- 1C. and O. Vogt Institute for Brain Research, Medical Faculty and University Hospital Düsseldorf, Henrich Heine University, Düsseldorf, Düsseldorf, Germany
- 2Institute for Biology-Neurobiology, Freie University of Berlin, Berlin, Germany
- 3Department of Psychiatry and Psychotherapy, University Hospital Bonn, Bonn, Germany
Adaptive neuroplasticity is a pivotal mechanism for healthy brain development and maintenance, as well as its restoration in disease- and age-associated decline. Management of mental disorders such as attention deficit hyperactivity disorder (ADHD) needs interventions stimulating adaptive neuroplasticity, beyond conventional psychopharmacological treatments. Physical exercises are proposed for the management of ADHD, and also depression and aging because of evoked brain neuroplasticity. Recent progress in understanding the mechanisms of muscle-brain cross-talk pinpoints the role of the myokine irisin in the mediation of pro-cognitive and antidepressant activity of physical exercises. In this review, we discuss how irisin, which is released in the periphery as well as derived from brain cells, may interact with the mechanisms of cellular autophagy to provide protein recycling and regulation of brain-derived neurotrophic factor (BDNF) signaling via glia-mediated control of BDNF maturation, and, therefore, support neuroplasticity. We propose that the neuroplasticity associated with physical exercises is mediated in part by irisin-triggered autophagy. Since the recent findings give objectives to consider autophagy-stimulating intervention as a prerequisite for successful therapy of psychiatric disorders, irisin appears as a prototypic molecule that can activate autophagy with therapeutic goals.
Graphical abstract: Physical activity results in irisin release. Irisin facilitates autophagy in the brain acting via glia activation. Autophagy activation favors maturation of BDNF and neuroplasticity.
1. Introduction
Neuroplasticity is a fundamental feature of neuronal tissue appearing as the ability of neurons to maintain and upgrade their connections and communications with respect to actual requirements (Gu and Kanai, 2014; Voss et al., 2017; Mateos-Aparicio and Rodríguez-Moreno, 2019). Neuroplasticity is a well-established mechanism of the brain to reorganize itself, both functionally and structurally and is recognized as a cause and/or accompanying trait of successful neuropsychiatric treatments. For example, the therapeutic mechanism of antidepressant activity has been shown to involve the modulation and induction of neuroplastic changes, including synaptogenesis (Castrén and Rantamäki, 2010). Changes in neuroplasticity seem to be a way to make up the neurobiological context in the brain, which could be essential to prevent precipitation, further development, or even recovery from a wide spectrum of psychiatric complications (McEwen and Chattarji, 2004; Nitsche et al., 2012).
Among the complex physiological factors that may interfere neuroplasticity, physical exercises (PEs) consistently gain attention as a supplementary or even standalone treatment of psychiatric diseases. Engagement of PEs is tempting considering their antidepressant and procognitive effects, which could involve the activation of neuroplasticity (Bettio et al., 2019; Di Liegro et al., 2019; Giménez-Meseguer et al., 2020; Swenson et al., 2020). Convenience to approach makes PEs an attractive therapeutic means, for instance in the management of depression, dementia, and attention deficit hyperactivity disorder (ADHD). However, a mechanical link between PEs and neuroplasticity is still not fully elucidated. Better understanding of molecular targets of PEs would serve the development of physiologically relevant and efficient pharmacological or genetic therapy.
One of PE’s consequences is an increase in autophagy both in peripheral organs and tissues and in the brain (Jang, 2020). Autophagy removes and helps to recycle unused macromolecules and organelles. In neurons, this fundamental function of autophagy is essential for the subcellular structural makeup required for neuroplasticity and synaptogenesis (Nikoletopoulou et al., 2017; Liang and Sigrist, 2018). Another important hallmark of PEs is the release of myokines (Jin et al., 2018). The myokine irisin initially garnered attention for its paracrine signaling leading to white to brown fat cell transformation and, upon its chronic action, to adaptation of muscle and bone tissue to increased physical load (Li H. et al., 2019; Waseem et al., 2022). However, effects of exogenous irisin (Cheng et al., 2021) or irisin gene knockdown (Islam et al., 2021) on animals’ behavior indicate that endogenous irisin stands behind activation of the muscle-brain axis and act as signaling molecule in the brain.
In the present paper, we would like to discuss the mechanism standing behind the beneficiary effects of activation of muscle-brain axis during physical activity in the frame of contemporary approaches of ADHD management and speculate if irisin signaling could be a critical component in the cross-talk between PE-induced autophagy and neuroplasticity.
2. The link between neuroplasticity and autophagy
2.1. Neuroplasticity phenomenon
Neuroplasticity is an ability of neuronal tissue to undergo functionally relevant structural and morphological changes in response to various kinds of environmental and endogenous stimuli (Gu and Kanai, 2014; Voss et al., 2017; Mateos-Aparicio and Rodríguez-Moreno, 2019). Basically, neuroplasticity mechanisms fortify functional connectivity between neurons supporting neuronal wiring. On the electrophysiological level, the phenomena of neuroplasticity can be seen as a long-term potentiation and long-term depression of neuronal activity; molecular events include changes in gene expression, post-translational modification of proteins, and changes in the activities and traffic of membrane receptors and proteins involved in signal transduction (Chen et al., 2021; Evans et al., 2021; Lee and Fields, 2021; Lim et al., 2022).
Neuroplasticity is essential for all forms of development and learning, and, therefore, in general, its high level is an adaptive feature of the brain (Compte et al., 2000; Spaak et al., 2017). In the majority of neuropsychiatric disorders, the decrease in neuroplasticity and synaptogenesis in the forebrain is often observed with no explicit nosological specificity (Goto et al., 2010; Bernardinelli et al., 2014; Vyas et al., 2016). In turn, an increase in neuroplasticity is regarded as an indicator of successful therapy across a variety of psychiatric disorders. Facilitation or strong maintenance of neuroplasticity, resulting from pharmacological interventions, is associated with an improvement of cognitive functions and top-down control of emotions (Kraus et al., 2017; Kugathasan et al., 2017). However, in a certain neurobiological context, for instance, during fear learning or development of drug dependence, neuroplasticity facilitation is maladaptive (Grimm et al., 2003; Lu et al., 2004). The region-specific increase in neuroplasticity occurs in the basal ganglia upon chronic stress and in depression (Mitra et al., 2005; Friedel et al., 2009).
Changes in neuronal wiring normally imply building up new synapses, however, they may occur without strong changes in neuronal morphology (Bhatt et al., 2009). Both ultrastructural changes and de novo synaptogenesis are supported by protein synthesis, reorganization of cellular milieu, and degradation of cellular components (Daskalaki et al., 2022; Kuijpers, 2022). As such, neuroplasticity implies a fine balance of ana- and cataplasticity that requires recycling and reutilization of macromolecules (Liang and Sigrist, 2018; Nikoletopoulou and Tavernarakis, 2018; Liang, 2019).
2.2. Autophagy as a major instrument of structural reorganization of cell
Autophagy is one of the most powerful mechanisms for maintaining protein homeostasis in the cell (He and Klionsky, 2009; Ohsumi, 2014; Anding and Baehrecke, 2017). It is a universal evolutionary conserved function, which is observed at every level of biological object complexity, from the single-cell to high-level organisms (Mizushima et al., 2004; Maday and Holzbaur, 2014; Hibshman et al., 2018). There are three types of autophagy: macroautophagy, microautophagy, and chaperone-mediated autophagy. Usually, the term autophagy refers to macroautophagy (Mijaljica et al., 2011; Kaushik and Cuervo, 2012).
Autophagy is a compartment-specific cellular milieu quality control mechanism to degrade and remove damaged organelles, misfolded proteins, or protein aggregates in a selective manner and reutilize the macromolecules after their decomposition (Mizushima and Klionsky, 2007; Naiki and Nagai, 2009; Mizushima and Komatsu, 2011; Wei et al., 2015). Thus, autophagy is protecting a cell from distress, such as starvation or chemical stress, and serving its survival during a period of restricted living conditions (Dikic, 2017; Bar-Yosef et al., 2019).
Autophagy is regulated by plenty of evolutionarily conserved autophagy related genes (atg) and respective regulatory proteins, for instance Unc-51 like autophagy activating kinase (ULK1) (homologue ATG1 in yeast) and Beclin1 (homologue ATG6 in yeast; He and Klionsky, 2009; Nakatogawa et al., 2009). These proteins are acting at the very early stages of proautophagosome formation and their inhibition prevents macroautophagy-dependent autophagy flux in the cell. Autophagy can also be controlled by many upstream signals in neurons. The mammalian target of rapamycin complex 1 (mTORC1) is a key suppressor of autophagy (Fukumoto et al., 2019). It supports a link between autophagy and a number of crucial intracellular signaling cascades, such as metabotropic receptor, grow factor receptor, glucocorticoid receptor signaling, as well as carbohydrate metabolism and differentiation (LiCausi and Hartman, 2018). Autophagy flux implies the formation of pro-autophagosomes, sequestration of cytoplasmic materials (autophagosome cargo) into double-membraned autophagosomes, their fusion with lysosomes or late endosomes, and degradation of cargos. The last that can be proved by a fact of sequestosome-1 degradation (also known as the ubiquitin-binding protein p62) (Mizushima and Komatsu, 2011). Cargo specificity is conferred by autophagic cargo receptors that recognize specific targets for degradation, as well as by microtubule-associated protein 1A/1B-light chain 3 (LC3), a protein that is anchored within the autophagosomal membrane (He and Klionsky, 2009). Autophagosomes are docked to cellular membranes with subsequent devastation and release of cargo into the extracellular space (Figure 1). Autophagy also coordinates with other systems of vesicular and molecular transport in the cell and with other fundamental mechanisms like apoptosis, often opposing the last one (Zhao Y. et al., 2022).
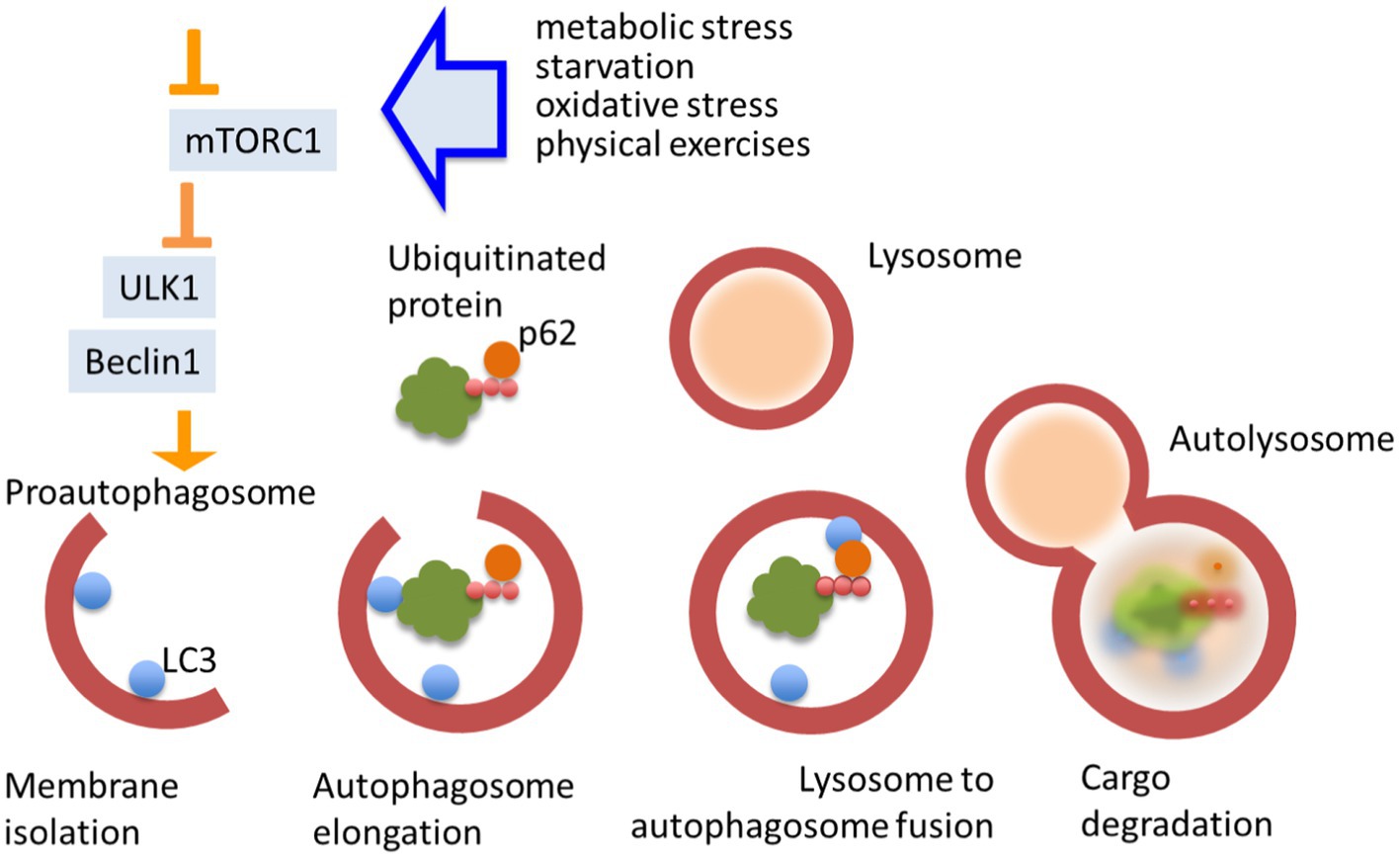
Figure 1. Autophagy flux. Autophagy is a pivotal mechanism of cellular homeostasis. It is triggered by chemical stress and its flux is regulated by multiple cellular signaling pathways. Autophagy strongly depends on the activity of mTORC1. Unc-51 like autophagy activating kinase (ULK1) and Becline1 are essential initiators of autophagosome formation. Cargo specificity is conferred by autophagic receptors that recognize specific target (proteins and other macromolecules), linked to p62, for degradation and microtubule-associated protein 1A/1B-light chain 3 LC3. Autolysosomes are docked to cellular membranes with subsequent devastation and release of cargo.
Overall, autophagy appears as a fundamental feature for providing a cell its ability to structurally reorganize and adapt.
2.3. Interlacement of autophagy with neuroplasticity
In neurons, autophagy influences core neuroplasticity mechanisms, both on presynaptic and postsynaptic levels. Autophagy may control vesicular release of neurotransmitters: activation of autophagy in atg7-KO mice resulted in an elevation of the evoked release of dopamine from slices of dorsal striatum (Hernandez et al., 2012). This effect may be mediated by rab26 adaptor proteins expressed on the synaptic vesicles (Binotti et al., 2015). The impairment of glutamate and gamma-aminobutyric acid (GABA)-mediated long-term plasticity in the neocortex and hippocampus was shown in mice deficient in Beclin1, the protein which is essential to initiate autophagosome formation. The changes in baseline synaptic transmission can be attributed to impairment in GABA and α-amino-3-hydroxy-5-methyl-4-isoxazolepropionic acid (AMPA) receptor trafficking and recycling due to a decrease in autophagy flux (Lalo et al., 2022). The deficits in synaptic plasticity [decrease in long-term depression in pyramidal neurons of Cornu Ammonis 1 (CA1) area of hippocampus] were observed under pharmacological inhibition of autophagosome formation or in conditions of post-translational suppression of atg5 expression (Kallergi et al., 2022). Interestingly, activation of autophagy may be more effective in changing the neuroplasticity phenomena at conditions of pathological cellular endophenotypes related to accumulation of pathoproteins in APP/PS1 model of Alzheimer’s disease, such as cellular senescence (Wang et al., 2021).
Autophagy contributes to neuroplasticity via engagement of the mechanism controlling the efficiency of brain-derived neurotrophic factor (BDNF) signaling. Autophagy governs intracellular trafficking of BDNF in neurons (Kononenko et al., 2017) and may be involved in BDNF expression in microglia (Tan et al., 2018). Additional mechanisms of autophagy-regulated neuroplasticity include the regulation of extracellular levels BDNF (Martinelli et al., 2021) and other signaling proteins (Zhang et al., 2015; Kimura et al., 2017).
Autophagy activation may evoke changes in the expression of BDNF due to the concurring needs in intracellular reorganization (Xu et al., 2020). In turn, an increase in BDNF expression leads to tyrosine kinase B (TrkB)-mediated increase in the activity of mTORC1, one of the key autophagy regulatory kinases (Kim and Guan, 2015).
The type of autophagy and the time-window of its engagement may be the critical factors determining the outcome of its activation. In certain contexts, a decrease in autophagy appears as a prerequisite for cognitive function improvement (Nikoletopoulou et al., 2017; Song et al., 2017; Tian et al., 2020). Nikoletopoulou et al. showed that BDNF signaling via the TrkB and the phosphatidylinositol-3′ kinase (PI3K)/Akt pathway suppresses autophagy in vivo in the mPFC and hypothalamus. The suppression of lytic autophagy was required for structural reorganization of synapses (increase of synaptogenesis markers PSD-95, PICK1, and SHANK3 levels) and for memory enhancement under conditions of nutritional stress (Nikoletopoulou et al., 2017). The decrease in lytic autophagy is not necessarily associated with a decrease in secretory autophagy and this uncoupling may occur, for instance under stress conditions or an increase in glucocorticoid receptor signaling (Martinelli et al., 2021). As a releasing mechanism, secretory autophagy acts as an important regulator of intercellular signaling. Lysosome fusion uncoupling appears as a way to regulate the quality of released substance and facilitate the unconventional secretion of proteins and peptides (Ponpuak et al., 2015; Kimura et al., 2017; Urano et al., 2018). Our study showed that activation of autophagy is required for release of cathepsins and accumulation of BDNF in the mouse brain upon stress signaling activation (Anderzhanova et al., 2020; Martinelli et al., 2021).
To sum, the accumulated experimental data indicate that autophagy, due to a strong ability to govern effective concentration of functionally active proteins inside and outside of a cell, effectively modulates neuroplasticity in neurons.
Because of the strong interaction between autophagy and neuroplasticity, it is not surprising that changes in autophagy emerged as a pathogenetic mechanism of neurological (first of all neurodegenerative) and some psychiatric disorders (Tang et al., 2021; Filippone et al., 2022; Sainani et al., 2022). In turn, facilitation of autophagy is more and more recognized and appreciated as an end effector of therapeutic means with pro-cognitive activity (Tomoda et al., 2020). One of the diseases there activation of autophagy may be a core of therapeutic intervention is ADHD (Hess et al., 2021).
2.4. Autophagy as a target mechanism of pro-cognitive treatment of ADHD
Physical activity and caloric restriction are two major factors leading to the activation of autophagy in organisms (He et al., 2012; Bareja et al., 2019; Chung and Chung, 2019; Escobar et al., 2019; Kou et al., 2019). The exercise-evoked regulation of autophagy includes both increased autophagy flux as well as an increase in expression of important autophagy genes potentially resulting in enhanced autophagy capacity. Remarkable, the increase in autophagy due to PEs is not specific to the periphery and can be seen in brain tissues as well (Jang, 2020).
In addition, calorie restriction and PEs are both associated with an improvement in cognitive performance in animals and humans (Witte et al., 2009; Yang et al., 2014; Törpel et al., 2018; Hugenschmidt et al., 2019; Yegla and Foster, 2019; Grigolon et al., 2020; Horowitz et al., 2020; Wilke, 2020). PEs are specifically proposed as a therapeutic means to treat ADHD (Corona, 2018; Vysniauske et al., 2020). The involvement of autophagy-related mechanisms in the realization of the effects of PEs is deducted from the effect of pharmacologically active compounds with more specific targets. For instance, modulation of autophagy is a component of the pharmacological activity of Rg2 compound, an active substance of ginseng, a plant with well-known adaptogenic action. Rg2 is a steroid glycoside that activates autophagy in an AMPK-ULK1-dependent and mTORC1-independent manner. Most notable of all benefits of Rg2 is a clinical improvement of ADHD symptoms (Niederhofer, 2009; Ko et al., 2014). Induction of autophagy by Rg2 has other benefits such as improvement of cognitive behaviors in mouse models of Alzheimer’s disease and prevention of high-fat diet-induced insulin resistance (Fan et al., 2016; Chung et al., 2018). Besides Rg2, Rg1 also shows autophagy-dependent anti-apoptotic effects via engagement of the AMPK/mTORC1 pathway (Yang et al., 2018), as well as Rd, which has anti-apoptotic and mitophagy inducing functions (Liu et al., 2015, 2018; González-Burgos et al., 2017). Another component in the autophagy induction pathway is the Akt/protein kinase B signaling pathway, more specifically, its downstream segment, the Akt-GSK3β interaction. Pharmacologically- and genetically-evoked inhibition of these kinases has been shown to facilitate autophagy (Roe and Ren, 2011; Qi et al., 2012; Zhang et al., 2019b). As we have shown earlier, the Akt-GSK3β pathway in the medial prefrontal cortex turn up as a specific target of the paradoxical calming activity of amphetamine in LAB mice, which were validated as a model of ADHD (Yen et al., 2013). This suggests that amphetamine, in the case of its paradoxical calming activity, might have the capability to facilitate autophagy specifically at conditions leading to an ADHD-like behavioral endophenotype (Ren et al., 2016; Mancinelli et al., 2017).
3. Physical exercises as an alternative to the "easy come-easy go" psychostimulant treatment of ADHD
3.1. Pros and cons for conventional treatment of ADHD with psychostimulants
Attention deficit hyperactivity disorder is a highly prevalent multifaceted neurodevelopmental disorder that affects around 5% of children and 2.5% of adults worldwide (Faraone et al., 2015). The insufficient control over executive cognitive functions and vigilance (Pievsky and McGrath, 2018; Agha et al., 2020; Krieger and Amador-Campos, 2021) is translated into inattention, impulsivity, and hyperactivity, the major behavioral ADHD endophenotype. Genetic polymorphism studies suggest the involvement of changes in glutamatergic transmission, expression of dopamine D4 receptors, and dopamine transporters in the pathophysiology of ADHD (Hawi et al., 2015; Naaijen et al., 2017; Bauer et al., 2018; Faraone and Larsson, 2019; Hai et al., 2020).
To date, the ADHD treatment implies the use of psychostimulants methylphenidate and amphetamines (Arnold et al., 1972). These drugs evoke a temporal paradoxical calming effect, thereby allowing one to achieve an appropriate control over executive function, leading to (i) adaptive behavior or (ii) increasing the possibility for appropriate cognitive activity by way of improvement in attention, goal-oriented behavior, and working memory and (iii) increased top-down control over limbic brain. However, the mechanism of paradoxical effect of psychostimulants is barely well understood (Zhuang et al., 2001; Zang et al., 2005; Yen et al., 2013, 2015; Cortese et al., 2018; Miller et al., 2018; Endres et al., 2019; Huang et al., 2019). As believed, the drugs indirectly modulate activity of dopaminergic neurons with respective decrease in hypervigilance and reinforcement of reward circuit (Yildiz et al., 2011; Ziereis and Jansen, 2015; Albertson et al., 2016; Peña and Shi, 2016; Cortese et al., 2018; MacQueen et al., 2018). A long-term treatment with psychostimulant in ADHD patients leads to improvement in neurocognitive performance (Tsai et al., 2013), improved cognitive training-induced morphological plasticity and neuroplasticity, and to volumetric increase or decrease in reduction of the cortex, right caudate nucleus, and cerebellum (Hoekzema et al., 2011; Frodl and Skokauskas, 2012; Spencer et al., 2013; Rubia, 2018). Due to a relatively short half-life, most psychostimulants, also with novel formulations with slow release (Adler et al., 2017), have to be taken once or twice daily and require—at least in adulthood—a long-term treatment. Despite efficiency, the use of psychostimulants continues to raise concerns due to side effects: reduced appetite, insomnia, headache, and irritability (Schep et al., 2010; Lakhan and Kirchgessner, 2012; Kis et al., 2020), neurotoxic effects (Anderzhanova et al., 2002; Steinkellner et al., 2011), as well as tolerance and dependence (Fleckenstein et al., 2009; Merkel and Kuchibhatla, 2009; Lakhan and Kirchgessner, 2012).
3.2. Physical exercises are pro-neuroplastic and procognitive in ADHD
Besides beneficiary effects related to the increase in cardiac output and metabolic rate, PEs gain more attention as a supplementary or even standalone treatment in ADHD management aiming at the facilitation of neurocognitive performance (Corona, 2018; Christiansen et al., 2019; Rassovsky and Alfassi, 2019; Mehren et al., 2020; Vysniauske et al., 2020). The onset of PEs action toward moderation of the core symptoms of ADHD, inattention and hypervigilance, appears to be immediate. Importantly, PEs have essentially no side effects (Lenz, 2012). When compared to amphetamines, which lead to improvements in defined symptoms of ADHD, PEs have, in addition to symptomatic improvements, further reaching more complex positive effects on mental, physical, and emotional wellbeing. Table 1 summaries the essential features of conventional psychostimulant and PE treatment of ADHD.
Physical exercises with intensities between 40 and 75% of maximal capacity are thought to have beneficial effects on memory, cognitive function, and neuroprotection (Dishman et al., 2006; Cotman et al., 2007; Mueller, 2007; Draganski and May, 2008). An increasing body of evidence supports that PEs induce remarkable functional and neuroanatomical plasticity in the mature brain, such as neurogenesis, angiogenesis, synaptic plasticity, and dendritic morphological remodeling (Bherer, 2015; Cassilhas et al., 2016; Phillips, 2017; Suarez-Manzano et al., 2018; Rassovsky and Alfassi, 2019). The hippocampus is one of the brain structures with the highest level of neuroplasticity (Bartsch and Wulff, 2015) and is also an area of neurogenesis in the sub-granular zone of the dentate gyrus. There are hypervolemic changes in the hippocampus (and also in amygdala) in kids with ADHD (Plessen et al., 2006). In addition, at least in the animal model of ADHD (spontaneously hypertensive rats), there is misbalanced glutamatergic signaling influencing norepinephrine release in the hippocampus (Howells and Russell, 2008; Shindo et al., 2022). Experimental studies in mice have demonstrated that PEs induce increases in volume and blood flow in the hippocampus (Firth et al., 2018; Tarkka et al., 2019; Broadhouse et al., 2020) and cortex (Chen et al., 2020). Interestingly, the hippocampus-specific effect may appear particularly as a consequence of moderate-to-vigorous physical activity (the level of which was proved by results of 7-day accelerometry), but not of cardiorespiratory fitness (Raichlen et al., 2019). Preclinical studies show that the effects of PEs on the brain are rather specific and attributable to changes in hippocampus function (Firth et al., 2018; Tarkka et al., 2019; Broadhouse et al., 2020). It is well known that the hippocampus is the gateway for the information that will be lately stored in cortex (Kol et al., 2020). Hippocampal neuroplasticity is an important factor of memory, navigation, and situational anxiety, which makes this brain region one of the most essential structures involved in the response to environmental changes (Morris et al., 2003; Bannerman et al., 2014). Therefore, the documented changes in the hippocampus after PEs also prove the beneficiary effect of PEs in ADHD.
Numerous studies associate the beneficiary effects of PEs with the upregulation of BDNF, an essential component of neuroplasticity (Kim et al., 2020; Müller et al., 2020; Nicolini et al., 2020; Park et al., 2020; Sugiyama et al., 2020), and with the transient increase in the availability of BDNF in the brain (Knaepen et al., 2010; Quan et al., 2020; Walsh et al., 2020). Since the mechanisms of PEs’ profitable effects may be similar to that of antidepressants (Björkholm and Monteggia, 2016; Castrén and Kojima, 2017), it is not surprising that PEs are beneficial in the improvement of regulation of emotional status (Zhang et al., 2019a; Liu et al., 2022). In addition to altering synapse wiring, PEs can induce neuroplasticity by acting on auxiliary functions that can lead to neuroplasticity, such as glial activation and angiogenesis (Morland et al., 2017; Li et al., 2021). Another hallmark of PEs is activation signaling along the muscle-brain axis due to increase in release of myokines (Jin et al., 2018).
Since the therapeutic efficacy of PEs is rather moderate, PEs are mostly suggested as a supplementary treatment (Seiffer et al., 2022). At the same time, action of PEs appears as an experimental paradigm to study interlacement between autophagy and neuroplasticity in the context of a search for pro-cognitive therapy. Elucidation of the mechanical link between PEs, autophagy, and neuroplasticity would serve to a hunt and development of more specific therapeutic approaches (Pedersen, 2019).
4. Irisin is linking PEs to neuroplasticity and autophagy in the brain
4.1. Irisin as a PEs proxy
Regular moderate-to-vigorous PEs are accompanied by changes across the whole body including cardiovascular adaptation and metabolic changes that definitely convey their beneficiary effects (Eijsvogels et al., 2016; Tofas et al., 2019; Larsen et al., 2020). Effects of PEs in the brain may also be directly attributed to muscle activation. Polypeptide myokines (cathepsin B, IL-6, decorin, BDNF, and irisin), which are released from myocytes upon their contractile activity, provide paracrine and endocrine signaling and mediate many changes across the body, including the brain (Kirk et al., 2020).
The myokine irisin is released by cleavage of membrane fibronectin type III domain-containing protein 5 (FNDC5). The expression of FNDC5/irisin in the body is rather ubiquitous, including the brain (Dun et al., 2013), and appears under the regulation of peroxisome proliferator-activated receptor-γ coactivator 1α (PGC-1α; Lourenco et al., 2019). Expression of PGC-1α, which was initially discovered as a coactivator of mitochondrial biogenesis (Goffart and Wiesner, 2003), is upregulated by the specific activity of myocytes in skeletal muscle (Figure 2).
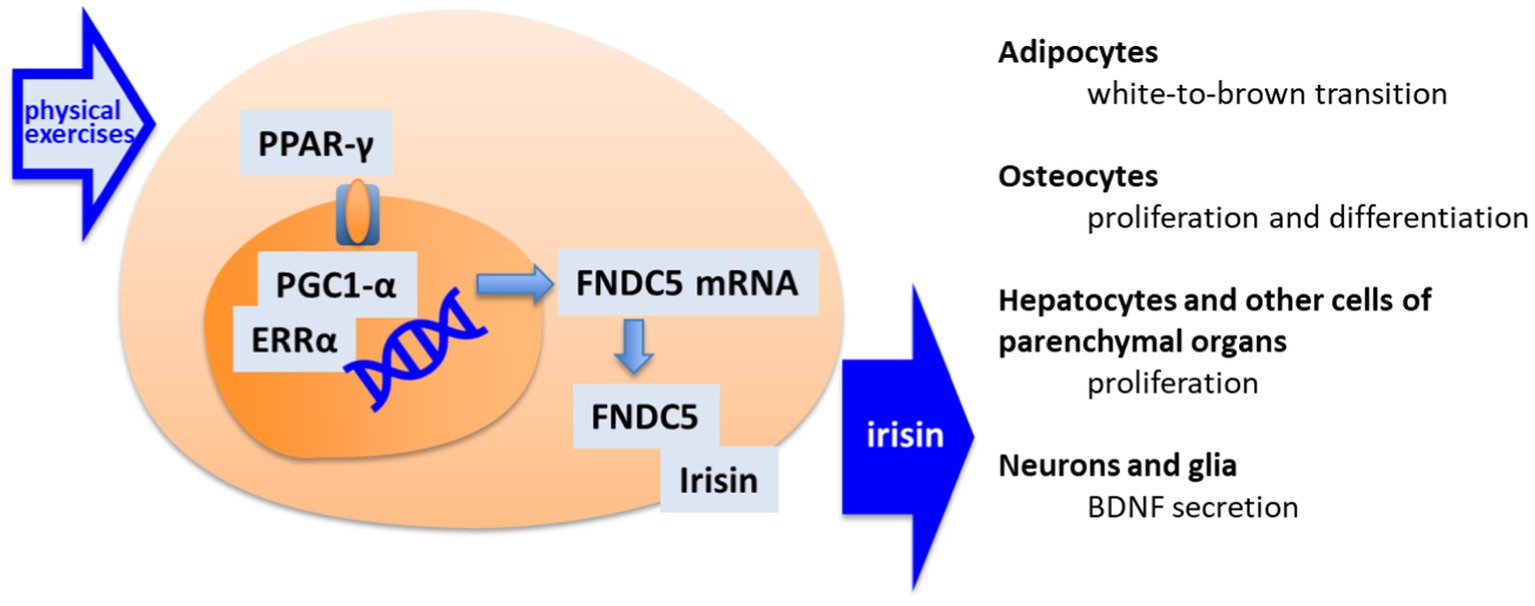
Figure 2. The pathway of irisin synthesis and its main cellular targets. By muscle activation, the peroxisome proliferator-activated receptor gamma (PPAR-γ) activates the PPAR receptors by giving rise to peroxisome proliferator-activated receptor gamma coactivator 1-alpha—estrogen-related receptor alpha (PGC1-α/ERRα) transcription factors for fibronectin type III domain-containing protein 5 (FNDC5) transcription. FNDC5 appears as precursor of irisin. Released Irisin accesses different organs, including brain, where it acts as an indirect stimulator of neuroplasticity.
Irisin initially garnered particular attention for its paracrine signaling leading to white to brown fat cell transformation (Li H. et al., 2019; Waseem et al., 2022). The brown adipocytes are better serving for the metabolic challenge during physical activity by sourcing carbohydrates to provide energy to cells (Cornish et al., 2020; Kirk et al., 2020; Vidal and Stanford, 2020). Irisin and other myokines are found in central circulation in human (Di Liegro et al., 2019). The endocrine effects of irisin allows relatively slow changes in organism and synchronization of changes in the liver, pancreas, gut, and kidney, which support metabolic and detoxification needs in response to physical load (Figure 1).
We would like to highlight two mechanisms, where irisin’s peripheral action appears in relation to activation of body–brain crosstalk. It is known that, PEs are able to restore the liver homeostasis having a therapeutic effect in models of NAFLD (Seo et al., 2021; De Nardo et al., 2022). Irisin may mediate these effects supporting appropriate lipogenesis in hepatocytes, as have been shown in in vitro experiment with application of exogenous irisin. Changes in lipid metabolism are achieved by increase in the expression lipid-reguating enzymes LXRα and SREBP-1 and the suppression of the pathological accumulation of lipids in hepatocytes. Irisn also downregulates oxidative stress in hepatocytes as was proved by the decrease in the expression of oxidative stress markers, NFκB, COX-2, p38 MAPK, TNF, and IL-6 (Park et al., 2015). Irisin also decreases expression of enzymes involved in regulation of inflammatory response in the liver (Xu et al., 2022). Appropriate biochemical landscape in hepatocyte is a prerequisite of normal synthesis and release of hepatokines (Jensen-Cody and Potthoff, 2021; Kim et al., 2021), which, in turn, may also positively influence brain (Cooper et al., 2018; Kiyohara et al., 2021; Zhao P. et al., 2022). Another example is the positive effect of irisin on the cardiac muscle. As had been shown, irisin has effect on expression of gene with cardioprotective functions (Alzoughool et al., 2022), improves heart conditions in a model of aging in mice (Hu et al., 2022), and works as cardiomyocyte protector both in vitro and in vivo (Cao et al., 2022). An overall expected therapeutic effect is stabilization of heart contractile activity and an adequate regulation of cardiac output. In turn, the stable system hemodynamic, which depends on heart activity, is essential to maintain all the brain functions, including supplementation of neuroplastic processes.
The proof of the relevance of irisin plasma levels to the effects of PE was particularly challenging, due to a lack of specific antibodies (Albrecht et al., 2015). However, recent studies using either LC–MS measurements of irisin in plasma (Jedrychowski et al., 2015) or applying extended validation of western blot measurements of plasma irisin (Löffler et al., 2015) proved the presence of free irisin in human blood and confirmed the positive correlation between PEs and circulating irisin in human (Tsuchiya et al., 2014; Jedrychowski et al., 2015; Ruan et al., 2018; Tari et al., 2019; Qiu et al., 2020). Preclinical studies also show the coincidence of physical activity and elevation in the plasma irisin levels in healthy subjects (Murao et al., 2019). Permanent PEs accomplished as daily swimming restore the impaired levels of FNDC5/irisin in blood in the animal’s models of Alzheimer’s disease (Lourenco et al., 2019). However, there were some concerns (Albrecht et al., 2015) on the reliability of irisin as an indicator or/and mediator of the effects of PEs because of reports of reductions of irisin plasma levels in association with PEs. For instance, an acute PE resulted in an elevation of plasma irisin, while 12-week training was associated with a decrease in plasma irisin (Norheim et al., 2014).
4.2. Brain-specific effects of irisin and its molecular targets in the brain
The brain emerges as an important target of irisin. It seems feasible that irisin is implemented in the realization of the mechanical link between exercises and changes in the neuronal cells leading, in analogy to peripheral effects, to adaptive adjustment of lipid metabolism, cellular excitation, and antioxidant systems in neurons and glia (Stranahan et al., 2010). Effects of exogenous irisin (Cheng et al., 2021) or irisin gene knockdown (Islam et al., 2021) on animals’ behavior indicate that it also provides a functionally relevant signaling in the brain.
Irisin is found in cerebrospinal fluid (CSF) in both humans and rats (Piya et al., 2014; Ruan et al., 2019). Irisin is a glycosylated protein, and, therefore, irisin released from myocytes may penetrate the blood–brain barrier (BBB; Otvos and Wade, 2014; Islam et al., 2021) and contribute to the brain/CSF pool of irisin. However, the neuronal tissue is another source of irisin. In the brain of mice, FNSC5/irisin expression is highest in the regions related to the regulation of locomotor activity, like the cerebellum, caudate, and putamen. It is also observed in the olfactory bulbs, ventral thalamus, medial vestibular nucleus, and CA1 region of the hippocampus (Dun et al., 2013; BrainStars, 2020). Interestingly, CSF and plasma irisin levels, each independently correlate with age, Alzheimer’s disease, and obesity (Lourenco et al., 2019; Ruan et al., 2019, 2020). These data suggest that FNDC5/irisin expression in the brain and peripheral organs are regulated separately, and the sizes of peripheral and central pools of irisin could be considered as independent measures.
The factors that influence the availability of brain-derived soluble irisin in the brain are generally not known. Experimental studies are showing interlacement of mechanisms regulating the FNDC5/irisin system and controlling behavioral responses. Chronic unpredictable stress leading to increased immobility correlates with the decrease in FNDC5/irisin expression in the brain (Wang and Pan, 2016). This observation suggests the involvement of glucocorticoid receptors in the regulation of FNDC5/irisin expression (Delezie and Handschin, 2018). Also, BDNF, which is an essential neurotrophin associated with neuroplasticity, can regulate FNDC5 levels by activation of negative feedback and, therefore, can reduce the bdnf gene’s expression (Wrann et al., 2013). The antidepressant effect of PEs was accompanied by an increase in neuron proliferation, differentiation, and survival and associated with an increase in the number of FNDC5-positive cells in the hippocampus (Siteneski et al., 2020).
The significance of irisin signaling for brain functions is supported by experimental studies. FNDC5/irisin expression is required to maintain the normal transcriptome of newborn neurons in the hippocampus. The genes with abnormal transcription were annotated to clusters associated with stem cell and neuron differentiation, neurotrophic and insulin signaling pathways, dendrite development, as well as neurodegenerative disorders (Islam et al., 2021). Irisin microinjected in the dental gyrus of the hippocampus of Wistar rats induced long-term potentiation and facilitated lipid peroxidation. Downregulation of FNDC5/irisin by shRNA impaired synaptic plasticity and memory in mice. This phenomenon corresponded to a decrease in field ePSPs in in vitro preparations from the brains (Mohammadi et al., 2019). As had been shown recently, the intravenous administration of irisin in spontaneously hypertensive rats, a model of ADHD (Botanas et al., 2016), resulted in normalization of blood pressure (Huo et al., 2020) that, together with changes in the paraventricular nucleus, suggest changes in ACHT-vasopressin system. The bilateral intra-hippocampal administration of irisin diminished the negative consequences of restraint stress in mice (Jodeiri Farshbaf et al., 2020). Notably, in this study, irisin showed gender-specific effects counteracting both neurobehavioral disturbances, like anxiety and memory impairment, and changes in vegetative system regulation in males, while only preventing the reduction in body weight in females (Jodeiri Farshbaf et al., 2020). Overexpression of irisin in the hippocampus (local injection of AAV8-irisin-FLAG) improved context-dependent memory in the paradigm of Pavlovian fear conditioning (Islam et al., 2021). Wang and Pan showed, that subcutaneous administration of irisin showed a dose-dependent improvement of behavior in a model of chronic unpredictable stress counteracting the depression-like endophenotype (Wang and Pan, 2016).
Post-translational modification of FNDC5 expression with siRNA resulted in a decrease of irisin but also in a decrease in the level of UCP2 (uncoupled protein 2 serving as inner mitochondrial membrane transporter; Pan et al., 2021), therefore, indicating possible changes in production of reactive oxygen species. Exogenous irisin showed a neuroprotective effect in models of neurodegenerative disorders (Lourenco et al., 2019; Zarbakhsh et al., 2019). Local administration of recombinant irisin, as well as expression of FNDC5-containing adenovirus vector in AβO-treated mice, restored memory in the mouse model of Alzheimer’s disease. Both positive effects corresponded to the normalization of diminished field ePSPs (Lourenco et al., 2019) in the experimental mice. An elegant approach to boost the pool in irisin in the periphery used an AAV vector-associated overexpression of FNDC5/irisin in the liver. The resulting increase in the availability of peripheral irisin improved the cognitive performance of mice in two transgenic models of Alzheimer’s disease (APP/PS1 and 5xFAD), which was also accompanied with reduced glia activation (Islam et al., 2021).
A study by Boström’s group showed an interaction between FNDC5 and BDNF systems, providing evidence for the influence of endurance PEs on PGC-1α/FNDC5-dependent expression of BDNF both in the peripheral organs and in the hippocampus of mice (Wrann et al., 2013). It is worth mentioning that the increase in FNDC5—BDNF system activity in the periphery is barely adding to the changes in brain BDNF immunoreactivity, because BDNF practically does not penetrate the BBB under healthy physiological conditions (Hernandez et al., 2022). A recent study showed that subcutaneous administration of irisin resulted in an increase of dendrite complexity in the CA1 and CA3 areas of the hippocampus in coincidence with upregulation of mRNA for PGC-1α, FNDC5, and BDNF, therefore, supporting the neurotrophic mechanism of irisin action (Mongrédien et al., 2019).
Results of in vitro experiments suggested that brain activity of irisin may be mediated by glial cells (Wang and Pan, 2016; Lourenco et al., 2019). The discovery of receptors specific to irisin, the integrin family receptor α1β1, and especially, αvβ5 (Kim et al., 2018), strongly confirmed the involvement of astrocytes, since these cells are known to express specifically integrin receptor αvβ5 (Milner et al., 1999). Subcutaneous irisin led to upregulation of mRNA for the astrocyte marker hevin (Mongrédien et al., 2019) and downregulation of tumor growth factor 1 (TGF-β1) mRNA, which would promote neuroplasticity due to respective changes in the release of these astrocyte signaling molecules (Tewari and Majumdar, 2012; Ota et al., 2013; Kim and Leem, 2019). All these remarkable discoveries give a new impulse to study the effects of irisin in the brain using glia as a model of irisin action.
However, despite the recent promising finding showing both the existence of integrin receptor αvβ5 for irisin and its effect on glial and neuronal activity and behavior, a mechanistic link between an increase in irisin content in brain and changes in brain function is still missing.
5. Autophagy as targets of irisin
Until present, the data on the molecular interaction underlying effect of irisin on autophagy are still very scattered. Analysis of the most validated intracellular targets of irisin reveals a few molecular nodes, at which the increase in irisin signaling influences autophagy. Experimental data prove such an interaction in cells of a non-neuronal lineage. Nonetheless, the respective intracellular pathways are also of a high importance in all cells and their engagement might predict the effect of irisin on autophagy in neurons and glia (Figure 3). Despite the involvement of established negative regulators, the available literature mainly reports an autophagy-activating effect of irisin, implying the existence of more active positive regulators such as mitogen-activated protein kinases (MAPKs). In astrocytes, an engagement of secondary messenger systems could be mediated by integrin receptor αvβ5. As had been shown, αvβ5 are involved in control of atg7-dependent autophagy in pulmonary endothelial cells (Zhang et al., 2019b).
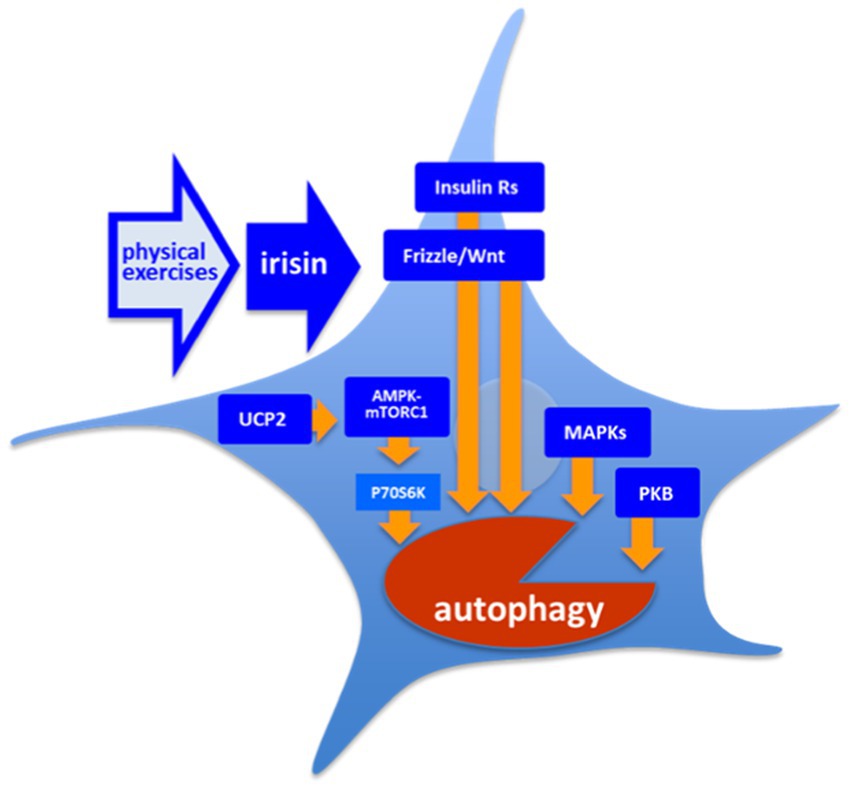
Figure 3. Molecular targets of irisin. There is a number of the intracellular cascades are shown to respond to irisin both in vitro and in vivo: However, the exact mechanism providing a coupling of free irisin and its intracellular targets is still unknown. AMPK, 5’ AMP-activated protein kinase; Frizzle/Wnt, key proteins of respective pathway; Insulin Rs, Trk insulin Receptors; mTORC1, mammalian target of rapamycin complex 1; PKB, protein kinase B (Akt); and UCP2, uncoupled protein 2.
Irisin leads to an increase in the activity of protein kinase B (PKB or Akt) in cardiomyoblasts, H9c2 cells, and in endothelial cells and, respectively, to an increase in activity of its downstream mTORC1 and supression of autophagy (Yang et al., 2013; Xie et al., 2015; Fu et al., 2016; Gao et al., 2021; Zajicek et al., 2022). Song et al. showed that exogenous irisin treatment (50–200 ng/ml, 24–48 h) decreased autophagy in H9c2 cells as it was seen by the decrease in LC3II/LC3I ratio. However, the authors showed that this negative effect depends in part on the PI3K/Akt signaling pathway (Song et al., 2021). As had been shown in cultured INS-1 cells, irisin activated 5’ AMP-activated protein kinase (AMPK)-mTORC1 cascade increasing survival of insulin producing cell (Lee et al., 2015; Li et al., 2018; Moon and Park, 2020). It has been shown that besides activation of AMPK-mTOR pathway, irisin led to mTORC1-independent activation of AMPK-ULK1 pathway, with both alleviating cardiac hypertrophy (Li et al., 2018; Yu et al., 2019). AMPK is also upregulated by uncoupled protein 2 (UCP2; Mao et al., 2021), which was shown to be decreased in absence of irisin in endothelial cells (Pan et al., 2021). Another pathway, which was highlighted with respect to irisin activity, is the AMPK/SIRT1/PGC-1α signaling cascade, however, this pathway was rather considered as an upstream regulator of FNDC5/irisin pathway (Li Q. et al., 2019). In addition, the Frizzle/Wnt pathway is also known to be modulated in presence of irisin, and in turn, to regulate autophagy flux in adipocytes (Petherick et al., 2013; Ma et al., 2019). The MAPKs-dependent pathway contributing to regulation of autophagy is also modulated by irisin (Mazur-Bialy et al., 2017; Li and Halterman, 2021). Worth mentioning, PEs are known to positively modulate Frizzle/Wnt signaling and MAPKs-signaling, as well as insulin signaling (Stranahan et al., 2010), which is, in turn, coupled to phosphatidylinositol 3-phosphate (PI3P)/PKB and MAPK pathways. Irisin (250 μg/kg, single dose) improves autophagy in aged hepatocytes in vivo via increasing telomerase activity. Moreover, the single administration of irisin results in increase in LC3II/LC3I ratio but also in the decrease in p62 expression, which may point to changes in the balance between secretory and lytic autophagy (Bi et al., 2020). Pang et al. showed that exogenous irisin (100 ng/ml, 72 h) leads to an increase in the levels of LC3II and p62, as well as to an increase to LC3II/LC3I ratio in vascular smooth muscle cells in mice. Notably, this effect goes along to the increase in levels of mRNAs of Map1lc3b, Becn1, Atg7, Atg5, and Lamp1, further highlighting epigenomic effects of irisin (Pang et al., 2022). Pan et al. showed that exogenous irisin (20 nM, 48 h) was diminishing the toxic effect of doxorubicin in the culture of endothelial cells. While irisin did not show effects when administered alone, this action was dependent on changes in autophagy flux, since cytoprotective combination of irisin and doxorubicin resulted in the prominent increase in p62 and a very moderate increase in LC3II/LC3I ratio (Pan et al., 2021). Irisin also activated Opa1-induced mitophagy to restore mitochondrial energy metabolism in cardiomyocytes (Xin and Lu, 2020).
The ability of irisin to activate the essential ubiquitous secondary messenger pathways, which are associated with regulation of autophagy, suggests a possibility for irisin to modulate autophagy in neuronal and glial cells.
5.1. Hypothesis: Irisin-evokes neuroplasticity by triggering autophagy
We propose that autophagy activation is a critical component of irisin action in the brain. Modulation of autophagy does not only support unspecific remodeling of cellular content but also evokes neuroplasticity. Our recent studies using FKBP51-KO mice as a model for impaired stress response give an idea of how irisin-stimulated autophagy leads to activation of neuroplasticity cascades, particularly to those required for BDNF signaling.
It is established that the stress-responsive co-chaperone FKBP51 (Binder, 2009; Touma et al., 2011) is required for autophagy-dependent antidepressant activity of amitriptyline and paroxetine (Gassen et al., 2014, 2015). Recently we have shown that FKBP51 expression in mice has a permissive role in acute ketamine antidepressant action. Moreover, FKBP51 expression is critical for the almost immediate increase in the extracellular mature BDNF (mBDNF) content induced by a single dose of ketamine (Anderzhanova et al., 2020) or acute stress (Martinelli et al., 2021). Moreover, the diminution of stress-evoked increase in extracellular mBDNF upon suppression of autophagy with ULK1 inhibitor was coincided with a decrease in release of autophagy cargo cathepsin D and matrix metalloproteinase 9 (MMP9). Analysis of the secretome of cultured astrocytes revealed that atg5-expression-dependent autophagy is critical for the release of MMP9. Furthermore, the in vivo secretome analysis showed that FKBP51-dependent increase in extracellular levels of mBDNF coincided with release of MMP9, but not pro-BDNF. Therefore, our data show that autophagy is a key for maturation of BDNF due to control of MMP9 secretion. As it appears, this mechanism is not constitutively active but is engaged on demand, during acute activation of stress-associated glucocorticoid receptor signaling or during acute action of the antidepressant ketamine.
We believe that irisin acts at astrocyte αvβ5 and, therefore, interferes with autophagy flux, and facilitates secretion of MMP9. At the same time, the activity-dependent release of pro-BDNF supplies the extracellular milieu with the MMP9 substrate. In sum, a stimulation of BDNF signaling becomes feasible in the activated structures, where irisin is expressed or perceived. The proposed scenario is taking into consideration that the overall effect of irisin depends on the pattern of αvβ5 integrin’s’ expression, and therefore its experimental proof requires discrimination of the effect of irisin in neurons and glial cells.
The proposed hypothesis, however, allows one to escape a question on the ultimate requirement of irisin upregulation in the neurons to upregulate BDNF expression since it relays only on an increase in irisin content, regardless of the source of such an increase. Secondly, the assumable mechanism incorporates astrocyte and neuron cross-talk in the implementation of neuroplasticity. Thirdly, the hypothesis integrates autophagy and neuroplasticity into one biologically relevant process of on-demand response of the brain to changes.
6. Conclusion
Active movement and physical activity are emerging not only as a part of a healthy lifestyle but also as an important therapeutic means to improve brain functioning. The mechanisms of PEs therapeutic activity were engaged in human natural history. At early stages of its evolution, Homo sapiens was adapting to nomad life in savannah. The new circumstances required a lot of running, goal-oriented behavior, high ability for spatial navigation, and good memory together with effectuation of coordinated activity in social groups for effective foraging and defense of a tribe as a whole. This adaptation was supported by respective neuroplastic and morphological changes in the brain (Lago et al., 2019; Duckworth et al., 2020), which were stabilized in the genome (Noakes and Spedding, 2012).
Beside supporting an evolutional process in the past, mechanisms which underly effects of PEs in the brain may be a paradigm to look for a novel treatment of psychopathological complications, for instance in ADHD management. In this review, we justified and proposed that PE-related neuroplasticity is mediated in part by irisin-triggered autophagy. Since the recent findings give the objectives to consider autophagy-stimulating therapy as a prerequisite for successful therapy of psychiatric disorders, irisin appears as a prototypic molecule that can activate autophagy with therapeutic goals. There is a decisive evidence gap in the knowledge of the mechanisms of action of irisin in the brain. Future study on a mechanical link between irisin and autophagy would improve our understanding of the mechanisms involved in physiologically relevant therapy of psychiatric disorders.
Author contributions
AA: original draft preparation, reviewing, and editing. MP and AM: writing and reviewing. AP: conceptualization and reviewing. EA: supervision, conceptualization, reviewing, and editing. All authors contributed to the article and approved the submitted version.
Funding
Publication fees funded by Klinik und Poliklinik für Psychiatrie und Psychotherapie Universitätsklinikums Bonn Venusberg-Campus 1 Gebäude 80/82 53127 Bonn to AP.
Conflict of interest
The authors declare that the research was conducted in the absence of any commercial or financial relationships that could be construed as a potential conflict of interest.
Publisher’s note
All claims expressed in this article are solely those of the authors and do not necessarily represent those of their affiliated organizations, or those of the publisher, the editors and the reviewers. Any product that may be evaluated in this article, or claim that may be made by its manufacturer, is not guaranteed or endorsed by the publisher.
References
Adler, L. A., Alperin, S., Leon, T., and Faraone, S. V. (2017). Pharmacokinetic and Pharmacodynamic properties of Lisdexamfetamine in adults with attention-deficit/hyperactivity disorder. J. Child Adolesc. Psychopharmacol. 27, 196–199. doi: 10.1089/cap.2016.0121
Agha, S. S., Zammit, S., Thapar, A., and Langley, K. (2020). Parent psychopathology and neurocognitive functioning in children with ADHD. J. Atten. Disord. 24, 1836–1846. doi: 10.1177/1087054717718262
Albertson, T. E., Chenoweth, J. A., Colby, D. K., and Sutter, M. E. (2016). The changing drug culture: use and misuse of cognition-enhancing drugs. FP Essent. 441, 25–29.
Albrecht, E., Norheim, F., Thiede, B., Holen, T., Ohashi, T., Schering, L., et al. (2015). Irisin—a myth rather than an exercise-inducible myokine. Sci. Rep. 5:8889. doi: 10.1038/srep08889
Alzoughool, F., Al-Zghoul, M. B., Ghanim, B. Y., Gollob, M., Idkaidek, N., and Qinna, N. A. (2022). The role of interventional Irisin on heart molecular physiology. Pharm. Basel Switz. 15:863. doi: 10.3390/ph15070863
Anderzhanova, E., Hafner, K., Genewsky, A. J., Soliman, A., Pöhlmann, M. L., Schmidt, M. V., et al. (2020). The stress susceptibility factor FKBP51 controls S-ketamine-evoked release of mBDNF in the prefrontal cortex of mice. Neurobiol. Stress 13:100239. doi: 10.1016/j.ynstr.2020.100239
Anderzhanova, E., Rayevsky, K. S., Saransaari, P., Riitamaa, E., and Oja, S. S. (2002). Effects of acute toxic doses of psychostimulants on extracellular levels of excitatory amino acids and taurine in rats: comparison of d-amphetamine and sydnocarb. Ann. N. Y. Acad. Sci. 965, 193–203. doi: 10.1111/j.1749-6632.2002.tb04161.x
Anding, A. L., and Baehrecke, E. H. (2017). Cleaning house: selective autophagy of organelles. Dev. Cell 41, 10–22. doi: 10.1016/j.devcel.2017.02.016
Arnold, L. E., Wender, P. H., McCloskey, K., and Snyder, S. H. (1972). Levoamphetamine and dextroamphetamine: comparative efficacy in the hyperkinetic syndrome. Assessment by target symptoms. Arch. Gen. Psychiatry 27, 816–822. doi: 10.1001/archpsyc.1972.01750300078015
Bannerman, D. M., Sprengel, R., Sanderson, D. J., McHugh, S. B., Rawlins, J. N. P., Monyer, H., et al. (2014). Hippocampal synaptic plasticity, spatial memory and anxiety. Nat. Rev. Neurosci. 15, 181–192. doi: 10.1038/nrn3677
Bareja, A., Lee, D. E., and White, J. P. (2019). Maximizing longevity and Healthspan: multiple approaches all converging on autophagy. Front. Cell Dev. Biol. 7:183. doi: 10.3389/fcell.2019.00183
Bartsch, T., and Wulff, P. (2015). The hippocampus in aging and disease: from plasticity to vulnerability. Neuroscience 309, 1–16. doi: 10.1016/j.neuroscience.2015.07.084
Bar-Yosef, T., Damri, O., and Agam, G. (2019). Dual role of autophagy in diseases of the central nervous system. Front. Cell. Neurosci. 13:196. doi: 10.3389/fncel.2019.00196
Bauer, J., Werner, A., Kohl, W., Kugel, H., Shushakova, A., Pedersen, A., et al. (2018). Hyperactivity and impulsivity in adult attention-deficit/hyperactivity disorder is related to glutamatergic dysfunction in the anterior cingulate cortex. world J. biol. psychiatry off. J. World Fed. Soc. Biol. Psychiatry 19, 538–546. doi: 10.1080/15622975.2016.1262060
Bernardinelli, Y., Nikonenko, I., and Muller, D. (2014). Structural plasticity: mechanisms and contribution to developmental psychiatric disorders. Front. Neuroanat. 8:123. doi: 10.3389/fnana.2014.00123
Bettio, L., Thacker, J. S., Hutton, C., and Christie, B. R. (2019). Modulation of synaptic plasticity by exercise. Int. Rev. Neurobiol. 147, 295–322. doi: 10.1016/bs.irn.2019.07.002
Bhatt, D. H., Zhang, S., and Gan, W.-B. (2009). Dendritic spine dynamics. Annu. Rev. Physiol. 71, 261–282. doi: 10.1146/annurev.physiol.010908.163140
Bherer, L. (2015). Cognitive plasticity in older adults: effects of cognitive training and physical exercise. Ann. N. Y. Acad. Sci. 1337, 1–6. doi: 10.1111/nyas.12682
Bi, J., Yang, L., Wang, T., Zhang, J., Li, T., Ren, Y., et al. (2020). Irisin improves autophagy of aged hepatocytes via increasing telomerase activity in liver injury. Oxidative Med. Cell. Longev. 2020, 1–13. doi: 10.1155/2020/6946037
Binder, E. B. (2009). The role of FKBP5, a co-chaperone of the glucocorticoid receptor in the pathogenesis and therapy of affective and anxiety disorders. Psychoneuroendocrinology 34, S186–S195. doi: 10.1016/j.psyneuen.2009.05.021
Binotti, B., Pavlos, N. J., Riedel, D., Wenzel, D., Vorbrüggen, G., Schalk, A. M., et al. (2015). The GTPase Rab 26 links synaptic vesicles to the autophagy pathway. elife 4:e05597. doi: 10.7554/eLife.05597
Björkholm, C., and Monteggia, L. M. (2016). BDNF—a key transducer of antidepressant effects. Neuropharmacology 102, 72–79. doi: 10.1016/j.neuropharm.2015.10.034
Botanas, C. J., Lee, H., de la Peña, J. B., Dela Peña, I. J., Woo, T., Kim, H. J., et al. (2016). Rearing in an enriched environment attenuated hyperactivity and inattention in the spontaneously hypertensive rats, an animal model of attention-deficit hyperactivity disorder. Physiol. Behav. 155, 30–37. doi: 10.1016/j.physbeh.2015.11.035
BrainStars (2020). FNDC5 expression in the brain [WWW document]. Available at: http://brainstars.org/probeset/1453135_at
Broadhouse, K. M., Singh, M. F., Suo, C., Gates, N., Wen, W., Brodaty, H., et al. (2020). Hippocampal plasticity underpins long-term cognitive gains from resistance exercise in MCI. NeuroImage Clin. 25:102182. doi: 10.1016/j.nicl.2020.102182
Cao, G., Yang, C., Jin, Z., Wei, H., Xin, C., Zheng, C., et al. (2022). FNDC5/irisin reduces ferroptosis and improves mitochondrial dysfunction in hypoxic cardiomyocytes by Nrf2/HO-1 axis. Cell Biol. Int. 46, 723–736. doi: 10.1002/cbin.11763
Cassilhas, R. C., Tufik, S., and de Mello, M. T. (2016). Physical exercise, neuroplasticity, spatial learning and memory. Cell. Mol. Life Sci. CMLS 73, 975–983. doi: 10.1007/s00018-015-2102-0
Castrén, E., and Kojima, M. (2017). Brain-derived neurotrophic factor in mood disorders and antidepressant treatments. Neurobiol. Dis. 97, 119–126. doi: 10.1016/j.nbd.2016.07.010
Castrén, E., and Rantamäki, T. (2010). The role of BDNF and its receptors in depression and antidepressant drug action: reactivation of developmental plasticity. Dev. Neurobiol. 70, 289–297. doi: 10.1002/dneu.20758
Chen, Z., Lan, W., Yang, G., Li, Y., Ji, X., Chen, L., et al. (2020). Exercise intervention in treatment of neuropsychological diseases: a review. Front. Psychol. 11:569206. doi: 10.3389/fpsyg.2020.569206
Chen, Q.-Y., Li, X.-H., and Zhuo, M. (2021). NMDA receptors and synaptic plasticity in the anterior cingulate cortex. Neuropharmacology 197:108749. doi: 10.1016/j.neuropharm.2021.108749
Cheng, Y., Cui, Y., Zhai, Y., Xin, W., Yu, Y., Liang, J., et al. (2021). Neuroprotective effects of exogenous Irisin in Kainic acid-induced status epilepticus. Front. Cell. Neurosci. 15:738533. doi: 10.3389/fncel.2021.738533
Christiansen, L., Beck, M. M., Bilenberg, N., Wienecke, J., Astrup, A., and Lundbye-Jensen, J. (2019). Effects of exercise on cognitive performance in children and adolescents with ADHD: potential mechanisms and evidence-based recommendations. J. Clin. Med. 8:841. doi: 10.3390/jcm8060841
Chung, K. W., and Chung, H. Y. (2019). The effects of calorie restriction on autophagy: role on aging intervention. Nutrients 11:2923. doi: 10.3390/nu11122923
Chung, J., Zhang, X., Allen, M., Wang, X., Ma, Y., Beecham, G., et al. (2018). Genome-wide pleiotropy analysis of neuropathological traits related to Alzheimer’s disease. Alzheimers Res. Ther. 10:22. doi: 10.1186/s13195-018-0349-z
Compte, A., Brunel, N., Goldman-Rakic, P. S., and Wang, X. J. (2000). Synaptic mechanisms and network dynamics underlying spatial working memory in a cortical network model. Cereb. Cortex 10, 910–923. doi: 10.1093/cercor/10.9.910
Cooper, C., Moon, H. Y., and van Praag, H. (2018). On the run for hippocampal plasticity. Cold Spring Harb. Perspect. Med. 8:a029736. doi: 10.1101/cshperspect.a029736
Cornish, S. M., Bugera, E. M., Duhamel, T. A., Peeler, J. D., and Anderson, J. E. (2020). A focused review of myokines as a potential contributor to muscle hypertrophy from resistance-based exercise. Eur. J. Appl. Physiol. 120, 941–959. doi: 10.1007/s00421-020-04337-1
Corona, J. C. (2018). Natural compounds for the Management of Parkinson’s disease and attention-deficit/hyperactivity disorder. Bio. Med. Res. Int. 2018:4067597. doi: 10.1155/2018/4067597
Cortese, S., Adamo, N., Del Giovane, C., Mohr-Jensen, C., Hayes, A. J., Carucci, S., et al. (2018). Comparative efficacy and tolerability of medications for attention-deficit hyperactivity disorder in children, adolescents, and adults: a systematic review and network meta-analysis. Lancet Psychiatry 5, 727–738. doi: 10.1016/S2215-0366(18)30269-4
Cotman, C. W., Berchtold, N. C., and Christie, L.-A. (2007). Exercise builds brain health: key roles of growth factor cascades and inflammation. Trends Neurosci. 30, 464–472. doi: 10.1016/j.tins.2007.06.011
Daskalaki, A.-D., Kallergi, E., Kolaxi, A., and Nikoletopoulou, V. (2022). Local biogenesis of autophagic vesicles in neuronal dendrites facilitates long-term synaptic depression. Autophagy 18, 2011–2012. doi: 10.1080/15548627.2022.2061757
De Nardo, W., Miotto, P. M., Bayliss, J., Nie, S., Keenan, S. N., Montgomery, M. K., et al. (2022). Proteomic analysis reveals exercise training induced remodelling of hepatokine secretion and uncovers syndecan-4 as a regulator of hepatic lipid metabolism. Mol. Metab. 60:101491. doi: 10.1016/j.molmet.2022.101491
Delezie, J., and Handschin, C. (2018). Endocrine crosstalk between skeletal muscle and the brain. Front. Neurol. 9:698. doi: 10.3389/fneur.2018.00698
Di Liegro, C. M., Schiera, G., Proia, P., and Di Liegro, I. (2019). Physical activity and brain health. Gene 10:720. doi: 10.3390/genes10090720
Dikic, I. (2017). Proteasomal and Autophagic degradation systems. Annu. Rev. Biochem. 86, 193–224. doi: 10.1146/annurev-biochem-061516-044908
Dishman, R. K., Berthoud, H.-R., Booth, F. W., Cotman, C. W., Edgerton, V. R., Fleshner, M. R., et al. (2006). Neurobiology of exercise. Obes. Silver Spring Md 14, 345–356. doi: 10.1038/oby.2006.46
Draganski, B., and May, A. (2008). Training-induced structural changes in the adult human brain. Behav. Brain Res. 192, 137–142. doi: 10.1016/j.bbr.2008.02.015
Duckworth, S. C., Higginbotham, C. S., Pederson, J. A., Rogers, R. R., Marshall, M. R., Williams, T. D., et al. (2020). Physical and cognitive performance during upper-extremity versus full-body exercise under dual tasking conditions. Percept. Mot. Skills 128, 338–352. doi: 10.1177/0031512520945088
Dun, S. L., Lyu, R.-M., Chen, Y.-H., Chang, J.-K., Luo, J. J., and Dun, N. J. (2013). Irisin-immunoreactivity in neural and non-neural cells of the rodent. Neuroscience 240, 155–162. doi: 10.1016/j.neuroscience.2013.02.050
Eijsvogels, T. M. H., Fernandez, A. B., and Thompson, P. D. (2016). Are there deleterious cardiac effects of acute and chronic endurance exercise? Physiol. Rev. 96, 99–125. doi: 10.1152/physrev.00029.2014
Endres, D., Tebartz van Elst, L., Maier, S. J., Feige, B., Goll, P., Meyer, S. A., et al. (2019). Neurochemical sex differences in adult ADHD patients: an MRS study. Biol. Sex Differ. 10:50. doi: 10.1186/s13293-019-0264-4
Escobar, K. A., Cole, N. H., Mermier, C. M., and Van Dusseldorp, T. A. (2019). Autophagy and aging: maintaining the proteome through exercise and caloric restriction. Aging Cell 18:e12876. doi: 10.1111/acel.12876
Evans, H. T., Blackmore, D., Götz, J., and Bodea, L.-G. (2021). De novo proteomic methods for examining the molecular mechanisms underpinning long-term memory. Brain Res. Bull. 169, 94–103. doi: 10.1016/j.brainresbull.2020.12.015
Fan, Y., Wang, N., Rocchi, A., Zhang, W., Vassar, R., Zhou, Y., et al. (2016). Identification of natural products with neuronal and metabolic benefits through autophagy induction. Autophagy 13, 41–56. doi: 10.1080/15548627.2016.1240855
Faraone, S. V., Asherson, P., Banaschewski, T., Biederman, J., Buitelaar, J. K., Ramos-Quiroga, J. A., et al. (2015). Attention-deficit/hyperactivity disorder. Nat. Rev. Dis. Primer 1:15020. doi: 10.1038/nrdp.2015.20
Faraone, S. V., and Larsson, H. (2019). Genetics of attention deficit hyperactivity disorder. Mol. Psychiatry 24, 562–575. doi: 10.1038/s41380-018-0070-0
Filippone, A., Esposito, E., Mannino, D., Lyssenko, N., and Praticò, D. (2022). The contribution of altered neuronal autophagy to neurodegeneration. Pharmacol. Ther. 238:108178. doi: 10.1016/j.pharmthera.2022.108178
Firth, J., Stubbs, B., Vancampfort, D., Schuch, F., Lagopoulos, J., Rosenbaum, S., et al. (2018). Effect of aerobic exercise on hippocampal volume in humans: a systematic review and meta-analysis. NeuroImage 166, 230–238. doi: 10.1016/j.neuroimage.2017.11.007
Fleckenstein, A. E., Volz, T. J., and Hanson, G. R. (2009). Psychostimulant-induced alterations in vesicular monoamine transporter-2 function: neurotoxic and therapeutic implications. Neuropharmacology 56, 133–138. doi: 10.1016/j.neuropharm.2008.07.002
Friedel, E., Schlagenhauf, F., Sterzer, P., Park, S. Q., Bermpohl, F., Ströhle, A., et al. (2009). 5-HTT genotype effect on prefrontal-amygdala coupling differs between major depression and controls. Psychopharmacology 205, 261–271. doi: 10.1007/s00213-009-1536-1
Frodl, T., and Skokauskas, N. (2012). Meta-analysis of structural MRI studies in children and adults with attention deficit hyperactivity disorder indicates treatment effects. Acta Psychiatr. Scand. 125, 114–126. doi: 10.1111/j.1600-0447.2011.01786.x
Fu, J., Han, Y., Wang, J., Liu, Y., Zheng, S., Zhou, L., et al. (2016). Irisin lowers blood pressure by improvement of endothelial dysfunction via AMPK-Akt-eNOS-NO pathway in the spontaneously hypertensive rat. J. Am. Heart Assoc. 5:e003433. doi: 10.1161/JAHA.116.003433
Fukumoto, K., Fogaça, M. V., Liu, R.-J., Duman, C., Kato, T., Li, X.-Y., et al. (2019). Activity-dependent brain-derived neurotrophic factor signaling is required for the antidepressant actions of (2R,6R)-hydroxynorketamine. Proc. Natl. Acad. Sci. U. S. A. 116, 297–302. doi: 10.1073/pnas.1814709116
Gao, X., Yu, M., Sun, W., Han, Y., Yang, J., Lu, X., et al. (2021). Lanthanum chloride induces autophagy in primary cultured rat cortical neurons through Akt/mTOR and AMPK/mTOR signaling pathways. Food Chem. Toxicol. Int. J. Publ. Br. Ind. Biol. Res. Assoc. 158:112632. doi: 10.1016/j.fct.2021.112632
Gassen, N. C., Fries, G. R., Zannas, A. S., Hartmann, J., Zschocke, J., Hafner, K., et al. (2015). Chaperoning epigenetics: FKBP51 decreases the activity of DNMT1 and mediates epigenetic effects of the antidepressant paroxetine. Sci. Signal. 8:ra119. doi: 10.1126/scisignal.aac7695
Gassen, N. C., Hartmann, J., Zschocke, J., Stepan, J., Hafner, K., Zellner, A., et al. (2014). Association of FKBP51 with priming of autophagy pathways and mediation of antidepressant treatment response: evidence in cells, mice, and humans. PLoS Med. 11:e1001755. doi: 10.1371/journal.pmed.1001755
Giménez-Meseguer, J., Tortosa-Martínez, J., and Cortell-Tormo, J. M. (2020). The benefits of physical exercise on mental disorders and quality of life in substance use disorders patients. Systematic review and meta-analysis. Int. J. Environ. Res. Public. Health 17:3680. doi: 10.3390/ijerph17103680
Goffart, S., and Wiesner, R. J. (2003). Regulation and co-ordination of nuclear gene expression during mitochondrial biogenesis. Exp. Physiol. 88, 33–40. doi: 10.1113/eph8802500
González-Burgos, E., Fernández-Moriano, C., Lozano, R., Iglesias, I., and Gómez-Serranillos, M. P. (2017). Ginsenosides Rd and re co-treatments improve rotenone-induced oxidative stress and mitochondrial impairment in SH-SY5Y neuroblastoma cells. Food Chem. Toxicol. Int. J. Publ. Br. Ind. Biol. Res. Assoc. 109, 38–47. doi: 10.1016/j.fct.2017.08.013
Goto, Y., Yang, C. R., and Otani, S. (2010). Functional and dysfunctional synaptic plasticity in prefrontal cortex: roles in psychiatric disorders. Biol. Psychiatry 67, 199–207. doi: 10.1016/j.biopsych.2009.08.026
Grigolon, R. B., Brietzke, E., Trevizol, A. P., McIntyre, R. S., and Mansur, R. B. (2020). Caloric restriction, resting metabolic rate and cognitive performance in non-obese adults: a post-hoc analysis from CALERIE study. J. Psychiatr. Res. 128, 16–22. doi: 10.1016/j.jpsychires.2020.05.018
Grimm, J. W., Lu, L., Hayashi, T., Hope, B. T., Su, T.-P., and Shaham, Y. (2003). Time-dependent increases in brain-derived neurotrophic factor protein levels within the mesolimbic dopamine system after withdrawal from cocaine: implications for incubation of cocaine craving. J. Neurosci. 23, 742–747. doi: 10.1523/JNEUROSCI.23-03-00742.2003
Gu, J., and Kanai, R. (2014). What contributes to individual differences in brain structure? Front. Hum. Neurosci. 8:262. doi: 10.3389/fnhum.2014.00262
Hai, T., Duffy, H., Lemay, J.-F., Swansburg, R., Climie, E. A., and MacMaster, F. P. (2020). Neurochemical correlates of executive function in children with attention-deficit/hyperactivity disorder. J. Can. Acad. Child Adolesc. Psychiatry. J. Acad. Can. Psychiatr. Enfant Adolesc. 29, 15–25.
Hawi, Z., Cummins, T. D. R., Tong, J., Johnson, B., Lau, R., Samarrai, W., et al. (2015). The molecular genetic architecture of attention deficit hyperactivity disorder. Mol. Psychiatry 20, 289–297. doi: 10.1038/mp.2014.183
He, C., and Klionsky, D. J. (2009). Regulation mechanisms and signaling pathways of autophagy. Annu. Rev. Genet. 43, 67–93. doi: 10.1146/annurev-genet-102808-114910
He, C., Sumpter, R. Jr., and Levine, B. (2012). Exercise induces autophagy in peripheral tissues and in the brain. Autophagy 8, 1548–1551. doi: 10.4161/auto.21327
Hernandez, D., Torres, C. A., Setlik, W., Cebrián, C., Mosharov, E. V., Tang, G., et al. (2012). Regulation of presynaptic neurotransmission by macroautophagy. Neuron 74, 277–284. doi: 10.1016/j.neuron.2012.02.020
Hernandez, L., Ward, L. J., Arefin, S., Ebert, T., Laucyte-Cibulskiene, A., Collaborators, G. O. I. N. G.-F. W. D., et al. (2022). Blood–brain barrier and gut barrier dysfunction in chronic kidney disease with a focus on circulating biomarkers and tight junction proteins. Sci. Rep. 12:4414. doi: 10.1038/s41598-022-08387-7
Hess, J. L., Radonjić, N. V., Patak, J., Glatt, S. J., and Faraone, S. V. (2021). Autophagy, apoptosis, and neurodevelopmental genes might underlie selective brain region vulnerability in attention-deficit/hyperactivity disorder. Mol. Psychiatry 26, 6643–6654. doi: 10.1038/s41380-020-00974-2
Hibshman, J. D., Leuthner, T. C., Shoben, C., Mello, D. F., Sherwood, D. R., Meyer, J. N., et al. (2018). Nonselective autophagy reduces mitochondrial content during starvation in Caenorhabditis elegans. Am. J. Physiol.-Cell Physiol. 315, C781–C792. doi: 10.1152/ajpcell.00109.2018
Hoekzema, E., Carmona, S., Ramos-Quiroga, J. A., Barba, E., Bielsa, A., Tremols, V., et al. (2011). Training-induced neuroanatomical plasticity in ADHD: a tensor-based morphometric study. Hum. Brain Mapp. 32, 1741–1749. doi: 10.1002/hbm.21143
Horowitz, A. M., Fan, X., Bieri, G., Smith, L. K., Sanchez-Diaz, C. I., Schroer, A. B., et al. (2020). Blood factors transfer beneficial effects of exercise on neurogenesis and cognition to the aged brain. Science 369, 167–173. doi: 10.1126/science.aaw2622
Howells, F. M., and Russell, V. A. (2008). Glutamate-stimulated release of norepinephrine in hippocampal slices of animal models of attention-deficit/hyperactivity disorder (spontaneously hypertensive rat) and depression/anxiety-like behaviours (Wistar-Kyoto rat). Brain Res. 1200, 107–115. doi: 10.1016/j.brainres.2008.01.033
Hu, C., Zhang, X., Hu, M., Teng, T., Yuan, Y.-P., Song, P., et al. (2022). Fibronectin type III domain-containing 5 improves aging-related cardiac dysfunction in mice. Aging Cell 21:e13556. doi: 10.1111/acel.13556
Huang, X., Wang, M., Zhang, Q., Chen, X., and Wu, J. (2019). The role of glutamate receptors in attention-deficit/hyperactivity disorder: from physiology to disease. Am. J. Med. genet. Part B Neuropsychiatr. Genet. Off. Publ. Int. Soc. Psychiatr. Genet. 180, 272–286. doi: 10.1002/ajmg.b.32726
Hugenschmidt, C. E., Leng, X., Lyles, M., Michael, L., Dougherty, A., Babcock, P., et al. (2019). Cognitive effects of adding caloric restriction to aerobic exercise training in older adults with obesity. Obes. Silver Spring Md 27, 1266–1274. doi: 10.1002/oby.22525
Huo, C.-J., Yu, X.-J., Sun, Y.-J., Li, H.-B., Su, Q., Bai, J., et al. (2020). Irisin lowers blood pressure by activating the Nrf2 signaling pathway in the hypothalamic paraventricular nucleus of spontaneously hypertensive rats. Toxicol. Appl. Pharmacol. 394:114953. doi: 10.1016/j.taap.2020.114953
Islam, M. R., Valaris, S., Young, M. F., Haley, E. B., Luo, R., Bond, S. F., et al. (2021). Exercise hormone irisin is a critical regulator of cognitive function. Nat. Metab. 3, 1058–1070. doi: 10.1038/s42255-021-00438-z
Jang, Y. (2020). Endurance exercise-induced expression of autophagy-related protein coincides with anabolic expression and neurogenesis in the hippocampus of the mouse brain. Neuroreport 31, 442–449. doi: 10.1097/WNR.0000000000001431
Jedrychowski, M. P., Wrann, C. D., Paulo, J. A., Gerber, K. K., Szpyt, J., Robinson, M. M., et al. (2015). Detection and quantitation of circulating human Irisin by tandem mass spectrometry. Cell Metab. 22, 734–740. doi: 10.1016/j.cmet.2015.08.001
Jensen-Cody, S. O., and Potthoff, M. J. (2021). Hepatokines and metabolism: deciphering communication from the liver. Mol. Metab. 44:101138. doi: 10.1016/j.molmet.2020.101138
Jin, Y., Sumsuzzman, D., Choi, J., Kang, H., Lee, S.-R., and Hong, Y. (2018). Molecular and functional interaction of the Myokine Irisin with physical exercise and Alzheimer’s disease. Molecules 23:3229. doi: 10.3390/molecules23123229
Jodeiri Farshbaf, M., Garasia, S., Moussoki, D. P. K., Mondal, A. K., Cherkowsky, D., Manal, N., et al. (2020). Hippocampal injection of the exercise-induced myokine irisin suppresses acute stress-induced neurobehavioral impairment in a sex-dependent manner. Behav. Neurosci. 134, 233–247. doi: 10.1037/bne0000367
Kallergi, E., Daskalaki, A.-D., Kolaxi, A., Camus, C., Ioannou, E., Mercaldo, V., et al. (2022). Dendritic autophagy degrades postsynaptic proteins and is required for long-term synaptic depression in mice. Nat. Commun. 13:680. doi: 10.1038/s41467-022-28301-z
Kaushik, S., and Cuervo, A. M. (2012). Chaperone-mediated autophagy: a unique way to enter the lysosome world. Trends Cell Biol. 22, 407–417. doi: 10.1016/j.tcb.2012.05.006
Kim, T.-W., Baek, K.-W., Yu, H. S., Ko, I.-G., Hwang, L., and Park, J.-J. (2020). High-intensity exercise improves cognitive function and hippocampal brain-derived neurotrophic factor expression in obese mice maintained on high-fat diet. J. Exerc. Rehabil. 16, 124–131. doi: 10.12965/jer.2040050.025
Kim, Y. C., and Guan, K.-L. (2015). mTOR: a pharmacologic target for autophagy regulation. J. Clin. Invest. 125, 25–32. doi: 10.1172/JCI73939
Kim, T. H., Hong, D.-G., and Yang, Y. M. (2021). Hepatokines and non-alcoholic fatty liver disease: linking liver pathophysiology to metabolism. Biomedicine 9:1903. doi: 10.3390/biomedicines9121903
Kim, M.-H., and Leem, Y.-H. (2019). The effects of peripherally-subacute treatment with irisin on hippocampal dendritogenesis and astrocyte-secreted factors. J. Exerc. Nutr. Biochem. 23, 32–35. doi: 10.20463/jenb.2019.0029
Kim, H., Wrann, C. D., Jedrychowski, M., Vidoni, S., Kitase, Y., Nagano, K., et al. (2018). Irisin mediates effects on bone and fat via αV integrin receptors. Cells 175, 1756–1768.e17. doi: 10.1016/j.cell.2018.10.025
Kimura, T., Jia, J., Kumar, S., Choi, S. W., Gu, Y., Mudd, M., et al. (2017). Dedicated SNAREs and specialized TRIM cargo receptors mediate secretory autophagy. EMBO J. 36, 42–60. doi: 10.15252/embj.201695081
Kirk, B., Feehan, J., Lombardi, G., and Duque, G. (2020). Muscle, bone, and fat crosstalk: the biological role of Myokines, Osteokines, and Adipokines. Curr. Osteoporos. Rep. 18, 388–400. doi: 10.1007/s11914-020-00599-y
Kis, B., Lücke, C., Abdel-Hamid, M., Heßmann, P., Graf, E., Berger, M., et al. (2020). Safety profile of methylphenidate under long-term treatment in adult ADHD patients–results of the COMPAS study. Pharmacopsychiatry 53, 263–271. doi: 10.1055/a-1207-9851
Kiyohara, A. C. P., Torres, D. J., Hagiwara, A., Pak, J., Rueli, R. H. L. H., Shuttleworth, C. W. R., et al. (2021). Selenoprotein P regulates synaptic zinc and reduces tau phosphorylation. Front. Nutr. 8:683154. doi: 10.3389/fnut.2021.683154
Knaepen, K., Goekint, M., Heyman, E. M., and Meeusen, R. (2010). Neuroplasticity—exercise-induced response of peripheral brain-derived neurotrophic factor: a systematic review of experimental studies in human subjects. Sports Med. Auckl. NZ 40, 765–801. doi: 10.2165/11534530-000000000-00000
Ko, H.-J., Kim, I., Kim, J.-B., Moon, Y., Whang, M.-C., Lee, K.-M., et al. (2014). Effects of Korean red ginseng extract on behavior in children with symptoms of inattention and hyperactivity/impulsivity: a double-blind randomized placebo-controlled trial. J. Child Adolesc. Psychopharmacol. 24, 501–508. doi: 10.1089/cap.2014.0013
Kol, A., Adamsky, A., Groysman, M., Kreisel, T., London, M., and Goshen, I. (2020). Astrocytes contribute to remote memory formation by modulating hippocampal-cortical communication during learning. Nat. Neurosci. 23, 1229–1239. doi: 10.1038/s41593-020-0679-6
Kononenko, N. L., Claßen, G. A., Kuijpers, M., Puchkov, D., Maritzen, T., Tempes, A., et al. (2017). Retrograde transport of Trk B-containing autophagosomes via the adaptor AP-2 mediates neuronal complexity and prevents neurodegeneration. Nat. Commun. 8:14819. doi: 10.1038/ncomms14819
Kou, X., Chen, D., and Chen, N. (2019). Physical activity alleviates cognitive dysfunction of Alzheimer’s disease through regulating the mTOR signaling pathway. Int. J. Mol. Sci. 20:1591. doi: 10.3390/ijms20071591
Kraus, C., Castrén, E., Kasper, S., and Lanzenberger, R. (2017). Serotonin and neuroplasticity—links between molecular, functional and structural pathophysiology in depression. Neurosci. Biobehav. Rev. 77, 317–326. doi: 10.1016/j.neubiorev.2017.03.007
Krieger, V., and Amador-Campos, J. A. (2021). Clinical presentations of attention-deficit/hyperactivity disorder (ADHD) in children and adolescents: comparison of neurocognitive performance. Child Neuropsychol. 27, 1024–1053. doi: 10.1080/09297049.2021.1917530
Kugathasan, P., Waller, J., Westrich, L., Abdourahman, A., Tamm, J. A., Pehrson, A. L., et al. (2017). In vivo and in vitro effects of vortioxetine on molecules associated with neuroplasticity. J. Psychopharmacol. 31, 365–376. doi: 10.1177/0269881116667710
Kuijpers, M. (2022). Keeping synapses in shape: degradation pathways in the healthy and aging brain. Neuronal. Signals 6:NS20210063. doi: 10.1042/NS20210063
Lago, T. R., Hsiung, A., Leitner, B. P., Duckworth, C. J., Balderston, N. L., Chen, K. Y., et al. (2019). Exercise modulates the interaction between cognition and anxiety in humans. Cognit. Emot. 33, 863–870. doi: 10.1080/02699931.2018.1500445
Lakhan, S. E., and Kirchgessner, A. (2012). Prescription stimulants in individuals with and without attention deficit hyperactivity disorder: misuse, cognitive impact, and adverse effects. Brain Behav. 2, 661–677. doi: 10.1002/brb3.78
Lalo, U., Nezis, I. P., and Pankratov, Y. (2022). Impact of autophagy impairment on experience- and diet-related synaptic plasticity. Int. J. Mol. Sci. 23:9228. doi: 10.3390/ijms23169228
Larsen, M. N., Madsen, M., Nielsen, C. M., Manniche, V., Hansen, L., Bangsbo, J., et al. (2020). Cardiovascular adaptations after 10 months of daily 12-min bouts of intense school-based physical training for 8-10-year-old children. Prog. Cardiovasc. Dis. 63, 813–817. doi: 10.1016/j.pcad.2020.05.011
Lee, P. R., and Fields, R. D. (2021). Activity-dependent gene expression in neurons. Neurosci. Rev. J. Bringing Neurobiol. Neurol. Psychiatry 27, 355–366. doi: 10.1177/1073858420943515
Lee, H. J., Lee, J. O., Kim, N., Kim, J. K., Kim, H. I., Lee, Y. W., et al. (2015). Irisin, a novel Myokine, regulates glucose uptake in skeletal muscle cells via AMPK. Mol. Endocrinol. 29, 873–881. doi: 10.1210/me.2014-1353
Lenz, T. L. (2012). A pharmacological/physiological comparison between ADHD medications and exercise: am. J. Lifestyle Med. 6, 306–308. doi: 10.1177/1559827612443346
Li, F., Geng, X., Yun, H. J., Haddad, Y., Chen, Y., and Ding, Y. (2021). Neuroplastic effect of exercise through astrocytes activation and cellular crosstalk. Aging Dis. 12, 1644–1657. doi: 10.14336/AD.2021.0325
Li, Y., and Halterman, M. W. (2021). The MAP kinase phosphatase MKP-1 modulates neurogenesis via effects on BNIP3 and autophagy. Biomol. Ther. 11:1871. doi: 10.3390/biom11121871
Li, Q., Jia, S., Xu, L., Li, B., and Chen, N. (2019). Metformin-induced autophagy and irisin improves INS-1 cell function and survival in high-glucose environment via AMPK/SIRT1/PGC-1α signal pathway. Food Sci. Nutr. 7, 1695–1703. doi: 10.1002/fsn3.1006
Li, R.-L., Wu, S.-S., Wu, Y., Wang, X.-X., Chen, H.-Y., Xin, J.-J., et al. (2018). Irisin alleviates pressure overload-induced cardiac hypertrophy by inducing protective autophagy via mTOR-independent activation of the AMPK-ULK1 pathway. J. Mol. Cell. Cardiol. 121, 242–255. doi: 10.1016/j.yjmcc.2018.07.250
Li, H., Zhang, Y., Wang, F., Donelan, W., Zona, M. C., Li, S., et al. (2019). Effects of irisin on the differentiation and browning of human visceral white adipocytes. Am. J. Transl. Res. 11, 7410–7421.
Liang, Y. (2019). Emerging concepts and functions of autophagy as a regulator of synaptic components and plasticity. Cells 8:34. doi: 10.3390/cells8010034
Liang, Y., and Sigrist, S. (2018). Autophagy and proteostasis in the control of synapse aging and disease. Curr. Opin. Neurobiol. 48, 113–121. doi: 10.1016/j.conb.2017.12.006
LiCausi, F., and Hartman, N. (2018). Role of mTOR complexes in neurogenesis. Int. J. Mol. Sci. 19:1544. doi: 10.3390/ijms19051544
Lim, S.-H., Lee, N.-Y., Ryu, J. Y., An, J. H., Lee, G. S., Min, S. S., et al. (2022). Phosphoproteome profiling of hippocampal synaptic plasticity. Biochem. Biophys. Res. Commun. 626, 92–99. doi: 10.1016/j.bbrc.2022.07.051
Liu, J., Gao, S., and Zhang, L. (2022). Effects of physical exercises on emotion regulation: a meta-analysis. Sports Med. doi: 10.1101/2022.07.04.22277120
Liu, C., Wang, J., Yang, Y., Liu, X., Zhu, Y., Zou, J., et al. (2018). Ginsenoside Rd ameliorates colitis by inducing p 62-driven mitophagy-mediated NLRP3 inflammasome inactivation in mice. Biochem. Pharmacol. 155, 366–379. doi: 10.1016/j.bcp.2018.07.010
Liu, Y., Zhang, R.-Y., Zhao, J., Dong, Z., Feng, D.-Y., Wu, R., et al. (2015). Ginsenoside Rd protects SH-SY5Y cells against 1-Methyl-4-phenylpyridinium induced injury. Int. J. Mol. Sci. 16, 14395–14408. doi: 10.3390/ijms160714395
Löffler, D., Müller, U., Scheuermann, K., Friebe, D., Gesing, J., Bielitz, J., et al. (2015). Serum irisin levels are regulated by acute strenuous exercise. J. Clin. Endocrinol. Metab. 100, 1289–1299. doi: 10.1210/jc.2014-2932
Lourenco, M. V., Frozza, R. L., de Freitas, G. B., Zhang, H., Kincheski, G. C., Ribeiro, F. C., et al. (2019). Exercise-linked FNDC5/irisin rescues synaptic plasticity and memory defects in Alzheimer’s models. Nat. Med. 25, 165–175. doi: 10.1038/s41591-018-0275-4
Lu, L., Grimm, J. W., Hope, B. T., and Shaham, Y. (2004). Incubation of cocaine craving after withdrawal: a review of preclinical data. Neuropharmacology 47, 214–226. doi: 10.1016/j.neuropharm.2004.06.027
Ma, E. B., Sahar, N. E., Jeong, M., and Huh, J. Y. (2019). Irisin exerts inhibitory effect on Adipogenesis through regulation of Wnt signaling. Front. Physiol. 10:1085. doi: 10.3389/fphys.2019.01085
MacQueen, D. A., Minassian, A., Kenton, J. A., Geyer, M. A., Perry, W., Brigman, J. L., et al. (2018). Amphetamine improves mouse and human attention in the 5-choice continuous performance test. Neuropharmacology 138, 87–96. doi: 10.1016/j.neuropharm.2018.05.034
Maday, S., and Holzbaur, E. L. F. (2014). Autophagosome biogenesis in primary neurons follows an ordered and spatially regulated pathway. Dev. Cell 30, 71–85. doi: 10.1016/j.devcel.2014.06.001
Mancinelli, R., Carpino, G., Petrungaro, S., Mammola, C. L., Tomaipitinca, L., Filippini, A., et al. (2017). Multifaceted roles of GSK-3 in cancer and autophagy-related diseases. Oxidative Med. Cell. Longev. 2017:4629495. doi: 10.1155/2017/4629495
Mao, J.-Y., Su, L.-X., Li, D.-K., Zhang, H.-M., Wang, X.-T., and Liu, D.-W. (2021). The effects of UCP2 on autophagy through the AMPK signaling pathway in septic cardiomyopathy and the underlying mechanism. Ann. Transl. Med. 9:259. doi: 10.21037/atm-20-4819
Martinelli, S., Anderzhanova, E. A., Bajaj, T., Wiechmann, S., Dethloff, F., Weckmann, K., et al. (2021). Stress-primed secretory autophagy promotes extracellular BDNF maturation by enhancing MMP9 secretion. Nat. Commun. 12:4643. doi: 10.1038/s41467-021-24810-5
Mateos-Aparicio, P., and Rodríguez-Moreno, A. (2019). The impact of studying brain plasticity. Front. Cell. Neurosci. 13:66. doi: 10.3389/fncel.2019.00066
Mazur-Bialy, A. I., Pocheć, E., and Zarawski, M. (2017). Anti-inflammatory properties of Irisin, mediator of physical activity, are connected with TLR4/MyD88 signaling pathway activation. Int. J. Mol. Sci. 18:E701. doi: 10.3390/ijms18040701
McEwen, B. S., and Chattarji, S. (2004). Molecular mechanisms of neuroplasticity and pharmacological implications: the example of tianeptine. Eur. Neuropsychopharmacol. 14, S497–S502. doi: 10.1016/j.euroneuro.2004.09.008
Mehren, A., Reichert, M., Coghill, D., Müller, H. H. O., Braun, N., and Philipsen, A. (2020). Physical exercise in attention deficit hyperactivity disorder – evidence and implications for the treatment of borderline personality disorder. Borderline Personal. Disord. Emot. Dysregulation 7:1. doi: 10.1186/s40479-019-0115-2
Merkel, R. L., and Kuchibhatla, A. (2009). Safety of stimulant treatment in attention deficit hyperactivity disorder: part I. Expert Opin. Drug Saf. 8, 655–668. doi: 10.1517/14740330903279956
Mijaljica, D., Prescott, M., and Devenish, R. J. (2011). Microautophagy in mammalian cells: revisiting a 40-year-old conundrum. Autophagy 7, 673–682. doi: 10.4161/auto.7.7.14733
Miller, E. M., Quintero, J. E., Pomerleau, F., Huettl, P., Gerhardt, G. A., and Glaser, P. E. A. (2018). Chronic methylphenidate alters tonic and phasic glutamate signaling in the frontal cortex of a freely-moving rat model of ADHD. Neurochem. Res. 44, 89–101. doi: 10.1007/s11064-018-2483-1
Milner, R., Huang, X., Wu, J., Nishimura, S., Pytela, R., Sheppard, D., et al. (1999). Distinct roles for astrocyte alphavbeta 5 and alphavbeta8 integrins in adhesion and migration. J. Cell Sci. 112, 4271–4279. doi: 10.1242/jcs.112.23.4271
Mitra, R., Jadhav, S., McEwen, B. S., Vyas, A., and Chattarji, S. (2005). Stress duration modulates the spatiotemporal patterns of spine formation in the basolateral amygdala. Proc. Natl. Acad. Sci. U. S. A. 102, 9371–9376. doi: 10.1073/pnas.0504011102
Mizushima, N., and Klionsky, D. J. (2007). Protein turnover via autophagy: implications for metabolism. Annu. Rev. Nutr. 27, 19–40. doi: 10.1146/annurev.nutr.27.061406.093749
Mizushima, N., and Komatsu, M. (2011). Autophagy: renovation of cells and tissues. Cells 147, 728–741. doi: 10.1016/j.cell.2011.10.026
Mizushima, N., Yamamoto, A., Matsui, M., Yoshimori, T., and Ohsumi, Y. (2004). In vivo analysis of autophagy in response to nutrient starvation using transgenic mice expressing a fluorescent autophagosome marker. Mol. Biol. Cell 15, 1101–1111. doi: 10.1091/mbc.e03-09-0704
Mohammadi, S., Oryan, S., Komaki, A., Eidi, A., and Zarei, M. (2019). Effects of intra-dentate gyrus microinjection of myokine irisin on long-term potentiation in male rats. Arq. Neuropsiquiatr. 77, 881–887. doi: 10.1590/0004-282X20190184
Mongrédien, R., Erdozain, A. M., Dumas, S., Cutando, L., Del Moral, A. N., Puighermanal, E., et al. (2019). Cartography of hevin-expressing cells in the adult brain reveals prominent expression in astrocytes and parvalbumin neurons. Brain Struct. Funct. 224, 1219–1244. doi: 10.1007/s00429-019-01831-x
Moon, J.-H., and Park, S.-Y. (2020). Prion peptide-mediated calcium level alteration governs neuronal cell damage through AMPK-autophagy flux. Cell Commun. Signal. CCS 18:109. doi: 10.1186/s12964-020-00590-1
Morland, C., Andersson, K. A., Haugen, Ø. P., Hadzic, A., Kleppa, L., Gille, A., et al. (2017). Exercise induces cerebral VEGF and angiogenesis via the lactate receptor HCAR1. Nat. Commun. 8:15557. doi: 10.1038/ncomms15557
Morris, R. G. M., Moser, E. I., Riedel, G., Martin, S. J., Sandin, J., Day, M., et al. (2003). Elements of a neurobiological theory of the hippocampus: the role of activity-dependent synaptic plasticity in memory. Philos. Trans. R. Soc. Lond. Ser. B Biol. Sci. 358, 773–786. doi: 10.1098/rstb.2002.1264
Mueller, P. J. (2007). Exercise training and sympathetic nervous system activity: evidence for physical activity dependent neural plasticity. Clin. Exp. Pharmacol. Physiol. 34, 377–384. doi: 10.1111/j.1440-1681.2007.04590.x
Müller, P., Duderstadt, Y., Lessmann, V., and Müller, N. G. (2020). Lactate and BDNF: key mediators of exercise induced neuroplasticity? J. Clin. Med. 9:1136. doi: 10.3390/jcm9041136
Murao, M., Imano, T., Akiyama, J., Kawakami, T., and Nakajima, M. (2019). Effect of single bout downhill running on the serum irisin concentrations in rats. Growth Factors Chur Switz. 37, 257–262. doi: 10.1080/08977194.2020.1742118
Naaijen, J., Bralten, J., Poelmans, G., IMAGE consortium, Glennon, J. C., Franke, B., et al. (2017). Glutamatergic and GABAergic gene sets in attention-deficit/hyperactivity disorder: association to overlapping traits in ADHD and autism. Transl. Psychiatry 7:e999. doi: 10.1038/tp.2016.273
Naiki, H., and Nagai, Y. (2009). Molecular pathogenesis of protein misfolding diseases: pathological molecular environments versus quality control systems against misfolded proteins. J. Biochem. 146, 751–756. doi: 10.1093/jb/mvp119
Nakatogawa, H., Suzuki, K., Kamada, Y., and Ohsumi, Y. (2009). Dynamics and diversity in autophagy mechanisms: lessons from yeast. Nat. Rev. Mol. Cell Biol. 10, 458–467. doi: 10.1038/nrm2708
Nicolini, C., Michalski, B., Toepp, S. L., Turco, C. V., D’Hoine, T., Harasym, D., et al. (2020). A single bout of high-intensity interval exercise increases corticospinal excitability, brain-derived neurotrophic factor, and Uncarboxylated Osteolcalcin in sedentary, healthy males. Neuroscience 437, 242–255. doi: 10.1016/j.neuroscience.2020.03.042
Niederhofer, H. (2009). Panax ginseng may improve some symptoms of attention-deficit hyperactivity disorder. J. Diet. Suppl. 6, 22–27. doi: 10.1080/19390210802687221
Nikoletopoulou, V., Sidiropoulou, K., Kallergi, E., Dalezios, Y., and Tavernarakis, N. (2017). Modulation of autophagy by BDNF underlies synaptic plasticity. Cell Metab. 26, 230–242.e5. doi: 10.1016/j.cmet.2017.06.005
Nikoletopoulou, V., and Tavernarakis, N. (2018). Regulation and roles of autophagy at synapses. Trends Cell Biol. 28, 646–661. doi: 10.1016/j.tcb.2018.03.006
Nitsche, M. A., Müller-Dahlhaus, F., Paulus, W., and Ziemann, U. (2012). The pharmacology of neuroplasticity induced by non-invasive brain stimulation: building models for the clinical use of CNS active drugs. J. Physiol. 590, 4641–4662. doi: 10.1113/jphysiol.2012.232975
Norheim, F., Langleite, T. M., Hjorth, M., Holen, T., Kielland, A., Stadheim, H. K., et al. (2014). The effects of acute and chronic exercise on PGC-1α, irisin and browning of subcutaneous adipose tissue in humans. FEBS J. 281, 739–749. doi: 10.1111/febs.12619
Ohsumi, Y. (2014). Historical landmarks of autophagy research. Cell Res. 24, 9–23. doi: 10.1038/cr.2013.169
Ota, Y., Zanetti, A. T., and Hallock, R. M. (2013). The role of astrocytes in the regulation of synaptic plasticity and memory formation. Neural Plast. 2013:185463. doi: 10.1155/2013/185463
Otvos, L., and Wade, J. D. (2014). Current challenges in peptide-based drug discovery. Front. Chem. 2:62. doi: 10.3389/fchem.2014.00062
Pan, J., Zhang, H., Lin, H., Gao, L., Zhang, H.-l., Zhang, J., et al. (2021). Irisin ameliorates doxorubicin-induced cardiac perivascular fibrosis through inhibiting endothelial-to-mesenchymal transition by regulating ROS accumulation and autophagy disorder in endothelial cells. Redox Biol. 46:102120. doi: 10.1016/j.redox.2021.102120
Pang, Q., Wang, P., Pan, Y., Dong, X., Zhou, T., Song, X., et al. (2022). Irisin protects against vascular calcification by activating autophagy and inhibiting NLRP3-mediated vascular smooth muscle cell pyroptosis in chronic kidney disease. Cell Death Dis. 13:283. doi: 10.1038/s41419-022-04735-7
Park, J., Cheon, W., and Kim, K. (2020). Effects of long-term endurance exercise and lithium treatment on neuroprotective factors in hippocampus of obese rats. Int. J. Environ. Res. Public Health 17:3317. doi: 10.3390/ijerph17093317
Park, M.-J., Kim, D.-I., Choi, J.-H., Heo, Y.-R., and Park, S.-H. (2015). New role of irisin in hepatocytes: the protective effect of hepatic steatosis in vitro. Cell. Signal. 27, 1831–1839. doi: 10.1016/j.cellsig.2015.04.010
Pedersen, B. K. (2019). Physical activity and muscle–brain crosstalk. Nat. Rev. Endocrinol. 15, 383–392. doi: 10.1038/s41574-019-0174-x
Peña, I. D., and Shi, W.-X. (2016). Chemogenetic approach to model hypofrontality. Med. Hypotheses 93, 113–116. doi: 10.1016/j.mehy.2016.05.032
Petherick, K. J., Williams, A. C., Lane, J. D., Ordóñez-Morán, P., Huelsken, J., Collard, T. J., et al. (2013). Autolysosomal β-catenin degradation regulates Wnt-autophagy-p 62 crosstalk. EMBO J. 32, 1903–1916. doi: 10.1038/emboj.2013.123
Phillips, C. (2017). Brain-derived neurotrophic factor, depression, and physical activity: making the Neuroplastic connection. Neural Plast. 2017:7260130. doi: 10.1155/2017/7260130
Pievsky, M. A., and McGrath, R. E. (2018). The neurocognitive profile of attention-deficit/hyperactivity disorder: a review of meta-analyses. Arch. Clin. Neuropsychol. Off. J. Natl. Acad. Neuropsychol. 33, 143–157. doi: 10.1093/arclin/acx055
Piya, M. K., Harte, A. L., Sivakumar, K., Tripathi, G., Voyias, P. D., James, S., et al. (2014). The identification of irisin in human cerebrospinal fluid: influence of adiposity, metabolic markers, and gestational diabetes. Am. J. Physiol. Endocrinol. Metab. 306, E512–E518. doi: 10.1152/ajpendo.00308.2013
Plessen, K. J., Bansal, R., Zhu, H., Whiteman, R., Amat, J., Quackenbush, G. A., et al. (2006). Hippocampus and amygdala morphology in attention-deficit/hyperactivity disorder. Arch. Gen. Psychiatry 63, 795–807. doi: 10.1001/archpsyc.63.7.795
Ponpuak, M., Mandell, M. A., Kimura, T., Chauhan, S., Cleyrat, C., and Deretic, V. (2015). Secretory autophagy. Curr. Opin. Cell Biol. 35, 106–116. doi: 10.1016/j.ceb.2015.04.016
Qi, Z.-F., Luo, Y.-M., Liu, X.-R., Wang, R.-L., Zhao, H.-P., Yan, F., et al. (2012). AKT/GSK3β-dependent autophagy contributes to the neuroprotection of limb remote ischemic postconditioning in the transient cerebral ischemic rat model. CNS Neurosci. Ther. 18, 965–973. doi: 10.1111/cns.12016
Qiu, S., Bosnyák, E., Zügel, M., Steinacker, J. M., and Schumann, U. (2020). Autonomic function may not modulate irisin release in healthy adults: findings from a randomized cross-over study. Arch. Endocrinol. Metab. 64, 201–204. doi: 10.20945/2359-3997000000243
Quan, H., Koltai, E., Suzuki, K., Aguiar, A. S., Pinho, R., Boldogh, I., et al. (2020). Exercise, redox system and neurodegenerative diseases. Biochim. Biophys. Acta Mol. basis Dis. 1866:165778. doi: 10.1016/j.bbadis.2020.165778
Raichlen, D. A., Klimentidis, Y. C., Bharadwaj, P. K., and Alexander, G. E. (2019). Differential associations of engagement in physical activity and estimated cardiorespiratory fitness with brain volume in middle-aged to older adults. Brain Imaging Behav. 14, 1994–2003. doi: 10.1007/s11682-019-00148-x
Rassovsky, Y., and Alfassi, T. (2019). Attention improves during physical exercise in individuals with ADHD. Front. Psychol. 9:2747. doi: 10.3389/fpsyg.2018.02747
Ren, F., Zhang, L., Zhang, X., Shi, H., Wen, T., Bai, L., et al. (2016). Inhibition of glycogen synthase kinase 3β promotes autophagy to protect mice from acute liver failure mediated by peroxisome proliferator-activated receptor α. Cell Death Dis. 7:e2151. doi: 10.1038/cddis.2016.56
Roe, N. D., and Ren, J. (2011). Akt2 knockout mitigates chronic iNOS inhibition-induced cardiomyocyte atrophy and contractile dysfunction despite persistent insulin resistance. Toxicol. Lett. 207, 222–231. doi: 10.1016/j.toxlet.2011.09.015
Ruan, Q., Huang, Y., Yang, L., Li, J., Gu, W., Bao, Z., et al. (2020). Associations of preoperative Irisin levels of paired cerebrospinal fluid and plasma with physical dysfunction and muscle wasting severity in residents of surgery wards. J. Nutr. Health Aging 24, 412–422. doi: 10.1007/s12603-020-1343-2
Ruan, Q., Huang, Y., Yang, L., Ruan, J., Gu, W., Zhang, X., et al. (2019). The effects of both age and sex on irisin levels in paired plasma and cerebrospinal fluid in healthy humans. Peptides 113, 41–51. doi: 10.1016/j.peptides.2019.01.004
Ruan, Q., Zhang, L., Ruan, J., Zhang, X., Chen, J., Ma, C., et al. (2018). Detection and quantitation of irisin in human cerebrospinal fluid by tandem mass spectrometry. Peptides 103, 60–64. doi: 10.1016/j.peptides.2018.03.013
Rubia, K. (2018). Cognitive neuroscience of attention deficit hyperactivity disorder (ADHD) and its clinical translation. Front. Hum. Neurosci. 12:100. doi: 10.3389/fnhum.2018.00100
Sainani, S. R., Pansare, P. A., Rode, K., Bhalchim, V., Doke, R., and Desai, S. (2022). Emendation of autophagic dysfuction in neurological disorders: a potential therapeutic target. Int. J. Neurosci. 132, 466–482. doi: 10.1080/00207454.2020.1822356
Schep, L. J., Slaughter, R. J., and Beasley, D. M. G. (2010). The clinical toxicology of metamfetamine. Clin. Toxicol. 48, 675–694. doi: 10.3109/15563650.2010.516752
Seiffer, B., Hautzinger, M., Ulrich, R., and Wolf, S. (2022). The efficacy of physical activity for children with attention deficit hyperactivity disorder: a meta-analysis of randomized controlled trials. J. Atten. Disord. 26, 656–673. doi: 10.1177/10870547211017982
Seo, D. Y., Park, S. H., Marquez, J., Kwak, H.-B., Kim, T. N., Bae, J. H., et al. (2021). Hepatokines as a molecular transducer of exercise. J. Clin. Med. 10:385. doi: 10.3390/jcm10030385
Shindo, T., Shikanai, H., Watarai, A., Hiraide, S., Iizuka, K., and Izumi, T. (2022). D-serine metabolism in the medial prefrontal cortex, but not the hippocampus, is involved in AD/HD-like behaviors in SHRSP/Ezo. Eur. J. Pharmacol. 923:174930. doi: 10.1016/j.ejphar.2022.174930
Siteneski, A., Olescowicz, G., Pazini, F. L., Camargo, A., Fraga, D. B., Brocardo, P. S., et al. (2020). Antidepressant-like and pro-neurogenic effects of physical exercise: the putative role of FNDC5/irisin pathway. J. Neural Transm. Vienna Austria 1996 127, 355–370. doi: 10.1007/s00702-020-02143-9
Song, X., Liu, B., Cui, L., Zhou, B., Liu, W., Xu, F., et al. (2017). Silibinin ameliorates anxiety/depression-like behaviors in amyloid β-treated rats by upregulating BDNF/Trk B pathway and attenuating autophagy in hippocampus. Physiol. Behav. 179, 487–493. doi: 10.1016/j.physbeh.2017.07.023
Song, R., Zhao, X., Cao, R., Liang, Y., Zhang, D.-Q., and Wang, R. (2021). Irisin improves insulin resistance by inhibiting autophagy through the PI3K/Akt pathway in H9c2 cells. Gene 769:145209. doi: 10.1016/j.gene.2020.145209
Spaak, E., Watanabe, K., Funahashi, S., and Stokes, M. G. (2017). Stable and dynamic coding for working memory in primate prefrontal cortex. J. Neurosci. 37, 6503–6516. doi: 10.1523/JNEUROSCI.3364-16.2017
Spencer, T. J., Brown, A., Seidman, L. J., Valera, E. M., Makris, N., Lomedico, A., et al. (2013). Effect of psychostimulants on brain structure and function in ADHD: a qualitative literature review of magnetic resonance imaging–based neuroimaging studies. J. Clin. Psychiatry 74, 902–917. doi: 10.4088/JCP.12r08287
Steinkellner, T., Freissmuth, M., Sitte, H. H., and Montgomery, T. (2011). The ugly side of amphetamines: short- and long-term toxicity of 3, 4-methylenedioxymethamphetamine (MDMA, ‘ecstasy’), methamphetamine and D-amphetamine. Biol. Chem. 392, 103–115. doi: 10.1515/BC.2011.016
Stranahan, A. M., Lee, K., Becker, K. G., Zhang, Y., Maudsley, S., Martin, B., et al. (2010). Hippocampal gene expression patterns underlying the enhancement of memory by running in aged mice. Neurobiol. Aging 31, 1937–1949. doi: 10.1016/j.neurobiolaging.2008.10.016
Suarez-Manzano, S., Ruiz-Ariza, A., De La Torre-Cruz, M., and Martínez-López, E. J. (2018). Acute and chronic effect of physical activity on cognition and behaviour in young people with ADHD: a systematic review of intervention studies. Res. Dev. Disabil. 77, 12–23. doi: 10.1016/j.ridd.2018.03.015
Sugiyama, A., Kato, H., Takakura, H., Osawa, S., Maeda, Y., and Izawa, T. (2020). Effects of physical activity and melatonin on brain-derived neurotrophic factor and cytokine expression in the cerebellum of high-fat diet-fed rats. Neuropsychopharmacol. Rep 40, 291–296. doi: 10.1002/npr2.12125
Swenson, S., Blum, K., McLaughlin, T., Gold, M. S., and Thanos, P. K. (2020). The therapeutic potential of exercise for neuropsychiatric diseases: a review. J. Neurol. Sci. 412:116763. doi: 10.1016/j.jns.2020.116763
Tan, X., Du, X., Jiang, Y., Botchway, B. O. A., Hu, Z., and Fang, M. (2018). Inhibition of autophagy in microglia alters depressive-like behavior via BDNF pathway in postpartum depression. Front. Psychol. 9:434. doi: 10.3389/fpsyt.2018.00434
Tang, M., Liu, T., Jiang, P., and Dang, R. (2021). The interaction between autophagy and neuroinflammation in major depressive disorder: from pathophysiology to therapeutic implications. Pharmacol. Res. 168:105586. doi: 10.1016/j.phrs.2021.105586
Tari, A. R., Norevik, C. S., Scrimgeour, N. R., Kobro-Flatmoen, A., Storm-Mathisen, J., Bergersen, L. H., et al. (2019). Are the neuroprotective effects of exercise training systemically mediated? Prog. Cardiovasc. Dis. 62, 94–101. doi: 10.1016/j.pcad.2019.02.003
Tarkka, I. M., Hautasaari, P., Pesonen, H., Niskanen, E., Rottensteiner, M., Kaprio, J., et al. (2019). Long-term physical activity May modify brain structure and function: studies in Young healthy twins. J. Phys. Act. Health 16, 637–643. doi: 10.1123/jpah.2018-0416
Tewari, S. G., and Majumdar, K. K. (2012). A mathematical model of the tripartite synapse: astrocyte-induced synaptic plasticity. J. Biol. Phys. 38, 465–496. doi: 10.1007/s10867-012-9267-7
Tian, A., Ma, X., Li, H., and Zhang, R. (2020). Dl-3n-butylphthalide improves spatial learning and memory in rats with vascular dementia by reducing autophagy via regulation of the mTOR signaling pathway. Exp. Ther. Med. 19, 1940–1946. doi: 10.3892/etm.2019.8402
Tofas, T., Draganidis, D., Deli, C. K., Georgakouli, K., Fatouros, I. G., and Jamurtas, A. Z. (2019). Exercise-induced regulation of redox status in cardiovascular diseases: the role of exercise training and detraining. Antioxidants 9:13. doi: 10.3390/antiox9010013
Tomoda, T., Yang, K., and Sawa, A. (2020). Neuronal autophagy in synaptic functions and psychiatric disorders. Biol. Psychiatry 87, 787–796. doi: 10.1016/j.biopsych.2019.07.018
Törpel, A., Herold, F., Hamacher, D., Müller, N., and Schega, L. (2018). Strengthening the brain—is resistance training with blood flow restriction an effective strategy for cognitive improvement? J. Clin. Med. 7:337. doi: 10.3390/jcm7100337
Touma, C., Gassen, N. C., Herrmann, L., Cheung-Flynn, J., Büll, D. R., Ionescu, I. A., et al. (2011). FK506 binding protein 5 shapes stress responsiveness: modulation of neuroendocrine reactivity and coping behavior. Biol. Psychiatry 70, 928–936. doi: 10.1016/j.biopsych.2011.07.023
Tsai, C.-S., Huang, Y.-S., Wu, C.-L., Hwang, F.-M., Young, K.-B., Tsai, M.-H., et al. (2013). Long-term effects of stimulants on neurocognitive performance of Taiwanese children with attention-deficit/hyperactivity disorder. BMC Psychiatry 13:330. doi: 10.1186/1471-244X-13-330
Tsuchiya, Y., Ando, D., Goto, K., Kiuchi, M., Yamakita, M., and Koyama, K. (2014). High-intensity exercise causes greater irisin response compared with low-intensity exercise under similar energy consumption. Tohoku J. Exp. Med. 233, 135–140. doi: 10.1620/tjem.233.135
Urano, Y., Mori, C., Fuji, A., Konno, K., Yamamoto, T., Yashirogi, S., et al. (2018). 6-Hydroxydopamine induces secretion of PARK7/DJ-1 via autophagy-based unconventional secretory pathway. Autophagy 14, 1943–1958. doi: 10.1080/15548627.2018.1493043
Vidal, P., and Stanford, K. I. (2020). Exercise-induced adaptations to adipose tissue thermogenesis. Front. Endocrinol. 11:270. doi: 10.3389/fendo.2020.00270
Voss, P., Thomas, M. E., Cisneros-Franco, J. M., and de Villers-Sidani, É. (2017). Dynamic brains and the changing rules of neuroplasticity: implications for learning and recovery. Front. Psychol. 8:1657. doi: 10.3389/fpsyg.2017.01657
Vyas, S., Rodrigues, A. J., Silva, J. M., Tronche, F., Almeida, O. F. X., Sousa, N., et al. (2016). Chronic stress and glucocorticoids: from neuronal plasticity to neurodegeneration. Neural Plast. 2016:6391686. doi: 10.1155/2016/6391686
Vysniauske, R., Verburgh, L., Oosterlaan, J., and Molendijk, M. L. (2020). The effects of physical exercise on functional outcomes in the treatment of ADHD: a meta-analysis. J. Atten. Disord. 24, 644–654. doi: 10.1177/1087054715627489
Walsh, E. I., Smith, L., Northey, J., Rattray, B., and Cherbuin, N. (2020). Towards an understanding of the physical activity-BDNF-cognition triumvirate: a review of associations and dosage. Ageing Res. Rev. 60:101044. doi: 10.1016/j.arr.2020.101044
Wang, H., Fu, J., Xu, X., Yang, Z., and Zhang, T. (2021). Rapamycin activates Mitophagy and alleviates cognitive and synaptic plasticity deficits in a mouse model of Alzheimer’s disease. J. Gerontol. A Biol. Sci. Med. Sci. 76, 1707–1713. doi: 10.1093/gerona/glab142
Wang, S., and Pan, J. (2016). Irisin ameliorates depressive-like behaviors in rats by regulating energy metabolism. Biochem. Biophys. Res. Commun. 474, 22–28. doi: 10.1016/j.bbrc.2016.04.047
Waseem, R., Shamsi, A., Mohammad, T., Hassan, M. I., Kazim, S. N., Chaudhary, A. A., et al. (2022). FNDC5/Irisin: physiology and pathophysiology. Molecules 27:1118. doi: 10.3390/molecules27031118
Wei, Y., An, Z., Zou, Z., Sumpter, R., Su, M., Zang, X., et al. (2015). The stress-responsive kinases MAPKAPK2/MAPKAPK3 activate starvation-induced autophagy through Beclin 1 phosphorylation. elife 4:e05289. doi: 10.7554/eLife.05289
Wilke, J. (2020). Functional high-intensity exercise is more effective in acutely increasing working memory than aerobic walking: an exploratory randomized, controlled trial. Sci. Rep. 10:12335. doi: 10.1038/s41598-020-69139-z
Witte, A. V., Fobker, M., Gellner, R., Knecht, S., and Flöel, A. (2009). Caloric restriction improves memory in elderly humans. Proc. Natl. Acad. Sci. U. S. A. 106, 1255–1260. doi: 10.1073/pnas.0808587106
Wrann, C. D., White, J. P., Salogiannnis, J., Laznik-Bogoslavski, D., Wu, J., Ma, D., et al. (2013). Exercise induces hippocampal BDNF through a PGC-1α/FNDC5 pathway. Cell Metab. 18, 649–659. doi: 10.1016/j.cmet.2013.09.008
Xie, C., Zhang, Y., Tran, T. D. N., Wang, H., Li, S., George, E. V., et al. (2015). Irisin controls growth, intracellular Ca2+ signals, and mitochondrial thermogenesis in Cardiomyoblasts. PloS One 10:e0136816. doi: 10.1371/journal.pone.0136816
Xin, T., and Lu, C. (2020). Irisin activates Opa1-induced mitophagy to protect cardiomyocytes against apoptosis following myocardial infarction. Aging 12, 4474–4488. doi: 10.18632/aging.102899
Xu, T.-T., Li, H., Dai, Z., Lau, G. K., Li, B.-Y., Zhu, W.-L., et al. (2020). Spermidine and spermine delay brain aging by inducing autophagy in SAMP8 mice. Aging 12, 6401–6414. doi: 10.18632/aging.103035
Xu, R., Liu, Q., Ma, X., and Hou, J. (2022). Irisin inhibits PCSK9 expression through activating AMPK-SREBP2 pathway. Biochem. Biophys. Res. Commun. 630, 77–83. doi: 10.1016/j.bbrc.2022.09.034
Yang, F., Chu, X., Yin, M., Liu, X., Yuan, H., Niu, Y., et al. (2014). mTOR and autophagy in normal brain aging and caloric restriction ameliorating age-related cognition deficits. Behav. Brain Res. 264, 82–90. doi: 10.1016/j.bbr.2014.02.005
Yang, W., Ju, J., Lee, K., Nam, K., Oh, S., and Shin, I. (2013). Protein kinase B/Akt1 inhibits autophagy by down-regulating UVRAG expression. Exp. Cell Res. 319, 122–133. doi: 10.1016/j.yexcr.2012.11.014
Yang, P., Ling, L., Sun, W., Yang, J., Zhang, L., Chang, G., et al. (2018). Ginsenoside Rg1 inhibits apoptosis by increasing autophagy via the AMPK/mTOR signaling in serum deprivation macrophages. Acta Biochim. Biophys. Sin. 50, 144–155. doi: 10.1093/abbs/gmx136
Yegla, B., and Foster, T. (2019). Effect of systemic inflammation on rat attentional function and Neuroinflammation: possible protective role for food restriction. Front. Aging Neurosci. 11:296. doi: 10.3389/fnagi.2019.00296
Yen, Y.-C., Anderzhanova, E., Bunck, M., Schuller, J., Landgraf, R., and Wotjak, C. T. (2013). Co-segregation of hyperactivity, active coping styles, and cognitive dysfunction in mice selectively bred for low levels of anxiety. Front. Behav. Neurosci. 7:103. doi: 10.3389/fnbeh.2013.00103
Yen, Y.-C., Gassen, N. C., Zellner, A., Rein, T., Landgraf, R., Wotjak, C. T., et al. (2015). Glycogen synthase kinase-3β inhibition in the medial prefrontal cortex mediates paradoxical amphetamine action in a mouse model of ADHD. Front. Behav. Neurosci. 9:67. doi: 10.3389/fnbeh.2015.00067
Yildiz, O., Sismanlar, S. G., Memik, N. C., Karakaya, I., and Agaoglu, B. (2011). Atomoxetine and methylphenidate treatment in children with ADHD: the efficacy, tolerability and effects on executive functions. Child Psychiatry Hum. Dev. 42, 257–269. doi: 10.1007/s10578-010-0212-3
Yu, Q., Kou, W., Xu, X., Zhou, S., Luan, P., Xu, X., et al. (2019). FNDC5/Irisin inhibits pathological cardiac hypertrophy. Clin. Sci. Lond. Engl. 133, 611–627. doi: 10.1042/CS20190016
Zajicek, A. S., Ruan, H., Dai, H., Skolfield, M. C., Phillips, H. L., Burnette, W. J., et al. (2022). Cylindromatosis drives synapse pruning and weakening by promoting macroautophagy through Akt-mTOR signaling. Mol. Psychiatry 27, 2414–2424. doi: 10.1038/s41380-022-01571-1
Zang, Y.-F., Jin, Z., Weng, X.-C., Zhang, L., Zeng, Y.-W., Yang, L., et al. (2005). Functional MRI in attention-deficit hyperactivity disorder: evidence for hypofrontality. Brain Dev. 27, 544–550. doi: 10.1016/j.braindev.2004.11.009
Zarbakhsh, S., Safari, M., Aldaghi, M. R., Sameni, H. R., Ghahari, L., Khaleghi Lagmouj, Y., et al. (2019). Irisin protects the substantia nigra dopaminergic neurons in the rat model of Parkinson’s disease. Iran. J. Basic Med. Sci. 22, 722–728. doi: 10.22038/ijbms.2019.33444.7987
Zhang, Y., Fu, R., Sun, L., Gong, Y., and Tang, D. (2019a). How does exercise improve implicit emotion regulation ability: preliminary evidence of mind-body exercise intervention combined with aerobic jogging and mindfulness-based yoga. Front. Psychol. 10:1888. doi: 10.3389/fpsyg.2019.01888
Zhang, M., Kenny, S. J., Ge, L., Xu, K., and Schekman, R. (2015). Translocation of interleukin-1β into a vesicle intermediate in autophagy-mediated secretion. eLife 4:e11205. doi: 10.7554/eLife.11205
Zhang, Y., Wang, J., Hui, B., Sun, W., Li, B., Shi, F., et al. (2019b). Pristimerin enhances the effect of cisplatin by inhibiting the miR-23a/Akt/GSK3β signaling pathway and suppressing autophagy in lung cancer cells. Int. J. Mol. Med. 43, 1382–1394. doi: 10.3892/ijmm.2019.4057
Zhao, Y., Luo, Y., Liu, Y., Lenahan, C., Wu, Q., and Chen, S. (2022). The role of autophagy and apoptosis in early brain injury after subarachnoid hemorrhage: an updated review. Mol. Biol. Rep. 49, 10775–10782. doi: 10.1007/s11033-022-07756-9
Zhao, P., Wei, Y., Sun, G., Xu, L., Wang, T., Tian, Y., et al. (2022). Fetuin-a alleviates neuroinflammation against traumatic brain injury-induced microglial necroptosis by regulating Nrf-2/HO-1 pathway. J. Neuroinflammation 19:269. doi: 10.1186/s12974-022-02633-5
Zhuang, X., Oosting, R. S., Jones, S. R., Gainetdinov, R. R., Miller, G. W., Caron, M. G., et al. (2001). Hyperactivity and impaired response habituation in hyperdopaminergic mice. Proc. Natl. Acad. Sci. U. S. A. 98, 1982–1987. doi: 10.1073/pnas.98.4.1982
Keywords: physical exercises, ADHD, autophagy, BDNF, neuroplacticity, irisin
Citation: Abdulghani A, Poghosyan M, Mehren A, Philipsen A and Anderzhanova E (2023) Neuroplasticity to autophagy cross-talk in a therapeutic effect of physical exercises and irisin in ADHD. Front. Mol. Neurosci. 15:997054. doi: 10.3389/fnmol.2022.997054
Edited by:
Ji Hu, ShanghaiTech University, ChinaReviewed by:
Judit Zsuga, University of Debrecen, HungaryChuang Wang, Ningbo University, China
Elizabeth Hernández-Echeagaray, Biomedicine Research Unit, Faculty of Higher Education Iztacala, National Autonomous University of Mexico, Mexico
Copyright © 2023 Abdulghani, Poghosyan, Mehren, Philipsen and Anderzhanova. This is an open-access article distributed under the terms of the Creative Commons Attribution License (CC BY). The use, distribution or reproduction in other forums is permitted, provided the original author(s) and the copyright owner(s) are credited and that the original publication in this journal is cited, in accordance with accepted academic practice. No use, distribution or reproduction is permitted which does not comply with these terms.
*Correspondence: Alhasan Abdulghani, ✉ YWxhYmQxMDFAdW5pLWR1ZXNzZWxkb3JmLmRl
‡Present addresses: Elmira Anderzhanova, BAU International University, Batumi, Georgia; Centre for Strategic Planning of FMBA of Russia, Moscow, Russia
†These authors have contributed equally to this work