- 1Graduate School, Tianjin University of Chinese Medicine, Tianjin, China
- 2Department of Clinical Pharmacology, The Second Affiliated Hospital of Tianjin University of Chinese Medicine, Tianjin, China
Glutamate plays an important role in excitotoxicity and ferroptosis. Excitotoxicity occurs through over-stimulation of glutamate receptors, specifically NMDAR, while in the non-receptor-mediated pathway, high glutamate concentrations reduce cystine uptake by inhibiting the System Xc-, leading to intracellular glutathione depletion and resulting in ROS accumulation, which contributes to increased lipid peroxidation, mitochondrial damage, and ultimately ferroptosis. Oxidative stress appears to crosstalk between excitotoxicity and ferroptosis, and it is essential to maintain glutamate homeostasis and inhibit oxidative stress responses in vivo. As researchers work to develop natural compounds to further investigate the complex mechanisms and regulatory functions of ferroptosis and excitotoxicity, new avenues will be available for the effective treatment of ischaemic stroke. Therefore, this paper provides a review of the molecular mechanisms and treatment of glutamate-mediated excitotoxicity and ferroptosis.
1. Introduction
Ischemic stroke is the primary cause of death and disability in Chinese adults, characterized by high morbidity, disability, mortality, and recurrence rate (Sturm et al., 2002; Gao J. et al., 2022). According to statistics, the age-standardized prevalence of stroke in China in 2013 was 1114.8 per 100,000, with an incidence rate of 246.8 per 100,000 and a mortality rate of 114.8 per 100,000 (Wang W. et al., 2017), The Continuous Stroke Surveillance Program in 31 Chinese provinces reported an annual increase of 8.3% in the incidence of first stroke in adults, from 189 cases per 100,000 people in 2002 to 379 cases per 100,000 people in 2013,with the incidence of ischaemic stroke and hemorrhagic stroke at 335 per 100,000 population and 44 per 100,000 population, respectively, in 2013 (He et al., 2022). In the United States, more than 795,000 people suffer a stroke each year, accounting for about one in 10 deaths in the United States, and is the leading cause of long-term disability in the country (Engler-Chiurazzi et al., 2017; Ho et al., 2019; Barthels and Das, 2020). By 2050, more than 150 million people worldwide will be 65 and over (Feigin et al., 2014; Thomazi et al., 2018; He and Zhou, 2020),the number of people suffering from stroke is expected to increase steadily in the coming decades as the population ages (Boudreau et al., 2013; Ji et al., 2022; Kevdzija et al., 2022; Tsao et al., 2022; Zhou et al., 2022).
The central premise of ischaemic stroke treatment is to limit infarction by rapid and effective recanalization of occluded vessels, leading to reperfusion of the ischaemic semidark zone, and there have been significant advances in the treatment of patients with ischaemic stroke over the last decade or so of research (Bivard et al., 2017; Malysz-Cymborska et al., 2021). Currently, drugs commonly used to treat ischaemic stroke include drugs to improve cerebral circulation, neuroprotective agents, and herbs to activate blood circulation and resolve blood stasis. The only thrombolytic medication that has received FDA approval is tissue fibrinogen activator (tPA), but its clinical application is restricted to a certain time window (Fukuta et al., 2017; Hu et al., 2022; Yoon et al., 2022). A recent meta-analysis of individual participant data on alteplase showed that, regardless of age or stroke severity, giving alteplase within 4–5 h of stroke onset significantly improved the overall odds of a good stroke prognosis, despite an increased risk of fatal intracranial hemorrhage within a few days of treatment, and the earlier the treatment, the greater the proportion of benefit. However, recanalisation success rates were lower with intravenous administration of alteplase, thus reducing overall efficacy (Emberson et al., 2014; Leiva-Salinas et al., 2016). Although numerous studies have shown that inflammation, oxidative stress, excitotoxicity, calcium overload, apoptosis, and disruption of the blood–brain barrier are causative mechanisms of ischaemic stroke, preclinical protective agents targeting one of these mechanisms have not been used in the clinic (Dirnagl et al., 1999; He et al., 2013). Therefore, there is an urgent need to better understand the physio-pathological mechanisms that regulate these complex molecular effects in order to facilitate the research and development of new drugs and improve patient prognosis (Zou et al., 2022). This article critically discusses the role of glutamate receptor-mediated excitotoxicity and cystine/glutamate antiporter-mediated ferroptosis in ischemic stroke, as shown in Figure 1.
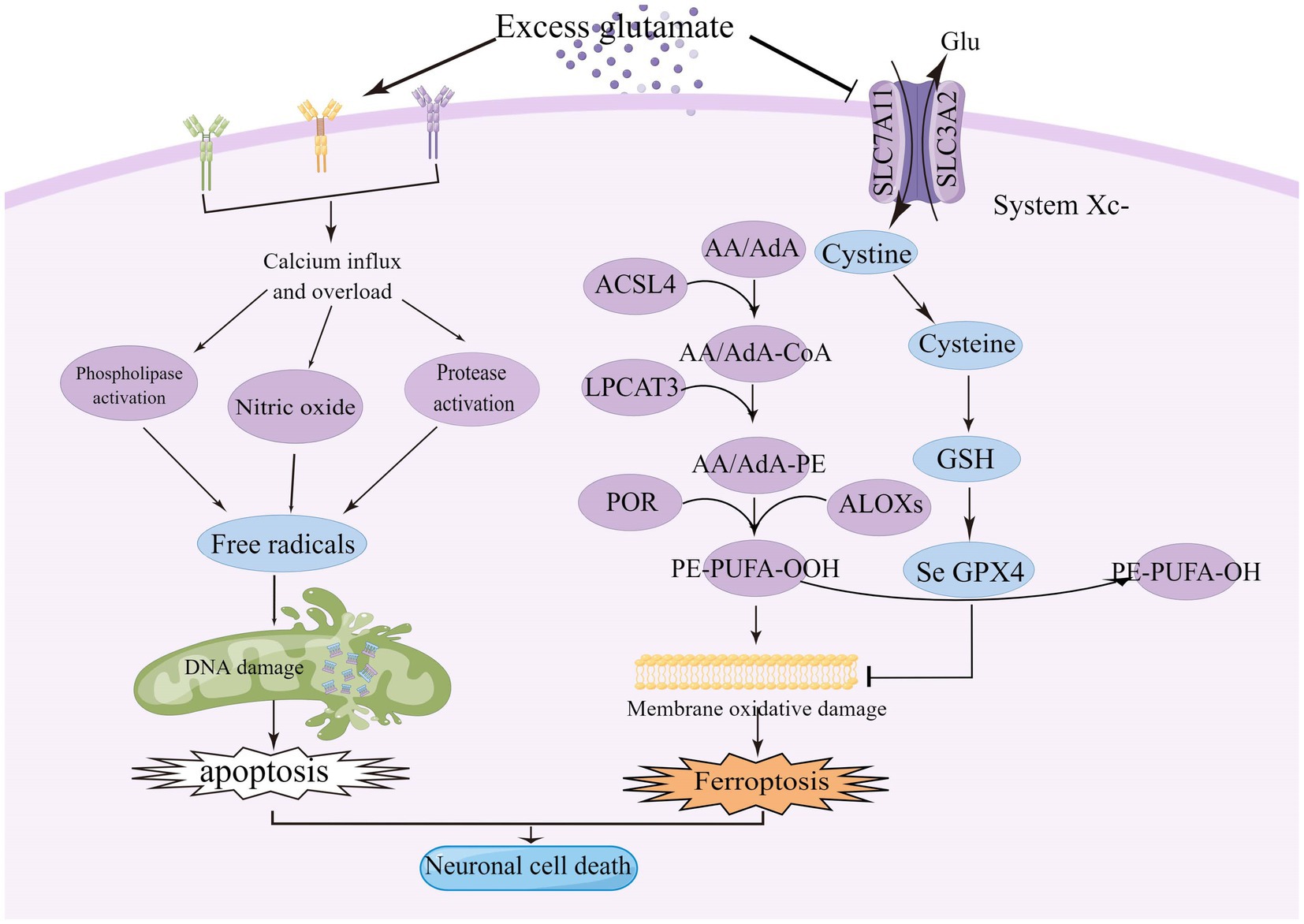
Figure 1. Excitotoxicity is caused by over-stimulation of glutamate receptors, particularly NMDAR, leading to high calcium influx, mitochondrial dysfunction, and DNA breakage. High levels of glutamate reduce the uptake of cystine via the Xc-system, leading to intracellular glutathione depletion resulting in the accumulation of reactive oxygen species (ROS), which increases lipid peroxidation, mitochondrial damage and ultimately ferroptosis. ACSL4, acyl-coenzyme A synthase long chain family member 4; System Xc-, cystine/glutamate reverse transporter; LPCAT3, lysophosphatidylcholine acyltransferase 3; AA, arachidonic acid; AdA, adrenoyl acid; ALOXs, lipoxygenases; CoA, coenzyme A; POR, cytochrome p450 oxidoreductase; GPX4, glutathione peroxidase 4; and GSH, glutathione.
2. Glutamate receptor-mediated excitotoxicity
2.1. The role of glutamate in synaptic transmission
Glutamate is the main excitatory neurotransmitter in the central nervous system (CNS) and is closely linked to synaptic activity, plasticity, cell death and survival, learning and memory, and pain perception (Byrnes et al., 2009; Arteaga Cabeza et al., 2021). Excitotoxicity, a toxic effect of excessive or prolonged glutamate activation of the receptor, was first studied by Dr. Olney (Wang S. et al., 2017; McCaughey-Chapman and Connor, 2022). Excitotoxicity, excessive and pathological stimulation of neurons, associated with neuronal death in many neurological diseases, including ischaemia, traumatic brain injury, and neurodegenerative diseases (Connolly et al., 2016; Krasil'nikova et al., 2019). All intercellular signaling is dependent on chemical signals, and glutamate is one of the most important intercellular chemical signals in the nervous system (Özel et al., 2014; Teng et al., 2016).
Glutamate is approximately 5–15 mmol/kg in brain tissue, 5–10 mM in neurons, and 30–50 μM in plasma, with glutamate concentrations fluctuating in response to body metabolism, diet, etc. (Bramham et al., 1990; Ottersen et al., 1990, 1992; Osen et al., 1995; Danbolt, 2001). Glutamate concentrations in neurons are highest at axon terminals, which means that axon terminals somehow restrict glutamate movement or local synthesis and utilization of glutamate, and glutaminase is responsible for glutamate synthesis in most neurons (Márquez et al., 2009; Barbano et al., 2020; Pietrancosta et al., 2020). Glutamate in neurons is concentrated in synaptic vesicles via the vesicular glutamate transporter (VGLUT) and released into the extracellular space when the neuron is depolarized (Takamori et al., 2000; Takamori, 2006). Glutamate concentrations are highest in teleneuron and up to 100 mM in synaptic vesicles (Riveros et al., 1986; Burger et al., 1989; Shupliakov et al., 1992). When an action potential reaches the presynaptic terminal, Ca2+ influx via voltage-gated calcium channels (VGCC) triggers the fusion of vesicles loaded with neurotransmitter with the cell membrane, thereby releasing neurotransmitter in the synaptic cleft (Nishimune et al., 2016; Liang et al., 2021; Tukker and Westerink, 2021; Fedorovich and Waseem, 2022). Glutamate is secreted into the synaptic gap where it can diffuse around the neuron and interact with surrounding targets (Clewett et al., 2017), closest to the axon terminal is the postsynaptic membrane, which contains a large number of membrane-associated proteins, these “postsynaptic densities (PSD)” can be seen under the electron microscope (Kennedy, 1997; Xu Y. et al., 2021), PSDs contains a large number of glutamate receptors, which bind to glutamate and then trigger the postsynaptic cell to complete the synaptic transmission of glutamate signals from the presynaptic to the postsynaptic cell (Guo and Cordeiro, 2008; Terauchi and Umemori, 2012; Katayama et al., 2017). The transport pattern of glutamate is shown in Figure 2.
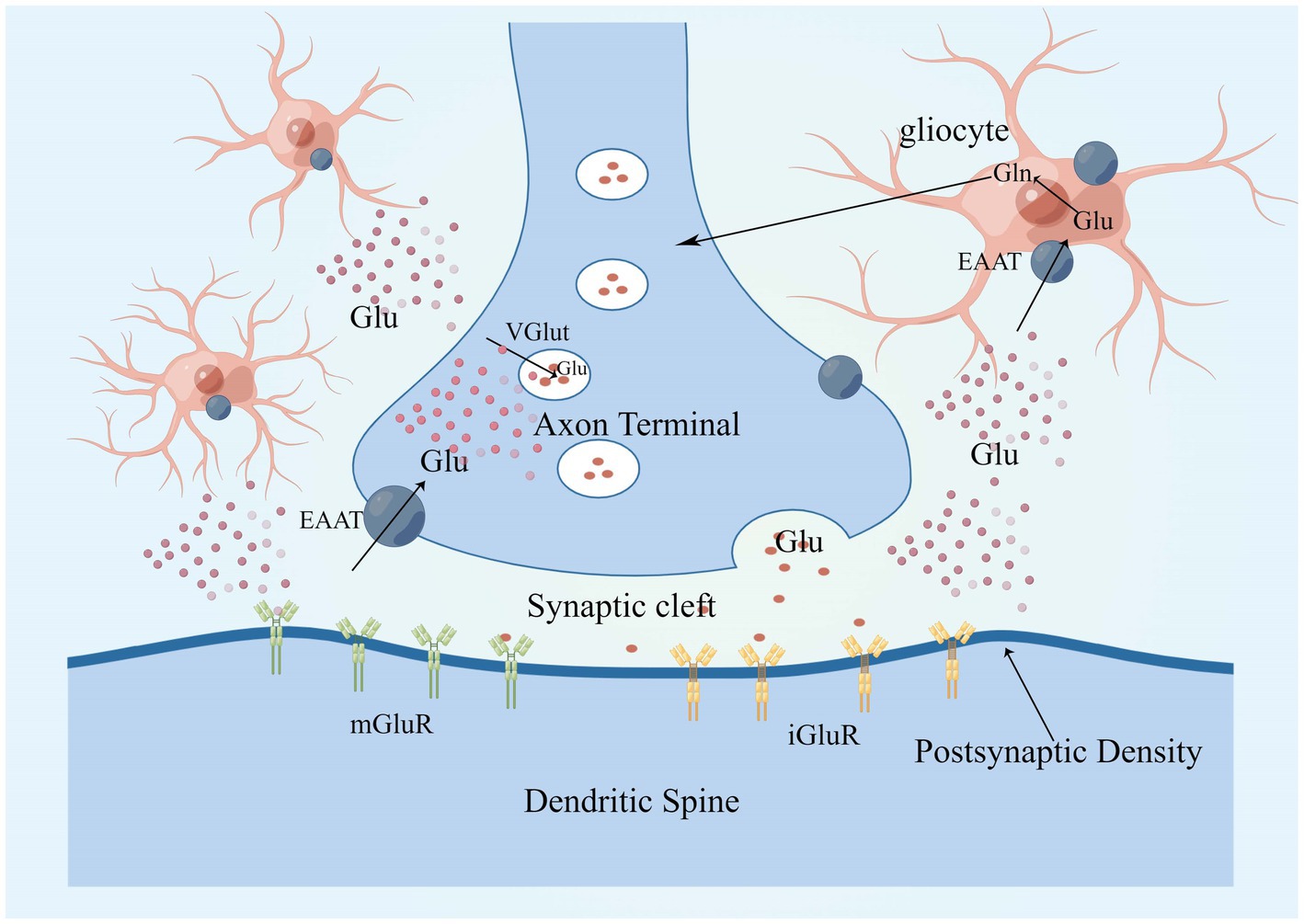
Figure 2. Glutamate in neurons is concentrated in synaptic vesicles via VGLUT and is released into the extracellular space in response to neuronal depolarization. There are two main classes of glutamate receptors, that is, mGluRs and iGluRs, glutamate clearance from the extracellular space takes place mostly through the high-affinity EAATs, EAAT 1 and 2 are mainly expressed in astrocytes. Glutamate enters glial cells via EAAT1 and EAAT2, where it is metabolized to glutamine, which is released into the extracellular space and converted to glutamate after uptake by neurons, completing a cycle. EAAT, excitatory amino acid transporter; mGluR, metabotropic glutamate receptor; iGluRs, ionotropic glutamate receptors; VGLUT, vesicular glutamate transporters; and PSD, postsynaptic density.
2.2. Type of glutamate receptor and mechanism of action
Excitotoxicity was one of the first mechanisms of ischemic cell death to be identified and one of the most intensively studied, with the term “excitotoxicity” describing the process by which excess glutamate overactivates NMDA receptors (NMDARs) and induces neuronal toxicity (Choi et al., 1988; Garthwaite et al., 1992). There are two types of glutamate receptors: ionotropic glutamate receptors (iGluRs), which are ligand-gated ion channels, and metabotropic glutamate receptors (mGluRs), which are G protein-coupled receptors (Pin and Duvoisin, 1995; Ferraguti and Shigemoto, 2006). The ionotropic receptors include kainate (KA) receptors, alpha-amino-3-hydroxy-5-methyl-4-isoxazole propionic acid (AMPA) receptors, and N-methyl-D-aspartate (NMDA) receptors (Takahashi, 2019; Burada et al., 2020). iGluRs are ligand-gated ion channels that allow cations such as calcium and potassium to cross the plasma membrane after glutamate binding to the receptor (Wei et al., 2011; Rocha-Ferreira and Hristova, 2016).
NMDA receptors require a basic NR1 subunit and one or more regulatory NR2 subunits (NR2A-D), and also NR3 subunits (NR3A-B), in some specific cases (Ye et al., 2013; Rebas et al., 2020). In the resting state, NMDAR channels are normally blocked by Mg2+, but when large amounts of glutamate accumulate, activated AMPAR causes partial depolarization of the postsynaptic membrane, sufficient to clear the Mg2+ on the NMDAR. Among the currently known ionotropic and metabotropic glutamate receptors, NMDAR play an important role in allowing excess Ca2+ inward flow, leading to ischemic cell death (Mao et al., 2022). Calcium overload activates a large number of downstream pro-death signals such as calpain activation, reactive oxygen species (ROS) production, and mitochondrial damage (Fujimura et al., 1998; Kristián and Siesjö, 1998; Eliasson et al., 1999; Lau and Tymianski, 2010), resulting in cell necrosis or apoptosis (Köhr, 2006; Shi et al., 2017; Yoo et al., 2017; Maher et al., 2018). GluN2A and GluN2B play opposite roles in ischaemic stroke, with activation of GluN2B leading to excitotoxicity and neuronal apoptosis, while activation of GluN2A protects neurons (Liu et al., 2007; Chen et al., 2008). Under stress conditions, NMDAR2A activates the PI3K/Akt kinase pathway, promoting the expression of cAMP response element binding protein (CREB) related genes and inhibiting the expression of pro-death genes, and Akt promotes cell survival by phosphorylating many downstream targets (Wu and Tymianski, 2018). Akt also inactivates the pro-apoptotic Bcl-2 family member BAD (Bcl2/Bcl-XL-antagonist causing cell death) by phosphorylation, thus stopping its interaction with and blockade of the pro-survival Bcl-2 family members Bcl-2 and Bcl-XL (Papadia and Hardingham, 2007). The JNK/p38 activator ASK1 is also inhibited by phosphorylation by Akt, and the activity of p53 is inhibited by Akt, resulting in reduced Bax expression (Kim et al., 2001; Yamaguchi et al., 2001). CREB target genes include the anti-apoptotic BTG2, the apoptotic p53 inhibitor BCL6, and the neurotrophic factor BDNF (Hardingham et al., 2002; Hardingham, 2009). During synaptic contact, these receptors are present in high density in a specific region of the postsynaptic membrane, which is closely associated with the presynaptic active zone of glutamate release (Sheng and Hoogenraad, 2007). PSD-95 was found to bind to NMDAR2B and intracellular neuronal nitric oxide synthase (nNOS) as part of a scaffold synaptic protein, and in the presence of intracellular calcium, PSD-95 plays a crucial role in the mechanism by which NMDAR activity triggers the production of nitric oxide production by nNOS and excitotoxicity (Cui et al., 2007; Forder and Tymianski, 2009; Abergel, 2020). NO combines with superoxide radicals to produce large amounts of nitrite, which leads to protein oxidation, lipid peroxidation, and DNA damage (Lipton et al., 1993), as shown in the Figure 3.
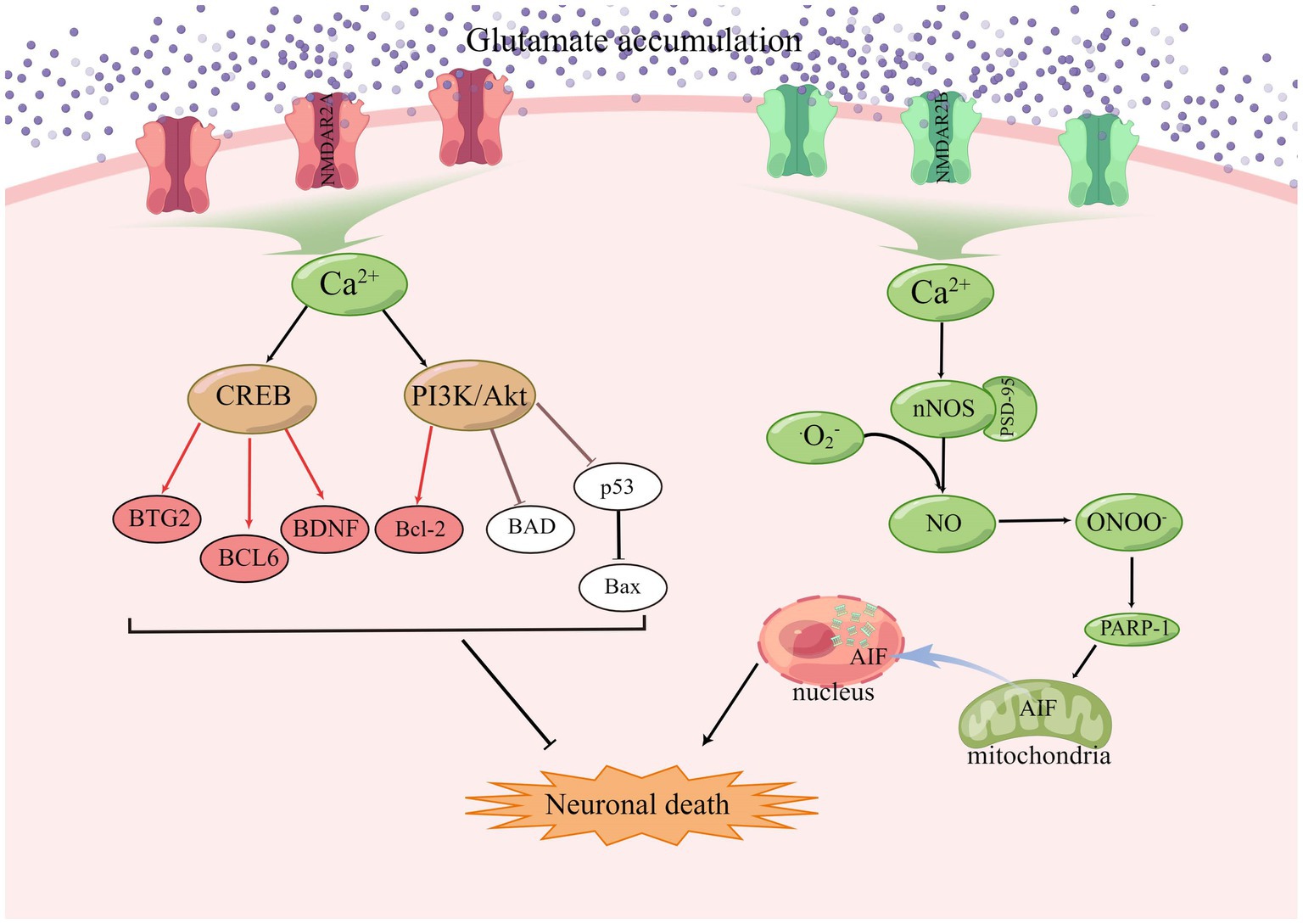
Figure 3. In ischaemic stroke, GluN2A and GluN2B play opposing roles, with GluN2B activation leading to excitotoxicity and apoptosis and GluN2A activation promoting cell survival.
AMPAR is constructed from four subunits (GluR1-4; Hwang and Lupica, 2020; Zhang et al., 2022). Under resting conditions, the NMDAR channel pores are blocked by Mg2+ ions and once sufficient membrane depolarization has been established, the Mg2+ block is removed, allowing the influx of cations (Lin et al., 2008; Olive, 2009).AMPAR activation increases Na+ influx into neurons, depolarizes membranes, and activates voltage-dependent Ca2+ channels and NMDARs (Andriessen et al., 2010), the substitution of a positively-charged arginine residue for a neutrally-charged glutamine residue at the apex of the membrane reentrant pore loop (M2) changes the conductance properties of channels containing an edited GluR2 subunit (Köhr et al., 1998; Hood and Emeson, 2012). Most GluR2 subunits expressed in the mature rat cochlea are edited form and therefore, when incorporated into AMPA receptors, render the GluR complex calcium impermeable (Carriedo et al., 1996; Graham et al., 2011; Basappa et al., 2012). Molecular cloning has identified five isoforms, named GluK1, GluK2, GluK3, GluK4, and GluK5 according to the new IUPHAR nomenclature, which form functional receptors in various combinations (Dingledine et al., 1999).
3. Excess glutamate accumulation can inhibit the cystine/glutamate reverse transporter and lead to ferroptosis
Since 2005, the Nomenclature Committee on Cell Death (NCCD) has updated the classification system and in 2018 introduced an updated version based on molecular mechanisms, in which cell death is divided into two parts, accidental cell death (ACD), and regulated cell death (RCD; Galluzzi et al., 2018; Čepelak et al., 2020). There are several types of RCD, including apoptotic and non-apoptotic (Shen et al., 2022). Ferroptosis cells often show a necrotic appearance, such as cell swelling, plasma membrane rupture, and mitochondrial damage, unlike apoptotic cells, which are characterized by membrane blistering and contraction (Hou et al., 2021). Ferroptosis is a newly identified form of cell death caused by iron-dependent lipid peroxidation. Which leads to cell membrane damage and the accumulation of reactive lipid hydroperoxides to lethal levels (Munro et al., 2022). Our original knowledge of the molecular mechanisms of ferroptosis stemmed from studies using small molecule compounds to selectively inhibit cancer cells with oncogenic RAS mutations (Chen et al., 2021; Andreani et al., 2022). Ca2+ plays a fundamental role in glutamate-mediated excitotoxicity or oxidation-mediated cell death, a form of programmed cell death similar to or possibly identical to ferroptosis (Tan et al., 2001; Maher et al., 2018). Inhibiting System Xc- and inactivating GSH peroxidase-4 (GPX4) causes cellular glutathione (GSH) depletion and impaired ROS scavenging, resulting in disruption of cellular redox homeostasis, accumulation of ROS in the lipid peroxidation or Fenton reaction, and ultimately cell death (Shi et al., 2021).
3.1. Characteristics of ferroptosis
Ferroptosis cells undergo morphological changes at both the cellular and ultrastructural levels: the plasma membrane loses its integrity, the cytoplasm becomes enlarged, the mitochondria become smaller than normal cells, the mitochondrial cristae shrink or disappear, the outer mitochondrial membrane ruptures and the membrane density increases (Dolma et al., 2003; Yagoda et al., 2007; Dixon et al., 2012; Friedmann Angeli et al., 2014; Vanden Berghe et al., 2014). Mitochondria are an important source of ROS. Recent studies have found that impaired mitochondrial function leading to ROS production, DNA stress, and metabolic reprogramming is responsible for lipid peroxidation and ferroptosis (Gao et al., 2019; Lee et al., 2020; Li et al., 2021). Ferroptosis is mainly associated with iron accumulation and lipid peroxidation. Excess iron combines with hydrogen peroxide in a Fenton reaction to produce large amounts of hydroxyl radicals, increasing oxidative damage. Iron also increases the activity of lipoxygenase (ALOX) or prolyl hydroxylase (PHD), further aggravating lipid peroxidation (Chen X. et al., 2020; Lin et al., 2021; Tang D. et al., 2021). Lipid peroxidation occurs as a free radical-driven reaction that primarily affects the metabolism of polyunsaturated fatty acids (PUFAs) in cell membranes (Gao Q. et al., 2022; Nie et al., 2022). Lipopolymer peroxidation products include the initial lipid hydroperoxide (LOOH) and the subsequent reactive aldehyde (MDA,4-HNE), which increase during ferroptosis (Nie et al., 2022). The PTGS2 gene encodes prostaglandin endoperoxide synthase (PTGS), a key enzyme in prostaglandin biosynthesis (Yang et al., 2014). Acyl-Coenzyme A synthase long chain family member 4 (ACSL4) is thought to be a specific biomarker and driver of ferroptosis as it is a key enzyme involved in fatty acid metabolism. Upregulation of ACSL4 leads to an increase in polyunsaturated fatty acid content in phospholipids, which are particularly susceptible to oxidative reactions and ultimately ferroptosis (Yuan et al., 2016; Doll et al., 2017). Activation of transcriptional pathways of genes responsible for antioxidant defense (GSH, CoQ10, and NRF2) and membrane repair (ESCRT-III) limits membrane damage during ferroptosis (Dixon et al., 2012; Sun et al., 2016; Bersuker et al., 2019; Doll et al., 2019; Dai et al., 2020). The dynamic balance between damage and resistance to damage determines the survival or death of cells.
3.2. Critical role of amino acid metabolism and lipid metabolism in ferroptosis
3.2.1. Amino acid metabolism
Cystine/glutamate reverse transporter (System Xc-) is an amino acid reverse transporter protein that mediates the inward flow of cystine and the outward flow of glutamate (Kagami et al., 2018; Wang et al., 2020; Marcoli et al., 2022). The cystine taken into the cell is reduced to cysteine, part of which participates in intracellular GSH synthesis and the other part flows out of the cell to be converted to cystine and re-involved in the System Xc- (Liu N. et al., 2020; Tang Z. et al., 2021). Glutathione is an antioxidant and an important indicator of oxidative stress in cells (Guo et al., 2012). When there is too much extracellular glutamate, it inhibits the function of the System Xc-, resulting in less cystine entering the cell, which is an excitatory neurotransmitter with neurotoxic and excitatory effects (Liao et al., 2018; Ratan, 2020). System Xc- mediates the uptake of cystine and the release of glutamate, thereby promoting the synthesis of GSH, which acts as a co-molecule with GPX-4 to assist in the scavenging of lipid peroxides to protect cells (Zhao et al., 2021). System Xc- is a heterodimeric protein consisting of one light chain and one heavy chain with a disulfide bond between the two chains (Chen et al., 2022; Wang Y. et al., 2022). The light chain subunit SLC7A11 is the primary transporter and is highly sensitive to cystine and glutamate, while the heavy chain subunit SLC3A2 acts essentially as a chaperone protein and plays an important role in the transport of SLC7A11 to the plasma membrane (Koppula et al., 2018). SLC7A11 is a 12-channel transmembrane protein with both its N and C termini in intracellular locations, whereas SLC3A2 is a single-transmembrane protein with its N terminus in intracellular locations and its c terminus in extracellular locations (Sato et al., 1999; Xu C. et al., 2021; Chen et al., 2022). In addition, Knockdown of SLC3A2 has been shown to result in a significant lowering of SLC7A11 protein levels, suggesting that SLC3A2 is critical in sustaining SLC7A11 protein stability (Nakamura et al., 1999; Shin et al., 2017; Koppula et al., 2018). Intracellular cysteine is an essential precursor of glutathione. Glutathione is a tripeptide synthesized by cysteine, glutamate and glycine (Koppula et al., 2018; Gan, 2019; Zhao et al., 2022). The biosynthesis of GSH involves two crucial steps, first by formation of gamma-glutamylcysteinyl linkage by formation of gamma-glutamyl cysteine, followed by the addition of glycine via glutathione synthase (GSS) to produce the tripeptide glutathione (Raza et al., 2022). Endogenous enzymes protect cells from damage caused by excess ROS, including superoxide dismutase which converts superoxide (O2−) to hydrogen peroxide (H2O2), glutathione peroxidase (GPX) which converts free H2O2 to water, glutathione reductase which converts glutathione disulfide to the sulfhydryl form and catalytic breakdown of H2O2 to water and oxygen by peroxidase (Mahmoud et al., 2014). Oxidation of glutathione by the action of GPX and reduction of glutathione by glutathione reductase (GR) at the expense of NADPH (Koppula et al., 2018). Thus, System Xc- is critically important for the uptake of cystine to produce cysteine for the maintenance of intracellular GSH levels.
Two transcription factors were identified that regulate SLC7A11, nuclear factor red lineage 2-related factor 2 (NRF2) and activating transcription factor 4 (ATF4). NRF2 is a master transcription factor that accounts for antioxidant responses (Becker et al., 2016; Kuo et al., 2022). Under normal physiological conditions, Nrf2 is ubiquitinated by the Keap1-Cullin3 ubiquitin ligase complex and is conventionally fragmented by the 26 s proteasome. In contrast, under oxidative stress conditions, ubiquitin ligase activity is blocked by modifying the cysteine residues in Keap1, thereby stabilizing and activating (Noguchi et al., 2018), stable NRF2 then translocates into the nucleus, binds to antioxidant response elements in the gene promoter region and regulates the transcription of a range of target genes involved in antioxidant defense and cellular redox maintenance (Ooi et al., 2018; Koppula et al., 2021a), Similarly, overexpression of NRF2 upregulated the expression levels of antioxidant genes such as SLC7A11 and promoted the synthesis of GSH (Shih et al., 2003). Consequently, SLC7A11 is one of the most important transcriptional targets that can mediate the anti-oxidant response.
Transcription factor ATF4 regulates the expression of genes involved in amino acid metabolism, redox homeostasis and endoplasmic reticulum stress response (Pakos-Zebrucka et al., 2016; Sazonova et al., 2021). Translation of ATF4 mRNA is silenced by two short UORFs located in the 5′ untranslated region (UTR). The kinase that is catalyzed by eIF2α phosphorylation is activated by various cellular stresses, such as amino acid deprivation, endoplasmic reticulum stress, and viral infection (Koppula et al., 2018; Scalise et al., 2020). Inhibition of eIF2alpha phosphorylation levels led to inhibition of ATF4 mRNA translation and decreased ATF4 protein levels, while increased eIF2alpha phosphorylation levels led to enhanced ATF4 mRNA translation and increased ATF4 protein (Pathak et al., 2019). One upstream kinase of eIF2α is general control non-repressor-2 (GCN2), which is activated by free tRNAs in the presence of amino acid deprivation (Scalise et al., 2020). Thus, during amino acid deletion, GCN2 phosphorylates eIF2α, leading to the inhibition of protein synthesis in general, while increasing the translation of the specific transcription factor ATF4 (Ferraz-Bannitz et al., 2021). ATF4 associates with amino acid response elements (AARE) and promotes the transcription of genes related to amino acid metabolism and stress response, in particular SLC7A11, thereby enabling cells to cope with amino acid-limited conditions (Koppula et al., 2021b). Indeed, SLC7A11 expression can be strongly induced by deprivation of a variety of amino acids, and SLC7A11 expression induced by amino acid deprivation is mainly mediated by ATF4 (Koppula et al., 2021b). In summary, these data support that amino acid deletion induces SLC7A11 expression through the GCN2-eIF2α-ATF4 signaling axis.
Several studies have shown that the above transcription factors regulate downstream biological effects, including ferroptosis, antioxidant, and nutrient-dependent, through the regulation of SLC7A11 expression. SLC7A11 inhibits ferroptosis by increasing intracellular cystine and promoting glutathione synthesis (Dixon et al., 2012). By increasing SLC7A11 expression, ATF4 and NRF2 at least partially inhibit ferroptosis, whereas p53 stimulates ferroptosis by repressing SLC7A11 expression (Jiang et al., 2015; Fan et al., 2017; Roh et al., 2017). A study showed that p53 inhibits cystine uptake and leads to ferroptosis by suppressing SLC7A11, a component of the cystine/glutamate countertransport protein. In addition, mutant p533KR is defective in p53-dependent cell cycle arrest, apoptosis and senescence, but retains the ability to inhibit SLC7A11 expression, thereby regulating cystine metabolism and ferroptosis (Jiang et al., 2015).
3.2.2. Lipid metabolism
Fatty acid metabolism is divided into anabolic and catabolic pathways, both of which are regulated by a variety of enzymes (Wakil and Abu-Elheiga, 2009). Fatty acid β-oxidation (FAO) in mitochondria normally consumes most of the fatty acids, leading to a reduction in lipid peroxidation. Cytoplasmic lipid droplets form the energy hub of almost all eukaryotic cells and when energy is available, they store energy in the form of esterified fatty acids and release them to local or distant tissues for oxidation (Goodman, 2019).
Lipids play an important role in cellular functions, including membrane formation, energy production, intra- and intercellular signaling, and the regulation of cell death. Oxidation of phospholipids contributes to ferroptosis in cells (Yang et al., 2016). Lipid peroxides are produced in cells by three main pathways: first, lipid ROS from iron via a non-enzymatic Fenton reaction, second, lipid peroxides from oxidation and esterification of PUFAs, and third, lipid peroxides from iron-catalyzed lipid autoxidation. AA is a PUFAs that can be converted to adrenal acid (AdA) by prolonged enzymes. The accumulation of oxygenated AA-PE and AdA-PE evokes intracellular ferroptosis. Free PUFAs can be ultimately converted to phosphatidylethanolamine (PE)-PUFAs-OOH by three important enzymes, ACSL4, lysophosphatidylcholine acyltransferase 3 (LPCAT3), and lipoxygenases (LOXs; Jiang et al., 2020). The formation of lipid peroxides involves the formation of AA-PE from phosphatidylethanolamine (PE), an essential component of cell membranes, and arachidonic acid (AA), a PUFA, catalyzed by ACSL4 and LPCAT3, which is then peroxidized by iron-dependent LOX to form AA- OH -PE, the major actuator of ferroptosis (Protchenko et al., 2021; Liu et al., 2022), this is shown in Figure 4. ACSL family made up of proteins on the endoplasmic reticulum and outer membrane, ACSLs are responsible for the formation of fatty acid acyl coenzyme a esters from free long-chain fatty acids. The ACSL family contains five enzymes, ACSL4 is one of a family of five isomers, but only ACSL4 has a specific effect on ferroptosis (Yan and Zhang, 2019; Capelletti et al., 2020).
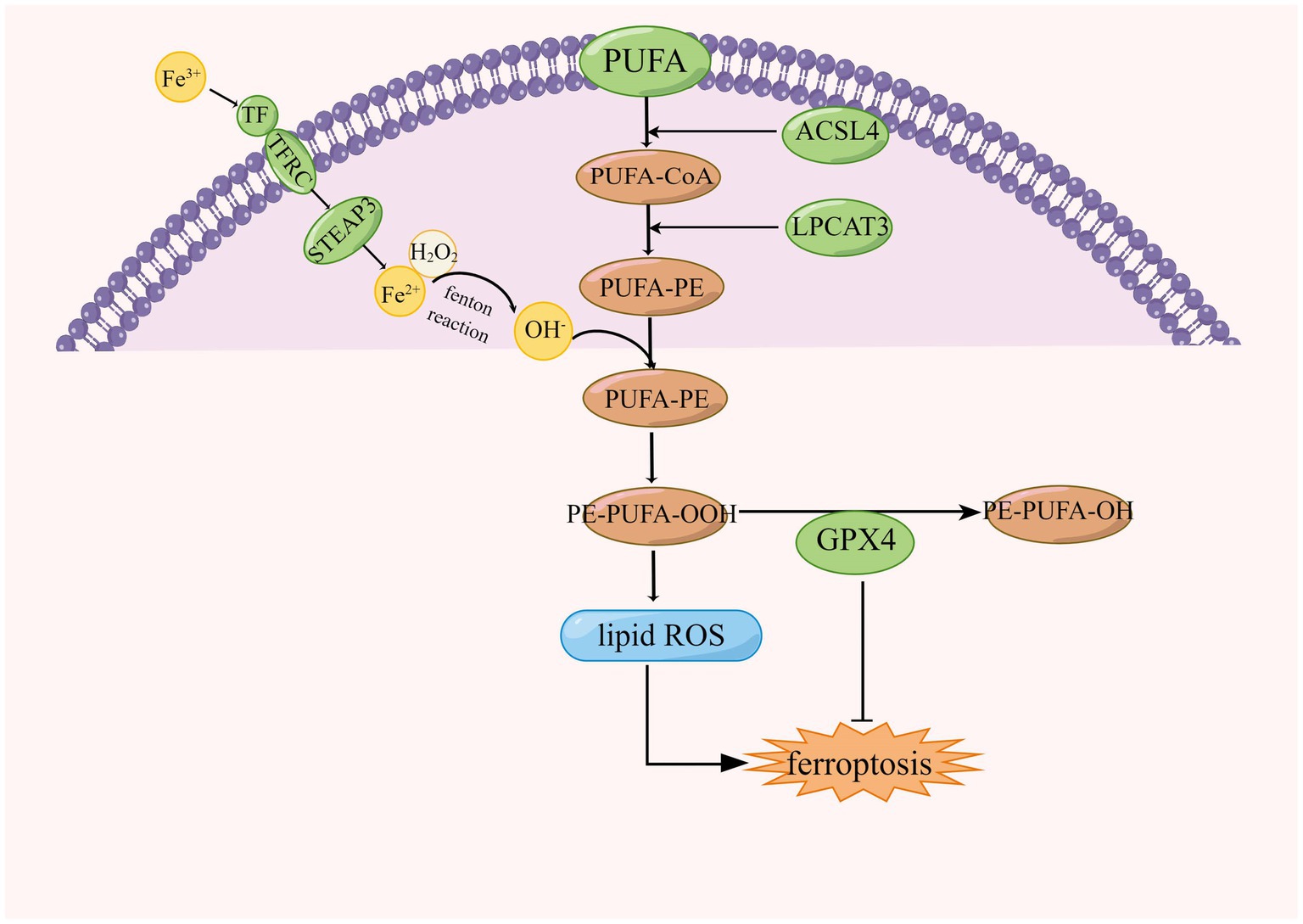
Figure 4. Regulation of lipid peroxidation in ferroptosis, ACSL4, acyl-coenzyme A synthase long chain family member 4; LPCAT3, lysophosphatidylcholine acyltransferase 3; CoA, coenzyme A; GPX4, glutathione peroxidase 4; and PUFA, polyunsaturated fatty acid.
4. Crosstalk between excitotoxicity and ferroptosis
Excitotoxicity is mainly due to excessive glutamate release during ischemia leading to excessive activation of NMDAR, which leads to intracellular calcium overload, ROS-induced oxidative stress, mitochondrial dysfunction, and impaired membrane permeability (Yoo et al., 2017), Ca2+ overload via glutamate receptor-induced cPLA2 activation produces neurotoxic metabolites such as prostaglandins, leukotrienes, ROS, and platelet-activating factor via AA and lysophospholipid metabolism. It is known to be particularly sensitive to ferroptosis as AA and ADA are the main substrates of lipid peroxidation (Liu Y. et al., 2020; She et al., 2020; Hong et al., 2022), this is shown in Figure 5. The ROS generation cascade also includes the reaction of superoxide with nitric oxide to form peroxynitrite, hydrogen peroxide catalyzed by peroxidase to form hypochlorous acid, and the Fenton reaction catalyzed by iron to form hydroxyl radicals (Lin et al., 2016; Griendling et al., 2021). Mitochondrial ROS are essential not only for apoptosis but also for ferroptosis, although the common mechanisms determining the relationship between the two different types of cell death remain obscure (Gao et al., 2019; Lee et al., 2020; Li et al., 2021; Tang Z et al., 2021). However, there appears to be crosstalk between oxidative stress and ferroptosis during the development of ischaemic stroke. Excess glutamate accumulates extracellularly during stroke, causing excessive NMDAR activation and neuroexcitotoxicity, as well as inducing NMDAR-mediated iron uptake (Cheah et al., 2006), as BBB dysfunction during stroke allows iron-containing substances to enter the brain and accumulate in areas of ischaemic brain tissue prior to neurodegeneration (Helal, 2008; DeGregorio-Rocasolano et al., 2019). Thus, crosstalk between iron and glutamate in neurons is a target for intervention that cannot be ignored.
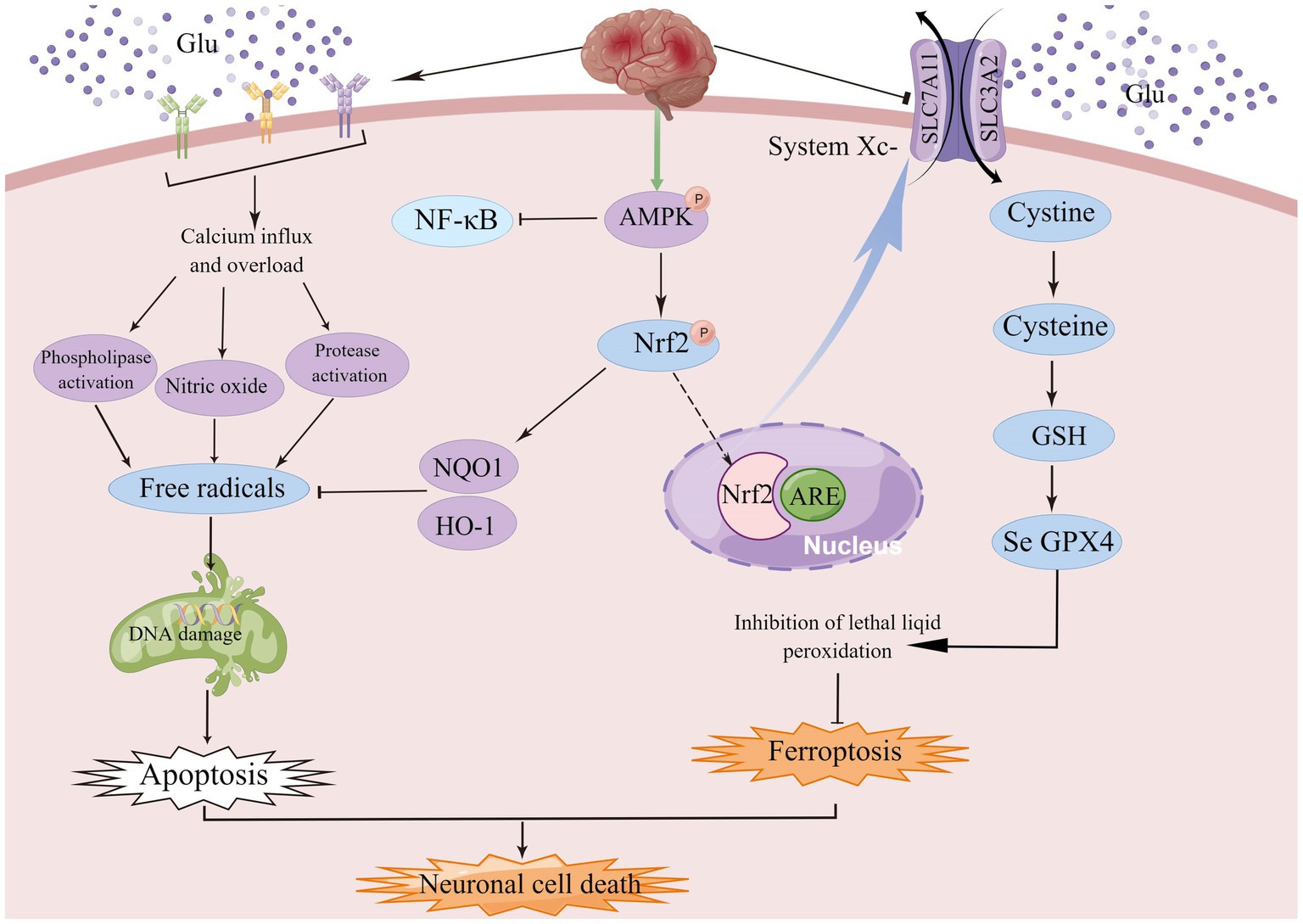
Figure 5. AMPK, a member of the serine/threonine kinase family, is an important endogenous defense factor against ischemia, and the Nrf2/ARE signaling pathway counteracts ischemia–reperfusion injury by inducing endogenous antioxidant defense factors and attenuating ROS production during reperfusion injury.
AMPK, a family member of serine/threonine kinases, is an invaluable endogenous defense factor against cerebral ischemia. During cerebral ischemia or hypoxia, the deprivation of energy and the consequent increase in the AMP/ATP ratio facilitates AMPK phosphorylation and initiates autophagy to bolster energy production (Jiang et al., 2014; Fang et al., 2018; Qin et al., 2022). AMPK activator A-769662 mimics the effects of silymarin and inhibits ROS production and neuronal cell death after OGD/R. In conclusion, these results suggest that silymarin-mediated neuroprotection may in part require activation of AMPK signaling (Xie et al., 2014). Under oxidative stress, Nrf2 is released from Keap1 and translocated to the nucleus, where it binds to the antioxidant response element (ARE) and upregulates the expression of NQO1 and HO-1 (Meng et al., 2014). The Nrf2/ARE signaling pathway counteracts ischemia–reperfusion injury by enhancing endogenous antioxidant defense factors and suppressing ROS production during reperfusion, which indicates that enhanced antioxidant properties can protect neurons (Young Park et al., 2019), and more importantly, NRF2 plays a key role in mediating iron/hemoglobin metabolism. NRF2 regulates the light and heavy chains of the iron storage protein ferritin (FTL/FTH1), and the iron transporter (SLC40A1) responsible for iron efflux from cells (Harada et al., 2011; Agyeman et al., 2012; Kerins and Ooi, 2018). NRF2 controls many of the enzymes that participate in glutathione synthesis and metabolism, including the catalytic and regulatory subunits of glutamate-cysteine ligase (GCLC/GCLM), glutathione synthase (GSS) and the subunit of the cystine/glutamate transporter xCT (SLC7A11), all of which are required for glutathione synthesis (Kwak et al., 2002; Sasaki et al., 2002). Among the multiple AMPK-related signaling pathways, the Nrf2 signaling pathway plays an important role in the regulation of genes and proteins with cytoprotective functions (Jiang et al., 2021). AMPK/NRF2 not only protects cells from oxidative stress damage, but also effectively regulates the expression of related genes to inhibit ferroptosis.
5. Treatment of ischemic stroke
5.1. Maintaining glutamate homeostasis
Glutamate is an important transmitter that plays a vital role in a variety of biological processes. Excess glutamate leads to over-stimulation of postsynaptic glutamatergic receptors, particularly NMDARs and AMPARs, allowing calcium to enter the cell, causing neuronal depolarisation and further neuronal death (Glotfelty et al., 2019), Inhibition of glutamate release, enhancement of glutamate clearance and blockade of glutamate receptors may be major directions for future stroke research. Methionine sulfoximine was found to be effective in inhibiting glutamate synthesis in mice (Ghoddoussi et al., 2010),Dextromethorphan can inhibit glutamate release by inhibiting presynaptic voltage-dependent calcium channels (VDCC; Lin et al., 2009). In terms of glutamate clearance, ceftriaxone effectively increases GLT expression in glial cells and enhances glutamate clearance (Lai et al., 2011). NMDAR inhibitors are widely studied drugs, and magnesium sulfate has shown a prominent role in protecting neurons from excitotoxicity by inhibiting NMDAR, reducing the transmission of the excitatory neurotransmitter glutamate, and reducing the inward flow of calcium ions (Ovbiagele et al., 2003). Memantine is a non-competitive NMDAR inhibitor. Memantine selectively blocks the over-activation of NMDAR in excitotoxicity and memantine increases the upregulation of brain-derived neurotrophic factor (BDNF) and glial cell-derived neurotrophic factor (Martínez-Coria et al., 2021). A meta-analysis showed no improvement in key outcome indicators and mortality in acute ischaemic stroke treated with magnesium sulfate (Avgerinos et al., 2019), it seems to be due to the fact that it is more difficult to treat effectively within the time window. Peritoneal dialysis has been demonstrated to decrease peripheral blood glutamate levels in rats with cerebral ischemia (Godino Mdel et al., 2013). Therefore, inhibition of glutamate synthesis, enhancement of glutamate clearance and inhibition of glutamate receptors play an important role in the protection of ischemic stroke.
5.2. Inhibition of calcium increase and oxidative stress
Calcium ions are a commonly present second messenger that regulates a variety of activities such as excitability, cytoplasmic division, motility, transcription and apoptosis in eukaryotic cells (Bootman, 2012; Carafoli and Krebs, 2016; Pchitskaya et al., 2018). The initial calcium influx following excitotoxic glutamate stimulation is known to trigger a secondary intracellular calcium overload, and this secondary response strongly correlates with neuronal death (Randall and Thayer, 1992; Tymianski et al., 1993). The plasma membrane sodium-calcium exchanger (NCX) is an essential modulator of intracellular calcium levels, using the force of sodium influx to expel calcium ions. The action of the NCX partially restores calcium ions to physiological levels following glutamate stimulation (White and Reynolds, 1995). Another major player in intracellular calcium homeostasis is the mitochondria, which can restore intracellular calcium concentrations by absorbing large amounts of calcium themselves (Valdinocci et al., 2019; Sanz-Morello et al., 2021), and by facilitating ATP-dependent calcium extrusion (Budd and Nicholls, 1996; White and Reynolds, 1996). Mitochondrial uptake of calcium in response to excitotoxic glutamate stimulation leads to ROS production (Castilho et al., 1999),excessive opening of the mitochondrial membrane permeability transition pore leads to a decrease in mitochondrial membrane potential (Castilho et al., 1999), induction of neuronal death (Stout et al., 1998; Ward et al., 2000). Therefore, inhibition of calcium increase and oxidative stress may be a therapeutic target in ischaemic stroke. Studies have demonstrated that the influx of calcium ions into cells during excitotoxicity is an essential pathway causing cell death, so interruption of the inward flow of extracellular calcium ions and decreasing the degree of calcium overload could theoretically protect neuronal cells to a large extent. Calcium antagonists have been proven in animal experiments to dramatically reduce the size of brain infarcts in rats and to have a protective neuronal effect (Zapater et al., 1997; Choi et al., 2011). Results of a meta-analysis show no effect of calcium antagonists on primary patient outcomes or death, and researchers show no evidence to support the use of calcium antagonists in patients with ischaemic stroke as beneficial (Zhang et al., 2019). 4,1-benzothiazoles are non-calcium antagonist drugs that reduce calcium levels in neurons by modulating mitochondria (Viejo et al., 2021). Calcium antagonists continue to be the subject of stroke research, although they have not achieved the desired results in clinical trials, probably because of intolerable side effects, low efficacy and short treatment windows. Uric acid is the final oxidation product of purine catabolism in the body and accounts for about two-thirds of the total antioxidant capacity of plasma (Becker, 1993). Uric acid has been shown to prevent glutamate-induced cell death in vitro and to inhibit ROS and RNS to reduce infarct size and improve prognosis in rodents after transient or permanent cerebral ischemia (Squadrito et al., 2000; Romanos et al., 2007; Onetti et al., 2015; Chamorro et al., 2016). Edaravone is an antioxidant drug that has been shown to scavenge the accumulation of free radicals and lipid peroxidation products in both clinical trials and basic experiments (Kasuya et al., 2014; Fidalgo et al., 2022).
5.3. Inhibition of ferroptosis
As ferroptosis is characterized by excessive lipid peroxidation, iron chelators, lipophilic antioxidants, and lipid peroxidation inhibitors can inhibit ferroptosis (Wang K. et al., 2022). Four types of ferroptosis inhibitors have been identified: GPX4 specifically catalyzes the loss of lipid peroxide oxidation activity in a GSH-dependent manner, FSP1 converts ubiquitin ketone on cell membranes to reduced ubiquitin, which can inhibit peroxidation and prevent iron droopy, GCH1/BH4 pathway is an endogenous antioxidant pathway, GCH1 protects cells from ferroptosis mainly through the antioxidant effect of BH4, and DHODH protects cells from ferroptosis in mitochondria by regulating the production of dihydrobisquinone in the inner mitochondrial membrane (Wang D. et al., 2022), this is shown in Figure 6. Some compounds inhibit ferroptosis directly or indirectly by targeting lipid peroxidation and iron metabolism (Chen G. et al., 2020). Both iron chelators (2,2′-pyridine, deferoxamine, deferoxamine mesylate) and inhibitors of lipid peroxides (Ferrostatin-1, Liproxstatin-1, Vitamin E) suppressed ferroptosis (Čepelak et al., 2020; Li et al., 2020). In additional, GSH, GPX4, heat shock protein β-1 and Nrf2 negatively modulate ferroptosis by restraining ROS production and repressing cellular uptake of iron (Sun et al., 2015; Qin et al., 2021). DFO, the most widely used iron chelator approved by the FDA, inhibits lipid peroxide chelation by inhibiting the Fenton reaction, and one study found that DFO effectively protects neurons by increasing the expression of hypoxia-inducible factor 1 (HIF-1; Baranova et al., 2007; Zhang et al., 2021). The widely used RTAs are ferrostatin-1 and liproxstatin-1, which can inhibit lipid peroxidation linked to ferroptosis (Han et al., 2020). ACSL4 is a crucial enzyme for AA and ADA esterification and is most probably an essential target for the inhibition of ferroptosis. Thiazolidinediones (TZNs) have been shown to potentially inhibit the activity of ACSL4 specifically and to prevent ferroptosis (Doll et al., 2017; Zhang et al., 2021).
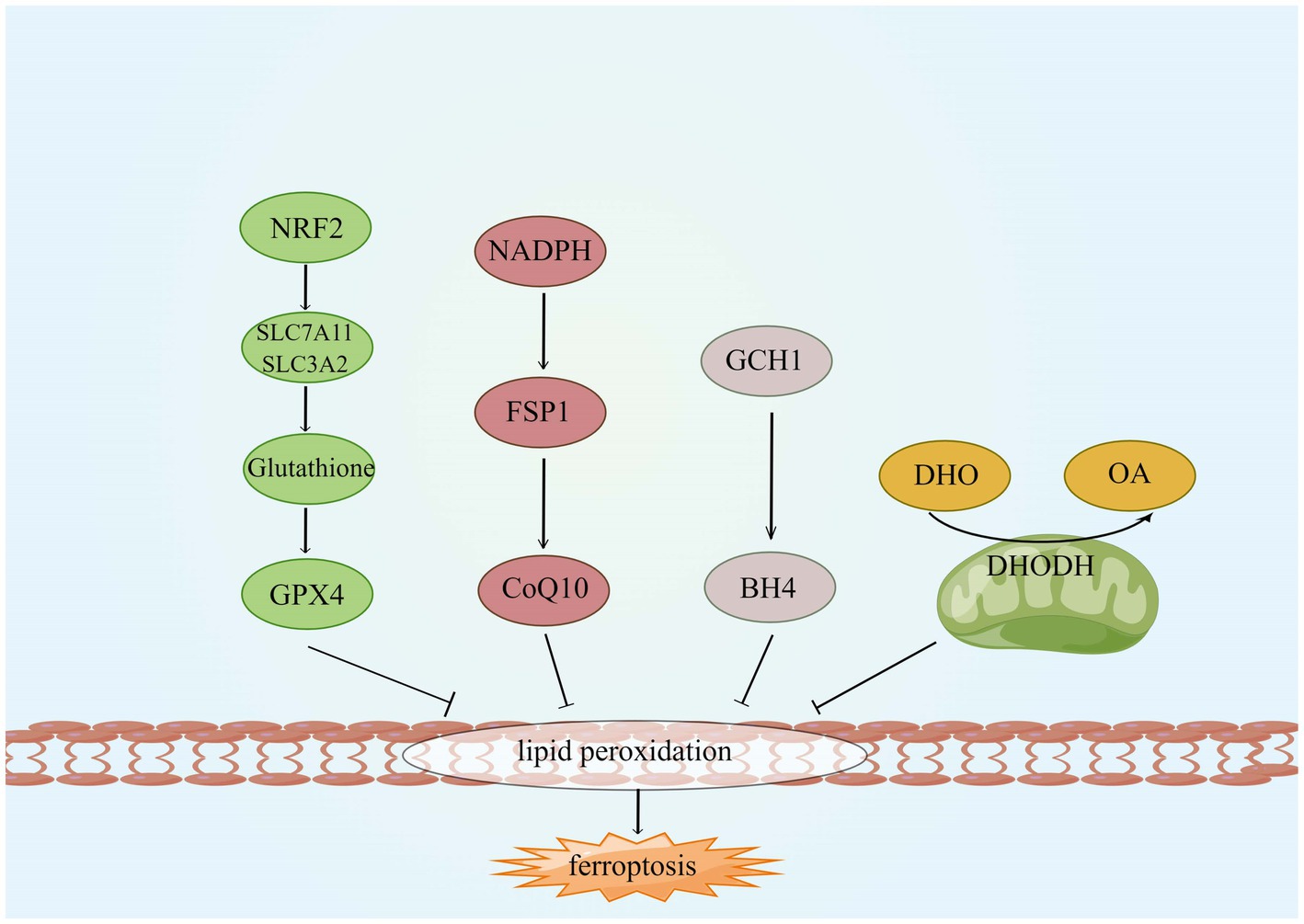
Figure 6. Four ferroptosis defense pathways. GCH1, GTP cyclohydrolyse-1; FSP1, ferroptosis suppressor protein 1; CoQ10, coenzyme Q; DHODH, dihydroorotate dehydrogenase; DHO, dihydroorotate; OA, orotate; GSH, glutathione; and BH4, tetrahydrobiopterin.
6. Conclusion and perspectives
Excessive accumulation of glutamate not only leads to excitotoxicity, but also to ferroptosis, Therefore, maintaining glutamate homeostasis is essential to inhibit excitotoxicity and ferroptosis. We can see a large number of articles using glutamate modeling to study the mechanism of excitotoxicity, most of which only look at the increase in calcium ions and mitochondrial dysfunction caused by excitotoxicity. In fact, the researchers used glutamate to create excitotoxic cell models that also caused ferroptosis, and excitotoxicity may be only part of the equation. Therefore it is also important to focus on neuronal ferroptosis when using glutamate for modeling in future studies. Neuronal excitotoxicity or ferroptosis can be effectively inhibited by compounds in most basic studies, particularly glutamate receptor inhibitors, but the role in clinical trials has been greatly reduced, probably mainly due to the failure to treat effectively within the time window in clinical trials. A number of influencing factors are essential, including informed patient consent, family cooperation and a well-established hospital system of care may all be influential in clinical trials. We remain confident in developing natural compounds that regulate both ferroptosis and excitotoxicity in future basic practice and further investigating their complex mechanisms and regulatory effects.
Author contributions
GF and YH conceived and designed this review. ML and JL contributed to the collection of literature and related information. GF and ML wrote the manuscript and examined the charts and the grammar of the manuscript. JL and YH provide guidance throughout the manuscript preparation process. All authors contributed to the article and approved the submitted version.
Funding
This project is supported by the Young Qihuang scholars program of National Administration of Traditional Chinese Medicine.
Acknowledgments
The authors thank to the editors and reviewers for their hard work and important comments. Picture was exported by FigDraw (www.figdraw.com).
Conflict of interest
The authors declare that the research was conducted in the absence of any commercial or financial relationships that could be construed as a potential conflict of interest.
Publisher’s note
All claims expressed in this article are solely those of the authors and do not necessarily represent those of their affiliated organizations, or those of the publisher, the editors and the reviewers. Any product that may be evaluated in this article, or claim that may be made by its manufacturer, is not guaranteed or endorsed by the publisher.
References
Abergel, E. (2020). The future of stroke interventions. Rambam Maimonid. Med. J. 11:e0018. doi: 10.5041/rmmj.10404
Agyeman, A. S., Chaerkady, R., Shaw, P. G., Davidson, N. E., Visvanathan, K., Pandey, A., et al. (2012). Transcriptomic and proteomic profiling of KEAP1 disrupted and sulforaphane-treated human breast epithelial cells reveals common expression profiles. Breast Cancer Res. Treat. 132, 175–187. doi: 10.1007/s10549-011-1536-9
Andreani, C., Bartolacci, C., and Scaglioni, P. P. (2022). Ferroptosis: a specific vulnerability of RAS-driven cancers? Front. Oncol. 12:923915. doi: 10.3389/fonc.2022.923915
Andriessen, T. M., Jacobs, B., and Vos, P. E. (2010). Clinical characteristics and pathophysiological mechanisms of focal and diffuse traumatic brain injury. J. Cell. Mol. Med. 14, 2381–2392. doi: 10.1111/j.1582-4934.2010.01164.x
Arteaga Cabeza, O., Zhang, Z., Smith Khoury, E., Sheldon, R. A., Sharma, A., Zhang, F., et al. (2021). Neuroprotective effects of a dendrimer-based glutamate carboxypeptidase inhibitor on superoxide dismutase transgenic mice after neonatal hypoxic-ischemic brain injury. Neurobiol. Dis. 148:105201. doi: 10.1016/j.nbd.2020.105201
Avgerinos, K. I., Chatzisotiriou, A., Haidich, A. B., Tsapas, A., and Lioutas, V. A. (2019). Intravenous magnesium sulfate in acute stroke. Stroke 50, 931–938. doi: 10.1161/strokeaha.118.021916
Baranova, O., Miranda, L. F., Pichiule, P., Dragatsis, I., Johnson, R. S., and Chavez, J. C. (2007). Neuron-specific inactivation of the hypoxia inducible factor 1 alpha increases brain injury in a mouse model of transient focal cerebral ischemia. J. Neurosci. 27, 6320–6332. doi: 10.1523/jneurosci.0449-07.2007
Barbano, M. F., Wang, H. L., Zhang, S., Miranda-Barrientos, J., Estrin, D. J., Figueroa-González, A., et al. (2020). VTA Glutamatergic neurons mediate innate defensive behaviors. Neuron 107, 368–382.e368. doi: 10.1016/j.neuron.2020.04.024
Barthels, D., and Das, H. (2020). Current advances in ischemic stroke research and therapies. Biochim. Biophys. Acta Mol. Basis Dis. 1866:165260. doi: 10.1016/j.bbadis.2018.09.012
Basappa, J., Graham, C. E., Turcan, S., and Vetter, D. E. (2012). The cochlea as an independent neuroendocrine organ: expression and possible roles of a local hypothalamic-pituitary-adrenal axis-equivalent signaling system. Hear. Res. 288, 3–18. doi: 10.1016/j.heares.2012.03.007
Becker, B. F. (1993). Towards the physiological function of uric acid. Free Radic. Biol. Med. 14, 615–631. doi: 10.1016/0891-5849(93)90143-i
Becker, A., Klapczynski, A., Kuch, N., Arpino, F., Simon-Keller, K., De La Torre, C., et al. (2016). Gene expression profiling reveals aryl hydrocarbon receptor as a possible target for photobiomodulation when using blue light. Sci. Rep. 6:33847. doi: 10.1038/srep33847
Bersuker, K., Hendricks, J. M., Li, Z., Magtanong, L., Ford, B., Tang, P. H., et al. (2019). The CoQ oxidoreductase FSP1 acts parallel to GPX4 to inhibit ferroptosis. Nature 575, 688–692. doi: 10.1038/s41586-019-1705-2
Bivard, A., Kleinig, T., Miteff, F., Butcher, K., Lin, L., Levi, C., et al. (2017). Ischemic core thresholds change with time to reperfusion: a case control study. Ann. Neurol. 82, 995–1003. doi: 10.1002/ana.25109
Bootman, M. D. (2012). Calcium signaling. Cold Spring Harb. Perspect. Biol. 4:a011171. doi: 10.1101/cshperspect.a011171
Boudreau, D. M., Guzauskas, G., Villa, K. F., Fagan, S. C., and Veenstra, D. L. (2013). A model of cost-effectiveness of tissue plasminogen activator in patient subgroups 3 to 4.5 hours after onset of acute ischemic stroke. Ann. Emerg. Med. 61, 46–55. doi: 10.1016/j.annemergmed.2012.04.020
Bramham, C. R., Torp, R., Zhang, N., Storm-Mathisen, J., and Ottersen, O. P. (1990). Distribution of glutamate-like immunoreactivity in excitatory hippocampal pathways: a semiquantitative electron microscopic study in rats. Neuroscience 39, 405–417. doi: 10.1016/0306-4522(90)90277-b
Budd, S. L., and Nicholls, D. G. (1996). Mitochondria, calcium regulation, and acute glutamate excitotoxicity in cultured cerebellar granule cells. J. Neurochem. 67, 2282–2291. doi: 10.1046/j.1471-4159.1996.67062282.x
Burada, A. P., Vinnakota, R., and Kumar, J. (2020). Cryo-EM structures of the ionotropic glutamate receptor GluD1 reveal a non-swapped architecture. Nat. Struct. Mol. Biol. 27, 84–91. doi: 10.1038/s41594-019-0359-y
Burger, P. M., Mehl, E., Cameron, P. L., Maycox, P. R., Baumert, M., Lottspeich, F., et al. (1989). Synaptic vesicles immunoisolated from rat cerebral cortex contain high levels of glutamate. Neuron 3, 715–720. doi: 10.1016/0896-6273(89)90240-7
Byrnes, K. R., Loane, D. J., and Faden, A. I. (2009). Metabotropic glutamate receptors as targets for multipotential treatment of neurological disorders. Neurotherapeutics 6, 94–107. doi: 10.1016/j.nurt.2008.10.038
Capelletti, M. M., Manceau, H., Puy, H., and Peoc'h, K. (2020). Ferroptosis in liver diseases: An overview. Int. J. Mol. Sci. 21:4908. doi: 10.3390/ijms21144908
Carafoli, E., and Krebs, J. (2016). Why calcium? How calcium became the best communicator. J. Biol. Chem. 291, 20849–20857. doi: 10.1074/jbc.R116.735894
Carriedo, S. G., Yin, H. Z., and Weiss, J. H. (1996). Motor neurons are selectively vulnerable to AMPA/kainate receptor-mediated injury in vitro. J. Neurosci. 16, 4069–4079. doi: 10.1523/jneurosci.16-13-04069.1996
Castilho, R. F., Ward, M. W., and Nicholls, D. G. (1999). Oxidative stress, mitochondrial function, and acute glutamate excitotoxicity in cultured cerebellar granule cells. J. Neurochem. 72, 1394–1401. doi: 10.1046/j.1471-4159.1999.721394.x
Čepelak, I., Dodig, S., and Dodig, D. (2020). Ferroptosis: regulated cell death. Arh. High. Rada Toksikol. 71, 99–109. doi: 10.2478/aiht-2020-71-3366
Chamorro, Á., Dirnagl, U., Urra, X., and Planas, A. M. (2016). Neuroprotection in acute stroke: targeting excitotoxicity, oxidative and nitrosative stress, and inflammation. Lancet Neurol. 15, 869–881. doi: 10.1016/S1474-4422(16)00114-9
Cheah, J. H., Kim, S. F., Hester, L. D., Clancy, K. W., Patterson, S. E. 3rd, Papadopoulos, V., et al. (2006). NMDA receptor-nitric oxide transmission mediates neuronal iron homeostasis via the GTPase Dexras1. Neuron 51, 431–440. doi: 10.1016/j.neuron.2006.07.011
Chen, G., Guo, G., Zhou, X., and Chen, H. (2020). Potential mechanism of ferroptosis in pancreatic cancer. Oncol. Lett. 19, 579–587. doi: 10.3892/ol.2019.11159
Chen, X., Kang, R., Kroemer, G., and Tang, D. (2021). Ferroptosis in infection, inflammation, and immunity. J. Exp. Med. 218:e20210518. doi: 10.1084/jem.20210518
Chen, M., Lu, T. J., Chen, X. J., Zhou, Y., Chen, Q., Feng, X. Y., et al. (2008). Differential roles of NMDA receptor subtypes in ischemic neuronal cell death and ischemic tolerance. Stroke 39, 3042–3048. doi: 10.1161/strokeaha.108.521898
Chen, X., Yu, C., Kang, R., and Tang, D. (2020). Iron metabolism in Ferroptosis. Front. Cell Dev. Biol. 8:590226. doi: 10.3389/fcell.2020.590226
Chen, Y. X., Zuliyaer, T., Liu, B., Guo, S., Yang, D. G., Gao, F., et al. (2022). Sodium selenite promotes neurological function recovery after spinal cord injury by inhibiting ferroptosis. Neural Regen. Res. 17, 2702–2709. doi: 10.4103/1673-5374.339491
Choi, D. W., Koh, J. Y., and Peters, S. (1988). Pharmacology of glutamate neurotoxicity in cortical cell culture: attenuation by NMDA antagonists. J. Neurosci. 8, 185–196. doi: 10.1523/jneurosci.08-01-00185.1988
Choi, S. K., Lee, G. J., Choi, S., Kim, Y. J., Park, H. K., and Park, B. J. (2011). Neuroprotective effects by nimodipine treatment in the experimental global ischemic rat model: real time estimation of glutamate. J. Kor. Neurosurg. Soc. 49, 1–7. doi: 10.3340/jkns.2011.49.1.1
Clewett, D., Sakaki, M., Huang, R., Nielsen, S. E., and Mather, M. (2017). Arousal amplifies biased competition between high and low priority memories more in women than in men: the role of elevated noradrenergic activity. Psychoneuroendocrinology 80, 80–91. doi: 10.1016/j.psyneuen.2017.02.022
Connolly, N. M., D'Orsi, B., Monsefi, N., Huber, H. J., and Prehn, J. H. (2016). Computational analysis of AMPK-mediated Neuroprotection suggests acute Excitotoxic bioenergetics and glucose dynamics are regulated by a minimal set of critical reactions. PLoS One 11:e0148326. doi: 10.1371/journal.pone.0148326
Cui, H., Hayashi, A., Sun, H. S., Belmares, M. P., Cobey, C., Phan, T., et al. (2007). PDZ protein interactions underlying NMDA receptor-mediated excitotoxicity and neuroprotection by PSD-95 inhibitors. J. Neurosci. 27, 9901–9915. doi: 10.1523/jneurosci.1464-07.2007
Dai, E., Meng, L., Kang, R., Wang, X., and Tang, D. (2020). ESCRT-III-dependent membrane repair blocks ferroptosis. Biochem. Biophys. Res. Commun. 522, 415–421. doi: 10.1016/j.bbrc.2019.11.110
Danbolt, N. C. (2001). Glutamate uptake. Prog. Neurobiol. 65, 1–105. doi: 10.1016/s0301-0082(00)00067-8
DeGregorio-Rocasolano, N., Martí-Sistac, O., and Gasull, T. (2019). Deciphering the iron side of stroke: neurodegeneration at the crossroads between iron Dyshomeostasis, Excitotoxicity, and Ferroptosis. Front. Neurosci. 13:85. doi: 10.3389/fnins.2019.00085
Dingledine, R., Borges, K., Bowie, D., and Traynelis, S. F. (1999). The glutamate receptor ion channels. Pharmacol. Rev. 51, 7–61.
Dirnagl, U., Iadecola, C., and Moskowitz, M. A. (1999). Pathobiology of ischaemic stroke: an integrated view. Trends Neurosci. 22, 391–397. doi: 10.1016/s0166-2236(99)01401-0
Dixon, S. J., Lemberg, K. M., Lamprecht, M. R., Skouta, R., Zaitsev, E. M., Gleason, C. E., et al. (2012). Ferroptosis: an iron-dependent form of nonapoptotic cell death. Cells 149, 1060–1072. doi: 10.1016/j.cell.2012.03.042
Doll, S., Freitas, F. P., Shah, R., Aldrovandi, M., da Silva, M. C., Ingold, I., et al. (2019). FSP1 is a glutathione-independent ferroptosis suppressor. Nature 575, 693–698. doi: 10.1038/s41586-019-1707-0
Doll, S., Proneth, B., Tyurina, Y. Y., Panzilius, E., Kobayashi, S., Ingold, I., et al. (2017). ACSL4 dictates ferroptosis sensitivity by shaping cellular lipid composition. Nat. Chem. Biol. 13, 91–98. doi: 10.1038/nchembio.2239
Dolma, S., Lessnick, S. L., Hahn, W. C., and Stockwell, B. R. (2003). Identification of genotype-selective antitumor agents using synthetic lethal chemical screening in engineered human tumor cells. Cancer Cell 3, 285–296. doi: 10.1016/s1535-6108(03)00050-3
Eliasson, M. J., Huang, Z., Ferrante, R. J., Sasamata, M., Molliver, M. E., Snyder, S. H., et al. (1999). Neuronal nitric oxide synthase activation and peroxynitrite formation in ischemic stroke linked to neural damage. J. Neurosci. 19, 5910–5918. doi: 10.1523/jneurosci.19-14-05910.1999
Emberson, J., Lees, K. R., Lyden, P., Blackwell, L., Albers, G., Bluhmki, E., et al. (2014). Effect of treatment delay, age, and stroke severity on the effects of intravenous thrombolysis with alteplase for acute ischaemic stroke: a meta-analysis of individual patient data from randomised trials. Lancet 384, 1929–1935. doi: 10.1016/S0140-6736(14)60584-5
Engler-Chiurazzi, E. B., Brown, C. M., Povroznik, J. M., and Simpkins, J. W. (2017). Estrogens as neuroprotectants: estrogenic actions in the context of cognitive aging and brain injury. Prog. Neurobiol. 157, 188–211. doi: 10.1016/j.pneurobio.2015.12.008
Fan, Z., Wirth, A. K., Chen, D., Wruck, C. J., Rauh, M., Buchfelder, M., et al. (2017). Nrf2-Keap1 pathway promotes cell proliferation and diminishes ferroptosis. Oncogene 6:e371. doi: 10.1038/oncsis.2017.65
Fang, W., Zhai, X., Han, D., Xiong, X., Wang, T., Zeng, X., et al. (2018). CCR2-dependent monocytes/macrophages exacerbate acute brain injury but promote functional recovery after ischemic stroke in mice. Theranostics 8, 3530–3543. doi: 10.7150/thno.24475
Fedorovich, S. V., and Waseem, T. V. (2022). The role of GRP81 lactate receptor in synaptic transmission regulation: does it enhance endocytosis? Neural Regen. Res. 17, 2657–2658. doi: 10.4103/1673-5374.335819
Feigin, V. L., Forouzanfar, M. H., Krishnamurthi, R., Mensah, G. A., Connor, M., Bennett, D. A., et al. (2014). Global and regional burden of stroke during 1990-2010: findings from the global burden of disease study 2010. Lancet 383, 245–254. doi: 10.1016/s0140-6736(13)61953-4
Ferraguti, F., and Shigemoto, R. (2006). Metabotropic glutamate receptors. Cell Tissue Res. 326, 483–504. doi: 10.1007/s00441-006-0266-5
Ferraz-Bannitz, R., Welendorf, C. R., Coelho, P. O., Salgado, W. Jr., Nonino, C. B., Beraldo, R. A., et al. (2021). Bariatric surgery can acutely modulate ER-stress and inflammation on subcutaneous adipose tissue in non-diabetic patients with obesity. Diabetol. Metab. Syndr. 13:19. doi: 10.1186/s13098-021-00623-w
Fidalgo, M., Ricardo Pires, J., Viseu, I., Magalhães, P., Gregório, H., Afreixo, V., et al. (2022). Edaravone for acute ischemic stroke—systematic review with meta-analysis. Clin. Neurol. Neurosurg. 219:107299. doi: 10.1016/j.clineuro.2022.107299
Forder, J. P., and Tymianski, M. (2009). Postsynaptic mechanisms of excitotoxicity: involvement of postsynaptic density proteins, radicals, and oxidant molecules. Neuroscience 158, 293–300. doi: 10.1016/j.neuroscience.2008.10.021
Friedmann Angeli, J. P., Schneider, M., Proneth, B., Tyurina, Y. Y., Tyurin, V. A., Hammond, V. J., et al. (2014). Inactivation of the ferroptosis regulator Gpx4 triggers acute renal failure in mice. Nat. Cell Biol. 16, 1180–1191. doi: 10.1038/ncb3064
Fujimura, M., Morita-Fujimura, Y., Murakami, K., Kawase, M., and Chan, P. H. (1998). Cytosolic redistribution of cytochrome c after transient focal cerebral ischemia in rats. J. Cereb. Blood Flow Metab. 18, 1239–1247. doi: 10.1097/00004647-199811000-00010
Fukuta, T., Asai, T., Yanagida, Y., Namba, M., Koide, H., Shimizu, K., et al. (2017). Combination therapy with liposomal neuroprotectants and tissue plasminogen activator for treatment of ischemic stroke. FASEB J. 31, 1879–1890. doi: 10.1096/fj.201601209R
Galluzzi, L., Vitale, I., Aaronson, S. A., Abrams, J. M., Adam, D., Agostinis, P., et al. (2018). Molecular mechanisms of cell death: recommendations of the nomenclature committee on cell death 2018. Cell Death Differ. 25, 486–541. doi: 10.1038/s41418-017-0012-4
Gan, B. (2019). DUBbing Ferroptosis in cancer cells. Cancer Res. 79, 1749–1750. doi: 10.1158/0008-5472.Can-19-0487
Gao, J., Liu, J., Yao, M., Zhang, W., Yang, B., and Wang, G. (2022). Panax notoginseng Saponins stimulates neurogenesis and neurological restoration after microsphere-induced cerebral embolism in rats partially via mTOR signaling. Front. Pharmacol. 13:889404. doi: 10.3389/fphar.2022.889404
Gao, M., Yi, J., Zhu, J., Minikes, A. M., Monian, P., Thompson, C. B., et al. (2019). Role of mitochondria in Ferroptosis. Mol. Cell 73, 354–363.e353. doi: 10.1016/j.molcel.2018.10.042
Gao, Q., Yin, X. D., Zhang, F., Zhu, Y. Z., and Li, Z. L. (2022). The regulatory effects of traditional Chinese medicine on Ferroptosis. Oxidative Med. Cell. Longev. 2022:4578381. doi: 10.1155/2022/4578381
Garthwaite, G., Williams, G. D., and Garthwaite, J. (1992). Glutamate toxicity: An experimental and theoretical analysis. Eur. J. Neurosci. 4, 353–360. doi: 10.1111/j.1460-9568.1992.tb00882.x
Ghoddoussi, F., Galloway, M. P., Jambekar, A., Bame, M., Needleman, R., and Brusilow, W. S. (2010). Methionine sulfoximine, an inhibitor of glutamine synthetase, lowers brain glutamine and glutamate in a mouse model of ALS. J. Neurol. Sci. 290, 41–47. doi: 10.1016/j.jns.2009.11.013
Glotfelty, E. J., Delgado, T., Tovar, Y. R. L. B., Luo, Y., Hoffer, B., Olson, L., et al. (2019). Incretin Mimetics as rational candidates for the treatment of traumatic brain injury. ACS Pharmacol. Transl. Sci. 2, 66–91. doi: 10.1021/acsptsci.9b00003
Godino Mdel, C., Romera, V. G., Sánchez-Tomero, J. A., Pacheco, J., Canals, S., Lerma, J., et al. (2013). Amelioration of ischemic brain damage by peritoneal dialysis. J. Clin. Invest. 123, 4359–4363. doi: 10.1172/jci67284
Goodman, J. M. (2019). LDAF1 holds the key to Seipin function. Dev. Cell 51, 544–545. doi: 10.1016/j.devcel.2019.11.009
Graham, C. E., Basappa, J., Turcan, S., and Vetter, D. E. (2011). The cochlear CRF signaling systems and their mechanisms of action in modulating cochlear sensitivity and protection against trauma. Mol. Neurobiol. 44, 383–406. doi: 10.1007/s12035-011-8203-3
Griendling, K. K., Camargo, L. L., Rios, F. J., Alves-Lopes, R., Montezano, A. C., and Touyz, R. M. (2021). Oxidative stress and hypertension. Circ. Res. 128, 993–1020. doi: 10.1161/circresaha.121.318063
Guo, L., and Cordeiro, M. F. (2008). Assessment of neuroprotection in the retina with DARC. Prog. Brain Res. 173, 437–450. doi: 10.1016/s0079-6123(08)01130-8
Guo, Y., Yang, X., Hakuna, L., Barve, A., Escobedo, J. O., Lowry, M., et al. (2012). A fast response highly selective probe for the detection of glutathione in human blood plasma. Sensors 12, 5940–5950. doi: 10.3390/s120505940
Han, C., Liu, Y., Dai, R., Ismail, N., Su, W., and Li, B. (2020). Ferroptosis and its potential role in human diseases. Front. Pharmacol. 11:239. doi: 10.3389/fphar.2020.00239
Harada, N., Kanayama, M., Maruyama, A., Yoshida, A., Tazumi, K., Hosoya, T., et al. (2011). Nrf2 regulates ferroportin 1-mediated iron efflux and counteracts lipopolysaccharide-induced ferroportin 1 mRNA suppression in macrophages. Arch. Biochem. Biophys. 508, 101–109. doi: 10.1016/j.abb.2011.02.001
Hardingham, G. E. (2009). Coupling of the NMDA receptor to neuroprotective and neurodestructive events. Biochem. Soc. Trans. 37, 1147–1160. doi: 10.1042/bst0371147
Hardingham, G. E., Fukunaga, Y., and Bading, H. (2002). Extrasynaptic NMDARs oppose synaptic NMDARs by triggering CREB shut-off and cell death pathways. Nat. Neurosci. 5, 405–414. doi: 10.1038/nn835
He, F., Blackberry, I., Yao, L., Xie, H., and Mnatzaganian, G. (2022). Geographical disparities in pooled stroke incidence and case fatality in mainland China, Hong Kong, and Macao: protocol for a systematic review and meta-analysis. JMIR Res. Protoc. 11:e32566. doi: 10.2196/32566
He, M., Zhang, B., Wei, X., Wang, Z., Fan, B., Du, P., et al. (2013). HDAC4/5-HMGB1 signalling mediated by NADPH oxidase activity contributes to cerebral ischaemia/reperfusion injury. J. Cell. Mol. Med. 17, 531–542. doi: 10.1111/jcmm.12040
He, Y., and Zhou, D. (2020). Can molecular targeting the TNFα-ERK-ETS1-IL27Rα pathway keep us young and healthy by protecting HSCs from aging? Blood Sci. 2, 148–149. doi: 10.1097/bs9.0000000000000060
Helal, G. K. (2008). Systemic administration of Zn2+ during the reperfusion phase of transient cerebral ischaemia protects rat hippocampus against iron-catalysed postischaemic injury. Clin. Exp. Pharmacol. Physiol. 35, 775–781. doi: 10.1111/j.1440-1681.2007.04858.x
Ho, K. C., Speier, W., Zhang, H., Scalzo, F., El-Saden, S., and Arnold, C. W. (2019). A machine learning approach for classifying ischemic stroke onset time from imaging. IEEE Trans. Med. Imaging 38, 1666–1676. doi: 10.1109/tmi.2019.2901445
Hong, M., Rong, J., Tao, X., and Xu, Y. (2022). The emerging role of Ferroptosis in cardiovascular diseases. Front. Pharmacol. 13:822083. doi: 10.3389/fphar.2022.822083
Hood, J. L., and Emeson, R. B. (2012). Editing of neurotransmitter receptor and ion channel RNAs in the nervous system. Curr. Top. Microbiol. Immunol. 353, 61–90. doi: 10.1007/82_2011_157
Hou, K., Shen, J., Yan, J., Zhai, C., Zhang, J., Pan, J. A., et al. (2021). Loss of TRIM21 alleviates cardiotoxicity by suppressing ferroptosis induced by the chemotherapeutic agent doxorubicin. EBioMedicine 69:103456. doi: 10.1016/j.ebiom.2021.103456
Hu, X., Wang, Y., Du, W., Liang, L. J., Wang, W., and Jin, X. (2022). Role of glial cell-derived oxidative stress in blood-brain barrier damage after acute ischemic stroke. Oxidative Med. Cell. Longev. 2022:7762078. doi: 10.1155/2022/7762078
Hwang, E. K., and Lupica, C. R. (2020). Altered Corticolimbic control of the nucleus Accumbens by long-term Δ (9)-tetrahydrocannabinol exposure. Biol. Psychiatry 87, 619–631. doi: 10.1016/j.biopsych.2019.07.024
Ji, X., Tian, L., Yao, S., Han, F., Niu, S., and Qu, C. (2022). A systematic review of body fluids biomarkers associated with early neurological deterioration following acute ischemic stroke. Front. Aging Neurosci. 14:918473. doi: 10.3389/fnagi.2022.918473
Jiang, L., Kon, N., Li, T., Wang, S. J., Su, T., Hibshoosh, H., et al. (2015). Ferroptosis as a p53-mediated activity during tumour suppression. Nature 520, 57–62. doi: 10.1038/nature14344
Jiang, M., Qiao, M., Zhao, C., Deng, J., Li, X., and Zhou, C. (2020). Targeting ferroptosis for cancer therapy: exploring novel strategies from its mechanisms and role in cancers. Transl. Lung Cancer Res. 9, 1569–1584. doi: 10.21037/tlcr-20-341
Jiang, W., Song, J., Zhang, S., Ye, Y., Wang, J., and Zhang, Y. (2021). CTRP13 protects H9c2 cells against hypoxia/Reoxygenation (H/R)-induced injury via regulating the AMPK/Nrf2/ARE signaling pathway. Cell Transplant. 30:9636897211033275. doi: 10.1177/09636897211033275
Jiang, T., Yu, J. T., Zhu, X. C., Wang, H. F., Tan, M. S., Cao, L., et al. (2014). Acute metformin preconditioning confers neuroprotection against focal cerebral ischaemia by pre-activation of AMPK-dependent autophagy. Br. J. Pharmacol. 171, 3146–3157. doi: 10.1111/bph.12655
Kagami, T., Yamade, M., Suzuki, T., Uotani, T., Tani, S., Hamaya, Y., et al. (2018). High expression level of CD44v8-10 in cancer stem-like cells is associated with poor prognosis in esophageal squamous cell carcinoma patients treated with chemoradiotherapy. Oncotarget 9, 34876–34888. doi: 10.18632/oncotarget.26172
Kasuya, A., Sakabe, J., and Tokura, Y. (2014). Potential application of in vivo imaging of impaired lymphatic duct to evaluate the severity of pressure ulcer in mouse model. Sci. Rep. 4:4173. doi: 10.1038/srep04173
Katayama, N., Yamamori, S., Fukaya, M., Kobayashi, S., Watanabe, M., Takahashi, M., et al. (2017). SNAP-25 phosphorylation at Ser187 regulates synaptic facilitation and short-term plasticity in an age-dependent manner. Sci. Rep. 7:7996. doi: 10.1038/s41598-017-08237-x
Kennedy, M. B. (1997). The postsynaptic density at glutamatergic synapses. Trends Neurosci. 20, 264–268. doi: 10.1016/s0166-2236(96)01033-8
Kerins, M. J., and Ooi, A. (2018). The roles of NRF2 in modulating cellular iron homeostasis. Antioxid. Redox Signal. 29, 1756–1773. doi: 10.1089/ars.2017.7176
Kevdzija, M., Bozovic-Stamenovic, R., and Marquardt, G. (2022). Stroke Patients' free-time activities and spatial preferences during inpatient recovery in rehabilitation centers. HERD 15, 96–113. doi: 10.1177/19375867221113054
Kim, A. H., Khursigara, G., Sun, X., Franke, T. F., and Chao, M. V. (2001). Akt phosphorylates and negatively regulates apoptosis signal-regulating kinase 1. Mol. Cell. Biol. 21, 893–901. doi: 10.1128/mcb.21.3.893-901.2001
Köhr, G. (2006). NMDA receptor function: subunit composition versus spatial distribution. Cell Tissue Res. 326, 439–446. doi: 10.1007/s00441-006-0273-6
Köhr, G., Melcher, T., and Seeburg, P. H. (1998). Candidate editases for GluR channels in single neurons of rat hippocampus and cerebellum. Neuropharmacology 37, 1411–1417. doi: 10.1016/s0028-3908(98)00149-x
Koppula, P., Olszewski, K., Zhang, Y., Kondiparthi, L., Liu, X., Lei, G., et al. (2021a). KEAP1 deficiency drives glucose dependency and sensitizes lung cancer cells and tumors to GLUT inhibition. iScience 24:102649. doi: 10.1016/j.isci.2021.102649
Koppula, P., Zhang, Y., Zhuang, L., and Gan, B. (2018). Amino acid transporter SLC7A11/xCT at the crossroads of regulating redox homeostasis and nutrient dependency of cancer. Cancer Commun. 38:12. doi: 10.1186/s40880-018-0288-x
Koppula, P., Zhuang, L., and Gan, B. (2021b). Cystine transporter SLC7A11/xCT in cancer: ferroptosis, nutrient dependency, and cancer therapy. Protein Cell 12, 599–620. doi: 10.1007/s13238-020-00789-5
Krasil'nikova, I., Surin, A., Sorokina, E., Fisenko, A., Boyarkin, D., Balyasin, M., et al. (2019). Insulin protects cortical neurons against glutamate Excitotoxicity. Front. Neurosci. 13:1027. doi: 10.3389/fnins.2019.01027
Kristián, T., and Siesjö, B. K. (1998). Calcium in ischemic cell death. Stroke 29, 705–718. doi: 10.1161/01.str.29.3.705
Kuo, T. T., Lin, L. C., Chang, H. Y., Chiang, P. J., Wu, H. Y., Chen, T. Y., et al. (2022). Quantitative proteome analysis reveals Melissa officinalis extract targets mitochondrial respiration in colon cancer cells. Molecules 27:4533. doi: 10.3390/molecules27144533
Kwak, M. K., Itoh, K., Yamamoto, M., and Kensler, T. W. (2002). Enhanced expression of the transcription factor Nrf2 by cancer chemopreventive agents: role of antioxidant response element-like sequences in the nrf2 promoter. Mol. Cell. Biol. 22, 2883–2892. doi: 10.1128/mcb.22.9.2883-2892.2002
Lai, P. C., Huang, Y. T., Wu, C. C., Lai, C. J., Wang, P. J., and Chiu, T. H. (2011). Ceftriaxone attenuates hypoxic-ischemic brain injury in neonatal rats. J. Biomed. Sci. 18:69. doi: 10.1186/1423-0127-18-69
Lau, A., and Tymianski, M. (2010). Glutamate receptors, neurotoxicity and neurodegeneration. Pflugers Arch. 460, 525–542. doi: 10.1007/s00424-010-0809-1
Lee, H., Zandkarimi, F., Zhang, Y., Meena, J. K., Kim, J., Zhuang, L., et al. (2020). Energy-stress-mediated AMPK activation inhibits ferroptosis. Nat. Cell Biol. 22, 225–234. doi: 10.1038/s41556-020-0461-8
Leiva-Salinas, C., Patrie, J. T., Xin, W., Michel, P., Jovin, T., and Wintermark, M. (2016). Prediction of early arterial recanalization and tissue fate in the selection of patients with the greatest potential to benefit from intravenous tissue-type plasminogen activator. Stroke 47, 397–403. doi: 10.1161/STROKEAHA.115.011066
Li, J., Cao, F., Yin, H. L., Huang, Z. J., Lin, Z. T., Mao, N., et al. (2020). Ferroptosis: past, present and future. Cell Death Dis. 11:88. doi: 10.1038/s41419-020-2298-2
Li, C., Zhang, Y., Liu, J., Kang, R., Klionsky, D. J., and Tang, D. (2021). Mitochondrial DNA stress triggers autophagy-dependent ferroptotic death. Autophagy 17, 948–960. doi: 10.1080/15548627.2020.1739447
Liang, C. Q., Zhang, G., Zhang, L., Chen, S. Y., Wang, J. N., Zhang, T. T., et al. (2021). Calmodulin Bidirectionally regulates evoked and spontaneous neurotransmitter release at retinal ribbon synapses. eNeuro 8:ENEURO.0257-20.2020. doi: 10.1523/eneuro.0257-20.2020
Liao, W. T., Liu, J., Zhou, S. M., Xu, G., Gao, Y. Q., and Liu, W. Y. (2018). UHPLC-QTOFMS-based Metabolomic analysis of the hippocampus in hypoxia preconditioned mouse. Front. Physiol. 9:1950. doi: 10.3389/fphys.2018.01950
Lin, C. H., Chen, P. S., and Gean, P. W. (2008). Glutamate preconditioning prevents neuronal death induced by combined oxygen-glucose deprivation in cultured cortical neurons. Eur. J. Pharmacol. 589, 85–93. doi: 10.1016/j.ejphar.2008.05.047
Lin, T. Y., Lu, C. W., and Wang, S. J. (2009). Inhibitory effect of glutamate release from rat cerebrocortical synaptosomes by dextromethorphan and its metabolite 3-hydroxymorphinan. Neurochem. Int. 54, 526–534. doi: 10.1016/j.neuint.2009.02.012
Lin, X., Wang, R., Zou, W., Sun, X., Liu, X., Zhao, L., et al. (2016). The influenza virus H5N1 infection can induce ROS production for viral replication and host cell death in A549 cells modulated by human cu/Zn superoxide dismutase (SOD1) overexpression. Viruses 8:13. doi: 10.3390/v8010013
Lin, L., Zhang, M. X., Zhang, L., Zhang, D., Li, C., and Li, Y. L. (2021). Autophagy, Pyroptosis, and Ferroptosis: new regulatory mechanisms for atherosclerosis. Front. Cell Dev. Biol. 9:809955. doi: 10.3389/fcell.2021.809955
Lipton, S. A., Choi, Y. B., Pan, Z. H., Lei, S. Z., Chen, H. S., Sucher, N. J., et al. (1993). A redox-based mechanism for the neuroprotective and neurodestructive effects of nitric oxide and related nitroso-compounds. Nature 364, 626–632. doi: 10.1038/364626a0
Liu, Y., Cao, X., He, C., Guo, X., Cai, H., Aierken, A., et al. (2022). Effects of Ferroptosis on male reproduction. Int. J. Mol. Sci. 23:7139. doi: 10.3390/ijms23137139
Liu, N., Lin, X., and Huang, C. (2020). Activation of the reverse transsulfuration pathway through NRF2/CBS confers erastin-induced ferroptosis resistance. Br. J. Cancer 122, 279–292. doi: 10.1038/s41416-019-0660-x
Liu, Y., Wong, T. P., Aarts, M., Rooyakkers, A., Liu, L., Lai, T. W., et al. (2007). NMDA receptor subunits have differential roles in mediating excitotoxic neuronal death both in vitro and in vivo. J. Neurosci. 27, 2846–2857. doi: 10.1523/jneurosci.0116-07.2007
Liu, Y., Zeng, L., Yang, Y., Chen, C., Wang, D., and Wang, H. (2020). Acyl-CoA thioesterase 1 prevents cardiomyocytes from doxorubicin-induced ferroptosis via shaping the lipid composition. Cell Death Dis. 11:756. doi: 10.1038/s41419-020-02948-2
Maher, P., van Leyen, K., Dey, P. N., Honrath, B., Dolga, A., and Methner, A. (2018). The role of ca(2+) in cell death caused by oxidative glutamate toxicity and ferroptosis. Cell Calcium 70, 47–55. doi: 10.1016/j.ceca.2017.05.007
Mahmoud, A. M., Yang, W., and Bosland, M. C. (2014). Soy isoflavones and prostate cancer: a review of molecular mechanisms. J. Steroid Biochem. Mol. Biol. 140, 116–132. doi: 10.1016/j.jsbmb.2013.12.010
Malysz-Cymborska, I., Golubczyk, D., Kalkowski, L., Kwiatkowska, J., Zawadzki, M., Głodek, J., et al. (2021). Intra-arterial transplantation of stem cells in large animals as a minimally-invasive strategy for the treatment of disseminated neurodegeneration. Sci. Rep. 11:6581. doi: 10.1038/s41598-021-85820-3
Mao, R., Zong, N., Hu, Y., Chen, Y., and Xu, Y. (2022). Neuronal death mechanisms and therapeutic strategy in ischemic stroke. Neurosci. Bull. 38, 1229–1247. doi: 10.1007/s12264-022-00859-0
Marcoli, M., Cervetto, C., Amato, S., Fiorucci, C., Maura, G., Mariottini, P., et al. (2022). Transgenic mouse overexpressing Spermine oxidase in Cerebrocortical neurons: astrocyte dysfunction and susceptibility to epileptic seizures. Biomol. Ther. 12:204. doi: 10.3390/biom12020204
Márquez, J., Tosina, M., de la Rosa, V., Segura, J. A., Alonso, F. J., Matés, J. M., et al. (2009). New insights into brain glutaminases: beyond their role on glutamatergic transmission. Neurochem. Int. 55, 64–70. doi: 10.1016/j.neuint.2009.02.022
Martínez-Coria, H., Arrieta-Cruz, I., Cruz, M.-E., and López-Valdés, H. E. (2021). Physiopathology of ischemic stroke and its modulation using memantine: evidence from preclinical stroke. Neural Regen. Res. 16, 433–439. doi: 10.4103/1673-5374.293129
McCaughey-Chapman, A., and Connor, B. (2022). Rat cortico-striatal sagittal organotypic slice cultures as ex vivo excitotoxic striatal lesion models. Heliyon 8:e10819. doi: 10.1016/j.heliyon.2022.e10819
Meng, X., Wang, M., Wang, X., Sun, G., Ye, J., Xu, H., et al. (2014). Suppression of NADPH oxidase- and mitochondrion-derived superoxide by Notoginsenoside R1 protects against cerebral ischemia-reperfusion injury through estrogen receptor-dependent activation of Akt/Nrf2 pathways. Free Radic. Res. 48, 823–838. doi: 10.3109/10715762.2014.911853
Munro, M. J., Wickremesekera, S. K., Tan, S. T., and Peng, L. (2022). Proteomic analysis of low- and high-grade human colon adenocarcinoma tissues and tissue-derived primary cell lines reveals unique biological functions of tumours and new protein biomarker candidates. Clin. Proteomics 19:27. doi: 10.1186/s12014-022-09364-y
Nakamura, E., Sato, M., Yang, H., Miyagawa, F., Harasaki, M., Tomita, K., et al. (1999). 4F2 (CD98) heavy chain is associated covalently with an amino acid transporter and controls intracellular trafficking and membrane topology of 4F2 heterodimer. J. Biol. Chem. 274, 3009–3016. doi: 10.1074/jbc.274.5.3009
Nie, Q., Hu, Y., Yu, X., Li, X., and Fang, X. (2022). Induction and application of ferroptosis in cancer therapy. Cancer Cell Int. 22:12. doi: 10.1186/s12935-021-02366-0
Nishimune, H., Badawi, Y., Mori, S., and Shigemoto, K. (2016). Dual-color STED microscopy reveals a sandwich structure of bassoon and piccolo in active zones of adult and aged mice. Sci. Rep. 6:27935. doi: 10.1038/srep27935
Noguchi, T., Suzuki, M., Mutoh, N., Hirata, Y., Tsuchida, M., Miyagawa, S., et al. (2018). Nuclear-accumulated SQSTM1/p62-based ALIS act as microdomains sensing cellular stresses and triggering oxidative stress-induced parthanatos. Cell Death Dis. 9:1193. doi: 10.1038/s41419-018-1245-y
Olive, M. F. (2009). Metabotropic glutamate receptor ligands as potential therapeutics for addiction. Curr. Drug Abuse Rev. 2, 83–98. doi: 10.2174/1874473710902010083
Onetti, Y., Dantas, A. P., Pérez, B., Cugota, R., Chamorro, A., Planas, A. M., et al. (2015). Middle cerebral artery remodeling following transient brain ischemia is linked to early postischemic hyperemia: a target of uric acid treatment. Am. J. Physiol. Heart Circ. Physiol. 308, H862–H874. doi: 10.1152/ajpheart.00001.2015
Ooi, S. L., Green, R., and Pak, S. C. (2018). N-Acetylcysteine for the treatment of psychiatric disorders: a review of current evidence. Biomed. Res. Int. 2018:2469486. doi: 10.1155/2018/2469486
Osen, K. K., Storm-Mathisen, J., Ottersen, O. P., and Dihle, B. (1995). Glutamate is concentrated in and released from parallel fiber terminals in the dorsal cochlear nucleus: a quantitative immunocytochemical analysis in Guinea pig. J. Comp. Neurol. 357, 482–500. doi: 10.1002/cne.903570311
Ottersen, O. P., Storm-Mathisen, J., Bramham, C., Torp, R., Laake, J., and Gundersen, V. (1990). A quantitative electron microscopic immunocytochemical study of the distribution and synaptic handling of glutamate in rat hippocampus. Prog. Brain Res. 83, 99–114. doi: 10.1016/s0079-6123(08)61244-3
Ottersen, O. P., Zhang, N., and Walberg, F. (1992). Metabolic compartmentation of glutamate and glutamine: morphological evidence obtained by quantitative immunocytochemistry in rat cerebellum. Neuroscience 46, 519–534. doi: 10.1016/0306-4522(92)90141-n
Ovbiagele, B., Kidwell, C. S., Starkman, S., and Saver, J. L. (2003). Neuroprotective agents for the treatment of acute ischemic stroke. Curr. Neurol. Neurosci. Rep. 3, 9–20. doi: 10.1007/s11910-003-0031-z
Özel, R. E., Ispas, C., Ganesana, M., Leiter, J. C., and Andreescu, S. (2014). Glutamate oxidase biosensor based on mixed ceria and titania nanoparticles for the detection of glutamate in hypoxic environments. Biosens. Bioelectron. 52, 397–402. doi: 10.1016/j.bios.2013.08.054
Pakos-Zebrucka, K., Koryga, I., Mnich, K., Ljujic, M., Samali, A., and Gorman, A. M. (2016). The integrated stress response. EMBO Rep. 17, 1374–1395. doi: 10.15252/embr.201642195
Papadia, S., and Hardingham, G. E. (2007). The dichotomy of NMDA receptor signaling. Neuroscientist 13, 572–579. doi: 10.1177/10738584070130060401
Pathak, S. S., Liu, D., Li, T., de Zavalia, N., Zhu, L., Li, J., et al. (2019). The eIF2α kinase GCN2 modulates period and rhythmicity of the circadian clock by translational control of Atf4. Neuron 104, 724–735. doi: 10.1016/j.neuron.2019.08.007
Pchitskaya, E., Popugaeva, E., and Bezprozvanny, I. (2018). Calcium signaling and molecular mechanisms underlying neurodegenerative diseases. Cell Calcium 70, 87–94. doi: 10.1016/j.ceca.2017.06.008
Pietrancosta, N., Djibo, M., Daumas, S., El Mestikawy, S., and Erickson, J. D. (2020). Molecular, structural, functional, and pharmacological sites for vesicular glutamate transporter regulation. Mol. Neurobiol. 57, 3118–3142. doi: 10.1007/s12035-020-01912-7
Pin, J. P., and Duvoisin, R. (1995). The metabotropic glutamate receptors: structure and functions. Neuropharmacology 34, 1–26. doi: 10.1016/0028-3908(94)00129-g
Protchenko, O., Baratz, E., Jadhav, S., Li, F., Shakoury-Elizeh, M., Gavrilova, O., et al. (2021). Iron chaperone poly rC binding protein 1 protects mouse liver from lipid peroxidation and Steatosis. Hepatology 73, 1176–1193. doi: 10.1002/hep.31328
Qin, Y., Qiao, Y., Wang, D., Tang, C., and Yan, G. (2021). Ferritinophagy and ferroptosis in cardiovascular disease: mechanisms and potential applications. Biomed. Pharmacother. 141:111872. doi: 10.1016/j.biopha.2021.111872
Qin, C., Yang, S., Chu, Y.-H., Zhang, H., Pang, X.-W., Chen, L., et al. (2022). Signaling pathways involved in ischemic stroke: molecular mechanisms and therapeutic interventions. Signal Transduct. Target. Ther. 7:215. doi: 10.1038/s41392-022-01064-1
Randall, R. D., and Thayer, S. A. (1992). Glutamate-induced calcium transient triggers delayed calcium overload and neurotoxicity in rat hippocampal neurons. J. Neurosci. 12, 1882–1895. doi: 10.1523/jneurosci.12-05-01882.1992
Ratan, R. R. (2020). The chemical biology of Ferroptosis in the central nervous system. Cell Chem. Biol. 27, 479–498. doi: 10.1016/j.chembiol.2020.03.007
Raza, A., Xie, W., Kim, K. H., Dronamraju, V. R., Williams, J., Vince, R., et al. (2022). Dipeptide of ψ-GSH inhibits oxidative stress and Neuroinflammation in an Alzheimer's disease mouse model. Antioxidants 11:1075. doi: 10.3390/antiox11061075
Rebas, E., Rzajew, J., Radzik, T., and Zylinska, L. (2020). Neuroprotective polyphenols: a modulatory action on neurotransmitter pathways. Curr. Neuropharmacol. 18, 431–445. doi: 10.2174/1570159x18666200106155127
Riveros, N., Fiedler, J., Lagos, N., Muñoz, C., and Orrego, F. (1986). Glutamate in rat brain cortex synaptic vesicles: influence of the vesicle isolation procedure. Brain Res. 386, 405–408. doi: 10.1016/0006-8993(86)90181-2
Rocha-Ferreira, E., and Hristova, M. (2016). Plasticity in the neonatal brain following hypoxic-Ischaemic injury. Neural Plast. 2016:4901014. doi: 10.1155/2016/4901014
Roh, J. L., Kim, E. H., Jang, H., and Shin, D. (2017). Nrf2 inhibition reverses the resistance of cisplatin-resistant head and neck cancer cells to artesunate-induced ferroptosis. Redox Biol. 11, 254–262. doi: 10.1016/j.redox.2016.12.010
Romanos, E., Planas, A. M., Amaro, S., and Chamorro, A. (2007). Uric acid reduces brain damage and improves the benefits of rt-PA in a rat model of thromboembolic stroke. J. Cereb. Blood Flow Metab. 27, 14–20. doi: 10.1038/sj.jcbfm.9600312
Sanz-Morello, B., Ahmadi, H., Vohra, R., Saruhanian, S., Freude, K. K., Hamann, S., et al. (2021). Oxidative stress in optic neuropathies. Antioxidants 10:1538. doi: 10.3390/antiox10101538
Sasaki, H., Sato, H., Kuriyama-Matsumura, K., Sato, K., Maebara, K., Wang, H., et al. (2002). Electrophile response element-mediated induction of the cystine/glutamate exchange transporter gene expression. J. Biol. Chem. 277, 44765–44771. doi: 10.1074/jbc.M208704200
Sato, H., Tamba, M., Ishii, T., and Bannai, S. (1999). Cloning and expression of a plasma membrane cystine/glutamate exchange transporter composed of two distinct proteins. J. Biol. Chem. 274, 11455–11458. doi: 10.1074/jbc.274.17.11455
Sazonova, M. A., Sinyov, V. V., Ryzhkova, A. I., Sazonova, M. D., Kirichenko, T. V., Khotina, V. A., et al. (2021). Some molecular and cellular stress mechanisms associated with neurodegenerative diseases and atherosclerosis. Int. J. Mol. Sci. 22:699. doi: 10.3390/ijms22020699
Scalise, M., Console, L., Rovella, F., Galluccio, M., Pochini, L., and Indiveri, C. (2020). Membrane transporters for amino acids as players of cancer metabolic rewiring. Cells 9:2028. doi: 10.3390/cells9092028
She, X., Lan, B., Tian, H., and Tang, B. (2020). Cross talk between Ferroptosis and cerebral ischemia. Front. Neurosci. 14:776. doi: 10.3389/fnins.2020.00776
Shen, S., Ji, C., and Wei, K. (2022). Cellular senescence and regulated cell death of tubular epithelial cells in diabetic kidney disease. Front. Endocrinol. 13:924299. doi: 10.3389/fendo.2022.924299
Sheng, M., and Hoogenraad, C. C. (2007). The postsynaptic architecture of excitatory synapses: a more quantitative view. Annu. Rev. Biochem. 76, 823–847. doi: 10.1146/annurev.biochem.76.060805.160029
Shi, J., Tang, M., Zhou, S., Xu, D., Zhao, J., Wu, C., et al. (2021). Programmed cell death pathways in the pathogenesis of idiopathic inflammatory myopathies. Front. Immunol. 12:783616. doi: 10.3389/fimmu.2021.783616
Shi, Z., Zhu, L., Li, T., Tang, X., Xiang, Y., Han, X., et al. (2017). Neuroprotective mechanisms of Lycium barbarum polysaccharides against ischemic insults by regulating NR2B and NR2A containing NMDA receptor signaling pathways. Front. Cell. Neurosci. 11:288. doi: 10.3389/fncel.2017.00288
Shih, A. Y., Johnson, D. A., Wong, G., Kraft, A. D., Jiang, L., Erb, H., et al. (2003). Coordinate regulation of glutathione biosynthesis and release by Nrf2-expressing glia potently protects neurons from oxidative stress. J. Neurosci. 23, 3394–3406. doi: 10.1523/jneurosci.23-08-03394.2003
Shin, C. S., Mishra, P., Watrous, J. D., Carelli, V., D'Aurelio, M., Jain, M., et al. (2017). The glutamate/cystine xCT antiporter antagonizes glutamine metabolism and reduces nutrient flexibility. Nat. Commun. 8:15074. doi: 10.1038/ncomms15074
Shupliakov, O., Brodin, L., Cullheim, S., Ottersen, O. P., and Storm-Mathisen, J. (1992). Immunogold quantification of glutamate in two types of excitatory synapse with different firing patterns. J. Neurosci. 12, 3789–3803. doi: 10.1523/jneurosci.12-10-03789.1992
Squadrito, G. L., Cueto, R., Splenser, A. E., Valavanidis, A., Zhang, H., Uppu, R. M., et al. (2000). Reaction of uric acid with peroxynitrite and implications for the mechanism of neuroprotection by uric acid. Arch. Biochem. Biophys. 376, 333–337. doi: 10.1006/abbi.2000.1721
Stout, A. K., Raphael, H. M., Kanterewicz, B. I., Klann, E., and Reynolds, I. J. (1998). Glutamate-induced neuron death requires mitochondrial calcium uptake. Nat. Neurosci. 1, 366–373. doi: 10.1038/1577
Sturm, J. W., Dewey, H. M., Donnan, G. A., Macdonell, R. A. L., McNeil, J. J., and Thrift, A. G. (2002). Handicap after stroke: how does it relate to disability, perception of recovery, and stroke subtype?: the north north East Melbourne stroke incidence study (NEMESIS). Stroke 33, 762–768. doi: 10.1161/hs0302.103815
Sun, X., Ou, Z., Chen, R., Niu, X., Chen, D., Kang, R., et al. (2016). Activation of the p62-Keap1-NRF2 pathway protects against ferroptosis in hepatocellular carcinoma cells. Hepatology 63, 173–184. doi: 10.1002/hep.28251
Sun, X., Ou, Z., Xie, M., Kang, R., Fan, Y., Niu, X., et al. (2015). HSPB1 as a novel regulator of ferroptotic cancer cell death. Oncogene 34, 5617–5625. doi: 10.1038/onc.2015.32
Takahashi, T. (2019). Novel synaptic plasticity enhancer drug to augment functional recovery with rehabilitation. Curr. Opin. Neurol. 32, 822–827. doi: 10.1097/wco.0000000000000748
Takamori, S. (2006). VGLUTs: 'exciting' times for glutamatergic research? Neurosci. Res. 55, 343–351. doi: 10.1016/j.neures.2006.04.016
Takamori, S., Rhee, J. S., Rosenmund, C., and Jahn, R. (2000). Identification of a vesicular glutamate transporter that defines a glutamatergic phenotype in neurons. Nature 407, 189–194. doi: 10.1038/35025070
Tan, S., Schubert, D., and Maher, P. (2001). Oxytosis: a novel form of programmed cell death. Curr. Top. Med. Chem. 1, 497–506. doi: 10.2174/1568026013394741
Tang, D., Chen, X., Kang, R., and Kroemer, G. (2021). Ferroptosis: molecular mechanisms and health implications. Cell Res. 31, 107–125. doi: 10.1038/s41422-020-00441-1
Tang, Z., Huang, Z., Huang, Y., Chen, Y., Huang, M., Liu, H., et al. (2021). Ferroptosis: the silver lining of cancer therapy. Front. Cell Dev. Biol. 9:765859. doi: 10.3389/fcell.2021.765859
Teng, L., Lei, H. M., Sun, F., An, S. M., Tang, Y. B., Meng, S., et al. (2016). Autocrine glutamatergic transmission for the regulation of embryonal carcinoma stem cells. Oncotarget 7, 49552–49564. doi: 10.18632/oncotarget.9973
Terauchi, A., and Umemori, H. (2012). Specific sets of intrinsic and extrinsic factors drive excitatory and inhibitory circuit formation. Neuroscientist 18, 271–286. doi: 10.1177/1073858411404228
Thomazi, R., Silveira, L. V. A., Boas, P., and Jacinto, A. F. (2018). Frequency of dementia among elderly admitted to a geriatrics inpatients sector of a Brazilian public hospital. Dement. Neuropsychol. 12, 35–39. doi: 10.1590/1980-57642018dn12-010005
Tsao, C. W., Aday, A. W., Almarzooq, Z. I., Alonso, A., Beaton, A. Z., Bittencourt, M. S., et al. (2022). Heart disease and stroke Statistics-2022 update: a report from the American Heart Association. Circulation 145, e153–e639. doi: 10.1161/CIR.0000000000001052
Tukker, A. M., and Westerink, R. H. S. (2021). Novel test strategies for in vitro seizure liability assessment. Expert Opin. Drug Metab. Toxicol. 17, 923–936. doi: 10.1080/17425255.2021.1876026
Tymianski, M., Charlton, M. P., Carlen, P. L., and Tator, C. H. (1993). Secondary Ca2+ overload indicates early neuronal injury which precedes staining with viability indicators. Brain Res. 607, 319–323. doi: 10.1016/0006-8993(93)91523-u
Valdinocci, D., Simões, R. F., Kovarova, J., Cunha-Oliveira, T., Neuzil, J., and Pountney, D. L. (2019). Intracellular and intercellular mitochondrial dynamics in Parkinson's disease. Front. Neurosci. 13:930. doi: 10.3389/fnins.2019.00930
Vanden Berghe, T., Linkermann, A., Jouan-Lanhouet, S., Walczak, H., and Vandenabeele, P. (2014). Regulated necrosis: the expanding network of non-apoptotic cell death pathways. Nat. Rev. Mol. Cell Biol. 15, 135–147. doi: 10.1038/nrm3737
Viejo, L., Rubio-Alarcón, M., Arribas, R. L., Moreno-Castro, M., Pérez-Marín, R., Braun-Cornejo, M., et al. (2021). Synthesis and biological assessment of 4,1-Benzothiazepines with Neuroprotective activity on the ca(2+) overload for the treatment of neurodegenerative diseases and stroke. Molecules 26:4473. doi: 10.3390/molecules26154473
Wakil, S. J., and Abu-Elheiga, L. A. (2009). Fatty acid metabolism: target for metabolic syndrome. J. Lipid Res. 50, S138–S143. doi: 10.1194/jlr.R800079-JLR200
Wang, W., Jiang, B., Sun, H., Ru, X., Sun, D., Wang, L., et al. (2017). Prevalence, incidence, and mortality of stroke in China: results from a Nationwide population-based survey of 480 687 adults. Circulation 135, 759–771. doi: 10.1161/CIRCULATIONAHA.116.025250
Wang, S., Liao, L., Wang, M., Zhou, H., Huang, Y., Wang, Z., et al. (2017). Pin1 promotes regulated necrosis induced by glutamate in rat retinal neurons via CAST/Calpain2 pathway. Front. Cell. Neurosci. 11:425. doi: 10.3389/fncel.2017.00425
Wang, L., Liu, Y., Du, T., Yang, H., Lei, L., Guo, M., et al. (2020). ATF3 promotes erastin-induced ferroptosis by suppressing system xc(). Cell Death Differ. 27, 662–675. doi: 10.1038/s41418-019-0380-z
Wang, K., Mei, S., Cai, M., Zhai, D., Zhang, D., Yu, J., et al. (2022). Ferroptosis-related long noncoding RNAs as prognostic biomarkers for ovarian cancer. Front. Oncol. 12:888699. doi: 10.3389/fonc.2022.888699
Wang, D., Tang, L., Zhang, Y., Ge, G., Jiang, X., Mo, Y., et al. (2022). Regulatory pathways and drugs associated with ferroptosis in tumors. Cell Death Dis. 13:544. doi: 10.1038/s41419-022-04927-1
Wang, Y., Tang, B., Zhu, J., Yu, J., Hui, J., Xia, S., et al. (2022). Emerging mechanisms and targeted therapy of Ferroptosis in neurological diseases and neuro-oncology. Int. J. Biol. Sci. 18, 4260–4274. doi: 10.7150/ijbs.72251
Ward, M. W., Rego, A. C., Frenguelli, B. G., and Nicholls, D. G. (2000). Mitochondrial membrane potential and glutamate excitotoxicity in cultured cerebellar granule cells. J. Neurosci. 20, 7208–7219. doi: 10.1523/jneurosci.20-19-07208.2000
Wei, X., Walia, V., Lin, J. C., Teer, J. K., Prickett, T. D., Gartner, J., et al. (2011). Exome sequencing identifies GRIN2A as frequently mutated in melanoma. Nat. Genet. 43, 442–446. doi: 10.1038/ng.810
White, R. J., and Reynolds, I. J. (1995). Mitochondria and Na+/Ca2+ exchange buffer glutamate-induced calcium loads in cultured cortical neurons. J. Neurosci. 15, 1318–1328. doi: 10.1523/jneurosci.15-02-01318.1995
White, R. J., and Reynolds, I. J. (1996). Mitochondrial depolarization in glutamate-stimulated neurons: an early signal specific to excitotoxin exposure. J. Neurosci. 16, 5688–5697. doi: 10.1523/jneurosci.16-18-05688.1996
Wu, Q. J., and Tymianski, M. (2018). Targeting NMDA receptors in stroke: new hope in neuroprotection. Mol. Brain 11:15. doi: 10.1186/s13041-018-0357-8
Xie, Z., Ding, S. Q., and Shen, Y. F. (2014). Silibinin activates AMP-activated protein kinase to protect neuronal cells from oxygen and glucose deprivation-re-oxygenation. Biochem. Biophys. Res. Commun. 454, 313–319. doi: 10.1016/j.bbrc.2014.10.080
Xu, C., Liu, Z., and Xiao, J. (2021). Ferroptosis: a double-edged sword in gastrointestinal disease. Int. J. Mol. Sci. 22:12403. doi: 10.3390/ijms222212403
Xu, Y., Song, X., Wang, D., Wang, Y., Li, P., and Li, J. (2021). Proteomic insights into synaptic signaling in the brain: the past, present and future. Mol. Brain 14:37. doi: 10.1186/s13041-021-00750-5
Yagoda, N., von Rechenberg, M., Zaganjor, E., Bauer, A. J., Yang, W. S., Fridman, D. J., et al. (2007). RAS-RAF-MEK-dependent oxidative cell death involving voltage-dependent anion channels. Nature 447, 864–868. doi: 10.1038/nature05859
Yamaguchi, A., Tamatani, M., Matsuzaki, H., Namikawa, K., Kiyama, H., Vitek, M. P., et al. (2001). Akt activation protects hippocampal neurons from apoptosis by inhibiting transcriptional activity of p53. J. Biol. Chem. 276, 5256–5264. doi: 10.1074/jbc.M008552200
Yan, N., and Zhang, J. J. (2019). The emerging roles of Ferroptosis in vascular cognitive impairment. Front. Neurosci. 13:811. doi: 10.3389/fnins.2019.00811
Yang, W. S., Kim, K. J., Gaschler, M. M., Patel, M., Shchepinov, M. S., and Stockwell, B. R. (2016). Peroxidation of polyunsaturated fatty acids by lipoxygenases drives ferroptosis. Proc. Natl. Acad. Sci. U. S. A. 113, E4966–E4975. doi: 10.1073/pnas.1603244113
Yang, W. S., SriRamaratnam, R., Welsch, M. E., Shimada, K., Skouta, R., Viswanathan, V. S., et al. (2014). Regulation of ferroptotic cancer cell death by GPX4. Cells 156, 317–331. doi: 10.1016/j.cell.2013.12.010
Ye, Q., Zhang, X., Huang, B., Zhu, Y., and Chen, X. (2013). Astaxanthin suppresses MPP(+)-induced oxidative damage in PC12 cells through a Sp1/NR1 signaling pathway. Mar. Drugs 11, 1019–1034. doi: 10.3390/md11041019
Yoo, S. J., Cho, B., Lee, D., Son, G., Lee, Y. B., Soo Han, H., et al. (2017). The erythropoietin-derived peptide MK-X and erythropoietin have neuroprotective effects against ischemic brain damage. Cell Death Dis. 8:e3003. doi: 10.1038/cddis.2017.381
Yoon, E. J., Choi, Y., Kim, T. M., Choi, E. K., Kim, Y. B., and Park, D. (2022). The Neuroprotective effects of Exosomes derived from TSG101-overexpressing human neural stem cells in a stroke model. Int. J. Mol. Sci. 23:9532. doi: 10.3390/ijms23179532
Young Park, S., Jin Kim, Y., Park, G., and Kim, H.-H. (2019). Neuroprotective effect of Dictyopteris divaricata extract-capped gold nanoparticles against oxygen and glucose deprivation/reoxygenation. Colloids Surf. B: Biointerfaces 179, 421–428. doi: 10.1016/j.colsurfb.2019.03.066
Yuan, H., Li, X., Zhang, X., Kang, R., and Tang, D. (2016). Identification of ACSL4 as a biomarker and contributor of ferroptosis. Biochem. Biophys. Res. Commun. 478, 1338–1343. doi: 10.1016/j.bbrc.2016.08.124
Zapater, P., Moreno, J., and Horga, J. F. (1997). Neuroprotection by the novel calcium antagonist PCA50938, nimodipine and flunarizine, in gerbil global brain ischemia. Brain Res. 772, 57–62. doi: 10.1016/s0006-8993(97)00838-x
Zhang, J., Liu, J., Li, D., Zhang, C., and Liu, M. (2019). Calcium antagonists for acute ischemic stroke. Cochrane Database Syst. Rev. 2:Cd001928. doi: 10.1002/14651858.CD001928.pub3
Zhang, Y., Lu, X., Tai, B., Li, W., and Li, T. (2021). Ferroptosis and its multifaceted roles in cerebral stroke. Front. Cell. Neurosci. 15:615372. doi: 10.3389/fncel.2021.615372
Zhang, J., Wang, X., Bernardi, R. E., Ju, J., Wei, S., and Gong, Z. (2022). Activation of AMPA receptors in the lateral Habenula produces anxiolytic effects in a rat model of Parkinson's disease. Front. Pharmacol. 13:821975. doi: 10.3389/fphar.2022.821975
Zhao, Y. Y., Yang, Y. Q., Sheng, H. H., Tang, Q., Han, L., Wang, S. M., et al. (2022). GPX4 plays a crucial role in Fuzheng Kang'ai decoction-induced non-small cell lung cancer cell Ferroptosis. Front. Pharmacol. 13:851680. doi: 10.3389/fphar.2022.851680
Zhao, W. K., Zhou, Y., Xu, T. T., and Wu, Q. (2021). Ferroptosis: opportunities and challenges in myocardial ischemia-reperfusion injury. Oxidative Med. Cell. Longev. 2021:9929687. doi: 10.1155/2021/9929687
Zhou, S. Y., Guo, Z. N., Zhang, D. H., Qu, Y., and Jin, H. (2022). The role of Pericytes in ischemic stroke: Fom cellular functions to therapeutic targets. Front. Mol. Neurosci. 15:866700. doi: 10.3389/fnmol.2022.866700
Keywords: neuroexcitotoxicity, ferroptosis, glutamate, cystine-glutamate antiporter, ischemic stroke
Citation: Fan G, Liu M, Liu J and Huang Y (2023) The initiator of neuroexcitotoxicity and ferroptosis in ischemic stroke: Glutamate accumulation. Front. Mol. Neurosci. 16:1113081. doi: 10.3389/fnmol.2023.1113081
Edited by:
Sara Anna Bonini, University of Brescia, ItalyReviewed by:
Franca Codazzi, San Raffaele Hospital (IRCCS), ItalyEun Hee Ahn, Hallym University, Republic of Korea
Copyright © 2023 Fan, Liu, Liu and Huang. This is an open-access article distributed under the terms of the Creative Commons Attribution License (CC BY). The use, distribution or reproduction in other forums is permitted, provided the original author(s) and the copyright owner(s) are credited and that the original publication in this journal is cited, in accordance with accepted academic practice. No use, distribution or reproduction is permitted which does not comply with these terms.
*Correspondence: Yuhong Huang, hyh101@126.com