- 1Department of Sleep Medicine and Metabolic Disorders, Medical University of Lodz, Lodz, Poland
- 2Department of Neurosurgery and Neuro-Oncology, Barlicki University Hospital, Medical University of Lodz, Lodz, Poland
- 3Department of Pediatrics, Oncology, and Hematology, Medical University of Lodz, Lodz, Poland
- 4Department of Affective and Psychotic Disorders, Medical University of Lodz, Lodz, Poland
Obstructive sleep apnea (OSA) is one of the most common sleep disorders, which is characterized by recurrent apneas and/or hypopneas occurring during sleep due to upper airway obstruction. Among a variety of health consequences, OSA patients are particularly susceptible to developing metabolic complications, such as metabolic syndrome and diabetes mellitus type 2. MicroRNAs (miRNAs) as epigenetic modulators are promising particles in both understanding the pathophysiology of OSA and the prediction of OSA complications. This review describes the role of miRNAs in the development of OSA-associated metabolic complications. Moreover, it summarizes the usefulness of miRNAs as biomarkers in predicting the aforementioned OSA complications.
1. Introduction
Obstructive sleep apnea (OSA) is a chronic breathing disorder, which presents with recurrent apneas and/or hypopneas during sleep (Arnold et al., 2017). As the result of neuromuscular factors (e.g., hypoglossal nerve and genioglossus muscle) and negative airway pressure the tongue falls backward leading to occlusion in the upper airway (Pham and Schwartz, 2015). Apnea is a cessation of breathing for at least 10 s, while hypopnea means a reduction in the airflow by at least 50% associated with a drop in arterial blood oxygen saturation of at least 3% or reduction of the airflow by 30% and desaturation by 4% (Krawczyk et al., 2013). The gold standard in diagnosing and assessing the severity of OSA is the nocturnal polysomnography (PSG) examination. Although PSG is a prominent diagnostic tool for detecting OSA, the study can be aggravating for patients and does not inform about the risk of metabolic complications. During the PSG examination, apnea-hypopnea index (AHI) defined as the number of apneas and hypopneas per hour of effective sleep, is calculated. This index shows the severity of the disease: 5 > AHI – no OSA, 15 > AHI ≥ 5 – mild, 30 > AHI ≥ 15 – moderate, and AHI ≥ 30 – severe OSA (Kapur et al., 2017). It is estimated that 14% of men and 5% of women experience mild, moderate, or severe forms of this condition in their lifetime, however, in some populations, the percentage can reach up to 80% (Patil et al., 2019). Unfortunately, a large proportion of moderate and severe OSA, remain undiagnosed (Young et al., 1993; Fleming et al., 2018). Obesity is considered the most prominent risk factor for developing OSA, followed by male sex, and older age (Liu et al., 2016). A higher prevalence of OSA is observed in patients who have hypertension (30–83%) (Logan et al., 2001), diabetes mellitus (40–69%) (Fallahi et al., 2019), or metabolic syndrome (55–81%) (Drager et al., 2009). Dominant symptoms of OSA are non-specific: snoring, drowsiness, excessive daytime sleepiness, and fatigue. OSA is dangerous not only because of chronically occurring hypoxia but also because of its possible complications, such as diabetes mellitus type 2 (T2DM), metabolic syndrome (MetS), cardiovascular diseases, asthma, idiopathic pulmonary fibrosis, cancer (Kong et al., 2016; Cao et al., 2022; Karuga et al., 2022; Salari et al., 2022; Wang C. et al., 2022; Wang et al., 2023). Continuous positive airway pressure (CPAP), a gold standard treatment in OSA, might delay or even eliminate symptoms and complications of this disorder (Fu et al., 2017; Bonsignore et al., 2019; Gabryelska et al., 2021b). However, individuals who are diagnosed with moderate or severe OSA might require additional treatment, for example, oral appliances, hypoglossal nerve stimulators, or upper-airway surgeries (Waters, 2019). Many papers link higher mortality in OSA with metabolic complications (Labarca et al., 2021; Su et al., 2021; Liu et al., 2022). Therefore, it is crucial to detect the early signs of OSA complications in order to optimize the treatment strategy (Kendzerska et al., 2014). Ongoing research focuses on finding a satisfactory biomarker to achieve this goal. The most promising candidates for OSA biomarkers include as follows: miRNA levels of ADAM29, FLRT2, and SLC18A3 determined in peripheral blood mononuclear cells, serum levels of Endocan and YKL-40, as well as plasma levels of IL-6 and Vimentin (Gaspar et al., 2022). Contemporary miRNA exhibits several advantages over other molecular biomarkers. It has been reported that noninvasive quantification of miRNA profiles is highly sensitive, robust, and cost-effective for the clinical management of different pathological conditions such as head and neck squamous cell carcinoma, heart failure, or osteoporosis Additionally, detecting differences in gene expression rather than in gene content becomes an effective and practical approach for associating molecular markers with the patient phenotype and disease outcome (Bock, 2009). There are miRNAs that are involved in OSA and the development of its complications (Malicki et al., 2022). Therefore, such miRNAs can reveal clinical value in order to specific OSA phenotypes diagnosis or act as a predictive factor of concrete complications development at an early stage of the disease (Gaspar et al., 2022). In the present study, we reviewed miRNAs that can be potential biomarkers of OSA metabolic consequences and should be investigated in the future. However, it should be mentioned that miRNA biomarkers have their limitations in both sensitivity and specificity. The blood sampling methods should be carefully considered as well as the selection criteria of the study group due to the influence of concomitant diseases miRNAs levels. In addition, each miRNA can have various expressions depending on the specimen (e.g., blood, urine, muscle tissue, exosomes).
MiRNAs are small, up to 30 bases in length, strands of ribonucleic acid, which are responsible for regulating the expression of many genes (Dutkowska et al., 2021). Based on the location miRNAs are generally divided into two groups – intracellular and extracellular. While intracellular mature miRNAs can be secreted from the cytoplasm and preserve high stability, extracellular molecules, e.g., packaged in exosomes or encapsulated in liposomes, are promising and more accessible for clinical diagnostics (Leung, 2015). MiRNAs can be used to detect the disease before its first manifestations and predict the response of the organism to the suggested treatment (Gabryelska et al., 2020b,c,d; Pozniak et al., 2022). The main role of miRNAs is cell-to-cell communication via post-transcriptional epigenetic mechanism leading to mRNA degradation or translation inhibition (Czarnecka et al., 2019). Their proper functioning is crucial for maintaining correct cell activity, such as apoptosis, stress response, proliferation, and metabolism. Any disruption in the regulatory properties of miRNAs can result in the pathogenesis of many disorders, including respiratory diseases and their complications (Dutkowska et al., 2021). Therefore, analyzing miRNAs, particularly as specific subsets, may be considered not only important for a better diagnosis and therapy but also as an essential factor for understanding the pathophysiology of OSA and its association with other disorders (Gabryelska et al., 2020a). In the current review, we explain the role of miRNAs in the development of OSA-related metabolic complications.
2. miRNAs in metabolic complications of OSA
2.1. OSA
A better insight into OSA-related miRNAs may lead not only to a better understanding of OSA but also to the development of new diagnostic and therapeutic strategies, as PSG examination is a good tool for diagnosing OSA but is expensive and uncomfortable for patients – they have to spend at least one night away from home. In addition, miRNA expression may affect gene expression, which can result in the development of complications, especially metabolic. Santamaria-Martos et al. (2019a) performed an analysis to determine miRNAs that separate OSA from non-OSA patients. Initially, 14 miRNAs (−181-a-2, −495, −451, −486, −660, −345, −340, −107, −486-3p, −133a, −181a, −let-7d, −199a, −199b) revealed different expression between these two groups. However, further validation with the qPCR method confirmed that only six of them might be suitable for clinical use (−181a, −199b, −345, −133a, −340, −486-3p). Khurana et al. published their cohort study where they noted the downregulation of miRNA-27 and let-7 in OSA patients (Khurana et al., 2020). Santamaria-Martos et al. (2019b) selected eight biomarker candidates: miRNA-106a, miRNA-186, miRNA-29a, miRNA-21, miRNA-103, miRNA-27a, miRNA-140, and miRNA-145. It was observed that the combination miRNA-106a/miRNA-186 was the most stable among all the candidates (Santamaria-Martos et al., 2019b). Although some of the above-mentioned miRNAs have not been described as dysregulated particularly in diabetes mellitus or metabolic syndrome, studies are needed to specify their levels and potential role in patients with OSA and concomitant metabolic complications. Common miRNAs between OSA and metabolic complications can include also miRNAs: −17-5p, −21-5p, −22-3p, −31, −126, −130, −155, −181, and − 199; their involvement is further presented in this review.
2.2. Metabolic syndrome
Metabolic syndrome (MetS), initially known as syndrome X, was first described by Reaven in 1988 after it was noticed that insulin resistance and hyperinsulinemia increase the risk of T2DM, hypertension, and coronary artery disease development (Reaven, 1988). According to the most common definition, MetS is a co-occurrence of abdominal obesity and at least two cardiometabolic risk factors such as hypertension, insulin resistance, hypertriglyceridemia, and low concentration of high-density cholesterol (Saklayen, 2018). MetS increases the risk of cardiovascular complications occurrence 2-fold and up to 5-fold in the case of T2DM (Samson and Garber, 2014). The prevalence of MetS has increased worldwide with averaging values at 30% in adults (Engin, 2017) and between 6 and 39% in children/teenagers (Weihe and Weihrauch-Blüher, 2019). MetS has a variety of anticipated miRNA biomarkers, which are summarized in Table 1. Furthermore, some of the miRNAs can have a gender-specific (Wang et al., 2013) or physical (Zhou et al., 2014) link in MetS patients. Obstructive sleep apnea is independently associated with MetS occurrence. Risk estimates are 6–9 times higher in patients with OSA compared to the general (non-OSA) population (Coughlin et al., 2004). It has been shown that CPAP reduces the risk of developing MetS in OSA patients (Phillips et al., 2011). Although the connection between OSA and MetS is not fully understood, studies are emphasizing the influence of intermittent hypoxia and sleep fragmentation (Khalyfa et al., 2018), abnormal sympathetic activation (Trombetta et al., 2013), and chronic inflammation (Drager et al., 2010; Turkiewicz et al., 2021; Kaczmarski et al., 2022). There are no conducted studies focusing on the relationship between patients with MetS and OSA in the context of miRNAs. Nevertheless, preliminary conclusions can be drawn from the available literature.
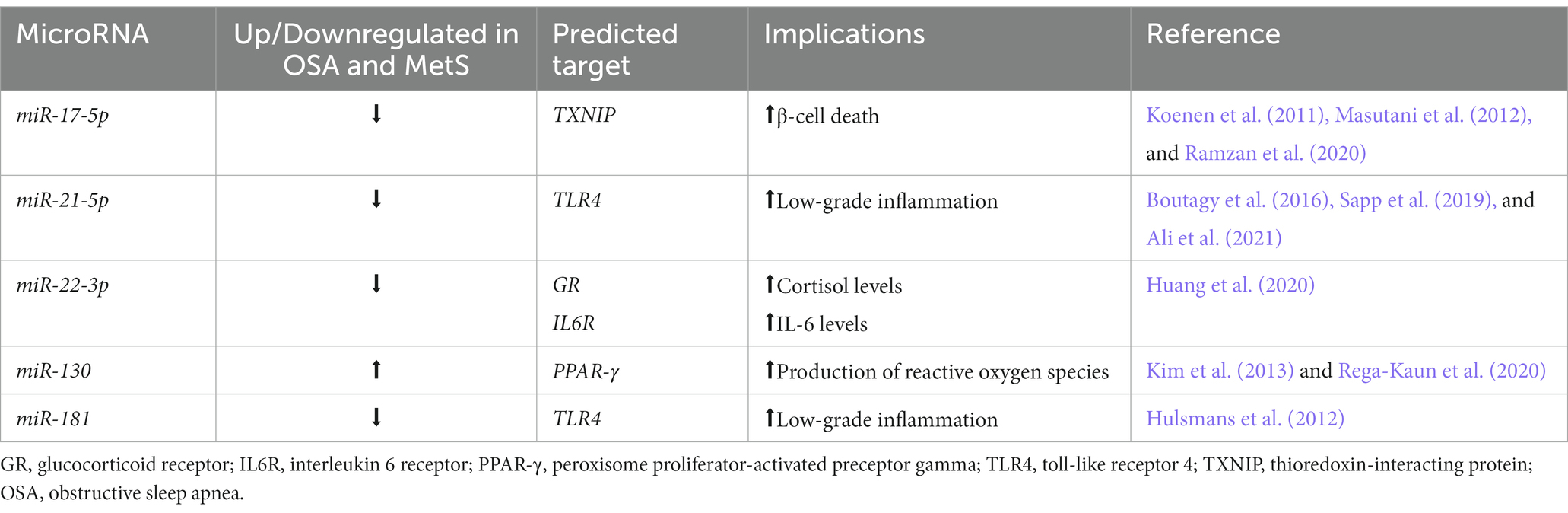
Table 1. The role of selected miRNAs in metabolic syndrome and obstructive sleep apnea pathogenesis.
The dysregulation of miRNA-181a presented in OSA may exacerbate inflammation in the case of MetS as a comorbid condition (Santamaria-Martos et al., 2019b). Hulsmans et al. study identified toll-like receptor (TLR) 4 as an inflammation factor associated with the levels of miRNA-181a in patients with both morbid obesity and MetS; the downregulation contained in CD14+ monocytes enhanced TLR/NF-κB signaling pathway, which was associated with chronic low-grade inflammation development (Hulsmans et al., 2012). The target gene may be a TLR4-interactor with leucine-rich repeats, a functional component of TLR4. The knockdown of TLR4 via siTLR4 has been shown to affect the miRNA-181a levels (Xie et al., 2013b). As the decreased levels of miRNA-181a in monocytes are associated with targeting IL-1 via binding the site of 3′-untranslated regions, the modulation of miRNA can alleviate systemic inflammation and thereupon decrease the severity of both MetS and OSA or even act as MetS development prevention (Xie et al., 2013a).
Altered miRNA-22-3p expression in OSA patients (Shao et al., 2021) can contribute to greater severity of MetS since it takes part in the development of the main features of the disease. MiRNA-22-3p has been reported to be downregulated in peripheral blood mononuclear cells (PBMCs) in MetS patients – the study disclosed a negative correlation with a variety of MetS components such as blood pressure, plasma triglyceride, and waist circumference, and a positive correlation with plasma high-density lipoprotein levels (Huang et al., 2020). Furthermore, OSA-related hypertension may arise from miRNA-22-3p downregulation that influences vascular smooth muscle cells (VSMCs) by targeting the methyl-CpG binding protein 2 gene (Shao et al., 2021). Excessive proliferation and migration of VSMCs impede the arterial intima balance, which is inherently connected with atherosclerosis and subsequent hypertension development, thus pointing at miRNA-22-3p dysregulation as a trigger for the development of hypertension.
The downregulation of miRNA-17-5p might be a novel biomarker of OSA or MetS, which is linked with obesity and impaired adipogenesis (Ramzan et al., 2020). Thioredoxin interacting protein (TXNIP) is an aspiring target of miRNA-17-5p which was described in metabolic disorders including obesity and insulin resistance (Koenen et al., 2011; Masutani et al., 2012). Exposing the genioglossus muscle cells to intermittent hypoxia led to a downregulation of miRNA-17-5p. Upregulation of miRNA-17-5p by miRNA mimics resulted in a strengthening of the muscle by regaining the mitochondrial function and cell proliferative capacity (Qin et al., 2019). Genioglossus plays an important role as an upper-airway dilator muscle, helping to maintain adequate oxygen supply during sleep. TXNIP may have an impact on tissue subjected to chronic intermittent hypoxia via TXNIP/NLRP3/IL-1β pathway and mediate mitochondrial dysfunction (Yan et al., 2021). Thus, the bidirectional relationship can be observed – downregulation of miRNA-17-5p occurring during OSA may exacerbate the likelihood of MetS occurrence through affecting fat accumulation, while downregulation happening in MetS may weaken the restoration power of genioglossus muscle and make the patient more susceptible to apnea development or cause a more severe course of coexisting OSA.
As mentioned before, some miRNAs might be used as potential diagnostic markers only in a specific group. Sapp et al. study revealed downregulation of miRNA-21-5p in postmenopausal African American women with MetS compared to a healthy group (Sapp et al., 2019). These results contrast with those found in a study by Doghish et al. where miRNA-21-5p levels were increased in adult Egyptian males with MetS (Doghish et al., 2021). In treatment-naive OSA patients, miRNA-21-5p was decreased and negatively correlated with oxygen desaturation index and AHI. Exposure of human monocytic THP-1 cell lines (cell model of human monocytes) to intermittent hypoxia with reoxygenation resulted in decreased levels of miRNA-21-5p, in vitro studies pointed out that miRNA-21-5p might target TNF-α/TLR4 pathway resulting in hypoxia-induced inflammation and cell apoptosis (Chen Y. C. et al., 2020). In turn, TLR4 activation in MetS may increase reactive oxygen species production, which has been related to inflammation, endothelial dysfunction, and metabolic impairment (Boutagy et al., 2016; Ali et al., 2021). The downregulation of miRNA-21-5p in OSA and MetS may indicate a self-perpetuating cycle of inflammation via activation of TLR4 as a pattern recognition receptor, and cell dysfunction. However, this was observed only in specific groups, prompting the need for further investigation.
Altered expression of miRNA-130 might be relevant to MetS and indicate metabolic aspects of OSA. In tissue exposed to chronic intermittent hypoxia, the major harmful factor of OSA, the miRNA-130 levels were upregulated (Zhang X. B. et al., 2020). The study of Rega-Kaun et al. (2020) showed that Roux-en-Y-Bariatric Surgery significantly reduced upregulated miRNA-130 levels in MetS patients, which exhibited clinical improvement of the disorder. Elevated miRNA-130 can especially affect islets of Langerhans via modulation of ATP/ADP concentration, thereby impairing the leading pancreatic function relying on glucose homeostasis regulation (Ofori et al., 2017). Additionally, in obese schoolchildren aged 12–18 miRNA-130 positively correlated with MetS risk factors (Al-Rawaf, 2019). Peroxisome proliferator-activated receptor gamma (PPAR-γ) was found to be a possible target of miRNA-130 – PPAR-γ might regulate adipocyte differentiation and promote both oxidative stress injury and proinflammatory response (Kim et al., 2013; Zhang Y. et al., 2017). Especially in a chronic intermittent hypoxic environment, PPAR-γ is in charge of neuroinflammation development and cognitive performance (Dong et al., 2018; Wang H. et al., 2021), endothelial cell regulation (Lian et al., 2021), and defense from the kidney (Zhang et al., 2019) or cardiac (Pai et al., 2022) injury. Nocturnal hypercapnia, another hallmark of OSA, can contribute to obesity and metabolic impairment through PPAR-γ dysregulation (Kikuchi et al., 2017). Overall, miRNA-130 can be a valuable predictor in children/adolescents of subsequent MetS and OSA development and a biomarker of treatment response in adults.
The role of chosen miRNAs in the development of OSA-related metabolic complications is presented in Figure 1.
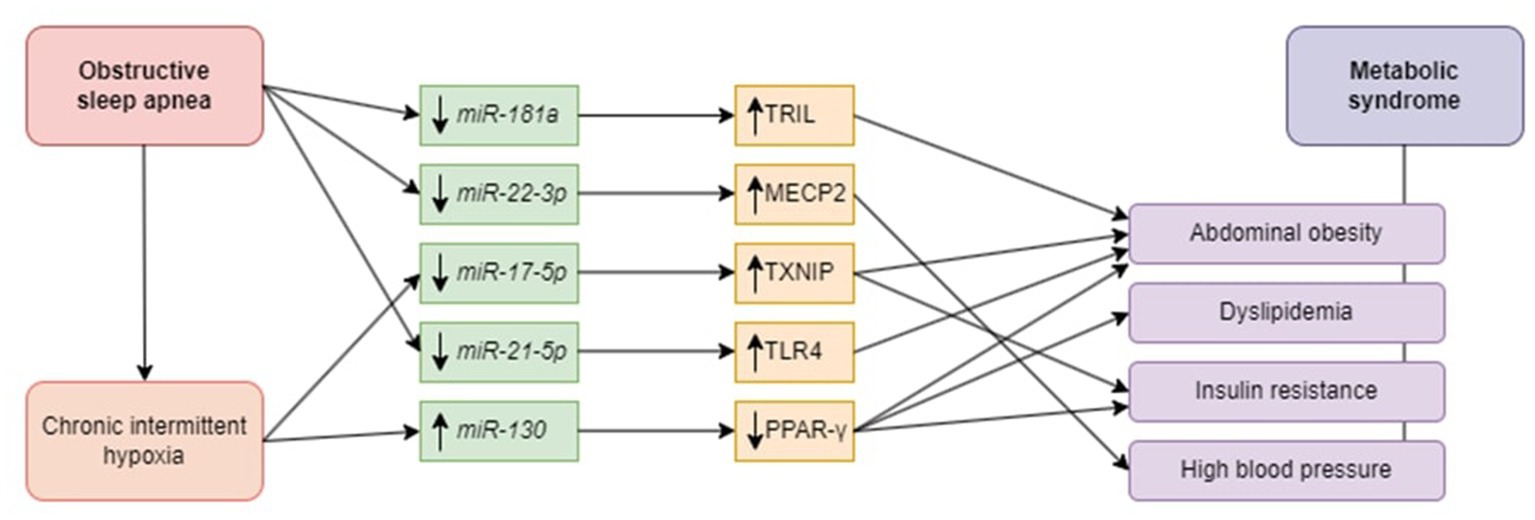
Figure 1. Potential role of microRNAs in the pathophysiology of metabolic syndrome in obstructive sleep apnea patients. Obstructive sleep apnea in general affects miR -181a, -22-3p, -17-5p, and via chronic intermittent hypoxia miR-21-5p and -130. Downregulation of miR-181a causes upregulation of TRIL and leads to abdominal obesity. Downregulation of miRNA-22-3p is associated with high blood pressure due to targeting MECP2. Downregulation of miR-17-5p triggers abdominal obesity and insulin resistance via TXNIP upregulation. miR-21-5p downregulation leads to the upregulation of TLR4, thereby contributing to abdominal obesity. Upregulation of miRNA-130 stimulates PPAR-γ, predisposing to abdominal obesity, dyslipidemia, and insulin resistance. miR, microRNA; TRIL, TLR4 interactor with leucine-rich repeats; MECP2, methyl-CpG binding protein 2; TXNIP, thioredoxin interacting protein; TLR4, toll-like receptor 4; PPAR- γ, peroxisome proliferator-activated receptor gamma.
2.3. Diabetes mellitus
Diabetes mellitus is a group of metabolic disorders characterized by chronic hyperglycemia resulting from defects in insulin action, insulin secretion, or both. T2DM is the most common type of diabetes mellitus. In 2017, approximately 6.28% of the world’s population was affected by T2DM and around 1 million deaths yearly can be attributed to T2DM alone(Khan et al., 2020). The risk factors for T2DM include both nonmodifiable factors (e.g., genetic predisposition, family history), and modifiable factors (e.g., obesity, unhealthy diet, and low physical activity) (Galicia-Garcia et al., 2020). There are many miRNAs that may have an impact on T2DM (see Table 2). They can inhibit insulin signaling, inhibit glucose uptake, promote insulin signaling, and reduce insulin secretion(Sekar et al., 2016; Szweda and Łaczmański, 2016; Rovira-Llopis et al., 2018; Deng and Guo, 2019; Kim and Zhang, 2019; Jankauskas et al., 2021). OSA is recognized as an independent risk factor for metabolic diseases, including T2DM (Gabryelska et al., 2020a). In the study of Mahmood et al., it was found that the prevalence of T2DM in OSA patients was 30.1%, while in the group without OSA only 18.6% (Mahmood et al., 2009). Moreover, Gabryelska et al. (2021a) showed that higher oxygen saturation in OSA patients is associated with the later onset of T2DM. As it was mentioned before the most effective form of treatment for OSA is CPAP, which generates air pressure in the upper airways preventing their collapse and eliminating the recurrent periods of hypoxia. Unfortunately, CPAP treatment might be ineffective in patients with T2DM (Labarca et al., 2018), however, it may slow down the progression of T2DM (Reutrakul and Mokhlesi, 2020). The coexistence of these two diseases is the subject of numerous scientific papers, which investigate possible mechanisms of this interaction, for example, mechanisms mediated via HIF-1α or sirtuin1 (SIRT1) (Reutrakul and Mokhlesi, 2017; Song et al., 2018; Gabryelska et al., 2020a). Nevertheless, the relationship between these two diseases is not fully understood. Some studies postulate the role of certain miRNAs in T2DM among OSA patients.
The study of Ren et al. (2018) disclosed the upregulation of miRNA-31 emerging from intermittent hypoxia that resulted in cardiac hypertrophy. As predicted, myocardial remodeling was affected by the miRNA-31/PKC-ε signaling pathway to some extent. In turn, in a separate study, the downregulation of miRNA-31 elicited a protective cardiac performance via miRNA-31/PKC-ε/NF-κB pathway (Wang et al., 2015). Despite being engaged in detrimental cardiac changes, the impact of miRNA-31 dysregulation on hypoxia-subjected tissues seems to be variable. Hypoxic diabetic adipose stem cells (ADSCs) engaged in soft tissue repair processes showed better responses to damage in comparison to non-hypoxic ADSCs (Wang J. et al., 2021). Upregulation of miRNA-31 in hypoxic ADSCs may account for accelerated wound healing via targeting factor-inhibiting HIF-1 (FIH-1) and epithelial membrane protein-1 (EMP-1) (Huang et al., 2021). Exosomic miRNA-31 overexpression in recalcitrant diabetic wounds contributed to augmented healing due to its proangiogenic and proliferative features. Likewise, FIH-1 was the estimated target gene (Yan et al., 2022). The study of Han et al. (2021) carried out on healthy skin samples showed a positive impact of overexpressed miRNA-31 on the cell migration as well. Although the upregulation of miRNA-31 in diabetic foot skin did not achieve statistical significance due to the variability of samples and the absence of severity-related group division, there is a strong presumption of the influence of miRNA-31 on skin dysfunction in the course of diabetes mellitus (Ramirez et al., 2015). Given the fact that OSA patients generally have worse progress in diabetic ulcer treatment, the protective effect of upregulated miRNA-31 on wound healing may work only in some cases or be a part of a specific subset responsible for the repair (Maltese et al., 2018). The same miRNA was upregulated in the retina (Kovacs et al., 2011) and periodontal ligament (Zhen et al., 2017) of diabetic rats, and in endothelial progenitor cells obtained from T2DM patients (Lian et al., 2018). Looking at the last two examples, a plausible common target of miRNA-31 is a special AT-rich sequence-binding protein 2 (Satb2), which has been reported to trigger vascular endothelial dysfunction and suppression of osteogenic differentiation in DM. Increased levels of transforming growth factor β (TGF-β) occurring in OSA (Hernández-Jiménez et al., 2017) can downregulate Satb2, thereby decreasing bone density via promoting osteoblast dysfunction (Freude et al., 2012). Upregulation of miRNA-31 and TGF-β demonstrated in OSA coupled with DM may be responsible for the higher incidence of vascular malfunction and poor bone remodeling.
Dysregulation of miRNA-155, which has been broadly described in the literature about diabetes and its complications, might be linked with OSA-related kidney deterioration. In renal tissue exposed to chronic intermittent hypoxia, the activation of the NLRP3 inflammasome pathway led to miRNA-155 upregulation. A prompt response to stimuli triggered hypoxia-induced renal injury due to the exacerbation of the inflammatory process (Wu et al., 2018). miRNA-155 upregulation was detected both in urine and renal tissue, indicating a possible application for this biomarker, albeit the direction of dysregulation may be dependent on the location of miRNA (Akhbari et al., 2019). miRNA-155 deficiency can favor the acetylation of nephrin, thus ameliorating diabetes-induced perturbation via restoring podocyte function(Lin et al., 2015). Potential corresponding targets in hyperglycemia-injured renal tissue comprise brain-derived neurotrophic factor (BDNF), which has been proven to contribute to kidney deterioration through autophagy attenuation, fibrosis progression, oxidative stress imbalance, and microinflammation (Gao et al., 2022). In several studies dysregulation of BDNF was observed among OSA patients (Arslan et al., 2021; Gabryelska et al., 2022, 2023; Gabryelska and Sochal, 2022). Furthermore, patients suffering from both OSA and T2DM may be more prone to develop impairment of kidney function as a long-term complication; however, this link has not been proven yet and shows the need for further research.
Alteration of miRNA-126 expression detected in OSA patients (Yang et al., 2018) might be involved in diabetes mellitus development and dysglycemia-related complications as well. In the foreseeable future, miRNA-126 dysregulation may serve as an accurate diagnostic biomarker of prediabetes (Liu et al., 2014) and T2DM (Dehghani et al., 2020) due to its close correlation. Except the potential ethnic variability (Weale et al., 2021), the progressive decline of miRNA can reflect the advancement of the disease and predict long-term all-cause mortality (Pordzik et al., 2021). Thus, miRNA-126 downregulation, occurring secondary to OSA, may exacerbate T2DM or even contribute to the onset of the disease. miRNA-126 downregulation has been reported to impair vascular performance in T2DM (Jansen et al., 2016). It plays an especially important role in the development of retinopathy through the enhancement of neovascularization; targeting vascular endothelial growth factor (VEGF), which in turn promotes migration and sprouting in retinal vascular endothelial cells via VEGF/PI3K/AKT signaling pathway. Additionally, in hypoxia-treated cells, miRNA-126 is a negative regulator of VEGF expression (Ye et al., 2013). It may partly explain why OSA subjects exhibit repercussions linked with eyes, such as increased retinal vessel tortuosity, declined vascular density, or pathological changes in the choroid (Nakayama et al., 2021). miRNA-126 downregulation is likewise associated with diabetic nephropathy (Al-Kafaji et al., 2016) and worse diabetic foot ulcer healing (Zhang J. et al., 2017). As diabetic patients with OSA are generally more vulnerable to developing diabetic retinopathy (Chang et al., 2018) and nephropathy (Misra and Shrivastava, 2016) or have impaired wound healing (Ramirez et al., 2015), these dependencies are of great importance.
miRNA-181a is one of the most hypoxia-sensitive miRNAs which is best illustrated by the example of tumor progression (Agrawal et al., 2014; Sun et al., 2015a; Macharia et al., 2021). Under these circumstances, the main mechanism of action is presumably aimed at angiogenesis promotion via targeting VEGF, which then induces tumor growth and subsequent metastasis development (Sun et al., 2015b; Silvestris et al., 2017). Dysregulation of miRNA-181a in response to hypoxia may participate in cardiovascular damage where the possible interplay between miRNA-181a, VEGF, and HIF1α seems to be important (Cuevas et al., 2014). The underlying role of miRNA-181a in cell damage and apoptosis via SIRT1 regulation can also be observed in injured cardiomyocytes (Qi et al., 2020; Song et al., 2021). miRNA-181a also plays a role in the development of cerebral ischemia, having a neuroprotective effect when upregulated. What is more, in OSA patients the expression of miRNA-181a is decreased and correlates with the AHI and arousal index. Among diabetic patients, the expression of miRNA-181a in the adipose tissue is reduced as well and a higher level of this is known to prevent insulin resistance induced via TNFα (Lozano-Bartolomé et al., 2018). The exact impact of miRNA-181a on diabetes mellitus development is not fully explored, presenting different data. In Gok et al. (2019) study the downregulation of miRNA-181a and simultaneous upregulation of SIRT1 were connected with the development of T2DM. However, Zhou et al. (2012) showed that the downregulation of miRNA-181a improves hepatic sensitivity via SIRT1 upregulation; SIRT1, as a gene with a positive therapeutic effect on glucose metabolism (Sun et al., 2007), may play a compensative role during diabetes mellitus development. Decreased levels of miRNA-181a were also noticed in diabetic complications including cardiomyopathy (Raut et al., 2016; Zhao S. F. et al., 2021) and nephropathy (Zha et al., 2019; Liang and Xu, 2020). As the case may be, in diabetic hearts miRNA-181 targets programmed cell death 4 (PDCD4), or TP53. Cardiomyocytes with decreased miRNA-181a levels are more prone to develop hypertrophy, inflammation, impaired angiogenesis, and undergo apoptosis. In diabetic kidney tissue, miRNA-181a targets may be a Kruppel-like factor 6 (KLF6) and early growth response factor-1 (EGR-1), which are involved in the disordered proliferation of the glomerular mesangial cells, tubulointerstitial fibrosis, and enhanced cell apoptosis. Overall, the downregulation of miRNA-181a observed in OSA may mean that non-OSA patients are more resistant to developing diabetic-related cardiomyopathy or nephropathy, but have less expanded compensatory mechanisms against impaired insulin sensitivity. In regard to other diabetic complications, it was discovered that the upregulation of miRNA-181a may be responsible for diabetic corneal nerve neuropathy in mice (Hu et al., 2020). Upregulated levels of miRNA-181a were also noticed in patients with gestational diabetes mellitus (Hromadnikova et al., 2020, 2022) and animal diabetic models with impaired wound and fracture healing (Takahara et al., 2018; He et al., 2019).
Another promising hypoxia-regulated molecule is miRNA-199a, its activity mainly focuses on the co-expression of two target genes, HIF-1α and SIRT1. HIF-1α compensation permits the cells to adapt to low oxygen conditions. In a hypoxic preconditioning miRNA-199a downregulation was associated with the upregulation of HIF-1α and SIRT1, resulting in the adaptation to external stimulus (Rane et al., 2009). A similar outcome was obtained after stimulation of the insulin receptor, which regulates the AKT pathway (Rane et al., 2010). SIRT1, besides maintaining metabolic homeostasis, acts as a regulator of HIF-1α. SIRT1 binds to the protein and deacetylates lysine 674, resulting in the suppression of HIF-1α transcriptional activity (Lim et al., 2010). Some authors describe SIRT1 as an indispensable element of HIF-1α activity due to its role in the positive regulation of the protein (Laemmle et al., 2012). In OSA patients Santamaria-Martos et al. (2019b) revealed downregulation of miRNA-199a. It was also seen that induction of miRNA-199a and ensuing HIF-1α downregulation can relieve OSA-related hypertension via oxidative stress injury reduction and suppression of inflammation (Guo et al., 2022). In diabetic subjects, a downregulation of miRNA-199a was observed in nephropathy and the inhibitor of nuclear factor kappa b kinase subunit beta (IKKβ) was identified as a potential target (Zhang R. et al., 2020). Additionally, IKKβ can play an important role in developing insulin resistance (Zhao L. et al., 2021) and atherosclerosis (Imamura et al., 2016) in OSA patients. Decreased levels of miRNA-199a were similarly present in diabetic-induced cardiomyopathy via targeting protein kinase B (AKT) and growth factors: vascular (VEGF), insulin-like (IGF), and acidic fibroblast (FGF-1) (Ahmed et al., 2021). Diabetic cataract was associated with miRNA-199a downregulation and its influence on the specific protein 1 (SP1) gene (Liu et al., 2020). In turn, diabetic retinopathy can partially result from miRNA-199a dysregulation associated with VEGF or FGF7 signaling (Wang L. et al., 2020; Zhou et al., 2021). In contrast, Yan et al. (2014) found out that miRNA-199a levels were upregulated in T2DM patients and due to the influence on GLUT4 expression might be involved in insulin resistance development. Overexpression of TNF-α presented in OSA may additionally enhance DM development by suppressing GLUT-4 expression and promoting insulin resistance through TNF-α/IKKβ/IKβ/NF-κB signaling pathway (Swaroop et al., 2012; Ji et al., 2021). Upregulation of miRNA-199a might be connected with decreased cell viability and enhanced apoptosis in pancreatic beta cells through the downregulation of SIRT1 (Lin et al., 2017). In accordance with other articles, a high level of miRNA-199a was observed in the placenta of gestational diabetes mellitus patients(Guan et al., 2022) and tissues obtained from patients with a diabetic foot ulcer (Wang H. et al., 2022). miRNA-199a expression in patients with diabetic neuropathy showed inconsistent results; the downregulation was associated with increased binding immunoglobulin protein expression levels (Hassani et al., 2023), while upregulation decreased the levels of SerpinE2 (Li et al., 2017).
Detection of described miRNAs with an altered expression profile in OSA patients may provide valuable info about a high risk of T2DM development as an OSA complication in such patients (Figure 2).
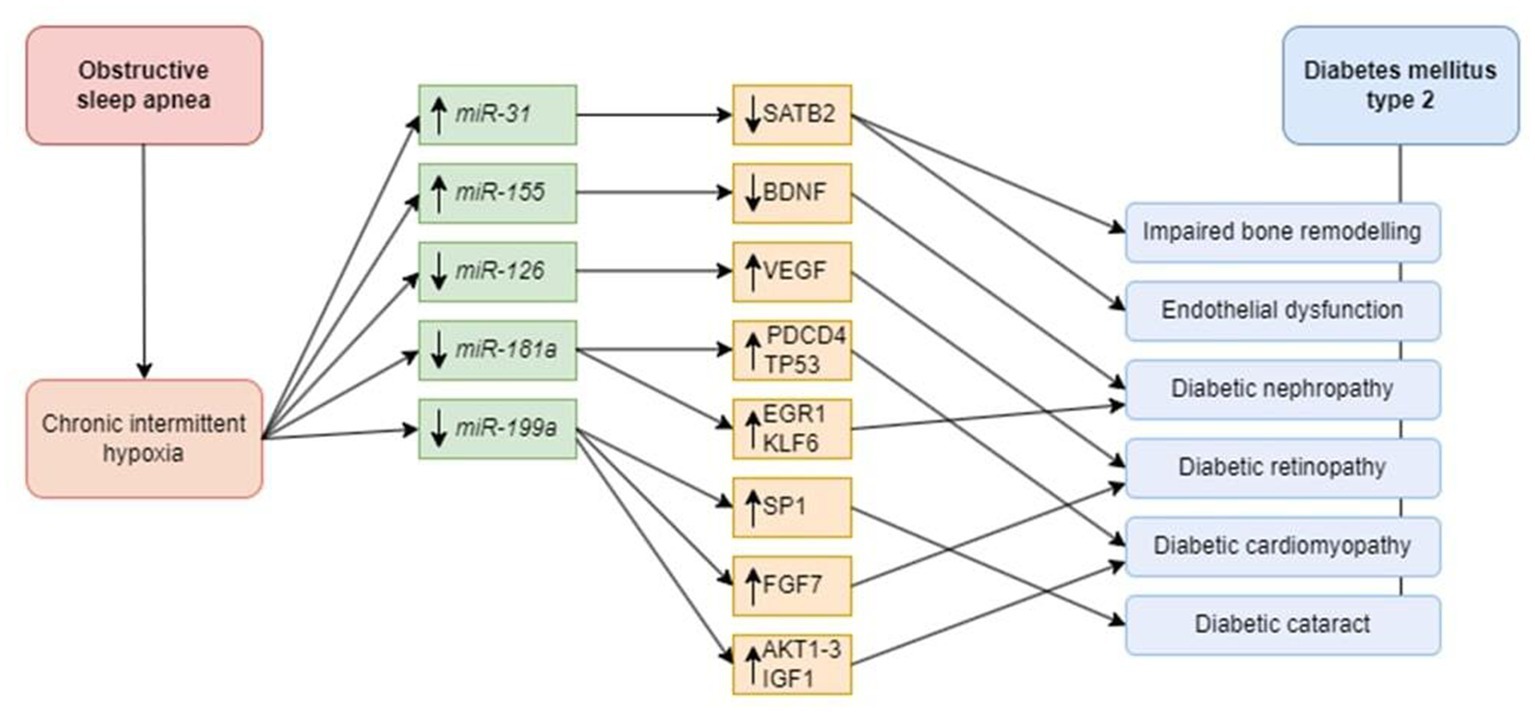
Figure 2. Possible role of microRNAs in the pathophysiology of diabetes mellitus and diabetic complications. Chronic intermittent hypoxia as the main consequence of obstructive sleep apnea affects miR-31 and -155, resulting in their downregulation, and miR-126, -181a, and -199a, leading to their upregulation. Dysregulation of microRNAs can predispose patients suffering both from OSA and DM to particular complications. Decreased level of SATB2 contributes to impaired bone remodeling and endothelial dysfunction. In turn, BDNF dysregulation is associated with diabetic nephropathy. Downregulation of the VEGF gene results in diabetic retinopathy development. Increased TP53 and PDCD4 lead to diabetic cardiomyopathy. Targeting EGR-1 and KLF6 can trigger diabetic nephropathy. An increase in SP1 is responsible for diabetic cataract. Another upregulated target, FGF7, can contribute to diabetic retinopathy development. Downregulation of AKT, IGF, and FGF-1 play important role in diabetic cardiomyopathy development. miR, microRNA; SATB2, special AT-rich sequence-binding protein 2; BDNF, brain-derived neurotrophic factor; VEGF, vascular endothelial growth factor; PDCD4, programmed cell death 4; TP53, tumor protein 53; EGR1, early growth response 1; KLF6, Kruppel-like factor 6; SP1, specific protein 1; FGF7, fibroblast growth factor 7; AKT, protein kinase B; IGF, insulin-like growth factor 1; FGF1, fibroblast growth factor 1.
3. Conclusion
OSA is associated with metabolic complications such as metabolic syndrome and T2DM. Many of the possible molecular pathways involved in the relationship between OSA and metabolic complications have been described in the literature. In this review, we summarized the available data about the role of miRNAs in OSA-related metabolic disorders development. The literature suggests that OSA alters the expression of miRNAs in the organism. It results in altered gene expression. The most important examples include miRNA-181a and miRNA-199a, which play an important role in the metabolic consequences of OSA development. Future miRNA investigations in the context of hypoxia should focus on the above-mentioned miRNAs. They can act not only as cheaper and more reliable OSA diagnostic markers but also valuable prognostic factors in patients suffering from OSA. For a better understanding of the relationship between OSA and metabolic complications, studies should also focus on the possible change in miRNA levels in response to implemented treatment of T2DM and MetS, and their mechanisms of action.
Author contributions
FFK and AG provided the overall concept and framework of the manuscript. JJ, MM, and FFK researched and identified appropriate articles and wrote the manuscript. JJ and MM were responsible for the visualization. AG, BS, PB, DS, and MS revised the manuscript. All authors have read and agreed to the published version of the manuscript.
Funding
The study was supported by National Science Centre, Poland, Preludium 20 grant no. 2021/41/N/NZ5/00486 (for FFK).
Conflict of interest
The authors declare that the research was conducted in the absence of any commercial or financial relationships that could be construed as a potential conflict of interest.
Publisher’s note
All claims expressed in this article are solely those of the authors and do not necessarily represent those of their affiliated organizations, or those of the publisher, the editors and the reviewers. Any product that may be evaluated in this article, or claim that may be made by its manufacturer, is not guaranteed or endorsed by the publisher.
References
Agrawal, R., Pandey, P., Jha, P., Dwivedi, V., Sarkar, C., and Kulshreshtha, R. (2014). Hypoxic signature of microRNAs in glioblastoma: insights from small RNA deep sequencing. BMC Genomics 15:686. doi: 10.1186/1471-2164-15-686
Ahmed, U., Ashfaq, U. A., Qasim, M., Ahmad, I., Ahmad, H. U., Tariq, M., et al. (2021). Dysregulation of circulating miRNAs promotes the pathogenesis of diabetes-induced cardiomyopathy. PLoS One 16:e0250773. doi: 10.1371/JOURNAL.PONE.0250773
Akhbari, M., Khalili, M., Shahrabi-Farahani, M., Biglari, A., and Bandarian, F. (2019). Expression level of circulating cell free miR-155 gene in serum of patients with diabetic nephropathy. Clin. Lab. 65, 1493–1499. doi: 10.7754/CLIN.LAB.2019.190209
Ali, S., Malloci, M., Safiedeen, Z., Soleti, R., Vergori, L., Vidal-Gómez, X., et al. (2021). LPS-enriched small extracellular vesicles from metabolic syndrome patients trigger endothelial dysfunction by activation of TLR4. Metabolism 118:154727. doi: 10.1016/J.METABOL.2021.154727
Al-Kafaji, G., Al-Mahroos, G., Al-Muhtaresh, H. A., Skrypnyk, C., Sabry, M. A., and Ramadan, A. R. (2016). Decreased expression of circulating microRNA-126 in patients with type 2 diabetic nephropathy: a potential blood-based biomarker. Exp. Ther. Med. 12, 815–822. doi: 10.3892/ETM.2016.3395
Al-Rawaf, H. A. (2019). Circulating microRNAs and adipokines as markers of metabolic syndrome in adolescents with obesity. Clin. Nutr. 38, 2231–2238. doi: 10.1016/J.CLNU.2018.09.024
Arnold, J., Sunilkumar, M., Krishna, V., Yoganand, S., Kumar, M., and Shanmugapriyan, D. (2017). Obstructive sleep apnea. J. Pharm. Bioallied Sci. 9:S26. doi: 10.4103/JPBS.JPBS_155_17
Arslan, B., Şemsi, R., İriz, A., and Sepici Dinçel, A. (2021). The evaluation of serum brain-derived neurotrophic factor and neurofilament light chain levels in patients with obstructive sleep apnea syndrome. Laryngoscope Investig Otolaryngol 6, 1466–1473. doi: 10.1002/LIO2.683
Bonsignore, M. R., Baiamonte, P., Mazzuca, E., Castrogiovanni, A., and Marrone, O. (2019). Obstructive sleep apnea and comorbidities: a dangerous liaison. Multidiscip Respirat Med 14, 1–12. doi: 10.1186/S40248-019-0172-9
Boutagy, N. E., McMillan, R. P., Frisard, M. I., and Hulver, M. W. (2016). Metabolic endotoxemia with obesity: is it real and is it relevant? Biochimie 124, 11–20. doi: 10.1016/J.BIOCHI.2015.06.020
Cao, Y., Ning, P., Li, Q., and Wu, S. (2022). Cancer and obstructive sleep apnea: an updated meta-analysis. Medicine 101:E28930. doi: 10.1097/MD.0000000000028930
Chang, A. C., Fox, T. P., Wang, S., and Wu, A. Y. (2018). Relationship between obstructive sleep apnea and the PRESENCE and severity of diabetic retinopathy. Retina 38, 2197–2206. doi: 10.1097/IAE.0000000000001848
Chen, Y. C., Hsu, P. Y., Su, M. C., Chin, C. H., Liou, C. W., Wang, T. Y., et al. (2020). miR-21-5p under-expression in patients with obstructive sleep apnea modulates intermittent hypoxia with re-oxygenation-induced-cell apoptosis and cytotoxicity by targeting pro-inflammatory TNF-α-TLR4 signaling. Int. J. Mol. Sci. 21:999. doi: 10.3390/IJMS21030999
Chen, X., Yu, X., Li, X., Li, L., Li, F., Guo, T., et al. (2020). MiR-126 targets IL-17A to enhance proliferation and inhibit apoptosis in high-glucose-induced human retinal endothelial cells. Biochem. Cell Biol. 98, 277–283. doi: 10.1139/BCB-2019-0174
Coughlin, S. R., Mawdsley, L., Mugarza, J. A., Calverley, P. M. A., and Wilding, J. P. H. (2004). Obstructive sleep apnoea is independently associated with an increased prevalence of metabolic syndrome. Eur. Heart J. 25, 735–741. doi: 10.1016/J.EHJ.2004.02.021
Cuevas, A., Saavedra, N., Cavalcante, M. F., Salazar, L. A., and Abdalla, D. S. P. (2014). Identification of microRNAs involved in the modulation of pro-angiogenic factors in atherosclerosis by a polyphenol-rich extract from propolis. Arch. Biochem. Biophys. 557, 28–35. doi: 10.1016/J.ABB.2014.04.009
Czarnecka, K. H., Szmyd, B., Barańska, M., Kaszkowiak, M., Kordiak, J., Antczak, A., et al. (2019). A strong decrease in TIMP3 expression mediated by the Presence of miR-17 and 20a enables extracellular matrix remodeling in the NSCLC lesion surroundings. Front. Oncol. 9:1372. doi: 10.3389/fonc.2019.01372
Dehghani, M., Aghaei Zarch, S. M., Vahidi Mehrjardi, M. Y., Nazari, M., Babakhanzadeh, E., Ghadimi, H., et al. (2020). Evaluation of miR-181b and miR-126-5p expression levels in T2DM patients compared to healthy individuals: relationship with NF-κB gene expression. Endocrinol Diabetes Nutr 67, 454–460. doi: 10.1016/J.ENDINU.2019.09.009
Deng, J., and Guo, F. (2019). Micrornas and type 2 diabetes. ExRNA 1, 1–5. doi: 10.1186/S41544-019-0038-5/TABLES/1
Doghish, A. S., Elsisi, A. M., Amin, A. I., and Abulsoud, A. I. (2021). Circulating miR-148a-5p and miR-21-5p as novel diagnostic biomarkers in adult Egyptian male patients with metabolic syndrome. Can. J. Diabetes 45, 614–618. doi: 10.1016/J.JCJD.2020.12.005
Dong, P., Zhao, J., Li, N., Lu, L., Li, L., Zhang, X., et al. (2018). Sevoflurane exaggerates cognitive decline in a rat model of chronic intermittent hypoxia by aggravating microglia-mediated neuroinflammation via downregulation of PPAR-γ in the hippocampus. Behav. Brain Res. 347, 325–331. doi: 10.1016/J.BBR.2018.03.031
Drager, L. F., Lopes, H. F., Maki-Nunes, C., Trombetta, I. C., Toschi-Dias, E., Alves, M. J. N. N., et al. (2010). The impact of obstructive sleep apnea on metabolic and inflammatory markers in consecutive patients with metabolic syndrome. PLoS One 5:e12065. doi: 10.1371/JOURNAL.PONE.0012065
Drager, L. F., Queiroz, E. L., Lopes, H. F., Genta, P. R., Krieger, E. M., and Lorenzi-Filho, G. (2009). Obstructive sleep apnea is highly prevalent and correlates with impaired glycemic control in consecutive patients with the metabolic syndrome. J. Cardiometab. Syndr. 4, 89–95. doi: 10.1111/J.1559-4572.2008.00046.X
Dutkowska, A., Szmyd, B., Kaszkowiak, M., Domańska-Senderowska, D., Pastuszak-Lewandoska, D., Brzeziańska-Lasota, E., et al. (2021). Expression of inflammatory interleukins and selected miRNAs in non-small cell lung cancer. Sci. Rep. 11 11, 5092–5011. doi: 10.1038/s41598-021-84408-1
Engin, A. (2017). The definition and prevalence of obesity and metabolic syndrome. Adv. Exp. Med. Biol. 960, 1–17. doi: 10.1007/978-3-319-48382-5_1
Fallahi, A., Jamil, D. I., Karimi, E. B., Baghi, V., and Gheshlagh, R. G. (2019). Prevalence of obstructive sleep apnea in patients with type 2 diabetes: a systematic review and meta-analysis. Diabetes Metab. Syndr. 13, 2463–2468. doi: 10.1016/J.DSX.2019.06.030
Fleming, W. E., Holty, J. E. C., Bogan, R. K., Hwang, D., Ferouz-Colborn, A. S., Budhiraja, R., et al. (2018). Use of blood biomarkers to screen for obstructive sleep apnea. Nat Sci Sleep 10, 159–167. doi: 10.2147/NSS.S164488
Freude, T., Braun, K. F., Haug, A., Pscherer, S., Stöckle, U., Nussler, A. K., et al. (2012). Hyperinsulinemia reduces osteoblast activity in vitro via upregulation of TGF-β. J. Mol. Med. (Berl) 90, 1257–1266. doi: 10.1007/S00109-012-0948-2
Fu, Y., Xia, Y., Yi, H., Xu, H., Guan, J., and Yin, S. (2017). Meta-analysis of all-cause and cardiovascular mortality in obstructive sleep apnea with or without continuous positive airway pressure treatment. Sleep Breath. 21, 181–189. doi: 10.1007/S11325-016-1393-1
Gabryelska, A., Chrzanowski, J., Sochal, M., Kaczmarski, P., Turkiewicz, S., Ditmer, M., et al. (2021a). Nocturnal oxygen saturation parameters as independent risk factors for type 2 diabetes mellitus among obstructive sleep apnea patients. J. Clin. Med. 10:3770. doi: 10.3390/JCM10173770
Gabryelska, A., Karuga, F. F., Szmyd, B., and Białasiewicz, P. (2020a). HIF-1α as a mediator of insulin resistance, T2DM, and its complications: potential links with obstructive sleep apnea. Front. Physiol. 11:1035. doi: 10.3389/FPHYS.2020.01035/BIBTEX
Gabryelska, A., and Sochal, M. (2022). Evaluation of HIF-1 involvement in the BDNF and ProBDNF signaling pathways among obstructive sleep apnea patients. Int. J. Molec. Sci. 23:14876. doi: 10.3390/IJMS232314876
Gabryelska, A., Sochal, M., Wasik, B., Szczepanowski, P., and Białasiewicz, P. (2021b). Factors affecting long-term compliance of CPAP treatment single Centre experience. J. Clin. Med. 11:139. doi: 10.3390/JCM11010139
Gabryelska, A., Stawski, R., Sochal, M., Szmyd, B., and Białasiewicz, P. (2020b). Influence of one-night CPAP therapy on the changes of HIF-1α protein in OSA patients: a pilot study. J. Sleep Res. 29:e12995. doi: 10.1111/JSR.12995
Gabryelska, A., Szmyd, B., Panek, M., Szemraj, J., Kuna, P., and Białasiewiczw, P. (2020c). Serum hypoxia–inducible factor–1α protein level as a diagnostic marker of obstructive sleep apnea. Pol Arch Intern Med 130, 158–160. doi: 10.20452/PAMW.15104
Gabryelska, A., Szmyd, B., Szemraj, J., Stawski, R., Sochal, M., and Białasiewicz, P. (2020d). Patients with obstructive sleep apnea present with chronic upregulation of serum HIF-1α protein. J. Clin. Sleep Med. 6, 1761–1768. doi: 10.5664/JCSM.8682
Gabryelska, A., Turkiewicz, S., Ditmer, M., Karuga, F. F., Strzelecki, D., Białasiewicz, P., et al. (2022). BDNF and proBDNF serum protein levels in obstructive sleep apnea patients and their involvement in insomnia and depression symptoms. J. Clin. Med. 11:7135. doi: 10.3390/JCM11237135
Gabryelska, A., Turkiewicz, S., Ditmer, M., and Sochal, M. (2023). Neurotrophins in the neuropathophysiology, course, and complications of obstructive sleep apnea; a narrative review. Int. J. Molec. Sci. 24:1808. doi: 10.3390/IJMS24031808
Galicia-Garcia, U., Benito-Vicente, A., Jebari, S., Larrea-Sebal, A., Siddiqi, H., Uribe, K. B., et al. (2020). Pathophysiology of type 2 diabetes mellitus. Int. J. Mol. Sci. 21, 1–34. doi: 10.3390/IJMS21176275
Gao, J., Liang, Z., Zhao, F., Liu, X., and Ma, N. (2022). Triptolide inhibits oxidative stress and inflammation via the microRNA-155-5p/brain-derived neurotrophic factor to reduce podocyte injury in mice with diabetic nephropathy. Bioengineered 13, 12275–12288. doi: 10.1080/21655979.2022.2067293
Gaspar, L. S., Santos-Carvalho, A., Santos, B., Carvalhas-Almeida, C., Barros-Viegas, A. T., Oliveiros, B., et al. (2022). Peripheral biomarkers to diagnose obstructive sleep apnea in adults: a systematic review and meta-analysis. Sleep Med. Rev. 64:101659. doi: 10.1016/j.smrv.2022.101659
Gok, O., Karaali, Z., Ergen, A., Ekmekci, S. S., and Abaci, N. (2019). Serum sirtuin 1 protein as a potential biomarker for type 2 diabetes: increased expression of sirtuin 1 and the correlation with microRNAs. J. Res. Med. Sci. 24:56. doi: 10.4103/JRMS.JRMS_921_18
Guan, C. Y., Cao, J. L., Zhang, L., Wang, X. Q., Ma, X., and Xia, H. F. (2022). miR-199a is upregulated in GDM targeting the MeCP2-Trpc3 pathway. Front. Endocrinol. (Lausanne) 13:917386. doi: 10.3389/FENDO.2022.917386
Guo, L., Tan, K., Luo, Q., and Bai, X. (2020). Dihydromyricetin promotes autophagy and attenuates renal interstitial fibrosis by regulating miR-155-5p/PTEN signaling in diabetic nephropathy. Bosn. J. Basic Med. Sci. 20, 372–380. doi: 10.17305/BJBMS.2019.4410
Guo, C., Zhang, M., Su, W., Xu, M., and Zhao, S. (2022). miR-199a-5p relieves obstructive sleep apnea syndrome-related hypertension by targeting HIF-1 α. J Immunol Res. 2022:7236647. doi: 10.1155/2022/7236647
Han, D., Liu, W., Li, G., and Liu, L. (2021). Circ_PRKDC knockdown promotes skin wound healing by enhancing keratinocyte migration via miR-31/FBN1 axis. J. Mol. Histol. 52, 681–691. doi: 10.1007/S10735-021-09996-8
Hassani, S. S., Karamali, N., Rajabinejad, M., Ashjari, D., Afshar Hezarkhani, L., Gorgin Karaji, A., et al. (2023). Dysregulation of long noncoding RNA NEAT1/miR-199a-5/BiP Axis in patients with diabetic neuropathy. Lab. Med. 54, 160–165. doi: 10.1093/LABMED/LMAC082
He, Z. Y., Wei, T. H., Zhang, P. H., Zhou, J., and Huang, X. Y. (2019). Long noncoding RNA-antisense noncoding RNA in the INK4 locus accelerates wound healing in diabetes by promoting lymphangiogenesis via regulating miR-181a/Prox1 axis. J. Cell. Physiol. 234, 4627–4640. doi: 10.1002/JCP.27260
Hernández-Jiménez, E., Cubillos-Zapata, C., Toledano, V., Pérez de Diego, R., Fernández-Navarro, I., Casitas, R., et al. (2017). Monocytes inhibit NK activity via TGF-β in patients with obstructive sleep apnoea. Eur. Respir. J. 49:1602456. doi: 10.1183/13993003.02456-2016
Hromadnikova, I., Kotlabova, K., Dvorakova, L., and Krofta, L. (2020). Diabetes mellitus and cardiovascular risk assessment in mothers with a history of gestational diabetes mellitus based on postpartal expression profile of MicroRNAs associated with diabetes mellitus and cardiovascular and cerebrovascular diseases. Int. J. Mol. Sci. 21:2437. doi: 10.3390/IJMS21072437
Hromadnikova, I., Kotlabova, K., and Krofta, L. (2022). Cardiovascular disease-associated MicroRNAs as novel biomarkers of first-trimester screening for gestational diabetes mellitus in the absence of other pregnancy-related complications. Int. J. Mol. Sci. 23:10635. doi: 10.3390/IJMS231810635
Hu, J., Huang, Y., Lin, Y., and Lin, J. (2020). Protective effect inhibiting the expression of miR-181a on the diabetic corneal nerve in a mouse model. Exp. Eye Res. 192:107925. doi: 10.1016/J.EXER.2020.107925
Huang, J., Yu, M., Yin, W., Liang, B., Li, A., Li, J., et al. (2021). Development of a novel RNAi therapy: engineered miR-31 exosomes promoted the healing of diabetic wounds. Bioact Mater 6, 2841–2853. doi: 10.1016/J.BIOACTMAT.2021.02.007
Huang, Y., Zhao, L., Yan, Y., Chen, J., Liu, P., Xv, W., et al. (2020). PBMCs to stress-associated miR-18a-5p and miR-22-3p ratios as new indicators of metabolic syndrome. Biomed. Res. Int. 2020, 1–10. doi: 10.1155/2020/8159342
Hulsmans, M., Sinnaeve, P., Van Der Schueren, B., Mathieu, C., Janssens, S., and Holvoet, P. (2012). Decreased miR-181a expression in monocytes of obese patients is associated with the occurrence of metabolic syndrome and coronary artery disease. J. Clin. Endocrinol. Metab. 97, E1213–E1218. doi: 10.1210/JC.2012-1008
Imamura, T., Poulsen, O., and Haddad, G. G. (2016). Intermittent hypoxia induces murine macrophage foam cell formation by IKK-β-dependent NF-κB pathway activation. J. Appl. Physiol. 1985, 670–677. doi: 10.1152/JAPPLPHYSIOL.00307.2016
Jankauskas, S. S., Gambardella, J., Sardu, C., Lombardi, A., and Santulli, G. (2021). Functional role of miR-155 in the pathogenesis of diabetes mellitus and its complications. Noncoding RNA 7:39. doi: 10.3390/NCRNA7030039
Jansen, F., Wang, H., Przybilla, D., Franklin, B. S., Dolf, A., Pfeifer, P., et al. (2016). Vascular endothelial microparticles-incorporated microRNAs are altered in patients with diabetes mellitus. Cardiovasc. Diabetol. 15:49. doi: 10.1186/S12933-016-0367-8
Ji, L., Liu, Y., Liu, P., Ji, G., He, J., Gan, Y., et al. (2021). Serum periostin and TNF-α levels in patients with obstructive sleep apnea-hypopnea syndrome. Sleep Breath. 25, 331–337. doi: 10.1007/S11325-020-02124-Y
Kaczmarski, P., Karuga, F. F., Szmyd, B., Sochal, M., Białasiewicz, P., Strzelecki, D., et al. (2022). The role of inflammation, hypoxia, and opioid receptor expression in pain modulation in patients suffering from obstructive sleep apnea. Molec Sci. 23:9080. doi: 10.3390/IJMS23169080
Kapur, V. K., Auckley, D. H., Chowdhuri, S., Kuhlmann, D. C., Mehra, R., Ramar, K., et al. (2017). Clinical practice guideline for diagnostic testing for adult obstructive sleep apnea: an American Academy of sleep medicine clinical practice guideline. J. Clin. Sleep Med. 13, 479–504. doi: 10.5664/JCSM.6506
Karuga, F. F., Kaczmarski, P., Szmyd, B., Białasiewicz, P., Sochal, M., and Gabryelska, A. (2022). The association between idiopathic pulmonary fibrosis and obstructive sleep apnea: a systematic review and Meta-analysis. J. Clin. Med. 11:5008. doi: 10.3390/JCM11175008
Kendzerska, T., Mollayeva, T., Gershon, A. S., Leung, R. S., Hawker, G., and Tomlinson, G. (2014). Untreated obstructive sleep apnea and the risk for serious long-term adverse outcomes: a systematic review. Sleep Med. Rev. 18, 49–59. doi: 10.1016/J.SMRV.2013.01.003
Khalyfa, A., Gozal, D., Masa, J. F., Marin, J. M., Qiao, Z., Corral, J., et al. (2018). Sleep-disordered breathing, circulating exosomes, and insulin sensitivity in adipocytes. Int. J. Obes. 42, 1127–1139. doi: 10.1038/S41366-018-0099-9
Khan, M. A. B., Hashim, M. J., King, J. K., Govender, R. D., Mustafa, H., and Kaabi, J.Al. (2020). Epidemiology of type 2 diabetes – global burden of disease and forecasted trends. J Epidemiol Glob Health 10:107. doi: 10.2991/JEGH.K.191028.001
Khurana, S., Waidha, K., Guleria, R., Sharda, S., and Bose, S. (2020). In-silico investigations of selective miRNA-gene targets and their validation studies in obstructive sleep apnea (OSA) patient cohorts. Comput. Biol. Chem. 87:107264. doi: 10.1016/J.COMPBIOLCHEM.2020.107264
Kikuchi, R., Tsuji, T., Watanabe, O., Yamaguchi, K., Furukawa, K., Nakamura, H., et al. (2017). Hypercapnia accelerates adipogenesis: a novel role of high CO2 in exacerbating obesity. Am. J. Respir. Cell Mol. Biol. 57, 570–580. doi: 10.1165/RCMB.2016-0278OC
Kim, C., Lee, H., Cho, Y. M., Kwon, O.-J., Kim, W., and Lee, E. K. (2013). TNFα-induced miR-130 resulted in adipocyte dysfunction during obesity-related inflammation. FEBS Lett. 587, 3853–3858. doi: 10.1016/j.febslet.2013.10.018
Kim, M., and Zhang, X. (2019). The profiling and role of miRNAs in diabetes mellitus. J Diabetes Clin Res 1:5. doi: 10.33696/DIABETES.1.003
Koenen, T. B., Stienstra, R., van Tits, L. J., de Graaf, J., Stalenhoef, A. F. H., Joosten, L. A. B., et al. (2011). Hyperglycemia activates caspase-1 and TXNIP-mediated IL-1beta transcription in human adipose tissue. Diabetes 60, 517–524. doi: 10.2337/DB10-0266
Kong, D. L., Qin, Z., Wang, W., Pan, Y., Kang, J., and Pang, J. (2016). Association between obstructive sleep apnea and metabolic syndrome: a meta-analysis. Clin. Invest. Med. 39, E161–E172. doi: 10.25011/CIM.V39I5.27148
Kovacs, B., Lumayag, S., Cowan, C., and Xu, S. (2011). MicroRNAs in early diabetic retinopathy in streptozotocin-induced diabetic rats. Invest. Ophthalmol. Vis. Sci. 52, 4402–4409. doi: 10.1167/IOVS.10-6879
Krawczyk, M., Flinta, I., Garncarek, M., Jankowska, E. A., Banasiak, W., Germany, R., et al. (2013). Sleep disordered breathing in patients with heart failure. Cardiol. J. 20, 345–355. doi: 10.5603/CJ.2013.0092
Labarca, G., Dreyse, J., Salas, C., Schmidt, A., Rivera, F., Letelier, F., et al. (2021). Risk of mortality among patients with moderate to severe obstructive sleep apnea and diabetes mellitus: results from the SantOSA cohort. Sleep Breath. 25, 1467–1475. doi: 10.1007/S11325-020-02283-Y
Labarca, G., Reyes, T., Jorquera, J., Dreyse, J., and Drake, L. (2018). CPAP in patients with obstructive sleep apnea and type 2 diabetes mellitus: systematic review and meta-analysis. Clin. Respir. J. 12, 2361–2368. doi: 10.1111/CRJ.12915
Laemmle, A., Lechleiter, A., Roh, V., Schwarz, C., Portmann, S., Furer, C., et al. (2012). Inhibition of SIRT1 impairs the accumulation and transcriptional activity of HIF-1α protein under hypoxic conditions. PLoS One 7:e33433. doi: 10.1371/JOURNAL.PONE.0033433
Leung, A. K. L. (2015). The whereabouts of microRNA actions: cytoplasm and beyond. Trends Cell Biol. 25, 601–610. doi: 10.1016/J.TCB.2015.07.005
Li, Y. B., Wu, Q., Liu, J., Fan, Y. Z., Yu, K. F., and Cai, Y. (2017). miR-199a-3p is involved in the pathogenesis and progression of diabetic neuropathy through downregulation of SerpinE2. Mol. Med. Rep. 16, 2417–2424. doi: 10.3892/MMR.2017.6874
Lian, N., Chen, M., Zhang, S., Chen, L., Huang, J., and Lin, Q. (2021). Decreased expression of PPARγ is associated with aortic endothelial cell apoptosis in intermittently hypoxic rats. Sleep Breath. 25, 2241–2250. doi: 10.1007/S11325-021-02319-X
Lian, W., Hu, X., Shi, R., Han, S., Cao, C., Wang, K., et al. (2018). MiR-31 regulates the function of diabetic endothelial progenitor cells by targeting Satb2. Acta Biochim. Biophys. Sin. Shanghai 50, 336–344. doi: 10.1093/ABBS/GMY010
Liang, X., and Xu, W. (2020). miR-181a-5p regulates the proliferation and apoptosis of glomerular mesangial cells by targeting KLF6. Exp. Ther. Med. 20, 1121–1128. doi: 10.3892/ETM.2020.8780
Lim, J. H., Lee, Y. M., Chun, Y. S., Chen, J., Kim, J. E., and Park, J. W. (2010). Sirtuin 1 modulates cellular responses to hypoxia by deacetylating hypoxia-inducible factor 1alpha. Mol. Cell 38, 864–878. doi: 10.1016/J.MOLCEL.2010.05.023
Lin, X., You, Y., Wang, J., Qin, Y., Huang, P., and Yang, F. (2015). MicroRNA-155 deficiency promotes nephrin acetylation and attenuates renal damage in hyperglycemia-induced nephropathy. Inflammation 38, 546–554. doi: 10.1007/S10753-014-9961-7
Lin, N., Zhao, G. J., Qin, L. L., Yang, Z., and Su, Q. (2017). Association between advanced oxidation protein products (AOPP) and vascular calcification in uremic patients. Eur. Rev. Med. Pharmacol. Sci. 21, 4147–4152.
Liu, Y., Gao, G., Yang, C., Zhou, K., Shen, B., Liang, H., et al. (2014). The role of circulating microRNA-126 (miR-126): a novel biomarker for screening prediabetes and newly diagnosed type 2 diabetes mellitus. Int. J. Mol. Sci. 15, 10567–10577. doi: 10.3390/IJMS150610567
Liu, X., Gong, Q., Yang, L., Liu, M., Niu, L., and Wang, L. (2020). microRNA-199a-5p regulates epithelial-to-mesenchymal transition in diabetic cataract by targeting SP1 gene. Mol. Med. 26:122. doi: 10.1186/S10020-020-00250-7
Liu, D., Myles, H., Foley, D. L., Watts, G. F., Morgan, V. A., Castle, D., et al. (2016). Risk factors for obstructive sleep apnea are prevalent in people with psychosis and correlate with impaired social functioning and poor physical health. Front. Psych. 7:139. doi: 10.3389/FPSYT.2016.00139
Liu, L., Su, X., Zhao, Z., Han, J., Li, J., Xu, W., et al. (2022). Association of metabolic syndrome with long-term cardiovascular risks and all-cause mortality in elderly patients with obstructive sleep apnea. Front Cardiovasc Med 8:813280. doi: 10.3389/FCVM.2021.813280
Logan, A. G., Perlikowski, S. M., Mente, A., Tisler, A., Tkacova, R., Niroumand, M., et al. (2001). High prevalence of unrecognized sleep apnoea in drug-resistant hypertension. J. Hypertens. 19, 2271–2277. doi: 10.1097/00004872-200112000-00022
Lozano-Bartolomé, J., Llauradó, G., Portero-Otin, M., Altuna-Coy, A., Rojo-Martínez, G., Vendrell, J., et al. (2018). Altered expression of miR-181a-5p and miR-23a-3p is associated with obesity and TNFα-induced insulin resistance. J. Clin. Endocrinol. Metab. 103, 1447–1458. doi: 10.1210/JC.2017-01909
Macharia, L. W., Muriithi, W., Heming, C. P., Nyaga, D. K., Aran, V., Mureithi, M. W., et al. (2021). The genotypic and phenotypic impact of hypoxia microenvironment on glioblastoma cell lines. BMC Cancer 21:1248. doi: 10.1186/S12885-021-08978-Z
Mahmood, K., Akhter, N., Eldeirawi, K., Önal, E., Christman, J. W., Carley, D. W., et al. (2009). Prevalence of type 2 diabetes in patients with obstructive sleep apnea in a multi-ethnic sample. J. Clin. Sleep Med. 5, 215–221. doi: 10.5664/jcsm.27489
Malicki, M., Karuga, F. F., Szmyd, B., Sochal, M., and Gabryelska, A. (2022). Obstructive sleep apnea, circadian clock disruption, and metabolic consequences. Meta 13:60. doi: 10.3390/metabo13010060
Maltese, G., Fountoulakis, N., Drakatos, P., Shah, D., Patel, K., Sharma, A., et al. (2018). Elevated obstructive sleep apnoea risk score is associated with poor healing of diabetic foot ulcers: a prospective cohort study. Diabet. Med. 35, 1494–1498. doi: 10.1111/DME.13780
Masutani, H., Yoshihara, E., Masaki, S., Chen, Z., and Yodoi, J. (2012). Thioredoxin binding protein (TBP)-2/Txnip and α-arrestin proteins in cancer and diabetes mellitus. J. Clin. Biochem. Nutr. 50, 23–34. doi: 10.3164/JCBN.11-36SR
Meng, S., Cao, J. T., Zhang, B., Zhou, Q., Shen, C. X., and Wang, C. Q. (2012). Downregulation of microRNA-126 in endothelial progenitor cells from diabetes patients, impairs their functional properties, via target gene Spred-1. J. Mol. Cell. Cardiol. 53, 64–72. doi: 10.1016/J.YJMCC.2012.04.003
Misra, A., and Shrivastava, U. (2016). Obstructive sleep apnea and diabetic nephropathy. Diabetes Technol. Ther. 18, 405–407. doi: 10.1089/DIA.2016.0147
Nakayama, L. F., Tempaku, P. F., Bergamo, V. C., Polizelli, M. U., Santos da Cruz, N. F., Bittencourt, L. R. A., et al. (2021). Obstructive sleep apnea and the retina: a review. J. Clin. Sleep Med. 17, 1947–1952. doi: 10.5664/JCSM.9312
Ofori, J. K., Salunkhe, V. A., Bagge, A., Vishnu, N., Nagao, M., Mulder, H., et al. (2017). Elevated miR-130a/miR130b/miR-152 expression reduces intracellular ATP levels in the pancreatic beta cell. Sci. Rep. 7:44986. doi: 10.1038/SREP44986
Pai, P. Y., Lin, Y. Y., Yu, S. H., Lin, C. Y., Liou, Y. F., Wu, X. B., et al. (2022). Angiotensin II receptor blocker irbesartan attenuates sleep apnea-induced cardiac apoptosis and enhances cardiac survival and sirtuin 1 upregulation. Sleep Breath. 26, 1161–1172. doi: 10.1007/S11325-021-02499-6
Patil, S. P., Ayappa, I. A., Caples, S. M., John Kimoff, R., Patel, S. R., and Harrod, C. G. (2019). Treatment of adult obstructive sleep apnea with positive airway pressure: an American Academy of sleep medicine systematic review, Meta-analysis, and GRADE assessment. J. Clin. Sleep Med. 15:301. doi: 10.5664/JCSM.7638
Pham, L. V., and Schwartz, A. R. (2015). The pathogenesis of obstructive sleep apnea. J. Thorac. Dis. 7:1358. doi: 10.3978/J.ISSN.2072-1439.2015.07.28
Phillips, C. L., Yee, B. J., Marshall, N. S., Liu, P. Y., Sullivan, D. R., and Grunstein, R. R. (2011). Continuous positive airway pressure reduces postprandial lipidemia in obstructive sleep apnea: a randomized, placebo-controlled crossover trial. Am. J. Respir. Crit. Care Med. 184, 355–361. doi: 10.1164/RCCM.201102-0316OC
Pordzik, J., Eyileten-Postuła, C., Jakubik, D., Czajka, P., Nowak, A., de Rosa, S., et al. (2021). MiR-126 is an independent predictor of long-term all-cause mortality in patients with type 2 diabetes mellitus. J. Clin. Med. 10:2371. doi: 10.3390/JCM10112371
Pozniak, T., Shcharbin, D., and Bryszewska, M. (2022). Circulating microRNAs in medicine. Int. J. Mol. Sci. 23:3996. doi: 10.3390/IJMS23073996
Qi, M., He, L., Ma, X., and Li, Z. (2020). MiR-181a-5p is involved in the cardiomyocytes apoptosis induced by hypoxia-reoxygenation through regulating SIRT1. Biosci. Biotechnol. Biochem. 84, 1353–1361. doi: 10.1080/09168451.2020.1750943
Qin, W., Zhang, Y., Deng, B., Liu, J., Zhang, H., and Jin, Z. (2019). MiR-17-5p modulates mitochondrial function of the genioglossus muscle satellite cells through targeting Mfn2 in hypoxia. J. Biol. Regul. Homeost. Agents 33, 753–761.
Ramirez, H. A., Liang, L., Pastar, I., Rosa, A. M., Stojadinovic, O., Zwick, T. G., et al. (2015). Comparative genomic, MicroRNA, and tissue analyses reveal subtle differences between non-diabetic and diabetic foot skin. PLoS One 10. doi: 10.1371/JOURNAL.PONE.0137133
Ramzan, F., D’Souza, R. F., Durainayagam, B. R., Milan, A. M., Markworth, J. F., Miranda-Soberanis, V., et al. (2020). Circulatory miRNA biomarkers of metabolic syndrome. Acta Diabetol. 57, 203–214. doi: 10.1007/S00592-019-01406-6
Rane, S., He, M., Sayed, D., Vashistha, H., Malhotra, A., Sadoshima, J., et al. (2009). Downregulation of miR-199a derepresses hypoxia-inducible factor-1alpha and Sirtuin 1 and recapitulates hypoxia preconditioning in cardiac myocytes. Circ. Res. 104, 879–886. doi: 10.1161/CIRCRESAHA.108.193102
Rane, S., He, M., Sayed, D., Yan, L., Vatner, D., and Abdellatif, M. (2010). An antagonism between the AKT and beta-adrenergic signaling pathways mediated through their reciprocal effects on miR-199a-5p. Cell. Signal. 22, 1054–1062. doi: 10.1016/J.CELLSIG.2010.02.008
Raut, S. K., Singh, G. B., Rastogi, B., Saikia, U. N., Mittal, A., Dogra, N., et al. (2016). miR-30c and miR-181a synergistically modulate p53-p21 pathway in diabetes induced cardiac hypertrophy. Mol. Cell. Biochem. 417, 191–203. doi: 10.1007/S11010-016-2729-7
Reaven, G. M. (1988). Banting lecture 1988. Role of insulin resistance in human disease. Diabetes 37, 1595–1607. doi: 10.2337/DIAB.37.12.1595
Rega-Kaun, G., Kaun, C., Jaegersberger, G., Prager, M., Hackl, M., Demyanets, S., et al. (2020). Roux-en-Y-bariatric surgery reduces markers of metabolic syndrome in morbidly obese patients. Obes. Surg. 30, 391–400. doi: 10.1007/S11695-019-04190-Y
Ren, J., Liu, W., Li, G. C., Jin, M., You, Z. X., Liu, H. G., et al. (2018). Atorvastatin attenuates myocardial hypertrophy induced by chronic intermittent hypoxia in vitro partly through miR-31/PKCε pathway. Curr Med Sci 38, 405–412. doi: 10.1007/S11596-018-1893-2
Reutrakul, S., and Mokhlesi, B. (2017). Obstructive sleep apnea and diabetes: a state of the art review. Chest 152, 1070–1086. doi: 10.1016/J.CHEST.2017.05.009
Reutrakul, S., and Mokhlesi, B. (2020). Can long-term treatment of obstructive sleep apnea with CPAP improve glycemia and prevent type 2 diabetes? Diabetes Care 43, 1681–1683. doi: 10.2337/DCI20-0014
Rezk, N. A., Sabbah, N. A., and Saad, M. S. S. (2016). Role of MicroRNA 126 in screening, diagnosis, and prognosis of diabetic patients in Egypt. IUBMB Life 68, 452–458. doi: 10.1002/IUB.1502
Rovira-Llopis, S., Escribano-Lopez, I., Diaz-Morales, N., Iannantuoni, F., Lopez-Domenech, S., Andújar, I., et al. (2018). Downregulation of miR-31 in diabetic nephropathy and its relationship with inflammation. Cell. Physiol. Biochem. 50, 1005–1014. doi: 10.1159/000494485
Saklayen, M. G. (2018). The global epidemic of the metabolic syndrome. Curr. Hypertens. Rep. 20:12. doi: 10.1007/S11906-018-0812-Z
Salari, N., Khazaie, H., Abolfathi, M., Ghasemi, H., Shabani, S., Rasoulpoor, S., et al. (2022). The effect of obstructive sleep apnea on the increased risk of cardiovascular disease: a systematic review and meta-analysis. Neurol. Sci. 43, 219–231. doi: 10.1007/S10072-021-05765-3
Samson, S. L., and Garber, A. J. (2014). Metabolic syndrome. Endocrinol. Metab. Clin. N. Am. 43, 1–23. doi: 10.1016/J.ECL.2013.09.009
Santamaria-Martos, F., Benítez, I., Ortega, F., Zapater, A., Giron, C., Pinilla, L., et al. (2019a). Circulating microRNA profile as a potential biomarker for obstructive sleep apnea diagnosis. Sci. Rep. 9:13456. doi: 10.1038/S41598-019-49940-1
Santamaria-Martos, F., Benítez, I., Zapater, A., Girón, C., Pinilla, L., Fernandez-Real, J. M., et al. (2019b). Identification and validation of circulating miRNAs as endogenous controls in obstructive sleep apnea. PLoS One 14:e0213622. doi: 10.1371/JOURNAL.PONE.0213622
Sapp, R. M., Shill, D. D., Dash, C., Hicks, J. C., Adams-Campbell, L. L., and Hagberg, J. M. (2019). Circulating microRNAs and endothelial cell migration rate are associated with metabolic syndrome and fitness level in postmenopausal African American women. Physiol. Rep. 7. doi: 10.14814/PHY2.14173
Sekar, D., Venugopal, B., Sekar, P., and Ramalingam, K. (2016). Role of microRNA 21 in diabetes and associated/related diseases. Gene 582, 14–18. doi: 10.1016/J.GENE.2016.01.039
Shao, H., Shen, P., and Chen, J. (2021). Expression profile analysis and image observation of miRNA in serum of patients with obstructive sleep apnea-hypopnea syndrome. Contrast Media Mol. Imaging 2021:9731502. doi: 10.1155/2021/9731502
Silvestris, N., Danza, K., Longo, V., Brunetti, O., Fucci, L., Argentiero, A., et al. (2017). Angiogenesis in adenosquamous cancer of pancreas. Oncotarget 8, 95773–95779. doi: 10.18632/ONCOTARGET.21319
Song, B., Wei, D., Yin, G., Song, X., Wang, S., Jia, S., et al. (2021). Critical role of SIRT1 upregulation on the protective effect of lncRNA ANRIL against hypoxia/reoxygenation injury in H9c2 cardiomyocytes. Mol. Med. Rep. 24:547. doi: 10.3892/MMR.2021.12186
Song, J., Yang, B., Jia, X., Li, M., Tan, W., Ma, S., et al. (2018). Distinctive roles of Sirtuins on diabetes, protective or detrimental? Front Endocrinol (Lausanne) 9:724. doi: 10.3389/FENDO.2018.00724
Su, X., Li, J. H., Gao, Y., Chen, K., Gao, Y., Guo, J. J., et al. (2021). Impact of obstructive sleep apnea complicated with type 2 diabetes on long-term cardiovascular risks and all-cause mortality in elderly patients. BMC Geriatr. 21:508. doi: 10.1186/S12877-021-02461-X
Sun, X., Charbonneau, C., Wei, L., Chen, Q., and Terek, R. M. (2015a). miR-181a targets RGS16 to promote chondrosarcoma growth, angiogenesis, and metastasis. Mol. Cancer Res. 13, 1347–1357. doi: 10.1158/1541-7786.MCR-14-0697
Sun, X., Wei, L., Chen, Q., and Terek, R. M. (2015b). MicroRNA regulates vascular endothelial growth factor expression in chondrosarcoma cells. Clin. Orthop. Relat. Res. 473, 907–913. doi: 10.1007/S11999-014-3842-0
Sun, C., Zhang, F., Ge, X., Yan, T., Chen, X., Shi, X., et al. (2007). SIRT1 improves insulin sensitivity under insulin-resistant conditions by repressing PTP1B. Cell Metab. 6, 307–319. doi: 10.1016/J.CMET.2007.08.014
Swaroop, J. J., Rajarajeswari, D., and Naidu, J. N. (2012). Association of TNF-α with insulin resistance in type 2 diabetes mellitus. Indian J. Med. Res. 135, 127–130. doi: 10.4103/0971-5916.93435
Szweda, N., and Łaczmański, Ł. (2016). miRNA in type 2 diabetes. Clin Diabetol 5, 100–106. doi: 10.5603/DK.2016.0016
Takahara, S., Lee, S. Y., Iwakura, T., Oe, K., Fukui, T., Okumachi, E., et al. (2018). Altered expression of microRNA during fracture healing in diabetic rats. Bone Joint Res 7, 139–147. doi: 10.1302/2046-3758.72.BJR-2017-0082.R1
Trombetta, I. C., Maki-Nunes, C., Toschi-Dias, E., Alves, M. J. N. N., Rondon, M. U. P. B., Cepeda, F. X., et al. (2013). Obstructive sleep apnea is associated with increased chemoreflex sensitivity in patients with metabolic syndrome. Sleep 36, 41–49. doi: 10.5665/SLEEP.2298
Turkiewicz, S., Ditmer, M., Sochal, M., Białasiewicz, P., Strzelecki, D., and Gabryelska, A. (2021). Obstructive sleep apnea as an acceleration trigger of cellular senescence processes through telomere shortening. Int. J. Molec. Sci. 22:12536. doi: 10.3390/IJMS222212536
Wang, X., Gao, Y., Yi, W., Qiao, Y., Hu, H., Wang, Y., et al. (2021). Inhibition of miRNA-155 alleviates high glucose-induced podocyte inflammation by targeting SIRT1 in diabetic mice. J. Diabetes Res. 2021, 1–11. doi: 10.1155/2021/5597394
Wang, L., Liu, W. X., and Huang, X. G. (2020). MicroRNA-199a-3p inhibits angiogenesis by targeting the VEGF/PI3K/AKT signalling pathway in an in vitro model of diabetic retinopathy. Exp. Mol. Pathol. 116:104488. doi: 10.1016/J.YEXMP.2020.104488
Wang, Y., Men, M., Yang, W., Zheng, H., and Xue, S. (2015). MiR-31 downregulation protects against cardiac ischemia/reperfusion injury by targeting protein kinase C epsilon (PKCε) directly. Cell. Physiol. Biochem. 36, 179–190. doi: 10.1159/000374062
Wang, C., Tan, J., Miao, Y., and Zhang, Q. (2022). Obstructive sleep apnea, prediabetes and progression of type 2 diabetes: a systematic review and meta-analysis. J. Diabetes Investig. 13, 1396–1411. doi: 10.1111/JDI.13793
Wang, Y. T., Tsai, P. C., Liao, Y. C., Hsu, C. Y., and Juo, S. H. H. (2013). Circulating microRNAs have a sex-specific association with metabolic syndrome. J. Biomed. Sci. 20:72. doi: 10.1186/1423-0127-20-72
Wang, H., Wang, X., Liu, X., Zhou, J., Yang, Q., Chai, B., et al. (2022). miR-199a-5p plays a pivotal role on wound healing via suppressing VEGFA and ROCK1 in diabetic ulcer foot. Oxidative Med. Cell. Longev. 2022:4791059. doi: 10.1155/2022/4791059
Wang, J., Wu, H., Peng, Y., Zhao, Y., Qin, Y., Zhang, Y., et al. (2021). Hypoxia adipose stem cell-derived exosomes promote high-quality healing of diabetic wound involves activation of PI3K/Akt pathways. J Nanobiotechnol 19:202. doi: 10.1186/S12951-021-00942-0
Wang, G., Wu, B., Zhang, B., Wang, K., and Wang, H. (2020). lncRNA CTBP1-AS2 alleviates high glucose-induced oxidative stress, ECM accumulation, and inflammation in diabetic nephropathy via miR-155-5p/FOXO1 axis. Biochem. Biophys. Res. Commun. 532, 308–314. doi: 10.1016/J.BBRC.2020.08.073
Wang, H., Xiong, W., Hang, S., Wang, Y., Zhang, S., and Liu, S. (2021). Depletion of SENP1-mediated PPARγ SUMOylation exaggerates intermittent hypoxia-induced cognitive decline by aggravating microglia-mediated neuroinflammation. Aging 13, 15240–15254. doi: 10.18632/AGING.203084
Wang, Y., Zheng, Z. J., Jia, Y. J., Yang, Y. L., and Xue, Y. M. (2018). Role of p53/miR-155-5p/sirt1 loop in renal tubular injury of diabetic kidney disease. J. Transl. Med. 16:146. doi: 10.1186/S12967-018-1486-7
Wang, D., Zhou, Y., Chen, R., Zeng, X., Zhang, S., Su, X., et al. (2023). The relationship between obstructive sleep apnea and asthma severity and vice versa: a systematic review and meta-analysis. Eur. J. Med. Res. 28:139. doi: 10.1186/S40001-023-01097-4
Waters, T. (2019). Alternative interventions for obstructive sleep apnea. Cleve. Clin. J. Med. 86, 34–41. doi: 10.3949/CCJM.86.S1.06
Weale, C. J., Matshazi, D. M., Davids, S. F. G., Raghubeer, S., Erasmus, R. T., Kengne, A. P., et al. (2021). MicroRNAs-1299, −126-3p and -30e-3p as potential diagnostic biomarkers for prediabetes. Diagnostics (Basel) 11:949. doi: 10.3390/DIAGNOSTICS11060949
Weihe, P., and Weihrauch-Blüher, S. (2019). Metabolic syndrome in children and adolescents: diagnostic criteria, therapeutic options and perspectives. Curr. Obes. Rep. 8, 472–479. doi: 10.1007/S13679-019-00357-X
Wu, X., Chang, S. C., Jin, J., Gu, W., and Li, S. (2018). NLRP3 inflammasome mediates chronic intermittent hypoxia-induced renal injury implication of the microRNA-155/FOXO3a signaling pathway. J. Cell. Physiol. 233, 9404–9415. doi: 10.1002/JCP.26784
Xie, W., Li, Z., Li, M., Xu, N., and Zhang, Y. (2013b). miR-181a and inflammation: miRNA homeostasis response to inflammatory stimuli in vivo. Biochem. Biophys. Res. Commun. 430, 647–652. doi: 10.1016/J.BBRC.2012.11.097
Xie, W., Li, M., Xu, N., Lv, Q., Huang, N., He, J., et al. (2013a). MiR-181a regulates inflammation responses in monocytes and macrophages. PLoS One 8. doi: 10.1371/JOURNAL.PONE.0058639
Xu, P., Guan, M. P., Bi, J. G., Wang, D., Zheng, Z. J., and Xue, Y. M. (2017). High glucose down-regulates microRNA-181a-5p to increase pro-fibrotic gene expression by targeting early growth response factor 1 in HK-2 cells. Cell. Signal. 31, 96–104. doi: 10.1016/J.CELLSIG.2017.01.012
Yan, C., Chen, J., Wang, C., Yuan, M., Kang, Y., Wu, Z., et al. (2022). Milk exosomes-mediated miR-31-5p delivery accelerates diabetic wound healing through promoting angiogenesis. Drug Deliv. 29, 214–228. doi: 10.1080/10717544.2021.2023699
Yan, S. T., Li, C. L., Tian, H., Li, J., Pei, Y., Liu, Y., et al. (2014). MiR-199a is overexpressed in plasma of type 2 diabetes patients which contributes to type 2 diabetes by targeting GLUT4. Mol. Cell. Biochem. 397, 45–51. doi: 10.1007/S11010-014-2170-8
Yan, Y. R., Zhang, L., Lin, Y. N., Sun, X. W., Ding, Y. J., Li, N., et al. (2021). Chronic intermittent hypoxia-induced mitochondrial dysfunction mediates endothelial injury via the TXNIP/NLRP3/IL-1β signaling pathway. Free Radic. Biol. Med. 165, 401–410. doi: 10.1016/J.FREERADBIOMED.2021.01.053
Yang, X., Niu, X., Xiao, Y., Lin, K., and Chen, X. (2018). miRNA expression profiles in healthy OSAHS and OSAHS with arterial hypertension: potential diagnostic and early warning markers. Respir. Res. 19:194. doi: 10.1186/S12931-018-0894-9
Ye, P., Liu, J., He, F., Xu, W., and Yao, K. (2013). Hypoxia-induced deregulation of miR-126 and its regulative effect on VEGF and MMP-9 expression. Int. J. Med. Sci. 11, 17–23. doi: 10.7150/IJMS.7329
Young, T., Palta, M., Dempsey, J., Skatrud, J., Weber, S., and Badr, S. (1993). The occurrence of sleep-disordered breathing among middle-aged adults. N. Engl. J. Med. 328, 1230–1235. doi: 10.1056/NEJM199304293281704
Zha, F., Qu, X., Tang, B., Li, J., Wang, Y., Zheng, P. X., et al. (2019). Long non-coding RNA MEG3 promotes fibrosis and inflammatory response in diabetic nephropathy via miR-181a/Egr-1/TLR4 axis. Aging 11, 3716–3730. doi: 10.18632/AGING.102011
Zhang, C., Dong, H., Chen, F., Wang, Y., Ma, J., and Wang, G. (2019). The HMGB1-RAGE/TLR-TNF-α signaling pathway may contribute to kidney injury induced by hypoxia. Exp. Ther. Med. 17, 17–26. doi: 10.3892/ETM.2018.6932
Zhang, X. B., Lin, X. L., Wu, X. Y., Zeng, Y. M., Chen, X. Y., Luo, X. B., et al. (2020). Differential expression of microRNAs in xenografted Lewis lung carcinomas subjected to intermittent hypoxia: a next-generation sequence analysis. Transl. Cancer Res. 9, 4354–4365. doi: 10.21037/TCR-19-2913
Zhang, R., Qin, L., and Shi, J. (2020). MicroRNA-199a-3p suppresses high glucose-induced apoptosis and inflammation by regulating the IKKβ/NF-κB signaling pathway in renal tubular epithelial cells. Int. J. Mol. Med. 46, 2161–2171. doi: 10.3892/IJMM.2020.4751
Zhang, J., Sun, X. J., Chen, J., Hu, Z. W., Wang, L., Gu, D. M., et al. (2017). Increasing the miR-126 expression in the peripheral blood of patients with diabetic foot ulcers treated with maggot debridement therapy. J. Diabetes Complicat. 31, 241–244. doi: 10.1016/J.JDIACOMP.2016.07.026
Zhang, Y., Zhang, C., Li, H., and Hou, J. (2017). Down-regulation of vascular PPAR-γ contributes to endothelial dysfunction in high-fat diet-induced obese mice exposed to chronic intermittent hypoxia. Biochem. Biophys. Res. Commun. 492, 243–248. doi: 10.1016/J.BBRC.2017.08.058
Zhao, L., Liu, Y., and Wang, X. (2021). TNF-α promotes insulin resistance in obstructive sleep apnea-hypopnea syndrome. Exp. Ther. Med. 21:568. doi: 10.3892/ETM.2021.10000
Zhao, S. F., Ye, Y. X., Xu, J. D., He, Y., Zhang, D. W., Xia, Z. Y., et al. (2021). Long non-coding RNA KCNQ1OT1 increases the expression of PDCD4 by targeting miR-181a-5p, contributing to cardiomyocyte apoptosis in diabetic cardiomyopathy. Acta Diabetol. 58, 1251–1267. doi: 10.1007/S00592-021-01713-X
Zhen, L., Jiang, X., Chen, Y., and Fan, D. (2017). miR-31 is involved in the high glucose-suppressed osteogenic differentiation of human periodontal ligament stem cells by targeting Satb2. Am. J. Transl. Res. 9:2384.
Zhou, B., Li, C., Qi, W., Zhang, Y., Zhang, F., Wu, J. X., et al. (2012). Downregulation of miR-181a upregulates sirtuin-1 (SIRT1) and improves hepatic insulin sensitivity. Diabetologia 55, 2032–2043. doi: 10.1007/S00125-012-2539-8
Zhou, L., Zhang, S., Zhang, L., Li, F., Sun, H., and Feng, J. (2021). MiR-199a-3p inhibits the proliferation, migration, and invasion of endothelial cells and retinal pericytes of diabetic retinopathy rats through regulating FGF7 via EGFR/PI3K/AKT pathway. J. Recept. Signal Transduct. Res. 41, 19–31. doi: 10.1080/10799893.2020.1783556
Keywords: microRNA, OSA, obstructive sleep apnea, diabetes, metabolic syndrome, metabolic complications
Citation: Karuga FF, Jaromirska J, Malicki M, Sochal M, Szmyd B, Białasiewicz P, Strzelecki D and Gabryelska A (2023) The role of microRNAs in pathophysiology and diagnostics of metabolic complications in obstructive sleep apnea patients. Front. Mol. Neurosci. 16:1208886. doi: 10.3389/fnmol.2023.1208886
Edited by:
Yajin Liao, University of South China, ChinaReviewed by:
Insup Choi, Icahn School of Medicine at Mount Sinai, United StatesGniewko Więckiewicz, Medical University of Silesia, Poland
Copyright © 2023 Karuga, Jaromirska, Malicki, Sochal, Szmyd, Białasiewicz, Strzelecki and Gabryelska. This is an open-access article distributed under the terms of the Creative Commons Attribution License (CC BY). The use, distribution or reproduction in other forums is permitted, provided the original author(s) and the copyright owner(s) are credited and that the original publication in this journal is cited, in accordance with accepted academic practice. No use, distribution or reproduction is permitted which does not comply with these terms.
*Correspondence: Filip Franciszek Karuga, ZmlsaXAua2FydWdhQGdtYWlsLmNvbQ==; Agata Gabryelska, YWdhdGEuZ2FicnllbHNrYUBnbWFpbC5jb20=