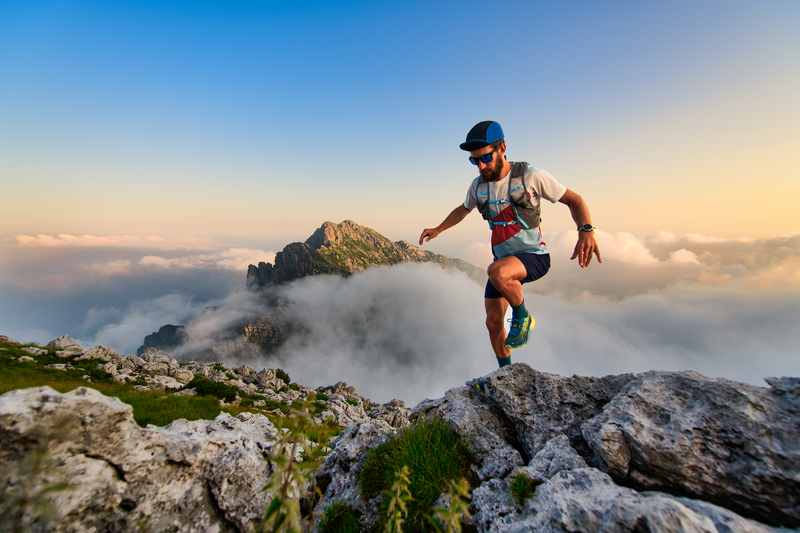
95% of researchers rate our articles as excellent or good
Learn more about the work of our research integrity team to safeguard the quality of each article we publish.
Find out more
MINI REVIEW article
Front. Mol. Neurosci. , 12 April 2023
Sec. Brain Disease Mechanisms
Volume 16 - 2023 | https://doi.org/10.3389/fnmol.2023.1152167
This article is part of the Research Topic What does it take to cure the brain? Studies Toward Genes, Proteins, Processes, and Rehabilitation View all 5 articles
Neonatal hypoxic-ischaemic events, which can result in long-term neurological impairments or even cell death, are among the most significant causes of brain injury during neurodevelopment. The complexity of neonatal hypoxic-ischaemic pathophysiology and cellular pathways make it difficult to treat brain damage; hence, the development of new neuroprotective medicines is of great interest. Recently, numerous neuroprotective medicines have been developed to treat brain injuries and improve long-term outcomes based on comprehensive knowledge of the mechanisms that underlie neuronal plasticity following hypoxic-ischaemic brain injury. In this context, understanding of the medicinal potential of cannabinoids and the endocannabinoid system has recently increased. The endocannabinoid system plays a vital neuromodulatory role in numerous brain regions, ensuring appropriate control of neuronal activity. Its natural neuroprotection against adult brain injury or acute brain injury also clearly demonstrate the role of endocannabinoid signalling in modulating neuronal activity in the adult brain. The goal of this review is to examine how cannabinoid-derived compounds can be used to treat neonatal hypoxic-ischaemic brain injury and to assess the critical function of the endocannabinoid system and its potential for use as a new neuroprotective treatment for neonatal hypoxic-ischaemic brain injury.
One of the major causes of impairment in newborns is neonatal hypoxic-ischaemic encephalopathy (HIE), which has serious long-term implications for child development (Barata et al., 2019; Zhou et al., 2023). At present, the incidence of perinatal asphyxia ranges between 0.5–1% of all live births, and substantial neurologic damage occurs in as many as 50–75% of these children (Torfs et al., 1990; Ferriero, 2004). Depending on the severity, location, and type of neurologic damage as well as the gestational age, impairments may include a variety of sensorimotor and cognitive abnormalities, which arise at various stages of development and have a considerable effect on children, their families, and society (Du Plessis and Volpe, 2002; Carli et al., 2004). Although neuroprotective treatment has improved, the development of neurological damage remains a substantial issue in HIE cases (Berger and Garnier, 2000).
Currently, neuroprotective measures, such as the rapid identification of affected neonates to enable the timely initiation of therapy, improved monitoring during the perinatal period, strict control during intensive care, and therapies that lessen the developing injury, are urgently needed to minimize the neurological effects of hypoxic-ischaemic brain damage (Shalak et al., 2003; Sanders et al., 2010). For instance, it is important to concentrate on the period directly after the hypoxic-ischaemic episode in neonatal insults because this is when therapeutic approaches can be effective in preventing brain damage. This time frame is typically brief and might range from 2 to 6 h. Therefore, rapid identification would enable easier application of various rescue treatments. Recent studies on neonates have revealed that hypothermia provides varying degrees of neuroprotection, either by preventing DNA breakage and apoptotic cell death after hypoxia-ischaemia (Esteve et al., 1999; Adachi et al., 2001) or by delaying the accumulation of intracellular calcium, decreasing the synthesis of nitric oxide, and decreasing the glutamate concentration in the synaptic space (Hashimoto et al., 2003; Zhu et al., 2004). The only currently available treatment for hypoxic-ischaemic injury in newborns is therapeutic hypothermia, which, despite advancements in its administration, is ineffective in approximately 50% of treated infants (Natarajan et al., 2016). In addition, this treatment has variable efficacy in asphyxiated children and is more effective in treating larger babies than smaller babies (Wyatt et al., 2007). Thus, the complicated pathophysiology of HIE makes treatment challenging and necessitates the development of multiple approaches (Juul and Ferriero, 2014).
Currently, alternative treatments focus on reducing brain damage caused by free radicals by using antioxidant compounds, such as allopurinol, which blocks xanthine oxidase (Palmer et al., 1993; Van Bel et al., 1998) and N-acetylcysteine activity, which reduces apoptosis and inflammation while increasing the intracellular level of glutathione to sequester free radicals (Jatana et al., 2006; Lee et al., 2008). Erythropoietin, which has antiapoptotic and angiogenic effects, is another frequently utilized antioxidant-related medication (Sola et al., 2005) and has been shown to promote neurogenesis and have neuroprotective effects in newborn rats (Chang et al., 2005; Gonzalez et al., 2007). Similarly, melatonin prevents brain damage and the subsequent development of sequelae (Carloni et al., 2008; Signorini et al., 2009). Additionally, substances with anti-inflammatory qualities have been investigated. These include second generation tetracyclines, which prevent microglia from being activated, approaches that increase the lifespan of neurons (Arvin et al., 2002; Jantzie et al., 2005), and statins, which reduce the expression of interleukin-1β and intercellular adhesion molecule 1 (Carloni et al., 2006, 2009). Due to the intricacy of neonatal hypoxic-ischaemic pathophysiology, there is presently no treatment specifically for perinatal brain injuries.
Recent research suggests that cannabinoids are highly effective neuroprotective agents in both acute neurodegenerative conditions, such as hypoxic-ischaemic encephalopathy or traumatic brain injury, and chronic conditions, such as multiple sclerosis (MS), Parkinson’s disease, and Alzheimer’s disease (AD) (Ben Amar, 2006; Maresz et al., 2007). Additionally, cannabinoids have anti-excitotoxic (Marsicano et al., 2003), anti-inflammatory (Chang et al., 2001), and vasodilatory effects (Parmentier-Batteur et al., 2002) and can regulate calcium homeostasis (Barha et al., 2011). Due to their ability to alter glial and neuronal responses, these chemicals have become recognized as neuroprotectants. According to recent research, several anti-inflammatory medications may enhance healing by encouraging neurogenesis after brain injury (Whitney et al., 2009). Because of its anti-inflammatory properties, cannabinoid receptor activation is an important neuroprotective therapy for neonatal hypoxic-ischaemic brain injury (Fernandez-Lopez et al., 2010). In this report, we concentrate on the function of cannabinoids and endocannabinoids and their potential to prevent brain damage caused by neonatal hypoxia and ischaemia.
In the 1960s, as marijuana use for recreational purposes increased, anecdotal reports suggested that cannabis could help people with Tourette syndrome, MS, and epilepsy (Cristino et al., 2019). Cannabis, the most widely used illegal recreational drug in the world, comprises approximately 80 phenolic compounds and terpenes, also known as “cannabinoids” (Izzo et al., 2009). As we know, major efforts have been made to pinpoint the chemical components that give marijuana and other cannabis flower preparations their euphoric, perception-altering, and potentially therapeutic effects (Kerai et al., 2018; Lucas et al., 2018). For instance, cannabinoids originating from plants are commonly referred to as phytocannabinoids, of which 9-tetrahydrocannabinol (THC), the main psychoactive ingredient in cannabis, is the most well-known (Izzo et al., 2009). The phytocannabinoid cannabidiol (CBD), in addition to THC, may have an important role in mediating the impact of cannabis on post-traumatic stress disorder (PTSD). While THC is known to exert effects by directly activating cannabinoid receptors, CBD is known to interact with a variety of neurochemical systems, most notably serotonergic and adenosine signalling, and thus its pharmacology is more complex (Carrier et al., 2006; Izzo et al., 2009; Rock et al., 2012). Since the psychoactive effects of THC limit its therapeutic potential and restrict its use in clinical investigations, CBD is more acceptable for clinical development, even for paediatric populations (Devinsky et al., 2016, 2017).
Two inhibitory G-protein-coupled receptors (GPCRs), cannabinoid receptor 1 (CB1) and cannabinoid receptor 2 (CB2), as well as two important endogenous ligands, N-arachidonoylethanolamine (anandamide/AEA) and 2-arachidonoylglycerol (2-AG), make up the majority of the endocannabinoid system. Additionally, fatty acid amide hydrolase (FAAH) and monoacylglyceride lipase (MAGL), which hydrolyse AEA and 2-AG, respectively, are metabolic enzymes that considerably influence endocannabinoid signalling (Meyer et al., 2018). The lipid membranes of postsynaptic neurons contain the precursors for AEA and 2-AG. To bind to endocannabinoid receptors in the presynaptic space and control the release of other neurotransmitters, such as glutamate, GABA, dopamine, serotonin, and acetylcholine, AEA and 2-AG are produced as needed and are retrogradely transported across the synaptic cleft (Lovinger, 2008; Jutras-Aswad et al., 2009; Katona and Freund, 2012) (Figure 1).
Figure 1. Simplified scheme representing endocannabinoid system-modulated synaptic transmission. The endocannabinoids AEA and 2-AG are not stored in vesicles but instead are synthesized de novo from phospholipid precursors through calcium-dependent mechanisms. N-acylphosphatidylethanolamine (NAPE) is hydrolysed by N-arachidonoyl-phosphatidylethanolamine-specific phospholipase D (NPLD) to yield AEA, and diacylglycerol (DAG) is converted to 2-AG by diacylglycerol lipase (DAGL). Both endogenous ligands traverse the synaptic cleft and activate presynaptic CB1 receptors, thereby regulating ion channels and ultimately suppressing neurotransmitter release. Endocannabinoid signalling is terminated following degradation by hydrolytic enzymes in the presynaptic and postsynaptic compartments. Primarily, AEA is converted to arachidonic acid (AA) and ethanolamine by fatty acid amide hydrolase (FAAH) localized to the postsynaptic cell, whereas 2-AG is hydrolysed presynaptically into AA and glycerol by monacylglycerol lipase (MAGL).
Accumulated studies have reported that endocannabinoids modulate the intensity and extent of neurotoxic processes (Barata et al., 2019; Gupta et al., 2020) and the inflammatory response (Chiurchiu et al., 2018; Marinelli et al., 2023) and promote cell survival (Viscomi et al., 2009). Synthetic cannabinoid agonists have shown considerable grey and white matter protection in animal studies of brain injury (Fernández-López et al., 2007). In large animal models of perinatal asphyxia, the cannabinoid WIN55212-2 administered immediately after HI protected against mitochondrial injury and prevented apoptosis (Alonso-Alconada et al., 2010). Cannabidiol given immediately after HI reduced neuronal injury, cerebral haemodynamic impairment, brain oedema and seizures and restored motor and behavioural performance 72 h after HI (Pazos et al., 2013). In rodent models of stroke, prolonged 7-day administration of the cannabinoid WIN55212-2 immediately after injury enhanced neuronal and oligodendrocyte recovery and regeneration in long-term (Fernandez-Lopez et al., 2010). Cannabinoids, however, achieve neuroprotection in part through hypothermia (Leker et al., 2003).
In 1992, AEA, the first described endocannabinoid, was discovered in the brain of a pig (Devane et al., 1992). In the mid-1990s, 2-AG, the second most studied endocannabinoid, was found in the intestines of canines (Sugiura et al., 1995). The most prevalent endocannabinoids in the central nervous system (CNS) and all peripheral tissues are AEA and 2-AG. The phospholipid precursors for AEA and 2-AG appear to be produced as needed in the somatodendritic compartment of neurons in response to calcium influx or activation of intracellular phospholipases. Although it is believed that endocannabinoids are instantly produced in response to specific stimuli, there is some evidence that they are transported through cells, stored, and even degraded in adiposomes, suggesting a complicated underlying mechanism of endocannabinoid signalling (Oddi et al., 2008; Kaczocha et al., 2010). 2-AG may be the predominant endogenous agonist of CB2 receptors, and AEA has a higher affinity for CB1 receptors (Pacher et al., 2006). Additionally, it is well known that tissues contain more 2-AG than AEA (Sugiura and Waku, 2002). Although AEA and 2-AG have well-established production and metabolism mechanisms, how these endocannabinoids are transported across the cell membrane remains unknown. Increasing evidence currently points to the possibility that cells can absorb AEA and 2-AG through protein transporter-mediated enhanced diffusion (Yates and Barker, 2009; Nicolussi and Gertsch, 2015).
The first endogenous CB1 receptor was initially discovered in samples from rat brains (Devane et al., 1988). The cerebral cortex, hippocampus, caudate-putamen, substantia nigra pars reticulata, globus pallidus, entopeduncular nucleus, cerebellum, and spinal cord all have high levels of CB1 receptor expression (Hu and Mackie, 2015). Presynaptic CB1 receptors are primarily found in neurons. Some evidence indicates that only a small percentage of postsynaptic CB1 receptors is found in the mitochondria’s exterior membrane (Benard et al., 2012), where it interferes with the respiratory chain and electron transport, altering brain metabolism and memory formation (Hebert-Chatelain et al., 2016). The CB1 receptors in astrocytes play a role in leptin signalling in the hypothalamus and the modulation of synaptic plasticity in the hippocampus (Bosier et al., 2013; Robin et al., 2018). In addition to stimulating adult progenitor stem cell proliferation and differentiation into neurons or astrocytes, activation of the CB1 receptor has a function in neurodegenerative diseases (Prenderville et al., 2015).
Immune and haematopoietic cells were the first cells to be identified to have the second major endogenous CB2 receptor (Munro et al., 1993; Galiègue et al., 1995). The widespread expression of CB2 receptors in immune cells indicates that endocannabinoids have a unique immunomodulatory function (Lynn and Herkenham, 1994). In addition to traditional immune tissues (thymus, bone marrow, and spleen), other peripheral organs, including the liver (Julien et al., 2005), pancreatic beta cells (Juan-Pico et al., 2006), bone (Ofek et al., 2006), myocardium (Montecucco et al., 2009), and vasculature (Rajesh et al., 2007), express CB2 receptors. According to research on neurological disorders, the main function of the CB2 receptor is immunological regulation. Studies on human brain samples have shown that microglia affected by disorders such as AD, MS, and amyotrophic lateral sclerosis (ALS) have high and specific expression of the CB2 receptor (Aymerich et al., 2018). Furthermore, adult neurogenesis is also stimulated by CB2 receptor activation (Palazuelos et al., 2012), and some data suggest that the CB2 receptor plays a role in controlling the permeability of the blood–brain barrier (BBB) (Chung et al., 2016). According to a study, healthy neurons show very little expression of the CB2 receptor, and CB2 receptor activation produces the opposite effect to that of CB1 receptor stimulation (Navarrete et al., 2013). However, some of these investigations relied on pharmacological or immunological methods that were later discovered to have low selectivity, making the results of these studies questionable (Soethoudt et al., 2017). Finally, it is unclear how CB2 receptors impact neuronal activity. According to one study, functional interaction between the sodium-bicarbonate transporter and the postsynaptic CB2 receptor lowers neuronal excitability in the CA3 and CA2 areas of the hippocampus (Stempel et al., 2016).
The essential involvement of cannabinoid and endocannabinoid system receptors in important developmental processes, such as neurogenesis, glial formation, neuronal migration, axonal elongation, fasciculation (axonal bundling), synaptogenesis, and synaptic pruning, has been extensively demonstrated in the literature (Berghuis et al., 2007; Mulder et al., 2008; Maccarrone et al., 2014). The major targets of THC are CB1 and CB2 receptors, with the CB1 receptor playing a considerable role in CNS development due to its widespread expression in the developing brain, unlike the CB2 receptor, which has a function that is mostly associated with cells of the microglial/macrophage lineage (Zurolo et al., 2010). In humans, CB1 receptors are present and are functional by the ninth gestational week, which coincides with the start of cortex development. In rodents, CB1 receptors are present and functional from gestational day 11 (Biegon and Kerman, 2001; Zurolo et al., 2010). CB1 receptors are temporarily present on white matter neuronal fibres in both rats and humans during the embryonic stages (Berrendero et al., 1999; Mato et al., 2003). The growth and migration of axons to their final location to establish neuronal pathways may reflect the effects of CB1 receptors on axons or their presence on nonneuronal cells (astrocytes and oligodendrocytes) that direct neuronal migration and axonal elongation. Numerous pluripotent cells carry the CB1 receptor, which controls cell division and proliferation (Maccarrone et al., 2014; De Salas-Quiroga et al., 2015), neural differentiation (Harkany et al., 2007). In postmitotic neurons, CB1 receptor expression and endocannabinoid signalling play crucial roles in the migration and differentiation of glutamatergic and GABAergic cortical cells, cholinergic basal forebrain neurons, GABAergic cerebellar cells, and hypothalamic neurons, according to studies conducted on rodents (Keimpema et al., 2013). Before reaching high levels in early adulthood, when it is ubiquitously expressed and becomes the most abundant GPCR, and the expression of the CB1 receptor is dynamic throughout postnatal development until adolescence (Wang et al., 2003; Mackie, 2005). The adult brain regions with the highest concentrations of CB1 receptors include the cerebral cortex, basal ganglia, hippocampus, and cerebellum (Mackie, 2005), and CB1 receptors are predominantly localized to the synapse on presynaptic terminals (Freund et al., 2003) of both glutamatergic and GABAergic cells (Marsicano and Lutz, 1999).
The two main ligands of the endocannabinoid system, AEA and 2-AG, exhibit divergent ontogenic bioavailability and diverse developmental trajectories. While increasing 2-AG levels throughout embryonic development are correlated with cell differentiation and axonal elongation in the brain, it has been shown that AEA is essential during the early stages of pregnancy for embryo implantation in the uterus (Maccarrone et al., 2014). In addition, 2-AG levels peak at postnatal day 1 and then remain constant until adolescence, when they fluctuate (with high levels during both early and late adolescence) before returning to normal levels in adulthood (Berrendero et al., 1999; Ellgren et al., 2008). In contrast, in the majority of the examined brain areas, AEA concentrations gradually rise from gestational day 21 and peak throughout adolescence (Ellgren et al., 2008; Lee et al., 2013).
Endocannabinoid signalling effects go well beyond neuromodulation and can even affect the survival of injured neurons. The ability of CB1 and CB2 receptors to communicate across multiple signalling pathways that regulate brain cell formation and maturation during developmental stages is reflected in cannabinoid regulation of neural cell survival (Galve-Roperh et al., 2013; Maccarrone et al., 2014). Therefore, throughout embryonic neurogenesis and during perinatal and adolescent brain development when gliogenesis, myelination and neuron circuit refinement take place, cannabinoid receptors, their downstream signalling pathways and endocannabinoid ligands are all active. Endocannabinoid signalling exerts important cellular plasticity effects that may have an impact on neuronal remodelling of the developing brain in addition to supporting neuronal homeostasis in the adult brain. We next briefly discuss the effects of CB1 and CB2 receptors signalling on neural cell plasticity during brain development (Figure 2).
Figure 2. Endocannabinoid system control of neurogenesis and neural cell fate in the immature brain. CB1 receptor expression is present in neural progenitors (NPs) and increases during neuronal proliferation, differentiation and maturation. In contrast, the CB2 receptor is present in NPs and is downregulated upon neuronal proliferation, differentiation and maturation. During neuronal development, CB1 and CB2 receptors control NP proliferation, neuroblast migration and neuron maturation. Under neuroinflammatory conditions, activation of CB1 receptors has been shown to restore adult neurogenesis and decrease the number of injured neurons.
CB1 receptors are expressed by cells ranging from neural progenitor (NP) cells to fully differentiated neurons with distinctly diverse functions. CB1 receptor signalling in NPs controls cell identity and proliferation, encouraging the shift from radial glial cells to intermediate progenitors (Diaz-Alonso et al., 2015). Later, the ability of CB1 receptor signalling to regulate NP proliferation was found to be conserved in adult neurogenic regions, where CB1 receptors govern the proliferation of hippocampal subgranular cells (Aguado et al., 2007). The CB1 receptor is also active in the subventricular zone and influences oligodendrogenesis and neurogenesis (Xapelli et al., 2013). Hemopressin, a CB1 receptor modulator, has been demonstrated to encourage SVZ-derived oligodendrogenesis in newborn mice (Xapelli et al., 2014). In addition, a study of genetic engineering of FAAH and diacylglycerol lipase (DAGL), the key enzymes responsible for AEA breakdown and 2-AG synthesis, respectively, confirmed that endocannabinoid signalling controls adult neurogenesis in a manner consistent with findings from endocannabinoid receptor knockout mouse models (Gao et al., 2010).
The vast majority of neuronal populations lack the CB2 receptor, and its function in normal physiological brain function is a current topic of study. However, the importance of CB2 receptor signalling has been shown in cases of neurodegenerative diseases and nervous system injury. CB2 receptors are mostly recognized for their capacity to regulate neuroinflammation, and their activation is linked to decreased levels of inflammatory cytokines, innate immunity, and infiltration of peripheral immune cells (Turcotte et al., 2016). Therefore, the CB2 receptor has neuroprotective effects that are primarily due to the regulation of the negative effects of inflammation. Previous studies have reported that inhibition of hippocampal neurogenesis can be prevented by the administration of a CB2 receptor agonist (Avraham et al., 2014); this treatment can also prevent inhibition of oligodendrogenesis in Borna Disease (BD) virus encephalitis (Solbrig et al., 2010). Additionally, notable examples of the positive effects of the CB2 receptor in models of acute inflammation include protection against ageing-related neuroinflammation and reduced neurogenesis (Goncalves et al., 2008; Marchalant et al., 2009). In the APP/PS1 experimental model of AD, CB2 receptor activation can reduce both cognitive decline and hippocampal neurogenesis impairment (Wu et al., 2017).
The CB2 receptor is also expressed in NPs, and in addition to indirect regulation of neurogenesis and neuroprotection, its activity regulates cell proliferation and neurogenesis in a cell-autonomous manner (Palazuelos et al., 2012). As their activity promotes neuroblast migration towards the damaged cortex, CB2 receptors are known to be involved in brain encephalopathies (Bravo-Ferrer et al., 2017). These studies have highlighted the role of endocannabinoid signalling, including that of both the CB1 and CB2 receptors, in neuroblast migration along the rostral migratory stream (Oudin et al., 2011). Overall, the role of endocannabinoid signalling in neuronal development and plasticity is demonstrated by the capacity of the CB1 receptor to connect to numerous signalling pathways involved in neural precursor cell proliferation, neuronal differentiation, and survival. Furthermore, the therapeutic effects of cannabinoids in the treatment of brain encephalopathies and injuries to the developing brain are explained by the complementary effects of CB2 receptor signalling on neural cell survival. Notably, the development of CB2 receptor-specific manipulation techniques can mitigate the negative effects of neuroinflammation without causing the side effects that are associated with typical neuronal CB1 receptor activity.
Several studies have proposed the involvement of the cannabinoid and endocannabinoid systems in a variety of activities, including the modulation of calcium homeostasis and excitability, regulation of immune and inflammatory responses (Klein, 2005), activation of cytoprotective signalling pathways (Pacher et al., 2006), and modulation of synaptic plasticity, excitatory glutamatergic transmissions (Freund et al., 2003) and their hypothermic and antioxidant properties (Hampson et al., 2000), although the precise neuroprotective mechanisms of cannabis are not fully understood. In this context, the cannabinoid and endocannabinoid system may additionally serve as a crucial neuroprotective mechanism in both acute and chronic neuronal hypoxic-ischaemic brain injury.
Numerous in vitro investigations have documented the neuroprotective properties of cannabis in connection with its antioxidant properties (Marsicano et al., 2002). Cannabis has shown these antioxidant-related neuroprotective effects in in vivo models of neurodegenerative disorders (De Lago and Fernández-Ruiz, 2007). Additionally, it has been shown to reduce body temperature (Pertwee et al., 1991). Studies on adult rats using various cannabinoids have shown that a considerable portion of the neuroprotective effect of these substances depends on the presence of hypothermic conditions, as returning the rat body temperature to a normal temperature decreases or even eliminates the positive effect (Leker et al., 2003). Additionally, hypothermia, the current gold standard of treatment is not an easily accessible and 100% curative therapy due to its limited availability and technical complications. There is definitely a need for combination cannabinoid receptor agonist therapies that are easily accessible and have additive neuroprotective effects (Gupta et al., 2020). Previous studies have observed that a single injection of the CB1 synthetic agonist HU-210 significantly reduced body temperature, conferring a strong neuroprotective effect in hypoxic-ischaemic rats, and this beneficial effect was lost when animals were treated with the selective CB1 antagonist SR141716 (Leker et al., 2003). The enhancement of hypothermia by stimulating the endocannabinoid systems or by combined therapies targeting the endocannabinoid system plus hypothermia may have beneficial outcomes in neonates, so these responses are currently under investigation in preclinical models (Lafuente et al., 2016; Barata et al., 2019). Furthermore, cannabinoids cause vasodilation in the brain (Golech et al., 2004), stabilize the BBB and are involved in neuron proliferative processes (Aguado et al., 2006). Cannabinoids improve the energy metabolism of astrocytes (Stella, 2004) and shield these glial cells from cytotoxic and proapoptotic stimuli after brain damage (Docagne et al., 2007).
Previous research has shown that CB1 receptor activation prevents acute stroke through several mechanisms, including the reduction of BBB disruption, a decrease in the volume of infarcted brain tissue, and the induction of hypothermia. These effects are all typically reversed by CB1 receptor antagonists (Chi et al., 2012). Additionally, animals subjected to CB1 receptor deletion have more severe strokes (Parmentier-Batteur et al., 2002), although one study revealed that CB1 receptor antagonists might offer protection in cases of temporary or permanent cerebral artery blockage (Muthian et al., 2004). Similarly, CB2 receptor activation decreases infarct volume and enhances neurological outcome and cerebral microcirculatory function in mice with middle cerebral artery blockage (Zarruk et al., 2012). In fact, palmitoylethanolamide and other N-acylethanolamines protected against transient focal cerebral ischaemia in rats and against the effects of middle cerebral artery occlusion in mice via mechanisms that did not require activation of the CB1 receptor but the CB2 receptor or TRPV1 (Franklin et al., 2003). Recent studies have shown that the anti-inflammatory and immunomodulatory effects of cannabis are mediated by CB2 receptors (Fernandez-Ruiz et al., 2007). Numerous studies have demonstrated the anti-inflammatory therapeutic potential of CB2 receptor activation in conditions affecting the central nervous system, including MS, traumatic brain injury, and AD (Mauler et al., 2003; Ni et al., 2004; Ramirez et al., 2005). The presence of CB2 receptors in inflammatory cells in the brain, including microglia (Maresz et al., 2005), has recently been demonstrated, and CB2 receptor expression is induced by hypoxia-ischaemia in the brain (Fernandez-Ruiz et al., 2008). Additionally, CB2 receptor agonists have demonstrated promising results in a variety of neonatal hypoxic-ischaemic brain injury paradigms, reducing cell death and modulating glutamate release, cytokine production, and the expression of cyclooxygenase-2 and iNOS. In an animal model of stroke, it was discovered that the CB2 receptor agonist O-1966 increased blood flow to the brain and reduced neuroinflammation (Sinor et al., 2000). In addition, CB2 receptor activation has been shown to reduce infarct size after middle cerebral artery occlusion and to decrease inflammation-dependent neurodegeneration, reducing the release of inflammatory cytokines and leukocyte adhesion to cerebral vessels (Zhang et al., 2007; Rivers and Ashton, 2010). These findings lend support to the idea that the protective effects of CB2 receptors are primarily due to their anti-inflammatory properties (Castillo et al., 2010). This offers new information on its potential application as a neuroprotective target following neonatal hypoxia.
However, the potential therapeutic effect of CB receptors on ischaemic disorders is far from clear in currently. For example, CB1R activation can promote either protective or toxic responses after brain ischaemia (Pellegrini-Giampietro et al., 2009), as these receptors can either promote the inhibition of glutamate (inducing neuroprotection) or the release of gamma-aminobutyric acid (thus amplifying the toxic response), leading to oxidative stress. In a recent report (Rivers-Auty et al., 2014), the CB2R-selective agonist GW405833 did not show a beneficial effect in a model of cerebral HI, although CB2R-induced neuroprotection has long been known to be related to its anti-inflammatory capacity. Thus, the antioxidant capacity and/or the anti-inflammatory effect developed by the endocannabinoid system after perinatal asphyxia remain a subject of investigation. Further studies should analyse the modulatory effect of CB receptors agonists on ROS and inflammatory cytokine production after HIE, which may contribute to illustrating the role of the cannabinoids and endocannabinoid system in HIE treatment.
Finally, numerous studies have suggested that using synthetic cannabis can lessen damage after brain injury (Fernández-López et al., 2007; Alonso-Alconada et al., 2012; Dai et al., 2014). A histopathological study specifically found that administering WIN55212 soon after recovery from hypoxia-ischaemia successfully reduced brain damage (Fernández-López et al., 2007). Additionally, WIN55212 was shown to prevent the death of apoptotic cells in every area examined by maintaining the integrity and activity of the mitochondria (Fernandez-Lopez et al., 2010) and to encourage neurogenesis in the subventricular zone, oligodendrogenesis, white matter remyelination, and neuroblast production after neonatal hypoxic-ischaemic episodes (Zhang et al., 2009).
Interest in cannabinoids and endocannabinoids as treatments to manage neonatal hypoxic-ischaemic encephalopathy is supported by the pharmacological characteristics of cannabinoids. In experimental HIE and brain insult models, the administration of cannabis has been shown to have neuroprotective effects. Cannabis preparations may mitigate some of the negative effects of HIE damage in the developing brain. Because cannabinoids have a complicated pharmacology that enables them to target various molecular effectors and receptors, the use of cannabinoid compounds with diverse pharmacological profiles will have distinct effects. Endocannabinoids safeguard the developing brain by inhibiting neuronal excitotoxicity, inflammation, and oxidative stress as well as by altering the fate of neurons and preventing neurodegeneration and harmful glial activation. These cannabis substances provide promising potential clinical applications and raise the possibility of better long-term benefit outcomes for these individuals.
JX and YZ prepared the first draft of the manuscript. LS collected academic papers and provided critical advice on the manuscript. The study was conceptualized by HW, who also supervised the work and reviewed the entire manuscript. All authors contributed to the article and approved the submitted version.
The authors acknowledge the Department of Paediatrics, Sichuan Academy of Medical Science and Sichuan Provincial People’s Hospital, and Department of Pathology, Affiliated Hospital of Hubei Polytechnic University for supporting our work.
The authors declare that the research was conducted in the absence of any commercial or financial relationships that could be construed as a potential conflict of interest.
All claims expressed in this article are solely those of the authors and do not necessarily represent those of their affiliated organizations, or those of the publisher, the editors and the reviewers. Any product that may be evaluated in this article, or claim that may be made by its manufacturer, is not guaranteed or endorsed by the publisher.
Adachi, M., Sohma, O., Tsuneishi, S., Takada, S., and Nakamura, H. (2001). Combination effect of systemic hypothermia and caspase inhibitor administration against hypoxic-ischemic brain damage in neonatal rats. Pediatr. Res. 50, 590–595. doi: 10.1203/00006450-200111000-00010
Aguado, T., Palazuelos, J., Monory, K., Stella, N., Cravatt, B., Lutz, B., et al. (2006). The endocannabinoid system promotes astroglial differentiation by acting on neural progenitor cells. J. Neurosci. 26, 1551–1561. doi: 10.1523/JNEUROSCI.3101-05.2006
Aguado, T., Romero, E., Monory, K., Palazuelos, J., Sendtner, M., Marsicano, G., et al. (2007). The CB1 cannabinoid receptor mediates excitotoxicity-induced neural progenitor proliferation and neurogenesis. J. Biol. Chem. 282, 23892–23898. doi: 10.1074/jbc.M700678200
Alonso-Alconada, D., Alvarez, A., Alvarez, F. J., Martinez-Orgado, J. A., and Hilario, E. (2012). The cannabinoid WIN 55212-2 mitigates apoptosis and mitochondrial dysfunction after hypoxia ischemia. Neurochem. Res. 37, 161–170. doi: 10.1007/s11064-011-0594-z
Alonso-Alconada, D., Alvarez, F. J., Alvarez, A., Mielgo, V. E., Goni-de-Cerio, F., Rey-Santano, M. C., et al. (2010). The cannabinoid receptor agonist WIN 55,212-2 reduces the initial cerebral damage after hypoxic-ischemic injury in fetal lambs. Brain Res. 1362, 150–159. doi: 10.1016/j.brainres.2010.09.050
Arvin, K. L., Han, B. H., Du, Y., Lin, S. Z., Paul, S. M., and Holtzman, D. M. (2002). Minocycline markedly protects the neonatal brain against hypoxic-ischemic injury. Ann. Neurol. 52, 54–61. doi: 10.1002/ana.10242
Avraham, H. K., Jiang, S., Fu, Y., Rockenstein, E., Makriyannis, A., Zvonok, A., et al. (2014). The cannabinoid CB (Zybura et al.) receptor agonist AM1241 enhances neurogenesis in GFAP/Gp120 transgenic mice displaying deficits in neurogenesis. Br. J. Pharmacol. 171, 468–479. doi: 10.1111/bph.12478
Aymerich, M. S., Aso, E., Abellanas, M. A., Tolon, R. M., Ramos, J. A., Ferrer, I., et al. (2018). Cannabinoid pharmacology/therapeutics in chronic degenerative disorders affecting the central nervous system. Biochem. Pharmacol. 157, 67–84. doi: 10.1016/j.bcp.2018.08.016
Barata, L., Arruza, L., Rodriguez, M. J., Aleo, E., Vierge, E., Criado, E., et al. (2019). Neuroprotection by cannabidiol and hypothermia in a piglet model of newborn hypoxic-ischemic brain damage. Neuropharmacology 146, 1–11. doi: 10.1016/j.neuropharm.2018.11.020
Barha, C. K., Ishrat, T., Epp, J. R., Galea, L. A., and Stein, D. G. (2011). Progesterone treatment normalizes the levels of cell proliferation and cell death in the dentate gyrus of the hippocampus after traumatic brain injury. Exp. Neurol. 231, 72–81. doi: 10.1016/j.expneurol.2011.05.016
Ben Amar, M. (2006). Cannabinoids in medicine: A review of their therapeutic potential. J. Ethnopharmacol. 105, 1–25. doi: 10.1016/j.jep.2006.02.001
Benard, G., Massa, F., Puente, N., Lourenco, J., Bellocchio, L., Soria-Gomez, E., et al. (2012). Mitochondrial CB (1) receptors regulate neuronal energy metabolism. Nat. Neurosci. 15, 558–564. doi: 10.1038/nn.3053
Berger, R., and Garnier, Y. (2000). Perinatal brain injury. J. Perinat. Med. 28, 261–285. doi: 10.1515/JPM.2000.034
Berghuis, P., Rajnicek, A. M., Morozov, Y. M., Ross, R. A., Mulder, J., Urban, G. M., et al. (2007). Hardwiring the brain: Endocannabinoids shape neuronal connectivity. Science 316, 1212–1216. doi: 10.1126/science.1137406
Berrendero, F., Sepe, N., Ramos, J. A., Di Marzo, V., and Fernández-Ruiz, J. J. (1999). Analysis of cannabinoid receptor binding and mRNA expression and endogenous cannabinoid contents in the developing rat brain during late gestation and early postnatal period. Synapse 33, 181–191. doi: 10.1002/(SICI)1098-2396(19990901)33:3<181::AID-SYN3>3.0.CO;2-R
Biegon, A., and Kerman, I. A. (2001). Autoradiographic study of pre-and postnatal distribution of cannabinoid receptors in human brain. NeuroImage 14, 1463–1468. doi: 10.1006/nimg.2001.0939
Bosier, B., Bellocchio, L., Metna-Laurent, M., Soria-Gomez, E., Matias, I., Hebert-Chatelain, E., et al. (2013). Astroglial CB1 cannabinoid receptors regulate leptin signaling in mouse brain astrocytes. Mol. Metab. 2, 393–404. doi: 10.1016/j.molmet.2013.08.001
Bravo-Ferrer, I., Cuartero, M. I., Zarruk, J. G., Pradillo, J. M., Hurtado, O., Romera, V. G., et al. (2017). Cannabinoid Type-2 receptor drives neurogenesis and improves functional outcome after stroke. Stroke 48, 204–212. doi: 10.1161/STROKEAHA.116.014793
Carli, G., Reiger, I., and Evans, N. (2004). One-year neurodevelopmental outcome after moderate newborn hypoxic ischaemic encephalopathy. J. Paediatr. Child Health 40, 217–220. doi: 10.1111/j.1440-1754.2004.00341.x
Carloni, S., Girelli, S., Buonocore, G., Longini, M., and Balduini, W. (2009). Simvastatin acutely reduces ischemic brain damage in the immature rat via Akt and CREB activation. Exp. Neurol. 220, 82–89. doi: 10.1016/j.expneurol.2009.07.026
Carloni, S., Mazzoni, E., Cimino, M., De Simoni, M. G., Perego, C., Scopa, C., et al. (2006). Simvastatin reduces caspase-3 activation and inflammatory markers induced by hypoxia-ischemia in the newborn rat. Neurobiol. Dis. 21, 119–126. doi: 10.1016/j.nbd.2005.06.014
Carloni, S., Perrone, S., Buonocore, G., Longini, M., Proietti, F., and Balduini, W. (2008). Melatonin protects from the long-term consequences of a neonatal hypoxic-ischemic brain injury in rats. J. Pineal Res. 44, 157–164. doi: 10.1111/j.1600-079X.2007.00503.x
Carrier, E. J., Auchampach, J. A., and Hillard, C. J. (2006). Inhibition of an equilibrative nucleoside transporter by cannabidiol: A mechanism of cannabinoid immunosuppression. Proc. Natl. Acad. Sci. U. S. A. 103, 7895–7900. doi: 10.1073/pnas.0511232103
Castillo, A., Tolon, M. R., Fernandez-Ruiz, J., Romero, J., and Martinez-Orgado, J. (2010). The neuroprotective effect of cannabidiol in an in vitro model of newborn hypoxic-ischemic brain damage in mice is mediated by CB (Zybura et al.) and adenosine receptors. Neurobiol. Dis. 37, 434–440. doi: 10.1016/j.nbd.2009.10.023
Chang, Y. H., Lee, S. T., and Lin, W. W. (2001). Effects of cannabinoids on LPS-stimulated inflammatory mediator release from macrophages: involvement of eicosanoids. J. Cell. Biochem. 81, 715–723. doi: 10.1002/jcb.1103
Chang, Y. S., Mu, D., Wendland, M., Sheldon, R. A., Vexler, Z. S., Mcquillen, P. S., et al. (2005). Erythropoietin improves functional and histological outcome in neonatal stroke. Pediatr. Res. 58, 106–111. doi: 10.1203/01.PDR.0000163616.89767.69
Chi, O. Z., Barsoum, S., Grayson, J., Hunter, C., Liu, X., and Weiss, H. R. (2012). Effects of cannabinoid receptor agonist WIN 55,212-2 on blood-brain barrier disruption in focal cerebral ischemia in rats. Pharmacology 89, 333–338. doi: 10.1159/000338755
Chiurchiu, V., van der Stelt, M., Centonze, D., and Maccarrone, M. (2018). The endocannabinoid system and its therapeutic exploitation in multiple sclerosis: Clues for other neuroinflammatory diseases. Prog. Neurobiol. 160, 82–100. doi: 10.1016/j.pneurobio.2017.10.007
Chung, Y. C., Shin, W. H., Baek, J. Y., Cho, E. J., Baik, H. H., Kim, S. R., et al. (2016). CB2 receptor activation prevents glial-derived neurotoxic mediator production, BBB leakage and peripheral immune cell infiltration and rescues dopamine neurons in the MPTP model of Parkinson's disease. Exp. Mol. Med. 48:e205. doi: 10.1038/emm.2015.100
Cristino, L., Bisogno, T., and Di Marzo, V. (2019). Cannabinoids and the expanded endocannabinoid system in neurological disorders. Nat. Rev. Neurol. 16, 9–29. doi: 10.1038/s41582-019-0284-z
Dai, Y., Li, W., Zhong, M., Chen, J., Liu, Y., Cheng, Q., et al. (2014). Preconditioning and post-treatment with cobalt chloride in rat model of perinatal hypoxic-ischemic encephalopathy. Brain Dev. 36, 228–240. doi: 10.1016/j.braindev.2013.04.007
De Lago, E., and Fernández-Ruiz, J. (2007). Cannabinoids and neuroprotection in motor-related disorders. CNS Neurol. Disord. Drug Targets 6, 377–387. doi: 10.2174/187152707783399210
De Salas-Quiroga, A., Diaz-Alonso, J., Garcia-Rincon, D., Remmers, F., Vega, D., Gomez-Canas, M., et al. (2015). Prenatal exposure to cannabinoids evokes long-lasting functional alterations by targeting CB1 receptors on developing cortical neurons. Proc. Natl. Acad. Sci. U. S. A. 112, 13693–13698. doi: 10.1073/pnas.1514962112
Devane, W. A., Dysarz, F. A. 3rd, Johnson, M. R., Melvin, L. S., and Howlett, A. C. (1988). Determination and characterization of a cannabinoid receptor in rat brain. Mol. Pharmacol. 34, 605–613.
Devane, W. A., Hanus, L., Breuer, A., Pertwee, R. G., Stevenson, L. A., Griffin, G., et al. (1992). Isolation and structure of a brain constituent that binds to the cannabinoid receptor. Science 258, 1946–1949. doi: 10.1126/science.1470919
Devinsky, O., Cross, J. H., Laux, L., Marsh, E., Miller, I., Nabbout, R., et al. (2017). Trial of Cannabidiol for drug-resistant seizures in the Dravet syndrome. N. Engl. J. Med. 376, 2011–2020. doi: 10.1056/NEJMoa1611618
Devinsky, O., Marsh, E., Friedman, D., Thiele, E., Laux, L., Sullivan, J., et al. (2016). Cannabidiol in patients with treatment-resistant epilepsy: An open-label interventional trial. Lancet Neurol. 15, 270–278. doi: 10.1016/S1474-4422(15)00379-8
Diaz-Alonso, J., Aguado, T., De Salas-Quiroga, A., Ortega, Z., Guzman, M., and Galve-Roperh, I. (2015). CB1 cannabinoid receptor-dependent activation of mTORC1/Pax6 signaling drives Tbr2 expression and basal progenitor expansion in the developing mouse cortex. Cereb. Cortex 25, 2395–2408. doi: 10.1093/cercor/bhu039
Docagne, F., Muneton, V., Clemente, D., Ali, C., Loria, F., Correa, F., et al. (2007). Excitotoxicity in a chronic model of multiple sclerosis: Neuroprotective effects of cannabinoids through CB1 and CB2 receptor activation. Mol. Cell. Neurosci. 34, 551–561. doi: 10.1016/j.mcn.2006.12.005
Du Plessis, A. J., and Volpe, J. J. (2002). Perinatal brain injury in the preterm and term newborn. Curr. Opin. Neurol. 15, 151–157. doi: 10.1097/00019052-200204000-00005
Ellgren, M., Artmann, A., Tkalych, O., Gupta, A., Hansen, H. S., Hansen, S. H., et al. (2008). Dynamic changes of the endogenous cannabinoid and opioid mesocorticolimbic systems during adolescence: THC effects. Eur. Neuropsychopharmacol. 18, 826–834. doi: 10.1016/j.euroneuro.2008.06.009
Esteve, J. M., Mompo, J., Garcia De La Asuncion, J., Sastre, J., Asensi, M., Boix, J., et al. (1999). Oxidative damage to mitochondrial DNA and glutathione oxidation in apoptosis: Studies in vivo and in vitro. FASEB J. 13, 1055–1064. doi: 10.1096/fasebj.13.9.1055
Fernández-López, D., Pazos, M. R., Tolón, R. M., Moro, M. A., Romero, J., Lizasoain, I., et al. (2007). The cannabinoid agonist WIN55212 reduces brain damage in an in vivo model of hypoxic-ischemic encephalopathy in newborn rats. Pediatr. Res. 62, 255–260. doi: 10.1203/PDR.0b013e318123fbb8
Fernandez-Lopez, D., Pradillo, J. M., Garcia-Yebenes, I., Martinez-Orgado, J. A., Moro, M. A., and Lizasoain, I. (2010). The cannabinoid WIN55212-2 promotes neural repair after neonatal hypoxia-ischemia. Stroke 41, 2956–2964. doi: 10.1161/STROKEAHA.110.599357
Fernandez-Ruiz, J., Pazos, M. R., Garcia-Arencibia, M., Sagredo, O., and Ramos, J. A. (2008). Role of CB2 receptors in neuroprotective effects of cannabinoids. Mol. Cell. Endocrinol. 286, S91–S96. doi: 10.1016/j.mce.2008.01.001
Fernandez-Ruiz, J., Romero, J., Velasco, G., Tolon, R. M., Ramos, J. A., and Guzman, M. (2007). Cannabinoid CB2 receptor: A new target for controlling neural cell survival? Trends Pharmacol. Sci. 28, 39–45. doi: 10.1016/j.tips.2006.11.001
Ferriero, D. M. (2004). Neonatal brain injury. N. Engl. J. Med. 351, 1985–1995. doi: 10.1056/NEJMra041996
Franklin, A., Parmentier-Batteur, S., Walter, L., Greenberg, D. A., and Stella, N. (2003). Palmitoylethanolamide increases after focal cerebral ischemia and potentiates microglial cell motility. J. Neurosci. 23, 7767–7775. doi: 10.1523/JNEUROSCI.23-21-07767.2003
Freund, T. F., Katona, I., and Piomelli, D. (2003). Role of endogenous cannabinoids in synaptic signaling. Physiol. Rev. 83, 1017–1066. doi: 10.1152/physrev.00004.2003
Galiègue, S., Mary, S., Marchand, J., Dussossoy, D., Carrière, D., Carayon, P., et al. (1995). Expression of central and peripheral cannabinoid receptors in human immune tissues and leukocyte subpopulations. Eur. J. Biochem. 232, 54–61. doi: 10.1111/j.1432-1033.1995.tb20780.x
Galve-Roperh, I., Chiurchiu, V., Diaz-Alonso, J., Bari, M., Guzman, M., and Maccarrone, M. (2013). Cannabinoid receptor signaling in progenitor/stem cell proliferation and differentiation. Prog. Lipid Res. 52, 633–650. doi: 10.1016/j.plipres.2013.05.004
Gao, Y., Vasilyev, D. V., Goncalves, M. B., Howell, F. V., Hobbs, C., Reisenberg, M., et al. (2010). Loss of retrograde endocannabinoid signaling and reduced adult neurogenesis in diacylglycerol lipase knock-out mice. J. Neurosci. 30, 2017–2024. doi: 10.1523/JNEUROSCI.5693-09.2010
Golech, S. A., Mccarron, R. M., Chen, Y., Bembry, J., Lenz, F., Mechoulam, R., et al. (2004). Human brain endothelium: Coexpression and function of vanilloid and endocannabinoid receptors. Brain Res. Mol. Brain Res. 132, 87–92. doi: 10.1016/j.molbrainres.2004.08.025
Goncalves, M. B., Suetterlin, P., Yip, P., Molina-Holgado, F., Walker, D. J., Oudin, M. J., et al. (2008). A diacylglycerol lipase-CB2 cannabinoid pathway regulates adult subventricular zone neurogenesis in an age-dependent manner. Mol. Cell. Neurosci. 38, 526–536. doi: 10.1016/j.mcn.2008.05.001
Gonzalez, F. F., Mcquillen, P., Mu, D., Chang, Y., Wendland, M., Vexler, Z., et al. (2007). Erythropoietin enhances long-term neuroprotection and neurogenesis in neonatal stroke. Dev. Neurosci. 29, 321–330. doi: 10.1159/000105473
Gupta, B., Hornick, M. G., Briyal, S., Donovan, R., Prazad, P., and Gulati, A. (2020). Anti-apoptotic and Immunomodulatory effect of CB2 agonist, JWH133, in a neonatal rat model of hypoxic-ischemic encephalopathy. Front. Pediatr. 8:65. doi: 10.3389/fped.2020.00065
Hampson, A. J., Grimaldi, M., Lolic, M., Wink, D., Rosenthal, R., and Axelrod, J. (2000). Neuroprotective antioxidants from marijuana. Ann. N. Y. Acad. Sci. 899, 274–282.
Harkany, T., Guzman, M., Galve-Roperh, I., Berghuis, P., Devi, L. A., and Mackie, K. (2007). The emerging functions of endocannabinoid signaling during CNS development. Trends Pharmacol. Sci. 28, 83–92. doi: 10.1016/j.tips.2006.12.004
Hashimoto, T., Yonetani, M., and Nakamura, H. (2003). Selective brain hypothermia protects against hypoxic-ischemic injury in newborn rats by reducing hydroxyl radical production. Kobe J. Med. Sci. 49, 83–91.
Hebert-Chatelain, E., Desprez, T., Serrat, R., Bellocchio, L., Soria-Gomez, E., Busquets-Garcia, A., et al. (2016). A cannabinoid link between mitochondria and memory. Nature 539, 555–559. doi: 10.1038/nature20127
Hu, S. S., and Mackie, K. (2015). Distribution of the Endocannabinoid system in the central nervous system. Handb. Exp. Pharmacol. 231, 59–93. doi: 10.1007/978-3-319-20825-1_3
Izzo, A. A., Borrelli, F., Capasso, R., Di Marzo, V., and Mechoulam, R. (2009). Non-psychotropic plant cannabinoids: New therapeutic opportunities from an ancient herb. Trends Pharmacol. Sci. 30, 515–527. doi: 10.1016/j.tips.2009.07.006
Jantzie, L. L., Cheung, P. Y., and Todd, K. G. (2005). Doxycycline reduces cleaved caspase-3 and microglial activation in an animal model of neonatal hypoxia-ischemia. J. Cereb. Blood Flow Metab. 25, 314–324. doi: 10.1038/sj.jcbfm.9600025
Jatana, M., Singh, I., Singh, A. K., and Jenkins, D. (2006). Combination of systemic hypothermia and N-acetylcysteine attenuates hypoxic-ischemic brain injury in neonatal rats. Pediatr. Res. 59, 684–689. doi: 10.1203/01.pdr.0000215045.91122.44
Juan-Pico, P., Fuentes, E., Bermudez-Silva, F. J., Javier Diaz-Molina, F., Ripoll, C., Rodriguez De Fonseca, F., et al. (2006). Cannabinoid receptors regulate ca(2+) signals and insulin secretion in pancreatic beta-cell. Cell Calcium 39, 155–162. doi: 10.1016/j.ceca.2005.10.005
Julien, B., Grenard, P., Teixeira-Clerc, F., Van Nhieu, J. T., Li, L., Karsak, M., et al. (2005). Antifibrogenic role of the cannabinoid receptor CB2 in the liver. Gastroenterology 128, 742–755. doi: 10.1053/j.gastro.2004.12.050
Jutras-Aswad, D., Dinieri, J. A., Harkany, T., and Hurd, Y. L. (2009). Neurobiological consequences of maternal cannabis on human fetal development and its neuropsychiatric outcome. Eur. Arch. Psychiatry Clin. Neurosci. 259, 395–412. doi: 10.1007/s00406-009-0027-z
Juul, S. E., and Ferriero, D. M. (2014). Pharmacologic neuroprotective strategies in neonatal brain injury. Clin. Perinatol. 41, 119–131. doi: 10.1016/j.clp.2013.09.004
Kaczocha, M., Glaser, S. T., Chae, J., Brown, D. A., and Deutsch, D. G. (2010). Lipid droplets are novel sites of N-acylethanolamine inactivation by fatty acid amide hydrolase-2. J. Biol. Chem. 285, 2796–2806. doi: 10.1074/jbc.M109.058461
Katona, I., and Freund, T. F. (2012). Multiple functions of endocannabinoid signaling in the brain. Annu. Rev. Neurosci. 35, 529–558. doi: 10.1146/annurev-neuro-062111-150420
Keimpema, E., Calvigioni, D., and Harkany, T. (2013). Endocannabinoid signals in the developmental programming of delayed-onset neuropsychiatric and metabolic illnesses. Biochem. Soc. Trans. 41, 1569–1576. doi: 10.1042/BST20130117
Kerai, A., Sim, T. F., and Emmerton, L. (2018). Medical cannabis: A needs analysis for people with epilepsy. Complement. Ther. Clin. Pract. 33, 43–48. doi: 10.1016/j.ctcp.2018.08.003
Klein, T. W. (2005). Cannabinoid-based drugs as anti-inflammatory therapeutics. Nat. Rev. Immunol. 5, 400–411. doi: 10.1038/nri1602
Lafuente, H., Pazos, M. R., Alvarez, A., Mohammed, N., Santos, M., Arizti, M., et al. (2016). Effects of Cannabidiol and hypothermia on short-term brain damage in new-born piglets after acute hypoxia-ischemia. Front. Neurosci. 10:323. doi: 10.3389/fnins.2016.00323
Lee, T. T., Hill, M. N., Hillard, C. J., and Gorzalka, B. B. (2013). Temporal changes in N-acylethanolamine content and metabolism throughout the peri-adolescent period. Synapse 67, 4–10. doi: 10.1002/syn.21609
Lee, T. F., Jantzie, L. L., Todd, K. G., and Cheung, P. Y. (2008). Postresuscitation N-acetylcysteine treatment reduces cerebral hydrogen peroxide in the hypoxic piglet brain. Intensive Care Med. 34, 190–197. doi: 10.1007/s00134-007-0880-z
Leker, R. R., Gai, N., Mechoulam, R., and Ovadia, H. (2003). Drug-induced hypothermia reduces ischemic damage: Effects of the cannabinoid HU-210. Stroke 34, 2000–2006. doi: 10.1161/01.STR.0000079817.68944.1E
Lovinger, D. M. (2008). Presynaptic modulation by endocannabinoids. Handb. Exp. Pharmacol. 184, 435–477. doi: 10.1007/978-3-540-74805-2_14
Lucas, C. J., Galettis, P., and Schneider, J. (2018). The pharmacokinetics and the pharmacodynamics of cannabinoids. Br. J. Clin. Pharmacol. 84, 2477–2482. doi: 10.1111/bcp.13710
Lynn, A. B., and Herkenham, M. (1994). Localization of cannabinoid receptors and nonsaturable high-density cannabinoid binding sites in peripheral tissues of the rat: Implications for receptor-mediated immune modulation by cannabinoids. J. Pharmacol. Exp. Ther. 268, 1612–1623.
Maccarrone, M., Guzman, M., Mackie, K., Doherty, P., and Harkany, T. (2014). Programming of neural cells by (endo)cannabinoids: From physiological rules to emerging therapies. Nat. Rev. Neurosci. 15, 786–801. doi: 10.1038/nrn3846
Mackie, K. (2005). Distribution of cannabinoid receptors in the central and peripheral nervous system. Handb. Exp. Pharmacol. 168, 299–325. doi: 10.1007/3-540-26573-2_10
Marchalant, Y., Brothers, H. M., Norman, G. J., Karelina, K., Devries, A. C., and Wenk, G. L. (2009). Cannabinoids attenuate the effects of aging upon neuroinflammation and neurogenesis. Neurobiol. Dis. 34, 300–307. doi: 10.1016/j.nbd.2009.01.014
Maresz, K., Carrier, E. J., Ponomarev, E. D., Hillard, C. J., and Dittel, B. N. (2005). Modulation of the cannabinoid CB2 receptor in microglial cells in response to inflammatory stimuli. J. Neurochem. 95, 437–445. doi: 10.1111/j.1471-4159.2005.03380.x
Maresz, K., Pryce, G., Ponomarev, E. D., Marsicano, G., Croxford, J. L., Shriver, L. P., et al. (2007). Direct suppression of CNS autoimmune inflammation via the cannabinoid receptor CB1 on neurons and CB2 on autoreactive T cells. Nat. Med. 13, 492–497. doi: 10.1038/nm1561
Marinelli, S., Marrone, M. C., Di Domenico, M., and Marinelli, S. (2023). Endocannabinoid signaling in microglia. Glia 71, 71–90. doi: 10.1002/glia.24281
Marsicano, G., Goodenough, S., Monory, K., Hermann, H., Eder, M., Cannich, A., et al. (2003). CB1 cannabinoid receptors and on-demand defense against excitotoxicity. Science 302, 84–88. doi: 10.1126/science.1088208
Marsicano, G., and Lutz, B. (1999). Expression of the cannabinoid receptor CB1 in distinct neuronal subpopulations in the adult mouse forebrain. Eur. J. Neurosci. 11, 4213–4225. doi: 10.1046/j.1460-9568.1999.00847.x
Marsicano, G., Moosmann, B., Hermann, H., Lutz, B., and Behl, C. (2002). Neuroprotective properties of cannabinoids against oxidative stress: Role of the cannabinoid receptor CB1. J. Neurochem. 80, 448–456. doi: 10.1046/j.0022-3042.2001.00716.x
Mato, S., Del Olmo, E., and Pazos, A. (2003). Ontogenetic development of cannabinoid receptor expression and signal transduction functionality in the human brain. Eur. J. Neurosci. 17, 1747–1754. doi: 10.1046/j.1460-9568.2003.02599.x
Mauler, F., Horváth, E., De Vry, J., Jäger, R., Schwarz, T., Sandmann, S., et al. (2003). BAY 38-7271: A novel highly selective and highly potent cannabinoid receptor agonist for the treatment of traumatic brain injury. CNS Drug Rev. 9, 343–358. doi: 10.1111/j.1527-3458.2003.tb00259.x
Meyer, H. C., Lee, F. S., and Gee, D. G. (2018). The role of the Endocannabinoid system and genetic variation in adolescent brain development. Neuropsychopharmacology 43, 21–33. doi: 10.1038/npp.2017.143
Montecucco, F., Lenglet, S., Braunersreuther, V., Burger, F., Pelli, G., Bertolotto, M., et al. (2009). CB (Zybura et al.) cannabinoid receptor activation is cardioprotective in a mouse model of ischemia/reperfusion. J. Mol. Cell. Cardiol. 46, 612–620. doi: 10.1016/j.yjmcc.2008.12.014
Mulder, J., Aguado, T., Keimpema, E., Barabás, K., Ballester Rosado, C. J., Nguyen, L., et al. (2008). Endocannabinoid signaling controls pyramidal cell specification and long-range axon patterning. Proc. Natl. Acad. Sci. U. S. A. 105, 8760–8765. doi: 10.1073/pnas.0803545105
Munro, S., Thomas, K. L., and Abu-Shaar, M. (1993). Molecular characterization of a peripheral receptor for cannabinoids. Nature 365, 61–65. doi: 10.1038/365061a0
Muthian, S., Rademacher, D. J., Roelke, C. T., Gross, G. J., and Hillard, C. J. (2004). Anandamide content is increased and CB1 cannabinoid receptor blockade is protective during transient, focal cerebral ischemia. Neuroscience 129, 743–750. doi: 10.1016/j.neuroscience.2004.08.044
Natarajan, G., Pappas, A., and Shankaran, S. (2016). Outcomes in childhood following therapeutic hypothermia for neonatal hypoxic-ischemic encephalopathy (HIE). Semin. Perinatol. 40, 549–555. doi: 10.1053/j.semperi.2016.09.007
Navarrete, F., Rodriguez-Arias, M., Martin-Garcia, E., Navarro, D., Garcia-Gutierrez, M. S., Aguilar, M. A., et al. (2013). Role of CB2 cannabinoid receptors in the rewarding, reinforcing, and physical effects of nicotine. Neuropsychopharmacology 38, 2515–2524. doi: 10.1038/npp.2013.157
Ni, X., Geller, E. B., Eppihimer, M. J., Eisenstein, T. K., Adler, M. W., and Tuma, R. F. (2004). Win 55212-2, a cannabinoid receptor agonist, attenuates leukocyte/endothelial interactions in an experimental autoimmune encephalomyelitis model. Mult. Scler. 10, 158–164. doi: 10.1191/1352458504ms1009oa
Nicolussi, S., and Gertsch, J. (2015). Endocannabinoid transport revisited. Vitam. Horm. 98, 441–485. doi: 10.1016/bs.vh.2014.12.011
Oddi, S., Fezza, F., Pasquariello, N., De Simone, C., Rapino, C., Dainese, E., et al. (2008). Evidence for the intracellular accumulation of anandamide in adiposomes. Cell. Mol. Life Sci. 65, 840–850. doi: 10.1007/s00018-008-7494-7
Ofek, O., Karsak, M., Leclerc, N., Fogel, M., Frenkel, B., Wright, K., et al. (2006). Peripheral cannabinoid receptor, CB2, regulates bone mass. Proc. Natl. Acad. Sci. U. S. A. 103, 696–701. doi: 10.1073/pnas.0504187103
Oudin, M. J., Gajendra, S., Williams, G., Hobbs, C., Lalli, G., and Doherty, P. (2011). Endocannabinoids regulate the migration of subventricular zone-derived neuroblasts in the postnatal brain. J. Neurosci. 31, 4000–4011. doi: 10.1523/JNEUROSCI.5483-10.2011
Pacher, P., Bátkai, S., and Kunos, G. (2006). The endocannabinoid system as an emerging target of pharmacotherapy. Pharmacol. Rev. 58, 389–462. doi: 10.1124/pr.58.3.2
Palazuelos, J., Ortega, Z., Diaz-Alonso, J., Guzman, M., and Galve-Roperh, I. (2012). CB2 cannabinoid receptors promote neural progenitor cell proliferation via mTORC1 signaling. J. Biol. Chem. 287, 1198–1209. doi: 10.1074/jbc.M111.291294
Palmer, C., Towfighi, J., Roberts, R. L., and Heitjan, D. F. (1993). Allopurinol administered after inducing hypoxia-ischemia reduces brain injury in 7-day-old rats. Pediatr. Res. 33, 405–411. doi: 10.1203/00006450-199304000-00018
Parmentier-Batteur, S., Jin, K., Mao, X. O., Xie, L., and Greenberg, D. A. (2002). Increased severity of stroke in CB1 cannabinoid receptor knock-out mice. J. Neurosci. 22, 9771–9775. doi: 10.1523/JNEUROSCI.22-22-09771.2002
Pazos, M. R., Mohammed, N., Lafuente, H., Santos, M., Martinez-Pinilla, E., Moreno, E., et al. (2013). Mechanisms of cannabidiol neuroprotection in hypoxic-ischemic newborn pigs: Role of 5HT(1A) and CB2 receptors. Neuropharmacology 71, 282–291. doi: 10.1016/j.neuropharm.2013.03.027
Pellegrini-Giampietro, D. E., Mannaioni, G., and Bagetta, G. (2009). Post-ischemic brain damage: The endocannabinoid system in the mechanisms of neuronal death. FEBS J. 276, 2–12. doi: 10.1111/j.1742-4658.2008.06765.x
Pertwee, R. G., Nash, K., and Trayhurn, P. (1991). Evidence that the hypothermic response of mice to delta-9-tetrahydrocannabinol is not mediated by changes in thermogenesis in brown adipose tissue. Can. J. Physiol. Pharmacol. 69, 767–770. doi: 10.1139/y91-114
Prenderville, J. A., Kelly, A. M., and Downer, E. J. (2015). The role of cannabinoids in adult neurogenesis. Br. J. Pharmacol. 172, 3950–3963. doi: 10.1111/bph.13186
Rajesh, M., Mukhopadhyay, P., Bátkai, S., Haskó, G., Liaudet, L., Huffman, J. W., et al. (2007). CB2-receptor stimulation attenuates TNF-alpha-induced human endothelial cell activation, transendothelial migration of monocytes, and monocyte-endothelial adhesion. Am. J. Physiol. Heart Circ. Physiol. 293, H2210–H2218. doi: 10.1152/ajpheart.00688.2007
Ramirez, B. G., Blazquez, C., Gomez Del Pulgar, T., Guzman, M., and De Ceballos, M. L. (2005). Prevention of Alzheimer's disease pathology by cannabinoids: Neuroprotection mediated by blockade of microglial activation. J. Neurosci. 25, 1904–1913. doi: 10.1523/JNEUROSCI.4540-04.2005
Rivers, J. R., and Ashton, J. C. (2010). The development of cannabinoid CBII receptor agonists for the treatment of central neuropathies. Cent. Nerv. Syst. Agents Med. Chem. 10, 47–64. doi: 10.2174/187152410790780145
Rivers-Auty, J. R., Smith, P. F., and Ashton, J. C. (2014). The cannabinoid CB2 receptor agonist GW405833 does not ameliorate brain damage induced by hypoxia-ischemia in rats. Neurosci. Lett. 569, 104–109. doi: 10.1016/j.neulet.2014.03.077
Robin, L. M., Oliveira Da Cruz, J. F., Langlais, V. C., Martin-Fernandez, M., Metna-Laurent, M., Busquets-Garcia, A., et al. (2018). Astroglial CB(1) receptors determine synaptic D-serine availability to enable recognition memory. Neuron 98, 935–944.e5. doi: 10.1016/j.neuron.2018.04.034
Rock, E. M., Bolognini, D., Limebeer, C. L., Cascio, M. G., Anavi-Goffer, S., Fletcher, P. J., et al. (2012). Cannabidiol, a non-psychotropic component of cannabis, attenuates vomiting and nausea-like behaviour via indirect agonism of 5-HT(1A) somatodendritic autoreceptors in the dorsal raphe nucleus. Br. J. Pharmacol. 165, 2620–2634. doi: 10.1111/j.1476-5381.2011.01621.x
Sanders, R. D., Manning, H. J., Robertson, N. J., Ma, D., Edwards, A. D., Hagberg, H., et al. (2010). Preconditioning and postinsult therapies for perinatal hypoxic-ischemic injury at term. Anesthesiology 113, 233–249. doi: 10.1097/ALN.0b013e3181dc1b84
Shalak, L. F., Laptook, A. R., Velaphi, S. C., and Perlman, J. M. (2003). Amplitude-integrated electroencephalography coupled with an early neurologic examination enhances prediction of term infants at risk for persistent encephalopathy. Pediatrics 111, 351–357. doi: 10.1542/peds.111.2.351
Signorini, C., Ciccoli, L., Leoncini, S., Carloni, S., Perrone, S., Comporti, M., et al. (2009). Free iron, total F-isoprostanes and total F-neuroprostanes in a model of neonatal hypoxic-ischemic encephalopathy: Neuroprotective effect of melatonin. J. Pineal Res. 46, 148–154. doi: 10.1111/j.1600-079X.2008.00639
Sinor, A. D., Irvin, S. M., and Greenberg, D. A. (2000). Endocannabinoids protect cerebral cortical neurons from in vitro ischemia in rats. Neurosci. Lett. 278, 157–160. doi: 10.1016/s0304-3940(99)00922-2
Soethoudt, M., Grether, U., Fingerle, J., Grim, T. W., Fezza, F., De Petrocellis, L., et al. (2017). Cannabinoid CB2 receptor ligand profiling reveals biased signalling and off-target activity. Nat. Commun. 8:13958. doi: 10.1038/ncomms13958
Sola, A., Wen, T. C., Hamrick, S. E., and Ferriero, D. M. (2005). Potential for protection and repair following injury to the developing brain: a role for erythropoietin? Pediatr. Res. 57, 110R–117R. doi: 10.1203/01.PDR.0000159571.50758.39
Solbrig, M. V., Fan, Y., Hermanowicz, N., Morgese, M. G., and Giuffrida, A. (2010). A synthetic cannabinoid agonist promotes oligodendrogliogenesis during viral encephalitis in rats. Exp. Neurol. 226, 231–241. doi: 10.1016/j.expneurol.2010.09.003
Stempel, A. V., Stumpf, A., Zhang, H. Y., Ozdogan, T., Pannasch, U., Theis, A. K., et al. (2016). Cannabinoid type 2 receptors mediate a cell type-specific plasticity in the hippocampus. Neuron 90, 795–809. doi: 10.1016/j.neuron.2016.03.034
Sugiura, T., Kondo, S., Sukagawa, A., Nakane, S., Shinoda, A., Itoh, K., et al. (1995). 2-Arachidonoylglycerol: A possible endogenous cannabinoid receptor ligand in brain. Biochem. Biophys. Res. Commun. 215, 89–97. doi: 10.1006/bbrc.1995.2437
Sugiura, T., and Waku, K. (2002). Cannabinoid receptors and their endogenous ligands. J. Biochem. 132, 7–12. doi: 10.1093/oxfordjournals.jbchem.a003200
Torfs, C. P., Van Den Berg, B., Oechsli, F. W., and Cummins, S. (1990). Prenatal and perinatal factors in the etiology of cerebral palsy. J. Pediatr. 116, 615–619. doi: 10.1016/S0022-3476(05)81615-4
Turcotte, C., Blanchet, M. R., Laviolette, M., and Flamand, N. (2016). The CB2 receptor and its role as a regulator of inflammation. Cell. Mol. Life Sci. 73, 4449–4470. doi: 10.1007/s00018-016-2300-4
Van Bel, F., Shadid, M., Moison, R. M., Dorrepaal, C. A., Fontijn, J., Monteiro, L., et al. (1998). Effect of allopurinol on postasphyxial free radical formation, cerebral hemodynamics, and electrical brain activity. Pediatrics 101, 185–193. doi: 10.1542/peds.101.2.185
Viscomi, M. T., Oddi, S., Latini, L., Pasquariello, N., Florenzano, F., Bernardi, G., et al. (2009). Selective CB2 receptor agonism protects central neurons from remote axotomy-induced apoptosis through the PI3K/Akt pathway. J. Neurosci. 29, 4564–4570. doi: 10.1523/JNEUROSCI.0786-09.2009
Wang, X., Dow-Edwards, D., Keller, E., and Hurd, Y. L. (2003). Preferential limbic expression of the cannabinoid receptor mRNA in the human fetal brain. Neuroscience 118, 681–694. doi: 10.1016/S0306-4522(03)00020-4
Whitney, N. P., Eidem, T. M., Peng, H., Huang, Y., and Zheng, J. C. (2009). Inflammation mediates varying effects in neurogenesis: Relevance to the pathogenesis of brain injury and neurodegenerative disorders. J. Neurochem. 108, 1343–1359. doi: 10.1111/j.1471-4159.2009.05886.x
Wu, J., Hocevar, M., Foss, J. F., Bie, B., and Naguib, M. (2017). Activation of CB2 receptor system restores cognitive capacity and hippocampal Sox2 expression in a transgenic mouse model of Alzheimer's disease. Eur. J. Pharmacol. 811, 12–20. doi: 10.1016/j.ejphar.2017.05.044
Wyatt, J. S., Gluckman, P. D., Liu, P. Y., Azzopardi, D., Ballard, R., Edwards, A. D., et al. (2007). Determinants of outcomes after head cooling for neonatal encephalopathy. Pediatrics 119, 912–921. doi: 10.1542/peds.2006-2839
Xapelli, S., Agasse, F., Grade, S., Bernardino, L., Ribeiro, F. F., Schitine, C. S., et al. (2014). Modulation of subventricular zone oligodendrogenesis: A role for hemopressin? Front. Cell. Neurosci. 8:59. doi: 10.3389/fncel.2014.00059
Xapelli, S., Agasse, F., Sarda-Arroyo, L., Bernardino, L., Santos, T., Ribeiro, F. F., et al. (2013). Activation of type 1 cannabinoid receptor (CB1R) promotes neurogenesis in murine subventricular zone cell cultures. PLoS One 8:e63529. doi: 10.1371/journal.pone.0063529
Yates, M. L., and Barker, E. L. (2009). Organized trafficking of anandamide and related lipids. Vitam. Horm. 81, 25–53. doi: 10.1016/S0083-6729(09)81002-9
Zarruk, J. G., Fernandez-Lopez, D., Garcia-Yebenes, I., Garcia-Gutierrez, M. S., Vivancos, J., Nombela, F., et al. (2012). Cannabinoid type 2 receptor activation downregulates stroke-induced classic and alternative brain macrophage/microglial activation concomitant to neuroprotection. Stroke 43, 211–219. doi: 10.1161/STROKEAHA.111.631044
Zhang, M., Martin, B. R., Adler, M. W., Razdan, R. K., Jallo, J. I., and Tuma, R. F. (2007). Cannabinoid CB(2) receptor activation decreases cerebral infarction in a mouse focal ischemia/reperfusion model. J. Cereb. Blood Flow Metab. 27, 1387–1396. doi: 10.1038/sj.jcbfm.9600447
Zhang, M., Martin, B. R., Adler, M. W., Razdan, R. J., Kong, W., Ganea, D., et al. (2009). Modulation of cannabinoid receptor activation as a neuroprotective strategy for EAE and stroke. J. Neuroimmune Pharmacol. 4, 249–259. doi: 10.1007/s11481-009-9148-4
Zhou, Y., Sun, L., and Wang, H. (2023). Ketogenic diet for neonatal hypoxic-ischemic encephalopathy. ACS Chem. Neurosci. 14, 1–8. doi: 10.1021/acschemneuro.2c00609
Zhu, C., Wang, X., Cheng, X., Qiu, L., Xu, F., Simbruner, G., et al. (2004). Post-ischemic hypothermia-induced tissue protection and diminished apoptosis after neonatal cerebral hypoxia-ischemia. Brain Res. 996, 67–75. doi: 10.1016/j.brainres.2003.10.013
Keywords: cannabinoids, endocannabinoid system, HIE, neuroprotective, brain development
Citation: Xiao J, Zhou Y, Sun L and Wang H and (2023) Role of integrating cannabinoids and the endocannabinoid system in neonatal hypoxic-ischaemic encephalopathy. Front. Mol. Neurosci. 16:1152167. doi: 10.3389/fnmol.2023.1152167
Received: 27 January 2023; Accepted: 16 March 2023;
Published: 12 April 2023.
Edited by:
Valentino Laquintana, University of Bari Aldo Moro, ItalyReviewed by:
Giuditta Gambino, University of Palermo, ItalyCopyright © 2023 Xiao, Zhou, Sun and Wang. This is an open-access article distributed under the terms of the Creative Commons Attribution License (CC BY). The use, distribution or reproduction in other forums is permitted, provided the original author(s) and the copyright owner(s) are credited and that the original publication in this journal is cited, in accordance with accepted academic practice. No use, distribution or reproduction is permitted which does not comply with these terms.
*Correspondence: Haichuan Wang, d2hjMTg1ODI1MjQxODJAMTYzLmNvbQ==
†These authors have contributed equally to this work
Disclaimer: All claims expressed in this article are solely those of the authors and do not necessarily represent those of their affiliated organizations, or those of the publisher, the editors and the reviewers. Any product that may be evaluated in this article or claim that may be made by its manufacturer is not guaranteed or endorsed by the publisher.
Research integrity at Frontiers
Learn more about the work of our research integrity team to safeguard the quality of each article we publish.