- 1CNC-Center for Neuroscience and Cell Biology, University of Coimbra, Coimbra, Portugal
- 2Center for Innovative Biomedicine and Biotechnology, University of Coimbra, Coimbra, Portugal
- 3Institute for Interdisciplinary Research, University of Coimbra, Doctoral Programme in Experimental Biology and Biomedicine (PDBEB), Coimbra, Portugal
- 4Instituto de Neurociencias de Castilla y León, University of Salamanca, Salamanca, Spain
- 5Institute of Biomedical Research of Salamanca (IBSAL), Salamanca, Spain
- 6Department of Cell Biology and Pathology, University of Salamanca, Salamanca, Spain
Stem cells have potential applications in the field of neurological diseases, as they allow for the development of new biological models. These models can improve our understanding of the underlying pathologies and facilitate the screening of new therapeutics in the context of precision medicine. Stem cells have also been applied in clinical tests to repair tissues and improve functional recovery. Nevertheless, although promising, commonly used stem cells display some limitations that curb the scope of their applications, such as the difficulty of obtention. In that regard, urine-derived cells can be reprogrammed into induced pluripotent stem cells (iPSCs). However, their obtaining can be challenging due to the low yield and complexity of the multi-phased and typically expensive differentiation protocols. As an alternative, urine-derived stem cells (UDSCs), included within the population of urine-derived cells, present a mesenchymal-like phenotype and have shown promising properties for similar purposes. Importantly, UDSCs have been differentiated into neuronal-like cells, auspicious for disease modeling, while overcoming some of the shortcomings presented by other stem cells for these purposes. Thus, this review assesses the current state and future perspectives regarding the potential of UDSCs in the ambit of neurological diseases, both for disease modeling and therapeutic applications.
1. Introduction
Neurological diseases encompass various categories of disorders, imposing a heavy burden on society (Thakur et al., 2016). Animal models are widely used to assess the etiopathogenesis of brain disorders and test potential treatments. However, animal models also have limitations, such as their limited genotypic variability, high cost, ethical implications, and the high complexity of the human brain, leading to different therapeutic effects (Shen, 2016; Białoń and Wąsik, 2022). In vitro models based on human samples are an alternative approach that can be more cost-effective than animal models. Recent advances in stem cell research have led to the use of stem cells in brain disease modeling and therapy. Stem cells have a critical advantage in that they not only provide models for understanding disease and a platform for drug discovery, but they may also have therapeutic applications on their own. Stem cells commonly used for brain disease modeling and/or therapy include embryonic stem cells (ESCs), neural stem cells (NSCs), mesenchymal stem cells (MSCs), endothelial progenitor cells (EPCs), and induced pluripotent stem cells (iPSCs). Although stem cells hold great promise for brain disease modeling and therapy, one of the biggest challenges associated with using stem cells is the high cost and low yield of the complex and multistep differentiation process required to generate brain cells, which can require invasive sample collection techniques in some cases (Fowler et al., 2020). New types of stem cells are being explored to overcome these limitations, such as urine-derived stem cells (UDSCs).
Several types of cells can be found in urine, namely epithelial cells, immune cells, and the subpopulations of UDSCs (Zhang et al., 2014; Arcolino et al., 2015; Manaph et al., 2018). UDSCs have emerged as a promising tool for stem cell research and therapy, given that they can be harvested from patients with minimal discomfort and without invasive procedures, while maintaining their genetic background (Bento et al., 2020). Importantly, UDSCs can be directly converted into other cell types, including brain cells, without the need for an intermediate conversion, which reduces costs, complexity, and risks of mutations in the process (Liu et al., 2013). Accordingly, recent data support the use of UDSCs as an in vitro cellular model for comprehensive precision medicine since they can allow the unveiling of patients’ specific disease biochemical mechanisms’ complexities. Moreover, they can also be used in therapy, either in their naturally occurring form or differentiated into other cell types.
In this review, we will tackle the current state of the art for UDSCs, and the future perspectives for their application in neurological diseases’ modeling and tentative therapeutic applications.
2. Current challenges in brain disease modeling and the emergence of stem cell research
Brain disorders represent a vast and intricate field within neuroscience, encompassing various categories of disorders, such as neurodegenerative, neurodevelopmental, cerebrovascular, infectious, tumoral, traumatic injury, and mental health disorders. Collectively, these disorders place a substantial demand on society’s capacity to overcome the economic and social burden (Thakur et al., 2016). In 2014, approximately 100 million people in the United States were affected by a neurological disorder, with an estimated cost of almost 789 trillion USD (Gooch et al., 2017). In Europe, neurological disorders were the third most common cause of premature death and disability in 2017, and their negative societal impact is expected to increase with the aging population (Deuschl et al., 2020). Therefore, finding solutions to mitigate the impact of neurological diseases is becoming increasingly crucial. The key to discovering new, translational findings is the development of effective models to gain a comprehensive understanding of diseases and design successful therapeutic approaches. However, modeling brain-associated pathologies is challenging due to the wide range of underlying causes, symptoms, and the variability of cellular and molecular effects. Hence, there are various types of models available, each with its own advantages and limitations.
Evaluating the causes of brain disorders and experimenting with potential treatments frequently makes use of animal models, which are extensively employed in research (Dawson et al., 2018). Animal models offer advantages such as the ability to study hindrances in a living, dynamic system, accounting for the functional interplay of different cell types and even organs that are not represented in cellular models. In addition, animal models allow researchers to account for various factors, including environmental or dietary alterations. These models can be created by genetically inducing brain changes specific to a given neurological disorder through germinal or neural cell-targeted mutations or pharmacologically inducing cellular degeneration using drug administration. Trauma can also be externally induced to simulate specific conditions. However, animal models have limitations. Human diseases that have complex etiologies or that are poorly understood may be misrepresented in animal models. Due to the way animal models are typically generated, diseases that do not naturally occur in animals may only be replicated by altering one of several intervening genes and overexpressing it. This may result in models that replicate the disease’s phenotype but not its underlying causes and induce the condition by mechanisms distinct from those at play in a real situation (McGraw et al., 2017). Another limitation of animal models is the limited genotypic variability of laboratory animals due to inbreeding, which can lead to a failure to represent the complexity of human genetic diversity. As a result, extrapolating results from animal models to humans may not always be successful, leading to the failure of clinical trials and the loss of considerable amounts of money, time, and lives. Even in the case of adequate representation, the high complexity of the human brain and its unique genetic and physiological dysfunctionality can lead to dissimilar therapeutic effects (Shen, 2016). In addition, animal models can be costly due to the expenses associated with acquiring and maintaining them, as well as running experiments (Białoń and Wąsik, 2022). Furthermore, modern societies are increasingly concerned about the ethical implications of using animals in scientific research. While ex vivo tissues from animals can also be used as a simplified model, they also require animal sacrifice.
An alternative approach to studying brain diseases involves using in vitro models based on human samples. While these models can also be expensive, techniques like 2D cell culture tend to be more cost-effective than animal models. While these simplified models do not account for systemic responses that may be involved in a specific disease, they do offer a more precise and focused characterization of cellular events. By using human cells, including patient-derived ones, researchers can study the disease in an autologous context. However, it’s important to note that brain cells collected in specific conditions, namely primary cultures, are often only available at more advanced stages of the disease, resulting in a poorer understanding of the disease’s earlier stages (Eichmüller and Knoblich, 2022).
In recent years, research has made remarkable progress in using stem cells for both modeling and as platforms for discovering new drugs. Additionally, stem cells have enormous potential for therapeutic applications, such as replacing damaged or dysfunctional brain cells or promoting specific biological responses, such as angiogenesis (Ryu et al., 2016). Commonly used stem cells for brain disease modeling and/or therapy include ESCs, NSCs, MSCs, EPCs, and iPSCs. Through specific differentiation protocols, these cells can be used to obtain different types of brain cells (Gage and Temple, 2013; Appaix, 2014; Terrigno et al., 2018). Despite the immense potential of stem cells, it is critical to recognize that they also come with significant limitations as models, varying in degree. One of the most significant hurdles associated with stem cell use is the high cost and low yield of the intricate and multistep differentiation process necessary for creating brain cells, which can also require invasive sample collection techniques in certain cases (Fowler et al., 2020). Additionally, incorrect differentiation into brain cells can lead to inaccurate modeling of the disease and limit their potential for therapeutic use (Strano et al., 2020). Moreover, there is a risk of tumorigenesis due to undesired mutations and potential teratoma formation in the cells generated through certain methods (Ganat et al., 2012). Another concern is the ethical considerations surrounding the use of embryonic stem cells, which require the destruction of human embryos. Despite these challenges, researchers are exploring novel types of stem cells to overcome these limitations and fully unlock the potential of stem cells for disease modeling and therapy.
Within the scope of novel disease modeling and treatment feasibility and to overcome several drawbacks, UDSCs are gaining increasing interest. They constitute a comprehensive in vitro cellular model and can be safely and easily isolated from patients’ urine samples. UDSCs are part of the group of body fluid-derived stem cells (BFDSCs). They offer an alternative to solid tissue-derived stem cells by also presenting characteristics of stemness, including self-renewal properties, the expression of stem cell surface markers, and multi-differentiation potential (Huang et al., 2023). BFDSCs encompasses different cell types that can be obtained from diverse sources, such as urine (Lang et al., 2013), synovial fluid (Li F. et al., 2020), menstrual fluid (Bozorgmehr et al., 2020), umbilical cord blood (Nguyen et al., 2022), peripheral blood (Pouryazdanpanah et al., 2018), breast milk (Mane et al., 2022), among others. In comparison with solid tissue-derived stem cells, BFDSCs collection is less invasive, and their isolation tends to be simpler. It bypasses the commonly used enzymatic digestion, which is otherwise applied to separate the desired cells from the extracellular matrix, using instead straightforward methods such as centrifugations to isolate the target cells fraction (Jia et al., 2018; Liu et al., 2018; Huang et al., 2023). Importantly, BFDSCs have shown significant therapeutic potential, aligning with their intrinsic biological properties. Urine sample collection is easy, fast, painless, and non-invasive, making it a safe method for obtaining UDSCs in relatively high numbers (Shi et al., 2022). Additionally, UDSCs can be obtained daily (limited by menstruation), from both healthy individuals and patients, regardless of their gender, without major ethical concerns. Furthermore, the isolation and maintenance of UDSCs are associated with significantly lower costs, adding to the appeal of using these cells. These advantages are not only applicable when compared to other sources of BFDSCs, such as those derived from breastmilk, umbilical cord blood, menstrual blood, or peripheral blood, but also when compared to alternative sources of stem cells, like adipose tissue or bone marrow mesenchymal stem cells, which may lack at least one of the aforementioned benefits. Among BFDSCs, UDSCs attributes make them an attractive option for diverse applications in precision medicine, in vitro pharmacological tests, regenerative medicine, and disease modeling (Bento et al., 2020).
3. Characterization of UDSCs
Urine includes different cell types, broadly classified as urine-derived cells, encompassing several cellular subpopulations such as podocytes and epithelial cells from the renal tubules, nephrons, ureters, bladder, and urethra and, among others, a fraction of stem cells (Arcolino et al., 2015; Manaph et al., 2018). Those stem cells (UDSCs) represent a minor portion of urine-derived cells. UDSCs exhibit mesenchymal stromal cell (MSC)-like properties (Zhang et al., 2008) and have the ability to proliferate and to differentiate into various cell lineages (Bento et al., 2020). UDSCs express MSC surface markers including CD24, CD44, CD73, CD90, and CD105 (Dominici et al., 2006; Lv et al., 2014), the pluripotent stem cell markers POU5F1, as well as Sox2, c-Myc, among others like the ESCs markers SSEA4, TRA 1–60, TRA 1–81, common to pluripotent stem cells (Dominici et al., 2006; Manaph et al., 2018). They are also characterized by the lack of expression of the hematopoietic surface markers CD14, CD20, CD34, and CD45 (Culenova et al., 2019, 2021; Figure 1).
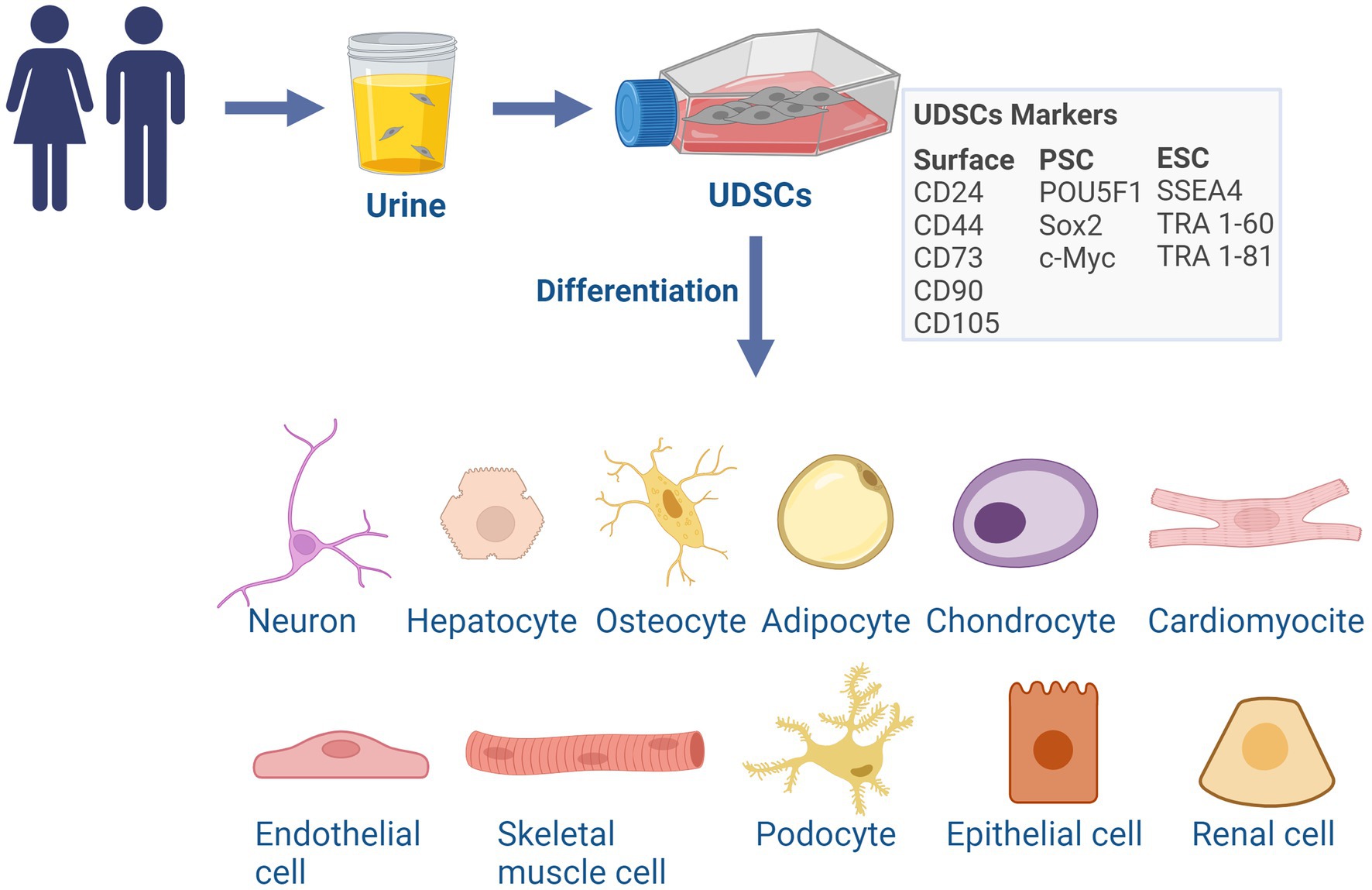
Figure 1. Characterization and differentiation potential of UDSCs. UDSCs can be isolated from the urine of healthy subjects or patients of all ages and have a similar phenotype to mesenchymal stroma cells (MSC). UDSCs express MSC surface markers, pluripotent and embryonic stem cell markers. UDSCs evidence a certain degree of pluripotency and can be directly differentiated into cells of the three germ layers: neurons, hepatocytes-like cells, osteocytes, adipocytes, chondrocytes, cardiomyocytes, endothelial cells and skeletal myogenic cells, renal cells, podocytes and tubular epithelial cells. ESC, embryonic stem cell; PSC, pluripotent stem cell. Created with BioRender.com.
The biological origins and the specific mechanisms underlying the generation and shedding of UDSCs are still unclear. It was proposed that they can be biosynthesized in the proximal and distal convoluted tubules in nephrons, in the renal cortex and papilla, in the ureter, urethra, and bladder (Dörrenhaus et al., 2000; Bharadwaj et al., 2013; Bento et al., 2020). In addition, they are possibly shed into urine as part of the process of physiological tissue reparation and turnover (Dörrenhaus et al., 2000; Zhang Y. Y. et al., 2001; Zhang Y. et al., 2008).
UDSCs can be isolated from the excreted urine (non-invasive) or collected with a catheter from the upper urinary tract (invasive) of healthy subjects or patients of all ages (Zhang et al., 2008; Bharadwaj et al., 2011). Despite urine normally being a sterile fluid while stored in the body, contaminations may happen sporadically, such as through exposure to a contaminated environment at the moment of collection or in the presence of a urinary tract infection, which most frequently occurs in women (Lang et al., 2013). Additionally, still within the biological system, cells may be exposed to excretion products naturally occurring in urine such as the metabolic waste molecules ammonia, urea and creatinine, the breakdown products of drugs, among others (Bouatra et al., 2013). However, to mitigate these shortcomings, a washing step in the isolation procedure with an antimicrobial solution (Manaph et al., 2018), such as phosphate buffered saline solution enriched with antibiotics (Kim et al., 2020), may be carried out to remove possible contaminants and further help remove vestiges of urine in cell culture, along with the toxic metabolites that may be present therein.
Due to their characteristic of being plastic-adherent in the absence of coating, UDSCs can be easily separated from other urinary-derived cells in culture. During a medium change, the remaining cells are washed away, leaving behind only the adherent UDSCs in culture (Culenova et al., 2021), without the need of special substrates. Of note, a single urine collection (~70 mL) yields an average of 3 millions UDSCs which can be frozen (in passage 3, p3), and then thawed and expanded for use in experiments. In this sense, Bharadwaj and colleagues (Bharadwaj et al., 2011) evidenced that the maximum population doublings of UDSCs derived from the upper urinary tract was 56.7, corresponding to a maximum passage of p14 (Bharadwaj et al., 2011), being, in practice, used until p6 for experiments. Importantly, UDSCs maintain their proliferation, self-renewal and multi-differentiation capacity following storage at 4°C for 24 h, thus enabling their handling in batches and improving the efficacy of their processing (Lang et al., 2013). Moreover, the isolation and culture of UDSCs are cost-effective in comparison with other stem cells. UDSCs can be at least half the price and up to seven times cheaper than other stem cells regarding their obtention and maintenance, namely due to costs inherent to sample collection of the latter, which often require the need of specialized invasive medical procedures and posterior requirement of expensive substrates for cell culture (Manaph et al., 2018). Notably, these cells present a low immunogenicity (Guan et al., 2014), and absence of tumorigenicity (Zhang et al., 2008; Bharadwaj et al., 2011; Chun et al., 2012; Bharadwaj et al., 2013; Lee et al., 2013).
When in culture and still in a stem cell state, UDSCs present a “spindle,” “rice-grain” or “cobblestone” shape (Bharadwaj et al., 2011; Lang et al., 2013; Schosserer et al., 2015; Chen C. Y. et al., 2018; Wan et al., 2018). The morphology of the cells can differ slightly depending on their origin along the urinary tract. According to Chen A. J. et al., (2020) and UDSCs obtained from the renal mesenchyme have a spindle-like morphology, while cells originated in the nephron tubules are thought to be the ones with a rice-like shape. In addition, numerous studies have highlighted differences between these two morphologically distinct cell types in terms of their expression of cell surface markers, motility, proliferative capacity, and longevity in culture (Zhou et al., 2012; Chen A. J. et al., 2020; Shi and Cheung, 2021). Under in vitro experimental conditions, both cell types express the stemness-related markers POU5F1 and c-Myc. However, cells from renal mesenchyme origin (spindle-like cells) present enhanced motility and proliferation rate (Chen A. J. et al., 2020).
Importantly, UDSCs are characterized by a multipotential of differentiation and can be directly differentiated into cells representative of the three germ layers, such as neurons (Bharadwaj et al., 2013; Guan J. J. et al., 2014; Kang et al., 2015; Kim et al., 2018; Xu et al., 2019; Liu et al., 2020), hepatocytes-like cells (Zhou et al., 2020), osteocytes, adipocytes, chondrocytes (Guan J. J. et al., 2014), cardiomyocytes (Guan X. et al., 2014), endothelial cells (Bharadwaj et al., 2013; Kang et al., 2015) and skeletal myogenic cells (Liu et al., 2013). Moreover, UDSCs have the capacity to differentiate into renal cells, podocytes and tubular epithelial cells (Lazzeri et al., 2015), an important feature for their potential for renal repair (Figure 1). This fact suggests a degree of pluripotency, although the true extent of this characteristic is still not fully comprehended. The complete range and full extent of their biological functions are still being debated, however, their role in the promotion of tissue regeneration, replacement, and immunomodulation is already scientifically recognized (Zhang et al., 2014; Wu et al., 2021).
For all these reasons, UDSCs may be employed to perform in vitro pharmacological tests, in disease modeling approaches, in regenerative medicine procedures, and for precision medicine (Bento et al., 2020).
4. Direct conversion of UDSCs into neuronal cells
The ideal model for studying the complexity of human brain diseases should be a reliable platform that can be easily obtained from every patient, without requiring invasive procedures or raising major ethical concerns. It should be capable of producing consistent results and accurately reflecting the genetic and epigenetic background of a specific disease, all while being cost-effective and efficient. Building such a model is a huge challenge. However, certain emerging cellular-based platforms, such as UDSCs, offer promising opportunities for this purpose. Research efforts are currently underway to comprehensively understand their capabilities and limitations as a neurological model, including exploring various strategies for their direct differentiation into neuronal cells. This section presents a discussion of the relevant studies published in this area, providing details on the different protocols (Table 1) and the specific role of each compound in the differentiation media (Table 2).
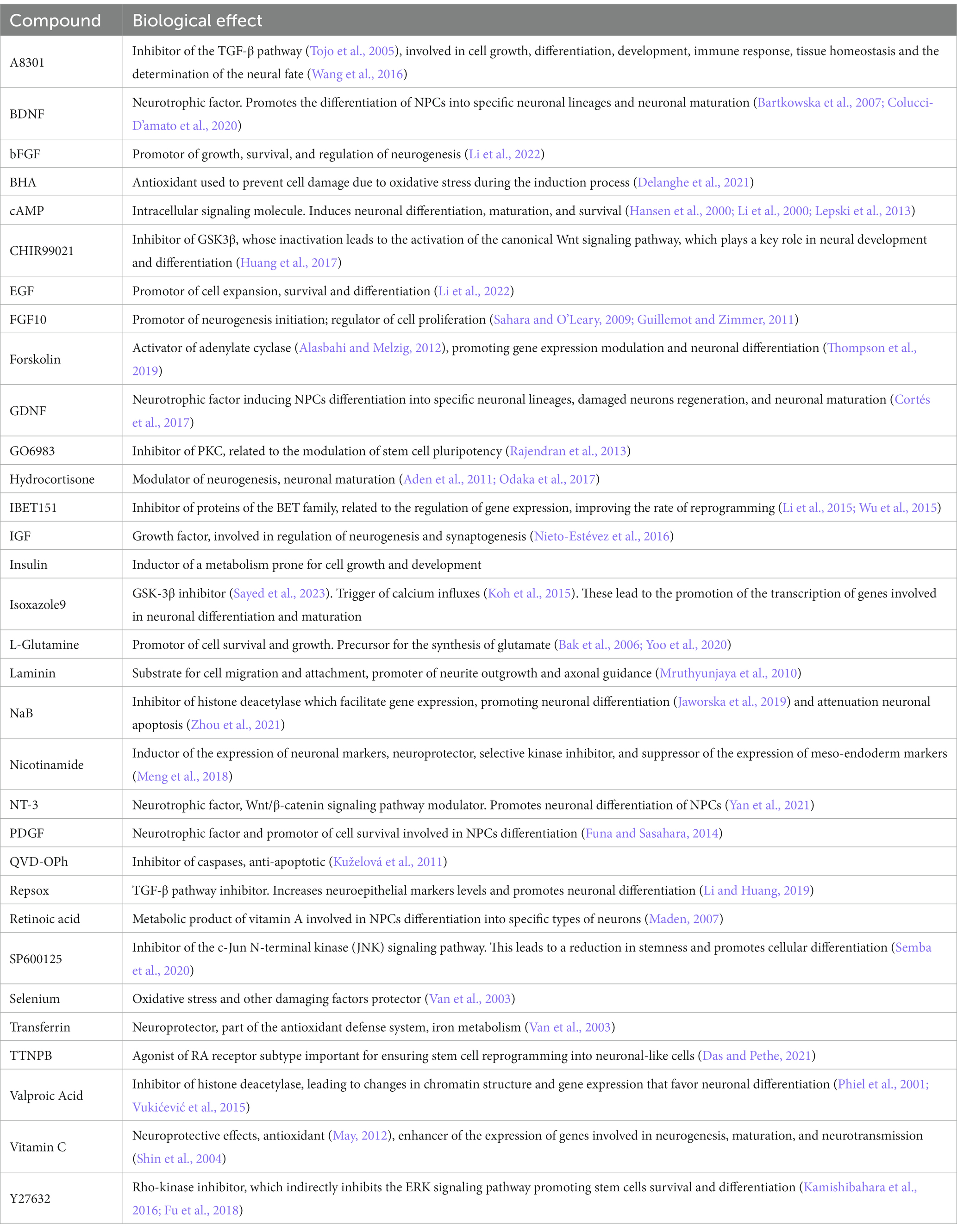
Table 2. Core compounds used among the existing protocols for the differentiation of UDSCs into neuron-like cells and main functions.
Several scientific reports have demonstrated the successful direct conversion of UDSCs into various cell types across different germline lineages. For instance, hepatocyte-like cells have been achieved (Zhou et al., 2020), along with the differentiation into osteocytes, adipocytes, and chondrocytes (Guan J. J. et al., 2014). Furthermore, different authors have accomplished the direct conversion of UDSCs into neuron-like cells using various approaches, resulting in cells with distinct characteristics (Bharadwaj et al., 2013; Guan J. J. et al., 2014; Kang et al., 2015; Kim et al., 2018; Xu et al., 2019; Liu et al., 2020).
In 2013, Bharadwaj and colleagues (Bharadwaj et al., 2013) used a multistep protocol to achieve the differentiation of UDSCs into neuron-like cells. At D3 (maximum days in culture), cells had already undergone morphological changes, including the emergence of extensions and processes. Forty % of cells in culture were expressing nestin, and NF200 (neurofilament 200), characteristic markers of neural progenitor cells (NPCs) and neuronal cells, respectively. This differentiation success rate indicates that there was still room for efficiency enhancement of the neuronal differentiation process, along with improved neuronal maturation (Bharadwaj et al., 2013).
The following year, another study achieved the transformation of UDSCs into NPCs (Guan J. J. et al., 2014). At D12, cells differentiated from UDSCs were expressing the Sox2 and Nestin (markers of NPCs) in higher levels than NSE (neuron-specific enolase) and β-III-tubulin, both neuronal markers. It is relevant to highlight that at D12, the maximum number of days that the authors maintained cells in culture, β-III-tubulin expression was residual, indicating that the obtained neuron-like cells were in an early stage of neuronal differentiation. Additionally, in this study, UDSCs were transplanted into a rat brain, being able to survive and migrate while expressing proteins characteristic of a neuronal phenotype, demonstrating the potential of UDSCs to differentiate into neuronal-like cells in the brain.
In another study (Kang et al., 2015), the capacity of UDSCs to differentiate into neuron-like cells was compared to the one from adipose tissue stem cells (ADSCs). The authors found that UDSCs have a better neurogenic differentiation rate than ADSCs, suggesting that these cells are more suitable to be converted into neurons (Kang et al., 2015).
Later, in 2018, Kim and colleagues (Kim et al., 2018) optimized a protocol to obtain mature neurons from UDSCs recurring to laminin coating and/or treatment with platelet-derived growth factor BB (PDGF-BB). After 14 days of neuronal induction, the authors observed an increase in the expression of early neuronal differentiation markers (nestin and β-III-tubulin) and mature neuronal cells [NeuN (hexaribonucleotide binding protein-3 or Fox-3), MAP2 (microtubule-associated protein 2), and NF-M (neurofilament marker)] in the PDGF-BB treated UDSCs cultured on laminin-coated plates. This suggests a synergistic effect between laminin and PDGF-BB to promote a higher degree of neuronal maturity.
A more recent investigation (Xu et al., 2019) pursued the differentiation of UDSCs into neuron-like cells through a distinct methodology. The study employed a direct reprogramming two-step neuronal induction approach, utilizing seven small molecules and growth factors. By establishing this protocol the authors were able to obtain a higher degree of neuronal maturation and conversion efficiency when compared to the previous reports. The alteration of the UDSCs morphology into a more neuron-like morphology was observed early in the application of the protocol, being visible after 24 h of NIM application. At D5, 58% of cells expressed β-III-tubulin. At D17, cells depicted a more extended neurite outgrowth with dendrite-like structures and apart from β-III-tubulin, also expressed MAP2, NeuN, Tau, SYN (synapsin), and NF-01, being negative to GFAP (glial fibrillary acidic protein). The expression of these neuronal markers and neuronal morphology was maintained through D30. At D17, the authors further characterized the neuronal type generated using this protocol and confirmed its glutamatergic nature, as evidenced by the expression of the glutamatergic marker glutamate. Additionally, markers associated with other neuron types, such as GABA (gamma-aminobutyric acid), HB9 (homeobox HB9), and TH (tyrosine hydroxylase), were rarely detected. To assess the functionality of the neurons derived from UDSCs, whole-cell patch-clamp recording was employed. Following the complete differentiation process, cells at D17 exhibited partial electrophysiological properties, displaying both outward and inward currents. However, neither at D17 nor at D47 were the cells able to generate action potentials. The study conducted by Xu and colleagues (Xu et al., 2019) represented a significant advancement in establishing a neuronal differentiation protocol for converting UDSCs. However, the successful conversion of UDSC into functional neurons has not yet been accomplished, underscoring the pressing requirement for additional research in this field.
Another differentiation approach, also recurring to small molecules, was performed by Liu and collaborators (Liu et al., 2020), who obtained neuron-like cells, with a discernible subset exhibiting GABAergic characteristics. By D6 cells were expressing the neuronal markers Tuj-1, MAP2, Tau, and PSA-Ncam (polysialylated neuronal cell adhesion molecule). At D14, a small subset of cells exhibited GABA positivity, indicative of GABAergic neurons. There was also no evidence of ChAT- or TH-positive cells (cholinergic or dopaminergic neurons, respectively), despite the majority of cells expressing typical neuron-specific genes such as Tau, MAP2, Ncam, and NeuN. The authors did not assess glutamate expression, unlike in the previous study, making it difficult to comprehend the similarities and differences between the neuron-like cells generated using these two protocols. Furthermore, the resultant cells displayed voltage-gated channels for Na+ and K+, characteristic of excitable cells, but lacked Ca+ currents, thereby failing to generate action potentials. Based on these findings, it can be concluded that the conversion of UDSCs in both studies (Xu et al., 2019; Liu et al., 2020) did not result in fully matured neurons.
Similar to the potential of UDSCs to differentiate into neuron-like cells, other cells found in urine hold valuable clinical applications as an important source for the conversion into neuronal cells and disease modeling. In line with this, various studies have reported the generation of disease-related neuronal cells through the reprogramming of urine cells. These methodologies aim to achieve neuronal cells through direct differentiation, or through urine cells reprograming into iPSCs for further neuronal differentiation; and involved the use of retroviruses (Zhang et al., 2016), episomal vectors (Liu et al., 2020), or a reprogramming medium supplemented with small molecules (Yi et al., 2018). Thus, small molecules can be used to differentiate urine-derived renal cells into neuron-like cells showing neuron-specific genes expression and voltage gated Na+ and K+ currents (Liu et al., 2020). Similarly, Zhang and colleagues were able to obtain induced-neurons expressing multiple neuron-specific proteins and capable of generating action potentials from urine cells from control individuals and Wilson’s disease patient, through the overexpression of the transcription factors Ascl1, Brn2, NeuroD, c-Myc, and Myt1l (Zhang et al., 2016). Importantly, iPSCs obtained from urine sample can be used to generate extended pluripotent stem cells (EPSCs) which could then be differentiated to neuronal progenitor cells (Hao et al., 2023). Moreover, urine-derived iPSCs previously reprogramed can also be differentiated into functional motor neurons capable of forming neuromuscular junctions in co-cultures with muscle cells (Yi et al., 2018). In the same line, urine-derived iPSCs from Down Syndrome individuals have been used to obtain cells differentiated into glutamatergic neurons (Lee et al., 2017). Furthermore, in a recent breakthrough, Teles e Silva and colleagues demonstrated the pioneering use of urine-derived iPSCs in producing human cerebral organoids to model Down Syndrome for the first time (Silva AL et al., 2023). Of note, these cerebral organoids faithfully replicate early features of human cortical development, encompassing the organization of neural progenitor zones, programmed differentiation of both excitatory and inhibitory neurons, and the presence of upper and deep-layer cortical neurons, along with astrocytes (Silva AL et al., 2023). Important hallmarks have been achieved, namely the obtention of mature neurons or neurons carrying the phenotype of a specific diseases. As such, studies of this nature might provide some insights that could prove useful in guiding future works that aim to achieve disease models of neurological diseases based on UDSCs due to the close nature between them (Figure 2).
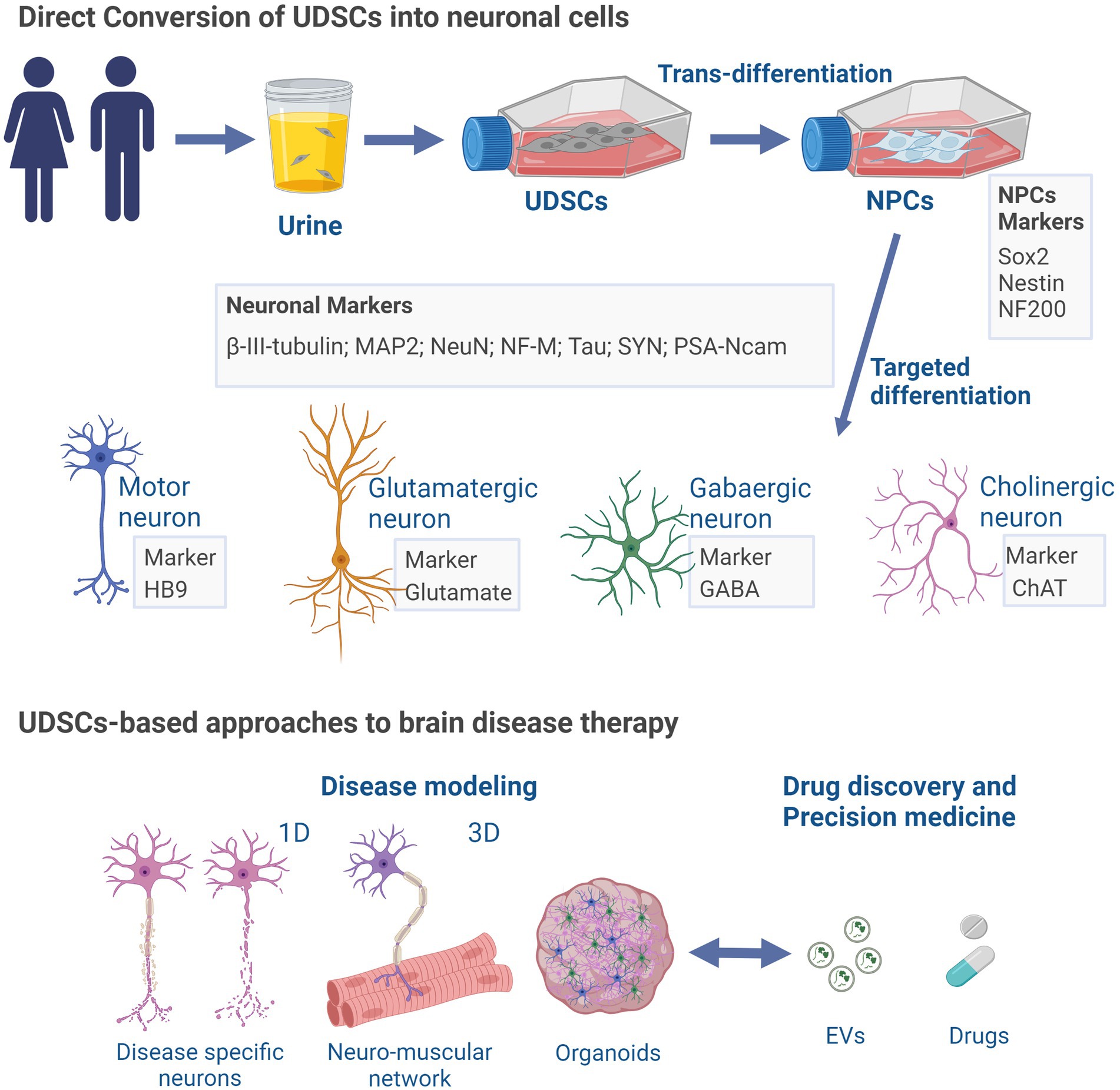
Figure 2. UDSCs-induced neuronal differentiation and brain disease applications. UDSCs isolated from the urine can be directly converted into neuron-like cells divided into different neuronal type depending on their specific markers (motor, glutamatergic, gabaergic or cholinergic). UDSCs-derived neurons may be employed in disease modeling approaches, to perform in vitro pharmacological tests, and for precision medicine. ChAT, Choline acetyltransferase; EVs, extracellular vesicles; GABA, gamma-aminobutyric acid; HB9 homeobox HB9; MAP2, microtubule-associated protein 2; NeuN, hexaribonucleotide binding protein-3; NFM, neurofilament marker; NF200, neurofilament 200; NPCs, neural progenitor cells; PSA-Ncam (polysialylated neuronal cell adhesion molecule); SYN, synapsin. Created with BioRender.com.
5. UDSCs-based approaches to brain disease therapy
5.1. UDSCs in vivo transplantation
The therapeutic potential of UDSCs for neurological diseases remains relatively unexplored, yet the limited findings obtained thus far hold promise. UDSCs engraftment or their secretomes have shown to be effective mostly in preclinical reports of renal and bladder diseases, such as acute kidney injury (Li X. et al., 2020), renal acute tubular injury (Grange et al., 2020) or bladder fibrosis (Wu et al., 2023), evidencing a consistent tissue (Li F. et al., 2020; Li X. et al., 2020) regeneration effect, mainly through their pro-angiogenic, anti-apoptotic and anti-inflammatory effects (Grange et al., 2020; Li F. et al., 2020; Li X. et al., 2020 Wu et al., 2023), over the living afflicted tissues. Within the field of neurological disorders, very few studies of UDSCs in vivo preclinical engraftment have been performed. Nevertheless, in the ones available in the literature, transplanted UDSCs have been shown to have multiple differentiation potential, chemotaxis ability, and an undeniable functional recovery and cellular neuroprotective effect. In more detail, UDSCs, when embedded in hydrogel scaffolds and transplanted into rat’s craniotomy-lesioned motor cortex, survive at least 3 weeks and present full in vivo differentiation potential into astrocyte resembling morphology cells, expressing neurogenesis-related markers such as GFAP, β-III-tubulin, and nestin. Importantly, transplanted cells were found not only surrounding the lesion site, but also functionally integrating hippocampus neural tissue, thus demonstrating migration abilities (Guan J. J. et al., 2014). In the context of spinal cord injury (SCI), in comparison with the control group, the transplant of UDSCs promoted motor functional recovery in a rat model of SCI (Chen H. et al., 2018). Additionally, the authors showed that the injection of UDSCs in conjugation with chondroitinase ABC, an enzyme involved in axon regeneration (Day et al., 2020), generated a synergistic effect on faster motor function recovery of the lesioned animals (Chen H. et al., 2018). Later, Muir and collaborators evidenced that functional recovery observed in the hUDSCs+ ChABC animals engrafted group was related to the increase mRNA expression levels of BDNF and nerve growth factor (NGF), both neuroprotective neurotrophins (Muir et al., 2017), and previously described as involved in post-lesion tissue repair (Hasan et al., 2017).
Importantly, UDSCs have a significant advantage over other stem cells, such as the absence of undesired immune response (le Blanc et al., 2003; Chun et al., 2012; Guan et al., 2015; Kang et al., 2015; Wu et al., 2018; Bento et al., 2020) and teratoma formation (Zhang et al., 2008; Bharadwaj et al., 2011; Chun et al., 2012; Bharadwaj et al., 2013; Lee et al., 2013). In particular, UDSCs, do not express HLA-DR glycoproteins (le Blanc et al., 2003; Guan et al., 2015; Kang et al., 2015; Chen L. et al. 2018; Wu et al., 2018; Bento et al., 2020) until around p7 in vitro, often responsible for triggering transplant rejection, as happening with hematopoietic (Gabbianelli et al., 1987; Park and Seo, 2012) and adipose stem cells (Dam et al., 2021). In addition, UDSCs isolated from healthy subjects, which retained a normal karyotype in vitro, did not induce an adverse response and scaffold rejection after transplantation into an animal model of traumatic brain injury (Guan J. J. et al., 2014).
By bridging the bench with the bedside, animal models provided direct evidence of the feasibility of transplanted stem cells’ regenerative properties, therefore, anticipating the efficacy and safety of specific clinical procedures. The existent studies using animal models and grafting strategies emphasize the necessity of exploring the therapeutic potential of UDSCs and the underlying cellular and molecular mechanisms in different neuropathological contexts.
5.2. UDSCs secretome-based approaches to brain diseases therapy
Similarly, to the therapeutic effects in the scope of direct UDSCs transplantation, preclinical studies employing UDSCs-derived secretome also show promising results for the treatment of brain disorders. UDSCs-related secretomes’ regenerative and immunomodulatory properties arose in several diseases, from urological (Grange et al., 2020; Li X. et al., 2020; Wu et al., 2023) to diabetic wound healing (Zhao et al., 2018). Several authors hypothesized that UDSCs therapeutic may depend on these cells’ ability to secrete various bioactive cargoes. Among them, we can highlight miRNAs, such as miR-26a (Ling et al., 2020), related to neurogenesis enhancement; miR-21-5p (Nasci et al., 2019) and miR-26a-5p (Wan et al., 2022), both involved in cell proliferation; growth factors such as VEGF (Jiang et al., 2016), IGF-1 and EGF (Zhu et al., 2018); DMBT1 and TIMP1 proteins (Chen C. Y. et al., 2020), angiogenin (Jiang et al., 2016), Klotho protein (Grange et al., 2020) and matrilin-3 (MATN3) (Zidan et al., 2021). Importantly, these biomolecules act as paracrine factors over recipient cells (He et al., 2018), exerting regulatory effects in several cellular mechanisms, including proliferation, differentiation, cell fate and survival, neuroprotection, neurogenesis, angiogenesis, and tissue regeneration. A great amount of these studies encompass the use of exosomes, a group of cup-shaped extracellular vesicles (EVs) (Guo et al., 2021), identifiable in biofluids and that shuttle multiple bioactive cargos, able to influence recipient cells. Therefore, we will focus in this section on the therapeutic use of UDSCs- derived EVs.
UDSCs have been also associated with the release of heterogeneous EVs, ranging from microvesicles (150 nm to 1 μm) to exosomes (60 to 150 nm) (Zidan et al., 2021). Importantly, like in the case of other EVs, the content of UDSCs-derived EVs often reflects their origin and the pathophysiological environment in which they were generated (Arcolino et al., 2015). Additionally, EVs from healthy UDSCs may be used for therapeutic purposes.
Anti-inflammatory properties of UDSCs-secreted EVs have been assessed, opening new possibilities for their therapeutic use in immunodeficiency scenarios (Zidan et al., 2021). Particularly, UDSCs-derived exosomes have been shown to contain immunomodulatory molecules such as the cytokines TGF-β1 (Jiang et al., 2016), B-cell activating factor (BAFF), A proliferation-inducing ligand (APRIL), interleukin-6 (IL–6), and the CD40 ligand protein (CD40L), which stimulate B cells to initiate the immune response without T cell proliferation stimulation, possibly due to the presence of immunosuppressive cytokines and miRNAs, such as miRNA146–5p (Zidan et al., 2021). Furthermore, miR-146a-5p has been identified to target the interleukin-1 receptor-associated kinase 1 (IRAK1) mRNA, which degradation results in nuclear factor (NF)-κB signaling inhibition (Li X. et al., 2020). Downregulation of IRAK1 may also be involved in the modulation of the phosphoinositide 3-kinase (PI3K)/Akt and the mitogen-activated protein kinase (MAPK) pathways, leading to a decrease in apoptosis and inflammatory cytokines in podocytes (Zhang et al., 2018).
Although studies are scarce, some articles demonstrate the benefits of using EVs from UDSCs, most notoriously exosomes, to improve some brain-related disorders. For instance, in a rat model of ischemic stroke, direct intravenous injection of exosomes derived from human UDSCs, 4 h after stroke induction, resulted in a reduction of infarct volume and enhancement of the subventricular zone (SVZ) endogenous neurogenesis, via miR-26a/HDAC6 signaling pathway activation, suggesting the neuroprotective effect of those EVs (Ling et al., 2020). Moreover, in a mouse model of Rett Syndrome, administration of UDSCs-derived exosomes improved the motor coordination, cognition, and behavioral symptoms presented by the animals (Pan et al., 2021). Importantly, this effect was related to the enhancement of neurogenesis in the SVZ, resulting from the integration of EVs enriched in miR-21-5p by the neural stem cells. miR-21-5p, a regulator of cell proliferation, mitochondrial respiration, and angiogenesis (Nasci et al., 2019) further inhibited the EPha4/TEK axis, resulting in neurogenesis enhancement, as evidenced by the increased expression of β-III-tubulin and DCX (doublecortin) (Pan et al., 2021). Following spinal cord injury, UDSCs-derived exosomes induce motor neurons functional recovery, concomitantly with local endothelial cells microvessel regeneration through ANGPTL3 (Angiopoietin-like 3)-mediated PI3K/AKT signaling pathway activation (Cao et al., 2021), which promotes angiogenesis process (Jiang et al., 2019). Additionally, Dan and colleagues recently suggested that UDSCs-derived exosomes prevented apoptosis of aging retinal ganglion cells (RGCs) (Dan et al., 2023). The authors exposed aging RGCs to UDSCs conditioned medium and observed the promotion of cell survival and proliferation (Dan et al., 2023). However, since the authors did not analyze the presence of exosomes in the culture medium used, it is not possible to assure that those were the mediators in the effects observed.
Overall, UDSCs-related therapies, whether they depend on a cell transplant or cell-derived secretome treatment, have shown promising results, both leading to tissue repair. Effects depend mainly on the activation of regeneration-associated signaling pathways, along with immunomodulatory properties, showing promise within this scope for future studies.
6. Future perspectives
UDSCs can be safely and easily isolated from patients’ urine samples, expanded in vitro, and differentiated into different cell types, including neuron-like cells (Bharadwaj et al., 2013; Guan J. J. et al., 2014; Kang et al., 2015; Xu et al., 2019; Liu et al., 2020). However, they still present some limitations due to difficult culturing conditions such as exposition to a wide range of contaminants (e.g., infections), as well as metabolic waste products, which can potentially affect them. Some of these disadvantages should be overcome by improving the current methodological procedures or developing new ones.
Despite being in its nascent stages, the study of UDSCs elicits optimism, primarily rooted in promising preclinical research showcasing their biocompatibility, engraftment, and in vivo differentiation potential. As stated in section 5, preclinical studies evaluating the therapeutic properties of UDSCs have been exclusively conducted in small animal models. Thus, further investigations are imperative to scrutinize UDSCs post-injection reprogramming prowess and functional efficacy.
Patient UDSCs, and specifically their derived EVs, can be engineered to carry specific cargo that will exert a neuroprotective activity over targeted cells or, the attenuation of disease-related dysfunctional signaling pathways (Drago et al., 2013). The direct delivery of these cargo and its amplification through the control induced-expression of surface antibodies, to be recognized by specific cell types (Bryniarski et al., 2013) renders a new field to be exploited.
7. Conclusion
UDSCs are notorious for their ease, non-invasive process of obtention, able to be simply isolated from any patient’s urine, without major ethical concerns, which makes them a promising alternative to other cell types that can be used for the modeling of neurological disorders and theranostics. Preclinical reports provide evidence of the therapeutic and modeling potential of these cells and their secretome for a multitude of central nervous system-related disorders. UDSCs may be directly reprogrammed into the desired cell type, without the need for pluripotency induction as for iPSCs. Importantly, UDSCs may differentiate in vitro and in vivo into neuronal cells and evidence immunomodulatory and neuroreparative properties. Thus, UDSCs hold great promise as a research field for the development of new brain therapies that could enhance the quality of life for patients with neurological diseases.
Author contributions
GA, CC, SM, and EF have reviewed the literature and wrote the first draft of the manuscript. PO, JV, SM, and EF edited and revised the manuscript. All authors contributed to manuscript revision, read, and approved the submitted version.
Funding
This work was financed by the European Regional Development Fund (ERDF), through the Centro 2020 Regional Operational Programme, through the COMPETE 2020 – Operational Programme for Competitiveness and Internationalisation and Portuguese national funds via FCT – Fundação para a Ciência e a Tecnologia, under the project PTDC/BTM-ORG/0055/2021, and UIDB/04539/2020, UIDP/04539/2020 and LA/P/0058/2020. CC is supported by FCT (Ref: 2022. 13281.BD). SM is supported by FCT (Ref: DL57/2016/CP1448/CT0027). GA is supported by FCT under a PhD fellowship from project PTDC/BTM-ORG/0055/2021. EF was supported by FCT under CEECIND/00322/2017 and is supported by FCT under 2022.00011.CEECIND.
Conflict of interest
The authors declare that the research was conducted in the absence of any commercial or financial relationships that could be construed as a potential conflict of interest.
Publisher’s note
All claims expressed in this article are solely those of the authors and do not necessarily represent those of their affiliated organizations, or those of the publisher, the editors and the reviewers. Any product that may be evaluated in this article, or claim that may be made by its manufacturer, is not guaranteed or endorsed by the publisher.
Abbreviations
ADSCs, Adipose tissue stem cells; BDNF, Brain-derived neurotrophic factor; bFGF, Basic fibroblast growth factor; DMEM, Dulbecco’s Modified Eagle Medium; EGF, Epidermal growth factor; EPCs, Endothelial progenitor cells; ESCs, Embryonic stem cells; EVs, Extracellular vesicles; FBS, Fetal bovine serum; FGF10, Fibroblast growth factor 10; GDNF, Glial cell-derived neurotrophic factor; GFAP, Glial fibrillary acidic protein; iPSCs, Induced pluripotent stem cells; KSFM, Keratinocyte Serum-Free Medium; MSCs, Mesenchymal stem cells; NEAA, Non-essential amino acids; NIM, Neuronal Induction Medium; NM, Neuronal Medium; NMM, Neuronal Maturation Media; NPCs, Neural progenitor cells; NSCs, Neural stem cells; RA, Retinoic acid; REGM, Renal Epithelial Cell Growth Medium; RGCs, Retinal ganglion cells; SVZ, Subventricular zone; UDSCs, Urine-derived stem cells; VPA, Valproic acid.
References
Aden, P., Paulsen, R. E., Mæhlen, J., Løberg, E. M., Goverud, I. L., Liestøl, K., et al. (2011). Glucocorticoids dexamethasone and hydrocortisone inhibit proliferation and accelerate maturation of chicken cerebellar granule neurons. Brain Res. 1418, 32–41. doi: 10.1016/j.brainres.2011.08.053
Alasbahi, R. H., and Melzig, M. F. (2012). Forskolin and derivatives as tools for studying the role of cAMP. Pharmazie 67, 5–13.
Appaix, F. (2014). Brain mesenchymal stem cells: the other stem cells of the brain? World J. Stem Cells. 6:134. doi: 10.4252/wjsc.v6.i2.134
Arcolino, F. O., Piella, A. T., Papadimitriou, E., Bussolati, B., Antonie, D. J., Murray, P., et al. (2015). Human urine as a noninvasive source of kidney cells. Stem Cells Int. 2015, 1–7. doi: 10.1155/2015/362562
Bak, L. K., Schousboe, A., and Waagepetersen, H. S. (2006). The glutamate/GABA-glutamine cycle: aspects of transport, neurotransmitter homeostasis and ammonia transfer. J. Neurochem. 98, 641–53. doi: 10.1111/j.1471-4159.2006.03913.x
Bartkowska, K., Paquin, A., Gauthier, A. S., Kaplan, D. R., and Miller, F. D. (2007). Trk signaling regulates neural precursor cell proliferation and differentiation during cortical development. Development 134, 4369–80. doi: 10.1242/dev.008227
Bento, G., Shafigullina, A. K., Rizvanov, A. A., Sardão, V. A., Macedo, M. P., and Oliveira, P. J. (2020). Urine-derived stem cells: applications in regenerative and predictive medicine. Cells 9:573. doi: 10.3390/cells9030573
Bharadwaj, S., Liu, G., Shi, Y., Markert, C., Andersson, K. E., Atala, A., et al. (2011). Characterization of urine-derived stem cells obtained from upper urinary tract for use in cell-based urological tissue engineering. Tissue Eng. Part A 17, 2123–2132. doi: 10.1089/ten.tea.2010.0637
Bharadwaj, S., Liu, G., Shi, Y., Wu, R., Yang, B., He, T., et al. (2013). Multipotential differentiation of human urine-derived stem cells: potential for therapeutic applications in urology. Stem Cells 31, 1840–1856. doi: 10.1002/stem.1424
Białoń, M., and Wąsik, A. (2022). Advantages and limitations of animal schizophrenia models. Int. J. Mol. Sci. 23:5968. doi: 10.3390/ijms23115968
Bouatra, S., Aziat, F., Mandal, R., Guo, A. C., Wilson, M. R., Knox, C., et al. (2013). The human urine metabolome. PLoS One 8:e73076. doi: 10.1371/journal.pone.0073076
Bozorgmehr, M., Gurung, S., Darzi, S., Nikoo, S., Kazemnejad, S., Zarnani, A. H., et al. (2020). Endometrial and menstrual blood mesenchymal stem/stromal cells: biological properties and clinical application. Front. Cell Dev. Biol. :8. doi: 10.3389/fcell.2020.00497
Bryniarski, K., Ptak, W., Jayakumar, A., Püllmann, K., Caplan, M. J., Chairoungdua, A., et al. (2013). Antigen-specific, antibody-coated, exosome-like nanovesicles deliver suppressor T-cell microRNA-150 to effector T cells to inhibit contact sensitivity. J. Allergy Clin. Immunol. 132, 170–81. doi: 10.1016/j.jaci.2013.04.048
Cao, Y., Xu, Y., Chen, C., Xie, H., Lu, H., and Hu, J. (2021). Local delivery of USC-derived exosomes harboring ANGPTL3 enhances spinal cord functional recovery after injury by promoting angiogenesis. Stem Cell Res Ther 12:20. doi: 10.1186/s13287-020-02078-8
Chen, C. Y., Du, W., Rao, S. S., Tan, Y. J., Hu, X. K., Luo, M. J., et al. (2020). Extracellular vesicles from human urine-derived stem cells inhibit glucocorticoid-induced osteonecrosis of the femoral head by transporting and releasing pro-angiogenic DMBT1 and anti-apoptotic TIMP1. Acta Biomater. 111, 208–220. doi: 10.1016/j.actbio.2020.05.020
Chen, L., Li, L., Xing, F., Peng, J., Peng, K., Wang, Y., et al. (2018). Human urine-derived stem cells: potential for cell-based therapy of cartilage defects. Stem Cells Int. 2018:14. doi: 10.1155/2018/4686259
Chen, H., Li, J., and Yan, H. (2018). The transplantation of human urine stem cells combined with chondroitinase ABC promotes brain-derived neurotrophic factor and nerve growth factor following spinal cord injury in rats. Int. J. Clin. Exp. Pathol. 11, 3858–3866.
Chen, A. J., Pi, J. K., Hu, J. G., Huang, Y. Z., Gao, H. W., Li, S. F., et al. (2020). Identification and characterization of two morphologically distinct stem cell subpopulations from human urine samples. Sci. China Life Sci. 63, 712–723. doi: 10.1007/s11427-018-9543-1
Chen, C. Y., Rao, S. S., Ren, L., Hu, X. K., Tan, Y. J., Hu, Y., et al. (2018). Exosomal DMBT1 from human urine-derived stem cells facilitates diabetic wound repair by promoting angiogenesis. Theranostics [Internet]. 8, 1607–1623. doi: 10.7150/thno.22958
Chun, S. Y., Kim, H. T., Lee, J. S., Kim, M. J., Kim, B. S., Kim, B. W., et al. (2012). Characterization of urine-derived cells from upper urinary tract in patients with bladder cancer. Urology [Internet]. 79, 1186.e1–1186.e7. doi: 10.1016/j.urology.2011.12.034
Colucci-D’amato, L., Speranza, L., and Volpicelli, F. (2020). Neurotrophic factor bdnf, physiological functions and therapeutic potential in depression, neurodegeneration and brain cancer. Int. J. Mol. Sci. 21:7777. doi: 10.3390/ijms21207777
Cortés, D., Carballo-Molina, O. A., Castellanos-Montiel, M. J., and Velasco, I. (2017). The non-survival effects of glial cell line-derived neurotrophic factor on neural cells. Front. Mol. Neurosci. 10:e00258. doi: 10.3389/fnmol.2017.00258
Culenova, M., Nicodemou, A., Novakova, Z. V., Debreova, M., Smolinská, V., Bernatova, S., et al. (2021). Isolation, culture and comprehensive characterization of biological properties of human urine-derived stem cells. Int. J. Mol. Sci. 22:12503. doi: 10.3390/ijms222212503
Culenova, M., Ziaran, S., and Danisovic, L. (2019). Cells involved in urethral tissue engineering: systematic review. Cell Transplant. 28, 1106–1115. doi: 10.1177/0963689719854363
Dam, P. T. M., Hoang, V. T., Bui, H. T. H., Hang, L. M., Hoang, D. M., Nguyen, H. P., et al. (2021). Human adipose-derived mesenchymal stromal cells exhibit high HLA-DR levels and altered cellular characteristics under a Xeno-free and Serum-free condition. Stem Cell Rev. Rep. 17, 2291–2303. doi: 10.1007/s12015-021-10242-7
Dan, Q. Q., Chen, L., Shi, L. L., Zhou, X., Wang, T. H., and Liu, H. (2023). Urine-derived mesenchymal stem cells-derived exosomes enhances survival and proliferation of aging retinal ganglion cells. BMC Struct. Biol. 24:8. doi: 10.1186/s12860-023-00467-4
Das, M., and Pethe, P. (2021). Differential expression of retinoic acid alpha and beta receptors in neuronal progenitors generated from human embryonic stem cells in response to TTNPB (a retinoic acid mimetic). Differentiation 121, 13–24. doi: 10.1016/j.diff.2021.08.001
Dawson, T. M., Golde, T. E., and Lagier-Tourenne, C. (2018). Animal models of neurodegenerative diseases. Nat. Neurosci. 21, 1370–1379. doi: 10.1038/s41593-018-0236-8
Day, P., Alves, N., Daniell, E., Dasgupta, D., Ogborne, R., Steeper, A., et al. (2020). Targeting chondroitinase ABC to axons enhances the ability of chondroitinase to promote neurite outgrowth and sprouting. PLoS One 15:e0221851. doi: 10.1371/journal.pone.0221851
Delanghe, T., Huyghe, J., Lee, S., Priem, D., Van, C. S., Gilbert, B., et al. (2021). Antioxidant and food additive BHA prevents TNF cytotoxicity by acting as a direct RIPK1 inhibitor. Cell Death Dis. 12:699. doi: 10.1038/s41419-021-03994-0
Deuschl, G., Beghi, E., Fazekas, F., Varga, T., Christoforidi, K. A., Sipido, E., et al. (2020). The burden of neurological diseases in Europe: an analysis for the global burden of disease study 2017. Lancet Public Health 5, e551–e567. doi: 10.1016/S2468-2667(20)30190-0
Dominici, M., Le, B. K., Mueller, I., Slaper-Cortenbach, I., Marini, F. C., Krause, D. S., et al. (2006). Minimal criteria for defining multipotent mesenchymal stromal cells. The International Society for Cellular Therapy position statement. Cytotherapy 8, 315–7. doi: 10.1080/14653240600855905
Dörrenhaus, A., Müller, J. I. F., Golka, K., Jedrusik, P., Schulze, H., and Föllmann, W. (2000). Cultures of exfoliated epithelial cells from different locations of the human urinary tract and the renal tubular system. Arch. Toxicol. 74, 618–626. doi: 10.1007/s002040000173
Drago, D., Cossetti, C., Iraci, N., Gaude, E., Musco, G., Bachi, A., et al. (2013). The stem cell secretome and its role in brain repair. Biochimie 95, 2271–85. doi: 10.1016/j.biochi.2013.06.020
Eichmüller, O. L., and Knoblich, J. A. (2022). Human cerebral organoids — a new tool for clinical neurology research. Nat. Rev. Neurol. 18, 661–680. doi: 10.1038/s41582-022-00723-9
Fowler, JL, Ang, LT, and Loh, KM. A critical look: Challenges in differentiating human pluripotent stem cells into desired cell types and organoids. 9, Hoboken: Wiley Interdisciplinary Reviews Developmental Biology. (2020).
Fu, P. C., Tang, R. H., Yu, Z. Y., Xie, M. J., Wang, W., and Luo, X. (2018). The rho-associated kinase inhibitors Y27632 and fasudil promote microglial migration in the spinal cord via the ERK signaling pathway. Neural Regen. Res. 13, 677–683. doi: 10.4103/1673-5374.230294
Funa, K., and Sasahara, M. (2014). The roles of PDGF in development and during neurogenesis in the normal and diseased nervous system. J. Neuroimmune Pharmacol. 9, 168–81. doi: 10.1007/s11481-013-9479-z
Gabbianelli, M., Boccoli, G., Petti, S., Cianetti, L., La valle, R., Ferbus, D., et al. (1987). Expression and in-vitro modulation of HLA antigens in Ontogenic development of human Hemopoietic system. Ann. N. Y. Acad. Sci. 511, 138–147. doi: 10.1111/j.1749-6632.1987.tb36244.x
Gage, F. H., and Temple, S. (2013). Neural stem cells: generating and regenerating the brain. Neuron 80, 588–601. doi: 10.1016/j.neuron.2013.10.037
Ganat, Y. M., Calder, E. L., Kriks, S., Nelander, J., Tu, E. Y., Jia, F., et al. (2012). Identification of embryonic stem cell-derived midbrain dopaminergic neurons for engraftment. J. Clin. Investig. 122, 2928–39. doi: 10.1172/JCI58767
Gooch, C. L., Pracht, E., and Borenstein, A. R. (2017). The burden of neurological disease in the United States: a summary report and call to action. Ann. Neurol. 81, 479–484. doi: 10.1002/ana.24897
Grange, C., Papadimitriou, E., Dimuccio, V., Pastorino, C., Molina, J., O’Kelly, R., et al. (2020). Urinary extracellular vesicles carrying klotho improve the recovery of renal function in an acute tubular injury model. Mol. Ther. 28, 490–502. doi: 10.1016/j.ymthe.2019.11.013
Guan, X., Mack, D. L., Moreno, C. M., Strande, J. L., Mathieu, J., Shi, Y., et al. (2014). Dystrophin-deficient cardiomyocytes derived from human urine: new biologic reagents for drug discovery. Stem Cell Res. 12, 467–80. doi: 10.1016/j.scr.2013.12.004
Guan, J. J., Niu, X., Gong, F. X., Hu, B., Guo, S. C., Lou, Y. L., et al. (2014). Biological characteristics of human-urine-derived stem cells: potential for cell-based therapy in neurology. Tissue Eng. Part A 20, 1794–1806. doi: 10.1089/ten.tea.2013.0584
Guan, J., Zhang, J., Li, H., Zhu, Z., Guo, S., Niu, X., et al. (2015). Human urine derived stem cells in combination with β-TCP can be applied for bone regeneration. PLoS One 10:e0125253. doi: 10.1371/journal.pone.0125253
Guillemot, F., and Zimmer, C. (2011). From cradle to grave: the multiple roles of fibroblast growth factors in neural development. Neuron 71, 574–88. doi: 10.1016/j.neuron.2011.08.002
Guo, Z., Su, W., Zhou, R., Zhang, G., Yang, S., Wu, X., et al. (2021). Exosomal MATN3 of urine-derived stem cells ameliorates intervertebral disc degeneration by Antisenescence effects and promotes NPC proliferation and ECM synthesis by activating TGF- β. Oxidative Med. Cell. Longev. 2021:2074610. doi: 10.1155/2021/2074610
Hansen, T. V. O., Rehfeld, J. F., and Nielsen, F. C. (2000). Cyclic AMP-induced neuronal differentiation via activation of p38 mitogen-activated protein kinase. J. Neurochem. 75, 1870–7. doi: 10.1046/j.1471-4159.2000.0751870.x
Hao, C., Chu, S., Quan, X., Zhou, T., Shi, J., Huang, X., et al. (2023). Establishing extended pluripotent stem cells from human urine cells. Cell Biosci. 13:38. doi: 10.1186/s13578-023-01051-1
Hasan, A., Deeb, G., Rahal, R., Atwi, K., Mondello, S., Marei, H. E., et al. (2017). Mesenchymal stem cells in the treatment of traumatic brain injury. Front. Neurol. 8:e0028. doi: 10.3389/fneur.2017.00028
He, C., Zheng, S., Luo, Y., and Wang, B. (2018). Exosome theranostics: biology and translational medicine. Theranostics 8, 237–255. doi: 10.7150/thno.21945
Huang, J., Guo, X., Li, W., and Zhang, H. (2017). Activation of Wnt/β-catenin signalling via GSK3 inhibitors direct differentiation of human adipose stem cells into functional hepatocytes. Sci. Rep. 7:7. doi: 10.1038/srep40716
Huang, R. L., Li, Q., Ma, J. X., Atala, A., and Zhang, Y. (2023). Body fluid-derived stem cells - an untapped stem cell source in genitourinary regeneration. Nat. Rev. Urol. doi: 10.1038/s41585-023-00787-2
Jaworska, J., Zalewska, T., Sypecka, J., and Ziemka-Nalecz, M. (2019). Effect of the HDAC inhibitor, sodium butyrate, on neurogenesis in a rat model of neonatal hypoxia–ischemia: potential mechanism of action. Mol. Neurobiol. 56, 6341–6370. doi: 10.1007/s12035-019-1518-1
Jia, Y., Xu, H., Li, Y., Wei, C., Guo, R., Wang, F., et al. (2018). A modified Ficoll-Paque gradient method for isolating mononuclear cells from the peripheral and umbilical cord blood of humans for biobanks and clinical laboratories. Biopreserv. Biobank 16, 82–91. doi: 10.1089/bio.2017.0082
Jiang, Z. Z., Liu, Y. M., Niu, X., Yin, J. Y., Hu, B., Guo, S. C., et al. (2016). Exosomes secreted by human urine-derived stem cells could prevent kidney complications from type i diabetes in rats. Stem Cell Res Ther 7:24. doi: 10.1186/s13287-016-0287-2
Jiang, S., Qiu, G. H., Zhu, N., Hu, Z. Y., Liao, D. F., and Qin, L. (2019). ANGPTL3: a novel biomarker and promising therapeutic target. J. Drug Target. 27, 876–884. doi: 10.1080/1061186X.2019.1566342
Kamishibahara, Y., Kawaguchi, H., and Shimizu, N. (2016). Rho kinase inhibitor Y-27632 promotes neuronal differentiation in mouse embryonic stem cells via phosphatidylinositol 3-kinase. Neurosci. Lett. 615, 44–9. doi: 10.1016/j.neulet.2016.01.022
Kang, H. S., Choi, S. H., Kim, B. S., Choi, J. Y., Park, G. B., Kwon, T. G., et al. (2015). Advanced properties of urine derived stem cells compared to adipose tissue derived stem cells in terms of cell proliferation, immune modulation and multi differentiation. J. Korean Med. Sci. 30, 1764–76. doi: 10.3346/jkms.2015.30.12.1764
Kim, J. Y., Chun, S. Y., Park, J. S., Chung, J. W., Ha, Y. S., Lee, J. N., et al. (2018). Laminin and platelet-derived growth factor-BB promote neuronal differentiation of human urine-derived stem cells. Tissue Eng. Regen. Med. 15, 195–209. doi: 10.1007/s13770-017-0102-x
Kim, K., Gil, M., Dayem, A. A., Choi, S., Kang, G. H., Yang, G. M., et al. (2020). Improved isolation and culture of urine-derived stem cells (USCs) and enhanced production of immune cells from the USC-derived induced pluripotent stem cells. J. Clin. Med. 9. doi: 10.3390/jcm9030827
Koh, S. H., Liang, A. C., Takahashi, Y., Maki, T., Shindo, A., Osumi, N., et al. (2015). Differential effects of isoxazole-9 on neural stem/progenitor cells, oligodendrocyte precursor cells, and endothelial progenitor cells. PLoS One 10:e0138724. doi: 10.1371/journal.pone.0138724
Kuželová, K., Grebeňová, D., and Brodská, B. (2011). Dose-dependent effects of the caspase inhibitor Q-VD-OPh on different apoptosis-related processes. J. Cell. Biochem. 112. doi: 10.1002/jcb.23263
Lang, R., Liu, G., Shi, Y., Bharadwaj, S., Leng, X., Zhou, X., et al. (2013). Self-renewal and differentiation capacity of urine-derived stem cells after urine preservation for 24 hours. PLoS One [Internet]. 8:e53980. doi: 10.1371/journal.pone.0053980
Lazzeri, E., Ronconi, E., Angelotti, M. L., Peired, A., Mazzinghi, B., Becherucci, F., et al. (2015). Human urine-derived renal progenitors for personalized modeling of genetic kidney disorders. J. Am. Soc. Nephrol. 26, 1961–1974. doi: 10.1681/ASN.2014010057
le Blanc, K., Tammik, C., Rosendahl, K., Zetterberg, E., and Ringdén, O. (2003). HLA expression and immunologic properties of differentiated and undifferentiated mesenchymal stem cells. Exp. Hematol. 31, 890–896. doi: 10.1016/S0301-472X(03)00110-3
Lee, A. S., Tang, C., Rao, M. S., Weissman, I. L., and Wu, J. C. (2013). Tumorigenicity as a clinical hurdle for pluripotent stem cell therapies. Nat. Med. 19, 998–1004. doi: 10.1038/nm.3267
Lee, Y. M., Zampieri, B. L., Scott-McKean, J. J., Johnson, M. W., and Costa, A. C. S. (2017). Generation of integration-free induced pluripotent stem cells from urine-derived cells isolated from individuals with down syndrome. Stem Cells Trans. Med. 6, 1465–1476. doi: 10.1002/sctm.16-0128
Lepski, G., Jannes, C. E., Nikkhah, G., and Bischofberger, J. (2013). cAMP promotes the differentiation of neural progenitor cells in vitro via modulation of voltage-gated calcium channels. Front. Cell. Neurosci. 7:e00155. doi: 10.3389/fncel.2013.00155
Li, F., Chen, J., Gong, M., Bi, Y., Hu, C., Zhang, Y., et al. (2020). Isolation and characterization of human synovial fluid-derived mesenchymal stromal cells from popliteal cyst. Stem Cells Int. 2020. doi: 10.1155/2020/7416493
Li, H., Gan, X., Pan, L., Zhang, Y., Hu, X., and Wang, Z. (2022). EGF/bFGF promotes survival, migration and differentiation into neurons of GFP-labeled rhesus monkey neural stem cells xenografted into the rat brain. Biochem. Biophys. Res. Commun. 620. doi: 10.1016/j.bbrc.2022.06.077
Li, Q., and Huang, Q. (2019). Single-cell qPCR demonstrates that Repsox treatment changes cell fate from endoderm to neuroectoderm and disrupts epithelialmesenchymal transition. PLoS One 14. doi: 10.1371/journal.pone.0223724
Li, X., Liao, J., Su, X., Li, W., Bi, Z., Wang, J., et al. (2020). Human urine-derived stem cells protect against renal ischemia/reperfusion injury in a rat model via exosomal miR-146a-5p which targets IRAK1. Theranostics 10. doi: 10.7150/thno.42153
Li, M., Wang, X., Meintzer, M. K., Laessig, T., Birnbaum, M. J., and Heidenreich, K. A. (2000). Cyclic AMP promotes neuronal survival by phosphorylation of glycogen synthase kinase 3β. Mol. Cell. Biol. 20. doi: 10.1128/MCB.20.24.9356-9363.2000
Li, X., Zuo, X., Jing, J., Ma, Y., Wang, J., Liu, D., et al. (2015). Small-molecule-driven direct reprogramming of mouse fibroblasts into functional neurons. Cell Stem Cell 17. doi: 10.1016/j.stem.2015.06.003
Ling, X., Zhang, G., Xia, Y., Zhu, Q., Zhang, J., Li, Q., et al. (2020). Exosomes from human urine-derived stem cells enhanced neurogenesis via miR-26a/HDAC6 axis after ischaemic stroke. J. Cell. Mol. Med. 24. doi: 10.1111/jcmm.14774
Liu, G, Deng, C, and Zhang, Y. Urine-derived stem cells: Biological characterization and potential clinical applications. (2013).
Liu, Y., Niu, R., Yang, F., Yan, Y., Liang, S., Sun, Y., et al. (2018). Biological characteristics of human menstrual blood-derived endometrial stem cells. J. Cell. Mol. Med. 22, 1627–1639. doi: 10.1111/jcmm.13437
Liu, G., Pareta, R. A., Wu, R., Shi, Y., Zhou, X., Liu, H., et al. (2013). Skeletal myogenic differentiation of urine-derived stem cells and angiogenesis using microbeads loaded with growth factors. Biomaterials 34, 1311–1326. doi: 10.1016/j.biomaterials.2012.10.038
Liu, D., Rychkov, G., Al-Hawwas, M., Manaph, N. P. A., Zhou, F., Bobrovskaya, L., et al. (2020). Conversion of human urine-derived cells into neuron-like cells by small molecules. Mol. Biol. Rep. 47. doi: 10.1007/s11033-020-05370-1
Lv, F. J., Tuan, R. S., Cheung, K. M. C., and Leung, V. Y. L. (2014). Concise review: the surface markers and identity of human mesenchymal stem cells. Stem Cells 32, 1408–19. doi: 10.1002/stem.1681
Maden, M. (2007). Retinoic acid in the development, regeneration and maintenance of the nervous system. Nat. Rev. Neurosci. 8. doi: 10.1038/nrn2212
Manaph, N. P. A., Al-Hawaas, M., Bobrovskaya, L., Coates, P. T., and Zhou, X. F. (2018). Urine-derived cells for human cell therapy. Stem Cell Res. Ther. 9:222. doi: 10.1186/s13287-018-0974-2
Mane, S., Taneja, S., Madala, J. S., Agarkhedkar, S., and Khetan, M. (2022). Study of stem cells in human Milk. Cureus 14:e23701. doi: 10.7759/cureus.23701
May, J. M. (2012). Vitamin C transport and its role in the central nervous system. Subcell. Biochem. 56, 85–103. doi: 10.1007/978-94-007-2199-9_6
McGraw, C. M., Ward, C. S., and Samaco, R. C. (2017). Genetic rodent models of brain disorders: perspectives on experimental approaches and therapeutic strategies. Am. J. Med. Genet. C Semin. Med. Genet. 175, 368–379. doi: 10.1002/ajmg.c.31570
Meng, Y., Ren, Z., Xu, F., Zhou, X., Song, C., Wang, V. Y. F., et al. (2018). Nicotinamide promotes cell survival and differentiation as kinase inhibitor in human pluripotent stem cells. Stem Cell Rep. 11, 1347–56. doi: 10.1016/j.stemcr.2018.10.023
Mruthyunjaya, S., Manchanda, R., Godbole, R., Pujari, R., Shiras, A., and Shastry, P. (2010). Laminin-1 induces neurite outgrowth in human mesenchymal stem cells in serum/differentiation factors-free conditions through activation of FAK-MEK/ERK signaling pathways. Biochem. Biophys. Res. Commun. 391, 43–8. doi: 10.1016/j.bbrc.2009.10.158
Muir, E., Raza, M., Ellis, C., Burnside, E., Love, F., Heller, S., et al. (2017). Trafficking and processing of bacterial proteins by mammalian cells: insights from chondroitinase ABC. PLoS One 12:e0186759. doi: 10.1371/journal.pone.0186759
Nasci, V. L., Chuppa, S., Griswold, L., Goodreau, K. A., Dash, R. K., and Kriegel, A. J. (2019). Mir-21-5p regulates mitochondrial respiration and lipid content in h9c2 cells. Am. J. Physiol. Heart Circ. Physiol. 316, H710–21. doi: 10.1152/ajpheart.00538.2017
Nguyen, L. T., Tran, N. T., Than, U. T. T., Nguyen, M. Q., Tran, A. M., Do, P. T. X., et al. (2022). Optimization of human umbilical cord blood-derived mesenchymal stem cell isolation and culture methods in serum- and xeno-free conditions. Stem Cell Res. Ther. 13, 1–19.
Nieto-Estévez, V., Defterali, Ç., and Vicario-Abejón, C. (2016). IGF-I: a key growth factor that regulates neurogenesis and synaptogenesis from embryonic to adult stages of the brain. Front. Neurosci. 10:e0052. doi: 10.3389/fnins.2016.00052
Odaka, H., Adachi, N., and Numakawa, T. (2017). Impact of glucocorticoid on neurogenesis. Neural Regen. Res. 12, 1028–1035. doi: 10.4103/1673-5374.211174
Pan, W., Xu, X., Zhang, M., and Song, X. (2021). Human urine-derived stem cell-derived exosomal miR-21-5p promotes neurogenesis to attenuate Rett syndrome via the EPha4/TEK axis. Lab. Investig. 101. doi: 10.1038/s41374-021-00574-w
Park, M., and Seo, J. J. (2012). Role of HLA in hematopoietic stem cell transplantation. Bone Marrow Res. 2012. doi: 10.1155/2012/680841
Phiel, C. J., Zhang, F., Huang, E. Y., Guenther, M. G., Lazar, M. A., and Klein, P. S. (2001). Histone deacetylase is a direct target of Valproic acid, a potent anticonvulsant, mood stabilizer, and teratogen. J. Biol. Chem. 276, 36734–36741. doi: 10.1074/jbc.M101287200
Pouryazdanpanah, N., Dabiri, S., Derakhshani, A., Vahidi, R., and Farsinejad, A. (2018). Peripheral blood-derived mesenchymal stem cells: growth factor-free isolation, molecular characterization and differentiation. Iran J. Pathol. 13:461.
Rajendran, G., Dutta, D., Hong, J., Paul, A., Saha, B., Mahato, B., et al. (2013). Inhibition of protein kinase c signaling maintains rat embryonic stem cell pluripotency. J. Biol. Chem. 288. doi: 10.1074/jbc.M113.455725
Ryu, S., Lee, S. H., Kim, S. U., and Yoon, B. W. (2016). Human neural stem cells promote proliferation of endogenous neural stem cells and enhance angiogenesis in ischemic rat brain. Neural Regen. Res. 11. doi: 10.4103/1673-5374.177739
Sahara, S., and O’Leary, D. D. M. (2009). Fgf10 regulates transition period of cortical stem cell differentiation to radial glia controlling generation of neurons and basal progenitors. Neuron 63. doi: 10.1016/j.neuron.2009.06.006
Sayed, S., Song, J., Wang, L., Muluh, T. A., Liu, B., Lin, Z., et al. (2023). Isoxazole 9 (ISX9), a small molecule targeting Axin, activates Wnt/β-catenin signalling and promotes hair regrowth. Br. J. Pharmacol. 180, 1748–65. doi: 10.1111/bph.16046
Schosserer, M., Reynoso, R., Wally, V., Jug, B., Kantner, V., Weilner, S., et al. (2015). Urine is a novel source of autologous mesenchymal stem cells for patients with epidermolysis bullosa. BMC. Res. Notes 8:767. doi: 10.1186/s13104-015-1686-7
Semba, T., Sammons, R., Wang, X., Xie, X., Dalby, K. N., and Ueno, N. T. (2020). Concise review: JNK signaling in stem cell self-renewal and differentiation. Int. J. Mol. Sci. 21:2613. doi: 10.3390/ijms21072613
Shen, H. H. (2016). Better models for brain disease. Proc. Natl. Acad. Sci. U. S. A. 113, 5461–4. doi: 10.1073/pnas.1615096113
Shi, T., and Cheung, M. (2021). Urine-derived induced pluripotent/neural stem cells for modeling neurological diseases. Cell Biosci. 11:85. doi: 10.1186/s13578-021-00594-5
Shi, Y., Liu, G., Wu, R., Mack, D. L., Sun, X. S., Maxwell, J., et al. (2022). Differentiation capacity of human urine-derived stem cells to retain telomerase activity. Front. Cell Dev. Biol. 10:890574. doi: 10.3389/fcell.2022.890574
Shin, D. M., Ahn, J. I., Lee, K. H., Lee, Y. S., and Lee, Y. S. (2004). Ascorbic acid responsive genes during neuronal differentiation of embryonic stem cells. Neuroreport 15, 1959–63. doi: 10.1097/00001756-200408260-00025
Silva AL, T. E., Yokota, B. Y., Sertié, A. L., and Zampieri, B. L. (2023). Generation of urine-derived induced pluripotent stem cells and cerebral organoids for modeling down syndrome. Stem Cell Rev. Rep. 19, 1116–1123. doi: 10.1007/s12015-022-10497-8
Strano, A., Tuck, E., Stubbs, V. E., and Livesey, F. J. (2020). Variable outcomes in neural differentiation of human PSCs Arise from intrinsic differences in developmental signaling pathways. Cell Rep. 31. doi: 10.1016/j.celrep.2020.107732
Terrigno, M., Busti, I., Alia, C., Pietrasanta, M., Arisi, I., D’Onofrio, M., et al. (2018). Neurons generated by mouse ESCs with hippocampal or cortical identity display distinct projection patterns when co-transplanted in the adult brain. Stem Cell Rep. 10. doi: 10.1016/j.stemcr.2018.01.010
Thakur, K. T., Albanese, E., Giannakopoulos, P., Jette, N., Linde, M., Prince, M. J., et al. (2016). Neurological disorders. Disease control priorities, third edition. Ment. Neurol. Subst. Use Disord. 4, 87–107. doi: 10.1596/978-1-4648-0426-7_ch5
Thompson, R., Casali, C., and Chan, C. (2019). Forskolin and IBMX induce neural Transdifferentiation of MSCs through downregulation of the NRSF. Sci. Rep. 9:2969. doi: 10.1038/s41598-019-39544-0
Tojo, M., Hamashima, Y., Hanyu, A., Kajimoto, T., Saitoh, M., Miyazono, K., et al. (2005). The ALK-5 inhibitor A-83-01 inhibits Smad signaling and epithelial-to-mesenchymal transition by transforming growth factor-β. Cancer Sci. 96. doi: 10.1111/j.1349-7006.2005.00103.x
Van, C. A., Van, C. C. M., Lagrou, A. R., and Manuel-Y-Keenoy, B. (2003). Transferrin modifications and lipid peroxidation: implications in diabetes mellitus. Free Radic. Res. 37. doi: 10.1080/10715760310001600390
Vukićević, V., Qin, N., Balyura, M., Eisenhofer, G., Wong, M. L., Licinio, J., et al. (2015). Valproic acid enhances neuronal differentiation of sympathoadrenal progenitor cells. Mol. Psych. 20, 941–950. doi: 10.1038/mp.2015.3
Wan, S., Bao, D., Li, J., Lin, K., Huang, Q., Li, Q., et al. (2022). Extracellular vesicles from hypoxic pretreated urine-derived stem cells enhance the proliferation and migration of chondrocytes by delivering miR-26a-5p. Cartilage 13. doi: 10.1177/19476035221077401
Wan, Q., Xiong, G., Liu, G., Shupe, T. D., Wei, G., Zhang, D., et al. (2018). Urothelium with barrier function differentiated from human urine-derived stem cells for potential use in urinary tract reconstruction. Stem Cell Res Ther 9. doi: 10.1186/s13287-018-1035-6
Wang, L., Li, X., Huang, W., Zhou, T., Wang, H., Lin, A., et al. (2016). TGFβ signaling regulates the choice between pluripotent and neural fates during reprogramming of human urine derived cells. Sci. Rep. 6:6. doi: 10.1038/srep22484
Wu, C., Chen, L., Huang, Y. Z., Huang, Y., Parolini, O., Zhong, Q., et al. (2018). Comparison of the proliferation and differentiation potential of human urine-, placenta decidua basalis-, and bone marrow-derived stem cells. Stem Cells Int. 2018, 1–11. doi: 10.1155/2018/7131532
Wu, T., Pinto, H. B., Kamikawa, Y. F., and Donohoe, M. E. (2015). The BET family member BRD4 interacts with OCT4 and regulates pluripotency gene expression. Stem Cell Rep. 4. doi: 10.1016/j.stemcr.2015.01.012
Wu, R., Soland, M., Liu, G., Shi, Y., Zhang, C., Tang, Y., et al. (2021). Functional characterization of the immunomodulatory properties of human urine-derived stem cells. Trans. Androl. Urol. 10. doi: 10.21037/tau-21-506
Wu, J., Wang, X., Fu, G., Feng, Y., Wang, Y., Zhang, G., et al. (2023). Exosomes from human urine-derived stem cells carry NRF1 to alleviate bladder fibrosis via regulating miR-301b-3p/TGFβR1 pathway. Mol. Cell. Biochem. 478. doi: 10.1007/s11010-022-4484-3
Xu, G., Wu, F., Gu, X., Zhang, J., You, K., Chen, Y., et al. (2019). Direct conversion of human urine cells to neurons by small molecules. Sci. Rep. 9. doi: 10.1038/s41598-019-53007-6
Yan, Z., Shi, X., Wang, H., Si, C., Liu, Q., and Du, Y. (2021). Neurotrophin-3 promotes the neuronal differentiation of BMSCs and improves cognitive function in a rat model of Alzheimer’s disease. Front. Cell. Neurosci. 15:15. doi: 10.3389/fncel.2021.629356
Yi, H., Xie, B., Liu, B., Wang, X., Xu, L., Liu, J., et al. (2018). Derivation and identification of motor neurons from human urine-derived induced pluripotent stem cells. Stem Cells Int. 2018. doi: 10.1155/2018/3628578
Yoo, H. C., Yu, Y. C., Sung, Y., and Han, J. M. (2020). Glutamine reliance in cell metabolism. Exp. Mol. Med. 52, 1496–1516. doi: 10.1038/s12276-020-00504-8
Zhang, Y., Chen, X., Yuan, L., Zhang, Y., Wu, J., Guo, N., et al. (2018). Down-regulation of IRAK1 attenuates podocyte apoptosis in diabetic nephropathy through PI3K/Akt signaling pathway. Biochem. Biophys. Res. Commun. 506. doi: 10.1016/j.bbrc.2018.09.175
Zhang, Y. Y., Ludwikowski, B., Hurst, R., and Frey, P. (2001). Expansion and long-term culture of differentiated normal rat urothelial cells in vitro. In Vitro Cell. Dev. Biol. Anim. 37, 419–429. doi: 10.1290/1071-2690(2001)037<0419:EALTCO>2.0.CO;2
Zhang, S. Z., Ma, L. X., Qian, W. J., Li, H. F., Wang, Z. F., Wang, H. X., et al. (2016). Modeling neurological disease by rapid conversion of human urine cells into functional neurons. Stem Cells Int. 2016:8. doi: 10.1155/2016/1929536
Zhang, Y., McNeill, E., Tian, H., Soker, S., Andersson, K. E., Yoo, J. J., et al. (2008). Urine derived cells are a potential source for urological tissue reconstruction. Urol. Surv. 180, 2226–2233. doi: 10.1016/j.juro.2008.07.023
Zhang, D., Wei, G., Li, P., Zhou, X., and Zhang, Y. (2014). Urine-derived stem cells: a novel and versatile progenitor source for cell-based therapy and regenerative medicine. Genes Dis. 1, 8–17. doi: 10.1016/j.gendis.2014.07.001
Zhao, T., Luo, D., Sun, Y., Niu, X., Wang, Y., Wang, C., et al. (2018). Human urine-derived stem cells play a novel role in the treatment of STZ-induced diabetic mice. J. Mol. Histol. 49, 419–28. doi: 10.1007/s10735-018-9772-5
Zhou, T., Benda, C., Dunzinger, S., Huang, Y., Ho, J. C., Yang, J., et al. (2012). Generation of human induced pluripotent stem cells from urine samples. Nat. Protoc. 7, 2080–9. doi: 10.1038/nprot.2012.115
Zhou, M., Shen, L., Qiao, Y., and Sun, Z. (2020). Inducing differentiation of human urine-derived stem cells into hepatocyte-like cells by coculturing with human hepatocyte L02 cells. J. Cell. Biochem. 121, 566–73. doi: 10.1002/jcb.29301
Zhou, Z., Xu, N., Matei, N., McBride, D. W., Ding, Y., Liang, H., et al. (2021). Sodium butyrate attenuated neuronal apoptosis via GPR41/Gβγ/PI3K/Akt pathway after MCAO in rats. J. Cereb. Blood Flow Metab. 41, 267–81. doi: 10.1177/0271678X20910533
Zhu, Q., Li, Q., Niu, X., Zhang, G., Ling, X., Zhang, J., et al. (2018). Extracellular vesicles secreted by human urine-derived stem cells promote ischemia repair in a mouse model of hind-limb ischemia. Cell. Physiol. Biochem. 47, 1181–92. doi: 10.1159/000490214
Keywords: neurological diseases, urine-derived stem cells, advanced therapies, exosomes, neuronal differentiation, regenerative medicine
Citation: Cavaleiro C, Afonso GJM, Oliveira PJ, Valero J, Mota SI and Ferreiro E (2023) Urine-derived stem cells in neurological diseases: current state-of-the-art and future directions. Front. Mol. Neurosci. 16:1229728. doi: 10.3389/fnmol.2023.1229728
Edited by:
Jean-Marc Taymans, Institut National de la Santé et de la Recherche Médicale (INSERM), FranceReviewed by:
Martin Chi Hang Cheung, The University of Hong Kong, Hong Kong SAR, ChinaAndrea Papait, Catholic University of the Sacred Heart, Italy
Copyright © 2023 Cavaleiro, Afonso, Oliveira, Valero, Mota and Ferreiro. This is an open-access article distributed under the terms of the Creative Commons Attribution License (CC BY). The use, distribution or reproduction in other forums is permitted, provided the original author(s) and the copyright owner(s) are credited and that the original publication in this journal is cited, in accordance with accepted academic practice. No use, distribution or reproduction is permitted which does not comply with these terms.
*Correspondence: Sandra I. Mota, c2FuZHJhLm1vdGFAY25jLnVjLnB0; Elisabete Ferreiro, ZWJmQGNuYy51Yy5wdA==
†These authors have contributed equally to this work and share first authorship
‡These authors have contributed equally to this work and share senior authorship