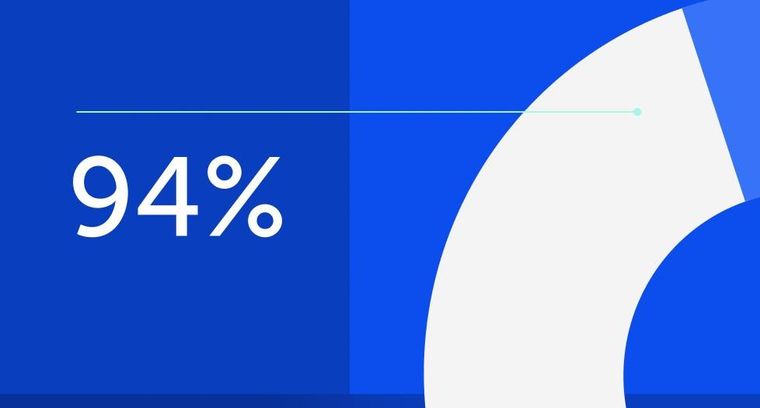
94% of researchers rate our articles as excellent or good
Learn more about the work of our research integrity team to safeguard the quality of each article we publish.
Find out more
REVIEW article
Front. Mol. Neurosci., 31 May 2023
Sec. Molecular Signalling and Pathways
Volume 16 - 2023 | https://doi.org/10.3389/fnmol.2023.1190324
This article is part of the Research Topic90th Anniversary of the 1932 Sherrington and Adrian Nobel Prize: Molecular Pathways of Synaptic Transmission RegulationView all 6 articles
Glutamate N-methyl-D-aspartate receptor (NMDAR) is critical for promoting physiological synaptic plasticity and neuronal viability. As a major subpopulation of the NMDAR, the GluN2B subunit-containing NMDARs have distinct pharmacological properties, physiological functions, and pathological relevance to neurological diseases compared with other NMDAR subtypes. In mature neurons, GluN2B-containing NMDARs are likely expressed as both diheteromeric and triheteromeric receptors, though the functional importance of each subpopulation has yet to be disentangled. Moreover, the C-terminal region of the GluN2B subunit forms structural complexes with multiple intracellular signaling proteins. These protein complexes play critical roles in both activity-dependent synaptic plasticity and neuronal survival and death signaling, thus serving as the molecular substrates underlying multiple physiological functions. Accordingly, dysregulation of GluN2B-containing NMDARs and/or their downstream signaling pathways has been implicated in neurological diseases, and various strategies to reverse these deficits have been investigated. In this article, we provide an overview of GluN2B-containing NMDAR pharmacology and its key physiological functions, highlighting the importance of this receptor subtype during both health and disease states.
The N-methyl-D-aspartate glutamate receptor (NMDAR) has been widely recognized as a key family of ligand-gated glutamate receptors (Paoletti et al., 2013). Although the NMDAR channel mediates postsynaptic depolarization in response to the excitatory neurotransmitter glutamate, it generally contributes little to the fast synaptic transmission, but mediates more complex physiological functions in the central nervous system (CNS; Paoletti et al., 2013). One of the central themes related to NMDAR function is that the receptors play a critical role in the bidirectional, activity-dependent changes of synaptic strength, which is known as synaptic plasticity (Collingridge et al., 2004; Paoletti et al., 2013). A second theme related to NMDAR function is that these receptors mediate neuronal survival and death during neuronal development and neuronal excitotoxicity, a delayed neuronal death process in response to the overexcitation of neurons (Tashiro et al., 2006; Wu and Tymianski, 2018; Ge et al., 2020). Based on the importance of neuronal plasticity and the regulation of neuronal survival and death during both physiological and pathological conditions, understanding the precise physiological functions of NMDARs is critical for discovering the fundamental processes related to learning and memory and the pathological mechanisms underlying multiple neurological diseases.
The diversity of NMDAR subunits plays a central role in mediating the complex functions of NMDARs (Paoletti et al., 2013). Genome sequencing experiments have first identified multiple NMDAR subunits, which are capable of assembling into various functional NMDAR subtypes, as demonstrated by subsequent biological and electrophysiological characterizations (Monyer et al., 1994; Sheng et al., 1994). More recently, the functional diversity of NMDAR subunits has been increasingly acknowledged, facilitated by the availability of pharmacological tools and genetic mice models to achieve targeted manipulation of each of the several different NMDAR subtypes (Liu et al., 2004; Brigman et al., 2010; Paoletti et al., 2013). These studies have identified NMDARs containing the GluN2B subunit as, arguably, the most widely expressed subtypes in forebrain neurons and the most important NMDAR subtype contributing to neuronal development, synaptic plasticity, and neuronal excitotoxicity (Sheng et al., 1994; Paoletti et al., 2013; Ge et al., 2020). Here, we will summarize the current understanding of the pharmacology, physiology, and pathology related to GluN2B-containing NMDARs with a focus on the more recent investigations.
The NMDARs are heterotetrameric complexes composed of eight different subunits in humans (and in rodents) including GluN1, GluN2(A-D), and GluN3(A/B; Collingridge et al., 2004). While the two GluN1 subunits are essential for the formation of functional NMDAR complexes, the remaining GluN2/3 subunits determine the receptor’s functional diversity (Paoletti et al., 2013). In the case of GluN2B-containing NMDARs, at least three different compositions have been studied using recombinant expression systems. These receptor subtypes include the diheteromeric GluN1/GluN2B (2B/2B)- (Hansen et al., 2014; Yi et al., 2019), triheteromeric GluN1/GluN2A/GluN2B (2A/2B)- (Hansen et al., 2014), and triheteromeric GluN1/GluN2B/GluN2D (2B/2D)-NMDARs (Yi et al., 2019; Figure 1). Despite some recent advancements in the pharmacological understanding of each receptor subtype, the proportion of diheteromeric and triheteromeric GluN2B-containing NMDARs expressed in CNS neurons is still a subject of debate.
Figure 1. Subtype diversity and structures of GluN2B-containing NMDARs. (A) The diheteromeric GluN2B/GluN2B (2B/2B)-NMDAR is composed of two GluN1 (N1) and two GluN2B (2B) subunits (left). Sideview (middle) and topview (right) of 2B/2B-NMDAR structure resolved from x-ray diffraction (PDB: 4PE5). (B) The triheteromeric GluN2A/GluN2B (2A/2B)-NMDAR is composed of two N1, one GluN2A (2A), and one 2B subunits (left). Sideview (middle) and topview (right) of 2A/2B-NMDAR structure resolved from electron microscopy (PDB: 5UOW). (C) The triheteromeric GluN2B/GluN2D (2B/2D)-NMDAR has two N1, one 2B, and one GluN2D (2D) subunits. Crystal structures were visualized using PyMOL academic version.
Although triheteromeric GluN2B-containing NMDARs may be an important subpopulation of GluN2B-containing NMDARs in CNS neurons, most physiological studies overlook their contribution, primarily due to the inability of currently used experimental techniques to separate them from diheteromeric 2B/2B-NMDARs. To further complicate this issue, when used at their typical working concentrations, subtype-specific antagonists that are known to almost fully block the diheteromeric 2B/2B-NMDAR currents generally block a proportion (~30%–60%) of the triheteromeric GluN2B-containing NMDARs currents (Hansen et al., 2014; Stroebel et al., 2018). Thus, reduction of NMDAR function by GluN2B-specific antagonists could theoretically be due to either a full blockade of diheteromeric 2B/2B-NMDAR function or a partial blockade of triheteromeric GluN2B-containing NMDAR function. Nevertheless, accumulating evidence supports that at least some diheteromeric 2B/2B-NMDARs are present throughout the life span of neurons, and they have channel functions and downstream signaling properties that are different from non-GluN2B-containing NMDARs. Consequently, the physiological functions that will be discussed in this review are thought to be contributed largely by the diheteromeric 2B/2B-NMDARs. It is important to acknowledge, however, that the same physiological functions may be contributed by triheteromeric GluN2B-containing NMDARs.
The GluN2B subunit shows distinct temporospatial expression patterns from other GluN2/3 subunits (Wenzel et al., 1997). Sharp contrasts have been drawn between the expression patterns of the GluN2B and GluN2A subunits. For example, the GluN2B subunit is by far the most expressed GluN2/3 subunit in embryonic murine brains; however, its expression peaks at around the first postnatal week, after which it is partially and gradually replaced by the increasingly expressed GluN2A subunit (Wenzel et al., 1997). In adult murine forebrain neurons, one commonly cited finding is that the GluN2B subunit is mainly located extrasynaptically, whereas the GluN2A subunit is primarily expressed at the neuronal synapses (Stocca and Vicini, 1998). Nevertheless, this model of subunit-dependent localization of NMDAR subtypes is likely too simplistic, since some studies have reported a substantial proportion of GluN2B-containing NMDAR-mediated synaptic currents in more mature neurons (Thomas et al., 2006; Pegasiou et al., 2020).
The temporospatial expression of diheteromeric vs. triheteromeric GluN2B-containing NMDAR subtypes is still a subject of immense debate. Based on the predominant expression of the GluN2B subunit during early postnatal days, it is reasonable to hypothesize that diheteromeric 2B/2B-NMDAR is the dominant NMDAR complex during early development (Wenzel et al., 1997). As the expression of the GluN2A subunit increases (particularly at the postsynaptic density), synaptic NMDARs in the cortical neurons may start to be populated by diheteromeric 2A/2A-or triheteromeric 2A/2B-NMDARs (Paoletti et al., 2013). Nevertheless, the exact composition of the NMDARs throughout the developmental time course has yet to be determined by using more sophisticated structural biology methods, such as using cryogenic electron microscopy (cryo-EM) to investigate the subunit stoichiometry of native NMDARs in the CNS (Zhao et al., 2019).
Some divalent cations, including Zn2+ and Mg2+, modulate the activities of NMDARs in a subtype-specific manner (Nowak et al., 1984; Paoletti et al., 2013). For example, Zn2+ is a more potent allosteric inhibitor on diheteromeric 2A/2A-NMDARs than diheteromeric 2B/2B-NMDARs, and, thus, a frequently used pharmacological tool for investigating GluN2A-containing NMDAR functions (Nozaki et al., 2011); however, its specificity for NMDARs containing the GluN2A subunit is limited by its similarly potent inhibition on triheteromeric 2A/2B-NMDAR currents and its modest efficacy of blocking (~50%) diheteromeric 2A/2A-NMDAR currents under physiological pH (Hansen et al., 2014). NMDAR subtypes also experience voltage-dependent channel blocking by Mg2+, and the Mg2+ sensitivity is roughly 10 times stronger for NMDARs containing the GluN2A/GluN2B subunits compared with those containing the GluN2C/GluN2D subunits (Paoletti et al., 2013). Importantly, the relief from Mg2+ inhibition of the GluN2A and/or GluN2B-containing NMDAR currents during repetitive or strong synaptic activities is critical for activity-dependent synaptic plasticity (Paoletti et al., 2013). The subtype-dependent modulation of the activities of GluN2B-containing and other NMDAR subtypes, by these divalent cations, are important physiological mechanisms for regulating neuronal functions.
Developing subtype-specific modulators for GluN2B-containing NMDARs has been a long-standing interest for both research and therapeutic purposes. Multiple GluN2B-containing NMDAR antagonists have been tested in preclinical studies and in clinical trials, most of which are analogous to a phenylethanolamine called ifenprodil, which binds to a GluN1-GluN2B subunit interface at the N-terminal domain (Karakas et al., 2011; Liu W. et al., 2020). Some of the antagonists in this class, such as Ro25-6981 and CP-101606, have achieved outstanding subtype specificity with over 1,000-fold higher affinity for diheteromeric GluN2B-containing NMDARs compared with other diheteromeric NMDAR subtypes (Chenard et al., 1995; Fischer et al., 1997). Although Ro25-6981, when applied at its working concentration, also blocks a proportion of the currents measured from recombinantly expressed triheteromeric 2A/2B-NMDARs (Hansen et al., 2014), its blocking effect on the 2A/2B-NMDAR currents is small (~30%) compared with that on diheteromeric 2B/2B-NMDAR currents (>90%). Therefore, this drug has been a useful tool for investigating the physiological functions of diheteromeric 2B/2B-NMDARs.
Several endogenous polyamines in the CNS, including spermine and spermidine, positively modulate the GluN2B-containing NMDAR currents (Williams et al., 1994). Importantly, the positive modulation is specific to the diheteromeric 2B/2B-NMDARs with little to no effect on other NMDAR subtypes, including the triheteromeric 2A/2B-NMDARs (Stroebel et al., 2014). It is important to note that polyamines generally have low potency toward modulating the diheteromeric 2B/2B-NMDAR currents (EC50 around hundreds of μM) and may also affect many other targets in the CNS at the concentration that is commonly used (Guerra et al., 2016). Further understanding of the structural biology of polyamine modulation on diheteromeric NMDARs, including the resolution of a crystal structure illustrating the binding of polyamines to 2B/2B-NMDARs, may facilitate the development of more potent and specific modulators to investigate the functions of diheteromeric 2B/2B-NMDARs.
It has been widely recognized that GluN2B-containing NMDARs often have distinct physiological functions compared with other NMDAR subtypes. Genetic knockout/knockin and pharmacological inhibition have been the most important approaches for studying the functions of GluN2B-containing NMDARs, and a wide range of physiological functions has been investigated (Paoletti et al., 2013). While a comprehensive discussion of GluN2B-containing NMDAR functions is out of the scope of this article, we will focus on the roles of this receptor subpopulation on synaptic plasticity and neuronal fate (particularly in terms of neuronal survival and death).
Long-term potentiation (LTP) has been acknowledged as a well-investigated form of activity-dependent synaptic plasticity in mammalian brains. Although the roles of GluN2B-containing NMDARs in LTP may be ambivalent overall, many earlier lines of evidence support the importance of this NMDAR subgroup in the induction of LTP (Shipton and Paulsen, 2014). First, compared with the GluN2A subunit, the GluN2B subunit has a much higher affinity for calcium calmodulin-dependent kinase II (CaMKII), a key protein kinase that is involved in LTP induction (Barria and Malinow, 2005). Knockin mice with the GluN2B subunit’s CaMKII binding site residues replaced by the analogous ones on the GluN2A subunit have severely impaired LTP (Barria and Malinow, 2005). Furthermore, genetic ablation of the GluN2B subunit in the hippocampal CA1 region abolishes LTP in a subset of hippocampal circuits, whereas transgenic mice overexpressing the GluN2B subunit have dramatically enhanced LTP in the hippocampal CA3-CA1 synapse (Tang et al., 1999; Akashi et al., 2009). Together, these data support the hypothesis that GluN2B-containing NMDARs and their associated protein kinases play essential roles in the induction of LTP.
Many recent studies examining the roles of GluN2B-containing NMDARs in LTP have generated mixed results (Shipton and Paulsen, 2014). For example, several lines of lately generated transgenic mice conditionally lacking the GluN2B subunit in the hippocampus only have minor impairment in a weak form of tetanus-induced LTP, but no impairment in a stronger form of LTP in the CA3-CA1 synapse (von Engelhardt et al., 2008; Brigman et al., 2010). Moreover, GluN2B-containing NMDAR antagonists such as Ro25-6981 do not affect high-frequency stimulation-induced LTP in many studies (see Liu et al., 2004; Fox et al., 2006; Papouin et al., 2012 but Bartlett et al., 2007; Berberich et al., 2007). Several factors, including the age of the animals (Ito et al., 1996), LTP induction protocol (von Engelhardt et al., 2008), and neural circuit and brain region (Akashi et al., 2009) may affect the roles of GluN2B-containing NMDAR in LTP induction. Overall, the requirement of the GluN2B subunit for the induction of LTP is likely situation-dependent.
Several factors will need to be considered when interpreting the roles of GluN2B-containing NMDARs in LTP. First, most current studies ignore the contribution of triheteromeric NMDARs, especially those containing both GluN2A and GluN2B subunits. One study provided evidence for the importance of triheteromeric 2A/2B-NMDARs in LTP using carefully designed interrogation of NMDAR subtype functions (Delaney et al., 2012), but exclusive isolation of the triheteromeric NMDAR functions remains challenging with current experimental approaches. Second, transgenic mice studies overexpressing or deleting GluN2B may alter the neuronal development and/or trigger molecular mechanisms to compensate for the changes in the GluN2B subunit expression, thereby misrepresenting the true physiological functions of the subunit (Ito et al., 1996; Tang et al., 1999). Third, multiple forms of NMDAR-dependent LTP may be present (Liu A. et al., 2020), but most experiments testing the roles of GluN2B-containing NMDARs in LTP used a single form of induction protocol limited to the same neural circuit, thus considering LTP as a unified phenomenon. Nevertheless, as illustrated by a thoughtfully designed study using both genetic and pharmacological manipulations (Foster et al., 2010), it is generally accepted that the C-terminal of the GluN2B subunit plays a scaffolding role for recruiting proteins that are critical for LTP induction, whereas the channel function specific for diheteromeric 2B/2B-NMDAR may not be required for the induction of LTP.
The NMDAR-dependent form of long-term depression (LTD)-most commonly studied at the hippocampal CA3-CA1 circuit-has been one of the best-characterized mechanisms of activity-dependent reduction in synaptic strength (Collingridge et al., 2010). Increasing amount of evidence suggests an important role of GluN2B-containing NMDARs in the induction of NMDAR-dependent LTD. One of the earliest studies in this field, by using differential activation of NMDAR subpopulations, found that synaptic NMDAR activation mediated LTP, while extrasynaptic NMDAR activation mediated LTD (Lu et al., 2001). Since the GluN2B subunit is predominant expressed in the extrasynaptic location in adult neurons (Stocca and Vicini, 1998), this evidence indirectly supports the importance of GluN2B-containing NMDARs in LTD.
Subsequent pharmacological studies using subtype-specific modulators found that Ro25-6981 was sufficient to block LTD in the CA3-CA1 synapse using acutely prepared brain slices derived from adult animals (see Liu et al., 2004; Fox et al., 2006; Papouin et al., 2012 but Bartlett et al., 2007; Morishita et al., 2007) and in vivo using anesthetized animals (Fox et al., 2006; Ge et al., 2010). Notably, both homozygote Cre-dependent GluN2B knockout and heterozygote loss-of-function GluN2B knockin in mice reliably abolish LTD (Ito et al., 1996; Brigman et al., 2010; Shin et al., 2020), providing the strongest evidence for the critical roles of the GluN2B subunit in LTD. Despite the inconsistent results derived from pharmacological studies, a major proportion of evidence, particularly from studies using in vivo models, supports an essential role of GluN2B-containing NMDARs in LTD induction.
GluN2B-containing NMDARs play important roles in a wide range of cognitive functions in rodent models, and this phenomenon is thought to be mediated by their roles in LTP and LTD (von Engelhardt et al., 2008; Brigman et al., 2010). For example, transgenic mice overexpressing the GluN2B subunit in forebrain neurons have improved object recognition memory (Tang et al., 1999), spatial memory (Tang et al., 1999), working memory (Cui et al., 2011), social recognition memory (Jacobs and Tsien, 2012), and motor skill learning (Duan et al., 2018). These enhanced learning abilities may be related to the enhanced LTP in the transgenic mice (Tang et al., 1999; Cui et al., 2011). By contrast, knocking out the GluN2B subunit in mice cortex and hippocampal CA1 regions impaired spatial learning, working memory, and trace fear conditioning (Brigman et al., 2010). Such learning deficits may be related to the impaired LTD (Brigman et al., 2010). Altogether, these pieces of evidence support the indispensable roles of GluN2B-containing NMDARs in many types of learning and memory and the possibility of memory enhancement by enhancing GluN2B-containing NMDAR functions. It should be noted that whether these effects on learning and memory, particularly those observed from the GluN2B subunit-overexpressed transgenic mice, are due to the functional (channel opening) and/or structural (recruiting critical molecules such as CaMKII) roles of GluN2B-containing NMDARs remains to be determined.
Recent behavioral experiments indicate that certain types of cognitive performances, especially those that require flexible adaptations to learned responses, are selectively affected by the manipulation of GluN2B-containing NMDAR functions. Pharmacological inhibition of GluN2B-containing NMDAR function impaired fear memory extinction (Milton et al., 2013), strategy shift during a discrimination task (Dalton et al., 2011), and reversal learning during Morris Water maze (Dong et al., 2013) in rodents, but not their performances on fear conditioning, visual discrimination, and initial spatial learning. Moreover, the impaired behavioral flexibility following GluN2B-antagonism may be mediated by the deficits in LTD, which could be critical for weakening the previously formed associations before new learning can takes place. Indeed, impairment of fear extinction learning in rats by a GluN2B-containing NMDAR antagonist is associated with their impaired LTD in an amygdala circuit (Dalton et al., 2012), while enhancement of spatial reversal learning in mice by NMDAR co-agonist D-serine is associated with their enhanced LTD in the hippocampus (Duffy et al., 2008). Although GluN2B-containing NMDARs have been implicated in other cognitive functions related to memory acquisition (Howland and Cazakoff, 2010; Dong et al., 2012), retrieval (Wong et al., 2007), and forgetting (Sachser et al., 2016), their role in learning tasks that require behavioral flexibility has been the most consistently replicated phenomenon across different behavioral assays and research settings.
Overactivation of NMDARs during pathological conditions is known to cause excitotoxic neuronal death (known as excitotoxicity), during which different NMDAR subtypes may have distinct, even opposite functions (Wu and Tymianski, 2018; Ge et al., 2020). A large body of literature has suggested that pathological overactivity of GluN2B-containing NMDARs is associated with neuronal death (Ge et al., 2020). For example, neurons expressing chimeric NMDARs with the C-terminal region of the GluN2A subunit replaced by that of the GluN2B subunit experienced increased susceptibility to NMDA-induced cell death, an in vitro model of excitotoxic neuronal injury (Martel et al., 2012). By contrast, transgenic mice with parts of the GluN2B C-terminal domain (CTD) region deleted or modified experienced decreased susceptibility to in vitro NMDA-induced cell death and an in vivo middle cerebral artery occlusion (MCAO) model of stroke (Martel et al., 2012; Vieira et al., 2016; Tang et al., 2018). Furthermore, antagonists that are selective for GluN2B-containing NMDARs, but not those that are preferential for GluN2A-containing NMDARs, protected neurons from excitotoxic death during multiple forms of neuronal injury (DeRidder et al., 2006; Liu et al., 2007; Chen et al., 2008). Although the strict “NMDAR subtype” hypothesis in neuronal survival and death has been challenged by some inconsistent findings (Papouin et al., 2012; Zhou et al., 2013), and by the recent evidence suggesting the roles of triheteromeric 2A/2B-NMDAR and other GluN2-containing NMDAR subtypes in neuronal death (Doyle et al., 2018; Ma et al., 2019), it is generally accepted that GluN2B-containing NMDARs, by coupling to multiple neuronal death signaling complexes, play a dominant role in mediating neuronal toxicity (Wu and Tymianski, 2018; Ge et al., 2020).
To conserve energy and maximize the efficiency of the cellular processes, neurons often use redundant molecules or signaling pathways to mediate multiple physiological functions, such as synaptic plasticity and neuronal fate, in parallel (see Bartlett and Wang, 2013; Sheng and Ertürk, 2014 for a comprehensive review of these signaling pathways). In most cases, synaptic long-term potentiation shares common signaling mechanisms with neuronal survival signaling, and synaptic long-term depression are mediated by similar processes as neuronal death signaling (Bartlett and Wang, 2013; Sheng and Ertürk, 2014). Largely in consistency with these hypotheses, many GluN2B-dependent signaling pathways regulate both synaptic plasticity and neuronal fate. Here, we will summarize several key protein complexes that associate with the GluN2B subunit and mediate the downstream signaling cascades following GluN2B-containing NMDAR activation.
The GluN2B-CaMKII has been the best-characterized GluN2B-dependent signaling complex by far, largely due to the abundance of CaMKII holoenzyme and its importance in synaptic plasticity, especially LTP, and the formation and maintenance of glutamatergic synapses (Lisman et al., 2012; Bayer and Schulman, 2019; Yasuda et al., 2022). During the induction of LTP, a high calcium concentration mediated by the calcium influx through the NMDAR activates calcium calmodulin (CaM), which triggers the CaMKII kinase activity, autophosphorylation of the threonine (Thr)286 residue, and binding to the GluN2B-subunit of the NMDAR (Strack and Colbran, 1998; Bayer et al., 2001; Barria and Malinow, 2005). The binding of CaMKII to GluN2B is critical for inducing the downstream signaling cascades, including the phosphorylation of multiple protein substrates for the CaMKII-dependent potentiation of the synaptic strength (Barria et al., 1997; Tomita et al., 2005; Figure 2). It is important to acknowledge that CaMKII also plays important roles in LTD (Bayer and Schulman, 2019). Notably, the effects of CaMKII on LTD may not be dependent on its binding with GluN2B, but on the autonomous activity of CaMKII (Pi et al., 2010). This autonomous activity is inhibited by a high concentration of CaM but favors a prolonged level of intracellular calcium at low to moderate concentrations (Bayer and Schulman, 2019), and is dependent on the autophosphorylation of Thr305/306 on CaMKII (Pi et al., 2010; Cook et al., 2021; Figure 2). Additionally, LTD stimuli in the excitatory synapse may result in CaMKII translocation to the inhibitory synapse and the subsequent inhibitory LTP (iLTP; Cook et al., 2021). Overall, the roles of CaMKII in bidirectional plasticity are highly consistent with the type of stimulation that is necessary to induce LTP (strong and brief) and LTD (moderate and long) in neurons.
Figure 2. The GluN2B-CaMKII and GluN2B-DAPK1 signaling complexes. (A) During high concentration of Ca2+, activated CaMKII (with Thr286 autophosphorylation) translocates and forms a complex with GluN2B-containing NMDARs. The association between CaMKII and the GluN2B subunit is required for CaMKII-mediated induction of LTP and the neuronal survival signaling. (B) During low to medium concentration of Ca2+, calcineurin dephysphorylates and activates DAPK1. The association between DAPK1 and the GluN2B subunit is required for DAPK1-mediated induction of LTD and the neuronal survival signaling. The low Ca2+ condition also favors the autonomous activity of CaMKII (with both Thr286 and Thr305 autophosphorylations), which is involved in the induction of LTD and the neuronal death signaling.
CaMKII has long been implicated in neuronal survival and death, but the direction to which it contributes to neuronal viability has been controversial (Coultrap et al., 2011). Recently, it has been found that a peptide inhibitor tat-CN21, which blocks the autonomous activity of CaMKII, is neuroprotective against glutamate-induced excitotoxicity when applied before or shortly after the insult, whereas CaMKII inhibitor KN-93, which does not block the autonomous activity of CaMKII, has no neuroprotective effect (Vest et al., 2010; Ashpole and Hudmon, 2011). These studies suggest that the autonomous activity of CaMKII, in addition to mediating LTD, may also mediate a critical signaling pathway underlying neuronal death. While the autonomous activity of CaMKII has been increasingly acknowledged as a target for neuroprotection, it is important to note that prolonged inhibition of CaMKII autonomous activity by tat-CN21 for longer than 8 h increased, rather than decreased the susceptibility of neurons to subsequence excitotoxic insults (Ashpole et al., 2012). Moreover, compared to wild-type mice, transgenic mice without CaMKII are more susceptible to ischemic neuron insults, suggesting that the same pathway may also be implicated in neuronal survival signaling (Waxham et al., 1996). Since CaMKII protein may be critical for both neuronal survival and neuronal death, further evidence clarifying the CaMKII-dependent neuronal survival signaling from neuronal death signaling pathways, including the involvement of GluN2B-CaMKII binding in the signaling, will be needed to optimize the CaMKII-based neuroprotective strategy.
The GluN2B-death-associated protein kinase 1 (DAPK1) complex plays a critical role in mediating downstream signaling cascades following GluN2B-containing NMDAR activation (Wu and Tymianski, 2018; Ge and Wang, 2022). To begin with, the GluN2B-DAPK1 has been discovered as a neuronal death signaling complex which rapidly assemblies upon the activation of extrasynaptic NMDAR during excitotoxic insult (Tu et al., 2010). Transgenic mice lacking the DAPK1 gene or with mutated GluN2B C-terminal region abolishing GluN2B-DAPK1 binding is resistant to ischemic insult during in vitro and in vivo models of stroke (see Tu et al., 2010; Tang et al., 2018 but McQueen et al., 2017), while interfering the formation of the GluN2B-DAKP1 complex using cell-penetrant peptides Tat-GluN2Bct and Tat-GluN2Bct-CTM is neuroprotective against mice models of ischemic stroke (Tu et al., 2010; Fan et al., 2014). Although conflicting evidence regarding the importance of this signaling pathway in neuronal death has been reported, especially regarding the requirement of GluN2B S1303 phosphorylation by DAPK1 during neuronal death signaling (McQueen et al., 2017; Tullis et al., 2021), the GluN2B-DAPK1 complex has still been well-recognized as one of the critical signaling pathways for NMDAR-receptor mediated excitotoxicity and, consequently, an important drug target for treating ischemic stroke.
Other than playing a central role in neuronal death signaling, the GluN2B-DAPK1 complex has recently been found to mediate NMDAR-dependent LTD (Goodell et al., 2017; Figure 2). Interestingly, the signaling of GluN2B-DAPK1 during LTD is dependent on the protein phosphatase calcineurin (Tu et al., 2010; Goodell et al., 2017), and this new evidence is highly coherent with the well-recognized roles of protein phosphatases in LTD. Moreover, DAPK1 competitively prevents the formation of the GluN2B-CaMKII complex, and this process blocks the induction of LTP by CaMKII and favors the induction of LTD (Goodell et al., 2017). The unique roles of DAPK1 in regulating GluN2B-CaMKII complex activity may be critical for determining the direction of synaptic plasticity (Bayer and Schulman, 2019). These studies illustrating the roles of the GluN2B-DAPK1 complex in LTD, together with the recent studies describing the roles of CaMKII in LTD, help provide a coherent explanation regarding the signaling pathways underlying NMDAR-dependent LTP and LTD (Figure 2).
The GluN2B-Ras Protein Specific Guanine Nucleotide Releasing Factor 1 (RasGRF1) complex mediates another highly important signaling pathway for the induction of LTD, whereas the GluN2A-RasGRF2 signaling favors the production of LTP (Li et al., 2006; Feig, 2011; Miller et al., 2013). RasGRF1 is a small GTPase exchange factor (GEF) that binds specifically to the C-terminal region of the GluN2B subunit, but not that of the GluN2A or GluN1 subunits (Krapivinsky et al., 2003). The binding of RasGRF1 with GluN2B-containing NMDARs allows it to function as a calcium sensor and an activator of the p38 mitogen-activated protein kinase (MAPK) signaling pathway, which plays critical roles in LTD (Kim et al., 2005; Falcicchia et al., 2020). Interestingly, transgenic mice lacking RasGRF1 are specifically impaired in NMDAR-dependent LTD but not LTP, further supporting that the activation of RasGRF1 is more critical for this form of synaptic plasticity (Li et al., 2006). Besides mediating an important signaling pathway for LTD, some evidence suggests that the GluN2B-RasGRF1 complex may regulate neuronal fate. In comparison with their wild-type counterparts, transgenic mice lacking both RasGRF1 and RasGRF2 are more susceptible to neuronal infarction induced by MCAO (Tian et al., 2004). Moreover, p38 MAPK, the downstream effector of RasGRF1, is critical for mediating neuronal excitotoxicity (Cao et al., 2004). Given that LTD and neuronal death often involve similar signaling cascades, the role of the GluN2B-RasGRF1 complex in neuronal fate, particularly in neuronal death signaling, may warrant further investigation.
The GluN2B-postsynaptic density protein 95 (PSD95)-neuronal nitric oxide synthase (nNOS) complex has been an established signaling complex mediating neuronal death and an important drug target for neuroprotection (Sattler et al., 1999; Wu and Tymianski, 2018; Ge and Wang, 2022). Multiple drugs or interfering peptides, including NA-1 (also known as Tat-NR2BC9; Aarts et al., 2002), Tat-N-dimer (Bach et al., 2012), and ZL006 (Zhou et al., 2010), were designed to disrupt the formation of this protein complex during neuronal injury, and these therapeutics achieved neuroprotection in rodent models of ischemic stroke (Zhou et al., 2010; Bach et al., 2012), traumatic brain injury (Qu et al., 2020), and epilepsy (Colciaghi et al., 2019). Notably, in a phase III clinical trial (NCT02930018), NA-1 effectively improved neurological outcomes and reduced the brain infarct volume in ischemic stroke patients undergoing endovascular treatment (Hill et al., 2020). This clinical evidence further validates the GluN2B-PSD95-nNOS complex as an important therapeutic target for neuroprotection and a key mediator of neuronal death signaling. Limited evidence also supports the role of GluN2B-PSD95-nNOS in synaptic plasticity. For example, disrupting this protein complex with ZL006 impaired LTP that was measured from the amygdala in rat brain slices (Li et al., 2018). Nevertheless, the definitive role of this protein complex in bidirectional synaptic plasticity has yet to be verified. Given that several other neuronal death signaling complexes have been implicated in synaptic depression, it may be worthwhile to further explore the roles of the GluN2B-PSD95-nNOS complex in LTD of the glutamatergic synapses.
While most of the signaling complexes associated with GluN2B-containing NMDARs are activated by calcium-dependent mechanisms, some investigators have found that GluN2B-containing NMDAR can mediate downstream signaling through a channel function-independent mechanism (Dore et al., 2016). It has been demonstrated that the glutamate binding to the NMDAR, without the channel opening, is sufficient to induce LTD (see Nabavi et al., 2013 but Babiec et al., 2014). This calcium-independent, metabotropic function of NMDARs may be predominantly mediated by GluN2B-containing NMDARs (Tamburri et al., 2013), although one study suggested that the GluN2A and GluN2B subunit contributed equally to this form of LTD (Wong and Gray, 2018). Moreover, the metabotropic function of GluN2B-containing NMDARs has been shown to mediate structural LTD during amyloid beta-induced synaptic loss (Kessels et al., 2013; Stein et al., 2015). In contrast to the widely accepted hypothesis that ion flux through the NMDARs is required for LTD, accumulating evidence suggests that the metabotropic function of NMDARs is sufficient for both structural and functional LTD.
Given that structural LTD involving synaptic loss and neuronal death are often mediated by similar molecular mechanisms (Sheng and Ertürk, 2014), it is reasonable to hypothesize that the metabotropic function of NMDARs may also be critical for neuronal death signaling. Indeed, NMDARs form a metabotropic signaling-dependent neuronal death signaling complex with the pannexin-1 (Panx1) channel. Blocking the channel function or dissociating this protein complex using an interfering peptide is neuronal protective against oxygen–glucose deprivation (OGD)-induced neuronal death, an in vitro model of ischemic stroke (Weilinger et al., 2016). Given that GluN2B-containing NMDAR is a critical NMDAR subtype mediating neuronal death signaling, it would be interesting to determine the contribution of the GluN2B subunit vs. other GluN2 subunits in the metabotropic NMDAR function-related neuronal death. Future studies should also illustrate the relative contribution of metabotropic and ionotropic NMDAR functions in neuronal death signaling using multiple neuronal toxicity models.
In addition to regulating synaptic plasticity and neuronal fate in mature neurons, GluN2B-containing NMDARs have been recognized to play critical roles in neuronal development (reviewed in Paoletti et al., 2013). Consequently, heterozygote loss of function of the GluN2B subunit has been associated with autism spectrum disorder (ASD) and related developmental disorders in both human patients and animal models (Liu et al., 2017; Vyklicky et al., 2018; Sceniak et al., 2019; Wang et al., 2022). Consistent with these results, several genetic mice models of ASD and ASD-related developmental disabilities all exhibit hypofunction of GluN2B (Peça et al., 2011; Li et al., 2015; Toft et al., 2016), suggesting the deficit of GluN2B function as a common postsynaptic mechanism that is associated with multiple neurodevelopmental diseases. In accordance with this evidence, early administration of an NMDAR co-agonist D-cycloserine in transgenic mice harboring an ASD-risk GluN2B mutation corrected the ASD-related synaptic deficits and behavioral abnormalities in adult mutant mice (Shin et al., 2020). This evidence indicates that correcting GluN2B-containing NMDAR hypofunction during the developmental period may be a viable strategy for treating ASD-related neurodevelopmental disorders.
In consistency with the critical role of GluN2B-containing NMDARs in synaptic plasticity and cognition, GluN2B hypofunction has been commonly associated with both intellectual disabilities in children and cognitive deficits during aging (Wang et al., 2014; Vyklicky et al., 2018). Pathogenic GluN2B variants have been nearly invariably associated with intellectual disability, which is diagnosed by severely low intelligence quotient (IQ) and limitation in daily activities (García-Recio et al., 2021). Moreover, the GluN2B subunit expression experiences age-dependent reduction. This decreased protein expression is conserved across species and is correlated with reduced cognitive performances (Wang et al., 2014; Pegasiou et al., 2020). Interestingly, both germline and virus-mediated overexpression of the GluN2B subunit enhanced learning and memory performances in aged mice, especially in the retention of long-term spatial memory (Cao et al., 2007; Brim et al., 2013). Together, these data indicate the critical role of GluN2B hypofunction in both normal and pathological memory decline, suggesting the potential for boosting GluN2B-NMDAR function for cognitive enhancement.
NMDAR hypofunction has been widely recognized to play a critical role in schizophrenia, but the contribution of GluN2B hypofunction to schizophrenia has yet to be determined (Pratt et al., 2012). Interestingly, impaired behavioral flexibility has been the most consistent cognitive deficit in schizophrenic patients (Floresco et al., 2009). This finding, together with the consistent role of GluN2B-containing NMDAR in tasks that require behavioral flexibility in rodent studies (Dalton et al., 2011; Marquardt et al., 2019), indicates the potential role of GluN2B-containing NMDAR in the cognitive deficits of schizophrenia. Nevertheless, a recent large-scale genetic study has identified the GluN2A, but not the GluN2B subunit as a key schizophrenia risk gene (Singh et al., 2022), suggesting a superior role of the GluN2A subunit in the pathogenesis of schizophrenia and a target for disease intervention.
Overactivation of GluN2B-containing NMDARs is a key pathogenetic mechanism for neuronal excitotoxicity, which may play critical roles in neuronal injury during stroke, traumatic brain injury, and epilepsy (Wu and Tymianski, 2018; Ge et al., 2020). In preclinical animal models, blocking GluN2B-containing NMDAR currents is effective against ischemic neuronal death in vitro and in vivo (DeRidder et al., 2006; Liu et al., 2007; Chen et al., 2008); however, the bench-to-bedside translation of these therapeutics has proven to be challenging, likely due to them causing unwanted side effects and having short therapeutic windows (Ge et al., 2020). Recently, there has been a strategic shift toward targeting the downstream signaling complex associated with the GluN2B subunit, with the hope of improving the therapeutic time window (Sun et al., 2015). Moreover, a recent study investigating the time course of GluN2B-containing NMDAR activation during stroke has suggested that the initial overactivation of GluN2B may be followed by a prolonged hypoactivity of GluN2B-containing NMDAR during stroke recovery (Liu et al., 2010). In line with these results, enhancing NMDAR activity using D-cycloserine post-stroke has been shown to promote functional recovery in rats after MCAO (Dhawan et al., 2011). Therefore, the timing of GluN2B-containing NMDAR activation during ischemic stroke needs to be carefully investigated. The activation, rather than inhibition of GluN2B-containing NMDAR activity may be an effective treatment strategy during stroke recovery.
GluN2B-containing NMDARs and their associated protein complexes may be critical targets for treating major depressive disorder (MDD; Paoletti et al., 2013; Ge and Wang, 2022). The hypothesis for targeting glutamate receptors in MDD derived at least partially from the discovery and approval of ketamine, an NMDAR channel blocker, as a rapid-onset antidepressant used for treatment-resistant MDD (McGirr et al., 2015). Recently, several studies focusing on ketamine’s mechanisms of action have demonstrated that its antagonism on the GluN2B-containing subtype of NMDARs is required for its antidepressant effects (Miller et al., 2014; Gerhard et al., 2020; Pothula et al., 2021a). These studies strongly support the possibility of targeting NMDARs, especially the GluN2B-containing subtype, for the treatment of MDD. It has been found subsequently that both NMDAR inhibitors and positive allosteric modulators (PAM) may produce antidepressant-like effects in rodent models (Li et al., 2011, 2021; Pothula et al., 2021a,b), raising the question regarding the direction of GluN2B-containing NMDAR dysregulation during MDD. One hypothesis is that the antidepressant effects of NMDAR antagonists and PAMs are mediated by different cell types; NMDAR antagonists inhibited the GluN2B-containing NMDAR function on interneurons (Pothula et al., 2021a), whereas NMDAR PAMs enhanced the GluN2B-containing NMDAR function on pyramidal neurons (Pothula et al., 2021b). Both mechanisms were implicated in the antidepressant effects of these NMDAR-targeting drugs. Overall, targeting GluN2B-containing NMDAR may produce ketamine-like rapid antidepressant effects and may lead to the development of a new class of antidepressant drugs for a sizeable proportion of patients who do not respond to the standard-of-care pharmacotherapies.
Dysregulated fear learning has been a key characteristic in patients suffering from post-traumatic stress disorder (PTSD). Since GluN2B-containing NMDARs play critical roles in fear memory stability (Mamou et al., 2006), fear extinction (Dalton et al., 2012), and fear memory generalization (Asim et al., 2020), dysfunction of GluN2B-containing NMDARs may underlie the symptoms of PTSD. Indeed, the GluN2B subunit expression in mice brains is elevated following a strong fear experience, and normalizing the GluN2B-containing NMDAR function may be critical for preventing the generalization of the learned fear response (Asim et al., 2020). Moreover, through a GluN2B-containing NMDAR-dependent mechanism, NMDAR PAM NYX-783 reduced the spontaneous recovery of fear following fear extinction training in rats (Lee et al., 2022). This drug has shown efficacy in treating patients with PTSD in a phase II clinical trial (NCT04044664). Although a direct link between dysfunction in synaptic plasticity and PTSD has not been rigorously established, current evidence suggests that targeting GluN2B-containing NMDAR-dependent plasticity may be an effective way for treating PTSD.
Neuronal degeneration or excitotoxicity, mediated by the overactivation of GluN2B-containing NMDARs, has been a common feature among multiple neurodegenerative diseases, including Alzheimer’s disease, Parkinson’s disease, and Huntington’s disease (Paoletti et al., 2013). Based on the roles of GluN2B-containing NMDAR in neuronal death signaling, inhibiting extrasynaptic, GluN2B-containing NMDAR function has frequently been tested as a strategy for preventing neuronal degeneration (Hardingham and Bading, 2010). However, the translation of these therapeutics to the clinic has mostly failed, raising the need for alternative approaches to reverse the synaptic dysfunction in these diseases (Liu W. et al., 2020). Recently, there has been a surge in developing NMDAR PAMs for improving synaptic plasticity, thus the cognitive symptoms in multiple neurodegenerative diseases (Hill et al., 2022; Lee et al., 2022). One of these drugs, known as SAGE-718, is being evaluated in phase II clinical trials for cognitive symptoms in Alzheimer’s disease (NCT05619692), Parkinson’s disease (NCT05318937), and Huntington’s disease (NCT05107128). The renewed interest in targeting NMDAR dysfunction may be critical for improving the functional outcomes in patients with neurodegenerative disease, especially considering the slow progress in the development of disease-modifying therapeutics in the past decades.
Understanding the pharmacological properties of GluN2B-containing NMDARs and having the tools to manipulate their functions, accordingly, has led to important discoveries related to these receptors (Paoletti et al., 2013; Ge et al., 2020). On the flip side, the lack of experimental techniques to specifically manipulate some receptor subpopulations, especially the triheteromeric GluN2B-containing NMDARs, has limited our understanding of their physiological functions. Although some recent studies have attempted to develop novel methods to investigate triheteromeric NMDARs (Stroebel et al., 2014), or to specifically target diheteromeric NMDARs (Khatri et al., 2014), further research is needed to develop more sophisticated tools to understand the full diversity of GluN2B-containing NMDAR functions.
Several GluN2B-containing NMDAR-associated signaling complexes have been identified, many of which mediate the crosstalk between the GluN2B subunit-mediated synaptic plasticity and neuronal fate (Wu and Tymianski, 2018; Ge and Wang, 2022); however, much less is known about the interaction between these signaling complexes and the relative contribution of each to these physiological functions. Future studies may use a network, multiomics approach to generate more coherent and unbiased representations of the GluN2B-dependent signaling pathways during synaptic plasticity and neuronal survival and death signaling. Moreover, with the increased appreciation of the metabotropic function of GluN2B-containing NMDARs, it would be critical to isolate the signaling pathways mediated by the ionotropic and metabotropic function of the receptor and describe the potential interactions between them.
Most previous studies have focused on inhibiting GluN2B-containing NMDAR function for treating neuropsychiatric diseases, but, recently, there has been a paradigm shift in the therapeutic strategy toward enhancing GluN2B-containing NMDAR function, particularly in an allosteric manner. This new approach has been investigated for improving stroke recovery, major depressive disorder, PTSD, and cognitive symptoms in neurodegenerative diseases (Dhawan et al., 2011; Pothula et al., 2021b; Lee et al., 2022). Future studies should further pinpoint the GluN2B-containing NMDAR dysfunction in neurological disorders to validate this approach. For instance, it would be critical to further understand the temporal dynamics, cell types, and brain regions that are involved in GluN2B-containing NMDAR dysfunction. With the recent advancement of NMDAR PAMs in clinical studies, future research may develop subtype-specific PAMs targeting GluN2B-containing NMDARs with potentially improved therapeutic profile for neurological diseases. Given the accumulating structural biology, preclinical, and clinical data for therapeutics targeting NMDARs, using computer-assisted, structural-based drug design methods combined with machine learning approaches may maximize the success rates of such drug discovery projects.
All authors listed have made a substantial, direct, and intellectual contribution to the work and approved it for publication.
This work was supported by CIHR Canada Foundation grant (FDN-154286).
The authors declare that the research was conducted in the absence of any commercial or financial relationships that could be construed as a potential conflict of interest.
All claims expressed in this article are solely those of the authors and do not necessarily represent those of their affiliated organizations, or those of the publisher, the editors and the reviewers. Any product that may be evaluated in this article, or claim that may be made by its manufacturer, is not guaranteed or endorsed by the publisher.
Aarts, M., Liu, Y., Liu, L., Besshoh, S., Arundine, M., Gurd, J. W., et al. (2002). Treatment of ischemic brain damage by perturbing NMDA receptor-PSD-95 protein interactions. Science 298, 846–850. doi: 10.1126/science.1072873
Akashi, K., Kakizaki, T., Kamiya, H., Fukaya, M., Yamasaki, M., Abe, M., et al. (2009). NMDA receptor GluN2B (GluRε2/NR2B) subunit is crucial for channel function, postsynaptic macromolecular organization, and actin cytoskeleton at hippocampal CA3 synapses. J. Neurosci. 29, 10869–10882. doi: 10.1523/JNEUROSCI.5531-08.2009
Ashpole, N. M., and Hudmon, A. (2011). Excitotoxic neuroprotection and vulnerability with CaMKII inhibition. Mol. Cell. Neurosci. 46, 720–730. doi: 10.1016/j.mcn.2011.02.003
Ashpole, N. M., Song, W., Brustovetsky, T., Engleman, E. A., Brustovetsky, N., Cummins, T. R., et al. (2012). Calcium/calmodulin-dependent protein kinase II (CaMKII) inhibition induces neurotoxicity via dysregulation of glutamate/calcium signaling and hyperexcitability. J. Biol. Chem. 287, 8495–8506. doi: 10.1074/jbc.M111.323915
Asim, M., Hao, B., Yang, Y.-H., Fan, B.-F., Xue, L., Shi, Y.-W., et al. (2020). Ketamine alleviates fear generalization through GluN2B-BDNF signaling in mice. Neurosci. Bull. 36, 153–164. doi: 10.1007/s12264-019-00422-4
Babiec, W. E., Guglietta, R., Jami, S. A., Morishita, W., Malenka, R. C., and O'Dell, T. J. (2014). Ionotropic NMDA receptor signaling is required for the induction of long-term depression in the mouse hippocampal CA1 region. J. Neurosci. 34, 5285–5290. doi: 10.1523/JNEUROSCI.5419-13.2014
Bach, A., Clausen, B. H., Møller, M., Vestergaard, B., Chi, C. N., Round, A., et al. (2012). A high-affinity, dimeric inhibitor of PSD-95 bivalently interacts with PDZ1-2 and protects against ischemic brain damage. Proc. Natl. Acad. Sci. 109, 3317–3322. doi: 10.1073/pnas.1113761109
Barria, A., and Malinow, R. (2005). NMDA receptor subunit composition controls synaptic plasticity by regulating binding to CaMKII. Neuron 48, 289–301. doi: 10.1016/j.neuron.2005.08.034
Barria, A., Muller, D., Derkach, V., Griffith, L. C., and Soderling, T. R. (1997). Regulatory phosphorylation of AMPA-type glutamate receptors by CaM-KII during long-term potentiation. Science 276, 2042–2045. doi: 10.1126/science.276.5321.2042
Bartlett, T. E., Bannister, N. J., Collett, V. J., Dargan, S. L., Massey, P. V., Bortolotto, Z. A., et al. (2007). Differential roles of NR2A and NR2B-containing NMDA receptors in LTP and LTD in the CA1 region of two-week old rat hippocampus. Neuropharmacology 52, 60–70. doi: 10.1016/j.neuropharm.2006.07.013
Bartlett, T. E., and Wang, Y. T. (2013). The intersections of NMDAR-dependent synaptic plasticity and cell survival. Neuropharmacology 74, 59–68. doi: 10.1016/j.neuropharm.2013.01.012
Bayer, K.-U., De Koninck, P., Leonard, A. S., Hell, J. W., and Schulman, H. (2001). Interaction with the NMDA receptor locks CaMKII in an active conformation. Nature 411, 801–805. doi: 10.1038/35081080
Bayer, K. U., and Schulman, H. (2019). CaM kinase: still inspiring at 40. Neuron 103, 380–394. doi: 10.1016/j.neuron.2019.05.033
Berberich, S., Jensen, V., Hvalby, Ø., Seeburg, P. H., and Köhr, G. (2007). The role of NMDAR subtypes and charge transfer during hippocampal LTP induction. Neuropharmacology 52, 77–86. doi: 10.1016/j.neuropharm.2006.07.016
Brigman, J. L., Wright, T., Talani, G., Prasad-Mulcare, S., Jinde, S., Seabold, G. K., et al. (2010). Loss of Glu N2B-containing NMDA receptors in CA1 hippocampus and cortex impairs long-term depression, reduces dendritic spine density, and disrupts learning. J. Neurosci. 30, 4590–4600. doi: 10.1523/JNEUROSCI.0640-10.2010
Brim, B., Haskell, R., Awedikian, R., Ellinwood, N., Jin, L., Kumar, A., et al. (2013). Memory in aged mice is rescued by enhanced expression of the GluN2B subunit of the NMDA receptor. Behav. Brain Res. 238, 211–226. doi: 10.1016/j.bbr.2012.10.026
Cao, X., Cui, Z., Feng, R., Tang, Y. P., Qin, Z., Mei, B., et al. (2007). Maintenance of superior learning and memory function in NR2B transgenic mice during ageing. Eur. J. Neurosci. 25, 1815–1822. doi: 10.1111/j.1460-9568.2007.05431.x
Cao, J., Semenova, M. M., Solovyan, V. T., Han, J., Coffey, E. T., and Courtney, M. J. (2004). Distinct requirements for p38α and c-Jun N-terminal kinase stress-activated protein kinases in different forms of apoptotic neuronal death. J. Biol. Chem. 279, 35903–35913. doi: 10.1074/jbc.M402353200
Chen, M., Lu, T.-J., Chen, X.-J., Zhou, Y., Chen, Q., Feng, X.-Y., et al. (2008). Differential roles of NMDA receptor subtypes in ischemic neuronal cell death and ischemic tolerance. Stroke 39, 3042–3048. doi: 10.1161/STROKEAHA.108.521898
Chenard, B., Bordner, J., Butler, T., Chambers, L., Collins, M., De Costa, D., et al. (1995). (1S, 2S)-1-(4-hydroxyphenyl)-2-(4-hydroxy-4-phenylpiperidino)-1-propanol: a potent new neuroprotectant which blocks N-methyl-d-aspartate responses. J. Med. Chem. 38, 3138–3145. doi: 10.1021/jm00016a017
Colciaghi, F., Nobili, P., Cipelletti, B., Cagnoli, C., Zambon, S., Locatelli, D., et al. (2019). Targeting PSD95-nNOS interaction by tat-N-dimer peptide during status epilepticus is neuroprotective in MAM-pilocarpine rat model. Neuropharmacology 153, 82–97. doi: 10.1016/j.neuropharm.2019.04.028
Collingridge, G. L., Isaac, J. T. R., and Wang, Y. T. (2004). Receptor trafficking and synaptic plasticity. Nat. Rev. Neurosci. 5, 952–962. doi: 10.1038/nrn1556
Collingridge, G. L., Peineau, S., Howland, J. G., and Wang, Y. T. (2010). Long-term depression in the CNS. Nat. Rev. Neurosci. 11, 459–473. doi: 10.1038/nrn2867
Cook, S. G., Buonarati, O. R., Coultrap, S. J., and Bayer, K. U. (2021). CaMKII holoenzyme mechanisms that govern the LTP versus LTD decision. Science. Advances 7:eabe2300. doi: 10.1126/sciadv.abe2300
Coultrap, S. J., Vest, R. S., Ashpole, N. M., Hudmon, A., and Bayer, K. U. (2011). CaMKII in cerebral ischemia. Acta Pharmacol. Sin. 32, 861–872. doi: 10.1038/aps.2011.68
Cui, Y., Jin, J., Zhang, X., Xu, H., Yang, L., Du, D., et al. (2011). Forebrain NR2B overexpression facilitating the prefrontal cortex long-term potentiation and enhancing working memory function in mice. PLoS One 6:e20312. doi: 10.1371/journal.pone.0020312
Dalton, G. L., Ma, L. M., Phillips, A. G., and Floresco, S. B. (2011). Blockade of NMDA GluN2B receptors selectively impairs behavioral flexibility but not initial discrimination learning. Psychopharmacology (Berl) 216, 525–535. doi: 10.1007/s00213-011-2246-z
Dalton, G. L., Wu, D. C., Wang, Y. T., Floresco, S. B., and Phillips, A. G. (2012). NMDA GluN2A and GluN2B receptors play separate roles in the induction of LTP and LTD in the amygdala and in the acquisition and extinction of conditioned fear. Neuropharmacology 62, 797–806. doi: 10.1016/j.neuropharm.2011.09.001
Delaney, A. J., Sedlak, P. L., Autuori, E., Power, J. M., and Sah, P. (2012). Synaptic NMDA receptors in basolateral amygdala principal neurons are triheteromeric proteins: physiological role of GluN2B subunits. J. Neurophysiol. 109, 1391–1402. doi: 10.1152/jn.00176.2012
DeRidder, M. N., Simon, M. J., Siman, R., Auberson, Y. P., Raghupathi, R., and Meaney, D. F. (2006). Traumatic mechanical injury to the hippocampus in vitro causes regional caspase-3 and calpain activation that is influenced by NMDA receptor subunit composition. Neurobiol. Dis. 22, 165–176. doi: 10.1016/j.nbd.2005.10.011
Dhawan, J., Benveniste, H., Luo, Z., Nawrocky, M., Smith, S. D., and Biegon, A. (2011). A new look at glutamate and ischemia: NMDA agonist improves long-term functional outcome in a rat model of stroke. Future Neurol. 6, 823–834. doi: 10.2217/fnl.11.55
Dong, Z., Bai, Y., Wu, X., Li, H., Gong, B., Howland, J. G., et al. (2013). Hippocampal long-term depression mediates spatial reversal learning in the Morris water maze. Neuropharmacology 64, 65–73. doi: 10.1016/j.neuropharm.2012.06.027
Dong, Z., Gong, B., Li, H., Bai, Y., Wu, X., Huang, Y., et al. (2012). Mechanisms of hippocampal long-term depression are required for memory enhancement by novelty exploration. J. Neurosci. 32, 11980–11990. doi: 10.1523/JNEUROSCI.0984-12.2012
Dore, K., Aow, J., and Malinow, R. (2016). The emergence of NMDA receptor metabotropic function: insights from imaging. Front Synapt Neurosci 8:20. doi: 10.3389/fnsyn.2016.00020
Doyle, S., Hansen, D. B., Vella, J., Bond, P., Harper, G., Zammit, C., et al. (2018). Vesicular glutamate release from central axons contributes to myelin damage. Nat. Commun. 9, 1–15. doi: 10.1038/s41467-018-03427-1
Duan, Y., Wang, Q., Zeng, Q., Wang, J., Chen, Z., Xu, M., et al. (2018). Striatal GluN2B involved in motor skill learning and stimulus-response learning. Neuropharmacology 135, 73–85. doi: 10.1016/j.neuropharm.2018.03.002
Duffy, S., Labrie, V., and Roder, J. C. (2008). D-serine augments NMDA-NR2B receptor-dependent hippocampal long-term depression and spatial reversal learning. Neuropsychopharmacology 33, 1004–1018. doi: 10.1038/sj.npp.1301486
Falcicchia, C., Tozzi, F., Arancio, O., Watterson, D. M., and Origlia, N. (2020). Involvement of p38 MAPK in synaptic function and dysfunction. Int. J. Mol. Sci. 21:5624. doi: 10.3390/ijms21165624
Fan, X., Jin, W. Y., Lu, J., Wang, J., and Wang, Y. T. (2014). Rapid and reversible knockdown of endogenous proteins by peptide-directed lysosomal degradation. Nat. Neurosci. 17, 471–480. doi: 10.1038/nn.3637
Feig, L. A. (2011). Regulation of neuronal function by Ras-GRF exchange factors. Genes Cancer 2, 306–319. doi: 10.1177/1947601911408077
Fischer, G., Mutel, V., Trube, G., Malherbe, P., Kew, J., Mohacsi, E., et al. (1997). Ro 25–6981, a highly potent and selective blocker of N-methyl-D-aspartate receptors containing the NR2B subunit. Characterization in vitro. J. Pharmacol. Exp. Ther. 283, 1285–1292.
Floresco, S. B., Zhang, Y., and Enomoto, T. (2009). Neural circuits subserving behavioral flexibility and their relevance to schizophrenia. Behav. Brain Res. 204, 396–409. doi: 10.1016/j.bbr.2008.12.001
Foster, K. A., McLaughlin, N., Edbauer, D., Phillips, M., Bolton, A., Constantine-Paton, M., et al. (2010). Distinct roles of NR2A and NR2B cytoplasmic tails in long-term potentiation. J. Neurosci. 30, 2676–2685. doi: 10.1523/JNEUROSCI.4022-09.2010
Fox, C. J., Russell, K. I., Wang, Y. T., and Christie, B. R. (2006). Contribution of NR2A and NR2B NMDA subunits to bidirectional synaptic plasticity in the hippocampus in vivo. Hippocampus 16, 907–915. doi: 10.1002/hipo.20230
García-Recio, A., Santos-Gómez, A., Soto, D., Julia-Palacios, N., García-Cazorla, À., Altafaj, X., et al. (2021). GRIN database: a unified and manually curated repertoire of GRIN variants. Hum. Mutat. 42, 8–18. doi: 10.1002/humu.24141
Ge, Y., Chen, W., Axerio-Cilies, P., and Wang, Y. T. (2020). NMDARs in cell survival and death: implications in stroke pathogenesis and treatment. Trends Mol. Med. 26, 533–551. doi: 10.1016/j.molmed.2020.03.001
Ge, Y., Dong, Z., Bagot, R. C., Howland, J. G., Phillips, A. G., Wong, T. P., et al. (2010). Hippocampal long-term depression is required for the consolidation of spatial memory. Proc. Natl. Acad. Sci. 107, 16697–16702. doi: 10.1073/pnas.1008200107
Ge, Y., and Wang, Y. T. (2022). Postsynaptic signaling at glutamatergic synapses as therapeutic targets. Curr. Opin. Neurobiol. 75:102585. doi: 10.1016/j.conb.2022.102585
Gerhard, D. M., Pothula, S., Liu, R.-J., Wu, M., Li, X.-Y., Girgenti, M. J., et al. (2020). GABA interneurons are the cellular trigger for ketamine’s rapid antidepressant actions. J. Clin. Invest. 130, 1336–1349. doi: 10.1172/JCI130808
Goodell, D. J., Zaegel, V., Coultrap, S. J., Hell, J. W., and Bayer, K. U. (2017). DAPK1 mediates LTD by making CaMKII/GluN2B binding LTP specific. Cell Rep. 19, 2231–2243. doi: 10.1016/j.celrep.2017.05.068
Guerra, G. P., Rubin, M. A., and Mello, C. F. (2016). Modulation of learning and memory by natural polyamines. Pharmacol. Res. 112, 99–118. doi: 10.1016/j.phrs.2016.03.023
Hansen, K. B., Ogden, K. K., Yuan, H., and Traynelis, S. F. (2014). Distinct functional and pharmacological properties of Triheteromeric Glu N1/Glu N2A/GluN2B NMDA receptors. Neuron 81, 1084–1096. doi: 10.1016/j.neuron.2014.01.035
Hardingham, G. E., and Bading, H. (2010). Synaptic versus extrasynaptic NMDA receptor signalling: implications for neurodegenerative disorders. Nat. Rev. Neurosci. 11, 682–696. doi: 10.1038/nrn2911
Hill, M. D., Blanco, M.-J., Salituro, F. G., Bai, Z., Beckley, J. T., Ackley, M. A., et al. (2022). SAGE-718: a first-in-class N-methyl-d-aspartate receptor positive allosteric modulator for the potential treatment of cognitive impairment. J. Med. Chem. 65, 9063–9075. doi: 10.1021/acs.jmedchem.2c00313
Hill, M. D., Goyal, M., Menon, B. K., Nogueira, R. G., McTaggart, R. A., Demchuk, A. M., et al. (2020). Efficacy and safety of nerinetide for the treatment of acute ischaemic stroke (ESCAPE-NA1): a multicentre, double-blind, randomised controlled trial. Lancet 395, 878–887. doi: 10.1016/S0140-6736(20)30258-0
Howland, J. G., and Cazakoff, B. N. (2010). Effects of acute stress and GluN2B-containing NMDA receptor antagonism on object and object–place recognition memory. Neurobiol. Learn. Mem. 93, 261–267. doi: 10.1016/j.nlm.2009.10.006
Ito, I., Sakimura, K., Mishina, M., and Sugiyama, H. (1996). Age-dependent reduction of hippocampal LTP in mice lacking N-methyl-d-aspartate receptor ϵ1 subunit. Neurosci. Lett. 203, 69–71. doi: 10.1016/0304-3940(95)12258-3
Jacobs, S. A., and Tsien, J. Z. (2012). Genetic overexpression of NR2B subunit enhances social recognition memory for different strains and species. PLoS One 7:e36387. doi: 10.1371/journal.pone.0036387
Karakas, E., Simorowski, N., and Furukawa, H. (2011). Subunit arrangement and phenylethanolamine binding in GluN1/GluN2B NMDA receptors. Nature 475, 249–253. doi: 10.1038/nature10180
Kessels, H. W., Nabavi, S., and Malinow, R. (2013). Metabotropic NMDA receptor function is required for β-amyloid–induced synaptic depression. Proc. Natl. Acad. Sci. 110, 4033–4038. doi: 10.1073/pnas.1219605110
Khatri, A., Burger, P. B., Swanger, S. A., Hansen, K. B., Zimmerman, S., Karakas, E., et al. (2014). Structural determinants and mechanism of action of a GluN2C-selective NMDA receptor positive allosteric modulator. Mol. Pharmacol. 86, 548–560. doi: 10.1124/mol.114.094516
Kim, M. J., Dunah, A. W., Wang, Y. T., and Sheng, M. (2005). Differential roles of NR2A-and NR2B-containing NMDA receptors in Ras-ERK signaling and AMPA receptor trafficking. Neuron 46, 745–760. doi: 10.1016/j.neuron.2005.04.031
Krapivinsky, G., Krapivinsky, L., Manasian, Y., Ivanov, A., Tyzio, R., Pellegrino, C., et al. (2003). The NMDA receptor is coupled to the ERK pathway by a direct interaction between NR2B and RasGRF1. Neuron 40, 775–784. doi: 10.1016/S0896-6273(03)00645-7
Lee, B., Pothula, S., Wu, M., Kang, H., Girgenti, M. J., Picciotto, M. R., et al. (2022). Positive modulation of N-methyl-D-aspartate receptors in the mPFC reduces the spontaneous recovery of fear. Mol. Psychiatry 27, 2580–2589. doi: 10.1038/s41380-022-01498-7
Li, Z., Cai, G., Fang, F., Li, W., Fan, M., Lian, J., et al. (2021). Discovery of novel and potent N-methyl-d-aspartate receptor positive allosteric modulators with antidepressant-like activity in rodent models. J. Med. Chem. 64, 5551–5576. doi: 10.1021/acs.jmedchem.0c02018
Li, J., Chai, A., Wang, L., Ma, Y., Wu, Z., Yu, H., et al. (2015). Synaptic P-Rex1 signaling regulates hippocampal long-term depression and autism-like social behavior. Proc. Natl. Acad. Sci. 112, E6964–E6972. doi: 10.1073/pnas.1512913112
Li, L. P., Dustrude, E. T., Haulcomb, M. M., Abreu, A. R., Fitz, S. D., Johnson, P. L., et al. (2018). PSD95 and nNOS interaction as a novel molecular target to modulate conditioned fear: relevance to PTSD. Transl. Psychiatry 8:155. doi: 10.1038/s41398-018-0208-5
Li, N., Liu, R.-J., Dwyer, J. M., Banasr, M., Lee, B., Son, H., et al. (2011). Glutamate N-methyl-D-aspartate receptor antagonists rapidly reverse behavioral and synaptic deficits caused by chronic stress exposure. Biol. Psychiatry 69, 754–761. doi: 10.1016/j.biopsych.2010.12.015
Li, S., Tian, X., Hartley, D. M., and Feig, L. A. (2006). Distinct roles for Ras-guanine nucleotide-releasing factor 1 (Ras-GRF1) and Ras-GRF2 in the induction of long-term potentiation and long-term depression. J. Neurosci. 26, 1721–1729. doi: 10.1523/JNEUROSCI.3990-05.2006
Lisman, J., Yasuda, R., and Raghavachari, S. (2012). Mechanisms of CaMKII action in long-term potentiation. Nat. Rev. Neurosci. 13, 169–182. doi: 10.1038/nrn3192
Liu, A., Ji, H., Ren, Q., Meng, Y., Zhang, H., Collingride, G., et al. (2020). The requirement of the C-terminal domain of glua1 in different forms of long-term potentiation in the hippocampus is age-dependent. Front Synapt Neurosci 12:588785. doi: 10.3389/fnsyn.2020.588785
Liu, W., Jiang, X., Zu, Y., Yang, Y., Liu, Y., Sun, X., et al. (2020). A comprehensive description of GluN2B-selective N-methyl-D-aspartate (NMDA) receptor antagonists. Eur. J. Med. Chem. 200:112447. doi: 10.1016/j.ejmech.2020.112447
Liu, Y., Wong, T. P., Aarts, M., Rooyakkers, A., Liu, L., Lai, T. W., et al. (2007). NMDA receptor subunits have differential roles in mediating excitotoxic neuronal death both in vitro and in vivo. J. Neurosci. 27, 2846–2857. doi: 10.1523/JNEUROSCI.0116-07.2007
Liu, L., Wong, T. P., Pozza, M. F., Lingenhoehl, K., Wang, Y., Sheng, M., et al. (2004). Role of NMDA receptor subtypes in governing the direction of hippocampal synaptic plasticity. Science 304, 1021–1024. doi: 10.1126/science.1096615
Liu, Z., Zhao, W., Xu, T., Pei, D., and Peng, Y. (2010). Alterations of NMDA receptor subunits NR1, NR2A and NR2B mRNA expression and their relationship to apoptosis following transient forebrain ischemia. Brain Res. 1361, 133–139. doi: 10.1016/j.brainres.2010.09.035
Liu, S., Zhou, L., Yuan, H., Vieira, M., Sanz-Clemente, A., Badger, J. D., et al. (2017). A rare variant identified within the GluN2B C-terminus in a patient with autism affects NMDA receptor surface expression and spine density. J. Neurosci. 37, 4093–4102. doi: 10.1523/JNEUROSCI.0827-16.2017
Lu, W.-Y., Man, H.-Y., Ju, W., Trimble, W. S., MacDonald, J. F., and Wang, Y. T. (2001). Activation of synaptic NMDA receptors induces membrane insertion of new AMPA receptors and LTP in cultured hippocampal neurons. Neuron 29, 243–254. doi: 10.1016/S0896-6273(01)00194-5
Ma, C.-L., Sun, H., Yang, L., Wang, X.-T., Gao, S., Chen, X.-W., et al. (2019). Wang G-h, Shi Z, Zheng Q-Y: acid-sensing ion channel 1a modulates NMDA receptor function through targeting NR1/NR2A/NR2B triheteromeric receptors. Neuroscience 406, 389–404. doi: 10.1016/j.neuroscience.2019.03.044
Mamou, C. B., Gamache, K., and Nader, K. (2006). NMDA receptors are critical for unleashing consolidated auditory fear memories. Nat. Neurosci. 9, 1237–1239. doi: 10.1038/nn1778
Marquardt, K., Josey, M., Kenton, J. A., Cavanagh, J. F., Holmes, A., and Brigman, J. L. (2019). Impaired cognitive flexibility following NMDAR-GluN2B deletion is associated with altered orbitofrontal-striatal function. Neuroscience 404, 338–352. doi: 10.1016/j.neuroscience.2019.01.066
Martel, M.-A., Ryan, T. J., Bell, K. F., Fowler, J. H., McMahon, A., Al-Mubarak, B., et al. (2012). The subtype of GluN2 C-terminal domain determines the response to excitotoxic insults. Neuron 74, 543–556. doi: 10.1016/j.neuron.2012.03.021
McGirr, A., Berlim, M., Bond, D., Fleck, M., Yatham, L., and Lam, R. (2015). A systematic review and meta-analysis of randomized, double-blind, placebo-controlled trials of ketamine in the rapid treatment of major depressive episodes. Psychol. Med. 45, 693–704. doi: 10.1017/S0033291714001603
McQueen, J., Ryan, T. J., McKay, S., Marwick, K., Baxter, P., Carpanini, S. M., et al. (2017). Pro-death NMDA receptor signaling is promoted by the GluN2B C-terminus independently of Dapk1. Elife 6:e17161. doi: 10.7554/eLife.17161
Miller, M. B., Yan, Y., Eipper, B. A., and Mains, R. E. (2013). Neuronal rho GEFs in synaptic physiology and behavior. Neuroscientist 19, 255–273. doi: 10.1177/1073858413475486
Miller, O. H., Yang, L., Wang, C.-C., Hargroder, E. A., Zhang, Y., Delpire, E., et al. (2014). GluN2B-containing NMDA receptors regulate depression-like behavior and are critical for the rapid antidepressant actions of ketamine. Elife 3:e03581. doi: 10.7554/eLife.03581
Milton, A. L., Merlo, E., Ratano, P., Gregory, B. L., Dumbreck, J. K., and Everitt, B. J. (2013). Double dissociation of the requirement for GluN2B-and GluN2A-containing NMDA receptors in the destabilization and restabilization of a reconsolidating memory. J. Neurosci. 33, 1109–1115. doi: 10.1523/JNEUROSCI.3273-12.2013
Monyer, H., Burnashev, N., Laurie, D. J., Sakmann, B., and Seeburg, P. H. (1994). Developmental and regional expression in the rat brain and functional properties of four NMDA receptors. Neuron 12, 529–540. doi: 10.1016/0896-6273(94)90210-0
Morishita, W., Lu, W., Smith, G. B., Nicoll, R. A., Bear, M. F., and Malenka, R. C. (2007). Activation of NR2B-containing NMDA receptors is not required for NMDA receptor-dependent long-term depression. Neuropharmacology 52, 71–76. doi: 10.1016/j.neuropharm.2006.07.005
Nabavi, S., Kessels, H. W., Alfonso, S., Aow, J., Fox, R., and Malinow, R. (2013). Metabotropic NMDA receptor function is required for NMDA receptor-dependent long-term depression. Proc. Natl. Acad. Sci. 110, 4027–4032. doi: 10.1073/pnas.1219454110
Nowak, L., Bregestovski, P., Ascher, P., Herbet, A., and Prochiantz, A. (1984). Magnesium gates glutamate-activated channels in mouse central neurones. Nature 307, 462–465. doi: 10.1038/307462a0
Nozaki, C., Vergnano, A. M., Filliol, D., Ouagazzal, A.-M., Le Goff, A., Carvalho, S., et al. (2011). Zinc alleviates pain through high-affinity binding to the NMDA receptor NR2A subunit. Nat. Neurosci. 14, 1017–1022. doi: 10.1038/nn.2844
Paoletti, P., Bellone, C., and Zhou, Q. (2013). NMDA receptor subunit diversity: impact on receptor properties, synaptic plasticity and disease. Nat. Rev. Neurosci. 14, 383–400. doi: 10.1038/nrn3504
Papouin, T., Ladépêche, L., Ruel, J., Sacchi, S., Labasque, M., Hanini, M., et al. (2012). Synaptic and extrasynaptic NMDA receptors are gated by different endogenous coagonists. Cells 150, 633–646. doi: 10.1016/j.cell.2012.06.029
Peça, J., Feliciano, C., Ting, J. T., Wang, W., Wells, M. F., Venkatraman, T. N., et al. (2011). Shank3 mutant mice display autistic-like behaviours and striatal dysfunction. Nature 472, 437–442. doi: 10.1038/nature09965
Pegasiou, C. M., Zolnourian, A., Gomez-Nicola, D., Deinhardt, K., Nicoll, J. A., Ahmed, A. I., et al. (2020). Age-dependent changes in synaptic NMDA receptor composition in adult human cortical neurons. Cereb. Cortex 30, 4246–4256. doi: 10.1093/cercor/bhaa052
Pi, H. J., Otmakhov, N., Lemelin, D., De Koninck, P., and Lisman, J. (2010). Autonomous CaMKII can promote either long-term potentiation or long-term depression, depending on the state of T305/T306 phosphorylation. J. Neurosci. 30, 8704–8709. doi: 10.1523/JNEUROSCI.0133-10.2010
Pothula, S., Kato, T., Liu, R.-J., Wu, M., Gerhard, D., Shinohara, R., et al. (2021a). Cell-type specific modulation of NMDA receptors triggers antidepressant actions. Mol. Psychiatry 26, 5097–5111. doi: 10.1038/s41380-020-0796-3
Pothula, S., Liu, R.-J., Wu, M., Sliby, A.-N., Picciotto, M. R., Banerjee, P., et al. (2021b). Positive modulation of NMDA receptors by AGN-241751 exerts rapid antidepressant-like effects via excitatory neurons. Neuropsychopharmacology 46, 799–808. doi: 10.1038/s41386-020-00882-7
Pratt, J., Winchester, C., Dawson, N., and Morris, B. (2012). Advancing schizophrenia drug discovery: optimizing rodent models to bridge the translational gap. Nat. Rev. Drug Discov. 11, 560–579. doi: 10.1038/nrd3649
Qu, W., Liu, N.-K., Wu, X., Wang, Y., Xia, Y., Sun, Y., et al. (2020). Disrupting nNOS–PSD95 interaction improves neurological and cognitive recoveries after traumatic brain injury. Cereb. Cortex 30, 3859–3871. doi: 10.1093/cercor/bhaa002
Sachser, R. M., Santana, F., Crestani, A. P., Lunardi, P., Pedraza, L. K., Quillfeldt, J. A., et al. (2016). Forgetting of long-term memory requires activation of NMDA receptors, L-type voltage-dependent Ca2+ channels, and calcineurin. Sci. Rep. 6, 1–9. doi: 10.1038/srep22771
Sattler, R., Xiong, Z., Lu, W.-Y., Hafner, M., MacDonald, J. F., and Tymianski, M. (1999). Specific coupling of NMDA receptor activation to nitric oxide neurotoxicity by PSD-95 protein. Science 284, 1845–1848. doi: 10.1126/science.284.5421.1845
Sceniak, M. P., Fedder, K. N., Wang, Q., Droubi, S., Babcock, K., Patwardhan, S., et al. (2019). An autism-associated mutation in GluN2B prevents NMDA receptor trafficking and interferes with dendrite growth. J. Cell Sci. 132:jcs232892. doi: 10.1242/jcs.232892
Sheng, M., Cummings, J., Roldan, L. A., Jan, Y. N., and Jan, L. Y. (1994). Changing subunit composition of heteromeric NMDA receptors during development of rat cortex. Nature 368, 144–147. doi: 10.1038/368144a0
Sheng, M., and Ertürk, A. (2014). Long-term depression: a cell biological view. Philosoph Transac R Soc B Biol Sci 369:20130138. doi: 10.1098/rstb.2013.0138
Shin, W., Kim, K., Serraz, B., Cho, Y. S., Kim, D., Kang, M., et al. (2020). Early correction of synaptic long-term depression improves abnormal anxiety-like behavior in adult GluN2B-C456Y-mutant mice. PLoS Biol. 18:e3000717. doi: 10.1371/journal.pbio.3000717
Shipton, O. A., and Paulsen, O. (2014). GluN2A and GluN2B subunit-containing NMDA receptors in hippocampal plasticity. Philosoph Transac R Soc B Biol Sci 369:20130163. doi: 10.1098/rstb.2013.0163
Singh, T., Poterba, T., Curtis, D., Akil, H., Al Eissa, M., Barchas, J. D., et al. (2022). Rare coding variants in ten genes confer substantial risk for schizophrenia. Nature 604, 509–516. doi: 10.1038/s41586-022-04556-w
Stein, I. S., Gray, J. A., and Zito, K. (2015). Non-ionotropic NMDA receptor signaling drives activity-induced dendritic spine shrinkage. J. Neurosci. 35, 12303–12308. doi: 10.1523/JNEUROSCI.4289-14.2015
Stocca, G., and Vicini, S. (1998). Increased contribution of NR2A subunit to synaptic NMDA receptors in developing rat cortical neurons. J. Physiol. 507, 13–24. doi: 10.1111/j.1469-7793.1998.013bu.x
Strack, S., and Colbran, R. J. (1998). Autophosphorylation-dependent targeting of calcium/calmodulin-dependent protein kinase II by the NR2B subunit of theN-methyl-D-aspartate receptor. J. Biol. Chem. 273, 20689–20692. doi: 10.1074/jbc.273.33.20689
Stroebel, D., Carvalho, S., Grand, T., Zhu, S., and Paoletti, P. (2014). Controlling NMDA receptor subunit composition using ectopic retention signals. J. Neurosci. 34, 16630–16636. doi: 10.1523/JNEUROSCI.2736-14.2014
Stroebel, D., Casado, M., and Paoletti, P. (2018). Triheteromeric NMDA receptors: from structure to synaptic physiology. Curr. Opin. Physio. 2, 1–12. doi: 10.1016/j.cophys.2017.12.004
Sun, Y., Zhang, L., Chen, Y., Zhan, L., and Gao, Z. (2015). Therapeutic targets for cerebral ischemia based on the signaling pathways of the GluN2B C terminus. Stroke 46, 2347–2353. doi: 10.1161/STROKEAHA.115.009314
Tamburri, A., Dudilot, A., Licea, S., Bourgeois, C., and Boehm, J. (2013). NMDA-receptor activation but not ion flux is required for amyloid-beta induced synaptic depression. PLoS One 8:e65350. doi: 10.1371/journal.pone.0065350
Tang, Y.-P., Shimizu, E., Dube, G. R., Rampon, C., Kerchner, G. A., Zhuo, M., et al. (1999). Genetic enhancement of learning and memory in mice. Nature 401, 63–69. doi: 10.1038/43432
Tang, N., Wu, J., Zhu, H., Yan, H., Guo, Y., Cai, Y., et al. (2018). Genetic mutation of GluN2B protects brain cells against stroke damages. Mol. Neurobiol. 55, 2979–2990. doi: 10.1007/s12035-017-0562-y
Tashiro, A., Sandler, V. M., Toni, N., Zhao, C., and Gage, F. H. (2006). NMDA-receptor-mediated, cell-specific integration of new neurons in adult dentate gyrus. Nature 442, 929–933. doi: 10.1038/nature05028
Thomas, C. G., Miller, A. J., and Westbrook, G. L. (2006). Synaptic and extrasynaptic NMDA receptor NR2 subunits in cultured hippocampal neurons. J. Neurophysiol. 95, 1727–1734. doi: 10.1152/jn.00771.2005
Tian, X., Gotoh, T., Tsuji, K., Lo, E. H., Huang, S., and Feig, L. A. (2004). Developmentally regulated role for Ras-GRFs in coupling NMDA glutamate receptors to Ras, Erk and CREB. EMBO J. 23, 1567–1575. doi: 10.1038/sj.emboj.7600151
Toft, A. K. H., Lundbye, C. J., and Banke, T. G. (2016). Dysregulated NMDA-receptor signaling inhibits long-term depression in a mouse model of fragile X syndrome. J. Neurosci. 36, 9817–9827. doi: 10.1523/JNEUROSCI.3038-15.2016
Tomita, S., Stein, V., Stocker, T. J., Nicoll, R. A., and Bredt, D. S. (2005). Bidirectional synaptic plasticity regulated by phosphorylation of stargazin-like TARPs. Neuron 45, 269–277. doi: 10.1016/j.neuron.2005.01.009
Tu, W., Xu, X., Peng, L., Zhong, X., Zhang, W., Soundarapandian, M. M., et al. (2010). DAPK1 interaction with NMDA receptor NR2B subunits mediates brain damage in stroke. Cells 140, 222–234. doi: 10.1016/j.cell.2009.12.055
Tullis, J. E., Buonarati, O. R., Coultrap, S. J., Bourke, A. M., Tiemeier, E. L., Kennedy, M. J., et al. (2021). GluN2B S1303 phosphorylation by CaMKII or DAPK1: no indication for involvement in ischemia or LTP. Iscience 24:103214. doi: 10.1016/j.isci.2021.103214
Vest, R. S., O'Leary, H., Coultrap, S. J., Kindy, M. S., and Bayer, K. U. (2010). Effective post-insult neuroprotection by a novel Ca2+/calmodulin-dependent protein kinase II (CaMKII) inhibitor. J. Biol. Chem. 285, 20675–20682. doi: 10.1074/jbc.M109.088617
Vieira, M. M., Schmidt, J., Ferreira, J. S., She, K., Oku, S., Mele, M., et al. (2016). Multiple domains in the C-terminus of NMDA receptor GluN2B subunit contribute to neuronal death following in vitro ischemia. Neurobiol. Dis. 89, 223–234. doi: 10.1016/j.nbd.2015.11.007
von Engelhardt, J., Doganci, B., Jensen, V., Hvalby, Ø., Göngrich, C., Taylor, A., et al. (2008). Contribution of hippocampal and extra-hippocampal NR2B-containing NMDA receptors to performance on spatial learning tasks. Neuron 60, 846–860. doi: 10.1016/j.neuron.2008.09.039
Vyklicky, V., Krausova, B., Cerny, J., Ladislav, M., Smejkalova, T., Kysilov, B., et al. (2018). Surface expression, function, and pharmacology of disease-associated mutations in the membrane domain of the human GluN2B subunit. Front. Mol. Neurosci. 11:110. doi: 10.3389/fnmol.2018.00110
Wang, X., Guo, Z., Mei, D., Zhang, Y., Zhao, S., Hu, S., et al. (2022). The GluN2B-Trp373 NMDA receptor variant is associated with autism-, epilepsy-related phenotypes and reduces NMDA receptor currents in rats. Neurochem. Res. 47, 1588–1597. doi: 10.1007/s11064-022-03554-8
Wang, D., Jacobs, S. A., and Tsien, J. Z. (2014). Targeting the NMDA receptor subunit NR2B for treating or preventing age-related memory decline. Expert Opin. Ther. Targets 18, 1121–1130. doi: 10.1517/14728222.2014.941286
Waxham, M. N., Grotta, J. C., Silva, A. J., Strong, R., and Aronowski, J. (1996). Ischemia-induced neuronal damage: a role for calcium/calmodulin-dependent protein kinase II. J. Cereb. Blood Flow Metab. 16, 1–6. doi: 10.1097/00004647-199601000-00001
Weilinger, N. L., Lohman, A. W., Rakai, B. D., Ma, E. M., Bialecki, J., Maslieieva, V., et al. (2016). Metabotropic NMDA receptor signaling couples Src family kinases to pannexin-1 during excitotoxicity. Nat. Neurosci. 19, 432–442. doi: 10.1038/nn.4236
Wenzel, A., Fritschy, J. M., Mohler, H., and Benke, D. (1997). NMDA receptor heterogeneity during postnatal development of the rat brain: differential expression of the NR2A, NR2B, and NR2C subunit proteins. J. Neurochem. 68, 469–478. doi: 10.1046/j.1471-4159.1997.68020469.x
Williams, K., Zappia, A. M., Pritchett, D. B., Shen, Y. M., and Molinoff, P. B. (1994). Sensitivity of the N-methyl-D-aspartate receptor to polyamines is controlled by NR2 subunits. Mol. Pharmacol. 45, 803–809.
Wong, J. M., and Gray, J. A. (2018). Long-term depression is independent of GluN2 subunit composition. J. Neurosci. 38, 4462–4470. doi: 10.1523/JNEUROSCI.0394-18.2018
Wong, T. P., Howland, J. G., Robillard, J. M., Ge, Y., Yu, W., Titterness, A. K., et al. (2007). Hippocampal long-term depression mediates acute stress-induced spatial memory retrieval impairment. Proc. Natl. Acad. Sci. 104, 11471–11476. doi: 10.1073/pnas.0702308104
Wu, Q. J., and Tymianski, M. (2018). Targeting NMDA receptors in stroke: new hope in neuroprotection. Mol. Brain 11, 1–14. doi: 10.1186/s13041-018-0357-8
Yasuda, R., Hayashi, Y., and Hell, J. W. (2022). CaMKII: a central molecular organizer of synaptic plasticity, learning and memory. Nat. Rev. Neurosci. 23, 666–682. doi: 10.1038/s41583-022-00624-2
Yi, F., Bhattacharya, S., Thompson, C. M., Traynelis, S. F., and Hansen, K. B. (2019). Functional and pharmacological properties of triheteromeric Glu N1/2B/2D NMDA receptors. J. Physiol. 597, 5495–5514. doi: 10.1113/JP278168
Zhao, Y., Chen, S., Swensen, A. C., Qian, W.-J., and Gouaux, E. (2019). Architecture and subunit arrangement of native AMPA receptors elucidated by cryo-EM. Science 364, 355–362. doi: 10.1126/science.aaw8250
Zhou, X., Ding, Q., Chen, Z., Yun, H., and Wang, H. (2013). Involvement of the GluN2A and GluN2B subunits in synaptic and extrasynaptic N-methyl-D-aspartate receptor function and neuronal excitotoxicity. J. Biol. Chem. 288, 24151–24159. doi: 10.1074/jbc.M113.482000
Keywords: NMDAR (NMDA receptor), GluN2B (NMDA receptor subunit NR2B), synaptic plasticity (LTP/LTD), neuronal death, neurological disorders
Citation: Ge Y and Wang YT (2023) GluN2B-containing NMDARs in the mammalian brain: pharmacology, physiology, and pathology. Front. Mol. Neurosci. 16:1190324. doi: 10.3389/fnmol.2023.1190324
Received: 20 March 2023; Accepted: 24 April 2023;
Published: 31 May 2023.
Edited by:
Miriam Kessi, Central South University, ChinaReviewed by:
Victor Anggono, The University of Queensland, AustraliaCopyright © 2023 Ge and Wang. This is an open-access article distributed under the terms of the Creative Commons Attribution License (CC BY). The use, distribution or reproduction in other forums is permitted, provided the original author(s) and the copyright owner(s) are credited and that the original publication in this journal is cited, in accordance with accepted academic practice. No use, distribution or reproduction is permitted which does not comply with these terms.
*Correspondence: Yu Tian Wang, eXR3YW5nQGJyYWluLnViYy5jYQ==
Disclaimer: All claims expressed in this article are solely those of the authors and do not necessarily represent those of their affiliated organizations, or those of the publisher, the editors and the reviewers. Any product that may be evaluated in this article or claim that may be made by its manufacturer is not guaranteed or endorsed by the publisher.
Research integrity at Frontiers
Learn more about the work of our research integrity team to safeguard the quality of each article we publish.