- 1Department of Neurophysiology and Chronobiology, Jagiellonian University, Krakow, Poland
- 2Florey Department of Neuroscience and Mental Health, The Florey Institute of Neuroscience and Mental Health, The University of Melbourne, Parkville, VIC, Australia
- 3Department of Anatomy and Physiology, The University of Melbourne, Parkville, VIC, Australia
- 4UP Medicine, Faculty of Health Sciences, CIBERSAM, ISCIII, Universitat Jaume I, Castellón de la Plana, Spain
- 5Department of Biology, University of Naples Federico II, Naples, Italy
The relaxins (RLNs) are a group of peptide hormone/neuromodulators that can regulate a wide range of physiological processes ranging from reproduction to brain function. All the family members have originated from a RLN3-like ancestor via different rounds of whole genome and gene specific duplications during vertebrate evolution. In mammals, including human, the divergence of the different family members and the emergence of new members led to the acquisition of specific functions for the various relaxin family peptide and associated receptor genes. In particular, in mammals, it was shown, that the role of RLN3 is correlated to the modulation of arousal, stress responses, emotion, social recognition, and other brain functions, positioning this gene/peptide as a potential therapeutic target for neuropsychiatric disorders. This review highlights the evolutionary conservation of relaxin family peptide and receptor gene expression and their associated brain neural circuits. In the zebrafish, the expression pattern of the different relaxin family members has specific features that are conserved in higher species, including a likely similar functional role for the ancestral RLN3-like gene. The use of different model organisms, particularly the zebrafish, to explore the diversification and conservation of relaxin family ligands and receptor systems, provides a relatively high-throughput platform to identify their specific conserved or differential neuromodulatory roles in higher species including human.
Overview of insulin-like/relaxin family peptides and receptors in mammals
Two relaxin genes (RLN1 and RLN2) have been identified in humans (Crawford et al., 1984) and higher primates (Arroyo et al., 2014), whereas other mammals have only one gene (Rln1). Other insulin-like/relaxin family member genes were subsequently identified in humans (and other species): insulin-like peptide 3 (INSL3) (Adham et al., 1993); insulin-like peptide 4 (INSL4) (Koman et al., 1996); insulin-like peptide 5 (INSL5) (Conklin et al., 1999); insulin-like peptide 6 (INSL6) (Lok et al., 2000); and the most recently discovered, relaxin-3 (RLN3) (or insulin-like peptide 7, INSL7) (Bathgate et al., 2002; Burazin et al., 2002; Tanaka et al., 2005).
It is now widely accepted that RXFP1, RXFP2, RXFP3, and RXFP4 are the cognate receptors for RLN, INSL3, RLN3, and INSL5, respectively (Hsu et al., 2002; Hsu, 2003; Liu et al., 2003; Halls et al., 2007).
Relaxin peptide is considered a pleiotropic hormone acting as a paracrine, autocrine, and endocrine factor to mediate matrix remodeling, with numerous roles in and independent of reproduction, such as vasodilator and anti-fibrotic properties (Nistri et al., 2007; Du et al., 2010; Halls et al., 2015; Samuel et al., 2017). The numerous hormonal effects of relaxin are in accordance with the broad expression of its cognate receptor, RXFP1 (see Novak et al., 2006; Giordano et al., 2012; Bathgate et al., 2013; Chen et al., 2020 for details).
A clear role in male and female reproduction has been established for INSL3/RXFP2 signaling, since both Insl3 and Rxfp2 knockout male mice display a cryptorchid phenotype (Nef and Parada, 1999; Zimmermann et al., 1999; Overbeek et al., 2001; Gorlov et al., 2002), and an essential role in ovarian follicle maturation has been described (Pelusi et al., 2013; Ivell and Anand-Ivell, 2018).
The expression of Insl5 and Rxfp4 mRNA has been detected in the pancreas, thymus, eye and in the gastrointestinal tract (Conklin et al., 1999; Grosse et al., 2014), particularly in the L-cells of distal gut (Billing et al., 2018, 2019); and INSL5 has been shown to function as an orexigenic hormone (Grosse et al., 2014), with a role in control of colonic propulsion (Diwakarla et al., 2020).
RLN3 is considered a neuropeptide in mammals, since Rln3/RLN3 mRNA/peptide is highly expressed in GABAergic neurons in the ventromedial pontine tegmentum, in an area known as the nucleus incertus (NI), and in the pontine raphe nucleus, the juxta-aqueductal ventral periaqueductal gray and the area adjacent to the substantia nigra in non-human primate, rat and mouse brain (Bathgate et al., 2002; Burazin et al., 2002; Liu et al., 2003; Tanaka et al., 2005; Ma et al., 2007, 2009; Smith et al., 2010). The cognate receptor for Rln3, Rxfp3 is widely expressed in many areas of the brain including the prefrontal and cingulate cortex, hippocampus, septum, thalamus, hypothalamus and the brainstem, and the pattern of RXFP3 expression largely overlaps NI efferent projections targets (Liu et al., 2003; Sutton et al., 2004; Smith et al., 2010). The broad expression of Rxfp3 in the brain is in line with suggested roles for Rln3/Rxfp3 signaling in modulation of stress responses, appetite, feeding and metabolism, motivation and reward, exploratory navigation, emotion (anxiety) and social recognition, memory and cognition, and sleep and circadian rhythm (Sutton et al., 2004; Gundlach et al., 2009; Smith et al., 2010; Tanaka, 2010; Callander et al., 2012; Ganella et al., 2013; Ma et al., 2013, 2017; Ryan et al., 2013; Kumar et al., 2017; de Ávila et al., 2018; Albert-Gasco et al., 2019; Kania et al., 2020).
There is little information available about the role of INSL4 and INSL6, and their target receptors are yet to be identified. Therefore, the following sections concentrate on the other relaxin family peptides, with a special focus on RLN3, a product of the ancestral relaxin family gene, as its expression and role in both fish and mammalian brain is well studied.
Insulin-like/relaxin family peptide and receptor systems in teleost fish and mammals
Based on sequence conservation, a RLN3-like gene emerged as the ancestral member of the relaxin peptide family (Wilkinson et al., 2005a,b). This hypothesis was later corroborated by studies of gene expression patterns in the developing zebrafish (Donizetti et al., 2008, 2009), that revealed a pattern of mRNA localization that closely resembled that of mammalian Rln3 mRNA in the mature brain of multiple species and developing mouse brain (Miyamoto et al., 2008). Subsequent phylogenetic, molecular evolutionary, and syntenic analyses established that teleosts contain orthologs of four relaxin family peptides (Good-Avila et al., 2009). A tripartite origin was proposed for the relaxin ligand/receptor system, whereby all vertebrate family members appear to have arisen from three genes in the ancestral vertebrate genome, one for the ligand (ancrln), and two for the receptors (ancrxfp1/2 and ancrxfp3/4) (Yegorov et al., 2014). During vertebrate evolution, the repertoire of ligands and receptors increased differently between tetrapods and teleost fish, with significantly higher retention rates of rxfp genes in the latter (Yegorov and Good, 2012).
In humans and mice, the receptor to ligand ratio is close to one, although binding promiscuity accounts for the observations that ligands can bind to multiple receptors (Bathgate et al., 2013, 2018). The co-evolution of rln/insl/rxfp peptide and receptor genes in teleosts is different, with a receptor-ligand ratio in zebrafish higher than in tetrapod species (Good et al., 2012; Alnafea et al., 2019). In teleosts, there are multiple receptors for some ligands (for comprehensive analysis of relaxin ligand-receptor phylogenetics and coevolution see Good et al., 2012; Yegorov et al., 2014). Two duplicated rln3 and insl5 paralogs are present in zebrafish together with seven rxfp3 paralogs (zebrafish lacks a rxfp4 gene, but has an additional copy of the rxfp3-3 gene, and rxfp3-3a3), while rln and insl3 are in single copy together with one copy of rxfp1 and three of rxfp2 (Good et al., 2012; Yegorov et al., 2014). Retention of such multiple copies of ligand and receptor genes may be a consequence of the sub-functionalization process and provides the opportunity to dissect the role in specific territories/processes of the mammalian ortholog.
Relaxin ligand/receptor system expression in the developing zebrafish
The study of the developmental expression pattern of relaxin family ligands and receptors in zebrafish provided some insights into their likely function in vertebrate embryogenesis, which is largely unexplored in mammals. Zebrafish possesses six genes for relaxin ligands and eleven for their putative receptors (Yegorov and Good, 2012). Most of them are expressed during embryonic development with many transcripts of maternal origin, and an expression level relatively higher at pharyngula and larval stages than early stages (Donizetti et al., 2008, 2009, 2010, 2015a,b; Fiengo et al., 2012, 2013; Venditti et al., 2018). The temporal expression pattern during zebrafish development offers an interesting insight into the evolution of duplicated genes. Regarding the paralog genes arising from the teleost-specific, third whole genome duplication, the zebrafish genome contains paralog genes for two relaxin family ligands, rln3 and insl5, and three receptors, rxfp2, rxfp3-2, and rxfp3-3. All the paralog genes display a different expression pattern, highlighting the divergence of their regulatory regions, and corroborated by their spatial expression pattern (see below).
Relaxin ligand/receptor system expression in the teleost fish brain
The distribution of rln3a expression during zebrafish development was in accordance with the embryonic expression pattern of the Rln3 gene during rat brain development (Miyamoto et al., 2008), with rln3 mRNA-expressing cells located in two bilateral columns near the fourth ventricle, which likely correspond to the nucleus incertus (NI) (Donizetti et al., 2008). Despite this conservation, some interesting differences between zebrafish and rat were also observed. In the developing and adult rat brain, Rln3 transcripts are reported to be predominantly located in the NI, whereas a smaller number of Rln3 mRNA-expressing neurons are present in other brain regions, such as periaqueductal gray (PAG), lateral substantia nigra and nucleus of the raphe pontis (Tanaka et al., 2005; Ma et al., 2007; Miyamoto et al., 2008; Blasiak et al., 2013). In zebrafish larva, rln3a gene expression appears first in the griseum centrale (GC), the homolog region of the mammalian PAG (Figure 1; Donizetti et al., 2008). The rln3b paralog gene is uniquely expressed in this territory during zebrafish brain development (Figure 1; Donizetti et al., 2009). Interestingly, the zebrafish rln gene, differently from the mammalian homolog Rln1, is expressed in the NI (Figure 1; Fiengo et al., 2012), probably as a consequence of an evolutionary conservation of regulatory elements for the expression in that territory associated with the ancestral gene. This expression pattern corroborated the hypothesis that the rln gene in fish is under different evolutionary pressures compared to the mammalian homolog gene, mimicking rln3 gene (Good-Avila et al., 2009), and highlighting the putative relevance of the rln3-like ancestral gene function for the NI.
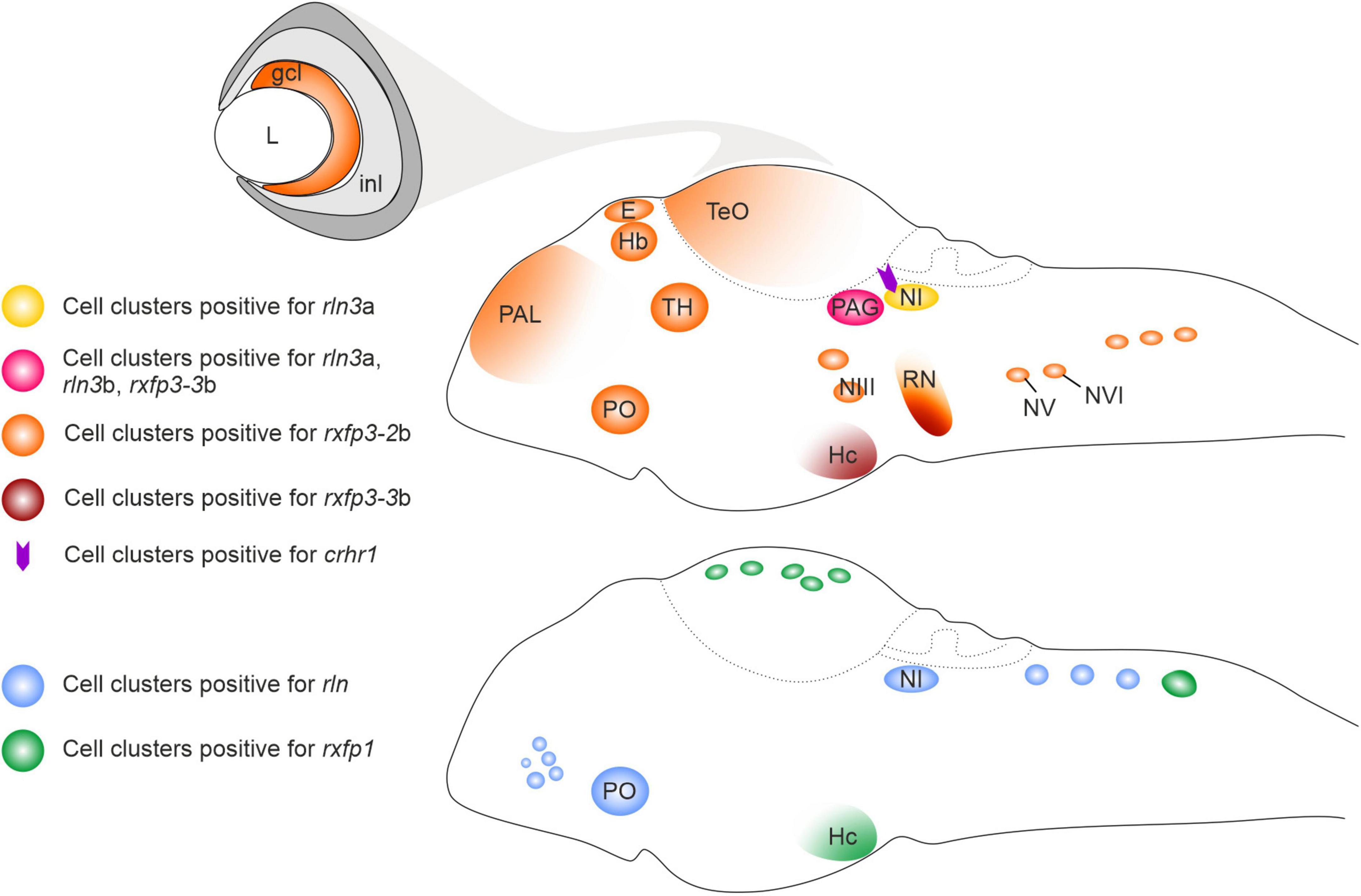
Figure 1. Schematic illustration of relaxin ligand and receptor expression patterns in the larval zebrafish brain. crhr1, corticotropin-releasing hormone type 1 receptor gene; ccl, ganglion cell layer; E, epiphysis; Hb, habenula; Hc, caudal hypothalamus; inl, inner cell layer; L, lens; NI, nucleus incertus; NIII, NIII cranial nerve nuclei; NV, NV cranial nerve nuclei; NVI, NVI cranial nerve nuclei; PAL, pallium; PAG, periaqueductal gray; PO, preoptic area; rln, relaxin; rln3a, relaxin peptide 3a gene; rln3b, relaxin peptide 3b gene; RN, raphe; rxfp1, relaxin family peptide type 1 receptor gene; rxfp3-2b, relaxin family peptide type 3 receptor 2b gene; rxfp3-3b, relaxin family peptide type 3 receptor 3b gene; TeO, optic tectum; TH, thalamus.
As predicted, relaxin family receptors display a wider expression in the developing zebrafish brain than their putative ligands (Figure 1; Donizetti et al., 2010, 2015a; Fiengo et al., 2013), highlighting an intricate network of functional connections between the different expression territories. At the larval stage, expression of rxfp1 has been observed in different brain regions, among them the post-optic area, posterior tuberculum, hypothalamus, and cell clusters in the optic tectum and the rhombencephalic region (Donizetti et al., 2010). Rxfp3-2b is the receptor gene that displayed the widest expression, with cell clusters distributed in several brain regions, such as forebrain, pallium, epiphysis, habenula, thalamic regions and optic tectum, posterior tuberculum area of hypothalamus, medulla oblongata, and cranial nerve nuclei (Fiengo et al., 2013). rxfp3-3b transcripts were detected in the PAG, raphe and hypothalamus (caudal region) at relatively high levels, while weak hybridization signals were evident in the epiphysis, preoptic area, pons region and in the posterior rhombencephalic region (Figure 1; Donizetti et al., 2015a). Overall, the expression patterns of rxfp3-2b and –3b generally overlap the expression pattern of their mammalian ortholog (Liu et al., 2005; Sutton et al., 2004; Ma et al., 2007; Smith et al., 2010).
Relaxins in the fish–functional implications
In zebrafish, rln3a is expressed in two neuron clusters belonging to the GC; and the rln3b gene is co-expressed with rln3a in one cell cluster, while rln is co-expressed with rln3a in the other cluster. The GC is already present in lampreys, the oldest currently living group of vertebrates, and the pattern of input and output connections of the GC/PAG is similar in lampreys, zebrafish and mammals (Olson et al., 2017). One of the major inputs to the GC in zebrafish, originates from the dorsal interpeduncular nucleus (dIPN), and the IPN is heavily innervated by the lateral part of the dorsal habenula (dHbL) (Okamoto et al., 2012). A very similar pattern of innervation is observed in mammals, where the medial habenula (mHb; homolog of dorsal habenula in fish) heavily innervates the IPN (Lima et al., 2017; Quina et al., 2017), and the PAG and the NI receive a strong innervation from the IPN (Goto et al., 2001; McLaughlin et al., 2017; Quina et al., 2017). Notably, the mHb-IPN pathway is one of the most evolutionarily preserved neural tracts in the forebrain from lamprey to human (Díaz et al., 2011; Stephenson-Jones et al., 2012; Villalón et al., 2012). The conservation of the GC/PAG region and its connectivity with other brain areas, suggests that a structure corresponding to the GC/PAG was present very early in vertebrate evolution and that the functions of the neuronal circuits associated with GC/PAG are similar in the vertebrate lineage.
In both fish and mammals, the GC/PAG and NI are embedded within neural circuits associated with aversion and stress-related behavior (Figure 2; Agetsuma et al., 2010; Okamoto et al., 2012; do Carmo Silva et al., 2018) and the Hb–IPN–GC/PAG/NI pathway has been suggested to control freezing and stress/aversion-related behaviors (Agetsuma et al., 2010; Yamaguchi et al., 2013). In rodents the RLN3/RXFP3 signaling system, with RLN3 neurons originating in the NI, has been implicated in the control of food intake, stress responses (including freezing), anxiety, addiction, locomotor activity, memory, and other arousal-related behaviors (Ma et al., 2013; Pereira et al., 2013; Blasiak et al., 2017; Kumar et al., 2017). Moreover, NI RLN3-synthetizing neurons have been shown to be directly sensitive to stress and arousal-related neurotransmitters, and to express corticotropin-releasing hormone type 1 receptors (CRHR1), orexin type 2 receptors (OX2), and melanin-concentrating hormone type 1 receptors (MCH1) (Blasiak et al., 2013, 2015; Ma et al., 2013, 2017; Kastman et al., 2016; Sabetghadam et al., 2018). Although these data relate to studies in rats, and the role of rln3a and rln3b expressing neurons in fish has not been verified, given the conservation of the relaxin family peptides and the predicted distribution of Rln3a and Rln3b peptides in corresponding, highly preserved structures in fish and mammalian brain (CG and PAG/NI, respectively), it is reasonable to consider that the role of these peptides is similar across these, and other vertebrate groups. Involvement of the RLN3/RXFP3 system in mammals and fish in stress-related behaviors is also supported by crhr1 expression in NI neurons in zebrafish (Donizetti et al., 2008). These data suggest, that in fish as in mammals, the RLN3/RXFP3 system may be involved in control of behavioral activity levels, and specifically in CRH/CRHR1 system-induced hyperactivity (Donizetti et al., 2008; Ma et al., 2013; Lu et al., 2020; Faught and Vijayan, 2022). Finally, the expression of rxfp3-2b in areas of fish brain that broadly overlap the expression pattern of its mammalian ortholog, RXFP3 in rodent brain (Fiengo et al., 2013; Ma et al., 2017), reinforces the proposed parallel role for the RLN3/RXFP3 system across vertebrates.
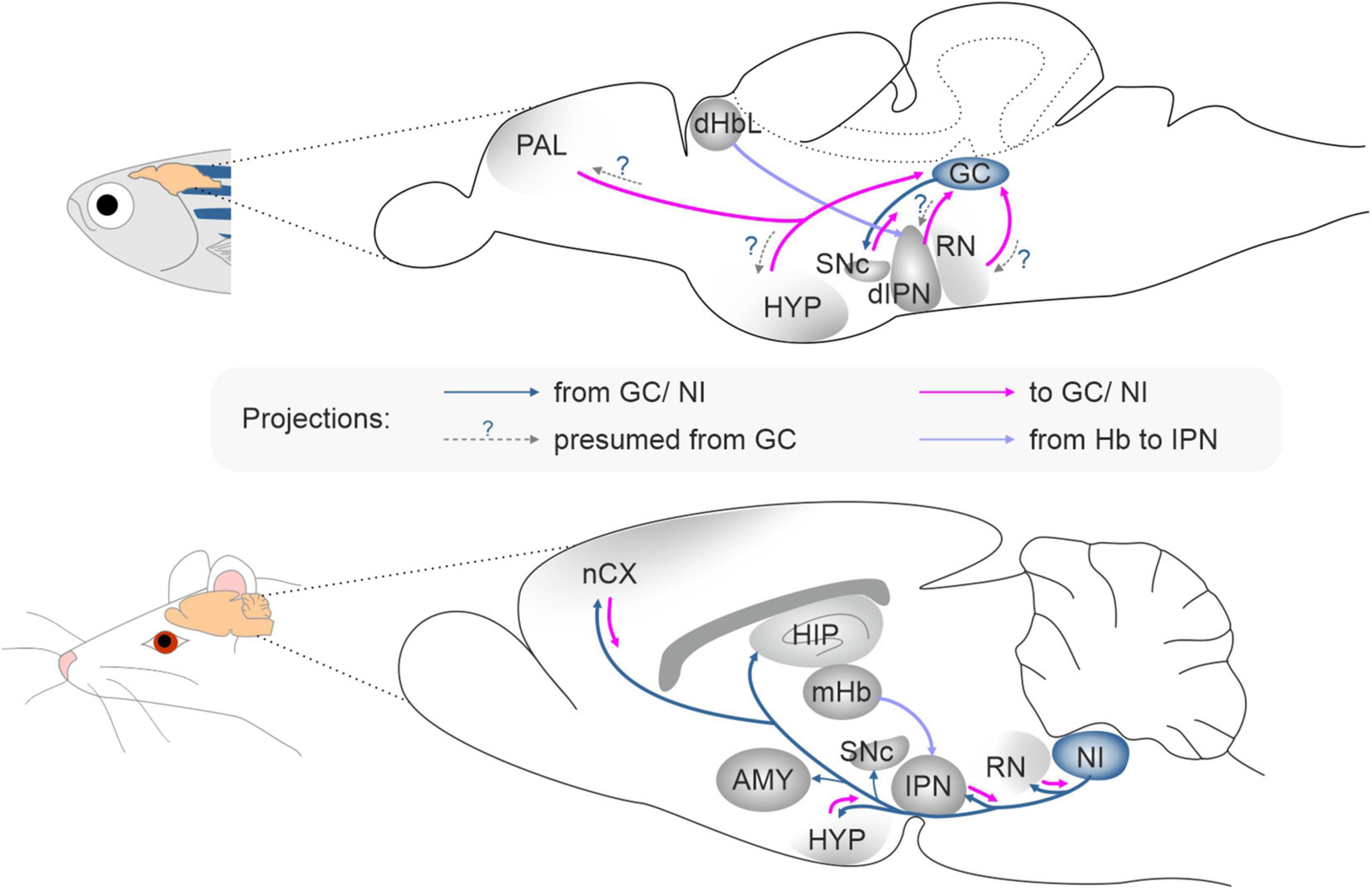
Figure 2. The connectivity pattern of the griseum centrale in zebrafish and the nucleus incertus in rat. AMY, amygdala; dHbL, dorsal habenula lateral part; dIPN, dorsal interpeduncular nucleus; GC, griseum centrale; HIP, hippocampus; HYP, hypothalamus; IPN, interpeduncular nucleus; nCX, neocortex; mHb, medial habenula; NI, nucleus incertus; PAL, pallium; RN, raphe nucleus; SNc, substantia nigra pars compacta.
Future directions
Relaxin family peptides and their receptors are crucial players in neural processes associated with survival and proper functioning in the environment, yet many questions regarding relaxinergic systems remain unanswered. The specific role and nature of relaxin family peptide signaling in structures such as amygdala, IPN, hippocampus and raphe nuclei, as well as the consequences of dysregulation of relaxin family peptides synthesis and their receptor functioning remain obscure and identify the need for expansion of current studies into new research areas using multiple techniques and tools. A high level of conservation of relaxin family peptides and their receptors, reflected by similarities in the structure of peptides and receptors, as well as their distribution in the brain in fish and mammals, makes the zebrafish an attractive experimental model for this field. Importantly, the very similar pattern of anatomical connections of structures shown to be part of the relaxinergic system in fish and mammals, is a strong predictor that these anatomical similarities are associated with functional commonalities in these groups of organisms. This particularly applies to the dHb/IPN/GC system, which in zebrafish was shown to be crucial for the control of learning, aversive behavior, directional-based decision making and social interactions (Agetsuma et al., 2010; Chou et al., 2016; Cherng et al., 2020; Palumbo et al., 2020); and is in line with data revealing that mammalian Hb/IPN/PAG/NI connections and interactions are involved in the control of the same processes (Ma et al., 2013; Pereira et al., 2013; Kumar et al., 2017).
Therefore, expanding research on relaxin peptides and their receptors in zebrafish provides a unique opportunity to answer further questions about their specific function in defined physiological processes, provide details of both anatomical and functional connections between relaxin peptide- and relaxin receptor-expressing brain structures, and determine the influence of relaxinergic system manipulations on animal behavior, including the involvement of relaxin family peptide and receptor systems in stress management. In this regard, zebrafish offers a powerful opportunity considering that the stress response system in fish is comparable to those of rodents and human. In fact, most stress-related genes show a high genetic homology between zebrafish and human/rodent analogs, together with similar neurochemical and neuroendocrine mechanisms related to physiological and behavioral stress responses (reviewed in de Abreu et al., 2021). In addition, the mammalian hypothalamic-pituitary-adrenal (HPA) axis, a well-known responder during stress, shares extensive structural and functional homologies with the hypothalamic-pituitary-interrenal (HPI) stress axis of zebrafish (Alsop and Vijayan, 2008, 2009a,b). In regard to stress research, a fruitful investigation would be the analysis of relaxin ligand/receptor gene expression under prenatal stress conditions. In particular, the zebrafish larva represents a valid complementary model to rodents (Steenbergen et al., 2011), whereby alterations in gene expression can be analyzed in relatively simple experimental paradigms (D’Agostino et al., 2019). However, one of the unresolved issues in zebrafish concerns the effective identification and localization of the various relaxin family peptides, to complement the anatomical expression of their corresponding mRNA species and provide a more complete picture of expression pattern regulation of these peptide/receptor systems. In addition, the profile of binding and activation of the receptors by key ligands also needs to be determined to gain information on the pairing relationships between ligands and receptors and to better elucidate the role of the different relaxins.
In terms of functional studies, the zebrafish offers a powerful platform to readily conduct loss-of-function studies and explore possible genetic compensation by paralog genes (reviewed in Salanga and Salanga, 2021). Currently, CRISPR/Cas9 technology represents a mature approach to generate gene knockout lines, and can be integrated with strategies to generate conditional KO lines (Hans et al., 2021), and it is potentially able to precisely label different alleles and follow the genotype of each allele and each cell (Li et al., 2019). A major advantage of using the zebrafish for functional studies of the relaxinergic system is the opportunity to focus on the larval stage when expression of the relaxin ligand/receptor genes is restricted to specific territories, but still largely reflects the expression in the adult zebrafish brain. Studies at this developmental stage are strategic considering that many methods can be applied in a high-throughput fashion, such as an automated system that can monitor and quantify multiple behavioral features in different experimental paradigms, including sleep (Tran and Prober, 2022) and drug effects (see e.g., Barros et al., 2008; Cornet et al., 2018). Therefore, these methods combined with genetic manipulations could accelerate the discovery of the roles of relaxinergic systems in zebrafish brain and help identify possible therapeutic approaches for clinical neuropsychiatric conditions.
Author contributions
AB and AD proposed the topic of the manuscript. AB, AG, ALG, FA, and AD reviewed the literature. AB, AG, and AD wrote the original draft. ALG and FO-B critically edited and revised the manuscript. AB, AG, ALG, and AD conducted the final editing. All authors approved the submitted version.
Funding
Research by FA and AD was supported by funds from University of Naples Federico II, “Ricerca Dipartimentale.” AD was supported by “Programma di scambi internazionali per mobilità di breve durata—anno 2017” from University of Naples Federico II. Research by AB and AG was supported by research grants from the National Science Centre, Poland (UMO-2018/30/E/NZ4/00687 to AB and UMO-2017/27/N/NZ4/01545 to AG). Research by FO-B was supported by the “Plan Nacional sobre Drogas” of the Spanish Ministry of Health Grant number 2020I012 and Spanish Ministry of Science and Innovation grant number RTI2018-095698-B-I00.
Conflict of interest
The authors declare that the research was conducted in the absence of any commercial or financial relationships that could be construed as a potential conflict of interest.
Publisher’s note
All claims expressed in this article are solely those of the authors and do not necessarily represent those of their affiliated organizations, or those of the publisher, the editors and the reviewers. Any product that may be evaluated in this article, or claim that may be made by its manufacturer, is not guaranteed or endorsed by the publisher.
References
Adham, I. M., Burkhardt, E., Benahmed, M., and Engel, W. (1993). Cloning of a cDNA for a novel insulin-like peptide of the testicular Leydig cells. J. Biol. Chem. 268, 26668–26672. doi: 10.1016/S0021-9258(19)74364-6
Agetsuma, M., Aizawa, H., Aoki, T., Nakayama, R., Takahoko, M., Goto, M., et al. (2010). The habenula is crucial for experience-dependent modification of fear responses in zebrafish. Nat. Neurosci. 13, 1354–1356. doi: 10.1038/nn.2654
Albert-Gasco, H., Sanchez-Sarasua, S., Ma, S., García-Díaz, C., Gundlach, A. L., Sanchez-Perez, A. M., et al. (2019). Central relaxin-3 receptor (RXFP3) activation impairs social recognition and modulates ERK-phosphorylation in specific GABAergic amygdala neurons. Brain Struct. Funct. 224, 453–469. doi: 10.1007/s00429-018-1763-5
Alnafea, H., Vahkal, B., Zelmer, C. K., Yegorov, S., Bogerd, J., and Good, S. V. (2019). Japanese medaka as a model for studying the relaxin family genes involved in neuroendocrine regulation: Insights from the expression of fish-specific rln3 and insl5 and rxfp3/4-type receptor paralogues. Mol. Cell Endocrinol. 487, 2–11. doi: 10.1016/j.mce.2019.01.017
Alsop, D., and Vijayan, M. (2009a). The zebrafish stress axis: molecular fallout from the teleost-specific genome duplication event. Gen. Comp. Endocrinol. 161, 62–66. doi: 10.1016/j.ygcen.2008.09.011
Alsop, D., and Vijayan, M. M. (2008). Development of the corticosteroid stress axis and receptor expression in zebrafish. Am. J. Physiol. Regul. Integr. Comp. Physiol. 294, R711–R719. doi: 10.1152/ajpregu.00671.2007
Alsop, D., and Vijayan, M. M. (2009b). Molecular programming of the corticosteroid stress axis during zebrafish development. Comp. Biochem. Physiol. A Mol. Integr. Physiol. 153, 49–54. doi: 10.1016/j.cbpa.2008.12.008
Arroyo, J. I., Hoffmann, F. G., and Opazo, J. C. (2014). Evolution of the relaxin/insulin-like gene family in anthropoid primates. Genome Biol. Evol. 6, 491–499. doi: 10.1093/gbe/evu023
Barros, T. P., Alderton, W. K., Reynolds, H. M., Roach, A. G., and Berghmans, S. (2008). Zebrafish: an emerging technology for in vivo pharmacological assessment to identify potential safety liabilities in early drug discovery. Br. J. Pharmacol. 154, 1400–1413. doi: 10.1038/bjp.2008.249
Bathgate, R. A., Halls, M. L., van der Westhuizen, E. T., Callander, G. E., Kocan, M., and Summers, R. J. (2013). Relaxin family peptides and their receptors. Physiol. Rev. 93, 405–480. doi: 10.1152/physrev.00001.2012
Bathgate, R. A., Samuel, C. S., Burazin, T. C., Layfield, S., Claasz, A. A., Reytomas, I. G., et al. (2002). Human relaxin gene 3 (H3) and the equivalent mouse relaxin (M3) gene Novel members of the relaxin peptide family. J. Biol. Chem. 277, 1148–1157. doi: 10.1074/jbc.M107882200
Bathgate, R. A. D., Kocan, M., Scott, D. J., Hossain, M. A., Good, S. V., Yegorov, S., et al. (2018). The relaxin receptor as a therapeutic target – perspectives from evolution and drug targeting. Pharmacol. Ther. 187, 114–132. doi: 10.1016/j.pharmthera.2018.02.008
Billing, L. J., Larraufie, P., Lewis, J., Leiter, A., Li, J., Lam, B., et al. (2019). Single cell transcriptomic profiling of large intestinal enteroendocrine cells in mice – Identification of selective stimuli for insulin-like peptide-5 and glucagon-like peptide-1 co-expressing cells. Mol. Metab. 29, 158–169. doi: 10.1016/j.molmet.2019.09.001
Billing, L. J., Smith, C. A., Larraufie, P., Goldspink, D. A., Galvin, S., Kay, R. G., et al. (2018). Co-storage and release of insulin-like peptide-5, glucagon-like peptide-1 and peptide YY from murine and human colonic enteroendocrine cells. Mol. Metab. 16, 65–75. doi: 10.1016/j.molmet.2018.07.011
Blasiak, A., Blasiak, T., Lewandowski, M. H., Hossain, M. A., Wade, J. D., and Gundlach, A. L. (2013). Relaxin-3 innervation of the intergeniculate leaflet of the rat thalamus – neuronal tract-tracing and in vitro electrophysiological studies. Eur. J. Neurosci. 37, 1284–1294. doi: 10.1111/ejn.12155
Blasiak, A., Gundlach, A. L., Hess, G., and Lewandowski, M. H. (2017). Interactions of circadian rhythmicity, stress and orexigenic neuropeptide systems: Implications for food intake control. Front. Neurosci. 20:127. doi: 10.3389/fnins.2017.00127
Blasiak, A., Siwiec, M., Grabowiecka, A., Blasiak, T., Czerw, A., Blasiak, E., et al. (2015). Excitatory orexinergic innervation of rat nucleus incertus – Implications for ascending arousal, motivation and feeding control. Neuropharmacology 99, 432–447. doi: 10.1016/j.neuropharm.2015.08.014
Burazin, T. C., Bathgate, R. A., Macris, M., Layfield, S., Gundlach, A. L., and Tregear, G. W. (2002). Restricted, but abundant, expression of the novel rat gene-3 (R3) relaxin in the dorsal tegmental region of brain. J. Neurochem. 82, 1553–1557. doi: 10.1046/j.1471-4159.2002.01114.x
Callander, G. E., Ma, S., Ganella, D. E., Wimmer, V. C., Gundlach, A. L., Thomas, W. G., et al. (2012). Silencing relaxin-3 in nucleus incertus of adult rodents: a viral vector-based approach to investigate neuropeptide function. PLoS One 7:e42300. doi: 10.1371/journal.pone.0042300
Chen, T. Y., Li, X., Hung, C. H., Bahudhanapati, H., Tan, J., Kass, D. J., et al. (2020). The relaxin family peptide receptor 1 (RXFP1): An emerging player in human health and disease. Mol. Genet. Genomic Med. 8:e1194. doi: 10.1002/mgg3.1194
Cherng, B. W., Islam, T., Torigoe, M., Tsuboi, T., and Okamoto, H. (2020). The dorsal lateral habenula-interpeduncular nucleus pathway is essential for left-right-dependent decision making in zebrafish. Cell Rep. 32:108143. doi: 10.1016/j.celrep.2020.108143
Chou, M. Y., Amo, R., Kinoshita, M., Cherng, B. W., Shimazaki, H., Agetsuma, M., et al. (2016). Social conflict resolution regulated by two dorsal habenular subregions in zebrafish. Science 352, 87–90. doi: 10.1126/science.aac9508
Conklin, D., Lofton-Day, C. E., Haldeman, B. A., Ching, A., Whitmore, T. E., Lok, S., et al. (1999). Identification of INSL5, a new member of the insulin superfamily. Genomics 60, 50–56. doi: 10.1006/geno.1999.5899
Cornet, C., Di Donato, V., and Terriente, J. (2018). Combining zebrafish and CRISPR/Cas9: Toward a more efficient drug discovery pipeline. Front. Pharmacol. 9:703. doi: 10.3389/fphar.2018.00703
Crawford, R. J., Hudson, P., Shine, J., Niall, H. D., Eddy, R. L., and Shows, T. B. (1984). Two human relaxin genes are on chromosome 9. EMBO J. 3, 2341–2345. doi: 10.1002/j.1460-2075.1984.tb02136.x
D’Agostino, S., Testa, M., Aliperti, V., Venditti, M., Minucci, S., Aniello, F., et al. (2019). Expression pattern dysregulation of stress- and neuronal activity-related genes in response to prenatal stress paradigm in zebrafish larvae. Cell Stress Chaperones. 24, 1005–1012. doi: 10.1007/s12192-019-01017-8
de Abreu, M. S., Demin, K. A., Giacomini, A. C. V. V., Amstislavskaya, T. G., Strekalova, T., Maslov, G. O., et al. (2021). Understanding how stress responses and stress-related behaviors have evolved in zebrafish and mammals. Neurobiol. Stress 15:100405. doi: 10.1016/j.ynstr.2021.100405
de Ávila, C., Chometton, S., Lenglos, C., Calvez, J., Gundlach, A. L., and Timofeeva, E. (2018). Differential effects of relaxin-3 and a selective relaxin-3 receptor agonist on food and water intake and hypothalamic neuronal activity in rats. Behav. Brain Res. 336, 135–144. doi: 10.1016/j.bbr.2017.08.044
Díaz, E., Bravo, D., Rojas, X., and Concha, M. L. (2011). Morphologic and immunohistochemical organization of the human habenular complex. J. Comp. Neurol. 519, 3727–3747. doi: 10.1002/cne.22687
Diwakarla, S., Bathgate, R. A. D., Zhang, X., Hossain, M. A., and Furness, J. B. (2020). Colokinetic effect of an insulin-like peptide 5-related agonist of the RXFP4 receptor. Neurogastroenterol. Motil. 32:e13796. doi: 10.1111/nmo.13796
do Carmo Silva, R. X., Lima-Maximino, M. G., and Maximino, C. (2018). The aversive brain system of teleosts: Implications for neuroscience and biological psychiatry. Neurosci. Biobehav. Rev. 95, 123–135. doi: 10.1016/j.neubiorev.2018.10.001
Donizetti, A., Fiengo, M., del Gaudio, R., Di Giaimo, R., Minucci, S., and Aniello, F. (2010). Characterization and developmental expression pattern of the relaxin receptor rxfp1 gene in zebrafish. Dev. Growth Differ. 52, 799–806. doi: 10.1111/j.1440-169X.2010.01215.x
Donizetti, A., Fiengo, M., Iazzetti, G., del Gaudio, R., Di Giaimo, R., Pariante, P., et al. (2015a). Expression analysis of five zebrafish RXFP3 homologues reveals evolutionary conservation of gene expression pattern. J. Exp. Zool. B. Mol. Dev. Evol. 324, 22–29. doi: 10.1002/jez.b.22591
Donizetti, A., Fiengo, M., del Gaudio, R., Iazzetti, G., Pariante, P., Minucci, S., et al. (2015b). Expression pattern of zebrafish rxfp2 homologue genes during embryonic development. J. Exp. Zool. B. Mol. Dev. Evol. 324, 605–613. doi: 10.1002/jez.b.22637
Donizetti, A., Fiengo, M., Minucci, S., and Aniello, F. (2009). Duplicated zebrafish relaxin-3 gene shows a different expression pattern from that of the co-orthologue gene. Dev. Growth Differ. 51, 715–722. doi: 10.1111/j.1440-169X.2009.01131.x
Donizetti, A., Grossi, M., Pariante, P., D’Aniello, E., Izzo, G., Minucci, S., et al. (2008). Two neuron clusters in the stem of postembryonic zebrafish brain specifically express relaxin-3 gene: first evidence of nucleus incertus in fish. Dev. Dyn 237, 3864–3869. doi: 10.1002/dvdy.21786
Du, X. J., Bathgate, R. A., Samuel, C. S., Dart, A. M., and Summers, R. J. (2010). Cardiovascular effects of relaxin: from basic science to clinical therapy. Nat. Rev. Cardiol. 7, 48–58. doi: 10.1038/nrcardio.2009.198
Faught, E., and Vijayan, M. M. (2022). Coordinated action of corticotropin-releasing hormone and cortisol shapes the acute stress-induced behavioural response in zebrafish. Neuroendocrinology 112, 74–87. doi: 10.1159/000514778
Fiengo, M., del Gaudio, R., Iazzetti, G., Di Giaimo, R., Minucci, S., Aniello, F., et al. (2013). Developmental expression pattern of two zebrafish rxfp3 paralogue genes. Dev. Growth Differ. 55, 766–775. doi: 10.1111/dgd.12093
Fiengo, M., Donizetti, A., del Gaudio, R., Minucci, S., and Aniello, F. (2012). Characterization, cDNA cloning and expression pattern of relaxin gene during embryogenesis of Danio rerio. Dev. Growth Differ. 54, 579–587. doi: 10.1111/j.1440-169X.2012.01361.x
Ganella, D. E., Callander, G. E., Ma, S., Bye, C. R., Gundlach, A. L., and Bathgate, R. A. (2013). Modulation of feeding by chronic rAAV expression of a relaxin-3 peptide agonist in rat hypothalamus. Gene Ther. 20, 703–716. doi: 10.1038/gt.2012.83
Giordano, N., Volpi, N., Franci, D., Corallo, C., Fioravanti, A., Papakostas, P., et al. (2012). Expression of RXFP1 in skin of scleroderma patients and control subjects. Scand. J. Rheumatol. 41, 391–395. doi: 10.3109/03009742.2012.669496
Good, S., Yegorov, S., Martijn, J., Franck, J., and Bogerd, J. (2012). New insights into ligand-receptor pairing and coevolution of relaxin family peptides and their receptors in teleosts. Int. J. Evol. Biol. 2012:310278. doi: 10.1155/2012/310278
Good-Avila, S. V., Yegorov, S., Harron, S., Bogerd, J., Glen, P., Ozon, J., et al. (2009). Relaxin gene family in teleosts: phylogeny, syntenic mapping, selective constraint, and expression analysis. BMC Evol. Biol. 9:293. doi: 10.1186/1471-2148-9-293
Gorlov, I. P., Kamat, A., Bogatcheva, N. V., Jones, E., Lamb, D. J., Truong, A., et al. (2002). Mutations of the GREAT gene cause cryptorchidism. Hum. Mol. Genet. 11, 2309–2318. doi: 10.1093/hmg/11.19.2309
Goto, M., Swanson, L. W., and Canteras, N. S. (2001). Connections of the nucleus incertus. J. Comp. Neurol. 438, 86–122. doi: 10.1002/cne.1303
Grosse, J., Heffron, H., Burling, K., Akhter Hossain, M., Habib, A. M., Rogers, G. J., et al. (2014). Insulin-like peptide 5 is an orexigenic gastrointestinal hormone. Proc. Natl. Acad. Sci. U.S.A. 111, 11133–11138. doi: 10.1073/pnas.1411413111
Gundlach, A. L., Ma, S., Sang, Q., Shen, P. J., Piccenna, L., Sedaghat, K., et al. (2009). Relaxin family peptides and receptors in mammalian brain. Ann. N Y Acad. Sci. 1160, 226–235. doi: 10.1111/j.1749-6632.2009.03956.x
Halls, M. L., Bathgate, R. A., Sutton, S. W., Dschietzig, T. B., and Summers, R. J. (2015). International Union of Basic and Clinical Pharmacology XCV. Recent advances in the understanding of the pharmacology and biological roles of relaxin family peptide receptors 1-4, the receptors for relaxin family peptides. Pharmacol. Rev. 67, 389–440. doi: 10.1124/pr.114.009472
Halls, M. L., van der Westhuizen, E. T., Bathgate, R. A., and Summers, R. J. (2007). Relaxin family peptide receptors – former orphans reunite with their parent ligands to activate multiple signalling pathways. Br. J. Pharmacol. 150, 677–691. doi: 10.1038/sj.bjp.0707140
Hans, S., Zöller, D., Hammer, J., Stucke, J., Spieß, S., Kesavan, G., et al. (2021). Cre-controlled CRISPR mutagenesis provides fast and easy conditional gene inactivation in zebrafish. Nat. Commun. 12:1125. doi: 10.1038/s41467-021-21427-6
Hsu, S. Y. (2003). New insights into the evolution of the relaxin-LGR signaling system. Trends Endocrinol. Metab. 14, 303–309. doi: 10.1016/s1043-2760(03)00106-1
Hsu, S. Y., Nakabayash, I. K., Nishi, S., Kumagai, J., Kudo, M., Sherwood, O. D., et al. (2002). Activation of orphan receptors by the hormone relaxin. Science 295, 671–674. doi: 10.1126/science.1065654
Ivell, R., and Anand-Ivell, R. (2018). Insulin-like peptide 3 (INSL3) is a major regulator of female reproductive physiology. Hum. Reprod. Update 24, 639–651. doi: 10.1093/humupd/dmy029
Kania, A., Szlaga, A., Sambak, P., Gugula, A., Blasiak, E., Micioni, et al. (2020). RLN3/RXFP3 signaling in the PVN inhibits magnocellular neurons via M-like current activation and contributes to binge eating behavior. J. Neurosci. 40, 5362–5375. doi: 10.1523/JNEUROSCI.2895-19.2020
Kastman, H. E., Blasiak, A., Walker, L., Siwiec, M., Krstew, E. V., Gundlach, A. L., et al. (2016). Nucleus incertus orexin-2 receptors mediate alcohol seeking in rats. Neuropharmacology 110, 82–91. doi: 10.1016/j.neuropharm.2016.07.006
Koman, A., Cazaubon, S., Couraud, P. O., Ullrich, A., and Strosberg, A. D. (1996). Molecular characterization and in vitro biological activity of placentin, a new member of the insulin gene family. J. Biol. Chem. 271, 20238–20241. doi: 10.1074/jbc.271.34.20238
Kumar, J. R., Rajkumar, R., Jayakody, T., Marwari, S., Hong, J. M., Ma, S., et al. (2017). Relaxin’ the brain: a case for targeting the nucleus incertus network and relaxin-3/RXFP3 system in neuropsychiatric disorders. Br. J. Pharmacol. 174, 1061–1076. doi: 10.1111/bph.13564
Li, W., Zhang, Y., Han, B., Li, L., Li, M., Lu, X., et al. (2019). One-step efficient generation of dual-function conditional knockout and geno-tagging alleles in zebrafish. Elife 8:e48081. doi: 10.7554/eLife.48081
Lima, L. B., Bueno, D., Leite, F., Souza, S., Gonçalves, L., Furigo, I. C., et al. (2017). Afferent and efferent connections of the interpeduncular nucleus with special reference to circuits involving the habenula and raphe nuclei. J. Comp. Neurol. 525, 2411–2442. doi: 10.1002/cne.24217
Liu, C., Chen, J., Kuei, C., Sutton, S., Nepomuceno, D., Bonaventure, P., et al. (2005). Relaxin-3/insulin-like peptide 5 chimeric peptide, a selective ligand for G protein-coupled receptor (GPCR)135 and GPCR142 over leucine-rich repeat-containing G protein-coupled receptor 7. Mol. Pharmacol. 67, 231–240. doi: 10.1124/mol.104.006700
Liu, C., Eriste, E., Sutton, S., Chen, J., Roland, B., Kuei, C., et al. (2003). Identification of relaxin-3/INSL7 as an endogenous ligand for the orphan G-protein-coupled receptor GPCR135. J. Biol. Chem. 278, 50754–50764. doi: 10.1074/jbc.M308995200
Lok, S., Johnston, D. S., Conklin, D., Lofton-Day, C. E., Adams, R. L., Jelmberg, A. C., et al. (2000). Identification of INSL6, a new member of the insulin family that is expressed in the testis of the human and rat. Biol. Reprod. 62, 1593–1599. doi: 10.1095/biolreprod62.6.1593
Lu, L., Ren, Y., Yu, T., Liu, Z., Wang, S., Tan, L., et al. (2020). Control of locomotor speed, arousal, and hippocampal theta rhythms by the nucleus incertus. Nat. Commun. 11:262. doi: 10.1038/s41467-019-14116-y
Ma, S., Blasiak, A., Olucha-Bordonau, F. E., Verberne, A. J., and Gundlach, A. L. (2013). Heterogeneous responses of nucleus incertus neurons to corticotrophin-releasing factor and coherent activity with hippocampal theta rhythm in the rat. J. Physiol. 591, 3981–4001. doi: 10.1113/jphysiol.2013.254300
Ma, S., Bonaventure, P., Ferraro, T., Shen, P. J., Burazin, T. C., Bathgate, R. A., et al. (2007). Relaxin-3 in GABA projection neurons of nucleus incertus suggests widespread influence on forebrain circuits via G-protein-coupled receptor-135 in the rat. Neuroscience 144, 165–190. doi: 10.1016/j.neuroscience.2006.08.072
Ma, S., Sang, Q., Lanciego, J. L., and Gundlach, A. L. (2009). Localization of relaxin-3 in brain of Macaca fascicularis: identification of a nucleus incertus in primate. J. Comp. Neurol. 517, 856–872. doi: 10.1002/cne.22197
Ma, S., Smith, C. M., Blasiak, A., and Gundlach, A. L. (2017). Distribution, physiology and pharmacology of relaxin-3/RXFP3 systems in brain. Br. J. Pharmacol. 174, 1034–1048. doi: 10.1111/bph.13659
McLaughlin, I., Dani, J. A., and De Biasi, M. (2017). The medial habenula and interpeduncular nucleus circuitry is critical in addiction, anxiety, and mood regulation. J. Neurochem. 142, 130–143. doi: 10.1111/jnc.14008
Miyamoto, Y., Watanabe, Y., and Tanaka, M. (2008). Developmental expression and serotonergic regulation of relaxin 3/INSL7 in the nucleus incertus of rat brain. Regul. Pept. 145, 54–59. doi: 10.1016/j.regpep.2007.08.010
Nef, S., and Parada, L. F. (1999). Cryptorchidism in mice mutant for Insl3. Nat. Genet. 22, 295–299. doi: 10.1038/10364
Nistri, S., Bigazzi, M., and Bani, D. (2007). Relaxin as a cardiovascular hormone: physiology, pathophysiology and therapeutic promises. Cardiovasc. Hematol. Agents Med. Chem. 5, 101–108. doi: 10.2174/187152507780363179
Novak, J., Parry, L. J., Matthews, J. E., Kerchner, L. J., Indovina, K., Hanley-Yanez, K., et al. (2006). Evidence for local relaxin ligand-receptor expression and function in arteries. FASEB J. 20, 2352–2362. doi: 10.1096/fj.06-6263com
Okamoto, H., Agetsuma, M., and Aizawa, H. (2012). Genetic dissection of the zebrafish habenula, a possible switching board for selection of behavioral strategy to cope with fear and anxiety. Dev. Neurobiol. 72, 386–394. doi: 10.1002/dneu.20913
Olson, I., Suryanarayana, S. M., Robertson, B., and Grillner, S. (2017). Griseum centrale, a homologue of the periaqueductal gray in the lamprey. IBRO Rep. 2, 24–30. doi: 10.1016/j.ibror.2017.01.001
Overbeek, P. A., Gorlov, I. P., Sutherland, R. W., Houston, J. B., Harrison, W. R., Boettger-Tong, H. L., et al. (2001). A transgenic insertion causing cryptorchidism in mice. Genesis 30, 26–35. doi: 10.1002/gene.1029
Palumbo, F., Serneels, B., Pelgrims, R., and Yaksi, E. (2020). The zebrafish dorsolateral habenula is required for updating learned behaviors. Cell Rep. 32:108054. doi: 10.1016/j.celrep.2020.108054
Pelusi, C., Fanelli, F., Pariali, M., Zanotti, L., Gambineri, A., and Pasquali, R. (2013). Parallel variations of insulin-like peptide 3 (INSL3) and antimüllerian hormone (AMH) in women with the polycystic ovary syndrome according to menstrual cycle pattern. J. Clin. Endocrinol. Metab. 98, E1575–E1582. doi: 10.1210/jc.2013-1107
Pereira, C. W., Santos, F. N., Sánchez-Pérez, A. M., Otero-García, M., Marchioro, M., Ma, S., et al. (2013). Electrolytic lesion of the nucleus incertus retards extinction of auditory conditioned fear. Behav. Brain Res. 247, 201–210. doi: 10.1016/j.bbr.2013.03.025
Quina, L. A., Harris, J., Zeng, H., and Turner, E. E. (2017). Specific connections of the interpeduncular subnuclei reveal distinct components of the habenulopeduncular pathway. J. Comp. Neurol. 525, 2632–2656. doi: 10.1002/cne.24221
Ryan, P. J., Büchler, E., Shabanpoor, F., Hossain, M. A., Wade, J. D., Lawrence, A. J., et al. (2013). Central relaxin-3 receptor (RXFP3) activation decreases anxiety- and depressive-like behaviours in the rat. Behav. Brain Res. 244, 142–151. doi: 10.1016/j.bbr.2013.01.034
Sabetghadam, A., Grabowiecka-Nowak, A., Kania, A., Gugula, A., Blasiak, E., Blasiak, T., et al. (2018). Melanin-concentrating hormone and orexin systems in rat nucleus incertus: Dual innervation, bidirectional effects on neuron activity, and differential influences on arousal and feeding. Neuropharmacology 139, 238–256. doi: 10.1016/j.neuropharm.2018.07.004
Salanga, C. M., and Salanga, M. C. (2021). Genotype to phenotype: CRISPR gene editing reveals genetic compensation as a mechanism for phenotypic disjunction of morphants and mutants. Int. J. Mol. Sci. 22:3472. doi: 10.3390/ijms22073472
Samuel, C. S., Royce, S. G., Hewitson, T. D., Denton, K. M., Cooney, T. E., and Bennett, R. G. (2017). Anti-fibrotic actions of relaxin. Br. J. Pharmacol. 174, 962–976. doi: 10.1111/bph.13529
Smith, C. M., Shen, P. J., Banerjee, A., Bonaventure, P., Ma, S., Bathgate, R. A., et al. (2010). Distribution of relaxin-3 and RXFP3 within arousal, stress, affective, and cognitive circuits of mouse brain. J. Comp. Neurol. 518, 4016–4045. doi: 10.1002/cne.22442
Steenbergen, P. J., Richardson, M. K., and Champagne, D. L. (2011). The use of the zebrafish model in stress research. Prog. Neuropsychopharmacol. Biol. Psychiatry 35, 1432–1451. doi: 10.1016/j.pnpbp.2010.10.010
Stephenson-Jones, M., Floros, O., Robertson, B., and Grillner, S. (2012). Evolutionary conservation of the habenular nuclei and their circuitry controlling the dopamine and 5-hydroxytryptophan (5-HT) systems. Proc. Natl. Acad. Sci. U.S.A. 109, E164–E173. doi: 10.1073/pnas.1119348109
Sutton, S. W., Bonaventure, P., Kuei, C., Roland, B., Chen, J., Nepomuceno, D., et al. (2004). Distribution of G-protein-coupled receptor (GPCR)135 binding sites and receptor mRNA in the rat brain suggests a role for relaxin-3 in neuroendocrine and sensory processing. Neuroendocrinology 80, 298–307. doi: 10.1159/000083656
Tanaka, M. (2010). Relaxin-3/insulin-like peptide 7, a neuropeptide involved in the stress response and food intake. FEBS J. 277, 4990–4997. doi: 10.1111/j.1742-4658.2010.07931.x
Tanaka, M., Iijima, N., Miyamoto, Y., Fukusumi, S., Itoh, Y., Ozawa, H., et al. (2005). Neurons expressing relaxin 3/INSL 7 in the nucleus incertus respond to stress. Eur. J. Neurosci. 21, 1659–1670. doi: 10.1111/j.1460-9568.2005.03980.x
Tran, S., and Prober, D. A. (2022). Validation of candidate sleep disorder risk genes using zebrafish. Front. Mol. Neurosci. 15:873520. doi: 10.3389/fnmol.2022.873520
Venditti, M., Donizetti, A., Fiengo, M., Fasano, C., Santillo, A., Aniello, F., et al. (2018). Temporal and spatial expression of insulin-like peptide (insl5a and insl5b) paralog genes during the embryogenesis of Danio rerio. J. Exp. Zool. B Mol. Dev. Evol. 330, 33–40. doi: 10.1002/jez.b.22787
Villalón, A., Sepúlveda, M., Guerrero, N., Meynard, M. M., Palma, K., and Concha, M. L. (2012). Evolutionary plasticity of habenular asymmetry with a conserved efferent connectivity pattern. PLoS One 7:e35329. doi: 10.1371/journal.pone.0035329
Wilkinson, T. N., Speed, T. P., Tregear, G. W., and Bathgate, R. A. (2005a). Evolution of the relaxin-like peptide family. BMC Evol. Biol. 5:14. doi: 10.1186/1471-2148-5-14
Wilkinson, T. N., Speed, T. P., Tregear, G. W., and Bathgate, R. A. (2005b). Evolution of the relaxin-like peptide family: from neuropeptide to reproduction. Ann. N Y Acad. Sci. 1041, 530–533. doi: 10.1196/annals.1282.079
Yamaguchi, T., Danjo, T., Pastan, I., Hikida, T., and Nakanishi, S. (2013). Distinct roles of segregated transmission of the septo-habenular pathway in anxiety and fear. Neuron 78, 537–544. doi: 10.1016/j.neuron.2013.02.035
Yegorov, S., Bogerd, J., and Good, S. V. (2014). The relaxin family peptide receptors and their ligands: new developments and paradigms in the evolution from jawless fish to mammals. Gen. Comp. Endocrinol. 209, 93–105. doi: 10.1016/j.ygcen.2014.07.014
Yegorov, S., and Good, S. (2012). Using paleogenomics to study the evolution of gene families: origin and duplication history of the relaxin family hormones and their receptors. PLoS One 7:e32923. doi: 10.1371/journal.pone.0032923
Keywords: griseum centrale, neuropsychiatric disorders, nucleus incertus, relaxins, stress response, zebrafish
Citation: Blasiak A, Gugula A, Gundlach AL, Olucha-Bordonau FE, Aniello F and Donizetti A (2022) Relaxin ligand/receptor systems in the developing teleost fish brain: Conserved features with mammals and a platform to address neuropeptide system functions. Front. Mol. Neurosci. 15:984524. doi: 10.3389/fnmol.2022.984524
Received: 02 July 2022; Accepted: 16 September 2022;
Published: 05 October 2022.
Edited by:
Jose G. Ortiz, University of Puerto Rico, Puerto RicoReviewed by:
Timothy Lynagh, University of Bergen, NorwayGuillermo Romero, University of Pittsburgh, United States
Daniele Bani, University of Florence, Italy
Copyright © 2022 Blasiak, Gugula, Gundlach, Olucha-Bordonau, Aniello and Donizetti. This is an open-access article distributed under the terms of the Creative Commons Attribution License (CC BY). The use, distribution or reproduction in other forums is permitted, provided the original author(s) and the copyright owner(s) are credited and that the original publication in this journal is cited, in accordance with accepted academic practice. No use, distribution or reproduction is permitted which does not comply with these terms.
*Correspondence: Aldo Donizetti, YWxkby5kb25pemV0dGlAdW5pbmEuaXQ=