1101/pdb.prot5439
Ripa, R., Dolfi, L., Terrigno, M., Pandolfini, L., Savino, A., Arcucci, V., et al. (2017). MicroRNA miR-29 controls a compensatory response to limit neuronal iron accumulation during adult life and aging. BMC Biol. 15:9. doi: 10.1186/s12915-017-0354-x
Rohm, M., May, C., Marcus, K., Steinbach, S., Theis, V., Theiss, C., et al. (2019). The microRNA miR-375-3p and the tumor suppressor NDRG2 are involved in sporadic amyotrophic lateral sclerosis. Cell. Physiol. Biochem. 52, 1412–1426. doi: 10.33594/000000099
Roos, R. A. (2010). Huntington’s disease: a clinical review. Orphanet J. Rare Dis. 5:40. doi: 10.1186/1750-1172-5-40
Rosenblum, L. T., and Trotti, D. (2017). EAAT2 and the molecular signature of amyotrophic lateral sclerosis. Adv. Neurobiol. 16, 117–136. doi: 10.1007/978-3-319-55769-4_6
Roser, A. E., Caldi Gomes, L., Schünemann, J., Maass, F., and Lingor, P. (2018). Circulating miRNAs as diagnostic biomarkers for Parkinson’s disease. Front. Neurosci. 12:625. doi: 10.3389/fnins.2018.00625
Russell, A. P., Wada, S., Vergani, L., Hock, M. B., Lamon, S., Léger, B., et al. (2013). Disruption of skeletal muscle mitochondrial network genes and miRNAs in amyotrophic lateral sclerosis. Neurobiol. Dis. 49, 107–117. doi: 10.1016/j.nbd.2012.08.015
Saba, R., Goodman, C. D., Huzarewich, R. L. C. H., Robertson, C., and Booth, S. A. (2008). A miRNA signature of prion induced neurodegeneration. PLoS One 3:e3652. doi: 10.1371/journal.pone.0003652
Salem, A., Wilson, C. J., Rutledge, B. S., Dilliott, A., Farhan, S., Choy, W.-Y., et al. (2022). Matrin3: disorder and ALS pathogenesis. Front. Mol. Biosci. 8:794646. doi: 10.3389/fmolb.2021.794646
Salta, E., Sierksma, A., Vanden Eynden, E., and De Strooper, B. (2016). miR-132 loss de-represses ITPKB and aggravates amyloid and TAU pathology in Alzheimer’s brain. EMBO Mol. Med. 8, 1005–1018. doi: 10.15252/emmm.201606520
Santa-Maria, I., Alaniz, M. E., Renwick, N., Cela, C., Fulga, T. A., Van Vactor, D., et al. (2015). Dysregulation of microRNA-219 promotes neurodegeneration through post-transcriptional regulation of tau. J. Clin. Invest. 125, 681–686. doi: 10.1172/JCI78421
Santos, A. L., and Lindner, A. B. (2017). Protein posttranslational modifications: roles in aging and age-related disease. Oxidative Med. Cell. Longev. 2017:e5716409, 1–19. doi: 10.1155/2017/5716409
Santosh, B., Varshney, A., and Yadava, P. K. (2015). Non-coding RNAs: biological functions and applications. Cell Biochem. Funct. 33, 14–22. doi: 10.1002/cbf.3079
Sawada, S., Akimoto, T., Takahashi, M., Sakurai, R., Shinkai, S., Ushida, T., et al. (2014). Effect of aging and sex on circulating MicroRNAs in humans. Adv. Aging Res. 3, 152–159. doi: 10.4236/aar.2014.32023
Schedin-Weiss, S., Winblad, B., and Tjernberg, L. O. (2014). The role of protein glycosylation in Alzheimer disease. FEBS J. 281, 46–62. doi: 10.1111/febs.12590
Schipper, H. M., Maes, O. C., Chertkow, H. M., and Wang, E. (2007). MicroRNA expression in Alzheimer blood mononuclear cells. Gene Regul. Syst. Biol. 1, 263–274. doi: 10.4137/GRSB.S361
Schonrock, N., Humphreys, D. T., Preiss, T., and Götz, J. (2012). Target gene repression mediated by miRNAs miR-181c and miR-9 both of which are Down-regulated by amyloid-β. J. Mol. Neurosci. 46, 324–335. doi: 10.1007/s12031-011-9587-2
Schonrock, N., Ke, Y. D., Humphreys, D., Staufenbiel, M., Ittner, L. M., Preiss, T., et al. (2010). Neuronal MicroRNA deregulation in response to Alzheimer’s disease amyloid-β. PLoS One 5:e11070. doi: 10.1371/journal.pone.0011070
Schwarzenbach, H., da Silva, A. M., Calin, G., and Pantel, K. (2015). Data normalization strategies for MicroRNA quantification. Clin. Chem. 61, 1333–1342. doi: 10.1373/clinchem.2015.239459
Sempere, L. F., Freemantle, S., Pitha-Rowe, I., Moss, E., Dmitrovsky, E., and Ambros, V. (2004). Expression profiling of mammalian microRNAs uncovers a subset of brain-expressed microRNAs with possible roles in murine and human neuronal differentiation. Genome Biol. 5:R13. doi: 10.1186/gb-2004-5-3-r13
Sethi, P., and Lukiw, W. J. (2009). Micro-RNA abundance and stability in human brain: specific alterations in Alzheimer’s disease temporal lobe neocortex. Neurosci. Lett. 459, 100–104. doi: 10.1016/j.neulet.2009.04.052
Seyedaghamiri, F., Rajabi, M., and Mohaddes, G. (2023). Targeting novel microRNAs in developing novel Alzheimer’s disease treatments. Neurochem. Res. 48, 26–38. doi: 10.1007/s11064-022-03734-6
Shang, R., Lee, S., Senavirathne, G., and Lai, E. C. (2023). microRNAs in action: biogenesis, function and regulation. Nat. Rev. Genet. 24, 816–833. doi: 10.1038/s41576-023-00611-y
Sharma, S., and Lu, H. C. (2018). microRNAs in neurodegeneration: current findings and potential impacts. J. Alzheimers Dis. Parkinsonism 8:420. doi: 10.4172/2161-0460.1000420
Sharma, P., Parihar, A., and Khan, R. (2024). “Sampling methods and biomarkers for early detection of neurodegenerative disorders” in Smart diagnostics for neurodegenerative disorders. eds. A. Parihar, R. Khan, and A. K. Srivastava (Academic Press), 39–59.
Sheinerman, K. S., Toledo, J. B., Tsivinsky, V. G., Irwin, D., Grossman, M., Weintraub, D., et al. (2017). Circulating brain-enriched microRNAs as novel biomarkers for detection and differentiation of neurodegenerative diseases. Alzheimers Res. Ther. 9:89. doi: 10.1186/s13195-017-0316-0
Sheinerman, K. S., and Umansky, S. R. (2013). Early detection of neurodegenerative diseases: circulating brain-enriched microRNA. Cell Cycle 12, 1–2. doi: 10.4161/cc.23067
Shi, Z., Chen, T., Yao, Q., Zheng, L., Zhang, Z., Wang, J., et al. (2017). The circular RNA ciRS-7 promotes APP and BACE1 degradation in an NF-κB-dependent manner. FEBS J. 284, 1096–1109. doi: 10.1111/febs.14045
Shi, Y., Lan, F., Matson, C., Mulligan, P., Whetstine, J. R., Cole, P. A., et al. (2004). Histone demethylation mediated by the nuclear amine oxidase homolog LSD1. Cell 119, 941–953. doi: 10.1016/j.cell.2004.12.012
Shi, Z., Wang, J., Yan, Z., You, Y., Li, C., Qian, X., et al. (2012). MiR-128 inhibits tumor growth and angiogenesis by targeting p70S6K1. PLoS One 7:e32709. doi: 10.1371/journal.pone.0032709
Shioya, M., Obayashi, S., Tabunoki, H., Arima, K., Saito, Y., Ishida, T., et al. (2010). Aberrant microRNA expression in the brains of neurodegenerative diseases: miR-29a decreased in Alzheimer disease brains targets neurone navigator 3. Neuropathol. Appl. Neurobiol. 36, 320–330. doi: 10.1111/j.1365-2990.2010.01076.x
Shlevkov, E., Kramer, T., Schapansky, J., LaVoie, M. J., and Schwarz, T. L. (2016). Miro phosphorylation sites regulate Parkin recruitment and mitochondrial motility. Proc. Natl. Acad. Sci. 113, E6097–E6106. doi: 10.1073/pnas.1612283113
Shu, Y., Qian, J., and Wang, C. (2020). Aberrant expression of microRNA-132-3p and microRNA-146a-5p in Parkinson’s disease patients. Open Life Sci. 15, 647–653. doi: 10.1515/biol-2020-0060
Shusharina, N., Yukhnenko, D., Botman, S., Sapunov, V., Savinov, V., Kamyshov, G., et al. (2023). Modern methods of diagnostics and treatment of neurodegenerative diseases and depression. Diagnostics 13:573. doi: 10.3390/diagnostics13030573
Siegel, S. R., Mackenzie, J., Chaplin, G., Jablonski, N. G., and Griffiths, L. (2012). Circulating microRNAs involved in multiple sclerosis. Mol. Biol. Rep. 39, 6219–6225. doi: 10.1007/s11033-011-1441-7
Silvestro, S., Bramanti, P., and Mazzon, E. (2019). Role of miRNAs in Alzheimer’s disease and possible Fields of application. Int. J. Mol. Sci. 20:3979. doi: 10.3390/ijms20163979
Simunkova, M., Alwasel, S. H., Alhazza, I. M., Jomova, K., Kollar, V., Rusko, M., et al. (2019). Management of oxidative stress and other pathologies in Alzheimer’s disease. Arch. Toxicol. 93, 2491–2513. doi: 10.1007/s00204-019-02538-y
Singh, R., Hussain, J., Kaur, A., Jamdare, B. G., Pathak, D., Garg, K., et al. (2023). The hidden players: shedding light on the significance of post-translational modifications and miRNAs in Alzheimer’s disease development. Ageing Res. Rev. 90:102002. doi: 10.1016/j.arr.2023.102002
Sinha, M., Ghose, J., and Bhattarcharyya, N. P. (2011). Micro RNA-214,-150,-146a and-125b target huntingtin gene. RNA Biol. 8, 1005–1021. doi: 10.4161/rna.8.6.16035
Sinha, M., Ghose, J., Das, E., and Bhattarcharyya, N. P. (2010). Altered microRNAs in STHdh(Q111)/Hdh(Q111) cells: miR-146a targets TBP. Biochem. Biophys. Res. Commun. 396, 742–747. doi: 10.1016/j.bbrc.2010.05.007
Smith, P., Al Hashimi, A., Girard, J., Delay, C., and Hébert, S. S. (2011). In vivo regulation of amyloid precursor protein neuronal splicing by microRNAs. J. Neurochem. 116, 240–247. doi: 10.1111/j.1471-4159.2010.07097.x
Smith, K. M., Guerau-de-Arellano, M., Costinean, S., Williams, J. L., Bottoni, A., Cox, G. M., et al. (2012). miR-29ab1-deficiency identifies a negative feedback loop controlling Th1 Bias that is dysregulated in multiple sclerosis. J. Immunol. 189, 1567–1576. doi: 10.4049/jimmunol.1103171
Smith-Vikos, T., Liu, Z., Parsons, C., Gorospe, M., Ferrucci, L., Gill, T. M., et al. (2016). A serum miRNA profile of human longevity: findings from the Baltimore longitudinal study of aging (BLSA). Aging 8, 2971–2987. doi: 10.18632/aging.101106
Smith-Vikos, T., and Slack, F. J. (2012). MicroRNAs and their roles in aging. J. Cell Sci. 125, 7–17. doi: 10.1242/jcs.099200
Søndergaard, H. B., Hesse, D., Krakauer, M., Sørensen, P. S., and Sellebjerg, F. (2013). Differential microRNA expression in blood in multiple sclerosis. Mult. Scler. J. 19, 1849–1857. doi: 10.1177/1352458513490542
Soni, D. K., and Biswas, R. (2021). Role of non-coding RNAs in post-transcriptional regulation of lung diseases. Front. Genet. 12:767348. doi: 10.3389/fgene.2021.767348
Soreq, L., Salomonis, N., Bronstein, M., Greenberg, D., Israel, Z., Bergman, H., et al. (2013). Small RNA sequencing-microarray analyses in Parkinson leukocytes reveal deep brain stimulation-induced splicing changes that classify brain region transcriptomes. Front. Mol. Neurosci. 6:10. doi: 10.3389/fnmol.2013.00010
Southwell, A. L., Smith, S. E. P., Davis, T. R., Caron, N. S., Villanueva, E. B., Xie, Y., et al. (2015). Ultrasensitive measurement of huntingtin protein in cerebrospinal fluid demonstrates increase with Huntington disease stage and decrease following brain huntingtin suppression. Sci. Rep. 5:12166. doi: 10.1038/srep12166
Sowell, E. R., Thompson, P. M., and Toga, A. W. (2004). Mapping changes in the human cortex throughout the span of life. Neuroscientist 10, 372–392. doi: 10.1177/1073858404263960
Spector, R., Robert Snodgrass, S., and Johanson, C. E. (2015). A balanced view of the cerebrospinal fluid composition and functions: focus on adult humans. Exp. Neurol. 273, 57–68. doi: 10.1016/j.expneurol.2015.07.027
Štětkářová, I., and Ehler, E. (2021). Diagnostics of amyotrophic lateral sclerosis: up to date. Diagnostics 11:231. doi: 10.3390/diagnostics11020231
Su, D., Chai, Y., Yang, J., Wang, X., Liu, Y., Ma, J., et al. (2021). Lentivirus-carried microRNA-195 rescues memory deficits of Alzheimer’s disease transgenic mouse by attenuating the generation of amyloid plaques. Front. Pharmacol. 12:633805. doi: 10.3389/fphar.2021.633805
Subramanian, S., Mahadevan, A., Satishchandra, P., and Shankar, S. K. (2016). Development of a dot blot assay with antibodies to recombinant "core" 14-3-3 protein: evaluation of its usefulness in diagnosis of Creutzfeldt-Jakob disease. Ann. Indian Acad. Neurol. 19, 205–210. doi: 10.4103/0972-2327.176867
Sun, J., Cheng, B., Su, Y., Li, M., Ma, S., Zhang, Y., et al. (2022). The potential role of m6A RNA methylation in the aging process and aging-associated diseases. Front. Genet. 13:869950. doi: 10.3389/fgene.2022.869950
Sun, F., Yu, M., Yu, J., Liu, Z., Zhou, X., Liu, Y., et al. (2018). miR-338-3p functions as a tumor suppressor in gastric cancer by targeting PTP1B. Cell Death Dis. 9:522. doi: 10.1038/s41419-018-0611-0
Suzuki, F., Okuno, M., Tanaka, T., and Sanuki, R. (2020). Overexpression of neural miRNAs miR-9/9* and miR-124 suppresses differentiation to Müller glia and promotes differentiation to neurons in mouse retina in vivo. Genes Cells 25, 741–752. doi: 10.1111/gtc.12809
Swarbrick, S., Wragg, N., Ghosh, S., and Stolzing, A. (2019). Systematic review of miRNA as biomarkers in Alzheimer’s disease. Mol. Neurobiol. 56, 6156–6167. doi: 10.1007/s12035-019-1500-y
Tabrizi, S. J., Flower, M. D., Ross, C. A., and Wild, E. J. (2020). Huntington disease: new insights into molecular pathogenesis and therapeutic opportunities. Nature reviews. Neurology 16, 529–546. doi: 10.1038/s41582-020-0389-4
Taj, A., Rehman, A., and Bajwa, S. Z. (2020). “Biomarkers and their role in detection of biomolecules” in Nanobiosensors: From Design to Applications. eds. A. Wu and W. S. Khan John Wiley & Sons, Ltd., 73–94.
Takahashi, I., Hama, Y., Matsushima, M., Hirotani, M., Kano, T., Hohzen, H., et al. (2015). Identification of plasma microRNAs as a biomarker of sporadic amyotrophic lateral sclerosis. Mol. Brain 8:67. doi: 10.1186/s13041-015-0161-7
Takeda, T., and Tanabe, H. (2016). Lifespan and reproduction in brain-specific miR-29-knockdown mouse. Biochem. Biophys. Res. Commun. 471, 454–458. doi: 10.1016/j.bbrc.2016.02.055
Tan, L., Yu, J.-T., and Tan, L. (2015). Causes and consequences of MicroRNA dysregulation in neurodegenerative diseases. Mol. Neurobiol. 51, 1249–1262. doi: 10.1007/s12035-014-8803-9
Tao, C. C., Hsu, W. L., Ma, Y. L., Cheng, S. J., and Lee, E. H. (2017). Epigenetic regulation of HDAC1 SUMOylation as an endogenous neuroprotection against Aβ toxicity in a mouse model of Alzheimer’s disease. Cell Death Different. 24, 597–614. doi: 10.1038/cdd.2016.161
Tarawneh, R., and Holtzman, D. M. (2012). The clinical problem of symptomatic Alzheimer disease and mild cognitive impairment. Cold Spring Harb. Perspect. Med. 2:a006148. doi: 10.1101/cshperspect.a006148
Tasca, E., Pegoraro, V., Merico, A., and Angelini, C. (2016). Circulating microRNAs as biomarkers of muscle differentiation and atrophy in ALS. Clin. Neuropathol. 35, 22–30. doi: 10.5414/NP300889
Tellinghuisen, J., and Spiess, A.-N. (2019). qPCR data analysis: better results through iconoclasm. Biomol. Detect. Quantif. 17:100084. doi: 10.1016/j.bdq.2019.100084
Terry, R. D., Masliah, E., Salmon, D. P., Butters, N., DeTeresa, R., Hill, R., et al. (1991). Physical basis of cognitive alterations in alzheimer’s disease: synapse loss is the major correlate of cognitive impairment. Ann. Neurol. 30, 572–580. doi: 10.1002/ana.410300410
Thamilarasan, M., Koczan, D., Hecker, M., Paap, B., and Zettl, U. K. (2012). MicroRNAs in multiple sclerosis and experimental autoimmune encephalomyelitis. Autoimmun. Rev. 11, 174–179. doi: 10.1016/j.autrev.2011.05.009
Tian, W., Yang, X., Yang, H., Lv, M., Sun, X., and Zhou, B. (2021). Exosomal miR-338-3p suppresses non-small-cell lung cancer cells metastasis by inhibiting CHL1 through the MAPK signaling pathway. Cell Death Dis. 12:1030. doi: 10.1038/s41419-021-04314-2
Tiribuzi, R., Crispoltoni, L., Porcellati, S., Di Lullo, M., Florenzano, F., Pirro, M., et al. (2014). miR128 up-regulation correlates with impaired amyloid β(1-42) degradation in monocytes from patients with sporadic Alzheimer’s disease. Neurobiol. Aging 35, 345–356. doi: 10.1016/j.neurobiolaging.2013.08.003
Toivonen, J. M., Manzano, R., Oliván, S., Zaragoza, P., García-Redondo, A., and Osta, R. (2014). MicroRNA-206: a potential circulating biomarker candidate for amyotrophic lateral sclerosis. PLoS One 9:e89065. doi: 10.1371/journal.pone.0089065
Tolosa, E., Garrido, A., Scholz, S. W., and Poewe, W. (2021). Challenges in the diagnosis of Parkinson’s disease. Lancet Neurol. 20, 385–397. doi: 10.1016/S1474-4422(21)00030-2
Tribolet, L., Kerr, E., Cowled, C., Bean, A. G. D., Stewart, C. R., Dearnley, M., et al. (2020). MicroRNA biomarkers for infectious diseases: from basic research to biosensing. Front. Microbiol. 11:1197. doi: 10.3389/fmicb.2020.01197
Tripathi, A., Volsko, C., Garcia, J. P., Agirre, E., Allan, K. C., Tesar, P. J., et al. (2019). Oligodendrocyte intrinsic miR-27a controls myelination and Remyelination. Cell Rep. 29, 904–919.e9. doi: 10.1016/j.celrep.2019.09.020
Trist, B. G., Genoud, S., Roudeau, S., Rookyard, A., Abdeen, A., Cottam, V., et al. (2022). Altered SOD1 maturation and post-translational modification in amyotrophic lateral sclerosis spinal cord. Brain: a. J. Neurol. 145, 3108–3130. doi: 10.1093/brain/awac165
Tysnes, O.-B., and Storstein, A. (2017). Epidemiology of Parkinson’s disease. J. Neural Transmis. 124, 901–905. doi: 10.1007/s00702-017-1686-y
Vahsen, B. F., Gray, E., Thompson, A. G., Ansorge, O., Anthony, D. C., Cowley, S. A., et al. (2021). Non-neuronal cells in amyotrophic lateral sclerosis—from pathogenesis to biomarkers. Nat. Rev. Neurol. 17, 333–348. doi: 10.1038/s41582-021-00487-8
Vallelunga, A., Ragusa, M., Di Mauro, S., Iannitti, T., Pilleri, M., Biundo, R., et al. (2014). Identification of circulating microRNAs for the differential diagnosis of Parkinson’s disease and multiple system atrophy. Front. Cell. Neurosci. 8:156. doi: 10.3389/fncel.2014.00156
Varendi, K., Kumar, A., Härma, M.-A., and Andressoo, J.-O. (2014). miR-1, miR-10b, miR-155, and miR-191 are novel regulators of BDNF. Cell. Mol. Life Sci. 71, 4443–4456. doi: 10.1007/s00018-014-1628-x
Vasudevan, S. (2012). Posttranscriptional upregulation by MicroRNAs. WIREs. RNA 3, 311–330. doi: 10.1002/wrna.121
Vaz, A. R., Vizinha, D., Morais, H., Colaço, A. R., Loch-Neckel, G., Barbosa, M., et al. (2021). Overexpression of miR-124 in motor neurons plays a key role in ALS pathological processes. Int. J. Mol. Sci. 22:6128. doi: 10.3390/ijms22116128
Velázquez-Cruz, A., Baños-Jaime, B., Díaz-Quintana, A., De la Rosa, M. A., and Díaz-Moreno, I. (2021). Post-translational control of RNA-binding proteins and disease-related dysregulation. Front. Mol. Biosci. 8:658852. doi: 10.3389/fmolb.2021.658852
Veremeyko, T., Yung, A. W. Y., Dukhinova, M., Strekalova, T., and Ponomarev, E. D. (2019). The role of neuronal factors in the epigenetic reprogramming of microglia in the Normal and diseased central nervous system. Front. Cell. Neurosci. 13:453. doi: 10.3389/fncel.2019.00453
Verma, P., Augustine, G. J., Ammar, M.-R., Tashiro, A., and Cohen, S. M. (2015). A neuroprotective role for microRNA miR-1000 mediated by limiting glutamate excitotoxicity. Nat. Neurosci. 18, 379–385. doi: 10.1038/nn.3935
Visser, H., and Thomas, A. D. (2021). MicroRNAs and the DNA damage response: How is cell fate determined? DNA Repair 108:103245. doi: 10.1016/j.dnarep.2021.103245
Viswambharan, V., Thanseem, I., Vasu, M. M., Poovathinal, S. A., and Anitha, A. (2017). miRNAs as biomarkers of neurodegenerative disorders. Biomark. Med 11, 151–167. doi: 10.2217/bmm-2016-0242
von Bernhardi, R. (2008). Neurodegenerative diseases: from molecular concepts to therapeutic targets. (Neurology - Laboratory and Clinical Research Developments Series). Nova Publishers.
Vrabec, K., Boštjančič, E., Koritnik, B., Leonardis, L., Dolenc Grošelj, L., Zidar, J., et al. (2018). Differential expression of several miRNAs and the host genes AATK and DNM2 in leukocytes of sporadic ALS patients. Front. Mol. Neurosci. 11:106. doi: 10.3389/fnmol.2018.00106
Vu, L. T., and Bowser, R. (2017). Fluid-based biomarkers for amyotrophic lateral sclerosis. Neurotherapeutics 14, 119–134. doi: 10.1007/s13311-016-0503-x
Wahid, F., Shehzad, A., Khan, T., and Kim, Y. Y. (2010). MicroRNAs: synthesis, mechanism, function, and recent clinical trials. Biochimica et Biophysica Acta (BBA) - molecular. Cell Res. 1803, 1231–1243. doi: 10.1016/j.bbamcr.2010.06.013
Walden, H., and Muqit, M. M. K. (2017). Ubiquitin and Parkinson’s disease through the looking glass of genetics. Biochem. J. 474, 1439–1451. doi: 10.1042/BCJ20160498
Waller, R., Goodall, E. F., Milo, M., Cooper-Knock, J., Da Costa, M., Hobson, E., et al. (2017). Serum miRNAs miR-206, 143-3p and 374b-5p as potential biomarkers for amyotrophic lateral sclerosis (ALS). Neurobiol. Aging 55, 123–131. doi: 10.1016/j.neurobiolaging.2017.03.027
Waller, R., Wyles, M., Heath, P. R., Kazoka, M., Wollff, H., Shaw, P. J., et al. (2018). Small RNA sequencing of sporadic amyotrophic lateral sclerosis cerebrospinal fluid reveals differentially expressed miRNAs related to neural and glial activity. Front. Neurosci. 11:731. doi: 10.3389/fnins.2017.00731
Wang, X. (2014). Composition of seed sequence is a major determinant of microRNA targeting patterns. Bioinformatics 30, 1377–1383. doi: 10.1093/bioinformatics/btu045
Wang, H., and Chen, Y.-H. (2021). microRNA biomarkers in clinical study. Biomol. Ther. 11:1810. doi: 10.3390/biom11121810
Wang, J., Chen, J., and Sen, S. (2016). MicroRNA as biomarkers and diagnostics. J. Cell. Physiol. 231, 25–30. doi: 10.1002/jcp.25056
Wang, B., Howel, P., Bruheim, S., Ju, J., Owen, L. B., Fodstad, O., et al. (2011). Systematic evaluation of three microRNA profiling platforms: microarray, beads Array, and quantitative real-time PCR Array. PLoS One 6:e17167. doi: 10.1371/journal.pone.0017167
Wang, W.-X., Huang, Q., Hu, Y., Stromberg, A. J., and Nelson, P. T. (2011). Patterns of microRNA expression in normal and early Alzheimer’s disease human temporal cortex: White matter versus gray matter. Acta Neuropathol. 121, 193–205. doi: 10.1007/s00401-010-0756-0
Wang, G., Huang, Y., Wang, L.-L., Zhang, Y.-F., Xu, J., Zhou, Y., et al. (2016). MicroRNA-146a suppresses ROCK1 allowing hyperphosphorylation of tau in Alzheimer’s disease. Sci. Rep. 6:26697. doi: 10.1038/srep26697
Wang, J., Li, H.-Y., Wang, H.-S., and Su, Z.-B. (2018). MicroRNA-485 modulates the TGF-β/Smads signaling pathway in chronic asthmatic mice by targeting Smurf2. Cell. Physiol. Biochem. 51, 692–710. doi: 10.1159/000495327
Wang, L., and Liang, Y. (2022). MicroRNAs as T lymphocyte regulators in multiple sclerosis. Front. Mol. Neurosci. 15:865529. doi: 10.3389/fnmol.2022.865529
Wang, Y., and Mandelkow, E. (2016). Tau in physiology and pathology. Nat. Rev. Neurosci. 17, 5–21. doi: 10.1038/nrn.2015.1
Wang, H., Peng, R., Wang, J., Qin, Z., and Xue, L. (2018). Circulating microRNAs as potential cancer biomarkers: the advantage and disadvantage. Clin. Epigenetics 10:59. doi: 10.1186/s13148-018-0492-1
Wang, M., Qin, L., and Tang, B. (2019). MicroRNAs in Alzheimer’s disease. Front. Genet. 10:153. doi: 10.3389/fgene.2019.00153
Wang, W.-X., Rajeev, B. W., Stromberg, A. J., Ren, N., Tang, G., Huang, Q., et al. (2008). The expression of MicroRNA miR-107 decreases early in Alzheimer’s disease and May accelerate disease progression through regulation of β-site amyloid precursor protein-cleaving enzyme 1. J. Neurosci. 28, 1213–1223. doi: 10.1523/JNEUROSCI.5065-07.2008
Wang, X., Tan, L., Lu, Y., Peng, J., Zhu, Y., Zhang, Y., et al. (2015). MicroRNA-138 promotes tau phosphorylation by targeting retinoic acid receptor alpha. FEBS Lett. 589, 726–729. doi: 10.1016/j.febslet.2015.02.001
Wang, Y., Veremeyko, T., Wong, A. H.-K., El Fatimy, R., Wei, Z., Cai, W., et al. (2017). Downregulation of miR-132/212 impairs S-nitrosylation balance and induces tau phosphorylation in Alzheimer’s disease. Neurobiol. Aging 51, 156–166. doi: 10.1016/j.neurobiolaging.2016.12.015
Wang, L., and Zhang, L. (2020). Circulating Exosomal miRNA as diagnostic biomarkers of neurodegenerative diseases. Front. Mol. Neurosci. 13:53. doi: 10.3389/fnmol.2020.00053
Weber, J. A., Baxter, D. H., Zhang, S., Huang, D. Y., How Huang, K., Jen Lee, M., et al. (2010). The MicroRNA Spectrum in 12 body fluids. Clin. Chem. 56, 1733–1741. doi: 10.1373/clinchem.2010.147405
Weidner, W. S., and Barbarino, P. (2019). P4-443: the state of the art of dementia research: new frontiers. Alzheimers Dement. 15:P1473. doi: 10.1016/j.jalz.2019.06.4115
Weinberg, R. B., Mufson, E. J., and Counts, S. E. (2015). Evidence for a neuroprotective microRNA pathway in amnestic mild cognitive impairment. Front. Neurosci. 9:430. doi: 10.3389/fnins.2015.00430
Wilkinson, K. A., and Henley, J. M. (2010). Mechanisms, regulation and consequences of protein SUMOylation. Biochem. J. 428, 133–145. doi: 10.1042/BJ20100158
Williams, A. H., Valdez, G., Moresi, V., Qi, X., McAnally, J., Elliott, J. L., et al. (2009). MicroRNA-206 delays ALS progression and promotes regeneration of neuromuscular synapses in mice. Science 326, 1549–1554. doi: 10.1126/science.1181046
Wirths, O., and Zampar, S. (2019). Emerging roles of N-and C-terminally truncated Aβ species in Alzheimer’s disease. Expert Opin. Ther. Targets 23, 991–1004. doi: 10.1080/14728222.2019.1702972
Witwer, K. W. (2015). Circulating MicroRNA biomarker studies: pitfalls and potential solutions. Clin. Chem. 61, 56–63. doi: 10.1373/clinchem.2014.221341
Wnuk, A., Rzemieniec, J., Staroń, J., Litwa, E., Lasoń, W., Bojarski, A., et al. (2019). Prenatal exposure to Benzophenone-3 impairs autophagy, disrupts RXRs/PPARγ signaling, and alters epigenetic and post-translational statuses in brain neurons. Mol. Neurobiol. 56, 4820–4837. doi: 10.1007/s12035-018-1401-5
Wu, S., Kim, T.-K., Wu, X., Scherler, K., Baxter, D., Wang, K., et al. (2016). Circulating MicroRNAs and life expectancy among identical twins. Ann. Hum. Genet. 80, 247–256. doi: 10.1111/ahg.12160
Wu, Q., Song, R., Ortogero, N., Zheng, H., Evanoff, R., Small, C. L., et al. (2012). The RNase III enzyme DROSHA is essential for microRNA production and spermatogenesis. J. Biol. Chem. 287, 25173–25190. doi: 10.1074/jbc.M112.362053
Wu, Z., Wang, S., Belmonte, J. C. I., Zhang, W., Qu, J., and Liu, G.-H. (2022). Emerging role of RNA m6A modification in aging regulation. Curr. Med. 1:8. doi: 10.1007/s44194-022-00009-8
Wu, Q., Ye, X., Xiong, Y., Zhu, H., Miao, J., Zhang, W., et al. (2016). The protective role of microRNA-200c in Alzheimer’s disease pathologies is induced by Beta amyloid-triggered endoplasmic reticulum stress. Front. Mol. Neurosci. 9:140. doi: 10.3389/fnmol.2016.00140
Xi, Y., Nakajima, G., Gavin, E., Morris, C. G., Kudo, K., Hayashi, K., et al. (2007). Systematic analysis of microRNA expression of RNA extracted from fresh frozen and formalin-fixed paraffin-embedded samples. RNA 13, 1668–1674. doi: 10.1261/rna.642907
Xu, W. L., von Strauss, E., Qiu, C. X., Winblad, B., and Fratiglioni, L. (2009). Uncontrolled diabetes increases the risk of Alzheimer’s disease: a population-based cohort study. Diabetologia 52, 1031–1039. doi: 10.1007/s00125-009-1323-x
Xu, X.-F., Wang, Y.-C., Zong, L., and Wang, X.-L. (2019). miR-151-5p modulates APH1a expression to participate in contextual fear memory formation. RNA Biol. 16, 282–294. doi: 10.1080/15476286.2019.1572435
Xu, Q., Zhao, Y., Zhou, X., Luan, J., Cui, Y., and Han, J. (2018). Comparison of the extraction and determination of serum exosome and miRNA in serum and the detection of miR-27a-3p in serum exosome of ALS patients. Intract. Rare Dis. Res. 7, 13–18. doi: 10.5582/irdr.2017.01091
Yang, Z., Li, T., Cui, Y., Li, S., Cheng, C., Shen, B., et al. (2019a). Elevated plasma microRNA-105-5p level in patients with idiopathic Parkinson’s disease: a potential disease biomarker. Front. Neurosci. 13:218. doi: 10.3389/fnins.2019.00218
Yang, Z., Li, T., Li, S., Wei, M., Qi, H., Shen, B., et al. (2019b). Altered expression levels of MicroRNA-132 and Nurr1 in peripheral blood of Parkinson’s disease: potential disease biomarkers. ACS Chem. Neurosci. 10, 2243–2249. doi: 10.1021/acschemneuro.8b00460
Yang, D., Li, T., Wang, Y., Tang, Y., Cui, H., Tang, Y., et al. (2012). miR-132 regulates the differentiation of dopamine neurons by directly targeting Nurr1 expression. J. Cell Sci. 125, 1673–1682. doi: 10.1242/jcs.086421
Yang, T. T., Liu, C. G., Gao, S. C., Zhang, Y., and Wang, P. C. (2018). The serum exosome derived MicroRNA−135a, −193b, and −384 were potential Alzheimer’s disease biomarkers. Biomed. Environ. Sci. 31, 87–96. doi: 10.3967/bes2018.011
Yang, J. X., Rastetter, R. H., and Wilhelm, D. (2016). “Non-coding RNAs: an introduction” in Non-coding RNA and the reproductive system. eds. D. Wilhelm and P. Bernard (Netherlands: Springer), 13–32.
Yang, D., Wang, W.-Z., Zhang, X.-M., Yue, H., Li, B., Lin, L., et al. (2014). MicroRNA expression aberration in Chinese patients with relapsing remitting multiple sclerosis. J. Mol. Neurosci. 52, 131–137. doi: 10.1007/s12031-013-0138-x
Yelick, J., Men, Y., Jin, S., Seo, S., Espejo-Porras, F., and Yang, Y. (2020). Elevated exosomal secretion of miR-124-3p from spinal neurons positively associates with disease severity in ALS. Exp. Neurol. 333:113414. doi: 10.1016/j.expneurol.2020.113414
Yi, R., Qin, Y., Macara, I. G., and Cullen, B. R. (2003). Exportin-5 mediates the nuclear export of pre-microRNAs and short hairpin RNAs. Genes Dev. 17, 3011–3016. doi: 10.1101/gad.1158803
Yin, L., Sun, Y., Wu, J., Yan, S., Deng, Z., Wang, J., et al. (2015). Discovering novel microRNAs and age-related nonlinear changes in rat brains using deep sequencing. Neurobiol. Aging 36, 1037–1044. doi: 10.1016/j.neurobiolaging.2014.11.001
Ying, S.-Y., Chang, D. C., and Lin, S.-L. (2008). The MicroRNA (miRNA): overview of the RNA genes that modulate gene function. Mol. Biotechnol. 38, 257–268. doi: 10.1007/s12033-007-9013-8
Yu, F., Jia, X., Du, F., Wang, J., Wang, Y., Ai, W., et al. (2013). microRNA-155 deficiency in bone marrow results in enhanced tumor metastasis. Mol. Cancer Res. 11, 923–936. doi: 10.1158/1541-7786.MCR-12-0686
Zakharova, M. N., and Abramova, A. A. (2021). Lower and upper motor neuron involvement and their impact on disease prognosis in amyotrophic lateral sclerosis. Neural Regen. Res. 17, 65–73. doi: 10.4103/1673-5374.314289
Zetterberg, H., and Blennow, K. (2006). Plasma Aβ in Alzheimer’s disease—up or down? Lancet Neurol. 5, 638–639. doi: 10.1016/S1474-4422(06)70503-8
Zhang, J., Cao, Z., Yang, G., You, L., Zhang, T., and Zhao, Y. (2019). MicroRNA-27a (miR-27a) in solid tumors: a review based on mechanisms and clinical observations. Front. Oncol. 9:893. doi: 10.3389/fonc.2019.00893
Zhang, X., Huang, X., Fang, C., Li, Q., Cui, J., Sun, J., et al. (2017). miR-124 regulates the expression of BACE1 in the Hippocampus under chronic cerebral Hypoperfusion. Mol. Neurobiol. 54, 2498–2506. doi: 10.1007/s12035-016-9845-y
Zhang, X., Ji, R., Liao, X., Castillero, E., Kennel, P. J., Brunjes, D. L., et al. (2018). MicroRNA-195 regulates metabolism in failing myocardium via alterations in Sirtuin 3 expression and mitochondrial protein acetylation. Circulation 137, 2052–2067. doi: 10.1161/CIRCULATIONAHA.117.030486
Zhang, W.-H., Jiang, L., Li, M., and Liu, J. (2023). MicroRNA-124: An emerging therapeutic target in central nervous system disorders. Exp. Brain Res. 241, 1215–1226. doi: 10.1007/s00221-022-06524-2
Zhang, R., Jin, H., and Lou, F. (2018). The Long non-coding RNA TP73-AS1 interacted with miR-142 to modulate brain glioma growth through HMGB1/RAGE pathway. J. Cell. Biochem. 119, 3007–3016. doi: 10.1002/jcb.26021
Zhang, W. T., Wang, Y. J., Yao, Y. F., Zhang, G. X., Zhang, Y. N., and Gao, S. S. (2022). Circulating microRNAs as potential biomarkers for the diagnosis of Parkinson’s disease: a meta-analysis. Neurologia. doi: 10.1016/j.nrl.2022.03.002
Zhang, Y., Xing, H., Guo, S., Zheng, Z., Wang, H., and Xu, D. (2016). MicroRNA-135b has a neuroprotective role via targeting of β-site APP-cleaving enzyme 1. Exp. Ther. Med. 12, 809–814. doi: 10.3892/etm.2016.3366
Zhang, H., Yang, H., Zhang, C., Jing, Y., Wang, C., Liu, C., et al. (2015). Investigation of MicroRNA expression in human serum during the aging process. J. Gerontol. 70, 102–109. doi: 10.1093/gerona/glu145
Zhang, F., Yao, Y., Miao, N., Wang, N., Xu, X., and Yang, C. (2022). Neuroprotective effects of microRNA 124 in Parkinson’s disease mice. Arch. Gerontol. Geriatr. 99:104588. doi: 10.1016/j.archger.2021.104588
Zhang, L., Zhang, J., Wang, K., and Wang, R. (2020). Serum microRNA-30c-5p and microRNA-373 expressions as potential biomarkers for Parkinson’s disease. All Life 13, 194–200. doi: 10.1080/26895293.2020.1741453
Zhao, Y., Alexandrov, P. N., Jaber, V., and Lukiw, W. J. (2016). Deficiency in the ubiquitin conjugating enzyme UBE2A in Alzheimer’s disease (AD) is linked to deficits in a natural circular miRNA-7 sponge (circRNA; ciRS-7). Genes 7:116. doi: 10.3390/genes7120116
Zhao, J., He, Z., and Wang, J. (2021). MicroRNA-124: a key player in microglia-mediated inflammation in neurological diseases. Front. Cell. Neurosci. 15:771898. doi: 10.3389/fncel.2021.771898
Zhao, Y., Samal, E., and Srivastava, D. (2005). Serum response factor regulates a muscle-specific microRNA that targets Hand2 during cardiogenesis. Nature 436, 214–220. doi: 10.1038/nature03817
Zhao, C., Sun, G., Li, S., and Shi, Y. (2009). A feedback regulatory loop involving microRNA-9 and nuclear receptor TLX in neural stem cell fate determination. Nat. Struct. Mol. Biol. 16, 365–371. doi: 10.1038/nsmb.1576
Zhao, N., Wang, R., Zhou, L., Zhu, Y., Gong, J., and Zhuang, S.-M. (2014). MicroRNA-26b suppresses the NF-κB signaling and enhances the chemosensitivity of hepatocellular carcinoma cells by targeting TAK1 and TAB3. Mol. Cancer 13:35. doi: 10.1186/1476-4598-13-35
Zhao, J., Yue, D., Zhou, Y., Jia, L., Wang, H., Guo, M., et al. (2017). The role of MicroRNAs in Aβ deposition and tau phosphorylation in Alzheimer’s disease. Front. Neurol. 8:342. doi: 10.3389/fneur.2017.00342
Zheng, J. C., and Chen, S. (2022). Translational neurodegeneration in the era of fast growing international brain research. Transl. Neurodegen. 11:1. doi: 10.1186/s40035-021-00276-9
Zheng, Q., Huang, T., Zhang, L., Zhou, Y., Luo, H., Xu, H., et al. (2016). Dysregulation of ubiquitin-proteasome system in neurodegenerative diseases. Front. Aging Neurosci. 8:303. doi: 10.3389/fnagi.2016.00303
Zhou, Y., Deng, J., Chu, X., Zhao, Y., and Guo, Y. (2019). Role of post-transcriptional control of Calpain by miR-124-3p in the development of Alzheimer’s disease. J. Alzheimers Dis. 67, 571–581. doi: 10.3233/JAD-181053
Zhou, Y., Wang, Z.-F., Li, W., Hong, H., Chen, J., Tian, Y., et al. (2018). Protective effects of microRNA-330 on amyloid β-protein production, oxidative stress, and mitochondrial dysfunction in Alzheimer’s disease by targeting VAV1 via the MAPK signaling pathway. J. Cell. Biochem. 119, 5437–5448. doi: 10.1002/jcb.26700
Zhou, X., Wen, W., Shan, X., Zhu, W., Xu, J., Guo, R., et al. (2016). A six-microRNA panel in plasma was identified as a potential biomarker for lung adenocarcinoma diagnosis. Oncotarget 8, 6513–6525. doi: 10.18632/oncotarget.14311
Zhou, F., Zhang, C., Guan, Y., Chen, Y., Lu, Q., Jie, L., et al. (2018). Screening the expression characteristics of several miRNAs in G93A-SOD1 transgenic mouse: altered expression of miRNA-124 is associated with astrocyte differentiation by targeting Sox2 and Sox9. J. Neurochem. 145, 51–67. doi: 10.1111/jnc.14229
Zhu, Q.-B., Unmehopa, U., Bossers, K., Hu, Y.-T., Verwer, R., Balesar, R., et al. (2016). MicroRNA-132 and early growth response-1 in nucleus basalis of Meynert during the course of Alzheimer’s disease. Brain 139, 908–921. doi: 10.1093/brain/awv383
Zhu, W., Zheng, D., Wang, D., Yang, L., Zhao, C., and Huang, X. (2021). Emerging roles of ubiquitin-specific protease 25 in diseases. Front. Cell Dev. Biol. 9:698751. doi: 10.3389/fcell.2021.698751
Zia, A., Farkhondeh, T., Sahebdel, F., Pourbagher-Shahri, A. M., and Samarghandian, S. (2022). Key miRNAs in modulating aging and longevity: a focus on signaling pathways and cellular targets. Curr. Mol. Pharmacol. 15, 736–762. doi: 10.2174/1874467214666210917141541
Zingale, V. D., Gugliandolo, A., and Mazzon, E. (2022). MiR-155: An important regulator of Neuroinflammation. Int. J. Mol. Sci. 23:90. doi: 10.3390/ijms23010090
Zong, Y., Wang, H., Dong, W., Quan, X., Zhu, H., Xu, Y., et al. (2011). miR-29c regulates BACE1 protein expression. Brain Res. 1395, 108–115. doi: 10.1016/j.brainres.2011.04.035
Zovoilis, A., Agbemenyah, H. Y., Agis-Balboa, R. C., Stilling, R. M., Edbauer, D., Rao, P., et al. (2011). microRNA-34c is a novel target to treat dementias. EMBO J. 30, 4299–4308. doi: 10.1038/emboj.2011.327
Zubenko, G. S., Zubenko, W. N., McPherson, S., Spoor, E., Marin, D. B., Farlow, M. R., et al. (2003). A collaborative study of the emergence and clinical features of the major depressive syndrome of Alzheimer’s disease. Am. J. Psychiatry 160, 857–866. doi: 10.1176/appi.ajp.160.5.857
Glossary
Keywords: neurodegenerative diseases, microRNA, biomarkers, nervous system, diagnostic tools, therapeutic tools, protein post-translational modifications, limitations
Citation: Azam HMH, Rößling RI, Geithe C, Khan MM, Dinter F, Hanack K, Prüß H, Husse B, Roggenbuck D, Schierack P and Rödiger S (2024) MicroRNA biomarkers as next-generation diagnostic tools for neurodegenerative diseases: a comprehensive review. Front. Mol. Neurosci. 17:1386735. doi: 10.3389/fnmol.2024.1386735
Edited by:
Gabriela Pavlinkova, Institute of Biotechnology (ASCR), CzechiaReviewed by:
Ravi Kiran Kasula, Georgetown University, United StatesGonçalo Garcia, Research Institute for Medicines (iMed.ULisboa), Portugal
Copyright © 2024 Azam, Rößling, Geithe, Khan, Dinter, Hanack, Prüß, Husse, Roggenbuck, Schierack and Rödiger. This is an open-access article distributed under the terms of the Creative Commons Attribution License (CC BY). The use, distribution or reproduction in other forums is permitted, provided the original author(s) and the copyright owner(s) are credited and that the original publication in this journal is cited, in accordance with accepted academic practice. No use, distribution or reproduction is permitted which does not comply with these terms.
*Correspondence: Stefan Rödiger, c3RlZmFuLnJvZWRpZ2VyQGItdHUuZGU=