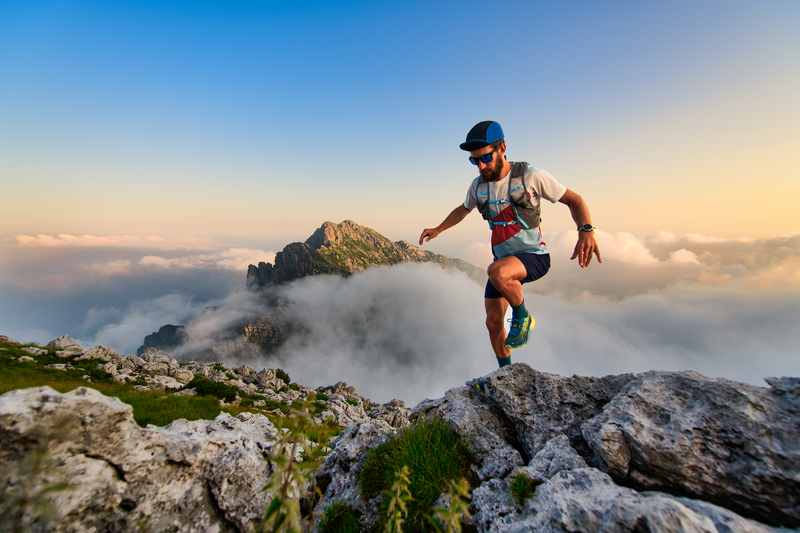
95% of researchers rate our articles as excellent or good
Learn more about the work of our research integrity team to safeguard the quality of each article we publish.
Find out more
ORIGINAL RESEARCH article
Front. Mol. Neurosci. , 04 January 2024
Sec. Brain Disease Mechanisms
Volume 16 - 2023 | https://doi.org/10.3389/fnmol.2023.1305949
This article is part of the Research Topic Astrocytes in neurological and neuropsychiatric disorders View all 4 articles
Microglia and astrocytes play an important role in the neuroinflammatory response and contribute to both the destruction of neighboring tissue as well as the resolution of inflammation following stroke. These reactive glial cells are highly heterogeneous at both the transcriptomic and functional level. Depending upon the stimulus, microglia and astrocytes mount a complex, and specific response composed of distinct microglial and astrocyte substates. These substates ultimately drive the landscape of the initiation and recovery from the adverse stimulus. In one state, inflammation- and damage-induced microglia release tumor necrosis factor (TNF), interleukin 1α (IL1α), and complement component 1q (C1q), together “TIC.” This cocktail of cytokines drives astrocytes into a neurotoxic reactive astrocyte (nRA) substate. This nRA substate is associated with loss of many physiological astrocyte functions (e.g., synapse formation and maturation, phagocytosis, among others), as well as a gain-of-function release of neurotoxic long-chain fatty acids which kill neighboring cells. Here we report that transgenic removal of TIC led to reduction of gliosis, infarct expansion, and worsened functional deficits in the acute and delayed stages following stroke. Our results suggest that TIC cytokines, and likely nRAs play an important role that may maintain neuroinflammation and inhibit functional motor recovery after ischemic stroke. This is the first report that this paradigm is relevant in stroke and that therapies against nRAs may be a novel means to treat patients. Since nRAs are evolutionarily conserved from rodents to humans and present in multiple neurodegenerative diseases and injuries, further identification of mechanistic role of nRAs will lead to a better understanding of the neuroinflammatory response and the development of new therapies.
Stroke is the second-leading cause of death worldwide, and one in four people who reach the age of 25 will have a stroke in their lifetime (Feigin et al., 2022). An ischemic stroke occurs when blood supply to a region of the brain ceases due to blockage of a blood vessel (usually by formation of a blood clot) and is one of the most common types of strokes. Current stroke therapies include intravenous and endovascular thrombolysis, and mechanical clot removal, but treatment only helps patients whose stroke has not completed prior to arriving at the hospital and endovascular therapies are not available at most hospitals. Current FDA approved treatments for ischemic stroke aim at returning blood flow to the affected area by breaking up the blood clot, with the only approved drug being recombinant tissue plasminogen activator (rt-PA). While the use of rt-PA is beneficial in stroke outcomes for some patients, there is a very small therapeutic window, with only 2% or less of ischemic stroke patients eligible to receive rt-PA (Wardlaw et al., 2012; Tseng et al., 2020; Xiong et al., 2022).
Initial cell death occurs within minutes of the onset of stroke, and these dead and dying cells make up the ischemic infarct core. Additional brain cells in the surrounding tissue, known as the peri-infarct area, are vulnerable during the acute and sub-acute period and their death leads to infarct expansion (Lakhan et al., 2009). Many pathophysiological mechanisms including oxidative stress, excitotoxity, hypoxia due to brain edema, and neuroinflammation all contribute to this cell death in the subacute period (Lakhan et al., 2009; Pantcheva et al., 2014; Khoshnam et al., 2017).
The neuroinflammatory response is complex and has both beneficial and detrimental attributes related to stroke recovery. In the CNS, the inflammatory response is primarily orchestrated by the release of cytokines from glial cells, such as microglia and astrocytes (Sun et al., 2015; Jayaraj et al., 2019). During post-stroke neuroinflammation, microglia alter their morphology, increase phagocytosis, and alter the types and levels cytokines they secrete (Stence et al., 2001; Zhang, 2019). During acute stages of ischemia, microglia may release anti-inflammatory cytokines, with a maintenance of proinflammatory cytokine release as the inflammatory response progresses (Hu et al., 2012). We and others have previously demonstrated that tumor necrosis factor (TNF), interleukin 1α (IL1α), and complement component 1q (C1q), together “TIC,” are released by microglia in the peri-infarct region following stroke, and are required and sufficient to transform astrocytes into a neurotoxic substate (Hu et al., 2012; Liddelow et al., 2017).
These neurotoxic reactive astrocytes (nRA) lose many normal physiological functions including their ability to induce and support synapses, reuptake and recycle glutamate, and downregulate phagocytosis pathways (Liddelow and Barres, 2017; Liddelow et al., 2017; Barbar et al., 2020; Guttenplan et al., 2021). On the other hand, nRAs have an intriguing gain-of-function ability, namely the release of fully saturated long chain free fatty acids which are sufficient to induce caspase-3 mediated lipoapoptotic neuron death (Guttenplan et al., 2021). In the context of stroke, this lipid-mediated cell death is potentially responsible for further expanding the infarct area.
To examine the role that these nRAs play in ischemic stroke, transgenic, triple knockout (TKO) mice unable to produce the TIC cytokines can be used. We previously used these TKO mice to determine that the release of the TIC cytokines by microglia are important in transforming astrocytes into the neurotoxic substate. In a mouse model of glaucoma, TKO mice uncovered that TIC cytokine-induced nRAs contributed to retinal ganglion cell neuron death (Guttenplan et al., 2020a; Sterling et al., 2020). The inhibition of microglia-derived TIC cytokines has also been achieved pharmacologically using a glucagon-like 1 peptide receptor agonist in the pre-formed alpha synuclein mouse model of Parkinson's disease. Here block of microglia-induced nRA reactivity was sufficient to decrease neuronal cell loss and improve motor outcomes (Yun et al., 2018). Given these promising results, and the localization of nRAs following stroke, we tested here whether TKO mice would have reduced astrogliosis, decreased infarct size, and improved motor outcomes after ischemic stroke compared to wild type (WT) mice.
Seventy-six female C57BL/6J mice (WT; Jackson Laboratory, Ellsworth, ME) and female transgenic mice (TNF−/−, IL1-α−/−, and C1q−/−; TKO) at approximately 8 weeks of age were used. Mice had ad libitum access to food and water, and were housed up to 5 animals per cage. Mice were housed at room temperature (22 ± 1°C) on a 12-h reverse light/dark cycle. All experimental procedures and protocols were approved by the Stanford University Institutional Animal Care and Use committee (IACUC).
To characterize the pathophysiological differences after stroke, the distal middle cerebral artery occlusion (dMCAO) model was used in the acute and subacute time points. Mice underwent permanent focal ischemia using the dMCAO model. WT (N = 15) and TKO (N = 16) mice were perfused 24 hours after dMCAO to examine the acute phase, and WT (N = 10) and TKO (N = 8) mice were perfused 7 days after dMCAO to examine the subacute phase (Figure 1A). The dMCAO model was selected for the acute and subacute timepoints because it is a more clinically relevant model for middle cerebral artery occlusion known for highly reproduceable cortical lesions (Doyle et al., 2012; Doyle and Buckwalter, 2014; Peterson et al., 2021). While long-term deficits have been demonstrated in the dMCAO model in at least one task (Lubjuhn et al., 2009), we have found that it does not reliably produce long-term behavioral deficits in mice (Doyle et al., 2012; Doyle and Buckwalter, 2014). While photothrombotic stroke is less pathologically or clinically relevant, it is a well-established model of ischemic stroke that results in significant motor deficits even at the chronic timepoint (Peterson et al., 2021). To characterize the pathological differences during the chronic time-point and test longitudinal motor outcomes, WT (N = 16) and TKO (N = 11) mice underwent a photothrombotic stroke over the motor cortex and behavior was assessed. Prior to sacrifice 30 days post-stroke, behavior assessments were performed prior to and 1-, 3-, 7-, 14-, and 28 days post-stroke. We assessed gross motor movement using the rotating beam task and fine motor movement was assessed using the tapered beam task and foot fault task. Animals were perfused with 0.1 M phosphate buffered saline 30 days after stroke and prepared for tissue processing and immunohistochemistry.
Figure 1. Experimental timeline and survival rate of animals 30 days after photothrombotic stroke. (A) Timeline showing 1- and 7-day sacrifice after dMCAO and 30-day sacrifice following photothrombotic stroke. Immunohistochemistry was performed to examine infarct volume (NeuN/cresyl violet), astrogliosis (GFAP), and microgliosis (CD68). Functional recovery assessment was performed up to 28 days after photothrombotic stroke include the rotating beam, tapered beam, and grid walk tasks. Survival rates were not significantly different between wildtype (WT) and Tnf−/−Il1a−/−C1q−/− triple knockout (TKO) mice 1 (B) or 7 days after dMCAO (C), or 30 days (D) after photothrombotic stroke (X2= 1.85, p = 0.17). IHC; immunohistochemistry, GFAP; glial fibrillary acidic protein, CD68; Cluster of Differentiation 68, d, day; dMCAO, distal middle cerebral artery occlusion; TKO, Tnf−/−Il1a−/−C1q−/− triple knockout mouse; WT, wild type.
Mice were anesthetized using 2% Isoflurane in 2 L/min oxygen and temperature was maintained at 37°C. For the 24 h and 7-day sacrifice timepoints, mice underwent a dMCAO to create a primary cortical infarct as described (Doyle and Buckwalter, 2014; Peterson et al., 2021). Briefly, an incision was made in the skin over the temporalis muscle to expose the medial cerebral artery through the skull. A craniotomy was performed, and the medial cerebral artery was then cauterized. The temporalis muscle and skin were replaced, and the incision closed. Buprenorphine (0.1 mg/kg) and Cefazolin (25 mg/kg) were injected subcutaneously.
To assess chronic inflammation and functional recovery, the photothrombotic stroke model was performed targeting the motor cortex. Mice were injected intraperitonially with Rose Bengal (10 mg/ml in sterile saline; 40 mg/kg of body weight), anesthetized, and then mounted on a stereotactic frame. A Metal Halide Fiber optic light (No. 56371, Edmund Optics) with a 1 mm diameter was directly placed over the right motor cortex (−0.5 mm anterior, +1.5 mm lateral to bregma) for 15 min. The incision was closed and a subcutaneous injection of Buprenorphine (0.1 mg/kg) and Cefazolin (25 mg/kg) was administered.
Motor behavior tasks were performed in WT and TKO mice 1-, 3-, 7-, 14-, and 28 days after photothrombotic surgery and included the rotating beam, tapered beam, and foot fault tasks described previously (Schaar et al., 2010; Cheng et al., 2014, 2016; Peterson et al., 2021). For all tasks, there was one habituation day and two pre-training days that occurred prior to stroke induction. For the rotating beam task, animals crossed a rotating beam (6 rpm) longitudinally and the distance covered before falling was recorded for four trials per session. For the tapered beam test, the number of contralateral foot faults made as they progressed down the beam for four trials per session was recorded. For the foot fault task, animals were allowed to freely roam for 2 min and the number of contralateral forelimb faults was recorded.
Mice were anesthetized with 87.5 mg/kg Ketamine/12.5 mg/kg Xylazine and transcardially perfused with 10 U/mL heparin in 0.9% NaCl before the brain was dissected and drop fixed in 4% paraformaldehyde in phosphate buffer overnight. The brain was then transferred to 30% sucrose in phosphate buffered saline (PBS). Coronal sections (40 μm) were sectioned using a freezing sliding microtome (Microm HM430), and sequentially placed in 16 tubes filled with cryoprotective medium (30% glycerin, 30% ethylene glycol, 40% 0.5 M sodium phosphate buffer) and stored at −20°C until processing.
Free floating tissue was stained using standard immunohistochemistry procedures. Briefly, sections were washed and agitated in 3% serum (donkey, EMD Millipore, Cat# 566460, rabbit, Vector, Cat# S-5000; goat, Vector, Cat# S-1000) prior to antibody incubation. Primary antibodies (NeuN, 1:20,000, Millipore, MAB377B; CD68, 1:1,000, Serotec, MCA1957S; GFAP, 1:10,000, Dako Z0334) were diluted in 0.3% Triton X in PBS and incubated for 12 h at RT. After washing in PBS, secondary antibodies (NeuN n/a biotinylated primary; anti-rabbit, 1:500, Vector, BA-1000; anti-rat, 1:500, Boster, BA1058) were incubated for 60 min at RT. We then applied the Avidin-Biotin Complex (ABC; Vector Laboratories, Cat# PK-4000) for 60 min, and then 3,3′-Diaminobenzidine substrate (VWR, Cat# 97062-970) was applied for 5 min. For cresyl violet counter staining, mounted tissue was rehydrated before being stained in cresyl violet for 5 min and dehydrated through graduated ethanol before cover slipping with entellan mounting medium.
To measure stroke volume, 10 coronal sections spaced exactly 320 μm apart were stained with NeuN, and then counter stained with cresyl violet (see above). Sections were imaged using a PathScan Enabler IV (Meyer Instruments, Housten, TX) and the infarct and hemisphere volumes traced using Fiji (version 2.9.0/1.53t) (Schindelin et al., 2012). To control for edema on the side ipsilateral of stroke that commonly occurs in the dMCAO model, the percent of infarcted hemisphere {infarcted area [contralateral hemisphere area/(ipsilateral area without infarct + infarct area)]/contralateral hemisphere X 100} was used (Nouraee et al., 2019; Peterson et al., 2021). The photothrombotic stroke model has little edema associated with it 29 days after stroke, so the direct infarct volume was used for measurements.
To image macrophages and astrocytes, five sections spaced 640 μm apart were immunostained with CD68 and GFAP; respectively. Two images were taken (Keyence microscope) at 40 × magnification in the peri-infarct cortex, one medial and one lateral to the stroke core. Each image was one view field (543 μm) from the stroke border. Each data point collected is the mean of 10 images (5 sections × 2 images) that have been thresholded for each animal. Fiji was used to convert the images to 8-bit color (black and white) and blindly quantified images at a uniform threshold encompassing the area covered (density) of the soma and processes using Fiji (Peterson et al., 2021).
GraphPad Prism (Software, 2023) and RStudio (Team, 2020) were used to perform graphing and statistical analysis. Significance was determined with a p < 0.05 and values are expressed as the mean ± standard error. Survival data was analyzed using the Kaplan-Meier method. Immunohistochemical data were analyzed using a one-tailed Student's t test for 2 groups. For the behavior tasks, a linear mixed effects model was analyzed using RStudio and the lme4 R package (Bates et al., 2015). Mice were specified as a random factor to help account for unequal group sizes due to mortality. A one-way analysis of variance (ANOVA) was performed to test the differences between models using the lmerTest package (Kuznetsova et al., 2017). Where differences were found, a Dunnett's post-hoc test was performed to examine differences by day.
Survival rates of animals following ischemia can be indicative of stroke severity. To test if TKO animals had altered survival compared to WT animals, we used the dMCAO model of stroke to examine both an acute (24 h) and subacute (7 days) timepoint. We found no difference in the survival of WT and TKO mice at either the acute (zero mortality, Figure 1B) or subacute period (p = 0.54, Figure 1C). We also assessed survival rates following the photothrombotic model of stroke and tracked 26 animals out to 30 days. We found no difference in the survival of WT and TKO mice over this time period, (p = 0.174, Figure 1D). The mortality rates differed by stroke model and timepoint and led to unbalanced N within and between the groups. Our data suggests that while TIC cytokines play important roles in inflammation, the removal of these pro-inflammatory cytokines does not increase the chance of surviving following stroke.
To examine how TIC cytokines influence the neuroinflammatory response after stroke, the area covered by both macrophages and astrocytes in TKO and WT mice was measured. To assess astrocyte cytoskeletal structure, we immunohistostained for GFAP in the peri-infarct area during the acute (24 h) and subacute (7 days) phases following dMCAO, and the chronic (30 days) phase after photothrombotic stroke (Figures 2A, C, E). There was no difference in the amount of GFAP immunoreactivity 24 h post-stroke between the TKO and WT mice (p = 0.104, Figure 2B), however TKO mice had significantly reduced GFAP levels compared to the WT mice 7 days post-stroke (p = 0.011) and 30 days post-stroke (p < 0.0001; Figures 2D, F). While many have reported that alterations in GFAP levels alone are indicative of changes in astrocyte reactivity, we would caution this interpretation from this data alone.
Figure 2. TNF, IL1α, and C1q knockout reduces GFAP+ cell number in the peri-infarct area 7- and 30-days post ischemic stroke. Low- (5x) and high-magnification (40x) representative images of GFAP+ cells in the infarct and peri-infarct area for wildtype (WT) and Tnf−/−Il1a−/−C1q−/− triple knockout (TKO) mice 1- (A), 7-days post-dMCAO (C) and 30-days post-photothrombotic stroke (E). (B) No difference was found in GFAP+ cells in the peri-infarct area 1 day after ischemia between the WT and TKO mice, t(29) = 1.29, p = 0.104 post-dMCAO. (D) TKO mice had significantly reduced GFAP+ cells in the peri-infarct area than WT mice 7 days post-dMCAO, t(12) = 2.64, p = 0.011. (F) TKO mice had significantly reduced GFAP+ cells in the peri-infarct area than WT mice 30 days post-photothrombotic stroke, t(14) = 5.03, p < 0.0001. * p < 0.05, ** p < 0.001, *** p < 0.0001. GFAP; glial fibrillary acidic protein, TKO, Tnf−/−Il1a−/−C1q−/− triple knockout mouse; WT, wild type; DV, dorsoventral; ML, mediolateral.
To examine how TIC cytokines collectively influence myeloid cells following ischemic stroke, we used CD68+ immunostaining and quantified cell coverage in the peri-infarct at 24 h, and 7 days following dMCAO, and 30 days after photothrombotic stroke (Figures 3A, C, E). There was no difference in macrophage response, measured by the density of CD68 immunostaining at 24 h post-stroke between the TKO and WT mice (p = 0.285, Figure 3B), but TKO mice had significantly reduced CD68 immunostaining compared to WT mice 7 days post-stroke (p = 0.001) and 30 days post-stroke (p = 0.0001, Figures 3D, F). Similar to the use of a single marker, GFAP, for astrocyte reactivity, we would caution the interpretation of changes in myeloid cell reactivity states using CD68 alone. Thus, removal of TIC cytokines in the more chronic stages following stroke appears to alter the proinflammatory environment. Not only that, but while the initial upregulation at 24 h is normal, the TKO mice do not propagate the inflammatory signal 7- and 30 days post-stroke.
Figure 3. TNF, IL1α, and C1q knockout reduces CD68+ cells in the peri-infarct area 7- and 30-days post ischemic stroke. Low- (5x) and high-magnification (40x) representative images of CD68+ cells in the infarct and peri-infarct area for wildtype (WT) and Tnf−/−Il1a−/−C1q−/− triple knockout (TKO) mice 1- (A), 7-days post-dMCAO (C), and 30-days post-photothrombotic stroke (E). (B) No difference was found in CD68+ cells in the peri-infarct area 1 day after ischemia between the WT and TKO mice, t(29) = 0.29, p = 0.285, post-dMCAO. (D) TKO mice had significantly reduced CD68+ cells in the peri-infarct area than WT mice 7 days post-dMCAO, t(15) = 3.51, p = 0.002. (F) TKO mice had significantly reduced CD68+ cells in the peri-infarct area than WT mice 30 days post-photothrombotic stroke, t(19) = 4.55, p = 0.0001. * p < 0.05, ** p < 0.001, *** p < 0.0001. CD68; Cluster of Differentiation 68, TKO, Tnf−/−Il1a−/−C1q−/− triple knockout mouse; WT, wild type; DV, dorsoventral; ML, mediolateral.
Together with GFAP staining, these data suggest that in the absence of TIC cytokines, minimal differences occur in astrocyte GFAP and myeloid cell CD68 protein levels immediately following stroke. However, there was a decrease in these astrocyte and myeloid cell markers from 1 week onwards suggesting that the inflammatory response resolves much more quickly in the TKO mice.
Given our discovery of altered astrocyte and myeloid cell responses in the absence of TIC cytokines, we next decided to measure infarct expansion in these animals. To assess how TIC cytokines can influence infarct volume during the acute, subacute, and chronic phases after stroke, we used immunohistochemistry of NeuN+ neurons with a cresyl violet counterstain to measure the gross infarct volume. TKO mice had significantly reduced infarct volumes 24 h (p = 0.002) and 7 days post-stroke (p < 0.0001; Figures 4A–C) using the dMCAO model. During the chronic phase of stroke, TKO mice were also found to have a significantly reduced infarct volume 30 days post photothrombotic stroke of the motor cortex (p = 0.008, Figures 4A, D). These data suggest that TIC cytokines directly, or TIC cytokine-induced nRAs are consequential to the viability of neurons and other cells in the infarct area following ischemic insult in the brain.
Figure 4. TNF, IL1α, and C1q knockout reduces infarct volume 1-, 7-, and 30-days post stroke. (A) Representative images of stroke volume using NeuN/cresyl violet stain of wildtype (WT) and Tnf−/−Il1a−/−C1a−/− triple knockout (TKO) mice 1-, 7-days post-dMCAO, and 30-days post-photothrombotic stroke. (B) TKO mice had significantly reduced infarct volume compared to WT mice 1-day post-dMCAO, t(29) = 2.09, p = 0.022. (C) TKO mice had significantly reduced infarct volume than WT mice 7 days post-dMCAO, t(12) = 4.64, p < 0.0001. (D) TKO mice had a significantly reduced infarct volume compared to the WT mice 30 days post-photothrombotic stroke, t(15) = 2.712, p = 0.008. * p < 0.05, ** p < 0.001, *** p < 0.0001. dMCAO, distal middle cerebral artery occlusion; TKO, Tnf−/−Il1a−/−C1q−/− triple knockout mouse; WT, wild type.
These data suggest that in line with our hypothesis, TIC induced nRAs play a key role in cell death and infarct expansion following stroke. Given these results, we next wanted to determine if this decrease in infarct expansion would correlate with improvements in motor function.
Since deletion of TIC cytokines decreased infarct volume following dMCAO, we hypothesized that this would lead to improved functional recovery following stroke in the photothrombotic stroke model. We examined motor function using the, a photothrombotic stroke of the motor cortex in adult mice. We quantified limb function contralateral to the stroke 1-, 3-, 7-, 14-, and 28 days post-stroke using a battery of behavioral tests. TKO mice traveled significantly longer on the rotating beam compared to WT mice 1- and 3 days post-stroke (p = 0.026, Figure 5A). TKO mice had significantly fewer contralateral limb errors on the tapered beam compared to WT mice 1-, 3-, 7-, and 14 days post-stroke (p = 0.0189, Figure 5B). This measures fine motor ability. There were no differences of contralateral forelimb foot faults made on the foot fault task between groups (p = 0.91, Figures 5A, C). These data suggest that in addition to decreasing astrocyte cytoskeletal rearrangement, myeloid cell responses, and reducing infarct expansion, deletion of TIC in mice improves their motor function after stroke.
Figure 5. TNF, IL1α, and C1q deletion reduces behavioral deficits after ischemic stroke. (A) Tnf−/−Il1a−/−C1q−/− triple knockout (TKO) mice a significantly further distance on the rotating beam as the wildtype (WT) mice, F(1, 24) = 0.3969, p = 0.026. A post-hoc analysis revealed that TKO mice made fewer errors 1- (p = 0.0358) and 3 days (p = 0.0244) after stroke. (B) TKO mice mad significantly fewer errors on the tapered beam task than WT mice, F(1, 24) = 6.342, p = 0.0189. A post-hoc analysis revealed that TKO mice made fewer errors 1- (p < 0.0124), 3- (p < 0.023), 7- (p < 0.0329), and 14 (p < 0.0453) days after stroke. (C) TKO mice and WT mice made a similar number of contralateral forelimb errors on the grid walk task, F(1, 32.008) = 0.015, p = 0.91. * p < 0.05. TKO, Tnf−/−Il1a−/−C1q−/− triple knockout mouse; WT, wild type.
We examined the role TIC cytokines in the neuroinflammatory response after stroke by using TKO mice with global deletion of these cytokines. These TKO mice had a reduced astrocyte and myeloid cell response after stroke, which translated to a reduction in infarct volume. TKO mice also had reduced motor behavior deficits when compared to WT mice. Previous research demonstrates that these cytokines play an important role in nRA formation, and our research suggests that nRAs also likely play a detrimental role after ischemic stroke (Liddelow et al., 2017; Yun et al., 2018; Guttenplan et al., 2020b). Inhibiting nRAs by targeting the release of these specific cytokines could lead to new stroke therapies aimed at reducing secondary damage caused by the neuroinflammatory response.
We were able to demonstrate a robust reduction in stroke size, which may be due to a direct effect on neurons resulting in less inflammation, or a reduction of neuroinflammation, which caused less neuronal injury. While we were not able to uncouple direct effects of TIC cytokines directly acting on neurons vs. nRA functions, the reduction in infarct volume of TKO mice from 24 h to 30 days remains both exciting and intriguing. TIC cytokines are primarily being produced by reactive microglia (Liddelow et al., 2017; Hernandez et al., 2023) following stroke, and could have direct actions of their own, on neurons for example, in addition to creating nRAs. These effects could be net positive or negative. TNF is largely thought of as a negative factor, but can also have beneficial effects in stroke (Pan and Kastin, 2007). TNF, IL-1, and C1q also play a dual role in regulating synaptic plasticity (Stellwagen and Malenka, 2006; Stevens et al., 2007; Steinmetz and Turrigiano, 2010; Erion et al., 2014; Liu et al., 2017; Nemeth and Quan, 2021) and may be involved with synaptic reorganization following acute stroke and trauma (Park and Bowers, 2010; Liguz-Lecznar and Kossut, 2013; O'Connor, 2013; Rizzo et al., 2018). However, since TIC cytokines are necessary and sufficient to induce nRAs (Liddelow et al., 2017), it is most likely that TKO mice are reducing the number and magnitude of nRAs, which is leading to this protective effect (Yun et al., 2018; Guttenplan et al., 2021).
Importantly, not all astrocytes after stroke will be neurotoxic, and they are unlikely to be identical. Recent evidence points to multiple transcriptomically-defined reactive astrocytes substates (Boghadadi et al., 2021; Hasel et al., 2021; Garcia-Bonilla et al., 2023). While these substates have not been defined at the functional level, it is most likely they play a key role in the modulation of both the inflammatory response, neuronal maintenance, and tissue preservation in the acute period after stroke.
Examining the level of astrocyte cytoskeletal elements 7- and 30 days post-stroke, TKO mice had reduced GFAP levels in astrocytes compared to WT mice. During the chronic phase of ischemic stroke, there are changes in astrocyte ramification and branch/process length (Wagner et al., 2012; Mestriner et al., 2015). A confound for the interpretation of these results is the use of a single marker, GFAP, which has increased levels following stroke—which may account for increased length and process measurements that are GFAP+ but not necessarily a change in the total number and length of processes in these astrocytes (if measured using a marker that has unaltered levels following ischemia). We have a similar caveat here.
By removing the TIC cytokines, we were able to reduce neuroinflammation during the sub-acute and chronic phases of stroke, and this translated to quicker motor recovery in TKO mice after stroke. Functional improvement was only detected during the acute and sub-acute phases, however in most tasks mice recovered to baseline. Thus, it is possible that nRAs only play a detrimental role in early functional outcomes. However, it is more likely that this is due to the motor tasks we used and the spontaneous recovery of function in both. This is typical for these models in the chronic phase (Moon et al., 2009; Balkaya et al., 2013; Lai et al., 2015).
Recent research has found that reducing nRAs after ischemic stroke with the drug semaglutide led to changes in infarct volume, neuroinflammation, and reduction of behavioral deficits up to 28 days after stroke (Zhang et al., 2022). With the improved functional recovery of TKO mice during the acute and sub-acute phases combined with the evidence that the neuroinflammatory response and infarct volume was decreased in TKO mice during all stages of stroke, it is probable that nRAs play a detrimental role in chronic functional recovery.
Using knockout mice to examine the role of removing TIC cytokines from interacting with the neuroinflammatory response after stroke does pose some limitations. The removal of these cytokines is not just from microglia, but from all cell types throughout the whole animal, and at all developmental stages (as a non-inducible, global knockout model was used). TNF plays an important role in modulating the host defense against infections, but becomes harmful with excessive and prolonged production (Bradley, 2008). Similarly, IL-1 family members are implicated in synaptic plasticity in multiple models (Erion et al., 2014; Nemeth and Quan, 2021). Developmentally, C1q is integral for correct synapse pruning by microglia (Stevens et al., 2007)—a mechanism that is also integral in neurodegenerative diseases like Alzheimer's (Hong et al., 2016). Therefore, removing these cytokines with integral physiological functions globally could introduce confounding variables as different pathways of the neuroinflammatory response are possibly being affected by the removal of these cytokines, not only the formation of nRAs.
There are several other factors outside the scope of this study that will be interesting to examine in the future. One such limitation to the current design includes the utilization of only female mice. We acknowledge that the best practice is to utilize both sexes when examining the neuroinflammatory mechanisms following stroke. While we have completed a more comprehensive mechanistic role of nRAs in both male and female mice in dMCAO and other models of neural insult (Liddelow et al., 2017) in this same transgenic cell line, the current study design lacks the fidelity to provide direct comparisons between the sexes with these dependent variables. Since there are well-defined sex differences in the response to stroke (Zhao et al., 2017) and other types of neural insult, it is warranted to follow up with the examination of these mechanisms in male mice as well.
Additionally, we have chosen to model stroke in animals that are 8 weeks of age. We recognize that animal models should mimic the human condition as closely as possible for improved translation into the clinic and this is a limitation. However, we were particularly interested in the cellular responses of stroke regardless of age and since the microglia-astrocyte signaling axis is altered in aged animals, we chose to remove this variable by using healthy, younger, adult animals. Additionally, since there are several co morbid conditions in aged animals and a reduced likelihood of animals to survive around 18 months of age.1 For these reasons, one important future direction would be to determine whether TICs have a similar effect in aged animals.
Future research should also examine potential therapeutics that target the release of these three immune factors, or intrinsic activation of nRAs, especially with clinically relevant, or potentially already approved compounds. Additional approaches include the use of astrocyte-specific conditional knockout of toxic fatty acid production enzymes, like ELOVL1, which we recently reported was protective following acute axonal injury in the optic nerve (Guttenplan et al., 2021). Further dissection of this pathway to identify how saturated long chain FFAs released from nRAs are affecting further astrocyte and microglial activation could lead to a more clinically relevant way to alter this pathway and reduce neuroinflammation after stroke. More appropriate genetic tools are already being used to investigate these pathways in other disease/trauma systems with cell-type specific and temporal control of nRA function (Guttenplan et al., 2021). Understanding this complex neuroinflammatory response following stroke would undoubtedly lead to therapies with a longer treatment window than current options.
The original contributions presented in the study are included in the article/supplementary material, further inquiries can be directed to the corresponding author.
The animal study was approved by Stanford Institutional Care and Use Committee UNCW Institutional Care and Use Committee. The study was conducted in accordance with the local legislation and institutional requirements.
KP: Data curation, Formal analysis, Investigation, Methodology, Software, Validation, Visualization, Writing—original draft, Writing—review & editing. AM: Conceptualization, Data curation, Formal analysis, Investigation, Methodology, Supervision, Writing—review & editing. EB: Data curation, Formal analysis, Investigation, Methodology, Writing—review & editing. MW: Data curation, Formal analysis, Investigation, Methodology, Writing—review & editing. KI: Data curation, Investigation, Methodology, Writing—review & editing. MB: Conceptualization, Data curation, Formal analysis, Funding acquisition, Investigation, Methodology, Project administration, Resources, Software, Supervision, Validation, Visualization, Writing—review & editing. SL: Conceptualization, Data curation, Formal analysis, Funding acquisition, Investigation, Methodology, Project administration, Resources, Software, Supervision, Validation, Visualization, Writing—original draft, Writing—review & editing. TP: Conceptualization, Data curation, Formal analysis, Funding acquisition, Investigation, Methodology, Project administration, Resources, Software, Supervision, Validation, Visualization, Writing—original draft, Writing—review & editing.
The author(s) declare financial support was received for the research, authorship, and/or publication of this article. This work was funded by the National Institute of Health NINDS F32NS089162 to TP, the American Heart Association Career Development Award (20CDA35310828) to TP. NIH NEI 5R01EY033353, the Cure Alzheimer's Fund, Alzheimer's Association, the National Multiple Sclerosis Society, Anonymous Donors, and the Parekh Center for Interdisciplinary Neurology to SL. Also, a Frontiers in Brain Health Award from AHA/Allen Frontiers Groups 19PABHI34580007 (MB) and the Leducq Stroke-IMPaCT Transatlantic Network of Excellence (MB).
SL declares ownership in AstronauTx Ltd, and sits on the Scientific Advisory Board of the Global BioAccess Fund, Tamborine, and Synapticure.
The remaining authors declare that the research was conducted in the absence of any commercial or financial relationships that could be construed as a potential conflict of interest.
The author(s) declared that they were an editorial board member of Frontiers, at the time of submission. This had no impact on the peer review process and the final decision.
All claims expressed in this article are solely those of the authors and do not necessarily represent those of their affiliated organizations, or those of the publisher, the editors and the reviewers. Any product that may be evaluated in this article, or claim that may be made by its manufacturer, is not guaranteed or endorsed by the publisher.
1. ^Life span as a biomarker. Available online at: https://www.jax.org/research-and-faculty/research-labs/the-harrison-lab/gerontology/life-span-as-a-biomarker.
Balkaya, M., Kröber, J. M., Rex, A., and Endres, M. (2013). Assessing post-stroke behavior in mouse models of focal ischemia. J. Cereb. Blood Flow Metab. 33, 330–338. doi: 10.1038/jcbfm.2012.185
Barbar, L., Jain, T., Zimmer, M., Kruglikov, I., Sadick, J. S., Wang, M., et al. (2020). CD49f is a novel marker of functional and reactive human iPSC-derived astrocytes Author links open overlay panel. Neuron 107, 436–453. doi: 10.1016/j.neuron.2020.05.014
Bates, D., Kliegl, R., Vasishth, S., and Baayen, H. (2015). Parsimonious mixed models. arXiv [Preprint]. arXiv:1506.04967.
Boghadadi, A. G., Spurrier, J., Teo, L., Li, M., Skarica, M., Cao, B., et al. (2021). NogoA-expressing astrocytes limit peripheral macrophage infiltration after ischemic brain injury in primates. Nat. Commun. 12, 6906. doi: 10.1038/s41467-021-27245-0
Bradley, J. R. (2008). TNF-mediated inflammatory disease. J. Pathol. 214, 149–160. doi: 10.1002/path.2287
Cheng, M. Y., Aswendt, M., and Steinberg, G. K. (2016). Optogenetic approaches to target specific neural circuits in post-stroke recovery. Neurotherapeutics 13, 325–340. doi: 10.1007/s13311-015-0411-5
Cheng, M. Y., Wang, E. H., Woodson, W. J., Wang, S., Sun, G., Lee, A. G., et al. (2014). Optogenetic neuronal stimulation promotes functional recovery after stroke. Proc. Natl. Acad. Sci. U S A. 111, 12913–12918. doi: 10.1073/pnas.1404109111
Doyle, K. P., and Buckwalter, M. S. (2014). “A mouse model of permanent focal ischemia: Distal middle cerebral artery occlusion,” in Cerebral Angiogenesis, ed. R. Milner (Totowa, NJ: Humana Press), 103–110. doi: 10.1007/978-1-4939-0320-7_9
Doyle, K. P., Fathali, N., Siddiqui, M. R., and Buckwalter, M. S. (2012). Distal hypoxic stroke: a new mouse model of stroke with high throughput, low variability and a quantifiable functional deficit. J. Neurosci. Method. 207, 31–40. doi: 10.1016/j.jneumeth.2012.03.003
Erion, J. R., Wosiski-Kuhn, M., Dey, A., Hao, S., Davis, C. L., Pollock, N. K., and amd Stranahan, A. M. (2014). Obesity elicits interleukin 1-mediated deficits in hippocampal synaptic plasticity. J. Neurosci. 34, 2618–2632. doi: 10.1523/JNEUROSCI.4200-13.2014
Feigin, V. L., Brainin, M., Norrving, B., Martins, S., Sacco, R. L., Hacke, W., et al. (2022). World Stroke Organization (WSO): global stroke fact sheet 2022. Int. J. Stroke 17, 18–29. doi: 10.1177/17474930211065917
Garcia-Bonilla, L., Shahanoor, Z., Sciortino, R., Nazarzoda, O., Racchumi, G., Iadecola, C., et al. (2023). Brain and blood single-cell transcriptomics in acute and subacute phases after experimental stroke. bioRxiv. doi: 10.1101/2023.03.31.535150
Guttenplan, K. A., Stafford, B. K., El-Danaf, R. N., Adler, D. I., Münch, A. E., Weigel, M. K., et al. (2020a). Neurotoxic reactive astrocytes drive neuronal death after retinal injury. Cell Rep. 31, 10776. doi: 10.1016/j.celrep.2020.107776
Guttenplan, K. A., Weigel, M. K., Adler, D. I., Couthouis, J., Liddelow, S. A., Gitler, A. D., et al. (2020b). Knockout of reactive astrocytes activating factors slows diseases progression in an ALS mouse model. Nat. Commun. 11, 3753. doi: 10.1038/s41467-020-17514-9
Guttenplan, K. A., Weigel, M. K., Prakash, P., Wijewardhane, P. R., Hasel, P., Rufen-Blanchette, U., et al. (2021). Neurotoxic reactive astrocytes induce cell death via saturated lipids. Nature 599, 102–107. doi: 10.1038/s41586-021-03960-y
Hasel, P., Rose, I. V. L., Sadick, J. S., Kim, R. D., and Liddelow, S. A. (2021). Neuroinflammatory astrocyte subtypes in the mouse brain. Nat. Neurosci. 24, 1475–1487. doi: 10.1038/s41593-021-00905-6
Hernandez, V. G., Lechtenberg, K. J., Peterson, T. C., Zhu, L., Lucas, T. A., Bradshaw, K. P., et al. (2023). Translatome analysis reveals microglia and astrocytes to be distinct regulators of inflammation in the hyperacute and acute phases after stroke. Glia 71, 1960–1984. doi: 10.1002/glia.24377
Hong, S., Beja-Glasser, V. F., Nfonoyim, B. M., Frouin, A., Li, S., Ramakrishnan, S., et al. (2016). Complement and microglia mediate early synapse loss in Alzheimer mouse models. Science 352, 712–716. doi: 10.1126/science.aad8373
Hu, X., Peiying, L., Guo, Y., Wang, H., Leak, R. K., Chen, S., et al. (2012). Microglia/macrophage polarization dynamics reveal novel mechanism of injury expansion after focal cerebral ischemia. Stroke 43, 3063–3070. doi: 10.1161/STROKEAHA.112.659656
Jayaraj, R. L., Aximullah, S., Beiram, R., Jalal, F. Y., and Rosenberg, G. A. (2019). Neuroinflammation: friend and foe for ischemic stroke. J. Neuroinflam. 16, 1–24. doi: 10.1186/s12974-019-1516-2
Khoshnam, S. E., Winlow, W., Farzaneh, M., Farbood, Y., and Moghaddam, H. F. (2017). Pathogenic mechanisms following ischemic stroke. Neurol. Sci. 38, 1167–1186. doi: 10.1007/s10072-017-2938-1
Kuznetsova, A., Brockhoff, P. B., and Christensen, R. H. B. (2017). lmerTest package: tests in linear mixed effects models. J. Statist. Softw. 82, 1–26. doi: 10.18637/jss.v082.i13
Lai, S., Panarese, A., Spalletti, C., Alia, C., Ghionzoli, A., Caleo, M., et al. (2015). Quantitative kinematic characterization of reaching impairments in mice after a stroke. Neurorehab. Neur. Rep. 29, 382–392. doi: 10.1177/1545968314545174
Lakhan, S. E., Kirchgessner, A., and Hofer, M. (2009). Inflammatory mechanisms in ischemic stroke: therapeutic approaches. J. Transl. Med. 7, 97. doi: 10.1186/1479-5876-7-97
Liddelow, S. A., and Barres, B. A. (2017). Reactive astrocytes: production, function, and therapeutic potential. Immunity 46, 957–967. doi: 10.1016/j.immuni.2017.06.006
Liddelow, S. A., Guttenplan, K. A., Clarke, L. E., Bennett, F. C., Bohlen, C. J., Schirmer, L., et al. (2017). Neurotoxic reactive astrocytes are induced by activated microglia. Nature 541, 481–487. doi: 10.1038/nature21029
Liguz-Lecznar, M., and Kossut, M. (2013). Influence of inflammation on poststroke plasticity. Neural Plast. 2013, 258582. doi: 10.1155/2013/258582
Liu, Y., Zhou, L.-J., Wang, J., Li, D., Ren, W.-J., Peng, J., et al. (2017). TNF-α differentially regulates synaptic plasticity in the hippocampus and spinal cord by microglia-dependent mechanisms after peripheral nerve injury. J. Neurosci. 37, 871–881. doi: 10.1523/JNEUROSCI.2235-16.2016
Lubjuhn, J., Gastens, A., vong Wilpert, G., Bargiotas, P., Herrmann, O., Murikinati, S., et al. (2009). Functional testing in a mouse stroke model induced by occlusion of the distal middle cerebral artery. J. Neurosci. Method 184, 95–103. doi: 10.1016/j.jneumeth.2009.07.029
Mestriner, R. G., Saur, L., Bagatini, P. B., Baptista, P. P. A., Vaz, S. P., Ferreira, K., et al. (2015). Astrocyte morphology after ischemic and hemorrhagic experimental stroke has no influence on the different recovery patterns. Behav. Brain Res. 278, 257–261. doi: 10.1016/j.bbr.2014.10.005
Moon, S.-K., Alverdashvili, M., Cross, A. R., and Whishaw, I. Q. (2009). Both compensation and recovery of skilled reaching following small photothrombotic stroke to motor cortex in the rat. Exper. Neurol. 218, 145–153. doi: 10.1016/j.expneurol.2009.04.021
Nemeth, D. P., and Quan, N. (2021). Modulation of neural networks by interleukin-1. Brain Plast. 7, 17–32. doi: 10.3233/BPL-200109
Nouraee, C., Fisher, M., Napoli, M. D., Salazar, P., Farr, T. D., Jafarli, A., et al. (2019). A brief review of edema-adjusted infarct volume measurement techniques for rodent focal cerebral ischemia models with practical recommendations. J. Vasc. Interv. Neurol. 10, 38–45.
O'Connor, J. (2013). Targeting tumour necrosis factor-α in hypoxia and synaptic signalling. Ir. J. Med. Sci. 182, 157–162. doi: 10.1007/s11845-013-0911-4
Pan, W., and Kastin, A. J. (2007). Tumor necrosis factor and stroke: role of the blood-brain barrier. Prog. Neurobiol. 83, 363–374. doi: 10.1016/j.pneurobio.2007.07.008
Pantcheva, P., Elias, M., Duncan, K., Borlongan, C. V., Tajiri, N., and Kaneko, Y. (2014). The role of DJ-1 in the oxidative stress cell death cascade after stroke. Neur. Regen. Res. 9, 1430–1433. doi: 10.4103/1673-5374.139458
Park, K. M., and Bowers, W. J. (2010). Tumor necrosis factor-alpha mediated signaling in neuronal homeostasis and dysfunction. Cell Signal. 22, 977–983. doi: 10.1016/j.cellsig.2010.01.010
Peterson, T. C., Lechtenberg, K. J., Piening, B. D., Lucas, T. A., Wei, E., Chaib, H., et al. (2021). Obesity drives delayed infarct expansion, inflammation, and distinct gene networks in a mouse stroke model. Transl. Stroke Res. 12, 331–346. doi: 10.1007/s12975-020-00826-9
Rizzo, F. R., Musella, A., De Vito, F., Fresegna, D., Bullitta, S., Vanni, V., et al. (2018). Tumor necrosis factor and interleukin-1β modulate synaptic plasticity during neuroinflammation. Neural Plast. 2018, 8430123. doi: 10.1155/2018/8430123
Schaar, K. L., Brenneman, M. M., and Savitz, S. I. (2010). Functional assessments in the rodent stroke model. Exper. Transl. Stroke Med. 2, 13. doi: 10.1186/2040-7378-2-13
Schindelin, J., Arganda-Carreras, I., Frise, E., Kaynig, V., Longair, M., Pietsch, T., et al. (2012). Fiji: an open-source platform for biological-image analysis. Nat. Method. 7, 676–682. doi: 10.1038/nmeth.2019
Software, G. (2023). Available online at: www.graphpad.com (accessed November 17, 2023).
Steinmetz, C., and Turrigiano, G. G. (2010). Tumor necrosis factor-α signaling maintains the ability of cortical synapses to express synaptic scaling. J. Neurosci. 30, 14685–14690. doi: 10.1523/JNEUROSCI.2210-10.2010
Stellwagen, D., and Malenka, R. C. (2006). Synaptic scaling mediated by glial TNF-alpha. Nature 440, 1054–1059. doi: 10.1038/nature04671
Stence, N., Waite, M., and Dailey, M. E. (2001). Dynamics of microglial activation: a confocal time-lapse analysis in hippocampal slices. Glia 33, 256–266. doi: 10.1002/1098-1136(200103)33:3<256::AID-GLIA1024>3.0.CO;2-J
Sterling, J. K., Adetunji, M. O., Guttha, S., Bargoud, A. R., Uyhazi, K. E., Ross, A. G., et al. (2020). GLP-1 Receptor agonist NLY01 reduces retinal inflammation and neuron death secondary to ocular hypertension. Cell Rep. 33, 108271. doi: 10.1016/j.celrep.2020.108271
Stevens, B., Allen, N. J., Vazquez, L. E., Howell, G. R., Christopherson, K. S., Nouri, N., et al. (2007). The classical complement cascade mediates CNS synapse elimination. Cell 132, 1164–1178. doi: 10.1016/j.cell.2007.10.036
Sun, M., Deng, B., Zhao, X., Gao, C., Yang, L., Hui Zhao, H., et al. (2015). Isoflurane preconditioning provides neuroprotection against stroke by regulating the expression of the TLR4 signalling pathway to alleviate microglial activation. Scient. Rep. 5, 11445. doi: 10.1038/srep11445
Team, R. (2020). RStudio: integrated development for R. RStudio. In (Version 2021.09.0) PBC. Available online at: http://www.rstudio.com/ (accessed November 17, 2023).
Tseng, Y.-J., Hu, R.-F., Lee, S.-T., Lin, Y.-l., Hsu, C.-L., Lin, S.-W., et al. (2020). Risk factors associated with outcomes of recombinant tissue plasminogen activator therapy in patients with acute ischemic stroke. Environ. Res. Public Health 17, 618. doi: 10.3390/ijerph17020618
Wagner, D.-C., Scheibe, J., Glocke, I., Weise, G., Deten, A., Boltze, J., et al. (2012). Object-based analysis of astroglial reaction and astrocyte subtype morphology after ischemic brain injury. Acta Neurobiol. Exp. 72, 79–87. doi: 10.55782/ane-2013-1923
Wardlaw, J. M., Murray, V., Berge, E., del Zoppo, G., Sandercock, P., Lindley, R. L., et al. (2012). Recombinant tissue plasminogen activator for acute ischaemic stroke: an updated systematic review and meta-analysis. Lancet 379, 2364–2372. doi: 10.1016/S0140-6736(12)60738-7
Xiong, Y., Wakhloo, A. K., and Fisher, M. (2022). Advances in acute ischemic stroke therapy. Circul. Res. 130, 1230–1251. doi: 10.1161/CIRCRESAHA.121.319948
Yun, S. P. Y., Kam, T.-I., Nikhil, P., Kim, S., Oh, y., Park, J.-S., et al. (2018). Block of A1 astrocyte conversion by microglia is neuroprotective in models of Parkinson's disease. Nat. Med. 24, 931–938. doi: 10.1038/s41591-018-0051-5
Zhang, Q., Liu, C., Shi, R., Zhou, S., Shan, H., Deng, L., et al. (2022). Blocking C3d+/GFAP+ A1 astrocyte conversion with semaglutide attenuates blood-brain barrier disruption in mice after ischemic stroke. Aging Dis. 13, 943–959. doi: 10.14336/AD.2021.1029
Zhang, S. (2019). Microglial activation after ischaemic stroke. Stroke Vasc. Neurol. 4, 196. doi: 10.1136/svn-2018-000196
Keywords: astrocyte, stroke, macrophage, recovery of function, infarct volume reduction
Citation: Prescott K, Münch AE, Brahms E, Weigel MK, Inoue K, Buckwalter MS, Liddelow SA and Peterson TC (2024) Blocking of microglia-astrocyte proinflammatory signaling is beneficial following stroke. Front. Mol. Neurosci. 16:1305949. doi: 10.3389/fnmol.2023.1305949
Received: 02 October 2023; Accepted: 01 December 2023;
Published: 04 January 2024.
Edited by:
Sadayuki Hashioka, Asahikawa Medical University, JapanReviewed by:
Angela Gomez-Arboledas, University of California, Irvine, United StatesCopyright © 2024 Prescott, Münch, Brahms, Weigel, Inoue, Buckwalter, Liddelow and Peterson. This is an open-access article distributed under the terms of the Creative Commons Attribution License (CC BY). The use, distribution or reproduction in other forums is permitted, provided the original author(s) and the copyright owner(s) are credited and that the original publication in this journal is cited, in accordance with accepted academic practice. No use, distribution or reproduction is permitted which does not comply with these terms.
*Correspondence: Todd C. Peterson, cGV0ZXJzb250QHVuY3cuZWR1
†ORCID: Kimberly Prescott orcid.org/0000-0002-4652-6724
Alexandra E. Münch orcid.org/0000-0002-9609-3277
Evan Brahms orcid.org/0000-0002-0073-3699
Maya K. Weigel orcid.org/0000-0003-0557-5172
Kenya Inoue orcid.org/0009-0004-0760-5341
Marion S. Buckwalter orcid.org/0000-0003-2807-2447
Shane A. Liddelow orcid.org/0000-0002-0840-1437
Todd C. Peterson orcid.org/0000-0001-5441-6157
Disclaimer: All claims expressed in this article are solely those of the authors and do not necessarily represent those of their affiliated organizations, or those of the publisher, the editors and the reviewers. Any product that may be evaluated in this article or claim that may be made by its manufacturer is not guaranteed or endorsed by the publisher.
Research integrity at Frontiers
Learn more about the work of our research integrity team to safeguard the quality of each article we publish.