- 1Department of Experimental and Clinical Pharmacology, Medical University of Lublin, Lublin, Poland
- 2Department of Animal Physiology and Pharmacology, Institute of Biological Sciences, Maria Curie-Skłodowska University, Lublin, Poland
- 3Canada East Spine Center, Saint John Regional Hospital, Horizon Health Center, Saint John, NB, Canada
- 4Department of Pharmacognosy with Medicinal Plants Garden, Medical University, Lublin, Poland
Traditionally, selected plant sources have been explored for medicines to treat convulsions. This continues today, especially in countries with low-income rates and poor medical systems. However, in the low-income countries, plant extracts and isolated drugs are in high demand due to their good safety profiles. Preclinical studies on animal models of seizures/epilepsy have revealed the anticonvulsant and/or antiepileptogenic properties of, at least some, herb preparations or plant metabolites. Still, there is a significant number of plants known in traditional medicine that exert anticonvulsant activity but have not been evaluated on animal models. Zebrafish is recognized as a suitable in vivo model of epilepsy research and is increasingly used as a screening platform. In this review, the results of selected preclinical studies are summarized to provide credible information for the future development of effective screening methods for plant-derived antiseizure/antiepileptic therapeutics using zebrafish models. We compared zebrafish vs. rodent data to show the translational value of the former in epilepsy research. We also surveyed caveats in methodology. Finally, we proposed a pipeline for screening new anticonvulsant plant-derived drugs in zebrafish (“from tank to bedside and back again”).
1. Introduction
1.1. Epilepsy
Epilepsy is a common neurological disease characterized by the occurrence of seizures caused by uncontrolled excessive discharges of neurons in the brain. The diagnosis of epilepsy requires two unprovoked or reflex seizure incidents at least 24 h apart. Epilepsy is also diagnosed in patients who have experienced only one seizure (unprovoked or reflex) if there is at least 60% likelihood of further seizure attacks to occur within the next 10 years, as well as in those patients who have been diagnosed with an epilepsy syndrome (Fisher et al., 2014). Epilepsy affects about 50 million people worldwide and remains an important problem for current medicine due to its varied etiology, complex clinical profile, and high morbidity and mortality rates (Duncan et al., 2006; World Health Organization, 2019). The disease can develop and manifest itself in people of all ages, from newborns to the elderly, although it is most commonly identified in children under the age of 10 and in adults over the age of 85 (Beghi, 2020). Every year, 61.4 per 100,000 people in the world develop epilepsy, and the incidence rate is significantly higher in low- and middle-income countries than in high-income countries, i.e., 139 vs. 48.9 per 100,000 people/year (World Health Organization, 2019; Beghi, 2020). One of the most important problems of seizure attacks is the increased risk of premature death, which in people with epilepsy is, on average, two or three times higher than in the general population (Trinka et al., 2023). There are several reasons for higher mortality, starting with sudden unexpected death in epilepsy or status epilepticus, ending with trauma, pneumonia, or suicide (Thurman et al., 2017). The standardized mortality rate ranges from 1.6 to 3.0 in high-income countries, while in low- and middle-income countries it reaches 19.8 (Thurman et al., 2017; Beghi, 2020; Trinka et al., 2023).
The first mentions of epilepsy and its treatment strategies were made at least 4,000 years ago, in Mesopotamia. Over the centuries, the understanding of epilepsy and the approach to its treatment have changed—from ineffective treatments of epilepsy involving skull trephination or bloodletting in ancient times, through a more religious approach in the Middle Ages, with epilepsy viewed as a sign of evil influence or occultism, to a modern approach due to the rapid development of medical sciences (Kaculini et al., 2021).
Epilepsy is not a homogenous disease. Its types can differ in terms of etiology and symptoms, as well as treatment response (Duncan et al., 2006; Scheffer et al., 2017). Pharmacotherapy is the basic treatment for epilepsy, and provides satisfactory seizure control and substantial improvements in the patient’s quality of life in most cases. The currently available antiseizure medications (ASMs) act through several main mechanisms, i.e., (1) the modulation of some voltage-gated ion channels, i.e., sodium (phenytoin, primidone, and eslicarbazepine), calcium (ethosuximide, pregabalin), and potassium (retigabine); (2) the potentiation of γ-aminobutyric acid (GABA) inhibitory neurotransmission (benzodiazepines, valproic acid, tiagabine, and vigabatrin); (3) the restriction of excessive glutamatergic neurotransmission (perampanel); and (4) neurotransmitters, i.e., glutamate and GABA release modulation (levetiracetam, brivaracetam, gabapentin, and pregabalin); (Sills and Rogawski, 2020; Łukasiuk and Lasoń, 2023). Nevertheless, approximately 30% of all patients with epilepsy continue to experience seizures despite using available ASMs as mono- or polytherapy (Guerreiro, 2016; Hakami, 2021). In addition, ASMs, especially first-generation drugs, cause some adverse effects, among which the most serious and troublesome are depression, anxiety, mood changes, cognitive dysfunction, sleep disturbances, and motor impairments. In many cases, such adverse effects are so disruptive to patient functioning that they decide to discontinue therapy—even if it effectively controls seizures (Abou-Khalil, 2016; Guerreiro, 2016; Hakami, 2021). An additional disadvantage of ASMs is that they only treat the symptoms of epilepsy, but do not cure the cause(s) of the disease—meaning that they do not prevent or inhibit epileptogenesis (the process that changes a healthy brain into the epileptic); (French and Perucca, 2020). All these problems confront modern medicine and compel the undertaking of the ambitious task to look for new therapeutics that will not only reduce seizure severity and/or frequency (and have less adverse effects), but will primarily be able to prevent epileptogenesis.
In the past decade, as the knowledge of the genetic background of the diseases and gene manipulation techniques has increased, many researchers have been developing genetically modified disease models to study drug response. Pharmacogenetic studies show how drug response is affected by genotype (Fontana et al., 2018). The rapid disease-gene discoveries have resulted in enormous development and understanding in the field of genetic epilepsy (Myers et al., 2019). Human genome sequencing has become much easier since 2003, which was when human gene sequencing was fully explored, and the gene panels, exomes and genomes available have led to higher diagnostic rates and a better understanding of the disease processes (Gonzaga-Jauregui et al., 2012). The development of recent technology has supported genetic discovery in epilepsy and the molecular mechanisms of many epileptic diseases have been increasingly understood, eventually providing targets for a precision medicine approach to epilepsy treatment (Kearney et al., 2019).
1.2. Plants as a source of anticonvulsants
Plants with anticonvulsant properties have been known for centuries and are also currently used in the treatment of epilepsy/seizures in many cultures, especially in low-income countries with poor medical systems (Aghdash, 2021; Birhan, 2022; Faheem et al., 2022). Anticonvulsant remedies of plant origin are traditionally administered in the form of infusions, decoctions or powders; however, numerous species are also ingested as regular food. Limited access to modern synthetic drugs makes herbal preparations the main, or even the only, treatment for the disease in the developing countries (Liu et al., 2017). Developed countries medicine is more skeptical to the use of herbal preparations. Nevertheless, there are several plant-derived drugs known to effectively treat patients suffering from epilepsy. A good example is valproic acid. This is a derivative of valeric acid—a compound naturally present in the roots of Valeriana officinalis. Valproic acid was first synthesized in 1882 by Beverly Burton, an American chemist, and it was initially used as a pharmacologically inert solvent in medical research. In 1962, scientists found that proconvulsant substances dissolved in valproic acid did not induce seizures. This finding led to approving valproic acid as an anticonvulsant drug in 1972, in France. Nowadays, this is one of the most commonly used drugs in diverse forms of epilepsy (Tomson et al., 2016). Another example of an ASM with strict plant origin is cannabidiol (CBD)—a cannabinoid naturally present in Cannabis spp. CBD was found to effectively inhibit seizures in patients with Dravet or Lennox–Gastaut syndromes—serious developmental and epileptic encephalopathies that are generally resistant to treatment (García-Peñas et al., 2021). The U.S. Food and Drug Administration (FDA) and the European Medicines Agency (EMA) have approved CBD to treat these syndromes even in 1- and 2-year-old children (Devinsky et al., 2017, 2018). Moreover, clinical trials of huperzine A, a compound isolated from the Chinese plant Huperzia serrata, are currently being conducted among adult patients with pharmacoresistant epilepsy (Clinicaltrials.gov/ Identifier: NCT03474770, NCT05518578).
Preclinical studies on animal models of seizures and epilepsy have revealed the anticonvulsant and/or antiepileptogenic properties of numerous herb preparations and plant-derived drugs. Indeed, some of the studied plants have been used in traditional ethnomedicine (Zhu et al., 2014; Kwon et al., 2019; Bertoncello and Bonan, 2021; Sharifi-Rad et al., 2021). However, there is a lack of robust results from preclinical studies that could objectively confirm or disaffirm their use as potential ASMs (Liu et al., 2017).
1.3. Zebrafish
Zebrafish (Danio rerio) is a small striped minnow natural to the freshwaters of the Indian subcontinent. Since their first scientific use in the 1960s, the popularity of zebrafish in biomedical sciences has been increasing at a rapid pace (Stewart et al., 2014; Crouzier et al., 2021; Al-Hamaly et al., 2023; Chang et al., 2023; Liu, 2023; Tesoriero et al., 2023). Zebrafish is a very popular organism for high-throughput screening studies (Baxendale et al., 2012; Baraban et al., 2013; Asad et al., 2023). Larval zebrafish have also gained popularity as an animal model for the study of the molecular mechanisms of brain diseases at early developmental stages and for testing potential therapeutic drugs (Grone and Baraban, 2015; Kundap et al., 2017).
There is definitely a long list of advantages of zebrafish use for biomedical studies, especially for epilepsy research (Stewart et al., 2014, 2015; Bertoncello and Bonan, 2021; Yaksi et al., 2021; D'Amora et al., 2023). Briefly, there is a high homology of zebrafish and human genomes, with at least one zebrafish orthologue existing for 70% of all human-disease related genes (Howe et al., 2013). There is also a high physiological similarity between zebrafish and humans in terms of molecular pathways, neurotransmitters, and receptors (Kalueff et al., 2014; Stewart et al., 2015; Jurisch-Yaksi et al., 2020). All this makes zebrafish an excellent model for genetic studies and for the creation of genetically altered animal models.
The zebrafish brain consists of the forebrain, the midbrain, the hindbrain, and the spinal cord, and brain structures like the cerebellum or the thalamus are homologous to human structures (Guo et al., 2012; Wullimann et al., 2012). There are, however, some differences between zebrafish and mammal brains, e.g., zebrafish lack the neocortex, and the telencephalon, in contrast to mammals, develops through eversion and not through invagination (Wullimann et al., 2012). The blood–brain barrier in zebrafish, however, is complex and similar to that of mammals. It starts to develop at 3 days post-fertilization (dpf) becoming mature at 10 dpf (Jeong et al., 2008; Fleming et al., 2013; Quiñonez-Silvero et al., 2020). This has to be taken into account when planning experiments using larvae at this stage of development.
From the practical point of view, zebrafish are quick and easy to breed, with low maintenance costs compared to rodents. The small size of zebrafish embryos and larvae substantially decreases the amounts of substances, drugs or extracts used during screening assays. In addition, the minute body size of the larvae allows the concomitant screening of up to 96 larval zebrafish within one time frame, which substantially speeds up the entire process of screening and makes it more efficient and less expensive. According to current European Union legislation, testing the larval zebrafish up to 120 h post-fertilization (hpf), i.e., the stage of being capable of independent feeding, does not require any ethical permission (Figure 1).
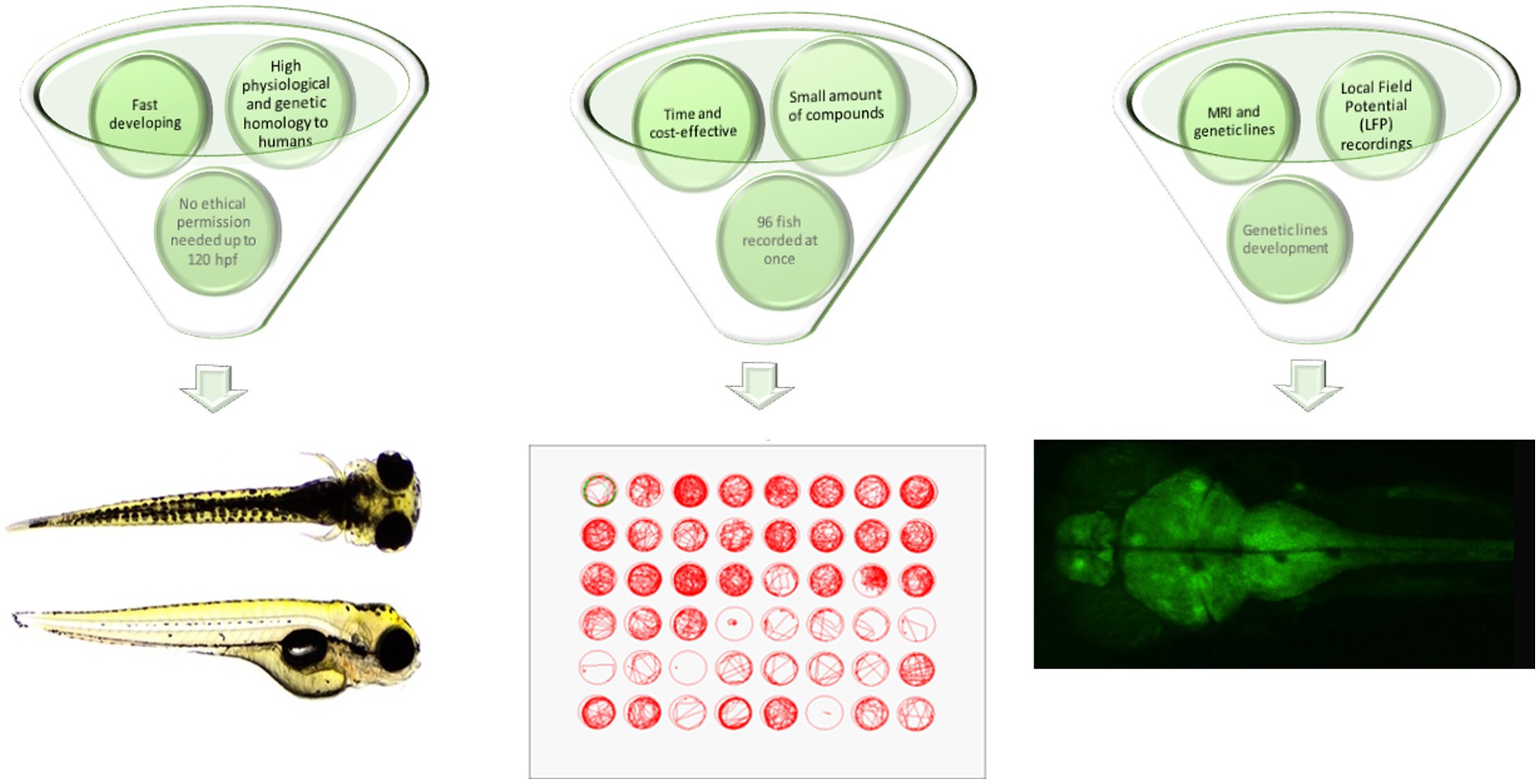
Figure 1. Advantages of larval zebrafish for high-throughput screening of plant-derived drugs with anticonvulsant properties—a summary. hpf, hours post fertilization; MRI, magnetic resonance imaging.
Zebrafish have been frequently employed as model organisms in epilepsy research (Gawel et al., 2020b; Crouzier et al., 2021; Yaksi et al., 2021). Currently, the best validated model of convulsions in larval zebrafish consists of animal exposure to pentylenetetrazole (PTZ; Table 1). Other proconvulsant agents (e.g., picrotoxin, kainic acid, or pilocarpine) are less validated and utilized rarely, but, similar to PTZ, they are still used more frequently in larvae than in adult zebrafish (Gawel et al., 2020b). Therefore, in most but not all cases, researchers employ the PTZ-induced seizure model as their first-choice screening platform (although it is worth noting that scn1Lab zebrafish mutants were utilized to identify anticonvulsant properties of clemizole; Baraban et al., 2013). Interestingly, PTZ added to the medium induces abrupt hyperlocomotion, both in larvae and adult zebrafish. One may assume that hyperlocomotion reflects clonic–tonic-like seizures in humans (Baraban et al., 2005; Wong et al., 2010; Afrikanova et al., 2013). Furthermore, pretreatment with anticonvulsant compounds decreases the speed and/or distance traveled by PTZ-exposed zebrafish (Baraban et al., 2005; Afrikanova et al., 2013). This approach has been widely adopted in the scientific community (Baxendale et al., 2012; Orellana-Paucar et al., 2012; Buenafe et al., 2013; Gawel et al., 2021).
Bertoncello and Bonan (2021) elaborated data concerning various plants and/or their isolated constituents that were investigated for antiseizure activity using zebrafish as a screening platform. In their review, the authors focused mostly on discovered drugs. Here, particular emphasis was placed on assessing the anticonvulsant effect(s) of the plant extract(s) or isolated plant-derived drug(s), estimated via different models of convulsions/seizures, which were recorded using multiple testing methods. In our study, we selected and evaluated only the most comprehensive papers in this field. That which contained too many gaps (e.g., lack of critical details of the methodology used, insufficient description of plant extraction, and invalid description of the locomotor activity assay used) were not included. This allowed us to identify and survey caveats, e.g., in a methodology which should be considered in future experiments to move the field further on. Moreover, we gathered and compared zebrafish and rodent data to show the translational value of zebrafish in epilepsy research. Finally, we proposed a pipeline for screening new anticonvulsant drugs of plant origin in zebrafish (“from tank to bedside and back again”).
2. Plant extracts and/or active constituents with anticonvulsant activity
2.1. Berberis spp.
Berberis spp. (Berberidaceae; barberry) are found mainly in the temperate zone of the northern hemisphere, and they are used both as a food flavor and in traditional medicine to treat insomnia, liver, and bronchial diseases, as well as afflictions of the urinary and gastrointestinal systems (Abdykerimova et al., 2020). Extracts from Berberis spp. have been found to display antimicrobial, anti-inflammatory and antinociceptive properties. The anticonvulsant properties of Berberis L. vulgaris and Berberis integerrima Bunge. extracts were previously demonstrated in rodent models of seizures (Hosseinzadeh et al., 2013; Khosravi Dehaghi et al., 2017; Supplementary Table 1), while PTZ-induced seizure assay in the zebrafish larvae was employed to evaluate the anticonvulsant potential of the Berberis sibirica root extract (Gawel et al., 2020a; Table 2).
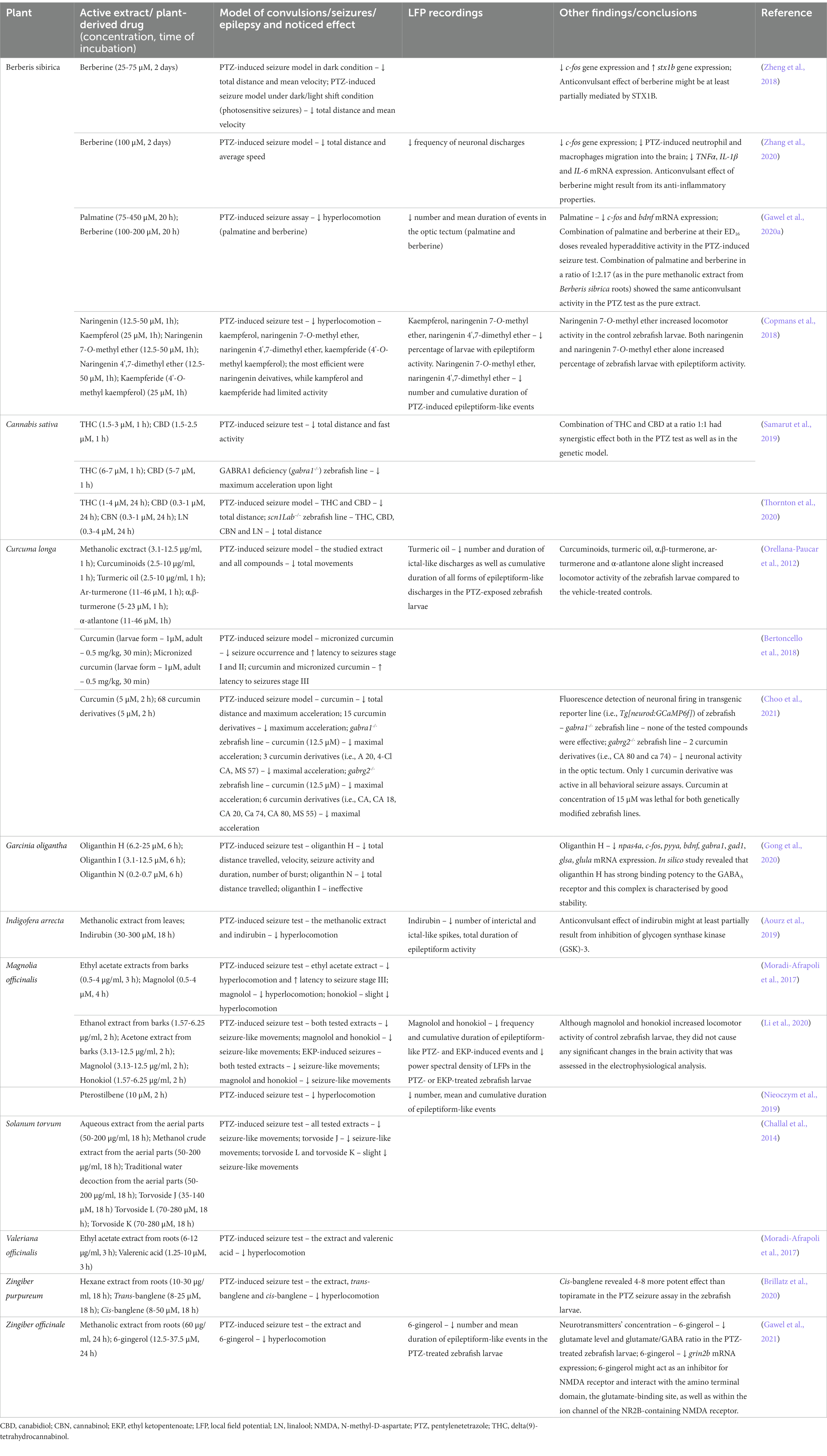
Table 2. Anticonvulsant effects of plant extracts or plant-derived drugs in different seizure or epilepsy models in zebrafish.
Berberine and palmatine (O,O-dimethyldemethyleneberberine) are the main isoquinoline alkaloids present in Berberis sibirica, and they are responsible for its pharmacological properties. These compounds are also identified in other plants from the Berberidaceae, Papaveraceae, and Ranunculaceae families (Tarabasz and Kukula-Koch, 2020; Zhang et al., 2020). Plants containing berberine and palmatine have been exploited for centuries in traditional oriental medicine as a remedy for various ailments and diseases. Therapeutic effects of berberine include lowering body temperature, detoxification, analgesic, spasmolytic, and antihypertensive activity (Filli et al., 2020). Palmatine has been reported to be used as a remedy in jaundice- and liver-related diseases, hypertension, inflammation, dysentery, as well as in infections of the urinary, gastrointestinal and respiratory tracts (Tarabasz and Kukula-Koch, 2020).
The anticonvulsant activity of berberine was first evaluated in rodent models of seizures and epilepsy (Shanbhag et al., 1970; Bhutada et al., 2010; Gao et al., 2014; Mojarad and Roghani, 2014; Sadeghnia et al., 2017; Sedaghat et al., 2017; Supplementary Table 1), and then was investigated in larval zebrafish seizure tests (Zheng et al., 2018; Zhang et al., 2020; Table 2). Zheng et al. (2018) demonstrated the anticonvulsant action of berberine in two variants of PTZ-induced seizure tests in larval zebrafish, i.e., in tests conducted in the dark condition, as well as under dark/light shift condition (four cycles of 5-min dark and 10-s light periods). Light stimulation was applied to modulate photosensitive seizures that are at least partially mediated by syntaxin 1b (stx1b) deficiency, and in zebrafish larvae, alkaloid concentration-dependently limited the PTZ-induced hyperlocomotion both under dark and light stimulation conditions. The behavioral observation was correlated with the reduction of c-fos expression and the recovery of stx1b expression in the brain of the PTZ-treated zebrafish larvae. The possibility of quickly adopting genetic modification in zebrafish larvae allowed the identification of potential mechanisms responsive to the anticonvulsant action of berberine, and in situ hybridization was used to show changes in the c-fos and stx1b expression in their brains (Zheng et al., 2018).
Previous studies have revealed that the anticonvulsant effect of berberine in the zebrafish larvae PTZ-induced seizure assay might also result from its anti-inflammatory properties. In addition to reducing the seizure-like hyperlocomotion and c-fos expression, berberine decreased the number of spikes in local field potential (LFP) recordings. Inflammatory reactions associated with the seizure activity, i.e., macrophages and neutrophils recruitment, and increased expression of some inflammatory markers (i.e., tumor necrosis factor α, interleukin 1β and 6), in the PTZ-treated zebrafish larvae, were substantially suppressed by the alkaloid (Zhang et al., 2020).
Recently, our research group has demonstrated the anticonvulsant effect of the Berberis sibrica radix extract; berberine and another isoquinoline alkaloid (palmatine) were isolated from this extract and studied by means of zebrafish larvae PTZ-induced seizure assay (Gawel et al., 2020a). In our study, berberine was used as a reference compound. The Berberis sibrica root extract and both studied alkaloids decreased hyperlocomotion in the PTZ-treated larvae. The analysis of the LFP recordings from the larval optic tectum confirmed the anticonvulsant effect of the studied drugs. Both alkaloids reduced the number of PTZ-induced epileptiform-like discharges, but only berberine shortened the mean duration of epileptiform-like events. In contrast, palmatine reduced the bdnf and c-fos mRNA expression in the PTZ-exposed larvae (Gawel et al., 2020a).
In our study, a mixture of palmatine and berberine combined at their ED16 concentrations (palmatine and berberine at a 1:2.17 ratio that resembled their distribution in the plant extract), was also studied (Gawel et al., 2020a). A hyper-additive interaction between these two alkaloids was noted and the combination employed revealed an anticonvulsant effect that was comparable to the total Berberis sibrica root extract. Those results suggest that the mechanisms of the anticonvulsant action of palmatine and berberine are distinct and these two alkaloids are probably the main active compounds that determine the anticonvulsant properties of the Berberis sibrica extract (Gawel et al., 2020a).
2.2. Bioflavonoids
Naringenin [5,7-dihydroxy-2-(4-hydroxyphenyl)chroman-4-one] is a flavonoid formed due to the hydrolysis of its naringin or narirutin glycone forms. It is mainly present in grapefruit juice, but might also be found in other citrus fruit (Wilcox et al., 2006). Naringenin has many proven pharmacological effects, among others, antioxidant, anti-inflammatory, antiulcer, hypocholesterolemic, antimutagenic, and neuroprotective activity (Ribeiro, 2011).
Kaempferol [3,5,7-trihydroxy-2-(4-hydroxyphenyl)chromen-4-one], a flavonoid present in many vegetables and fruit, e.g., broccoli, cabbage, beans, tomato, strawberries, and grapes, can also be found in plants used in traditional medicine, e.g., in Ginkgo biloba, Tilia spp., Equisetum spp., as well as in Crinum jagus. The last is commonly used in Cameroon as a traditional remedy for epilepsy, convulsions, and psychoses (Calderón-Montaño et al., 2011; Taiwe et al., 2016). Kaempferol has a wide range of pharmacological effects, inter alia, antioxidant, anti-inflammatory, neuroprotective, antidiabetic, antiosteoporotic, anxiolytic, analgesic and antiallergic activities (Calderón-Montaño et al., 2011).
In a study by Copmans et al. (2018) (Table 2), naringenin, kaempferol, and their methylated derivatives, i.e., naringenin 7-O-methyl ether, naringenin 4′,7-dimethyl ether and kaempferide (4’-O-methyl kaempferol), were investigated via zebrafish larvae PTZ-evoked hyperlocomotion assay. Here, naringenin 7-O-methyl ether and naringenin 4′,7-dimethyl ether were found to be the most efficient in attenuating seizure-like behavior, as they reduced PTZ-induced hyperlocomotion to ca. 20–30% of the control PTZ-evoked activity. Kaempferol, kaempferide, and naringenin had markedly weaker anticonvulsant effects. It cannot be excluded that differences in the activity of the studied drugs, especially in the case of naringenin, were due to their different bioavailability and insufficient time of incubation, i.e., 1 h was not long enough to penetrate the blood–brain barrier (Guarin et al., 2021).
To verify behavioral results, LFP recordings were obtained. Naringenin 7-O-methyl ether, naringenin 4′,7-dimethyl ether, and kaempferol were found to reduce the percentage of larvae with epileptiform-like activity in the LFP recordings. Additionally, the studied naringenin derivatives reduced the number and cumulative duration of the PTZ-induced epileptiform-like events. Since naringenin 7-O-methyl ether itself increased larval locomotion, in comparison to the vehicle-treated control group, the larvae pre-incubated in the studied compounds alone were also submitted to a LFP recordings assay. Interestingly, the researchers noted that naringenin 7-O-methyl ether, as well as naringenin alone, induced the epileptiform-like events recorded in the larval optic tectum. As a follow up, naringenin 4′,7-dimethyl ether was also investigated via intravenous (iv) PTZ and 6-Hz psychomotor seizure tests in mice. In both tests, this compound exhibited anticonvulsant properties. The results obtained in the zebrafish larvae and mouse tests suggest that naringenin 4′,7-dimethyl ether might be considered a new drug candidate for epilepsy treatment, although in-depth analysis to determine the mechanisms of its action is needed (Copmans et al., 2018).
2.3. Cannabis sativa
Cannabis sativa L. (Cannabaceae), also called “hemp” or “marihuana,” has been a commonly used medicinal plant since ancient times. It is believed that Cannabis sativa is native to the alpine foothills of the Himalayas. Nowadays, Cannabis sativa is cultivated all over the world and used in the food, textile, and pharmaceutical industries (ElSohly et al., 2017). Interest in using Cannabis sativa and cannabis-based products in epilepsy treatment has grown rapidly in recent years (Gaston and Friedman, 2017). One of the phytocannabinoids that are deprived of the psychoactive activity, i.e., CBD, was approved for the treatment of seizures in Dravet and Lennox–Gastaut syndrome patients (Morano et al., 2020).
Although the anticonvulsant properties of Cannabis sativa isolates have been known for centuries and used by local herbalists, and they have been confirmed in numerous studies using rodent models of seizures and epilepsy (Chesher and Jackson, 1974; Elisabetsky et al., 1999; Elisabetsky and Brum, 2003; Shirazi-zand et al., 2013; Gobira et al., 2015; Kaplan et al., 2017; Klein et al., 2017; Vilela et al., 2017; Patra et al., 2019; Anderson et al., 2020; Gray and Whalley, 2020; Zou et al., 2020; Costa et al., 2021; Supplementary Table 1), there are also some studies that evaluated the protective properties of selected phytocannabinoids using zebrafish larvae (Samarut et al., 2019; Thornton et al., 2020; Table 2).
Using zebrafish larvae to study the therapeutic properties of cannabis-derived medicaments is highly reasonable since all important endocannabinoid-related genes are expressed in the zebrafish larvae. Moreover, a high level of similarity between the cannabinoid system in zebrafish and other vertebrates makes zebrafish an appropriate model for studying the therapeutic properties and mechanism of action of phytocannabinoids (Rodriguez-Martin et al., 2007). The expression of cannabinoid receptor 1 (CB-1), the major target for the cannabinoids, in the zebrafish larvae begins as early as 24 hpf and its increased expression in the brain structures is observed by 48 hpf (Lam et al., 2006).
Samarut et al. (2019) employed two zebrafish larvae models of seizures, i.e., PTZ-induced seizure test and gabra1−/− zebrafish mutants (Table 1) to evaluate the single and combined effects of CBD and Δ-9-tetrahydrocannabinol (THC; Table 2). In the study, both CBD and THC alone substantially reduced the seizure-like activity in the PTZ-induced and genetic models. However, higher concentrations of the studied compounds had to be administered to reduce seizures in gabra1−/− mutants. The obtained results suggest that the anticonvulsant effect produced by the studied phytocannabinoids, especially by THC, might at least partially result from their sedative activity. The co-exposure of both drugs tested at their low ineffective concentrations, at a 1:1 ratio, resulted in a synergistic anticonvulsant effect in both models (Samarut et al., 2019).
In a study by Thornton et al. (2020) (Table 2), five cannabis-derived compounds, i.e., CBD, THC, cannabidivarin, cannabinol, and linalool, were investigated using PTZ-induced seizure model and scn1Lab−/− zebrafish mutants (Table 1). This study confirmed the results that had been previously presented by Samarut et al. (2019) on the anticonvulsant effect of CBD and THC in the PTZ-induced seizure test. However, there were some differences concerning the effective concentrations of the studied compounds, especially CBD, which was effective at relatively low concentrations in comparison to the results obtained by Samarut et al. (2019). These differences might have resulted from differences in time exposure to the studied compounds—in the study presented by Samarut et al. (2019), zebrafish were incubated for 1 h, while in the study conducted by Thornton et al. (2020), the incubation time was 24 h. Cannabidivarin, cannabinol, and linalool did not affect seizure-like activity in the zebrafish larvae PTZ-induced seizure assay (Thornton et al., 2020). Moreover, CBD and THC reduced the seizure activity in the scn1Lab−/− zebrafish larvae. The anticonvulsant effect of cannabinol and linalool was also noted in the scn1Lab−/− mutants. While the efficacy of CBD in Dravet syndrome models had been known earlier, the efficacy of cannabinol and linalool was demonstrated in this study for the first time (Thornton et al., 2020).
2.4. Curcuma longa
Curcuma longa L. (Zingiberaceae; turmeric) is a herb native to South Asia. Due to its intensive flavor and yellow color, turmeric rhizome became an important spice in the kitchens of South Asia, Iran, China, Polynesia, and Thailand. For at least 2,500 years, turmeric has also been a common and widely used herbal constituent in traditional South Asia and Chinese medicine as a remedy for plenty of diseases. The main active constituent of turmeric is curcumin (2–5%) which possesses antioxidant, antimicrobial, anti-inflammatory, antiangiogenic, antimutagenic, and antiplatelet properties (Kocaadam and Şanlier, 2017). Recent studies have also demonstrated the anticonvulsant properties of curcumin (Agarwal et al., 2011; Reeta et al., 2011; Ahmad, 2013; Akula and Kulkarni, 2014; Arbabi Jahan et al., 2018; Dhir, 2018; Supplementary Table 1), though the clinical relevance of this indication is doubtful due to very low absorption of curcumin from the gastrointestinal tract (Lopresti, 2018). However, it is very likely that curcumin is not the only constituent of Curcuma longa with anticonvulsant properties because another relevant constituent of turmeric—turmeric oil, shows some therapeutic properties which also include neuroprotective and antiseizure activities (Dohare et al., 2008; Zhang L. et al., 2017; Supplementary Table 1).
Orellana-Paucar et al. (2012) (Table 2) revealed the anticonvulsant activity of the methanolic extract of turmeric utilizing the larval zebrafish PTZ-induced seizure model. To identify active constituents of turmeric, a curcuminoids mixture (98% of curcumin) of turmeric and turmeric oil was also examined. They found that the curcuminoids mixture was more efficient in attenuating PTZ-induced seizure behavior than turmeric oil alone, which revealed an anticonvulsant effect only at the highest concentration tested. Interestingly, both the curcuminoids mixture and turmeric oil alone slightly increased zebrafish locomotor activity compared to the control group (Orellana-Paucar et al., 2012).
Active constituents of turmeric oil, i.e., ar-turmerone, α,β-turmerone, and α-atlantone, also displayed anticonvulsant action, but they increased the locomotor activity in the control zebrafish. To verify whether turmeric oil itself has proconvulsant activity, LFP recordings were obtained. The analysis revealed that it did not cause any epileptiform-like discharges and it reduced the number and duration of ictal-like events in the PTZ-exposed larvae. Finally, stage of the study, the anticonvulsant potential of turmeric oil and its two main constituents, i.e., ar-turmerone and α,β-turmerone, was confirmed via timed iv PTZ test in mice (Orellana-Paucar et al., 2012).
To sum up, the study conducted by Orellana-Paucar et al. (2012) revealed, for the first time, that the anticonvulsant properties of Curcuma longa are not only due to curcumin presence, but they also depend on other compounds, i.e., ar-turmerone, α,β-turmerone, and α-atlantone. Importantly, in this study, the effects of the turmeric-derived compounds tested were verified carefully using both behavioral studies in zebrafish larvae, as well as LFPs analysis, and they involved a conventional seizure model in mice.
Since low bioavailability limits the therapeutic utility of curcumin (Lopresti, 2018), Bertoncello et al. (2018) (Table 2) used the micronized form of this compound that was prepared by supercritical carbon dioxide processing. The effect of the micronized curcumin in the PTZ-induced seizure tests in larvae and adult zebrafish was compared to the effect of curcumin in its natural form, as well as to valproic acid (classic ASM). In adult zebrafish, the studied compounds (administered intraperitoneally) exhibited some protective activity, but only valproate and micronized curcumin reduced the occurrence of tonic–clonic-like seizures. This paper demonstrates some interesting data, but the final confirmation of the superior anticonvulsant activity of micronized curcumin over curcumin must be checked in an LFP assay since the seizure score assay in larval zebrafish, as employed in this paper, is of low objectivity. It would also be recommended to investigate whether, and to what extent, the micronized form of curcumin is able to penetrate biological membranes better than curcumin.
Choo et al. (2021) (Table 2) analyzed the anticonvulsant properties of new synthetic analogs of curcumin using PTZ-induced hyperlocomotion assay as an initial screening platform. Among the 68 analogs tested, 15 substantially reduced the total distance traveled by and/or the maximum acceleration of PTZ-exposed larvae. The effective compounds were then tested in two zebrafish larvae genetic models of epilepsy, i.e., gabra1−/− and gabrg2−/− (Table 1). In the study, three of the selected derivatives of this polyphenol abrogated the behavioral manifestation of seizures in gabra1a−/− mutants, six of them showed anticonvulsant effect in gabrg2−/− zebrafish, and only one was effective in both genetic models. To complement behavioral observations in mutants, hit compounds were additionally tested in a transgenic reporter line, i.e., Tg[neurod:GCaMP6f], which allowed detecting neuronal firing. Here, mutants and wild-type larvae were first incubated in curcumin derivatives and then laser stimuli were used to evoke the excitation of post-mitotic neurons in the larval optic tectum. Unfortunately, none of the selected analogs was able to rescue the neuronal firing in gabra1a−/− mutants and only two of them decreased fluorescence intensity upon laser stimuli in gabrg2−/− larvae (Choo et al., 2021).
In conclusion, the obtained data have confirmed that the PTZ-induced seizure assay is a good standard for the initial screening of compounds, but it may yield false-positive results. Thus, e.g., neuronal firing assay or LFP recordings, should be performed. Nonetheless, the paper by Choo et al. (2021) revealed the anticonvulsant potential of compounds that were synthesized based on the chemical structure of naturally occurring compounds.
2.5. Garcinia oligantha
Garcinia oligantha Merr. (Guttiferae) is a shrub commonly present in the forests of the Guangdong and Hainan provinces in China. In traditional folk medicine, Garcinia oligantha is used for body detoxification and as a remedy for inflammation (Lin F. et al., 2021). Moreover, cytotoxic properties of Garcinia oligantha extracts and xanthones derived from this plant have been reported (Tang et al., 2016). Interestingly, there are no reports regarding the use of this plant in traditional medicine for epilepsy treatment or experimental studies reporting its anticonvulsant properties.
Gong et al. (2020) (Table 2) studied the anticonvulsant properties of three xanthones isolated from Garcinia oligantha leaves, i.e., oliganthin H, oliganthin I, and oliganthin N. The compounds were evaluated via PTZ-induced seizure test in zebrafish larvae. Of the three compounds tested, the strongest anticonvulsant effect was produced by oliganthin H—the lower concentrations tested prolonged seizure latency, while the highest concentration tested additionally reduced the total distance traveled. A substantial reduction of PTZ-induced seizure-like activity was also caused by oliganthin N, though only at the maximum concentration tested. Oliganthin I did not inhibit PTZ-induced activity, but was able to prolong seizure latency. Since oliganthin H was the most potent compound, its anticonvulsant activity was precisely analyzed. Quantitative reverse transcription PCR (RT-qPCR) analysis revealed that it normalized the expression of some genes related to the neuronal activity, GABA, and glutamate neurotransmission, i.e., npas4a, c-fos, pyya, bdnf, gabra1, gad1, glsa, and glula genes. Moreover, a molecular docking study demonstrated that oliganthin H has a strong binding potency toward the GABAA receptor and this complex is characterized by good stability. The obtained results indicate that the anticonvulsant effect of oliganthin H involves the GABA/glutamate system (Gong et al., 2020).
2.6. Indigofera arrecta
Indigofera arrecta Hochst. ex A.Rich. (Natal indigo) is a member of the Leguminaceae family and it naturally occurs in savannah regions. It is found, inter alia, in Tropical Africa, Saudi Arabia, Eastern and Southern Africa. The great availability of this plant in Africa results in its intensive use by traditional healers, e.g., as a soothing agent for venomous insect and snake bites, and as an antiseptic, healing, and antipruritic agent (Gerometta et al., 2020). There are also reports regarding the use of phytomedicines derived from Indigofera arrecta in the treatment of epilepsy (among other places, in the Congo), anxiety and other nervous system diseases (Amponsah, 2014; Aourz et al., 2019; Table 2). Indirubin [3-(3-oxo-1H-indol-2-ylidene)-1H-indol-2-one], a compound naturally present in Indigofera arrecta, acts as an inhibitor of glycogen synthase kinase-3 (GSK-3); (Aourz et al., 2019), cyclin dependent kinases type 1, 2, and 5 (Canavese et al., 2012), as well as an activator of the aryl hydrocarbon receptor (AhR); (Adachi et al., 2001).
Aourz et al. (2019) (Table 2) demonstrated the anticonvulsant activity of the methanolic extract obtained from Indigofera arrecta leaves in the larval zebrafish PTZ-induced seizure assay. Subsequently, indirubin, an active metabolite, was isolated and evaluated in the seizure test. It was found to reduce PTZ-induced hyperlocomotion, as well as the duration of epileptiform-like activity and the number of interictal and ictal-like spikes (Aourz et al., 2019). Moreover, rodent models of seizures and epilepsy, namely the 6-Hz-induced psychomotor seizure and iv PTZ tests in mice, as well as the pilocarpine model of epilepsy in rats, were applied to evaluate the anticonvulsant effect of indirubin (Supplementary Table 1). Although it did not show anticonvulsant activity in the iv PTZ test in mice, it dose-dependently prevented 6-Hz- and pilocarpine-induced seizures. The experiments in which a panel of compounds with different known affinities toward GSK-3, cyclin dependent kinases type 1, 2, and 5, as well as AhR, was used pointed out GSK-3 as a target in the mechanisms of anticonvulsant action of indirubin. To further strengthen these findings, Aourz et al. (2019) induced transient knockdown of gsk-3ß in larval zebrafish using an antisense morpholino oligomer, and showed that the lack of gsk-3ß exhibited a protective effect against PTZ-evoked seizures.
A study conducted by Aourz et al. (2019) is particularly relevant for a few reasons. First, zebrafish larvae were used as an animal model to point out, for the first time, GSK-3 as a new target for epilepsy treatment. Using a morpholino oligomer for the transient knockdown of gsk-3ß in larval zebrafish, the authors proved the hypothesis concerning the target for indirubin. Different inter-species models of seizures were combined, and the utility of larval zebrafish for the initial screening of plant metabolites with potential anticonvulsant activity was highlighted.
2.7. Magnolia officinalis
Bark extracts of different magnolia species, including Magnolia officinalis Rehder & Wilson (Magnoliaceae), have been used in traditional Chinese and Japanese medicine. Health-promoting properties of Magnolia officinalis include mainly antioxidant and anti-inflammatory action, but sedative, anxiolytic, antidepressant and antiepileptic activities have also been reported (Martínez et al., 2006; Chen et al., 2011; Lee et al., 2011; Han et al., 2015; Poivre and Duez, 2017; Vega-García et al., 2019). The two major biologically active compounds of the Magnolia officinalis bark are magnolol and honokiol—structurally related neolignans with similar pharmacokinetics. Both compounds display antiseizure properties, and exert GABAergic and cannabimimetic activity (Alexeev et al., 2012; Chen et al., 2012; Woodbury et al., 2013; Sarrica et al., 2018; Lin Y. et al., 2021; Supplementary Table 1).
Anticonvulsant properties of the Magnolia officinalis bark extracts and their active compounds, i.e., magnolol and honokiol, were tested both in the larval zebrafish PTZ-induced hyperlocomotion assay (Moradi-Afrapoli et al., 2017; Li et al., 2020; Table 2) and in the ethyl ketopentenoate (EKP) model of pharmacoresistant seizures (Zhang Y. et al., 2017; Table 1). A study by Moradi-Afrapoli et al. (2017) revealed that the ethyl acetate extract of the Magnolia officinalis bark concentration-dependently reduced the PTZ-induced seizure-like behavior. Magnolol was identified as the main ingredient of the extract, and a significant anticonvulsant effect in the PTZ-induced seizure assay was noted for this compound (Moradi-Afrapoli et al., 2017).
Li et al. (2020) studied three extracts (i.e., water, ethanol, and acetone) from the Magnolia officinalis bark in the PTZ-induced seizure test in the zebrafish larvae. Only two, i.e., ethanol and acetone extracts, revealed concentration-dependent anticonvulsant effects. Those two extracts also effectively reduced EKP-induced seizure-like behavior. The active ingredients of Magnolia officinalis, i.e., magnolol and honokiol, brought about a substantial (over 40%) reduction in PTZ-induced seizure movement at the highest concentrations tested, while in the EKP-induced seizure test, both compounds displayed concentration-dependent anticonvulsant effects at all concentrations tested. In addition to the behavioral results, both magnolol and honokiol reduced the frequency, as well as duration of epileptiform-like discharges in the PTZ- or EKP-treated zebrafish larvae. Power spectral density analysis of electrophysiological signals also revealed that these compounds attenuated PTZ- and EKP-increased LFP power. A study presented by Li et al. (2020) not only confirmed previous reports concerning the anticonvulsant effects of Magnolia officinalis extracts and their ingredients in the zebrafish larvae PTZ-induced seizure assay (Moradi-Afrapoli et al., 2017), but also complimented them by the data from the EKP-induced seizure test, which is thought to be an acute model of pharmacoresistant seizures (Zhang Y. et al., 2017). Of note, the results obtained in the behavioral tests were confirmed by an in-depth analysis of electrophysiological signals (Li et al., 2020).
2.8. Pterostilbene
Pterostilbene [4-(3,5-dimethoxystyryl)phenol] is a stilbenoid—a naturally dimethylated analog of resveratrol. Originally, it was isolated from sandalwood and was later also identified in blueberries and grapes. Pterostilbene has numerous health-promoting properties, including anti-inflammatory, antioxidant, antitumor, neuroprotective, and antidiabetic activity (Chang et al., 2012; Teng et al., 2021). Recent studies have suggested its influence on cognition, anxiety-like behavior, and other neuronal functions (Al Rahim et al., 2013; Poulose et al., 2015).
Our research team has demonstrated the anticonvulsant activity of pterostilbene in the larval zebrafish PTZ-induced seizure assay (Nieoczym et al., 2019; Table 2). Pterostilbene substantially limited PTZ-induced hyperlocomotion and reduced both the total and mean duration of the epileptiform-like events registered from optic tectum (Nieoczym et al., 2019). The anticonvulsant effect of pterostilbene was confirmed in three acute seizure tests in mice, i.e., in the maximal electroshock seizure (MES) threshold test, the psychomotor 6 Hz-induced seizure threshold test, as well as the iv PTZ test (Supplementary Table 1). The mechanism of anticonvulsant action of ptereostilbene has not been explained yet (Nieoczym et al., 2019).
2.9. Solanum torvum
Solanum torvum Tw. (Solanaceae) is a shrub commonly found in South India, Malaysia, China, Philippines, Thailand, West Indies, and Tropical America (Balachandran et al., 2015). In Thailand, it is known as “Turkey berry” and in India as “wild brinjal” (Yuan et al., 2016; Vanti et al., 2020). In India and Africa, Solanum torvum is cultivated for its edible fruit. The fruit, leaves, and roots of this plant are commonly used in traditional Cameroonian and Chinese medicine as a remedy for fever, hypertension, gastralgia, or furuncle (Mohan et al., 2009; Yuan et al., 2016). Antiviral, antioxidant, analgesic and anti-inflammatory activities of extracts prepared from this plant have been demonstrated (Balachandran et al., 2015).
Three different formulations, i.e., the aqueous extract, the methanol crude extracts and traditional water decoction from the aerial parts of Solanum torvum revealed anticonvulsant activity in the PTZ-induced seizure assay in larval zebrafish (Challal et al., 2014; Table 2). Since the composition of methanolic and aqueous extracts is very similar to the preparations used in traditional herbal medicine, findings from the seizure test justify the use of Solanum torvum extracts in epilepsy treatment.
In the composition of the methanolic extract, some steroid glycosides were found and six ingredients were identified, i.e., tervoside J, L and K, paniculonin B and A, and (22R,23S,25S)-3β,6α,23-trihydroxy-5α-spirostane-6-O-β-dxylopyranosyl-(1 → 3)-O-β-D-quinovopyranoside. These compounds, as well as solanolide (an aglycone obtained by the acid hydrolysis of the methanolic extract), were also evaluated for anticonvulsant activity. Substantial and concentrations-dependent anticonvulsant action was reported for torvoside J, while torvoside L and K only induced a tenuous effect (Challal et al., 2014).
An important advantage of the study conducted by Challal et al. (2014) is the detailed analysis of the chemical composition of Solanum torvum extracts and the identification of biologically active compounds. However, the anticonvulsant activity of the studied extracts and compounds was identified only through evaluating zebrafish larvae behavior and was not verified by any deeper phenotyping.
2.10. Zingiber purpureum and Zingiber officinale
Different species of the Zingiberacae family have been used as herbal medicines since ancient times. Plants belonging to the genus Zingiber (ginger) are present mainly in India, Japan, China, South Korea, Indo-China, and Southeast Asia, and they are especially popular in these regions. Phenolic acids and terpene compounds are the main active ingredients of Zingiber spp. and are responsible for their pharmacological and biological properties. Ginger extracts have revealed antimicrobial, antioxidant, anti-inflammatory properties, they are also used to attenuate motion sickness, gastrointestinal and menstrual disorders, asthma and headache (Gupta and Sharma, 2014; Kukula-Koch et al., 2018; Khan et al., 2019; Shahrajabian et al., 2019; Kausar et al., 2021).
Extracts from two plants from the genus Zingiber, i.e., Zingiber purpureum Ridl. and Zingiber officinale, Rosc. were studied in the zebrafish larvae PTZ-induced seizure test (Brillatz et al., 2020; Gawel et al., 2021; Table 2). Of the four Zingiber purpureum extracts tested, i.e., hexane, ethyl acetate, ethanol, and aqueous, the hexane extract exerted the most potent anticonvulsant effect in PTZ-treated zebrafish larvae. Fifteen fractions obtained from this extract were evaluated in the zebrafish larvae PTZ assay and one, a mixture of trans-banglene and cis-banglene, showed an especially potent anticonvulsant effect, as it reduced PTZ-induced hyperlocomotion by as much as 68%. When tested separately, the strongest anticonvulsant effect was noted for cis-banglene. Interestingly, this compound revealed a 4–8 times more potent anticonvulsant effect than topiramate (a broad-spectrum ASM). The mixture of cis-bangelene and trans-banglene (50/50 ratio) decreased both the seizure score and mortality in the PTZ-induced seizure test in mice (Brillatz et al., 2020).
Studies of the anticonvulsant potential of plants belonging to the genus Zingiber have been recently expanded by our research group (Gawel et al., 2021). We noted that methanolic extract from the Zingiber officinale rhizome reduced PTZ-induced hyperlocomotion in the zebrafish larvae. Subsequently, 6-gingerol was identified as the active ingredient of the studied extract and was separately evaluated. We found that it reduced the seizure-like behavior and decreased the number and mean duration of epileptiform-like events in the LFP recordings. Biochemical and molecular studies that were conducted to find the possible mechanism of anticonvulsant action of 6-gingerol also revealed that it affected mainly glutamatergic neurotransmission. Primarily, it lowered the glutamate level in the PTZ-treated zebrafish larvae and thus restored the glutamate/GABA ratio and balance between the excitatory and inhibitory neurotransmission in the brain. Secondly, 6-gingerol decreased the expression of the grin2b gene that encodes the subunit of the N-methyl-D-aspartate (NMDA) receptor and thus additionally mitigated excitatory glutamatergic neurotransmission. Molecular docking analysis showed that the studied compound might also interact with the ATD- and glutamate-binding site, as well as within the ion channel in the NMDA receptor complex (Gawel et al., 2021).
The studies presented by Brillatz et al. (2020) and by our group (Gawel et al., 2021) provide evidence for the anticonvulsant properties of ginger extracts and their active ingredients. We also aimed to verify the possible mechanisms of 6-gingerol anticonvulsant activity.
3. Studies regarding anticonvulsant effects of plant-derived drugs in zebrafish vs. rodents—a comparison
In Table 3, we have selected and gathered data from Table 2 (zebrafish assays) and Supplementary Table 1 (rodent assays) to compare zebrafish vs. rodent studies. Most of the experiments on the anticonvulsant effect of plant metabolites were carried out in the PTZ model, both in zebrafish and rodents. Therefore, in this review, the zebrafish vs. rodent comparison refers primarily to PTZ-induced seizures. Generally, the results obtained from studies utilizing zebrafish and mouse models are qualitatively comparable at least in most of the cited reports. Consistent anticonvulsant effects were found for ar-turmerone, α,β-turmerone, CBD, curcumin, kaempferol, magnolol, naringenin 4′,7-dimethyl ether and pterostilbene (for details, see Table 3). However, it is worth emphasizing that only the CBD and curcumin results are based on studies conducted in different independent laboratories. In many others, these are often only single investigations, the results of which have not been validated by other authors, which makes it challenging, at least at this state of knowledge, to draw unequivocal conclusions and to make categorical recommendations. Incompatibility is noted for berberine, which is active in the PTZ-induced seizure model in zebrafish and ineffective in rodent PTZ assays. Inconsistencies were also noted for indirubin, linalool and naringenin, with only single reports available, which again makes it difficult to draw generalized conclusions. The reason for these differences is unknown and further research is needed.
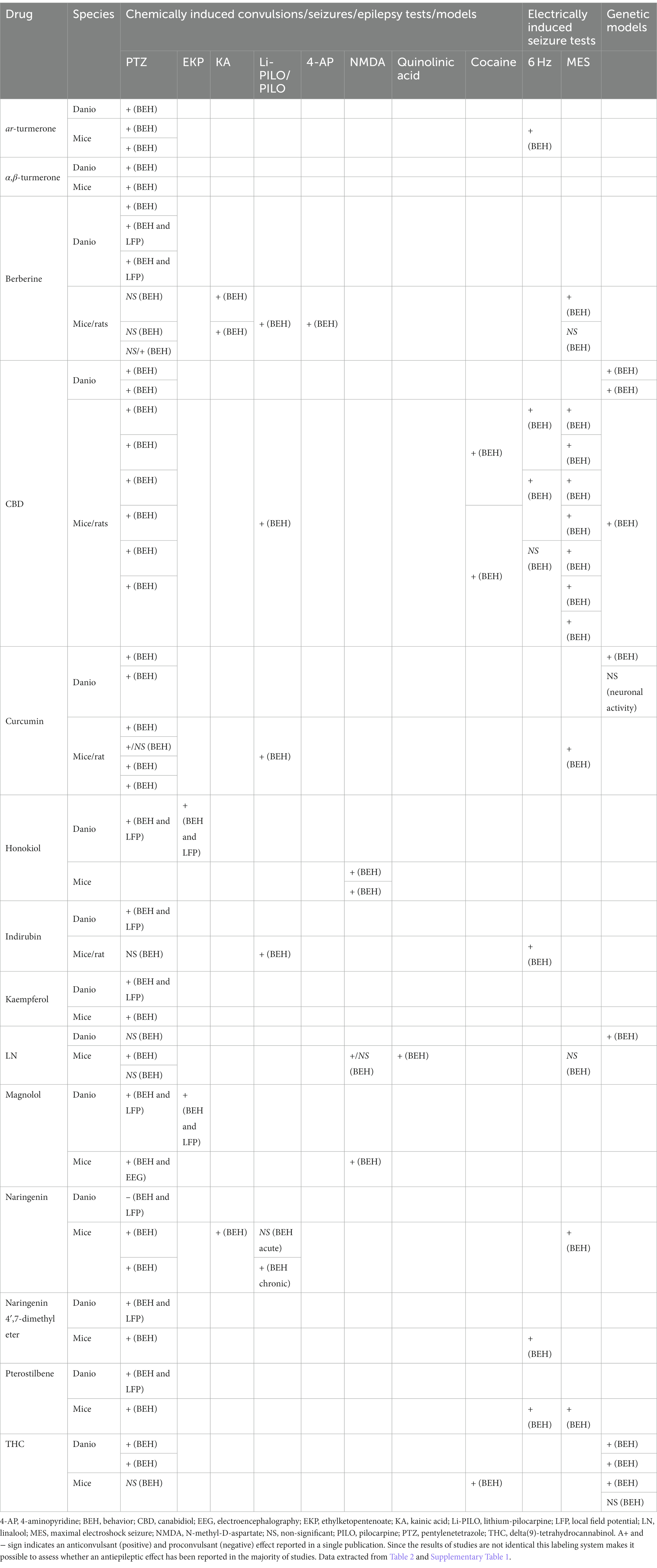
Table 3. Anticonvulsant effects of plant-derived drugs in different seizure or epilepsy models in zebrafish and rodents—a comparison.
It is interesting (and surprising) to compare the effectiveness of plant metabolites in the zebrafish PTZ-induced seizure model vs. electrically induced seizures (6 Hz and MES) in rodents because there is an almost perfect agreement of all analyzed plant substances. The only exception is naringenin, though it is the only single result (Table 3). It is worth noting that such a high compatibility applies to both anticonvulsant activity and the lack of anticonvulsant effect. If it is confirmed in a larger group of plant metabolites, it could be predicted that the PTZ-induced seizure model in zebrafish might replace the PTZ test and the electric convulsion tests performed on rodents as a screening platform.
A meaningful advantage of zebrafish lies in the ease, compared to rodents, of obtaining genetically altered strains. A comparison of the anticonvulsant effect of the analyzed plant metabolites in genetic models vs. PTZ and electric convulsions indicates a very high convergence of the results obtained in zebrafish and rodents, which additionally provides a positive validation of the genetic models in zebrafish for high-throughput screening purposes.
4. Methodology: challenges
For the past 15 years, a tremendous increase in the use of zebrafish in biomedical sciences has been observed. Epilepsy was one of the first central nervous system diseases that were studied using zebrafish as a model. Baraban et al. (2005) were first to describe the PTZ-induced seizure assay in zebrafish larvae, and Orellana-Paucar et al. (2012) were one of the first to employ this test for the screening of plant constituents. Since then, a number of new plant-derived anticonvulsant drug leads have been discovered (Bertoncello and Bonan, 2021). The PTZ-induced model of seizures described by Baraban et al. (2005) is still widely used as a first-choice screening platform. However, a discovery of Yaksi et al. (2021) shed light on problems in its application. In this paper, the authors pointed out that relying only on the locomotor assessment as a readout of anticonvulsant activity may, in a small number of cases, yield false-positive results. We, therefore, recommend evaluating hit molecules from the group of screened compounds by means of different complementing techniques—ideally, LFPs recorded from the larval optic tectum (Baraban et al., 2005; Afrikanova et al., 2013; Gawel et al., 2020b,c) or assessing neuronal activity using bioluminescence, for example, employing transgenic zebrafish expressing fluorescent photoprotein GFP-apoAequorin [Tg(elavl3:eGFP-apoAequorin); Zhang Y. et al., 2017]. Alternatively, transgenic lines encoding calcium indicators [e.g., Tg(elavl3:GCaMP6s)] may be used for this purpose (Choo et al., 2021).
Confocal imaging of GCaMP expressing zebrafish larvae with LFP recording has been applied to study epileptic-like events in the whole brain (Kettunen, 2020). Monitoring the neuronal network for dynamic activities provides an important understanding of seizure initiation and propagation. A recent study has revealed the rapid propagation of seizure activity from anterior-to-posterior brain regions using GCaMP imaging in the central nervous system of zebrafish, which was performed in zebrafish brains treated with PTZ, and which was also observed for its behavior activity and LFP recording (Liu and Baraban, 2019).
Calcium imaging has also become a very useful tool, similar to brain LFP recordings, to assess the level of neuronal firing in epileptic and normal zebrafish brains. It was used to record neuronal activity in gabrga1 and gabrg2 mutant zebrafish brains (Samarut et al., 2018; Liao et al., 2019). However, if none of the above-mentioned methods is feasible, at least the c-fos oncogene (a marker of neuronal activity) or epilepsy-specific marker (e.g., npas4 or sestrin) expression in zebrafish brain using RT-qPCR (Klarić et al., 2014; Johnson et al., 2015; Shan et al., 2018; Zhang et al., 2020; Gawel et al., 2020a) or whole-mount in situ hybridization (Zheng et al., 2018) should be performed. For adult zebrafish, RT-qPCR (Mazumder et al., 2019; Gawel et al., 2020c) or whole-mount in situ hybridization (Ariyasiri et al., 2021) of such genes can be undertaken.
One should remember that the PTZ-induced seizure assay in zebrafish is considered to be an equivalent of tonic–clonic-like seizures in humans. Thus, other pharmacological models should be applied for initial screening purposes. As mentioned, some have been occasionally used (e.g., pilocarpine or picrotoxin) in larval zebrafish (Gawel et al., 2020b), but they have not been employed for high-throughput screening purposes so far. Therefore, the validation of these chemical models is mandatory. At the same time, the EKP-induced seizure model, which is considered to be a model of pharmacoresistant epilepsy (Zhang Y. et al., 2017), has been recently successfully applied by Li et al. (2020) and pointed out magnolol and honokiol as compounds with anticonvulsant potency. Nevertheless, one should bear in mind that EKP is not commercially available. Despite its limited use so far, it has the potential to become a standard chemoconvulsant for the initial screening of extracts/compounds, comparable to the PTZ assay.
Another challenge that must be met is the need to implement zebrafish genetic models of epilepsy as routine, commonly available models to expand screening possibilities. Until now, many mutations in genes have been identified as causing epilepsy, but the genotype–phenotype correlation has not been well understood (Hortopan et al., 2010). Some research groups have developed zebrafish lines carrying mutations in epilepsy-causing genes, showing that mutant zebrafish undergo specific types of spontaneous seizures under stress conditions (Copmans et al., 2017). The GABA receptor loss of function α and γ subunit produce light reflex seizures in zebrafish larvae. As researchers were studying epilepsy behavior in mutant zebrafish, they surprisingly learned that the different mutations in the same gene produced different types of seizures under light (stress) condition (Samarut et al., 2018). This shows that not all mutations produce the same type of epilepsy, and each type of epilepsy requires an appropriate personalized ASMs treatment (Liao et al., 2019). Precision medicine is a therapeutic approach that is individually selected for each patient and that ideally targets molecular pathomechanisms of the disease (Balestrini et al., 2021). In our understanding, epilepsy offers an excellent, yet extraordinarily challenging, opportunity for treatment personalization, given the very large pool of genes which have been discovered in the past decade (Nabbout and Kuchenbuch, 2020). This modification, which is now possible in zebrafish, proves to be an easy high-throughput drug screen which can now be possible for each genetic phenotype (Cunliffe et al., 2015). For example, Thornton et al. (2020) used a zebrafish model of Dravet syndrome (scn1Lab−/−) whereas Choo et al. (2021) employed gabra1a and gabrg2 knockouts to point out some cannabis constituents or curcumin analogs with antiseizure activity, respectively. All the above-mentioned mutants are in fact very good for initial screening purposes because of their clear phenotype: all those mutants have disturbed basic or stimulated (light) locomotor activity, and compounds with anticonvulsant activity counteract the behavioral impairments.
Another important issue which should be considered is the use of appropriate solvents or carriers for plant extracts or isolated molecules. This requires special attention because most molecules do not dissolve in water. If the molecule of interest is not soluble in the water, in most cases scientists, use dimethyl sulfoxide (DMSO) as a polar solvent. It has been shown that up to 2–2.5% DMSO was safe for embryos or larval zebrafish of the AB strain (Maes et al., 2012), albeit its safety was based only on morphological descriptors and one zebrafish line. A concentration of 1% DMSO is usually used for screening (Copmans et al., 2018; Aourz et al., 2019; Brillatz et al., 2020), but for genetic lines, it may be decreased to 0.1%. As a rule of thumb, the lowest possible concentration should always be used. This is especially true taking into account the data showing that DMSO itself induces seizures in both rodents (Kovács et al., 2011) and humans (Bauwens et al., 2005; Maral et al., 2018). It cannot be excluded that higher concentrations of DMSO in larval zebrafish might affect LFP recordings, thus, it is highly recommended to use as low concentrations as possible to avoid this interference.
Alternatively, if the molecule of interest is not soluble in DMSO, other solvents, e.g., ethanol, might be used with caution. It was found, however, that ethanol in intermediate and high concentrations produced hyper- and hypoactivity of larvae (Lockwood et al., 2004; Guo et al., 2015), respectively, which can interfere with the final outcome derived from the locomotor activity assessment of PTZ-induced seizures—leading to false-positive results and incorrect conclusions. Another solvent, acetone, is also contraindicated since it has been found to exert anticonvulsant activity in rodents (Likhodii et al., 2003). However, this has not yet been analyzed in zebrafish. Acetonitrile has been shown to be very toxic for larvae, while polyethylene glycol (PEG-400), methanol and propylene glycol have been found to be quite well tolerated (Maes et al., 2012). Furthermore, methanol did not affect the locomotor activity of larvae up to 1.5% (Lockwood et al., 2004). Nevertheless, all the above-mentioned solvents, except for DMSO, have been used occasionally, so they should be approached with caution.
At this moment, there is less data regarding drug pharmacokinetics in zebrafish than in rodents. There are, however, some scarce pharmacokinetic data for the known ASMs in adult zebrafish (Pieróg et al., 2021a,b). Nevertheless, most screening experiments, for obvious practical and ethical reasons, have been conducted in larval zebrafish. In those studies, zebrafish larvae have been usually exposed to the tested compounds for 18 h (Challal et al., 2014; Aourz et al., 2019; Brillatz et al., 2020) or for longer periods (Gawel et al., 2020a, 2021; Thornton et al., 2020), or sometimes for 2–3 h (Moradi-Afrapoli et al., 2017; Nieoczym et al., 2019; Choo et al., 2021). Guarin et al. (2021) compared the pharmacokinetics of different fluorescent dyes in zebrafish embryos after immersion or intra-yolk microinjection. Here, they found out that the intrabody absorption of dyes depended on the lipophilicity of the molecule, i.e., the more lipophilic compound, the better absorption. For less lipophilic molecules, microinjections seem to give better results. Since the lipophilicity of the investigated compound may strongly affect the outcome due to low brain penetration, in the case of less lipophilic compounds, longer incubation time is required (at least 24 h or preferably 48 h). Therefore, it seems reasonable to prolong the incubation time in larval zebrafish.
Thus far, most of the published data have focused on searching for new drug leads of plant origin without any follow up. However, first attempts have appeared to give insight into the molecular mechanisms of action of those “hits.” In larval zebrafish, the most frequently used technique for the initial evaluation of the mechanisms of action is RT-qPCR (Orellana-Paucar et al., 2013; Gong et al., 2020; Zhang et al., 2020; Gawel et al., 2021). Among the investigated genes, protooncogene c-fos, a general marker of neuronal activity, is one of the most often studied. However, there are some discrepancies between laboratories in terms of the duration of PTZ exposure before its overexpression is observed. Some authors have observed changes after 30 min (Gong et al., 2020; Zhang et al., 2020), others after 45 min (Gawel et al., 2020a) or 90 min (Gawel et al., 2021) of PTZ exposure. Interestingly, changes observed after 3 min (Zhang et al., 2020) were also described. One should consider that c-fos is expressed earlier than other markers, thus longer incubation in PTZ seems to be better for other genes. It is known that protein analysis is superior to the analysis of gene expression, but some antibodies available in the market are not dedicated to zebrafish, which might be one of the reasons why RT-qPCR continues to be more often conducted (Orellana-Paucar et al., 2013; Gawel et al., 2020c, 2021; Gong et al., 2020; Zhang et al., 2020).
Recently, we have validated the method of neurotransmitter analysis (GABA, glutamate, dopamine, and serotonin), but one pooled sample used for determination purposes consisted of 100 larvae (Gawel et al., 2021). When we collected samples consisting of smaller numbers of larvae, the neurotransmitter levels were below the detection limit. Leclercq et al. (2015) analyzed the neurotransmitter levels in larval zebrafish with 6 heads collected per sample, but GABA and glutamate were the only parameters analyzed in this study. Recently, a few more groups have analyzed neurotransmitters and neurochemicals levels (Tufi et al., 2016; Heylen et al., 2021; Jeong et al., 2022), but these proved methodologically different from the above-mentioned papers. In summary, the neurotransmitter analysis method applied in larval zebrafish studies should be further validated.
Other methods that have been described in literature include the transient knockdown of gene encoding targets of the compound of interest, e.g., stx1b (Zheng et al., 2018) and gsk-3ß (Aourz et al., 2019). The recruitment of macrophages and neutrophils in the MPO:GFP line zebrafish (the green fluorescent protein expressed in neutrophils driven by the myeloperoxidase promoter) after exposure to PTZ was also described (Zhang et al., 2020), but there have been few reports on this topic so far.
In relation to all the above-mentioned limitations and challenges, Figure 2 presents a proposed pipeline for different laboratories specializing in studies devoted to screening plant-derived drugs with potential anticonvulsant properties using zebrafish, to make the obtained data both comparable and repeatable.
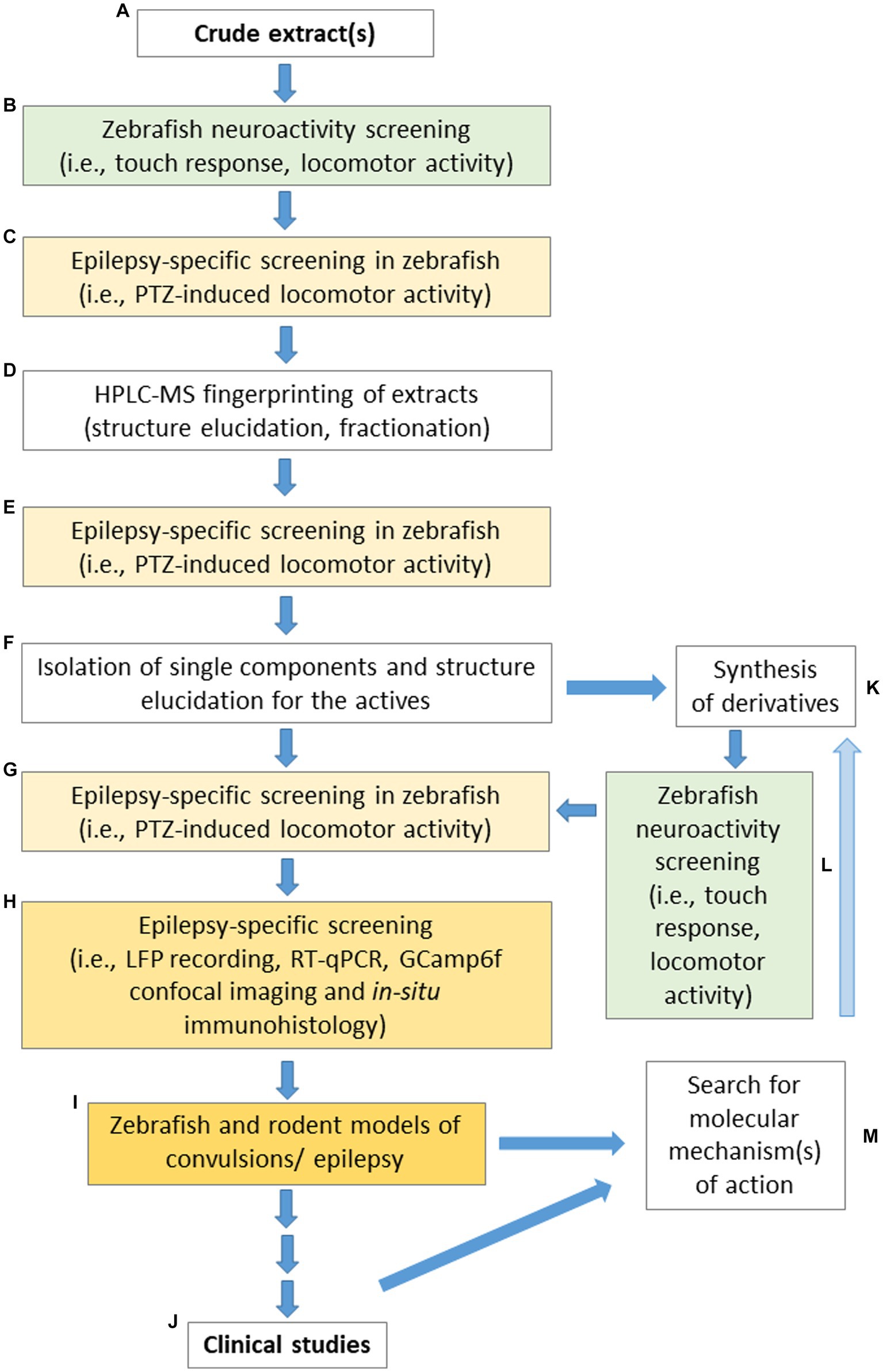
Figure 2. “From tank to bedside and back again in epilepsy”—the suggested pipeline for the search of active components extracted from plant material suitable for the application in anticonvulsant/antiepileptic therapies. The following steps are recommended: (A) optimized extraction protocol; (B) initial neuroactivity screening; (C) epilepsy-specific screening in the PTZ-induced seizure assay performed on zebrafish; (D) fingerprinting of extracts by HPLC-MS and the extract fractionation by modern chromatographic techniques (e.g., Flash/CPC/CPE/prep-HPLC/CE/MPLC/prep-TLC) to obtain active fractions or single isolates; (E) examination of the fractions and isolates in the PTZ-induced seizure assay; (F) bio guided fractionation to obtain single molecules or small groups of compounds; (G) further biological activity screening in the PTZ-induced seizure assay; (H) conduction of other experiments (e.g., LFP recordings or in situ hybridization) to confirm the promising behavioral observations; (I) testing of selected promising metabolites in a range of zebrafish and rodent models of convulsions to verify their activity; (J) referral of drug candidates for clinical trials; (K) synthesis of derivatives; (L) initial neuroactivity screening of newly synthesized derivatives; (M) use of data obtained from preclinical and clinical studies to search FIGURE 2 (Continued)for the molecular mechanism of action which will be utilized to synthesize new improved derivatives (K), screening these for their anticonvulsant activity (L,G–J,M). The steps are highlighted in colors: the green background indicates the first set of behavioral tests that assess the effect of the tested compounds on the neuroactive performance of zebrafish; the yellow background indicates a set of tests oriented to study anticonvulsant effects; more intense color indicates more sophisticated procedures. LFP, local field potential; PTZ, pentylenetetrazole; RT-qPCR, quantitative reverse transcription PCR.
5. Conclusion
This article contains a review of recent findings and challenges linked to searching for plant-derived drugs with anticonvulsant potency in zebrafish-based screening assays. The conducted analysis confirms the usefulness of Danio rerio models (both PTZ and genetic) in epilepsy research. The example of berberine shows that the zebrafish model revealed its anticonvulsant effect better than the PTZ convulsive model in rodents, in which it proved ineffective. In our opinion, zebrafish as a screening tool has a chance to bring a breakthrough in identifying new drug leads of plant origin, which might be used as new anticonvulsants. However, more work is advisable to implement new models of seizures/epilepsy, as well to validate the techniques/conditions used in different laboratories. The next step, therefore, should be to study the mechanisms of the anticonvulsant activity of the hit molecules, as otherwise those substances have a lower chance of entering clinical trials.
Author contributions
KG: conceptualization and supervision. BK, DN, UK, and KG: writing of original draft. DN, UK, WK-K, WAT, and KG: review and editing. DN, KK-T, and WAT: tables. DN, UK, and KG: figures. All authors contributed to the article and approved the submitted version.
Funding
This paper was partially supported by the DS 448 from Medical University of Lublin, Poland and by National Science Center, Poland within OPUS grant (project no 2021/41/B/NZ4/00337).
Conflict of interest
The authors declare that the research was conducted in the absence of any commercial or financial relationships that could be construed as a potential conflict of interest.
Publisher’s note
All claims expressed in this article are solely those of the authors and do not necessarily represent those of their affiliated organizations, or those of the publisher, the editors and the reviewers. Any product that may be evaluated in this article, or claim that may be made by its manufacturer, is not guaranteed or endorsed by the publisher.
Supplementary material
The Supplementary material for this article can be found online at: https://www.frontiersin.org/articles/10.3389/fnmol.2023.1221665/full#supplementary-material
References
Abdykerimova, S., Sakipova, Z., Nakonieczna, S., Koch, W., Biernasiuk, A., Grabarska, A., et al. (2020). Superior antioxidant capacity of Berberis iliensis—HPLC-Q-TOF-MS based phytochemical studies and spectrophotometric determinations. Antioxidants 9:504. doi: 10.3390/antiox9060504
Abou-Khalil, B. W. (2016). Antiepileptic Drugs. Continuum (Minneap Minn) 22, 132–156. doi: 10.1212/con.0000000000000289
Adachi, J., Mori, Y., Matsui, S., Takigami, H., Fujino, J., Kitagawa, H., et al. (2001). Indirubin and indigo are potent aryl hydrocarbon receptor ligands present in human urine. J. Biol. Chem. 276, 31475–31478. doi: 10.1074/jbc.C100238200
Afrikanova, T., Serruys, A. S., Buenafe, O. E., Clinckers, R., Smolders, I., De Witte, P. A., et al. (2013). Validation of the zebrafish pentylenetetrazol seizure model: locomotor versus electrographic responses to antiepileptic drugs. PLoS One 8:e54166. doi: 10.1371/journal.pone.0054166
Agarwal, N. B., Jain, S., Agarwal, N. K., Mediratta, P. K., and Sharma, K. K. (2011). Modulation of pentylenetetrazole-induced kindling and oxidative stress by curcumin in mice. Phytomedicine 18, 756–759. doi: 10.1016/j.phymed.2010.11.007
Aghdash, N. S. (2021). Herbal medicine in the treatment of epilepsy. Curr. Drug Targets 22, 356–367. doi: 10.2174/1389450121999201001152221
Ahmad, M. (2013). Protective effects of curcumin against lithium-pilocarpine induced status epilepticus, cognitive dysfunction and oxidative stress in young rats. Saudi J. Biol. Sci. 20, 155–162. doi: 10.1016/j.sjbs.2013.01.002
Akula, K. K., and Kulkarni, S. K. (2014). Effect of curcumin against pentylenetetrazol-induced seizure threshold in mice: possible involvement of adenosine A1 receptors. Phytother. Res. 28, 714–721. doi: 10.1002/ptr.5048
Al Rahim, M., Rimando, A. M., Silistreli, K., and El-Alfy, A. T. (2013). Anxiolytic action of pterostilbene: involvement of hippocampal ERK phosphorylation. Planta Med. 79, 723–730. doi: 10.1055/s-0032-1328553
Alexeev, M., Grosenbaugh, D. K., Mott, D. D., and Fisher, J. L. (2012). The natural products magnolol and honokiol are positive allosteric modulators of both synaptic and extra-synaptic GABA(A) receptors. Neuropharmacology 62, 2507–2514. doi: 10.1016/j.neuropharm.2012.03.002
Al-Hamaly, M. A., Turner, L. T., Rivera-Martinez, A., Rodriguez, A., and Blackburn, J. S. (2023). Zebrafish Cancer avatars: A translational platform for analyzing tumor heterogeneity and predicting patient outcomes. Int. J. Mol. Sci. 24:2288. doi: 10.3390/ijms24032288
Amponsah, D. N. H. (2014). Determination of the presence of flavonoids in the leaves, seeds and branches of the matured plant, Indigofera Arrecta. Int. J. Sci. Technol. Res. 3
Anderson, L. L., Low, I. K., Mcgregor, I. S., and Arnold, J. C. (2020). Interactions between cannabidiol and Δ(9) -tetrahydrocannabinol in modulating seizure susceptibility and survival in a mouse model of Dravet syndrome. Br. J. Pharmacol. 177, 4261–4274. doi: 10.1111/bph.15181
Aourz, N., Serruys, A.-S. K., Chabwine, J. N., Balegamire, P. B., Afrikanova, T., Edrada-Ebel, R., et al. (2019). Identification of GSK-3 as a potential therapeutic entry point for epilepsy. ACS Chem. Neurosci. 10, 1992–2003. doi: 10.1021/acschemneuro.8b00281
Arbabi Jahan, A., Rad, A., Ghanbarabadi, M., Amin, B., and Mohammad-Zadeh, M. (2018). The role of serotonin and its receptors on the anticonvulsant effect of curcumin in pentylenetetrazol-induced seizures. Life Sci. 211, 252–260. doi: 10.1016/j.lfs.2018.09.007
Ariyasiri, K., Choi, T.-I., Gerlai, R., and Kim, C.-H. (2021). Acute ethanol induces behavioral changes and alters c-fos expression in specific brain regions, including the mammillary body, in zebrafish. Prog. Neuro-Psychopharmacol. Biol. Psychiatry 109:110264. doi: 10.1016/j.pnpbp.2021.110264
Asad, A., Shahidan, N. O., De La Vega De León, A., Wiggin, G. R., Whitfield, T. T., and Baxendale, S. (2023). A screen of pharmacologically active compounds to identify modulators of the Adgrg6/Gpr126 signalling pathway in zebrafish embryos. Basic Clin. Pharmacol. Toxicol. doi: 10.1111/bcpt.13923
Balachandran, C., Emi, N., Arun, Y., Yamamoto, Y., Ahilan, B., Sangeetha, B., et al. (2015). In vitro anticancer activity of methyl caffeate isolated from Solanum torvum Swartz. Fruit. Chem. Biol. Interact. 242, 81–90. doi: 10.1016/j.cbi.2015.09.023
Balestrini, S., Chiarello, D., Gogou, M., Silvennoinen, K., Puvirajasinghe, C., Jones, W. D., et al. (2021). Real-life survey of pitfalls and successes of precision medicine in genetic epilepsies. J. Neurol. Neurosurg. Psychiatry 92, 1044–1052. doi: 10.1136/jnnp-2020-325932
Baraban, S. C., Dinday, M. T., and Hortopan, G. A. (2013). Drug screening in Scn1a zebrafish mutant identifies clemizole as a potential Dravet syndrome treatment. Nat. Commun. 4:2410. doi: 10.1038/ncomms3410
Baraban, S. C., Taylor, M. R., Castro, P. A., and Baier, H. (2005). Pentylenetetrazole induced changes in zebrafish behavior, neural activity and c-fos expression. Neuroscience 131, 759–768. doi: 10.1016/j.neuroscience.2004.11.031
Bauwens, D., Hantson, P., Laterre, P. F., Michaux, L., Latinne, D., De Tourtchaninoff, M., et al. (2005). Recurrent seizure and sustained encephalopathy associated with dimethylsulfoxide-preserved stem cell infusion. Leuk. Lymphoma 46, 1671–1674. doi: 10.1080/10428190500235611
Baxendale, S., Holdsworth, C. J., Meza Santoscoy, P. L., Harrison, M. R., Fox, J., Parkin, C. A., et al. (2012). Identification of compounds with anti-convulsant properties in a zebrafish model of epileptic seizures. Dis. Model. Mech. 5, 773–784. doi: 10.1242/dmm.010090
Beghi, E. (2020). The epidemiology of epilepsy. Neuroepidemiology 54, 185–191. doi: 10.1159/000503831
Bertoncello, K. T., Aguiar, G. P. S., Oliveira, J. V., and Siebel, A. M. (2018). Micronization potentiates curcumin’s anti-seizure effect and brings an important advance in epilepsy treatment. Sci. Rep. 8:2645. doi: 10.1038/s41598-018-20897-x
Bertoncello, K. T., and Bonan, C. D. (2021). Zebrafish as a tool for the discovery of anticonvulsant compounds from botanical constituents. Eur. J. Pharmacol. 908:174342. doi: 10.1016/j.ejphar.2021.174342
Bhutada, P., Mundhada, Y., Bansod, K., Dixit, P., Umathe, S., and Mundhada, D. (2010). Anticonvulsant activity of berberine, an isoquinoline alkaloid in mice. Epilepsy Behav. 18, 207–210. doi: 10.1016/j.yebeh.2010.03.007
Birhan, Y. S. (2022). Medicinal plants utilized in the management of epilepsy in Ethiopia: ethnobotany, pharmacology and phytochemistry. Chin. Med. 17:129. doi: 10.1186/s13020-022-00686-5
Brillatz, T., Kubo, M., Takahashi, S., Jozukuri, N., Takechi, K., Queiroz, E. F., et al. (2020). Metabolite profiling of Javanese ginger Zingiber purpureum and identification of Antiseizure metabolites via a Low-cost open-source zebrafish bioassay-guided isolation. J. Agric. Food Chem. 68, 7904–7915. doi: 10.1021/acs.jafc.0c02641
Buenafe, O. E., Orellana-Paucar, A., Maes, J., Huang, H., Ying, X., De Borggraeve, W., et al. (2013). Tanshinone IIA exhibits anticonvulsant activity in zebrafish and mouse seizure models. ACS Chem. Neurosci. 4, 1479–1487. doi: 10.1021/cn400140e
Calderón-Montaño, J. M., Burgos-Morón, E., Pérez-Guerrero, C., and López-Lázaro, M. (2011). A review on the dietary flavonoid kaempferol. Mini-Rev. Med. Chem. 11, 298–344. doi: 10.2174/138955711795305335
Canavese, M., Santo, L., and Raje, N. (2012). Cyclin dependent kinases in cancer. Cancer Biol. Ther. 13, 451–457. doi: 10.4161/cbt.19589
Challal, S., Buenafe, O. E., Queiroz, E. F., Maljevic, S., Marcourt, L., Bock, M., et al. (2014). Zebrafish bioassay-guided microfractionation identifies anticonvulsant steroid glycosides from the Philippine medicinal plant Solanum torvum. ACS Chem. Neurosci. 5, 993–1004. doi: 10.1021/cn5001342
Chang, C., Li, H., and Zhang, R. (2023). Zebrafish facilitate non-alcoholic fatty liver disease research: tools, models and applications. Liver Int. 43, 1385–1398. doi: 10.1111/liv.15601
Chang, J., Rimando, A., Pallas, M., Camins, A., Porquet, D., Reeves, J., et al. (2012). Low-dose pterostilbene, but not resveratrol, is a potent neuromodulator in aging and Alzheimer's disease. Neurobiol. Aging 33, 2062–2071. doi: 10.1016/j.neurobiolaging.2011.08.015
Chen, C. R., Tan, R., Qu, W. M., Wu, Z., Wang, Y., Urade, Y., et al. (2011). Magnolol, a major bioactive constituent of the bark of Magnolia officinalis, exerts antiepileptic effects via the GABA/benzodiazepine receptor complex in mice. Br. J. Pharmacol. 164, 1534–1546. doi: 10.1111/j.1476-5381.2011.01456.x
Chen, C. R., Zhou, X. Z., Luo, Y. J., Huang, Z. L., Urade, Y., and Qu, W. M. (2012). Magnolol, a major bioactive constituent of the bark of Magnolia officinalis, induces sleep via the benzodiazepine site of GABA(A) receptor in mice. Neuropharmacology 63, 1191–1199. doi: 10.1016/j.neuropharm.2012.06.031
Chesher, G. B., and Jackson, D. M. (1974). Anticonvulsant effects of cannabinoids in mice: drug interactions within cannabinoids and cannabinoid interactions with phenytoin. Psychopharmacologia 37, 255–264. doi: 10.1007/BF00421539
Choo, B. K. M., Kundap, U. P., Faudzi, S. M. M., Abas, F., Shaikh, M. F., and Samarut, É. (2021). Identification of curcumin analogues with anti-seizure potential in vivo using chemical and genetic zebrafish larva seizure models. Biomed. Pharmacother. 142:112035. doi: 10.1016/j.biopha.2021.112035
Copmans, D., Orellana-Paucar, A. M., Steurs, G., Zhang, Y., Ny, A., Foubert, K., et al. (2018). Methylated flavonoids as anti-seizure agents: Naringenin 4′,7-dimethyl ether attenuates epileptic seizures in zebrafish and mouse models. Neurochem. Int. 112, 124–133. doi: 10.1016/j.neuint.2017.11.011
Copmans, D., Siekierska, A., and De Witte, P. A. (2017). “Zebrafish Models of Epilepsy and Epileptic Seizures,” in Models of Seizures and Epilepsy, ed. A. Pitkanen, P. S. Buckmaster, A. S. Galanopoulou, S. L. Moshe, (Academic Press), 369–384.
Costa, A. M., Senn, L., Anceschi, L., Brighenti, V., Pellati, F., and Biagini, G. (2021). Antiseizure effects of fully characterized non-psychoactive Cannabis sativa L. extracts in the repeated 6-Hz corneal stimulation test. Pharmaceuticals (Basel) 14. doi: 10.3390/ph14121259
Crouzier, L., Richard, E. M., Sourbron, J., Lagae, L., Maurice, T., and Delprat, B. (2021). Use of zebrafish models to boost research in rare genetic diseases. Int. J. Mol. Sci. 22. doi: 10.3390/ijms222413356
Cunliffe, V. T., Baines, R. A., Giachello, C. N., Lin, W.-H., Morgan, A., Reuber, M., et al. (2015). Epilepsy research methods update: understanding the causes of epileptic seizures and identifying new treatments using non-mammalian model organisms. Seizure 24, 44–51. doi: 10.1016/j.seizure.2014.09.018
D'amora, M., Galgani, A., Marchese, M., Tantussi, F., Faraguna, U., De Angelis, F., et al. (2023). Zebrafish as an innovative tool for epilepsy modeling: state of the art and potential future directions. Int. J. Mol. Sci. 24:7702. doi: 10.3390/ijms24097702
Devinsky, O., Cross, J. H., and Wright, S. (2017). Trial of Cannabidiol for drug-resistant seizures in the Dravet syndrome. N. Engl. J. Med. 377, 699–700. doi: 10.1056/NEJMc1708349
Devinsky, O., Patel, A. D., Cross, J. H., Villanueva, V., Wirrell, E. C., Privitera, M., et al. (2018). Effect of Cannabidiol on drop seizures in the Lennox-Gastaut syndrome. N. Engl. J. Med. 378, 1888–1897. doi: 10.1056/NEJMoa1714631
Dhir, A. (2018). Curcumin in epilepsy disorders. Phytother. Res. 32, 1865–1875. doi: 10.1002/ptr.6125
Dohare, P., Varma, S., and Ray, M. (2008). Curcuma oil modulates the nitric oxide system response to cerebral ischemia/reperfusion injury. Nitric Oxide 19, 1–11. doi: 10.1016/j.niox.2008.04.020
Duncan, J. S., Sander, J. W., Sisodiya, S. M., and Walker, M. C. (2006). Adult epilepsy. Lancet 367, 1087–1100. doi: 10.1016/S0140-6736(06)68477-8
Elisabetsky, E., and Brum, L. (2003). Linalool as active component of traditional remedies: anticonvulsant properties and mechanisms of action. Curare 26, 45–52. doi: 10.17660/ActaHortic.1999.501.36
Elisabetsky, E., Brum, L. F., and Souza, D. O. (1999). Anticonvulsant properties of linalool in glutamate-related seizure models. Phytomedicine 6, 107–113. doi: 10.1016/s0944-7113(99)80044-0
Elsohly, M. A., Radwan, M. M., Gul, W., Chandra, S., and Galal, A. (2017). Phytochemistry of Cannabis sativa L. Prog. Chem. Org. Nat. Prod. 103, 1–36. doi: 10.1007/978-3-319-45541-9_1
Faheem, M., Ameer, S., Khan, A. W., Haseeb, M., Raza, Q., Ali Shah, F., et al. (2022). A comprehensive review on antiepileptic properties of medicinal plants. Arab. J. Chem. 15:103478. doi: 10.1016/j.arabjc.2021.103478
Filli, M., Ibrahim, A., Kesse, S., Aquib, M., Boakye-Yiadom, K., Farooq, M., et al. (2020). Synthetic berberine derivatives as potential new drugs. Br. J. Pharm. Sci. 58:e18835. doi: 10.1590/s2175-97902020000318835
Fisher, R. S., Acevedo, C., Arzimanoglou, A., Bogacz, A., Cross, J. H., Elger, C. E., et al. (2014). ILAE official report: A practical clinical definition of epilepsy. Epilepsia 55, 475–482. doi: 10.1111/epi.12550
Fleming, A., Diekmann, H., and Goldsmith, P. (2013). Functional characterisation of the maturation of the blood-brain barrier in larval zebrafish. PLoS One 8:e77548. doi: 10.1371/journal.pone.0077548
Fontana, B. D., Mezzomo, N. J., Kalueff, A. V., and Rosemberg, D. B. (2018). The developing utility of zebrafish models of neurological and neuropsychiatric disorders: a critical review. Exp. Neurol. 299, 157–171. doi: 10.1016/j.expneurol.2017.10.004
French, J. A., and Perucca, E. (2020). Time to start calling things by their own names? The case for Antiseizure medicines. Epilepsy Curr. 20, 69–72. doi: 10.1177/1535759720905516
Gao, F., Gao, Y., Liu, Y. F., Wang, L., and Li, Y. J. (2014). Berberine exerts an anticonvulsant effect and ameliorates memory impairment and oxidative stress in a pilocarpine-induced epilepsy model in the rat. Neuropsychiatr. Dis. Treat. 10, 2139–2145. doi: 10.2147/ndt.S73210
García-Peñas, J. J., Gil Nagel-Rein, A., Sánchez-Carpintero, R., and Villanueva-Haba, V. (2021). Cannabidiol for the treatment of Lennox-Gastaut syndrome and Dravet syndrome: experts' recommendations for its use in clinical practice in Spain. Rev. Neurol. 73, S1–S8. doi: 10.33588/rn.73S01.2021250
Gaston, T. E., and Friedman, D. (2017). Pharmacology of cannabinoids in the treatment of epilepsy. Epilepsy Behav. 70, 313–318. doi: 10.1016/j.yebeh.2016.11.016
Gawel, K., Kukula-Koch, W., Banono, N. S., Nieoczym, D., Targowska-Duda, K. M., Czernicka, L., et al. (2021). 6-Gingerol, a major constituent of Zingiber officinale Rhizoma, exerts anticonvulsant activity in the Pentylenetetrazole-induced seizure model in larval zebrafish. Int. J. Mol. Sci. 22. doi: 10.3390/ijms22147745
Gawel, K., Kukula-Koch, W., Nieoczym, D., Stepnik, K., Van Der Ent, W., Banono, N. S., et al. (2020a). The influence of Palmatine isolated from Berberis sibirica Radix on Pentylenetetrazole-induced seizures in zebrafish. Cells :9. doi: 10.3390/cells9051233
Gawel, K., Langlois, M., Martins, T., Van Der Ent, W., Tiraboschi, E., Jacmin, M., et al. (2020b). Seizing the moment: zebrafish epilepsy models. Neurosci. Biobehav. Rev. 116, 1–20. doi: 10.1016/j.neubiorev.2020.06.010
Gawel, K., Turski, W. A., Van Der Ent, W., Mathai, B. J., Kirstein-Smardzewska, K. J., Simonsen, A., et al. (2020c). Phenotypic characterization of larval zebrafish (Danio rerio) with partial knockdown of the cacna1a gene. Mol. Neurobiol. 57, 1904–1916. doi: 10.1007/s12035-019-01860-x
Gerometta, E., Grondin, I., Smadja, J., Frederich, M., and Gauvin-Bialecki, A. (2020). A review of traditional uses, phytochemistry and pharmacology of the genus Indigofera. J. Ethnopharmacol. 253:112608. doi: 10.1016/j.jep.2020.112608
Gobira, P. H., Vilela, L. R., Gonçalves, B. D. C., Santos, R. P. M., De Oliveira, A. C., Vieira, L. B., et al. (2015). Cannabidiol, a Cannabis sativa constituent, inhibits cocaine-induced seizures in mice: possible role of the mTOR pathway and reduction in glutamate release. Neurotoxicology 50, 116–121. doi: 10.1016/j.neuro.2015.08.007
Gong, G., Chen, H., Kam, H., Chan, G., Tang, Y. X., Wu, M., et al. (2020). In vivo screening of Xanthones from Garcinia oligantha identified Oliganthin H as a novel natural inhibitor of convulsions. J. Nat. Prod. 83, 3706–3716. doi: 10.1021/acs.jnatprod.0c00963
Gonzaga-Jauregui, C., Lupski, J. R., and Gibbs, R. A. (2012). Human genome sequencing in health and disease. Annu. Rev. Med. 63:35. doi: 10.1146/annurev-med-051010-162644
Gray, R. A., and Whalley, B. J. (2020). The proposed mechanisms of action of CBD in epilepsy. Epileptic Disord. 22, 10–15. doi: 10.1684/epd.2020.1135
Grone, B. P., and Baraban, S. C. (2015). Animal models in epilepsy research: legacies and new directions. Nat. Neurosci. 18, 339–343. doi: 10.1038/nn.3934
Guarin, M., Ny, A., De Croze, N., Maes, J., Léonard, M., Annaert, P., et al. (2021). Pharmacokinetics in zebrafish embryos (ZFE) following immersion and Intrayolk administration: a fluorescence-based analysis. Pharmaceuticals (Basel) 14. doi: 10.3390/ph14060576
Guerreiro, C. A. (2016). Epilepsy: is there hope? Indian J. Med. Res. 144, 657–660. doi: 10.4103/ijmr.IJMR_1051_16
Guo, N., Lin, J., Peng, X., Chen, H., Zhang, Y., Liu, X., et al. (2015). Influences of acute ethanol exposure on locomotor activities of zebrafish larvae under different illumination. Alcohol 49, 727–737. doi: 10.1016/j.alcohol.2015.08.003
Guo, S., Wagle, M., and Mathur, P. (2012). Toward molecular genetic dissection of neural circuits for emotional and motivational behaviors. Dev. Neurobiol. 72, 358–365. doi: 10.1002/dneu.20927
Gupta, S. K., and Sharma, A. (2014). Medicinal properties of Zingiber officinale roscoe—A review. IOSR J. Pharm. Biol. Sci. 9, 124–129.
Hakami, T. (2021). Efficacy and tolerability of antiseizure drugs. Ther. Adv. Neurol. Disord. 14:17562864211037430. doi: 10.1177/17562864211037430
Han, J.-Y., Ahn, S.-Y., Yoo, J. H., Nam, S.-Y., Hong, J. T., and Oh, K.-W. (2015). Alleviation of Kainic acid-induced brain barrier dysfunction by 4-O-Methylhonokiol in in vitro and in vivo models. Biomed. Res. Int. 2015:893163. doi: 10.1155/2015/893163
Heylen, L., Pham, D. H., De Meulemeester, A. S., Samarut, É., Skiba, A., Copmans, D., et al. (2021). Pericardial injection of Kainic acid induces a chronic epileptic state in larval zebrafish. Front. Mol. Neurosci. 14:753936. doi: 10.3389/fnmol.2021.753936
Hortopan, G. A., Dinday, M. T., and Baraban, S. C. (2010). Zebrafish as a model for studying genetic aspects of epilepsy. Dis. Model. Mech. 3, 144–148. doi: 10.1242/dmm.002139
Hosseinzadeh, H., Ramezani, M., Shafaei, H., and Taghiabadi, E. (2013). Anticonvulsant effect of Berberis integerrima L. Root Extracts in Mice. J. Acupunct. Merid. Stud. 6, 12–17. doi: 10.1016/j.jams.2012.07.018
Howe, K., Clark, M. D., Torroja, C. F., Torrance, J., Berthelot, C., Muffato, M., et al. (2013). The zebrafish reference genome sequence and its relationship to the human genome. Nature 496, 498–503. doi: 10.1038/nature12111
Jeong, S., Jang, S., Kim, S. S., Bae, M. A., Shin, J., Lee, K. B., et al. (2022). Size-dependent seizurogenic effect of polystyrene microplastics in zebrafish embryos. J. Hazard. Mater. 439:129616. doi: 10.1016/j.jhazmat.2022.129616
Jeong, J. Y., Kwon, H. B., Ahn, J. C., Kang, D., Kwon, S. H., Park, J. A., et al. (2008). Functional and developmental analysis of the blood-brain barrier in zebrafish. Brain Res. Bull. 75, 619–628. doi: 10.1016/j.brainresbull.2007.10.043
Johnson, M. R., Behmoaras, J., Bottolo, L., Krishnan, M. L., Pernhorst, K., Santoscoy, P. L. M., et al. (2015). Systems genetics identifies Sestrin 3 as a regulator of a proconvulsant gene network in human epileptic hippocampus. Nat. Commun. 6:6031. doi: 10.1038/ncomms7031
Jurisch-Yaksi, N., Yaksi, E., and Kizil, C. (2020). Radial glia in the zebrafish brain: functional, structural, and physiological comparison with the mammalian glia. Glia 68, 2451–2470. doi: 10.1002/glia.23849
Kaculini, C. M., Tate-Looney, A. J., and Seifi, A. (2021). The history of epilepsy: from ancient mystery to modern misconception. Cureus 13:e13953. doi: 10.7759/cureus.13953
Kalueff, A. V., Stewart, A. M., and Gerlai, R. (2014). Zebrafish as an emerging model for studying complex brain disorders. Trends Pharmacol. Sci. 35, 63–75. doi: 10.1016/j.tips.2013.12.002
Kaplan, J. S., Stella, N., Catterall, W. A., and Westenbroek, R. E. (2017). Cannabidiol attenuates seizures and social deficits in a mouse model of Dravet syndrome. Proc. Natl. Acad. Sci. U. S. A. 114, 11229–11234. doi: 10.1073/pnas.1711351114
Kausar, T., Anwar, S., Hanan, E., Yaseen, M., Aboelnaga, S., and Azad, Z. R. A. A. (2021). Therapeutic role of ginger (Zingiber officinale)—A review. J. Pharm. Res. Int., 9–16. doi: 10.9734/jpri/2021/v33i29B31584
Kearney, H., Byrne, S., Cavalleri, G. L., and Delanty, N. (2019). Tackling epilepsy with high-definition precision medicine: a review. JAMA Neurol. 76, 1109–1116. doi: 10.1001/jamaneurol.2019.2384
Kettunen, P. (2020). Calcium imaging in the zebrafish. Calc. Signal., 901–942. doi: 10.1007/978-3-030-12457-1_36
Khan, M., Ullah, S., Azhar, M., Komal, W., Muhammad, W., and Karbaschi, P. (2019). A Mini-review on the therapeutic potential of Zingiber officinale (ginger). J. Nat. Prod. 15, 1–6. doi: 10.37532/0974-7508.2019.15(1).125
Khosravi Dehaghi, N., Zeraati, M., Kamali, J., Ghorbani Nohooji, M., and Rahimzadeh, M. (2017). Effects of Berberis vulgaris fractions on PTZ induced seizure in male rats. Res. J. Pharmacog. 4:101.
Klarić, T., Lardelli, M., Key, B., Koblar, S., and Lewis, M. (2014). Activity-dependent expression of neuronal PAS domain-containing protein 4 (npas4a) in the developing zebrafish brain. Front. Neuroanat. 8:148. doi: 10.3389/fnana.2014.00148
Klein, B. D., Jacobson, C. A., Metcalf, C. S., Smith, M. D., Wilcox, K. S., Hampson, A. J., et al. (2017). Evaluation of Cannabidiol in animal seizure models by the epilepsy therapy screening program (ETSP). Neurochem. Res. 42, 1939–1948. doi: 10.1007/s11064-017-2287-8
Kocaadam, B., and Şanlier, N. (2017). Curcumin, an active component of turmeric (Curcuma longa), and its effects on health. Crit. Rev. Food Sci. Nutr. 57, 2889–2895. doi: 10.1080/10408398.2015.1077195
Kovács, Z., Czurkó, A., Kékesi, K. A., and Juhász, G. (2011). The effect of intraperitoneally administered dimethyl sulfoxide on absence-like epileptic activity of freely moving WAG/Rij rats. J. Neurosci. Methods 197, 133–136. doi: 10.1016/j.jneumeth.2011.02.005
Kukula-Koch, W., Koch, W., Czernicka, L., Głowniak, K., Asakawa, Y., Umeyama, A., et al. (2018). MAO-A inhibitory potential of terpene constituents from ginger rhizomes-A bioactivity guided fractionation. Molecules 23:1301. doi: 10.3390/molecules23061301
Kundap, U. P., Kumari, Y., Othman, I., and Shaikh, M. F. (2017). Zebrafish as a model for epilepsy-induced cognitive dysfunction: A pharmacological, biochemical and behavioral approach. Front. Pharmacol. 8:515. doi: 10.3389/fphar.2017.00515
Kwon, J. Y., Jeon, M.-T., Jung, U. J., Kim, D. W., Moon, G. J., and Kim, S. R. (2019). Perspective: therapeutic potential of flavonoids as alternative medicines in epilepsy. Adv. Nutr. 10, 778–790. doi: 10.1093/advances/nmz047
Lam, C. S., Rastegar, S., and Strähle, U. (2006). Distribution of cannabinoid receptor 1 in the CNS of zebrafish. Neuroscience 138, 83–95. doi: 10.1016/j.neuroscience.2005.10.069
Leclercq, K., Afrikanova, T., Langlois, M., De Prins, A., Buenafe, O. E., Rospo, C. C., et al. (2015). Cross-species pharmacological characterization of the allylglycine seizure model in mice and larval zebrafish. Epilepsy Behav. 45, 53–63. doi: 10.1016/j.yebeh.2015.03.019
Lee, Y. J., Lee, Y. M., Lee, C. K., Jung, J. K., Han, S. B., and Hong, J. T. (2011). Therapeutic applications of compounds in the Magnolia family. Pharmacol. Ther. 130, 157–176. doi: 10.1016/j.pharmthera.2011.01.010
Li, J., Copmans, D., Partoens, M., Hunyadi, B., Luyten, W., and De Witte, P. (2020). Zebrafish-based screening of Antiseizure plants used in traditional Chinese medicine: Magnolia officinalis extract and its constituents Magnolol and Honokiol exhibit potent anticonvulsant activity in a therapy-resistant epilepsy model. ACS Chem. Neurosci. 11, 730–742. doi: 10.1021/acschemneuro.9b00610
Liao, M., Kundap, U., Rosch, R. E., Burrows, D. R. W., Meyer, M. P., Ouled Amar Bencheikh, B., et al. (2019). Targeted knockout of GABA-A receptor gamma 2 subunit provokes transient light-induced reflex seizures in zebrafish larvae. Dis. Model. Mech. 12:dmm040782. doi: 10.1242/dmm.040782
Likhodii, S. S., Serbanescu, I., Cortez, M. A., Murphy, P., Snead, O. C. 3rd, and Burnham, W. M. (2003). Anticonvulsant properties of acetone, a brain ketone elevated by the ketogenic diet. Ann. Neurol. 54, 219–226. doi: 10.1002/ana.10634
Lin, Y., Li, Y., Zeng, Y., Tian, B., Qu, X., Yuan, Q., et al. (2021). Pharmacology, toxicity, bioavailability, and formulation of Magnolol: an update. Front. Pharmacol. 12:632767. doi: 10.3389/fphar.2021.632767
Lin, F., Luo, B.-S., Cheng, Z., Li, P., and Long, C. (2021). Ethnobotanical study on Garcinia (Clusiaceae) in China. Acta Soc. Bot. Pol. 90, 1–18. doi: 10.5586/asbp.9012
Liu, Y. (2023). Zebrafish as a model organism for studying pathologic mechanisms of neurodegenerative diseases and other neural disorders. Cell. Mol. Neurobiol. doi: 10.1007/s10571-023-01340-w
Liu, J., and Baraban, S. C. (2019). Network properties revealed during multi-scale calcium imaging of seizure activity in zebrafish. Eneuro 6:ENEU19.2019. doi: 10.1523/ENEURO.0041-19.2019
Liu, W., Ge, T., Pan, Z., Leng, Y., Lv, J., and Li, B. (2017). The effects of herbal medicine on epilepsy. Oncotarget 8, 48385–48397. doi: 10.18632/oncotarget.16801
Lockwood, B., Bjerke, S., Kobayashi, K., and Guo, S. (2004). Acute effects of alcohol on larval zebrafish: a genetic system for large-scale screening. Pharmacol. Biochem. Behav. 77, 647–654. doi: 10.1016/j.pbb.2004.01.003
Lopresti, A. L. (2018). The problem of curcumin and its bioavailability: could its gastrointestinal influence contribute to its overall health-enhancing effects? Adv. Nutr. 9, 41–50. doi: 10.1093/advances/nmx011
Łukasiuk, K., and Lasoń, W. (2023). Emerging molecular targets for anti-epileptogenic and epilepsy modifying drugs. Int. J. Mol. Sci. 24. doi: 10.3390/ijms24032928
Maes, J., Verlooy, L., Buenafe, O. E., De Witte, P. A., Esguerra, C. V., and Crawford, A. D. (2012). Evaluation of 14 organic solvents and carriers for screening applications in zebrafish embryos and larvae. PLoS One 7:e43850. doi: 10.1371/journal.pone.0043850
Maral, S., Albayrak, M., Pala, C., Yildiz, A., Sahin, O., and Ozturk, H. B. (2018). Dimethyl sulfoxide-induced tonic-Clonic seizure and cardiac arrest during infusion of autologous peripheral blood stem cells. Cell Tissue Bank. 19, 831–832. doi: 10.1007/s10561-018-9718-x
Martínez, A. L., Domínguez, F., Orozco, S., Chávez, M., Salgado, H., González, M., et al. (2006). Neuropharmacological effects of an ethanol extract of the Magnolia dealbata Zucc. Leaves in mice. J. Ethnopharmacol. 106, 250–255. doi: 10.1016/j.jep.2006.01.003
Mazumder, A. G., Kumari, S., and Singh, D. (2019). Anticonvulsant action of a selective phosphatidylinositol-3-kinase inhibitor LY294002 in pentylenetetrazole-mediated convulsions in zebrafish. Epilepsy Res. 157:106207. doi: 10.1016/j.eplepsyres.2019.106207
Mohan, M., Jaiswal, B. S., and Kasture, S. (2009). Effect of Solanum torvum on blood pressure and metabolic alterations in fructose hypertensive rats. J. Ethnopharmacol. 126, 86–89. doi: 10.1016/j.jep.2009.08.008
Mojarad, T. B., and Roghani, M. (2014). The anticonvulsant and antioxidant effects of Berberine in Kainate-induced temporal lobe epilepsy in rats. Basic Clin Neurosci 5, 124–130.
Moradi-Afrapoli, F., Ebrahimi, S. N., Smiesko, M., and Hamburger, M. (2017). HPLC-based activity profiling for GABA(A) receptor modulators in extracts: validation of an approach utilizing a larval zebrafish locomotor assay. J. Nat. Prod. 80, 1548–1557. doi: 10.1021/acs.jnatprod.7b00081
Morano, A., Fanella, M., Albini, M., Cifelli, P., Palma, E., Giallonardo, A. T., et al. (2020). Cannabinoids in the treatment of epilepsy: current status and future prospects. Neuropsychiatr. Dis. Treat. 16, 381–396. doi: 10.2147/ndt.S203782
Myers, K., Johnstone, D., and Dyment, D. (2019). Epilepsy genetics: current knowledge, applications, and future directions. Clin. Genet. 95, 95–111. doi: 10.1111/cge.13414
Nabbout, R., and Kuchenbuch, M. (2020). Impact of predictive, preventive and precision medicine strategies in epilepsy. Nat. Rev. Neurol. 16, 674–688. doi: 10.1038/s41582-020-0409-4
Nieoczym, D., Socała, K., Gawel, K., Esguerra, C. V., Wyska, E., and Wlaź, P. (2019). Anticonvulsant activity of Pterostilbene in zebrafish and mouse acute seizure tests. Neurochem. Res. 44, 1043–1055. doi: 10.1007/s11064-019-02735-2
Orellana-Paucar, A. M., Afrikanova, T., Thomas, J., Aibuldinov, Y. K., Dehaen, W., De Witte, P. A., et al. (2013). Insights from zebrafish and mouse models on the activity and safety of ar-turmerone as a potential drug candidate for the treatment of epilepsy. PLoS One 8:e81634. doi: 10.1371/journal.pone.0081634
Orellana-Paucar, A. M., Serruys, A. S., Afrikanova, T., Maes, J., De Borggraeve, W., Alen, J., et al. (2012). Anticonvulsant activity of bisabolene sesquiterpenoids of Curcuma longa in zebrafish and mouse seizure models. Epilepsy Behav. 24, 14–22. doi: 10.1016/j.yebeh.2012.02.020
Patra, P. H., Barker-Haliski, M., White, H. S., Whalley, B. J., Glyn, S., Sandhu, H., et al. (2019). Cannabidiol reduces seizures and associated behavioral comorbidities in a range of animal seizure and epilepsy models. Epilepsia 60, 303–314. doi: 10.1111/epi.14629
Pieróg, M., Socała, K., Doboszewska, U., Wyska, E., Guz, L., Szopa, A., et al. (2021a). Effects of classic antiseizure drugs on seizure activity and anxiety-like behavior in adult zebrafish. Toxicol. Appl. Pharmacol. 415:115429. doi: 10.1016/j.taap.2021.115429
Pieróg, M., Socała, K., Doboszewska, U., Wyska, E., Guz, L., Szopa, A., et al. (2021b). Effects of new antiseizure drugs on seizure activity and anxiety-like behavior in adult zebrafish. Toxicol. Appl. Pharmacol. 427:115655. doi: 10.1016/j.taap.2021.115655
Poivre, M., and Duez, P. (2017). Biological activity and toxicity of the Chinese herb Magnolia officinalis Rehder & E. Wilson (Houpo) and its constituents. J Zhejiang Univ Sci B 18, 194–214. doi: 10.1631/jzus.B1600299
Poulose, S. M., Thangthaeng, N., Miller, M. G., and Shukitt-Hale, B. (2015). Effects of pterostilbene and resveratrol on brain and behavior. Neurochem. Int. 89, 227–233. doi: 10.1016/j.neuint.2015.07.017
Quiñonez-Silvero, C., Hübner, K., and Herzog, W. (2020). Development of the brain vasculature and the blood-brain barrier in zebrafish. Dev. Biol. 457, 181–190. doi: 10.1016/j.ydbio.2019.03.005
Reeta, K. H., Mehla, J., Pahuja, M., and Gupta, Y. K. (2011). Pharmacokinetic and pharmacodynamic interactions of valproate, phenytoin, phenobarbitone and carbamazepine with curcumin in experimental models of epilepsy in rats. Pharmacol. Biochem. Behav. 99, 399–407. doi: 10.1016/j.pbb.2011.05.011
Ribeiro, M. H. (2011). Naringinases: occurrence, characteristics, and applications. Appl. Microbiol. Biotechnol. 90, 1883–1895. doi: 10.1007/s00253-011-3176-8
Rodriguez-Martin, I., De Velasco, E. M. F., and Rodriguez, R. E. (2007). Characterization of cannabinoid-binding sites in zebrafish brain. Neurosci. Lett. 413, 249–254. doi: 10.1016/j.neulet.2006.11.057
Sadeghnia, H. R., Taji, A. R., Forouzanfar, F., and Hosseinzadeh, H. (2017). Berberine attenuates convulsing behavior and extracellular glutamate and aspartate changes in 4-aminopyridine treated rats. Iran. J. Basic Med. Sci. 20, 588–593. doi: 10.22038/ijbms.2017.8756
Samarut, É., Nixon, J., Kundap, U. P., Drapeau, P., and Ellis, L. D. (2019). Single and synergistic effects of Cannabidiol and Δ-9-tetrahydrocannabinol on zebrafish models of neuro-hyperactivity. Front. Pharmacol. 10:226. doi: 10.3389/fphar.2019.00226
Samarut, É., Swaminathan, A., Riché, R., Liao, M., Hassan-Abdi, R., Renault, S., et al. (2018). γ-Aminobutyric acid receptor alpha 1 subunit loss of function causes genetic generalized epilepsy by impairing inhibitory network neurodevelopment. Epilepsia 59, 2061–2074. doi: 10.1111/epi.14576
Sarrica, A., Kirika, N., Romeo, M., Salmona, M., and Diomede, L. (2018). Safety and toxicology of Magnolol and Honokiol. Planta Med. 84, 1151–1164. doi: 10.1055/a-0642-1966
Scheffer, I. E., Berkovic, S., Capovilla, G., Connolly, M. B., French, J., Guilhoto, L., et al. (2017). ILAE classification of the epilepsies: position paper of the ILAE Commission for Classification and Terminology. Epilepsia 58, 512–521. doi: 10.1111/epi.13709
Sedaghat, R., Taab, Y., Kiasalari, Z., Afshin-Majd, S., Baluchnejadmojarad, T., and Roghani, M. (2017). Berberine ameliorates intrahippocampal kainate-induced status epilepticus and consequent epileptogenic process in the rat: underlying mechanisms. Biomed. Pharmacother. 87, 200–208. doi: 10.1016/j.biopha.2016.12.109
Shahrajabian, M. H., Sun, W., and Cheng, Q. (2019). Clinical aspects and health benefits of ginger (Zingiber officinale) in both traditional Chinese medicine and modern industry. Acta Agric. Scand. Sec. B. Soil Plant Sci. 69, 546–556. doi: 10.1080/09064710.2019.1606930
Shan, W., Nagai, T., Tanaka, M., Itoh, N., Furukawa-Hibi, Y., Nabeshima, T., et al. (2018). Neuronal PAS domain protein 4 (Npas4) controls neuronal homeostasis in pentylenetetrazole-induced epilepsy through the induction of Homer1a. J. Neurochem. 145, 19–33. doi: 10.1111/jnc.14274
Shanbhag, S. M., Kulkarni, H. J., and Gaitonde, B. B. (1970). Pharmacological actions of BERBERINE on the central nervous system. Jpn. J. Pharmacol. 20, 482–487. doi: 10.1254/jjp.20.482
Sharifi-Rad, J., Quispe, C., Herrera-Bravo, J., Martorell, M., Sharopov, F., Tumer, T. B., et al. (2021). A pharmacological perspective on plant-derived bioactive molecules for epilepsy. Neurochem. Res. 46, 2205–2225. doi: 10.1007/s11064-021-03376-0
Shirazi-Zand, Z., Ahmad-Molaei, L., Motamedi, F., and Naderi, N. (2013). The role of potassium BK channels in anticonvulsant effect of cannabidiol in pentylenetetrazole and maximal electroshock models of seizure in mice. Epilepsy Behav. 28, 1–7. doi: 10.1016/j.yebeh.2013.03.009
Sills, G. J., and Rogawski, M. A. (2020). Mechanisms of action of currently used antiseizure drugs. Neuropharmacology 168:107966. doi: 10.1016/j.neuropharm.2020.107966
Stewart, A. M., Braubach, O., Spitsbergen, J., Gerlai, R., and Kalueff, A. V. (2014). Zebrafish models for translational neuroscience research: from tank to bedside. Trends Neurosci. 37, 264–278. doi: 10.1016/j.tins.2014.02.011
Stewart, A. M., Ullmann, J. F., Norton, W. H., Parker, M. O., Brennan, C. H., Gerlai, R., et al. (2015). Molecular psychiatry of zebrafish. Mol. Psychiatry 20, 2–17. doi: 10.1038/mp.2014.128
Taiwe, G. S., Tchoya, T. B., Menanga, J. R., Dabole, B., and De Waard, M. (2016). Anticonvulsant activity of an active fraction extracted from Crinum jagus L. (Amaryllidaceae), and its possible effects on fully kindled seizures, depression-like behaviour and oxidative stress in experimental rodent models. J. Ethnopharmacol. 194, 421–433. doi: 10.1016/j.jep.2016.10.023
Tang, Y.-X., Fu, W.-W., Wu, R., Tan, H.-S., Shen, Z.-W., and Xu, H.-X. (2016). Bioassay-guided isolation of Prenylated Xanthone derivatives from the leaves of Garcinia oligantha. J. Nat. Prod. 79, 1752–1761. doi: 10.1021/acs.jnatprod.6b00137
Tarabasz, D., and Kukula-Koch, W. (2020). Palmatine: A review of pharmacological properties and pharmacokinetics. Phytother. Res. 34, 33–50. doi: 10.1002/ptr.6504
Teng, W.-L., Huang, P.-H., Wang, H.-C., Tseng, C.-H., and Yen, F.-L. (2021). Pterostilbene attenuates particulate matter-induced oxidative stress, inflammation and aging in keratinocytes. Antioxidants. doi: 10.3390/antiox10101552
Tesoriero, C., Greco, F., Cannone, E., Ghirotto, F., Facchinello, N., Schiavone, M., et al. (2023). Modeling human muscular dystrophies in zebrafish: mutant lines, transgenic fluorescent biosensors, and phenotyping assays. Int. J. Mol. Sci. 24:8314. doi: 10.3390/ijms24098314
Thornton, C., Dickson, K. E., Carty, D. R., Ashpole, N. M., and Willett, K. L. (2020). Cannabis constituents reduce seizure behavior in chemically-induced and scn1a-mutant zebrafish. Epilepsy Behav. 110:107152. doi: 10.1016/j.yebeh.2020.107152
Thurman, D. J., Logroscino, G., Beghi, E., Hauser, W. A., Hesdorffer, D. C., Newton, C. R., et al. (2017). The burden of premature mortality of epilepsy in high-income countries: a systematic review from the mortality task force of the international league against epilepsy. Epilepsia 58, 17–26. doi: 10.1111/epi.13604
Tomson, T., Battino, D., and Perucca, E. (2016). The remarkable story of valproic acid. Lancet Neurol. 15:141. doi: 10.1016/s1474-4422(15)00398-1
Trinka, E., Rainer, L. J., Granbichler, C. A., Zimmermann, G., and Leitinger, M. (2023). Mortality, and life expectancy in epilepsy and status epilepticus—current trends and future aspects. Front. Epidemiol. 3
Tufi, S., Leonards, P., Lamoree, M., De Boer, J., Legler, J., and Legradi, J. (2016). Changes in neurotransmitter profiles during early zebrafish (Danio rerio) development and after pesticide exposure. Environ. Sci. Technol. 50, 3222–3230. doi: 10.1021/acs.est.5b05665
Vanti, G. L., Kurjogi, M., Basavesha, K. N., Teradal, N. L., Masaphy, S., and Nargund, V. B. (2020). Synthesis and antibacterial activity of solanum torvum mediated silver nanoparticle against Xxanthomonas axonopodis pv.Punicae and Ralstonia solanacearum. J. Biotechnol. 309, 20–28. doi: 10.1016/j.jbiotec.2019.12.009
Vega-García, A., Santana-Gómez, C. E., Rocha, L., Magdaleno-Madrigal, V. M., Morales-Otal, A., Buzoianu-Anguiano, V., et al. (2019). Magnolia officinalis reduces the long-term effects of the status epilepticus induced by kainic acid in immature rats. Brain Res. Bull. 149, 156–167. doi: 10.1016/j.brainresbull.2019.04.003
Vilela, L. R., Lima, I. V., Kunsch, É. B., Pinto, H. P. P., De Miranda, A. S., Vieira, É. L. M., et al. (2017). Anticonvulsant effect of cannabidiol in the pentylenetetrazole model: pharmacological mechanisms, electroencephalographic profile, and brain cytokine levels. Epilepsy Behav. 75, 29–35. doi: 10.1016/j.yebeh.2017.07.014
Wilcox, L., Borradaile, N., and Huff, M. (2006). Antiatherogenic properties of Naringenin, a Citrus flavonoid. Cardiovasc. Drug Rev. 17, 160–178. doi: 10.1111/j.1527-3466.1999.tb00011.x
Wong, K., Stewart, A., Gilder, T., Wu, N., Frank, K., Gaikwad, S., et al. (2010). Modeling seizure-related behavioral and endocrine phenotypes in adult zebrafish. Brain Res. 1348, 209–215. doi: 10.1016/j.brainres.2010.06.012
Woodbury, A., Yu, S. P., Wei, L., and Garcia, P. (2013). Neuro-modulating effects of Honokiol: a review. Front. Neurol. 4:130. doi: 10.3389/fneur.2013.00130
World Health Organization (2019). Epilepsy: A Public Health Imperative: Summary. Geneva: World Health Organization
Wullimann, M. F., Rupp, B., and Reichert, H. (2012). Neuroanatomy of the Zebrafish Brain. A Topological Atlas. Basel: Birkhäuser
Yaksi, E., Jamali, A., Diaz Verdugo, C., and Jurisch-Yaksi, N. (2021). Past, present and future of zebrafish in epilepsy research. FEBS J. 288, 7243–7255. doi: 10.1111/febs.15694
Yuan, P., Guo, F., Zheng, K., Chen, K., Jia, Q., and Li, Y. (2016). Nine sesquiterpenes from Solanum torvum. Nat. Prod. Res. 30, 1682–1689. doi: 10.1080/14786419.2015.1135142
Zhang, Y., Vanmeert, M., Siekierska, A., Ny, A., John, J., Callewaert, G., et al. (2017). Inhibition of glutamate decarboxylase (GAD) by ethyl ketopentenoate (EKP) induces treatment-resistant epileptic seizures in zebrafish. Sci. Rep. 7:7195. doi: 10.1038/s41598-017-06294-w
Zhang, B., Wang, L., Ji, X., Zhang, S., Sik, A., Liu, K., et al. (2020). Anti-inflammation associated protective mechanism of Berberine and its derivatives on attenuating Pentylenetetrazole-induced seizures in zebrafish. J. NeuroImmune Pharmacol. 15, 309–325. doi: 10.1007/s11481-019-09902-w
Zhang, L., Yang, Z., Wei, J., Su, P., Chen, D., Pan, W., et al. (2017). Contrastive analysis of chemical composition of essential oil from twelve Curcuma species distributed in China. Ind. Crop. Prod. 108, 17–25. doi: 10.1016/j.indcrop.2017.06.005
Zheng, Y. M., Chen, B., Jiang, J. D., and Zhang, J. P. (2018). Syntaxin 1B mediates Berberine's roles in epilepsy-like behavior in a Pentylenetetrazole-induced seizure zebrafish model. Front. Mol. Neurosci. 11:378. doi: 10.3389/fnmol.2018.00378
Zhu, H. L., Wan, J. B., Wang, Y. T., Li, B. C., Xiang, C., He, J., et al. (2014). Medicinal compounds with antiepileptic/anticonvulsant activities. Epilepsia 55, 3–16. doi: 10.1111/epi.12463
Keywords: zebrafish, mice, plant, plant-derived drugs, convulsion, epilepsy, screening, methodology
Citation: Knap B, Nieoczym D, Kundap U, Kusio-Targonska K, Kukula-Koch W, Turski WA and Gawel K (2023) Zebrafish as a robust preclinical platform for screening plant-derived drugs with anticonvulsant properties—a review. Front. Mol. Neurosci. 16:1221665. doi: 10.3389/fnmol.2023.1221665
Edited by:
Han Wang, Soochow University, ChinaReviewed by:
Karen Mruk, East Carolina University, United StatesAleksandra Siekierska, KU Leuven, Belgium
Copyright © 2023 Knap, Nieoczym, Kundap, Kusio-Targonska, Kukula-Koch, Turski and Gawel. This is an open-access article distributed under the terms of the Creative Commons Attribution License (CC BY). The use, distribution or reproduction in other forums is permitted, provided the original author(s) and the copyright owner(s) are credited and that the original publication in this journal is cited, in accordance with accepted academic practice. No use, distribution or reproduction is permitted which does not comply with these terms.
*Correspondence: Kinga Gawel, a2luZ2FnYXdlbEB1bWx1Yi5wbA==
†ORCID: Bartosz Knap, https://orcid.org/0000-0002-5161-3494
Dorota Nieoczym, https://orcid.org/0000-0002-9446-7343
Uday Kundap, https://orcid.org/0000-0003-4791-4646
Kamila Kusio-Targonska, https://orcid.org/0000-0003-2667-6749
Wirginia Kukula-Koch, https://orcid.org/0000-0001-7076-600X
Waldemar A. Turski, https://orcid.org/0000-0002-5805-5106
Kinga Gawel, https://orcid.org/0000-0001-8857-847X