- 1Department of Biological Sciences, Faculty of Pharmaceutical Sciences, Teikyo University, Tokyo, Japan
- 2Department of Molecular Biology, Faculty of Pharmaceutical Sciences, Teikyo University, Tokyo, Japan
Serotonin (5-hydroxytryptamine, 5-HT) is a phylogenetically conserved neurotransmitter and modulator. Neurons utilizing serotonin have been identified in the central nervous systems of all vertebrates. In the central serotonergic system of vertebrate species examined so far, serotonergic neurons have been confirmed to exist in clusters in the brainstem. Although many serotonin-regulated cognitive, behavioral, and emotional functions have been elucidated in mammals, equivalents remain poorly understood in non-mammalian vertebrates. The purpose of this review is to summarize current knowledge of the anatomical organization and molecular features of the avian central serotonergic system. In addition, selected key functions of serotonin are briefly reviewed. Gene association studies between serotonergic system related genes and behaviors in birds have elucidated that the serotonergic system is involved in the regulation of behavior in birds similar to that observed in mammals. The widespread distribution of serotonergic modulation in the central nervous system and the evolutionary conservation of the serotonergic system provide a strong foundation for understanding and comparing the evolutionary continuity of neural circuits controlling corresponding brain functions within vertebrates. The main focus of this review is the chicken brain, with this type of poultry used as a model bird. The chicken is widely used not only as a model for answering questions in developmental biology and as a model for agriculturally useful breeding, but also in research relating to cognitive, behavioral, and emotional processes. In addition to a wealth of prior research on the projection relationships of avian brain regions, detailed subdivision similarities between avian and mammalian brains have recently been identified. Therefore, identifying the neural circuits modulated by the serotonergic system in avian brains may provide an interesting opportunity for detailed comparative studies of the function of serotonergic systems in mammals.
1. Introduction
Serotonin (5-hydroxytryptamine, 5-HT) is an ancient signaling molecule (Hay-Schmidt, 2000). Serotonin utilization is not only evolutionarily conserved in vertebrates and invertebrates of the animal kingdom; it has also been identified in plants (for further information, see the review by Erland et al., 2016). In the animal kingdom, numerous studies have suggested that the neuromodulatory functions of serotonin are implicated in a wide range of processes, including cognition, behavior, and emotion, and that these associations are also evolutionarily conserved (Jacobs and Azmitia, 1992; Lucki, 1998; Kandel et al., 2000; Bacque-Cazenave et al., 2020). In invertebrates, one of the most notable examples of the effects of serotonergic function on behavior is its effect on rapidly inducing notorious swarming and gregarious behavior in desert locusts (Schistocerca gregaria) (Anstey et al., 2009; Rogers and Ott, 2015). In vertebrates and especially humans, the serotonergic system has received much attention as a pharmacological target because it has been implicated in many brain functions associated with psychiatric disorders (Belmaker and Agam, 2008; Ravindran and Stein, 2010). Researchers have sought to understand, at the neural circuit level, the brain regions involved in mammalian cognitive, behavioral, and emotional processing, as well as the specific cells modulated by serotonin within these regions (Olivier, 2015; Strac et al., 2016; Yagishita, 2020). For example, the serotonergic system is implicated in the regulation of fear-related behaviors in mammals by modulating the neural microcircuits of the amygdala via several serotonergic receptors (LeDoux, 2000; Deakin and Graeff, 2013; Duvarci and Pare, 2014; Janak and Tye, 2015; Tovote et al., 2015; Bocchio et al., 2016; Lawther et al., 2020). However, it remains to be elucidated whether the neural circuits involved in cognitive, behavioral, and emotional processing, as well as their relationships with serotonergic modulation, are evolutionary conserved across vertebrates. To address this issue, it is necessary to clarify such neural circuits in a range of vertebrate animals other than mammals.
At the conceptual level, serotonergic systems display remarkable conservation across vertebrate species (Parent, 1981), suggesting evolutionary conserved roles in brain functions. However, the macro structure of vertebrate brains is diverse and lineage dependent, limiting the availability of information about which neural circuits are involved in cognition, behavior, and emotion (Pessoa et al., 2019). Birds represent unique model animals for elucidating the evolutionary continuity of the neural basis of cognition, behavior, and emotion because of the availability of information on several neural circuits associated with behaviors (Jarvis et al., 2005; Rosa Salva et al., 2015; Martinez-Garcia and Lanuza, 2018; Papini et al., 2019). As a result of the efforts of many scientific investigators over the years, the avian research community currently has access to a wealth of resources including behavior-related brain lesion data (Benowitz, 1980; Matsushima et al., 2003), comprehensive brain region projection relationships (Axer et al., 2016; Herold et al., 2019; Stacho et al., 2020), a revised consensus on brain nomenclature (Reiner et al., 2004), and several excellent brain atlases (e.g., for chicken, Kuenzel and Masson, 1988; Puelles et al., 2018).
In this review, we summarize the currently available information on avian brain serotonergic systems. This review consists of four sections. First, we briefly introduce well-studied serotonergic systems in the mammalian brain for comparison with those of birds. Second, we discuss the extent of homology between avian and mammalian brains. There is some controversy about the correspondence between bird and mammal brains, but here we focus on homologies. Third, we describe serotonergic systems in chicken brains, mainly based on our recent molecular dissection work in chicks (Fujita et al., 2019, 2020, 2022a,b,c). Fourth, we briefly summarize the functions of serotonergic systems in bird brains, focused at the level of gene function.
2. A brief introduction to serotonergic systems in mammals
There are two independent serotonergic systems in mammals. On the one hand, the central serotonergic system functions as a central neurotransmitter, whereas on the other the peripheral serotonergic system works as a peripheral signaling molecule. The proportion of serotonin contained in the central nervous system is small; most serotonin is located in the gut, where it contributes to important physiological functions such as motility, secretion, and vasodilation (Erspamer, 1937; Erspamer and Asero, 1952; Spohn and Mawe, 2017). Importantly, the central and peripheral serotonergic systems are completely independent, since serotonin molecules cannot cross the blood–brain barrier (Xu et al., 2014; Mosienko et al., 2020).
In the central nervous system, serotonergic neurons are present in cell groups in the raphe nuclei of the brainstem. Cell groups containing serotonergic neurons have historically been called groups B1–B9 (Dahlstroem and Fuxe, 1964; Soiza-Reilly and Gaspar, 2020). Among these serotonergic cell groups, the dorsal raphe (DR: B6 and B7) and median raphe (MR: B5 and B8) provide ascending innervation to the forebrain and midbrain (Figure 1A; Olivier, 2015; Cohen and Grossman, 2020; Soiza-Reilly and Gaspar, 2020). Given that the neural projections from the DR and MR cover almost all areas of the forebrain, including the amygdala, nucleus accumbens (NAc), bed nucleus of the stria terminalis (BNST), olfactory bulb (OB), prefrontal cortex (PFC), hippocampal formation (HF), and periaqueductal gray (PAG) (Figure 1A), and are involved in the regulation of many cognitive, behavioral, and emotional states and processes such as learning, reward, aggression, impulsivity, anxiety, and mood, the DR and MR serotonergic systems have been the subjects of intensive research (Olivier, 2015; Lawther et al., 2020; Liu et al., 2020; O'leary et al., 2020; Yagishita, 2020; Awasthi et al., 2021; Beyeler et al., 2021).
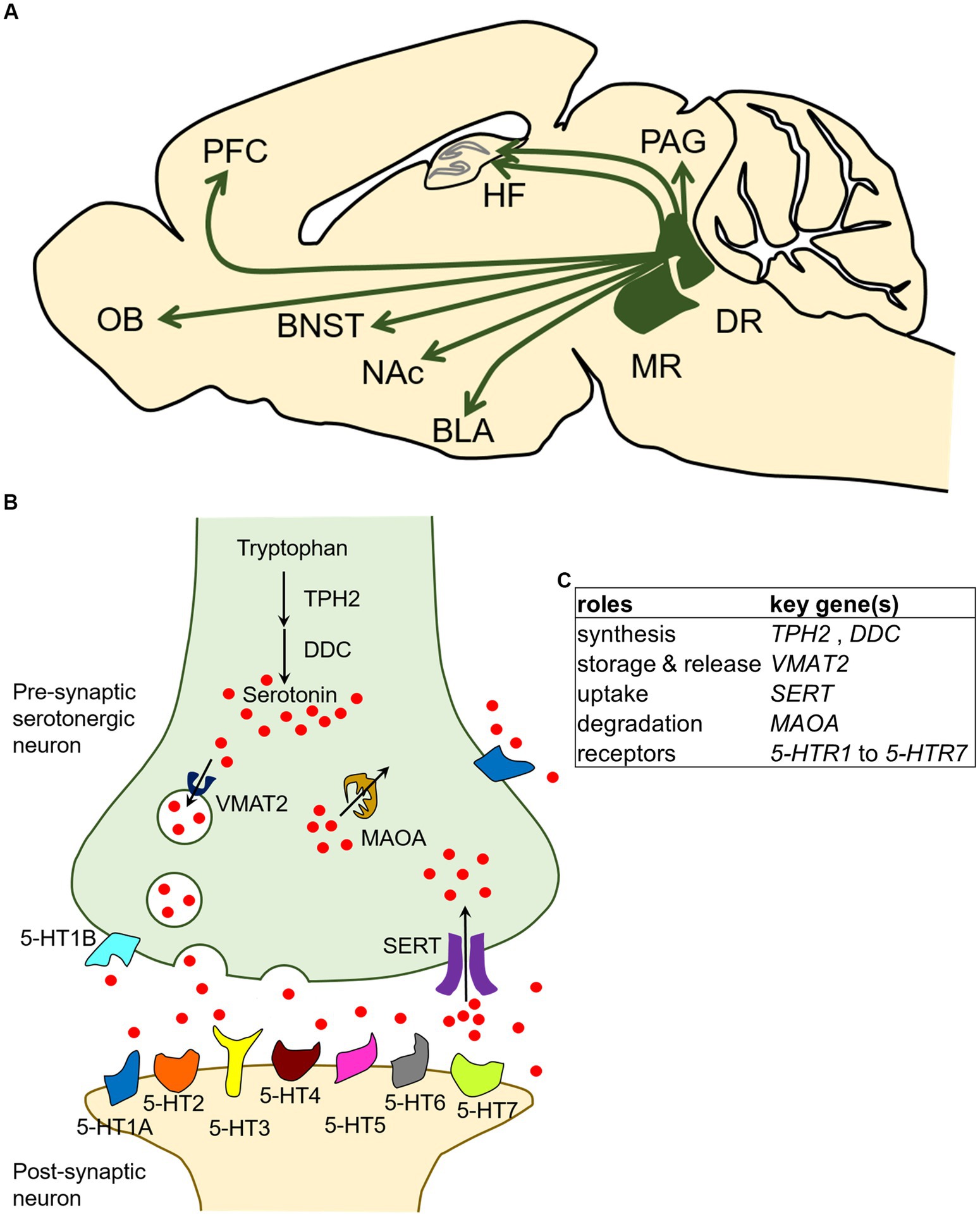
Figure 1. Localization and broad brain projections of the dorsal raphe and median raphe nuclei in the mammalian central serotonergic system and the molecular characteristics of serotonergic neurons. (A) Serotonergic neurons of the central serotonergic system are localized to the raphe nuclei of the brainstem, and the DR and MR send extensive projections throughout the forebrain and midbrain. The model diagram represents a sagittal section of a rodent, and it should be noted that the projected brain regions may not all be observable on the same sagittal plane. Arrows indicate the presence of projections. BLA, basolateral amygdala; BNST, bed nucleus of the stria terminalis; DR, dorsal raphe nucleus; HF, hippocampal formation; MR, median raphe nucleus; NAc, nucleus accumbens; OB, olfactory bulb; PAG, periaqueductal gray; PFC, prefrontal cortex. (B) In serotonergic neurons, serotonin is synthesized by the hydroxylation of tryptophan by tryptophan hydroxylase 2 (TPH2) followed by decarboxylation by DOPA decarboxylase (DDC). Serotonin is packaged into storage vesicles by vesicular monoamine transporter 2 (VMAT2) and released into the synaptic cleft. The released serotonin is mediated by serotonin receptors (5-HTR1 to 5-HTR7) present on the membranes of presynaptic and postsynaptic neurons, and signals are transmitted to cells. Serotonin in the synaptic cleft is taken up by serotonin transporter (SERT) and degraded by monoamine oxidase A (MAOA). (C) Summary table of characteristic genetic influences of serotonergic neurons schematized in panel (B).
In the serotonergic neuron, serotonin is synthetized in a two-step process: conversion of the amino acid tryptophan (Trp) to 5-hydroxytryptophan (5-HTP), and subsequent decarboxylation of 5-HTP to 5-HT (serotonin). The first, rate-limiting step is mediated by tryptophan hydroxylase 2 (Tph2) (Walther et al., 2003; Walther and Bader, 2003). The second step is mediated by the aromatic amino acid decarboxylase (Aadc), also called DOPA decarboxylase (Ddc) because it is involved in dopamine biosynthesis. The synthesized serotonin is then stored in secretory vesicles by vesicular monoamine transporter 2 (Vmat2) and the vesicles later transport serotonin to the presynaptic terminal for release (Schuldiner et al., 1995). The serotonin released in the synaptic cleft is taken up by the presynaptic neuron through the serotonin transporter (Sert), which is encoded by the solute carrier family 6 member 4 (Slc6a4) gene (Hoffman et al., 1991; Lesch et al., 1993). After the reuptake of serotonin into the serotonergic neurons, monoamine oxidase A (Maoa) catalyzes the oxidative deamination of 5-HT (Youdim et al., 2006). Among the proteins involved in serotonin synthesis, storage, release, uptake, and degradation, Ddc, Vmat2, and Maoa are involved in non-serotonin monoaminergic neuron processes and are thus expressed in both serotonergic and non-serotonergic neurons (Lein et al., 2007; Ng et al., 2009, 2010). In contrast, Tph2 and Sert are useful as molecular markers of serotonergic neurons because they function exclusively in the serotonergic neurons at least in the central nervous system of adult mammals. It should be noted that Sert is transiently expressed in non-serotonergic neurons in several brain regions during development (Lebrand et al., 1996; Narboux-Nême et al., 2008). In addition, the released serotonin acts on its downstream targets through multiple receptors called 5-HT receptors (5-HTRs) which exist on the cell membrane. To date, 14 distinct receptors have been identified and classified into seven groups, namely 5-HTR1 to 5-HTR7, based on their functional, structural, and biochemical characteristics. All 5-HTRs are G protein-coupled receptors (GPCRs) except for 5-HTR3 family members, which are ligand-gated ion channels (Hannon and Hoyer, 2008; Marin et al., 2020). An overview of the characteristic molecular mechanisms of serotonergic neurons described above is presented in Figures 1B,C. In terms of molecular components, the monoaminergic (including serotonergic) systems are thought to be largely conserved in vertebrates (Yamamoto and Vernier, 2011).
3. The avian brain
The field of comparative neuroscience has revealed that the basic organization of the brain is shared across vertebrates. The vertebrate brain consists of the forebrain, midbrain, and hindbrain. Although the midbrain and hindbrain tend to be relatively well conserved, the forebrain has undergone major evolutionary changes. The telencephalon, which occupies most of the forebrain, can be divided into the pallium and subpallium (Striedter, 2005). In the avian pallium, the dorsal ventricular ridge (DVR), which consists of the mesopallium, nidopallium, and arcopallium, is a large proportion of the entire brain (Figure 2; Aboitiz et al., 2003; Molnár, 2011). The macro structure of the adult avian pallium looks quite different from that of mammals, making it difficult to understand its correspondence with the adult mammalian pallium, a historically controversial subject (Karten, 1997; Puelles, 2001; Jarvis et al., 2005). Regarding the macro structural differences between the adult pallium of birds and mammals, for example, while most mammalian cortex exhibits a six-layered structure, most superficial areas of avian pallium appear to lack an obvious layered structure and are instead organized into clusters of neurons (nuclei) (Karten, 2015). In order to investigate whether the neural circuits processing specific cognitive, behavioral, emotional processes are evolutionarily conserved or not, it is necessary to understand the homology of brain regions between birds and mammals.
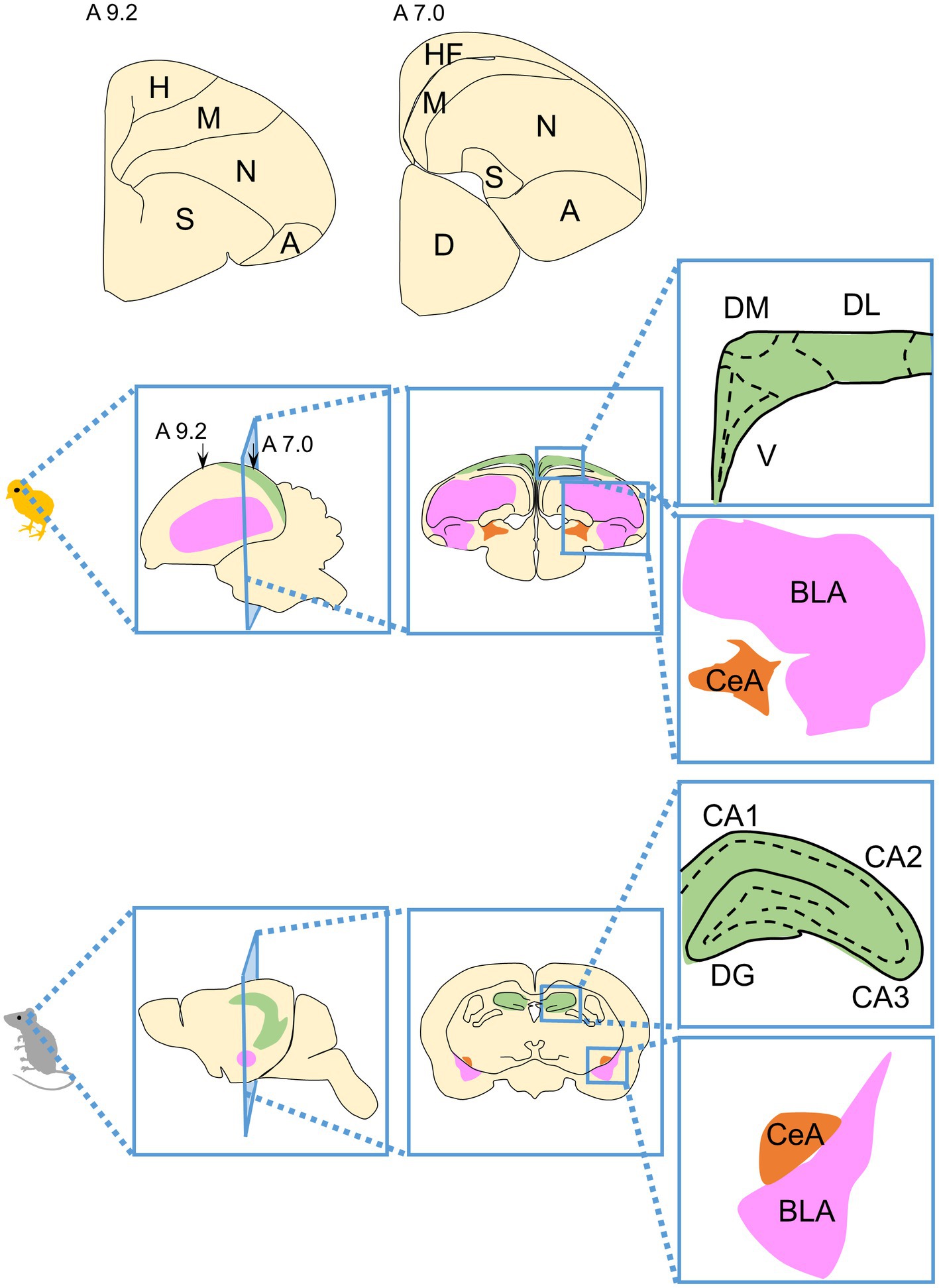
Figure 2. Schematic drawings of the structure of the avian telencephalon and its correspondence with the mammalian telencephalon. The avian telencephalon contains the pallium and subpallium. The largest portion of the pallium is the dorsal ventricular ridge (DVR) consisting of the mesopallium (M), nidopallium (N), and arcopallium (A). Schematic representations of hemisphere sections are shown above, with section levels according to the atlas of Kuenzel and Masson (1988). A schematic diagram of the brain structures of a chicken (as an example of an avian brain) and mouse (as an example of a mammalian brain) is shown. Portions considered to be homologous regions are indicated by the same color code. Green indicates the HF, magenta indicates the BLA, and orange indicates the CeA in the brains of both birds and mammals. A, arcopallium; BLA, basolateral amygdala; CA1, cornu ammonis field 1, CA2, cornu ammonis field2; CA3, cornu ammonis field 3; CeA, central nucleus of the amygdala; D, diencephalon; DG, dentate gyrus; DL, dorsal lateral region; DM, dorsal medial region; H, hyperpallium; HF, hippocampal formation; M, mesopallium; N, nidopallium; S, striatum; V, V-shaped complex.
One of the most commonly used methods to study homology is to compare expression regions of genes that are highly conserved in broad animal phyla during early development. Such research has led to the creation of a field referred to as evolutionary developmental biology (Evo-Devo), and one of the best-known discoveries in this field is that many of the key genes involved in developmental patterning, called ‘toolkit genes,’ are conserved across all bilaterally symmetric animal phyla. These toolkit genes consist of a small set of master regulatory genes, including transcription factors and signal transduction molecules (Carroll, 2008; Carroll et al., 2013). Studies have been performed to elucidate homologous regions of vertebrate pallium by combining expression regions of regulatory genes conserved in vertebrates in morphogenesis at early embryonic stages and morphological landmarks (Fernandez et al., 1998; Puelles et al., 2000). Currently, the embryonic pallium has been divided into four (Puelles et al., 2017) or six (Desfilis et al., 2018) components, it has been clarified that pallium derivatives correspond to adult brain regions. Here, we refer to the proposed pallium divisions as the medial, dorsal, dorsolateral/lateral, and ventral/ventrocaudal pallial divisions (Pessoa et al., 2019). The brain structures derived from each component of the pallium in birds and mammals have been proposed as follows: the medial pallium gives rise to the hippocampal formation in birds and mammals; the dorsal pallium gives rise to the hyperpallium in birds and the neocortex in mammals; the dorsolateral/lateral pallium give rise to the mesopallium in birds and the claustro-insular region, orbitofrontal cortex rostrally, and perirhinal/lateral entorhinal cortex caudally in mammals; and the ventral/ventrocaudal pallium give rise to the arcopallium and nidopallium in birds and the olfactory cortex and pallial amygdala, which is part of amygdala, in mammals (Puelles, 2001; Moreno and González, 2006; Medina and Abellán, 2009; Puelles et al., 2017; Medina et al., 2017a; Desfilis et al., 2018). Depending on the species, pallium-derived brain structures may undergo different developmental trajectories after late development and may acquire a different cytoarchitecture, neurochemical features, and connectivity (Puelles and Medina, 2002; Striedter, 2005; Medina et al., 2017a). Therefore, it is difficult to understand the homology of brain regions between different species by comparing the properties of the adult traits and terminal functional molecules alone. The results of recent single-cell RNA sequencing analyses strongly support the homologous relationship between the avian and mammalian brain regions described above, based on similarities such as the combinatorial profiles of transcription factors that determine cell properties (Tosches et al., 2018; Colquitt et al., 2021).
In addition, homologies are beginning to reveal correspondences between subdivisions of avian and mammalian brain structures. Here, we briefly discuss the current state of two structures recognized for their important contributions to cognitive, behavioral, and emotional processing: the hippocampal formation (HF) and amygdala (Figure 2).
We first discuss HF. All mammalian HFs are well conserved for macroscopic histological features (Hevner, 2016). They are composed of similarly complex and intertwined three-layered subdivisions, known as the Ammon’s horns or Cornu ammonis (CA) fields 1 (CA1), CA2, CA3, dentate gyrus (DG), and the subiculum (Hevner, 2016; Medina et al., 2017a). As a connection relationship within such subdivisions of HF, a neural circuit called “trisynaptic circuit” is considered to be important. The trisynaptic ciruit involves subdivisions of the entorhinal cortex (EC), DG, CA3, and CA1, and consists of projections from EC to DG, DG to CA3, and CA3 to CA1(Sloviter and Lomo, 2012). Among these projections, the DG to CA3 projection is called the “mossy fiber” and is a well known anatomical landmark of the HF. On the other hand, avian HFs occupy a large area of the caudal surface of the pallium with a high density of neurons, with a layered structure that is not readily observable macro-anatomically and subdivision that is similarly not clearly observable (Atoji and Wild, 2006; Herold et al., 2015; Striedter, 2016). Furthermore, the mossy fiber-like structures seen in the mammalian DG have not been found in avian HF (Faber et al., 1989; Montagnese et al., 1993, 1996; Tombol et al., 2000; Herold et al., 2014). To date, several subdivisions have been proposed for avian HFs, using several methods and criteria, such as histology, immunohistochemistry, projection relationships, and developmental origin (Kuenzel and Masson, 1988; Atoji and Wild, 2004, 2006; Suarez et al., 2006; Gupta et al., 2012; Abellan et al., 2014; Herold et al., 2014; Atoji et al., 2016; Medina et al., 2017a; Puelles et al., 2018). However, there is controversy over homologous regions within avian HFs for mammalian DG and CA fields, and it is difficult to say that a one-to-one correspondence between avian HFs and mammalian HFs have been established for subdivision within HFs (Atoji and Wild, 2004, 2006; Kempermann, 2012; Abellan et al., 2014; Herold et al., 2014; Atoji et al., 2016; Hevner, 2016; Striedter, 2016; Medina et al., 2017a). Briefly, there are three possibilities for homology with subdivisions of mammalian HFs for the subdivision of avian HFs. A first possibility is that the V-shaped complex (V) in the avian HF corresponds to the mammalian DG (Atoji and Wild, 2004; Suarez et al., 2006; Gupta et al., 2012; Herold et al., 2014; Atoji et al., 2016; Puelles et al., 2018). A second possibility is that the dorsal medial region (DM) in the avian HF corresponds to the mammalian DG (Montagnese et al., 1996; Szekely, 1999). A third possibility is that the avian HF does not have a homolog of the mammalian DG. The underlying idea is that it has been so long since birds and mammals diverged that it is difficult to compare HF subdivisions between birds and mammals in the first place. In other words, the idea is that the DG is a uniquely acquired trait of mammalian HF (Hevner, 2016; Striedter, 2016). Recently, neurons with a function similar to “place cells” that selectively fire when passing through a specific place have been identified in the HF of birds (Payne et al., 2021). In mammals, place cells are recognized as pyramidal neurons in the CA1 and CA3 as well as granule cells in the DG of the trisynaptic circuit (O'Keefe and Dostrovsky, 1971; Muller et al., 1987; Jung and Mcnaughton, 1993; Wilson and Mcnaughton, 1993). This finding raises the possibility that a neural circuit corresponding to the trisynaptic circuit exists in the HF of birds. The current state of the avian HF, especially in chickens, was recently reviewed (see Morandi-Raikova and Mayer, 2022).
We second discuss the amygdala. The mammalian amygdala is a brain region composed of a heterogeneous assembly of nuclei derived from both the subpallial nuclei and pallial nuclei. The amygdala has been investigated as one of the regions responsible for processing emotions, including learned fear (LeDoux, 2000; Medina et al., 2017b; Martinez-Garcia and Lanuza, 2018). Under laboratory conditions, the critical neural microcircuits responsible for learned fear have been revealed using Pavlovian fear conditioning paradigms. Among the subdivisions of the amygdala, the lateral nucleus (LA), basal nucleus (BA), and basomedial (BM) nucleus together constitute such a neural circuit with the involvement of the basolateral complex of the amygdala (BLA). In this circuit, the LA receives sensory projections from outside of the amygdala and projects to the BA, which projects to the central nucleus of the amygdala (CeA) to provide output to the brainstem (LeDoux, 2000; Duvarci and Pare, 2014; Janak and Tye, 2015; Tovote et al., 2015).
On the other hand, it has been proposed that regions homologous to the avian pallial amygdala are the arcopallium and nidopallium, as described above, but there is no consensus among the avian research community.
Historically, the homologous region of the avian pallial amygdala has often been referred to as the arcopallium, and in recent years the avian amygdala tends to be treated as the arcopallium/amygdala complex, which combines the arcopallium, posterior pallial amygdala, nucleus taeniae of the amygdala (TnA), and subpallial amygdaloid area (Herold et al., 2018). Regarding the nidopallium, previous studies have functionally associated it with the mammalian PFC, so few attempts have been made to associate the nidopallium with the mammalian amygdala (Karten, 1997, 2013; Güntürkün, 2005; Butler et al., 2011; Dugas-Ford et al., 2012; Herold et al., 2018). Although no previous studies using the Pavlovian fear conditioning paradigm have been conducted to examine the functions involved in the arcopallium and nidopallium, several studies using the pigeon appetite conditioning paradigm have been conducted to implicate parts of the arcopallium and nidopallium in extinction learning (Lissek and Güntürkün, 2005; Lengersdorf et al., 2014, 2015; Starosta et al., 2017; Gao et al., 2018, 2019a,b; Güntürkün et al., 2020). In avian, the medial and central amygdaloid structures and output projections from amygdala have been unveiled (Abellán and Medina, 2009; Vicario et al., 2014; Hanics et al., 2017).
In summary, the crude homology between avian and mammalian pallium is grossly apparent. However, the correspondence between brain structural subregions remains unclear due to the divergence of adult brain morphology. In the future, the development of single-cell transcriptomics that traces the developmental processes of avian pallium will clarify the homology between the brain subregions of birds and mammals. In addition, a future task is to clarify the extent to which neural circuits between homologous regions of the brains of birds and mammals share common cognitive, behavioral, and emotional functions.
4. Molecular dissections of the serotonergic system in the chick brain
The central serotonergic system of vertebrates has a conserved form in which serotonergic neurons reside in the raphe nuclei of the brainstem, where the DR and MR send neuronal projections throughout the forebrain and midbrain (Parent, 1981). The distribution of serotonergic neurons in the central serotonergic system of vertebrates other than mammals has been identified to an extent. In fish, for example, the locations and projections of serotonergic neurons have been comprehensively described by generating a transgenic zebrafish line expressing GFP under control of the regulatory elements of pet1, referred to as the serotonergic neuron specific marker gene of the raphe (Lillesaar et al., 2009). In addition, the distribution and function of serotonergic neurons in fish have already been reviewed (Lillesaar, 2011). In amphibians, the organization of central serotonergic neurons has been described in several species, including salamander (Dubé and Parent, 1982; Clairambault et al., 1994; Dicke et al., 1997) and frog (van Mier et al., 1986; Bhat and Ganesh, 2023). In reptiles, the location of serotonergic neurons has been described in several species, such as turtle (Ueda et al., 1983), lizard (Smeets and Steinbusch, 1988), snake (Challet et al., 1991), and crocodile (Rodrigues et al., 2008). In birds, the distribution of serotonergic neurons has been described in multiple species, including chicken (Ikeda and Goto, 1971; Dube and Parent, 1981; Parent, 1981; Yamada, 1984), pigeon (Fuxe and Ljunggren, 1965; Challet et al., 1996; Meneghelli et al., 2009), and quail (Cozzi et al., 1991). In the vertebrates examined previously, the presence of serotonergic neurons as cell groups in the brainstem, specifically the DR and MR, has been confirmed. However, for many non-mammalian vertebrates, the anatomical information is not well organized, and hence the one-to-one correspondence with mammalian nuclei is not always obvious. In addition, to the best of our knowledge, detailed properties such as the molecular characteristics of the central serotonergic system neurons of non-mammalian vertebrate remain largely unknown. Furthermore, our understanding of the distribution of neurons that are modulated by serotonin (that is, those expressing 5-HTRs) in non-mammalian vertebrae is limited. Therefore, using the chicken (Gallus gallus domesticus) as an avian model, which is easy to manage, we attempted to molecularly elucidate the central serotonergic system of the DR and MR. Below, we summarize the details of the distribution of serotonergic neurons in the DR and MR and the molecular heterogeneity of serotonergic neurons (Fujita et al., 2022b). We also summarize the expression regions of most 5-HTR genes in the telencephalon (Fujita et al., 2020, 2022a), and the potential for serotonergic regulation of the dopaminergic system (Fujita et al., 2022c). All data presented below are based on results from post-hatched day 1 (P1) chick brain samples in controlled growing conditions (Yamaguchi et al., 2008a,b). The histological terminology used in these studies, and the histological atlas primarily used, is the terminology of the avian brain nomenclature consortium (Reiner et al., 2004), and the atlas of Kuenzel and Masson (1988), respectively. In addition, the atlas of Puelles et al. (2018) was also used for the histological position of several brain structures.
4.1. The distribution and heterogeneity of the serotonergic neurons in the chick DR and MR
Previous studies have described the anatomical position of the raphe nuclei (Kuenzel and Masson, 1988; Puelles et al., 2018), the distribution of monoamines based on fluorescence histochemistry (Ikeda and Goto, 1971; Dube and Parent, 1981; Parent, 1981), and the distribution of serotonergic neurons based on immunohistochemistry using an anti-serotonin antiserum (Yamada, 1984), as well as information about their developmental processes (Wallace, 1985; Sako et al., 1986; Okado et al., 1992; Huang X. et al., 2019) in chicken. Several studies have used chickens to describe the distribution of the brainstem cell groups containing cells with characteristics of serotonergic neurons. In addition, based on their anatomical position, possible correspondences between these chick cell groups and mammalian serotonergic cell groups have been proposed (Ikeda and Goto, 1971; Dube and Parent, 1981; Yamada, 1984). However, in the above-mentioned studies, the serotonergic cell groups were without consideration of the mammalian serotonergic cell groups, and hence, the group numbers do not necessarily match between mammals and birds. Therefore, we used the terms chicken DR and MR as follows: the DR for “B5” (Ikeda and Goto, 1971), “Group 5” (Yamada, 1984), and “DR nucleus” (Puelles et al., 2018); and the MR for “B6” and “B7” (Ikeda and Goto, 1971), “Group 6” and “Group 10” (Yamada, 1984), “rhombomere 1 median raphe nucleus (r1MnR),” “rhombomere 2 median raphe nucleus (r2MnR),” and “caudal linear nucleus of the raphe (CLi)” (Puelles et al., 2018).
First, the distribution of cell bodies of serotonergic neurons in the brainstem, including the chicken DR and MR, was analyzed using in situ hybridization (ISH) to determine the gene expression distribution of the chicken orthologues of the mammalian serotonergic neuron markers TPH2 and SERT (Fujita et al., 2022b). TPH2- and SERT-expressing cells were found to be densely distributed in a lateral spread form from the mesencephalon to the medulla oblongata in the DR (equivalent to A2.0 to A1.0 in the Kuenzel and Masson Atlas). In the MR, they were found to be distributed slightly more towards the mesencephalon side than the DR (equivalent to A2.4 to A1.0 in the Kuenzel and Masson Atlas) from the mesencephalon to the medulla oblongata (Fujita et al., 2022b). A comparison of the expression patterns of these genes using serial sections of the brainstem showed a well-matched distribution (Fujita et al., 2022b), which corresponded well with the distribution of serotonergic neurons described in previous studies (Ikeda and Goto, 1971; Dube and Parent, 1981; Yamada, 1984), suggesting that these two genes are useful markers for specifically visualizing serotonergic neurons in chick brains. There is considerable heterogeneity among serotonergic neurons between and within the DR and MR in relation to multiple aspects, including their developmental origin, connectivity, electrophysiological properties, molecular organization, and behavioral functions in mammals (Calizo et al., 2011; Alonso et al., 2013; Okaty et al., 2019, 2020; Ren et al., 2019; Huang K. W. et al., 2019; Soiza-Reilly and Gaspar, 2020). In addition, developmental and projection target heterogeneity of serotonergic neurons also exist in fish (Gaspar and Lillesaar, 2012), suggesting that the existence of heterogeneity in serotonergic neurons is evolutionarily conserved in vertebrates. As an example of the molecular heterogeneity of serotonergic neurons, such neurons are known to express 5-HTRs on their own and are subject to serotonergic modulation (Beyeler et al., 2021; De Deurwaerdere and Di Giovanni, 2021). There is heterogeneity between and within the DR and MR in which 5-HTR is expressed in each serotonergic neuron (Okaty et al., 2019, 2020; Ren et al., 2019; Huang K. W. et al., 2019).
Moreover, the expression of most 5-HTR orthologues was examined in the chick DR and MR. This revealed that 5-HTR1A, 5-HTR1B, 5-HTR1D, 5-HTR1E, and 5-HTR5A were differently expressed in chick DR and MR serotonergic neurons with partial overlap, indicating the heterogeneity in chick serotonergic neurons (Fujita et al., 2022b). Furthermore, the set of 5-HTRs expressed in the serotonergic neurons of chicken DR and MR is similar to that of the mammalian DR and MR, suggesting that the molecular organization of serotonergic neurons is evolutionarily conserved. The exception is that the set of 5-HTRs in the genome is not strictly identical from species to species, notable examples are the absence of orthologues in the genome: 5-HTR5B is absent from the chicken genome (International Chicken Genome Sequencing Consortium, 2004) and 5-Htr1e is absent from the mouse genome (Vilaró et al., 2020). Evolutionary conservation of the molecular organization of serotonergic neurons is also supported by studies showing similarities in gene expression during brainstem development in chickens and mice (Cambronero and Puelles, 2000; Alonso et al., 2013; Watson et al., 2019). In the future, further research is needed to clarify the heterogeneity, including projection destination preferences and their electrophysiological heterogeneity, in avian DR and MR serotonergic neurons in order to better understand the degree of conservation of the serotonergic system.
4.2. Expression of 5-HTRs in the chick telencephalon
Little is known about the distribution of neurons that are modulated by serotonin in the avian telencephalon. To date, for example, the site of action of 5-HTR1A in the pigeon brain has been studied using the selective radioligand [3H]-8-hydroxy-2-(di-n-propylamino)tetralin ([3H]-8-OH-DPAT) for 5-HTR1A (Dietl and Palacios, 1988; Waeber et al., 1989; Herold et al., 2012, 2014; dos Santos et al., 2015; Herold et al., 2018). We have previously comprehensively examined the expression regions of the above 5-HTR orthologues in the chick whole telencephalon (Fujita et al., 2020, 2022a). Notably, the strongest and most widespread signals, including strong signals in the striatum, were obtained from 5-HTR1B, while 5-HTR1A and 5-HTR3A had a sparse distribution of expressing cells in all brain regions examined. These expression patterns appear to be similar to those characteristic of 5-HTR1A, 5-HTR1B, and 5-HTR3A expression patterns in the mouse brain (Lein et al., 2007; Ng et al., 2009, 2010). Therefore, in order to compare the gross characteristics of the brain regions where serotonin regulation occurs in birds and mammals, we summarized the 5-HTR expression regions in the chick brain and existing information about 5-HTR expression data in mice (Lein et al., 2007; Ng et al., 2009, 2010; Vilaró et al., 2020). A comparison of the information with a focus on homologous regions is shown in Figure 3A. From the ventral pallium/ventrocaudal pallium-derived brain structures, the arcopallium and nidopallium in chicks and the olfactory cortex and pallial amygdala in mice showed the most commonality in the presence or absence of the detection of 5-HTR family genes. This fact is consistent with the widely accepted fact that the avian arcopallium is homologous and functionally conserved with part of the mammalian pallial amygdala (Medina et al., 2017b; Martinez-Garcia and Lanuza, 2018), and suggests that there are molecular commonalities in serotonergic regulation between birds and mammals. However, for DP-derived brain structures, the hyperpallium in chicks and neocortex in mice, opposite features were observed (Figure 3A). For example, the orthologues of 5-HTR2A and 5-HTR2C have characteristic expression patterns in the mammalian neocortex; however, no significant signal was detected in the chick hyperpallium (Fujita et al., 2020). Given that the neocortex is a highly specialized brain structure only in mammals, such a result does not deserve to be surprising. On the other hand, there is a consensus about the homology of the MP-derived HFs in both birds and mammals (Reiner et al., 2004; Herold et al., 2015; Striedter, 2016), and the ancient origin of HFs has been strongly corroborated by tissue dissected bulk transcriptome study (Belgard et al., 2013) and recent single-cell transcriptome study (Tosches et al., 2018). We compared the functions of each subdivision between avian and mammalian HFs from the viewpoint of the molecular basis of serotonin regulation, but there was no clear correspondence (Figure 3B).
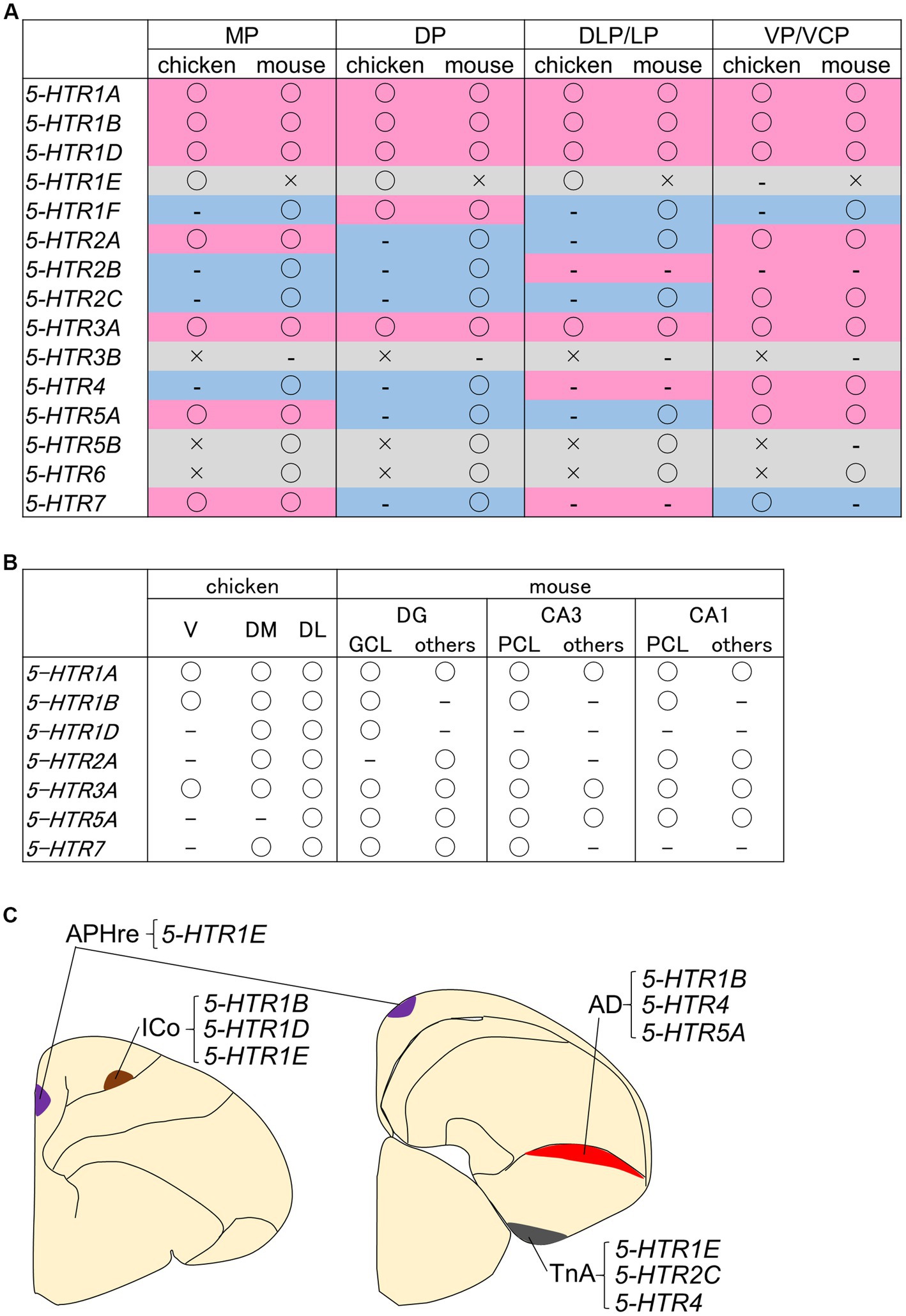
Figure 3. Summaries of 5-HTRs expression information in the chicken pallium. Tables summarizing the pallium (A) and HF subdivisions (B) in context of whether the 5-HTR family genes (and their orthologs) are present in chicken and mouse genomes. The brain structures derived from each pallium is as follows: the MP gives rise to the HF in birds and mammals; the DP gives rise to the hyperpallium in birds and the neocortex in mammals; the DLP/LP gives rise to the mesopallium in birds and the claustro-insular region, orbitofrontal cortex rostrally, and perirhinal/lateral entorhinal cortex caudally in mammals; and the VP/VCP gives rise to the arcopallium and nidopallium in birds and the olfactory cortex and pallial amygdala in mammals (Puelles, 2001; Moreno and González, 2006; Medina and Abellán, 2009; Puelles et al., 2017; Medina et al., 2017a; Desfilis et al., 2018). Please refer to the main text for more details on pallium homology. “○” indicates that it has been detected, “–” indicates that it has not been detected, and “×” indicates that it does not exist in the genome or has not been examined. These data are based on Fujita et al. (2020, 2022a) for chickens and on expression information from previous studies, including parts confirmed using the Allen brain atlas (Lein et al., 2007; Ng et al., 2009, 2010; Tanaka et al., 2012; Luo et al., 2019; Vilaró et al., 2020). The background colors in the table of panel (A) represent the following: magenta, common detection or non-detection in chicken and mouse; blue, divergent results between chicken and mouse; and light gray, comparison not possible because an “×” is included in one of the genomes. Note that the data for chickens were obtained by the chemical coloring in situ hybridization method, and the detection methods for mice are not necessarily the same. (C) Schematic representation of neuronal information with characteristic combinations of 5-HTRs expression in the chicken telencephalon. Purple indicates APHre, brown indicates ICo, red indicates AD, and gray indicates TnA. AD, dorsal arcopallium; APHre, ectopic part of the rostral area parahippocampalis; CA1, cornu ammonis field 1, CA3, cornu ammonis field 3; DG, dentate gyrus; DL, dorsal lateral region; DLP/LP, dorsolateral pallium/lateral pallium; DM, dorsal medial region; DP, dorsal pallium; GCL, granule cell layer; ICo, intercalated core nucleus; MP, medial pallium; PCL, pyramidal cell layer; TnA, nucleus taeniae of the amygdala; V, V-shaped complex; VP/VCP, ventral pallium/ventrocaudal pallium.
In the mammalian HF, a large number of glutamatergic excitatory neurons are distributed in the DG granule cell layer, CA3 pyramidal cell layer, and CA1 pyramidal cell layer, which have high cell densities, and it is widely recognized that neuron populations in these layers constitute a trisynaptic circuit (Sloviter and Lomo, 2012; Hevner, 2016; Medina et al., 2017a). On the other hand, in general and except for developmental processes, cell types in avian HFs are not well understood at the molecular level, making it difficult to compare cell types with mammalian cell types. Some neuronal markers, such as calbindin, CaMKII, and DCX, which are used from a neurochemical point of view and in immunohistochemistry, are also used in the avian HF (Suarez et al., 2006; Melleu et al., 2013; Herold et al., 2019; Rook et al., 2023). A particularly interesting study using HF cell type markers by Atoji et al. (2016) showed that the orthologue of the DG granule cell population marker, prox1, was not only available as a developmental chicken DG region marker (Gupta et al., 2012; Abellan et al., 2014), but was also expressed in adult pigeon V. Since the avian V has been proposed to be equivalent to the mammalian DG (Atoji and Wild, 2004; Suarez et al., 2006; Gupta et al., 2012; Herold et al., 2014; Atoji et al., 2016; Puelles et al., 2018), this raised the possibility that PROX1 could also be used as a marker for corresponding cells in the DG granule cell population marker in adult birds. To better understand the characteristics of serotonin-regulated neural circuits in the avian HF, it is necessary to increase the available markers for cell types within the avian HF. In the mouse HF, the 5-HTR1B gene is selectively and strongly expressed in the CA1 pyramidal layer (Lein et al., 2007; Ng et al., 2009, 2010), whereas in the chick HF, 5-HTR1B-expressing cells are sparsely distributed in the V and DM, and abundantly distributed throughout the DL (Fujita et al., 2020, 2022a). For example, if avian HF 5-HTR1B-expressing cells can be cell typed, it may be possible to identify the distribution of neurons in the avian HF that share similarities in serotonergic control with mammalian CA1 pyramidal neurons.
In addition, several brain regions (nuclei) that exhibit characteristic 5-HTRs expression patterns in the chick have been identified. First, the intercalated core nucleus (ICo) of the hyperpallium strongly expresses 5-HTR1B, 5-HTR1D, and 5-HTR1E (Fujita et al., 2020, 2022a; Figure 3C). The ICo has been recently named by Puelles et al., 2018 to describe the nucleus, first noted for its zinc-rich property (Faber et al., 1989) and subsequently noted to express of ENC1 (García-Calero and Puelles, 2009), ZENK (Zapka et al., 2010), and NR4A2 genes (Puelles et al., 2016; Fujita et al., 2019). Since there is little information on how the ICo is in involved in brain functions such as cognition, behavior, and emotion, it is expected that attention will focus on serotonergic regulation prior to advances in our understanding. Second, 5-HTR1E was found to be highly selectively expressed in a part of the DM subdivision of the HF, an ectopic part of the rostral area parahippocampalis (APHre) characterized by LEF1 expression (Abellan et al., 2014; Figure 3C). LEF1 is a transcription factor required for the generation of granule cells in the mammalian DG (Galceran et al., 2000). A significant LEF1-expressing population during the development of HFs is commonly observed in both chickens and mice (Gupta et al., 2012; Abellan et al., 2014). However, in the adult HF, there is a significant LEF1-expressing population in the APHre in post-hatched chicken, whereas LEF1 expression is not significant in the adult HF of mice (Lein et al., 2007; Ng et al., 2009, 2010). As such, the APHre may represent a unique cellular population within birds and be a region key to understanding the functional correspondence between avian and mammalian HFs. In chickens, the projection relationship of the APHre and related functions are completely unknown, but in the HF of pigeons, several excellent descriptions of the intra-HF projection relationships have been published (Atoji and Wild, 2004, 2006; Atoji et al., 2016; Herold et al., 2019; Rook et al., 2023). The subdivision chicken APHre (Abellan et al., 2014) appears to correspond to the parvocellular hippocampal area (Pa) (Atoji and Wild, 2004) or the dorsal dorsomedial (DMd) region (Herold et al., 2014) in pigeons, but detailed correspondence information remains to be elucidated. Confirming whether the Pa or DMd in pigeons and the APHre in chickens can be regarded as homologs and unifying terminology will be the first step toward understanding the functions of the APHre in birds. Third, 5-HTR1B, 5-HTR4, and 5-HTR5A are expressed in the dorsal arcopallium (AD), while 5-HTR2C, 5-HTR4, and 5-HTR1E are enriched in the TnA (Figure 3C). Previous studies on the distribution of serotonergic projections and terminals using anti-serotonin antiserum or antibody have shown that the AD and TnA of avian brains, including chickens (Yamada and Sano, 1985; Metzger et al., 2002) and pigeons (Challet et al., 1996), were commonly enriched with serotonergic nerve terminals. However, it was revealed that the AD and TnA express different 5-HTR family genes, and focusing on the differences in their molecular compositions will help clarify the mode of serotonergic regulation that the AD and TnA receive.
Taken together, our previous work provided a comprehensive overview of 5-HTR gene expression patterns in the chick telencephalon (Fujita et al., 2020, 2022a). For example, mammalian 5-Htr4 is known to have multiple splice variants (Bockaert et al., 2006; Marin et al., 2020), and it has been clarified that the expression site differs depending on the variant (Vilaró et al., 2005). As multiple isoforms of chicken 5-HTR4 are registered in the database, it is possible that there are differences in the expression site for each of these isoforms. To make a more detailed molecular comparison of serotonergic regulation in mammalian and avian brains, it is important to increase the information on the expression distribution of avian receptors. However, 5-HTRs for whom characteristic expression patterns have not been clarified may exhibit low levels of expression. Therefore, in order to elucidate the detailed distribution, it may be necessary to use highly sensitive methods, such as using radioisotopes. Few prior studies have demonstrated the existence of the neural circuits involved in cognitive, behavioral, and emotional functions in bird brain regions. Information on the expression population of 5-HTR genes provides a useful molecular basis for studying the role of neural circuits in birds in the future.
4.3. Serotonergic modulation of the other modulatory system in chicks
In addition to the central serotonergic system, the dopaminergic system is another important modulatory system known to be involved in motivation-related behavior. In addition, the central serotonergic system and the dopaminergic system interact with each other in mammals (Boureau and Dayan, 2011; De Deurwaerdere and Di Giovanni, 2017, 2021; Yagishita, 2020; Beyeler et al., 2021; Peters et al., 2021). The midbrain dopaminergic nuclei receive the projections from the DR including serotonergic neurons (Ogawa et al., 2014; Beier et al., 2015; Ogawa and Watabe-Uchida, 2018). Furthermore, recently, it has been clarified how dopaminergic neurons undergo serotonergic regulation at the neural circuit level and which 5-HTRs are involved in mice (Wang et al., 2019; Peters et al., 2021). Interaction between the serotonergic and dopaminergic systems appears to occur in birds (Matsunami et al., 2012), but the molecular basis of this interaction was previously unknown. Through comprehensive examination of the expression of the 5-HTRs probe set described above, we revealed that 5-HTR1A and 5-HTR1B are expressed in chick dopaminergic nuclei (Fujita et al., 2022c). Therefore, it was suggested that the dopaminergic system is also regulated by serotonin through 5-HTR1A and 5-HTR1B in birds, supporting the importance of the interaction between the serotonergic and dopaminergic systems. Unlike in mammals, the 5-HTR1A- and 5-HTR1B-expressing cells and dopaminergic neuron marker expression did not overlap, and 5-HTRs expressed directly in dopaminergic neurons could not be detected. It would be interesting to clarify serotonergic regulation of dopaminergic neurons in birds using methods with higher detection sensitivity and through pharmacological examination of dopaminergic neurons. Analysis of the molecular basis of interactions between modulatory systems in birds is nascent, and further analysis is required in the future.
5. Central serotonergic system functions in birds
The central serotonergic system in mammals has been demonstrated to be involved in a wide variety of cognitive, behavioral, and emotional processes, including mood, fear, anxiety, appetite, aggression, impulsivity, reward and learning (Jacobs and Azmitia, 1992; Lucki, 1998; Yagishita, 2020). These neuromodulatory functions of serotonin have also been investigated in birds. For example, behavioral-pharmacological studies have revealed the involvement of serotonergic regulation in aggression in pigeons (Fachinelli et al., 1989) and foraging behavior in chickens (Matsunami et al., 2012). In addition, associations with feather-pecking behavior, during which chickens on a poultry farm peck their feathers together sometimes resulting in cannibalism in extreme cases (Savory, 1995), have also been examined through combining analyses of behavior and brain serotonin levels (Kops et al., 2017). For an excellent review on the relationship between feather-pecking behavior and the serotonergic system the reader is referred to De Haas and Van Der Eijk (2018).
Although specific gene functions in the serotonergic system are not clearly linked to specific cognitive, behavioral, and emotional processes in birds, studies are beginning to focus on expression of the 5-HTR genes. For example, a previous study linked high expression of 5-HTR2C in the right cerebral hemisphere with susceptibility to being a victim of feather pecking (Yao et al., 2017), and another study correlated the expression levels of 5-HTR2A and 5-HTR2B in the caudal region of the left telencephalon with individual variations in cognition using reversal learning (Boddington et al., 2020). Association studies between SERT polymorphism and behaviors, of which there are a relatively large number, are now discussed.
In humans, the promoter region of the SERT gene has a repetitive sequence insertion/deletion (IN/DEL) polymorphism, known as the SERT polymorphic region (SERTPR, or 5-HT transporter (5-HTT)-linked polymorphic region, 5-HTTLPR) that affects its expression level; it has been repeatedly indicated that this polymorphism is associated with many psychiatric states such as depression, anxiety, and suicidal behavior (Serretti et al., 2006; Canli and Lesch, 2007; Murphy et al., 2008). In chickens, a functional polymorphism considered analogous to human SERTPR has been discovered and shown to have associations to body weight gain and locomotion activity (Phi-Van et al., 2014). Subsequently, the same chicken SERTPR polymorphism was shown to be associated with feed intake (Kjaer and Phi-Van, 2016) and fear-related behaviors (Krause et al., 2017, 2019; Phi Van et al., 2018; Table 1). However, it should be noted that the behavioral assays used to measure fear-related behaviors in chickens have not been standardized and are not necessarily widely supported, unlike the common assay systems used in mammals for fear conditioning (LeDoux, 2000) and anxiety-related behaviors (reviewed in Allsop et al., 2014; O'leary et al., 2020). As a notable example, tonic immobility (TI), a paradigm often chosen in behavioral studies to observe indicators of fear in chickens, is thought to represent a form of reproducible hypnotic state (Gallup et al., 1971; Gallup, 1974). The bird TI has a long history of being used as an index of fear and, since it can be observed with good reproducibility, it is undoubtedly highly useful as an indicator of certain behaviors (Gallup, 1973; Fureix and Meagher, 2015). However, it is unclear whether the TI in birds should be compared to fear-related behavior in mammals. Elucidating the neural circuits that control TI in chickens will advance what kind of behavioral and emotional states TI represents, and thus what emotional states it should be compared to in mammals. In addition, there are also interesting examples of association studies combining the genotyping of SERT polymorphisms and ecological observations as well as behavioral tests in wild birds. Several SERT polymorphisms have been associated with behavioral traits, such as the performance of novel object tests and urban or rural habitat differences in great tits (Parus major) (Riyahi et al., 2015, 2022; Timm et al., 2018, 2019; Grunst et al., 2021; Thys et al., 2021), blackbirds (Turdus erula) (Müller et al., 2013), and dunnocks (Prunella modularis) (Holtmann et al., 2016), and have been linked to animal personalities such as boldness and aggression (Table 1). However, associations between SERT polymorphisms and behavioral traits have not always yielded consistent results across birds and populations. For example, no association was detected between SERT polymorphisms and behavioral tests using Seychelles warbler (Acrocephalus sechellensis) (Edwards et al., 2015), no SERT polymorphism was detected with black swans (Cygnus atratus) (Van Dongen et al., 2015), and when using great tits Timm et al. (2019) and Thys et al. (2021) had conflicting results regarding female aggression. The discrepancies in these results appear to be at least in part due to differences in species and populations, as well as the difficulty in collecting a sample size that enhances statistical power, due to the use of wild animals. More research in the field is required to facilitate a future meta-analysis that could elucidate the issue.
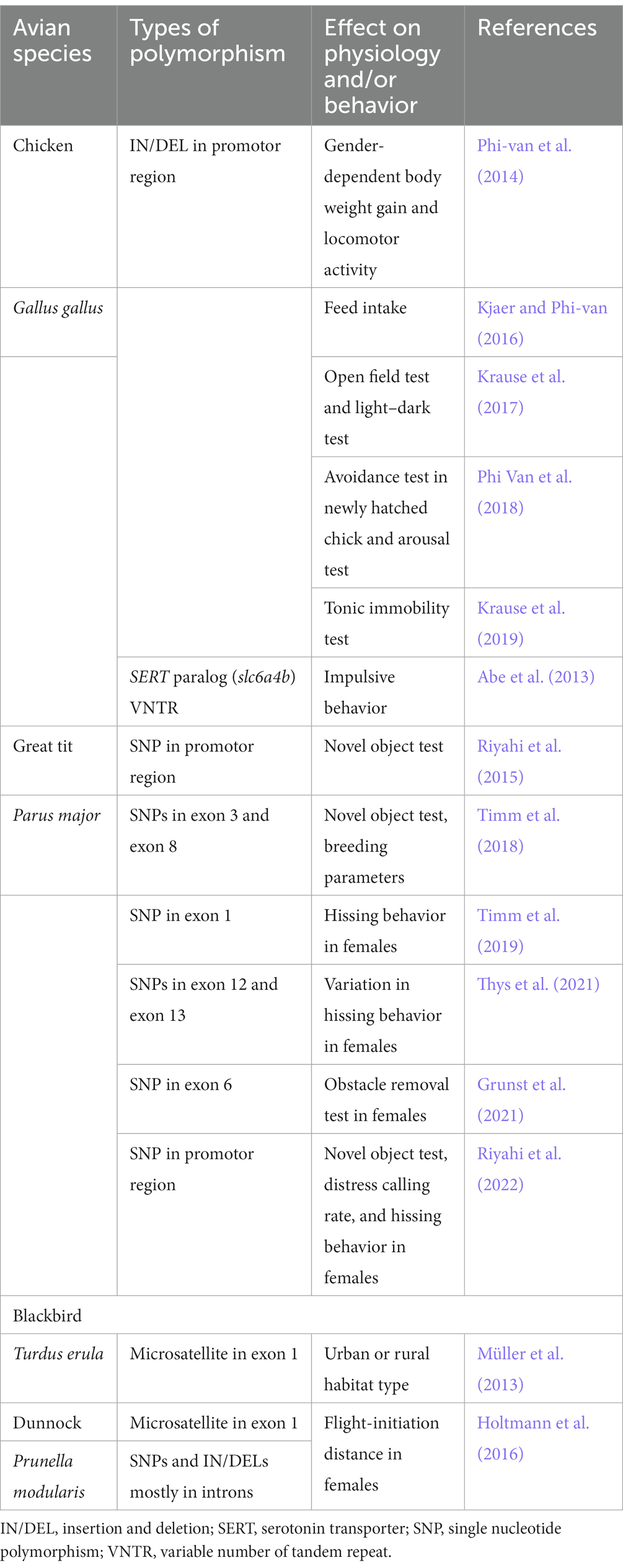
Table 1. Overview of association studies between SERT polymorphisms and physiology and behavior in birds.
In addition to its role as a neuromodulator, there is increasing evidence that serotonin acts as a signaling molecule involving many aspects of neural development such as regulating cell proliferation, neuronal differentiation, neurite outgrowth, and synaptogenesis in mammals (Daubert and Condron, 2010; Wirth et al., 2017; Whitaker-Azmitia, 2020). In birds, serotonin levels in the brain and expression levels of serotonergic system-related genes during the development of serotonergic neurons have been clarified (Huang X. et al., 2019), and it has been shown that injecting serotonin externally to embryos affects neurodevelopment in chickens (Huang et al., 2021). As Huang et al. (2021) pointed out, in contrast to mammals that receive serotonin from the placenta during development, birds develop independently within eggs. Therefore, avian embryos may provide a unique model to investigate the role of serotonin as a morphogen in neurogenesis.
6. Conclusion and future perspectives
The anatomical organization of the avian central serotonergic system has been noted to be highly similar within vertebrates. In this review, we highlight that the molecular properties of the avian serotonergic system are also similar to those of the mammalian serotonergic system, supporting evolutionary conservation of the central serotonergic system in vertebrates. Going forward, it will be important to understand in detail the projection destinations of avian serotonergic neurons and to elucidate the functions involved at the neural circuitry level. Optogenetics, a revolutionary method for demonstrating the functions involved at the neural circuitry level, is becoming available in birds using viral vectors (Roberts et al., 2012; Rook et al., 2021). By combining such viral vectors with avian transgenic technology (Motono et al., 2010; Tsujino et al., 2019; Hagihara et al., 2020), it will be possible not only to comprehensively visualize targeted neural projection relationships, but also to elucidate the functions of avian neural circuits at the cellular level. Birds have emerged as unique model organisms for understanding the evolutionary continuity of the neural circuits responsible for cognition, behavior, and emotion. In addition to understanding the detailed correspondence between avian and mammalian brain structure, and between avian behavior assay systems and mammalian behavior, a neural circuitry level understanding of avian behavior regulated by the central serotonergic system could provide an opportunity for comparison with the neural circuits revealed in mammals. Such comparison of the neural circuits responsible for cognition, behavior, and emotion between birds and mammals will facilitate our understanding of the evolutionary continuity of the neural circuits.
Author contributions
TF and SY designed the study and prepared the first draft, figures, and table. TF, NA, CM, KH, and SY wrote the paper. All authors have reviewed and accepted the final version of the manuscript.
Funding
This work was supported by a Teikyo University Research Encouragement Grant (TF), Grants-in-Aid for Scientific Research from the Japan Society for the Promotion of Science (SY, 24590096, 15K07945, 18K06667; NA, 24790089, 20K06915; CM, 20K16472 and KH, 26440182, 17K07492, 20K06747), the Uehara Memorial Foundation (SY), the Sagawa Foundation for Promotion of Cancer Research (SY), and a Grant-in-Aid for Scientific Research on Innovative Areas “Memory dynamism” (26115522), “Adaptive circuit shift” (15H01449), and “Evolinguistics” (20H05012) from the Ministry of Education, Culture, Sports, Science, and Technology (KH), the Naito Foundation (KH), and the Japan Foundation for Applied Enzymology (KH).
Acknowledgments
The authors would like to thank Editage (www.editage.com) for English language editing.
Conflict of interest
The authors declare that the research was conducted in the absence of any commercial or financial relationships that could be construed as a potential conflict of interest.
Publisher’s note
All claims expressed in this article are solely those of the authors and do not necessarily represent those of their affiliated organizations, or those of the publisher, the editors and the reviewers. Any product that may be evaluated in this article, or claim that may be made by its manufacturer, is not guaranteed or endorsed by the publisher.
References
Abe, H., Takeuchi, H. A., Yamada, S., Nakamura, A., Nagao, K., Nirasawa, K., et al. (2013). Characterization of the intronic VNTR polymorphisms found in a paralog of chicken serotonin transporter gene. Anim. Sci. J. 84, 281–288. doi: 10.1111/asj.12011
Abellan, A., Desfilis, E., and Medina, L. (2014). Combinatorial expression of Lef1, Lhx2, Lhx5, Lhx9, Lmo3, Lmo4, and Prox1 helps to identify comparable subdivisions in the developing hippocampal formation of mouse and chicken. Front. Neuroanat. 8:59. doi: 10.3389/fnana.2014.00059
Abellán, A., and Medina, L. (2009). Subdivisions and derivatives of the chicken subpallium based on expression of LIM and other regulatory genes and markers of neuron subpopulations during development. J. Comp. Neurol. 515, 465–501. doi: 10.1002/cne.22083
Aboitiz, F., Morales, D., and Montiel, J. (2003). The evolutionary origin of the mammalian isocortex: towards an integrated developmental and functional approach. Behav. Brain Sci. 26, 535–552. doi: 10.1017/S0140525X03000128
Allsop, S. A., Vander Weele, C. M., Wichmann, R., and Tye, K. M. (2014). Optogenetic insights on the relationship between anxiety-related behaviors and social deficits. Front. Behav. Neurosci. 8:241. doi: 10.3389/fnbeh.2014.00241
Alonso, A., Merchan, P., Sandoval, J. E., Sanchez-Arrones, L., Garcia-Cazorla, A., Artuch, R., et al. (2013). Development of the serotonergic cells in murine raphe nuclei and their relations with rhombomeric domains. Brain Struct. Funct. 218, 1229–1277. doi: 10.1007/s00429-012-0456-8
Anstey, M. L., Rogers, S. M., Ott, S. R., Burrows, M., and Simpson, S. J. (2009). Serotonin mediates behavioral gregarization underlying swarm formation in desert locusts. Science 323, 627–630. doi: 10.1126/science.1165939
Atoji, Y., Sarkar, S., and Wild, J. M. (2016). Proposed homology of the dorsomedial subdivision and V-shaped layer of the avian hippocampus to Ammon's horn and dentate gyrus, respectively. Hippocampus 26, 1608–1617. doi: 10.1002/hipo.22660
Atoji, Y., and Wild, J. M. (2004). Fiber connections of the hippocampal formation and septum and subdivisions of the hippocampal formation in the pigeon as revealed by tract tracing and kainic acid lesions. J. Comp. Neurol. 475, 426–461. doi: 10.1002/cne.20186
Atoji, Y., and Wild, J. M. (2006). Anatomy of the avian hippocampal formation. Rev. Neurosci. 17, 3–15. doi: 10.1515/revneuro.2006.17.1-2.3
Awasthi, J. R., Tamada, K., Overton, E. T., and Takumi, T. (2021). Comprehensive topographical map of the serotonergic fibers in the male mouse brain. J. Comp. Neurol. 529, 1391–1429. doi: 10.1002/cne.25027
Axer, M., Strohmer, S., Grassel, D., Bucker, O., Dohmen, M., Reckfort, J., et al. (2016). Estimating fiber orientation distribution functions in 3D-polarized light imaging. Front. Neuroanat. 10:40. doi: 10.3389/fnana.2016.00040
Bacque-Cazenave, J., Bharatiya, R., Barriere, G., Delbecque, J. P., Bouguiyoud, N., Di Giovanni, G., et al. (2020). Serotonin in animal cognition and behavior. Int. J. Mol. Sci. 21, 1649. doi: 10.3390/ijms21051649
Beier, K. T., Steinberg, E. E., Deloach, K. E., Xie, S., Miyamichi, K., Schwarz, L., et al. (2015). Circuit architecture of VTA dopamine neurons revealed by systematic input-output mapping. Cells 162, 622–634. doi: 10.1016/j.cell.2015.07.015
Belgard, T. G., Montiel, J. F., Wang, W. Z., Garcia-Moreno, F., Margulies, E. H., Ponting, C. P., et al. (2013). Adult pallium transcriptomes surprise in not reflecting predicted homologies across diverse chicken and mouse pallial sectors. Proc. Natl. Acad. Sci. U. S. A. 110, 13150–13155. doi: 10.1073/pnas.1307444110
Belmaker, R. H., and Agam, G. (2008). Major depressive disorder. N. Engl. J. Med. 358, 55–68. doi: 10.1056/NEJMra073096
Benowitz, L. (1980). “Functional organization of the avian telencephalon” in Comparative Neurology of the Telencephalon. ed. S. O. E. Ebbesson (Boston, MA: Springer), 389–421.
Beyeler, A., Ju, A., Chagraoui, A., Cuvelle, L., Teixeira, M., Di Giovanni, G., et al. (2021). Multiple facets of serotonergic modulation. Prog. Brain Res. 261, 3–39. doi: 10.1016/bs.pbr.2021.02.002
Bhat, S. K., and Ganesh, C. B. (2023). Organization of serotonergic system in Sphaerotheca breviceps (Dicroglossidae) tadpole brain. Cell Tissue Res. 391, 67–86. doi: 10.1007/s00441-022-03709-7
Bocchio, M., Mchugh, S. B., Bannerman, D. M., Sharp, T., and Capogna, M. (2016). Serotonin, amygdala and fear: assembling the puzzle. Front. Neural Circ. 10:24. doi: 10.3389/fncir.2016.00024
Bockaert, J., Claeysen, S., Bécamel, C., Dumuis, A., and Marin, P. (2006). Neuronal 5-HT metabotropic receptors: fine-tuning of their structure, signaling, and roles in synaptic modulation. Cell Tissue Res. 326, 553–572. doi: 10.1007/s00441-006-0286-1
Boddington, R., Gómez Dunlop, C. A., Garnham, L. C., Ryding, S., Abbey-Lee, R. N., Kreshchenko, A., et al. (2020). The relationship between monoaminergic gene expression, learning, and optimism in red junglefowl chicks. Anim. Cogn. 23, 901–911. doi: 10.1007/s10071-020-01394-z
Boureau, Y. L., and Dayan, P. (2011). Opponency revisited: competition and cooperation between dopamine and serotonin. Neuropsychopharmacology 36, 74–97. doi: 10.1038/npp.2010.151
Butler, A. B., Reiner, A., and Karten, H. J. (2011). Evolution of the amniote pallium and the origins of mammalian neocortex. Ann. N. Y. Acad. Sci. 1225, 14–27. doi: 10.1111/j.1749-6632.2011.06006.x
Calizo, L. H., Akanwa, A., Ma, X., Pan, Y.-Z., Lemos, J. C., Craige, C., et al. (2011). Raphe serotonin neurons are not homogenous: electrophysiological, morphological and neurochemical evidence. Neuropharmacology 61, 524–543. doi: 10.1016/j.neuropharm.2011.04.008
Cambronero, F., and Puelles, L. (2000). Rostrocaudal nuclear relationships in the avian medulla oblongata: a fate map with quail chick chimeras. J. Comp. Neurol. 427, 522–545. doi: 10.1002/1096-9861(20001127)427:4<522::AID-CNE3>3.0.CO;2-Y
Canli, T., and Lesch, K.-P. (2007). Long story short: the serotonin transporter in emotion regulation and social cognition. Nat. Neurosci. 10, 1103–1109. doi: 10.1038/nn1964
Carroll, S. B. (2008). Evo-devo and an expanding evolutionary synthesis: a genetic theory of morphological evolution. Cells 134, 25–36. doi: 10.1016/j.cell.2008.06.030
Carroll, S.B., Grenier, J.K., and Weatherbee, S.D. (2013). From DNA to diversity: molecular genetics and the evolution of animal design. Hoboken, NJ John Wiley & Sons.
Challet, E., Miceli, D., Pierre, J., Repérant, J., Masicotte, G., Herbin, M., et al. (1996). Distribution of serotonin-immunoreactivity in the brain of the pigeon (Columba livia). Anat. Embryol. 193, 209–227. doi: 10.1007/BF00198325
Challet, E., Pierre, J., Repérant, J., Ward, R., and Miceli, D. (1991). The serotoninergic system of the brain of the viper, Vipera aspis. An immunohistochemical study. J. Chem. Neuroanat. 4, 233–248. doi: 10.1016/0891-0618(91)90015-5
Clairambault, P., Christophe, N., Pairault, C., Herbin, M., Ward, R., and Reperant, J. (1994). Organization of the serotoninergic system in the brain of two amphibian species, Ambystoma mexicanum (Urodela) and Typhlonectes compressicauda (Gymnophiona). Anat. Embryol. 190, 87–99. doi: 10.1007/BF00185849
Cohen, J. Y., and Grossman, C. D. (2020). “Dorsal raphe serotonergic neurons regulate behavior on multiple timescales” in Handbook of behavioral neuroscience. eds. C. P. Cunningham and K. A. Müller (Amsterdam: Elsevier), 521–529.
Colquitt, B. M., Merullo, D. P., Konopka, G., Roberts, T. F., and Brainard, M. S. (2021). Cellular transcriptomics reveals evolutionary identities of songbird vocal circuits. Science 371:eabd9704. doi: 10.1126/science.abd9704
Cozzi, B., Viglietti-Panzica, C., Aste, N., and Panzica, G. C. (1991). The serotoninergic system in the brain of the Japanese quail. An immunohistochemical study. Cell Tissue Res. 263, 271–284. doi: 10.1007/BF00318769
Dahlstroem, A., and Fuxe, K. (1964). Evidence for the existence of monoamine-containing neurons in the central nervous system. I. Demonstration of monoamines in the cell bodies of brain stem neurons. Acta Physiol. Scand. Suppl. 232, 231–255.
Daubert, E. A., and Condron, B. G. (2010). Serotonin: a regulator of neuronal morphology and circuitry. Trends Neurosci. 33, 424–434. doi: 10.1016/j.tins.2010.05.005
De Deurwaerdere, P., and Di Giovanni, G. (2017). Serotonergic modulation of the activity of mesencephalic dopaminergic systems: therapeutic implications. Prog. Neurobiol. 151, 175–236. doi: 10.1016/j.pneurobio.2016.03.004
De Deurwaerdere, P., and Di Giovanni, G. (2021). 5-HT interaction with other neurotransmitters: an overview. Prog. Brain Res. 259, 1–5. doi: 10.1016/bs.pbr.2021.01.001
De Haas, E. N., and Van Der Eijk, J. A. J. (2018). Where in the serotonergic system does it go wrong? Unravelling the route by which the serotonergic system affects feather pecking in chickens. Neurosci. Biobehav. Rev. 95, 170–188. doi: 10.1016/j.neubiorev.2018.07.007
Deakin, J. F. W., and Graeff, F. G. (2013). 5-HT and mechanisms of defence. J. Psychopharmacol. 5, 305–315. doi: 10.1177/026988119100500414
Desfilis, E., Abellan, A., Sentandreu, V., and Medina, L. (2018). Expression of regulatory genes in the embryonic brain of a lizard and implications for understanding pallial organization and evolution. J. Comp. Neurol. 526, 166–202. doi: 10.1002/cne.24329
Dicke, U., Wallstein, M., and Roth, G. (1997). 5-HT-like immunoreactivity in the brains of plethodontid and salamandrid salamanders (Hydromantes italicus, Hydromantes genei, Plethodon jordani, Desmognathus ochrophaeus, Pleurodeles waltl): an immunohistochemical and biocytin double-labelling study. Cell Tissue Res. 287, 513–523. doi: 10.1007/s004410050775
Dietl, M., and Palacios, J. M. (1988). Receptor autoradiography as a tool for the study of the phylogeny of the basal ganglia. J. Recept. Res. 8, 521–532. doi: 10.3109/10799898809049009
Dos Santos, T. S., Krüger, J., Melleu, F. F., Herold, C., Zilles, K., Poli, A., et al. (2015). Distribution of serotonin 5-HT1A-binding sites in the brainstem and the hypothalamus, and their roles in 5-HT-induced sleep and ingestive behaviors in rock pigeons (Columba livia). Behav. Brain Res. 295, 45–63. doi: 10.1016/j.bbr.2015.03.059
Dube, L., and Parent, A. (1981). The monoamine-containing neurons in avian brain: I. A study of the brain stem of the chicken (Gallus domesticus) by means of fluorescence and acetylcholinesterase histochemistry. J. Comp. Neurol. 196, 695–708. doi: 10.1002/cne.901960413
Dubé, L., and Parent, A. (1982). The organization of monoamine-containing neurons in the brain of the salamander, Necturus maculosus. J. Comp. Neurol. 211, 21–30. doi: 10.1002/cne.902110104
Dugas-Ford, J., Rowell, J. J., and Ragsdale, C. W. (2012). Cell-type homologies and the origins of the neocortex. Proc. Natl. Acad. Sci. U. S. A. 109, 16974–16979. doi: 10.1073/pnas.1204773109
Duvarci, S., and Pare, D. (2014). Amygdala microcircuits controlling learned fear. Neuron 82, 966–980. doi: 10.1016/j.neuron.2014.04.042
Edwards, H. A., Hajduk, G. K., Durieux, G., Burke, T., and Dugdale, H. L. (2015). No association between personality and candidate gene polymorphisms in a wild bird population. PLoS One 10:e0138439. doi: 10.1371/journal.pone.0138439
Erland, L. A., Turi, C. E., and Saxena, P. K. (2016). Serotonin: an ancient molecule and an important regulator of plant processes. Biotechnol. Adv. 34, 1347–1361. doi: 10.1016/j.biotechadv.2016.10.002
Erspamer, V. (1937). Experimental research on the biological significance of enterochromaffin cells. Arch. Fisiol. 37, 156–169.
Erspamer, V., and Asero, B. (1952). Identification of enteramine, the specific hormone of the enterochromaffin cell system, as 5-hydroxytryptamine. Nature 169, 800–801. doi: 10.1038/169800b0
Faber, H., Braun, K., Zuschratter, W., and Scheich, H. (1989). System-specific distribution of zinc in the chick brain. A light- and electron-microscopic study using the Timm method. Cell Tissue Res. 258, 247–257. doi: 10.1007/BF00239445
Fachinelli, C., Sargo, S., Bataller, R., and Echandía, E. R. (1989). Effect of 5-HTP and ketanserine on the aggressive reaction induced by food competition in dominant and submissive pigeons (Columba livia). Behav. Brain Res. 35, 265–270. doi: 10.1016/S0166-4328(89)80146-9
Fernandez, A. S., Pieau, C., Reperant, J., Boncinelli, E., and Wassef, M. (1998). Expression of the Emx-1 and Dlx-1 homeobox genes define three molecularly distinct domains in the telencephalon of mouse, chick, turtle and frog embryos: implications for the evolution of telencephalic subdivisions in amniotes. Development 125, 2099–2111. doi: 10.1242/dev.125.11.2099
Fujita, T., Aoki, N., Fujita, E., Matsushima, T., Homma, K. J., and Yamaguchi, S. (2019). The chick pallium displays divergent expression patterns of chick orthologues of mammalian neocortical deep layer-specific genes. Sci. Rep. 9, 20400. doi: 10.1038/s41598-019-56960-4
Fujita, T., Aoki, N., Mori, C., Fujita, E., Matsushima, T., Homma, K. J., et al. (2020). The dorsal arcopallium of chicks displays the expression of orthologs of mammalian fear related serotonin receptor subfamily genes. Sci. Rep. 10, 21183. doi: 10.1038/s41598-020-78247-9
Fujita, T., Aoki, N., Mori, C., Fujita, E., Matsushima, T., Homma, K. J., et al. (2022a). Chick hippocampal formation displays subdivision- and layer-selective expression patterns of serotonin receptor subfamily genes. Front. Physiol. 13:882633. doi: 10.3389/fphys.2022.882633
Fujita, T., Aoki, N., Mori, C., Fujita, E., Matsushima, T., Homma, K. J., et al. (2022b). Serotonergic neurons in the Chick brainstem express various serotonin receptor subfamily genes. Front. Physiol. 12:815997. doi: 10.3389/fphys.2021.815997
Fujita, T., Aoki, N., Mori, C., Serizawa, S., Kihara-Negishi, F., Homma, K. J., et al. (2022c). Dopaminergic nuclei in the chick midbrain express serotonin receptor subfamily genes. Front. Physiol. 13:1030621. doi: 10.3389/fphys.2022.1030621
Fureix, C., and Meagher, R. K. (2015). What can inactivity (in its various forms) reveal about affective states in non-human animals? A review. Appl. Anim. Behav. Sci. 171, 8–24. doi: 10.1016/j.applanim.2015.08.036
Fuxe, K., and Ljunggren, L. (1965). Cellular localization of monoamines in the upper brain stem of the pigeon. J. Comp. Neurol. 125, 355–381. doi: 10.1002/cne.901250306
Galceran, J., Miyashita-Lin, E. M., Devaney, E., Rubenstein, J. L. R., and Grosschedl, R. (2000). Hippocampus development and generation of dentate gyrus granule cells is regulated by LEF1. Development 127, 469–482. doi: 10.1242/dev.127.3.469
Gallup, G. G. (1973). Tonic immobility in chickens: is a stimulus that signals shock more aversive than the receipt of shock? Anim. Learn. Behav. 1, 228–232. doi: 10.3758/BF03199080
Gallup, G. G. (1974). Animal hypnosis: factual status of a fictional concept. Psychol. Bull. 81:836. doi: 10.1037/h0037227
Gallup, G. G., Nash, R. F., and Wagner, A. M. (1971). The tonic immobility reaction in chickens: response characteristics and methodology. Behav. Res. Methods Instrum. 3, 237–239. doi: 10.3758/BF03208389
Gao, M., Lengersdorf, D., Stüttgen, M. C., and Güntürkün, O. (2018). NMDA receptors in the avian amygdala and the premotor arcopallium mediate distinct aspects of appetitive extinction learning. Behav. Brain Res. 343, 71–82. doi: 10.1016/j.bbr.2018.01.026
Gao, M., Lengersdorf, D., Stüttgen, M. C., and Güntürkün, O. (2019a). Transient inactivation of the visual-associative nidopallium frontolaterale (NFL) impairs extinction learning and context encoding in pigeons. Neurobiol. Learn. Mem. 158, 50–59. doi: 10.1016/j.nlm.2019.01.012
Gao, M., Pusch, R., and Güntürkün, O. (2019b). Blocking NMDA-receptors in the pigeon’s medial striatum impairs extinction acquisition and induces a motoric disinhibition in an appetitive classical conditioning paradigm. Front. Behav. Neurosci. 13:153. doi: 10.3389/fnbeh.2019.00153
García-Calero, E., and Puelles, L. (2009). Enc1 expression in the chick telencephalon at intermediate and late stages of development. J. Comp. Neurol. 517, 564–580. doi: 10.1002/cne.22164
Gaspar, P., and Lillesaar, C. (2012). Probing the diversity of serotonin neurons. Philos. Transact. R. Soc. B 367, 2382–2394. doi: 10.1098/rstb.2011.0378
Grunst, A. S., Grunst, M. L., Staes, N., Thys, B., Pinxten, R., and Eens, M. (2021). Serotonin transporter (SERT) polymorphisms, personality and problem-solving in urban great tits. Sci. Rep. 11:24270. doi: 10.1038/s41598-021-03466-7
Güntürkün, O. (2005). The avian ‘prefrontal cortex’ and cognition. Curr. Opin. Neurobiol. 15, 686–693. doi: 10.1016/j.conb.2005.10.003
Güntürkün, O., Stüttgen, M. C., Starosta, S., Pusch, R., Gao, M., Nitsche, M., et al. (2020). Beyond the classic extinction network: a wider, comparative view. e-Neuroforum 26, 161–169. doi: 10.1515/nf-2020-0015
Gupta, S., Maurya, R., Saxena, M., and Sen, J. (2012). Defining structural homology between the mammalian and avian hippocampus through conserved gene expression patterns observed in the chick embryo. Dev. Biol. 366, 125–141. doi: 10.1016/j.ydbio.2012.03.027
Hagihara, Y., Okuzaki, Y., Matsubayashi, K., Kaneoka, H., Suzuki, T., Iijima, S., et al. (2020). Primordial germ cell-specific expression of eGFP in transgenic chickens. Genesis 58:e23388. doi: 10.1002/dvg.23388
Hanics, J., Teleki, G., Alpár, A., Székely, A. D., and Csillag, A. (2017). Multiple amygdaloid divisions of arcopallium send convergent projections to the nucleus accumbens and neighboring subpallial amygdala regions in the domestic chicken: a selective pathway tracing and reconstruction study. Brain Struct. Funct. 222, 301–315. doi: 10.1007/s00429-016-1219-8
Hannon, J., and Hoyer, D. (2008). Molecular biology of 5-HT receptors. Behav. Brain Res. 195, 198–213. doi: 10.1016/j.bbr.2008.03.020
Hay-Schmidt, A. (2000). The evolution of the serotonergic nervous system. Proc. Biol. Sci. 267, 1071–1079. doi: 10.1098/rspb.2000.1111
Herold, C., Bingman, V. P., Ströckens, F., Letzner, S., Sauvage, M., Palomero-Gallagher, N., et al. (2014). Distribution of neurotransmitter receptors and zinc in the pigeon (Columba livia) hippocampal formation: a basis for further comparison with the mammalian hippocampus. J. Comp. Neurol. 522, 2553–2575. doi: 10.1002/cne.23549
Herold, C., Coppola, V. J., and Bingman, V. P. (2015). The maturation of research into the avian hippocampal formation: recent discoveries from one of the nature's foremost navigators. Hippocampus 25, 1193–1211. doi: 10.1002/hipo.22463
Herold, C., Palomero-Gallagher, N., Güntürkün, O., and Zilles, K. (2012). Serotonin 5-HT1A receptor binding sites in the brain of the pigeon (Columba livia). Neuroscience 200, 1–12. doi: 10.1016/j.neuroscience.2011.10.050
Herold, C., Paulitschek, C., Palomero-Gallagher, N., Güntürkün, O., and Zilles, K. (2018). Transmitter receptors reveal segregation of the arcopallium/amygdala complex in pigeons (Columba livia). J. Comp. Neurol. 526, 439–466. doi: 10.1002/cne.24344
Herold, C., Schlomer, P., Mafoppa-Fomat, I., Mehlhorn, J., Amunts, K., and Axer, M. (2019). The hippocampus of birds in a view of evolutionary connectomics. Cortex 118, 165–187. doi: 10.1016/j.cortex.2018.09.025
Hevner, R. F. (2016). Evolution of the mammalian dentate gyrus. J. Comp. Neurol. 524, 578–594. doi: 10.1002/cne.23851
Hoffman, B. J., Mezey, E., and Brownstein, M. J. (1991). Cloning of a serotonin transporter affected by antidepressants. Science 254, 579–580. doi: 10.1126/science.1948036
Holtmann, B., Grosser, S., Lagisz, M., Johnson, S., Santos, E., Lara, C., et al. (2016). Population differentiation and behavioural association of the two ‘personality’ genes DRD 4 and SERT in dunnocks (Prunella modularis). Mol. Ecol. 25, 706–722. doi: 10.1111/mec.13514
Huang, X., Kuang, S., Applegate, T. J., Lin, T.-L., and Cheng, H.-W. (2019). The development of the serotonergic and dopaminergic systems during chicken mid-late embryogenesis. Mol. Cell. Endocrinol. 493:110472. doi: 10.1016/j.mce.2019.110472
Huang, X., Kuang, S., Applegate, T. J., Lin, T.-L., and Cheng, H.-W. (2021). Prenatal serotonin fluctuation affects serotoninergic development and related neural circuits in chicken embryos. Neuroscience 473, 66–80. doi: 10.1016/j.neuroscience.2021.08.011
Huang, K. W., Ochandarena, N. E., Philson, A. C., Hyun, M., Birnbaum, J. E., Cicconet, M., et al. (2019). Molecular and anatomical organization of the dorsal raphe nucleus. elife 8, e46464. doi: 10.7554/eLife.46464
Ikeda, H., and Goto, J. (1971). Distribution of monoamine-containing cells in the central nervous system of the chicken. Jpn. J. Pharmacol. 21, 763–784. doi: 10.1016/S0021-5198(19)36177-3
International Chicken Genome Sequencing Consortium (2004). Sequence and comparative analysis of the chicken genome provide unique perspectives on vertebrate evolution. Nature 432, 695–716. doi: 10.1038/nature03154
Jacobs, B. L., and Azmitia, E. C. (1992). Structure and function of the brain serotonin system. Physiol. Rev. 72, 165–229. doi: 10.1152/physrev.1992.72.1.165
Janak, P. H., and Tye, K. M. (2015). From circuits to behaviour in the amygdala. Nature 517, 284–292. doi: 10.1038/nature14188
Jarvis, E. D., Gunturkun, O., Bruce, L., Csillag, A., Karten, H., Kuenzel, W., et al. (2005). Avian brains and a new understanding of vertebrate brain evolution. Nat. Rev. Neurosci. 6, 151–159. doi: 10.1038/nrn1606
Jung, M., and Mcnaughton, B. (1993). Spatial selectivity of unit activity in the hippocampal granular layer. Hippocampus 3, 165–182. doi: 10.1002/hipo.450030209
Kandel, E.R., Schwartz, J.H., Jessell, T.M., Siegelbaum, S., Hudspeth, A.J., and Mack, S. (2000). Principles of neural science. New York McGraw-hill.
Karten, H. J. (1997). Evolutionary developmental biology meets the brain: the origins of mammalian cortex. Proc. Natl. Acad. Sci. U. S. A. 94, 2800–2804. doi: 10.1073/pnas.94.7.2800
Karten, H. J. (2013). Neocortical evolution: neuronal circuits arise independently of lamination. Curr. Biol. 23, R12–R15. doi: 10.1016/j.cub.2012.11.013
Karten, H. J. (2015). Vertebrate brains and evolutionary connectomics: on the origins of the mammalian 'neocortex'. Philos. Trans. R. Soc. Lond. Ser. B Biol. Sci. 370:20150060. doi: 10.1098/rstb.2015.0060
Kempermann, G. (2012). PERSPECTIVES new neurons for 'survival of the fittest'. Nat. Rev. Neurosci. 13, 727–736. doi: 10.1038/nrn3319
Kjaer, J. B., and Phi-Van, L. (2016). Evidence for the association of a deleted variant in the 5′-flanking region of the chicken serotonin transporter (5-HTT) gene with a temporary increase in feed intake and growth rate. Animals 6:63. doi: 10.3390/ani6100063
Kops, M. S., Kjaer, J. B., Güntürkün, O., Westphal, K. G. C., Korte-Bouws, G. A. H., Olivier, B., et al. (2017). Brain monoamine levels and behaviour of young and adult chickens genetically selected on feather pecking. Behav. Brain Res. 327, 11–20. doi: 10.1016/j.bbr.2017.03.024
Krause, E. T., Kjaer, J. B., Dudde, A., Schrader, L., and Phi-Van, L. (2019). Fear but not social behaviour is affected by a polymorphism in the 5′-flanking region of the serotonin transporter (5-HTT) gene in adult hens. Behav. Brain Res. 361, 50–53. doi: 10.1016/j.bbr.2018.12.029
Krause, E. T., Kjaer, J. B., and Lüders, C. (2017). A polymorphism in the 5′-flanking region of the serotonin transporter (5-HTT) gene affects fear-related behaviors of adult domestic chickens. Behav. Brain Res. 330, 92–96. doi: 10.1016/j.bbr.2017.04.051
Kuenzel, W.J., and Masson, M. (1988). A stereotaxic atlas of the brain of the Chick (Gallus domesticus). Baltimore, MD Johns Hopkins University Press
Lawther, A. J., Hale, M. W., and Lowry, C. A. (2020). Serotonin and the neurobiology of anxious states. Handb. Behav. Neurosci. 31, 505–520.
Lebrand, C., Cases, O., Adelbrecht, C., Doye, A., Alvarez, C., El Mestikawy, S., et al. (1996). Transient uptake and storage of serotonin in developing thalamic neurons. Neuron 17, 823–835. doi: 10.1016/S0896-6273(00)80215-9
LeDoux, J. E. (2000). Emotion circuits in the brain. Annu. Rev. Neurosci. 23, 155–184. doi: 10.1146/annurev.neuro.23.1.155
Lein, E. S., Hawrylycz, M. J., Ao, N., Ayres, M., Bensinger, A., Bernard, A., et al. (2007). Genome-wide atlas of gene expression in the adult mouse brain. Nature 445, 168–176. doi: 10.1038/nature05453
Lengersdorf, D., Marks, D., Uengoer, M., Stüttgen, M. C., and Güntürkün, O. (2015). Blocking NMDA-receptors in the pigeon’s “prefrontal” caudal nidopallium impairs appetitive extinction learning in a sign-tracking paradigm. Front. Behav. Neurosci. 9:85. doi: 10.3389/fnbeh.2015.00085
Lengersdorf, D., Stüttgen, M. C., Uengoer, M., and Güntürkün, O. (2014). Transient inactivation of the pigeon hippocampus or the nidopallium caudolaterale during extinction learning impairs extinction retrieval in an appetitive conditioning paradigm. Behav. Brain Res. 265, 93–100. doi: 10.1016/j.bbr.2014.02.025
Lesch, K. P., Wolozin, B. L., Estler, H. C., Murphy, D. L., and Riederer, P. (1993). Isolation of a Cdna-encoding the human brain-serotonin transporter. J. Neur. Trans. Gen. Sect. 91, 67–72. doi: 10.1007/BF01244919
Lillesaar, C. (2011). The serotonergic system in fish. J. Chem. Neuroanat. 41, 294–308. doi: 10.1016/j.jchemneu.2011.05.009
Lillesaar, C., Stigloher, C., Tannhäuser, B., Wullimann, M. F., and Bally-Cuif, L. (2009). Axonal projections originating from raphe serotonergic neurons in the developing and adult zebrafish, Danio rerio, using transgenics to visualize raphe-specific pet1 expression. J. Comp. Neurol. 512, 158–182. doi: 10.1002/cne.21887
Lissek, S., and Güntürkün, O. (2005). Out of context: NMDA receptor antagonism in the avian'prefrontal cortex'impairs context processing in a conditional discrimination task. Behav. Neurosci. 119:797. doi: 10.1037/0735-7044.119.3.797
Liu, Z. X., Lin, R., and Luo, M. M. (2020). Reward contributions to serotonergic functions. Annu. Rev. Neurosci. 43, 141–162. doi: 10.1146/annurev-neuro-093019-112252
Lucki, I. (1998). The spectrum of behaviors influenced by serotonin. Biol. Psychiatry 44, 151–162. doi: 10.1016/S0006-3223(98)00139-5
Luo, X., Munoz-Pino, E., Francavilla, R., Vallee, M., Droit, A., and Topolnik, L. (2019). Transcriptomic profile of the subiculum-projecting VIP GABAergic neurons in the mouse CA1 hippocampus. Brain Struct. Funct. 224, 2269–2280. doi: 10.1007/s00429-019-01883-z
Marin, P., Becamel, C., Chaumont-Dubel, S., Vandermoere, F., Bockaert, J., and Claeysen, S. (2020). “Classification and signaling characteristics of 5-HT receptors: toward the concept of 5-HT receptosomes” in Handbook of behavioral neuroscience. ed. B. L. Müller Caj (Amsterdam: Elsevier), 91–120.
Martinez-Garcia, F., and Lanuza, E. (2018). Evolution of vertebrate survival circuits. Curr. Opin. Behav. Sci. 24, 113–123. doi: 10.1016/j.cobeha.2018.06.012
Matsunami, S., Ogura, Y., Amita, H., Izumi, T., Yoshioka, M., and Matsushima, T. (2012). Behavioural and pharmacological effects of fluvoxamine on decision-making in food patches and the inter-temporal choices of domestic chicks. Behav. Brain Res. 233, 577–586. doi: 10.1016/j.bbr.2012.05.045
Matsushima, T., Izawa, E.-I., Aoki, N., and Yanagihara, S. (2003). The mind through chick eyes: memory, cognition and anticipation. Zool. Sci. 20, 395–408. doi: 10.2108/zsj.20.395
Medina, L., and Abellán, A. (2009). Development and evolution of the pallium. Semin. Cell Dev. Biol. 20, 698–711.
Medina, L., Abellan, A., and Desfilis, E. (2017a). Contribution of genoarchitecture to understanding hippocampal evolution and development. Brain Behav. Evol. 90, 25–40. doi: 10.1159/000477558
Medina, L., Abellán, A., Vicario, A., Castro-Robles, B., and Desfilis, E. (2017b). “The Amygdala” in Evolution of nervous systems. ed. J. Kaas , vol. 1. 2nd ed (Oxford: Elsevier), 427–478.
Melleu, F., Santos, T., Lino-De-Oliveira, C., and Marino-Neto, J. (2013). Distribution and characterization of doublecortin-expressing cells and fibers in the brain of the adult pigeon (Columba livia). J. Chem. Neuroanat. 47, 57–70. doi: 10.1016/j.jchemneu.2012.10.006
Meneghelli, C., Rocha, N. H., Mengatto, V., Hoeller, A. A., Santos, T. S., Lino-De-Oliveira, C., et al. (2009). Distribution of tryptophan hydroxylase-immunoreactive neurons in the brainstem and diencephalon of the pigeon (Columba livia). J. Chem. Neuroanat. 38, 34–46. doi: 10.1016/j.jchemneu.2009.03.007
Metzger, M., Toledo, C., and Braun, K. (2002). Serotonergic innervation of the telencephalon in the domestic chick. Brain Res. Bull. 57, 547–551. doi: 10.1016/S0361-9230(01)00688-8
Molnár, Z. (2011). Evolution of cerebral cortical development. Brain Behav. Evol. 78, 94–107. doi: 10.1159/000327325
Montagnese, C. M., Geneser, F. A., and Krebs, J. R. (1993). Histochemical distribution of zinc in the brain of the Zebra finch (Taenopygia guttata). Anat. Embryol. 188, 173–187. doi: 10.1007/BF00186251
Montagnese, C. M., Krebs, J. R., and Meyer, G. (1996). The dorsomedial and dorsolateral forebrain of the zebra finch, Taeniopygia guttata: a Golgi study. Cell Tissue Res. 283, 263–282. doi: 10.1007/s004410050537
Morandi-Raikova, A., and Mayer, U. (2022). Spatial cognition and the avian hippocampus: research in domestic chicks. Front. Psychol. 13:1005726. doi: 10.3389/fpsyg.2022.1005726
Moreno, N., and González, A. (2006). The common organization of the amygdaloid complex in tetrapods: new concepts based on developmental, hodological and neurochemical data in anuran amphibians. Prog. Neurobiol. 78, 61–90. doi: 10.1016/j.pneurobio.2005.12.005
Mosienko, V., Bader, M., and Alenina, N. (2020). “The serotonin-free brain: behavioral consequences of Tph2 deficiency in animal models” in Handbook of behavioral neuroscience (Amsterdam: Elsevier), 601–607.
Motono, M., Yamada, Y., Hattori, Y., Nakagawa, R., Nishijima, K.-I., and Iijima, S. (2010). Production of transgenic chickens from purified primordial germ cells infected with a lentiviral vector. J. Biosci. Bioeng. 109, 315–321. doi: 10.1016/j.jbiosc.2009.10.007
Muller, R. U., Kubie, J. L., and Ranck, J. B. (1987). Spatial firing patterns of hippocampal complex-spike cells in a fixed environment. J. Neurosci. 7, 1935–1950. doi: 10.1523/JNEUROSCI.07-07-01935.1987
Müller, J. C., Partecke, J., Hatchwell, B. J., Gaston, K. J., and Evans, K. L. (2013). Candidate gene polymorphisms for behavioural adaptations during urbanization in blackbirds. Mol. Ecol. 22, 3629–3637. doi: 10.1111/mec.12288
Murphy, D. L., Fox, M. A., Timpano, K. R., Moya, P. R., Ren-Patterson, R., Andrews, A. M., et al. (2008). How the serotonin story is being rewritten by new gene-based discoveries principally related to SLC6A4, the serotonin transporter gene, which functions to influence all cellular serotonin systems. Neuropharmacology 55, 932–960. doi: 10.1016/j.neuropharm.2008.08.034
Narboux-Nême, N., Pavone, L. M., Avallone, L., Zhuang, X., and Gaspar, P. (2008). Serotonin transporter transgenic (SERTcre) mouse line reveals developmental targets of serotonin specific reuptake inhibitors (SSRIs). Neuropharmacology 55, 994–1005. doi: 10.1016/j.neuropharm.2008.08.020
Ng, L., Bernard, A., Lau, C., Overly, C. C., Dong, H. W., Kuan, C., et al. (2009). An anatomic gene expression atlas of the adult mouse brain. Nat. Neurosci. 12, 356–362. doi: 10.1038/nn.2281
Ng, L., Lau, C., Sunkin, S. M., Bernard, A., Chakravarty, M. M., Lein, E. S., et al. (2010). Surface-based mapping of gene expression and probabilistic expression maps in the mouse cortex. Methods 50, 55–62. doi: 10.1016/j.ymeth.2009.10.001
Ogawa, S. K., Cohen, J. Y., Hwang, D., Uchida, N., and Watabe-Uchida, M. (2014). Organization of Monosynaptic Inputs to the serotonin and dopamine neuromodulatory systems. Cell Rep. 8, 1105–1118. doi: 10.1016/j.celrep.2014.06.042
Ogawa, S. K., and Watabe-Uchida, M. (2018). Organization of dopamine and serotonin system: anatomical and functional mapping of monosynaptic inputs using rabies virus. Pharmacol. Biochem. Behav. 174, 9–22. doi: 10.1016/j.pbb.2017.05.001
Okado, N., Sako, H., Homma, S., and Ishikawa, K. (1992). Development of serotoninergic system in the brain and spinal cord of the chick. Prog. Neurobiol. 38, 93–123. doi: 10.1016/0301-0082(92)90036-E
Okaty, B. W., Commons, K. G., and Dymecki, S. M. (2019). Embracing diversity in the 5-HT neuronal system. Nat. Rev. Neurosci. 20, 397–424. doi: 10.1038/s41583-019-0151-3
Okaty, B. W., Sturrock, N., Escobedo Lozoya, Y., Chang, Y., Senft, R. A., Lyon, K. A., et al. (2020). A single-cell transcriptomic and anatomic atlas of mouse dorsal raphe Pet1 neurons. elife 9, e55523. doi: 10.7554/eLife.55523
O'Keefe, J., and Dostrovsky, J. (1971). The hippocampus as a spatial map: preliminary evidence from unit activity in the freely-moving rat. Brain Res. 34, 171–175. doi: 10.1016/0006-8993(71)90358-1
O'leary, O. F., Codagnone, M. G., and Cryan, J. F. (2020). “Revisiting the behavioral genetics of serotonin: relevance to anxiety and depression” in Handbook of behavioral neuroscience (Amsterdam: Elsevier), 665–709.
Olivier, B. (2015). Serotonin: a never-ending story. Eur. J. Pharmacol. 753, 2–18. doi: 10.1016/j.ejphar.2014.10.031
Papini, M. R., Penagos-Corzo, J. C., and Perez-Acosta, A. M. (2019). Avian emotions: comparative perspectives on fear and frustration. Front. Psychol. 9:2707. doi: 10.3389/fpsyg.2018.02707
Parent, A. (1981). Comparative anatomy of the serotoninergic systems. J. Physiol. Paris 77, 147–156.
Payne, H. L., Lynch, G. F., and Aronov, D. (2021). Neural representations of space in the hippocampus of a food-caching bird. Science 373, 343–348. doi: 10.1126/science.abg2009
Pessoa, L., Medina, L., Hof, P. R., and Desfilis, E. (2019). Neural architecture of the vertebrate brain: implications for the interaction between emotion and cognition. Neurosci. Biobehav. Rev. 107, 296–312. doi: 10.1016/j.neubiorev.2019.09.021
Peters, K. Z., Cheer, J. F., and Tonini, R. (2021). Modulating the neuromodulators: dopamine, serotonin, and the endocannabinoid system. Trends Neurosci. 44, 464–477. doi: 10.1016/j.tins.2021.02.001
Phi Van, V. D., Krause, E. T., and Phi-Van, L. (2018). Modulation of fear and arousal behavior by serotonin transporter (5-HTT) genotypes in newly hatched chickens. Front. Behav. Neurosci. 12:284. doi: 10.3389/fnbeh.2018.00284
Phi-Van, L., Holtz, M., Kjaer, J. B., Van Phi, V. D., and Zimmermann, K. (2014). A functional variant in the 5′-flanking region of the chicken serotonin transporter gene is associated with increased body weight and locomotor activity. J. Neurochem. 131, 12–20. doi: 10.1111/jnc.12799
Puelles, L. (2001). Thoughts on the development, structure and evolution of the mammalian and avian telencephalic pallium. Philos. Trans. R. Soc. Lond. B 356, 1583–1598. doi: 10.1098/rstb.2001.0973
Puelles, L., Ayad, A., Alonso, A., Sandoval, J. E., Martinez-De-La-Torre, M., Medina, L., et al. (2016). Selective early expression of the orphan nuclear receptor Nr4a2 identifies the claustrum homolog in the avian mesopallium: impact on sauropsidian/mammalian pallium comparisons. J. Comp. Neurol. 524, 665–703. doi: 10.1002/cne.23902
Puelles, L., Kuwana, E., Puelles, E., Bulfone, A., Shimamura, K., Keleher, J., et al. (2000). Pallial and subpallial derivatives in the embryonic chick and mouse telencephalon, traced by the expression of the genes Dlx-2, Emx-1, Nkx-2.1, Pax-6, and Tbr-1. J. Comp. Neurol. 424, 409–438. doi: 10.1002/1096-9861(20000828)424:3<409::AID-CNE3>3.0.CO;2-7
Puelles, L., Martinez-De-La-Torre, M., Martinez, S., Watson, C., and Paxinos, G. (2018). The chick brain in stereotaxic coordinates and alternate stains: featuring neuromeric divisions and mammalian homologies Academic Press, London.
Puelles, L., and Medina, L. (2002). Field homology as a way to reconcile genetic and developmental variability with adult homology. Brain Res. Bull. 57, 243–255. doi: 10.1016/S0361-9230(01)00693-1
Puelles, L., Sandoval, J., Ayad, A., Del Corral, R., Alonso, A., Ferran, J., et al. (2017). “The pallium in reptiles and birds in the light of the updated tetrapartite pallium model” in Evolution of nervous systems. ed. J. Kaas (Oxford: Academic Press)
Ravindran, L. N., and Stein, M. B. (2010). The pharmacologic treatment of anxiety disorders: a review of progress. J. Clin. Psychiatry 71, 839–854. doi: 10.4088/JCP.10r06218blu
Reiner, A., Perkel, D. J., Bruce, L. L., Butler, A. B., Csillag, A., Kuenzel, W., et al. (2004). Revised nomenclature for avian telencephalon and some related brainstem nuclei. J. Comp. Neurol. 473, 377–414. doi: 10.1002/cne.20118
Ren, J., Isakova, A., Friedmann, D., Zeng, J., Grutzner, S. M., Pun, A., et al. (2019). Single-cell transcriptomes and whole-brain projections of serotonin neurons in the mouse dorsal and median raphe nuclei. elife 8:e49424. doi: 10.7554/eLife.49424
Riyahi, S., Carrillo-Ortiz, J. G., Uribe, F., Calafell, F., and Senar, J. C. (2022). Risk-taking coping style correlates with SERT SNP290 polymorphisms in free-living great tits. J. Exp. Biol. 225:jeb243342. doi: 10.1242/jeb.243342
Riyahi, S., Sánchez-Delgado, M., Calafell, F., Monk, D., and Senar, J. C. (2015). Combined epigenetic and intraspecific variation of the DRD4 and SERT genes influence novelty seeking behavior in great tit Parus major. Epigenetics 10, 516–525. doi: 10.1080/15592294.2015.1046027
Roberts, T. F., Gobes, S. M., Murugan, M., Ölveczky, B. P., and Mooney, R. (2012). Motor circuits are required to encode a sensory model for imitative learning. Nat. Neurosci. 15, 1454–1459. doi: 10.1038/nn.3206
Rodrigues, S.-L., Maseko, B. C., Ihunwo, A. O., Fuxe, K., and Manger, P. R. (2008). Nuclear organization and morphology of serotonergic neurons in the brain of the Nile crocodile, Crocodylus niloticus. J. Chem. Neuroanat. 35, 133–145. doi: 10.1016/j.jchemneu.2007.08.007
Rogers, S. M., and Ott, S. R. (2015). Differential activation of serotonergic neurons during short- and long-term gregarization of desert locusts. Proc. Biol. Sci. 282:20142062. doi: 10.1098/rspb.2014.2062
Rook, N., Stacho, M., Schwarz, A., Bingman, V. P., and Güntürkün, O. (2023). Neuronal circuits within the homing pigeon hippocampal formation. J. Comp. Neurol., 531, 790–813. doi: 10.1002/cne.25462
Rook, N., Tuff, J. M., Isparta, S., Masseck, O. A., Herlitze, S., Güntürkün, O., et al. (2021). AAV1 is the optimal viral vector for optogenetic experiments in pigeons (Columba livia). Communicat. Biol. 4:100. doi: 10.1038/s42003-020-01595-9
Rosa Salva, O., Mayer, U., and Vallortigara, G. (2015). Roots of a social brain: developmental models of emerging animacy-detection mechanisms. Neurosci. Biobehav. Rev. 50, 150–168. doi: 10.1016/j.neubiorev.2014.12.015
Sako, H., Kojima, T., and Okado, N. (1986). Immunohistochemical study on the development of serotoninergic neurons in the chick: I. Distribution of cell bodies and fibers in the brain. J. Comp. Neurol. 253, 61–78. doi: 10.1002/cne.902530106
Savory, C. (1995). Feather pecking and cannibalism. Worlds Poult. Sci. J. 51, 215–219. doi: 10.1079/WPS19950016
Schuldiner, S., Shirvan, A., and Linial, M. (1995). Vesicular neurotransmitter transporters: from bacteria to humans. Physiol. Rev. 75, 369–392. doi: 10.1152/physrev.1995.75.2.369
Serretti, A., Calati, R., Mandelli, L., and De Ronchi, D. (2006). Serotonin transporter gene variants and behavior: a comprehensive review. Curr. Drug Targets 7, 1659–1669. doi: 10.2174/138945006779025419
Sloviter, R. S., and Lomo, T. (2012). Updating the lamellar hypothesis of hippocampal organization. Front. Neural Circ. 6:102. doi: 10.3389/fncir.2012.00102
Smeets, W. J., and Steinbusch, H. W. (1988). Distribution of serotonin immunoreactivity in the forebrain and midbrain of the lizard Gekko gecko. J. Comp. Neurol. 271, 419–434. doi: 10.1002/cne.902710309
Soiza-Reilly, M., and Gaspar, P. (2020). “From B1 to B9: a guide through hindbrain serotonin neurons with additional views from multidimensional characterization” in Handbook of behavioral neuroscience. eds. C. P. Müller and K. A. Cunningham (Amsterdam: Elsevier), 23–40.
Spohn, S. N., and Mawe, G. M. (2017). Non-conventional features of peripheral serotonin signalling – the gut and beyond. Nat. Rev. Gastroenterol. Hepatol. 14, 412–420. doi: 10.1038/nrgastro.2017.51
Stacho, M., Herold, C., Rook, N., Wagner, H., Axer, M., Amunts, K., et al. (2020). A cortex-like canonical circuit in the avian forebrain. Science 369, eabc5534. doi: 10.1126/science.abc5534
Starosta, S., Bartetzko, I., Stüttgen, M. C., and Güntürkün, O. (2017). Integration of contextual cues into memory depends on “prefrontal” N-methyl-D-aspartate receptors. Neurobiol. Learn. Mem. 144, 19–26. doi: 10.1016/j.nlm.2017.05.012
Strac, D. S., Pivac, N., and Muck-Seler, D. (2016). The serotonergic system and cognitive function. Transl. Neurosci. 7, 35–49. doi: 10.1515/tnsci-2016-0007
Striedter, G. F. (2016). Evolution of the hippocampus in reptiles and birds. J. Comp. Neurol. 524, 496–517. doi: 10.1002/cne.23803
Suarez, J., Davila, J. C., Real, M. A., Guirado, S., and Medina, L. (2006). Calcium-binding proteins, neuronal nitric oxide synthase, and GABA help to distinguish different pallial areas in the developing and adult chicken. I. Hippocampal formation and hyperpallium. J. Comp. Neurol. 497, 751–771. doi: 10.1002/cne.21004
Szekely, A. D. (1999). The avian hippocampal formation: subdivisions and connectivity. Behav. Brain Res. 98, 219–225. doi: 10.1016/S0166-4328(98)00087-4
Tanaka, K. F., Samuels, B. A., and Hen, R. (2012). Serotonin receptor expression along the dorsal-ventral axis of mouse hippocampus. Philos. Trans. R. Soc. B Biol. Sci. 367, 2395–2401. doi: 10.1098/rstb.2012.0038
Thys, B., Grunst, A. S., Staes, N., Pinxten, R., Eens, M., and Grunst, M. L. (2021). The serotonin transporter gene and female personality variation in a free-living passerine. Sci. Rep. 11:8577. doi: 10.1038/s41598-021-88225-4
Timm, K., Koosa, K., and Tilgar, V. (2019). The serotonin transporter gene could play a role in anti-predator behaviour in a forest passerine. J. Ethol. 37, 221–227. doi: 10.1007/s10164-019-00593-7
Timm, K., Van Oers, K., and Tilgar, V. (2018). SERT gene polymorphisms are associated with risk-taking behaviour and breeding parameters in wild great tits. J. Exp. Biol. 221:jeb171595. doi: 10.1242/jeb.171595
Tombol, T., Davies, D. C., Nemeth, A., Sebesteny, T., and Alpar, A. (2000). A comparative Golgi study of chicken (Gallus domesticus) and homing pigeon (Columba livia) hippocampus. Anat. Embryol. 201, 85–101. doi: 10.1007/PL00008235
Tosches, M. A., Yamawaki, T. M., Naumann, R. K., Jacobi, A. A., Tushev, G., and Laurent, G. (2018). Evolution of pallium, hippocampus, and cortical cell types revealed by single-cell transcriptomics in reptiles. Science 360, 881–888. doi: 10.1126/science.aar4237
Tovote, P., Fadok, J. P., and Luthi, A. (2015). Neuronal circuits for fear and anxiety. Nat. Rev. Neurosci. 16, 317–331. doi: 10.1038/nrn3945
Tsujino, K., Okuzaki, Y., Hibino, N., Kawamura, K., Saito, S., Ozaki, Y., et al. (2019). Identification of transgene integration site and anatomical properties of fluorescence intensity in a EGFP transgenic chicken line. Develop. Growth Differ. 61, 393–401. doi: 10.1111/dgd.12631
Ueda, S., Takeuchi, Y., and Sano, Y. (1983). Immunohistochemical demonstration of serotonin neurons in the central nervous system of the turtle (Clemmys japonica). Anat. Embryol. 168, 1–19. doi: 10.1007/BF00305395
Van Dongen, W. F., Robinson, R. W., Weston, M. A., Mulder, R. A., and Guay, P.-J. (2015). Variation at the DRD4 locus is associated with wariness and local site selection in urban black swans. BMC Evol. Biol. 15, 1–11. doi: 10.1186/s12862-015-0533-8
Van Mier, P., Joosten, H., Van Rheden, R., and Ten Donkelaar, H. (1986). The development of serotonergic raphespinal projections in Xenopus laevis. Int. J. Dev. Neurosci. 4, 465–475. doi: 10.1016/0736-5748(86)90028-6
Vicario, A., Abellán, A., Desfilis, E., and Medina, L. (2014). Genetic identification of the central nucleus and other components of the central extended amygdala in chicken during development. Front. Neuroanat. 8:90. doi: 10.3389/fnana.2014.00090
Vilaró, M. T., Cortés, R., and Mengod, G. (2005). Serotonin 5-HT4 receptors and their mRNAs in rat and guinea pig brain: distribution and effects of neurotoxic lesions. J. Comp. Neurol. 484, 418–439. doi: 10.1002/cne.20447
Vilaró, M., Cortés, R., Mengod, G., and Hoyer, D. (2020). “Distribution of 5-HT receptors in the central nervous system: an update” in Handbook of behavioral neuroscience (Amsterdam: Elsevier), 121–146.
Waeber, C., Hoyer, D., and Palacios, J. (1989). 5. HT 1 receptors in the vertebrate brain: regional distribution examined by autoradiography. Naunyn Schmiedeberg's Arch. Pharmacol. 340, 486–494. doi: 10.1007/BF00260602
Wallace, J. A. (1985). An immunocytochemical study of the development of central serotoninergic neurons in the chick embryo. J. Comp. Neurol. 236, 443–453. doi: 10.1002/cne.902360403
Walther, D. J., and Bader, M. (2003). A unique central tryptophan hydroxylase isoform. Biochem. Pharmacol. 66, 1673–1680. doi: 10.1016/S0006-2952(03)00556-2
Walther, D. J., Peter, J. U., Bashammakh, S., Hortnagl, H., Voits, M., Fink, H., et al. (2003). Synthesis of serotonin by a second tryptophan hydroxylase isoform. Science 299:76. doi: 10.1126/science.1078197
Wang, H. L., Zhang, S. L., Qi, J., Wang, H. K., Cachope, R., Mejias-Aponte, C. A., et al. (2019). Dorsal raphe dual serotonin-glutamate neurons drive reward by establishing excitatory synapses on VTA Mesoaccumbens dopamine neurons. Cell Rep. 26:1128. doi: 10.1016/j.celrep.2019.01.014
Watson, C., Bartholomaeus, C., and Puelles, L. (2019). Time for radical changes in brain stem nomenclature-applying the lessons from developmental gene patterns. Front. Neuroanat. 13:10. doi: 10.3389/fnana.2019.00010
Whitaker-Azmitia, P. M. (2020). “Serotonin and development” in Handbook of behavioral neuroscience. eds. C. P. Müller and K. A. Cunningham (Amsterdam: Elsevier), 413–435.
Wilson, M. A., and Mcnaughton, B. L. (1993). Dynamics of the hippocampal ensemble code for space. Science 261, 1055–1058. doi: 10.1126/science.8351520
Wirth, A., Holst, K., and Ponimaskin, E. (2017). How serotonin receptors regulate morphogenic signalling in neurons. Prog. Neurobiol. 151, 35–56. doi: 10.1016/j.pneurobio.2016.03.007
Xu, C. J., Zhang, P., Dai, T. L., Niu, X. Y., Wang, J. L., Jin, M. S., et al. (2014). Evaluation of blood-brain barrier permeability in tryptophan hydroxylase 2-knockout mice. Exp. Ther. Med. 8, 1467–1470. doi: 10.3892/etm.2014.1938
Yagishita, S. (2020). Transient and sustained effects of dopamine and serotonin signaling in motivation-related behavior. Psychiatry Clin. Neurosci. 74, 91–98. doi: 10.1111/pcn.12942
Yamada, H. (1984). Immunohistochemical studies on the serotonin neuron system in the brain of the chicken (Gallus domesticus). I. The distribution of the neuronal somata. Biogenic Amines 1, 83–94.
Yamada, H., and Sano, Y. (1985). Immunohistochemical studies on the serotonin neuron system in the brain of the chicken (Gallus domesticus). 2. The distribution of the nerve-fibers. Biogenic Amines 2, 21–36.
Yamaguchi, S., Fujii-Taira, I., Katagiri, S., Izawa, E., Fujimoto, Y., Takeuchi, H., et al. (2008a). Gene expression profile in cerebrum in the filial imprinting of domestic chicks (Gallus gallus domesticus). Brain Res. Bull. 76, 275–281. doi: 10.1016/j.brainresbull.2008.02.002
Yamaguchi, S., Fujii-Taira, I., Murakami, A., Hirose, N., Aoki, N., Izawa, E., et al. (2008b). Up-regulation of microtubule-associated protein 2 accompanying the filial imprinting of domestic chicks (Gallus gallus domesticus). Brain Res. Bull. 76, 282–288. doi: 10.1016/j.brainresbull.2008.02.010
Yamamoto, K., and Vernier, P. (2011). The evolution of dopamine systems in chordates. Front. Neuroanat. 5:21. doi: 10.3389/fnana.2011.00021
Yao, J., Wang, X., Yan, H., Cai, X., Wang, M., Tu, Y., et al. (2017). Enhanced expression of serotonin receptor 5-hydroxytryptamine 2C is associated with increased feather damage in Dongxiang blue-shelled layers. Behav. Genet. 47, 369–374. doi: 10.1007/s10519-017-9839-1
Youdim, M. B., Edmondson, D., and Tipton, K. F. (2006). The therapeutic potential of monoamine oxidase inhibitors. Nat. Rev. Neurosci. 7, 295–309. doi: 10.1038/nrn1883
Keywords: serotonin, serotonergic system, serotonergic receptor, avian brain, homology
Citation: Fujita T, Aoki N, Mori C, Homma KJ and Yamaguchi S (2023) Molecular biology of serotonergic systems in avian brains. Front. Mol. Neurosci. 16:1226645. doi: 10.3389/fnmol.2023.1226645
Edited by:
Wei Meng, Jiangxi Science and Technology Normal University, ChinaReviewed by:
Skirmantas Janusonis, University of California, Santa Barbara, United StatesYousuke Tsuneoka, Toho University, Japan
Copyright © 2023 Fujita, Aoki, Mori, Homma and Yamaguchi. This is an open-access article distributed under the terms of the Creative Commons Attribution License (CC BY). The use, distribution or reproduction in other forums is permitted, provided the original author(s) and the copyright owner(s) are credited and that the original publication in this journal is cited, in accordance with accepted academic practice. No use, distribution or reproduction is permitted which does not comply with these terms.
*Correspondence: Shinji Yamaguchi, c2hpbmppLXlAcGhhcm0udGVpa3lvLXUuYWMuanA=