- 1Department of Physiology and Biophysics, University of California, Irvine, Irvine, CA, United States
- 2Department of Pharmaceutical Sciences, University of California, Irvine, Irvine, CA, United States
- 3Department of Biomedical Engineering, University of California, Irvine, Irvine, CA, United States
- 4Department of Neurobiology and Behavior, University of California, Irvine, Irvine, CA, United States
- 5Center for the Neurobiology of Learning and Memory, University of California, Irvine, Irvine, CA, United States
- 6UCI Mind, University of California, Irvine, Irvine, CA, United States
Dysfunction in dopamine (DA) signaling contributes to neurological disorders ranging from drug addiction and schizophrenia to depression and Parkinson’s Disease. How might impairment of one neurotransmitter come to effect these seemingly disparate diseases? One potential explanation is that unique populations of DA-releasing cells project to separate brain regions that contribute to different sets of behaviors. Though dopaminergic cells themselves are spatially restricted to the midbrain and constitute a relatively small proportion of all neurons, their projections influence many brain regions. DA is particularly critical for the activity and function of medial prefrontal cortical (mPFC) ensembles. The midbrain and mPFC exhibit reciprocal connectivity – the former innervates the mPFC, and in turn, the mPFC projects back to the midbrain. Viral mapping studies have helped elucidate the connectivity within and between these regions, which likely have broad implications for DA-dependent behaviors. In this review, we discuss advancements in our understanding of the connectivity between the mPFC and midbrain DA system, focusing primarily on rodent models.
Introduction
The prefrontal cortex (PFC) is critical for a variety of executive functions, including motivation, attention, decision-making, inhibitory control, and memory (Toshiyuki and Goldman-Rakic, 1991; Miller, 1999; Bechara et al., 2000; Ridderinkhof et al., 2004; Euston et al., 2012; Laubach et al., 2015). In rodents, deficits in PFC signaling have been linked to impaired performance on memory acquisition and consolidation (Bontempi et al., 1999; Tronel and Sara, 2003). Likewise, deficits in homologous regions in primates, such as the dorsolateral PFC and anterior cingulate cortices, interfere with memory and decision making (Amiez et al., 2006; Oyama et al., 2021).
Proper functioning of the medial prefrontal cortical (mPFC) hinges upon a delicate balance of neuromodulator input. Among these, the most well-characterized neuromodulators include acetylcholine (ACh), serotonin (5-HT), norepinephrine (NE), and dopamine (DA), each of which exerts important and varied effects on mPFC function. For example, disruptions to cholinergic input from the basal forebrain have detrimental outcomes on attention (Robbins et al., 1989; Pang et al., 1993; Voytko et al., 1994; McGaughy et al., 2002; Dalley, 2004; Newman and McGaughy, 2008; Hasselmo and Sarter, 2011), whereas impaired serotonergic innervation from the medial and dorsal raphe nuclei has opposing effects (Schmitt et al., 2000; Gallagher et al., 2003; Wingen et al., 2007). In contrast, NE and DA appear to have synergistic effects – prior studies have suggested that prefrontal cortical NE transmission is necessary for DA release in the mPFC (Ventura et al., 2003, 2005, 2007), and DA can be taken up through the NE transporter (Sesack et al., 1998; Morón et al., 2002). Disruptions in the transmission of either neuromodulator lead to impairments in working memory (Brozoski et al., 1979; Williams and Goldman-Rakic, 1995; Arnsten, 2004; Ramos and Arnsten, 2007; Vijayraghavan et al., 2007; Levy, 2009; Arnsten and Jin, 2014). Altogether, the differential input of these neuromodulators likely helps to fine-tune prefrontal processing in a context-dependent manner.
Each of these four neuromodulators innervate overlapping but distinct spatial domains of the mPFC, with significant anatomical differences across species (Berger et al., 1991; Coppola et al., 2018). Both factors likely impact subsequent behavioral differences in the effects of different neuromodulators. For example, locus coeruleus (LC) NE neurons in the brainstem broadly target the entire cerebral cortex and serve as the main source of NE transmission to the mPFC. DA innervation, on the other hand, is much more spatially restricted, with midbrain ventral tegmental area (VTA) cells primarily innervating the mPFC. Expansion of this dopaminergic midbrain-to-cortical, or “mesocortical pathway,” in higher-order mammals is thought to play a role in the increasing complexity of decision-making skills found in higher-order mammals. Primates exhibit larger, re-organized terminal fields, different co-localization of neuropeptides, and developmental differences in circuit maturation relative to rodents (Berger et al., 1991). In this mini-review, we will focus primarily on viral mapping studies linking the mesocortical DA system and its downstream target, the mPFC.
Role of dopamine in the medial prefrontal cortex
Of the major dopaminergic pathways in the brain, the mesocorticolimbic system, which comprises the mesocortical and mesolimbic system, is a key regulator of motivation, reward, and aversive behavioral responses. The mesolimbic pathway, which transmits DA from the midbrain to the ventral striatum, has historically garnered more attention from the scientific community; its role in reward-related cognition is well-characterized. In contrast, the mesocortical limb is less well-understood, but has been implicated in behavioral responses to aversive cues (Thierry et al., 1976; Abercrombie et al., 1989; Mantz et al., 1989) and therefore serves as a promising target for understanding how integration of different environmental cues can influence behavioral outcomes in the face of aversive stimuli.
Dopamine signaling within the mPFC has been shown to play a role in a variety of neuropsychiatric disorders, such as schizophrenia, post-traumatic stress disorder, and attention deficit hyperactivity disorder (Deutch and Young, 1995; Okubo et al., 1997; Lindström et al., 1999; Granon et al., 2000; Arnsten and Dudley, 2005; Howes and Kapur, 2009; Pitman et al., 2012; Grace, 2016; Lee et al., 2016). However, defining the exact signals carried by DA in the mPFC has proven to be complicated, as studies have shown that mesocortical DA cells are activated by both aversive and rewarding stimuli (Thierry et al., 1976; Mantz et al., 1989; Finlay et al., 1995; Ahn and Phillips, 1999; Bassareo et al., 2002; Lammel et al., 2011; St. Onge et al., 2012; Kim et al., 2016; Ellwood et al., 2017). Moreover, while the overall anatomy of the dopaminergic system is well-preserved across all mammals and birds, there are differences between species that may give rise to species-specific functions. Given the heterogeneity of cell types, neurotransmitters, receptor expression, and projections within both the mPFC and ventral midbrain, careful mapping of each structure may provide insight into how communication with one another regulates behavior.
Features of VTADA→mPFC cells
Midbrain DA cells project to a variety of forebrain sites, including the nucleus accumbens (NAc), dorsal striatum, amygdala, and mPFC. In each output region, DA appears to facilitate distinct behavioral adaptations. One potential mechanism for the heterogeneity in output responses is that each output region comprises a unique combination of the five possible DA receptors. Another possibility is that separate populations of VTADA cells project to each of these forebrain sites. Studies examining DA release indicate that DA release in each of these regions is different, favoring the latter hypothesis. For example, while DA release in the NAc primarily occurs in response to rewarding stimuli, DA release in the mPFC is largely a response to aversive stimuli or stressful events (Abercrombie et al., 1989; Bassareo et al., 2002; Lammel et al., 2014). Using retrobead injections into multiple forebrain sites, retrograde mapping studies labeled distinct groups of cells in the VTA that could be defined by their projection sites, further reinforcing this hypothesis (Lammel et al., 2008). In addition to being identified by their output sites, these VTA cells have unique electrophysiological properties. DA cells projecting to the mPFC, for example, are (1) located in the medial aspect of the VTA, (2) have lower expression levels of the dopamine transporter, DAT, than DA cells projecting to the nucleus accumbens lateral shell (NAcLat) or dorsal striatum, (3) have broad action potential waveforms with no after-hyperpolarizations and a relatively high maximal firing frequency (∼25 Hz), and (4) do not express D2 autoreceptors (Lammel et al., 2008). Relative to DA cells projecting to the NAcLat, mPFC-projecting VTADA cells receive preferential inputs from the lateral habenula (LHb); moreover, activation of LHb inputs to the VTA resulted in conditioned place aversion that could be reversed by infusion of the D1 antagonist SCH23390 (Lammel et al., 2012). In addition, VTADA→mPFC cells were not significantly activated in mice that received a reward but were activated following an aversive tail shock, lending further support toward the hypothesis that VTADA→mPFC cells signal aversion (Kim et al., 2016).
Recently, we performed comprehensive input-output viral-genetic mapping experiments of VTADA subpopulations, including the mPFC. In contrast to the previous retrograde mapping strategy, we retrogradely targeted VTADA→mPFC cells using an intersectional viral-genetic strategy and labeled neurons, including their entire axonal arbors, with GFP. Consistent with previous studies, we found that VTADA→mPFC cells largely had distinct arborization patterns relative to other VTADA subpopulations. However, we did observe significant overlap, predominantly with VTADA cells projecting to the medial shell of the nucleus accumbens (NAcMed) and amygdala (Beier et al., 2019). Altogether, our results indicated that VTADA cells projecting to the mPFC, NAcMed, and amygdala share a closer set of outputs with one another than with VTADA→NAcLat cells. Interestingly, this also applies to the gene expression and electrophysiological properties of these cells, as identified previously (Lammel et al., 2008).
When assessing the global input patterns of VTADA→mPFC cells, we found that they were highly similar to that of VTADA→NAcMed and VTADA→amygdala cells, further reinforcing the similarity of these cells (Beier et al., 2015). A deeper dive into the input-output connectivity of each VTADA subpopulation suggested that VTADA→mPFC cells receive preferential inputs from the dorsal raphe (DR) relative to other VTADA subtypes (Derdeyn et al., 2022). However, a logistic regression model found that the identity of VTADA→mPFC cells could not be predicted well by inputs, indicating that these cells did not have a highly unique global input pattern relative to the other VTADA subpopulations. Instead, the identity of VTADA→mPFC cells could be decoded relatively well by their location in the dorsomedial aspect of the VTA (Derdeyn et al., 2022). This means that while VTADA→mPFC cells share a high degree of similarity with VTADA→NAcMed and VTADA→amygdala cells, they possess several distinctive features, such as their preferential response to aversive stimuli and lack of D2 autoreceptors.
Species differences between rodents and primates in VTADA→mPFC cells
Relative to the VTA, the PFC is dramatically expanded in primates compared to rodents. Most of this increase stems from the expansion of sensory association cortices, which is thought to have contributed to higher-level information processing and therefore more complex behavioral outcomes (Pandya and Yeterian, 1996). Most neuroanatomists agree that the human PFC can be divided into the dorsolateral (dlPFC), ventrolateral (vlPFC), dorsomedial (dmPFC), ventromedial (vmPFC), anterior cingulate cortex (ACC), and orbitofrontal (OFC) regions, with some debate over the topographic mapping of subdivisions onto various Brodmann areas. Though the precise mapping of specific prefrontal nuclei found in rodent models onto the primate brain is still unclear, most agree the mPFC in rodents comprises the ACC, prelimbic cortex (PL), and infralimbic cortex (IL), though some studies also include the secondary motor cortex (M2) (Figure 1).
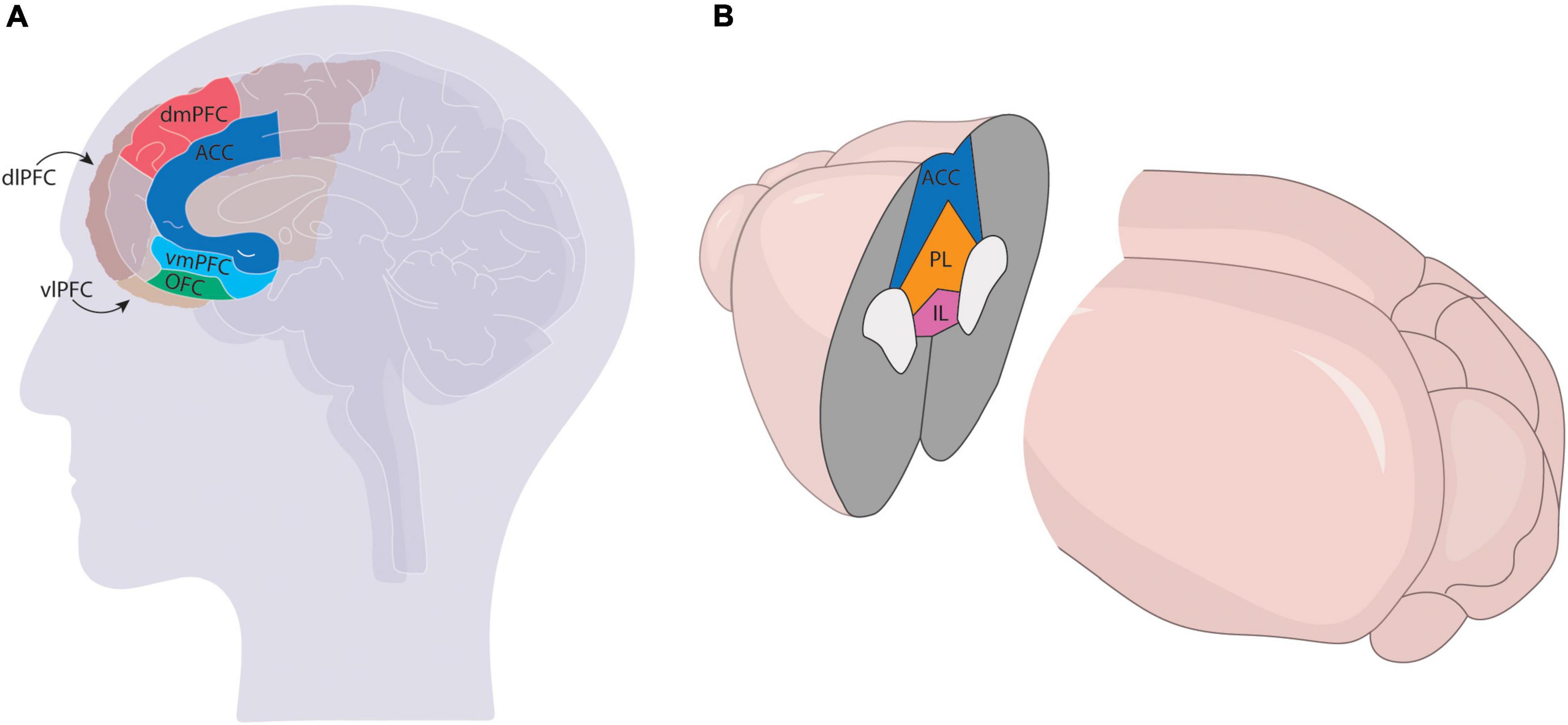
Figure 1. Comparison of mPFC in the primate (A) and rodent (B) brains. The mPFC has more subdivisions in primates [ventrolateral (vlPFC), ventromedial (vmPFC) dorsolateral (dlPFC), dorsomedial (dmPFC), anterior cingulate (ACC), and orbitofrontal (OFC)] and comprises a larger region of the cortex than the rodent brain, which generally only comprises the infralimbic (IL), prelimbic (PL), and ACC regions.
Though dopaminergic innervation of the cortex shares some similar features across mammalian species, the laminar distribution and extent of innervation varies significantly, in a manner that is potentially suggestive of higher-level cognitive capacity. For example, studies in cats, monkeys, and humans report a similar bilaminar pattern of D1-specific binding of [3H]SCH23390 in the cerebral cortex that is absent in rats (Lidow et al., 1991), though overall dopaminergic innervation of the cortex appears more homogenous across cortical layers in primates relative to rodents (Williams and Goldman-Rakic, 1993; vander Weele et al., 2018). Laminar distribution of receptors also varies across species, with cortical layer 1 expressing high dopaminergic receptor density in primates but not in rodents. In addition, primates exhibit denser dopaminergic innervation relative to rodents throughout the brain (Thierry et al., 1973; Descarries et al., 1987; Lewis and Sesack, 1997; Bentivoglio and Morelli, 2005). Regions such as the motor, premotor, and supplementary motor area are highly innervated in primates whereas the equivalent regions in rats receive little to no dopaminergic projections (Berger et al., 1991).
Reciprocal connectivity from the medial prefrontal cortex to the midbrain
Circuit mapping studies have long pointed to reciprocal connectivity between the VTA and mPFC (Lindvall et al., 1984; Sesack and Pickel, 1992; Carr and Sesack, 1998). However, it remains unclear whether mPFC cells that receive input from VTADA cells are modulated by the same mesocortical dopamine neurons to which they project. Previous electron microscopy work examining inputs from the mPFC in the VTA of rat brains suggested that the mPFC sent inputs onto VTADA→mPFC cells and VTAGABA→NAc cells, but not VTADA→NAc cells (Carr and Sesack, 2000). However, our viral-genetic mapping experiments have found the opposite to be true – the mPFC preferentially provides input onto VTADA→NAcLat cells. We observed this bias toward VTADA→NAcLat cells to be true for anterior cortex inputs generally, as well as the mPFC specifically (Beier et al., 2015, 2019). This could be because VTADA→NAcLat and VTADA→mPFC cells are located in different areas of the VTA that were incompletely sampled in the Carr and Sesack study (Lammel et al., 2008; Beier et al., 2015), whereas RABV mapping samples broader areas of the VTA. It is also possible that whereas electron microscopy is a powerful method, it is less well-suited for large-scale connectivity analysis than RABV-based mapping. Furthermore, stimulation of frontal cortex inputs to the midbrain was reinforcing, and this reinforcement was blocked by infusion of the D1/D2 non-selective antagonist flupentixol in NAcLat, indicating that the functional output of this circuit was to trigger DA release into the NAc and not the mPFC (Beier et al., 2015). This result was supported by a separate study demonstrating that activating inputs from the frontal cortex to the midbrain was locomotor-activating and led to DA release in the striatum, and that this effect could be blocked by the D2 receptor antagonist haloperidol (Kim et al., 2015). Moreover, burst stimulation from VTADA neurons does not alter the mean firing rate of mPFC→VTA neurons, further suggesting that these neurons may not receive direct modulation from VTADA neurons (Au-Young et al., 1999). Unlike mPFC neurons, which exhibit sustained increases during the delay period of a classical delay task, primate A10 (VTA in primates) cells increased in firing rate only during the presentation of the cue light (Ljungberg et al., 1991; Schultz et al., 1993), suggesting that local mechanisms and not DA release by VTADA→mPFC cells may be responsible for modulation of mPFC neurons. While this does not rule out ultrastructural connections between the mPFC and VTADA→mPFC cells that were not detected by RABV mapping, that the behavioral results align with our viral-genetic mapping approach indicates that mPFC activation functionally leads to DA release in the NAc and is reinforcing.
Medial prefrontal cortex cell inputs
Several studies have mapped the input landscape onto different sets of mPFC cells. DeNardo et al. (2015) mapped and compared inputs to layer 5 neurons between the mPFC and barrel cortex. Both regions shared a similar distribution of local inputs, though mPFC L5 neurons received both a greater proportion of inputs from layer 1 vs. layer 3 cells as well as approximately 2.5-fold greater inputs from local GABAergic interneurons than comparable cells in the barrel cortex. This increased interneuron presence suggests that mPFC L5 cells are under stronger inhibitory control – potentially important in providing feedforward inhibition onto L5 output cells.
While their local input distribution may have been similar, the long-range input connectivity of the mPFC and barrel cortex differed substantially. L5 cells in the barrel cortex received about 79% of their total inputs from local neurons, whereas the majority of inputs to mPFC L5 cells arose from non-local brain sites such as other prefrontal areas including the agranular insula, and the dorsal thalamus subcortical regions such as the amygdala and hypothalamus. In total, roughly ∼60% of inputs to the mPFC L5 cells arise from cortical inputs, though these are distributed across the frontal cortex and in the contralateral hemisphere.
More recently, Ährlund-Richter et al. (2019) mapped inputs to four different cell types in the mPFC: three interneuron types (parvalbumin, somatostatin, VIP), as well as mostly excitatory neurons defined by the expression of the CamKII promoter. As in the DeNardo study, the majority of inputs to the mPFC arose from the cortex, with additional significant input from the thalamus and hypothalamus. As is typical of viral mapping studies examining inputs from intermingled cell types, the authors observed that inputs arose from largely overlapping regions providing quantitatively similar input. However, the Ährlund-Richter study noted a much higher proportion of inputs from other PFC sites than DeNardo’s (approximately ∼50% compared to ∼18%, respectively). This discrepancy could potentially be attributed to the former’s viral strategy, which may have resulted in off-target labeling of neurons near the injection site, while the DeNardo paper used a version of TVA that limits off-target labeling in the absence of Cre recombination. Therefore, while the two studies largely agree on the identity of regions that provide direct input to the mPFC – which are consistent with older studies using classical tracers (Hoover and Vertes, 2007) – they bring into question the precise quantitative contribution of these input regions to different cell types. However, while the Ährlund-Richter study included an analysis of laminar distribution for local GABAergic interneuron interconnectivity, they did not perform the same analysis when mapping whole-brain inputs to the mPFC. This is a significant omission, as it may explain a relatively high fraction of the variance in inputs, as observed in other comparable studies (DeNardo et al., 2015; Wall et al., 2016).
Ventral tegmental area interconnectivity
While local microcircuit connectivity onto VTADA→mPFC cells has not yet been mapped, we recently performed an analysis that allowed us to link local inputs to putative VTADA→mPFC cells by examining brain regions with similar variance across brains quantified for RABV labeling (Beier, 2022). Our results indicated that VTADA→mPFC cells receive preferential inputs relative to other VTADA cells from several midline structures – the interpeduncular nucleus (IPN), raphe magnus, and supramammillary nucleus – as well as other regions, such as the superior colliculus, anterior tegmental nucleus, pedunculopontine tegmental nucleus, pontine reticular nucleus, reticulotegmental nucleus, microcellular tegmental nucleus, retrorubal field, and dorsal nucleus of the lateral lemniscus. Notably, this list includes inputs from neither the VTA nor the rostrotegmental nucleus, which contain GABA neurons thought to oppose the action of reward-related DA cells. Though we also identified robust DA-DA interconnectivity in the VTA, VTADA→mPFC cells lack D2 autoreceptors and thus are unlikely to be strongly influenced by DA release from other DA cells. However, at this point, little is known about how local inputs to VTADA→mPFC cells may contribute to their unique function.
Open questions
There remain many unanswered questions about the role of midbrain and mPFC connections in adaptive and pathological behaviors. For one, while some of the studies discussed in this review have attempted to define the input landscape to different mPFC subpopulations, their findings were not entirely in agreement (DeNardo et al., 2015; Ährlund-Richter et al., 2019). A more careful and comprehensive analysis of mPFC cell types that includes starter cell spatial information could help resolve these discrepancies. Furthermore, it remains unclear how input connectivity to the mPFC relates to the outputs of different mPFC subpopulations. We recently developed a method, Tracing the Relationships between Inputs and Outputs (TRIO) that could be used to map the inputs to output-defined mPFC cells (Beier et al., 2015; Lerner et al., 2015; Schwarz et al., 2015).
While further connectivity mapping would certainly help define the link between the mPFC and VTA, to understand how DA influences mPFC outputs, we also need to consider the potential impact of DA receptor heterogeneity in different mPFC cell populations. All DA receptors (D1-D5) are found in the mPFC with varying levels of expression. While the broad expression patterns of DA receptors have been mapped, their relation to subtypes of glutamatergic and GABAergic neurons remains unclear. Combining viral mapping studies with single nucleus RNA sequencing could help illuminate the constellation of DA receptor expression in the mPFC. Moreover, while the mesocortical pathway is thought to be the source of DA in the mPFC, it is possible DA may also be released from NE cells in the locus coeruleus – a phenomenon recently reported in the hippocampus (Takeuchi et al., 2016). Because mesocortical DA cells largely innervate deeper layers of the mPFC whereas NE cells innervate more superficial layers, DA release from NE cells would expand the direct influence of DA throughout the mPFC.
Author contributions
MH and KB wrote the manuscript. Both authors contributed to the article and approved the submitted version.
Funding
This work was supported by the NIH (R00 DA041445 and DP2 AG067666), Tobacco Related Disease Research Program (T31KT1437 and T31P1426), Alzheimer’s Association (AARG-NTF-20-685694), and New Vision Research (CCAD2020-002) to KB and T31DT1729 and T32GM008620 to MH.
Conflict of interest
The authors declare that the research was conducted in the absence of any commercial or financial relationships that could be construed as a potential conflict of interest.
Publisher’s note
All claims expressed in this article are solely those of the authors and do not necessarily represent those of their affiliated organizations, or those of the publisher, the editors and the reviewers. Any product that may be evaluated in this article, or claim that may be made by its manufacturer, is not guaranteed or endorsed by the publisher.
References
Abercrombie, E. D., Keefe, K. A., DiFrischia, D. S., and Zigmond, M. J. (1989). Differential effect of stress on in vivo dopamine release in striatum, nucleus accumbens, and medial frontal cortex. J. Neurochem. 52, 1655–1658. doi: 10.1111/j.1471-4159.1989.tb09224.x
Ahn, S., and Phillips, A. G. (1999). Dopaminergic correlates of sensory-specific satiety in the medial prefrontal cortex and nucleus accumbens of the rat. J. Neurosci. 19, 1–6. doi: 10.1523/jneurosci.19-19-j0003.1999
Ährlund-Richter, S., Xuan, Y., van Lunteren, J. A., Kim, H., Ortiz, C., Pollak Dorocic, I., et al. (2019). A whole-brain atlas of monosynaptic input targeting four different cell types in the medial prefrontal cortex of the mouse. Nat. Neurosci. 22, 657–668. doi: 10.1038/s41593-019-0354-y
Amiez, C., Joseph, J. P., and Procyk, E. (2006). Reward encoding in the monkey anterior cingulate cortex. Cereb. Cortex 16, 1040–1055. doi: 10.1093/cercor/bhj046
Arnsten, A. F. T. (2004). Adrenergic targets for the treatment of cognitive deficits in schizophrenia. Psychopharmacology 174, 25–31. doi: 10.1007/s00213-003-1724-3
Arnsten, A. F. T., and Dudley, A. G. (2005). Methylphenidate improves prefrontal cortical cognitive function through α2 adrenoceptor and dopamine D1 receptor actions: relevance to therapeutic effects in Attention Deficit Hyperactivity Disorder. Behav. Brain Funct. 1, 1–9. doi: 10.1186/1744-9081-1-2
Arnsten, A. F. T., and Jin, L. E. (2014). Molecular influences on working memory circuits in dorsolateral prefrontal cortex. Prog. Mol. Biol. Transl. Sci. 122, 211–231. doi: 10.1016/B978-0-12-420170-5.00008-8
Au-Young, S. M. W., Shen, H., and Yang, C. R. (1999). Medial prefrontal cortical output neurons to the ventral tegmental area (VTA) and their responses to burst-patterned stimulation of the VTA: neuroanatomical and in vivo electrophysiological analyses. Synapse 34, 245–255.
Bassareo, V., De Luca, M. A., and Di Chiara, G. (2002). Differential expression of motivational stimulus properties by dopamine in nucleus accumbens shell versus core and prefrontal cortex. J. Neurosci. 22, 4709–4719. doi: 10.1523/jneurosci.22-11-04709.2002
Bechara, A., Tranel, D., and Damasio, H. (2000). Characterization of the decision-making deficit of patients with ventromedial prefrontal cortex lesions. Brain 123, 2189–2202. doi: 10.1093/brain/123.11.2189
Beier, K. T. (2022). Modified viral-genetic mapping reveals local and global connectivity relationships of ventral tegmental area dopamine cells. bioRxiv [Preprint]. doi: 10.1101/2022.01.18.476718
Beier, K. T., Gao, X. J., Xie, S., DeLoach, K. E., Malenka, R. C., and Luo, L. (2019). Topological organization of ventral tegmental area connectivity revealed by viral-genetic dissection of input-output relations. Cell Rep. 26, 159.e6–167.e6. doi: 10.1016/j.celrep.2018.12.040
Beier, K. T., Steinberg, E. E., Deloach, K. E., Xie, S., Miyamichi, K., Schwarz, L., et al. (2015). Circuit architecture of VTA dopamine neurons revealed by systematic input-output mapping. Cell 162, 622–634. doi: 10.1016/j.cell.2015.07.015
Bentivoglio, M., and Morelli, M. (2005). Chapter I The organization and circuits of mesencephalic dopaminergic neurons and the distribution of dopamine receptors in the brain. Handb. Chem. Neuroanat. 21, 1–107. doi: 10.1016/S0924-8196(05)80005-3
Berger, B., Gaspar, P., and Verney, C. (1991). Dopaminergic innervation of the cerebral cortex: unexpected differences between rodents and primates. Trends Neurosci. 14, 21–27. doi: 10.1016/0166-2236(91)90179-x
Bontempi, B., Laurent-Demir, C., Destrade, C., and Jaffard, R. (1999). Time-dependent reorganization of brain circuitry underlying long-term memory storage. Nature 400, 671–675. doi: 10.1038/23270
Brozoski, T. J., Brown, R. M., Rosvold, H. E., and Goldman, P. S. (1979). Cognitive deficit caused by regional depletion of dopamine in prefrontal cortex of rhesus monkey. Science 205, 929–932. doi: 10.1126/science.112679
Carr, D. B., and Sesack, S. R. (1998). Callosal terminals in the rat prefrontal cortex: synaptic targets and association with GABA-immunoreactive structures. Synapse 29, 193–205.
Carr, D. B., and Sesack, S. R. (2000). Projections from the rat prefrontal cortex to the ventral tegmental area: target specificity in the synaptic associations with mesoaccumbens and mesocortical neurons. J. Neurosci. 20, 3864–3873.
Coppola, G., di Renzo, A., Tinelli, E., di Lorenzo, C., Scapeccia, M., Parisi, V., et al. (2018). Resting state connectivity between default mode network and insula encodes acute migraine headache. Cephalalgia 38, 846–854. doi: 10.1177/0333102417715230
Dalley, J. W. (2004). Cortical cholinergic function and deficits in visual attentional performance in rats following 192 IgG-saporin-induced lesions of the medial prefrontal cortex. Cerebr. Cortex 14, 922–932. doi: 10.1093/cercor/bhh052
DeNardo, L. A., Berns, D. S., DeLoach, K., and Luo, L. (2015). Connectivity of mouse somatosensory and prefrontal cortex examined with trans-synaptic tracing. Nat. Neurosci. 84, 778–789. doi: 10.1038/nn.4131
Derdeyn, P., Hui, M., Macchia, D., and Beier, K. (2022). Uncovering the connectivity logic of the ventral tegmental area. Front. Neural Circ. 15:799688.
Descarries, L., Lemay, B., Doucet, G., and Berger, B. (1987). Regional and laminar density of the dopamine innervation in adult rat cerebral cortex. Neuroscience 21, 807–824. doi: 10.1016/0306-4522(87)90038-8
Deutch, A. Y., and Young, C. D. (1995). “A model of the stress-induced activation of prefrontal cortical dopamine systems: coping and the development of post-traumatic stress disorder,” in Neurobiological and Clinical Consequences of Stress: From Normal Adaptation to Post-Traumatic Stress Disorder, eds M. J. Friedman, D. S. Charney, and A. Y. Deutch (Philadelphia, PA: Lippincott Williams & Wilkins Publishers), 163–175.
Ellwood, I. T., Patel, T., Wadia, V., Lee, A. T., Liptak, A. T., Bender, K. J., et al. (2017). Tonic or phasic stimulation of dopaminergic projections to prefrontal cortex causes mice to maintain or deviate from previously learned behavioral strategies. J. Neurosci. 37, 8315–8329. doi: 10.1523/JNEUROSCI.1221-17.2017
Euston, D. R., Gruber, A. J., and McNaughton, B. L. (2012). The role of medial prefrontal cortex in memory and decision making. Neuron 76, 1057–1070. doi: 10.1016/j.neuron.2012.12.002
Finlay, J. M., Zigmond, M. J., and Abercrombie, E. D. (1995). Increased dopamine and norepinephrine release in medial prefrontal cortex induced by acute and chronic stress: effects of diazepam. Neuroscience 64, 619–628. doi: 10.1016/0306-4522(94)00331-X
Gallagher, P., Massey, A. E., Young, A. H., and McAllister-Williams, R. H. (2003). Effects of acute tryptophan depletion on executive function in healthy male volunteers. BMC Psychiatry 3:10. doi: 10.1186/1471-244X-3-10
Grace, A. A. (2016). Dysregulation of the dopamine system in the pathophysiology of schizophrenia and depression. Nat. Rev. Neurosci. 17, 524–532. doi: 10.1038/nrn.2016.57
Granon, S., Passetti, F., Thomas, K. L., Dalley, J. W., Everitt, B. J., and Robbins, T. W. (2000). Enhanced and impaired attentional performance after infusion of D1 dopaminergic receptor agents into rat prefrontal cortex. J. Neurosci. 20, 1208–1215. doi: 10.1523/jneurosci.20-03-01208.2000
Hasselmo, M. E., and Sarter, M. (2011). Modes and models of forebrain cholinergic neuromodulation of cognition. Neuropsychopharmacology 36, 52–73. doi: 10.1038/npp.2010.104
Hoover, W. B., and Vertes, R. P. (2007). Anatomical analysis of afferent projections to the medial prefrontal cortex in the rat. Brain Struct. Funct. 212, 149–179. doi: 10.1007/s00429-007-0150-4
Howes, O. D., and Kapur, S. (2009). The dopamine hypothesis of schizophrenia: version III - The final common pathway. Schizophr. Bull. 35, 549–562. doi: 10.1093/schbul/sbp006
Kim, C. K., Yang, S. J., Pichamoorthy, N., Young, N. P., Kauvar, I., Jennings, J. H., et al. (2016). Simultaneous fast measurement of circuit dynamics at multiple sites across the mammalian brain. Nat. Methods 13, 325–328. doi: 10.1038/nmeth.3770
Kim, I. H., Rossi, M. A., Aryal, D. K., Racz, B., Kim, N., Uezu, A., et al. (2015). Spine pruning drives antipsychotic-sensitive locomotion via circuit control of striatal dopamine. Nat. Neurosci. 18, 883–891. doi: 10.1038/nn.4015
Lammel, S., Hetzel, A., Häckel, O., Jones, I., Liss, B., and Roeper, J. (2008). Unique properties of mesoprefrontal neurons within a dual mesocorticolimbic dopamine system. Neuron 57, 760–773. doi: 10.1016/j.neuron.2008.01.022
Lammel, S., Ion, D. I., Roeper, J., and Malenka, R. C. (2011). Report projection-specific modulation of dopamine neuron synapses by aversive and rewarding stimuli. Neuron 70, 855–862. doi: 10.1016/j.neuron.2011.03.025
Lammel, S., Lim, B. K., and Malenka, R. C. (2014). Reward and aversion in a heterogeneous midbrain dopamine system. Neuropharmacology 76, 351–359. doi: 10.1016/j.neuropharm.2013.03.019
Lammel, S., Lim, B. K., Ran, C., Huang, K. W., Betley, M. J., Tye, K. M., et al. (2012). Input-specific control of reward and aversion in the ventral tegmental area. Nature 491, 212–217. doi: 10.1038/nature11527
Laubach, M., Caetano, M. S., and Narayanan, N. S. (2015). Mistakes were made: neural mechanisms for the adaptive control of action initiation by the medial prefrontal cortex. J. Physiol. Paris 109, 104–117. doi: 10.1016/j.jphysparis.2014.12.001
Lee, J. C., Wang, L. P., and Tsien, J. Z. (2016). Dopamine rebound-excitation theory: putting brakes on PTSD. Front. Psychiatry 7:163. doi: 10.3389/fpsyt.2016.00163
Lerner, T. N., Shilyansky, C., Davidson, T. J., Evans, K. E., Beier, K. T., Zalocusky, K. A., et al. (2015). Intact-brain analyses reveal distinct information carried by SNc dopamine subcircuits. Cell 162, 635–647. doi: 10.1016/j.cell.2015.07.014
Levy, F. (2009). Dopamine vs noradrenaline: inverted-U effects and ADHD theories. Austral. New Zeal. J. Psychiatry 43, 101–108. doi: 10.1080/00048670802607238
Lewis, D. A., and Sesack, S. R. (1997). Chapter VI Dopamine systems in the primate brain. Handb. Chem. Neuroanat. 13, 263–375. doi: 10.1016/S0924-8196(97)80008-5
Lidow, M. S., Goldman-Rakic, P. S., Gallager, D. W., and Rakic, P. (1991). Distribution of dopaminergic receptors in the primate cerebral cortex: quantitative autoradiographic analysis using [3H]raclopride, [3H]spiperone and [3H]SCH23390. Neuroscience 40, 657–71. doi: 10.1016/0306-4522(91)90003-7
Lindström, L. H., Gefvert, O., Hagberg, G., Lundberg, T., Bergström, M., Hartvig, P., et al. (1999). Increased dopamine synthesis rate in medial prefrontal cortex and striatum in schizophrenia indicated by L-(β-11C) DOPA and PET. Biol. Psychiatry 46, 681–688. doi: 10.1016/S0006-3223(99)00109-2
Lindvall, O., Björklund, A., and Skagerberg, G. (1984). Selective histochemical demonstration of dopamine terminal systems in rat di- and telecephalon: new evidence for dopaminergic innervation of hypothalamic neurosecretory nuclei. Brain Res. 306, 19–30. doi: 10.1016/0006-8993(84)90352-4
Ljungberg, T., Apicella, P., and Schultz, W. (1991). Responses of monkey midbrain dopamine neurons during delayed alternation performance. Brain Res. 567, 337–341. doi: 10.1016/0006-8993(91)90816-E
Mantz, J., Thierry, A. M., and Glowinski, J. (1989). Effect of noxious tail pinch on the discharge rate of mesocortical and mesolimbic dopamine neurons: selective activation of the mesocortical system. Brain Res. 476, 377–381. doi: 10.1016/0006-8993(89)91263-8
McGaughy, J., Dalley, J. W., Morrison, C. H., Everitt, B. J., and Robbins, T. W. (2002). Selective behavioral and neurochemical effects of cholinergic lesions produced by intrabasalis infusions of 192 IgG-saporin on attentional performance in a five-choice serial reaction time task. J. Neurosci. 22, 1905–1913. doi: 10.1523/JNEUROSCI.22-05-01905.2002
Miller, E. K. (1999). The prefrontal cortex: complex neural properties for complex behavior. Neuron 22, 15–17. doi: 10.1016/S0896-6273(00)80673-X
Morón, J. A., Brockington, A., Wise, R. A., Rocha, B. A., and Hope, B. T. (2002). Dopamine uptake through the norepinephrine transporter in brain regions with low levels of the dopamine transporter: evidence from knock-out mouse lines. J. Neurosci. 22, 389–395.
Newman, L. A., and McGaughy, J. (2008). Cholinergic deafferentation of prefrontal cortex increases sensitivity to cross-modal distractors during a sustained attention task. J. Neurosci. 28, 2642–2650. doi: 10.1523/JNEUROSCI.5112-07.2008
Okubo, Y., Suhara, T., Suzuki, K., Kobayashi, K., Inoue, O., Terasaki, O., et al. (1997). Decreased prefrontal dopamine D1 receptors in schizophrenia revealed by PET. Nature 385, 634–636. doi: 10.1038/385634a0
Oyama, K., Hori, Y., Nagai, Y., Miyakawa, N., Mimura, K., Hirabayashi, T., et al. (2021). Chemogenetic dissection of the primate prefronto-subcortical pathways for working memory and decision-making. Sci. Adv. 7:eabg4246. doi: 10.1126/sciadv.abg4246
Pandya, D. N., and Yeterian, E. H. (1996). Comparison of prefrontal architecture and connections. Philos. Trans. R. Soc. Lond. B Biol. Sci. 351, 1423–1432. doi: 10.1098/rstb.1996.0127
Pang, K., Williams, M. J., Egeth, H., and Olton, D. S. (1993). Nucleus basalis magnocellularis and attention: effects of muscimol infusions. Behav. Neurosci. 107, 1031–1038. doi: 10.1037/0735-7044.107.6.1031
Pitman, R. K., Rasmusson, A. M., Koenen, K. C., Shin, L. M., Orr, S. P., Gilbertson, M. W., et al. (2012). Biological studies of post-traumatic stress disorder. Nat. Rev. Neurosci. 13, 769–787. doi: 10.1038/nrn3339
Ramos, B. P., and Arnsten, A. F. T. (2007). Adrenergic pharmacology and cognition: focus on the prefrontal cortex. Pharmacol. Ther. 113, 523–536. doi: 10.1016/j.pharmthera.2006.11.006
Ridderinkhof, K. R., Van Den Wildenberg, W. P. M., Segalowitz, S. J., and Carter, C. S. (2004). Neurocognitive mechanisms of cognitive control: the role of prefrontal cortex in action selection, response inhibition, performance monitoring, and reward-based learning. Brain Cogn. 56, 129–140. doi: 10.1016/j.bandc.2004.09.016
Robbins, T. W., Everitt, B. J., Marston, H. M., Wilkinson, J., Jones, G. H., and Page, K. J. (1989). Comparative effects of ibotenic acid- and quisqualic acid-induced lesions of the substantia innominata on attentional function in the rat: further implications for the role of the cholinergic neurons of the nucleus basalis in cognitive processes. Behav. Brain Res. 35, 221–240. doi: 10.1016/S0166-4328(89)80143-3
Schmitt, J. A. J., Jorissen, B. L., Sobczak, S., van Boxtel, M. P. J., Hogervorst, E., Deutz, N. E. P., et al. (2000). Tryptophan depletion impairs memory consolidation but improves focussed attention in healthy young volunteers. J. Psychopharmacol. 14, 21–29. doi: 10.1177/026988110001400102
Schultz, W., Apicella, P., and Ljungberg, T. (1993). Responses of monkey dopamine neurons to reward and conditioned stimuli during successive steps of learning a delayed response task. J. Neurosci. 13, 900–913. doi: 10.1523/JNEUROSCI.13-03-00900.1993
Schwarz, L. A., Miyamichi, K., Gao, X. J., Beier, K. T., Weissbourd, B., DeLoach, K. E., et al. (2015). Viral-genetic tracing of the input-output organization of a central noradrenaline circuit. Nature 524, 88–92. doi: 10.1038/nature14600
Sesack, S. R., Hawrylak, V. A., Matus, C., Guido, M. A., and Levey, A. I. (1998). Dopamine axon varicosities in the prelimbic division of the rat prefrontal cortex exhibit sparse immunoreactivity for the dopamine transporter. J. Neurosci. 18, 2697–2708. doi: 10.1523/JNEUROSCI.18-07-02697.1998
Sesack, S. R., and Pickel, V. M. (1992). Prefrontal cortical efferents in the rat synapse on unlabeled neuronal targets of catecholamine terminals in the nucleus accumbens septi and on dopamine neurons in the ventral tegmental area. J. Comp. Neurol. 320, 145–160. doi: 10.1002/cne.903200202
St. Onge, J. R., Ahn, S., Phillips, A. G., and Floresco, S. B. (2012). Dynamic fluctuations in dopamine efflux in the prefrontal cortex and nucleus accumbens during risk-based decision making. J. Neurosci. 32, 16880–16891. doi: 10.1523/JNEUROSCI.3807-12.2012
Takeuchi, T., Duszkiewicz, A. J., Sonneborn, A., Spooner, P. A., Yamasaki, M., Watanabe, M., et al. (2016). Locus coeruleus and dopaminergic consolidation of everyday memory. Nature 537, 357–362. doi: 10.1038/nature19325
Thierry, A. M., Blanc, G., Sobel, A., Stinus, L., and Glowinski, J. (1973). Dopaminergic terminals in the rat cortex. Science 182, 499–501. doi: 10.1126/science.182.4111.499
Thierry, A. M., Tassin, J. P., Blanc, G., and Glowinski, J. (1976). Selective activation of the mesocortical DA system by stress. Nature 263, 242–244. doi: 10.1038/263242a0
Toshiyuki, S., and Goldman-Rakic, P. S. (1991). D1 dopamine receptors in prefrontal cortex: involvement in working memory. Science 251, 947–950. doi: 10.1126/science.1825731
Tronel, S., and Sara, S. J. (2003). Blockade of NMDA receptors in prelimbic cortex induces an enduring amnesia for odor-reward associative learning. J. Neurosci. 23, 5472–5476.
vander Weele, C. M., Siciliano, C. A., Matthews, G. A., Namburi, P., Izadmehr, E. M., Espinel, I. C., et al. (2018). Dopamine enhances signal-to-noise ratio in cortical-brainstem encoding of aversive stimuli. Nature 563, 397–401. doi: 10.1038/s41586-018-0682-1
Ventura, R., Alcaro, A., and Puglisi-Allegra, S. (2005). Prefrontal cortical norepinephrine release is critical for morphine-induced reward, reinstatement and dopamine release in the nucleus accumbens. Cereb. Cortex 15, 1877–1886. doi: 10.1093/cercor/bhi066
Ventura, R., Cabib, S., Alcaro, A., Orsini, C., and Puglisi-Allegra, S. (2003). Norepinephrine in the prefrontal cortex is critical for amphetamine-induced reward and mesoaccumbens dopamine release. J. Neurosci. 23, 1879–1885.
Ventura, R., Morrone, C., and Puglisi-Allegra, S. (2007). Prefrontal/accumbal catecholamine system determines motivational salience attribution to both reward- and aversion-related stimuli. Proc. Natl. Acad. Sci. U.S.A. 104, 5181–5186. doi: 10.1073/pnas.0610178104
Vijayraghavan, S., Wang, M., Birnbaum, S. G., Williams, G. V., and Arnsten, A. F. T. (2007). Inverted-U dopamine D1 receptor actions on prefrontal neurons engaged in working memory. Nat. Neurosci. 10, 376–384. doi: 10.1038/nn1846
Voytko, M. L., Olton, D. S., Richardson, R. T., Gorman, L. K., Tobin, J. R., and Price, D. L. (1994). Basal forebrain lesions in monkeys disrupt attention but not learning and memory. J. Neurosci. 14, 167–186.
Wall, N. R., De La Parra, M., Sorokin, J. M., Taniguchi, H., Huang, Z. J., and Callaway, E. M. (2016). Brain-wide maps of synaptic input to cortical interneurons. J. Neurosci. 36, 4000–4009. doi: 10.1523/JNEUROSCI.3967-15.2016
Williams, G. V., and Goldman-Rakic, P. S. (1995). Modulation of memory fields by dopamine Dl receptors in prefrontal cortex. Nature 376, 572–575. doi: 10.1038/376572a0
Williams, S. M., and Goldman-Rakic, P. S. (1993). Characterization of the dopaminergic innervation of the primate frontal cortex using a dopamine-specific antibody. Cerebr. Cortex 3, 199–222. doi: 10.1093/cercor/3.3.199
Keywords: prefrontal cortex, dopamine, ventral midbrain, Rabies, connectivity
Citation: Hui M and Beier KT (2022) Defining the interconnectivity of the medial prefrontal cortex and ventral midbrain. Front. Mol. Neurosci. 15:971349. doi: 10.3389/fnmol.2022.971349
Received: 16 June 2022; Accepted: 05 July 2022;
Published: 22 July 2022.
Edited by:
Mihaela D. Iordanova, Concordia University, CanadaReviewed by:
Tamara Boto, Trinity College Dublin, IrelandCopyright © 2022 Hui and Beier. This is an open-access article distributed under the terms of the Creative Commons Attribution License (CC BY). The use, distribution or reproduction in other forums is permitted, provided the original author(s) and the copyright owner(s) are credited and that the original publication in this journal is cited, in accordance with accepted academic practice. No use, distribution or reproduction is permitted which does not comply with these terms.
*Correspondence: Kevin T. Beier, a2JlaWVyQHVjaS5lZHU=