- 1Institute of Visual Neuroscience and Stem Cell Engineering, Wuhan University of Science and Technology, Wuhan, China
- 2College of Life Sciences and Health, Wuhan University of Science and Technology, Wuhan, China
- 3Hubei Province Key Laboratory of Occupational Hazard Identification and Control, Wuhan University of Science and Technology, Wuhan, China
Microglia are the primary resident retinal macrophages that monitor neuronal activity in real-time and facilitate angiogenesis during retinal development. In certain retinal diseases, the activated microglia promote retinal angiogenesis in hypoxia stress through neurovascular coupling and guide neovascularization to avascular areas (e.g., the outer nuclear layer and macula lutea). Furthermore, continuously activated microglia secrete inflammatory factors and expedite the loss of the blood-retinal barrier which causes irreversible damage to the secondary death of neurons. In this review, we support microglia can be a potential cellular therapeutic target in retinopathy. We briefly describe the relevance of microglia to the retinal vasculature and blood-retinal barrier. Then we discuss the signaling pathway related to how microglia move to their destinations and regulate vascular regeneration. We summarize the properties of microglia in different retinal disease models and propose that reducing the number of pro-inflammatory microglial death and conversing microglial phenotypes from pro-inflammatory to anti-inflammatory are feasible for treating retinal neovascularization and the damaged blood-retinal barrier (BRB). Finally, we suppose that the unique properties of microglia may aid in the vascularization of retinal organoids.
1. Introduction
Microglia, which are derived from the yolk sac, are the primary resident mononuclear macrophages in the retina and are regarded as the first line of active immune defenders. They express many pattern recognition receptors and immune receptors, such as AT1R, AT2R, and TβRII, empowering their function of guiding blood vessel formation, guaranteeing the survival of neurons, monitoring neuronal activity, pruning synapses of neurons, sustaining density of dendritic spines and clearing apoptotic cells or protein aggregates (Takahashi et al., 2005; Parkhurst et al., 2013; Cramer et al., 2022; He et al., 2022; Figure 1). Retinal microglia mainly present high branch structures to guarantee retinal homeostasis. When the balance of the retinal microenvironment is broken, microglia change their phenotypes, such as amoeboid mode, and stay in an activated state. Activated microglia are one of the essential neurotoxic mediators of neuroinflammation (Deng et al., 2009; Zhao et al., 2015). They rapidly move to damage sites and secrete pro-inflammatory factors (e.g., IL-1β, IL-6, TNF-α), chemokines (e.g., CCL2, CCL4, CXCL10) resulting in secondary damage to neuronal death, thereby accelerating the disease progression of patients (Wu et al., 2021; Puthenparampil et al., 2022).
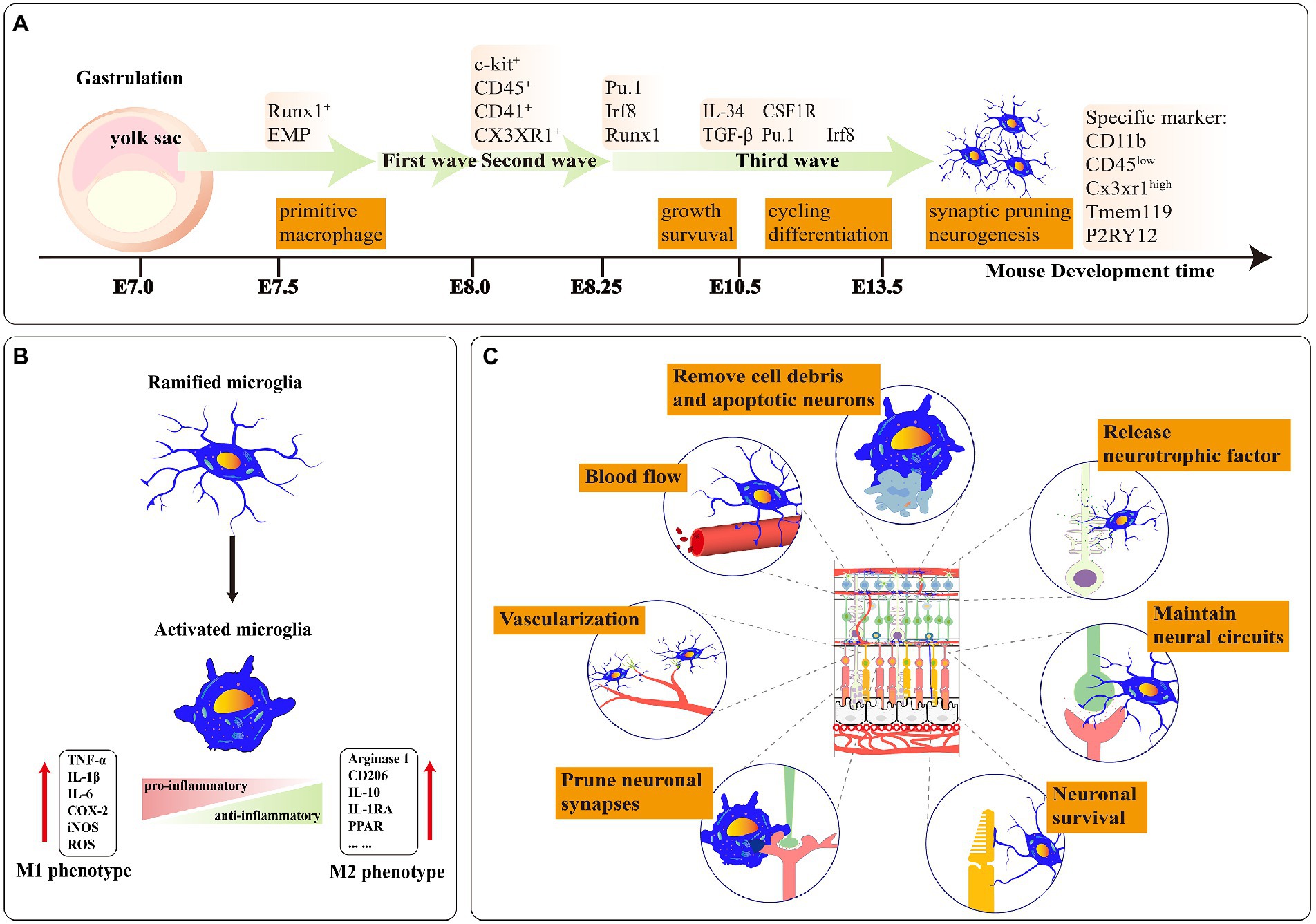
Figure 1. (A) A stepwise microglia developmental program in the retina. Mature microglia maintain a stable population derived from self-renewal. E-embryo. (B) Ramified microglia can be activated to two polarized phenotypes: M1 and M2. The polarization of M1 microglia increased the expression level of proinflammatory factors. Moreover, the polarization of M2 microglia expressed a high level of anti-inflammatory factors. (C) The functions of microglia in healthy retina tissue: Phagocytosis of apoptotic cells and debris; releasing neurotrophic factors to neurons; maintaining neural circuits; ensuring the survival of neurons; pruning neuronal synapses; promoting angiogenesis in retinal development; controlling the retinal vascular blood flow rate.
The retina, a highly active tissue member of the central nervous system, requires high oxygen consumption and metabolic demands. The retinal vascular systems transport nutrients to the retina and break down metabolites. In retinal diseases, such as proliferative diabetic retinopathy and retinopathy of prematurity, neovascularization exacerbate the loss of sight. Microglia participate in neovascularization and present co-localization with the neovascular plexus. According to recently published papers, researchers classified microglia into pro-inflammatory and anti-inflammatory based on the classification method of macrophages (Cano-Cano et al., 2021). The conversation from the pro-inflammatory microglia to the anti-inflammatory-microglia effectively reduces the formation of neovascularization (Zhou et al., 2021). In addition, endothelial cells cooperate with other types of cells and form the blood-retinal barrier (BRB) which strictly limits the exchange of various molecules and segregates the plasma and the tissue. Activated microglia promote the dysfunction of BRB which further aggravates plasma leakage and the death of cells. And it is possible that interventions targeting microglia could alleviate neovascularization and the damage to the blood-retinal barrier, thereby helping patients preserve their sight.
This review narrates the correlation of microglia with retinal neovascularization and the blood-retinal barrier. Targeting microglial activation may represent an attractive therapeutic method in retinopathy. Microglia involve in retinal vascularization during normal retinal development. Under some retinopathy, microglia participate in pathological angiogenesis, particularly in hypoxia conditions. The large number of inflammatory factors released by continuously activated microglia can accelerate the loss of vision and lead to the death of retinal endothelial cells, pericytes, retinal pigment epithelium (RPE), and neurons. Pro-inflammatory microglial death is fatal to the retinal disease process. Hence, we recapitulate three microglial signaling pathways we are more interested in. They may monitor retinal vascular function and decipher how microglia migrate near blood vessels through intercellular signaling crosstalk. This evidence demonstrates the vital role of microglia in the retinal vasculature and BRB. Finally, we summarize the research progress of microglia induced by iPSC (iMG) which is a reliable method to predict the molecular mechanisms of retinal microglia in vitro. And the unique properties of microglia may contribute to vascularizing human retinal organoids.
2. Retina microglia, retina vessel, and blood-retinal barrier
2.1. Microglia in retina
2.1.1. Derivation and localization of retinal microglia
Yolk sac progenitor cells in gastrula ultimately evolve into retinal microglia through three developmental waves from differentiation to primitive bone marrow cells. At mouse E7.0–7.5, the yolk sac progenitor cells in the blood land specifically express the RUNX family transcription factor 1 (Runx1) and differentiate to C-kit+ early bone marrow erythroid progenitor cells. At mouse E8.0, bone marrow erythroid progenitor cells enter the first wave of growth and precisely express CD41 and CD45. At E8.25, CD41+ and CD45+ cells access the second wave of development and further distinguish to the precursor microglia under the regulation of transcription factors such as SPi-1 proto-oncogene (PU.1), interferon regulatory factor (Irf8) and Runx1. At mouse E9.5, the precursor microglia enter the central nervous system (CNS) supported by Sodium/Calcium exchanger protein (NCX-1) and then colonize in the retina (Ginhoux et al., 2010; Prinz et al., 2021). At mouse E10.5, these cells eventually differentiate into the naive microglia under the stimulus factor of transforming growth factor beta (Tgfβ), colony stimulating factor 1 receptor (CSF1R), and interleukin 34 (IL34). EMR1/ADGRE1 (F4/80+) microglial population can be identified in the retina at E11.5 (Ajami et al., 2007; Tay et al., 2017). At mouse E12.5, the number of F4/80+ cell populations steadily increases and then proliferates and migrates from the central area to the retinal periphery (Santos et al., 2008). At mouse E14.5, microglia possess the function of synaptic pruning and shaping neural circuits (Figure 1A).
2.1.2. Microglial colonization and recolonization after ablation
Microglia progressively migrate to the whole retina during development and are finally located in ganglion cell layer (GCL), inner plexiform layer (IPL), outer plexiform layer (OPL), and nerve fiber layer (NFL) layers (Lee et al., 2008). Microglial CSF1R is one of the basic receptors to colonize the retina (Ginhoux et al., 2010). CSF1R, as a tyrosine kinase transmembrane receptor, has two activating ligands (Wei et al., 2010); (1) CSF-1, known as macrophage colony stimulating factor (M-CSF), plays an essential role in regulating the proliferation, differentiation, and survival of macrophages (Sherr et al., 1985); (2) IL34, mainly produced by glial cells and neurons, considers a substitute ligand for CSF-1 (Wei et al., 2010). When CSF-1 and IL34 bind to CSF1R, they encourage non-covalent dimerization of the receptor chains and transphosphorylation of tyrosine residues (Yu et al., 2008). And CSF-1 and IL34 have different affinities for D2 and D3 protein domains from CSF1R (Stanley and Chitu, 2014). Furthermore, unlikely microglia in OPL, microglia depend on IL-34 secreted by other neurons to localize in IPL and have the feature of assisting cone and rod in transferring chemical signals (Greter et al., 2012).
The neuronal regeneration capacity is limited in the damaged adult mammalian retina (Jorstad et al., 2017). Nevertheless, microglia repopulate the retina via individual mitosis or migration of the microglia/macrophage outside the retina to guarantee population quantity (Zhang et al., 2018). Huang et al. used PLX5622 to eliminate nearly all endogenous microglia in the retina of Cx3cr1+/GFP transgenic mice and discovered two microglial refilling ways: The epibiotic microglia of the optic nerve enter the retinal optic disc and then refill from the retinal center to retinal periphery; another microglial refill way derived from corpus ciliare/iris is in the opposite direction with above (Huang et al., 2018a). In addition, the newly recolonized microglia compulsively own similar phenotypes to the endogenous microglia in function, morphology and proliferation under the influence of the retina-tissue environment (Jin et al., 2017; McPherson et al., 2019).
2.1.3. The classification of retinal microglia
Retinal neuronal activity can affect microglial phenotypes (Anderson et al., 2022). The polarization phenotypes of retinal microglia present two extreme states: pro-inflammatory (M1) and anti-inflammatory (M2; Figure 1B). M1-microglia stay in “Classical activation” which secretes high levels of TNF-α, IL-1β, ROS, etc. Retinal IL-1β is primarily expressed by microglia and adding small doses of exogenous IL-1β increases neuronal survival ability in the excitotoxic condition (Todd et al., 2019). Appropriate activation of M1 microglia also facilitates axonal regeneration in trauma sites (Kigerl et al., 2009). However, continued activation of M1 microglia leads to irreversible neuronal damage (Tang and Le, 2016). In RD10, activated infiltrating microglia continuously secreted IL-1β which further accelerated the degeneration of the rod (Zhao et al., 2015).
The microglial phenotypes are in dynamic alternation during the disease process. M2 microglia can be subdivided into “alternative activation” and “acquired deactivation,” hinging on the activation environment and stimulation factors (Walker and Lue, 2015; Siddiqui et al., 2016). Alternative activated M2 microglia are heavily linked with functions such as anti-inflammatory repair and extracellular matrix reconstitution. In contrast, acquired deactivated M2 microglia convert their phenotype in response to anti-inflammatory factors (e.g., TGFβ1, IL-4) from the environment (Caruso et al., 2020; Chen et al., 2022). M2 microglia increase phagocytosis of erythrocytes and tissue debris, which facilitates hematoma regression (Lan et al., 2017). M1 transformation to M2 microglia alleviates the degeneration of photoreceptors in the mouse model RD1 (Zhou et al., 2018; Figure 1B). The conversion of the M1/ M2 phenotype in the appropriate period of acute or chronic retinopathy may provide better therapeutic benefits.
However, with the discovery of the biomarker, retinal microglia likewise generated different subsets of species, and their classification should not be limited to M1 or M2 in the fully polarized state (Wieghofer et al., 2021). In the meanwhile, Liu et al., had pointed out that a proliferative retinopathy-associated subset of microglia presented to perivascular newborn tufts in the oxygen-induced model (Liu Z. et al., 2022). Therefore, a rational classification of these microglia subsets and naming them with specialized nomenclature is necessary.
2.2. Microglia correlation with retinal vasculature and BRB in retina
Microglial branches contact retinal blood vessels, secrete nutritional factors and angiogenic factors, control the apoptosis of pericytes and endothelial cells, and timely eliminate redundancy vessel debris which plat an outstanding significance to maintaining retinal function (Ritter et al., 2006; Jolivel et al., 2015; Harsing et al., 2021). They regulate vessel diameter and blood flow velocity through neurovascular coupling (Császár et al., 2021; Mills et al., 2021). Astrocyte and Müller cells are also members of neurovascular coupling. Astrocytes mainly contact the superficial vascular plexus and the regulation ability of Müller cells is principally limited in the intermediate vascular plexus in stable conditions (Biesecker et al., 2016).
IPL and OPL are the main oxygen-consuming layers (Yu and Cringle, 2001). In these layers, microglial branches co-locate with blood vessels which is sufficient to justify the importance of microglia to the retinal blood vessel and the BRB. Therefore, in this section, we briefly introduce the structure of retina and then summarize the relevance of microglia to the retinal neovascularization and BRB.
2.2.1. Structure of retina
Retina, as a functional unit of the CNS, is mainly composed of six types of neurons (rod, cone, amacrine, bipolar, horizontal and ganglion) which convert light from the external environment into neural chemical signal and then transfer it to the brain through the optic nerve. And there are three species of glia cells; thereinto, astrocyte and Müller provide retina nutrition and supporting function. Microglia, as the third type of glia in the retina, supervises the homeostasis of the retinal environment in real time and resists foreign microorganism invasion. In structure, the retina is divided into: (1) ONL: cytons of cone and rod; (2) OPL: synapses of cones, rods and horizontal; (3) INL: cytons of bipolar, horizontal and amacrine and Müller; (4) IPL: synapses of bipolar, amacrine cells, retinal ganglion cells and Müller; (5) GCL: cytons of retinal ganglion cells (Figure 2).
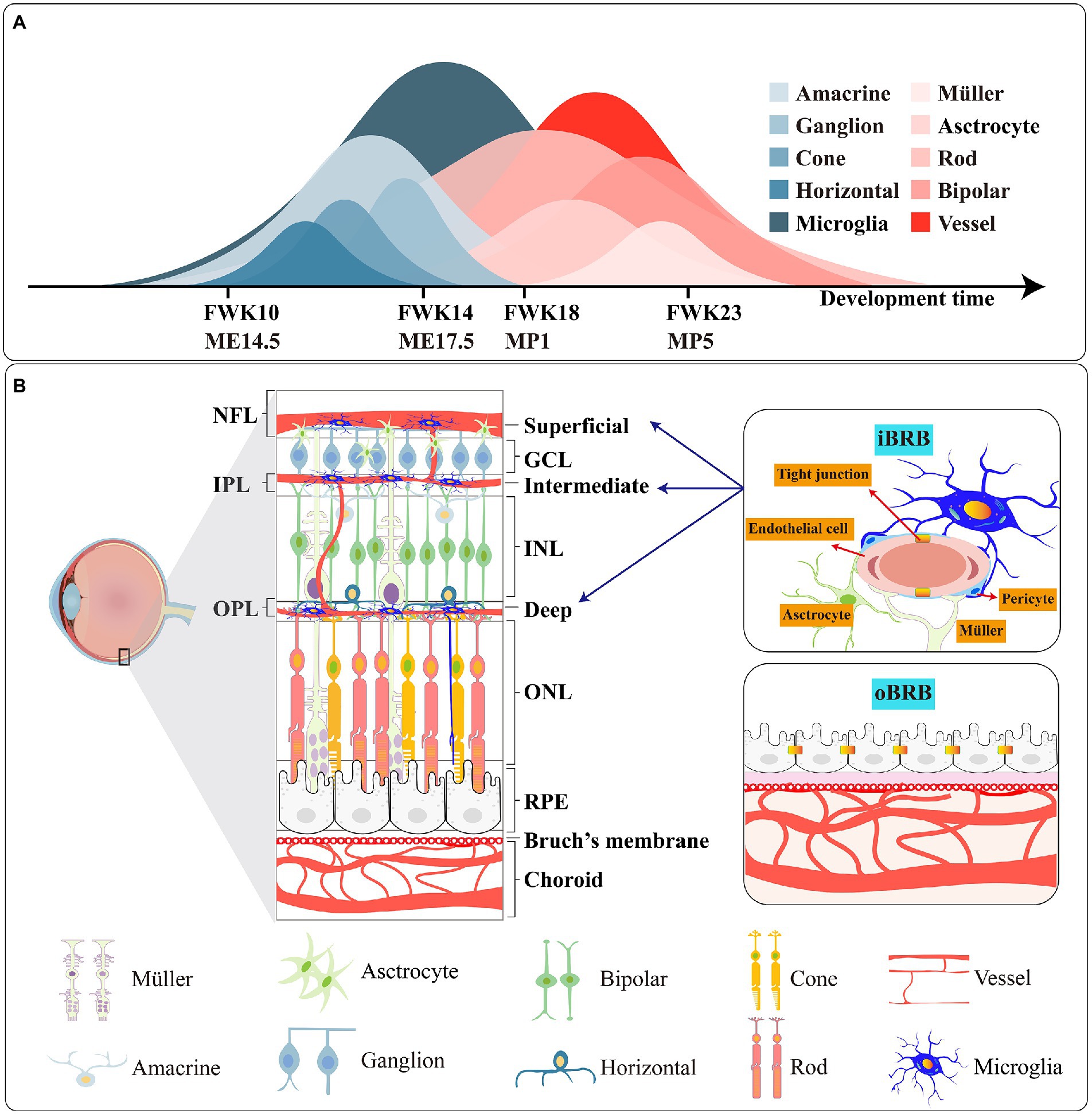
Figure 2. (A) Genesis of retinal cells during the development of the human and mouse. FWK-fetal week; ME-mouse embryo; MP-mouse postnatally. (B) Schematic picture of the retina, the iBRB and oBRB. The main types of tight junction protiens are different in iBRB and oBRB.
2.2.2. Retinal vasculature
The retina of humans, mice and rats have formed three kinds of vasculature systems in the long course of evolution. And the sequence of their development is choroidal vasculature - hyaloid vasculature-retinal vasculature (Lutty and McLeod, 2018). Retina, as a high oxygen consumption and metabolic demand tissue in the CNS (Kooragayala et al., 2015), has a dual blood supply vascular system: (1) Retinal vasculature: Central artery and vein radially extend along retinal exterior and then form arterioles and venules. Arterioles expand to the IPL and OPL layers and then continue to nurture capillaries. Blood backflow to venules along the capillaries eventually returns to the central vein and leaves the visual system through the optic nerve, and completes the blood cycle of vision. At 14 weeks of gestation in humans, the retinal vasculature starts to develop and enters the epilogue at 23 weeks (Hasegawa et al., 2008). Unlike humans, the retinal vasculature of the mouse comes to maturity about 1–2 weeks after postnatal development, and the time of maturation varies between strains (Stahl et al., 2010). After the development of the retinal vascular system, three layers of vascular plexus are differentiated in the GCL, IPL, and OPL, respectively called superficial vascular plexus, intermediate vascular plexus, and deep vascular plexus. And the growth rate of the deep is preferred to the middle (Fruttiger, 2007). (2) Choroid vasculature also consists of three blood plexuses. The outermost plexus is called Haller’s layer, the middle one is the Sattler layer, and the innermost anterior is the capillaries (Lutty and McLeod, 2018). The anterior capillaries allow plasma to congregate the surface of Bruch’s membrane, and vesicles in RPE deliver nutrients and oxygen from plasma to the rod and cone.
Pathological angiogenesis is the leading irreversible cause of blindness among potentially blinding eye diseases. Angiogenesis is a process defined as forming new blood vessels on basis of existing capillaries under the combined action of angiogenic factors and endothelial cells. Endothelial cells consolidate their proliferation and transferability under the action of vascular endothelial growth factor (VEGF), insulin like growth factor 1 (IGF1) and Notch family receptors and their ligands; some endothelial cells grow the filopodia and become endothelial tip cells (Gerhardt et al., 2003; Cao et al., 2017). Mature neovascularization connects with the initial vessels to participate in blood circulation and become a section of the vascular system (Zoya Tahergorabil, 2012). In multiple retinal diseases, such as RAP, the neovascularization extending to the avascular area aggravated vision loss in patients (Spaide, 2013). And the activated microglia are involved in angiogenesis during disease onset.
2.2.3. BRB
BRB is vital to retinal function. Sharing resemblances with the blood–brain barrier in the brain, BRB protects the retina and isolates pathogens and microorganisms. The BRB consists of the inner blood-retinal barrier (iBRB) and the outer blood-retinal barrier (oBRB). Endothelial cells in the iBRB safeguard the integrity of the iBRB under the action of the neurovascular unit formed by microglia, Müller cells, astrocytes and pericytes (O'Leary and Campbell, 2021). And the oBRB is composed of choroid, Bruch’s membrane and RPE (Figure 2B).
In angiodynamic researches, fluorescence angiography (VFA) is commonly utilized to examine the half-rise, half-fall and offset time of blood flow filling in retinal arteries, veins and capillaries after intravenous injection of drugs to check the damage of the blood-retinal barrier, vascular leakage and angiogenesis (Hui et al., 2014).
2.2.4. Microglial relationship with retinal vasculature
Microglia settle in the retina through the optic nerve, vitreous body, and ciliary body before retinal vasculature matures (Diaz-Araya et al., 1995). Their ramifications contact endothelial stalk cells and filopodia on tip cells in the period of mouse retinal vasculature development (Checchin et al., 2006). And microglia may lead tip cells to clarify the direction of neovascularization which is ultimately limited in retinal GCL, IPL and OPL layers (Haupt et al., 2019). Sharing resemblances with the brain, after treatment with lipopolysaccharide, the primary microglia up-regulate the VEGF-A and PDGF-BB expression level of RMEC which promotes the ability of tubes formation, migration and proliferation (Li et al., 2014; Ding et al., 2018). After using PLX5622 to deplete microglia, retinal choroidal vessels begin to atrophy, and RPE present dysfunction (Yang et al., 2020). Microglia and retinal vessels are positively correlated in terms of quantity during development (Checchin et al., 2006; Zeng et al., 2022). The diminished number of retinal microglia result in sparse vascular density during the second and fourth postnatal days in CSF−/− deficient mice (Rymo et al., 2011). Exogenous microglia within intravitreal injection promotes the decreasing density and area of vascular triggered by resident microglia depletion during the retina development process (Checchin et al., 2006). Activated pro-inflammatory microglia are involved in the remodeling of retinal vasculature in the PKD model (Chen et al., 2021). This evidence is sufficient to demonstrate the indispensable role of microglia in adjacent retinal vasculature (Figures 3A–D).
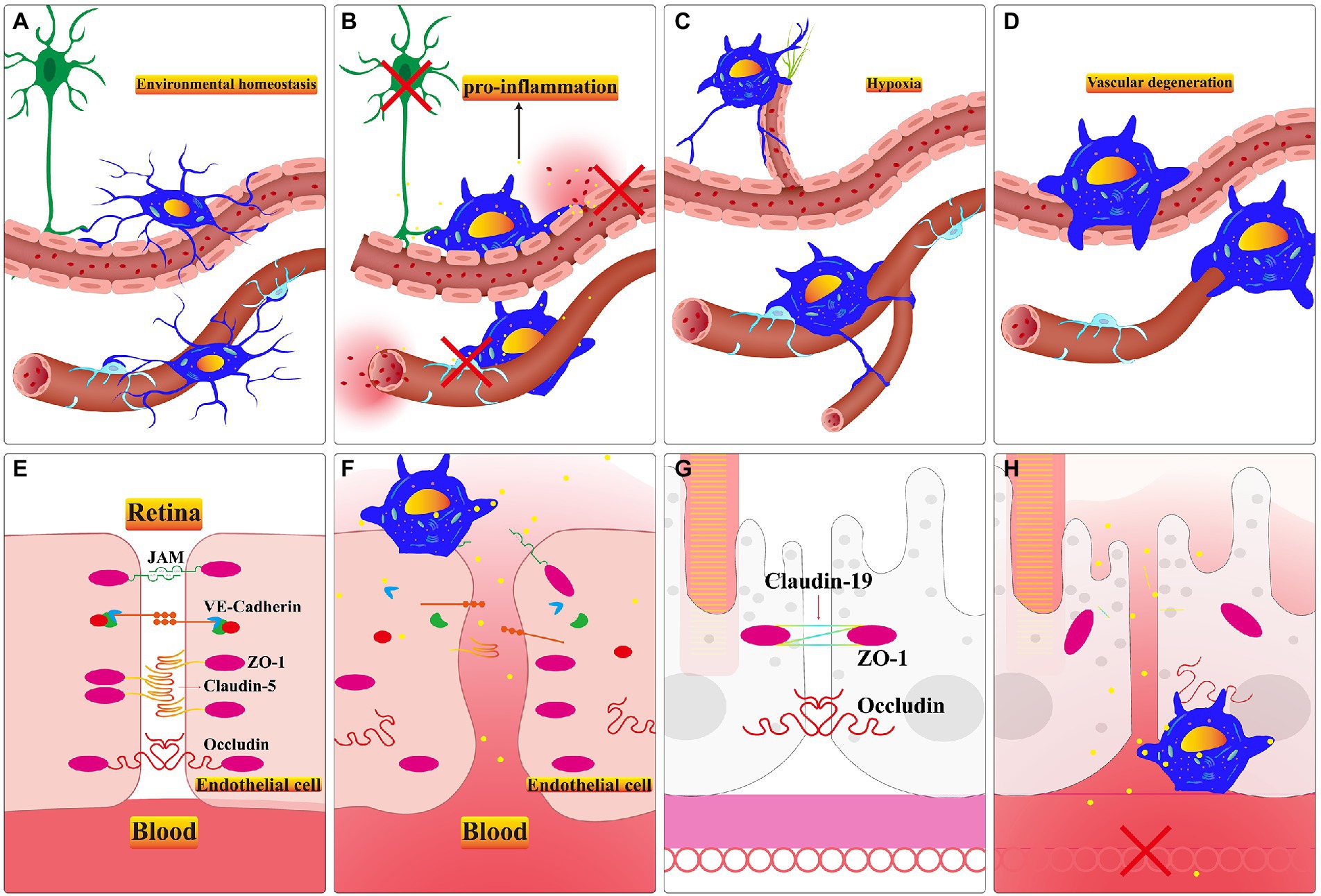
Figure 3. (A) Microglia monitor the retinal pericytes and endothelial cells and regulate the rate of retinal blood flow in healthy retina. B-D. The microglial responses in different retinal dysfunction environments. (B) Activated microglia promote apoptosis of neuronal cells, endothelial cells, and pericytes. Persistent activation of microglia alters the permeability of endothelial cells and promotes the appearance of vascular leakage. (C) Microglia guide the formation of neovascularization when the retina stays in a comparatively hypoxic environment. (D) In an inflammatory environment, activated microglia phagocytose the neovascular plexus or engulf apoptotic endothelial cells. (E) The major types of tight juctional molecules between endothelial cells of the iBRB in healthy retina. (F) Inflammatory factors secreted by activated microglia facilitate the downregulation of tight junctional molecules between endothelial cells, and vascular leakage occurs. (G) The significant types of tight junctional molecules between RPE cells of the oBRB in healthy retina. (H) Inflammatory factors secreted by activated microglia accelerate the downregulation of tight junctional molecules between RPE cells, capillary leakage, and RPE cells apoptosis. Choroidal neovascularization invasion to subretinal space in a relatively hypoxic environment.
2.2.5. Microglial relationship with BRB
Altered endothelial cell permeability is the central mechanism of BRB dysfunction during retinopathy (Sun et al., 2021). The defective BRB accelerates the death of the cone leading to swift vision degeneration (Ivanova et al., 2019). Targeting activated microglia is a crucial factor in repairing damaged BRB. They recruit neutrophil infiltration, aggravating the damage to the blood-retina barrier and optic nerve cell death in the retinal vein occlusion model (Jovanovic et al., 2020). Microglia can be activated by hyperglycemia in the retinal environment (Zhang et al., 2019). And the activated microglia engulf endothelial cells which exacerbates the phenomenon of acellular capillaries and albumin leakage in the diabetic model (Xie et al., 2021). Inflammatory factors secreted by activated microglia aggravate the damage of BRB (Usui-Ouchi et al., 2020). Pro-inflammatory microglia activate the TLR4/MyD88/NF-κB p65 signaling axis and the NF-κB p65 nuclear translocation promotes the inflammatory factors resulting in the BRB breakdown (Fang et al., 2021). In the co-culture system, unstimulated microglia promote the expression of endothelial tight junction protein (TJP), Zonula Ocluden-1 (ZO-1) and Occludin (Mehrabadi et al., 2017). However, activated microglia secrete IL-1β which stimulates VEGF releasing; subsequently, VEGF can down-regulate the expression of ZO-1 and Claudin-5 in endothelial cells in hypoxia conditions (Inada et al., 2021). And the ablation of activated microglia alleviates innate immunity stimulated by LPS and protects retinal BRB integrity through up-regulating Occludin, ZO1 and Claudin-5 (Kokona et al., 2018; Figures 3E–H).
In conclusion, activated microglia produce inflammatory factors and participate in the damage of BRB. In the hypoxia condition, microglia assist neovascularization and guide them incorrect positioning which causes the secondary lesions and accelerates the disorder progression in patients. Therefore, profoundly investigating the characteristics of microglia under pathological conditions may become a key to treating retinal diseases. After this, we choose one system and two targets we are more interested in. They contribute to retinal vasoconstriction, neovascularization and microglial cytoactive.
3. Potential targets of microglia in retinopathy
3.1. The microglial renin-angiotensin system contributes to retinal vasoconstriction and angiogenesis
Renin-angiotensin system was initially considered a humoral system that governed blood pressure and water-sodium homeostasis. In recent studies, researchers find that renin-angiotensin system has the function of regulating ocular circulation and balancing intraocular pressure. The upregulation of the renin-angiotensin system contributes to the formation of blood vessels in the developing retina (Sarlos and Wilkinson-Berka, 2005). Dysregulation of the renin-angiotensin system is commonly seen in retinopathy, such as ROP. Blocking the renin-angiotensin system could postpone neovascularization forming (Misrak Tadesse et al., 2001).
In the renin-angiotensin system, renin, a rate-limiting enzyme which the precursor is pro-renin, disassembles angiotensinogen (Agt) encoded by the AGT gene to angiotensin I (Ang I). Ang I subsequently hydrolyze to effector molecule angiotensin II (Ang II) under angiotensin-converting enzyme (ACE). In the model of proliferative Diabetic Retinopathy (DR) and Age-Related Macular Degeneration (AMD), Ang II upregulates the secretion of angiogenic factors and growth factors, provokes microglial activation and leads to hypertension (Moravski et al., 2000; Shi et al., 2010; Hatzopoulos et al., 2014). And up-regulating the expression of retinal Ang II promotes the expression of TNF-α secreted by the activated glial cells which contributes to the death of RGC (Jeon et al., 2022). Activated microglia secrete Agt and Ang II resulting in angiogenesis during tissue injury or disease (Mills et al., 2021).
However, the functions are dissimilar when Ang II binds with various receptors. The common receptors of Ang II are angiotensin subtype-1 receptor (AT1R), Ang II type 2 (AT2R) and MAS-R (Figure 4A).
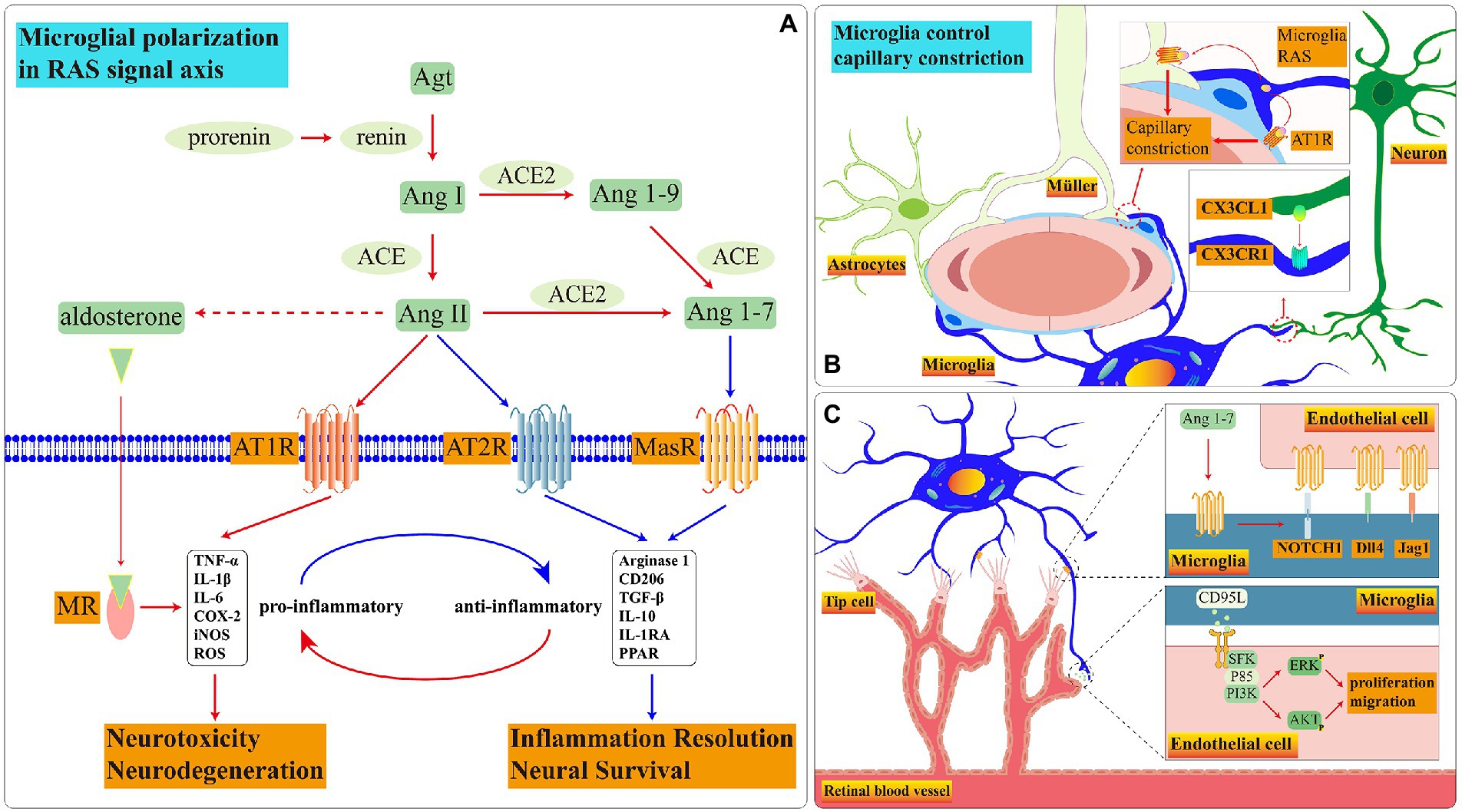
Figure 4. Schematic diagram of RAS signal pathways in microglia. (A) During disease onset, the RAS in microglia is imbalanced. Ang II is biased towards binding to AT1R and MR which promote microglia to pro-inflammatory functions. Among these receptors, the presence and function of AT2R in retinal microglia remain to be demonstrated. (B) The crosstalk among three glia cells controls blood flow rate through RAS. (C) Microglial MasR controls blood vessel formation during retinal development.
3.1.1. Renin-angiotensin-aldosterone (RAAS) and AT1R
The human retina has locally subsisting RAAS systems (Wagner et al., 1996). RAAS controls vasoconstriction, regulates the release of vascular endothelial growth factor (VEGF) to participate in angiogenesis and increase vascular permeability. Moreover, AT1R, as an essential receptor with cardiovascular homeostasis, facilitates the efficacy of Ang II. And Ang II participates in aldosterone release under the action of aldosterone synthase (Maning et al., 2017). Aldosterone further binds to mineralocorticoid receptor (MR) regulating internal water-electrolyte balance and influencing cardiovascular diseases (Waanders et al., 2011). In addition, the aldosterone with the intravitreal injection can mimic clinical symptoms of central serous chorioretinopathy (CSC; Yu et al., 2022).
Retinal microglia have RAAS. In healthy conditions, Microglial CX3CR1 receives neuronal CX3CL1 signal which may govern the velocity of retina blood flow through RAAS (Mills et al., 2021; Figure 4B). When the exogenous Ang II binds to AT1R on the surface of N9(a microglia cell line) and primary microglia, the ROCK activation stimulates the NOX activation via P38 (Rodriguez-Perez et al., 2015). NOX is one of ROS manufacturers which facilitates the process of retinopathy (Ahmad et al., 2021). In addition, the binding of Ang II to AT1R motivates the translocation process of NF-кβ and STAT3 which accelerates the TNF-α releasing (Abadir et al., 2011). Adding AT1R antagonist effectively reverses microglial inflammatory phenotype and reduces the release of inflammatory factors stimulated by exogenous Ang II (Phipps et al., 2018). AT1R up-regulates at 12 h after ischemia, the candesartan, as an AT1R antagonist, effectively reduces the inflammation (Fukuda et al., 2010). The AT1R antagonist diminishes NF-Kβ nuclear translocation and STAT3 phosphorylation which declines the release of TNF-α, IL-10, ROS and nitrite accumulation in BV2 microglia treated with LPS (Bhat et al., 2016).
Microglia also express MR and aldosterone synthase. Microglial density significantly up-regulates in both REN-2 transgenic rats (rats overexpress renin and Ang II) and the Oxygen-Induced Retinopathy(OIR) model; Valsartan (an antagonist of AT1R) and Spironolactone (an antagonist of MR) effectively diminish the VEGF, CCL5 and IFN-γ secreted by the primary microglia in vitro hypoxia condition (Rana et al., 2020). Activated microglia can promote retinal neovascularization in some cases (Hatzopoulos et al., 2014). Using FAD286 (an aldosterone synthase inhibitor) reduces the density of Iba1+ microglia and inhibits approximately 89% of neovascularization and 67% of neovascular tufts in the OIR model (Deliyanti et al., 2012).
According to the clues above, we can summarize microglia may regulate the velocity of retina blood flow through RAAS in healthy conditions. However, in chronic diseases, RAAS converts microglia to the pro-inflammatory phenotype, which releases inflammatory factors and injures the integrity of BRB. Reactive microglia promote angiogenesis when the survival environment situates in relative hypoxia.
3.1.2. Ace/Ang II/AT2R
The ACE/Ang II/AT2R signaling pathway is associated with anti-angiogenesis, anti-inflammatory and partly balances the influence of RAAS (Tao et al., 2016; Carey, 2017). The expression of AT2R decreases with age and is lower than AT1R in the adult mouse retinas (Yoon et al., 2016; Verma et al., 2019). The up-regulation of AT2R effectively prevents damage to the optic nerve and the polymorphism of G/A at the 1,675 site may be related to arteriolar diameter (Kurihara et al., 2006; Liu et al., 2011). Moreover, the activation of the AngII-AT2R signaling pathway can reverse the microglial inflammatory phenotype (Gao W. et al., 2022).
Utilizing exogenous Ang II activates the GSK3β through upregulating the phosphorylation of Y216 and downregulating the phosphorylation of S9 which degrade the NRF2 expression and inhibit microglial antioxidant capacity; the escalation of Ang II concurrently leads to mitochondrial dysfunction through stimulating phosphorylation of PKCα/β and P-PKCδ, activating phosphorylation of NOX-2p47phox and generating ROS accumulation (Bhat et al., 2019). AT2R binding to AngII stimulates the activation of PP2A (Guimond and Gallo-Payet, 2012). PP2A guarantees neuronal survival and silences PKC/ERK/NF-кB signaling pathway to prevent inflammatory cascade reaction stimulated by LPS (Egger et al., 2003; Bononi et al., 2011). CGP42112A, as an AT2R agonist, induces PP2A activation by declining the p-Y307-PP2A expression and inhibits NOX-2 activation by declining the P-S345-P47phox expression; in addition, CGP42112A reduces the ROS production induced by Ang II and converts microglial phenotype from pro-inflammatory to anti-inflammatory (Bhat et al., 2019). Delaying administration of C21 (an agonist of AT2R) after 3 days post-stroke aggrandizes the number of anti-inflammatory microglia and effectively improves the rate of survivability, sensorimotor and cognitive deficits (Jackson et al., 2020; Sumners et al., 2020). Adding CGP42112 boosts the proliferation ability of microglial; the newborn cells predominantly present the ramified structure in peripheral infarct and the area of the infarct is diminished after 3 days of stoke; however, using PD4123319 (an AT2R antagonist) reverses the protective function of CGP42112 (McCarthy et al., 2012). We speculate that CGP42112 may promote anti-inflammatory microglia which maintain neuronal survivability. And in the central infarct core, the continuous release of inflammatory factors stimulates microglia to form different phenotypes with microglia in the peripheral infarct area. Whereafter, McCarthy et al. find that under the C21 stimulation, microglia releases brain derived neurotrophic factor (BDNF) which protects neuron survival and improves vasodilation (McCarthy et al., 2014). In addition, the upregulation of VEGF stimulated by PD123319 promotes the proliferation and migration ability of endothelial cells; on the contrary, CGP42112A selectively inhibits vascularization driven by VEGF in the OIR model (Carbajo-Lozoya et al., 2012).
Therefore, we speculate there may be a similar mechanism to brain-derived microglia in retinal microglia. Retinal microglia may preserve neuronal survival and blood vessels through the ACE/Ang II/AT2R signaling pathway which is expected to treat neoangiogenic retinopathy.
3.1.3. ACE2/Ang1-7/MasR
ACE2/Ang-(1–7)/MasR promotes vasodilation and has demonstrated anti-inflammatory properties (Liu et al., 2016). ACE2, a homolog of ACE, hydrolyzes Ang I and Ang II to Ang1-7; Ang1-7 binds to Mas receptor (MasR) which is encoded by MAS1 proto-oncogene (Mas1; Jiang et al., 2013). In oxidative stress injury induced by Ang II, the Ang1-7 activation protects the survival of dopaminergic neurons, alleviates microglia-induced inflammatory responses and enhances the survival rate of hypertensive stroke rats model (Regenhardt et al., 2014; Liu et al., 2016; Costa-Besada et al., 2018).
Retinal microglia express MasR which gradually decreases with age (Tuhina Prasad and Li, 2014). In primary retinal microglia, the expression of MasR is up-regulated following the hypoxia condition which is a necessary element to stimulate angiogenesis in retinal development process (Gariano and Gardner, 2005; Foulquier et al., 2019). In MAS1 deficient mice model, the rate of angiogenesis is slower than in controls, and the number of perivascular microglia and filopodia of endothelial tip cells is significantly decreased; under the stimulating of MAS agonist AVE0991, the mRNA expression level of IL-10, Notch1, Dll4, and Jag1 are increased (Foulquier et al., 2019). The contact points between microglial ramifications and endothelial cells activate the Notch1 signal which facilitates tip cells to recruit microglia during retinal development in newborn mice (Outtz et al., 2011). Microglia can stimulate vessel sprouting and branching of aortic ring explants through soluble factors and angiogenic factors; in turn, aortic ring explants recruit microglia to migrate toward them (Rymo et al., 2011). Therefore, we have reasonable doubt to suspect that microglia interact with endothelial cells and guide the tip cells in the neovascular to migrate toward the retinal periphery through the MAS signaling pathway during retinal development (Figure 4C).
The primary microglia of the brain also express MasR (Rivas-Santisteban et al., 2021). Nevertheless, renin-angiotensin system becomes unbalanced under the long-term stimulation of LPS in the brain; when ACE2/ Ang(1–7)/MasR signal axis is stimulated by AVE0991, microglia convert their phenotype from pro-inflammatory to anti-inflammatory (Dang et al., 2021). Utilizing DIZE, an ACE2 activator, diminishes inflammation of activated microglia, up-regulates cell survival proteins and down-regulates neuronal apoptotic proteins through stimulating the ACE2/ Ang (1–7)/MasR signal axis in the 6-OHDA induced Parkinson’s model; nevertheless, the A-779, a MasR antagonist, balances the protective affection of DIZE (Gupta et al., 2022). MasR can form a dimer with AT1R or AT2R. The expression of these dimers in striatal microglia is higher than those in cerebral neurons; when microglia are treated with IFN-γ and LPS, the expression of AT1R-MasR is up-regulated and the expression of AT2R-MasR is down-regulated; Rivas et al. propose the possibility of forming heterotrimer among AT1R, AT2R and MasR (Rivas-Santisteban et al., 2021).
Based on the above clues, we consider the dysfunction of the microglial renin-angiotensin system loses the ability to control retinal vasoconstriction in retinal vascular malformation diseases which reduces the adequate oxygen supply to retinal neurons leading to the deterioration of vision. The molecular relationship among AT1R, AT2R, and MasR needs to be further verified in retinal microglia (Figure 4).
3.2. Microglial CD39 may provide anti-inflammatory treatment and protect BBB integrity during retinopathy
In the mammalian retina, ATP and adenosine (ADO) are crucial molecules that participate in vascular remodeling and form the neurovascular coupling (Zeiner et al., 2019; Losenkova et al., 2022). Abnormally elevated expression of ATP is a common phenomenon in many retinopathies. There are an abundant supply of adenosine triphosphate (ATP) ecto-nucleotidases near retinal blood vessels which are classified into three main types: nucleoside triphosphate diphosphohydrolase 1 (NTPDase1/CD39), NTPDase2 and ecto-5′-nucleotidase (CD73). Thereinto, CD39 is an extracellular enzyme encoded by ENTPD1 which hydrolyzes ATP to adenosine diphosphate (ADP) and adenosine (ADO) by cooperating with CD73. And retinal CD39 are mainly produced by microglia and endothelial cells. At the early stage of retinopathy, the CD39 expression is significantly up-regulated to maintain the balance between ATP and ADO; when retinal disease develops in the advanced stage, the number of CD39+ microglia decrease prominently and the balance between ATP and ADO is broken; The overladen ATP in the retinal microenvironment mediate inflammatory reaction that leads to neuronal death and M1 microglial activation (Lu et al., 2015; Hu et al., 2017; Rodrigues-Neves et al., 2018).
In addition to eliminating the redundant ATP in the internal environment, CD39 can remodel the anti-inflammatory properties of macrophages (Villacampa et al., 2015; Jakovljevic et al., 2019). CD39 and CD73 synergistically inhibit the multiplication capacity of T cells and decrease the production of inflammatory factors in the EAU mouse model (Chen et al., 2016). Similarly, in type I diabetic hypothalamus, the expression of CD39 in microglia and blood vessels is diminished by 30%, and the integrity of the blood–brain barrier is impaired; Minocycline can effectively promote the expression of CD39 and the pro-inflammatory microglial activation is decreased (Bulavina et al., 2013; Hu et al., 2015).
The ADO, the hydrolysate of ATP, participates in the microglial contracting branches process and recruits them to migrate toward the lesion area (Matyash et al., 2017; Lim et al., 2018). The microglia migration capacity decreases significantly in CD39-deficient mice; after adding exogenous ecto-nucleotidases or ADO, microglial migration ability recovered (Farber et al., 2008).Microglial CD39 mitigates neuronal overactivation through the ATP/AMP/ADO/A1R signaling axis (Badimon et al., 2020). ADO can bind to different receptors and perform different functions. Adenosine A1 receptor (A1R) and A2aR are involved in regulating angiogenesis, blood flow rate and inflammatory response. For example, in the diabetic retina, the ADO releasing and formation stimulated by Triamcinolone promotes the A1R activation which prevents cells from osmotic swelling and results in ion efflux through potassium and chloride channels (Wurm et al., 2008). And the A2aR activation can inhibit phagocytic activity and migration capacity of primary microglia (Ingwersen et al., 2016). The downstream response mechanism of retinal microglia receiving ADO signals is still unclear and needs further exploration (Figure 5A).
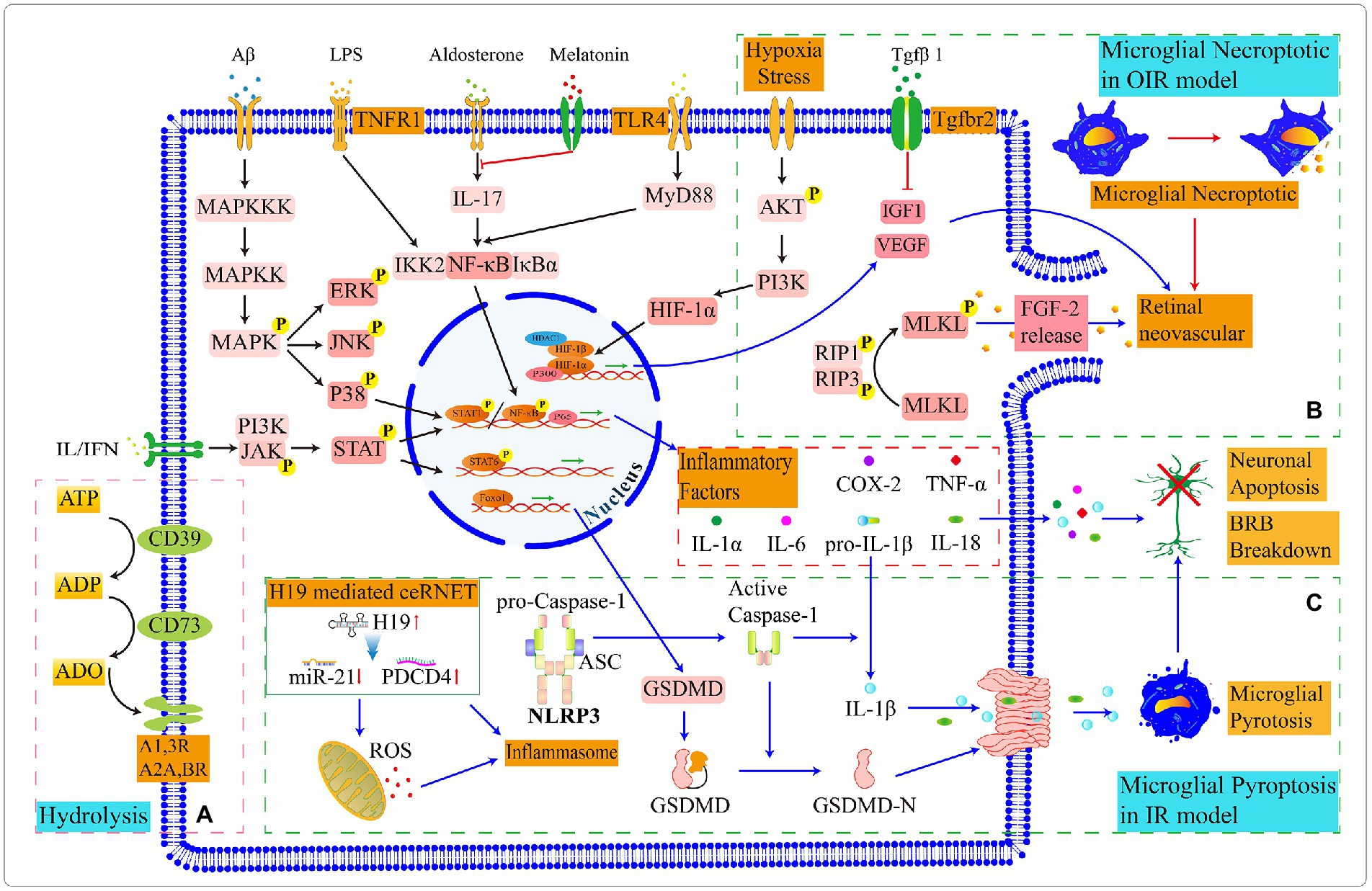
Figure 5. Schematic diagram of partly signal pathways in retinal microglia. (A) Microglia express CD39 and CD73 that progressively hydrolyze ATP to ADO together. (B) In the OIR model, microglial necrosis promotes the leakage of FGF, which stimulates retinal neoangiogenesis. Microglia express proangiogenic factors to promote retinal vascular proliferation, and the binding of (C) In the IR model, a competing endogenous RNA network (ceRNET) stimulates microglial pyroptosis. The maturation of pro-caspase1 in inflammasome and the activation and assembly of GSDMD protiens lead to the leak age of inflammatory factors, which ultimately result in the apoptosis of retinal neurons and damage to the BRB. The switching status of microglial signal pathways in different retinal environments and the target genes in upstream and downstream need to be further explored.
Interestingly, microglia create a spatially arranged network in the retinal parenchyma and form a local “purinergic junctions” system with CD39low/CD73− neuronal cell bodies and CD39high/CD73− retinal blood vessels through their CD39high/CD73low branches (Losenkova et al., 2022). Further exploring the mechanisms through which a broad spectrum of soluble and membrane-binding enzymes synergistically regulates purine levels in the retina may serve as potential therapeutic targets for the treatment of retinopathy (Zeiner et al., 2019). Increasing the number of CD39+ microglia subset may contribute to the restoration of the retinal barrier and alleviate inflammation and reduce retinal vascularization.
3.3. Microglial Tgfβ1 and its receptor TβRII involve in vascular remodeling and cellular phenotype conversion
Transforming growth factor beta (Tgfβ), as a multifunctional cytokine which is associated with AMD susceptibility, is vital to sustain the specificity of microglia, ensure the stability of retinal vascular endothelial cells and BBB (Walshe et al., 2009; Braunger et al., 2013; Bohlen et al., 2017; Zarkada et al., 2021). TGFβ can up-regulate SNAIL through AKT and polarize macrophages to anti-inflammatory phenotype; when blocking TGFβ/SNAIL signal transduction, they promote the output of inflammatory factors and convert their phenotype to pro-inflammatory (Zhang et al., 2016). The Tgfβ up-regulation inhibits pro-inflammatory microglial activation and reduces the TNF-α and IL-6 releasing in microglia during retinopathy (Kim et al., 2004; Taylor et al., 2017; Yang et al., 2021).
In mammals, three members of the Tgfβ isoform family encoded by independent genes are identified: Tgfβ1, Tgfβ2 and Tgfβ3 (Anderson et al., 1995). Tgfβ1, as a potential therapeutic target, reduces microglia-mediated neuroinflammation and improves outcomes of intracerebral hemorrhage after acute injury (Taylor et al., 2017). Although the expression of Tgfβ1 is the lowest one of the Tgfβ family in the adult retina, the ability of Tgfβ1 has been proven to induce microglial conversion from the pro-inflammatory phenotype to anti-inflammatory phenotype which is related to neuroprotection and the anti-inflammatory treatment (Tosi et al., 2018; Caruso et al., 2020). In Rho−/− and PDE6βmut/mut mouse model of retinitis pigmentosa, AAV8-mediated supplementation of Tgfβ1 effectively prolongs the cone degeneration time through up-regulating Spp1 and down-regulating Gas6 of microglia, thereby indicating the therapeutic potential of precisely polarizing pro-inflammatory to anti-inflammatory microglia (Wang et al., 2020). And Tgfβ1 can also promote the up-regulation of CD73, inhibit the proliferation of activated T cells and reduce inflammation of the internal environment in the EAU model (Chen et al., 2016). Surprisingly, Tgfβ1 expressed by microglia frequently associates with vascular remodeling. In the microglial autocrine Tgfβ1 signaling pathway, Kindlin3, as an intracellular adapter molecule, is associated with microglial polarization; when Kindlin3 is knockout, high myosin contractility contributes to ERK phosphorylation and further promotes the overexpression of Tgfβ1 which results in angiogenesis (Dudiki et al., 2020).
The Tgfβ receptor consists of two structurally similar sub-families (TβRI and TβRII) and a transmembrane proteoglycan (beta-glycan/TβRIII). Thereinto, TβRII belongs to the serine and threonine transferase receptor, which is highly expressed in retinal microglia and endothelial cells (Ma et al., 2019). After Tgfβ ligation to TβRII, SMAD2 and SMAD3 are phosphorylated and form complexes with SMAD4 which transfer to the nucleus and further promote the transcription of target genes (Javelaud and Mauviel, 2004). In TβRII–deficient condition, the retina presents a critically pathological change in structure and function, such as the shortage of pericyte differentiation and retinal capillaries, which leads to microaneurysms, hemorrhages, microglial activation and proliferative retinopathy (Braunger et al., 2015). According to the collaborative genome-wide association study, TβRII, as a receptor for Tgfβ signaling pathway, was also associated with AMD susceptibility (Fritsche et al., 2013).
Nevertheless, because of the postnatal lethality of TGFβ1-deficient mice or lethal embryonic phenotypes of TβRII-deficient mice, was limited to investigate how TGFβ1 regulates microglial activation through TβRII in adults (Shull et al., 1992; Oshima et al., 1996). Therefore, Zoller et al. constructed Cx3cr1CreERT2:Tgfbr2fl/fl mouse model to persuade the conditional deletion of TβRII in adult microglia and found that TβRII-deficient microglia change the original morphology, up-regulate microglial activation and increase phosphorylation of TAK1 (Zoller et al., 2018). The ablation of TβRII in retinal microglia induces the secondary apoptosis of Müller and reduces mRNA expression level of microglial ‘sensome’ transcripts, such as Siglech (Hickman et al., 2013; Ma et al., 2019). Although TβRII-deficient microglia do not induce obvious changes in the retinal structure of adults, they promote pathological angiogenesis after laser injury (Ma et al., 2019). Similarly, in OIR, TβRII-deficient microglia secrete chemokines and up-regulate Igf1 expression which exacerbates retinal neovascularization plexus forming (Usui-Ouchi et al., 2022; Figure 5B).
According to these shreds of evidence, we draw partly microglial signaling pathways we are more interested in (Figure 5). Then we speculate that retinal TGFβ1 promotes the up-regulation of CD73; CD39 in microglia or endothelial cells cooperates with CD73 to hydrolyze ATP to ADP and ADO; and the hydrolysis production ADO can recruit microglia. In retinal vascular diseases, such as diabetic retinopathy and retinopathy of prematurity, the expression of ATP and ADO are upregulated. This may partly explain why microglia appear in adjacent vessel and injury sites. Intracellular molecular mechanisms in microglia responding to ADO need to be explored in depth. In addition, the Tgfβ1/TβRII signaling pathway in microglia effectively decreases microglial activation and exhibits a protective effect on neurons in retinopathy. And the disruption of the Tgfβ1/TβRII signaling pathway in microglia accelerates pathological angiogenesis during retinal injury and hypoxic processing. The relevance of Tgfβ1 to microglia CD39 and the renin-angiotensin system is currently unknown.
4. Effect of microglia on retinal vasculature and BRB in various retinopathy models
4.1. Microglia in diabetic retinopathy
DR, characterized by the apoptosis of pericytes and endothelial cells as an early clinical feature, results in the damage of BRB integrity and leakage of plasma. Although microglia may be a member of the BRB and act as a secondary protective barrier for extravasated proteins, we cannot ignore the inflammatory response induced by activated microglia. Microglia is the main source of retinal TNF-α after retinal injury. At 3 h of TNF-α intravitreal injections, endothelial cells appeared necrotic and revascularization began at 24 h injection (Claudio et al., 1994). The massive IFN-γ and IL-6 in DR activate microglial STAT3 phosphorylation which stimulates TNF-α secretion, suppresses the kinase activity in AKT/p70S6 signal axis and leads to the apoptosis of pericytes. Under IL-6 stimulation, the activated microglia recruit to the vicinity of RPE and secrete TNF-α to downregulate the expression of ZO-1 and Occludin in the RPE which disrupt the integrity of oBRB; whereas using STAT3 inhibitors modify the influence of microglia to RPE (Jo et al., 2019). With a similar mechanism, the activated microglia increase the permeability of endothelial cells in iBRB (Yun et al., 2017).
In addition, the normal retina responds to light stimulation through neurovascular coupling which expands the blood flow per unit time; whereas in the early stages of type I and II diabetes mellitus, patients normally present a deferred reaction under the stimulation of flickering (Lim et al., 2014). Microglial branches contact the pericytes, endothelial cells, and neuronal synapses in the retinal vasculature and regulate retinal vasoconstriction through the renin-angiotensin system; nevertheless, microglia lost this ability in the early STZ-induced mouse model (Mills et al., 2021). Asiatic acid upregulates the protein arginine (Arg-1) expression and reduces NF-κB p65 nuclear translocation in microglia; the microglial phenotype is reversed and alleviates the disease process of DR (Fang et al., 2021). Erythropoietin protects the BRB by increasing phosphorylation of the Src/Akt/cofilin signaling axis to inhibit microglia engulfing endothelial cells (Xie et al., 2021). CD5-2, as a novel oligonucleotide-based drug, enlarges the VE-cadherin transcription which is silenced by miR-27a in endothelial cells and defends the integrity of BRB and preserves the coverage of pericytes; the activation state of microglia is suppressed after treatment with CD5-2 (Ting et al., 2019). Melatonin can inhibit microglia activation through the PI3K/Akt/Stat3/NF-κB signal axis and protect pericytes from apoptosis (Tang L. et al., 2022). And MicroRNA-93-5p can restrain M1 microglia activation by silencing STAT3 (Wang et al., 2021).
4.2. Microglia in age-related macular degeneration
AMD is a neurodegenerative disease of retinal macular degeneration which is associated with age and can mainly divide into three types: (1) Geographic atrophy; (2) choroidal neovascularization; (3) retinal angiomatous proliferation (RAP). In healthy human retinas, the macular region has no blood vessels on account of the abundantly antiangiogenic factors expressed by cells (Lutty and McLeod, 2018). And the high density of cones in this area indicates more susceptibility to oxidative stress.
Quite unlike humans, the retina of mice does not have macular structures. Hence, external assistance is needed to establish disease models and explore the mechanisms involved in macular degeneration. For example, the NRL-deficient transgenic model assists us in researching retinal degenerative diseases related to cones and decreases the interference of rods (Samardzija et al., 2014). Barben et al., based on this model and knocked out the von Hippel Lindau protein to mimic the degenerative death mechanism of cone under chronic hypoxic conditions in AMD and found that microglia infiltrate to the fundus and the upregulation of H1F-1A, VEGF, and Fgf2 related to neovascularization during early retinal development (Barben et al., 2018). In contrast, the sterile 1% NaIO3 can mimic geographic atrophy by injecting the tail vein (Enzbrenner et al., 2021). And in the 5XFAD mouse model, the presence of deposits on the fundus can mimic types 2 and 3 AMD while using the low-dose efavirenz reduces microglial activation, neovascular formation, and accumulations of amyloid β plaques in focus (El-Darzi et al., 2022).
The microglial number in perivascular and subretinal increases with age. The increased number of microglia and lipofuscin deposition synergistically contribute to the risk of the AMD process (Xu et al., 2008). And the structurally altered HTRA1 contributes to the Tgfβ signaling in autocrine microglia which downregulates phosphorylation of SMAD2 and similarly increases the risk of AMD (Friedrich et al., 2015). Moreover, the high-fat diet activates the immune response of retinal microglia; IL-1β released by the activated microglia provokes the cellular iron sequestration reaction which sparks the toxic accumulation of iron in RPE cells; subsequently, the RPE presents oxidative stress and electrophysiological dysfunction facilitating the process of AMD (Sterling et al., 2022).
4.3. Microglia in other types of retinal diseases
Autoimmune uveitis (Au) is a chronic inflammatory intraocular disease mediated by the autoimmune system. In AU, the abnormal activation of microglia increase the production of inducible nitric oxide synthase (iNOS) which accelerates retinal degeneration and the loss of iBRB integrity, the leakage and abnormal proliferation of capillaries. In the EAU mouse model, the addition of Icariin upregulates PRDX3 which transfers the microglia phenotype from pro-inflammatory to anti-inflammatory and alleviates the state of illness (Wang et al., 2022). Thereinto, PRDX3, a primary isoform of six peroxidases in mitochondria, swiftly scavenges abnormal accumulation of H2O2 to decrease apoptosis and damage caused by oxidative stress (Rebelo et al., 2021).
In addition, CSC is characterized by the dilation and leakage of choroidal vasculature leading to the accumulation of subretinal fluid and serous detachment of the neurosensory retina which can mimic by using aldosterone with the intravitreal injection (Zhao et al., 2012; Yu et al., 2022). CSC may be associated with inappropriate activation of MR (Zhao et al., 2017). The melatonin through intraperitoneal injection rescues microglial infiltration mediated by activation of the IL-17A/NF-kb signaling pathway and significantly reduces CX3CR1 and cyclooxygenase 2 secreted by the activated microglia in the CSC mouse model (Yu et al., 2022).
4.4. Microglia in oxygen-induced retinopathy (OIR)
Microglia are involved in neoangiogenesis and are activated prior to neovascularization in the central avascular region during OIR (Ritter et al., 2006; Fischer et al., 2011). At the beginning of the OIR model construction, mice are exposed to an oxygen-rich environment and the capillaries appear to atrophy; when the survival environment returns to the normoxia, neovascularization occurs in the retina. At postnatal 12 days, the NF-κβ/STAT3 signaling pathway is activated in pro-inflammatory microglia which are presented in the central and peripheral neovascular plexus of the retina; and at postnatal 17 days, anti-inflammatory microglia gradually emerge (Li et al., 2021). During this period, the decreased number of microglia can exacerbate retinal vascular degeneration (Liu J. et al., 2022). Microglia activate the RIP1/ RIP3 signaling pathway to promote the phosphorylation of MLKL which translocate to the cytoplasmic membrane and regulates the activation of ion channels leading to necroptotic of microglia; the necrotic microglia release FGF2 and HIF-1A which stimulate retinal neoangiogenesis; and the knockdown of RIP3 effectively alleviate angiogenesis (Kubota et al., 2009).
The deletion of CCN1, a gene that encodes the extracellular matrix-associated integrin-binding protein, exacerbates the pro-inflammatory responses of microglia and leads to the malformation of retinal vasculature in the OIR model (Yan et al., 2015). And the administration of Celastrol reverses the activation of the miR-17-5p/HIF-1α/VEGF signaling pathway which reduces retinal neovascularization by inhibiting the microglial activation and inflammation; the proliferation, migration and tube formation ability of human retinal microvascular endothelial cells are also inhibited in vitro culture (Zhao et al., 2022). In addition, KC7F2, a novel molecular compound, reduces retinal angiogenesis by reducing the co-localization ratio between microglia and neovascularization and inhibits the activation of the HIF1α/VEGF signaling pathway in human umbilical vein endothelial cells (Tang X. et al., 2022).
4.5. Microglia in ischemia–reperfusion (IR) model
The retinal IR model is another common disease model used in experiments to mimic glaucoma, DR and retinal arterial obstruction (Osborne et al., 2004). The activation of immune cells and cytokines can mediate the onset of inflammation and tissue damage; thereinto, microglia are also activated in the early injury process (Zhang et al., 2005). The permeability is quickly increased in vascular endothelial cells accompanied by a strong sterile inflammatory response leading to the BRB breakdown (Muthusamy et al., 2014). On the 13th day of modeling, the average thickness of the GCL and IPL layers decreases by 36 and 5% is reduced in OPL layers; on the 28th day, the overall retinal thickness diminishes by about 10% compared with the control; in addition, after 2 days of IR, the up-regulation of Occludin pSer490 stimulates the Occludin degradation through ubiquitination predicting that TJPs initiate hydrolysis in retinal endothelial cells and inflammation gradually subsides by the fourth week of IR modeling; the activated microglia engulf optic nerve cells and participate in vascular permeability; the administration of minocycline can improve the iBRB repair and reduce the abnormal activation of microglia in the early stage (Abcouwer et al., 2021).
Long non-coding RNA(lncRNA)-H19 is a key target of IR-induced inflammation; lncRNA-H19 reduces the miR-21 and promotes the PDCD4 expression in the competing endogenous RNA network which activates inflammasome; subsequently, the activated inflammasome prompt the caspase-1 maturation and induce the microglial pyroptosis through GSDMD protein; Microglial IL-1β and IL18 release to the retina and participates in inflammatory response (Wan et al., 2020; Figure 5C).
The inflammatory response from inappropriately activated microglia can exacerbate the retinopathy and the death of pro-inflammatory microglia at later stages is fatal to the disease. Therefore, transferring pro-inflammatory microglia to anti-inflammatory microglia is feasible (Table 1).
5. Microglia and induced pluripotent stem cell (iPSC) technology
The advent of single-cell sequencing and fate mapping techniques promises to enlarge the understanding of microglial diversity and provide a novel vision of disease-related heterogeneity and plasticity of microglia responses (Elmore et al., 2014; Huang et al., 2018a,b). However, the specific genes in microglia are instantly down-regulated when transferred from vivo to the extracorporeal culture environment (Gosselin et al., 2017). Establishing induced pluripotent stem cell-derived microglia (iMG) provides a new effective method to explore the microglial characteristic in the pathological condition, regenerative therapeutic and the intercellular co-relationship during the developing retina. iMG shows comparable signatures with purified human fetal microglia in the same culture condition which can respond to LPS and IFN-γ stimulation and possess professional phagocytosis (Muffat et al., 2016). The iMGs cocultured with retinal organoids furnish a foundation for future research to investigate molecular signaling mechanisms (Bartalska et al., 2022).
In self-formed ectodermal autonomous multi-zone (SEAM), a two-dimensional model of human induced pluripotent stem cells of ocular cells, iMGs enhance the expression of VEGF-A under the stimulus of Tgfβ1 (Hayashi et al., 2016; Shiraki et al., 2022). Hematopoietic progenitor cells induced by pluripotent stem cells can be converted into iMGs and PSC-derived macrophages in different culture conditions; iMGs and their coculture with retinal organoids promote mutual differentiation; the differentiated iMGs migrate to the beneath photoreceptor cell layer under the coculture with retinal organoids and further differentiate into resident microglia which can promote the migration of photoreceptor precursors and may contribute to the function of pruning synapses; retinal organoids up-regulate pivotal genes which are related to development, such as SIX3, SIX6, OTX2, HES1 and DKK3 under the addition of iMGs (Gao M. L. et al., 2022). In addition, with the help of iMGs, Micklisch et al. discover that human microglia express the age-related maculopathy susceptibility 2 (ARMS2) transcripts which can cooperate with properdin and intensify the ability of complement activation to eliminate apoptotic and necrotic cells and its polymorphism is associated with AMD susceptibility (Micklisch et al., 2017). Similarly, iMGs, with the deficit of ADAM metallopeptidase domain 17 (ADAM17), present similar phenomena with ADAM17−/− Drosophila which leads to the accumulation of lipid droplet and the semblable clinical features is semblable with age-dependent degeneration of the retina (Muliyil et al., 2020).
Therefore, iMGs can be an effective reference that is vital to analyze the function of retinal microglia and potential therapeutic targets. The unique cellular properties of microglia may help retinal organoids build vascular networks.
6. Perspectives
Following the deep reform in single-cell RNA sequencing technology, the exploration of new targets has also been effectively explored in these years and we have generated preliminary knowledge about microglia. For example, based on previous Rna-seq sequencing data, we know the macroglial subsets specifically express the diazepam-binding inhibitor (DBI) which can restore microglial inflammatory response to the baseline (Menon et al., 2019). Translocator Protein (TPSO) in microglia is upregulated after inflammation activation. And DBI negatively regulates microglial activation by binding to TSPO and limits the extent of the inflammatory responses at the onset which facilitates the regression of inflammation (Wang M. et al., 2014). DBI-TSPO signaling pathway exerts anti-inflammatory and neuroprotective effects which provide clues for the research of anti-VEGF drugs (Gao S. et al., 2022). Although intravitreal injection of VEGF-inhibiting drugs is a breakthrough treatment for retinal neovascularization, the therapy targeting VEGF is not widely available for all patients (Ip et al., 2015). And repeated injections of anti-VEGF drugs are a safety concern for high-risk patients including premature infants, diabetes, and cardiovascular diseases (Usui-Ouchi and Friedlander, 2019). Based on the findings of Liu et al., there is a subset of microglia associated with neovascularization during pathological retinal angiogenesis which expresses IGF1 (Liu Z. et al., 2022). This subset may be a breakthrough to treat proliferative diabetic retinopathy and retinopathy of prematurity. CD39, Tgfβ and the renin-angiotensin system are undoubtedly potential molecular targets in terms of blocking microglia recruitment and reducing neovascularization. Hence, it is necessary to investigate the relevance of the three in retinopathy. Furthermore, we need to explore the microglial subset that induces blood vessel formation during normal retinal development, as such cells may contribute to vascularizing human retinal organoids. In addition, in the stem cell transplantation technology, the absence of microglia and chondroitin sulfate proteoglycans facilitate the migration of exogenous Müller; although the use of chondroitinase ABC and erythropoietin reduce inflammation to some extent, it still presents immune rejection at the fourth week of transplantation (Bull et al., 2008; Singhal et al., 2008). According to the RNA-seq data of Huang et al., the regenerated microglia after ablation show no significant up-regulation of inflammation-related genes (Huang et al., 2018a). Therefore, the two conjectures: stem cell transplantation technology combined with the melting regeneration properties of microglia and modified microglia for drug delivery to the retina are expected to be a promising treatment for retinal inflammation, alleviate angiogenesis and protect neurons and BRB.
Microglia have considerable potential as the only cells that can regenerate without limits in the steady-state environment of the retina. Microglia have been discovered for a century, but the mechanisms of molecular need to be further elucidated in detail.
Author contributions
Original draft preparation was conducted by XF and SF. Writing, reviewing and editing was completed by HQ, LY, and KY. Visualization and figure creation was completed by CZ. All authors contributed to the article and approved the submitted version.
Funding
This work was supported by National Natural Science Foundation of China (no. 31970930), Hubei Natural Science Foundation (no. 2020CFA069, no. 2018CFB434), and Neuroscience Team Development Project of Wuhan University of Science and Technology (no. 1180002).
Acknowledgments
We thank the members of Yao laboratory for their kind suggestion and technical assistance.
Conflict of interest
The authors declare that the research was conducted in the absence of any commercial or financial relationships that could be construed as a potential conflict of interest.
Publisher’s note
All claims expressed in this article are solely those of the authors and do not necessarily represent those of their affiliated organizations, or those of the publisher, the editors and the reviewers. Any product that may be evaluated in this article, or claim that may be made by its manufacturer, is not guaranteed or endorsed by the publisher.
Abbreviations
6-OHDA, 6-Hydroxydopamine; ACE2, Angiotensin converting enzyme 2; AMD, Age-related macular degeneration; AT1R, Angiotensin Ii type 1 receptor; AVE, Masr agonist, Ave0991; CCL5, C-C motif chemokine ligand 5; CNS, Central nervous system; CSC, Central serous chorioretinopathy; CSF-1R, Macrophage colony stimulating factor-1 receptor; CX3CR1, C-X3-C motif chemokine receptor 1; DAT, Dopamine transporters; DIZE, Diminazene aceturate Ace2 activator; DR, Diabetic retinopathy; EAU, Experimental autoimmune uveitis; ENTPD, Ectonucleoside triphosphate diphosphohydrolase; GCL, Ganglion cell layer; GSDMD, Pore-forming protein gasdermind; HIF-1Α, Hypoxia-inducible factor; IBA1, Ionized calcium binding adaptor protein 1; IFN-γ, Interferon gamma; IGF1, Insulin like growth factor 1; IL-1β, Interleukin-1β; INL, Inner nuclear layer; IOP, Intraocular pressure; IPL, Inner plexiform layer; IR, Ischemia–reperfusion injury; iBRB, Inner blood-retinal barrier; MAPK, Mitogen-activated protein kinase; MR, Mineralocorticoid receptor; NF-кB, Nuclear factor-kappa B; NMDA, N-methyl-D-aspartate; NOX, Nicotinamide adenine dinucleotide phosphate oxidase; NRF2, Nfe2-like bzip transcription factor 2; NV, Neovascularization; OIR, Oxygen-induced retinopathy; ONL, Outer nuclear layer; OPL, Outer plexiform layer; oBRB, Outer blood-retinal barrier; PD, Parkinson’s disease; PKC, Protein kinase C; PKD, Olycystic kidney disease; PP2A, Protein phosphatase 2a; RAP, Retinal angiomatous proliferation; BRB, Blood-retinal barrier; RD10, Retinitis pigmentosa; RMEC, Retinal microvascular endothelial cells; ROCK, RhoA/rhokinase; ROS, Reactive oxygen species; RPE, Retinal pigment epithelium; STAT3, Signal transducer and activator of transcription proteins 3; STZ, Streptozotocin; Tgfβ, Transforming growth factor beta; TH, Tyrosine hydroxylase; TNF-α, Tumor necrosis factor-α; TPSO, Translocator protein; VEGF, Vascular endothelial growth factor; ZO-1, Zonula ocluden-1.
References
Abadir, P. M., Walston, J. D., Carey, R. M., and Siragy, H. M. (2011). Angiotensin II type-2 receptors modulate inflammation through signal transducer and activator of transcription proteins 3 phosphorylation and TNFalpha production. J. Interf. Cytokine Res. 31, 471–474. doi: 10.1089/jir.2010.0043
Abcouwer, S. F., Lin, C. M., Shanmugam, S., Muthusamy, A., Barber, A. J., and Antonetti, D. A. (2013). Minocycline prevents retinal inflammation and vascular permeability following ischemia-reperfusion injury. J. Neuroinflammation 10:149. doi: 10.1186/1742-2094-10-149
Abcouwer, S. F., Shanmugam, S., Muthusamy, A., Lin, C. M., Kong, D., Hager, H., et al. (2021). Inflammatory resolution and vascular barrier restoration after retinal ischemia reperfusion injury. J. Neuroinflammation 18:186. doi: 10.1186/s12974-021-02237-5
Ahmad, A., Nawaz, M. I., Siddiquei, M. M., and Abu El-Asrar, A. M. (2021). Apocynin ameliorates NADPH oxidase 4 (NOX4) induced oxidative damage in the hypoxic human retinal Muller cells and diabetic rat retina. Mol. Cell. Biochem. 476, 2099–2109. doi: 10.1007/s11010-021-04071-y
Ajami, B., Bennett, J. L., Krieger, C., Tetzlaff, W., and Rossi, F. M. (2007). Local self-renewal can sustain CNS microglia maintenance and function throughout adult life. Nat. Neurosci. 10, 1538–1543. doi: 10.1038/nn2014
Anderson, D. H., Guerin, C. J., Hageman, G. S., Pfeffer, B. A., and Flanders, K. C. (1995). Distribution of transforming growth factor-beta isoforms in the mammalian retina. J. Neurosci. Res. 42, 63–79. doi: 10.1002/jnr.490420108
Anderson, S. R., Roberts, J. M., Ghena, N., Irvin, E. A., Schwakopf, J., Cooperstein, I. B., et al. (2022). Neuronal apoptosis drives remodeling states of microglia and shifts in survival pathway dependence. elife 11:76564. doi: 10.7554/eLife.76564
Badimon, A., Strasburger, H. J., Ayata, P., Chen, X., Nair, A., Ikegami, A., et al. (2020). Negative feedback control of neuronal activity by microglia. Nature 586, 417–423. doi: 10.1038/s41586-020-2777-8
Barben, M., Schori, C., Samardzija, M., and Grimm, C. (2018). Targeting Hif1a rescues cone degeneration and prevents subretinal neovascularization in a model of chronic hypoxia. Mol. Neurodegener. 13:12. doi: 10.1186/s13024-018-0243-y
Bartalska, K., Hubschmann, V., Korkut-Demirbas, M., Cubero, R. J. A., Venturino, A., Rossler, K., et al. (2022). A systematic characterization of microglia-like cell occurrence during retinal organoid differentiation. iScience 25:104580. doi: 10.1016/j.isci.2022.104580
Bhat, S. A., Goel, R., Shukla, R., and Hanif, K. (2016). Angiotensin receptor blockade modulates NFkappaB and STAT3 signaling and inhibits glial activation and Neuroinflammation better than angiotensin-converting enzyme inhibition. Mol. Neurobiol. 53, 6950–6967. doi: 10.1007/s12035-015-9584-5
Bhat, S. A., Sood, A., Shukla, R., and Hanif, K. (2019). AT2R activation prevents microglia pro-inflammatory activation in a NOX-dependent manner: inhibition of PKC activation and p47(phox) phosphorylation by PP2A. Mol. Neurobiol. 56, 3005–3023. doi: 10.1007/s12035-018-1272-9
Bian, M., Du, X., Cui, J., Wang, P., Wang, W., Zhu, W., et al. (2016). Celastrol protects mouse retinas from bright light-induced degeneration through inhibition of oxidative stress and inflammation. J. Neuroinflammation 13:50. doi: 10.1186/s12974-016-0516-8
Biesecker, K. R., Srienc, A. I., Shimoda, A. M., Agarwal, A., Bergles, D. E., Kofuji, P., et al. (2016). Glial cell calcium signaling mediates capillary regulation of blood flow in the retina. J. Neurosci. 36, 9435–9445. doi: 10.1523/JNEUROSCI.1782-16.2016
Bohlen, C. J., Bennett, F. C., Tucker, A. F., Collins, H. Y., Mulinyawe, S. B., and Barres, B. A. (2017). Diverse requirements for microglial survival, specification, and function revealed by defined-medium cultures. Neuron 94, 759–773.e8. doi: 10.1016/j.neuron.2017.04.043
Bononi, A., Agnoletto, C., De Marchi, E., Marchi, S., Patergnani, S., Bonora, M., et al. (2011). Protein kinases and phosphatases in the control of cell fate. Enzyme Res 2011:329098. doi: 10.4061/2011/329098
Braunger, B. M., Leimbeck, S. V., Schlecht, A., Volz, C., Jagle, H., and Tamm, E. R. (2015). Deletion of ocular transforming growth factor beta signaling mimics essential characteristics of diabetic retinopathy. Am. J. Pathol. 185, 1749–1768. doi: 10.1016/j.ajpath.2015.02.007
Braunger, B. M., Pielmeier, S., Demmer, C., Landstorfer, V., Kawall, D., Abramov, N., et al. (2013). TGF-beta signaling protects retinal neurons from programmed cell death during the development of the mammalian eye. J. Neurosci. 33, 14246–14258. doi: 10.1523/JNEUROSCI.0991-13.2013
Bulavina, L., Szulzewsky, F., Rocha, A., Krabbe, G., Robson, S. C., Matyash, V., et al. (2013). NTPDase1 activity attenuates microglial phagocytosis. Purinergic Signal 9, 199–205. doi: 10.1007/s11302-012-9339-y
Bull, N. D., Limb, G. A., and Martin, K. R. (2008). Human Muller stem cell (MIO-M1) transplantation in a rat model of glaucoma: survival, differentiation, and integration. Invest. Ophthalmol. Vis. Sci. 49, 3449–3456. doi: 10.1167/iovs.08-1770
Busch, S., Kannt, A., Kolibabka, M., Schlotterer, A., Wang, Q., Lin, J., et al. (2014). Systemic treatment with erythropoietin protects the neurovascular unit in a rat model of retinal neurodegeneration. PLoS One 9:e102013. doi: 10.1371/journal.pone.0102013
Cano-Cano, F., Alcalde-Estevez, E., Gomez-Jaramillo, L., Iturregui, M., Sanchez-Fernandez, E. M., Garcia Fernandez, J. M., et al. (2021). Anti-inflammatory (M2) response is induced by a sp(2)-Iminosugar glycolipid sulfoxide in diabetic retinopathy. Front. Immunol. 12:632132. doi: 10.3389/fimmu.2021.632132
Cao, J., Ehling, M., Marz, S., Seebach, J., Tarbashevich, K., Sixta, T., et al. (2017). Polarized actin and VE-cadherin dynamics regulate junctional remodelling and cell migration during sprouting angiogenesis. Nat. Commun. 8:2210. doi: 10.1038/s41467-017-02373-8
Carbajo-Lozoya, J., Lutz, S., Feng, Y., Kroll, J., Hammes, H. P., and Wieland, T. (2012). Angiotensin II modulates VEGF-driven angiogenesis by opposing effects of type 1 and type 2 receptor stimulation in the microvascular endothelium. Cell. Signal. 24, 1261–1269. doi: 10.1016/j.cellsig.2012.02.005
Carey, R. M. (2017). Update on angiotensin AT2 receptors. Curr. Opin. Nephrol. Hypertens. 26, 1–96. doi: 10.1097/MNH.0000000000000304
Caruso, S. M., Ryu, J., Quinn, P. M., and Tsang, S. H. (2020). Precision metabolome reprogramming for imprecision therapeutics in retinitis pigmentosa. J. Clin. Invest. 130, 3971–3973. doi: 10.1172/JCI139239
Checchin, D., Sennlaub, F., Levavasseur, E., Leduc, M., and Chemtob, S. (2006). Potential role of microglia in retinal blood vessel formation. Invest. Ophthalmol. Vis. Sci. 47, 3595–3602. doi: 10.1167/iovs.05-1522
Chen, Y., Lin, J., Schlotterer, A., Kurowski, L., Hoffmann, S., Hammad, S., et al. (2021). MicroRNA-124 alleviates retinal vasoregression via regulating Microglial Polarization. Int. J. Mol. Sci. 22:11068. doi: 10.3390/ijms222011068
Chen, D., Peng, C., Ding, X. M., Wu, Y., Zeng, C. J., Xu, L., et al. (2022). Interleukin-4 promotes microglial polarization toward a neuroprotective phenotype after retinal ischemia/reperfusion injury. Neural Regen. Res. 17, 2755–2760. doi: 10.4103/1673-5374.339500
Chen, X., Shao, H., Zhi, Y., Xiao, Q., Su, C., Dong, L., et al. (2016). CD73 pathway contributes to the immunosuppressive ability of mesenchymal stem cells in intraocular autoimmune responses. Stem Cells Dev. 25, 337–346. doi: 10.1089/scd.2015.0227
Claudio, L., Martiney, J. A., and Brosnan, C. F. (1994). Ultrastructural Studies of the Blood-Retina Barrier After Exposure to Interleukin-1β or Tumor Necrosis Factor-α. Lab Invest. 70, 850–861.
Costa-Besada, M. A., Valenzuela, R., Garrido-Gil, P., Villar-Cheda, B., Parga, J. A., Lanciego, J. L., et al. (2018). Paracrine and Intracrine angiotensin 1-7/mas receptor Axis in the substantia Nigra of rodents, monkeys, and humans. Mol. Neurobiol. 55, 5847–5867. doi: 10.1007/s12035-017-0805-y
Cramer, T., Gill, R., Thirouin, Z. S., Vaas, M., Sampath, S., Martineau, F., et al. (2022). Cross-talk between GABAergic postsynapse and microglia regulate synapse loss after brain ischemia. Sci. Adv. 8:eabj0112. doi: 10.1126/sciadv.abj0112
Császár, E., Lénárt, N., Cserép, C., Környei, Z., Fekete, R., Pósfai, B., et al. (2021). Microglia control cerebral blood flow and neurovascular coupling via P2Y12R-mediated actions. bioRxiv. doi: 10.1101/2021.02.04.429741
Dang, R., Yang, M., Cui, C., Wang, C., Zhang, W., Geng, C., et al. (2021). Activation of angiotensin-converting enzyme 2/angiotensin (1-7)/mas receptor axis triggers autophagy and suppresses microglia proinflammatory polarization via forkhead box class O1 signaling. Aging Cell 20:e13480. doi: 10.1111/acel.13480
Deliyanti, D., Miller, A. G., Tan, G., Binger, K. J., Samson, A. L., and Wilkinson-Berka, J. L. (2012). Neovascularization is attenuated with aldosterone synthase inhibition in rats with retinopathy. Hypertension 59, 607–613. doi: 10.1161/HYPERTENSIONAHA.111.188136
Deng, Y. Y., Lu, J., Ling, E. A., and Kaur, C. (2009). Monocyte chemoattractant protein-1 (MCP-1) produced via NF-kappaB signaling pathway mediates migration of amoeboid microglia in the periventricular white matter in hypoxic neonatal rats. Glia 57, 604–621. doi: 10.1002/glia.20790
Diaz-Araya, C. M., Provis, J. M., and Penfold, P. L. (1995). Ontogeny and cellular expression of MHC and leucocyte antigens in human retina. Glia 15, 458–470. doi: 10.1002/glia.440150409
Ding, X., Gu, R., Zhang, M., Ren, H., Shu, Q., Xu, G., et al. (2018). Microglia enhanced the angiogenesis, migration and proliferation of co-cultured RMECs. BMC Ophthalmol. 18:249. doi: 10.1186/s12886-018-0886-z
Dudiki, T., Meller, J., Mahajan, G., Liu, H., Zhevlakova, I., Stefl, S., et al. (2020). Microglia control vascular architecture via a TGFbeta1 dependent paracrine mechanism linked to tissue mechanics. Nat. Commun. 11:986. doi: 10.1038/s41467-020-14787-y
Egger, T., Schuligoi, R., Wintersperger, A., Amann, R., Malle, E., and Sattler, W. (2003). Vitamin E (alpha-tocopherol) attenuates cyclo-oxygenase 2 transcription and synthesis in immortalized murine BV-2 microglia. Biochem. J. 370, 459–467. doi: 10.1042/BJ20021358
El-Darzi, N., Mast, N., Buchner, D. A., Saadane, A., Dailey, B., Trichonas, G., et al. (2022). Low-dose anti-HIV drug Efavirenz mitigates retinal vascular lesions in a mouse model of Alzheimer's disease. Front. Pharmacol. 13:902254. doi: 10.3389/fphar.2022.902254
Elmore, M. R., Najafi, A. R., Koike, M. A., Dagher, N. N., Spangenberg, E. E., Rice, R. A., et al. (2014). Colony-stimulating factor 1 receptor signaling is necessary for microglia viability, unmasking a microglia progenitor cell in the adult brain. Neuron 82, 380–397. doi: 10.1016/j.neuron.2014.02.040
Enzbrenner, A., Zulliger, R., Biber, J., Pousa, A. M. Q., Schafer, N., Stucki, C., et al. (2021). Sodium iodate-induced degeneration results in local complement changes and inflammatory processes in murine retina. Int. J. Mol. Sci. 22:9218. doi: 10.3390/ijms22179218
Fang, M., Wan, W., Li, Q., Wan, W., Long, Y., Liu, H., et al. (2021). Asiatic acid attenuates diabetic retinopathy through TLR4/MyD88/NF-kappaB p65 mediated modulation of microglia polarization. Life Sci. 277:119567. doi: 10.1016/j.lfs.2021.119567
Farber, K., Markworth, S., Pannasch, U., Nolte, C., Prinz, V., Kronenberg, G., et al. (2008). The ectonucleotidase cd39/ENTPDase1 modulates purinergic-mediated microglial migration. Glia 56, 331–341. doi: 10.1002/glia.20606
Fischer, F., Martin, G., and Agostini, H. T. (2011). Activation of retinal microglia rather than microglial cell density correlates with retinal neovascularization in the mouse model of oxygen-induced retinopathy. J. Neuroinflammation 8:120. doi: 10.1186/1742-2094-8-120
Foulquier, S., Caolo, V., Swennen, G., Milanova, I., Reinhold, S., Recarti, C., et al. (2019). The role of receptor MAS in microglia-driven retinal vascular development. Angiogenesis 22, 481–489. doi: 10.1007/s10456-019-09671-3
Friedrich, U., Datta, S., Schubert, T., Plossl, K., Schneider, M., Grassmann, F., et al. (2015). Synonymous variants in HTRA1 implicated in AMD susceptibility impair its capacity to regulate TGF-beta signaling. Hum. Mol. Genet. 24, 6361–6373. doi: 10.1093/hmg/ddv346
Fritsche, L. G., Chen, W., Schu, M., Yaspan, B. L., Yu, Y., Thorleifsson, G., et al. (2013). Seven new loci associated with age-related macular degeneration. Nat. Genet. 45, 433–439. doi: 10.1038/ng.2578
Fruttiger, M. (2007). Development of the retinal vasculature. Angiogenesis 10, 77–88. doi: 10.1007/s10456-007-9065-1
Fukuda, K., Hirooka, K., Mizote, M., Nakamura, T., Itano, T., and Shiraga, F. (2010). Neuroprotection against retinal ischemia–reperfusion injury by blocking the angiotensin II type 1 receptor. Investigat. Opthalmol. Vis. Sci. 51, 3629–3638. doi: 10.1167/iovs.09-4107
Gao, S., Li, N., Wang, Y., Lin, Z., Zhu, Y., Xu, J., et al. (2022). Inhibition of vascular endothelial growth factor alleviates neovascular retinopathy with regulated neurotrophic/proinflammatory cytokines through the modulation of DBI-TSPO signaling. FASEB J. 36:e22367. doi: 10.1096/fj.202101294RRR
Gao, W., Shen, L., Long, D. D., Pan, T. T., Wang, D., Chai, X. Q., et al. (2022). Angiotensin II type 2 receptor pharmacological agonist, C21, reduces the inflammation and pain hypersensitivity in mice with joint inflammatory pain. Int. Immunopharmacol. 110:108921. doi: 10.1016/j.intimp.2022.108921
Gao, M. L., Zhang, X., Han, F., Xu, J., Yu, S. J., Jin, K., et al. (2022). Functional microglia derived from human pluripotent stem cells empower retinal organ. Sci. China Life Sci. 65, 1057–1071. doi: 10.1007/s11427-021-2086-0
Gariano, R. F., and Gardner, T. W. (2005). Retinal angiogenesis in development and disease. Nature 438, 960–966. doi: 10.1038/nature04482
Gerhardt, H., Golding, M., Fruttiger, M., Ruhrberg, C., Lundkvist, A., Abramsson, A., et al. (2003). VEGF guides angiogenic sprouting utilizing endothelial tip cell filopodia. J. Cell Biol. 161, 1163–1177. doi: 10.1083/jcb.200302047
Ginhoux, F., Greter, M., Leboeuf, M., Nandi, S., See, P., Gokhan, S., et al. (2010). Fate mapping analysis reveals that adult microglia derive from primitive macrophages. Science 330, 841–845. doi: 10.1126/science.1194637
Gosselin, D., Skola, D., Coufal, N. G., Holtman, I. R., Schlachetzki, J. C. M., Sajti, E., et al. (2017). An environment-dependent transcriptional network specifies human microglia identity. Science 356:eaal3222. doi: 10.1126/science.aal3222
Greter, M., Lelios, I., Pelczar, P., Hoeffel, G., Price, J., Leboeuf, M., et al. (2012). Stroma-derived interleukin-34 controls the development and maintenance of langerhans cells and the maintenance of microglia. Immunity 37, 1050–1060. doi: 10.1016/j.immuni.2012.11.001
Grotegut, P., Perumal, N., Kuehn, S., Smit, A., Dick, H. B., Grus, F. H., et al. (2020). Minocycline reduces inflammatory response and cell death in a S100B retina degeneration model. J. Neuroinflammation 17:375. doi: 10.1186/s12974-020-02012-y
Guimond, M. O., and Gallo-Payet, N. (2012). The angiotensin II type 2 receptor in brain functions: an update. Int. J. Hypertens. 2012:351758, 1–18. doi: 10.1155/2012/351758
Gupta, S., Tiwari, V., Tiwari, P., Parul Mishra, A., Hanif, K., and Shukla, S. (2022). Angiotensin-converting enzyme 2 activation mitigates behavioral deficits and Neuroinflammatory burden in 6-OHDA induced experimental models of Parkinson's disease. ACS Chem. Neurosci. 13, 1491–1504. doi: 10.1021/acschemneuro.1c00797
Harsing, L. G., Szenasi, G., Zelles, T., and Koles, L. (2021). Purinergic-Glycinergic interaction in neurodegenerative and Neuroinflammatory disorders of the retina. Int. J. Mol. Sci. 22:6209. doi: 10.3390/ijms22126209
Hasegawa, T., McLeod, D. S., Prow, T., Merges, C., Grebe, R., and Lutty, G. A. (2008). Vascular precursors in developing human retina. Invest. Ophthalmol. Vis. Sci. 49, 2178–2192. doi: 10.1167/iovs.07-0632
Hatzopoulos, K. M., Vessey, K. A., Wilkinson-Berka, J. L., and Fletcher, E. L. (2014). The vasoneuronal effects of AT1 receptor blockade in a rat model of retinopathy of prematurity. Invest. Ophthalmol. Vis. Sci. 55, 3957–3970. doi: 10.1167/iovs.13-13532
Haupt, F., Krishnasamy, K., Napp, L. C., Augustynik, M., Limbourg, A., Gamrekelashvili, J., et al. (2019). Retinal myeloid cells regulate tip cell selection and vascular branching morphogenesis via notch ligand Delta-like 1. Sci. Rep. 9:9798. doi: 10.1038/s41598-019-46308-3
Hayashi, R., Ishikawa, Y., Sasamoto, Y., Katori, R., Nomura, N., Ichikawa, T., et al. (2016). Co-ordinated ocular development from human iPS cells and recovery of corneal function. Nature 531, 376–380. doi: 10.1038/nature17000
He, D., Xu, H., Zhang, H., Tang, R., Lan, Y., Xing, R., et al. (2022). Disruption of the IL-33-ST2-AKT signaling axis impairs neurodevelopment by inhibiting microglial metabolic adaptation and phagocytic function. Immunity 55, 159–173.e9. doi: 10.1016/j.immuni.2021.12.001
Hickman, S. E., Kingery, N. D., Ohsumi, T. K., Borowsky, M. L., Wang, L. C., Means, T. K., et al. (2013). The microglial sensome revealed by direct RNA sequencing. Nat. Neurosci. 16, 1896–1905. doi: 10.1038/nn.3554
Hu, P., Hunt, N. H., Arfuso, F., Shaw, L. C., Uddin, M. N., Zhu, M., et al. (2017). Increased Indoleamine 2,3-dioxygenase and Quinolinic acid expression in microglia and Muller cells of diabetic human and rodent retina. Invest. Ophthalmol. Vis. Sci. 58, 5043–5055. doi: 10.1167/iovs.17-21654
Hu, P., Thinschmidt, J. S., Caballero, S., Adamson, S., Cole, L., Chan-Ling, T., et al. (2015). Loss of survival factors and activation of inflammatory cascades in brain sympathetic centers in type 1 diabetic mice. Am. J. Physiol. Endocrinol. Metab. 308, E688–E698. doi: 10.1152/ajpendo.00504.2014
Huang, Y., Xu, Z., Xiong, S., Qin, G., Sun, F., Yang, J., et al. (2018a). Dual extra-retinal origins of microglia in the model of retinal microglia repopulation. Cell Discov 4:9. doi: 10.1038/s41421-018-0011-8
Huang, Y., Xu, Z., Xiong, S., Sun, F., Qin, G., Hu, G., et al. (2018b). Repopulated microglia are solely derived from the proliferation of residual microglia after acute depletion. Nat. Neurosci. 21, 530–540. doi: 10.1038/s41593-018-0090-8
Hui, F., Nguyen, C. T., Bedggood, P. A., He, Z., Fish, R. L., Gurrell, R., et al. (2014). Quantitative spatial and temporal analysis of fluorescein angiography dynamics in the eye. PLoS One 9:e111330. doi: 10.1371/journal.pone.0111330
Inada, M., Xu, H., Takeuchi, M., Ito, M., and Chen, M. (2021). Microglia increase tight-junction permeability in coordination with Muller cells under hypoxic condition in an in vitro model of inner blood-retinal barrier. Exp. Eye Res. 205:108490. doi: 10.1016/j.exer.2021.108490
Ingwersen, J., Wingerath, B., Graf, J., Lepka, K., Hofrichter, M., Schroter, F., et al. (2016). Dual roles of the adenosine A2a receptor in autoimmune neuroinflammation. J. Neuroinflammation 13:48. doi: 10.1186/s12974-016-0512-z
Ip, M. S., Domalpally, A., Sun, J. K., and Ehrlich, J. S. (2015). Long-term effects of therapy with ranibizumab on diabetic retinopathy severity and baseline risk factors for worsening retinopathy. Ophthalmology 122, 367–374. doi: 10.1016/j.ophtha.2014.08.048
Ivanova, E., Alam, N. M., Prusky, G. T., and Sagdullaev, B. T. (2019). Blood-retina barrier failure and vision loss in neuron-specific degeneration. JCI Insight 4:e126747. doi: 10.1172/jci.insight.126747
Jackson, L., Dong, G., Althomali, W., Sayed, M. A., Eldahshan, W., Baban, B., et al. (2020). Delayed Administration of Angiotensin II type 2 receptor (AT2R) agonist compound 21 prevents the development of post-stroke cognitive impairment in diabetes through the modulation of microglia polarization. Transl. Stroke Res. 11, 762–775. doi: 10.1007/s12975-019-00752-5
Jakovljevic, M., Lavrnja, I., Bozic, I., Milosevic, A., Bjelobaba, I., Savic, D., et al. (2019). Induction of NTPDase1/CD39 by reactive microglia and macrophages is associated with the functional state during EAE. Front. Neurosci. 13:410. doi: 10.3389/fnins.2019.00410
Javelaud, D., and Mauviel, A. (2004). Mammalian transforming growth factor-βs: Smad signaling and physio-pathological roles. Int. J. Biochem. Cell Biol. 36, 1161–1165. doi: 10.1016/s1357-2725(03)00255-3
Jeon, S. J., Huh, J., Jeong, E., Park, C. K., and Park, H. Y. L. (2022). Angiotensin II related glial cell activation and necroptosis of retinal ganglion cells after systemic hypotension in glaucoma. Cell Death Dis. 13:323. doi: 10.1038/s41419-022-04762-4
Jiang, T., Gao, L., Lu, J., and Zhang, Y. D. (2013). ACE2-Ang-(1-7)-mas Axis in brain: a potential target for prevention and treatment of ischemic stroke. Curr. Neuropharmacol. 11, 209–217. doi: 10.2174/1570159X11311020007
Jin, N., Gao, L., Fan, X., and Xu, H. (2017). Friend or foe? Resident microglia vs bone marrow-derived microglia and their roles in the retinal degeneration. Mol. Neurobiol. 54, 4094–4112. doi: 10.1007/s12035-016-9960-9
Jo, D. H., Yun, J. H., Cho, C. S., Kim, J. H., Kim, J. H., and Cho, C. H. (2019). Interaction between microglia and retinal pigment epithelial cells determines the integrity of outer blood-retinal barrier in diabetic retinopathy. Glia 67, 321–331. doi: 10.1002/glia.23542
Jolivel, V., Bicker, F., Biname, F., Ploen, R., Keller, S., Gollan, R., et al. (2015). Perivascular microglia promote blood vessel disintegration in the ischemic penumbra. Acta Neuropathol. 129, 279–295. doi: 10.1007/s00401-014-1372-1
Jorstad, N. L., Wilken, M. S., Grimes, W. N., Wohl, S. G., VandenBosch, L. S., Yoshimatsu, T., et al. (2017). Stimulation of functional neuronal regeneration from Muller glia in adult mice. Nature 548, 103–107. doi: 10.1038/nature23283
Jovanovic, J., Liu, X., Kokona, D., Zinkernagel, M. S., and Ebneter, A. (2020). Inhibition of inflammatory cells delays retinal degeneration in experimental retinal vein occlusion in mice. Glia 68, 574–588. doi: 10.1002/glia.23739
Kigerl, K. A., Gensel, J. C., Ankeny, D. P., Alexander, J. K., Donnelly, D. J., and Popovich, P. G. (2009). Identification of two distinct macrophage subsets with divergent effects causing either neurotoxicity or regeneration in the injured mouse spinal cord. J. Neurosci. 29, 13435–13444. doi: 10.1523/JNEUROSCI.3257-09.2009
Kim, W. K., Hwang, S. Y., Oh, E. S., Piao, H. Z., Kim, K. W., and Han, I. O. (2004). TGF-beta1 represses activation and resultant death of microglia via inhibition of phosphatidylinositol 3-kinase activity. J. Immunol. 172, 7015–7023. doi: 10.4049/jimmunol.172.11.7015
Kokona, D., Ebneter, A., Escher, P., and Zinkernagel, M. S. (2018). Colony-stimulating factor 1 receptor inhibition prevents disruption of the blood-retina barrier during chronic inflammation. J. Neuroinflammation 15:340. doi: 10.1186/s12974-018-1373-4
Kooragayala, K., Gotoh, N., Cogliati, T., Nellissery, J., Kaden, T. R., French, S., et al. (2015). Quantification of oxygen consumption in retina ex vivo demonstrates limited reserve capacity of photoreceptor mitochondria. Invest. Ophthalmol. Vis. Sci. 56, 8428–8436. doi: 10.1167/iovs.15-17901
Krady, J. K., Basu, A., Allen, C. M., Xu, Y., LaNoue, K. F., Gardner, T. W., et al. (2005). Minocycline reduces proinflammatory cytokine expression, microglial activation, and caspase-3 activation in a rodent model of diabetic retinopathy. Diabetes 54, 1559–1565. doi: 10.2337/diabetes.54.5.1559
Kubota, Y., Takubo, K., Shimizu, T., Ohno, H., Kishi, K., Shibuya, M., et al. (2009). M-CSF inhibition selectively targets pathological angiogenesis and lymphangiogenesis. J. Exp. Med. 206, 1089–1102. doi: 10.1084/jem.20081605
Kurihara, T., Ozawa, Y., Shinoda, K., Nagai, N., Inoue, M., Oike, Y., et al. (2006). Neuroprotective effects of angiotensin II type 1 receptor (AT1R) blocker, telmisartan, via modulating AT1R and AT2R signaling in retinal inflammation. Invest. Ophthalmol. Vis. Sci. 47, 5545–5552. doi: 10.1167/iovs.06-0478
Lan, X., Han, X., Li, Q., Yang, Q. W., and Wang, J. (2017). Modulators of microglial activation and polarization after intracerebral haemorrhage. Nat. Rev. Neurol. 13, 420–433. doi: 10.1038/nrneurol.2017.69
Lee, J. E., Liang, K. J., Fariss, R. N., and Wong, W. T. (2008). Ex vivo dynamic imaging of retinal microglia using time-lapse confocal microscopy. Invest. Ophthalmol. Vis. Sci. 49, 4169–4176. doi: 10.1167/iovs.08-2076
Li, Y., Liu, D. X., Li, M. Y., Qin, X. X., Fang, W. G., Zhao, W. D., et al. (2014). Ephrin-A3 and ephrin-A4 contribute to microglia-induced angiogenesis in brain endothelial cells. Anat Rec (Hoboken) 297, 1908–1918. doi: 10.1002/ar.22998
Li, J., Yu, S., Lu, X., Cui, K., Tang, X., Xu, Y., et al. (2021). The phase changes of M1/M2 phenotype of microglia/macrophage following oxygen-induced retinopathy in mice. Inflamm. Res. 70, 183–192. doi: 10.1007/s00011-020-01427-w
Lim, H. M., Heo, W., Han, J. W., Lee, M. G., and Kim, J. Y. (2018). NPP1 is responsible for potent extracellular ATP hydrolysis as NTPDase1 in primary cultured murine microglia. Purinergic Signal 14, 157–166. doi: 10.1007/s11302-018-9601-z
Lim, L. S., Ling, L. H., Ong, P. G., Foulds, W., Tai, E. S., Wong, E., et al. (2014). Dynamic responses in retinal vessel caliber with flicker light stimulation in eyes with diabetic retinopathy. Invest. Ophthalmol. Vis. Sci. 55, 5207–5213. doi: 10.1167/iovs.14-14301
Liu, Y. P., Kuznetsova, T., Thijs, L., Jin, Y., Schmitz, B., Brand, S. M., et al. (2011). Are retinal microvascular phenotypes associated with the 1675G/a polymorphism in the angiotensin II type-2 receptor gene? Am. J. Hypertens. 24, 1300–1305. doi: 10.1038/ajh.2011.151
Liu, M., Shi, P., and Sumners, C. (2016). Direct anti-inflammatory effects of angiotensin-(1-7) on microglia. J. Neurochem. 136, 163–171. doi: 10.1111/jnc.13386
Liu, Z., Shi, H., Xu, J., Yang, Q., Ma, Q., Mao, X., et al. (2022). Single-cell transcriptome analyses reveal microglia types associated with proliferative retinopathy. JCI Insight 7:e160940. doi: 10.1172/jci.insight.160940
Liu, J., Tsang, J. K. W., Fung, F. K. C., Chung, S. K., Fu, Z., and Lo, A. C. Y. (2022). Retinal microglia protect against vascular damage in a mouse model of retinopathy of prematurity. Front. Pharmacol. 13:945130. doi: 10.3389/fphar.2022.945130
Losenkova, K., Takeda, A., Ragauskas, S., Cerrada-Gimenez, M., Vahatupa, M., Kaja, S., et al. (2022). CD73 controls ocular adenosine levels and protects retina from light-induced phototoxicity. Cell. Mol. Life Sci. 79:152. doi: 10.1007/s00018-022-04187-4
Lu, W., Hu, H., Sevigny, J., Gabelt, B. T., Kaufman, P. L., Johnson, E. C., et al. (2015). Rat, mouse, and primate models of chronic glaucoma show sustained elevation of extracellular ATP and altered purinergic signaling in the posterior eye. Invest. Ophthalmol. Vis. Sci. 56, 3075–3083. doi: 10.1167/iovs.14-15891
Lutty, G. A., and McLeod, D. S. (2018). Development of the hyaloid, choroidal and retinal vasculatures in the fetal human eye. Prog. Retin. Eye Res. 62, 58–76. doi: 10.1016/j.preteyeres.2017.10.001
Ma, W., Silverman, S. M., Zhao, L., Villasmil, R., Campos, M. M., Amaral, J., et al. (2019). Absence of TGFbeta signaling in retinal microglia induces retinal degeneration and exacerbates choroidal neovascularization. elife 8:e42049. doi: 10.7554/eLife.42049
Maning, J., Negussie, S., Clark, M. A., and Lymperopoulos, A. (2017). Biased agonism/antagonism at the AngII-AT1 receptor: implications for adrenal aldosterone production and cardiovascular therapy. Pharmacol. Res. 125, 14–20. doi: 10.1016/j.phrs.2017.05.009
Matyash, M., Zabiegalov, O., Wendt, S., Matyash, V., and Kettenmann, H. (2017). The adenosine generating enzymes CD39/CD73 control microglial processes ramification in the mouse brain. PLoS One 12:e0175012. doi: 10.1371/journal.pone.0175012
McCarthy, C. A., Vinh, A., Broughton, B. R., Sobey, C. G., Callaway, J. K., and Widdop, R. E. (2012). Angiotensin II type 2 receptor stimulation initiated after stroke causes neuroprotection in conscious rats. Hypertension 60, 1531–1537. doi: 10.1161/HYPERTENSIONAHA.112.199646
McCarthy, C. A., Vinh, A., Miller, A. A., Hallberg, A., Alterman, M., Callaway, J. K., et al. (2014). Direct angiotensin AT2 receptor stimulation using a novel AT2 receptor agonist, compound 21, evokes neuroprotection in conscious hypertensive rats. PLoS One 9:e95762. doi: 10.1371/journal.pone.0095762
McPherson, S. W., Heuss, N. D., Lehmann, U., Roehrich, H., Abedin, M., and Gregerson, D. S. (2019). The retinal environment induces microglia-like properties in recruited myeloid cells. J. Neuroinflammation 16:151. doi: 10.1186/s12974-019-1546-9
McVicar, C. M., Hamilton, R., Colhoun, L. M., Gardiner, T. A., Brines, M., Cerami, A., et al. (2011). Intervention with an erythropoietin-derived peptide protects against neuroglial and vascular degeneration during diabetic retinopathy. Diabetes 60, 2995–3005. doi: 10.2337/db11-0026
Mehrabadi, A. R., Korolainen, M. A., Odero, G., Miller, D. W., and Kauppinen, T. M. (2017). Poly(ADP-ribose) polymerase-1 regulates microglia mediated decrease of endothelial tight junction integrity. Neurochem. Int. 108, 266–271. doi: 10.1016/j.neuint.2017.04.014
Menon, M., Mohammadi, S., Davila-Velderrain, J., Goods, B. A., Cadwell, T. D., Xing, Y., et al. (2019). Single-cell transcriptomic atlas of the human retina identifies cell types associated with age-related macular degeneration. Nat. Commun. 10:4902. doi: 10.1038/s41467-019-12780-8
Micklisch, S., Lin, Y., Jacob, S., Karlstetter, M., Dannhausen, K., Dasari, P., et al. (2017). Age-related macular degeneration associated polymorphism rs10490924 in ARMS2 results in deficiency of a complement activator. J. Neuroinflammation 14:4. doi: 10.1186/s12974-016-0776-3
Mills, S. A., Jobling, A. I., Dixon, M. A., Bui, B. V., Vessey, K. A., Phipps, J. A., et al. (2021). Fractalkine-induced microglial vasoregulation occurs within the retina and is altered early in diabetic retinopathy. Proc. Natl. Acad. Sci. U. S. A. 118:e2112561118. doi: 10.1073/pnas.2112561118
Misrak Tadesse, Y. Y., Yossuck, P., and Higgins, R. D. (2001). Captopril improves retinal neovascularization via endothelin-1. Invest. Ophthalmol. Vis. Sci. 42, 1867–1872.
Moravski, C. J., Kelly, D. J., Cooper, M. E., Gilbert, R. E., Bertram, J. F., Shahinfar, S., et al. (2000). Retinal neovascularization is prevented by blockade of the renin-angiotensin system. Hypertension 36, 1099–1104. doi: 10.1161/01.hyp.36.6.1099
Muffat, J., Li, Y., Yuan, B., Mitalipova, M., Omer, A., Corcoran, S., et al. (2016). Efficient derivation of microglia-like cells from human pluripotent stem cells. Nat. Med. 22, 1358–1367. doi: 10.1038/nm.4189
Muliyil, S., Levet, C., Dusterhoft, S., Dulloo, I., Cowley, S. A., and Freeman, M. (2020). ADAM17-triggered TNF signalling protects the ageing Drosophila retina from lipid droplet-mediated degeneration. EMBO J. 39:e104415. doi: 10.15252/embj.2020104415
Muthusamy, A., Lin, C.-M., Shanmugam, S., Lindner, H. M., Abcouwer, S. F., and Antonetti, D. A. (2014). Ischemia–reperfusion injury induces Occludin phosphorylation/ubiquitination and retinal vascular permeability in a VEGFR-2-dependent manner. J. Cereb. Blood Flow Metab. 34, 522–531. doi: 10.1038/jcbfm.2013.230
O'Leary, F., and Campbell, M. (2021). The blood-retina barrier in health and disease. FEBS J. doi: 10.1111/febs.16330
Osborne, N. N., Casson, R. J., Wood, J. P., Chidlow, G., Graham, M., and Melena, J. (2004). Retinal ischemia: mechanisms of damage and potential therapeutic strategies. Prog. Retin. Eye Res. 23, 91–147. doi: 10.1016/j.preteyeres.2003.12.001
Oshima, M., Oshima, H., and Taketo, M. M. (1996). TGF-beta receptor type II deficiency results in defects of yolk sac hematopoiesis and vasculogenesis. Dev. Biol. 179, 297–302. doi: 10.1006/dbio.1996.0259
Outtz, H. H., Tattersall, I. W., Kofler, N. M., Steinbach, N., and Kitajewski, J. (2011). Notch1 controls macrophage recruitment and notch signaling is activated at sites of endothelial cell anastomosis during retinal angiogenesis in mice. Blood 118, 3436–3439. doi: 10.1182/blood-2010-12-327015
Ozaki, E., Delaney, C., Campbell, M., and Doyle, S. L. (2022). Minocycline suppresses disease-associated microglia (DAM) in a model of photoreceptor cell degeneration. Exp. Eye Res. 217:108953. doi: 10.1016/j.exer.2022.108953
Parkhurst, C. N., Yang, G., Ninan, I., Savas, J. N., Yates, J. R. 3rd, Lafaille, J. J., et al. (2013). Microglia promote learning-dependent synapse formation through brain-derived neurotrophic factor. Cells 155, 1596–1609. doi: 10.1016/j.cell.2013.11.030
Phipps, J. A., Vessey, K. A., Brandli, A., Nag, N., Tran, M. X., Jobling, A. I., et al. (2018). The role of angiotensin II/AT1 receptor signaling in regulating retinal microglial activation. Invest. Ophthalmol. Vis. Sci. 59, 487–498. doi: 10.1167/iovs.17-22416
Prinz, M., Masuda, T., Wheeler, M. A., and Quintana, F. J. (2021). Microglia and central nervous system-associated macrophages-from origin to disease modulation. Annu. Rev. Immunol. 39, 251–277. doi: 10.1146/annurev-immunol-093019-110159
Puthenparampil, M., Torresin, T., Franciotta, S., Marin, A., De Napoli, F., Mauceri, V. A., et al. (2022). Hyper-reflecting foci in multiple sclerosis retina associate with macrophage/microglia-derived cytokines in cerebrospinal fluid. Front. Immunol. 13:852183. doi: 10.3389/fimmu.2022.852183
Rana, I., Suphapimol, V., Jerome, J. R., Talia, D. M., Deliyanti, D., and Wilkinson-Berka, J. L. (2020). Angiotensin II and aldosterone activate retinal microglia. Exp. Eye Res. 191:107902. doi: 10.1016/j.exer.2019.107902
Rebelo, A. P., Eidhof, I., Cintra, V. P., Guillot-Noel, L., Pereira, C. V., Timmann, D., et al. (2021). Biallelic loss-of-function variations in PRDX3 cause cerebellar ataxia. Brain 144, 1467–1481. doi: 10.1093/brain/awab071
Regenhardt, R. W., Mecca, A. P., Desland, F., Ritucci-Chinni, P. F., Ludin, J. A., Greenstein, D., et al. (2014). Centrally administered angiotensin-(1-7) increases the survival of stroke-prone spontaneously hypertensive rats. Exp. Physiol. 99, 442–453. doi: 10.1113/expphysiol.2013.075242
Ritter, M. R., Banin, E., Moreno, S. K., Aguilar, E., Dorrell, M. I., and Friedlander, M. (2006). Myeloid progenitors differentiate into microglia and promote vascular repair in a model of ischemic retinopathy. J. Clin. Invest. 116, 3266–3276. doi: 10.1172/JCI29683
Rivas-Santisteban, R., Lillo, J., Munoz, A., Rodriguez-Perez, A. I., Labandeira-Garcia, J. L., Navarro, G., et al. (2021). Novel interactions involving the mas receptor show potential of the renin-angiotensin system in the regulation of microglia activation: altered expression in parkinsonism and dyskinesia. Neurotherapeutics 18, 998–1016. doi: 10.1007/s13311-020-00986-4
Roche, S. L., Ruiz-Lopez, A. M., Moloney, J. N., Byrne, A. M., and Cotter, T. G. (2018). Microglial-induced Muller cell gliosis is attenuated by progesterone in a mouse model of retinitis pigmentosa. Glia 66, 295–310. doi: 10.1002/glia.23243
Roche, S. L., Wyse-Jackson, A. C., Ruiz-Lopez, A. M., Byrne, A. M., and Cotter, T. G. (2017). Fractalkine-CX3CR1 signaling is critical for progesterone-mediated neuroprotection in the retina. Sci. Rep. 7:43067. doi: 10.1038/srep43067
Rodrigues-Neves, A. C., Aires, I. D., Vindeirinho, J., Boia, R., Madeira, M. H., Goncalves, F. Q., et al. (2018). Elevated pressure changes the purinergic system of microglial cells. Front. Pharmacol. 9:16. doi: 10.3389/fphar.2018.00016
Rodriguez-Perez, A. I., Borrajo, A., Rodriguez-Pallares, J., Guerra, M. J., and Labandeira-Garcia, J. L. (2015). Interaction between NADPH-oxidase and rho-kinase in angiotensin II-induced microglial activation. Glia 63, 466–482. doi: 10.1002/glia.22765
Rymo, S. F., Gerhardt, H., Wolfhagen Sand, F., Lang, R., Uv, A., and Betsholtz, C. (2011). A two-way communication between microglial cells and angiogenic sprouts regulates angiogenesis in aortic ring cultures. PLoS One 6:e15846. doi: 10.1371/journal.pone.0015846
Samardzija, M., Caprara, C., Heynen, S. R., Willcox DeParis, S., Meneau, I., Traber, G., et al. (2014). A mouse model for studying cone photoreceptor pathologies. Invest. Ophthalmol. Vis. Sci. 55, 5304–5313. doi: 10.1167/iovs.14-14789
Santos, A. M., Calvente, R., Tassi, M., Carrasco, M. C., Martin-Oliva, D., Marin-Teva, J. L., et al. (2008). Embryonic and postnatal development of microglial cells in the mouse retina. J. Comp. Neurol. 506, 224–239. doi: 10.1002/cne.21538
Sarlos, S., and Wilkinson-Berka, J. L. (2005). The renin-angiotensin system and the developing retinal vasculature. Investigat. Opthalmol. Vis. Sci. 46:1069. doi: 10.1167/iovs.04-0885
Sasahara, M., Otani, A., Oishi, A., Kojima, H., Yodoi, Y., Kameda, T., et al. (2008). Activation of bone marrow-derived microglia promotes photoreceptor survival in inherited retinal degeneration. Am. J. Pathol. 172, 1693–1703. doi: 10.2353/ajpath.2008.080024
Scholz, R., Sobotka, M., Caramoy, A., Stempfl, T., Moehle, C., and Langmann, T. (2015). Minocycline counter-regulates pro-inflammatory microglia responses in the retina and protects from degeneration. J. Neuroinflammation 12:209. doi: 10.1186/s12974-015-0431-4
Shen, W., Chung, S. H., Irhimeh, M. R., Li, S., Lee, S. R., and Gillies, M. C. (2014). Systemic administration of erythropoietin inhibits retinopathy in RCS rats. PLoS One 9:e104759. doi: 10.1371/journal.pone.0104759
Sherr, C. J., Rettenmier, C. W., Sacca, R., Roussel, M. F., Look, A. T., and Stanley, E. R. (1985). The c-fms proto-oncogene product is related to the receptor for the mononuclear phagocyte growth factor, CSF 1. Cells 41, 665–676. doi: 10.1016/s0092-8674(85)80047-7
Shi, P., Diez-Freire, C., Jun, J. Y., Qi, Y., Katovich, M. J., Li, Q., et al. (2010). Brain microglial cytokines in neurogenic hypertension. Hypertension 56, 297–303. doi: 10.1161/HYPERTENSIONAHA.110.150409
Shiraki, N., Maruyama, K., Hayashi, R., Oguchi, A., Murakawa, Y., Katayama, T., et al. (2022). PAX6-positive microglia evolve locally in hiPSC-derived ocular organoids. Stem Cell Reports 17, 221–230. doi: 10.1016/j.stemcr.2021.12.009
Shull, M. M., Ormsby, I., Kier, A. B., Pawlowski, S., Diebold, R. J., Yin, M., et al. (1992). Targeted disruption of the mouse transforming growth factor-beta 1 gene results in multifocal inflammatory disease. Nature 359, 693–699. doi: 10.1038/359693a0
Siddiqui, T. A., Lively, S., and Schlichter, L. C. (2016). Complex molecular and functional outcomes of single versus sequential cytokine stimulation of rat microglia. J. Neuroinflammation 13:66. doi: 10.1186/s12974-016-0531-9
Singhal, S., Lawrence, J. M., Bhatia, B., Ellis, J. S., Kwan, A. S., Macneil, A., et al. (2008). Chondroitin sulfate proteoglycans and microglia prevent migration and integration of grafted Muller stem cells into degenerating retina. Stem Cells 26, 1074–1082. doi: 10.1634/stemcells.2007-0898
Spaide, R. F. (2013). Clinical manifestations of choroidal neovascularization in AMD. Age Relat. Macular Degener. Berlin, Heidelberg: Springer. 111–119. doi: 10.1007/978-3-642-22107-1_7
Stahl, A., Connor, K. M., Sapieha, P., Chen, J., Dennison, R. J., Krah, N. M., et al. (2010). The mouse retina as an angiogenesis model. Invest. Ophthalmol. Vis. Sci. 51, 2813–2826. doi: 10.1167/iovs.10-5176
Stanley, E. R., and Chitu, V. (2014). CSF-1 receptor signaling in myeloid cells. Cold Spring Harb. Perspect. Biol. 6. doi: 10.1101/cshperspect.a021857
Sterling, J. K., Baumann, B., Foshe, S., Voigt, A., Guttha, S., Alnemri, A., et al. (2022). Inflammatory adipose activates a nutritional immunity pathway leading to retinal dysfunction. Cell Rep. 39:110942. doi: 10.1016/j.celrep.2022.110942
Sumners, C., Alleyne, A., Rodriguez, V., Pioquinto, D. J., Ludin, J. A., Kar, S., et al. (2020). Brain angiotensin type-1 and type-2 receptors: cellular locations under normal and hypertensive conditions. Hypertens. Res. 43, 281–295. doi: 10.1038/s41440-019-0374-8
Sun, L., Wang, R., Hu, G., Liu, H., Lv, K., Duan, Y., et al. (2021). Single cell RNA sequencing (scRNA-Seq) deciphering pathological alterations in streptozotocin-induced diabetic retinas. Exp. Eye Res. 210:108718. doi: 10.1016/j.exer.2021.108718
Takahashi, K., Rochford, C. D., and Neumann, H. (2005). Clearance of apoptotic neurons without inflammation by microglial triggering receptor expressed on myeloid cells-2. J. Exp. Med. 201, 647–657. doi: 10.1084/jem.20041611
Takeda, A., Shinozaki, Y., Kashiwagi, K., Ohno, N., Eto, K., Wake, H., et al. (2018). Microglia mediate non-cell-autonomous cell death of retinal ganglion cells. Glia 66, 2366–2384. doi: 10.1002/glia.23475
Tang, X., Cui, K., Lu, X., Wu, P., Yu, S., Yang, B., et al. (2022). A novel hypoxia-inducible factor 1alpha inhibitor KC7F2 attenuates oxygen-induced retinal neovascularization. Invest. Ophthalmol. Vis. Sci. 63:13. doi: 10.1167/iovs.63.6.13
Tang, Y., and Le, W. (2016). Differential roles of M1 and M2 microglia in neurodegenerative diseases. Mol. Neurobiol. 53, 1181–1194. doi: 10.1007/s12035-014-9070-5
Tang, L., Zhang, C., Lu, L., Tian, H., Liu, K., Luo, D., et al. (2022). Melatonin maintains inner blood-retinal barrier by regulating microglia via inhibition of PI3K/Akt/Stat3/NF-kappaB signaling pathways in experimental diabetic retinopathy. Front. Immunol. 13:831660. doi: 10.3389/fimmu.2022.831660
Tao, L., Qiu, Y., Fu, X., Lin, R., Lei, C., Wang, J., et al. (2016). Angiotensin-converting enzyme 2 activator diminazene aceturate prevents lipopolysaccharide-induced inflammation by inhibiting MAPK and NF-kappaB pathways in human retinal pigment epithelium. J. Neuroinflammation 13:35. doi: 10.1186/s12974-016-0489-7
Tay, T. L., Mai, D., Dautzenberg, J., Fernandez-Klett, F., Lin, G. S., Datta, M., et al. (2017). A new fate mapping system reveals context-dependent random or clonal expansion of microglia. Nat. Neurosci. 20, 793–803. doi: 10.1038/nn.4547
Taylor, R. A., Chang, C. F., Goods, B. A., Hammond, M. D., Mac Grory, B., Ai, Y., et al. (2017). TGF-beta1 modulates microglial phenotype and promotes recovery after intracerebral hemorrhage. J. Clin. Invest. 127, 280–292. doi: 10.1172/JCI88647
Ting, K. K., Zhao, Y., Shen, W., Coleman, P., Yam, M., Chan-Ling, T., et al. (2019). Therapeutic regulation of VE-cadherin with a novel oligonucleotide drug for diabetic eye complications using retinopathy mouse models. Diabetologia 62, 322–334. doi: 10.1007/s00125-018-4770-4
Todd, L., Palazzo, I., Suarez, L., Liu, X., Volkov, L., Hoang, T. V., et al. (2019). Reactive microglia and IL1beta/IL-1R1-signaling mediate neuroprotection in excitotoxin-damaged mouse retina. J. Neuroinflammation 16:118. doi: 10.1186/s12974-019-1505-5
Toonen, J. A., Solga, A. C., Ma, Y., and Gutmann, D. H. (2017). Estrogen activation of microglia underlies the sexually dimorphic differences in Nf1 optic glioma-induced retinal pathology. J. Exp. Med. 214, 17–25. doi: 10.1084/jem.20160447
Tosi, G. M., Neri, G., Caldi, E., Fusco, F., Bacci, T., Tarantello, A., et al. (2018). TGF-beta concentrations and activity are down-regulated in the aqueous humor of patients with neovascular age-related macular degeneration. Sci. Rep. 8:8053. doi: 10.1038/s41598-018-26442-0
Tuhina Prasad, A. V., and Li, Q. (2014). Expression and cellular localization of the mas receptor in the adult and developing mouse retina. Mol. Vis. 20:1443.
Usui-Ouchi, A., Eade, K., Giles, S., Ideguchi, Y., Ouchi, Y., Aguilar, E., et al. (2022). Deletion of Tgfbeta signal in activated microglia prolongs hypoxia-induced retinal neovascularization enhancing Igf1 expression and retinal leukostasis. Glia 70, 1762–1776. doi: 10.1002/glia.24218
Usui-Ouchi, A., and Friedlander, M. (2019). Anti-VEGF therapy: higher potency and long-lasting antagonism are not necessarily better. J. Clin. Invest. 129, 3032–3034. doi: 10.1172/JCI129862
Usui-Ouchi, A., Usui, Y., Kurihara, T., Aguilar, E., Dorrell, M. I., Ideguchi, Y., et al. (2020). Retinal microglia are critical for subretinal neovascular formation. JCI Insight 5:e137317. doi: 10.1172/jci.insight.137317
Verma, A., Zhu, P., de Kloet, A., Krause, E., Sumners, C., and Li, Q. (2019). Angiotensin receptor expression revealed by reporter mice and beneficial effects of AT2R agonist in retinal cells. Exp. Eye Res. 187:107770. doi: 10.1016/j.exer.2019.107770
Villacampa, N., Almolda, B., Vilella, A., Campbell, I. L., Gonzalez, B., and Castellano, B. (2015). Astrocyte-targeted production of IL-10 induces changes in microglial reactivity and reduces motor neuron death after facial nerve axotomy. Glia 63, 1166–1184. doi: 10.1002/glia.22807
Vincent, J. A., and Mohr, S. (2007). Inhibition of caspase-1/interleukin-1beta signaling prevents degeneration of retinal capillaries in diabetes and galactosemia. Diabetes 56, 224–230. doi: 10.2337/db06-0427
Waanders, F., de Vries, L. V., van Goor, H., Hillebrands, J. L., Laverman, G. D., Bakker, S. J., et al. (2011). Aldosterone, from (patho)physiology to treatment in cardiovascular and renal damage. Curr. Vasc. Pharmacol. 9, 594–605. doi: 10.2174/157016111796642689
Wagner, J., Jan Danser, A. H., Derkx, F. H., de Jong, T. V., Paul, M., Mullins, J. J., et al. (1996). Demonstration of renin mRNA, angiotensinogen mRNA, and angiotensin converting enzyme mRNA expression in the human eye: evidence for an intraocular renin-angiotensin system. Br. J. Ophthalmol. 80, 159–163. doi: 10.1136/bjo.80.2.159
Walker, D. G., and Lue, L. F. (2015). Immune phenotypes of microglia in human neurodegenerative disease: challenges to detecting microglial polarization in human brains. Alzheimers Res. Ther. 7:56. doi: 10.1186/s13195-015-0139-9
Walshe, T. E., Saint-Geniez, M., Maharaj, A. S., Sekiyama, E., Maldonado, A. E., and D'Amore, P. A. (2009). TGF-beta is required for vascular barrier function, endothelial survival and homeostasis of the adult microvasculature. PLoS One 4:e5149. doi: 10.1371/journal.pone.0005149
Wan, P., Su, W., Zhang, Y., Li, Z., Deng, C., Li, J., et al. (2020). LncRNA H19 initiates microglial pyroptosis and neuronal death in retinal ischemia/reperfusion injury. Cell Death Differ. 27, 176–191. doi: 10.1038/s41418-019-0351-4
Wang, L. L., Chen, H., Huang, K., and Zheng, L. (2012). Elevated histone acetylations in Muller cells contribute to inflammation: a novel inhibitory effect of minocycline. Glia 60, 1896–1905. doi: 10.1002/glia.22405
Wang, Y., Chen, S., Wang, J., Liu, Y., Chen, Y., Wen, T., et al. (2021). MicroRNA-93/STAT3 signalling pathway mediates retinal microglial activation and protects retinal ganglion cells in an acute ocular hypertension model. Cell Death Dis. 12:41. doi: 10.1038/s41419-020-03337-5
Wang, G., Li, X., Li, N., Wang, X., He, S., Li, W., et al. (2022). Icariin alleviates uveitis by targeting peroxiredoxin 3 to modulate retinal microglia M1/M2 phenotypic polarization. Redox Biol. 52:102297. doi: 10.1016/j.redox.2022.102297
Wang, K., Peng, B., and Lin, B. (2014). Fractalkine receptor regulates microglial neurotoxicity in an experimental mouse glaucoma model. Glia 62, 1943–1954. doi: 10.1002/glia.22715
Wang, M., Wang, X., Zhao, L., Ma, W., Rodriguez, I. R., Fariss, R. N., et al. (2014). Macroglia-microglia interactions via TSPO signaling regulates microglial activation in the mouse retina. J. Neurosci. 34, 3793–3806. doi: 10.1523/JNEUROSCI.3153-13.2014
Wang, S. K., Xue, Y., and Cepko, C. L. (2020). Microglia modulation by TGF-beta1 protects cones in mouse models of retinal degeneration. J. Clin. Invest. 130, 4360–4369. doi: 10.1172/JCI136160
Wei, S., Nandi, S., Chitu, V., Yeung, Y. G., Yu, W., Huang, M., et al. (2010). Functional overlap but differential expression of CSF-1 and IL-34 in their CSF-1 receptor-mediated regulation of myeloid cells. J. Leukoc. Biol. 88, 495–505. doi: 10.1189/jlb.1209822
Wieghofer, P., Hagemeyer, N., Sankowski, R., Schlecht, A., Staszewski, O., Amann, L., et al. (2021). Mapping the origin and fate of myeloid cells in distinct compartments of the eye by single-cell profiling. EMBO J. 40:e105123. doi: 10.15252/embj.2020105123
Wu, J., Gao, G., Shi, F., Xie, H., Yang, Q., Liu, D., et al. (2021). Activated microglia-induced neuroinflammatory cytokines lead to photoreceptor apoptosis in Abeta-injected mice. J. Mol. Med. (Berl) 99, 713–728. doi: 10.1007/s00109-021-02046-6
Wurm, A., Iandiev, I., Hollborn, M., Wiedemann, P., Reichenbach, A., Zimmermann, H., et al. (2008). Purinergic receptor activation inhibits osmotic glial cell swelling in the diabetic rat retina. Exp. Eye Res. 87, 385–393. doi: 10.1016/j.exer.2008.07.004
Xie, H., Zhang, C., Liu, D., Yang, Q., Tang, L., Wang, T., et al. (2021). Erythropoietin protects the inner blood-retinal barrier by inhibiting microglia phagocytosis via Src/Akt/cofilin signalling in experimental diabetic retinopathy. Diabetologia 64, 211–225. doi: 10.1007/s00125-020-05299-x
Xin, H., Zhou, F., Liu, T., Li, G. Y., Liu, J., Gao, Z. Z., et al. (2012). Icariin ameliorates streptozotocin-induced diabetic retinopathy in vitro and in vivo. Int. J. Mol. Sci. 13, 866–878. doi: 10.3390/ijms13010866
Xu, H., Chen, M., Manivannan, A., Lois, N., and Forrester, J. V. (2008). Age-dependent accumulation of lipofuscin in perivascular and subretinal microglia in experimental mice. Aging Cell 7, 58–68. doi: 10.1111/j.1474-9726.2007.00351.x
Xu, Y., Cui, K., Li, J., Tang, X., Lin, J., Lu, X., et al. (2020). Melatonin attenuates choroidal neovascularization by regulating macrophage/microglia polarization via inhibition of RhoA/ROCK signaling pathway. J. Pineal Res. 69:e12660. doi: 10.1111/jpi.12660
Xu, Y., Lu, X., Hu, Y., Yang, B., Tsui, C. K., Yu, S., et al. (2018). Melatonin attenuated retinal neovascularization and neuroglial dysfunction by inhibition of HIF-1alpha-VEGF pathway in oxygen-induced retinopathy mice. J. Pineal Res. 64:e12473. doi: 10.1111/jpi.12473
Xu, X.-J., Wang, S.-M., Jin, Y., Hu, Y.-T., Feng, K., and Ma, Z.-Z. (2017). Melatonin delays photoreceptor degeneration in a mouse model of autosomal recessive retinitis pigmentosa. J. Pineal Res. 63. doi: 10.1111/jpi.12428
Yan, L., Lee, S., Lazzaro, D. R., Aranda, J., Grant, M. B., and Chaqour, B. (2015). Single and compound Knock-outs of MicroRNA (miRNA)-155 and its Angiogenic gene target CCN1 in mice Alter vascular and Neovascular growth in the retina via resident microglia. J. Biol. Chem. 290, 23264–23281. doi: 10.1074/jbc.M115.646950
Yang, P., Chen, L., Shi, Y., Zhou, F., Tian, H., Li, J., et al. (2021). Progesterone alters the activation and typing of the microglia in the optic nerve crush model. Exp. Eye Res. 212:108805. doi: 10.1016/j.exer.2021.108805
Yang, X., Zhao, L., Campos, M. M., Abu-Asab, M., Ortolan, D., Hotaling, N., et al. (2020). CSF1R blockade induces macrophage ablation and results in mouse choroidal vascular atrophy and RPE disorganization. elife 9:e55564. doi: 10.7554/eLife.55564
Ye, D., Xu, Y., Shi, Y., Fan, M., Lu, P., Bai, X., et al. (2022). Anti-PANoptosis is involved in neuroprotective effects of melatonin in acute ocular hypertension model. J. Pineal Res. 73:e12828. doi: 10.1111/jpi.12828
Yoon, H. E., Kim, E. N., Kim, M. Y., Lim, J. H., Jang, I. A., Ban, T. H., et al. (2016). Age-associated changes in the vascular renin-angiotensin system in mice. Oxidative Med. Cell. Longev. 2016, 1–14. doi: 10.1155/2016/6731093
Yu, W., Chen, J., Xiong, Y., Pixley, F. J., Dai, X. M., Yeung, Y. G., et al. (2008). CSF-1 receptor structure/function in MacCsf1r−/− macrophages: regulation of proliferation, differentiation, and morphology. J. Leukoc. Biol. 84, 852–863. doi: 10.1189/jlb.0308171
Yu, D.-Y., and Cringle, S. J. (2001). Oxygen distribution and consumption within the retina in vascularised and avascular retinas and in animal models of retinal disease. Prog. Retin. Eye Res. 20, 175–208. doi: 10.1016/s1350-9462(00)00027-6
Yu, S., Cui, K., Wu, P., Wu, B., Lu, X., Huang, R., et al. (2022). Melatonin prevents experimental central serous chorioretinopathy in rats. J. Pineal Res. 73:e12802. doi: 10.1111/jpi.12802
Yun, J. H., Park, S. W., Kim, K. J., Bae, J. S., Lee, E. H., Paek, S. H., et al. (2017). Endothelial STAT3 activation increases vascular leakage through downregulating tight junction proteins: implications for diabetic retinopathy. J. Cell. Physiol. 232, 1123–1134. doi: 10.1002/jcp.25575
Zarkada, G., Howard, J. P., Xiao, X., Park, H., Bizou, M., Leclerc, S., et al. (2021). Specialized endothelial tip cells guide neuroretina vascularization and blood-retina-barrier formation. Dev. Cell 56, 2237–2251.e6. doi: 10.1016/j.devcel.2021.06.021
Zeiner, J., Loukovaara, S., Losenkova, K., Zuccarini, M., Korhonen, A. M., Lehti, K., et al. (2019). Soluble and membrane-bound adenylate kinase and nucleotidases augment ATP-mediated inflammation in diabetic retinopathy eyes with vitreous hemorrhage. J. Mol. Med. (Berl) 97, 341–354. doi: 10.1007/s00109-018-01734-0
Zeng, Y., Zhang, X., Mi, L., Gan, Y., Su, Y., Li, M., et al. (2022). Characterization of macrophage-like cells in retinal vein occlusion using En face optical coherence tomography. Front. Immunol. 13:855466. doi: 10.3389/fimmu.2022.855466
Zhang, C., Lam, T. T., and Tso, M. O. (2005). Heterogeneous populations of microglia/macrophages in the retina and their activation after retinal ischemia and reperfusion injury. Exp. Eye Res. 81, 700–709. doi: 10.1016/j.exer.2005.04.008
Zhang, T., Ouyang, H., Mei, X., Lu, B., Yu, Z., Chen, K., et al. (2019). Erianin alleviates diabetic retinopathy by reducing retinal inflammation initiated by microglial cells via inhibiting hyperglycemia-mediated ERK1/2-NF-kappaB signaling pathway. FASEB J. 33, 11776–11790. doi: 10.1096/fj.201802614RRR
Zhang, F., Wang, H., Wang, X., Jiang, G., Liu, H., Zhang, G., et al. (2016). TGF-beta induces M2-like macrophage polarization via SNAIL-mediated suppression of a pro-inflammatory phenotype. Oncotarget 7, 52294–52306. doi: 10.18632/oncotarget.10561
Zhang, Y., Zhao, L., Wang, X., Ma, W., Lazere, A., Qian, H. H., et al. (2018). Repopulating retinal microglia restore endogenous organization and function under CX3CL1-CX3CR1 regulation. Sci. Adv. 4:eaap8492. doi: 10.1126/sciadv.aap8492
Zhao, M., Celerier, I., Bousquet, E., Jeanny, J. C., Jonet, L., Savoldelli, M., et al. (2012). Mineralocorticoid receptor is involved in rat and human ocular chorioretinopathy. J. Clin. Invest. 122, 2672–2679. doi: 10.1172/JCI61427
Zhao, K., Jiang, Y., Zhang, J., Shi, J., Zheng, P., Yang, C., et al. (2022). Celastrol inhibits pathologic neovascularization in oxygen-induced retinopathy by targeting the miR-17-5p/HIF-1alpha/VEGF pathway. Cell Cycle 21, 2091–2108. doi: 10.1080/15384101.2022.2087277
Zhao, M., Rodriguez-Villagra, E., Kowalczuk, L., Le Normand, M., Berdugo, M., Levy-Boukris, R., et al. (2017). Tolerance of high and low amounts of PLGA microspheres loaded with mineralocorticoid receptor antagonist in retinal target site. J. Control. Release 266, 187–197. doi: 10.1016/j.jconrel.2017.09.029
Zhao, L., Zabel, M. K., Wang, X., Ma, W., Shah, P., Fariss, R. N., et al. (2015). Microglial phagocytosis of living photoreceptors contributes to inherited retinal degeneration. EMBO Mol. Med. 7, 1179–1197. doi: 10.15252/emmm.201505298
Zhou, T., Huang, Z., Zhu, X., Sun, X., Liu, Y., Cheng, B., et al. (2018). Alpha-1 antitrypsin attenuates M1 microglia-mediated Neuroinflammation in retinal degeneration. Front. Immunol. 9:1202. doi: 10.3389/fimmu.2018.01202
Zhou, L., Xu, Z., Oh, Y., Gamuyao, R., Lee, G., Xie, Y., et al. (2021). Myeloid cell modulation by a GLP-1 receptor agonist regulates retinal angiogenesis in ischemic retinopathy. JCI Insight 6. doi: 10.1172/jci.insight.93382
Zhou, J., Yang, J., Dai, M., Lin, D., Zhang, R., Liu, H., et al. (2020). A combination of inhibiting microglia activity and remodeling gut microenvironment suppresses the development and progression of experimental autoimmune uveitis. Biochem. Pharmacol. 180:114108. doi: 10.1016/j.bcp.2020.114108
Zoller, T., Schneider, A., Kleimeyer, C., Masuda, T., Potru, P. S., Pfeifer, D., et al. (2018). Silencing of TGFbeta signalling in microglia results in impaired homeostasis. Nat. Commun. 9:4011. doi: 10.1038/s41467-018-06224-y
Keywords: microglia, retinopathy, retinal neovascularization, blood-retinal barrier, retinal inflammation
Citation: Fu X, Feng S, Qin H, Yan L, Zheng C and Yao K (2023) Microglia: The breakthrough to treat neovascularization and repair blood-retinal barrier in retinopathy. Front. Mol. Neurosci. 16:1100254. doi: 10.3389/fnmol.2023.1100254
Edited by:
Ashley S. Harms, University of Alabama at Birmingham, United StatesReviewed by:
Francesca Massenzio, Università di Bologna, ItalyMirko H. H. Schmidt, Dresden University of Technology, Germany
Copyright © 2023 Fu, Feng, Qin, Yan, Zheng and Yao. This is an open-access article distributed under the terms of the Creative Commons Attribution License (CC BY). The use, distribution or reproduction in other forums is permitted, provided the original author(s) and the copyright owner(s) are credited and that the original publication in this journal is cited, in accordance with accepted academic practice. No use, distribution or reproduction is permitted which does not comply with these terms.
*Correspondence: Kai Yao, ✉ a3lhbzIxQG91dGxvb2suY29t
†These authors have contributed equally to this work