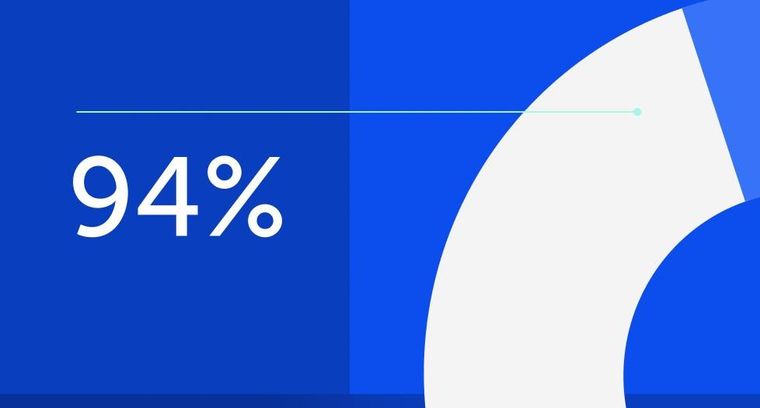
94% of researchers rate our articles as excellent or good
Learn more about the work of our research integrity team to safeguard the quality of each article we publish.
Find out more
REVIEW article
Front. Mol. Neurosci., 03 April 2023
Sec. Brain Disease Mechanisms
Volume 16 - 2023 | https://doi.org/10.3389/fnmol.2023.1076016
This article is part of the Research TopicAdvances in Brain Disorders: from Mechanisms to Therapeutic TargetsView all 21 articles
An ischemic stroke occurs when the blood supply is obstructed to the vascular basin, causing the death of nerve cells and forming the ischemic core. Subsequently, the brain enters the stage of reconstruction and repair. The whole process includes cellular brain damage, inflammatory reaction, blood–brain barrier destruction, and nerve repair. During this process, the proportion and function of neurons, immune cells, glial cells, endothelial cells, and other cells change. Identifying potential differences in gene expression between cell types or heterogeneity between cells of the same type helps to understand the cellular changes that occur in the brain and the context of disease. The recent emergence of single-cell sequencing technology has promoted the exploration of single-cell diversity and the elucidation of the molecular mechanism of ischemic stroke, thus providing new ideas and directions for the diagnosis and clinical treatment of ischemic stroke.
Stroke threatens human life and is one of the world’s most common causes of disability and death (Feigin et al., 2022). According to the Global Burden of Diseases 2019, the burden of stroke has increased significantly from 1990 to 2019 (70.0% increase in stroke events and 43.0% increase in stroke-related deaths) (Owolabi et al., 2022). Ischemic stroke accounts for 87% of all strokes globally (Virani et al., 2020). Acute ischemic stroke (AIS) is caused by a blockade of the local cerebral blood supply caused by various reasons, leading to hypoxia, ischemic changes in brain tissue, and a corresponding loss of nerve function. Since there is no effective treatment for neurological impairment caused by AIS except thrombolysis, early and accurate detection of ischemic injury is essential for initiating appropriate acute intervention and preventing recurrence strategies.
The current diagnosis of AIS is still based on clinical and neuroimaging evaluation (Bustamante et al., 2017). Therefore, it is essential to elucidate the pathophysiology of its pathogenesis further. The heterogeneity of cells in the central nervous system (CNS) has made it challenging to define the role of whole-brain cell subgroups in AIS pathogenesis and progression, which involves complex interactions between neurons, glial cells, and other cell types (Brioschi et al., 2021; Shen et al., 2021). Therefore, identifying changes in neuronal functions, transcription factors, and molecular pathways related to the above cellular processes might help to develop new therapeutic strategies. Therefore, it is necessary to understand each cell and its subgroup caused by AIS comprehensively. The traditional research method was to study the multicellular population and obtain the average value of a particular parameter. This method leads to a loss of heterogeneity information and many other “important details.” The population study can only obtain dominant cellular information for some complex tissues, such as highly heterogeneous solid tumor tissues. Some cells are ignored in the study because of their small size. Additionally, various microorganisms in nature are not only limited in number but also can not be expanded and cultured by traditional methods. Conventional techniques are of limited use for the analysis of these microorganisms, and therefore, single-cell RNA sequencing came into existence.
Single-cell sequencing mainly includes single-cell genome sequencing, single-cell epigenome sequencing, and single-cell transcriptome sequencing (scRNA-seq). There are currently many single-cell sequencing methods, such as Smart-seq, Drop-seq, and 10× genomics. Most have the same workflow: single cell isolation and collection, cell lysis, reverse transcription of RNA, cDNA amplification, library preparation, sequencing, and data analysis. The outstanding advantages of single-cell sequencing technology are that it can detect cell specificity and intercellular differences, explore the cooperative operation mode between cells, and study tissue heterogeneity from the perspective of a cell atlas. A combination of multi-omics analysis, cell and molecular imaging, and other technologies allows the generation of more accurate cell maps, deepens the understanding of disease development laws, helps find new targets for disease treatment and explores the cell development process. This article reviews the application of single-cell sequencing technology in AIS and its molecular basis in the pathological process of AIS.
After an ischemic stroke, there are two zones of injury: the infarct core area and the ischemic penumbra (Heiss, 2000). Several brain cells in the ischemic penumbra, either dormant or semi-dormant, maintain the integrity of their morphology because of collateral arteries in the supply area after ischemia. Although these cells can continue to live in the brain for several hours, they cannot perform their original normal functions (Tang et al., 2022). Therefore, it is important to explicit the mechanism of ischemic cell death for the progression from penumbra towards irreversible injury. Guo et al. (2021) used scRNA-seq to comprehensively map the cell types of the ischemic penumbra in the middle cerebral artery occlusion (MCAO) mouse model and determined 24 cell clusters. All cells were divided into 13 major cell sets, and their marker genes have been confirmed in other articles. These cells include neurons (MAP2; Chen M.L. et al., 2021 and TBR1; Yook et al., 2019), astrocytes (GFAP; Wang et al., 2020 and ALDOC; Zeisel et al., 2015), microglia (HEXB8; Sousa et al., 2018), oligodendrocytes (PLP1; Marques et al., 2016), oligodendrocyte precursor cells (PDGFRA; Alessandrini et al., 2020), endothelial cells (ITM2A; Cegarra et al., 2022), pericytes (Desmin and PDGFR-β; Smyth et al., 2018), macrophages (CD163; Skytthe et al., 2020), B cells (MS4A1 and CD79A; Lerman et al., 2022), T cells (CD3, CD4, CD5, CD8; Martinez-Lage et al., 2019), monocytes (CD14 and CD16; Calderon et al., 2017), ependymal cells (TTR; Gokce et al., 2016), and fibroblasts (COL1A1; Bonney et al., 2022). Among them, most cells were microglia, astrocytes, and oligodendrocytes. The team of Lin and others obtained 3,186 mouse cerebrovascular cell samples from the GSE98816 dataset by examining the cerebrovascular-related databases (Kundishora et al., 2021). ScRNA-seq cell cluster analysis showed ten cell clusters and four cell subgroups, including endothelial cells, fibroblasts, oligodendrocytes, and microglia. After analyzing the differential expression of related genes in cell subgroups, it was the first time to find that calcium signaling pathway-related genes [AC079305.10, BCL10, BCL2 Related Protein A1 (BCL2A1), BRE-AS1, Dynein Light Chain LC8-Type 2 (DYNLL2), Epiregulin (EREG), and Prostaglandin-Endoperoxide Synthase 2 (PTGS2)] and transcription factors [Jun, Interferon Regulatory Factor 9 (IRF9), ETS Variant Transcription Factor 5 (ETV5), and Peroxisome Proliferator Activated Receptor Alpha (PPARa)] play a key role in AIS. Many cellular functions are regulated by calcium signals that are generated by different signaling pathways, and participate in the occurrence and development of many diseases (Madreiter-Sokolowski et al., 2020) such as Parkinson’s disease, Alzheimer’s disease, etc. Jun was identified to be associated with hypoxia in endothelial cells (Kundishora et al., 2021), PPARA is a transcription factor that regulates genes involved in fatty acid metabolism and activates hepatic autophagy, it is also an important factor regulating autophagy in the clearance of Aβ in Alzheimer’s disease (Luo et al., 2020). AIS leads to a significant increase in monocyte-derived cells (the proportion of cells changed from 2 to 16%), neutrophils, and pericytes, while endothelial cells and fibroblasts decreased slightly. The expression of specific ischemic injury-related genes, such as Glycerol-3-Phosphate Dehydrogenase 1 (GPD1) in oligodendrocytes, C-C Motif Chemokine Ligand 11 (CCL11) in pericytes, CD72 Molecule (CD72) and Leukocyte Immunoglobulin Like Receptor B4 (LILRB4) in microglia, were all upregulated (Zheng et al., 2021). Rusu et al. identify GPD1 as a specific marker for dormant and chemoresistant brain tumor stem cells (BTSCs) and show that targeting GPD1 disrupts BTSC maintenance and extends survival (Rusu et al., 2019). CCL11 is important in the regulation of colitis and associated carcinogenesis (Polosukhina et al., 2021). These genes are expressed in ischemic stroke and other diseases. Biomarkers are signal indicators that are abnormal due to environmental pollutants at different biological levels (molecules, cells, individuals, etc.) before organisms are seriously damaged. It can provide early warning of serious toxic injury. The onset of ischemic stroke is acute, and the pathophysiological process is complex. Currently, the commonly used clinical biomarkers do not have the high specificity of the AIS, In the future, we can use single-cell sequencing technology to seek more specific biomarkers in ischemic stroke. Neurons in the cerebral cortex are mainly excitatory neurons and interneurons (Bandler et al., 2017). Excitatory neurons originate from the precursor cells of the developing cerebral cortex, while interneurons originate from the ganglion eminence (Shi Y. et al., 2021). By RNA sequencing, Zhong et al. (2018) analyzed more than 2,300 single cells in the developing human prefrontal cortex from 8 to 26 weeks of gestation, further dividing the excitatory neurons into seven subtypes. Among them, the 13 weeks of gestation is the critical period for the migration of the newly formed neurons. In the prefrontal cortex, the growth related gene expression of neurons increases at 16 weeks of gestation, and the functional gene expression, such as genes related to calcium input, increases at 19 weeks. At 19–26 weeks of gestation, genes related to axonogenesis were expressed in the prefrontal cortex, followed by genes related to synapse formation at 23–26 weeks, indicating that the initial formation of neural connections occurs between 19 and 26 weeks of gestation.
Interneurons were further divided into eight subtypes, and cell populations expressing interneuron markers, such as Transcription Termination Factor 1 (TTF1), LIM Homeobox 6 (Lhx6), and Distal-Less Homeobox 1 (DLX1), persisted throughout development. Calbindin 2 (Calb2) + and Somatostatin (SST) + interneurons appeared earlier, followed by Calbindin 1 (Calb1)+, Cholecystokinin (CCK)+, and Vasoactive Intestinal Peptide (VIP) + interneurons. Overall, the developmental peak of excitatory neurons appeared at 16 weeks of gestation, and that of interneurons appeared at 26 weeks. There are potential subtype-specific marker genes in each neuronal cluster (Zhong et al., 2018). Chen et al. (2017) used scRNA-seq to demonstrate the diversity of hypothalamic cells. They identified 15 glutamatergic neuron subtypes (Glu1-Glu15), 18 γ-aminobutyric acid (GABA)-ergic neuron subtypes (GABA1-GABA18), and one histaminergic neuron cluster (Hasta) expressing high levels of Histidine Decarboxylase (HDC). Kiss1 and Pomc represent Glu11 and Glu13 cell clusters, respectively, while Vip and Agouti Related Neuropeptide (Agrp) represent GABA9 and GABA15 cell clusters, consistent with their roles in controlling neuronal differentiation and identity. Recent studies have shown that RNA binding proteins CUGBP Elav-Like Family Member 1/2 (CELF1/2), Muscleblind Like Splicing Regulator 2 (Mbnl2), and KH RNA Binding Domain Containing, Signal Transduction Associated 3 (Khdrbs3) are preferentially expressed and more active in glutamatergic neurons (Feng et al., 2021). In contrast, ELAV Like RNA Binding Protein 2 (ELAVL2) and QK are preferentially expressed and more active in GABAergic neurons, indicating the hierarchical regulation of alternative splicing between different neuronal cell types and providing a basis to specify the identity and function of neurons. Single-cell sequencing studies revealed the heterogeneity among different neuronal and non-neuronal cells in various brain regions (Zeisel et al., 2015). Neuronal changes in some neurological diseases have not been described in detail. Moreover, the changes and migration of neuronal cells in the ischemic penumbra have not been reported, and therefore, further research is needed for a better understanding (Table 1).
Under normal circumstances, the human CNS is separated from the peripheral immune system through a complete blood–brain barrier. The nerve cells die of ischemia within a few minutes after an ischemic stroke, releasing “danger signals” and activating the innate immune response in the brain. Promote the production of neurotoxic substances, such as inflammatory cytokines, chemokines, reactive oxygen species and nitric oxide (Shi et al., 2019), and the destruction of the blood–brain barrier is mediated, resulting in a series of inflammatory cascades. At the same time, the expression of adhesion molecules in the cerebral vascular endothelial cells increases, and immune-inflammatory cells such as polymorphonuclear neutrophils, lymphocytes, and monocyte macrophages enter the brain tissue through vascular endothelial cells. By recognizing the antigens exposed by the CNS in the brain, the immune-inflammatory cells activate the adaptive immune response, further mediating the secondary injury of neurons and aggravating the neurological defect. At the same time, to reduce the damage mentioned earlier, the body initiates peripheral immunosuppressive response after the stroke through negative feedback, thereby increasing the incidence of post-stroke infection (Iadecola et al., 2020). Different immune cells play different roles after the occurrence of AIS. Understanding the type, migration, and transformation of cells would help explore the pathogenesis of AIS further and find new intervention targets.
Microglia play a key role in brain development, immune defense, and maintenance of CNS homeostasis (Lehnardt, 2010). Common immunological markers of microglia include CD45, CD68, HLA-DR, and IBA-1, which are slowly updated at an average rate of 28% per year. Only 2% of microglia are believed to proliferate at a specific time (Askew et al., 2017; Réu et al., 2017). Previous studies exhibited a wide range of microglial DEGs between large tissues and single cells (>50%), indicating that microglia are a core player in ischemic stroke inflammation. Meanwhile, microglia have 157 unique DEGs in all cell types, ranking first, followed by monocyte-derived cells, oligodendrocytes, endothelial cells, and CNS-related macrophages (Zheng et al., 2021). Guo et al. also confirmed that microglia accounted for the most significant number of cells after MCAO induction. Using scRNA-seq, they also showed that microglia exhibit polarization and differentiation in two different progression trajectories 24 h after MCAO (Guo et al., 2021). The most enriched signatures in subclusters 3, 4, 9, and 10 were the hypoxia pathway, as well as TNF-α, IL-6, and IL-2 genes and pathways related to inflammation. Compared with the previous M1/M2 dichotomy of microglia polarization, it would be of great significance to further study the multi-polarization of microglia by using single-cell technology. On the other hand, Li et al. found six subpopulations of microglia in aged rats after ischemia–reperfusion (Li et al., 2022). MG5 and MG6 were the main subpopulations after stroke. In MG5 cells, the expression of Top2a, Stmn1, Mki67, and Cdk1 was significantly upregulated, indicating that MG5 cells were in a highly proliferative state. Compared with MG1, expression of the steady-state genes of microglia (such as P2ry12, Tmem119, Cx3cr1, and Hexb) were downregulated in MG5. While MG6 represented a unique microglial state that appears only after stroke, was close to neutrophils on the uniform manifold approximation and projection (UMAP) map, and expressed high levels of Cxcr2, S100a8, Il1b, and Mmp9, showing a unique “neutrophil-like” phenotype and participating in the inflammatory response. Zheng et al. detected CCL7 and CCL12 in microglia, providing evidence for the molecular and cellular basis of inflammatory response after MCAO induction. They showed that the release of damage-associated molecular patterns (DAMPs) in damaged tissues initiated the secretion of these chemokines and cytokines by glial cells (Li P. et al., 2018; Zheng et al., 2021). CCL22 and many other inflammatory factors further exacerbated brain injury by enhancing cytotoxicity. Chemotactic factors direct the migration of immune cells, multipotent stem cells, and progenitor cells under physiologic and pathologic conditions. CCL7 is also highly expressed in the tumor microenvironment of various cancers, including colorectal cancer, breast cancer, oral cancer, renal cancer, and gastric cancer (Lee and Cho, 2020). CCL12 and CCL20 also contributes to the progression of many cancers, such as liver cancer, breast cancer (Chen et al., 2020; Li B.H. et al., 2020), etc. Similarly, some studies showed that in the MCAO group, the intercellular interactions dominated by microglia and macrophages increased significantly after AIS, especially the interactions between microglia and other immune cells, astrocytes, pericytes, and oligodendrocytes (Zheng et al., 2021). These findings imply that 24 h before the onset of AIS might be a good window for intervention to help cells survive.
Compared with microglia, the polarization of peripheral macrophages seems complete, indicating that microglia and blood immune cells in the brain have different activation periods after AIS (Zubova et al., 2022). It has been recently found that the infiltration and polarization of macrophages can be detected in the early stage of AIS, thus promoting the progress of inflammation (Bernstein and Rom, 2020). Zheng et al. identified six macrophage subpopulations in the MCAO group, all expressing core characteristic genes LYV1, CD163, MRC1, and CBR2 (Zheng et al., 2021). According to the changes in the cell proportion of the six subpopulations after ischemia injury, it was found that the fourth and fifth subpopulations of macrophages were mainly from the MCAO group. The MHCII-related antigen-presenting molecules of the fourth subpopulation (such as H2-Aa, H2-Ab1, and Cd74) were higher. The fifth subpopulation was characterized by increased expression of genes related to oxidative phosphorylation and respiratory electron transport chain, such as Cox7b, Cox8a, and Uqcr11. Therefore, obstructing the initial recruitment of peripheral immune cells might be an effective measure to alleviate stroke inflammation.
Neutrophils are short-lived but influential immune cells that can provide an early and robust inflammatory response after tissue injury, including AIS (Aronowski and Roy-O'reilly, 2019). Neutrophils are also one of the most abundant cell populations in the injured brain, and their number rapidly peaks at the lesion site 1 to 3 days after the occurrence of AIS (Grønberg et al., 2013). At the same time, Neutrophils could also worsen AIS through multiple mechanisms, including physical blockade within microvessels to reduce cerebral blood flow further and direct entry into the brain parenchyma, followed by the release of particles containing antimicrobial enzymes and chemical components, such as MMP9 that may further damage brain tissue. scRNA-seq provides a unique insight into the cellular heterogeneity of inflammatory response after AIS, revealing immune cell subpopulations with different functions in the pathophysiology of ischemic stroke and seeking a better target for inflammatory intervention in the subsequent stroke.
Cho et al. used individual peripheral blood mononuclear cells (PBMCs) prepared using ddSEQ (Illumina BioRad) and sequenced on the Illumina NovaSeq 6000 platform (Shi X. et al., 2021). They found that the overall gene expression of NK cells in AIS patients showed a strong increase in cell activity and a significant decrease in the number of CD14+ monocytes subdivided into dendritic cells and CD14+ monocytes associated with NK cells. Patients with mild to moderate AIS show a slight increase in the proportion of NK cells in the blood on day 7 (Yan et al., 2009), but patients with moderate to severe stroke do not exhibit any changes in the proportion of NK cells (Peterfalvi et al., 2009; Jiang et al., 2017). Reduced NK cell numbers are associated with reduced cytokine levels in the blood. Cytokine deficiency in the blood of patients with ischemic stroke leads to immunosuppression and post-stroke infection (Wang et al., 2019). This is a severe complication leading to poor outcomes of AIS, and previous reports have also shown that NK cells are involved in this process (Chen et al., 2019; Wang et al., 2019). Gene set variation analysis (GSVA) showed that oligodendrocytes contained nine subpopulations rich in IL-6, complement system, TNF-α Pathway, and KRAS signaling, indicating that serum-and glucocorticoid-inducible kinases 3 (SGK3) from oligodendrocytes may play an essential role in regulating oligodendrocyte viability and inflammatory response in the acute phase of ischemic stroke (Inoue et al., 2016).
Zheng et al. detected an upregulation of CCL7 and CCL12 in microglia, CCL4 and CDKN1a in astrocytes, and CCL4 in ependymal cells, providing evidence for the molecular and cellular basis of inflammatory response after MCAO. They showed that the release of DAMPs in damaged tissues initiates the secretion of these chemokines and cytokines by glial cells (Li P. et al., 2018; Zheng et al., 2021). CCL7, CCL12 and CCL4 are proinflammatory chemokines belonging to the CC family, and their expression is not specific in ischemic stroke. Increase in mRNA and protein levels of CCL4 in the animal model of temporal lobe epilepsy (Guzik-Kornacka et al., 2011). Subsequently, many infiltrating monocytes and lymphocytes were characterized by increased expression of CCRL2, CXCL3, CCL7, and CCL22, and many other inflammatory factors further exacerbated brain injury by enhancing cell excitotoxicity. Furthermore, AIS reduces the correlation between fibroblasts and other cells (Zheng et al., 2021). Similarly, 24 h after ischemia–reperfusion, astrocytes can act as a signal amplifier to release inflammatory signals such as cytokines and attract assistance from distal sites (Figure 1; Ma et al., 2022).
Figure 1. The inflammatory reaction of ischemic stroke. MG, microglia; Cxcr2, cxc chemokine receptor 2; Il1b, interleukin-1 beta; Mmp-9, matrix metallopeptidase 9; P2ry12, recombinant purinergic receptor P2Y, G protein poupled 12; Tmem119, transmembrane protein 119; Cx3cr1, c-x3-c motif chemokine receptor 1; Hexb, hexosaminidase subunit beta; Top2A, DNA topoisomerase II alpha; Stmn1, stathmin1; Mki67, marker of proliferation ki-67; TNF-α, tumor necrosis factor-α; IL-6, interleukin- 6; IL-2, interleukin-2; Cdk1, cyclin-dependent kinases 1; Cox7b, cytochrome c oxidase subunit 7b; Cox8a, cytochrome c oxidase subunit 8a; Uqcr11, ubiquinol-cytochrome c reductase, complex III subunit XI; H2-Aa, histocompatibility 2, class II antigen A, alpha; H2-Ab1, histocompatibility 2, class II antigen A, beta 1; Mrc1, mannose receptor C-Type 1; Cbr2, carbonyl reductase 2; NE, neutrophil; Wfdc17, WAP four-disulfide core domain 17; Ifitm1, interferon induced transmembrane protein 1; Fxyd5, FXYD Domain Containing Ion Transport Regulator 5; Rps27, Ribosomal Protein S27; Csf3r, colony stimulating factor 3 receptor; Chil3, Chitinase-3-like protein 3; Ltf, lactotransferrin; Lyz2, lysozyme 2; Cybb, cytochrome b-245 beta chain; Ly6g, lymphocyte antigen 6 complex locus G6D; NK, natural kill cell; Mono, monocyte; Ccrl2, c-c motif chemokine receptor like 2; Cxcl3, c-x-c motif chemokine ligand 3; Ccl7, c-c motif chemokine ligand 7; Ccl4, c-c motif chemokine ligand 4; Cdkn1a, cyclin dependent kinase inhibitor 1a. Figure was created with Biorender.com.
We have checked the Figure 1 carefully and corrected the “I11b” into “Il1b” in the MG6 part.
The blood–brain barrier (BBB) is a highly selective semi-permeable border of brain microvascular endothelial cells regulated by tight junctions. Pericytes, basement membranes, and glial cells induce and maintain the essential functions of the blood–brain barrier. The complex they form governs the movement of molecules, ions, and cells between the blood and CNS through interaction so that the blood–brain barrier can strictly regulate CNS homeostasis (Daneman and Prat, 2015; Profaci et al., 2020). This complex also plays an essential role in maintaining the physiological function of neurons and protecting the CNS from toxins, pathogens, inflammation, and damage. After the occurrence of AIS, activated immune cells successively reach the ischemic area through the breakdown of the blood–brain barrier. They could play a double-edged sword role by destroying or protecting the integrity of the blood–brain barrier (Li Y. et al., 2018).
Endothelial cells (ECs) are critical cellular components of BBB. Brain ECs establish a continuous complex of tight and adhesive junctions along the EC-EC contact, providing a size-selective barrier that can further express different inflow and outflow transporters. Brain ECs also have a deficient level of vesicle transport, further limiting the passage of blood-derived water-soluble molecules of various sizes in the blood (Daneman and Prat, 2015). Zheng et al. Identified six endothelial cell subpopulations in the MCAO group using scRNA-seq. Ischemia-induced inflammation and oxidative stress increased the death of endothelial cells, thus decreasing the cell proportion of the MCAO group. In contrast, the cells of BBB-related clusters increase, including a series of BBB functional disorder module-related gene expressions, such as ADAMTS4, UPP1, TIMP1, and PDLIM1 (Munji et al., 2019).
Moreover, BBB enriched endothelial cell subpopulation 3 highly expresses the KRAS gene and participates in the regulation of the RAS/MAPK pathway, which is closely related to the apoptosis of cerebral microvascular endothelial cells in AIS (Yong et al., 2019). ScRNA-seq of human glioblastoma showed that ECs in the surrounding tissues have a quiescent phenotype characterized by high expression of BBB-enriched genes, including SLC2A1 and KLF2 (Xie et al., 2021). KLF2 is a crucial transcription factor that coordinates a gene network that promotes EC response to blood flow (Dekker et al., 2006) and is one of the top ten enriched genes in the brain EC cluster. GLUT1 encoded by SLC2A1 is highly expressed in BBB ECs and promotes glucose transport across the BBB (Yong et al., 2019). Depleting GLUT1 in adult brain ECs leads to inflammation and activation of extracellular matrix-related genes (Veys et al., 2020). These results contradict the common belief that glioblastoma EC has a partially intact BBB phenotype characterized by the downregulation of transporter genes. At the same time, the expression of linker molecules remains normal or increased, and the vascular marker of BBB destruction—plasmalemma vesicle-associated protein (PLVAP) is in a high expression state.
The glial cells in the CNS mainly include astrocytes, microglia, and oligodendrocytes. Different glial cells are interconnected to neurons and surrounding blood vessels, forming a complex information exchange network (Huang et al., 2019). Glial cells support nerve transmission, maintain extracellular ion balance, insulate axons, and accelerate electrical signal transmission (Allen and Lyons, 2018). Guo et al. showed that in the MCAO group, Cyr61 in astrocytes and Sgk3 in oligodendrocytes were overexpressed (Guo et al., 2021). These genes might be potential therapeutic targets in this stage of AIS. Overexpression of the CYR61 gene may contribute to the survival of astrocytes after AIS. Although there is a lack of research on AIS, previous studies have shown that the CYR61 gene is closely related to stress and tumor cell proliferation (Sun et al., 2020). A study examining the relationship between astrocytes and BBB in heat stroke rats showed that astrocytes, but not neuronal DEGs, were rich in clusters of leukocyte chemotaxis and cytokine signals as cytokines/chemokines, toll-like receptors, and NF-κB signaling pathway (Niu et al., 2017). These chemokine-related genes were not found in neurons but combined into a regulatory sub-network in the protein–protein interaction network of astrocyte DEGs. In primary cultured astrocytes, scRNA-seq and qPCR showed upregulation of C6, CCL3, and CCR1 after heat stress but downregulation in heat stroke rats (Niu et al., 2017), while the transcriptional levels of CCL3 and CCR1 were downregulated in heat stroke rats (Audet et al., 2016). Glial cells are one of the essential components of BBB. Further exploring the regulatory mechanism of BBB injury might be one of the targets of AIS intervention in the future.
Other immune cells also play an essential role in BBB injury. Neutrophils cause the breakdown of the blood–brain barrier by releasing MMP9 and other substances, further aggravating neuroinflammation (Amantea et al., 2009). MMP9 has the ability to degrade the extracellular matrix components and has important role in the pathophysiological functions (Mondal et al., 2020). Some studies have shown that blood neutrophils in AIS patients increase and are closely related to the severity of AIS, infarct volume, and worse neurological function (Kim et al., 2012). In addition, peripheral macrophages are generally recruited to the lesion through the damaged BBB within 24 h (Jian et al., 2019). Previous studies have shown that MMP9 is highly expressed in microglia, leading to hydrolysis and vascular damage, thereby causing changes in BBB (Rosenkranz et al., 2020). Another study showed that MMPs could induce the physical destruction of BBB by digesting BBB matrix proteins (Ji et al., 2017). Guo et al. showed that MMP9 and MMP8 were overexpressed in macrophages of the MCAO group (Guo et al., 2021), indicating that in addition to microglia in the ischemic penumbra, the breakdown of BBB may also be caused by macrophages recruited from the periphery. Compared with microglia and macrophages, subpopulations of astrocytes and oligodendrocytes showed less polarization in the early stage of AIS in scRNA-seq analysis. Inflammatory mediators such as cytokines and chemokines are released through the extracellular BBB when astrocyte gap junctions are damaged during AIS (Ma et al., 2018). The mechanism of cerebral edema after cerebral ischemia is complex, caused by the interaction between many factors, and can lead to high mortality. Therefore, effective treatment measures are necessary to improve the functional prognosis of patients with cerebral ischemia (Figure 2).
Figure 2. Blood–brain barrier disruption in ischemic stroke. EC, endothelial cell; IFIT3, interferon induced protein with tetratricopeptide repeats 3; Isg15, interferon-stimulated gene 15; USP18, ubiquitin-specific protease 18; Cyr61, cysteine-rich protein 61; Sgk3, serum and glucocorticoid induced kinase; MMP-9, matrix metallopeptidase 9; MMP-8, matrix metallopeptidase 8; NF-κB, nuclear factor kappa-B; NMDA receptor, N-methyl-D-aspartic acid receptor; BBB, blood–brain barrier. Figure was created with Biorender.com.
In the recovery stage of ischemic stroke, microglia, astrocytes, and NG2 glia proliferate highly, forming reactive gliosis and glial scars in the lesion area (Wanner et al., 2013). Glial scars have traditionally been thought to hinder axon regeneration and myelin sheath regeneration (Fawcett and Asher, 1999). However, there is increasing evidence that the formation of glial scars also contributes to CNS axons regeneration (Rolls et al., 2009; Anderson et al., 2016).
Studies show that microglia and astrocytes are activated in mice with ischemic brain injury to form glial scars (Shi X. et al., 2021). The phagocytic capacity of these glial cells was enhanced in the scar area, and more synapses were phagocytized. The phagocytic ability of microglia was more substantial than that of astrocytes. High-resolution transmission electron microscopy further confirmed that synapses existed in the cell bodies of microglia and astrocytes. The GSVA results of the sixth and eighth subpopulations of microglia by Guo et al. showed that these subpopulations are MG2 type, mainly rich in the KRAS signaling pathway (Heiss, 2000), and are closely related to the survival of cancer cells (Saad et al., 2019), and may also be related to repair, neurogenesis, axon remodeling and angiogenesis (Hu et al., 2015).
ScRNA-seq analysis revealed ten different astrocyte subpopulations with different cellular functional characteristics, and synaptic pruning-related processes significantly upregulated the astrocyte transcriptomic characteristics of subpopulation three. During the post-AIS repair and remodeling phase, reactive microglial proliferation and astrogliosis actively phagocytize synapses through MEGF10 and MERTK-related pathways and inhibit microglial proliferation or astrogliosis-mediated synaptic phagocytosis by improving the prognosis of AIS mice (Shi X. et al., 2021). MEGF10-knockout mice show defective long-term synaptic plasticity and impaired formation of hippocampal memories (Lee et al., 2021). Previous studies have shown that hypoxia and focal cerebral ischemia increase the number of neural stem/progenitor cells (NS/PCS) in the hippocampal and subependymal area of MCAO mice after 1 month with an increase in neuroblasts. The canonical Wnt signaling pathway is involved in this process (Knotek et al., 2020). Kraska et al. evaluated the effect of the classical Wnt signaling pathway on the differentiation potential of NS/PCS under physiological conditions and after ischemia (Kriska et al., 2021). They showed that focal cerebral ischemia increased the expression of target genes and cell type-specific proteins in the Wnt signaling pathway, affected the electrophysiological characteristics of differentiated NS/PCS, and promoted neurogenesis. ScRNA-seq provided an essential clue for analyzing the role of the Wnt signaling pathway in patients with ischemic stroke. A recent study found that astrocytes play an independent neuroprotective role 12 h after ischemia–reperfusion, mainly manifested by the activation of oxidative phosphorylation, gap junction and tight junction, ferroptosis, and other pathways (Ma et al., 2022).
Using scRNA-seq and flow cytometry, Shi et al. showed that the number of Treg cells in the brain significantly increased from 1 to 5 weeks after MCAO mouse modeling (Shi L. et al., 2021). Treg cells-derived osteopontin acts through integrin receptors on microglia to enhance the repair activity of microglia, thus promoting oligodendrocytes regeneration and white matter repair in the chronic phase of AIS. A recent study further confirmed that Treg cells contribute to the recovery of the CNS in the chronic phase (>1 week) after the initial ischemic injury. The number of Treg cells in the ischemic brain increases for at least 1 month after stroke. These accumulated Treg cells are thought to promote functional recovery after stroke by inhibiting astrocyte proliferation (Ito et al., 2019). Additionally, Treg cell depletion inhibits the proliferation of neural stem cells 4 days after stroke, indicating the involvement of Treg cells in neurogenesis (Wang et al., 2015). These findings reveal that Treg cells are neural repair targets for AIS recovery. In the early stage of ischemia, endothelial progenitor cells can replace the damaged vascular endothelial cells and remodel the blood–brain barrier. The release of various nutritional factors can also protect other damaged cells. In the recovery period of cerebral infarction, vascular regeneration, neuroprotection, and nerve regeneration complement each other, and its potential mechanisms include neovascularization, which provides blood flow with nutrients. Endothelial progenitor cells can secrete chemical factors such as SDF-1 and VEGF to create a microenvironment suitable for nerve regeneration and survival (Chen B. et al., 2021). In addition, endogenous neural stem cells migrate to the periphery of the infarct along the newly formed blood vessels, promoting nerve regeneration (Figure 3).
Figure 3. Nerve repair in ischemic stroke. MEGF10, multiple EGF like domains 10; MERTK, MER proto-oncogene, tyrosine kinase. Figure was created with Biorender.com.
There are still many limitations in single-cell sequencing, which cause data bias and distortion: (1) Batch effect during technical operation (Li X. et al., 2020); (2) Cells get lost during preparation of single cell suspension (Xin et al., 2016); (3) Low cell activity leads to the failure of the reverse transcription (Li L.C. et al., 2018); (4) RNA with low abundance gets lost during reverse transcription (Wang et al., 2016); (5) Changes in gene transcriptome during the preparation of single cell suspension (Wang et al., 2016). Since the advent of single-cell sequencing, new dimension reduction methods have been emerging, such as single-cell harmful binomial matrix decomposition (Sun et al., 2019), single-cell bimodal clustering analysis (Kim et al., 2018), and new feature methods to speed up the analysis and processing speed and reduce the batch effect in experiments (Li X. et al., 2020). When using single-cell sequencing to analyze data, one should treat the analysis results dialectically and conduct experimental verification in various ways. Although single-cell sequencing can provide much information, the interaction between cells, the localization of stem cells in tissues, and the epigenetic modification of genes are still unclear. Therefore, follow-up research is needed by combining single-cell sequencing with metabolomics, spatial transcriptome, and epigenetic modification of genes.
In general, single-cell sequencing provides new information and direction for the molecular basis of AIS through dimensionality reduction and bioinformatics analysis and broadens our vision of AIS cell heterogeneity and cell expression-specific genes. Identifying specific genes expressed by cells can promote the application of molecular imaging research toward monitoring dynamic disease in AIS patients. It will open up a new field for exploring the pathogenesis of AIS and drug development based on cell subtype-specific molecules and lay a foundation for further research on the human brain after AIS.
XS: conceptualization and writing – original draft preparation. ML: conducting a research and investigation process and specifically performing the evidence collection. KS: presentation of the published work, specifically data presentation. YL: writing – reviewing and editing. ZG: writing – reviewing and editing and acquisition of the financial support for the project leading to this publication. All authors contributed to the article and approved the submitted version.
This study was supported by the special fund project for doctoral training program of Lanzhou University Second Hospital (no. YJS-BD-05); the clinical Research Center for neurological diseases of Gansu Province (no. 2020-0411-SFC-0025); and Cuiying Technology Innovation Project of Lanzhou University Second Hospital (no. CY2022-QN-A05).
The authors declare that the research was conducted in the absence of any commercial or financial relationships that could be construed as a potential conflict of interest.
All claims expressed in this article are solely those of the authors and do not necessarily represent those of their affiliated organizations, or those of the publisher, the editors and the reviewers. Any product that may be evaluated in this article, or claim that may be made by its manufacturer, is not guaranteed or endorsed by the publisher.
Alessandrini, F., Ceresa, D., Appolloni, I., Pagani, F., Poliani, P. L., Marubbi, D., et al. (2020). Glioblastoma models driven by different mutations converge to the proneural subtype. Cancer Lett. 469, 447–455. doi: 10.1016/j.canlet.2019.11.010
Allen, N. J., and Lyons, D. A. (2018). Glia as architects of central nervous system formation and function. Science 362, 181–185. doi: 10.1126/science.aat0473
Amantea, D., Nappi, G., Bernardi, G., Bagetta, G., and Corasaniti, M. T. (2009). Post-ischemic brain damage: pathophysiology and role of inflammatory mediators. FEBS J. 276, 13–26. doi: 10.1111/j.1742-4658.2008.06766.x
Anderson, M. A., Burda, J. E., Ren, Y., Ao, Y., O’Shea, T. M., Kawaguchi, R., et al. (2016). Astrocyte scar formation aids central nervous system axon regeneration. Nature 532, 195–200. doi: 10.1038/nature17623
Aronowski, J., and Roy-O'reilly, M. A. (2019). Neutrophils, the felons of the brain. Stroke 50, e42–e43. doi: 10.1161/STROKEAHA.118.021563
Askew, K., Li, K., Olmos-Alonso, A., Garcia-Moreno, F., Liang, Y., Richardson, P., et al. (2017). Coupled proliferation and apoptosis maintain the rapid turnover of microglia in the adult brain. Cell Rep. 18, 391–405. doi: 10.1016/j.celrep.2016.12.041
Audet, G. N., Dineen, S. M., Quinn, C. M., and Leon, L. R. (2016). Altered hypothalamic inflammatory gene expression correlates with heat stroke severity in a conscious rodent model. Brain Res. 1637, 81–90. doi: 10.1016/j.brainres.2016.01.048
Bandler, R. C., Mayer, C., and Fishell, G. (2017). Cortical interneuron specification: the juncture of genes, time and geometry. Curr. Opin. Neurobiol. 42, 17–24. doi: 10.1016/j.conb.2016.10.003
Bernstein, D. L., and Rom, S. (2020). Let-7g* and mi R-98 reduce stroke-induced production of proinflammatory cytokines in mouse brain. Front. Cell Dev. Biol. 8:632. doi: 10.3389/fcell.2020.00632
Bonney, S. K., Sullivan, L. T., Cherry, T. J., Daneman, R., and Shih, A. Y. (2022). Distinct features of brain perivascular fibroblasts and mural cells revealed by in vivo two-photon imaging. J. Cereb. Blood Flow Metab. 42, 966–978. doi: 10.1177/0271678X211068528
Brioschi, S., Wang, W. L., Peng, V., Wang, M., Shchukina, I., Greenberg, Z. J., et al. (2021). Heterogeneity of meningeal B cells reveals a lymphopoietic niche at the CNS borders. Science 373:eabf9277. doi: 10.1126/science.abf9277
Bustamante, A., López-Cancio, E., Pich, S., Penalba, A., Giralt, D., García-Berrocoso, T., et al. (2017). Blood biomarkers for the early diagnosis of stroke: the stroke-Chip study. Stroke 48, 2419–2425. doi: 10.1161/STROKEAHA.117.017076
Calderon, T. M., Williams, D. W., Lopez, L., Eugenin, E. A., Cheney, L., Gaskill, P. J., et al. (2017). Dopamine increases CD14(+)CD16(+) monocyte transmigration across the blood brain barrier: implications for substance abuse and HIV Neuropathogenesis. J. Neuroimmune Pharmacol. 12, 353–370. doi: 10.1007/s11481-017-9726-9
Cegarra, C., Chaves, C., Déon, C., do, T. M., Dumas, B., Frenzel, A., et al. (2022). Exploring ITM2A as a new potential target for brain delivery. Fluids Barriers CNS 19:25. doi: 10.1186/s12987-022-00321-3
Chen, C., Ai, Q. D., Chu, S. F., Zhang, Z., and Chen, N. H. (2019). NK cells in cerebral ischemia. Biomed. Pharmacother. 109, 547–554. doi: 10.1016/j.biopha.2018.10.103
Chen, B., Banton, M. C., Singh, L., Parkinson, D. B., and Dun, X. P. (2021). Single cell transcriptome data analysis defines the heterogeneity of peripheral nerve cells in homeostasis and regeneration. Front. Cell. Neurosci. 15:624826. doi: 10.3389/fncel.2021.624826
Chen, M. L., Hong, C. G., Yue, T., Li, H. M., Duan, R., Hu, W. B., et al. (2021). Inhibition of mi R-331-3p and mi R-9-5p ameliorates Alzheimer's disease by enhancing autophagy. Theranostics 11, 2395–2409. doi: 10.7150/thno.47408
Chen, W., Qin, Y., and Liu, S. (2020). CCL20 signaling in the tumor microenvironment. Adv. Exp. Med. Biol. 1231, 53–65. doi: 10.1007/978-3-030-36667-4_6
Chen, R., Wu, X., Jiang, L., and Zhang, Y. (2017). Single-cell RNA-Seq reveals hypothalamic cell diversity. Cell Rep. 18, 3227–3241. doi: 10.1016/j.celrep.2017.03.004
Cho, Y. E., Lee, H., Bae, H. R., Kim, H., Yun, S., Vorn, R., et al. (2022). Circulating immune cell landscape in patients who had mild ischaemic stroke. Stroke Vasc Neurol 7, 319–327. doi: 10.1136/svn-2021-001224
Daneman, R., and Prat, A. (2015). The blood-brain barrier. Cold Spring Harb. Perspect. Biol. 7:a020412. doi: 10.1101/cshperspect.a020412
Dekker, R. J., Boon, R. A., Rondaij, M. G., Kragt, A., Volger, O. L., Elderkamp, Y. W., et al. (2006). KLF2 provokes a gene expression pattern that establishes functional quiescent differentiation of the endothelium. Blood 107, 4354–4363. doi: 10.1182/blood-2005-08-3465
Fawcett, J. W., and Asher, R. A. (1999). The glial scar and central nervous system repair. Brain Res. Bull. 49, 377–391. doi: 10.1016/S0361-9230(99)00072-6
Feigin, V., Brainin, M., Norrving, B., Martins, S., Sacco, R. L., Hacke, W., et al. (2022). World stroke organization (WSO): global stroke fact sheet 2022. Int. J. Stroke 17, 18–29. doi: 10.1177/17474930211065917
Feng, H., Moakley, D. F., Chen, S., McKenzie, M. G., Menon, V., and Zhang, C. (2021). Complexity and graded regulation of neuronal cell-type-specific alternative splicing revealed by single-cell RNA sequencing. Proc. Natl. Acad. Sci. U. S. A. 118:e2013056118. doi: 10.1073/pnas.2013056118
Gokce, O., Stanley, G. M., Treutlein, B., Neff, N. F., Camp, J. G., Malenka, R. C., et al. (2016). Cellular taxonomy of the mouse striatum as revealed by single-cell RNA-Seq. Cell Rep. 16, 1126–1137. doi: 10.1016/j.celrep.2016.06.059
Grønberg, N. V., Johansen, F. F., Kristiansen, U., and Hasseldam, H. (2013). Leukocyte infiltration in experimental stroke. J. Neuroinflammation 10:115. doi: 10.1186/1742-2094-10-115
Guo, K., Luo, J., Feng, D., Wu, L., Wang, X., Xia, L., et al. (2021). Single-cell RNA sequencing with combined use of bulk RNA sequencing to reveal cell heterogeneity and molecular changes at acute stage of ischemic stroke in mouse cortex penumbra area. Front. Cell Dev. Biol. 9:624711. doi: 10.3389/fcell.2021.624711
Guzik-Kornacka, A., Sliwa, A., Plucinska, G., and Lukasiuk, K. (2011). Status epilepticus evokes prolonged increase in the expression of CCL3 and CCL4 mRNA and protein in the rat brain. Acta Neurobiol. Exp. (Wars) 71, 193–207.
Heiss, W. D. (2000). Ischemic penumbra: evidence from functional imaging in man. J. Cereb. Blood Flow Metab. 20, 1276–1293. doi: 10.1097/00004647-200009000-00002
Hu, X., Leak, R. K., Shi, Y., Suenaga, J., Gao, Y., Zheng, P., et al. (2015). Microglial and macrophage polarization—new prospects for brain repair. Nat. Rev. Neurol. 11, 56–64. doi: 10.1038/nrneurol.2014.207
Huang, L., Nakamura, Y., Lo, E. H., and Hayakawa, K. (2019). Astrocyte signaling in the neurovascular unit after central nervous system injury. Int. J. Mol. Sci. 20:282. doi: 10.3390/ijms20020282
Iadecola, C., Buckwalter, M. S., and Anrather, J. (2020). Immune responses to stroke: mechanisms, modulation, and therapeutic potential. J. Clin. Invest. 130, 2777–2788. doi: 10.1172/JCI135530
Inoue, K., Sakuma, E., Morimoto, H., Asai, H., Koide, Y., Leng, T., et al. (2016). Serum-and glucocorticoid-inducible kinases in microglia. Biochem. Biophys. Res. Commun. 478, 53–59. doi: 10.1016/j.bbrc.2016.07.094
Ito, M., Komai, K., Mise-Omata, S., Iizuka-Koga, M., Noguchi, Y., Kondo, T., et al. (2019). Brain regulatory T cells suppress astrogliosis and potentiate neurological recovery. Nature 565, 246–250. doi: 10.1038/s41586-018-0824-5
Ji, B., Zhou, F., Han, L., Yang, J., Fan, H., Li, S., et al. (2017). Sodium Tanshinone IIA sulfonate enhances effectiveness Rt-PA treatment in acute ischemic stroke patients associated with ameliorating blood-brain barrier damage. Transl. Stroke Res. 8, 334–340. doi: 10.1007/s12975-017-0526-6
Jian, Z., Liu, R., Zhu, X., Smerin, D., Zhong, Y., Gu, L., et al. (2019). The involvement and therapy target of immune cells after ischemic stroke. Front. Immunol. 10:2167. doi: 10.3389/fimmu.2019.02167
Jiang, C., Kong, W., Wang, Y., Ziai, W., Yang, Q., Zuo, F., et al. (2017). Changes in the cellular immune system and circulating inflammatory markers of stroke patients. Oncotarget 8, 3553–3567. doi: 10.18632/oncotarget.12201
Kim, J., Song, T. J., Park, J. H., Lee, H. S., Nam, C. M., Nam, H. S., et al. (2012). Different prognostic value of white blood cell subtypes in patients with acute cerebral infarction. Atherosclerosis 222, 464–467. doi: 10.1016/j.atherosclerosis.2012.02.042
Kim, J., Stanescu, D. E., and Won, K. J. (2018). Cell BIC: bimodality-based top-down clustering of single-cell RNA sequencing data reveals hierarchical structure of the cell type. Nucleic Acids Res. 46:e124. doi: 10.1093/nar/gky698
Knotek, T., Janeckova, L., Kriska, J., Korinek, V., and Anderova, M. (2020). Glia and neural stem and progenitor cells of the healthy and ischemic brain: the workplace for the Wnt signaling pathway. Genes (Basel) 11:804. doi: 10.3390/genes11070804
Kriska, J., Janeckova, L., Kirdajova, D., Honsa, P., Knotek, T., Dzamba, D., et al. (2021). Wnt/β-catenin signaling promotes differentiation of ischemia-activated adult neural stem/progenitor cells to neuronal precursors. Front. Neurosci. 15:628983. doi: 10.3389/fnins.2021.628983
Kundishora, A. J., Peters, S. T., Pinard, A., Duran, D., Panchagnula, S., Barak, T., et al. (2021). DIAPH1 variants in non-east Asian patients with sporadic Moyamoya disease. JAMA Neurol. 78, 993–1003. doi: 10.1001/jamaneurol.2021.1681
Lee, Y. S., and Cho, Y. B. (2020). CCL7 signaling in the tumor microenvironment. Adv. Exp. Med. Biol. 1231, 33–43. doi: 10.1007/978-3-030-36667-4_4
Lee, J. H., Kim, J. Y., Noh, S., Lee, H., Lee, S. Y., Mun, J. Y., et al. (2021). Astrocytes phagocytose adult hippocampal synapses for circuit homeostasis. Nature 590, 612–617. doi: 10.1038/s41586-020-03060-3
Lehnardt, S. (2010). Innate immunity and neuroinflammation in the CNS: the role of microglia in toll-like receptor-mediated neuronal injury. Glia 58, 253–263. doi: 10.1002/glia.20928
Lerman, I., Bawany, F., Whitt, W., Esaa, F., Yon, J., Babkowski, N., et al. (2022). Prominent B-cell signature differentiates discoid from subacute cutaneous lupus erythematosus. J. Invest. Dermatol. 142, 2885–2895.e2. doi: 10.1016/j.jid.2022.03.033
Li, B. H., Garstka, M. A., and Li, Z. F. (2020). Chemokines and their receptors promoting the recruitment of myeloid-derived suppressor cells into the tumor. Mol. Immunol. 117, 201–215. doi: 10.1016/j.molimm.2019.11.014
Li, X., Lyu, J., Li, R., Jain, V., Shen, Y., del Águila, Á., et al. (2022). Single-cell transcriptomic analysis of the immune cell landscape in the aged mouse brain after ischemic stroke. J. Neuroinflammation 19:83. doi: 10.1186/s12974-022-02447-5
Li, P., Stetler, R. A., Leak, R. K., Shi, Y., Li, Y., Yu, W., et al. (2018). Oxidative stress and DNA damage after cerebral ischemia: potential therapeutic targets to repair the genome and improve stroke recovery. Neuropharmacology 134, 208–217. doi: 10.1016/j.neuropharm.2017.11.011
Li, X., Wang, K., Lyu, Y., Pan, H., Zhang, J., Stambolian, D., et al. (2020). Deep learning enables accurate clustering with batch effect removal in single-cell RNA-seq analysis. Nat. Commun. 11:2338. doi: 10.1038/s41467-020-15851-3
Li, L. C., Yu, X. X., Zhang, Y. W., Feng, Y., Qiu, W. L., and Xu, C. R. (2018). Single-cell transcriptomic analyses of mouse pancreatic endocrine cells. J. Vis. Exp. 139:58000. doi: 10.3791/58000-v
Li, Y., Zhu, Z. Y., Huang, T. T., Zhou, Y. X., Wang, X., Yang, L. Q., et al. (2018). The peripheral immune response after stroke-a double edge sword for blood-brain barrier integrity. CNS Neurosci. Ther. 24, 1115–1128. doi: 10.1111/cns.13081
Lin, W., Wang, Y., Chen, Y., Wang, Q., Gu, Z., and Zhu, Y. (2021). Role of calcium signaling pathway-related gene regulatory networks in ischemic stroke based on multiple WGCNA and single-cell analysis. Oxidative Med. Cell. Longev. 2021:8060477. doi: 10.1155/2021/8060477
Luo, R., Su, L. Y., Li, G., Yang, J., Liu, Q., Yang, L. X., et al. (2020). Activation of PPARA-mediated autophagy reduces Alzheimer disease-like pathology and cognitive decline in a murine model. Autophagy 16, 52–69. doi: 10.1080/15548627.2019.1596488
Ma, D., Feng, L., Cheng, Y., Xin, M., You, J., Yin, X., et al. (2018). Astrocytic gap junction inhibition by carbenoxolone enhances the protective effects of ischemic preconditioning following cerebral ischemia. J. Neuroinflammation 15:198. doi: 10.1186/s12974-018-1230-5
Ma, H., Zhou, Y., Li, Z., Zhu, L., Li, H., Zhang, G., et al. (2022). Single-cell RNA-sequencing analyses revealed heterogeneity and dynamic changes of metabolic pathways in astrocytes at the acute phase of ischemic stroke. Oxidative Med. Cell. Longev. 2022, 1–22. doi: 10.1155/2022/1817721
Madreiter-Sokolowski, C. T., Thomas, C., and Ristow, M. (2020). Interrelation between ROS and Ca2+ in aging and age-related diseases. Redox Biol. 36:101678. doi: 10.1016/j.redox.2020.101678
Marques, S., Zeisel, A., Codeluppi, S., van Bruggen, D., Mendanha Falcão, A., Xiao, L., et al. (2016). Oligodendrocyte heterogeneity in the mouse juvenile and adult central nervous system. Science 352, 1326–1329. doi: 10.1126/science.aaf6463
Martinez-Lage, M., Lynch, T. M., Bi, Y., Cocito, C., Way, G. P., Pal, S., et al. (2019). Immune landscapes associated with different glioblastoma molecular subtypes. Acta Neuropathol. Commun. 7:203. doi: 10.1186/s40478-019-0803-6
Mondal, S., Adhikari, N., Banerjee, S., Amin, S. A., and Jha, T. (2020). Matrix metalloproteinase-9 (MMP-9) and its inhibitors in cancer: A minireview. Eur J Med Chem 194:112260. doi: 10.1016/j.ejmech.2020.112260
Munji, R. N., Soung, A. L., Weiner, G. A., Sohet, F., Semple, B. D., Trivedi, A., et al. (2019). Profiling the mouse brain endothelial transcriptome in health and disease models reveals a core blood-brain barrier dysfunction module. Nat. Neurosci. 22, 1892–1902. doi: 10.1038/s41593-019-0497-x
Niu, B., Zhang, T., Hu, H., and Cao, B. (2017). Transcriptome sequencing reveals astrocytes as a therapeutic target in heat-stroke. Neurosci. Bull. 33, 627–640. doi: 10.1007/s12264-017-0156-8
Owolabi, M. O., Thrift, A. G., Mahal, A., Ishida, M., Martins, S., Johnson, W. D., et al. (2022). Primary stroke prevention worldwide: translating evidence into action. Lancet Public Health 7, e74–e85. doi: 10.1016/S2468-2667(21)00230-9
Peterfalvi, A., Molnar, T., Banati, M., Pusch, G., Miko, E., Bogar, L., et al. (2009). Impaired function of innate T lymphocytes and NK cells in the acute phase of ischemic stroke. Cerebrovasc. Dis. 28, 490–498. doi: 10.1159/000236527
Polosukhina, D., Singh, K., Asim, M., Barry, D. P., Allaman, M. M., Hardbower, D. M., et al. (2021). CCL11 exacerbates colitis and inflammation-associated colon tumorigenesis. Oncogene 40, 6540–6546. doi: 10.1038/s41388-021-02046-3
Profaci, C. P., Munji, R. N., Pulido, R. S., and Daneman, R. (2020). The blood-brain barrier in health and disease: important unanswered questions. J. Exp. Med. 217:e20190062. doi: 10.1084/jem.20190062
Réu, P., Khosravi, A., Bernard, S., Mold, J. E., Salehpour, M., Alkass, K., et al. (2017). The lifespan and turnover of microglia in the human brain. Cell Rep. 20, 779–784. doi: 10.1016/j.celrep.2017.07.004
Rolls, A., Shechter, R., and Schwartz, M. (2009). The bright side of the glial scar in CNS repair. Nat. Rev. Neurosci. 10, 235–241. doi: 10.1038/nrn2591
Rosenkranz, S. C., Shaposhnykov, A., Schnapauff, O., Epping, L., Vieira, V., Heidermann, K., et al. (2020). TRPV4-mediated regulation of the blood brain barrier is abolished during inflammation. Front. Cell Dev. Biol. 8:849. doi: 10.3389/fcell.2020.00849
Rusu, P., Shao, C., Neuerburg, A., Acikgöz, A. A., Wu, Y., Zou, P., et al. (2019). GPD1 specifically Marks dormant glioma stem cells with a distinct metabolic profile. Cell Stem Cell 25, 241–257.e8. doi: 10.1016/j.stem.2019.06.004
Saad, M. I., Alhayyani, S., Mcleod, L., Yu, L., Alanazi, M., Deswaerte, V., et al. (2019). ADAM17 selectively activates the IL-6 trans-signaling/ERK MAPK axis in KRAS-addicted lung cancer. EMBO Mol. Med. 11:e9976. doi: 10.15252/emmm.201809976
Shen, X. Y., Gao, Z. K., Han, Y., Yuan, M., Guo, Y. S., and Bi, X. (2021). Activation and role of astrocytes in ischemic stroke. Front. Cell. Neurosci. 17:755955 doi: 10.3389/fncel.2021.755955
Shi, X., Luo, L., Wang, J., Shen, H., Li, Y., Mamtilahun, M., et al. (2021). Stroke subtype-dependent synapse elimination by reactive gliosis in mice. Nat. Commun. 12:6943. doi: 10.1038/s41467-021-27248-x
Shi, L., Sun, Z., Su, W., Xu, F., Xie, D., Zhang, Q., et al. (2021). Treg cell-derived osteopontin promotes microglia-mediated white matter repair after ischemic stroke. Immunity 54, 1527–1542.e8. doi: 10.1016/j.immuni.2021.04.022
Shi, K., Tian, D. C., Li, Z. G., Ducruet, A. F., Lawton, M. T., and Shi, F. D. (2019). Global brain inflammation in stroke. Lancet Neurol. 18, 1058–1066. doi: 10.1016/S1474-4422(19)30078-X
Shi, Y., Wang, M., Mi, D., Lu, T., Wang, B., Dong, H., et al. (2021). Mouse and human share conserved transcriptional programs for interneuron development. Science 374:eabj6641. doi: 10.1126/science.abj6641
Skytthe, M. K., Graversen, J. H., and Moestrup, S. K. (2020). Targeting of CD163(+) macrophages in inflammatory and malignant diseases. Int. J. Mol. Sci. 21:5497. doi: 10.3390/ijms21155497
Smyth, L. C. D., Rustenhoven, J., Scotter, E. L., Schweder, P., Faull, R. L. M., Park, T. I. H., et al. (2018). Markers for human brain pericytes and smooth muscle cells. J. Chem. Neuroanat. 92, 48–60. doi: 10.1016/j.jchemneu.2018.06.001
Sousa, C., Golebiewska, A., Poovathingal, S. K., Kaoma, T., Pires-Afonso, Y., Martina, S., et al. (2018). Single-cell transcriptomics reveals distinct inflammation-induced microglia signatures. EMBO Rep. 19:e46171. doi: 10.15252/embr.201846171
Sun, S., Chen, Y., Liu, Y., and Shang, X. (2019). A fast and efficient count-based matrix factorization method for detecting cell types from single-cell RNAseq data. BMC Syst. Biol. 13:28. doi: 10.1186/s12918-019-0699-6
Sun, J., Zhang, W., Tan, Z., Zheng, C., Tang, Y., Ke, X., et al. (2020). Zika virus promotes CCN1 expression via the CaMKIIα-CREB pathway in astrocytes. Virulence 11, 113–131. doi: 10.1080/21505594.2020.1715189
Tang, H., Li, Y., Tang, W., Zhu, J., Parker, G. C., and Zhang, J. H. (2022). Endogenous neural stem cell-induced neurogenesis after ischemic stroke: processes for brain repair and perspectives. Transl. Stroke Res. doi: 10.1007/s12975-022-01078-5 Epub ahead of print. Erratum in: Transl Stroke Res.
Veys, K., Fan, Z., Ghobrial, M., Bouché, A., García-Caballero, M., Vriens, K., et al. (2020). Role of the GLUT1 glucose transporter in postnatal CNS angiogenesis and blood-brain barrier integrity. Circ. Res. 127, 466–482. doi: 10.1161/CIRCRESAHA.119.316463
Virani, S. S., Alonso, A., Benjamin, E. J., Bittencourt, M. S., Callaway, C. W., Carson, A. P., et al. (2020). American Heart Association Council on epidemiology and prevention statistics committee and stroke statistics subcommittee heart disease and stroke Statistics-2020 update: a report from the American Heart Association. Circulation 141, e139–e596. doi: 10.1161/CIR.0000000000000757
Wang, J., Sareddy, G. R., Lu, Y., Pratap, U. P., Tang, F., Greene, K. M., et al. (2020). Astrocyte-derived estrogen regulates reactive Astrogliosis and is neuroprotective following ischemic brain injury. J. Neurosci. 40, 9751–9771. doi: 10.1523/JNEUROSCI.0888-20.2020
Wang, Y. J., Schug, J., Won, K. J., Liu, C., Naji, A., Avrahami, D., et al. (2016). Single-cell transcriptomics of the human endocrine pancreas. Diabetes 65, 3028–3038. doi: 10.2337/db16-0405
Wang, J., Xie, L., Yang, C., Ren, C., Zhou, K., Wang, B., et al. (2015). Activated regulatory T cell regulates neural stem cell proliferation in the subventricular zone of normal and ischemic mouse brain through interleukin 10. Front. Cell. Neurosci. 9:361. doi: 10.3389/fncel.2015.00361
Wang, Y., Zhang, J. H., Sheng, J., and Shao, A. (2019). Immunoreactive cells after cerebral ischemia. Front. Immunol. 10:2781. doi: 10.3389/fimmu.2019.02781
Wanner, I. B., Anderson, M. A., Song, B., Levine, J., Fernandez, A., Gray-Thompson, Z., et al. (2013). Glial scar borders are formed by newly proliferated, elongated astrocytes that interact to corral inflammatory and fibrotic cells via STAT3-dependent mechanisms after spinal cord injury. J. Neurosci. 33, 12870–12886. doi: 10.1523/JNEUROSCI.2121-13.2013
Xie, Y., He, L., Lugano, R., Zhang, Y., Cao, H., He, Q., et al. (2021). Key molecular alterations in endothelial cells in human glioblastoma uncovered through single-cell RNA sequencing. JCI Insight 6:e150861. doi: 10.1172/jci.insight.150861
Xin, Y., Kim, J., Ni, M., Wei, Y., Okamoto, H., Lee, J., et al. (2016). Use of the Fluidigm C1 platform for RNA sequencing of single mouse pancreatic islet cells. Proc. Natl. Acad. Sci. U. S. A. 113, 3293–3298. doi: 10.1073/pnas.1602306113
Yan, J., Greer, J. M., Etherington, K., Cadigan, G. P., Cavanagh, H., Henderson, R. D., et al. (2009). Immune activation in the peripheral blood of patients with acute ischemic stroke. J. Neuroimmunol. 206, 112–117. doi: 10.1016/j.jneuroim.2008.11.001
Yong, Y. X., Yang, H., Lian, J., Xu, X. W., Han, K., Hu, M. Y., et al. (2019). Up-regulated micro RNA-199b-3p represses the apoptosis of cerebral microvascular endothelial cells in ischemic stroke through down-regulation of MAPK/ERK/EGR1 axis. Cell Cycle 18, 1868–1881. doi: 10.1080/15384101.2019.1632133
Yook, C., Kim, K., Kim, D., Kang, H., Kim, S. G., Kim, E., et al. (2019). A TBR1-K228E mutation induces Tbr1 upregulation, altered cortical distribution of interneurons, increased inhibitory synaptic transmission, and autistic-like behavioral deficits in mice. Front. Mol. Neurosci. 12:241. doi: 10.3389/fnmol.2019.00241
Zeisel, A., Muñoz-Manchado, A. B., Codeluppi, S., Lönnerberg, P., La Manno, G., Juréus, A., et al. (2015). Brain structure. Cell types in the mouse cortex and hippocampus revealed by single-cell RNA-seq. Science 347, 1138–1142. doi: 10.1126/science.aaa1934
Zheng, K., Lin, L., Jiang, W., Chen, L., Zhang, X., Zhang, Q., et al. (2021). Single-cell RNA-seq reveals the transcriptional landscape in ischemic stroke. J. Cereb. Blood Flow Metab. 42, 56–73. doi: 10.1177/0271678X211026770
Zhong, S., Zhang, S., Fan, X., Wu, Q., Yan, L., Dong, J., et al. (2018). A single-cell RNA-seq survey of the developmental landscape of the human prefrontal cortex. Nature 555, 524–528. doi: 10.1038/nature25980
Keywords: neuron, immune cell, glial, single-cell sequencing, ischemic stroke
Citation: Shen X, Li M, Shao K, Li Y and Ge Z (2023) Post-ischemic inflammatory response in the brain: Targeting immune cell in ischemic stroke therapy. Front. Mol. Neurosci. 16:1076016. doi: 10.3389/fnmol.2023.1076016
Received: 21 October 2022; Accepted: 13 March 2023;
Published: 03 April 2023.
Edited by:
Arianna Bellucci, University of Brescia, ItalyReviewed by:
Abhijit Maji, University of Texas Southwestern Medical Center, United StatesCopyright © 2023 Shen, Li, Shao, Li and Ge. This is an open-access article distributed under the terms of the Creative Commons Attribution License (CC BY). The use, distribution or reproduction in other forums is permitted, provided the original author(s) and the copyright owner(s) are credited and that the original publication in this journal is cited, in accordance with accepted academic practice. No use, distribution or reproduction is permitted which does not comply with these terms.
*Correspondence: Zhaoming Ge, MTM4OTMyODUxMjBAMTYzLmNvbQ==; Yongnan Li, bHluZ3lxMjAwNkBmb3htYWlsLmNvbQ==
†These authors have contributed equally to this work
Disclaimer: All claims expressed in this article are solely those of the authors and do not necessarily represent those of their affiliated organizations, or those of the publisher, the editors and the reviewers. Any product that may be evaluated in this article or claim that may be made by its manufacturer is not guaranteed or endorsed by the publisher.
Research integrity at Frontiers
Learn more about the work of our research integrity team to safeguard the quality of each article we publish.