- Department of Neurology, The Ohio State University College of Medicine, Columbus, OH, United States
Remyelination biology and the therapeutic potential of restoring myelin sheaths to prevent neurodegeneration and disability in multiple sclerosis (MS) has made considerable gains over the past decade with many regeneration strategies undergoing tested in MS clinical trials. Animal models used to investigate oligodendroglial responses and regeneration of myelin vary considerably in the mechanism of demyelination, involvement of inflammatory cells, neurodegeneration and capacity for remyelination. The investigation of remyelination in the context of aging and an inflammatory environment are of considerable interest for the potential translation to progressive multiple sclerosis. Here we review how remyelination is assessed in mouse models of demyelination, differences and advantages of these models, therapeutic strategies that have emerged and current pro-remyelination clinical trials.
Introduction
Multiple sclerosis (MS) is a chronic demyelinating, inflammatory and neurodegenerative disease of the central nervous system (CNS). Remyelination is a regenerative process by which oligodendrocytes restore myelin sheaths to demyelinated axons. Evidence from animal models indicate that remyelination can restore neuronal conduction (Smith et al., 1979, 1981), promote functional recovery (Jeffery and Blakemore, 1997; Jeffery et al., 1999; Liebetanz and Merkler, 2006; Duncan et al., 2009; Mei et al., 2016a) and protect axons from degeneration (Irvine and Blakemore, 2008; Mei et al., 2016a). Myelination and connection of the oligodendrocyte-axonal unit provides metabolic support and reciprocal signaling that promotes axonal function and survival (Nave, 2010; Simons and Nave, 2015; Thornton and Hughes, 2020; Duncan et al., 2021).
Remyelination is robust in many animal models that have been used to study remyelination, which is somewhat discordant with the heterogeneous patterns of oligodendrocyte loss, demyelination and remyelination in human pathology-based studies of MS tissue (Lucchinetti et al., 1999, 2000; Lassmann et al., 2001; Pittock and Lucchinetti, 2007). The mechanisms of remyelination failure in MS are likely complex (Franklin, 2002; Franklin and Ffrench-Constant, 2008) and may depend on lesion stage (Heß et al., 2020) and disease duration (Goldschmidt et al., 2009). Remyelination failure in human MS lesions may involve mechanisms related to oligodendrocyte apoptosis and phagocytosis (Prineas and Parratt, 2012), paucity of oligodendrocyte progenitor cells (OPCs) (Kuhlmann et al., 2008; Boyd et al., 2013; Cui et al., 2013), quiescent OPCs (Wolswijk, 1998), impaired differentiation into mature oligodendrocytes (Kuhlmann et al., 2008), and impaired contact of differentiated oligodendrocytes with demyelinated axons (Chang et al., 2002).
The inflammatory environment can modulate oligodendroglial properties including oligodendroglial survival, migration, differentiation, axon engagement and remyelination (Antel et al., 2019; Greenhalgh et al., 2020). Advances in molecular techniques have revealed transcriptional diversity in glial cell types (Zia et al., 2020; Schirmer et al., 2021) including oligodendroglia in experimental inflammatory mouse models (Falcão et al., 2018; Kirby et al., 2019; Meijer et al., 2022; Harrington et al., 2023; Hou et al., 2023) and human MS (Jäkel et al., 2019; Schirmer et al., 2019; Absinta et al., 2021), suggesting that oligodendroglia may have immunoregulatory roles in MS (Zeis et al., 2016; Harrington et al., 2020). Oligodendroglia subgroups also demonstrate distinct spatial and functional responses neuronal activity (Marisca et al., 2020) and injury (Floriddia et al., 2020) in animal models. The inflammatory environment, anatomical and subregional location, sex and age may influence the heterogeneity of oligodendroglia in MS lesions (Seeker and Williams, 2022) and could impact remyelination capacity and these factors need to be considered when modeling MS in animal models.
In animal models, aging influences oligodendroglial properties (Koutsoudaki et al., 2020; Perdaens and van Pesch, 2021; Zhang et al., 2021; Rawji et al., 2023) and progenitor-driven remyelination declines with aging (Shields et al., 1999; Sim et al., 2002) and can vary depending on regional origin of OPCs (Crawford et al., 2016). Impaired remyelination with aging can be rejuvenated with young myeloid-derived macrophages (Ruckh et al., 2012) and with fasting or metformin treatment (Neumann et al., 2019). Chronological aging is strongly associated with the development of clinical and pathological features of progressive MS (Zuo et al., 2022; Graves et al., 2023). Longer disease duration and older age is associated with higher numbers of inactive lesions and smoldering lesions (Frischer et al., 2015). Microglial activation and cortical demyelination found in progressive MS tissue (Kutzelnigg et al., 2005; Howell et al., 2011) as well as B cell follicular structures and meningeal inflammation (Howell et al., 2011) are all factors that may influence remyelination capacity in progressive MS and are not well recapitulated in mouse models.
Animal studies have revealed that newly born oligodendrocytes efficiently generate myelin sheaths (Bacmeister et al., 2020; Neely et al., 2022) and while mature surviving oligodendrocytes can generate myelin sheaths they are less efficient (Bacmeister et al., 2020; Neely et al., 2022; Mezydlo et al., 2023) and rarely restore internodes (Mezydlo et al., 2023). The differences between remyelination capacity of surviving mature oligodendrocytes and newly generated oligodendrocytes in response to demyelination likely has implications in which subsets of oligodendroglia are capable of remyelinating in human MS (Franklin et al., 2021). Radiocarbon dating with the genomic integration of 14C has been used to investigate the age of oligodendroglia within MS lesions and this study indicated limited production of new oligodendrocytes within shadow plaques that may have undergone remyelination suggesting that mature surviving oligodendrocytes contribute to subsequent remyelination in human MS lesions (Yeung et al., 2019). Post-mortem tissue analysis is limited by the inability to determine lesion age and extent of remyelination, and assumptions made about human oligodendrocyte progenitor properties, such as for carbon dating studies that OPCs must divide (and incorporate 14C) prior to differentiation despite rodent studies indicating that OPCs can directly differentiate into mature oligodendrocytes without cell division (Hughes et al., 2013). The contribution of newly born oligodendrocytes compared to surviving mature oligodendrocytes to remyelination in human MS will be difficult to determine definitively based on human post-mortem tissue analysis.
Remyelination and pro-regenerative strategies remain a major unmet need in the treatment of MS. Studying remyelination in human MS patients has been impaired by the limited array of tools to measure remyelination in humans and improved clinical measures need to be developed for incorporation into clinical trials to facilitate testing of remyelination therapies (Hill et al., 2022). While many animal models exist for investigating remyelination, this review will focus primarily on in vivo rodent models in which remyelination has been clearly demonstrated while highlighting limitations and advantages to consider in relation to human MS and a discussion of remyelinating therapies in clinical trials for MS.
Identifying remyelination
Remyelination in animal models is determined through assessment of myelin sheath thickness and internode length, which is based on the early observations from animal models that remyelinated myelin sheaths are thinner than expected for axonal diameter (Blakemore, 1973, 1974; Bunge et al., 1961; Figure 1A) and internode length is shorter (Gledhill and McDonald, 1977). Myelin sheath thickness is best quantified by transmission electron microscopy (TEM) of 50–90 nm resin embedded sections and g-ratio analysis (ratio of myelinated axon to the axon alone) (Blakemore and Franklin, 2008). Remyelination of small diameter axons is difficult to determine as small axons with thinner myelin sheaths at baseline are not discernable from remyelinated axons (Stidworthy et al., 2003; Bai et al., 2016). Utilization of 3D-TEM techniques have demonstrated that reduced internodal length can be used as a readout of early remyelination in the corpus callosum, however, with time internodal length is restored to distances seen pre-demyelinating insult (Bai et al., 2016). Areas of remyelination can also be difficult to determine over time as myelin sheath thickness and remodeling closely resembles the surrounding normal appearing white matter (Neumann et al., 2020). Longitudinal intravital microscopy of oligodendroglia (Hughes et al., 2018; El Waly et al., 2020; Orthmann-Murphy et al., 2020; Bottes and Jessberger, 2021; Call and Bergles, 2021; Figure 1B) and genetic labeling strategies for lineage tracing of oligodendrocyte progenitors and subsequent myelin sheath generation (Mei et al., 2016a; Figure 1C) are alternative methods that have been utilized for assessment of remyelination and dynamics and patterns of myelin sheath formation. The complexities of accurately assessing remyelination need to be carefully considered when using animal models.
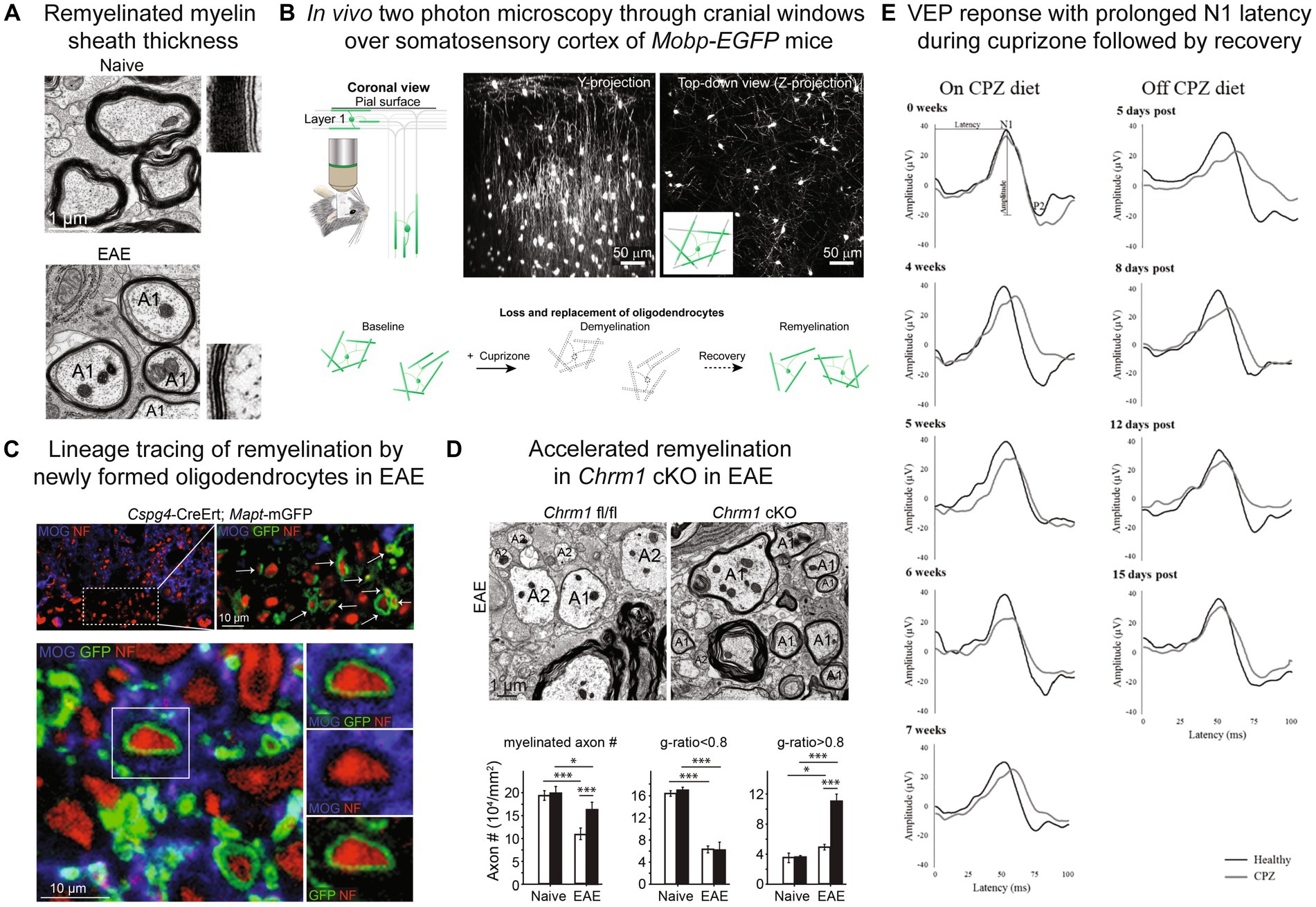
Figure 1. Remyelination assessment in mouse models. (A) Myelin sheath visualization by electron microscopy with thinner myelin sheaths in remyelinated axons (A1) in EAE compared to naïve spinal cord. (B) In vivo two photon microscopy through cranial windows over the somatosensory cortex of Mobp-EGFP mice allows for visualization of loss and replacement of oligodendrocytes and myelin sheaths. (C) Lineage tracing approach to visualize myelin sheaths from newly born oligodendrocytes utilizing Cspg4-CreER™; Mapt-mGFP mice and tamoxifen injection prior to EAE induction. New myelin sheaths generated by OPCs express mGFP reporter (green) and wrap neurofilament positive axons (red). (D) Electron microscopy of conditional knockout of muscarinic acetylcholine receptor Chrm1 in oligodendrocytes (Chrm1 cKO: Cnp-Cre+; Chrm1fl/fl) in EAE results in reduced axonal loss and enhanced remyelination in spinal cord indicated by significantly increased axons with g-ratios >0.8. Black bars indicate Chrm1 cKO and white bars Cnp-Cre-; Chrm1fl/fl control. A1-remyelinated axon, A2-demyelinated axon. (E) Longitudinal visual evoked potential (VEP) waveforms during and off cuprizone (CPZ) diet. N1 latency is prolonged on cuprizone diet and recovers after return to normal diet. Black lines-healthy/normal diet mice, gray lines-cuprizone treated mice. (A,C,D) Reproduced from Mei et al. (2016a), accelerated remyelination during inflammatory demyelination prevents axonal loss and improves functional recovery, eLife © Creative Commons. (B) Reproduced from Orthmann-Murphy et al. (2020), remyelination alters the pattern of myelin in the cerebral cortex, eLife © Creative Commons. (E) Reproduced from Marenna et al. (2022), visual evoked potentials to monitor myelin cuprizone-induced functional changes, Frontiers in Neuroscience © Creative Commons.
Toxin-mediated demyelination models
Experimental models of demyelination based on the use of toxins, while these models may not recapitulate the autoimmune pathobiology of MS, they offer the advantage of stereotyped demyelination and remyelination process which has been invaluable in the investigation of the molecular mechanisms involved in remyelination.
Focal toxins-lysolecithin and ethidium bromide
Focal demyelinating agents can be injected into the spinal cord dorsal and ventrolateral funiculi, caudal cerebellar peduncle, corpus callosum, optic nerve and subcortical white matter to create focal demyelinated lesions. Focal toxin models offer the advantage of a synchronized short demyelinating process compared to systemic toxins such as cuprizone in which demyelination is protracted and occurring in an environment that may be influenced by continued toxin exposure.
Lysolecithin or lysophosphatidylcholine (LPC) is an endogenous lysophospholipid that can be used to generate a focal demyelinating lesion by injection into white matter tracts and demyelination results through disruption of oligodendroglial cell membranes (Hall, 1972; Plemel et al., 2018) leading to oligodendroglial cell loss (McKay et al., 1998; Plemel et al., 2018). Lysolecithin-mediated demyelination is not selective for oligodendroglia. Astrocyte loss and markers of axonal injury are notable within the lesion core (Plemel et al., 2018). Generation of larger lesions or poor surgical technique can result in axonal degeneration (Blakemore and Franklin, 2008). In young animals remyelination occurs rapidly and the majority of axons are remyelinated by oligodendrocytes and Schwann cells derived from OPCs (Zawadzka et al., 2010). After the primary myelinopathy injury a secondary inflammatory response occurs which is characterized by microglial and macrophage infiltration and activation and reactive astrogliosis (Plemel et al., 2018). T cells also appear to play a role in lysolecithin-induced remyelination with Rag1 knockout and depletion of CD4 and CD8 T cells demonstrating impaired remyelination (Bieber et al., 2003).
In the lysolecithin model, myelin (Kotter et al., 2006) and extracellular matrix components such as chondroitin sulfate proteoglycans (CSPGs) (Lau et al., 2012) within the lesion can impair oligodendrocyte differentiation. Infiltrating macrophages play an important role in influencing OPC recruitment into lesions (Kotter et al., 2005) and depletion of macrophages during early stages of remyelination impairs remyelination (Kotter et al., 2001). Oligodendroglia in remyelinating lesions express transforming growth factor (TGF) β member activin-A and activin-A released from inflammatory microglia and macrophages may promote oligodendrocyte differentiation and remyelination (Miron et al., 2013).
Ethidium bromide, a DNA-intercalating agent, injected into white matter tracts creates a focal demyelinating lesion with a larger area of demyelination compared to lysolecithin (Blakemore and Franklin, 2008). Ethidium bromide, opposed to a primary myelinopathy observed in lysolecithin, is directly cytotoxic and induces cell death of oligodendrocytes and astrocytes (Blakemore, 1982). Vacuoles and splitting within the myelin sheath lamellae can occur (Yajima and Suzuki, 1979). Schwann cells have a notable contribution to remyelination in ethidium bromide lesions (Blakemore, 1982; Graça and Blakemore, 1986; Reynolds and Wilkin, 1993; Woodruff and Franklin, 1999). Ethidium bromide injections in the rat cervical spinal cord have been useful in functional studies assessing the role of remyelination on neuronal function (Jeffery and Blakemore, 1997) and cerebellar peduncle injections have facilitated investigation of repeated demyelinating events within the same site (Penderis et al., 2003).
Combining X-irradiation with ethidium bromide injection has allowed for the investigation of transplanted progenitor cells in a lesion environment devoid of endogenous remyelination potential (Blakemore et al., 2002). Neonatal OPCs (Blakemore et al., 2002), adult OPCs (Talbott et al., 2006) and adult neural stem/progenitor cells (Mothe and Tator, 2008) transplanted into ethidium bromide/X-irradiated lesions can differentiate into mature oligodendrocytes and Schwann cells capable of remyelination (Talbott et al., 2006; Mothe and Tator, 2008).
Aged animals exhibit slower remyelination after lysolecithin and ethidium bromide injection (Shields et al., 1999) and aged animals have impaired myelin debris clearance within lesions (Graça and Blakemore, 1986; Ruckh et al., 2012; Natrajan et al., 2015; Cantuti-Castelvetri et al., 2018). Agonism of retinoid X receptor gamma (RXRγ) signaling promotes macrophage clearance of myelin debris and improves remyelination efficiency in aged animals (Huang et al., 2011; Natrajan et al., 2015). Parabiotic recruitment of young monocytes (Ruckh et al., 2012) and caloric restriction and fasting mimetic metformin (Neumann et al., 2019) can accelerate remyelination in aged animals. Extracellular matrix stiffening with aging can also impair OPC differentiation and remyelination in focal toxin models (Segel et al., 2019).
Systemic toxins-cuprizone
Cuprizone, bis-cyclohexanone-oxaldihydrazone, was first used as an animal model of demyelination in the 1960s (Carlton, 1966, 1969; Carlton, 1967). Cuprizone ingestion results in demyelination of white matter tracts including the corpus callosum, thalamus, anterior commissure and cerebellar peduncles as well as cortical gray matter (Blakemore, 1972). The mechanisms of cuprizone-induced demyelination are complex and multifactorial with evidence for contributions from primary oligodendrocyte cell death due to mitochondrial dysfunction and reactive oxygen species, oligodendrocyte cell death from toxic factors released by microglia and astrocytes, and direct attack of oligodendrocytes by innate immune cells (Matsushima and Morell, 2001; Kipp et al., 2009; Praet et al., 2014; Zirngibl et al., 2022). Cuprizone can be administered for acute and chronic durations, with 3 weeks commonly used for intravital microscopy studies (Bacmeister et al., 2020; Orthmann-Murphy et al., 2020), 4–6 weeks for acute demyelination histological studies and 12 weeks or longer for chronic demyelination studies (Kipp et al., 2009; Zirngibl et al., 2022). Oligodendrocyte apoptosis, microglial activation and reactive astrogliosis occur within the first 2 weeks of cuprizone ingestion (Buschmann et al., 2012; Wergeland et al., 2012; Zhan et al., 2020) and regional variability in degree of microglial and astrocyte activation (Gudi et al., 2009; Goldberg et al., 2015) may influence demyelination and oligodendrocyte responses. Regional differences are also seen in the extent of demyelination and oligodendrocyte loss (Gudi et al., 2009; Yang et al., 2009; Wergeland et al., 2012; Schmidt et al., 2013; Hochstrasser et al., 2019; Zhan et al., 2020), OPC proliferation (Gudi et al., 2009) and remyelination (Stidworthy et al., 2003). Axonal spheroids are present after acute cuprizone exposure (Goldberg et al., 2015) and notable axonal degeneration occurs with chronic cuprizone exposure and persists even after the remyelination period (Lindner et al., 2009). Axonal degeneration varies with cuprizone concentration, mouse strain and age (Irvine and Blakemore, 2006). Acute single and repeated cuprizone exposure both result in late onset locomotor dysfunction, brain atrophy and callosal axonal loss despite remyelination (Manrique-Hoyos et al., 2012).
Myelin loss occurs after several weeks of cuprizone ingestion and peaks at 4–5 weeks (Hiremath et al., 1998; Matsushima and Morell, 2001). One of the challenges of cuprizone-mediated demyelination is early OPC proliferative response during cuprizone ingestion resulting in endogenous remyelination even in the presence of cuprizone (Mason et al., 2000; Matsushima and Morell, 2001; Gudi et al., 2009; Zirngibl et al., 2022). Combining a rapamycin, a mammalian target of rapamycin (mTOR) inhibitor, with cuprizone ingestion can suppress spontaneous remyelination during cuprizone treatment (Sachs et al., 2014; Bai et al., 2016).
Manipulation of astrocyte and microglial responses after cuprizone-mediated demyelination can influence remyelination. Ablation of astrocytes after chronic cuprizone treatment results in improved oligodendrocyte density, remyelination and motor functional outcomes (Madadi et al., 2019). Astrocyte secretion of cytokines such as tumor necrosis factor alpha and lymphotoxins can influence demyelination and remyelination (Arnett et al., 2001; Plant et al., 2005, 2007). Astrocytes can also promote microglial accumulation and activation (Skripuletz et al., 2013). Microglial depletion reduces cuprizone-mediated demyelination and injection of colony stimulating factor 1 (CSF1) induces focal demyelination (Marzan et al., 2021) suggesting an important role for microglia in mediating demyelination during cuprizone ingestion. Microglial MER proto-oncogene tyrosine kinase (MERTK) signaling (Shen et al., 2021), triggering receptor expressed on myeloid cells 2 (TREM2) signaling (Cantoni et al., 2015; Poliani et al., 2015; Cignarella et al., 2020) and colony stimulating factor 1 (CSF1) signaling (Laflamme et al., 2018) facilitate myelin debris clearance and remyelination after cuprizone-mediated demyelination. Trem2 deficiency results in reduction of a subset of oligodendroglia that is induced in response to demyelination which may be due to delay in myelin debris clearance and induction of this subset of oligodendroglia (Hou et al., 2023).
The role of T cells in cuprizone-mediated demyelination is unclear. T cells are present in cuprizone ingestion (Remington et al., 2007) and CD8 T cells accumulate in the corpus callosum during cuprizone treatment and express activation markers (Kaddatz et al., 2021). Interleukin-17 (IL-17) secreted by T cells during cuprizone may play a role in activating microglia and mediating demyelination (Kang et al., 2012; Zimmermann et al., 2018).
Toxin models have greatly facilitated the investigation of pathways involved in oligodendroglial proliferation, recruitment, differentiation and remyelination. Promising mechanisms that are under investigation in clinical trials for remyelination in MS that have emerged from investigation of remyelination in focal and systemic toxin models include LINGO-1 antagonism (Mi et al., 2009), Nogo-A antagonism (Ineichen et al., 2017), RXR agonism (Huang et al., 2011; Natrajan et al., 2015), muscarinic receptor antagonism (Mei et al., 2014; Chen et al., 2017), semaphorin 3A (Piaton et al., 2011; Syed et al., 2011), sex hormone estrogen and testosterone supplementation (Patel et al., 2013), estrogen receptor modulators (Sicotte et al., 2007; Gonzalez et al., 2016; Rankin et al., 2019; Voskuhl et al., 2019), thyroid hormone (Bai et al., 2016; Hartley et al., 2019; Rosato-Siri et al., 2021; Pagnin et al., 2022) and metabolism modulation (Berghoff et al., 2017; Neumann et al., 2019).
The major advantages of toxin models are the robust remyelination response with stereotyped kinetics, separation of the demyelinating process from the regenerative process, minimal axonal degeneration, and decline of remyelination with aging that have allowed for discovery of targets that accelerate repair in an aged environment. While focal toxin models have these advantages, the short demyelinating insult and robust remyelination response are limitations. Diffuse CNS demyelination and white matter injury induced by cuprizone ingestion offers the advantage of an environment with prolonged oligodendroglial loss, neuronal stress and subsequent neurodegeneration, which may allow for the investigation of pathways that prevent neuronal degeneration and allow for the ability to assess motor (Manrique-Hoyos et al., 2012; Lubrich et al., 2022) and physiological outcomes (Bando et al., 2008; Cordano et al., 2022; Marenna et al., 2022). One of the potential limitations of toxin-induced demyelinating models is the absence of inflammatory niches and microenvironments found in human MS lesions such as ectopic lymphoid follicles (Negron et al., 2020) and absence of robust T and B cell responses in these toxin-mediated mouse models. However, progressive MS lesions have a paucity of inflammatory infiltrates (Frischer et al., 2009) and mechanisms involved in neurodegeneration and remyelination in progressive MS may be independent of ongoing inflammatory activity that may be best modeled in chronic demyelination models such as cuprizone with the presence of late neurodegeneration and motor deficits (Manrique-Hoyos et al., 2012). Glial heterogeneity present in toxin-mediated models (Hou et al., 2023) should be compared to glial transcriptional heterogeneity found in MS tissue (Jäkel et al., 2019; Schirmer et al., 2019; Absinta et al., 2021) to better delineate whether toxin-mediated models recapitulate glial populations present in MS lesions.
Experimental autoimmune encephalomyelitis models
Experimental autoimmune encephalomyelitis (EAE) models are one of the most commonly used models to investigate the immunopathogenesis of MS (Gold et al., 2006; Baxter, 2007; Denic et al., 2011; Rangachari and Kuchroo, 2013; Simmons et al., 2013; Lassmann and Bradl, 2017). Immunization with emulsions of CNS tissue evolved into immunization with encephalitogenic antigens or adoptive transfer of myelin specific T cells (Baxter, 2007). EAE models can be generally classified as active (immunization of CNS peptides) or passive (adoptive transfer of encephalitogenic T cells) and they offer different advantages for the investigation of disease mechanisms of repair and remyelination.
Active immunization
Immunization with a CNS antigen and adjuvant is used to induce active EAE in rodents and the combination of peptide and mouse strain used influences the disease course and pathology (Gold et al., 2006; Rangachari and Kuchroo, 2013; Simmons et al., 2013; Lassmann and Bradl, 2017). C57BL/6 mice were found to be susceptible to EAE with immunization with myelin oligodendrocyte glycoprotein (MOG)35–55 peptide (Mendel et al., 1995) which has facilitated the use of transgenic lines in investigation of disease mechanisms. In the MOG35-55 EAE model, demyelination is secondary to axonal injury and degeneration mediated by the adaptive and innate immune system (Kim et al., 2006; Soulika et al., 2009; Nikić et al., 2011) and does not involve a cytolytic auto-antibody response (Bourquin et al., 2003) which needs to be considered when utilizing this model for investigating mechanisms of demyelination and neurodegeneration. For modeling primary antibody-mediated demyelination in immunization models, C57BL/6 mice immunized with human MOG (Oliver et al., 2003) or rats with MOG1-125 peptide can be used to generate lesions with primary demyelination and axonal sparing (Storch et al., 1998). Cortical subpial demyelination can be modeled in rats with MOG immunization (Storch et al., 2006) or sub-clinical MOG immunization followed by injection of tumor necrosis factor (TNF) and IFN-γ overlying or in superficial cortical layers (Merkler et al., 2006; Gardner et al., 2013).
A limited degree of remyelination has been demonstrated in active EAE by the use of genetic lines labeling myelin sheaths generated from OPCs (Cspg4-CreER™; Mapt-mGFP) (Mei et al., 2016a) and transmission electron microscopy g-ratio analysis (Figures 1A,D). Significant axonal loss occurs during EAE and a large proportion of axons remain demyelinated during late EAE, 26% in spinal cord ventral white matter analyzed in this study (Mei et al., 2016a). Despite the significant degree of neurodegeneration and modest amount of remyelination, conditional knockout of M1 muscarinic receptor in oligodendrocytes (Figure 1D; Mei et al., 2016a), pharmacological antagonism of muscarinic receptors (Cordano et al., 2022) and K-opioid receptor agonism (Du et al., 2016) were able to enhance remyelination in MOG35-55 EAE. Visual evoked potentials can be used as biomarker for remyelination in EAE and cuprizone (Cordano et al., 2022). Immunization with proteolipid protein (PLP)139–151 peptide in SJL/J mice results in an acute phase of clinical disability similar to MOG35-55 but with partial clinical remission followed periods of fluctuating clinical scores in what has been termed “relapsing remitting” clinical course (Rangachari and Kuchroo 2013). Remyelination has been demonstrated by electronic microscopy at acute phase peak disease course with treatment with muscarinic antagonist benztropine in this EAE model (Deshmukh et al., 2013). The relapsing remitting clinical course of the SJL/J PLP139-151 EAE model may be useful for modeling remyelination in relapsing remitting MS however the degree of axons with high g-ratios, either demyelinated or remyelinated, normalizes during remission phase (Deshmukh et al., 2013) and whether this is due to a combination of loss of demyelinated axons or subsequent remyelination is unclear. Electrophysiological analysis of visual evoked potentials could be used as a biomarker for remyelinating in different clinical phases of the SJL/J PLP139-151 EAE model but ongoing inflammatory activity and neurodegeneration may limit this assessment.
Active immunization models have many features that differ from human MS pathobiology and clinical course. The robust inflammatory response and resulting secondary demyelination, early neurodegeneration (Jones et al., 2008), sparing of cerebrum, and predominance of CD4 T cells and lack of significant CD8 T cell infiltrate are all features of active EAE that do not correlate well with human MS pathobiology. The monophasic chronic disease course of MOG35-55 EAE does not resemble the clinical course of relapsing remitting or progressive MS. The high degree of neurodegeneration and minimal remyelination after the acute EAE phase represent challenges for the utilization of these models in assessing remyelination strategies.
Adoptive transfer
Encephalitogenic T cells from CNS antigen immunized mice or myelin-specific T cells isolated from T cell receptor transgenic lines can be used to induce neuroinflammatory disease upon adoptive transfer into naive hosts (Paterson, 1960; Ben-Nun et al., 1981). A major advantage of adoptive transfer models is the avoidance of the priming and immune expansion phase that occurs in the periphery in immunization models that can contribute to the neuroinflammatory response. Adoptive transfer of myelin-specific CD4 T cells has been the most widely used model to generate EAE. Adoptive transfer of myelin basic protein (MBP)-specific CD8 T cells from C3H mice can induce EAE (Huseby et al., 2001). Through the utilization of transgenic lines in which CNS resident cells express foreign antigens, EAE can also be induced by adoptive transfer of CD8 T cells specific for the foreign antigen (Cabarrocas et al., 2003; Na et al., 2008; Saxena et al., 2008). These models may offer the advantage of induction of primary demyelination directly targeting oligodendrocytes (Na et al., 2008; Saxena et al., 2008) which may facilitate the exploration of mechanisms involved in CD8-mediated pathogenesis in MS, as CD8 T cells outnumber CD4 T cells in MS lesions (Booss et al., 1983; Hauser et al., 1986; Babbe et al., 2000) and CD8 infiltrates correlate with the degree of axonal degeneration in MS lesions (Bitsch et al., 2000; Kuhlmann et al., 2002).
Adoptive transfer of myelin-reactive Th17 cells after acute cuprizone ingestion has been used as a model to investigate oligodendroglial responses in the setting of T cell mediated inflammation (Baxi et al., 2015, 2017; Kirby et al., 2019) and has the advantage of perivascular inflammatory and corpus callosum infiltrates and may allow for investigation of therapeutics that target pathways activated in oligodendroglia in the context of inflammation. Whether adoptive transfer models can be used to investigate remyelination remains to be determined, but these models offer the advantage of the ability to bypass the priming phase of active EAE and investigate therapeutic effects of compounds or genetic manipulations in the absence of a priming process.
Genetic demyelination models
Genetic models that trigger oligodendrocyte ablation or ectopic expression of interferon gamma (IFN-γ) have been used to investigate demyelination and remyelination. Initial studies of transgenic mice with targeted expression of IFN-γ from the myelin basic protein (MBP) promotor (Corbin et al., 1996) and glial acidic fibrillary protein (GFAP) promoter (LaFerla et al., 2000) demonstrated forced expression of IFN-γ during development results in CNS hypomyelination and abnormal cerebellar development. Combining GFAP promotor-driven expression of a tetracycline-controlled transactivator (tTA) (Gfap-tTA) with tetracycline response element (TRE) upstream of IFN-γ sequence (TRE-IFN-γ) in a double transgenic line allows for temporal regulation of CNS IFN-γ expression upon removal of doxycycline (Lin et al., 2004). CNS IFN-γ expression during cuprizone-mediated demyelination with removal of doxycycline during cuprizone exposure in Gfap-tTA; TRE-IFN-γ mice resulted in reduced differentiated oligodendrocytes and impaired remyelination (Lin et al., 2006). Prolongation of the integrated stress response (ISR) during cuprizone-mediated demyelination with CNS IFN-γ expression enhanced mature oligodendrocyte generation and remyelination (Chen et al., 2021) suggesting a beneficial role of oligodendrocyte ISR signaling in promoting remyelination.
Oligodendrocyte ablation through the combination of an inducible conditional mature oligodendrocyte Cre line (Plp-CreER™) with a diphtheria toxin subunit A (DTA) floxed stop reporter line (ROSA26-eGFP-DTA) results in oligodendrocyte apoptosis upon exposure to tamoxifen (Traka et al., 2010). Rapid oligodendrocyte loss occurs within the first week after tamoxifen injection and demyelination peaks at 5 weeks. Mice develop clinical symptoms of ataxia, tremor, hind-limb paralysis with some degree of lethality. At 10 weeks post-tamoxifen oligodendrocytes regenerate, remyelination and axonal numbers are comparable to controls, and axonal conduction assessed by spinal somatosensory evoked potentials are restored (Traka et al., 2010). Recovered animals develop a secondary fatal immune-mediated phase of demyelination that occurs around 40 weeks post-tamoxifen accompanied by focal inflammatory lesions, extensive myelin and axonal loss and the presence of MOG-specific T cells in lymphoid organs that are encephalitogenic when transferred into naïve recipients (Traka et al., 2016). This secondary immune-mediated demyelination was inhibited by tolerization with MOG35-55 after the initial remyelination phase. The DTA model may be useful for investigation of strategies to prevent development of a secondary adaptive immune response and investigate pathways to accelerate OPC maturation and subsequent remyelination in the setting of complete loss of mature oligodendrocytes.
Loss of function of transcription factor myelin gene regulatory factor (MYRF) that induces expression of mature myelin genes (Emery et al., 2009) in mature oligodendrocytes (Plp-CreER™; Myrffl/fl) results in oligodendrocyte death, widespread demyelination, microglia and macrophage reactivity, axonal damage and incomplete remyelination (Koenning et al., 2012). Subsequent development of a secondary immune-mediated demyelination has not been reported in Myrf conditional knockout, possibly due to CNS restriction of Myrf expression to oligodendrocytes whereas Proteolipid protein 1 (Plp1) is expressed in Schwann cells which may contribute to the development of secondary autoimmunity. CNS penetrant thyroid hormone receptor mimetic, sobetirome, promoted OPC proliferation, remyelination and motor recovery in Myrf conditional knockouts (Hartley et al., 2019). While the severity of oligodendrocyte loss and demyelination in genetic oligodendrocyte ablation models may be a limitation, these models have the advantage of quantifiable motor recovery outcomes and secondary robust OPC response that can be modulated.
Viral models
Chronic encephalomyelitis viral mouse models share pathogenic features similar to MS (Oleszak et al., 2004; Lane and Hosking, 2010; Pike et al., 2022) and may provide insight into the induction of CNS autoimmunity and pathways that are activated in CNS resident populations in response to anti-viral inflammatory responses.
Theiler’s murine encephalomyelitis virus
Theiler’s murine encephalomyelitis virus (TMEV) is a single-stranded RNA picornavirus that causes flaccid myelitis in mice (Theiler, 1934) and can be used to induce an acute encephalomyelitis demyelinating disease through intracerebral infection. BeAn and Daniel’s TMEV strains can cause a biphasic disease process characterized by an acute infectious phase and viral clearance followed by a secondary progressive chronic demyelinating myelitis phase in susceptible mouse strains (Oleszak et al., 2004). Oligodendrocytes, astrocytes and macrophages are viral reservoirs during chronic infection (Rodriguez et al., 1983; Lipton et al., 1995) and demyelination occurs within areas of microglia and macrophage activation generating demyelinating lesions with variable axonal injury and remyelination that varies depending on mouse strain (Bieber et al., 2005). Macrophages and microglia play a critical role in immune-mediated demyelination in the TMEV model and mouse strain differences in susceptibility to demyelination may be due to differences in macrophages and microglia (Dal Canto et al., 1996). The TMEV model offers the advantage of a cytotoxic axonal injury mechanism (Rivera-Quiñones et al., 1998; Howe et al., 2007) which may facilitate the investigation of immune-mediated mechanisms of axonal injury and neurodegeneration.
Mouse hepatitis virus
Mouse hepatitis virus (MHV) is a positive-strand RNA virus with neurotropic strains that can be used to induce a chronic demyelinating disease through intracranial or intranasal inoculation of susceptible mouse strains. An acute encephalomyelitis phase is followed by a secondary phase of demyelination and remyelination (Bender and Weiss, 2010; Lane and Hosking, 2010). MHV-specific T cells appear to instigate demyelination (Wu et al., 2000; Dandekar et al., 2001, 2004; Dandekar and Perlman, 2002). Diffuse macrophage and microglial activation and upregulation of oxidative stress pathways in the MHV model resembles changes seen in MS tissue (Schuh et al., 2014). Microglia play a critical role in the clearance of myelin debris and facilitating remyelination (Sariol et al., 2020). Oligodendrocytes that survive the acute MHV infection have prolonged MHC class I expression (Pan et al., 2020). Viral encephalomyelitis models offer the ability to investigate microglial, astrocyte and oligodendrocyte secondary responses to an inflammatory viral insult and subsequent repair mechanisms.
Remyelination therapies-from mouse to human
High-throughput screens that evaluate the ability of compounds to promote oligodendrocyte differentiation in vitro have been used to identify pathways with remyelination potential (Joubert et al., 2010; Deshmukh et al., 2013; Mei et al., 2014; Najm et al., 2015; Porcu et al., 2015; Lariosa-Willingham et al., 2016; Mei et al., 2016b). Many pathways and compounds that have demonstrated improved remyelination in animal models have moved to human MS clinical trials (Table 1).
Assessment of remyelination in MS clinical trials has been challenging, with few validated clinical tools to assess remyelination in humans (Hill et al., 2022). Measurement of visual pathway conduction speed with visual evoked potential (VEP) has allowed for assessment of the change in P100 latency which correlates with remyelination in autoimmune encephalomyelitis (Cordano et al., 2022) and toxin (Marenna et al., 2022) mouse models (Figure 1E). Multifocal VEP (mf-VEP) compared to full-field VEP (ff-VEP) may be a more accurate measure of remyelination after optic neuritis (Pihl-Jensen et al., 2017) and is a secondary outcome measure in NCT05131828-CCMR Two trial. Opicinumab treatment after first time acute optic neuritis significantly reduced ff-VEP latency in the per-protocol population at 32 weeks in the RENEW trial (Cadavid et al., 2017). Opicinumab combined with interferon beta-1a did not demonstrate a significant improvement in disability at 72 weeks in SYNERGY trial, however univariate analysis suggested that younger age, shorter disease duration and higher baseline brain volumes may be associated with improved disability (Cadavid et al., 2019). The ReBUILD clemastine placebo crossover trial in relapsing remitting MS (RRMS) with chronic optic neuropathy demonstrated significantly improved ff-VEP latency while on clemastine treatment (Green et al., 2017). New MRI techniques such as myelin water fraction (MWF), diffusion tensor imaging (DTI) and magnetization transfer ratio (MTR) are currently being developed to assess remyelination (Hill et al., 2022). MTR has shown promise as an imaging measure of remyelination with significant MTR lesion change in GSK239512 (Schwartzbach et al., 2017) and in gray matter and brainstem lesions in bexarotene (Brown et al., 2021). Continued development of clinical techniques to assess remyelination and thoughtful design of patient inclusion criteria and outcome measures are critical for designing clinical trials for remyelination therapies in MS. Rigorous pre-clinical testing of remyelinating pathways and compounds in animal models has facilitated the success of recent clinical trials and allowed for multiple promising therapies for myelin repair for MS.
Conclusion
Animal models of MS with demonstrated remyelination capacity vary considerable in their mechanisms of demyelination, inflammatory infiltrates, degree of ongoing inflammatory activity, axonal loss and neurodegeneration and extent of remyelination. Focal toxin models offer the advantage of stereotyped remyelination after a short single demyelinating insult which has allowed for the investigation of factors that promote or inhibit this robust reparative response. A prolonged demyelinating insult predominated by corpus callosum and cortical demyelination and subsequent neurodegeneration and motor decline can be modelled with chronic cuprizone exposure and may share some features of the neurodegenerative process in progressive MS. Both cuprizone and EAE models induce inflammatory subsets of glia that are found in MS tissue and further investigation of how these subsets of glia contribute to ongoing inflammatory activity and promote or inhibit repair may offer insight into potential mechanisms to modulate remyelination in inflammatory settings. While no single animal model recapitulates the pathobiology of MS, considerations of the limitations and advantages of each model should be taken into account when investigating remyelination and translating animal model findings to human MS.
Author contributions
DP and EH contributed to writing the manuscript. EF contributed to researching and generating the clinical trial table. All authors contributed to the article and approved the submitted version.
Conflict of interest
The authors declare that the research was conducted in the absence of any commercial or financial relationships that could be construed as a potential conflict of interest.
Publisher’s note
All claims expressed in this article are solely those of the authors and do not necessarily represent those of their affiliated organizations, or those of the publisher, the editors and the reviewers. Any product that may be evaluated in this article, or claim that may be made by its manufacturer, is not guaranteed or endorsed by the publisher.
References
Absinta, M., Maric, D., Gharagozloo, M., Garton, T., Smith, M. D., Jin, J., et al. (2021). A lymphocyte-microglia-astrocyte axis in chronic active multiple sclerosis. Nature 597, 709–714. doi: 10.1038/s41586-021-03892-7
Antel, J. P., Lin, Y. H., Cui, Q. L., Pernin, F., Kennedy, T. E., Ludwin, S. K., et al. (2019). Immunology of oligodendrocyte precursor cells in vivo and in vitro. J. Neuroimmunol. 331, 28–35. doi: 10.1016/j.jneuroim.2018.03.006
Arnett, H. A., Mason, J., Marino, M., Suzuki, K., Matsushima, G. K., and Ting, J. P. Y. (2001). TNF alpha promotes proliferation of oligodendrocyte progenitors and remyelination. Nat. Neurosci. 4, 1116–1122. doi: 10.1038/nn738
Babbe, H., Roers, A., Waisman, A., Lassmann, H., Goebels, N., Hohlfeld, R., et al. (2000). Clonal expansions of CD8(+) T cells dominate the T cell infiltrate in active multiple sclerosis lesions as shown by micromanipulation and single cell polymerase chain reaction. J. Exp. Med. 192, 393–404. doi: 10.1084/jem.192.3.393
Bacmeister, C. M., Barr, H. J., McClain, C. R., Thornton, M. A., Nettles, D., Welle, C. G., et al. (2020). Motor learning promotes remyelination via new and surviving oligodendrocytes. Nat. Neurosci. 23, 819–831. doi: 10.1038/s41593-020-0637-3
Bai, C. B., Sun, S., Roholt, A., Benson, E., Edberg, D., Medicetty, S., et al. (2016). A mouse model for testing remyelinating therapies. Exp. Neurol. 283, 330–340. doi: 10.1016/j.expneurol.2016.06.033
Bando, Y., Takakusaki, K., Ito, S., Terayama, R., Kashiwayanagi, M., and Yoshida, S. (2008). Differential changes in axonal conduction following CNS demyelination in two mouse models. Eur. J. Neurosci. 28, 1731–1742. doi: 10.1111/j.1460-9568.2008.06474.x
Baxi, E. G., DeBruin, J., Jin, J., Strasburger, H. J., Smith, M. D., Orthmann-Murphy, J. L., et al. (2017). Lineage tracing reveals dynamic changes in oligodendrocyte precursor cells following cuprizone-induced demyelination. Glia 65, 2087–2098. doi: 10.1002/glia.23229
Baxi, E. G., DeBruin, J., Tosi, D. M., Grishkan, I. V., Smith, M. D., Kirby, L. A., et al. (2015). Transfer of myelin-reactive th17 cells impairs endogenous remyelination in the central nervous system of cuprizone-fed mice. J. Neurosci. 35, 8626–8639. doi: 10.1523/JNEUROSCI.3817-14.2015
Baxter, A. G. (2007). The origin and application of experimental autoimmune encephalomyelitis. Nat. Rev. Immunol. 7, 904–912. doi: 10.1038/nri2190
Bender, S. J., and Weiss, S. R. (2010). Pathogenesis of murine coronavirus in the central nervous system. J. Neuroimmune Pharmacol. 5, 336–354. doi: 10.1007/s11481-010-9202-2
Ben-Nun, A., Wekerle, H., and Cohen, I. R. (1981). The rapid isolation of clonable antigen-specific T lymphocyte lines capable of mediating autoimmune encephalomyelitis. Eur. J. Immunol. 11, 195–199. doi: 10.1002/eji.1830110307
Berghoff, S. A., Gerndt, N., Winchenbach, J., Stumpf, S. K., Hosang, L., Odoardi, F., et al. (2017). Dietary cholesterol promotes repair of demyelinated lesions in the adult brain. Nat. Commun. 8:14241. doi: 10.1038/ncomms14241
Bieber, A. J., Kerr, S., and Rodriguez, M. (2003). Efficient central nervous system remyelination requires T cells. Ann. Neurol. 53, 680–684. doi: 10.1002/ana.10578
Bieber, A. J., Ure, D. R., and Rodriguez, M. (2005). Genetically dominant spinal cord repair in a murine model of chronic progressive multiple sclerosis. J. Neuropathol. Exp. Neurol. 64, 46–57. doi: 10.1093/jnen/64.1.46
Bitsch, A., Schuchardt, J., Bunkowski, S., Kuhlmann, T., and Brück, W. (2000). Acute axonal injury in multiple sclerosis. Correlation with demyelination and inflammation. Brain J. Neurol. 123, 1174–1183. doi: 10.1093/brain/123.6.1174
Blakemore, W. F. (1972). Observations on oligodendrocyte degeneration, the resolution of status spongiosus and remyelination in cuprizone intoxication in mice. J. Neurocytol. 1, 413–426. doi: 10.1007/BF01102943
Blakemore, W. F. (1973). Remyelination of the superior cerebellar peduncle in the mouse following demyelination induced by feeding cuprizone. J. Neurol. Sci. 20, 73–83. doi: 10.1016/0022-510x(73)90119-6
Blakemore, W. F. (1974). Pattern of remyelination in the CNS. Nature 249, 577–578. doi: 10.1038/249577a0
Blakemore, W. F. (1982). Ethidium bromide induced demyelination in the spinal cord of the cat. Neuropathol. Appl. Neurobiol. 8, 365–375. doi: 10.1111/j.1365-2990.1982.tb00305.x
Blakemore, W. F., Chari, D. M., Gilson, J. M., and Crang, A. J. (2002). Modelling large areas of demyelination in the rat reveals the potential and possible limitations of transplanted glial cells for remyelination in the CNS. Glia 38, 155–168. doi: 10.1002/glia.10067
Blakemore, W. F., and Franklin, R. J. M. (2008). Remyelination in experimental models of toxin-induced demyelination. Curr. Top. Microbiol. Immunol. 318, 193–212. doi: 10.1007/978-3-540-73677-6_8
Booss, J., Esiri, M. M., Tourtellotte, W. W., and Mason, D. Y. (1983). Immunohistological analysis of T lymphocyte subsets in the central nervous system in chronic progressive multiple sclerosis. J. Neurol. Sci. 62, 219–232. doi: 10.1016/0022-510x(83)90201-0
Bottes, S., and Jessberger, S. (2021). Live imaging of remyelination in the adult mouse corpus callosum. Proc. Natl. Acad. Sci. U. S. A. 118:e2025795118. doi: 10.1073/pnas.2025795118
Bourquin, C., Schubart, A., Tobollik, S., Mather, I., Ogg, S., Liblau, R., et al. (2003). Selective unresponsiveness to conformational B cell epitopes of the myelin oligodendrocyte glycoprotein in H-2b mice. J. Immunol. 171, 455–461. doi: 10.4049/jimmunol.171.1.455
Boyd, A., Zhang, H., and Williams, A. (2013). Insufficient OPC migration into demyelinated lesions is a cause of poor remyelination in MS and mouse models. Acta Neuropathol. 125, 841–859. doi: 10.1007/s00401-013-1112-y
Brown, J. W. L., Cunniffe, N. G., Prados, F., Kanber, B., Jones, J. L., Needham, E., et al. (2021). Safety and efficacy of bexarotene in patients with relapsing-remitting multiple sclerosis (CCMR one): a randomised, double-blind, placebo-controlled, parallel-group, phase 2a study. Lancet Neurol. 20, 709–720. doi: 10.1016/S1474-4422(21)00179-4
Bunge, M. B., Bunge, R. P., and Ris, H. (1961). Ultrastructural study of remyelination in an experimental lesion in adult cat spinal cord. J. Biophys. Biochem. Cytol. 10, 67–94. doi: 10.1083/jcb.10.1.67
Buschmann, J. P., Berger, K., Awad, H., Clarner, T., Beyer, C., and Kipp, M. (2012). Inflammatory response and chemokine expression in the white matter corpus callosum and gray matter cortex region during cuprizone-induced demyelination. J. Mol. Neurosci. 48, 66–76. doi: 10.1007/s12031-012-9773-x
Cabarrocas, J., Bauer, J., Piaggio, E., Liblau, R., and Lassmann, H. (2003). Effective and selective immune surveillance of the brain by MHC class I-restricted cytotoxic T lymphocytes. Eur. J. Immunol. 33, 1174–1182. doi: 10.1002/eji.200323492
Cadavid, D., Balcer, L., Galetta, S., Aktas, O., Ziemssen, T., Vanopdenbosch, L., et al. (2017). Safety and efficacy of opicinumab in acute optic neuritis (RENEW): a randomised, placebo-controlled, phase 2 trial. Lancet Neurol. 16, 189–199. doi: 10.1016/S1474-4422(16)30377-5
Cadavid, D., Mellion, M., Hupperts, R., Edwards, K. R., Calabresi, P. A., Drulović, J., et al. (2019). Safety and efficacy of opicinumab in patients with relapsing multiple sclerosis (SYNERGY): a randomised, placebo-controlled, phase 2 trial. Lancet Neurol. 18, 845–856. doi: 10.1016/S1474-4422(19)30137-1
Call, C. L., and Bergles, D. E. (2021). Cortical neurons exhibit diverse myelination patterns that scale between mouse brain regions and regenerate after demyelination. Nat. Commun. 12:4767. doi: 10.1038/s41467-021-25035-2
Cantoni, C., Bollman, B., Licastro, D., Xie, M., Mikesell, R., Schmidt, R., et al. (2015). TREM2 regulates microglial cell activation in response to demyelination in vivo. Acta Neuropathol. 129, 429–447. doi: 10.1007/s00401-015-1388-1
Cantuti-Castelvetri, L., Fitzner, D., Bosch-Queralt, M., Weil, M. T., Su, M., Sen, P., et al. (2018). Defective cholesterol clearance limits remyelination in the aged central nervous system. Science 359, 684–688. doi: 10.1126/science.aan4183
Carlton, W. W. (1966). Response of mice to the chelating agents sodium diethyldithiocarbamate, alpha-benzoinoxime, and biscyclohexanone oxaldihydrazone. Toxicol. Appl. Pharmacol. 8, 512–521. doi: 10.1016/0041-008x(66)90062-7
Carlton, W. W. (1967). Studies on the induction of hydrocephalus and spongy degeneration by cuprizone feeding and attempts to antidote the toxicity. Life Sci. 6, 11–19. doi: 10.1016/0024-3205(67)90356-6
Carlton, W. W. (1969). Spongiform encephalopathy induced in rats and guinea pigs by cuprizone. Exp. Mol. Pathol. 10, 274–287. doi: 10.1016/0014-4800(69)90057-4
Chang, A., Tourtellotte, W. W., Rudick, R., and Trapp, B. D. (2002). Premyelinating oligodendrocytes in chronic lesions of multiple sclerosis. N. Engl. J. Med. 346, 165–173. doi: 10.1056/NEJMoa010994
Chen, Y., Kunjamma, R. B., Weiner, M., Chan, J. R., and Popko, B. (2021). Prolonging the integrated stress response enhances CNS remyelination in an inflammatory environment. eLife 10, 1–21. doi: 10.7554/eLife.65469
Chen, Y., Zhen, W., Guo, T., Zhao, Y., Liu, A., Rubio, J. P., et al. (2017). Histamine receptor 3 negatively regulates oligodendrocyte differentiation and remyelination. PLoS One 12:e0189380. doi: 10.1371/journal.pone.0189380
Cignarella, F., Filipello, F., Bollman, B., Cantoni, C., Locca, A., Mikesell, R., et al. (2020). TREM2 activation on microglia promotes myelin debris clearance and remyelination in a model of multiple sclerosis. Acta Neuropathol. 140, 513–534. doi: 10.1007/s00401-020-02193-z
Corbin, J. G., Kelly, D., Rath, E. M., Baerwald, K. D., Suzuki, K., and Popko, B. (1996). Targeted CNS expression of interferon-γ in transgenic mice leads to Hypomyelination, reactive gliosis, and abnormal cerebellar development. Mol. Cell. Neurosci. 7, 354–370. doi: 10.1006/mcne.1996.0026
Cordano, C., Sin, J. H., Timmons, G., Yiu, H. H., Stebbins, K., Guglielmetti, C., et al. (2022). Validating visual evoked potentials as a preclinical, quantitative biomarker for remyelination efficacy. Brain J. Neurol. 145, 3943–3952. doi: 10.1093/brain/awac207
Crawford, A. H., Tripathi, R. B., Richardson, W. D., and Franklin, R. J. M. (2016). Developmental origin of oligodendrocyte lineage cells determines response to demyelination and susceptibility to age-associated functional decline. Cell Rep. 15, 761–773. doi: 10.1016/j.celrep.2016.03.069
Cui, Q.-L., Kuhlmann, T., Miron, V. E., Leong, S. Y., Fang, J., Gris, P., et al. (2013). Oligodendrocyte progenitor cell susceptibility to injury in multiple sclerosis. Am. J. Pathol. 183, 516–525. doi: 10.1016/j.ajpath.2013.04.016
Dal Canto, M. C., Kim, B. S., Miller, S. D., and Melvold, R. W. (1996). Theiler’s murine encephalomyelitis virus (TMEV)-induced demyelination: A model for human multiple sclerosis. Methods 10, 453–461. doi: 10.1006/meth.1996.0123
Dandekar, A. A., Anghelina, D., and Perlman, S. (2004). Bystander CD8 T-cell-mediated demyelination is interferon-gamma-dependent in a coronavirus model of multiple sclerosis. Am. J. Pathol. 164, 363–369. doi: 10.1016/s0002-9440(10)63126-4
Dandekar, A. A., and Perlman, S. (2002). Virus-induced demyelination in nude mice is mediated by gamma delta T cells. Am. J. Pathol. 161, 1255–1263. doi: 10.1016/s0002-9440(10)64402-1
Dandekar, A. A., Wu, G. F., Pewe, L., and Perlman, S. (2001). Axonal damage is T cell mediated and occurs concomitantly with demyelination in mice infected with a neurotropic coronavirus. J. Virol. 75, 6115–6120. doi: 10.1128/JVI.75.13.6115-6120.2001
Denic, A., Johnson, A. J., Bieber, A. J., Warrington, A. E., Rodriguez, M., and Pirko, I. (2011). The relevance of animal models in multiple sclerosis research. Pathophysiology 18, 21–29. doi: 10.1016/j.pathophys.2010.04.004
Deshmukh, V. A., Tardif, V., Lyssiotis, C. A., Green, C. C., Kerman, B., Kim, H. J., et al. (2013). A regenerative approach to the treatment of multiple sclerosis. Nature 502, 327–332. doi: 10.1038/nature12647
du, C., Duan, Y., Wei, W., Cai, Y., Chai, H., Lv, J., et al. (2016). Kappa opioid receptor activation alleviates experimental autoimmune encephalomyelitis and promotes oligodendrocyte-mediated remyelination. Nat. Commun. 7:11120. doi: 10.1038/ncomms11120
Duncan, I. D., Brower, A., Kondo, Y., Curlee, J. F. Jr., and Schultz, R. D. (2009). Extensive remyelination of the CNS leads to functional recovery. Proc. Natl. Acad. Sci. U. S. A. 106, 6832–6836. doi: 10.1073/pnas.0812500106
Duncan, G. J., Simkins, T. J., and Emery, B. (2021). Neuron-oligodendrocyte interactions in the structure and integrity of axons. Front. Cell Dev. Biol. 9:653101. doi: 10.3389/fcell.2021.653101
el Waly, B., Buttigieg, E., Karakus, C., Brustlein, S., and Debarbieux, F. (2020). Longitudinal Intravital microscopy reveals axon degeneration concomitant with inflammatory cell infiltration in an LPC model of demyelination. Front. Cell. Neurosci. 14:165. doi: 10.3389/fncel.2020.00165
Emery, B., Agalliu, D., Cahoy, J. D., Watkins, T. A., Dugas, J. C., Mulinyawe, S. B., et al. (2009). Myelin gene regulatory factor is a critical transcriptional regulator required for CNS myelination. Cells 138, 172–185. doi: 10.1016/j.cell.2009.04.031
Falcão, A. M., van Bruggen, D., Marques, S., Meijer, M., Jäkel, S., Agirre, E., et al. (2018). Disease-specific oligodendrocyte lineage cells arise in multiple sclerosis. Nat. Med. 24, 1837–1844. doi: 10.1038/s41591-018-0236-y
Floriddia, E. M., Lourenço, T., Zhang, S., van Bruggen, D., Hilscher, M. M., Kukanja, P., et al. (2020). Distinct oligodendrocyte populations have spatial preference and different responses to spinal cord injury. Nat. Commun. 11:5860. doi: 10.1038/s41467-020-19453-x
Franklin, R. J. M. (2002). Why does remyelination fail in multiple sclerosis? Nat. Rev. Neurosci. 3, 705–714. doi: 10.1038/nrn917
Franklin, R. J. M., and Ffrench-Constant, C. (2008). Remyelination in the CNS: from biology to therapy. Nat. Rev. Neurosci. 9, 839–855. doi: 10.1038/nrn2480
Franklin, R. J. M., Frisén, J., and Lyons, D. A. (2021). Revisiting remyelination: towards a consensus on the regeneration of CNS myelin. Semin. Cell Dev. Biol. 116, 3–9. doi: 10.1016/j.semcdb.2020.09.009
Frischer, J. M., Bramow, S., Dal-Bianco, A., Lucchinetti, C. F., Rauschka, H., Schmidbauer, M., et al. (2009). The relation between inflammation and neurodegeneration in multiple sclerosis brains. Brain J. Neurol. 132, 1175–1189. doi: 10.1093/brain/awp070
Frischer, J. M., Weigand, S. D., Guo, Y., Kale, N., Parisi, J. E., Pirko, I., et al. (2015). Clinical and pathological insights into the dynamic nature of the white matter multiple sclerosis plaque. Ann. Neurol. 78, 710–721. doi: 10.1002/ana.24497
Gardner, C., Magliozzi, R., Durrenberger, P. F., Howell, O. W., Rundle, J., and Reynolds, R. (2013). Cortical grey matter demyelination can be induced by elevated pro-inflammatory cytokines in the subarachnoid space of MOG-immunized rats. Brain J. Neurol. 136, 3596–3608. doi: 10.1093/brain/awt279
Gledhill, R. F., and McDonald, W. I. (1977). Morphological characteristics of central demyelination and remyelination: a single-fiber study. Ann. Neurol. 1, 552–560. doi: 10.1002/ana.410010607
Gold, R., Linington, C., and Lassmann, H. (2006). Understanding pathogenesis and therapy of multiple sclerosis via animal models: 70 years of merits and culprits in experimental autoimmune encephalomyelitis research. Brain J. Neurol. 129, 1953–1971. doi: 10.1093/brain/awl075
Goldberg, J., Clarner, T., Beyer, C., and Kipp, M. (2015). Anatomical distribution of Cuprizone-induced lesions in C57BL6 mice. J. Mol. Neurosci. 57, 166–175. doi: 10.1007/s12031-015-0595-5
Goldschmidt, T., Antel, J., Konig, F. B., Bruck, W., and Kuhlmann, T. (2009). Remyelination capacity of the MS brain decreases with disease chronicity. Neurology 72, 1914–1921. doi: 10.1212/WNL.0b013e3181a8260a
Gonzalez, G. A., Hofer, M. P., Syed, Y. A., Amaral, A. I., Rundle, J., Rahman, S., et al. (2016). Tamoxifen accelerates the repair of demyelinated lesions in the central nervous system. Sci. Rep. 6:31599. doi: 10.1038/srep31599
Graça, D. L., and Blakemore, W. F. (1986). Delayed remyelination in rat spinal cord following ethidium bromide injection. Neuropathol. Appl. Neurobiol. 12, 593–605. doi: 10.1111/j.1365-2990.1986.tb00162.x
Graves, J. S., Krysko, K. M., Hua, L. H., Absinta, M., Franklin, R. J. M., and Segal, B. M. (2023). Ageing and multiple sclerosis. Lancet Neurol. 22, 66–77. doi: 10.1016/S1474-4422(22)00184-3
Green, A. J., Gelfand, J. M., Cree, B. A., Bevan, C., Boscardin, W. J., Mei, F., et al. (2017). Clemastine fumarate as a remyelinating therapy for multiple sclerosis (ReBUILD): a randomised, controlled, double-blind, crossover trial. Lancet 390, 2481–2489. doi: 10.1016/S0140-6736(17)32346-2
Greenberg, B. M., Bowen, J. D., Alvarez, E., Rodriguez, M., Caggiano, A. O., Warrington, A. E., et al. (2022). A double-blind, placebo-controlled, single-ascending-dose intravenous infusion study of rHIgM22 in subjects with multiple sclerosis immediately following a relapse. Multiple Sclerosis J 8:205521732210914. doi: 10.1177/20552173221091475
Greenhalgh, A. D., David, S., and Bennett, F. C. (2020). Immune cell regulation of glia during CNS injury and disease. Nat. Rev. Neurosci. 21, 139–152. doi: 10.1038/s41583-020-0263-9
Gudi, V., Moharregh-Khiabani, D., Skripuletz, T., Koutsoudaki, P. N., Kotsiari, A., Skuljec, J., et al. (2009). Regional differences between grey and white matter in cuprizone induced demyelination. Brain Res. 1283, 127–138. doi: 10.1016/j.brainres.2009.06.005
Hall, S. M. (1972). The effect of injections of lysophosphatidyl choline into white matter of the adult mouse spinal cord. J. Cell Sci. 10, 535–546. doi: 10.1242/jcs.10.2.535
Harrington, E. P., Bergles, D. E., and Calabresi, P. A. (2020). Immune cell modulation of oligodendrocyte lineage cells. Neurosci. Lett. 715:134601. doi: 10.1016/j.neulet.2019.134601
Harrington, E. P., Catenacci, R. B., Smith, M. D., Heo, D., Miller, C. E., Meyers, K. R., et al. (2023). MHC class I and MHC class II reporter mice enable analysis of immune oligodendroglia in mouse models of multiple sclerosis. eLife 12:e82938. doi: 10.7554/eLife.82938
Hartley, M. D., Banerji, T., Tagge, I. J., Kirkemo, L. L., Chaudhary, P., Calkins, E., et al. (2019). Myelin repair stimulated by CNS-selective thyroid hormone action. JCI insight 4:e126329. doi: 10.1172/jci.insight.126329
Hauser, S. L., Bhan, A. K., Gilles, F., Kemp, M., Kerr, C., and Weiner, H. L. (1986). Immunohistochemical analysis of the cellular infiltrate in multiple sclerosis lesions. Ann. Neurol. 19, 578–587. doi: 10.1002/ana.410190610
Heß, K., Starost, L., Kieran, N. W., Thomas, C., Vincenten, M. C. J., Antel, J., et al. (2020). Lesion stage-dependent causes for impaired remyelination in MS. Acta Neuropathol. 140, 359–375. doi: 10.1007/s00401-020-02189-9
Hill, M. F. E., Cunniffe, N. G., and Franklin, R. J. M. (2022). Seeing is believing: identifying remyelination in the central nervous system. Curr. Opin. Pharmacol. 66:102269. doi: 10.1016/j.coph.2022.102269
Hiremath, M. M., Saito, Y., Knapp, G. W., Ting, J. P. Y., Suzuki, K., and Matsushima, G. K. (1998). Microglial/macrophage accumulation during cuprizone-induced demyelination in C57BL/6 mice. J. Neuroimmunol. 92, 38–49. doi: 10.1016/s0165-5728(98)00168-4
Hochstrasser, T., Rühling, S., Hecher, K., Fabisch, K. H., Chrzanowski, U., Brendel, M., et al. (2019). Stereological investigation of regional brain volumes after acute and chronic Cuprizone-induced demyelination. Cells 8:1024. doi: 10.3390/cells8091024
Hou, J., Zhou, Y., Cai, Z., Terekhova, M., Swain, A., Andhey, P. S., et al. (2023). Transcriptomic atlas and interaction networks of brain cells in mouse CNS demyelination and remyelination. Cell Rep. 42:112293. doi: 10.1016/j.celrep.2023.112293
Howe, C. L., Adelson, J. D., and Rodriguez, M. (2007). Absence of perforin expression confers axonal protection despite demyelination. Neurobiol. Dis. 25, 354–359. doi: 10.1016/j.nbd.2006.10.001
Howell, O. W., Reeves, C. A., Nicholas, R., Carassiti, D., Radotra, B., Gentleman, S. M., et al. (2011). Meningeal inflammation is widespread and linked to cortical pathology in multiple sclerosis. Brain J. Neurol. 134, 2755–2771. doi: 10.1093/brain/awr182
Huang, J. K., Jarjour, A. A., Nait Oumesmar, B., Kerninon, C., Williams, A., Krezel, W., et al. (2011). Retinoid X receptor gamma signaling accelerates CNS remyelination. Nat. Neurosci. 14, 45–53. doi: 10.1038/nn.2702
Hughes, E. G., Kang, S. H., Fukaya, M., and Bergles, D. E. (2013). Oligodendrocyte progenitors balance growth with self-repulsion to achieve homeostasis in the adult brain. Nat. Neurosci. 16, 668–676. doi: 10.1038/nn.3390
Hughes, E. G., Orthmann-Murphy, J. L., Langseth, A. J., and Bergles, D. E. (2018). Myelin remodeling through experience-dependent oligodendrogenesis in the adult somatosensory cortex. Nat. Neurosci. 21, 696–706. doi: 10.1038/s41593-018-0121-5
Huseby, E. S., Liggitt, D., Brabb, T., Schnabel, B., Öhlén, C., and Goverman, J. (2001). A pathogenic role for myelin-specific CD8(+) T cells in a model for multiple sclerosis. J. Exp. Med. 194, 669–676. doi: 10.1084/jem.194.5.669
Ineichen, B. V., Kapitza, S., Bleul, C., Good, N., Plattner, P. S., Seyedsadr, M. S., et al. (2017). Nogo-A antibodies enhance axonal repair and remyelination in neuro-inflammatory and demyelinating pathology. Acta Neuropathol. 134, 423–440. doi: 10.1007/s00401-017-1745-3
Irvine, K.-A., and Blakemore, W. F. (2006). Age increases axon loss associated with primary demyelination in cuprizone-induced demyelination in C57BL/6 mice. J. Neuroimmunol. 175, 69–76. doi: 10.1016/j.jneuroim.2006.03.002
Irvine, K. A., and Blakemore, W. F. (2008). Remyelination protects axons from demyelination-associated axon degeneration. Brain J. Neurol. 131, 1464–1477. doi: 10.1093/brain/awn080
Jäkel, S., Agirre, E., Mendanha Falcão, A., van Bruggen, D., Lee, K. W., Knuesel, I., et al. (2019). Altered human oligodendrocyte heterogeneity in multiple sclerosis. Nature 566, 543–547. doi: 10.1038/s41586-019-0903-2
Jeffery, N. D., and Blakemore, W. F. (1997). Locomotor deficits induced by experimental spinal cord demyelination are abolished by spontaneous remyelination. Brain J. Neurol. 120, 27–37. doi: 10.1093/brain/120.1.27
Jeffery, N. D., Crang, A. J., O'Leary, M. T., Hodge, S. J., and Blakemore, W. F. (1999). Behavioural consequences of oligodendrocyte progenitor cell transplantation into experimental demyelinating lesions in the rat spinal cord. Eur. J. Neurosci. 11, 1508–1514. doi: 10.1046/j.1460-9568.1999.00564.x
Jones, M. V., Nguyen, T. T., DeBoy, C. A., Griffin, J. W., Whartenby, K. A., Kerr, D. A., et al. (2008). Behavioral and pathological outcomes in MOG 35-55 experimental autoimmune encephalomyelitis. J. Neuroimmunol. 199, 83–93. doi: 10.1016/j.jneuroim.2008.05.013
Joubert, L., Foucault, I., Sagot, Y., Bernasconi, L., Duval, F., Alliod, C., et al. (2010). Chemical inducers and transcriptional markers of oligodendrocyte differentiation. J. Neurosci. Res. 88, 2546–2557. doi: 10.1002/jnr.22434
Kaddatz, H., Joost, S., Nedelcu, J., Chrzanowski, U., Schmitz, C., Gingele, S., et al. (2021). Cuprizone-induced demyelination triggers a CD8-pronounced T cell recruitment. Glia 69, 925–942. doi: 10.1002/glia.23937
Kang, Z., Liu, L., Spangler, R., Spear, C., Wang, C., Gulen, M. F., et al. (2012). IL-17-induced Act1-mediated signaling is critical for cuprizone-induced demyelination. J. Neurosci. 32, 8284–8292. doi: 10.1523/JNEUROSCI.0841-12.2012
Kim, J. H., Budde, M. D., Liang, H.-F., Klein, R. S., Russell, J. H., Cross, A. H., et al. (2006). Detecting axon damage in spinal cord from a mouse model of multiple sclerosis. Neurobiol. Dis. 21, 626–632. doi: 10.1016/j.nbd.2005.09.009
Kipp, M., Clarner, T., Dang, J., Copray, S., and Beyer, C. (2009). The cuprizone animal model: new insights into an old story. Acta Neuropathol. 118, 723–736. doi: 10.1007/s00401-009-0591-3
Kirby, L., Jin, J., Cardona, J. G., Smith, M. D., Martin, K. A., Wang, J., et al. (2019). Oligodendrocyte precursor cells present antigen and are cytotoxic targets in inflammatory demyelination. Nat. Commun. 10:3887. doi: 10.1038/s41467-019-11638-3
Koenning, M., Jackson, S., Hay, C. M., Faux, C., Kilpatrick, T. J., Willingham, M., et al. (2012). Myelin gene regulatory factor is required for maintenance of myelin and mature oligodendrocyte identity in the adult CNS. J. Neurosci. Off. J. Soc. Neurosci. 32, 12528–12542. doi: 10.1523/JNEUROSCI.1069-12.2012
Kotter, M. R., Li, W.-W., Zhao, C., and Franklin, R. J. M. (2006). Myelin impairs CNS remyelination by inhibiting oligodendrocyte precursor cell differentiation. J. Neurosci. Off. J. Soc. Neurosci. 26, 328–332. doi: 10.1523/JNEUROSCI.2615-05.2006
Kotter, M. R., Setzu, A., Sim, F. J., van Rooijen, N., and Franklin, R. J. M. (2001). Macrophage depletion impairs oligodendrocyte remyelination following lysolecithin-induced demyelination. Glia 35, 204–212. doi: 10.1002/glia.1085
Kotter, M. R., Zhao, C., van Rooijen, N., and Franklin, R. J. M. (2005). Macrophage-depletion induced impairment of experimental CNS remyelination is associated with a reduced oligodendrocyte progenitor cell response and altered growth factor expression. Neurobiol. Dis. 18, 166–175. doi: 10.1016/j.nbd.2004.09.019
Koutsoudaki, P. N., Papadopoulos, D., Passias, P.-G., Koutsoudaki, P., and Gorgoulis, V. G. (2020). Cellular senescence and failure of myelin repair in multiple sclerosis. Mech. Ageing Dev. 192:111366. doi: 10.1016/j.mad.2020.111366
Kuhlmann, T., Lingfeld, G., Bitsch, A., Schuchardt, J., and Brück, W. (2002). Acute axonal damage in multiple sclerosis is most extensive in early disease stages and decreases over time. Brain J. Neurol. 125, 2202–2212. doi: 10.1093/brain/awf235
Kuhlmann, T., Miron, V., Cuo, Q., Wegner, C., Antel, J., and Bruck, W. (2008). Differentiation block of oligodendroglial progenitor cells as a cause for remyelination failure in chronic multiple sclerosis. Brain J. Neurol. 131, 1749–1758. doi: 10.1093/brain/awn096
Kutzelnigg, A., Lucchinetti, C. F., Stadelmann, C., Brück, W., Rauschka, H., Bergmann, M., et al. (2005). Cortical demyelination and diffuse white matter injury in multiple sclerosis. Brain J. Neurol. 128, 2705–2712. doi: 10.1093/brain/awh641
LaFerla, F. M., Sugarman, M. C., Lane, T. E., and Leissring, M. A. (2000). Regional hypomyelination and dysplasia in transgenic mice with astrocyte-directed expression of interferon-γ. J. Molecular Neurosci. 15, 45–60. doi: 10.1385/JMN:15:1:45
Laflamme, N., Cisbani, G., Préfontaine, P., Srour, Y., Bernier, J., St-Pierre, M. K., et al. (2018). mCSF-induced microglial activation prevents myelin loss and promotes its repair in a mouse model of multiple sclerosis. Front. Cell. Neurosci. 12:178. doi: 10.3389/fncel.2018.00178
LaGanke, C., Samkoff, L., Edwards, K., Jung Henson, L., Repovic, P., Lynch, S., et al. (2017). Safety/tolerability of the anti-semaphorin 4D antibody VX15/2503 in a randomized phase 1 trial. Neurol. Neuroimmunol. Neuroinflammation 4:e367. doi: 10.1212/NXI.0000000000000367
Lane, T. E., and Hosking, M. P. (2010). The pathogenesis of murine coronavirus infection of the central nervous system. Crit. Rev. Immunol. 30, 119–130. doi: 10.1615/critrevimmunol.v30.i2.20
Lariosa-Willingham, K. D., Rosler, E. S., Tung, J. S., Dugas, J. C., Collins, T. L., and Leonoudakis, D. (2016). A high throughput drug screening assay to identify compounds that promote oligodendrocyte differentiation using acutely dissociated and purified oligodendrocyte precursor cells. BMC. Res. Notes 9:419. doi: 10.1186/s13104-016-2220-2
Lassmann, H., and Bradl, M. (2017). Multiple sclerosis: experimental models and reality. Acta Neuropathol. 133, 223–244. doi: 10.1007/s00401-016-1631-4
Lassmann, H., Brück, W., and Lucchinetti, C. (2001). Heterogeneity of multiple sclerosis pathogenesis: implications for diagnosis and therapy. Trends Mol. Med. 7, 115–121. doi: 10.1016/s1471-4914(00)01909-2
Lau, L. W., Keough, M. B., Haylock-Jacobs, S., Cua, R., Döring, A., Sloka, S., et al. (2012). Chondroitin sulfate proteoglycans in demyelinated lesions impair remyelination. Ann. Neurol. 72, 419–432. doi: 10.1002/ana.23599
Liebetanz, D., and Merkler, D. (2006). Effects of commissural de-and remyelination on motor skill behaviour in the cuprizone mouse model of multiple sclerosis. Exp. Neurol. 202, 217–224. doi: 10.1016/j.expneurol.2006.05.032
Lin, W., Kemper, A., Dupree, J. L., Harding, H. P., Ron, D., and Popko, B. (2006). Interferon-gamma inhibits central nervous system remyelination through a process modulated by endoplasmic reticulum stress. Brain J. Neurol. 129, 1306–1318. doi: 10.1093/brain/awl044
Lin, W., Kemper, A., McCarthy, K. D., Pytel, P., Wang, J. P., Campbell, I. L., et al. (2004). Interferon-gamma induced medulloblastoma in the developing cerebellum. J. Neurosci. Off. J. Soc. Neurosci. 24, 10074–10083. doi: 10.1523/JNEUROSCI.2604-04.2004
Lindner, M., Fokuhl, J., Linsmeier, F., Trebst, C., and Stangel, M. (2009). Chronic toxic demyelination in the central nervous system leads to axonal damage despite remyelination. Neurosci. Lett. 453, 120–125. doi: 10.1016/j.neulet.2009.02.004
Lipton, H. L., Twaddle, G., and Jelachich, M. L. (1995). The predominant virus antigen burden is present in macrophages in Theiler’s murine encephalomyelitis virus-induced demyelinating disease. J. Virol. 69, 2525–2533. doi: 10.1128/JVI.69.4.2525-2533.1995
Lubrich, C., Giesler, P., and Kipp, M. (2022). Motor behavioral deficits in the Cuprizone model: validity of the Rotarod test paradigm. Int. J. Mol. Sci. 23:11342. doi: 10.3390/ijms231911342
Lucchinetti, C., Brück, W., Parisi, J., Scheithauer, B., Rodriguez, M., and Lassmann, H. (2000). Heterogeneity of multiple sclerosis lesions: implications for the pathogenesis of demyelination. Ann. Neurol. 47, 707–717. doi: 10.1002/1531-8249(200006)47:6<707::aid-ana3>3.0.co;2-q
Lucchinetti, C., Brück, W., Parisi, J., Scheithauer, B., Rodriguez, M., and Lassmann, H. (1999). A quantitative analysis of oligodendrocytes in multiple sclerosis lesions. A study of 113 cases. Brain J. Neurol. 122, 2279–2295. doi: 10.1093/brain/122.12.2279
Madadi, S., Pasbakhsh, P., Tahmasebi, F., Mortezaee, K., Khanehzad, M., Boroujeni, F. B., et al. (2019). Astrocyte ablation induced by La-aminoadipate (L-AAA) potentiates remyelination in a cuprizone demyelinating mouse model. Metab. Brain Dis. 34, 593–603. doi: 10.1007/s11011-019-0385-9
Manrique-Hoyos, N., Jürgens, T., Grønborg, M., Kreutzfeldt, M., Schedensack, M., Kuhlmann, T., et al. (2012). Late motor decline after accomplished remyelination: impact for progressive multiple sclerosis. Ann. Neurol. 71, 227–244. doi: 10.1002/ana.22681
Marenna, S., Huang, S.-C., Dalla Costa, G., d’Isa, R., Castoldi, V., Rossi, E., et al. (2022). Visual evoked potentials to monitor myelin cuprizone-induced functional changes. Front. Neurosci. 16:820155. doi: 10.3389/fnins.2022.820155
Marisca, R., Hoche, T., Agirre, E., Hoodless, L. J., Barkey, W., Auer, F., et al. (2020). Functionally distinct subgroups of oligodendrocyte precursor cells integrate neural activity and execute myelin formation. Nat. Neurosci. 23, 363–374. doi: 10.1038/s41593-019-0581-2
Marzan, D. E., Brügger-Verdon, V., West, B. L., Liddelow, S., Samanta, J., and Salzer, J. L. (2021). Activated microglia drive demyelination via CSF1R signaling. Glia 69, 1583–1604. doi: 10.1002/glia.23980
Mason, J. L., Jones, J. J., Taniike, M., Morell, P., Suzuki, K., and Matsushima, G. K. (2000). Mature oligodendrocyte apoptosis precedes IGF-1 production and oligodendrocyte progenitor accumulation and differentiation during demyelination/remyelination. J. Neurosci. Res. 61, 251–262. doi: 10.1002/1097-4547(20000801)61:3<251::AID-JNR3>3.0.CO;2-W
Matsushima, G. K., and Morell, P. (2001). The neurotoxicant, cuprizone, as a model to study demyelination and remyelination in the central nervous system. Brain Pathol. 11, 107–116. doi: 10.1111/j.1750-3639.2001.tb00385.x
McKay, J. S., Blakemore, W. F., and Franklin, R. J. (1998). Trapidil-mediated inhibition of CNS remyelination results from reduced numbers and impaired differentiation of oligodendrocytes. Neuropathol. Appl. Neurobiol. 24, 498–506. doi: 10.1046/j.1365-2990.1998.00148.x
Mei, F., Fancy, S. P. J., Shen, Y.-A. A., Niu, J., Zhao, C., Presley, B., et al. (2014). Micropillar arrays as a high-throughput screening platform for therapeutics in multiple sclerosis. Nat. Med. 20, 954–960. doi: 10.1038/nm.3618
Mei, F., Lehmann-Horn, K., Shen, Y. A. A., Rankin, K. A., Stebbins, K. J., Lorrain, D. S., et al. (2016a). Accelerated remyelination during inflammatory demyelination prevents axonal loss and improves functional recovery. eLife 5:e18246. doi: 10.7554/eLife.18246
Mei, F., Mayoral, S. R., Nobuta, H., Wang, F., Desponts, C., Lorrain, D. S., et al. (2016b). Identification of the kappa-opioid receptor as a therapeutic target for oligodendrocyte Remyelination. J. Neurosci. Off. J. Soc. Neurosci. 36, 7925–7935. doi: 10.1523/JNEUROSCI.1493-16.2016
Meijer, M., Agirre, E., Kabbe, M., van Tuijn, C. A., Heskol, A., Zheng, C., et al. (2022). Epigenomic priming of immune genes implicates oligodendroglia in multiple sclerosis susceptibility. Neuron 110, 1193–1210.e13. doi: 10.1016/j.neuron.2021.12.034
Mendel, I., Kerlero de Rosbo, N., and Ben-Nun, A. (1995). A myelin oligodendrocyte glycoprotein peptide induces typical chronic experimental autoimmune encephalomyelitis in H-2b mice: fine specificity and T cell receptor V beta expression of encephalitogenic T cells. Eur. J. Immunol. 25, 1951–1959. doi: 10.1002/eji.1830250723
Merkler, D., Ernsting, T., Kerschensteiner, M., Brück, W., and Stadelmann, C. (2006). A new focal EAE model of cortical demyelination: multiple sclerosis-like lesions with rapid resolution of inflammation and extensive remyelination. Brain J. Neurol. 129, 1972–1983. doi: 10.1093/brain/awl135
Mezydlo, A., Treiber, N., Ullrich Gavilanes, E. M., Eichenseer, K., Ancău, M., Wens, A., et al. (2023). Remyelination by surviving oligodendrocytes is inefficient in the inflamed mammalian cortex. Neuron 111, 1748–1759.e8. doi: 10.1016/j.neuron.2023.03.031
Mi, S., Miller, R. H., Tang, W., Lee, X., Hu, B., Wu, W., et al. (2009). Promotion of central nervous system remyelination by induced differentiation of oligodendrocyte precursor cells. Ann. Neurol. 65, 304–315. doi: 10.1002/ana.21581
Miron, V. E., Boyd, A., Zhao, J.-W., Yuen, T. J., Ruckh, J. M., Shadrach, J. L., et al. (2013). M2 microglia and macrophages drive oligodendrocyte differentiation during CNS remyelination. Nat. Neurosci. 16, 1211–1218. doi: 10.1038/nn.3469
Mothe, A. J., and Tator, C. H. (2008). Transplanted neural stem/progenitor cells generate myelinating oligodendrocytes and Schwann cells in spinal cord demyelination and dysmyelination. Exp. Neurol. 213, 176–190. doi: 10.1016/j.expneurol.2008.05.024
Na, S.-Y., Cao, Y., Toben, C., Nitschke, L., Stadelmann, C., Gold, R., et al. (2008). Naive CD8 T-cells initiate spontaneous autoimmunity to a sequestered model antigen of the central nervous system. Brain J. Neurol. 131, 2353–2365. doi: 10.1093/brain/awn148
Najm, F. J., Madhavan, M., Zaremba, A., Shick, E., Karl, R. T., Factor, D. C., et al. (2015). Drug-based modulation of endogenous stem cells promotes functional remyelination in vivo. Nature 522, 216–220. doi: 10.1038/nature14335
Natrajan, M. S., de la Fuente, A. G., Crawford, A. H., Linehan, E., Nuñez, V., Johnson, K. R., et al. (2015). Retinoid X receptor activation reverses age-related deficiencies in myelin debris phagocytosis and remyelination. Brain J. Neurol. 138, 3581–3597. doi: 10.1093/brain/awv289
Nave, K.-A. (2010). Myelination and support of axonal integrity by glia. Nature 468, 244–252. doi: 10.1038/nature09614
Neely, S. A., Williamson, J. M., Klingseisen, A., Zoupi, L., Early, J. J., Williams, A., et al. (2022). New oligodendrocytes exhibit more abundant and accurate myelin regeneration than those that survive demyelination. Nat. Neurosci. 25, 415–420. doi: 10.1038/s41593-021-01009-x
Negron, A., Stüve, O., and Forsthuber, T. G. (2020). Ectopic lymphoid follicles in multiple sclerosis: centers for disease control? Front. Neurol. 11:607766. doi: 10.3389/fneur.2020.607766
Neumann, B., Baror, R., Zhao, C., Segel, M., Dietmann, S., Rawji, K. S., et al. (2019). Metformin restores CNS Remyelination capacity by rejuvenating aged stem cells. Cell Stem Cell 25, 473–485.e8. doi: 10.1016/j.stem.2019.08.015
Neumann, B., Foerster, S., Zhao, C., Bodini, B., Reich, D. S., Bergles, D. E., et al. (2020). Problems and pitfalls of identifying Remyelination in multiple sclerosis. Cell Stem Cell 26, 617–619. doi: 10.1016/j.stem.2020.03.017
Nikić, I., Merkler, D., Sorbara, C., Brinkoetter, M., Kreutzfeldt, M., Bareyre, F. M., et al. (2011). A reversible form of axon damage in experimental autoimmune encephalomyelitis and multiple sclerosis. Nat. Med. 17, 495–499. doi: 10.1038/nm.2324
Oleszak, E. L., Chang, J. R., Friedman, H., Katsetos, C. D., and Platsoucas, C. D. (2004). Theiler’s virus infection: a model for multiple sclerosis. Clin. Microbiol. Rev. 17, 174–207. doi: 10.1128/CMR.17.1.174-207.2004
Oliver, A. R., Lyon, G. M., and Ruddle, N. H. (2003). Rat and human myelin oligodendrocyte glycoproteins induce experimental autoimmune encephalomyelitis by different mechanisms in C57BL/6 mice. J. Immunol. 171, 462–468. doi: 10.4049/jimmunol.171.1.462
Orthmann-Murphy, J., Call, C. L., Molina-Castro, G. C., Hsieh, Y. C., Rasband, M. N., Calabresi, P. A., et al. (2020). Remyelination alters the pattern of myelin in the cerebral cortex. elife 9:e56621. doi: 10.7554/eLife.56621
Pagnin, M., Dekiwadia, C., Petratos, S., and Richardson, S. J. (2022). Enhanced re-myelination in transthyretin null mice following cuprizone mediated demyelination. Neurosci. Lett. 766:136287. doi: 10.1016/j.neulet.2021.136287
Pan, R., Zhang, Q., Anthony, S. M., Zhou, Y., Zou, X., Cassell, M., et al. (2020). Oligodendrocytes that survive acute coronavirus infection induce prolonged inflammatory responses in the CNS. Proc. Natl. Acad. Sci. U. S. A. 117, 15902–15910. doi: 10.1073/pnas.2003432117
Patel, R., Moore, S., Crawford, D. K., Hannsun, G., Sasidhar, M. V., Tan, K., et al. (2013). Attenuation of corpus callosum axon myelination and remyelination in the absence of circulating sex hormones. Brain Pathol. 23, 462–475. doi: 10.1111/bpa.12029
Paterson, P. Y. (1960). Transfer of allergic encephalomyelitis in rats by means of lymph node cells. J. Exp. Med. 111, 119–136. doi: 10.1084/jem.111.1.119
Penderis, J., Shields, S. A., and Franklin, R. J. M. (2003). Impaired remyelination and depletion of oligodendrocyte progenitors does not occur following repeated episodes of focal demyelination in the rat central nervous system. Brain J. Neurol. 126, 1382–1391. doi: 10.1093/brain/awg126
Perdaens, O., and van Pesch, V. (2021). Molecular mechanisms of Immunosenescene and Inflammaging: relevance to the Immunopathogenesis and treatment of multiple sclerosis. Front. Neurol. 12:811518. doi: 10.3389/fneur.2021.811518
Piaton, G., Aigrot, M.-S., Williams, A., Moyon, S., Tepavcevic, V., Moutkine, I., et al. (2011). Class 3 semaphorins influence oligodendrocyte precursor recruitment and remyelination in adult central nervous system. Brain J. Neurol. 134, 1156–1167. doi: 10.1093/brain/awr022
Pihl-Jensen, G., Schmidt, M. F., and Frederiksen, J. L. (2017). Multifocal visual evoked potentials in optic neuritis and multiple sclerosis: A review. Clin. Neurophysiol. 128, 1234–1245. doi: 10.1016/j.clinph.2017.03.047
Pike, S. C., Welsh, N., Linzey, M., and Gilli, F. (2022). Theiler’s virus-induced demyelinating disease as an infectious model of progressive multiple sclerosis. Front. Mol. Neurosci. 15:1019799. doi: 10.3389/fnmol.2022.1019799
Pittock, S. J., and Lucchinetti, C. F. (2007). The pathology of MS: new insights and potential clinical applications. Neurologist 13, 45–56. doi: 10.1097/01.nrl.0000253065.31662.37
Plant, S. R., Arnett, H. A., and Ting, J. P.-Y. (2005). Astroglial-derived lymphotoxin-alpha exacerbates inflammation and demyelination, but not remyelination. Glia 49, 1–14. doi: 10.1002/glia.20089
Plant, S. R., Iocca, H. A., Wang, Y., Thrash, J. C., O'Connor, B. P., Arnett, H. A., et al. (2007). Lymphotoxin beta receptor (Lt betaR): dual roles in demyelination and remyelination and successful therapeutic intervention using Lt betaR-Ig protein. J. Neurosci. Off. J. Soc. Neurosci. 27, 7429–7437. doi: 10.1523/JNEUROSCI.1307-07.2007
Plemel, J. R., Michaels, N. J., Weishaupt, N., Caprariello, A. V., Keough, M. B., Rogers, J. A., et al. (2018). Mechanisms of lysophosphatidylcholine-induced demyelination: A primary lipid disrupting myelinopathy. Glia 66, 327–347. doi: 10.1002/glia.23245
Poliani, P. L., Wang, Y., Fontana, E., Robinette, M. L., Yamanishi, Y., Gilfillan, S., et al. (2015). TREM2 sustains microglial expansion during aging and response to demyelination. J. Clin. Invest. 125, 2161–2170. doi: 10.1172/JCI77983
Porcu, G., Serone, E., de Nardis, V., di Giandomenico, D., Lucisano, G., Scardapane, M., et al. (2015). Clobetasol and Halcinonide act as smoothened agonists to promote myelin gene expression and RxRγ receptor activation. PloS One 10:e0144550. doi: 10.1371/journal.pone.0144550
Pozzilli, C., de Giglio, L., Barletta, V. T., Marinelli, F., Angelis, F. D., Gallo, V., et al. (2015). Oral contraceptives combined with interferon β in multiple sclerosis. Neurol. Neuroimmunol. Neuroinflammation 2:e120. doi: 10.1212/NXI.0000000000000120
Praet, J., Guglielmetti, C., Berneman, Z., van der Linden, A., and Ponsaerts, P. (2014). Cellular and molecular neuropathology of the cuprizone mouse model: clinical relevance for multiple sclerosis. Neurosci. Biobehav. Rev. 47, 485–505. doi: 10.1016/j.neubiorev.2014.10.004
Prineas, J. W., and Parratt, J. D. E. (2012). Oligodendrocytes and the early multiple sclerosis lesion. Ann. Neurol. 72, 18–31. doi: 10.1002/ana.23634
Rangachari, M., and Kuchroo, V. K. (2013). Using EAE to better understand principles of immune function and autoimmune pathology. J. Autoimmun. 45, 31–39. doi: 10.1016/j.jaut.2013.06.008
Rankin, K. A., Mei, F., Kim, K., Shen, Y. A. A., Mayoral, S. R., Desponts, C., et al. (2019). Selective estrogen receptor modulators enhance CNS Remyelination independent of estrogen receptors. J. Neurosci. Off. J. Soc. Neurosci. 39, 2184–2194. doi: 10.1523/JNEUROSCI.1530-18.2019
Rawji, K. S., Neumann, B., and Franklin, R. J. M. (2023). Glial aging and its impact on central nervous system myelin regeneration. Ann. NY. Acad. Sci. 1519, 34–45. doi: 10.1111/nyas.14933
Remington, L. T., Babcock, A. A., Zehntner, S. P., and Owens, T. (2007). Microglial recruitment, activation, and proliferation in response to primary demyelination. Am. J. Pathol. 170, 1713–1724. doi: 10.2353/ajpath.2007.060783
Reynolds, R., and Wilkin, G. P. (1993). Cellular reaction to an acute demyelinating/remyelinating lesion of the rat brain stem: localisation of GD3 ganglioside immunoreactivity. J. Neurosci. Res. 36, 405–422. doi: 10.1002/jnr.490360407
Rivera-Quiñones, C., McGavern, D., Schmelzer, J. D., Hunter, S. F., Low, P. A., and Rodriguez, M. (1998). Absence of neurological deficits following extensive demyelination in a class I-deficient murine model of multiple sclerosis. Nat. Med. 4, 187–193. doi: 10.1038/nm0298-187
Rodriguez, M., Leibowitz, J. L., and Lampert, P. W. (1983). Persistent infection of oligodendrocytes in Theiler’s virus-induced encephalomyelitis. Ann. Neurol. 13, 426–433. doi: 10.1002/ana.410130409
Rosato-Siri, M. V., Marziali, L. N., Mattera, V., Correale, J., and Pasquini, J. M. (2021). Combination therapy of apo-transferrin and thyroid hormones enhances remyelination. Glia 69, 151–164. doi: 10.1002/glia.23891
Ruckh, J. M., Zhao, J.-W., Shadrach, J. L., van Wijngaarden, P., Rao, T. N., Wagers, A. J., et al. (2012). Rejuvenation of regeneration in the aging central nervous system. Cell Stem Cell 10, 96–103. doi: 10.1016/j.stem.2011.11.019
Sachs, H. H., Bercury, K. K., Popescu, D. C., Narayanan, S. P., and Macklin, W. B. (2014). A new model of cuprizone-mediated demyelination/remyelination. ASN Neuro 6:1759091414551955. doi: 10.1177/1759091414551955
Sariol, A., Mackin, S., Allred, M.-G., Ma, C., Zhou, Y., Zhang, Q., et al. (2020). Microglia depletion exacerbates demyelination and impairs remyelination in a neurotropic coronavirus infection. Proc. Natl. Acad. Sci. U. S. A. 117, 24464–24474. doi: 10.1073/pnas.2007814117
Saxena, A., Bauer, J., Scheikl, T., Zappulla, J., Audebert, M., Desbois, S., et al. (2008). Cutting edge: multiple sclerosis-like lesions induced by effector CD8 T cells recognizing a sequestered antigen on oligodendrocytes. J. Immunol. 181, 1617–1621. doi: 10.4049/jimmunol.181.3.1617
Schirmer, L., Schafer, D. P., Bartels, T., Rowitch, D. H., and Calabresi, P. A. (2021). Diversity and function of glial cell types in multiple sclerosis. Trends Immunol. 42, 228–247. doi: 10.1016/j.it.2021.01.005
Schirmer, L., Velmeshev, D., Holmqvist, S., Kaufmann, M., Werneburg, S., Jung, D., et al. (2019). Neuronal vulnerability and multilineage diversity in multiple sclerosis. Nature 573, 75–82. doi: 10.1038/s41586-019-1404-z
Schmidt, T., Awad, H., Slowik, A., Beyer, C., Kipp, M., and Clarner, T. (2013). Regional heterogeneity of cuprizone-induced demyelination: topographical aspects of the midline of the corpus callosum. J Molecular Neurosci 49, 80–88. doi: 10.1007/s12031-012-9896-0
Schuh, C., Wimmer, I., Hametner, S., Haider, L., van Dam, A. M., Liblau, R. S., et al. (2014). Oxidative tissue injury in multiple sclerosis is only partly reflected in experimental disease models. Acta Neuropathol. 128, 247–266. doi: 10.1007/s00401-014-1263-5
Schwartzbach, C. J., Grove, R. A., Brown, R., Tompson, D., Then Bergh, F., and Arnold, D. L. (2017). Lesion remyelinating activity of GSK239512 versus placebo in patients with relapsing-remitting multiple sclerosis: a randomised, single-blind, phase II study. J. Neurol. 264, 304–315. doi: 10.1007/s00415-016-8341-7
Seeker, L. A., and Williams, A. (2022). Oligodendroglia heterogeneity in the human central nervous system. Acta Neuropathol. 143, 143–157. doi: 10.1007/s00401-021-02390-4
Segel, M., Neumann, B., Hill, M. F. E., Weber, I. P., Viscomi, C., Zhao, C., et al. (2019). Niche stiffness underlies the ageing of central nervous system progenitor cells. Nature 573, 130–134. doi: 10.1038/s41586-019-1484-9
Shen, K., Reichelt, M., Kyauk, R. V., Ngu, H., Shen, Y. A. A., Foreman, O., et al. (2021). Multiple sclerosis risk gene Mertk is required for microglial activation and subsequent remyelination. Cell Rep. 34:108835. doi: 10.1016/j.celrep.2021.108835
Shields, S. A., Gilson, J. M., Blakemore, W. F., and Franklin, R. J. M. (1999). Remyelination occurs as extensively but more slowly in old rats compared to young rats following gliotoxin-induced CNS demyelination. Glia 28, 77–83. doi: 10.1002/(sici)1098-1136(199910)28:1<77::aid-glia9>3.0.co;2-f
Sicotte, N. L., Giesser, B. S., Tandon, V., Klutch, R., Steiner, B., Drain, A. E., et al. (2007). Testosterone treatment in multiple sclerosis: a pilot study. Arch. Neurol. 64, 683–688. doi: 10.1001/archneur.64.5.683
Sicotte, N. L., Liva, S. M., Klutch, R., Pfeiffer, P., Bouvier, S., Odesa, S., et al. (2002). Treatment of multiple sclerosis with the pregnancy hormone estriol. Ann. Neurol. 52, 421–428. doi: 10.1002/ana.10301
Sim, F. J., Zhao, C., Penderis, J., and Franklin, R. J. M. (2002). The age-related decrease in CNS remyelination efficiency is attributable to an impairment of both oligodendrocyte progenitor recruitment and differentiation. J. Neurosci. Off. J. Soc. Neurosci. 22, 2451–2459. doi: 10.1523/JNEUROSCI.22-07-02451.2002
Simmons, S. B., Pierson, E. R., Lee, S. Y., and Goverman, J. M. (2013). Modeling the heterogeneity of multiple sclerosis in animals. Trends Immunol. 34, 410–422. doi: 10.1016/j.it.2013.04.006
Simons, M., and Nave, K.-A. (2015). Oligodendrocytes: myelination and axonal support. Cold Spring Harb. Perspect. Biol. 8:a020479. doi: 10.1101/cshperspect.a020479
Skripuletz, T., Hackstette, D., Bauer, K., Gudi, V., Pul, R., Voss, E., et al. (2013). Astrocytes regulate myelin clearance through recruitment of microglia during cuprizone-induced demyelination. Brain J. Neurol. 136, 147–167. doi: 10.1093/brain/aws262
Smith, K. J., Blakemore, W. F., and McDonald, W. I. (1979). Central remyelination restores secure conduction. Nature 280, 395–396. doi: 10.1038/280395a0
Smith, K. J., Blakemore, W. F., and McDonald, W. I. (1981). The restoration of conduction by central remyelination. Brain J. Neurol. 104, 383–404. doi: 10.1093/brain/104.2.383
Soulika, A. M., Lee, E., McCauley, E., Miers, L., Bannerman, P., and Pleasure, D. (2009). Initiation and progression of axonopathy in experimental autoimmune encephalomyelitis. J. Neurosci. Off. J. Soc. Neurosci. 29, 14965–14979. doi: 10.1523/JNEUROSCI.3794-09.2009
Stidworthy, M. F., Genoud, S., Suter, U., Mantei, N., and Franklin, R. J. M. (2003). Quantifying the early stages of remyelination following cuprizone-induced demyelination. Brain Pathol. 13, 329–339. doi: 10.1111/j.1750-3639.2003.tb00032.x
Storch, M. K., Bauer, J., Linington, C., Olsson, T., Weissert, R., and Lassmann, H. (2006). Cortical demyelination can be modeled in specific rat models of autoimmune encephalomyelitis and is major histocompatibility complex (MHC) haplotype-related. J. Neuropathol. Exp. Neurol. 65, 1137–1142. doi: 10.1097/01.jnen.0000248547.13176.9d
Storch, M. K., Stefferl, A., Brehm, U., Weissert, R., Wallström, E., Kerschensteiner, M., et al. (1998). Autoimmunity to myelin oligodendrocyte glycoprotein in rats mimics the spectrum of multiple sclerosis pathology. Brain Pathol. 8, 681–694. doi: 10.1111/j.1750-3639.1998.tb00194.x
Syed, Y. A., Hand, E., Möbius, W., Zhao, C., Hofer, M., Nave, K. A., et al. (2011). Inhibition of CNS remyelination by the presence of semaphorin 3A. J. Neurosci. Off. J. Soc. Neurosci. 31, 3719–3728. doi: 10.1523/JNEUROSCI.4930-10.2011
Talbott, J. F., Cao, Q., Enzmann, G. U., Benton, R. L., Achim, V., Cheng, X. X., et al. (2006). Schwann cell-like differentiation by adult oligodendrocyte precursor cells following engraftment into the demyelinated spinal cord is BMP-dependent. Glia 54, 147–159. doi: 10.1002/glia.20369
Theiler, M. (1934). Spontaneous encephalomyelitis of mice--a new virus disease. Science 80:122. doi: 10.1126/science.80.2066.122-a
Thornton, M. A., and Hughes, E. G. (2020). Neuron-oligodendroglia interactions: activity-dependent regulation of cellular signaling. Neurosci. Lett. 727:134916. doi: 10.1016/j.neulet.2020.134916
Traka, M., Arasi, K., Avila, R. L., Podojil, J. R., Christakos, A., Miller, S. D., et al. (2010). A genetic mouse model of adult-onset, pervasive central nervous system demyelination with robust remyelination. Brain J. Neurol. 133, 3017–3029. doi: 10.1093/brain/awq247
Traka, M., Podojil, J. R., McCarthy, D. P., Miller, S. D., and Popko, B. (2016). Oligodendrocyte death results in immune-mediated CNS demyelination. Nat. Neurosci. 19, 65–74. doi: 10.1038/nn.4193
Voskuhl, R. R., Itoh, N., Tassoni, A., Matsukawa, M. A., Ren, E., Tse, V., et al. (2019). Gene expression in oligodendrocytes during remyelination reveals cholesterol homeostasis as a therapeutic target in multiple sclerosis. Proc. Natl. Acad. Sci. U. S. A. 116, 10130–10139. doi: 10.1073/pnas.1821306116
Wergeland, S., Torkildsen, Ø., Myhr, K.-M., Mørk, S. J., and Bø, L. (2012). The cuprizone model: regional heterogeneity of pathology. APMIS: acta pathologica, microbiologica, et immunologica. Scandinavica 120, 648–657. doi: 10.1111/j.1600-0463.2012.02882.x
Wolswijk, G. (1998). Chronic stage multiple sclerosis lesions contain a relatively quiescent population of oligodendrocyte precursor cells. J. Neurosci. Off. J. Soc. Neurosci. 18, 601–609. doi: 10.1523/JNEUROSCI.18-02-00601.1998
Woodruff, R. H., and Franklin, R. J. (1999). Demyelination and remyelination of the caudal cerebellar peduncle of adult rats following stereotaxic injections of lysolecithin, ethidium bromide, and complement/anti-galactocerebroside: a comparative study. Glia 25, 216–228. doi: 10.1002/(sici)1098-1136(19990201)25:3<216::aid-glia2>3.0.co;2-l
Wooliscroft, L., Altowaijri, G., Hildebrand, A., Samuels, M., Oken, B., Bourdette, D., et al. (2020). Phase I randomized trial of liothyronine for remyelination in multiple sclerosis: A dose-ranging study with assessment of reliability of visual outcomes. Mult. Scler. Relat. Disord. 41:102015. doi: 10.1016/j.msard.2020.102015
Wu, G. F., Dandekar, A. A., Pewe, L., and Perlman, S. (2000). CD4 and CD8 T cells have redundant but not identical roles in virus-induced demyelination. J. Immunol. 165, 2278–2286. doi: 10.4049/jimmunol.165.4.2278
Yajima, K., and Suzuki, K. (1979). Ultrastructural changes of oligodendroglia and myelin sheaths induced by ethidium bromide. Neuropathol. Appl. Neurobiol. 5, 49–62. doi: 10.1111/j.1365-2990.1979.tb00613.x
Yang, H.-J., Wang, H., Zhang, Y., Xiao, L., Clough, R. W., Browning, R., et al. (2009). Region-specific susceptibilities to cuprizone-induced lesions in the mouse forebrain: implications for the pathophysiology of schizophrenia. Brain Res. 1270, 121–130. doi: 10.1016/j.brainres.2009.03.011
Yeung, M. S. Y., Djelloul, M., Steiner, E., Bernard, S., Salehpour, M., Possnert, G., et al. (2019). Dynamics of oligodendrocyte generation in multiple sclerosis. Nature 566, 538–542. doi: 10.1038/s41586-018-0842-3
Zawadzka, M., Rivers, L. E., Fancy, S. P. J., Zhao, C., Tripathi, R., Jamen, F., et al. (2010). CNS-resident glial progenitor/stem cells produce Schwann cells as well as oligodendrocytes during repair of CNS demyelination. Cell Stem Cell 6, 578–590. doi: 10.1016/j.stem.2010.04.002
Zeis, T., Enz, L., and Schaeren-Wiemers, N. (2016). The immunomodulatory oligodendrocyte. Brain Res. 1641, 139–148. doi: 10.1016/j.brainres.2015.09.021
Zhan, J., Mann, T., Joost, S., Behrangi, N., Frank, M., and Kipp, M. (2020). The Cuprizone model: dos and do nots. Cells 9:843. doi: 10.3390/cells9040843
Zhang, X., Huang, N., Xiao, L., Wang, F., and Li, T. (2021). Replenishing the aged brains: targeting oligodendrocytes and myelination? Front. Aging Neurosci. 13:760200. doi: 10.3389/fnagi.2021.760200
Zia, S., Rawji, K. S., Michaels, N. J., Burr, M., Kerr, B. J., Healy, L. M., et al. (2020). Microglia diversity in health and multiple sclerosis. Front. Immunol. 11:588021. doi: 10.3389/fimmu.2020.588021
Zimmermann, J., Emrich, M., Krauthausen, M., Saxe, S., Nitsch, L., Heneka, M. T., et al. (2018). IL-17A promotes granulocyte infiltration, myelin loss, microglia activation, and behavioral deficits during Cuprizone-induced demyelination. Mol. Neurobiol. 55, 946–957. doi: 10.1007/s12035-016-0368-3
Zirngibl, M., Assinck, P., Sizov, A., Caprariello, A. V., and Plemel, J. R. (2022). Oligodendrocyte death and myelin loss in the cuprizone model: an updated overview of the intrinsic and extrinsic causes of cuprizone demyelination. Mol. Neurodegener. 17:34. doi: 10.1186/s13024-022-00538-8
Keywords: demyelination, oligodendrocyte, OPC, multiple sclerosis, remyelination
Citation: Packer D, Fresenko EE and Harrington EP (2023) Remyelination in animal models of multiple sclerosis: finding the elusive grail of regeneration. Front. Mol. Neurosci. 16:1207007. doi: 10.3389/fnmol.2023.1207007
Edited by:
Jay S. Schneider, Thomas Jefferson University, United StatesReviewed by:
Greg J. Duncan, Oregon Health and Science University, United StatesMaria Traka, Midwestern University, United States
Copyright © 2023 Packer, Fresenko and Harrington. This is an open-access article distributed under the terms of the Creative Commons Attribution License (CC BY). The use, distribution or reproduction in other forums is permitted, provided the original author(s) and the copyright owner(s) are credited and that the original publication in this journal is cited, in accordance with accepted academic practice. No use, distribution or reproduction is permitted which does not comply with these terms.
*Correspondence: Em P. Harrington, RW0uSGFycmluZ3RvbkBvc3VtYy5lZHU=