- 1Department of Pharmacology and Toxicology, Medical College of Wisconsin, Milwaukee, WI, United States
- 2Neuroscience Research Institute, Medical College of Wisconsin, Milwaukee, WI, United States
Conventional inter-neuronal communication conceptualizes the wired method of chemical synapses that physically connect pre-and post-synaptic neurons. In contrast, recent studies indicate that neurons also utilize synapse-independent, hence “wireless” broadcasting-type communications via small extracellular vesicles (EVs). Small EVs including exosomes are secreted vesicles released by cells and contain a variety of signaling molecules including mRNAs, miRNAs, lipids, and proteins. Small EVs are subsequently absorbed by local recipient cells via either membrane fusion or endocytic processes. Therefore, small EVs enable cells to exchange a “packet” of active biomolecules for communication purposes. It is now well established that central neurons also secrete and uptake small EVs, especially exosomes, a type of small EVs that are derived from the intraluminal vesicles of multivesicular bodies. Specific molecules carried by neuronal small EVs are shown to affect a variety of neuronal functions including axon guidance, synapse formation, synapse elimination, neuronal firing, and potentiation. Therefore, this type of volume transmission mediated by small EVs is thought to play important roles not only in activity-dependent changes in neuronal function but also in the maintenance and homeostatic control of local circuitry. In this review, we summarize recent discoveries, catalog neuronal small EV-specific biomolecules, and discuss the potential scope of small EV-mediated inter-neuronal signaling.
1. Introduction
EVs are traditionally classified based on their size, cargo molecules, and originating cell populations (Fowler, 2019). EVs are called by many names − exosomes, microvesicles, ectosomes, shedding vesicles, microparticles, etc. Exosomes (50–200 nm in diameter) are derived from the intraluminal vesicles (ILVs) of multivesicular bodies (MVBs). Ectosomes, also called microvesicles, are generated by the shedding of the direct outward budding of the plasma membrane, whose sizes are in the range of ~100 nm to 1 μm in diameter. However, as EVs are highly heterogenous, even at the single cell levels (Zabeo et al., 2017), it has become harder to define specific categories. In this minireview, we use a term small EVs for EVs smaller than 200 nm in diameter (Thery et al., 2018), which include exosomes.
Exosomes were first discovered nearly 40 years ago and had long been considered as a mere cellular waste disposal mechanism (Trams et al., 1981; Thery, 2011). However, recent major discoveries argue their role as active messengers for cellular communication: exosomes contain many bioactive molecules (especially mRNA and microRNAs) that are re-uptaken by cells (Valadi et al., 2007). Since then, the signaling function of EVs are well established in immune responses and cancer cell biology (Thery et al., 2009).
The vertebrate brain contains various types of non-neuronal cells including astrocytes, oligodendrocytes, and microglia. It is well known that small EVs and exosomes secreted from these non-neuronal cells affect various neuronal functions. Since the first demonstration that postmitotic neurons secrete exosomes by Sadoul’s group (Faure et al., 2006), significant advances have been made in neuronal EV research (Budnik et al., 2016; Fowler, 2019). Now, neuronal exosomes are implicated in a variety of processes including neurogenesis, axon guidance, synaptogenesis, synapse elimination, neuroprotection, mRNA expression, synaptic plasticity, and inflammation (Korkut et al., 2009; Escudero et al., 2014; Gong et al., 2016; Lee et al., 2018; Sharma et al., 2019; Vilcaes et al., 2021; Antoniou et al., 2023). Brain EVs are also implicated for the spread of pathogenic molecules such as amyloid β (Aβ), amyloid precursor proteins (APP), prions, tau, and α-synuclein, which a recent review extensively covered (Delpech et al., 2019). Also, we note that EVs are actively developed as biomarkers for specific diseases and vehicles for drug delivery. However, in this review, we will limit our discussion to the recent discoveries on the signaling function of neuronal small EVs under normal healthy conditions, focusing specifically on cargo molecules of small EVs.
2. Signaling molecules present in neuronal EVs and their functions
Since the discovery that Wnts are secreted on EVs (Korkut et al., 2009), more than a dozen of signaling proteins and other molecules (miRNAs, mRNAs, and lipid messengers) have been identified to be secreted via EVs by neurons (see Table 1; Figure 1) and the number is only expected to increase (Chen et al., 2022).
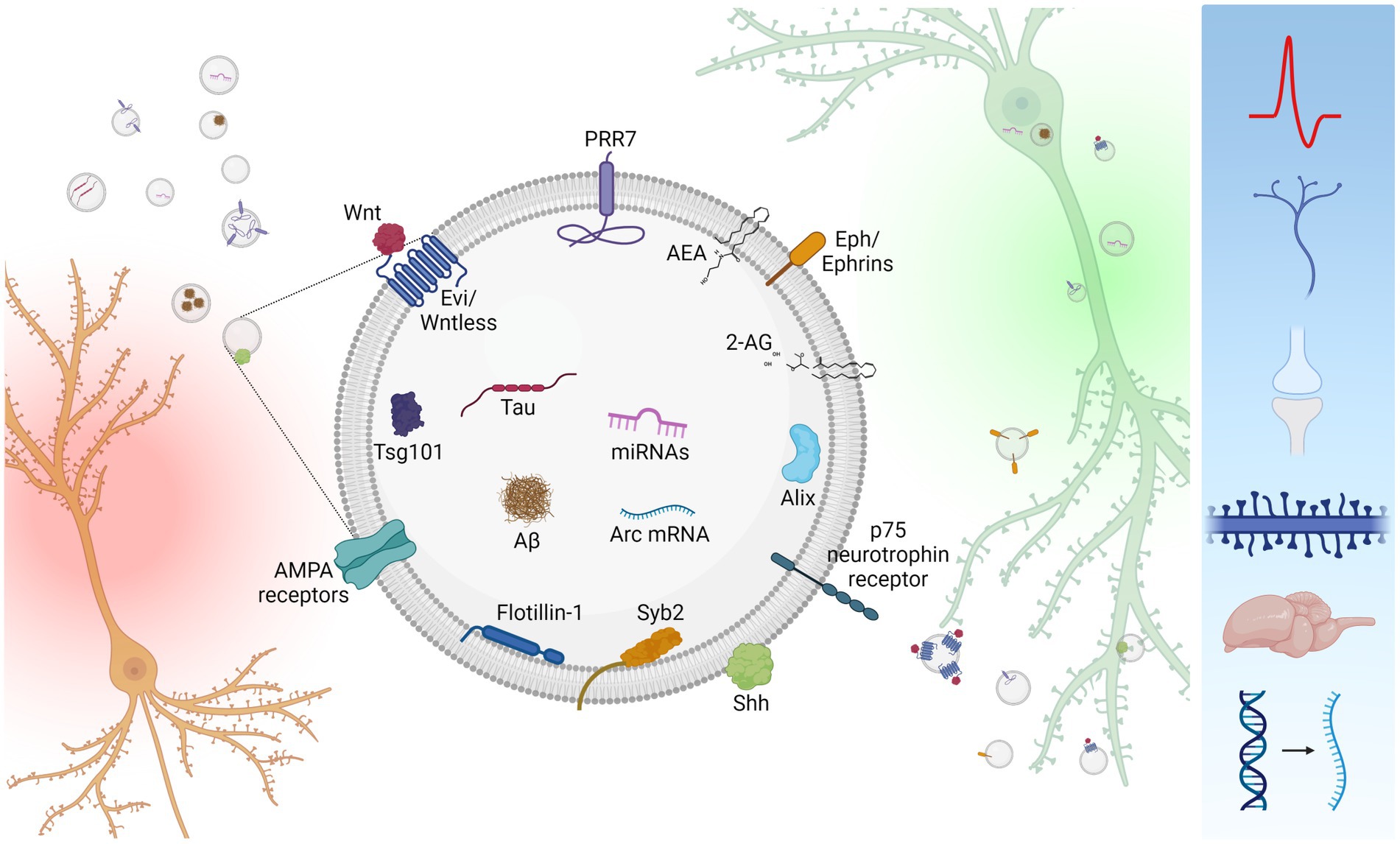
Figure 1. Inter-neuronal signaling mediated by neuronal small EVs. Neurons (orange) secrete small EVs carrying a variety of signaling molecules (center). However, neuronal small EVs are heterogenous in size and molecular composition, and single small EVs unlikely carry all these molecules. Secreted small EVs are absorbed by recipient neurons (green) either via endocytosis or membrane fusion. Uptaken small EVs affect a variety of cellular processes including neuronal firing, axon guidance, synapse formation, dendritic spine maintenance, synapse elimination, brain development, and mRNA expression (right blue box). Created with BioRender.com.
2.1. Wnts and Evi/Wntless
Wnt signaling controls myriads of fundamental biological processes during development and adult life (Clevers and Nusse, 2012). Wnts are one of the first molecules identified to be secreted on EVs and has signaling function during development (Greco et al., 2001; Korkut et al., 2009; Gross and Boutros, 2013). Wnts are hydrophobic protein and thus its secretion mechanism has been in question. EVs serve as carriers for the extracellular dissemination of Wnts (Zhang and Wrana, 2014). In Drosophlia neuromuscular junction (NMJ), EVs containing Wnts and Wnt carrier protein Evi/Wntless are involved in the development of NMJs (Korkut et al., 2009). Wnt signaling is also important for synaptogenesis, synapse and dendritic maintenance as well as spatial learning and memory (Dickins and Salinas, 2013; Chen et al., 2017). Recent studies indicate a critical role for Wnts in the formation of hippocampal long-term potentiation (LTP) (McLeod et al., 2018), raising an important question as to whether the effect is mediated by exosomal Wnts.
2.2. Proline rich 7
Proline rich 7 (PRR7) is a proline-rich type 1 transmembrane protein, first identified as a protein enriched in the postsynaptic density (Murata et al., 2005). Recent studies indicate that PRR7 is secreted by neurons on exosomes in an activity-dependent manner and functions as a novel Wnt inhibitor in synapse regulation by inhibiting Wnt secretion on exosomes (Lee et al., 2018). Importantly, `exosomes carrying high levels of PRR7 are absorbed by neurons and were shown to be necessary and sufficient to induce excitatory synapse loss in recipient neurons. These findings highlight the signaling function of neuronal exosomes in synapse maintenance in the central neurons. The zebrafish ortholog of PRR7, Ottogi (Otg), also functions as a Wnt inhibitor during development (Kim et al., 2017). Otg inhibits Wnt signaling by blocking the surface expression of Frizzled receptors. Interestingly, Otg only has cell-autonomous effects, suggesting that it may not be secreted on exosomes.
2.3. p75 neurotrophin receptor
The neurotrophin receptor p75, also known as nerve growth factor receptor, mediates multifaceted signaling pathways of neurite outgrowth, neuronal survival, and death (Ibanez and Simi, 2012). One study showed that p75 undergoes endosomal trafficking to MVBs and is subsequently released by exosomes (Escudero et al., 2014). These authors suggested an interesting possibility of transferring p75 signaling complexes from one cell to another. However, it remains to be determined whether exosomal p75 can induce neurotrophin signaling in recipient cells.
2.4. Eph and ephrins
Eph receptor tyrosine kinase and their membrane-tethered ephrin ligands play crucial roles in axon guidance and specific synapse formation (Klein and Kania, 2014). Interestingly, Gong et al., showed that dissociated motor cortex neurons secrete biologically active Eph and ephrins on exosomes that can cause growth cone collapse, suggesting the potential role of exosomal Eph and ephrins in neuronal development and synapse physiology (Gong et al., 2016).
2.5. AMPA receptors
AMPA receptors are major glutamate-gated ion channels and the main excitatory postsynaptic potential generator in the brain (Bassani et al., 2013). Thus, AMPA receptors control synaptic transmission and are the main substrates of synaptic plasticity (Shepherd and Huganir, 2007; Kessels and Malinow, 2009). Interestingly, unlike NMDA receptors, AMPA receptors are one of the consistently found molecules in neuronal exosome preparations (Faure et al., 2006; Lachenal et al., 2011; Lee et al., 2018). These findings suggest that exosomal AMPA receptors may contribute to neuronal excitability of recipient neurons. However, currently the role of exosomal AMPA receptors are completely unknown.
2.6. Synaptobrevin 2
Synaptobrevin 2 (Syb2; also called VAMP2) belongs to N-ethylmaleimide-sensitive factor (NSF)-attachment factor receptor (SNARE) proteins that are involved in synaptic vesicle fusion. Syb2 was found from small EV preparations purified from culture supernatant of cultured rat hippocampal neurons, along with other SNARE proteins, syntaxin-1 and synaptotagmin-5 (Vilcaes et al., 2021). Remarkably, the authors showed that small EVs containing syb2 are absorbed by neurons and selectively enhance inhibitory synaptic transmission in a CD81-dependent manner, indicating that neuronal EVs can potentially regulate the excitability of neurons. However, it remains to be determined whether the exosomal secretion of syb2 is a regulated (activity-dependent) process.
2.7. Arc protein and mRNA
The activity-regulated cytoskeleton associated protein (Arc) is a neuron-specific protein that is critical for synaptic plasticity and memory formation (Korb and Finkbeiner, 2011). Two groups found that Arc mRNA is encapsulated in retrovirus-like gag protein Arc and secreted via small EVs for trans-synaptic traffic in synaptic boutons of the neuromuscular junction or inter-neuronal RNA transfer (Ashley et al., 2018; Pastuzyn et al., 2018). Although small EV-carried Arc mRNAs are shown to be functionally active, their contribution to synaptic plasticity awaits further investigation.
2.8. microRNAs
MicroRNAs (miRNAs) are short (19–24 nucleotide) noncoding single-stranded RNAs that function in post-transcriptional gene silencing (Saliminejad et al., 2019). Goldie et al. first showed that depolarized SH-SY5Y human neuroblast cells secrete miRNAs in exosomes (Goldie et al., 2014). Since then, several reports indicate that neurons also secrete various species of miRNAs including miR-124, miR-21-5p, and miR132, which are subsequently absorbed by microglia, astrocytes, or endothelial cells. These exosome-derived miRNAs influence a variety of processes in the recipient cells, including the modulation of microglial activity (Veremeyko et al., 2019), pro-inflammatory responses (Simeoli et al., 2017), gene transcription in astrocytes (Men et al., 2019), and brain vascular integrity (Xu et al., 2017). Most recently, it was shown that brain-derived neurotrophic factor (BDNF) promotes the sorting of miRNAs to neuronal exosomes, which enhances excitatory synapse formation in recipient neurons (Antoniou et al., 2023).
2.9. Endocannabinoids
Endocannabinoids (eCBs) are lipid messengers that modulate synaptic functions in both short-term and long-term forms of plasticity (Castillo et al., 2012). Interestingly, it was reported that microglia secrete small EVs containing N-arachidonylethanolamine (AEA) on their surface (Gabrielli et al., 2015). The AEA-containing small EVs can induce type 1 eCB receptor (CB1)-mediated signaling and inhibit presynaptic transmission, suggesting an active signaling function of small EV-carried AEA. Moreover, more recent studies showed that cocaine induces the secretion of another form of eCB, anandamide (2-AG), via small EVs in the midbrain of mice (Nakamura et al., 2019). It is unclear at this point whether neurons also secrete AEA and 2-AG on EVs. However, since neurons actively synthesize eCBs and eCBs have such profound neuromodulatory function on neurons, further studies on the possibility are warranted.
3. Neuronal EV secretion mechanisms
Importantly, exosome secretion by neurons could be a regulated process that is induced by high K+-induced depolarization, GABAAR antagonists, and/or blocked by NMDA receptor blockers (Faure et al., 2006; Lachenal et al., 2011; Wang et al., 2017; Lee et al., 2018; Kumar et al., 2020), indicating that it is an activity-dependent phenomenon and thus has signaling function. We also note here that neuronal EV secretion could also be a constitutive process not dependent on activity (Vilcaes et al., 2021).
Studies done in other types of cells indicate that multiple routes for ILV biosynthesis exist (Blanchette and Rodal, 2020), including well-studied the Endosomal Sorting Complex Required for Transport (ESCRT)-dependent process and ceramide/lipid-dependent process (Trajkovic et al., 2008; Babst, 2011). In the case of neuronal exosomes and small EVs, cargo molecules such as Eph, ephrins and Shh are shown to be dependent on ESCRTs for their EV secretion. Interestingly, ESCRT-III protein Chmp1a is specifically required for the secretion of Shh via small EVs (Coulter et al., 2018). On the other hand, small EV secretion of neuronal miRNAs was shown to be dependent on ceramide (Antoniou et al., 2023). These findings suggest that different cargo molecules undergo distinct exosomal sorting pathways that require specific ESCRT proteins and/or lipids in neurons.
Rab GTPases play critical function in vesicular traffic inside cells (Stenmark, 2009). It was shown that different species of Rab proteins are required for the exosomal secretion of specific cargo proteins in neurons: for example, Wnts require Rab11 but not ceramide (Koles et al., 2012; Beckett et al., 2013). On the other hand, the exosomal secretion of PRR7 requires Rab27b not Rab11 (Lee et al., 2018).
Neuronal exosomes are shown to be released at soma and dendrites (Lachenal et al., 2011; Kumar et al., 2020), which is consistent with the MVB localization in neurons (Von Bartheld and Altick, 2011). However, it is worth to point out that there remains a possibility of axonal release since small EVs carrying Wnts are secreted at axon terminals in the neuromuscular junctions of Drosophilia (Korkut et al., 2009).
Membrane fusion events are controlled by Ca2+. Likewise, neuronal exosome secretion was also shown to require Ca2+ (Lachenal et al., 2011). However, it remains to be identified what specific steps of exosome secretion are controlled by Ca2+: for example, initial endocytic events of cargo molecules and/or exocytosis processes required for the membrane fusion of MVBs to the PM. Interestingly, the knockdown of VAMP3 specifically attenuated fibroblast growth factor 2-induced neuronal exosome release (Kumar et al., 2020), suggesting that specific SNARE proteins might be involved in the EV secretion.
4. Neuronal EV uptake mechanisms
In contrast to exosome secretion mechanisms, the mechanisms by which neurons uptake neuronal exosomes are much less understood. Several studies suggest that EV uptake depends on both the origin of EVs and recipient cells (Fowler, 2019). Interestingly, neuronal exosomes seem to be preferentially uptaken by neurons, indicating their aptitude for inter-neuronal communication. For example, studies using GFP-fused tetanus toxin C-terminal protein showed that neuronal exosomes are uptaken and endocytosed specifically by neurons and not by astroglia cells (Chivet et al., 2014). Moreover, our studies using PRR7-containing neuronal exosomes also showed preferential absorption by neurons through a process of membrane fusion (Lee et al., 2018). Similarly, APP-and Syb2-conbtaining neuronal exosomes were also shown to undergo membrane fusion (Laulagnier et al., 2018; Vilcaes et al., 2021). On the other hand, EVs containing miRNAs are uptaken by neurons in a dynamin-dependent manner, suggesting endocytosis-dependent mechanism (Antoniou et al., 2023). Therefore, it seems neurons uptake exosomes by both endocytosis and membrane fusion mechanisms. However, it remains to be identified whether specific cargo-molecules in exosomes and neuronal surface molecules mediate the selective neuronal uptake of neuronal exosomes via different mechanisms.
Synaptic clefts of hippocampal neurons are ~24 nm in average width (Zuber et al., 2005), which is crowded with cell adhesion molecules and other receptors. Therefore, synaptic clefts seem too small for EV to freely enter and diffuse in. Considering this space limitation, neuronal exosomes are most likely uptaken at non-synaptic sites, including extra-synaptic membrane area in dendrites, axons, and soma. Further studies are necessary to determine the initial EV contact sites of neurons, also where the endocytosis and/or membrane fusion events are initiated.
5. Extracellular space (ECS) and small EV signaling range
Neurons and other cells in the brain are separated by ECS. ECS occupies almost one-fifth of brain volume and serves as a reservoir of ions and a physical corridor for diffusional transport of substances including nutrients (Hrabetova et al., 2018). Since EVs are secreted to ECS, for them to move freely around and spread in the brain tissue, intercellular space should be larger than the size of EVs. Intriguingly, contrary to the conventional idea based on the images of fixed brain tissue observed under electron microscope, state-of-the-art imaging techniques reveal that ECS is larger than people previously thought (Korogod et al., 2015; Tonnesen et al., 2018). ECS size ranges between 80 and 270 nm (ave. 150 ± 40 nm) (Godin et al., 2017), which is big enough to allow exosomes or small EVs to move around in the brain. Moreover, ECS is not static but rather dynamic, and changes during neuronal activity, sleep, and disease courses (Binder et al., 2004; Ding et al., 2016; Hrabetova et al., 2018).
The effective signaling ranges of extracellular signaling molecules can be juxtacrine (direct neighbors), paracrine (over several cells), or long-range endocrine (Muller and Schier, 2011). Considering all findings on the brain ECS and neuronal EVs, it is likely that neuronal small EVs under normal conditions are absorbed by the immediate neighboring neurons (juxtacrine) and in an autocrine manner. Under certain conditions that allow the expansion of ECS and/or excessive secretion, small neuronal EVs diffuse further away before they are uptaken by neurons. In support of this long-range signaling, exosomes injected either directly into the brain or peripherally were found throughout the brains (Alvarez-Erviti et al., 2011; Yang et al., 2021). On the other hand, aging causes the shrinkage of ECS and thus may limit small EV travel, hampering the exosome-mediated intercellular communication.
6. Future directions
In summary, EVs, especially small EVs including exosomes, provide novel mechanisms for synaptic connection-independent inter-neuronal communication, which affect diverse neuronal functions. However, many important questions remain.
1. It is known that not all exosomes are created equal and rather diverse subpopulations of exosomes are released by the same cells (Bobrie et al., 2012; Zabeo et al., 2017), indicating heterogeneity of EVs. Then, do single neurons also release discrete populations of EVs that contain different cargo molecules for signaling purposes? Then, how diverse are the molecular compositions of single EVs?
2. Do individual neurons utilize multiple distinct mechanisms for the secretion of exosomes containing different cargos?
3. What are the mechanisms by which neuronal exosomes are specifically absorbed by neurons? Is this uptake process also activity-dependent or constitutive?
4. Lastly, what are the physiological functions of molecules carried on EVs?
Almost all, if not all, molecules found in EVs are abundantly present inside cells and not specific to EVs. Therefore, it is very difficult to understand the specific function of these EV-carried molecules, distinguished from their cell-autonomous roles. Considering that most of EV studies used a large amount of purified but heterogenous EVs for the examination of their biological effects, future studies should be focused on the molecule-specific role in EV-mediated communication.
Answering these questions likely requires some technical innovations that allow singe EV proteomics and RNAseq studies. Despite these challenges, better understating of small EV-mediated inter-neuronal communication will reveal another important layer of plasticity mechanisms operating in the brain.
Author contributions
DN and SL wrote and edited the manuscript and prepared the Figure illustration. All authors contributed to the article and approved the submitted version.
Funding
The works in the Author’s lab are supported by NIH MH119105 and AG073610 grant (to SL).
Conflict of interest
The authors declare that the research was conducted in the absence of any commercial or financial relationships that could be construed as a potential conflict of interest.
Publisher’s note
All claims expressed in this article are solely those of the authors and do not necessarily represent those of their affiliated organizations, or those of the publisher, the editors and the reviewers. Any product that may be evaluated in this article, or claim that may be made by its manufacturer, is not guaranteed or endorsed by the publisher.
References
Alvarez-Erviti, L., Seow, Y., Yin, H., Betts, C., Lakhal, S., and Wood, M. J. (2011). Delivery of siRNA to the mouse brain by systemic injection of targeted exosomes. Nat. Biotechnol. 29, 341–345. doi: 10.1038/nbt.1807
Antoniou, A., Auderset, L., Kaurani, L., Sebastian, E., Zeng, Y., Allahham, M., et al. (2023). Neuronal extracellular vesicles and associated microRNAs induce circuit connectivity downstream BDNF. Cell Rep. 42:112063. doi: 10.1016/j.celrep.2023.112063
Ashley, J., Cordy, B., Lucia, D., Fradkin, L. G., Budnik, V., and Thomson, T. (2018). Retrovirus-like gag protein Arc1 binds RNA and traffics across synaptic Boutons. Cells 172, 262–274.e11. doi: 10.1016/j.cell.2017.12.022
Babst, M. (2011). MVB vesicle formation: ESCRT-dependent, ESCRT-independent and everything in between. Curr. Opin. Cell Biol. 23, 452–457. doi: 10.1016/j.ceb.2011.04.008
Bassani, S., Folci, A., Zapata, J., and Passafaro, M. (2013). AMPAR trafficking in synapse maturation and plasticity. Cell. Mol. Life Sci. 70, 4411–4430. doi: 10.1007/s00018-013-1309-1
Beckett, K., Monier, S., Palmer, L., Alexandre, C., Green, H., Bonneil, E., et al. (2013). Drosophila S2 cells secrete wingless on exosome-like vesicles but the wingless gradient forms independently of exosomes. Traffic 14, 82–96. doi: 10.1111/tra.12016
Binder, D. K., Papadopoulos, M. C., Haggie, P. M., and Verkman, A. S. (2004). In vivo measurement of brain extracellular space diffusion by cortical surface photobleaching. J. Neurosci. Off. J. Soc. Neurosci. 24, 8049–8056. doi: 10.1523/JNEUROSCI.2294-04.2004
Blanchette, C. R., and Rodal, A. A. (2020). Mechanisms for biogenesis and release of neuronal extracellular vesicles. Curr. Opin. Neurobiol. 63, 104–110. doi: 10.1016/j.conb.2020.03.013
Bobrie, A., Colombo, M., Krumeich, S., Raposo, G., and Thery, C. (2012). Diverse subpopulations of vesicles secreted by different intracellular mechanisms are present in exosome preparations obtained by differential ultracentrifugation. J. Extracell. Vesicl. 1:18397. doi: 10.3402/jev.v1i0.18397
Budnik, V., Ruiz-Canada, C., and Wendler, F. (2016). Extracellular vesicles round off communication in the nervous system. Nat. Rev. Neurosci. 17, 160–172. doi: 10.1038/nrn.2015.29
Castillo, P. E., Younts, T. J., Chavez, A. E., and Hashimotodani, Y. (2012). Endocannabinoid signaling and synaptic function. Neuron 76, 70–81. doi: 10.1016/j.neuron.2012.09.020
Chen, T. Y., Gonzalez-Kozlova, E., Soleymani, T., La Salvia, S., Kyprianou, N., Sahoo, S., et al. (2022). Extracellular vesicles carry distinct proteo-transcriptomic signatures that are different from their cancer cell of origin. iScience 25:104414. doi: 10.1016/j.isci.2022.104414
Chen, C. M., Orefice, L. L., Chiu, S. L., LeGates, T. A., Hattar, S., Huganir, R. L., et al. (2017). Wnt5a is essential for hippocampal dendritic maintenance and spatial learning and memory in adult mice. Proc. Natl. Acad. Sci. U. S. A. 114, E619–E628. doi: 10.1073/pnas.1615792114
Chivet, M., Javalet, C., Laulagnier, K., Blot, B., Hemming, F. J., and Sadoul, R. (2014). Exosomes secreted by cortical neurons upon glutamatergic synapse activation specifically interact with neurons. J. Extracell. Vesicl. 3:24722. doi: 10.3402/jev.v3.24722
Clevers, H., and Nusse, R. (2012). Wnt/beta-catenin signaling and disease. Cells 149, 1192–1205. doi: 10.1016/j.cell.2012.05.012
Coulter, M. E., Dorobantu, C. M., Lodewijk, G. A., Delalande, F., Cianferani, S., Ganesh, V. S., et al. (2018). The ESCRT-III protein CHMP1A mediates secretion of sonic hedgehog on a distinctive subtype of extracellular vesicles. Cell Rep. 24:e978, 973–986.e8. doi: 10.1016/j.celrep.2018.06.100
Danzer, K. M., Kranich, L. R., Ruf, W. P., Cagsal-Getkin, O., Winslow, A. R., Zhu, L., et al. (2012). Exosomal cell-to-cell transmission of alpha synuclein oligomers. Mol Neurodegener 7:42.
Delpech, J. C., Herron, S., Botros, M. B., and Ikezu, T. (2019). Neuroimmune crosstalk through extracellular vesicles in health and disease. Trends Neurosci. 42, 361–372. doi: 10.1016/j.tins.2019.02.007
Dickins, E. M., and Salinas, P. C. (2013). Wnts in action: from synapse formation to synaptic maintenance. Front. Cell. Neurosci. 7:162. doi: 10.3389/fncel.2013.00162
Ding, F., O'Donnell, J., Xu, Q., Kang, N., Goldman, N., and Nedergaard, M. (2016). Changes in the composition of brain interstitial ions control the sleep-wake cycle. Science 352, 550–555. doi: 10.1126/science.aad4821
Escudero, C. A., Lazo, O. M., Galleguillos, C., Parraguez, J. I., Lopez-Verrilli, M. A., Cabeza, C., et al. (2014). The p75 neurotrophin receptor evades the endolysosomal route in neuronal cells, favouring multivesicular bodies specialised for exosomal release. J. Cell Sci. 127, 1966–1979. doi: 10.1242/jcs.141754
Faure, J., Lachenal, G., Court, M., Hirrlinger, J., Chatellard-Causse, C., Blot, B., et al. (2006). Exosomes are released by cultured cortical neurones. Mol. Cell. Neurosci. 31, 642–648. doi: 10.1016/j.mcn.2005.12.003
Fowler, C. D. (2019). NeuroEVs: characterizing extracellular vesicles generated in the neural domain. J. Neurosci. Off. J. Soc. Neurosci. 39, 9262–9268. doi: 10.1523/JNEUROSCI.0146-18.2019
Gabrielli, M., Battista, N., Riganti, L., Prada, I., Antonucci, F., Cantone, L., et al. (2015). Active endocannabinoids are secreted on the surface of microglial microvesicles. Springerplus 4:L29. doi: 10.1186/2193-1801-4-S1-L29
Godin, A. G., Varela, J. A., Gao, Z., Danne, N., Dupuis, J. P., Lounis, B., et al. (2017). Single-nanotube tracking reveals the nanoscale organization of the extracellular space in the live brain. Nat. Nanotechnol. 12, 238–243. doi: 10.1038/nnano.2016.248
Goldie, B. J., Dun, M. D., Lin, M., Smith, N. D., Verrills, N. M., Dayas, C. V., et al. (2014). Activity-associated miRNA are packaged in Map1b-enriched exosomes released from depolarized neurons. Nucleic Acids Res. 42, 9195–9208. doi: 10.1093/nar/gku594
Gong, J., Korner, R., Gaitanos, L., and Klein, R. (2016). Exosomes mediate cell contact-independent ephrin-Eph signaling during axon guidance. J. Cell Biol. 214, 35–44. doi: 10.1083/jcb.201601085
Greco, V., Hannus, M., and Eaton, S. (2001). Argosomes: a potential vehicle for the spread of morphogens through epithelia. Cells 106, 633–645. doi: 10.1016/S0092-8674(01)00484-6
Gross, J. C., and Boutros, M. (2013). Secretion and extracellular space travel of Wnt proteins. Curr. Opin. Genet. Dev. 23, 385–390. doi: 10.1016/j.gde.2013.02.017
Guix, F. X., Corbett, G. T., Cha, D. J., Mustapic, M., Liu, W., Mengel, D., et al. (2018). Detection of Aggregation-Competent Tau in Neuron-Derived Extracellular Vesicles. Int. J. Mol. Sci. 19:663. doi: 10.3390/ijms19030663
Guo, B. B., Bellingham, S. A., and Hill, A. F. (2015). The neutral sphingomyelinase pathway regulates packaging of the prion protein into exosomes. J. Bio. Chemist. 290, 3455–3467.
Hrabetova, S., Cognet, L., Rusakov, D. A., and Nagerl, U. V. (2018). Unveiling the extracellular space of the brain: from super-resolved microstructure to in vivo function. J. Neurosci. Off. J. Soc. Neurosci. 38, 9355–9363. doi: 10.1523/JNEUROSCI.1664-18.2018
Ibanez, C. F., and Simi, A. (2012). p75 neurotrophin receptor signaling in nervous system injury and degeneration: paradox and opportunity. Trends Neurosci. 35, 431–440. doi: 10.1016/j.tins.2012.03.007
Kessels, H. W., and Malinow, R. (2009). Synaptic AMPA receptor plasticity and behavior. Neuron 61, 340–350. doi: 10.1016/j.neuron.2009.01.015
Kim, H. T., Lee, M. S., Jeong, Y. M., Ro, H., Kim, D. I., Shin, Y. H., et al. (2017). Ottogi inhibits Wnt/beta-catenin signaling by regulating cell membrane trafficking of Frizzled8. Sci. Rep. 7:13278. doi: 10.1038/s41598-017-13429-6
Klein, R., and Kania, A. (2014). Ephrin signalling in the developing nervous system. Curr. Opin. Neurobiol. 27, 16–24. doi: 10.1016/j.conb.2014.02.006
Koles, K., Nunnari, J., Korkut, C., Barria, R., Brewer, C., Li, Y., et al. (2012). Mechanism of Evi-exosome release at synaptic boutons. J. Biol. Chem. 287, 16820–16834. doi: 10.1074/jbc.M112.342667
Korb, E., and Finkbeiner, S. (2011). Arc in synaptic plasticity: from gene to behavior. Trends Neurosci. 34, 591–598. doi: 10.1016/j.tins.2011.08.007
Korkut, C., Ataman, B., Ramachandran, P., Ashley, J., Barria, R., Gherbesi, N., et al. (2009). Trans-synaptic transmission of vesicular Wnt signals through Evi/Wntless. Cells 139, 393–404. doi: 10.1016/j.cell.2009.07.051
Korogod, N., Petersen, C. C., and Knott, G. W. (2015). Ultrastructural analysis of adult mouse neocortex comparing aldehyde perfusion with cryo fixation. elife 4:e05793. doi: 10.7554/eLife.05793
Kumar, R., Tang, Q., Muller, S. A., Gao, P., Mahlstedt, D., Zampagni, S., et al. (2020). Fibroblast growth factor 2-mediated regulation of neuronal exosome release depends on VAMP3/Cellubrevin in hippocampal neurons. Adv. Sci. (Weinh) 7:1902372. doi: 10.1002/advs.201902372
Lachenal, G., Pernet-Gallay, K., Chivet, M., Hemming, F. J., Belly, A., Bodon, G., et al. (2011). Release of exosomes from differentiated neurons and its regulation by synaptic glutamatergic activity. Mol. Cell. Neurosci. 46, 409–418. doi: 10.1016/j.mcn.2010.11.004
Laulagnier, K., Javalet, C., Hemming, F. J., Chivet, M., Lachenal, G., Blot, B., et al. (2018). Amyloid precursor protein products concentrate in a subset of exosomes specifically endocytosed by neurons. Cell. Mol. Life Sci. 75, 757–773. doi: 10.1007/s00018-017-2664-0
Lee, S. H., Shin, S. M., Zhong, P., Kim, H. T., Kim, D. I., Kim, J. M., et al. (2018). Reciprocal control of excitatory synapse numbers by Wnt and Wnt inhibitor PRR7 secreted on exosomes. Nat. Commun. 9:3434. doi: 10.1038/s41467-018-05858-2
Miranda, A. M., Lasiecka, Z. M., Xu, Y., Neufeld, J., Shahriar, S., Simoes, S., et al. (2018). Neuronal lysosomal dysfunction releases exosomes harboring APP C-terminal fragments and unique lipid signatures. Nat. Comm. 9:291.
McLeod, F., Bossio, A., Marzo, A., Ciani, L., Sibilla, S., Hannan, S., et al. (2018). Wnt signaling mediates LTP-dependent spine plasticity and AMPAR localization through Frizzled-7 receptors. Cell Rep. 23, 1060–1071. doi: 10.1016/j.celrep.2018.03.119
Men, Y., Yelick, J., Jin, S., Tian, Y., Chiang, M. S. R., Higashimori, H., et al. (2019). Exosome reporter mice reveal the involvement of exosomes in mediating neuron to astroglia communication in the CNS. Nat. Commun. 10:4136. doi: 10.1038/s41467-019-11534-w
Muller, P., and Schier, A. F. (2011). Extracellular movement of signaling molecules. Dev. Cell 21, 145–158. doi: 10.1016/j.devcel.2011.06.001
Murata, Y., Doi, T., Taniguchi, H., and Fujiyoshi, Y. (2005). Proteomic analysis revealed a novel synaptic proline-rich membrane protein (PRR7) associated with PSD-95 and NMDA receptor. Biochem. Biophys. Res. Commun. 327, 183–191. doi: 10.1016/j.bbrc.2004.11.154
Nakamura, Y., Dryanovski, D. I., Kimura, Y., Jackson, S. N., Woods, A. S., Yasui, Y., et al. (2019). Cocaine-induced endocannabinoid signaling mediated by sigma-1 receptors and extracellular vesicle secretion. elife 8:e47209. doi: 10.7554/eLife.47209
Pastuzyn, E. D., Day, C. E., Kearns, R. B., Kyrke-Smith, M., Taibi, A. V., McCormick, J., et al. (2018). The neuronal gene arc encodes a repurposed Retrotransposon gag protein that mediates intercellular RNA transfer. Cells 172:e218. doi: 10.1016/j.cell.2017.12.024
Podvin, S., Jones, A., Liu, Q., Aulston, B., Mosier, C., Ames, J., et al. (2021). Mutant Presenilin 1 Dysregulates Exosomal Proteome Cargo Produced by Human-Induced Pluripotent Stem Cell Neurons. ACS Omega 6, 13033–13056.
Reilly, P., Winston, C. N., Baron, K. R., Trejo, M., Rockenstein, E. M., Akers, J. C., et al. (2017). Novel human neuronal tau model exhibiting neurofibrillary tangles and transcellular propagation. Neurobio. Dis. 106, 222–234.
Saliminejad, K., Khorram Khorshid, H. R., Soleymani Fard, S., and Ghaffari, S. H. (2019). An overview of microRNAs: biology, functions, therapeutics, and analysis methods. J. Cell. Physiol. 234, 5451–5465. doi: 10.1002/jcp.27486
Sardar Sinha, M., Ansell-Schultz, A., Civitelli, L., Hildesjo, C., Larsson, M., Lannfelt, L., et al. (2018). Alzheimer’s disease pathology propagation by exosomes containing toxic amyloid-beta oligomers. Acta Neuro 136, 41–56.
Sharma, P., Mesci, P., Carromeu, C., McClatchy, D. R., Schiapparelli, L., Yates, J. R., et al. (2019). Exosomes regulate neurogenesis and circuit assembly. Proc. Natl. Acad. Sci. U. S. A. 116, 16086–16094. doi: 10.1073/pnas.1902513116
Shepherd, J. D., and Huganir, R. L. (2007). The cell biology of synaptic plasticity: AMPA receptor trafficking. Annu. Rev. Cell Dev. Biol. 23, 613–643. doi: 10.1146/annurev.cellbio.23.090506.123516
Simeoli, R., Montague, K., Jones, H. R., Castaldi, L., Chambers, D., Kelleher, J. H., et al. (2017). Exosomal cargo including microRNA regulates sensory neuron to macrophage communication after nerve trauma. Nat. Commun. 8:1778. doi: 10.1038/s41467-017-01841-5
Stenmark, H. (2009). Rab GTPases as coordinators of vesicle traffic. Nat. Rev. Mol. Cell Biol. 10, 513–525. doi: 10.1038/nrm2728
Thery, C. (2011). Exosomes: secreted vesicles and intercellular communications. F1000 Biol. Rep. 3:15. doi: 10.3410/B3-15
Thery, C., Ostrowski, M., and Segura, E. (2009). Membrane vesicles as conveyors of immune responses. Nat. Rev. Immunol. 9, 581–593. doi: 10.1038/nri2567
Thery, C., Witwer, K. W., Aikawa, E., Alcaraz, M. J., Anderson, J. D., Andriantsitohaina, R., et al. (2018). Minimal information for studies of extracellular vesicles 2018 (MISEV2018): a position statement of the International Society for Extracellular Vesicles and update of the MISEV2014 guidelines. J. Extracell. Vesicl. 7:1535750. doi: 10.1080/20013078.2018.1535750
Tonnesen, J., Inavalli, V., and Nagerl, U. V. (2018). Super-resolution imaging of the extracellular space in living brain tissue. Cells 172:e1115, 1108–1121.e15. doi: 10.1016/j.cell.2018.02.007
Trajkovic, K., Hsu, C., Chiantia, S., Rajendran, L., Wenzel, D., Wieland, F., et al. (2008). Ceramide triggers budding of exosome vesicles into multivesicular endosomes. Science 319, 1244–1247. doi: 10.1126/science.1153124
Trams, E. G., Lauter, C. J., Salem, N. Jr., and Heine, U. (1981). Exfoliation of membrane ecto-enzymes in the form of micro-vesicles. Biochim. Biophys. Acta 645, 63–70. doi: 10.1016/0005-2736(81)90512-5
Valadi, H., Ekstrom, K., Bossios, A., Sjostrand, M., Lee, J. J., and Lotvall, J. O. (2007). Exosome-mediated transfer of mRNAs and microRNAs is a novel mechanism of genetic exchange between cells. Nat. Cell Biol. 9, 654–659. doi: 10.1038/ncb1596
Vella, L. J., Sharples, R. A., Lawson, V. A., Masters, C. L., Cappai, R., and Hill, A. F. (2007). Packaging of prions into exosomes is associated with a novel pathway of PrP processing. J. Pathol. 211, 582–590.
Veremeyko, T., Kuznetsova, I. S., Dukhinova, M. A. W. Y. Y., Kopeikina, E., Barteneva, N. S., and Ponomarev, E. D. (2019). Neuronal extracellular microRNAs miR-124 and miR-9 mediate cell-cell communication between neurons and microglia. J. Neurosci. Res. 97, 162–184. doi: 10.1002/jnr.24344
Vilcaes, A. A., Chanaday, N. L., and Kavalali, E. T. (2021). Interneuronal exchange and functional integration of synaptobrevin via extracellular vesicles. Neuron 109:e975, 971–983.e5. doi: 10.1016/j.neuron.2021.01.007
Von Bartheld, C. S., and Altick, A. L. (2011). Multivesicular bodies in neurons: distribution, protein content, and trafficking functions. Prog. Neurobiol. 93, 313–340. doi: 10.1016/j.pneurobio.2011.01.003
Wang, Y., Balaji, V., Kaniyappan, S., Kruger, L., Irsen, S., Tepper, K., et al. (2017). The release and trans-synaptic transmission of tau via exosomes. Mol. Neurodegener. 12:5. doi: 10.1186/s13024-016-0143-y
Winston, C. N., Goetzl, E. J., Baker, L. D., Vitiello, M. V., and Rissman, R. A. (2018). Growth Hormone-Releasing Hormone Modulation of Neuronal Exosome Biomarkers in Mild Cognitive Impairment. J. Alzheimer’s Dis. 66, 971–981.
Xu, B., Zhang, Y., Du, X. F., Li, J., Zi, H. X., and Bu, J. W. (2017). Neurons secrete miR-132-containing exosomes to regulate brain vascular integrity. Cell Res. 27, 882–897. doi: 10.1038/cr.2017.62
Yang, J., Luo, S., Zhang, J., Yu, T., Fu, Z., Zheng, Y., et al. (2021). Exosome-mediated delivery of antisense oligonucleotides targeting alpha-synuclein ameliorates the pathology in a mouse model of Parkinson's disease. Neurobiol. Dis. 148:105218. doi: 10.1016/j.nbd.2020.105218
Yuyama, K., Sun, H., Mitsutake, S., and Igarashi, Y. (2012). Sphingolipid-modulated exosome secretion promotes the clearance of amyloid-beta by microglia. J. Bio. Chemist. 287, 10977–10989. doi: 10.1074/jbc.M111.324616
Zabeo, D., Cvjetkovic, A., Lasser, C., Schorb, M., Lotvall, J., and Hoog, J. L. (2017). Exosomes purified from a single cell type have diverse morphology. J. Extracell. Vesicl. 6:1329476. doi: 10.1080/20013078.2017.1329476
Zhang, L., and Wrana, J. L. (2014). The emerging role of exosomes in Wnt secretion and transport. Curr. Opin. Genet. Dev. 27, 14–19. doi: 10.1016/j.gde.2014.03.006
Keywords: extracellular vesicles, exosomes, neuron–neuron communication, synapse-independent, neuronal plasticity
Citation: Nieves Torres D and Lee SH (2023) Inter-neuronal signaling mediated by small extracellular vesicles: wireless communication? Front. Mol. Neurosci. 16:1187300. doi: 10.3389/fnmol.2023.1187300
Edited by:
Jean-Marc Taymans, Institut National de la Santé et de la Recherche Médicale (INSERM), FranceReviewed by:
Ruud Toonen, VU Amsterdam, NetherlandsCopyright © 2023 Nieves Torres and Lee. This is an open-access article distributed under the terms of the Creative Commons Attribution License (CC BY). The use, distribution or reproduction in other forums is permitted, provided the original author(s) and the copyright owner(s) are credited and that the original publication in this journal is cited, in accordance with accepted academic practice. No use, distribution or reproduction is permitted which does not comply with these terms.
*Correspondence: Sang H. Lee, c2hsZWVAbWN3LmVkdQ==